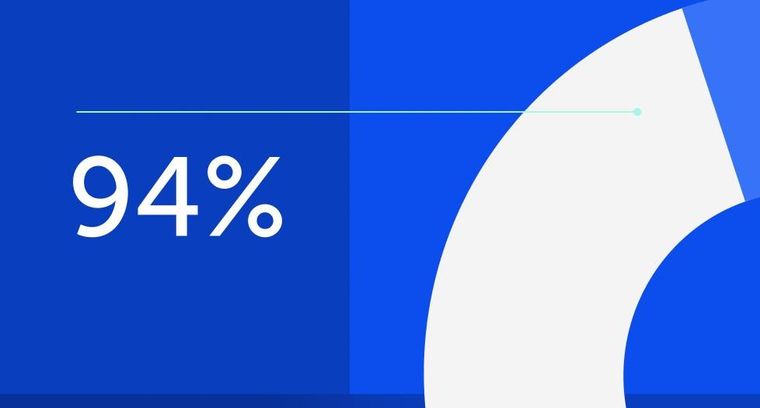
94% of researchers rate our articles as excellent or good
Learn more about the work of our research integrity team to safeguard the quality of each article we publish.
Find out more
REVIEW article
Front. Earth Sci., 20 March 2020
Sec. Hydrosphere
Volume 8 - 2020 | https://doi.org/10.3389/feart.2020.00064
This article is part of the Research TopicConnecting Mountain Hydroclimate Through the American CordillerasView all 19 articles
The Andes is the longest cordillera in the world and extends from northern South America to the southern extreme of the continent (from 11°N to 53°S). The Andes runs through seven countries and is characterized by a wide variety of ecosystems strongly related to the contrasting climate over its eastern and western sides, as well as along its latitudinal extension. This region faces very high potential impacts of climate change, which could affect food and water security for about 90 million people. In addition, climate change represents an important threat on biodiversity, particularly in the tropical Andes, which is the most biodiverse region on Earth. From a scientific and societal view, the Andes exhibits specific challenges because of its unique landscape and the fragile equilibrium between the growing population and its environment. In this manuscript, we provide an updated review of the most relevant scientific literature regarding the hydroclimate of the Andes with an integrated view of the entire Andes range. This review paper is presented in two parts. Part I is dedicated to summarize the scientific knowledge about the main climatic features of the Andes, with emphasis on mean large-scale atmospheric circulation, the Andes-Amazon hydroclimate interconnections and the most distinctive diurnal and annual cycles of precipitation. Part II, which is also included in the research topic “Connecting Mountain Hydroclimate Through the American Cordilleras,” focuses on the hydroclimate variability of the Andes at the sub-continental scale, including the effects of El Niño-Southern Oscillation.
The Andes is the longest mountain chain in the world (∼7240 km) and the highest within the inner tropics. The Andes extends over South America with a south-north direction from Patagonia to Venezuela. The complexity of the topography and the relative low density/short period of the meteorological network over the Andes have been an inconvenience for a proper characterization of the main atmospheric circulation patterns and their relationships with the main hydroclimatic features, including the spatio-temporal characterization of precipitation over the whole of the Andes chain. Sustainable development in the Andes is currently menaced by changes ranging from local and regional (land use and deforestation; e.g., Nobre et al., 2016; Aide et al., 2019) to global (climate change; Magrín et al., 2014), which in turn would affect its climate and water resources, from glaciers retreat (Rabatel et al., 2013) to water supply and ecosystem services (Vuille et al., 2018).
During the last two decades, a few studies have summarized the main features of the atmospheric circulation over particular regions in South America, including Barros et al. (2000), focusing on the La Plata Basin in Southeastern South America, and Garreaud and Aceituno (2007), focusing on the subtropical/extratropical part of the continent. Over equatorial and tropical part of South America, several studies have reviewed the main mechanisms associated to the South America Monsoon System (SAMS) (Zhou and Lau, 1998; Vera et al., 2006; Marengo et al., 2012), whereas Vuille et al. (2012) provide a review of changes in this monsoon circulation during the past two millennia. Reviews of the hydroclimate of the Amazon basin have been provided by Figueroa and Nobre (1990), Espinoza et al. (2009b), and more recently by Marengo and Espinoza (2016), with particular focus on extreme hydrological events. At the regional level, Poveda et al. (2006) and Garreaud et al. (2003) have provided review papers on the climate of Northern South America/Southern Mesoamerica and the South American Altiplano, respectively. However, to our knowledge, we still lack of integrated reviews and assessments of the hydroclimate over the entire Andes range.
This contribution makes part of a series of review papers focused on diverse aspects of climate, weather, hydrology and cryosphere of the Andes cordillera. Such reviews are being prepared to provide a state-of-the-art synthesis of current knowledge, as part of the ANDEX initiative. ANDEX is a prospective Regional Hydrological Project (RHP) of the Global Energy and Water Cycle Experiment (GEWEX)1 project of the World Climate Research Programme (WCRP)2. Three international workshops have been held among researchers from Andean countries and elsewhere to discuss the existing knowledge about the Andes hydroclimatology, to identify the research community working on the relevant scientific disciplines and applications, and to start identifying major research gaps. The ANDEX foundational meeting was held in Medellín, Colombia, on 5–7 December 2017; the second one in Santiago, Chile, on 22–24 October 2018; and the third workshop took place in Quito, Ecuador, on 21–24 April 2019. As a result of these activities, three overarching questions have been defined to guide the ANDEX scientific agenda, as follows3 :
(i) What are the main physical processes driving the water and energy budgets of the Andes (as a singular cordillera), at a broad range of spatial and temporal scales, and their interactions with the Pacific and Atlantic Oceans and major river basins of South America?
(ii) How climate change, deforestation and land use changes are affecting the hydroclimatological functioning of the Andes across the altitudinal and latitudinal gradients, from glaciers to paramos, punas, cloud montane forests, rainforests, dry forests, and deserts?
(iii) What is the scientific basis underpinning the sustainable development of the Andean region?
In particular, this review aims to assess and discuss recent progress in the knowledge of the Andes hydroclimate, under an integrated view from the Patagonia to the northern Andes, including for the first time all latitudinal ranges: north tropical (north of 8°S), south tropical (8°S–27°S), subtropical (27°S–37°S) and extratropical (south of 37°S). This review considers the interactions Andes-Amazonas, which play a key role controlling hydroclimate over the Andes. This comprehensive approach has allowed better describing the differences and connections among regions, including water and energy fluxes. Also, this manuscript highlights the main research gaps that need to be addressed in order to improve our current understanding of Andean hydroclimate. This manuscript is presented in two parts. Part I is dedicated to summarize (i) the mean large-scale atmospheric circulation that characterize the hydroclimate of the Andes, including zonal flow impinging the Andes, the low-level jets observed over the Andes and the hydroclimatic connectivity between the Amazon and the Andes; and (ii) the regular cycles of precipitation, including the most distinctive diurnal and annual cycles of precipitation. Part II addresses the hydroclimate variability of the Andes at the sub-continental scale, including interannual variability and its relationship with the El Niño-Southern Oscillation (ENSO). Recent progress in the knowledge about impacts of the ENSO on the Andean hydroclimate are also reviewed by Poveda et al. (submitted) in the paper “High Impact Weather Events in the Andes,” submitted to this special issue (“Connecting Mountain Hydroclimate Through the American Cordilleras”).
The atmospheric circulation over South America and adjacent oceans are major drivers of weather and climate over the Andes, including mean climatic conditions and regular cycles. These large-scale features are in turn affected by the Andean topography, vegetation patterns, the gradually-varying boundary conditions provided by the Pacific and Atlantic oceans, the tropical-extratropical interactions, the Amazon-Andes connection, among other regional-to-local interactions. In the next sections, these main features are described and discussed.
Figure 1 shows the long-term mean upper and lower tropospheric wind field in South America for January and July from the NCEP-NCAR reanalysis, a rather coarse dataset but fine enough to describe the large-scale circulation interacting with the Andes (see also Garreaud, 2009). To complement the isobaric maps, Figure 2 displays the latitude-height cross section of the zonal flow at 70°W. Broadly speaking, the mid- and upper-level circulation atop and over the Andes is mostly zonal, with westerly flow from 25°S southward and easterly flow at low latitudes. During the austral winter [June-July-August (JJA)], mid-level westerlies reach ∼10°S and the upper-level jet stream is located over the subtropical Andes, at about 30°S. During the summer [December–January–February (DJF)], the zonal flow over South America is disrupted by the Bolivian High (see section 3) but since it is centered over the Altiplano, the flow atop of the cordillera remains mostly easterly (from 20°N to 20°S) or westerly (from 25°S southward, with an upper-level jet stream located over the extratropical Andes at about 45°S). The narrowness and elevation of the mountain range preclude the formation of large-scale stationary waves, because most of the low- and mid-level flow impinging the Andes is blocked and there is little deflection of the horizontal streamlines over the sloping terrain (that otherwise will generate topographic Rossby waves, e.g., Holton, 2002). On the other hand, the interaction of the strong westerly winds with the extratropical Andes (south of 37°S) is capable to produce large-amplitude gravity waves propagating into the stratosphere where they deposit their momentum (e.g., Jiang et al., 2013).
Figure 1. (a,b) Long-term mean (1981–2020) winds at 200 hPa (arrows) and precipitation (shades). (c,d) Long-term mean (1981–2020) winds at 925 hPa (arrows) over blue marble. Data from NCEP/NCAR reanalysis.
Figure 2. Cross-section of long-term-mean (1981–2020) zonal wind (U) at 70°W for (A) austral winter (JJA) and (B) summer (DJF). Gray area indicates mean Andes height averaged at 80–60°W. Contours indicate U = 0, 15, and 30 m/s. Data from NCEP-NCAR Reanalysis.
Baroclinic disturbances embedded in the mid-latitude westerly wind belt are responsible for most of the rain and snowfall in the subtropical and extratropical Andes (particularly, central-southern Chile, and western Argentina) due to the spill-over effect. The axis of the southern hemisphere storm track intersect the extratropical Andes at 45–50°S year round (Figure 1; Berbery and Vera, 1996; Inatsu and Hoskins, 2004; Hoskins and Hodges, 2005) and recent studies have shown that climate change is responsible for a poleward shift of the storm track (Bengtsson et al., 2006; Chang et al., 2012) with important consequences for the Chile/western Argentina hydroclimate (Boisier et al., 2018).
Near-surface, westerly flow continues to impinge against the Andes at mid-latitudes and easterly flow near the equator. The trans-Andean flow at mid-latitudes -responsible for some of the most marked precipitation gradients on Earth (see Part II)- is possible because of the strong flow and the relatively low topography, even though some blocking occurs at the lowest levels (Viale and Garreaud, 2015). The trans-Andean flow at low latitudes is more difficult to occur given the altitude of the mountains in this region. Indeed, the eastern slopes of the south Tropical Andes (which cover Bolivia, Peru, and Ecuador) act as a topographic barrier for the warm and moist flux from the Amazon region. Moisture advection from the Amazon region is predominant during the austral summer (Figure 1a). However, at low latitudes (north of 5°S), where the altitude of the mountains is lower, trans-Andean flows are predominant all year around, producing a complex rainfall regime over this region (Laraque et al., 2007; Espinoza et al., 2009b; Segura et al., 2019).
Because of the topographic blocking, the low-level flow adjacent to the subtropical Andes is mostly meridional: southerly winds to the west and northerly winds to the east. This meridional flow pattern is stronger in the austral summer and in quasi-geostrophic balance with the subtropical Pacific anticyclone and the continental low (Seluchi et al., 2003), whose maintenance is partially driven by the Andes (Rodwell and Hoskins, 2001; Takahashi and Battisti, 2007). Near to the western flank of the subtropical Andes, the flow becomes northerly, with a well-defined jet at about 700 hPa within 30 km from the mountain surface (Rutllant and Garreaud, 2004; Garreaud, 2009). The low-level, terrain-parallel flow close to both sides of the Andes often organize in low-level jets (LLJs) described in detail in section Low Level Jets at the Andes Foothills.
The relevance of the Andes cordillera in organizing the tropospheric flow at the continental scale (and so the moisture transport among other consequences) can also be appreciated in numerical experiments in which the Andes is removed, as presented in Insel et al. (2010) and Garreaud et al. (2010). Of particular relevance, the presence of the Andes is instrumental for the existence of the low-level jet east of the Andes, the South American Low-Level jet (SALLJ; see section LLJs to the East of the Andes), that transport humidity from the Amazonian rainforest into the southern plains of the continent, and so it is fundamental for inducing the occurrence of the SAMS during austral summer. The SAMS corresponds to a monsoonal circulation over South America, mainly Bolivia, Peru, Brazil and northern Argentina, covering the Amazon rainforest (e.g., Zhou and Lau, 1998), that exhibits four dominant features: (i) an upper troposphere anticyclone (at 200–300 hPa), located over Bolivia, known as the Bolivian High (see section Regular Cycles of Precipitation); (ii) a northwest-southwest oriented band of cloudiness over the southeast of the continent, known as the South Atlantic Convergence Zone (SACZ); (iii) the occurrence of high surface temperatures over the Atlantic Ocean prior to the wet season onset; and (iv) the presence of the SALLJ to the east of the Andes (see section LLJs to the East of the Andes) (Gan et al., 2004; Vera et al., 2006; Carvalho et al., 2011; Marengo et al., 2012). The evolution of the SAMS is also related to the location and intensity of the Atlantic Intertropical Convergence Zone (ITCZ). For instance, during the onset and mature phases of the SAMS (early-mid austral summer), the ITCZ is confined to the Atlantic region between 5 and 8°N (Zhou and Lau, 1998). During the retreat phase of the SAMS (late austral summer-early austral fall), the deep convection of the Atlantic ITCZ is relatively weak and shows a northward migration (Vera et al., 2006). Therefore, atmospheric circulation over the Andes is partly connected to the dynamics of the SAMS, as discussed throughout the next subsections.
The Amazon-Andes transition region is characterized by a unique landscape and the fragile equilibrium between the growing population and its environment (Myers et al., 2000; Vuille et al., 2018). This region shows an exceptional biodiversity and biogeographical patterns, which are the result of the interplay between contrasted climates and the complexity of Andean topography (Hoorn et al., 2010; Rangel et al., 2018). The eastern Andes and the Amazon River basin constitute an entangled biogeophysical system. The Amazon region exports water vapor and nutrients to the Andes through the moisture-laden trade winds (e.g., Garreaud, 1999; Poveda et al., 2006, 2014; Zemp et al., 2017). The ascent of these winds owing to the orographic effect of the Andes, favors convection and high intensity rainfall rates over the eastern piedmont (e.g., Espinoza et al., 2009b, 2015; Chavez and Takahashi, 2017; Junquas et al., 2018; Kumar et al., 2019), which in turn produces the river runoff draining sediments, pollutants, and nutrients downstream to the Amazon lowlands (Poveda et al., 2006; McClain and Naiman, 2008; Espinoza et al., 2009a; Moquet et al., 2011; Molina-Carpio et al., 2017; Builes-Jaramillo and Poveda, 2018).
The eastern flank of the tropical Andes is the wettest region in the Amazonia (e.g., Figueroa and Nobre, 1990; Espinoza et al., 2009b). From the diurnal to the interdecadal timescale, rainfall variability in the Amazon-Andes transition region is the result of the interplay between regional atmospheric circulation, lowland-highland temperature contrast and the complex Andean topography (Ronchail and Gallaire, 2006; Espinoza et al., 2015; Segura et al., 2016; Chavez and Takahashi, 2017; Junquas et al., 2018). These features produce high rainfall rates of around 6000–7000 mm/year, generally observed at around 400–2000 m.a.s.l (Johnson, 1976; Giovannettone and Barros, 2009; Poveda et al., 2014; Espinoza et al., 2015; Chavez and Takahashi, 2017). As a consequence, the Andean region has the largest runoff per unit area of the Amazon basin (Moquet et al., 2011; Builes-Jaramillo and Poveda, 2018; Figure 3) and the extreme hydrological events that occur over this region can have impacts over the entire Amazon basin, as observed during the exceptional 2014 flood in the upper Madeira River basin (Espinoza et al., 2014; Ovando et al., 2016). Studies have documented the key role of the hydrological variability of the Andean-Amazon rivers, such as the Madeira, Ucayali, Marañón and Caquetá-Japura for a broad understanding of hydrological variability of the entire Amazon basin at the seasonal and interannual time scales, including long-term hydrological trends (e.g., Roche and Fernandez Jáuregui, 1988; Espinoza et al., 2009a; Lavado-Casimiro et al., 2013; Molina-Carpio et al., 2017; Wongchuig-Correa et al., 2017; Barichivich et al., 2018), atmospheric and surface water balances (Builes-Jaramillo and Poveda, 2018, Figure 3) and the dynamics of the sediments and nutrients at the Amazon basin scale (Guyot et al., 2007; Moquet et al., 2011; Armijos et al., 2013; Bouchez et al., 2017; Vauchel et al., 2017). Multiannual rainfall variability in the Amazon basin, including extreme hydroclimatic events, has been mainly attributed to the sea surface temperatures (SST) variations in both the tropical Pacific and Atlantic oceans, with seasonal and regional specificities. In addition, a reduced vegetation cover in the southern Amazon also contributes to a more frequent occurrence of longer dry seasons over this region (for more details see Debortoli et al., 2015; Marengo and Espinoza, 2016; Barichivich et al., 2018; Builes-Jaramillo and Poveda, 2018; Espinoza et al., 2019; Leite-Filho et al., 2019; Ruiz-Vasquez et al., submitted, among others).
Figure 3. Illustration of the components of surface water balance and atmospheric water balance based on the observations (OBS) and reanalysis (ERA) datasets in the Andes and Amazonia subregions of the Amazon River basin. P, precipitation; E, actual evapotranspiration; R, runoff; C, moisture convergence. Values are in units of mm.y–1. The arrow convention on the bottom left represents 1000 mm.y–1. Source: Builes-Jaramillo and Poveda (2018). © American Geophysical Union. Reprinted by permission from John Wiley and Sons.
Builes-Jaramillo and Poveda (2018) provide recent estimates of water balance components in the Andes-Amazon transition zone (Figure 3). Despite receiving less precipitation, runoff is higher in the Andean part of the Amazon basin than the Amazon lowlands (Moquet et al., 2011). This difference could be related to the spatial variability of rainfall over the Andes-Amazon region, where the rainiest zones are observed (“hotspots”), as well as the steep topography that favors runoff (Espinoza et al., 2014). High rainfall rates on the eastern slopes of the Andes cause strong erosion, particularly over the south tropical Andes, mainly related to climate variability (Pepin et al., 2013; Lowman and Barros, 2014). The erosion of the eastern flank of the tropical Andes provides nearly 100% of the suspended sediment load observed in the Amazon Basin (estimated between 750 and 1200 Mt/year), with a major contribution from southern Amazon rivers such as the Ucayali and Madeira. For instance, the average suspended sediment production of the Madeira River Andean catchment has recently been estimated at 640 Mt/year (Vauchel et al., 2017), but almost a third of that load is deposited in the floodplains before reaching the Amazon (Guyot et al., 2007; Armijos et al., 2013; Santini et al., 2014; Vauchel et al., 2017; Espinoza-Villar et al., 2018; Ayes-Rivera et al., 2019). Andean rivers also control the Amazon basin hydrochemistry, in particular due to the salt rocks and carbonates present in the Marañón and Ucayali basins (Moquet et al., 2011; Bouchez et al., 2017).
The Atlantic Ocean and the Amazon rainforest are, respectively, the main oceanic and continental atmospheric moisture sources for most of the north tropical Andes (north of 8°S) and neighboring regions (Arias et al., 2015; Hoyos et al., 2017; Zemp et al., 2017; Figures 3, 4). On the other hand, the Amazon rainforest is a major supply of humidity for neighboring regions, such as tropical South America, the La Plata river basin and the Andes cordillera (e.g., Berbery and Barros, 2002; Martinez and Dominguez, 2014; Poveda et al., 2014; Arias et al., 2015; Molina et al., 2019; Ruiz-Vasquez et al., submitted). In a recent study, Staal et al. (2018) estimates that around 25–50% of total annual rainfall observed in the tropical Andes might be originated by Amazon tree transpiration transported westward by dominant trade winds (Figure 4). For instance, Rodriguez et al. (2018) suggest that environmental change (mainly land use change) in the southern and southeastern Amazon basin has reduced its capacity to regulate low flows. Also, according to previous works, Amazonian rainforest is the main source of atmospheric moisture for the subtropical Andes (e.g., Garreaud, 1999; Garreaud et al., 2003; Falvey and Garreaud, 2005; Sorí et al., 2017; Segura et al., 2020).
Figure 4. (A) Fraction of total precipitation during the extended dry season (June–November) that last evaporated from the ocean, calculated from atmospheric moisture tracking (1989–2005). Arrows represent vertically integrated moisture fluxes. Source: Zemp et al. (2017), © Nature Communications. CC licence. (B) Fraction of mean annual rainfall that has been transpired by trees in the Amazon basin. Source: Staal et al. (2018). © Nature Climate Change. Reprinted by permission from Springer Nature.
Consequently, the high rate of Amazonian land-cover change probably will affect the entire hydrological cycle over both the Amazon basin and the Andes. In fact, the existence of two-way biogeophysical feedbacks between the Andes and the Amazon is difficult to overstate. Massive amounts of water vapor are evapotranspired into the atmosphere by the Amazonian rainforest, which are condensed and converted into rainfall (precipitation recycling), and later on evapotranspired several times within the region (Figure 3). Such cascade of water vapor is then transported by the trade winds from eastern to central and western Amazonia (Figure 4A), to be lifted by the orography of the Andes and then condensed and converted into rainfall. This explains that the rainiest regions of the Amazon river basin are located along the piedmonts of the Andes of Colombia, Ecuador, Peru, and Bolivia (Espinoza et al., 2009b), which in turn feed the extraordinarily high precipitation recycling rates in the Andes (Zemp et al., 2014; Staal et al., 2018). Such high amounts of rainfall along the Andes feed the rivers flowing to the low-lying Amazonia (Figure 3). Therefore, deforestation of the low-lying Amazon would reduce the recycling of precipitation, but also the flux of water vapor exported to the Andes, and the surface runoff and sediments exported from the Andes to the low-lying Amazonia, increasing the risks of drought, tree mortality and fires. Such hydrological disruption also threatens the supply of water that is transported from the low-lying Amazon to the tropical Andean glaciers, already undergoing a fast retreat owing to global warming (Sagredo and Lowell, 2012; Rabatel et al., 2013), which in turn impact the ecological dynamics of paramos, yungas, punas, and Andean forests (cloud, montane, and rainforests) (Zimmer et al., 2018), and put in peril the water supply for major cities like Bogotá, Lima, Quito, and La Paz, and hundreds of cities and towns along the Andes.
During the DJF season (austral summer), the 200 hPa westerlies over South America are mainly observed south of 22°S. Also, the extreme north of the continent is dominated by westerlies (Figure 5). An upper-level anticyclonic circulation (around 200–300 hPa), referred as the Bolivian High, is observed over the tropical South American continent (centered at around 15°S–65°W). Downstream, over northeastern Brazil, an upper level cyclonic circulation is observed in complement to the Bolivian High and a convergence region is defined over the Peruvian and Ecuadorian coasts (Virji, 1981; Lenters and Cook, 1997).
Figure 5. 200 hPa winds for 3 days in austral summer depicting an absent Bolivian High (BH; A), a Bolivian High near its climatological position (B) and an intense, broad Bolivian High centered well to the south of its mean position (C). Wind data from NCEP-NCAR Reanalysis.
Initial studies suggested a thermal origin for the Bolivian High, supported by the strong sensible heating and the liberation of latent heat over the Altiplano and the tropical Andes during the austral summer (Schwerdtfeger, 1961; Gutman and Schwerdtfeger, 1965). Later, a comprehensive modeling study by Lenters and Cook (1997) suggested that the Bolivian High is a dynamical response to the heating of the upper levels of the troposphere produced by the deep convection over the central-western Amazonia. Diabatic heating over the Andes and the Altiplano does not seem crucial for the development of the Bolivian High, while the Andes organize the low-level circulation and convection over tropical South America. Hence, the Bolivian High is now recognized as one of the most prominent regional responses of the tropospheric circulation associated with the strong convection over the Amazon observed during the SAMS in austral summer (e.g., Vera et al., 2006). The Bolivian High contributes actively to the precipitation over the Altiplano and the south tropical Andes during the austral summer because the easterly winds in the upper troposphere favor the transport of moist air from the Amazon lowlands toward the tropical Andes (Garreaud, 1999; Segura et al., 2019). In addition, the Bolivian High is crucial to the strengthening of the SACZ during the DJF season, due to vorticity advection observed upward (Figueroa et al., 1995). During the March-April-May (MAM) season, the southern hemisphere westerlies return to encompass the subtropics and a couple of anticyclones is observed over the tropical part of the continent, in accordance with the convection over the equator.
The Bolivian High experiences strong synoptic and intraseasonal variability (Figure 5) in its intensity, position and extent. While the Bolivian High mean-state has been firmly related to convection over the Amazon, it is unclear how both elements are connected at higher frequencies. A confounding factor is extratropical variability that can impact both upper-level winds and low-level conditions affecting continental convection. Of particular relevance is a sub-monthly scale dipole of convection that is often observed in southeastern South America between the La Plata basin and southern Brazil (e.g., Nogués-Paegle and Mo, 1997; Díaz and Aceituno, 2003). Earlier studies indicate that this dipole is related to distinct changes in intensity and position of the Bolivian High (Vera and Vigliarolo, 2000).
Prominent LLJs are observed at both sides of the Andes and at different ranges of latitude, which are induced by mechanical blocking of impinging flow and/or diabatic heating in the mountain slopes. These LLJs are important because they transport vast quantities of moisture along large meridional distances, except for the case of the jets to the west of the Andes at subtropical latitudes. A schematic representation of these LLJs is presented in Figure 6 and we now examine their main features.
Figure 6. Schematic figure of South America depicting the principal LLJs at both sides of the Andes cordillera.
One of the LLJs to the east to the Andes circulates over northern South America from the Tropical North Atlantic through Guyana and eastern Venezuela. It becomes a LLJ blowing over the Venezuela-Colombian Llanos (plains) of the Orinoco River, so-called Corriente de los Andes Orientales (CAO), the Llanos jet, the Orinoco jet, or the Eastern Andes Jet (Montoya et al., 2001; Torrealba and Amador, 2010; Jiménez-Sánchez et al., 2019; see Figure 6). The easterly flow of the CAO reaches the eastern piedmont of the Andes as the northernmost leg of the SALLJ (Figure 6). The SALLJ is characterized by northerly surface (850 hPa) wind speeds higher than 12 ms–1 (Marengo et al., 2004). This LLJ reaches regions of Bolivia, Paraguay, Southern Brazil, Uruguay and Argentina (Byerle and Peagle, 2002; Campetella and Vera, 2002; Wang and Fu, 2002; Vera et al., 2006; Moraes-Arraut et al., 2012; Poveda et al., 2014) where it delivers vast quantities of moisture transported from the Atlantic or the Amazon basin, feeding severe weather well into subtropical latitudes (e.g., Salio et al., 2007). The SALLJ is promoted by the diabatic heating released in the SAMS region (Vera et al., 2006). This LLJ attracted considerable attention in the early 2000’s when a major field experiment (SALLJEX) was conducted in the summer of 2003, mostly over Bolivia and Paraguay4.
Over the Colombian and Venezuelan Llanos region, the CAO exhibits a clear annual cycle with peak velocities during DJF (8–12 ms–1) and lower velocities during JJA (2–3 ms–1). It is worth noticing that the annual cycle of the CAO wind strength is negatively correlated with rainfall over the Colombian Llanos (Rueda, 2014; Jiménez-Sánchez et al., 2019) and the western Amazon basin (Espinoza et al., 2018) and positively correlated with rainfall over the south tropical Andes and southern Amazonia, where annual rainfall reaches 6000 mm (e.g., Figueroa and Nobre, 1990; Garreaud et al., 2003; Espinoza et al., 2009b; Giovannettone and Barros, 2009; Segura et al., 2019). At the eastern flank of the south tropical Andes, the moisture fluxes provided by the CAO produce high rainfall rates (5000–7000 mm yr–1) over the Andes-Amazon transition zone of Peru and Bolivia (Poveda et al., 2014; Espinoza et al., 2015; Chavez and Takahashi, 2017).
One of the rainiest places on Earth (Lloró; 5°30′N, 76°32′W) is situated alongside the Pacific coast of Colombia, witnessing mean annual precipitation rates reaching 13,000 mm (Snow, 1976; Meisner and Arkin, 1987; Poveda and Mesa, 1999, 2000; Adler et al., 2000; Mapes et al., 2003; Sakamoto et al., 2011; Zuluaga and Houze, 2015; Jaramillo et al., 2017; King et al., 2017). Such world record precipitation is explained by the dynamics of the Chocó low-level jet (hereafter, Chocó Jet), enhanced by atmosphere-ocean-land surface interactions (Poveda and Mesa, 1999, 2000). The Chocó Jet is a singular southwesterly circulation feature acting over the easternmost tropical Pacific, with origin off the Chilean coast (Sakamoto et al., 2011; Arias et al., 2015; Hoyos et al., 2017). Once the southeasterly trade winds cross the Equator over the eastern Pacific, they enter onshore in the form of a LLJ, which is then lifted up due to the orographic effect of the western north tropical Andes, and interacts aloft with the warmer easterly trade winds to increase deep convection (López and Howell, 1967; Poveda and Mesa, 2000; Mapes et al., 2003; Warner et al., 2003; Poveda et al., 2006; Jaramillo et al., 2017). Figure 7 depicts the mean seasonal vertical distribution of 925 hPa zonal winds in the tropical Americas with data from MERRA-2 Reanalysis (Gelaro et al., 2017). The Choco jet exhibits a distinctive annual cycle with higher velocities during September–October-November (SON), and lower during February-March, transporting higher amounts of moisture during SON (∼90 ms–1 g kg–1), and minimum values during March, according to data from the NCEP/NCAR Reanalysis (Poveda et al., 2006, 2014; Rueda and Poveda, 2006). The Choco jet reaches southern Central America during June to November (Durán-Quesada et al., 2010). The meridional migration exhibited by this LLJ, characterized by a further north location during SON, is related to the seasonal migration of the ITCZ (Sierra et al., 2018). It is also associated with the dynamics of Mesoscale Convective Systems (MCS) off the Colombian Pacific (Velasco and Fritsch, 1987; Poveda and Mesa, 2000; Mapes et al., 2003; Zuluaga and Poveda, 2004; Mejia and Poveda, 2005; Zuluaga and Houze, 2015; Jaramillo et al., 2017; King et al., 2017).
Figure 7. Vertical distribution of mean (1980–2019) zonal winds (m s−1) based on ERA 5 at 79–80°W during (A) December–February (DJF), (B) March–May (MAM), (C) June–August (JJA), and (D) September–November (SON). Positive values indicate westerly flow while negative values indicate easterly flow. CLLJ indicates the easterly blowing Caribbean LLJ crossing Central America. The westerly blowing Choco jet is most evident from May through November. Images provided by the NOAA/ESRL Physical Sciences Division, Boulder Colorado from their web site at https://www.esrl.noaa.gov/psd/.
A fourth LLJ in the Andean region forms over the southeastern Pacific, close to the Andean western slope (Garreaud and Muñoz, 2005; Muñoz and Garreaud, 2005). It has been shown that this LLJ is produced by the ageostrophic wind balance between pressure, Coriolis and friction forces within the Marine Boundary Layer. The geostrophic balance is broken down by both the Andes cordillera and a coastal range (Takahashi, 2012). While this southerly LLJ does not transport considerable amounts of moisture, it drives the upwelling of cold but nutrient reach water masses along north-central Chile and southern Peru. Weakening of this southerly LLJ can result in the onset of a Coastal El Niño with dramatic consequences along the Peruvian and Ecuadorian coasts (Garreaud, 2018). This was particularly observed during 2016–2017, when the weakening of this LLJ was remotely forced by anomalously intense deep convection in the far-western Pacific that triggered Rossby wave trains extending across the South Pacific, further inducing the occurrence of a Coastal El Niño event in 2017 (Garreaud, 2018).
Regular cycles of precipitation over the Andes are associated with complex interactions between the above-described large-scale atmospheric features (e.g., latitudinal oscillation of the ITCZ and the westerlies) and local patterns, such as the Andean orography, local circulations (e.g., diurnal thermally driven circulations, upslope, and downslope moisture transport) and temperature gradients. Based on the current scientific literature, this section provides a synthesis of diurnal and seasonal cycles of precipitation across the entire Andes.
The most dominant feature of tropical climatology is the strong amplitude of the diurnal cycle of temperature, which in turn is associated with the formation and development of shallow and deep convective processes generating rainfall. Orography exerts a strong role to control and focus local circulation and temperature gradients, and therefore tropical Andean rainfall exhibits a strong spatiotemporal variability. The diurnal cycle of rainfall in the north tropical Andes of Colombia (north of 8°S) has been studied by Poveda et al. (2001,a,b,c,2005). Figure 8 exemplifies the strong variability in the hour of preferential rains over the north tropical Andes of Colombia, which can be unimodal (diurnal) or bimodal (semi-diurnal). The phase of the diurnal cycle of rainfall in the north tropical Andes of Colombia also exhibits a strong spatial variability with respect to the month of the year. Dedicated studies are needed to further understand the physical mechanisms and dynamic and thermodynamic processes that explain such strong spatial variability in the diurnal cycle of rainfall within the seasonal march.
Figure 8. Seasonal march of the diurnal cycle of rainfall at 17 selected stations in the north tropical Andes of Colombia (north of 8°S). The diurnal cycle is defined from 0700 to 0700 local standard time (LST), and interpolated isolines indicate percent of total daily rainfall, with the color scale shown at the bottom. Boundaries of the neighboring (left) Cauca and (right) Magdalena River valleys are shown in white. The inset at the bottom left shows details of rain gauges located in the western flank of the central range of the Colombian Andes. Source: Poveda et al. (2005). © American Meteorological Society. Used with permission.
In most of the south tropical Andes (mainly in southern Peru and Bolivia), a clear diurnal cycle has been identified, characterized by maximum precipitation values observed during the day (night) in the western (eastern) flank of the highlands (Chavez and Takahashi, 2017; Junquas et al., 2018; Saavedra et al., 2020). In the eastern valleys of the Amazon-Andes transition region, the maximum (minimum) precipitation is observed during the night (day). This is the case for the valleys in the Cuzco region (Figure 9; Junquas et al., 2018). On the other side, a thermally-driven early afternoon maximum (Garreaud, 1999) is frequently observed over the hillslopes, associated to upslope flows that mobilize water vapor from the valley floor. This local circulation pattern has been related to the strong precipitation spatial variability and gradients that occur in many Andean valleys (Molina-Carpio et al., 2019). The use of high-resolution climate models has been proposed to study diurnal variability and thus its relationship with spatial variability at local scales (Junquas et al., 2018).
Figure 9. Mean December-February precipitation estimated by the precipitation radar of the Tropical Rainfall Measuring Mission (TRMM-2A25) for the 2000–2014 period (mm/day) in the Peruvian Andes (Cuzco region), corresponding to (a) all times step mean, (b) daytime mean [7–19 h Local Time (LT)], (c) nighttime mean (19–7 h LT), (d) 3 h times-step mean. Bold black contours show 500 and 3500 m orography limits. The black boxes indicated in (a) define the West (W), Center (C), and North (N) boxes. In (d), the indicated time-step titles are 10 h (7–10 h mean), 13 h (10–13 h mean), 16 h (13–16 h mean), 19 h (16–19 h mean), 22 h (19–22 h mean), 1 h (22–1 h mean), 4 h (1–4 h mean), 7 h (4–7 h mean). In (e) the location of the main cities and rivers are indicated. Source: Junquas et al. (2018). © Climate Dynamics. Reprinted by permission from Springer Nature.
Over the Altiplano, the maximum precipitation is observed in the early afternoon (13 h LT) associated with the maximum outgoing longwave radiation, the radiative heating and the upslope flow development in both sides of the Andes (Garreaud, 1999; Garreaud et al., 2009). However, the diurnal cycle of convection and rainfall along the eastern piedmont of the south tropical Andes, one of the rainiest places along the cordillera, is out of phase with the diurnal cycle over the Andes, with maximum occurring at late night/dawn, related to the diurnal thermal valley/mountain circulations, which produce cold air descending downslope and convergence of low-level moisture in the piedmont (Killeen et al., 2007; Chavez and Takahashi, 2017; Junquas et al., 2018).
Further south, the western subtropical Andes have little convective activity during summer, but it is enhanced when rainfall occurs in the late afternoon (Viale and Garreaud, 2014). On the eastern side of the subtropical Andes, a clear summer diurnal cycle is present right at the foothills (Romatschke and Houze, 2013), which corresponds with one of the places with the most intense convection in the world (Zipser et al., 2006; Rasmussen et al., 2014; Liu and Zipser, 2015). Some of these convective systems propagate east characterizing their diurnal life cycle (Rasmussen et al., 2016). The winter rainfall over the subtropical and extratropical Andes have no diurnal cycle given its frontal nature (Saavedra and Foppiano, 1992). Likewise, the annual cycle of rainfall from 35°S southward is relatively small although one can discern a preference for the austral winter months (Viale and Garreaud, 2015).
The latitudinal oscillation of the ITCZ is the principal modulator of the annual cycle of rainfall along the north tropical South America (Mejia et al., 1999; Hastenrath, 2002; Poveda et al., 2006; Killeen et al., 2007; Espinoza et al., 2009b; Alvarez-Villa et al., 2011; Sierra et al., 2015). Thus, the annual cycle of precipitation in western and central Colombia is mostly bimodal, exhibiting two wet seasons (MAM and SON), and two drier seasons (DJF and JJA) (Bendix and Lauer, 1992; Mejia et al., 1999; Ricaurte et al., 2019; Figure 10). On the other hand, the annual cycle of rainfall in the Caribbean region of Colombia, over the north tropical Andes (north of 8°S), is mostly unimodal, and thus it cannot be explained only by the ITCZ migration. In particular, precipitation over this region is influenced by the occurrence of synoptic disturbances associated to the African easterly waves and the Caribbean LLJ (Poveda et al., 2006; Arias et al., 2015; Giraldo-Cárdenas et al., 2017).
Figure 10. Mean annual cycle of precipitation in different rain gauges over the north tropical Andes of Colombia (north of 8°S). Adapted from Ricaurte et al. (2019).
Over the equatorial Andes, the eastern slope is characterized by a unimodal rainfall annual cycle with maximum values in June–August (Figures 11e,g). This pattern is related to an intensification of the westward moisture advection from the equatorial Amazon basin and orographic uplift forced by the Andean topography (Bendix and Lauer, 1992; Rollenbeck and Bendix, 2011; Campozano et al., 2016). Two wet seasons are identified in the highest part of the equatorial Andes (e.g., Guaslán station in Figure 11g), corresponding to the February–April and the October–December seasons (Bendix and Lauer, 1992; Laraque et al., 2007; Espinoza et al., 2009b; Rollenbeck and Bendix, 2011; Campozano et al., 2016, 2018).
Figure 11. (a–g) Rainfall regimes in eight stations in the south tropical Andes-Amazon region. Adapted from: Espinoza et al. (2009b). © International Journal of Climatology. Reprinted by permission from John Wiley and Sons. (h) Relative difference (PSD12-6; shaded) between the maximum spectral value of monthly precipitation from 10 to 16.67 months (defined as the power spectrum density-PSD12-unimodal index) and the maximum spectral value between 5 and 8 months (defined as the PSD6 bimodal index). PSD12 and PSD6 are computed using Equation 3 in Segura et al. (2019) and CHIRPS data set. (i) Relative difference between the harmonic of 12 months and the harmonic of 6 month (C12-C6) estimated by using in situ precipitation of 206 rain-gauge stations located in Peru and Ecuador and Equation 6 from Segura et al. (2019). Topography contours of the altitude of 350 and 2000 m.a.s.l are plotted in black solid lines. Source: Segura et al. (2019). © Climate Dynamics. Reprinted by permission from Springer Nature.
Over the south tropical Andes/Altiplano, where unimodal rainfall regime predominates (Figure 11h), the rainy season extends from November to March with its peak in January, when the establishment of the Bolivian High brings easterly, moist-laden winds from the interior of the continent (Garreaud, 1999). The rest of the year, the south tropical Andes are dominated by westerly flow that brings dry air from the mid-level troposphere over the southeast Pacific (Figures 11a–d). In an early study (Garreaud and Wallace, 1998), the frequency of cold clouds was used as a proxy of convection, but more recent climatologists have considered surface rain data, satellite/radar data and more recently lightning data (e.g., Giovannettone and Barros, 2009; Chavez and Takahashi, 2017; Junquas et al., 2018; Kumar et al., 2019).
In a recent study, Segura et al. (2019) identify three zones over the tropical Andes in relation to seasonal rainfall regime using the Climate Hazards group Infrared Precipitation with Stations (CHIRPS) monthly data (Figures 11h,i): the equatorial Andes (EA; 5°S-1°N), the transition zone (TZ; 8°S–5°S) and the southern tropical Andes (STA, 20°S–8°S). For this purpose, the maximum spectral precipitation value between 10 and 16.67 months is defined as the unimodal index (PSD12) and the value between 5 and 8 months as the bimodal index (PSD6). Thus, the unimodal and bimodal indices allow us to estimate the relative difference between the unimodal and bimodal regime (PSD12-6, see Equation 3 in Segura et al., 2019). The EA and the TZ are characterized by a same wet season in the February–April period, associated with the seasonal variations of the ITCZ and the Walker cell (i.e., southerly displacement of the ITCZ and the weakened Walker Cell during this season). In addition, strong westward moisture transport from the equatorial Amazon is observed during the October–November wet season in the TZ.
Atop and over the western side of the subtropical and extratropical Andes most of the rainfall is produced by frontal systems in winter crossing the continent (Saavedra and Foppiano, 1992), especially fronts associated with atmospheric rivers (Viale et al., 2018). The fronts are rooted in extratropical cyclones that drift in the westerly wind belt and the collocated storm track. These two features of the general circulation remain between 45 and 55°S year round, producing a precipitation annual cycle of low seasonality over the extratropical Andes. Yet, the meridional displacement of the upper-level jet stream does favor more precipitation during the austral winter (JJA) over the subtropical Andes, causing the wet season in central-southern Chile (Rutllant and Fuenzalida, 1991; Viale and Garreaud, 2015; Viale et al., 2019, Figure 12). The mean annual precipitation has a strong latitudinal gradient between 25 and 40°S (Falvey and Garreaud, 2007; Garreaud, 2009). In Santiago (Chile), at 33°S just west of the Andes, more than 80% of the annual rainfall is concentrated between May and September. On the contrary, the eastern slopes of the subtropical Andes receive most of the precipitation in the austral summer due to convective activity over western Argentina (Viale et al., 2019). South of 40°S, the annual cycle of precipitation over the austral Andes exhibits a low seasonality and it is rather similar between the western and eastern slopes, despite the large difference in precipitation totals at each side of the mountain range (Viale et al., 2019; Figure 12).
Figure 12. Mean annual precipitation in different rain gauges of the Andes at subtropical (35°S) and extratropical (43°S) latitudes. In both cases, the figure is a west-east cross section including topography (gray shaded), annual mean precipitation (circles) in rain gauges (crosses). The boxes at the top indicate the fraction of precipitation that falls in austral winter (April–September). Adapted from Viale et al. (2019).
Sustainable development in the Andes is currently menaced by multiscale changes, which affect its climate and water resources from glaciers retreat to ecosystem services and water supply for about 90 million people. Under this context, is crucial to improve our understanding about large-scale controls of the Andes hydroclimate and its interactions with regional and local processes, and therefore enable adaptation to high impacts events related to climate variability and climate change. This manuscript presents a review of the recent progress in the understanding of the Andes hydroclimate based on a unified overview from southern Patagonia to the northern part of the Andes in South America. This section is dedicated to summarizing the most relevant issues stated in the manuscript and the associated research gaps. Two major topics have been summarized in Part I of this paper:
(i) The mean large-scale atmospheric circulation that characterizes the hydroclimate of the Andes
Over the Andes, the mid- and upper-level circulation is mostly zonal, with westerly flow southward of 25°S and easterly flow at low latitudes. However, during the austral winter, mid-level westerlies reach around 10°S and the upper-level jet stream is located over the subtropical Andes (27–37°S). At low latitudes (north of 5°S), trans-Andean flows are predominant all year around, producing a complex rainfall regime over this region (north of Peru, Ecuador and Colombia). During the austral summer, when the mature phase of the South American Monsoon System (SAMS) occurs, the mountains of the south tropical Andes (8–27°S) act as a topographic barrier for the warm and moist flux from the Amazonian region. These flows are crucial for moisture advection to the tropical Andes; however, studies suggest that environmental change (e.g., land use change) in the south and southeastern Amazon basin has reduced its capacity to regulate low flows. Further observational and modeling analysis are necessary to improve our understanding of biosphere-atmosphere interactions involving Amazon rainforest and water cycle in the Andes.
Low-level jets (LLJs) are distinctly observed at both sides of the Andes and at different ranges of latitude, which are induced by mechanical blocking of impinging flow and/or diabatic heating in the mountain slopes. At the western side of the Andes, the Chilean and Peruvian LLJ blows equatorward along the subtropical Andes (27–37°S) over the dry Pacific coast of central-northern Chile and Peru, while a LLJ blows poleward along the extratropical Andes (south of 37°S) due to blocking effect of the barrier on low-level winds associated with frontal precipitation system. Some climatological studies (Falvey and Garreaud, 2007; Viale and Garreaud, 2015) suggested that the blocking effect of the extratropical Andes largely modulate precipitation over the Andes and adjacent low lands, but their physical mechanisms are still little understood and deserve further research in future. At the north tropical Andes (north of 8°S), the Chocó LLJ is a singular southwesterly circulation feature acting over the easternmost tropical Pacific and is enhanced by atmosphere-ocean-land surface interactions over the Pacific coast of Colombia. At the northern part of the eastern flank of the Andes, the so-called Corriente de los Andes Orientales (CAO), Llanos jet, Orinoco jet, or Eastern Andes Jet circulates from the Tropical North Atlantic through Guyana and eastern Venezuela reaching the northernmost leg of the South American low-level jet (SALLJ). The SALLJ blows poleward over regions of Bolivia, Paraguay, Southern Brazil, Uruguay and Argentina, where it delivers vast quantities of moisture originated in the Atlantic or over the Amazon basin, feeding severe weather well into subtropical latitudes. From reanalysis data, the SALLJ intensity, frequency (Jones, 2019), and moisture flux (Montini et al., 2019) have shown an increase in the last decades in most of the seasons, affecting precipitation in the eastern Andes. Further studies are needed to understand how these changes will be projected in a warmer climate, which will require improvements in global models to better representing the low-level circulation in the region (Barros and Doyle, 2018). In addition, interactions between large-scale atmospheric features (Intertropical Convergence Zone (ITCZ), SAMS, Bolivian High, Hadley, and Walker cell, etc.), LLJs in South America, biosphere-atmosphere exchanges and atmospheric mechanisms related to local rainfall in the Andes remain little understood. Modeling and observational initiatives on multiscale atmospheric interactions deserve further attention in future research, including both land cover changes and climate change.
(ii) The regular cycles of precipitation (diurnal and annual cycles)
The above-mentioned large-scale atmospheric mechanisms, the latitudinal migration of the ITCZ, the westerlies, the complex Andean orography, as well as local circulations and temperature gradients, are the main factors that explain regular cycles of precipitation over the Andes. The annual cycle of precipitation in the western and central Colombian Andes is mostly bimodal, exhibiting two wet (drier) seasons in March–April–May and September–October–November [December–January–February and June–July–August (JJA)]. On the other hand, the annual cycle of rainfall in the eastern and northern Colombian Andes, is mostly unimodal, and thus cannot be explained only by the ITCZ migration but also by the synoptic activity related to the easterly waves over the Atlantic Ocean. Over the equatorial Andes, a unimodal regime with a peak in the JJA period is associated with the intensification of the westward moisture transport from the equatorial Amazon and a forced orographic uplift. However, over the high altitudes, two wet periods are observed in February–April and in October–December, in relation to the latitudinal migration of the ITCZ. However, what determines that the bimodality of the annual cycle is limited to the Andean region of Colombia, Ecuador and northern Peru deserves clarifications in future studies (Ricaurte et al., 2019; Segura et al., 2019). Over the south tropical Andes (8–27°S), the rainy season extends from November to March, when the establishment of the Bolivian High brings easterly, moist-laden winds from the interior of the continent. Dry conditions are observed the rest of the year. Over the western side of the Andes, south of 25°S, most of the rainfall is produced by frontal systems crossing the continent. The meridional displacement of the upper-level jet stream does favor more precipitation during the austral winter (JJA) over the subtropical Andes (27–37°S) causing the wet season in central-southern Chile and central-western Argentina. By the contrary, the eastern slopes of the subtropical Andes receive most of the precipitation in the austral summer due to convective activity over western Argentina. This region is part of today’s active research (Seneviratne and Stephens, 2014), as several aspects of the multiscale nature of convection and its impact in the hydrology are not well understood (Varble et al., 2017). The better understanding of how convection initiates, the efficient generation of hail and its propagation, would help to improve forecasting in the Andean region. South of 40°S, the annual cycle is gradually reduced poleward and rather similar between the western and eastern slopes of the Andes, due to the nature of precipitation is not convective and midlatitude frontal precipitation within the westerly circulation and the storm tracks dominate there. Further work is also needed to evaluate how the annual cycle of precipitation (rainfall and snowfall) will change in a warmer climate at different latitudinal and altitudinal ranges of the Andes cordillera. If more model simulations are available, an assessment on the main changes on the annual cycle characteristics would give insights on the impact and tools to evaluate adaptation for sustainable development.
The very high spatial variability of the diurnal cycle of precipitation along the Andes is the result of the interplay among large-scale and local circulation patterns and the complex Andean orography. In particular, diurnal cycles associated to local circulation features in the tropical Andes have been poorly studied (e.g., diurnal thermally driven circulations related to the topography and propagation of off coast convective rainfall). One of the rainiest spots in the World (in Colombia) has been recently center of a field experiment performed to study diverse aspects of the Choco Jet and its effect in convection (Mejia and Poveda, 2005; Yepes et al., 2019). This unique observational data will help to characterize the variability of the diurnal cycle of precipitation and wind circulations in the mesoscale, and the dominant mechanisms of westerly propagation of convection offshore (Yepes et al., submitted). Furthermore, the diurnal cycle of local circulations over the eastern and western Andean slopes are especially interesting as they can be related to the strong precipitation spatial variability and gradients that occur in many Andean valleys, which usually are not identified by the meteorological networks and satellite data. This results in a poor estimation of the precipitation and large residuals (usually negatives) in water balances, even for big Andean basins (Espinoza et al., 2015; Builes-Jaramillo and Poveda, 2018). This research topic could be addressed with the help of high-resolution (both spatial and temporal) climate models that use the relatively scarce sub-daily meteorological data available (e.g., Junquas et al., 2018; Kumar et al., 2019; Saavedra et al., 2020).
Regarding the high relevance of meteorological data in regions of complex topography such as the Andes, recent progress in hydroclimate data in the Andes is reviewed in a specific study by Condom et al. (unpublished), submitted to this special issue (“Connecting Mountain Hydroclimate Through the American Cordilleras”).
Due to this strong interdependence, the Amazon-Andes connectivity (Figures 3, 4) represents a specific challenge for the scientific community, which is particularly important in a climate change context and amidst the intensification of seasonal extreme floods and droughts in Amazonia (Gloor et al., 2013; Marengo and Espinoza, 2016; Barichivich et al., 2018; Espinoza et al., 2018; Wang et al., 2018; Haghtalab et al., submitted), including changes in the land surface, related to climate and human forcing activity (Nobre et al., 2016; Aragão et al., 2018; Aide et al., 2019). Thus, changes in Amazon water cycle could locally affect diurnal and annual cycles of precipitation in the Andes (e.g., Segura et al., 2020), including the hydroclimatological functioning of the glaciers, paramos, punas, cloud montane forests, rainforests, dry forests and deserts. Considering that the annual cycle of precipitation and evapotranspiration is an important research topic for the Andes economical activities, it is crucial to better identify atmospheric mechanisms involving Amazon-Andes connectivity in terms of evapotranspiration, moisture transport and local convection.
In Part II of this manuscript, which is also included in this special issue, we review the hydroclimate variability of the Andes at the sub-continental scale, including the effects of El Niño-Southern Oscillation.
All authors contributed to the conception, discussion, and refinement of this manuscript.
The authors declare that the research was conducted in the absence of any commercial or financial relationships that could be construed as a potential conflict of interest.
This review manuscript has been achieved as part of the ANDEX program (www.gewex.org/project/andex/), which is a prospective Regional Hydroclimate Project (RHP) of the GEWEX Hydroclimatology Panel (GHP). JE was supported by the French AMANECER-MOPGA project funded by ANR and IRD (ref. ANR-18-MPGA-0008). PA was supported by the Universidad de Antioquia through the Grant CODI PRG2017-16264. LS would like to acknowledge the financial support from Tri-agency Institutional Programs Secretariat of Canada through the Global Water Futures Program, Canada First Research Excellence Fund. MM was supported by the Consejo Nacional de Investigaciones Científicas y Técnicas (CONICET), Argentina. MV was supported by FONDECYT 11151009 and FONCYT PICT2016-1666. Finally, this article has the support of UNESCO’s Intergovernmental Hydrological Programme through the Working Group Hydrogeomorphology of the Andes-Amazon Basin in which participate PA, JE, GP, and JM-C. The views presented in this paper are those of the authors and do not compromise the Working Group or UNESCO in any way.
Adler, R. F., Huffman, G. J., Bolvin, D. T., Curtis, S., and Nelkin, E. J. (2000). Tropical rainfall distributions determined using TRMM combined with other satellite and rain gauge information. J. Appl. Meteorol. 39, 2007–2023. doi: 10.1175/1520-0450(2001)040<2007:trddut>2.0.co;2
Aide, T. M., Grau, H. R., Graesser, J., Andrade-Nunez, M. J., Araoz, E., and Barros, A. P. (2019). Woody vegetation dynamics in the tropical and subtropical Andes from 2001 to 2014: satellite image interpretation and expert validation. Glob. Change Biol. 5, 2112–2126. doi: 10.1111/gcb.14618
Alvarez-Villa, O. D., Vélez, J. I., and Poveda, G. (2011). Improved long-term mean annual rainfall fields for Colombia. Int. J. Climatol. 31, 2194–2212. doi: 10.1002/joc.2232
Aragão, L. E. O., Anderson, L. O., Fonseca, M. G., Rosan, T. M., Vedovato, L. B., and Wagner, F. H. (2018). 21st Century drought-related fires counteract the decline of Amazon deforestation carbon emissions. Nat. Commun. 9:536. doi: 10.1038/s41467-017-02771-y
Arias, P. A., Martínez, J. A., and Vieira, S. C. (2015). Moisture sources to the 2010-2012 anomalous wet season in northern South America. Clim. Dyn. 45, 2861–2884. doi: 10.1007/s00382-015-2511-7
Armijos, E., Crave, A., Vauchel, P., Fraizy, P., Santini, W., Moquet, J. S., et al. (2013). Suspended sediment dynamics in the Amazon River of Peru. J. South Am. Earth Sci. 44, 75–84. doi: 10.1073/pnas.1907842116
Ayes-Rivera, I., Armijos Cardenas, E., Espinoza-Villar, R., Espinoza, J. C., Molina-Carpio, J., Ayala, J. M., et al. (2019). Decline of fine suspended sediments in the madeira river basin (2003–2017). Water 11:514. doi: 10.3390/w11030514
Barichivich, J., Gloor, E., Peylin, P., Brienen, R. J. W., Schönegartn, J., Espinozan, J. C., et al. (2018). Recent intensification of Amazon flooding extremes driven by strengthened Walker circulation. Sci. Adv. 4:eaat8785. doi: 10.1126/sciadv.aat8785
Barros, V., Gonzalez, M., Liebmann, B., and Camilloni, I. (2000). Influence of the South Atlantic convergence zone and South Atlantic Sea surface temperature on interannual summer rainfall variability in Southeastern South America. Theor. Appl. Climatol. 67, 123–133. doi: 10.1007/s007040070002
Barros, V. R., and Doyle, M. E. (2018). Low-level circulation and precipitation simulated by CMIP5 GCMS over southeastern South America. Int. J. Climatol. 38, 5476–5490. doi: 10.1002/joc.5740
Bendix, J., and Lauer, W. (1992). Die Niederschlagsjahreszeiten in Ecuador undihre klimadynamische Interpretation (Rainy Seasons in Ecuador and Their Climate-Dynamic Interpretation). Erdkunde 2, 118–134.
Bengtsson, L., Hodges, K. I., and Roeckner, E. (2006). Storm tracks and climate change. J. Clim. 19, 3518–3543. doi: 10.1175/jcli3815.1
Berbery, E. H., and Barros, V. (2002). The hydrologic cycle of the La Plata basin in South America. J. Hydrometeorol. 3, 630–645. doi: 10.1175/1525-7541(2002)003<0630:thcotl>2.0.co;2
Berbery, E. H., and Vera, C. (1996). Characteristics of the Southern Hemisphere Winter Storm Track with Filtered and Unfiltered Data. J. Atmos. Sci. 53, 468–481. doi: 10.1175/1520-0469(1996)053<0468:cotshw>2.0.co;2
Boisier, J. P., Alvarez-Garreton, C., Cordero, R. R., Damian, A., Gallardo, L., Garreaud, R. D. et al. (2018). Anthropogenic drying in Central-Southern Chile evidenced by long term observations and climate model simulations. Elem. Sci. Anthropocene 6:74. doi: 10.1525/elementa.328
Bouchez, J., Moquet, J. S., Espinoza, J. C., Martinez, J. M., Guyot, J. L., Lagane, C., et al. (2017). River mixing in the Amazon as a driver of concentration discharge relationships. Water Resour. Res. 53, 8660–8685. doi: 10.1002/2017WR020591
Builes-Jaramillo, L. A., and Poveda, G. (2018). Conjoint analysis of surface and atmospheric water balances in the Andes-Amazon system. Water Resour. Res. 54, 3472–3489. doi: 10.1029/2017WR021338
Byerle, L. A., and Peagle, J. (2002). Description of the seasonal cycle of low-level flows flanking the Andes and their interannual variability. Meteorologica 27, 71–88.
Campetella, C. M., and Vera, C. (2002). The influence of the Andes mountains on the South American low-level flow. Geophys. Res. Lett. 29:1826. doi: 10.1029/2002GL015451
Campozano, L., Célleri, R., Trachte, K., Bendix, J., and Samaniego, E. (2016). Rainfall and cloud dynamics in the Andes: a Southern Ecuador case study. Adv. Meteorol. 2016:3192765.
Campozano, L., Trachte, K., Célleri, R., Samaniego, E., Bendix, J., Albuja, C., et al. (2018). Climatology and teleconnections of mesoscale convective systems in an Andean basin in southern Ecuador: the case of the Paute basin. Adv. Meteorol. 2018, 1–13. doi: 10.1155/2018/4259191
Carvalho, L. M., Silva, A. E., Jones, C., Liebmann, B., Dias, P. L. S., and Rocha, H. R. (2011). Moisture transport and intraseasonal variability in the South America monsoon system. Clim. Dyn. 36, 1865–1880. doi: 10.1007/s00382-010-0806-2
Chang, E. K. M., Guo, Y., and Xia, X. (2012). CMIP5 multimodel ensemble projection of storm track change under global warming. J. Geophys. Res. 117:D23118. doi: 10.1029/2012JD018578
Chavez, S. P., and Takahashi, K. (2017). Orographic rainfall hotspots in the Andes-Amazon transition according to the TRMM precipitation radar and in situ data. J. Geophys. Res. 122, 5870–5882. doi: 10.1002/2016JD026282
Debortoli, N. S., Dubreuil, V., Funatsu, B., Delahaye, F., Henke de Oliveira, C., Rodrigues-Filho, S., et al. (2015). Rainfall patterns in the Southern Amazon: a chronological perspective (1971– 2010). Clim. Change 132, 251–264. doi: 10.1007/s10584-015-1415-1
Díaz, A., and Aceituno, P. (2003). Atmospheric circulation anomalies during episodes of enhanced and reduced convective cloudiness over Uruguay. J. Clim. 16, 3171–3185. doi: 10.1175/1520-0442(2003)016<3171:acadeo>2.0.co;2
Durán-Quesada, A. M., Gimeno, L., Amador, J. A., and Nieto, R. (2010). Moisture sources for Central America: Identification of moisture sources using a Lagrangian analysis technique. J. Geophys. Res. 115:D05103. doi: 10.1029/2009JD012455
Espinoza, J. C., Chavez, S., Ronchail, J., Junquas, C., Takahashi, K., and Lavado, W. (2015). Rainfall hotspots over the southern tropical Andes: spatial distribution, rainfall intensity and relations with largescale atmospheric circulation. Water Resour. Res. 51, 3459–3475. doi: 10.1002/2014wr016273
Espinoza, J. C., Marengo, J. A., Ronchail, J., Molina, J., Noriega, L., and Guyot, J. L. (2014). The extreme 2014 flood in south-western Amazon basin: the role of tropical-subtropical south Atlantic SST gradient. Environm. Res. Lett. 9:124007. doi: 10.1088/1748-9326/9/12/124007
Espinoza, J. C., Guyot, J. L., Ronchail, J., Cochonneau, G., Filizola, N., Fraizy, P., et al. (2009a). Contrasting regional discharge evolutions in the Amazon basin (1974-2004). J. Hydrol. 375, 297–311. doi: 10.1016/j.jhydrol.2009.03.004
Espinoza, J. C., Ronchail, J., Guyot, J. L., Cochonneau, G., Naziano, F., Lavado, W., et al. (2009b). Spatio-temporal rainfall variability in the Amazon basin countries (Brazil, Peru, Bolivia, Colombia, and Ecuador). Int. J. Climatol. 29, 1574–1594. doi: 10.1002/joc.1791
Espinoza, J. C., Ronchail, J., Marengo, J. A., and Segura, H. (2018). Contrasting North–South changes in Amazon wet-day and dry-day frequency and related atmospheric features (1981–2017). Clim. Dyn. 116, 1–18. doi: 10.1007/s00382-018-4462-2
Espinoza, J. C., Sörensson, A., Ronchail, J., Molina-Carpio, J., Segura, H., Gutierrez-Cori, O., et al. (2019). Regional hydro-climatic changes in the Southern Amazon Basin (Upper Madeira Basin) during the 1982–2017 period. J. Hydrol. Reg. Stud. 26:10063. doi: 10.1016/j.ejrh.2019.100637
Espinoza-Villar, R., Martinez, J. M., Armijos, E., Espinoza, J. C., Filizola, N., Dos Santos, A., et al. (2018). Spatio-temporal monitoring of suspendent sediment in Solimões River (2000–2014). C. R. Geosci. 350, 4–12. doi: 10.1016/j.crte.2017.05.001
Falvey, M., and Garreaud, R. D. (2005). Moisture variability over the South American Altiplano during the South American low level jetexperiment (SALLJEX) observing season. J. Geophy. Res. 110:D22105. doi: 10.1029/2005JD006152
Falvey, M., and Garreaud, R. (2007). Wintertime precipitation episodes in central Chile: associated meteorological conditions and orographic influences. J. Hydrometeorol. 8, 171–193. doi: 10.1175/JHM562.1
Figueroa, S. N., and Nobre, C. A. (1990). Precipitation distribution over central and western tropical South America. Climanalise 6, 36–40.
Figueroa, S. N., Satyamurty, P., and Da Silva Dias, P. L. (1995). Simulations of the summer circulation over the South American region with an Eta coordinate model. J. Atmos. Sci. 52, 1573–1584. doi: 10.1175/1520-0469(1995)052<1573:sotsco>2.0.co;2
Gan, M. A., Kousky, V. E., and Ropelewski, C. F. (2004). The South America monsoon circulation and its relationship to rainfall over west-central Brazil. J. Clim. 17, 47–66. doi: 10.1175/1520-0442(2004)017<0047:tsamca>2.0.co;2
Garreaud, R., Molina, A., and Farias, M. (2010). Andean uplift and Atacama Hyperaridity: a climate modeling perspective. Earth Planet. Sci. Lett. 292, 39–50. doi: 10.1016/j.epsl.2010.01.017
Garreaud, R., and Muñoz, R. (2005). The low-level jet off the subtropical west coast of South America: structure and variability. Mon. Weather Rev. 133, 2246–2261. doi: 10.1175/mwr2972.1
Garreaud, R., Vuille, M., and Clement, A. (2003). The climate of the Altiplano: observed current conditions and mechanisms of past changes. Palaeogeogr. Palaeoclimatol. Palaeoecol. 194, 5–22. doi: 10.1016/s0031-0182(03)00269-4
Garreaud, R., and Wallace, J. M. (1998). Summertime incursions of midlatitude air into subtropical and tropical South America. Mon. Weather Rev. 126, 2713–2733. doi: 10.1175/1520-0493(1998)126<2713:siomai>2.0.co;2
Garreaud, R. D. (1999). Multiscale analysis of the summertime precipitation over the Central Andes. Mon. Weather Rev. 127, 901–921. doi: 10.1175/1520-0493(1999)127<0901:maotsp>2.0.co;2
Garreaud, R. D. (2009). The Andes climate and weather. Adv. Geosci. 22, 3–11. doi: 10.5194/adgeo-22-3-2009
Garreaud, R. D. (2018). A plausible atmospheric trigger for the 2017 coastal El Niño. Int. J. Climatol. 38, 1296–1302.
Garreaud, R. D., and Aceituno, P. (2007). “Atmospheric circulation over South America: mean features and variability,” in The Physical Geography of South America, eds T. Veblen, K. Young, and A. Orme (Oxford: Oxford University Press), 45–66.
Garreaud, R. D., Vuille, M., Compagnucci, R., and Marengo, J. (2009). Present-day South American climate. Palaeogeogr. Palaeoclimatol. Palaeoecol. 281, 180–195. doi: 10.1016/j.palaeo.2007.10.032
Gelaro, R., McCarty, W., Suárez, M. J., Todling, R., Molod, A., and Takacs, L. (2017). MERRA-2 overview: the Modern-Era retrospective analysis for research and applications, version 2 (MERRA-2). J. Clim. 30, 5419–5454. doi: 10.1175/JCLI-D-16-0758.1
Giovannettone, J. P., and Barros, A. P. (2009). Probing regional orographic controls of precipitation and cloudiness in the central Andes using satellite data. J. Hydrometeorol. 10, 167–182. doi: 10.1175/2008jhm973.1
Giraldo-Cárdenas, S., Arias, P. A., and Vieira, S. C. (2017). “The African Easterly Waves over Northern South America,” in Proceedings of the 2nd International Electronic Conference on Atmospheric Sciences, Vol. 1, Basel, 165. doi: 10.3390/ecas2017-04151
Gloor, M., Brienen, R. J. W., Galbraithm, D., Feldpauschm, T. R., Schöngartm, J., Guyotm, J. L., et al. (2013). Intensification of the Amazon hydrological cycle over the last two decades. Geophys. Res. Lett. 40, 1–5. doi: 10.1002/grl.50377
Gutman, G., and Schwerdtfeger, W. (1965). The role of latent and sensible heat for the development of a high pressure system over the subtropical Andes in the summer. Meteorol. Rund. 18, 69–75.
Guyot, J.-L., Bazan, H., Fraizy, P., Ordonez, J. J., Armijos, E., and Laraque, A. (2007). “Suspended sediment yields in the Amazon basin of Peru: a first estimation,” in Water Quality and Sediment Behaviour of the Future: Predictions for the 21st Century, eds B. W. Webb and D. De Boer (Wallingford: AISH), 3–10.
Hastenrath, S. (2002). The Intertropical Convergence Zone of the eastern Pacific revisited. Int. J. Climatol. 22, 347–356. doi: 10.1002/joc.739
Hoorn, C., Wesseling, P., ter Steege, H., Bermudez, M. A., Mora, A., Sevink, J., et al. (2010). Amazonia through time: andean uplift, climate change, landscape evolution, and biodiversity. Science 330, 927–931. doi: 10.1126/science.1194585
Hoskins, B. J., and Hodges, K. I. (2005). A new perspective on Southern Hemisphere storm tracks. J. Clim. 18, 4108–4129. doi: 10.1175/jcli3570.1
Hoyos, F. I, Dominguez, J., Cañón-Barriga, J. A., Martínez, R., Nieto, L., and Gimeno et al. (2017). Moisture origin and transport processes in Colombia, northern South America. Clim. Dyn. 50, 971–990. doi: 10.1007/s00382-017-3653-6
Inatsu, M., and Hoskins, B. J. (2004). The zonal asymmetry of the Southern Hemisphere winter storm track. J. Clim. 17, 4882–4891.
Insel, N., Poulsen, C. J., and Ehlers, T. A. (2010). Influence of the Andes mountains on South American moisture transport, convection, and precipitation. Clim. Dyn. 35, 1477–1492. doi: 10.1007/s00382-009-0637-1
Jaramillo, L., Poveda, G., and Mejía, J. F. (2017). Mesoscale convective systems and other precipitation features over the tropical Americas and surrounding seas as seen by TRMM. Intern. J. Climatol 37, 380–397. doi: 10.1002/joc.5009
Jiang, Q., Doyle, J. D., Reinecke, A., Smith, R. B., and Eckermann, S. D. (2013). A modeling study of stratospheric waves over the Southern Andes and Drake Passage. J. Atmos. Sci. 70, 1668–1689. doi: 10.1175/JAS-D-12-0180.1
Jiménez-Sánchez, G., Markowski, P. M., Jewtoukoff, V., Young, G. S., and Stensrud, D. J. (2019). The Orinoco low-level jet: an investigation of its characteristics and evolution using the WRF model. J. Geophys. Res. Atmos. 124, 10696–10711. doi: 10.1029/2019JD030934
Johnson, A. M. (1976). “The climate of Peru, Bolivia and Ecuador,” in Climates of Central and South America, World Survey of Climatology, Vol. 12, ed. W. Schwerdtfeger (Amsterdam: Elsevier), 147–218.
Jones, C. (2019). Recent changes in the South America low-level jet. npj Clim. Atmos. Sci. 2, 1–8. doi: 10.1038/s41612-019-0077-5
Junquas, C., Takahashi, K., Condom, T., Espinoza, J. C., Chavez, S., Sicart, J. E., et al. (2018). Understanding the influence of orography over the precipitation diurnal cycle and the associated atmospheric processes in the central Andes. Clim. Dyn. 50, 3995–4017. doi: 10.1007/s00382-017-3858-8
Killeen, T. J., Douglas, M., Consiglio, T., Jorgensen, P. M., and Mejía, J. (2007). Dry spots and wet spots in the Andean hotspot. J. Biogeogr. 34, 1357–1373. doi: 10.1111/j.1365-2699.2006.01682.x
King, M. J., Wheeler, M. C., and Lane, T. P. (2017). Mechanisms linking global 5-day waves to tropical convection. J. Atmos. Sci. 74:36793702. doi: 10.1175/JASD-17-0101.1
Kumar, S., Vidal, Y.-S., Moya-Álvarez, A. S., and Martínez-Castro, D. (2019). Effect of the surface wind flow and topography on precipitating cloud systems over the Andes and associated Amazon basin: GPM observations. Atmos. Res. 225, 193–208. doi: 10.1016/j.atmosres.2019.03.027
Laraque, A., Ronchail, J., Cochonneau, G., Pombosa, R., and Guyot, J. L. (2007). Heterogeneous distribution of rainfall and discharge regimes in the Ecuadorian Amazon basin. J. Hydrometeorol. 8, 1364–1381. doi: 10.1175/2007jhm784.1
Lavado-Casimiro, W., Labat, D., Ronchail, J., Espinoza, J. C., and Guyot, J. L. (2013). Trends in rainfall and temperature in the Peruvian Amazon-Andes basin over the last 40 years (1965-2007). Hydrol. Processes 41, 2944–2957. doi: 10.1002/hyp.9418
Leite-Filho, A. T., de Sousa Pontes, V. Y., and Costa, M. H. (2019). Effects of deforestation on the onset of the rainy season and the duration of dry spells in southern Amazonia. J. Geophys. Res. Atmos. 124, 5268–5281. doi: 10.1029/2018jd029537
Lenters, J.-D., and Cook, K.-H. (1997). On the origin of the Bolivian high and related circulation features of the South American climate. J. Atmos. Sci. 54, 656–677.
Liu, C., and Zipser, E. J. (2015). The global distribution of largest, deepest, and most intense precipitation systems. Geophys. Res. Lett. 42, 3591–3595. doi: 10.1002/2015gl063776
López, M. E., and Howell, W. E. (1967). Katabatic winds in the Equatorial Andes. J. Atmos. Sci. 24, 29–35. doi: 10.1175/1520-0469(1967)024<0029:kwitea>2.0.co;2
Lowman, E. L., and Barros, A. P. (2014). Investigating links between climate and orography in the central Andes: coupling erosion and precipitation using a physical-statistical model. JGR Earth Surf. 119, 1322–1353. doi: 10.1002/2013JF002940
Magrín, G. O., Marengo, J. A., Boulanger, J.-P., Buckeridge, M. S., Castellanos, E., Poveda, G., et al. (2014). “Central and South America,” in Climate Change 2014: Impacts, Adaptation, and Vulnerability. Part B: Regional Aspects. Contribution of Working Group II to the Fifth Assessment Report of the Intergovernmental Panel on Climate Change, eds V. R. Barros, C. B. Field, D. J. Dokken, M. D. Mastrandrea, K. J. Mach, T. E. Bilir, et al. (Cambridge: Cambridge University Press), 1499–1566.
Mapes, B. E., Warner, T. T., Xu, M., and Negri, A. J. (2003). Diurnal patterns of rainfall in northwestern South America. Part I: Observations and context. Mon. Weather Rev. 131, 799–812. doi: 10.1175/1520-0493(2003)131<0799:dporin>2.0.co;2
Marengo, J. A., and Espinoza, J. C. (2016). Extreme seasonal droughts and floods in Amazonia: causes, trends and impacts. Int. J. Climatol. 36, 1033–1050. doi: 10.1002/joc.4420
Marengo, J. A., Liebmann, B., Grimm, A. M., Misra, V., Silva Dias, P. L. D., Cavalcanti, I. F. A., et al. (2012). Recent developments on the South American monsoon system. Int. J. Climatol. 32, 1–21.
Marengo, J. A., Soares, W. R., Saulo, C., and Nicolini, M. (2004). Climatology of the low-level jet east of the Andes as derived from NCEP-NCAR reanalyses: characteristics and temporal variability. J. Clim. 17, 2261–2280. doi: 10.1175/1520-0442(2004)017<2261:cotlje>2.0.co;2
Martinez, J. A., and Dominguez, F. (2014). Sources of Atmospheric Moisture for the La Plata River Basin. J. Clim. 27, 6737–6753. doi: 10.1175/JCLI-D-14-00022.1
McClain, M. E., and Naiman, R. J. (2008). Andean influences on the biogeochemistry and ecology of the Amazon river. Bioscience 58, 325–338. doi: 10.1641/b580408
Meisner, B. N., and Arkin, P. A. (1987). Spatial and annual variations in the diurnal cycle of large scale tropical convective cloudiness and precipitation. Mon. Weather Rev. 115, 2009–2030.
Mejia, J. F., Mesa, O., Poveda, G., and Velez, J. I. (1999). Distribución espacial y ciclos anual y semianual de la precipitación en Colombia. Dyna 127, 7–14.
Mejia, J. F., and Poveda, G. (2005). Atmospheric environments of mesoscale convective systems over Colombia during 1999 using TRMM radar and microwave products and NCEP/NCAR reanalysis [in Spanish]. Rev. Acad. Colomb. Cienc. 29:495514.
Molina, R., Salazar, J. F., Martínez, J. A., Villegas, J. C., and Arias, P. A. (2019). Exponential growth of precipitation along a “forest-fed moisture conveyor belt” above the Amazon. J. Geophys. Res. Atmos. 124, 2589–2599. doi: 10.1029/2018jd029534
Molina-Carpio, J., Espinoza, J. C., Coritza, E., Salcedo, F., Farfán, C., Mamani, L., et al. (2019). Climate and spatial variability of the humid upper Andes. Ecol. Boliv. 54, 40–56.
Molina-Carpio, J., Espinoza, J. C., Vauchel, P., Ronchail, J., Gutierrez, B., Guyot, J. L., et al. (2017). The hydroclimatology of the upper Madeira River basin: spatio-temporal variability and trends (1967-2013). Hydrol. Sci. J. 62, 911–927. doi: 10.1080/02626667.2016.1267861
Montini, T. L., Jones, C., and Carvalho, L. M. V. (2019). The South American Low-Level Jet: a new climatology, variability, and changes. J. Geophys. Res. Atmos. 124, 1200–1218. doi: 10.1029/2018jd029634
Montoya, G., Pelkowski, J., and Eslava, J. A. (2001). On the northeasterlies trade winds and the existence of a low-level jet along the eastern Andes piedemont [In Spanish]. Rev. Acad. Colomb. Cienc. 96, 363–370.
Moquet, J.-S., Crave, A., Viers, J., Selyer, P., Armijos, J., and Bourrel, L. (2011). Chemical weathering and atmospheric/soil CO2 uptake in the Andean and Foreland Amazon basins. Chem. Geol. 287, 1–26. doi: 10.1016/j.chemgeo.2011.01.005
Moraes-Arraut, J., Nobre, C. A., Barbosa, H. M., Obregon, G., and Marengo, J. A. (2012). Aerial rivers and lakes: looking at large-scale moisture transport and its relation to Amazonia and to subtropical rainfall in South America. J. Clim 25, 543–556. doi: 10.1175/2011JCLI4189.1
Muñoz, R., and Garreaud, R. (2005). Dynamics of the low-level jet off the subtropical west coast of South America. Mon. Weather Rev. 133, 3661–3677. doi: 10.1175/mwr3074.1
Myers, N., Mittermeier, R. A., Mittermeier, C. G., da Fonseca, C. A. B., and Kent, J. (2000). Biodiversity hotspots for conservation priorities. Nature 403, 853–858. doi: 10.1038/35002501
Nobre, C. A., Sampaio, G., Borma, L. S., Castilla-rubio, J. C., Silva, J. S., and Cardoso, M. (2016). Land-use and climate change risks in the Amazon and the need of a novel sustainable development Paradigm. Proc. Natl. Acad. Sci. U.S.A. 113, 10759–10768. doi: 10.1073/pnas.1605516113
Nogués-Paegle, J., and Mo, K. (1997). Alternating Wet and Dry Conditions over South America during Summer. Mon. Weather Rev. 125, 279–291. doi: 10.1175/1520-0493(1997)125<0279:awadco>2.0.co;2
Ovando, A., Tomasella, J., Rodriguez, D. A., Martinez, J. M., Siqueira-Junior, J. L., Pinto, G. L. N., et al. (2016). Extreme flood events in the Bolivian Amazon wetlands. J. Hydrol. Region. Stud. 5, 293–308. doi: 10.1016/j.ejrh.2015.11.004
Pepin, E., Guyot, J.-L., Armijos, E., Bazan, H., Fraisy, P., Moquet, J. S., et al. (2013). Climatic control on eastern Andean denudation rates (Central cordillera from Ecuador to Bolivia). J. South Am. Earth Sci. 44, 85–93. doi: 10.1016/j.jsames.2012.12.010
Poveda, G., Jaramillo, L., and Vallejo, L. F. (2014). Seasonal precipitation patterns along pathways of South American low-level jets and aerial rivers. Water Resour. Res. 50, 98–118. doi: 10.1002/2013WR014087
Poveda, G., and Mesa, O. J. (1999). The CHOCO low-level jet and two others jets over Colombia: climatology and variability during ENSO [in Spanish]. Rev. Acad. Colomb. Cienc. 23, 517–528.
Poveda, G., and Mesa, O. J. (2000). On the existence of Lloró (the rainiest locality on Earth): enhanced ocean-atmosphere-land interaction by a low-level jet. Geophys. Res. Lett. 27, 1675–1678. doi: 10.1029/1999gl006091
Poveda, G., Mesa, O. J., Agudelo, P. A., Álvarez, J. F., Arias, P. A., Moreno, H. A., et al. (2002a). “Diagnóstico del ciclo diurno de precipitación en los Andes tropicales de Colombia,” in Proceedings of the Memorias XX Congreso Latinoamericano de Hidráulica, ISBN 959-7160-17-X, Ciudad de La Habana.
Poveda, G., Mesa, O. J., Agudelo, P. A., Álvarez, J. F., Arias, P. A., Moreno, H. A., et al. (2002b). “Influencia del ENSO, oscilación Madden-Julian, ondas del este, huracanes y fases de la luna en el ciclo diurno de la precipitación en los Andes tropicales de Colombia,” in Proceedings of the Memorias XX Congreso Latinoamericano de Hidráulica, ISBN 959-7160-17-X, Ciudad de La Habana.
Poveda, G., Mesa, O. J., Salazar, L. F., Arias, P. A., Moreno, H. A., Vieira, S. C., et al. (2005). Diurnal cycle of precipitation in the tropical Andes of Colombia. Mon. Weather Rev. 133, 228–240. doi: 10.1175/mwr-2853.1
Poveda, G., Mesa, O. J., Toro, V. G., Agudelo, P. A., Álvarez, J. F., Arias, P. A., et al. (2002c). “Diagnóstico del ciclo anual y efectos del ENSO sobre la intensidad máxima de lluvias de duración entre 1 y 24 horas en los Andes de Colombia,” in Proceedings of the Memorias XX Congreso Latinoamericano de Hidráulica, ISBN 959-7160-17-X, Ciudad de La Habana.
Poveda, G., Moreno, H. A., Vieira, S. C., Agudelo, P., Arias, P. A., Salazar, L. F., et al. (2001). “Caracterización del ciclo diurno de la precipitación en los Andes tropicales de Colombia,” in Proceedings of the Memorias IX Congreso Latinoamericano e Ibérico de Meteorología, Buenos Aires, 7–11.
Poveda, G., Waylen, P. R., and Pulwarty, R. (2006). Modern climate variability in northern South America and southern Mesoamerica. Palaeogeogr. Palaeoclimatol. Palaeoecol. 234, 3–27. doi: 10.1016/j.palaeo.2005.10.031
Rabatel, A., Francou, B., Soruco, A., Gomez, J., Cáceres, B., Ceballos, J. L., et al. (2013). Current state of glaciers in the tropical Andes: a multi-century perspective on glacier evolution and climate change. Cryosphere 7, 81–102. doi: 10.5194/tc-7-81-2013
Rangel, T. F., Edwards, N. R., Holden, P. B., Diniz-Filho, J. A. F., Gosling, W. D., and Coelho, M. T. P. (2018). Modeling the ecology and evolution of biodiversity: Biogeographical cradles, museums, and graves. Science 361:eaar5452. doi: 10.1126/science.aar5452
Rasmussen, K. L., Chaplin, M. M., Zuluaga, M. D., and Houze, R. A. (2016). Contribution of extreme convective storms to rainfall in South America. J. Hydrometeorol. 17, 353–367. doi: 10.1175/jhm-d-15-0067.1
Rasmussen, K. L., Zuluaga, M. D., and Houze, R. A. (2014). Severe convection and lightning in subtropical South America. Geophys. Res. Lett. 41, 7359–7366. doi: 10.1002/2014gl061767
Ricaurte, L. F., Patiño, J. E., and Junk, W. J. (2019). A classification system for Colombian wetlands: an essential step forward in open environmental policy-making. Wetlands 39, 971–990. doi: 10.1007/s13157-019-01149-8
Roche, M. A., and Fernandez Jáuregui, C. (1988). Water resources, salinity and salt yields of the rivers of the Bolivian Amazon. J. Hydrol. 101, 305–331. doi: 10.1016/0022-1694(88)90042-x
Rodriguez, D. A., Tomasella, J., and Linhares, C. (2018). Is the forest conversion to pasture affecting the hydrological response of Amazonian catchments? Signals in the Ji-Paraná Basin. Hydrol. Processes 24, 1254–1269. doi: 10.1002/hyp.7586
Rodwell, M. J., and Hoskins, B. J. (2001). Subtropical anticyclones and summer monsoons. J. Clim. 14, 3192–3211. doi: 10.1038/srep21346
Rollenbeck, R., and Bendix, J. (2011). Rainfall distribution in the Andes of southern Ecuador derived from blending weather radar data and meteorological field observations. Atmos. Res. 99, 277–289. doi: 10.1016/j.atmosres.2010.10.018
Romatschke, U., and Houze, R. A. (2013). Characteristics of precipitating convective systems accounting for the summer rainfall of tropical and subtropical South America. J. Hydrometeorol. 14, 25–46. doi: 10.1175/jhm-d-12-060.1
Ronchail, J., and Gallaire, R. (2006). ENSO and rainfall along the Zongo Valley (Bolivia) from the Altiplano to the Amazon Basin. Int. J. Climatol. 26, 1223–1236. doi: 10.1002/joc.1296
Rueda, D. C. (2014). Caracterización de la corriente en chorro de bajo nivel de los llanos orientales colombianos. Master’s thesis, Universidad Nacional de Colombia, Bogotá.
Rueda, O. A., and Poveda, G. (2006). Variabilidad espacial y temporal del Chorro del Chocó y su efecto en la hidroclimatología del Pacífico Colombiano. Meteorol. Colomb. 10, 132–145.
Rutllant, J., and Fuenzalida, H. (1991). Synoptic aspects of the central Chile rainfall variability associated with the Southern Oscillation. Int. J. Climatol 11, 63–76. doi: 10.1002/joc.3370110105
Rutllant, J. A., and Garreaud, R. D. (2004). Episodes of strong flow down the western slope of the subtropical Andes. Mon. Weather Rev. 132, 611. doi: 10.1175/1520-0493(2004)132<0611:eosfdt>2.0.co;2
Saavedra, M., Junquas, C., Espinoza, J. C., and Silva, Y. (2020). Impacts of topography and land use change on the air surface temperature and precipitation over the central Peruvian Andes. Atmos. Res. 234:104711.
Saavedra, N., and Foppiano, A. J. (1992). Monthly mean pressure model for Chile. Int. J. Climatol. 12, 469–480. doi: 10.2196/10226
Sagredo, E., and Lowell, T. (2012). Climatology of Andean glaciers: a framework to understand glacier response to climate change. Glob. Planet. Chang. 86–87, 101–109. doi: 10.1016/j.gloplacha.2012.02.010
Sakamoto, M., Ambrizzi, T., and Poveda, G. (2011). Moisture sources and life cycle of convective systems over western Colombia. Adv. Meteorol. 2011:890759. doi: 10.1155/2011/890759
Salio, P., Nicolini, M., and Zipser, E. J. (2007). Mesoscale convective systems over southeastern South America and their relationship with the South American low-level jet. Mon. Weather Rev. 135, 1290–1309. doi: 10.1175/mwr3305.1
Santini, W., Martinez, J. M., Espinoza Villar, R., Cochonneau, G., Vauchel, P., Moquet, J. S., et al. (2014). “Sediment budget in the Ucayali River basin, an Andean tributary of the Amazon River,” in Sediment Dynamics from the Summit to the Sea, ed. Y. J. Xu (Wallingford: AISH), 320–325. doi: 10.5194/piahs-367-320-2015
Schwerdtfeger, W. C. (1961). Stromings und Temperatufeld der freien Atmosphare uber den Andes. Meteorol. Rund. 14, 1–6.
Segura, H., Espinoza, J. C., Junquas, C., Lebel, T., Vuille, M., and Garreaud, R. (2020). Recent changes in the precipitation-driving processes over the southern tropical Andes/western Amazon. Clim. Dyn. doi: 10.1007/s00382-020-05132-6
Segura, H., Espinoza, J. C., Junquas, C., and Takahashi, K. (2016). Evidencing Decadal and Interdecadal Hydroclimatic Variability over the Central Andes. Environ. Res. Lett. 11:094016. doi: 10.1088/1748-9326/11/9/094016
Segura, H., Junquas, C., Espinoza, J. C., Vuille, M., Jauregui, Y. R., Rabatel, A., et al. (2019). New insights into the rainfall variability in the tropical Andes on seasonal and interannual time scales. Clim. Dyn. 53, 405–426. doi: 10.1007/s00382-018-4590-8
Seluchi, M. E., Saulo, A. C., NicoLini, M., and Satyamurthy, P. (2003). The northwestern Argentinean low: A study of two typical events. Mon. Weather Rev. 131, 2361–2378. doi: 10.1175/1520-0493(2003)131<2361:tnalas>2.0.co;2
Seneviratne, S. I., and Stephens, G. (2014). “7th International Scientific Conference on the Global Water and Energy Cycle,” in Proceedings of the Highlights of the 7th International GEWEX Conference and Pan-GEWEX Meeting 3.
Sierra, J. P., Arias, P. A., and Vieira, S. C. (2015). Precipitation over Northern South America and Its Seasonal Variability as Simulated by the CMIP5 Models. Adv. Meteorol. 2015:634720.
Sierra, J. P., Arias, P. A., Vieira, S. C., and Agudelo, J. (2018). How well do CMIP5 models simulate the low-level jet in western Colombia? Clim. Dyn. 51, 2247–2265. doi: 10.1007/s00382-017-4010-5
Snow, J. W. (1976). “The climate of northern South America,” in Climates of Central and South America, ed. W. Schwerdtfeger (Amsterdam: Elsevier), 295–403.
Sorí, R., Marengo, J., Nieto, R., Drumond, A., and Gimeno, L. (2017). “Drought and wet episodes in Amazonia: the role of atmospheric moisture transport,” in Proceedings of the 1st International Electronic Conference on Hydrological Cycle (CHyCle-2017), Basel.
Staal, A., Tuinenburg, O., Bosmans, J., and Dekker, S. C. (2018). Forest-rainfall cascades buffer against drought across the Amazon. Nat. Clim. Chang. 8, 539–543. doi: 10.1038/s41558-018-0177-y
Takahashi, K. (2012). Thermotidal and land-heating forcing of the diurnal cycle of oceanic surface winds in the eastern tropical Pacific. Geophys. Res. Lett. 39:L15809. doi: 10.1029/2011GL050692
Takahashi, K., and Battisti, D. (2007). Processes controlling the mean Tropical Pacific precipitation pattern. Part II: the SPCZ and the Southeast Pacific Dry Zone. J. Clim. 20, 5696–5706. doi: 10.1175/2007jcli1656.1
Torrealba, E. R., and Amador, J. A. (2010). La corriente en chorro de bajo nivel sobre los Llanos Venezolanos de Sur America. Rev. Climatol. 10, 1–20.
Varble, A., Steve, N., Paola, S., Edward, Z., Susan, V. D. H., and Greg, M. (2017). Cloud, Aerosol and Complex Terrain Interactions (CACTI) Preliminary Science Plan. Washington, DC: United States Department of Energy.
Vauchel, P., Santini, W., Guyot, J. L., Moquet, J. S., Martinez, J. M., and Espinoza, J. C. (2017). A reassessment of the suspended sediment load in the Madeira River Basin from the Andes of Peru and Bolivia to the Amazon River in Brazil, based on 10 years of data from the HYBAM monitoring programme. J. Hydrol. 553, 35–48. doi: 10.1016/j.jhydrol.2017.07.018
Velasco, I., and Fritsch, J. M. (1987). Mesoscale convective complexes in the Americas. J. Geophys. Res. 92, 9591–9613. doi: 10.1002/9781118782071.ch7
Vera, C., Higgins, W., Amador, J., Ambrizzi, T., Garreaud, R., and Gochis, D. (2006). Toward a unified view of the American monsoon systems. J. Clim. 19, 4977–5000. doi: 10.1175/jcli3896.1
Vera, C. S., and Vigliarolo, P. K. (2000). A diagnostic study of cold-air outbreaks over South America. Mon. Weather Rev. 128, 3–24. doi: 10.1175/1520-0493(2000)128<0003:ADSOCA>2.0.CO;2
Viale, M., Bianchi, E., Cara, L., Ruiz, L. E., Villalba, R., and Pitte, P. (2019). Contrasting Climates at Both Sides of the Andes in Argentina and Chile. Front. Environ. Sci. 7:69. doi: 10.3389/fenvs.2019.00069
Viale, M., and Garreaud, R. (2015). Orographic effects of the subtropical and extratropical Andes on upwind precipitating clouds. J. Geophys. Res. Atmos. 120, 4962–4974. doi: 10.1002/2014jd023014
Viale, M., and Garreaud, R. D. (2014). Summer precipitation events over the western slopes of the subtropical Andes. Mon. Weather Rev. 142, 1074–1092. doi: 10.1175/mwr-d-13-00259.1
Viale, M., Valenzuela, R., Garreaud, R., and Ralph, F. M. (2018). Impacts of Atmospheric Rivers on Precipitation in Southern South America. J. Hydrometeorol. 19, 1671–1687. doi: 10.1111/gcb.14128
Virji, H. (1981). A preliminary study of summertime tropospheric circulation patterns over South America estimated from cloud winds. Mon. Weather Rev. 109, 599–610. doi: 10.1175/1520-0493(1981)109<0599:apsost>2.0.co;2
Vuille, M., Burns, S. J., Taylor, B. L., Cruz, F. W., Bird, B. W., Abbott, M. B., et al. (2012). A review of the South American monsoon history as recorded in stable isotopic proxies over the past two millennia. Clim. Past 8, 1309–1321. doi: 10.5194/cp-8-1309-2012
Vuille, M., Carey, M., Huggel, C., Buytaert, W., Rabatel, A., Jacobsen, D., et al. (2018). Rapid decline of snow and ice in the tropical Andes – impacts, uncertainties and challenges ahead. Earth Rev. 176, 195–213. doi: 10.1016/j.earscirev.2017.09.019
Wang, H., and Fu, R. (2002). Cross-equatorial flow and seasonal cycle of precipitation over South America. J. Clim. 15, 1591–1608. doi: 10.1175/1520-0442(2002)015<1591:cefasc>2.0.co;2
Wang, X.-Y., Li, X., Zhu, J., and Tanajura, C. (2018). The strengthening of Amazonian precipitation during the wet season driven by tropical sea surface temperature forcing. Environ. Res. Lett. 13:094015. doi: 10.1088/1748-9326/aadbb9
Warner, T. T., Mapes, B. E., and Xu, M. (2003). Diurnal patterns of rainfall in northwestern South America. Part II: Model simulations. Mon. Weather Rev. 131, 813–829. doi: 10.1175/1520-0493(2003)131<0813:dporin>2.0.co;2
Wongchuig-Correa, S., de Paiva, R. C. D., Espinoza, J. C., and Collischonn, W. (2017). Multi-decadal hydrological retrospective: case study of Amazon floods and droughts. J. Hydrol. 549, 667–684. doi: 10.1016/j.jhydrol.2017.04.019
Yepes, J., Poveda, G., Mejía, J. F., Moreno, L., and Rueda, C. (2019). CHOCO-JEX. A Research Experiment Focused on the Chocó Low-Level Jet over the Far Eastern Pacific and Western Colombia. Bull. Am. Meteorol. Soc. 100, 779–796. doi: 10.1175/bams-d-18-0045.1
Zemp, D. C., Schleussner, C. F., Barbosa, H. M., Hirota, M., Montade, V., Sampaio, G., et al. (2017). Self-amplified Amazon forest loss due to vegetation-atmosphere feedbacks. Nat. Commun. 8:14681. doi: 10.1038/ncomms14681
Zemp, D. C., Schleussner, C. F., Barbosa, H. M. J., van der Ent, R. J., Donges, J. F., Heink, J., et al. (2014). On the importance of cascading moisture recycling in South America. Atmos. Chem. Phys. 14, 13337–13359. doi: 10.5194/acp-14-13337-2014
Zhou, J., and Lau, K. M. (1998). Does a monsoon climate exist over South America? J. Climate 11, 1020–1040. doi: 10.1371/journal.pone.0199457
Zimmer, A., Meneses, R. I., Rabatel, A., Soruco, A., Dangles, O., and Anthelme, F. (2018). Time lag between glacial retreat and upward migration alters tropical alpine communities. Perspect. Plant Ecol. Evol. Syst. 30, 89–102. doi: 10.1016/j.ppees.2017.05.003
Zipser, E. J., Cecil, D. J., Liu, C., Nesbitt, S. W., and Yorty, D. P. (2006). Where are the most intense thunderstorms on Earth? Bull. Am. Meteorol. Soc. 87, 1057–1071. doi: 10.1038/ncomms12786
Zuluaga, M. D., and Houze, R. A. (2015). Extreme Convection of the Near-Equatorial Americas, Africa, and Adjoining Oceans as seen by TRMM. Mon. Weather Rev. 143, 298–316. doi: 10.1175/mwr-d-14-00109.1
Keywords: Sou nailing, Andes (South America), atmospheric circulation, rainfall variability, hydrological cycle
Citation: Espinoza JC, Garreaud R, Poveda G, Arias PA, Molina-Carpio J, Masiokas M, Viale M and Scaff L (2020) Hydroclimate of the Andes Part I: Main Climatic Features. Front. Earth Sci. 8:64. doi: 10.3389/feart.2020.00064
Received: 16 October 2019; Accepted: 20 February 2020;
Published: 20 March 2020.
Edited by:
Wouter Buytaert, Imperial College London, United KingdomReviewed by:
Patricio Javier Crespo, Universidad de Cuenca, EcuadorCopyright © 2020 Espinoza, Garreaud, Poveda, Arias, Molina-Carpio, Masiokas, Viale and Scaff. This is an open-access article distributed under the terms of the Creative Commons Attribution License (CC BY). The use, distribution or reproduction in other forums is permitted, provided the original author(s) and the copyright owner(s) are credited and that the original publication in this journal is cited, in accordance with accepted academic practice. No use, distribution or reproduction is permitted which does not comply with these terms.
*Correspondence: Jhan Carlo Espinoza, amhhbi1jYXJsby5lc3Bpbm96YUBpcmQuZnI=
Disclaimer: All claims expressed in this article are solely those of the authors and do not necessarily represent those of their affiliated organizations, or those of the publisher, the editors and the reviewers. Any product that may be evaluated in this article or claim that may be made by its manufacturer is not guaranteed or endorsed by the publisher.
Research integrity at Frontiers
Learn more about the work of our research integrity team to safeguard the quality of each article we publish.