- 1IANIGLA, Institute of Snow, Glaciology and Environmental Science, Mendoza, Argentina
- 2Cátedra Edafología, Facultad de Ciencias Agrarias, Universidad Nacional de Cuyo, Mendoza, Argentina
This paper reviews the genesis of a chaotic deposit in the Blanco River basin (BRB) in the Argentine Andes that has been interpreted to be either of debris flow or glacial origin. A detailed sedimentological and geomorphic study of the deposit was undertaken to determine its origin. Glacial landforms in the source area of the deposit contrast markedly to those in neighboring valleys of the BRB. The lack of moraines and the presence only of rock glaciers in the headwaters of the Angostura Valley suggest that a glacier might have been destroyed by a rock avalanche in the Late Pleistocene. The deposit itself has the characteristics of rapid deposition by a huge (≤105 m3) rock avalanche sourced on the eastern slope of Mount Plata (5,956 m asl). The rock avalanche traveled a distance of 26.6 km and descended 4,700 m in altitude. The optically stimulated luminescence (OSL) ages on alluvial sediments associated with the landslide deposits suggest that the rock avalanche occurred ∼35–39 ka ago. The rock avalanche may have been triggered by an earthquake, given that the active Carrera fault system extends across the basin and there is a cluster of seven Late Pleistocene rock avalanches in the region.
Introduction
Researchers often argue about the origin of chaotic sedimentary deposits in high mountains (Abele, 1984; Hewitt, 1999; Hewitt et al., 2011). Landslide deposits assumed to be of glacial origin have evoked erroneous paleoclimatic interpretations. In the Central Andes, for example, landslide deposits originally interpreted to be glacial have been reinterpreted in recent years (Antinao and Gosse, 2009; Sepúlveda and Moreiras, 2013; Hermanns et al., 2015; Moreiras and Sepúlveda, 2015).
Near the area of this study, such reinterpretation began much earlier with the seminal work of Dessanti (1946) and Polanski (1953, 1963, 1961), who debated the genesis of a chaotic deposit called the Quemado Conglomerate, which extends down to 1,200 m asl. If the conglomerate were glacial in origin, glaciers would have to have reached the foothills of the Andes. Polanski (1953, 1963) emphatically argued that Pleistocene glaciers could not have reached below 1,800 m asl, whereas Groeber (1951, 1955) defended the existence of such extensive advances. This discussion continued two decades later when the “cenoglomerate,” a chaotic mass of boulders lacking internal structure in the Blanco River basin (BRB) which Polanski (1966) interpreted to be the deposit of a huge debris flow, was reinterpreted as till of the Angostura glacial advance (Wayne and Corte, 1983; Wayne, 1984). Based on relative methods (e.g., rock varnish on blocks, degree of weathering, and soil development), these authors proposed an Early Pleistocene age for a glacier that reached 1,500 m asl in the BRB. Later, Wayne (1990) returned to Polanski’s original viewpoint.
This paper reviews and reconsiders the deposits described by Polanski (1966) and Wayne (1984, 1990) based on new detailed stratigraphic studies of the “cenoglomerate” in the BRB. As the source of the deposit has never been clear, I analyzed landforms in the formerly glaciated headwaters of the basin. Globally, main efforts for deciphering landform genesis are focused on characterizing the deposit features, while a general geomorphological study for understanding the genesis of certain landform is overlooked. This study highlights the necessity of integrating sedimentology and geomorphology when attempting to discriminate glacial and landslide.
Setting of the Blanco River Basin
The Blanco River basin (BRB) has an area of 150 km2 and is located on the eastern slope of the Plata Range in the Argentine Central Andes. The slopes are steep, and the peaks reach to more than 5,000 m asl; the elevations drop gradually to the north (Figure 1). The basin is drained by the Blanco River and its tributaries (Angostura and Vallecitos rivers), and the southern Alto Las Vegas sub-catchment is drained by the Mulas River (Drovandi et al., 2010; Massone et al., 2016) (Figure 1). The Blanco River, with a mean annual flow of 1 m3/s (Massone et al., 2016), has season variability due to snowmelt during late spring and summer.
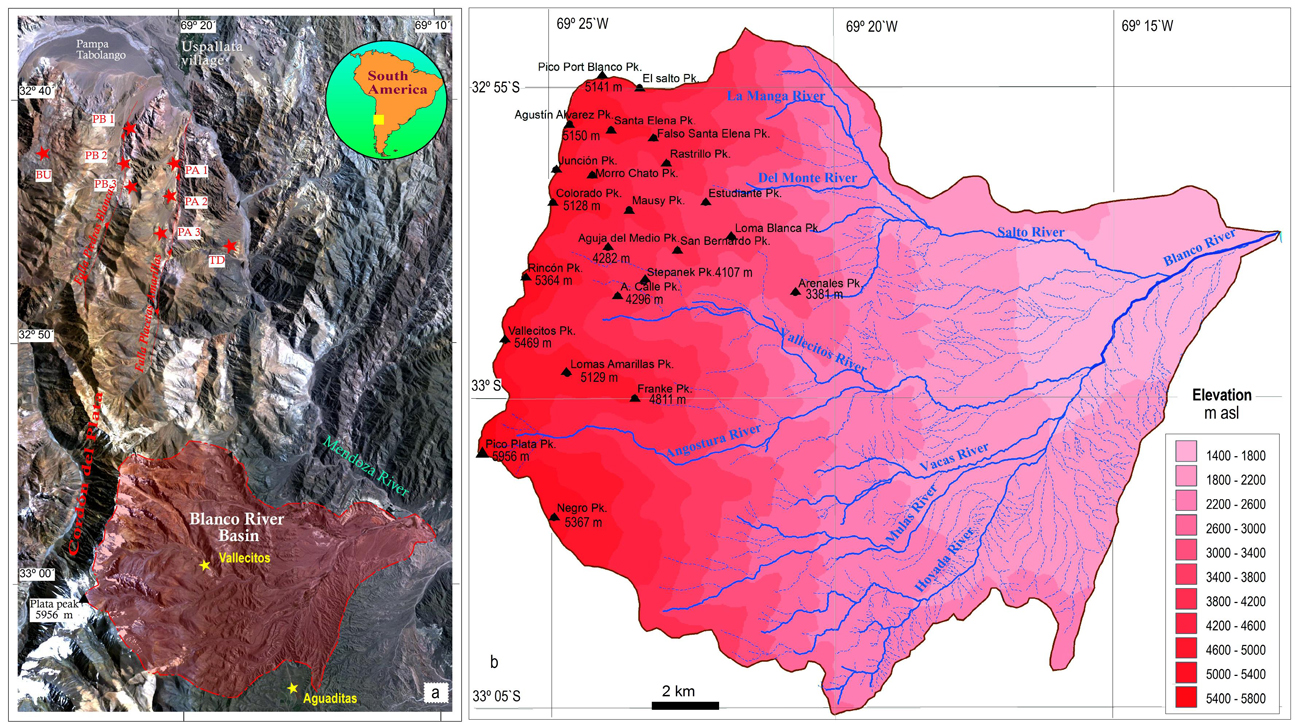
Figure 1. Study area location at 33°S: (a) the Blanco River Basin (BRB) on the eastern slope of the Plata Range (polygon in red) and its relative position with a cluster of rock avalanches studied in the northern extreme of Plata Range. Rock avalanches are represented by red stars: BU, Burro rock avalanche; PB, Piedras Blancas rock avalanches: PB1, PB2, PB3; PA, Placetas Amarillas: PA1, PA2, PA3; TD, Tigre Dormido rock avalanche. Yellow stars indicate meteorological stations of Vallecitos and Aguaditas. (b) Digital elevation model of the Plata Range showing highest peaks and the catchment area of the BRB integrated by the main central branch of the Blanco River including the Angostura and Vallecitos rivers, the sub-catchments the Alto Manantiales integrated by the Salto, Manga, del Monte and Chacay rivers, and the southern subcatchment denominated Alto Las Vegas integrated by Mulas river.
This part of the Andes is semiarid. The climate is forced by the Southern Arid Diagonal, which prevents tropical air masses from the Atlantic from reaching the region. Westerlies from the Pacific Ocean, however, generate snowfall of about 240 mm/year (snow water equivalent) in mountain areas during the South Hemisphere winter (June–August) and rainfall in the foothills during summer (December–February) (Norte, 1995). The Vallecitos (2,470 m asl) and the Las Aguaditas (2,200 m asl) meteorological stations report a mean annual temperature of 5 and 7.6°C and a mean annual precipitation of 294 and 450 mm, respectively (Wayne, 1984). The mean annual temperature is below 0°C at about 3,200 m asl, which is the lower limit of Andean permafrost (Corte and Grosso, 1993). The permafrost reaches depths of about 90 cm at 4,500 m asl (Trombotto, 1991, 2002; Trombotto and Borzotta, 2009). Rock glaciers attest to the periglacial conditions at these high elevations (Brenning et al., 2005; Drewes et al., 2018). Glaciers reach down to 4,700 m asl in some valleys; debris-covered glaciers are common due to the arid environment conditions in the Central Andes (Corte and Espizúa, 1981; Espizúa, 1982; Corte and Grosso, 1993; Buk, 2002).
Geological Framework and Neotectonic Activity
The Plata Range is part of the Andean Frontal Cordillera morphotectonic unit and was uplifted during the Andean Orogeny 12–18 Ma ago (Giambiagi et al., 2003). The basement of the Frontal Cordillera comprises Devonian metasedimentary rocks of the Vallecitos Series (Heredia et al., 2012) and Carboniferous–Permian marine pelite of the Loma de los Morteritos and El Plata formations (Caminos, 1965) (Figure 2). The basement rocks are overlain by a thick sequence of Permo-Triassic volcanic rocks of the Choiyoi Group, which were erupted in an extensional environmental during a final stage of subduction (Llambías et al., 1993; Kleiman and Japas, 2009). Granitic rocks were also intruded into the crust at this time. The Choiyoi Group also includes sedimentary units (Tambillos and Mal Pais formations). The sequence continues with a thick pile of Triassic continental fluvial deposits and subordinate volcanic rocks emplaced within the Cuyo rift basin (Rio Mendoza, Potrerillos, Cacheuta, and Rio Blanco formations). These Triassic rocks are overlain by conglomerates of the Jurassic Papagallos Formation. Paleogene and Lower Neogene rocks are mainly represented by sandstones of the Mariño Formation in lower piedmont areas (Caminos, 1965). This Mariño Formation is overlain by a thin succession of poorly stratified coarse conglomerate of the Mogotes Formation of late Pliocene age (Irigoyen et al., 2000, 2002).
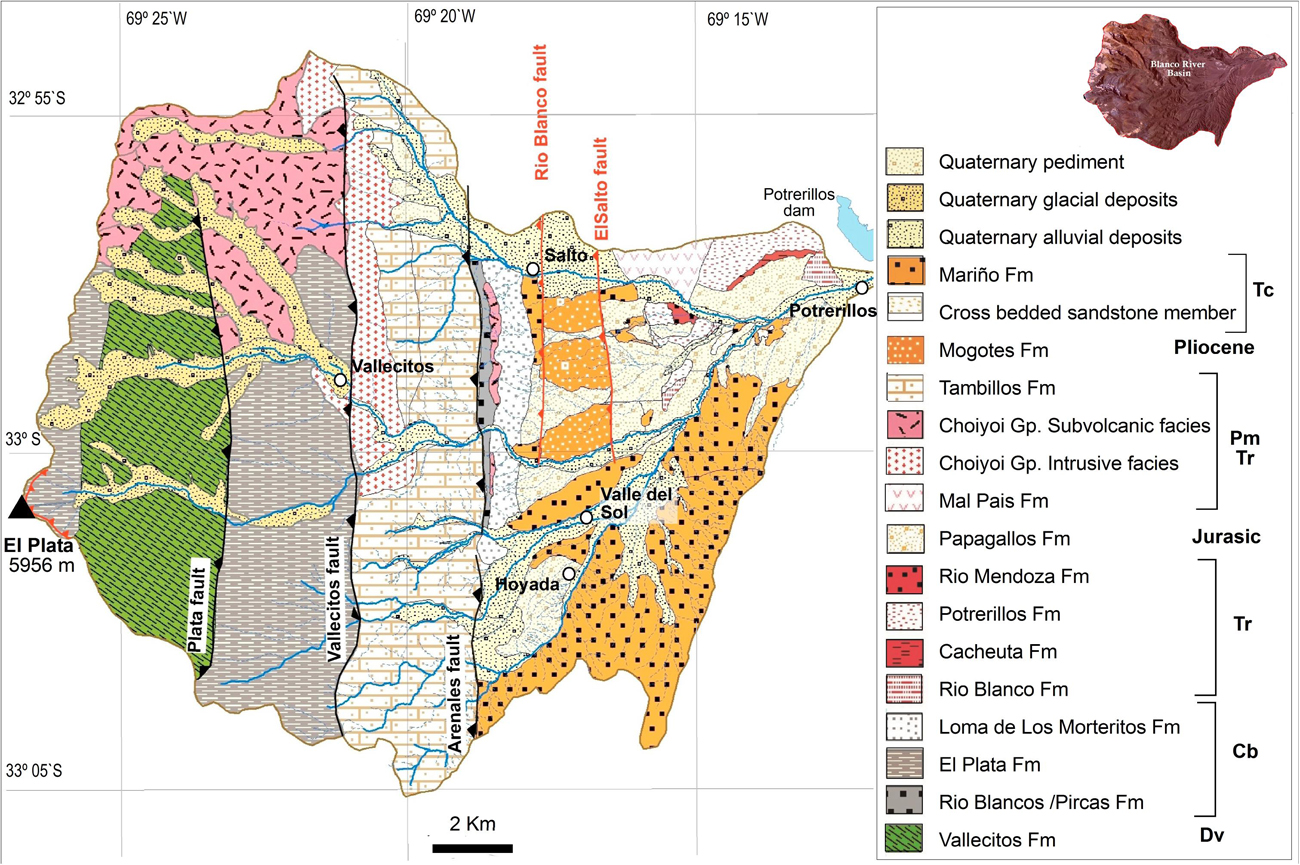
Figure 2. Quaternary, Tertiary (Tc), Pliocene, Jurassic, Permo-Triassic (PmTr), Triassic (Tr), Carboniferous (Cb), and Devonian (Dv) lithological units outcropping in the BRB with the main structures of the Carrera fault system responsible for the Frontal Cordillera uplift. Rio Blanco (RBF) and Salto (SF) active faults are mapped in red color. The probable source area of the Plata rock avalanche is indicated in red line the headwater of the Angostura Valley at the Plata Mount (5,956 m asl). Dots indicate main villages.
Quaternary deposits are present within BRB valleys and in the piedmont region. Moraines have been identified in the higher parts of some valleys (Wayne and Corte, 1983; Wayne, 1984; Moreiras et al., 2017). Recently, some of these moraines have been dated using surface exposure cosmogenic methods as ∼8–25 ka and are probably linked to the Last Glacial Maximum. One moraine in the Mulas River valley yielded an age of ∼40 ka (Moreiras et al., 2017). Near the study area, the Penitentes glaciation was dated ∼30 ka in the Mendoza River valley (Espizúa and Bigazzi, 1998; Espizúa, 1999); however, Late Pleistocene climatic conditions remain uncertain for the region.
The Carrera fault system extends along the eastern margin of the Plata Range and is the main structure responsible for the uplift of the Frontal Cordillera (Caminos, 1965) (Figure 1). This system comprises north-trending imbricated reverse faults with eastern vergence (Polanski, 1972). The Arenales fault thrusts granitic intrusive rocks over the volcanic rocks of Choiyoi Group, and the Médanos fault displaces rocks as young as Neogene (Folguera et al., 2004; Casa et al., 2010). The Rio Blanco fault offsets Pliocene and Quaternary strata, and the El Salto fault has uplifted three pediment levels of Quaternary age (Casa et al., 2010). The offset Quaternary alluvial fans (Cortés et al., 1999, 2006; Borgnia, 2004; Casa, 2005, 2009; Casa et al., 2010) and a cluster of seven Late Pleistocene rock avalanches in the northern Plata Range (Moreiras, 2006; Fauqué et al., 2009; Moreiras et al., 2015) provide evidence that the Carrera fault system remains active (Figure 2). Different levels of alluvial terraces have been identified in the lower piedmont portion of the study area (Polanski, 1963) and have been attributed to the uplift of the Frontal Cordillera (Rodríguez and Barton, 1993; Casa, 2005).
Methodology
The motivation for this study was understanding the genesis of a chaotic deposit that had been previously and ambiguously interpreted to be till (Wayne and Corte, 1983; Wayne, 1984) or a debris flow deposit (Polanski, 1961, 1966). To resolve this disagreement, I initially carried out a geospatial analysis of the study area based on remotely sensed imagery. A geomorphological map of the main Quaternary deposits and landforms was done using pairs of 1,963 airphotos, Landsat satellite images, and Google Earth imagery. The map shows bare and debris-covered glacier ice, rock glaciers, and snow patches, following the classification of Janke et al. (2015) (Figure 3). Moraines and outwash and alluvial deposits were also mapped. The map was verified during the field sessions carried out between 2012 and 2015.
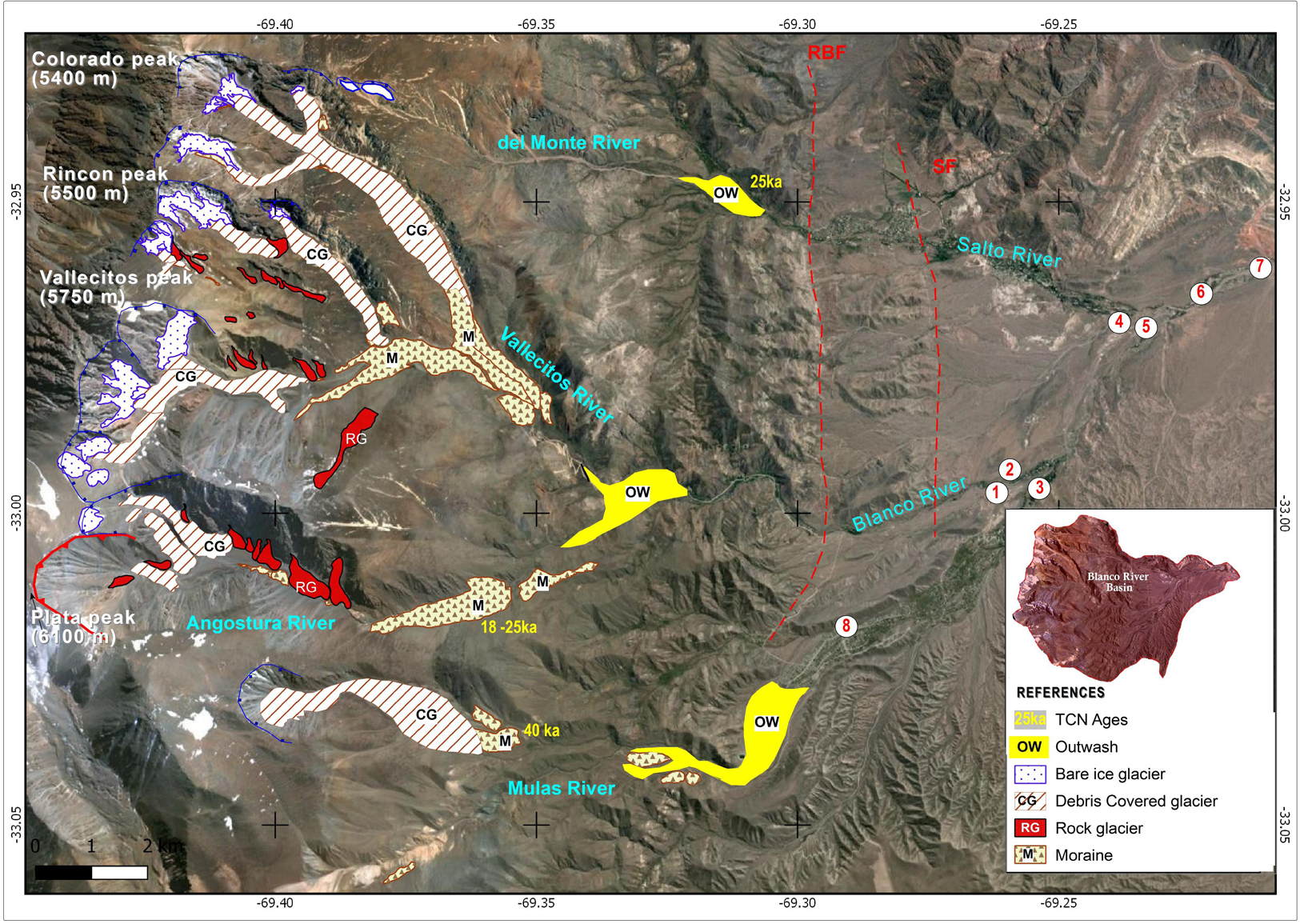
Figure 3. Google image showing main landform of the BRB: bare ice glaciers; debris-covered glaciers, rock glaciers, moraines, and outwash deposits. The 10 Be ages of the Salto outwash and moraines of Angostura and Mulas rivers are in yellow letters (after Moreiras et al., 2017). Note that the Angostura valley has preserved relict moraines at the headwaters but uncovered glacier in the highest peak of the Plata peak (5,956 m asl) is missed. RBF, Rio Blanco fault and SF, Salto fault mapped in red lines. Number indicates places where the rock avalanche outcrops.
The cenoglomerate chaotic deposit was studied in the field using methods taken from Abele (1984); Hewitt (1999), and Reznichenko et al. (2012). Specifically, observations were made in terms of fabric, grain size, boulder lithology, size, and roundness, matrix percentage, stratigraphic context, and the presence or the absence of striations.
Stratigraphic profiles were described at different locations within the BRB (Figure 3) in an effort to find possible facies differences along the depositional path. Even though rock avalanche deposits are chaotic in nature, they typically exhibit characteristics indicative of rapid motion, fragmentation, and abrupt deposition, for example, jigsaw-like fracturing of large clasts, inverse grading, sheared or mylonitized layers, mixed facies, a matrix with fractal-like particle size distribution, and deformed or liquefied substrates (e.g., Cruden and Hungr, 1986; Gates, 1987; Yarnold and Lombard, 1989; Dunning, 2006; Prager et al., 2012; Weidinger et al., 2014; Wang et al., 2015, 2018; Dufresne et al., 2016; Strom and Abdrakhmatov, 2018; Zeng et al., 2019). The principal morphological parameters of the cenoglomerate were estimated, and its isolated remnants were mapped to establish the original extent of the deposit and to provide a rough estimate of its volume.
The alluvial sediments underlying and overlying the cenoglomerate were dated using optically stimulated luminescence (OSL) techniques to establish the age of the deposit. Radiocarbon dating was not possible because the sediments on the BRB lack organic matter. OSL dating determines the time since the last exposure to daylight of quartz or feldspar grains in unheated sediments (Aitken, 1998; Rhodes, 2011). I collected samples for OSL dating by cleaning a vertical exposure and then inserting plastic tubes 25 cm long and 70 mm in diameter horizontally into the sediment by hammering. The ends of the tubes were sealed with aluminum foil and the tubes were wrapped with a black plastic bag. In the lab, sediment immediately surrounding the primary sample was collected to calculate the ß contribution to the dose rate, and a second sub-sample was taken 10–30 cm from the primary sample to calculate the Y dose rate component (Antinao et al., 2013).
Sand-sized grains were analyzed because silt is more likely to move vertically within a profile, a process that increases the chances of mixed-age grain populations within a given stratum (e.g., Berger et al., 2004). Both quartz and feldspar grains were dated. Values of water (relation between of the weight of wet sample/dry sample) were estimated based on data for similar sediments in similar climatic settings, with an estimated uncertainty of ±0.02 (Antinao et al., 2013). The potassium value (K2O) for material around the quartz grains was established with an estimated uncertainty set to ±0.05%. The value of K2O was set to zero for dose rate calculations in the case of quartz separates, while the value of K2O at 10 ± 2% was used for the feldspar data. Total (Ct) and thorium (Cth) count rates were established from finely powered samples by the thick-source-alpha-particle-counting (Huntley and Wintle, 1981). These values were inserted directly into the age equations of Berger (1988), with the internal dose rate components set to zero. Cu is equal to the total count (Ct) minus the thorium count (Cth). A cosmic ray component, basically a function of burial depth, was estimated using the algorithm of Prescott and Hutton (1994).
The dose rate was calculated using the conversion factors given by Adamiec and Aitken (1998) and the equations of Berger (1988). Attenuation of ß radiation across the sand grain was accounted for by using attenuation factors from Aitken (1985). An estimated small internal dose rate in quartz of 0.05 ± 0.03 Gy/ka was added to the calculated dose rate (Table 1).
The ages reported here stem mainly from single-grain aliquots, indicated by “single grain” in Table 2. A grain size attenuation factor for the beta dose was taken from Mejdahl (1979). The preheating temperature for the single-aliquot regenerative dose approach was held for 10 s (Murray and Wintle, 2000). A signal-readout temperature of 125°C was employed for quartz, whereas sequential measurements at 50°C (whole disc. IRSL) and 225°C (post-IR single-grain IRSL) were used for feldspar. The dose estimation used for age determination is based on the minimum age model (MAM) or the central age model (Galbraith et al., 1999).
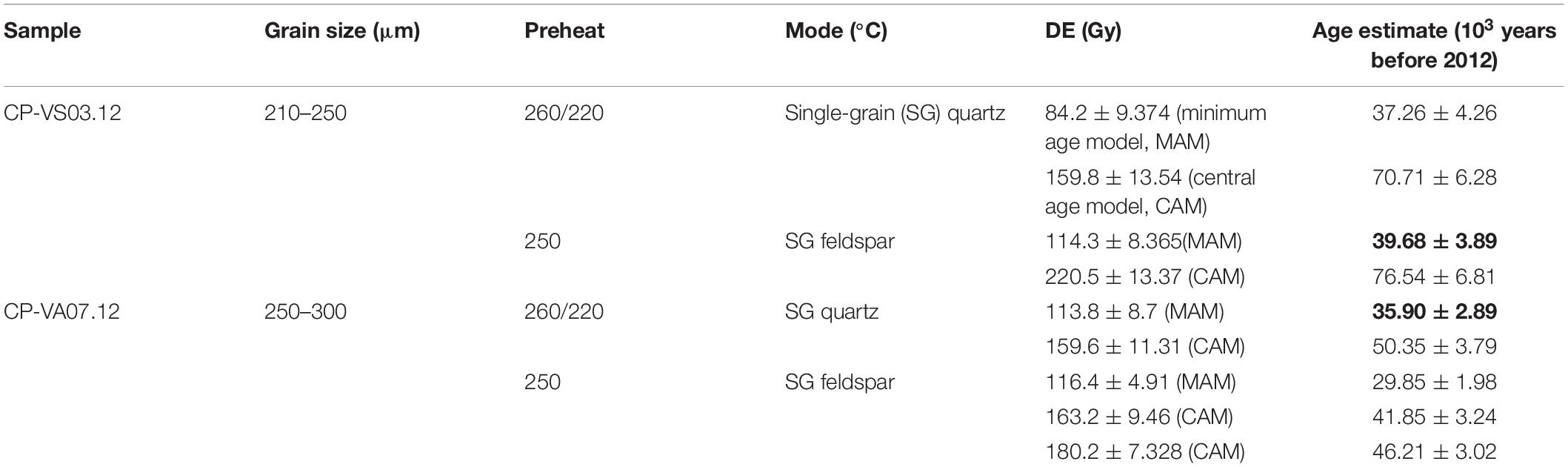
Table 2. Single-grain equivalent dose (DE) and estimated ages for the sand samples (preferred age in bold).
Results
Deposit Sedimentology and Stratigraphy
The deposit that motivated this study has been described as a greenish–grayish chaotic deposit found along the main branch of the Blanco River (site 6, Figure 3). However, the deposit also crops out elsewhere in the BRB, including Angostura Valley (sites 1–3), Blanco River valley (sites 4–7), and along the Vacas River (site 8) (Figure 3). The deposit is up to 8.5 m thick at higher sites in the Angostura Valley but is generally much thinner (<3.5 m) in the Blanco Valley where it is unconformably overlain by alluvial fan sediments. The clasts in the deposit are up to 3 m in size (mean diameter = 0.5 m) and are mainly derived from the Carboniferous El Plata Formation. More than 98% of blocks are gray sandstone, conglomerate, and phyllite of this unit. The clasts of an altered gabbro, although only about 2% of the deposit, contribute to the greenish matrix color. The clasts are angular to subangular and show no evidence of having been rounded during transport. They bear no striations or other evidence of a glacial origin. Although the deposit is typically massive, inverse grading and weak bedding were noted in some outcrops. The matrix content ranges from 20 to 40%. The matrix is dominantly sand-sized, but gravel was noted in some sections. The chaotic deposits comprise two main lithofacies. The finer facies (“a”) has a brownish-grayish color, with clasts <0.5 m in size, and a high matrix content. It forms the lowest part of the deposit at some sites. In contrast, lithofacies “b” has a greenish–grayish color, with larger blocks (up to 3 m).
Site 1, Stratigraphic Profile A ∼1,780 m asl
This section is a road cut at the margin of the Angostura Valley and was created during the construction of the road to Vallecitos (Figure 4a), which is basal glaciofluvial gravel consisting mainly of clasts of sedimentary and metamorphic rocks derived from Carboniferous and Devonian formations that crop out in the headwaters of the valley. It is overlain by the massive chaotic deposit, which here is 2–3 m thick and contains blocks of the Carboniferous El Plata Formation up to 2.5 m in size within a sandy matrix. The chaotic deposit is covered by 0.7 m of alluvium with carbonate-coated clasts (Figure 5).
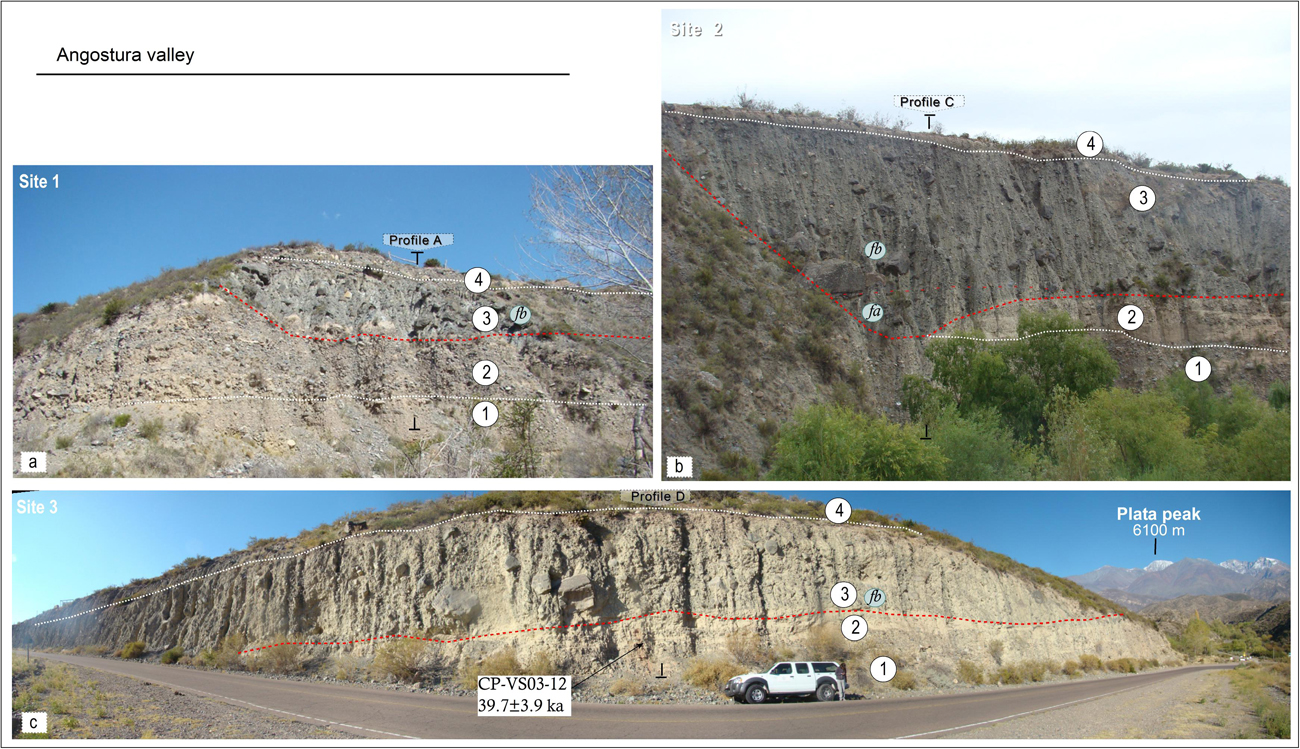
Figure 4. Cross sections along the Angostura Valley where the Plata rock avalanche outcrops: (a) Cross section at site 1 where the profile A was described on the right margin of the Angostura valley. Units: 1. corresponds to basal fluvio-glacial deposits, 2. alluvial deposits, 3. the Plata rock avalanche, and 4. A 0.7 m thick- alluvial layer (see Figure 5). The profile B was described 300 m from profile A. (b) Cross section at site 2 where the profile C was described on the left margin of the Angostura valley. Units: 1. gravel alluvial deposits, 2. finer alluvial deposits, 3. the Plata rock avalanche with erosive basal contact, and 4. alluvial layer (see Figure 5). (c) Cross-section at site 3 just in front site 2 where the profile D was described on the right margin of the Angostura valley. Units: 1. gravel alluvial deposits, 2. finer alluvial deposits from where the sample CP.VS 03.12 was taken, 3. the Plata rock avalanche, and 4. alluvial layer (see Figure 5). Facies a (fa) and facies b (fb).
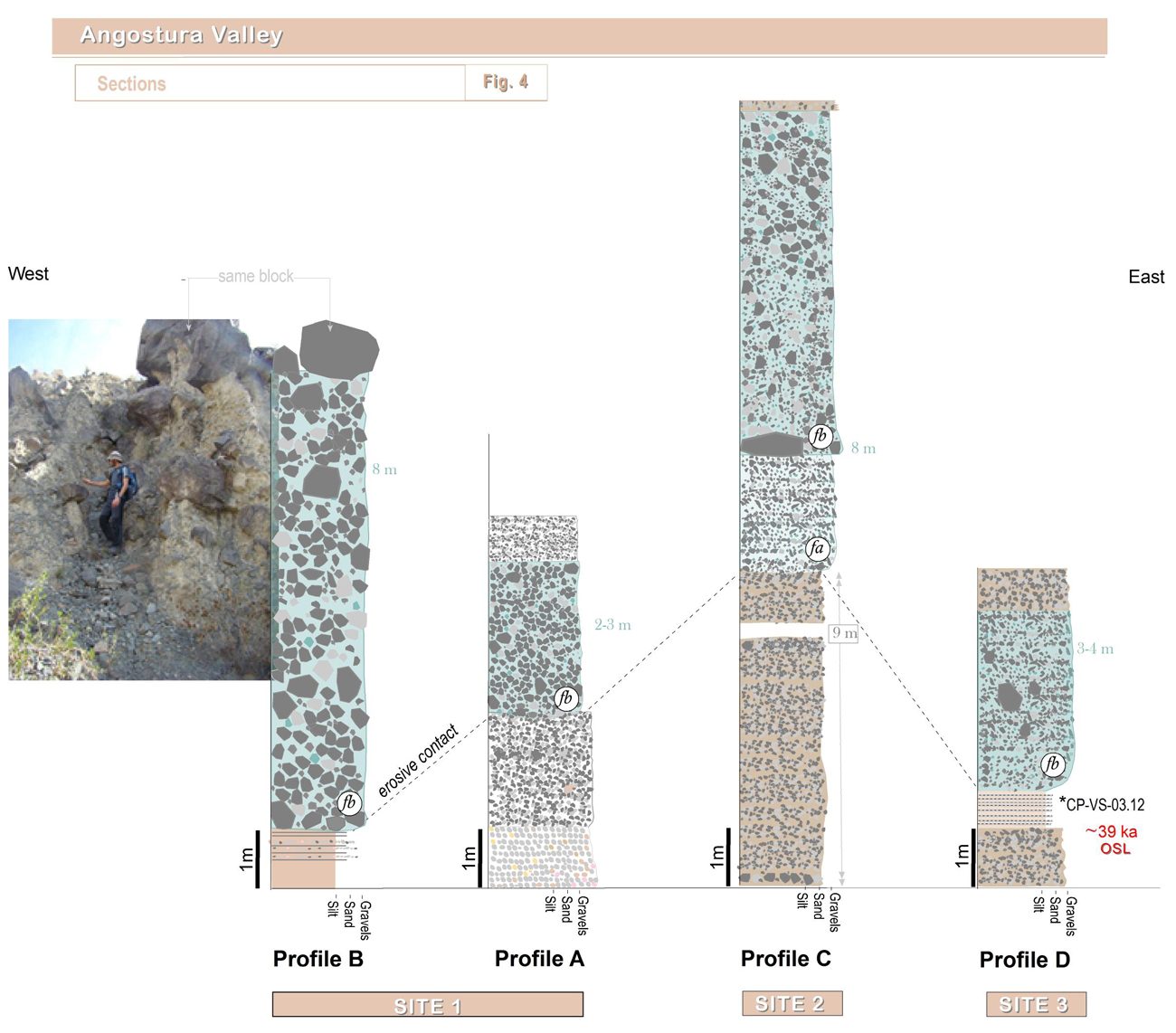
Figure 5. Graph of sedimentological profiles described in sites 1, 2, and 3 along the Angostura Valley showing presence of facie a (fa) or facie b (fb) (see pictures in Figure 4). The photo on the left corresponds to the top of the profile B with a person as scale.
Site 1, Stratigraphic Profile B ∼1,800 m asl
This natural exposure is 300 m upstream of profile A. The chaotic deposit is 8 m thick at this site (Figure 5), and the fluvial deposits noted at the previous site are absent here. Blocks within the chaotic deposit reach up to 2.5 m in size and lie within a sandy matrix that constitutes 20–40% of the deposit. Most of the blocks are El Plata breccia, sandstone, and pelites; some clasts of sedimentary rocks derived from the Loma de los Morteritos Formation are also present, as are pebbles (1%) of granite and silicified rocks 2–4 cm in diameter. The deposit is massive but shows the same inverse grading with the largest blocks near the top of the exposure.
Site 2, Stratigraphic Profile C ∼1,750 m asl
Section C is a 17-m-high natural exposure in an incised Quaternary alluvial terrace in the Angostura Valley (Figure 4b), which is up to 9 m of coarse fluvial gravel and with clasts up to 0.5 m in diameter forming the lower half of the exposure. This sequence changes laterally: an upper finer muddy layer overlies a coarser layer (Figure 5). The chaotic deposit, which is 8 m thick here, overlies the alluvium across an erosional contact. It comprises the two lithofacies mentioned above: basal facies with high matrix content and blocks < 0.5 m in diameter (lithofacies a) and an upper grayish-green facies with less matrix content, mainly sand, and larger blocks (up to 2 m in size) (lithofacies b). The contact between the two facies is gradational. No stratification or block imbrication is visible in the section.
Site 3, Stratigraphic Profile D ∼1,750 m asl
Site 3 is an exposure along the road to Valle del Sol in the Angostura Valley. About 1 m of alluvium (Figures 4c, 5) is present at the base of the exposure. It consists of intercalated layers of sand and silt with some gravel lenses with heterogeneous compositions (pelite, volcanite, and metamorphic rocks) (2–4 cm in diameter). Sample CP-VS03.12 was extracted from this unit for OSL dating. The alluvium is overlain across an erosional contact by 3–4 m of the chaotic deposit, with boulders reaching 2 m across and a sandy matrix that constitutes 10–20% of the mass. The chaotic deposit is unconformably overlain by alluvium consisting of sub-rounded clasts of Devonian metamorphic rocks (mainly gneiss), rhyolite, andesite, and granite, as well as clasts of the same composition as the blocks in the underlying chaotic deposit (Figure 5).
Site 4, Stratigraphic Profile E ∼1,580 m asl
This exposure is located along the Blanco River at the junction of the roads to El Salto and Vallecitos. At this site, the chaotic deposit is 4.5–6 m thick and has a marked greenish–grayish color (Figure 6a). The blocks are 0.4–0.7 m in size and are set in a sandy matrix that forms 40% of the deposit. The chaotic deposit lies within a paleochannel incised into older alluvium and is covered by up to 1.5 m of reddish alluvium across an irregular contact (Figure 7). The upper alluvium unit was sampled for OSL dating (sample CP-SA07.12).
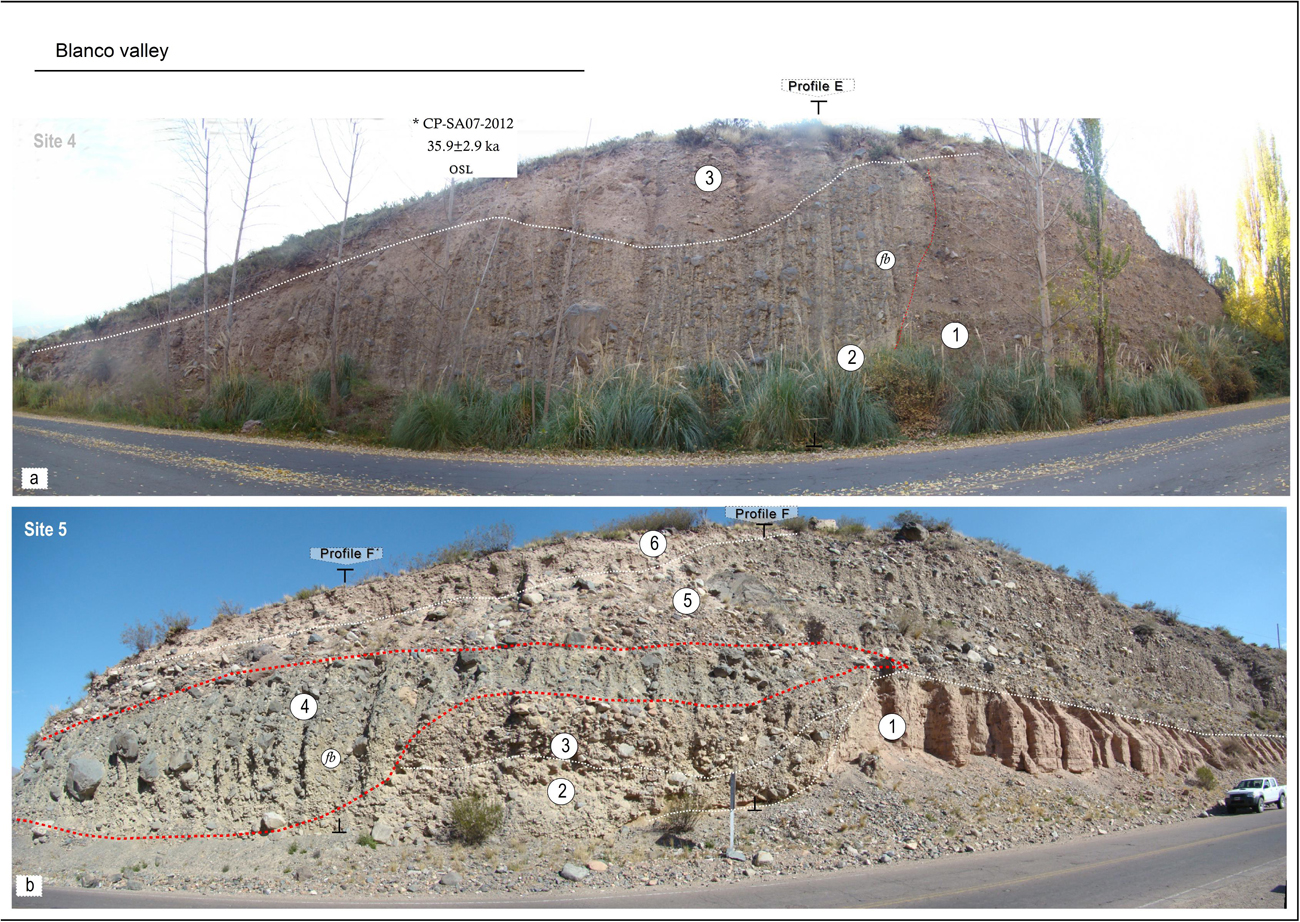
Figure 6. Cross sections along the Blanco River where the Plata rock avalanche outcrops: (a) Cross section at site 4 where unit 1 corresponds to gravel alluvial deposits, 2. the Plata rock avalanche with apparent vertical lamination due to vertical erosion (fb is facie b), and 3. finer alluvial deposits where the sample CP-SA07.12 was taken for OSL dating. (b) Cross section in site 5 where unit 1 corresponds to Tertian outcroppings, 2. and 3. paleo-channel deposits, 4. the rock avalanche, 5. outwash deposit, and 6. alluvial deposits. See profiles on Figure 7.
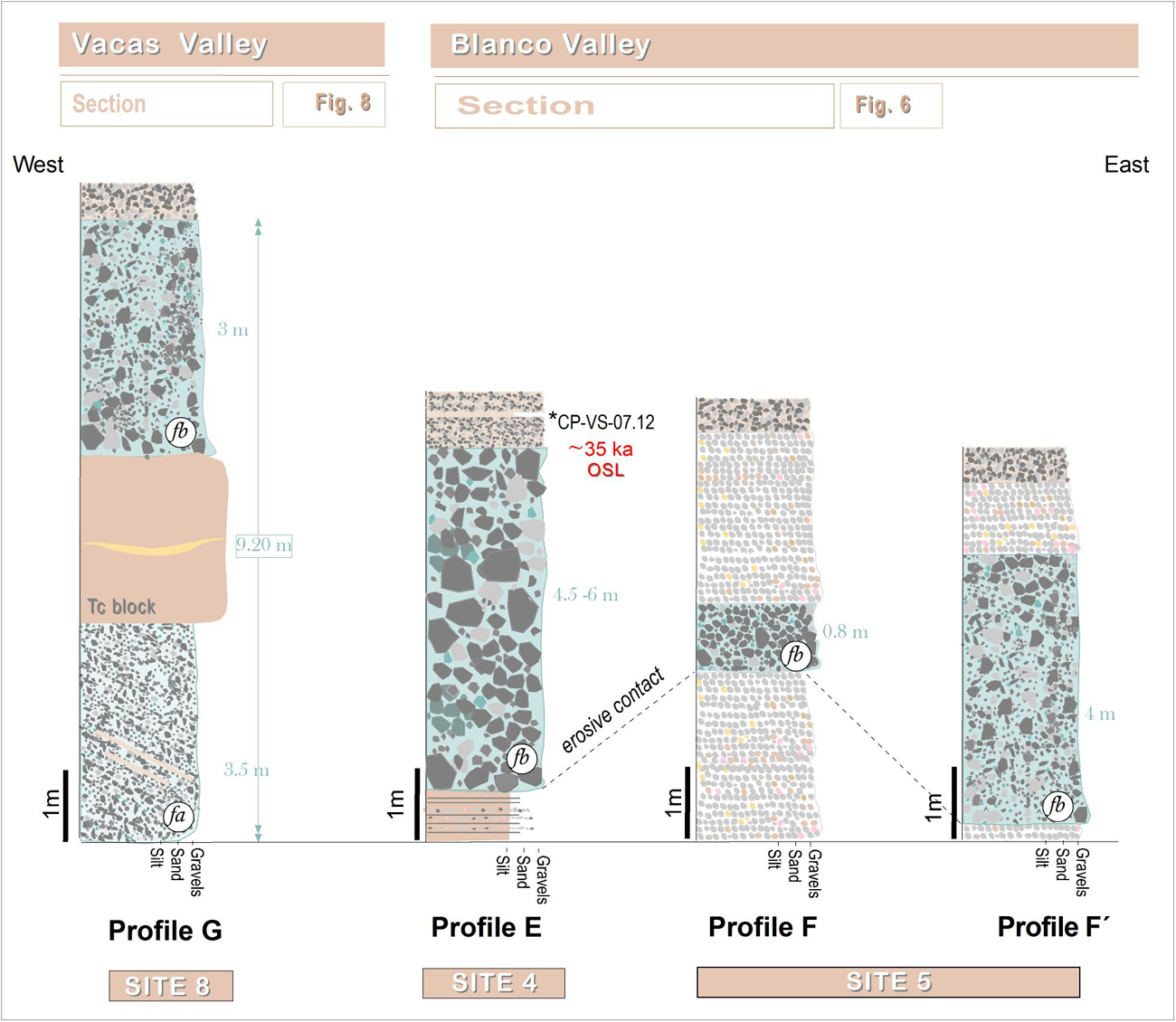
Figure 7. Graph of sedimentological profiles described in sites 4 and 5 along the Blanco Valley (see pictures in the Figure 6) and profile G described in site 8 along the Vacas valley (see Figure 8). Tc block corresponds to the block of Tertiary rocks engulfing by the facie a (fa) and covered by the facie b (fb).
Site 5, Stratigraphic Profile F ∼1,540 m asl
Up to 2.4 m of glaciofluvial gravel containing rounded and sub-rounded boulders up to 0.60 m in diameter form the lower part of the road cut at site 5 in the Blanco Valley (Figure 6b). This deposit lies within a paleochannel incised into the Tertiary Mariño Formation. The boulders are mainly volcanic and intrusive rocks of the Permo-Triassic Choiyoi Group (andesite, gabbro, rhyolite, and granite) but include some Carboniferous pelite and sandstone. About 30% of the deposit is a sandy matrix.
The glaciofluvial unit is overlain by 0.8 m of the chaotic deposit with its typical greenish–grayish color. Blocks up to 2 m in size are set in a matrix that constitutes 40% of the deposit. About 98% of the blocks are sedimentary rocks of the Plata Formation. The chaotic deposit is overlain successively by 1–2.5 m of glaciofluvial gravel with rounded boulders of a variety of lithologies and 0.4 m of alluvial fan deposits (Figure 7).
The chaotic deposit shows a thicker thickness (4 m) in section F’, described to be 10 m from previous section F (Figure 6b). Here the boulder sizes are up 2 m in diameter, predominating the largest blocks near the top of the unit, and the matrix content reaches 35%. A 1-m-thick fluvial glacial level and 0.7-m-thick alluvial deposits are overlying the chaotic deposit (Figures 6b, 7).
Site 8, Stratigraphic Profile G ∼1,800 m asl
This 11-m-high section is located at the north margin of the Vacas valley (Figure 8). About 3.5 m of the greenish–grayish chaotic deposit (lithofacies a), similar to the deposit at site 2, marks the base of the section. The clasts are smaller (<0.5 m) and the matrix content is higher (40%) than at previously described sections. Some of the clasts are Tertiary siltstone and sandstone. The deposit is stratified, and the clasts are imbricated and inclined toward the west. A 2.7-m block of reddish Tertiary sedimentary rock separates this unit from 3 m of lithofacies b (Figure 7). The basal unit dips toward the west and east adjacent to the large Tertiary block; it appears that the block was flowing on this basal unit. The upper part of the chaotic deposit (lithofacies b) is massive and contains clasts of Carboniferous rocks 0.3–0.5 m in size. It is overlain at the top of the exposure by 0.6–1 m of alluvium.
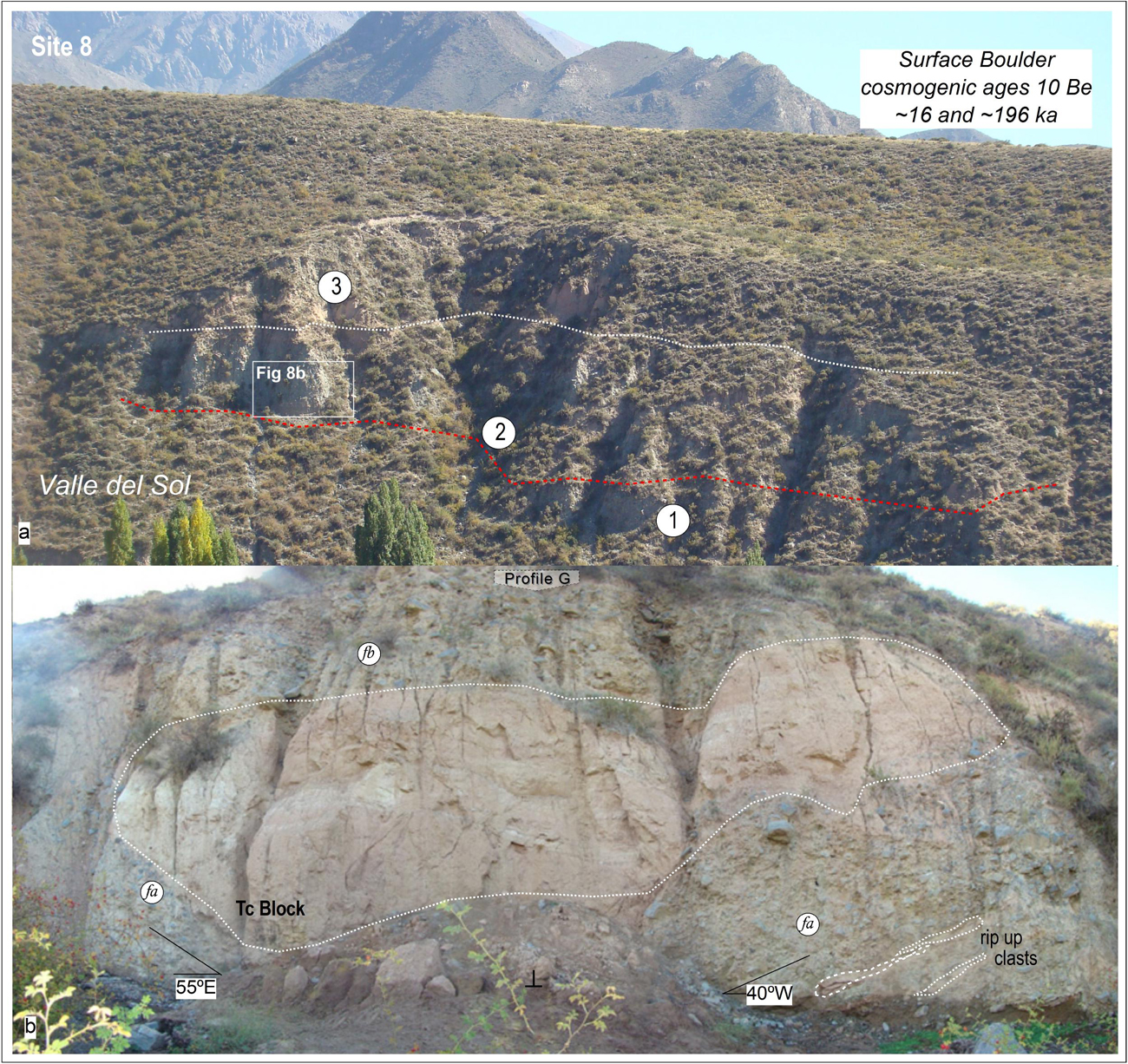
Figure 8. Site 8 just in from of the Valle del Sol village. (a) Panoramic view of alluvial deposits along the Vacas Valley where 1. corresponds to alluvial deposits, 2. rock avalanche, and 3. alluvial deposits previously dated by cosmogenic nucleoids on surficial blocks (10Be) ∼16 and ∼196 ka. (b) Detail of the rock avalanche deposit where a block of Tertian rocks (Tc block) is engulfed by the rock avalanches facies (fa) and facies (fb). Note that the basal layers are dipping in contrary directions containing rip up clasts.
Source Area
The source area of the chaotic deposit is not obvious. The composition of the deposit matches the widespread occurrence of Carboniferous rocks on the east slope of El Plata Peak (5,956 m asl), but the headwaters of the Angostura Valley lack a characteristic spoon-shaped scar. Furthermore, the chaotic deposits appear to be absent in the headwaters of the Angostura Valley (Figures 3, 9). Different types of glaciers flow off this massif; rather, glaciers occupy the head of valleys of the BRB. In the Vallecitos Valley, Vallecitos, Rincon, Coloradas, and Stepanek (bare ice) glaciers are located above 4,700 m asl and cover a total area of 2.3 km2, but the debris-covered glaciers flowing down along this valley have a greater area of 5.6 km2 as with other dry environment mountains. Farther south, the only ice landform is the Lomas de los Morteritos Glacier in the headwaters of the Mulas Valley. This debris-covered glacier lies at 3,400 m asl, with a length of 2.7 km (Figure 3). In both valleys of the BRB, rock glaciers cover smaller areas (<0.86 km2) and represent not more than 10% of glaciated areas in each sub-basin (Table 3).
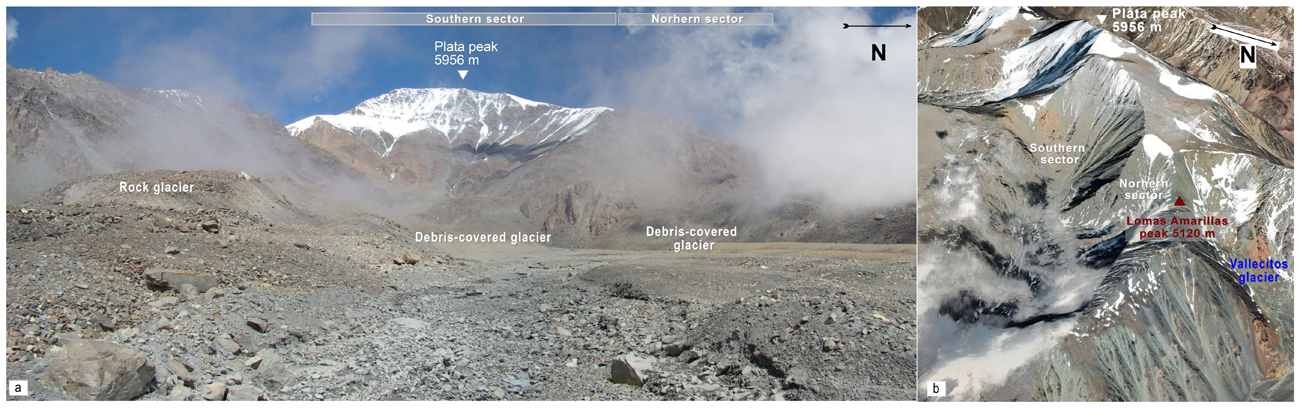
Figure 9. Source area. (a) Picture of Plata peak showing predomination of ice covered glacier and debris rock glaciers in the southern sector of the headwater of the Angostura Valley where rock avalanche remains are lacking. (b) Google 3D stereographic view of the Plata peak without glaciers in the southern sector.

Table 3. Areas occupied by different types of glaciers in the main sub-basins of the Blanco River basin.
Glacier ice cover in the headwaters of the Angostura Valley differs from north to south, with larger glaciers to the north and smaller ones and rock glaciers to the south. A bare ice glacier is at the top of the northern headwaters, and two big debris-covered glaciers occupied the whole valley downstream, with practically no rock glaciers. This seems anomalous given that the southern headwaters initiate below the highest peak of the study area (El Plata, 5,656 m asl), where only a debris-covered glacier was identified.
There is no doubt that all the high valleys in the BRB supported glaciers at the LGM (Moreiras et al., 2017). However, the lack of a large glacier in the southern headwaters of the Angostura Valley is evidence that a rock avalanche was generated in this sector and removed the glacier during the collapse. The material detachment surely changed the original aspect of the slope preventing the further accumulation of snow/ice and the generation of a niche or circus what restricted the ensuing regrowth of a glacier. However, the debris-covered glacier just below this area could indicate the restoration of the glacial environment in this southern headwater of the Angostura valley.
Origin, Volume, and Emplacement of the Chaotic Deposit
The sedimentological and stratigraphic evidence presented above support the idea that the chaotic “cenoglomerate” is not a glacial deposit but rather the product of a rock avalanche. After the rock mass collapsed, debris was channelized into the Angostura Valley and streamed down the Blanco River. Part of the streaming debris overtopped a topographic barrier and entered the southern parallel valley of the Vacas River (site 8) (Figure 8).
Assuming that the east face of El Plata Peak was the source area of the rock avalanche, the debris traveled at least 26.6 km over a vertical range of 4,700 m. The calculated fahrboschung of 0.17 indicates high mobility and an excessive travel distance compared to many other rock avalanches worldwide (Korup et al., 2007; Hewitt, 2009; Yang et al., 2019).
Only a rough estimate of the volume of the rock avalanche is possible because only isolated eroded remnants of the deposit exist and any material in the source area was removed. The maximum observed thickness of the rock avalanche deposit is 9.2 m, but at many sites < 0.6 m is exposed. Assuming a very conservative area covered by rock avalanche of 64,000 m2, based on the surface of preserved deposits, and a mean thickness of the deposit of 5 m, a minimum volume of 3.2 × 105 m3 was obtained. Other estimates, obtained by the mean thickness, travel distance (26.6 km), and assuming valley widths of 100 or 200 m, range from 1.46 to 2.9 × 107 m3. These volumes are relatively small compared with those of neighboring rock avalanches, which reach up to 109 m3 (Moreiras et al., 2015), and thus may be underestimates.
Age of Rock Avalanche
The Plata rock avalanche deposit overlies outwash and alluvium deposits and underlies alluvium along the Blanco River, neither of which has been previously dated. Polanski (1966) assumed a Late Pleistocene age based on the assumed association of the deposit with a dated tephra in overlying alluvial sediments. In contrast, Wayne (1984) considered that the deposit was a product of an Early Pleistocene glacial advance.
The rock avalanche deposit overlies alluvium, including silt and sand layers at sites 1, 2, and 3 (Figures 4, 5). A sample of underlying fine sediments at site 3 (CP-VS03.12) yielded OSL ages of 39.7 ± 3.9 and 76.54 ± 6.81 ka (on feldspar) (CP-VS03.12), depending on the model used (Table 2). A second OSL sample collected from a sandy layer in alluvium overlying the rock avalanche deposit at site 4 (CP-SA07.12) yielded ages of 35.90 ± 2.89 ka or 50.35 ± 3.79 ka (on quartz), depending on the model used.
The dose rates for most terrestrial sediments are 2–4 Gy/ka (Aitken, 1985, 1998). The measured dose rate for quartz in our samples ranges from 2.2 to 3.1 Gy/ka and for feldspar from 2.9 to 3.9 Gy/ka (Table 1). Both quartz and feldspar mineral grains have been utilized. Quartz was favored initially, but due to the relatively low proportion of sensitive grains obtained in the first runs, feldspar experiments were added to the suite of analyses (Antinao et al., 2013). The ages obtained from feldspar by the MAM (Galbraith et al., 1999) were preferred for the CP-VS03.12 sample as a larger fraction of feldspars was sensitive compared to the fraction displayed by quartz. The age obtained from quartz was still preferred for the CP-SA07.12 sample since about 3–5% of the analyzed grains of the total feldspars showed saturation (i.e., the accumulated dose cannot be related to a finite artificial dose), indicating that these grains, although sensitive, have not been bleached. Coupled together, these data indicate that probably an analysis with a MAM would yield an equivalent dose identifiable with the event that buried the alluvial deposits (Table 2).
Conclusion
Wayne (1990) concluded that there were four glacial advances in the BRB, as supported by his interpretation of the deposits as tills. He noted glacial micromorphology on quartz grains, the presence of striated quartzite boulders, boulder fabrics that he attributed to glacial processes, valley walls polished by larger glaciers, and the large size of most of the cirques. However, according to this study, the Angostura till of Wayne and Corte (1983) and Wayne (1984), said to be a nearly monolithic deposit, is associated with a huge rock avalanche. Polanski (1966) and, later, Wayne (1990) attributed this event to a debris flow. However, the monolithic composition, with debris derived nearly entirely (98%) from the Carboniferous El Plata Formation (98%), the matrix content (20–40%), the dominance of subangular to angular blocks, the local presence of inverse grading, the absence of striations on clasts, and the presence of two related lithofacies are more consistent with a rock avalanche origin.
Rock avalanches are powerful mass movements resulting from the sudden and catastrophic failure of large rock masses that rapidly fragment and move as rapid flows downslope (Cruden and Varnes, 1996; Hungr and Evans, 2004; Strom and Korup, 2006; Hungr et al., 2014). The Plata rock avalanche mobilized at least 3.2 × 105 m3 of debris and traveled up to 26.6 km from the source. The debris became channelized along the Blanco River but had enough energy to cross over to the Vacas Valley. A block 12 m wide and 5 m high floated on the streaming debris (Figure 8).
Since the pioneer work of Yarnold and Lombard (1989), typical facies models of rock avalanches describe a disturbed basal facies influenced by interactions with the substrate over which the landslide flows, overlain by a mixed zone with a higher content of finer material, and then a boulder cap known as a “carapace” (Prager et al., 2012; Weidinger et al., 2014; Wang et al., 2015; Dufresne et al., 2016; Strom and Abdrakhmatov, 2018; Zeng et al., 2019). This entire set of facies was not observed at any of the studied sections, but the lithofacies a at sites 2 and 8 share some similarities to the mixed zone mentioned above. Ripped-up clasts were observed as well in this basal facies. This weakly stratified facies resembles debris deposited by rock avalanches in the Tien Shan in Central Asia (e.g., Ornok, Yashilkul landslides; Strom and Abdrakhmatov, 2018). Lithofacies b has larger blocks and less matrix content than lithofacies a. Notably, at site 8, a large block of Tertiary rock at the base of facies b “rides” on much finer facies and debris. Although inverse grading is noted in lithofacies b (e.g., profile B at site 1), the typical carapace facies (Dufresne et al., 2016) was not observed, perhaps because it was subsequently removed by erosion.
Characterization of the rock avalanche deposit was helpful for understanding the provenance and the behavior of the landslide. The rock avalanche was initiated on the eastern slope of El Plata Peak, even though a typical source scar is lacking. The geomorphological study likewise reveals that the distribution of glaciers in the valleys of the BRB is not uniform. Even though the Plata Range is sufficiently high to support glaciers, they are poorly developed in the southern sector of the headwaters of the Angostura Valley. The lack of bare ice glaciers in this southern area could be explained by the generation of a great rock slope collapse. Even though the modified steep hillslope aspect of the east face of El Plata hindered the generation of a new glacier accumulation zone, the glacial environment could have been later restituted. A debris-covered glacier places just below the inferred source area of the rock avalanche and moraines associated with the LMG (∼18–25 ka) remain in this valley downstream.
The rock avalanche generated in the south face of the Aconcagua peak (∼11 ka) (Fauqué et al., 2009) modified the previous cirque area, generating a hanging wall glacier that remains until today. The lower Horcones inferior glacier, which is a reconstructed debris-covered glacier, is disconnected from the accumulation zone. This glacier is fed by snow avalanches coming from the south face of the Aconcagua peak. In the study area, the debris-covered glacier in the southern headwater of Angostura valley is disconnected from an “accumulation zone” in the eastern hillslope of the Plata peak (Figure 9). Besides that, a cirque morphology is absent in this hillslope.
Another evidence of the possible glacier contribution is that the Plata rock avalanche traveled 26.6 km, widely exceeding the distance of typical rock avalanches. This run-out is much longer than rock avalanches in the northern Plata Range (<10 km). The H/L ratio (∼0.17) is low, even if excess mobility is forced by the larger volume (Hsu, 1975; Hungr et al., 2005). A likely cause of this extraordinary run-out is that the rock avalanche incorporated glacier ice that melted and lubricated the debris material, increasing the erosional power and the distance traveled. Rock avalanches that overrun glaciers show more mobility (Evans and Clague, 1988; Sosio et al., 2012; De Blasio, 2014; Deline et al., 2015; Yang et al., 2019). The existence of a glacier in the headwaters of the Angostura Valley when the landslide happened is plausible as a moraine ∼40 ka was dated in the nearby Las Mulas basin. Similarly, the long run-out of Horcones rock avalanche in the Argentine Andes (13 km) has been attributed to the collapse of a rock mass onto a glacier below the south face of Aconcagua Peak (6,958 m asl) (Fauqué et al., 2009; Hermanns et al., 2015).
Optically stimulated luminescence dating of alluvial sediments above and below the rock avalanche deposit placed the event between about 40 and 36 ka. These ages are within the envelope of 10Be ages of ∼16 and ∼169 ka previously obtained on boulders on the alluvial fan covering the rock avalanche in the Vacas Valley (site 8) (Moreiras et al., 2017). Evidence for climate forcing of the rock avalanche is weak as evidences for warmer or wetter conditions between 40 and 35 ka have not been found in the region. The collapse was possibly caused by an earthquake as alluvial deposits associated with the Plata rock avalanche are offset by the Rio Blanco and Salto faults belonging to the Carrera Fault system. A cluster of seven rock avalanches in the northern Plata Range is likewise associated with the same fault system (Moreiras et al., 2015).
Data Availability Statement
All datasets generated for this study are included in the article/supplementary material.
Author Contributions
The author confirms being the sole contributor of this work and has approved it for publication.
Funding
This research was funded by the Universidad Nacional de Cuyo through Projects SeCTyP 2011-2013 06/A519 and ANLAC Program Res 571/15.
Conflict of Interest
The authors declare that the research was conducted in the absence of any commercial or financial relationships that could be construed as a potential conflict of interest.
Acknowledgments
We used QGis for data processing. OSL dating was done in the DRI lab. I thank J. Antinao for dating and P. Rodriguez, C. Lauro, and L. Mastrantonio for help with fieldwork. Gregory Carling and two reviewers helped to improve this manuscript.
References
Abele, G. (1984). Derrumbes de montaña y morrenas en los Andes chilenos. Rev. Geogr. Norte Grande 11, 17–30.
Antinao, J. L., and Gosse, J. (2009). Large rockslides in the Southern Central An-des of Chile (32–34.5°S): tectonic control and significance for Quaternary landscape evolution. Geomorphology 104, 117–133. doi: 10.1016/j.geomorph.2008.08.008
Antinao, J. L., Huenupi, E., Baker, S., and Lewis, A. (2013). “Luminescence dating of alluvial deposits,” in Desert Research Institute Final Report, (Vallecitos), 20.
Berger, G. W. (1988). “Dating quaternary events by luminescence,” in Dating Quaternary Sediments, Special Paper, Vol. 227, ed. D. J. Easterbrook (Boulder, CO: Geological Society of America), 13–50.
Berger, G. W., Henderson, T. K., Banerjee, D., and Nials, F. L. (2004). Photonic dating of prehistoric irrigation canals at Phoenix. Arizona. Geoarchaeol. 19, 1–19. doi: 10.1002/gea.10101
Borgnia, M. (2004). Neotectónica del Piedemonte Oriental del Cordón del Plata al Norte del río BLANCO, Provincia de Mendoza. MSc thesis, Universidad de Buenos Aires, Buenos Aires. 43.
Brenning, A., Trombotto, D., and Schroeder, H. (2005). Zur verbreitung von blockgletschern in den semiariden anden (Chile/Argentinien, 32-35°S): Bedeutung, Regionale Trends und der Einfluss des Reliefs. Berl. Geograph. Arbeiten 100, 19–28.
Buk, E. (2002). “Hidrología de ambientes periglaciales. cuenca morenas coloradas–vallecitos, cordón del plata, cordillera frontal, mendoza,” in IANIGLA, 1973-2003: 30 Años de Investigación Básica y Aplicada en Ciencias Ambientales, eds D. Trombotto and R. Villalba (Mendoza), 60–65.
Caminos, R. (1965). Geología de la vertiente oriental del Cordón del Plata, Cordillera Frontal de Mendoza. Asoc. Geol. Argent. Rev. 20, 351–392.
Casa, A. L. (2005). Geología y Neotectónica del Piedemonte Oriental del Cordón del Plata en los Alrededores de El Santo. Msc thesis. Universidad de Buenos Aires, Buenos Aires, 174.
Casa, A. L. (2009). Sistema de fallas de La Carrera (AR-86; AR-114; AR-115; AR-116; AR-156). In: atlas de deformaciones cuaternarias de los andes. proyecto multinacional andino: geociencia para las comunidades andinas. atlas de deformaciones cuaternarias de los andes. Serv. Naci. Geol. Minería Publ. Geol. Mult. 7, 118–123.
Casa, A. L., Cortés, J. M., and Borgnia, M. (2010). Evidencias de deformación pleistocena en el sistema de fallas de La Carrera (32° 40’ – 33° 15’ LS), Cordillera Frontal, Mendoza. Revi. Asoc. Geol. Argent. 67, 91–104.
Corte, A. E., and Espizúa, L. (1981). Inventario de Glaciares de la Cuenca del Río Mendoza. Mendoza: Instituto Argentino de Nivologia y Glaciología, 64.
Corte, A. E., and Grosso, E. (1993). “Geocriología,” in Geología y Recursos Naturales de Mendoza. XII Congreso Geologico Argentino y II Congreso de Exploración d Hidrocarburos, Vol. 16, ed. V. A. Ramos (Medoza: Relatorio), 205–216.
Cortés, J. M., Casa, A., Pasini, M., Yamín, M. G., and Terrizzano, C. (2006). Fajas oblicuas de deformación neotectónica en precordillera y Cordillera Frontal (31°30’-33°30’ LS): controles paleotectónicos. Rev. Asoc. Geol. Argent. 61, 639–646.
Cortés, J. M., Vinciguerra, P., Yamín, M., and Pasini, M. M. (1999). “Tectónica Cuaternaria de la fregión Andina del Nuevo Cuyo (28° - 38° LS),” in Geología Argentina. Subsecretaría de Minería de la Nación, Vol. 29, ed. R. Caminos (Anales: Servicio Geológico Minero Argentino), 760–778.
Cruden, D. M., and Hungr, O. (1986). The debris of the Frank slide and theories of rockslide-avalanche mobility. Can. J. Earth Sci. 23, 425–432. doi: 10.1139/e86-044
Cruden, D. M., and Varnes, D. J. (1996). Landslide types and processes. U.S. Natl. Acad. Sci. Trans. Res. Board Special Rep. 247, 36–75.
De Blasio, F. V. (2014). Friction and dynamics of rock avalanches travelling on glaciers. Geomorphology 213, 88–98. doi: 10.1016/j.geomorph.2014.01.001
Deline, P., Hewitt, K., Reznichenko, N., and Shugar, D. (2015). “Rock avalanches onto glaciers,” in Landslide Hazards, Risks and Disasters. Amsterdam, eds F. John, J. F. Shroder, and T. Davies (Amsterdam: Elsevier), 263–319. doi: 10.1016/b978-0-12-396452-6.00009-4
Dessanti, R. N. (1946). Hallazgo de depósitos glaciales en las Huayquerías de San Carlos. Rev. Asoc. Geol. Argenti. I 1, 270–284.
Drewes, J., Moreiras, S., and Korup, O. (2018). Permafrost activity and atmospheric warming in the Argentinian Andes. Geomorphology 323, 13–24. doi: 10.1016/j.geomorph.2018.09.005
Drovandi, A., Zuluaga, J., Filippini, M., Consoli, D., Valdes, A., Nacif, C., et al. (2010). “Evaluación de la calidad del agua en el Embalse Potrerillos y Cuenca del Río Blanco, Provincia de Mendoza,” in Proceedings, III Congreso Internacional sobre Gestión y Tratamiento Integral del Agua, Córdoba, 1–39.
Dufresne, A., Bösmeier, A., and Prager, C. (2016). Sedimentology of rock avalanche deposits – Case study and review. Earth Sci. Rev. 163, 234–259. doi: 10.1016/j.earscirev.2016.10.002
Dunning, S. A. (2006). The grain size distribution of rock-avalanche deposits in valley confined settings. Italian J. Eng. Geol. Environ. Special Issue 1, 117–121.
Espizúa, L. E. (1982). Glacier and moraine inventory on the eastern slopes of cordon del plata and cordon del portillo, central andes, argentina. in: tills and related deposits. Proc. INQUA Sympos. Argent. 1982, 381–395.
Espizúa, L. E. (1999). Chronology of late pleistocene glacier advances in the río mendoza valley, argentina. Global Plane. Change 22, 193–200. doi: 10.1016/s0921-8181(99)00036-3
Espizúa, L. E., and Bigazzi, G. (1998). Fission-track dating of the Punta de Vaca glaciation in the río mendoza valley, Argentina. Q. Sci. Rev. 17, 755–760. doi: 10.1016/s0277-3791(97)00056-5
Evans, S. G., and Clague, J. J. (1988). “Catastrophic rock avalanches in glacial environments. Landslides,” in Proceedings, 5th Symposium on Landslides, Vol. 2, Lausanne, 1153–1158.
Fauqué, L., Hermanns, R., Hewitt, K., Rosas, M., Wilson, C., Baumann, V., et al. (2009). Mega-landslide in the southern wall of the Aconcagua and its relationship with deposits assigned to Pleistocene glaciations. Rev. Asoc. Geol. Argent. 65, 691–712.
Folguera, A., Etcheverría, M., Pazos, P., Giambiagi, L., Fauqué, L., Cortés, J., et al. (2004). Hoja geológica 3369-15, potrerillos, provincia de mendoza. Serv. Geol. Min. Argent. Bolet. 301:135.
Galbraith, R. F., Roberts, R. G., Laslett, G. M., Yoshida, H., and Olley, J. M. (1999). Optical dating of single and multiple grains of quartz from Jinmium rock shelter, northern Australia: part i, experimental design and statistical models. Archaeometry 41, 339–364. doi: 10.1111/j.1475-4754.1999.tb00987.x
Gates, W. C. B. (1987). The fabric of rockslide avalanche deposits. Bull. Assoc. Eng Geol. 24, 389–402. doi: 10.2113/gseegeosci.xxiv.3.389
Giambiagi, L. B., Ramos, V. A., Godoy, E., Alvarez, P. P., and Orts, S. (2003). Cenozoic deformation and tectonic style of the Andes, between 33° and 34° south latitude. Tectonics 22, 1041–1059. doi: 10.1029/2001TC001354
Groeber, P. (1951). La Alta Cordillera entre las Latitudes 34° y 29°30’. Museo Argent. Bernard. Riv. Revi. Cien. Geol. 1:352.
Groeber, P. (1955). Bosquejo paleogeográfico de los glaciares del Diamante y Atuel. Rev. Asoc. Geol. Argent. 9, 89–108.
Heredia, N., Farias, P., García-Sansegundo, J., and Giambiagi, L. (2012). The basement of the andean frontal cordillera in the cordón del plata (Mendoza, Argentina): geodynamic evolution. Andean Geol. 39, 242–257. doi: 10.5027/andgeoV39n2-a03
Hermanns, R., Fauqué, F., and Wilson, C. (2015). “36Cl terrestrial cosmogenic nuclide dating suggests late Pleistocene to early Holocene mass movements on the south face of Aconcagua mountain and in the Las Cuevas – Horcones valleys, Central Andes, Argentina,” in Geodynamic Processes in the Andes of Central Chile and Argentina, Vol. 399, eds S. A. Sepúlveda, L. B. Giambiagi, S. M. Moreiras, L. Pinto, M. Tunik, G. D. Hoke, et al. (London: Geological Society of London), 345–368. doi: 10.1144/sp399.19
Hewitt, K. (1999). Quaternary moraines vs. catastrophic rock avalanches in the Karakoram Himalaya, Northern Pakistan. Q. Res. 51, 220–237. doi: 10.1006/qres.1999.2033
Hewitt, K. (2009). Glacially conditioned rock-slope failures and disturbance-regime landscapes, Upper Indus Basin, northern Pakistan. Geol. Soc. Special Publ. 320, 235–255. doi: 10.1144/sp320.15
Hewitt, K., Gosse, J., and Clague, J. J. (2011). Rock avalanches and the pace of late Quaternary development of river valleys in the Karakoram Himalaya. Geol. Soc. Am. Bull. 123, 1836–1850. doi: 10.1130/b30341.1
Hsu, K. J. (1975). Catastrophic debris streams (sturzstroms) generated by rockfalls. Geol. Soc. Am. Bull. 86, 129–140.
Hungr, O., Corominas, J., and Eberhardt, E. (2005). “Estimating landslide motion mechanism, travel distance and velocity,” in Landslide Risk Management, Balkema. Proceedings of the International Conference on Landslide risk Management, ed. O. Hungr (Vancouver), 99–128.
Hungr, O., and Evans, S. G. (2004). Entrainment of debris in rock avalanches: an analysis of a long run-out mechanism. Geol. Soc. Am. Bul. 116, 1240–1252. doi: 10.1130/B25362.1
Hungr, O., Leroueil, S., and Picarelli, L. (2014). The Varnes classification of landslide types, an update. Landslides 11, 167–194. doi: 10.1007/s10346-013-0436-y
Huntley, D. J., and Wintle, A. G. (1981). The use of alpha scintillation counting for measuring Th-230 and Pa-231 contents of ocean sediments. Can. J. Earth Sci. 18, 419–432. doi: 10.1139/e81-036
Irigoyen, M. V., Buchan, K. L., and Brown, R. L. (2000). Magnetostratigraphy of Neogene Andean foreland-basin strata, lat 33°S, Mendoza Province, Argentina. Geol. Soc. Am. Bull. 112, 803–816. doi: 10.1130/0016-7606(2000)112<803:monafs>2.0.co;2
Irigoyen, M. V., Buchan, K. L., Villeneuve, M. E., and Brown, R. L. (2002). Cronología y significado tectónico de los estratos sinorogénicos neógenos aflorantes en la región de Cacheuta-Tupungato, Provincia de Mendoza. Rev. Asoc. Geol. Argen. 57, 3–18.
Janke, J. R., Bellisario, A. C., and Ferrando, F. A. (2015). Classification of debris-covered glaciers and rock glaciers in the Andes of central Chile. Geomorphology 241, 98–121. doi: 10.1016/j.geomorph.2015.03.034
Kleiman, L. E., and Japas, M. S. (2009). The Choiyoi volcanic province at 34°S-36°S (San Rafael, Mendoza, Argentina): implications for the Late Palaeozoic evolution of the southwestern margin of Gondwana. Tectonophysics 473, 283–299. doi: 10.1016/j.tecto.2009.02.046
Korup, O., Clague, J. J., Hermanns, R., Hewitt, K., Strom, A. L., and Weidinger, J. T. (2007). Giant landslides, topography, and erosion. Earth Planet. Sci. Let. 261, 578–589. doi: 10.1016/j.epsl.2007.07.025
Llambías, E. J., Kleiman, L. E., and Salvarredi, J. A. (1993). “El magmatismo Gondwánico,” in Geología y Recursos Naturales de Mendoza. 12° Congreso Geológico Argentino y 2° Congreso de Exploración de Hidrocarburos, ed. V. A. Ramos (Mendoza: Relatorio), 53–64.
Massone, H., Martinez, D., Vich, A., Londoño, M. Q., Trombotto, D., and Grondona, S. (2016). Snowmelt contribution to the sustainability of the irrigated Mendoza’s oasis, Argentina: an isotope study. Environ. Earth Sci. 75:520.
Mejdahl, V. (1979). Thermoluminescence dating: beta-dose attenuation in quartz grains. Archaeometry 21, 61–72. doi: 10.1111/j.1475-4754.1979.tb00241.x
Moreiras, S. M. (2006). Chronology of a Pleistocene rock avalanche probable linked to neotectonic, Cordon del Plata (Central Andes). Mendoza Argent. Q. Int. 148, 138–148. doi: 10.1016/j.quaint.2005.11.009
Moreiras, S. M., Hermanns, R. L., and Fauqué, L. (2015). Cosmogenic dating of rock avalanches constraining quaternary stratigraphy and regional neotectonics in the argentine central andes (32° S). Q. Sci. Rev. 112, 45–58. doi: 10.1016/j.quascirev.2015.01.016
Moreiras, S. M., Páez, M. S., Lauro, C., and Jeanneret, P. (2017). First cosmogenic ages for glacial deposits from the Plata range (33° S): new inferences for quaternary landscape evolution in the Central Andes. Q. Int. 438, 50–64. doi: 10.1016/j.quaint.2016.08.041
Moreiras, S. M., and Sepúlveda, S. A. (2015). “Megalandslides in the andes of central chile and argentina (32°-34°s) and potential hazards,” in Geodynamic Processes in the Andes of Central Chile and Argentina, Vol. 399, eds S. A. Sepúlveda, L. B. Giambiagi, S. M. Moreiras, L. Pinto, M. Tunik, G. D. Hoke, et al. (London: Geological Society of London), 329–344. doi: 10.1144/sp399.18
Murray, A. S., and Wintle, A. G. (2000). Luminescence dating of quartz using an improved single aliquot regenerative-dose protocol. Radiat. Meas. 32, 57–73. doi: 10.1016/s1350-4487(99)00253-x
Norte, F. (1995). Condiciones Meteorológicas Favorables a la Contaminación Atmosférica en Mendoza, eds E. Martinez Carretero and A. Dalmasso (Mendoza Ambiental), 197–206.
Polanski, J. (1953). Supuestos englazamientos en la llanura pedemontana de Mendoza. Rev. Asoc. Geol. Argent. 8, 195–213.
Polanski, J. (1963). Estratigrafía, neotectónica y geomorfología del Pleistoceno pedemontano entre los ríos Diamante y Mendoza. Rev. Asoc. Geol. Argent. 17, 127–349.
Polanski, J. (1966). Flujos Rápidos de Escombros Rocosos en Zonas áridas y Volcánicas. Argentina: Universitaria de Buenos Aires, 67.
Polanski, J. (1972). Descripción Geológica de la Hoja 24a-b, Cerro Tupungato, Provincia de Mendoza. Dir. Nacion. Geol. Min. Bolet. 124:114.
Prager, C., Zangerl, C., and Kerschner, H. (2012). “Sedimentology and mechanics of major rock avalanches: implications from (pre-) historic sturzstrom deposits (Tyrolean Alps, Austria),” in Landslides and Engineered Slopes, Protecting Society through Improved Understanding, Vol. 2, eds E. Eberhardt, C. Froese, K. Turner, and S. Leroueil (Boca Raton, FL: CRC Press), 895–900.
Prescott, J. R., and Hutton, J. T. (1994). Cosmic ray contributions to dose rates for luminescence and ESR dating: large depths and long-term time variations. Radiat. Meas. 23, 497–500. doi: 10.1016/1350-4487(94)90086-8
Reznichenko, N. V., Davies, T. R. H., Shulmeister, J., and Larsen, S. H. (2012). A new technique for identifying rock avalanche-sourced sediment in moraines and some paleoclimatic implications. Geology 49, 319–322. doi: 10.1130/g32684.1
Rhodes, E. J. (2011). Optically stimulated luminescence dating of sediments over the past 200,000 years. Annu. Rev. Earth Planet. Sci. 39, 461–488. doi: 10.1146/annurev-earth-040610-133425
Rodríguez, E., and Barton, M. (1993). “El cuaternario de la llanura,” in Geología y Recursos Naturales de Mendoza. Relatorio del XII Congreso Geológico Argentino y II Congreso de Exploración de Hidrocarburos, ed. V. Ramos (Mendoza), 173–194.
Sepúlveda, S. A., and Moreiras, S. M. (2013). Large volume landslides in the central Andes of Chile and Argentina (32°-34°S) and related hazards. Bull. J. Eng. Geol. Environ. 6, 287–294. doi: 10.4408/IJEGE.2013-06.B-26
Sosio, R., Crosta, G. B., Chen, J. H., and Hungr, O. (2012). Modelling rock avalanche propagation onto glaciers. Q. Sci. Rev. 47, 23–40. doi: 10.1016/j.quascirev.2012.05.010
Strom, A. L., and Korup, O. (2006). Extremely large rockslides and rock avalanches in the Tien Shan mountains, Kyrgyzstan. Landslides 3, 125–136. doi: 10.1007/s10346-005-0027-7
Strom, A. L., and Abdrakhmatov, K. (2018). “Structure and grain-size composition of large-scale bedrock landslides deposits,” in Rockslides and Rock Avalanches in Central Asia: Distribution, Morphology and Internal Structure, ed. M. Le Fleur (Amsterdam: Elsevier), 458.
Trombotto, D. (1991). Untersuchungen zum Periglazialen Formenschatz und zu Periglazialen Sedimenten in der “Lagunita del Plata”, Mendoza, Argentinien. Heidelber. Geograph. Arbeit. 90:171.
Trombotto, D. (2002). “Present cryogenic environment and palaeopermafrost in Southern South America,” in IANIGLA, 1973-2003: 30 años de Investigación Básica y Aplicada en Ciencias Ambientales. ZETA, eds D. Trombotto and R. Villalba Mendoza, 65–70.
Trombotto, D., and Borzotta, E. (2009). Indicators of present global warming through changes in active layer-thickness, estimation of thermal diffusivity and geomorphological observations in the Morenas Coloradas rock glacier. Argentina. Cold Regions Sci. Technol. 55, 321–330. doi: 10.1016/j.coldregions.2008.08.009
Wang, Y. F., Cheng, Q. G., Lin, Q. W., Li, K., and Yang, H. F. (2018). Insights into the kinematics and dynamics of the Luanshibao rock avalanche (Tibetan Plateau, China) based on its complex surface landforms. Geomorphology 317, 170–183. doi: 10.1016/j.geomorph.2018.05.025
Wang, Y. F., Cheng, Q. G., and Zhu, Q. (2015). Surface microscopic examination of quartz grains from rock avalanche basal facies. Can. Geotech. J. 52, 167–181. doi: 10.1139/cgj-2013-0284
Wayne, W. J. (1984). The quaternary succession in the rio blanco basin, cordon del plata, mendoza province, argentina: an application of multiple relative-dating techniques. Dev. Palaeontol. Stratigr. 7, 389–406. doi: 10.1016/s0920-5446(08)70083-9
Wayne, W. J. (1990). The diamictons of rio blanco basin, Cordon del Plata, Mendoza. Q. South Am. Antarct. Penin. 2, 8–31.
Wayne, W. J., and Corte, A. E. (1983). Multiple glaciations of the Cordon del Plata, Mendoza, Argentina. Palaeogeogr., Paleoclimato. Paleoecol. 42, l85–l209.
Weidinger, J. T., Korup, O., Munack, H., Altenberger, U., Dunning, S. A., Tippelt, G., et al. (2014). Giant rockslides from the inside. Earth Planet. Sci. Lett. 389, 62–73. doi: 10.1016/j.epsl.2013.12.017
Yang, Q., Su, Z., Cheng, Q., Ren, Y., and Caid, F. (2019). High mobility of rock-ice avalanches: insights from small flume tests of gravel-ice mixtures. Eng. Geol. 260, 105–260.
Yarnold, J. C., and Lombard, J. P. (1989). “A facies model for large rock-avalanche deposits formed in dry climate,” in Conglomerates in Basin Analysis: A Symposium Dedicated to A.O. Woodford, Pacific Section, Vol. 62, eds I. P. Colburn, L. Patrick, X. Abbott, and J. Minch (Tulsa, OK: SEPM), 9–31.
Keywords: rock avalanche, Late Pleistocene, Central Andes, Argentina, sedimentology
Citation: Moreiras SM (2020) The Plata Rock Avalanche: Deciphering the Occurrence of This Huge Collapse in a Glacial Valley of the Central Andes (33° S). Front. Earth Sci. 8:267. doi: 10.3389/feart.2020.00267
Received: 28 January 2020; Accepted: 15 June 2020;
Published: 17 July 2020.
Edited by:
John Joseph Clague, Simon Fraser University, CanadaReviewed by:
Fabio Matano, National Research Council (CNR), ItalyCristina Maria Pinto Gama, University of Évora, Portugal
Copyright © 2020 Moreiras. This is an open-access article distributed under the terms of the Creative Commons Attribution License (CC BY). The use, distribution or reproduction in other forums is permitted, provided the original author(s) and the copyright owner(s) are credited and that the original publication in this journal is cited, in accordance with accepted academic practice. No use, distribution or reproduction is permitted which does not comply with these terms.
*Correspondence: Stella M. Moreiras, bW9yZWlyYXNAbWVuZG96YS1jb25pY2V0LmdvYi5hcg==