- 1Aix Marseille Univ, CNRS, Minist Culture, LAMPEA, Aix-en-Provence, France
- 2CASEs Research Group, Department of Humanities, Universitat Pompeu Fabra, Barcelona, Spain
- 3Department of Anthropology, University of North Carolina, Wilmington, NC, United States
- 4School of Geography, Archaeology and Environmental Studies, University of the Witwatersrand, Johannesburg, South Africa
- 5Department of Archaeology, Simon Fraser University, Burnaby, BC, Canada
- 6ICREA - Passeig Lluís Companys 23, Barcelona, Spain
C4 crops such as sorghum (Sorghum bicolor) and finger millet (Eleusine coracana) have played a significant role in the economic livelihood in arid and semi-arid zones of tropical and sub-tropical Africa since prehistoric times. However, to date, our knowledge of their past management practices is limited. Stable isotope analysis of archaeobotanical remains has been recognized as a valuable tool for reconstructing past agricultural practices, e.g. water management, and fertilization. Nonetheless, our limited understanding of the isotopic variability of C4 plants calls for further research on modern plant before application to archaeobotanical remains. In this paper, we aim to enhance our understanding of modern C4 botanical remains' isotopic variability by analyzing sorghum and finger millet plants. These crops were cultivated according to traditional local practices and collected from ten villages located in the Konso Zone (South Ethiopia) and Tigray Regional State (North Ethiopia), where they are among the daily ingredients for food, and traditional alcoholic and non-alcoholic drinks. We analyzed carbon and nitrogen stable isotopes of seeds and biosilica content in chaff, as it has been suggested that a relationship can exist between silicon and C:N. Carbon isotope values show significant variability, positively correlated with altitude. By demonstrating the sensitivity of C4 grain carbon stable isotope to altitude variations, which are likely connected to water availability, this study offers invaluable insights for the accurate assessment of isotopic values derived from ancient C4 crops. The absence of significant correlations with δ15N suggests that nitrogen isotope values may be less effective for understanding environmental variations in this kind of context. This highlights the limitations of nitrogen isotope data for interpreting ancient agricultural practices and underscores the importance of relying more on carbon isotopes for insights related to environmental conditions and altitude. Furthermore, we confirm that the amount of assimilated carbon may depend also on the biosilica content, which is in turn modulated by environmental parameters such as water availability or soil silicon levels.
1 Introduction
Recent advances in isotope analysis of botanical remains are unraveling the complex relationships between plants and their environment through time. The analysis of stable isotopes in archaeobotanical remains, i.e. seeds, phytoliths, charcoal have proven to be useful to infer environmental and anthropogenic effects on growing conditions, preserving information about water sources, and nutrient cycles. Applications span from the reconstruction of paleoclimate, to crop growing conditions and past agricultural practices, extending to crop provenancing and ecological adaptation (Araus et al., 1997; Aguilera et al., 2008; Bogaard et al., 2013; Masi et al., 2013, 2014; Riehl et al., 2014; Styring et al., 2017; Varalli et al., 2023b).
In the framework of the ERC Starting Grant RAINDROPS, we are performing phytolith and stable isotopes analysis of botanical remains from different geographical areas and time periods for both methodological and archaeological advances (Varalli et al., 2023a; D'Agostini et al., 2024). Specifically, we combine experimentally controlled data of macro-remains (carbon and nitrogen isotopes on grains) with ethnographic evidence to create a methodological framework for the assessment of crop water availability. This approach has been developed for three of the major tropical and sub-tropical cereals, both in prehistory and today—finger millet (Eleusine coracana L. Gaertn.), sorghum (Sorghum bicolor L. Moench) and pearl millet [Cenchrus americanus L. Morrone, syn. Pennisetum glaucum (L.) R.Br.]—and is applied to selected key ethnographical settings, i.e. Konso, Tigray (Ethiopia), Karthoum State (Sudan), Sindh (Pakistan) as well as archaeological settings, i.e. Farafra (Egypt), Bando Qubo, Taloor Je Bhitt (Pakistan), Al Khiday, Mouweis (Sudan), Schroda, Mutaba (South Africa) (Varalli et al., 2024). The combination of stable isotope analysis of experimental and ethnographic reference material allows us to better understand past human-plant relationships as well as to contribute to the present debate of resilient ecosystems.
In this paper we present a pilot study focusing on stable isotope data obtained from modern grains of sorghum and finger millet cultivated at different altitudes in two Ethiopian regions. These crops were grown according to traditional local practices and collected from ten villages located in the Konso Zone (South Ethiopia) and Tigray Regional State (North Ethiopia), where they are among the staple ingredients used in the preparation of food, and traditional alcoholic and non-alcoholic drinks. By analyzing carbon and nitrogen stable isotopes in grains, our objective is to investigate the variability in isotopic values among plants cultivated under similar environmental conditions and agricultural practices but at different altitudes. To obtain reliable information about the growing conditions of the crops under study, we pair the analysis of the isotope composition of the grains with the analysis of the biosilica content in the chaff. According to the most recent literature (e.g. Liu et al., 2023), C:N ratio could influence biosilica that consequently mirrors the productive capacity of crops. Indeed, by analyzing biosilica content in chaff, we want to test the relationship between silicon and C:N. The results of this study offer invaluable insights for the accurate assessment of isotopic values derived from ancient C4 crops.
2 C4 plants and environmental adaptation
2.1 C4 photosynthesis and isotope discrimination
C4 photosynthesis is a highly efficient carbon fixation pathway that enhances photosynthetic performance under conditions of high light intensity, high temperatures, and limited water availability. Unlike the C3 pathway, where CO2 is fixed by the enzyme Ribulose-1,5-bisphosphate carboxylase/oxygenase (RuBisCo) in mesophyll cells, C4 plants utilize a distinct mechanism involving two types of cells to incorporate atmospheric CO2: mesophyll and bundle-sheath cells. Initially, CO2 is fixed into a four-carbon compound by the enzyme phosphoenolpyruvate (PEP) carboxylase in the mesophyll cells. This four-carbon compound is then transported to the bundle-sheath cells, where CO2 is released and refixed by RuBisCo, thereby entering the Calvin cycle. The carbon isotope fractionation between CO2 and the final photosynthetic product can be positive, negative, or zero. This variation depends on the ratio of intercellular to ambient CO2 partial pressure (pi/pa), temperature, and leakiness (ϕ) of the bundle sheath cells (Farquhar et al., 1989; Williams et al., 2001). The spatial separation of the initial CO2 fixation and the Calvin cycle minimizes photorespiration, a wasteful process that occurs when RuBisCo reacts with O2 instead of CO2. This adaptation provides C4 plants with a significant advantage in hot and arid environments, contributing to their dominance in many tropical and subtropical grasslands and savannas. As a consequence, C4 plants exhibit less carbon isotope discrimination compared to C3 plants, resulting in higher δ13C values, with an average of −28‰ in C3 plants and −12.5‰ in C4 plants (Cerling et al., 1998; Kohn, 2010). The effects of the environmental factors on carbon stable isotopes have been widely studied in C3 plants and it has been demonstrated that they can strongly affect the carbon isotopic values (Farquhar et al., 1982, 1989; Körner et al., 1991). The influence of the environmental factors on C4 plants is less clear and results are often contradictory. Even though some research attests that the influence of environmental factors on the 13C fractionation of C4 plants is negligible, more studies show that factors such as light intensity (Buchmann et al., 1996; Kromdijk et al., 2008; Ubierna et al., 2013), water availability (Buchmann et al., 1996; Ghannoum et al., 2002; Weiguo et al., 2005; Reid et al., 2018; Lightfoot et al., 2020; Sanborn et al., 2021), salinity (Bowman et al., 1989), latitude (An et al., 2015; Yang and Li, 2015), and altitude (Wang et al., 2008) affect C4 plants, although the relationship is not always linear (Swap et al., 2004; An et al., 2015). Indeed, one of the most debated topics is the relationship between δ13C values and water availability, encompassing studies on both precipitation and irrigation in controlled experiments. Some studies find positive correlation between rainfall regional gradients or cumulative annual precipitation and δ13C values (Schulze et al., 1996; Murphy and Bowman, 2009; An et al., 2015; Yang and Li, 2015; Reid et al., 2018), while others demonstrate negative correlations (Weiguo et al., 2005). In experimental cultivation where irrigation was controlled in greenhouse conditions or in screen houses, δ13C values were mostly positively correlated with water availability: this result has been attested in different C4 species such as Zea mays (Dercon et al., 2006), Pennisetum glaucum (Sanborn et al., 2021), Setaria italica (Lightfoot et al., 2020; Dong et al., 2022), while Panicum miliaceum is negatively correlated, even if not statistically significant (Dong et al., 2022). However, when Panicum miliaceum was sampled in nature and environmental factors were measured, δ13C was positively correlated with precipitation (Yang and Li, 2015). The relationship between δ13C and altitude is another controversial issue. Even though it seems that different photosynthetic pathways (C3 vs. C4) do not affect the relative importance of environmental contributions to the carbon isotope discrimination and gas exchange altitudinal variations (Wang et al., 2008), the effect of altitude on C4 plants is still little explored and the relationship between altitude and δ13C is unclear. For example, an analysis of Setaria viridis found a positive correlation between δ13C and altitude (Wang et al., 2008). In contrast, an analysis of Panicum miliaceum recorded a negative correlation, while no correlation was found for Setaria italica (Yang and Li, 2015).
2.2 Nitrogen uptake isotope discrimination
Nitrogen isotope ratios for non-nitrogen-fixing plants are dictated by sources including ammonium (NH4+) and nitrate (NO3–) in soil. The δ15N value of a plant depends on the proportion of these nitrogen sources and the discrimination factors affecting each in the plant (Evans, 2001). For most C3 and C4 plants (non-nitrogen- fixing), soil is the major source of N. Soil δ15N values are influenced by soil composition (Makarov, 2009), system openness (Handley et al., 1999), salinity (van Groenigen and van Kessel, 2002), soil age and depth (Nadelhoffer et al., 1996), water availability (e.g. rainfall) (Amundson, 2003) and altitude (Liu and Wang, 2010). Generally, soil δ15N values increase as 15N-depleted nitrogen compounds are lost through nitrification, ammonia volatilization, and leaching. This is the case, for example, in hot and arid regions like the deserts of southern Africa, where the δ15N values in soil, plants, and animals are enriched due to reduced rainfall (Shearer et al., 1978; Heaton et al., 1986; Heaton, 1987; Sealy et al., 1987; Schulze et al., 1991; Evans and Ehleringer, 1993, 1994). Plant δ15N values are positively correlated with temperature, and in addition negative correlations with annual precipitation have been shown (Handley et al., 1999; Amundson, 2003). Nitrogen isotopic discrimination in plants is influenced by soil nutrient availability, reflecting N cycling on various scales (Tilman, 1988; Nadelhoffer and Fry, 1994), and by the surrounding environment, including anthropic activity. Anthropogenic activities, such as agriculture, affect plant δ15N values, sometimes even masking the natural δ15N variability induced by environmental factors (Amundson, 2003). The use of fertilizers, such as manure and excrement, causes important changes in plant δ15N values, leading to an enrichment in 15N (Choi et al., 2006). Experimental research on different crops shows an increase in δ15N values when manure is used, in both C3 (Fraser et al., 2011) and C4 crops (Christensen et al., 2022; Dong et al., 2022). Differences in N-isotope fractionation between C3 and C4 plants are not well established. C4 species show more efficient nitrogen use than C3 plants (Schmitt and Edwards, 1981; Sage and Pearcy, 1987; Makino et al., 2003), which may cause δ15N to differ between the two pathways (Murphy and Bowman, 2009). Similar to carbon, among the limited experimental studies conducted on C4 plants, some research illustrates a positive relationship between grains δ15N values and cumulative annual precipitation in Pennisetum glaucum (Reid et al., 2018), while other research attests that water availability has no discernible effect on grains and leaf δ15N values of the same species (Sanborn et al., 2021). Concerning the altitude, it seems that different photosynthetic pathways do not affect isotopic results and in general δ15N values decrease with increasing elevation (Liu and Wang, 2010). Precipitation and temperature seem to be the main factors influencing δ15N variation in plants with altitude. Lower δ15N values at higher altitudes are attributed to reduced mineralization and net nitrification rates caused by lower temperatures and abundant rainfall (Liu and Wang, 2010).
2.3 Biosilica in C4 plants
Silica (SiO2), most commonly encountered in nature as quartz, comprises over 10% of the Earth's crust. Plants absorb silicic acid Si(OH)4, through their root systems, together with water, minerals and nutrients and deposit it in the aerial parts forming phytoliths. When it comes to mechanisms of silica absorption, plants can be classified as active, passive or rejecter (Tubaña and Heckman, 2015) depending on the concentration of silicic acid in the xylem compared to that in the soil. Most C4 plants are considered high silica accumulators with silica concentrations that can reach up to 10% of the dried weight. This means that the amount of silicic acid absorbed depends on its availability in the soil, the physiological state of the plant, and the genetic makeup. When silicic acid absorbed passively through transpiration is in too low concentrations, the density of silica transporters increases, driving an active absorption process (Ma and Yamaji, 2008). Si absorption in plants has been linked to increased growth rates in crop plants (e.g. Greger et al., 2018) and higher grain yield (e.g., D'Agostini et al., 2023), thereby promoting both plant growth and reproduction. Si compounds in both C3 and C4 cereals have been proven to significantly enhance plant photosynthesis (Goto et al., 2003; Cornelis and Delvaux, 2016) and chlorophyll content (Verma et al., 2019), potentially influencing leaf nitrogen (N) and carbon (C) content and altering C:N stoichiometry (Liu et al., 2023). Indeed, photosynthetic capacity is generally closely correlated with leaf nitrogen and phosphorus content because both largely affect the CO2 assimilation capacity of plants by altering the concentration of enzymes and ATP (Li et al., 2018; Zhang et al., 2018). Consequently, assessing the biosilica content in the plant and its possible relationship with carbon and nitrogen content can provide insights into the plant's productivity and photosynthetic rate relative to its growth environment. Additionally, it contributes important information to understanding the mechanisms of carbon and nitrogen discrimination in C4 plants, which, as mentioned above, remains a highly debated topic. Therefore, we expect to observe a correlation between biosilica content and C:N ratios, which may vary depending on environmental conditions. A higher silicon content can reduce the need for energy resources used for chemical defense or structure, allowing the plant to allocate more carbon to growth (C in grains and leaves). Similarly, under water stress conditions, C4 plants with higher biosilica levels might show a different carbon allocation compared to those with lower biosilica levels, thereby demonstrating that phytoliths and δ13C are not independent proxies.
3 The studied areas
The Konso Zone, located ~570 km southwest of Addis Ababa, is part of the South Ethiopian Regional State (Figure 1). The Konso people (Konsitas) reside in the Konso highlands, a mountainous area that blocks the Great East African Rift Valley. Tigray Regional State, the northernmost region in Ethiopia (Figure 1), is primarily inhabited by Tigrayans, Irob, and Kunama people. The Tekezze River, originating in the central Ethiopian highlands near Mount Qachen in Lasta-Lalibella, flows west into Amhara and then north into Tigray, serving as a natural boundary between Ethiopia and Eritrea.
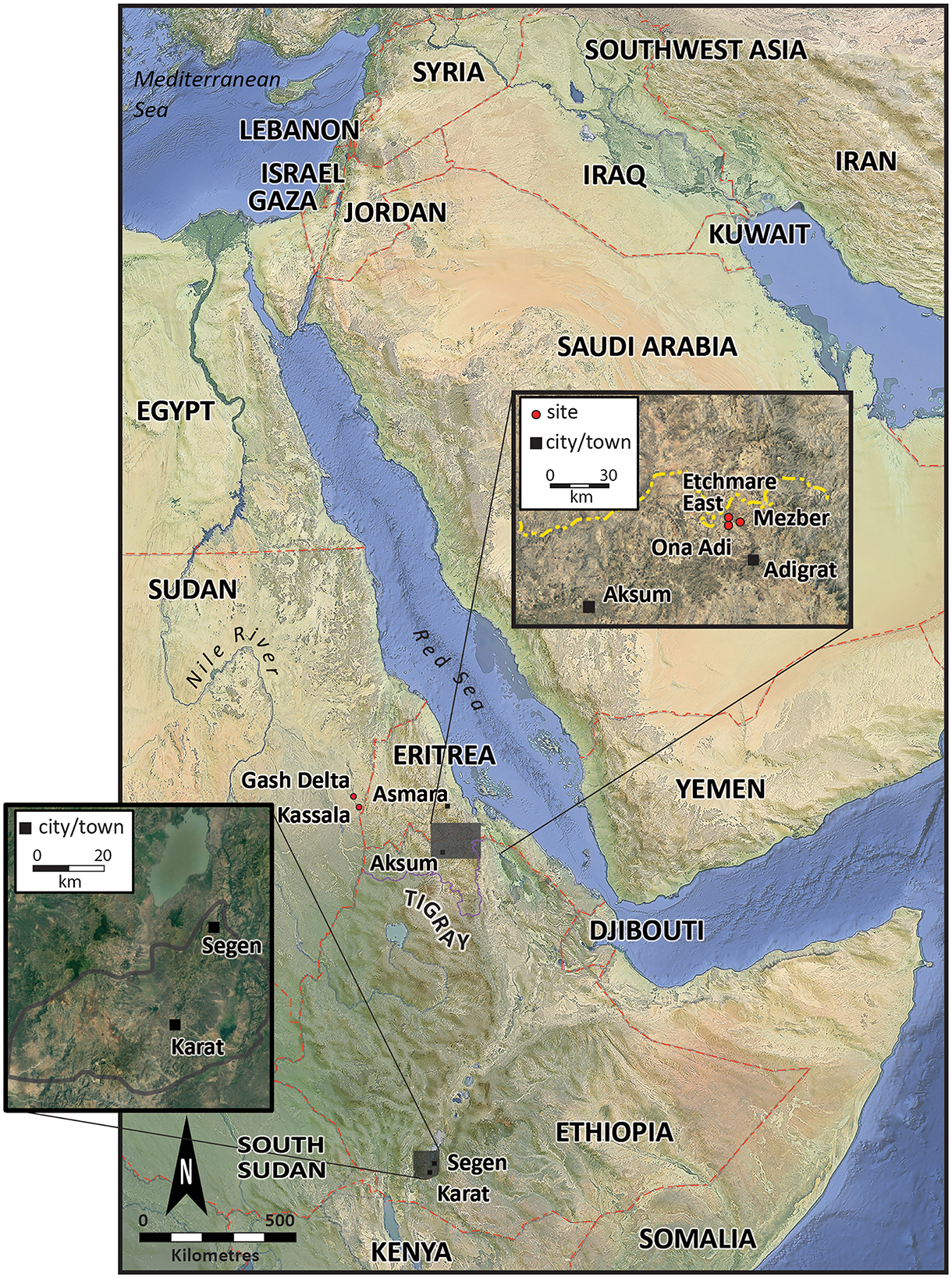
Figure 1. Map of Ethiopia with the Konso Zone and Adigrat in East Tigray and major archaeological sites in the region (map after Beldados et al., 2023).
Ethiopia has five major agroclimatic zones, classified based on altitude, temperature, and rainfall. These zones are important for determining agricultural potential and land use: berha: below 500 m a.s.l. (Lowlands, Hot Arid Zone—dominated by desert and semi-desert areas, with sparse vegetation. Limited agricultural potential, mainly suitable for pastoralism and drought-resistant crops); kola: 500–1,500 m a.s.l. (Lowlands, Subtropical Zone—mixed agriculture. Predominant crops are sorghum, millets, sesame, cowpeas, and groundnuts. Livestock rearing is common); woina dega: 1,500–2,300 m a.s.l. (Midlands Subtropical Zone—moderate temperatures, good for a variety of crops such as t'ef, maize, wheat and pulses. This zone supports both agriculture and livestock); dega: 2,300–3,200 m a.s.l. [Highland Cool Zone—cooler climate, ideal for crops like barley, wheat, and pulses. Livestock rearing (cattle, sheep) is also important here]; wurich: above 3,000 m a.s.l. [Highland Cold Zone—harsh, cold conditions with limited agricultural activity (barley). Mostly suitable for livestock grazing, particularly sheep and goats] (Pankhurst, 1961).
3.1 Environment and agriculture in Konso
The Konso landscape includes three of the five major agroclimatic zones: berha, kola, and woina daga. Kola makes up 70% of the territory, while woina daga and berha account for 20% and 10%, respectively (Yeshambel, 2013; Fikadu, 2023). Crop agriculture and animal husbandry have been the main means of livelihood in Konso. Konsitas rarely engage in traditional oxen plow agriculture because most of their land is hilly, rocky, small in size, and fragmented (Watson, 2009). Instead, farmers cultivate the terraced land using single-edged and double-edged composite hoes. These composite tools help prepare the soil by pulling out roots, rocks, shrubs, and bushes. Poor soil conditions, lack of adequate cultivable land, and unpredictable and insufficient annual rainfall have been critical challenges for farmers in the region. To overcome some of these challenges, Konso farmers have adopted terracing, irrigation, insulating, multiple cropping, and the planting and use of trees and shrubs (Förch, 2003; Amesias, 2023; Beldados et al., 2023). To conserve soil and water in their agricultural fields, farmers construct terraces on almost all of their land, using traditional field stone mortarless construction methods. This terracing technique conserves the soil from wind and water erosion, facilitates deep drainage and provides cultivable spaces on steep slopes (Tadesse, 2010; Tamiru, 2014). Most of the terraced farm lands are cropped year-round without fertilization. Instead, farmers keep some herbaceous nitrogen-fixing trees such as Ipomoea kituiensis Vatke, Lamiaceae, Asteraceae, Solanaceae and Rubiaceae in their farm land to convert nitrogen into a usable agricultural component.
With the progressive demographic increase at higher elevations and with the need of expanding commercial farms and productivity, Konso farmers recently started to occupy the adjacent lowlands (1200 and 800 m a.s.l) along the Segen River. Here, over the last two decades, farmers have cultivated sorghum, millets, and maize for commercial and household use. Ox-plow agriculture, irrigation and soil terracing are the main features of farming in the lowlands.
Unique aspects of agricultural practice in Konso, in comparison with other neighboring regions, include intercropping and the cultivation of C4 crops in a difficult hilly landscape (Hallpike, 1972; Watson, 2009). A single household owns on the average farming plots on the order of 20m X 10m, where up to 12 crop varieties are planted. The most common species cultivated are chickpea, cowpea, bean, hyacinth bean, pigeon pea, mung beans and peas. Moringa stanopetela (Baker f.) Cufod. (popularly known as moringa) widely grows in Konso and is used as staple food and medicinal plant. Furthermore, oil crops (sunflower, castor, and linseed), root crops (pumpkin, yams, sweet potatoes, and tomatoes), cash crops (coffee, cotton, tobacco, and maize), and fruits (papaya, mango, banana, orange, lemon, and avocado) are also cultivated in the area (Amesias, 2023; Fikadu, 2023). Sorghum and millets are the main C4 crops that grow in Konso in both mid and lower elevations over an altitudinal range of one thousand meters, between 700 and 1,700 m a.sl. These crops are well-adapted to the dry and semi-desert environmental conditions of the area and contribute significantly to the local diet.
The average annual rainfall of the study area is about 570 mm. Konso is characterized by prolonged dry seasons and two poor-quality rainy seasons: the most intense from mid-February to the end of April, followed by the small rainy season from May to June. Fifty-seven percent of the total annual rain falls from February to April and of this, 62% is most often concentrated in April (UNDP Situation Report, 1999). There are two planting seasons in the area: belg (February-May) and meher (October-November, Hagaya months). During belg, farmers plant and cultivate crops including sorghum, t'ef, maize, haricot bean, barley and wheat, accounting for 65%−75% of the yearly crop harvest. Ratooning is a traditional technique employed which permits a second sorghum cultivation during meher (UNDP Situation Report, 1999; Förch, 2003).
3.2 Sorghum in Konso
Sorghum [Sorghum bicolor (L.) Moench] is a staple crop in the drier parts of tropical Africa and it has been cultivated and consumed since prehistoric times. According to archaeobotanical studies, the oldest evidence for the domestication of sorghum in settlement contexts dates back to the last 6,000 years in the eastern Sahel region of Sudan (Winchell et al., 2018). However, even though the wild progenitor of sorghum is widely attested in Sudan (Beldados and Costantini, 2011; Beldados, 2015; Beldados et al., 2018), its cultivation in Ethiopia is more recent. The earliest evidence of carbonized grains of domesticated sorghum in Ethiopia is from the D-site of Aksum, dated to ca. 500–700 CE, and from the Tomb of the Brick Arches, dated to ca. 200–550 CE (Boardman, 2000). From post Aksumite and early medieval contexts, sorghum grains were recovered from the Islamic trade center of Harlaa (6th to 15th centuries CE) in Dire Dawa in east Ethiopia (Siraj, 2021; Beldados et al., 2024). Sorghum grains were also recovered in a context dated to the 12th and 13th centuries CE (Firie, 2023) in the Christian site of Gannata Mariam, adjacent to the World Heritage Site of Lalibella, in north central Ethiopia.
At present, 17 varieties of sorghum are cultivated in Konso and are characterized by different colors, texture, size, and productivity. Out of the 17 varieties, we selected 6 that are the most widely available, productive, and fast-ripening. These varieties are commonly stored in every farming household, whereas others are more difficult to obtain. Agronomists overseeing farming practices in the region also encourage farmers to prioritize these varieties. In addition, both agronomists and government agents provide free seeds to farmers to promote their cultivation (Table 1, Figure 2). Sorghum is intercropped with perennial legumes such as beans, bush beans, lablab bean, pigeon pea and is consumed as kitcha (flat bread made from wheat flour), dama (balls of sorghum flour boiled and stuck together usually accompanied with halako of boiled moringa leaves) and chaqqa (traditional fermented alcoholic drink which is strong and full of carbohydrates).
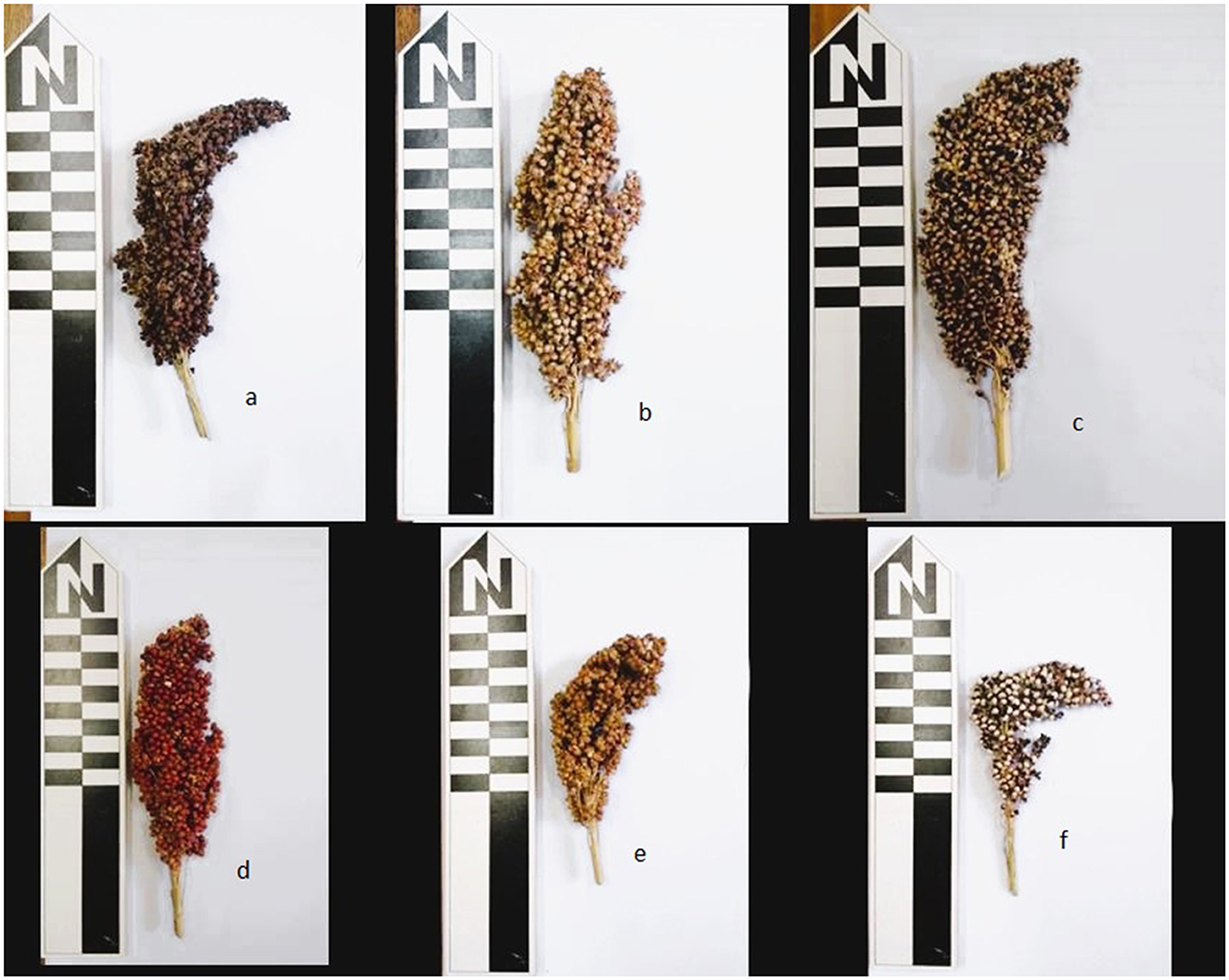
Figure 2. Varieties of sorghum growing in Konso included in this study: (a) Shuleya, (b) Gambella, (c) Kulishayta, (d) Kodhano, (e) Hoyriya, and (f) Harketta.
3.3 Environment and agriculture in Tigray
The Tigrayan landscape includes four agroclimatic zones: kola, woina dega, dega and wurich (Haftom et al., 2019). Despite this classification, environmental conditions in Tigray are particularly influenced by differences in altitude and topography, resulting in microclimatic variations across small and large expanses. The mean annual temperature ranges from 15 to 25°C, and mean annual rainfall ranges between 500 and 750 mm (Hagos, 2003; Hadgu et al., 2013). Both agriculture and livestock are key economic activities in Tigray Regional State. Agriculture is the primary source of food and livelihood for about 80% of the population, with 75% of the people in Tigray being farmers. These farmers still rely on traditional methods to cultivate their land: oxen-drawn plows, hand-sowing seeds, hand and hoe weeding, and rain-fed crops (Biagetti et al., 2022). This traditional farming system has sustained the region for centuries. The main cereal crops cultivated in Tigray are barley (Hordeum vulgare L.), wheat (Triticum aestivum L.), t'ef [Eragrostis tef (Zucc.) Trotter], sorghum [Sorghum bicolor (L.) Moench.], and finger millet (Eleusine coracana Gaertn.). The two common C3 plants, barley and wheat, are grown in almost all districts. Maize (Zea mays L.) is cultivated in low-lying areas below 1,900 m a.s.l. Additionally, leguminous plants such as horse bean (Vicia faba L.), field pea (Pisum sativum L.), grass pea (Lathyrus sativus L.), lentil (Lens culinaris Medik.), chickpea (Cicer arietinum L.), and fenugreek (Trigonella foenum-graecum L.) are cultivated to minimize cereal crop failures (D'Andrea et al., 2018; Tsegaye, 2019).
Unlike the Konso Zone, the study of past and present agriculture in Tigray has been widely investigated. The earliest evidence of agriculture in the region is dated to 1,600 BCE. Archaeobotanical research from the Mezber site near Adigrat revealed macro-remains of mainly C3 temperate cereals, legumes and oil crops including wheat, barley, lentils, flax (Linum usitatissimum L.) and weeds of cultivated cereals (Lolium sp.). In addition, two possible t'ef grains were identified in deposits dating from 400 BCE to CE 25 (Beldados et al., 2023; D'Andrea et al., 2023; Ruiz-Giralt and Beldados, 2024). Microbotanical investigations from sediments identified C4 tropical/sub-tropical crops such as t'ef, sorghum and finger millet or wild grasses. Phytolith and starch analyses from artifacts from the site of Ona Adi further confirmed the presence of C3 and C4 plants, including wheat, barley, millets, lentils, and various root crops (Ruiz-Giralt et al., 2023a; Meresa et al., 2024).
Archaeological investigations in Central Tigray, specifically at the Aksum World Heritage Site, have yielded diverse botanical findings. Boardman (2000) recovered remains of hulled barley, linseed, emmer, lentil, pea, and sorghum from sites D and K during the Aksumite period (c. 150–330 CE). These sites also provided insights into agricultural practices, revealing the cultivation of C4 plants such as sorghum alongside C3 plants, legumes, and oil plants. D'Andrea et al. (2008) conducted further studies at Bieta Giyorgis, documenting plant remains spanning the Proto-Aksumite (400–50 BCE), Early to Classic Aksumite (50 BCE-CE 340), and Post-Aksumite (700–900 CE) phases. Significant findings included free-threshing wheat, barley, t'ef, lentil, and flax during the Aksumite phase, along with a variety of wild plants. These archaeological excavations provide valuable insights into the agricultural practices and botanical diversity of the Aksumite civilization in Central Tigray (Boardman, 2000; D'Andrea et al., 2008).
3.4 Sorghum and millets cultivation in Tigray Regional State
Similar to Konso, C4 plants such as sorghum and millets are widely cultivated in Tigray. The crops are known for their drought resistant nature and can grow in diverse environmental conditions, including in places with poor soils and unpredictable rainfall. In the highlands and adjacent lowlands of the northern horn of Africa these crops are well adapted to the semi-desert environmental conditions (Ruiz-Giralt et al., 2023a). This region is also considered to be the domestication milieu of finger millet (Fuller and Hildebrand, 2013; Fuller, 2014).
Results of microbotanical analyses from the sites of Mezber and Ona Adi in Eastern Tigray identified C4 tropical plants of African origin as Andropogoneae, Eragrostideae and Panicoideae in a context dated to 1,600 BCE and subsequently in chronological phases of Early Pre-Aksumite (850–750 BCE), Middle Pre-Aksumite (600–400 BCE) and Late Pre-Aksumite (400 BCE-1 CE) periods (Ruiz-Giralt et al., 2023b). In the western part of Tigray, sorghum and millet grains were identified at Aksum from Classic Aksumite contexts (Boardman, 2000) and finger millet from Bieta Giorgis during the Early Aksumite phase (D'Andrea et al., 2008). All these archaeobotanical studies from the region indicate that sorghum and millets have deep rooted cultivation and domestication history comparable with the C3 plants of Southwest Asian origin.
Sorghum and finger millet are part of the daily cuisine in Tigray. The crops are consumed in the form of injera (flat bread baked in a griddle), bread, porridge, kollo (roasted cereals, mainly sorghum). Both of the crops are also consumed as part of the components in the preparation of local/traditional alcoholic drinks like suwa (mild alcoholic drink) and araki (strong alcoholic drinks). On the basis of color, two types of sorghum are cultivated in the region: red and white. Sorghum and millets are grown at an elevational range of 900–2,500 m a.s.l. in places where rainfall ranges from 500 to 1,200 mm per annum (Terefe and Beldados, 2021).
4 Materials and methods
The botanical assemblage presented in this paper includes grains and chaff from 11 plants of sorghum and finger millet (Table 2). Six sorghum plants are from a local farmer in Dokato, Konso. The fields are located at 1,523 m. asl (Gambella, and Shuleya) and at 995 m. a.s.l (Hargitet, Kodhano, Kulisheyda and Hoyriya). All Konso plants were planted in February 2021 and harvested in June 2021. The remaining four plants of sorghum and finger millet are from a local market in Adigrat, in Tigray. All Adigrat fields (Nech Meshila, Keyih Meshila, Bicha Meshila, Meshila and Keyih Degussa) are located at 2,457 m. a.s.l. Both sorghum and finger millet from Adigrat were planted in June 2016 and harvested in November 2016. According to the farmers, the plants collected both in Konso and in Adigrat were not irrigated but only rain-fed. Authorization to export and analyze the samples was granted by the Federal Democratic Republic of Ethiopia, Ethiopian Biodiversity Institute (Reference number: E.B.I 71/4051/2015; May 9, 2023). The authorization to import the samples into Spain was provided by the Ministerio de Agricultura, Pesca y Alimentación (Spanish Government). Table 3 lists the environmental data recorded in Konso (year 2021) and in Adigrat (year 2016) provided by the Ethiopian Meteorological Agency (https://www.ethiomet.gov.et). The table includes the maximum and minimum temperatures (Tmax and Tmin), precipitation, and relative humidity (RH) for the growing season, while the data for the whole year are in Supplementary information 1. Figure 3 shows the maximum and minimum temperatures and precipitation recorded in the two areas during the years of cultivation.
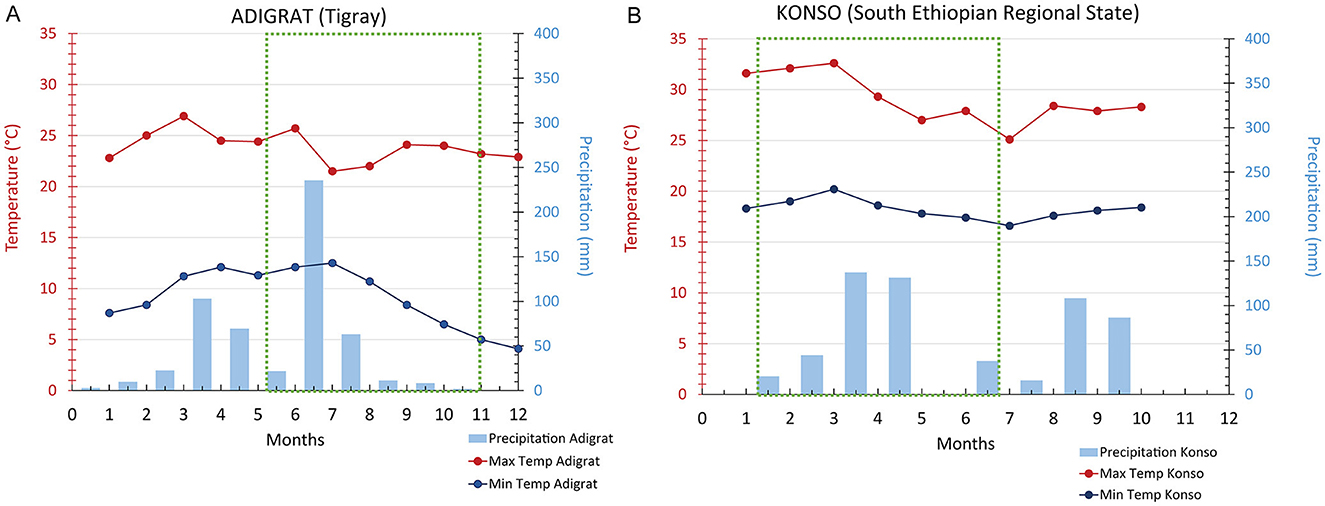
Figure 3. Adigrat (A) and Konso (B) maximum and minimum temperatures and precipitation recorded in the years of cultivation of the plants. Green square: period included between plantation and harvesting.
Samples were prepared for both isotopic and biosilica analysis at the Laboratory of Environmental Archaeology of Pompeu Fabra University, Barcelona (Spain).
4.1 Stable isotope analysis
Each plant corresponds to a sample, and each sample is composed of seven grains, except for finger millet, for which more than 40 grains were selected due to their small size. The grains of each sample were weighted and ground by hand in an agate mortar and homogenized. Carbon and nitrogen isotope analysis were undertaken using a Europa Scientific ANCA-SL elemental analyzer (EA), with zero-blank autosampler, coupled to a Europa Scientific 20–20 isotope ratio mass spectrometer (IRMS) at Iso-Analytical (Sercon Ltd., Crewe, UK). The powdered and homogenized grains were weighted (1.5 ± 0.1 mg) in tin capsules (8 × 5 mm), sealed, and loaded together with standards into the Europa Scientific elemental analyzer. A subset representing 20% of the samples was analyzed in duplicate. Carbon and nitrogen isotope values are calibrated to V-PDB and AIR using IA-R001 standard (wheat flour, δ13CV − PDB = −26.43‰ ± 0.08, δ15NAIR = 2.55‰ ± 0.22). Carbon and nitrogen measurements uncertainty was monitored using two check standards: IA-R045/IA-R005 (mixture of ammonium sulfate and beet sugar, δ13CV − PDB = −26.03‰ ± 0.11, δ15NAIR = −4.71‰ ± 0.07) and IA-R046/IA-R006 (mixture of ammonium sulfate and cane sugar, δ13CV − PDB = −11.64‰ ± 0.03, δ15NAIR = 22.04‰ ± 0.06). IA-R001 is calibrated against and traceable to IAEA-CH-6 (sucrose, δ13CV − PDB = −10.45‰) and IAEA-N-1 (ammonium sulfate, δ15NAIR = 0.40‰). IA-R005 and IA-R006 are calibrated against and traceable to IAEA-CH-6. IA-R045 and IA-R046 are calibrated against and traceable to IAEA-N-1. IAEA-CH-6 and IAEA-N-1 are inter-laboratory comparison standards distributed by the International Atomic Energy Agency, Vienna.
The isotope ratios are reported as “delta” defined according to International Union of Pure and Applied Chemistry (IUPAC):
where Rs and Rst are the isotope ratios 15N/14N and 13C/12C of the isotopic abundances of 15N, 14N and 13C, 12C and ‰ = 10−3. Using the calculation provided by Szpak et al. (2017) based on repeated measurements of calibration standards, check standards and sample replicates, the precision [u(RW)] of the measurements are estimated to be ± 0.05‰ for δ13C and ± 0.06‰ for δ15N values. Accuracy or systematic errors [U(bias)] are determined to be ±0.08‰ for δ13C and ± 0.17‰ for δ15N values, on the basis of the difference between the observed and known δ values of the check standards and the long–term standard deviations of these check standards. The total analytical uncertainties [u(c)] are estimated to be ± 0.10‰ for δ13C, ± 0.18‰ for δ15N values (Supplementary information 2: excel calibration calculations).
4.2 Biosilica analysis
Extractions were performed on a subset of chaff samples (n = 7) that exhibited an adequate quantity of material for analysis (Table 4). Chaff samples are from the same panicles from which the isotopic measurements of the seeds were taken. The samples underwent an extraction process following a protocol that integrates a dry ashing technique followed by wet oxidation. The procedure has been published step by step in D'Agostini et al. (2022). The results are reported as the weight in grams of biosilica extracted from the dry tissue and as a percentage of the total tissue weight.
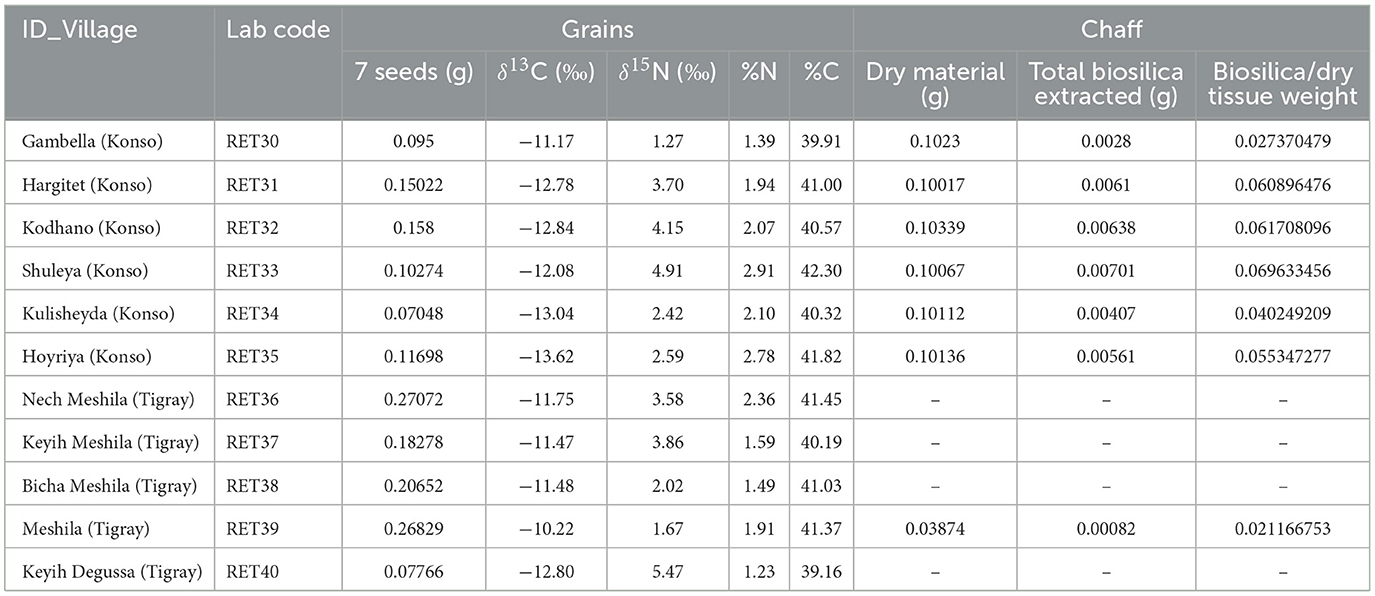
Table 4. Sorghum and finger millet grains stable isotope results and chaff samples analyzed with their respective weights in grams used for extractions.
5 Results
5.1 Carbon and nitrogen isotope results
The amount of carbon (%C) and nitrogen (%N) and the stable isotope values from sorghum and finger millet are shown in Figure 4 and presented in Table 4. The %C span from 39.16 and 42.30 (40.83% ± 0.91%) and the %N spans from 1.23 and 2.91 (1.98% ± 0.55%). δ15N values span from 1.27‰ to 5.47‰ (3.24‰ ± 1.35‰) with a range of 4.2‰ and δ13C values span from −13.62‰ to −10.22‰ (−12.11‰ ± 1.00‰), with a range of 3.4‰. Examining the distribution of sorghum values, the δ15N ranges of plants cultivated at different altitudes largely overlap. In contrast, the δ13C ranges show almost no overlap and significantly increase with altitude. Correlations between the isotopic values and maximum, minimum and average temperatures, total precipitation, altitude and relative humidity recorded during the growing period of the plants were evaluated using the Tau B Kendall test (Table 5). The δ13C values show a significant positive correlation with altitude (Tau B Kendall = 0.569, p = 0.027, n = 11, Table 5), which is even stronger when only sorghum grains are considered (Tau B Kendall = 0.685, p = 0.012, n = 10, Figure 5). The δ15N values show no correlation with any of the environmental factors or the altitude of the sites (Table 5, Figure 4). Particular attention is due to finger millet Keyih Degussa from Adigrat (RET 40): even though only one sample is available, results are interesting because they show an opposite trend compared to the samples of sorghum from the same altitude. This is because the δ13C value falls in the range of the samples from the site placed at the lowest altitude, and the δ15N value is the highest value of all samples analyzed.
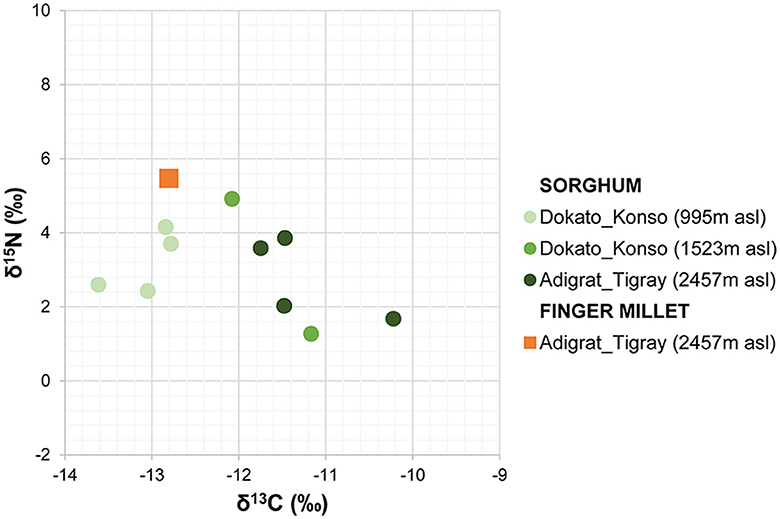
Figure 4. Stable carbon and nitrogen isotope values of finger millet and sorghum from Adigrat and Konso.
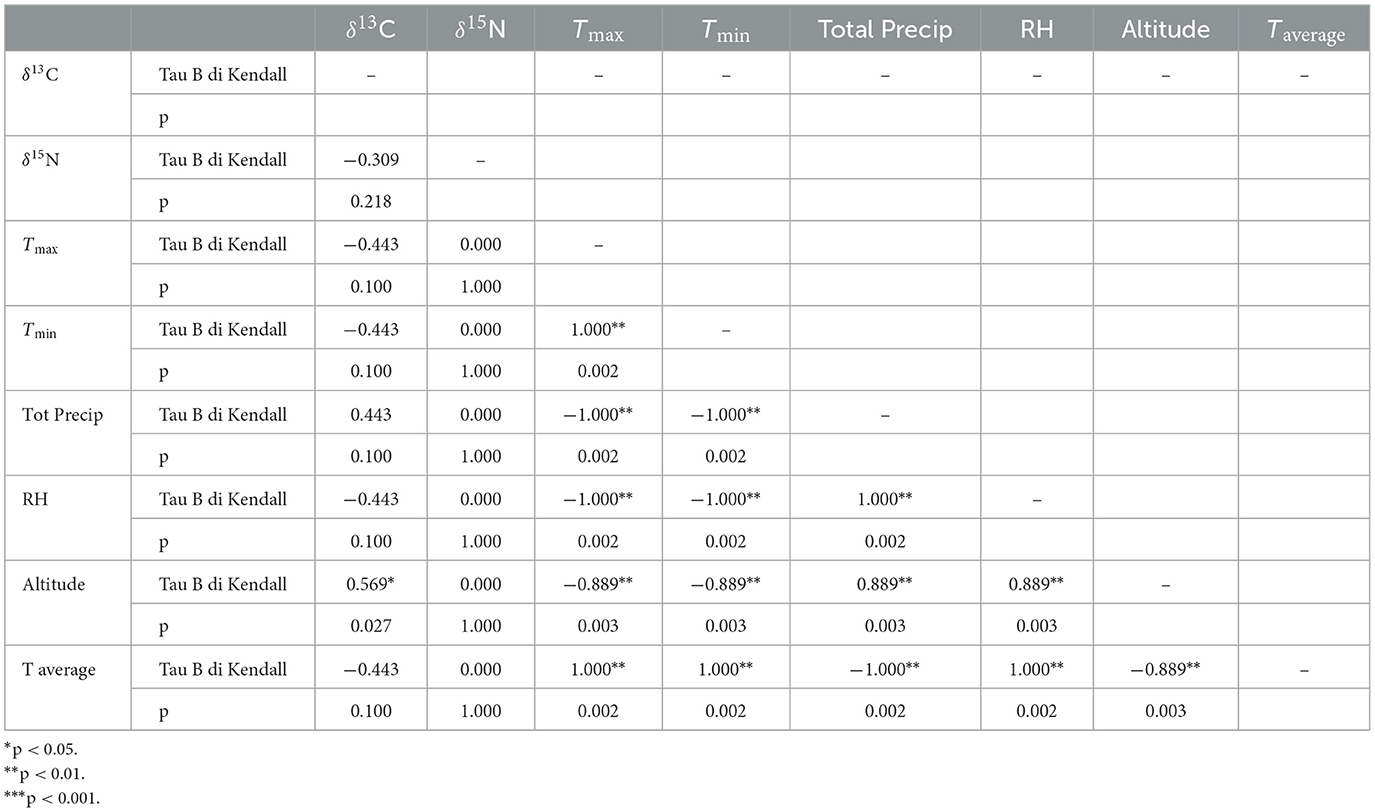
Table 5. Correlation of sorghum and finger millet grains between δ13C and δ15N and the environmental factors.
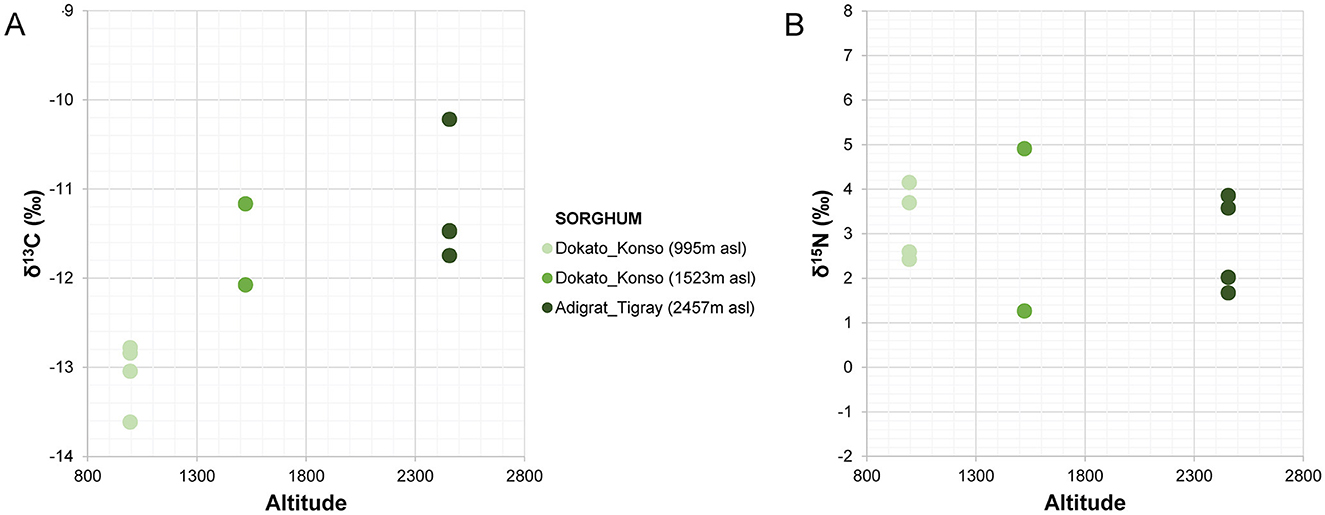
Figure 5. Stable carbon (A) and nitrogen (B) isotope values of sorghum from Adigrat and Konso plotted according to the altitude.
5.2 Biosilica results
The extracted biosilica ranges from ~2% to ~7% of the total dry weight, showing some variability despite the samples belonging to the same species and sharing the same C4 photosynthetic pathway. The highest values were observed in the Shuleya variant (RET 33) with a total of 6.96% (w/w) of biosilica, while the lowest value corresponds to the Meshila (RET 39) with 2.11% (w/w). Complete results can be found in Table 4. In Figure 6, the biosilica data are shown in comparison to the C:N data. The graph indicates that the samples with high C:N ratio have low biosilica content (RET 30 and 39 with the highest C:N ratio and the lowest biosilica content), while RET 33 with the highest biosilica content have the lowest C:N ratio. Figure 6 shows a general negative relationship between biosilica and C:N.
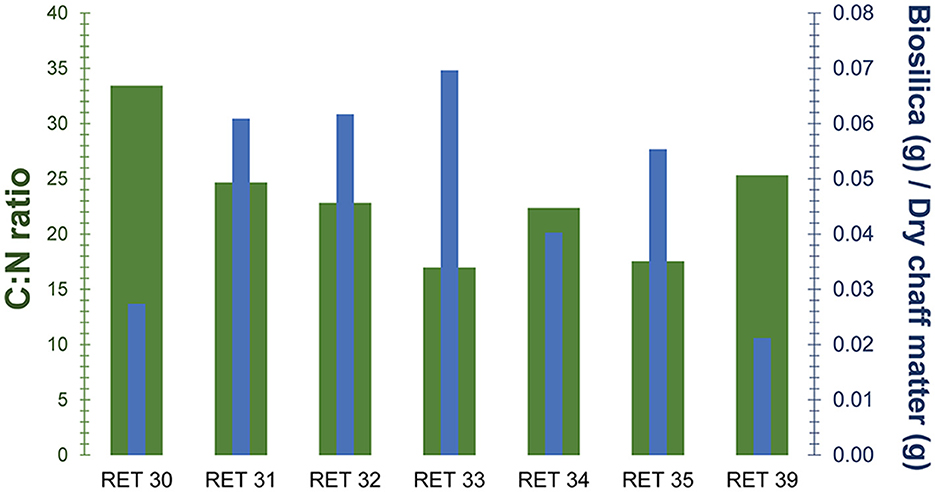
Figure 6. Quantity of biosilica extracted (blue columns) in relation to the weight of material extracted compared the C:N ratio (green columns) for each of the analyzed samples.
6 Discussion
Stable nitrogen and carbon isotopes in plants serve as valuable indicators for reconstructing past agriculture practice, paleoclimate and ecological processes. Additionally, observations regarding biosilica and its relation to carbon and nitrogen content have been recently demonstrated to provide information on plant development, and consequently on the environment where the plants grow. This pilot study provides an opportunity to explore the variability of δ15N and δ13C values in grains, as well as the biosilica content in the corresponding chaff (coming from the same panicles), across different altitudes in arid environments. It is to be highlighted, moreover, that the samples from Tigray, constitute the only samples available for the area so far and for the near future, due to the post-war local conditions that hinder research at the time of writing. As such, this research offers valuable insights and proposes directions for future studies.
In a mountainous country such as Ethiopia, altitude is the main factor influencing differences in crop production. Researchers use altitude to identify the five main Ethiopian agro-ecological zones (see Section 3). This is the rationale behind establishing five distinct types of agro-climatic zones, locally termed as wurch (extremely cold highlands), dega (permanently cold highlands), woina dega (mid-elevation or temperate-like), kola (hot lower elevations), and bereha (very hot and desert-like) (Pankhurst, 1961). An example of the influence of altitude on micro-scale climate can be seen in the types of crops cultivated. C3 plants are typically grown at higher elevations with cooler weather, whereas C4 plants are predominantly grown at hotter, lower elevations and in the valleys.
The plants analyzed are from fields where traditional C4 crops are grown by local farmers in the Konso and Adigrat regions of Ethiopia. Although Konso and Adigrat are not geographically close, both areas are characterized by aridity and dryness for most of the year, except during the brief rainy seasons, when the crops are grown. During these periods, farmers cultivate their crops, which are predominantly rain-fed. Environmental differences observed at varying altitudes include temperature variations, with Konso experiencing higher maximum and minimum temperatures than Adigrat. Accordingly, the Konso Zone experiences relatively higher temperatures as its geographic altitudinal set up is dominantly of kola. Konso records temperatures of 29.8°C (max) and 18.6°C (min), with an average of 24.2°C, while Adigrat records 23.4°C (max) and 9.2°C (min), with an average of 16.3°C. We also expect that different altitudes will exhibit varying vapor pressures and moisture levels (Gamachu, 1988). Relative humidity and rainfall levels (for the cultivation period) slightly increase with altitude, considering that our Konso study areas are located between 995 and 1,523 m a.s.l. while the Adrigrat study area is located at 2,457 m a.s.l. Indeed, in this research, we considered the annual rainfall and the specific rainfall during the cultivation months as two separate parameters, and we only compared monthly precipitation with the isotopic data under examination. Concentrating the focus on the cultivation periods, Konso has a relative humidity of 60% and a total rainfall of 332 mm, compared to Adigrat's 62% relative humidity and 342 mm of rainfall, resulting in 10 mm difference in precipitation and 2% difference in humidity between the two areas. However, when considering the entire annual rainfall, Konso had higher precipitation than Adigrat, with a total of 581 mm vs. 549 mm. This implies that outside the cultivation period we considered, Konso generally received more rainwater than Adigrat. This shows that it is crucial to consider the precise timing of the growing periods of the plants analyzed, rather than relying on average annual values. Using general annual data can bias interpretations, particularly in isotope analysis of crop remains and when in a year there is more than one rainy season utilized for cultivation.
The carbon isotope values of the sorghum plants exhibit a wide distribution, from −13.62‰ to −10.22‰, indicating quite a significant variability among different areas under cultivation. Each altitude group is clearly distinct from the others (Figure 5), thus explaining the observed variability. Plants at lower altitudes (995 m a.s.l.) show lower δ13C values (−13.62‰ < δ13C < −12.78‰), while plants at higher altitudes (2,457 m a.s.l.) display higher δ13C values (−11.75‰ < δ13C < −10.22‰). The intermediate group, located at 1,523 m a.s.l., consists of RET 30 and RET 33. The first sample, RET 33 from Shuleya (a variety that is mostly cultivated on a terraced land), fits between the two previous groups (δ13C = −12.08‰), while the second, RET 30 from Gambella, falls in the range of the results for the highest altitude plants (δ13C = −11.17‰). Although caution is necessary when interpreting data from just one sample, a possible reason why this sample fits within the range of the field placed at the highest elevation could be the specific geographical position of the cultivation of this sorghum variety. Indeed, Gambella is cultivated in a valley near the Yalda-Toume River. The Yalda-Toume River flows in a relatively low-lying area and runs almost all year-round, serving as a tributary to the Segen River. This flow possibly provides additional soil moisture for cultivation, which can potentially contribute to an increase in δ13C values, placing the samples closer to those from Tigray in terms of relative humidity and water availability. Moreover, when testing the correlation between altitude and all the sorghum δ13C values, a significant positive correlation exists (Tau B Kendall = 0.685, p = 0.012, n = 10). This indicates that, despite the presence of outliers, δ13C values tend to increase with altitude. On the other hand, no significant correlations are observed between any of the recorded environmental parameters and δ13C values. However, when the sample from Gambella is excluded, not only does the correlation between δ13C values and altitude become stronger (Tau B Kendall = 0.816, p = 0.005, n = 9), but significant positive correlations also appear between RH, total precipitation, and δ13C values (Tau B Kendall = 0.745, p = 0.014, n = 9) and significant negative correlations are found between Tmax, Tmin, Taverage, and δ13C values (Tau B Kendall = −0.745, p = 0.014, n = 9).
The positive correlation between relative humidity and water availability (including both precipitation and controlled watering in experimental studies) and δ13C values has been observed in many studies, such as those by Wang et al. (2006), Reid et al. (2018), Lightfoot et al. (2020), Sanborn et al. (2021), An et al. (2015), and Yang and Li (2015). Moreover, the negative correlation between temperature and δ13C values is consistent with the available literature published so far (Reid et al., 2018). Thus, the increase in altitude, and the related decreased partial pressure of CO2 and O2, is likely associated with lower drought stress because, at higher altitudes, temperature decreases and precipitation and RH increase, leading to an increase in δ13C values. δ13C responds to environmental factors primarily through changes in the ratio of intercellular to ambient partial pressure of CO2 (pi/pa), regulated by the opening or closing of leaf stomata (Körner and Diemer, 1987; Körner and Larcher, 1988; Körner et al., 1989). The ci/ca ratio typically decreases with stomatal closure caused by drought stress (Farquhar et al., 1989). In C4 plants, the relationship between δ13C and water availability cannot be directly estimated due to the leakiness of CO2 and HCO3- from bundle sheath cells to mesophyll cells, determined by the plant's physiological pathways (Farquhar et al., 1989; Williams et al., 2001; Sage, 2004). However, the leakiness effect on δ13C in C4 can be detected and is also evident in our experimental data presented in this study.
This study indicates that altitude seems to play an important role in influencing the δ13C values in plants. A positive correlation between altitude and δ13C values was also attested by Wang et al. (2008) on Setaria viridis L. Thell. When plants were collected from a wide altitudinal range and then grown in the same environment, the greenhouse progenies no longer displayed any significant change with the altitude of origin as recorded in situ. Photosynthetic rate, stomatal conductance, the ratio of intercellular to ambient CO2 and intrinsic water use efficiency for S. viridis progenies were also not significantly related to the altitude of origin. This implies that altitudinal variations in carbon isotope values are principally correlated to changes in environmental factors, rather than by genetic adaptations to different altitudes (Wang et al., 2008). This fact is of significant importance for both paleoenvironmental and archaeological reconstruction since it confirms that δ13C values are directly relatable to environmental parameters, while at the same time suggesting that predicting environmental characteristics based on either C4 or C3 signals predetermined by specific δ13C values could be potentially misleading, as genetics proves to be less effective compared to the effects of the environmental setting.
Supporting the fact that carbon assimilation is directly related to environmental parameters and not genetic ones, are the data obtained from the study of biosilica. In fact, the correlation analysis in our study confirms a negative relationship between δ13C and biosilica despite all the samples belonging to the same species and the same photosynthetic pathway, that could have indicated a genetic control over it. These consistent findings align with prior research on biosilica and assimilated carbon (e.g. Liu et al., 2023) and suggest a potential adaptive strategy in sorghum, where the defense/structural mechanism associated with biosilica deposition in the form of phytoliths could confer competitive advantages over carbon based structures when carbon assimilation is lower. In our study, the sample with the lowest C:N assimilation rate, RET 33, also has the highest biosilica accumulation. Conversely, RET 30 and RET 39, with very high C:N ratios have the lowest biosilica accumulation. These preliminary results suggest a connection between biosilica and carbon content, which are typically considered independent. They also show that the amount of assimilated carbon may partially depend on the biosilica content, which is in turn modulated by environmental parameters such as water availability or soil silicon levels (Olivera-Viciedo et al., 2024).
Finger millet needs to be discussed separately, because it belongs to the NAD-ME C4 subtype while sorghum belongs to the NADP-ME C4 subtype (Hattersley, 1982). The NADP-ME subtype has suberized lamellae around bundle sheath cells, which decrease leakiness, and there are fewer grana in the bundle sheath chloroplasts, whereas the NAD-ME subtype has more grana and lacks suberized lamellae. These morphological differences explain why NADP-ME C4 subtype species usually have significantly higher δ13C values than their NAD-ME counterparts (Hattersley, 1982; Schulze et al., 1996). These differences reflect how each subtype has adapted to its environmental setting due to their different water use efficiencies (WUE) and drought tolerances (Ghannoum, 2009). Under water stress, the NAD-ME subtype demonstrates higher WUE compared to the NADP-ME subtype (Ghannoum et al., 2002). Moreover, the NADP-ME subtype is typically found in mesic environments, while the NAD-ME subtype is prevalent in drier areas (Hattersley, 1982; Schulze et al., 1996). Indeed, the finger millet RET 40 from Keyih Degussa, cultivated at 2,457 m a.s.l., has a δ13C value of −12.80‰, which is lower than those recorded for sorghum plants, that are NADP-ME, cultivated at the same altitude.
When examining nitrogen isotope values, the overall variability is relatively low (~4‰), with all data ranging between 1.27‰ and 5.47‰ without showing any distinct patterns, despite the analysis of different species. Even though distinct agricultural practices can be recorded between Konso and Tigray (hoe vs. plow), they do not affect the δ15N values, in fact the δ15N values from the two zones overlap and no significant differences exist between the two geographical regions. Additionally, the results further confirm that neither Konso nor Tigray practiced manuring, as the δ15N values are below the recognized threshold for which fertilization is commonly indicated (Fraser et al., 2011). No relationships are evident between the nitrogen isotope values and temperature, relative humidity, and precipitation recorded at different altitudes, whether considering both species, only sorghum, or sorghum excluding the Gambella sample. This result suggests two possible scenarios: (1) the measured environmental factors we considered do not influence the δ15N values, as it has been observed in other studies, such as those by Sanborn et al. (2021) and Lightfoot et al. (2020); or (2) the differences in the measured environmental parameters at various altitudes are not significant enough to impact nitrogen isotope values meaningfully. An increase in temperature has been demonstrated to positively affect δ15N values by Reid et al. (2018) and Liu and Wang (2010), while an increased watering showed a negative effect on δ15N values in Liu and Wang (2010), Craine et al. (2009), and Amundson (2003). Our data support the interpretation that the trends globally observed in other studies are mainly a consequence of larger differences in environmental parameters affecting soil δ15N rather than nitrogen isotope fractionation, as noted by Sanborn et al. (2021). According to the hypothesis by Liu and Wang (2010), it is likely that the temperature and precipitation in Adigrat were not low and abundant enough to cause lower mineralization and lower net nitrification rates that would negatively affect δ15N values. These results, however, once again highlight how difficult it is to use specific ranges of nitrogen and carbon isotopes to identify differences in floristic composition (e.g., the presence or absence of C4 plants), as various species are highly dependent on environmental effects that vary from region to region depending on the intensity of the phenomenon.
7 Conclusion
This pilot study analyzed carbon and nitrogen stable isotopes from grains and silicon content from chaff of sorghum and finger millet plants cultivated at different altitudes in Konso and Tigray regions of Ethiopia. The results demonstrate a positive correlation between δ13C and altitude, and excluding possible outliers, δ13C also correlated positively with precipitation and relative humidity and negatively correlated with temperature. This suggests that while there were minor environmental differences across altitudes, factors such as temperature, relative humidity, and precipitation of the different altitudes influenced δ13C values in sorghum. In contrast, no significant correlations were found for nitrogen, indicating that these environmental parameters either did not affect nitrogen isotope values or their differences were not pronounced enough to influence δ15N values. Further studies with a more comprehensive dataset, including additional variables like light intensity, are needed to confirm these patterns. However, by providing baseline data on the isotopic variability of modern C4 crops like sorghum and finger millet, this study significantly aids in interpreting isotopic signatures from ancient plant remains, particularly on four key aspects: (1) Understanding C4 plant isotopic variability: the study enhances our understanding of how carbon and nitrogen isotopes respond to environmental factors such as altitude and water availability; (2) Water management and climate reconstruction: the correlation between carbon isotopes and altitude, likely reflecting water availability, offers a tool to deduce ancient water management strategies and climate conditions. This is particularly useful in arid and semi-arid zones regions where water control would have been crucial for agricultural productivity; (3) Limits of nitrogen isotope values in environmental interpretation: the lack of significant correlations with δ15N indicates that nitrogen isotope data may not be as useful for understanding environmental variations in this context. However, this information helps to understand the limits of nitrogen isotopes when interpreting ancient agricultural practices and suggests they should rely more on carbon isotopes for environmental and altitude-related insights. (4) Relationship between carbon and biosilica: we showed that the amount of assimilated carbon may partially depend on the biosilica content, which is in turn modulated by environmental parameters such as water availability or soil silicon levels.
Data availability statement
The original contributions presented in the study are included in the article/Supplementary material, further inquiries can be directed to the corresponding author.
Author contributions
AV: Conceptualization, Data curation, Formal analysis, Investigation, Methodology, Visualization, Writing – original draft, Writing – review & editing. AB: Conceptualization, Data curation, Funding acquisition, Investigation, Resources, Visualization, Writing – original draft, Writing – review & editing. FD'A: Conceptualization, Data curation, Formal analysis, Investigation, Methodology, Visualization, Writing – original draft, Writing – review & editing. MM: Resources, Writing – review & editing. CD'A: Funding acquisition, Resources, Writing – review & editing. CL: Conceptualization, Data curation, Funding acquisition, Investigation, Resources, Writing – review & editing.
Funding
The author(s) declare financial support was received for the research, authorship, and/or publication of this article. This work was funded by the ERC Starting Grant RAINDROPS (G.A. no. 759800) under the Horizon 2020 program of the European Commission. CASEs is a Quality Research Group funded by the Government of Catalonia (SGR00950-2021). Additional funding has been provided by the Social Sciences and Humanities Research Council of Canada, Insight Grant No. 435-2018-0445.
Acknowledgments
The authors would like to acknowledge Ian Begley (Iso-Analytics) for the helpful exchanges about the isotopic analyses. The authors would like to thank Shannon Wood for editing Figure 1.
Conflict of interest
The authors declare that the research was conducted in the absence of any commercial or financial relationships that could be construed as a potential conflict of interest.
Publisher's note
All claims expressed in this article are solely those of the authors and do not necessarily represent those of their affiliated organizations, or those of the publisher, the editors and the reviewers. Any product that may be evaluated in this article, or claim that may be made by its manufacturer, is not guaranteed or endorsed by the publisher.
Supplementary material
The Supplementary Material for this article can be found online at: https://www.frontiersin.org/articles/10.3389/fearc.2024.1473056/full#supplementary-material
Supplementary information 1 | Environmental data recorded in Konso (year 2021) and in Adigrat (year 2016) provided by the Ethiopian Meteorological Agency (https://www.ethiomet.gov.et).
Supplementary information 2 | Calibration tables (estimation using Szpak et al., 2017).
References
Aguilera, M., Araus, J. L., Voltas, J., Rodríguez-Ariza, M. O., Molina, F., Rovira, N., et al. (2008). Stable carbon and nitrogen isotopes and quality traits of fossil cereal grains provide clues on sustainability at the beginnings of Mediterranean agriculture. Rapid Commun. Mass Spectrom. 22, 1653–1663. doi: 10.1002/rcm.3501
Amesias, A. (2023). Ethnoarchaeological and Archaeobotanical Study of Wild Edible Plants in Konso Zone, Southern Ethiopia. Addis Ababa: Department of Archaeology and Heritage Studies, Addis Ababa University.
Amundson, R. (2003). Global patterns of the isotopic composition of soil and plant nitrogen. Global Biogeochem. Cycles 17:1031. doi: 10.1029/2002GB001903
An, C. B., Dong, W., Li, H., Zhang, P., Zhao, Y., Zhao, X., et al. (2015). Variability of the stable carbon isotope ratio in modern and archaeological millets: evidence from northern China. J. Archaeol. Sci. 53, 316–322. doi: 10.1016/j.jas.2014.11.001
Araus, J. L., Febrero, A., Buxó, R., Rodríguez-Ariza, M. O., Molina, F., Camalich, M. D., et al. (1997). Identification of ancient irrigation practices based on the carbon isotope discrimination of plant seeds: a case study from the South-East Iberian Peninsula. J. Archaeol. Sci. 24, 729–740. doi: 10.1006/jasc.1997.0154
Beldados, A. (2015). Paleoethnobotanical Study of Ancient Food Crops and the Environmental Context in North-East Africa, 6000 BC–AD 200/300. Ann Arbor, MI: University of Michigan Press. doi: 10.30861/9781407313573
Beldados, A., and Costantini, L. (2011). Sorghum Exploitation at Kassala and Its Environs, North Eastern Sudan in the Second and First Millennia BC. Nyame akuma. Available at: https://www.semanticscholar.org/paper/Sorghum-Exploitation-at-Kassala-and-Its-Environs%2C-Beldados-Costantini/f384ac1354f489bf20e2f79381e8a95bdec3a12e (accessed May 14, 2024).
Beldados, A., Manzo, A., Murphy, C., Stevens, C. J., and Fuller, D. Q. (2018). “Evidence of sorghum cultivation and possible pearl millet in the second millennium BC at Kassala, Eastern Sudan,” in Plants and People in the African Past, eds. A. M. Mercuri, A. C. D'Andrea, R. Fornaciari, and A. Höhn (Cham: Springer International Publishing), 503–528. doi: 10.1007/978-3-319-89839-1_22
Beldados, A., Siraj, E. H., Insoll, T., Eshetu, Z., and Angestrom, F. (2024). Archaeobotany, plant economy, palaeoenvironment and religious identity in medieval Eastern Ethiopia the results of analysis from Harlaa, Dire Dawa region. Ann. Ethiop. 35, 59–96.
Beldados, A., Yohannes, Z., Habir, M., Teke, F., Zelalem, F., Zelalem, B., et al. (2023). Newly discovered Yalda-Toume Valley Plio-Pleistocene Site, Konso Zone, Ethiopia. Rass. Studi Ethiop. 7, 289–300.
Biagetti, S., Ruiz-Giralt, A., Madella, M., Khalid Magzoub, M., Meresa, Y., Gebreselassie, M. H., et al. (2022). No rain, no grain? Ethnoarchaeology of sorghum and millet cultivation in dryland environments of Sudan, Pakistan, and Ethiopia. Ethnoarchaeology 13, 80–104. doi: 10.1080/19442890.2022.2059994
Boardman, S. J. (2000). “Archaeobotany,” in Archaeology at Aksum, Ethiopia, ed. D. W. Phillipson (London: The British Institute in Eastern Africa and The Society of Antiquaries), 1993–1997, 363–470.
Bogaard, A., Fraser, R., Heaton, T. H. E., Wallace, M., Vaiglova, P., Charles, M., et al. (2013). Crop manuring and intensive land management by Europe's first farmers. Proc. Natl. Acad. Sci. USA. 110, 12589–12594. doi: 10.1073/pnas.1305918110
Bowman, W. D., Hubick, K. T., von Caemmerer, S., and Farquhar, G. D. (1989). Short-term changes in leaf carbon isotope discrimination in salt- and water-stressed c(4) grasses. Plant Physiol. 90, 162–166. doi: 10.1104/pp.90.1.162
Buchmann, N., Brooks, J. R., Rapp, K. D., and Ehleringer, J. R. (1996). Carbon isotope composition of C4 grasses is influenced by light and water supply. Plant Cell Environ. 19, 392–402. doi: 10.1111/j.1365-3040.1996.tb00331.x
Cerling, T. E., Ehleringer, J. R., and Harris, J. M. (1998). Carbon dioxide starvation, the development of C4 ecosystems, and mammalian evolution. Philos. Trans. R. Soc. Lond. B. Biol. Sci. 353, 159–171.
Choi, W. J., Arshad, M. A., Chang, S. X., and Kim, T. H. (2006). Grain 15N of crops applied with organic and chemical fertilizers in a four-year rotation. Plant Soil 284, 165–174. doi: 10.1007/s11104-006-0038-8
Christensen, B. T., Jensen, J. L., Dong, Y., and Bogaard, A. (2022). Manure for millet: Grain δ15N values as indicators of prehistoric cropping intensity of Panicum miliaceum and Setaria italica. J. Archaeol. Sci. 139:105554. doi: 10.1016/J.JAS.2022.105554
Cornelis, J., and Delvaux, B. (2016). Soil processes drive the biological silicon feedback loop. Br. Ecol. Soc. 1, 1298–1310. doi: 10.1111/1365-2435.12704
Craine, J. M., Elmore, A. J., Aidar, M. P. M., Bustamante, M., Dawson, T. E., Hobbie, E. A., et al. (2009). Global patterns of foliar nitrogen isotopes and their relationships with climate, mycorrhizal fungi, foliar nutrient concentrations, and nitrogen availability. New Phytol. 183, 980–992. doi: 10.1111/j.1469-8137.2009.02917.x
D'Agostini, F., Frick, D. A., Varalli, A., Madella, M., and Lancelotti, C. (2024). Silicon stable isotopes of phytoliths to reconstruct past water availability in Sorghum biolor. Oral presentation at the Isotopes in Biogenic Silica conference (IBiS), Louvain-La-Neuve.
D'Agostini, F., Ruiz-Pérez, J., Madella, M., Vadez, V., Kholova, J., and Lancelotti, C. (2023). Phytoliths as indicators of plant water availability: The case of millets cultivation in the Indus Valley civilization. Rev. Palaeobot. Palynol. 309:104783. doi: 10.1016/J.REVPALBO.2022.104783
D'Agostini, F., Ruiz-Pérez, J., Madella, M., Vadez, V., and Lancelotti, C. (2022). Phytolith extraction and counting procedure for modern plant material rich in silica skeletons V.2. Protocols.io. doi: 10.17504/protocols.io.q26g74mb8gwz/v2
D'Andrea, A. C., Perry, L., Nixon-Darcus, L., Fahmy, A. G., and Attia, E. A. E. (2018). “A pre-aksumite culinary practice at the mezber site, Northern Ethiopia,” in Plants and People in the African Past: Progress in African Archaeobotany, eds. A. M. Mercuri, A. C. D'Andrea, R. Fornaciari, and A. Höhn (Cham: Springer International Publishing), 453–478. doi: 10.1007/978-3-319-89839-1_20
D'Andrea, A. C., Schmidt, P. R., and Curtis, M. C. (2008). “Paleobotanical analysis and agricultural economy in early first millennium BCE sites around Asmara,” in The Archaeology of Ancient Eritrea, eds. P. Ridgway Schmidt, Y. Hameso, and M. C. Curtis (Trenton: Red Sea Press), 207–216.
D'Andrea, A. C., Welton, L., Manzo, A., Woldekiros, H. S., Brandt, S. A., et al. (2023). The Pre-Aksumite Period: indigenous origins and development in the Horn of Africa. Azania: Archaeol. Res. Afr. 58, 329–392. doi: 10.1080/0067270X.2023.2236484
Dercon, G., Clymans, E., Diels, J., Merckx, R., and Deckers, J. (2006). Differential 13C isotopic discrimination in maize at varying water stress and at low to high nitrogen availability. Plant Soil 282, 313–326. doi: 10.1007/s11104-006-0001-8
Dong, Y., Bi, X., Wu, R., Belfield, E. J., Harberd, N. P., Christensen, B. T., et al. (2022). The potential of stable carbon and nitrogen isotope analysis of foxtail and broomcorn millets for investigating ancient farming systems. Front. Plant Sci. 13:1018312. doi: 10.3389/FPLS.2022.1018312
Evans, R. D. (2001). Physiological mechanisms influencing plant nitrogen isotope composition. Trends Plant Sci. 6, 121–126. doi: 10.1016/S1360-1385(01)01889-1
Evans, R. D., and Ehleringer, J. R. (1993). A break in the nitrogen cycle in aridlands? Evidence from δ 15N of soils. Oecologia 94, 314–317.
Evans, R. D., and Ehleringer, J. R. (1994). Water and nitrogen dynamics in an arid woodland. Oecologia 99, 233–242. doi: 10.1007/BF00627735
Farquhar, G. D., Ehleringer, J. R., and Hubick, K. T. (1989). Carbon isotope discrimination and photosynthesis. Annu. Rev. Plant Physiol. Plant Mol. Biol. 40, 503–537.
Farquhar, G. D., O'Leary, M. H., and Berry, J. A. (1982). On the relationship between carbon isotope discrimination and the intercellular carbon dioxide concentration in leaves. Aust. J. Plant Physiol. 9, 121–137. doi: 10.1071/PP9820121
Fikadu, A. (2023). Continuity and Change in Traditional Terracing and Agricultural Economy in Medieval Konso, Southern Ethiopia. Addis Ababa: Department of Archaeology and Heritage Studies, Addis Ababa University.
Firie, T. (2023). Reconstructing the Subsistence Strategy of Ancient Populations from the Medieval Site of Gennete Maryam, Lalibela, through Archaeobotanical and Ethno-Archaeological Approaches. Aksum: Department of Archaeology and Heritage Management, Aksum University.
Förch, W. (2003). Case study: the agricultural system of the Konso in South-Western Ethiopia. FWU Water Resour. Publications 1:278.
Fraser, R. A., Bogaard, A., Heaton, T., Charles, M., Jones, G., Christensen, B. T., et al. (2011). Manuring and stable nitrogen isotope ratios in cereals and pulses: towards a new archaeobotanical approach to the inference of land use and dietary practices. J. Archaeol. Sci. 38, 2790–2804. doi: 10.1016/j.jas.2011.06.024
Fuller, D. Q. (2014). “Finger millet: origins and development,” in Encyclopedia of Global Archaeology, ed. C. Smith (New York, NY: Springer), 2783–2785. doi: 10.1007/978-1-4419-0465-2_2314
Fuller, D. Q., and Hildebrand, E. (2013). “Domesticating plants in Africa,” in The Oxford Handbook of African Archaeology, eds. P. Mitchell and P. J. Lane (Oxford: Oxford University Press), 506–525. doi: 10.1093/oxfordhb/9780199569885.013.0035
Gamachu, D. (1988). Some patterns of altitudinal variation of climatic elements in the mountainous regions of Ethiopia. Mt. Res. Dev. 8, 131–138. doi: 10.2307/3673439
Ghannoum, O. (2009). C4 photosynthesis and water stress. Ann. Bot. 103, 635–644. doi: 10.1093/aob/mcn093
Ghannoum, O., Von Caemmerer, S., and Conroy, J. P. (2002). The effect of drought on plant water use efficiency of nine NAD-ME and nine NADP-ME Australian C4 grasses. Funct. Plant Biol. 29, 1337–1348. doi: 10.1071/FP02056
Goto, M., Ehara, H., Karita, S., Takabe, K., Ogawa, N., Yamada, Y., et al. (2003). Protective effect of silicon on phenolic biosynthesis and ultraviolet spectral stress in rice crop. Plant Sci. 164, 349–356. doi: 10.1016/S0168-9452(02)00419-3
Greger, M., Landberg, T., and Vaculík, M. (2018). Silicon influences soil availability and accumulation of mineral nutrients in various plant species. Plants 7:41. doi: 10.3390/plants7020041
Hadgu, G., Tesfaye, K., Mamo, G., and Kassa, B. (2013). Trend and variability of rainfall in Tigray, Northern Ethiopia: Analysis of meteorological data and farmers' perception. Available at: http://hdl.handle.net/10883/3354 (accessed July 29, 2024).
Haftom, H., Haftu, A., Goitom, K., and Meseret, H. (2019). Agroclimatic zonation of Tigray region of Ethiopia based on aridity index and traditional agro-climatic zones. J. Agrometeorol. 21, 176–181. doi: 10.54386/jam.v21i2.229
Hagos, F. (2003). Poverty, Institutions, Peasant Behavior and Conservation Investment in Northern Ethiopia. Oslo: Department of Economics and Social Sciences Agricultural University of Norway.
Hallpike, C. R. (1972). The Konso Of Ethiopia. Oxford: Clarendon Press. Available at: https://www.hallpike.com/book/the-konso-of-ethiopia/ (accessed May 23, 2024).
Handley, L. L., Austin, A. T., Robinson, D., Scrimgeour, C. M., Raven, J. A., Heaton, T. H. E., et al. (1999). The 15N natural abundance (δ 15N) of ecosystem samples reflects measures of water availability. Aust. J. Plant Physiol. 26, 185–199. doi: 10.1071/PP98146
Hattersley, P. (1982). δ13 values of C4 types in grasses. Funct. Plant Biol. 9, 139–154. doi: 10.1071/PP9820139
Heaton, T. H. E. (1987). The 15N/14N ratios in plants in South Africa and Namibia: relationship to climate and coastal saline environments. Oecologia 74, 236–246.
Heaton, T. H. E., Vogel, H., Von la Chevallerie, G., and Gollet, G. (1986). Climatic influence on the isotopic composition of bone nitrogen. Nature 322, 822–823.
Kohn, M. J. (2010). Carbon isotope compositions of terrestrial C3 plants as indicators of (paleo)ecology and (paleo)climate. Proc. Natl. Acad. Sci. USA. 107, 19691–19695. doi: 10.1073/pnas.1004933107
Körner, C., and Diemer, M. (1987). In situ photosynthesis responses to light, temperature and carbon dioxide in herbaceous plants from low and high altitude. Funct. Ecol. 1, 179–194. doi: 10.2307/2389420
Körner, C., Neumayer, M., Menendez-Riedl, S. P., and Smeets-Scheel, A. (1989). Functional morphology of mountain plants. Flora 182, 353–383. doi: 10.1016/S0367-2530(17)30426-7
Körner, Ch., Farquhar, G. D., and Wong, S. C. (1991). Carbon isotope discrimination by plants follows latitudinal and altitudinal trends. Oecologia 88, 30–40.
Kromdijk, J., Schepers, H. E., Albanito, F., Fitton, N., Carroll, F., Jones, M. B., et al. (2008). Bundle sheath leakiness and light limitation during C4 leaf and canopy CO2 uptake. Plant Physiol. 148, 2144–2155. doi: 10.1104/pp.108.129890
Li, X., Brestic, M., Tan, D.-X., Zivcak, M., Zhu, X., Liu, S., et al. (2018). Melatonin alleviates low PS I-limited carbon assimilation under elevated CO2 and enhances the cold tolerance of offspring in chlorophyll b-deficient mutant wheat. J. Pineal Res. 64:e12453. doi: 10.1111/jpi.12453
Lightfoot, E., Ustunkaya, M. C., Przelomska, N., O'Connell, T. C., Hunt, H. V., Jones, M. K., et al. (2020). Carbon and nitrogen isotopic variability in foxtail millet (Setaria italica) with watering regime. Rapid Commun. Mass Spectrom. 34:e8615. doi: 10.1002/RCM.8615
Liu, X., Tang, X., Compson, Z. G., Huang, D., Zou, G., Luan, F., et al. (2023). Silicon supply promotes differences in growth and C:N:P stoichiometry between bamboo and tree saplings. BMC Plant Biol. 23:443. doi: 10.1186/s12870-023-04443-0
Liu, X., and Wang, G. (2010). Measurements of nitrogen isotope composition of plants and surface soils along the altitudinal transect of the eastern slope of Mount Gongga in southwest China. Rapid Commun. Mass Spectrom. 24, 3063–3071. doi: 10.1002/rcm.4735
Ma, J. F., and Yamaji, N. (2008). Functions and transport of silicon in plants. Cell. Mol. Life Sci. 65, 3049–3057. doi: 10.1007/s00018-008-7580-x
Makarov, M. I. (2009). The nitrogen isotopic composition in soils and plants: its use in environmental studies (a review). Eurasian Soil Sc. 42, 1335–1347. doi: 10.1134/S1064229309120035
Makino, A., Sakuma, H., Sudo, E., and Mae, T. (2003). Differences between maize and rice in N-use efficiency for photosynthesis and protein allocation. Plant Cell Physiol. 44, 952–956. doi: 10.1093/pcp/pcg113
Masi, A., Sadori, L., Balossi Restelli, F., Baneschi, I., and Zanchetta, G. (2014). Stable carbon isotope analysis as a crop management indicator at Arslantepe (Malatya, Turkey) during the late chalcolithic and early Bronze age. Veg. Hist. Archaeobot. 23, 751–760. doi: 10.1007/s00334-013-0421-3
Masi, A., Sadori, L., Baneschi, I., Siani, A. M., and Zanchetta, G. (2013). Stable isotope analysis of archaeological oak charcoal from eastern Anatolia as a marker of mid-Holocene climate change. Plant Biol. 15, 83–92. doi: 10.1111/j.1438-8677.2012.00669.x
Meresa, Y., Ruiz-Girault, A., Beldados, A., Lancelotti, C., and D'Andrea, A. C. (2024). Pre-Aksumite and Aksumite Agricultural Economy at Ona Adi, Tigrai (Ethiopia): First Look at a 1000-Year History. Afr. Archaeol. Rev. 41, 239–268. doi: 10.1007/s10437-024-09595-4
Murphy, B. P., and Bowman, D. M. J. S. (2009). The carbon and nitrogen isotope composition of Australian grasses in relation to climate. Funct. Ecol. 23, 1040–1049. doi: 10.1111/j.1365-2435.2009.01576.x
Nadelhoffer, K., Shaver, G., Fry, B., Giblin, A., Johnson, L., and McKane, R. (1996). 15N natural abundances and N use by tundra plants. Oecologia 107, 386–394. doi: 10.1007/BF00328456
Nadelhoffer, K. J., and Fry, B. (1994). “Nitrogen isotope studies in forest ecosystems.,” in Stable Isotopes in Ecology and Environmental Science, eds. K. Lajtha and R. Michener (Boston:Wiley), 23–44.
Olivera-Viciedo, D., Oliveira, K. S., de Mello Prado, R., Habermann, E., Martínez, C. A., and de Moura Zanine, A. (2024). Silicon uptake and utilization on Panicum maximum grass modifies C:N:P stoichiometry under warming and soil water deficit. Soil Till. Res. 235:105884. doi: 10.1016/j.still.2023.105884
Pankhurst, K. P. (1961). An Introduction to the Economic History of Ethiopia from Early Times to 1800. London: Lalibela House.
Reid, R. E. B., Lalk, E., Marshall, F., and Liu, X. (2018). Carbon and nitrogen isotope variability in the seeds of two African millet species: Pennisetum glaucum and Eleusine coracana. Rapid Commun. Mass Spectrom. 32, 1693–1702. doi: 10.1002/rcm.8217
Riehl, S., Pustovoytov, K. E., Weippert, H., Klett, S., and Hole, F. (2014). Drought stress variability in ancient Near Eastern agricultural systems evidenced by δ13C in barley grain. Proc. Natl. Acad. Sci. USA. 111, 12348–12353. doi: 10.1073/pnas.1409516111
Ruiz-Giralt, A., and Beldados, A. (2024). The development of crop production in the northern Horn of Africa: a review of the archaeobotanical evidence. Azania: Archaeol. Res. Afr. 59, 183–212. doi: 10.1080/0067270X.2024.2316518
Ruiz-Giralt, A., Biagetti, S., Madella, M., and Lancelotti, C. (2023a). Small-scale farming in drylands: new models for resilient practices of millet and sorghum cultivation. PLoS ONE 18:e0268120. doi: 10.1371/journal.pone.0268120
Ruiz-Giralt, A., Nixon-Darcus, L., D'Andrea, A. C., Meresa, Y., Biagetti, S., and Lancelotti, C. (2023b). On the verge of domestication: early use of C4 plants in the Horn of Africa. Proc. Nat. Acad. Sci. 120:e2300166120. doi: 10.1073/pnas.2300166120
Sage, R. F. (2004). The evolution of C4 photosynthesis. New Phytol. 161, 341–370. doi: 10.1111/j.1469-8137.2004.00974.x
Sage, R. F., and Pearcy, R. W. (1987). The nitrogen use efficiency of C3 and C4 plants: I. Leaf nitrogen, growth, and biomass partitioning in Chenopodium album (L.) and Amaranthus retroflexus (L.). Plant Physiol. 84, 954–958.
Sanborn, L. H., Reid, R. E. B., Bradley, A. S., and Liu, X. (2021). The effect of water availability on the carbon and nitrogen isotope composition of a C4 plant (pearl millet, Pennisetum glaucum). J. Archaeol. Sci. Rep. 38:103047. doi: 10.1016/j.jasrep.2021.103047
Schmitt, M. R., and Edwards, G. E. (1981). Photosynthetic capacity and nitrogen use efficiency of maize, wheat, and rice: a comparison between C3 and C4 photosynthesis. J. Exp. Bot. 32, 459–466. doi: 10.1093/jxb/32.3.459
Schulze, E.-D., Gebauer, G., Ziegler, H., and Lange, O. L. (1991). Estimates of nitrogen fixation by trees on an aridity gradient in Namibia. Oecologia 88, 451–455. doi: 10.1007/BF00317592
Schulze, E. D., Ellis, R., Schulze, W., Trimborn, P., and Ziegler, H. (1996). Diversity, metabolic types and δ13C carbon isotope ratios in the grass flora of Namibia in relation to growth form, precipitation and habitat conditions. Oecologia 106, 352–369. doi: 10.1007/BF00334563
Sealy, J., van der Merwe, N. J., Thorp, J. A. L., and Lanham, J. L. (1987). Nitrogen isotopic ecology in southern Africa: implications for environmental and dietary tracing. Geochim. Cosmochim. Acta 51, 2707–2717.
Shearer, G., Kohl, D. H., and Chien, S.-H. (1978). The nitrogen-15 abundance in a wide variety of soils. Soil Sci. Soc. Am. J. 42, 899–902. doi: 10.2136/sssaj1978.03615995004200060013x
Siraj, E. H. (2021). An Archaeological Investigation of the Archaeobotanical Remains from the Site of Harlaa, Eastern Ethiopia. Addis Ababa: Addis Ababa University.
Styring, A. K., Charles, M., Fantone, F., Hald, M. M., McMahon, A., Meadow, R. H., et al. (2017). Isotope evidence for agricultural extensification reveals how the world's first cities were fed. Nat. Plants 3:17076. doi: 10.1038/nplants.2017.7
Swap, R. J., Aranibar, J. N., Dowty, P. R., Gilhooly, W. P., and Macko, S. A. (2004). Natural abundance of 13C and 15N in C3 and C4 vegetation of southern Africa: patterns and implications. Glob. Change Biol. 10, 350–358. doi: 10.1111/j.1365-2486.2003.00702.x
Szpak, P., Metcalfe, J. Z., and Macdonald, R. A. (2017). Best practices for calibrating and reporting stable isotope measurements in archaeology. J. Archaeol. Sci. Rep. 13, 609–661. doi: 10.1016/J.JASREP.2017.05.007
Tadesse, M. (2010). Living with Adversity and Vulnerability. Uppsala: Swedish University of Agricultural Sciences.
Tamiru, K. (2014). Indigenous Land Management Practices in Konso: South Ethiopia. Available at: https://www.semanticscholar.org/paper/Indigenous-Land-Management-Practices-in-Konso%3A-Karse/8238df60b297ac85c6f6ce5202e620152e6a1897 (accessed May 23, 2024).
Terefe, T., and Beldados, A. (2021). Ethnoarchaeological and experimental charring studies related to the cultivation of finger millet (Eleusine Coracana [L.] Gaertn.) in Northwestern Ethiopia. Ethnoarchaeology 13, 105–122. doi: 10.1080/19442890.2022.2076979
Tilman, D. (1988). Plant Strategies and the Dynamics of Structure of Plant Communities. Princeton, NJ: Princeton University Press.
Tsegaye, B. (2019). Effect of land use and land cover changes on soil erosion in Ethiopia. Int. J. Agric. Sci. Food Technol. 5, 026–034. doi: 10.17352/2455-815X.000038
Tubaña, B. S., and Heckman, J. R. (2015). “Silicon in soils and plants,” in Silicon and Plant Diseases, eds. F. A. Rodrigues and L. E. Datnoff (Cham: Springer International Publishing), 7–51. doi: 10.1007/978-3-319-22930-0_2
Ubierna, N., Sun, W., Kramer, D. M., and Cousins, A. B. (2013). The efficiency of C4 photosynthesis under low light conditions in Zea mays, Miscanthus x giganteus and Flaveria bidentis. Plant Cell Environ. 36, 365–381. doi: 10.1111/j.1365-3040.2012.02579.x
UNDP Situation Report (1999). Ethiopia: Rapid Assessment Report: Konso Special Wereda, SNNPR - Ethiopia | ReliefWeb. Available at: https://reliefweb.int/report/ethiopia/ethiopia-rapid-assessment-report-konso-special-wereda-snnpr (accessed May 23, 2024).
van Groenigen, J.-W., and van Kessel, C. (2002). Salinity-induced patterns of natural abundance carbon-13 and nitrogen-15 in plant and soil. Soil Sci. Soc. Am. J. 66, 489–498. doi: 10.2136/sssaj2002.4890
Varalli, A., D'Agostini, F., Jiménez-Arteaga, C., Haidgen, S., Bouchaud, C., Antonites, A., et al. (2024). “Unravelling past agricultural systems in arid environments: advances and limits in isotopic analysis and modelling,” in 29th Annual Meeting of the European Association of Archaeologists (Rome).
Varalli, A., D'Agostini, F., Madella, M., Fiorentino, G., and Lancelotti, C. (2023a). Charring effects on stable carbon and nitrogen isotope values on C4 plants: inferences for archaeological investigations. J. Archaeol. Sci. 156:105821. doi: 10.1016/j.jas.2023.105821
Varalli, A., Peake, R., Auxiette, G., Balter, V., Delattre, V., Gouge, P., et al. (2023b). Insights into the frontier zone of Upper Seine Valley (France) during the Bronze Age through subsistence strategies and dietary patterns. Archaeol. Anthropol. Sci. 2023 15:3 15, 1–28. doi: 10.1007/S12520-023-01721-8
Verma, K. K., Singh, R. K., Song, Q. Q., Singh, P., Zhang, B.-Q., Song, X.-P., et al. (2019). Silicon Alleviates drought stress of sugarcane plants by improving antioxidant responses. BJSTR 17, 12580–12586. doi: 10.26717/BJSTR.2019.17.002957
Wang, G., Han, J., Faiia, A., Tan, W., Shi, W., and Liu, X. (2008). Experimental measurements of leaf carbon isotope discrimination and gas exchange in the progenies of plantago depressa and Setaria viridis collected from a wide altitudinal range. Physiol. Plant. 134, 64–73. doi: 10.1111/j.1399-3054.2008.01102.x
Wang, G., Han, J., Zhou, L., Xiong, X., Tan, M., Wu, Z., et al. (2006). Carbon isotope ratios of C4 plants in loess areas of North China. Sci. China Ser. D 49, 97–102. doi: 10.1007/s11430-004-5238-6
Watson, E. E. (2009). Living Terraces in Ethiopia: Konso Landscape, Culture and Development. Boydell & Brewer. Available at: https://www.jstor.org/stable/10.7722/j.ctt81fh5 (accessed May 23, 2024).
Weiguo, L., Xiahong, F., Youfeng, N., Qingle, Z., Yunning, C., and Zhisheng, A. N. (2005). δ13C variation of C3 and C4 plants across an Asian monsoon rainfall gradient in arid northwestern China. Glob. Chang. Biol. 11, 1094–1100. doi: 10.1111/j.1365-2486.2005.00969.x
Williams, D. G., Gempko, V., Fravolini, A., Leavitt, S. W., Wall, G. W., Kimball, B. A., et al. (2001). Carbon isotope discrimination by Sorghum bicolor under CO2 enrichment and drought. New Phytol. 150, 285–293. doi: 10.1046/j.1469-8137.2001.00093.x
Winchell, F., Brass, M., Manzo, A., Beldados, A., Perna, V., Murphy, C., et al. (2018). On the origins and dissemination of domesticated sorghum and pearl millet across africa and into india: a view from the butana group of the Far Eastern Sahel. Afr. Archaeol. Rev. 35, 483–505. doi: 10.1007/s10437-018-9314-2
Yang, Q., and Li, X. (2015). Investigation of the controlled factors influencing carbon isotope composition of foxtail and common millet on the Chinese Loess Plateau. Sci. China Earth Sci. 58, 2296–2308. doi: 10.1007/s11430-015-5181-8
Yeshambel, M. (2013). Indigenous knowledge practices in soil conservation at Konso People, South Western Ethiopia. J. Agric. Environ. Sci. 2, 1–10.
Keywords: traditional agriculture, sorghum, finger millet, nitrogen and carbon stable isotopes, Ethiopia
Citation: Varalli A, Beldados A, D'Agostini F, Mvimi M, D'Andrea C and Lancelotti C (2024) Isotopic analysis of modern sorghum and finger millet from different altitudes in Ethiopia: implications for ancient farming practices. Front. Environ. Archaeol. 3:1473056. doi: 10.3389/fearc.2024.1473056
Received: 30 July 2024; Accepted: 11 October 2024;
Published: 06 November 2024.
Edited by:
Darren R. Gröcke, Durham University, United KingdomReviewed by:
Rachel E. B. Reid, Virginia Tech, United StatesLukasz Pospieszny, University of Gdansk, Poland
Copyright © 2024 Varalli, Beldados, D'Agostini, Mvimi, D'Andrea and Lancelotti. This is an open-access article distributed under the terms of the Creative Commons Attribution License (CC BY). The use, distribution or reproduction in other forums is permitted, provided the original author(s) and the copyright owner(s) are credited and that the original publication in this journal is cited, in accordance with accepted academic practice. No use, distribution or reproduction is permitted which does not comply with these terms.
*Correspondence: Alessandra Varalli, YWxlc3NhbmRyYS52YXJhbGxpQHVuaXYtYW11LmZy