- 1wildFIRE Lab, University of Exeter, Exeter, United Kingdom
- 2Geography Department, Swansea University, Swansea, United Kingdom
Here, we explore how charcoal formation under different heating regimes and circumstances leads to chars of different physical properties. In order to do this, we have undertaken (1) carefully controlled laboratory experiments that replicate the different heating regimes that might be experienced during a wildfire and (2) two experimental wildfires where heat variations were monitored across the burn from which resulting charcoal has been studied. The charcoal properties were assessed using charcoal reflectance that measures the light reflected back from the charcoals structure and which links to changes in its structural properties. We find that increased total heat released during combustion positively correlates with increased charcoal reflectance and that this is evidenced from both our laboratory experiments and experimental wildfires. Charcoals that related to lower total heat release were found to have more lignin remaining than those subjected to greater heating indicating that charcoals formed in lower energy regimes are likely to be more susceptible to post-fire degradation. We conclude that charcoal reflectance may make a useful metric with which to determine the distribution of energy delivery across a burned area and that this may be utilized to inform both variations in fire severity and enable the prediction of long-term C budgeting for different types of wildfire.
Introduction
Charcoals are a key component of the continuum of products that comprise the fire-derived organic matter pool, known as pyrogenic carbon (PyC) (Bird et al., 2015; Santín et al., 2016). The properties of charcoals relate not only to the nature of the fuel from which they are formed, but also to the characteristics of the fire that generated them (Ascough et al., 2008; McBeath et al., 2013; Michelotti and Miesel, 2015; Belcher and Hudspith, 2016; Hudspith et al., 2017b). These properties should also determine how different charcoals are then subsequently modified chemically and physically in the post fire environment (Harvey et al., 2012; Santín et al., 2017). However, because fire is a complex chemical reaction that also relates to the physical state of the fuel it has been difficult to disentangle how the nature of fire might impact the production of different charcoals and therefore to what extent individual fire and fuel characteristics might lead to charcoals with varying levels of resistance to biological and physical degradation or to combustion in subsequent fires (e.g., Doerr et al., 2018).
Building this understanding is important because most wildfires are considered ‘net zero carbon emission events’, where the carbon released to the atmosphere is balanced by the re-uptake of C from the atmosphere by regrowth of the ecosystem. Notwithstanding this, PyC products that remain following a fire can indeed act as a carbon sink (Santín et al., 2015), but they are not yet fully considered or included in carbon fluxes and budgeting models (Santín et al., 2016). The charcoal fraction of PyC is known to have the potential to add to geologically long carbon stores as indicated by the presence of fossil charcoals found in deposits as old as 410 Ma (Belcher et al., 2013). This long-term presence of charcoals makes it tempting to assume that charcoal must be rather resistant to biotic and abiotic degradation over the long term. However, charcoal is not pure carbon and contains a variety of compounds that have been shown to have lower or greater reactivity (Ascough et al., 2010) and therefore ought to determine the likelihood of a charcoal’s long-term preservation and carbon burial potential (Bird et al., 2015). In order to further understand the contribution of charcoals in carbon budgeting we need to explore how differences in fuel type and fire behavior influence the production and properties of charcoals.
To address this, methodologies need to be developed and applied that link the nature of the fuel and the dynamics of combustion to charcoal formation. Measurement of the reflective properties of charcoals has been an area of study for decades (e.g., Jones et al., 1991). This is achieved by embedding charcoal in resin, polishing the sample and studying the charcoal under oil in reflected light with a photometric measurement taken of the fraction of incident light radiation reflected from the samples surface (e.g., Belcher and Hudspith, 2016; Hudspith et al., 2017a). More highly reflecting chars (Figure 1A) are thought to contain more stable, well organized graphite-like domains while lower reflecting chars (Figure 1B) are characterized by a more complex disorganized phase containing a greater proportion of less stable aliphatic phases (Cohen-Ofri et al., 2006; Ascough et al., 2008).
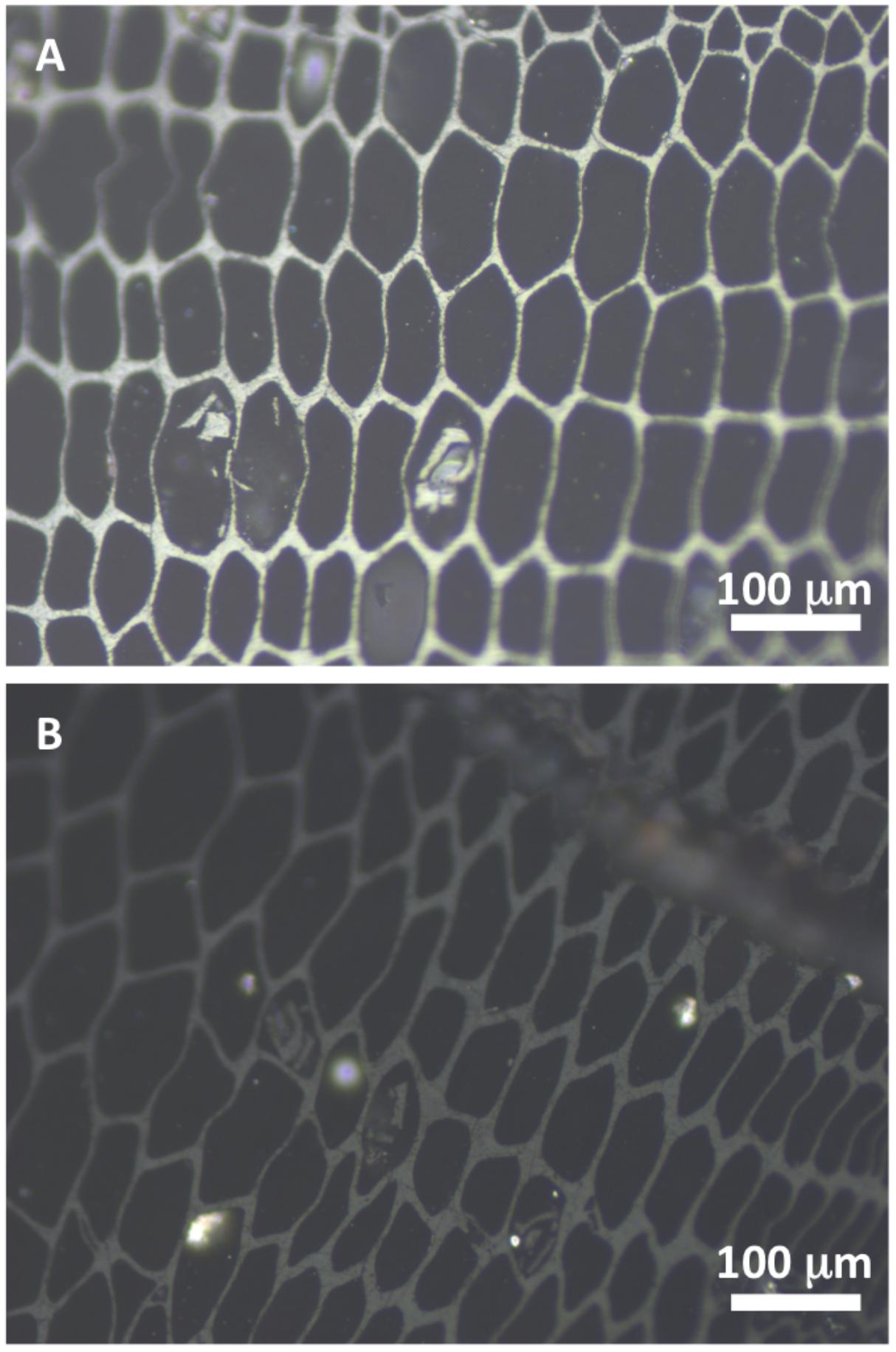
FIGURE 1. Shows charred western red cedar as studied in reflected light. (A) Shows higher reflecting cell walls (bright gray) and (B) shows lower reflecting cell walls (darker gray).
Previous research using oven formed charcoals has linked the formation of varying reflectance with the temperature of their formation (McParland et al., 2009; Ascough et al., 2010). However, more recently Belcher and Hudspith (2016) argued that charcoals produced in ovens relate to a single heat flux/temperature while charcoals formed in wildfires are the product of varying heat fluxes and energy exchanges that cannot be described by a single temperature. They found that charcoal surface reflectance increased between char extracted at peak heat release rate (pHRR; peak fire intensity) and at the end of flaming combustion, leading to the conclusion that charcoal reflectance must continually evolve throughout the combustion process. Oven based charring fails to capture the transitions in energy release that occurs during a wildfire and therefore the progressive development of reflectance as combustion proceeds (Belcher and Hudspith, 2016). Along these same lines, Santín et al. (2017) have proven that wildfire and man-made PyC sequestration potential and physio-chemical properties differ, even when produced from the same original materials. Recently, changes in the cellular level structural properties in charcoals have been observed to relate to energy transfer, where the energy transfer through a char layer leads firstly to the fusion of cell walls in the plant material and as heating continues refracturing along the middle lamella of the cell walls causing fragmentation (Hudspith and Belcher, 2017). This is also in contrast to charcoals produced in ovens that appear to reach only the cell wall fusion stage (Hudspith and Belcher, 2017). This evidence supports the idea that charcoal reflectance should change throughout the phases of combustion that allow for pyrolysis, however, to date no research has been able to provide satisfactory understanding about the relationship between how fuel and fire properties relate to the formation of charcoal reflectance.
Despite the lack of knowledge on the formation of different charcoal reflectance in wildfires as opposed to ovens, there has been previous research that compares their conditions of formation, reflectance and recalcitrance. Large differences have been shown between oxidation experiments of charcoals generated at 300°C compared to those generated at 400°C or greater (oven formed), leading to the conclusion that oxidative resistance is positively correlated with production temperature (Ascough et al., 2011). While, we disagree that single temperatures are representative of wildfires, these results indicate that charcoal generated at higher temperatures appear to lose aliphatics, oxygenated functional groups of cellulose and lignin, and become dominated by aromatic carbon=carbon bonds (Keiluweit et al., 2010; Ascough et al., 2011; Santín et al., 2017). Preliminary work linking charcoal reflectance and reactive/oxidative properties of chars indicates that low reflecting chars (formed at 300°C) appear less recalcitrant than more highly reflecting chars (generated at 400°C or greater) (Ascough et al., 2010).
Here, we explore the relationship between a range of measurable fuel (wood bulk-density) and fire properties both in the lab [heat release rate (HRR), total heat release (THR)] and in field scale experimental fires (variations in temperature over time) to begin to develop a better understanding of charcoal reflectance. We then explore the possibility of whether charcoal reflectance also relates to the degradative/oxidative properties of the remaining charcoal. The aim being that with further development, it might be possible to link fire behavior, charcoal reflectance and the recalcitrance of the charcoals produced to better estimate the contribution of PyC to long term carbon pools for different wildfire types.
Materials and Methods
Laboratory Fire Experiments
There have been many analyses linking charcoal reflectance with oven temperature during oven-based pyrolysis experiments (Jones et al., 1991; McParland et al., 2009; Ascough et al., 2010). In order to improve upon these experiments and find linkages between the properties of wildfires, such as fire intensity and total energy release we have used an iCone Calorimeter (Fire Testing Technology, East Grinstead, United Kingdom), that better replicates the combustion processes that occur in the natural environment compared to oven heating. The iCone allows for charring to occur in ambient pressure and oxygen conditions in which the samples (described below) were exposed to a heat flux of 50 kW.m2 in the iCone calorimeter, following ISO 5660–1/ASTM E1354 (International Organization for Standardization (ISO) 2015/(ASTM International, 2016). This mimicked the heat flux from an approaching fire front and began to decompose the wood samples, generating pyrolysis gases (pyrolysate) as the surface began to char (pyrolyse). Once the volume of pyrolysate released was sufficient, a spark igniter ignited the pyrolysate, leading to flaming ignition. During combustion, the iCone calorimeter measured the time taken for each sample to ignite (time to ignition, TTI), the rate of heat release throughout the burn, enabling it to quantifying HRR, pHRR, and THR during the flaming phase of the fire.
To investigate variations in charcoal reflectance we undertook two sets of experiments: the first examined one fuel type, western red cedar (WRC) wood (Thuja plicata) and subjected it to four different flaming durations; the second considered different bulk density woods [four species; Oak (Quercus robur), Beech (Fagus sylvatica), Eucalyptus (Eucalyptus sp.) and Balsa (Ochroma pyramidale) for two flaming durations. In all cases samples were dried at 45°C until fully dry (until no further mass loss was observed) and left in a drying oven until ready for testing.
WRC Experiments
9.5 cm × 9.5 cm × 3 cm blocks of WRC were placed in the standard iCone calorimeter holder of same length and width dimensions, the sample was then raised up flush to the surface of the holder using ceramic wool beneath the wood. The samples were tested using an incident heat flux of 50 kW.m-2 and ignited after a mean time of 24 s. Samples were then allowed to flame for 5, 10, 15, and 20 min, with duplicates of each flaming duration run. The TTI, HRR, and THR were measured throughout the duration of flaming combustion. Following the allotted time, the sample was taken away from the heat, wrapped tightly in foil and buried in sand to exclude oxygen and cease the combustion process. This allowed the collection of a suite of charcoal samples that were representative of the different flaming durations.
Bulk Density Experiments
A similar protocol was followed using different bulk density woods. Eucalyptus, Oak, Beech, and Balsa wood were tested, representing densities of 0.8, 0.74, 0.7, and 0.16 × 103 kg.m3, respectively. The samples were subject to an incident heat flux of 40 kW.m-2 and one set heated for 4 min and a second set heated until flaming ceased naturally on each sample. In both cases, duplicates of each sample were run. As before TTI, HRR and THR were measured. The samples were removed and oxygen excluded in the same way.
Experimental Field Scale Fires
Two fires in the boreal forest (Northwest Territories, Canada) were studied: (A) a high-intensity crown fire and (B) a low-intensity surface fire.
The high-intensity crown fire (Triangle plot) was an experimental fire conducted on 15/6/2015 as part of the Canadian Boreal Community FireSmart Project (61°34′55′′ N; 117°10′13′′ W) and aimed at simulating wildfire conditions. The experimental site was a mature stand of black spruce (Picea mariana) and jack pine (Pinus banksiana). The understory was very sparse, the forest floor composed of litter, mosses and lichens (<10 cm depth), with some downed wood present (see Santín et al., 2015 for details). The fire was fast moving with a total burn time of <5 min and flame lengths >5 m above canopy height. Fireline intensity was estimated as ∼8,000–12,000 kW.m-1, which is within the range typical of high-intensity boreal crown fires in Canada (de Groot et al., 2009). The fire resulted in the complete consumption of the canopy, small branches and the understory.
The low-intensity surface fire (Pine Point) was a slow-moving, backing surface wildfire (Fire 28, Hay River 60°49′38′′ N; 114°24′28′′ W). It affected a mixed black spruce and jack pine stand of comparable stand characteristics to the high intensity fire, but with an organic soil layer of up 50 cm depth. This lightning-caused wildfire burnt across the site on 29/6/2017 during calm atmospheric conditions advancing at <5 m.h-1. This allowed sufficient time to place samples, thermocouples and data loggers ahead of the advancing fire. This surface fire led to the patchy smoldering combustion of the forest floor to a depth of up to 30 cm and the “torching” of a few individual trees.
The same WRC wood used in the laboratory experiments, but in blocks of 3 cm × 3 cm × 3 cm, and pieces of wood of the native Jack Pine (JP) (Pinus banksiana) (small twigs of 2–3 cm diameter and 2–4 cm length) were exposed to these two contrasting fire conditions. 15 samples of each type were placed onto the forest floor < 24 h prior to each fire. In the Triangle plot, the wood pieces were placed randomly within an area of 15 m × 15 m. In Pine Point, the wood pieces were placed 2 m apart along a transect parallel to the fire front. Within <12 h after the fires, samples were relocated (unless completely consumed) and placed in sealed containers for subsequent laboratory analysis.
In order to remove consideration of variations of moisture content in our experiments the WRC and JP were dried in an oven at 40°C until no further mass loss was observed, and stored in sealed bags until deposited in the burn ahead of the fires. By standardizing the fuel moisture of the samples our approach was to be able to look at how fire behavior directly influenced the char properties by limiting any variations in fuel moisture of the items that will be collected for analysis.
To provide a temperature-duration record for each individual wood sample, K-type thermocouples were attached directly to the surface of the wood pieces, connected to data loggers (Lascar, Easylog) that were buried in the adjacent soil. Due to the patchy nature of the low-intensity fire in Pine Point, not all samples were exposed to burning and, in some cases, the combustion of the organic soil led to the destruction and associated data loss of some of the loggers. For more details on the characteristics of the fires, sites, and experimental settings, see Doerr et al. (2018). Following the fires, the integrated area under each thermocouple curve was calculated for the duration each sample spent at >300°C. This served as a guide to the total heating experienced by the sample throughout the duration of the fire.
Preparation of Charcoal Samples and Measurement of Charcoal Reflectance
The laboratory generated charcoal blocks were cut into sections approximately 2 cm wide and 3 cm deep and embedded in resin with the surface exposed to flaming facing the upper surface of the resin block, and this surface polished ready for analysis in reflected light (c.f. Belcher and Hudspith, 2016). The samples subject to 20 min flaming duration were additionally embedded on their side and the side/depth profile polished, allowing measurements with depth from the surface to the base of the char layer to be observed and the reflective properties measured. The field charcoal samples varied in their remains if the samples were <1 cm3 the entire sample was embedded in resin with the most charred side polished for analysis. If larger samples were available approximately half the sample was embedded in resin and again the most charred side polished for analysis.
Both the polished laboratory and wildfire charcoals were then analyzed under oil of refractive index 1.514 (at 23°C), using a Zeiss Axio-Scope A1 optical microscope, with a TIDAS-MSP 200 microspectrometer, at the wildFIRE Lab at the University of Exeter. Samples were studied using a ×50 objective (with ×32 eyepiece magnification), and reflectance measurements were obtained manually using MSP200 v 3.47 software. The system was calibrated with three synthetic reflectance standards, where reflectance is denoted as Ro) Strontium Titanite (5.41% Ro), Gadolinium Gallium Garnet (GGG) (1.719% Ro) and spinel (0.42% Ro). Manual reflectance measurements were taken at cell wall junctions across the polished surface of the charcoal. Thirty measurements were taken of each charcoal sample. Representative color micrographs were taken using an AxioCam 105 color 5-megapixel eyepiece camera attached to the reflectance microscope, and Zeiss Zen software (e.g., Figure 1)
Exploration of Laboratory Produced Charcoal Reactivity and Thermal Recalcitrance
Any lignin remaining in the WRC charcoals subject to flaming for 5 and 20 min was analyzed using the acetyl bromide method (Moreira-Vilar et al., 2014). Char was carefully scraped from the upper surface of the charcoals, so as to capture the same surface that had been analyzed for charcoal reflectance; two samples were collected from each flaming duration. 2 mg of each char sample was incubated in acetyl bromide (0.5 ml, 25% v/v in acetic acid) at 50°C for 2 h. After incubation, sodium hydroxide (0.9 ml, 2 M) and hydroxylamine hydrochloride (0.1 ml, 1 M) were added, along with acetic acid (5 ml). The lignin content of the extracts was then quantified by measuring the absorbance at 280 nm using a UV-1600PC Spectrophotometer (VWR, Leicestershire, United Kingdom) (three replicates of each sample).
The reactivity of the chars was considered using a Federal Aviation Administration (United States, FAA) Microcalorimeter (Fire Testing Technology, East Grinstead, United Kingdom) that was developed to allow direct measurements of HRR in respect to material properties and chemical composition of materials (Lyon and Walters, 2002). The FAA microcalorimeter is a pyrolysis combustion flow calorimeter and was used to reproduce the solid-state and gas phase processes of flaming combustion by reheating the char in an inert gas stream (Nitrogen gas) where upon the remaining volatile gases are driven off and oxidized at high-temperature in excess oxygen. The microcalorimeter then measures the rate of heat release based on the oxygen consumption history of the fuel (Lyon and Walters, 2002). Approximately 3 mg was scraped from the charred surface of the WRC samples that had been exposed to flaming combustion for 5, 10, 15, and 20 min and tested in the microcalorimeter. The samples were re-heated using a heating program that ramped up to 750°C at a rate of 3°C per second. The volatile gases released during heating were combusted to estimate the remaining reactivity of the char. Each sample was analyzed in triplicate. The microcalorimeter in this case was utilized to consider the reactivity of the charred samples, where the nature of the compounds in the char determines the temperature at which the maximum decomposition rate of the solid fuel occurs and the time taken for each sample to reach pHRR. We anticipated that (a) those samples containing a more reactive fraction would reach their maximum rate of decomposition more rapidly and at a lower temperature, as lignocellulosic structures should be easier to break down than large polyaromatic compounds (Drysdale, 2011) and (b) that samples containing a higher abundance of lignocellulosic compounds would have a lower pHRR than those containing abundance polyaromatics (Drysdale, 2011).
Results
Formation of and Variations in Charcoal Reflectance
The HRR and THR were measured during all laboratory experiments. Figure 2 provides a summary overview of HRR profiles, THR and the range of surface Ro% generated during flaming combustion tests of WRC. Figure 3 reports the changes in reflectance that resulted from the range of flame durations tested in the lab and shows that surface Ro% increases throughout flaming combustion and markedly following 20 min of flaming. The mean surface Ro% was found to be positively correlated to THR during flaming combustion (r2 = 0.852, Figure 4A). THR was also shown to correlate with mean char depth (r2 = 0.914; Figure 4B). When charcoal reflectance was measured at depth intervals throughout the char layer, the reflectance could be seen to decrease with depth through the char profile (Figure 5).
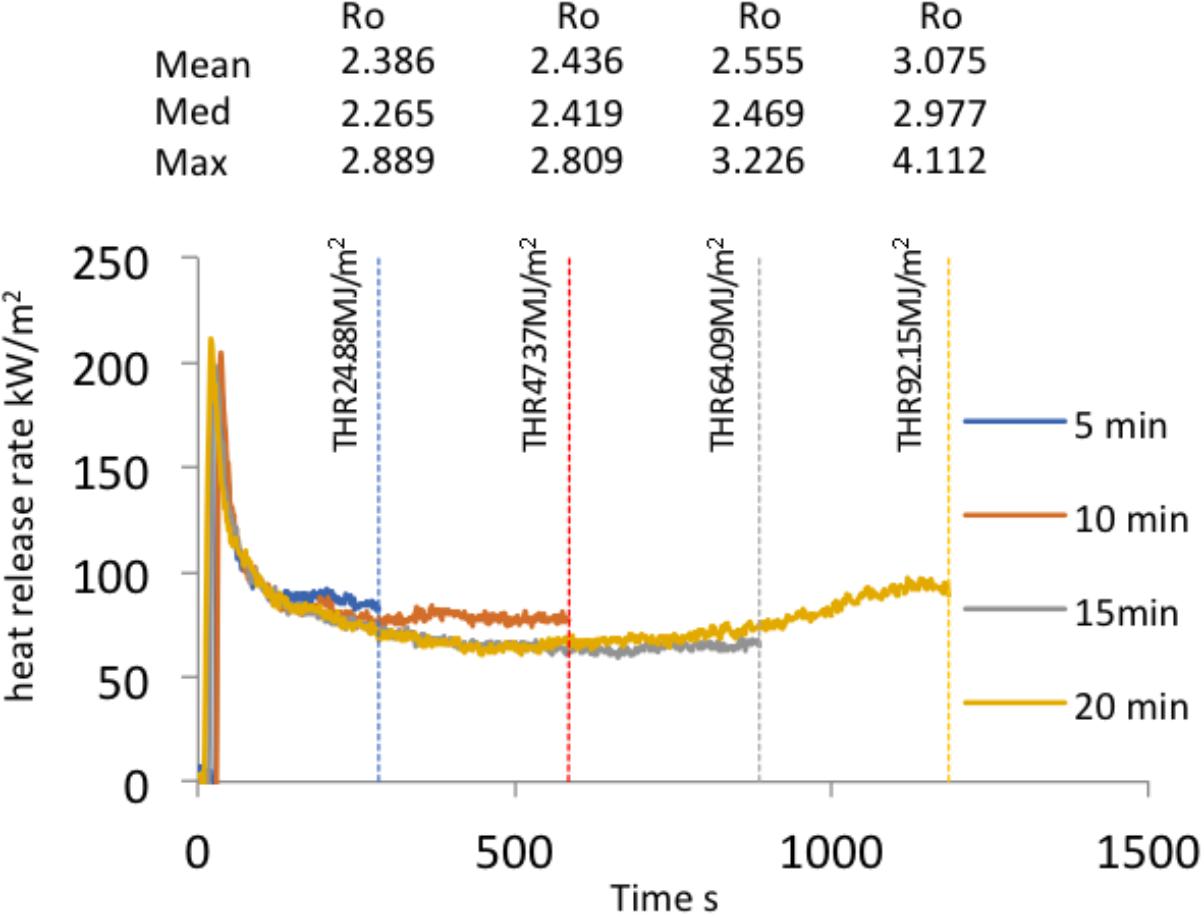
FIGURE 2. Summary overview of heat release rate profiles, total heat release and the range of surface Ro% generated during flaming combustion tests of WRC. Blue line flaming for 5 min, orange line 10 min, gray line 15 min, and yellow line 20 min.
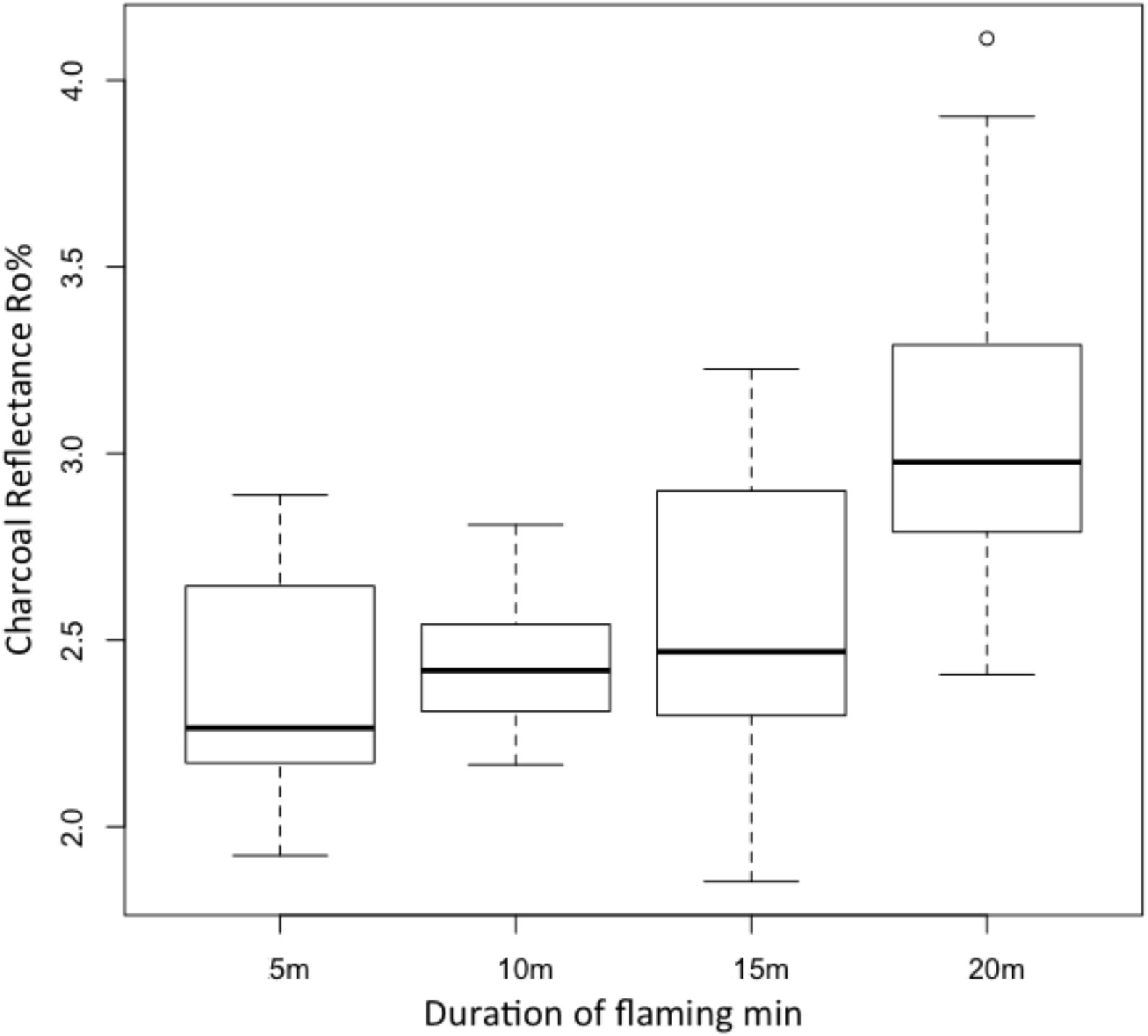
FIGURE 3. Box plot of the range of measured charcoal reflectance values from WRC subject to 5, 10, 15, and 20 min flaming combustion where n = 90 for each box.
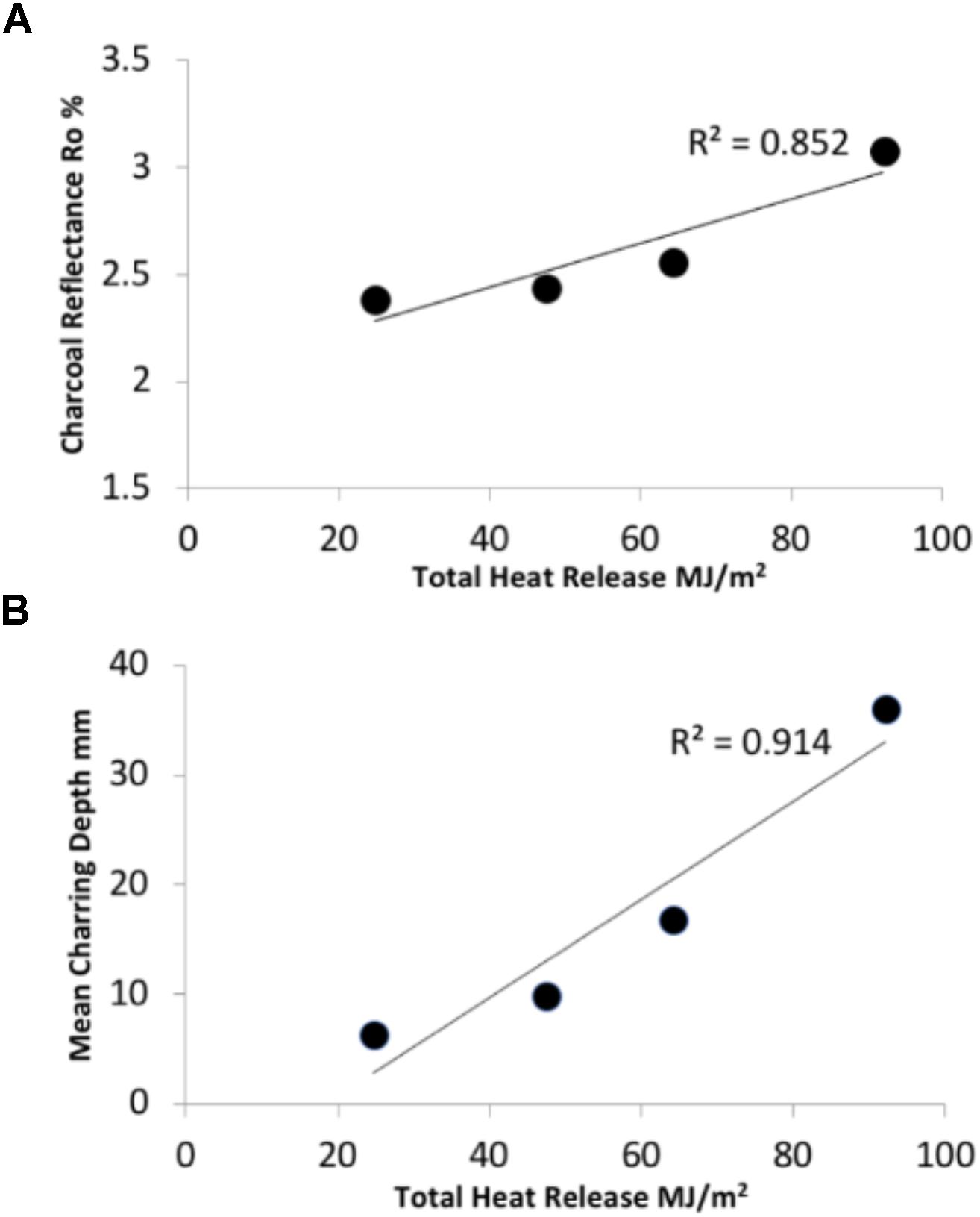
FIGURE 4. Graphs indicating strong positive correlations between the total heat released during flaming combustion of WRC in respect to (A) charcoal reflectance and (B) mean charring depth attained. The mean of 90 measurements is represented by each data point on both graphs.
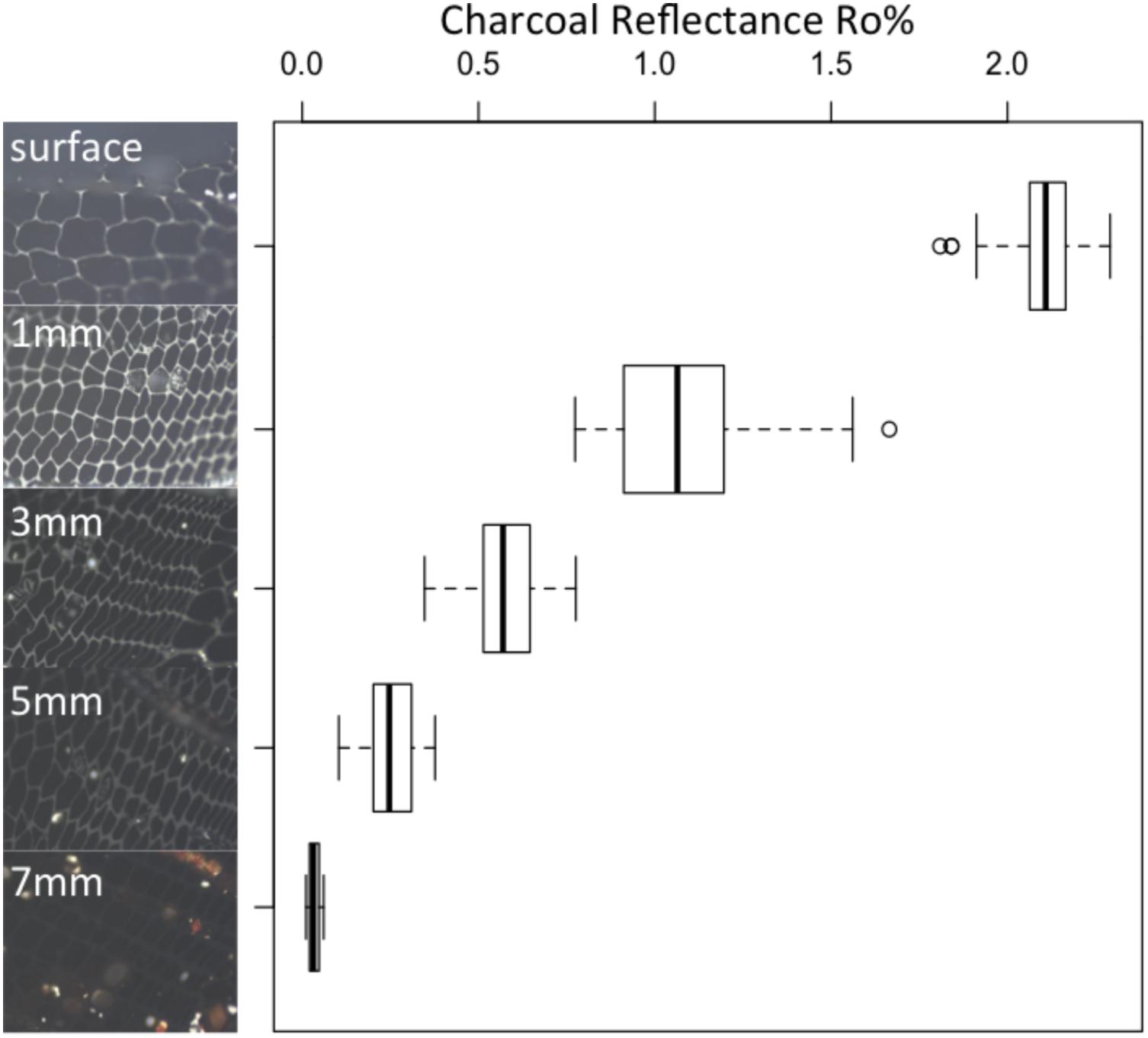
FIGURE 5. Reflectance images and box plots (n = 90 for each box) showing the variations in charcoal reflectance with depth in a charred block of WRC. Indicating that charcoal reflectance decreases away from the heated sample surface and into the char layer.
When woods of different bulk densities were considered over two flaming durations both the duration of flaming and the bulk density of the wood influenced mean surface Ro% (Figure 6A). As in the WRC experiments the mean surface Ro% from each species was positively correlated with THR (r2 = 0.836, Figure 6B).
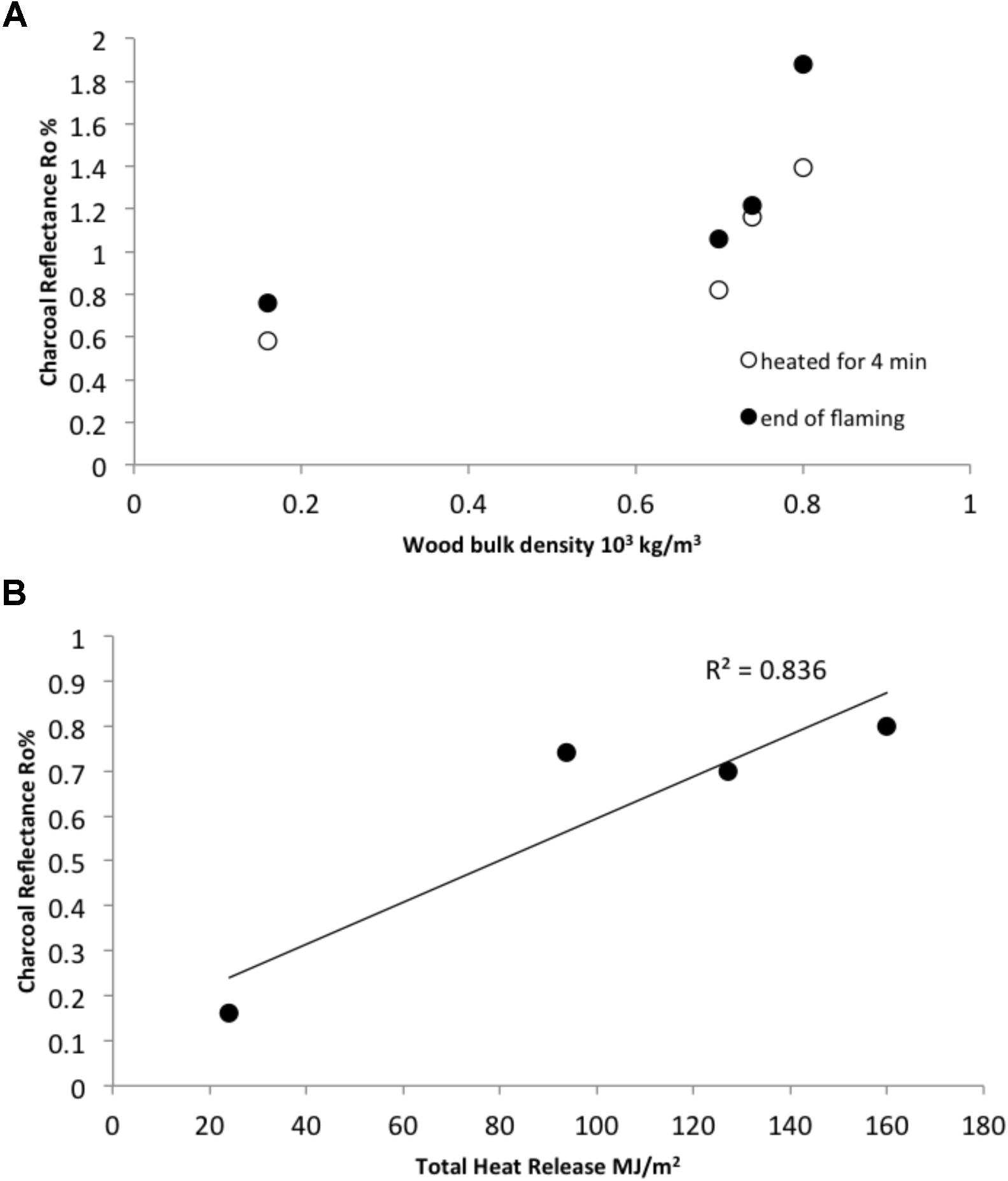
FIGURE 6. The influence of wood bulk density on charcoal reflectance. (A) Indicates bulk density and charcoal reflectance for samples subject to 4 min of flaming combustion and sampled at the end of flaming combustion. (B) The mean total heat released measured at the end of flaming combustion four different bulk density woods and the corresponding mean charcoal reflectance attained for each bulk density set. The mean of 90 measurements is represented by each data point on both graphs.
Field Scale Experimental Fires
As explained in the methodology, WRC and Pinus banksiana samples were also exposed to two different fire behaviors in the two experimental fires. At the Triangle plot site, which experienced a high intensity fast-moving crown fire, the mean maximum temperature recorded from the thermocouples attached to the wood pieces was 842°C (range of max temperatures recorded from the loggers was 661–1,002°C, the upper limit may have been higher as this is typically the upper point up to which type K thermocouples read). Mean integrated area under the thermocouple curves >300°C) was 50,306°C s (range 21,406–122,798°C s).
At the Pine Point site, which was subject to a low intensity, slow burning surface fire, the mean maximum temperature recorded from the thermocouples was 482°C (range of maximum temperatures recorded was 40–791.5°C). Mean energy release (area integrated under the thermocouple curves >300°C) was 22,090°C s (range 0–99,327°C s).
Correspondingly, the resulting surface Ro% for both species (WRC and Pinus banksiana) was significantly different from the two fires (p = 0.00291). The high intensity crown fire at the Triangle site produced more highly reflecting chars than the slower moving surface fire at Pine Point (Figures 7A,B). Pinus banksiana generated slightly lower surface Ro% than WRC (Figure 7C). The integrated area under the thermocouple temperature profile curves produce an estimate of the energy release that was >300°C, during the field scale fires and serve as a comparator to our measurements of THR taken in the laboratory. The difference in surface Ro% appears to relate to the different total energy release from the fires (Kruskall–Wallis p = 0.01287).
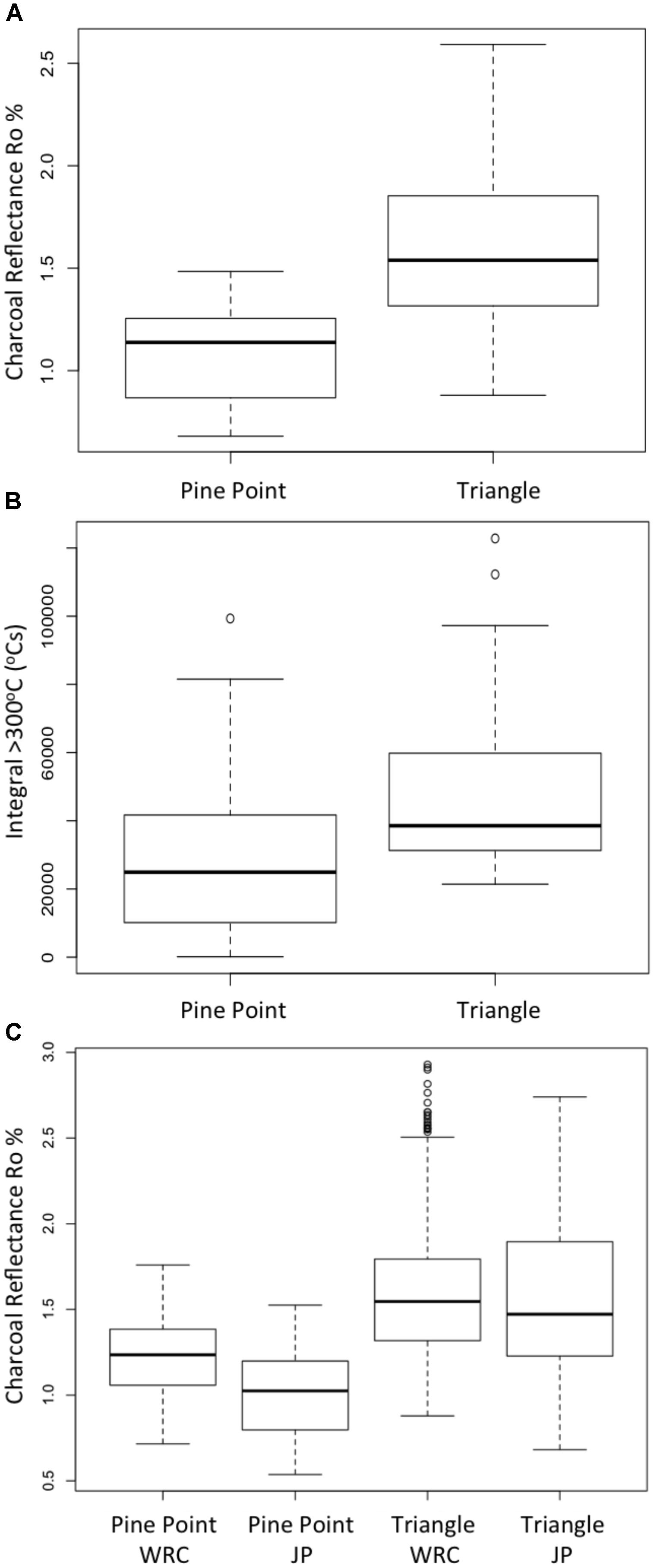
FIGURE 7. Box plots (n = 30 for each boxes) exploring the distribution of charcoal reflectance and the integral >300°C for the fires at the Pine Point and Triangle sites, Canada (A) shows the range of reflectance values from WRC samples for the two sites. (B) Indicates the integrated area under the thermal couple curve >300°C (as an estimate of energy flux to the fuel). (C) Shows charcoal reflectance variations between WRC and Jack Pine (JP) (Pinus banksiana) for both sites.
Preliminary Exploration of Variations in Charcoal Recalcitrance
We used two approaches to preliminarily assess the recalcitrance/reactivity of the charcoals formed in our laboratory experiments. On re-heating in the FAA microcalorimeter the WRC char samples that had been subject to flaming combustion for 5 min had a mean maximum temperature of decomposition around half that of the other chars (Figure 8A). They also reached pHRR twice as rapidly as those previously subject to flaming for 10, 15, or 20 min (Figure 8B). However, pHRR revealed a positive correlation with their original duration of heating (r2 = 0.888, Figure 8C). When the remaining lignin content of the chars was examined the samples subject to flaming for 5 min were found to contain up to three times the amount of lignin than the chars analyzed after 20 min of flaming (Figure 9, p = 0.004).
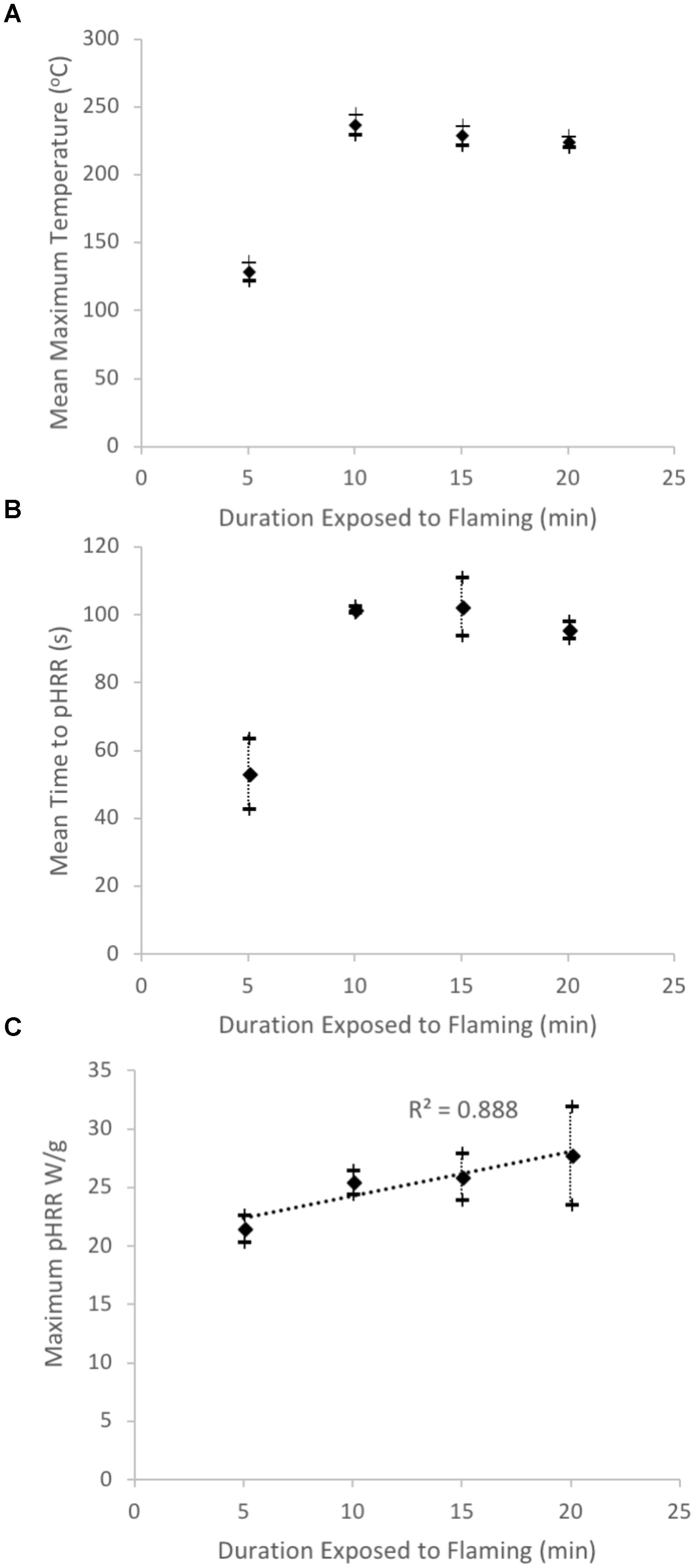
FIGURE 8. Thermal reactivity of chars as measured in the microcalorimeter showing results from the analysis of four sets of charcoal formed during flaming combustion in the iCone calorimeter for 5, 10, 15, and 20 min. (A) The mean temperature at which the charcoals reached their maximum rate of decomposition during re-heating in the microcalorimeter. (B) The mean time taken for the charcoals to reach peak heat release rate (pHRR) in the microcalorimeter and (C) shows a strong positive relationship between the duration of heating over which the samples were originally formed in the iCone calorimeter and the maximum pHRR attained on re-heating in the microcalorimeter.
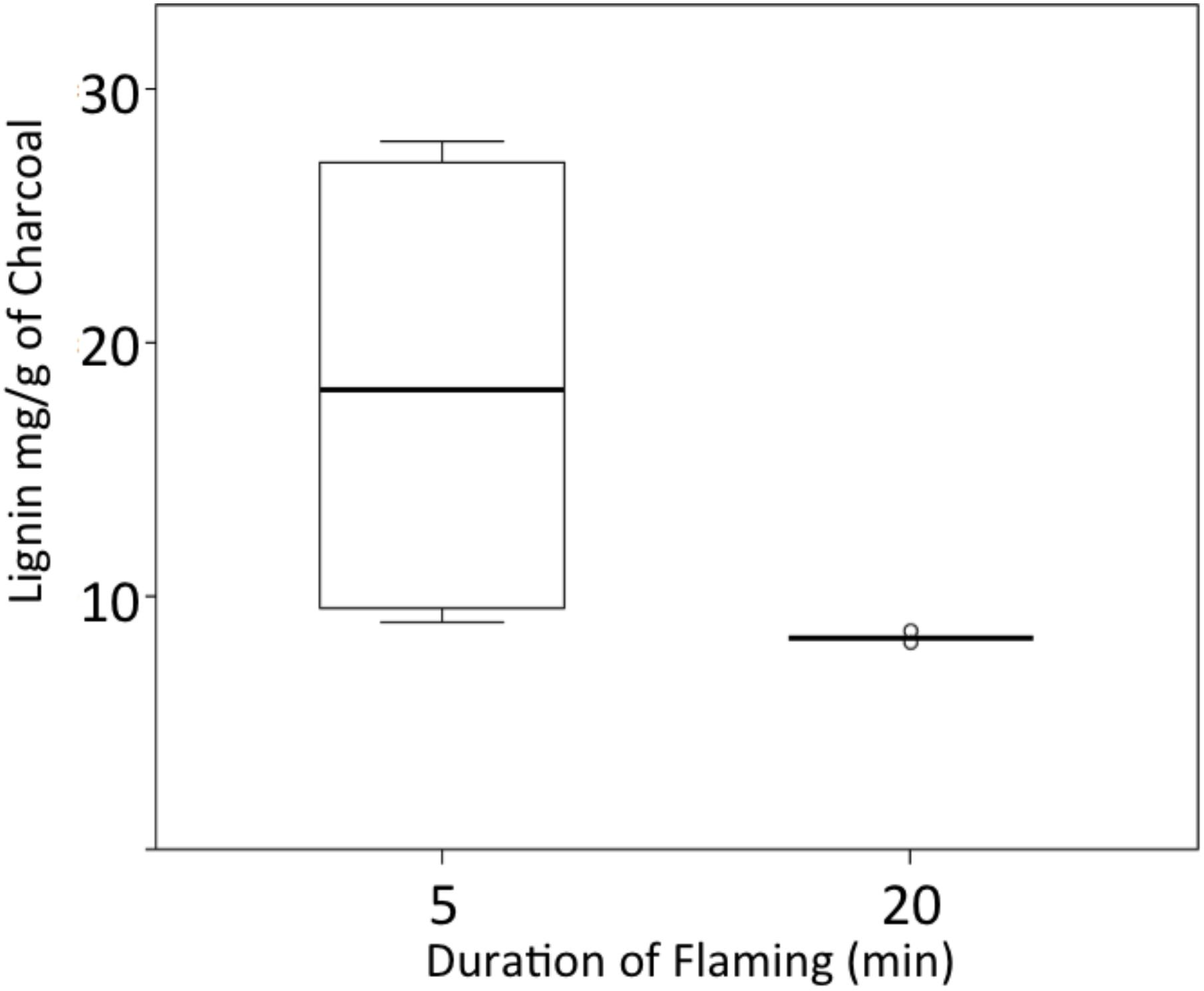
FIGURE 9. Amount of lignin remaining in chars formed over two different flame durations (n = 6 per box).
Discussion
The Formation of Charcoal Reflectance
The formation of charcoal in wildfires is affected by the properties of the fuel, which in turn, together with terrain and atmospheric conditions, influence the combustion dynamics. Charcoal yields are known to depend on the rate and amount of heating that relates to the imposed heat flux and the supply of oxygen at the fuel surface (Kashiwagi et al., 1987). These interact to alter the rate and volume of volatile gas release, which are required to initiate flaming combustion. In the case of wildfires, the imposed heat flux would be the approaching and passing fireline. Figure 10A provides a schematic of a fire and highlights the exchanges of energy that occur during the formation of charcoal reflectance during flaming combustion. The iCone calorimeter used in our laboratory experiments measures the HRR per unit area as a function of the external radiant heat flux (the cone heater, used to decompose the virgin and generate pyrolysate). It then calculates the THR from ignition to the point at which we removed samples, and therefore charring must relate to the rate of heat release and duration of heat supplied by the flame. The formation of charcoal reflectance must directly relate to the net heat transfer through the fuels surface and must be equal to the absorbed external radiant flux (the cone heater), plus the heat transfer from the flame (see Figure 10A). Our laboratory experiments indicate that these properties influence charcoal reflectance, where THR (heat release over time) and fuel density (which itself influences THR), both showed strong correlations with surface charcoal reflectance and the depth of charring (e.g., Figures 4–6). A substantial volume of previous research has linked increasing charcoal reflectance with increasing oven temperatures during the formation of the char (e.g., Scott and Glasspool, 2007; McParland et al., 2009; Ascough et al., 2010) leading to the suggestion that Ro% can be used to estimate wildfire temperature. The energy field around a burning solid fuel is illustrated in Figure 10A and described above; and indicates why most oven-based charcoal forming experiments (that often include the formation of charcoal in sealed metal tubes (McParland et al., 2009), do not capture the mechanism of char formation during flaming combustion in wildfires. Table 1 compares the typical charcoal reflectance generated at similar temperatures in an oven and in the iCone and contrasts these to the mean Ro% resulting from the wildfires studied. Here, we monitored the surface temperature of the fuel during combustion in the iCone and found the mean surface temperature to be ∼586°C which we compare to oven produced chars formed at 600°C. Scott and Glasspool (2007) produced chars in an oven at 600°C for 1,200 s (the minimum duration they explored), the iCone charcoal reflectances were found to be 22% lower than those generated in an oven over the same duration (Table 1).
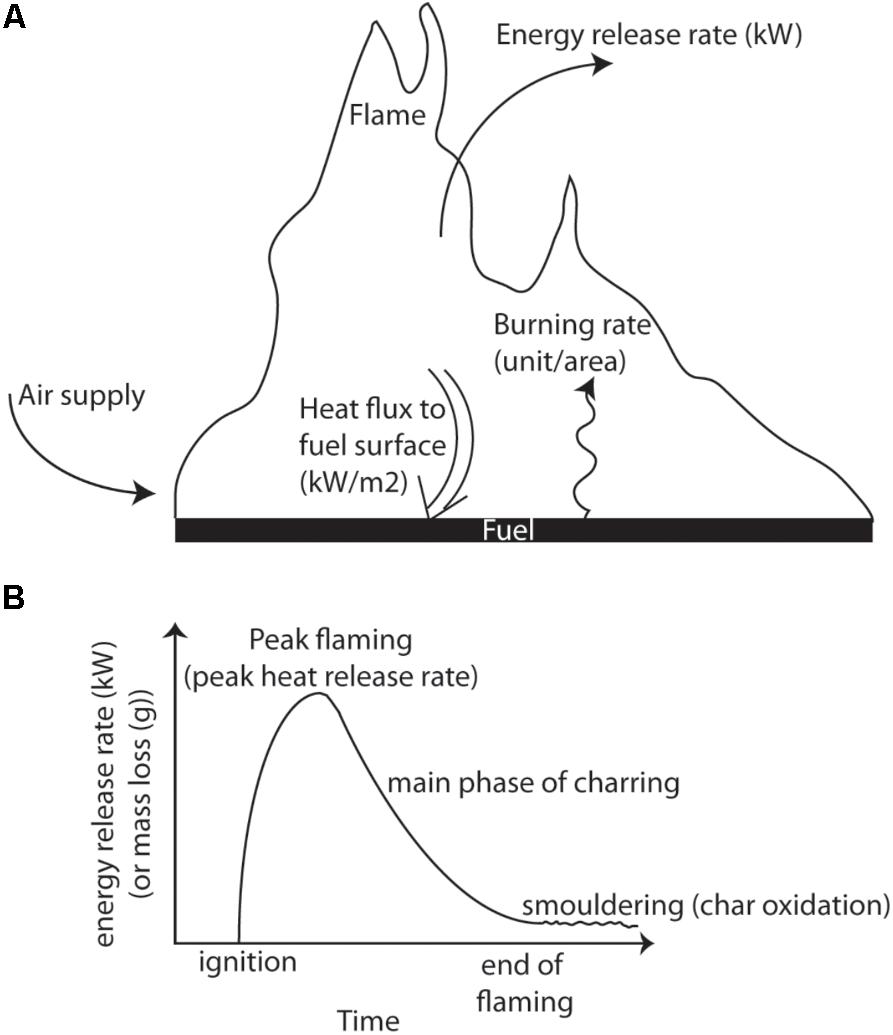
FIGURE 10. (A) Schematic of the directions of energy release and energy fluxes associated with flaming combustion of a solid fuel. (B) Schematic heat release rate profile highlighting key transitions during combustion.
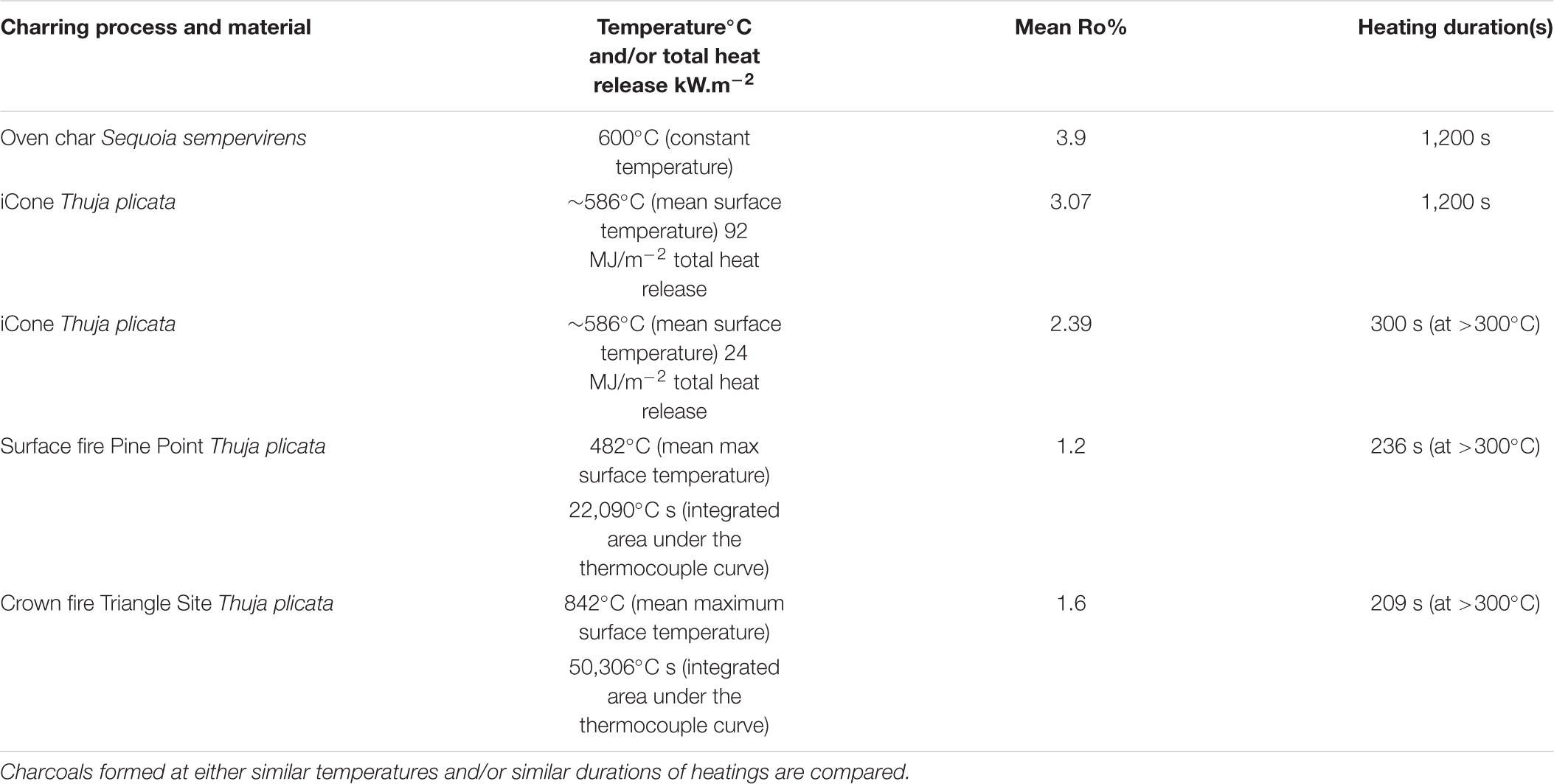
TABLE 1. Comparison of charcoal reflectance and heating regimes for oven char (taken from Scott and Glasspool, 2007), and those generated in the laboratory and the field in this research.
Higher reflectance should be expected in oven formed chars because oven based experiments are designed to generate perfect char. The oven setting minimizes heat losses and oxygen is excluded by either placing the samples in metal tubes (Scott and Glasspool, 2007) or burying samples in sand (Crawford and Belcher, 2016). This prevents oxidation of the volatile gases released (i.e., no flaming combustion) and oxidation of the solid fuel (char consumption). As such this poorly replicates char formation in a wildfire. A similar conclusion was reached by Santín et al. (2017) when comparing wildfire charcoals and slow-pyrolysis biochars, the latter produced in pyrolytic ovens. The heating regime is also significantly different. Wood placed in an oven takes ∼15 min to match the temperature of the oven (Scott and Glasspool, 2007) and does not ignite (because oxygen is excluded). In contrast the WRC tested in the iCone takes a mean of 30 s to ignite using a radiant heat flux of 50 kW.m-2. A small amount of charring occurs during the period of pre-heating ahead of ignition, which is also characteristic of pre-heating from an approaching fire-front during wildfires. Once flaming combustion is established (e.g., after ∼30 s of pre-heating) this then leads to the main charring phase in the iCone calorimeter.
However, we note that the mean reflectance for all durations of heating in our laboratory experiments using WRC, yielded higher Ro% than were observed in either of the wildfires studied (compare Figure 3 to Figure 7A and see Table 1). The mean from the charcoals formed by 5 min (300 s) flaming combustion in the laboratory experiments was 2.39 Ro% (range 1.92–2.89) while for 20 min (1,200 s) it was 3.07 Ro% (range 2.41–4.11) (Figure 3 and Table 1). This indicates that the surface reflectance of our laboratory char is on average higher than that observed in either of the wildfires (Figure 7A). This appears to relate to the fact that the duration of heating in the both the wildfires studied, as determined from the thermocouple readings taken from the WRC blocks in the field (Table 1) was ∼100 s shorter (e.g., between total heating of 209 and 236 s) than our shortest laboratory experiments (e.g., 300 s). The higher mean reflectance of charcoals formed by the Triangle site fire experienced a shorter duration of heating but double the amount of ‘energy’ as represented by the integrated area under the thermocouples curves when compared to the fire at Pine Point (Table 1). This highlights that Ro% not only relates to the duration of heating but also the flux of heat, hence charcoal reflectance must relate to the balance of energy released over time and the total energy it receives.
The importance of quantifying energy as opposed to a single temperature can be illustrated by considering a single candle; this candle burns producing a given temperature and the energy release of a single candle. However, if 10 candles are lit, they will still produce the same measurable temperature, but they will release 10 times the amount of energy that the single candle did. Hence the single temperature and typically relatively long duration of heating utilized in oven charring is not appropriate for considering the development of charcoal reflectance in wildfires. A passing fireline delivers a transient energy flux to the fuel present in a wildfire. The fuel is pre-heated, ignites rapidly leading to peak energy release which then declines and eventually decays over time (related to the fuel load available) (see Figure 2). In the case of our laboratory experiments each WRC block experiences approximately the same TTI (mean 30 s) and a similar pHRR (∼ 200 kW.m-2) (Figure 2). The differences in Ro% then relate to a combination of duration of burning (e.g., 5 –20 min) and the amount of energy delivered over this period, which relates well to THR. Char forming fuels rapidly reach a peak burning flux and the maximum point of energy release from flaming, then their energy release rate decays over time (Figures 2, 10B). Therefore, the mean HRR decreases while the surface charcoal Ro% increases over time. This decay is due to the insulative properties of the forming char layer that protects the virgin fuel beneath from the heat flux to the surface of the fuel (Moghtaderi et al., 2006) and slows volatile release (until it eventually ceases). This process is captured in the Ro% measurements taken through the depth of the char layer (e.g., Figures 4B, 5). While the entire char layer appears blackened to the naked eye, Ro% declines significantly throughout the profile of the char layer. Here, the time taken for the heat to penetrate through the forming char layer will vary with HRR, the duration over which the heat is applied as well as the insulative properties of the char layer being formed.
Because mean HRR decreases as combustion proceeds (e.g., Figure 2), the rate of increase in Ro% also decreases with the duration of heating. By standardizing Ro% as a function of time (Ro%.s) it can be seen that samples of the same fuel under the same conditions, but heated for longer will have a lower mean HRR and Ro%.s (Figure 11). Figure 11 shows that the mean rate of Ro% increase is strongly positively correlated with mean HRR (r2 = 0.919). These data are taken from the experiments that allowed WRC to burn for 5, 10, 15, and 20 min; the rate of Ro% increase for both Canadian wildfires are also indicated on Figure 11 and reveals that, despite our laboratory experiments allowing heating for longer durations that the Ro%.s for the Canadian fires falls within a similar HRR-Ro% rate space. Our results indicate the rate of increase in Ro% decreases with time despite Ro% increasing with increased burn duration. Therefore, the final Ro% and the rate of change in Ro% between different fires is a balance between the HRR kW/m-2 and the duration that materials are exposed to flaming; the end product of which (%Ro) is best related to THR. Future work should seek to undertake laboratory experiments under the shorter durations to those observed in wildfires to improve upon this relationship and gain a better understanding of the relationship between duration, THR and surface Ro%. Despite this, our results presented here indicate that, in respect to wildfires, THR is a better correlate with Ro% than temperature because THR is a more meaningful description of the energy release from a wildfire. We note, however, that because different wood densities were found to show clear differences in their reflective properties, that variations in the Ro% and THR relationship should be considered within species and not between species.
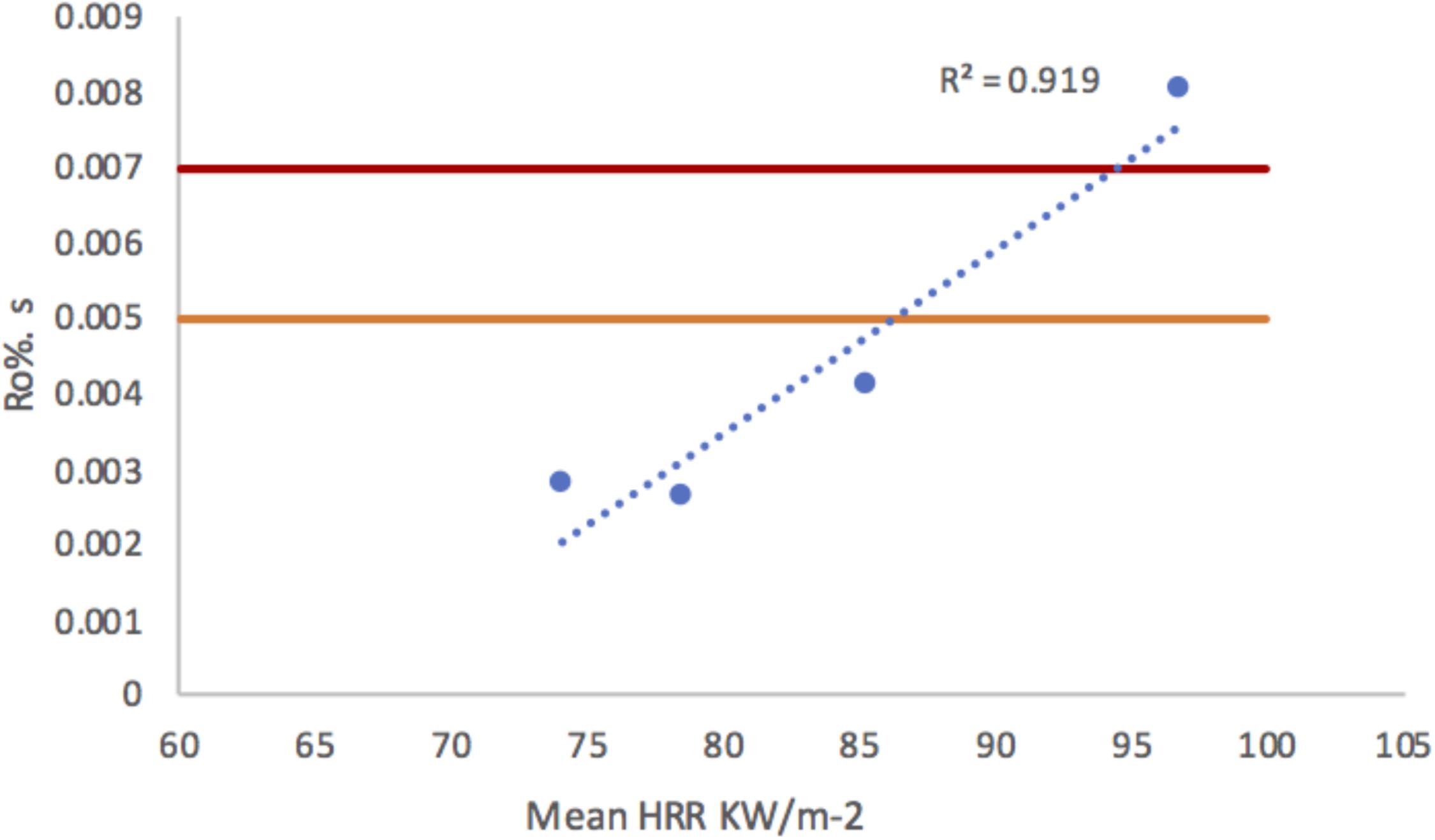
FIGURE 11. Graph of mean heat release and standardized charcoal reflectance as a function of time (Ro%.s) indicating a strong positive correlation.
Charcoal Reactivity and Likely Resistance to Future Degradation
Wood charcoal is the most common form of PyC found after wildfires because wood contains a complex mixture of polymers of high molecular weight, including cellulose, hemicellulose and lignin. As a passing fireline heats the plant materials, hemicellulose and cellulose decompose more rapidly than lignin. Cellulose is suggested to leave just 5% charcoal, highlighting why leaf material typically leaves much less charcoal and more ash, while fuels with higher lignin contents (such as wood) leave greater volumes of charred material (15–25% of the original material; Drysdale, 2011). In oven formed charcoals, those produced at or above 400°C have been suggested to appear homogeneous, largely aromatic and recalcitrant when compared to those formed at 300°C (Ascough et al., 2011). It has been indicated that the size of polyaromatic units in lower temperature charcoals is smaller and retain incompletely converted lignocellulosic structures (Knicker et al., 2010; Ascough et al., 2011). Our laboratory results are consistent with these observations where the samples that were subject to flaming for 5 min contained three times the amount of lignin (greater reactivity) than those subject to heating for 20 min (lower reactivity) (Figure 9). Our microcalorimetry results support the notion that smaller aromatic molecules remain in the chars formed during 5 min, where these were observed to require a shorter period of re-heating in the microcalorimeter before pHRR was reached and that this was observed to occur at lower temperatures (Figure 8) (e.g., lower thermal recalcitrance). Interestingly the chars subject to flaming for 10, 15, and 20 min reveal little difference in their reaction time to reheating in the microcalorimeter, suggesting that there is a critical threshold in changes in the chemical structure of charcoals formed between 5 and 10 min flame durations, or in the case of WRC (in these experiments) between ∼25 kW.m-2 and ∼47 kW.m-2 THR. However, their pHRR did increase with previous heating duration, where we anticipate that more highly aromatic molecules are capable of delivering higher heat release than shorter chained aliphatic fractions.
Our laboratory results indicate that charcoals formed over short periods of heating and leading to low THR will be more susceptible to post fire oxidative processes owing to the presence of incompletely degraded lignin fragments and the predominance of shorted chained aromatic molecules. While, those formed above a critical threshold of energy transfer, contain little reactive lignocellulosic substances and a large fraction of polyaromatic compounds. The rise in median Ro% between the WRC samples subject to flaming for 5 min (25 kW.m-2 THR) as compared to 10 min (47 kW.m-2 THR) (Figure 3) indicates that Ro% may show some relevance toward estimating the long-term resistance of charcoals produced by wildfires. However, although reflectance continued to evolve between 10 and 20 min heating at 47–92 kW.m-2 THR, likely increasing the ordering of polyaromatic microcrystalline domains, we did not observe a decrease in reactivity during subsequent heating in the microcalorimeter (Figure 8). However, there was an observable increase in the charcoals pHRR, implying that the those previously subject to longer duration heating (and THR) can be related to increased surface Ro% (Figure 8). Where increasing Ro% must relate to increased amounts of larger aromatic molecules, leading to more recalcitrant polyaromatic charcoals.
Our comparison of charcoal reflectance between two different wildfires at Triangle (high-intensity) and Pine Point (low-intensity) has revealed promising results. The crown fire at the Triangle site produced a mean reflectance of 1.58 Ro% (both wood species) while, the surface fire at Pine Point produced a mean reflectance of 1.11 Ro%. This seems to make intuitive sense because crown fires typically burn more intensely than surface and ground fires in this region. This is evidenced by the double mean integrated area under the thermocouple curve, measured at the surface by the fire at the Triangle site, 50,306 compared to 22,327°C s at Pine Point. The WRC samples placed at the two sites came from the same tree as were used in the laboratory experiments and were all of equal moisture content when deployed into the fires, allowing direct comparison between the behavior of these samples in the lab and in the wildfires. WRC surface Ro% measured at the Triangle site ranged 1.07–2.59, while at Pine Point it ranged 0.87–1.48 (Figure 7A). In terms of charcoal reactivity/recalcitrance, our laboratory experiments suggest that the Pine Point fire would have produced charcoal that remained more reactive (due to the lower Ro%), while those from the Triangle fire are likely to be more recalcitrant owing to their higher Ro%. This is in agreement with the results of Doerr et al. (2018), where Ro% has been proven to be positively related to several indicators of chemical recalcitrance and the properties of these same fires. For example, the temperature at which 50% of the total energy was released showed positive correlations with Ro% (r2= 0.77) where the percentage of carbon in the charred remains was also found to increase with Ro% (Doerr et al., 2018). Doerr et al. (2018) also report that previously generated charcoals exposed to a second fire (the two fires in this study) increased their thermal recalcitrance particularly in the high-intensity fire at the Triangle site. Therefore, charcoals with higher reflectances will not only be more chemically resistance to post-fire degradative processes but can become increasingly resistant if subject to subsequent high intensity fires.
In summary, our results that link THR and Ro% are intriguing because they imply that charcoal reflectance may have utility in determining the distribution of energy delivery across a burned area. This could provide useful links to fire severity that describes the immediate impacts of heat pulses above ground and below ground, including, for example, tree mortality and soil and duff consumption (Keeley, 2009). With further development, Ro% measurements might have the potential to enable estimation of what fraction of the charcoals produced by a fire that might be considered reactive versus recalcitrant and therefore which will be more resistant to degradation over the long-term in the post fire environment. Future research should additionally consider involving methods that are better placed to identify the compounds/molecular groups (such as FTIR, e.g., see Ascough et al., 2011) that form in higher energy regimes and closely link these to observed transitions in surface Ro%. Such approaches may be suitable for considering fire characteristics and the contribution of char to C-cycling over the longer-term, including utility in both historic, pre-historic, and paleontological analyses (e.g., Hudspith et al., 2015). In all cases, however, it is critical that further research is conducted using charcoals generated by flaming combustion and/or instrumented field-scale fires if we are to move forward our understanding of charcoal reactivity and resistance to degradation in respect to fire dynamics and charcoal reflectance.
Author Contributions
CB, SD, CS, and SN conceived the work. SD and CS carried out the field experimentation. CB, VH, SN, and MG conducted the various charring experiments. CB, VH, and SN carried out the reflectance analyses and RD the lignin analyses. All authors contributed to the interpretation of the data and to drafting the manuscript.
Funding
Fieldwork and sample analysis was supported a Leverhulme Trust Grant to SD (RPG-2014-095) and a European Research Council Starter Grant ERC-2013-StG-335891-ECOFLAM to CB. SN received funding from the Natural Environment Research Council (NE/L002434/1) as part of the GW4+ Doctoral Training Partnership and CS by a Sêr Cymru Fellowship co-funded by European Union’s Horizon 2020 research and innovation programme (Marie Skłodowska-Curie Grant Agreement No. 663830).
Conflict of Interest Statement
The authors declare that the research was conducted in the absence of any commercial or financial relationships that could be construed as a potential conflict of interest.
Acknowledgments
Special thanks go to Westly Steed and his Department of Environment and Natural Resources, Government of the North-West Territories, Canada and also the staff of FPInnovations, Alberta Environment and Sustainable Resource Development for supporting the fieldwork.
References
Ascough, P., Bird, M. I., Wormald, P., Snape, C. E., and Apperley, D. (2008). Influence of pyrolysis variables and starting material on charcoal stable isotopic and molecular characteristics. Geochim. Cosmochim. Acta 72, 6090–6102. doi: 10.1016/j.gca.2008.10.009
Ascough, P. L., Bird, M. I., Scott, A. C., Collinson, M. E., Cohen-Ofri, I., Snape, C. E., et al. (2010). Charcoal reflectance measurements: implications for structural characterization and assessment of diagenetic alteration. J. Archaeol. Sci. 37, 1590–1599. doi: 10.1016/j.jas.2010.01.020
Ascough, P. L., Bird, M. I., Francis, S. M., Thornton, B., Midwood, A. J., Scott, A. C., et al. (2011). Variability in oxidative degradation of charcoal: influence of production conditions and environmental exposure. Geochim. Cosmochim. Acta 75, 2361–2378. doi: 10.1016/j.gca.2011.02.002
ASTM International (2016). E1354: Standard Test Method for heat and Visible Smoke Release Rates for Materials and Products Using an Oxygen Consumption Calorimeter. West Conshohocken, PA: ASTM International.
Belcher, C. M., and Hudspith, V. A. (2016). The formation of charcoal reflectance and its potential use in post-fire assessments. Int. J. Wildl. Fire 25, 775–779. doi: 10.1071/WF15185
Belcher, C. M., Collinson, M. E., and Scott, A. C. (2013). “A 450-million-year history of fire,” in Fire Phenomena and the Earth System an Interdisciplinary Guide to Fire Science, ed. C. M. Belcher (Hoboken, NJ: Wiley), 229–249. doi: 10.1002/9781118529539.ch12
Bird, M. I., Wynn, J. G., Saiz, G., Wurster, C. M., and McBeath, A. (2015). The pyrogenic carbon cycle. Annu. Rev. Earth Planet. Sci. 43, 273–298. doi: 10.1146/annurev-earth-060614-105038
Cohen-Ofri, I., Weiner, L., Boaretto, E., Mintz, G., and Weiner, S. (2006). Modern and fossil charcoal: aspects of structure and diagenesis. J. Archaeol. Sci. 33, 428–439. doi: 10.1016/J.JAS.2005.08.008
Crawford, A. J., and Belcher, C. M. (2016). Area-volume relationships for fossil charcoal and their relevance for fire history reconstruction. Holocene 26, 822–826. doi: 10.1177/0959683615618264
de Groot, W. J., Pritchard, J. M., and Lynham, T. J. (2009). Forest floor fuel consumption and carbon emissions in Canadian boreal forest fires. Can. J. For. Res. 39, 367–382. doi: 10.1139/X08-192
Drysdale, D. (2011). An Introduction to Fire Dynamics, 3rd Edn. Hoboken, NJ: Wiley. doi: 10.1002/9781119975465
Doerr, S. H., Santin, C., Merino, A. G., Belcher, C. M., and Baxter, G. (2018). Fire as a removal mechanism of pyrogenic carbon from the environment: effects of fire and pyrogenic carbon characteristics. Front. Earth Sci. 6:127. doi: 10.3389/feart.2018.00127
Harvey, O. R., Kuo, L. J., Zimmerman, A. R., Louchouarn, P., Amonette, J. E., and Herbert, B. E. (2012). An index-based approach to assessing recalcitrance and soil carbon sequestration potential of engineered black carbons (biochars). Environ. Sci. Technol. 46, 1415–1421. doi: 10.1021/es2040398
Hudspith, V. A., and Belcher, C. M. (2017). Observations of the structural changes that occur during charcoalification: implications for identifying charcoal in the fossil record. Palaeontology 60, 503–510. doi: 10.1111/pala.12304
Hudspith, V. A., Belcher, C. M., Kelly, R., and Hu, F. S. (2015). Charcoal reflectance reveals early Holocene boreal deciduous forests burned at high intensities. PLoS One 10:e0120835. doi: 10.1371/JOURNAL.PONE.0120835
Hudspith, V. A., Belcher, C. M., Barnes, J., Dash, C. B., Kelly, R., and Hu, F. S. (2017a). Charcoal reflectance suggests heating duration and fuel moisture affected burn severity in four Alaskan tundra wildfires. Int. J. Wildl. Fire 26, 306–316. doi: 10.1071/WF16177
Hudspith, V. A., Hadden, R. M., Bartlett, A. I., and Belcher, C. M. (2017b). Does fuel type influence the amount of charcoal produced in wildfires? Implications for the fossil record. Palaeontology 61, 159–171. doi: 10.1111/pala.12341
Jones, T., Scott, A. C., and Cope, M. (1991). Reflectance measurements against temperature of formation for modern charcoals and their implications for the study of fusain. Bulletin Soc. Ge′ol. France 162, 193–200.
Kashiwagi, T., Ohlemiller, T. J., and Werner, K. (1987). Effects of external radiant heat flux and ambient oxygen concentration on non-flaming gasification rates and evolved products in white pine. Combust. Flame 69, 331–345. doi: 10.1016/0010-2180(87)90125-8
Keeley, J. E. (2009). Fire intensity, fire severity and burn severity: a brief review and suggested usage. Int. J. Wildl. Fire 18, 116–126. doi: 10.1071/WF07049
Keiluweit, M., Nico, P. S., Johnson, M., and Kleber, M. (2010). Dynamic molecular structure of plant biomass-derived black carbon (biochar). Environ. Sci. Technol. 44, 1247–1253. doi: 10.1021/es9031419
Knicker, H., Muller, P., and Hilscher, A. (2010). How useful is chemical oxidation with dichromate for the determination of “Black Carbon” in fire-affected soils? Geoderma 142, 178–196. doi: 10.1016/j.geoderma.2007.08.010
Lyon, R. E., and Walters, R. (2002). “A microscale combustion calorimeter,” in Federal Aviation Administration William J. Hughes Technical Centre. Report No. DOT/FAA/AR-01/117. Washington, DC: US Department of Transport.
McBeath, A. V., Smernik, R. J., and Krull, E. S. (2013). A demonstration of the high variability of chars produced from wood in bushfires. Org. Geochem. 55, 38–44. doi: 10.1016/j.orggeochem.2012.11.006
McParland, L. C., Collinson, M. E., Scott, A. C., and Campbell, G. (2009). The use of reflectance values for the interpretation of natural and anthropogenic charcoal assemblages. Archaeol. Anthropol. Sci. 1, 249–261. doi: 10.1007/S12520-009-0018-Z
Michelotti, L., and Miesel, J. (2015). Source material and concentration of wildfire-produced pyrogenic carbon influence post-fire soil nutrient dynamics. Forests 6, 1325–1342. doi: 10.3390/f6041325
Moghtaderi, B., Novozhilov, V., Flectcher, D. F., and Kent, J. H. (2006). A new correlation for bench-scale piloted ignition of wood. Fire Saf. J. 29, 41–59. doi: 10.1016/S0379-7112(97)00004-0
Moreira-Vilar, F. C., Ferro, A. P., and Finger-Teixeira, A. (2014). The acetyl bromide method is faster, simpler and presents best recovery of lignin in different herbaceous tissues than klason and thioglycolic acid methods. PLoS One 9:e110000. doi: 10.1371/journal.pone.0110000
Santín, C., Doerr, S. H., Preston, C. M., and González-Rodríguez, G. (2015). Pyrogenic organic matter production from wildfires: a missing sink in the global carbon cycle. Glob. Chang. Biol. 21, 1621–1633. doi: 10.1111/gcb.12800
Santín, C., Doerr, S. H., Kane, E. S., Masiello, C. A., Ohlson, M., de la Rosa, J. M., et al. (2016). Towards a global assessment of pyrogenic carbon from vegetation fires. Glob. Chang. Biol. 22, 76–91. doi: 10.1111/gcb.12985
Santín, C., Doerr, S. H., Merino, A., Bucheli, T. D., Bryant, R., Ascough, P., et al. (2017). Carbon sequestration potential and physicochemical properties differ between wildfire charcoals and slow-pyrolysis biochars. Sci. Rep. 7:11233. doi: 10.1038/s41598-017-10455-2
Keywords: charcoal reflectance, fire-behavior, charcoal degradation, fire-severity, pyrolysis
Citation: Belcher CM, New SL, Santín C, Doerr SH, Dewhirst RA, Grosvenor MJ and Hudspith VA (2018) What Can Charcoal Reflectance Tell Us About Energy Release in Wildfires and the Properties of Pyrogenic Carbon? Front. Earth Sci. 6:169. doi: 10.3389/feart.2018.00169
Received: 25 April 2018; Accepted: 28 September 2018;
Published: 25 October 2018.
Edited by:
Francien Peterse, Utrecht University, NetherlandsReviewed by:
Gustavo Saiz, Imperial College London, United KingdomWilliam Patrick Gilhooly III, Indiana University–Purdue University Indianapolis, United States
Copyright © 2018 Belcher, New, Santín, Doerr, Dewhirst, Grosvenor and Hudspith. This is an open-access article distributed under the terms of the Creative Commons Attribution License (CC BY). The use, distribution or reproduction in other forums is permitted, provided the original author(s) and the copyright owner(s) are credited and that the original publication in this journal is cited, in accordance with accepted academic practice. No use, distribution or reproduction is permitted which does not comply with these terms.
*Correspondence: Claire M. Belcher, Yy5iZWxjaGVyQGV4ZXRlci5hYy51aw==