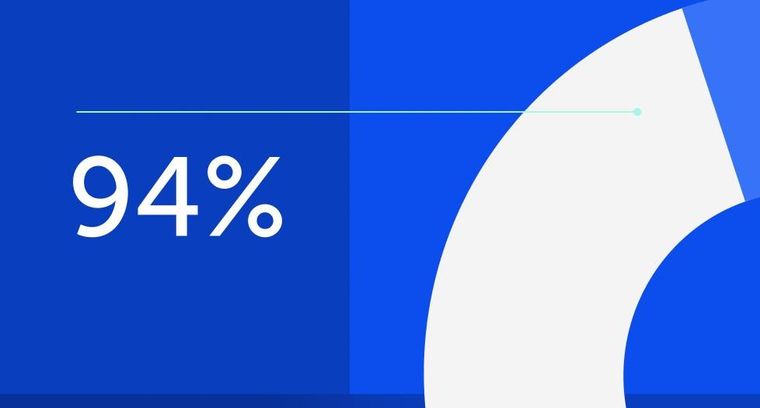
94% of researchers rate our articles as excellent or good
Learn more about the work of our research integrity team to safeguard the quality of each article we publish.
Find out more
SPECIALTY GRAND CHALLENGE article
Front. Drug Deliv., 11 July 2022
Sec. Vaccine Delivery
Volume 2 - 2022 | https://doi.org/10.3389/fddev.2022.964298
The worldwide pandemic of coronavirus disease 2019 (COVID-19), caused by severe acute respiratory syndrome coronavirus 2 (SARS-CoV-2), has come at immense social and economic costs, both to individuals and societies. For vaccine research, the COVID-19 pandemic has been a scientifically highly exciting period with remarkable collaborative achievements, which have been referred to as the medical parallel to the moon landing, and it has underlined that vaccines are as important as ever for global public health care. It is indisputable that vaccines have contributed to reducing deaths and severe illness. The pandemic has provided a unique opportunity for testing and comparing different COVID-19 vaccine platforms (Frederiksen et al., 2020), which were developed and approved at an unprecedented pace. Although the COVID-19 pandemic is not over yet, we are moving beyond the emergency response and are adapting to live alongside with the virus, while strategies are being developed for handling the lasting threat of SARS-CoV-2. It is time for reflection on what we have learned from the COVID-19 vaccine rollout, and what grand challenges that must be addressed in the vaccine delivery field. Some of the most important challenges are presented below.
In the race to develop COVID-19 vaccines, the mRNA vaccines became the frontrunners (Pardi et al., 2018; Wadhwa et al., 2020; Dolgin, 2021). Two different mRNA vaccines, i.e., Comirnaty® (Pfizer/BioNTech, New York City, NY, United States) and Spikevax® (Moderna, Cambridge, MA, United States), were shown to be highly safe and efficacious (Polack et al., 2020; El Sahly et al., 2021), and they received emergency approval for mass vaccination less than 1 year after the sequencing of the full SARS-CoV-2 genome (Zhou et al., 2020; Zhu et al., 2020). This unprecedented fast development time can be attributed to: 1) very efficient collaborations between governments, manufacturers, and regulatory authorities, and 2) the distinct advantages of this vaccine type over traditional vaccine platforms (Hogan and Pardi, 2022). Bioinformatics is used for rapid design of antigen-encoding mRNA, which for the COVID-19 vaccines was based on the sequence of the SARS-CoV-2 genome (Zhou et al., 2020; Zhu et al., 2020) and previous experience from vaccine development against Middle East respiratory syndrome coronavirus (Pallesen et al., 2017). The COVID-19 mRNA vaccines contain nucleoside-modified mRNA encoding prefusion-stabilized SARS-CoV-2 spike (S) protein, encapsulated in lipid nanoparticles (LNPs). The LPNs protect the mRNA against degradation and transport it to the cell cytosol, where it is in situ translated into protein antigen, which subsequently induces protective immune responses. The LNP design is based on the LNP technology developed for systemic liver targeting of short interfering RNA used in the drug Onpattro® (Alnylam® Pharmaceuticals, Cambridge, MA, United States), which was approved in 2018 for treatment of polyneuropathies induced by hereditary transthyretin amyloidosis (Adams et al., 2018; Akinc et al., 2019). To date, more than one billion COVID-19 mRNA vaccine doses have been administered, and the mRNA vaccine platform has been established as highly efficacious and safe, fast to develop, and versatile, because it is easily upgraded with new antigens, when new virus variants emerge (Chaudhary et al., 2021), and it can rapidly be scaled up for mass production. This achievement represents a true breakthrough, because it is the first time that a nucleic acid-based drug has been used globally. However, first-generation mRNA vaccines have a number of weaknesses, e.g., waining vaccine effectiveness and systemic side effects like aches and inflammation, especially in male adolescents and young adults, who more frequently experience for example rare cases of myocarditis and/or pericarditis (Barda et al., 2021; Mevorach et al., 2021; Oster et al., 2022; Patone et al., 2022; Wong et al., 2022). Hence, there is room for improvement in the design of next-generation RNA vaccines (Altmann and Boyton, 2022). It is interesting how broadly applicable the mRNA vaccine platform is for pathogens other than SARS-CoV-2, and it will likely play a key role in the future pandemic preparedness.
In the COVID-19 vaccine race, vaccines based on the adenovirus platform were developed at a slighty slower pace than the mRNA vaccines. Adenovirus-based vaccines encoding the S protein were tested and found safe and efficacious (Jacob-Dolan and Barouch, 2022). These included Vaxzevria®/COVISHIELDTM from Oxford/AstraZeneca and Jcovden® from Janssen/Johnson & Johnson. Although adenovirus vectors had been used in the past for vaccines against other viruses, they had never been produced, distributed, or administered to humans at such a large scale. During the initial COVID-19 vaccine roll-out, the rare but severe, and in some cases even fatal, side effect vaccine-induced immune thrombotic thrombocytopenia was observed (Greinacher et al., 2021; Klok et al., 2022), which led to restricted use of adenoviral vector vaccines in many jurisdictions. Although millions of doses have been administered to date, the development of adenovirus-based vaccines with improved patient safety is warranted.
A number of whole inactivated virus and protein-based COVID-19 vaccines have also been developed and obtained approval (Hotez and Bottazzi, 2022). These conventional vaccine platforms have far lower manufacturing costs and are easier to produce and store than mRNA vaccines. The inactivated virus vaccines include CoronaVac (Sinovac, China), BBIBP-CorV (Sinopharm, China), and Covaxin® (Bharat Biotech, India), which are widely used in many low- and middle-income countries. The traditional recombinant protein-based subunit vaccines have a longer development time, but the common belief in the field is that the subunit vaccine technology is safe and efficacious, and has a real long-term potential, because the immunity that subunit vaccines induces is expected to have a longer duration. In addition, the platform is suitable for the developing world, because it is much cheaper and more stable than for example mRNA vaccines. We only recently started to gain more widespread clinical experience with this COVID-19 vaccine platform, for example the approved subunit vaccine Nuvaxovid® from Novavax (Rockville, MA, United States) containing recombinant S protein formulated with the Matrix-MTM adjuvant (Heath et al., 2021), and clinical data are currently emerging for the COVID-19 vaccine from Sanofi-GlaxoSmithKline, which includes recombinant S protein formulated with the squalene oil-based adjuvant AS03 (Pavot et al., 2022).
Despite the success of the COVID-19 vaccines, it has become clear that there is a need for improved vaccine technologies in the toolbox of the health authorities. For example, it has become evident during the COVID-19 vaccine rollout that vaccine effectiveness for all applied vaccine platforms is waning over time due to fading immunity (Feikin et al., 2022). This rather short duration of protective immunity has necessitated frequent booster doses. However, frequent revaccination several times a year of large population groups is not a sustainable strategy, because it may likely cause vaccination fatigue and it may not be immunologically beneficial. Annual COVID-19 revaccinations with monovalent or multivalent variant-updated vaccines, like for the seasonal flu prophylaxis, is a viable option, which is under investigation. For example, variant adapted versions of Comirnaty® and Spikevax® are currently tested in clinical trials as potential vaccine booster approaches for the fall 2022 season. Hence, a key question is how to design longer-lasting COVID-19 vaccines and vaccination strategies that induce more durable immunity.
During the COVID-19 pandemic, we have witnessed the frequent emergence and rapid spread of new SARS-CoV-2 variants, e.g., the highly transmissible delta and omicron variants, and coronaviruses are expected to emerge again in the future due to viral drift and recombination variants, potentially posing a threat to global health and economy. It is highly unlikely that coronaviruses can be eradicated, because they have a rapid mutation rate due to the low fidelity of RNA polymerase, and they are zoonotic viruses with a virus reservoir in mammals that can be the source of future zoonotic outbreaks and pandemics. Coronaviruses will continue to circulate with periodic outbreaks and endemics. Therefore, another pertinent grand challenge is the urgent need for safe universal vaccines, or pan-coronavirus vaccines, that can induce more broadly protective immunity (Dolgin, 2022). For example, for both COVID-19 and influenza, there is a need for universal vaccines that protect against all coronaviruses and influenza viruses (Giurgea et al., 2020; Morens et al., 2022). Vaccine development against respiratory viruses is challenging. Currently, the only approved vaccines against respiratory viruses are those against influenza, but despite decades of research, existing flu vaccines have limited effectiveness against seasonal influenza, which provides a need for new flu vaccines. More knowledge is required about virus ecosystems to identify cross-reactive and cross-protective epitopes, which can be targets for universal vaccine development, and more knowledge is needed about the requirements for induction of protective immunity against coronaviruses. Ongoing clinical trials investigate multivalent and variant-targeted COVID-19 vaccines, and data from these trials will guide the regulatory and health authorities in how to design vaccination strategies against COVID-19 for the near future. However, to date, it remains unknown whether and how more broadly and permanently protective immunity can be achieved, and whether it can prevent emergence of immune escape variants of SARS-CoV-2.
It is also clear that the currently used COVID-19 vaccines offer little protection against transmission of SARS-CoV-2. The reason for this is suggested to be that systemically administered vaccines fail to induce protective mucosal immune responses (Russell et al., 2020). Non-systemic respiratory viruses, e.g., influenza viruses and SARS-CoV-2, primarily infect epithelial cells on mucosal surfaces and have limited contact with the systemic immune system. Hence, systemically administered vaccines elicit incomplete and transient immunity, which does not protect against reinfection and disease, which may cause breakthrough infections. Mucosal vaccines, which are administered directly on mucosal sites, offer the potential to trigger robust protective immune responses at the predominant sites of pathogen infection (Lycke, 2012; Lavelle and Ward, 2022). In principle, the induction of adaptive immunity at mucosal sites, involving secretory antibody responses and tissue-resident T cells, has the capacity to prevent an infection from becoming established in the first place, rather than only curtailing infection and protecting against the development of disease symptoms. However, the progress in the field of mucosal vaccines has not been as fast as in the field of parenteral vaccines, and to date, mucosal vaccines comprise solely live attenuated and inactivated whole-cell preparations, and no mucosal adjuvants has been approved. Hence, there is an unmet medical need for safe and efficacious mucosal adjuvants and delivery strategies that can potentiate mucosal immune responses against co-administered antigen(s) at mucosal surfaces, e.g., in the airways, in the gastrointestinal tract, and in the genital tract. To achieve this, knowledge is needed about how to design vaccines and delivery strategies to induce and maintain mucosal immune responses. Also, we need to identify systemic and mucosal correlates of protection against clinical disease after infection and vaccination.
Goal three of the 17 Sustainable Development Goals of the United Nations is to ensure healthy lives and promote well-being for all at all ages. A goal three target is “to support the research and development of vaccines and medicines for the communicable and noncommunicable diseases that primarily affect developing countries, provide access to affordable essential medicines and vaccines”. Hence, an important grand challenge is equal access to vaccines for all, i.e., affordable vaccines that also can be used in low-income countries. Although COVID-19 represents a global health crisis, we must not forget that we still lack safe and efficacious, yet affordable prophylactic vaccines against the world’s leading infectious disease killers, e.g., malaria, tuberculosis, and AIDS. The diseases represent so-called difficult targets, which are challenging to develop vaccines against, and it requires will from governments and funding bodies to support the collaborative research efforts that are needed to develop such vaccines.
An important grand challenge is also the design of thermostable vaccine dosage forms that can be stored, shipped and distributed independently of an expensive cold-chain, for example to remote areas in low-income countries. Most vaccines are formulated as liquid dosages forms that require storage at cold temperature. For example, Spikevax® remains stable at −20°C for up to 6 months, whereas Comirnaty® needs to be stored at −70°C, which requires specialized freezers and shipping conditions. Solid dosages forms are often manufactured using drying processes like freeze drying or spray drying, and addition of cryo- or lyoprotectants, e.g., trehalose, sucrose and mannitol, is needed to stabilize vaccines during drying and in the solid state and preserve vaccine stability and potency (Crommelin et al., 2021). More basic knowledge is required about how to stabilize complex vaccines in the solid state to ensure long-term vaccine stability at ambient temperature.
Finally, a grand challenge still remains as to how to design therapeutic cancer vaccines that can educate the immune system to recognize and kill cancer cells. Therapeutic cancer vaccines should induce cytotoxic CD8+ T-cells that can kill cancer cells, but more knowledge is needed with respect to selection of tumor-associated antigen(s), adjuvant design, and how to overcome the immunosuppressive microenvironment of tumors (Sahin et al., 2017). The mRNA vaccine platform in particular appears to have a huge potential for the treatment of cancer (Pardi et al., 2018; Van Hoecke et al., 2021). An interesting approach is personalized cancer vaccines directed against neoantigens unique to each patient. Remaining challenges, among others, also include how to design: 1) single-dose vaccines that are highly efficacious in one dose and induces robust and lifelong immunity, and 2) needle-free vaccine delivery technologies with improved access and compliance.
As Speciality Editor, it is my pleasure to welcome you to the Vaccine Delivery Section of Frontiers in Drug Delivery. My ambition is that this Section will become an important forum for scientific communication and discussion of vaccine delivery aspects to address exciting grand challenges and translate new vaccine delivery technologies to the clinic.
CF wrote the article in its entirety.
The vaccine research from the Foged laboratory has been funded by the Independent Research Fund Denmark (Grant numbers 4184-00422 and 9041-00198B).
The author declares that the research was conducted in the absence of any commercial or financial relationships that could be construed as a potential conflict of interest.
All claims expressed in this article are solely those of the authors and do not necessarily represent those of their affiliated organizations, or those of the publisher, the editors and the reviewers. Any product that may be evaluated in this article, or claim that may be made by its manufacturer, is not guaranteed or endorsed by the publisher.
Adams, D., Gonzalez-Duarte, A., O’Riordan, W. D., Yang, C.-C., Ueda, M., Kristen, A. V., et al. (2018). Patisiran, an RNAi Therapeutic, for Hereditary Transthyretin Amyloidosis. N. Engl. J. Med. 379 (1), 11–21. doi:10.1056/NEJMoa1716153
Akinc, A., Maier, M. A., Manoharan, M., Fitzgerald, K., Jayaraman, M., Barros, S., et al. (2019). The Onpattro Story and the Clinical Translation of Nanomedicines Containing Nucleic Acid-Based Drugs. Nat. Nanotechnol. 14 (12), 1084–1087. doi:10.1038/s41565-019-0591-y
Altmann, D. M., and Boyton, R. J. (2022). COVID-19 Vaccination: The Road Ahead. Science 375 (6585), 1127–1132. doi:10.1126/science.abn1755
Barda, N., Dagan, N., Ben-Shlomo, Y., Kepten, E., Waxman, J., Ohana, R., et al. (2021). Safety of the BNT162b2 mRNA Covid-19 Vaccine in a Nationwide Setting. N. Engl. J. Med. 385 (12), 1078–1090. doi:10.1056/NEJMoa2110475
Chaudhary, N., Weissman, D., and Whitehead, K. A. (2021). mRNA Vaccines for Infectious Diseases: Principles, Delivery and Clinical Translation. Nat. Rev. Drug Discov. 20 (11), 817–838. doi:10.1038/s41573-021-00283-5
Crommelin, D. J. A., Anchordoquy, T. J., Volkin, D. B., Jiskoot, W., and Mastrobattista, E. (2021). Addressing the Cold Reality of mRNA Vaccine Stability. J. Pharm. Sci. 110 (3), 997–1001. doi:10.1016/j.xphs.2020.12.006
Dolgin, E. (2021). How COVID Unlocked the Power of RNA Vaccines. Nature 589 (7841), 189–191. doi:10.1038/d41586-021-00019-w
Dolgin, E. (2022). Pan-coronavirus Vaccine Pipeline Takes Form. Nat. Rev. Drug Discov. 21 (5), 324–326. doi:10.1038/d41573-022-00074-6
El Sahly, H. M., Baden, L. R., Essink, B., Doblecki-Lewis, S., Martin, J. M., Anderson, E. J., et al. (2021). Efficacy of the mRNA-1273 SARS-CoV-2 Vaccine at Completion of Blinded Phase. N. Engl. J. Med. 385 (19), 1774–1785. doi:10.1056/NEJMoa2113017
Feikin, D. R., Higdon, M. M., Abu-Raddad, L. J., Andrews, N., Araos, R., Goldberg, Y., et al. (2022). Duration of Effectiveness of Vaccines against SARS-CoV-2 Infection and COVID-19 Disease: Results of a Systematic Review and Meta-Regression. Lancet 399 (10328), 924–944. doi:10.1016/S0140-6736(22)00152-0
Frederiksen, L. S. F., Zhang, Y., Foged, C., and Thakur, A. (2020). The Long Road toward COVID-19 Herd Immunity: Vaccine Platform Technologies and Mass Immunization Strategies. Front. Immunol. 11, 1817. doi:10.3389/fimmu.2020.01817
Giurgea, L. T., Han, A., and Memoli, M. J. (2020). Universal Coronavirus Vaccines: the Time to Start Is Now. npj Vaccines 5 (1), 43. doi:10.1038/s41541-020-0198-1
Greinacher, A., Selleng, K., Palankar, R., Wesche, J., Handtke, S., Wolff, M., et al. (2021). Insights in ChAdOx1 nCoV-19 Vaccine-Induced Immune Thrombotic Thrombocytopenia. Blood 138 (22), 2256–2268. doi:10.1182/blood.2021013231
Heath, P. T., Galiza, E. P., Baxter, D. N., Boffito, M., Browne, D., Burns, F., et al. (2021). Safety and Efficacy of NVX-CoV2373 Covid-19 Vaccine. N. Engl. J. Med. 385 (13), 1172–1183. doi:10.1056/NEJMoa2107659
Hogan, M. J., and Pardi, N. (2022). mRNA Vaccines in the COVID-19 Pandemic and beyond. Annu. Rev. Med. 73 (1), 17–39. doi:10.1146/annurev-med-042420-112725
Hotez, P. J., and Bottazzi, M. E. (2022). Whole Inactivated Virus and Protein-Based COVID-19 Vaccines. Annu. Rev. Med. 73 (1), 55–64. doi:10.1146/annurev-med-042420-113212
Jacob-Dolan, C., and Barouch, D. H. (2022). COVID-19 Vaccines: Adenoviral Vectors. Annu. Rev. Med. 73 (1), 41–54. doi:10.1146/annurev-med-012621-102252
Klok, F. A., Pai, M., Huisman, M. V., and Makris, M. (2022). Vaccine-induced Immune Thrombotic Thrombocytopenia. Lancet Haematol. 9 (1), e73–e80. doi:10.1016/S2352-3026(21)00306-9
Lavelle, E. C., and Ward, R. W. (2022). Mucosal Vaccines - Fortifying the Frontiers. Nat. Rev. Immunol. 22 (4), 236–250. doi:10.1038/s41577-021-00583-2
Lycke, N. (2012). Recent Progress in Mucosal Vaccine Development: Potential and Limitations. Nat. Rev. Immunol. 12 (8), 592–605. doi:10.1038/nri3251
Mevorach, D., Anis, E., Cedar, N., Bromberg, M., Haas, E. J., Nadir, E., et al. (2021). Myocarditis after BNT162b2 mRNA Vaccine against Covid-19 in Israel. N. Engl. J. Med. 385 (23), 2140–2149. doi:10.1056/NEJMoa2109730
Morens, D. M., Taubenberger, J. K., and Fauci, A. S. (2022). Universal Coronavirus Vaccines - an Urgent Need. N. Engl. J. Med. 386 (4), 297–299. doi:10.1056/NEJMp2118468
Oster, M. E., Shay, D. K., Su, J. R., Gee, J., Creech, C. B., Broder, K. R., et al. (2022). Myocarditis Cases Reported after mRNA-Based COVID-19 Vaccination in the US from December 2020 to August 2021. JAMA 327 (4), 331–340. doi:10.1001/jama.2021.24110
Pallesen, J., Wang, N., Corbett, K. S., Wrapp, D., Kirchdoerfer, R. N., Turner, H. L., et al. (2017). Immunogenicity and Structures of a Rationally Designed Prefusion MERS-CoV Spike Antigen. Proc. Natl. Acad. Sci. U.S.A. 114 (35), E7348–E7357. doi:10.1073/pnas.1707304114
Pardi, N., Hogan, M. J., Porter, F. W., and Weissman, D. (2018). mRNA Vaccines - a New Era in Vaccinology. Nat. Rev. Drug Discov. 17 (4), 261–279. doi:10.1038/nrd.2017.243
Patone, M., Mei, X. W., Handunnetthi, L., Dixon, S., Zaccardi, F., Shankar-Hari, M., et al. (2022). Risks of Myocarditis, Pericarditis, and Cardiac Arrhythmias Associated with COVID-19 Vaccination or SARS-CoV-2 Infection. Nat. Med. 28 (2), 410–422. doi:10.1038/s41591-021-01630-0
Pavot, V., Berry, C., Kishko, M., Anosova, N. G., Huang, D., Tibbitts, T., et al. (2022). Protein-based SARS-CoV-2 Spike Vaccine Booster Increases Cross-Neutralization against SARS-CoV-2 Variants of Concern in Non-human Primates. Nat. Commun. 13 (1), 1699. doi:10.1038/s41467-022-29219-2
Polack, F. P., Thomas, S. J., Kitchin, N., Absalon, J., Gurtman, A., Lockhart, S., et al. (2020). Safety and Efficacy of the BNT162b2 mRNA Covid-19 Vaccine. N. Engl. J. Med. 383 (27), 2603–2615. doi:10.1056/NEJMoa2034577
Russell, M. W., Moldoveanu, Z., Ogra, P. L., and Mestecky, J. (2020). Mucosal Immunity in COVID-19: A Neglected but Critical Aspect of SARS-CoV-2 Infection. Front. Immunol. 11. doi:10.3389/fimmu.2020.611337
Sahin, U., Derhovanessian, E., Miller, M., Kloke, B.-P., Simon, P., Löwer, M., et al. (2017). Personalized RNA Mutanome Vaccines Mobilize Poly-specific Therapeutic Immunity against Cancer. Nature 547 (7662), 222–226. doi:10.1038/nature23003
Van Hoecke, L., Verbeke, R., Dewitte, H., Lentacker, I., Vermaelen, K., Breckpot, K., et al. (2021). mRNA in Cancer Immunotherapy: beyond a Source of Antigen. Mol. Cancer 20 (1), 48. doi:10.1186/s12943-021-01329-3
Wadhwa, A., Aljabbari, A., Lokras, A., Foged, C., and Thakur, A. (2020). Opportunities and Challenges in the Delivery of mRNA-Based Vaccines. Pharmaceutics 12 (2), 102. doi:10.3390/pharmaceutics12020102
Wong, H.-L., Hu, M., Zhou, C. K., Lloyd, P. C., Amend, K. L., Beachler, D. C., et al. (2022). Risk of Myocarditis and Pericarditis after the COVID-19 mRNA Vaccination in the USA: a Cohort Study in Claims Databases. Lancet 399 (10342), 2191–2199. doi:10.1016/S0140-6736(22)00791-7
Zhou, P., Yang, X.-L., Wang, X.-G., Hu, B., Zhang, L., Zhang, W., et al. (2020). A Pneumonia Outbreak Associated with a New Coronavirus of Probable Bat Origin. Nature 579 (7798), 270–273. doi:10.1038/s41586-020-2012-7
Keywords: vaccines, delivery, mucosal vaccines, mRNA vaccines, adjuvants, administration route, universal vaccines, COVID-19
Citation: Foged C (2022) Grand Challenges in Vaccine Delivery: Lessons Learned From the COVID-19 Vaccine Rollout. Front. Drug. Deliv. 2:964298. doi: 10.3389/fddev.2022.964298
Received: 08 June 2022; Accepted: 20 June 2022;
Published: 11 July 2022.
Edited and reviewed by:
David J. Brayden, University College Dublin, IrelandCopyright © 2022 Foged. This is an open-access article distributed under the terms of the Creative Commons Attribution License (CC BY). The use, distribution or reproduction in other forums is permitted, provided the original author(s) and the copyright owner(s) are credited and that the original publication in this journal is cited, in accordance with accepted academic practice. No use, distribution or reproduction is permitted which does not comply with these terms.
*Correspondence: Camilla Foged, Y2FtaWxsYS5mb2dlZEBzdW5kLmt1LmRr
Disclaimer: All claims expressed in this article are solely those of the authors and do not necessarily represent those of their affiliated organizations, or those of the publisher, the editors and the reviewers. Any product that may be evaluated in this article or claim that may be made by its manufacturer is not guaranteed or endorsed by the publisher.
Research integrity at Frontiers
Learn more about the work of our research integrity team to safeguard the quality of each article we publish.