- 1Department of Art and Culture, History, Antiquity, Faculty of Humanities, Vrije Universiteit of Amsterdam, Amsterdam, Netherlands
- 2Earth and Climate Cluster, Faculty of Science, Vrije Universiteit of Amsterdam, Amsterdam, Netherlands
- 3Department of Natural Sciences and Environmental Health, University of South-Eastern Norway, Bø, Norway
- 4Mediterranean Institute for Agriculture, Environment and Development (MED) and Institute for Global Change and Sustainability Associated Labs (CHANGE), Universidade de Évora, Évora, Portugal
- 5Departamento de Paisagem, Ambiente e Ordenamento, Colégio Luís António Verney, Universidade de Évora, Évora, Portugal
- 6Departamento de Geografía Física y Análisis Geográfico Regional, Facultad de Geografía e Historia, Universidad de Sevilla, Sevilla, Spain
- 7Spanish Association for the Study of Quaternary (AEQUA), Sevilla, Spain
Introduction: Enacting transitions toward more sustainable management and use of land, energy, and natural resources poses multiple challenges for human societies. Such transitions have been a constant throughout human history and therefore there is a need to learn from them and apply that knowledge to current land-use policies and management. Significant human impact on landscape and environment in Cantabrian Spain has been documented in alignment with the Neolithization (ca. 7,000 cal BP). While the classic approach of identifying cultural phases based on historical and archaeological data has been extensively studied, much less is understood on how such phases are dependent upon increasing anthropogenic influence on the environment.
Methods: Cantabrian Spain is well-known for its long mining history. Key processes historically shaping landscapes in the region include the implementation of mining/metallurgy industries and extraction of forest resources. These historical processes were characterized, respectively using heavy metal pollution contents (Hg, Zn, Cd, As, Ni, REE, Pb, and 206 Pb/207 Pb) and total arboreal pollen percentages in peat bogs, providing global trends of human impact on the environment. These trends were then compared to climate (temperature and precipitation) and natural vegetation evolution modeling through time.
Results: Results show seven phases of major human impact on the environment: (1) the Copper phase ca. 4,400–4,100 cal BP, (2) the Middle Bronze phase ca. 3,500–3,150 cal BP, (3) the Iron phase ca. 2,800–2,500 cal BP, (4) the Roman phase ca. 2,200–1,750 cal BP, (5) the Medieval phase ca. 1,250–1,000 cal BP, (6) the Colonial phase ca. 650–400 cal BP, and (7) the Industrial phase ca. 150 cal BP-Present.
Discussion: Four phases are tightly related to substantial changes in land use and subsistence strategies: (1) Production, with the appearance of productive economies during the Neolithic, (2) Specialization, with the appearance of specialized activities and trade during the Middle Bronze phase, (3) Urbanization, with the first urban centers during the Roman phase, and (4) Globalization, with worldwide colonialism and capitalism economies during the Colonial phase.
Introduction
Rising sea-level and increasing global temperatures driven by greenhouse gas emissions, biodiversity loss, and depletion of primary resources are urgent challenges that human societies are currently facing (IPCC, 2022). Major changes in our energy systems and landscape management are needed to render our society more sustainable. But such challenges are not new; landscape changes in Europe have been well-documented since the Mid-Holocene, starting with the foundation of the first farming communities. Expansion of cropland and pastures, loss of wilderness and wild land, land use intensification, urbanization and industrialization are key processes related to the historical human transformation of landscapes (Ellis and Ramankutty, 2008; Ellis, 2015).
One way to describe major changes in human societies is through the use of energy. Energy is available in primary resources such as wood, coal, food, etc., and can be stocked, converted or used to produce useful work in numerous activities (heating, farming, metallurgy, etc.). Energy use largely changed through time along with knowledge and technological evolution (Sieferle, 2001; Burke, 2009; Fischer-Kowalski et al., 2014; Krausmann et al., 2016). The framework of Energy Regimes (ERs) considers resources and energy sources that sustain the development of human societies, and explores the inter-relations between human societies and their environment (Vlachogianni and Valavanidis, 2013). ERs are independent from time and cultural sequences, allowing comparison from past and modern societies. Four ERs are currently acknowledged: hunter-gatherer, agrarian, industrial and low-carbon (Goudsblom, 1992; Sieferle, 2001; Fischer-Kowalski and Haberl, 2007; Simmons, 2008; Burke, 2009; Valavanidis and Vlachogianni, 2013). If the theoretical framework of ERs has been developed for more than 30 years, its application in concrete case study is a novel approach (Martinez et al., 2022, 2023). ERs can be addressed with geoarchaeological proxies to explore land-use strategies and the impact of anthropic activities on the environment.
The complex ways in which climate has influenced vegetation and cultural changes during the Holocene have been widely debated (DeMenocal, 2001; Roberts et al., 2011; Lillios et al., 2016; Griffiths and Robinson, 2018). Coincidences between climatic changes and cultural developments or major societal disruptions have been detected (Berglung, 2003; Arbogast et al., 2006; Turney et al., 2006; Schmid et al., 2015) along with the influence of climate and human activities on landscape and vegetation evolution (Martínez-Cortizas et al., 2005; Jalut et al., 2009; López-Merino et al., 2012; Silva-Sánchez et al., 2014; Silva-Sánchez, 2015; Sánchez-Morales, 2023).
A wealth of studies on landscape transformations in relation to human activities in Northern Spain have already provided a well-documented landscape evolution storyline throughout the Holocene. Nonetheless, the focal point of such research line is often limited to specific time periods or cultural entities, and considers very specific thematics, such as fire activity (Pérez-Obiol et al., 2016; Carracedo et al., 2018; Uzquiano, 2018), agrarian activity (López-Merino et al., 2010, 2011; Fernández Mier et al., 2014), vegetation and environmental reconstitution (Schellekens et al., 2011; Irabien et al., 2015; Pérez-Díaz et al., 2016b), climate evolution (Muñoz-Sobrino et al., 2005; Mighall et al., 2006, 2023), or human land-use (López-Merino et al., 2014; Pérez-Díaz et al., 2018; González-Álvarez, 2019a,b). All studies acknowledge the difficulty to disentangle anthropogenic influences on landscape changes from natural ones (Mighall et al., 2023).
In this work, we focus on the identification of major changes in the history of human impact on the environment in Northern Spain along the Holocene. This region is well-known for its long history of mining and metallurgy (Comendador-Rey et al., 2017), and its well-documented vegetation history and exploitation by human groups (Sánchez-Morales, 2023). Northern Spain hosts the largest metal ore belt in Western Europe which has been extensively exploited since the late Prehistory (Comendador-Rey et al., 2017; Fernández et al., 2018). It also possesses a substantial amount of wetlands, lakes and caves that act as natural archives for pollen accumulation, which can provide insights into forest cover evolution (López-Sáez et al., 2006; Sánchez-Morales, 2023). Hence, to disentangle these complex histories, Heavy metal content (HMC) and Total Tree Cover (TTC) have been used as indicators of human mining and metallurgy activities and forest exploitation, respectively. Previous interdisciplinary studies in Europe taking into account geochemical, palynological and archaeological/historical proxies to identify phases of human impact (e.g., Wick et al., 2003; Lomas-Clarke and Barber, 2006; Pontevedra-Pombal et al., 2013; Wacnik et al., 2016; Bajard et al., 2022; Robles et al., 2022) are multiplying and provided interesting results. The goals of this paper are: (1) to propose an interdisciplinary synthesis of, and confront available information, on HMC and TTC in Northern Spain, (2) to identify key periods of major changes in human impact history in this region, (3) to confront our results with cultural contexts from archaeological and historical literature, and (4) to develop and test the ERs framework for agrarian societies based on our multi-proxy analysis.
Geographic and temporal settings
Our geographic focus is on Cantabrian Spain, which is spread across the NUTS-2 regions (Eurostat, 2020) of Asturias, Cantabria, and the Basque Country (provinces of Biscay and Gipuzkoa; Figure 1). The study area covers a surface of ca. 20,000 km2 which is dominated by karstic limestone lithology (IGME and LNEG, 2015), alterning with metamorphic substrates (such as shales or sandstones) and some granite outcrops (Vera, 2004). The collision of European and Iberian tectonic plates during the Alpine orogeny resulted in a high intensity of crustal deformations and a very sharp topography (Amigo et al., 2017). The study area fits fully within the Eurosiberian biogeographic region (Rivas-Martinez, 2007), under a wet and temperate climate with abundant rainfall, even during summer, and a low yearly variation of temperatures (Amigo et al., 2017; Díaz González and Penas, 2017). These conditions favored the development of deciduous forests dominated by taxa such as Quercus and Fagus, among others, with the presence of evergreen species (i.e., Ilex and Pinus, among others), grasslands, and heathlands in higher altitudes (Amigo et al., 2017; Díaz González and Penas, 2017). Warm and wet conditions together with topographic and geomorphological caracteristics are favorable to the formation of numerous peatlands in flat mountain tops (Ramil-Rego et al., 1996; Izco et al., 2001). The study area is limited to the north by the Bay of Biscay and to the south by the ridge line of the Cantabrian mountain range. The westernmost major waterway is the Navia river in Asturias, which is located over limestone karst terrains and marks the western boundary of the study area. Its eastern boundary is delimited by the Bidasoa river in Gipuzkoa, which is the easternmost major waterway that discharges into the Bay of Biscay. The study area is marked by a dissected terrain with inner valleys and mountains consecutively following each other over very short distances (Mata Olmo and Sanz Herráiz, 2004). The Cantabrian range consists in rugged and youthful mountains, which are some of the most recently formed and steep in the Iberian Peninsula (Meléndez Hevia, 2004).
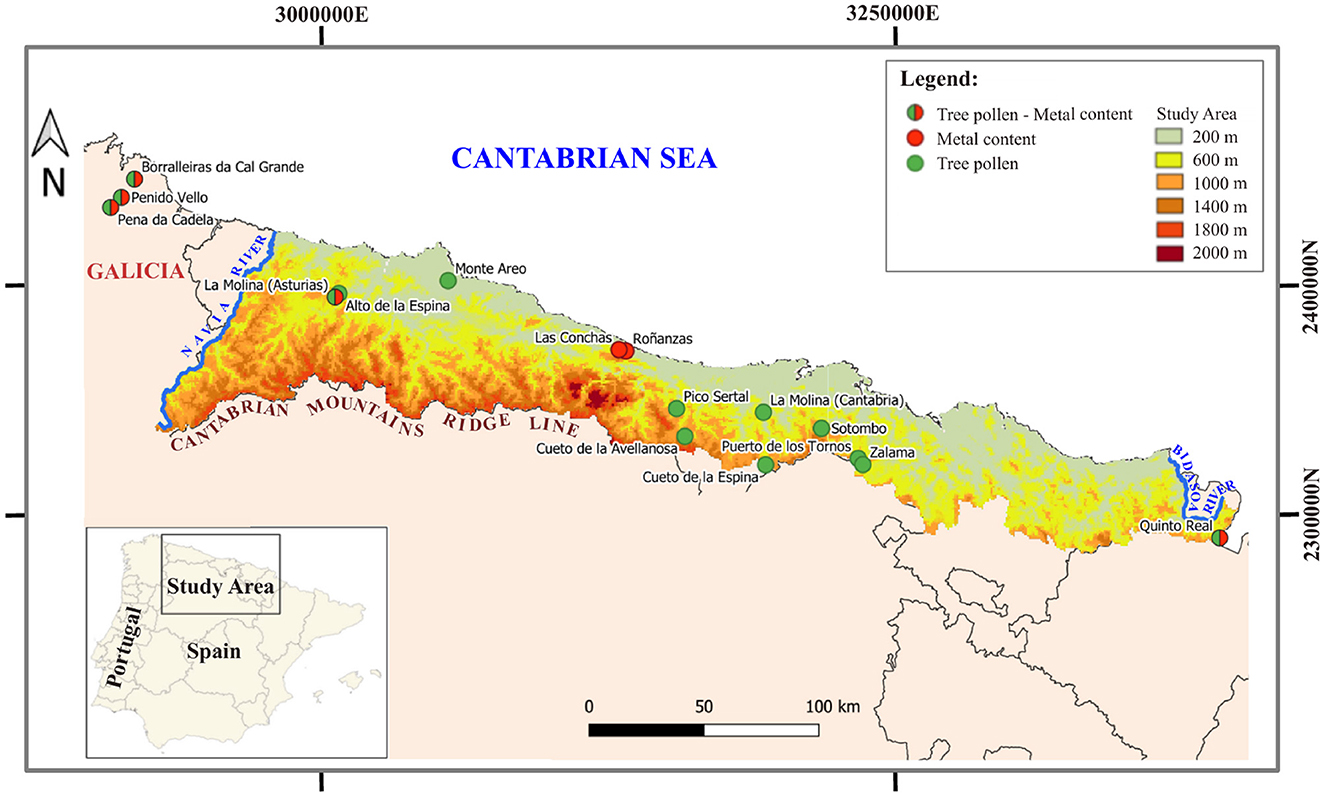
Figure 1. Study area in Northern Spain with localization of peat bogs with tree pollen (green) and metal content (red) data.
In this work we investigate the impact of anthropogenic activities on the landscape during the Holocene, stretching between the Neolithic Agricultural (ca. 7,000 cal BP), and the Industrial Revolutions (ca. 150 cal BP). This time period therefore encompasses a long succession of cultural phases and socio-political regimes (Martín, 1975; Marín Suárez, 2011; Lillios, 2019) as well as unstable climatic sequences of warm/wet and cold/dry periods (Bond et al., 2001; Martín-Chivelet et al., 2011; Walker et al., 2012; Baldini et al., 2019). A synthesis of the successions of cultural phases and climatic sequences, retrieved from the literature, is provided in Figure 2. Both anthropogenic and climatic sequences have influenced the configuration and evolution of landscapes during the Holocene, making it particularly challenging to separate one source from the other (Ellis et al., 2021). Moreover, landscape evolution is closely linked to phases of development and disruptions of the evolution of human societies. The adoption of productive economies totally sifted prior land-use strategies by hunter-gatherers (Martinez et al., 2023), facilitating many other societal innovations and/or disruptions during late prehistoric and historical times that could have important consequences on land-use and landscapes. Among those societal innovations, settlement of Bronze Age populations into villages led to unprecedented land clearing with more cultivated and managed landscapes (Ontañón-Peredo, 1995; Amigo et al., 2017). Another major disruption is the colonization of other continents by Iberian political authorities from the fifteenth century AD onward, leading to the introduction of many foreign wood, plant and fiber resources into the region, and significantly affecting the local biodiversity (Amigo et al., 2017).
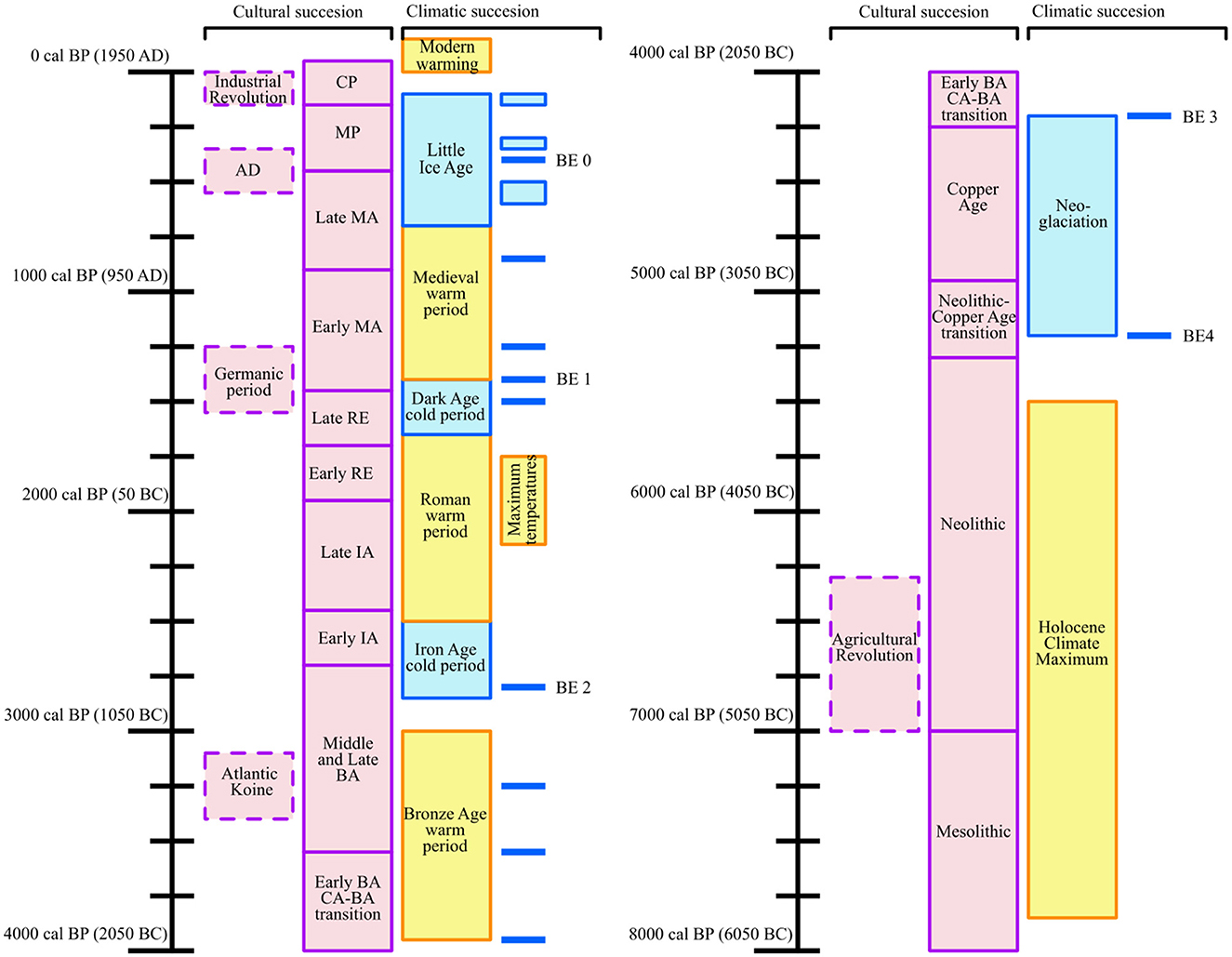
Figure 2. Cultural phases and climate sequences extracted from the literature (8,000 cal BP-Present day). The timeline is separated into two columns; (1) from present day to 4,000 cal BP on the left, and (2) from 4,000 cal BP to 8,000 cal BP on the right. Thick lines represents short cold events. Abbreviations for climate sequences: BE, Bond Event. Sources for climate sequence: Holocene Climate Maximum dates (López-Merino et al., 2010); Neoglaciation dates (Baldini et al., 2019); Bronze Age warm period, Iron Age cold period, Roman warm period, Dark Ages cold period, Medieval warm period, Little Ice Age and Modern warming dates, and all short cold events (Martín-Chivelet et al., 2011); Bond events dates (Bond et al., 2001). BE0: 0.4 ka event cal BP; BE1: 1.4 ka event cal BP; BE2: 2.8 ka event cal BP; BE3: 4.2 ka event cal BP; BE4: 5.2 ka event cal BP. Abbreviation for cultural phases: CA, Copper Age; BA, Bronze Age; IA, Iron Age; RE, Roman Empire; MA, Middle Ages; MP, Modern Period; AD, Age of Discoveries; CP, Contemporary Period. Sources for cultural sequence: Neolithic starting date (Fano et al., 2015); Agricultural Revolution dates (Martinez et al., 2022); Neolithic ending date and Copper Age dates (Pérez-Obiol et al., 2016); Early BA dates (there is no clear boundary between the end of the CA and the beginning of the Early BA; Blanco-González et al., 2018; Lillios, 2019); Atlantic Koine dates (Pontevedra-Pombal et al., 2013); Late BA-Early IA transition dates (Pérez-Obiol et al., 2016); Early IA-Late IA transition, Late IA-Early RE transition, and Early RE-Late RE transition dates (López-Merino et al., 2014); Late RE-Early MA transition, Early MA-Late MA transition, and Late MA-MP transitions dates, and Germanic period (also called the late Roman-medieval); dates (Martín, 1975); AD dates (Arnold, 2021); Industrial Revolution date (Leorri et al., 2014); CP dates (Blumenberg, 2008).
Methodology
In order to disentangle complex histories of cultural influences on the landscape over deep time history, two main methods are presented relating to geochemistry and palynology. Secondly, modeling and interpolation methodologies are given on climate simulations (temperature and precipitation) and natural forest cover.
HMC (stipulated using geochemical signals) and TTC (stipulated using palynological signals), as they evolve through time, are sound proxies for studying human activities in agrarian societies (Martínez-Cortizas et al., 2009). Both geochemical and palynological signals have been retrieved from cores in ombrotrophic and minerotrophic peat bogs. Ombrotrophic peat bogs are hydrologically isolated from the soil and receive atmospheric inputs only, whilst minerotrophic peat bogs are also influenced by the geochemistry of the soil (Martínez-Cortizas et al., 2002a; Kylander et al., 2005). Hence, ombrotrophic peat bogs are valuable sedimentary archives for detecting atmospheric pollution in heavy metals and pollen deposition, and thus signals from such archives have been preferential in our research work.
The evolution through time of HMC and TTC can be influenced by anthropogenic activities as well as by climatic parameters (Mighall et al., 2023). Hence, both HMC and TTC records were confronted with models of natural vegetation, and with temperature and precipitation indexes in order to refine the extent of climatic and anthropogenic influence on both indicators.
Heavy metal content
HMC content considered various geochemical elements described in Table 1. Lead (Pb) has been a major pollutant since the Copper Age and remains immobile in peat bogs (MacKenzie et al., 1997; Weiss et al., 1999), making it a valuable element to investigate human impact. Nickel (Ni), zinc (Zn), arsenic (As), cadmium (Cd) are largely present in metal dust caused by copper, tin, iron, and gold extraction (Pontevedra-Pombal et al., 2013), and hence are good proxies for mining activities. Mercury (Hg) derives from aerosols released by various anthropogenic activities (Roos-Barraclough et al., 2002; Moreno et al., 2005).
There is a limited number of HMC records from peat bogs within the time frame considered in this study in Cantabrian Spain. A total of 10 geochemical signals in seven peat bogs could be retrieved from the literature (description of the geochemical signals and their sources are given in Table 1). More records of geochemical signals exist (i.e., Martínez-Cortizas et al., 1997a,b, 1999, 2020; Galop et al., 2001; Moreno et al., 2009; Rauch et al., 2010), but either present signals with inseparable natural and anthropogenic sources, or their results have been updated and refined by more recent studies. Indeed, the technique and methodology to identify and measure the anthropogenic extent of HMC evolved along the last 30 years and has recently been managed to discern between natural and anthropogenic sources. Hence, for each geochemical signal we considered the most recent and precise measures (enrichment factor of any given element), and when available, the direct anthropogenic pollution signal.
Three peat bogs (Penido Vello, Pena da Cadela, and Borralleiras da Cal Grande) out of seven with geochemical signals are located out with our case study area, but are nonetheless placed at < 65 km from its limits and still within the Eurosiberian biogeographic region (Figure 1). Moreover, these geochemical signals are clear with respect to both their local and regional provenance (Galop et al., 2001; Gallego et al., 2019), making these three peat bogs a relevant complement to the four others placed within the study area.
For each record of geochemical signal (Table 1), sequences of increasing pollution both during high and low pollution phases were highlighted and reported on a unique time scale with a time resolution of 50 years (Figure 3). Periods of major changes were then identified, either caused by changing human behavior or by changing climate.
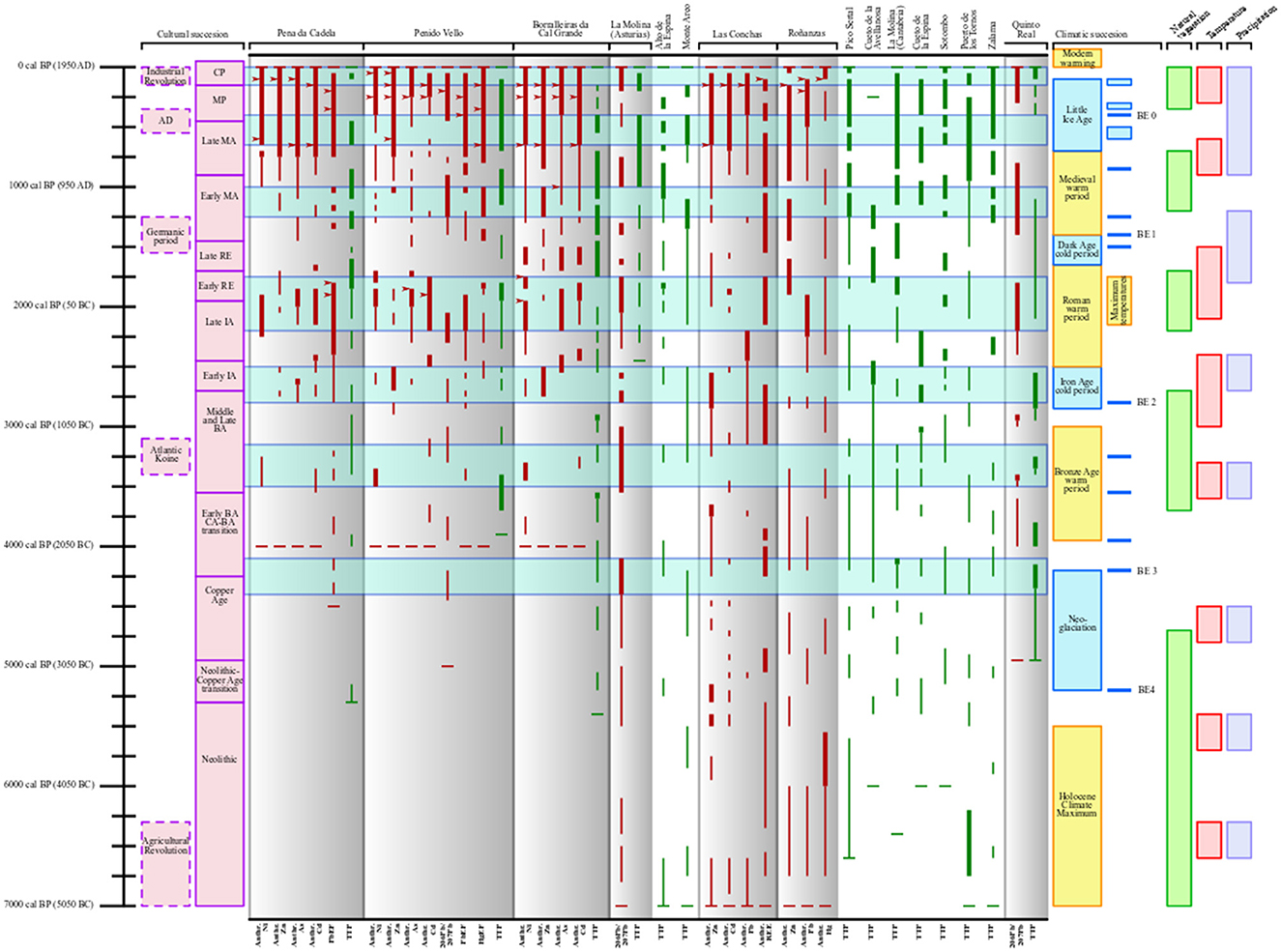
Figure 3. Synthesis of heavy metal pollution and Total Tree Cover signals for the 16 cores. Thin red lines: episodes of increased metal pollution during a phase of low pollution. Thick red line: episodes of increased metal pollution during phase of high pollution. Thin green lines: episodes of forest retreat during a phase of high forest cover. Thick green line: episodes of forest retreat during a phase of lower forest cover. Absence of lines: phases of stagnation or recovery (for both metal pollution and forest cover). Arrow heads: dramatic peaks or accelerations of the signal. On the right side, phases of increasing natural vegetation cover, temperature and precipitation are represented, respectively by green, red and blue bands. Absence of bands: phases of stagnation or decreasing natural vegetation cover, temperature and precipitation. Cal BP, years calibrated before present; BC, years calibrated before Christ; Anthr, anthropogenic; Ni, nickel; Zn, zinc; As, arsenic; Cd, cadmium; Pb, lead; Hg, mercury; REE, rare earth element; PbEF, enrichment factor of lead; HgEF, enrichment factor of mercury; 206 Pb/207 Pb; lead isotope ratio; TTP, Total Tree Pollen; BE, Bond Event. BE0: 0.4 ka event cal BP; BE1: 1.4 ka event cal BP; BE2: 2.8 ka event cal BP; BE3: 4.2 ka event cal BP; BE4: 5.2 ka event cal BP.
Total Tree Cover
TTC information in Northern Spain has recently been synthesized by Sánchez-Morales (2023). From this work, 14 relevant pollen records were identified and selected for use in the present study (Table 2) based on the following criteria: (1) pollen records are located within the study area or in peat bogs where HMC records also exist, (2) they cover most of the timespan of agrarian societies, and (3) a precise chronology of the evolution of total tree pollen accumulation has been set for each of these. All sources for each of these records are provided in Table 2. Records from Penido Vello, Pena da Cadela, and Borralleiras da Cal Grande peat bogs are situated out with, but close to the study area, and were finally included in our study because they also provided HMC information additionally complying with the same requirements in Section 3.1 above.
As for HMC records, sequences of decline of forest cover, both during phases of high and low forest cover, were highlighted and reported based on the same timescale as the increasing metal content sequences (Figure 3). Total tree pollen records in pollen diagrams across the study area provided information on the forest cover at regional scale. Pollen deposition in peat bogs can have local origins, potentially leading to biases in the regional representation of forest cover in certain historical periods. Another limitation to this proxy is the extent to which taxa may be over-/under represented in the records regarding local ecologies.
Modeling and interpretation
Climate simulations
Models of temperature and precipitation indexes were confronted to natural and current forest cover signals in order to enhance the identification of anthropogenic influence over forest evolution.
Climate simulations were performed with the iLOVECLIM model, an Earth system model of intermediate complexity. The version of the iLOVECLIM used in this study includes an atmospheric component (ECBilt), an oceanic general circulation model (CLIO), and a simple land vegetation model (VECODE; Roche et al., 2014). An interactive downscaling was utilized to increase the resolution from 55 to 25 km for the overall territory of Europe (Quiquet et al., 2018). Transient simulations of temperature and precipitation for the Holocene (spanning from 7,200 cal BP to Present) were also performed for our case study area. This time period was divided into 24 time windows of 300 years each. The simulations were performed using the appropriate climate forcings; orbital forcings (Berger, 1978), atmospheric trace gas concentrations of CO2, CH4, and N2O (Raynaud et al., 2000), which vary annually for the simulation period. Constant ice sheet configurations were prescribed while the solar constant and aerosol levels were kept fixed at pre-industrial levels. The downscaling applied to the model increased the spatial variability and the results of temperature and precipitation agrees better with proxy based data as compared to the low resolution version of the model (Arthur et al., 2023).
The temperatures index corresponds to the yearly mean of temperatures and is expressed in degree Celsius (°C; Figure 4). The precipitation index corresponds to the yearly amount of precipitation and is expressed in meters per year (m/yr; Figure 5). The results of the climate simulation are given in Figure 4.
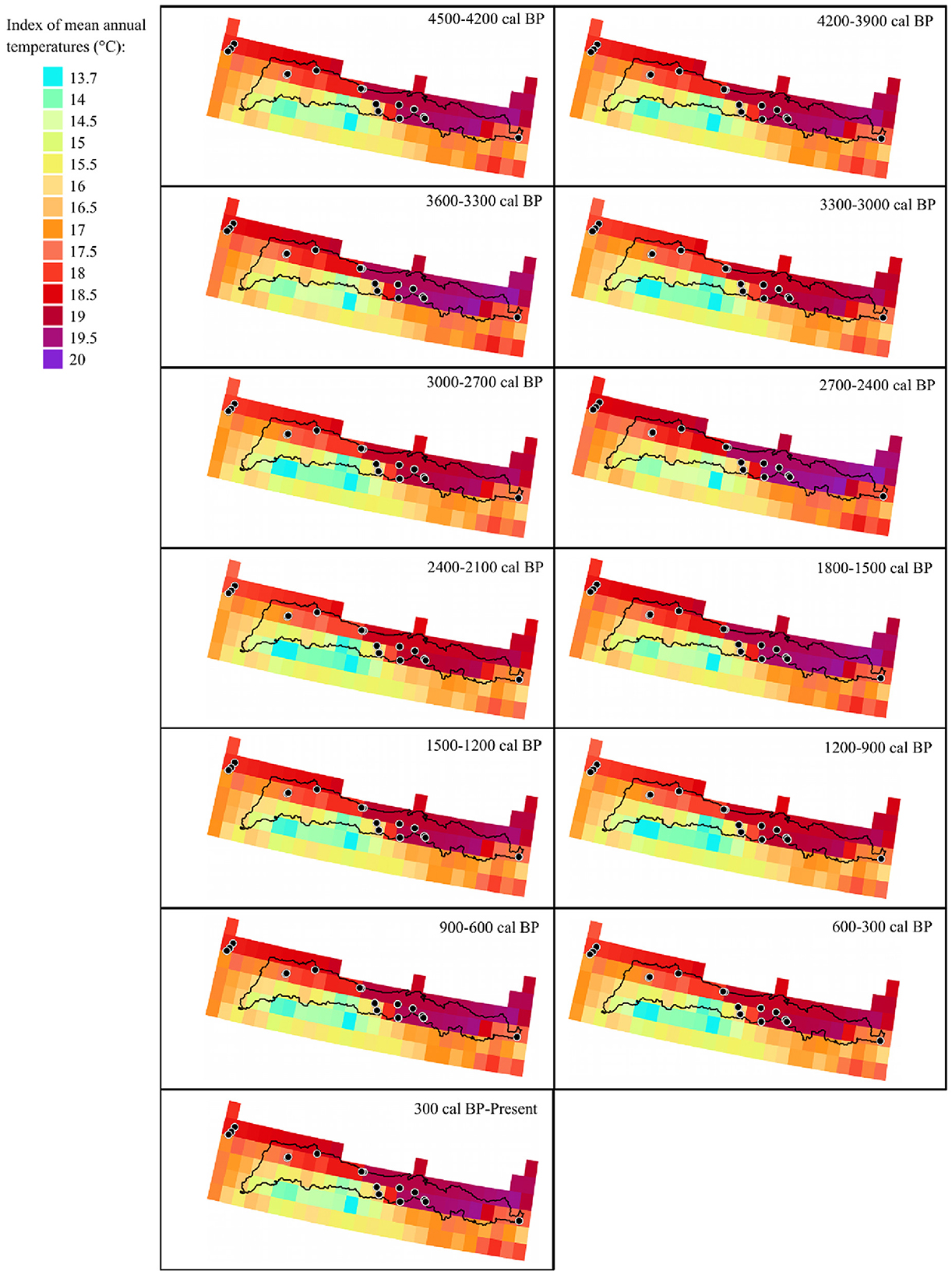
Figure 4. Modeling of annual mean of temperature index across the study area from 4,500 cal BP to Present, expressed in degree Celsius (°C). Only time windows with dating crossing episodes of major changes in agrarian societies are represented.
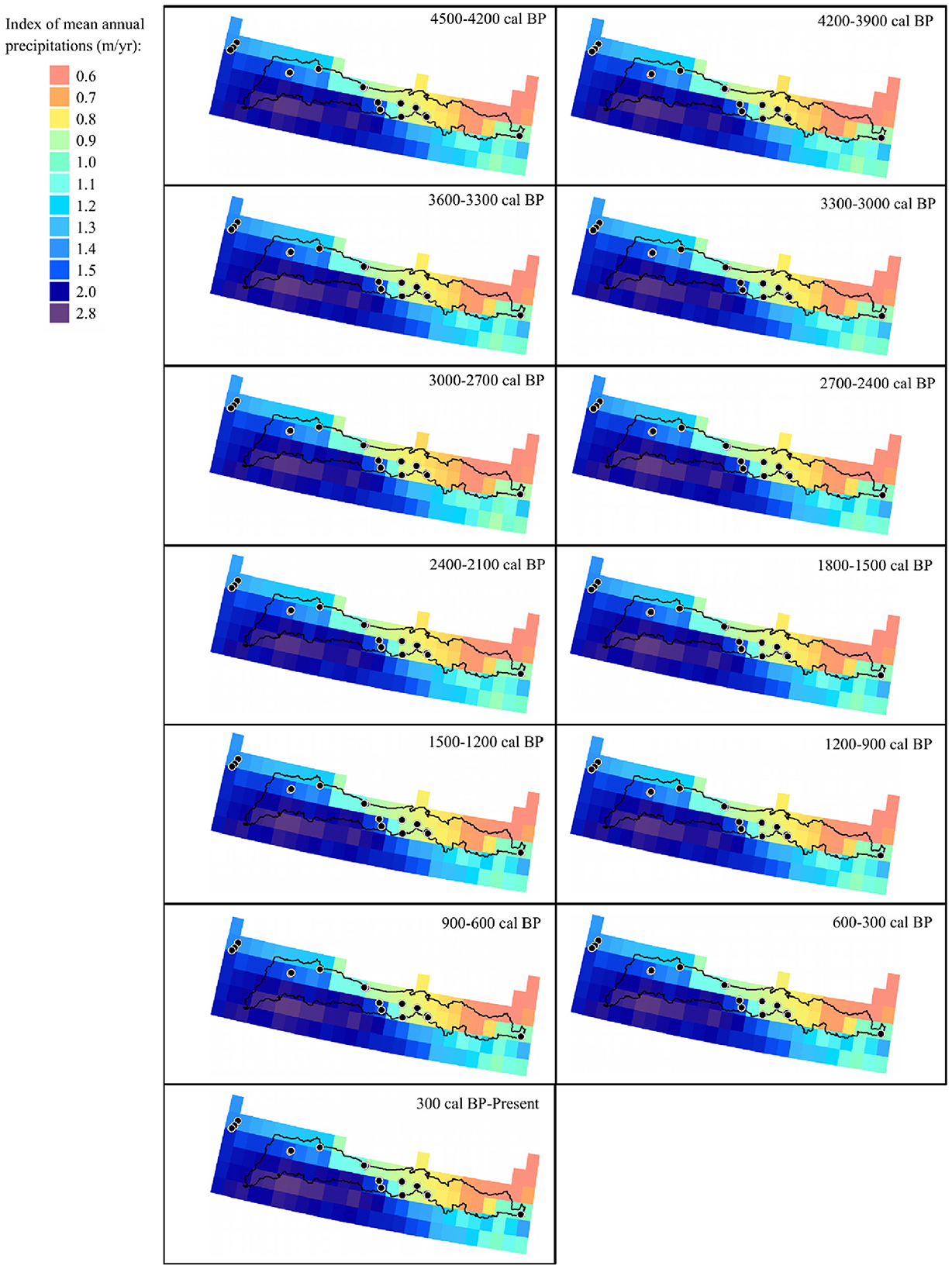
Figure 5. Modeling of annual mean of precipitation indexes across the study area from 4,500 cal BP to Present, expressed in meters per year (m/yr). Only time windows with dating crossing episodes of major changes in agrarian societies are represented.
Natural forest cover
The evolution of natural forest cover through time was modeled to provide a baseline to compare with the observed forest cover obtained from pollen diagrams. The difference between both signals would allow us to refine the anthropogenic influence over forest cover through time.
Natural forest cover was modeled using the CARAIB digital vegetation model (Warnant et al., 1994; Otto et al., 2002; Laurent et al., 2008; Dury et al., 2011; François et al., 2011). The CARAIB-simulated dataset represents the potential distribution of natural vegetation driven solely by climate conditions (Zapolska et al., 2023a). CARAIB simulated vegetation dynamics based on climatic variables, generated via the iLOVECLIM model (Goosse et al., 2010), revised by Roche (2013) and further expanded upon by Quiquet et al. (2018) using an integrated online interactive downscaling approach (Quiquet et al., 2018). To ensure higher reliability of the modeled results, climate variables were subjected to correction of bias by applying the CDF-t bias correction technique (Vrac, 2018; Zapolska et al., 2023b). The specificities of the modeling setup, along with the application of the CDF-t technique within this framework, were previously described and validated by Zapolska et al. (2023a,b).
CARAIB outputs used in this study include distribution of fractions of nine plant functional types for the period 7,200 BP—Present, and describe the hypothetical distribution of plant functional types that could exist in our study area under environmental conditions naturally changing through time, which allowed for the consideration of absence of influence by human activities and other disturbances (Zapolska et al., 2023a). A spatial resolution of 25 km was applied, and the time period of 7,200 years was divided into 16 time windows of 500 years each, starting from 7,200 cal BP up to Present. The period from 700 cal BP to Present was further divided into 3 time windows: 700–350 cal BP, 350–100 cal BP, and 100–0 cal BP. Finally, the forest cover is expressed in a percentage of land covered by forest. The results of the natural vegetation cover are given in Figure 6.
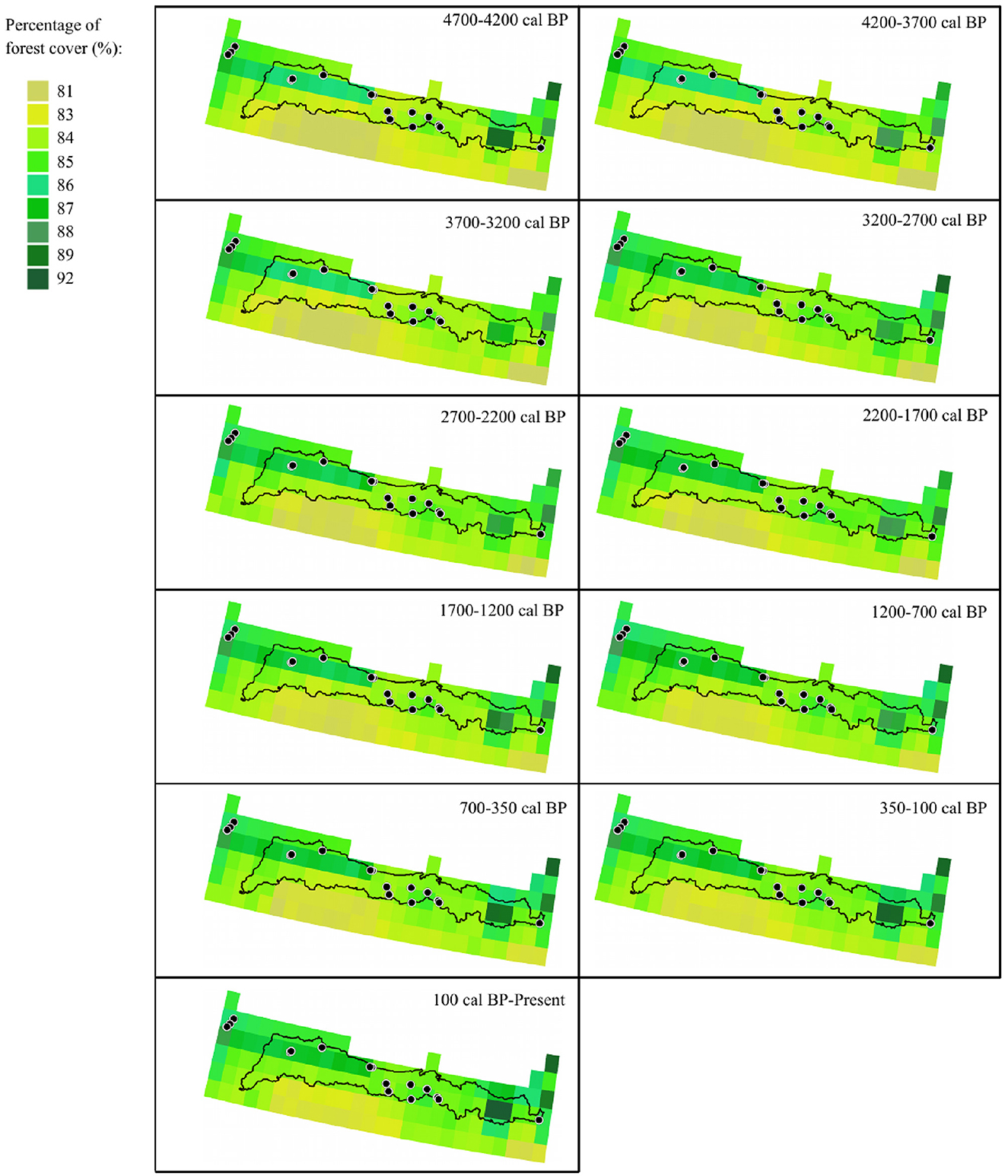
Figure 6. Modeling of natural forest cover across the study area from 4,700 cal BP to Present, expressed in percentages of covered surface (%). Only time windows with dating crossing episodes of major changes in agrarian societies are represented.
Interpolation
The datasets created by the models cover the whole of Europe at a grid cell resolution of 0.25 × 0.25° (~20 × 27.75 km at the latitude of the study area). This is significantly coarser than the resolution of the Digital Elevation Model (DEM) of the study region (EU-DEM, 2016), which has a grid cell size of 790 × 790 meters. In practice, this means that some model grid cells that are positioned in the ocean partially overlap with the coastal area in the DEM. While the climate simulations provide valid results for the “ocean cells,” this is not the case for the natural forest cover, which is only modeled for the land area. To provide natural forest cover values for the ocean cells that partially overlap with the coastal areas in the DEM, the grids were re-interpolated using QGIS 3.32 (QGIS Development Team, 2023). First, the grids were clipped to the extent of the study area. Then, the non-empty grid cells were converted to points located at the grid cell center, with the modeled value for natural forest cover as their attribute. These points were then used as input for an Inverse Distance Weighted (IDW) interpolation (Shepard, 1968) of natural forest cover values for the ocean cells that are overlapping with the coastal areas. IDW interpolation is suitable for this, as it produces smooth surfaces with gradual transitions between points. For the interpolation, the standard distance coefficient (power parameter, or p) of 2 was used. However, it has to be kept in mind that different settings of p may produce different outcomes.
Results
In this section the evolution of HMC and TTC through time will be described and confronted with the results from the models of natural forest cover, and temperature and precipitation indexes (Figure 3). A total of 16 peat bogs provided 25 records of HMC and 14 records of TTC. Among those, 18 records (eight HMC and 10 TTC) have been dated from as early as Neolithic times, five records (three HMC and two TTC) from the Neolithic-Copper Age transition and the Copper Age, 15 records (14 HMC and one TTC) from the Bronze Age, and one TTC record from the Iron Age. All records ended with the year 1950 AD, which corresponds to the Present (0 cal BP).
Globally, Metal Pollution (MP) and Forest Retreat (FR) episodes increased both in number and intensity through time, in all records from all peat bogs, punctuated with short phases of recovery (Figure 3). Most of the events of increased MP and FR are synchronous across the study area. A general, unprecedented acceleration (most often more than 10 or 15 times the basal value, see sources cited in Table 1) of all MP signals started around 750–550 cal BP, while globally the FR gradually worsened from ca. 2,000 cal BP. The natural vegetation model shows very few variations through time, with a forest cover spread between 82% (lowest values for the period 7,200–6,700 cal BP, during the early phase of the Neolithic) and 87% (the highest values for the most recent period, ca. 100 cal BP-Present; Figure 6). Temperature and precipitation show very similar trends over time. Differences in means of annual temperature vary much more between each site (about 3°C of difference) than through time for the same site (about 1°C of difference; Figure 4). Local environment and ecological inertia as well as geographic settings of the sites such as altitude, orientation, etc. are likely to be the main influence for the differences in temperatures and precipitation. Two precipitation regimes are clearly distinct between the east (below 1 m/yr) and the west (above 1 m/yr) of the study area, with the exceptions of the sites of Pico Sertal and Cueto de la Avellanosa situated in the middle, which have the highest rainfall (between 1.45 and 1.54 m/yr; Figure 5). Nonetheless, they both follow the same evolutionary trend through time. The regional trend shows highest temperatures for the Neolithic periods of 6,600–6,300 and 5,700–5,400 cal BP during the Holocene Climate Maximum, and the lowest temperatures for the Late Iron Age (2,400–2,100 cal BP; Figure 3). This time period is situated at the very beginning of the Roman Warm period, which is still under the influence of the previous period (Iron Age Cold period) as the temperatures improved very gradually (Martín-Chivelet et al., 2011). Highest precipitation in the eastern part of the study area occurred during the period of 600–300 cal BP (coincident with the Little Ice Age and the Age of Discoveries), whilst it occurred during the Neolithic periods of 6,600–6,300 and 5,700–5,400 cal BP (coincident with the maximum of temperatures) in the western part of the study area. The lowest rainfall across the whole region occurred during a short period (6,000–5,700 cal BP) during the Holocene Climate Maximum.
Neolithic, copper age, and bronze age
All records of heavy metal content from the Neolithic show episodes of increase in MP, whilst only three records of total tree cover out of 10 from this period show significant episodes of FR (Figure 3). However, natural forest cover was globally increasing. This period was mainly characterized by the Holocene Climate Maximum, which was marked by two phases of both increasing temperature and precipitation (6,600–6,300 and 5,700–5,400 cal BP). Interestingly, a sharp decrease of rainfall (globally a reduction of 0.06 m/yr) occurred in the period 6,000–5,700 cal BP between the two periods of increased precipitation, but does not seem to be correlated with a specific episode of MP or FR. If natural environmental factors have certainly influenced the evolution of forest cover (López-Merino et al., 2010), anthropogenic disturbances (e.g., forest fires, slash-and-burn practices, agropastoral activities, etc.) have also impacted the evolution of forest cover (Pérez-Díaz et al., 2016b; Pérez-Obiol et al., 2016).
As early as the transitional phase to the Copper Age, episodes of both MP and FR occurred more often in all records, but were dispersed through time. This time period is coincident with the Neoglaciation (ca. 5,400–4,200 cal BP; Baldini et al., 2019), when the climate was less favorable to forest growth, and might be the main cause of FR, albeit the influence of agropastoral activities. An episode of increasing temperature and precipitation occurred between 4,800 and 4,500 cal BP (globally +0.15°C and +0.03 m/yr) but does not seem to have had much influence over MP and FR episodes. The joint contribution of both climate worsening and agropastoral activities can explain the increase in FR episodes. In fact, both result in a reduction of forest cover and a subsequent increase in soil erosion, leading to the deposit of geochemical element such as Pb in peat bog reservoirs (Gallego et al., 2013). MP episodes are partly due to the first recorded mining activities in the region (Monna et al., 2004; Jouffroy-Bapicot et al., 2007; Martínez-Cortizas et al., 2016; Gallego et al., 2019), and to agropastoral activities as cereal pollen have also been identified (López-Merino, 2009; Carrión et al., 2015; Martínez-Cortizas et al., 2016). In the case of Las Conchas, the presence of lutites in the peat bog, which are sedimentary rocks rich in rare earth elements, might have enhanced MP signals (Gallego et al., 2019).
Simultaneous occurrences of MP and FR took place around the boundary between the Copper Age and the Bronze Age, between 4,400 and 4,100 cal BP, probably related to the cold and dry Bond Event 3, also called the 4.2 ka event (Bond et al., 2001). At the same time, large mines such as El Aramo, El Milagro and La Profunda among others were exploited for the extraction of azurite and malachite (both being copper ores; de Blas Cortina, 2005). This time period is also marked by a decline of the modeled natural vegetation and a reduction in temperature and precipitation. If episodes of FR seemed to end after the 4.2 ka event, MP episodes lasted during most of the Early Bronze Age, with already some high intensity pollution, such as the lead isotope ratio (fall from 1.185 to 1.175) in La Molina (Asturias). The period of forest recovery after the 4.2 ka event seemed to be hampered by new episodes of FR toward the end of the Early Bronze Age, in Borralleiras da Cal Grande (Mighall et al., 2006), in Cueto de la Espina (Ródriguez-Coterón, 2022), and in Zalama (Pontevedra-Pombal et al., 2018). Although the 4,400–4,100 cal BP phase was influenced by the end of the Neoglaciation, the widespread and simultaneous occurrence of both MP and FR provoked by mining and agropastoral activities, drove us to identify this period as the first phase (the Copper phase, 4,400–4,100 cal BP) of major anthropic impact on the landscape (Table 3).
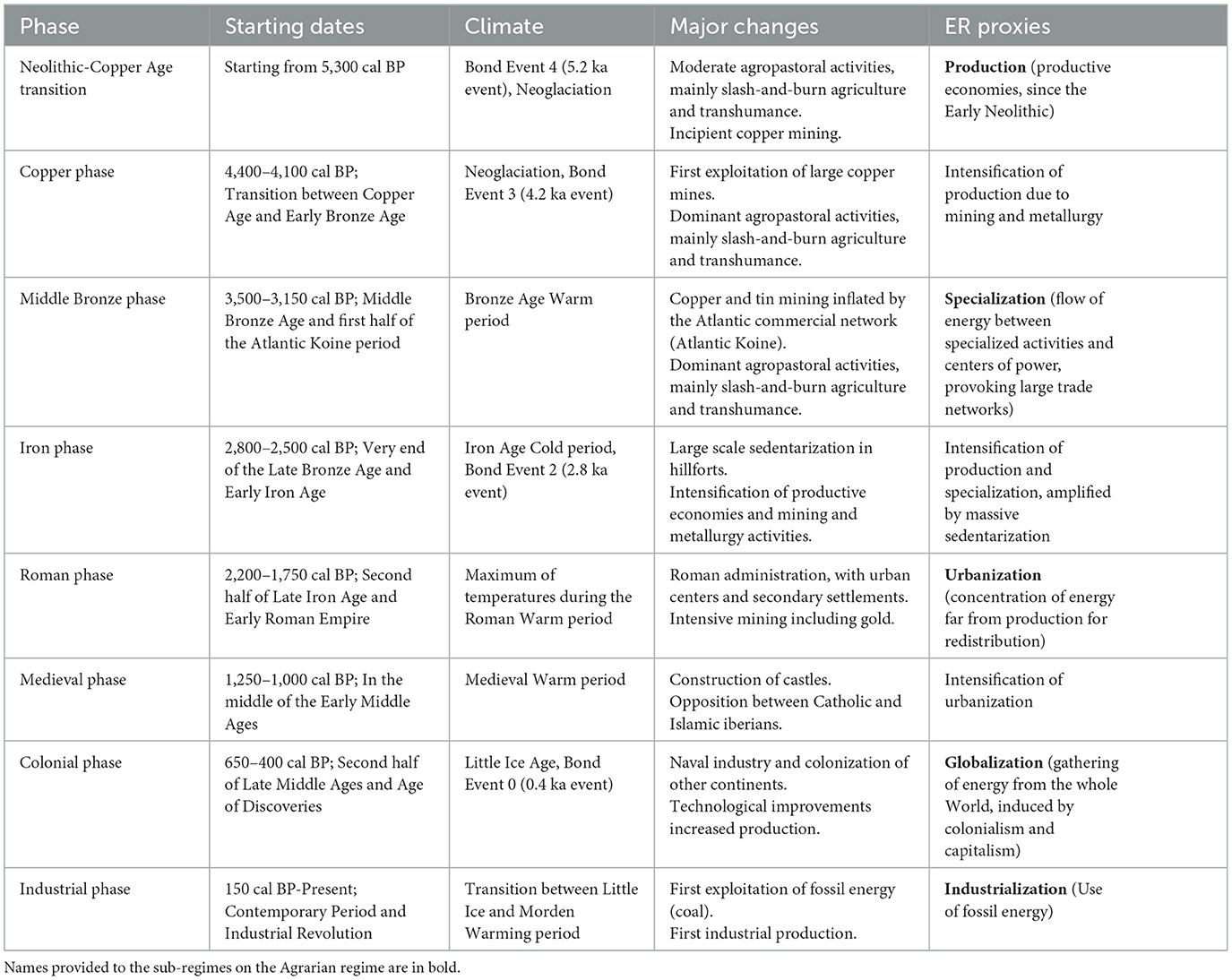
Table 3. Synthesis of major changes in human societies inferred from the intensification of their impact on landscapes (heavy metal pollution and forest decline) in Northern Spain.
The next phase of intense, simultaneous MP and FR occurred during the Middle Bronze Age, coincident with the Atlantic Koine period, between 3,500 and 3,150 cal BP (Figure 3). The Atlantic Koine, also called the Channel Bronze Age, was a period when a commercial network developed between societies from Northern Europe, the British Isles, Brittany and the northwest and west facades of the Iberian Peninsula (Cunliffe, 2001; Needham, 2007). Episodes of intense MP and FR appeared now in more cores than during the previous Copper-Bronze Age transition, with a particularly long lasting high level of MP in La Molina (Asturias). MP signals are particularly high for Penido Vello and Borralleiras da Cal Grande as Ni increased by 50 and 300%, respectively (Pontevedra-Pombal et al., 2013), and in La Molina (Asturias) where lead isotope ratio decreased from 1.190 to 1.165 (Martínez-Cortizas et al., 2016).
At this time period, FR occurred whilst the natural forest cover slightly increased across the whole study area (+0.005%). The mean temperature and the precipitation also increased during this phase (+0.3°C and +0.03 m/yr respectively), which is coherent with the warm and wet Bronze Age Warm period that influenced the climate in the region (Martín-Chivelet et al., 2011). This new commercial network likely stimulated local economies, and in the case of Northern Spain, copper and tin mining (de Blas Cortina, 1996; Comendador-Rey et al., 2006), and provoked this global phase of MP and FR in a time when forest cover should have been increasing. Thus, we identify this period as the second phase (the Middle Bronze phase, 3,500–3,150 cal BP) of major anthropic impact on the landscape (Table 3).
During the Late Bronze Age, FR episodes seem to be more scattered through time. MP episodes are almost non-existent, except for the long lasting MP signals in Las Conchas and Roñanzas peat bogs, the former one being quite intense (anthropogenic REE more than doubled, from 0.49 to 1.1 mg/kg) and continuing until the Iron Age (Gallego et al., 2019).
Iron Age and Roman Empire
The Early Iron Age started with the second Bond Event also called the 2.8 ka event (Bond et al., 2001), and was characterized by the Iron Age Cold Period. The natural vegetation cover and the temperature show a phase of stagnation (and negligible increase of temperature) at this time, while rainfall increased (+0.04 m/yr). This result is inconsistent with the dry and cold climate described in the literature (Bond et al., 2001; Martín-Chivelet et al., 2011; Baldini et al., 2019), but can be explained by the fact that our model provides time windows of 500 years for temperature and precipitation, which might fade shorter cold and dry events (the Iron Age Cold period lasted for ca. 350 years). Another source of error is likely the flat trend of the radiocarbon calibration date, also called the Hallstatt Plateau), which hampers any precise dating during a time period coinciding with the Early Iron Age (Alonso Matthias, 2002; Rubinos and Alonso Matthias, 2002).
During the Early Iron Age between 2,800 and 2,500 cal BP, both MP and FR episodes seem much more frequent than during the previous period, as they occurred in almost all cores, but they appear dispersed through time. Important cultural changes occurred during this period, notably the large-scale sedentism of human groups who now settled into castros, which were small hillforts (Marín Suárez, 2009, 2011). Consequently, productive economies started to significantly increase, when transhumance and seasonal settlements were preferred in earlier periods (Marín Suárez, 2009). Mining and metallurgy then intensified to sustain this new economy (Marín Suárez, 2011). Although the climate probably enhanced MP and FR records, about half of the signals now happened during phases of already high proportions of MP (e.g., 28 mg/kg of Zn in Las Conchas for a baseline value of 0.5 mg/kg prior to the Early Iron Age, see Gallego et al., 2019) and FR (e.g., 50% of forest cover in Quinto Real, see Monna et al., 2004), which is a significant intensification compared to the previous periods. Hence, we identify this period as the third phase (the Iron phase, 2,800–2,500 cal BP) of major anthropic impact on the landscape (Table 3).
The start of the Late Iron Age is coincident with the start of the Roman Warm Period, which covered this period and the subsequent Early Roman Empire (Martín-Chivelet et al., 2011). The first half of the Late Iron Age is marked by a global forest recovery and a diminution of metal content (e.g., general decrease of 25% of Ni content, see Pontevedra-Pombal et al., 2013) in peat bogs (Figure 3), with the exception of lead content in all records of heavy metal content, and the lasting episodes of FR in Pico Sertal, Cueto de la Espina, and Zalama peat bogs. This decrease in mining and metallurgy activities has also been observed in southern Spain (García-Alix et al., 2013), and is likely induced by the collapse of the traditional Atlantic commercial network related to the introduction of iron ore (González-Ruibal, 2004; Marín Suárez, 2011). It is possible that the still high Pb values are due to the increasing agropastoral activities, and that FR differences reflect local effects.
The second half of the Late Iron Age is marked by another iteration of simultaneous and unprecedented intensity of MP episodes lasting until the end of the Early Roman Empire period, between 2,200 and 1,750 cal BP. Peaks of pollution are visible during the Early Roman Empire period; at 1,900 and 1,800 cal BP in Pena da Cadela (+100% compared to the beginning of this phase; Martínez-Cortizas et al., 2002b), at 1,900 and 1,850 cal BP in Penido Vello (+400% compared to the beginning of this phase; Pontevedra-Pombal et al., 2013), and at 1,950 and 1,750 cal BP in Borralleiras da Cal Grande (+150% compared to the beginning of this phase; Pontevedra-Pombal et al., 2013). Climate modeling shows increasing temperatures (+0.15°C globally) for this period, which is also coincident with a moderate phase of expansion of natural forest cover (+0.015 % globally). This is consistent with the maximum of temperatures during the Roman Warm Period (Martín-Chivelet et al., 2011). Thus, this major phase of increasing MP and FR in a climate globally favorable to forest expansion indicates that anthropic activities must have been intensifying. Iron metallurgy appeared and intensified rapidly during the Late Iron Age, principally leading to the development of warlike equipment (Torres-Martínez, 2010; Marín Suárez, 2011). Iron was indeed preferentially used by men in increasingly bellicose societies (Fanjul and Marín, 2006). The Cantabrian Wars (1,979–1,969 cal BP, i.e., 29–19 BC) led to the conquest of Northern Spain by the Roman (Costa-García, 2017, 2018), and intense gold mining developed immediately afterward under the Roman influence (Marín Suárez, 2011). A particularly important gold belt is situated in Galicia and western Asturias (Lewis and Jones, 1970), and has been largely exploited by the Romans (Spiering et al., 2000). Thus, we identify this period as the fourth phase (the Roman phase, 2,200–1,750 cal BP) of major anthropic impact on the landscape (Table 3).
Under the Late Roman Empire and during the Early phase of the Middle Ages, both MP and FR signals were very dispersed through time. This phase of recovery coincides with the end of the Roman Warm period and the following Dark Age Cold period (Martín-Chivelet et al., 2011), ending with the Bond Event 1, also called the 1.4 ka event (Bond et al., 2001). The natural forest cover modeling shows a reduction in forest cover, while the climate modeling shows an increase in temperature and precipitation. These results are in contradiction with the setting of the Dark Age Cold period that took place at that time (Martín-Chivelet et al., 2011). Once again, long time windows (500 years) might be fading shorter temperature and rainfall episodes, as it encompasses both the end of the Roman Warm period and the beginning of the Dark Ages Cold period.
Middle ages, modern period, and contemporary period
After the demise of the Roman Empire, Northern Spain was left under the influence of the warring Germanic Sueves, Vandals, Alan, and Visigoth tribes (Sánchez-Morales, 2023), leading to the later establishing the Visigoth Kingdom during the early stage of the Early Middle Ages (Collins, 2004). This new cultural phase is coincident with the establishment of the Medieval Warm period (Martín-Chivelet et al., 2011). The climate modeling shows a decrease in temperature (−0.2°C) and natural forest cover (−0,003%) and an increase in precipitation (+0.01 m/yr) for this time period. It is possible that the early stage of the Early Middle Ages period is still under the influence of the previous Dark Age Cold period, and that the coarse resolution of our time windows likely overestimated the decrease of temperature and natural forest cover. MP and FR signals remained infrequent and dispersed through time during this period, continuing the trend of recovery already set in the Late Roman Empire.
Starting from the mid-Early Middle Ages, at the end of the Germanic Period (also called the late Roman-medieval period), a new phase of simultaneous MP and FR episodes occurred between 1,250 and 1,000 cal BP. MP signals are globally 2–3 times less intense than during the Roman phase (Martínez-Cortizas et al., 2000, 2002b; Monna et al., 2004; Pontevedra-Pombal et al., 2013), and forest cover globally declined to reach 20–30% (see sources cited in Table 2). The temperature was pursuing its decreasing trend, but rainfall started to decrease while natural forest cover started to increase (+0.003%). Once more, the coarse resolution of our time windows likely overestimates the impact of a short cold and dry event just at the beginning of this new phase, at 1,250 cal BP (Martín-Chivelet et al., 2011). Nonetheless, it is likely that this short event enhanced the synchronous MP and FR episodes. MP and FR episodes in a global context of natural forest expansion indicates that anthropogenic impact on landscape was intense. This period is exactly coincident with the appearance of first prominent castles, which construction likely needed a large amount of resources (Martínez and Mantecón Callejo, 2012; Sánchez-Morales, 2023). It also coincides with the Islamic Period of the Iberian Peninsula, when Muslim controlled most of it and were warring against catholic kingdoms from Northern Spain and Western Europe (Martín, 1975). Thus, we identify this period as the fifth phase (the Medieval phase, 1,250–1,000 cal BP) of major anthropogenic impact on the landscape (Table 3).
A short disruption marks the end of this phase, coincident with the transition between the Early and the Late Middle Ages, but both MP and FR episodes resume during the Late Middle Ages in almost all peat bogs. Most peat bogs show a low forest cover already, and about half of the MP episodes occurred in phases of high level of pollution. However, MP and FR signals do not appear to be simultaneous. At that time, the natural vegetation cover continued the increasing trend that started during the Early Middle Ages, and both temperature (+0.3°C) and precipitation (between +0.01 m/yr and +0.03 m/yr) started to increase.
It is not before the second half of the Late Middle Ages that high levels of pollution are also attested in most of the MP records. Starting from 650 cal BP, almost all MP signals are constantly increasing, and from this point onward, only tipping points in MP acceleration curves (instead of starting MP and FR episodes as in earlier periods) may indicate changes in major anthropogenic impact. Several MP episodes in Pena da Cadela (Pontevedra-Pombal et al., 2013), Penido Vello (Martínez-Cortizas et al., 2000; Pontevedra-Pombal et al., 2013), Borralleiras da Cal Grande (Pontevedra-Pombal et al., 2013), and Las Conchas (Gallego et al., 2019) suddenly accelerated from 650 cal BP onward. This is coincident with the beginning of the Little Ice Age (Martín-Chivelet et al., 2011). Climate modeling show a reduction of 0.2°C and a negligible reduction of natural forest cover for this period (−0.015%), but rainfall reached the highest values (from 0.82 to 0.95 m/yr for the eastern part of the study area; from 1.07 to 1.58 m/yr for the western part). This new phase of widespread, simultaneous and intense MP and FR episodes occurred between 650 and 400 cal BP and encompassed almost the whole Age of Discoveries (Arnold, 2021). At that time, the naval industry was developing rapidly as the Spanish Empire started to exploit colonies to sustain its economy (Ceballos Cuerno, 2001). The naval industry was very demanding in both wood and products of metallurgy, partly explaining the intense MP and FR episode for this period (Corbera Millán, 1999; Ceballos Cuerno, 2001), together with the climate worsening (Martín-Chivelet et al., 2011). Moreover, traditional foundries functioning close to sources of wood to produce charcoal, iron ore and waterways (Martinez, 2003) were being replaced by hydraulic foundries, marking a significant technological evolution (Urteaga, 1999). Thus, we identify this period as the sixth phase (the Colonial phase, 650–400 cal BP) of major anthropogenic impact on the landscape (Table 3).
The dramatic MP acceleration that started with the 650 cal BP tipping point continued until Present-day. Nonetheless, a very short disruption in FR signals appeared at 400 cal BP, synchronous with both the Bond Event 0 (also called te 0.4 ka event; Bond et al., 2001) and the transition to the Modern Period (Martín, 1975). This is also corresponding to a period of crises for Spain, which was at war with the Netherlands and lost its Gran Armada to England (Fernández Izquierdo, 1989), and which was lacking good quality wood resources for shipbuilding because of centuries of wood exploitation, forcing it to start displacing shipyard activities to the Indies and the Americas (Torres Ramírez, 1981). It is worth noting that 2 peaks of MP appeared at 400 cal BP in Pena da Cadela and Penido Vello, and one at 350 cal BP in Penido Vello. Those peaks are observed in PbEF and HgEF signals, which are not anthropogenic signals but encompass both natural and anthropogenic enrichment factors (Martínez-Cortizas et al., 2000, 2002b). Thus, it is likely that those peaks are overestimated.
During the following Modern Period, the results of our models show the beginning of the last phase of expansion of natural forest cover (coming to the highest values, up to 87%) and increasing temperature (+ 0.3°C) until Present-day. In the western half of the study area, the precipitation are continuing their increasing trend started in the previous period (up to 1.41 m/yr), while they are slightly decreasing in the eastern half of the study area (down to 0.83 m/yr). Two new tipping points in the acceleration curves of MP occurred ca. 250 and ca. 150 cal BP. The 250 cal BP event might be related to a renewed, intense shipbuilding activity to tackle the growing influence of the corsairs (Fernández Izquierdo, 1989), while the 150 cal BP marked the beginning of the Industrial Revolution (Sánchez Fernández et al., 2018), the end of the Little Ice Age (Martín-Chivelet et al., 2011), and the transition to the Contemporary Period (Blumenberg, 2008). For this 150 cal BP tipping point, the MP acceleration is of an unprecedented intensity. Globally, MP values reached dramatically high values for the most recent times, from 15 times the background values such as Cd and Zn signals in Las Conchas (Gallego et al., 2019), to almost 200 fold greater than the background values such as Pb signals in Penido Vello (Martínez-Cortizas et al., 2000; Kylander et al., 2005). Pontevedra-Pombal et al. (2013) estimated that 97% of total heavy metal deposition in peat bogs were from anthropogenic sources. More dramatic again is the global, exponential acceleration of MP deposition that continues up to the twenty-first century AD, caused by the Industrial Revolution and the Great Acceleration of human pressure after the Second World War (Irabien et al., 2015). The first mentions of the use of coal in Northern Spain dated from 1,593 AD (ca. 350 cal BP; Casal y Julian, 1762) and then from 1,789 AD (ca. 150 cal BP; Sánchez Fernández et al., 2018), the first coal exploitation started between 150 and 50 cal BP (Sánchez Fernández et al., 2018). Coal exploitation was intense from 100 cal BP as it was used in the already dominant steel industry (Muñiz Sánchez, 2011). Moreover, Asturias became the center of coal and steel industries in Spain ca. 50 cal BP (Ojeda Gutiérrez, 1994). Thus, we identify this period as the seventh phase (the Industrial phase, 150 cal BP-Present) of major anthropogenic impact on the landscape (Table 3).
Contrary to the MP signals, FR episodes appeared shortly disrupted ca. 50 cal BP, in a context of minimum forest cover globally between 45 and 30% of forest cover (see all sources in Table 2), with the minimum at 10% in Penido Vello (Muñoz-Sobrino et al., 2009) since the beginning of the records (the model of natural forest cover shows that 84 to 87% of the study area should be covered by forest). This short forest recovery was likely favored both by political choices of forest preservation (by reduction of grazing pressure and tree establishment; Rozas, 2002) and by the end of the Little Ice Age and the transition toward the Modern Warming period (Martín-Chivelet et al., 2011), as shown by the increasing temperature, precipitation and natural forest cover in the results of our models.
Major phases of anthropogenic impact
The disentanglement of complex histories of cultural influences on the landscape over deep time history in northern Spain has delivered some interesting results. Human impact over time is waxing and waning over most of the deep time period, clearly waxing in the Roman Age, while steeply all over increasing with unprecedented continuity since the Late Middle Ages (Figure 3). Up to seven phases of major changes in human societies and impact on landscapes were identified based on HMC and TTC records (Table 3). Here we will discuss how these phases of major change are considered within the framework of ER, and how they led to the identification of four sub-regimes within the Agrarian regime based on subsistence strategies: (1) Production, (2) Specialization, (3) Urbanization, and (4) Globalization.
ER2a: production
The framework of ERs has recently been investigated and tested for hunter-gatherer societies until the adoption of productive economies, i.e., ER1, also called the Standard Solar regime (Martinez et al., 2022, 2023). Hunter-gatherer societies were sustained by solar energy, as they lived on the consumption of wild plants (growing with sun energy) and animals (either herbivores feeding on plants, or carnivores feeding on herbivores). Thus, solar energy was harnessed, but not controlled, by hunter-gatherers during ER1 (Martinez et al., 2023). The Agrarian regime (i.e., ER2) differs from the ER1 in that human societies function on a strategy of production: they are able to control solar energy. The appearance of productive economies (agropastoral activities, transhumance, and cattle raising) induced one major change in energy use, as solar energy is now willingly concentrated into cereal and leguminose fields and cattle raising (Martinez et al., 2023).
The transition to the Agrarian regime occurred ca. 5,300 cal BP, corresponding to the start of the Neolithic-Copper Age transition, based on statistical analysis of Mesolithic and Neolithic archaeological remains such as ceramics, plant and faunal domestic taxa, burials and megalithic structures (Martinez et al., 2023). Although the oldest evidence of domestic taxa (either cereal or animals) dated from the Early Neolithic (Peña-Chocarro et al., 2005; López-Merino et al., 2010), they remained anecdotal until the transition to the Copper Age (Ontañón-Peredo, 2003; Cubas et al., 2016). Some works have identified the first human impact on the landscape through reduction of forest cover (due to the use of fire for forest clearing, eg. slash-and-burn practices) starting from 6,300 cal BP (Pérez-Díaz et al., 2016b; Carracedo et al., 2018). But this impact appears to be synchronous with the starting point of a new trend of increasing cold and dry conditions, hence reducing its significance (Figure 3). The 5,300 cal BP transition occurred right before the Bond Event 4 (5.2 ka event) and similarly corresponds to the transition to a cold and dry period (Neoglaciation; Bond et al., 2001; Martín-Chivelet et al., 2011).
What led Martinez et al. (2023) to set the date 5,300 cal BP as the starting point of the Agrarian regime was the related significant increase of productive economies and the appearance of mining activities (copper ores) and metallurgy, although it remained anecdotal until the transition to the Bronze Age (Ontañón-Peredo, 1995). These new activities consumed energy that was produced by the community (e.g., metabolic energy coming from food), but in return did not produce directly useful energy to sustain themselves. Thus, the notion of specialization and flow of energies between individuals has been used to describe such specialized activities (Renfrew, 1969; Martinez et al., 2023). However, flows of energy were already existent with the various European and Mediterranean trade networks (e.g., obsidian and stone axes) during the Neolithic (Robb and Farr, 2005; Peruchetti et al., 2020). Trade at that time and during the Copper Age consisted in a mutually satisfactory exchange between neighboring groups only, which did not imply any commercial value (Renfrew, 1969), and thus, specialization did not represent a subsistence strategy for the communities, as it will at a later time (see next section).
Figure 3 shows no significant impact of anthropogenic activities on the landscape before the period of 4,400–4,100 cal BP, which we identified in the previous section as the Copper phase of major impact, coincident with a transitional phase between the Copper Age and the Early Bronze Age. Another significant change at this time was the first exploitation of large mines such as El Aramo, El Milagro, and La Profunda (de Blas Cortina, 2005), indicating an intensification of productive activities. Copper production in northern Spain largely exceeded the regional needs and the surplus was traded with closest neighbors, such as in the northern Meseta (Ontañón-Peredo, 2003). Once again, specialization and trade were not used as a proper subsistence strategy (Renfrew, 1969), but more likely were part of the “package” of goods with cultural identity value, which were exchanged along with the incipient expansion of the Bell Beaker (Rojo Guerra et al., 2005; Ortega et al., 2021).
The energy system and subsistence strategies did not change over this time period from the Early Neolithic to the Early Bronze Age. The strategy of production intensified with the first mining/metallurgy activities, and both “specialization” and “trade” did not have any subsistence value. Three remarkable events occurred during the ER2a regime, namely the 6,300 cal BP forest decline, the 5,300 cal BP incipient mining activity, and the Copper phase of major impact ca. 4,400–4,100 cal BP. But all three occurred under cold and dry climate periods that might have amplified them, thus reducing their significance. Consequently, we define the first phase of the Agrarian regime (ER2a) as a regime of production strategy, starting from the Early Neolithic, ca. 7,000 cal BP (Martinez et al., 2023). It includes the Neolithization process during the Early Neolithic, which corresponds to the incipient phase of the production strategy, and the Copper Age, corresponding to a phase of intensification of the production strategy, notably due to copper mining.
ER2b: specialization
During the Atlantic Koine ca. 3,500–3,150 cal BP (Figure 3), the climate was warm and wet, and thus, favorable to a phase of recovery instead of a phase of metal pollution and forest decline. A substantial commercial network developed between societies from Northern Europe, the British Isles, Brittany and the northwest and west facades of the Iberian Peninsula (Cunliffe, 2001; Needham, 2007). Northern Spain was rich in copper and tin, both ores being essential to the bronze industry (Earle et al., 2015; Vandkilde, 2016). Moreover, tin was not as current as copper in Europe, making it a valuable resource (Gómez Ramos, 1999; Earle et al., 2015). Northern Spain communities were still semi-sedentary living on seasonal agropastoral activities and transhumance, and were not organized under the control of an elite (Sánchez-Morales, 2023). However, such organization (central power controlled by an elite) was not necessary for communities to take part in this commercial network (Earle et al., 2015).
By contrast to the previous period, the strategy of specialization now consists in a subsistence strategy with trade gaining a commercial value (Earle, 2002), hence indicating a change in the energy regime. The system now has energy sources (e.g., mining community) and recipients (e.g., commercial partner), linked by energy flows (e.g., metal objects). Beyond northern Spain and the Atlantic Koine, a proto-historic, global commercial network expands over Europe, Northern Africa, and Western and Central Asia, and is called the Bronzization (Vandkilde, 2016). The incipient phase of the Bronzization occurred at 4,150 cal BP, which coincides with the phase of non-commercial specialization and trade described in the previous section (Vandkilde, 2016). However, Bronzization significantly increased and expanded from 3,550 cal BP, coincidentally with the start of the Atlantic Koine (Vandkilde, 2016). This critical increase of human impact from the Bronze Age onward has also been identified in the Mediterranean (Mercuri et al., 2019).
With such a large scale, many products were traded across Europe (e.g., metal ores, salt, amber, lapis, etc., see Earle et al., 2015). The Bronzization provoked many specialized activities, including professional traders (Vandkilde, 2016). Many technological innovations developed in this regard (e.g., road construction, boat technology, etc.) in order to compress the traveling time (Johansen et al., 2003; Anthony, 2007; Ling, 2008), involving specialized personnel and expertise in return (e.g., miners, craftsmen, warriors, etc.; Earle et al., 2015). Interestingly, the Bronzization started to decline ca. 3,150 cal BP (Vandkilde, 2016), coincidentally with the end of the Middle Bronze phase of impact (Figure 3), further strengthening our view that the Atlantic Koine may be the local (Atlantic Europe) expression of the Eurasian Bronzization.
From the end of the Late Bronze Age and during the Early Iron Age, a new phase of major human impact occurred (the Iron phase, ca. 2,800–2,500 cal BP; Table 3). This phase started with the Iron Age Cold period and the Bond Event 2 (2.8 ka event), which likely amplified it. Major cultural change occurred during this period, expressed by the start of a massive sedentarization process with the appearance of hillfort settlements called castros (Parcero Oubiña, 2002; Marín Suárez, 2009, 2011). Interestingly, the start of this Iron phase is coincident with the conclusion of the Bronzization that occurred ca. 2,750 cal BP (Vandkilde, 2016). Although sedentarization is a prerequisite to urbanization and complex societies, it developed in northern Spain during the collapse of the Bronze Age World, while already existing complex societies (e.g., in the Eastern Mediterranean) collapsed along with the Bronzization (Earle et al., 2015). The castros were first situated on trading roads from the previous cultural phase (Marín Suárez, 2009), and hence, productive economies started to really develop from this point onwards (Marín Suárez, 2009), likely enhancing both the production and the specialization strategies. Thus, the sedentarization process related to the Iron phase of major impact intensified the production and specialization strategies, but did not bring any new subsistence strategies to northern Spain societies. Consequently, we define the second phase of the Agrarian regime (ER2b, starting from the Middle Bronze Age, ca. 3,500–3,150 cal BP) as a regime of specialization strategy, which adds to the production strategy of ER2a. It includes both the Middle Bronze phase of major impact during the Middle Bronze Age, which is characterized by the appearance of the specialization strategy, and the Iron phase of major impact, corresponding to a phase of intensification of production and specialization strategies, notably due to the sedentarization process.
ER2c: urbanization
The Roman phase of major impact (ca. 2,200–1,750 cal BP) started during the second half of the Late Iron Age and covered the Early Roman Empire period (Figure 3). It occurred during the maximum temperatures of the Roman Warm period, a climate that was favorable to a phase of recovery. Thus, this phase must have been very intense. The first iron objects were found in northern Spain during the Late Iron Age, starting from ca. 2,450 cal BP (Camino Mayor, 2002; Villa Valdés, 2002), but the first iron mining activities were recorded during the Early Roman Empire period (Marín Suárez, 2011). Iron deposits were very common in northern Spain, and hence settlements were never too distant from them, facilitating their exploitation (Marín Suárez, 2011). Although iron exploitation was new, it did not have any implication for the energy system, as it was still part of production and specialization strategies.
The Romanization started with the Cantabrian Wars that took place between 1,979 and 1,969 cal BP (i.e., 29–19 BC; Costa-García, 2017, 2018). An important cultural change from the Early Roman period was the start of gold mining, which was immediate after the Romanization (Marín Suárez, 2011) and severely impacted the landscape (Sánchez Palencia et al., 2011). Mining activities and areas were then directly administered by the Romans but using local population for labor (Orejas et al., 2002). However, as for iron mining in the previous periods, gold mining did not change the main subsistence strategy in the energy system.
Before the Romanization, the castros were small and dispersed through the study area (Marín Suárez, 2011). Castros communities were characterized by the absence of an elite or ruling class, and organized in broader egalitarian societies, which translates in archaeological context in the absence of prestige goods (González Ruibal, 2012). Such societies are called deep rural communities (Jedrej, 1995). A new, hierarchical administrative system appeared after the Roman conquest (Sastre Prats and Currás, 2019), with the notable creation of political and economic urban centers ex novo (Díaz and Menéndez-Bueyes, 2005). In this work, we refer to the definition of urban center proposed by Weber (1966) and Capel (1975): a concentration of population with a centralized government, with a functional economic and political specialization. Consequently, some urban centers had influence over secondary satellite settlements (e.g., vici, villae; Pérez-Losada, 1996). Urbanization is particularly difficult to define (Ruiz-Zapatero et al., 2020), as theoretical concepts (e.g., in Currás and Sastre Prats, 2019) need to incorporate archaeological data (Ruiz-Zapatero et al., 2020). In this work, we understand urbanization from two distinct definitions. Han (2016) argued that urbanization emerged from “external connections, heterogeneity and aggregation,” which could refer to trade, specialized activities and urban centers. To this definition, we could add another characteristic proposed by Gaydarska (2017), who stated that urbanization implies the proliferation and the sustainability of urban centers. The urbanization phenomenon represents an important change in energy management. For the first time, products (hence energy) from secondary settlements are gathered in urban centers, where they are concentrated and redistributed, as trade flows channeled from the urban center to secondary, rural settlements (Ruiz-Zapatero et al., 2020).
After the collapse of the Roman Empire, feudalism developed through successive political power in northern Spain (e.g., Visigoth Kingdom, Kingdom of Asturias), starting from ca. 1,450 cal BP (Collins, 2004; Sánchez-Morales, 2023). Political centers gained more and more influence and controlled larger territories (Martín, 1975). Thus, Early Middle Ages societies witnessed an intensification of the urbanization strategy, but did not adopt any new subsistence strategy. This is in this context that the Medieval phase of major impact occurred, ca. 1,250–1,000 cal BP. At that time, the Christian Kingdom of Asturias was constantly opposed to the muslim kingdoms occupying the Iberian Peninsula, and thus, the economy needed to sustain the constant war effort (Martín, 1975). One particular indicator to this is the spread of castles during this period, which construction likely needed a large amount of energy and resources (Martínez and Mantecón Callejo, 2012; Sánchez-Morales, 2023), hence intensifying the production, specialization and urbanization strategies. Consequently, we define the third phase of the Agrarian regime (ER2c), starting from the Early Roman Empire period (ca. 1,950 cal BP) as a regime of urbanization strategy, which adds to the production (ER2a) and specialization (ER2b) strategies. The start of ER2c occurred during the second half of the Roman phase of major impact (ca. 2,200–1,750 cal BP), which the first half was also marked by the sedentarization process, prerequisite to urbanization. ER2c also includes the Early Middle Ages, which corresponds to a phase of increased production, specialization, and urbanization strategies.
ER2d: globalization
The Colonial phase of major impact (ca. 650–400 cal BP) started during the second half of the Late Middle Ages and covered the Age of Discoveries (Figure 3). It is coincident with the beginning of the Little Ice Age, hence it is possible that this phase may be amplified by the cold climate. At that time, the Spanish economy was recovering after a century of crises (wars, plague, civil conflicts between 750 and 650 cal BP; Casado Alonso and Ruiz, 2019). Because of favorable politics to transhumance activities, large herds of sheeps deteriorated the forest over the Iberian Peninsula, and forest damage prevention as well as reforestoration were ineffective (Valbuena-Carabaña et al., 2010). This political decision was made to link the Spanish economy to the international trade of wool (Valbuena-Carabaña et al., 2010). However, wood resources were needed for charcoal production and the naval industry (Corbera Millán, 1999; Ceballos Cuerno, 2001), used either in metallurgy or foundry activities (Corbera Millán, 2011).
From this point onwards, economics and finance played a dominant role in the organization of societies, supported by many technological innovations (Urteaga, 1999). Inter-continental trade networks in hierarchical societies led to accumulation of wealth by some and poverty of others, paving the way to capitalism (Sieferle, 2001). The major influence of capitalism on human societies and subsistence strategies (called the Capitalocene) had also been investigated by Moore (2017, 2018), who identified its origin linked with colonialism during a time period corresponding to our Colonial phase of major impact. This inequality is also visible in the energy system, with discrepancies in energy flows and concentration (Moore, 2017, 2018). Colonialism is the result of this capitalist approach, pushing the Kingdom of Castile to always accumulate more resources and riches from other continents (Moore, 2017, 2018). This thirst of foreign resources likely fed the increased naval industry. By gathering energy and resources from all continents to sustain its economy, Spain entered in a globalization strategy, in which foreign energy and resources became necessary to sustain its development.
Interestingly, this period is coincident with the Orbis hypothesis (Lewis and Maslin, 2015). The Orbis hypothesis stipulates that because of the wars and diseases as well as famine and enslavement, brought by the colonization of the Americas, a huge decline in American population (90% of the population, from 61 to 6 millions in a course of 150 years), and its subsequent land abandonments, allowed a major episode of forest recovery, which is also visible with a sudden dip in the curve of global CO2 (Lewis and Maslin, 2015). This is exactly coincident with the Colonial phase of major impact (650–400 cal BP) and the following recovery period (350 cal BP; Figure 3).
The last phase of major impact is the Industrial Revolution ca. 150 cal BP-Present (Table 3). It is characterized by the exploitation of fossil energies such as coal for iron and steel industries (Torres Villanueva, 1991; Leorri et al., 2014), which are not sustainable. However, the use of fossil fuel is part of ER3, which is out of the scope of this paper. It is worth noting that our results showed that from ca. 650 cal BP onwards, MP and FR are only increasing, and only the tipping points of acceleration can identify new episodes of significant anthropic impact (at 650 cal BP and at 150 cal BP; Figure 3). The 650 cal BP acceleration is one of two dates exactly coinciding with recent propositions for the beginning of the Anthropocene based on global markers such as records of animal fossils and CO2 content (Lewis and Maslin, 2015). The other date is 1964 AD, i.e., right after 0 cal BP (Present Day), and corresponds to the nuclear bomb spike (Lewis and Maslin, 2015), but is not considered in our work. Since our results for heavy metal content are similar to other studies in Europe (e.g., many references across Europe are cited in Pontevedra-Pombal et al., 2013) and globally (Settle and Patterson, 1980; Nriagu, 1983, 1996), we hypothesize that this proxy may be used to further strengthen those dates in the debate to define the Anthropocene. Consequently, we define the fourth phase of the Agrarian regime (ER2d), starting from the Late Middle Ages (ca. 650 cal BP) as a regime of globalization strategy, which adds to the production (ER2a), specialization (ER2b) and urbanization (ER2c) strategies. The start of ER2d occurred during the second half of the Colonial phase of major impact (ca. 650–400 cal BP).
Conclusion
The unprecedented, high-resolution, inter-disciplinary investigation of HMC and TTC in peat bogs, and climate (temperature and precipitation) and natural vegetation models allowed us to highlight and identify seven phases of major, synchronous anthropogenic impact on landscapes in northern Spain: (1) the Copper phase ca. 4,400–4,100 cal BP, (2) the Middle Bronze phase ca. 3,500–3,150 cal BP, (3) the Iron phase ca. 2,800–2,500 cal BP, (4) the Roman phase ca. 2,200–1,750 cal BP, (5) the Medieval phase ca. 1,250–1000 cal BP, (6) the Colonial phase ca. 650-400 cal BP, and (7) the Industrial phase ca. 150 cal BP-Present. The results are coherent with previous research in both the Iberian Peninsula and in Europe (Monna et al., 2004; Pontevedra-Pombal et al., 2013).
HMC in peat bogs is a proxy that can easily be applied in other case study areas as HMC are similar at both local and global scale. TTC in peat bogs are much more subject to local discrepancies, as pollen data mainly record local vegetation, but our results show that a macro-scale analysis provides a global signal consistent with HMC. In addition, climate and natural vegetation simulations allowed us to refine the extent of natural vs. anthropogenic influence over changes in landscape. Thus, the focus on anthropogenic impact on landscapes provides valuable information that could potentialy lead to new methodologies to quantify the evolution and development of human activities through time. In turn, such quantified investigation could have potential to help unravel the debate on the origins of the Anthropocene, as our results already show some coherence with recent advances on the Anthropocene (Lewis and Maslin, 2015).
The confrontation of the results with archaeological and historical literature allowed us to document each phase of major impact. However, not all of them are related to major changes in human subsistence strategies. We identified four main subsistence strategies that agrarian societies developed over time, corresponding to four sub-regimes of the ER2: (1) Production (ER2a), with the first occurrences of productive economies during the Neolithic (agropastoral activities) and implying the control over solar energy, (2) Specialization (ER2b), with the first occurrences of specialization of activities and trade networks during the Middle Bronze phase, implying significant flow of energy within a society, (3) Urbanization (ER2c), with the first occurrences of urban centers and complex societies during the Roman phase, implying a complex system of stockage and distribution of energy within a society, and (4) Globalization (ER2d), with the first occurrences of worldwide trade, technological improvements, colonialism and capitalism economies during the Colonial phase, implying the globalization of the energy system over the entire world.
The framework of Energy Regimes as developed in this work and in previous research (Martinez et al., 2022, 2023) shows potential to provide a functioning analysis of the development of human societies and their subsistence strategies through time. Further research is needed to better understand the causes and consequences for each transition, but such investigation could provide invaluable information on the transitional phase between each ER, and hence, some insight on our current transition to a low-carbon society and its possible consequences. If we cannot directly compare our society with past ones, ERs focus on energy systems in a given society and hence make such comparison possible.
Data availability statement
The original contributions presented in the study are included in the article/supplementary material, further inquiries can be directed to the corresponding author.
Author contributions
AM: Conceptualization, Investigation, Methodology, Project administration, Resources, Software, Visualization, Writing – original draft, Writing – review & editing. AZ: Methodology, Resources, Software, Writing – original draft, Writing – review & editing. FA: Methodology, Resources, Software, Writing – original draft. PV: Methodology, Resources, Software, Writing – original draft, Writing – review & editing. SK: Funding acquisition, Supervision, Validation, Writing – review & editing. JM-R: Supervision, Writing – review & editing. CB: Supervision, Writing – review & editing. PF: Supervision, Writing – review & editing.
Funding
The author(s) declare financial support was received for the research, authorship, and/or publication of this article. The research presented in this paper has been undertaken within the project TERRANOVA: the European Landscape Learning Initiative, funded by the European Union's Horizon 2020 research and innovation programme, Marie Skłodowska-Curie Actions–Innovative Training Networks MSCA-ITN, under the grant agreement No: 813904. Funding for JM-R was obtained through Projects UIDB/05183/2020 (MED) and LA/P/0121/2020 (CHANGE-LAB), both of which are funded by the Portuguese National Foundation for Science and Technology (FCT). Works by CB and PF were founded by the AEQUA (Asociación Española para el Estudio del Cuaternario). This research is included in TERRANOVA's Work Package 2 (WP2): Exploring past environments and energy regimes, focusing on the reconstruction of past land-use strategies and energy regimes. The names of the Energy Regimes have been elaborated by Emily Vella from the Department of Archaeology and Ancient History of the University of Uppsala, and member of TERRANOVA's WP2. The results and interpretations of this work reflect the views only of the authors, and the European Union cannot be held responsible for any use which may be made of the information contained therein.
Conflict of interest
The authors declare that the research was conducted in the absence of any commercial or financial relationships that could be construed as a potential conflict of interest.
Publisher's note
All claims expressed in this article are solely those of the authors and do not necessarily represent those of their affiliated organizations, or those of the publisher, the editors and the reviewers. Any product that may be evaluated in this article, or claim that may be made by its manufacturer, is not guaranteed or endorsed by the publisher.
References
Alonso Matthias, F. (2002). “Fechas de Carbono 14 en los castros asturianos,” in Los poblados fortificados del NO de la Península Ibérica: formación y desarrollo de la cultura castreña, eds. M. A. de Blas Cortina and A. Villa Valdés (Navia: Ayuntamiento de Navia), 337–344.
Amigo, J., Rodríguez-Guitián, M. A., Pradinho Honrado, J. J., and Alves, P. (2017). “The lowlands and midlands of Northwestern Atlantic Iberia,” in The Vegetation of the Iberian Peninsula, Volume 1, ed. J. Loidi (Cham: Springer), 191–250.
Anthony, D. W. (2007). The Horse, the Wheel, and Languages: How Bronze-Age Riders From the Eurasian Steppes Shaped the Modern World (Princeton, NJ: Princeton University Press), 568.
Arbogast, R. M., Jacomet, S., Magny, M., and Schibler, J. (2006). The significance of climate fluctuations for lake level changes and shifts in subsistence economy during the late Neolithic (4300-2400 BC) in central Europe. Veget. Hist. Archaeobot. 15, 403–418. doi: 10.1007/s00334-006-0053-y
Arthur, F., Roche, D., Fyfe, R., Quiquet, A., and Renssen, H. (2023). Simulations of the Holocene climate in Europe using an interactive downscaling within the iLOVECLIM model (version 1.1). Clim. Past 19, 87–106. doi: 10.5194/cp-19-87-2023
Bajard, M., Ballo, E., Høeg, H. I., Bakke, J., Støren, E., Loftsgarden, K., et al. (2022). Climate adaptation of pre-Viking societies. Quat. Sci. Rev. 278:107374. doi: 10.1016/j.quascirev.2022.107374
Baldini, L. M., Baldini, J. U., McDermott, F., Arias, P., Cueto, M., Fairchild, I. J., et al. (2019). North Iberian temperature and rainfall seasonality over the Younger Dryas and Holocene. Quat. Sci. Rev. 226, 105–998. doi: 10.1016/j.quascirev.2019.105998
Berger, A. L. (1978). Long-term variations of daily insolation and Quaternary climatic changes. J. Atmos. Sci. 35, 2362–2367. doi: 10.1175/1520-0469(1978)035<2362:LTVODI>2.0.CO;2
Berglung, B. E. (2003). Human impact and climate changes-Synchronous events and a casual link? Quat. Int. 105, 7–12. doi: 10.1016/S1040-6182(02)00144-1
Blanco-González, A., Lillios, K. T., López-Sáez, J. A., and Drake, B. L. (2018). Cultural, demographic and environmental dynamics of the copper and early bronze age in Iberia (3300-1500 BC): towards an interregional multiproxy comparison at the time of the 4.2 ky BP event. J. World Prehist. 31, 1–79. doi: 10.1007/s10963-018-9113-3
Bond, G., Kromer, B., Beer, J., Muscheler, R., Evans, M. N., Showers, W., et al. (2001). Persistent solar influence on North Atlantic climate during the Holocene. Science 294, 2130–2136. doi: 10.1126/science.1065680
Burke, E. (2009). “The big story. Human history, energy regimes, and the environment,” in The Environment and World History, eds. E. Burke and K. Pomeranz (Berkeley, CA: University of California Press), 33–53.
Camino Mayor, J. (2002). “Algunos comentarios sobre las pautas territoriales y sociales de los Castros del Oriente de Asturias,” in Los poblados fortificados del NO de la Península Ibérica: formación y desarrollo de la cultura castreña, eds. M. A. de Blas Cortina and A. Villa Valdés (Navia: Ayuntamiento de Navia),139–157.
Carracedo, V., Cunill, R., García-Codron, J. C., Pèlachs, A., Pérez-Obiol, R., and Soriano, J. M. (2018). History of fires and vegetation since the Neolithic in the Cantabrian Mountains (Spain). Land Degrad. Dev. 29, 2060–2072. doi: 10.1002/ldr.2891
Carrión, J. S., Fernández, S., González-Sampériz, P., López-Merino, L., Peña, L., and Burjachs, F. (2015). Cinco millones de años de cambio florístico y vegetal en la Península Ibérica e Islas Baleares (Madrid: Ministerio de Economía y Competitividad de Madrid), 1017.
Casado Alonso, H., and Ruiz, T. F. (2019). Economic life in late medieval and early modern Spain, 1085–1815. Oxf. Res. Encycl. Econ. Fin. 13:481. doi: 10.1093/acrefore/9780190625979.013.481
Casal y Julian, G. (1762). Historia natural, y médica del Principado de Asturias. Edition del II Centenario, 1900 (Oviedo: Disputación Provincial de Oviedo), 440.
Ceballos Cuerno, C. (2001). Arozas y ferrones: las ferrerías de Cantabria en el Antiguo Régimen (Santander: Editorial, Universidad de Cantabria), 408.
Comendador-Rey, B., Meunier, E., Figueiredo, E., Lackinger, A., Fonte, J., Fernández Fernández, C., et al. (2017). “Northwestern Iberian tin mining from Bronze age to modern times: an overview,” in The Tinworking Landscape of Dartmoor in a European Context—Preshitory to 20th Century. Paper Presented at a Conference in Tavistock, Devon, 6-11 May 2016, ed. P. Newman (Devon: Dartmoor Tinworking Research Group), 133–153.
Comendador-Rey, B., Reboreda, S., Kockelmann, W., Macdonald, M., Bell, T., and Pantos, M. (2006). “The source of tin: early bronzes technology and the route to the land's end in North-Western Iberia,” in Proceedings for the International Symposium on Science and Technology in Homeric Epics, ed. S. Paipetis (Olympia: Springer), 1–19.
Corbera Millán, M. (1999). “Ferrerías en Cantabria,” in Hierro al mar. Minas, bosques, ferrerías, astilleros y arsenales 'litoral atlántico', ed. L. Azurmendi Perez (Madrid: Instituto de Estudios Cántabros, Asociación Tajamar), 45–51.
Corbera Millán, M. (2011). “Sobre los agentes de la deforestación en Cantabria entre los siglos XVI y XIX,” in La evolución del paisaje vegetal y el uso del fuego en la cordillera cantábrica, eds. F. J. Ezquerra Boticario and E. Rey van den Bercken (Valladolid: Fundación Patrimonio Natural de Castilla y León), 171–184.
Costa-García, J. M. (2017). “Rediscovering the Roman Conquest of the North-western Iberian Peninsula,” in Conflict Archaeology. Materialities of Collective Violence from Prehistory to Late Antiquity, eds. M. Fernández-Götz and N. Roymans (Oxford: Routledge), 141–151.
Costa-García, J. M. (2018). “Roman camp and fort design in Hispania: an approach to the distribution, morphology and settlement pattern of Roman Military Sites during the Early Empire,” in Proceedings of the 23rd International Limes Congress in Ingolstadt 2015, Limes XXIII, Ingolstadt, Germany, 12-23 September 2015, volume 2, eds. C. S. Sommer and S. Matešić (Mainz: Nünnerich-Asmus), 986–993.
Cubas, M., Altuna, J., Álvarez-Fernández, E., Armendariz, A., Fano, M. Á., López-Dóriga, I. L., et al. (2016). Re-evaluating the Neolithic: the impact and the consolidation of farming practices in the Cantabrian Region (Northern Spain). J. World Prehist. 29, 79–116. doi: 10.1007/s10963-016-9091-2
Cunliffe, B. (2001). Facing the Ocean. The Atlantic and its peoples. 8000 BC-AD 1500 (Oxford: Oxford University Press), 608.
Currás, B. X., and Sastre Prats, I. (2019). Alternative Iron Age: Social Theory From Archaeological Analysis (London: Routledge), 389.
de Blas Cortina, M. A. (1996). La primera minería metálica del N. peninsular: las indicaciones del C-14 y la cronología prehistórica de la explotaciones cupríferas del Aramo y El Milagro. Complutum Extra. 6, 217–226.
de Blas Cortina, M. A. (2005). Un témoignage probant de l'exploitation préhistorique du cuivre dans le nord de la péninsule ibérique: le complexe minier de l'Aramo. Mémoires de la Société Préhistorique Française. 37, 195–205.
DeMenocal, P. B. (2001). Cultural response to climate change during the late Holocene. Science 292, 667–673. doi: 10.1126/science.1059287
Díaz González, T. E., and Penas, Á. (2017). “The high moutain area of Northwestern Spain: the Cantabrian Range, the Galician-Leonese Mountains and the Bierzo Trench,” in The Vegetation of the Iberian Peninsula, Volume 1, ed. J. Loidi (Cham: Springer), 251–321.
Díaz, P. C., and Menéndez-Bueyes, R. (2005). “The Cantabrian basin in the fourth and fifth centuries: from imperial province to periphery,” in Hispania in Late Antiquity: Current Approaches, eds. K. Bowes and M. Kulikowski (Leiden: E. J. Brill), 265–298.
Dury, M., Hambuckers, A., Warnant, P., Henrot, A., Favre, E., Ouberdous, M., et al. (2011). Responses of European foerst ecosystems to 21st century climate: assessing changes in interannual variability and fire intensity. iforest Biogeosci. For. 4, 82–99. doi: 10.3832/ifor0572-004
Earle, T. (2002). Bronze Age Economics. The Beginning of Political Economies (Cambridge: Westview Press), 432.
Earle, T., Ling, J., Uhnér, C., Stos-Gale, Z., and Melheim, L. (2015). The political economy and metal trade in Bronze Age Europe: understanding regional variability in terms of comparative advantages and articulations. Eur. J. Archaeol. 18, 633–657. doi: 10.1179/1461957115Y.0000000008
Ellis, E. C. (2015). Ecology in an anthropogenic biosphere. Ecol. Monogr. 85, 287–331. doi: 10.1890/14-2274.1
Ellis, E. C., Gauthier, N., Klein Goldewijk, K., Bliege Bird, R., Boivin, N., Díaz, S., et al. (2021). People have shaped most of terrestrial nature for at least 12,000 years. Proc. Natl. Acad. Sci. U. S. A. 118:e2023483118. doi: 10.1073/pnas,.2023483118
Ellis, E. C., and Ramankutty, N. (2008). Putting people in the map: anthropogenic biomes of the world. Front. Ecol. Environ. 6, 439–447. doi: 10.1890/070062
EU-DEM (2016). European Digital Elevation Model, version 1.1. Retrieved from the Land Monitoring Service of the Copernicus Programme. Available online at: https://land.copernicus.eu/imagery-in-situ/eu-dem/eu-dem-v1.1/view (accessed July 4, 2022).
Eurostat (2020). Statistical Regions in the European Union and Partner Countries. NUTS and Statistical Regions 2021. Luxembourg: Publications Office of the European Union.
Fanjul, A., and Marín, C. (2006). La metalurgia del hierro en la Asturias castreña: nuevos datos y estado de la cuestión. Trabajos de Prehistoria. 63, 113–131. doi: 10.3989/tp.2006.v63.i1.7
Fano, M. Á., Cubas, M., and Wood, R. (2015). The first farmers in Cantabrian Spain: contribution of numerical chronology to understand an historical process. Quat. Int. 364, 153–161. doi: 10.1016/j.quaint.2014.09.026
Fernández Izquierdo, F. (1989). “Astilleros y construcción naval en la España anterior a la Ilustración,” in España y el ultramar hispánico hasta la Ilustración: Volume I Jornadas de histori marítima, ed. Instituto de Historia y Cultura Naval (Madrid: Instituto de Historia y Cultura Naval), 35–62.
Fernández Mier, M., Fernández Fernández, J., Alonso González, P., López-Sáez, J. A., Pérez-Díaz, S., and Hernández Beloqui, B. (2014). The investigation of currently inhabited villages of medieval origin: Agrarian archaeology in Asturias (Spain). Quat. Int. 346, 41–55. doi: 10.1016/j.quaint.2014.01.032
Fernández, S., Cotos-Yáñes, T., Roca-Pardiñas, J., and Ordoñez, C. (2018). Geographically weighted principal components analysis to assess diffuse pollution sources of soil heavy metals. Application to rough mountain areas in Northwest Spain. Geoderma 311, 120–129. doi: 10.1016/j.geoderma.2016.10.012
Fischer-Kowalski, M., and Haberl, H. (2007). “Conceptualizing, observing and comparing socioecological transitions,” in Socioecological Transitions and Global Change: Trajectories of Social Metabolism and Land Use, eds. M. Fischer-Kowalski and H. Haberl (Cheltenham: Edward Elgar), 1–30.
Fischer-Kowalski, M., Krausmann, F., and Pallua, I. (2014). A sociometabolic reading of the Anthropocene: modes of subsistence, population size and human impact on Earth. Anthropocene Rev. 1, 8–33. doi: 10.1177/2053019613518033
François, L., Utescher, T., Favre, E., Henrot, A. J., Warnant, P., Micheels, A., et al. (2011). Modelling Late Miocene vegetation in Europe: results of the CARAIB model and comparison with palaeovegetation data. Palaeogeogr. Palaeoclimatol. Palaeoecol. 304, 359–378. doi: 10.1016/j.palaeo.2011.01.012
Gallego, J. L. R., Ortiz, J. E., Sánchez-Palencia, Y., Baragaño, D., Borrego, A. G., and Torres, T. (2019). A multivariate examination of the timing and accumulation of potentially toxic elements at Las Conchas bog (NW Spain). Environ. Pollut. 254:113048. doi: 10.1016/j.envpol.2019.113048
Gallego, J. L. R., Ortiz, J. E., Sierra, C., Torres, T., and Llamas, J. F. (2013). Multivariate study of trace element distribution in the geological record of Rañanzas peat bog (Asturias, N. Spain). Paleoenvironmental evolution and human activities over the last 8000 cal yr BP. Sci. Tot. Environ. 454–455, 16–29. doi: 10.1016/j.scitotenv.2013.02.083
Galop, D., Tual, M., Monna, F., Dominik, J., Beyrie, A., and Marembert, F. (2001). Cinq millénaires de métallurgie en montagne basque. Les apports d'une démarche intégrée alliant palynologie et géochimie isotopique du plomb. Sud-Ouest Européen 11, 3–15. doi: 10.3406/rgpso.2001.2763
García-Alix, A., Jiménez-Espejo, F. J., Lozano, J. A., Jiménez-Moreno, G., Martínez-Ruiz, F., García Sanjuán, L., et al. (2013). Anthropogenic impact and lead pollution throughout the Holocene in Southern Iberia. Sci. Tot. Environ. 449, 451–460. doi: 10.1016/j.scitotenv.2013.01.081
Gaydarska, B. (2017). Introduction: European Prehistory and urban studies. J. World Prehist. 30, 177–188. doi: 10.1007/s10963-017-9104-9
Gómez Ramos, P. (1999). Obtención de metals en la Prehistoria de la Peninsula Ibérica. (Oxford: Archaeo Press), 225.
González Ruibal, A. (2012). “The politics of identity: ethnicity and the economy of power in Iron Age Northwest Iberia,” in Landscape, Ethnicity, Identity in the Archaic Mediterranean Area, eds. S. Stoddart and G. Cifani (Oxford: Oxbow Books), 245–266.
González-Álvarez, D. (2019a). “Humanizing the western Cantabrian Mountains in northwestern Iberia: a diachronic perspective on the exploitation of the uplands during Late Prehistory,” in Hitorical Ecologies, Heterarchies and Transtemporal Landscapes, eds. C. Ray and M. Fernández-Götz (New York, NY: Routledge), 156–175.
González-Álvarez, D. (2019b). The need to understand the cultural biographies of alpine and subalpine landscapes during the later prehistory: upland archaeology in the Cantabrian mountains. Cuadernos de Investigación Geográfica 45, 146–165. doi: 10.18172/cig.3824
González-Ruibal, A. (2004). Facing two seas: Mediterranean and Atlantic contacts in the north-est of Iberia in the first millennium BC. Oxford J. Archaeol. 23, 287–317. doi: 10.1111/j.1468-0092.2004.00213.x
Goosse, H., Brovkin, V., Fichefet, T., Haarsma, R., Huybrechts, P., Jongma, J., et al. (2010). Description of the Earth system model of intermediate complexity LOVECLIM version 1.2. Geosci. Model Dev. 3, 603–633. doi: 10.5194/gmd-3-603-2010
Griffiths, S., and Robinson, E. (2018). The 8.2 ka BP climate change event and human population resilience in northwest Atlantic Europe. Quat. Int. 465, 251–257. doi: 10.1016/j.quaint.2017.10.017
Han, H. P. (2016). “Cities between de-territorialization and networking: on the dynamics of urbanization and social change context,” in Eurasia at the Dawn of History: Urbanization and Social Change, eds. M. Fernández-Götz and D. Krausse (Cambridge: Cambridge University Press), 169–180.
IGME and LNEG (2015). Mapa Geologico de Espanha e Portugal à escala 1:1 000 000. 3rd Edn. Madrid: Laboratorio Nacional de Energía y Geología.
IPCC (2022). Climate Change 2022: Mitigation of Climate Change. Contribution of Working Group II to the sixth assessment report of the Intergovernmental Panel on Climate Change. Cambridge: Cambridge University Press.
Irabien, M. J., García-Artola, A., Cearreta, A., and Leorri, E. (2015). Chemostratigraphic and lithostratigraphic signatures of the Anthropocene in estuarine areas from the eastern Cantabrian coast (N. Spain). Quat. Int. 364, 196–205. doi: 10.1016/j.quaint.2014.08.056
Izco, J., Díaz-Varela, R., Martínez-Sánchez, S., Rodríguez-Guitián, M. A., Ramil-Rego, P., and Pardo-Gamundi, I. (2001). Análisis y valoracíon de la Sierra de O Xistral: un modelo de aplicacíon de la Directiva Hábitat en Galicia (Santiago de Compostela: Consellería de Medio Ambiente. Xunta de Galicia), 161.
Jalut, G., Dedoubat, J. J., Fontugne, M., and Otto, T. (2009). Holocene circum-Mediterranean vegetation changes: climate forcing and human impact. Quat. Int. 200, 4–18. doi: 10.1016/j.quaint.2008.03.012
Jedrej, M. C. (1995). Ingessana: The Religious Institutions of a People of the Sudan-Ethiopian Borderland (Leiden: E. J. Brill), 136.
Johansen, K. L., Laursen, S. T., and Holst, M. K. (2003). Spatial patterns of social organization in the Early Bronze Age of South Scandinavia. J. Anthropol. Archaeol. 23, 33–55. doi: 10.1016/j.jaa.2003.10.002
Jouffroy-Bapicot, I., Pulido, M., Baron, S., Galop, D., Monna, F., Lavoie, M., et al. (2007). Environmental impact of early palaeometallurgy: pollen and geochemical analysis. Veget. Hist. Archaeobot. 16, 251–258. doi: 10.1007/s00334-006-0039-9
Krausmann, F., Weisz, H., and Eisenmenger, N. (2016). “Transition in sociometabolic regimes throughout human history,” in Social Ecology: Society-Nature Relations Across Time and Space, eds. H. Haberl, M. Fischer-Kowalski, F. Krausmann and V. Winiwarter (New York, NY: Springer International Publishing), 63–92.
Kylander, M. E., Weiss, D. J., Martínez-Cortizas, A., Spiro, B., Garcia-Sanchez, R., and Coles, B. J. (2005). Refining the pre-industrial atmospheric Pb isotope evolution curve in Europe using an 8,000 year old peat core from NW Spain. Earth Planet. Sci. Lett. 240, 467–485. doi: 10.1016/j.epsl.2005.09.024
Laurent, J. M., François, L. M., Bar-Hen, A., Bel, L., and Cheddadi, R. (2008). European bioclimatic affinity groups: Data-model comparisons. Glob. Planet Change. 61, 28–40. doi: 10.1016/j.gloplacha.2007.08.017
Leorri, E., Mitra, S., Irabien, M. J., Zimmerman, A. R., Blake, W. H., and Cearreta, A. (2014). A 700 year record of combustion-derived pollution in northern Spain: tools to identify the Holocene/Anthropocene transition in coastal environments. Sci. Tot. Environ. 470–471, 240–247. doi: 10.1016/j.scitotenv.2013.09.064
Lewis, P. R., and Jones, G. D. B. (1970). Roman gold-mining in north-west Spain. J. Roman Stud. 60, 169–185. doi: 10.1017/S0075435800043343
Lewis, S. L., and Maslin, M. A. (2015). Defining anthropocene. Nature 519, 171–180. doi: 10.1038/nature14258
Lillios, K. T. (2019). The Archaeology of the Iberian Peninsula: From the Paleolithic to the Bronze Age (Cambridge: Cambridge University Press), 387.
Lillios, K. T., Blanco-González, A., Drake, B. L., and López-Sáez, J. A. (2016). Mid-late Holocene climate, demography, and cultural dynamics in Iberia: a multi-proxy approach. Quat. Sci. Rev. 135, 138–153. doi: 10.1016/j.quascirev.2016.01.011
Ling, J. (2008). Elevated Rock Art. Towards a Maritime Understanding of Bronze Age Rock Art in Northern Bohuslän, Sweden. GOTARC. Series B, Gothenburg Archaeological Theses; no. 49 (Göteborg: Göteborg Universitet. Institutionen för arkeologi), 271.
Lomas-Clarke, S. H., and Barber, K. E. (2006). Human impact signals from peat bogs - a combined palynological and geochemical approach. Veget. Hist. Archaeobot. 16, 419–429. doi: 10.1007/s00334-006-0085-3
López-Merino, L. (2009). Paleoambiente y antropización en Asturias durante el Holoceno (Madrid: Universidad Autónoma de Madrid), 274.
López-Merino, L., Martínez-Cortizas, A., and López-Sáez, J. A. (2010). Early agriculture and palaeoenvironmental history in the North of the Iberian Peninsula: a multi-proxy analysis of the Monte Areo mire (Asturias, Spain). J. Archaeol. Sci. 37, 1978–1988. doi: 10.1016/j.jas.2010.03.003
López-Merino, L., Martínez-Cortizas, A., and López-Sáez, J. A. (2011). Human-induced changes on wetlands: a study case from NW Iberia. Quat. Sci. Rev. 30, 2745–2754. doi: 10.1016/j.quascirev.2011.06.004
López-Merino, L., Martínez-Cortizas, A., Reher, G. S., López-Sáez, J. A., Mighall, T. M., and Bindler, R. (2014). Reconstructing the impact of human activities in a NW Iberian Roman mining landscape for the last 2,500 years. J. Archaeol. Sci. 50, 208–218. doi: 10.1016/j.jas.2014.07.016
López-Merino, L., Sánchez, N. S., Kaal, J., López-Sáez, J. A., and Martínez-Cortizas, A. (2012). Post-disturbance vegetation dynamics during the Late Pleistocene and the Holocene: an example from NW Iberia. Glob. Planet. Change 92, 58–70. doi: 10.1016/j.gloplacha.2012.04.003
López-Sáez, J. A., López-García, P., and López-Merino, L. (2006). “El impacto humano en la Cordillera Cantábrica: Estudios palinológicos durante el Holoceno Medio,” in Miscelánea en homaje a Victoria Cabrera, eds. J. M. Maíllo-Fernández and E. Baquedano-Pérez (Madrid: Museo Arqueológico Regional), 123–130.
MacKenzie, A. B., Farmer, J. G., and Sugden, C. L. (1997). Isotopic evidence of the relative retention and mobility of lead and radiocesium in Scottish ombrotrophic peats. Sci. Tot. Environ. 36, 115–127. doi: 10.1016/S0048-9697(97)00139-3
Marín Suárez, C. (2009). De nómadas a castreños. Los orígenes de la Edad de Hierro en Asturias, Entemu. 16, 21–46.
Marín Suárez, C. (2011). De nómadas a castreños: el primer milenio antes de la era en el sector centro-occidental de la Cordillera Cantábrica. Tesis doctoral (Madrid: Universidad Complutense de Madrid, Facultad de Geografía e Historia, Departamento de Prehistoria), 750.
Mariscal, B. (1983). Estudio polínico de la turbera del Cueto de la Avellanosa, Polaciones (Cantabria). Cadernos do Laboratorio Xeolóxico de Laxe. 5, 205–226.
Martín-Chivelet, J., Muñoz-García, M. B., Edwards, R. L., Turrero, M. J., and Ortega, A. I. (2011). Land surface temperature changes in Northern Iberia since 4,000 yr BP, based on δ13C of speleothems. Glob. Planet. Change 77, 1–12. doi: 10.1016/j.gloplacha.2011.02.002
Martinez, A., Kluiving, S., Muños-Rojas, J., Borja Barrera, C., and Fraile Jurado, P. (2022). From hunter-gatherer subsistence strategies to the Agricultural Revolution: disentangling energy regimes as a complement to cultural phases in Northern Spain. Holocene 32, 884–896. doi: 10.1177/09596836221095990
Martinez, A., Kluiving, S., Muños-Rojas, J., Borja Barrera, C., Fraile Jurado, P., Roldán-Muñoz, M. E., et al. (2023). Energy regimes help tackle limitations with the prehistoric cultural phases approach to learn about sustainable transitions: archaeological evidence from northern Spain. J. Quat. Sci. 38, 921–937. doi: 10.1002/jqs.3522
Martinez, J. M. (2003). La metalurgie prehidráulica del hierro: aproximación a las ferrerías secas en Cantabria. Sautola Revista del Instituto de prehistoria y Arqueología Sautola. 9, 393–408.
Martínez, J. M., and Mantecón Callejo, L. (2012). “Aproximación a las fortificaciones de cronología altomedieval en Cantabria,” in Los castillos altomedievales en el Noroeste de la Península Ibérica, eds. J. A. Quirós Castillo and J. M. Tejado Sebastián (Bilbao: Servicio Editorial de la Universidad del País Vasco),99–122.
Martínez-Cortizas, A., Costa-Casais, M., and López-Sáez, J. A. (2009). Environmental change in NW Iberia between 7000 and 500 cal BC. Quat. Int. 200, 77–89. doi: 10.1016/j.quaint.2008.07.012
Martínez-Cortizas, A., García-Rodeja, E., Pontevedra-Pombal, X., Nóvoa Muñoz, J. C., Weiss, D., and Cheburkin, A. (2002b). Atmospheric Pb deposition in Spain during the last 4,600 years recorded by two ombrotrophic peat bogs and implications for the ise of peat as archive. Sci. Tot. Environ. 292, 33–44. doi: 10.1016/S0048-9697(02)00031-1
Martínez-Cortizas, A., García-Rodeja, E., and Weiss. (2002a). Peat bog archives of atmospheric metal deposition. Sci. Tot. Environ. 292, 1–5. doi: 10.1016/S0048-9697(02)00024-4
Martínez-Cortizas, A., López-Costas, O., Orme, L., Mighall, T., Kylander, M. E., Bindler, R., et al. (2020). Holocene atmospheric dust deposition in NW Spain. Holocene 30, 507–518. doi: 10.1177/0959683619875193
Martínez-Cortizas, A., López-Merino, L., Bindler, R., Mighall, T. M., and Kylander, M. E. (2013). Atmospheric Pb pollution in N Iberia during the late Iron Age/Roman times reconstructed using high-resolution record of La Molina mire (Asturias, Spain). J. Paleolimnol. 50, 71–86. doi: 10.1007/s10933-013-9705-y
Martínez-Cortizas, A., López-Merino, L., Bindler, R., Mighall, T. M., and Kylander, M. E. (2016). Early atmospheric metal pollution provides evidence for Chalcolithic/Bronze Age mining and metallurgy in Southwestern Europe. Sci. Tot. Environ. 545–546, 398–406. doi: 10.1016/j.scitotenv.2015.12.078
Martínez-Cortizas, A., Mighall, T., Pontevedra-Pombal, X., Nóvoa Muñoz, J. C., Peiteado Varela, E., and Piñeiro Rebolo, R. (2005). Linking changes in atmospheric dust deposition, vegetation change and human activities in northwest Spain during the last 5,300 years. Holocene 15, 698–706. doi: 10.1191/0959683605hl834rp
Martínez-Cortizas, A., Nóvoa Muñoz, J. C., Pontevedra-Pombal, X., García-Rodeja, E., and Llana Rodríguez, C. (1997b). Paleocontaminación. Evidencias de contaminación atmosférica antrópica en Galicia durante los últimos 4,000 años. Gallaecia 16, 7–22.
Martínez-Cortizas, A., Pontevedra-Pombal, X., García-Rodeja, E., Nóvoa Muñoz, J. C., and Shotyk, W. (1999). Mercury in a Spanish peat bog: archive of climate change and atmospheric metal deposition. Science 284, 939–942. doi: 10.1126/science.284.5416.939
Martínez-Cortizas, A., Pontevedra-Pombal, X., Nóvoa Muñoz, J. C., and García-Rodeja, E. (1997a). Four thousand years of atmospheric Pb, Cd and Zn deposition recorded by the ombrotrophic peat bog og Penido Vello (Northwestern Spain). Water Air Soil Pollut. 100, 387–403. doi: 10.1023/A:1018312223189
Martínez-Cortizas, A., Pontevedra-Pombal, X., Nóvoa Muñoz, J. C., and García-Rodeja, E. (2000). Turberas de montaña del noroeste de la Península Ibérica. Edafologia 7, 1–29.
Mata Olmo, R., and Sanz Herráiz, C. (2004). Atlas de los paisajes de España. Cartografía 1:250.000 (Original: 1.000.000) Unidades de Paisaje. Madrid: Ministerio de Medio Ambiente.
Meléndez Hevia, I. (2004). Geología de España: una historia de seiscientos millones de años (Madrid: Ediciones Rueda), 277.
Mercuri, A. M., Florenzano, A., Burjachs, F., Giardini, M., Kouli, K., Masi, A., et al. (2019). From influence to impact: the multifunctional land-use in Mediterranean prehistoru emerging from plynology of archaeological sites (8.0-2.8 ka BP). Holocene 29, 830–846. doi: 10.1177/0959683619826631
Mighall, T. M., Martínez-Cortizas, A., Biester, H., and Turner, S. E. (2006). Proxy climate and vegetation changes during the last five millennia in NW Iberia: Pollen and non-pollen palynomorph data from two ombrotrophic peat bogs in the North Western Iberian Peninsula. Rev. Palaeobot. Palynol. 141, 203–223. doi: 10.1016/j.revpalbo.2006.03.013
Mighall, T. M., Martínez-Cortizas, A., Silva-Sánchez, N., López-Costas, O., and López-Merino, L. (2023). Climate change, fire and human activity drive vegetation change during the last eight millennia in the Xistral mountains of NW Iberia. Quaternary 6:5. doi: 10.3390/quat6010005
Monna, F., Galop, D., Carozza, L., Tual, M., Beyrie, A., Marembert, F., et al. (2004). Environmental impact of early Basque mining and smelting recorded in a high ash minerogenic peat deposit. Sci. Tot. Environ. 327, 197–214. doi: 10.1016/j.scitotenv.2004.01.010
Moore, J. W. (2017). The Capitalocene, Part I: on the nature and origins of our ecological crisis. J. Peasant Stud. 44, 594–630. doi: 10.1080/03066150.2016.1235036
Moore, J. W. (2018). The Capitalocene, Part II: accumulation by appropriation and the centrality of unpaid work/energy. J. Peasant Stud. 45, 237–279. doi: 10.1080/03066150.2016.1272587
Moreno, L., Gallego, J. L. R., Ortiz, J. E., Torres, T., and Sierra, C. (2009). Distribución de elementos traza en el registro de la turbera de Roñanzas (Asturias, España). Geogaceta 46, 123–126.
Moreno, T., Higueras, P., Jones, T., McDonald, I., and Gibbons, W. (2005). Size fractionation in mercury-bearing airborne particles (HgMP10) at Almadén, Spain: implications for inhalation hazard around old mines. Atmos. Environ. 39, 6409–6419. doi: 10.1016/j.atmosenv.2005.07.024
Muñiz Sánchez, J. (2011). Administrar minas, cuerpos y mentes. Los ingenieros del siglo XIX. Una fuente fundamental para la historia social de Asturias. Historia Trabajo y Sociedad 2, 11–32.
Muñoz-Sobrino, C., Ramil-Rego, P., Gómez-Orellana, L., and Díaz Varela, R. A. (2005). Palynological data on major Holocene climatic events in NW Iberia. Boreas 34, 381–400. doi: 10.1080/03009480510013006
Muñoz-Sobrino, C., Ramil-Rego, P., Gómez-Orellana, L., Ferreiro da Costa, J., and Díaz Varela, R. A. (2009). Climatic and human effects of the post-glacial dynamics of Fagus sylvatica L. in NW Iberia. Plant Ecol. 203, 317–340. doi: 10.1007/s11258-008-9552-5
Needham, S. (2007). Late Neolithic and Bronze Age Connections With Europe. London: Unpublished paper presented at South East Research Framework Resource Assessment Seminars.
Nriagu, J. O. (1996). A history of global pollution. Science 272, 223–224. doi: 10.1126/science.272.5259.223
Núñez, S. (2018). Dinámicas socio-ecológicas, resiliencia y vulnerabilidad en un paisaje atlántico montañoso: la Región Cantábrica durante el Holoceno (Santander: Universidad de Cantabria), 266.
Ojeda Gutiérrez, G. (1994). “La economía asturiana en el siglo XIX: una visión de conjunto,” in Historia de la economía asturiana, Volume 1, eds. J. Vázquez García and G. Ojeda (Oviedo: Editorial Prensa Asturiana), 33–48.
Ontañón-Peredo, R. (1995). El Neolítico final y el Calcolítico en Cantabria. Cuadernos de Sección. Prehistoria-Arqueología 6, 81–103.
Ontañón-Peredo, R. (2003). Caminos hacia la complejidad. El Calcolítico en la región cantábrica (Santander: Universidad de Cantabria), 397.
Orejas, A., Sánchez Palencia, F. J., and Sastre Prats, I. (2002). “Los castros y la ocupación romana en zonas mineras del NO de la Península Ibérica,” in Los poblados fortificados del NO de la Península Ibérica: formación y desarrollo de la cultura castreña, eds. M. A. de Blas Cortina and A. Villa Valdés (Navia: Ayuntamiento de Navia), 241–259.
Ortega, L. A., Alonso-Fernández, C., Guede, I., Zuluaga, M. C., Alonso-Olazabal, A., and Jiménez-Echevarría, J. (2021). Strontium and oxygen isotopes to trace mobility routes during the Bell Beaker period in the north of Spain. Sci. Rep. 11:19553. doi: 10.1038/s41598-021-99002-8
Otto, D., Rasse, D., Kaplan, J., Warnant, P., and François, L. (2002). Biospheric carbon stocks reconstructed at the Last Glacial Maximum: comparison between general circulation models using prescribed and computed sea surface temperatures. Glob. Planet. Change 33, 117–138. doi: 10.1016/S0921-8181(02)00066-8
Parcero Oubiña, C. (2002). La construcción del paisaje social en la Edad del Hierro del Noroeste Ibérico (Ortigueira: Fundación Ortegalia. Monografías de Arqueoloxía, Historia y Patrimonio), 299.
Peña-Chocarro, L., Peña, L. Z., Gazólaz, J. G., Morales, M. G., Sesma, J. S., and Straus, L. G. (2005). The spread of agriculture in northern Iberia: new archaeobotanical data from El Mirón cave (Cantabria) and the open-air site of Los Cascajos (Navarra). Veget. Hist. Archaeobot. 14, 268–278. doi: 10.1007/s00334-005-0078-7
Pérez-Díaz, S., López-Sáez, J. A., Núñez de la Fuente, S., and Ruiz-Alonso, M. (2018). Early farmers, megalithic builders and the shaping of the cultural landscapes during the Holocene in Northern Iberian mountains. A palaeoenvironmental perspective. J. Archaeol. Sci. 18, 463–474. doi: 10.1016/j.jasrep.2018.01.043
Pérez-Díaz, S., López-Sáez, J. A., Pontevedra-Pombal, X., Souto-Souto, M., and Galop, D. (2016b). 8,000 years of vegetation history in the northern Iberian Peninsula inferred from the palaeoenvironmental study of the Zalama ombrotrophic bog (Basque-Cantabrian Mountains, Spain). Boreas 45, 658–672. doi: 10.1111/bor.12182
Pérez-Díaz, S., Núñez, S., Frochoso, M., González, R., Allende, F., and López, J. A. (2016a). Seis mil años de gestión dinámica antrópica en el entorno del Parque Natural de los Collados de Asón (Cordillera Cantábrica Oriental). Cuaternario y Geomorfología 30, 49–74. doi: 10.17735/cyg.v30i3-4.49677
Pérez-Losada, F. (1996). “Hacia una definición de los asentamientos rurales en la Gallaecia: poblados (vici) y casas de campo (villae),” in Los Finisterres Atlánticos en la Antigüedad. Época prerromana y romana, ed. C. Fernández Ochoa (Madrid: Sociedad Editorial Electa-Ayuntamiento de Gijón), 189–197.
Pérez-Obiol, R., García-Codron, J. C., Pèlachs, A., Pérez-Haase, A., and Soriano, J. M. (2016). Landscape dynamics and fire activity since 6740 cal yr BP in the Cantabrian region (La Molina peat bog, Puente Viesgo, Spain). Quat. Sci. Rev. 135, 65–78. doi: 10.1016/j.quascirev.2016.01.021
Peruchetti, L., Montero-Riuz, I., and Bray, P. (2020). Mapping archaeometallurgical data of the Iberian Copper Age: different ways to look at a big picture. J. Archaeol. Sci. 119:105165. doi: 10.1016/j.jas.2020.105165
Pontevedra-Pombal, X., Mighall, T. M., Nóvoa-Muñoz, J. C., Peiteado-Varela, E., Rodríguez-Racedo, J., García-Rodeja, E., et al. (2013). Five thousand years of atmospheric Ni, Zn, As, and Cd deposition recorded in bogs from NW Iberia: prehistoric and historic anthropogenic contributions. J. Archaeol. Sci. 40, 764–777. doi: 10.1016/j.jas.2012.07.010
Pontevedra-Pombal, X., Souto, M., Castro, D., López-Sáez, J. A., Pérez-Díaz, S., Fraga, M. I., et al. (2018). “Origin and evolution of the blanket bog of Zalama (Montes de Ordunte, Northern Iberian Peninsula),” in Inventory, Value and Restoration of Peatlands and Mires: Recent Contributions, eds. J. M. Fernández-Garcia and F. J. Pérez (Bizkaia: Hazi Foundation), 223–236.
QGIS Development Team (2023). QGIS Geographic Information System, v. 3.32 (Lima). QGIS Association. Available online at: https://www.qgis.org (accessed July 4, 2023).
Quiquet, A., Roche, D. M., Dumas, C., and Paillard, D. (2018). Online dynamical downscaling of temperature and precipitation within the iLOVECLIM model (version 1.1). Geosci. Model Dev. 11, 453–466. doi: 10.5194/gmd-11-453-2018
Ramil-Rego, P., Rodríguez-Guitián, M. A., and Rodríguez-Oubiña, J. (1996). “Valoración de los humedales continentales del NW Ibérico: caracterización hidrológica y vegetacional de las turberas de las Sierras Septentrionales de Galicia,” in Avances en la reconstrucción paleoambiental de las áreas de monta éna lucenses, eds. A. Pérez-Alberti and A. Martínez-Cortizas (Lugo: Monografías G.E.P. n. 1, Diputación Provincial de Lugo), 166–187.
Rauch, S., Peucker-Ehreenbrink, B., Kylander, M. E., Weiss, D. J., Martínez-Cortizas, A., Heslop, D., et al. (2010). Anthropogenic forcings on the surficial osmium cycle. Environ. Sci. Technol. 44, 881–887. doi: 10.1021/es901887f
Raynaud, D., Barnola, J. M., Chappellaz, J., Blunier, T., Indermuhle, A., and Stauffer, B. (2000). The ice record of greenhouse gases: a view in the context of future changes. Quat. Sci. Rev. 19, 9–17. doi: 10.1016/S0277-3791(99)00082-7
Renfrew, C. (1969). Trade and culture process in European Prehistory. Curr. Anthropol. 10, 151–169. doi: 10.1086/201066
Rivas-Martinez, S. (2007). Mapa de series, geoseries y geopermaseries de vegetación de España. Memoria del mapa de la vegetación potencial de España, parte 1. Itinera Geobotanica 17, 5–435.
Robb, J. E., and Farr, R. H. (2005). “Substances in motion: neolithic mediterranean “trade”,” in The Archaeology of Mediterranean Prehistory, eds. E. Blake and A. B. Knapp (London: Blackwell Publishing), 352.
Roberts, N., Eastwood, W. J., Kuzucuoglu, C., Fiorentino, G., and Caracuta, V. (2011). Climatic, vegetation and cultural change in the eastern Mediterranean during the mid-Holocene environmental transition. Holocene 21, 147–162. doi: 10.1177/0959683610386819
Robles, M., Peyron, O., Brugiapaglia, E., Ménot, G., Dugerdil, L., Ollivier, V., et al. (2022). Impact of climate changes on vegetation and human societies during the Holocene in the South Caucasus (Vanevan, Armenia): a multiproxy approach including pollen, NPPs and brGDGTs. Quat. Sci. Rev. 277:107297. doi: 10.1016/j.quascirev.2021.107297
Roche, D. M. (2013). δ18O water isotope in the iLOVECLIM model (version 1.0)—part 1: implementation and verification. Geosci. Model Dev. 6, 1481–1491. doi: 10.5194/gmd-6-1481-2013
Roche, D. M., Dumas, C., Bügelmayer, M., Charbit, S., and Ritz, C. (2014). Adding a dynamical cryosphere to iLOVECLIM (version 1.0): coupling with GRISLI ice-sheet model. Geosci. Model Dev. 7, 1377–1394. doi: 10.5194/gmd-7-1377-2014
Ródriguez-Coterón, S. (2022). El papel del fuego en la configuración del paisaje vegetal de la sierra del escudo (Montaña Cantábrica central) durante los últimos 6000 años (Santander: Universidad de Cantabria), 334.
Rojo Guerra, M., Garrido-Pena, R., and García-Martínez de Lagrán, I. (2005). El Campaniforme en la Península Ibérica y su contexto europeo (Valladolid: Universidad de Valladolid), 612.
Roos-Barraclough, F., Givelet, N., Martínez-Cortizas, A., Goodsite, M. E., Biester, H., and Shotyk, W. (2002). An analytical protocol for the determination of total mercury concentrations in solid peat samples. Sci. Tot. Environ. 292, 129–139. doi: 10.1016/S0048-9697(02)00035-9
Rozas, V. (2002). Regeneration patterns, dendroecology, and forest-use history in an old-growth beech-oak lowland forest in Northern Spain. For. Ecol. Manag. 182, 175–194. doi: 10.1016/S0378-1127(03)00070-7
Rubinos, A., and Alonso Matthias, F. (2002). “Las aplicaciones del carbono-14,” in El Castiellu de Llagú. Un castro astur en los orígenes de Oviedo, eds. L. Berrocal Rangel, P. Martínez-Seco and C. Ruiz Triviño (Madrid: Real Academia de la Historia—Principado de Asturias), 297–303.
Ruiz-Zapatero, G., Álvarez-Sanchís, J. R., and Rodríguez-Hernández, J. (2020). Urbanism in Iron Age Iberia. Two worlds in contact. J. Urb. Archaeol. 1, 123–150. doi: 10.1484/J.JUA.5.120913
Sánchez Fernández, L. V., Neri Vela, R., Cobo Barquín, J. C., and Hernández Martín, E. (2018). Condiciones de vida en las cuencas mineras centrales de Asturias durante la fase expansiva de la revolución industrial asturiana (1885-1907) a través de las Topografías Médicas. Ería 38, 367–384. doi: 10.17811/er.3.2018.367-384
Sánchez Palencia, F. J., López-Sáez, J. A., Reher Díez, G. S., and López-Merino, L. (2011). “La minería romana en León y Asturias, su importancia en la configuración de los paisajes,” in La evolución del paisaje vegetal y el uso del fuego en la cordillera cantábrica, eds. F. J. Ezquerra Boticario and E. Rey van den Bercken (Valladolid: Fundación Patrimonio Natural de Castilla y León), 125–138.
Sánchez-Morales, M. (2023). “Palaeoecological review of the climatic, cultural and plant landscape dynamics of the Cantabrian region,” in El paisaje de las montañas atlánticas: avances en el conocimiento geohistórico y ambiental, ed. M. A. Poblete Piedrabuena (Oviedo: Servicio de Publicaciones de la Universidad de Oviedo), 13–86.
Sastre Prats, I., and Currás, B. X. (2019). “Aggregation and dispersal: rural landscapes of the Northwestern Iberian Peninsula from the Iron Age to the Early Roman Empire,” in Coming Together. Comparative Approaches to Population Aggregation and Early Urbanization, ed. A. Gyucha (Albany: State University of New York), 295–320.
Schellekens, J., Buurman, P., Fraga, I., and Martínez-Cortizas, A. (2011). Holocene vegetation and hydrologic changes inferred from molecular vegetation markers in peat, Penido Vello (Galicia, Spain). Palaeogeogr. Palaeoclimatol. Palaeoecol. 299, 56–69. doi: 10.1016/j.palaeo.2010.10.034
Schmid, B. V., Büntgen, U., Easterday, W. R., Ginzler, C., Walløe, L., Bramanti, B., et al. (2015). Climate-driven introduction of the Black Death and successive plague reintroductions into Europe. Proc. Natl. Acad. Sci. U. S. A. 112, 3020–3025. doi: 10.1073/pnas.1412887112
Settle, D., and Patterson, C. (1980). Lead in albacore: guide to lead pollutions in Americans. Science 207, 1167–1176. doi: 10.1126/science.6986654
Shepard, D. (1968). “A two-dimensional interpolation function for irregularly-spaced data,” in ACM '68: Proceedings of the 1968 23rd ACM National Conference (New York, NY: Association for Computing Machinery), 517–524.
Sieferle, R. P. (2001). The Subterranean Forest: Energy Systems and the Industrial Revolution. Cambridge: White Horse Press.
Silva-Sánchez, N. (2015). Mining and metallurgical activities in N Iberia and their link to forest evolution using environmental archives (centuries AD V to XI). Estudos do Quaternário 12, 15–26. doi: 10.30893/eq.v0i12.104
Silva-Sánchez, N., Martínez-Cortizas, A., and López-Merino, L. (2014). Linking forest cover, soil erosion and mire hydrology to late-Holocene human activity and climate in NW Spain. Holocene 24, 714–725. doi: 10.1177/0959683614526934
Simmons, I. G. (2008). Global Environmental History: 10,000 BC to ad 2000. Edinburgh: University Press.
Spiering, E. D., Pevida, L. R., Maldonado, C., González, S., García, J., Varela, A., et al. (2000). The gold belt of Western Asturias and Galicia (NW Spain). J. Geochem. Explor. 71, 89–101. doi: 10.1016/S0375-6742(00)00147-3
Torres Ramírez, B. (1981). La Armada de Barlovento (Sevilla: Escuela de Estudios Hispano-Americanos de Sevilla), 374.
Torres Villanueva, E. T. (1991). Barcos, carbón y mineral de hierro. Los vapores de Sota y Aznary los orígenes de la moderna flota mercante de Bilbao, 1889-1900. Revista de Historia Económica 9, 11–32. doi: 10.1017/S0212610900002603
Torres-Martínez, J. F. (2010). “El medio ambiente, la “construcción” del territorio y la obtención de recursos en la Edad de Hierro en Cantabria,” in Castros y castra en Cantabria: fortificaciones desde los orígenes de la Edad del Hierro a las guerras con Roma: catálogo, revisión y puesta al día, eds. M. L. Serna Gancedo, A. Martínez Veasco and V. Fernández Acebo (Santander: Acanto), 74–109.
Turney, C. S., Baillie, M., Palmer, J., and Brown, D. (2006). Holocene climatic change and past Irish societal response. J. Archaeol. Sci. 33, 34–38. doi: 10.1016/j.jas.2005.05.014
Urteaga, M. (1999). “La industria del hierro en Guipuzkoa,” in Hierro al mar. Minas, bosques, ferrerías, astilleros y arsenales 'litoral atlántico', ed. L. Azurmendi Perez (Madrid: Instituto de Estudios Cántabros, Asociación Tajamar),38–45.
Uzquiano, P. (2018). Vegetation, firewood exploitation and human settlement in northern Spain in relation to Holocene climate and cultural dynamics. Quat. Int. 463, 414–424. doi: 10.1016/j.quaint.2016.10.034
Valavanidis, A., and Vlachogianni, T. (2013). Homo sapiens' energy dependence and use throughout human history and evolution. Sci. Adv. Environ. Toxicol. Ecotoxicol. 1, 1–32.
Valbuena-Carabaña, M., López de Heredia, U., Fuentes-Utrilla, P., González-Doncel, I., and Gil, L. (2010). Historical and recent changes in the Spanish forests: a socio-economic process. Rev. Palaeobot. Palynol. 162, 492–506. doi: 10.1016/j.revpalbo.2009.11.003
Vandkilde, H. (2016). Bronzization: the Bronze age as pre-modern globalization. Praehistorische Zeltschrift 91, 103–123. doi: 10.1515/pz-2016-0005
Vera, J. A. (2004). Geología de España (Madrid: Sociedad Geológica de España. Instituto Geológico y Minero de España), 890.
Villa Valdés, A. (2002). Sobre la secuencia cronoestratigráfica de los castros asturianos (ss. VIII a. C. - II d. C.). Trabajos de Prehistoria 59, 149–162. doi: 10.3989/tp.2002.v59.i2.203
Vlachogianni, T., and Valavanidis, A. (2013). Energy and environmental impact on the biosphere energy flow, storage and conversion in human civilization. Am. J. Educ. Res. 1, 68–78. doi: 10.12691/education-1-3-2
Vrac, M. (2018). Multivariate bias adjustment of high-dimensional climate simulations: the Rank Resampling for Distributions and Dependencies (R2D2) bias correction. Hydrol. Earth Syst. Sci. 22, 3175–3196. doi: 10.5194/hess-22-3175-2018
Wacnik, A., Tylmann, W., Bonk, A., Goslar, T., Enters, D., Meyer-Jacob, C., et al. (2016). Determining the responses of vegetation to natural processes and human impacts in north-eastern Poland during the last millennium: combined pollen, geochemical and historical data. Veget. Hist. Archaeobot. 25, 479–498. doi: 10.1007/s00334-016-0565-z
Walker, M. J. C., Berkelhammer, M., Björck, S., Cwynar, L. C., Fisher, D. A., Long, A. J., et al. (2012). Formal subdivision of the Holocene Series/Epoch: a Discussion Paper by a Working Group of INTIMATE (Integration of ice-core, marine and terrestrial records) and the Subcommission on Quaternary Stratigraphy (International Commission on Stratigraphy). J. Quat. Sci. 27, 649–659. doi: 10.1002/jqs.2565
Warnant, P., François, L., Strivay, D., and Gérard, J. C. (1994). CARAIB: a global model of terrestrial biological productivity. Glob. Biogeochem. Cycl. 8, 255–270. doi: 10.1029/94GB00850
Weiss, D. J., Shotyk, W., Appleby, P. G., Kramers, J. D., and Cheburkin, A. (1999). Atmospheric Pb deposition since the industrial revolution recorded by five Swiss peat profiles: enrichment factors, fluxes, isotopic composition, and sources. Environ. Sci. Technol. 33, 1340–1352. doi: 10.1021/es980882q
Wick, L., Lemcke, G., and Sturm, M. (2003). Evidence of Lateglacial and Holocene climatic change and human impact in eastern Anatolia: high-resolution pollen, charcoal, isotopic and geochemical records from the laminated sediments of Lake Van, Turkey. Holocene 13, 665–675. doi: 10.1191/0959683603hl653rp
Zapolska, A., Serge, M. A., Mazier, F., Quiquet, A., Renssen, H., Vrac, M., et al. (2023a). More than agriculture: analyzing time-cumulative human impact on European land-cover of second half of the Holocene. Quat. Sci. Rev. 314:108227. doi: 10.1016/j.quascirev.2023.108227
Zapolska, A., Vrac, M., Quiquet, A., Extier, T., and Arthur, F. (2023b). Improving biome and climate modelling for a set of past climate conditions: evaluating bias correction using the CDF-t approach Improving biome and climate modelling for a set of past climate conditions : evaluating bias correction using the CDF-t approach. Environ. Res. 2:accbe2. doi: 10.1088/2752-5295/accbe2
Keywords: Cantabrian Spain, deforestation, metal pollution, climate evolution, energy regimes, Holocene
Citation: Martinez A, Zapolska A, Arthur F, Verhagen P, Kluiving S, Muñoz-Rojas J, Borja Barrera C and Fraile Jurado P (2024) Identifying major phases in the use of land, energy and changing landscapes by agrarian societies (7,000 cal BP-Present) in Cantabrian Spain, based on cultural changes and anthropogenic signals. Front. Environ. Archaeol. 3:1339172. doi: 10.3389/fearc.2024.1339172
Received: 15 November 2023; Accepted: 02 February 2024;
Published: 26 February 2024.
Edited by:
Laurent Lespez, Université Paris-Est Créteil Val de Marne, FranceReviewed by:
Anna Maria Mercuri, University of Modena and Reggio Emilia, ItalySebastián Pérez-Díaz, University of Cantabria, Spain
Copyright © 2024 Martinez, Zapolska, Arthur, Verhagen, Kluiving, Muñoz-Rojas, Borja Barrera and Fraile Jurado. This is an open-access article distributed under the terms of the Creative Commons Attribution License (CC BY). The use, distribution or reproduction in other forums is permitted, provided the original author(s) and the copyright owner(s) are credited and that the original publication in this journal is cited, in accordance with accepted academic practice. No use, distribution or reproduction is permitted which does not comply with these terms.
*Correspondence: Alexandre Martinez, YS5mLm1hcnRpbmV6QHZ1Lm5s