- 1Research Group for Biogeochemistry of Aquatic Ecosystems, Laboratório de Ciências Ambientais, Centro de Biociências e Biotecnologia Universidade Estadual do Norte Fluminense, Campos dos Goytacazes, Brazil
- 2Research Group for Marine Geochemistry (ICBM—MPI Bridging Group), Institute for Chemistry and Biology of the Marine Environment, Carl von Ossietzky University, Oldenburg, Germany
Rivers annually carry 25–28 Tg carbon in the form of pyrogenic dissolved organic matter (dissolved black carbon, DBC) into the ocean, which is equivalent to about 10% of the entire riverine land-ocean flux of dissolved organic carbon (DOC). The objective of this study was to identify the main processes behind the release and turnover of DBC on a riverine catchment scale. As a model system, we chose the headwater-to-ocean continuum of Paraíba do Sul River (Brazil), the only river system with long-term DBC flux data available. The catchment was originally covered by Atlantic rain forest (mainly C3 plants) which was almost completely destroyed over the past centuries by slash-and-burn. As a result, large amounts of wood-derived charcoal reside in the soils. Today, fire-managed pasture and sugar cane (both dominated by C4 plants) cover most of the catchment area. Water samples were collected along the river, at the main tributaries, and also along the salinity gradient in the estuary and up to 35 km offshore during three different seasons. DBC was determined on a molecular level as benzenepolycarboxylic acids (BPCAs). Stable carbon isotopes (δ13C) were determined in solid phase extractable DOC (SPE-DOC) to distinguish C4 and C3 sources. Our results clearly show a relationship between hydrology and DBC concentrations in the river, with highest DBC concentrations and fluxes in the wet season (flux of 770 moles s−1 in 2013 and 59 moles s−1 in 2014) and lowest in the dry season (flux of 27 moles s−1). This relationship indicates that DBC is mainly mobilized from the upper soil horizons during heavy rainfalls. The relationship between DBC concentrations and δ13C-SPE-DOC indicated that most of DBC in the river system originated from C3 plants, i.e., from the historic burning event of the Atlantic rain forest. A conservative mixing model could largely reproduce the observed DBC fluxes within the catchment and the land to ocean continuum. Comparably slight deviations from conservative mixing were accompanied by changes in the molecular composition of DBC (i.e., the ratio of benzenepenta- to benzenehexacarboxylic acid) that are indicative for photodegradation of DBC.
Introduction
Forest fires produce airborne combustion products and charred residues on and in the ground (Preston and Schmidt, 2006). Charred materials include a wide range of compounds, from dehydrated sugars formed at low charring temperature to highly-condensed graphite-like material produced at high temperatures, and secondary condensation products like soot (Santín et al., 2016). Also fuel characteristics are important, charcoal from woody and soft plant tissues often have different levels of condensation and oxygen content (Forbes et al., 2006; Schneider et al., 2010; Ding et al., 2014). The entire continuum of charred material is considered pyrogenic organic matter, of which the most condensed fraction is commonly referred to as black carbon (BC; Forbes et al., 2006).
Black carbon, which is largely derived from high-temperature woody chars, has received large attention in the literature, because it is more resistant to further biological and chemical degradation than the biomolecular precursors (Forbes et al., 2006). As a consequence, BC is ubiquitous in soils, sediments, and aquatic environments (Forbes et al., 2006; Jaffé et al., 2013). Important removal mechanisms of BC from soils are solubilization and subsequent transport in the dissolved phase (Dittmar et al., 2012a) and lateral transport of BC particles in the landscape (Major et al., 2010). During degradation in soils, oxygen atoms can be introduced into condensed aromatic structures of charcoal (Abiven et al., 2011). The resulting carboxylated molecular subunits partially dissolve in water and migrate through the soil as dissolved BC (DBC; Cheng and Lehmann, 2009). There is a significant time lag between wildfire induced BC production, incorporation into soils, and the actual release of DBC to aquatic systems (Dittmar et al., 2012a; Ding et al., 2014). Microbial reworking of charcoal may be required to enhance the translocation of soil BC to DBC (Ding et al., 2014). This translocation process can explain the presence of DBC in dissolved organic matter (DOM) in rivers, estuaries, and the ocean (Kim et al., 2004; Mannino and Harvey, 2004; Ziolkowski and Druffel, 2010). Global export of DBC from land to ocean amounts to ~27 Tg carbon year−1, which is equivalent to 10% of the entire riverine dissolved organic carbon (DOC) flux (Jaffé et al., 2013). DBC is distributed throughout the ocean and may impact biogeochemical processes on a global scale (Ziolkowski and Druffel, 2010). Even in the most remote basins of the deep ocean, ~2% of DOM contains a heat-induced molecular signature (Dittmar and Koch, 2006; Dittmar and Paeng, 2009).
Very little is known on how DBC behaves in aquatic environments. While there is evidence that DBC is very stable in the deep ocean having conservative, salt-like properties (Dittmar and Paeng, 2009; Ziolkowski and Druffel, 2010), it is very susceptible to UV radiation in sunlit waters (Stubbins et al., 2012). Once exposed to sunlight, most of DBC is lost from seawater (Stubbins et al., 2012) and river water (Riedel et al., 2016). It is unclear what proportion of DBC that is introduced from soils and groundwater into the rivers eventually survives riverine transport from the headwaters to the estuaries and from there into the open ocean. The objective of this study was to fill this gap of knowledge for one of the best studied rivers in this context. Paraíba do Sul River (PSR) in Brazil is the only river system for which long-term DBC flux data are available (Dittmar et al., 2012a). In this river, DBC annual export exceeds by far present BC production rates, and charcoal still residing in the soils after historic forest fires is the most likely source of DBC in the river today. PSR drains an area formerly covered entirely by Brazilian Atlantic Forest. Its original area comprised 1.3 million km2, but nowadays, only 12–15% of its original extension remains as secondary forest distributed as isolated, disconnected patches (Ribeiro et al., 2009; Fundação SOS Mata Atlântica Instituto Nacional de Pesquisas Espaciais, 2011; Lira et al., 2012). Deforestation occurred mainly between 1850 and 1970 via slash-and-burn (Warren, 1995). Today, 74% of the watershed is covered by fire-managed grassland and, in the area close to the coastal region, fire-managed sugar cane plantations. DBC concentration in PSR fluctuates with seasons, with highest concentrations during wet seasons and lowest ones during dry seasons, excluding direct deposition as a significant source (Dittmar et al., 2012a).
In this study we tested the hypothesis that due to limited light penetration and bio-recalcitrant properties, DBC is funneled unmodified within the PSR from the headwaters to the estuary and into the open ocean. We focused on the polycyclic aromatic fraction of DBC that can be quantified in natural waters with help of molecular proxies, i.e., benzenepolycarboxylic acids that are released from DBC during nitric acid oxidation (Hammes et al., 2007; Dittmar, 2008). We tested for conservative behavior of DBC in the headwaters-to-ocean continuum in three sampling campaigns, two in the rainy and one in the dry season.
Furthermore, we searched for chemical evidence that DBC in PSR is indeed mainly released from charcoal produced in historic forest fires and not primarily derived from today's fire management practice, as deduced previously from budget calculations (Dittmar et al., 2012a). We took advantage of the fact that the historic Atlantic forest vegetation was composed mainly of C3 plants, while today's pastures and sugar cane plantations are dominated by plants with the C4 photosynthetic pathway. Both types of plants carry a distinct carbon isotopic signature (δ13C) in their organic tissue. We determined δ13C on bulk SPE-DOM along the headwater-to-ocean continuum, which provided us with information about the main sources of DOM, of which DBC is a significant fraction.
Materials and Methods
Study Area
The PSR watershed occupies an area of 57,300 km2 in the states of São Paulo, Minas Gerais and Rio de Janeiro, located between 20°26′ and 23°28′S latitude and 41°00′ and 46°30′W longitude (Ovalle et al., 2013). The headwaters of the PSR are formed by the confluence of the Paraitinga and Paraibuna Rivers. The total length of the river channel is ~1150 km. The PSR basin can be divided into three macro-sectors (Figure 1A): (1) An upper basin sector with an area of 7,300 km2, where the river descends from an altitude of around 1800 to 600 m through narrow and embedded valleys carved out of crystalline rocks; (2) A middle basin sector with an area of 27,500 km2 and average elevation of 510 m, this sector is most influenced by industry, meanly for steel, chemicals, food, and paper; (3) A lower basin sector with an area of 22,500 km2 that is mainly occupied by coastal plain with numerous riverine meanders and islands.
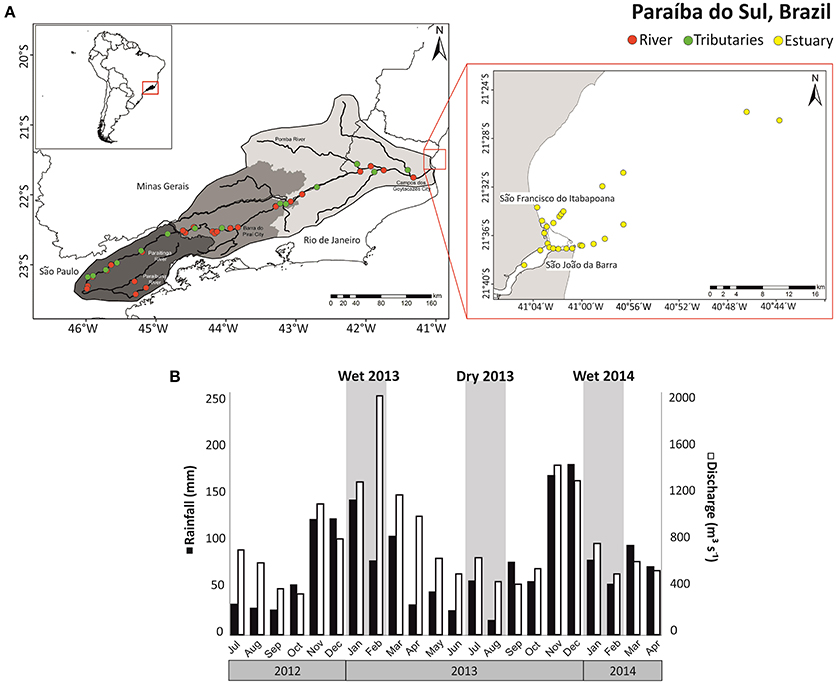
Figure 1. (A) Map showing the Paraíba do Sul (Brazil) basin and the sampling locations in the river (red), tributaries (green), and estuary (yellow); (B) discharge of PSR (white) and rainfall at Campos (black) during the times series of 2012-2014, highlighting (gray) the seasons when sampling was performed (wet 2013, 2014, and dry 2013).
The area within the PSR watershed is highly urbanized and industrialized, with about 5 million inhabitants. The PSR is used for supplying drinking water to over 14 million people. Close to Barra do Piraí city, ~160 m3 s−1 of water is diverted from PSR for water supply to Rio de Janeiro city. There is a strong seasonality in water discharge in PSR. The high and low water periods range from December to February and from June to August, respectively. Major industrial areas are concentrated in the middle and upper sector basin and in the sub-basins of the Pomba and Paraibuna Rivers (Ovalle et al., 2013). In the lower basin sector, extensive farming prevails, especially sugar cane production. In addition, 47 different reservoirs and hydroelectric dams with varying sizes influence the hydrology of the river system throughout the basin (Ovalle et al., 2013). The Paraíba do Sul estuary is located at the coastal plain formed by the PSR delta in the North of Rio de Janeiro state, near São João da Barra city (Souza et al., 2010).
Sampling
Samples from rivers, estuary, and adjacent ocean (Figure 1A) were collected during the wet seasons in January 2013 and February 2014. Average fluvial discharge in the lower reaches of PSR (Campos dos Goytacazes) was 1875 m3 s−1 in January 2013 and 719 m3 s−1 in February 2014. Sampling in the dry season was done in July and August of 2013, with an average discharge of 478 m3 s−1. The discharge value was estimated using river velocity and cross-sectional area measurements (General Oceanic model 2030 current meter). Surface water samples (3 L) were collected in acid rinsed bottles at 24 sites along the main channel of the PSR (Figure 1A, red symbols) and 14 sites at the PSR tributaries (Figure 1A, green symbols). In the estuary and adjacent ocean up to 35 km offshore, 24 samples were collected along the salinity gradient in the wet season of 2013, and 21 sites during the other campaigns (Figure 1A, yellow symbols). The PSR and tributaries were sampled from bridges while the estuary and ocean were sampled from a trawler. All samples were retrieved from the water surface with acid-rinsed buckets. We collected superficial soil samples in the forest and pasture sites (three samples at each site) to constrain the end-members used in the two-source isotopic model as C3 plants (forest site) and C4 plants (pasture site).
Sample Processing
Immediately after sampling, samples were filtered through pre-combusted GF/F filters (Whatman, nominal pore size 0.7 μm). After filtration, samples were acidified with HCl (32%, analytical grade) to pH 2 and DOM was isolated from the water samples via solid-phase extraction (SPE; Dittmar et al., 2008). In brief, filtered and acidified samples were passed by gravity through solid-phase cartridges (1 g PPL, Agilent). The cartridges were desalted with 0.01 mol L−1 HCl, dried with a stream of N2, and DOM was eluted with 8 mL of methanol (HPLC grade). The DOC extraction efficiency was determined for each sample by evaporating an aliquot of the methanol extract to dryness, re-dissolving it in ultrapure water at pH 2, and relating the DOC concentration of this solution to that of the original sample. On average among all samples (n = 177), 45% (SE ± 13%) of DOC was recovered by the SPE. Extraction efficiency did not systematically vary along the river and along the salinity gradient. Soil samples were freeze-dried and the fraction >2.0 mm removed by sieving.
Dissolved Organic Carbon and Stable Carbon Isotope Determination
The concentration of DOC in filtered samples was determined by the high-temperature catalytic oxidation method on an automated TOC analyzer (Shimadzu TOC 5000), using five calibration solutions spanning the concentration range of the samples. All DOC data reported are the mean of three replicate injections, for which the coefficient of variance was <5%. Procedural blanks, including the filtration step, were obtained using ultrapure water. These blank samples did not contain any detectable amounts of DOC. The detection limit for DOC was 5 μM, and the analytical accuracy (relative to the reference material) and precision (replicate injections) were within ± 1 μM. The deep sea reference material provided by D. Hansell (University of Miami, USA) was repeatedly analyzed in each run to control accuracy.
The stable carbon isotope composition of SPE-DOM was determined following an established protocol (Seidel et al., 2015). In brief, an aliquot of 800–1600 μL of SPE-DOM extract, corresponding to ~20 μg of SPE-DOC, was dried under N2 flux. Then, it was re-dissolved in 50 μL of methanol, transferred into Sn combustion capsules (Elemental) and dried in an oven at 60°C for 24 h. Soil samples were weighed (10 mg) in Sn combustion capsules (Elemental). The isotopic composition was analyzed on an elemental analyzer (Flash 2000) coupled to an isotope-ratio mass spectrometer Delta V Advantage (Thermo Scientific, Germany). Stable carbon isotope ratio is expressed as δ13C (%) relative to the Pee Dee Belemnite (PDB) standard reference.
Dissolved Black Carbon
The benzenepolycarboxylic acids (BPCAs) method (Dittmar, 2008) was used to quantify the condensed polyaromatic fraction of DBC. This method is the most sensitive and unequivocal method for the determination of BC in fluvial and marine DOM (Dittmar et al., 2012b). Moreover, the proportions of the different detectable BPCAs are indicative of the extent of condensation and size of the polycyclic aromatics. For example, char produced at 1000°C is typically highly condensed and BPCAs released by nitric acid oxidation are basically composed of benzenehexacarboxylic acid. Charcoal produced at 200°C is characterized by a low number of condensed aromatic rings, and BPCAs are less carboxylated compared to high-temperature chars (Schneider et al., 2010). For BPCA analysis, 300–500 μL of the methanol extracts, corresponding to 1–10 μmol of SPE-DOC, were transferred into 2 mL glass ampoules, evaporated to dryness in an oven at 60°C and dissolved in 0.5 mL of concentrated HNO3 (65%). The ampoules were flame sealed, placed in a stainless-steel pressure bomb and kept for 9 h at 170°C in a furnace. After the ampoules had cooled, the HNO3 was evaporated to dryness in a speed vacuum centrifuge (60°C, Christ RV2-18). Samples were dissolved in 100 μL of phosphate buffer at pH 7.2 (Na2HPO4 and NaH2PO4, each 0.5 mM) and analyzed on an ultrahigh performance liquid chromatography system (Waters Acquity UPLC), equipped with a photodiode array light-absorbance detector. BPCAs were identified in accordance to retention time and absorbance spectra (220–380 mm). Quantification was performed using the absorbance signal at 240 mm and an external calibration. The injection volume was 1 μl. BPCA concentrations were converted into DBC concentrations after the equation of Dittmar (2008), with the slight modification outlined in Stubbins et al. (2015), where the most robustly quantified B6CA and B5CA are used for estimating DBC. For the equations we refer to Stubbins et al. (2015).
Two Source Isotopic Model
To estimate the contribution of C4 plant derived organic matter to PSR and tributaries DOM, we used a linear two-source mixing model (Martinelli et al., 2002):
where δ13Csample is the isotopic composition of SPE-DOM in a given sample, δ13CC3soil (−29.4 ± 0.4%) is the isotopic composition of the forest soil and δ13CC4soil (−14.9 ± 0.3%) is the isotopic composition of the pasture soil. An underlying assumption of our calculations is that there are only two main sources of SPE-DOM. Other sources, like sewage or autochthonous production by algae reduce the accuracy of our calculations. Also isotope fractionation during DOM decomposition is not considered in our model.
Hydrological and Conservative Mixing Models
Daily water discharge data are available for the lower reach of PSR, at the city of Campos dos Goytacazes. We used electric conductivity as a tracer to backwards calculate the water discharge of the tributaries and at each sampling point of PSR. Electrical conductivity was determined in situ with a WTW portable probe calibrated directly before each measurement. Under the reasonable assumption that electrical conductivity behaves conservatively during mixing, the relative proportion of water discharge of a tributary and the mainstream before and after the effluent was calculated, based on the principles of mass conservation:
Q is the water discharge after the tributary (Qafter), before the tributary (Qbefore), and of the tributary (Qtributary); and σ is the electrical conductivity at the respective position. For example, the water discharge in Campos dos Goytacazes (Qafter) was 1875 m3 s−1 in January 2013 and σafter at that site was 65 μS cm−1. From the electrical conductivity of the next upstream tributary (σtributary; 73 μS cm−1) and the river sample before the tributary (σbefore; 59 μS cm−1), we calculated a water discharge of 803 m3 s−1 from the tributary and 1071 m3 s−1 from the PSR upstream the tributary. Loss of water (e.g., through evaporation) and unknown sources of water (e.g., groundwater inputs) are sources of errors in this model.
As a second step we calculated the respective fluxes of DBC (FDBC) as water discharge multiplied by DBC concentration (cDBC) at each site.
DBC fluxes were then compared to the theoretical DBC fluxes for ideal conservative behavior at each site. Deviations of the measured fluxes from these conservative fluxes indicate additional source and sink terms along the river. Conservative fluxes were calculated on the assumption that DBC behaves like electrical conductivity in the conservative case. Consequently, the theoretical, conservative DBC flux at a given station is the DBC flux of the uppermost station in PSR, plus the additive flux of each tributary upstream of a given station:
The theoretical, conservative DBC concentration (cDBC, conservative) at each station is:
Similar calculations were done for the estuary. The proportion of freshwater (%fresh) and seawater (%sea) in each estuarine sample was calculated from electrical conductivity. We considered the samples taken in PSR in Campos dos Goytaczes (freshwater) and the outermost marine station (seawater) as endmembers for these calculations. Based on the principles of mass conservation:
cDBC,fresh and cDBC,sea are the DBC concentrations of the endmembers.
In the conservative case, the freshwater flux of DBC throughout the entire estuary is equal to the DBC flux in the lowermost riverine station:
The real flux of freshwater-derived DBC in the estuary is approximated from the deviations of DBC concentration from conservative mixing:
Results
DOC and DBC concentrations were highly variable between sites and seasons in the main stem of the river, the tributaries, and the estuary. The DOC concentration in the PSR system ranged between 168 and 894 μmol L−1 in riverine samples, 72–529 μmol L−1 in estuarine samples and 143–1381 μmol L−1 in tributaries (Figure 2A, Tables S1–S3). Riverine and estuarine DOC concentrations were distinctly higher during the wet season of 2013, while during the dry season of 2013 and the wet season of 2014 DOC concentrations were lower (Student's t-test, p < 0.01, Figure 2A, Tables S1–S3). In contrast, DOC concentrations in the tributaries were not significantly different between both wet seasons, but differed between wet and dry seasons (Student's t-test, p < 0.05, Figure 2A, Tables S1–S3). DOC concentrations in the estuary decreased with distance offshore. In all the sampling periods, DOC concentrations slightly deviated from conservative mixing in the river and estuary (Figure 2A), but the resulting DBC fluxes were not significantly different from those calculated from the conservative mixing model. The stable carbon isotopic composition (δ13C) of SPE-DOM ranged between −29.0 and −23.5% in the main stem of PSR, and from −28.0 to −21.3% in the tributaries. In the estuary, δ13C increased from a minimum of −25.7% inshore to −20.0% in the marine endmember offshore (Figure 2B, Tables S1–S3). Riverine and estuarine δ13C were more depleted (more negative δ13C values) in the wet season of 2013 (Student's t-test, p < 0.05, Figure 2B), followed by the wet season of 2014 and finally of dry season of 2013 (Student's t-test, p < 0.05, Figure 2B). The δ13C of forest soil sample was −29.4 ± 0.4% whilst it was −14.9 ± 0.3% for the pasture soil sample.
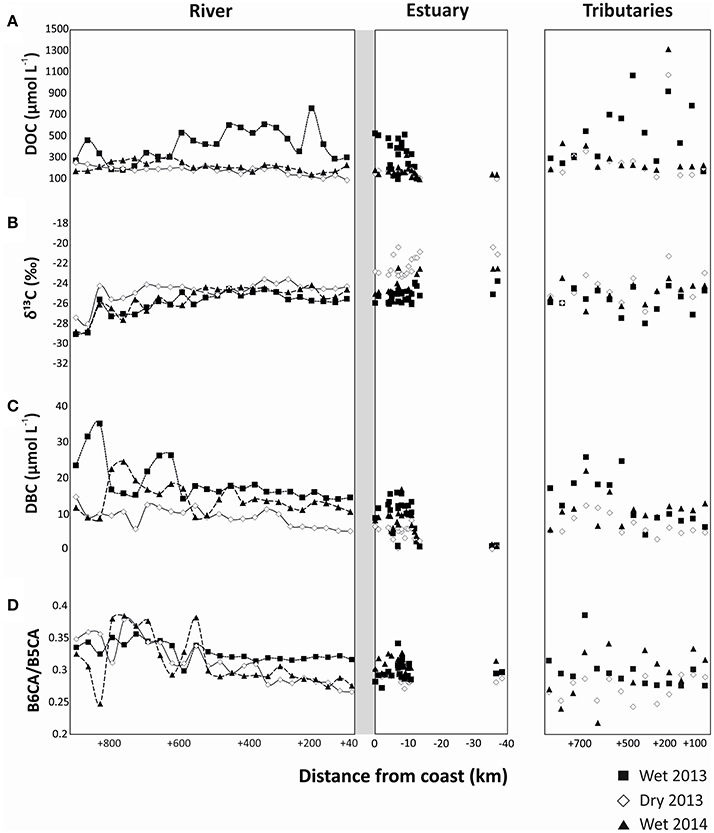
Figure 2. Spatial distributions of (A) DOC concentrations, (B) δ13C of SPE-DOC, (C) DBC concentrations, and (D) B6CA/B5CA ratio in the river, estuary and tributaries of Paraíba do Sul during the different sampling seasons.
DBC concentrations in PSR ranged between 5 and 35 μmol L−1, with significantly higher values during the wet season of 2013 compared to the other sampling campaigns (Student's t-test, p < 0.01, Figure 2C, Table S1). DBC concentrations in the tributaries ranged from 3 to 26 μmol L−1, and were similar in both wet seasons, but distinctly different between wet and dry seasons (Student's t-test, p < 0.01, Figure 2C, Table S2). Estuarine DBC concentrations ranged from 0.3 to 17 μmol L−1, strongly decreasing from in- to offshore; they were similar in both wet seasons, and distinctly different between wet and dry seasons (Student's t-test, p < 0.01, Figure 2C, Table S3). The concentration ratio of benzenehexa- to benzenepentacarboxylic acids (B6CA/B5CA) in PSR ranged between 0.27 and 0.36, in the tributaries between 0.22 and 0.34 and in the estuary between 0.27 and 0.34 (Figure 2D, Tables S1–S3), with a decreasing trend from in- to offshore.
Discussion
The Source of DBC
The PSR catchment area was once covered by Atlantic forest. Due to almost complete destruction via the slash-and-burn practice until the mid-1970's large amounts of charcoal had been deposited in the soils of the catchment. DBC is slowly released when charcoal ages in soils (Ding et al., 2013). Simultaneous microbial oxidation of soil organic matter and charcoal likely results in a strong relationship between DOC and DBC concentrations in the rivers draining the area (Ding et al., 2013). Consistent with this concept, statistically significant correlations between DBC and DOC concentrations were observed for PSR, tributaries, and estuary (Figure 3). Similar relationships were previously found in intertidal systems (Dittmar et al., 2012b), intermittent grassland streams (Ding et al., 2013), and fluvial systems (Dittmar et al., 2012a; Jaffé et al., 2013; Stubbins et al., 2015). In our study, the slope of the resulting regressions indicated that the DOC pool in the river contained 12.2% of DBC, followed by the estuary with 11.8% and the tributaries with 10.5%, which is close to the previously reported global riverine average (Jaffé et al., 2013).
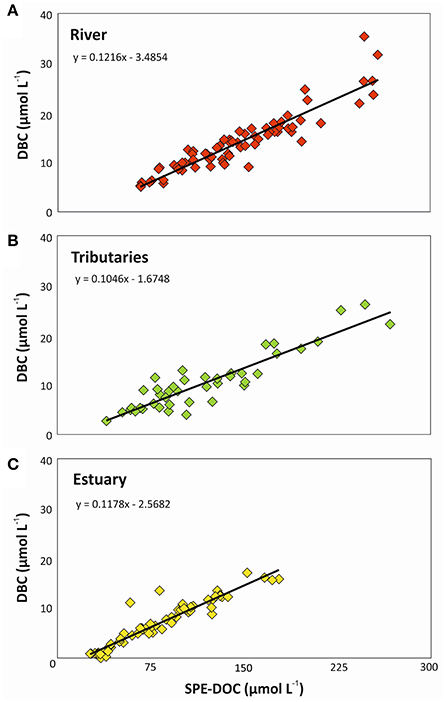
Figure 3. Geometric mean regression between SPE-DOC and DBC concentrations in (A) Paraíba do Sul river, (B) tributaries, and (C) estuary.
It was proposed that DBC in PSR is largely derived from historic charcoal deposits in the soils, and only to a minor degree from today's fire-management practice (Dittmar et al., 2012a). The historic forest vegetation was composed mainly of C3 plants, while today's pastures and sugar cane plantations are dominated by C4 plants. DBC concentrations in the river system inversely correlated with the percentage of DOC that was derived from C4 plants (Figure 4; Figure S1). The higher the contribution of C3 plants the higher was the concentration of DBC in the river. The relationship between all samples presented a strong negative correlation (rs = −0.605, p < 0.001, n = 114) indicating that historical fire events from Atlantic Forest represented a more important source of DBC to the river water today than recent burning activities.
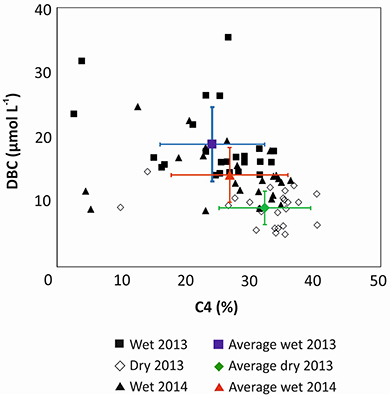
Figure 4. Relationship between the estimated percentage of C4-derived carbon in SPE-DOC (derived from δ13C) and DBC concentrations in Paraíba do Sul river and tributaries.
The source of DOC shifted between the seasons and was consistent with DBC concentrations. During the rainy season, DBC concentrations were high, and the DOC was largely derived from historic carbon sources (C3) with more depleted δ13C values. In the dry season, DBC concentration was lower and the contribution of today's vegetation (C4) was higher, with δ13C values more enriched. The identification of C3 or C4 plants with carbon isotopic composition was possible due the marked differences in δ13C values between each kind of plants. C3 plants have δ13C values depleted close to −31%, while C4 plants have δ13C values less depleted close to −14% (Kruche et al., 2002). This observation is probably a reflection of water flow paths. During the rainy season, upper soil horizons, where most (historic) charcoal deposits are flushed. Deeper groundwater that fuels the river during base flow seems to be less influenced by soil-derived DOM. In accordance, in the Amazon Forest, the upper soil horizons (upper 60 cm) contain higher amounts of BC and char than deeper horizons (Glaser et al., 2001). The char in soil in the Amazon had an apparent radiocarbon age of 1775 ± 325 years (Glaser et al., 2001), indicating a long residence time of char in tropical soils. In addition, enhanced in-situ production by algae during the dry season caused by deeper light penetration should be taken into account. Algae are potentially another source of isotopically heavy and DBC-poor DOC to the river. Nevertheless, the historic C3 vegetation is apparently the predominant source of bulk DOC and associated DBC to the river system.
Processing of DBC in the River-to-Ocean Continuum
In the headwaters-to-ocean continuum of PSR, the DBC concentrations varied over one order of magnitude. Within the main river, the DBC concentrations matched those reported earlier for PSR in Campos dos Goytacazes (Dittmar et al., 2012a), and are within the range of global rivers (Jaffé et al., 2013) and coastal wetlands (Dittmar et al., 2012b; Ding et al., 2014). The much lower concentrations offshore are consistent with the low DBC concentrations reported for the sea surface in the Gulf of Mexico (Dittmar, 2008) and the Southern Indian Ocean (Dittmar and Paeng, 2009).
To identify potential sources and sinks along the PSR and estuary we compare the observed trends with those expected from conservative mixing, i.e., a scenario in which DBC has salt-like characteristics in the headwater-to-ocean continuum. In all sampling campaigns a cumulative net-removal of DBC was observed from the headwaters down to the lower reaches of the riverine system (300 km inland, Figure 5). This apparent non-conservative behavior of DBC was restricted to few sites and the introduced deviation propagated downstream. An apparent net-removal of DBC persisted all the way to the estuary in the dry season of 2013, but was counteracted or even outbalanced by inputs of DBC in the lower reaches of the river during the wet seasons. In the estuary, a net-input was observed in both rainy seasons; in the dry season, DBC mixed conservatively in the estuary and inner shelf. Overall, the slight deviations of DBC concentration from the conservative mixing model had comparatively little effect on DBC fluxes in the rivers system that were dominated by the large discharge of few tributaries in the lower reaches of PSR (Figure 6).
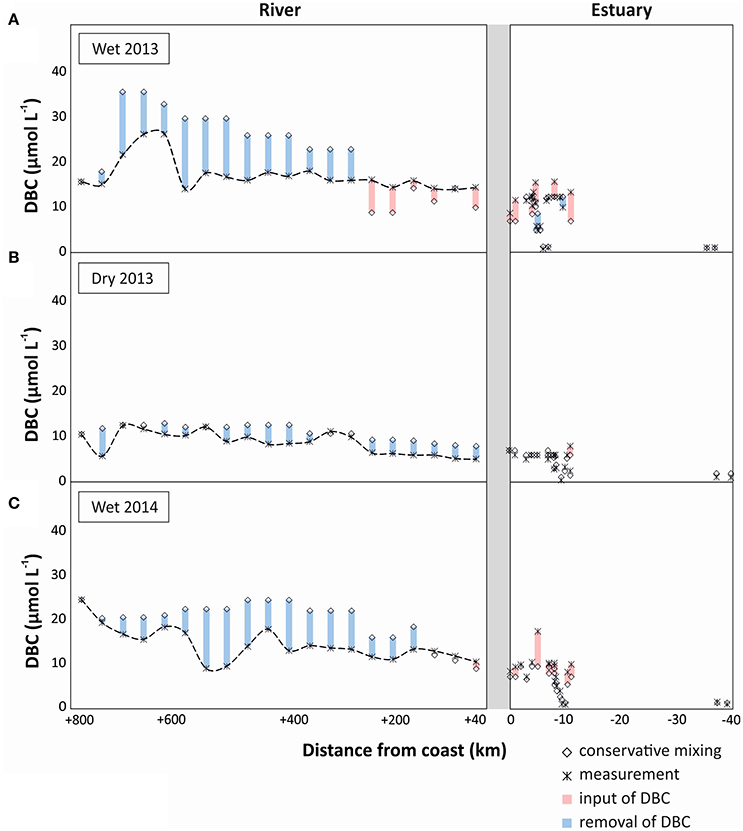
Figure 5. DBC concentration along the headwaters-to-ocean continuum of Paraíba do Sul (measured concentration and conservative mixing model), indicating potential inputs of DBC (in red; real measurement > conservative mixing model value) and potential sinks of DBC (in blue; conservative mixing model value > real measurement). (A) wet season 2013, (B) dry season 2013, and (C) wet season 2014.
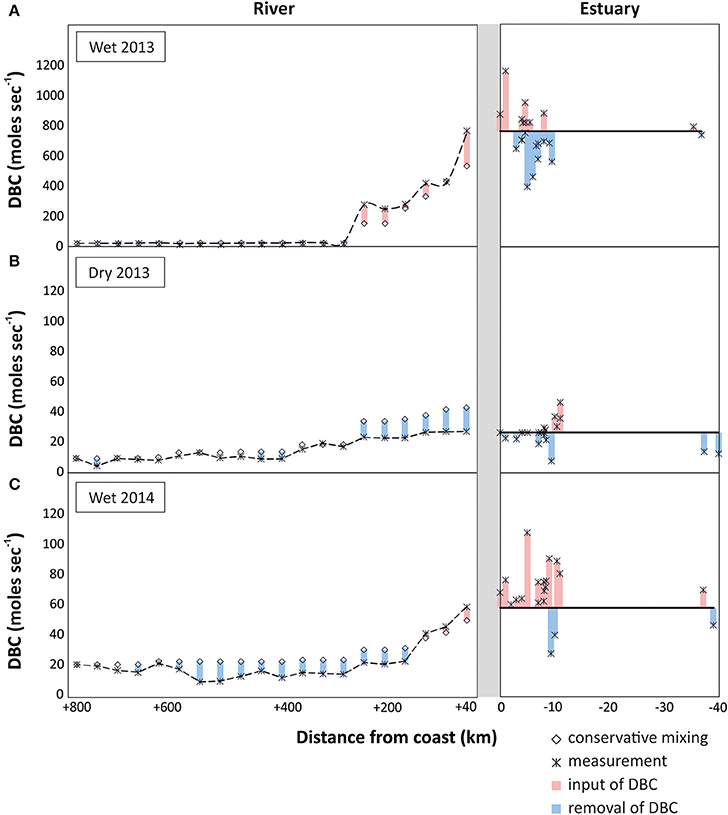
Figure 6. Flux of DBC (moles s−1) in the headwaters-to-ocean continuum of Paraíba do Sul system, indicating potential inputs of DBC (in red; real measurement > conservative mixing model value) and potential sinks of DBC (in blue; conservative mixing model value > real measurement), for the different sampled seasons: (A) wet season 2013, (B) dry season 2013, and (C) wet season 2014. Note different scales for DBC fluxes.
In principle, two explanations can be put forward for a non-conservative behavior of DBC in the river-to-ocean continuum. First, unknown sources of water with different DBC to conductivity ratios than the sampled tributaries introduce errors in our mass balance calculations. Deep groundwater is generally poor in DBC (Dittmar et al., 2012a; Stubbins et al., 2015). With increased rainfall, the hydrological pathway also incorporates active soil layers that are rich in organic compounds and charcoal (Guggenberger et al., 2008; Stubbins et al., 2015). At base flow during the dry season, deep groundwater is the main source of water to PSR and the DBC concentrations are consequently lower than during the rainy season when upper soil horizons are flushed (Dittmar et al., 2012a). Lateral input of groundwater into the tributaries is indirectly considered in our mass balance model, but direct inputs of groundwater into the main stem not. Depending on the source of groundwater, these undetected inputs may cause net-inputs from upper soil horizons (rainy seasons) or an apparent net-removal due to dilution with deep groundwater (dry season). Because we observed an overall consistent pattern of net-removal of DBC during wet and dry seasons we consider methodological artifacts as an unlikely reason for the observed net-removal. About 23–40% of DBC was lost along the transect from river to estuary during the various seasons.
DBC consists of condensed organic compounds, and is thus sensible to photooxidation (Stubbins et al., 2008; Spencer et al., 2009). This could be an important sink for DBC in the riverine system, and explain the observed net-removal. One way to assess whether photooxidation indeed occurred is provided by changes of the B6CA/B5CA ratio. Benzenehexacarboxylic acid (B6CA) is indicative of highly condensed aromatics, whereas benzenepolycarboxylic acids with a lower number of carboxylic substitutes are indicative of molecules with a lower number of condensed rings in their core structure (Schneider et al., 2010). Thus, the B6CA/B5CA ratio was proposed as a measure for the degree of condensation of DBC (Stubbins et al., 2012). Highly condensed structures are preferentially degraded by irradiation, and 28 days of exposure to sunlight of North Atlantic Deep Water caused a decrease of B6CA/B5CA from 0.32 to 0.23 (Stubbins et al., 2012). Similarly in our study, apparent loss of DBC was associated with a decrease of B6CA/B5CA (Figure 2D). In the dry season of 2013 and the wet season of 2014, the B6CA/B5CA ratio decreased from 0.38 to 0.27 along the river, which is a clear indication for photodegradation. In the wet season of 2013, the change in B6CA/B5CA was not as pronounced, as there was a net-input of DBC in the lower reaches of the river. In the lower reaches of PSR and next to the estuary, there are extensive fire-managed sugar cane plantations located in direct vicinity of the estuary. These areas of sugar cane plantations have been managed since 1538 (Oscar, 1985). It is plausible that lateral inputs from these areas especially during the rainy season caused the observed net-inputs in the corresponding areas. DBC from upper soil horizons has not been exposed to sunlight yet, which is consistent with the relatively high B6CA/B5CA in the respective area and season. Furthermore, during the wet season of 2013, the load of suspended particles in the river was almost one order of magnitude higher than during the other campaigns at station Campos dos Goytacazes (120 mg L−1, compared to 15 mg L−1, unpublished data). Particles shade the water and reduce photodegradation. In addition, DBC may desorb from the particles thereby contributing to the apparent net-input of DBC in the lower reaches of the river during the rainy season of 2013.
In the rainy seasons, DBC concentrations were higher than during the dry season. In conjunction with the higher water discharge, DBC fluxes were even more enhanced during the rainy seasons (Figure 6). This cumulative effect of higher DBC concentration and higher water discharge was most pronounced during the wet season of 2013. During this campaign water discharge was more than one order of magnitude higher at the lowest reaches of PSR compared to the other sampling campaigns, resulting in correspondingly high fluxes of DBC from the river to the ocean. The flux of DBC fluctuated in the estuary, but there was no indication for net-removal of DBC. Fluctuations are likely due to spatial heterogeneity of DBC concentration and local inputs from resuspended sediments, tidal creeks and groundwater discharge. Overall, it appears that a large fraction of the DBC survived transport through the estuary and onto the inner shelf, and possibly over larger scales in the ocean.
Conclusions
This study presents the first data about the DBC spatial and seasonal behavior in a tropical basin. As model system, we chose the Paraíba do Sul system (Brazil), the only river system for which long-term DBC flux data exist. Our study indicates that hydrology plays an important role in DBC dissolution and migration, with highest DBC concentrations in the wet season and lowest in the dry season. This relationship suggests that DBC is mainly mobilized from the upper soil horizons during heavy rainfalls. Therefore, lateral transport to the ocean seems to be an important removal mechanism for BC in soils. A direct relationship between DOC and DBC concentrations was observed, indicating fire-altered carbon as an intrinsic component of the DOC pool. The similarities in the mechanisms of DOC and DBC stabilization and loss, suggested that DOC may contribute to the mobilization of soil BC to the aquatic systems. In addition, a statistically significant relationship between DBC and δ13C-SPE-DOC is consistent with previous literature suggestion (Dittmar et al., 2012a), that DBC in the PSR system is derived from aged charcoal, produced during the Atlantic forest destruction and historically accumulated in soils. Future studies should be directed toward compound-specific carbon isotope analysis on BPCAs to unambiguously confirm the source and age of DBC.
A simple mixing model could largely reproduce the observed DBC fluxes within the catchment and the headwaters-to-ocean continuum. Photooxidation likely removed some DBC along the course of the river-to-ocean-continuum. High water discharge and increased DBC concentrations had a cumulative effect on DBC flux during the rainy seasons. We found no evidence for net DBC removal in the estuary or inner shelf, thus large-scale transport in the ocean is likely. Anticipated temperature increase and changes in the water cycle may result in an increase of fire frequency. As global climate change effects promote extreme dry and wet seasons, the DBC export may increase proportionally and alter the size of the refractory DOM pool in the deep ocean.
Author Contributions
All authors contributed to the design of the study. JM and MA analyzed samples for stable carbon isotopic composition. JM and JN analyzed samples for dissolved black carbon. TD, CR, and JM conducted modeling studies. All authors contributed to data interpretation and writing of the manuscript. JM and GG built the figures.
Funding
INCT-TMCOcean on the Continent-Ocean Materials Transfer (CNPq: 573.601/08-9). CR received financial support from CNPq (506.750/2013-2) and FAPERJ (E-26/111.616/2011 and E-26/201.188/2014). The Science without Border (CNPq CSF 400.963/2012-4) provided financial support for JM 1 year in a sandwich program at the University of Oldenburg; MA 1 year of Post Doctor position in Environmental Sciences Laboratory (UENF) and TD 3 months as a Visiting Professor at Universidade Estadual do Norte Fluminense.
Conflict of Interest Statement
The authors declare that the research was conducted in the absence of any commercial or financial relationships that could be construed as a potential conflict of interest.
The reviewer AC and handling Editor declared their shared affiliation, and the handling Editor states that the process nevertheless met the standards of a fair and objective review.
Acknowledgments
The authors are grateful to the Laboratório de Ciências Ambientais of the Centro de Biociências e Biotecnologia at the Universidade Estadual do Norte Fluminense and the University of Oldenburg Research Group for Marine Geochemistry (ICBM—MPI Bridging Group), Institute for Chemistry and Biology of the Marine Environment (ICBM) for the use of its facilities. We thank Thiago Rangel, Diogo Quitete for sampling assistance and Ina Ulber and Matthias Friebe for assistance in the lab.
Supplementary Material
The Supplementary Material for this article can be found online at: http://journal.frontiersin.org/article/10.3389/feart.2017.00011/full#supplementary-material
References
Abiven, S., Hengartner, P., Schneider, M. P. W., Singh, N., and Schmidt, M. W. I. (2011). Pyrogenic carbon soluble fraction is larger and more aromatic in aged charcoal than in fresh charcoal. Soil Biol. Biochem. 43, 1615–1617. doi: 10.1016/j.soilbio.2011.03.027
Cheng, C. H., and Lehmann, J. (2009). Ageing of black carbon along a temperature gradient. Chemosphere 75, 1021–1027. doi: 10.1016/j.chemosphere.2009.01.045
Ding, Y., Cawlley, K. M., Cunha, C. N., and Jaffé, R. (2014). Environmental dynamics of dissolved black carbon in wetlands. Biogeochemistry 119, 259–273. doi: 10.1007/s10533-014-9964-3
Ding, Y., Yamashita, Y., Dodds, W. K., and Jaffé, R. (2013). Dissolved black carbon in grassland streams: is there an effect of recent fire history? Chemosphere 90, 2557–2562. doi: 10.1016/j.chemosphere.2012.10.098
Dittmar, T. (2008). The molecular level determination of black carbon in marine dissolved organic matter. Organ. Geochem. 39, 396–407. doi: 10.1016/j.orggeochem.2008.01.015
Dittmar, T., and Koch, B. P. (2006). Thermogenic organic matter dissolved in the abyssal ocean. Mar. Chem. 102, 208–217. doi: 10.1016/j.marchem.2006.04.003
Dittmar, T., Koch, B., Hertkorn, N., and Kattner, G. (2008). A simple and efficient method for the solid-phase extraction of dissolved organic matter (SPE-DOM) from seawater. Limnol. Oceanogr. Methods 6, 230–235. doi: 10.4319/lom.2008.6.230
Dittmar, T., and Paeng, J. (2009). A heat-induced molecular signature in marine dissolved organic matter. Nat. Geosci. 2, 175–179. doi: 10.1038/ngeo440
Dittmar, T., Paeng, J., Gihring, T. M., Suryaputra, I. G. N. A., and Huettel, M. (2012b). Discharge of dissolved black carbon from a fire-affected intertidal system. Limnol. Oceanogr. 57, 1171–1181. doi: 10.4319/lo.2012.57.4.1171
Dittmar, T., Rezende, C. E., Manecki, M., Niggemann, J., Ovalle, A. R. C., Stubbis, A., et al. (2012a). Continuous flux of dissolved black carbon from a vanished tropical forest biome. Nat. Geosci. 5, 618–622. doi: 10.1038/ngeo1541
Forbes, M. S., Raison, R. J., and Skjemstad, J. O. (2006). Formation, transformation and transport of black carbon (charcoal) in terrestrial and aquatic ecosystems. Sci. Total Environ. 370, 190–206. doi: 10.1016/j.scitotenv.2006.06.007
Fundação SOS Mata Atlântica Instituto Nacional de Pesquisas Espaciais (2011). Atlas dos Remansecentes Florestais da Mata Atlântica, Período 2008–2010.
Glaser, B., Haumaier, L., Guggenberger, G., and Zech, W. (2001). The ‘Terra Preta’ phenomenon: a model for sustainable agriculture in the humid tropics. Naturwissenschaffen 88, 37–41. doi: 10.1007/s001140000193
Guggenberger, G., Rodionov, A., Shibistova, O., Grabe, M., Kasansky, O., Fuchs, H., et al. (2008). Storage and mobility of black carbon in permafrost soils of the forest tundra ecotone in Northern Siberia. Glob. Change Biol. 14, 1397–1381. doi: 10.1111/j.1365-2486.2008.01568.x
Hammes, K., Schmidt, M. W. I., Smernik, R. J., Currie, L. A., Ball, W. P., Nguyen, T. H., et al. (2007). Comparison of quantification methods to measure fire-derived (black/elemental) carbon in soils and sediments using reference materials from soil, water, sediment and the atmosphere. Glob. Biogeochem. Cycles 21:GB3016. doi: 10.1029/2006GB002914
Jaffé, R., Ding, Y., Niggemann, J., Vähätalo, A. V., Stubbins, A., Spencer, R. G. M., et al. (2013). Global charcoal mobilization from soils via dissolution and riverine transport to the oceans. Science 340, 345–347. doi: 10.1126/science.1231476
Kim, S., Kaplan, L. A., Benner, R., and Hatcher, P. G. (2004). Hydrogen-deficient molecules in natural riverine water sample – evidence for existence of black carbon in DOM. Mar. Chem. 92, 225–234. doi: 10.1016/j.marchem.2004.06.042
Kruche, A. V., Martinelli, L. A., Victoria, R. L., Bernades, M. C., Camargo, P. B., Ballester, M. V., et al. (2002). Compositional of particulate and dissolved organic matter in a disturbed watershed of southeast Brazil (Piracicaba River basin). Water Res. 36, 2743–2752. doi: 10.1016/S0043-1354(01)00495-X
Lira, P. K., Tambosi, L. R., Ewers, R. M., and Metzger, J. P. (2012). Land-use and land-cover change in Atlantic Forest landscapes. Forest Ecol. Manage. 15, 80–89. doi: 10.1016/j.foreco.2012.05.008
Major, J., Lehmann, J., Rondon, M., and Goodale, C. (2010). Fate of soil-applied black carbon: downward migration, leaching and soil respiration. Glob. Change Biol. 16, 1366–1379. doi: 10.1111/j.1365-2486.2009.02044.x
Mannino, A., and Harvey, H. R. (2004). Black carbon in estuarine and coastal ocean dissolved organic matter. Limnol. Oceanogr. 49, 735–740. doi: 10.4319/lo.2004.49.3.0735
Martinelli, L. A., Camargo, P. B., Lara, L. B. L. S., Victoria, R. L., and Artaxo, P. (2002). Stable carbon and nitrogen isotopic composition of bulk aerosol particles in a C4 landscape of southeast Brazil. Atmos. Environ. 36, 2427–2432. doi: 10.1016/S1352-2310(01)00454-X
Oscar, J. (1985). Escravidão and Engenhos: Campos, São João da Barra, Macaé, e São Fidélis. Rio de Janeiro: Rio de Janeiro Achiame.
Ovalle, A. R. C., Silva, C. F., Rezende, C. E., Gatts, C. E. N., Suzuki, M. S., and Figueiredo, R. O. (2013). Long-term trends in hydrochemistry in the Paraíba do Sul River, southeastern Brazil. J. Hydrol. 481, 191–203. doi: 10.1016/j.jhydrol.2012.12.036
Preston, C. M., and Schmidt, M. W. I. (2006). Black (pyrogenic) carbon in boreal forests: a synthesis of current knowledge and uncertainties. Biogeosciences 3, 211–271. doi: 10.5194/bg-3-397-2006
Ribeiro, M. C., Metzger, J. P., Martensen, A. C., Ponzoni, F. J., and Hirota, M. M. (2009). The Brazilian Atlantic Forest: how much is left, and how the remaining forest distributed? Implications for conservation. Biol. Conserv. 142, 1141–1153. doi: 10.1016/j.biocon.2009.02.021
Riedel, T., Zark, M., Vähätalo, A. V., Niggemann, J., Spencer, R. G. M., Hernes, P. J., et al. (2016). Molecular signatures of biogeochemical transformations in dissolved organic matter from ten World Rivers. Front. Earth Sci. 4:85. doi: 10.3389/feart.2016.00085
Santín, C., Doerr, S. H., Kane, E. S., Masiello, C. A., Ohlson, M., Rosa, J. M., et al. (2016). Towards a global assessment of pyrogenic carbon from vegetation fires. Glob. Change Biol. 22, 76–91. doi: 10.1111/gcb.12985
Schneider, M. P. W., Hilf, M., Vogt, U. F., and Schmidt, M. W. I. (2010). The benzene polycarboxylic acid (BPCA) pattern of wood pyrolyzed between 200 C° and 1000 C°. Organ. Geochem. 41, 1082–1088. doi: 10.1016/j.orggeochem.2010.07.001
Seidel, M., Yager, P. L., Ward, N. D., Carpenter, E. J., Gomes, H. R., Kruske, A. L., et al. (2015). Molecular-level changes of dissolved organic matter along the Amazon River-to-ocean continuum. Mar. Chem. 177, 218–231. doi: 10.1016/j.marchem.2015.06.019
Souza, T. A., Godoy, J. M., Godoy, M. L., Moreira, I., Carvalho, Z. L., Salomão, M. S., et al. (2010). Use of multitracers of the study of water mixing in the Paraíba do Sul River estuary. J. Environ. Radioact. 101, 564–570. doi: 10.1016/j.jenvrad.2009.11.001
Spencer, R. G. M., Stubbins, A., Hernes, P. J., Baker, A., Mopper, K., Aufdenkampe, A. K., et al. (2009). Photochemical degradation of dissolved organic matter and dissolved lignin phenols from the Congo River. J. Geophys. Res. 114:G03010. doi: 10.1029/2009jg000968
Stubbins, A., Hubbard, V., Uher, G., Law, C. S., Upstill-Goddard, R. C., et al. (2008). Relating carbon monoxide photoproduction to dissolved organic matter functionality. Environ. Sci. Technol. 42, 3271–3276. doi: 10.1021/es703014q
Stubbins, A., Niggemann, J., and Dittmar, T. (2012). Photo-lability of deep ocean dissolved black carbon. Biogeosciences 9, 1661–1670. doi: 10.5194/bg-9-1661-2012
Stubbins, A., Spencer, R. G. M., Mann, P. J., Holmes, R. M., McClelland, J. W., Niggemann, J., et al. (2015). Utilizing colored dissolved organic matter to derive dissolved black carbon export by Artic Rivers. Front. Earth Sci. 3:63. doi: 10.3389/feart.2015.00063
Warren, D. (1995). With Broadax and Firebrand. The Destruction of the Brazilian Atlantic forest. California: University of California Press.
Keywords: headwaters-to-ocean, black carbon, dissolved black carbon, dissolved organic carbon, Paraíba do Sul River
Citation: Marques JSJ, Dittmar T, Niggemann J, Almeida MG, Gomez-Saez GV and Rezende CE (2017) Dissolved Black Carbon in the Headwaters-to-Ocean Continuum of Paraíba Do Sul River, Brazil. Front. Earth Sci. 5:11. doi: 10.3389/feart.2017.00011
Received: 12 November 2016; Accepted: 31 January 2017;
Published: 27 February 2017.
Edited by:
Samuel Abiven, University of Zurich, SwitzerlandReviewed by:
Philippa Louise Ascough, Scottish Universities Environmental Research Centre, UKAlysha Coppola, University of Zurich, Switzerland
Copyright © 2017 Marques, Dittmar, Niggemann, Almeida, Gomez-Saez and Rezende. This is an open-access article distributed under the terms of the Creative Commons Attribution License (CC BY). The use, distribution or reproduction in other forums is permitted, provided the original author(s) or licensor are credited and that the original publication in this journal is cited, in accordance with accepted academic practice. No use, distribution or reproduction is permitted which does not comply with these terms.
*Correspondence: Jomar S. J. Marques, am9tYXIudWVuZkBnbWFpbC5jb20=