- Department of Life Sciences, University of Modena and Reggio Emilia, Modena, Italy
Traditional de novo drug discovery, which typically presents an 11% approval rate from phase I trials and even higher failure rates in fields like neurodegeneration, often requires two to three billion dollars and 10–17 years per new drug. In contrast, drug repurposing can reduce risks and bring drugs to the market in 3–12 years, with an average of $300 million investment. In this article, we will outline how drug repurposing can accelerate the discovery of drugs derived from natural and synthetic products. The vast amount of chemical, biological, structural, and clinical data available in public repositories will greatly facilitate drug discovery, without the need to start a discovery campaign from scratch. In the big data era, data mining and artificial intelligence will play major roles in both drug repurposing and drug discovery. This article will provide valuable insights into how drug repurposing can support drug discovery and vice versa, emphasizing its impact in addressing unmet medical needs, achieving cost-effectiveness, and enabling faster market access. Despite legal and regulatory challenges, the cost-effectiveness, and the potential to give new life to compounds already in the pipeline make drug repurposing a crucial complement to traditional drug discovery in the era of precision medicine.
1 Introduction
Drug repurposing (DR), often referred to as drug repositioning, is a drug discovery and development strategy that has recently gained growing interest in both academic and industrial research (March-Vila et al., 2017). Coined in 2004 by T. T. Ashburn and K. B. Thor (Ashburn and Thor, 2004), it is defined as “the process of finding new uses outside the scope of the original medical indication for existing drugs”. DR represents a viable alternative (or complement) to traditional de novo drug discovery, which is a lengthy and costly process often hampered by high failure rates. This novel drug development process involves identifying new therapeutic indications for approved drugs or synthetic and natural products in advanced preclinical settings. Drug repurposing reduces time, risks and costs associated with traditional discovery pipelines, as de-risked compounds have already passed safety and toxicity studies (March-Vila et al., 2017). Rational drug repurposing leverages integrated in silico approaches, such as modeling, data mining, and machine learning to facilitate the selection of candidates from existing drugs and discover new uses (Langedijk et al., 2015). As pointed out by J. Langedijk et al. (Langedijk et al., 2015), the term “existing drugs” can encompass approved drugs, withdrawn medications or treatments no longer produced for commercial reasons, and drug candidates undergoing clinical trials. A specific case of drug repurposing, often referred to as drug rescue, concerns drugs that did not pass clinical trials because their efficacy was inferior to those already on the market (Langedijk et al., 2015).
The term “new uses” extends beyond the repurposing of a drug for a pharmacological target and/or a pathology different from those for which it was originally developed. Indeed, it can also include: i) addressing new patient populations (e.g., the pediatric one); ii) developing new dosage forms, and; iii) exploring new routes of administration, or lines of treatment (Langedijk et al., 2015). In addition, applications of on-target and off-target repositioning are quite distinct. In the former, a known mechanism of action is applied to a new therapeutic indication, while the latter aims to elucidate the mechanism of action of known compounds through the analysis of undesirable side effects. In the following paragraphs, we will dissect the advantages, disadvantages and opportunities embedded in rational DR, with the aim of understanding how this approach can complement and accelerate traditional drug discovery. Examples from the fields of natural products and compounds obtained through organic synthesis will be presented. Their discussion will provide a more concrete understanding of how drug repurposing may help drug discovery. Finally, this review will offer insights on the computational methods applied to DR, aiming to offer a clearer picture of the opportunities and challenges provided by this innovative strategy (Figure 1).
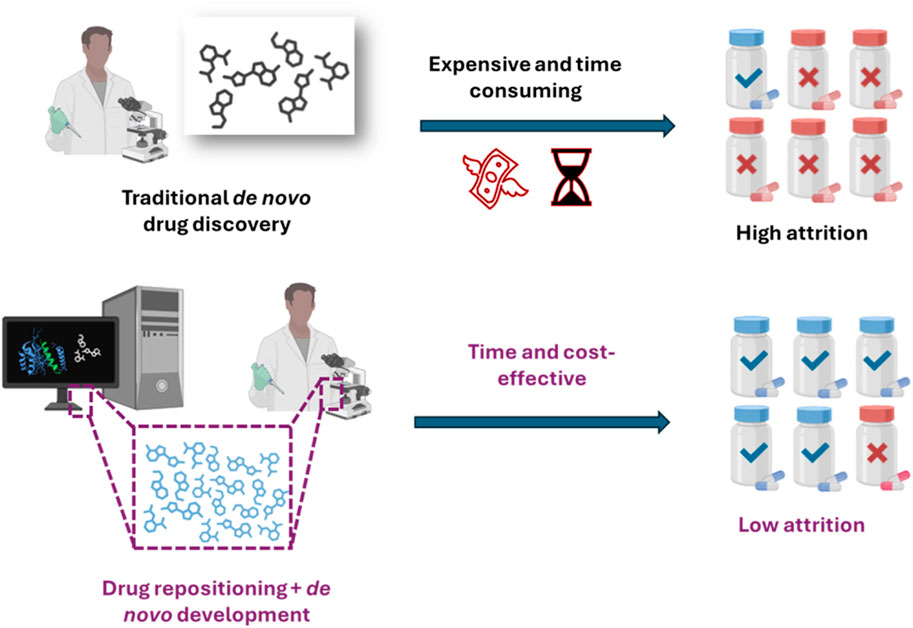
Figure 1. The integrated approach of drug repurposing and traditional de novo drug development increases the cost-effectiveness of the whole drug discovery process, by assuring lower attrition rates and saving time.
2 Famous drug repurposing examples
Many of the so-called blockbuster drugs have been brought to success through repurposing procedures. Most of these examples have originated from serendipity, retrospective clinical observation, or pharmacological mechanism analysis (Pushpakom et al., 2019). All these approaches are in agreement with the concept that if a drug for a certain indication shows an unexpected off-target activity or side effect, it opens the possibility of repurposing it for a second indication, which may take over from the first, becoming the indication par excellence. A well-known example in this regard is the case of minoxidil. In the 1950s, Upjohn Company developed a compound with supposed anti-ulcer action (Ashique et al., 2020). Although the activity was not confirmed in trials on dogs, the compound proved to be a powerful vasodilator. A series of analogues has then been synthesized, leading to the antihypertensive drug minoxidil (Ashique et al., 2020). Interestingly, in the following decades, minoxidil manifested hypertrichosis side effects (Gottlieb et al., 1972). Several mechanisms have been proposed to explain this effect, including local vasodilation of the scalp, with increased oxygen and nutrient supply; upregulation of the vascular endothelial growth factor VEGF expression in the dermal papilla, responsible for the cyclic rhythm of follicular activity; and the opening of potassium channels, resulting in the prolongation of the follicular anagen phase (Ishida et al., 2016). Currently, its topical formulations represent the treatment of choice for alopecia, resulting in sales of $1.5 billion in 2022 (Global Market Insights, 2024a, https://www.gminsights.com/industry-analysis/minoxidil-market, accessed on 20 June 2024). Another famous example comes with sildenafil, developed by Pfizer in the 1980s as an antihypertensive and anti-anginal drug (Ghofrani et al., 2006). Being a phosphodiesterase type 5 (PDE-5) inhibitor, the compound was intended to relax the smooth muscles of the coronary arteries, but this effect was not observed in healthy volunteers (Ashburn and Thor, 2004). In the 1990s the drug was repurposed as a treatment for erectile dysfunction following clinical observations (Ashburn and Thor, 2004). Remarkably, sildenafil accounted for a market worth over $2.9 billion in 2023 (Coherent Market Insights, 2024; https://www.coherentmarketinsights.com/industry-reports/sildenafil-drug-market, accessed on 20 June 2024), and, in 2005, it was repositioned once more as a treatment for pulmonary arterial hypertension (Galiè et al., 2005). Another extraordinary rescue is represented by the case of thalidomide. The compound was marketed in the 1950s as a sedative and anti-nausea drug intended for pregnant women (Ashburn and Thor, 2004). It was distributed in 46 countries before being withdrawn due to its devastating teratogenic effects (i.e., phocomelia) and severe multiple malformations. By chance, the drug was accidently present in the medicine cabinet of the University Hospital of Marseille, where, in 1964, a doctor administered it to a patient suffering from erythema nodosum leprosum (ENL), who had not slept for weeks due to severe pain. The remedy had an effect far beyond the hoped-for relief, and it could cure his condition (Ashburn and Thor, 2004). Subsequent studies involving 4,552 patients with the same condition showed a complete remission within 2 weeks in 99% of cases. Remarkably, the drug is still the sole treatment for ENL (Upputuri et al., 2020). Later, it was discovered that thalidomide acts as a tumor necrosis factor α (TNF-α) inhibitor with antiangiogenic effects, which justified its off-label use in multiple myeloma (Ashburn and Thor, 2004). Subsequently, the derivative lenalidomide was synthesized, which is now a first-line drug for the treatment of this neoplastic disease. In 2023, lenalidomide accounted for a $18 billion market (Global Market Insights, 2024b; https://www.gminsights.com/industry-analysis/lenalidomide-market, accessed on 20 June 2024).
3 Advantages over traditional drug discovery
Traditional de novo drug discovery involves high costs, long timelines, and high attrition rates. Nearly nine out of 10 drug candidates fail during clinical trials (Van Norman, 2016). In particular, out of 100 drugs that enter phase I, 60% advance to phase II, 21% reach phase III, 13% are submitted to the regulatory authorities, and only 11% obtain approval (Van Norman, 2016). The failure rate can be even higher in fields like neurodegenerative diseases, where the development of new drugs has a nearly 100% failure rate (Cummings et al., 2014). Drug repositioning based on compounds that have successfully completed phase I clinical trials imply lower failure rates, at least in terms of safety, during subsequent clinical efficacy studies targeting new indications. In particular, this involves de-risked compounds (Pushpakom et al., 2019), where the approval rate rises to 30% (Hernandez et al., 2017), depending on the stage of lead optimization, drug development, and resolution of pharmacokinetic and formulation issues. Indeed, the most significant cost savings occur during preclinical and clinical phases I and II (Pushpakom et al., 2019). This strategy is also beneficial from a regulatory point of view. A huge amount of data needs to be provided when compiling registration dossiers, whose collection is faster when information from the literature and previous studies on the concerned compound is already available from the outset. Whoever submits the marketing authorization (MA) application to regulatory agencies can be authorized to perform only those studies necessary by the indication switching (Hernandez et al., 2017). Furthermore, if the new indication meets the requirements of novelty, inventive originality, reproducibility, and applicability, it becomes possible to protect the intellectual property of the discovery. For instance, the European Medical Agency (EMA) can grant up to 10 years of exclusivity to pediatric indications and formulations of already authorized products (Article 30 of Regulation EC 1901/2006), as well as for repositioned drugs, if they are orphan (Toumi and Rémuzat, 2017). All these factors make drug repurposing a viable route to extend the commercial life of drugs. Moreover, drug repurposing is also a strategic choice for highly fatal diseases, such as cancer, or rare and orphan diseases, for which it may occasionally be the sole viable approach to obtain therapeutic options quickly and at reduced costs (Pushpakom et al., 2019). Indeed, a repositioning campaign, from the identification of a novel potential drug application to its introduction on the market, requires on average 6.5 years and an investment of about $300 million (Nosengo, 2016). This stands in contrast to the 13–15 years and $two to three billion investment required for a research program aimed at the discovery of a new chemical entity (Nosengo, 2016). Finally, it is important to note that repositioning may involve not only drugs already on the market, but also the identification of new therapeutic indications for molecules of pharmaceutical interest. This encompasses both natural products and molecules of synthetic origin, as discussed below.
4 Disadvantages of drug repurposing
While drug repurposing is an advantageous approach, the process still faces some challenges. Firstly, using known drugs implies the need to evaluate related intellectual properties, which may potentially hamper the ability to patent repurposed drugs. Other major challenges concern prices and sales (Aboy et al., 2022). The lack of patentability reduces profit opportunities, discouraging pharmaceutical companies from pursuing this strategy (Van Der Pol et al., 2023). Initiatives aimed at facilitating drug repurposing through streamlining the marketing authorization process and allocating dedicated funds have been proposed (Van Der Pol et al., 2023). However, re-patenting a known drug is possible only if its therapeutic activity identified by repurposing is unknown. Yet, many of the potential uses found by pharmaceutical repositioning may already be documented in the literature and in clinical practice. Secondly, access to inherent pharmacovigilance and clinical trial data in therapeutic repositioning has long been limited by commercial and confidentiality issues. To overcome this limitation, the EMA made all clinical trial data public since October 2016 (Ishida et al., 2016). Moreover, if a higher dosage of the repositioned drug is required, or if a different route of administration is used than the original, it may be necessary to undergo phase I clinical trials, thereby increasing costs (Pritchard et al., 2017). Remarkably, seven government-run programs in the United States, European Union and United Kingdom have recently been established to help organizations to reduce costs, or to mitigate one or more of these drug repurposing challenges (Liddicoat et al., 2024).
5 Repurposing products of natural origin
Natural products, including compounds from plants and marine origin (Azab et al., 2016; Cornara et al., 2017; Rastelli et al., 2020; Atanasov et al., 2021), have been widely used for millennia (Bernardini et al., 2018). A well-known example is curcumin, which is a polyphenol compound extracted from the rhizome of Curcuma Longa, used widely in traditional medicine (Seca and Pinto, 2018). Initially used as a food spice, curcumin has recently gained attention for its anti-inflammatory, antioxidant, antitumor and neuroprotective activities (Wilken et al., 2011; Nelson et al., 2017). Traditional medicine, still representing a source of healthcare for many people (Yuan et al., 2016), has already contributed to the search for new therapeutic entities (Bernardini et al., 2018; Chaachouay and Zidane, 2024). Natural products are characterized by unique properties, thanks to their significant structural diversity (Mazumder et al., 2018; Atanasov et al., 2021). According to the Food and Drug Administration (FDA), around 40% of recently approved drugs are derived from or inspired by natural products, with a peak of 64% for antihypertensive and 74% for anticancer drugs (Yuan et al., 2016; Seca and Pinto, 2018; Newman and Cragg, 2020). The main limitations associated with natural products are the risk of extinction of plant species (Atanasov et al., 2021), low yields, low bioavailability, and complexity of extraction and purification processes (Atanasov et al., 2021). While a semi-synthetic route may offer a potential solution, its implementation may be problematic due to the structural complexity of these compounds, such as the presence of chiral atoms and labile functional groups (Atanasov et al., 2021). Despite progress in this area, the full potential of natural compounds in drug discovery and repurposing has yet to be exploited. In particular, most of the compounds are still in the pre-clinical stages, and very few entered in clinical trials. A list with the main repurposed natural compounds is presented in Table 1. Among them, only cannabidiol (CBD) is used in therapy (Blessing et al., 2015), while all the other natural derivatives are still under preclinical or clinical evaluation.
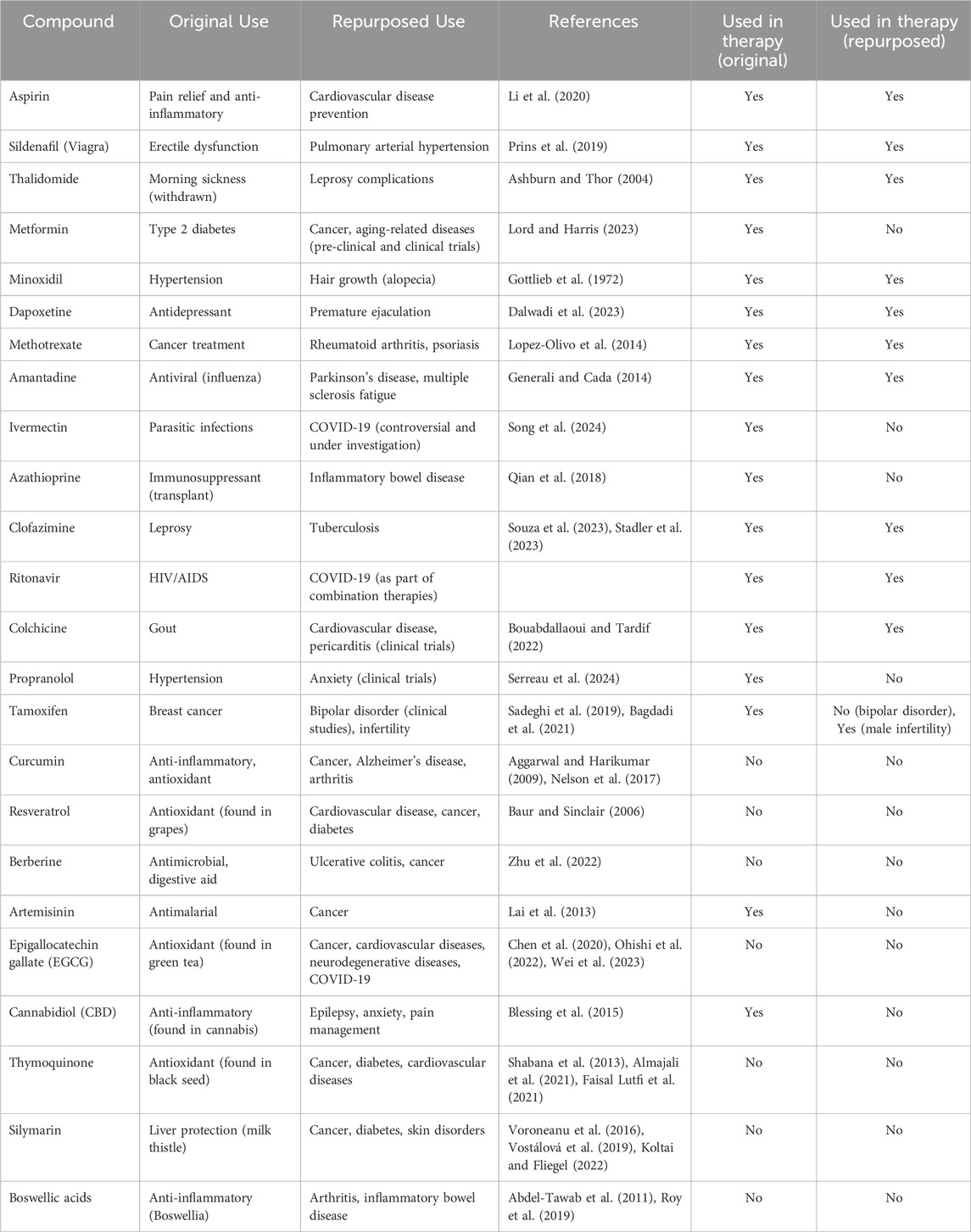
Table 1. A list of the main natural and synthetic repurposed compounds is presented. In the table, it is also clarified whether the drug is currently approved or is under clinical investigation.
6 Repurposing products of synthetic origin
In recent years, repurposing approaches have been extended to include the identification of novel therapeutic applications, not only for approved drugs but also for preclinical candidates and, more generally, products of synthetic origin (Blakemore et al., 2018). While these applications extend beyond the original definition of drug repurposing (Ashburn and Thor, 2004), they allow for a more through exploitation of compounds that are already available and have a known biological profile. In fact, significant efforts are usually needed to develop new synthetic pathways to obtain compounds with novel scaffolds or to introduce substituents on known scaffolds for structure-activity relationship (SAR) studies. However, these approaches often do not guarantee success (Sun et al., 2022). This is in line with the observation that, despite an increase in the number of synthesized molecules over the years, there has been little corresponding rise in FDA-approved drugs (Brown and Boström, 2016).
Repositioning already synthesized and tested compounds that were discarded for not fulfilling their original purpose can be a strategic approach, especially considering the challenges associated with their synthetic accessibility (Blakemore et al., 2018). Practical examples of this strategy can be found in the ReFRAME (Janes et al., 2018) and the LigAdvisor (Pinzi et al., 2021) webservers, which are specifically dedicated to drug repurposing. The CURE Drug Repurposing Collaboratory (CDRC) has developed a digital platform called CURE ID, which assists healthcare providers and researchers in finding new uses for existing approved drugs (Critical Path Institute, CURE Drug Repurposing Collaboratory, 2023; https://c-path.org/programs/cdrc/). By leveraging existing chemical and structural information, the repurposing of already synthesized compounds can significantly accelerate the de novo design of novel therapeutics. Moreover, structural analogues and reaction intermediates identified through these approaches can serve as valuable starting points for predicting new drug-target associations (Wołos et al., 2022). However, it is also important to note that any structural modification of known drugs results in new chemical entities, which must undergo comprehensive preclinical and clinical assessments for safety and efficacy before approval. Remarkably, the more information available on these candidates, the easier and faster their optimization will be toward the new therapeutic area.
A list with the main synthetic repurposed compounds is presented in Table 1.
7 Drug repurposing via computational approaches
Drug repurposing often occurred through serendipity or the observation of unexpected side effects (Ashburn and Thor, 2004). For this reason, predicting repositioning hypotheses in a rational and cost-effective manner would be highly valuable (Park, 2019). In this context, advances in medicinal chemistry, alongside those in “omics” sciences (i.e., metabolomics, proteomics, genomics, transcriptomics), present new opportunities in drug discovery. In particular, the amount of data generated through these new technologies is growing exponentially, resulting in the so-called “big data” era (March-Vila et al., 2017). Such data available to users has gained increasing attention as a source to extract information of interest for drug discovery, e.g., through data mining (Lavecchia, 2015). Examples of valuable data sources include the DrugBank, which contains information on about 12,000 approved and investigational drug-like compounds (Knox et al., 2024); the Protein Data Bank, with three-dimensional structures of over 220,000 biomolecules both alone and in complex with their ligands (Berman, 2000); and ChEMBL, one of the largest reference databases for biological activity annotations, covering more than 15 million activity data points for nearly two million individual compounds (Gaulton et al., 2017). The possibility of using computational approaches for mining and analyzing large amounts of data with extremely limited resources is becoming increasingly important (Li et al., 2016). Practical applications of computational workflows for drug repositioning have been reviewed (March-Vila et al., 2017; Pinzi et al., 2019). Importantly, the integration of different approaches has proven to be an excellent approach to overcoming the inherent weaknesses of individual methods (March-Vila et al., 2017). For example, ligand-based approaches are based on the underlying paradigm that similarity between compounds may be indicative of a similarity in biological activities (Bajorath, 2017). As such, the possibility of quantitatively evaluate the compounds’ similarity with respect to molecules with known biological activities is highly attractive. This approach enables the high-throughput screening of large public databases. However, it is important to consider that small structural differences can sometimes result in markedly different and unexpected activity profiles, commonly referred to as “activity cliffs” (Stumpfe and Bajorath, 2012). Additionally, these approaches do not allow for significant departures from the starting chemical space when used as stand-alone methods. Structure-based approaches use structural data for macromolecular targets, typically obtained experimentally or through computational approaches (Jumper et al., 2021). These approaches help evaluating whether ligands possess the required complementarity with the intended biological target (Ferreira et al., 2015). Molecular docking is widely used to simulate the interactions of a ligand with a macromolecular target, predicting its most likely orientation and bound conformation (Ferreira et al., 2015). Furthermore, the poses obtained through docking can undergo post-docking procedures that leverage free energy calculations to evaluate more accurately ligand binding scores (Rastelli et al., 2009). Machine learning (ML) approaches are a sub-field of artificial intelligence (AI) that rely on the identification of patterns and inferences to build statistical models capable of performing a wide range of tasks (Lavecchia, 2015). In the field of drug repurposing, ML approaches are particularly valuable as they efficiently leverage vast amounts of data while simultaneously providing far-from-obvious predictions (Vamathevan et al., 2019; Urbina et al., 2021). In general, machine learning methods include supervised methods, wherein ML models are built from a “labeled” dataset (i.e., the required outcome is already known), and unsupervised methods, where the data for model training lacks pre-determined labels (Vamathevan et al., 2019). In the context of drug repurposing, supervised approaches have been used, for example, in classification tasks for predicting drug-target and/or drug-disease associations (Nickel et al., 2014; Tinivella et al., 2021; Liu et al., 2022). Additionally, applications of unsupervised approaches have been recently employed for systematic drug repurposing, as reported by S.S. Madugula et al. (Madugula et al., 2021). In particular, in their study, the authors performed extensive Principal Component Analyses (PCA) followed by k-means clustering on molecular properties of about 1,600 approved drugs, identifying more than 1,400 drug repurposing candidates against more than 300 diseases. While the application of AI in the context of DR is still at its infancy, these examples clearly demonstrate the potential of this innovative approach to uncovering a significantly larger number of DR opportunities, especially when integrated with other in silico methods.
8 Conclusion
Drug repurposing plays a key role in reducing the financial burden and accelerating the market entry time of new drugs (Ashburn and Thor, 2004; Pushpakom et al., 2019). Another key feature regards the safety profile of repurposed drugs, because the toxicity profiles of approved or investigational drugs are well established. This helps to understand how the drugs behave in in vivo settings decreasing the risk of failure and enhancing the chances of success by up to 30%. These features represent a marked improvement over the traditional de novo drug discovery approach, where the success of a drug entering the market from scratch is often lower than 10% (Hay et al., 2014). Another way DR can effectively support drug discovery is by providing treatments for unmet targets or medical needs. Neglected, orphan or rare diseases are often overlooked by industries due to the limited commercial potential. Repurposing can bring new therapies to fill this medical gap, as shown by the employment of clofazimine as possible anti-trypanosome agent, pyronaridine against Chagas disease (Yamey, 2002; Hernandez et al., 2019) or ataluren in Shwachman-Diamond syndrome (Bezzerri et al., 2018). However, intellectual property issues may hamper the patentability of repurposed drugs. Furthermore, there are still cases where new phase I trials are required, particularly if higher dosages or different administration routes are necessary (Pritchard et al., 2017). Despite these limitations, DR can play a pivotal role in accelerating drug discovery and development. Chemical, biological, structural, and clinical data in public repositories can significantly facilitate drug discovery, without the need to start a discovery campaign from scratch. Big data mining and AI offer unprecedented opportunities in this respect. In many cases, repurposing can extend the commercial viability of drugs and offer new incomes, by opening new markets when a new indication is found (Mishra et al., 2024). At the same time, insights from de novo drug discovery help repurposing efforts by identifying novel applications for previously discarded compounds, creating a synergistic relationship that enhances the overall efficiency of the discovery and development process. This effect is strengthened by the advent of data mining and ML, enabling the analysis of extensive public databases, predicting new drug-target interactions, and uncovering valuable insights from structural data (Berman, 2000; Lavecchia, 2015; Li et al., 2016; Gaulton et al., 2017; Knox et al., 2024). In this way, natural and synthetic compounds investigated in preclinical and clinical settings may offer new and unexpected applications, providing solutions to unmet therapeutical needs (Bernardini et al., 2018; Janes et al., 2018). Although hampered by intellectual property limitations and potential additional trial requirements, drug repurposing can efficiently aid drug discovery by providing a faster and less risky route for developing new treatments. The synergistic interplay between repurposing and traditional drug discovery underscores the critical role drug repositioning can play in the modern pharmaceutical landscape.
Author contributions
LP: Conceptualization, Writing–original draft, Writing–review and editing. NB: Conceptualization, Writing–original draft, Writing–review and editing. GR: Conceptualization, Writing–original draft, Writing–review and editing, Funding acquisition, Project administration.
Funding
The author(s) declare that financial support was received for the research, authorship, and/or publication of this article. The research leading to these results has received funding from the European Union - NextGenerationEU through the Italian Ministry of University and Research under PNRR–M4C2-I1.3 Project PE_00000019 “HEAL ITALIA” to GR and NB The views and opinions expressed are those of the authors only and do not necessarily reflect those of the European Union or the European Commission. Neither the European Union nor the European Commission can be held responsible for them. LP would like to thank the Italian funding programme Fondo Sociale Europeo REACT-EU—PON “Ricerca e Innovazione” 2014–2020—Azione IV.4 “Dottorati e contratti di ricerca su tematiche dell’innovazione” for supporting his research.
Conflict of interest
The authors declare that the research was conducted in the absence of any commercial or financial relationships that could be construed as a potential conflict of interest.
The author(s) declared that they were an editorial board member of Frontiers, at the time of submission. This had no impact on the peer review process and the final decision.
Publisher’s note
All claims expressed in this article are solely those of the authors and do not necessarily represent those of their affiliated organizations, or those of the publisher, the editors and the reviewers. Any product that may be evaluated in this article, or claim that may be made by its manufacturer, is not guaranteed or endorsed by the publisher.
References
Abdel-Tawab, M., Werz, O., and Schubert-Zsilavecz, M. (2011). Boswellia serrata: an overall assessment of in vitro, preclinical, pharmacokinetic and clinical data. Clin. Pharmacokinet. 50, 349–369. doi:10.2165/11586800-000000000-00000
Aboy, M., Liddell, K., Jordan, M., Crespo, C., and Liddicoat, J. (2022). European patent protection for medical uses of known products and drug repurposing. Nat. Biotechnol. 40, 465–471. doi:10.1038/s41587-022-01269-3
Aggarwal, B. B., and Harikumar, K. B. (2009). Potential therapeutic effects of curcumin, the anti-inflammatory agent, against neurodegenerative, cardiovascular, pulmonary, metabolic, autoimmune and neoplastic diseases. Int. J. Biochem. Cell Biol. 41, 40–59. doi:10.1016/j.biocel.2008.06.010
Almajali, B., Al-Jamal, H. A. N., Taib, W. R. W., Ismail, I., Johan, M. F., Doolaanea, A. A., et al. (2021). Thymoquinone, as a novel therapeutic candidate of cancers. Pharmaceuticals 14, 369. doi:10.3390/ph14040369
Ashburn, T. T., and Thor, K. B. (2004). Drug repositioning: identifying and developing new uses for existing drugs. Nat. Rev. Drug Discov. 3, 673–683. doi:10.1038/nrd1468
Ashique, S., Sandhu, N. K., Haque, S. N., and Koley, K. (2020). A systemic review on topical marketed formulations, natural products, and oral supplements to prevent androgenic alopecia: a review. Nat. Prod. Bioprospect. 10, 345–365. doi:10.1007/s13659-020-00267-9
Atanasov, A. G., Zotchev, S. B., Dirsch, V. M., and Supuran, C. T. (2021). Natural products in drug discovery: advances and opportunities. Nat. Rev. Drug Discov. 20, 200–216. doi:10.1038/s41573-020-00114-z
Azab, A., Nassar, A., and Azab, A. (2016). Anti-inflammatory activity of natural products. Molecules 21, 1321. doi:10.3390/molecules21101321
Bagdadi, N., Azab, A. N., and Shvartsur, R. (2021). The use of tamoxifen as a potential treatment for bipolar disorder. Psychiatry Clin. Psychopharmacol. 31, 344–352. doi:10.5152/pcp.2021.21817
Bajorath, J. (2017). Molecular similarity concepts for informatics applications. Methods Mol. Biol. 1526, 231–245. doi:10.1007/978-1-4939-6613-4_13
Baur, J. A., and Sinclair, D. A. (2006). Therapeutic potential of resveratrol: the in vivo evidence. Nat. Rev. Drug Discov. 5, 493–506. doi:10.1038/nrd2060
Berman, H. M., Westbrook, J., Feng, Z., Gilliland, G., Bhat, T. N., Weissig, H., et al. (2000). The protein Data Bank. Nucleic Acids Res. 28, 235–242. doi:10.1093/nar/28.1.235
Bernardini, S., Tiezzi, A., Laghezza Masci, V., and Ovidi, E. (2018). Natural products for human health: an historical overview of the drug discovery approaches. Nat. Prod. Res. 32, 1926–1950. doi:10.1080/14786419.2017.1356838
Bezzerri, V., Bardelli, D., Morini, J., Vella, A., Cesaro, S., Sorio, C., et al. (2018). Ataluren-driven restoration of Shwachman-Bodian-Diamond syndrome protein function in Shwachman-Diamond syndrome bone marrow cells. Am. J. Hematol. 93, 527–536. doi:10.1002/ajh.25025
Blakemore, D. C., Castro, L., Churcher, I., Rees, D. C., Thomas, A. W., Wilson, D. M., et al. (2018). Organic synthesis provides opportunities to transform drug discovery. Nat. Chem. 10, 383–394. doi:10.1038/s41557-018-0021-z
Blessing, E. M., Steenkamp, M. M., Manzanares, J., and Marmar, C. R. (2015). Cannabidiol as a potential treatment for anxiety disorders. Neurotherapeutics 12, 825–836. doi:10.1007/s13311-015-0387-1
Bouabdallaoui, N., and Tardif, J.-C. (2022). Repurposing colchicine for heart disease. Annu. Rev. Pharmacol. Toxicol. 62, 121–129. doi:10.1146/annurev-pharmtox-052120-020445
Brown, D. G., and Boström, J. (2016). Analysis of past and present synthetic methodologies on medicinal chemistry: where have all the new reactions gone? miniperspective. J. Med. Chem. 59, 4443–4458. doi:10.1021/acs.jmedchem.5b01409
Chaachouay, N., and Zidane, L. (2024). Plant-derived natural products: a source for drug discovery and development. DDC 3, 184–207. doi:10.3390/ddc3010011
Chen, B.-H., Hsieh, C.-H., Tsai, S.-Y., Wang, C.-Y., and Wang, C.-C. (2020). Anticancer effects of epigallocatechin-3-gallate nanoemulsion on lung cancer cells through the activation of AMP-activated protein kinase signaling pathway. Sci. Rep. 10, 5163. doi:10.1038/s41598-020-62136-2
Coherent Market Insights (2024). Available at: https://www.coherentmarketinsights.com/industry-reports/sildenafil-drug-market (accessed on June 20, 2024).
Cornara, L., Biagi, M., Xiao, J., and Burlando, B. (2017). Therapeutic properties of bioactive compounds from different honeybee products. Front. Pharmacol. 8, 412. doi:10.3389/fphar.2017.00412
Cummings, J. L., Morstorf, T., and Zhong, K. (2014). Alzheimer’s disease drug-development pipeline: few candidates, frequent failures. Alzheimers Res. Ther. 6, 37. doi:10.1186/alzrt269
Dalwadi, S. M., Hunt, A., Bonnen, M. D., and Ghebre, Y. T. (2023). Computational approaches for drug repurposing in oncology: untapped opportunity for high value innovation. Front. Oncol. 13, 1198284. doi:10.3389/fonc.2023.1198284
Faisal Lutfi, M., Abdel-Moneim, A.-M. H., Alsharidah, A. S., Mobark, M. A., Abdellatif, A. A. H., Saleem, I. Y., et al. (2021). Thymoquinone lowers blood glucose and reduces oxidative stress in a rat model of diabetes. Molecules 26, 2348. doi:10.3390/molecules26082348
Ferreira, L., Dos Santos, R., Oliva, G., and Andricopulo, A. (2015). Molecular docking and structure-based drug design strategies. Molecules 20, 13384–13421. doi:10.3390/molecules200713384
Galiè, N., Ghofrani, H. A., Torbicki, A., Barst, R. J., Rubin, L. J., Badesch, D., et al. (2005). Sildenafil citrate therapy for pulmonary arterial hypertension. N. Engl. J. Med. 353, 2148–2157. doi:10.1056/NEJMoa050010
Gaulton, A., Hersey, A., Nowotka, M., Bento, A. P., Chambers, J., Mendez, D., et al. (2017). The ChEMBL database in 2017. Nucleic Acids Res. 45, D945-D954–D954. doi:10.1093/nar/gkw1074
Generali, J. A., and Cada, D. J. (2014). Amantadine: multiple sclerosis–related fatigue. Hosp. Pharm. 49, 710–712. doi:10.1310/hpj4908-710
Ghofrani, H. A., Osterloh, I. H., and Grimminger, F. (2006). Sildenafil: from angina to erectile dysfunction to pulmonary hypertension and beyond. Nat. Rev. Drug Discov. 5, 689–702. doi:10.1038/nrd2030
Global Market Insights (2024a). Available at: https://www.gminsights.com/industry-analysis/minoxidil-market (accessed on June 20, 2024).
Global Market Insights (2024b). Available at: https://www.gminsights.com/industry-analysis/lenalidomide-market (accessed on June 20, 2024).
Gottlieb, T. B., Katz, F. H., and Chidsey, C. A. (1972). Combined therapy with vasodilator drugs and beta-adrenergic blockade in hypertension: a comparative study of minoxidil and hydralazine. Circulation 45, 571–582. doi:10.1161/01.CIR.45.3.571
Hay, M., Thomas, D. W., Craighead, J. L., Economides, C., and Rosenthal, J. (2014). Clinical development success rates for investigational drugs. Nat. Biotechnol. 32, 40–51. doi:10.1038/nbt.2786
Hernandez, H. W., Soeung, M., Zorn, K. M., Ashoura, N., Mottin, M., Andrade, C. H., et al. (2019). High throughput and computational repurposing for neglected diseases. Pharm. Res. 36, 27. doi:10.1007/s11095-018-2558-3
Hernandez, J. J., Pryszlak, M., Smith, L., Yanchus, C., Kurji, N., Shahani, V. M., et al. (2017). Giving drugs a second chance: overcoming regulatory and financial hurdles in repurposing approved drugs as cancer therapeutics. Front. Oncol. 7, 273. doi:10.3389/fonc.2017.00273
Ishida, J., Konishi, M., Ebner, N., and Springer, J. (2016). Repurposing of approved cardiovascular drugs. J. Transl. Med. 14, 269. doi:10.1186/s12967-016-1031-5
Janes, J., Young, M. E., Chen, E., Rogers, N. H., Burgstaller-Muehlbacher, S., Hughes, L. D., et al. (2018). The ReFRAME library as a comprehensive drug repurposing library and its application to the treatment of cryptosporidiosis. Proc. Natl. Acad. Sci. U.S.A. 115, 10750–10755. doi:10.1073/pnas.1810137115
Jumper, J., Evans, R., Pritzel, A., Green, T., Figurnov, M., Ronneberger, O., et al. (2021). Highly accurate protein structure prediction with AlphaFold. Nature 596, 583–589. doi:10.1038/s41586-021-03819-2
Knox, C., Wilson, M., Klinger, C. M., Franklin, M., Oler, E., Wilson, A., et al. (2024). DrugBank 6.0: the DrugBank knowledgebase for 2024. Nucleic Acids Res. 52, D1265–D1275. doi:10.1093/nar/gkad976
Koltai, T., and Fliegel, L. (2022). Role of silymarin in cancer treatment: facts, hypotheses, and questions. J. Evid. Based Complement. Altern. Med. 27, 2515690X211068826. doi:10.1177/2515690X211068826
Lai, H. C., Singh, N. P., and Sasaki, T. (2013). Development of artemisinin compounds for cancer treatment. Invest. New Drugs 31, 230–246. doi:10.1007/s10637-012-9873-z
Langedijk, J., Mantel-Teeuwisse, A. K., Slijkerman, D. S., and Schutjens, M.-H. D. B. (2015). Drug repositioning and repurposing: terminology and definitions in literature. Drug Discov. Today 20, 1027–1034. doi:10.1016/j.drudis.2015.05.001
Lavecchia, A. (2015). Machine-learning approaches in drug discovery: methods and applications. Drug Discov. Today 20, 318–331. doi:10.1016/j.drudis.2014.10.012
Li, J., Zheng, S., Chen, B., Butte, A. J., Swamidass, S. J., and Lu, Z. (2016). A survey of current trends in computational drug repositioning. Brief. Bioinform. 17, 2–12. doi:10.1093/bib/bbv020
Li, X., Rousseau, J. F., Ding, Y., Song, M., and Lu, W. (2020). Understanding drug repurposing from the perspective of biomedical entities and their evolution: bibliographic research using aspirin. JMIR Med. Inf. 8, e16739. doi:10.2196/16739
Liddicoat, J., Hamidzadeh, A., Liddell, K., Schito, M., Simon, D., Aboy, M., et al. (2024). New government drug repurposing programs: opportunities and uncertainties. Sci. Transl. Med. 16, eadl0998. doi:10.1126/scitranslmed.adl0998
Liu, J., Peng, D., Li, J., Dai, Z., Zou, X., and Li, Z. (2022). Identification of potential Parkinson’s disease drugs based on multi-source data fusion and convolutional neural network. Molecules 27, 4780. doi:10.3390/molecules27154780
Lopez-Olivo, M. A., Siddhanamatha, H. R., Shea, B., Tugwell, P., Wells, G. A., and Suarez-Almazor, M. E. (2014). Methotrexate for treating rheumatoid arthritis. Cochrane Database Syst. Rev. 2014, CD000957. doi:10.1002/14651858.CD000957.pub2
Lord, S. R., and Harris, A. L. (2023). Is it still worth pursuing the repurposing of metformin as a cancer therapeutic? Br. J. Cancer 128, 958–966. doi:10.1038/s41416-023-02204-2
Madugula, S. S., John, L., Nagamani, S., Gaur, A. S., Poroikov, V. V., and Sastry, G. N. (2021). Molecular descriptor analysis of approved drugs using unsupervised learning for drug repurposing. Comput. Biol. Med. 138, 104856. doi:10.1016/j.compbiomed.2021.104856
March-Vila, E., Pinzi, L., Sturm, N., Tinivella, A., Engkvist, O., Chen, H., et al. (2017). On the integration of in silico drug design methods for drug repurposing. Front. Pharmacol. 8, 298. doi:10.3389/fphar.2017.00298
Mazumder, A., Cerella, C., and Diederich, M. (2018). Natural scaffolds in anticancer therapy and precision medicine. Biotechnol. Adv. 36, 1563–1585. doi:10.1016/j.biotechadv.2018.04.009
Mishra, A. S., Vasanthan, M., and Malliappan, S. P. (2024). Drug repurposing: a leading strategy for new threats and targets. ACS Pharmacol. Transl. Sci. 7, 915–932. doi:10.1021/acsptsci.3c00361
Nelson, K. M., Dahlin, J. L., Bisson, J., Graham, J., Pauli, G. F., and Walters, M. A. (2017). The essential medicinal chemistry of curcumin: miniperspective. J. Med. Chem. 60, 1620–1637. doi:10.1021/acs.jmedchem.6b00975
Newman, D. J., and Cragg, G. M. (2020). Natural products as sources of new drugs over the nearly four decades from 01/1981 to 09/2019. J. Nat. Prod. 83, 770–803. doi:10.1021/acs.jnatprod.9b01285
Nickel, J., Gohlke, B.-O., Erehman, J., Banerjee, P., Rong, W. W., Goede, A., et al. (2014). SuperPred: update on drug classification and target prediction. Nucleic Acids Res. 42, W26–W31. doi:10.1093/nar/gku477
Ohishi, T., Hishiki, T., Baig, M. S., Rajpoot, S., Saqib, U., Takasaki, T., et al. (2022). Epigallocatechin gallate (EGCG) attenuates severe acute respiratory coronavirus disease 2 (SARS-CoV-2) infection by blocking the interaction of SARS-CoV-2 spike protein receptor-binding domain to human angiotensin-converting enzyme 2. PLoS ONE 17, e0271112. doi:10.1371/journal.pone.0271112
Park, K. (2019). A review of computational drug repurposing. Transl. Clin. Pharmacol. 27, 59–63. doi:10.12793/tcp.2019.27.2.59
Pinzi, L., Lherbet, C., Baltas, M., Pellati, F., and Rastelli, G. (2019). In silico repositioning of cannabigerol as a novel inhibitor of the enoyl acyl carrier protein (ACP) reductase (InhA). Molecules 24, E2567. doi:10.3390/molecules24142567
Pinzi, L., Tinivella, A., Gagliardelli, L., Beneventano, D., and Rastelli, G. (2021). LigAdvisor: a versatile and user-friendly web-platform for drug design. Nucleic Acids Res. 49, W326–W335. doi:10.1093/nar/gkab385
Prins, K. W., Thenappan, T., Weir, E. K., Kalra, R., Pritzker, M., and Archer, S. L. (2019). Repurposing medications for treatment of pulmonary arterial hypertension: what’s old is new again. JAHA 8, e011343. doi:10.1161/JAHA.118.011343
Pritchard, J.-L. E., O’Mara, T. A., and Glubb, D. M. (2017). Enhancing the promise of drug repositioning through genetics. Front. Pharmacol. 8, 896. doi:10.3389/fphar.2017.00896
Pushpakom, S., Iorio, F., Eyers, P. A., Escott, K. J., Hopper, S., Wells, A., et al. (2019). Drug repurposing: progress, challenges and recommendations. Nat. Rev. Drug Discov. 18, 41–58. doi:10.1038/nrd.2018.168
Qian, X., Wang, T., Shen, J., and Ran, Z. (2018). Low dose of azathioprine is effective to induce and maintain remission in active Crohn disease: a prospective observational study. Medicine 97, e11814. doi:10.1097/MD.0000000000011814
Rastelli, G., Degliesposti, G., Del Rio, A., and Sgobba, M. (2009). Binding estimation after refinement, a new automated procedure for the refinement and rescoring of docked ligands in virtual screening. Chem. Biol. Drug. Des. 73, 283–286. doi:10.1111/j.1747-0285.2009.00780.x
Rastelli, G., Pellati, F., Pinzi, L., and Gamberini, M. C. (2020). Repositioning natural products in drug discovery. Molecules 25, 1154. doi:10.3390/molecules25051154
Roy, N. K., Parama, D., Banik, K., Bordoloi, D., Devi, A. K., Thakur, K. K., et al. (2019). An update on pharmacological potential of boswellic acids against chronic diseases. Int. J. Mol. Sci. 20, 4101. doi:10.3390/ijms20174101
Sadeghi, S., Reza Talebi, A., Shahedi, A., Reza Moein, M., and Abbasi-sarcheshmeh, A. (2019). Effects of different doses of tamoxifen on the sperm parameters and chromatin quality in mice: an experimental model. IJRM 17, 279–286. doi:10.18502/ijrm.v17i4.4553
Seca, A., and Pinto, D. (2018). Plant secondary metabolites as anticancer agents: successes in clinical trials and therapeutic application. Int. J. Mol. Sci. 19, 263. doi:10.3390/ijms19010263
Serreau, R., Amirouche, A., Benyamina, A., and Berteina-Raboin, S. (2024). Propranolol hydrochloride psychiatric effectiveness and oxidative stress: an update. Oxygen 4, 139–149. doi:10.3390/oxygen4020009
Shabana, A., El-Menyar, A., Asim, M., Al-Azzeh, H., and Al Thani, H. (2013). Cardiovascular benefits of black cumin (nigella sativa). Cardiovasc. Toxicol. 13, 9–21. doi:10.1007/s12012-012-9181-z
Song, Z., Shi, S., and Zhang, Y. (2024). Ivermectin for treatment of COVID-19: a systematic review and meta-analysis. Heliyon 10, e27647. doi:10.1016/j.heliyon.2024.e27647
Souza, K. M., Carrasco, G., Rojas-Cortés, R., Michel Barbosa, M., Bambirra, E. H. F., Castro, J. L., et al. (2023). Effectiveness of nirmatrelvir-ritonavir for the treatment of patients with mild to moderate COVID-19 and at high risk of hospitalization: systematic review and meta-analyses of observational studies. PLoS ONE 18, e0284006. doi:10.1371/journal.pone.0284006
Stadler, J. A. M., Maartens, G., Meintjes, G., and Wasserman, S. (2023). Clofazimine for the treatment of tuberculosis. Front. Pharmacol. 14, 1100488. doi:10.3389/fphar.2023.1100488
Stumpfe, D., and Bajorath, J. (2012). Exploring activity cliffs in medicinal chemistry: miniperspective. J. Med. Chem. 55, 2932–2942. doi:10.1021/jm201706b
Sun, D., Gao, W., Hu, H., and Zhou, S. (2022). Why 90% of clinical drug development fails and how to improve it? Acta Pharm. Sin. B 12, 3049–3062. doi:10.1016/j.apsb.2022.02.002
Tinivella, A., Pinzi, L., and Rastelli, G. (2021). Prediction of activity and selectivity profiles of human Carbonic Anhydrase inhibitors using machine learning classification models. J. Cheminform. 13, 18. doi:10.1186/s13321-021-00499-y
Toumi, M., and Rémuzat, C. (2017). Value added medicines: what value repurposed medicines might bring to society? J. Mark. Access Health Policy 5, 1264717. doi:10.1080/20016689.2017.1264717
Upputuri, B., Pallapati, M. S., Tarwater, P., and Srikantam, A. (2020). Thalidomide in the treatment of erythema nodosum leprosum (ENL) in an outpatient setting: a five-year retrospective analysis from a leprosy referral centre in India. PLoS Negl. Trop. Dis. 14, e0008678. doi:10.1371/journal.pntd.0008678
Urbina, F., Puhl, A. C., and Ekins, S. (2021). Recent advances in drug repurposing using machine learning. Curr. Opin. Chem. Biol. 65, 74–84. doi:10.1016/j.cbpa.2021.06.001
Vamathevan, J., Clark, D., Czodrowski, P., Dunham, I., Ferran, E., Lee, G., et al. (2019). Applications of machine learning in drug discovery and development. Nat. Rev. Drug Discov. 18, 463–477. doi:10.1038/s41573-019-0024-5
Van Der Pol, K. H., Aljofan, M., Blin, O., Cornel, J. H., Rongen, G. A., Woestelandt, A.-G., et al. (2023). Drug repurposing of generic drugs: challenges and the potential role for government. Appl. Health Econ. Health Policy 21, 831–840. doi:10.1007/s40258-023-00816-6
Van Norman, G. A. (2016). Drugs, devices, and the FDA: Part 1: an overview of approval processes for drugs. J. Am. Coll. Cardiol. Basic Trans. Sci. 1, 170–179. doi:10.1016/j.jacbts.2016.03.002
Voroneanu, L., Nistor, I., Dumea, R., Apetrii, M., and Covic, A. (2016). Silymarin in type 2 diabetes mellitus: a systematic review and meta-analysis of randomized controlled trials. J. Diabetes Res. 2016, 5147468. doi:10.1155/2016/5147468
Vostálová, J., Tinková, E., Biedermann, D., Kosina, P., Ulrichová, J., and Rajnochová Svobodová, A. (2019). Skin protective activity of silymarin and its flavonolignans. Molecules 24, 1022. doi:10.3390/molecules24061022
Wei, X.-Y., Zeng, Y.-F., Guo, Q.-H., Liu, J.-J., Yin, N., Liu, Y., et al. (2023). Cardioprotective effect of epigallocatechin gallate in myocardial ischemia/reperfusion injury and myocardial infarction: a meta-analysis in preclinical animal studies. Sci. Rep. 13, 14050. doi:10.1038/s41598-023-41275-2
Wilken, R., Veena, M. S., Wang, M. B., and Srivatsan, E. S. (2011). Curcumin: a review of anti-cancer properties and therapeutic activity in head and neck squamous cell carcinoma. Mol. Cancer. 10, 12. doi:10.1186/1476-4598-10-12
Wołos, A., Koszelewski, D., Roszak, R., Szymkuć, S., Moskal, M., Ostaszewski, R., et al. (2022). Computer-designed repurposing of chemical wastes into drugs. Nature 604, 668–676. doi:10.1038/s41586-022-04503-9
Yamey, G., and Torreele, E. (2002). The world’s most neglected diseases. BMJ 325, 176–177. doi:10.1136/bmj.325.7357.176
Yuan, H., Ma, Q., Ye, L., and Piao, G. (2016). The traditional medicine and modern medicine from natural products. Molecules 21, 559. doi:10.3390/molecules21050559
Keywords: drug repurposing, drug discovery, natural products, organic compounds, in silico approaches
Citation: Pinzi L, Bisi N and Rastelli G (2024) How drug repurposing can advance drug discovery: challenges and opportunities. Front. Drug Discov. 4:1460100. doi: 10.3389/fddsv.2024.1460100
Received: 05 July 2024; Accepted: 30 July 2024;
Published: 20 August 2024.
Edited by:
Simone Brogi, University of Pisa, ItalyReviewed by:
Amiram Goldblum, Hebrew University of Jerusalem, IsraelCopyright © 2024 Pinzi, Bisi and Rastelli. This is an open-access article distributed under the terms of the Creative Commons Attribution License (CC BY). The use, distribution or reproduction in other forums is permitted, provided the original author(s) and the copyright owner(s) are credited and that the original publication in this journal is cited, in accordance with accepted academic practice. No use, distribution or reproduction is permitted which does not comply with these terms.
*Correspondence: Giulio Rastelli, Z2l1bGlvLnJhc3RlbGxpQHVuaW1vcmUuaXQ=