- 1Department of Chemical and Biological Engineering, The Hong Kong University of Science and Technology, Hong Kong, China
- 2Institute for Nanoscience and Nanotechnology, Sharif University of Technology, Tehran, Iran
The eye is a hard-to-treat organ due to its poor regenerative capacity and susceptibility to inflammation; as a result, it has an immune privilege mechanism. In the case of ocular degenerative disorders, chronic and uncontrolled ocular inflammations can overcome this immune response to initiate and exacerbate tissue degeneration, ultimately leading to blindness. Recent landmark discoveries on the key roles of the ocular innate immune system in regulating acute and chronic inflammations as well as tissue fibrosis and homeostasis have shed light on the value of novel treatment interventions in modulating ocular immune responses at the molecular, cellular, and tissue levels. This strategy can be attained by using therapeutics to target resident phagocytes and antigen-presenting cells, namely, microglia and dendritic cells, as well as infiltrating neutrophils and macrophages. Biomaterials are foreign materials to the host and interact with innate immune cells. To leverage such intrinsic immunomodulatory properties, biomaterials such as implants, injectable depots, and nano/micro particles can be used alone as a treatment or with different payloads as carriers in immune-related ocular disorders. This article discusses how physicochemical properties such as biodegradability, size, shape, and charge affect biomaterials’ interaction with the eye’s innate immune system, therefore influencing outcomes towards pro- or anti-inflammatory responses. Knowledge about the eye’s immunological response is required for designing tolerogenic biomaterials including intraocular lenses, cellular scaffolds, therapeutic molecule depots, or carriers of gene therapies. The discussion presented in this review will shed light on the potential use of biomaterials to direct immune responses toward favorable treatment outcomes.
1 Introduction
Since eye tissue, which serves as our window to the outside world, lacks the ability to regenerate, immune-related inflammation poses a serious risk to the eye by potentially damaging its tissue, leading to vision loss. As a result, ocular tissue employs an immune privilege strategy to actively prevent any inflammation by providing an immunosuppressive environment (Niederkorn, 2019; Murakami et al., 2020). The term “immune privilege” comes from Medawar and others’ definition in the 1940s (Medawar, 1948; Taylor, 2016), where the ocular anti-inflammatory mechanism of having a private microenvironment for improving the anti-inflammatory response and tolerating the ocular immune cell’s function and balancing it was discussed. Eye tissue, in homeostatic conditions, has an immunoregulatory function (Dick et al., 2003). This inherent ocular immune tolerance, however, is compromised by several degenerative disorders including uveitis, diabetic retinopathy (DR), dry eye disease (DED), age-related macular degeneration (AMD), and choroidal neovascularization (CNV) (Perez and Caspi, 2015; Murakami et al., 2020; Gilger and Hirsch, 2022).
The body’s immune system response battles danger agents in two ways: the innate immune response and the adaptive immune response (Dempsey et al., 2003). In this way, the innate immune system acts as a first defender during the first days of the inflammation process or injury. If the innate immune cells are activated and do not regulate by proper signaling pathways after treatment, chronic inflammation and even visual impairment may occur (Murakami et al., 2020).
Different innate immune cells, including neutrophils, microglia/macrophages, and dendritic cells in the eye, play essential roles in the aforementioned types of diseases. The infiltration and activity of innate immune cells change depending on the type of ocular disorder, its location, and the milieu supplied by the cells (reviewed in detail by (Murakami et al. (2020)), in the form of inflammatory induction or suppression. When eyes are infected or injured, different proinflammatory cytokines and chemokines (such as tumor necrosis factor-alpha (TNF-
The biocompatibility of a material is defined by William’s dictionary as “the ability of a material to perform with an appropriate host response in a specific application” (Donaruma, 1988; Williams, 1999). Biomaterials can act alone as a treatment or can be combined with other modalities to provide therapeutic intervention. The roles of biomaterials in immunomodulation in ocular therapy can be classified as follows. The first approach is to use biomaterials to construct carriers of therapeutic agents while the biomaterial itself is intended to passively and inertly affect the immune response (Garzón et al., 2022). The second approach is similar but more intriguing. Also for constructing carriers, the biomaterial is designed to interact with the immune system, to promote the immunogenic or immunotolerant effect, depending on the application, and to augment the effect of the cargo (Im, 2020; Gao et al., 2022). For these two approaches, the immunomodulatory cargoes include steroids, proteins, and nucleic acids. In a third approach, biomaterials are used to make ophthalmic devices such as ocular lenses and ocular inserts (Kwon et al., 2020). The functions and longevities of these devices in part depend on their interaction with the immune system. The last approach is to use biomaterials to support cell therapy. There are several reviews on the design criteria of immunomodulatory biomaterials in tissue engineering (Zolnik et al., 2010; Andorko and Jewell, 2017; Li H. et al., 2022; Mitarotonda et al., 2022). One possible therapeutic intervention is to direct the response of immune cells by using immunomodulatory biomaterials alone or in combination with engineered cells. An overview of these approaches is summarized in Figure 1. The biomaterials can be designed to participate in the immunogenic or immunosuppressive pathways based on their physicochemical properties such as form, size, shape, charge, hydrophilicity/hydrophobicity, degradability, and mechanical strength. In this review, we will focus on the interaction of biomaterials with the eye’s innate immune system, and consider how they can influence, positively or negatively, the treatment of inflammatory ocular diseases.
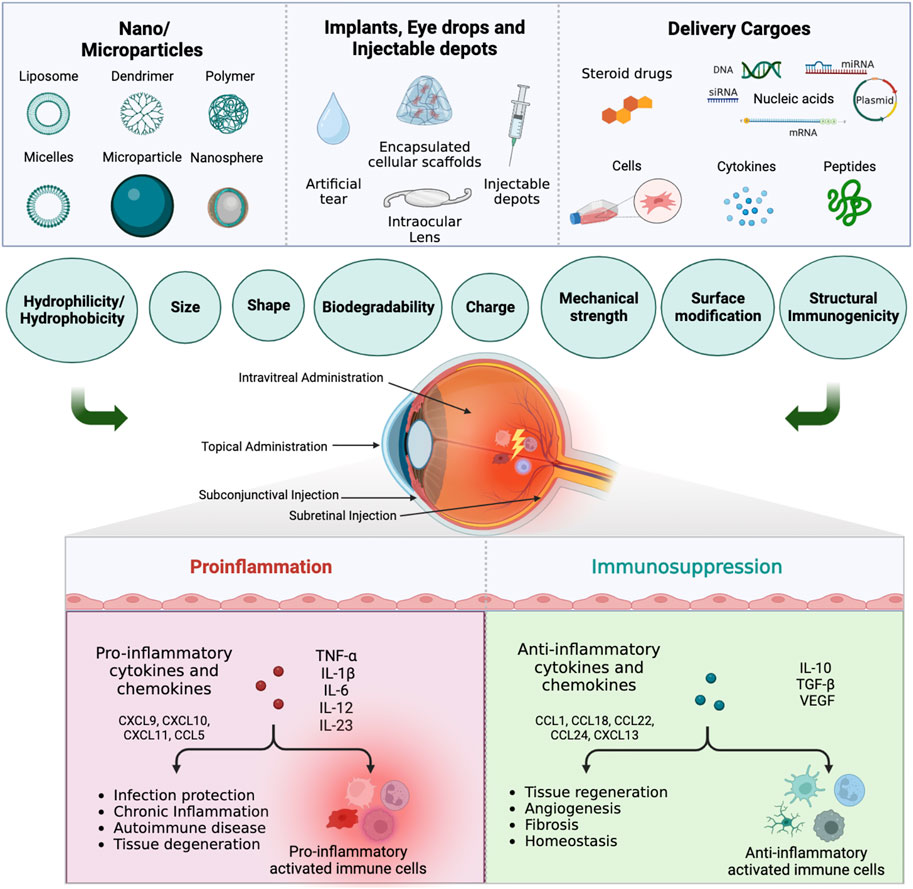
FIGURE 1. Immunomodulatory drug delivery systems for ocular innate immune cells immunotherapy. Biomaterials such as nanoparticles, microparticles, implants, eye drops, and injectable depots, with or without immunomodulatory cargoes, can be used to treat ocular diseases via different administration routes. Depending on the physiochemical properties, the biomaterials can promote or suppress the response from proinflammatory innate immune cells, which will in turn affect the therapeutic outcome (Created in BioRender.com).
2 The innate immune system in the eye
Eye tissue has been recognized as an immune-privileged organ for 150 years. The first long-term survival of mouse skin implanted into a dog eye’s anterior chamber was described by a Dutch ophthalmologist (van Dooremaal, 1873). Zirm carried out the first successful human corneal transplant a few years later, in 1905 (Zirm, 1906; Niederkorn, 2019). At the time of these observations, neither the human immune system nor the fundamentals of implant rejection had been studied. By inserting rabbit skin into an allogenic rabbit’s anterior chamber, Medawar further demonstrated in 1948 the distinct immunologic characteristics of the eye (Medawar, 1948). Immune privilege is an action in the homeostatic condition of the eye that modulates the induction and progression of inflammation to prevent any excessive inflammation that could degenerate tissue (Murakami et al., 2020). Ocular immune privilege consists of an immunosuppressive microenvironment with different components including transforming growth factor-β (TGF-
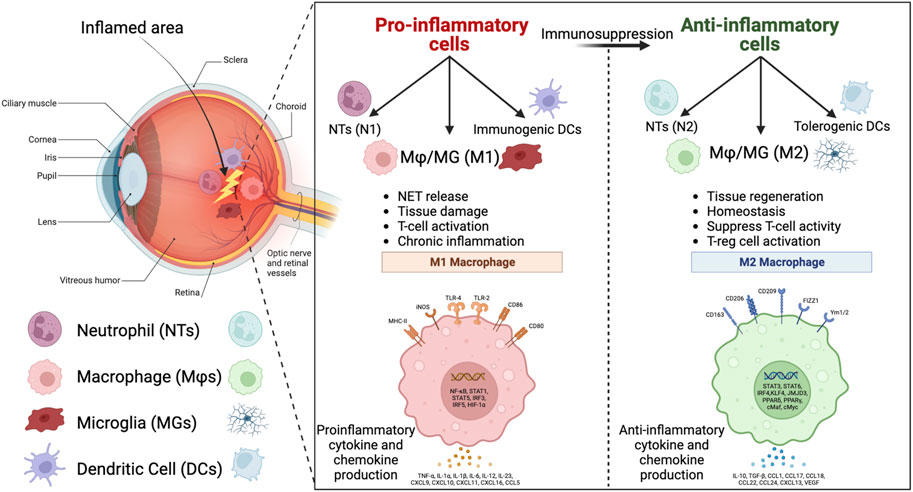
FIGURE 2. Eye tissue and the key innate immune cells in the inflamed area. The pro-inflammatory innate immune cells, including N1 neutrophils (NTs), M1 macrophages/microglia (MΦ/MG), and immunogenic dendritic cells (DCs), with proinflammatory functions that are listed in the left window, can be re-educated by immunomodulatory biomaterials to anti-inflammatory cells, including N2 NTs, M2 MΦ/MG, and tolerogenic DCs, with anti-inflammatory functions that are listed in the right window (Created in BioRender.com).
2.1 Key players as innate immune cells in the eye
2.1.1 Neutrophils
The main players in acute inflammation and the first line of fighting any danger, including pathogens, inflammation, and injury in the innate immune system, are neutrophils. Neutrophils’ lifespan has been shown to be very short (less than 24 h) in mice and humans, although recently, a number of studies showed that the lifespan of neutrophils can be increased by inflammatory cytokines and signals that induce the adaptive immune system and aggravate the inflammation for up to 5.4 days (Pillay et al., 2010; Kolaczkowska and Kubes, 2013). They are the first immune cells that detect any inflammation that occurs, by sensing the pathogen-associated molecular pattern (PAMP) present in microbes and the danger-associated molecular pattern (DAMP) present in injured tissues. Neutrophils play a critical role in the pro-inflammatory function through phagocytosis and by secreting pro-inflammatory cytokines to induce an innate and adaptive immune response (Ghosh et al., 2019). Neutrophils also release neutrophil extracellular traps (NETs). NETs are composed of nuclear DNA, histones, and other granular proteins. NETs are induced by nitric oxide, cytokines, and other autoantibodies to prevent inflammation progression. However, it has been shown that chronic aggregation of NETs can cause autoimmune, allergic, chronic diseases, tissue damage, and immune rejection to implants (Kolaczkowska and Kubes, 2013; Mahajan et al., 2021; Mun et al., 2021; Yıldız and Yıldız, 2022). For example, Mahajan et al. (2021) found that NETs aggregated after ocular surface inflammation, resulting in meibomian gland dysfunction (MGD) due to blockage. Although it has been shown that NETs are useful to prevent inflammation progression in the eye rheum, they are harmful to corneal diseases, uveitis, and diabetic retinopathy and contribute to poor prognosis of these diseases (Estúa-Acosta et al., 2019). Neutrophils perform diversely in inflammation and injury conditions by regulating acute inflammation and repair processes, autoimmune diseases, and chronic inflammatory diseases, based on the type of cytokines and special receptors that exist in the inflamed environment (Estúa-Acosta et al., 2019; Liew and Kubes, 2019; Tan et al., 2020). The signaling pathways of an inflamed environment can result in different phenotypes of neutrophils: N1, which represents the proinflammatory phase, and N2, which represents the anti-inflammatory phase (Cuartero et al., 2013; García-Culebras et al., 2018).
2.1.2 Microglia/macrophages
Macrophages are key players in the innate immune system, fighting any infection and danger in the tissue through phagocytosis and by inducing regeneration and fibrosis afterward. They function in a spectrum between the M1 proinflammatory phase and the M2 anti-inflammatory phase (Tan et al., 2020). The interferon-gamma (IFN-
Retina tissue in the eye possesses resident immune cells known as glial cells, which are categorized into three main groups: astrocytes, microglia, and Müller glia. Microglia cells are myeloid-derived populations in the retina that manage the immune response and environmental cues by interacting with retinal cells and maintaining the retinal cell’s homeostatic function and neuronal homeostasis (Tan et al., 2020; Guo et al., 2022; Wang and Cepko, 2022). They play a critical role in interacting with retinal cells and recruiting neutrophils and macrophages for further functions during eye injuries and inflammation (Minghetti et al., 2008; Tan et al., 2020). The resting state of microglia cells is achieved by intraretinal signaling and cytokines secretion such as TGF-
Microglia activation is linked to several inflammatory and degenerative ocular disorders, including retinitis pigmentosa (RP), AMD, glaucoma, DR, and uveitis in the eye. This is due to their status as resident immune cells in the retina, which makes them very sensitive to changes in cell phenotype, ligand-receptor interactions, and environmental signals. For instance, it has been shown that in experimental AMD, aging CX3CR1 deficiency causes microglia to migrate and accumulate in the subretinal space because it lacks the CX3CR-1-CX3CL1 complex, which physiologically maintains microglia homeostasis (Penfold et al., 2001; Minghetti et al., 2008). Arroba et al. studied the polarization dynamics of microglia in DR in in vitro and in vivo in mice and found that the microglia are initially in the M2 phase, but at more advanced stages of DR development, their phase shifts to M1 and chronic pro-inflammatory (Arroba et al., 1862). Since the progression of RP, AMD, glaucoma, DR, and uveitis has been related to the activation of microglia, these cell types are of great interest as a target for treating these diseases. There are two effective ways to accomplish this goal: either preventing their pro-inflammatory response or reprogramming them via gene therapy to downregulate the inflammatory response and immune cell recruitment (Arroba et al., 1862; Minghetti et al., 2008; Guo et al., 2022; Wang and Cepko, 2022).
The dynamics of microglia activation and repolarization to homeostasis, with Iba-1 (for M1 phase) and P2Y12 (for M2 phase) as markers, was monitored in an ocular hypertension (OHT)-induced glaucoma mouse model by Ramírez et al. (2020). The authors showed that at first (day 0), the damaged tissue released ATP signals and P2Y12 was upregulated. After a few hours, the P2Y12 expression was downregulated, and this decreasing signal was the most sensitive alarm for the transition from the resting to the M1 phase of microglia with Iba-1+. After 24 h, the Iba-1+ cells showed P2Y12 expression elevation. On the 3rd and 5th days, downregulation of P2Y12 expression was observed. Based on their collected data, the authors stated that the inflammation peaked and was strongest on the 3rd and 5th days after induction. Then, P2Y12 expression increased slowly from day 8 to reach the naïve eye amount on the 15th day for this animal model. The same group conducted a different time point investigation on the levels of proinflammatory cytokines (IFN-
2.1.3 Dendritic cells
Dendritic cells (DCs), similar to macrophages, are phagocytic and are able to present antigens to immune effector cells. The distinctive feature of DCs is their migration to lymph nodes to activate naïve lymphocytes, thereby bridging innate and adaptive immunity. Most DCs are found in the corneal and conjunctival epithelium, thereby serving as the first line of defense on the ocular surface. In the posterior ocular section, DCs can be mostly found in the retina and choroidal space (McMenamin et al., 2019). Once activated, most ocular DCs migrate to cervical lymph nodes to trigger downstream immune responses. There are several subtypes of DCs that can be found in the eye, such as conventional DCs (cDCs), Langerhans cells (LCs), plasmacytoid DCs (pDCs); they all play key roles in maintaining immune tolerance and resolving post-inflammation challenges.
In mice, cDCs can be classified into types 1 and 2. cDC1 can be identified as CD4−CD8α+CD11b-CD11c+ and cDC2 as CD4+CD8α-CD11b+CD11c+. cDCs recognize both extracellular and intracellular pathogens; of which cDC1 can efficiently cross-present exogenous antigens on MHC-I molecules to CD8+ T cells, whereas cDC2 activates CD4+ T cells, and trigger TH2 and TH17 immune responses. On the other hand, pDCs are distinguished from cDCs by the expression of CD45RA, lymphocyte antigen 6 complex (Ly6C), sialic acid binding immunoglobulin-like lectin H (Siglec-H), and CD317. These cells are important in anti-viral immunity and systemic autoimmunity, because they can sense intracellular self and non-self nucleic acids via TLRs pathways and produce type I and III interferons (Musumeci et al., 2019). In addition, LCs are another subtype of DC, which are considered to be potent APC. Under physiological conditions, their population frequency is 5%. However, during a disease/inflammation-challenged state, their population is replenished by infiltrating monocytes and 10%–20% of LCs migrate to the lymph nodes (Merad et al., 2008). LCs play a crucial role in maintaining immune resolution post-infection and tolerance. In the disease context of allergic contact dermatitis, it was observed that LCs can effectively induce anergy and apoptosis of CD8+ T cells while activating ICOS+ CD4+ FoxP3+ Treg cells (Kaplan et al., 2005).
Resident DCs are considered “immature” during the resting state. During the pathogen infection/inflammation state, DCs undergo maturation, expressing higher level of MHC-molecules, the co-stimulatory molecules CD86, CD83, CD40, and producing pro-inflammatory cytokines such as IL-12, IL-6, and TNF-α. Indeed, the activation of CD86+ DC participated in the onset and progression of dry eye disease (Maruoka et al., 2018). In addition, DCs were also found to promote the progression of anterior uveitis (Lin et al., 2019).
Under physiological conditions, ocular cells can actively participate in the suppression of DC immunogenic activation to maintain an anti-inflammatory state in the ocular environment. For instance, retinal pigment epithelial cells can produce IL-1Ra to suppress DC activation. Corneal stromal cells can produce TGF-β1 to inhibit DC activity while promoting corneal wound healing (Hamrah et al., 2003; Lu et al., 2012; Sugita et al., 2013; Morante-Palacios et al., 2021). In fact, DCs can also be activated into tolerogenic phenotypes. These DCs can produce anti-inflammatory cytokines such as IL-10, TGF-β, and indoleamine 2, 3-dioxygenase (IDO) to suppress T-cell activity and function in maintaining ocular tolerance. Tolerogenic DCs can be generated in vitro with the co-culture of stromal cells or by treatment with immunosuppressive agents such as IL-10, TGF-β, vitamin D receptor agonists, and vasoactive intestinal peptide (VIP) (Dempsey et al., 2003; Hu and Wan, 2011; Haneklaus et al., 2012; Perez and Caspi, 2015; Puri et al., 2022). Although tolerogenic DCs demonstrated therapeutic strengths in autoimmune diseases, the exploration of their potential in the ocular field is still under investigation.
3 Application of biomaterials in immunomodulation of the innate immune system
Biomaterials are widely used as carriers or scaffolds for ocular therapeutic entities, such as small molecular drugs, biomacromolecules such as proteins or nucleic acids and living cells. The carriers however have been known to actively interact with the immune system. The resulting host reaction as a result of the implanted materials is coined “foreign body response” (FBR). Biomaterial-induced FBR can lead to cargo clearance and elimination, countering therapeutic intentions. However, if properly gauged by appropriate material selection and design, the immune reaction can be utilized for pro-healing responses. Therefore, the design of biomaterials generally adopts strategies to 1) mitigate or 2) leverage FBR depending on the therapeutic goals in the eye. Indeed, this can be achieved with the high responsivity and plasticity of the above-mentioned innate immune cells in response to the exogenous biomaterials’ cues. With the appropriate selection of biomaterials and platform characteristics, these cells can be strategically manipulated and programmed toward a pro- or anti-immunomodulatory response to facilitate the overall therapeutic outcome.
Understanding the dynamics of FBR, that is, the biomaterials-innate immune cell interplay, is critical for this goal. The activation mechanism of innate immune cells by biomaterials is mainly governed by pattern-recognition receptors (PRRs). PRRs are present on the plasma membrane surface or in the cytoplasm and can sense a broad range of damage and pathogenic cues. PRRs consist of TLRs, nod-like receptors (NLRs), and inflammasomes. TLRs exist on the surface or in the endosomal compartment of antigen-presenting cells (APCs) including microglia, macrophages, and dendritic cells, and recognize a broad range of microbial molecules such as proteins, nucleic acids, and LPS, etc. NLRs are intracellular receptors with inflammasome subunits. Inflammasomes are the complex of these proteins, act as receptors of the innate immune system, and are responsible for the inflammatory response by cysteine-aspartic acid protease-1 (caspase-1) induction to produce the proinflammatory cytokines IL-1
The activation of PRRs begins with the detection of foreign patterns including DAMP and PAMP and the secretion of signaling pathways to combat harmful agents (Andorko and Jewell, 2017). PAMPs and DAMPs can take in diverse forms; for instance, polysaccharides, peptides, glycopeptides, lipopeptides, LPSs, and nucleic acids, etc., which are derived from microbes or damaged host cells fragments during invasion and tissue injuries. The eye’s innate immune system is extremely sensitive in detecting these molecular entities. Once the cells are activated, they secrete proinflammatory cytokines to initiate FBR. Similarly, the surfaces of biomaterials and delivery platforms also exhibit physicochemical features that resemble PAMPs. Therefore, FBR is inevitable with the introduction of ocular implants, injectable depots, and nano/micro particles. On the other hand, several TLRs are expressed by different ocular tissues, such as the cornea, conjunctiva, retinal pigment epithelial cells, and the uvea, to protect and isolate the eyeball from any specific PAMP (Wakefield et al., 2010). The uvea, for instance, is particularly susceptible to LPS-mediated TLR4, and acute anterior uveitis has been observed, based on TLR4 cell response to LPS (Yu and Hazlett, 2006). The ocular innate immune system, however, is also perceptive to anything that poses a threat to the host tissue through endogenous DAMPs. These molecules are released from the injured tissue or dead cells’ intracellular or extracellular regions including ATP, high mobility group protein B1 (HMGB1), and other molecules derived from injured tissue (Mahaling et al., 2022). According to the review by Mahaling et al. (2022)), these DAMPs are linked to inflammations brought on by age, increased ocular pressure, oxidative stress, ischemia, stress, and environmental factors, etc., in retinal illnesses.
A vast diversity of biomaterials has been explored and commercialized in ophthalmic drug delivery systems, mostly aiming to circumvent FBR. Conversely, with a deeper dive into the ocular immune response and biomaterial interplay and dissections, we can better understand the active role of biomaterials, and derive potential therapeutic approaches in ocular regenerative medicine and immune interventions. This can be achieved by targeting the FBR cascade, in terms of the distribution and timing of the key immune cellular and molecular players, using biomaterials and delivery devices. Moreover, learning from the natural defense mechanism against pathogens, the biomaterial polymer structure’s repeating units, size, hydrophobicity, and patterning can be fine-tuned to mitigate or engage innate immune cell activation (Kakizawa et al., 2017). In the next section, we discuss several biomaterial systems, such as implants and injectable depots, nano/micro particles, and hybrid systems, as well as their physiochemical requirements for directing ocular innate immune cells to the desired anti- or pro-inflammatory phenotypes.
3.1 Implants and injectable depots
FBR is inevitable with the introduction of ocular implants and injectable depots. In ocular therapy, biomaterials can be used to implant cells, create therapeutic implants, and create injectable depots. During the initial stage of FBR, the acute inflammatory response generates pro-inflammatory cytokines to promote inflammatory cell infiltration, extracellular matrix (ECM), vascular remodeling, and perfusion. This can lead to an increase in ocular pressure, oxidative stress, ischemia, stress, and retinal illnesses, all of which are detrimental to the ocular structure and function. The chronic inflammation process often leads to deposition of the collagenous matrix surrounding the implants, causing rejection. Particularly for the intraocular lens (IOL), intravitreal drug depots, retinal prostheses, other long-term ocular implants, and fibrotic encapsulation not only result in device wastage but also vision compromise (Anderson et al., 2008; Veiseh and Vegas, 2019). Therefore, the timing and dynamics of FBR are important targets for biomaterial development.
An anti-FBR approach is rather common in ocular applications, especially for extended, controlled delivery platforms and prostheses. Biomaterials and delivery platforms are often used in an “immune stealth” or anti-inflammatory context to inhibit immune recognition and suppress the deposition of proteins and cells; thereby extending the half-life and bioavailability of therapeutic entities. Polyethylene glycol (PEG) is one of the most popular stealth materials in suppressing non-specific protein deposition on hydrophilic polymer coated devices. In some applications, it is useful for the biomaterial to mimic the ECM of the tissue, in terms of biochemical and biomechanical properties, to suppress the immune activation. The major compositions of ocular ECM are hyaluronic acid and collagens. For this ECM-mimicking purpose, the use of ECM-derived components and analogs, such as hyaluronic acid, fibrin, and collagen, as well as decellularized tissue, has been extensively explored (Rowley et al., 2019). These materials are commonly used in the development of intravitreal implants and corneal grafts. Moreover, ECM-derived peptides, including arginyl-glycyl-aspartic acid (RGD), matrix metalloproteinase (MMP)-sensitive peptides, or leukocyte-associated immunoglobulin-like receptor-1 (LAIR-1) ligand, are also incorporated on synthetic materials to improve the biocompatibility of the implants (Rowley et al., 2019). The design parameters of biomaterials for the anti-FBR approach is further discussed in the following section.
3.1.1 Hydrogels and polymeric depots
Hydrogels are widely used as delivery devices and supporting scaffolds in the ocular field. Hydrogels are 3D crosslinked hydrophilic polymers. They can hold small hydrophilic molecular drugs, and bioactive molecules such as proteins and nucleic acids. They can protect labile cargoes from tissue clearance and enzymatic degradation. Controllable hydrogel architecture design and degradability can help provide spatial and temporally controlled release of cargoes. More importantly, hydrogels also possess mechanical and optical characteristics that are compatible with those of the eyes.
For anterior ocular treatments, hydrogels are commonly used in the preparation of artificial tears, corneal regeneration, and IOL fabrication to provide structural support. Non-etheless, implantation sites such as the ocular surface and epithelial and stromal regions are populated with innate immune cells. They are sensitive to environmental anomalies triggered by the implants, eye drops, and injectables, subsequently inducing a cascade inflammatory response. Indeed, corneal haze and posterior capsular opacification (PCO) are common post-surgical complications in artificial corneal and IOL transplants, respectively.
On the other hand, for the ocular posterior, hydrogels can be used as vitreous substitutes to regulate intraocular pressure and structures. Delivery depots can protect laden drugs and therapeutic cells while providing controlled release of the therapeutic molecules directly to the diseased sites, circumventing the blood-retinal barrier. With appropriate biomaterial selection, FBR can be suppressed. For instance, a single intravitreal injection of in-situ crosslinkable hyaluronate (HA)/dextran hydrogel, which was designed to provide sustained release of anti-VEGF for 6-months, showed no inflammation of the ocular structures (Yu et al., 2015; Yu et al., 2019). In addition, the prolonged presence of thermosensitive methoxy-poly (ethylene glycol)-block-poly (lactic-co-glycolic acid) (mPEG-PLGA-BOX) hydrogel in the vitreal region, providing extended release of anti-VEGF for 42 days, also avoided inflammation in the posterior ocular region (Hu et al., 2019). However, another study that adopted PolyActive™, degradable PEG-polybutylene terephthalate-based (PEG-PBT-based) microparticles, to deliver anti-VEGF, observed inflammation at both acute initial and later stages of the treatment, suggesting that degradation products from the microparticles could trigger immunogenic responses in the eye (Adamson et al., 2016). The co-delivery of immunosuppressive signals also inhibited FBR against the long-term device. For instance, the commercial intravitreal injectable Ozurdex® is a degradable poly (lactide-co-glycolide) (PLGA) matrix for extended release of dexamethasone to circumvent FBR and actively suppress inflammatory events of uveitis.
Therefore, it is crucial to maintain ocular immune tolerance, that is, anti-inflammatory and anti-FBR responses, in order to improve the lifetime and optimal performance of the delivery device (Allyn et al., 2022). The general design strategies of implants and injectables often aim to 1) reduce non-specific protein and cellular adhesion or design anti-fouling properties to circumvent FBR, 2) introduce a delivery system with tissue-matching properties, and 3) mask receptors associated with pro-inflammatory pathway activation for suppressing innate immune activations. In the first approach, biomaterials and delivery platforms are often used in an “immune stealth” or anti-inflammatory context to inhibit immune recognition and to suppress the deposition of proteins and cells, thereby extending the half-life and bioavailability of therapeutic entities. PEG is amongst the most popular stealth material in suppressing non-specific protein deposition on hydrophilic polymer-coated devices.
3.1.2 Encapsulated cell implants
3.1.2.1 Immunomodulatory cells
For decades, it has been demonstrated that mesenchymal stem cells (MSCs) are potential immunomodulators of the innate and adaptive immune systems (by inhibiting Th1 and Th17 and inducing the Treg and M2 macrophage phases) for various ocular inflammatory disorders such as corneal angiogenesis, neovascularization, autoimmune uveitis, and autoimmune DED (Lee et al., 2015; Song et al., 2018; Oh and Lee, 2021; Li Y. et al., 2022).
For targeted delivery to the eye, MSCs can be injected naked or encapsulated in a polymeric scaffold such as hydrogels to promote ocular tissue regeneration or suppress degeneration. The hydrogels can serve as a supporting matrix to separate the cells’ cargo from the host tissue. In addition, they will support cell growth and functions, while the meshwork can facilitate sufficient exchange of materials between implanted cells and their host tissues, ensuring that laden cells are functioning properly. The choice of biomaterial is critical in the second strategy, to achieve immunomodulatory and biocompatibility goals using different administration routes such as topical or contact lenses or injectable scaffolds.
3.1.2.2 Implants for encapsulating engineered cells
Based on previous works on several anti-inflammatory applications using MSCs and bandage contact lenses, the amniotic membrane (AM) is the most employed scaffold in the topical administration of MSCs in ocular surface therapy (Abu-Ain and Webber, 2010). AM exhibited remarkable anti-inflammatory and immunomodulatory effects (Parolini et al., 2009; Orozco Morales et al., 2019; Beeken et al., 2021). However, due to donor variability and the danger of disease transmission, this procedure lacks standard safety regulations. Synthetic and natural hydrogels can be employed as substitutes for AMs in this application. For instance, fibrin gel was used in a study as the carrier of rabbit MSCs and was transplanted on the surface of damaged rabbit cornea; it can be differentiated to corneal epithelial cells and inhibited inflammation in the area (Gu et al., 2009). Another scaffold that has been used is polylactic acid (PLA) nanofibers for seeding bone marrow-derived MSCs (BM-MSCs), adipose tissue-derived MSCs (Ad-MSCs), or limbal epithelial stem cells (LSCs) for the treatment of corneal optical properties after alkali burns that precede the inflammatory response (Cejka et al., 2016). BM-MSCs nanofibers and LSCs nanofibers are capable of suppressing corneal inflammation and neovascularization by suppressing MMP9, iNOS, and VEGF in the cornea. Polyamide 6/12 nanofiber scaffold (Zajicova et al., 2010) is another example of using hydrogels with MSCs in ocular surface inflammations; it significantly reduced the local inflammatory response in various ocular surface injuries.
Counterintuitively, the inflammatory response triggered by biomaterials can be leveraged to accelerate tissue regeneration, involving MSC and LSC. Polysaccharide has been shown to accelerate the tissue repair process by stimulating the inflammatory phase, with increased activation of macrophages, infiltrating cells, and fibroblasts (Matica et al., 2019). In this way, the acute inflammatory phase can be soon taken over by the proliferative phase to facilitate rapid re-epithelization and wound closure. The timing and dynamics of the inflammatory response are important targets for biomaterial development. Particularly, at the early stage of inflammation, intervention using biomaterials can promote pro-healing responses, predominantly for the delivery of therapeutic entities for ocular tissue regeneration.
Besides the use of MSC-encapsulated implants as an immunomodulatory treatment, there is another intriguing cellular therapeutic strategy that has been tested in clinical trials for eye disorders. In this strategy, cells are genetically transfected before encapsulation, and by then loading cells inside the polymeric implant, the engineered cells are capable of producing the targeted protein for a prolonged time (Zhang et al., 2011; Wong et al., 2017; Belhaj et al., 2020). The polymeric implant is permeable, allowing the therapeutic drug to diffuse while protecting cells from the host’s immunological responses. As a result, the selection of biomaterial as a protective carrier is critical to avoid inducing an unwanted immune response, or, even one step better, inducing an anti-inflammatory response. Neurotech is a pioneer in this technology, having developed the NT-501 device to provide sustained delivery of ciliary neurotrophic factor (CNTF; a growth factor shown to decrease photoreceptor degeneration in RP animal models) to treat retinal degenerative diseases such as RP, AMD, and glaucoma (Zhang et al., 2011; Kauper et al., 2012a; Kauper et al., 2012b; Chew et al., 2015).
Early clinical trials employed polymeric matrix-based systems, such as NT-501 (Renexus®), which is composed of a semi-permeable, non-biodegradable polysulfone scaffold, with polyethylene terephthalate as an internal matrix, to support CNTF-producing ARPE-19 cells. However, this cell-encapsulating device measured 1 mm in diameter and 6 mm in length, and requires surgical insertion in the vitreal region. NT-501 is currently under stage 2 clinical trials for glaucoma, and no device-related adverse events have been reported so far (Zhang et al., 2011; Wong et al., 2017). This method may be used for long-term delivery of different proteins and polypeptides such as VEGF-antagonist, inhibitory domain of factor H (complement receptor 2 and factor H (CR2-fH)) as a complement inhibitor of CNV, or anti-inflammatory cytokine target to suppress inflammation in inflammatory diseases (Adamson et al., 2016; Belhaj et al., 2020).
To improve patient compliance and ease of use, several hydrogel alternatives have been proposed, using a less-invasive injection procedure in encapsulated cell therapy. Immune-compatible materials, such as alginate and collagen-based hydrogels have been developed to deliver cells. Studies demonstrated minimal host-cells attachment on the hydrogel surface, the presence of living cell colonies, and the generation of active biomolecules over an extended number of days of implantation (Wikström et al., 2008; Wong et al., 2017; Belhaj et al., 2020). For instance, in one animal study, ARPE-19 cells were transfected to express the gene of choice and encapsulated in alginate polymer using a microencapsulation method coupled with electrospray to generate an encapsulated cell alginate capsule. The size of the capsule was controlled by the alginate concentration and the voltage of the electrospray. The results showed that a size of 150
3.1.3 Implants and injectable depots design parameters
3.1.3.1 Mechanical strength
Ocular tissues exhibit different mechanical strengths, with the cornea at 3.8 MPa, IOL at 2.5–6 MPa, and vitreous 20–50 Pa. Tissue stiffness is subject to differences in species, age, and disease states (Nickerson et al., 2004; Formisano et al., 2021). Corneas and IOLs undergo substantial mechanical loading and stretch as part of their normal functions in maintaining ocular pressure and visual acuity. Resident innate immune cells have been exposed and habituated to these mechanical stimuli during physiological conditions (Liu and Li, 2021; Du et al., 2022). However, during diseased conditions, such as during glaucoma, the change in intraocular pressure and mechanical environment may induce pro-inflammatory activation of resident immune cells and may exacerbate disease progression. In fact, mechanical sensors, transient receptor potential vanilloid (TRPV), pannexin-1, and P2X7 can be found on retinal ganglion cells (RGCs) and glial (Müller) cells in posterior ocular structures (Križaj et al., 2014). The activation of mechanosensors may result in the release of pro-inflammatory mediators, such as IL-1β, which can in turn promote the activation of nuclear factor kappa B (NF-κB) in microglia. On the other hand, the corneal epithelium, which comprises the major ocular anterior surface, is able to sense shear stress. Abnormalities in mechanical properties can trigger the activation of resident innate immune cells (Liu and Li, 2021). Pathological conditions may result in dry eye disease.
Therefore, hydrogel stiffness should match tissue stiffness depending on the implantation site, to help maintain ocular structures and immune responses. The mechanical strength of hydrogels can be tuned by changing the polymer concentration, molecular weight of polymers, and crosslinking densities, as well as by incorporating nanomaterials and composites. For instance, in dry eye symptom relief, our group reported a HA-based soft hydrogel, which matched the mechanical properties of the tear film and provided surface lubrication for an extended period of time, without frequent corneal instillation. Moreover, when combined with cyclosporine, an immunosuppressant, the treatment regime significantly improved the symptoms of dry eye diseases in canine clinical studies, in comparison to cyclosporine treatment alone (Yu et al., 2021). It was reported that hard methacrylate-gelatin (GelMA) hydrogel, with a measured strength of 29 kPa, can induce M1 differentiation of macrophages in vitro and severe FBR responses in vivo, in contrast to softer alternatives with strengths of 2 and 10 kPa (Zhuang et al., 2020). A similar trend was observed in the THP-1 macrophage line culture on collagen-coated polyacrylamide gels, with higher M1 polarization on hard gels (323 kPa) than softer gels (11 kPa and 88 kPa). It was also demonstrated that the hydrogel stiffness modulated macrophage migration behavior, with a higher spreading area and slower movement on the stiffer gel. Moreover, it was reported that macrophages exhibit lower phagocytic ability on a stiffer gel (Sridharan et al., 2019; Li and Bratlie, 2021).
Soft hydrogels are generally preferred in ocular applications and are favored in the structural design of the ocular surface and intravitreal implants, with minimal adverse immune responses. However, tissue engineering for corneas and IOLs requires hydrogel scaffolds with much higher mechanical strengths to withstand ocular structural changes and functions. To accommodate these mechanical needs, other design modifications, such as surface hydrophobicity and topography are pursued to suppress immune activation.
3.1.3.2 Surface chemistry: Charge and hydrophobicity
In the case of cornea and IOL implantations, hydrophobic materials are generally preferred in order to resist swelling, lens epithelial cell engagement, and migration from the peripheral to the visual region (Zhao et al., 2017). Non-etheless, PCO and corneal haze are common among patients after surgery, both of which are characterized by the FBR of prostheses, resulting in secondary visual impairment (Pintwala et al., 2014; Tamura et al., 2017). Indeed, it is generally accepted that a hydrophobic surface can promote non-specific protein deposition and initiation of FBR. Hunter et al. (1981) were the first researchers who worked on the adjuvant efficiency of the polymer backbone structure. It has been shown that different inflammatory and immune responses are stimulated based on the physiochemical properties (hydrophile-lipophile balance) of the biomaterial and that the hydrophobic part of the molecule can stimulate the innate immune cells and cause inherent immunogenicity by PAMP or DAMP (Seong and Matzinger, 2004; Andorko and Jewell, 2017). The process is characterized by the fusion of macrophages to form giant body cells to facilitate the clearance of foreign materials. The cells also secrete immunomodulatory cytokines such as TNF-α, IL-1, and TGF-β which can further promote nearby fibroblasts to secrete fibrinogen that surrounds the prostheses to isolate them from the host tissue environment.
Common strategies to bypass FBR include modifying the surface charge and hydrophobicity of implants. Hydrophilic PEG and anionic and zwitterionic polymers are often selected as coating materials for such purposes. There are several ways to modify the implant surface, and these generally involve physical or chemical methods, as discussed in a previous review (Song et al., 2020). The physical methods include electrospinning/spraying, spin coating, dip coating, and layer-by-layer (LbL) polymer assembly; chemical methods generally involve radical polymerization, 3-aminopropyltriethoxylsilane (APTES) and glutaraldehyde, carbodiimide coupling, or “click” chemistry. Indeed, studies on surface modification of the commercial artificial corneas Keraklear, Korea Seoul-type, and T-style keratoprostheses demonstrated that coatings of hydrophobic poly (methyl methacrylate) (PMMA) or poly (2-hydroxyethyl methacrylate) (PHEMA) with PEG, a hydrophilic polymer, can effectively resist immune cell engagement and suppress the secretion of pro-inflammatory cytokines (Kim et al., 2002; Xiang et al., 2015; Farid et al., 2020). As well as PEG, implant surface coatings with zwitterionic polymer brushes, such as phosphorylcholine and sulfobetaine, have also been utilized, and results demonstrated superior capabilities in suppressing FBR (Han et al., 2017; Wang et al., 2021). Other natural hydrophilic polymers, such as anionic HA, have been employed together with lysozyme in PMMA surface coating; they exhibited anti-bacterial properties and reduced the adhesion of cells and bacteria in in vitro culture (Wang et al., 2014). Moreover, a silicone-based hydrogel with a surface LbL assembly of alginate and chitosan was developed to achieve sustained release of the immunosuppressant diclofenac to suppress post-surgical complications related to IOLs (Silva et al., 2016). The hydrogel surface charge can also influence the treatment outcomes of several posterior ocular diseases. In the case of glaucoma, the suprachoroidal injection of HA hydrogel produced a shorter retention time and showed minor inflammation and fibrosis at the injection site. However, the application of the zwitterionic hydrogel polycarboxybetaine significantly suppressed inflammation and prolonged the reduction of intraocular pressure (IOP) via suprachoroidal space expansion (Hao et al., 2022).
3.1.3.3 Surface topography and porosity
On top of the choice of hydrophilic or hydrophobic material chemistry, the surface roughness or topography of a hydrogel can also affect the wetting state, protein adsorption, and cell interactions. There is increasing evidence that hydrogel surface topography can modulate cell behaviors such as adhesion, migration, proliferation, and differentiation. During fabrication, hydrogel surface patterning can be achieved, up to micron and sub-micron resolutions, with the appropriate choice of materials and pattern design to counter the swelling behavior while preserving the pattern fidelity. The fabrication methods often include casting, nanoimprinting, 3D printing, electrospinning, multiphoton patterning, lithographic patterning, and swelling-induced patterning (Cui et al., 2021).
Surface patterning of hydrogels can influence the wettability of materials. In the case of micropillar array design, the spacing and height interfere with the water contact angle and protein adsorption, as well as cell-material interactions. A high throughput screening approach using a diverse library of 2,176 micropatterns was recently developed to study the relationship between monocyte-derived macrophages and topography. The study revealed that diameters of 5–10 μm favored macrophage attachment, and smaller, denser micropillars pattern can instruct M2 phenotype polarization (Vassey et al., 2020). Another study also reported that micropillars with 2 μm spacing and 4.5 μm in height are able to resist silicone hydrogel wettability as well as protein and cell adhesion (Papenburg et al., 2010). On the other hand, PVA with 2 μm gratings and a concave lens topography of 1.8 μm can promote endothelial cell adhesion on PVA hydrogel, compared with wider (10 μm) gratings, pillars, and convex lens textures (McWhorter et al., 2013). In general, surface texture design often aims to mimic niche tissue topographical features to better direct cell behaviors for optimal delivery device performance and therapeutic efficacy. IOLs with surface micropatterning are able to resist PCO. The presence of micron-sized isotropic elements arranged in a symmetric and regular pattern can resist cell adhesion and migration. It was suggested that the texture interfered with the formation of focal adhesion within cells, which hampers cell adhesion and spreading. Indeed, the study demonstrated that ridge(R)/grooves(G) patterns with sizes comparable to cells, e.g., R5G10 with a gradient spacing, can also significantly suppress fibroblast cell adhesion and promote directional cell migration on the culture surface (Kwon et al., 2017).
Conversely, anisotropic geometries, such as gratings, can promote cell differentiation. It was observed that macrophages in the pro-healing M2 state exhibit extended or oblong morphology, in comparison to M1. Several studies demonstrated that by controlling macrophage morphology using micropatterning methods, macrophages can be polarized into the M2 state without the addition of cytokines. The cells cultured on confined micropatterned fibronectin lines 20 μm in width were able to produce higher levels of IL-4 and IL-13 and resisted the effect of M1-inducing LPS stimulus (McWhorter et al., 2013). In addition, a honeycomb-like surface pattern 90 nm in size can also promote M2 differentiation, with highest expressions observed for CD206, IL-4, IL-10, and growth factors, which support tissue regeneration. It was suggested that the honeycomb-like texture can promote filopodia formation, which is associated with RhoA/ROCK signaling pathways, and can induce M2 polarization (Zhu et al., 2021). Moreover, a study reported that fibrous meshes of gelatin hydrogel nanofibers mimicking ECM promoted macrophage adhesion and differentiation into M2 phenotypes, with upregulation of CD206 expression and downregulation of IL-1β and IL-8 in in vitro culture (Taskin et al., 2021).
The porosity of hydrogel implants can also be tuned to modulate immune cell behaviors. Under physiological conditions, cells reside in porous ECM which provides optimal mechanical cues and spaces for biochemical cue exchange and cell-cell communications. In pathological challenges, ECM polymer backbones can support immune cell infiltration to wound sites. The design of microporous hydrogels aims to promote immune cell infiltration for local antigen delivery or immunomodulation. This can be achieved by introducing degradable porogens during hydrogel fabrication. A study reported an alginate-based porous hydrogel system incorporating rapidly degradable alginate beads into a non-degrading bulk alginate hydrogel. The authors further adjusted the porosity of the hydrogel by adjusting the percentage of porogens. The study revealed that a system with 50% porogen significantly promoted DC infiltration and maintained the immature phenotype, compared with 25% and 70% porosity (Verbeke et al., 2015).
The size of pores can also be adjusted to protect cargoes from immune cell-mediated clearance while preserving materials exchange between the carrier and host tissue environments. For instance, nanopores can be introduced to cell-laden hydrogels to ensure that the cargo survives and functions. Indeed, an alginate hydrogel with a pore size of 600 nm was developed to encapsulate mesenchymal stem cells and was able to resist pro-inflammatory immune cell infiltration (Moshaverinia et al., 2015). Such hydrogel design could promote stem cell survival and the maintenance of phenotypes to support tissue regeneration.
3.1.3.4 Degradability
Biodegradable hydrogels have received increasing attention for the design of drug delivery systems for spatiotemporally controlled release, protection from physiological degradation and clearance, and improved patient compliance. Hydrogel drug depots can be injected into the vitreal space to provide extended release and to improve the bioavailability of therapeutics for posterior ocular disease treatment. Drug depots are often designed to match the optical and mechanical properties of tissue sites to preserve visual functions. Moreover, degradable drug depots are more favored in intravitreal injections to circumvent the invasive surgical retrieval of non-degradable implants from the eye.
Generally, depending on the hydrogel design, the depots can undergo different types of biodegradation mechanisms, namely, solubilization, chemical hydrolysis, and enzymatic degradation. More importantly, the drug release kinetics can be controlled by the hydrogel degradation rate. There are several strategies that have been developed to regulate hydrogel degradation kinetics, which involve physical and chemical factors. For instance, physical interventions include changing the polymer concentration, the molecular weights of hydrogel precursors, hydrogel size, architecture, and microstructure. On the other hand, chemical methods include introducing functional groups to modify polymer charge or hydrophobicity, the nature of crosslinkers, and crosslinking density (Kong et al., 2004; Jain et al., 2017; Lau et al., 2021).
Non-etheless, the long-term presence of hydrogel depots in the intravitreal space is often associated with FBR, and the design of degradable depot formulations can induce chronic inflammation, thereby hampering the immune compatibility of the delivery device. In fact, an undesirable immune response has been observed in vitreous substitutes that consisted of HA and PEG. In the case of PEG, despite being known for its excellent anti-fouling properties in bypassing FBR, its in vivo degradation has been shown to induce the generation of anti-PEG antibodies and local inflammatory responses (Reid et al., 2015; Chen et al., 2021). Moreover, for HA hydrogels, the choice of molecular weights during preparation is crucial in regulating its immunomodulating properties. Generally, higher HA molecular weights (>500 kDa) are preferred, as they can induce anti-inflammatory responses by promoting macrophage M2 polarization and tolerogenic dendritic cell induction. Our group observed that a higher HA molecular weight of 670 kDa could avoid inflammatory responses when the polymers were used to coat lipid-based nanoparticles to improve intravitreal retention in rabbit eyes. Non-etheless, with the use of small HA molecular weights such as 36 kDa and 120 kDa, inflammation is observed in posterior ocular structures (Xiaonan, 2020). The underlying mechanism is due to HA interactions with PAMP/DAMP receptors on innate immune cells, in which the high molecular weight biopolymer exerts competitive engagement of TLR2 and/or TLR4 receptors of the immune cells, resulting in signaling inhibition of pro-inflammatory pathways governed by Myeloid differentiation primary response 88 (MyD88) and NF-κB. The smaller molecular weight alternatives, on the other, hand can act as TLR agonists (Østerholt et al., 2011; Rayahin et al., 2015; Gebe et al., 2017). A recent study used zwitterionic hydrogel composed of polycarboxybetaine and demonstrated its superior anti-fouling ability against proteins and cells. The material was then used as a vitreous substitute in a rabbit model, with no visible adverse effect (He et al., 2021) (Figure 3).
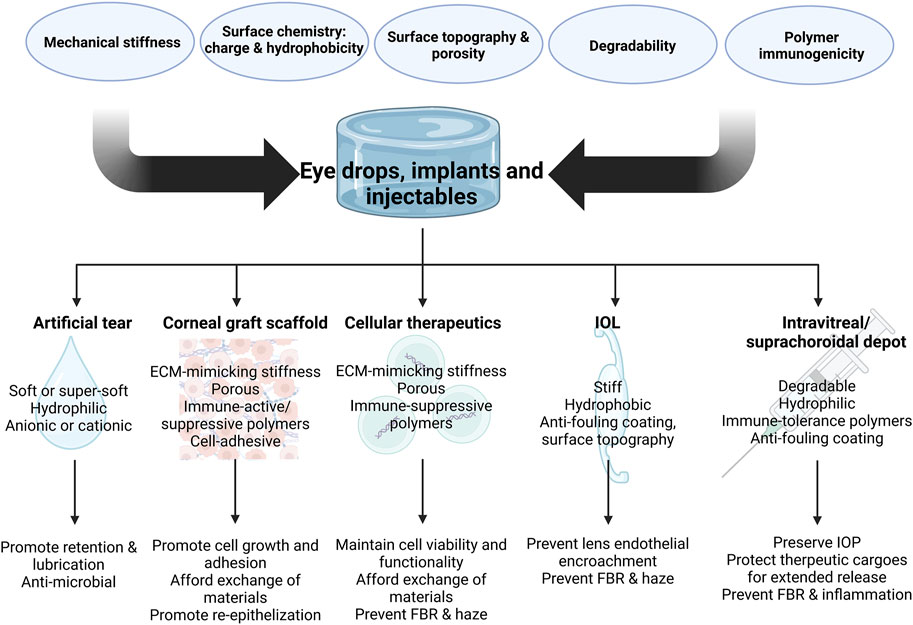
FIGURE 3. Design parameters of implants and injectable depots for various ocular applications and key features for desired therapeutic outcomes (Created in BioRender.com).
3.2 Nano/micro particles
For years, nano/micro particles have been used as common drug delivery systems in the eye and could be a potential drug delivery carrier for ophthalmic applications. Nanoparticles (NPs) are considered to have a size under 1000 nm and microparticles (MPs) between 1 micron and 1 mm. MPs can be used as microcapsules (a core drug with a polymeric layer surrounding it) or microspheres (drugs dispersed throughout the polymer matrix), based on their structure (Herrero-Vanrell et al., 2014). Microcapsules are also referred to as ocular implants and these have already been covered in the section on implants (Zimmer and Kreuter, 1995; Adamson et al., 2016).
MPs are usually used in ocular applications for prolonged delivery (at least 1 week) of proteins, peptides, and biomolecules. PLGA is the most commonly used polymer in this area (Cohen et al., 1991; Carrasquillo et al., 2003; Gavini et al., 2004; Mandal et al., 2018). For instance, Gavini et al. (2004) developed vancomycin-encapsulated PLGA microspheres for topical ocular delivery, and showed a high concentration of peptide drug in the rabbit aqueous humor just after 3 h of topical administration. Albumin, gelatin, PLA, polyanhydrides (Ron et al., 1993), and cyclodextrins (Davis and Brewster, 2004) are other biodegradable polymers that are used as microspheres. For the sustained release of pilocarpine, which is used to lower intraocular pressure, Rathod et al. developed pilocarpine-loaded egg albumin microspheres as an eye drop (Rathod and Deshpande, 2008). Another work on the transscleral delivery of PLGA microspheres encapsulated with pegaptanib sodium (an RNA aptamer (EYE001) that inhibits VEGF) showed sustained delivery of EYE001 over 20 days on the surface of the sclera to treat choroidal and retinal diseases such as wet AMD (Carrasquillo et al., 2003). The administration route and the type of polymer significantly alter the inflammatory response of innate immune cells in microsphere ocular delivery systems. For instance, it has been demonstrated that intravitreal injection of PLA and PLGA microparticles may increase inflammatory responses due to their propensity to aggregate, making them more suitable for use as depots for the simultaneous delivery of several biomolecules loaded into nanoparticles (Herrero-Vanrell et al., 2014).
It has been demonstrated in the literature that NPs are favored over MPs in circumstances of prolonged drug delivery and targeted delivery to deeper ocular layers (Zimmer and Kreuter, 1995; Andrés-Guerrero et al., 2015; Huang and Chau, 2019a). The use of NPs in the treatment of ocular disorders is a promising new tactic that may pave the way for more successful ocular therapy and penetration for targeting posterior parts of the eye. They may target the cornea, retina, and choroid, and exhibit several advantages for use in the treatment of eye disorders. These systems are capable of transporting various cargoes. For instance, the use of NPs may make it easier and more effective to distribute hydrophobic anti-inflammatory drugs such as corticosteroids and unstable nucleic acids such as mRNA. Furthermore, since they can pass through intricate ocular barriers such as ocular-retinal barriers and blood-retinal barriers of the eye, their delivery route through difficult-to-access areas of the eye is facilitated. Additionally, they sustain the long-term release of immunomodulatory medications for chronic ocular inflammatory conditions (Diebold and Calonge, 2010; Huang and Chau, 2019a; Tang et al., 2022). Various nano/microparticles that have been employed in immunomodulatory ocular applications are listed in Table 1 as a summary. Smart biomaterials, on the other hand, may be created to target specific tissue locations by employing optical, electrical, or mechanical targeting properties. They have several uses in biological imaging, targeting, smart drug delivery systems, and anti-inflammatory eye treatments (Ren et al., 2019; Luo et al., 2020).
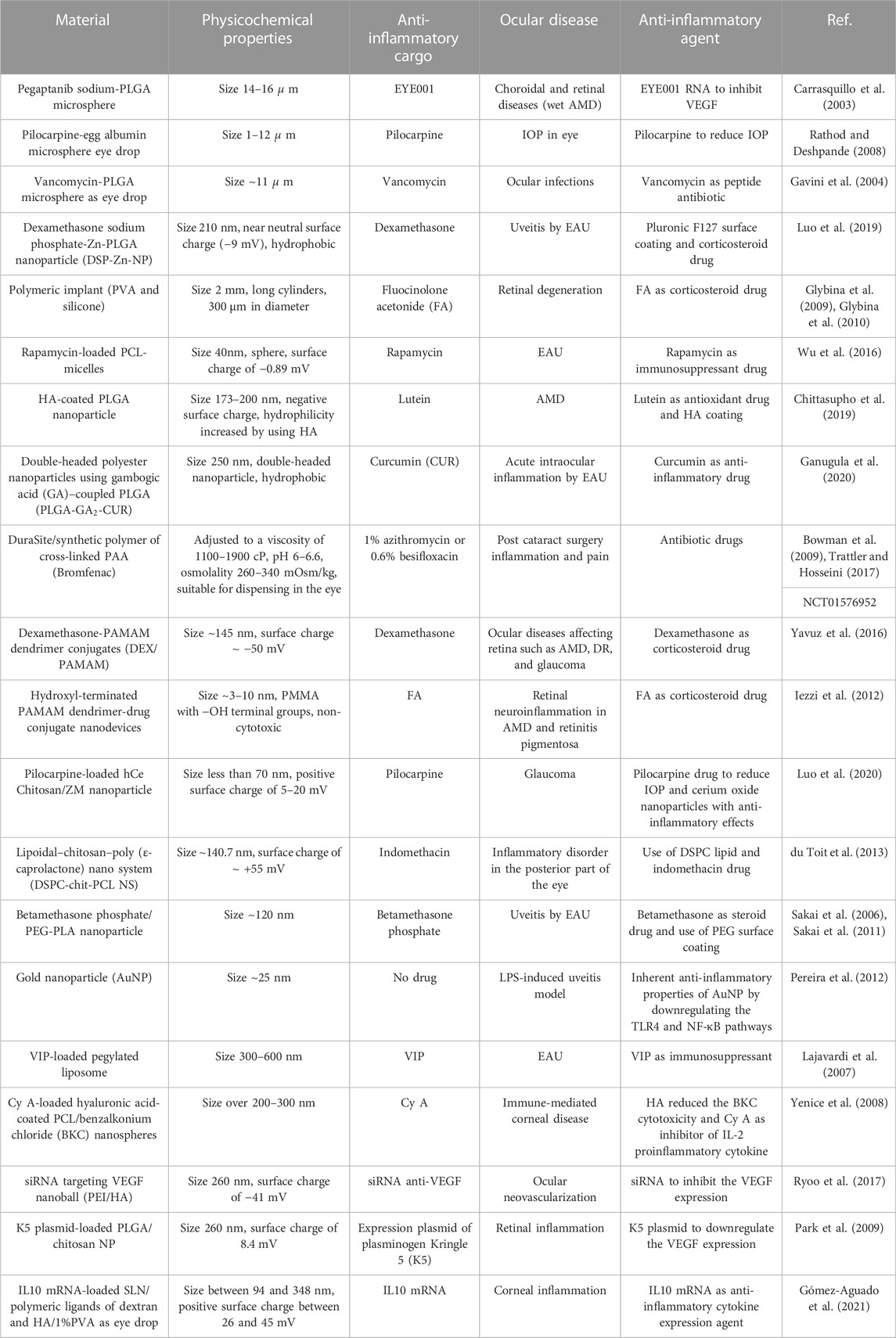
TABLE 1. Summary of different micro/nano particles used in the immunomodulation of ocular disorders.
3.2.1 Nanoparticle design parameters
Immune cells may respond in either a pro- or anti-inflammatory manner depending on the physiochemical characteristics of nanoparticles, including their size, shape, chemical composition, and surface charge (Figure 4). Therefore, by taking these variables into account, nanoparticles can be designed to regulate the immune response. The impact of these parameters is discussed in the following sections.
3.2.1.1 Size
The size of the particle is a key characteristic that drives the innate immune cell response, and as the particle size grows, the innate immune cells’ inflammatory and phagocytosis responses are amplified. The main disadvantages of corneal topical applications are drug bioavailability over time and increasing corneal penetration. Studies have indicated that the nanoparticle size should be less than 200 nm to allow easy uptake via endocytosis in the conjunctiva and cornea. Particle size is directly associated with penetration through the barriers of the cornea. A 180 nm gelatin nanoparticle, used as an eye drop in rabbit eye, for instance, could be retained in the cornea for a long time as a result of being taken up by ocular epithelial cells (Tseng et al., 2013; Soiberman et al., 2017; Tsai et al., 2018; Souto et al., 2019).
The literature also mentions the possibility of injecting positively charged particles with a size range of 50–350 nm into the vitreous body. Notably, for particles bigger than 350 nm, the size impact may be a significant component affecting their distribution, but for particles smaller than 350 nm, the charge effect is a significant parameter (Tsai et al., 2018; Mobaraki et al., 2020). A previous review paper by our group, on intravitreal nanoparticles for retinal delivery (Huang and Chau, 2019a), also noted that nanoparticles, after passing the internal limiting membrane (ILM), are directed to inner layers via endocytosis. To determine whether a particle with a particular size and charge may pass through the vitreous and ILM barriers or not, it is crucial to consider the size-cut off for nanoparticles passing through those barriers. Peynshaert et al. provide an in-depth discussion on drug delivery barriers in the posterior segment of the eye (Peynshaert et al., 2018; Peynshaert et al., 2019).
On the other hand, for the treatment of posterior ocular diseases, particles and implants larger than 2
Regarding the immunogenicity and cellular uptake mechanism of smaller nanoparticles, there are still several unanswered problems. Therefore, a crucial factor in identifying whether the innate immune cell interaction pathway is pro- or anti-inflammatory is particle size. In 1998 (D’orazio and Niederkorn, 1998) an intriguing experiment was carried out by D’Orazio et al. The authors investigated the ocular APC response to various antigen types, as well as how the type of antigen affected anterior chamber-associated immune deviation (ACAID), which is a defense mechanism for controlling and avoiding immunologically induced damage. Ovalbumin (OVA) antigen was used as a model antigen. It arrived in two forms: soluble and particulate, and it was passively absorbed on the surface of latex (polystyrene) particles with a size of 460 nm. The authors demonstrated that particulate antigens can stimulate APCs, increasing IL12 production and Th1 activation, whereas soluble antigens activate the ACAID pathway, increasing IL10 production and Th2 regulatory cells (D’Orazio et al., 2001).
3.2.1.2 Chemical structure
Another factor that may affect whether the response of the innate immune system is pro- or anti-inflammatory, as previously mentioned, is the intrinsic chemical structure of the nanoparticle. Natural biomaterials such as gelatin (Vandervoort and Ludwig, 2004; Mathurm and Gilhotra, 2011), chitosan (Marõ´a et al., 2003; Enríquez de Salamanca et al., 2006; Tsukamoto et al., 2013; Li et al., 2009; Aşık et al., 2013), collagen (Luis et al., 2016; Simpson et al., 2021; Song et al., 2021), albumin (Zimmer et al., 1994; Tiwari et al., 2021), alginate (Shafie and Fayek, 2013; Kostenko et al., 2022), and HA (de La Fuente et al., 2008; Yenice et al., 2008; Rayahin et al., 2015; Gebe et al., 2017; Guter and Breunig, 2017; Casey-Power et al., 2022) are frequently used as anti-inflammatory agents in eye treatment because they do not cause an inflammatory response from the innate immune system (Sharma et al., 2016). They come in the form of nanoparticles, nano emulsions, nano capsules, liposomes, and lipid nanoparticles. Although these biomaterials have been used in numerous previous studies as carriers to support drug release, enhance ocular penetration, lengthen ocular retention, and improve ocular bioavailability, future research could focus on the materials’ interaction with ocular innate immune cells to suppress the inflammatory phase of macrophages, without the use of steroid drugs.
Chitosan, a common nanomaterial in ocular research, has been proven in several studies to exhibit minimal immunogenicity for innate immune cells, as previously stated. Chitosan is bioadhesive, can enhance ocular penetration, and does not cause an inflammatory response, according to several experiments (Marõ´a et al., 2003; Enríquez de Salamanca et al., 2006). Furthermore, HA as a nanoparticle or coating of polymeric nanoparticles has shown substantial potential in reprogramming ocular innate immune cells (Yenice et al., 2008; Guter and Breunig, 2017; You et al., 2020). A higher HA molecular weight, as previously noted, may trigger an anti-inflammatory response of innate immune cells (Casey-Power et al., 2022).
On the other hand, PLGA (Casey et al., 2019; Ganugula et al., 2020; Luo et al., 2020), PLA (Sakai et al., 2006; Sakai et al., 2011), poly-caprolactone (PCL) (Yenice et al., 2008; du Toit et al., 2013), polyacrylic acid (PAA) (Trattler and Hosseini, 2017), and poly (amidoamine) (PAMAM) (Iezzi et al., 2012; Yavuz et al., 2016) are the most commonly used synthetic biomaterials as carriers of anti-inflammatory medications in ophthalmic therapy. According to Casey et al. (2019), PLGA and PLA particles have broad-acting mechanisms that inhibit TLR signaling by programming innate immune cells, and they exhibit inherent immunomodulatory properties depending on their physiochemical properties. The authors declared that more research into the inherent immunomodulatory properties of polymer-based particles is necessary since they have the potential to treat a wide range of human disorders via abnormal TLR activation (Casey et al., 2019). Similar to natural biomaterials, they come in form of dendrimers, nano capsules, and nano emulsions. Nevertheless, the fact that they degrade in vivo raises further questions regarding their immunogenicity, and more research is required to ascertain how different types of materials impact immunity. For instance, in order to prevent the development of experimental autoimmune uveitis (EAU), Wu et al. created PCL-micelles loaded with rapamycin that were 40 nm in size, spherical in form, and with −0.89 mV charge (Wu et al., 2016). Another study aimed to reduce uveitis inflammation by employing PLGA nanoparticles (210 nm) containing dexamethasone (Luo et al., 2019). The cytotoxicity of several materials, including PLGA, PCL, and PEG-PLGA, toward the retinal cell line (ARPE-19) was also tested by Lin et al. The authors found that PEG-PLGA had the lowest cytotoxicity over 6 days, in comparison to the other materials, which exhibited different cytotoxicities (Lin et al., 2016). Another group claimed that surface modification of synthetic polymers reduces their cytotoxicity and subsequent immune responses since HA-coating on PLGA nanoparticles did not exhibit any cytotoxic impact on RPE cells (Chittasupho et al., 2019).
Aside from these two groups, there are other nanomaterials with inherent immunosuppressive functions (anti-inflammatory, anti-angiogenesis, anti-bacterial, and anti-oxidative stress) that have been shown to influence ocular inflammation, including cerium oxide NPs for AMD treatment (Tisi et al., 2019; Luo et al., 2020), gold NPs for uveitis and AMD (Pereira et al., 2012; Singh et al., 2020), and silver NPs for fungal keratitis (Shi et al., 2021). For instance, Pereira et al. (2012) studied the inflammatory response of gold nanoparticles (AuNP) in endotoxin-induced uveitis and showed that the topical use of AuNP reduces inflammation by downregulating the TLR4 and NF-
3.2.1.3 Shape
The shape of the material and the way it affects how nanomaterials interact with innate immune cells is another crucial factor. There is no assurance that the innate immune system will not react negatively to the nanoparticles. Nanoparticle size and shape have the potential to stimulate macrophages to phagocytose and to consume and frustrate them, causing them to secrete an increasing amount of pro-inflammatory cytokines, eventually causing chronic inflammation, or even to stimulate DCs to mature and activate T cells (Bartneck et al., 2010; Niikura et al., 2013; Andorko and Jewell, 2017). According to Bartneck et al., macrophage absorption of gold nanorods is higher than that of nanospheres via the macropinocytosis process; nanorods elicited a stronger inflammatory response in vivo even after surface modification with polyethylene oxide (PEO) (Bartneck et al., 2010; Andorko and Jewell, 2017). Another intriguing work by Niikura et al. (2013) reported that the size and shape of gold nanoparticles (AuNPs) in vitro and in vivo may be used to tailor the responses of innate immune cells such as macrophages and DCs. Cells exposed to rod-shaped nanoparticles released cytokines via pro-inflammatory inflammasome pathways, such as IL-1
3.2.1.4 Surface charge
Another crucial factor is surface charge, which is particularly important for nanoparticles due to concerns regarding delivery, corona protein aggregation, and stability. Researchers have discovered that nanoparticles with a charge lower than −30 mV may have an anti-inflammatory impact on the immune system. A higher amount of negatively charged particles causes a lower immunogenic response and they may be immunosuppressive (Andorko and Jewell, 2017; Li H. et al., 2022; Wen et al., 2016; Getts et al., 2014). Despite the fact that positively charged particles may be more easily taken up by cells than negatively charged particles, they will indeed be more cytotoxic and trigger an inflammatory response in the cells (Zhu et al., 2019). Similarly, our group noted that surface charge plays an important role in the biodistribution of nanoparticles, while simultaneously triggering an immunogenic response (Huang and Chau, 2019a).
Yavuaz et al. used −50 mV negatively charged dexamethasone-PAMAM dendrimer conjugates for various ocular diseases affecting retinae such as AMD, DR, and glaucoma. The authors demonstrated that these dendrimers can effectively permeate to the back of the eye and release anti-inflammatory drugs in a sustained manner; however, the clearance time was quick. The authors suggested that the use of cationic dendrimers to determine the impact of surface charge on clearance time be taken into account (Yavuz et al., 2016). Additional information on the immunological characteristics of various engineered nanomaterials is discussed in the review of Dobrovolskaia (Dobrovolskaia and McNeil, 2007). Generation 3.5 PAMAM conjugated to glucosamine-negative dendrimers is one example that can inhibit human macrophages and DCs’ proinflammatory response and is immunosuppressive (Shaunak et al., 2004).
Lipid-based nanomaterials are another category of nanoparticles that have been widely used for ocular treatment, based on their controllable surface charge, especially as non-viral gene delivery systems. Due to their tendency to disassemble and aggregate, these materials are less stable in the eye compared to polymeric nanoparticles (Huang and Chau, 2019a). Lipid-based nanoparticles (LNPs) encapsulated with the model drug small interfering RNA (siRNA) were studied by our group to determine the impact of charge on intraocular distribution. We discovered that LNPs with neutral or negative charges exhibit problems with rapid clearance, whereas LNPs with a positive charge of 35 mV can diffuse through the retina and deliver the siRNA (Huang and Chau, 2019a; Huang and Chau, 2019b; Huang and Chau, 2021). In a different study conducted by our group, we found that siRNA-encapsulated LNPs with a positive surface charge of +33 mV did not cause activation of microglia cells or an inflammatory response (Xiaonan, 2020).
The particle clearance time and immunogenic reactions should be taken into account in ocular studies. The circulation and retention period of nanoparticles inside the eye can be prolonged by adopting a stealth technique that coats the nanoparticles with a hydrophilic substance called PEG. One example of this use is the systemic administration of PEG-stealth-PLA nanoparticles encapsulated with betamethasone for targeting the inflamed uvea and retina in a rat with EAU. The authors demonstrated that these stealth NPs reduced inflammation by the first day after administration, and their effects remained for 2 weeks. This period was significantly greater than their earlier experiment, which employed betamethasone/PLA NPs alone, which presented quick systemic phagocytosis clearance (Sakai et al., 2006; Sakai et al., 2011).
In order to inhibit the TLR-mediated innate immune pathway, Casey et al. (2019) used cargo-free nanoparticles of PLA and PLGA with different coatings of poly (ethylene-alt-maleic anhydride) (PEMA) and poly (vinyl alcohol) (PVA), different molecular weights, and different charges. The authors demonstrated that the PEMA coating caused a larger immunosuppression effect compared with the PVA coating. (Figure 4). The downregulation of pro-inflammatory cytokines and the suppression of TLR-mediated inflammation were accomplished by altering the physicochemical characteristics of the nanomaterials, such as the charge, molecular weight, and polymer composition.
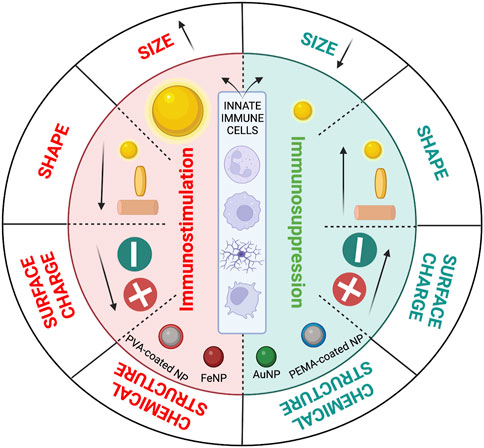
FIGURE 4. The effect of different nanoparticle design parameters on immune system response. Nanoparticles’ size, shape, surface charge, and chemical structure can lead to immunogenic or immunosuppressive responses from innate immune cells (from top to bottom: neutrophils, macrophages, microglia, and dendritic cells) (Created in BioRender.com).
3.2.2 Immunomodulatory cargoes for nanoparticle
Using nanoparticles is the most common method for treating challenging ocular disorders related to the posterior part of the eye. By penetrating beyond ocular barriers, they can reach and distribute in the posterior segment. Based on the importance of chronic inflammatory diseases affecting the posterior region of the eye (e.g., glaucoma, AMD, and DR), the most common immunomodulatory cargoes used in nanoparticles are discussed in the following section. In light of this information, more efficient treatment strategies for chronic inflammatory eye disorders may be suggested, taking into account these potential cargoes and understanding the criteria for designing immunomodulatory nanoparticles.
3.2.2.1 Steroids
The most common immunosuppressive agents are steroids. They have been used in a large number of commercial anti-inflammatory ocular drugs such as Bromfenac and INVELTYS™, both of which contain cross-linked PAA or chitosan polymer; they are widely used for treating post-ocular surgery inflammation, reducing post-surgery pain, and DED (Bowman et al., 2009; Schopf et al., 2015; Trattler and Hosseini, 2017; Kim et al., 2018; Gupta and Venkateswaran, 2021). Steroidal immunosuppressants have several drawbacks; they require high administration dosages, frequent injections in chronic ocular inflammatory diseases that lead to defects in the innate immune system, and other long-term side effects such as cataracts and IOP in the eye. By using immunomodulatory biomaterials as carriers instead of traditional inert carriers and alternative immunosuppressive drugs instead of steroids, such as anti-inflammatory cytokines, anti-inflammatory peptides, and nucleic acids, it is possible to overcome these problems in the eye.
3.2.2.2 Biomolecules (proteins and peptides)
Another type of cargo used in ocular immunomodulatory treatment includes biomolecules such as proteins and peptides. However, there are drawbacks to employing these cargoes, for example, cytokine’s short half-life and biomolecules’ poor ocular bioavailability. Different immunosuppressive biomolecules such as TGF-
As previously mentioned, TNF-
A recent study was conducted on the dynamics of microglia polarization and their regulation by an anti-inflammatory mixture of cytokines IL4/IL13 or the antitumor agent bicyclic nojirimycin derivative (1R)-1-dodecylsulfinyl-5N,6O-oxomethylidenenojirimycin (R-DS-ONJ) in a mouse DR model. The authors reported that using M2 cytokines or R-DS-ONJ as a modulating agent in the environment of activated microglia cells reduced retinal degeneration and inflammatory progression both in vitro and in vivo (Arroba et al., 1862). Further research can be conducted in this area to determine if inflammatory microglia targeting can be used as a therapeutic method to postpone or stop the impairment of visual function in DR.
3.2.2.3 Nucleic acids
Gene therapy for ocular inflammation may be an excellent option, even in clinical trials, and may use DNA, RNA, aptamers, and oligonucleotides. The stability of nucleic acid and the efficiency of its targeted delivery to minimize off-target effects are two challenges in this approach (Guzman-Aranguez et al., 2013; Raghunath and Perumal, 2015).
Viral and non-viral vectors are the two main types of nucleic acid delivery methods. Although viral vectors have been widely used as nucleic acid delivery methods, their immunogenicity, which may result in inflammation by inducing an immune response, is a major concern when employing them in clinical studies (Bordet and Behar-Cohen, 2019). These viruses include adenoviruses (AVs), adeno-associated viruses (AAVs), and lentiviruses. AAVs have been used in several studies on ocular gene therapy and chronic ocular disorders, although it has been demonstrated that they produce a mild immune response (Timmers et al., 2020). The first FDA-approved ocular gene therapy product, Luxturna®, also used an AAV2 vector and targeted diseases caused by the mutation of RPE65 genes such as retinitis pigmentosa (Russell et al., 2017). In this way, the type of vector, route of administration, and viral dosage cause different immune responses. For instance, the intravitreal route showed a higher immune response in comparison to other routes, and subretinal injection showed a lower immune response (Wasnik and Thool, 2022). An interesting study on the immunomodulation of uveitis by gene therapy is the use of a mutant serotype 8 adeno-associated virus (AAV8) (Y733F)-chicken β-actin (CBA)-MIF vector to express the macrophage migration inhibitory factor (MIF), which is an important cytokine for regulating macrophage function and T cell activation (Yang et al., 2016). It has been shown that MIF is critical for modulating ocular inflammation in EAU, linked to the Notch signaling pathway; this may be a promising therapeutic approach for uveitis.
Recently, Gilger and Hirsch (2022) reviewed the potential of using the human leukocyte antigen G (HLA-G) gene in AAV vectors for ocular inflammatory treatments. HLA-G is an important anti-inflammatory agent that can suppress the inflammatory function of innate immune cells such as neutrophils, dendritic cells, macrophages, and natural killer cells. The authors stated that, based on the anti-vascular performance of the HLA-G, it could be a great target for the treatment of different ocular diseases including corneal inflammation, corneal graft rejection, DED, uveitis, AMD, and DR (Gilger and Hirsch, 2022).
Nevertheless, based on concerns regarding the immunogenicity of viral vectors (Bordet and Behar-Cohen, 2019), non-viral vectors (with low immunogenic risk) are alternatives for gene therapy, especially in immunomodulatory approaches. LNPs, nano polymers, and self-assembly peptides with nucleic acid are the most common non-viral delivery systems in this area. There are several studies and clinical trials on ocular gene therapy for immunomodulatory approaches using siRNA, miRNA, mRNA, DNA, and plasmid DNA (Ghoraba et al., 2022).
The use of siRNA in different ocular inflammatory diseases was reported for silencing different innate immune cell-mediated inflammatory genes (Guzman-Aranguez et al., 2013). For instance, SYL040012 (bamosiran) is a commercial siRNA topical drug used to suppress the β-Adrenergic Receptor 2 as well as to lower intraocular pressure in glaucoma (Pintor, 2012; Bordet and Behar-Cohen, 2019; Ghoraba et al., 2022). For treating neovascularization-related disorders such as retinopathy and AMD, an important key regulatory agent is VEGF. Inhibition of VEGF was achieved by using recombinant AAV-mediated soluble VEGF receptor 1 (sFlt-1) expression (Bainbridge et al., 2002; Rota et al., 2004; Shen et al., 2006) or siRNA-targeting VEGF (Reich et al., 2003; Jiang et al., 2009; Ashikari et al., 2010). It was shown that neovascularization was reduced in the retina and choroidal, respectively. Interestingly, Ryoo et al. (2017) developed a novel siRNA-based anti-VEGF nanoball that was composed of siRNA-targeting VEGF as the core hydrogel with a coating of branched PEI and HA, achieved by applying an electrical force, for the treatment of choroidal neovascularization in AMD. The authors demonstrated excellent therapeutic results over 2 weeks as a result of intravitreal injection and targeting efficiency in the sub-retinal space via CD44 receptor endocytosis. AAV-mediated gene delivery of pigment epithelium derived factor (PEDF) reduced neovascularization in the choroid (Mori et al., 2002). The potential role of RTP801 in retinopathy was also studied, and the results indicated that by inhibition of its expression, the development of retinopathy was significantly reduced (Brafman et al., 2004).
miRNA is also used for regulating inflammatory-mediated genes in different diseases in the eye (Raghunath and Perumal, 2015). For instance, in AMD treatment, it has been shown that the upregulation of miR-9, miR-125b, miR-146a, and miR-155, alone or in combination, regulates the innate immune system and inflammation signals via the NF-
Messenger RNA (mRNA) is another type of nucleic acid that has been widely used in gene therapy. It allows faster protein translation as it does not require trafficking of the cargo to the cell nucleus in comparison to DNA. A primary anti-inflammatory cytokine is IL10, and delivery of the IL10 gene to the disorders causing ocular inflammation may be an effective therapy. To treat ocular inflammation, one method employed is to distribute IL10 mRNA using solid lipid nanoparticles (SLN) and various polymeric ligands such as dextran or HA for long-term stability (Gómez-Aguado et al., 2021). In this study, the authors used mRNA and plasmid DNA (pDNA) for de novo production of IL10 and showed that the mRNA systems induced a higher expression of IL10 in corneal epithelial cells than pDNA systems, and that they suppress corneal inflammation.
3.3 Hybrid systems of biomaterial carriers and different cargoes
New drug delivery systems that combine several drug delivery techniques such as hydrogel and nano systems, have shown intriguing results in recent years, leading to the development of more intelligent and controlled drug delivery systems. In ophthalmic drug delivery systems, it is important to reduce side effects and increase treatment efficiency and bioavailability by minimal injection or surgery in the eye (Sharma et al., 2016). One example of these hybrid scaffolds is provided by du Toit et al. (2013). The hybrid system employed was an indomethacin anti-inflammatory non-steroidal drug encapsulated in a lipoidal-chitosan-PCL nano system with a size of 140.7 nm and a positive surface charge for targeting the inflammatory disorder in the posterior part of the eye. The authors demonstrated that, in comparison to the chitosan-PCL nano system alone, the composite lipoidal nano system suppressed the inflammation by decreasing the amount of NF-
4 Conclusion
In ocular inflammatory illnesses, innate immune cells play a key role in removing the inflammatory pathogen or infection and subsequent regeneration of the inflamed region by homeostatic action and fibrosis development. Since the eye is extremely sensitive to inflammation and injury, it is critical to maintain its homeostasis and to avoid any chronic or harmful inflammation that can lead to ocular disorders such as DED, uveitis, glaucoma, AMD, as well as any other chronic inflammation that can result in eye tissue degeneration and blindness. Further research regarding the eye’s immunological response is required for designing tolerogenic biomaterials that act as intraocular lenses, cellular scaffolds, therapeutic molecule depots, or carriers of gene therapies. The discussion presented in this article sheds light on the potential use of biomaterials to direct immune responses toward favorable treatment outcomes.
Biomaterials can be utilized as manipulating agents for interacting with ocular innate immune cells and directing pro- or anti-inflammatory responses by using them either cargo-less or cargo-loaded. In this review, we discussed several physiochemical features of biomaterials such as mechanical strength, size, shape, charge, wettability, and biodegradability, as well as how each of these factors might program the fate of the innate immune cells’ phase by inhibiting or inducing special receptors and signaling pathways. These physiochemical parameters also affect the biodistribution and the interaction between the target cells and the cargoes. While previous efforts have focused on the intended action of the carrier, the influence of these parameters on the immune response has been neglected. They are often “discovered” as a surprise or treated as an unexpected side effect.
However, one of the most difficult issues to address is determining how to identify the influence of each physiochemical feature on the underlying biomaterial-related immune response and what cross-talks exist between them. More study and deeper understanding are required to determine the precise cell-biomaterial interaction mechanism by combining these physiochemical features and determining the effect of combining them on innate immune system programming. In the future, machine learning and artificial intelligence (AI) will be useful to forecast the ocular innate immune system’s reaction to a given biomaterial with defined features. A combination of gene therapies and immunomodulatory biomaterials carriers could be also a promising method for programming targeted overacting pro-inflammatory innate immune cells in a downregulating manner to retain their anti-inflammatory action, without side-effects on other immune cells’ fighting action.
Overall, there is significant therapeutic potential in ocular inflammatory diseases by focusing on triggering the anti-inflammatory phase of ocular-activated innate immune cells and providing homeostasis and tissue regeneration signals to the eye via immunomodulatory biomaterials that can sustain the release of novel therapeutic agents such as nucleic acids, biomolecules, or MSCs for an extended period of time with only one administration.
Author contributions
All authors listed have made a substantial, direct, and intellectual contribution to the work and approved it for publication.
Acknowledgments
The authors acknowledge the use of BioRender to generate Figures 1–4.
Conflict of interest
The authors declare that the research was conducted in the absence of any commercial or financial relationships that could be construed as a potential conflict of interest.
Publisher’s note
All claims expressed in this article are solely those of the authors and do not necessarily represent those of their affiliated organizations, or those of the publisher, the editors and the reviewers. Any product that may be evaluated in this article, or claim that may be made by its manufacturer, is not guaranteed or endorsed by the publisher.
References
Abu-Ain, M. S., and Webber, S. K. (2010). The biological bandage contact lens: A novel technique for using the amniotic membrane in the treatment of persistent corneal epithelial defects. Eye 24 (7), 1306–1307. doi:10.1038/eye.2010.1
Adamson, P., Wilde, T., Dobrzynski, E., Sychterz, C., Polsky, R., Kurali, E., et al. (2016). Single ocular injection of a sustained-release anti-VEGF delivers 6months pharmacokinetics and efficacy in a primate laser CNV model. J. Control Release 244, 1–13. doi:10.1016/j.jconrel.2016.10.026
Ahn, H., Kim, J., Jeung, E. B., and Lee, G. S. (2014). Dimethyl sulfoxide inhibits NLRP3 inflammasome activation. Immunobiology 219 (4), 315–322. doi:10.1016/j.imbio.2013.11.003
Allyn, M. M., Luo, R. H., Hellwarth, E. B., and Swindle-Reilly, K. E. (2022). Considerations for polymers used in ocular drug delivery. Front. Med. (Lausanne) 8, 787644. doi:10.3389/fmed.2021.787644
Anderson, J. M. (2003). Biological responses to materials. Annu. Rev. Mater. Res. 31, 81–110. doi:10.1146/annurev.matsci.31.1.81
Anderson, J. M., Rodriguez, A., and Chang, D. T. (2008). Foreign body reaction to biomaterials. Semin. Immunol. 20 (2), 86–100. doi:10.1016/j.smim.2007.11.004
Andorko, J. I., and Jewell, C. M. (2017). Designing biomaterials with immunomodulatory properties for tissue engineering and regenerative medicine. Bioeng. Transl. Med. 2 (2), 139–155. doi:10.1002/btm2.10063
Andrés-Guerrero, V., Zong, M., Ramsay, E., Rojas, B., Sarkhel, S., Gallego, B., et al. (2015). Novel biodegradable polyesteramide microspheres for controlled drug delivery in Ophthalmology. J. Control. Release 211, 105–117. doi:10.1016/j.jconrel.2015.05.279
Arroba, A. I., Alcalde-Estevez, E., García-Ramírez, M., Cazzoni, D., de la Villa, P., Sánchez-Fernández, E. M., et al. (1862). Modulation of microglia polarization dynamics during diabetic retinopathy in db/db mice. Biochimica Biophysica Acta (BBA) - Mol. Basis Dis. 1862 (9), 1663–1674. doi:10.1016/j.bbadis.2016.05.024
Ashikari, M., Tokoro, M., Itaya, M., Nozaki, M., and Ogura, Y. (2010). Suppression of laser-induced choroidal neovascularization by nontargeted siRNA. Invest. Ophthalmol. Vis. Sci. 51 (7), 3820–3824. doi:10.1167/iovs.09-5121
Aşık, M. D., Doğan Aşık, M., Uğurlu, N., Yülek, F., Tuncer, S., Türk, M., et al. (2013). Ketorolac tromethamine loaded chitosan nanoparticles as a nanotherapeutic system for ocular diseases. Hacettepe J. Biol. Chem. 41 (1), 81–86.
Bainbridge, J. W. B., Mistry, A., de Alwis, M., Paleolog, E., Baker, A., Thrasher, A. J., et al. (2002). Inhibition of retinal neovascularisation by gene transfer of soluble VEGF receptor sFlt-1. Gene Ther. 9 (5), 320–326. doi:10.1038/sj.gt.3301680
Bartneck, M., Keul, H. A., Singh, S., Czaja, K., Bornemann, J., Bockstaller, M., et al. (2010). Rapid uptake of gold nanorods by primary human blood phagocytes and immunomodulatory effects of surface chemistry. ACS Nano 4 (6), 3073–3086. doi:10.1021/nn100262h
Bauernfeind, F., Rieger, A., Schildberg, F. A., Knolle, P. A., Schmid-Burgk, J. L., and Hornung, V. (2012). NLRP3 inflammasome activity is negatively controlled by miR-223. J. Immunol. 189 (8), 4175–4181. doi:10.4049/jimmunol.1201516
Beeken, L. J., Ting, D. S. J., and Sidney, L. E. (2021). Potential of mesenchymal stem cells as topical immunomodulatory cell therapies for ocular surface inflammatory disorders. Stem Cells Transl. Med. 10 (1), 39–49. doi:10.1002/sctm.20-0118
Belhaj, M., Annamalai, B., Parsons, N., Shuler, A., Potts, J., and Rohrer, B. (2020). Encapsulated cell technology for the delivery of biologics to the mouse eye. J. Vis. Exp. 2020 (157). doi:10.3791/60162
Blume, Z. I., Lambert, J. M., Lovel, A. G., and Mitchell, D. M. (2020). Microglia in the developing retina couple phagocytosis with the progression of apoptosis via P2RY12 signaling. Dev. Dyn. 249 (6), 723–740. doi:10.1002/dvdy.163
Bordet, T., and Behar-Cohen, F. (2019). Ocular gene therapies in clinical practice: Viral vectors and nonviral alternatives. Drug Discov. Today 24 (8), 1685–1693. doi:10.1016/j.drudis.2019.05.038
Bowman, L. M., Si, E., Pang, J., Archibald, R., and Friedlaender, M. (2009). Development of a topical polymeric mucoadhesive ocular delivery system for azithromycin. J. Ocul. Pharmacol. Ther. 25 (2), 133–139. doi:10.1089/jop.2008.0066
Brafman, A., Mett, I., Shafir, M., Gottlieb, H., Damari, G., Gozlan-Kelner, S., et al. (2004). Inhibition of oxygen-induced retinopathy in RTP801-deficient mice. Invest. Ophthalmol. Vis. Sci. 45 (10), 3796–3805. doi:10.1167/iovs.04-0052
Camelo, S., Lajavardi, L., Bochot, A., Goldenberg, B., Naud, M. C., Brunel, N., et al. (2009). Protective effect of intravitreal injection of vasoactive intestinal peptide-loaded liposomes on experimental autoimmune uveoretinitis. J. Ocul. Pharmacol. Ther. 25 (1), 9–21. doi:10.1089/jop.2008.0074
Carrasquillo, K. G., Ricker, J. A., Rigas, I. K., Miller, J. W., Gragoudas, E. S., and Adamis, A. P. (2003). Controlled delivery of the anti-VEGF aptamer EYE001 with poly(lactic-co-glycolic)acid microspheres. Invest. Ophthalmol. Vis. Sci. 44 (1), 290–299. doi:10.1167/iovs.01-1156
Casey, L. M., Kakade, S., Decker, J. T., Rose, J. A., Deans, K., Shea, L. D., et al. (2019). Cargo-less nanoparticles program innate immune cell responses to toll-like receptor activation. Biomaterials 218, 119333. doi:10.1016/j.biomaterials.2019.119333
Casey-Power, S., Ryan, R., Behl, G., McLoughlin, P., Byrne, M. E., and Fitzhenry, L. (2022). Hyaluronic acid: Its versatile use in ocular drug delivery with a specific focus on hyaluronic acid-based polyelectrolyte complexes. Pharmaceutics 14 (7), 1479. doi:10.3390/pharmaceutics14071479
Cejka, C., Holan, V., Trosan, P., Zajicova, A., Javorkova, E., and Cejkova, J. (2016). The favorable effect of mesenchymal stem cell treatment on the antioxidant protective mechanism in the corneal epithelium and renewal of corneal optical properties changed after alkali burns. Oxid. Med. Cell Longev. 2016, 5843809. doi:10.1155/2016/5843809
Cha, B. H., Shin, S. R., Leijten, J., Li, Y. C., Singh, S., Liu, J. C., et al. (2017). Integrin-mediated interactions control macrophage polarization in 3D hydrogels. Adv. Healthc. Mater 6 (21), 1700289. doi:10.1002/adhm.201700289
Chen, B. M., Cheng, T. L., and Roffler, S. R. (2021). Polyethylene glycol immunogenicity: Theoretical, clinical, and practical aspects of anti-polyethylene glycol antibodies. ACS Nano 15, 14022–14048. doi:10.1021/acsnano.1c05922
Chen, M., Luo, C., Zhao, J., Devarajan, G., and Xu, H. (2019). Immune regulation in the aging retina. Prog. Retin Eye Res. 69, 159–172. doi:10.1016/j.preteyeres.2018.10.003
Chew, E. Y., Clemons, T. E., Peto, T., Sallo, F. B., Ingerman, A., Tao, W., et al. (2015). Ciliary neurotrophic factor for macular telangiectasia type 2: Results from a phase 1 safety trial. Am. J. Ophthalmol. 159 (4), 659–666.e1. doi:10.1016/j.ajo.2014.12.013
Chinnery, H. R., Mcmenamin, P. G., and Dando, S. J. (1947). Macrophage physiology in the eye. Pflugers Arch. 469 (3-4), 501–515. doi:10.1007/s00424-017-1947-5
Chittasupho, C., Posritong, P., and Ariyawong, P. (2019). Stability, cytotoxicity, and retinal pigment epithelial cell binding of hyaluronic acid-coated PLGA nanoparticles encapsulating lutein. AAPS PharmSciTech 20 (1), 4–13. doi:10.1208/s12249-018-1256-0
Cohen, S., Yoshioka, T., Lucarelli, M., Hwang, L. H., and Langer, R. (1991). Controlled delivery systems for proteins based on poly(lactic/glycolic acid) microspheres. Pharm. Res. 8 (6), 713–720. doi:10.1023/a:1015841715384
Cuartero, M. I., Ballesteros, I., Moraga, A., Nombela, F., Vivancos, J., Hamilton, J. A., et al. (2013). N2 neutrophils, novel players in brain inflammation after stroke: Modulation by the pparγ agonist rosiglitazone. Stroke 44 (12), 3498–3508. doi:10.1161/STROKEAHA.113.002470
Cui, L., Yao, Y., and Yim, E. K. F. (2021). The effects of surface topography modification on hydrogel properties. Apl. Bioeng. 5 (3), 031509. doi:10.1063/5.0046076
Dick, A. D., Carter, D., Robertson, M., Broderick, C., Hughes, E., Forrester, J. v., et al. (2003). Control of myeloid activity during retinal inflammation. J. Leukoc. Biol. 74 (2), 161–166. doi:10.1189/jlb.1102535
Das, S., Ahmad, Z., Suryawanshi, A., and Kumar, A. (2022). Innate immunity dysregulation in aging eye and therapeutic interventions. Ageing Res. Rev. 82, 101768. doi:10.1016/j.arr.2022.101768
Davis, M. E., and Brewster, M. E. (2004). Cyclodextrin-based pharmaceutics: Past, present and future. Nat. Rev. Drug Discov. 3 (12), 1023–1035. doi:10.1038/nrd1576
de La Fuente, M., Seijo, B., and Alonso, M. J. (2008). Novel hyaluronic acid-chitosan nanoparticles for ocular gene therapy. Invest. Ophthalmol. Vis. Sci. 49 (5), 2016–2024. doi:10.1167/iovs.07-1077
Dempsey, P. W., Vaidya, S. A., and Cheng, G. (2003). The Art of War: Innate and adaptive immune responses. Cell. Mol. Life Sci. CMLS 1260 (12), 2604–2621. doi:10.1007/s00018-003-3180-y
Diebold, Y., and Calonge, M. (2010). Applications of nanoparticles in ophthalmology. Prog. Retin Eye Res. 29 (6), 596–609. doi:10.1016/j.preteyeres.2010.08.002
Dobrovolskaia, M. A., and McNeil, S. E. (2007). Immunological properties of engineered nanomaterials. Nat. Nanotechnol. 2 (8), 469–478. doi:10.1038/nnano.2007.223
Domala, A., Bale, S., and Godugu, C. (2019). Protective effects of nanoceria in imiquimod induced psoriasis by inhibiting the inflammatory responses. Nanomedicine 15 (1), 5–22. doi:10.2217/nnm-2018-0515
Donaruma, L. G. (1988). Definitions in biomaterials, D. F. Williams, Ed., Elsevier, Amsterdam, 1987, 72 pp. JPoSL 26, 414.
D’Orazio, T. J., Mayhew, E., and Niederkorn, J. Y. (2001). Ocular immune privilege promoted by the presentation of peptide on tolerogenic B cells in the spleen. II. Evidence for presentation by qa-1. J. Immunol. 166 (1), 26–32. doi:10.4049/jimmunol.166.1.26
D’orazio, T. J., and Niederkorn, J. Y. (1998). The nature of antigen in the eye has a profound effect on the cytokine milieu and resultant immune response. Eur. J. Immunol. 28 (5), 1544–1553. doi:10.1002/(SICI)1521-4141(199805)28:05<1544::AID-IMMU1544>3.0.CO;2-P
Du, H., Bartleson, J. M., Butenko, S., Alonso, V., Liu, W. F., Winer, D. A., et al. (2022). Tuning immunity through tissue mechanotransduction. Nat. Rev. Immunol., 1–15. doi:10.1038/s41577-022-00761-w
du Toit, L. C., Govender, T., Carmichael, T., Kumar, P., Choonara, Y. E., and Pillay, V. (2013). Design of an anti-inflammatory composite nanosystem and evaluation of its potential for ocular drug delivery. J. Pharm. Sci. 102 (8), 2780–2805. doi:10.1002/jps.23650
Eitan, E., Hutchison, E., Greig, N., Tweedie, D., Celik, H., Ghosh, S., et al. (2015). Combination therapy with lenalidomide and nanoceria ameliorates CNS autoimmunity. Exp. Neurol. 273, 151–160. Elsevier. doi:10.1016/j.expneurol.2015.08.008
Enríquez de Salamanca, A., Diebold, Y., Calonge, M., García-Vazquez, C., Callejo, S., Vila, A., et al. (2006). Chitosan nanoparticles as a potential drug delivery system for the ocular surface: Toxicity, uptake mechanism and in vivo tolerance. science 47 (4), 1416–1425. doi:10.1167/iovs.05-0495
Estúa-Acosta, G. A., Zamora-Ortiz, R., Buentello-Volante, B., García-Mejía, M., and Garfias, Y. (2019). Neutrophil extracellular traps: Current perspectives in the eye. Cells 8 (9), 979. doi:10.3390/cells8090979
Fan, W., Huang, W., Chen, J., Li, N., Mao, L., and Hou, S. (2022). Retinal microglia: Functions and diseases. Immunology 166 (3), 268–286. doi:10.1111/imm.13479
Farid, M., Sabeti, S., and Minckler, D. S. (2020). Histopathological study of an explanted novel artificial corneal device. Cornea 39 (7), 915–918. doi:10.1097/ICO.0000000000002261
Fernández-Albarral, J. A., Salazar, J. J., Hoz, R., Marco, E. M., Martín-Sánchez, B., Flores-Salguero, E., et al. (2021). Retinal molecular changes are associated with neuroinflammation and loss of RGCs in an experimental model of glaucoma. Int. J. Mol. Sci. 22 (4), 2066.
Formisano, N., van der Putten, C., Grant, R., Sahin, G., Truckenmüller, R. K., Bouten, C. V. C., et al. (2021). Mechanical properties of bioengineered corneal stroma. Adv. Healthc. Mater 10 (20), e2100972. doi:10.1002/adhm.202100972
Ganugula, R., Arora, M., Lepiz, M. A., Niu, Y., Mallick, B. K., Pflugfelder, S. C., et al. (2020). Systemic anti-inflammatory therapy aided by double-headed nanoparticles in a canine model of acute intraocular inflammation. Sci. Adv. 6 (35), eabb7878. doi:10.1126/sciadv.abb7878
Gao, J., Yu, X., Wang, X., He, Y., and Ding, J. (2022). Biomaterial–related cell microenvironment in tissue engineering and regenerative medicine. Engineering 13, 31–45. doi:10.1016/j.eng.2021.11.025
García-Culebras, A., Durán-Laforet, V., Peña-Martínez, C., Ballesteros, I., Pradillo, J. M., Díaz-Guzmán, J., et al. (2018). Myeloid cells as therapeutic targets in neuroinflammation after stroke: Specific roles of neutrophils and neutrophil–platelet interactions. J. Cereb. Blood Flow Metabolism 38 (12), 2150–2164. doi:10.1177/0271678X18795789
Garzón, H., Suárez, L. J., Muñoz, S., Cardona, J., Fontalvo, M., and Alfonso-Rodríguez, C. A. (2022). Biomaterials used for periodontal disease treatment: Focusing on immunomodulatory properties. Int. J. Biomater. 2022, 7693793. doi:10.1155/2022/7693793
Gavini, E., Chetoni, P., Cossu, M., Alvarez, M. G., Saettone, M. F., and Giunchedi, P. (2004). PLGA microspheres for the ocular delivery of a peptide drug, vancomycin using emulsification/spray-drying as the preparation method: In vitro/in vivo studies. Eur. J. Pharm. Biopharm. 57 (2), 207–212. doi:10.1016/j.ejpb.2003.10.018
Gebe, J. A., Yadava, K., Ruppert, S. M., Marshall, P., Hill, P., Falk, B. A., et al. (2017). Modified high-molecular-weight hyaluronan promotes allergen-specific immune tolerance. Am. J. Respir. Cell Mol. Biol. 56 (1), 109–120. doi:10.1165/rcmb.2016-0111OC
Getts, D. R., Terry, R. L., Getts, M. T., Deffrasnes, C., Müller, M., Vreden, C., et al. (2014). Therapeutic inflammatory monocyte modulation using immune-modifying microparticles. Sci. Transl. Med. 6 (219), 219ra7. doi:10.1126/scitranslmed.3007563
Ghasemi, H., Ghazanfari, T., Yaraee, R., Owlia, P., Hassan, Z. M., and Faghihzadeh, S. (2012). Roles of IL-10 in ocular inflammations: A review. Ocul. Immunol. Inflamm. 20 (6), 406–418. doi:10.3109/09273948.2012.723109
Ghoraba, H. H., Akhavanrezayat, A., Karaca, I., Yavari, N., Lajevardi, S., Hwang, J., et al. (2022). Ocular gene therapy: A literature review with special focus on immune and inflammatory responses. Clin. Ophthalmol. 16, 1753–1771. doi:10.2147/OPTH.S364200
Ghosh, S., Padmanabhan, A., Vaidya, T., Watson, A. M., Bhutto, I. A., Hose, S., et al. (2019). Neutrophils homing into the retina trigger pathology in early age-related macular degeneration. Commun. Biol. 2 (1), 348. doi:10.1038/s42003-019-0588-y
Gilger, B. C., and Hirsch, M. L. (2022). Therapeutic applications of adeno-associated virus (AAV) gene transfer of HLA-G in the eye. Int. J. Mol. Sci. 23 (7), 3465. doi:10.3390/ijms23073465
Ginhoux, F., Lim, S., Hoeffel, G., Low, D., and Huber, T. (2013). Origin and differentiation of microglia. Front. Cell Neurosci. 7, 45. doi:10.3389/fncel.2013.00045
Glybina, I. v., Kennedy, A., Ashton, P., Abrams, G. W., and Iezzi, R. (2010). Intravitreous delivery of the corticosteroid fluocinolone acetonide attenuates retinal degeneration in S334ter-4 rats. Invest. Ophthalmol. Vis. Sci. 51 (8), 4243–4252. doi:10.1167/iovs.09-4492
Glybina, I. v., Kennedy, A., Ashton, P., Abrams, G. W., and Iezzi, R. (2009). Photoreceptor neuroprotection in RCS rats via low-dose intravitreal sustained-delivery of fluocinolone acetonide. Invest. Ophthalmol. Vis. Sci. 50 (10), 4847–4857. doi:10.1167/iovs.08-2831
Gomes dos Santos, A. L., Bochot, A., Doyle, A., Tsapis, N., Siepmann, J., Siepmann, F., et al. (2006). Sustained release of nanosized complexes of polyethylenimine and anti-TGF-β2 oligonucleotide improves the outcome of glaucoma surgery. J. Control. Release 112 (3), 369–381. doi:10.1016/j.jconrel.2006.02.010
Gómez-Aguado, I., Rodríguez-Castejón, J., Beraza-Millor, M., Vicente-Pascual, M., Rodríguez-Gascón, A., Garelli, S., et al. (2021). Mrna-based nanomedicinal products to address corneal inflammation by interleukin-10 supplementation. Pharmaceutics 13 (9), 1472. doi:10.3390/pharmaceutics13091472
Gu, S., Xing, C., Han, J., Tso, M. O. M., and Hong, J. (2009). Differentiation of rabbit bone marrow mesenchymal stem cells into corneal epithelial cells in vivo and ex vivo. Mol. Vis. 15, 99–107.
Guo, L., Choi, S., Bikkannavar, P., and Cordeiro, M. F. (2022). Microglia: Key players in retinal ageing and neurodegeneration. Front. Cell Neurosci. 16, 804782. doi:10.3389/fncel.2022.804782
Gupta, P. K., and Venkateswaran, N. (2021). The role of KPI-121 0.25% in the treatment of dry eye disease: Penetrating the mucus barrier to treat periodic flares. Ther. Adv. Ophthalmol. 13, 251584142110127. doi:10.1177/25158414211012797
Gupta, V., and Sahu, P. K. (2001). Topical cyclosporin A in the management of vernal keratoconjunctivitis. Eye 15 (1), 39–41. doi:10.1038/eye.2001.10
Guter, M., and Breunig, M. (2017). Hyaluronan as a promising excipient for ocular drug delivery. Eur. J. Pharm. Biopharm. 113, 34–49. doi:10.1016/j.ejpb.2016.11.035
Guzman-Aranguez, A., Loma, P., and Pintor, J. (2013). Small-interfering RNAs (siRNAs) as a promising tool for ocular therapy. Br. J. Pharmacol. 170 (4), 730–747. doi:10.1111/bph.12330
Hamrah, P., Liu, Y., Zhang, Q., and Dana, M. R. (2003). The corneal stroma is endowed with a significant number of resident dendritic cells. Invest. Ophthalmol. Vis. Sci. 44 (2), 581–589. doi:10.1167/iovs.02-0838
Han, Y., Xu, X., Tang, J., Shen, C., Lin, Q., and Chen, H. (2017). Bottom-up fabrication of zwitterionic polymer brushes on intraocular lens for improved biocompatibility. Int. J. Nanomedicine 12, 127–135. doi:10.2147/IJN.S107491
Haneklaus, M., Gerlic, M., Kurowska-Stolarska, M., Rainey, A.-A., Pich, D., McInnes, I. B., et al. (2012). Cutting edge: miR-223 and EBV miR-BART15 regulate the NLRP3 inflammasome and IL-1β production. J. Immunol. 189 (8), 3795–3799. doi:10.4049/jimmunol.1200312
Hao, H., He, B., Yu, B., Yang, J., Xing, X., and Liu, W. (2022). Suprachoroidal injection of polyzwitterion hydrogel for treating glaucoma. Biomater. Adv. 142, 213162. SSRN Electronic Journal. doi:10.1016/j.bioadv.2022.213162
He, B., Yang, J., Liu, Y., Xie, X., Hao, H., Xing, X., et al. (2021). An in situ-forming polyzwitterion hydrogel: Towards vitreous substitute application. Bioact. Mater 6 (10), 3085–3096. doi:10.1016/j.bioactmat.2021.02.029
He, X. T., Li, X., Xia, Y., Yin, Y., Wu, R. X., Sun, H. H., et al. (2019). Building capacity for macrophage modulation and stem cell recruitment in high-stiffness hydrogels for complex periodontal regeneration: Experimental studies in vitro and in rats. Acta Biomater. 88, 162–180. doi:10.1016/j.actbio.2019.02.004
Herrero-Vanrell, R., Bravo-Osuna, I., Andrés-Guerrero, V., Vicario-de-la-Torre, M., and Molina-Martínez, I. T. (2014). The potential of using biodegradable microspheres in retinal diseases and other intraocular pathologies. Prog. Retin Eye Res. 42, 27–43. doi:10.1016/j.preteyeres.2014.04.002
Hirst, S. M., Karakoti, A. S., Tyler, R. D., Sriranganathan, N., Seal, S., and Reilly, C. M. (2009). Anti-inflammatory properties of cerium oxide nanoparticles. Wiley Online Libr. 5 (24), 2848–2856. doi:10.1002/smll.200901048
Hu, C. C., Chiu, Y. C., Chaw, J. R., Chen, C. F., and Liu, H. W. (2019). Thermo-responsive hydrogel as an anti-VEGF drug delivery system to inhibit retinal angiogenesis in Rex rabbits. Technol. Health Care 27, 153–163. doi:10.3233/THC-199015
Hu, J., and Wan, Y. (2011). Tolerogenic dendritic cells and their potential applications. Immunology 132 (3), 307. doi:10.1111/j.1365-2567.2010.03396.x
Huang, X., and Chau, Y. (2021). Enhanced delivery of siRNA to retinal ganglion cells by intravitreal lipid nanoparticles of positive charge. Mol. Pharm. 18 (1), 377–385. doi:10.1021/acs.molpharmaceut.0c00992
Huang, X., and Chau, Y. (2019a). Intravitreal nanoparticles for retinal delivery. Drug Discov. Today 24 (8), 1510–1523. doi:10.1016/j.drudis.2019.05.005
Huang, X., and Chau, Y. (2019b). Investigating impacts of surface charge on intraocular distribution of intravitreal lipid nanoparticles. Exp. Eye Res. 186, 107711. doi:10.1016/j.exer.2019.107711
Hunter, R., Strickland, F., and Kézdy, F. (1981). The adjuvant activity of nonionic block polymer surfactants. I. The role of hydrophile-lipophile balance. J. Immunol. 127 (3), 1244–1250. doi:10.4049/jimmunol.127.3.1244
Iezzi, R., Guru, B. R., Glybina, I. v., Mishra, M. K., Kennedy, A., and Kannan, R. M. (2012). Dendrimer-based targeted intravitreal therapy for sustained attenuation of neuroinflammation in retinal degeneration. Biomaterials 33 (3), 979–988. doi:10.1016/j.biomaterials.2011.10.010
Im, G. (2020). Biomaterials in orthopaedics: The past and future with immune modulation. Biomater. Res. 24 (1), 1–4.
Ito, D., Imai, Y., Ohsawa, K., Nakajima, K., Fukuuchi, Y., and Kohsaka, S. (1998). Microglia-specific localisation of a novel calcium binding protein, Iba1. Mol. Brain Res. 57 (1), 1–9. doi:10.1016/s0169-328x(98)00040-0
Jain, E., Hill, L., Canning, E., Sell, S. A., and Zustiak, S. P. (2017). Control of gelation, degradation and physical properties of polyethylene glycol hydrogels through the chemical and physical identity of the crosslinker. J. Mater Chem. B 5 (14), 2679–2691. doi:10.1039/c6tb03050e
Ji, Y. W., Byun, Y. J., Choi, W., Jeong, E., Kim, J. S., Noh, H., et al. (2013). Neutralization of ocular surface TNF-α reduces ocular surface and lacrimal gland inflammation induced by in vivo dry eye. Invest. Ophthalmol. Vis. Sci. 54 (12), 7557–7566. doi:10.1167/iovs.12-11515
Jiang, J., O-Bo, X. I. A., Hui-Zhuo, X. U., Xiong, Y. U., Song, W. T., Xiong, S. I. Q. I., et al. (2009). Inhibition of retinal neovascularization by gene transfer of small interfering RNA targeting HIF-1alpha and VEGF. J. Cell Physiol. 218 (1), 66–74. doi:10.1002/jcp.21566
Kakizawa, Y., Lee, J. S., Bell, B., and Fahmy, T. M. (2017). Precise manipulation of biophysical particle parameters enables control of proinflammatory cytokine production in presence of TLR 3 and 4 ligands. Acta Biomater. 57, 136–145. doi:10.1016/j.actbio.2017.01.025
Kaplan, D. H., Jenison, M. C., Saeland, S., Shlomchik, W. D., and Shlomchik, M. J. (2005). Epidermal Langerhans cell-deficient mice develop enhanced contact hypersensitivity. Immunity 23 (6), 611–620. doi:10.1016/j.immuni.2005.10.008
Kauper, K., Ling, V., Elliot, S., McGovern, C., Sherman, S., Dean, B., et al. (2012b). Long-term, sustained intraocular delivery of a VEGF antagonist using encapsulated cell technology implant for the treatment of choroidal neovascular diseases. Invest. Ophthalmol. Vis. Sci. 53 (14), 455.
Kauper, K., McGovern, C., Sherman, S., Heatherton, P., Rapoza, R., Stabila, P., et al. (2012a). Two-year intraocular delivery of ciliary neurotrophic factor by encapsulated cell technology implants in patients with chronic retinal degenerative diseases. Invest. Ophthalmol. Vis. Sci. 53 (12), 7484–7491. doi:10.1167/iovs.12-9970
Kim, M. K., Lee, J. L., Wee, W. R., and Lee, J. H. (2002). Seoul-type keratoprosthesis: Preliminary results of the first 7 human cases. Archives Ophthalmol. 120 (6), 761–766. doi:10.1001/archopht.120.6.761
Kim, T., Sall, K., Holland, E. J., Brazzell, R. K., Coultas, S., and Gupta, P. K. (2018). Safety and efficacy of twice daily administration of KPI-121 1% for ocular inflammation and pain following cataract surgery. Clin. Ophthalmol. 13, 69–86. doi:10.2147/OPTH.S185800
Kolaczkowska, E., and Kubes, P. (2013). Neutrophil recruitment and function in health and inflammation. Nat. Rev. Immunol. 13 (3), 159–175. doi:10.1038/nri3399
Kong, H. J., Alsberg, E., Kaigler, D., Lee, K. Y., and Mooney, D. J. (2004). Controlling degradation of hydrogels via the size of cross-linked junctions. Adv. Mater 16 (21), 1917–1921. doi:10.1002/adma.200400014
Kostenko, A., Swioklo, S., and Connon, C. J. (2022). Alginate in corneal tissue engineering. Biomed. Mater. 17 (2), 022004. doi:10.1088/1748-605X/ac4d7b
Križaj, D., Ryskamp, D. A., Tian, N., Tezel, G., Mitchell, C. H., Slepak, V. Z., et al. (2014). From mechanosensitivity to inflammatory responses: New players in the pathology of glaucoma. Curr. Eye Res. 39 (2), 105–119. doi:10.3109/02713683.2013.836541
Kubota, I., Sakamoto, T., Kawano, Y.-I., Tsutsumi, T., Hisatomi, T., Komiyama, S., et al. (2022). Immunoregulatory role of ocular macrophages: The macrophages produce RANTES to suppress experimental autoimmune uveitis. J. Immunol. Ref. 171, 2652–2659. doi:10.4049/jimmunol.171.5.2652
Kutty, R. K., Nagineni, C. N., Samuel, W., Vijayasarathy, C., Jaworski, C., Duncan, T., et al. (2013). Differential regulation of microRNA-146a and microRNA-146b-5p in human retinal pigment epithelial cells by interleukin-1β, tumor necrosis factor-α, and interferon-γ. Mol. Vis. 19, 737–750.
Kwon, S., Kim, S. H., Khang, D., and Lee, J. Y. (2020). Potential therapeutic usage of nanomedicine for glaucoma treatment. Int. J. Nanomedicine. 15, 5745–5765.
Kwon, C., Kim, Y., and Jeon, H. (2017). Collective migration of lens epithelial cell induced by differential microscale groove patterns. J. Funct. Biomater. 8 (3), 34. doi:10.3390/jfb8030034
Lajavardi, L., Bochot, A., Camelo, S., Goldenberg, B., Naud, M. C., Behar-Cohen, F., et al. (2007). Downregulation of endotoxin-induced uveitis by intravitreal injection of vasoactive intestinal peptide encapsulated in liposomes. Invest. Ophthalmol. Vis. Sci. 48 (7), 3230–3238. doi:10.1167/iovs.06-1305
Lallemand, F., Felt-Baeyens, O., Besseghir, K., Behar-Cohen, F., and Gurny, R. (2003). Cyclosporine A delivery to the eye: A pharmaceutical challenge. Eur. J. Pharm. Biopharm. 56 (3), 307–318. doi:10.1016/s0939-6411(03)00138-3
Langmann, T. (2007). Microglia activation in retinal degeneration. J. Leukoc. Biol. 81 (6), 1345–1351. doi:10.1189/jlb.0207114
Lau, C. M. L., Jahanmir, G., Yu, Y., and Chau, Y. (2021). Controllable multi-phase protein release from in-situ hydrolyzable hydrogel. J. Control. Release 335, 75–85. doi:10.1016/j.jconrel.2021.05.006
Lechner, J., Chen, M., Hogg, R. E., Toth, L., Silvestri, G., Chakravarthy, U., et al. (2017). Peripheral blood mononuclear cells from neovascular age-related macular degeneration patients produce higher levels of chemokines CCL2 (MCP-1) and CXCL8 (IL-8). J. Neuroinflammation 14 (1), 42. doi:10.1186/s12974-017-0820-y
Lee, H. J., Ko, J. H., Jeong, H. J., Ko, A. Y., Kim, M. K., Wee, W. R., et al. (2015). Mesenchymal stem/stromal cells protect against autoimmunity via CCL2-dependent recruitment of myeloid-derived suppressor cells. J. Immunol. 194 (8), 3634–3645. doi:10.4049/jimmunol.1402139
Li, H., Yang, Y. G., and Sun, T. (2022). Nanoparticle-based drug delivery systems for induction of tolerance and treatment of autoimmune diseases. Front. Bioeng. Biotechnol. 10, 889291. doi:10.3389/fbioe.2022.889291
Li, J., Jiang, X., Li, H., Gelinsky, M., Gu, Z., Li, J., et al. (2021). Tailoring materials for modulation of macrophage fate. Adv. Mater. 33 (12), e2004172. doi:10.1002/adma.202004172
Li, N., Zhuang, C., Wang, M., Sun, X., Nie, S., and Pan, W. (2009). Liposome coated with low molecular weight chitosan and its potential use in ocular drug delivery. Int. J. Pharm. 379 (1), 131–138. doi:10.1016/j.ijpharm.2009.06.020
Li, Y., Ren, X., Zhang, Z., Duan, Y., Li, H., Chen, S., et al. (2022). Effect of small extracellular vesicles derived from IL-10-overexpressing mesenchymal stem cells on experimental autoimmune uveitis. Stem Cell Res. Ther. 13 (1), 100–115. doi:10.1186/s13287-022-02780-9
Li, Y. Y., Cui, J. G., Dua, P., Pogue, A. I., Bhattacharjee, S., and Lukiw, W. J. (2011). Differential expression of miRNA-146a-regulated inflammatory genes in human primary neural, astroglial and microglial cells. Neurosci. Lett. 499 (2), 109–113. doi:10.1016/j.neulet.2011.05.044
Li, Z., and Bratlie, K. M. (2021). Effect of RGD functionalization and stiffness of gellan gum hydrogels on macrophage polarization and function. Mater. Sci. Eng. C 128, 112303. doi:10.1016/j.msec.2021.112303
Liew, P. X., and Kubes, P. (2019). The neutrophil’s role during health and disease. Physiol. Rev. 99 (2), 1223–1248. doi:10.1152/physrev.00012.2018
Lin, H., Yue, Y., Maidana, D. E., Bouzika, P., Atik, A., Matsumoto, H., et al. (2016). Drug delivery nanoparticles: Toxicity comparison in retinal pigment epithelium and retinal vascular endothelial cells. Semin. Ophthalmol. 31, 1–9. doi:10.3109/08820538.2015.1114865
Lin, W., Liu, T., Wang, B., and Bi, H. (2019). The role of ocular dendritic cells in uveitis. Immunol. Lett. 209, 4–10. doi:10.1016/j.imlet.2019.03.016
Liu, J., and Li, Z. (2021). Resident innate immune cells in the cornea. Front. Immunol. 12, 620284. doi:10.3389/fimmu.2021.620284
Lopez, P. F., Grossniklaus, H. E., Lambert, H. M., Aaberg, T. M., Capone, A., Sternberg, P., et al. (1991). Pathologic features of surgically excised subretinal neovascular membranes in age-related macular degeneration. Am. J. Ophthalmol. 112 (6), 647–656. doi:10.1016/s0002-9394(14)77270-8
Lu, J. M., Song, X. J., Wang, H. F., Li, X. L., and Zhang, X. R. (2012). Murine corneal stroma cells inhibit LPS-induced dendritic cell maturation partially through TGF-β2 secretion in vitro. Mol. Vis. 18, 2255–2264.
Luis, D., Kieran, F., Marc, F.-Y., Manus, B., Abhay, P., and Dimitrios, Z. (2016). Collagen cross-linking increases scaffold stability while modulates pro-inflammatory macrophage response. Front. Bioeng. Biotechnol. 4. doi:10.3389/conf.fbioe.2016.01.02202
Lukiw, W. J., Surjyadipta, B., Dua, P., and Alexandrov, P. N. (2012). Common micro RNAs (miRNAs) target complement factor H (CFH) regulation in Alzheimer’s disease (AD) and in age-related macular degeneration (AMD). Int. J. Biochem. Mol. Biol. 3 (1), 105–116.
Luo, L. J., Nguyen, D. D., and Lai, J. Y. (2020). Dually functional hollow ceria nanoparticle platform for intraocular drug delivery: A push beyond the limits of static and dynamic ocular barriers toward glaucoma therapy. Biomaterials 243, 119961. doi:10.1016/j.biomaterials.2020.119961
Luo, L., Yang, J., Oh, Y., Hartsock, M. J., Xia, S., Kim, Y. C., et al. (2019). Controlled release of corticosteroid with biodegradable nanoparticles for treating experimental autoimmune uveitis. J. Control. Release 296, 68–80. doi:10.1016/j.jconrel.2019.01.018
Lutty, G. A., Cao, J., and McLeod, D. S. (1997). Relationship of polymorphonuclear leukocytes to capillary dropout in the human diabetic choroid. Am. J. Pathology 151 (3), 707–714.
Mahajan, A., Hasíková, L., Hampel, U., Grüneboom, A., Shan, X., Herrmann, I., et al. (2021). Aggregated neutrophil extracellular traps occlude Meibomian glands during ocular surface inflammation. Ocul. Surf. 20, 1–12. doi:10.1016/j.jtos.2020.12.005
Mahaling, B., Low, S. W. Y., Beck, M., Kumar, D., Ahmed, S., Connor, T. B., et al. (2022). Damage-associated molecular patterns (DAMPs) in retinal disorders. Int. J. Mol. Sci. 23 (5), 2591. doi:10.3390/ijms23052591
Mandal, A., Pal, D., Agrahari, V., My Trinh, H., Joseph, M., Mitra, A. K., et al. (2018). Ocular delivery of proteins and peptides: Challenges and novel formulation approaches. Adv. Drug Deliv. Rev. 126, 67–95. doi:10.1016/j.addr.2018.01.008
Marõ´a, M., Alonso, J., Sá Nchez, A., Alonso, M. J., Mar|´a, M., and Sá, A. (2003). The potential of chitosan in ocular drug delivery. Wiley Online Libr. 55 (11), 1451–1463. doi:10.1211/0022357022476
Maruoka, S., Inaba, M., and Ogata, N. (2018). Activation of dendritic cells in dry eye mouse model. Invest. Ophthalmol. Vis. Sci. 59 (8), 3269–3277. doi:10.1167/iovs.17-22550
Mathurm, M., and Gilhotra, R. M. (2011). Glycerogelatin-based ocular inserts of aceclofenac: Physicochemical, drug release studies and efficacy against prostaglandin E₂-induced ocular inflammation. Drug Deliv. 18 (1), 54–64. doi:10.3109/10717544.2010.509366
Matica, M. A., Aachmann, F. L., Tøndervik, A., Sletta, H., and Ostafe, V. (2019). Chitosan as a wound dressing starting material: Antimicrobial properties and mode of action. Int. J. Mol. Sci. 20 (23), 5889. doi:10.3390/ijms20235889
McMenamin, P. G., Saban, D. R., and Dando, S. J. (2019). Immune cells in the retina and choroid: Two different tissue environments that require different defenses and surveillance. Prog. Retin Eye Res. 70, 85–98. doi:10.1016/j.preteyeres.2018.12.002
McWhorter, F. Y., Wang, T., Nguyen, P., Chung, T., and Liu, W. F. (2013). Modulation of macrophage phenotype by cell shape. Proc. Natl. Acad. Sci. U. S. A. 110 (43), 17253–17258. doi:10.1073/pnas.1308887110
Medawar, P. B. (1948). Immunity to homologous grafted skin; the fate of skin homografts transplanted to the brain, to subcutaneous tissue, and to the anterior chamber of the eye. Br. J. Exp. Pathol. 29 (1), 58–69.
Merad, M., Ginhoux, F., and Collin, M. (2008). Origin, homeostasis and function of Langerhans cells and other langerin-expressing dendritic cells. Nat. Rev. Immunol. 8 (12), 935–947. doi:10.1038/nri2455
Mérida, S., Palacios, E., Navea, A., and Bosch-Morell, F. (2015). Macrophages and uveitis in experimental animal models. Mediat. Inflamm. 2015, 671417. doi:10.1155/2015/671417
Minghetti, L., Malchiodi-Albedi, F., Matteucci, A., and Bernardo, A. (2008). PPAR-γ, microglial cells, and ocular inflammation: New venues for potential therapeutic approaches. PPAR Res. 2008, 295784. doi:10.1155/2008/295784
Mitarotonda, R., Giorgi, E., Eufrasio-da-Silva, T., Dolatshahi-Pirouz, A., Mishra, Y. K., Khademhosseini, A., et al. (2022). Immunotherapeutic nanoparticles: From autoimmune disease control to the development of vaccines. Biomater. Adv. 135, 212726. doi:10.1016/j.bioadv.2022.212726
Mobaraki, M., Soltani, M., Harofte, S. Z., Zoudani, E. L., Daliri, R., Aghamirsalim, M., et al. (2020). Pharmaceutics biodegradable nanoparticle for cornea drug delivery: Focus review. Pharmaceutics 12, 1232. doi:10.3390/pharmaceutics12121232
Morante-Palacios, O., Fondelli, F., Ballestar, E., and Martínez-Cáceres, E. M. (2021). Tolerogenic dendritic cells in autoimmunity and inflammatory diseases. Trends Immunol. 42 (1), 59–75. doi:10.1016/j.it.2020.11.001
Mori, K., Gehlbach, P., Yamamoto, S., Duh, E., Zack, D. J., Li, Q., et al. (2002). AAV-mediated gene transfer of pigment epithelium-derived factor inhibits choroidal neovascularization. Invest. Ophthalmol. Vis. Sci. 43 (6), 1994–2000.
Morillas, A. G., Besson, V. C., Lerouet, D., and Aranda, C. (2021). Molecular sciences microglia and neuroinflammation: What place for P2RY12? Int. J. Mol. Sci. 22 (4), 1636. doi:10.3390/ijms22041636
Moshaverinia, A., Chen, C., Xu, X., Ansari, S., Zadeh, H. H., Schricker, S. R., et al. (2015). Regulation of the stem cell–host immune system interplay using hydrogel coencapsulation system with an anti-inflammatory drug. Adv. Funct. Mat. 25 (15), 2296–2307. Wiley Online Library. doi:10.1002/adfm.201500055
Mosser, D. M., and Edwards, J. P. (2008). Exploring the full spectrum of macrophage activation. Nat. Rev. Immunol. 8 (12), 958–969. doi:10.1038/nri2448
Mun, Y., Hwang, J. S., and Shin, Y. J. (2021). Role of neutrophils on the ocular surface. Int. J. Mol. Sci. 22 (19), 10386. doi:10.3390/ijms221910386
Murakami, Y., Ishikawa, K., Nakao, S., and Sonoda, K. H. (2020). Innate immune response in retinal homeostasis and inflammatory disorders. Prog. Retin Eye Res. 74, 100778. doi:10.1016/j.preteyeres.2019.100778
Musumeci, A., Lutz, K., Winheim, E., and Krug, A. B. (2019). What makes a PDC: Recent advances in understanding plasmacytoid DC development and heterogeneity. Front. Immunol. 10, 1222. doi:10.3389/fimmu.2019.01222
Nickerson, C. S., Karageozian, H. L., Park, J., and Kornfield, J. A. (2004). The mechanical properties of the vitreous humor. Invest. Ophthalmol. Vis. Sci. 45 (13), 37.
Niederkorn, J. Y. (2019). The eye sees eye to eye with the immune system: The 2019 proctor lecture. Invest. Ophthalmol. Vis. Sci. 60 (13), 4489–4495. doi:10.1167/iovs.19-28632
Niikura, K., Matsunaga, T., Suzuki, T., Kobayashi, S., Yamaguchi, H., Orba, Y., et al. (2013). Gold nanoparticles as a vaccine platform: Influence of size and shape on immunological responses in vitro and in vivo. ACS Nano 7 (5), 3926–3938. doi:10.1021/nn3057005
Nussenblatt, R. B., and Palestine, A. G. (1986). Cyclosporine: Immunology, pharmacology and therapeutic uses. Surv. Ophthalmol. 31 (3), 159–169. doi:10.1016/0039-6257(86)90035-4
Oh, J. Y., and Lee, R. H. (2021). Mesenchymal stromal cells for the treatment of ocular autoimmune diseases. Prog. Retin Eye Res. 85, 100967. doi:10.1016/j.preteyeres.2021.100967
Ohsawa, K., Imai, Y., Kanazawa, H., Sasaki, Y., and Kohsaka, S. (2000). Involvement of Iba1 in membrane ruffling and phagocytosis of macrophages/microglia. J. Cell Sci. 113 (17), 3073–3084. doi:10.1242/jcs.113.17.3073
Orozco Morales, M. L., Marsit, N. M., McIntosh, O. D., Hopkinson, A., and Sidney, L. E. (2019). Anti-inflammatory potential of human corneal stroma-derived stem cells determined by a novel in vitro corneal epithelial injury model. World J. Stem Cells 11 (2), 84–99. doi:10.4252/wjsc.v11.i2.84
Østerholt, H., Lundeland, B. S., Sonerud, T., Saugstad, O. D., and Nakstad, B. (2011). Effects of hyaluronic acid on expression of TLR2 and TLR4 on cord blood monocytes. Pediatr. Res. 70 (5), 476. doi:10.1038/pr.2011.701
Papenburg, B. J., Rodrigues, E. D., Wessling, M., and Stamatialis, D. (2010). Insights into the role of material surface topography and wettability on cell-material interactions. Soft Matter 6 (18), 4377–4388. doi:10.1039/b927207k
Park, K., Chen, Y., Hu, Y., Mayo, A. S., Kompella, U. B., Longeras, R., et al. (2009). Nanoparticle-mediated expression of an angiogenic inhibitor ameliorates ischemia-induced retinal neovascularization and diabetes-induced retinal vascular leakage. Diabetes 58 (8), 1902–1913. doi:10.2337/db08-1327
Parolini, O., Soncini, M., Evangelista, M., and Schmidt, D. (2009). Amniotic membrane and amniotic fluid-derived cells: Potential tools for regenerative medicine? Regen. Med. 4 (2), 275–291. doi:10.2217/17460751.4.2.275
Penfold, P. L., Madigan, M. C., Gillies, M. C., and Provis, J. M. (2001). Immunological and aetiological aspects of macular degeneration. Prog. Retin Eye Res. 20 (3), 385–414. doi:10.1016/s1350-9462(00)00025-2
Pereira, D. V., Petronilho, F., Pereira, H. R. S. B., Vuolo, F., Mina, F., Possato, J. C., et al. (2012). Effects of gold nanoparticles on endotoxin-induced uveitis in rats. Invest. Ophthalmol. Vis. Sci. 53 (13), 8036–8041. doi:10.1167/iovs.12-10743
Perez, V. L., and Caspi, R. R. (2015). Immune mechanisms in inflammatory and degenerative eye disease. Trends Immunol. 36 (6), 354–363. doi:10.1016/j.it.2015.04.003
Peynshaert, K., Devoldere, J., de Smedt, S. C., and Remaut, K. (2018). In vitro and ex vivo models to study drug delivery barriers in the posterior segment of the eye. Adv. Drug Deliv. Rev. 126, 44–57. doi:10.1016/j.addr.2017.09.007
Peynshaert, K., Devoldere, J., Minnaert, A. K., de Smedt, S. C., and Remaut, K. (2019). Morphology and composition of the inner limiting membrane: Species-specific variations and relevance toward drug delivery research. Drug Deliv. Res. 44 (5), 465–475. doi:10.1080/02713683.2019.1565890
Pillay, J., den Braber, I., Vrisekoop, N., Kwast, L. M., de Boer, R. J., Borghans, J. A. M., et al. (2010). In vivo labeling with 2H2O reveals a human neutrophil lifespan of 5.4 days. Blood 116 (4), 625–627. doi:10.1182/blood-2010-01-259028
Pintor, J. (2012). Silencing beta2-adrenergic receptors reduces intraocular pressure: A new approach for glaucoma therapy: A new approach to glaucoma therapy. Ann. R. Natl. Acad. Pharm. 78 (2), 230–240.
Pintwala, R., Postnikoff, C., Molladavoodi, S., and Gorbet, M. (2014). Coculture with intraocular lens material-activated macrophages induces an inflammatory phenotype in lens epithelial cells. J. Biomater. Appl. 29 (8), 1119–1132. doi:10.1177/0885328214552711
Pogue, A. I., and Lukiw, W. J. (2018). Up-regulated pro-inflammatory MicroRNAs (miRNAs) in alzheimer’s disease (AD) and age-related macular degeneration (AMD). Cell Mol. Neurobiol. 38 (5), 1021–1031. doi:10.1007/s10571-017-0572-3
Puri, S., Kenyon, B. M., and Hamrah, P. (2022). Immunomodulatory role of neuropeptides in the cornea. Biomedicines 10 (8), 1985. doi:10.3390/biomedicines10081985
Raghunath, A., and Perumal, E. (2015). Micro-RNAs and their roles in eye disorders. Ophthalmic Res. 53 (4), 169–186. doi:10.1159/000371853
Ramírez, A. I., de Hoz, R., Fernández-Albarral, J. A., Salobrar-Garcia, E., Rojas, B., Valiente-Soriano, F. J., et al. (2020). Time course of bilateral microglial activation in a mouse model of laser-induced glaucoma. Sci. Rep. 10 (1), 4890. doi:10.1038/s41598-020-61848-9
Rathod, S., and Deshpande, S. (2008). Albumin microspheres as an ocular delivery system for pilocarpine nitrate. Indian J. Pharm. Sci. 70 (2), 193–197. doi:10.4103/0250-474X.41454
Rayahin, J. E., Buhrman, J. S., Zhang, Y., Koh, T. J., and Gemeinhart, R. A. (2015). High and low molecular weight hyaluronic acid differentially influence macrophage activation. ACS Biomater. Sci. Eng. 1 (7), 481–493. doi:10.1021/acsbiomaterials.5b00181
Reeves, A. R. D., Spiller, K. L., Freytes, D. O., Vunjak-Novakovic, G., and Kaplan, D. L. (2015). Controlled release of cytokines using silk-biomaterials for macrophage polarization. Biomaterials 73, 272–283. doi:10.1016/j.biomaterials.2015.09.027
Reich, S., Fosnot, J., Kuroki, A., Tang, W., and Vis, X. Y.-M. (2003). Small interfering RNA (siRNA) targeting VEGF effectively inhibits ocular neovascularization in a mouse model. Mol. Vis. 9, 210–216.
Reichenbach, A., and Bringmann, A. (2020). Glia of the human retina. Glia 68 (4), 768–796. doi:10.1002/glia.23727
Reid, B., Gibson, M., Singh, A., Taube, J., Furlong, C., Murcia, M., et al. (2015). PEG hydrogel degradation and the role of the surrounding tissue environment. J. Tissue Eng. Regen. Med. 9 (3), 315–318. doi:10.1002/term.1688
Ren, N., Sun, R., Xia, K., Zhang, Q., Li, W., Wang, F., et al. (2019). DNA-based hybrid hydrogels sustain water-insoluble ophthalmic therapeutic delivery against allergic conjunctivitis. ACS Appl. Mater Interfaces 11 (30), 26704–26710. doi:10.1021/acsami.9b08652
Riabov, V., Salazar, F., Htwe, S. S., Gudima, A., Schmuttermaier, C., Barthes, J., et al. (2017). Generation of anti-inflammatory macrophages for implants and regenerative medicine using self-standing release systems with a phenotype-fixing cytokine cocktail formulation. Acta Biomater. 53, 389–398. doi:10.1016/j.actbio.2017.01.071
Ron, E., Turek, T., Mathiowitz, E., Chasin, M., Hageman, M., and Langer, R. (1993). Controlled release of polypeptides from polyanhydrides. Proc. Natl. Acad. Sci. U. S. A. 90 (9), 4176–4180. doi:10.1073/pnas.90.9.4176
Rota, R., Riccioni, T., Zaccarini, M., Lamartina, S., del Gallo, A., Fusco, A., et al. (2004). Marked inhibition of retinal neovascularization in rats following soluble-flt-1 gene transfer. J. Gene Med. 6 (9), 992–1002. doi:10.1002/jgm.586
Rowley, A. T., Nagalla, R. R., Wang, S. W., and Liu, W. F. (2019). Extracellular matrix-based strategies for immunomodulatory biomaterials engineering. Adv. Healthc. Mater 8 (8), e1801578. doi:10.1002/adhm.201801578
Rumelt, S., Bersudsky, V., Blum-Hareuveni, T., and Rehany, U. (2002). Systemic cyclosporin A in high failure risk, repeated corneal transplantation. Br. J. Ophthalmol. 86 (9), 988–992. doi:10.1136/bjo.86.9.988
Russell, S., Bennett, J., Wellman, J. A., Chung, D. C., Yu, Z. F., Tillman, A., et al. (2017). Efficacy and safety of voretigene neparvovec (AAV2-hRPE65v2) in patients with RPE65-mediated inherited retinal dystrophy: A randomised, controlled, open-label, phase 3 trial. Lancet 390 (10097), 849–860. doi:10.1016/S0140-6736(17)31868-8
Ryoo, N. K., Lee, J., Lee, H., Hong, H. K., Kim, H., Lee, J. B., et al. (2017). Therapeutic effects of a novel siRNA-based anti-VEGF (siVEGF) nanoball for the treatment of choroidal neovascularization. Nanoscale 9 (40), 15461–15469. doi:10.1039/c7nr03142d
Sakai, T., Ishihara, T., Higaki, M., Akiyama, G., and Tsuneoka, H. (2011). Therapeutic effect of stealth-type polymeric nanoparticles with encapsulated betamethasone phosphate on experimental autoimmune uveoretinitis. Invest. Ophthalmol. Vis. Sci. 52 (3), 1516–1521. doi:10.1167/iovs.10-5676
Sakai, T., Kohno, H., Ishihara, T., Higaki, M., Saito, S., Matsushima, M., et al. (2006). Treatment of experimental autoimmune uveoretinitis with poly(lactic acid) nanoparticles encapsulating betamethasone phosphate. Exp. Eye Res. 82 (4), 657–663. doi:10.1016/j.exer.2005.09.003
Schanen, B. C., Das, S., Reilly, C. M., Warren, W. L., Self, W. T., Seal, S., et al. (2013). Immunomodulation and T helper TH₁/TH₂ response polarization by CeO₂ and TiO₂ nanoparticles. PLoS One 8 (5), e62816. doi:10.1371/journal.pone.0062816
Schirmer, L., Atallah, P., Werner, C., and Freudenberg, U. (2016). StarPEG-heparin hydrogels to protect and sustainably deliver IL-4. Adv. Healthc. Mater 5 (24), 3157–3164. doi:10.1002/adhm.201600797
Schopf, L. R., Popov, A. M., Enlow, E. M., Bourassa, J. L., Ong, W. Z., Nowak, P., et al. (2015). Topical ocular drug delivery to the back of the eye by mucus-penetrating particles. Transl. Vis. Sci. Technol. 4 (3), 11. doi:10.1167/tvst.4.3.11
Schroder, S., Palinski, W., and Schmid-Schonbein, G. W. (1991). Activated monocytes and granulocytes, capillary nonperfusion, and neovascularization in diabetic retinopathy. Am. J. Pathology 139 (1), 81–100.
Seong, S. Y., and Matzinger, P. (2004). Hydrophobicity: An ancient damage-associated molecular pattern that initiates innate immune responses. Nat. Rev. Immunol. 4 (6), 469–478. doi:10.1038/nri1372
Serda, M., Becker, F. G., Cleary, M., Team, R. M., Holtermann, H., The, D., et al. (1990). Update on topical cyclosporin A: Background, immunology, and pharmacology. Cornea 9 (3), 184–195. doi:10.1097/00003226-199007000-00002
Shafie, M., and Fayek, H. (2013). Formulation and evaluation of betamethasone sodium phosphate loaded nanoparticles for ophthalmic delivery. J. Clin. Exp. Ophthalmol. 4, 273. doi:10.4172/2155-9570.1000273
Sharma, A. K., Arya, A., Sahoo, P. K., and Majumdar, D. K. (2016). Overview of biopolymers as carriers of antiphlogistic agents for treatment of diverse ocular inflammations. Mater. Sci. Eng. C 67, 779–791. doi:10.1016/j.msec.2016.05.060
Shaunak, S., Thomas, S., Gianasi, E., Godwin, A., Jones, E., Teo, I., et al. (2004). Polyvalent dendrimer glucosamine conjugates prevent scar tissue formation. Nat. Biotechnol. 22 (8), 977–984. doi:10.1038/nbt995
Shen, J., Samul, R., Silva, R. L., Akiyama, H., Liu, H., Saishin, Y., et al. (2006). Suppression of ocular neovascularization with siRNA targeting VEGF receptor 1. Gene Ther. 13 (3), 225–234. doi:10.1038/sj.gt.3302641
Shi, H., Ding, J., Chen, C., Yao, Q., Zhang, W., Fu, Y., et al. (2021). Antimicrobial action of biocompatible silver microspheres and their role in the potential treatment of fungal keratitis. ACS Biomater. Sci. Eng. 7 (11), 5090–5098. doi:10.1021/acsbiomaterials.1c00815
Silva, D., Pinto, L. F. V., Bozukova, D., Santos, L. F., Serro, A. P., and Saramago, B. (2016). Chitosan/alginate based multilayers to control drug release from ophthalmic lens. Colloids Surf. B Biointerfaces 147, 81–89. doi:10.1016/j.colsurfb.2016.07.047
Simpson, F. C., McTiernan, C. D., Islam, M. M., Buznyk, O., Lewis, P. N., Meek, K. M., et al. (2021). Collagen analogs with phosphorylcholine are inflammation-suppressing scaffolds for corneal regeneration from alkali burns in mini-pigs. Commun. Biol. 4, 608. doi:10.1038/s42003-021-02108-y
Singh, R., Batoki, J. C., Ali, M., Bonilha, V. L., and Anand-Apte, B. (2020). Inhibition of choroidal neovascularization by systemic delivery of gold nanoparticles. Nanomedicine 28, 102205. doi:10.1016/j.nano.2020.102205
Soiberman, U., Kambhampati, S. P., Wu, T., Mishra, M. K., Oh, Y., Sharma, R., et al. (2017). Subconjunctival injectable dendrimer-dexamethasone gel for the treatment of corneal inflammation. Biomaterials 125, 38–53. doi:10.1016/j.biomaterials.2017.02.016
Song, H. B., Park, S. Y., Ko, J. H., Park, J. W., Yoon, C. H., Kim, D. H., et al. (2018). Mesenchymal stromal cells inhibit inflammatory lymphangiogenesis in the cornea by suppressing macrophage in a TSG-6-dependent manner. Mol. Ther. 26 (1), 162–172. doi:10.1016/j.ymthe.2017.09.026
Song, J., Winkeljann, B., and Lieleg, O. (2020). Biopolymer-based coatings: Promising strategies to improve the biocompatibility and functionality of materials used in biomedical engineering. Adv. Mater Interfaces 7 (17), 2000850. doi:10.1002/admi.202000850
Song, Y., Overmass, M., Fan, J., Hodge, C., Sutton, G., Lovicu, F. J., et al. (2021). Application of collagen I and IV in bioengineering transparent ocular tissues. Front. Surg. 8, 639500. doi:10.3389/fsurg.2021.639500
Sonoda, K.-H., Sasa, Y., Qiao, H., Tsutsumi, C., Hisatomi, T., Komiyama, S., et al. (2003). Immunoregulatory role of ocular macrophages: The macrophages produce RANTES to suppress experimental autoimmune uveitis. J. Immunol. 171 (5), 2652–2659. doi:10.4049/jimmunol.171.5.2652
Souto, E. B., Dias-Ferreira, J., López-Machado, A., Ettcheto, M., Cano, A., Espuny, A. C., et al. (2019). Advanced formulation approaches for ocular drug delivery: State-Of-The-Art and recent patents. Pharmaceutics 11 (9), 460. doi:10.3390/pharmaceutics11090460
Sridharan, R., Cavanagh, B., Cameron, A. R., Kelly, D. J., and O’Brien, F. J. (2019). Material stiffness influences the polarization state, function and migration mode of macrophages. Acta Biomater. 89, 47–59. doi:10.1016/j.actbio.2019.02.048
Sugita, S., Kawazoe, Y., Imai, A., Usui, Y., Iwakura, Y., Isoda, K., et al. (2013). Mature dendritic cell suppression by IL-1 receptor antagonist on retinal pigment epithelium cells. Invest. Ophthalmol. Vis. Sci. 54 (5), 3240–3249. doi:10.1167/iovs.12-11483
Sugita, S., Usui, Y., Horie, S., Futagami, Y., Aburatani, H., Okazaki, T., et al. (2009). T-cell suppression by programmed cell death 1 ligand 1 on retinal pigment epithelium during inflammatory conditions. Invest. Ophthalmol. Vis. Sci. 50 (6), 2862–2870. doi:10.1167/iovs.08-2846
Tamura, T., Ishikawa, N., Tanaka, S., Hayashi, Y., Miyamoto, T., and Saika, S. (2017). Histopathological analyses of the differences in foreign body cell reactions against intraocular lenses according to the period of implantation. J. Eye Cataract Surg. 3. doi:10.21767/2471-8300.100017
Tan, W., Zou, J., Yoshida, S., Jiang, B., and Zhou, Y. (2020). The role of inflammation in age-related macular degeneration. Int. J. Biol. Sci. 16 (15), 2989–3001. doi:10.7150/ijbs.49890
Tang, Z., Fan, X., Chen, Y., and Gu, P. (2022). Ocular nanomedicine. Adv. Sci. 9 (15), 2003699. doi:10.1002/advs.202003699
Taskin, M. B., Tylek, T., Blum, C., Böhm, C., Wiesbeck, C., and Groll, J. (2021). Inducing immunomodulatory effects on human macrophages by multifunctional NCO-sP(EO-stat-PO)/Gelatin hydrogel nanofibers. ACS Biomater. Sci. Eng. 7 (7), 3166–3178. doi:10.1021/acsbiomaterials.1c00232
Taylor, A. W., and Ng, T. F. (2018). Negative regulators that mediate ocular immune privilege. J. Leukoc. Biol. 103 (6), 1179–1187. doi:10.1002/JLB.3MIR0817-337R
Taylor, A. W. (2016). Ocular immune privilege and transplantation. Front. Immunol. 7, 37. doi:10.3389/fimmu.2016.00037
Taylor, A. W. (2007). Ocular immunosuppressive microenvironment. Chem. Immunol. Allergy 92, 71–85. doi:10.1159/000099255
Timmers, A. M., Newmark, J. A., Turunen, H. T., Farivar, T., Liu, J., Song, C., et al. (2020). Ocular inflammatory response to intravitreal injection of adeno-associated virus vector: Relative contribution of genome and capsid. Hum. Gene Ther. 31 (1–2), 80–89. doi:10.1089/hum.2019.144
Tisi, A., Passacantando, M., Lozzi, L., Riccitelli, S., Bisti, S., and Maccarone, R. (2019). Retinal long term neuroprotection by Cerium Oxide nanoparticles after an acute damage induced by high intensity light exposure. Exp. Eye Res. 182, 30–38. doi:10.1016/j.exer.2019.03.003
Tiwari, R., Sethiya, N. K., Gulbake, A. S., Mehra, N. K., Murty, U. S. N., and Gulbake, A. (2021). A review on albumin as a biomaterial for ocular drug delivery. Int. J. Biol. Macromol. 191, 591–599. doi:10.1016/j.ijbiomac.2021.09.112
Toscano, M. A., Commodaro, A. G., Ilarregui, J. M., Bianco, G. A., Liberman, A., Serra, H. M., et al. (2006). Galectin-1 suppresses autoimmune retinal disease by promoting concomitant Th2- and T regulatory-mediated anti-inflammatory responses. J. Immunol. 176 (10), 6323–6332. doi:10.4049/jimmunol.176.10.6323
Trattler, W., and Hosseini, K. (2017). Twice-daily vs. Once-daily dosing with 0.075% bromfenac in DuraSite: Outcomes from a 14-day phase 2 study. Ophthalmol. Ther. 6 (2), 277–284. doi:10.1007/s40123-017-0102-x
Tsai, C. H., Wang, P. Y., Lin, I. C., Huang, H., Liu, G. S., and Tseng, C. L. (2018). Ocular drug delivery: Role of degradable polymeric nanocarriers for ophthalmic application. Int. J. Mol. Sci. 19, 2830. doi:10.3390/ijms19092830
Tseng, C. L., Chen, K. H., Su, W. Y., Lee, Y. H., Wu, C. C., and Lin, F. H. (2013). Cationic gelatin nanoparticles for drug delivery to the ocular surface: In vitro and in vivo evaluation. J. Nanomater 2013, 1–11. doi:10.1155/2013/238351
Tsukamoto, T., Hironaka, K., Fujisawa, T., Yamaguchi, D., Tahara, K., Tozuka, Y., et al. (2013). Preparation of bromfenac-loaded liposomes modified with chitosan for ophthalmic drug delivery and evaluation of physicochemical properties and drug release profile. Asian J. Pharm. Sci. 8 (2), 104–109. doi:10.1016/j.ajps.2013.07.013
van Dooremaal, J. C. (1873). Die Entwicklung der in fremden grund versetzten lebenden geweba. Albr. Graefes Arch Ophthalmol 19, 358–373.
Vandervoort, J., and Ludwig, A. (2004). Preparation and evaluation of drug-loaded gelatin nanoparticles for topical ophthalmic use. Eur. J. Pharm. Biopharm. 57 (2), 251–261. doi:10.1016/S0939-6411(03)00187-5
Vassey, M. J., Figueredo, G. P., Scurr, D. J., Vasilevich, A. S., Vermeulen, S., Carlier, A., et al. (2020). Immune modulation by design: Using topography to control human monocyte attachment and macrophage differentiation. Adv. Sci. 7 (11), 1903392. doi:10.1002/advs.201903392
Veiseh, O., and Vegas, A. J. (2019). Domesticating the foreign body response: Recent advances and applications. Adv. Drug Deliv. Rev. 144, 148–161. doi:10.1016/j.addr.2019.08.010
Verbeke, C. S., Mooney, D. J., Verbeke, C. S., Mooney John A Paulson, D. J., and Mooney, D. J. (2015). Injectable, pore-forming hydrogels for in vivo enrichment of immature dendritic cells. Adv. Healthc. Mater 4 (17), 2677–2687. doi:10.1002/adhm.201500618
Wakefield, D., Gray, P., Chang, J., di Girolamo, N., and McCluskey, P. (2010). The role of PAMPs and DAMPs in the pathogenesis of acute and recurrent anterior uveitis. Br. J. Ophthalmol. 94 (3), 271–274. doi:10.1136/bjo.2008.146753
Wang, B., Lin, Q., Jin, T., Shen, C., Tang, J., Han, Y., et al. (2014). Surface modification of intraocular lenses with hyaluronic acid and lysozyme for the prevention of endophthalmitis and posterior capsule opacification. RSC Adv. 5 (5), 3597–3604. doi:10.1039/c4ra13499k
Wang, R., Xia, J., Tang, J., Liu, D., Zhu, S., Wen, S., et al. (2021). Surface modification of intraocular lens with hydrophilic poly(sulfobetaine methacrylate) brush for posterior capsular opacification prevention. J. Ocul. Pharmacol. Ther. 37 (3), 172–180. doi:10.1089/jop.2020.0134
Wang, S. K., and Cepko, C. L. (2022). Targeting microglia to treat degenerative eye diseases. Front. Immunol. 13, 843558. doi:10.3389/fimmu.2022.843558
Wasnik, V. B., and Thool, A. R. (2022). Ocular gene therapy: A literature review with focus on current clinical trials. Cureus 14 (9), e29533. doi:10.7759/cureus.29533
Wen, Y., Waltman, A., Han, H., and Collier, J. H. (2016). Switching the immunogenicity of peptide assemblies using surface properties. ACS Nano 10 (10), 9274–9286. doi:10.1021/acsnano.6b03409
Wikström, J., Elomaa, M., Syväjärvi, H., Kuokkanen, J., Yliperttula, M., Honkakoski, P., et al. (2008). Alginate-based microencapsulation of retinal pigment epithelial cell line for cell therapy. Biomaterials 29 (7), 869–876. doi:10.1016/j.biomaterials.2007.10.056
Woźniak, A., Malankowska, A., Nowaczyk, G., Grześkowiak, B. F., Tuśnio, K., Słomski, R., Zaleska-Medynska, A., and Jurga, S. (2017). Size and shape-dependent cytotoxicity profile of gold nanoparticles for biomedical applications. J Mater Sci Mater Med 28 (6), 92. doi:10.1007/s10856-017-5902-y
Wong, F. S. Y., Tsang, K. K., and Lo, A. C. Y. (2017). Delivery of therapeutics to posterior eye segment: Cell-encapsulating systems. Neural Regen. Res. 12 (4), 576–577. doi:10.4103/1673-5374.205093
Wu, W., He, Z., Zhang, Z., Yu, X., Song, Z., and Li, X. (2016). Intravitreal injection of rapamycin-loaded polymeric micelles for inhibition of ocular inflammation in rat model. Int. J. Pharm. 513 (1–2), 238–246. doi:10.1016/j.ijpharm.2016.09.013
Xiang, J., Sun, J., Hong, J., Wang, W., Wei, A., Le, Q., et al. (2015). T-style keratoprosthesis based on surface-modified poly (2-hydroxyethyl methacrylate) hydrogel for cornea repairs. Mater. Sci. Eng. C 50, 274–285. doi:10.1016/j.msec.2015.01.089
Xiaonan, H. (2020). Engineered nanoparticles for retinal targeted delivery - xiaonan Huang - google books. Hong Kong: Hong Kong University of Science and Technology.
Yang, H., Zheng, S., Mao, Y., Chen, Z., Zheng, C., Li, H., et al. (2016). Modulating of ocular inflammation with macrophage migration inhibitory factor is associated with notch signalling in experimental autoimmune uveitis. Clin. Exp. Immunol. 183 (2), 280–293. doi:10.1111/cei.12710
Yavuz, B., Bozdağ Pehlivan, S., Sümer Bolu, B., Nomak Sanyal, R., Vural, İ., and Ünlü, N. (2016). Dexamethasone – PAMAM dendrimer conjugates for retinal delivery: Preparation, characterization and in vivo evaluation. J. Pharm. Pharmacol. 68 (8), 1010–1020. doi:10.1111/jphp.12587
Yenice, İ., Mocan, M., Palaska, E., Bochotd, A., Bilensoy, E., Vurala, I., et al. (2008). Hyaluronic acid coated poly-ɛ-caprolactone nanospheres deliver high concentrations of cyclosporine A into the cornea. Exp. Eye Res. 87, 162–167. Elsevier. doi:10.1016/j.exer.2008.04.002
Yerramothu, P., Vijay, A. K., and Willcox, M. D. P. (2018). Inflammasomes, the eye and anti-inflammasome therapy. Eye 32 (3), 491–505. doi:10.1038/eye.2017.241
Yıldız, M. B., and Yıldız, E. (2022). Evaluation of serum neutrophil-to-lymphocyte ratio in corneal graft rejection after low-risk penetrating keratoplasty. Int. Ophthalmol. 42 (1), 57–63. doi:10.1007/s10792-021-01999-4
You, N., Chu, S., Cai, B., Gao, Y., Hui, M., Zhu, J., et al. (2020). Bioactive hyaluronic acid fragments inhibit lipopolysaccharide-induced inflammatory responses via the Toll-like receptor 4 signaling pathway. Front. Med. 15 (2), 292–301. doi:10.1007/s11684-020-0806-5
Yu, F.-S. X., and Hazlett, L. D. (2006). Toll-like receptors and the eye. Invest. Ophthalmol. Vis. Sci. 47 (4), 1255–1263. doi:10.1167/iovs.05-0956
Yu, Y., Chow, D. W. Y., Lau, C. M. L., Zhou, G., Back, W., Xu, J., et al. (2021). A bioinspired synthetic soft hydrogel for the treatment of dry eye. Bioeng. Transl. Med. 6 (3), e10227. doi:10.1002/btm2.10227
Yu, Y., Lau, L. C. M., Lo, A. C., and Chau, Y. (2015). Injectable chemically crosslinked hydrogel for the controlled release of bevacizumab in vitreous: A 6-month in vivo study. Transl. Vis. Sci. Technol. 4 (2), 5. doi:10.1167/tvst.4.2.5
Yu, Y., Lin, X., Wang, Q., and Chau, Y. (2019). Long-term therapeutic effect in nonhuman primate eye from a single injection of anti-VEGF controlled release hydrogel. Bioeng. Transl. Med. 4 (2), e10128. doi:10.1002/btm2.10128
Zajicova, A., Pokorna, K., Lencova, A., Krulova, M., Svobodova, E., Kubinova, S., et al. (2010). Treatment of ocular surface injuries by limbal and mesenchymal stem cells growing on nanofiber scaffolds. Cell Transpl. 19 (10), 1281–1290. doi:10.3727/096368910X509040
Zhang, K., Hopkins, J. J., Heier, J. S., Birch, D. G., Halperin, L. S., Albini, T. A., et al. (2011). Ciliary neurotrophic factor delivered by encapsulated cell intraocular implants for treatment of geographic atrophy in age-related macular degeneration. Proc. Natl. Acad. Sci. U. S. A. 108 (15), 6241–6245. doi:10.1073/pnas.1018987108
Zhao, Y., Yang, K., Li, J., Huang, Y., and Zhu, S. (2017). Comparison of hydrophobic and hydrophilic intraocular lens in preventing posterior capsule opacification after cataract surgery: An updated meta-analysis. Medicine 96, e8301. doi:10.1097/MD.0000000000008301
Zhou, Q., Xiao, X., Wang, C., Zhang, X., Li, F., Zhou, Y., et al. (2012). Decreased microRNA-155 expression in ocular behcet’s disease but not in vogt koyanagi harada syndrome. Invest. Ophthalmol. Vis. Sci. 53 (9), 5665–5674. doi:10.1167/iovs.12-9832
Zhou, R., Horai, R., Mattapallil, M. J., and Caspi, R. R. (2011). A new look at immune privilege of the eye: Dual role for the vision-related molecule retinoic acid. J. Immunol. 187 (8), 4170–4177. doi:10.4049/jimmunol.1101634
Zhu, S., Gong, L., Li, Y., Xu, H., Gu, Z., and Zhao, Y. (2019). Safety assessment of nanomaterials to eyes: An important but neglected issue. Adv. Sci. 6 (16), 1802289. doi:10.1002/advs.201802289
Zhu, Y., Liang, H., Liu, X., Wu, J., Yang, C., Wong, T. M., et al. (2021). Regulation of macrophage polarization through surface topography design to facilitate implant-to-bone osteointegration. Sci. Adv. 7 (14), eabf6654. doi:10.1126/sciadv.abf6654
Zhuang, Z., Zhang, Y., Sun, S., Li, Q., Chen, K., An, C., et al. (2020). Control of matrix stiffness using methacrylate-gelatin hydrogels for a macrophage-mediated inflammatory response. ACS Biomater. Sci. Eng. 6 (5), 3091–3102. doi:10.1021/acsbiomaterials.0c00295
Zimmer, A. K., Maincent, P., Thouvenot, P., and Kreuter, J. (1994). Hydrocortisone delivery to healthy and inflamed eyes using a micellar polysorbate 80 solution or albumin nanoparticles. Int. J. Pharm. 110 (3), 211–222. doi:10.1016/0378-5173(94)90243-7
Zimmer, A., and Kreuter, J. (1995). Microspheres and nanoparticles used in ocular delivery systems. Adv. Drug Deliv. Rev. 16 (1), 61–73. doi:10.1016/0169-409x(95)00017-2
Zirm, E. (1906). Eine erfolgreiche totale Keratoplastik. Albr. Graefes Arch. für Ophthalmol. 64 (3), 580–593. doi:10.1007/bf01949227
Zolnik, B. S., González-Fernández, Á., Sadrieh, N., and Dobrovolskaia, M. A. (2010). Minireview: Nanoparticles and the immune system. Endocrinology 151 (2), 458–465. doi:10.1210/en.2009-1082
Glossary
NTs Neutrophils
MG Microglia
Mφs Macrophages
DCs Dendritic Cells
cDC conventional DC
pDC plasmacytoid DC
MSCs Mesenchymal stem cells
BM-MSCs Bone marrow-derived MSCs
LCs Langerhans cells
AM-MSCs Adipose tissue-derived MSCs
LSCs Limbal epithelial stem cells
APCs Antigen-presenting cells
ECM Extracellular matrix
AMD Age-related macular degeneration
DED Dry eye disease
DR Diabetic retinopathy
EAU Experimental autoimmune uveitis
OHT Ocular hypertension
IOP Intraocular pressure
RP Retinitis pigmentosa
RGCs Retinal ganglion cells
MGD Meibomian gland dysfunction
DAMP Danger-associated molecular pattern
PAMP Pathogen-associated molecular pattern
NETs Neutrophil extracellular traps
ACAID Anterior chamber-associated immune deviation
ADP/ATP Adenosine di/tri-phosphate
NF-κB Nuclear factor kappa B
IOL Intraocular lens
FA Fluocinolone acetonide
FBR Foreign body response
HLA-G Human leukocyte antigen G
HMGB1 High mobility group protein B1
LPS Lipopolysaccharides
IDO Indoleamine 2, 3-dioxygenase
PEDF Pigment epithelium derived factor
PRR Pattern-recognition receptors
LAIR-1 Leukocyte-associated immunoglobulin-like receptor-1
RANTES Regulated upon activation, normal T cell expressed and secreted
Ly6C Lymphocyte antigen 6 complex
OVA Ovalbumin
HA Hyaluronate
GelMA Methacrylate-gelatin
AVs Adenoviruses
AAVs Adeno-associated viruses
mRNA Messenger RNA
miRNA Micro RNA
siRNA small interfering RNA
pDNA Plasmid DNA
NPs Nanoparticles
MPs Microparticles
LNPs Lipid-based nanoparticles
SLN Solid lipid nanoparticles
AuNPs Gold nanoparticle
AI Artificial intelligence
AM Amniotic membrane
AIM2 Absent in melanoma 2
TNF-⍺ Tumor necrosis factor-alpha
IFN-γ Interferon gamma
TGF-β Transforming growth factor-β
iNOS inducible nitric oxide synthase
IL- Interleukin-
P2Y12 P2 purinergic receptor
Caspase-1 Cysteine-aspartic acid protease-1
Iba-1 Ionized calcium-binding adapter molecule 1
APTES Aminopropyltriethoxylsilane
ARG Arginase
ILM Internal limiting membrane
MCP-1 Monocyte chemoattractant protein-1
IgG Immunoglobulin G
CCL- C-C motif chemokine ligand
CCR- C-C chemokine receptor
CD- Cluster of differentiation
CD200R Cluster of differentiation-200 receptor
MHCII Major histocompatibility complex class II
CNTF Ciliary neurotrophic factor
CNV Choroidal neovascularization
COX- Cyclooxygenase
CR2-fH Complement receptor 2 and factor H
CXCL- C-X-C motif chemokine ligand
Cy A Cyclosporine A
PD-L1 Programmed cell death-ligand 1
NLR Nod-like receptors
VEGF Vascular endothelial growth factor
TLR- Toll-like receptor
ROS Reactive oxygen species
TRPV Transient receptor potential vanilloid
K 5 Kringle 5
VIP Vasoactive intestinal peptide
LBL Layer-by-layer
Siglec-H Sialic acid binding immunoglobulin-like lectin H
MIF Migration inhibitory factor
MMP Matrix metalloproteinase
MyD88 Myeloid differentiation primary response 88
PCO Posterior capsular opacification
RGD Arginyl-glycyl-aspartic acid
PAA Polyacrylic acid
PAMAM Poly (amidoamine)
mPEG-PLGA-BOX Methoxy-poly (ethylene glycol)-block-poly (lactic-co-glycolic acid)
PBT Polybutylene terephthalate
PCL Poly-caprolactone
PEG Polyethylene glycol
PEI Polyethylenimine
PEMA Poly (ethylene-alt-maleic anhydride)
PEO Polyethylene oxide
PHEMA Poly (2-hydroxyethyl methacrylate)
PLA Polylactic acid
PLGA Poly(lactide-co-glycolide)
PMMA Poly (methyl methacrylate)
PVA Poly (vinyl alcohol)
Keywords: biomaterials, eye, immune-related disorders, innate immune system, immunomodulation
Citation: Rafiei M, Chung JT and Chau Y (2023) Roles of biomaterials in modulating the innate immune response in ocular therapy. Front. Drug Deliv. 3:1077253. doi: 10.3389/fddev.2023.1077253
Received: 22 October 2022; Accepted: 26 January 2023;
Published: 15 February 2023.
Edited by:
Raj Thakur, Queen’s University Belfast, United KingdomReviewed by:
Katrien Remaut, Ghent University, BelgiumMarta Vicario-de-la-Torre, Complutense University of Madrid, Spain
Copyright © 2023 Rafiei, Chung and Chau. This is an open-access article distributed under the terms of the Creative Commons Attribution License (CC BY). The use, distribution or reproduction in other forums is permitted, provided the original author(s) and the copyright owner(s) are credited and that the original publication in this journal is cited, in accordance with accepted academic practice. No use, distribution or reproduction is permitted which does not comply with these terms.
*Correspondence: Ying Chau, S2V5Y2hhdUB1c3QuaGs=