- 1GEOMAR Helmholtz Centre for Ocean Research Kiel, Kiel, Germany
- 2Instituto Dom Luiz (IDL), Faculdade de Ciências da Universidade de Lisboa, Lisbon, Portugal
- 3Earth Sciences Department, Royal Holloway, University of London, Egham, United Kingdom
- 4Dipartimento di Scienze della Terra, Università di Firenze, Firenze, Italy
- 5Centre for Earth Evolution and Dynamics (CEED), University of Oslo, Oslo, Norway
- 6Department of Environmental Systems Science, ETH Zürich, Zurich, Switzerland
- 7IPMA- Portuguese Institute for Atmosphere and Ocean, Lisbon, Portugal
- 8Institut Français de Recherche pour l’Exploitation de la Mer (IFREMER), Département Ressources Physiques et Ecosystèmes de Fond de Mer, Unité des Géosciences Marines, Plouzané, France
- 9Aix Marseille Univ, CNRS, IRD, INRA, Coll France, CEREGE, Aix-en-Provence, France
- 10Department of Geosciences, University of Bremen, Bremen, Germany
- 11CNRS, IUEM, Laboratoire Géosciences Océan, University of Western Brittany, Brest, France
- 12Barcelona-CSI, Institut de Ciències del Mar (CSIC), Barcelona, Spain
- 13Eawag, Swiss Federal Institute of Aquatic Science and Technology, Dübendorf, Switzerland
- 14CNR, Institute of Marine Sciences (ISMAR), Bologna, Italy
- 15Istituto Nazionale di Oceanografia e di Geofisica Sperimentale, Trieste, Italy
- 16Department of Earth Sciences, University of Geneva, Geneva, Switzerland
- 17EMCOL and Faculty of Mining, Department of Geological Engineering, İstanbul Technical University, Istanbul, Turkey
- 18MARUM – Center for Marine Environmental Sciences, University of Bremen, Bremen, Germany
- 19Hellenic Centre for Marine Research, Institute of Oceanography, Anavyssos, Greece
- 20LOCEAN, UPMC, Sorbonne Université, Paris, France
- 21Integrated Geochemical Interpretation Ltd., The Granary, Hallsannery, Bideford, United Kingdom
Marine transform faults and associated fracture zones (MTFFZs) cover vast stretches of the ocean floor, where they play a key role in plate tectonics, accommodating the lateral movement of tectonic plates and allowing connections between ridges and trenches. Together with the continental counterparts of MTFFZs, these structures also pose a risk to human societies as they can generate high magnitude earthquakes and trigger tsunamis. Historical examples are the Sumatra-Wharton Basin Earthquake in 2012 (M8.6) and the Atlantic Gloria Fault Earthquake in 1941 (M8.4). Earthquakes at MTFFZs furthermore open and sustain pathways for fluid flow triggering reactions with the host rocks that may permanently change the rheological properties of the oceanic lithosphere. In fact, they may act as conduits mediating vertical fluid flow and leading to elemental exchanges between Earth’s mantle and overlying sediments. Chemicals transported upward in MTFFZs include energy substrates, such as H2 and volatile hydrocarbons, which then sustain chemosynthetic, microbial ecosystems at and below the seafloor. Moreover, up- or downwelling of fluids within the complex system of fractures and seismogenic faults along MTFFZs could modify earthquake cycles and/or serve as “detectors” for changes in the stress state during interseismic phases. Despite their likely global importance, the large areas where transform faults and fracture zones occur are still underexplored, as are the coupling mechanisms between seismic activity, fluid flow, and life. This manuscript provides an interdisciplinary review and synthesis of scientific progress at or related to MTFFZs and specifies approaches and strategies to deepen the understanding of processes that trigger, maintain, and control fluid flow at MTFFZs.
Introduction to Marine Transform Faults and Fracture Zones (MTFFZs)
Transform faults are one of the three basic types of plate boundaries in plate tectonics. They are so called “conservative” plate boundaries as – unlike at divergent spreading centers and convergent subduction/collision zones – plate material is neither created nor destroyed. Kinematically, transform faults are strike-slip faults that usually link the other two types of plate boundaries. The discovery of transform plate boundaries (Wilson, 1965) played a fundamental role in the development of the theory of plate tectonics as they were recognized to follow the trace of “small circles” according to the spherical Eulerian geometry (Morgan, 1968). Transform faults can be oceanic or continental, depending on the type of crust they crosscut. On the ocean floor transform faults are in most cases linked to plate growth structures (e.g., Gerya, 2010, 2013a,b; Giustiniani et al., 2015; for exceptions see Hey et al., 1980) as they commonly accommodate the progressive horizontal movements between two adjacent mid-ocean ridges – ridge-ridge transform faults (RRTF; Figure 1). Besides the most abundant RRTF, there are also ridge-trench (e.g., Mendocino Transform Fault) and trench-trench (e.g., Alpine Fault or the North Scotia Ridge) transform faults.
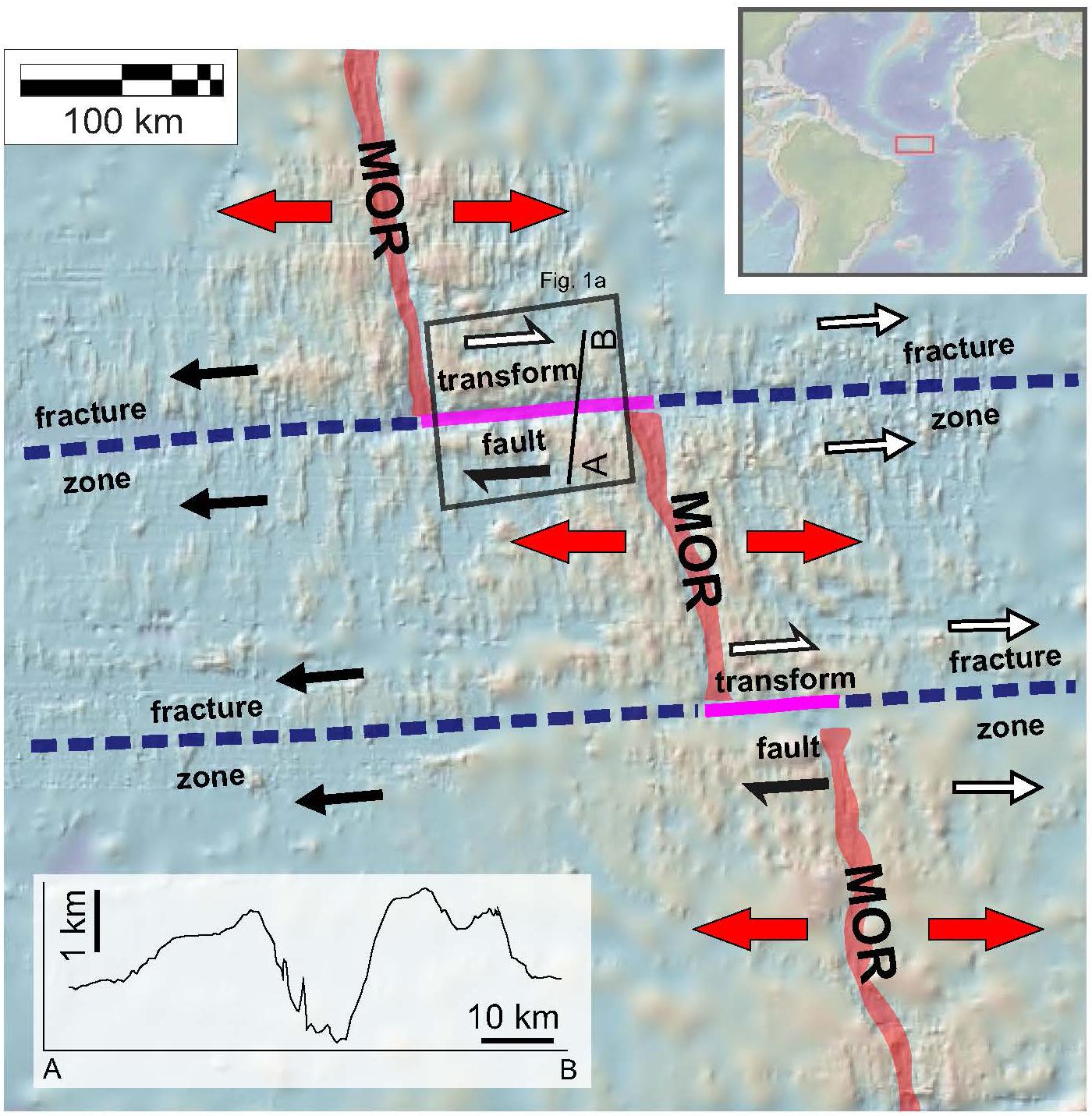
Figure 1. Bathymetric map of the Central Atlantic showing main morphological features of typical ridge-ridge transform fault sections (RRTF; bathymetric data were from GeoMapApp; Ryan et al., 2009).
Oceanic transform faults have a strong morphological signature on the ocean floor with narrow valleys and steep walls that can reach more than 2,000 m of relief (Figure 1) and water depths of >5000 m [Vema > 5100 m, Cannat et al., 1991; Romanche Trench > 7700 m, Bonatti et al., 1994; see overviews by Hekinian (1982) and Wolfson-Schwehr and Boettcher (2019)]. However, this characteristic morphology usually continues for thousands of kilometers to “scar” the ocean floor beyond the active plate boundary segment between mid-ocean ridges (see Figure 2). These scars, called fracture zones, were known as morphological features even before transform faults were recognized, and they correspond to the intra-plate traces of oceanic transform faults on the sides of mid-ocean ridges. Transform fault/fracture zone systems can extend for several thousand kilometers on the ocean floor and can show large offsets – the Romanche Fracture Zone in the Central Atlantic, for example, stretches over 5,000 km and reaches about 950 km of displacement (e.g., Hekinian, 1982; Wolfson-Schwehr and Boettcher, 2019; and online compilations1,2).
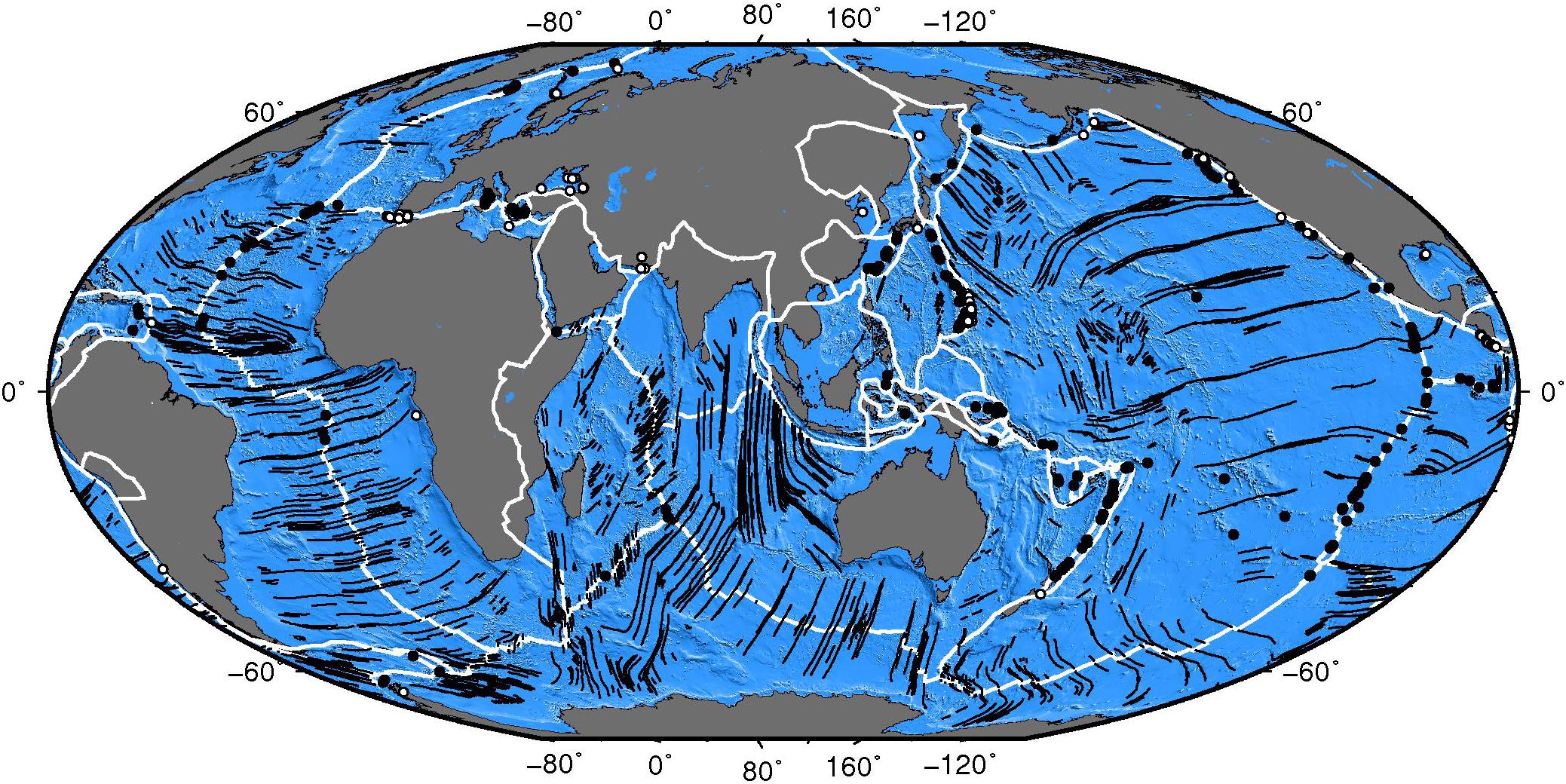
Figure 2. Global bathymetric map with major plate boundaries highlighting active transform faults and oceanic fracture zones (Bird, 2003; Müller et al., 2008; Wessel et al., 2015). Active sites of seafloor hydrothermal venting (solid black circles; Beaulieu and Szafranski, 2018) as well as low temperature seeps (white circles; Hensen et al., 2018) are indicated.
Fracture zones were historically viewed as tectonically inactive structures (Isacks et al., 1968). We now recognize that several of these fracture zones are seismically active (Lay, 2019), and have been generating large-scale earthquakes like the Sumatra-Wharton Basin Earthquake in 2012 (Mb8.6; Hill et al., 2015) or along the Atlantic Azores-Gibraltar Fracture Zone in 1755 (Great Lisbon Earthquake; Mw > 8.5; Gutscher, 2004) and in 1941 (M8.4; Lay, 2019). Oceanic transform plate boundaries may also act as pathways for hydrothermal circulation. A well-known example where off-axis seismicity is linked to hydrothermal fluid flow is the Lost City Hydrothermal Field (Atlantis Massif, Central North Atlantic), where faulting in combination with serpentinization-driven expansion of uplifted mantle rocks provides open pathways for hydrothermal circulation and associated water-rock interactions (Kelley et al., 2001). Serpentinization produces high concentrations of CH4 and H2 in the fluids (e.g., Kelley et al., 2001) that provide energy for chemosynthetic biota at the seafloor, and possibly below (see Figure 3). Hydrothermal fluids of deep crustal origin have also been suggested to play a role in mud volcanism (Hensen et al., 2015).
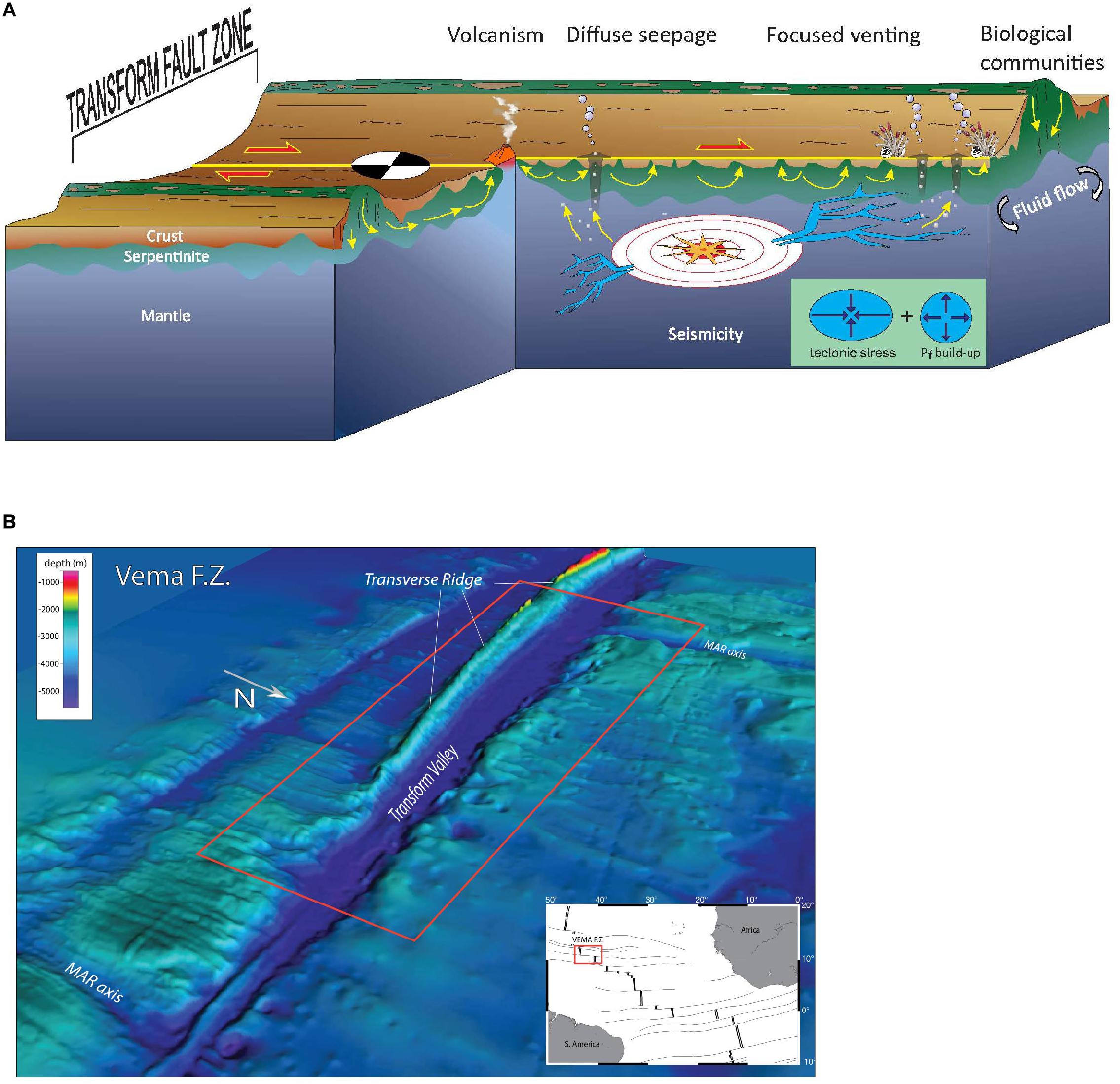
Figure 3. (A) Sketch illustrating the interaction of seismicity, fluid-flow, geochemical reactions, and life at and below the seafloor at MTFFZs inspired by (B) a 3D model of the Vema transform fault in the Equatorial Atlantic (based on multibeam bathymetric data collected during the PRIMAR-S19 Cruise; Fabretti et al., 1998; Bonatti et al., 2003). The large red rectangle indicates a typical location for the area sketched in the upper panel.
Three decades of continuous ocean exploration have led to the awareness that subsurface fluid-related processes (e.g., fluid formation, fluid-rock interaction, fluid transport) are key phenomena affecting and controlling geodynamic processes, because fluids affect almost every physical, chemical, mechanical, and thermal property of the seabed and upper crust (e.g., Judd and Hovland, 2007). Yet, an integrated understanding of how fluid-related geodynamic, geochemical, and biological processes interact is still missing, in particular in transform faults and fracture zones. The aim of this review is thus to present an overview of how crustal structure, fault stability, heat flow, fluid generating processes, geochemical conditions, and microbial life interact at Marine Transform Faults and associated Fracture Zones (MTFFZ). In the context of this manuscript, MTFFZ comprise typical oceanic transform faults and fracture zones, but also include other types of submerged (i.e., marine) parts of continental strike-slip faults/boundaries (e.g., the North Anatolian Fault (NAF) in the Marmara Sea). Terrestrial settings are generally excluded; exceptions are made with respect to specific findings where appropriate in the context. Overall, this manuscript is inspired by many discussions during the EU-funded network FLOWS3; 2013–2018). The objective of FLOWS was to merge the expertise of a large number of research groups and further interdisciplinary knowledge on how seep fluid (bio)geochemistry relates to seismicity.
Geodynamics of Marine Transform Faults and Fracture Zones (MTFFZs)
Tectonics and Lithospheric Structure
The key characteristic of transform faults is that they are plate boundaries and thus cut through the lithosphere (see e.g., Bartolome et al., 2012 showing deep strike-slip seismicity nucleating at lithospheric mantle depths of 10 s of kms; see also Gerya, 2016 for a more general review). Continental transform faults may re-use pre-existing fault zones such as sutures and often have multiple sub-parallel strands that define wide areas of strike-slip deformation (see recent reviews by Norris and Toy, 2014; Gerya, 2016; Şengör et al., 2019). In oceanic environments transform faults are typically associated with serpentinization, because large vertical and horizontal temperature gradients and existing, fault-related pathways allow the circulation of large volumes of sea water through the oceanic mantle (e.g., Francis, 1981; Ebert et al., 1983; Cannat et al., 1991; Escartin and Cannat, 1999; Früh-Green et al., 2016; Figure 3). Although some volcanism might be present – for example in “leaky” transform faults – transform faults are commonly characterized by absent volcanism. The inside corner of a ridge-transform intersection is a region where crustal-scale normal faulting often shows the intrusive lower crust and lenses of upper mantle material along exposed fault surfaces known as oceanic core complexes (Cann et al., 1997). Core complexes can be associated with hydrothermal fields in which unusual, serpentine-linked geochemical reactions occur, leading to the formation of carbonate-dominated vent systems such as the Lost City Hydrothermal Field in the North Atlantic. Although fluid percolation arguably has a profound effect on the strength of the lithosphere, the depth distribution and lateral extent of serpentinization in transform faults is yet to be determined.
RRTFs on the oceanic crust represent stable features. In contrast to these oceanic systems, spectacular surface expressions of transform faults on land, such as the San-Andreas Fault in Southern California, typically arise from changes in plate configuration, allowing them to grow or shrink with time (Atwater and Menard, 1970). Transform plate boundaries can also connect ridges and orogenic belts de facto intersecting both oceanic and continental crust. An example is the Owen Fracture Zone system connecting the Carlsberg Ridge to the Himalayan Orogeny. This fault structure is generally characterized by broad and discontinuous zones of deformation. In cases where a strike-slip fault terminates within a continent (e.g., East Antarctica; Storti et al., 2007), the maximum offset can be located in its center – while “typical” transform faults have constant offsets throughout their entire length.
Despite the fact that transform faults are often defined as conservative plate boundaries, horizontal movement in a system of strike-slip faults may locally cause (i) uplift and folding due to lateral transpression (crustal shortening perpendicular to the fault plane) and has been hypothesized as a precursor for subduction initiation (Casey and Dewey, 1984), (ii) transtension (crustal extension perpendicular to the fault plane) associated with volcanic activity (=leaky transform fault; Garfunkel, 1981), (iii) or the formation of pull-apart basins (e.g., Lonsdale, 1986).
Transform faults often lie within a transform valley, in which their surface expression is a zone only tens of meters wide (Maia, 2019). The limited dimensions of these narrow valleys, the accumulation of tens of kilometers to hundreds of kilometers of displacement over millions of years, and the young, hot, and rheologically weak lithosphere of RRTFs would suggest the development of a fairly smooth, primary fault strand. Transforms are therefore commonly envisaged as single well-localized faults, even though a closer examination often reveals a more complex geometry with multiple strands (Ligi et al., 2002). Fracture zones maintain the surface expression of transform faults and these valleys become major structures that can span over entire ocean basins (Figure 2). Spacing of transform faults intersecting mid-ocean ridges correlates with spreading rates: transform faults and fracture zones are more widely spaced in fast spreading centers like in the Pacific (600 ± 300 km) than in slow spreading centers like in the Atlantic (400 ± 200 km), while overlaps exist (Macdonald, 1998).
Transform faults are structures that originate to relieve thermal stress (Sandwell, 1986). Along transform faults, lithospheres of different age, and thus with different thermal regimes, get in contact, which is evoked as the primary reason for the possible reactivation of fracture zones. The different negative buoyancies of differently aged plates may, in fact, impart some physical imbalance between the two sides of the fracture zone that may persist during their entire lifetime, keeping the fracture zone “active.” Furthermore, the fact that transform faults are generally orthogonal to spreading centers means that their strike-slip motion does not obey the classical Anderson’s theory of faulting. At the extensional mid-ocean ridges normal faulting events predominate at ridge segments (Lay and Wallace, 1995), which according to Anderson means that on the horizontal plane the maximum principal stress is perpendicular to the strike of the transform fault. Transform plate boundaries are dominated by strike slip faults (thus strike slip events characterize transform faults; Lay and Wallace, 1995). According to Anderson’s theory of faulting the maximum principal stress should be at an angle of 30–45 degrees to them, but in fact is perpendicular to their strike. Faults that move under an unfavorable stress are typically weak, enable fluid circulation, and thus stimulate mineral transformation processes, which, specifically at transform faults, is in agreement with the occurrence of serpentinization. However, although sustained by observations or modeling, all these processes are still poorly known as transform faults and fracture zones have not been thoroughly investigated and their evolution is poorly understood (see Gerya, 2016 and references therein).
Tectonic Analog and Numerical Modeling
Analog models of transform faults have been carried out since the 1970s, including models with and without accreting plates. Examples of models of transform faulting with non-accreting material have been attempted by Dauteuil et al. (2002) and Marques et al. (2007). Examples of thermal+dynamical models with accreting plates are the ones by Oldenburg and Brune (1972, 1975) using freezing wax that reproduced ridge orthogonal transform faults (see Gerya, 2012, 2016 for a detailed review and discussion of this type of experiment). Interestingly, freezing wax experiments are the ones that reproduce best the main characteristics of RRTF, such as the development of “inactive” fracture zones, overlapping spreading centers, rotating microplates and transform faults orthogonal to spreading centers. These models showed that the stability of transform faults is dependent on the thermal state and strain-rate of the system and it is controlled by the almost lack of shear strength of the fault. Moreover, transform faults did not develop in experiments using paraffin suggesting that transform faults are highly dependent on rheology. Analog models with the injection of fluids have also been carried out to study the role of magmatic intrusions and diapirism, most of them using silicone putty (e.g., Corti et al., 2005) or vegetable oil (e.g., Galland et al., 2006). Analog models were also carried out to understand the tectonic consequences of fluid overpressure and seepage forces, demonstrating that gradients in fluid overpressure cause seepage forces, capable of modifying the total stress distribution and the geometry of the faults (Mourgues and Cobbold, 2003, 2006).
Progress in numerical modeling of transform faults has been recently reviewed by Gerya (2012, 2016). Numerical modeling efforts also began in the late 1970s. Due to computer limitations, the first models idealized the lithosphere as a “plane stress” membrane in which horizontal stresses were uniform with depth. This approximation allowed a ridge-transform-ridge system to be approximated with a 2-D model. Early investigations showed that ridge and transform boundaries needed to be much weaker than the lithosphere itself for their geometry to be “plate-like,” and also highlighted that stresses become concentrated in the “inside corner” at a ridge-transform intersection (Fujita and Sleep, 1978). The tensile stresses associated with resistance to extension at a ridge needed to be ∼3–5 times higher than shear stresses associated with slip on the transform in order to explain the observed toward-the-transform rotation of diking and normal faults near the ridge-transform intersection (Morgan and Parmentier, 1984). Full 3-D models of stresses and deformation along an oceanic transform were not explored until the 21st century. The first models assumed that the rheological structure of the transform was 2-D, e.g., invariant along the transform, and focused on continental transform systems (Sobolev et al., 2005). Gerya (2016) summarize major efforts toward a better understanding of the evolutionary generation of spreading-parallel transform faults from initially oblique rifted offsets.
Fault Behavior and Seismicity
Transform faults are seismically active (Figures 1, 3) and shearing is typically associated with the hydration of the upper lithospheric units driving the serpentinization of ultramafic rocks. Interestingly, earthquakes are more frequent along the active strike-slip transform segment than along the adjacent normal faulting mid-ocean ridge segments (Hanson and Bowman, 2005).
The seismogenic activity of transform faults in oceanic lithosphere has specific characteristics (Wolfson-Schwehr and Boettcher, 2019). It is generally acknowledged that the base of the seismogenic zone is limited by the 600°C isotherm (Abercrombie and Ekström, 2001; Boettcher and Jordan, 2004). For RRTF, this depth will thus depend on fault length, rate and on the intensity of hydrothermal convection (Roland et al., 2010). In general, RRTF also do not produce great earthquakes (>M8); in particular, if they cut intermediate- to fast-spreading ridges (>40 mm/yr), and have a low seismic coupling ratio (seismic moment release rate versus moment release rate expected from fault kinematics and locking depth; Bird and Kagan, 2004). A component of long-term creep on these transform faults may account for this relatively low level of seismic activity. Examples are known from the repeated occurrence of small earthquakes (e.g., Mendocino Fracture Zone; Materna et al., 2018) as well as from the relative regular occurrence of larger earthquakes on moderate and fast-moving segments (Boettcher and McGuire, 2009). From the seismological point of view, early work showed the anomalously large excitation of long-period waves by ocean transform earthquakes. Some transform earthquakes exhibit long-period seismic anomalies that are best explained by episodes of slow, smooth deformation, several hundred seconds before the high-frequency origin time (Kanamori and Stewart, 1976; Okal and Stewart, 1982; Ihmlé and Jordan, 1994). Because the possibility of anomalously slow rupture preceding normal speed rupture could be useful for short-term prediction, the seismological behavior of oceanic transform faults prior to large earthquakes has recently become the subject of active investigation. It is meanwhile recognized that large earthquakes on RRTFs are commonly preceded by foreshocks and by precursory changes in the seismic properties of the fault zone that could be linked to fluid-related processes (e.g., Dziak et al., 2003; McGuire et al., 2005, 2012). Hydrothermal fluids become (at constant temperature) significantly more compressible with decreasing pressure (Bischoff and Rosenbauer, 1985). Hence, the observed seismic precursors near the intersection of the mid-ocean ridge and the oceanic transform faults could be caused by an increase in fluid compressibility in response to fault core dilatancy just before rupture (Géli et al., 2014). In contrast, transforms in old oceanic lithosphere can produce great earthquakes, such as in the Gloria Fault in the Azores-Gibraltar Fracture Zone (Bezzeghoud et al., 2014) or in the Macquarie Fault Zone (Romanowicz, 1992). Fracture zones were historically thought to be seismically inactive, because the two segments flanking each fracture zone are part of the same plate and move in the same direction. Yet, seismologists have now documented major earthquakes on these structures (e.g., Bohnenstiehl et al., 2004; Lay, 2019). Examples include rupture events in highly stressed lithosphere in the diffuse deformation zone of the India/Australia plate boundary (Robinson et al., 2001; Bohnenstiehl et al., 2004; Hill et al., 2015; Lay, 2019) and in the Pacific Plate in the vicinity of the Alaska subduction and Yakutat terrane collision (Lahr et al., 1988; Lay, 2019). Moreover, the subduction of highly hydrated fracture zones and subsequent dewatering at depth may have an important role in changing the coupling between the lower and the upper plates, with potential consequences for interplate seismicity and back-arc volcanism (Dzierma et al., 2012) or the formation of fluid escape structures in the forearc (see section “Pore Fluid Geochemistry of Seafloor Seeps”). In addition, altered mantle rocks along transform faults or fracture zone may be transferred within subduction systems by plate convergence processes, enhancing shear processes and disruption of the lithosphere during the final closure of the oceans. Inherited discontinuities constituted by fracture zones might also control neotectonic activity, seismicity and location/reactivation of seismogenic structures, e.g., Eastern Mediterranean Sea (Granot, 2016) and Ionian Sea (Polonia et al., 2017).
Marine faults, unlike their continental equivalents, are characterized by the presence of a permanent supply of seawater from above, which is expected to influence geochemical processes within the fault zone itself. Heterogeneity in fault zone properties attributed to interaction with fluids and associated mantle hydration and serpentinization, has been proposed to control the segmentation of moderate and fast-moving RRTFs, and – as previously mentioned – the occurrence of foreshock swarms (e.g., McGuire et al., 2012; Géli et al., 2014). The characteristics of slow and reactivated transforms are less well known. Drilling through plate boundary faults e.g., (NanTroSEIZE; IODP 314/315/316, 338, Kinoshita et al., 2009; Strasser et al., 20144; J-Fast, IODP 343, Chester et al., 2013; SAFOD, Zoback et al., 2011) has shown that the composition of the gouge material in the fault core is an important controlling factor on frictional properties, and on frictional instability leading to earthquakes. Laboratory experiments further showed the complexity of frictional dependence on velocity and temperature, and that this complexity could explain earthquake sequences observed in subduction zones (e.g., Noda et al., 2017). The case of the Tohoku 2011 earthquake has notably suggested that seismic rupture, once initiated, can propagate in smectite rich fault gouge that displays velocity weakening at high slip velocities (Ujiie et al., 2013). Hydrated chrysotile (a serpentine group mineral) can display low friction coefficients and velocity strengthening behavior at low velocities, comparable to that of smectite (Moore et al., 1997). However, frictional properties of serpentinite-bearing fault gouge is complicated by serpentine mineral group polymorphism (antigorite being the most common) and by the presence of other minerals such as saponite, talc or brucite formed under hydrothermal conditions (Moore and Rymer, 2007; Holdsworth et al., 2011; Sone et al., 2012). In partially serpentinized mantle, or weathered oceanic crust, highly variable frictional behaviors are thus expected depending on mineral composition and temperature. In addition, thermo-mechanical coupling with the fluid phase may involve dilatancy strengthening as well as fault gouge weakening by fluid pressure increase from gouge compaction and/or thermal pressurization. However, serpentinite fault gouges may be more permeable than clay gouges, limiting thermal pressurization effect on friction (Sone et al., 2012). Moreover, below the hydrated zone, the wide range of temperature of the seismogenic zone in oceanic lithosphere (up to 600°C) may result in important variations of fluid compressibility with further consequences of fault stability (Géli et al., 2014). The diversity of behaviors observed at oceanic transform faults – and notably the apparent dependency on plate age and fault slip rate – can only be understood by integrating thermal regime, compositional variations, properties of materials, and interaction with the fluid phase.
Examples From the Southern European Margin
Based on the general information provided so far, this section is dedicated to a more detailed, exemplary description of two different types of MTFFZs, located at the southern European plate margin. These two prominent transform systems are the oceanic Azores-Gibraltar Fracture Zone, which includes the Gloria Fault and the SWIM Fault Zone, forming large parts of the in the central eastern Atlantic, and the submerged, continental NAF running through the Sea of Marmara and the northern Aegean Sea (Figure 4). Both systems have been thoroughly and interdisciplinarily investigated in the past and are target areas of ongoing research work. Below, the following sections provide a general description and generic characteristics of these areas, while subsequent sections will pick up on specific findings related to fluid geochemistry and life.
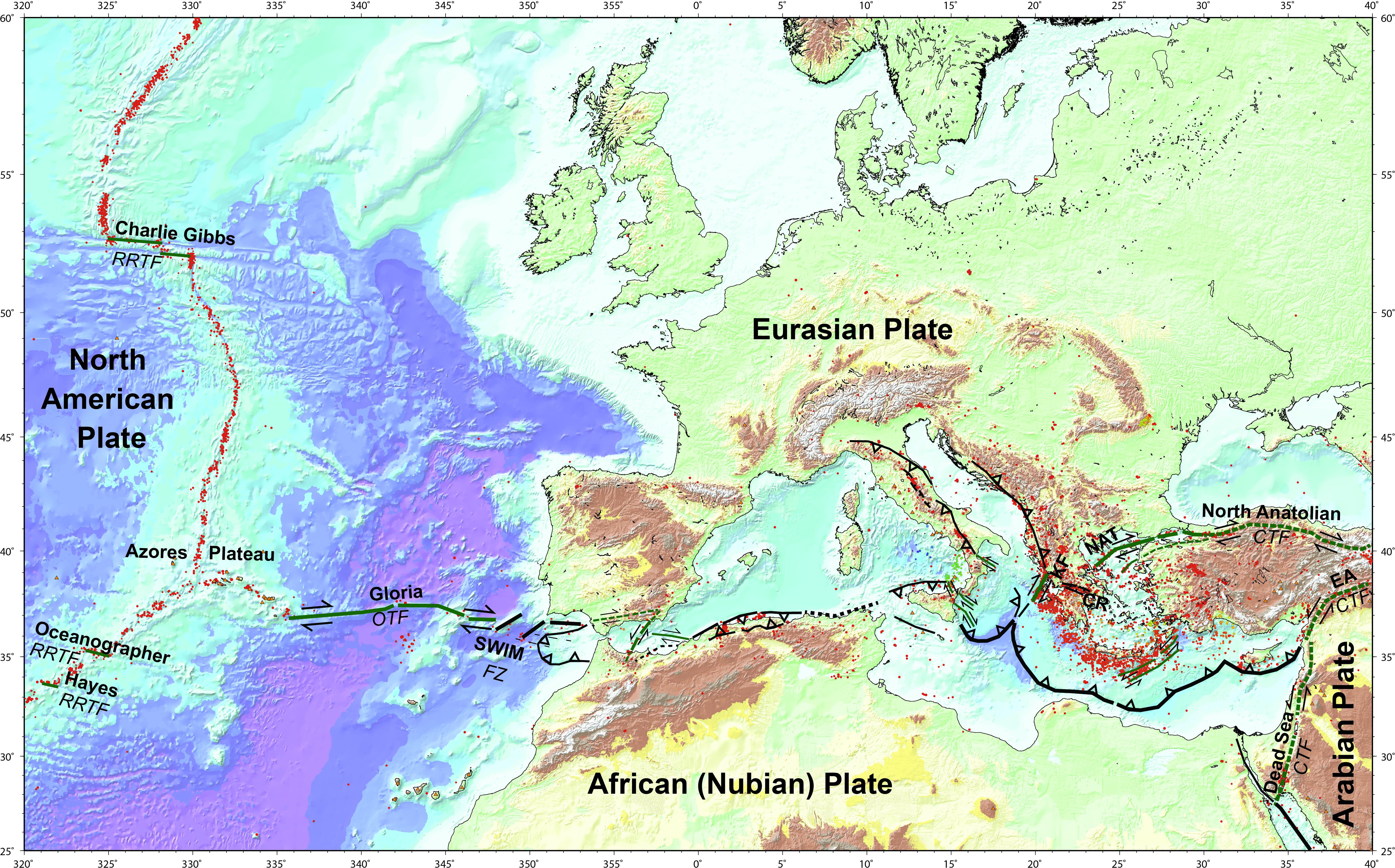
Figure 4. Relief, seismicity (small red dots), plate boundaries and major active faults in the European – Mediterranean region, including the Azores and NE Atlantic. Sense of motion shown on major faults by arrows and symbols (thrust teeth, normal barbs). RRTF, Ridge Ridge Transform Fault; OTF, Oceanic Transform Fault (shown as solid green lines), CTF, Continental Transform Fault (shown as tightly dashed green lines). SWIM FZ – South West Iberian Margin Fault Zone, EA – East Anatolian, NAT – North Aegean Trough, CR – Corinth Rift, KF – Kephalonia Fault. Dashed black lines indicate uncertain/debated faults or plate boundary segments.
Azores-Gibraltar Fracture Zone (AGFZ)
The term AGFZ has been used for decades to designate the Africa-Eurasia plate boundary in the Atlantic Ocean. It extends for more than 1500 km from the eastern tip of the Azores plateau to the Strait of Gibraltar, accomodating a relative interplate displacement of ∼ 4.5 mm/yr (Figures 4, 5; Bird, 2003; Neres et al., 2016). The lithospheric structure, geometry, kinematics and tectonics of this plate boundary are not simple. Instead, the AGFZ can be subdivided in two major segments: (i) the Gloria Fault and (ii) the Southwest Iberia Margin Fault Zone (SWIM FZ). The Gloria Fault is ∼800 km long and sub-divided in three near-linear segments connecting the Azores plateau to the Madeira-Tore Rise (Figure 5).
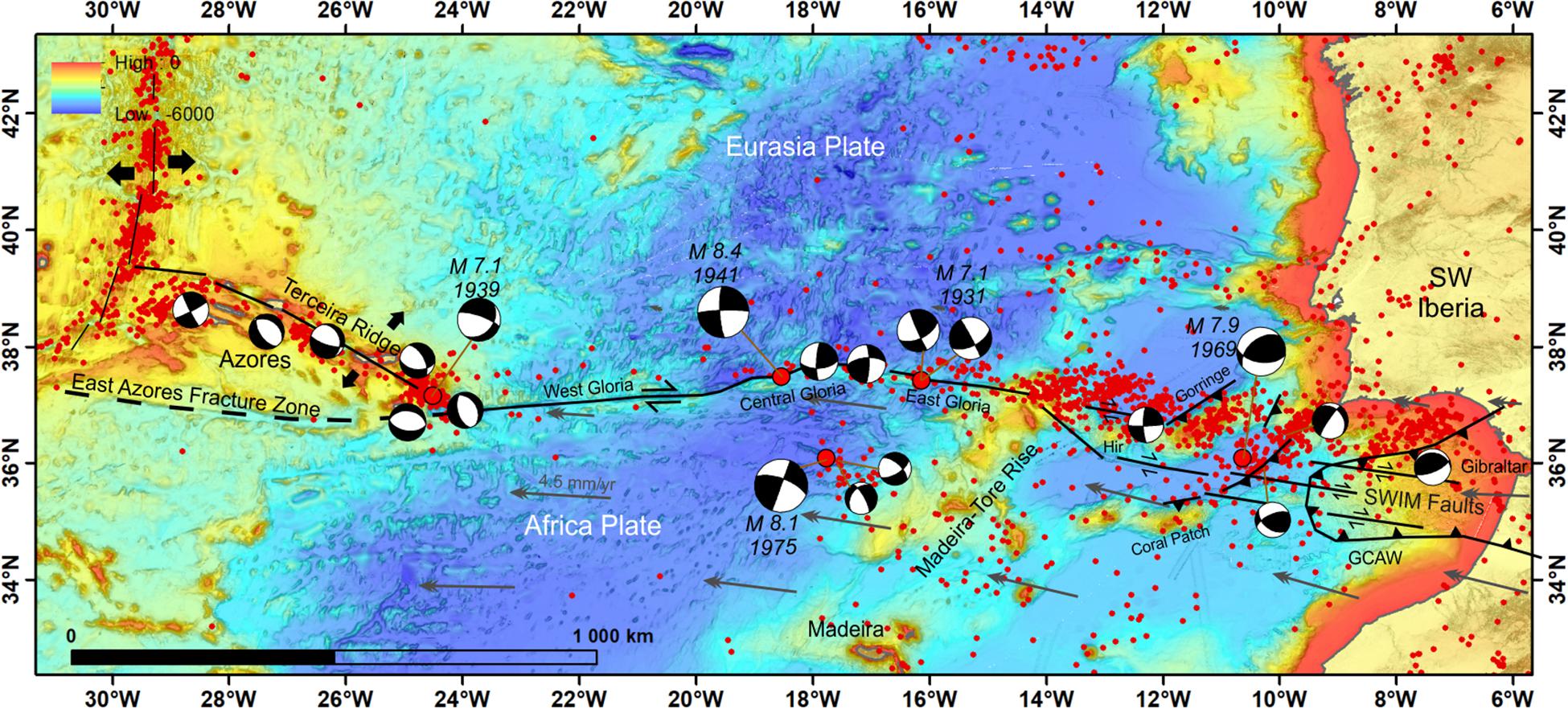
Figure 5. Bathymetric map of the AGFZ region showing major structural units and seismic events. Hir, Hirondelle Seamount; GCAW, Gulf of Cadiz Accretionary Wedge; Red dots are epicenters of M > 4 earthquakes from the International Seismological Centre (ISC) for the period 1970–2017. Focal mechanisms from the database compiled by Custódio et al. (2016) (M > 5.5). Gray arrows represent vectors from the relative Africa-Eurasia velocity field as modeled by the neotectonic model of Neres et al. (2016).
The Eastern and Western segments are sub-parallel to the movement of Africa with respect to Eurasia, at a small angle that generates transpression along a 50 km wide narrow belt of deformation. The Central segment is at a high angle to the motion of Africa with respect to Eurasia, creating a restraining bend of compressive deformation. The Gloria Fault West segment is characterized by a record of low seismic activity, which may be an expression fault locking or slow creeping. The Central and Eastern segments have a record of high to very high magnitude earthquakes, including the M8.4 event of 1941 and the M7.1 of 1931 (Figure 5). The 1941 event was, until the 2012 Sumatra earthquake in the Indo-Australian plate, the largest strike-slip event ever recorded. Interestingly, another very high magnitude event (M8.1) was recorded 200 km south of the plate boundary, probably reactivating an old fracture zone (Lay, 2019). This suggests that the deformation may be distributed over a wide zone rather than localized along a single fault trace.
Recent work on the western segment by Batista et al. (2017) showed the existence of a 4 km thick layer between 12 to 16 km below sea level suggesting the existence of hydrated mantle and implying seawater circulation along fractures to depths of 12 km below seafloor. Faults imaged in shallow reflection seismic profiles are compatible with a present day active transpressive flower-structure that is responsible for creating a linear positive ridge along the whole length of the Gloria fault.
To the East of the Madeira-Tore Rise (MTR) the Africa-Eurasia plate boundary has been described as diffuse on the basis that tectonic deformation is accommodated along several active structures within a 200 km wide (∼600 km long) zone of transpressive deformation between two main thrust systems (Sartori et al., 1994): the Gorringe Bank thrust that involves lithospheric mantle (Sallarès et al., 2013) and the Coral Patch Seamount thrust that involves oceanic crust (Martínez-Loriente et al., 2014). The main dextral strike-slip movement, caused by the oblique motion of Africa with respect to Eurasia, is materialized by the existence of a set of faults, the SWIM Faults, which seem to mark the present-day plate boundary between the MTR and the Strait of Gibraltar (Rosas et al., 2009; Zitellini et al., 2009). However, these faults do not show significant seismicity, which may suggest creeping, related with rheological weakening induced by fluid circulation and mantle hydration (Silva et al., 2017). The SWIM Faults control the location of mud volcanoes within and off the Gulf of Cadiz Accretionary Wedge. Fluid geochemistry of mud volcanoes in this area suggest that seawater circulation along the SWIM Faults reach the oceanic basement, ca. 5 km below seafloor (see section “Pore Fluid Geochemistry of Seafloor Seeps”; Hensen et al., 2015). The SWIM strike-slip faults propagate into crustal thrust faults and the intersections of these faults are also the loci of seismicity clusters (Silva et al., 2017). A recent study shows evidence for large-scale, tsunamigenic landslides in the Quaternary at the Hirondelle seamount (Hs), which is bound by splays of the SWIM Faults (Omira et al., 2016).
The SW Iberian Margin is characterized by shallow to deep earthquakes of low to moderate magnitude with a variety of focal mechanisms (Mw < 5.5; Buforn et al., 1991; Stich et al., 2003, 2007; Custódio et al., 2016). Notwithstanding, this region is also the source of the largest and most destructive earthquakes that have affected Western Europe (AD 1531, 1722, 1755, and 1969; Fukao, 1973). The 1755 Great Lisbon Earthquake (Mw > 8.5) destroyed Lisbon and was accompanied by tsunamis that devastated the SW Iberian and NW African coasts (Baptista et al., 1998; Baptista and Miranda, 2009). On the basis of geological evidence, geophysical data and tsunami modeling, different geodynamic models and mechanisms have been proposed for the source of the Lisbon Earthquake (cf. Gutscher, 2004; Gutscher et al., 2002, 2006; Gràcia et al., 2003; Zitellini et al., 2004, 2009; Stich et al., 2007; Terrinha et al., 2009). However, none of these models satisfactorily accounts for the estimated magnitude of the earthquake and tsunami arrival times at the different localities onshore, which suggests interference of various processes from mantle to surface, such as multiple rupture across the lithosphere and crust and slip along inter-connected strike-slip and thrust faults (Rosas et al., 2009; Silva et al., 2017) and large scale landsliding in the epicentral area (Gràcia et al., 2003; Terrinha et al., 2003; Lo Iacono et al., 2012; Omira et al., 2016). The AD 1969 thrust earthquake (M 7.9) that occurred within the flat Horseshoe Abyssal Plain can be a recent reactivation of fracture zone-thrust fault interference (Figure 5; Rosas et al., 2016).
The North Anatolian Fault in the Sea of Marmara and the Aegean Sea
The NAF is a right lateral, strike-slip fault separating the Eurasian and the Anatolian Plates. The NAF extends over 1500 km, from the Eurasia-Anatolia-Arabia triple junction near Karliova (∼41°E) in eastern Turkey into the North Aegean Trough in the Aegean Sea and terminates in the 1600 m deep Sporadhes Basin at the eastern margin of the mainland of Greece (Figure 6; e.g., McKenzie, 1978; Armijo et al., 1999; Barka, 1999; Le Pichon et al., 2001, 2015; Şengör et al., 2014 Çağatay and Uçarkuş, 2019).
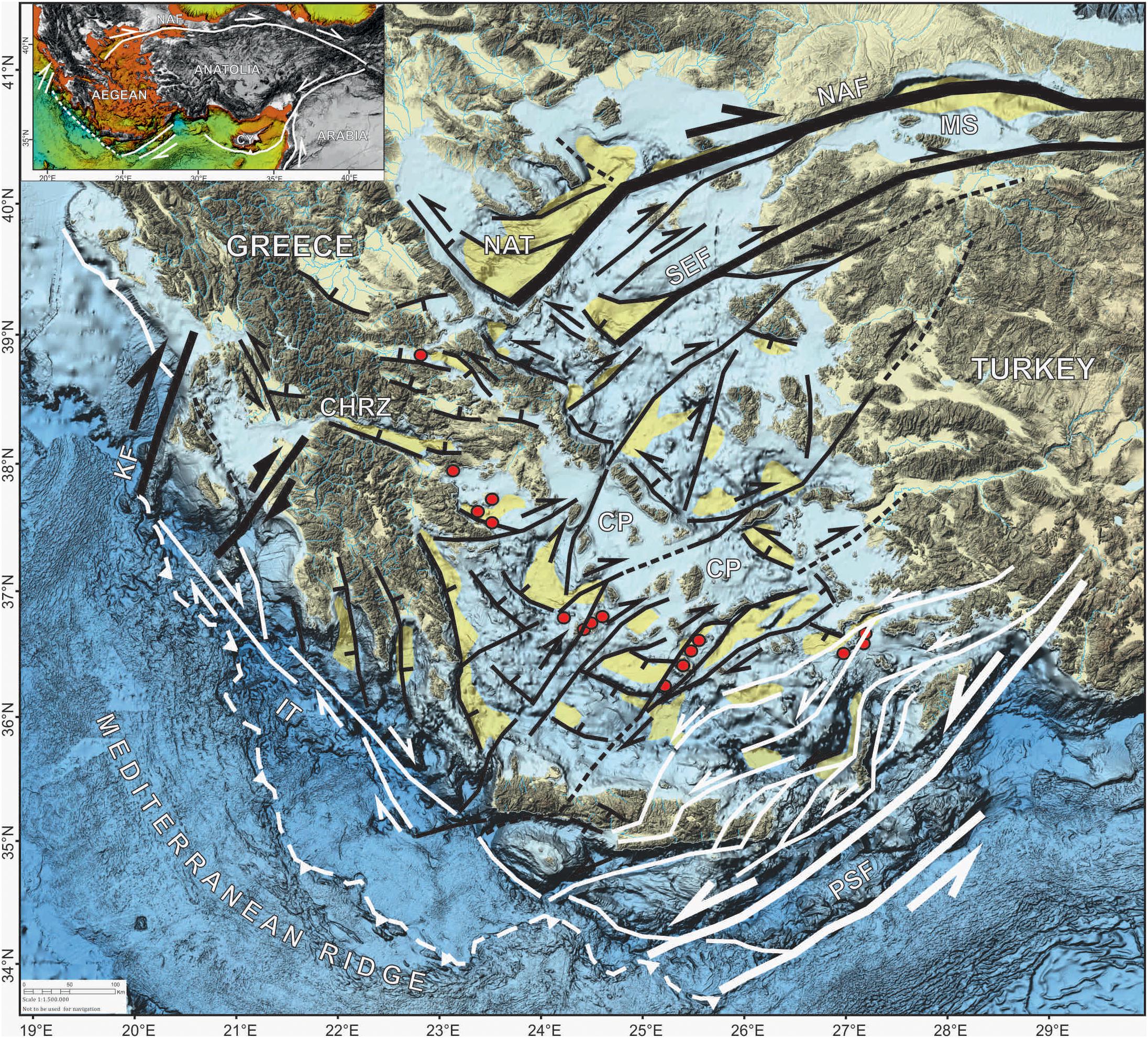
Figure 6. Tectonic and kinematic map of the NAF and the Aegean region based on interpretations by Sakellariou and Tsampouraki-Kraounaki (2019). The NAF, the Skyros-Edremit Fault (SEF) and major dextral, NE-SW trending fault zones in the Aegean along with their conjugate faults are drawn in black. The Pliny and Strabo Fault zones (PSF) and the sinistral fault zones in the Southeast Aegean are drawn in white. Yellow: Plio-Quaternary basins. Red dots: volcanoes of the South Aegean Volcanic Arc. KF: Kephallinia Fault; CP: Cyclades Plateau; IT: Ionian Trench; MS: Marmara Sea.
The North Aegean Trough has been very early associated with the westward prolongation of the NAF and considered to represent the northern boundary of the deforming Aegean region (McKenzie, 1978; Le Pichon and Angelier, 1979, 1981; Armijo et al., 1999). The main NAF zone consists of many segments running along the southern steep margin of the North Aegean Trough and give rise to transpressional ridges and transtensional basins between them (Sakellariou et al., 2018; Sakellariou and Tsampouraki-Kraounaki, 2019). GPS campaigns have confirmed the dextral shear along the NAF in the North Aegean Sea with strike-slip motion rates between 7 and 21 mm per year decreasing from east to west (Müller et al., 2013). Within the dominant NE-SW dextral shear regime, the Mw 6.4 Skyros 2001 earthquake related to strike-slip motion along the NAF in the North Aegean Trough (Ganas et al., 2005 and references therein). Moreover, the propagation of the NAF into the Northern Aegean Sea and the interaction with the Hellenic subduction in the Southern Aegean has strongly influenced the distribution of the volcanic centers (Armijo et al., 1999; Sakellariou and Tsampouraki-Kraounaki, 2019; Figure 6).
The submerged segment within the NAF is considered to be a natural laboratory for studying the coupling between fluid motion and fault slip (seismic and aseismic). In addition, the NAF is set along a former oceanic suture that holds ophiolitic bodies, which may influence both mechanical properties and fluid geochemistry (Tryon et al., 2010; Burnard et al., 2012). Since 1939, the NAF has produced a unique sequence of M > 7 earthquakes, starting from eastern Anatolia and propagating to the west toward Istanbul (e.g., Armijo et al., 1999; Parsons et al., 2000). Prior to this sequence, which ended in 1999 with the devastating earthquakes of Izmit and Düzce (causing more than 20,000 casualties at the eastern end of the Sea of Marmara) the fault ruptured in 1912. This earlier rupture developed in the west at the transition into the North Aegean, leaving the Marmara section of the NAF as the only part of the fault not having generated a major earthquake in the past 250 years. The Istanbul-Marmara region between the 1912 and 1999 ruptures is thus generally considered to represent a seismic gap with a potential for earthquake generation of up to M7.4 (Aochi and Ulrich, 2015 and references therein). As a consequence, the assessment of a seismic hazard for the densely populated (>15 million) greater Istanbul region is a matter of continuous investigation. Probabilistic interseismic models constrained by published GPS data sets, suggest that the Ganos and Çınarcık segments are locked, while creep is detected in the central portion of the Marine Marmara Fault (MMF; Ergintav et al., 2014; Klein et al., 2017). Submarine, acoustic-based geodetic data rather suggest locking along the Central segment (Sakic et al., 2016) and creeping along the Western High segment (Yamamoto et al., 2018). Thus, further geodetic studies are needed to provide a more complete picture.
Submarine paleoseismological techniques have been extensively applied at various key locations along the two submerged branches of the NAF in the Sea of Marmara. These reveal an average slip rate of 10 mm/year over the last ∼10 ka and long-term sedimentary records of earthquakes in the Sea of Marmara (e.g., Polonia et al., 2004; Sarı and Çağatay, 2006; McHugh et al., 2006, 2014; Gasperini et al., 2010, 2011; Drab et al., 2012; Çağatay et al., 2012). Slip rates are smaller than those determined by the GPS geodetic measurements, which are 18–20 mm/yr for the Northern branch (i.e., the MMF) and 5–7 mm/yr for the middle branch (McClusky et al., 2000; Meade et al., 2002). The discrepancy between the geological and geodetic slip rates could be explained by (i) changing rates of deformation over time, (ii) the deformation being accommodated by other faults in the region, (iii) or by the difficulties in modeling GPS data due to the water cover. Along with submarine paleoseismology, the correlation between the seismoturbidite records and the historical records over the last 2500 years reveals an average recurrence time of 250–300 years for the northern branch of the NAF (Sarı and Çağatay, 2006; McHugh et al., 2006, 2014; Beck et al., 2007; Drab et al., 2012; Çağatay et al., 2012), in agreement with the geodetic measurements (McClusky et al., 2000; Meade et al., 2002). The extensive seismological studies conducted over the last two decades have considerably improved our knowledge of the seismicity along the NAF. Since 2011, the identification of a precursory sequence of slow slip occurring at the base of the brittle crust, 44 min prior to the Mw 7.6 Izmit earthquake (Bouchon et al., 2011), has fostered the acquisition of new data in search of seismic tremors. The seismicity along the MMF is now well known to exhibit strong lateral variability (Schmittbuhl et al., 2016).
The Marmara Fault System is also characterized by an intense fluid activity at the seabed (Halbach et al., 2004; Armijo et al., 2005; Kuşçu et al., 2005; Géli et al., 2008; Zitter et al., 2008; Dupré et al., 2015) since it provides a major pathway for the seafloor expulsion of thermogenic hydrocarbons generated in Eocene-Oligocene Thrace Basin source rocks (Bourry et al., 2009; Ruffine et al., 2018a), possibly generating gas-induced seismicity (Batsi et al., 2018; Géli et al., 2018). These aspects will be discussed in more depth subsequently in Section “Pore Fluid Geochemistry of Seafloor Seeps” along with more detailed information of seep locations and fluid sources.
Heat and Fluid Flow at Marine Transform Faults and Fracture Zones (MTFFZs)
Based on the structural and geodynamic framework provided above, this chapter compiles the current knowledge on heat and focused fluid flow at MTFFZs, depicting some of the most interesting regions, and highlighting important processes and mechanisms affecting fluid geochemistry, and how they stimulate and sustain chemosynthetic life in the deep sea.
Heat Flow
As the lithosphere created at mid-ocean ridges (MORs) cools and subsides during lateral seafloor spreading, heat flow from the lithosphere to the ocean decreases. Models of a conductively cooling lithosphere predict that heat flow decreases linearly with the inverse square root of age, and that at the same time the depth of the ocean basins (subsidence) increases linearly with square root of age [further details in Davis and Elderfield (2004); Hasterok (2013); and references therein]. Heat flow at ridge flanks is highly variable but systematically lower than predicted by conductive cooling models. This heat deficit on ridge flanks is attributed to an incomplete or thin sediment cover, which allows a direct exchange of energy and mass between ocean and upper crust. Once the sediment cover is continuous and the hydraulic impedance of the sediment layer is above a critical threshold (about tens of meters), advective exchange between ocean and crust is significantly reduced (Spinelli et al., 2004). Consequently, at about a crustal age of about 65 ± 10 Ma, observed and predicted heat flow values are approximately equal, which indicates that crustal permeability is reduced below a level where advection of fluids is no longer possible (Manning and Ingebritsen, 1999). This crustal age is commonly referred to as “sealing age” (Stein and Stein, 1994). Heat flow on ocean crust >100 Ma is generally uniform (45–50 mW m-2; Davis et al., 1984; Lister et al., 1990) suggesting that the thermal structure of the lithosphere has stabilized in terms of energy input at its base from the convecting asthenosphere.
Investigations on the well-sedimented ridge flank in the Cascadia Basin (NE Pacific) revealed that faults and seamounts may act as places where seawater recharges into or discharges from the upper crust (Fisher, 2005). Recharge sites are characterized by a wide and clear depression in heat flow around the site (cooling halo) whereas discharge sites show very localized heat flow values up to a magnitude higher than regional values. Fisher (2005) summarized this concept of off-axis hydrothermal circulation and termed it “hydrothermal siphon,” a widespread phenomenon on Pacific crust (Hutnak et al., 2007; Kuhn et al., 2017; Villinger et al., 2017) as well as on Atlantic crust (Lucazeau et al., 2006; Le Gal et al., 2018). Moreover, a comparison of observed heat flow pattern and modeled heat flow based on known sediment thickness (depth to basement) reveals the pattern and strength of hydrothermal circulation in the upper crust. Therefore, seafloor heat flow measurements are a powerful, and currently the only tool to image hydrothermal circulation in the upper crust (Davis et al., 1997; Fisher and Harris, 2010; Le Gal et al., 2018).
Due to the structural weakness and permeability of transform faults and fracture zones, significant seafloor heat flow anomalies and possibly hydrothermal activity are to be expected. However, only very few detailed heat flow surveys across a fracture zone or a transform fault have been made up to now (von Herzen et al., 1970; Kolandaivelu et al., 2017). Published data from Khutorskoi and Polyak (2017) from the Vema fracture zone in the equatorial North Atlantic indicate remarkably uniform and high heat flow, with values between 100 and 250 mW m-2 (coinciding with similar results by Langseth and Hobart, 1976). Rather than focussing on MTFFZs, most of the offshore data from the Global Heat Flow Database (data from 1965 to 1985; Figure 7) were collected in order to characterize the marine heat flow pattern in conjunction with plate tectonics, and hence the cooling of the oceanic lithosphere. Only very few profiles were dedicated to fracture zones and transform faults. The latest compilation and interpretation of such data sets was recently published (Khutorskoi and Polyak, 2017), however, the original articles from which the data came from do not contain locations of these measurements.
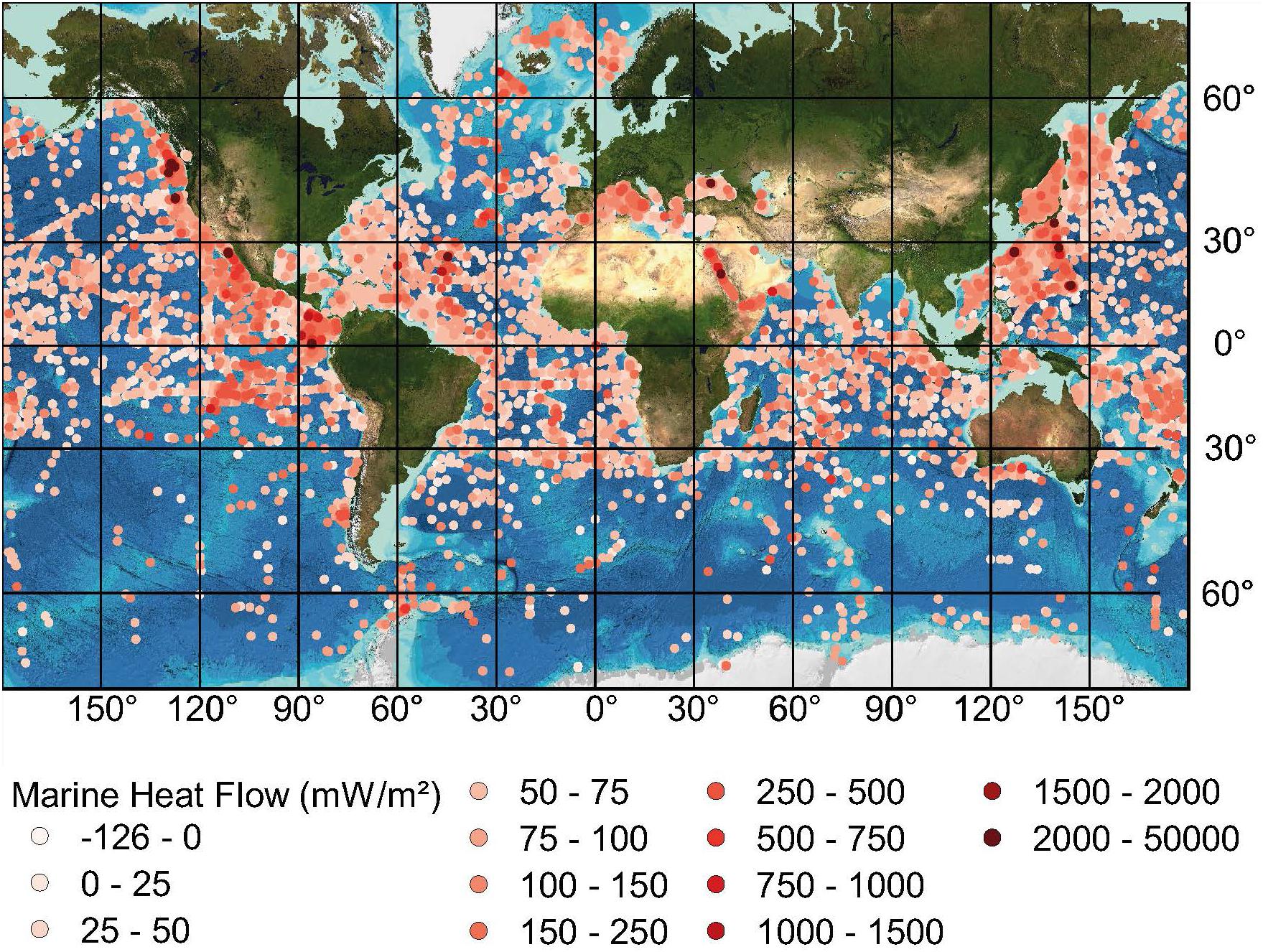
Figure 7. Global distribution of heat flow measurements at water depths >500 m (Global Heat Flow Compilation Group, 2013; https://doi.pangaea.de/10.1594/ PANGAEA.810104). Highest values are found at active spreading centers. To date, no systemic deviations along/across MTFFZs have been observed (background map is reproduced from the GEBCO world map 2014, www.gebco.net).
Compared to direct heat flow measurements, more research has been done involving modeling of heat flow at MTFFZs. Numerous articles on numerical or analytical models on the thermal-mechanical structure and behavior of a transform fault were published in the last 30 years with Roland et al. (2010) being the latest one containing a rich bibliography of research done in this field. Their 3D numerical model incorporates viscoplastic rheology, shear heating, and hydrothermal circulation in the upper crust and predicts elevated heat flow across transform faults. The model of Roland et al. (2010) was applied to the Blanco Transform Fault (Northeast Pacific) and the results are constrained by maximum earthquake depth, which the authors associate with the 600°C isotherms. Gerya (2012) and Gerya (2013a,b) reviewed the modeling of ridge segmentation and hence the development of transform faults and fracture zones. The emphasis of their modeling attempts is, however, on the seafloor expression of the underlying processes and the comparison with the observed bathymetry.
Overall, the presented literature and database search revealed that heat flow investigations at transform faults and fracture zones are a neglected research field. Yet, none of the published numerical models calculate seafloor heat flow, which would be, apart from the maximum depth of earthquakes, another helpful constraint for the models.
Pore Fluid Geochemistry of Seafloor Seeps
Fluid discharge at the seafloor is known from hot vents at mid-ocean ridges (e.g., Elderfield and Schultz, 1996; Von Damm, 2013; German and Seyfried, 2014), cold seeps and mud volcanoes at continental margins (e.g., Suess, 2014; Mazzini and Etiope, 2017), serpentinite-hosted fluid systems (e.g., Mottl et al., 2004; Okumura et al., 2016), as well as low-temperature discharge at seamounts (Wheat and Mottl, 2000; Wheat and Fisher, 2008). This distribution is well documented by numerous publications and illustrated in Figure 2, while reported findings from transform plate boundaries remain sparse (e.g., Martin et al., 1997). Hydrothermal circulation has been recognized as an important factor in global geochemical cycles and exchange processes between the lithosphere and the hydrosphere (e.g., Elderfield and Schultz, 1996; Davis and Elderfield, 2004). Flow rates at cold seeps are much lower and geochemical deviations from seawater, typically depending on the geological setting, source depth, etc., are mostly less pronounced when compared to hot vents. Whereas hydrothermal activity at spreading axes and ridge flanks has been relatively well investigated (e.g., Von Damm, 1990; Elderfield et al., 1999; Humphris and Klein, 2018; Le Gal et al., 2018), almost nothing is known about crust-seawater exchange on old oceanic crust.
As detailed above, heat flow data imply a general decline of hydrothermal circulation at crustal ages of >60 Ma due to cooling, cementation of pore space, and sealing by increasing sediment coverage. Due to this paradigm the vast area between spreading axes and the continents has largely remained unexplored with regard to fluid exchange and fluid seepage. Research over the past 15 years has, however, shown that hydrothermal circulation can even occur in oceanic crust that is older than 60 Ma (Von Herzen, 2004; Fisher and Von Herzen, 2005). Yet, a hydrological connection that results in significant exchange of energy between the permeable upper crust and the seafloor may only develop under specific conditions where seamounts or active faults provide suitable pathways through otherwise impermeable sediment layers.
In addition to seamounts, favorable structural settings for such fluid exchange between the crustal lithosphere and hydrosphere include transform faults and fracture zones. Currently, most examples are from active transform fault settings in young oceanic crust. Examples range from low-temperature hydrothermal systems with barite chimneys and associated mineralizations (Blanco Fracture Zone; Hein et al., 1999), or cold seeps with vigorous methane flow from the subsurface (Mendocino Transform Fault: Stakes et al., 2002; Guaymas Basin: Paull et al., 2007). In the Atlantic, shallow fluid circulation through ultramafic oceanic crust at the Lost City Hydrothermal Field (Atlantis Massif: Kelley et al., 2001, 2005) causes active serpentinization and thus an energy source for chemosymbiotic fauna (see section “Chemosynthesis-Based Ecosystems”). Indications for chemoautotrophic life have also been reported for the active Vema transform fault (Cannat et al., 1991; Krylova et al., 2010). Recently, this evidence was confirmed by pore water anomalies along an E-W transect, indicating the advection of methane-rich fluids in this area (Figure 8; Devey et al., 2018).
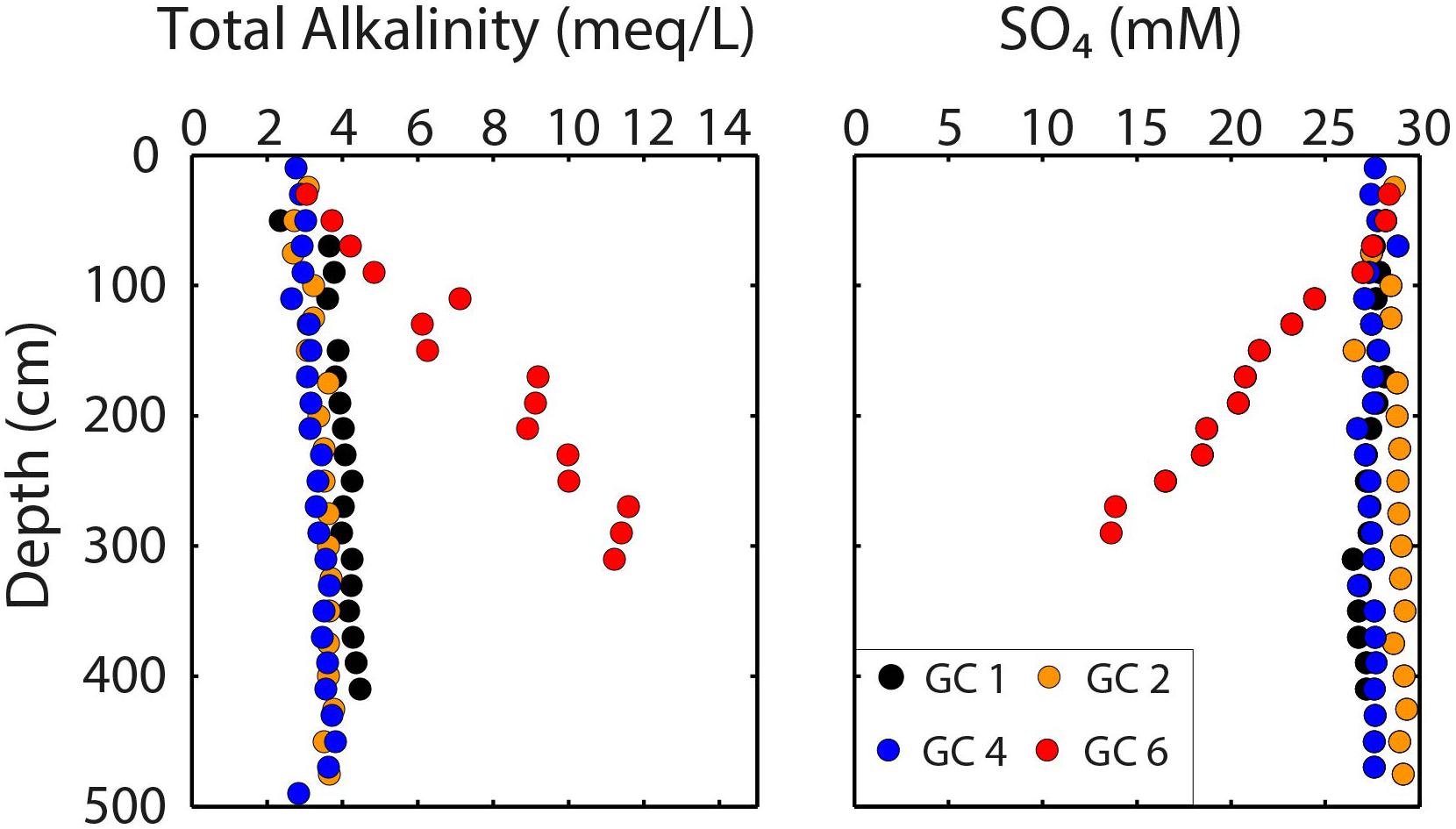
Figure 8. Pore water profiles of total alkalinity [from Devey et al. (2018)] and SO4 along an E-W transect on the Vema TFFZ (for locations see Figure 9).
Some recent evidence for fluid flow through older crust and discharge related to MTFFZs comes from deep-sea mud volcanoes in the Gulf of Cadiz (Eastern AGFZ; Figure 6). During RV Meteor cruise M86/5 in 2012, previously unknown mud volcanoes were discovered at great water depth of about 4500 m in a geo-tectonically atypical area, located roughly 90 km west of the deformation front of the accretionary wedge of the Gulf of Cadiz (Figure 9). Seismic data suggest that fluid flow is mediated by a >400-km-long strike-slip fault system proposed to represent a nascent plate boundary between Africa and Eurasia, reactivating old (Jurassic) oceanic fracture zones (Zitellini et al., 2009). In addition, geochemical data of pore fluids sampled at the mud volcanoes reveal that those fluids carry diagenetic signals from the oldest stratigraphic units and have at least partially interacted with the oceanic basement, hence, implying the existence of a hydrological connection from the seafloor to the oceanic crust (Scholz et al., 2009, 2010; Hensen et al., 2015; Schmidt et al., 2018; Nuzzo et al., 2019). The exact mechanism causing fluid migration is not known yet, but the recent findings (Hensen et al., 2015; Schmidt et al., 2018) indicate active fluid percolation through old (>140 Ma) oceanic crust, potentially induced by nearby crustal highs and adding further evidence to the notion that fluid circulation can continue at a crustal age of >65 Ma.
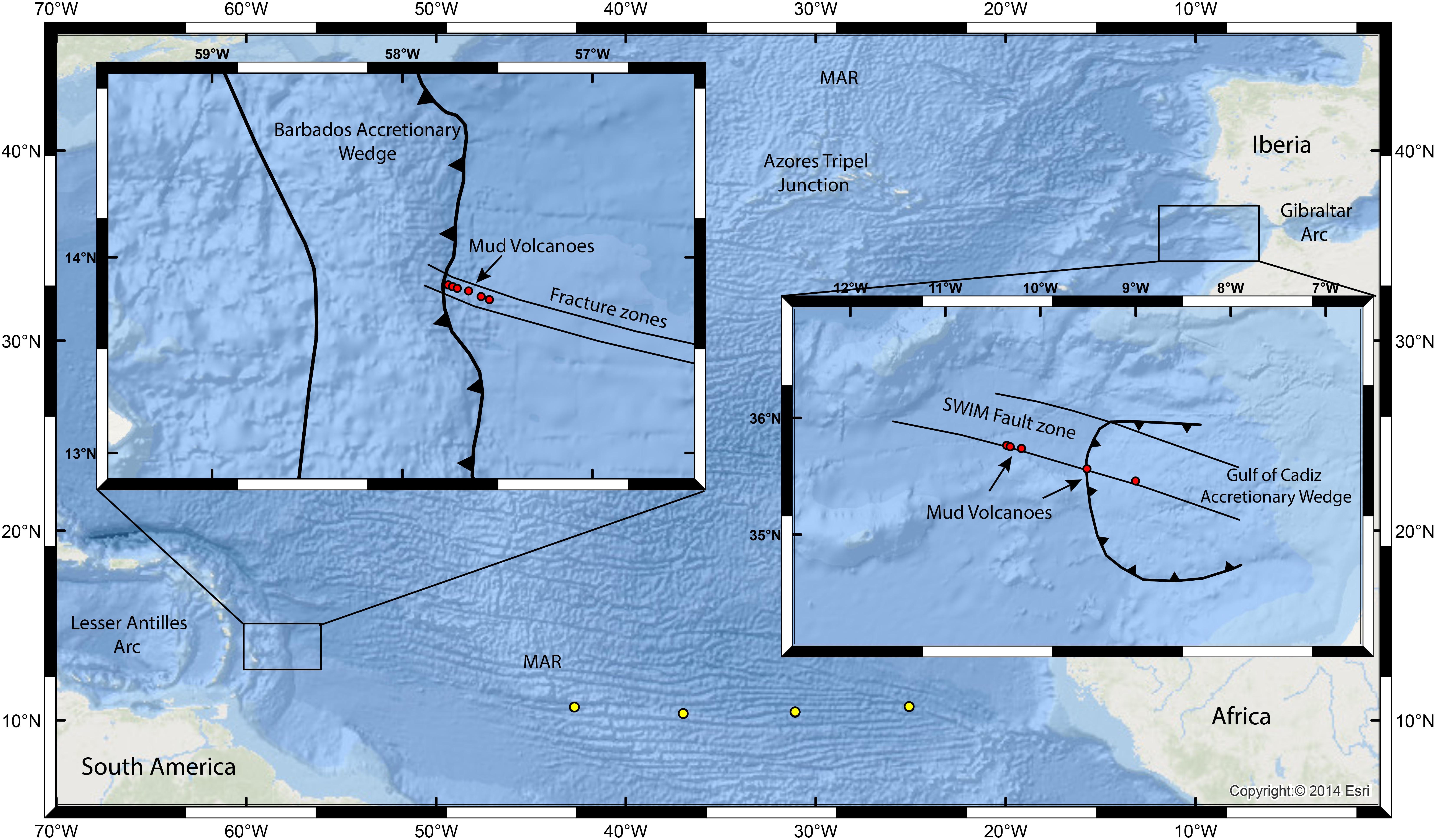
Figure 9. Mud volcanoes related to active strike-slip faulting in the western Gulf of Cadiz and aligned along the Mercurus Fracture zone offshore Barbados [adapted from Godón et al. (2004); Hensen et al. (2015)]. Yellow dots along the Vema TFFZ indicate the location of gravity cores shown in Figure 8 (bathymetric map was created using ArcGIS® software by Esri (www.esri.com).
Mud volcanoes expelling geochemically distinct fluids off the Barbados accretionary wedge (Dia et al., 1995; Godón et al., 2004) are found in a tectonic setting comparable to that in the Gulf of Cadiz. The mud volcanoes are aligned along the Mercurus fracture zone, which is running westward into the accretionary wedge of the subduction zone (Figure 9). By now, fluid mobilization and geochemical signals have been ascribed to subduction-related dewatering processes and associated mineral-water interactions. However, Sumner and Westbrook (2001) hypothesized that the mud volcanism along the Mercurus fracture zone was initiated by changes in plate motion along this fracture. Thus, processes similar to those in the Gulf of Cadiz might drive or at least contribute to the emergence of mud volcanoes off the Barbados accretionary wedge.
The fluid geochemistry of the mud volcanoes in Gulf of Cadiz and off Barbados is illustrated in Figure 10. Due to their location near continental margins, fluid geochemistry in both systems is dominated by sediment-water reactions (such as clay-mineral dehydration), illustrated by pronounced B-enrichment and Cl-depletion (e.g., Hensen et al., 2004). Similar B-enrichments are known from sediment-hosted hydrothermal systems, such as the Guaymas Basin. Here the dilution component is lacking, because B is extracted from sediments by percolation of saline, and thus Cl-rich, hydrothermal water. In non-sedimented systems the concentration of B is typically close to seawater or even depleted due to serpentinization. The lithospheric imprint becomes obvious by low (non-radiogenic) 87Sr/86Sr ratios and is most pronounced in hot hydrothermal systems. In the Gulf of Cadiz, the 87Sr/86Sr values are a combined signal from ancient (Mesozoic) carbonates and oceanic crust. For Barbados, there are no specific investigations available, but the interpretation of the 87Sr/86Sr is further complicated by widespread abundance of volcanogenic sediments.
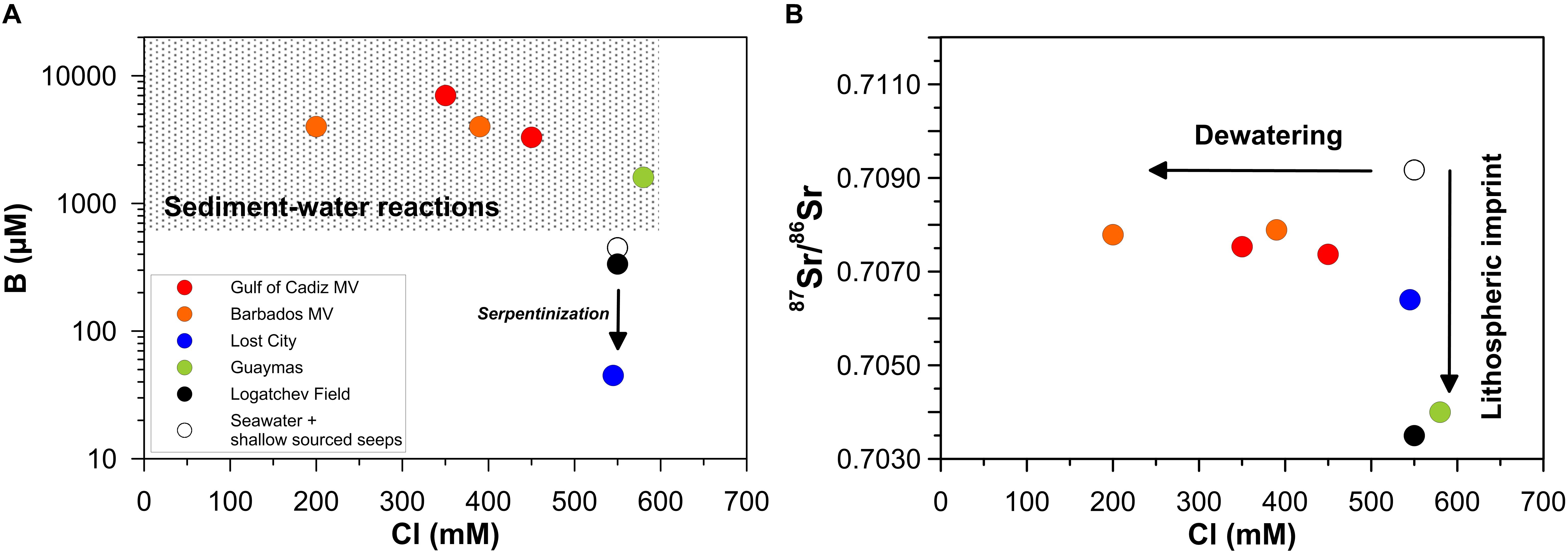
Figure 10. Cross plots of B vs. Cl (A) and 87Sr/86Sr vs. Cl (B) of fluid endmembers from mud volcanoes in the Gulf of Cadiz and off Barbados compared to endmembers from different hydrothermal systems; Lost City, off-axis, serpentinization; Logatchev Field, ridge-crest, high temperature; Guaymas, sediment hosted, high temperature. Data from: Von Damm et al. (1985), Palmer (1991), Godón et al. (2004), Schmidt et al. (2007), Foustoukos et al. (2008), Scholz et al. (2009), Hensen et al. (2015) and Seyfried et al. (2015).
Intense fluid emissions at the seafloor have also been detected in the continental margin setting along the NAF in the Sea of Marmara. In the shallow domain, within the Izmit Bay, the intimate link between fluid (gas) release and earthquake has been evidenced from seafloor inspections after the 1999 Izmit earthquake where sediment fluidization and cracks have been observed at locations above gas-charged sediments (Kuşçu et al., 2005; Gasperini et al., 2012). However, direct evidence for the coupling of seismicity and fluid flow remains difficult to prove (Embriaco et al., 2014; findings and state of research of this aspect are further explored in section “Fluid Flow and Chemical Indicators Related to Seismic Activity”). In the deeper parts of the Sea of Marmara, the nature and sources of fluids and gases were investigated during a number of cruises within the past two decades, specifically dedicated to a deeper understanding of how geodynamic processes along the NAF may control fluid flow in the subsurface (e.g., Géli et al., 2008; Bourry et al., 2009; Dupré et al., 2010, 2015; Grall et al., 2018a,b; Ruffine et al., 2018a,b; Figure 11). Emitted hydrocarbon gases indicate that deeper thermogenic sources are tapped in the western basin while microbial gases prevail in the eastern basin. In the western basin, there is also evidence for mantle-derived helium (Burnard et al., 2012; Ruffine et al., 2018a) and pore water data shown by Tryon et al. (2010) allow speculations about ongoing serpentinization in the deeper subsurface. Aseismic slip in the western Sea of Marmara may effectively maintain high permeability conduits in the NAF zone. In contrast, the long-term seismic quiescence (since 1766) and recent low seismicity along the Istanbul segment suggest that this segment is currently locked, providing a primary factor for the relative scarce gas emissions. Because hydrocarbon occurrence is a widespread phenomenon at ocean margins, the relation between gas emissions, seismicity and crustal creeping opens new perspectives for improving our understanding of fault behavior and geohazard assessment in gas-prone, submarine environments.
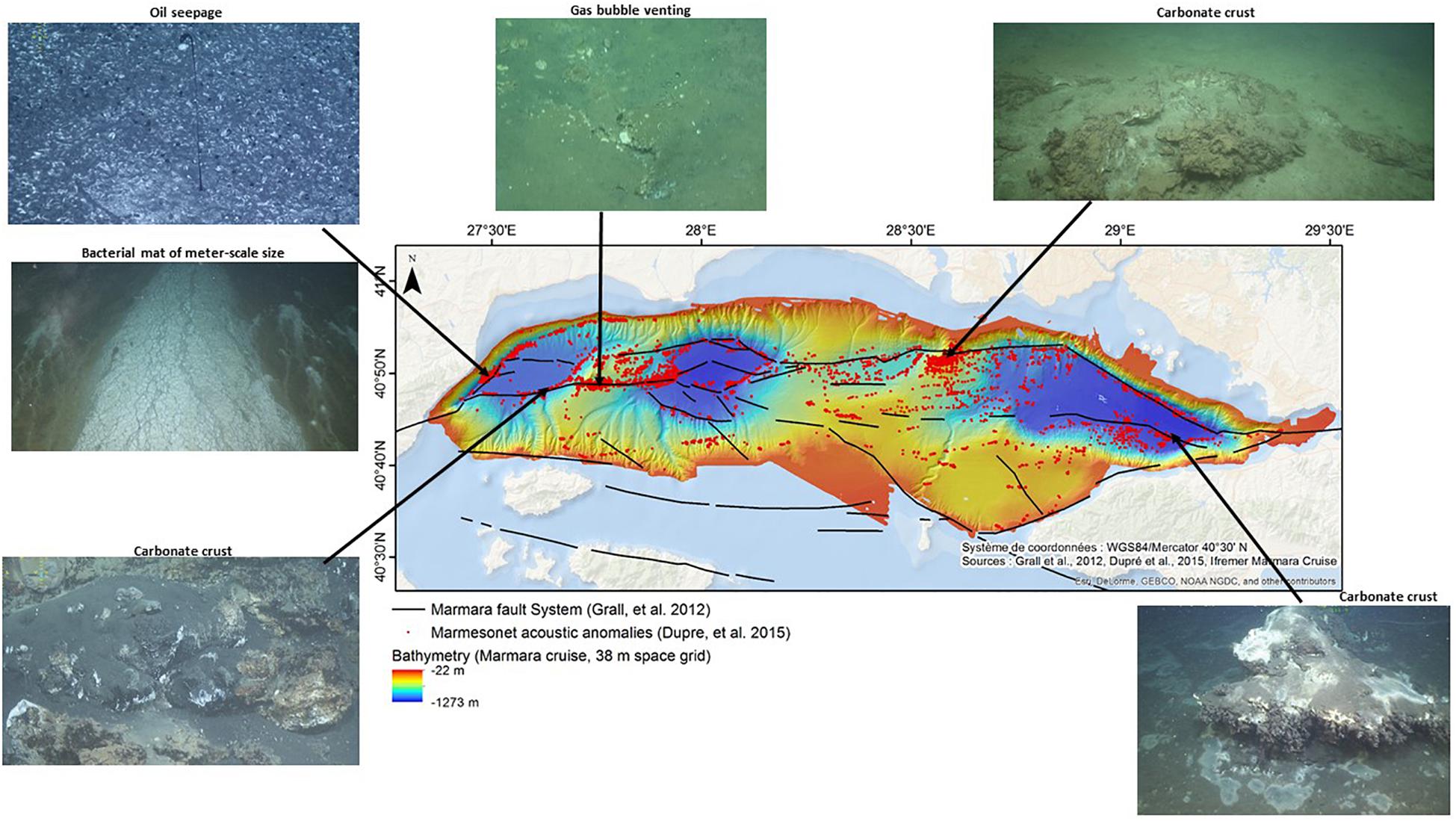
Figure 11. Bathymetric map of the Sea of Marmara highlighting the link between structural features and gas emissions [data from Grall et al. (2012, 2018a), Ruffine and Geli (2014), Dupré et al. (2018)]. A large variety of carbonate crusts and seeps (oil and gas) are widespread along the fault network. Fluid discharge sustains the development of bacterial mats at the seafloor.
Reports of mud volcanoes in deep-sea settings related to MTFFZs are still quite rare, but existing findings encourage further exploration. Evidence for methane-rich fluid venting has been reported e.g., from the eastern part of the Mendocino fracture zone in the Northeast Pacific (Stakes et al., 2002). Globally, transform-type plate boundaries are of similar length as convergent plate boundaries (∼50,000 km; Bird, 2003), which are known for intense seepage activity. Recently, Coogan and Gillis (2018) provided evidence that fluid fluxes at off-axis hydrothermal systems are likely important factors modulating ocean chemistry, but additional studies are indispensable to substantiate current estimates. Taking also into account the vast stretches of fracture zones, MTFFZ might bear a considerable potential for fluid discharge at the seafloor. However, based on the available data, we can only speculate about the role of MTFFZs as pathways for fluid exchange between the lithosphere and the deep ocean. Hence, systematic efforts are required to estimate the potential impact on geochemical cycles and life in the abyssal ocean.
Chemosynthesis-Based Ecosystems
The global impact of transform faults and fracture zones on life is still poorly understood. Several studies have shown that transform faults act as barriers to seafloor invertebrate dispersal (Johnson et al., 2006), including the dispersal of larvae of hydrothermal vent macrofauna (Young et al., 2008). This is because transform faults act as topographic breaks to the ridge axes formed during seafloor spreading, and as such interfere with the predominant along-axis water flow in spreading centers. The resulting cross-axis water flow through the transform fault and fracture zone can obstruct genetic exchange between adjacent sides of the ridge axis, and thus promote genetic drift and speciation, both among typical deep sea macrobenthos and highly specialized chemosymbiotic hydrothermal vent macrofauna (O’Mullan et al., 2001; Hurtado et al., 2004).
In locations where lithosphere-derived fluids are actively transported to the seafloor, transform faults are furthermore hotspots of life. First evidence for the support of chemosynthetic, biological communities by abiotically produced chemical compounds at transform faults came from the Lost City Hydrothermal Field. Fluids advecting out of the seafloor in this location are characterized by high pH (9–11), moderately warm temperatures within the temperature limits of life (40–90°C), and high concentrations of H2 (Kelley et al., 2001, 2005) produced by subseafloor serpentinization (Früh-Green et al., 2003). High concentrations of dissolved hydrocarbons, in particular methane, and organic acids, in particular formate, are also present in these fluids (Kelley et al., 2001, 2005; Lang et al., 2018), and have been explained with Fischer-Tropsch-Type or Sabatier-Type synthesis reactions involving serpentinization-derived H2 and mantle-derived inorganic carbon that is released by the weathering of host rocks (McCollom and Seewald, 2007; Prokurowski et al., 2008; Bradley and Summons, 2010). Analyses of methane isotopologues have suggested methane in aqueous fluids of the Lost City Hydrothermal Field to be formed under equilibrium temperature conditions around 310°C (Wang et al., 2018). In contrast, methane-carbon isotope analyses from lower temperature onshore serpentinizing environments such as found in Costa Rica and Turkey revealed that methane may be also partially formed biologically (Crespo-Medina et al., 2017; Zwicker et al., 2018). Evidence is based on carbon isotope fractionation being severely reduced under high partial pressure of H2 and its availability (Penning et al., 2005; Londry et al., 2008), thus eventually mimicking abiotic or thermogenic methane formation. Furthermore, under conditions similar to those found in the subsurface of the Lost City Hydrothermal Field, abiotic amino acid synthesis is thermodynamically favorable (Shock, 1990; Schulte and Shock, 1995). Aliphatic amino acids could be synthesized through Strecker-type reactions involving cyanide (HCN) and aldehydes or ketones (Holm and Neubeck, 2009; Konn et al., 2015), while aromatic amino acids could be abiotically produced via Friedel-Crafts reactions involving aromatic hydrocarbons as reactants and iron-rich saponite clays as catalysts (Ménez et al., 2018). Both types of abiotic amino acid synthesis reactions may help sustain chemoorganotrophic life at and below the seafloor of the Lost City Hydrothermal Field and in other ultramafic systems.
The mixing of deeply sourced fluids that are rich in electron donors, such as H2, methane, and organic acids, with bottom seawater containing high concentrations of the electron acceptors O2 and sulfate sustains a high biomass of aerobic and anaerobic H2-, methane-, and sulfur-metabolizing microbial communities in seafloor carbonate chimneys of the Lost City Hydrothermal Field (Kelley et al., 2005; Brazelton et al., 2006, 2012). Archaeal communities are dominated by H2-consuming methanogens and Anaerobic Methane-oxidizing archaea (ANMEs). Stable carbon isotope compositions of diagnostic archaeal lipid biomarkers imply extraordinary isotopic fractionations during carbon fixation in this environment (Bradley et al., 2009; Méhay et al., 2013). Sulfate-reducing bacteria also play an important role, possibly being the main consumers of abiotically produced formate (Lang et al., 2018). Macrofauna that are characteristic of high-temperature, “black smoker-type” hydrothermal vent environments (e.g., large tubeworms, Pompeii worms, mussels) are absent; instead a high biomass of sponges and corals is present (Kelley et al., 2001).
In addition to the Lost City Hydrothermal Field, several other locations within MTFFZs have been discovered, in which deeply sourced fluids are supporting abundant life at the seafloor. On the Gorda Escarpment in the Mendocino Fracture Zone off northern California, thriving chemosynthetic microbial and macrofaunal communities occur in areas where low-temperature methane-rich fluids are venting at exposed basement rock (Stakes et al., 2002). Macrofaunal communities are dominated by siboglinid tube worms (Lamellibrachia barhami) and vesicomyid clams. Both types of macrofauna are also found in cold seep environments along continental margins and forearcs, and are known to harbor aerobic sulfide-oxidizing bacteria as endosymbionts (Stakes et al., 2002; Levin, 2005; Dubilier et al., 2008; Ohara et al., 2012; Onishi et al., 2018). Along the Sonora Margin Transform Fault of the Guaymas Basin, there are cold seeps where dissolved inorganic carbon and methane of thermogenic origin are transported to the seafloor (Paull et al., 2007). Here, areas at the base of carbonate formations also have a high biomass of chemosymbiotic vestimentiferan tube worms and vesicomyid clams, whereas authigenic carbonates in places with fluid seepage are covered by sulfide-oxidizing microbial mats (Beggiatoa spp.) and harbor abundant populations of gastropods (Paull et al., 2007). Nearby sediments are also covered by Beggiatoa, which here coexist with a high diversity and abundance of ANMEs (Vigneron et al., 2013). Within cold seeps of the Sea of Marmara, high abundances of macro- and meiofauna, including symbiont-bearing bivalves (Vesicomyidae, Lucinidae, Mytilidae), are present at carbonate crusts and within soft sediments (Ritt et al., 2010). Methane seepage supports a high biomass of ANMEs (ANME-2a and -2c) and symbiotic sulfate-reducing Deltaproteobacteria (Desulfosarcina, -coccus, and -bulbus) in shallow subsurface sediments (Chevalier et al., 2011, 2013). High abundances of macrofauna (Siboglinidae) and high rates of anaerobic oxidation of methane coupled to sulfate reduction have furthermore been found in mud volcanoes of the Gulf of Cadiz (Niemann et al., 2006; Sommer et al., 2009). Yet, despite their locations in MTFFZs, most of the methane transported to the seafloor in both the Mendocino Fracture Zone and the Sonora Margin Transform Fault is likely of biogenic or thermogenic sedimentary rather than abiotic crustal origin.
The limited number of published studies already shows clearly that transport of deeply sourced fluids containing high concentrations of microbial energy substrates to the seafloor in MTFFZs can locally sustain a high diversity and biomass of microbial and macrofaunal life. Yet, the importance of serpentinization and other deep rock-fluid reactions as a source of these energy substrates in MTFFZs remains elusive, and more exploratory research on MTFFZs, including fracture zones that are located far off-axis relative to oceanic spreading centers, is needed. An additional important research aim will be to investigate the distribution of life below the seafloor in MTFFZs. Published studies indicate that microbial methane production and sulfate reduction – possibly supported by serpentinization – can occur at moderate temperatures (65° C) in olivine-rich, young (3.5 Ma) subseafloor basalt on the Juan de Fuca ridge flank (Lever et al., 2013). Similarly, active methane- and acetate-producing microbial communities have been linked to the turnover of H2 produced by serpentinization in deep subseafloor sediments of the Kumano mud volcano in the Nankai accretionary complex (Ijiri et al., 2018). Yet, currently nothing is known about the presence or activities of microbial communities in the subsurface of MTFFZs. In some places, these subsurface microorganisms could be an important but unknown sink for abiotically produced H2, methane, and organic acids, and consume these compounds before they reach the seafloor. As in other subseafloor environments (Hoehler and Jørgensen, 2013; Lever et al., 2015; LaRowe et al., 2017), the distribution of life in subseafloor environments within MTFFZs is most likely limited by the balance of catabolic energy obtained from the transformation of chemical compounds and the energetic cost of survival; the latter is largely driven by temperature-controlled rates of biomolecule damage, and other factors, e.g., pH and salinity, which affect the energetic cost of maintaining cellular homeostasis (Head et al., 2003; Harrison et al., 2013; Lever et al., 2015). Future investigations will reveal how the combination of locally high energy inputs, e.g., in areas of active fluid flow, affects the ability of microorganisms to cope with subsurface stressors, such as elevated temperature, highly alkaline pH, fluid compositions very different from those within cells, and whether a yet undiscovered, uniquely adapted, or globally important deep biosphere exists below the seafloor in MTFFZs.
Coupling of Seismicity and Fluid Flow
In the previous sections, we provided an overview on the current knowledge of fluid flow related processes at MTFFZs. Although, promising findings have been made, it is also obvious that large knowledge gaps exist, which need to be filled. This section provides an overview on the general relationship between seismic activity, faulting, fluid flow, and geochemical activity beyond the evidence from MTFFZs, also exploring the potential use of geochemical signals as precursors for seismic activity.
Fluid Flow and Chemical Indicators Related to Seismic Activity
The release of seismic energy and the associated static stress variations imposed by large magnitude earthquakes may cause abrupt changes in the near- and far-field of the fault. Hydrothermal systems, mud volcanoes, and magmatic volcanoes are geological environments particularly sensitive to dynamic and static stress variations because of their near-critical state at depth. Pore pressure deviating from hydrostatic conditions is a key condition to determine whether incoming seismic energy and long-term variations of the local stress field may promote a response. Triggered activity occurs over a range of timescales and distances spanning from seconds to decades and from the near- to the far-field (Hill et al., 2002; Delle Donne et al., 2010; Mazzini and Etiope, 2017; Miller and Mazzini, 2018).
The effect of earthquakes on pore pressures may cause processes such as rectified diffusion, gas exsolution, and/or unclogging of migration pathways (Hill et al., 1995; Manga and Brodsky, 2006). Reported phenomena are changes in groundwater flow rates (e.g., Tsunogai and Wakita, 1995; Brodsky, 2003), temperature fluctuations at mid ocean ridges (Johnson et al., 2000), water level changes in deep wells (Roeloffs et al., 2003), or variations in geyser periodicity (Husen et al., 2004). Examples of fault reactivation have been reported, among others, on Iceland after the Mw 6.6 2000 earthquake (Pagli, 2003), in Indonesia after the Mw 6.3 Yogyakarta earthquake (Mazzini et al., 2009), and in Costa Rica after the Mw 7.6 Nicoya earthquake (Lupi et al., 2014). In the Costa Rican forearc, the occurrence of seismic swarms in the subducting plate has been related to upward fluid flow eventually feeding seafloor seepage structures (Thorwart et al., 2014). Triggered fault slips are frequently connected to elevated fluid pressures at depth that induce rapid fluid flow along the fault plane (Miller et al., 2004; Lupi et al., 2011a) and may result in phenomena like flash vaporization of interstitial fluids (Weatherley and Henley, 2013).
In this context, seismically active areas have been studied in the past decades with the goal of associating gas anomalies to the occurrence of seismic events, e.g., earthquakes have been related to the release of seafloor hydrocarbons (Mau et al., 2007; Tsunogai et al., 2012; Fischer et al., 2013; Geersen et al., 2016). The most commonly investigated concentrations of elements were radon, helium, hydrogen, mercury, carbon dioxide, and several other volatiles (King, 1986). Geochemical anomalies preceding seismic activity, e.g., at fault junctions or above fractured reservoirs, may last from a few hours to several days (Claesson et al., 2007; Walia et al., 2009; Skelton et al., 2014). For instance, co-seismic geochemical variations in springs and gaseous vents were recorded during the 1997 seismic crisis in Italy (Miller et al., 2004). In Taiwan, Yang et al. (2006) identified significant variations in CO2 and CH4 emissions from mud pools along regional fracture zones so that monitoring of CO2 and CH4 seems to represent a potentially valuable tool for detecting intimate relationships between seismic activity and fluids. Helium (Sano et al., 1986, 2014), argon (Mamyrin and Tolstikhin, 1984) and radon concentrations (Fu et al., 2008; Attanasio and Maravalle, 2016) have been used in continental settings to analyze the response of terrestrial fluid emanation with respect to seismic activity. It is important to note in this context that the relationship between gas emissions and earthquakes seems to rely mostly on the gas flux variability (Kita et al., 1982; Field and Jennings, 1987; Hirose et al., 2011). Sano et al. (2014) reported 3He anomalies in relation to the catastrophic 2011 Tohoku-Oki earthquake (M9.0) suggesting the emission of mantle fluids along the subduction zone offshore Japan. Enrichment in 3He was also measured in gases from hot springs after the 1984 Nagano earthquake (M6.8; Sano et al., 1986) and related to deep-seated fluids being forced upward by earthquake-induced tectonic stress. However, the opposite pattern (4He enrichment, resulting in lower 3He/4He ratios) was observed after the 1995 Kobe earthquake (M7.2) and attributed to the generation of micro-fractures releasing radiogenic 4He from the rock matrix. Hence, the interpretation of volatile anomalies in relation to seismic events depends strongly on the geological setting and the available geochemical information. For instance, terrigeneous fluids in sediments of Lake Van (Turkey) located at the eastward extension of the NAF showed crustal He enrichments prior to the occurrence of the devastating M7.2 earthquake in 2011 (Tomonaga et al., 2011, 2012, 2014). Recently, Sano et al. (2016) could relate radiogenic 4He anomalies to the strain change in an active strike-slip fault system produced by the 2016 Kumamoto earthquake (M7.3), emphazising the potential use of such monitoring approach in other geological settings.
Mud volcanoes seem to be geological phenomena particularly sensitive to external forcing. The onset of mud volcanism is in fact often related to seismic activity, with many examples of earthquakes affecting or triggering onshore and offshore mud volcanoes (e.g., Kopf and Deyhle, 2002; Brodsky, 2003; Husen et al., 2004; Hieke, 2004; Manga and Brodsky, 2006; Mellors et al., 2007; Manga et al., 2009; Lupi et al., 2013; Miller and Mazzini, 2018). Bonini (2009) pointed out that the Nirano mud volcanic field in Italy showed a remarkable increase of fluid emission after the 15th of May 1873 earthquake that occurred at the Pede-Apennines margin, near Reggio-Emilia. Lupi et al. (2015) showed precursory seismic signals in the same region occurring before the 2013 Lunigiana earthquake. Mazzini and Etiope (2017) report on several recent onshore and offshore mud volcanic eruptions related to seismic activity. Specifically, the spectacular Lusi eruption (NE Java), following the 2006 M6.3 Yogyakarta strike-slip earthquake, represents one of the most recent events of a newborn, sediment-hosted hydrothermal system (Mazzini et al., 2012) triggered by a remote earthquake (Miller and Mazzini, 2018).
Among the most spectacular, the newly formed mud island offshore of Gwadar (Pakistan) erupting few hours after the September 2013 M7.7 earthquake occurring 410 km far from the coast. Dynamic triggering was also observed in a mud volcano in the Marmara Sea after the May 24, 2014, Mw 6.9 strike-slip earthquake that occurred in the northern Aegean Sea, about 250 km away (Lupi et al., 2018).
In addition to observations from fluid seepage sites, numerical modeling provides a powerful tool to identify links between fluid migration, active faults and earthquakes. Interconnected high permeability zones (e.g., fractures) may efficiently transfer pore fluids from deep sources toward the seafloor. For accurate simulations of the fluid system in response to pressure pulses, it is important to use realistic physical parametrization (e.g., permeability, capillary entry pressure) and mass transport mechanisms, like Darcy flow in porous media or Stokes flow in fractured media. Recently, basin-scale, numerical models of this type have been used to constrain fluid sources of mud volcanoes in the Gulf of Cadiz (Schmidt et al., 2018). Enhancing this type of model will result in the performance of realistic simulations on how deep fluid signals are transferred to the seafloor and an assessment of the response time between seismic events and fluid pulses prior and after the seismic events. Sophisticated modeling approaches including the three-dimensional representation of fault planes (e.g., Burwicz et al., 2017) already provide realistic predictions of fluid migration in natural geological settings in tectonically active regions.
Archives of Seafloor Fluid Discharge
At mud volcanoes or, more generally, cold seeps, authigenic carbonate crusts and chimneys are often precipitated due to the anaerobic oxidation of methane, the latter mediated by the presence of microbial communities such as sulfate-reducing bacteria and methanotrophic archea, leading to sequestration of methane-derived carbon (Chevalier et al., 2011; Akhoudas et al., 2018). Their size varies from a few centimeters to more than one-meter, with a variety of shapes and variable spatial extent, and they are mainly composed of calcite, Mg-calcite, aragonite, and dolomite (e.g., Zitter et al., 2008; Pierre et al., 2012; Çağatay et al., 2018; Ruffine et al., 2018b). Locations of carbonate-chimneys were first detected by use of shipborne multibeam echosounders, further constrained by microbathymetry AUV surveys, and finally by ROV or manned submersible dives allowing for visual observation and sampling (e.g., Zitter et al., 2008; Dupré et al., 2010, 2015; Gasperini et al., 2012).
Authigenic carbonate precipitates can be considered as archives for the activity of fluid flow and may thus provide important tools for deciphering the long-term relationship between fluid geochemistry and seismic activity (e.g., dating of seismic events). With these properties, the precipitates could be even more useful than seismo-turbidite archives that were described above for the Sea of Marmara (e.g., Drab et al., 2012; Çağatay et al., 2012; McHugh et al., 2014) and other tectonically active regions such as the Calabrian Arc Subduction Complex, SW Iberia, and New Zealand (e.g., Gràcia et al., 2010; Pouderoux et al., 2012; Polonia et al., 2013). Hence, extending this paleo-perspective on the coupling of seismicity to changes in geochemistry and intensity of fluid flow, authigenic precipitates may serve as long-term evolving recorder. Depending on setting and type, especially methane-derived authigenic carbonates (MDACs) may provide well-preserved archives of tectonically driven variations in fluid emanation (e.g., Kutterolf et al., 2008; Liebetrau et al., 2010, 2014; Magalhães et al., 2012; Teichert et al., 2018; Çağatay et al., 2018) as well as for major environmental changes in bottom and pore water chemistry (e.g., Bayon et al., 2009; Cremiere et al., 2013). However, the use of authigenic carbonates as paleo-earthquake and paleo-fluid geochemistry archives requires systematic studies involving U-Th dating, stable isotope and biomarker analyses (Çağatay et al., 2018). In this regard, a high-resolution multi-proxy study carried out by Teichert et al. (2018) in the Sea of Marmara provides a promising example for the use of authigenic carbonates as archives of past seismic activity. As future perspective, carbonate archives from different MTFFZs could be used to get new insights into their evolution and impact on chemical fluxes. In addition to these high sensitivity calibration attempts, paleorecords provide a useful approach to improve the systematics of major control processes and their impact in time and space. More examples as described for the Sea of Marmara are available for other long-term tectonically active regions like Calabrian Arc Subduction Complex, SW Iberia and New Zealand, e.g., Polonia et al. (2015), Gràcia et al. (2010), and Pouderoux et al. (2012), respectively.
In summary, to date, no geochemical proxies have been unequivocally identified which can be used either as records of past seismic events or as precursors for seismic activity. This is a scientific endeavor for future studies in order to develop more robust relationships between seismically imposed dynamic stress and the release of fluids from depth. Currently, available results, but also technical approaches are quite limited. Below, we address a number of useful developments that could help bridging the knowledge gap.
Seismic Imaging of Geological Structures and Subsurface Fluids
As it can be deduced from the previous sections, seismic records are of utmost importance for the fundamental understanding of subsurface structures and related geological processes. Seismic processing is typically used for characterizing variations in lithology and petrophysical properties. The imaging of complex geological structures, like faults or the occurrence of overpressured fluids requires sophisticated processing schemes, such as wave equation datuming (WED) or amplitude versus offset (AVO; e.g., Tinivella et al., 2005, 2017; Giustiniani et al., 2015, 2018; Figure 12). The AVO technique can be used to detect the presence of anomalous fluid content, mainly free gas (e.g., Ostrander, 1984), and anomalous pore pressures (e.g., Tinivella, 2002). Essentially, AVO analyses provide information about the compressional (P) and shear wave (S) velocity (V) contrasts at interfaces, which give indications about the pore fluid content. The joint analysis of both parameters yields information about the main geological features and the fluid presence and circulation (e.g., Accaino et al., 2005; Tinivella et al., 2005). Moreover, it is possible to estimate the pore pressure by comparing the seismic and the theoretical velocity and the resulting density decrease (Tinivella et al., 2005). More recently, full-waveform inversion (FWI) has been applied to extract petrophysical information from shallow seismic data, showing promising application also for crustal data (Virieux and Operto, 2009). Figure 12 shows an example of joint use of different seismic analysis to detect fluid presence in anomalous pressure condition. Another approach of high potential for assessing pore fluid dynamics is the analysis of the dynamics of seismicity clouds and seismic swarms, from which the propagation of pore pressure and hydraulic permeability can be inferred (e.g., Shapiro, 2000). An application of this method to marine tectonic settings can be found in Thorwart et al. (2014) providing evidence for the coupling of seismicity and fluid flow (see section “Fluid Flow and Chemical Indicators Related to Seismic Activity”).
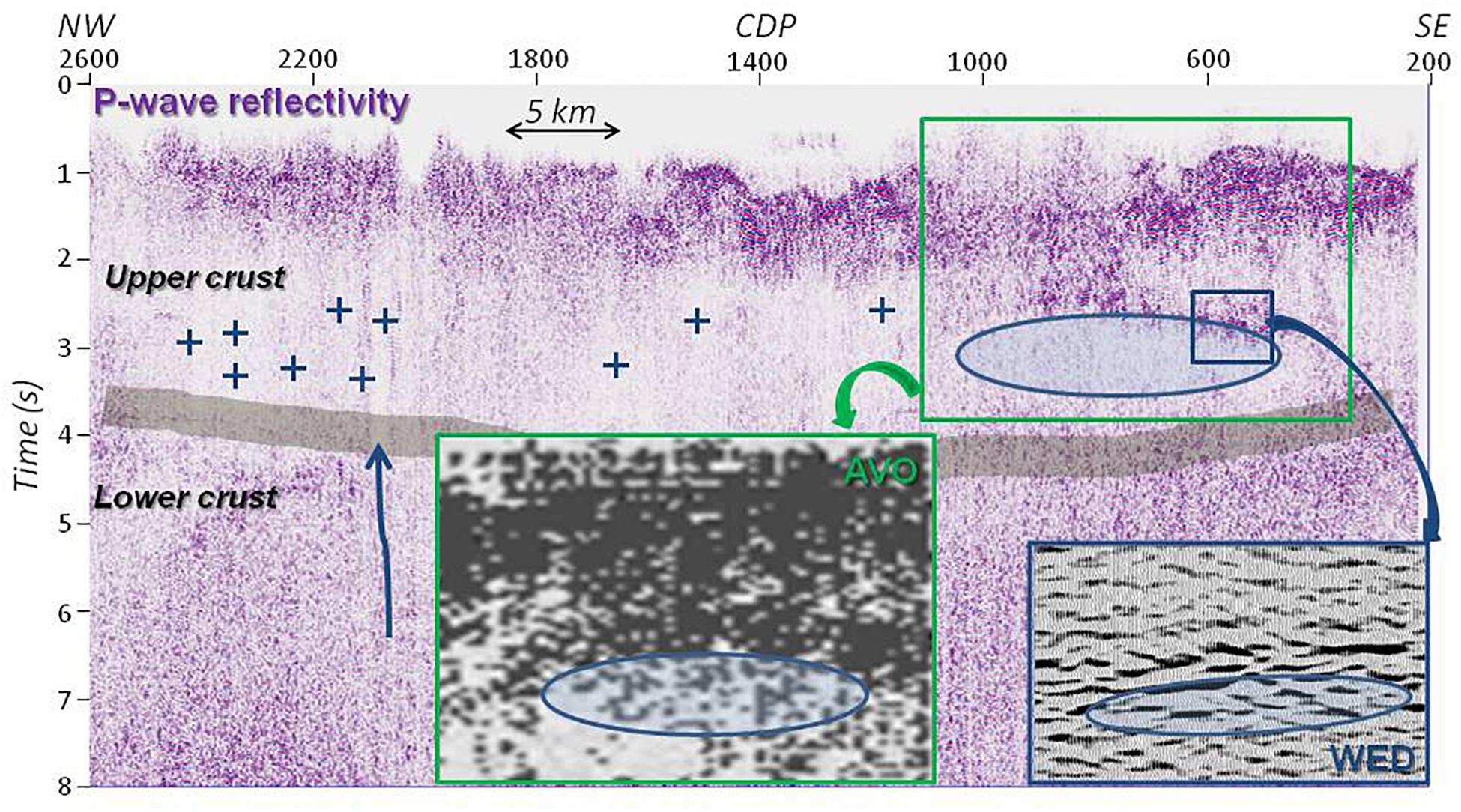
Figure 12. Example of P-wave reflectivities from the Tuscan geothermal field [data from Tinivella et al. (2005)] demonstrating the advantage of the joint use of seismic methods. A combined analysis of P- and S-wave reflectivities (AVO) within the green-framed area of the P-wave reflectivity plot shows general evidence for fluid overpressure (white spots). The high P-wave amplitude contrast is well imaged in the seismic line after the application of WED [blue box; data from Giustiniani et al., 2015)]. Light-blue zones: fluids. Blue arrow: vertical channel. Blue crosses: magmatic intrusions. The gray area indicates a regional reflecting zone related to lithologic change and may correspond to the brittle-ductile transition zone.
In summary, specific seismic processing schemes offer the opportunity of differentiating between lithology and fluid content as well as fluid-rock interactions. The improvement of such techniques, specifically in combination with geochemical methods is clearly suitable to strengthen our current knowledge on structural control on fluid mobilization processes and its relation to seismicity.
Sea-Floor Observatories and Emerging Monitoring Technologies
The deep ocean still remains a frontier for scientific exploration and discovery. Although the operation of research vessels has been providing a wealth of scientific knowledge, these platforms are clearly limited with respect to the exploration of processes at and below the deep seafloor over extended intervals of time. To address the frontier, research challenges in oceanographic science for the 21st century, permanent, multidisciplinary seafloor observatory networks are required for recording long-term time series measurements.
Considerable efforts in developing seafloor observatory networks have been made over the last two decades (Favali et al., 2015). In Japan, most advanced submarine cabled observatory networks for earthquake and tsunami observation have been established (DONET: Nankai Trough; S-Net: Japan Trench5; Kaneda, 2014; Kanazawa et al., 2015; Kamogawa et al., 2016). In Canada (e.g., Barnes et al., 2015), a regional cabled Ocean Observatory (Neptune Canada) for ocean sciences has been established in the Northeast Pacific Ocean6. In the United States, other observatories are also operated, as part of the Ocean Observatory Initiative (OOI: oceanobservatories.org). In Europe, the European Multidisciplinary Seafloor and water column Observatory (EMSO, emso.eu) is a relatively new science infrastructure under European Research Infrastructure Consortium (ERIC; Best et al., 2016). It consists of a system of distributed, regional facilities placed at key sites around Europe, from the Atlantic (Azores), through the Mediterranean to the Black Sea (e.g., Ruhl et al., 2011). Overall, ongoing activities clearly document the progress in monitoring technologies, which is important for many branches within ocean sciences, such as marine eco-systems, climate change, and geohazards. The understanding of seismic fault activity is one of the main challenges facing Earth Science. On land, substantial progress has been made by combining geophysical instrumentation (seismometers, GPS, tiltmeters) with the use of remote sensing facilities). Almost nothing comparable to this exists for the deep ocean. Except at specific sites (e.g., offshore Japan and in the NW Pacific Cascadia subduction zone), observing stations are deployed on land, far away from the areas where active processes are at work.
In submarine nearshore environments, amphibious approaches are a useful alternative (e.g., Guerrero Seismic Gap, off Mexico; Cruz-Atienza et al., 2018). Alternative solutions to fixed cabled observatories are in progress and will likely be more affordable and versatile. Overall, the emergence of new technologies offers a promising future, as for instance:
(1) Telecommunication optical fiber cables can detect seismic events when combined with state-of-the-art frequency metrology techniques (“distributed acoustic sensing,” DAS; e.g., Lindsey et al., 2017; Marra et al., 2018 and references herein).
(2) Laser reflectometry using Brillouin Optical Time Domain Reflectometry (BOTDR), commonly used for the monitoring of engineering structures can measure very small strains (<1 mm) at very large distances (10–200 km). This technique is currently tested witin the FOCUS project to demonstrate the feasibility of measuring small (1–2 cm) displacements across the tectonically active North Alfeo fault offshore eastern Sicily (Gutscher et al., 2018).
(3) New developments in high-resolution Absolute Pressure Gauges and Triaxial Accelerometers, based on in-situ calibration allows long-term measurements of geodesy7; Davis et al., 2017).
(4) In situ Raman sensor technology has been proven to monitor methane and other organic geochemical tracers subsea (e.g., Kolomijeca et al., 2013).
(5) Dissolved gases in seawater and seeping fluids can be determined by using in situ sensors operated by ROV, AUV, Seafloor-Landers, and from towed platforms (e.g., Linke et al., 2015; Schmidt et al., 2015).
(6) Mass spectrometry is a valuable tool for detecting and monitoring seeping fluids from the seafloor (e.g., Sommer et al., 2015). In situ, mass spectrometry for gas analyses at the seafloor is currently under development (e.g., Gentz, 2015).
(7) Noble gases have been shown to be a useful and robust tracer for fluid flow, fluid sources, and potentially as precursor for seismic activity, but state-of-the-art sampling approaches only allow collection of a very limited number of samples. Recently, mass spectrometers with a membrane inlet separating gases from water have been tested successfully (GE-MIMS; Mächler et al., 2012, 2014). Experimental developments in this field of research are currently pursued (Brennwald et al., 2016) and aim at the combined acquisition and integration in short- and long-term observatories (e.g., autonomously operated seafloor instruments).
Perspective
This manuscript has been developed to provide a comprehensive overview of the state-of-the-art and current research at MTFFZs beyond a geodynamic viewpoint. The key motivation was to provide an integrated view on current research linking plate tectonics, fluid flow, and life at MTFFZs. MTTFZs cover vast stretches of the seafloor in the deep oceans and offer an exciting field of research for cross-disciplinary studies, which is currently underrepresented in the research landscape. A number of findings suggest that the deep-sea areas between the spreading axis and the continental margins are more active in terms of heat and fluid flow than previously thought. To date, existing evidence, e.g., along oceanic fracture zones, comes from isolated observations, such as seismic records or heat flow measurements, indicating deviations from theory or empirically derived patterns. A deeper understanding of such regional or localized anomalies now requires comprehensive, multidisciplinary monitoring and research approaches. These may include investigations on seismic activity coupled with groundtruthing techniques through heat flow measurements, geochemical, and/or microbiological studies. An important first step to overcome was the conduction of interdisciplinary, sea-going expeditions. Given that MTFFZs cover vast areas of the seafloor, a comprehensive investigation even of some key regions will require long-lasting efforts, which will be supported by ongoing technological developments and new monitoring concepts e.g., for simultaneous records of seismic activity and fluid flow. This article aims at stimulating such future interdisciplinary research along MTFFZs in order to further our understanding of the still underexplored regions of the deep ocean.
Data Availability
All datasets generated for this study are included in the manuscript and/or the supplementary files.
Author Contributions
All authors actively contributed to various parts of the manuscript and agree with its contents. CH, JD, PV, AM, ML, PT, LG, PH, HV, JM, MS, and M-AG developed the concept of the manuscript. Figures were provided and or developed by AM, PV, M-AG, JD, LG, LGa, RB, CH, LB, DS, HV, CS, MS, LR, and UT.
Funding
This study was the outcome of interdisciplinary research and intense, pan-European, scientific exchange within the EU-funded COST Action FLOWS (ES1301; https://www.flows-cost.eu/) since 2013, supported by COST (European Cooperation in Science and Technology; www.cost.eu).
Conflict of Interest Statement
The authors declare that the research was conducted in the absence of any commercial or financial relationships that could be construed as a potential conflict of interest.
Acknowledgments
The authors wish to thank all members of the FLOWS consortium (https://www.flows-cost.eu/de/members) for fruitful discussions and ongoing knowledge exchange. Additional thanks for fruitful collaboration go to colleagues of the InterRidge working group on Oceanic Transform Faults (https://www.interridge.org/WG_Transform_Faults), the Deep Carbon Observatory (https://deepcarbon.net/), and the MARSITE Programme [EU Grant agreement N° (308417)]. The manuscript benefited from critical and insightful comments by Shinsuke Kawagucci and Wolfgang Rabbel. JD acknowledges an FCT Researcher contract, an exploratory project grant ref. IF/00702/2015, and the FCT project UID/ GEO/50019/2013-IDL. LG acknowledges the bilateral ANR/TÜBITAK collaborative research project MAREGAMI (ANR-16-CE03-0010-02 and Tübitak Project 116Y371). AM acknowledges the European Research Council under the Seventh Framework Programme (grant agreement 308126, LUSI LAB) and the Research Council of Norway (Centers of Excellence funding scheme 223272).
Footnotes
- ^ https://en.wikipedia.org/wiki/Fracture_zone
- ^ https://www.britannica.com/science/submarine-fracture-zone
- ^ https://www.flows-cost.eu/
- ^ http://publications.iodp.org/index.html
- ^ https://www.jamstec.go.jp/donet/e/rd/index.html
- ^ http://www.oceannetworks.ca/observatories/pacific
- ^ http://paroscientific.com/pdf/P20_Seismic_Oceanic_Sensors_(SOS).pdf
References
Abercrombie, R. E., and Ekström, G. (2001). Earthquake slip on oceanic transform faults. Nature 410, 74–77. doi: 10.1038/35065064
Accaino, F., Tinivella, U., Rossi, G., and Nicolich, R. (2005). “Geofluid evidence from analysis of deep crustal seismic data (Southern Tuscany, Italy), structures in the continental crust and geothermal resources,” in Journal of Volcanology and Geothermal Research, Vol. 148, eds D. Liotta and G. Ranalli (Duivendrecht: Elsevier), 46–59.
Akhoudas, C., Chevalier, N., Blanc-Valleron, M. M., Klein, V., Mendez-Millan, M., Demang, J., et al. (2018). Methane derived stromatolitic carbonate crust from an active fluid seepage in the western basin of the Sea of Marmara: mineralogical, isotopic and molecular geochemical characterization. Deep Sea Res. II 153, 110–120. doi: 10.1016/j.dsr2.2017.12.022
Aochi, H., and Ulrich, T. (2015). A probable earthquake scenario near Istanbul determined from dynamic simulations. Bull. Seismol. Soc. Am. 105, 1468–1475. doi: 10.1785/0120140283
Armijo, R., Meyer, B., Hubert, A., and Barka, A. (1999). Westward propagation of the north Anatolian into the northern Aegean: timing and kinematics. Geology 27, 267–270. doi: 10.1130/0091-7613 (1999)027<0267:WPOTNA>2.3.CO;2
Armijo, R., Pondard, N., Meyer, B., Mercier de Lepinay, B., Uçarkus, G., Malavieille, J., et al. (2005). Submarine fault scarps in the Sea of Marmara pull-apart (North Anatolian Fault): implications for seismic hazard in Istanbul. Geochem. Geophys. Geosyst. 6:Q06009. doi: 10.1029/2004GC000896
Attanasio, A., and Maravalle, M. (2016). Some considerations between radon and earthquakes in the crater of L’Aquila. Nat. Hazards 81, 1971–1979. doi: 10.1007/s11069-016-2169-4
Atwater, T., and Menard, H. W. (1970). Magnetic lineations in the Northeast Pacific. Earth Planet. Sci. Lett. 7, 445–450. doi: 10.1016/0012-821X(70)90089-0
Baptista, M. A., and Miranda, J. M. (2009). Revision of the Portuguese catalog of tsunamis. Nat. Hazards Earth Syst. Sci. 9, 25–42. doi: 10.5194/nhess-9-25-2009
Baptista, M. A., Miranda, P. M. A., Miranda, J. M., and Victor, L. M. (1998). Constrains on the source of the 1755 Lisbon tsunami inferred from numerical modelling of historical data. J. Geodyn. 25, 159–174. doi: 10.1016/S0264-3707(97)00020-3
Barka, A. A. (1999). The 17 August 1999 Izmit earthquake. Science 285, 1858–1859. doi: 10.1126/science.285.5435.1858
Barnes, C. R., Best, M. M. R., Johnson, F. R., and Pirenne, B. (2015). “NEPTUNE Canada: installation and initial operation of the world’s first regional cabled ocean observatory,” in Seafloor Observatories: A New Vision of the Earth from the Abyss, eds P. Favali, L. Beranzoli, and A. De Santis (Berlin: Springer), 415–437. doi: 10.1007/978-3-642-11374-1_16
Bartolome, R., Gràcia, E., Stich, D., Martínez-Loriente, S., Klaeschen, D., Mancilla, F. L., et al. (2012). Evidence for active strike-slip faulting along the Eurasia-Africa convergence zone: implications for seismic hazards on the SW Iberian Margin. Geology 40, 495–498. doi: 10.1130/G33107.1
Batista, L., Hubscher, C., Terrinha, P., Matias, L., Afilhado, A., and Ludmann, T. (2017). Crustal structure of the Eurasia–Africa plate boundary across the Gloria Fault, North Atlantic Ocean. Geophys. J. Int. 209, 713–729. doi: 10.1093/gji/ggx050
Batsi, E., Lomax, A., Tary, J. B., Klingelhoefer, F., Riboulot, V., Murphy, M., et al. (2018). An alternative view of the microseismicity along the western main marmara fault. Bull. Seismol. Soc. Am. 108, 2650–2674. doi: 10.1785/0120170258
Bayon, G., Henderson, G. M., and Bohn, M. (2009). U-Th stratigraphy of a cold seep carbonate crust. Chem. Geol. 260, 47–56. doi: 10.1016/j.chemgeo.2008.11.020
Beaulieu, S. E., and Szafranski, K. (2018). InterRidge Global Database of Active Submarine Hydrothermal Vent Fields, Version 3.4. World Wide Web Electronic Publication. Available at: http://vents-data.interridge.org/
Beck, C., Mercier de Lépinay, B., Schneider, J.-L., Cremer, M., Çağatay, N., Wendenbauma, E., et al. (2007). Late Quaternary co-seismic sedimentation in the Sea of Marmara’s deep basins. Sediment. Geol. 199, 65–89. doi: 10.1016/j.sedgeo.2005.12.031
Best, M. M. R., Favali, P., Beranzoli, L., Blandin, J., Çağğatay, M. N., Cannat, M., et al. (2016). The EMSO-ERIC pan-european consortium: data benefits and lessons learned as the legal entity forms. Mar. Technol. Soc. J. 50, 8–15. doi: 10.4031/MTSJ.50.3.13
Bezzeghoud, M., Adam, C., Buforn, E., Borges, J. F., and Caldeira, B. (2014). Seismicity along the Azores-Gibraltar region and global plate kinematics. J. Seismol. 18, 205–220. doi: 10.1007/s10950-013-9416-x
Bird, P. (2003). An updated digital model of plate boundaries. Geochem. Geophys. Geosyst. 4:1027. doi: 10.1029/2001GC000252
Bird, P., and Kagan, Y. Y. (2004). Plate-tectonic analysis of shallow seismicity: apparent boundary width, beta, corner magnitude, coupled lithosphere thickness, and coupling in seven tectonic settings. Bull. Seismol. Soc. Am. 94, 2380–2399. doi: 10.1785/0120030107
Bischoff, J. L., and Rosenbauer, R. J. (1985). An empirical equation of state for hydrothermal seawater (3.2 percent NaCl). Am. J. Sci. 285, 725–763. doi: 10.2475/ajs.285.8.725
Boettcher, M. S., and Jordan, T. H. (2004). Earthquake scaling relations for mid-ocean ridge transform faults. J. Geophys. Res. 109:B12302. doi: 10.1029/2004JB003110
Boettcher, M. S., and McGuire, J. J. (2009). Scaling relations for seismic cycles on mid-ocean ridge transform faults. Geophys. Res. Lett. 36:L21301. doi: 10.1029/2009GL040115
Bohnenstiehl, D. R., Tolstoy, M., and Chapp, E. (2004). Breaking into the plate: a 7.6 Mw fracture-zone earthquake adjacent to the Central Indian Ridge. Geophys. Res. Lett. 31:L02615. doi: 10.1029/2003gl018981
Bonatti, E., Ligi, M., Brunelli, D., Cipriani, A., Fabretti, P., Ferrante, V., et al. (2003). Mantle thermal pulses below the Mid-Atlantic Ridge and temporal variations in the formation of oceanic lithosphere. Nature 423, 499–505. doi: 10.1038/nature01594
Bonatti, E., Ligi, M., Gasperini, L., Peyve, A., Raznitsin, Y., and Chen, Y. J. (1994). Transform migration and vertical tectonics at the Romanche fracture zone, equatorial Atlantic. J. Geophys. Res. Solid Earth 99, 21779–21802. doi: 10.1029/94JB01178
Bonini, M. (2009). Mud volcano eruptions and earthquakes in the Northern Apennines and Sicily, Italy. Tectonophysics 474, 723–735. doi: 10.1016/j.tecto.2009.05.018
Bouchon, M., Karabulut, H., Aktar, M., Ozalabey, S., Schmittbuhl, J., and Bouin, M.-P. (2011). Extended nucleation of the 1999 M 7.6 izmit earthquake. Science 331, 877–880. doi: 10.1126/science.1197341
Bourry, C., Chazallon, B., Charlou, J. L., Pierre Donval, J., Ruffine, L., Henry, P., et al. (2009). Free gas and gas hydrates from the Sea of Marmara, Turkey: chemical and structural characterization. Chem. Geol. 264, 197–206. doi: 10.1016/j.chemgeo.2009.03.007
Bradley, A. S., Hayes, J. M., and Summons, R. E. (2009). Extraordinary 13C enrichment of diether lipids at the Lost City Hydrothermal Field indicates a carbon-limited ecosystem. Geochim. Cosmochim. Acta 73, 102–118. doi: 10.1016/j.gca.2008.10.005
Bradley, A. S., and Summons, R. E. (2010). Multiple origins of methane at the Lost City Hydrothermal Field. Earth Planet. Sci. Lett. 297, 34–41. doi: 10.1016/j.epsl.2010.05.034
Brazelton, W. J., Nelson, B., and Schrenk, M. O. (2012). Metagenomic evidence for H2 oxidation and H2 production by serpentinite-hosted subsurface microbial communities. Front. Microbiol. 2:268. doi: 10.3389/fmicb.2011.00268
Brazelton, W. J., Schrenk, M. O., Kelley, D. S., and Baross, J. A. (2006). Methane- and sulfur-metabolizing microbial communities dominate the Lost City Hydrothermal Field ecosystem. Appl. Environ. Microbiol. 72, 6257–6270. doi: 10.1128/AEM.00574-06
Brennwald, M. S., Schmidt, M., Oser, J., and Kipfer, R. (2016). A portable and autonomous mass spectrometric system for on-site environmental gas analysis. Environ. Sci. Technol. 50, 13455–13463. doi: 10.1021/acs.est.6b03669
Brodsky, E. E. (2003). A mechanism for sustained groundwater pressure changes induced by distant earthquakes. J. Geophys. Res. 108:2390. doi: 10.1029/2002JB002321
Buforn, E., Udias, A., and Madariaga, R. (1991). Intermediate and deep earthquakes in Spain. Pure Appl. Geophys. 136, 375–393. doi: 10.1007/BF00878576
Burnard, P., Bourlange, S., Henry, P., Géli, L., Tryon, M. D., Natal’in, B., et al. (2012). Constraints on fluid origins and migration velocities along the Marmara Main Fault (Sea of Marmara, Turkey) using helium isotopes. Earth Planet. Sci. Lett. 341–344, 68–78. doi: 10.1016/j.epsl.2012.05.042
Burwicz, E., Reichel, T., Wallmann, K., Haeckel, M., and Hensen, C. (2017). 3-D basin-scale reconstruction of natural gas hydrate system of the Green Canyon, Gulf of Mexico. Geochem. Geophys. Geosyst. 18, 1959–1985. doi: 10.1002/2017GC006876
Çağatay, M. N., Erel, L., Bellucci, L. G., Polonia, A., Gasperini, L., Eriş, K. K., et al. (2012). Sedimentary earthquake records in the Ýzmit Gulf, Sea of Marmara, Turkey. Sediment. Geol. 282, 347–359. doi: 10.1016/j.sedgeo.2012.10.001
Çağatay, M. N., and Uçarkuş, G. (2019). “Morphotectonics of the Sea of Marmara: basins and highs on the North Anatolian continental transform plate boundary,” in Transform Plate Boundaries and Fracture Zones, ed. J. C. Duarte (Amsterdam: Elsevier), 397–416. doi: 10.1016/B978-0-12-812064-4.00016-5
Çağatay, M. N., Yıldız, G., Bayon, G., Ruffine, L., and Henry, P. (2018). Seafloor authigenic carbonate crusts along the submerged part of the North Anatolian Fault in the Sea of Marmara: mineralogy, geochemistry, textures and genesis. Deep Sea Res. Part II 153, 92–109. doi: 10.1016/j.dsr2.2017.09.003
Cann, J. R., Blackman, D. K., Smith, D. K., McAllister, E., Janssen, B., Mello, S., et al. (1997). Corrugated slip surfaces formed at ridge-transform intersections on the Mid-Atlantic Ridge. Nature 385, 329–332. doi: 10.1038/385329a0
Cannat, M., Mamaloukas-Frangoulis, V., Auzende, J.-M., Bideau, D., Bonatti, E., Honnorez, J., et al. (1991). A geological cross-section of the Vema Fracture Zone transverse ridge, Atlantic Ocean. J. Geodyn. 13, 97–118. doi: 10.1016/0264-3707(91)90034-C
Casey, J. F., and Dewey, J. F. (1984). Initiation of subduction zones along transform and accreting plate boundaries, triple-junction evolution, and forearc spreading centres - Implications for ophiolitic geology and obduction. Geol. Soc. Spec. Publ. 13, 269–290. doi: 10.1144/GSL.SP.1984.013.01.22
Chester, F. M., Mori, J., Eguchi, N., and Toczko, S. (2013). Proc. IODP, 343/343T. Tokyo: Integrated Ocean Drilling Program Management International. doi: 10.2204/iodp.proc.343343T.2013
Chevalier, N., Bouloubassi, I., Birgel, D., Crémière, A., Taphanel, M. H., and Pierre, C. (2011). Authigenic carbonates at cold seeps in the Marmara Sea (Turkey): a lipid biomarker and stable carbon and oxygen isotope investigation. Mar. Geol. 288, 112–121. doi: 10.1016/j.margeo.2011.08.005
Chevalier, N., Bouloubassi, I., Birgel, D., Taphanel, M.-H., and López-García, P. (2013). Microbial methane turnover at Marmara Sea cold seeps: a combined 16S rRNA and lipid biomarker investigation. Geobiology 11, 55–71. doi: 10.1111/gbi.12014
Claesson, L., Skelton, A., Graham, C., and Morth, C. M. (2007). The timescale and mechanisms of fault sealing and water-rock interaction after an earthquake. Geofluids 7, 427–440. doi: 10.1111/j.1468-8123.2007.00197.x
Coogan, L. A., and Gillis, K. M. (2018). Low-temperature alteration of the seafloor: impacts on ocean chemistry. Ann. Rev. Earth Planet. Sci. 46, 21–45. doi: 10.1146/annurev-earth-082517-010027
Corti, G., Bonini, M., Innocenti, F., and Manetti, P. (2005). Extension of a continental lithosphere weakened by impregnation of asthenospheric melts: analogue modelling approach. Ofioliti 30:159.
Cremiere, A., Bayon, G., Ponzevera, E., and Pierre, C. (2013). Paleo-environmental controls on cold seep carbonate authigenesis in the Sea of Marmara. Earth Planet. Sci. Lett. 376, 200–211. doi: 10.1016/j.epsl.2013.06.029
Crespo-Medina, M., Twing, K. I., Snchez-Murillo, R., Brazelton, W. J., McCollom, T. M., and Schrenk, M. O. (2017). Methane dynamics in a tropical serpentinizing environment: the Santa Elena Ophiolite, Costa Rica. Front. Microbiol. 8:916. doi: 10.3389/fmicb.2017.00916
Cruz-Atienza, V. M., Ito, Y., Kostoglodov, V., Hjörleifsdóttir, V., Iglesias, A., Tago, J., et al. (2018). A seismogeodetic mphibious network in the Guerrero seismic gap, Mexico. Seismol. Res. Lett. 89, 1435–1449. doi: 10.1785/0220170173
Custódio, S., Lima, V., Vales, D., Cesca, S., and Carrilho, F. (2016). Imaging active faulting in a region of distributed deformation from the joint clustering of focal mechanisms and hypocentres: application to the Azores–western Mediterranean region. Tectonophysics 676, 70–89. doi: 10.1016/j.tecto.2016.03.013
Dauteuil, O., Bourgeois, O., and Mauduit, T. (2002). Lithosphere strength controls oceanic transform zone structure: insights from analogue models. Geophys. J. Int. 150, 706–714. doi: 10.1046/j.1365-246X.2002.01736.x
Davis, E. E., and Elderfield, H. (2004). Hydrology of the Oceanic Lithosphere, eds E. Davis and H. Elderfield (Cambridge: Combridge University Press), 706. doi: 10.1016/j.epsl.2017.01.042
Davis, E. E., Heesemann, M., Lambert, A., and He, J. (2017). Seafloor tilt induced by ocean tidal loading inferred from broadband seismometer data from the Cascadia subduction zone and Juan de Fuca Ridge. Earth Planet. Sci. Lett. 463, 243–252. doi: 10.1016/j.epsl.2017.01.042
Davis, E. E., Kelin, W., Jiangheng, H., Chapman, D. S., Villinger, H., and Rosenberger, A. (1997). An unequivocal case for high Nusselt number hydrothermal convection in sediment-buried igneous oceanic crust’. Earth Planet. Sci. Lett. 146, 137–150. doi: 10.1016/S0012-821X(96)00212-9
Davis, E. E., Lister, C. R., and Sclater, J. G. (1984). Towards determining the thermal state of old ocean lithosphere: heat-flow measurements from the Blake–Bahama outer ridge, north-westem Atlantic. Geophys. J. R. Astron. Soc. 78, 507–545. doi: 10.1111/j.1365-246X.1984.tb01962.x
Delle Donne, D., Harris, A. J. L., Ripepe, M., and Wright, R. (2010). Earthquake-induced thermal anomalies at active volcanoes. Geology 38, 771–774. doi: 10.1130/G30984.1
Devey, C. W., Augustin, N., Brandt, A., Brenke, N., Köhler, J., Lins, L., et al. (2018). Habitat characterization of the Vema Fracture Zone and Puerto Rico Trench. Deep Sea Res. Part II Top. Stud. Oceanogr. 148, 7–20. doi: 10.1016/j.dsr2.2018.02.003
Dia, A. N., Castrec, M., Boulègue, J., and Boudou, J. P. (1995). Major and trace element and Sr isotope constraints on fluid circulations in the Barbados accretionary complex. Part I: fluid origin. Earth Planet. Sci. Lett. 134, 69–85. doi: 10.1016/0012-821X(95)00102-I
Drab, L., Hubert Ferrari, A., Schmidt, S., and Martinez, P. (2012). The earthquake sedimentary record in the western part of the Sea of Marmara, Turkey. Nat. Hazards Earth Syst. Sci. 12, 1235–1254. doi: 10.5194/nhess-12-1235-2012
Dubilier, N., Bergin, C., and Lott, C. (2008). Symbiotic diversity in marine animals: the art of harnessing chemosynthesis. Nat. Rev. Microbiol. 6, 725–740. doi: 10.1038/nrmicro1992
Dupré, S., Scalabrin, C., Augustin, J.-M., Guérin, C., Ogor, A., and Clouet, H. (2018). Distribution Spatiale des Sorties de Fluides en mer de Marmara (4 Novembre au 14 Decembre 2009). Barcelona: SEANOE. doi: 10.17882/54983
Dupré, S., Scalabrin, C., Grall, C., Augustin, J.-M., Henry, P., Şengör, A. M. C., et al. (2015). Tectonic and sedimentary controls on widespread gas emissions in the Sea of Marmara: results from systematic, shipborne multibeam echo sounder water column imaging. J. Geophys. Res. Solid Earth 120, 2891–2912. doi: 10.1002/2014JB011617
Dupré, S., Woodside, J., Klaucke, I., Mascle, J., and Foucher, J. P. (2010). Widespread active seepage activity on the Nile Deep Sea Fan (offshore Egypt) revealed by high-definition geophysical imagery. Mar. Geol. 275, 1–19. doi: 10.1016/j.margeo.2010.04.003
Dziak, R. P., Chadwick, W. W. Jr., Fox, C. G., and Embley, R. W. (2003). Hydrothermal temperature changes at the Southern Juan de Fuca Ridge associated with Mw 6.2 Blanco Transform Earthquake. Geology 31, 119–122. doi: 10.1130/0091-7613(2003)031<0119:HTCATS>2.0.CO;2
Dzierma, Y., Rabbel, W., Thorwart, M., Koulakov, I., Wehrmann, H., Hoernle, K., et al. (2012). Seismic velocity structure of the slab and continental plate in the region of the 1960 Valdivia (Chile) slip maximum - Insights into fluid release and plate coupling. Earth Planet. Sci. Lett. 331–332, 164–176. doi: 10.1016/j.epsl.2012.02.006
Ebert, R., Bideau, D., and Hekinian, R. (1983). Ultramafic and mafic rocks from the Garret transform fault near 13°30’S on the East Pacific Rise: igneous petrology. Earth Planet. Sci. Lett. 65, 107–125. doi: 10.1016/0012-821X(83)90193-0
Elderfield, H., and Schultz, A. (1996). Mid-Ocean Ridge hydrothermal fluxes and the chemical composition of the ocean. Ann. Rev. Earth Planet. Sci. 24, 191–224. doi: 10.1146/annurev.earth.24.1.191
Elderfield, H., Wheat, C. G., Mottl, M. J., Monnin, C., and Spiro, B. (1999). Fluid and geochemical transport through oceanic crust: a transect across the eastern flank of the Juan de Fuca Ridge. Earth Planet. Sci. Lett. 172, 151–165. doi: 10.1016/S0012-821X(99)00191-0
Embriaco, D., Marinaro, G., Frugoni, F., Monna, S., Etiope, G., Gasperini, L., et al. (2014). Monitoring of gas and seismic energy release by multiparametric benthic observatory along the North Anatolian Fault in the Sea of Marmara (NW Turkey). Geophys. J. Int. 196, 850–866. doi: 10.1093/gji/ggt436
Ergintav, S., Reilinger, R. E., Çakmak, R., Floyd, M., Cakir, Z., Doğan, U., et al. (2014). Istanbul’s earthquake hot spots: geodetic constraints on strain accumulation along faults in the Marmara seismic gap. Geophys. Res. Lett. 41, 5783–5788. doi: 10.1002/2014GL060985
Escartin, J., and Cannat, M. (1999). Ultramafic exposures and gravity signature of the lithosphere near the Fifteen-Twenty Fracture Zone (Mid-Altlantic Ridge, 14°-16.5°N). Earth Planet. Sci. Lett. 171, 411–424. doi: 10.1016/S0012-821X(99)00169-7
Fabretti, P., Bonatti, E., Peyve, A., Brunelli, D., Cipriani, A., Dobrolubova, X., et al. (1998). First results of cruise S19 (PRIMAR Project): petrological and structural investigations of the Vema Transverse Ridge (equatorial Atlantic). G. Geol. 60, 3–16.
Favali, P., Beranzoli, L., and De Santis, A. (2015). Seafloor Observatories: A New Vision of the Earth from the Abyss. Berlin: Springer. doi: 10.1007/978-3-642-11374-1
Field, M. E., and Jennings, A. E. (1987). Seafloor gas seeps triggered by a northern California earthquake. Mar. Geol. 77, 39–51. doi: 10.1016/0025-3227(87)90082-X
Fischer, D., Mogollon, J. M., Strasser, M., Pape, T., Bohrmann, G., Fekete, N., et al. (2013). Subduction zone earthquake as potential trigger of submarine hydrocarbon seepage. Nat. Geosci. 6, 647–651. doi: 10.1038/ngeo1886
Fisher, A. T. (2005). Marine hydrogeology: recent accomplishments and future opportunities. Hydrogeol. J. 13, 69–97. doi: 10.1007/s10040-004-0400-y
Fisher, A. T., and Harris, R. N. (2010). Using seafloor heat flow as a tracer to map subseafloor fluid flow in the ocean crust. Geofluids 10, 142–160.
Fisher, A. T., and Von Herzen, R. P. (2005). Models of hydrothermal circulation within 106 Ma seafloor: constraints on the vigor of fluid circulation and crustal properties, below the Madeira Abyssal Plain. Geochem. Geophys. Geosyst. 6:Q11001. doi: 10.1029/2005GC001013
Foustoukos, D. I., Savov, I. P., and Janecky, D. R. (2008). Chemical and isotopic constraints on water/rock interactions at the Lost City Hydrothermal Field, 30°N Mid-Atlantic Ridge. Geochim. Cosmochim. Acta 72, 5457–5474. doi: 10.1016/j.gca.2008.07.035
Francis, T. J. G. (1981). Serpentinization faults and their role in the tectonics of slow spreading ridges. J. Geophys. Res. Solid Earth 86, 11616–11622. doi: 10.1029/JB086iB12p11616
Früh-Green, G. L., Kelley, D. S., Bernasconi, S. M., Karson, J. A., Ludwig, K. A., Butterfield, D. A., et al. (2003). 30,000 years of hydrothermal activity at the Lost City Vent Field. Science 301, 495–498. doi: 10.1126/science.1085582
Früh-Green, G. L., Orcutt, B. N., Green, S. L., and Cotterill, C. (2016). “Atlantis massif serpentinization and life,” in Proceedings of the International Ocean Discovery Program, (College Station, TX: International Ocean Discovery Program), 357. doi: 10.14379/iodp.proc.357.2017
Fu, C.-C., Yang, T. F., Du, J., Walia, V., Chen, Y.-G., Liu, T.-K., et al. (2008). “Variations of helium and radon concentrations in soil gases from an active fault zone in southern Taiwan,” in Proceedings of the 23rd International Conference on Nuclear Tracks in Solids, Vol. 43, Beijing, S348–S352. doi: 10.1016/j.radmeas.2008.03.035
Fujita, K., and Sleep, N. H. (1978). Membrane stresses near mid-ocean ridge-transform intersections. Tectonophysics 50, 207–221. doi: 10.1016/0040-1951(78)90136-1
Fukao, Y. (1973). Thrust faulting at a lithospheric plate boundary, the Portugal earthquake of 1969. Earth Planet. Sci. Lett. 18, 205–216. doi: 10.1016/0012-821X(73)90058-7
Galland, O., Cobbold, P. R., Hallot, E., de Bremend d’Ars, J., and Delavaud, G. (2006). Use of vegetable oil and silica powder for scale modelling of magmatic intrusion in a deforming brittle crust. Earth Planet. Sci. Lett. 243, 786–804. doi: 10.1016/j.epsl.2006.01.014
Ganas, A., Drakatos, G., Pavlides, S. B., Stavrakakis, G. N., Ziazia, M., Sokos, E., et al. (2005). The 2001 Mw = 6.4 Skyros earthquake, conjugate strike-slip faulting and spatial variation in stress within the central Aegean Sea. J. Geodyn. 39, 61–77. doi: 10.1016/j.jog.2004.09.001
Garfunkel, Z. (1981). Internal structure of the Dead Sea leaky transform (rift) in relation to plate kinematics. Tectonophysics 80, 81–108. doi: 10.1016/0040-1951(81)90143-8
Gasperini, L., Polonia, A., Bortoluzzi, G., Henry, P., Le Pichon, X., Tryon, M., et al. (2010). How far did the surface rupture of the 1999 İzmit earthquake reach in Sea of Marmara? Tectonics 30:TC1010. doi: 10.1029/2010TC002726
Gasperini, L., Polonia, A., Çağatay, M. N., Bortoluzzi, G., and Ferrante, V. (2011). Geological slip rates along the North Anatolian Fault in the Marmara region. Tectonics 30:TC6001. doi: 10.1029/2011TC002906
Gasperini, L., Polonia, A., Del Bianco, F., Etiope, G., Marinaro, G., Favali, P., et al. (2012). Gas seepage and seismogenic structures along the North Anatolian Fault in the eastern Sea of Marmara. Geochem. Geophys. Geosyst. 13:Q10018. doi: 10.1029/2012GC004190
Geersen, J., Scholz, F., Linke, P., Schmidt, M., Lange, D., Behrmann, J. H., et al. (2016). Fault zone controlled seafloor methane seepage in the rupture area of the 2010 Maule earthquake, Central Chile. Geochem. Geophys. Geosyst. 17, 4802–4813. doi: 10.1002/2016GC006498
Géli, L., Henry, P., Grall, C., Tary, J. B., Lomax, A., Batsi, E., et al. (2018). Gas and seismicity within the Istanbul seismic gap. Sci. Rep. 8:6819. doi: 10.1038/s41598-018-23536-7
Géli, L., Henry, P., Zitter, T., Dupré, S., Tryon, M., Çağatay, M. N., et al. (2008). Gas emissions and active tectonics within the submerged section of the North Anatolian Fault zone in the Sea of Marmara. Earth Planet. Sci. Lett. 274, 34–39. doi: 10.1016/j.epsl.2008.06.047
Géli, L., Piau, J.-M., Maury, V., Fitzenz, D., Dziak, R., Coutellier, Q., et al. (2014). Seismic precursors linked to highly compressible fluids at oceanic transform faults. Nat. Geosci. 7, 757–761. doi: 10.1038/ngeo2244
Gentz, T. (2015). “Improvements in under water mass spectrometry,” in Workshop on Harsh-Environment Mass Spectrometry Proceedings of the Meeting 13 September 2015 - 16 September 2015, (Baltimore, MD: University of Maryland School of Pharmacy).
German, C. R., and Seyfried, W. E. (2014). “8.7 - hydrothermal processes,” in Treatise on Geochemistry, 2nd Edn, eds H. D. Holland and K. K. Turekian (Oxford: Elsevier), 191–233. doi: 10.1016/B978-0-08-095975-7.00607-0
Gerya, T. V. (2010). Dynamical instability produces transform faults at mid-ocean ridges. Science 329, 1047–1050. doi: 10.1126/science.1191349
Gerya, T. V. (2012). Origin and models of oceanic transform faults. Tectonophysics 522–523, 34–54. doi: 10.1016/j.tecto.2011.07.006
Gerya, T. V. (2013a). Initiation of transform faults at rifted continental margins: 3D petrological–thermomechanical modeling and comparison to the Woodlark Basin. Petrology 21, 550–560. doi: 10.1134/S0869591113060039
Gerya, T. V. (2013b). Three-dimensional thermomechanical modeling of oceanic spreading initiation and evolution. Phys. Earth Planet. Interiors 214, 35–52. doi: 10.1016/j.pepi.2012.10.007
Gerya, T. V. (2016). “Origin, evolution, seismicity, and models of oceanic and continental transform boundaries,” in Plate Boundaries and Natural Hazards, AGU Geophysical Monograph Series 219, eds J. C. Duarte and W. P. Schellart (Hoboken, NJ: Wiley), 39–76.
Giustiniani, M., Tinivella, U., and Nicolich, R. (2015). Reflection seismic sections across the Geothermal Province of Tuscany from reprocessing CROP profiles. Geothermics 53, 498–507. doi: 10.1016/j.geothermics.2014.09.003
Giustiniani, M., Tinivella, U., and Nicolich, R. (2018). Crustal structure of central sicily. Tectonophysics 722, 299–313. doi: 10.1016/j.tecto.2017.08.034
Global Heat Flow Compilation Group (2013). Component Parts of the World Heat Flow Data Collection. Bremen: PANGAEA.
Godón, A., Jendrzejewski, N., Castrec-Rouelle, M., Dia, A., Pineau, F., Boulegue, J., et al. (2004). Origin and evolution of fluids from mud volcanoes in the Barbados accretionary complex. Geochim. Cosmochim. Acta 68, 2153–2165. doi: 10.1016/j.gca.2003.08.021
Gràcia, E., Dañbeitia, J., Vergés, J., and Bartolomé, R. (2003). Crustal architecture and tectonic evolution of the Gulf of Cadiz (SW Iberian margin) at the convergence of the Eurasian and African plates. Tectonics 22:1033. doi: 10.1029/2001TC901045
Gràcia, E., Vizcaino, A., Escutia, C., Asioli, A., Rodés, A., Pallàs, R., et al. (2010). Holocene earthquake record offshore Portugal (SW Iberia): testing turbidite paleoseismology in a slow-convergence margin. Quat. Sci. Rev. 29, 1156–1172. doi: 10.1016/j.quascirev.2010.01.010
Grall, C., Dupré, S., Guerin, C., Normand, A., Gaillot, A., Fleury, J., et al. (2018a). Processed AsterX AUV Data from the Sea of Marmara: High-Resolution Bathymetry and Seafloor Backscatter Images. Barcelona: SEANOE. doi: 10.17882/55744
Grall, C., Henry, P., Dupré, S., Géli, L., Scalabrin, C., Zitter, T. A. C., et al. (2018b). Upward migration of gas in an active tectonic basin: an example from the sea of Marmara. Deep Sea Res. Part II Top. Stud. Oceanogr. 153, 17–35. doi: 10.1016/j.dsr2.2018.06.007
Grall, C., Henry, P., Tezcan, D., Mercier de Lepinay, B., Bécel, A., Géli, L., et al. (2012). Heat flow in the Sea of Marmara Central Basin: possible implications for the tectonic evolution of the North Anatolian fault. Geology 40, 3–6. doi: 10.1130/G32192.1
Granot, R. (2016). Palaeozoic oceanic crust preserved beneath the eastern Mediterranean. Nat. Geosci. 9, 701–705. doi: 10.1038/ngeo2784
Gutscher, M.-A. (2004). What caused the great lisbon earthquake? Science 305, 1247–1248. doi: 10.1126/science.1101351
Gutscher, M.-A., Baptista, M. A., and Miranda, J. M. (2006). The Gibraltar Arc seismogenic zone (part 2): constraints on a shallow east dipping fault plane source for the 1755 Lisbon earthquake provided by tsunami modeling and seismic intensity. Tectonophysics 426, 153–166. doi: 10.1016/j.tecto.2006.02.025
Gutscher, M.-A., Malod, J., Rehault, J.-P., Contrucci, I., Klingelhoefer, F., Mendes-Victor, L., et al. (2002). Evidence for active subduction beneath Gibraltar. Geology 30, 1071–1074. doi: 10.1130/0091-7613(2002)030<1071:EFASBG>2.0.CO;2
Gutscher, M.-A., Royer, J.-Y., Graindorge, D., Murphy, S., Klingelhoefer, F., Cattaneo, A., et al. (2018). Benefitting from Cabled Observatories to Study Active Submarine Faults: the FOCUS Project (FOCUS = Fiber Optic Cable Use for Seafloor Studies of Earthquake Hazard and Deformation). Vienna: EGU General Assembly.
Halbach, P., Holzbecher, E., Reichel, T., and Moche, R. (2004). Migration of the sulphate - methane reaction zone in marine sediments of the Sea of Marmara - can this mechanisms be tectonically induced? Chem. Geol. 205, 73–82. doi: 10.1016/j.chemgeo.2003.12.013
Hanson, J. A., and Bowman, J. R. (2005). Indian Ocean ridge seismicity observed with a permanent hydroacoustic network. Geophys. Res. Lett. 32:L06301. doi: 10.1029/2004GL021931
Harrison, J. P., Gheeraert, N., Tsigelnitskiy, D., and Cockell, C. S. (2013). The limits of life under multiple extremes. Trends Microbiol. 21, 204–212. doi: 10.1016/j.tim.2013.01.006
Hasterok, D. (2013). Global patterns and vigor of ventilated hydrothermal circulation through young seafloor. Earth Planet. Sci. Lett. 380, 12–20. doi: 10.1016/j.epsl.2013.08.016
Head, I. M., Jones, D. M., and Larter, S. R. (2003). Biological activity in the deep subsurface and the origin of heavy oil. Nature 426, 344–352. doi: 10.1038/nature02134
Hein, J. R., Koski, R. A., Embley, R. W., Reid, J., and Chang, S.-W. (1999). Diffuse-flow hydrothermal field in an oceanic fracture zone setting, Northeast Pacific: deposit composition. Explor. Min. Geol. 8, 299–322.
Hekinian, R. (1982). Petrology of the ocean floor. chapter 7 oceanic fracture zones. Elsevier Oceanogr. Ser. 33, 219–222, 223, 227–249. doi: 10.1016/S0422-9894(08)70949-8
Hensen, C., Mazzini, A., and Bartolome, R. (2018). Data Compilation of Locations of Seafloor Seeps. Bremen: PANGAEA.
Hensen, C., Scholz, F., Nuzzo, M., Valadares, V., Gràcia, E., Terrinha, P., et al. (2015). Strike-slip faults mediate the rise of crustal-derived fluids and mud volcanism in the deep sea. Geology 43, 339–342. doi: 10.1130/G36359.1
Hensen, C., Wallmann, K., Schmidt, M., Ranero, C. R., and Suess, E. (2004). Fluid expulsion related to mud extrusion off Costa Rica continental margin - a window to the subducting slab. Geology 32, 201–204. doi: 10.1130/G20119.1
Hey, R., Duennebier, F. K., and Morgan, W. J. (1980). Propagating rifts on midocean ridges. J. Geophys. Res. 85, 3647–3658. doi: 10.1029/JB085iB07p03647
Hieke, W. (2004). The August 27, 1886 earthquake in Messenia (Peloponnesus) and reported flames over the Ionian Sea—a Mediterranean Ridge gas escape event? Mar. Geol. 207, 259–265. doi: 10.1016/j.margeo.2004.03.007
Hill, D., Johnston, M., Langbein, J., and Bilham, R. (1995). Response of long valley caldera to the M = 7.3 landers, California, earthquake. Geophys. Res. Lett. 100, 12985–13005. doi: 10.1029/95JB00860
Hill, D., Pollitz, F., and Newhall, C. (2002). Earthquake-volcano interactions. Phys. Today 55, 41–47. doi: 10.1063/1.1535006
Hill, E. M., Yue, H., Barbot, S., Lay, T., Tapponnier, P., Hermawan, I., et al. (2015). The 2012 Mw 8.6 Wharton Basin sequence: a cascade of great earthquakes generated by near-orthogonal, young, oceanic mantle faults. J. Geophys. Res. Solid Earth 120, 3723–3747. doi: 10.1002/2014JB011703
Hirose, T., Kawagucci, S., and Suzuki, K. (2011). Mechanoradical H2 generation during simulated faulting: implications for an earthquake-driven subsurface biosphere. Geophys. Res. Lett. 38:L17303. doi: 10.1029/2011GL048850
Hoehler, T. M., and Jørgensen, B. B. (2013). Microbial life under extreme energy limitation. Nature Rev. Microbiol. 11, 83–94. doi: 10.1038/nrmicro2939
Holdsworth, R. E., van Diggelen, E. W. E., Spiers, C. J., de Bresser, J. H. P., Walker, R. J., and Bowen, L. (2011). Fault rocks from the SAFOD core samples: implications for weakening at shallow depths along the San Andreas Fault, California. J. Struct. Geol. 33, 132–144. doi: 10.1016/j.jsg.2010.11.010
Holm, N. G., and Neubeck, A. (2009). Reduction of nitrogen compounds in oceanic basement and its implications for HCN formation and abiotic organic synthesis. Geochem. Trans. 10, 9–20. doi: 10.1186/1467-4866-10-9
Humphris, S. E., and Klein, F. (2018). Progress in deciphering the controls on the geochemistry of fluids in seafloor hydrothermal systems. Annu. Rev. Mar. Sci. 10, 315–343. doi: 10.1146/annurev-marine-121916-063233
Hurtado, L. A., Lutz, R. A., and Vrijenhoek, R. C. (2004). Distinct patterns of genetic differentiation among annelids of eastern Pacific hydrothermal vents. Mol. Ecol. 13, 2603–2615. doi: 10.1111/j.1365-294X.2004.02287.x
Husen, S., Wiemer, S., and Smith, R. B. (2004). Remotely triggered seismicity in the Yellowstone National Park region by the 2002 Mw 7.9 Denali Fault earthquake, Alaska. Bull. Seismol. Soc. Am. 94, S317–S331. doi: 10.1785/0120040617
Hutnak, M., Fisher, A. T., Stein, C. A., Harris, R., Wang, K., Silver, E., et al. (2007). “The thermal state of 18–24 ma upper lithosphere subducting below the nicoya peninsula, northern costa rica margin,” in Interplate Subduction Zone Seismogenesis, MARGINS Theoretical Institute, eds T. Dixon and C. Moore (New York, NY: Columbia University Press), 86–122.
Ihmlé, P., and Jordan, T. H. (1994). Teleseismic search for slow precursors to large earthquakes. Science 266, 1547–1551. doi: 10.1126/science.266.5190.1547
Ijiri, A., Inagaki, F., Kubo, Y., Adhikari, R. R., Hattori, S., Hoshino, T., et al. (2018). Deep-biosphere methane production stimulated by geofluids in the Nankai accretionary complex. Sci. Adv. 4:eaao4631. doi: 10.1126/sciadv.aao4631
Isacks, B., Oliver, J., and Sykes, L. R. (1968). Seismology and the new global tectonics. J. Geophys. Res. 73, 5855–5899. doi: 10.1029/JB073i018p05855
Johnson, H. P., Hutnak, M., Dziak, R. R. P., Fox, C. C. G., Urcuyo, I., Cowen, J. P. J., et al. (2000). Earthquake-induced changes in a hydrothermal system on the Juan de Fuca mid-ocean ridge. Nature 407, 174–177. doi: 10.1038/35025040
Johnson, S. B., Young, C. R., Jones, W. J., Warén, A., and Vrijenhoek, R. C. (2006). Migration, isolation, and speciation of hydrothermal vent limpets (Gastropoda; Lepetodrilidae) across the Blanco Transform Fault. Biol. Bull. 210, 140–157. doi: 10.2307/4134603
Judd, A. G., and Hovland, M. (2007). Seabed Fluid Flow. The Impact on Geology, Biology and the Marine Environment. Cambridge: Cambridge University Press, 293. doi: 10.1017/CBO9780511535918
Kamogawa, M., Orihara, Y., Tsurudome, C., Tomida, Y., Kanaya, T., Ikeda, D., et al. (2016). A possible space-based tsunami early warning system using observations of the tsunami ionospheric hole. Sci. Rep. 6:37989. doi: 10.1038/srep37989
Kanamori, H., and Stewart, G. S. (1976). Mode of the strain release along the gibbs fracture zone, mid-atlantic ridge. Phys. Earth Planet. Int. 11, 312–332. doi: 10.1016/0031-9201(76)90018-2
Kanazawa, T., Uehira, K., Mochizuki, M., Shinbo, T., Noguchi, S., and Fujimoto, H. (2015). “The Japan Trench earthquake and tsunami monitoring network project of cable-linked 150 seafloor observatories: the S-net project,” in Proceedings of the CTBTO Science and Technology Conference 2015, Vienna, 22–26.
Kaneda, Y. (2014). Introduction to ocean floor networks and their scientific application. Mar. Geophys. Res. 35, 177–180. doi: 10.1007/s11001-014-9232-x
Kelley, D. S., Karson, J. A., Blackman, D. K., Früh-Green, G. L., Butterfield, D. A., Lilley, M. D., et al. (2001). An off-axis hydrothermal vent field near the Mid-Atlantic Ridge at 30°N. Science 412, 145–149.
Kelley, D. S., Karson, J. A., Früh-Green, G. L., Yoerger, D. R., Shank, T. M., Butterfield, D. A., et al. (2005). A serpentinite-hosted ecosystem: the Lost City Hydrothermal Field. Science 307, 1428–1434. doi: 10.1126/science.1102556
Khutorskoi, M. D., and Polyak, B. G. (2017). Special features of heat flow in transform faults of the North Atlantic and Southeast Pacific. Geotectonics 51, 152–162. doi: 10.1134/S0016852117010022
King, C.-Y. (1986). Gas geochemistry applied to earthquake prediction: an overview. J. Geophys. Res. 91, 12269–12281. doi: 10.1029/JB091iB12p12269
Kinoshita, M., Tobin, H., Ashi, J., Kimura, G., Lallemant, S., Screaton, E. J., et al. (2009). Proc. IODP, 314/315/316. Washington, DC: Integrated Ocean Drilling Program Management International. doi: 10.2204/iodp.proc.314315316.2009
Kita, I., Matsuo, S., and Wakita, H. (1982). H2 generation by reaction between H2O and crushed rock: an experimental study on H2 degassing from the active fault zone. J. Geophys. Res. Solid Earth 87, 10789–10795. doi: 10.1029/JB087iB13p10789
Klein, E., Duputel, Z., Masson, F., Yavasoglu, H., and Agram, P. (2017). Aseismic slip and seismogenic coupling in the Marmara Sea: what can we learn from onland Geodesy? Geophys. Res. Lett. 44, 3100–3108. doi: 10.1002/2017GL072777
Kolandaivelu, K. P., Harris, R. N., Lowell, R. P., Alhamad, A., Gregory, E. P. M., and Hobbs, R. W. (2017). Analysis of a conductive heat flow profile in the Ecuador Fracture Zone. Earth Planet. Sci. Lett. 467, 120–127. doi: 10.1016/j.epsl.2017.03.024
Kolomijeca, A., Roland, F., Leon, P., and Kronfeldt, H.-D. (2013). Autonomous In-situ Raman sensor, suitable for surface enhanced Raman spectroscopy (SERS) for detection of chemicals in the Sea. Univers. J. Appl. Sci. 1, 38–43. doi: 10.13189/ujas.2013.010202
Konn, C., Charlou, J. L., Holm, N. G., and Mousis, O. (2015). The production of methane, hydrogen, and organic compounds in ultramafic-hosted hydrothermal vents of the Mid-Atlantic Ridge. Astrobiology 15, 381–399. doi: 10.1089/ast.2014.1198
Kopf, A., and Deyhle, A. (2002). Back to the roots: boron geochemistry of mud volcanoes and its implications for mobilization depth and global B cycling. Chem. Geol. 192, 195–210. doi: 10.1016/S0009-2541(02)00221-8
Krylova, E. M., Sahling, H., and Janssen, R. (2010). Abyssogena: a new genus of the family Vesicomyidae (Bivalvia) from deep-water vents and seeps. J. Molluscan Stud. 76, 107–132. doi: 10.1093/mollus/eyp052
Kuhn, T., Versteegh, G. J. M., Villinger, H., Dohrmann, I., Heller, C., Koschinsky, A., et al. (2017). Widespread seawater circulation in 18–22 Ma oceanic crust: impact on heat flow and sediment geochemistry. Geology 45, 799–802. doi: 10.1130/G39091.1
Kuşçu,İ., Okamura, M., Matsuoka, H., Gökaşan, E., Awata, Y., Tur, H., et al. (2005). Seafloor gas seeps and sediment failures triggered by the August 17, 1999 earthquake in the Eastern part of the Gulf of İzmit, Sea of Marmara, NW Turkey. Mar. Geol. 215, 193–214. doi: 10.1016/j.margeo.2004.12.002
Kutterolf, S., Liebetrau, V., Mörz, T., Freundt, A., Hammerich, T., and Garbe-Schönberg, D. (2008). Lifetime and cyclicity of fluid venting at forearc mound structures determined by tephrostratigraphy and radiometric dating of authigenic carbonates. Geology 36, 707–710. doi: 10.1130/G24806A.1
Lahr, J. C., Page, R. A., Stephens, C. D., and Christensen, D. H. (1988). Unusual earthquakes in the Gulf of Alaska and fragmentation of the Pacific Plate. Geophys. Res. Lett. 15, 1483–1486. doi: 10.1029/GL015i013p01483
Lang, S. O., Früh-Green, G. L., Bernasconi, S. M., Brazelton, W. J., Schrenk, M. O., and McGonigle, J. M. (2018). Deeply-sourced formate fuels sulfate reducers but not methanogens at Lost City Hydrothermal Field. Sci. Rep. 8:755. doi: 10.1038/s41598-017-19002-5
Langseth, M. G., and Hobart, M. A. (1976). Interpretation of heat flow measurements in the Vema Fracture Zone. Geophys. Res. Lett. 3, 241–244. doi: 10.1029/GL003i005p00241
LaRowe, D. E., Burwicz, E., Arndt, S., Dale, A. W., and Amend, J. P. (2017). Temperature and volume of global marine sediments. Geology 45, 275–278. doi: 10.1130/G38601.1
Lay, T. (2019). “Chapter 4 - reactivation of oceanic fracture zones in large intraplate earthquakes?,” in Transform Plate Boundaries and Fracture Zones, ed. J. C. Duarte (Amsterdam: Elsevier), 89–104. doi: 10.1016/B978-0-12-812064-4.00004-9
Le Gal, V., Lucazeau, F., Cannat, M., Poort, J., Monnin, C., Battani, A., et al. (2018). Heat flow, morphology, pore fluids and hydrothermal circulation in a typical Mid-Atlantic Ridge flank near Oceanographer Fracture Zone. Earth Planet. Sci. Lett. 482, 423–433. doi: 10.1016/j.epsl.2017.11.035
Le Pichon, X., and Angelier, J. (1979). The Hellenic arc and trench system: a key to the neotectonic evolution of the eastern Mediterranean area. Tectonophysics 60, 1–42. doi: 10.1016/0040-1951(79)90131-8
Le Pichon, X., and Angelier, J. (1981). The Aegean Sea. Philos. Trans. R. Soc. Lond. 300, 357–372. doi: 10.1098/rsta.1981.0069
Le Pichon, X., Şengör, A. M. C., Demirbağ, E., Rangin, C., Imren, C., Armijo, R., et al. (2001). The active main Marmara fault: comparative anatomy of a continental transform fault in a marine setting. Earth Planet. Sci. Lett. 192, 595–616. doi: 10.1016/S0012-821X(01)00449-6
Le Pichon, X., Şengör, A. M. C., Kende, J., İmren, C., Henry, P., Grall, C., et al. (2015). Propagation of a strike slip plate boundary within an extensional environment: the westward propagation of the North Anatolian Fault. Can. J. Earth Sci. 53, 1416–1439. doi: 10.1139/cjes-2015-0129
Lever, M. A., Rogers, K., Lloyd, K. G., Overmann, J. O., Schink, B., Thauer, R. K., et al. (2015). Microbial life under extreme energy limitation: a synthesis of laboratory- and field-based investigations. FEMS Microbiol. Rev. 39, 688–728. doi: 10.1093/femsre/fuv020
Lever, M. A., Rouxel, O., Alt, J. C., Shimizu, N., Ono, S., Coggon, R. M., et al. (2013). Evidence for microbial carbon and sulfur cycling in deeply buried ridge flank basalt. Science 339, 1305–1308. doi: 10.1126/science.1229240
Levin, L. A. (2005). Ecology of cold seep sediments: interactions of fauna with flow, chemistry and microbes. Oceanogr. Mar. Biol. Ann. Rev. 43, 1–46. doi: 10.1201/9781420037449.ch1
Liebetrau, V., Augustin, N., Kutterolf, S., Schmidt, M., Eisenhauer, A., Garbe-Schönberg, D., et al. (2014). Cold-seep-driven carbonate deposits at the Central American forearc: contrasting evolution and timing in escarpment and mound settings. Int. J. Earth Sci. 103, 1845–1872. doi: 10.1007/s00531-014-1045-2
Liebetrau, V., Eisenhauer, A., and Linke, P. (2010). Cold seep carbonates and associated coldwater corals at the Hikurangi Margin, New Zealand: new insights into fluid pathways, growth structures and geochronology. Mar. Geol. 272, 307–318. doi: 10.1016/j.margeo.2010.01.003
Ligi, M., Bonatti, E., Gasperini, L., and Poliakov, A. N. B. (2002). Oceanic broad mutifault transform plate boundaries. Geology 30, 11–14. doi: 10.1130/0091-7613(2002)030<0011:OBMTPB>2.0.CO;2
Lindsey, N. J., Martin, E. R., Dreger, D. S., Freifeld, B., Cole, S., James, S. R., et al. (2017). Fiber-optic network observations of earthquake wavefields. Geophys. Res. Lett. 44, 11,792–11,799. doi: 10.1002/2017GL075722
Linke, P., Schmidt, M., Rohleder, M., Al-Barakati, A., and Al-Farawati, R. (2015). Novel online digital video and high-speed data broadcasting via standard coaxial cable onboard marine operating vessels. Mar. Technol. Soc. J. 49, 7–18. doi: 10.4031/MTSJ.49.1.2
Lister, C. R. J., Sclater, G., Davis, E. E., Villinger, H., and Nagihara, S. (1990). Heat flow maintained in ocean basins of great age: investigations in the north-equatorial West Pacific. Geophys. J. Int. 102, 603–630. doi: 10.1111/j.1365-246X.1990.tb04586.x
Lo Iacono, C., Gràcia, E., Zaniboni, F., Pagnoni, G., Tinti, S., Bartolomé, R., et al. (2012). Large, deepwater slope failures: implications for landslide-generated tsunamis. Geology 40, 931–934. doi: 10.1130/G33446.1
Londry, K. L., Dawson, K. G., Grover, H. D., Summons, R. E., and Bradley, A. S. (2008). Stable carbon isotope fractionation between substrates and products of Methanosarcina barkeri. Org. Geochem. 39, 608–621. doi: 10.1016/j.orggeochem.2008.03.002
Lonsdale, P. (1986). Tectonic and magmatic ridges in the Eltanin fault system, South Pacific. Mar. Geophys. Res. 8, 203–242. doi: 10.1007/BF00305484
Lucazeau, F., Bonneville, A., Escartìn, J., Von Herzen, R. P., Gouze, P., Carton, H., et al. (2006). Heat-flow variations on a slowly accreting ridge: constraints on the hydrothermal and conductive cooling for the lucky strike segment (mid Atlantic ridge, 37°N). Geochem. Geophys. Geosyst. 7:Q07011. doi: 10.1029/2005GC001178
Lupi, M., Fallahi, M. J., Mazzini, A., Polonia, A., D’Alessandro, A., D’Anna, G., et al. (2018). “Seismic activity of mud volcanoes in the Marmara Sea, Turkey,” in Proceedings of the 20th EGU General Assembly, EGU2018 (Vienna: Geophysical Research Abstracts), 14482.
Lupi, M., Fuchs, F., and Pacheco, J. F. J. (2014). Fault reactivation due to the M 7.6 Nicoya earthquake at the Turrialba-Irazú volcanic complex, Costa Rica: effects of dynamic stress triggering. Geophys. Res. Lett. 41, 4142–4148. doi: 10.1002/2014GL059942
Lupi, M., Geiger, S., and Graham, C. M. (2011a). Numerical simulations of seismicity-induced fluid flow in the Tjörnes Fracture Zone, Iceland. J. Geophys. Res. 116:B07101. doi: 10.1029/2010JB007732
Lupi, M., Ricci, T., Suski, B., Kenkel, J., Fuchs, F., Miller, S. A., et al. (2015). Subsurface fluid distribution and possible seismic precursory signal at the Salse di Nirano mud volcanic field, Italy. Geophys. J. Int. 204, 907–917. doi: 10.1093/gji/ggv454
Lupi, M., Saenger, E. H., Fuchs, F., and Miller, S. A. (2013). Lusi mud eruption triggered by geometric focusing of seismic waves. Nat. Geosci. 6, 642–646. doi: 10.1038/ngeo2239
Macdonald, K. C. (1998). Linkages between faulting, volcanism, hydrothermal activity and segmentation on fast spreading centers. Geophys. Monogr. Ser. 106, 27–58. doi: 10.1029/GM106p0027
Mächler, L., Brennwald, M. S., and Kipfer, R. (2012). Membrane inlet mass spectrometer for the quasi-continuous on-site analysis of dissolved gases in groundwater. Environ. Sci. Technol. 46, 8288–8296. doi: 10.1021/es3004409
Mächler, L., Brennwald, M. S., Tyroller, L., Livingstone, D. M., and Kipfer, R. (2014). Conquering the outdoors with on-site mass spectrometry. Chimia 68, 155–159. doi: 10.2533/chimia.2014.155
Magalhães, V. H., Pinheiro, L. M., Ivanov, M. K., Kozlova, E., Blinova, V., Kolganova, J., et al. (2012). Formation processes of methane-derived authigenic carbonates from the Gulf of Cadiz. Sediment. Geol. 243–244, 155–168. doi: 10.1016/j.sedgeo.2011.10.013
Maia, M. (2019). “Topographic and morphologic evidences of deformation at oceanic transform faults: far-field and local-field stresses,” in Transform Plate Boundaries and Fracture Zones, ed. J. C. Duarte (Amsterdam: Elsevier), 61–87. doi: 10.1016/B978-0-12-812064-4.00003-7
Mamyrin, B. A., and Tolstikhin, I. N. (1984). “Helium isotopes in nature,” in Developments in Geochemistry, Vol. 3, ed. W. S. Fyfe (Amsterdam: Elsevier).
Manga, M., and Brodsky, E. (2006). Seismic triggering of eruptions in the far field: volcanoes and geysers. Annu. Rev. Earth Planet. Sci. 34, 263–291. doi: 10.1146/annurev.earth.34.031405.125125
Manga, M., Brumm, M., and Rudolph, M. L. (2009). Earthquake triggering of mud volcanoes. Mar. Pet. Geol. 26, 1785–1798. doi: 10.1016/j.marpetgeo.2009.01.019
Manning, C. E., and Ingebritsen, S. (1999). Permeability of the continental crust: implications of geothermal data and metamorphic systems. Rev. Geophys. 37, 127–150. doi: 10.1029/1998RG900002
Marques, F. O., Cobbold, P. R., and Lourenço, N. (2007). Physical models of rifting and transform faulting, due to ridge push in a wedge-shaped oceanic lithosphere. Tectonophysics 443, 37–52. doi: 10.1016/j.tecto.2007.07.002
Marra, G., Clivati, C., Luckett, R., Tampellini, A., Kronjäger, J., Wright, L., et al. (2018). Ultrastable laser interferometry for earthquake detection with terrestrial and submarine cables. Science 361, 486–490. doi: 10.1126/science.aat4458
Martin, J. B., Orange, D. L., Lorenson, T. D., and Kvenvolden, K. A. (1997). Chemical and isotopic evidence of gas influenced flow at a transform plate boundary: monterey Bay, California. J. Geophys. Res. 102, 24903–24915. doi: 10.1029/97JB02154
Martínez-Loriente, S., Sallarès, V., Gràcia, E., Bartolome, R., Dañobeitia, J. J., and Zitellini, N. (2014). Seismic and gravity constraints on the nature of the basement in the Africa-Eurasia plate boundary: new insights for the geodynamic evolution of the SW Iberian margin. J. Geophys. Res. Solid Earth 119, 127–149. doi: 10.1002/2013JB010476
Materna, K., Taira, T. A., and Bürgmann, R. (2018). Aseismic transform fault slip at the mendocino triple junction from characteristically repeating earthquakes. Geophys. Res. Lett. 45, 699–707. doi: 10.1002/2017GL075899
Mau, S., Rehder, G., Arroyo, I. G., Gossler, J., and Suess, E. (2007). Indications of a link between seismotectonics and CH4 release from seeps off Costa Rica. Geochem. Geophys. Geosyst. 8:Q04003. doi: 10.1029/2006GC001326
Mazzini, A., and Etiope, G. (2017). Mud volcanism: an updated review. Earth Sci. Rev. 168, 81–112. doi: 10.1016/j.earscirev.2017.03.001
Mazzini, A., Etiope, G., and Svensen, H. (2012). A new hydrothermal scenario for the 2006 Lusi eruption, Indonesia - Insights from gas geochemistry. Earth Planet. Sci. Lett. 317, 305–318. doi: 10.1016/j.epsl.2011.11.016
Mazzini, A., Nermoen, A., Krotkiewski, M., Podladchikov, Y., Planke, S., and Svensen, H. (2009). Strike-slip faulting as a trigger mechanism for overpressure release through piercement structures - Implications for the Lusi mud volcano, Indonesia. Mar. Petrol. Geol. 26, 1751–1765. doi: 10.1016/j.marpetgeo.2009.03.001
McClusky, S., Balassanian, S., Barka, A., Demir, C., Ergintav, S., Georgiev, I., et al. (2000). Global Positioning System constraints on plate kinematics and dynamics in the eastern Mediterranean and Caucasus. J. Geophys. Res. 105, 5695–5719. doi: 10.1029/1999JB900351
McCollom, T. M., and Seewald, J. S. (2007). Abiotic synthesis of organic compounds in deep-sea hydrothermal environments. Chem. Rev. 107, 382–401. doi: 10.1021/cr0503660
McGuire, J. J., Boettcher, M. S., and Jordan, T. H. (2005). Foreshock sequences and short-term earthquake predictability on East Pacific Rise transform faults. Nature 434, 457–461. doi: 10.1038/nature03377
McGuire, J. J., Collins, J. A., Gouédard, P., Roland, E., Lizarralde, D., Boettcher, M. S., et al. (2012). Variations in earthquake rupture properties along the Gofar transform fault, East Pacific Rise. Nat. Geosci. 5, 336–341. doi: 10.1038/ngeo1454
McHugh, C. M. G., Braudy, N., Çağatay, M. N., Sorlien, C., Cormier, M.-H., Seeber, L., et al. (2014). Seafloor fault ruptures along the North Anatolia Fault in the Marmara Sea, Turkey: link with the adjacent basin turbidite record. Mar. Geol. 353, 65–83. doi: 10.1016/j.margeo.2014.03.005.0025-3227/
McHugh, C. M. G., Seeber, L., Cormier, M. H., Dutton, J., Çağatay, M. N., Polonia, A., et al. (2006). Submarine earthquake geology along the North Anatolia Fault in the Marmara Sea, Turkey: a model for transform basin sedimentation. Earth Planet. Sci. Lett. 248, 661–668. doi: 10.1016/j.epsl.2006.05.038
McKenzie, D. P. (1978). Active tectonics of the Alpine Himalayan Belt, the Aegean Sea and surrounding regions. Geophys. J. Int. 55, 217–252. doi: 10.1111/j.1365-246X.1978.tb04759.x
Meade, B. J., Hager, B. H., McClusky, S., Reilinger, R., Ergintav, S., Lenk, O., et al. (2002). Estimates of seismic potential in the marmara sea region from block models of secular deformation constrained by global positioning system measurements. Bull. Seismol. Soc. Am. 92, 208–215. doi: 10.1785/0120000837
Méhay, S., Früh-Green, G. L., Lang, S. Q., Bernasconi, S. M., Brazelton, W. J., Schrenk, M. O., et al. (2013). Record of archaeal activity at the serpentinite hosted Lost City Hydrothermal Field. Geobiology 11, 570–592. doi: 10.1111/gbi.12062
Mellors, R., Kilb, D., Aliyev, A., Gasanov, A., and Yetirmishli, G. (2007). Correlations between earthquakes and large mud volcano eruptions. J. Geophys. Res. 112:B04304. doi: 10.1029/2006JB004489
Ménez, B., Pisapia, C., Andreani, M., Jamme, F., Vanbellingen, Q. P., Brunelle, A., et al. (2018). Abiotic synthesis of amino acids in the recesses of the oceanic lithosphere. Nature 564, 59–63. doi: 10.1038/s41586-018-0684-z
Miller, S. A., Collettini, C., Chiaraluce, L., Cocco, M., Barchi, M., and Kaus, B. J. P. (2004). Aftershocks driven by a high-pressure CO2 source at depth. Nature 427, 724–727. doi: 10.1038/nature02251
Miller, S. A., and Mazzini, A. (2018). More than ten years of Lusi: a review of facts, coincidences, and past and future studies. Mar. Petrol. Geol. 90, 10–25. doi: 10.1016/j.marpetgeo.2017.06.019
Moore, D. E., Lockner, D. A., Ma, S., Summers, R., and Byerlee, J. D. (1997). Strengths of serpentinite gouges at elevated temperatures. J. Geophys. Res. Solid Earth 102, 14787–14801. doi: 10.1029/97JB00995
Moore, D. E., and Rymer, M. J. (2007). Talc-bearing serpentinite and the creeping section of the San Andreas fault. Nature 448, 795–797. doi: 10.1038/nature06064
Morgan, J. P., and Parmentier, E. M. (1984). Lithospheric stress near a ridge-transform intersection. Geophys. Res. Lett. 11, 113–116. doi: 10.1029/GL011i002p00113
Morgan, W. J. (1968). Rises, trenches, great faults, and crustal blocks. J. Geophys. Res. 73, 1959–1981. doi: 10.1029/JB073i006p01959
Mottl, M. J., Wheat, C. G., Fryer, P., Gharib, J., and Martin, J.-B. (2004). Chemistry of springs across the Mariana forearc shows progressive devolatilization of the subducting plate. Geochim. Cosmochim. Acta 68, 4915–4933. doi: 10.1016/j.gca.2004.05.037
Mourgues, R., and Cobbold, P. R. (2003). Some tectonic consequences of fluid overpressures and seepage forces as demonstrated by sandbox modeling. Tectonophysics 376, 75–97. doi: 10.1016/S0040-1951(03)00348-2
Mourgues, R., and Cobbold, P. R. (2006). Sandbox experiments on gravitational spreading and gliding in the presence of fluid overpressures. J. Struct. Geol. 28, 887–901. doi: 10.1016/j.jsg.2005.12.013
Müller, M. D., Geiger, A., Kahle, H.-G., Veis, G., Billiris, H., Paradissis, D., et al. (2013). Velocity and deformation fields in the North Aegean domain, Greece, and implications for fault kinematics, derived from GPS data 1993–2009. Tectonophysics 597–598, 34–49. doi: 10.1016/j.tecto.2012.08.003
Müller, R. D., Sdrolias, M., Gaina, C., and Roest, W. R. (2008). Age spreading rates and spreading asymmetry of the world’s ocean crust. Geochem. Geophys. Geosyst. 9:Q04006. doi: 10.1029/2007GC001743
Neres, M., Carafa, M. M. C., Fernandes, R. M. S., Matias, L., Duarte, J. C., Barba, S., et al. (2016). Lithospheric deformation in the Africa-Iberia plate boundary: improved neotectonic modeling testing a basal-driven Alboran plate. J. Geophys. Res. Solid Earth 121, 6566–6596. doi: 10.1002/2016JB013012
Niemann, H., Duarte, J., Hensen, C., Omoregie, E., Magalhães, V. H., Elvert, M., et al. (2006). Microbial methane turnover at mud volcanoes of the Gulf of Cadiz. Geochim. Cosmochim. Acta 70, 5336–5355. doi: 10.1016/j.gca.2006.08.010
Noda, H., Sawai, M., and Shibazaki, B. (2017). Earthquake sequence simulations with measured properties for JFAST core samples. Philos. Trans. R. Soc. A Math. Phys. Eng. Sci. 375:20160003. doi: 10.1098/rsta.2016.0003
Norris, R. J., and Toy, V. G. (2014). Continental transforms: a view from the Alpine Fault. J. Struct. Geol. 64, 3–31. doi: 10.1016/j.jsg.2014.03.003
Nuzzo, M., Tomonaga, Y., Schmidt, M., Valadares, V., Faber, E., Piñero, E., et al. (2019). Formation and migration of hydrocarbons in deeply buried sediments of the Gulf of Cadiz convergent plate boundary - Insights from the hydrocarbon and helium isotope geochemistry of mud volcano fluids. Mar. Geol. 410, 56–69. doi: 10.1016/j.margeo.2019.01.005
Ohara, Y., Reagan, M. K., Fujikura, K., Watanabe, H., Michibayashi, K., Ishii, T., et al. (2012). A serpentinite-hosted ecosystem in the Southern Mariana Forearc. Proc. Natl. Acad. Sci. U.S.A. 109, 2831–2835. doi: 10.1073/pnas.1112005109
Okal, E. A., and Stewart, L. M. (1982). Slow earthquakes along oceanic fracture zones: evidence for asthenospheric flow away from hotspots? Earth Planet. Sci. Lett. 57, 75–87. doi: 10.1016/0012-821X(82)90174-1
Okumura, T., Ohara, Y., Stern, R. J., Yamanaka, T., Onishi, Y., Watanabe, H., et al. (2016). Brucite chimney formation and carbonate alteration at the Shinkai Seep Field, a serpentinite-hosted vent system in the southern Mariana forearc. Geochem. Geophys. Geosyst. 17, 3775–3796. doi: 10.1002/2016GC006449
Oldenburg, D. W., and Brune, J. N. (1972). Ridge transform fault spreading pattern in freezing wax. Science 178, 301–304. doi: 10.1126/science.178.4058.301
Oldenburg, D. W., and Brune, J. N. (1975). An Explanation for the orthogonality of ocean ridges and transform faults. J. Geophys. Res. 80, 2575–2585. doi: 10.1029/JB080i017p02575
Omira, R., Ramalho, I., Terrinha, P., Baptista, M. A., Batista, L., and Zitellini, N. (2016). Deep-water seamounts, a potential source of tsunami generated by landslides? – The Hirondelle seamount, NE Atlantic. Mar. Geol. 379, 267–280. doi: 10.1016/j.margeo.2016.06.010
O’Mullan, G. D., Maas, P. A. Y., Lutz, R. A., and Vrijenhoek, R. C. (2001). A hybrid zone between hydrothermal vent mussels (Bivalvia: Mytilidae) from the Mid-Atlantic Ridge. Mol. Ecol. 10, 2819–2831. doi: 10.1046/j.0962-1083.2001.01401.x
Onishi, Y., Yamanaka, T., Okumura, T., Kawagucci, S., Watanabe, H. K., and Ohara, Y. (2018). Evaluation of nutrient and energy sources of the deepest known serpentinite-hosted ecosystem using stable carbon, nitrogen, and sulfur isotopes. PLoS One 13:e0199000. doi: 10.1371/journal.pone.0199000
Ostrander, W. J. (1984). Plane-wave reflection coefficients for gas sands at non-normal angles of incidence. Geophysics 49, 1637–1648. doi: 10.1190/1.1441571
Pagli, C. (2003). Triggered fault slip on June 17, 2000 on the Reykjanes Peninsula, SW-Iceland captured by radar interferometry. Geophys. Res. Lett. 30:1273. doi: 10.1029/2002GL015310
Palmer, M. R. (1991). Boron isotope systematics of hydrothermal fluids and tourmalines: a synthesis. Chem. Geol. 94, 111–121. doi: 10.1016/S0009-2541(10)80023-3
Parsons, T., Toda, S., Stein, R. S., Barka, A., and Dieterich, J. H. (2000). Heightenined odds of large earthquake near Istanbul: an interaction-based probability calculation. Science 288, 661–666. doi: 10.1126/science.288.5466.661
Paull, C. K., Ussler, W. I. I. I., Peltzer, E. T., Brewer, P. G., Keaten, R., Mitts, P. J., et al. (2007). Authigenic carbon entombed in methane-soaked sediments from northeastern transform margin of the Guaymas Basin, Gulf of California. Deep Sea Res. II 54, 1240–1267. doi: 10.1016/j.dsr2.2007.04.009
Penning, H., Plugge, C. M., Galand, P. E., and Conrad, R. (2005). Variation of carbon isotope fractionation in hydrogenotrophic methanogenic microbial cultures and environmental samples at different energy status. Glob. Chang. Biol. 11, 2103–2113. doi: 10.1111/j.1365-2486.2005.01076.x
Pierre, C., Blanc-Valleron, M. M., Demange, J., Boudouma, O., Foucher, J. P., Pape, T., et al. (2012). Authigenic carbonates from active methane seeps offshore southwest Africa. Geo Mar. Lett. 32, 501–513. doi: 10.1007/s00367-012-0295-x
Polonia, A., Gasperini, L., Amorosi, A., Bonatti, E., Çağatay, N., Capotondi, L., et al. (2004). Holocene slip rate of the North Anatolian Fault beneath the Sea of Marmara. Earth Planet. Sci. Lett. 227, 411–426. doi: 10.1016/j.epsl.2004.07.042
Polonia, A., Panieri, G., Gasperini, L., Gasparotto, G., Bellucci, L. G., and Torelli, L. (2013). Turbidite paleoseismology in the Calabrian Arc Subduction Complex (Ionian Sea). Geochem. Geophys. Geosyst. 14, 112–140. doi: 10.1029/2012GC004402
Polonia, A., Romano, S., Çağatay, M. N., Capotondi, L., Gasparotto, G., Gasperini, L., et al. (2015). Are repetitive slumpings during sapropel S1 related to paleo-earthquakes? Mar. Geol. 361, 41–52. doi: 10.1016/j.margeo.2015.01.001
Polonia, A., Torelli, L., Gasperini, L., Cocchi, L., Muccini, F., Bonatti, E., et al. (2017). Lower plate serpentinite diapirism in the Calabrian Arc subduction complex. Nat. Commun. 8:2172. doi: 10.1038/s41467-017-02273-x
Pouderoux, H., Lamarche, G., and Proust, J.-N. (2012). Building an 18 000-year-long paleo-earthquake record from detailed deep-sea turbidite characterisation in Poverty Bay, New Zealand. Nat. Hazards Earth Syst. Sci. 12, 2077–2101. doi: 10.5194/nhess-12-2077-2012
Prokurowski, G., Lilley, M. D., Seewald, J. S., Früh-Green, G. L., Olson, E. J., Lupton, J. E., et al. (2008). Abiogenic hydrocarbon production at Lost City Hydrothermal Field. Science 319, 604–607. doi: 10.1126/science.1151194
Ritt, B., Sarrazin, J., Caprais, J.-C., Noël, P., Gauthier, O., Pierre, C., et al. (2010). First insights into the structure and environmental setting of cold-seep communities in the Marmara Sea. Deep Sea Res. Part I Oceanogr. Res. Pap. 57, 1120–1136. doi: 10.1016/j.dsr.2010.05.011
Robinson, D. P., Henry, C., Das, S., and Woodhouse, J. H. (2001). Simultaneous rupture along two conjugate planes of the Wharton Basin earthquake. Science 292, 1145–1148. doi: 10.1126/science.1059395
Roeloffs, E., Sneed, M., Galloway, D. L., Sorey, M. L., Farrar, C. D., Howle, J. F., et al. (2003). Water-level changes induced by local and distant earthquakes at Long Valley caldera, California. J. Volcanol. Geotherm. Res. 127, 269–303. doi: 10.1016/S0377-0273(03)00173-2
Roland, E., Behn, M. D., and Hirth, G. (2010). Thermal-mechanical behavior of oceanic transform faults: implications for the spatial distribution of seismicity. Geochem. Geophys. Geosyst. 11:Q07001. doi: 10.1029/2010GC003034
Romanowicz, B. (1992). Strike-slip earthquakes on quasi-vertical transcurrent faults: inferences for general scaling relations. Geophys. Res. Lett. 19, 481–484. doi: 10.1029/92GL00265
Rosas, F. M., Duarte, J., Terrinha, P., Valadares, V., and Matias, L. (2009). Morphotectonic characterization of major bathymetric lineaments in NW Gulf of Cadiz (Africa-Iberia plate boundary): insights from analogue modelling experiments. Mar. Geol. 261, 33–47. doi: 10.1016/j.margeo.2008.08.002
Rosas, F. M., Duarte, J. C., Terrinha, P., and Schellart, W. P. (2016). “Seismic potential of thrust-wrench tectonic interference between major active faults offshore SW iberia: a new explanation for the 1755 great lisbon earthquake?,” in Plate Boudaries and Natural Hazards, eds J. C. Duarte and W. P. Schellart (New Jersey, NJ: Wiley-Blackwell). doi: 10.1002/9781119054146.ch9
Ruffine, L., Donval, J.-P., Croguennec, C., Burnard, P., Luc, H., Germain, Y., et al. (2018a). Multiple gas reservoirs are responsible for the gas emissions along the Marmara fault network. Deep Sea Res. Part II 153, 48–60. doi: 10.1016/j.dsr2.2017.11.011
Ruffine, L., and Geli, L. (2014). MARSITECruise Report. 2014 1st–13th November – Leg 2. Issy-les-Moulineaux: Ifremer. doi: 10.13155/56480
Ruffine, L., Ondreas, H., Blanc-Valleron, M.-M., Teichert, B. M. A., Scalabrin, C., Rinnert, E., et al. (2018b). Multidisciplinary investigation on cold seeps with vigorous gas emissions in the Sea of Marmara (MarsiteCruise): strategy for site detection and sampling and first scientific outcome. Deep Sea Res. Part II Top. Stud. Oceanogr. 153, 36–47. doi: 10.1016/j.dsr2.2018.03.006
Ruhl, H. A., André, M., Beranzoli, L., Çağatay, M. N., Colaço, A., Cannat, M., et al. (2011). Societal need for improved understanding of climate change, anthropogenic impacts, and geo-hazard warning drive development of ocean observatories in European Seas. Prog. Oceanogr. 91, 1–33. doi: 10.1016/j.pocean.2011.05.001
Ryan, W. B. F., Carbotte, S. M., Coplan, J. O., O’Hara, S., Melkonian, A., Arko, R., et al. (2009). Global multi-resolution topography synthesis. Geochem. Geophys. Geosyst. 10:Q03014. doi: 10.1029/2008GC002332
Sakellariou, D., Rousakis, G., Morfis, I., Panagiotopoulos, I., Ioakim, C., Trikalinou, G., et al. (2018). “Deformation and kinematics at the termination of the North Anatolian Fault: the North Aegean Trough horsetail structure,” in Proceedings of the 9th International INQUA Meeting on Paleoseismology, Active Tectonics and Archeoseismology, 25-27 June 2018, Possidi. <doi>
Sakellariou, D., and Tsampouraki-Kraounaki, K. (2019). “Chapter 14 - plio-quaternary extension and strike-slip tectonics in the aegean,” in Transform Plate Boundaries and Fracture Zones, ed. J. C. Duarte (Amsterdam: Elsevier), 339–374. doi: 10.1016/B978-0-12-812064-4.00014-1
Sakic, P., Piété, H., Ballu, V., Royer, J.-Y., Kopp, H., Lange, D., et al. (2016). No significant steady state surface creep along the North Anatolian Fault offshore Istanbul: results of 6 months of seafloor acoustic ranging. Geophys. Res. Lett. 43, 6817–6825. doi: 10.1002/2016GL069600
Sallarès, V., Martínez-Loriente, S., Prada, M., Gràcia, E., Ranero, C., Gutscher, M.-A., et al. (2013). Seismic evidence of exhumed mantle rock basement at the Gorringe Bank and the adjacent Horseshoe and Tagus abyssal plains (SW Iberia). Earth Planet. Sci. Lett. 365, 120–131. doi: 10.1016/j.epsl.2013.01.021
Sandwell, D. (1986). Thermal stress and the spacings of transform faults. J. Geophys. Res. 91, 6405–6417. doi: 10.1029/JB091iB06p06405
Sano, Y., Hara, T., Takahata, N., Kawagucci, S., Honda, M., Nishio, Y., et al. (2014). Helium anomalies suggest a fluid pathway from mantle to trench during the 2011 Tohoku-Oki earthquake. Nat. Commun. 5:3084. doi: 10.1038/ncomms4084
Sano, Y., Nakamura, Y., Wakita, H., Notsu, K., and Kobayashi, Y. (1986). 3He/4He ratio anomalies associated with the 1984 Western Nagano Earthquake: possibly induced by a diapiric magma. J. Geophys. Res. Solid Earth 91, 12291–12295. doi: 10.1029/JB091iB12p12291
Sano, Y., Takahata, N., Kagoshima, T., Shibata, T., Onoue, T., and Zhao, D. (2016). Groundwater helium anomaly reflects strain change during the 2016 Kumamoto earthquake in Southwest Japan. Sci. Rep. 6:37939. doi: 10.1038/srep37939
Sarı, E., and Çağatay, M. N. (2006). Turbidites and their association with past earthquakes in the deep Çinarcik Basin of the Marmara Sea. Geo Mar. Lett. 26, 69–76. doi: 10.1007/s00367-006-0017-359
Sartori, R., Torelli, L., Zitellini, N., Peis, D., and Lodolo, E. (1994). Eastern segment of the Azores–Gibraltar line (central–eastern Atlantic); an oceanic plate boundary with diffuse compressional deformation. Geology 22, 555–558. doi: 10.1130/0091-7613(1994)022<0555:ESOTAG>2.3.CO;2
Schmidt, C., Burwicz, E., Hensen, C., Wallmann, K., Martínez-Loriente, S., and Gràcia, E. (2018). Genesis of mud volcano fluids in the Gulf of Cadiz using a novel basin-scale model approach. Geochim. Cosmochim. Acta 243, 186–204. doi: 10.1016/j.gca.2018.09.011
Schmidt, K., Koschinsky, A., Garbe-Schönberg, D., de Carvalho, L. M., and Seifert, R. (2007). Geochemistry of hydrothermal fluids from the ultramafic-hosted Logatchev hydrothermal field, 15°N on the Mid-Atlantic Ridge: temporal and spatial investigation. Chem. Geol. 242, 1–21. doi: 10.1016/j.chemgeo.2007.01.023
Schmidt, M., Linke, P., Sommer, S., Esser, D., and Cherednichenko, S. (2015). Natural CO2 seeps offshore Panarea - a test site for subsea CO2 leak detection technology. Mar. Technol. Soc. J. 49, 19–30. doi: 10.4031/MTSJ.49.1.3
Schmittbuhl, J., Karabulut, H., Lenligné, O., and Bouchon, M. (2016). Seismicity distribution and locking depth along the Main Marmara Fault, Turkey. Geochem. Geophys. Geosyst. 17, 954–965. doi: 10.1002/2015GC006120
Scholz, F., Hensen, C., Lu, Z., and Fehn, U. (2010). Controls on the 129I/I ratio of deep-seated marine interstitial fluids: ’old’ organic versus fissiogenic 129-iodine. Earth Planet. Sci. Lett. 294, 27–36. doi: 10.1016/j.epsl.2010.02.034
Scholz, F., Hensen, C., Reitz, A., Romer, R. L., Liebetrau, V., Meixner, A., et al. (2009). Isotopic evidence (87Sr/86Sr, δ7Li) for alteration of the oceanic crust at deep-rooted mud volcanoes in the Gulf of Cadiz, NE Atlantic Ocean. Geochim. Cosmochim. Acta 73, 5444–5459. doi: 10.1016/j.gca.2009.06.004
Schulte, M., and Shock, E. (1995). Thermodynamics of Strecker synthesis in hydrothermal systems. Orig. Life Evol. Biosph. 25, 161–173. doi: 10.1007/BF01581580
Şengör, A. M. C., Grall, C., İmren, C., Le Pichon, X., Görür, N., Henry, P., et al. (2014). The geometry of the North Anatolian transform fault in the Sea of Marmara and its temporal evolution: implications for the development of intracontinental transform faults. Can. J. Earth Sci. 51, 222–242. doi: 10.1139/cjes-2013-0160
Şengör, A. M. C., Zabcı, C., and Natal’in, B. A. (2019). “Chapter 9 - continental transform faults: congruence and incongruence with normal plate kinematics,” in Transform Plate Boundaries and Fracture Zones, ed. J. C. Duarte (Amsterdam: Elsevier), 169–247. doi: 10.1016/B978-0-12-812064-4.00009-8
Seyfried, W. E., Pester, N. J., Tutolo, B. M., and Ding, K. (2015). The Lost City hydrothermal system: constraints imposed by vent fluid chemistry and reaction path models on subseafloor heat and mass transfer processes. Geochim. Cosmochim. Acta 163, 59–79. doi: 10.1139/cjes-2013-0160
Shapiro, S. A. (2000). An inversion for fluid transport properties of three-dimensionally heterogeneous rocks using induced microseismicity. Geophys. J. Int. 143, 931–936. doi: 10.1046/j.1365-246X.2000.00264.x
Shock, E. L. (1990). Geochemical constraints on the origin of organic compounds in hydrothermal systems. Orig. Life Evol. Biosph. 20, 331–367. doi: 10.1007/BF01808115
Silva, S., Terrinha, P., Matias, L., Duarte, J. C., Roque, C., Ranero, C. R., et al. (2017). Micro-seismicity in the Gulf of Cadiz: is there a link between micro-seismicity, high magnitude earthquakes and active faults? Tectonophysics 717, 226–241.
Skelton, A., Andrén, M., Kristmannsdóttir, H., Stockmann, G., Mörth, C.-M., Sveinbjörnsdóttir, Á., et al. (2014). Changes in groundwater chemistry before two consecutive earthquakes in Iceland. Nat. Geosci. 7, 752–756. doi: 10.1038/ngeo2250
Sobolev, S. V., Petrunin, A., Garfunkel, Z., and Babeyko, A. Y. (2005). Thermo-mechanical model of the Dead Sea Transform. Earth Planet. Sci. Lett. 238, 78–95. doi: 10.1016/j.epsl.2005.06.058
Sommer, S., Linke, P., Pfannkuche, O., Schleicher, T., Schneider, V., Deimling, J., et al. (2009). Seabed methane emissions and the habitat of frenulate tubeworms on the Captain Arutyunov mud volcano (Gulf of Cadiz). Mar. Ecol. Prog. Ser. 382, 69–86. doi: 10.3354/meps07956
Sommer, S., Schmidt, M., and Linke, P. (2015). Continuous inline tracking of dissolved methane plume at a blowout site in the Northern North Sea UK - water column stratification impedes immediate methane release into the atmosphere. Mar. Petrol. Geol. 68, 766–775. doi: 10.1016/j.marpetgeo.2015.08.020
Sone, H., Shimamoto, T., and Moore, D. E. (2012). Frictional properties of saponite-rich gouge from a serpentinite-bearing fault zone along the Gokasho-Arashima Tectonic Line, central Japan. J. Struct. Geol. 38, 172–182. doi: 10.1016/j.jsg.2011.09.007
Spinelli, G. A., Giambalvo, E. R., and Fisher, A. T. (2004). “Sediment permeability, distribution and influence on fluxes in oceanic basement,” in Hydrology of the Oceanic Lithosphere, eds E. E. Davis and H. Elderfield (Cambridge: Cambridge University Press), 151–188.
Stakes, D. S., Trehu, A. M., Goffredi, S. K., Naehr, T. H., and Duncan, R. A. (2002). Mass wasting, methane venting, and biological communities on the Mendocino transform fault. Geology 30, 407–410. doi: 10.1130/0091-7613(2002)030<0407:MWMVAB>2.0.CO;2
Stein, C. A., and Stein, S. (1994). Constraints on hydrothermal heat flux through the oceanic lithosphere from global heat flow. J. Geophys. Res. 99, 3081–3095. doi: 10.1029/93JB02222
Stich, D., Ammon, C. J., and Morales, J. (2003). Moment-tensor solutions for small and moderate earthquakes in the Ibero-Maghreb region. J. Geophys. Res. 108, 21–48. doi: 10.1029/2002JB002057
Stich, D., Mancilla, F. D. L., Pondrelli, S., and Morales, J. (2007). Source analysis of the February 12th 2007, Mw 6.0 horseshoe earthquake: implications for the 1755 Lisbon earthquake. Geophys. Res. Lett. 34:L12308. doi: 10.1029/2007GL030012
Storti, F., Salvini, F., Rossetti, F., and Morgan, J. P. (2007). Intraplate termination of transform faulting within the Antarctic continent. Earth Planet. Sci. Lett. 260, 115–126. doi: 10.1016/j.epsl.2007.05.020
Strasser, M., Dugan, B., Kanagawa, K., Moore, G. F., Toczko, S., and Maeda, L. (2014). Proc. IODP. Yokohama: Integrated Ocean Drilling Program, 338. doi: 10.2204/iodp.proc.338.2014
Suess, E. (2014). Marine cold seeps and their manifestations: geological control, biogeochemical criteria and environmental conditions. Int. J. Earth Sci. 103, 1889–1916. doi: 10.1007/s00531-014-1010-0
Sumner, R. H., and Westbrook, G. K. (2001). Mud diapirism in front of the Barbados accretionary wedge: the influence of fracture zones and North America - South America plate motions. Mar. Petrol. Geol. 18, 591–613. doi: 10.1016/S0264-8172(01)00010-1
Teichert, B. M. A., Chevalier, N., Gussone, N., Bayon, G., Ponzevera, E., Ruffine, L., et al. (2018). Sulfate-dependent anaerobic oxidation of methane at a highly dynamic bubbling site in the Eastern Sea of Marmara (Çinarcik Basin). Deep Sea Res. Part II 153, 79–91. doi: 10.1016/j.dsr2.2017.11.014
Terrinha, P., Matias, L., Vicente, J., Duarte, J., Luíse, J., Pinheiro, L., et al. (2009). Morphotectonics and strain partitioning at the Iberia–Africa plate boundary from multibeam and seismic reflection data. Mar. Geol. 267, 156–174. doi: 10.1016/j.margeo.2009.09.012
Terrinha, P., Pinheiro, L. M., Henriet, J.-P., Matias, L., Ivanov, M., Monteiro, J. H., et al. (2003). Tsunamigenic-seismogenic structures, neotectonics, sedimentary processes and slope instability on the southwest Portuguese Margin. Mar. Geol. 195, 55–73. doi: 10.1016/S0025-3227(02)00682-5
Thorwart, M., Dzierma, Y., Rabbel, W., and Hensen, C. (2014). Seismic swarms, fluid flow and hydraulic conductivity in the forearc offshore North Costa Rica and Nicaragua. Int. J. Earth Sci. 103, 1789–1799. doi: 10.1007/s00531-013-0960-y
Tinivella, U. (2002). The seismic response to overpressure versus gas hydrate and free gas concentration. J. Seismic Explor. 11, 283–305.
Tinivella, U., Accaino, F., Rossi, G., and Nicolich, R. (2005). Petrophysical analysis of CROP-18 crustal seismic data. Boll. Soc. Geol. Ital. 3, 205–211.
Tinivella, U., Giustiniani, M., and Vargas-Cordero, I. (2017). Wave equation datuming applied to seismic data in shallow water environment and post-critical water bottom reflection. Energies 10:1414. doi: 10.3390/en10091414
Tomonaga, Y., Blättler, R., Brennwald, M. S., and Kipfer, R. (2012). Interpreting noble-gas concentrations as proxies for salinity and temperature in the world’s largest soda lake (Lake Van, Turkey). J. Asian Earth Sci. 59, 99–107. doi: 10.1016/j.jseaes.2012.05.011
Tomonaga, Y., Brennwald, M. S., and Kipfer, R. (2011). Spatial distribution and flux of terrigenic He dissolved in the sediment pore water of Lake Van (Turkey). Geochim. Cosmochim. Acta 75, 2848–2864. doi: 10.1016/j.gca.2011.02.038
Tomonaga, Y., Brennwald, M. S., Meydan, A. F., and Kipfer, R. (2014). Noble gases in the sediments of Lake Van – solute transport and palaeoenvironmental reconstruction. Quat. Sci. Rev. 104, 117–126. doi: 10.1016/j.quascirev.2014.09.005
Tryon, M. D., Henry, P., Çağatay, M. N., Zitter, T. A. C., Géli, M. L., Gasperini, L., et al. (2010). Pore fluid chemistry of the North Anatolian Fault Zone in the Sea of Marmara: a diversity of sources and processes. Geochem. Geophys. Geosyst. 11:Q0ad03. doi: 10.1029/2010GC003177
Tsunogai, U., Maegawa, K., Sato, S., Komatsu, D. D., Nakagawa, F., Toki, T., et al. (2012). Coseimic massive methane release from a submarine mud volcano. Earth Planet. Sci. Lett. 341–344, 79–85. doi: 10.1016/j.epsl.2012.06.004
Tsunogai, U., and Wakita, H. (1995). Precursory chemical changes in ground water: kobe Earthquake, Japan. Science 269, 61–63. doi: 10.1126/science.269.5220.61
Ujiie, K., Tanaka, H., Saito, T., Tsutsumi, A., Mori, J. J., Kameda, J., et al. (2013). Low coseismic shear stress on the tohoku-oki megathrust determined from laboratory experiments. Science 342, 1211–1214. doi: 10.1126/science.1243485
Vigneron, A., Cruaud, P., Pignet, P., Caprais, J.-C., Cambon-Bonavita, M.-A., Godfroy, A., et al. (2013). Archaeal and anaerobic methane oxidizer communities in the Sonora Margin cold seeps, Guaymas Basin (Gulf of California). ISME J. 7, 1595–1608. doi: 10.1038/ismej.2013.18
Villinger, H. W., Pichler, T., Kaul, N., Stephan, S., Pälike, H., and Stephan, F. (2017). Formation of hydrothermal pits and the role of seamounts in the Guatemala Basin (equatorial East Pacific) from heat flow, seismic, and core studies. Geochem. Geophys. Geosyst. 18, 369–383. doi: 10.1002/2016GC006665
Virieux, J., and Operto, S. (2009). An overview of full-waveform inversion in exploration geophysics. Geophysics 74, WCC1–WCC26. doi: 10.1190/1.3238367
Von Damm, K. L. (1990). Seafloor hydrothermal activity: black smoker chemistry and chimneys. Ann. Rev. Earth Planet. Sci. 18, 173–204. doi: 10.1146/annurev.ea.18.050190.001133
Von Damm, K. L. (2013). “Controls on the chemistry and temporal variability of seafloor hydrothermal fluids,” in Seafloor Hydrothermal Systems: Physical, Chemical, Biological, and Geological Interactions, eds S. E. Humphris, R. A. Zierenberg, L. S. Mullineaux, and R. E. Thomson (Hoboken, NJ: Wiley).
Von Damm, K. L., Edmond, J. M., Measures, C. I., and Grant, B. (1985). Chemistry of submarine hydrothermal solutions at Guaymas Basin, Gulf of California. Geochim. Cosmochim. Acta 49, 2221–2237. doi: 10.1016/0016-7037(85)90223-6
Von Herzen, R. (2004). “Evidence for continuing hydrothermal circulation in old crust,” in Hydrogeology of the Oceanic Lithosphere, eds E. E. Davis and H. Elderfield (Cambridge: Cambridge University Press), 414–447.
von Herzen, R., Simmons, G., and Folinsbee, A. (1970). Heat Flow between the Caribbean Sea and the Mid-Atlantic Ridge. J. Geophys. Res. 75, 1973–1984. doi: 10.1029/JB075i011p01973
Walia, V., Yang, T. F., Hong, W.-L., Lin, S.-J., Fu, C.-C., Wen, K.-L., et al. (2009). Geochemical variation of soil-gas composition for fault trace and earthquake precursory studies along the Hsincheng fault in NW Taiwan. Appl. Radiat. Isot. 67, 1855–1863. doi: 10.1016/j.apradiso.2009.07.004
Wang, D. T., Reeves, E. P., McDermott, J. M., Seewald, J. S., and Ono, S. (2018). Clumped isotopologue constraints on the origin of methane at seafloor hot springs. Geochim. Cosmochim. Acta 223, 141–158. doi: 10.1016/j.gca.2017.11.030
Weatherley, D. K., and Henley, R. W. (2013). Flash vaporization during earthquakes evidenced by gold deposits. Nat. Geosci. 6, 294–298. doi: 10.1038/ngeo1759
Wessel, P., Matthews, K. J., Müller, R. D., Mazzoni, A., Whittaker, J. M., Myhill, R., et al. (2015). Semiautomatic fracture zone tracking. Geochem. Geophys. Geosyst. 16, 2462–2472. doi: 10.1002/2015GC005853
Wheat, C. G., and Fisher, A. T. (2008). Massive, low-temperature hydrothermal flow from a basaltic outcrop on 23 Ma seafloor of the Cocos Plate: chemical constraints and implications. Geochem. Geophys. Geosyst. 9:Q12O14. doi: 10.1029/2008GC002136
Wheat, C. G., and Mottl, M. J. (2000). Composition of pore and spring waters from Baby Bare: global implications of geochemical fluxes from a ridge flank hydrothermal system. Geochim. Cosmochim. Acta 64, 629–642. doi: 10.1016/S0016-7037(99)00347-6
Wilson, J. T. (1965). A new class of faults and their bearing on bontinental drift. Nature 207, 343–347. doi: 10.1038/207343a0
Wolfson-Schwehr, M., and Boettcher, M. S. (2019). “Chapter 2 - global characteristics of oceanic transform fault structure and seismicity,” in Transform Plate Boundaries and Fracture Zones, ed. J. C. Duarte (Amsterdam: Elsevier), 21–59. doi: 10.1016/B978-0-12-812064-4.00002-5
Yamamoto, R., Kido, M., Ohta, Y., Takahashi, N., Yamamoto, Y., Ozener, H., et al. (2018). “Partial creep revealed by seafloor geodetic observation along the North Anatolian Fault, beneath the Sea of Marmara,” in Proceedings of the 20th EGU General Assembly, EGU2018, Conference Held 4-13 April, 2018, Vienna.
Yang, T. F., Fu, C.-C., Walia, V., Chen, C.-H., Chyi, L. L., Liu, T.-K., et al. (2006). Seismo-geochemical variations in SW Taiwan: multi-parameter automatic gas monitoring results. Pure Appl. Geophys. 163, 693–709. doi: 10.1007/s00024-006-0040-3
Young, C. R., Fujio, S., and Vrijenhoek, R. C. (2008). Directional dispersal between mid-ocean ridges: deep-ocean circulation and gene flow in Ridgeia piscesae. Mol. Ecol. 17, 1718–1731. doi: 10.1111/j.1365-294X.2008.03609.x
Zitellini, N., Gràcia, E., Matias, L., Terrinha, P., Abreu, M. A., DeAlteriis, G., et al. (2009). The quest for the Africa-Eurasia plate boundary west of the Strait of Gibraltar. Earth Planet. Sci. Lett. 280, 13–50. doi: 10.1016/j.epsl.2008.12.005
Zitellini, N., Rovere, M., Terrinha, P., Chierici, F., Matias, L., and Team, B. (2004). Neogene through quaternary tectonic reactivation of SW iberian passive margin. Pure Appl. Geophys. 161, 565–587. doi: 10.1007/s00024-003-2463-4
Zitter, T. A. C., Henry, P., Aloisi, G., Delaygue, G., Çagatay, M. N., Mercier de Lepinay, B., et al. (2008). Cold seeps along the main Marmara Fault in the Sea of Marmara (Turkey). Deep Sea Res. Part I 55, 552–570. doi: 10.1016/j.dsr.2008.01.002
Zoback, M. D., Hickman, S., and Ellsworth, W. (2011). Scientific drilling into the san andreas fault zone – an overview of SAFOD’s first five years. Sci. Drill. 11, 14–28. doi: 10.2204/iodp.sd.11.02.2011
Keywords: transform faults, fractures zones, coupling of seismicity and fluid flow, microbial life, heat flow, fluid geochemistry, seafloor observation systems, seismic precursors
Citation: Hensen C, Duarte JC, Vannucchi P, Mazzini A, Lever MA, Terrinha P, Géli L, Henry P, Villinger H, Morgan J, Schmidt M, Gutscher M-A, Bartolome R, Tomonaga Y, Polonia A, Gràcia E, Tinivella U, Lupi M, Çağatay MN, Elvert M, Sakellariou D, Matias L, Kipfer R, Karageorgis AP, Ruffine L, Liebetrau V, Pierre C, Schmidt C, Batista L, Gasperini L, Burwicz E, Neres M and Nuzzo M (2019) Marine Transform Faults and Fracture Zones: A Joint Perspective Integrating Seismicity, Fluid Flow and Life. Front. Earth Sci. 7:39. doi: 10.3389/feart.2019.00039
Received: 22 November 2018; Accepted: 18 February 2019;
Published: 19 March 2019.
Edited by:
Alessandro Tibaldi, University of Milano-Bicocca, ItalyReviewed by:
Shinsuke Kawagucci, Japan Agency for Marine-Earth Science and Technology, JapanWolfgang Rabbel, University of Kiel, Germany
Copyright © 2019 Hensen, Duarte, Vannucchi, Mazzini, Lever, Terrinha, Géli, Henry, Villinger, Morgan, Schmidt, Gutscher, Bartolome, Tomonaga, Polonia, Gràcia, Tinivella, Lupi, Çağatay, Elvert, Sakellariou, Matias, Kipfer, Karageorgis, Ruffine, Liebetrau, Pierre, Schmidt, Batista, Gasperini, Burwicz, Neres and Nuzzo. This is an open-access article distributed under the terms of the Creative Commons Attribution License (CC BY). The use, distribution or reproduction in other forums is permitted, provided the original author(s) and the copyright owner(s) are credited and that the original publication in this journal is cited, in accordance with accepted academic practice. No use, distribution or reproduction is permitted which does not comply with these terms.
*Correspondence: Christian Hensen, Y2hlbnNlbkBnZW9tYXIuZGU=