- 1Dental Biomedical Sciences Ph.D. Program, University of Maryland School of Dentistry, Baltimore, MD, United States
- 2Department of Restorative Dental Sciences, College of Dentistry, Umm Al-Qura University, Makkah, Saudi Arabia
- 3Department of Restorative Dental Sciences, College of Dentistry, King Saud University, Riyadh, Saudi Arabia
- 4Department of Restorative Dental Sciences, College of Dentistry, Imam Abdulrahman Bin Faisal University, Dammam, Saudi Arabia
- 5Biomaterials and Tissue Engineering Division, Department of Advanced Oral Sciences and Therapeutics, University Maryland School of Dentistry, Baltimore, MD, United States
- 6Center for Stem Cell Biology and Regenerative Medicine, University of Maryland School of Medicine, Baltimore, MD, United States
- 7Marlene and Stewart Greenebaum Cancer Center, University of Maryland School of Medicine, Baltimore, MD, United States
- 8Division of Operative Dentistry, Department of General Dentistry, University of Maryland School of Dentistry, Baltimore, MD, United States
The management and treatment of dental and craniofacial injuries have continued to evolve throughout the last several decades. Limitations with autograft, allograft, and synthetics created the need for more advanced approaches in tissue engineering. Calcium phosphate cements (CPC) are frequently used to repair bone defects. Since their discovery in the 1980s, extensive research has been conducted to improve their properties, and emerging evidence supports their increased application in bone tissue engineering. This review focuses on the up-to-date performance of calcium phosphate cement (CPC) scaffolds and upcoming promising dental and craniofacial bone regeneration strategies. First, we summarized the barriers encountered in CPC scaffold development. Second, we compiled the most up to date in vitro and in vivo literature. Then, we conducted a systematic search of scientific articles in MEDLINE and EMBASE to screen the related studies. Lastly, we revealed the current developments to effectively design CPC scaffolds and track the enhanced viability and therapeutic efficacy to overcome the current limitations and upcoming perspectives. Finally, we presented a timely and opportune review article focusing on the significant potential of CPC scaffolds for dental and craniofacial bone regeneration, which will be discussed thoroughly. CPC offers multiple capabilities that may be considered toward the oral defects, expecting a future outlook in nanotechnology design and performance.
Introduction
Following the increase in life expectancies, systematic diseases, congenital disabilities, trauma, tumor removal, and other causes of bone tissue loss (1, 2), the need for bone repair in craniofacial, dental, and orthopedic fields has increased. Each year, ~200,000 craniofacial fractures require bone transplantation in the U.S.A., with an economic burden of $2B. Appropriate restoration of the form and function of the missing skeletal tissue is an unprecedented challenge. Defects in the craniofacial bones represent a significant emotional and economic burden as their restoration/regeneration often requires multiple complex bone grafting procedures. To address this limitation, scaffold-mediated exogenous cell transplantation and growth factors/hormone delivery are two widely-studied alternatives to conventional autologous grafts, the “gold standard.” However, for therapeutic translation, both approaches encounter various barriers, including safety concerns (3–5).
The use of bone grafts to treat bone defects or regenerate a site remains a significant health challenge (6). Though autografts and allografts are used clinically, they have drawbacks such as morbidity at the donor site, disease transmission risks, and harvesting limitation (7). Different bone grafting materials have been introduced to overcome these limitations, including biodegradable alloplastic materials such as fiber-reinforced bioactive glass materials and polymer-based polyether ketone, xenogeneic bone materials, and bioactive degradable ceramics materials like calcium phosphate and hydroxyapatite (8, 9).
Calcium phosphate ceramics have been shown to express improved bone formation and the ability to function as bone grafting substitute materials due to their osteoconductive properties and excellent biocompatibility (10–12). The literature provides a historical perspective on calcium phosphate cement (CPC) performance in many medical, craniofacial, oral surgery applications, involving augmentation of craniofacial skeletal defects, ridge recontraction, sinus augmentation, periodontal bone defects regeneration, and tooth defect regeneration (11, 13–15).
In order for prefabricated bioceramic bone grafting materials to fit into the bone defect, the operator has to modify the graft shape to the desired size or modify the surgical site. These modifications could increase postoperative trauma, bone loss, and surgical time (16). On the other hand, calcium phosphate cement (CPC) delivers intimate adaptation to bone defect contours by their ability to be injected and placed in situ (13). Calcium phosphate cement (CPC) consists of a combination of tetracalcium phosphate [TTCP: Ca4(PO4)2O] and dicalcium phosphate anhydrous (DCPA:CaHPO4). The CPC paste consists of CPC powder with an aqueous liquid. The resultant paste is a self-hardening paste that forms a resorbable scaffold (17). Due to its desirable biological and mechanical properties, CPC is very promising for various clinical applications. As a result, in 1996, the Food and Drug Administration approved CPC use for craniofacial defects treatments in humans (18).
The original CPC formulation was introduced in 1988 by Brown and Chow (19). A plethora of CPC formulations have been explored for their potential as a grafting material. The design is detailed-oriented, and any minor changes in the CPC formulation affect the distribution and alignment of crystalline forms in the structure (19). The Food and Drug Administration approved at least three formulations for clinical use: Bone Source®, Alpha-BSM®, and Skeletal Repair Systems (S.R.S.)®.
Since the first FDA-approved CPC for human use, a broad scope of clinical uses, including orthopedic, craniofacial, and trauma treatment, has been endorsed worldwide (20). However, intraoral clinical use of CPC has been considerably deficient. Correspondingly, scientific research focused on intraoral applications of CPC's use is also relatively scarce (20). Nevertheless, CPC scaffolds can be used with outstanding outcomes in many dental procedures due to calcium phosphate's high osteoconductivity, self-hardening properties, excellent adaptation to bone defects, and gradual resorption and replacement with a new bone (2, 6, 12). In several current publications, various in vivo and in vitro studies on the application of CPC for intraoral applications can be observed (21–23).
Therefore, this study aimed to review evidence on calcium phosphate cement (CPC) scaffolds for dental and craniofacial applications and summarize the pre-clinical, in vivo studies and the limited clinical studies on using CPC scaffolds treatment of oral bone defects.
Materials and Methods
Study Design
A scoping review research technique was conducted for this review. The scoping review aims to map the currently existing literature on a complex topic or a particular topic with gaps in the literature, including the primary research characteristics, nature, and volume. Hence, the methodology used of literature scoping was carried on calcium phosphate cement (CPC) scaffolds for dental and craniofacial bone regeneration (19). For our scoping review, a 5-stage framework was adopted following Arskey and O'Malley's design (20), and the recommendations conducted by Levac, Colquhoun, and O'Brien were embraced (21). This approach includes a team with an iterative process for study selection and qualitative data assessment. The five stages involved: research question identification, identification of relevant results, selection of studies, data charting, and results reporting.
Stage I: Identification of Research Question
The aim was to answer two key questions for this research: “What are the main types of calcium phosphate cement (CPC) scaffolds that are mostly investigated for dental/ craniofacial regeneration” and “What are the modifications and additive components for those scaffolds or cements that imparted enhanced performance?”
Stage II: Identification of Pertinent Studies
A research library supported the databases search for subject terms, keywords, and text words related to studies that evaluated bone regeneration using calcium phosphate cement (CPC) scaffolds for dental and craniofacial applications. Two independent researchers reviewed the related articles (RA and HM) using EMBASE, and Medline (OVID) databases. The search strategy performed for MEDLINE was followed for EMBASE and revised properly to account for the syntax rules and vocabulary differences.
Terms searched were related to the calcium phosphate cement (CPC) scaffolds used and involved but were not limited to calcium phosphate or CaPo4, dental or craniofacial, scaffolds, cements, regeneration, or repair. To ensure the quality assessment of the found resources. The searches were limited to peer-reviewed journals. Searches were also limited to English language articles from 2000 to 2020. Figure 1 demonstrates the studies' search procedure using PRISMA flowchart.
Stage III: Selection of Studies
The criteria for studies inclusion were established by researchers (RA and HM) throughout stages II and III. The following inclusion criteria were followed: (1) references from peer-reviewed journals; (2) references that studied calcium phosphate materials for bone regeneration (including cements and scaffolds); (3) references that evaluated the bone regeneration of calcium phosphate cements or scaffolds for dental and craniofacial applications; (4) references with the publication year 2000-current; (5) references with the English language were selected.
Exclusion criteria were: (1) references with publication year prior to 2000; (2) references published in languages other than English. Screening of the references was made by reading the abstracts and titles. Then it was determined by each independent reviewer if the reference were to be considered for revision of the full text. Based on this screening, a final agreement was reached following the divergences of reviewers, and the assessment of full-text stages was established.
Stage IV: Data Charting
A spreadsheet software was used to create a template for data extraction. The template was established and reviewed by each author. The reviewers were calibrated on answering the research questions and recording the variables to be extracted. The extracted data from all studies included was made by (RA, HM, and AAB).
Stage V: Extraction of Data and Results Reporting
The extracted data from the included references were done by the first three authors (RA, HM, and AAB). Data were arranged according to (1) name; (2) date published; (3) country of origin; (4) study type (in vivo or in vitro); (5) calcium phosphate type used; (6) other materials incorporated into the cement or scaffold; and (7) any other modifications made to the calcium phosphate scaffolds and the reason behind any modifications.
Results
Articles' Distribution
Out of the 401 studies, 36 articles that investigated the potential use of CPC for dental tissue engineering and regeneration were included. Table 1 illustrates the detailed characteristics and outcomes of the included studies. Figure 2A illustrates the increasing number of the published articles related to the subject from 2006 and 2020. 38.88% of the studies were conducted in the United States of America (U.S.A.), 30.57% were published in China and Japan, and 19.44% were published in European countries as shown in Figure 2B.
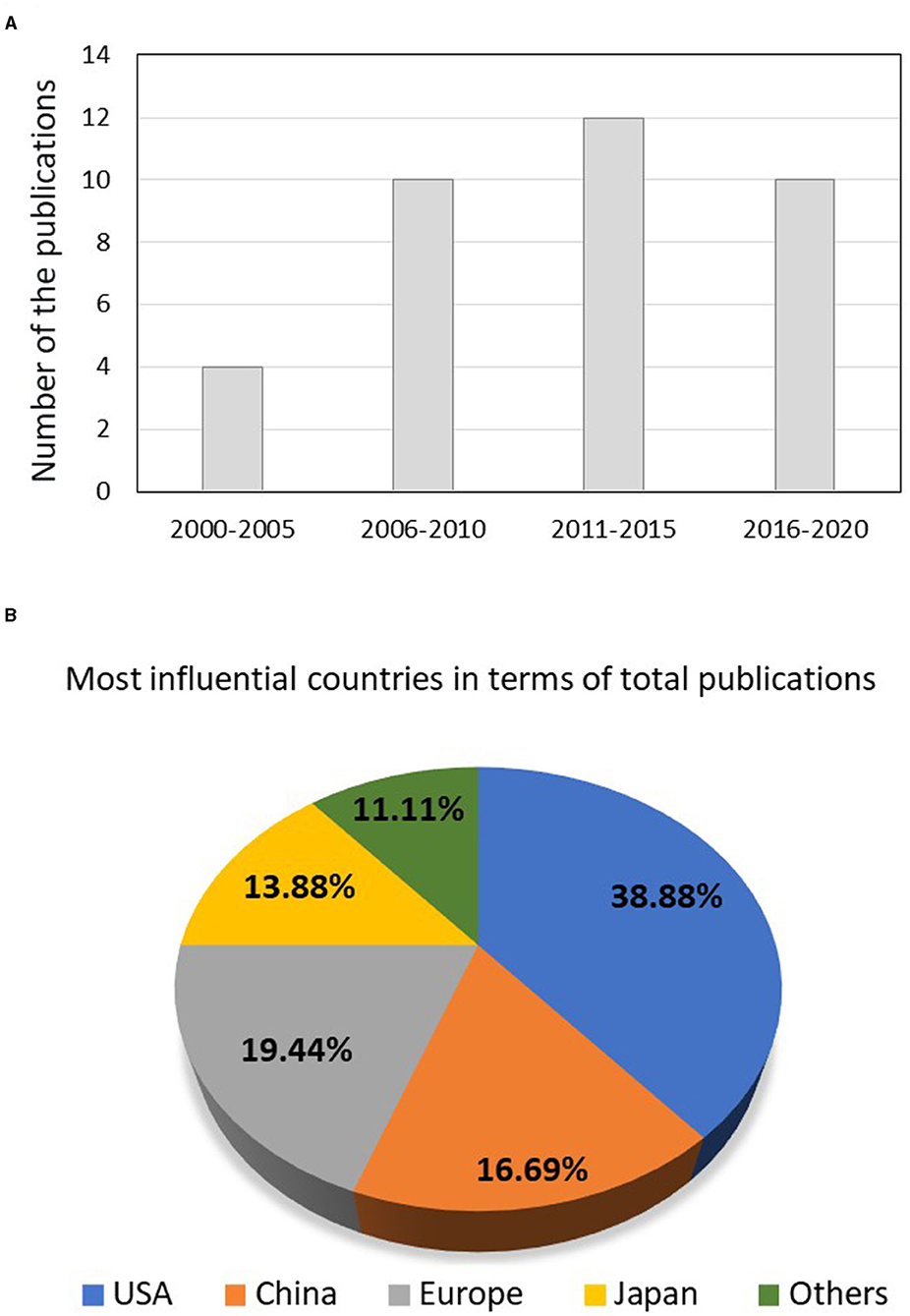
Figure 2. (A) The number of publications performed from 2000 to till 2021 concerning the use of calcium phosphate scaffolds in dentistry and (B) the most influential countries in terms of total publications.
The Type of the Study and the Investigated CPC
Most of the investigations were in vivo studies (58.33%) that utilized an animal model to study the effect of the CPC on tissue engineering and regeneration. Only 5.60% of the articles were clinical studies, while the remaining were in vitro, as displayed in Figure 3A. The most investigated CPC was tricalcium phosphate TCP (35.16%), in its two forms α-TCP and β-TCP, followed by the TTCP+DCPA mixture (32.43%) as displayed in Figure 3B. TTCP was used alone in around 10.81% of the included studies. The use of other calcium phosphate compounds such as biphasic calcium phosphate and calcium sulfate dehydrate was also reported. 35.13% of the studies focused on bone regeneration in general, 24.32% on periodontal and soft tissue regeneration, and 18.91% on alveolar bone regeneration, as displayed in Figure 3C.
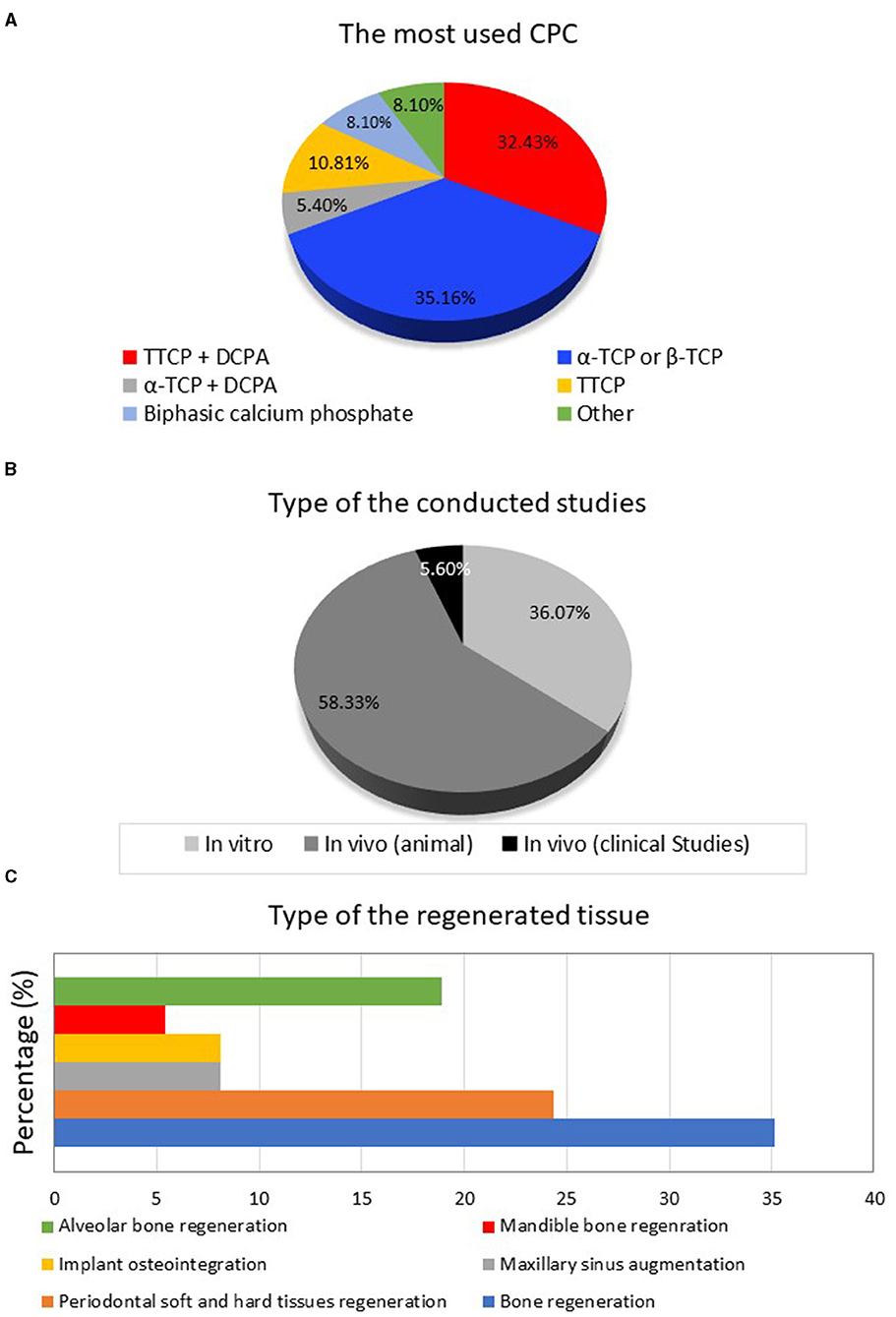
Figure 3. (A) The types of the included studies, (B) the different types of calcium phosphate compounds, and (C) the targeted regenerated dental tissues.
Adjunctive Incorporated Materials
Twenty seven out of the 36 studies had incorporated other materials with the CPC compounds. 21.27% of the incorporated materials are represented by growth factors, platelet-rich plasma (PRP), or proteins. Mesenchymal, stem, and differentiated cells composed 10.63% of the incorporated materials, and 19.14% were represented by polymeric materials, as illustrated in Figure 4A. Other materials such as hydrogels, clot-forming materials, hard or soft tissue derivatives, peptides, and fibers were also reported. Around 72.43% of these adjunctive materials were incorporated to improve tissue engineering and bone regeneration capabilities, as shown in Figure 4B.
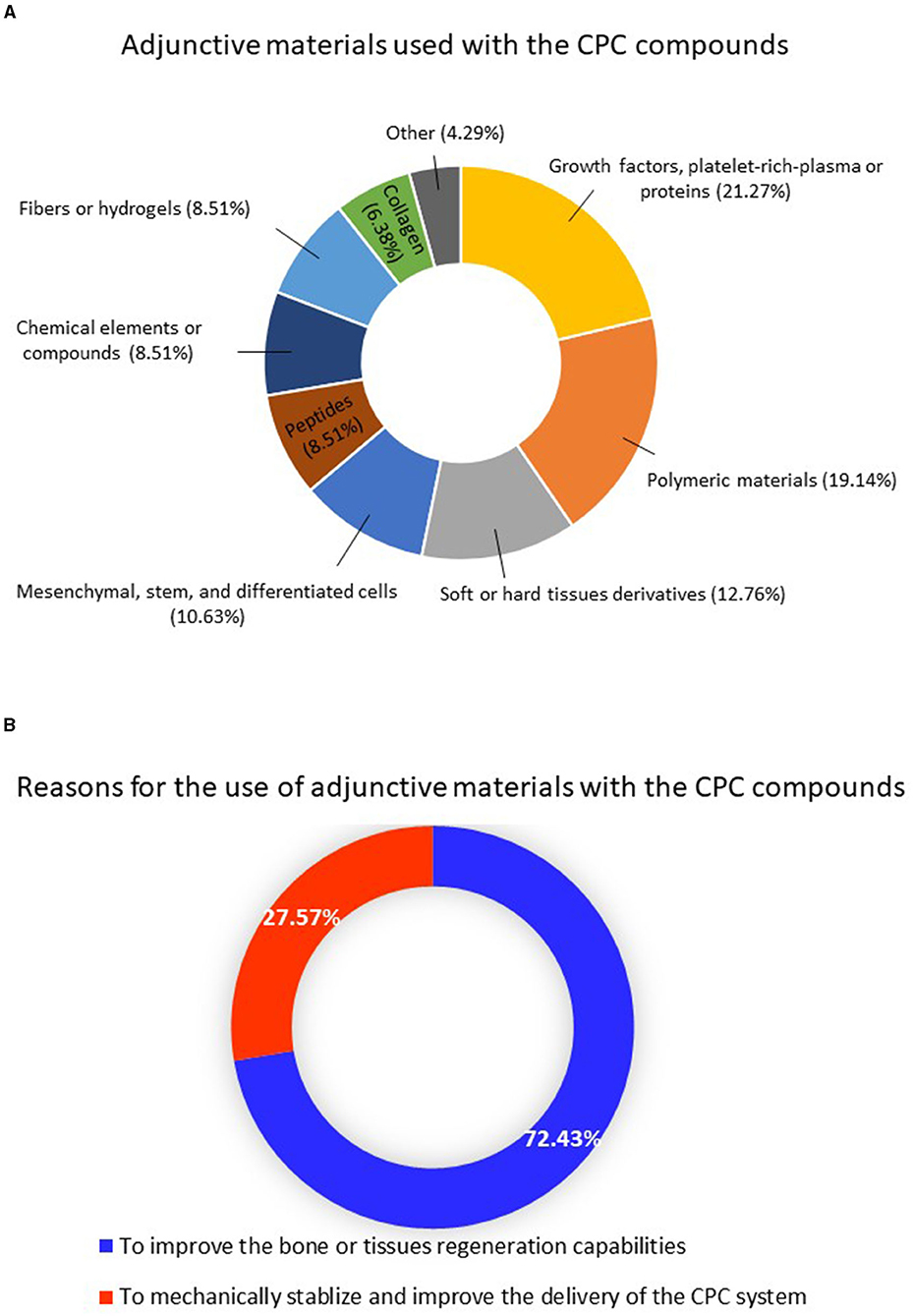
Figure 4. (A) Distribution of the adjunctive materials used with the calcium phosphate compounds and (B) the reasons for using these materials.
Discussion
This study sought to review evidence on calcium phosphate cement (CPC) scaffolds for dental applications and summarizes the pre-clinical, in vivo studies and the limited clinical studies on the use of CPC scaffolds to treat dental bone defects. This report's findings have elicited a better understanding of: (1) The main types of calcium phosphate cements scaffolds used in the dental/craniofacial field; (2) What are the modifications and additive components for those scaffolds or cements that imparted enhanced performance over nearly 20 years of research.
CPC shows promising bone replacement capability, osteoconductivity, self-hardening properties and is shown to be used in several intraoral procedures, including bone ridge augmentation, implant grafting, periodontal regeneration, and sinus lift augmentation (Figure 5) (20, 31, 41, 51). An appropriate CPC type should be used to achieve optimum clinical outcomes. Each type of CPC has a different resorption rate, and each proposed clinical use required a different resorption rate. For some applications such as periodontal bone defect repairs and sinus lift, the capability of the grafted cement to be replaced quickly by bone is highly desirable.
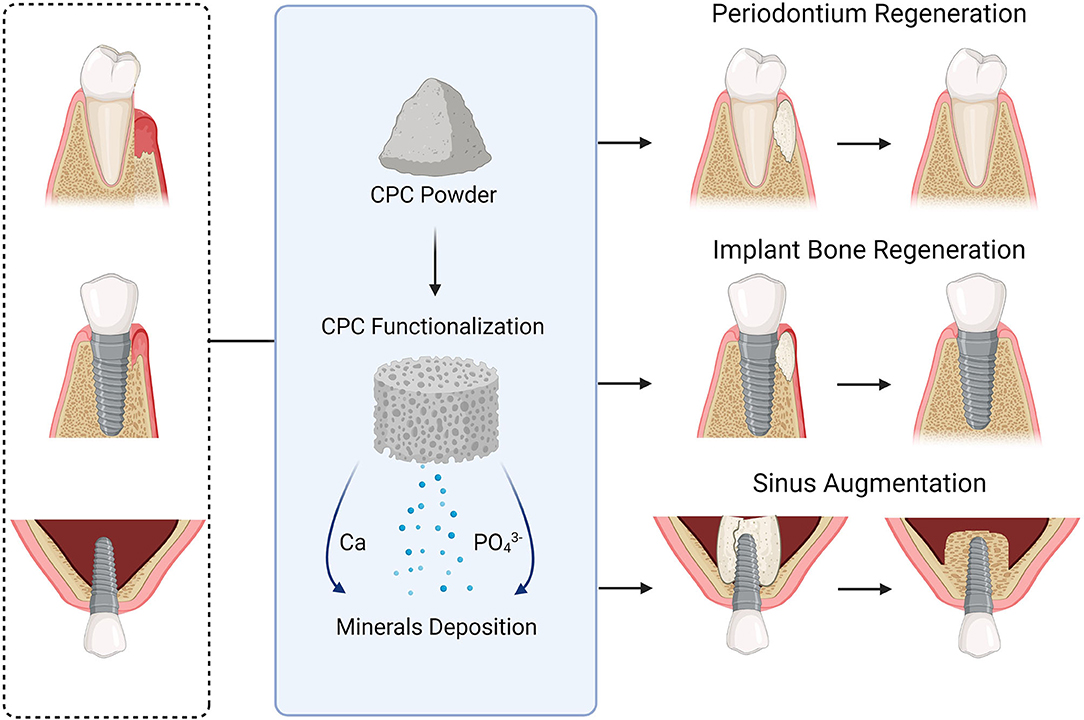
Figure 5. A schematic drawing illustrates some calcium phosphate compounds applications to regenerate soft and hard dental tissues.
On the other hand, other uses such as cranioplasty, graft stability, and integrity are more important than rapid resorption and replacement by bone (20). Using a different type of calcium phosphate as the significant element of CPC could provide an applicable method for formulating a cement with a different range of resorption rates. Depending on the cement setting reaction, different types of cement systems demonstrate different pH setting characteristics. Therefore, a CPC with a specific pH level should be used to gain compatibility with other components, such as antibiotics, growth factors, and osteoinductive factors (2).
In this scoping review, the most investigated CPC composition was TCP (35.13%), in its two forms, α-TCP, and β-TCP. TCP is a resorbable phase of calcium phosphate with a Ca/P ratio of 1.5. It has also been shown to help in bone growth. TCP cement helps in maintaining bony defect spaces and permits bone growth on their surface or into channels, pores, or pipes, and primarily acts as osteoconductive materials (57). The α and β phases have the same chemical composition, but they have different crystalline structures and solubility. The α-TCP has a monoclinic crystalline structure, and the β-TCP has a rhombohedral crystalline structure.
Both types of TCP are stable at room temperature in the absence of humidity; however, α-TCP has shown lower stability of the crystal lattice compared to β-TCP in a density functional study. Therefore, α-TCP can be hydrolyzed and be more reactive in aqueous systems than β-TCP. Similarly, α-TCP is used as a component of CPC, although α-TCP never occurs in biological calcifications (58, 59). In the field of dentistry, α-TCP is used primarily as a fine powder to prepare calcium phosphate cements due to its high solubility and reactivity, which makes it ideally used as injectable biodegradable cements (59). However, the main drawbacks that limit the use of α-TCP in its pure form in biomedical applications are its rapid resorption rate which is faster than the formation of a new bone, and its limited mechanical properties (60).
Meanwhile, β-TCP has a resorption rate slower than α-TCP. Therefore, β-TCP preserves the structural stability for a longer time compared to α-TCP. Also, it has excellent cell adhesion and biomineralization attributes. However, β-TCP has several drawbacks when used as an osteoconductive cement. First, the new bone absorption rate does not entirely match with the β-TCP absorption rate. As a result, new bone absorption is slightly faster than β-TCP absorption. Second, it has slight brittleness and poor mechanical properties, which limits its use in weight-bearing areas. Moreover, β-TCP lacks osteogenicity and osteoinductivity (2, 20, 58). Previous in vivo results showed very limited osteogenesis outcomes of β-TCP (61).
To improve the physical and biological properties and address the limitations of β-TCP, some adjunctive materials have been used to form β-TCP based CPC. These adjunctive materials involve osteogenic materials (bone marrow and mesenchymal stem cells), osteoconductive materials [hydroxyapatite (HA) and poly-caprolactone (PCL)], and bone-induced materials [platelet-rich plasma (PRP), and bone morphogenetic protein-2 (BMP-2)]. Moreover, to regulate β-TCP resorption rate, the activity of osteoclasts or osteoblasts can be regulated by the addition of metal ions such as Si and Zn (58).
In this scoping study, TCP was most commonly used to treat periodontal defects, followed by alveolar bone and bony defects around the dental implant. β-TCP was commonly used for alveolar bone defects and dental implant defects regeneration, while α-TCP was mainly used for periodontal regeneration. None of the studies compared the regeneration outcome of α-TCP and β-TCP. More studies are needed to find the best TCP-based CPC type for treating each intraoral defect type.
The second most investigated CPC type was the TTCP+DCPA mixture. TTCP is the most basic calcium phosphate and the most soluble calcium phosphate below a pH of 5 (62). TTCP shows poor biodegradability despite its high solubility and remains unreacted in an aqueous environment at room temperature for a long time. TTCP forms a thin, insoluble HA layer coating its particles and inhibiting further degradation, and this could be attributed to its low reactivity (63). On the other hand, DCPA is considered an acidic calcium phosphate. When mixing DCPA with TTCP, an acid-base reaction to form a poorly-crystalline hydroxyapatite is created (64). Under neutral pH environments, both types are much more soluble than HA.
Moreover, a slurry containing a mixture of DCPA-TTCP can generate a constant amount of HA precipitation without changing the composition of the solution (2). Previous observation found that aqueous pastes of DCPA+TTCP mixtures converted to a solid mass when remaining in test tubes for several hours. As a result, the first type of self-hardening cement that formed HA as a by-product and consisted of only calcium phosphates was discovered (64). This DCPA+TTCP composition became the first commercially available CPC for use in humans and received approval from the US Food and Drug Administration in 1996 (20).
Traditional DCPA+TTCP based CPC has self-setting properties and is an in situ hardening paste that can be injected or sculpted to bony defects during surgery. However, traditional DCPA+TTCP based CPC showed limited mechanical, physical, and biological properties (65, 66). Therefore, different approaches have been used to overcome these limitations, such as the incorporation of chitosan, absorbable fibers, biofunctionalization, mannitol porogens, gas-foaming agents, and alginate microbeads (65–67). These methods enhanced the CPC's setting time, mechanical strength, degradability, macroporosity, delivery of cells, growth factors, and improved cell attachments.
Different methods to enhance traditional CPC properties have been reported (27, 33, 43, 55). Chitosan had been used with DCPA+TTCP based CPC and could create a non-rigid cement that possesses high strength and durability compared to traditional CPC (27). Other studies showed that encapsulation of pluripotent-derived mesenchymal stem cells and BMP-2 on DCPA+TTCP based CPC significantly enhanced bone regeneration and achieved a 2–3 fold increase in bone regeneration compared to CPC control without cell delivery (33). When human dental pulp stem cells and human bone marrow stem cells were seeded into DCPA+TTCP based CPC in vitro, it showed excellent cell attachment, osteogenic differentiation, mineralization, and new bone and blood vessels were formed. In addition, seeding stem cells into CPC increased new bone formation and new blood vessel density (43). Moreover, in another study, the osteogenic differentiation of human dental pulp stem cells was considerably improved via iron oxide nanoparticles incorporation into CPC. The incorporation of iron oxide nanoparticles into the CPC scaffold significantly enhanced osteogenic differentiation and bone mineral synthesis (55).
Another promising calcium phosphate grafting material is biphasic calcium phosphate (BCP). It consists of hydroxyapatite (HA) and ß-tricalcium phosphate (ß-TCP). The chemical composition of BCP mimics the inorganic part of the natural bone matrix. ß-TCP degrades faster and has a different resorption pattern than HA, since HA is rigid, brittle, and has limited resorption after application (56, 68). For effective graft remodeling, there should be an appropriate balance between the resorption rate of the graft materials and the growth of new bone formation. BCP with a HA to ß-TCP ratio of 60:40 shows the slowest resorption rate of BCP currently used in clinics, whereas a bone graft with 100% ß-TCP has the fastest resorption rate and may resorb before new bone formation (53).
Most recently, Naujokat et al. evaluated the osseointegration of dental implants in ectopic engineered bone flaps in three different scaffold materials: HA, BCP, and titanium. Radiographic, histological, and histomorphometric analysis showed that all implants exhibited sufficient primary stability, and the success rate was 100%. The bone-to-implant contact ratios (BICs), the inter-thread bone densities, and the peri-implant bone-scaffold densities were higher in BCP than HA and titanium. The BIC exhibited a strong correlation (r = 0.76) with the density of the peri-implant bone scaffolds. However, BCP was not reported to be used as a calcium phosphate cement; it was reported to be used as a scaffold only (53, 68).
It is essential to highlight that most of the included reports were in vivo studies that applied an animal model. Such an observation indicates that different animal models are well-described in the literature. As a result, future investigations are encouraged to design randomized clinical trials to illustrate the clinical effectiveness of CPC scaffolds among patients. In addition, some limitations were found in the reported investigations, such as controls that involved no intervention (31). Therefore, the studied CPC scaffolds should be compared to a control with gold-standard intervention concerning the targeted dental bone in order to identify the clinical advantages of such treatment. Furthermore, most of the investigations were conducted using one type of CPC. While other factors such as the site of application and the compound's pH may play a role in selecting the CPC compound, studies that compared different types of CPC materials are needed.
The use of adjunctive materials to improve the stability or the regeneration capabilities was reported in most of the included studies. Such findings indicate that the CPC materials could be tuned to different nanoplatforms, stem cells, or growth factors to elicit better clinical outcomes. Bone regeneration is typically a long process; therefore, other materials to support the stability and control the release of the CPC compounds are essential to improve its success (69). In Table 1, it can be observed that using adjunctive material with the CPC compounds was, most of the time, beneficial in improving the bone regeneration capabilities compared to the use of CPC alone. More studies are needed to investigate and apply the new advancements in CPC technologies and focuses on innovative biological synergies of CPCs on the different applications of the oral cavity procedure Figures 6, 7 (70). The major current progress in CPCs, involving stem cell delivery, 3D printing, growth factor, drug delivery, and pre- vascularization of CPC scaffolds, is a promising field to enhance the success rate of bone and periodontal regeneration in the oral cavity. Future investigations are encouraged to explore different strategies and approaches to support the bone regeneration of the CPC materials. In this scoping review, only Medline and Embrace databases were used. As a result, more databases are recommended to add more eligible articles and create a more comprehensive search. Future meta-analysis is recommended to critically evaluate and statistically combine results of studies or trials of comparable outcome.
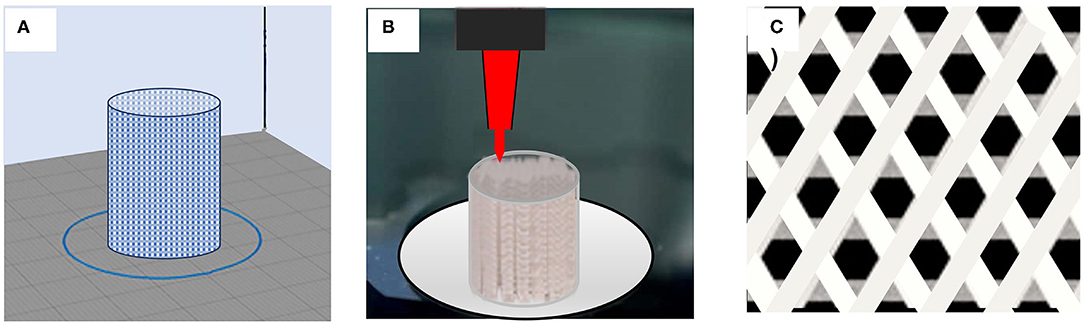
Figure 6. Illustration of structure and fabrication process of a 3D-printed calcium phosphate scaffold. (A,B) Design and printing stage of a calcium phosphate scaffold. (C) 3D-printed scaffolds structure.
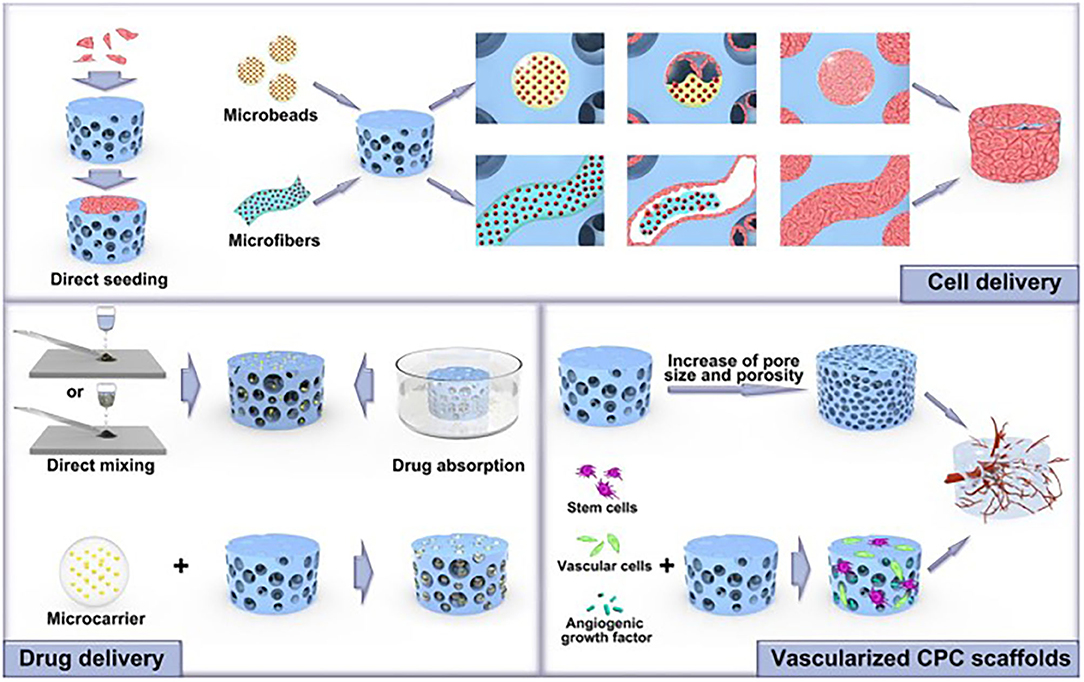
Figure 7. Various promising new advancements in CPC technologies and their major biological properties could be applied in the dental field to enhance bone and periodontal regeneration success rates. Adapted from Xu et al. (70).
Concluding Remarks
Within the outcomes of this scoping review, researchers could use the existing information on CPC combined with the new innovative methods to develop a new CPC scaffold type that can fully meet each dental use requirement. However, more in vivo and in vitro studies need to be conducted to determine the functional efficacy of the material for each dental application. CPC was successfully used in different oral applications, including periodontal regeneration, alveolar ridge augmentation, sinus lifting graft, and dental implant-related bony defects. For each clinical application, the requirements of the CPC material vary significantly. Therefore, it should not be expected that only one CPC formulation can be universally efficient. More studies are needed in order to draw a clear map of the necessary properties of each CPC materials type to meet the essential requirement for each dental application.
Data Availability Statement
The original contributions presented in the study are included in the article/Supplementary Material, further inquiries can be directed to the corresponding authors.
Author Contributions
All authors contributed in desgining and writing this manuscript. All authors have read and agreed to the published version of the manuscript.
Conflict of Interest
The authors declare that the research was conducted in the absence of any commercial or financial relationships that could be construed as a potential conflict of interest.
Publisher's Note
All claims expressed in this article are solely those of the authors and do not necessarily represent those of their affiliated organizations, or those of the publisher, the editors and the reviewers. Any product that may be evaluated in this article, or claim that may be made by its manufacturer, is not guaranteed or endorsed by the publisher.
Supplementary Material
The Supplementary Material for this article can be found online at: https://www.frontiersin.org/articles/10.3389/fdmed.2021.743065/full#supplementary-material
References
1. Lavik E, Langer R. Tissue engineering: current state and perspectives. Appl Microbiol Biotechnol. (2004) 65:1–8. doi: 10.1007/s00253-004-1580-z
2. Bohner M, Gbureck U, Barralet JE. Technological issues for the development of more efficient calcium phosphate bone cements: a critical assessment. Biomaterials. (2005) 26:6423–9. doi: 10.1016/j.biomaterials.2005.03.049
3. Mikos AG, Herring SW, Ochareon P, Elisseeff J, Lu HH, Kandel R, et al. Engineering complex tissues. Tissue Eng. (2006) 12:3307–39. doi: 10.1089/ten.2006.12.3307
4. Bohner M. Design of ceramic-based cements and putties for bone graft substitution. Eur Cell Mater. (2010) 20:1–12. doi: 10.22203/eCM.v020a01
5. Mao JJ, Stosich MS, Moioli EK, Lee CH, Fu SY, Bastian B, et al. Facial reconstruction by biosurgery: cell transplantation versus cell homing. Tissue Eng Part B Rev. (2010) 16:257–62. doi: 10.1089/ten.teb.2009.0496
6. Meinel L, Karageorgiou V, Fajardo R, Snyder B, Shinde-Patil V, Zichner L, et al. Bone tissue engineering using human mesenchymal stem cells: effects of scaffold material and medium flow. Ann Biomed Eng. (2004) 32:112–22. doi: 10.1023/B:ABME.0000007796.48329.b4
7. Ginebra MP, Traykova T, Planell JA. Calcium phosphate cements as bone drug delivery systems: a review. J Control Release. (2006) 113:102–10. doi: 10.1016/j.jconrel.2006.04.007
8. Lappalainen O-P, Karhula SS, Haapea M, Kauppinen S, Finnilä M, Saarakkala S, et al. Micro-CT analysis of bone healing in rabbit calvarial critical-sized defects with solid bioactive glass, tricalcium phosphate granules or autogenous bone. J Oral Maxillofac Res. (2016) 7:e4. doi: 10.5037/jomr.2016.7204
9. Lappalainen O-P, Karhula S, Haapea M, Kyllönen L, Haimi S, Miettinen S, et al. Bone healing in rabbit calvarial critical-sized defects filled with stem cells and growth factors combined with granular or solid scaffolds. Childs Nerv Syst. (2016) 32:681–8. doi: 10.1007/s00381-016-3017-2
10. Haddad AJ, Peel SAF, Clokie CML, Sándor GKB. Closure of rabbit calvarial critical-sized defects using protective composite allogeneic and alloplastic bone substitutes. J Craniofac Surg. (2006) 17:926–34. doi: 10.1097/01.scs.0000230615.49270.d1
11. Bittermann GKP, Janssen NG, van Leeuwen M, van Es RJJ. One-year volume stability of human facial defects filled with a β-tricalcium phosphate-hydroxyl apatite mixture (Atlantik). J Craniofac Surg. (2014) 25:372–4. doi: 10.1097/SCS.0000000000000636
12. Clokie CML, Moghadam H, Jackson MT, Sandor GKB. Closure of critical sized defects with allogenic and alloplastic bone substitutes. J Craniofac Surg. (2002) 13:111–21; discussion 122–3. doi: 10.1097/00001665-200201000-00026
13. Oortgiesen DAW, Meijer GJ, Bronckers ALJJ, Walboomers XF, Jansen JA. Regeneration of the periodontium using enamel matrix derivative in combination with an injectable bone cement. Clin Oral Investig. (2013) 17:411–21. doi: 10.1007/s00784-012-0743-z
14. Costantino PD, Friedman CD, Jones K, Chow LC, Sisson GA. Experimental hydroxyapatite cement cranioplasty. Plast Reconstr Surg. (1992) 90:174–85; discussion 186–91. doi: 10.1097/00006534-199290020-00003
15. Shindo ML, Costantino PD, Friedman CD, Chow LC. Facial skeletal augmentation using hydroxyapatite cement. Arch Otolaryngol Head Neck Surg. (1993) 119:185–90. doi: 10.1001/archotol.1993.01880140069012
16. Laurencin CT, Ambrosio AM, Borden MD, Cooper JA. Tissue engineering: orthopedic applications. Annu Rev Biomed Eng. (1999) 1:19–46. doi: 10.1146/annurev.bioeng.1.1.19
17. Xu HHK, Simon CG. Self-hardening calcium phosphate composite scaffold for bone tissue engineering. J Orthop Res. (2004) 22:535–43. doi: 10.1016/j.orthres.2003.09.010
18. Friedman CD, Costantino PD, Takagi S, Chow LC. BoneSource hydroxyapatite cement: a novel biomaterial for craniofacial skeletal tissue engineering and reconstruction. J Biomed Mater Res. (1998) 43:428–32. doi: 10.1002/(SICI)1097-4636(199824)43:4<428::AID-JBM10>3.0.CO;2-0
19. Ambard AJ, Mueninghoff L. Calcium phosphate cement: review of mechanical and biological properties. J Prosthodont. (2006) 15:321–8. doi: 10.1111/j.1532-849X.2006.00129.x
20. Chow LC. Next generation calcium phosphate-based biomaterials. Dent Mater J. (2009) 28:1–10. doi: 10.4012/dmj.28.1
21. Shirakata Y, Oda S, Kinoshita A, Kikuchi S, Tsuchioka H, Ishikawa I. Histocompatible healing of periodontal defects after application of an injectable calcium phosphate bone cement. A preliminary study in dogs. J Periodontol. (2002) 73:1043–53. doi: 10.1902/jop.2002.73.9.1043
22. Oortgiesen DAW, Walboomers XF, Bronckers ALJJ, Meijer GJ, Jansen JA. Periodontal regeneration using an injectable bone cement combined with BMP-2 or FGF-2. J Tissue Eng Regen Med. (2014) 8:202–9. doi: 10.1002/term.1514
23. Ohayon L. Maxillary sinus floor augmentation using biphasic calcium phosphate: a histologic and histomorphometric study. Int J Oral Maxillofac Implants. (2014) 29:1143–8. doi: 10.11607/jomi.3422
24. Sugawara A, Fujikawa K, Kusama K, Nishiyama M, Murai S, Takagi S, et al. Histopathologic reaction of a calcium phosphate cement for alveolar ridge augmentation. J Biomed Mater Res. (2002) 61:47–52. doi: 10.1002/jbm.10010
25. Fujikawa K, Sugawara A, Kusama K, Nishiyama M, Murai S, Takagi S, et al. Fluorescent labeling analysis and electron probe microanalysis for alveolar ridge augmentation using calcium phosphate cement. Dent Mater J. (2002) 21:296–305. doi: 10.4012/dmj.21.296
26. Sorensen RG, Wikesjö UME, Kinoshita A, Wozney JM. Periodontal repair in dogs: evaluation of a bioresorbable calcium phosphate cement (Ceredex) as a carrier for rhBMP-2. J Clin Periodontol. (2004) 31:796–804. doi: 10.1111/j.1600-051X.2004.00544.x
27. Xu HHK, Takagi S, Sun L, Hussain L, Chow LC, Guthrie WF, et al. Development of a nonrigid, durable calcium phosphate cement for use in periodontal bone repair. J Am Dent Assoc. (2006) 137:1131–8. doi: 10.14219/jada.archive.2006.0353
28. Masago H, Shibuya Y, Munemoto S, Takeuchi J, Umeda M, Komori T, et al. Alveolar ridge augmentation using various bone substitutes–a web form of titanium fibers promotes rapid bone development. Kobe J Med Sci. (2007) 53:257–63.
29. Shirakata Y, Yoshimoto T, Goto H, Yonamine Y, Kadomatsu H, Miyamoto M, et al. Favorable periodontal healing of 1-wall infrabony defects after application of calcium phosphate cement wall alone or in combination with enamel matrix derivative: a pilot study with canine mandibles. J Periodontol. (2007) 78:889–98. doi: 10.1902/jop.2007.060353
30. Xu HHK, Weir MD, Simon CG. Injectable and strong nano-apatite scaffolds for cell/growth factor delivery and bone regeneration. Dent Mater. (2008) 24:1212–22. doi: 10.1016/j.dental.2008.02.001
31. Arisan V, Ozdemir T, Anil A, Jansen JA, Ozer K. Injectable calcium phosphate cement as a bone-graft material around peri-implant dehiscence defects: a dog study. Int J Oral Maxillofac Implants. (2008) 23:1053–62.
32. Aral A, Yalçin S, Karabuda ZC, Anil A, Jansen JA, Mutlu Z. Injectable calcium phosphate cement as a graft material for maxillary sinus augmentation: an experimental pilot study. Clin Oral Implants Res. (2008) 19:612–7. doi: 10.1111/j.1600-0501.2007.01518.x
33. Fei Z, Hu Y, Wu D, Wu H, Lu R, Bai J, et al. Preparation and property of a novel bone graft composite consisting of rhBMP-2 loaded PLGA microspheres and calcium phosphate cement. J Mater Sci Mater Med. (2008) 19:1109–16. doi: 10.1007/s10856-007-3050-5
34. Um Y-J, Jung U-W, Chae G-J, Kim C-S, Lee Y-K, Cho K-S, et al. The effects of hydroxyapatite/calcium phosphate glass scaffold and its surface modification with bovine serum albumin on 1-wall intrabony defects of beagle dogs: a preliminary study. Biomed Mater. (2008) 3:044113. doi: 10.1088/1748-6041/3/4/044113
35. Mellonig JT, Valderrama P, Cochran DL. Clinical and histologic evaluation of calcium-phosphate bone cement in interproximal osseous defects in humans: a report in four patients. Int J Periodontics Restorative Dent. (2010) 30:121–7.
36. Weir MD, Xu HHK. Culture human mesenchymal stem cells with calcium phosphate cement scaffolds for bone repair. J Biomed Mater Res B Appl Biomater. (2010) 93:93–105. doi: 10.1002/jbm.b.31563
37. Thein-Han W, Xu HHK. Collagen-calcium phosphate cement scaffolds seeded with umbilical cord stem cells for bone tissue engineering. Tissue Eng Part A. (2011) 17:2943–54. doi: 10.1089/ten.tea.2010.0674
38. Wang L, Zou D, Zhang S, Zhao J, Pan K, Huang Y. Repair of bone defects around dental implants with bone morphogenetic protein/fibroblast growth factor-loaded porous calcium phosphate cement: a pilot study in a canine model. Clin Oral Implants Res. (2011) 22:173–81. doi: 10.1111/j.1600-0501.2010.01976.x
39. Thein-Han W, Liu J, Xu HHK. Calcium phosphate cement with biofunctional agents and stem cell seeding for dental and craniofacial bone repair. Dent Mater. (2012) 28:1059–70. doi: 10.1016/j.dental.2012.06.009
40. Shih T-C, Teng N-C, Wang P-D, Lin C-T, Yang J-C, Fong S-W, et al. In vivo evaluation of resorbable bone graft substitutes in beagles: histological properties. J Biomed Mater Res A. (2013) 101:2405–11. doi: 10.1002/jbm.a.34540
41. Shirakata Y, Yoshimoto T, Takeuchi N, Taniyama K, Noguchi K. Effects of EMD in combination with bone swaging and calcium phosphate bone cement on periodontal regeneration in one-wall intrabony defects in dogs. J Periodontal Res. (2013) 48:37–43. doi: 10.1111/j.1600-0765.2012.01499.x
42. Khojasteh A, Behnia H, Hosseini FS, Dehghan MM, Abbasnia P, Abbas FM. The effect of PCL-TCP scaffold loaded with mesenchymal stem cells on vertical bone augmentation in dog mandible: a preliminary report. J Biomed Mater Res B Appl Biomater. (2013) 101:848–54. doi: 10.1002/jbm.b.32889
43. Chen W, Zhou H, Weir MD, Tang M, Bao C, Xu HHK. Human embryonic stem cell-derived mesenchymal stem cell seeding on calcium phosphate cement-chitosan-RGD scaffold for bone repair. Tissue Eng Part A. (2013) 19:915–27. doi: 10.1089/ten.tea.2012.0172
44. Tang M, Chen W, Liu J, Weir MD, Cheng L, Xu HHK. Human induced pluripotent stem cell-derived mesenchymal stem cell seeding on calcium phosphate scaffold for bone regeneration. Tissue Eng Part A. (2014) 20:1295–305. doi: 10.1089/ten.tea.2013.0211
45. Chen W, Thein-Han W, Weir MD, Chen Q, Xu HHK. Prevascularization of biofunctional calcium phosphate cement for dental and craniofacial repairs. Dent Mater. (2014) 30:535–44. doi: 10.1016/j.dental.2014.02.007
46. Wang L, Wang P, Weir MD, Reynolds MA, Zhao L, Xu HHK. Hydrogel fibers encapsulating human stem cells in an injectable calcium phosphate scaffold for bone tissue engineering. Biomed Mater. (2016) 11:065008. doi: 10.1088/1748-6041/11/6/065008
47. Wang T, Wu D, Li Y, Li W, Zhang S, Hu K, et al. Substance P incorporation in calcium phosphate cement for dental alveolar bone defect restoration. Mater Sci Eng C Mater Biol Appl. (2016) 69:546–53. doi: 10.1016/j.msec.2016.07.014
48. Wongsupa N, Nuntanaranont T, Kamolmattayakul S, Thuaksuban N. Biological characteristic effects of human dental pulp stem cells on poly-ε-caprolactone-biphasic calcium phosphate fabricated scaffolds using modified melt stretching and multilayer deposition. J Mater Sci Mater Med. (2017) 28:25. doi: 10.1007/s10856-016-5833-z
49. Zhao W, Lu J-Y, Hao Y-M, Cao C-H, Zou D-R. Maxillary sinus floor elevation with a tissue-engineered bone composite of deciduous tooth stem cells and calcium phosphate cement in goats. J Tissue Eng Regen Med. (2017) 11:66–76. doi: 10.1002/term.1867
50. Kamal M, Andersson L, Tolba R, Al-Asfour A, Bartella AK, Gremse F, et al. Bone regeneration using composite non-demineralized xenogenic dentin with beta-tricalcium phosphate in experimental alveolar cleft repair in a rabbit model. J Transl Med. (2017) 15:263. doi: 10.1186/s12967-017-1369-3
51. Carlisle P, Guda T, Silliman DT, Burdette AJ, Talley AD, Alvarez R, et al. Localized low-dose rhBMP-2 is effective at promoting bone regeneration in mandibular segmental defects. J Biomed Mater Res B Appl Biomater. (2019) 107:1491–503. doi: 10.1002/jbm.b.34241
52. Xia Y, Chen H, Zhang F, Wang L, Chen B, Reynolds MA, et al. Injectable calcium phosphate scaffold with iron oxide nanoparticles to enhance osteogenesis via dental pulp stem cells. Artif Cells Nanomed Biotechnol. (2018) 46:423–33. doi: 10.1080/21691401.2018.1428813
53. Helder MN, van Esterik FAS, Kwehandjaja MD, Ten Bruggenkate CM, Klein-Nulend J, Schulten EAJM. Evaluation of a new biphasic calcium phosphate for maxillary sinus floor elevation: micro-CT and histomorphometrical analyses. Clin Oral Implants Res. (2018) 29:488–98. doi: 10.1111/clr.13146
54. Fakheran O, Birang R, Schmidlin PR, Razavi SM, Behfarnia P. Retro MTA and tricalcium phosphate/retro MTA for guided tissue regeneration of periodontal dehiscence defects in a dog model: a pilot study. Biomater Res. (2019) 23:14. doi: 10.1186/s40824-019-0163-0
55. Xia Y, Guo Y, Yang Z, Chen H, Ren K, Weir MD, et al. Iron oxide nanoparticle-calcium phosphate cement enhanced the osteogenic activities of stem cells through WNT/β-catenin signaling. Mater Sci Eng C Mater Biol Appl. (2019) 104:109955. doi: 10.1016/j.msec.2019.109955
56. Naujokat H, Açil Y, Harder S, Lipp M, Böhrnsen F, Wiltfang J. Osseointegration of dental implants in ectopic engineered bone in three different scaffold materials. Int J Oral Maxillofac Surg. (2020) 49:135–42. doi: 10.1016/j.ijom.2019.04.005
57. Al-Sanabani JS, Madfa AA, Al-Sanabani FA. Application of calcium phosphate materials in dentistry. Int J Biomater. (2013) 2013:876132. doi: 10.1155/2013/876132
58. Liu B, Lun D. Current application of β-tricalcium phosphate composites in orthopaedics. Orthop Surg. (2012) 4:139–44. doi: 10.1111/j.1757-7861.2012.00189.x
59. Carrodeguas RG, De Aza S. α-Tricalcium phosphate: synthesis, properties and biomedical applications. Acta Biomater. (2011) 7:3536–46. doi: 10.1016/j.actbio.2011.06.019
60. Eliaz N, Metoki N. Calcium phosphate bioceramics: a review of their history, structure, properties, coating technologies and biomedical applications. Materials (Basel). (2017) 10:334. doi: 10.3390/ma10040334
61. Orii H, Sotome S, Chen J, Wang J, Shinomiya K. Beta-tricalcium phosphate (beta-TCP) graft combined with bone marrow stromal cells (MSCs) for posterolateral spine fusion. J Med Dent Sci. (2005) 52:51–7.
62. Bohner M, Merkle HP, Lemaître J. In vitro aging of a calcium phosphate cement. J Mater Sci Mater Med. (2000) 11:155–62. doi: 10.1023/a:1008927624493
63. Gbureck U, Dembski S, Thull R, Barralet JE. Factors influencing calcium phosphate cement shelf-life. Biomaterials. (2005) 26:3691–7. doi: 10.1016/j.biomaterials.2004.09.036
64. Chow LC, Takagi S. A natural bone cement-a laboratory novelty led to the development of revolutionary new biomaterials. J Res Natl Inst Stand Technol. (2001) 106:1029–33. doi: 10.6028/jres.106.053
65. Xu HH, Quinn JB, Takagi S, Chow LC, Eichmiller FC. Strong and macroporous calcium phosphate cement: effects of porosity and fiber reinforcement on mechanical properties. J Biomed Mater Res. (2001) 57:457–66. doi: 10.1002/1097-4636(20011205)57:3<457::aid-jbm1189>3.0.co;2-x
66. Zhao L, Weir MD, Xu HHK. An injectable calcium phosphate-alginate hydrogel-umbilical cord mesenchymal stem cell paste for bone tissue engineering. Biomaterials. (2010) 31:6502–10. doi: 10.1016/j.biomaterials.2010.05.017
67. Xu HHK, Takagi S, Quinn JB, Chow LC. Fast-setting calcium phosphate scaffolds with tailored macropore formation rates for bone regeneration. J Biomed Mater Res A. (2004) 68:725–34. doi: 10.1002/jbm.a.20093
68. Kesseli FP, Lauer CS, Baker I, Mirica KA, Van Citters DW. Identification of a calcium phosphoserine coordination network in an adhesive organo-apatitic bone cement system. Acta Biomater. (2020) 105:280–9. doi: 10.1016/j.actbio.2020.01.007
69. Wang P, Zhao L, Chen W, Liu X, Weir MD, Xu HHK. Stem cells and calcium phosphate cement scaffolds for bone regeneration. J Dent Res. (2014) 93:618–25. doi: 10.1177/0022034514534689
Keywords: calcium phosphate, cement, scaffold, repair, regeneration, dental, craniofacial
Citation: Alsahafi RA, Mitwalli HA, Balhaddad AA, Weir MD, Xu HHK and Melo MAS (2021) Regenerating Craniofacial Dental Defects With Calcium Phosphate Cement Scaffolds: Current Status and Innovative Scope Review. Front. Dent. Med. 2:743065. doi: 10.3389/fdmed.2021.743065
Received: 17 July 2021; Accepted: 10 August 2021;
Published: 30 August 2021.
Edited by:
Amerigo Giudice, University Magna Graecia of Catanzaro, ItalyReviewed by:
Mohammed El-Awady Grawish, Mansoura University, EgyptThanaphum Osathanon, Chulalongkorn University, Thailand
Copyright © 2021 Alsahafi, Mitwalli, Balhaddad, Weir, Xu and Melo. This is an open-access article distributed under the terms of the Creative Commons Attribution License (CC BY). The use, distribution or reproduction in other forums is permitted, provided the original author(s) and the copyright owner(s) are credited and that the original publication in this journal is cited, in accordance with accepted academic practice. No use, distribution or reproduction is permitted which does not comply with these terms.
*Correspondence: Mary Anne S. Melo, bW1lbG9AdW1hcnlsYW5kLmVkdQ==; Michael D. Weir, bWljaGFlbC53ZWlyQHVtYXJ5bGFuZC5lZHU=; Hockin H. K. Xu, aHh1QHVtYXJ5bGFuZC5lZHU=