- School of Pharmacy, University of Eastern Finland, Kuopio, Finland
Topical ophthalmic instillation is an appealing strategy to deliver drugs to the back of the eye to treat retinal diseases such as neovascular age-related macular degeneration, diabetic retinopathy, retinal vein occlusion, and glaucomatous optic neuropathy. It has several advantages such as being non-invasive and user-friendly, e.g., allowing self-administration. However, the main obstacle has been how to achieve therapeutic drug concentrations in the retina due to the eye’s protective mechanisms, flows, and barriers. Less than 4% of the instilled drug dose enters the anterior chamber, and much less is expected to reach the posterior segment. It is crucial to understand a drug’s topical pharmacokinetics in humans and how one can extrapolate data from rabbits to humans. In this review, the available data on the retina and vitreous drug concentrations from pharmacokinetics studies conducted in human patients and rabbits have been compiled, together with the critical physiological factors to be considered for this route of administration. Improvements in the design of preclinical studies are suggested to increase their translatability to the treatment of human patients. Finally, the current status of clinical trials with topical ophthalmic formulations intended to treat the back of the eye is depicted. At present, no topical ophthalmic formulations to treat neovascular age-related macular degeneration or other retinal neurodegenerative illnesses have reached the market.
1 Introduction
Topical instillation is the most common route of administration for ophthalmic drugs (Hopkins, 2007a; Neervannan, 2021). It is a non-invasive form of administration, and the patients can administer the drops by themselves. Ophthalmic topical drugs are indicated for several diseases of the anterior segment of the eye; acute states such as conjunctivitis, keratitis, and iritis; or chronic conditions, for example, dry eye and glaucoma (Hopkins, 2007b). However, topical administration is currently being actively investigated to be applied in the treatment of ocular posterior segment diseases, i.e., retinal diseases, such as age-related macular degeneration (AMD), diabetic retinopathy (DR), diabetic macular edema (DME), retinal vein occlusion (RVO), and optic neuropathy glaucoma disease (Adams et al., 2018; Askew et al., 2018; Joussen et al., 2019; Samanta et al., 2020; Sripetch and Loftsson, 2021). The prevalence of these vision-threatening illnesses is increasing with the aging of the population. For example, AMD is expected to affect more than 280 million people worldwide by 2040 (Wong et al., 2014). The neovascular or wet AMD (nAMD) is an advanced form of the disease with a severe loss of central vision due to formation of abnormal, leaky blood vessels in the macula of the retina. Macular edema can also result in patients developing advanced DR or RVO diseases.
The current therapy to treat nAMD (and DR, DME, and RVO) involves intravitreal injections of anti-angiogenic agents targeting the vascular endothelial growth factor (VEGF), which is a major contributor to the development of neovessels in the macula. Regular intravitreal injections are required to retain the nAMD patient’s vision; the dosing regimen depends on the phase of the treatment, the drug, and the patient, but is relatively frequent (Khanna et al., 2019). For this reason, the number of intravitreal injections has increased all over the world, from around 0.6 million in 2006 up to 22.3 million in 2017 (about a 40-fold increase in 10 years) (del Amo, 2022; O’Rourke and Wilson, 2021; Market Scope). This is clearly a heavy burden for both patients and healthcare systems, so alternative therapy strategies are actively being investigated, with topical administration being one of them.
Along with AMD and DR, glaucoma is one of the most prevalent diseases leading to vision loss in aging populations, and it is expected to affect more than 110 million people by 2040 (Tham et al., 2014). In this disease, the optic head nerve or disc is progressively damaged, with a deterioration of the retinal ganglion cells in this region and loss of the visual field. At present, glaucoma is treated with topical medications that decrease intraocular pressure (IOP) by targeting tissues in the anterior segment of the eye (i.e., ciliary body and trabecular meshwork). However, neuroprotective drug treatments acting in the posterior segment of the eye are also being investigated to prevent the death of cells in the optic disc (Chua and Goldberg, 2010; Shalaby et al., 2022).
Topical administration is an appealing route to treat these chronic neurodegenerative diseases in the posterior segment of the eye. This interest is reflected in the increasing number of reviews recently published, sharing the same phrase: “Topical drug delivery to the posterior segment of the eye” (Rodrigues et al., 2018; Sripetch and Loftsson, 2021; Löscher et al., 2022), focusing on nAMD, DR, DME, RVO, and glaucomatous optic neuropathy therapies; similarly, this review focuses on the previously mentioned diseases. However, although this route may seem attractive, it faces many drug delivery problems since the pharmacokinetic experiments of topical drugs indicate that very low concentrations of the drug actually reach the neural retina (Sigurdsson et al., 2005; Holló et al., 2006; Johannsdottir et al., 2018).
In the present publication, the pharmacokinetic processes of topical ophthalmic compounds, focusing on small molecular weight drugs has been reviewed. The biologic drugs for topical instillation are not of significance in the ophthalmic market because of their poor penetration into the eye due to their large molecular size.
Moreover, topical ophthalmic drug delivery systems for treatment of retinal diseases (e.g., contact lenses, liposomes, nanoparticles) have not yet reached the stage where they could undergo clinical trials. For this reason, they are beyond the scope of the present review. However, the pharmacokinetic processes described within the present publication can contribute to understanding the fate of the fraction of the drug that has been solubilized and released from these more sophisticated systems.
A description of the relevant anatomical barriers and physiological parameters after topical drug administration is provided for both human and rabbit eyes. Rabbits have been extensively used as experimental animals in studies attempting to clarify ocular pharmacokinetics (del Amo et al., 2015; Maurice et al., 1984); the rabbit’s eye displays many anatomical and physiological similarities to the human eye. In addition, in this review, details of how the non-corneal absorption route could be exploited as the access route of a drug to the posterior segment of the eye are presented.
Additionally, the sparse posterior pharmacokinetic data of topical ophthalmic drugs presently available in the literature are reviewed, from both rabbits and humans. Some limitations of extrapolating the existing rabbit pharmacokinetic data toward advancing retinal treatment in patients are considered, and description of areas where there is scope for improvement is given. The same strategies can be applied to other animal models. Last, the past and the present clinical trials which have investigated “Topical drug delivery to the posterior segment of the eye” are reviewed and discussed.
2 Topical pharmacokinetics of ophthalmic formulations
Broadly, topical ophthalmic formulations include liquid, semi-solid, and solid forms (Hopkins, 2007a; Alghamdi et al., 2020). However, the present work focuses on liquid formulations, one of the most common ophthalmic formulations including both solutions and suspensions. If any of the ophthalmic formulations discussed within this review contain adjuvants (such as solubilizers or viscosity enhancers), their names will also be detailed since they affect the free (solubilized) drug pharmacokinetics.
After the administration of an eye drop onto the ocular surface, the drug’s fate is determined by how it copes with the eye’s protective mechanisms, flows, and barriers. The free drug’s passage through the eye is subjected to the following pharmacokinetic events: 1) drug clearance from the ocular surface (including non-productive absorption), 2) drug absorption into the inner tissues of the eye (productive absorption), and 3) drug distribution within the eye and clearance from the ocular tissues into the systemic circulation. These processes are described in the following sections.
2.1 Drug clearance from the ocular surface
A schematic representation of the anterior part of the eye is shown in Figure 1, including the lacrimal secretion and drainage systems and the drug clearance paths from the ocular surface.
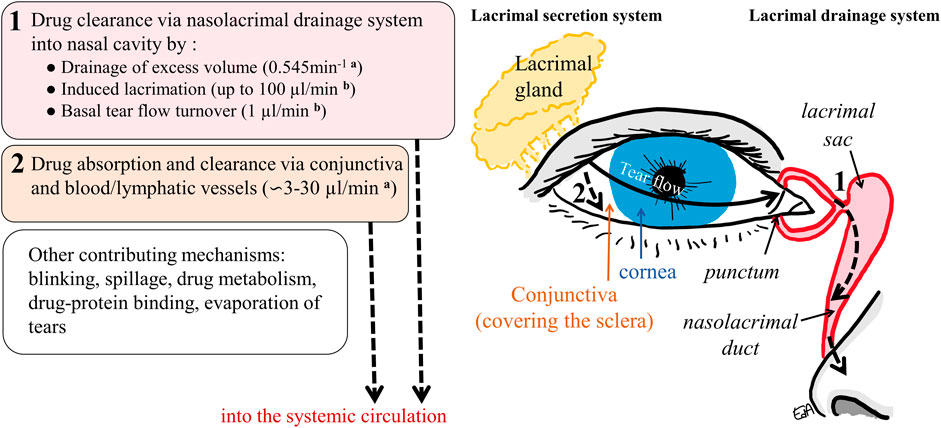
FIGURE 1. Schematic representation of the anterior part of the eye and the drug clearance routes from the ocular surface: 1) via nasolacrimal drainage and 2) via the conjunctival membrane and the blood/lymphatic vessels into the systemic circulation (a: in rabbits, b: in humans).
The tear volume present in the cul-de-sac of the human eye has been estimated to be 6.2 µl (Mishima et al., 1966), while the instilled volume of an ophthalmic formulation drop may be up to nine times this volume; eye drops have volumes ranging from 25 to 56 µl (Lederer and Harold, 1986). Upon instillation, the excess volume is rapidly drained into the nasolacrimal system with most of the drug washed out from the ocular surface until it achieves a physiological tear volume of 6.2 µl. Moreover, a lacrimation reflex may be induced, with an increase in the tear turnover rate. The tear flow value may increase to 50 μl/min in humans, and the maximum has been estimated to be not greater than 100 μl/min (Mishima et al., 1966; Sahlin and Chen, 1996).
Other mechanisms such as blinking may also increase solution drainage and spillage, and some of the formulation’s properties can increase the lacrimation reflex (formulations with a non-physiological pH of 7.4 and/or tonicity of 300 mOsm/kg) (Urtti and Salminen, 1993; Järvinen et al., 1995). It is also well known that the smaller the drop volume, the less drug that will be lost via the drainage mechanism (Chrai et al., 1973). Eventually, the increased tear flow slows down until it reaches its basal value (1.2 μL/min) a few minutes after instillation (Mishima et al., 1966). The initial drainage and induced lacrimation, along with the basal tear flow, contribute to drug dilution and clearance via the nasolacrimal drainage system. This comprises the puncta, canaliculi, lacrimal sac, and the nasolacrimal duct, which empties into the nasal cavity (Figure 1), where the drug will be absorbed mainly in the nasal mucosa epithelium and thus reaches the systemic circulation. To a lesser extent, drug absorption may happen further down in the epithelia of the nasopharynx or gastrointestinal tract (Urtti and Salminen, 1993).
Another important route of drug clearance is via permeation through the conjunctival membrane (i.e. non-productive absorption). This translucent tissue covers the exposed eyeball from the region limiting the cornea (limbus) to the lining of the inner part of the eyelids. It is larger and more permeable than the cornea and rich in blood and lymphatic vessels (Ramsay et al., 2018; Remington and Goodwin, 2021). Rabbit conjunctiva allows permeation of molecules of 20 kDa (∼3.2 nm) through the paracellular pore (Huang et al., 1989), with a surface area ∼nine times the corneal counterpart (Watsky et al., 1988). Moreover, metabolism may take place for drugs that are substrates of esterases (as observed in rabbit and porcine conjunctiva) (Heikkinen et al., 2018; Hammid et al., 2021). Most of the drugs absorbed in the conjunctiva will reach the conjunctival vessels and be effectively cleared into the systemic circulation.
Overall, most of the instilled drug reaches the systemic circulation via the nasolacrimal route or the non-productive conjunctival absorption, and these dominate over the productive absorption and may well lead to toxicity (Urtti and Salminen, 1993; Järvinen et al., 1995).
These processes are schematically presented in Figure 1, which also displays some physiological values related to the human or the rabbit eye. In rabbits, the physiological volume of tear fluid is estimated to be around 7.5 µl, with a tear turnover flow of 0.53 μl/min, while the rate constant of drainage for an instilled volume of 25 μl has been estimated to be 0.545 min−1 (Chrai et al., 1973). Based on rabbit experimental data, some estimates for drug conjunctival clearances have been determined; these vary depending on the physicochemical properties of the compounds: 2.9 μl/min (for inulin), 10.4 μl/min (for timolol), and 32 μL/min (for dexamethasone) (Ahmed et al., 1987; Balla et al., 2022).
2.2 Into the eye: productive absorption
The anatomy of the eye is shown in Figure 2 depicting the anterior segment tissues (cornea, aqueous humor, iris, ciliary body, and lens), posterior segment tissues (vitreous humor, retina, and choroid), and the blood-ocular barriers (blood-aqueous barrier, BAB, and blood-retinal barrier, BRB).
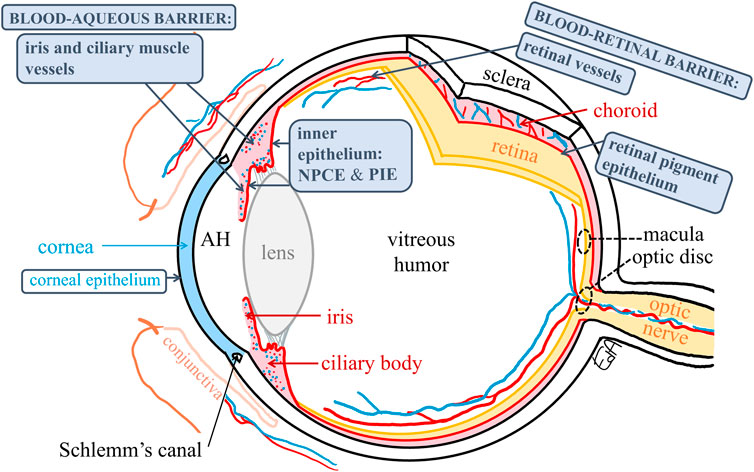
FIGURE 2. Schematic representation of the anatomy of the eye depicting the blood–ocular barriers and the target tissues for treatments of macular edema and glaucoma (macula and optic disc, respectively) (dashed circles). The ocular membranes containing tight junctions are shown in boxes. AH: aqueous humor, NPCE: non-pigmented ciliary epithelium, and PIE: posterior iris epithelium.
The fraction of drug that escapes from the “first-pass-clearance” (2.1) may permeate into the eye via two routes: the corneal route (across the cornea) and the non-corneal route (across the conjunctiva-sclera).
2.2.1 Corneal absorption route
The cornea is a transparent and avascular tissue in front of the eye and comprises the corneal epithelium, Bowman layer, stroma, Descemet membrane, and endothelium. The relative thickness of the corneal epithelium compared to the stroma and endothelium is 1:10:0.1. It contains tight intercellular junctions which represent the main barrier to the penetration of topical compounds (Figure 2). For example, inulin (5 kDa, ∼1.2 nm) cannot diffuse through the intercellular spaces of the rabbit corneal epithelium (Huang et al., 1989). Extensive drug permeability studies have been carried out with excised cornea tissue from different animal species (see data collections (Prausnitz and Noonan, 1998; Kidron et al., 2010; Loch et al., 2012; Ramsay et al., 2018)). Lipophilic drugs cross the cornea more effectively than hydrophilic compounds, and the permeabilities in the investigated species follow the trend of rabbit > porcine > bovine (Loch et al., 2012; Ramsay et al., 2018), with human corneal permeation expected between rabbit and porcine values, possibly because of differences in corneal thicknesses (Agarwal and Rupenthal, 2016).
Once the drug reaches the aqueous humor, it distributes to the surrounding tissues (cornea, lens, and iris-ciliary body) or is cleared via aqueous humor outflow through the trabecular meshwork into Schlemm’s canal, reaching the venous and lymphatic capillaries and then the systemic circulation (Figure 3). The aqueous humor outflow value for rabbits is 0.18 ml/h (3 μl/min) (Barany and Kinsey, 1949) and 0.14 ml/h (2 μL/min) for humans (Brubaker, 1982).
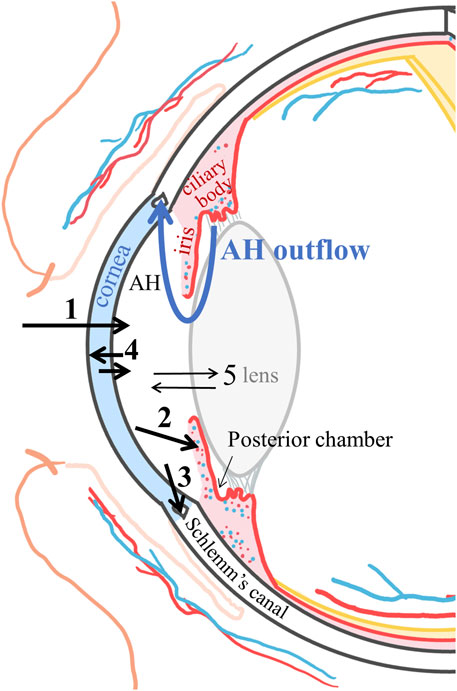
FIGURE 3. Schematic representation of the corneal route pharmacokinetics. 1: drug corneal absorption into the aqueous humor (AH); 2: drug distribution into the iris and ciliary body and clearance into the vessels and the systemic circulation; 3: clearance by the aqueous humor flow via the trabecular meshwork into Schlemm’s canal reaching the venous and lymphatic systems; 4: distribution to the cornea; and 5: distribution to the lens.
A drug that distributes into the vascularized iris-ciliary body tissue can permeate into the blood vessels. The blood flow in rabbit iris is 3.72 ml/h, and in the ciliary body, it is 4.91 ml/h (Nilsson and Alm, 2012). The corresponding values in monkeys are 1.02 ml/h and 5.34 ml/h, respectively (Alm and Bill, 1973). The iris-ciliary muscle vessels constitute the outer BAB (excluding the ciliary process vessels). Therefore, the clearance will be faster for lipophilic drugs more able to cross the BAB than hydrophilic drugs (Figure 2) (Fayyaz et al., 2020a; Fayyaz et al., 2020b). Drug diffusion from the iris-ciliary body back to the aqueous humor is unlikely for lipophilic drugs but may occur for compounds that are hydrophilic or have low permeability properties across biological membranes (e.g., atenolol or brinzolamide) (Fayyaz et al., 2020a; Naageshwaran et al., 2021).
The drug can also back-diffuse from aqueous humor into the cornea (Araie et al., 1982; Acheampong et al., 1995a; Fayyaz et al., 2020b). This has been observed for lipophilic drugs, when the drug exposure (area under the curve of the concentration-time profile, AUC) was measured in the corneal epithelium for three topical beta-blockers in the rabbit eye, and the most lipophilic drug, betaxolol, had a seven times higher AUC0 to ∞ than the more hydrophilic compound, atenolol (Fayyaz et al., 2021). In addition, some fraction of the drug in the cornea may diffuse laterally to the sclera.
Intracameral pharmacokinetic studies (drug injection into the anterior chamber) can provide a better understanding of topical drug kinetics and allow the calculation of the absolute aqueous humor bioavailability of topical drugs; this has been demonstrated to be typically less than 4% (Fayyaz et al., 2020b; Naageshwaran et al., 2021; Naageshwaran et al., 2022). After intracameral injection, the same kinetic pathways shown in Figure 3 are present with the obvious exclusion of pathway 1 (corneal absorption). Intracameral lipophilic drugs also have to undergo corneal partitioning (Naageshwaran et al., 2022; del Amo et al., 2022), while lens partitioning is less relevant (del Amo et al., 2022), for example, the AUC0–5h cornea/aqueous humor for acetaminophen was five times and for brimonidine seven times the corresponding AUC0–5h lens/aqueous humor. Interestingly, the same drugs injected in the vitreous humor (using a dose to acquire similar initial concentrations) achieved three times higher lens partitioning than after intracameral administration (del Amo et al., 2022), showing that lens access is more favorable from the vitreous than from the anterior chamber.
Even though drug distribution from the aqueous humor into the vitreous has been proposed after topical administration (Boddu et al., 2014; Sripetch and Loftsson, 2021; Löscher et al., 2022), this path seems unlikely. Drug diffusion from the aqueous humor through the pupil into the posterior chamber would be in the opposite direction to the aqueous humor flow, and the lens further acts as a barrier, limiting the drug’s circulation (Schoenwald et al., 1997; Fayyaz et al., 2021). Moreover, the drug lenticular distribution is observed only in the outermost lens layers capsule, epithelium (only present anteriorly), and the external cortex fibers (Heikkinen et al., 2018). Therefore, a drug is not expected to cross the lens and reach the vitreous, but back-diffusion into the anterior chamber is more likely.
2.2.2 Non-corneal absorption route
Topically applied ophthalmic drugs may be absorbed via the non-corneal absorption route crossing the bulbar conjunctiva and the anterior sclera into the uveal tract, with the drug reaching the iris-ciliary body bypassing the aqueous humor (Ahmed and Patton, 1985; Ahmed and Patton, 1987) (Figure 4). This route is important for drugs with poor permeation across the cornea, such as hydrophilic drugs (Fayyaz et al., 2021) and large molecules such as inulin (5 kDa, ∼1.2 nm) (Ahmed and Patton, 1985; Ahmed and Patton, 1987).
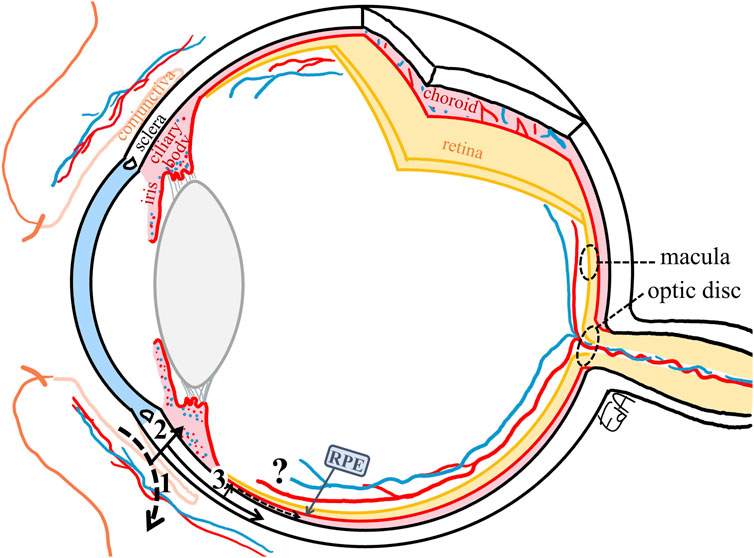
FIGURE 4. Schematic representation of the non-productive conjunctival absorption of a drug (1) and the non-corneal absorption route with drug permeation through the conjunctiva and sclera, (2) reaching the iris-ciliary body, from the sclera to the choroid (3), only a small fraction may cross the RPE (retinal pigment epithelium) to penetrate the peripheral retina. The target tissues for the treatments of macular edema and glaucoma (macula and optic disc, respectively) are marked with dashed circles.
The sclera is the connective tissue containing primary collagen fibrils that protect and support the eye. The relative scleral permeability of hydrophilic polyethylene glycol oligomers (0.2–1 kDa) compared to the cornea and bulbar conjunctiva was 10:1: ∼20 (Hämäläinen et al., 1997), while for the small lipophilic drug, timolol, the relative relationship was different, 5:1:2 (Ahmed et al., 1987). The scleral permeabilities of a set of compounds with a wide range of molecular weights (0.3–150 kDa, 0.5–8.25 nm) were similar between rabbits and humans but greater than that in bovine sclera (Ambati et al., 2000).
After crossing the anterior sclera, the drug then diffuses into the uveal tract, the vascularized and melanin-containing layer, that comprises the iris, ciliary body (anterior uvea), and choroid (posterior uvea), with the latter providing nutrition to the outer retina. These routes of drug diffusion are depicted in Figure 4. The most probable path for the topical drugs to enter the posterior tissues is via the non-corneal route as described previously by Ahmed and Patton, (1985); Ahmed and Patton, (1987), that is, the drug permeates across the anterior sclera through the suprachoroidal space and reaches the choroidal tissue. The choroid is a vascular and connective tissue layer that consists primarily of large and highly fenestrated blood vessels, with the highest blood flow per unit weight compared to any other tissue in the body (Alm and Bill, 1973), being 62 ml/h in rabbits (Nilsson and Alm, 2012) and 43 ml/h in humans (Sebag et al., 1994). Therefore, most of the drug that enters the choroid will be cleared by the choroidal blood flow, and only a small fraction may cross the outer BRB, that is, passing through the retinal pigment epithelium (RPE) to penetrate the peripheral inner retina.
2.3 Further inside
The main mechanism of drug movement through the ocular tissues via the non-corneal route is by paracellular or transcellular diffusion according to the concentration gradient, until the drug enters the blood vasculature of the uveal tract. It is unlikely that a drug may diffuse along the sclera (pure diffusion without convective transfer, i.e., ocular fluid flow) to the target tissues at the back of the eye (Figure 4: from 3 to the macula or optic disc).
A simplification of the ocular venous drainage is shown in Figure 5, although in reality, the eye’s vascular organization is far more complicated. The orbital vascular bed is also highly complex, with extensive interindividual variations (Hayreh, 2006). Furthermore, additional ocular flows may be present, and these may also affect the ocular drug distribution in the posterior segment. For example, aqueous humor flow from the anterior chamber into the ciliary muscle has been described to reach the supraciliary and suprachoroidal spaces and then further into the choroidal vessels and vortex veins (Sherman et al., 1978). Interestingly, Ahmed et al. (Ahmed and Patton, 1985; Ahmed and Patton, 1987) proposed that drug back-diffusion from the local ocular vasculature to the surrounding posterior tissues may occur, before the drug exits the eye from the vortex and anterior ciliary veins (Figure 5). However, the contribution of such a route is difficult to measure, and the authors concluded that its impact was not meaningful according to the complicated experiments they conducted (Ahmed and Patton, 1985; Ahmed and Patton, 1987).
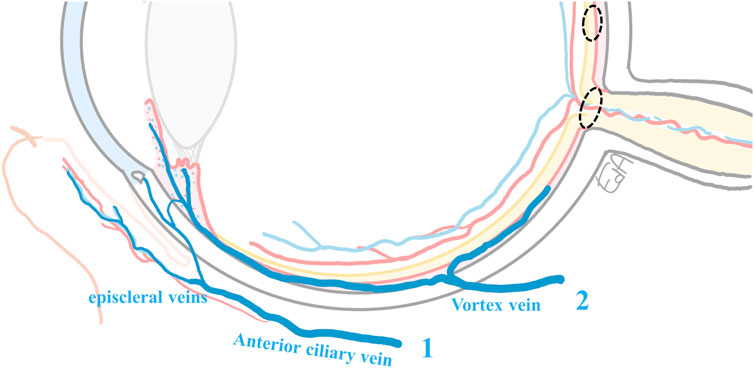
FIGURE 5. Schematic representation of the ocular venous drainage: 1) from conjunctiva and Schlemm’s canal vasculature draining to the episcleral veins and the anterior ciliary vein, and 2) from the iris, ciliary body vessels, and the choriocapillaris, blood drains into the vortex vein before exiting equatorially from the eye. The ciliary body blood also partly drains into the episcleral veins. The anterior ciliary veins and the vortex vein, posteriorly within the sclera, reach the inferior and the superior ophthalmic veins of the orbit (Chiou et al., 1989; Anand-Apte and Hollyfield, 2010). The target tissues for the treatments of macular edema and glaucoma are marked with dashed circles.
Ahmed et al. also investigated the effect of drug return from the systemic circulation after a single-drop instillation of two radioactively labeled compounds, timolol and inulin, in albino rabbits. Most of the tissue levels measured in the contralateral eye corresponded to a mere 1% of those in the dosed eye (at 20 min’ post-instillation when the maximum concentrations were observed in the ocular tissues). The ocular drug levels after i.v. injection of the same dose were also compared; there was only 5% of the concentration observed in the topically treated eye for timolol (at 20 min), and the extent of inulin return was even less than for timolol because of the difficulty of inulin to penetrate the blood-ocular barriers (Ahmed and Patton, 1985; Ahmed and Patton, 1987). In another topical pharmacokinetic experiment with timolol, now carried out in pigmented rabbits, the drug accumulated in the iris-ciliary body in the untreated contralateral eye after a topical multi-dose dosage (twice for 6 days, at 4h after the last drop) (Huupponen et al., 2009). Timolol is a melanin binder, and this is a relevant factor impacting the pharmacokinetics of drugs topically administered to the eye. Drugs with an affinity for melanin can be retained longer in the ocular melanin-containing cells of the uveal tract and RPE, and this impacts their topical pharmacokinetics and pharmacodynamics (Rimpelä et al., 2018a).
Last, ocular metabolism may influence the pharmacokinetics of topical ophthalmic drugs. However, in a recent comprehensive study of ocular pharmacokinetics in rabbit, the metabolism of four drugs was quantified (acetaminophen, brimonidine, cefuroxime axetil, and sunitinib) in six different ocular tissues (del Amo et al., 2022); metabolism was relevant only for those drugs that were a substrate of esterases (cefuroxime axetil), while enzymatic activity for those drugs metabolized by sulfotransferase (acetaminophen), aldehyde oxidase (brimonidine), or CYP3A (sunitinib) was found to be very low. In fact, the metabolite exposure in most ocular tissues was 12–103 times smaller than the exposure to the parent drug, so the impact of metabolism on drug clearance from the eye could be considered negligible (del Amo et al., 2022).
3 From preclinical studies to clinics: considerations and questions still to be answered
Animal models are necessary to acquire knowledge of retinal disease in patients and clarify the pharmacokinetics and pharmacodynamics of new topical ophthalmic therapies. Commonly, rodents and larger animals, mostly rabbits and monkeys, are used for those purposes (Rodrigues et al., 2018; Löscher et al., 2022). The pharmacodynamic or efficacy studies are typically carried out in rodents (e.g., oxygen-induced mouse retinopathy and rat laser-induced choroidal neovascularization for nAMD). However, it is well known that positive efficacy results in rodents cannot be directly translated to patients due to the anatomical and physiological differences between mice/rat eyes and the human eye (del Amo et al., 2017; Rodrigues et al., 2018; Löscher et al., 2022). Therefore, further investigations are required in larger animals. For example, when developing regorafenib eye drops for nAMD, monkey laser-induced choroidal neovascularization was used along with the rodent models (Boettger et al., 2015; Klar et al., 2015). However, despite the positive efficacy result obtained also in monkeys (25 µl drop twice daily for 2 weeks, 20 mg/ml), the clinical trial of topical regorafenib (40-50 μL twice daily for 2 weeks, 20 mg/ml) revealed a lack of efficacy in nAMD patients, and the trial was discontinued (NCT02222207). The most likely reason for the termination was insufficient drug exposure in the retina of the patients (Joussen et al., 2019). This highlights that not only pharmacodynamic but also pharmacokinetic studies in larger animals are required, taking into account how challenging it is to obtain an appropriate animal model for many ocular diseases. Later, Horita et al. observed that topical regorafenib treatment was effective in laser-induced choroidal neovascularization in rats but not in monkeys, as the pharmacokinetic studies on the latter species showed concentrations of regorafenib in the retina that were below the limit of quantification (BLQ<3 ng/g) 4 h after the instillation of a regorafenib 50 µl drop (17.1 mg/ml) (Horita et al., 2019).
Further regarding the preclinical pharmacokinetic studies performed on rabbits, it is surprising to note that a common procedure when investigating topical retinal treatments is the pooling together of choroid and retina tissues and then measuring the average drug concentration in both tissues (Shikamura et al., 2016; Askew et al., 2018; Horita et al., 2019; Huang et al., 2021). However, this makes it difficult to conclude the real drug exposure exclusively in the neural retina. In several extensive studies on ocular distribution after topical drug solution instillation, choroid-retina concentrations have been typically measured (Araie et al., 1982; Acheampong et al., 1995b; Acheampong et al., 2002) or alternatively only vitreous concentrations have been analyzed from the posterior segment (Urtti et al., 1990; Fayyaz et al., 2021).
When comparing drug concentrations observed in the vitreous humor and the tear fluid, there is a major difference, i.e., humor concentration is as low as 1/10,000 of the concentration in the tear fluid (Urtti et al., 1990; Fayyaz et al., 2021). The vitreal levels may provide an estimate of the free drug concentration available at the back of the eye since binding levels of drugs in the vitreous seem to be relatively low (Schauersberger and Jager, 2002; Petternel et al., 2004; Rimpelä et al., 2018b; Kompella et al., 2019).
A compilation of topical pharmacokinetics studies of ophthalmic formulations in rabbits and patients is presented in Table 1 (as a color image in the Supplementary Material), where concentrations of the drug in the vitreous and, when available, in the neural retina are presented (corresponding to the maximum reported concentration in rabbits and the available values from patients).
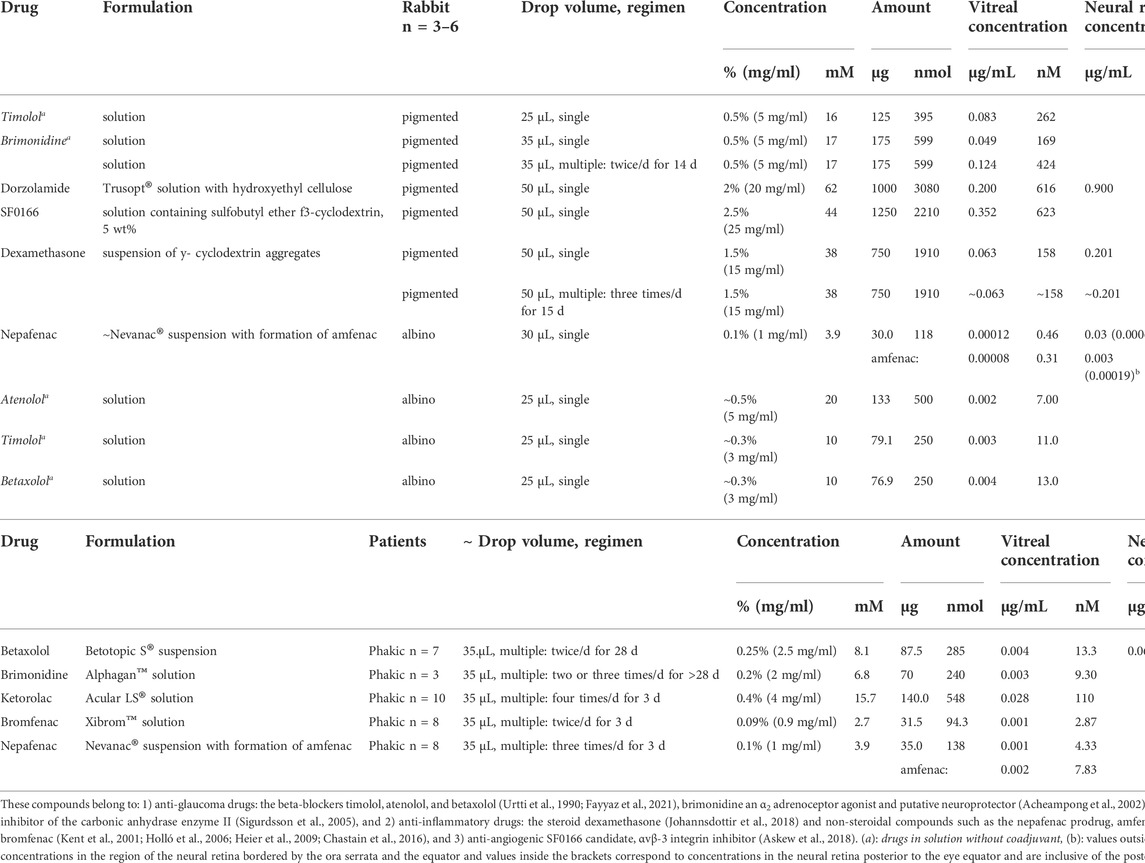
TABLE 1. Drug concentration in vitreous humor and retina after topical ophthalmic administration in rabbits (the maximum reported value in the concentration-time profile, n = 3–6) and in patients who underwent enucleation or vitrectomy surgery for a therapeutic need (the concentrations measured post surgery after a multi-dose administration of the commercial formulation, n = 3–10).
The rabbit study collection is non-exhaustive but intends to be representative of the variety of drugs investigated in albino and pigmented eyes (Urtti et al., 1990; Acheampong et al., 2002; Sigurdsson et al., 2005; Chastain et al., 2016; Askew et al., 2018; Johannsdottir et al., 2018; Fayyaz et al., 2021), while the patient data are comprehensive and obtained from patients who underwent enucleation or vitrectomy surgery for therapeutic reasons (Kent et al., 2001; Holló et al., 2006; Heier et al., 2009). Only patients with a healthy status of the lens (phakic) were selected since it is known that the lack of a lens affects ocular pharmacokinetics (Maurice et al., 1984; Mandell et al., 1993). The concentrations shown in Table 1 correspond to post-surgery measurements after a multi-dose administration of commercial formulations, with the last drop instillation made within 2–12 h before the surgery.
From rabbit studies, among all drugs shown in Table 1, the highest measured concentration in the neural retina was obtained for dorzolamide, 2.8 µM (0.9 μg/ml) after instillation of the highest dose (3 µmol) (Sigurdsson et al., 2005). Drug retinal concentrations were higher than the vitreal ones for the same drug, and a similar trend can be seen after intracameral pharmacokinetic studies (del Amo et al., 2022), supporting the idea of drug penetration from the non-corneal route probably from the retina to the vitreal cavity.
Brimonidine multi-dose was the treatment that showed the highest concentration in vitreous per nmol-dose among all the rabbit studies (Acheampong et al., 2002). When this treatment is compared to the dosing schedule in patients (Kent et al., 2001) (2.5 higher doses in rabbits than in patients, at similar dose intervals), the rabbit vitreal concentrations were as much as 46 times higher than those achieved in humans. This emphasizes the impact of the anatomical differences between rabbit and human eyes, in line with the expectation of higher ocular posterior drug exposure in the smaller eye of the rabbit.
The effect of melanin on drug distribution in the posterior ocular tissues was evident when the concentrations of timolol in pigmented and non-pigmented rabbits were compared (Urtti et al., 1990; Fayyaz et al., 2021). The beta-blockers timolol, atenolol, and betaxolol are melanin binders, as is brimonidine (Pelkonen et al., 2017; Rimpelä et al., 2018a), leading to longer retention of these drugs in the pigmented tissues. Similarly, the multi-dose administration of a topical dexamethasone formulation did not lead to higher levels in the posterior tissues than a single dose (Johannsdottir et al., 2018) because dexamethasone is a relatively low melanin binder (Pelkonen et al., 2017). This was not the case for brimonidine, which resulted in a higher drug concentration after the multi-dose regimen in the pigmented rabbit (Acheampong et al., 2002), despite administration of a three times smaller nmol-dose as compared to dexamethasone and less frequent dosing. Melanin binding can influence drug pharmacokinetics and for this reason, it is also important to carry out topical pharmacokinetic studies in pigmented rabbits, especially when the drug candidate targeting the back of the eye is known to bind to melanin.
Another crucial aspect when designing pharmacokinetic studies concerns the dissection of the ocular tissue. The neural retina should be dissected without the presence of RPE or choroid, and importantly, drug exposure in the target tissue of the disease investigated must be measured. For nAMD, this means the macula that corresponds in the rabbit to the visual streak of the rabbit’s retina (Yu and Cringle, 2004), while for glaucoma, the retina around the optic head nerve is the region to be dissected separately from the rest of the neural retina. While this will provide useful information on whether the concentrations are at a therapeutical level in the target sites, it is rarely carried out. Exceptionally, Chastain et al. (2016) investigated the ocular distribution of the prodrug nepafenac and its more active form, amfenac, separately in the peripheral and central retina of albino rabbit eyes. In that experiment, an eye drop of a nepafenac suspension was instilled with an identical composition to the commercial formulation Nevanac®, but only extremely low levels of nepafenac and amfenac were detected in the central retina (Table 1).
Among the few drug concentrations available from clinical studies (Kent et al., 2001; Holló et al., 2006; Heier et al., 2009), there are only data from betaxolol in the neural retina, and it is in the nM range (213 nM, 0.07 μg/ml) (Table 1) (Holló et al., 2006). Ketorolac was found to have the highest concentration in the vitreous (110 nM), and it has also displayed the highest vitreal concentration/dose ratio in the clinical data (Heier et al., 2009). The vitreal concentration of betaxolol in patients after multi-dose administration (Holló et al., 2006) was almost identical to the value obtained in albino rabbits after a single administration of a similar dose (Fayyaz et al., 2021). This is evidence of a relatively lower drug exposure in the eyes of human patients than in albino rabbits, considering the multi-dose regimen and betaxolol retention in the pigmented tissues which were present in the clinical study. It would be expected that higher betaxolol concentrations would be present in the posterior segment of a pigmented rabbit eye after single- and multi-dose instillations, as was reported in pharmacokinetic studies with brimonidine (Kent et al., 2001; Acheampong et al., 2002).
Another aspect to be taken into account when analyzing drug concentrations in the ocular tissues is to measure unbound drug concentrations. Only the free drug will exert an effect, and this is especially important for drugs that bind to melanin (Acheampong et al., 2002; Rimpelä et al., 2018a) or to other ocular sites, e.g., sunitinib. Not only is sunitinib a high melanin binder, but it also underwent high ocular partition to several ocular sites in albino rabbit pharmacokinetic studies e.g., to the cornea, iris-ciliary body, and retina after intracameral and intravitreal injections (del Amo et al., 2022).
A major challenge that hampers the interpretation of pharmacokinetic experiments is knowing the real therapeutic concentration of the drug in vivo. It is well known that a drug’s IC50 values obtained from in vitro assays are lower than in the in vivo situation, and multiplying its value by a factor of 100 to avoid underestimation is typically carried out (as shown in patent WO 2014/152959 Al) (Astafieva et al., 2014). More research is needed to obtain reliable in vitro–in vivo extrapolations to define the clinical therapeutic concentrations in the ocular target sites.
Regarding pharmacodynamic studies in rabbits, one should notice that there is a paucity of reliable disease animal models for nAMD in rabbits. While neovascularization induced by laser in rabbits is almost impossible, after intravitreal angiogenic factor injection it is only temporary (Lau et al., 2018), and it is important to demonstrate if neovascularization is present in the untreated control disease animal when conducting an efficacy study.
The pharmacokinetic translatability from rabbit to human eyes after topical ophthalmic administration has not yet been established, while for other routes, e.g., intravitreal is available (del Amo et al., 2017; del Amo and Urtti, 2015). Ideally, such an investigation would allow the estimation of scaling factors to account for the anatomical dimensional differences between rabbit and human eyes and the difference in relevant physiological parameters affecting topical pharmacokinetics and drug distribution to the back of the eye. Unfortunately, this type of data is not available yet, and therefore, one should be cautious even when interpreting in vivo data generated from rabbits.
4 Clinical trials on topically administered drugs to treat the posterior segment
Currently, three topical ophthalmic formulations are reported on ClinicalTrials.gov (ClinicalTrials.gov) as potential therapies for posterior segment illnesses, mainly nAMD and DR; these are presented in Table 2 with their corresponding national clinical trial (NCT) numbers. There are two ophthalmic suspensions (PAN-90806 and OCS-01) and one ophthalmic solution (SF0166, now OTT166).
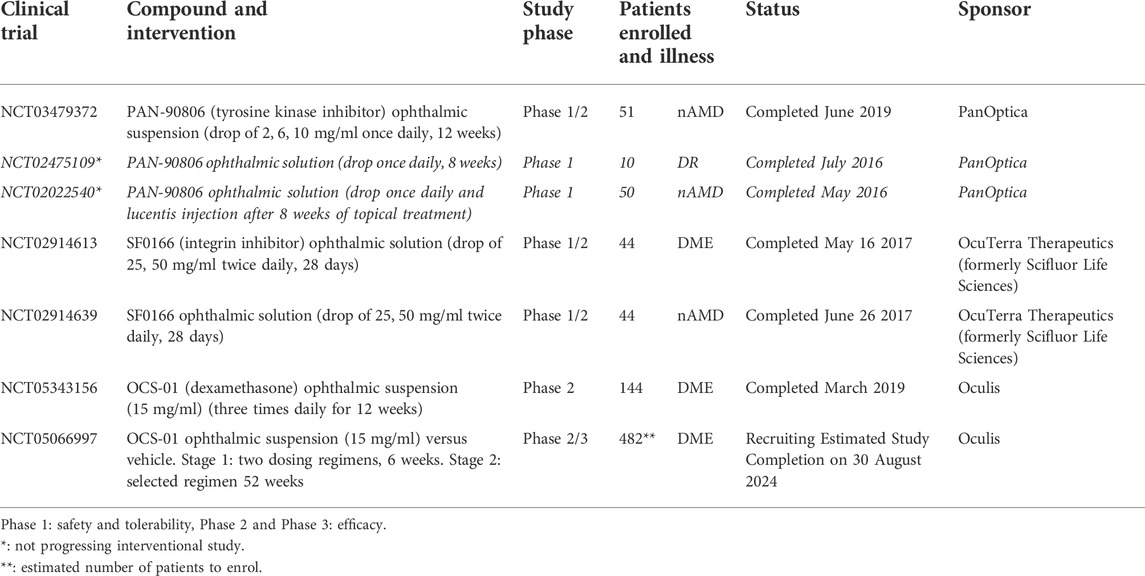
TABLE 2. Recent or ongoing clinical trials (ClinicalTrials.gov) of topical ophthalmic formulations to treat illnesses in the posterior segment (nAMD, DR, and DME).
PAN-90806 is a tyrosine kinase inhibitor that blocks the angiogenic activation of receptor tyrosine kinase VEGF receptor 2. It is being investigated for the treatment of neovascular eye diseases and specifically for nAMD with a once-a-day topical dosing regimen. From previous clinical trials with the solution formulation (NCT02475109, NCT02022540, Table 2), a side-effect was identified, punctate keratopathy, due to the off-target inhibition of corneal epithelial epidermal growth factor receptor (Rush et al., 2016). Punctate keratopathy can be minimized by the addition of nanomolar concentrations of menadione, a synthetic analog of vitamin K3, in the topical formulation without losing the anti-angiogenic efficacy of the compound (Rush et al., 2016). A new suspension formula was developed and used in a new clinical trial with 51 patients (NCT03479372, Table 2). No major safety issues were observed, and half of the patients (at all doses) did not need rescue therapy within 1 month after the 3-month topical treatment. Most of the patients (23 out of 26) showed clinical stabilization or improvement as reported in the company’s news bulletin (PanOptica, 2022). Presently, no new follow-up clinical trial has yet been registered.
SF0166 is a small molecule that inhibits the actions of one of the key integrin subtypes αvβ-3 that contributes to the progression of retinal diseases (Slack et al., 2022). Due to the blockade of αvβ-3, the activation signal for multiple growth factors (VEGF, fibroblast growth factor, and platelet-derived growth factor) is blocked, preventing neovascularization. The anti-integrin molecule is announced as being administered as a solution to the patients. However, it is unclear if this is an identical formulation as applied in the rabbit preclinical studies where the formulation contained 5 wt% sulfobutyl ether β-cyclodextrin to solubilize the drug (50 mg/ml, Table 1), whereas no coadjuvant was reported in those formulations used in the monkey and mouse preclinical trials (Askew et al., 2018). Although the clinical trial data (NCT02914613 and NCT02914639) seemed to indicate safety and the desired effect, (nine of the 42 patients presented a decrease in central retinal thickness and improvement of visual acuity of five letters), no further developments of this compound or new clinical trials have been reported at present, in web news (SciFluor, 2022).
OCS-01 is an ophthalmic suspension of dexamethasone at a concentration of 1.5% (15 mg/ml), where the drug and γ-cyclodextrins formed complexes by self-assembly, resulting in a mix of nano- and micro-particle aggregates (Johannsdottir et al., 2018). In the clinical trial of NCT05343156, 144 patients were recruited for OCS-01 treatment three times a day for 12 weeks. In the treated arm, 91 out of 99 patients showed a reduction in the central retinal thickness versus the placebo arm (-53.7 μm vs. -16.9 μm, p = 0.0115). The drug proved to be safe for the 12 weeks of trial, although the incidence of increased IOP, as expected, was more common in the treated than in the vehicle arm. The next phase of the study (NCT05066997, Table 2) aims to evaluate the efficacy and safety of the OCS-01 ophthalmic suspension in a larger group of DME patients.
Previously, several other small molecular weight drugs have been investigated in clinical trials, in patients or healthy volunteers, aiming to treat the posterior segment after topical instillation, but they have not proceeded to the market. Some examples are brimonidine, squalamine lactate or MSI-1256F, FOV2304, mecamylamine or ATG003, and dexamethasone formulated with cyclodextrin or in combination with iontophoresis. Additionally, several tyrosine kinase inhibitors have been investigated, such as acrizanib (or LHA510), regorafenib, and pazopanib, and the TG100801 prodrug that is converted into TG100572 by the ocular esterases. It does seem that all of these interventional studies have been discontinued or terminated (Table 3). Even though Table 3 is not intended to be a comprehensive list of the discontinued clinical trials registered in ClinicalTrials.gov (ClinicalTrials.gov), it does highlight the number of cases that fail at this stage of testing. They include drugs with different mechanisms of action and therapeutic indications, with some candidates even having a lengthy clinical trial history (e.g., squalamine lactate from 2004 until 2016). Unfortunately, reports of negative results in clinical trials are mainly missing, along with a lack of standardization and the sketchy quality of clinical data reporting in general (Varner, 2015). Similarly, the related preclinical supporting data may not be published. In summary, these are obstacles curtailing future advances in the search for new ocular therapies and impairing translation from preclinical studies to clinical trials.
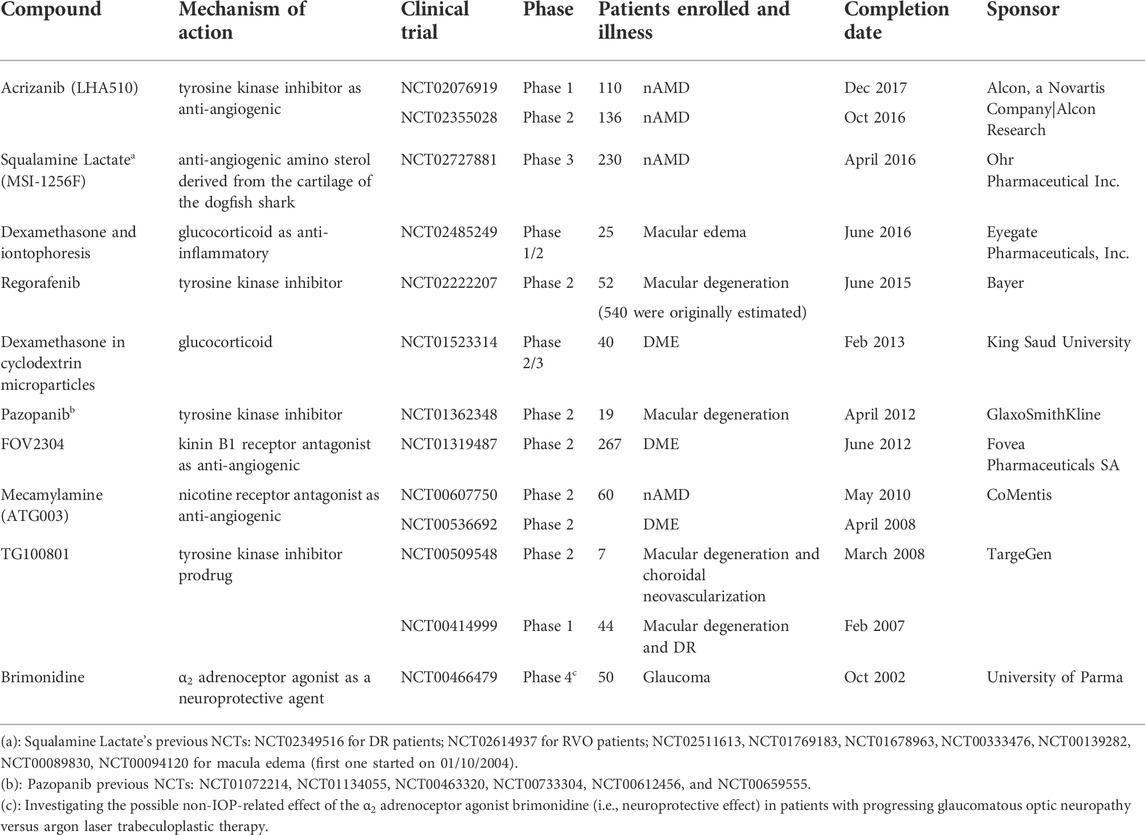
TABLE 3. Terminated or not progressing clinical trials to treat posterior segment illnesses (nAMD, DR, DME, or macula edema and glaucoma) after topical administration.
5 Conclusions
Treating retinal neurodegenerative diseases with topical administration is an attractive option not only for pharmaceutical companies but also for many patients. Although some trace concentrations of drugs have been measured in the retina after topical administration, presently, only three topical formulations are undergoing clinical trials, with only one having reached phase III. More relevant information can be extracted from well-designed preclinical studies that may allow better translatability to patient care. Additionally, publishing negative results of previously terminated clinical trials would help improve our understanding of the potential of the topical route to treat retinal diseases.
There are still many questions to be answered: is there a therapeutic concentration of the drug in the macula or optic disc? Are the drug’s safety and potency adequate? Will compliance with topical ophthalmic therapy be an issue?
It is evident that only drugs that are extremely potent, ocular-specific, and safe will be potential successful candidates for topical application if they are to exert an effect at the back of the eye (it has to be stressed that 50–100% of the topical dose may reach systemic circulation, with the risk of serious side effects).
Up to now, no topically administered drug has been approved for the treatment of nAMD, DR, DME, RVO, or optic neuropathy associated with glaucoma disease.
Author contributions
EdA contributed to the conception and design of the review, analyses of the literature, drawing and elaborating the figures, preparing the tables and calculations, and writing from the first to the last version of the manuscript.
Funding
This study was supported by the Emil Aaltonen Foundation and the strategic funding of the University of Eastern Finland.
Acknowledgments
I would like to thank Lecturer Dr. Veli-Pekka Ranta (School of Pharmacy, University of Eastern Finland) for the critical reading and stimulating discussions during the preparation of this review, Vicente Serra (photographer, Kuopio Municipality) for his technical advice on the figures, Lecturer emeritus Dr. Ewen MacDonald (School of Pharmacy, University of Eastern Finland) for checking the language of the manuscript, and the reviewers for their valuable comments.
Conflict of interest
The author declares that the research was conducted in the absence of any commercial or financial relationships that could be construed as a potential conflict of interest.
Publisher’s note
All claims expressed in this article are solely those of the authors and do not necessarily represent those of their affiliated organizations, or those of the publisher, the editors, and the reviewers. Any product that may be evaluated in this article, or claim that may be made by its manufacturer, is not guaranteed or endorsed by the publisher.
Supplementary material
The Supplementary Material for this article can be found online at: https://www.frontiersin.org/articles/10.3389/fddev.2022.954771/full#supplementary-material
Abbreviations
AH, aqueous humor; AMD, age-related macular degeneration; AUC, area under the curve of the concentration-time profile; BAB, blood–aqueous barrier; BRB, blood–retinal barrier; DME, diabetic macular edema; DR, diabetic retinopathy; ICB, iris-ciliary body; IOP, intraocular pressure; nAMD, neovascular age-related macular degeneration; NCT, National Clinical Trial; NPCE, non-pigmented ciliary epithelium; PIE, posterior iris epithelium; RPE, retinal pigment epithelium; RVO, retinal vein occlusion; VEGF, vascular endothelial growth factor; VH, vitreous humor.
References
Acheampong, A. A., Breau, A., Shackleton, M., Luo, W., Lam, S., and Tang-Liu, D. D. S. (1995). Comparison of concentration-time profiles of levobunolol and timolol in anterior and posterior ocular tissues of albino rabbits. J. Ocul. Pharmacol. Ther. 11 (4), 489–502. doi:10.1089/jop.1995.11.489
Acheampong, A. A., Shackleton, M., John, B., Burke, J., Wheeler, L., and Tang-Liu, D. (2002). Distribution of brimonidine into anterior and posterior tissues of monkey, rabbit, and rat eyes. Drug Metab. Dispos. 30 (4), 421–429. doi:10.1124/dmd.30.4.421
Acheampong, A. A., Shackleton, M., and Tang-Liu, D. D. S. (1995). Comparative ocular pharmacokinetics of brimonidine after a single dose application to the eyes of albino and pigmented rabbits. Drug Metab. Dispos. 23 (7), 708–712.
Adams, C. M., Anderson, K., Artman, G., Bizec, J. C., Cepeda, R., Elliott, J., et al. (2018). The discovery of N-(1-Methyl-5-(trifluoromethyl)-1H-pyrazol-3-yl)-5-((6- ((methylamino)methyl)pyrimidin-4-yl)oxy)-1H-indole-1-carboxamide (acrizanib), a VEGFR-2 inhibitor specifically designed for topical ocular delivery, as a therapy for neovascular age-related macular degeneration. J. Med. Chem. 61 (4), 1622–1635. doi:10.1021/acs.jmedchem.7b01731
Agarwal, P., and Rupenthal, I. D. (2016). In vitro and ex vivo corneal penetration and absorption models. Drug Deliv. Transl. Res. 6, 634–647.
Ahmed, I., Gokhale, R. D., Shah, M. V., and Patton, T. F. (1987). Physicochemical determinants of drug diffusion across the conjunctiva, sclera, and cornea. J. Pharm. Sci. 76 (8), 583–586. doi:10.1002/jps.2600760802
Ahmed, I., and Patton, T. F. (1987). Disposition of timolol and inulin in the rabbit eye following corneal versus non-corneal absorption. Int. J. Pharm. X. 38 (1–3), 9–21. doi:10.1016/0378-5173(87)90092-5
Ahmed, I., and Patton, T. F. (1985). Importance of the noncorneal absorption route in topical ophthalmic drug delivery. Investig. Ophthalmol. Vis. Sci. 26 (4), 584–587.
Alghamdi, E. A. S., Al Qahtani, A. Y., Sinjab, M. M., and Alyahya, K. M. (2020). “Topical ophthalmic drug forms,” in Extemporaneous ophthalmic preparations (Berlin, Germany: Springer International Publishing), 17–18.
Alm, A., and Bill, A. (1973). Ocular and optic nerve blood flow at normal and increased intraocular pressures in monkeys (Macaca irus): A study with radioactively labelled microspheres including flow determinations in brain and some other tissues. Exp. Eye Res. 15 (1), 15–29. doi:10.1016/0014-4835(73)90185-1
Ambati, J., Canakis, C. S., Miller, J. W., Gragoudas, E. S., Edwards, A., Weissgold, D. J., et al. (2000). Diffusion of high molecular weight compounds through sclera. Investig. Ophthalmol. Vis. Sci. 41 (5), 1181–1185.
Anand-Apte, B., and Hollyfield, J. G. (2010). “Developmental anatomy of the retinal and choroidal vasculature,” in Encyclopedia of the eye (Amsterdam, Netherlands: Elsevier), 9–15.
Araie, M., Takase, M., Sakai, Y., Ishii, Y., Yokoyama, Y., and Kitagawa, M. (1982). Beta-adrenergic blockers: Ocular penetration and binding to the uveal pigment. Jpn. J. Ophthalmol. 26 (3), 248–263.
Askew, B. C., Furuya, T., and Edwards, D. S. (2018). Ocular distribution and pharmacodynamics of SF0166, a topically administered αvβ3 integrin antagonist, for the treatment of retinal diseases. J. Pharmacol. Exp. Ther. 366 (2), 244–250. doi:10.1124/jpet.118.248427
Astafieva, I., Horvath, J., Farinas, K. C., Bueche, B., and Erickson, S. (2014). Systems for sustained intraocular delivery of low solubility compounds from a port delivery system implant [Internet]. World intellectual property organisation No 2014/152959 Al. Available at: https://patentscope.wipo.int/search/en/detail.jsf?docId=WO2014152959.
Balla, A., Ruponen, M., Valtari, A., Toropainen, E., Tuomainen, M., Alvarez-Lorenzo, C., et al. (2022). Understanding dexamethasone kinetics in the rabbit tear fluid: Drug release and clearance from solution, suspension and hydrogel formulations. Eur. J. Pharm. Biopharm. 172, 53–60. doi:10.1016/j.ejpb.2022.01.005
Barany, E., and Kinsey, V. E. (1949). The rate of flow of aqueous humor*. Am. J. Ophthalmol. 32 (6), 177–188. doi:10.1016/s0002-9394(14)78371-0
Boddu, S., Gupta, H., and Patel, S. (2014). Drug delivery to the back of the eye following topical administration: An update on research and patenting activity. Recent Pat. Drug Deliv. Formul. 8 (1), 27–36. doi:10.2174/1872211308666140130093301
Boettger, M. K., Klar, J., Richter, A., and Degenfeld, G. V. (2015). Topically administered regorafenib eye drops inhibit grade IV lesions in the non-human primate laser CNV model. Investig. Ophthalmol. Vis. Sci. 56 (7), 2294. Available at: https://iovs.arvojournals.org/article.aspx?articleid=2332060.
Brubaker, R. F. (1982). The flow of aqueous humor in the human eye. Trans. Am. Ophthalmol. Soc. 80, 391–474.
Chastain, J. E., Sanders, M. E., Curtis, M. A., Chemuturi, N. V., Gadd, M. E., Kapin, M. A., et al. (2016). Distribution of topical ocular nepafenac and its active metabolite amfenac to the posterior segment of the eye. Exp. Eye Res. 145, 58–67. doi:10.1016/j.exer.2015.10.009
Chiou, F. Y., Li, B. H. P., Ruiz-Razura, A., and Chiou, G. C. Y. (1989). Microvascular access to the uveal tract in the rabbit eye for ocular pharmacologic studies. Ophthalmic Res. 21 (6), 406–413. doi:10.1159/000266930
Chrai, S. S., Patton, T. F., Mehta, A., and Robinson, J. R. (1973). Lacrimal and instilled fluid dynamics in rabbit eyes. J. Pharm. Sci. 62 (7), 1112–1121. doi:10.1002/jps.2600620712
Chua, B., and Goldberg, I. (2010). Neuroprotective agents in glaucoma therapy: Recent developments and future directions. Expert Rev. Ophthalmol. 5, 627–636. doi:10.1586/eop.10.55
ClinicalTrials.gov. Available at: https://clinicaltrials.gov.
del Amo, E. M., Hammid, A., Tausch, M., Toropainen, E., Sadeghi, A., Valtari, A., et al. (2022). Ocular metabolism and distribution of drugs in the rabbit eye: Quantitative assessment after intracameral and intravitreal administrations. Int. J. Pharm. 613, 121361. doi:10.1016/j.ijpharm.2021.121361
del Amo, E. M. (2022). Pharmacokinetic models: Indispensable tools for ophthalmic drug development. ONdrugDelivery 2022, 59–63. Available at: https://www.ondrugdelivery.com/pharmacokinetic-models-indispensable-tools-for-opthalmic-drug-development/(Accessed June 2022).
del Amo, E. M., Rimpelä, A. K., Heikkinen, E., Kari, O. K., Ramsay, E., Lajunen, T., et al. (2017). Pharmacokinetic aspects of retinal drug delivery. Prog. Retin. Eye Res. 57, 134–185. doi:10.1016/j.preteyeres.2016.12.001
del Amo, E. M., and Urtti, A. (2015). Rabbit as an animal model for intravitreal pharmacokinetics: Clinical predictability and quality of the published data. Exp. Eye Res. 137, 111–124. doi:10.1016/j.exer.2015.05.003
del Amo, E. M., Vellonen, K. S., Kidron, H., and Urtti, A. (2015). Intravitreal clearance and volume of distribution of compounds in rabbits: In silico prediction and pharmacokinetic simulations for drug development. Eur. J. Pharm. Biopharm. 95 (2), 215–226. doi:10.1016/j.ejpb.2015.01.003
Fayyaz, A., Ranta, V. P., Toropainen, E., Vellonen, K. S., Ricci, G. D. A., Reinisalo, M., et al. (2020a). Ocular intracameral pharmacokinetics for a cocktail of timolol, betaxolol, and atenolol in rabbits. Mol. Pharm. 17 (2), 588–594. doi:10.1021/acs.molpharmaceut.9b01024
Fayyaz, A., Ranta, V. P., Toropainen, E., Vellonen, K. S., Valtari, A., Puranen, J., et al. (2020b). Topical ocular pharmacokinetics and bioavailability for a cocktail of atenolol, timolol and betaxolol in rabbits. Eur. J. Pharm. Sci. 155, 105553. doi:10.1016/j.ejps.2020.105553
Fayyaz, A., Vellonen, K. S., Ranta, V. P., Toropainen, E., Reinisalo, M., Valtari, A., et al. (2021). Ocular pharmacokinetics of atenolol, timolol and betaxolol cocktail: Tissue exposures in the rabbit eye. Eur. J. Pharm. Biopharm. 166, 155–162. doi:10.1016/j.ejpb.2021.06.003
Hämäläinen, K. M., Kananen, K., Auriola, S., Kontturi, K., and Urtti, A. (1997). Characterization of paracellular and aqueous penetration routes in cornea, conjunctiva, and sclera. Investig. Ophthalmol. Vis. Sci. 38 (3), 627–634.
Hammid, A., Fallon, J. K., Lassila, T., Salluce, G., Smith, P. C., Tolonen, A., et al. (2021). Carboxylesterase activities and protein expression in rabbit and pig ocular tissues. Mol. Pharm. 18 (3), 1305–1316. doi:10.1021/acs.molpharmaceut.0c01154
Hayreh, S. S. (2006). “Orbital vascular anatomy,” in Eye (Berlin, Germany: Nature Publishing Group), 1130–1144.
Heier, J. S., Awh, C. C., Busbee, B. G., Waterbury, L. D., Daniel, P., Stoller, G. L., et al. (2009). Vitreous nonsteroidal antiinflammatory drug concentrations and prostaglandin E2 levels in vitrectomy patients treated with ketorolac 0.4%, bromfenac 0.09%, and nepafenac 0.1%. Retina 29 (9), 1310–1313. doi:10.1097/IAE.0b013e3181b094e6
Heikkinen, E. M., del Amo, E. M., Ranta, V. P., Urtti, A., Vellonen, K. S., and Ruponen, M. (2018). Esterase activity in porcine and albino rabbit ocular tissues. Eur. J. Pharm. Sci. 123, 106–110. doi:10.1016/j.ejps.2018.07.034
Holló, G., Whitson, J. T., Faulkner, R., McCue, B., Curtis, M., Wieland, H., et al. (2006). Concentrations of betaxolol in ocular tissues of patients with glaucoma and normal monkeys after 1 month of topical ocular administration. Investig. Ophthalmol. Vis. Sci. 47 (1), 235–240. doi:10.1167/iovs.05-0945
Hopkins, G. (2007a). “Ophthalmic dosage forms,” in Ophthalmic drugs: Diagnostic and therapeutic uses (Amsterdam, Netherlands: Elsevier), 67–81.
Hopkins, G. (2007b). “Indications and contraindications for ophthalmic drugs,” in Ophthalmic drugs:diagnostic and therapeutic uses (Amsterdam, Netherlands: Elsevier), 257–277.
Horita, S., Watanabe, M., Katagiri, M., Nakamura, H., Haniuda, H., Nakazato, T., et al. (2019). Species differences in ocular pharmacokinetics and pharmacological activities of regorafenib and pazopanib eye-drops among rats, rabbits and monkeys. Pharmacol. Res. Perspect. 7 (6), e00545. doi:10.1002/prp2.545
Huang, A. J. W., Tseng, S. C. G., and Kenyon, K. R. (1989). Paracellular permeability of corneal and conjunctival epithelia. Investig. Ophthalmol. Vis. Sci. 30 (4), 684–689.
Huang, W. C., Cheng, F., Chen, C. C., Kuo, P. H., Wang, Y. J., Yin, S. C., et al. (2021). A novel eye drop formulation for potential treatment of neovascular age-related macular degeneration. Transl. Vis. Sci. Technol. 10 (14), 23. doi:10.1167/tvst.10.14.23
Huupponen, R., Kaila, T., Salminen, L., and Urtti, A. (2009). The pharmacokinetics of ocularly applied timolol in rabbits. Acta Ophthalmol. 65 (1), 63–66. doi:10.1111/j.1755-3768.1987.tb08493.x
Järvinen, K., Järvinen, T., and Urtti, A. (1995). Ocular absorption following topical delivery. Adv. Drug Deliv. Rev. 16, 3–19.
Johannsdottir, S., Jansook, P., Stefansson, E., Kristinsdottir, I. M., Fulop, Z., Asgrimsdottir, G. M., et al. (2018). Topical drug delivery to the posterior segment of the eye: Dexamethasone concentrations in various eye tissues after topical administration for up to 15 days to rabbits. J. Drug Deliv. Sci. Technol. 45, 449–454. doi:10.1016/j.jddst.2018.04.007
Joussen, A. M., Wolf, S., Kaiser, P. K., Boyer, D., Schmelter, T., Sandbrink, R., et al. (2019). The developing regorafenib eye drops for neovascular age-related macular degeneration (DREAM) study: An open-label phase II trial. Br. J. Clin. Pharmacol. 85 (2), 347–355. doi:10.1111/bcp.13794
Kent, A. R., Nussdorf, J. D., David, R., Tyson, F., Small, D., and Fellows, D. (2001). Vitreous concentration of topically applied brimonidine tartrate 0.2%. Ophthalmology 108 (4), 784–787. doi:10.1016/s0161-6420(00)00654-0
Khanna, S., Komati, R., Eichenbaum, D. A., Hariprasad, I., Ciulla, T. A., and Hariprasad, S. M. (2019). Current and upcoming anti-VEGF therapies and dosing strategies for the treatment of neovascular AMD: a comparative review. BMJ Open Ophthalmol. 4 (1). doi:10.1136/bmjophth-2019-000398
Kidron, H., Vellonen, K. S., Del Amo, E. M., Tissari, A., and Urtti, A. (2010). Prediction of the corneal permeability of drug-like compounds. Pharm. Res. 27, 1398–1407. doi:10.1007/s11095-010-0132-8
Klar, J., Boettger, M. K., Freundlieb, J., Keldenich, J., Elena, P-P., and Degenfeld, G. V. (2015). Effects of the multi-kinase inhibitor regorafenib on ocular neovascularization. Investig. Ophthalmol. Vis. Sci. 56 (7), 246. Available at: https://iovs.arvojournals.org/article.aspx?articleid=2332243.
Kompella, U. B., Domb, A., Urtti, A., Jayagopal, A., Wilson, C. G., and Tang-Liu, D. (2019). ISOPT clinical hot topic panel discussion on ocular drug delivery. J. Ocular Pharmacol. Ther. 35, 457–465. doi:10.1089/jop.2018.0138
Lau, C. M. L., Yu, Y., Jahamir, G., and Chau, Y. (2018). Controlled release technology for anti-angiogenesis treatment of posterior eye diseases: Current status and challenges. Adv. Drug Deliv. Rev. 126, 145–161. doi:10.1016/j.addr.2018.03.013
Lederer, C. M., and Harold, R. E. (1986). Drop size of commercial glaucoma medications. Am. J. Ophthalmol. 101 (6), 691–694. doi:10.1016/0002-9394(86)90771-3
Loch, C., Zakelj, S., Kristl, A., Nagel, S., Guthoff, R., Weitschies, W., et al. (2012). Determination of permeability coefficients of ophthalmic drugs through different layers of porcine, rabbit and bovine eyes. Eur. J. Pharm. Sci. 47 (1), 131–138. doi:10.1016/j.ejps.2012.05.007
Löscher, M., Seiz, C., Hurst, J., and Schnichels, S. (2022). Topical drug delivery to the posterior segment of the eye. Pharmaceutics 14.
Mandell, B. A., Meredith, T. A., Aguilar, E., El-Massry, A., Sawant, A., and Gardner, S. (1993). Effects of inflammation and surgery on amikacin levels in the vitreous cavity. Am. J. Ophthalmol. 115 (6), 770–774. doi:10.1016/s0002-9394(14)73646-3
Market Scope. Trusted Partner of Industry and Medical Professionals for Ophthalmic Market Data, Primary Research, Independent Perspective, & Objective Analysis. Available at: http://market-scope.com.
Maurice, D. M., and Mishima, S. (1984). “Ocular pharmacokinetics,” in Pharmacology of the eye. Editor M. Sears (Berlin-Heidelberg: Springer-Verlag), 19–116.
Mishima, S., Gasset, A., Klyce, S. D., and Baum, J. L. (1966). Determination of tear volume and tear flow. Investig. Ophthalmol. 5 (3), 264–276.
Naageshwaran, V., Ranta, V-P., Toropainen, E., Tuomainen, M., Gum, G., Xie, E., et al. (2022). Topical pharmacokinetics of dexamethasone suspensions in the rabbit eye: Bioavailability comparison. Int. J. Pharm. 615, 121515. doi:10.1016/j.ijpharm.2022.121515
Naageshwaran, V., Ranta, V. P., Gum, G., Bhoopathy, S., Urtti, A., and del Amo, E. M. (2021). Comprehensive ocular and systemic pharmacokinetics of brinzolamide in rabbits after intracameral, topical, and intravenous administration. J. Pharm. Sci. 110 (1), 529–535. doi:10.1016/j.xphs.2020.09.051
Neervannan, S. (2021). “Introduction and history of ophthalmic product development,” in Ophthalmic product development: From bench to bedside (Berlin, Germany: Springer), 3–13.
Nilsson, S. F. E., and Alm, A. (2012). “Determination of ocular blood flows with the microsphere method,” in Ocular blood flow (Berlin, Germany: Springer Berlin Heidelberg), 25–47.
O’Rourke, M., and Wilson, C. G. (2021). “The development and commercialization of sustained-release ocular drug delivery delivery technologies,” in Controlled release society focus group – ocular webinar, feb 2021.
PanOptica (2022). PanOptica anti-VEGF eye drop shows promise in treatment of neovascular (wet) AMD | business wire [internet]. Available at: https://www.businesswire.com/news/home/20191010005814/en/PanOptica-Anti-VEGF-Eye-Drop-Shows-Promise-in-Treatment-of-Neovascular-Wet-AMD (Accessed Jun 24, 2022).
Pelkonen, L., Tengvall-Unadike, U., Ruponen, M., Kidron, H., del Amo, E. M., Reinisalo, M., et al. (2017). Melanin binding study of clinical drugs with cassette dosing and rapid equilibrium dialysis inserts. Eur. J. Pharm. Sci. 109, 162–168. doi:10.1016/j.ejps.2017.07.027
Petternel, V., Krepler, K., Schauersberger, J., and Wedrich, A. (2004). Fosfomycin in human vitreous: –in–vitro investigation of the protein binding of fosfomycin in human vitreous –fosfomycin levels in the vitreous cavity after intravenous administration. Investig. Ophthalmol. Vis. Sci. 45 (13), 4930. Available at: https://iovs.arvojournals.org/article.aspx?articleid=2410444.
Prausnitz, M. R., and Noonan, J. S. (1998). Permeability of cornea, sclera, and conjunctiva: A literature analysis for drug delivery to the eye. J. Pharm. Sci. 87 (12), 1479–1488. doi:10.1021/js9802594
Ramsay, E., del Amo, E. M., Toropainen, E., Tengvall-Unadike, U., Ranta, V. P., Urtti, A., et al. (2018). Corneal and conjunctival drug permeability: Systematic comparison and pharmacokinetic impact in the eye. Eur. J. Pharm. Sci. 119, 83–89. doi:10.1016/j.ejps.2018.03.034
Remington, L. A., and Goodwin, D. (2021). “Clinical anatomy and physiology of the visual system,” in Clinical anatomy and physiology of the visual system. 4th Edition, 288.
Rimpelä, A. K., Reinisalo, M., Hellinen, L., Grazhdankin, E., Kidron, H., Urtti, A., et al. (2018). Implications of melanin binding in ocular drug delivery. Adv. Drug Deliv. Rev. 126, 23–43.
Rimpelä, A. K., Reunanen, S., Hagström, M., Kidron, H., and Urtti, A. (2018). Binding of small molecule drugs to porcine vitreous humor. Mol. Pharm. 15 (6), 2174–2179. doi:10.1021/acs.molpharmaceut.8b00038
Rodrigues, G. A., Lutz, D., Shen, J., Yuan, X., Shen, H., Cunningham, J., et al. (2018). Topical drug delivery to the posterior segment of the eye: Addressing the challenge of preclinical to clinical translation. Pharm. Res. 35 (12), 245. doi:10.1007/s11095-018-2519-x
Rush, J. S., Bingaman, D. P., Chaney, P. G., Wax, M. B., and Ceresa, B. P. (2016). Administration of menadione, vitamin K3, ameliorates off-target effects on corneal epithelial wound healing due to receptor tyrosine kinase inhibition. Investig. Ophthalmol. Vis. Sci. 57 (14), 5864–5871. doi:10.1167/iovs.16-19952
Sahlin, S., and Chen, E. (1996). Evaluation of the lacrimal drainage function by the drop test. Am. J. Ophthalmol. 122 (5), 701–708. doi:10.1016/s0002-9394(14)70490-8
Samanta, A., Aziz, A. A., Jhingan, M., Singh, S. R., Khanani, A. M., and Chhablani, J. (2020). Emerging therapies in neovascular age-related macular degeneration in 2020. Asia Pac J. Ophthalmol. (Phila) 9, 250–259. doi:10.1097/APO.0000000000000291
Schauersberger, J., and Jager, W. (2002). -vitro investigation of the protein binding of different antibiotics in the human vitreous. Investig. Ophthalmol. Vis. Sci. 43 (13), 1853. Available at: https://iovs.arvojournals.org/article.aspx?articleid=2419133.
Schoenwald, R. D., Deshpande, G. S., Rethwisch, D. G., and Barfknecht, C. F. (1997). Penetration into the anterior chamber via the conjunctival/scleral pathway. J. Ocul. Pharmacol. Ther. 13 (1), 41–59. doi:10.1089/jop.1997.13.41
SciFluor (2022). SciFluor announces positive top-line results of phase 1/2 study of SF0166 eye drops to treat wet age-related macular degeneration | business wire [internet]. Available at: https://www.businesswire.com/news/home/20171218005625/en/SciFluor-Announces (Accessed Jun 24, 2022).
Sebag, J., Tang, M., Brown, S., Sadun, A. A., and Charles, M. A. (1994). Effects of pentoxifylline on choroidal blood flow in nonproliferative diabetic retinopathy. Angiology 45 (6), 429–433. doi:10.1177/000331979404500603
Shalaby, W. S., Ahmed, O. M., Waisbourd, M., and Katz, L. J. (2022). A review of potential novel glaucoma therapeutic options independent of intraocular pressure. Surv. Ophthalmol. 67, 1062. doi:10.1016/j.survophthal.2021.12.003
Sherman, S. H., Green, K., and Laties, A. M. (1978). The fate of anterior chamber flurescein in the monkey eye. 1. The anterior chamber outflow pathways. Exp. Eye Res. 27 (2), 159–173. doi:10.1016/0014-4835(78)90086-6
Shikamura, Y., Yamazaki, Y., Matsunaga, T., Sato, T., Ohtori, A., and Tojo, K. (2016). Hydrogel ring for topical drug delivery to the ocular posterior segment. Curr. Eye Res. 41 (5), 653–661. doi:10.3109/02713683.2015.1050738
Sigurdsson, H. H., Stefánsson, E., Gudmundsdóttir, E., Eysteinsson, T., Thorsteinsdóttir, M., and Loftsson, T. (2005). Cyclodextrin formulation of dorzolamide and its distribution in the eye after topical administration. J. Control. Release 102 (1), 255–262. doi:10.1016/j.jconrel.2004.10.004
Slack, R. J., Macdonald, S. J. F., Roper, J. A., Jenkins, R. G., and Hatley, R. J. D. (2022). Emerging therapeutic opportunities for integrin inhibitors. Nat. Rev. Drug Discov. 21, 60–78. doi:10.1038/s41573-021-00284-4
Sripetch, S., and Loftsson, T. (2021). Topical drug delivery to the posterior segment of the eye: Thermodynamic considerations. Int. J. Pharm. 597, 120332. doi:10.1016/j.ijpharm.2021.120332
Tham, Y. C., Li, X., Wong, T. Y., Quigley, H. A., Aung, T., and Cheng, C. Y. (2014). Global prevalence of glaucoma and projections of glaucoma burden through 2040: A systematic review and meta-analysis. Ophthalmology 121 (11), 2081–2090. doi:10.1016/j.ophtha.2014.05.013
Urtti, A., Pipkin, J. D., Rork, G., Sendo, T., Finne, U., and Repta, A. J. (1990). Controlled drug delivery devices for experimental ocular studies with timolol 2. Ocular and systemic absorption in rabbits. Int. J. Pharm. X. 61 (3), 241–249. doi:10.1016/0378-5173(90)90215-p
Urtti, A., and Salminen, L. (1993). Minimizing systemic absorption of topically administered ophthalmic drugs. Surv. Ophthalmol. 37 (6), 435–456. doi:10.1016/0039-6257(93)90141-s
Varner, P. (2015). Ophthalmic pharmaceutical clinical trials: Design considerations. Clin. Investig. (Lond). 5 (5), 457–475. doi:10.4155/cli.15.5
Watsky, M. A., Jablonski, M. M., and Edelhauser, H. F. (1988). Comparison of conjunctival and corneal surface areas in rabbit and human. Curr. Eye Res. 7 (5), 483–486. doi:10.3109/02713688809031801
Wong, W. L., Su, X., Li, X., Cheung, C. M. G., Klein, R., Cheng, C-Y., et al. (2014). Global prevalence of age-related macular degeneration and disease burden projection for 2020 and 2040: A systematic review and meta-analysis. Lancet. Glob. Health 2 (2), e106–16. doi:10.1016/S2214-109X(13)70145-1
Keywords: topical ophthalmic pharmacokinetics, non-corneal absorption route, retina, neovascular age-related macular degeneration, macula edema, rabbit, clinical trials
Citation: del Amo EM (2022) Topical ophthalmic administration: Can a drug instilled onto the ocular surface exert an effect at the back of the eye?. Front. Drug. Deliv. 2:954771. doi: 10.3389/fddev.2022.954771
Received: 27 May 2022; Accepted: 08 July 2022;
Published: 08 September 2022.
Edited by:
Christian Waeber, University College Cork, IrelandReviewed by:
Tais Gratieri, University of Brasilia, BrazilSmrithi Padmakumar, Scientist/Spark Therapeutics, United States
Copyright © 2022 del Amo. This is an open-access article distributed under the terms of the Creative Commons Attribution License (CC BY). The use, distribution or reproduction in other forums is permitted, provided the original author(s) and the copyright owner(s) are credited and that the original publication in this journal is cited, in accordance with accepted academic practice. No use, distribution or reproduction is permitted which does not comply with these terms.
*Correspondence: Eva M. del Amo, ZXZhLmRlbGFtb0B1ZWYuZmk=