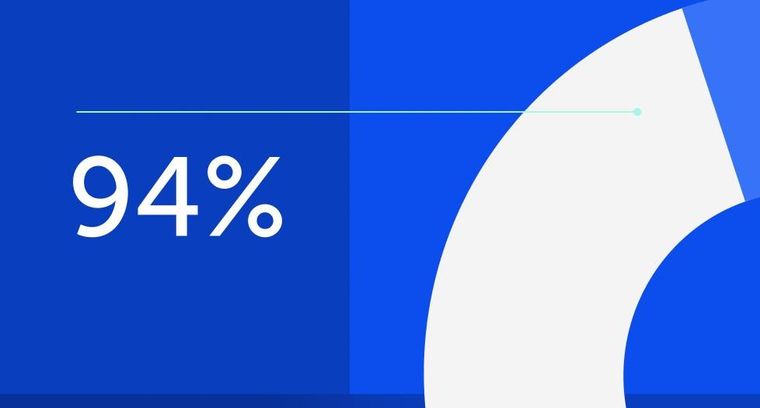
94% of researchers rate our articles as excellent or good
Learn more about the work of our research integrity team to safeguard the quality of each article we publish.
Find out more
ORIGINAL RESEARCH article
Front. Drug Deliv., 06 April 2022
Sec. Respiratory Drug Delivery
Volume 2 - 2022 | https://doi.org/10.3389/fddev.2022.864922
This article is part of the Research TopicStrategies to overcome the barriers to effective inhaled treatmentsView all 7 articles
Treprostinil palmitil (TP) is a prodrug of treprostinil that has been formulated as an inhaled powder, termed TPIP, for evaluation in patients with pulmonary arterial hypertension. In these characterization studies we investigated the aerosol performance of TPIP in response to changes in capsule fill, device resistance, and inspiratory flow rate to enable selection of an inhaler for clinical use. Capsules containing 8, 16 or 32 mg of TPIP (80, 160, or 320 μg TP, respectively) were evaluated using four commercially-available, breath-actuated RS01 devices (Plastiape, S. p.A., Osnago, Italy) with low, medium, high or ultra-high inspiratory resistances, creating 12 different capsule and device configurations for evaluation. Aerosol characterization was performed using the next generation impactor at compendial conditions of 23°C and 35% relative humidity and a flow rate corresponding to a 4 kPa pressure drop. The aerosol mass median aerodynamic diameter, geometric standard deviation, fine particle fraction, emitted dose and fine particle dose (FPD) were calculated from the in vitro impactor data. The TP emitted dose at 4 kPa exceeded 75% for all 12 capsule and device configurations. The FPD, an estimate of the respirable dose, varied between 61.0 and 70.6% of the loaded TP dose for all four devices with the 8 and 16 mg TPIP capsule dose. For the 32 mg TPIP capsule dose, the FPD remained above 61.0% for the high and ultra-high resistance devices but decreased to 48.5 and 52.6% for the low and medium resistance devices, respectively. Based on this initial data, the high resistance device was selected for additional characterization studies at 40 and 80 L/min corresponding to pressure drops of 1.4 and 5.4 kPa. The FPD was relatively insensitive to changes in flow rate, providing an expectation of a consistent total lung dose of TP under scenarios simulating variability in how the device is used. Based on these findings, the high resistance device was chosen for further development in human clinical trials.
Pulmonary arterial hypertension (PAH) is a life-threatening and rare disease characterized by a progressive increase in pulmonary vascular resistance due to narrowing of the pulmonary arteries that inexorably leads to right heart failure and death for most patients (Chapman et al., 2020). Most approved therapeutic interventions in PAH have been directed towards reducing pulmonary vasoconstriction and include the prostanoids, endothelin receptor antagonists, and phosphodiesterase five inhibitors, but none have been successful in reversing the pathology of the disease (Corboz et al., 2017).
Prostanoids are arguably the most effective therapies for PAH by inhibiting further vascular remodeling, increasing pulmonary vasodilation and providing general anti-inflammatory and antifibrotic activities (Corboz et al., 2018; Chapman et al., 2020). Of the approved prostanoids, treprostinil (TRE) and iloprost are available in inhaled formats, which provide high drug levels at the target organ (i.e., the lung) immediately after delivery. However, due to their rapid elimination from the lung, they must be readministered frequently to maintain effect: inhaled TRE (Tyvaso) is labeled for administration four times daily while inhaled iloprost (Ventavis) is administered six to nine times daily.
To overcome the pulmonary barriers for inhaled drugs with poor pharmacokinetics and extend the duration of pharmacological effect, formulation strategies (Cipolla and Gonda, 2011) as well as prodrug strategies (Chen et al., 2021; Plaunt et al., 2022) have been explored. Both strategies have been applied to TRE including the recent development of a prodrug of TRE, treprostinil palmitil (TP), initially formulated as a lipid nanoparticle to provide prolonged exposure of TP in the lung fluid after inhalation (Leifer et al., 2018). The TP prodrug consists of a 16-carbon alkyl chain covalently attached to TRE through an ester bond. Once deposited in the lung, the nanoparticle is designed to slowly release TP and generate TRE through the action of endogenous lung esterases. The sustained presence of TP and the formation of TRE in the lungs was confirmed in two species in single dose PK studies of a nebulized TP inhalation solution (TPIS) delivered by nose-only inhalation to rats and via face masks to beagle dogs (Corboz et al., 2017; Chapman et al., 2018).
Inhalation administration of TP may not only provide prolonged TRE activity in the lung, allowing for a reduction in the frequency of administration compared to TRE, but may also reduce peak TRE plasma levels and reduce the adverse effects associated with systemic exposure to TRE (Corboz et al., 2017; Chapman et al., 2018). In vivo studies in rats and dogs demonstrated that inhaled TPIS provided sustained vasodilation with plasma levels of TRE that were ∼60-fold and ∼550-fold lower, respectively, vs systemic levels of intravenous TRE required to generate a comparable vasodilatory effect (Chapman et al., 2018). In a rat sugen/hypoxia model of PAH, once-daily inhaled administration of TPIS improved the pulmonary vascular hemodynamics, reduced the increase in right heart size, enhanced cardiac performance and attenuated the histological changes compared to the positive control (Corboz et al., 2021a).
Regarding side effects, in a guinea pig model, evidence of cough was not observed with inhaled TP until it was administered at a ten-fold higher lung dose as compared to the lung dose for inhaled TRE that provoked cough (Chapman et al., 2021a). Studies were also performed in a hypoxia rat model to assess another concern with inhaled or continuous infusion of TRE–tachyphylaxis, or the desensitization of the TRE receptors that requires a continual escalation of dose to maintain efficacy. There was no evidence of tachyphylaxis with repeat daily dosing of TPIS for 32 days, the length of the study, in contrast to TRE infusion where it was observed after 16 days (Chapman et al., 2021b).
A spray-dried inhaled powder formulation of TP, termed treprostinil palmitil inhalation powder (TPIP), has been developed for delivery using a dry powder inhaler (DPI). The transition to a DPI format was made because of the potential to reduce administration time with improved ease of use for the patient, which could lead to greater patient satisfaction compared to nebulizer delivery. However, the choice of the DPI device for delivery of the TPIP powder is a complex one, as there are many DPI formats available including capsule-based single dose devices or multidose devices that either store powder in a reservoir or utilize blister strips containing individual powder doses. We decided to use a capsule-based DPI, the commercially available RS01 device, because of its simplicity of use and more rapid product development times compared to the multidose devices. The RS01 DPI pierces the capsule, and upon inspiration, the capsule rotates releasing the powder that deaggregates as it enters the inhalational air stream. There are multiple RS01 device designs with varying resistances to airflow, and for the same inspiratory effort, the air flow rate will decrease as the device resistance increases. This enables the drug developer to select the device that most effectively disperses their powder formulation at an inspiratory effort that can be effectively achieved by their patient population (Huynh et al., 2015; Sahay et al., 2021).
Inhaled TPIP demonstrated a similar PK profile to TPIS in rats with a half-life in the lung of ∼5–7 h over the 24-h study (Gauani et al., 2021). Furthermore, consistent with the earlier data reported for TPIS in a sugen/hypoxia rat model of PAH, TPIP demonstrated favorable efficacy compared to oral selexipag, and inhaled and intravenous TRE (Corboz et al., 2021b). In this paper, we evaluated the in vitro performance of TPIP utilizing breath-actuated RS01 DPI devices with varying resistances. The goal was to identify the RS01 variant with relatively stable delivery performance for the different capsule doses to be evaluated in phase 1 dose escalation human clinical studies. The high resistance RS01 DPI was chosen for more extensive characterization at flow rates both above and below the compendial standard to understand the sensitivity of the aerosol performance to variations in user operation. Based upon this in vitro data, the high resistance RS01 device was ultimately selected for use in the phase 1 clinical dose escalation studies.
Four Plastiape RS01 Model 7 DPIs (Plastiape S. p.A., Osnago, Italy) were selected for evaluation (Figure 1). They differed with respect to their device resistance: Ultra-high Resistance (UHR, code 239700005AA), High Resistance (HR, code 239700002AA), Medium Resistance (MR, code 239700006AA) and Low Resistance (LR, code 239700001AB). The pressure drop across each device containing an empty capsule was measured as a function of flow rate between 30 and 120 L/min (LPM) (Supplementary Figure S1).
TPIP composed of 1.0% TP, ∼69.7% mannitol and ∼29.3% leucine was manufactured at GLP production scale by Bend Research Inc. (Bend, OR). After spray drying, TPIP powder is characterized with a white physical appearance composed of collapsed spheres by scanning electron microscope, a tap density of ∼0.52 g/cm3 and a bulk density of ∼0.25 g/cm3. The powder contained 0.38% moisture and the particle size distribution by volume was characterized by laser diffraction (Malvern Mastersizer 3,000 with the Aero S Dry Powder Disperser Unit, Malvern, PA) with a D10 of 0.3 µm, D50 of 1.7 µm, D90 of 3.8 µm, and a Span represented by ((D90-D10)/D50) of 2.0. Size #3 Harro-filled Vcaps® Plus Hypromellose capsules (Capsugel Manufacturing Inc., Greenwood, SC) were filled with 8, 16 or 32 mg of TPIP, representing a loaded dose of 80, 160 or 320 μg TP, respectively.
Aerosol characterization was performed using the NGI (MSP Corp., Shoreview, MN) at environmental conditions of 23°C and 35% RH. In the aerosol characterization studies, each of the four DPIs was tested with each of the three capsule doses, in triplicate, for a total of 36 NGI experiments. The performance of the UHR, HR and MR devices was evaluated by drawing 4 L of air through the DPI at a flow rate of 40, 60 and 85 LPM, respectively, corresponding to the 4 kPa air pressure drop recommended by the compendia. The LR DPI was tested at 100 LPM, corresponding to a 3.4 kPa pressure drop, slightly below the compendial value of 4 kPa, but this represented the highest calibrated flow rate for the NGI.
Additional NGI testing was performed in triplicate for the HR device with all three different capsule fill weights at a lower flow rate of 40 LPM (corresponding to a 1.4 kPa pressure drop) and a higher flow rate of 80 LPM (corresponding to a 5.4 kPa pressure drop) to compare the aerosol performance relative to that at 60 LPM. These represented 18 additional NGI experiments.
The aerosol performance of each device/capsule configuration was characterized for TP with respect to the emitted dose (ED), mass median aerodynamic diameter (MMAD), geometric standard deviation (GSD), fine particle fraction <5 µm (FPF) and fine particle dose <5 µm (FPD) as described below. The total recovery of TP was also measured to provide assurance that each experiment was conducted acceptably.
TP was quantified by HPLC with mass spectrometry detector using an 8-point internal standard log-log calibration range of 4–500 ng/mL TP. The sample injection volume was 20 µl with separation conducted at an isocratic flow rate of 1.0 ml/min using a mobile phase of 85:15 acetonitrile:water containing 1% formic acid. The C8 HPLC column was equilibrated at 35°C.
An Agilent 6,130 single quad mass spectrometer was used as follows: The spray chamber was operated at a drying gas flow of 7 L/min, a nebulizer pressure of 35 psig, a vaporizer temperature of 175°C, a drying gas temperature of 225°C, a capillary voltage of 2,000 V, a corona current of 4.0 µA and a charging voltage of 2,000 V.
The TP in the powder recovered from the individual NGI stage cups, throat/adapter, pre-separator, and residual powder left in the device and capsule was extracted using 75% IPA and then further diluted with 40% acetonitrile, 40% methanol and 20% water prior to evaluation by HPLC.
After quantitation of TP in the residual powder in the capsule and DPI, and from the components of the NGI, the total recovery of TP was expressed relative to the nominal loaded dose of TP in the capsule. The emitted dose represents the percentage of TP recovered in the NGI relative to the total recovery of TP. The aerosol MMAD, GSD, FPF and FPD were calculated following USP and Ph. Eur compendial methods [European Pharmacopoeia, 2008; United States Pharmacopeia and National Formulary (USP 35/NF-31), 2012]. A linear regression analysis was performed on the log normal plot of the cumulative proportion of drug vs the cut-off diameter associated with each NGI stage. The MMAD was calculated as the midpoint in particle size based on TP recovery. The GSD was calculated as the square root of the ratio of the aerodynamic diameter associated with 84.1% of the cumulative TP mass to that for 15.9% of the cumulative TP mass. The FPF was calculated as the percentage of TP in particles with an aerodynamic diameter less than 5 µm. The FPD was calculated as the mass of TP in particles with an aerodynamic diameter less than 5 µm.
The cut-off diameters of each NGI stage varied with flow rate. At 40 LPM, the cut-off diameters for stages 1–7 were 10.03, 5.51, 3.45, 2.01, 1.17, 0.70, and 0.45 µm, respectively. At 60 LPM, the cut-off diameters for stages 1–7 were 8.06, 4.46, 2.82, 1.66, 0.94, 0.55 and 0.34 µm, respectively. At 80 LPM, the cut-off diameters for stages 1–7 were 6.90, 3.84, 2.44, 1.45, 0.81, 0.46 and 0.28 µm, respectively. At 85 LPM, the cut-off diameters for stages 1–7 were 6.68, 3.72, 2.37, 1.41, 0.78, 0.45 and 0.27 µm, respectively. At 100 LPM, the cut-off diameters for stages 1–7 were 6.12, 3.42, 2.18, 1.31, 0.72, 0.40 and 0.24 µm, respectively.
Mean values of ED, FPF, and FPD were compared for each of the combinations of the four Plastiape devices (UHR, HR, MR, LR) with capsules filled with three different TPIP doses (8, 16 or 32 mg) using a one-way Analysis of Variance (ANOVA) in Microsoft Excel. A statistically significant difference was noted for p-values < 0.05. Using Microsoft Excel, the best fit linear regression analysis of the mean FPD response for each combination tested is represented in Figures 3, 5. For the FPD response to capsule dose in Figure 3C, the linear regression analysis was forced through zero.
The TP aerosol particle size distribution profiles for the UHR, HR, MR, and LR DPI devices are shown in Figures 2A,B,C, for the 8, 16 and 32 mg TPIP capsules, respectively. The TP aerosol particle size distribution profiles are polydisperse for the LR, MR and HR devices with the UHR device showing a trend towards a less polydisperse profile. The values for the total mass recoveries, emitted dose, MMAD, GSD, FPF, and FPD for the UHR, HR, MR, and LR devices loaded with 8, 16 and 32 mg TPIP capsules are shown in Table 1. The mean total recovery of TP ranged between 90 and 100% for the 12 conditions indicating that the experimental procedure was well executed.
FIGURE 2. TP Aerosol Particle Size Distribution Profiles from the Next Generation Impactor (NGI). The profiles show the recovery of TP (mean ± SD) from each stage of the NGI with respect to its stage cut-off diameter for the ultra-high resistance (UHR, blue), high resistance (HR, orange), medium resistance (MR, gray) and low resistance (LR, yellow) devices. (A): 8 mg TPIP capsules. (B): 16 mg TPIP capsules. (C): 32 mg TPIP capsules.
TABLE 1. TPIP aerosol particle size distribution characteristics as a function of device resistance.
The ED was independent of capsule dose ranging from 76 to 86% for the 8 mg capsule, 76–88% for the 16 mg capsule, and 82–89% for the 32 mg capsule. However, the ED for the UHR device was lower than for the other three devices ranging from 76 to 82% vs 83–89% for the HR device, 85–86% for the MR device, and 81–88% for the LR device. This suggests that the lower inhalation flow rate of 40 LPM for the UHR device may not have been adequate to completely empty the capsule in one 4 L inhalation.
The MMADs increased with capsule dose but remained within the respirable range with MMADs close to 1 µm for the 8 mg capsule and increasing to 1.6–2.0 µm for the 32 mg capsule. For the 32 mg capsule, the MMAD was highest for the UHR device suggesting that the 40 LPM flow rate was not as effective at dispersing the powder as the higher flow rates used for the lower resistance devices. The GSDs were all above three for the HR, MR and LR devices indicating significant polydispersity in aerosol particle size. The UHR device had lower polydispersity with GSD values ranging between 2.4 and 2.7 for the three capsule doses. The MMAD and GSD data together suggest that while there was incomplete dispersion of the powder for the highest capsule dose, because the primary powder particles are very small, the percentage of TP in the respirable range is relatively unaffected by capsule dose.
The FPF decreased with capsule load ranging from 80 to 85% for the 8 mg capsule and decreasing to 71–81% for the 16 mg capsule and 58–76% for the 32 mg capsule (Figure 3A). The FPF was lower for the MR and LR devices at each capsule dose compared to the UHR and HR devices, and this was especially evident for the 32 mg capsule dose with the FPF decreasing to 62 and 58%, respectively. The FPD was uniform for all four devices for the 8 and 16 mg capsule doses, ranging between 51 and 56 μg TP for the 8 mg dose and 98–104 μg TP for the 16 mg dose (Figure 3B). The FPD was dose proportional for the UHR and HR devices with an FPD of 197–200 µg for the 32 mg capsule dose; however, for the MR and LR devices the FPD decreased to 168 and 155 μg, respectively (Figure 3C). The statistical analysis of the FPD values indicated no statistical difference among the DPI devices for the 8 and 16 mg capsule doses with p values of 0.167 and 0.089, respectively (Table 2). However, for the 32 mg capsule dose, there was a significant difference among these devices with a p value of 0.002 (Table 2).
FIGURE 3. (A): TP Fine Particle Fraction (FPF) and (B): TP Fine Particle Dose (FPD) as a Function of Device Resistance for the 8 mg (blue), 16 mg (orange), and 32 mg (gray) Capsule Dose. (C): TP FPD as a Function of Capsule Dose for Each Device. Trend lines are only shown for the UHR and HR DPIs in 3c. The ultra-high resistance (UHR), the high resistance (HR), the medium resistance (MR) and the low resistance (LR) devices were tested at 40, 60, 85 and 100 LPM, respectively.
TABLE 2. Trend Analysis of the Aerosol FPD as a Function of Flow Rate (associated with data in Figure 3B).
Based on the totality of the aerosol characterization data for the four devices, the HR device was selected for further analysis at flow rates that deviated from the compendial target of 4 kPa. The TP aerosol particle size distribution profile for the4device operated at 1.4, 4 and 5.4 kPa (corresponding to 40, 60 and 80 LPM, respectively) is shown in Figures 4A,B,C, for the 8, 16 and 32 mg TPIP capsules, respectively. Not surprisingly, the TP aerosol particle size distribution profiles for the HR device remained polydisperse when increased to the 80 LPM flow rate but became less polydisperse at 40 LPM, similar to what was observed for the UHR device at 40 LPM. The values for the total recovery, emitted dose, MMAD, GSD, FPF, and FPD are shown in Table 3. The mean total recovery of TP ranged between 94 and 105% for the nine conditions indicating that the experimental procedure was well executed.
FIGURE 4. TP Aerosol Distribution Profiles from the Next Generation Impactor (NGI). The profiles show the recovery of TP (mean ± SD) from each stage of the NGI with respect to its stage cut-off diameter for high resistance (HR) device operated at 40 LPM (blue), 60 LPM (orange), and 80 LPM (gray). (A): 8 mg TPIP capsules. (B): 16 mg TPIP capsules. (C): 32 mg TPIP capsules.
TABLE 3. TPIP aerosol particle size distribution performance characteristics for the high resistance DPI as a function of flow rate.
The ED was relatively independent of capsule dose ranging from 78 to 86% for the 8 mg capsule, 83–87% for the 16 mg capsule, and 88–89% for the 32 mg capsule and no reduction in ED was observed at the lower flow rate of 40 LPM (p = 0.106). As observed in the earlier studies, the MMADs increased with capsule dose but remained within the respirable range with MMADs close to 1 µm for the 8 mg capsule and increasing to 1.8–2 µm for the 32 mg capsule. For all three capsule doses, the MMAD was slightly higher at the lower flow rate of 40 LPM. The GSDs were all above 3 for at the 60 and 80 LPM flow rate, indicating significant polydispersity in aerosol particle size but decreased to below 3 at 40 LPM, consistent with what was observed for the UHR device at 40 LPM.
The FPF decreased with capsule load ranging from 81 to 86% for the 8 mg capsule dose and decreasing to 74–81% for the 16 mg capsule dose and 60–73% for the 32 mg capsule dose (Table 3). The FPF was lowest for each capsule dose at 80 LPM and highest for each capsule dose at 40 LPM. The FPD was uniform at all three flow rates for the 8 and 16 mg capsule doses, ranging between 54 and 56 μg TP for the 8 mg dose and 104–109 μg TP for the 16 mg dose (Figure 5). The FPD was dose proportional for the HR device at 40 and 60 LPM with an FPD of 197–207 µg for the 32 mg capsule dose; however, at 80 LPM the FPD decreased to 168 µg. The statistical analysis of the FPD values indicated no statistical difference over 40 to 80 LPM flow rate range for the 8 and 16 mg capsule doses with p values of 0.537 and 0.108, respectively (Table 4). However, for the 32 mg capsule dose, there was a significant difference over the flow rate range with a p value of 0.004.
FIGURE 5. TP Fine Particle Dose (FPD) as a function of Device Resistance and TPIP Capsule Dose for the HR Device. The dotted lines show the change in FPD for the 8 mg capsule dose (blue), 16 mg capsule dose (orange), and 32 mg capsule dose (gray) as a function of flow rate with the high resistance device tested at 40, 60 and 80 LPM.
TABLE 4. Trend Analysis of the Aerosol FPD as a Function of Flow Rate for the HR Device (associated with data in Figure 5).
There are multiple device technologies that can be used to deliver a drug via inhalation including metered dose inhalers (MDIs), DPIs, soft mist inhalers and nebulizers (Anderson et al., 2022). Each delivery format has distinct attributes, and the optimum choice of delivery format (and device within that format) for clinical development depends on multiple factors including the target dose to the lung, the drug’s therapeutic window, the ease of formulation development, the stability of the drug, the target patient population, and their capability to use the device correctly (Cipolla et al., 2010; Laube et al., 2011). TP was initially formulated as an inhaled suspension (TPIS) for nebulized delivery (Leifer et al., 2018) as that provided the most rapid development path for confirmation of clinical effect. However, to provide improved patient convenience, efforts were conducted in parallel to generate both an inhaled MDI product (Plaunt et al., 2021) and a DPI product (TPIP). TPIP was selected over the MDI product for subsequent clinical investigation in phase 1 dose escalation studies. The choice of the specific RS01 device to deliver the TPIP powder was established based on the in vitro studies summarized in this manuscript.
When a new dry powder formulation like TPIP is developed, the characteristics of the powder must be studied in concert with the choice of DPI, taking into consideration the capability of the target patient population to use that device. Using the selected DPI, the patient’s inhalation flow rate must be able to effectively deaggregate and disperse the powder to ensure that a consistent dose is emptied from the capsule, and that the particles are of a uniform size to avoid significant deposition in the oropharyngeal region so that the drug reaches the target organ, the lung (de Boer et al., 1996; Clark, 2015). The primary particle size of TPIP was designed to be between 1 and 2 microns, to facilitate delivery to the airways and deep lung even if TPIP were incompletely dispersed upon inspiration.
In this study, the in vitro performance of TPIP was evaluated utilizing RS01 DPIs with varying resistances. The performance of the TPIP powder in all four devices was excellent, with emitted doses exceeding 76% across all device and capsule dose configurations with MMADs between 1 and 2 µm. These attributes led to high fine particle fractions exceeding 70% for the HR and UHR devices with all capsule doses and for the LR and MR devices with the 8 and 16 mg capsules, but not with the 32 mg capsule. With respect to FPD, both the HR and UHR devices provided a dose proportional response over the 8, 16 and 32 mg capsule doses, which is a desirable attribute when evaluating dose response in a clinical setting. For the 32 mg capsule dose, the variability in FPD for the UHR device (8.3% RSD) was greater than that for the HR device (3.1% RSD), resulting in the selection of the HR device for further characterization studies evaluating its robustness to changes in flow rate.
The aerosol performance of TPIP in the RS01 devices compares favorably to published data for other micronized and spray-dried powders. Huynh et al. (2015) evaluated 20 mg micronized mannitol (median size of 5 µm) in the LR and HR devices. The ED for micronized mannitol was 73.8 and 72.5%, respectively, lower than that observed for all capsule doses of TPIP. The micronized mannitol study was not designed to report FPFs due to its large primary size. Yazdi and Smyth (2016) evaluated jet milled ibuprofen with a median size of 1.9 µm in the HR RS01 device. For the 10 and 25 mg ibuprofen capsule doses, the ED was 70 and 72%, respectively, lower than the 83% ED for the 8 and 16 mg TPIP doses. The FPF for the ibuprofen doses ranged between 76 and 80%, comparable to the TPIP FPF that ranged between 78 and 85%. Buttini et al. (2018) evaluated capsules containing 32 mg of spray dried tobramycin powder (median size of 2.4 µm) in a HR RS01 device. The tobramycin ED of 63% was lower than that for TPIP, but the FPF of 82% was comparable. Finally, Shetty et al. (2018) evaluated 10 mg capsules containing spray dried colistin and ciprofloxacin (median size of 1.4 µm) in a LR RS01 device. The 50:50 colistin:ciprofloxacin formulation had the best aerosol performance with an FPF of 67%; however, it was still lower than the FPF of 85% for the 8 mg TPIP capsule. In summary, the performance of TPIP in the RS01 devices is comparable or superior to that reported for other inhaled powders in the RS01 devices, likely owing to its small primary particle size and good dispersibility. The good dispersibility is attributed to the presence of ∼30% leucine in the formulation, which yields spray dried particles with low surface energy, reduced interparticle interactions (Chew et al., 2005) and superior protection against exposure to humidity (Wang et al., 2021).
DPIs have become increasingly popular because of their ease of use. However, due to their distinct designs and operating mechanisms, they have different resistances and therefore will produce different peak inspiratory flow rates upon inhalation by the same subject (Clark and Hollingworth, 1993). For breath-actuated DPIs like the ones evaluated in this study, the flow rate that the patient utilizes during inhalation is responsible for providing the turbulent energy to disperse the powder particles and empty the powder from the device. For most passive DPIs, patient use instructions guide the patient to first exhale, and then inhale forcefully on the DPI from the beginning of inhalation. This guidance generally leads to a faster rate of acceleration of inspiratory flow, which helps to ensure that the timing of capsule emptying occurs near the peak inspiratory flow (Clark et al., 2020). Peak inspiratory flow is often cited as the key predictor of clinical efficacy (Azouz et al., 2015). However, the ability to achieve a peak flow sufficient for effective DPI use is controlled by the subject’s ability to generate an adequate inspiratory pressure (Clark et al., 2020). The USP and other compendia recommend a 4 kPa pressure drop to evaluate inhaler device performance, and this is supported by a review of the literature: in healthy adults, maximum inspiratory pressures exceed 4 kPa out to 80 years of age (Clark, 2015). In our in vitro studies with TPIP, a pressure drop of 4 kPa was utilized to compare the performance of the RS01 DPIs, but after selecting the HR RS01 device for further testing, its performance was measured using a higher (5.4 kPa, equivalent to 80 LPM) and lower pressure drop (1.4 kPa, equivalent to 40 LPM) to simulate variability in patient use.
In those in vitro studies at both extremes of flow rates (40 and 80 LPM), the capsule emptying performance was not compromised, with EDs exceeding 78% for the HR RS01 device for all three capsule doses. While the MMAD slightly increased for all three TPIP capsule doses at the lower flow rate, the FPF values increased, instead of decreasing. Thus, at the low flow rate of 40 LPM, the FPD had even greater consistency across all three capsule doses ranging from 65 to 68% vs. 61–71% under the compendial testing at 60 LPM. At the higher flow rate of 80 LPM, while the FPD ranged from 65 to 68% for the 8 and 16 mg capsule doses, it declined to 53% for the 32 mg capsule dose due to the reduction in FPF. In summary, the performance of TPIP in the HR RS01 device is generally robust with respect to deviations in inspiratory flow, especially for lower inspiratory pressures that may arise when a user executes a more comfortable inhalation maneuver.
While FPD is generally used to reflect the respirable dose, that portion of the drug that is likely to be inhaled and avoid deposition in the upper respiratory tract, a more precise metric termed the impaction parameter considers the influence of flow rate on particle size (Weers et al., 2019). For an aerosol particle to deposit in the lung, it needs to first avoid deposition in the upper respiratory tract, which primarily occurs by inertial impaction and is governed by flow rate multiplied by the square of the particle diameter (Weers et al., 2019). Thus, at higher inspiratory flow rates, inertial impaction can increase even with no change in FPD, leading to lower lung deposition. With an MMAD varying between 1 and 2 µm over an inspiratory flow rate of 40–80 LPM, the impaction parameter for TPIP remains low suggesting that FPD may indeed be a good approximation of lung dose. Based on the FPDs for the HR RS01 device operated at 60 LPM, the lung dose of TP may be close to ∼57, 104 and 197 µg for the 8, 16 and 32 mg capsule strengths, respectively. After conversion of TP to the active moiety (TRE) in the lung, the equivalent lung dose of TRE would be ∼36, 66 and 125 μg, respectively. In comparison, the approved dose of Tyvaso® (treprostinil inhalation solution, United Therapeutics Corp., Research Triangle Park, NC) is 54 μg TRE QID [package insert1]. Recognizing such differences as dosing interval, fine particle dose, and the kinetics of the conversion of TP to TRE, we believe that these TPIP capsule strengths will allow us to reach a therapeutic (and safe) effect in subjects with PAH.
One limitation of this study is that it represents only in vitro data. In vivo studies will be needed to confirm whether a dose proportional exposure is observed in actual use, first with healthy subjects, which has already been conducted and confirmed, followed by those with disease. The clinical populations proposed for evaluation of TPIP, a prodrug of TRE, may include the same ones already indicated for treatment with inhaled TRE; i.e., PAH and pulmonary hypertension due to interstitial lung disease (PH-ILD) (Waxman et al., 2021), as well as those populations under clinical evaluation with inhaled TRE including pulmonary hypertension due to chronic obstructive pulmonary disease (PH-COPD, trial NCT03496623) and idiopathic pulmonary fibrosis (IPF, trials NCT04708782 and NCT04905693). To our knowledge, there are no published studies evaluating the inspiratory capability of patients specifically with PH-ILD or PH-COPD, but there have been studies in ILD and COPD, generally, and these can be used as comparators.
In a study of 41 patients with IPF, one of the more common types of ILD, the mean peak inspiratory pressure observed was 9.4 kPa (Nishiyama et al., 2005), comparable to healthy adults. Numerous studies have demonstrated the preservation of inspiratory force in ILD patients with a varied etiology including interstitial pneumonia, nonspecific interstitial pneumonia, fibrotic nonspecific interstitial pneumonia, acute interstitial pneumonia, lymphocytic interstitial pneumonia, fibrotic hypersensitivity pneumonitis, extrinsic allergic alveolitis, undifferentiated connective tissue disease and unspecified. In those studies, the mean peak inspiratory pressures were 10.0 kPa (n = 14, Garcia Rio et al., 2003), 9.3 kPa (n = 25, Walterspacher et al., 2013), 9.3 kPa (n = 30, Watanabe et al., 2013), and 7.9 kPa (n = 30, Pietro et al., 2017), evidencing little to no loss in the force generating capacity of the inspiratory muscles of these ILD patients. Turning to COPD, Clark (2015) reviewed the literature and reported that while there was a slight decrease in maximum inspiratory pressure for COPD subjects relative to controls, the mean values from those studies ranged between 5.5 and 7.0 kPa. Thus, the studies in ILD, PAH and COPD reported maximum inspiratory pressures that all exceed the compendial value of 4 kPa.
In contrast, PAH disease is associated with weakness in inspiratory and expiratory muscles (Meyer et al., 2005). Respiratory muscle dysfunction has been reported in subjects with idiopathic PAH, leading to mean reductions in their maximal inspiratory pressures of ∼35% in both men and women compared to healthy subjects (Meyer et al., 2005). This could potentially lead to generation of inadequate inspiratory pressures to inhale a lung dose or a reduction in their ability to effectively use a DPI. However, Faria-Urbina et al. (2021) evaluated both the LR and MR RS01 devices in subjects with PAH who were guided to forcefully inhale from the DPI. While PAH subjects were able to generate adequate inspiratory flow with both devices, a 20% greater inspiratory effort was achieved using the MR device (3.2 kPa) vs the LR device (2.6 kPa), implying that greater turbulent energy was available to disperse the drug particles in the MR device. Sahay et al. (2021) compared the inspiratory flow patterns of subjects with PAH using the LR and HR RS01 devices. Given instructions to inhale maximally, the PAH subjects achieved mean peak inspiratory pressures that were 76% higher for the HR device (6.5 kPa) vs the LR device (3.7 kPa), and the inspiratory pressure for the HR device exceeded the compendial value of 4 kPa. In contrast, when provided with instructions to inhale comfortably, the mean peak inspiratory pressure was 2.0 kPa, which is still greater than the lowest setting of 1.4 kPa that was utilized to aerosolize TPIP in the HR RS01 DPI. Notably, even at an inspiratory pressure of 1.4 kPa, the TPIP FPD values were comparable to those generated at the compendial value of 4 kPa.
Thus, the literature data on subjects with PAH, IPF, ILD and COPD suggests that they will be able to achieve adequate inspiratory pressures to effectively disperse and inhale the TPIP powder from the HR RS01 device, even if they choose to inhale comfortably, rather than forcefully.
The original contributions presented in the study are included in the article/Supplementary Material, further inquiries can be directed to the corresponding author.
Conceptualization: HG, ZL, VM, and DC. Methodology and execution: HG, TB, and ZL. Interpretation of the data: HG, ZL, and DC. Supervision: WP and ES. Drafting the manuscript: HG, ZL, and DC. Review and Editing: HG, TB, ZL, VM WP, ES, and DC. All authors contributed to the article and approved the submitted version.
Authors HG, TB, ZL, VM, WP, ES, and DC are currently employed by Insmed Incorporated. The analyses presented in this publication were funded by Insmed Incorporated, Bridgewater, NJ.
All claims expressed in this article are solely those of the authors and do not necessarily represent those of their affiliated organizations, or those of the publisher, the editors and the reviewers. Any product that may be evaluated in this article, or claim that may be made by its manufacturer, is not guaranteed or endorsed by the publisher.
The authors are forever indebted to Richard W. Chapman who was instrumental in leading the efforts characterizing the biological activity of treprostinil palmitil and who recently passed away in December of 2021. The authors acknowledge Michel Corboz and Andy Stautberg who contributed to the review of this manuscript.
The Supplementary Material for this article can be found online at: https://www.frontiersin.org/articles/10.3389/fddev.2022.864922/full#supplementary-material
1https://www.accessdata.fda.gov/drugsatfda_docs/label/2021/022387s017lbl.pdf.
Anderson, S., Atkins, P., Bäckman, P., Cipolla, D., Clark, A., Daviskas, E., et al. (2022). Inhaled Medicines: Past, Present, and Future. Pharmacol. Rev. 74 (1), 48–118. doi:10.1124/pharmrev.120.000108
Azouz, W., Chetcuti, P., Hosker, H. S. R., Saralaya, D., Stephenson, J., and Chrystyn, H. (2015). The Inhalation Characteristics of Patients when They Use Different Dry Powder Inhalers. J. Aerosol Med. Pulm. Drug Deliv. 28 (1), 35–42. doi:10.1089/jamp.2013.1119
Buttini, F., Balducci, A. G., Colombo, G., Sonvico, F., Montanari, S., Pisi, G., et al. (2018). Dose Administration Maneuvers and Patient Care in Tobramycin Dry Powder Inhalation Therapy. Int. J. Pharmaceutics 548 (1), 182–191. doi:10.1016/j.ijpharm.2018.06.006
Chapman, R. W., Li, Z., Corboz, M. R., Gauani, H., Plaunt, A. J., Konicek, D. M., et al. (2018). Inhaled Hexadecyl-Treprostinil Provides Pulmonary Vasodilator Activity at Significantly Lower Plasma Concentrations Than Infused Treprostinil. Pulm. Pharmacol. Ther. 49, 104–111. doi:10.1016/j.pupt.2018.02.002
Chapman, R. W., Corboz, M. R., Malinin, V. S., Plaunt, A. J., Konicek, D. M., Li, Z., et al. (2020). An Overview of the Biology of a Long-Acting Inhaled Treprostinil Prodrug. Pulm. Pharmacol. Ther. 65, 102002. doi:10.1016/j.pupt.2021.102002
Chapman, R. W., Corboz, M. R., Fernandez, C., Sullivan, E., Stautberg, A., Plaunt, A. J., et al. (2021a). Characterisation of Cough Evoked by Inhaled Treprostinil and Treprostinil Palmitil. ERJ Open Res. 7, 00592–02020. doi:10.1183/23120541.00592-2020
Chapman, R. W., Li, Z., Chun, D., Gauani, H., Malinin, V., Plaunt, A. J., et al. (2021b). Treprostinil Palmitil, an Inhaled Long-Acting Pulmonary Vasodilator, Does Not Show Tachyphylaxis with Daily Dosing in Rats. Pulm. Pharmacol. Ther. 66, 101983. doi:10.1016/j.pupt.2020.101983
Chen, K.-J., Plaunt, A. J., Leifer, F. G., Kang, J. Y., and Cipolla, D. (2021). Recent Advances in Prodrug-Based Nanoparticle Therapeutics. Eur. J. Pharmaceutics Biopharmaceutics 165, 219–243. doi:10.1016/j.ejpb.2021.04.025
Chew, N. Y. K., Shekunov, B. Y., Tong, H. H. Y., Chow, A. H. L., Savage, C., Wu, J., et al. (2005). Effect of Amino Acids on the Dispersion of Disodium Cromoglycate Powders. J. Pharm. Sci. 94 (10), 2289–2300. doi:10.1002/jps.20426
Cipolla, D. C., and Gonda, I. (2011). Formulation Technology to Repurpose Drugs for Inhalation Delivery. Drug Discov. Today Ther. Strateg. 8 (3-4), 123–130. doi:10.1016/j.ddstr.2011.07.001
Cipolla, D., Chan, H.-K., Schuster, J., and Farina, D. (2010). Personalizing Aerosol Medicine: Development of Delivery Systems Tailored to the Individual. Ther. Deliv. 1 (5), 667–682. doi:10.4155/tde.10.54
Clark, A. R., and Hollingworth, A. M. (1993). The Relationship between Powder Inhaler Resistance and Peak Inspiratory Conditions in Healthy Volunteers - Implications for In Vitro Testing. J. Aerosol Med. 6 (2), 99–110. doi:10.1089/jam.1993.6.99
Clark, A. R., Weers, J. G., and Dhand, R. (2020). The Confusing World of Dry Powder Inhalers: It Is All about Inspiratory Pressures, Not Inspiratory Flow Rates. J. Aerosol Med. Pulm. Drug Deliv. 33 (1), 1–11. doi:10.1089/jamp.2019.1556
Clark, A. (2015). The Role of Inspiratory Pressures in Determining the Flow Rates Though Dry Powder Inhalers; A Review. Cpd 21 (27), 3974–3983. doi:10.2174/1381612821666150820105800
Corboz, M. R., Li, Z., Malinin, V., Plaunt, A. J., Konicek, D. M., Leifer, F. G., et al. (2017). Preclinical Pharmacology and Pharmacokinetics of Inhaled Hexadecyl-Treprostinil (C16TR), a Pulmonary Vasodilator Prodrug. J. Pharmacol. Exp. Ther. 363 (3), 348–357. doi:10.1124/jpet.117.242099
Corboz, M. R., Zhang, J., LaSala, D., DiPetrillo, K., Li, Z., Malinin, V., et al. (2018). Therapeutic Administration of Inhaled INS1009, a Treprostinil Prodrug Formulation, Inhibits Bleomycin-Induced Pulmonary Fibrosis in Rats. Pulm. Pharmacol. Ther. 49, 95–103. doi:10.1016/j.pupt.2018.01.012
Corboz, M. R., Plaunt, A. J., Malinin, V., Li, Z., Gauani, H., Chun, D., et al. (2021a). Treprostinil Palmitil Inhibits the Hemodynamic and Histopathological Changes in the Pulmonary Vasculature and Heart in an Animal Model of Pulmonary Arterial Hypertension. Eur. J. Pharmacol. 916, 174484. doi:10.1016/j.ejphar.2021.174484
Corboz, M. R., Plaunt, A. J., Malinin, V., Li, Z., Gauani, H., Chun, D., et al. (2021b). Beneficial Effects of Treprostinil Palmitil in a Sugen/Hypoxia Rat Model of Pulmonary Arterial Hypertension; a Comparison With Inhaled and Intravenous Treprostinil and Oral Selexipag. Am. J. Resp. Crit. Care Med. 203, A3670.
de Boer, A. H., Winter, H. M. I., and Lerk, C. F. (1996). Inhalation Characteristics and Their Effects on In Vitro Drug Delivery from Dry Powder Inhalers Part 1. Inhalation Characteristics, Work of Breathing and Volunteers' Preference in Dependence of the Inhaler Resistance. Int. J. Pharmaceutics 130 (2), 231–244. doi:10.1016/0378-5173(95)04326-8
European Pharmacopoeia (2008). European Pharmacopoeia (Ph. Eur.), 7th Edition, 2.9.18. Preparations for Inhalation: Aerodynamic Assessment of Fine Particles. London, United Kingdom: Monograph 20918.
Faria-Urbina, M., Ung, K. T., Lawler, L., Zisman, L. S., and Waxman, A. B. (2021). Inspiratory Flow Patterns with Dry Powder Inhalers of Low and Medium Flow Resistance in Patients with Pulmonary Arterial Hypertension. Pulm. Circ. 11 (2), 20458940211012591. doi:10.1177/20458940211012591
García-Río, F., Pino, J. M., Ruiz, A., Díaz, S., Prados, C., and Villamor, J. (2003). Accuracy of Noninvasive Estimates of Respiratory Muscle Effort during Spontaneous Breathing in Restrictive Diseases. J. Appl. Phys 95 (4), 1542–1549. doi:10.1152/japplphysiol.01010.2002
Gauani, H., Chun, D., Plaunt, A. J., Malinin, V. S., Corboz, M. R., Chapman, R. W., et al. (2021). “Evaluation of the Pharmacokinetic Profile of Inhaled Treprostinil Palmitil Inhalation Powder (TPIP) in Rats,” in RDD Europe 2021. Editors R. N. Dalby, J. Peart, J. D. Suman, P. M. Young, and D. Traini (River Grove, IL: DHI Publishing), 191–196.
Huynh, B. K., Chen, Y., Fletcher, D. F., Young, P., Zhu, B., and Traini, D. (2015). An Investigation into the Powder Release Behavior from Capsule-Based Dry Powder Inhalers. Aerosol Sci. Tech. 49, 902–911. doi:10.1080/02786826.2015.1082532
Laube, B. L., Janssens, H. M., de Jongh, F. H. C., Devadason, S. G., Dhand, R., Diot, P., et al. (2011). What the Pulmonary Specialist Should Know about the New Inhalation Therapies. Eur. Respir. J. 37 (6), 1308–1417. doi:10.1183/09031936.00166410
Leifer, F., Konicek, D., Chen, K.-J., Plaunt, A., Salvail, D., Laurent, C., et al. (2018). Inhaled Treprostinil-Prodrug Lipid Nanoparticle Formulations Provide Long-Acting Pulmonary Vasodilation. Drug Res. (Stuttg) 68 (11), 605–614. doi:10.1055/s-0044-100374
Meyer, F. J., Lossnitzer, D., Kristen, A. V., Schoene, A. M., Kübler, W., Katus, H. A., et al. (2005). Respiratory Muscle Dysfunction in Idiopathic Pulmonary Arterial Hypertension. Eur. Respir. J. 25 (1), 125–130. doi:10.1183/09031936.04.00095804
Nishiyama, O., Taniguchi, H., Kondoh, Y., Kimura, T., Ogawa, T., Watanabe, F., et al. (2005). Quadriceps Weakness Is Related to Exercise Capacity in Idiopathic Pulmonary Fibrosis. Chest 127 (6), 2028–2033. doi:10.1378/chest.127.6.2028
Pietro, K. M., Ricardo, G., Rui, G. P. N. D., Marcelo, B. G., Fernando, F. G., Bruno, H., et al. (2017). Relationship of Pectoralis Muscle Area and Skeletal Muscle Strength with Exercise Tolerance and Dyspnea in Interstitial Lung Disease. Sarcoidosis Vasc. Diffuse Lung Dis. 34 (3), 200–208. doi:10.36141/svdld.v34i3.5384
Plaunt, A. J., Islam, S., Macaluso, T., Gauani, H., Baker, T., Chun, D., et al. (2021). Development and Characterization of Treprostinil Palmitil Inhalation Aerosol for the Investigational Treatment of Pulmonary Arterial Hypertension. Int. J. Mol. Sci. 22, 548. doi:10.3390/ijms22020548
Plaunt, A. J., Nguyen, T. L., Corboz, M. R., Malinin, V. S., and Cipolla, D. C. (2022). Strategies to Overcome Biological Barriers Associated with Pulmonary Drug Delivery. Pharmaceutics 14 (2), 302. doi:10.3390/pharmaceutics14020302
Sahay, S., Holy, R., Lyons, S., Parsley, E., Maurer, M., and Weers, J. (2021). Impact of Human Behavior on Inspiratory Flow Profiles in Patients with Pulmonary Arterial Hypertension Using AOS™ Dry Powder Inhaler Device. Pulm. Circ. 11 (1), 2045894020985345. doi:10.1177/2045894020985345
Shetty, N., Ahn, P., Park, H., Bhujbal, S., Zemlyanov, D., Cavallaro, A., et al. (2018). Improved Physical Stability and Aerosolization of Inhalable Amorphous Ciprofloxacin Powder Formulations by Incorporating Synergistic Colistin. Mol. Pharmaceutics 15 (9), 4004–4020. doi:10.1021/acs.molpharmaceut.8b00445
United States Pharmacopeia and National Formulary (USP 35/NF-31) (2012) General Chapter <601> Aerosol, Nasal Sprays, Metered-Dose Inhalers, and Dry Powder Inhalers.
Walterspacher, S., Schlager, D., Walker, D. J., Müller-Quernheim, J., Windisch, W., and Kabitz, H.-J. (2013). Respiratory Muscle Function in Interstitial Lung Disease. Eur. Respir. J. 42 (1), 211–219. doi:10.1183/09031936.00109512
Wang, Z., Wang, H., and Vehring, R. (2021). Leucine Enhances the Dispersibility of Trehalose-Containing spray-dried Powders on Exposure to a High-Humidity Environment. Int. J. Pharmaceutics 601, 120561. doi:10.1016/j.ijpharm.2021.120561
Watanabe, F., Taniguchi, H., Sakamoto, K., Kondoh, Y., Kimura, T., Kataoka, K., et al. (2013). Quadriceps Weakness Contributes to Exercise Capacity in Nonspecific Interstitial Pneumonia. Respir. Med. 107 (4), 622–628. doi:10.1016/j.rmed.2012.12.013
Waxman, A., Restrepo-Jaramillo, R., Thenappan, T., Ravichandran, A., Engel, P., Bajwa, A., et al. (2021). Inhaled Treprostinil in Pulmonary Hypertension Due to Interstitial Lung Disease. N. Engl. J. Med. 384 (4), 325–334. doi:10.1056/nejmoa2008470
Weers, J., Kadrichu, N., and Rao, N. (2019). “Is Aerodynamic Diameter a Good Metric for Understanding Regional Lung Deposition?,” in RDD Europe 2019. Editors R. N. Dalby, J. Peart, J. D. Suman, P. M. Young, and D. Traini (River Grove, IL: DHI Publishing), 59–66.
Yazdi, A. K., and Smyth, H. D. C. (2016). Carrier-free High-Dose Dry Powder Inhaler Formulation of Ibuprofen: Physicochemical Characterization and In Vitro Aerodynamic Performance. Int. J. Pharmaceutics 511 (1), 403–414. doi:10.1016/j.ijpharm.2016.06.061
ANOVA Analysis of variance
CMC Chemistry, manufacturing and controls
COPD chronic obstructive pulmonary disease
CV Coefficient of variation
DPI Dry powder inhaler
ED Emitted dose
FPD Fine particle dose (less than 5 µm)
FPF Fine particle fraction (less than 5 µm)
GSD Geometric standard deviation
HPLC High performance liquid chromatography
HR High resistance DPI
ILD Interstitial lung disease
IP Induction port (of the NGI)
IPF Idiopathic pulmonary fibrosis
LD Loaded dose (of treprostinil palmitil)
LPM Liters/min
LR Low resistance DPI
MMAD Mass median aerodynamic diameter
MOC Micro-orifice collector (of the NGI)
MR Medium resistance DPI
NGI Next generation impactor
PAH Pulmonary arterial hypertension
PH-COPD Pulmonary hypertension due to chronic obstructive pulmonary disease
PH-ILD Pulmonary hypertension due to interstitial lung disease
QID Quarter in die (Four times daily)
RH Relative humidity
RSD Relative standard deviation
SD Standard Deviation
TP Treprostinil palmitil
TPIP Treprostinil palmitil inhalation powder
TPIS Treprostinil palmitil inhalation suspension
TRE Treprostinil
UHR Ultra-high resistance DPI
VMD Volume median diameter
Keywords: device performance, dry powder inhaler, drug delivery, aerosol characterization, treprostinil palmitil, fine particle dose, pulmonary arterial hypertension, interstitial lung disease
Citation: Gauani H, Baker T, Li Z, Malinin VS, Perkins W, Sullivan E and Cipolla D (2022) Evaluation and Selection of the Inhaler Device for Treprostinil Palmitil Inhalation Powder. Front. Drug. Deliv. 2:864922. doi: 10.3389/fddev.2022.864922
Received: 29 January 2022; Accepted: 01 March 2022;
Published: 06 April 2022.
Edited by:
Philip Chi Lip Kwok, The University of Sydney, AustraliaCopyright © 2022 Gauani, Baker, Li, Malinin, Perkins, Sullivan and Cipolla. This is an open-access article distributed under the terms of the Creative Commons Attribution License (CC BY). The use, distribution or reproduction in other forums is permitted, provided the original author(s) and the copyright owner(s) are credited and that the original publication in this journal is cited, in accordance with accepted academic practice. No use, distribution or reproduction is permitted which does not comply with these terms.
*Correspondence: David Cipolla, RGF2aWQuQ2lwb2xsYUBpbnNtZWQuY29t
Disclaimer: All claims expressed in this article are solely those of the authors and do not necessarily represent those of their affiliated organizations, or those of the publisher, the editors and the reviewers. Any product that may be evaluated in this article or claim that may be made by its manufacturer is not guaranteed or endorsed by the publisher.
Research integrity at Frontiers
Learn more about the work of our research integrity team to safeguard the quality of each article we publish.