- 1Department of Environment, Centre de Recherches Océanologiques, Abidjan, Côte d'Ivoire
- 2Meise Botanic Garden, Meise, Belgium
- 3Ecosystem Management Research Group, Department of Biology, University of Antwerp, Antwerp, Belgium
Lagoons are shallow aquatic environments that typically show a broad variety in colonization by macrophytes. We present the biogenic silica (BSi) data obtained from 11 macrophyte species randomly collected in three small lagoons (Ono, Kodjoboue, and Hebe) of Ivory Coast during 12 consecutive months. BSi concentrations were different between species and between lagoons with average values ranging from 2 to 36 mg g−1. The highest values were found in Hebe and Kodjoboue lagoons due to the dominance of emergent plant species belonging to Poaceae and Cyperaceae families. However, because total plant coverage was low (5% of the lagoon surface), the total BSi stock in vegetation was low (0.2 and 6.1 t, respectively). Oppositely, lower BSi concentrations were found in plants from Ono lagoon, yet the abundance of macrophytes covered 66% of its surface area which resulted in a larger vegetation BSi stock (17.4 t). Dissolved silica in surface water varied seasonally between 1.7 and 10.8 mg L−1, and variation is assumed to be linked to diatom blooms rather than to macrophyte uptake. Sediment data showed that the three lagoons store a large quantity of BSi in their sediments with values ranging from 2 to 8 t BSi ha−1. Because of macrophyte influence in these lagoons, macrophyte phytoliths were expected to contribute significantly to this sediment BSi stock. However, microscopic analysis revealed that this stock is absolutely dominated by diatom frustules and sponge spicules rather than plant phytoliths. We conclude that macrophytes in these lagoons contribute only marginally to BSi storage in sediments but that fragile phytogenic silica structures may affect local silica cycling.
Introduction
Lagoons are transitional water systems between land and ocean, characterized by a shallow depth (<5 m) and occasional water exchanges with the adjacent ocean (Boynton et al., 1996). They are subjected worldwide to increased nutrient inputs due to anthropogenic activities such as land use change, effluent disposal and aquaculture (Caumette et al., 1996). The resulting eutrophication is characterized by enhanced phytoplankton blooms and increased macrophyte biomass (Sidinei et al., 2001). Macrophytes provide food and shelter for aquatic organisms and regulate nutrient and element cycling dynamics within these systems (Rejmankova, 2011). The distribution of different aquatic macrophyte species and growth forms in lagoons is related in various ways to the physical characteristics of the system (depth, bottom slope, type of sediments, wind regime…), to riverine inputs of nutrients, and to the frequency of salt ocean water intrusions (Kjerfve and Magil, 1989; Aloo et al., 2013).
Aquatic macrophytes are also known to store significant amounts of silica (SiO2), thus potentially affecting silica storage, fluxes and turnover rates in freshwater aquatic ecosystems (Schoelynck and Struyf, 2016). Silica in plants is present in an amorphous and hydrated form (SiO2·nH2O), usually referred to as biogenic silica (BSi) and mostly deposited as phytoliths (siliceous plant bodies) or associated with cell wall molecules (Broadley et al., 2012). Studies have demonstrated the significant roles of plant silicification in general (i.e., not only aquatic plants, but also terrestrial vegetation) on the silica cycle. It is well-established that 55–113 Tmol Si yr−1 is fixed globally in the terrestrial biosphere (Avg. 84 ± 29 Tmol Si yr−1; Carey and Fulweiler, 2012). This annual ecosystem biogeochemical uptake exceeds the annual export from continents to the ocean through physicochemical weathering of silicates by two orders of magnitude (Conley, 2002), and thus exerts a strong control on global land-ocean interactions in the silica cycle. Specifically for freshwater aquatic ecosystems, studies have pointed to a significant effect of phytoliths on the silica cycle, and strong potential storage of phytolith-silica in soils and sediments (Cary et al., 2005; Struyf et al., 2007). The carbon occluded in the phytoliths of wetland plant species potentially even forms an important sink to consider in the carbon cycle (Li et al., 2013). Exemplary to this is the strong (permanent) silica sink in the Okavango Delta sediments (Botswana) that can be associated with a dominance of silica-rich tropical giant-grasses such as Cyperus papyrus L. (Struyf et al., 2015; Mosimane et al., 2017; Schoelynck et al., 2017). BSi accumulation in macrophytes, and thus potentially in sediments, varies strongly between species. Aquatic vegetation shows apparent plasticity regarding silica uptake, adaptive to silica availability, water and wind dynamics, light interception, herbivory and nutrient stress (Schoelynck and Struyf, 2016). High silica uptake results in high BSi concentrations in plant litter (Struyf et al., 2005; Carey and Fulweiler, 2014), which can impact on aquatic decomposition processes (Schaller and Struyf, 2013) and thus delays in and/or reductions of the transfer of dissolved silica (DSi) from land to ocean. Besides macrophytes, diatoms (Bacillariophyta, unicellular algae), testate amoebae (polyphyletic assemblage of protozoa with a shell) and sponges (Porifera) also contribute significantly to the BSi storage in wetland ecosystems [see Puppe et al. (2015) for a classification of BSi pools in terrestrial ecosystems]. All diatom species and a large number of the testate amoebae synthesize amorphous siliceous shells (Aoki et al., 2007; Smol and Stoermer, 2010) or have an endoskeleton stiffened by (sponge) spicules (Maldonado et al., 2010).
It has been shown that wetlands, as is the case for many other nutrients, play an important role in the biogeochemical silica cycle (Struyf and Conley, 2009). In temperate and subarctic climates, both riparian (e.g., Struyf et al., 2009, 2010) and tidal wetlands (e.g., Struyf et al., 2006; Müller et al., 2013) play an essential role in Si cycling, but tropical wetlands have not yet received similar attention. Tropical rivers deliver about 70–80% of the global DSi load into the ocean (Beusen et al., 2009), implying it is crucial to assess environmental factors that can influence its transport. Studies have indicated the potential influence of Si uptake by giant grasses and sedges (e.g., Ding et al., 2008; Cardinal et al., 2010) on Si-isotope biogeochemistry in tropical rivers, but did not consider the importance of the wetlands in large scale Si balances.
In this study, we hypothesized that macrophyte phytoliths play an important role in silica storage in three tropical lagoons of Ivory Coast. The observed plant species are typical for eutrophic waters of numerous tropical rivers, streams, reservoirs, and natural lakes. After vegetation senescence, plant BSi (phytoliths) may contribute to the BSi stock in lagoon sediments. We quantified this contribution by counting the relative abundance of plant phytoliths, diatom frustules and sponge spicules using a light microscope. After dissolution, DSi may again be taken up by vegetation or be transported downstream. Therefore, we made conservative estimates of the storage of BSi in the vegetation and sediments and compare it between the different lagoons.
Materials and Methods
Description of the Study Area
Kodjoboue, Ono and Hebe are three small lagoons located in the South-East of Ivory Coast with a joined surface area of about 1,150 ha (Figure 1). These lagoons are mainly fed by ground water seepage and flow into the Comoé River before reaching the Atlantic Ocean through different small channels. The catchment area of Ono lagoon has been dominated by pineapple cultivation since 1960 while the watersheds of Hebe and Kodjoboue lagoons are influenced by palm and coconut crops. Today, the three lagoons behave as freshwater systems due to the absence of seawater input since the opening of the Vridi channel in the near of Abidjan in 1950, that discharges most of the flow. The lagoons became gradually dominated by freshwater macrophytes following the reduced salt water inputs. The development of plants is particularly high in Ono lagoon where it starts hampering water transport and fishery activities. Between 1986 and 1989, only Salvinia molesta D.S.Mitch. and Eichhornia crassipes (Mart.) Solms were reported occasionally along the shores of Ono lagoon (Amon et al., 1991; Guiral and N'da, 1991). Today, Ono lagoon is 66% covered by different macrophyte species while the two other lagoons remain more or less in their original state with (emergent) plants limited to the littoral zones. The climate of the area is sub-equatorial with bimodal character. Two rainy seasons, a long rainy season (April–July) and a short rainy season (October to November), are intercepted by two dry periods: a long dry season (December–March) and a short dry season (August–September). The average annual precipitation is 1,704 mm (referred to the years 1970–2014); average annual temperature is 26.3°C with March as the hottest month (27.8°C) and August as the coolest month (24.3°C) of the year. Humidity is high with average values of 85%.
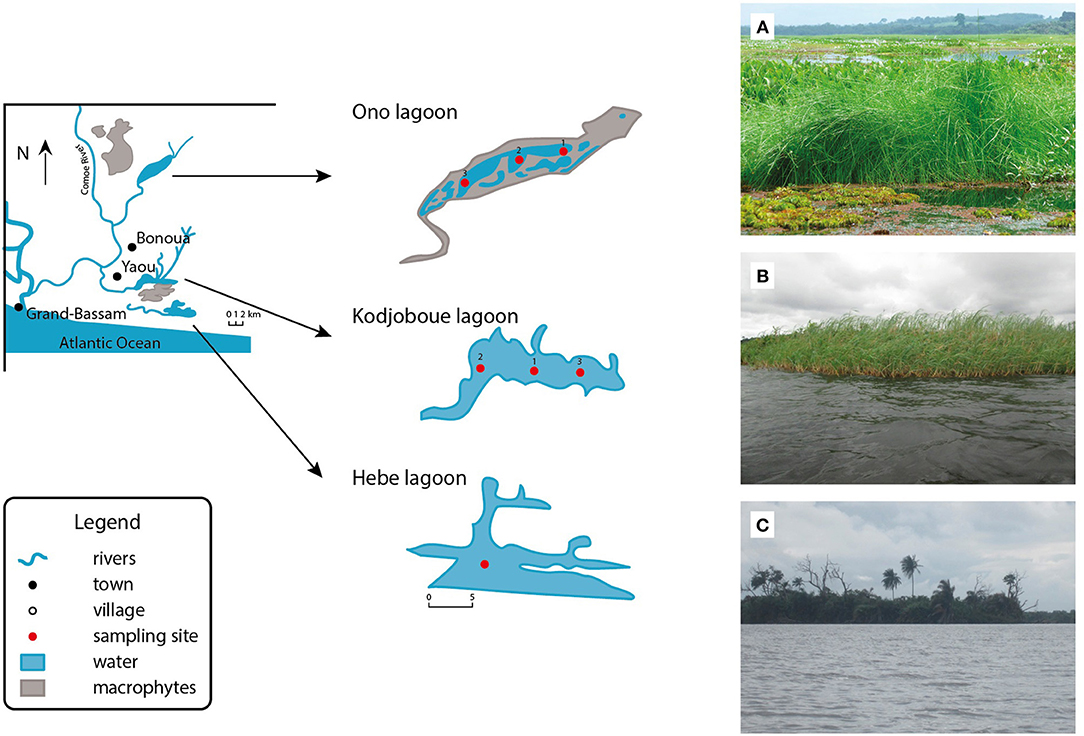
Figure 1. Map showing the location of the different lagoons with a photo impression of their macrophyte cover (A, Ono; B, Kodjoboue; C, Hebe).
Sampling and Analytical Techniques
The following physicochemical parameters were measured in situ with a probe (Ap-5000, AQUAREAD Limited, Broadstairs, UK): depth (m), pH, temperature (°C), and electric conductivity (μS cm−1). Subsurface waters (depth ~30 cm) were sampled with a 1.7 L Niskin bottle. Water samples for DSi analyses were filtered through Sartorius cellulose acetate filters and refiltered through 0.2 μm pore size polysulfone filters. DSi concentrations (mg L−1) were determined with a spectrophotometer (DR 6000) according to standard techniques (Grasshoff et al., 1983). DSi samples were collected only in Ono and Kodjoboue lagoons during eight sampling campaigns at three different stations in each lagoon (Figure 1). For Ono lagoon, station 1 was close to dense Echinochloa pyramidalis (Lam.) Hitchc. and Chase vegetation, station 2 was close to Hydrilla verticillata (L.f.) Royle vegetation, and station 3 was devoid of macrophytes and located in the main channel connecting Ono lagoon to the Comoé River. In Kodjoboue lagoon, station 1 was located in the center of the lagoon, station 2 was further to the west and close to the main channel, and station 3 was to the east. All three stations were devoid of macrophytes because plants are limited to the littoral zones.
In parallel, sediment samples were randomly collected at three occasions (April, July, and September) in Ono and Kodjoboue lagoons (same locations as for water samples) and one time (April) in Hebe lagoon (sampling station devoid of macrophytes because plants are limited to the littoral zones). Sediment samples were taken in the organic rich top layer of the sediment using a sediment corer of 3.3 cm diameter and 15 cm length. Sediment cores were subsampled at three different depths (0–5, 5–10, and 10–15 cm). Sediment samples were dried at 75°C for 5 days and manually homogenized afterwards. Sediment subsamples of 30 mg were mixed with 25 mL of NaOH solution (0.5 M) and incubated in a water bath maintained at 85°C for 5 h. Subsamples taken at 3, 4, and 5 h were filtered through 0.45 μm nitrocellulose Chromafil syringe filters (A-45/25) and analyzed for silica using the spectrophotometric molybdate—blue method (Grasshoff et al., 1983) on a colorimetric segmented flow analyser (SAN++, Skalar, Breda, The Netherlands). Sediment BSi concentration is derived from the intercept of the linear regression between sampling time and measured concentrations, as adapted from DeMaster (1981).
Macrophytes were collected randomly on a monthly base from October 2015 to September 2016 in the three lagoons (12 sampling campaigns). Free floating macrophytes were collected with a net by sweeping a demarcated quadrant (surface area: 0.315 m2). The other plants were cut manually from a fixed area of 1 m2. After collection, macrophytes were thoroughly rinsed to remove sediments, algae and macroinvertebrates, sorted per species and total fresh mass was weighted. A subsample of ±100 g fresh mass of each plant species was oven dried at 75°C for 5 days and weighed again. This conversion factor between fresh and dry mass of the subsample enabled to calculate total macrophyte dry mass per unit surface area. The dried samples were then ground to 300 μm. BSi was extracted from 25–30 mg of dried plant material by incubation in a 0.5 M NaOH solution at 80°C for 5 h (DeMaster, 1981), and analyzed for silica using the spectrophotometric molybdate—blue method (Grasshoff et al., 1983) on a colorimetric segmented flow analyser (SAN++, Skalar, Breda, The Netherlands).
Diatom, Sponge Spicule, and Phytolith Fixation Protocol
The presence/absence of BSi particles (primarily diatom frustules, sponge spicules and phytoliths) was determined in 12 sediment samples and 6 (non-rinsed) dominant plant samples (see Table 1 for species list) across all three lagoons (0–15 cm depth). Samples were prepared for light microscopy following the method described in Van der Werf (1955). Small parts of the sample were cleaned by adding 37% H2O2 and heating to 80°C for about 1 h. The reaction was completed by addition of saturated KMnO4. Following digestion and centrifugation (three times 10 min at 3,700 rpm), cleaned material was diluted with distilled water to avoid excessive concentrations of BSi particles on the object slides. Cleaned material was mounted in Naphrax. The object slides were analyzed using an Olympus BX53 microscope, equipped with Differential Interference Contrast (Nomarski) optics and the Olympus UC30 Imaging System. The variation in BSi particles in both sediment and plant samples was visualized and BSi particles were quantified (up to 100 particles) in sediment samples on random transects at 400x magnification and the relative abundance of each group (diatoms, sponge spicules, and phytoliths) was determined. Diatom species were identified up to genus level using Lange-Bertalot et al. (2017) and phytolith morphotypes were described according to Madella et al. (2005).
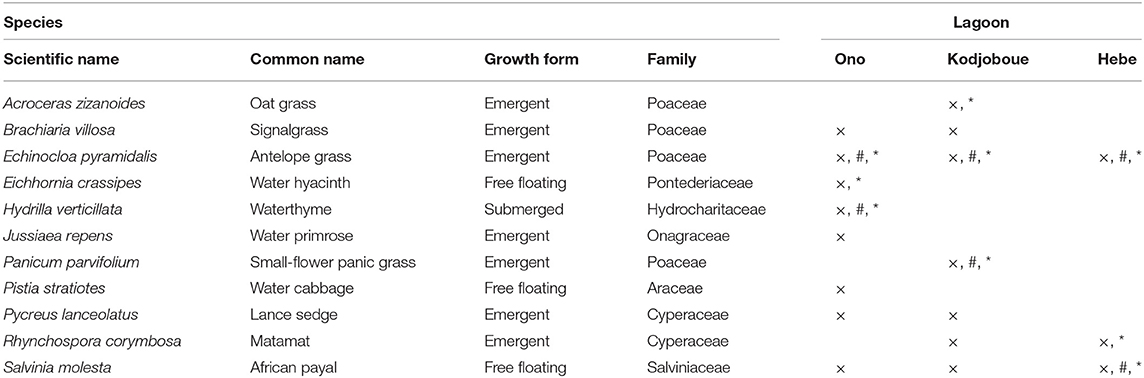
Table 1. Characteristics and occurrence of macrophyte species collected in the three lagoons (×, presence of the species; #, species used for phytolith visualization; *, species' values used in BSi stock calculation).
Stock Calculations
To calculate the BSi stocks in macrophyte biomass, we selected the dominant macrophyte species from each lagoon (see Table 1 for species list) and multiplied the average calculated dry biomass of each plant species (g m−2) with their respective average BSi concentration (mg g−1). These values were converted to t of BSi by multiplying with the respective vegetation coverage to obtain the total stock in vegetation in each lagoon. For Ono lagoon, the area covered by macrophytes was obtained by a satellite image of 2016 (Landsat 8-OLI/TIRS) using ArcGIS. For the two other lagoons, we did not get the images but field observations (Dr. Egnankou from the SOS Forêts, pers. obs.) show that the area covered by macrophytes is small and represents only about 1 and 5% for Hebe and Kodjoboue lagoon, respectively. To calculate the BSi stocks in the sediment, BSi concentrations in sediments were converted to t of BSi by multiplying the average concentrations (mg g−1) by the density of the sediment (g m−3), a depth of 10 cm (ca. rooting depth of macrophytes) and by the total surface area of the respective lagoon.
Statistical Analyses
By means of an Anova One Way test, average values of macrophyte BSi were statistically compared between species within the same lagoon, and average values of water DSi were statistically compared between months within the same lagoon [using Prism 5.00 (GraphPad)]. P-values are not explicitly mentioned hereafter but “significant(ly)” refers to p < 0.05 and “not significant(ly)” refers to p ≥ 0.05. Using linear regression, we did not find relationships between plant BSi, sediment BSi and water DSi, hence these results were excluded from the text.
Results
Water Quality Parameters and DSi Concentrations
In general, pH was low with average values below 7 (Table 2). Conductivity was also low with average values ranging from 13.5 to 36.7 μS cm−1 showing the highest values in Hebe lagoon and the lowest in Kodjoboue lagoon. Both parameters are characteristic for freshwater systems and demonstrate the present-day absence of marine influence. Temperature varied slightly between the three lagoons with average values ranging from 27.5 to 29.9°C. DSi showed a strong temporal variability with a similar pattern in Ono and Kodjoboue lagoons (Figure 2). The highest values were observed between March and April and the lowest in September. When comparing the two lagoons, the highest DSi values were generally observed in Ono lagoon. Overall, DSi concentrations ranged from 1.7 to 10.8 mg L−1.
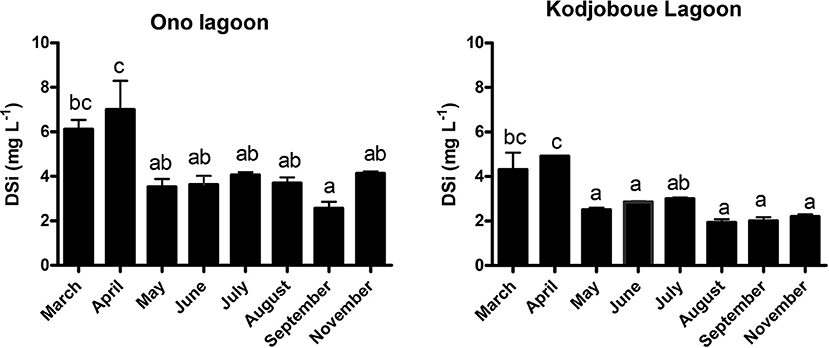
Figure 2. DSi concentrations (mg L−1) in the water column of Ono and Kodjoboue lagoons. Data represent averages of different sample points (n = 3) and error bars indicate standard deviation. Different letters indicate a significant difference (p < 0.05).
Biogenic Silica Accumulation in Macrophytes
Eight plant species were found in Ono lagoon, seven in Kodjoboue lagoon and only three in Hebe lagoon (Table 1). The most dominant species were Echinocloa pyramidalis followed by Hydrilla verticillata and Eichhornia crassipes for Ono lagoon, E. pyramidalis, Panicum parvifolium, and Acroceras zizanoides for Kodjoboue lagoon, and Salvinia molesta, E. pyramidalis, and Rhynchospora corymbosa for Hebe lagoon. All other species were less abundant.
BSi concentrations ranged from 0.8 to 54.9 mg g−1 with the lowest concentration found in E. crassipes in Ono lagoon and the highest found in S. molesta in Kodjoboue lagoon (Figure 3). Generally, free floating species had low BSi concentrations and much lower than submerged and emergent species. Among the emergent species, Brachiaria villosa and A. zizanioides showed the highest values while the lowest were found in Jussiaea repens. BSi concentrations of B. villosa were significantly higher than those of the other plants collected in Ono lagoon. In contrast, the BSi concentrations of A. zizanioides in Kodjoboue lagoon did not differ significantly than those of S. molesta (a free-floating species), P. parvifolium and B. villosa. For the single submerged macrophyte (H. verticillata) found in Ono lagoon, the concentrations were relatively high (5.1–19.8 mg g−1) and similar to those found in emergent species. In Hebe lagoon, BSi concentrations found in R. corymbosa were significantly higher than those obtained in S. molesta and E. pyramidalis.
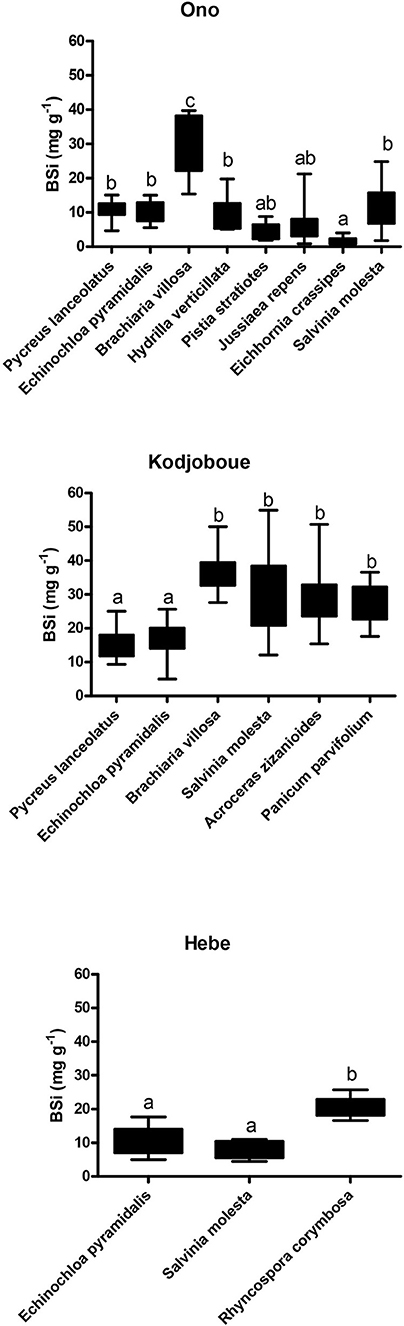
Figure 3. Box-plots showing average BSi concentrations in the different macrophytes for the three lagoons. The vertical bars refer to the minimum/maximum values and the different letters indicate a significant difference (p < 0.05).
Ono lagoon stored less BSi per m2 macrophyte biomass than the two other lagoons. Average values for the three lagoons were 0.054, 0.102, and 0.288 t BSi ha−1 for Ono, Hebe and Kodjoboue, respectively. By extrapolating these values to the total surface covered by macrophytes in each lagoon, the BSi stored in macrophyte biomass was 0.2, 6.1, and 17.4 t for Hebe, Kodjoboue and Ono, respectively.
Biogenic Silica Accumulation in Sediment
The concentrations of BSi generally decreased with depth (Table 3): the highest values were found near the subsurface (0–5 cm) while the lowest occurred at the bottom of the sediment core. The lowest values were observed in July in Ono lagoon. Overall, average BSi concentrations in sediments ranged from 49.6 to 89.4 mg g−1. The BSi stock in the sediments over 10 cm depth was higher in Kodjoboue lagoon (8.2 t BSi ha−1) in comparison to those observed in Hebe (4.2 t BSi ha−1) and Ono (5.5 t BSi ha−1). Extrapolating these values to the total surface of each lagoon, the BSi stock in the sediments was 1,025, 2,651, and 3,477 t for Hebe, Ono and Kodjoboue, respectively.
Characterization of the BSi Particles in Sediment and Plant Samples
All sediment samples were dominated by diatom frustules, mainly belonging to the genera Aulacoseira (>90%), Pinnularia (2–3%), Eunotia (2–3%), and Diadesmis (<1%). In the samples from Kodjoboue lagoon, several sponge spicules were observed (Figure 4a, arrow). Only megascleres were found. Gemmoscleres and microscleres were never observed. In Ono and Hebe lagoons, the amount of spicules was extremely low and spicules were usually not included in the 100 particle-counts. Phytoliths were only very rarely observed (Figure 4b). In the samples from Ono lagoon, no phytoliths were observed, even when scanning an entire object slide. In Hebe lagoon and Kodjoboue lagoon, occasionally, only fragments of phytoliths were recorded after scanning entire object slides but never during counting. The observed phytolith fragments were not identifiable. Intact shells of testate amoebae were not found, but very rarely idiosomes (siliceous platelets synthesized by testate amoebae for shell construction) were observed (Figures 4c,d).
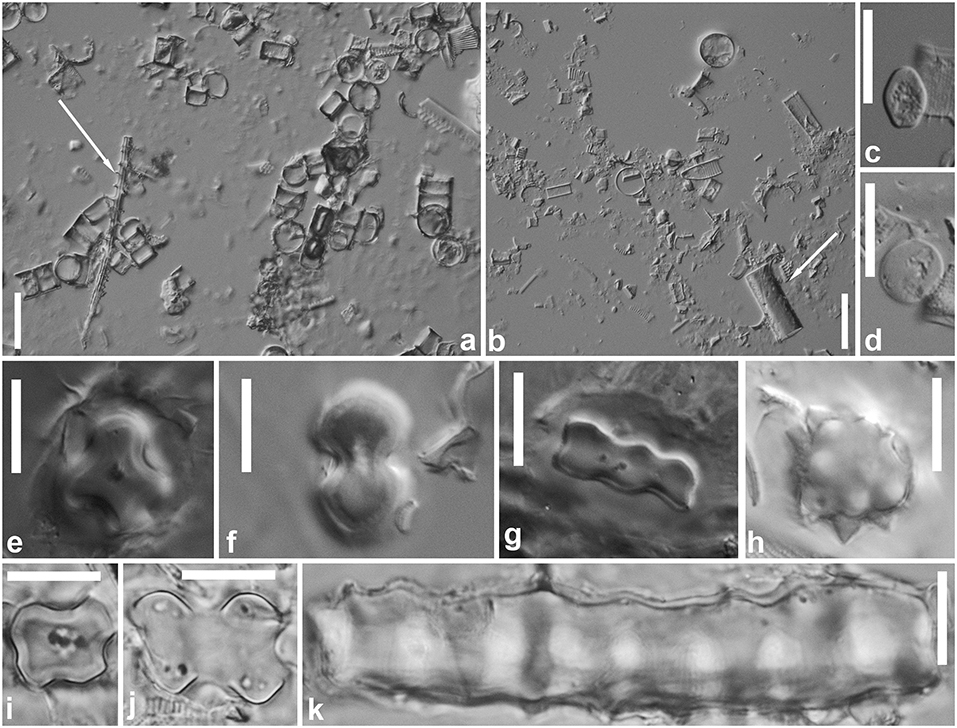
Figure 4. Silica particles in the sediment and plant samples. Sediment samples originate from Kodjoboue lagoon, and are exemplary to all examined samples from the three lagoons. (a) Large number of diatom valves, mainly belonging to the genus Aulacoseira and one sponge spicule (megasclere, see arrow). (b) Shows a few broken diatom valves and one phytolith (see arrow). Plant samples originate from dominant plant species from across all three lagoons. (c,d) Show testate amoeba idiosomes. (e–h) Show different phytoliths found in Echinochloa pyramidalis samples: short cell bilobate (e,f), cylindrical polylobate (g), and globular echinate (h) phytoliths were observed. (i–k) Show different phytoliths found in a Panicum parviflorum sample: short cell cross (i), short cell bilobate (j), and long cell echinate (k) phytoliths were observed. Scale bar represents 20 μm for panels (a,b) and 10 μm for panels (c–k).
In plant samples, phytoliths were observed in E. pyramidalis (Figures 4e–h) and P. parvifolium (Figures 4i–k) samples whereas in H. verticillata and S. molesta samples, no phytoliths were found. Most phytoliths were short bilobate or polylobate cells (Figures 4e–g,j). Occasionally, short cell cross (Figure 4i), globular and long echinate phytoliths (Figures 4h,k) were observed.
Discussion
The Role of Macrophytes and Diatoms in Lagoon BSi Storage
BSi accumulation in vegetation varied per lagoon and was dependent on plant species dominance [see Prychid et al. (2003) and Hodson et al. (2005) for BSi contents in plants related to their phylogenetic position]. The BSi stock in macrophytes ranged from 0.054 to 0.288 t BSi ha−1, which is situated between values reported for submerged macrophytes in the Okavango Delta channels (0.008 t BSi ha−1; Schoelynck et al., 2017) and values reported for emergent wetland vegetation in the Okavango Delta (ranging from 0.010 to 1.600 t BSi ha−1; Struyf et al., 2015). The highest BSi concentrations were found in macrophytes collected from Kodjoboue lagoon and were related to the dominance of emergent species (A. zizanioides, B. villosa, R. corymbosa, E. pyramidalis, P. lanceolatus, and P. parvifolium) belonging to the Poaceae and Cyperaceae families. Species of these two families are often characterized by high BSi contents (Ma and Takahashi, 2002) and were also identified as a macrophyte growth form with high BSi content in Schoelynck and Struyf (2016). The generally lower BSi concentrations found in Ono lagoon were counterbalanced by the high macrophyte coverage giving this lagoon the highest BSi stock in vegetation of all three lagoons: 17.4 t. We did not find a relationship between plant BSi and sediment BSi. Other studies (e.g. in the Okavango Delta, Struyf et al., 2015) identified an impact of vegetation on phytolith input to the soils and thus on BSi content, but results are not always consistent. In saltmarshes, de Bakker et al. (1999) found no relation between plant BSi content and sediment BSi content. Carey and Fulweiler (2014) concluded that silica uptake in saltmarsh plants is not always directly linked to sediment BSi and sediment DSi, since active uptake mechanisms stimulated by multiple abiotic factors (e.g., hydrological stress) can strongly impact plant silica uptake.
Light microscopy of the lagoon sediments revealed that the majority of BSi particles in the sediment of all lagoons comprise almost entirely of diatom frustules, occasionally sponge spicules and almost never phytolith particles. Tychoplanktonic diatoms such as Aulacoseira species form long filaments of large, heavily silicified, cylindrical cells, and are known to bloom mainly in spring and autumn (Siver and Kling, 1997). The light microscopy investigated samples in the current study were taken in November 2017 and April 2018, most likely in the middle of large Aulacoseira blooms. During this sampling period, stimulated by the nutrient rich character of these waters, the shallow depths and the abundant sunlight, Aulacoseira blooms may have produced large amounts of frustules (Conley et al., 1993). Additionally, non-rinsed plant samples were also dominated by large amounts of epiphytic diatoms, mainly belonging to the genus Eunotia, typically found in acidic environments such as these lagoons (Van de Vijver, pers. obs.). It is thus clear that diatoms could have depleted the plant-available DSi in the water rapidly, before the plants could take up the necessary silica to produce phytoliths. This can be observed in Figure 2 where the amount of DSi strongly decreased between April and May suggesting either a dilution effect in the rainy season (resulting in lower concentrations; Meunier et al., 2011), or an increased uptake by organisms compared to other months (Rabosky and Sorhannus, 2009).
The fact that we do find BSi in plants but rarely phytoliths, may be explained by different deposition mechanisms. Rapid deposition of silica results in lumen and cell wall phytoliths, whereas slow deposition results in silica deposition in intercellular spaces or in an extracellular (cuticular) layer (reviewed by Prychid et al., 2003; Hodson, 2019). These non-homogeneous masses can be i.a. a silica ring formed around the periphery of the cell, deposited on a dispersed organic matrix, or laid down as deposits within the cell wall or between the cellulose wall and the plasma membrane or in cortical intercellular spaces within the cell (Prychid et al., 2003). In general, these fragile phytogenic silica structures as well as very small phytoliths (<5 μm) are difficult to trace in sediments, but they potentially play an important role in silica cycling (Meunier et al., 2017; Puppe et al., 2017). Additionally, given the very large number of epiphytic diatoms on non-rinsed plant samples, it cannot be excluded that a fraction of plant-silica in our results is actually diatom-silica, even though plants were washed thoroughly before analysis, according to protocol. Furthermore, it could be hypothesized that plant organic matter may float away through the outlets of the lagoons, or accumulate on the shores, hence not contributing to the sediment silica storage (cf. Struyf et al., 2015). Alternatively, Puppe et al. (2017) suggest the formation of a layer of coarse organic matter on top of sediments from which phytoliths cannot be released easily and hence are missing in underneath sediment layers. We have no arguments supporting either of these hypotheses, none of our sediment cores contained such a distinct organic top layer and samples were visually homogeneous organic rich (fine material) over the entire depth of the core (15 cm).
Sediments as a BSi Sink
Overall, the BSi stocks in sediments of the three lagoons ranged from 4.2 × 103 kg ha−1 to 8.2 × 103 kg ha−1 and were much higher than those found in the vegetation, suggesting that lagoon sediments are important sites for long-term BSi storage. Similar studies on African wetland BSi accumulation are scarce. The best studied ecosystem in this regard is the Okavango Delta (Botswana) where similar values were found: between 3.8 × 103 kg ha−1 and 36 × 103 kg ha−1 in the riparian wetlands [depending on flooding frequency and in top 5 cm of sediment; Struyf et al. (2015)] and between 0.2 × 103 kg ha−1 and 1.5 × 103 kg ha−1 in the main channels [depending on vegetation cover and in top 10 cm of sediment; Schoelynck et al. (2017)]. Since phytoplankton production in the Delta is limited by low ambient nutrient concentrations, Okavango sediment BSi particles mainly originate from dead terrestrial and aquatic plant fragments (Struyf et al., 2015). In case of the analyzed groundwater-fed Ivory Coast lagoons, most of the BSi is produced within the lagoons and not brought in by any river. Overland runoff and topsoil erosion as in the Nyong basin in Cameroon (Cary et al., 2005) can potentially introduce allochthonous BSi particles, especially by transformation of an area from pristine to agricultural land use (cf. Smis et al., 2011). However, we have no indication at the moment that this is a significant process in the analyzed lagoons and no phytoliths with confirmed terrestrial origin were found in corresponding sediment samples. The investigated lagoons sediment BSi pool is thus dominated by diatom frustules, occasional sponge spicules and phytolith fragments, and an unquantified amount of fragile phytogenic silica structures and small phytoliths (<5 μm). Entire shells of testate amoebae were not observed probably because the organic cement, which glues the idiosomes (i.e., the building blocks of the shell) together, was destroyed during the H2O2 sample preparation step. But also single idiosomes were very rare in our samples, although other studies showed that they can be quite abundant in sediment samples in general (cf. Douglas and Smol, 1987; Cary et al., 2005).
Implications for the Ecosystem Silica Cycling
The in situ produced BSi is deposited locally due to limited exchange with the adjacent coastal ocean (Boynton et al., 1996). This accumulation strongly influences BSi cycling and storage in the sediments. In general, plant and phytoplankton BSi is returned in detritus to soil and sediments, where decomposition processes and pedogenic transformations can result in either stored BSi or re-dissolved DSi (Frings et al., 2014). As the analyzed lagoons have an acidic pH (<6.4), the preservation of diatom frustules and phytoliths can be assumed to be quite good (Flower and Ryves, 2009) making silica only very slowly available again for uptake. The average DSi concentration for the Comoé river draining these lagoons is with 2.86 mg L−1 rather low (Koné et al., 2008). Invertebrate fauna might be important contributors to remineralisation processes in general as was reported for other nutrients in Taabo Lake, which is similar to the studied lagoons (Kouamé et al., 2011). Invertebrate fauna, especially burrowers, are known to amplify BSi cycling in shallow environments like coastal lagoons and estuaries (Viaroli et al., 2013). The presumed fragile phytogenic silica in the plants (potentially explaining the relatively high plant BSi concentrations) may be very important in such conditions as they may be able to dissolve more easily than homogeneous masses (i.e., phytoliths and diatom frustules) and can hence contribute to local silica cycling. Plants may then be an important source of silica availability in the lagoons, although this warrants further investigation.
Generally, BSi stored in wetland sediments is several orders of magnitude more soluble than mineral silicates (Farmer et al., 2005) and its temporal or permanent storage vs. recycling or downstream transport exhibits an important control on silica export toward the ocean (e.g., Derry et al., 2005; Fulweiler and Nixon, 2005; Sommer et al., 2006), which is crucial for the silica cycling in these (sub) tropical regions (Beusen et al., 2009). Decreasing availability of silica compared to other nutrients can negate any competitive advantage the diatoms have and can lead to nuisance and toxic blooms of green and blue green algae in coastal ecosystems (Cloern, 2001). Diatoms need an optimal nutrient ratio of C:Si:N:P = 106:15:16:1, and diatom growth will cease when DSi supplies are depleted, allowing other phytoplankton classes to proliferate using any excess of N and P (Anderson and Burkholder, 2002). Because diatoms are primary producers at the base of the food-web, any change in that production might have knock-on effects to the entire ecosystem. Phytoplankton assemblages at Ivory Coast coastal environment show that, although diatoms are the most species-rich, cyanobacteria are the most abundant (up to 90% of the phytoplankton biomass), and silica was identified as predominant abiotic factor controlling phytoplankton dynamics (Osemwegie et al., 2016). Expected human population rise in the greater Abidjan area however, will probably put more pressure on local water bodies and nutrient levels may increase to levels which could escalate to dramatic levels of eutrophication [projections made for the nearby (and larger) Ebrié Lagoon which receives water from the Comoé River; (Scheeren et al., 2004)].
Conclusion
It can be concluded that relatively recent colonization of the lagoons by aquatic vegetation had no major effect yet on the lagoons' sediment BSi storage that is still dominated by phytoplankton, entirely comparable to any other open water system lacking plants. Perhaps it is just a matter of time before plants start having an impact here. Values reach up to 8.2 t BSi ha−1, which is similar to other tropical wetlands and may exhibit an important control on fluxes toward the tropical coastal zone. Relatively high plant BSi concentrations are likely explained by non-homogeneous fragile phytogenic silica structures that may be more able to dissolve and contribute to local silica cycling, rather than the more homogeneous diatom frustules. Using other techniques are advised measuring or visualizing these fragile phytogenic silica structures to prove this hypothesis.
Author Contributions
YK and JS conceived the program research. YK collected the samples. BV analyzed the BSi particles in sediment and plant samples. YK, JS, and BV wrote the paper. JS and BV read and approved the final manuscript and we are pretty confident that YK would have agreed with our corrections after revision.
Conflict of Interest
The authors declare that the research was conducted in the absence of any commercial or financial relationships that could be construed as a potential conflict of interest.
Acknowledgments
The authors thank the Director of the Centre de Recherches Océanologiques d'Abidjan (CRO), B. Diara, and E. K. Sessou from the University of Félix Houphouët Boigny for assisting with the field work, Dr. Christine Cocquyt (Meise Botanic Garden) for her help in identifying silica particles in the sediment samples, and Dr. Eric Struyf (UAntwerpen) for proofreading the manuscript. JS is a postdoctoral fellow of FWO (project no. 12H8616N).
References
Aloo, P., Ojwang, W., Omondi, R., Njiru, J. M., and Oyugi, D. (2013). A review of the impacts of invasive aquatic weeds on the biodiversity of some tropical water bodies with special reference to Lake Victoria (Kenya). Biodivers. J. 4, 471–482. Available online at: http://www.biodiversityjournal.com/pdf/4(4)_471-482.pdf
Amon, K. J. B., Guiral, D., Sankaré, Y., Kaba, N., and N'da, E. (1991). Remote mapping of the expansion of the macrophytes invading the Ebrié lagoon system (Côte d'Ivoire). J. Ivoir. Océanol. Limnol. 2, 11–24.
Anderson, P. G., and Burkholder, J. (2002). Harmful algal blooms and eutrophication nutrient sources, composition and consequences. Est. Coast 25, 704–726. doi: 10.1007/BF02804901
Aoki, Y., Hoshino, M., and Matsubara, T. (2007). Silica and testate amoebae in a soil under pine–oak forest. Geoderma 142, 29–35. doi: 10.1016/j.geoderma.2007.07.009
Beusen, A. H. W., Bouwman, A. F., Durr, H. H., Dekkers, A. L. M., and Hartmann, J. (2009). Global patterns of dissolved silica export to the coastal zone: Results from a spatially explicit global model. Glob. Biogeochem. Cycles 23, 1–13. doi: 10.1029/2008GB003281
Boynton, W. R., Hagy, J. D., Murray, L., Stokes, C., and Kemp, W. M. (1996). A comparative analysis of eutrophication patterns in a temperate coastal lagoon. Estuaries 19, 408–421. doi: 10.2307/1352459
Broadley, M. R., Brown, P., Cakmak, I., Ma, J. F., Rengel, Z., and Zhao, F. (2012). “Beneficial elements,” in Marschner's Mineral Nutrition of Higher Plants, ed P. Marschner (London, UK: Elsevier, 249–269.
Cardinal, D., Gaillardet, J., Hughes, H. J., Opfergelt, S., and André, L. (2010). Contrasting silicon isotope signatures in rivers from the Congo Basin and the specific behaviour of organic-rich waters. Geophys. Res. Lett. 37:L12403. doi: 10.1029/2010GL043413
Carey, J. C., and Fulweiler, R. W. (2012). The terrestrial silica pump. PLoS ONE 7:e52932. doi: 10.1371/journal.pone.0052932
Carey, J. C., and Fulweiler, R. W. (2014). Silica uptake by Spartina—evidence of multiple modes of accumulation from salt marshes around the world. Front. Plant Sci. 5:186. doi: 10.3389/fpls.2014.00186
Cary, L., Alexandre, A., Meunier, J.-D., Boeglin, J.-L., and Braun, J.-J. (2005). Contribution of phytoliths to the suspended load of biogenic silica in the Nyong basin rivers (Cameroon). Biogeochemistry 74, 101–114. doi: 10.1007/s10533-004-2945-1
Caumette, P., Castel, J., and Herbert, R. (1996). Coastal Lagoon Eutrophication and Anaerobic Processes: Nitrogen and Sulfur Cycles and Population Dynamics in Coastal Lagoons: A Research Programme of the Environment Programme of the EC (DG XII). Dordrecht: Kuwer Academic Publishers.
Cloern, J. E. (2001). Our evolving conceptual model of the coastal eutrophication problem. Mar. Ecol. Prog. Ser. 210, 223–253. doi: 10.3354/meps210223
Conley, D. J. (2002). Terrestrial ecosystems and the global biogeochemical silica cycle. Glob. Biogeochem. Cycles 16, 1–8. doi: 10.1029/2002GB001894
Conley, D. J., Schelske, C. L., and Stoermer, E. F. (1993). Modification of the biogeochemical cycle of silica with eutrophication. Mar. Ecol. Prog. Ser. 101, 179–192. doi: 10.3354/meps101179
de Bakker, N. V. J., Hemminga, M. A., and Van Soelen, J. (1999). The relationship between silicon availability, and growth and silicon concentration of the salt marsh halophyte Spartina anglica. Plant Soil 215, 19–27. doi: 10.1023/A:1004751902074
DeMaster, D. J. (1981). The supply and removal of silica from the marine environment. Geochim. Cosmochim. Acta 45, 1715–1732. doi: 10.1016/0016-7037(81)90006-5
Derry, L. A., Kurtz, A. C., Ziegler, K., and Chadwick, O. A. (2005). Biological control of terrestrial silica cycling and export fluxes to watersheds. Nature 433, 728–731. doi: 10.1038/nature03299
Ding, T. P., Zhou, J. X., Wan, D. F., Chen, Z. Y., Wang, C. Y., and Zhang, F. (2008). Silicon isotope fractionation in bamboo and its significance to the biogeochemical cycle of silicon. Geochim. Cosmochim. Acta 72, 1381–1395. doi: 10.1016/j.gca.2008.01.008
Douglas, M. S., and Smol, J. P. (1987). Siliceous protozoan plates in lake sediments. Hydrobiologia 154, 13–23. doi: 10.1007/BF00026827
Farmer, V. C., Delbos, E., and Miller, J. D. (2005). The role of phytolith formation and dissolution in controlling concentrations of silica in soil solutions and streams. Geoderma 127, 71–79. doi: 10.1016/j.geoderma.2004.11.014
Flower, R. J., and Ryves, D. B. (2009). Diatom preservation: differential preservation of sedimentary diatoms in two saline lakes. Acta Bot. Croat. 68, 381–399. Available online at: https://www.researchgate.net/publication/286305244_Diatom_preservation_Differential_preservation_of_sedimentary_Diatoms_in_two_saline_lakes
Frings, P., De La Rocha, C., Struyf, E., van Pelt, D., Schoelynck, J., Murray Hudson, M., et al. (2014). Tracing silicon cycling in the Okavango Delta, a sub-tropical flood-pulse wetland using silicon isotopes. Geochim. Cosmochim. Acta 142, 132–148. doi: 10.1016/j.gca.2014.07.007
Fulweiler, R. W., and Nixon, S. (2005). Terrestrial vegetation and the seasonal cycle of dissolved silica in a southern New England coastal river. Biogeochemistry 74, 115–130. doi: 10.1007/s10533-004-2947-z
Grasshoff, K., Ehrhardt, M., and Krelling, K. (1983). Methods of Seawater Analysis. Weinheim: Verlag Chemie.
Guiral, D., and N'da, E. (1991). Les macrophytes aquatiques des berges lagunaires. Ivoir. Océanol. Limnol. 2, 25–40.
Hodson, M. J. (2019). The relative importance of cell wall and lumen phytoliths in carbon sequestration in soil: a hypothesis. Front. Earth Sci. 7:167. doi: 10.3389/feart.2019.00167
Hodson, M. J., White, P. J., Mead, A., and Broadley, M. R. (2005). Phylogenetic variation in the silicon composition of plants. Ann. Bot. 96, 1027–1046. doi: 10.1093/aob/mci255
Kjerfve, B., and Magil, K. E. (1989). Geographic and hydrodynamic characteristics of shallow coastal lagoons. Mar. Geol. 88, 187–199. doi: 10.1016/0025-3227(89)90097-2
Koné, Y. J. M., Abril, G., Kouadio, K. N., Delille, B., and Borges, A. V. (2008). Seasonal variability of carbon dioxide in the rivers and lagoons of Ivory Coast (West Africa). Est. Coasts 32, 246–260. doi: 10.1007/s12237-008-9121-0
Kouamé, M. K., Dietoa, M. Y., Edia, E. O., Da Costa, S. K., Ouattara, A., and Gourène, G. (2011). Macroinvertebrate communities associated with macrophyte habitats in a tropical man-made lake (Lake Taabo, Côte d'Ivoire). Knowl. Manage. Aquatic Ecosyst. 400, 1–18. doi: 10.1051/kmae/2010035
Lange-Bertalot, H., Hofmann, G., Werum, M., and Cantonati, M. (2017). Freshwater Benthic Diatoms of Central Europe: Over 800 Common Species Used in Ecological Assessment. English Edition With Updated taxonomy and Added Species. Schmitten-Oberreifenberg: Koeltz Botanical Books.
Li, Z. M., Song, Z. L., and Li, B. L. (2013). The production and accumulation of phytolith-occluded carbon in Baiyangdian reed wetland of China. Appl. Geochem. 37, 117–124. doi: 10.1016/j.apgeochem.2013.07.012
Ma, J. F., and Takahashi, E. (2002). Soil, Fertiliser, and Plant Silicon Research in Japan. Amsterdam: Elsevier Science.
Madella, M., Alexandre, A., and Ball, T. (2005). International code for phytolith nomenclature 1.0. Ann. Bot. 96, 253–260. doi: 10.1093/aob/mci172
Maldonado, M., Riesgo, A., Bucci, A., and Rutzler, K. (2010). Revisiting silicon budgets at a tropical continental shelf: silica standing stocks in sponges surpass those in diatoms. Limnol. Oceanogr. 55, 2001–2010. doi: 10.4319/lo.2010.55.5.2001
Meunier, J. D., Barboni, D., Anwar-Ul-Haq, M., Levard, C., Chaurand, P., Vidal, V., et al. (2017). Effect of phytoliths for mitigating water stress in durum wheat. New Phytol. 15, 229–239. doi: 10.1111/nph.14554
Meunier, J. D., Braun, J. J., Riotte, J., Kumar, C., and Sekhar, M. (2011). Importance of weathering and human perturbations on the riverine transport of Si. Appl. Geochem. 26, S360–S362. doi: 10.1016/j.apgeochem.2011.03.060
Mosimane, K., Struyf, E., Gondwe, M., Frings, P., van Pelt, D., Wolski, P., et al. (2017). Variability in chemistry of surface and soil waters of an evapotranspiration-dominated flood-pulsed wetland: solute processing in the Okavango Delta, Botswana. Wat. SA. 43, 104–115. doi: 10.4314/wsa.v43i1.13
Müller, F., Struyf, E., Wanner, A., Hartmann, J., and Jensen, K. (2013). A comprehensive study of silica pools and fluxes in Wadden Sea salt marshes. Est. Coast 36, 1150–1164. doi: 10.1007/s12237-013-9621-4
Osemwegie, I., Niamien-Ebrottié, J. E., Koné, M. Y. J., Ouattara, A., Biemi, J., and Reichert, B. (2016). Characterization of phytoplankton assemblages in a tropical coastal environment using Kohonen self-organizing map. Afr. J. Ecol. 55, 487–499. doi: 10.1111/aje.12379
Prychid, C., Rudall, P. J., and Gregory, M. (2003). Systematics and biology of silica bodies in monocotyledons. Botan. Rev. 69, 377–440. doi: 10.1663/0006-8101(2004)069[0377:SABOSB]2.0.CO;2
Puppe, D., Ehrmann, O., Kaczorek, D., Wanner, M., and Sommer, M. (2015). The protozoic Si pool in temperate forest ecosystems – Quantification, abiotic controls and interactions with earthworms. Geoderma 243–244, 196–204. doi: 10.1016/j.geoderma.2014.12.018
Puppe, D., Höhn, A., Kaczorek, D., Wanner, M., Wehrhan, M., and Sommer, M. (2017). How big is the influence of biogenic silicon pools on short-term changes in water-soluble silicon in soils? Implications from a study of a 10-year-old soil–plant system. Biogeoscience 14, 5239–5252. doi: 10.5194/bg-14-5239-2017
Rabosky, D. L., and Sorhannus, U. (2009). Diversity dynamics of marine planktonic diatoms across the Cenozoic. Nature 457, 183–186. doi: 10.1038/nature07435
Rejmankova, E. (2011). The role of macrophytes in wetland ecosystems. J. Ecol. Field Biol. 34, 333–345. doi: 10.5141/JEFB.2011.044
Schaller, J., and Struyf, E. (2013). Silicon controls microbial decay and nutrient release of grass litter during aquatic decomposition. Hydrobiologia 709, 201–212. doi: 10.1007/s10750-013-1449-1
Scheeren, P., Kroeze, C., Janssen, F. J. J. G., Hordijk, L., and Ptasinski, K. (2004). Integrated water pollution assessment of the Ebrié Lagoon, Ivory Coast, West Africa. J. Mar. Syst. 44, 1–17. doi: 10.1016/j.jmarsys.2003.08.002
Schoelynck, J., Schaller, J., Murray-Hudson, M., Frings, P. J., Conley, D. J., van Pelt, D., et al. (2017). The trapping of organic matter within plant patches in the channels of the Okavango Delta: a matter of quality. Aquat. Sci. 79, 661–674. doi: 10.1007/s00027-017-0527-2
Schoelynck, J., and Struyf, E. (2016). Silicon in aquatic vegetation. Funct. Ecol. 30, 1323–1330. doi: 10.1111/1365-2435.12614
Sidinei, M. T., Enrich-Prast, A., Gonçalves, J. F. Jr., Dos Santos, A. M., and Esteves, F. A. (2001). Metabolism and gaseous exchanges in two coastal lagoons from Rio de Janeiro with distinct limnological characteristics. Braz. Arch. Biol. Technol. 44, 433–438. doi: 10.1590/S1516-89132001000400015
Siver, P. A., and Kling, H. (1997). Morphological observations of Aulacoseira using scanning electron microscopy. Can. J. Botan. 75, 1807–1835. doi: 10.1139/b97-894
Smis, A., Van Damme, S., Struyf, E., Clymans, W., Van Wesemael, B., Frot, E., et al. (2011). A trade-off between dissolved and amorphous silica transport during peak flow events (Scheldt river basin, Belgium): impacts of precipitation intensity on terrestrial Si dynamics in strongly cultivated catchments. Biogeochemistry 106, 475–487. doi: 10.1007/s10533-010-9527-1
Smol, J. P., and Stoermer, E. F. (2010). The Diatoms: Applications for the Environmental and Earth Sciences. Camebridge, UK: Cambridge University Press.
Sommer, M., Kaczorek, D., Kuzyakov, Y., and Breuer, J. (2006). Silicon pools and fluxes in soils and landscapes- a review. J. Plant. Nutr. Soil Sci. 169, 582–582. doi: 10.1002/jpln.200690016
Struyf, E., and Conley, D. (2009). Silica: an essential nutrient in wetland biogeochemistry. Front. Ecol. Environ. 7, 88–94. doi: 10.1890/070126
Struyf, E., Dausse, A., Van Damme, S., Bal, K., Gribsholt, B., Boschker, H. T. S., et al. (2006). Tidal marshes and biogenic silica recycling at the land-sea interface. Limnol. Oceanogr. 51, 838–846. doi: 10.4319/lo.2006.51.2.0838
Struyf, E., Mörth, M., Humborg, C., and Conley, D. J. (2010). An enormous amorphous silica pool in boreal wetlands. J. Geophys. Res. 115:G04008. doi: 10.1029/2010JG001324
Struyf, E., Mosimane, K., Van Pelt, D., Murray-Hudson, M., Meire, P., Patrick Frings, P., et al. (2015). The role of vegetation in the Okavango Delta silica sink. Wetlands 35, 171–181. doi: 10.1007/s13157-014-0607-1
Struyf, E., Opdekamp, W., Backx, H., Jacobs, S., Conley, D. J., and Meire, P. (2009). Vegetation and proximity to the river control amorphous Si storage in a riparian wetland (Bierbza National Park, Poland). Biogeosciences 6, 623–631. doi: 10.5194/bg-6-623-2009
Struyf, E., Van Damme, S., Gribsholt, B., Bal, K., Beauchard, O., Middelburg, J. J., et al. (2007). Phragmites australis and silica cycling in tidal wetlands. Aquat. Bot. 87, 134–140. doi: 10.1016/j.aquabot.2007.05.002
Struyf, E., Van Damme, S., Gribsholt, B., Middelburg, J. J., and Meire, P. (2005). Biogenic silica in tidal freshwater marsh sediments and vegetation (Schelde estuary, Belgium). MEPS 303, 51–60. doi: 10.3354/meps303051
Van der Werf, A. (1955). New method of concentrating and cleaning diatoms and other organisms. Proc. Int. Assoc. Theor. Appl. Limnol. 12, 276–277. doi: 10.1080/03680770.1950.11895297
Keywords: lagoons, phytogenic silica, sediment, diatom frustules, phytoliths, tropical wetland
Citation: Koné YJ-M, Van de Vijver B and Schoelynck J (2019) The Role of Macrophytes in Biogenic Silica Storage in Ivory Coast Lagoons. Front. Earth Sci. 7:248. doi: 10.3389/feart.2019.00248
Received: 18 December 2018; Accepted: 04 September 2019;
Published: 26 September 2019.
Edited by:
Martin John Hodson, Oxford Brookes University, United KingdomReviewed by:
Joanna Carey, Marine Biological Laboratory (MBL), United StatesDaniel Puppe, Leibniz Center for Agricultural Landscape Research (ZALF), Germany
Copyright © 2019 Koné, Van de Vijver and Schoelynck. This is an open-access article distributed under the terms of the Creative Commons Attribution License (CC BY). The use, distribution or reproduction in other forums is permitted, provided the original author(s) and the copyright owner(s) are credited and that the original publication in this journal is cited, in accordance with accepted academic practice. No use, distribution or reproduction is permitted which does not comply with these terms.
*Correspondence: Jonas Schoelynck, am9uYXMuc2Nob2VseW5ja0B1YW50d2VycGVuLmJl
†This manuscript is dedicated to a young and promising scientist who passed away too early