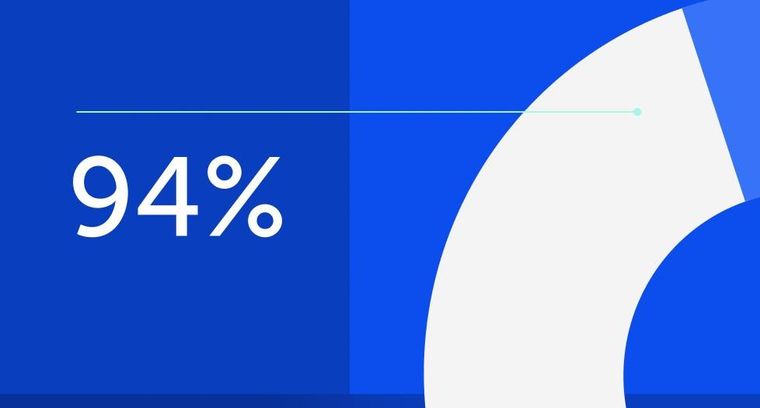
94% of researchers rate our articles as excellent or good
Learn more about the work of our research integrity team to safeguard the quality of each article we publish.
Find out more
PERSPECTIVE article
Front. Dent. Med., 27 July 2022
Sec. Systems Integration
Volume 3 - 2022 | https://doi.org/10.3389/fdmed.2022.974862
This article is part of the Research TopicBone and Metabolic ActivitiesView all 10 articles
A better understanding of the role of mineralized tissues and their associated factors in governing whole-body metabolism should be of value toward informing clinical strategies to treat mineralized tissue and metabolic disorders, such as diabetes and obesity. This perspective provides evidence suggesting a role for the arginine-glycine-aspartic acid (RGD) region, a sequence identified in several proteins secreted by bone cells, as well as other cells, in modulating systemic metabolic activity. We focus on (a) two of the SIBLING (small integrin-binding ligand, N-linked glycoprotein) family genes/proteins, bone sialoprotein (BSP) and osteopontin (OPN), (b) insulin-like growth factor-binding protein-1 & 2 (IGFBP-1, IGFBP-2) and (c) developmental endothelial locus 1 (DEL1) and milk fat globule–EGF factor-8 (MFG-E8). In addition, for our readers to appreciate the mounting evidence that a multitude of bone secreted factors affect the activity of other tissues, we provide a brief overview of other proteins, to include fibroblast growth factor 23 (FGF23), phosphatase orphan 1 (PHOSPHO1), osteocalcin (OCN/BGLAP), tissue non-specific alkaline phosphatase (TNAP) and acidic serine aspartic-rich MEPE-associated motif (ASARM), along with known/suggested functions of these factors in influencing energy metabolism.
Existing data provide credible evidence that proteins produced by mineralized tissues affect the activity of tissues at distant sites (1–4). However, the specific role of these proteins at distant sites has been elusive, with supportive evidence that factors secreted by skeletal tissues may modulate metabolic activity. A better understanding of the role of mineralized tissues and their associated factors in governing whole-body metabolism should be of value toward informing clinical strategies to treat mineralized tissue and metabolic disorders, such as diabetes and obesity.
This perspective provides evidence suggesting a role for the arginine-glycine-aspartic acid (RGD) region, a sequence identified in several proteins secreted by bone cells, as well as other cells, in modulating systemic metabolic activity. We focus on a) two of the SIBLING (small integrin-binding ligand, N-linked glycoprotein) family genes/proteins, bone sialoprotein (BSP) and osteopontin (OPN), (b) insulin-like growth factor-binding protein-1 & 2 (IGFBP-1, IGFBP-2) and (c) developmental endothelial locus 1 (DEL1) and milk fat globule–EGF factor-8 (MFG-E8) (Table 1 and below). In addition, for our readers to appreciate the mounting evidence that a multitude of bone secreted factors, not just those with RGD regions, affect the activity of other tissues, we provide a table (Table 2) to include the proteins, fibroblast growth factor 23 (FGF23), phosphatase orphan 1 (PHOSPHO1), osteocalcin (OCN/BGLAP), tissue non-specific alkaline phosphatase (TNAP) and acidic serine aspartic-rich MEPE-associated motif (ASARM), along with known/suggested functions of these factors, with an emphasis on their role in monitoring metabolic activity. This latter table provides a brief review with references provided for more detailed information.
Nutrient metabolism is essential for the survival, growth, and development of all living systems. The whole-body metabolic homeostasis of higher organisms relies on precise sensing of the energy state of the body and coordinated response of multiple organs to nutritional demands and environmental changes. The central nervous system plays an important role in regulating all aspects of metabolism, including energy intake, absorption, utilization, and storage (50, 51). One-way key metabolic tissues, such as the liver, muscle, adipose tissue, pancreas, and gut, communicate with the brain and each other is via secreted factors, including protein hormones, cytokines, small molecules, metabolites, and extracellular vesicles (52–57). Dysregulation of these inter-organ communications contributes to the pathogenesis of metabolic diseases, including obesity, type 2 diabetes, dyslipidemia, fatty liver disease, and cardiovascular diseases.
Increasing evidence suggests that adipose tissues play a central role in systemic metabolism, providing storage and release of energy from white fat, expending energy to generate heat in brown and beige fat, and secreting a diverse group of bioactive mediators, collectively called “adipokines” (53, 58, 59). Adipose tissue dysfunction in genetic and high-fat diets induced obesity or in lipodystrophy causes toxic lipid accumulation in the liver, skeletal muscle, and other tissues, leading to systemic insulin resistance (60, 61). Adipose tissue inflammation and misbalance between secretion of pro- and anti-inflammatory adipokines also contribute to the pathogenesis of metabolic dysfunction in obesity (62, 63).
Many studies have shown that alterations of glucose and lipid metabolism influence bone homeostasis (64). Skeletal tissue growth and remodeling are energy consuming processes tightly coupled with the regulation of systemic energy metabolism and reproduction (65). Numerous hormones, such as estrogen, testosterone, parathyroid hormone, insulin, adipokines (e.g., leptin, resistin, adiponectin, TNFα), vitamin D, as well as neuropeptides modulate bone metabolic activity (4, 54, 64, 66–70). Recent studies have emphasized the role of the bone marrow adipose tissue, located in close proximity to skeletal lineage cells, in bone metabolism (71–74). Expansion of this depot, observed in aging, obesity, diabetes, anorexia nervosa, is often inversely associated with bone mineral density. As bone marrow adipocytes and osteoblasts share a common precursor, mesenchymal stem cells, imbalance between adipogenesis and osteogenesis may contribute to bone loss under pathological conditions. This broad overview underscores the complex interactions between tissues required to modulate metabolic activity including mineralized tissues, which are the focus of this perspective.
Before moving forward with the major focus of this perspective, the potential role of the tripeptide motif, RGD, in regulating metabolic activity, a brief review of the activities known and proposed for RGD-integrin-binding mediated cell functions is provided for contextual purposes. The readers are referred to a few of many excellent reviews, and references therein: (75–77).
The interest in defining the functions of RGD peptides and associated integrins was spurred on by early studies suggesting that such molecules may serve as therapeutic targets for numerous diseases. These studies demonstrated that the RGD region of proteins via interactions with their selective cell surface integrins promotes cell migration, adhesion, and signal transduction, with changes in cell proliferation and differentiation over a life span. Further investigations have proposed roles for RGD-integrin binding to include but not limited to modulating cancer cells e.g., progression, metastasis, angiogenesis, to controlling diseases such as sepsis, fibrosis, neurological disorders, cardiovascular diseases, and viral infections, to monitoring disease progression (diagnostic/imaging tools) and controlling wound healing. At a mechanistic level, studies have shown that in addition to RGD-integrin binding mediating adhesion to extracellular matrix molecules, this interaction results in bidirectional signaling across membranes thus functioning as controllers of cellular processing (78, 79). Some interactions result in multiprotein clustering, forming focal adhesions, the sites of integrin-facilitated cell signaling. This elicits a cascade of phosphorylating events of downstream molecules, most notably focal adhesion kinase (FAK), mitogen-activated protein kinase (MAPK), phosphoinositide kinase (PI3K)/Akt, and extracellular signal regulated kinase (ERK) (76, 77). This brief synopsis, demonstrating the varied mechanistic aspects of RGD-integrin mediated cell behavior, sets the stage for the discussion below, to consider how specific proteins and linked integrins, may influence metabolic activity, both locally and at distant sites. Below we consider six RGD containing proteins and their known and proposed functions in modulating metabolic activity, summarized in Table 1 (8, 9, 14, 19).
In the late 1900s the role of the RGD region of proteins, specifically OPN and BSP, found in high concentrations in mineralized tissues and their associated receptors, primarily integrins, was receiving notable attention. Since these early years, there have been considerable advances in defining the function of the RGD region in proteins of mineralized tissues, as well as other tissues.
As members of the SIBLING family, OPN and BSP contain several highly conserved functional motifs, including a C-terminal RGD-integrin binding domain known to promote cell adhesion, migration, and signaling (5, 6, 80, 81). OPN and BSP, first identified in bone, were proposed to modulate mineralization, where OPN is considered to act as an inhibitor of nucleation and BSP as the crystal nucleator (10, 82–89). It is now recognized that the expression of these genes/proteins is not limited to mineralized tissues, as discussed below.
OPN: In addition to OPN's role via the RGD region in modulating cell behavior, OPN's inhibitory effect on mineralization has been ascribed to its negatively charged phosphorylated serine residues, to its modulation of osteoclasts, and to its ability to regulate pyrophosphate metabolism (90, 91). Soon after the discovery of OPN it was found to be expressed ubiquitously and to exhibit a variety of functions dependent on the specific cells/tissues/organs expressing OPN. Relevant to this perspective, OPN has been shown to have a role within bone as a regulator of bone mass (92), as well as a role in modulating systemic metabolic activity as described below.
OPN, also considered as a pro-inflammatory cytokine, has been shown to modulate immune cell responses, with an important role in advancing inflammation within many tissues including adipose, cardiovascular and renal tissues, and associated with obesity, insulin resistance and type 2 diabetes (11, 12, 93–96). In this regard Dai and co-workers provided data revealing the intricacies in defining the role of OPN as a regulator of bone metabolic activity vs. systemic metabolic activity (13). Data from their mouse studies demonstrate that OPN is secreted by adipose tissue (epididymal white adipose tissues) macrophages and selectively circulates to bone marrow, activating osteoclasts to degrade bone and modulating bone marrow-derived macrophages. In the latter case, the macrophages engulf lipid deposits released from adipocytes in the bone marrow and through a complex array of elegantly designed studies the authors showed that OPN-neutralizing antibodies ameliorated high fat diet assisted bone loss in these mice. Mouse studies focused on determining OPN's role in metabolic dysregulation in the liver found that OPN regulated cross talk between cholesterol (CHOL) and phosphatidylcholine (PC) metabolism via enhancing expression of cholesterol 7 alpha-hydroxylase (CYP7A1). This finding suggested that OPN, by disrupting PC and CHOL metabolism, may contribute to non–alcoholic fatty liver progression in nonobese patients (97). Further studies by other groups confirmed a role for OPN in modulating age-related nonalcoholic fatty liver disease (98). These studies provide evidence that OPN, regardless of where expressed, affects metabolic activity via pro-inflammatory events, and in many cases through integrin signaling pathways; however, a specific role of the RGD region in mediating these events was not discussed. In a study by Chen et al., focused on determining the factors involved in differentiation of mesenchymal precursor cells along an adipogenesis vs. osteogenesis pathway, the authors provided evidence that OPN-integrin links (via αvβ1) may be involved in this process (99). They further showed that the ratio of total fat weight to body weight was significantly higher in OPN-deficient mice at 5 weeks and 12 months vs. wild-type mice, reinforcing a role for OPN in modulating systemic metabolic activity and confirming results of other researchers related to the role of OPN in modulating obesity (100, 101).
Collectively, impressive data exist supporting a role for OPN in affecting systemic metabolic activity, although further studies are warranted to determine if the RGD region of OPN is involved.
BSP: BSP is more selective to mineralized tissues and most studies have focused on defining the mechanisms controlling BSP function in mineralized tissues. Data from studies using BSP-deficient mice reveal that BSP is a modulator of mineralization. The reader is refereed to several articles and references thereof for details on tooth/bone phenotype of BSP-deficient mice (7, 102–106). In brief, BSP-deficient mice have alteration in bone homeostasis and mineralization (hypomineralized) and defects in the region of the periodontium, to include impairments in formation of cementum and surrounding alveolar bone, resulting in a disorganized periodontal ligament (PDL) region, malocclusion and exfoliation of teeth, similar to mice and humans with alkaline phosphatase mutations (30). To define the role of the RGD domain of BSP in controlling periodontal tissues, Nagasaki et al. generated BSP-KAE knock-in (KI) mice, substituting a non-function KAE (lysine-alanine-glutamic acid) sequence for the RGD. The results emphasized the importance of the RGD region for forming and maintaining the PDL region, but not for promoting mineralization (107).
To the surprise of Nagasaki et al. the BSP-RGD non-functionalized mice exhibited increased body weight and energy intake with age (after 13 weeks postnatal) vs. wild-type mice. Accordingly, weight of epididymal fat pad and size of white and brown adipocytes were increased (108).
Brief reviews of metabolic functions of other RGD containing proteins, insulin-like growth factor-binding proteins 1 and 2 (IGFBP-1, IGFBP-2), developmental endothelial locus-1 (DEL-1) and milk fat globule–EGF factor-8 (MFG-E8) are provided below, with references to reviews for more extensive reports on their various roles.
Insulin-like growth factor-binding proteins 1 and 2 (IGFBP 1 and 2) are members of a highly conserved family of six IGFBPs, numbered IGFBP1 through 6 in vertebrates, that modulate the actions of insulin growth factors (IGFs) and play vital roles in regulating several cellular processes (15, 17, 109, 110). The IGFs act as both endocrine hormones and autocrine/paracrine growth factors, binding to the IGFBPs or IGF-1 receptor. While the IGFBPs share about 50% homology with each other, each has specific structural features and plays distinct roles locally and systemically. In addition to the role in modulating circulating and local levels on IGF via IGF-IGFBP binding, IGFBPs also have activities independent of IGF binding. IGFBP-1 and 2 are the smallest in size, 25kDa and 31kDa respectively, and are the only IGFBPs that have RGD sequences. Interestingly, IGFBP1 and 2 are reported to serve as markers of autoimmune diseases such as Type 1 diabetes mellitus and rheumatoid arthritis (110).
For IGFBP 1 and 2, their RGD region allows them to influence cell adhesion, migration and signaling (18, 111, 112). Data from mouse studies suggest that the RGD motifs of both IGFBP1 and 2, signaling through specific integrins to include α5β1, affect energy metabolism (111, 112). Further studies by Haywood et al., using both in vitro and in vivo models (the latter, metabolic profiling of obese mice), evaluated whether the RGD domain of IGFBP1 could affect insulin sensitivity, insulin secretion and whole-body glucose regulation (16). Their results suggest that the RGD-integrin binding domain via cell signaling enhanced insulin sensitivity and secretion and administration of RGD synthetic peptide to obese mice could improve glucose clearance and insulin sensitivity. The authors conclude that the RGD domain of IGFBP-1 may hold promise as a therapeutic approach to insulin resistance. In another study, using IGFBP-2 transgenic mice (female), Reyer et al. showed that an IGFBP-2-RGD dependent mechanism was associated with impaired glucose clearance and regulation of GLUT4 translocation in muscle (18).
Developmental endothelial locus-1 (DEL-1) and milk fat globule–EGF factor-8 (MFG-E8) are structurally related proteins consisting of N-terminal EGF-like repeats and C-terminal discoidin I-like domains (20, 25). An RGD motif present in the second EGF-like repeat enables both proteins to interact with integrins such as αvβ3, αvβ5, although DEL-1 has also been shown to bind to non-RGD-binding integrins (αLβ2, αMβ2). DEL-1 and MFG-E8 generally behave as anti-inflammatory and pro-resolving proteins, in large part due to their capacity to promote efferocytosis (26, 113). Additionally, DEL-1 inhibits inflammation by regulating neutrophil recruitment and T regulatory cell function (27, 114). DEL-1 and MFG-E8 have been shown to promote tissue healing (21, 28) and bone homeostasis through effects on both osteoblasts and osteoclasts (22, 28, 29, 115, 116). In this regard, local administration of recombinant DEL-1 or MFG-E8 in the gingiva of non-human primates inhibits periodontal bone loss (29, 117). Despite sharing several functions, DEL-1 and MFG-E8 mediate non-redundant roles in in vivo experimental models, presumably owing to their expression in different tissues, often by different cell types, and/or their regulation by distinct transcription factors (20, 26, 113, 118). It is also possible that these “cousin” proteins may be involved in metabolic regulation. MFG-E8 was reported to enhance the uptake of fatty acids by adipocytes and this function required an intact RGD motif since the effect was mediated by MFG-E8 interaction with αvβ3 or αvβ5 integrins (23, 24). In the same study, MFG-E8-deficient mice were protected from diet-induced obesity (23). It is currently uncertain whether DEL-1 shares a similar metabolic function, although its ability to interact with RGD-binding integrins warrants relevant investigation. Interestingly in that regard, EDIL3, the gene encoding DEL-1, has been associated with susceptibility to childhood obesity (119) and is overexpressed in diet-induced obesity in mice as well as in obese humans (120).
Coupled with the above evidence providing a role for the RGD region of proteins being involved in modulating energy metabolism are studies highlighting that RGD-binding integrins may serve as therapeutic targets for controlling metabolic activity and diseases, such as diabetic retinopathy (121), sepsis (122) and inflammatory disorders (123).
While this perspective highlights the RGD motif of proteins and their role in controlling metabolic activity, the fact that many genes/proteins associated with mineralized tissues have been reported to affect systemic metabolic activity provides additional support for the concept that mineralized tissues, through direct or indirect mechanisms, are important players in controlling whole-body homeostasis. Table 2 provides a brief overview of some of these factors, with additional references within these tables (31–49), for more detailed information.
This perspective provides data from studies over decades clearly showing that factors secreted by mineralized tissues as well as other tissues influence bone metabolic activity and also systemic metabolic activity. Yet, there remain missing pieces of the puzzle related to the mechanistic aspects for the genes and associated proteins affecting bone/systemic metabolic activity. Featured in this perspective is the need to consider the role of RGD-associated proteins in monitoring metabolic activity locally and systemically, with more answers to be forthcoming as investigations continue along this line of research. Such studies are important to better understand whether therapeutics targeting specific proteins and/or the specific RGD region may be attractive treatments for controlling obesity and associated diseases.
The original contributions presented in the study are included in the article/supplementary material, further inquiries can be directed to the corresponding author.
KN, OG, GH, and MS, contributed to this perspective from discussions on the outline of topics to be included, to working on and reviewing the text and tables, to finalizing the manuscript for peer review. All authors agree to be accountable for the content of the work. All authors contributed to the article and approved the submitted version.
KN and MS were supported by the NIAMS/NIH Intramural Program. OG was supported by the NIDDK/NIH Intramural Program. GH is supported by the NIDCR/NIH Grants DE026152 and DE028561.
The authors wish to acknowledge the numerous researchers, over many decades, for their important contributions toward advancing our understanding of the role of mineralized tissues in modulating local and systemic metabolic activity.
The authors declare that the research was conducted in the absence of any commercial or financial relationships that could be construed as a potential conflict of interest.
All claims expressed in this article are solely those of the authors and do not necessarily represent those of their affiliated organizations, or those of the publisher, the editors, and the reviewers. Any product that may be evaluated in this article, or claim that may be made by its manufacturer, is not guaranteed or endorsed by the publisher.
1. Wei J, Ferron M, Clarke CJ, Hannun YA, Jiang H, Blaner WS, et al. Bone-specific insulin resistance disrupts whole-body glucose homeostasis via decreased osteocalcin activation. J Clin Invest. (2014) 124:1–13. doi: 10.1172/JCI72323
2. Confavreux CB, Levine RL, Karsenty G. A paradigm of integrative physiology, the crosstalk between bone and energy metabolisms. Mol Cell Endocrinol. (2009) 310:21–9. doi: 10.1016/j.mce.2009.04.004
3. Goettsch C, Strzelecka-Kiliszek A, Bessueille L, Quillard T, Mechtouff L, Pikula S, et al. TNAP as a therapeutic target for cardiovascular calcification: a discussion of its pleiotropic functions in the body. Cardiovasc Res. (2022) 118:84–96. doi: 10.1093/cvr/cvaa299
4. Fulzele K, Riddle RC, DiGirolamo DJ, Cao X, Wan C, Chen D, et al. Insulin receptor signaling in osteoblasts regulates postnatal bone acquisition and body composition. Cell. (2010) 142:309–19. doi: 10.1016/j.cell.2010.06.002
5. Bellahcène A, Castronovo V, Ogbureke KU, Fisher LW, Fedarko NS. Small integrin-binding ligand N-linked glycoproteins (SIBLINGs): multifunctional proteins in cancer. Nat Rev Cancer. (2008) 8:212–26. doi: 10.1038/nrc2345
6. Bellahcène A, Bonjean K, Fohr B, Fedarko NS, Robey FA, Young MF, et al. Bone sialoprotein mediates human endothelial cell attachment and migration and promotes angiogenesis. Circ Res. (2000) 86:885–91. doi: 10.1161/01.RES.86.8.885
7. Foster BL, Ao M, Willoughby C, Soenjaya Y, Holm E, Lukashova L, et al. Mineralization defects in cementum and craniofacial bone from loss of bone sialoprotein. Bone. (2015) 78:150–64. doi: 10.1016/j.bone.2015.05.007
8. Wu K, Feng J, Lyu F, Xing F, Sharma S, Liu Y, et al. Exosomal miR-19a and IBSP cooperate to induce osteolytic bone metastasis of estrogen receptor-positive breast cancer. Nat Commun. (2021) 12:5196. doi: 10.1038/s41467-021-25473-y
9. Chen Y, Qin Y, Dai M, Liu L, Ni Y, Sun Q, et al. IBSP, a potential recurrence biomarker, promotes the progression of colorectal cancer via Fyn/β-catenin signaling pathway. Cancer Med. (2021) 10:4030–45. doi: 10.1002/cam4.3959
10. Foster BL, Ao M, Salmon CR, Chavez MB, Kolli TN, Tran AB, et al. Osteopontin regulates dentin and alveolar bone development and mineralization. Bone. (2018) 107:196–207. doi: 10.1016/j.bone.2017.12.004
11. Nomiyama T, Perez-Tilve D, Ogawa D, Gizard F, Zhao Y, Heywood EB, et al. Osteopontin mediates obesity-induced adipose tissue macrophage infiltration and insulin resistance in mice. J Clin Invest. (2007) 117:2877–88. doi: 10.1172/JCI31986
12. Kiefer FW, Zeyda M, Gollinger K, Pfau B, Neuhofer A, Weichhart T, et al. Neutralization of osteopontin inhibits obesity-induced inflammation and insulin resistance. Diabetes. (2010) 59:935–46. doi: 10.2337/db09-0404
13. Dai B, Xu J, Li X, Huang L, Hopkins C, Wang H, et al. Macrophages in epididymal adipose tissue secrete osteopontin to regulate bone homeostasis. Nat Commun. (2022) 13:427. doi: 10.1038/s41467-021-27683-w
14. Zhao H, Chen Q, Alam A, Cui J, Suen KC, Soo AP, et al. The role of osteopontin in the progression of solid organ tumour. Cell Death Dis. (2018) 9:356. doi: 10.1038/s41419-018-0391-6
15. Hoeflich A, Russo VC. Physiology and pathophysiology of IGFBP-1 and IGFBP-2 - consensus and dissent on metabolic control and malignant potential. Best Pract Res Clin Endocrinol Metab. (2015) 29:685–700. doi: 10.1016/j.beem.2015.07.002
16. Haywood NJ, Cordell PA, Tang KY, Makova N, Yuldasheva NY, Imrie H, et al. Insulin-like growth factor binding protein 1 could improve glucose regulation and insulin sensitivity through its RGD domain. Diabetes. (2017) 66:287–99. doi: 10.2337/db16-0997
17. Li T, Forbes ME, Fuller GN, Li J, Yang X, Zhang W. IGFBP2: integrative hub of developmental and oncogenic signaling network. Oncogene. (2020) 39:2243–57. doi: 10.1038/s41388-020-1154-2
18. Reyer A, Schindler N, Ohde D, Walz C, Kunze M, Tuchscherer A, et al. The RGD sequence present in IGFBP-2 is required for reduced glucose clearance after oral glucose administration in female transgenic mice. Am J Physiol Endocrinol Metab. (2015) 309:E409–17. doi: 10.1152/ajpendo.00168.2015
19. Zhang X, Gu HF, Frystyk J, Efendic S, Brismar K, Thorell A. Analyses of IGFBP2 DNA methylation and mRNA expression in visceral and subcutaneous adipose tissues of obese subjects. Growth Horm IGF Res. (2019) 45:31–6. doi: 10.1016/j.ghir.2019.03.002
20. Aziz M, Jacob A, Matsuda A, Wang P. Review: milk fat globule-EGF factor 8 expression, function and plausible signal transduction in resolving inflammation. Apoptosis. (2011) 16:1077–86. doi: 10.1007/s10495–011–0630–0
21. Bu HF, Zuo XL, Wang X, Ensslin MA, Koti V, Hsueh W, et al. Milk fat globule-EGF factor 8/lactadherin plays a crucial role in maintenance and repair of murine intestinal epithelium. J Clin Invest. (2007) 117:3673–83. doi: 10.1172/JCI31841
22. Abe T, Shin J, Hosur K, Udey MC, Chavakis T, Hajishengallis G. Regulation of osteoclast homeostasis and inflammatory bone loss by MFG-E8. J Immunol. (2014) 193:1383–91. doi: 10.4049/jimmunol.1400970
23. Khalifeh-Soltani A, McKleroy W, Sakuma S, Cheung YY, Tharp K, Qiu Y, et al. Mfge8 promotes obesity by mediating the uptake of dietary fats and serum fatty acids. Nat Med. (2014) 20:175–83. doi: 10.1038/nm.3450
24. Ren Y, Liu W, Zhang J, Bi J, Fan M, Lv Y, et al. MFG-E8 maintains cellular homeostasis by suppressing endoplasmic reticulum stress in pancreatic exocrine acinar cells. Front Cell Dev Biol. (2021) 9:803876. doi: 10.3389/fcell.2021.803876
25. Hajishengallis G, Chavakis T. DEL-1-regulated immune plasticity and inflammatory disorders. Trends Mol Med. (2019) 25:444–59. doi: 10.1016/j.molmed.2019.02.010
26. Kourtzelis I, Li X, Mitroulis I, Grosser D, Kajikawa T, Wang B, et al. DEL-1 promotes macrophage efferocytosis and clearance of inflammation. Nat Immunol. (2019) 20:40–9. doi: 10.1038/s41590-018-0249-1
27. Li X, Colamatteo A, Kalafati L, Kajikawa T, Wang H, Lim J-H, et al. The DEL-1/β3 integrin axis promotes regulatory T cell responses during inflammation resolution. J Clin Invest. (2020) 130:6261–77. doi: 10.1172/JCI137530
28. Yuh DY, Maekawa T, Li X, Kajikawa T, Bdeir K, Chavakis T, et al. The secreted protein DEL-1 activates a β3 integrin-FAK-ERK1/2-RUNX2 pathway and promotes osteogenic differentiation and bone regeneration. J Biol Chem. (2020) 295:7261–73. doi: 10.1074/jbc.RA120.013024
29. Shin J, Maekawa T, Abe T, Hajishengallis E, Hosur K, Pyaram K, et al. DEL-1 restrains osteoclastogenesis and inhibits inflammatory bone loss in nonhuman primates. Sci Transl Med. (2015) 7:307ra155. doi: 10.1126/scitranslmed.aac5380
30. Millán JL, Whyte MP. Alkaline phosphatase and hypophosphatasia. Calcif Tissue Int. (2016) 98:398–416. doi: 10.1007/s00223-015-0079-1
31. Briolay A, Bessueille L, Magne D. TNAP: a new multitask enzyme in energy metabolism. Int J Mol Sci. (2021) 22:10470. doi: 10.3390/ijms221910470
32. Sun Y, Rahbani JF, Jedrychowski MP, Riley CL, Vidoni S, Bogoslavski D, et al. Mitochondrial TNAP controls thermogenesis by hydrolysis of phosphocreatine. Nature. (2021) 593:580–5. doi: 10.1038/s41586-021-03533-z
33. Bessueille L, Briolay A, Como J, Mebarek S, Mansouri C, Gleizes M, et al. Tissue-nonspecific alkaline phosphatase is an anti-inflammatory nucleotidase. Bone. (2020) 133:115262. doi: 10.1016/j.bone.2020.115262
34. Krishnamurthy VR, Baird BC, Wei G, Greene T, Raphael K, Beddhu S. Associations of serum alkaline phosphatase with metabolic syndrome and mortality. Am J Med. (2011) 124:566.e1–7. doi: 10.1016/j.amjmed.2010.11.030
35. Graser S, Liedtke D, Jakob F. TNAP as a new player in chronic inflammatory conditions and metabolism. Int J Mol Sci. (2021) 22:919. doi: 10.3390/ijms22020919
36. Bacchetta J, Bardet C, Prié D. Physiology of FGF23 and overview of genetic diseases associated with renal phosphate wasting. Metabolism. (2020) 103S:153865. doi: 10.1016/j.metabol.2019.01.006
37. Bhattacharyya N, Chong WH, Gafni RI, Collins MT. Fibroblast growth factor 23: state of the field and future directions. Trends Endocrinol Metab. (2012) 23:610–8. doi: 10.1016/j.tem.2012.07.002
38. Minisola S, Peacock M, Fukumoto S, Cipriani C, Pepe J, Tella SH, et al. Tumour-induced osteomalacia. Nat Rev Dis Primers. (2017) 3:17044. doi: 10.1038/nrdp.2017.44
39. Wei J, Karsenty G. An overview of the metabolic functions of osteocalcin. Curr Osteoporos Rep. (2015) 13:180–5. doi: 10.1007/s11914-015-0267-y
40. Ferron M, McKee MD, Levine RL, Ducy P, Karsenty G. Intermittent injections of osteocalcin improve glucose metabolism and prevent type 2 diabetes in mice. Bone. (2012) 50:568–75. doi: 10.1016/j.bone.2011.04.017
41. Ferron M, Hinoi E, Karsenty G, Ducy P. Osteocalcin differentially regulates beta cell and adipocyte gene expression and affects the development of metabolic diseases in wild-type mice. Proc Natl Acad Sci U S A. (2008) 105:5266–70. doi: 10.1073/pnas.0711119105
42. Ducy P, Desbois C, Boyce B, Pinero G, Story B, Dunstan C, et al. Increased bone formation in osteocalcin-deficient mice. Nature. (1996) 382:448–52. doi: 10.1038/382448a0
43. Suchacki KJ, Morton NM, Vary C, Huesa C, Yadav MC, Thomas BJ, et al. PHOSPHO1 is a skeletal regulator of insulin resistance and obesity. BMC Biol. (2020) 18:149. doi: 10.1186/s12915-020-00880-7
44. Zweifler LE, Ao M, Yadav M, Kuss P, Narisawa S, Kolli TN, et al. Role of PHOSPHO1 in periodontal development and function. J Dent Res. (2016) 95:742–51. doi: 10.1177/0022034516640246
45. Houston B, Stewart AJ, Farquharson C. PHOSPHO1-A novel phosphatase specifically expressed at sites of mineralisation in bone and cartilage. Bone. (2004) 34:629–37. doi: 10.1016/j.bone.2003.12.023
46. McKee MD, Yadav MC, Foster BL, Somerman MJ, Farquharson C, Millán JL. Compounded PHOSPHO1/ALPL deficiencies reduce dentin mineralization. J Dent Res. (2013) 92:721–7. doi: 10.1177/0022034513490958
47. Rowe PS. Regulation of bone-renal mineral and energy metabolism: the PHEX, FGF23, DMP1, MEPE ASARM pathway. Crit Rev Eukaryot Gene Expr. (2012) 22:61–86. doi: 10.1615/CritRevEukarGeneExpr.v22.i1.50
48. Rowe PS, de Zoysa PA, Dong R, Wang HR, White KE, Econs MJ, et al. MEPE, a new gene expressed in bone marrow and tumors causing osteomalacia. Genomics. (2000) 67:54–68. doi: 10.1006/geno.2000.6235
49. Salmon B, Bardet C, Khaddam M, Naji J, Coyac BR, Baroukh B, et al. MEPE-derived ASARM peptide inhibits odontogenic differentiation of dental pulp stem cells and impairs mineralization in tooth models of X-linked hypophosphatemia. PLoS ONE. (2013) 8:e56749. doi: 10.1371/journal.pone.0056749
50. Kim KS, Seeley RJ, Sandoval DA. Signalling from the periphery to the brain that regulates energy homeostasis. Nat Rev Neurosci. (2018) 19:185–96. doi: 10.1038/nrn.2018.8
51. Jais A, Brüning JC. Arcuate nucleus-dependent regulation of metabolism-pathways to obesity and diabetes mellitus. Endocr Rev. (2022) 43:314–28. doi: 10.1210/endrev/bnab025
52. Priest C, Tontonoz P. Inter-organ cross-talk in metabolic syndrome. Nat Metab. (2019) 1:1177–88. doi: 10.1038/s42255-019-0145-5
53. Funcke JB, Scherer PE. Beyond adiponectin and leptin: adipose tissue-derived mediators of inter-organ communication. J Lipid Res. (2019) 60:1648–84. doi: 10.1194/jlr.R094060
54. Yang Q, Vijayakumar A, Kahn BB. Metabolites as regulators of insulin sensitivity and metabolism. Nat Rev Mol Cell Biol. (2018) 19:654–72. doi: 10.1038/s41580-018-0044-8
55. Liu S, Alexander RK, Lee CH. Lipid metabolites as metabolic messengers in inter-organ communication. Trends Endocrinol Metab. (2014) 25:356–63. doi: 10.1016/j.tem.2014.05.002
56. Crewe C, Scherer PE. Intercellular and interorgan crosstalk through adipocyte extracellular vesicles. Rev Endocr Metab Disord. (2022) 23:61–9. doi: 10.1007/s11154-020-09625-x
57. Romero A, Eckel J. Organ crosstalk and the modulation of insulin signaling. Cells. (2021) 10:2082. doi: 10.3390/cells10082082
58. Rosen ED, Spiegelman BM. What we talk about when we talk about fat. Cell. (2014) 156:20–44. doi: 10.1016/j.cell.2013.12.012
59. Sakers A, De Siqueira MK, Seale P, Villanueva CJ. Adipose-tissue plasticity in health and disease. Cell. (2022) 185:419–46. doi: 10.1016/j.cell.2021.12.016
60. Czech MP. Mechanisms of insulin resistance related to white, beige, and brown adipocytes. Mol Metab. (2020) 34:27–42. doi: 10.1016/j.molmet.2019.12.014
61. Mann JP, Savage DB. What lipodystrophies teach us about the metabolic syndrome. J Clin Invest. (2019) 129:4009–21. doi: 10.1172/JCI129190
62. Ouchi N, Parker JL, Lugus JJ, Walsh K. Adipokines in inflammation and metabolic disease. Nat Rev Immunol. (2011) 11:85–97. doi: 10.1038/nri2921
63. Lee YS, Olefsky J. Chronic tissue inflammation and metabolic disease. Genes Dev. (2021) 35:307–28. doi: 10.1101/gad.346312.120
64. Suzuki A, Minamide M, Iwaya C, Ogata K, Iwata J. Role of metabolism in bone development and homeostasis. Int J Mol Sci. (2020) 21:8992. doi: 10.3390/ijms21238992
65. Karsenty G, Khosla S. The crosstalk between bone remodeling and energy metabolism: a translational perspective. Cell Metab. (2022) 34:805–17. doi: 10.1016/j.cmet.2022.04.010
66. Wee NK, Kulkarni RN, Horsnell H, Baldock PA. The brain in bone and fuel metabolism. Bone. (2016) 82:56–63. doi: 10.1016/j.bone.2015.10.020
67. Parimisetty A, Dorsemans AC, Awada R, Ravanan P, Diotel N. Lefebvre d'Hellencourt C. Secret talk between adipose tissue and central nervous system via secreted factors-an emerging frontier in the neurodegenerative research. J Neuroinflammation. (2016) 13:67. doi: 10.1186/s12974-016-0530-x
68. Thommesen L, Stunes AK, Monjo M, Grøsvik K, Tamburstuen MV, Kjøbli E, et al. Expression and regulation of resistin in osteoblasts and osteoclasts indicate a role in bone metabolism. J Cell Biochem. (2006) 99:824–34. doi: 10.1002/jcb.20915
69. Ducy P, Amling M, Takeda S, Priemel M, Schilling AF, Beil FT, et al. Leptin inhibits bone formation through a hypothalamic relay: a central control of bone mass. Cell. (2000) 100:197–207. doi: 10.1016/S0092-8674(00)81558-5
70. Lewis JW, Edwards JR, Naylor AJ, McGettrick HM. Adiponectin signalling in bone homeostasis, with age and in disease. Bone Res. (2021) 9:1. doi: 10.1038/s41413-020-00122-0
71. Horowitz MC, Berry R, Holtrup B, Sebo Z, Nelson T, Fretz JA, et al. Bone marrow adipocytes. Adipocyte. (2017) 6:193–204. doi: 10.1080/21623945.2017.1367881
72. Pachón-Peña G, Bredella MA. Bone marrow adipose tissue in metabolic health. Trends Endocrinol Metab. (2022) 33:401–8. doi: 10.1016/j.tem.2022.03.003
73. Li J, Liu X, Zuo B, Zhang L. The role of bone marrow microenvironment in governing the balance between osteoblastogenesis and adipogenesis. Aging Dis. (2016) 7:514–25. doi: 10.14336/AD.2015.1206
74. Ning K, Liu S, Yang B, Wang R, Man G, Wang DE, et al. Update on the effects of energy metabolism in bone marrow mesenchymal stem cells differentiation. Mol Metab. (2022) 58:101450. doi: 10.1016/j.molmet.2022.101450
75. Ruoslahti E. RGD and other recognition sequences for integrins. Annu Rev Cell Dev Biol. (1996) 12:697–715. doi: 10.1146/annurev.cellbio.12.1.697
76. Ludwig BS, Kessler H, Kossatz S, Reuning U. RGD-binding integrins revisited: how recently discovered functions and novel synthetic ligands (Re-)shape an ever-evolving field. Cancers (Basel). (2021) 13:1711. doi: 10.3390/cancers13071711
77. Nieberler M, Reuning U, Reichart F, Notni J, Wester HJ, Schwaiger M, et al. Exploring the role of RGD-recognizing integrins in cancer. Cancers (Basel). (2017) 9:116. doi: 10.3390/cancers9090116
78. Zhu J, Springer TA. Complete integrin headpiece opening in eight steps. J Cell Biol. (2013) 201:1053–68. eng. doi: 10.1083/jcb.201212037
79. Harburger DS, Calderwood DA. Integrin signalling at a glance. J Cell Sci. (2009) 122:159–63. doi: 10.1242/jcs.018093
80. Fisher LW, Torchia DA, Fohr B, Young MF, Fedarko NS. Flexible structures of SIBLING proteins, bone sialoprotein, and osteopontin. Biochem Biophys Res Commun. (2001) 280:460–5. doi: 10.1006/bbrc.2000.4146
81. Fisher LW, Fedarko NS. Six genes expressed in bones and teeth encode the current members of the SIBLING family of proteins. Connect Tissue Res. (2003) 44 Suppl. 1:33–40. doi: 10.1080/03008200390152061
82. Fisher LW, Whitson SW, Avioli LV, Termine JD. Matrix sialoprotein of developing bone. J Biol Chem. (1983) 258:12723–7. doi: 10.1016/S0021-9258(17)44236-0
83. Somerman MJ, Sauk JJ, Foster RA, Norris K, Dickerson K, Argraves WS. Cell attachment activity of cementum: bone sialoprotein II identified in cementum. J Periodontal Res. (1991) 26:10–6. doi: 10.1111/j.1600-0765.1991.tb01620.x
84. Somerman MJ, Prince CW, Sauk JJ, Foster RA, Butler WT. Mechanism of fibroblast attachment to bone extracellular matrix: role of a 44 kilodalton bone phosphoprotein. J Bone Miner Res. (1987) 2:259–65. doi: 10.1002/jbmr.5650020313
85. Oldberg A, Franzén A, Heinegård D. Cloning and sequence analysis of rat bone sialoprotein (osteopontin) cDNA reveals an Arg-Gly-Asp cell-binding sequence. Proc Natl Acad Sci U S A. (1986) 83:8819–23. doi: 10.1073/pnas.83.23.8819
86. Hunter GK, Goldberg HA. Nucleation of hydroxyapatite by bone sialoprotein. Proc Natl Acad Sci U S A. (1993) 90:8562–5. doi: 10.1073/pnas.90.18.8562
87. Fisher LW, McBride OW, Termine JD, Young MF. Human bone sialoprotein. Deduced protein sequence and chromosomal localization. J Biol Chem. (1990) 265:2347–51. doi: 10.1016/S0021-9258(19)39982-X
88. Hunter GK, Hauschka PV, Poole AR, Rosenberg LC, Goldberg HA. Nucleation and inhibition of hydroxyapatite formation by mineralized tissue proteins. Biochem J. (1996) 317:59–64. doi: 10.1042/bj3170059
89. Gericke A, Qin C, Spevak L, Fujimoto Y, Butler WT, Sørensen ES, et al. Importance of phosphorylation for osteopontin regulation of biomineralization. Calcif Tissue Int. (2005) 77:45–54. doi: 10.1007/s00223-004-1288-1
90. Sørensen ES, Højrup P, Petersen TE. Posttranslational modifications of bovine osteopontin: identification of twenty-eight phosphorylation and three O-glycosylation sites. Protein Sci. (1995) 4:2040–9. doi: 10.1002/pro.5560041009
91. Harmey D, Johnson KA, Zelken J, Camacho NP, Hoylaerts MF, Noda M, et al. Elevated skeletal osteopontin levels contribute to the hypophosphatasia phenotype in Akp2(-/-) mice. J Bone Miner Res. (2006) 21:1377–86. doi: 10.1359/jbmr.060619
92. Si J, Wang C, Zhang D, Wang B, Zhou Y. Osteopontin in bone metabolism and bone diseases. Med Sci Monit. (2020) 26:e919159. doi: 10.12659/MSM.919159
93. Kahles F, Findeisen HM, Bruemmer D. Osteopontin: a novel regulator at the cross roads of inflammation, obesity and diabetes. Mol Metab. (2014) 3:384–93. doi: 10.1016/j.molmet.2014.03.004
94. Lancha A, Rodríguez A, Catalán V, Becerril S, Sáinz N, Ramírez B, et al. Osteopontin deletion prevents the development of obesity and hepatic steatosis via impaired adipose tissue matrix remodeling and reduced inflammation and fibrosis in adipose tissue and liver in mice. PLoS ONE. (2014) 9:e98398. doi: 10.1371/journal.pone.0098398
95. Denhardt DT, Noda M, O'Regan AW, Pavlin D, Berman JS. Osteopontin as a means to cope with environmental insults: regulation of inflammation, tissue remodeling, and cell survival. J Clin Invest. (2001) 107:1055–61. doi: 10.1172/JCI12980
96. Icer MA, Gezmen-Karadag M. The multiple functions and mechanisms of osteopontin. Clin Biochem. (2018) 59:17–24. doi: 10.1016/j.clinbiochem.2018.07.003
97. Nuñez-Garcia M, Gomez-Santos B, Buqué X, García-Rodriguez JL, Romero MR, Marin JJG, et al. Osteopontin regulates the cross-talk between phosphatidylcholine and cholesterol metabolism in mouse liver. J Lipid Res. (2017) 58:1903–15. doi: 10.1194/jlr.M078980
98. Gómez-Santos B, Saenz de. Urturi D, Nuñez-García M, Gonzalez-Romero F, Buque X, Aurrekoetxea I, et al. Liver osteopontin is required to prevent the progression of age-related nonalcoholic fatty liver disease. Aging Cell. (2020) 19:e13183. doi: 10.1111/acel.13183
99. Chen Q, Shou P, Zhang L, Xu C, Zheng C, Han Y, et al. An osteopontin-integrin interaction plays a critical role in directing adipogenesis and osteogenesis by mesenchymal stem cells. Stem Cells. (2014) 32:327–37. doi: 10.1002/stem.1567
100. Kiefer FW, Neschen S, Pfau B, Legerer B, Neuhofer A, Kahle M, et al. Osteopontin deficiency protects against obesity-induced hepatic steatosis and attenuates glucose production in mice. Diabetologia. (2011) 54:2132–42. doi: 10.1007/s00125-011-2170-0
101. Chapman J, Miles PD, Ofrecio JM, Neels JG Yu JG, Resnik JL, et al. Osteopontin is required for the early onset of high fat diet-induced insulin resistance in mice. PLoS ONE. (2010) 5:e13959. doi: 10.1371/journal.pone.0013959
102. Bouleftour W, Boudiffa M, Wade-Gueye NM, Bouët G, Cardelli M, Laroche N, et al. Skeletal development of mice lacking bone sialoprotein (BSP)–impairment of long bone growth and progressive establishment of high trabecular bone mass. PLoS ONE. (2014) 9:e95144. doi: 10.1371/journal.pone.0095144
103. Malaval L, Wade-Guéye NM, Boudiffa M, Fei J, Zirngibl R, Chen F, et al. Bone sialoprotein plays a functional role in bone formation and osteoclastogenesis. J Exp Med. (2008) 205:1145–53. doi: 10.1084/jem.20071294
104. Foster BL, Soenjaya Y, Nociti FH, Holm E, Zerfas PM, Wimer HF, et al. Deficiency in acellular cementum and periodontal attachment in bsp null mice. J Dent Res. (2013) 92:166–72. doi: 10.1177/0022034512469026
105. Bouleftour W, Bouet G, Granito RN, Thomas M, Linossier MT, Vanden-Bossche A, et al. Blocking the expression of both bone sialoprotein (BSP) and osteopontin (OPN) impairs the anabolic action of PTH in mouse calvaria bone. J Cell Physiol. (2015) 230:568–77. doi: 10.1002/jcp.24772
106. Bouleftour W, Juignet L, Verdière L, Machuca-Gayet I, Thomas M, Laroche N, et al. Deletion of OPN in BSP knockout mice does not correct bone hypomineralization but results in high bone turnover. Bone. (2019) 120:411–22. doi: 10.1016/j.bone.2018.12.001
107. Nagasaki K, Chavez MB, Nagasaki A, Taylor JM, Tan MH, Ma M, et al. The bone sialoprotein RGD domain modulates and maintains periodontal development. J Dent Res. (2022) 101:220345221100794. doi: 10.1177/00220345221100794
108. Taylor J, Nagasaki A, Kear B, Brock S, Ma Y, Gavrilova O, et al. The BSP-RGD motif affects PDL organization and age-related obesity 2021 IADR/AADR/CADR general session. Bethesda, MD: Archives IADR.
109. Allard JB, Duan C. IGF-binding proteins: why do they exist and why are there so many? Front Endocrinol (Lausanne). (2018) 9:117. doi: 10.3389/fendo.2018.00117
110. Ding H, Wu T. Insulin-like growth factor binding proteins in autoimmune diseases. Front Endocrinol (Lausanne). (2018) 9:499. doi: 10.3389/fendo.2018.00499
111. Jones JI, Gockerman A, Busby WH, Wright G, Clemmons DR. Insulin-like growth factor binding protein 1 stimulates cell migration and binds to the alpha 5 beta 1 integrin by means of its Arg-Gly-Asp sequence. Proc Natl Acad Sci U S A. (1993) 90:10553–7. doi: 10.1073/pnas.90.22.10553
112. Feng N, Zhang Z, Wang Z, Zheng H, Qu F, He X, et al. Insulin-like growth factor binding protein-2 promotes adhesion of endothelial progenitor cells to endothelial cells via integrin α5β1. J Mol Neurosci. (2015) 57:426–34. doi: 10.1007/s12031-015-0589-3
113. Hanayama R, Tanaka M, Miyasaka K, Aozasa K, Koike M, Uchiyama Y, et al. Autoimmune disease and impaired uptake of apoptotic cells in MFG-E8-deficient mice. Science. (2004) 304:1147–50. doi: 10.1126/science.1094359
114. Eskan MA, Jotwani R, Abe T, Chmelar J, Lim JH, Liang S, et al. The leukocyte integrin antagonist Del-1 inhibits IL-17-mediated inflammatory bone loss. Nat Immunol. (2012) 13:465–73. doi: 10.1096/fj.201701238R
115. Michalski MN, Seydel AL, Siismets EM, Zweifler LE, Koh AJ, Sinder BP, et al. Inflammatory bone loss associated with MFG-E8 deficiency is rescued by teriparatide. FASEB J. (2018) 32:3730–41.
116. Albus E, Sinningen K, Winzer M, Thiele S, Baschant U, Hannemann A, et al. Milk fat globule-epidermal growth factor 8 (MFG-E8) is a novel anti-inflammatory factor in rheumatoid arthritis in mice and humans. J Bone Miner Res. (2016) 31:596–605. doi: 10.1002/jbmr.2721
117. Kajikawa T, Meshikhes F, Maekawa T, Hajishengallis E, Hosur KB, Abe T, et al. Milk fat globule epidermal growth factor 8 inhibits periodontitis in non-human primates and its gingival crevicular fluid levels can differentiate periodontal health from disease in humans. J Clin Periodontol. (2017) 44:472–83. doi: 10.1111/jcpe.12707
118. Maekawa T, Hosur K, Abe T, Kantarci A, Ziogas A, Wang B, et al. Antagonistic effects of IL-17 and D-resolvins on endothelial Del-1 expression through a GSK-3beta-C/EBPbeta pathway. Nat Commun. (2015) 6:8272. doi: 10.1038/ncomms9272
119. Glessner JT, Bradfield JP, Wang K, Takahashi N, Zhang H, Sleiman PM, et al. A genome-wide study reveals copy number variants exclusive to childhood obesity cases. Am J Hum Genet. (2010) 87:661–6. doi: 10.1016/j.ajhg.2010.09.014
120. Cobb LP, Siamakpour-Reihani S, Zhang D, Qin X, Owzar K, Zhou C, et al. Obesity and altered angiogenic-related gene expression in endometrial cancer. Gynecol Oncol. (2021) 163:320–6. doi: 10.1016/j.ygyno.2021.08.010
121. Van Hove I, Hu TT, Beets K, Van Bergen T, Etienne I, Stitt AW, et al. Targeting RGD-binding integrins as an integrative therapy for diabetic retinopathy and neovascular age-related macular degeneration. Prog Retin Eye Res. (2021) 85:100966. doi: 10.1016/j.preteyeres.2021.100966
122. Nader D, Curley GF, Kerrigan SW. A new perspective in sepsis treatment: could RGD-dependent integrins be novel targets? Drug Discov Today. (2020) 25:2317–25. doi: 10.1016/j.drudis.2020.09.038
Keywords: bone, mineralized tissues, arginine-glycine-aspartic acid (RGD), metabolic activity, endocrinology, obesity
Citation: Nagasaki K, Gavrilova O, Hajishengallis G and Somerman MJ (2022) Does the RGD region of certain proteins affect metabolic activity?. Front. Dent. Med. 3:974862. doi: 10.3389/fdmed.2022.974862
Received: 21 June 2022; Accepted: 04 July 2022;
Published: 27 July 2022.
Edited by:
Thanaphum Osathanon, Chulalongkorn University, ThailandReviewed by:
Yorimasa Ogata, Nihon University, JapanCopyright © 2022 Nagasaki, Gavrilova, Hajishengallis and Somerman. This is an open-access article distributed under the terms of the Creative Commons Attribution License (CC BY). The use, distribution or reproduction in other forums is permitted, provided the original author(s) and the copyright owner(s) are credited and that the original publication in this journal is cited, in accordance with accepted academic practice. No use, distribution or reproduction is permitted which does not comply with these terms.
*Correspondence: Martha J. Somerman, bWFydGhhLnNvbWVybWFuQG5paC5nb3Y=
Disclaimer: All claims expressed in this article are solely those of the authors and do not necessarily represent those of their affiliated organizations, or those of the publisher, the editors and the reviewers. Any product that may be evaluated in this article or claim that may be made by its manufacturer is not guaranteed or endorsed by the publisher.
Research integrity at Frontiers
Learn more about the work of our research integrity team to safeguard the quality of each article we publish.