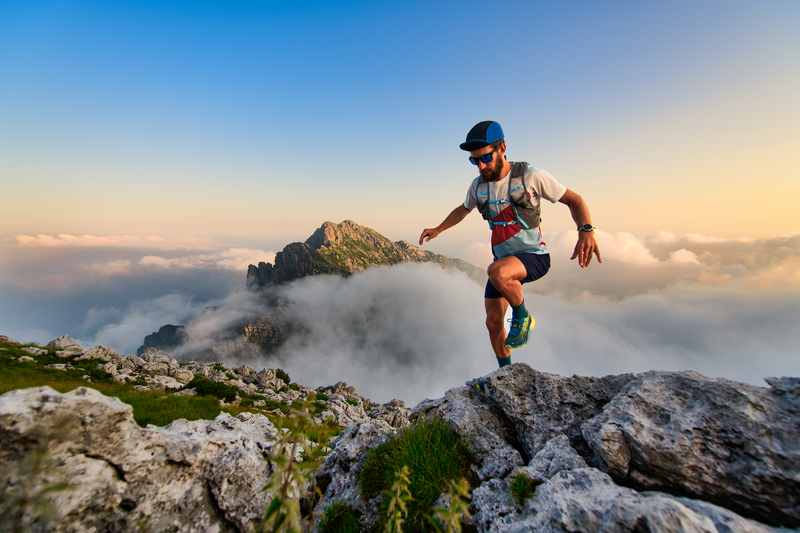
95% of researchers rate our articles as excellent or good
Learn more about the work of our research integrity team to safeguard the quality of each article we publish.
Find out more
ORIGINAL RESEARCH article
Front. Earth Sci. , 06 January 2016
Sec. Biogeoscience
Volume 3 - 2015 | https://doi.org/10.3389/feart.2015.00089
This article is part of the Research Topic Tropical forest ecosystem responses to increasing nutrient availability View all 10 articles
Tropical ecosystems have an important role in global change scenarios, in part because they serve as a large terrestrial carbon pool. Carbon protection is mediated by soil aggregation processes, whereby biotic and abiotic factors influence the formation and stability of aggregates. Nutrient additions may affect soil structure indirectly by simultaneous shifts in biotic factors, mainly roots, and fungal hyphae, but also via impacts on abiotic soil properties. Here, we tested the hypothesis that soil aggregation will be affected by nutrient additions primarily via changes in arbuscular mycorrhizal fungal (AMF) hyphae and root length in a pristine tropical forest system. Therefore, the percentage of water-stable macroaggregates (> 250 μm) (WSA) and the soil mean weight diameter (MWD) was analyzed, as well as nutrient contents, pH, root length, and AMF abundance. Phosphorus additions significantly increased the amount of WSA, which was consistent across two different sampling times. Despite a positive effect of phosphorus additions on extra-radical AMF biomass, no relationship between WSA and extra-radical AMF nor roots was revealed by regression analyses, contrary to the proposed hypothesis. These findings emphasize the importance of analyzing soil structure in understudied tropical systems, since it might be affected by increasing nutrient deposition expected in the future.
Soils represent a complex system due to the interactions of roots, fungi, fauna, and bacteria embedded within the soil structural environment. The hierarchical structure of micro- and macroaggregates in soils provides microhabitats, but also is important for gas exchange and water infiltration, protection against erosion, biogeochemical cycling, and especially the protection of soil organic carbon (C) (Six et al., 2000a; Jastrow et al., 2007). Soils represent one of the largest terrestrial C pools, which is of particular interest in the light of future global change scenarios (Kimble et al., 1990; Batjes, 1996). Tropical systems, in particular, play an important role as C sink, since tropical forests hold an estimated 20% of the world's terrestrial vegetation and soil C pool (Jobbagy and Jackson, 2000; Jimenez and Lal, 2006). However, tropical forests are highly endangered by deforestation, land use change, fertilization but also increased nutrient deposition by anthropogenic activities (Gullison et al., 2007; Galloway et al., 2008; Mahowald et al., 2008). Increases in nutrient deposition have been shown to affect plant diversity, ecosystem productivity, soil community composition as well as nutrient cycling (Treseder, 2008; Bobbink et al., 2010; Isbell et al., 2013; Wilcke et al., 2013b; Camenzind et al., 2014), and thus also directly or indirectly may have an impact on soil aggregation processes.
Studies analyzing the impact of nutrient additions on soil aggregation traditionally strongly focus on agricultural systems (e.g., Wang et al., 2011; Guo et al., 2012; Ortas and Lal, 2012). By contrast, to our knowledge only very few experiments have been conducted in natural systems, covering a limited range of ecosystem types like temperate grasslands (Van Groeningen et al., 2002; Wilson et al., 2009) or dry tropical savanna (Tripathi et al., 2008). The majority of these studies report positive effects of nutrient additions on soil aggregation, often accompanied by increases in root and arbuscular mycorrhizal fungal (AMF) hyphal abundance (Latif et al., 1992; Nobrega et al., 2001; Wilson et al., 2009; Alguacil et al., 2010). The latter are important factors in the process of aggregate formation, though abiotic factors likewise may play an important role: Fertilization studies focusing on abiotic soil parameters also demonstrated interrelations with pH and the proportion of exchangeable cations and electrostatic binding agents (Denef et al., 2002; Graham et al., 2002).
Generally, the formation of soil structure is a complex interaction of biotic and abiotic factors (Tisdall and Oades, 1982). A wide range of parameters involved in this process has been revealed—e.g., soil organic matter content, extracellular polysaccharides, humic substances, pH, and electrostatic binding agents, roots, fungal hyphae, macrofauna, microarthropods, or microbial biomass (Jastrow and Miller, 1991; Denef et al., 2002; Six et al., 2004)—though the relative importance of respective factors depends on soil type and study system (Six et al., 2000b; Denef et al., 2002; Denef and Six, 2005). Especially in highly weathered and acidic tropical soils pH and related Fe and Al contents, which affect electrostatic interactions and mineral-mineral bonding capacities, play an important role (Denef et al., 2002; Six et al., 2002; Denef and Six, 2005). However, across biomes, roots and fungal hyphae, especially mycorrhizal hyphae, have been shown to be one of the main factors involved in macroaggregate formation (Miller and Jastrow, 1990; Jastrow et al., 1998; Rillig and Mummey, 2006). Roots may indirectly affect aggregate stability by impacts on soil water regimes, support of soil microbial communities and dead root decomposition, but also directly via physical enmeshment of soil particles and the exudation of organic cementing agents (Angers and Caron, 1998; Hallett et al., 2009). Likewise, mycorrhizal fungal hyphae have been shown to have effects either indirectly, via influences on soil microbial communities, plant growth and root architecture as well as rapid hyphal turnover as C source, but also directly by physical hyphal enmeshment and the secretion of extracellular compounds (Rillig et al., 2002; Leifheit et al., 2014b), even though the precise mechanisms remain unknown.
Beside their predominant role in the formation of soil macroaggregates, AMF and roots may indeed be an important factor mediating changes in soil structure in the context of increased nutrient deposition by anthropogenic activities, since their abundance is affected by changes in nutrient availability. The main role of AMF—characterized as obligate biotrophic plant symbionts associated with more than 80% of land plants (Wang and Qiu, 2006)—represents improved nutrient uptake for the plant in exchange for photosynthetic C (Smith and Read, 2008). Thus, due to their involvement in nutrient uptake, roots and AMF can be affected by nutrient additions as predicted by the functional equilibrium model (Ericsson, 1995; Johnson, 2010): Under the assumption that plants invest more into structures associated with the acquisition of the most limiting resource, the release of soil nutrient deficiency by nutrient additions will result in an allocation shift toward aboveground structures. Therefore, a net negative effect of nutrient additions on root and AMF abundance is predicted, as has been shown in several experimental approaches mainly conducted in temperate areas (reviewed in Treseder, 2004). However, a large variability of possible outcomes was found also in response to nitrogen (N) vs. phosphorus (P) additions, mainly depending on the primary soil nutrient status (Treseder and Allen, 2002). In this context, the frequent observation of positive responses in AMF abundance is mainly explained by a primary nutrient limitation of the fungus itself (Bolan et al., 1984; Olsson et al., 1997; Johnson et al., 2003).
In this study, we aimed at expanding the knowledge on soil aggregation in a so far understudied biome, the pristine tropical forest. Effects of N and P additions on soil aggregation (measured as percentage of water-stable macroaggregates (WSA) and mean weight diameter (MWD) determined by dry-sieving) were analyzed in relation to biotic factors, namely root and AMF abundance, as well as abiotic factors in a tropical premontane forest located in southern Ecuador (Bendix et al., 2013). This study is part of a multidisciplinary nutrient manipulation experiment (NUMEX), which investigates the effects of moderate nutrient additions on this diverse and fragile ecosystem, in the context of increased nutrient depositions expected in the future (Phoenix et al., 2006; Mahowald et al., 2008; Wilcke et al., 2013a). Detailed responses in intra- and extra-radical AMF abundance as well as root length to N and P additions across an elevational transect in this area have been analyzed previously, also including amongst others this study site (own unpublished data). However, interrelations with other soil processes have not been evaluated. Here, we tested the hypothesis that soil aggregation will be affected by nutrient additions via indirect effects of changes in AMF (especially the extraradical part) and root abundance. Additionally, changes in basic soil properties were analyzed to include other potential influencing factors.
The study area is part of the Podocarpus National Park in the Cordillera Real, the eastern range of the South Ecuadorian Andes (Beck et al., 2008; Bendix et al., 2013). Plots are located in the Bombuscaro Area (4°11′S, 78°96′W) at 990–1100 m a.s.l. close to the city of Zamora, in the province of Zamora-Chinchipe. This area is part of the “Tropical Andes” hotspot of biodiversity (Myers et al., 2000) characterized by its outstanding number of plant species (Homeier et al., 2008) as well as of other organism groups, e.g., birds (Orme et al., 2005) and moths (Brehm et al., 2005). The forest is characterized as evergreen premontane rainforest (Homeier et al., 2008) with a mean annual precipitation of 2230 mm year−1 and a mean annual temperature of 19.4°C without pronounced seasonality (Moser et al., 2007). The rainforest at this altitude gets up to 40 m high, with common tree families of Fabaceae, Melastomataceae, Moraceae, Myristicaceae, Rubiaceae, and Sapotaceae (Homeier et al., 2013). The soil is characterized as Dystric Alumic Acrisol developed over deeply-weathered granitic rock (Graefe et al., 2008; Martinson et al., 2013). At this elevational site no organic layer is formed on top of mineral soil and soil texture is described as sandy loam. Furthermore, soils are characterized by a pH of 4.6, C/N ratios of 9.3 (± 0.4) (total C-values of 6.0 (± 0.1) mg g−1) and bulk densities of 0.84 (± 0.1) g cm−3 (Martinson et al., 2013).
Within the pristine forest a nutrient manipulation experiment (NUMEX) was set up, adding N and P in a two-factor randomized block design (Homeier et al., 2012). Nutrient additions started in 2008, with relatively low nutrient applications of 50 kg ha−1 year−1 of N (as urea) and 10 kg ha−1 year−1 P (as NaH2PO4·H2O) split into two applications per year. Four blocks were set up, each including one 20 × 20 m plot per treatment (control (Ctr), N, P, and NP). Additionally, within the plots six subplots of 2 × 2 m were randomly installed to facilitate coordinated sampling schemes.
In May 2010 a preliminary survey on soil structure was conducted, based on composite samples taken from each plot. For this purpose samples of the upper 5 cm of soil were taken from three randomly chosen subplots with a soil corer (Ø 5 cm), litter was removed. Samples from each plot were mixed, resulting in an overall amount of 16 samples—four per treatment.
In February 2013 again soil samples were taken from each plot, this time keeping samples originating from three different subplots separate to increase resolution and account for the strong spatial variability, resulting in an overall amount of 48 samples. Soil was sampled from the upper 15 cm of soil with a soil corer (Ø 5 cm), litter was removed.
Soil samples were immediately dried at 40°C, transferred to the Freie Universität Berlin and stored at room temperature.
The percentage of water-stable macroaggregates (WSA) was determined using an Eijkelkamp wet-sieving apparatus (Eijkelkamp Agrisearch Equipment, Giesbeek, the Netherlands) following the descriptions developed by Kemper and Rosenau (1986). Five grams of dry soil (10.0 g were used in the case of soil samples collected in May 2010) were rewetted by capillary action—after thorough examination the insertion of 5.0 g (carefully taken to represent an adequate subsample of the soil core) turned out to give better results due to the relatively small sieve sizes. After 5 min of sieving, the amount of water-stable macroaggregates (> 250 μm) was determined based on soil material kept on the 250 μm mesh—after an additional correction for coarse matter (Leifheit et al., 2014a). Additionally, the mean weight diameter (MWD) was calculated based on soil dry-sieving, dividing soil structure in its different size classes using a series of stacked sieves (2 mm, 1 mm, 250 μm, 53 μm) (Kemper and Rosenau, 1986; Barto et al., 2010). The resulting MWD represents the sum of proportions of soil material kept on every sieve size class, multiplied by the corresponding average size class (the highest average size class was estimated as 3.5 mm). The following formula was used:
where D is the mean diameter of each size fraction (in mm), W the proportion of total soil weight occurring in this fraction and n the number of size fractions.
For root length analyses, we used soil samples taken at the same time and adjacent to soil cores determined for AMF and soil structure analyses. These samples were transferred to University of Göttingen and stored at 4°C. For root length analyses, samples were soaked in water and adhering soil material was removed using a 0.25 mm sieve. Live fine roots were separated under the stereomicroscope based on color, root elasticity, and the degree of cohesion of cortex, periderm and stele (Persson, 1978; Leuschner et al., 2001). Root length (of live roots) was analyzed using WinRhizo (version 2007, Regent Instrument Inc., Quebec, Canada).
The percentage of AMF root colonization was quantified based on roots stained with 0.05% Trypan Blue by a modified staining protocol (Phillips and Hayman, 1970; Camenzind and Rillig, 2013). Roots were extracted with tweezers from soil and a standardized subset of roots was stained (approximately 30 root pieces of 1–2 cm). AMF root colonization was counted at 200x magnification under a stereomicroscope using the magnified intersections method by McGonigle et al. (1990). Besides the percentage of AMF root colonization, based on root length analyses a value for total AMF colonized root length was calculated.
Hyphae were extracted from 8.0 g of soil based on a modified aqueous filtration extraction method (Bardgett, 1991). Sodium hexametaphosphate solution (35 g L−1) was added to rewetted soil to detach hyphae from soil material for 1 h. Soil material was decanted on a 20 μm mesh (this mesh size was proven to avoid hyphal loss (Camenzind and Rillig, 2013) and transferred to 200 ml Erlenmeyer flasks. A defined amount of supernatant was mounted on a filter paper (pore size < 0.45 μm) by vacuum filtration and stained with 0.05% Trypan Blue. Hyphal length was counted with a grid-line intersect method at 200x magnification under a stereomicroscope (Rillig et al., 1999). AMF hyphae were defined as non-regularly septate hyphae with characteristic unilateral angular projections, that are stained dark- to light-blue by Trypan Blue (Mosse, 1959); all other hyphae were categorized as non-AMF hyphae.
The neutral lipid fatty acid (NLFA) 16:1ω5 has been shown to be a good signature of extra-radical AMF (Olsson et al., 1997; Olsson, 1999; Olsson and Johansen, 2000). Therefore, we extracted neutral lipid fatty acids from 5.0 g of soil following the protocol of van Aarle and Olsson (2003). Neutral lipids were purified from other lipids by silica column fractionation (Bond Elut, Varian Inc., Palo Alto, CA, USA) with chloroform as carrier, and subjected to a mild alkaline methanolysis. The abundance of NLFA 16:1ω5 was quantified on a gas chromatograph with a flame ionization detector and a 50 m HP5 capillary column, as described by Frostegård et al. (1993).
Total soil C and N contents were determined on milled soil with an Elemental Analyzer (EuroEA, HekaTech, Germany).
Total concentrations of P, Ca, Al, Fe, K, Mg, and Zn as well as soil pH were analyzed on separate soil samples taken in February 2013 (important elements which were included in this study were preselected by principal component analysis, selecting for ecologically relevant variables and excluding collinear predictors (Caruso et al., 2012; Camenzind et al., 2014). Samples were obtained from all six subplots (taken with a soil corer of 3.5 cm diameter), pooled separately for each plot and transferred to University of Tübingen and Freie Universität Berlin for further analyses. Here, the soil material was dried at 40°C. Element concentrations were determined based on milled soil using a microwave (Start 1500, MLS, Germany) pressure digestion with HNO3 and H2O2 as digestion agents. Base elements were determined with an Element Analyzer (ICP-OES 5300 DV, Perkin Elmer, Germany). Soil pH was analyzed in a 1:5 v:v suspension of soil and deionized water.
All statistical analyses were conducted in R version 3.1.2 (R Core Team, 2014). In order to account for the underlying block design, linear mixed-effects models were applied including Block and Plot as random effects. The function lme() from the package “nlme” was used (Pinheiro et al., 2014), fitting the model by maximizing the restricted log-likelihood (Zuur et al., 2009). All models were tested for the underlying assumptions of normality and homogeneity. In case these were not met, data were log, or square root transformed. Effects of nutrient additions on WSA, MWD and measured biotic and abiotic factors were analyzed by two-way linear mixed effects models, with N and P as fixed factors. For graphical illustrations, one-way analyses were included to assess single treatment effects in comparison to control plots. Linear correlations among WSA (or MWD) and respective biotic and abiotic predictor variables were determined using linear mixed-effects model. In case of certain abiotic variables being only present at plot level, the arithmetic mean of WSA (and MWD) per plot was calculated and used for correlation analyses. In order to account for the simultaneous impact of interacting variables on soil aggregation, multiple linear regression analyses were conducted. Here, only biotic variables, which were all measured at the same scale (subplot level), were included in the analyses. To select for the most important variables, stepwise model selection by backward elimination was performed based on the Akaike's Information Criterion (AIC). Regression analyses again were based on linear mixed effects models in order to include Block and Plot as random effects.
The amount of WSA was significantly affected by P additions in samples from 2010 and 2013 (Figure 1, Table 1). In 2010 effects were strongest in the NP treatment (average increase of 13.1%), whereas in 2013 both, P and NP additions similarly affected the amount of WSA (average increase of 4.1 and 3.9%, respectively) (Figure 1). Effect size clearly differed among different sampling dates, which seems to be driven by differences among control plots: In 2010 average WSA in control plots was 82.3 ± 3.3%, whereas in 2013 it was 90.9 ± 1.4%. However, variability was much higher in soil samples collected in 2010 (Figure 1).
Figure 1. Responses of the amount of water-stable macroaggregates as well as soil mean weight diameter to N and P additions in a tropical premontane forest soil. Bars represent mean values, error bars the respective standard error. Asterisks indicate significant differences compared to the control treatment (one-way linear mixed-effects model; P < 0.05).
Table 1. Responses of soil aggregation as well as biotic and abiotic soil factors to N and P additions, as well as the interaction between N and P additions (N:P) (two-way linear mixed-effects models, F-values (P-values) are presented).
MWD was on average 2.34 ± 0.1 mm (only control samples included), though these values were not coarse matter corrected. MWD was not significantly affected by nutrient additions, however, in both P treatments there was a positive trend (Figure 1).
AMF clearly represented the dominant mycorrhizal type in this system, though colonization rates were comparably low, with an average of 25 (± 4.8)%. AM hyphal length was on average 12.4 (± 1.6) m cm−3 soil−1 and the amount of AMF biomarker NLFA 16:1ω5 was 2.08 (± 0.17) nmol cm−3 soil−1. AMF intra- and extra-radical abundance was in general positively affected by P additions (Table 1). The amount of the AMF biomarker NLFA 16:1ω5 was significantly positively affected by P additions [average amount in the P and NP treatment, respectively: 2.8 (± 0.2) and 2.3 (± 0.2) nmol cm−3 soil−1], but also the percentage of AMF root colonization showed a marginally significant positive response [average amount in the P and NP treatment, respectively: 29.0 (± 4) and 34.3 (± 5)%]. By contrast, N additions exerted no or even weak negative effects, as observed in the case of AMF hyphal length (Table 1). Root length was not affected by nutrient additions [average root length in the control treatment: 0.76 (± 0.16) cm cm−3 soil−1].
The total amount of phosphorus in the soil was positively affected by P additions, whereas none of the other measured abiotic parameters showed a response (Table 1).
In order to find correlations of WSA (and MWD) with biotic and abiotic factors simple as well as multiple regression analyses were conducted. As indicated by simple linear regression, none of the biotic factors was significantly related to the amount of WSA (Table 2), and also not to MWD (data not shown). Furthermore, stepwise multiple regression analyses based on AIC model selection revealed no biotic factor correlated with the amount of WSA, with the lowest AIC obtained by the null model. However, concerning abiotic factors, regression analyses revealed a significantly negative correlation of WSA and C:N ratio, driven by the positive correlation of WSA and total N values, since total C was only marginally significantly correlated with WSA (Table 2). Furthermore, total amounts of P also showed a marginally significant positive correlation with WSA (Table 2).
Table 2. Results of simple linear regression analyses of biotic and abiotic predictor variables with the amount of water-stable macroaggregates (based on linear mixed-effects models including Block and Plot as random effects).
In summary, in this pristine tropical premontane forest P additions increased the amount of WSA in soil, whereas N additions alone did not exert an effect. Concomitant analyses of mainly biotic, but also some abiotic factors did not reveal a correlation with biotic factors, but only a negative relation of WSA and soil C:N ratios as well as a correlation with treatment associated increases in total amounts of P in the soil. Furthermore, the increase in WSA was not reflected in an increase in MWD, which potentially indicates that these changes do not solely affect the number of macroaggregates, but rather their stability.
In general, the increase in WSA following nutrient additions is in line with most findings from previous fertilization studies. As reported here, P additions have been shown to increase soil aggregation (Nobrega et al., 2001; Gil et al., 2009; Alguacil et al., 2010; Ortas and Lal, 2012), but also N additions (Latif et al., 1992; Tripathi et al., 2008; Wilson et al., 2009; Guo et al., 2012) as well as the application of multi-component fertilizers (Denef et al., 2002; Hati et al., 2006; Huo et al., 2008). As proposed here, many studies focused on the indirect impact of AMF hyphal length and root abundance in this context. For example, irrespective of the study system Wilson et al. (2009; natural field experiment—temperate grassland), Alguacil et al. (2010; agricultural field experiment—tropical savanna) and Nobrega et al. (2001; greenhouse experiment—tropical Oxisol) explained the observed increase in soil aggregation by the concurrent positive effect of nutrient additions on AMF abundance. Other studies related the observed changes in soil aggregation to root abundance (Latif et al., 1992; Jung et al., 2011) or both (Nobrega et al., 2001). However, here we did not find any direct correlation of either AMF intra- or extra-radical abundance or root length with the amount of WSA. Though the amount of AMF biomarker NLFA 16:1ω5 was significantly increased by P additions (also the percentage of AMF root colonization was marginally significantly affected), we did not detect a linear correlation of these parameters, also confirmed by stepwise multiple linear regression analyses. This lack of direct correlation corresponds to the result that NLFA 16:1ω5 was only affected by single P additions (statistics not presented), in contrast to the positive effect on WSA which was strongest in the NP treatment. Nevertheless, this parallel increase of NLFA 16:1ω5, intra-radical AMF abundance and WSA following P additions might point toward a partial involvement of AMF in soil aggregation in this system, potentially depending on additional factors (Denef and Six, 2005).
Apart from its impact on soil structure, the overall weak responses to N and P additions of AMF and root abundance is rather unexpected (Treseder, 2004; Johnson, 2010), based on a comparison with previous studies (Gower and Vitousek, 1989; Treseder, 2004; Wright et al., 2011). In the case of AMF, the reported increase in extraradical AMF abundance in response to P additions is rather unexpected considering the theory of reduced plant investment toward mycorrhizal structures following nutrient applications (Treseder, 2004; Johnson, 2010). However, there have been several previous reports on increased abundance following P additions, which is mainly discussed in the light of primary nutrient limitation of the fungus itself (Bolan et al., 1984; Olsson et al., 1997; Treseder and Allen, 2002; own unpublished data). Nevertheless, the overall weak response of “nutrient uptake structures” may be related to rather low nutrient inputs compared to other fertilization experiments (Treseder and Vitousek, 2001; Johnson et al., 2003; Wright et al., 2011). Additionally, the abundance of AMF was relatively low with average root colonization values of 25%, potentially indicating a low AMF dependency of plants in this system (Powell et al., 2009; Reinhart et al., 2012).
Though we did not detect (treatment related) correlating factors clearly explaining the observed increase in soil aggregation, the repeated finding of these patterns at different sampling times substantiates the reported treatment effects. Differences in the strength of effect sizes among sampling times might be associated with a strong initial response attenuating in the long-term (Comins and McMurtrie, 1993; Norby et al., 2007), though this may only partly explain differences among control plots. Regarding the experimental design, lower sample sizes in 2010 may have resulted in stronger random deviations causing high effect sizes (Barto and Rillig, 2012). Additionally, different conditions among sampling times may have directly or indirectly affected the amount of WSA in the soil, e.g., regarding rainfall events: February to May 2010 represented considerably drier months than the comparative period in 2013 (Thorsten Peters, personal communication). The rather low effect size in 2013 might be another reason for the inability to detect direct causal agents responsible for the increase in WSA, since also smaller changes may have caused the observed deviations. Nevertheless, in 2010 similarly no correlating factors have been detected despite the inclusion of several biotic and abiotic measurements (the same parameters as presented here were tested; data not shown).
Soil aggregation processes involve complex interactions among biotic and abiotic agents (Jastrow et al., 1998; Six et al., 2004; Barto et al., 2010). Beside the large evidence for the involvement of hyphae and roots in aggregate formation (Rillig and Mummey, 2006; Hallett et al., 2009), other studies previously likewise reported a lack of correlation among these factors (Degens et al., 1994; Eviner and Chapin, 2002; Piotrowski et al., 2004). Additionally, in tropical soils knowledge on soil aggregation processes is scarce, mainly based on agricultural systems (Feller and Beare, 1997; Cardoso and Kuyper, 2006). Within the available literature on tropical soils partly AMF (Nobrega et al., 2001) and also roots (Six et al., 2002) have been mentioned as influential factors. However, especially in highly weathered and acidic soils mainly pH and related Fe and Al contents, which affect electrostatic interactions and mineral-mineral bonding capacities, seem to have a strong impact (Denef et al., 2002; Six et al., 2002; Denef and Six, 2005). Nevertheless, in our study site different factors seem to play a more predominant role. The significantly positive correlation of WSA with total N values (though the direction of causality remains hidden) might indicate a predominant role of microbial activity in this system (Tripathi et al., 2008), whereas the marginally significant positive relation with total C points toward an involvement of other soil organic matter and C pools (Feller and Beare, 1997; Piccolo and Mbagwu, 1999; Six et al., 2000b). Macrofauna represents another potential candidate, e.g., springtails (Siddiky et al., 2012), but also direct and indirect interactions of plant and microbial communities might play a role (Gransee and Wittenmayer, 2000; Eviner and Chapin, 2002; Piotrowski et al., 2004). Finally, even though we did not detect effects at the level of abundances of biotic factors, it is possible that community composition, and with this community-level trait shifts, were responsible for the observed effects. For example, even though AMF abundance did not explain effects, community composition, likely to change in response to fertilization in this system (Camenzind et al., 2014), might explain it, if for example a shift toward AM fungal phylotypes with increased soil aggregation abilities, determined by species-level trait values (Lehmann and Rillig, 2015; Rillig et al., 2015), occurred.
In conclusion, our data show an increase in WSA following P additions, which is not related to changes in AMF and root abundances, factors which have been previously described as important aggregating agents. This inability to detect but also predict underlying mechanisms of soil aggregation highlights existing knowledge gaps on soil biotic and abiotic interactions in tropical forest soils. Soil structure represents a very important ecosystem component, especially in tropical forests which serve as large terrestrial C pool and are prone to erosion due to high rainfall events. In contrast to N deposition, potential future P depositions due to anthropogenic activities are still under debate: Mahowald et al. (2005, 2008) proposed increasing depositions at least in certain areas including the Andes, which was partly detected also in the study area (Jörg Bendix and Wolfgang Wilcke, personal communication). Furthermore, nutrient availability is affected by increasing land use intensity in the tropics. Therefore, consequences of P additions on soil aggregation processes, which are indicated by the presented data, might have an impact on other ecosystem processes, since soil structure represents such a crucial component of ecosystem functioning.
The authors declare that the research was conducted in the absence of any commercial or financial relationships that could be construed as a potential conflict of interest.
Financial support was provided by the Deutsche Forschungsgemeinschaft (DFG FOR816). We thank the Ministerio de Ambiente del Ecuador for the research permit and Nature and Culture International (NCI) in Loja for granting access to the San Francisco reserve and the research station.
Alguacil, M. D., Lozano, Z., Campoy, M. J., and Roldan, A. (2010). Phosphorus fertilisation management modifies the biodiversity of AM fungi in a tropical savanna forage system. Soil Biol. Biochem. 42, 1114–1122. doi: 10.1016/j.soilbio.2010.03.012
Angers, D. A., and Caron, J. (1998). Plant-induced changes in soil structure: processes and feedbacks. Biogeochemistry 42, 55–72. doi: 10.1023/a:1005944025343
Bardgett, R. D. (1991). The use of the membrane-filter technique for comparative measurements of hyphal lengths in different grassland sites. Agric, Ecosyst. Environ. 34, 115–119. doi: 10.1016/0167-8809(91)90099-j
Barto, E. K., Alt, F., Oelmann, Y., Wilcke, W., and Rillig, M. C. (2010). Contributions of biotic and abiotic factors to soil aggregation across a land use gradient. Soil Biol. Biochem. 42, 2316–2324. doi: 10.1016/j.soilbio.2010.09.008
Barto, E. K., and Rillig, M. C. (2012). Dissemination biases in ecology: effect sizes matter more than quality. Oikos 121, 228–235. doi: 10.1111/j.1600-0706.2011.19401.x
Batjes, N. H. (1996). Total carbon and nitrogen in the soils of the world. Eur. J. Soil Sci. 47, 151–163. doi: 10.1111/j.1365-2389.1996.tb01386.x
Beck, E., Bendix, J., Kottke, I., Makeschin, F., and Mosandl, R. (2008). Gradients in a Tropical Mountain Ecosystem of Ecuador. Berlin; Heidelberg; New York, NY: Springer
Bendix, J., Beck, E., Bräuning, A., Makeschin, F., Mosandl, R., Scheu, S., et al. (2013). Ecosystem Services, Biodiversity and Environmental Change in a Tropical Mountain Ecosystem of South Ecuador. Heidelberg; New York, NY; Dordrecht; London: Springer.
Bobbink, R., Hicks, K., Galloway, J., Spranger, T., Alkemade, R., Ashmore, M., et al. (2010). Global assessment of nitrogen deposition effects on terrestrial plant diversity: a synthesis. Ecol. Appl. 20, 30–59. doi: 10.1890/08-1140.1
Bolan, N. S., Robson, A. D., and Barrow, N. J. (1984). Increasing phosphorus supply can increase the infection of plant roots by vesicular arbuscular mycorrhizal fungi. Soil Biol. Biochem. 16, 419–420. doi: 10.1016/0038-0717(84)90043-9
Brehm, G., Pitkin, L., Hilt, N., and Fiedler, K. (2005). Montane Andean rain forests are a global diversity hotspot of geometrid moths. J. Biogeogr. 32, 1621–1627. doi: 10.1111/j.1365-2699.2005.01304.x
Camenzind, T., Hempel, S., Homeier, J., Horn, S., Velescu, A., Wilcke, W., et al. (2014). Nitrogen and phosphorus additions impact arbuscular mycorrhizal abundance and molecular diversity in a tropical montane forest. Glob. Change Biol. 20, 3646–3659. doi: 10.1111/gcb.12618
Camenzind, T., and Rillig, M. C. (2013). Extraradical arbuscular mycorrhizal fungal hyphae in an organic tropical montane forest soil. Soil Biol. Biochem. 64, 96–102. doi: 10.1016/j.soilbio.2013.04.011
Cardoso, I. M., and Kuyper, T. W. (2006). Mycorrhizas and tropical soil fertility. Agric. Ecosyst. Environ. 116, 72–84. doi: 10.1016/j.agee.2006.03.011
Caruso, T., Taormina, M., and Migliorini, M. (2012). Relative role of deterministic and stochastic determinants of soil animal community: a spatially explicit analysis of oribatid mites. J. Animal Ecol. 81, 214–221. doi: 10.1111/j.1365-2656.2011.01886.x
Comins, H. N., and McMurtrie, R. E. (1993). Long-term response of nutrient-limited forests to CO2 enrichment—equilibrium behavior of plant-soil models. Ecol. Appl. 3, 666–681. doi: 10.2307/1942099
Degens, B. P., Sparling, G. P., and Abbott, L. K. (1994). The contribution from hyphae, roots and organic-carbon constituents to the aggregation of a sandy loam under long-term clover-based and grass pastures. Eur. J. Soil Sci. 45, 459–468. doi: 10.1111/j.1365-2389.1994.tb00531.x
Denef, K., and Six, J. (2005). Clay mineralogy determines the importance of biological versus abiotic processes for macroaggregate formation and stabilization. Eur. J. Soil Sci. 56, 469–479. doi: 10.1111/j.1365-2389.2004.00682.x
Denef, K., Six, J., Merckx, R., and Paustian, K. (2002). Short-term effects of biological and physical forces on aggregate formation in soils with different clay mineralogy. Plant Soil 246, 185–200. doi: 10.1023/a:1020668013524
Ericsson, T. (1995). Growth and shoot-root ratio of seedlings in relation to nutrient availability. Plant Soil 168, 205–214. doi: 10.1007/bf00029330
Eviner, V. T., and Chapin, F. S. (2002). The influence of plant species, fertilization and elevated CO2 on soil aggregate stability. Plant Soil 246, 211–219. doi: 10.1023/a:1020657107687
Feller, C., and Beare, M. H. (1997). Physical control of soil organic matter dynamics in the tropics. Geoderma 79, 69–116. doi: 10.1016/s0016-7061(97)00039-6
Frostegård, A., Tunlid, A., and Bååth, E. (1993). Phospholipid fatty-acid composition, biomass and activity of microbial communities from two soil types experimentally exposed to different heavy-metals. Appl. Environ. Microbiol. 59, 3605–3617.
Galloway, J. N., Townsend, A. R., Erisman, J. W., Bekunda, M., Cai, Z. C., Freney, J. R., et al. (2008). Transformation of the nitrogen cycle: recent trends, questions, and potential solutions. Science 320, 889–892. doi: 10.1126/science.1136674
Gil, S. V., Becker, A., Oddino, C., Zuza, M., Marinelli, A., and March, G. (2009). Field trial assessment of biological, chemical, and physical responses of soil to tillage intensity, fertilization, and grazing. Environ. Manage. 44, 378–386. doi: 10.1007/s00267-009-9319-3
Gower, S. T., and Vitousek, P. M. (1989). Effects of nutrient amendments on fine root biomass in a primary successional forest in Hawaii. Oecologia 81, 566–568. doi: 10.1007/bf00378970
Graefe, S., Hertel, D., and Leuschner, C. (2008). Fine root dynamics along a 2,000-m elevation transect in South Ecuadorian mountain rainforests. Plant Soil 313, 155–166. doi: 10.1007/s11104-008-9688-z
Graham, M. H., Haynes, R. J., and Meyer, J. H. (2002). Changes in soil chemistry and aggregate stability induced by fertilizer applications, burning and trash retention on a long-term sugarcane experiment in South Africa. Eur. J. Soil Sci. 53, 589–598. doi: 10.1046/j.1365-2389.2002.00472.x
Gransee, A., and Wittenmayer, L. (2000). Qualitative and quantitative analysis of water-soluble root exudates in relation to plant species and development. J. Plant Nutr. Soil Sci. 163, 381–385. doi: 10.1002/1522-2624(200008)163:4<381::aid-jpln381>3.0.co;2-7
Gullison, R. E., Frumhoff, P. C., Canadell, J. G., Field, C. B., Nepstad, D. C., Hayhoe, K., et al. (2007). Tropical forests and climate policy. Science 316, 985–986. doi: 10.1126/science.1136163
Guo, S. L., Wu, J. S., Coleman, K., Zhu, H. H., Li, Y., and Liu, W. Z. (2012). Soil organic carbon dynamics in a dryland cereal cropping system of the Loess Plateau under long-term nitrogen fertilizer applications. Plant Soil 353, 321–332. doi: 10.1007/s11104-011-1034-1
Hallett, P. D., Feeney, D. S., Bengough, A. G., Rillig, M. C., Scrimgeour, C. M., and Young, I. M. (2009). Disentangling the impact of AM fungi versus roots on soil structure and water transport. Plant Soil 314, 183–196. doi: 10.1007/s11104-008-9717-y
Hati, K. M., Mandal, K. G., Misra, A. K., Ghosh, P. K., and Bandyopadhyay, K. K. (2006). Effect of inorganic fertilizer and farmyard manure on soil physical properties, root distribution, and water-use efficiency of soybean in Vertisols of central India. Bioresour. Technol. 97, 2182–2188. doi: 10.1016/j.biortech.2005.09.033
Homeier, J., Hertel, D., Camenzind, T., Cumbicus, N. L., Maraun, M., Martinson, G. O., et al. (2012). Tropical andean forests are highly susceptible to nutrient inputs-rapid effects of experimental N and p addition to an ecuadorian montane forest. PLoS ONE 7:e47128. doi: 10.1371/journal.pone.0047128
Homeier, J., Leuschner, C., Bräuning, A., Cumbicus, N. L., Hertel, D., Matrinson, G. O., et al. (2013). “Effects of Nutrient Addition on the Productivity of Montane Forests and Implications for the Carbon Cycle,” in Ecosystem Services, Biodiversity and Environmental Change in a Tropical Mountain Ecosystem of South Ecuador, eds J. Bendix, E. Beck, A. Bräuning, F. Makeschin, R. Mosandl, S. Scheu et al. (Berlin; Heidelberg: Springer-Verlag), 315–329.
Homeier, J., Werner, F. A., Gradstein, S. R., Breckle, S. W., and Richter, M. (2008). “Potential vegetation and floristic composition of Andean forests in South Ecuador, with a focus on the RBSF,” in Ecological Studies, eds E. Beck, J. Bendix, I. Kottke, F. Makeschin and R. Mosandl (New York, NY: Springer), 87–100.
Huo, L., Wu, T. Y., Lin, H. M., Cao, S. Y., and Tang, W. X. (2008). Effects of long-term fertilization on water-stable aggregates in calcic kastanozem of Loess Plateau. Ying Yong Sheng Tai Xue Bao 19, 545–550.
Isbell, F., Reich, P. B., Tilman, D., Hobbie, S. E., Polasky, S., and Binder, S. (2013). Nutrient enrichment, biodiversity loss, and consequent declines in ecosystem productivity. Proc. Natl. Acad. Sci. U.S.A. 110, 11911–11916. doi: 10.1073/pnas.1310880110
Jastrow, J. D., Amonette, J. E., and Bailey, V. L. (2007). Mechanisms controlling soil carbon turnover and their potential application for enhancing carbon sequestration. Clim. Change 80, 5–23. doi: 10.1007/s10584-006-9178-3
Jastrow, J. D., and Miller, R. M. (1991). Methods for assessing the effects of biota on soil structure. Agric. Ecosyst. Environ. 34, 279–303. doi: 10.1016/0167-8809(91)90115-e
Jastrow, J. D., Miller, R. M., and Lussenhop, J. (1998). Contributions of interacting biological mechanisms to soil aggregate stabilization in restored prairie. Soil Biol. Biochem. 30, 905–916. doi: 10.1016/s0038-0717(97)00207-1
Jimenez, J. J., and Lal, R. (2006). Mechanisms of C sequestration in soils of Latin America. CRC Crit. Rev. Plant Sci. 25, 337–365. doi: 10.1080/0735268060094240
Jobbagy, E. G., and Jackson, R. B. (2000). The vertical distribution of soil organic carbon and its relation to climate and vegetation. Ecol. Appl. 10, 423–436. doi: 10.2307/2641104
Johnson, N. C. (2010). Resource stoichiometry elucidates the structure and function of arbuscular mycorrhizas across scales. New Phytol. 185, 631–647. doi: 10.1111/j.1469-8137.2009.03110.x
Johnson, N. C., Rowland, D. L., Corkidi, L., Egerton-Warburton, L. M., and Allen, E. B. (2003). Nitrogen enrichment alters mycorrhizal allocation at five mesic to semiarid grasslands. Ecology 84, 1895–1908. doi: 10.1890/0012-9658(2003)084[1895:neamaa]2.0.co;2
Jung, J. Y., Lal, R., Jastrow, J. D., and Tyler, D. D. (2011). Nitrogenous fertilizer effects on soil structural properties under switchgrass. Agric. Ecosyst. Environ. 141, 215–220. doi: 10.1016/j.agee.2011.01.016
Kemper, W., and Rosenau, R. (1986). “Aggregate stability and size distribution,” in Methods of Soil Analysis (Part I), ed A. Klute (Madison, WI: American Society of Agronomy), 425–442.
Kimble, J., Levine, E., and Stewart, B. (1990). Soil Management and Greenhouse Effect. Boca Raton, FL: CRC Press.
Latif, M. A., Mehuys, G. R., MacKenzie, A. F., Alli, I., and Faris, M. A. (1992). Effects of legumes on soil physical quality in a maize crop. Plant Soil 140, 15–23. doi: 10.1007/bf00012802
Lehmann, A., and Rillig, M. C. (2015). Understanding mechanisms of soil biota involvement in soil aggregation: a way forward with saprobic fungi. Soil Biol. Biochem. 88, 298–302. doi: 10.1016/j.soilbio.2015.06.006
Leifheit, E. F., Verbruggen, E., and Rillig, M. C. (2014a). Rotation of hyphal in-growth cores has no confounding effects on soil abiotic properties. Soil Biol. Biochem. 79, 78–80. doi: 10.1016/j.soilbio.2014.09.006
Leifheit, E. F., Veresoglou, S. D., Lehmann, A., Morris, E. K., and Rillig, M. C. (2014b). Multiple factors influence the role of arbuscular mycorrhizal fungi in soil aggregation-a meta-analysis. Plant Soil 374, 523–537. doi: 10.1007/s11104-013-1899-2
Leuschner, C., Hertel, D., Coners, H., and Buttner, V. (2001). Root competition between beech and oak: a hypothesis. Oecologia 126, 276–284. doi: 10.1007/s004420000507
Mahowald, N., Jickells, T. D., Baker, A. R., Artaxo, P., Benitez-Nelson, C. R., Bergametti, G., et al. (2008). Global distribution of atmospheric phosphorus sources, concentrations and deposition rates, and anthropogenic impacts. Glob. Biogeochem. Cycles 22:Gb4026. doi: 10.1029/2008gb003240
Mahowald, N. M., Artaxo, P., Baker, A. R., Jickells, T. D., Okin, G. S., Randerson, J. T., et al. (2005). Impacts of biomass burning emissions and land use change on Amazonian atmospheric phosphorus cycling and deposition. Glob. Biogeochem. Cycles 19:Gb4030. doi: 10.1029/2005gb002541
Martinson, G. O., Corre, M. D., and Veldkamp, E. (2013). Responses of nitrous oxide fluxes and soil nitrogen cycling to nutrient additions in montane forests along an elevation gradient in southern Ecuador. Biogeochemistry 112, 625–636. doi: 10.1007/s10533-012-9753-9
McGonigle, T. P., Miller, M. H., Evans, D. G., Fairchild, G. L., and Swan, J. A. (1990). A new method which gives an objective-measure of colonization of roots by vesicular arbuscular mycorrhizal fungi. New Phytol. 115, 495–501. doi: 10.1111/j.1469-8137.1990.tb00476.x
Miller, R. M., and Jastrow, J. D. (1990). Hierarchy of root and mycorrhizal fungal interactions with soil aggregation. Soil Biol. Biochem. 22, 579–584. doi: 10.1016/0038-0717(90)90001-g
Moser, G., Hertel, D., and Leuschner, C. (2007). Altitudinal change in LAI and stand leaf biomass in tropical montane forests: a transect shady in Ecuador and a pan-tropical meta-analysis. Ecosystems 10, 924–935. doi: 10.1007/s10021-007-9063-6
Mosse, B. (1959). Observations on the extramatrical mycelium of a vesicular-arbuscular endophyte. Trans. Br. Mycol. Soc. 42, 439–448.
Myers, N., Mittermeier, R. A., Mittermeier, C. G., da Fonseca, G. A., and Kent, J. (2000). Biodiversity hotspots for conservation priorities. Nature 403, 853–858. doi: 10.1038/35002501
Nobrega, J. C. A., De Lima, J. M., Curi, N., Siqueira, J. O., and Da Motta, P. E. F. (2001). Aggregate stability in two cropped and no-cropped Oxisols as affected by phosphate addition and mycorrhiza. Pesqui. Agropecu. Bras. 36, 1425–1435. doi: 10.1590/S0100-204X2001001100014
Norby, R., Rustad, L., Dukes, J., Ojima, D., Parton, W., Del Grosso, S., et al. (2007). “Ecosystem responses to warming and interacting global change factors,” in Terrestrial Ecosystems in a Changing World, eds J. Canadell, D. Pataki, and L. Pitelka (New York, NY: Springer Science & Business Media), 23–36.
Olsson, P. A. (1999). Signature fatty acids provide tools for determination of the distribution and interactions of mycorrhizal fungi in soil. FEMS Microbiol. Ecol. 29, 303–310. doi: 10.1111/j.1574-6941.1999.tb00621.x
Olsson, P. A., Baath, E., and Jakobsen, I. (1997). Phosphorus effects on the mycelium and storage structures of an arbuscular mycorrhizal fungus as studied in the soil and roots by analysis of fatty acid signatures. Appl. Environ. Microbiol. 63, 3531–3538.
Olsson, P. A., and Johansen, A. (2000). Lipid and fatty acid composition of hyphae and spores of arbuscular mycorrhizal fungi at different growth stages. Mycol. Res. 104, 429–434. doi: 10.1017/s0953756299001410
Orme, C. D. L., Davies, R. G., Burgess, M., Eigenbrod, F., Pickup, N., Olson, V. A., et al. (2005). Global hotspots of species richness are not congruent with endemism or threat. Nature 436, 1016–1019. doi: 10.1038/nature03850
Ortas, I., and Lal, R. (2012). Long-term phosphorus application impacts on aggregate-associated carbon and nitrogen sequestration in a vertisol in the mediterranean Turkey. Soil Sci. 177, 241–250. doi: 10.1097/SS.0b013e318245d11c
Persson, H. (1978). Root dynamics in a young scots pine stand in Central Sweden. Oikos 30, 508–519. doi: 10.2307/3543346
Phillips, J. M., and Hayman, D. S. (1970). Improved procedures for clearing roots and staining parasitic and vesicular-arbuscular mycorrhizal fungi for rapid assessment of infection. Trans. Br. Mycol. Soc. 55, 158–161.
Phoenix, G. K., Hicks, W. K., Cinderby, S., Kuylenstierna, J. C. I., Stock, W. D., Dentener, F. J., et al. (2006). Atmospheric nitrogen deposition in world biodiversity hotspots: the need for a greater global perspective in assessing N deposition impacts. Glob. Change Biol. 12, 470–476. doi: 10.1111/j.1365-2486.2006.01104.x
Piccolo, A., and Mbagwu, J. S. C. (1999). Role of hydrophobic components of soil organic matter in soil aggregate stability. Soil Sci. Soc. Am. J. 63, 1801–1810.
Pinheiro, J., Bates, D., Debroy, S., Sarkar, D., and R Core Team (2014). nlme: Linear and Nonlinear Mixed Effects Models. R package version 3.1-117. Available online at: http://CRAN.R-project.org/package=nlme
Piotrowski, J. S., Denich, T., Klironomos, J. N., Graham, J. M., and Rillig, M. C. (2004). The effects of arbuscular mycorrhizas on soil aggregation depend on the interaction between plant and fungal species. New Phytol. 164, 365–373. doi: 10.1111/j.1469-8137.2004.01181.x
Powell, J. R., Parrent, J. L., Hart, M. M., Klironomos, J. N., Rillig, M. C., and Maherali, H. (2009). Phylogenetic trait conservatism and the evolution of functional trade-offs in arbuscular mycorrhizal fungi. Proc. R. Soc. B Biol. Sci. 276, 4237–4245. doi: 10.1098/rspb.2009.1015
R Core Team (2014). R: A Language and Environment for Statistical Computing. Vienna: R Foundation for Statistical Computing.
Reinhart, K. O., Wilson, G. W., and Rinella, M. J. (2012). Predicting plant responses to mycorrhizae: integrating evolutionary history and plant traits. Ecol. Lett. 15, 689–695. doi: 10.1111/j.1461-0248.2012.01786.x
Rillig, M. C., Aguilar-Trigueros, C. A., Bergmann, J., Verbruggen, E., Veresoglou, S. D., and Lehmann, A. (2015). Plant root and mycorrhizal fungal traits for understanding soil aggregation. New Phytol. 205, 1385–1388. doi: 10.1111/nph.13045
Rillig, M. C., Field, C. B., and Allen, M. F. (1999). Soil biota responses to long-term atmospheric CO2 enrichment in two California annual grasslands. Oecologia 119, 572–577. doi: 10.1007/s004420050821
Rillig, M. C., and Mummey, D. L. (2006). Mycorrhizas and soil structure. New Phytol. 171, 41–53. doi: 10.1111/j.1469-8137.2006.01750.x
Rillig, M. C., Wright, S. F., and Eviner, V. T. (2002). The role of arbuscular mycorrhizal fungi and glomalin in soil aggregation: comparing effects of five plant species. Plant Soil 238, 325–333. doi: 10.1023/a:1014483303813
Siddiky, M. R. K., Schaller, J., Caruso, T., and Rillig, M. C. (2012). Arbuscular mycorrhizal fungi and collembola non-additively increase soil aggregation. Soil Biol. Biochem. 47, 93–99. doi: 10.1016/j.soilbio.2011.12.022
Six, J., Bossuyt, H., Degryze, S., and Denef, K. (2004). A history of research on the link between (micro)aggregates, soil biota, and soil organic matter dynamics. Soil Tillage Res. 79, 7–31. doi: 10.1016/j.still.2004.03.008
Six, J., Elliott, E. T., and Paustian, K. (2000a). Soil macroaggregate turnover and microaggregate formation: a mechanism for C sequestration under no-tillage agriculture. Soil Biol. Biochem. 32, 2099–2103. doi: 10.1016/s0038-0717(00)00179-6
Six, J., Elliott, E. T., and Paustian, K. (2000b). Soil structure and soil organic matter: II. A normalized stability index and the effect of mineralogy. Soil Sci. Soc. Am. J. 64, 1042–1049.
Six, J., Feller, C., Denef, K., Ogle, S. M., Sa, J. C. D., and Albrecht, A. (2002). Soil organic matter, biota and aggregation in temperate and tropical soils—Effects of no-tillage. Agronomie 22, 755–775. doi: 10.1051/agro:2002043
Tisdall, J. M., and Oades, J. M. (1982). Organic matter and water-stable aggregates in soil. J. Soil Sci. 33, 141–163.
Treseder, K. K. (2004). A meta-analysis of mycorrhizal responses to nitrogen, phosphorus, and atmospheric CO2 in field studies. New Phytol. 164, 347–355. doi: 10.1111/j.1469-8137.2004.01159.x
Treseder, K. K. (2008). Nitrogen additions and microbial biomass: a meta-analysis of ecosystem studies. Ecol. Lett. 11, 1111–1120. doi: 10.1111/j.1461-0248.2008.01230.x
Treseder, K. K., and Allen, M. F. (2002). Direct nitrogen and phosphorus limitation of arbuscular mycorrhizal fungi: a model and field test. New Phytol. 155, 507–515. doi: 10.1046/j.1469-8137.2002.00470.x
Treseder, K. K., and Vitousek, P. M. (2001). Effects of soil nutrient availability on investment in acquisition of N and P in Hawaiian rain forests. Ecology 82, 946–954. doi: 10.1890/0012-9658(2001)082[0946:eosnao]2.0.co;2
Tripathi, S. K., Kushwaha, C. P., and Singh, K. P. (2008). Tropical forest and savanna ecosystems show differential impact of N and P additions on soil organic matter and aggregate structure. Glob. Change Biol. 14, 2572–2581. doi: 10.1111/j.1365-2486.2008.01702.x
van Aarle, I. M., and Olsson, P. A. (2003). Fungal lipid accumulation and development of mycelial structures by two arbuscular mycorrhizal fungi. Appl. Environ. Microbiol. 69, 6762–6767. doi: 10.1128/AEM.69.11.6762-6767.2003
Van Groeningen, K. J., Harris, D., Horwath, W. R., Hartwig, U. A., and Van Kessel, C. (2002). Linking sequestration of C-13 and N-15 in aggregates in a pasture soil following 8 years of elevated atmospheric CO2. Glob. Change Biol. 8, 1094–1108. doi: 10.1046/j.1365-2486.2002.00527.x
Wang, B., and Qiu, Y. L. (2006). Phylogenetic distribution and evolution of mycorrhizas in land plants. Mycorrhiza 16, 299–363. doi: 10.1007/s00572-005-0033-6
Wang, W., Chen, W. C., Wang, K. R., Xie, X. L., Yin, C. M., and Chen, A. L. (2011). Effects of long-term fertilization on the distribution of carbon, nitrogen and phosphorus in water-stable aggregates in paddy soil. Agric. Sci. China 10, 1932–1940. doi: 10.1016/s1671-2927(11)60194-6
Wilcke, W., Leimer, S., Peters, T., Emck, P., Rollenbeck, R., Trachte, K., et al. (2013a). The nitrogen cycle of tropical montane forest in Ecuador turns inorganic under environmental change. Glob. Biogeochem. Cycles 27, 1194–1204. doi: 10.1002/2012gb004471
Wilcke, W., Leimer, S., and Valarezo, C. (2013b). “Environmental change: increased nitrogen availability and litterfall,” in Tropical Mountain Forest (TMF) Newsletter, ed E. Schwarz-Weig (Marburg: Laboratory for Climatology and Remote Sensing (LCRS), University of Marburg; DFG Research Unit), 816. doi: 10.5678/lcrs/for816.cit.1233
Wilson, G. W., Rice, C. W., Rillig, M. C., Springer, A., and Hartnett, D. C. (2009). Soil aggregation and carbon sequestration are tightly correlated with the abundance of arbuscular mycorrhizal fungi: results from long-term field experiments. Ecol. Lett. 12, 452–461. doi: 10.1111/j.1461-0248.2009.01303.x
Keywords: arbuscular mycorrhizal fungi, tropical forest, Ecuador, soil aggregation, fertilization, global change
Citation: Camenzind T, Papathanasiou HJ, Förster A, Dietrich K, Hertel D, Homeier J, Oelmann Y, Olsson PA, Suárez JP and Rillig MC (2016) Increases in Soil Aggregation Following Phosphorus Additions in a Tropical Premontane Forest are Not Driven by Root and Arbuscular Mycorrhizal Fungal Abundances. Front. Earth Sci. 3:89. doi: 10.3389/feart.2015.00089
Received: 25 August 2015; Accepted: 08 December 2015;
Published: 06 January 2016.
Edited by:
Samuel Abiven, University of Zurich, SwitzerlandReviewed by:
Safya Menasseri-Aubry, Agrocampus Ouest, FranceCopyright © 2016 Camenzind, Papathanasiou, Förster, Dietrich, Hertel, Homeier, Oelmann, Olsson, Suárez and Rillig. This is an open-access article distributed under the terms of the Creative Commons Attribution License (CC BY). The use, distribution or reproduction in other forums is permitted, provided the original author(s) or licensor are credited and that the original publication in this journal is cited, in accordance with accepted academic practice. No use, distribution or reproduction is permitted which does not comply with these terms.
*Correspondence: Tessa Camenzind, dGVzc2FjQHplZGF0LmZ1LWJlcmxpbi5kZQ==
Disclaimer: All claims expressed in this article are solely those of the authors and do not necessarily represent those of their affiliated organizations, or those of the publisher, the editors and the reviewers. Any product that may be evaluated in this article or claim that may be made by its manufacturer is not guaranteed or endorsed by the publisher.
Research integrity at Frontiers
Learn more about the work of our research integrity team to safeguard the quality of each article we publish.