- School of Biochemistry and Immunology, Trinity Biomedical Sciences Institute, Trinity College Dublin, Dublin, Ireland
Mitochondrial metabolites and their derivatives have been the focus of recent efforts to develop new anti-inflammatory therapeutics. The widely used therapeutic agents dimethyl fumarate (DMF) and metformin have anti-inflammatory properties and have been shown to target metabolism. The mitochondrial metabolites succinate, itaconate, and fumarate have multiple immunomodulatory effects and present interesting therapeutic possibilities for immune and inflammatory diseases. Mitochondrial DNA and double-stranded RNA have also been shown to be highly inflammatory, acting via specific pattern recognition receptors (PRRs) such as cGAS and TLR9 for mitochondrial DNA, RIG-I, MDA5 for mitochondrial double stranded RNA, and TLR7 for mitochondrial single stranded RNA. These recent discoveries are changing our view of mitochondria suggesting that they are at the heart of multiple inflammatory diseases and provide opportunities for the development of new anti-inflammatory therapeutics.
Introduction
Inflammation is essential for defence against pathogens and the immune response to tissue injury. During inflammation, immune cells are activated to a proinflammatory state, and cytokines such as interleukin (IL)-1, IL6, and tumour necrosis factor alpha (TNF-α) are produced. They bind to specific receptors, and various cell signalling pathways are activated, which stimulate the expression of specific immune and inflammatory genes. However, excessive inflammation can be harmful and cause further damage. Healthy tissue and cells can be injured by overactivated immune cells, which then leads to autoinflammatory and autoimmune diseases (Broderick and Hoffman, 2022; Pisetsky, 2023). To alleviate the adverse response of excessive inflammation, many different anti-inflammatory drugs can be deployed. However, the inadequate responses highlight the need for better anti-inflammatory therapeutics.
When immune cells enter an inflammatory state, metabolic pathways are modulated and have a crucial impact on cell function (ONeill et al., 2016). Activated immune cells require rapid energy and building blocks for cytokine production and cell proliferation. During inflammation, the rate of aerobic glycolysis is upregulated in inflammatory cells. In Krebs cycle and oxidative phosphorylation are rewired in both lymphoid and myeloid cells leading to the accumulation of specific metabolites that are capable of modulating inflammatory responses (Xu et al., 2022). Also, reactive oxygen species (ROS) are produced and can have multiple inflammatory effects on cells (Lampropoulou et al., 2016; Beach et al., 2020).
In recent years, many discoveries have been made on the immunoregulatory role of mitochondria (Nakahira et al., 2010; Hooftman and ONeill, 2019; Monfort-Ferré et al., 2022; Hooftman et al., 2023). Two anti-inflammatory therapeutics already in use, dimethyl fumarate (DMF) and metformin, in fact, target mitochondria. In this review, we make the case for targeting specific mitochondrial metabolites or mitochondrially-derived nucleic acids as a compelling approach for the new anti-inflammatory therapeutics.
Existing anti-inflammatory drugs either derived from or that target mitochondria
DMF
DMF is a derivative of the Krebs Cycle metabolite fumarate. It was first developed as a drug for psoriasis (Schweckendiek, 1959). It was hypothesised that in psoriasis there is a disturbance in Krebs Cycle that triggers the symptoms so supplementation with DMF or other fumaric acid esters might restore the disrupted metabolism and ameliorate the excessive inflammation. Although this hypothesis was not confirmed, clinical trials on psoriasis patients showed a positive effect (Schweckendiek, 1966). DMF was then redeveloped as a drug against relapsing-remitting multiple sclerosis (RRMS) (Fox et al., 2012; Meissner et al., 2012). However, the effect of DMF treatment on immune cells was still not entirely defined. Scientists then started to investigate the immunomodulatory impact of DMF. Multiple studies have demonstrated that DMF can target both adaptive and innate immune cells. DMF treatment reduces the number of B cells particularly memory B cells in RRMS patients (Smith et al., 2017). Proinflammatory cytokine production by B cells is also decreased. Another study found that the circulating T cell population declined after treatment with DMF, and the number of Th2 cells increased while the more inflammatory Th1 and Th17 cell populations decreased (Wu et al., 2017). DMF induces macrophages to polarize towards a more immunoregulator M2 type and inhibits dendritic cell activation (Peng et al., 2012; Han et al., 2016).
Because of its electrophilicity, DMF can modify cysteine residues on target proteins in a process termed succination which influences protein function. DMF was shown to inhibit the glycolytic enzyme glyceraldehyde 3-phosphate dehydrogenase (GAPDH) in the experimental autoimmune encephalomyelitis (EAE) model of mouse and also in MS patients as part of its anti-inflammatory function (Kornberg et al., 2018). By reducing GAPDH activity, DMF downregulated aerobic glycolysis in activated immunes and ameliorated inflammation. Other than targeting metabolism, several immunoregulatory proteins, such as NRF2 and NLRP3, have been identified as major targets of DMF and fumarate (He et al., 2016; Schulze-Topphoff et al., 2016). However, DMF has a higher reactivity to cysteine than fumarate and therefore induces different responses (Kulkarni et al., 2019). DMF dampens NLRP3 activation by stimulating the NRF2 pathway (Shi et al., 2022). After NRF2 reduces ROS production and proinflammatory signals, the NLRP3 activity is consequently downregulated. DMF has been also shown to inhibit the formation of M1/K63 hybrid polyubiquitin chains by cysteine modification at the active cite of multiple ubiquitin enzymes (McGuire et al., 2016). This effect prevented TLR downstream signalling transduction independent of NRF2 activation. There is evidence showing that DMF can induce anti-inflammatory ubiquitination. DMF causes phosphorylation of NLRP3 through protein kinase A signalling (Shi et al., 2022). Phosphorylated NLRP3 is then ubiquitinated and degraded. Fumarate can control gene expression via epigenetic modifications as can DMF (Wentzel et al., 2017; Maltby et al., 2018). Both can modify DNA methylation via inhibition of DNA demethylases and inhibit gene expression. A study on NK cells showed DMF hypermethylates the Gasdermin (GSDM) D gene and prevents its expression, thereby preventing the inflammatory form of cell death termed pyroptosis (Muhammad et al., 2019). On the other hand, the cysteine residue on GSDMD protein can be succinated by DMF, and this modification prevents the interaction between caspase and GSDMD thereby inhibiting pyroptosis (Humphries et al., 2020).
Metformin
Metformin is a widely prescribed drug for the treatment of type 2 diabetes. It lowers blood glucose and increases insulin sensitivity. Complex I in the electron transport chain (ETC) is a major target. Unlike other inhibitors, such as rotenone, metformin reversibly inhibits Complex I and is well tolerated by cells (Wheaton et al., 2014). It is possible that a more profound inhibitor of complex I could be too toxic. Most of anti-inflammatory responses caused by metformin is via activating AMP-activated protein kinase (AMPK). Complex I inhibition decreases the ATP/Adenosine monophosphate (AMP) ratio. With the help of liver kinase B1 (LKB1), metformin, therefore, activates AMPK (Bailey, 2017). AMPK regulates various pathways to maintain the energy balance in cells. When it is activated, glucose uptake and metabolism are upregulated, and insulin resistance is also ameliorated (Gunton et al., 2003). In the liver, metformin also inhibits gluconeogenesis (Stumvoll et al., 1995; Song et al., 2001). Apart from glucose metabolism, metformin alters lipid and amino acid metabolism. Metformin reduces lipid synthesis and export through AMPK pathways (Jeppesen et al., 1994; Fullerton et al., 2013). Metformin also exhibits potent immune regulatory functions by targeting AMPK activation. Studies found that metformin-treated cells modulated key inflammatory regulators like PTEN, nuclear factor kappa-light-chain-enhancer of activated B cells (NFκB), Poly [ADP-ribose] polymerase 1 (PARP1), and NLRP3 activity through AMPK function (Hattori et al., 2006; Kim and Choi, 2012; Li et al., 2016; Ke et al., 2019). These effects then regulate various pathways and dampen inflammation. Proinflammatory cytokine production like TNF-α, IL-1, and IL-6 are reduced by metformin regulating these pathways (Hyun et al., 2013). Besides, metformin prevented IL-1 production also through inhibiting complex I activity to decrease ROS. This consequently boosted the production of the anti-inflammatory cytokine IL-10 (Kelly et al., 2015). The ability of metformin to reduce glucose levels inhibits the production of advanced glycation end products (AGEs). The receptor of AGEs (RAGE) activation and downstream proinflammatory effects were subsequently prevented (Zheng et al., 2011; Ishibashi et al., 2012; Byun et al., 2017). Overall, the targeting of complex I in mitochondria by metformin is likely to be critical for its anti-inflammatory effects.
Krebs Cycle metabolites and inflammation
Succinate
Succinate plays a critical role in regulating proinflammatory responses. Succinate levels are increased in multiple inflammatory diseases (Monfort-Ferré et al., 2022; Xu et al., 2022). Glutamine-dependent anaplerosis and GABA (γ-aminobutyric acid) shunt activity increase in M1 macrophages which leads to the build-up of succinate (Monfort-Ferré et al., 2022). IL-1β production has been shown to be regulated by succinate. As succinate accumulates, it impairs prolyl hydroxylase (PHD), thereby activating HIF-1α. IL-1β is a HIF-1α dependent gene and can be induced by succinate. Succinate oxidation by succinate dehydrogenase (SDH) can also drive reverse electron transport (RET) via complex I, generating mitochondrial ROS to promote HIF-1α activation (Guzy et al., 2008; Tannahill et al., 2013; Yin and ONeill, 2021). In T cells, a recent study has found that accumulation of succinate reduced CD4 T cell proliferation and promoted proinflammatory Th1 and Th17 cells by altering gene transcription (Chen X. et al., 2022). Succinate has also been shown to regulate protein function by direct modification. One example of this is succinylation of pyruvate kinase 2 (PKM2). This drives PKM2 translocation into the nucleus, where in macrophages it boosts HIF-1α gene expression. The desuccinylation enzyme SIRT5 is downregulated in LPS stimulated macrophage, which then allows succinate to modify PKM2 (Qi et al., 2019).
Succinate also can be secreted and bind to a specific receptor on cells called SUCNR1, also known as GPR91 (He et al., 2004). The study showed this receptor is widely expressed and increases blood pressure after binding succinate. After binding, similar to other GPCRs, SUCNR1 converts phosphatidylinositol bisphosphate (PIP2) to inositol trisphosphate (IP3) and diacylglycerol (DAG) (Ariza et al., 2012). Then, IP3 and DAG activate downstream signalling and processes like calcium mobilization and activation of protein kinase C (PKC). Many proinflammatory proteins are then activated by these two pathways. Dendritic cells can be stimulated to a more active state after succinate-SUCNR1 binding (Rubic et al., 2008). Their migration and antigen presenting ability are enhanced compared by succinate.
SUCNR1 expression is upregulated in LPS treated macrophages, which increases IL-1β production by activating the HIF-1α pathway (Littlewood-Evans et al., 2016). The SUCNR1 deficient mouse model of arthritis has reduced knee swelling and less activated macrophages in the joint. IL-1β levels are significantly lower in SUCNR1 deficient mice compared to control. SUCNR1 deficient macrophages also produce less IL-1β after incubation with synovial fluid (SF) from rheumatoid arthritis (RA) patients (Littlewood-Evans et al., 2016). Inhibiting SUCNR1 with antagonist might therefore have promise as a treatment for RA.
However, several studies also show the opposite effect of SUCNR1 activation under certain conditions. In diet-induced obesity mice, succinate treatment induces anti-inflammatory M2 macrophages and reduces inflammation in adipose tissue (Keiran et al., 2019). Cancer cells release succinate, which then binds to SUCNR1 on macrophages. These macrophages then convert to tumour-associated macrophages (TAMs) to dampen their tumour killing effects (Wu et al., 2020). IL-6 secreted by succinate-induced TAMs also promotes cancer cell migration. More studies are required to clarify SUCNR1 effects under different circumstances.
Because SDH is required for RET and mitochondrial ROS production, targeting SDH is a potential anti-inflammatory strategy. Inhibiting SDH activity could prevent RET during inflammation. Malonate ester prodrugs, dimethyl malonate (DMM) and diacetoxymethyl malonate (MAM) have been shown to competitively inhibit succinate binding with SDH (Xu et al., 2022). In LPS activated macrophages, DMM reduces ROS production and promotes anti-inflammatory cytokine IL-1RA and IL-10 production (Yang et al., 2019). Expression of many anti-inflammatory genes are increased after DMM treatment. In a mouse model of sepsis, DMM treatment reduces IL-1β level in serum. The anti-inflammatory properties of DMM have been also studied in multiple models of inflammation (Table 1). Recently, DMM has been shown to ameliorate the inflammation in model of Alzheimer’s disease and systemic inflammatory response syndrome (SIRS) through inhibition of RET. All these studies indicate the potential of DMM targeting SDH as an anti-inflammatory therapeutics.
Another group of inhibitors target the ubiquinone binding site on SDH, and, among them, thenoyltrifluoroacetone (TTFA) has been used most frequently (Moreno et al., 2020). TTFA also reduce ROS production and proinflammatory cytokine production (Hop et al., 2017; Zhao et al., 2019). Of note, both DMM and TTFA have been shown to dampen severe inflammation caused by bacterial infection (Hop et al., 2017; Li et al., 2019). However, more studies are required to have a better understanding on the anti-inflammatory property of TTFA and the difference between these two types of SDH inhibitors.
Blocking SUCNR1 also has potential as an anti-inflammatory strategy, and several studies have identified different SUCNR1 antagonists (Figure 1). In 2011, four compounds (2c, 4c, 5g, 7e) were reported to have therapeutic potential inhibiting SUCNR1 (Bhuniya et al., 2011). As mentioned above, in a study of RA, IL-1β production is decreased after 4c treatment on cells incubated with SF from RA patients (Littlewood-Evans et al., 2016). With the help of Structure-based mutagenesis and radioligand-binding studies, a small molecule called NF-56-EJ40 was identified as a SUCNR1 inhibitor (Haffke et al., 2019). It has a similar structure with the compounds in the previous study as the biphenyl structure is crucial for the interaction with SUCNR1. However, the study showed that its structure allows the molecule to interact with SUCNR1 in a multivalent ligand-binding mode. NF-56-EJ40 inhibits SUCNR1 by a different in a study of atherosclerosis-induced chronic inflammation, the serum level of IL-1β and succinate was higher in coronary heart disease patients. NF-56-EJ40 inhibited IL-1β production in both macrophages and human umbilical vein endothelial cells (HUVECs) (Monfort-Ferré et al., 2022). Later, a novel zwitterion antagonist was developed based on NF-56-EJ40 (Velcicky et al., 2020). Two conformations allow this molecule to have both higher permeability and efficacy. In a study of periodontitis, researchers found that patients with periodontitis possessed a higher succinate level and expressed more SUCNR1 on the gingival tissue cells. They then synthesized a small molecule, termed 7a, and blocked succinate-SUCNR1 binding, which blocked inflammation (Guo et al., 2022). The difference between 7a and previous compounds is that 7a contains a naphthyridine connected to a benzine ring instead of biphenyl, which might also interact similarly with SUCNR1.
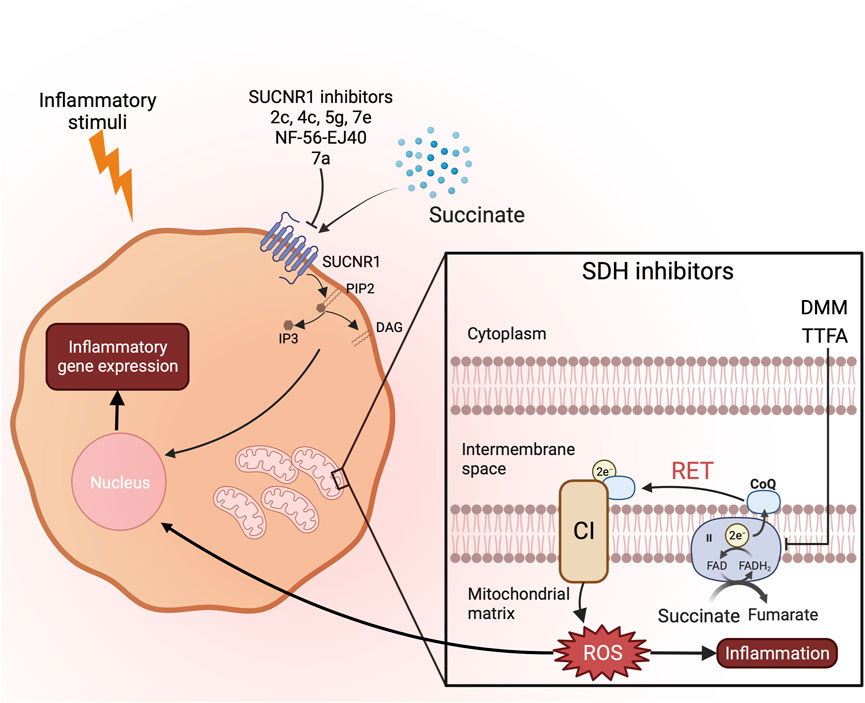
FIGURE 1. Possible therapeutics targeting succinate induced inflammation. To reduce inflammation caused by succinate accumulation, there are two different strategies. SDH inhibitors can prevent RET and ROS production limiting inflammation. SUCNR1 inhibitors prevent succinate from binding to its receptor and downstream proinflammatory signalling pathways induced by second messengers DAG and IP3.
Itaconate
Itaconate is mainly produced from M1 macrophages. Aconitate decarboxylase 1 (ACOD1), encoded by immunoresponsive gene 1 (Irg1), converts cis-aconitate to itaconate after proinflammatory signals. Itaconate has multiple functions in dealing with different pathogens and regulating signalling pathways. The anti-bacterial function of itaconate was first identified as it can inhibit isocitrate lyase and block the glyoxylate shunt, which is critical for bacterial growth (Rittenhouse and McFadden, 1974; McFadden and Purohit, 1977; Ruetz et al., 2019). Li and others discovered that itaconate enhances the bacterial killing ability of macrophages by alkylating transcription factor EB (TFEB) (Zhang Z. et al., 2022). After modification, this transcription factor is activated and enhances lysosomal generation. Itaconate also has anti-viral properties. A study revealed that itaconate its cell permeable derivative 4-octyl itaconate (4OI) ameliorates neuron cell death caused by Receptor interacting protein kinases-1 (RIP1) and 3 (RIP3) after Zika virus infection (Daniels et al., 2019). Itaconate 4OI also prevent influenza A replication by blocking exportin-1 (XPO1) and nuclear export of viral ribonucleoproteins (Waqas et al., 2023).
Apart from its role in host defence, itaconate has also been extensively studied in the context of inflammation, and 4-OI has been studied in different animal models shown in Table 2. Itaconate deficient mice have higher proinflammatory cytokines, like IL-1 and TNF-α, after LPS stimulation (Lampropoulou et al., 2016). Itaconate and its derivatives, 4-OI and dimethyl itaconate (DI), reduce the interferon response and excessive inflammation caused by infection with influenza A virus (Sohail et al., 2022). They also have the potential in relieving damaging inflammatory responses caused by SARS-CoV2 (Olagnier et al., 2020).
Itaconate and its derivatives carry out most of their function by protein post-translational modification and have immunomodulatory properties. Itaconate and most particularly its derivatives regulate several important pathways in immune cells by alkylating cysteine residue on proteins (Hooftman and ONeill, 2019). Like DMF, itaconate and 4-OI can alkylate the cysteine on Kelch-like ECH associated protein (KEAP1) and cause its degradation (Mills et al., 2018). This degradation consequently releases and activates NRF2, which acts as an anti-inflammatory and antioxidant transcription factor. After activation, NRF2 translocates into the cell nucleus and binds to antioxidant responsive elements (ARE). Many antioxidant proteins like glutathione S-transferase (GST) and heme oxygenase-1 (HMOX1) are upregulated by NRF2 and subsequently reduce ROS and inflammatory responses in cells. Studies showed that activating NRF2 and antioxidant proteins in LPS stimulated macrophages can reduce the production of inflammatory cytokines such as IL-6 and TNF-α (Bambouskova et al., 2018; Luo et al., 2018). These changes then dampen the activity of HIF-1α and reduce proinflammatory cytokines like IL-1β secretion. 4OI enhanced survival and clinical score and reduction of proinflammatory cytokines in serum through the NRF2 pathway in a mouse model of sepsis. NRF2 was shown to dampen the interferon response, and 4OI was shown to suppress type I interferon production by activating NRF2 (Ryan et al., 2022). 4OI and DI regulate tissue factor production and coagulopathy after SARS-CoV2 infection through this pathway (Ryan et al., 2023). NRF2 also downregulates IκB kinase (IKK) by inducing its degradation and prevents NFκB activation (Helou et al., 2019). Itaconate and its derivatives have been shown to have therapeutic effects in multiple disease models such as SARS-CoV2, pulmonary fibrosis, and osteoarthritis through NRF2 function (Olagnier et al., 2020; Zhang P. et al., 2022; Wu et al., 2023a). Other than NRF2, itaconate and 4-OI alkylates multiple cysteines on Stimulator of interferon gene (STING) protein and prevents its phosphorylation (Li W. et al., 2023). 4OI inhibiting STING can ameliorate the symptoms of sepsis in mouse models. The production of cytokines like interferon-beta (IFN-β) and TNF-α is downregulated after STING inhibition. Another recent study showed that 4-OI alkylated Cys91 of STING and prevented its palmitoylation, which ameliorated cGAS-STING-mediated autoimmune response (Su et al., 2023). For autoinflammatory diseases like cryopyrin-associated periodic syndrome (CAPS), 4-OI downregulates NLRP3 activity by cysteine modification (Hooftman et al., 2020). This modification prevents NIMA-Related Kinase 7 (NEK7) from binding to NLPR3 and inflammasome formation. Another study showed that GSDMD is also modified by 4OI preventing pyroptosis and inflammatory tissue damage (Bambouskova et al., 2021).
Itaconate and 4OI also modulate inflammation by targeting metabolic pathways enzymes in macrophages. Itaconate alkylates the cysteine in GAPDH (Liao et al., 2019). This change reduces its activity which ameliorates aerobic glycolysis in proinflammatory macrophages. A recent study also showed that itaconate and DI can irreversibly bind to carnitine/acylcarnitine carrier (CAC) (Giangregorio et al., 2023). This change could affect fatty acid oxidation and reduce ROS production during inflammation, but further study is required to prove this. In M2 macrophages, itaconate and 4-OI target Janus Kinase 1 (JAK1) and Signal Transducer and Activator of Transcription 6 (STAT6) (Runtsch et al., 2022). 4OI is capable of alkylating multiple cysteines on JAK1 and eventually prevents its phosphorylation and dimerization. This study showed itaconate and its derivatives also alleviated symptoms of asthma in a mouse model. Itaconate has also been found to modify lysine via itaconylation (Liu et al., 2023). After treating cells with LPS or itaconate, an increased itaconylation is observed. However, the effect of the modification still needs further study.
Besides protein modification, itaconate modulates immune responses through other routes. Itaconate can competitively bind to SDH and reduce ROS generation from RET (Lampropoulou et al., 2016). Itaconate and 4OI can also inhibit the PI3K/AKT/mTOR signalling pathway by blocking ROS production (Xin et al., 2020; Pan et al., 2022). This then ameliorates acute lung injury and osteoarthritis in mouse models. Another study found that 4OI reduces ROS production and mitochondrial DNA (mtDNA) release in alveolar macrophages alleviating acute respiratory distress syndrome (Wu et al., 2023b).
Itaconate also prevents IL-6 expression by causing electrophilic stress in cells (Bambouskova et al., 2018). ATF3 expression is increased in proinflammatory macrophages after itaconate and DI treatment. This transcription factor then inhibits IκBζ production, which is critical for IL-6 gene expression. In a psoriasis model, administration of DI dampens IL-17-mediated IκBζ induction in cells, which then reduces the severity of this disease (Bambouskova et al., 2018). Itaconate modifies TET2 and inhibits its catalytic domain (Chen L.-L. et al., 2022). This consequently suppresses histone and DNA demethylation.
An itaconate receptor termed OXGR1 has been recently identified. This receptor is a GPCR and acts similarly to SUCNR1. During pulmonary infection, itaconate is secreted by activated macrophages. It then binds to OXGR1 on epithelial cells and induces mucus secretion as a primary innate defence mechanism (Zeng et al., 2023).
Since itaconate has so many impact on cell functions, a specific delivery to the target tissue would have more therapeutic potential. The research showed that artificial cells containing itaconate can be taken up by macrophages in the liver. This is then able to reverse acute liver failure (ALF) in mouse model (Yin et al., 2023). NLRP3 activity and IL-1 production were inhibited in these macrophages, which protected mice from acetaminophen (APAP)-induced ALF.
However, some studies also show the ACOD1 activity sometimes can have some side effects and promote inflammation. Macrophages can be polarized to M1 phenotype after itaconate treatment (Ganta et al., 2017). Respiratory syncytial virus (RSV)-infected A549 epithelial cells and lung tissues from RSV infected mice have been shown an upregulation of ACOD1 (Ren et al., 2016). This is related to ROS and proinflammatory cytokine production, which then leads to tissue damage. By silencing ACOD1 expression, RSV infected mice had less ROS and proinflammatory cytokine and reduced immune cell infiltration caused lung injury. Itaconate has also been shown to increase IL-1β production, which is through the inhibition of aconitase (ACO) 1 and ACO2 and activation of NLRP3 (Liu et al., 2021). ACOD1 also boosted the TNF signalling pathway and cytokine production in sepsis. Treating cell with 4OI, likely to be, had no effect on TNF-α secretion, which indicates this effect is itaconate-independent (Wu et al., 2022). More studies are therefore needed on itaconate and its derivatives as therapeutics against inflammatory diseases.
Fumarate
Most recently, new information has emerged on fumarate and inflammation. Fumarate is one of the most upregulated metabolites in macrophages activated with LPS (Hooftman et al., 2023). FH inhibition with a small molecule inhibitor causes the building up of fumarate in cells, which in turn induces protein succination. FH inhibition also causes an increased mitochondrial membrane potential (MMP) leading to ROS production (Hooftman et al., 2023). FH inhibition and fumarate accumulation drive macrophages to a more proinflammatory state. Fumarate reduces c-fos and AP1 complex activity, likely through succination, and thereby blocks production of anti-inflammatory cytokine IL-10. Consequently, TNF-α secretion increases after FH inhibition. Importantly, in FH deficient mice and cells treated with the FH inhibitor, mtRNA is released into the cytosol after treatment with LPS. TLR7, Retinoic acid-inducible gene I (RIG-I), and melanoma differentiation-associated protein 5 (MDA5) then induce IFN-β production. This pattern is not seen in macrophages treated with DMF, which indicates the effect is induced by mitochondrial disturbance rather than succination. Reduced FH activity has been shown in several diseases. In Systemic lupus erythematosus (SLE) patients, autoantibodies to double stranded RNA (dsRNA) are observed (Davis et al., 1975). Reduced ADAR1 RNA-editing activity results in dsRNA-mediated interferon response. This reduced editing has been reported in multiple inflammatory pathologies such as MS, coronary artery disease, and SLE, and may be a common event in inflammatory diseases (Li et al., 2022). Also, a study found an abnormal increase in IFN-β production in SLE patients. This is caused by mitochondrial ROS induced mitochondrial antiviral-signalling protein (MAVS) oligomerization (Buskiewicz et al., 2016). The peripheral blood mononuclear cells (PBMCs) derived from these patients have decreased FH expression. Similar chronic inflammatory effects are observed in Hereditary leiomyomatosis and renal cell cancer (HLRCC) patients who have FH deficiency (Zecchini et al., 2023). It might be possible to ameliorate the symptoms of these diseases by restoring the normal activity of FH (Figure 2).
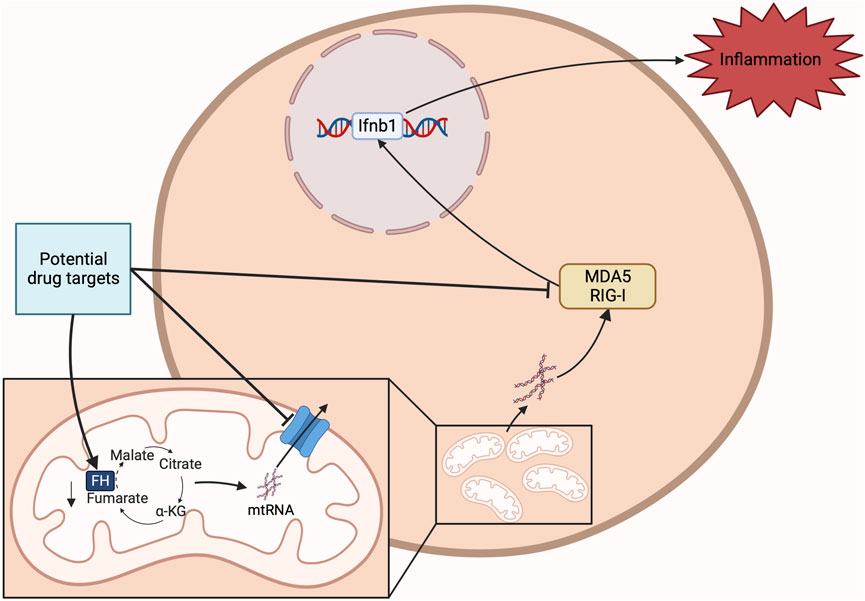
FIGURE 2. Potential drug target against FH deficiency. FH deficiency induces inflammation mainly through mtRNA release and binding to MDA5 and RIG-I. Restoring FH or targeting MDA5 and RIG-I or the reduction of mtRNA release have potential for the development of anti-inflammatory therapeutics.
The potential of targeting mitochondrial nucleic acid sensors and release in inflammation
The above study on FH and reports of mtDNA and mtRNA in inflammatory diseases indicate that cytosolic nucleic acid signalling PRRs might be of interest as targets for anti-inflammatory therapeutics. A study showed that NLRP3 interacts with mtDNA (Figure 3) (Nakahira et al., 2010). Caspase 1 activation and IL-1β and IL-18 secretion are observed in these abnormal apoptotic cells. The link between mitochondrial stress and caspase 1 activation is through mtDNA binding with NLRP3. Researchers in this study generated mtDNA depleted cells, known as ρ0 cells, with ethidium bromide (EtBr). In ρ0 cells, NLRP3 proinflammatory pathway is significantly downregulated. There is evidence of NLRP3 mediating mtDNA release as less cytosolic mtDNA are detected in NLRP3 deficient cells after stimulation. NLRP3 and caspase 1 activity were also shown to be dependent on mitochondrial ROS production. Another study has demonstrated that ROS oxidizes mtDNA in apoptotic macrophages after encountering danger signals (Shimada et al., 2012). This modification increases the affinity of mtDNA for NLRP3 and IL-1β and IL-18 production. In contrast, their expression is completely blocked in ρ0 cells. DNA glycosylase 8-oxoguanine glycosylase (OGG1) regulates mtDNA oxidation and consequently NLRP3 activation (Tumurkhuu et al., 2016). OGG1-deficient mice have higher level of oxidized mtDNA, which leads to increased NLRP3 activation and IL-1β and IL-18 production. There is evidence that mtDNA increases in macrophages after LPS stimulation (Zhong et al., 2018). This is through MyD88 and TRIF activated interferon regulatory factor 1 (IRF1). This transcription factor then binds and upregulates the expression of mitochondrial deoxyribonucleotide kinase called UMP-CMPK2, which is a rate-limiting enzyme for mtDNA production. Inhibition of UMP-CMPK2 substantially abrogates NLRP3 activation and IL-1β secretion. Research that aims to find small molecules targeting UMP-CMPK2 is currently ongoing (gossamer bio, 2022). Mitochondrial genome maintenance exonuclease 1 (Mgme1) is also shown to be involved in mtDNA synthesis as silencing its expression reduces mtDNA levels and inflammasome activation in cells (Xian et al., 2022). An intracellular orphan receptor Nur77 is activated by both LPS and mtDNA and activates NLRP3 in a non-canonical way. The depletion of mtDNA caused by ethidium bromide reduces its interaction with Nur77 and NLRP3 activation (Zhu et al., 2023).
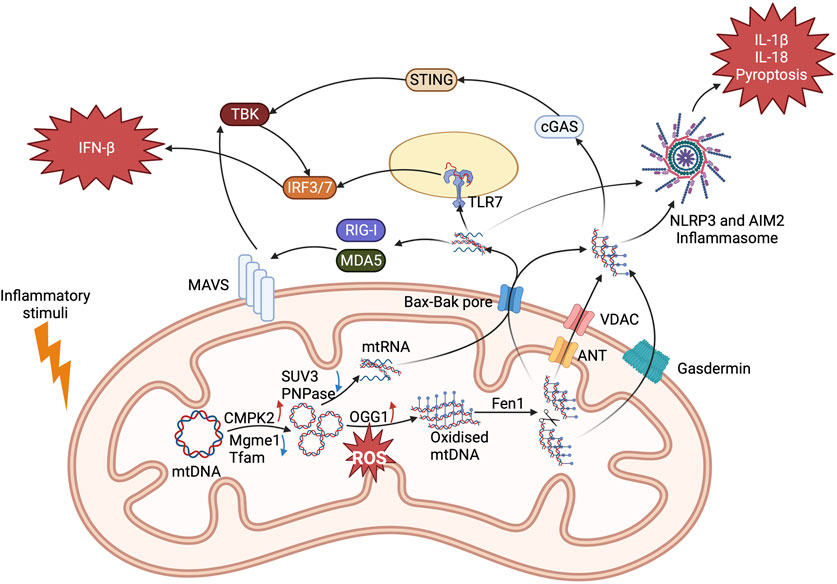
FIGURE 3. Mechanisms of mitochondrial nucleic acids activating proinflammatory pathways. mtDNA and mtRNA production are upregulated in response to inflammatory signals and cell stress. mtDNA is first oxidised by OGG1 and then cut by FEN1 which allows it to leave mitochondria through ANT (IMM) and VDAC (OMM) formed mPTP. When mtDNA enters the cytosol, it binds to NLRP3 and cGAS to induce proinflammatory IL-1β and IFN-β secretion. For mtRNA, surveillance proteins PNPase and SUV3 are downregulated to cause its accumulation. They leave mitochondrial through Bak and Bax pores. RNA sensing PRRs RIG-I, MDA5, and TLR7 are activated to cause IFN-β secretion. They also activate AIM2 to induce IL-1β and IL-18 secretion and pyroptosis. Each has therapeutic potential for inflammatory diseases.
Absent in melanoma 2 (AIM2) protein has also been reported to be involved in mtDNA sensing and inflammasome activation (Dang et al., 2017). Cholesterol 25-hydroxylase (Ch25h) deficient macrophages have increased cholesterol levels compared to controls. Excessive cholesterol causes mitochondrial stress and mtDNA release. This is then sensed by AIM2 and induces IL-1β and IL-18 production. This pathway is downregulated by EtBr induced ρ0 cells. In a mouse model of nonalcoholic fatty liver disease, AIM2 binds to mtDNA and causes pyroptosis and IL-1β production (Xu et al., 2021). mtDNA can also activate the cGAS-STING inflammatory pathway. The loss of Mitochondrial transcription factor A (TFAM) can cause instability and release of mtDNA, which then binds to cGAS to induce IFN-β expression (West et al., 2015). A higher resistance against virus infection was shown in TFAM-deficient mice as they had stronger innate immune responses. However, in apoptosis deficient mice, this pathway is activated and causes proinflammatory cell death (Rongvaux et al., 2014; White et al., 2014). In endothelial cells, mtDNA release induced the cGAS-STING pathway activation leading to YAP signalling and downstream cyclin D inhibition. This effect prevents cell proliferation and exaggerates the inflammatory response acute lung injury (Huang et al., 2020). In Amyotrophic lateral sclerosis patients, mtDNA and cGAS interaction induce neurodegeneration and inflammation (Yu et al., 2020). Studies found that mtDNA release can activate both NLRP3 and cGAS-STING pathway and induce both proinflammatory IL-1β and IFN-β secretion (Liu et al., 2022; Xian et al., 2022).
As mentioned above, for mtRNA, there are several different PRRs recognize single stranded RNA and short dsRNA, while MDA5 recognizes long dsRNA. Both trigger MAVS oligomerization and TBK1 activation. mtRNA has been shown to activate both RIG-I and MDA5 to increase interferon production and IRF7 activation (Dhir et al., 2018; Tigano et al., 2021). RNA helicase SUV3 and polynucleotide phosphorylase PNPase controls the formation of double strand mtRNA (dsmtRNA). Once they are depleted in cells, more dsmtRNA is detected by MDA5. Protein kinase RNA-activated (PKR) is also a mitochondrial RNA sensor. It binds to dsRNA and induces downstream ROS and pro-inflammatory cytokines production (Kim et al., 2018; Kim et al., 2020). A mouse model of osteoarthritis has elevated cytosolic mtRNA and phosphorylated PKR levels are detected (Kim et al., 2022). 2′-C-methyladenosine (2-CM) induced mtRNA depletion can effectively reduce the production of inflammatory senescence-associated secretory phenotype (SASP) factors, which is PKR activation dependent. This study also showed that mtRNA is released into extracellular space, which is then taken up by adjacent cells to activate MDA5, RIGI, and TLR3 signalling pathways. Recently, TLR7, which binds to single strand RNA, is also shown to bind mtRNA and activate IFN-β expression. Mitochondrial RNA polymerase inhibitor IMT1 reduced cytosolic mtRNA concentration and downregulated IFN-β production. This pathway probably plays a part in proinflammatory pathogenicity in SLE (Hooftman et al., 2023).
In order to bind cytosolic PRRs, mtDNA and mtRNA translocate through the mitochondrial double membrane (Figure 3). Preventing this should be anti-inflammatory. Different mechanisms of mitochondrial nucleic acid release have been reported. The mitochondrial permeability transition pore (mPTP), which is composed of mitochondrial inner membrane (MIM) adenine nucleotide translocase (ANT) and cyclophilin D and mitochondrial outer membrane (MOM) VDAC, allows for mtDNA release. This was first discovered using cyclosporin A (CsA) to inhibit calcium influx induced by non-specific mPTP opening and fragmented mtDNA release (Patrushev et al., 2004). Later, a study demonstrated that only mtDNA fragments under 700 base pairs were released through mPTP (García and Chávez, 2007). NLRP3 activation and inflammatory responses are also linked with PTP opening causing mtDNA release (Xian et al., 2022). Protein prohibitin 1 (PHB1) also showed evidence of regulating MIM mPTP as PHB1 deficient mice increase mtDNA release and proinflammatory cytokine production (Liu et al., 2022). On the MOM, VDAC is responsible for mtDNA entering cytosol (Kim et al., 2019). The N-terminus of VDAC can bind mtDNA to form a stable oligomer during activation. In VDAC knockdown cells or cells treated with VDAC inhibitor Vbit4, cytosolic mtDNA concentration is reduced significantly, and the activation of IFN-β response is also downregulated. In a mouse model of SLE inflammation was attenuated after administration of Vbit4 to prevent mtDNA release. In another study, the mitochondrial calcium uniport (MCU) triggered calcium influx inducing ANT and cyclophilin D activation leading to VDAC oligomerization (Xian et al., 2022). The same study showed that an endonuclease called flap-structure-specific endonuclease 1 (Fen1) can regulate this pathway. FEN1 fragments oxidized mtDNA to 500 to 600 base pair length that enables them pass through activated mPTP into the cytosol.
There is also evidence of Bak and Bax oligomerization inducing mtDNA and mtRNA release. mtDNA is released through apoptosis-activated Bax and Bak pores and stimulates cGAS-STING pathway activation (Rongvaux et al., 2014; White et al., 2014). The Bcl-2 inhibitor ABT-737 also induce Bax and Bak oligomerization and triggers an increase in IFN-β expression. A follow-up study illustrated that mtDNA leaves mitochondria through Bax and Bak pore by herniation of MIM (McArthur et al., 2018). Some hernias lose their integrity and allow mtDNA to enter the cytosol to cause cGAS-STING dependent IFN-β secretion. Bak and Bax exports mtRNA as well. dsmtRNA can be released through Bax and Bak after inhibition of SUV3 and PNPase (Dhir et al., 2018). The MIM herniation induced by mitochondrial DNA double strand breaks contains dsmtRNA (Tigano et al., 2021). Mice or patients with kidney injury showed an increased expression of Bax and cytosolic mtRNA (Doke et al., 2023). Treatment with NAD attenuates mitochondrial dysfunction and Bax oligomerization, which then reduces mtRNA release.
GSDMs can also be inserted in the mitochondrial membrane and trigger mtDNA release into the cytosol, which then activates PRRs and inflammatory pathways. The N-terminus of GSDMD is cleaved and plugged in to mitochondrial MOM that allows mtDNA to escape to the cytosol (Huang et al., 2020; Zhu et al., 2023). GSDMD deficient mice possess a lower cytosolic mtDNA levels and dampened inflammatory responses. In neurons, GSDME has a similar effect in inducing mtDNA and cytochrome C release, which might be the cause of neurodegeneration (Neel et al., 2023). Other proteins might also be involved in this process. Sorting Nexin 9 (SNX9) has been shown to play a role in mtDNA containing mitochondrial-derived vesicles (MDV) formation after LPS stimulation in FH deficient mice (Zecchini et al., 2023). By knockdown of SNX9, cytosolic mtDNA level is reduced, which further downregulates the cGAS-STING pathway and downstream interferon proinflammatory responses.
Although these studies provide some insights into mitochondrial nucleic acid releasing, more research is required to give a clear picture of how these mechanisms are orchestrated and regulated.
Concluding remarks
Various aspects of mitochondria therefore have potential as novel targets for anti-inflammatory therapeutics. Targeting succinate, mimicking itaconate with derivatives and modulating fumarate and downstream consequences of FH repression all looked promising. Also targeting the release or sensing of mitochondrial nucleic acid has potential in inflammatory disease, perhaps most notably in interferonopathies like SLE. Following the lead of DMF and metformin, mitochondrial targeting could hold great promise for new therapies against immune and inflammatory diseases.
Author contributions
YM: Writing–original draft. LO’N: Supervision, Writing–review and editing.
Funding
The author(s) declare financial support was received for the research, authorship, and/or publication of this article. This work is supported by the Science Foundation Ireland (19/FFP/6507) and the European Research Council (Metabinnate 834370).
Conflict of interest
The authors declare that the research was conducted in the absence of any commercial or financial relationships that could be construed as a potential conflict of interest.
Publisher’s note
All claims expressed in this article are solely those of the authors and do not necessarily represent those of their affiliated organizations, or those of the publisher, the editors and the reviewers. Any product that may be evaluated in this article, or claim that may be made by its manufacturer, is not guaranteed or endorsed by the publisher.
References
Ariza, A. C., Deen, P. M., and Robben, J. H. (2012). The succinate receptor as a novel therapeutic target for oxidative and metabolic stress-related conditions. Front. Endocrinol. 3, 22. doi:10.3389/fendo.2012.00022
Bailey, C. J. (2017). Metformin: historical overview. Diabetologia 60, 1566–1576. doi:10.1007/s00125-017-4318-z
Bambouskova, M., Gorvel, L., Lampropoulou, V., Sergushichev, A., Loginicheva, E., Johnson, K., et al. (2018). Electrophilic properties of itaconate and derivatives regulate the iκbζ–atf3 inflammatory axis. Nature 556, 501–504. doi:10.1038/s41586-018-0052-z
Bambouskova, M., Potuckova, L., Paulenda, T., Kerndl, M., Mogilenko, D. A., Lizotte, K., et al. (2021). Itaconate confers tolerance to late NLRP3 inflammasome activation. Cell Rep. 34, 108756. doi:10.1016/j.celrep.2021.108756
Beach, T. E., Prag, H. A., Pala, L., Logan, A., Huang, M. M., Gruszczyk, A. V., et al. (2020). Targeting succinate dehydrogenase with malonate ester prodrugs decreases renal ischemia reperfusion injury. Redox Biol. 36, 101640. doi:10.1016/j.redox.2020.101640
Behera, J., Nasme, F., Zhang, Y.-T., Smolenkova, I., and Tyagi, N. (2023). 4-octyl itaconate ameliorate cognitive impairment in 3xTg mouse model of alzheimer’s disease. Physiology 38. doi:10.1152/physiol.2023.38.s1.5732886
Bhuniya, D., Umrani, D., Dave, B., Salunke, D., Kukreja, G., Gundu, J., et al. (2011). Discovery of a potent and selective small molecule HGPR91 antagonist. Bioorg. Med. Chem. Lett. 21, 3596–3602. doi:10.1016/j.bmcl.2011.04.091
Broderick, L., and Hoffman, H. M. (2022). IL-1 and autoinflammatory disease: biology, pathogenesis and therapeutic targeting. Nat. Rev. Rheumatol. 18, 448–463. doi:10.1038/s41584-022-00797-1
Buskiewicz, I. A., Montgomery, T., Yasewicz, E. C., Huber, S. A., Murphy, M. P., Hartley, R. C., et al. (2016). Reactive oxygen species induce virus-independent MAVS oligomerization in systemic lupus erythematosus. Sci. Signal. 9, ra115. doi:10.1126/scisignal.aaf1933
Byun, K., Yoo, Y., Son, M., Lee, J., Jeong, G.-B., Park, Y. M., et al. (2017). Advanced glycation end-products produced systemically and by macrophages: a common contributor to inflammation and degenerative diseases. Pharmacol. Ther. 177, 44–55. doi:10.1016/j.pharmthera.2017.02.030
Chen, L.-L., Morcelle, C., Cheng, Z.-L., Chen, X., Xu, Y., Gao, Y., et al. (2022b). Itaconate inhibits Tet DNA dioxygenases to dampen inflammatory responses. Nat. Cell Biol. 24, 353–363. doi:10.1038/s41556-022-00853-8
Chen, X., Sunkel, B., Wang, M., Kang, S., Wang, T., Gnanaprakasam, J. N., et al. (2022a). Succinate dehydrogenase/complex II is critical for metabolic and epigenetic regulation of T cell proliferation and inflammation. Sci. Immunol. 7, eabm8161. doi:10.1126/sciimmunol.abm8161
Chouchani, E. T., Pell, V. R., Gaude, E., Aksentijević, D., Sundier, S. Y., Robb, E. L., et al. (2014). Ischaemic accumulation of succinate controls reperfusion injury through mitochondrial Ros. Nature 515, 431–435. doi:10.1038/nature13909
Dang, E. V., McDonald, J. G., Russell, D. W., and Cyster, J. G. (2017). Oxysterol restraint of cholesterol synthesis prevents AIM2 inflammasome activation. Cell 171, 1057–1071. doi:10.1016/j.cell.2017.09.029
Daniels, B. P., Kofman, S. B., Smith, J. R., Norris, G. T., Snyder, A. G., Kolb, J. P., et al. (2019). The nucleotide sensor ZBP1 and kinase RIPK3 induce the enzyme IRG1 to promote an antiviral metabolic state in neurons. Immunity 50, 64–76. doi:10.1016/j.immuni.2018.11.017
Davidson, S. M., Ferdinandy, P., Andreadou, I., Bøtker, H. E., Heusch, G., Ibáñez, B., et al. (2019). Multitarget strategies to reduce myocardial ischemia/reperfusion injury: JACC Review Topic of the Week. J. Am. Coll. Cardiol. 73, 89–99. doi:10.1016/j.jacc.2018.09.086
Davis, P., Cunnington, P., and Hughes, G. R. (1975). Double-stranded RNA antibodies in systemic lupus erythematosus. Ann. Rheumatic Dis. 34, 239–243. doi:10.1136/ard.34.3.239
Dhir, A., Dhir, S., Borowski, L. S., Jimenez, L., Teitell, M., Rötig, A., et al. (2018). Mitochondrial double-stranded RNA triggers antiviral signalling in humans. Nature 560, 238–242. doi:10.1038/s41586-018-0363-0
Doke, T., Mukherjee, S., Mukhi, D., Dhillon, P., Abedini, A., Davis, J. G., et al. (2023). NAD+ precursor supplementation prevents mtrna/rig-i-dependent inflammation during kidney injury. Nat. Metab. 5, 414–430. doi:10.1038/s42255-023-00761-7
Fan, K., Chen, K., Zan, X., Zhi, Y., Zhang, X., Zhang, X., et al. (2023). Negative regulation of pro-apoptotic AMPK/JNK pathway by itaconate in mice with Fulminant Liver Injury. Cell Death Dis. 14, 486. doi:10.1038/s41419-023-06001-w
Fan, K., Zan, X., Zhi, Y., Yang, Y., Hu, K., Zhang, X., et al. (2022). Immune response gene 1 deficiency impairs NRF2 activation and aggravates liver fibrosis in mice. Biochem. Biophysical Res. Commun. 607, 103–109. doi:10.1016/j.bbrc.2022.03.110
Fox, R. J., Miller, D. H., Phillips, J. T., Hutchinson, M., Havrdova, E., Kita, M., et al. (2012). Placebo-controlled phase 3 study of Oral BG-12 or Glatiramer in multiple sclerosis. N. Engl. J. Med. 367, 1087–1097. doi:10.1056/nejmoa1206328
Fullerton, M. D., Galic, S., Marcinko, K., Sikkema, S., Pulinilkunnil, T., Chen, Z.-P., et al. (2013). Single phosphorylation sites in ACC1 and ACC2 regulate lipid homeostasis and the insulin-sensitizing effects of metformin. Nat. Med. 19, 1649–1654. doi:10.1038/nm.3372
Ganta, V. C., Choi, M. H., Kutateladze, A., Fox, T. E., Farber, C. R., and Annex, B. H. (2017). A microrna93–interferon regulatory factor-9–immunoresponsive gene-1–itaconic acid pathway modulates M2-like macrophage polarization to revascularize ischemic muscle. Circulation 135, 2403–2425. doi:10.1161/circulationaha.116.025490
García, N., and Chávez, E. (2007). Mitochondrial DNA fragments released through the permeability transition pore correspond to specific gene size. Life Sci. 81, 1160–1166. doi:10.1016/j.lfs.2007.08.019
Giangregorio, N., Tonazzi, A., Console, L., Scalise, M., and Indiveri, C. (2023). Inhibition of the mitochondrial carnitine/acylcarnitine carrier by Itaconate through irreversible binding to cysteine 136: possible pathophysiological implications. Biomolecules 13, 993. doi:10.3390/biom13060993
gossamer bio (2022). Identification of novel inhibitor(s) targeting NLRP3 109 - gossamer bio. Available at: https://www.gossamerbio.com/wp-content/uploads/Poster-CYT0122.pdf.
Gunton, J. E., Delhanty, P. J., Takahashi, S.-I., and Baxter, R. C. (2003). Metformin rapidly increases insulin receptor activation in human liver and signals preferentially through insulin-receptor substrate-2. J. Clin. Endocrinol. Metabolism 88, 1323–1332. doi:10.1210/jc.2002-021394
Guo, Y., Xu, F., Thomas, S. C., Zhang, Y., Paul, B., Sakilam, S., et al. (2022). Targeting the succinate receptor effectively inhibits periodontitis. Cell Rep. 40, 111389. doi:10.1016/j.celrep.2022.111389
Guzy, R. D., Sharma, B., Bell, E., Chandel, N. S., and Schumacker, P. T. (2008). Loss of the SDHB, but not the SDHA, subunit of complex II triggers reactive oxygen species-dependent hypoxia-inducible factor activation and tumorigenesis. Mol. Cell. Biol. 28, 718–731. doi:10.1128/mcb.01338-07
Haffke, M., Fehlmann, D., Rummel, G., Boivineau, J., Duckely, M., Gommermann, N., et al. (2019). Structural basis of species-selective antagonist binding to the succinate receptor. Nature 574, 581–585. doi:10.1038/s41586-019-1663-8
Han, R., Xiao, J., Zhai, H., and Hao, J. (2016). Dimethyl fumarate attenuates experimental autoimmune neuritis through the nuclear factor erythroid-derived 2-related factor 2/hemoxygenase-1 pathway by altering the balance of M1/M2 macrophages. J. Neuroinflammation 13, 97. doi:10.1186/s12974-016-0559-x
Hattori, Y., Suzuki, K., Hattori, S., and Kasai, K. (2006). Metformin inhibits cytokine-induced nuclear factor kappaB activation via AMP-activated protein kinase activation in vascular endothelial cells. Hypertension 47, 1183–1188. doi:10.1161/01.hyp.0000221429.94591.72
He, S., Zhao, Y., Wang, G., Ke, Q., Wu, N., Lu, L., et al. (2023). 4-octyl itaconate attenuates glycemic deterioration by regulating macrophage polarization in mouse models of type 1 diabetes. Mol. Med. 29, 31. doi:10.1186/s10020-023-00626-5
He, W., Miao, F. J.-P., Lin, D. C.-H., Schwandner, R. T., Wang, Z., Gao, J., et al. (2004). Citric acid cycle intermediates as ligands for orphan G-protein-coupled receptors. Nature 429, 188–193. doi:10.1038/nature02488
He, Y., Zeng, M. Y., Yang, D., Motro, B., and Núñez, G. (2016). Nek7 is an essential mediator of NLRP3 activation downstream of potassium efflux. Nature 530, 354–357. doi:10.1038/nature16959
Helou, D. G., Martin, S. F., Pallardy, M., Chollet-Martin, S., and Kerdine-Römer, S. (2019). NRF2 involvement in chemical-induced skin innate immunity. Frontiers 2019, 01004. doi:10.3389/fimmu.2019.01004
Hooftman, A., Angiari, S., Hester, S., Corcoran, S. E., Runtsch, M. C., Ling, C., et al. (2020). The immunomodulatory metabolite ITACONATE modifies NLRP3 and inhibits inflammasome activation. Cell Metab. 32, 468–478. doi:10.1016/j.cmet.2020.07.016
Hooftman, A., and ONeill, L. A. J. (2019). The immunomodulatory potential of the metabolite Itaconate. Trends Immunol. 40, 687–698. doi:10.1016/j.it.2019.05.007
Hooftman, A., Peace, C. G., Ryan, D. G., Day, E. A., Yang, M., McGettrick, A. F., et al. (2023). Macrophage fumarate hydratase restrains mtrna-mediated interferon production. Nature 615, 490–498. doi:10.1038/s41586-023-05720-6
Hop, H. T., Reyes, A. W., Huy, T. X., Arayan, L. T., Min, W., Lee, H. J., et al. (2017). Activation of NF-KB-mediated TNF-induced antimicrobial immunity is required for the efficient Brucella abortus clearance in raw 264.7 cells. Front. Cell. Infect. Microbiol. 7, 437. doi:10.3389/fcimb.2017.00437
Huang, L. S., Hong, Z., Wu, W., Xiong, S., Zhong, M., Gao, X., et al. (2020). MtDNA activates cgas signaling and suppresses the yap-mediated endothelial cell proliferation program to promote inflammatory injury. Immunity 52, 475–486.e5. doi:10.1016/j.immuni.2020.02.002
Humphries, F., Shmuel-Galia, L., Ketelut-Carneiro, N., Li, S., Wang, B., Nemmara, V. V., et al. (2020). Succination inactivates Gasdermin D and blocks pyroptosis. Science 369, 1633–1637. doi:10.1126/science.abb9818
Hyun, B., Shin, S., Lee, A., Lee, S., Song, Y., Ha, N.-J., et al. (2013). Metformin down-regulates TNF-α secretion via suppression of scavenger receptors in macrophages. Immune Netw. 13, 123–132. doi:10.4110/in.2013.13.4.123
Ishibashi, Y., Matsui, T., Takeuchi, M., and Yamagishi, S. (2012). Metformin inhibits advanced glycation end products (ages)-induced renal tubular cell injury by suppressing reactive oxygen species generation via reducing receptor for ages (rage) expression. Hormone Metabolic Res. 44, 891–895. doi:10.1055/s-0032-1321878
Jeppesen, J., Zhou, M.-Y., Chen, Y.-D. I., and Reaven, G. M. (1994). Effect of metformin on postprandial lipemia in patients with fairly to poorly controlled NIDDM. Diabetes Care 17, 1093–1099. doi:10.2337/diacare.17.10.1093
Ke, Y., Wang, C., Zhang, J., Zhong, X., Wang, R., Zeng, X., et al. (2019). The role of parps in inflammation—and metabolic—related diseases: molecular mechanisms and beyond. Cells 8, 1047. doi:10.3390/cells8091047
Keiran, N., Ceperuelo-Mallafré, V., Calvo, E., Hernández-Alvarez, M. I., Ejarque, M., Núñez-Roa, C., et al. (2019). SUCNR1 controls an anti-inflammatory program in macrophages to regulate the metabolic response to obesity. Nat. Immunol. 20, 581–592. doi:10.1038/s41590-019-0372-7
Kelly, B., Tannahill, G. M., Murphy, M. P., and O’Neill, L. A. J. (2015). Metformin inhibits the production of reactive oxygen species from NADH:ubiquinone oxidoreductase to limit induction of interleukin-1β (il-1β) and boosts interleukin-10 (IL-10) in lipopolysaccharide (lps)-activated macrophages. J. Biol. Chem. 290, 20348–20359. doi:10.1074/jbc.m115.662114
Kim, J., Gupta, R., Blanco, L. P., Yang, S., Shteinfer-Kuzmine, A., Wang, K., et al. (2019). VDAC oligomers form mitochondrial pores to release mtdna fragments and promote lupus-like disease. Science 366, 1531–1536. doi:10.1126/science.aav4011
Kim, S., Lee, K., Choi, Y. S., Ku, J., Kim, H., Kharbash, R., et al. (2022). Mitochondrial double-stranded RNAS govern the stress response in chondrocytes to promote osteoarthritis development. Cell Rep. 40, 111178. doi:10.1016/j.celrep.2022.111178
Kim, S., Lee, K., Choi, Y. S., Ku, J., Lee, Y. J., and Kim, Y. (2020). Mitochondrial dsrnas activate PKR and TLR3 to promote chondrocyte degeneration in osteoarthritis. bioRxiv. doi:10.1101/2020.06.17.156323
Kim, S. A., and Choi, H. C. (2012). Metformin inhibits inflammatory response via AMPK–PTEN pathway in vascular smooth muscle cells. Biochem. Biophysical Res. Commun. 425, 866–872. doi:10.1016/j.bbrc.2012.07.165
Kim, Y., Park, J., Kim, S., Kim, M., Kang, M.-G., Kwak, C., et al. (2018). PKR senses nuclear and mitochondrial signals by interacting with endogenous double-stranded RNAS. Mol. Cell 71, 1051–1063. doi:10.1016/j.molcel.2018.07.029
Kornberg, M. D., Bhargava, P., Kim, P. M., Putluri, V., Snowman, A. M., Putluri, N., et al. (2018). Dimethyl fumarate targets GAPDH and aerobic glycolysis to modulate immunity. Science 360, 449–453. doi:10.1126/science.aan4665
Kulkarni, R. A., Bak, D. W., Wei, D., Bergholtz, S. E., Briney, C. A., Shrimp, J. H., et al. (2019). A chemoproteomic portrait of the oncometabolite fumarate. Nat. Chem. Biol. 15, 391–400. doi:10.1038/s41589-018-0217-y
Lampropoulou, V., Sergushichev, A., Bambouskova, M., Nair, S., Vincent, E. E., Loginicheva, E., et al. (2016). Itaconate links inhibition of succinate dehydrogenase with macrophage metabolic remodeling and regulation of inflammation. Cell Metab. 24, 158–166. doi:10.1016/j.cmet.2016.06.004
Li, A., Zhang, S., Li, J., Liu, K., Huang, F., and Liu, B. (2016). Metformin and resveratrol inhibit DRP1-mediated mitochondrial fission and prevent ER stress-associated NLRP3 inflammasome activation in the adipose tissue of diabetic mice. Mol. Cell. Endocrinol. 434, 36–47. doi:10.1016/j.mce.2016.06.008
Li, Q., Gloudemans, M. J., Geisinger, J. M., Fan, B., Aguet, F., Sun, T., et al. (2022). RNA editing underlies genetic risk of common inflammatory diseases. Nature 608 (7923), 569–577. doi:10.1038/s41586-022-05052-x
Li, R., Yang, W., Yin, Y., Zhang, P., Wang, Y., and Tao, K. (2021). Protective role of 4-octyl itaconate in murine LPS/D-GALN-induced acute liver failure via inhibiting inflammation, oxidative stress, and apoptosis. Oxidative Med. Cell. Longev. 2021, 9932099–9932111. doi:10.1155/2021/9932099
Li, W., Li, Y., Kang, J., Jiang, H., Gong, W., Chen, L., et al. (2023a). 4-octyl itaconate as a metabolite derivative inhibits inflammation via alkylation of Sting. Cell Rep. 42, 112145. doi:10.1016/j.celrep.2023.112145
Li, Y., Chen, X., Zhang, H., Xiao, J., Yang, C., Chen, W., et al. (2020). 4-Octyl itaconate alleviates lipopolysaccharide-induced acute lung injury in mice by inhibiting oxidative stress and inflammation. Drug Des. Dev. Ther. 14, 5547–5558. doi:10.2147/dddt.s280922
Li, Y., Jia, A., Wang, Y., Dong, L., Wang, Y., He, Y., et al. (2019). Immune effects of glycolysis or oxidative phosphorylation metabolic pathway in protecting against bacterial infection. J. Cell. Physiology 234, 20298–20309. doi:10.1002/jcp.28630
Li, Y., Li, B., Xiao, X., Qian, Q., Wang, R., Lyu, Z., et al. (2023b). Itaconate inhibits CD103+ TRM cells and alleviates hepatobiliary injury in mouse models of primary sclerosing cholangitis. Hepatology. doi:10.1097/hep.0000000000000549
Liao, S.-T., Han, C., Xu, D.-Q., Fu, X.-W., Wang, J.-S., and Kong, L.-Y. (2019). 4-octyl itaconate inhibits aerobic glycolysis by targeting gapdh to exert anti-inflammatory effects. Nat. Commun. 10, 5091. doi:10.1038/s41467-019-13078-5
Littlewood-Evans, A., Sarret, S., Apfel, V., Loesle, P., Dawson, J., Zhang, J., et al. (2016). GPR91 senses extracellular succinate released from inflammatory macrophages and exacerbates rheumatoid arthritis. J. Exp. Med. 213 (9), 1655–1662. doi:10.1084/jem.20160061
Liu, D., Xiao, W., Li, H., Zhang, Y., Yuan, S., Li, C., et al. (2023). Discovery of itaconate-mediated lysine acylation. J. Am. Chem. Soc. 145, 12673–12681. doi:10.1021/jacs.3c02332
Liu, H., Fan, H., He, P., Zhuang, H., Liu, X., Chen, M., et al. (2022). Prohibitin 1 regulates mtdna release and downstream inflammatory responses. EMBO J. 41, e111173. doi:10.15252/embj.2022111173
Liu, X., Shi, B., Suo, R., Xiong, S., Wang, X., Liang, X., et al. (2021). Itaconate regulates macrophage function through stressful iron-sulfur cluster disrupting and iron metabolism rebalancing. FASEB J. 35, e21936. doi:10.1096/fj.202100726rr
Luo, J.-F., Shen, X.-Y., Lio, C. K., Dai, Y., Cheng, C.-S., Liu, J.-X., et al. (2018). Activation of nrf2/HO-1 pathway by nardochinoid C inhibits inflammation and oxidative stress in lipopolysaccharide-stimulated macrophages. Front. Pharmacol. 9, 911. doi:10.3389/fphar.2018.00911
Maassen, S., Coenen, B., Ioannidis, M., Harber, K., Grijpstra, P., Van den Bossche, J., et al. (2023). Itaconate promotes a wound resolving phenotype in pro-inflammatory macrophages. Redox Biol. 59, 102591. doi:10.1016/j.redox.2022.102591
Maltby, V. E., Lea, R. A., Ribbons, K. A., Sanders, K. A., Kennedy, D., Min, M., et al. (2018). DNA methylation changes in CD4+ T cells isolated from multiple sclerosis patients on dimethyl fumarate. Multiple Scler. J. - Exp. Transl. Clin. 4, 205521731878782. doi:10.1177/2055217318787826
McArthur, K., Whitehead, L. W., Heddleston, J. M., Li, L., Padman, B. S., Oorschot, V., et al. (2018). Bak/Bax macropores facilitate mitochondrial herniation and mtdna efflux during apoptosis. Science 359, eaao6047. doi:10.1126/science.aao6047
McFadden, B. A., and Purohit, S. (1977). Itaconate, an isocitrate lyase-directed inhibitor in pseudomonas Indigofera. J. Bacteriol. 131, 136–144. doi:10.1128/jb.131.1.136-144.1977
McGuire, V. A., Ruiz-Zorrilla Diez, T., Emmerich, C. H., Strickson, S., Ritorto, M. S., Sutavani, R. V., et al. (2016). Dimethyl fumarate blocks pro-inflammatory cytokine production via inhibition of TLR induced M1 and K63 Ubiquitin Chain Formation. Sci. Rep. 6, 31159. doi:10.1038/srep31159
Meissner, M., Valesky, E. M., Kippenberger, S., and Kaufmann, R. (2012). Dimethyl fumarate - only an anti-psoriatic medication? JDDG J. der Deutschen Dermatologischen Gesellschaft 10, 793–801. doi:10.1111/j.1610-0387.2012.07996.x
Mills, E. L., Ryan, D. G., Prag, H. A., Dikovskaya, D., Menon, D., Zaslona, Z., et al. (2018). Itaconate is an anti-inflammatory metabolite that activates Nrf2 via alkylation of KEAP1. Nature 556, 113–117. doi:10.1038/nature25986
Monfort-Ferré, D., Caro, A., Menacho, M., Martí, M., Espina, B., Boronat-Toscano, A., et al. (2022). The gut microbiota metabolite succinate promotes adipose tissue browning in crohn’s disease. J. Crohn’s Colitis 16, 1571–1583. doi:10.1093/ecco-jcc/jjac069
Moreno, C., Santos, R. M., Burns, R., and Zhang, W. C. (2020). Succinate dehydrogenase and ribonucleic acid networks in cancer and other diseases. Cancers 12, 3237. doi:10.3390/cancers12113237
Muhammad, J. S., Jayakumar, M. N., Elemam, N. M., Venkatachalam, T., Raju, T. K., Hamoudi, R. A., et al. (2019). Gasdermin D hypermethylation inhibits pyroptosis and LPS-induced il-1β release from NK92 cells. ImmunoTargets Ther. 8, 29–41. doi:10.2147/itt.s219867
Nakahira, K., Haspel, J. A., Rathinam, V. A., Lee, S.-J., Dolinay, T., Lam, H. C., et al. (2010). Autophagy proteins regulate innate immune responses by inhibiting the release of mitochondrial DNA mediated by the NALP3 inflammasome. Nat. Immunol. 12, 222–230. doi:10.1038/ni.1980
Neel, D. V., Basu, H., Gunner, G., Bergstresser, M. D., Giadone, R. M., Chung, H., et al. (2023). Gasdermin-e mediates mitochondrial damage in axons and neurodegeneration. Neuron 111, 1222–1240.e9. doi:10.1016/j.neuron.2023.02.019
Olagnier, D., Farahani, E., Thyrsted, J., Blay-Cadanet, J., Herengt, A., Idorn, M., et al. (2020). SARS-cov2-mediated suppression of Nrf2-signaling reveals potent antiviral and anti-inflammatory activity of 4-octyl-itaconate and dimethyl fumarate. Nat. Commun. 11, 4938. doi:10.1038/s41467-020-18764-3
ONeill, L. A., Kishton, R. J., and Rathmell, J. (2016). A guide to immunometabolism for immunologists. Nat. Rev. Immunol. 16 (9), 553–565. doi:10.1038/nri.2016.70
Pan, X., Shan, H., Bai, J., Gao, T., Chen, B., Shen, Z., et al. (2022). Four-octyl itaconate improves osteoarthritis by enhancing autophagy in chondrocytes via PI3K/AKT/mtor signalling pathway inhibition. Commun. Biol. 5, 641. doi:10.1038/s42003-022-03592-6
Patrushev, M., Kasymov, V., Patrusheva, V., Ushakova, T., Gogvadze, V., and Gaziev, A. (2004). Mitochondrial permeability transition triggers the release of mtdna fragments. Cell. Mol. Life Sci. 61, 3100–3103. doi:10.1007/s00018-004-4424-1
Peng, H., Guerau-de-Arellano, M., Mehta, V. B., Yang, Y., Huss, D. J., Papenfuss, T. L., et al. (2012). Dimethyl fumarate inhibits dendritic cell maturation via nuclear factor ΚB (NF-ΚB) and extracellular signal-regulated kinase 1 and 2 (ERK1/2) and mitogen stress-activated kinase 1 (MSK1) signaling. J. Biol. 287, 28017–28026. doi:10.1074/jbc.M112.383380
Peng, X., Su, S., Zeng, J., Xie, K., Yang, X., Xian, G., et al. (2022). 4-octyl itaconate suppresses the osteogenic response in aortic valvular interstitial cells via the NRF2 pathway and alleviates aortic stenosis in mice with direct wire injury. Free Radic. Biol. Med. 188, 404–418. doi:10.1016/j.freeradbiomed.2022.06.246
Pisetsky, D. S. (2023). Pathogenesis of autoimmune disease. Nat. Rev. Nephrol. 19, 509–524. doi:10.1038/s41581-023-00720-1
Qi, H., Ning, X., Yu, C., Ji, X., Jin, Y., McNutt, M. A., et al. (2019). Succinylation-dependent mitochondrial translocation of PKM2 promotes cell survival in response to nutritional stress. Cell Death Dis. 10, 170. doi:10.1038/s41419-018-1271-9
Ren, K., Lv, Y., Zhuo, Y., Chen, C., Shi, H., Guo, L., et al. (2016). Suppression of IRG-1 reduces inflammatory cell infiltration and lung injury in respiratory syncytial virus infection by reducing production of reactive oxygen species. J. Virology 90, 7313–7322. doi:10.1128/jvi.00563-16
Rittenhouse, J. W., and McFadden, B. A. (1974). Inhibition of isocitrate lyase from pseudomonas Indigofera by itaconate. Archives Biochem. Biophysics 163, 79–86. doi:10.1016/0003-9861(74)90456-1
Rongvaux, A., Jackson, R., Harman, C. C. D., Li, T., West, A. P., de Zoete, M. R., et al. (2014). Apoptotic caspases prevent the induction of type I interferons by mitochondrial DNA. Cell 159, 1563–1577. doi:10.1016/j.cell.2014.11.037
Rubic, T., Lametschwandtner, G., Jost, S., Hinteregger, S., Kund, J., Carballido-Perrig, N., et al. (2008). Triggering the succinate receptor GPR91 on dendritic cells enhances immunity. Nat. Immunol. 9, 1261–1269. doi:10.1038/ni.1657
Ruetz, M., Campanello, G. C., Purchal, M., Shen, H., McDevitt, L., Gouda, H., et al. (2019). Itaconyl-COA forms a stable biradical in methylmalonyl-COA mutase and derails its activity and Repair. Science 366, 589–593. doi:10.1126/science.aay0934
Runtsch, M. C., Angiari, S., Hooftman, A., Wadhwa, R., Zhang, Y., Zheng, Y., et al. (2022). Itaconate and itaconate derivatives target JAK1 to suppress alternative activation of macrophages. Cell Metab. 34, 487–501.e8. doi:10.1016/j.cmet.2022.02.002
Ryan, D. G., Knatko, E. V., Casey, A. M., Hukelmann, J. L., Dayalan Naidu, S., Brenes, A. J., et al. (2022). Nrf2 activation reprograms macrophage intermediary metabolism and suppresses the type I interferon response. iScience 25, 103827. doi:10.1016/j.isci.2022.103827
Ryan, T. A., Hooftman, A., Rehill, A. M., Johansen, M. D., Brien, E. C., Toller-Kawahisa, J. E., et al. (2023). Dimethyl fumarate and 4-octyl itaconate are anticoagulants that suppress tissue factor in macrophages via inhibition of type I Interferon. Nat. Commun. 14, 3513. doi:10.1038/s41467-023-39174-1
Sangineto, M., Ciarnelli, M., Cassano, T., Radesco, A., Moola, A., Bukke, V. N., et al. (2023). Metabolic reprogramming in inflammatory microglia indicates a potential way of targeting inflammation in alzheimer’s disease. Redox Biol. 66, 102846. doi:10.1016/j.redox.2023.102846
Schulze-Topphoff, U., Varrin-Doyer, M., Pekarek, K., Spencer, C. M., Shetty, A., Sagan, S. A., et al. (2016). Dimethyl fumarate treatment induces adaptive and innate immune modulation independent of NRF2. Proc. Natl. Acad. Sci. 113, 4777–4782. doi:10.1073/pnas.1603907113
Schweckendiek, W. (1966). Behandlung von psoriasis mit lipoidloslichen fumarsaureverbindungen. Med. heute, 219–220.
Shi, F., Ni, S., Luo, S., Hu, B., Xu, R., Liu, S., et al. (2022). Dimethyl fumarate ameliorates autoimmune hepatitis in mice by blocking NLRP3 inflammasome activation. Int. Immunopharmacol. 108, 108867. doi:10.1016/j.intimp.2022.108867
Shi, F., Yuan, L., Wong, T., Li, Q., Li, Y., Xu, R., et al. (2023). Dimethyl fumarate inhibits necroptosis and alleviates systemic inflammatory response syndrome by blocking the RIPK1-ripk3-MLKL axis. Pharmacol. Res. 189, 106697. doi:10.1016/j.phrs.2023.106697
Shimada, K., Crother, T. R., Karlin, J., Dagvadorj, J., Chiba, N., Chen, S., et al. (2012). Oxidized mitochondrial DNA activates the NLRP3 inflammasome during apoptosis. Immunity 36, 401–414. doi:10.1016/j.immuni.2012.01.009
Smith, M. D., Martin, K. A., Calabresi, P. A., and Bhargava, P. (2017). Dimethyl fumarate alters B-cell memory and cytokine production in ms patients. Ann. Clin. Transl. Neurology 4, 351–355. doi:10.1002/acn3.411
Sohail, A., Iqbal, A. A., Sahini, N., Chen, F., Tantawy, M., Waqas, S. F. H., et al. (2022). Itaconate and derivatives reduce interferon responses and inflammation in influenza A virus infection. PLOS Pathog. 18, e1010219. doi:10.1371/journal.ppat.1010219
Song, S., Andrikopoulos, S., Filippis, C., Thorburn, A. W., Khan, D., and Proietto, J. (2001). Mechanism of fat-induced hepatic gluconeogenesis: effect of metformin. Am. J. Physiology-Endocrinology Metabolism 281, E275–E282. doi:10.1152/ajpendo.2001.281.2.e275
Stumvoll, M., Nurjhan, N., Perriello, G., Dailey, G., and Gerich, J. E. (1995). Metabolic effects of metformin in non-insulin-dependent diabetes mellitus. N. Engl. J. Med. 333, 550–554. doi:10.1056/nejm199508313330903
Su, C., Cheng, T., Huang, J., Zhang, T., and Yin, H. (2023). 4-octyl itaconate restricts sting activation by blocking its palmitoylation. Cell Rep. 42, 113040. doi:10.1016/j.celrep.2023.113040
Sun, G., Zhang, R., Liu, C., Meng, W., and Pang, Q. (2022). Itaconate attenuates neuroinflammation and exerts dopamine neuroprotection in Parkinson’s disease through inhibiting NLRP3 inflammasome. Brain Sci. 12, 1255. doi:10.3390/brainsci12091255
Sun, X., Zhang, B., Pan, X., Huang, H., Xie, Z., Ma, Y., et al. (2019). Octyl itaconate inhibits osteoclastogenesis by suppressing hrd1 and activating Nrf2 signaling. FASEB J. 33, 12929–12940. doi:10.1096/fj.201900887rr
Tannahill, G. M., Curtis, A. M., Adamik, J., Palsson-McDermott, E. M., McGettrick, A. F., Goel, G., et al. (2013). Succinate is an inflammatory signal that induces IL-1β through HIF-1α. Nature 496, 238–242. doi:10.1038/nature11986
Tian, F., Wang, Z., He, J., Zhang, Z., and Tan, N. (2020). 4-octyl ITACONATE protects against renal fibrosis via inhibiting TGF-β/SMAD pathway, autophagy and reducing generation of reactive oxygen species. Eur. J. Pharmacol. 873, 172989. doi:10.1016/j.ejphar.2020.172989
Tigano, M., Vargas, D. C., Tremblay-Belzile, S., Fu, Y., and Sfeir, A. (2021). Nuclear sensing of breaks in mitochondrial DNA enhances immune surveillance. Nature 591, 477–481. doi:10.1038/s41586-021-03269-w
Tumurkhuu, G., Shimada, K., Dagvadorj, J., Crother, T. R., Zhang, W., Luthringer, D., et al. (2016). ogg1 -dependent DNA repair regulates NLRP3 inflammasome and prevents atherosclerosis. Circulation Res. 119, e76–e90. doi:10.1161/circresaha.116.308362
Velcicky, J., Wilcken, R., Cotesta, S., Janser, P., Schlapbach, A., Wagner, T., et al. (2020). Discovery and optimization of novel SUCNR1 inhibitors: design of zwitterionic derivatives with a salt bridge for the improvement of Oral Exposure. J. Med. Chem. 63, 9856–9875. doi:10.1021/acs.jmedchem.0c01020
Waqas, F. H., Shehata, M., Elgaher, W. A., Lacour, A., Kurmasheva, N., Begnini, F., et al. (2023). NRF2 activators inhibit influenza A virus replication by interfering with nucleo-cytoplasmic export of viral rnps in an Nrf2-independent manner. PLOS Pathog. 19, e1011506. doi:10.1371/journal.ppat.1011506
Weiss, J. M., Palmieri, E. M., Gonzalez-Cotto, M., Bettencourt, I. A., Megill, E. L., Snyder, N. W., et al. (2023). Itaconic acid underpins hepatocyte lipid metabolism in non-alcoholic fatty liver disease in male mice. Nat. Metab. 5, 981–995. doi:10.1038/s42255-023-00801-2
Wentzel, J. F., Lewies, A., Bronkhorst, A. J., van Dyk, E., du Plessis, L. H., and Pretorius, P. J. (2017). Exposure to high levels of fumarate and succinate leads to apoptotic cytotoxicity and altered global DNA methylation profiles in vitro. Biochimie 135, 28–34. doi:10.1016/j.biochi.2017.01.004
West, A. P., Khoury-Hanold, W., Staron, M., Tal, M. C., Pineda, C. M., Lang, S. M., et al. (2015). Mitochondrial DNA stress primes the antiviral innate immune response. Nature 520, 553–557. doi:10.1038/nature14156
Wheaton, W. W., Weinberg, S. E., Hamanaka, R. B., Soberanes, S., Sullivan, L. B., Anso, E., et al. (2014). Metformin inhibits mitochondrial complex I of cancer cells to reduce tumorigenesis. eLife 3, e02242. doi:10.7554/elife.02242
White, M. J., McArthur, K., Metcalf, D., Lane, R. M., Cambier, J. C., Herold, M. J., et al. (2014). Apoptotic caspases suppress mtdna-induced sting-mediated type I IFN production. Cell 159, 1549–1562. doi:10.1016/j.cell.2014.11.036
Wu, J.-Y., Huang, T.-W., Hsieh, Y.-T., Wang, Y.-F., Yen, C.-C., Lee, G.-L., et al. (2020). Cancer-derived succinate promotes macrophage polarization and cancer metastasis via succinate receptor. Mol. Cell 77, 213–227.e5. doi:10.1016/j.molcel.2019.10.023
Wu, Q., Wang, Q., Mao, G., Dowling, C. A., Lundy, S. K., and Mao-Draayer, Y. (2017). Dimethyl fumarate selectively reduces memory T cells and shifts the balance between th1/th17 and th2 in multiple sclerosis patients. J. Immunol. 198, 3069–3080. doi:10.4049/jimmunol.1601532
Wu, R., Liu, J., Wang, N., Zeng, L., Yu, C., Chen, F., et al. (2022). Aconitate decarboxylase 1 is a mediator of polymicrobial sepsis. Sci. Transl. Med. 14, eabo2028. doi:10.1126/scitranslmed.abo2028
Wu, Y., Xu, W., Zheng, L., Wang, S., Wei, J., Liu, M., et al. (2023b). 4-octyl itaconate ameliorates alveolar macrophage pyroptosis against Ards via rescuing mitochondrial dysfunction and suppressing the CGAS/sting pathway. Int. Immunopharmacol. 118, 110104. doi:10.1016/j.intimp.2023.110104
Wu, Y., Zhang, Y., Jiang, F., He, S., Zhang, Y., Chen, D., et al. (2023a). 4-OI ameliorates bleomycin-induced pulmonary fibrosis by activating Nrf2 and suppressing macrophage-mediated epithelial-mesenchymal transition. Inflamm. Res. 72, 1133–1145. doi:10.1007/s00011-023-01733-z
Xian, H., Watari, K., Sanchez-Lopez, E., Offenberger, J., Onyuru, J., Sampath, H., et al. (2022). Oxidized DNA fragments exit mitochondria via MPTP- and VDAC-dependent channels to activate NLRP3 inflammasome and Interferon signaling. Immunity 55, 1370–1385.e8. doi:10.1016/j.immuni.2022.06.007
Xiao, H., Dong, Y., Wan, D., Wan, J., Huang, J., Tang, L., et al. (2023). Injectable hydrogel loaded with 4-octyl itaconate enhances cartilage regeneration by regulating macrophage polarization. Biomaterials Sci. 11, 2445–2460. doi:10.1039/d2bm01894b
Xin, Y., Zou, L., and Lang, S. (2020). 4-octyl itaconate (4-OI) attenuates lipopolysaccharide-induced acute lung injury by suppressing PI3K/AKT/NF-ΚB signaling pathways in mice. Exp. Ther. Med. 21, 141. doi:10.3892/etm.2020.9573
Xu, J., Pan, H., Xie, X., Zhang, J., Wang, Y., and Yang, G. (2018). Inhibiting succinate dehydrogenase by dimethyl malonate alleviates brain damage in a rat model of cardiac arrest. Neuroscience 393, 24–32. doi:10.1016/j.neuroscience.2018.09.041
Xu, J., Zheng, Y., Zhao, Y., Zhang, Y., Li, H., Zhang, A., et al. (2022). Succinate/IL-1β signaling axis promotes the inflammatory progression of endothelial and exacerbates atherosclerosis. Front. Immunol. 13, 817572. doi:10.3389/fimmu.2022.817572
Xu, L., Cai, J., Li, C., Yang, M., Duan, T., Zhao, Q., et al. (2023). 4-octyl itaconate attenuates LPS-induced acute kidney injury by activating Nrf2 and inhibiting STAT3 signaling. Mol. Med. 29, 58. doi:10.1186/s10020-023-00631-8
Xu, L., Zhou, J., Che, J., Wang, H., Yang, W., Zhou, W., et al. (2021). Mitochondrial DNA enables AIM2 inflammasome activation and hepatocyte pyroptosis in nonalcoholic fatty liver disease. Am. J. Physiology-Gastrointestinal Liver Physiology 320, G1034–G1044. doi:10.1152/ajpgi.00431.2020
Yang, W., Wang, Y., Wang, T., Li, C., Shi, L., Zhang, P., et al. (2023). Protective effects of IRG1/itaconate on acute colitis through the inhibition of gasdermins-mediated pyroptosis and inflammation response. Genes and Dis. 10, 1552–1563. doi:10.1016/j.gendis.2022.05.039
Yang, W., Wang, Y., Zhang, P., Wang, T., Li, C., Tong, X., et al. (2022). Hepatoprotective role of 4-octyl Itaconate in concanavalin a-induced autoimmune hepatitis. Mediat. Inflamm. 2022, 5766434–5766517. doi:10.1155/2022/5766434
Yang, Y., Shao, R., Tang, L., Li, L., Zhu, M., Huang, J., et al. (2019). Succinate dehydrogenase inhibitor dimethyl malonate alleviates lps/d-galactosamine-induced acute hepatic damage in mice. Innate Immun. 25, 522–529. doi:10.1177/1753425919873042
Ye, M., Zhao, Y., Wang, Y., Xie, R., Tong, Y., Sauer, J.-D., et al. (2022). NAD(H)-loaded nanoparticles for efficient sepsis therapy via modulating immune and vascular homeostasis. Nat. Nanotechnol. 17, 880–890. doi:10.1038/s41565-022-01137-w
Yi, Z., Deng, M., Scott, M. J., Fu, G., Loughran, P. A., Lei, Z., et al. (2020). Immune-responsive gene 1/itaconate activates nuclear factor erythroid 2–related factor 2 in hepatocytes to protect against liver ischemia–reperfusion injury. Hepatology 72, 1394–1411. doi:10.1002/hep.31147
Yin, M., and ONeill, L. A. (2021). The role of the electron transport chain in immunity. FASEB J. 35, e21974. doi:10.1096/fj.202101161r
Yin, N., Zhang, W., Sun, X.-X., Wei, R., Yang, Q., He, F., et al. (2023). Artificial cells delivering itaconic acid induce anti-inflammatory memory-like macrophages to reverse acute liver failure and prevent reinjury. Cell Rep. Med. 4, 101132. doi:10.1016/j.xcrm.2023.101132
Yu, C.-H., Davidson, S., Harapas, C. R., Hilton, J. B., Mlodzianoski, M. J., Laohamonthonkul, P., et al. (2020). TDP-43 triggers mitochondrial DNA release via MPTP to activate CGAS/sting in Als. Cell 183, 636–649. doi:10.1016/j.cell.2020.09.020
Zecchini, V., Paupe, V., Herranz-Montoya, I., Janssen, J., Wortel, I. M., Morris, J. L., et al. (2023). Fumarate induces vesicular release of mtdna to drive innate immunity. Nature 615, 499–506. doi:10.1038/s41586-023-05770-w
Zeng, Y.-R., Song, J.-B., Wang, D., Huang, Z.-X., Zhang, C., Sun, Y.-P., et al. (2023). The immunometabolite ITACONATE stimulates OXGR1 to promote mucociliary clearance during the pulmonary innate immune response. J. Clin. Investigation 133, e160463. doi:10.1172/jci160463
Zhang, P., Wang, X., Peng, Q., Jin, Y., Shi, G., Fan, Z., et al. (2022a). Four-octyl Itaconate protects chondrocytes against H2O2-induced oxidative injury and attenuates osteoarthritis progression by activating Nrf2 signaling. Oxidative Med. Cell. Longev. 2022, 2206167–2206213. doi:10.1155/2022/2206167
Zhang, Q., Bai, X., Wang, R., Zhao, H., Wang, L., Liu, J., et al. (2022). 4-Octyl itaconate inhibits lipopolysaccharide (LPS)-induced osteoarthritis via activating Nrf2 signalling pathway. J. Cell. Mol. Med. 26, 1515–1529. doi:10.1111/jcmm.17185
Zhang, Z., Chen, C., Yang, F., Zeng, Y.-X., Sun, P., Liu, P., et al. (2022b). Itaconate is a lysosomal inducer that promotes antibacterial innate immunity. Mol. Cell 82, 2844–2857.e10. doi:10.1016/j.molcel.2022.05.009
Zhao, J., Xu, S., and Liu, J. (2019). Fibrinopeptide a induces c-reactive protein expression through the ros-erk1/2/p38-nf-κb signal pathway in the human umbilical vascular endothelial cells. J. Cell. Physiology 234, 13481–13492. doi:10.1002/jcp.28027
Zheng, Z., Chen, H., Li, J., Li, T., Zheng, B., Zheng, Y., et al. (2011). Sirtuin 1–mediated cellular metabolic memory of high glucose via the LKB1/AMPK/Ros pathway and therapeutic effects of metformin. Diabetes 61, 217–228. doi:10.2337/db11-0416
Zhong, Z., Liang, S., Sanchez-Lopez, E., He, F., Shalapour, S., Lin, X., et al. (2018). New mitochondrial DNA synthesis enables NLRP3 inflammasome activation. Nature 560, 198–203. doi:10.1038/s41586-018-0372-z
Keywords: immunometabolism, mitochondrial nucleotide release, succinate, itaconate, fumarate, mtDNA, mtRNA
Citation: Min Y and O’Neill LAJ (2023) Targeting mitochondrial metabolites and nucleic acids as an anti-inflammatory strategy. Front. Drug Discov. 3:1294454. doi: 10.3389/fddsv.2023.1294454
Received: 14 September 2023; Accepted: 29 November 2023;
Published: 15 December 2023.
Edited by:
Ahmed Sheriff, Charité University Medicine Berlin, GermanyReviewed by:
Sambit Kumar Nanda, AstraZeneca, United StatesFélix A. Urra, University of Chile, Chile
Copyright © 2023 Min and O’Neill. This is an open-access article distributed under the terms of the Creative Commons Attribution License (CC BY). The use, distribution or reproduction in other forums is permitted, provided the original author(s) and the copyright owner(s) are credited and that the original publication in this journal is cited, in accordance with accepted academic practice. No use, distribution or reproduction is permitted which does not comply with these terms.
*Correspondence: Luke A. J. O’Neill, bGFvbmVpbGxAdGNkLmll