- 1Department of Anaesthesiology, Intensive Care, Emergency and Pain Medicine, University Hospital Würzburg, Würzburg, Germany
- 2Department of Anaesthesiology, The Affiliated Luohu Hospital of Shenzhen University, Shenzhen University, Shenzhen, China
- 3Institute of Experimental Neurosurgery, Charité—Universitätsmedizin Berlin, Berlin, Germany
- 4Laboratory of Molecular Biology, Institute of Physiotherapy and Health Sciences, Academy of Physical Education, Katowice, Poland
The blood-brain barrier (BBB) is a highly specialized structure that separates the brain from the blood and allows the exchange of molecules between these two compartments through selective channels. The breakdown of the BBB is implicated in the development of severe neurological diseases, especially stroke and traumatic brain injury. Oxygen-glucose deprivation is used to mimic stroke and traumatic brain injury in vitro. Pathways that trigger BBB dysfunction include an imbalance of oxidative stress, excitotoxicity, iron metabolism, cytokine release, cell injury, and cell death. MicroRNAs are small non-coding RNA molecules that regulate gene expression and are emerging as biomarkers for the diagnosis of central nervous system (CNS) injuries. In this review, the regulatory role of potential microRNA biomarkers and related therapeutic targets on the BBB is discussed. A thorough understanding of the potential role of various cellular and linker proteins, among others, in the BBB will open further therapeutic options for the treatment of neurological diseases.
1 Introduction
Globally, cerebrovascular diseases, such as ischemic stroke and traumatic brain injury (TBI) are the leading cause of death or long-term disability (Obermeier et al., 2013). Although cerebrovascular diseases of different entity cause different initial events of brain injury, it has been shown that there is an overlap of events downstream of blood-brain barrier (BBB) breakdown after the onset of disorder. It is therefore crucial that mechanisms contributing to BBB disruption reveal new therapeutic targets and strategies that preserve BBB integrity. The role of the BBB is to control homeostasis in the CNS and to protect it from physical, chemical, and biological damage. A highly dynamic and complex structure, the BBB consists of highly specialized endothelial cells (ECs) that form the thin capillaries. Together with pericytes, astrocytes, microglia and neurons, ECs form a cellular unit called the neurovascular unit (NVU) and regulate the transport of substances from blood to brain and vice versa (Shi and Liu, 2007; Jiang et al., 2018). Furthermore, the barrier function of brain ECs is tightly regulated by the intricate interactions of tightly linked proteins such as tight junctions (TJs) and adherens junctions (AJs) (Obermeier et al., 2013).
BBB breakdown takes place during the acute phase of ischemic stroke (Jiang et al., 2018). Tissue damage and/or death occurs primarily as a result of hypoxia (oxygen deficiency) and glucose deprivation (hypoglycemia) and is highly dependent on the extent and duration of the disruption in blood supply. Although oxygen levels are restored after reperfusion (reoxygenation), there is an increase in the secretion of various cytokines that exacerbate the ischemic injury and thereby cause further cellular damage (Lambertsen et al., 2012). During ischemia, switching to anaerobic metabolism in cells leads to a decrease in ATP levels and intracellular pH. Disturbances in transport of ions dependent on ATP are responsible for the excess of calcium concentration in the cytoplasm and in the mitochondria, with all consequences including cell death by necrotic, apoptotic or autophagic mechanism (Kalogeris et al., 2012).
MicroRNAs (miRNAs, miRs) are involved in all processes including cell proliferation, cell differentiation and apoptosis, cell metabolism, and cellular responses under physiological and pathological conditions (Chandran et al., 2017; Mitra et al., 2017; Hicks et al., 2018). To date, miRNAs have been studied as biomarkers for a variety of diseases, including stroke and TBI (Borlongan and Emerich, 2003; Abbott et al., 2010; Yin et al., 2014; Keaney et al., 2015; Curtaz et al., 2020a; Curtaz et al., 2022). However, various studies have shown that targeting changes in BBB functions can alleviate the adverse effects of thrombolytic drugs, extend the treatment window and improve patient prognosis. Therefore, a potential benefit of a targeted opening of the BBB, i.e., by miRNAs, would facilitate delivery of therapies to the brain and should be considered when considering treatment strategies (Borlongan and Emerich, 2003).
This article provides an overview of BBB dysfunction after ischemic injury and highlights recent advances in our understanding the underlying regulatory mechanisms, with a particular focus on the role of miRNAs. We provide an overview of changes in BBB-related cellular components and junctional proteins associated with oxygen-glucose deprivation (OGD) and bring new ideas and new targets for a deeper understanding of brain injury arising from cerebrovascular disintegration.
2 Blood-Brain barrier structure and what is known on the BBB dysfunction in the context of ischemia and/or stroke
Under normal physiological conditions, adjacent ECs and their TJs ensure the paracellular impermeability of the BBB (Tietz and Engelhardt, 2015; Liebner et al., 2018). However, after the onset of OGD, ECs respond to ischemia and potentially harmful substances released by the vasculature. This leads to cytoskeletal rearrangements, increased cytosis, and altered TJ proteins, which in turn progressively lead to BBB dysfunction. The BBB forms a barrier at three different levels. First, it is a physical barrier that blocks the paracellular transport of polar substances (including ions) between adjacent ECs (Stamatovic et al., 2016). Second, different types of transporter proteins have a broad affinity for lipophilic substances and pump them out of ECs or transport them specifically (Pardridge, 2012). Third, various enzymes act to metabolize the substances and form the enzymatic barrier (Decleves et al., 2011). Prominent pathological features of ischemic and hemorrhagic stroke are characterized by structural disruption and increased permeability of TJs and are often associated with poor prognosis (Prakash and Carmichael, 2015).
TJs are composed of transmembrane proteins that close the cell gap (e.g., Claudin-1,-5, -3, -12, occludin, junctional adhesion molecule-A), cytoplasmic scaffolding proteins that physically support the TJs (e.g., zonula occludens proteins ZO-1-3) and the actin cytoskeleton (Stamatovic et al., 2016). Ischemia affects TJ proteins at several levels: structure (e.g., through protein phosphorylation), distribution (e.g., through internalization) and expression (e.g., through degradation), resulting in destruction of the TJs. For example, claudin-5, occludin and ZO-1 undergo phosphorylation and other post-translational modifications in response to ischemic and inflammatory conditions. Such modifications affect protein-protein interactions within the TJs (e.g., claudin: claudin and claudin: ZO-1), resulting in instability, internalization of the protein via endocytosis, and potential lysosomal degradation. Many, but not all, studies have shown reduced expression of TJ proteins after cerebral ischemia, due to reduced transcription or increased protein degradation (Kleinschnitz et al., 2011; Neuhaus et al., 2012; Blecharz-Lang et al., 2018; Burek et al., 2019; Gabbert et al., 2020; Ittner et al., 2020; Rosing et al., 2020).
BBB hyperpermeability that occurs during and after brain ischemia is mainly caused by the mediators of the inflammatory process, which stimulate phosphorylation of TJs proteins. Significant phosphorylation of Tyr, Thr and Ser residues in the ZO-1 protein was observed in brain endothelial cells exposed to various cytokines and chemokines: tumor necrosis factor (TNF)-α, IL-6 and monocyte chemotactic protein 1 (MCP1)/CCL2 (Rochfort and Cummins, 2015; Salvador et al., 2015; Ittner et al., 2020). In concomitant cultures of endothelial cells and monocytes, activation of Rho/ROCK signaling pathway followed by phosphorylation of Ser and Tyr residues in occludin and claudin-5 was observed (Yamamoto et al., 2008); it allows facilitated migration of monocytes across the BBB. The dynamic behavior of TJ proteins in epithelial cells and ECs of peripheral organs has been extensively studied (Stamatovic et al., 2017). Incorporation of proteins that form TJs into cell membranes and subsequent transport followed by their degradation is involved in TJ recycling and modulation of BBB functions (Stamatovic et al., 2017). Diverse studies have shown, i.e., that occludin phosphorylation status of serine/threonine affects BBB permeability. Furthermore, ubiquitination of occludin and interactions with other cytokines such as TNF-α, IL-1ß, IFN-γ, and HGF (Capaldo and Nusrat, 2009; Murakami et al., 2009; Sundstrom et al., 2009) can affect BBB function by affecting the expression of occludin. In the OGD environment, BBB integrity can be affected by the activation of MAPK, PKC, ERK1/2 (Zhao et al., 2019) signaling pathways, and miRNAs such as miR-210 regulating occludin levels (Ma et al., 2017) (Table 1).
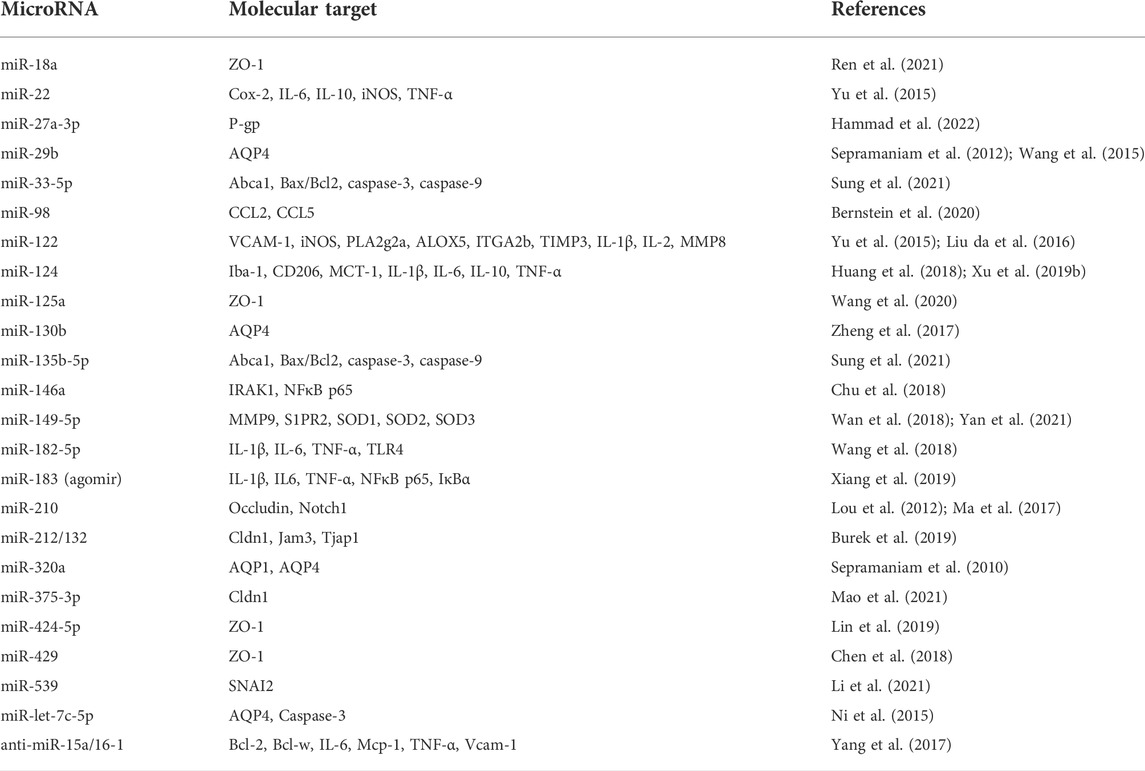
TABLE 1. MicroRNAs interfering with blood-brain barrier function in brain ischemic injury and in vitro model of oxygen-glucose deprivation.
As it was shown, ischemia also induces the expression of claudin-1 in BMECs. It occurs long after stroke and is accompanied by TJ instability and BBB leakage. Targeting Claudin-1 with claudin-1 peptides improves brain endothelial barrier permeability and results in a significant neurological recovery after stroke (Zwanziger et al., 2012; Dithmer et al., 2017; Sladojevic et al., 2019). Jam-3 belongs to the superfamily of immunoglobulins found not only in TJs of polarized cells but also on cell membranes of leukocytes (Wyss et al., 2012). Several members of the JAM family interact with scaffolding proteins containing PDZ domains (e.g., ZO-1) to regulate cell-cell contact maturation and the production of junctional complexes, such as TJ and AS junctions (Keiper et al., 2005; Ebnet, 2017). Recent studies have shown that Jam-3 stimulates human BMEC migration in vitro via the activation of src, p38, and PI3K signaling pathways (Rabquer et al., 2010) and its expression is directly regulated by the hypoxia-induced miRNA-212/132 (Burek et al., 2019) (Table 1). ZO-1 plays an important role in maintaining the integrity of the BBB. The functionality of ZO-1 can be regulated by its expression level and phosphorylation status (Halder et al., 2018). Stimulation by factors such as hypoxia can cause a decrease in ZO-1 expression and thus an impairment of BBB maintenance. For example, the pro-inflammatory cytokines TNF-α and IL-6 increase ZO-1 phosphorylation and reduces the integrity of BMECs (Ittner et al., 2020).
Astrocytes and glial cells are crucial components of the BBB that maintain the integrity of the BBB and the tight junctions between endothelial cells (Abbott et al., 2006; Sweeney et al., 2019). Astrocytes form an additional barrier at the endothelium by forming gab junctions and tight junctions between adjacent astrocytic protrusions. The astrocytic end-feet are tightly connected to the endothelium through specific interactions between the basal lamina and ECM (Xu et al., 2019a). Ischemic and/or traumatic brain injuries are responsible for the activation of astrocytes, which transform to their reactive form involved in numerous processes. The role of activated astrocytes varies over time after injury and in different parts of the CNS (Shen et al., 2021). Astrocyte swelling has been found to be one of the earliest responses after cerebral ischemia. Translocation of the water channel protein aquaporin 4 (AQP4) to the cell surface, facilitation of water influx along an osmotic gradient (ionic edema), and increased glutamate and lactate uptake have been proposed as possible mechanisms involved in astrocyte swelling (Larsen and MacAulay, 2017; Patabendige et al., 2021). AQP4 is widely expressed throughout the brain, particularly predominately at the astrocyte end-foot, where it selectively regulates blood-brain water flux and maintains cerebral water balance (Nagelhus and Ottersen, 2013). AQP4-deficient mice have reduced cytotoxic cerebral edema after ischemic stroke (Manley et al., 2000). Interestingly, astrocyte AQP4 is upregulated in the delayed phase after ischemia, which could be an additional mechanism involved in BBB repair (Tourdias et al., 2011). Over 80% of glutamate transporter proteins, particularly excitatory amino acid transporter 2 (EAAT2), are located on astrocytes, making them a major site of NVU glutamate uptake. In cerebral ischemia, glutamate uptake by astrocytes is essential for neuroprotection and for the prevention of extracellular glutamate rise into excitotoxic levels (Rosenberg et al., 1992; Petr et al., 2015). The swelling of astrocytes can compress the blood vessels in the ischemic area and exacerbate the lack of vascular perfusion (Sykova, 2001). Astrocytes may promote BBB disruption after ischemic stroke. In the co-culture of ECs and astrocytes, EC-derived macrovesicles stimulate increased apoptosis in OGD-treated astrocytes, which was accompanied by disruption of BBB and downregulation of occludin and claudin-5 (Pan et al., 2016). In addition, post-ischemic neurons stimulate astrocytes to synthesize VEGF that contributes to an increase in endothelial permeability and the loss of TJs proteins (Gabbert et al., 2020; Ittner et al., 2020). Knocked down VEGF expression in astrocytes suppressed the effects of OGD-treated neurons on BBB integrity (Li et al., 2014). Astrocytes are also a source of matrix metalloproteinases (MMPs), whose increased activity have been associated with the degradation of TJs and extracellular matrix after ischemia (Mun-Bryce and Rosenberg, 1998). Furthermore, an inhibition of MMPs prevented the loss of TJ proteins in the focal ischemia model (Yang et al., 2007). Activation of astrocytes can be accompanied by microglial activation and the secretion of some inflammatory factors such as transforming growth factor-α (TGF-α), IL-6, leukemia inhibitory factor (LIF), and TNF-α (Shen et al., 2021). In addition, post-ischemic neurons and ECs are also involved in astrocyte activation by releasing cytokines to regulate astrocyte activation and proliferation.
Pericytes have been described as capillary multifunctional mural cells with the highest density at cerebral capillaries within the NVU of the BBB (Brown et al., 2019). Pericytes are critical for regulation of cerebral blood flow, expression of TJs, and maintenance of BBB integrity (Liu et al., 2012; Oztop-Cakmak et al., 2017). Besides supporting cells for capillaries, pericytes have been suggested to play an important role in endothelial cell function and cerebral blood flow modulation (Bergers and Song, 2005; Armulik et al., 2010). After cerebral ischemia, pericytes respond rapidly and show protective or deleterious features such as contraction, migration, detachment from the microvascular wall, or even cell death (Hall et al., 2014; Fernandez-Klett and Priller, 2015; Brown et al., 2019). These changes observed in pericytes after stroke appear to be induced by an influx of intracellular calcium and increase of oxidative stress. Similar to ECs or astrocytes, pericytes release MMP9, causing a massive disruption of TJ proteins and BBB permeability (Brown et al., 2019). During middle cerebral artery occlusion (MCAO) in rats, pericytes detach and migrate from the basement membrane just 1 hour after occlusion. Three hours after injury, the gap between the pericytes and the basement membrane was even larger (Duz et al., 2007). In addition to mesenchymal properties of pericytes, post-ischemic pericytes can differentiate into major components of the NVU, supporting the hypothesis that pericytes may contribute to both neurogenesis and vasculogenesis at the site of brain injury (Nakagomi et al., 2015). These morphological changes and microvascular wall detachment were proposed to be the first step in the loss of BBB integrity. After MCAO, capillary reflux can be impaired by inducing pericytes contraction in the microvessels, resulting in obstruction of blood flow. Since the changes in the morphology of the pericytes persisted even after the restoration of the circulation in the cerebral vessels, it may be responsible for the development of brain pathology. Reactive oxygen and nitrogen species in microvessels play a crucial role in pericytes dysfunction in addition to their role in BBB damage (Yemisci et al., 2009; Dalkara et al., 2011). Inhibition of oxidative-nitrative stress by a suppression of peroxynitrite attenuates pericyte contraction after MCAO in mice (Yemisci et al., 2009). In addition to oxidative stress, ischemia-induced inflammation can further exacerbate pericyte-mediated BBB dysfunction. In co-cultures of pericyte cells and microvascular-derived fibroblasts, pro-inflammatory stimuli induce widespread changes in the expression of interleukins, chemokines and cell adhesion molecule genes in pericytes (Persidsky et al., 2016). It has been suggested that reducing pericyte-mediated inflammation may be beneficial in restoring BBB function and reducing inflammation in CNS injuries (Yemisci et al., 2009). The lack of a specific indicator of pericytes due to their multipotent (self-renewing) properties limits the study of these cells. Platelet-derived growth factor receptor-beta (PDGFRβ) and neural/glial antigen 2 (NG2), meaning the receptor and co-receptor for PDGF, respectively, are commonly used as relatively specific markers for pericytes (Jiang et al., 2018). The elevated endothelial PDGF-β during ischemia is associated with an increase in microvessel diameter and blood supply due to dilated pericytes (Arimura et al., 2012). Stark et al. (2013) showed the active involvement of NG2+ pericytes in the immune response to inflammatory mediators. Namely, NG2+ pericytes control the pattern and potency of leukocyte infiltration into the CNS through upregulation of Intercellular Adhesion Molecule 1 (ICAM-1) and synthesis of chemotactic macrophage migration inhibitory factor (MIF) (Stark et al., 2013). Recently, post-ischemic brain pericytes have been reported to adopt a microglial phenotype. In human brain tissue obtained from stroke patients, activated pericytes were found to express microglia-specific markers such as ionized calcium-binding adapter molecule 1 (Iba-1), CD11b and galectin-3 (GAL-3) (Ozen et al., 2014). Consistent with the results mentioned above, PDGFRβ+ perivascular cells isolated from areas of ischemia showed upregulation of microglia-specific markers (e.g., Iba1, CD11b) and stem cells markers (e.g., nestin, c-myc, Klf4, and Sox2), suggesting that pericytes can function as microglia-generating stem cells with potential phagocytic capacity (Sakuma et al., 2016). Furthermore, in the adult spinal cord, pericytes have been identified as a source of scar-forming cells in CNS injury. Also, after spinal cord injury, PDGFRβ+ cells co-express stromal cell markers and produce scar tissue distinct from glial scars (Fernandez-Klett et al., 2013).
Microglia are specialized CNS macrophages responsible for orchestration of the innate immune response, tissue development and maintaining homeostasis (Kettenmann et al., 2011). These primary CNS immune cells play a crucial role in brain and retinal vasculature development and are involved in vascular sprouting, migration and anastomosis (Arnold and Betsholtz, 2013; Harry, 2013). As an important component of the NVU, microglia also regulate BBB development actively communicating with ECs (da Fonseca et al., 2014). Microglia are present in vascular junctions and apical cells of the pontine endothelium and synergistically promote cerebrovascular network formation in combination with VEGF-induced vascular sprouting (Fantin et al., 2010). Microglia may increase vascular sprouting by secreting soluble factors rather than by direct contact with EC, as shown by organotypic aortic ring culture (Rymo et al., 2011). Under physiological conditions, microglia are inactive, with small cell bodies and highly ramified branching processes. After injury or invasion by pathogens, microglia transform into active phagocytic microglia, migrate and accumulate at the site of injury (Fu et al., 2014). After ischemic brain injury, microglia are rapidly activated, followed by morphological and genetic changes in these cells (Kettenmann et al., 2011; Liu et al., 2020). Microglia/macrophages are activated in two states of polarization: the pro-inflammatory phenotype (known as M1 microglia), and the anti-inflammatory phenotype (M2 microglia) (Liu et al., 2020; Rodriguez-Gomez et al., 2020). Activated microglia play a dual role in ischemic brain injury as well as in disrupting BBB integrity, possibly due to their phenotypic polarization (Kang et al., 2020). These highly dynamic cells produce large amounts of cytokines and chemokines. Moreover, microglial cells are able to increase the level of adhesion molecules on the surface of endothelial cells, which increases the likelihood of infiltration by leukocytes (da Fonseca et al., 2014). It has been postulated that dynamic changes in microglia and astrocyte phenotype are crucial in determining their deleterious or beneficial character at specific stages of CNS injury (Lan et al., 2017). Beneficial effects of activated microglia have been associated with phagocytosis of cellular debris and suppression of inflammatory responses (Sierra et al., 2010; Herzog et al., 2019). Neuroinflammation induced by MCAO or lipopolysaccharide (LPS) administration in vitro modulates the direction of microglia polarization. Microglia are more sensitive to pathogens or damage and therefore impair BBB function. Initially activated pro-inflammatory microglia produce common inflammatory cytokines such as IL-1 and TNF-α, leading to activation of astrocytes and increase in the permeability of BMECs (Nishioku et al., 2010; Huang et al., 2020). During CNS injury, microglia/macrophages are continuously activated, leading to distinct effects on the structure of BBB and NVU as the M1/M2 phenotype is switched and exchanged between the activated microglia and astrocytes (Kirkley et al., 2017). Pro-inflammatory microglia also lead to P-glycoprotein (P-gp) dysfunction in the outer cerebral cortex through activation of NADPH oxidase (Matsumoto et al., 2012) and a concomitant reduction in the efflux of neurotoxic substances from the CNS. However, microglial cells are also involved in the defense mechanisms against neuroinflammation, such as e.g., vascular remodelling that helps restore CNS functions and alleviate neurological symptoms (Yang et al., 2015). These microglia can also promote angiogenesis through the production of VEGF, IL-8 and microtubule-associated protein (MAP9) (Medina et al., 2011; Willenborg et al., 2012; Zajac et al., 2013).
2.1 The effect of miRNA on BBB dysfunction
MiRNAs regulate the function of the BBB in health and disease. Recent studies have shown that miR-98 directly targets the production of the pro-inflammatory cytokines CCL2 and CCL5 (Bernstein et al., 2020) and is implicated in the attraction of leukocyte adhesion and migration across the BBB (Schober, 2008). This is accompanied by an exacerbation of BBB dysfunction due to activation of small Rho GTPases followed by actin cytoskeletal rearrangements and redistribution of TJ proteins. Moreover, miR-375-3p can additionally affect the vascular barrier by targeting claudin-1 to promote metastasis in small cell lung cancer (Mao et al., 2021). The opposing effect can be induced by miR-155, which inhibits OGD-induced barrier dysfunction in human primary BMECs (Pena-Philippides et al., 2018). In addition, miR-429 inhibits the expression of ZO-1, which markedly worsens BBB function (Chen et al., 2018). Under hypoxic circumstances in vitro, miR-125a-5p, miR-18a, and miR-424-5p increase BBB permeability by downregulation of ZO-1 expression (Lin et al., 2019; Wang et al., 2020; Ren et al., 2021). In contrast, after OGD exposure, the expression of miR-15a was shown to be significantly increased in brain EC cultures as well in the bloodstream 1 day after ischemic stroke. Additionally, while miR-15a could post-transcriptionally enhance or repress its direct downstream target BCL-2, selective miR-15a transgene overexpression in ECs resulted in decreased brain capillary density, significantly enhanced the cerebral infarction area, and neurological deficits in mice 7 days after MCAO. These findings may indicate that miR-15a has a meaningful impact on post-ischemically-induced cerebral angiogenesis (Yin et al., 2014). Furthermore, the hypoxia-induced miR-210 is significantly upregulated in ischemic cerebral cortex of adult rats, where the Notch1 signaling is hyperactivated. These results could be verified in vitro in HUVEC cells. It was observed here, that an overexpression of miR-210 led to Notch1 signaling activation followed by the increase of EC migration and the formation of capillary-like structures. MiR-210 may therefore be responsible for post-ischemic neovascularization (Lou et al., 2012). Moreover, miR-210 was observed to regulate the angiogenesis of brain vessels under physiological conditions (Zeng et al., 2014).
Mechanisms of action of miRNA-based therapeutic are established anti-inflammatory activities such as an inhibition of astrocyte activation, cytokine secretion, and leukocyte extravasation (Sun et al., 2018). For example, altered expression of some miRNAs, either increased (i.e., miR-22 and miR-122) or decreased (miR-15a/16-1), is responsible for decreased expression of some pro-inflammatory factors in the ischemic brain: TNF-α, IL-6, monocyte chemoattractant protein-1 (MCP-1), cytochrome c oxidase (COX-2), inducible nitric oxide synthase (iNOS), and Vascular cell adhesion protein-1 (VCAM-1) in the ischemic brain (Yu et al., 2015; Liu da et al., 2016; Yang et al., 2017). Exosome-mediated delivery of miR-124-3p promoted M2 microglia polarization and suppressed tissue inflammation after TBI (Huang et al., 2018), and reduced lesion core after MCAO in mice (Hamzei Taj et al., 2016a). Interestingly, it was also found that an overexpression of miR-29b can reduce BBB disruption after ischemic stroke by downregulating AQP4 (Wang et al., 2015). In vitro experiments identified miR-130 as a strong transcriptional repressor of the AQP4 M1 isoform. In addition, in vivo studies have shown that anti-miR-130a upregulated the AQP4 M1 transcript (Sepramaniam et al., 2012). AQP4 is also a potential target for the regulation by miR-130b. Thereby, miR-130b accelerates the downregulation of AQP4 in primary astrocytes under normoxic and OGD conditions (Zheng et al., 2017). In contrast, anti-miR-320a treatment upregulates the expression of this water channel protein after stroke, which is associated with a reduction in cerebral edema (Sepramaniam et al., 2010). A few studies have examined the significance of astrocyte-enriched expression of miRNAs after ischemia and stroke. For example, miR-181a is overexpressed in the infarct core (Ouyang et al., 2012a) and associated with increased mitochondrial dysfunction and apoptosis in astrocytes (Ouyang et al., 2012b). Treatment with miR-181a antagomir reduced the loss of hippocampal CA1 neurons after forebrain ischemia (Moon et al., 2013). In addition, inhibition of miR-181a resulted in an improvement of 17β-estradiol-mediated stroke protection in females, in part by increasing estrogen receptor-α production (Stary et al., 2017). Intriguingly, astrocyte-enriched miR-29a changed in the opposite direction compared to miR-181a following transient forebrain ischemia, decreasing in hippocampal CA1 neurons and increasing in DG (Ouyang et al., 2013).
In rat pericytes after MCAO, miR149-5p increased expression of sphingosine-1-phosphate receptor 2 (S1PR2), superoxide dismutase (SOD) 1-3 and significantly decreased the expression of MMP9, thereby alleviating BBB permeability and playing a protective role for neurons (Yan et al., 2021). Furthermore, ICV injection of antagomir-149-5p attenuated BBB permeability and improved MCAO outcomes in rats (Wan et al., 2018). In BMECs, miR-539 bound to the zink-finger transcription factor SNAI2 and was involved in the regulation of EC permeability by affecting the SNAI2/MMP9 signaling pathway, suggesting that circulating miR-539 is a potential marker for predicting BBB integrity after ischemic stroke (Li et al., 2021).
Modulation of miRNAs has been reported to contribute to post-stoke pathology, including the regulation of neuroinflammation. Numerous miRNAs are involved in the molecular action of different microglia phenotypes (Lian et al., 2020). Differential expressions levels of miRNAs (e.g., miR-210, miR-155, miR-373, miR-689, and miR-182-5p) have been previously reported after LPS stimulation of microglial cells in vitro (Freilich et al., 2013; Wang et al., 2018). Similarly, different expression of certain miRNAs (such as miR-183, miR-146a) has been reported in the plasma and brain of animals exposed to experimental stroke (Chu et al., 2018; Xiang et al., 2019). In the work by Zhao et al. (2013) the authors showed that overexpression of miR-424 reduced brain injury after ischemic stroke in the mouse MCAO model by suppressing microglial activity. In addition, miR-let-7c-5p has been associated with anti-inflammatory properties promoting neuroprotection after ischemic stroke by inhibiting microglial activation and translational repression of caspase-3 (Zhao et al., 2013; Ni et al., 2015). One of the miRNAs almost exclusively expressed in the CNS is miR-124. Intracerebral injection of miR-124 in focal cerebral ischemia in mice modulated microglia/macrophage activation of the M2 phenotype, thus contributing to the recovery of brain cell function (Hamzei Taj et al., 2016b). Stimulation of mouse microglial cell line BV-2 with IL-4 in an ischemic conditions resulted in the subsequent release of miRNA-26 containing exosomes (Tian et al., 2019). It caused the formation of the tubes by endothelial cells in culture as well as angiogenesis in the MCAO model of stroke. The authors suggest that miRNA-26a, which has already been implicated in angiogenesis, may be responsible for new vessel formation after stroke and that such stimulation of a pre-existing pool of microglia in brain could be a promising therapeutic approach.
3 Transport pathways and their regulation by ischemia and/or stroke
The transporter barrier of the BBB tightly regulates the transport of substances in and out of the brain. Some substances (e.g., oxygen, carbon dioxide, nitrogen monoxide and lipophilic substances such as hormones) can pass through the BBB unhindered by diffusion. Other substances depend on transporter proteins that facilitate their passage (Figure 1). An alteration of the expression level of BBB transport proteins or the modulation of their activity are observed in CNS ischemic injury and act as an important element of the cellular response to oxygen and nutrient deprivation (Nilles et al., 2022).
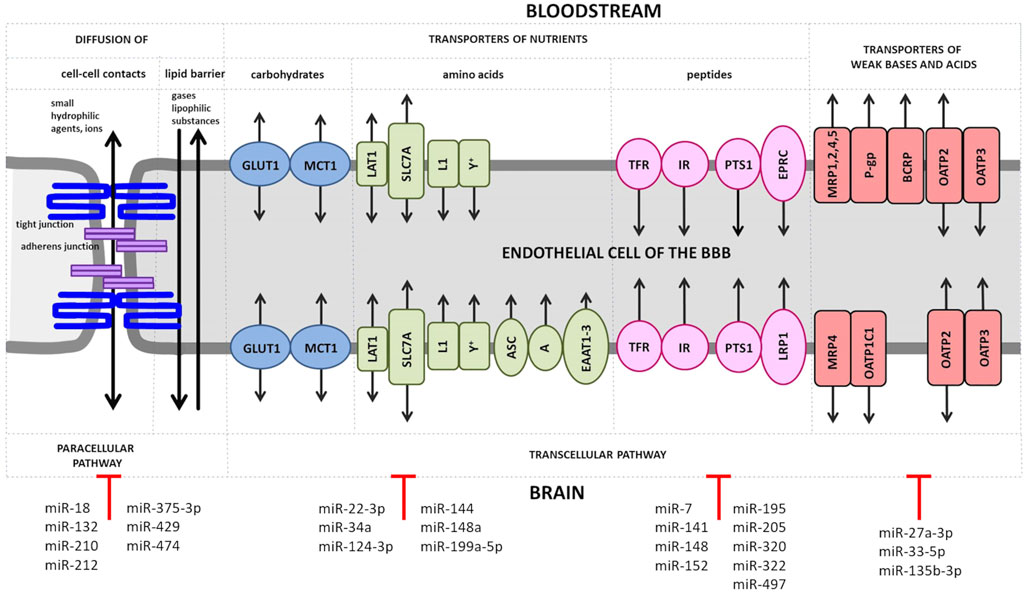
FIGURE 1. Transport of solutes across the BBB. Small ions and hydrophilic substances up to 800 Da permeate the endothelial layer of the BBB in both directions through tight junctions such as claudins by the paracellular pathway. At the basolateral site of these substances have also to pass the adherens junction. Small lipophilic compounds, such as drugs, and gases, like oxygen or carbon dioxide, diffuse transcellularly across the lipid monolayer. Transporters for carbohydrates are expressed at both, luminal and abluminal membrane of the BBB endothelium and include the following: glucose transporter 1 (GLUT1), lactate transporter monocarboxylate transporter 1 (MCT1), L-type amino acid transporter 1 (LAT1), solute carrier family 2 facilitated glucose transporter member 1 (SLC2A1) and L1 and y + transporters for large neutral and cationic essential amino acids. Non-essential amino acid transporters (the alanine-, serine- and cysteine-preferring system (ASC), the alanine-preferring system (A)) and the excitatory amino acid transporters 1–3 (EAAT1-3) are located at the abluminal side. Transporters of peptides or proteins including the transferrin receptors (TFRs), the insulin receptors (IRs) and the peptide transport system 1 (PTS1) for encephalins are expressed at both EC membranes, while the endothelial protein C receptor (EPCR) for activated protein C (APC) and the low density lipoprotein receptor-related protein 1 (LRP1) for amyloid-β are found at the luminal or abluminal side, respectively. The ATP-binding cassette (ABC) efflux transporters include multidrug resistance protein 1 (MRP1, 2, 4, 5, of which only MRP4 is present at both membrane sides of BBB ECs) and further the solute carrier organic anion transporter family member 1C1 (OATP1C1), the breast cancer resistance protein (BRCP) and the organic anion transporters/organic anion transporter peptides (OAT/OATPs). MicroRNAs (miRs), which have been shown to directly regulate genes encoding tight and adherens junction proteins and various transporter proteins, are shown.
There are multiple proteins expressed in BMECs to ensure the supply of the brain with nutrients (Figure 1). Glucose is passively transported by the glucose transporter 1 (GLUT1/SLC2A1) in a concentration-dependent manner (Blecharz et al., 2015). GLUT1 is upregulated by ischemia and hypoglycemia and contributes to edema formation in stroke by co-transporting water (Loike et al., 1992; Wright and Turk, 2004; Vemula et al., 2009; MacAulay and Zeuthen, 2010). The presence of a second glucose transporter at the BBB, the sodium-dependent glucose transporter (SGLT1 encoded by the SLC5A1 gene), is controversial (Elfeber et al., 2004; Vemula et al., 2009). Sglt1 has been shown to be markedly upregulated after OGD in BMECs (Neuhaus et al., 2012).
ECs possess a number of ATP-binding cassette proteins (ABC), which represent a large group of membrane pumps that actively efflux molecules from the cells (Helms et al., 2016; Tornabene and Brodin, 2016). Efflux pumps therefore play a key role in the absorption, distribution and elimination of endo- and xenobiotics or in reducing the permeability of blood-tissue barriers (Chen et al., 2016; Du et al., 2018). Depending on the location of the efflux pump, they can promote transport into the brain and protect the brain from potentially neurotoxic endogenous or lipophilic xenobiotic substances (Loscher and Potschka, 2005). The neuroprotective role of ABC transporters concurrently determines the difficulty for delivery of some drugs to the CNS. Namely, increased expression of transporters enhances neuroprotection, but at the expense of drug distribution in CNS; and conversely—reduced activity of ABC transporters decreases neuroprotection and provides improved drug access to the brain (Miller, 2015). The dominant ABC transporters at the BBB is P-gp, also known as multidrug resistance protein 1 - MDR1/ABCB1), breast cancer resistance protein (BCRPP/ABCG2), and the multidrug resistance-associated proteins (MRPs) (Demeule et al., 2002; Miller, 2015).
An alternated expression and functional changes of ABC transporters were observed after hypoxia or ischemia (Tornabene and Brodin, 2016; Burek et al., 2020). In a model of focal cerebral ischemia, the activity and expression of the brain luminal transporter P-gp was increased in mice (Spudich et al., 2006) as well in rats (DeMars et al., 2017) being consistent with in vitro studies showing that OGD treatment was able to upregulate P-gp both at mRNA and protein level (Neuhaus et al., 2014; DeMars et al., 2017). At the same time, the abluminal transporter ABCC1, which carries its substrates in the opposite direction, from blood to the brain, was found to be decreased in capillary extracts in response to MCAO (Kilic et al., 2008). In contrast to the in vitro study mentioned above, no changes in P-gp expression under hypoxia were observed in two independent studies (Patak et al., 2011; Lindner et al., 2012) using the immortalized human BMECs (hCMEC/D3). According to the authors, the lack of changes in P-gp expression may be due to the lost ability of hCMEC/D3 to respond to hypoxia (Lindner et al., 2012), or this cell line may not be suitable model for studying the EC response to ischemia in vitro (Patak et al., 2011).
Receptor-mediated endocytosis and exocytosis are responsible for the passage of macromolecules such as insulin, leptin, low-density lipoproteins and transferrin through the intact BBB (Blecharz et al., 2015). The transferrin receptor (TfR) is an iron-binding receptor that delivers iron to cells. TfR-mediated brain iron uptake remains partially functional after onset of ischemia and shows complete recovery after reperfusion (Hao and Bickel, 2013). In addition, expression of TfR was upregulated in hypoxic BMECs (Tornabene et al., 2019).
3.1 The effect of miRNA on transport pathways
Several studies have described the direct regulation of glucose transporters by miRNAs in different tissues, e.g., miR-144 upregulated GLUT1 and glucose uptake (Liu et al., 2016), while miR-148a, -199a-5p, -34a, downregulated GLUT 1 and GLUT1/SLC2A1 was confirmed to be a direct target of these miRNAs (Qiang et al., 2020; Tiemin et al., 2020; Xu et al., 2022). Monocarboxylate transporter 1 (MCT1/SLC16A1) being responsible for the transport of a broad range of monocarboxylates is expressed in BMECs (Hoshi et al., 2013; Curtaz et al., 2020b). Its expression was found to be significantly increased in BMECs after MCAO (Zhang et al., 2005). SLC16A1 is a direct target of miR-22-3p and miR-124-3p (Xu et al., 2019b; Xue et al., 2022). Interestingly, in the rat model of cerebral ischemia, miR-124-3p showed a time-dependent biphasic effects on MCT-1 expression and protective effects against ischemic brain injury (Xu et al., 2019b).
Studies have repeatedly shown that the ABC transporter family of efflux pumps may be regulated by miRNAs (Haenisch et al., 2014). P-gp is the most studied member within the ABC transporter family. In addition, P-gp expression is tightly regulated by differential molecules including miRNAs. Moreover, P-gp can regulate the intracellular expression of miRNAs (Lopes-Rodrigues et al., 2014). In a very recent study, Hammad et al. (2022) showed that the expression and activity of P-gp in hCMEC/D3 is modulated by miR-27a-3p. In addition, complex interactions between Pg-p, miRNAs and other cellular proteins have been characterized in several tumor cell lines describing the key mechanism of drug resistance within tumors (Haenisch et al., 2014; Lopes-Rodrigues et al., 2014).
There is accumulating evidence that miRNAs may contribute to the pathogenesis of cerebral ischemia and that the ischemic preconditioning (IPC) paradigm may exert neuroprotective properties by acting as both positive and negative regulators of cell survival (Bell et al., 2017; Vasudeva and Munshi, 2020). In the mouse model of ischemic stroke, miR-33-5p and miR-135b-5b were downregulated by earlier exposure to IPC. Neuroprotective effects of inhibition of these two miRNAs was found under in vitro OGD conditions. In addition, inhibition of miR-33-5p and miR-135b-5b increased the ABCA1 level (Sung et al., 2021). Additionally, it was recently reported that ABCA1 knockout mice showed increased BBB leakage, white matter/axonal damage and functional deficits after MCAO compared to healthy wild type mice (Cui et al., 2015).
TFRC encoding TfR is directly regulated by miR-7, -141, -148a, -152, and -320 in various type of cancers, where high iron levels are required for cell proliferation (Schaar et al., 2009; Kindrat et al., 2016; Miyazawa et al., 2018; Babu and Muckenthaler, 2019). The low-density lipoprotein receptor (LDLR) family is responsible for the transport of low-density lipoproteins (LDLs) such as apolipoprotein (Apo) B and E via endocytosis (Hladky and Barrand, 2018). Low-density lipoprotein receptor-related protein 1 (LRP1) is direct target of miR-205 (Song and Bu, 2009). Another protein ensuring receptor-mediated endocytosis and being highly expressed at the BBB and other tissues is the insulin receptor (INSR). It guarantees insulin signaling to modulate cellular functions either in health or disease (Tashima, 2022). INSR is a direct target of miRNA-195, -322, -497 (Wei et al., 2016; Geng et al., 2020). The direct regulation of cellular receptors at the BBB by miRNA can be used for the development of therapeutics or the generation of new tools for drug delivery to the CNS.
4 Discussion
In summarizing the existing literature, we were able to depict the current knowledge and improve the understanding of the impact of miRs on the normal BBB and the whole NVU function as well as on the role of these molecules under ischemic conditions. To maintain the BBB integrity and ensure a permanent homeostasis inside the brain appear to be essential in the treatment of numerous cerebrovascular disorders including ischemic stroke. Functional changes in the cellular architecture of the BBB result in the disruption of the BBB. At the cellular level, ECs, pericytes, astrocytes and microglia composing the NVU are affected by ischemia and influence each other. Structurally, alterations in the expression and distribution of TJ and AJ proteins, then, the local microenvironment, transport systems, enzymes and finally, the extracellular matrix, can ultimately lead to the passage of harmful serum components and immune cells through the BBB, disrupting CNS homeostasis and damaging the surrounding brain parenchyma. The role of miRNAs and their specific direct targets at the BBB needs however to be further explored in future studies as the full picture of miRNA-mediated BBB regulation is still insufficiently described. In addition, ischemia-induced miRNA changes in all NVU components should be intensively studied to identify further useful and specific biomarkers, including therapeutic miRNA. In future BBB studies, more effort should be put into studying proteins and signaling pathways specifically associated with certain cell types composing NVU, hence they may provide further useful indications and insights into the miRNA-mediated regulation of all BBB functions, including their involvement in pharmacokinetics of CNS drugs. Cell-specific proteins and signaling pathways involved in cerebral ischemia and their influence on the EC barrier should be also considered in the context of miRNAs in future BBB studies, hence they may provide further useful indications and insights into the miRNA-mediated regulation of BBB permeability and facilitation of drug delivery to the CNS. Finally, there is an urgent need to identify further precise strategies facilitating the recovery and repair of the BBB after ischemic stroke giving the functional recovery of the affected patients and improving their prognosis. As depicted in this review article, there is an objective evidence suggesting that miRNAs may serve as highly potent clinical therapeutics in the treatment of stroke and its respective consequential damages in future. Further studies to test the clinical effectiveness of miRNAs in the context of cerebrovascular diseases are therefore essential to extend our knowledge on these molecules.
Author contributions
AS, KB-L, MN-C, and MB wrote the manuscript and critically revised the manuscript, AM, PM critically revised the manuscript. All author have read and approved the last version of the manuscript.
Conflict of interest
The authors declare that the research was conducted in the absence of any commercial or financial relationships that could be construed as a potential conflict of interest.
Publisher’s note
All claims expressed in this article are solely those of the authors and do not necessarily represent those of their affiliated organizations, or those of the publisher, the editors and the reviewers. Any product that may be evaluated in this article, or claim that may be made by its manufacturer, is not guaranteed or endorsed by the publisher.
Abbreviations
A, alanine-preferring system; ABC, ATP-binding cassette; APC, activated protein C; ASC, alanine-, serine- and cysteine-preferring system; BCRP, breast cancer resistance protein; EAAT1-3, excitatory amino acid transporters 1–3; EPCR, endothelial protein C receptor; GLUT1, glucose transporter 1; IR, insulin receptors; LAT1, L-type amino acid transporter 1; LRP1, lipoprotein receptor-related protein 1; MCT1, monocarboxylate transporter 1; MRP, multidrug resistance protein; OATP, organic anion transporters/organic anion transporter peptide; PTS1, peptide transport system 1; SLC2A1, solute carrier family 2 facilitated glucose transporter member 1; TFR, transferrin receptors.
References
Abbott, N. J., Ronnback, L., and Hansson, E. (2006). Astrocyte-endothelial interactions at the blood-brain barrier. Nat. Rev. Neurosci. 7, 41–53. doi:10.1038/nrn1824
Abbott, N. J., Patabendige, A. A., Dolman, D. E., Yusof, S. R., and Begley, D. J. (2010). Structure and function of the blood-brain barrier. Neurobiol. Dis. 37, 13–25. doi:10.1016/j.nbd.2009.07.030
Arimura, K., Ago, T., Kamouchi, M., Nakamura, K., Ishitsuka, K., Kuroda, J., et al. (2012). PDGF receptor beta signaling in pericytes following ischemic brain injury. Curr. Neurovasc. Res. 9, 1–9. doi:10.2174/156720212799297100
Armulik, A., Genove, G., Mae, M., Nisancioglu, M. H., Wallgard, E., Niaudet, C., et al. (2010). Pericytes regulate the blood-brain barrier. Nature 468, 557–561. doi:10.1038/nature09522
Arnold, T., and Betsholtz, C. (2013). The importance of microglia in the development of the vasculature in the central nervous system. Vasc. Cell 5, 4. doi:10.1186/2045-824X-5-4
Babu, K. R., and Muckenthaler, M. U. (2019). miR-148a regulates expression of the transferrin receptor 1 in hepatocellular carcinoma. Sci. Rep. 9, 1518. doi:10.1038/s41598-018-35947-7
Bell, J. D., Cho, J. E., and Giffard, R. G. (2017). MicroRNA changes in preconditioning-induced neuroprotection. Transl. Stroke Res. 8, 585–596. doi:10.1007/s12975-017-0547-1
Bergers, G., and Song, S. (2005). The role of pericytes in blood-vessel formation and maintenance. Neuro. Oncol. 7, 452–464. doi:10.1215/S1152851705000232
Bernstein, D. L., Zuluaga-Ramirez, V., Gajghate, S., Reichenbach, N. L., Polyak, B., Persidsky, Y., et al. (2020). miR-98 reduces endothelial dysfunction by protecting blood-brain barrier (BBB) and improves neurological outcomes in mouse ischemia/reperfusion stroke model. J. Cereb. Blood Flow. Metab. 40, 1953–1965. doi:10.1177/0271678X19882264
Blecharz, K. G., Colla, R., Rohde, V., and Vajkoczy, P. (2015). Control of the blood-brain barrier function in cancer cell metastasis. Biol. Cell 107, 342–371. doi:10.1111/boc.201500011
Blecharz-Lang, K. G., Prinz, V., Burek, M., Frey, D., Schenkel, T., Krug, S. M., et al. (2018). Gelatinolytic activity of autocrine matrix metalloproteinase-9 leads to endothelial de-arrangement in Moyamoya disease. J. Cereb. Blood Flow. Metab. 38, 1940–1953. doi:10.1177/0271678X18768443
Borlongan, C. V., and Emerich, D. F. (2003). Facilitation of drug entry into the CNS via transient permeation of blood brain barrier: laboratory and preliminary clinical evidence from bradykinin receptor agonist, cereport. Brain Res. Bull. 60, 297–306. doi:10.1016/s0361-9230(03)00043-1
Brown, L. S., Foster, C. G., Courtney, J. M., King, N. E., Howells, D. W., and Sutherland, B. A. (2019). Pericytes and neurovascular function in the healthy and diseased brain. Front. Cell. Neurosci. 13, 282. doi:10.3389/fncel.2019.00282
Burek, M., Konig, A., Lang, M., Fiedler, J., Oerter, S., Roewer, N., et al. (2019). Hypoxia-induced MicroRNA-212/132 alter blood-brain barrier integrity through inhibition of tight junction-associated proteins in human and mouse brain microvascular endothelial cells. Transl. Stroke Res. 10, 672–683. doi:10.1007/s12975-018-0683-2
Burek, M., Burmester, S., Salvador, E., Moller-Ehrlich, K., Schneider, R., Roewer, N., et al. (2020). Kidney ischemia/reperfusion injury induces changes in the drug transporter expression at the blood-brain barrier in vivo and in vitro. Front. Physiol. 11, 569881. doi:10.3389/fphys.2020.569881
Capaldo, C. T., and Nusrat, A. (2009). Cytokine regulation of tight junctions. Biochim. Biophys. Acta 1788, 864–871. doi:10.1016/j.bbamem.2008.08.027
Chandran, R., Sharma, A., Bhomia, M., Balakathiresan, N. S., Knollmann-Ritschel, B. E., and Maheshwari, R. K. (2017). Differential expression of microRNAs in the brains of mice subjected to increasing grade of mild traumatic brain injury. Brain Inj. 31, 106–119. doi:10.1080/02699052.2016.1213420
Chen, Z., Shi, T., Zhang, L., Zhu, P., Deng, M., Huang, C., et al. (2016). Mammalian drug efflux transporters of the ATP binding cassette (ABC) family in multidrug resistance: A review of the past decade. Cancer Lett. 370, 153–164. doi:10.1016/j.canlet.2015.10.010
Chen, L., Xue, Y., Zheng, J., Liu, X., Liu, J., Chen, J., et al. (2018). MiR-429 regulated by endothelial monocyte activating polypeptide-II (EMAP-II) influences blood-tumor barrier permeability by inhibiting the expressions of ZO-1, occludin and claudin-5. Front. Mol. Neurosci. 11, 35. doi:10.3389/fnmol.2018.00035
Chu, B., Zhou, Y., Zhai, H., Li, L., Sun, L., and Li, Y. (2018). The role of microRNA-146a in regulating the expression of IRAK1 in cerebral ischemia-reperfusion injury. Can. J. Physiol. Pharmacol. 96, 611–617. doi:10.1139/cjpp-2017-0586
Cui, X., Chopp, M., Zacharek, A., Karasinska, J. M., Cui, Y., Ning, R., et al. (2015). Deficiency of brain ATP-binding cassette transporter A-1 exacerbates blood-brain barrier and white matter damage after stroke. Stroke 46, 827–834. doi:10.1161/STROKEAHA.114.007145
Curtaz, C. J., Schmitt, C., Blecharz-Lang, K. G., Roewer, N., Wockel, A., and Burek, M. (2020). Circulating MicroRNAs and blood-brain-barrier function in breast cancer metastasis. Curr. Pharm. Des. 26, 1417–1427. doi:10.2174/1381612826666200316151720
Curtaz, C. J., Schmitt, C., Herbert, S. L., Feldheim, J., Schlegel, N., Gosselet, F., et al. (2020). Serum-derived factors of breast cancer patients with brain metastases alter permeability of a human blood-brain barrier model. Fluids Barriers CNS 17, 31. doi:10.1186/s12987-020-00192-6
Curtaz, C. J., Reifschlager, L., Strahle, L., Feldheim, J., Feldheim, J. J., Schmitt, C., et al. (2022). Analysis of microRNAs in exosomes of breast cancer patients in search of molecular prognostic factors in brain metastases. Int. J. Mol. Sci. 23, 3683. doi:10.3390/ijms23073683
da Fonseca, A. C., Matias, D., Garcia, C., Amaral, R., Geraldo, L. H., Freitas, C., et al. (2014). The impact of microglial activation on blood-brain barrier in brain diseases. Front. Cell. Neurosci. 8, 362. doi:10.3389/fncel.2014.00362
Dalkara, T., Gursoy-Ozdemir, Y., and Yemisci, M. (2011). Brain microvascular pericytes in health and disease. Acta Neuropathol. 122, 1–9. doi:10.1007/s00401-011-0847-6
Decleves, X., Jacob, A., Yousif, S., Shawahna, R., Potin, S., and Scherrmann, J. M. (2011). Interplay of drug metabolizing CYP450 enzymes and ABC transporters in the blood-brain barrier. Curr. Drug Metab. 12, 732–741. doi:10.2174/138920011798357024
DeMars, K. M., Yang, C., Hawkins, K. E., McCrea, A. O., Siwarski, D. M., and Candelario-Jalil, E. (2017). Spatiotemporal changes in P-glycoprotein levels in brain and peripheral tissues following ischemic stroke in rats. J. Exp. Neurosci. 11, 1179069517701741. doi:10.1177/1179069517701741
Demeule, M., Regina, A., Jodoin, J., Laplante, A., Dagenais, C., Berthelet, F., et al. (2002). Drug transport to the brain: key roles for the efflux pump P-glycoprotein in the blood-brain barrier. Vasc. Pharmacol. 38, 339–348. doi:10.1016/s1537-1891(02)00201-x
Dithmer, S., Staat, C., Muller, C., Ku, M. C., Pohlmann, A., Niendorf, T., et al. (2017). Claudin peptidomimetics modulate tissue barriers for enhanced drug delivery. Ann. N. Y. Acad. Sci. 1397, 169–184. doi:10.1111/nyas.13359
Du, D., Wang-Kan, X., Neuberger, A., van Veen, H. W., Pos, K. M., Piddock, L. J. V., et al. (2018). Multidrug efflux pumps: structure, function and regulation. Nat. Rev. Microbiol. 16, 523–539. doi:10.1038/s41579-018-0048-6
Duz, B., Oztas, E., Erginay, T., Erdogan, E., and Gonul, E. (2007). The effect of moderate hypothermia in acute ischemic stroke on pericyte migration: an ultrastructural study. Cryobiology 55, 279–284. doi:10.1016/j.cryobiol.2007.08.009
Ebnet, K. (2017). Junctional adhesion molecules (JAMs): cell adhesion receptors with pleiotropic functions in cell physiology and development. Physiol. Rev. 97, 1529–1554. doi:10.1152/physrev.00004.2017
Elfeber, K., Kohler, A., Lutzenburg, M., Osswald, C., Galla, H. J., Witte, O. W., et al. (2004). Localization of the Na+-D-glucose cotransporter SGLT1 in the blood-brain barrier. Histochem. Cell Biol. 121, 201–207. doi:10.1007/s00418-004-0633-9
Fantin, A., Vieira, J. M., Gestri, G., Denti, L., Schwarz, Q., Prykhozhij, S., et al. (2010). Tissue macrophages act as cellular chaperones for vascular anastomosis downstream of VEGF-mediated endothelial tip cell induction. Blood 116, 829–840. doi:10.1182/blood-2009-12-257832
Fernandez-Klett, F., and Priller, J. (2015). Diverse functions of pericytes in cerebral blood flow regulation and ischemia. J. Cereb. Blood Flow. Metab. 35, 883–887. doi:10.1038/jcbfm.2015.60
Fernandez-Klett, F., Potas, J. R., Hilpert, D., Blazej, K., Radke, J., Huck, J., et al. (2013). Early loss of pericytes and perivascular stromal cell-induced scar formation after stroke. J. Cereb. Blood Flow. Metab. 33, 428–439. doi:10.1038/jcbfm.2012.187
Freilich, R. W., Woodbury, M. E., and Ikezu, T. (2013). Integrated expression profiles of mRNA and miRNA in polarized primary murine microglia. PLoS One 8, e79416. doi:10.1371/journal.pone.0079416
Fu, R., Shen, Q., Xu, P., Luo, J. J., and Tang, Y. (2014). Phagocytosis of microglia in the central nervous system diseases. Mol. Neurobiol. 49, 1422–1434. doi:10.1007/s12035-013-8620-6
Gabbert, L., Dilling, C., Meybohm, P., and Burek, M. (2020). Deletion of protocadherin gamma C3 induces phenotypic and functional changes in brain microvascular endothelial cells in vitro. Front. Pharmacol. 11, 590144. doi:10.3389/fphar.2020.590144
Geng, H., Song, Q., Cheng, Y., Li, H., Yang, R., Liu, S., et al. (2020). MicroRNA 322 aggravates dexamethasone-induced muscle atrophy by targeting IGF1R and INSR. Int. J. Mol. Sci. 21, E1111. doi:10.3390/ijms21031111
Haenisch, S., Werk, A. N., and Cascorbi, I. (2014). MicroRNAs and their relevance to ABC transporters. Br. J. Clin. Pharmacol. 77, 587–596. doi:10.1111/bcp.12251
Halder, S. K., Kant, R., and Milner, R. (2018). Hypoxic pre-conditioning suppresses experimental autoimmune encephalomyelitis by modifying multiple properties of blood vessels. Acta Neuropathol. Commun. 6, 86. doi:10.1186/s40478-018-0590-5
Hall, C. N., Reynell, C., Gesslein, B., Hamilton, N. B., Mishra, A., Sutherland, B. A., et al. (2014). Capillary pericytes regulate cerebral blood flow in health and disease. Nature 508, 55–60. doi:10.1038/nature13165
Hammad, S., Mabondzo, A., Hamoudi, R., and Harati, R. (2022). Regulation of P-glycoprotein by miR-27a-3p at the brain endothelial barrier. J. Pharm. Sci. 111, 1470–1479. doi:10.1016/j.xphs.2021.10.021
Hamzei Taj, S., Kho, W., Riou, A., Wiedermann, D., and Hoehn, M. (2016). MiRNA-124 induces neuroprotection and functional improvement after focal cerebral ischemia. Biomaterials 91, 151–165. doi:10.1016/j.biomaterials.2016.03.025
Hamzei Taj, S., Kho, W., Aswendt, M., Collmann, F. M., Green, C., Adamczak, J., et al. (2016). Dynamic modulation of microglia/macrophage polarization by miR-124 after focal cerebral ischemia. J. Neuroimmune Pharmacol. 11, 733–748. doi:10.1007/s11481-016-9700-y
Hao, J., and Bickel, U. (2013). Transferrin receptor mediated brain uptake during ischemia and reperfusion. J. Pharm. Pharm. Sci. 16, 541–550. doi:10.18433/j3b303
Harry, G. J. (2013). Microglia during development and aging. Pharmacol. Ther. 139, 313–326. doi:10.1016/j.pharmthera.2013.04.013
Helms, H. C., Abbott, N. J., Burek, M., Cecchelli, R., Couraud, P. O., Deli, M. A., et al. (2016). In vitro models of the blood-brain barrier: An overview of commonly used brain endothelial cell culture models and guidelines for their use. J. Cereb. Blood Flow. Metab. 36, 862–890. doi:10.1177/0271678X16630991
Herzog, C., Pons Garcia, L., Keatinge, M., Greenald, D., Moritz, C., Peri, F., et al. (2019). Rapid clearance of cellular debris by microglia limits secondary neuronal cell death after brain injury in vivo. Development 146, dev174698. doi:10.1242/dev.174698
Hicks, S. D., Johnson, J., Carney, M. C., Bramley, H., Olympia, R. P., Loeffert, A. C., et al. (2018). Overlapping MicroRNA expression in saliva and cerebrospinal fluid accurately identifies pediatric traumatic brain injury. J. Neurotrauma 35, 64–72. doi:10.1089/neu.2017.5111
Hladky, S. B., and Barrand, M. A. (2018). Elimination of substances from the brain parenchyma: efflux via perivascular pathways and via the blood-brain barrier. Fluids Barriers CNS 15, 30. doi:10.1186/s12987-018-0113-6
Hoshi, Y., Uchida, Y., Tachikawa, M., Inoue, T., Ohtsuki, S., and Terasaki, T. (2013). Quantitative atlas of blood-brain barrier transporters, receptors, and tight junction proteins in rats and common marmoset. J. Pharm. Sci. 102, 3343–3355. doi:10.1002/jps.23575
Huang, S., Ge, X., Yu, J., Han, Z., Yin, Z., Li, Y., et al. (2018). Increased miR-124-3p in microglial exosomes following traumatic brain injury inhibits neuronal inflammation and contributes to neurite outgrowth via their transfer into neurons. FASEB J. 32, 512–528. doi:10.1096/fj.201700673R
Huang, Y., Chen, S., Luo, Y., and Han, Z. (2020). Crosstalk between inflammation and the BBB in stroke. Curr. Neuropharmacol. 18, 1227–1236. doi:10.2174/1570159X18666200620230321
Ittner, C., Burek, M., Stork, S., Nagai, M., and Forster, C. Y. (2020). Increased catecholamine levels and inflammatory mediators alter barrier properties of brain microvascular endothelial cells in vitro. Front. Cardiovasc. Med. 7, 73. doi:10.3389/fcvm.2020.00073
Jiang, X., Andjelkovic, A. V., Zhu, L., Yang, T., Bennett, M. V. L., Chen, J., et al. (2018). Blood-brain barrier dysfunction and recovery after ischemic stroke. Prog. Neurobiol. 163-164, 144–171. doi:10.1016/j.pneurobio.2017.10.001
Kalogeris, T., Baines, C. P., Krenz, M., and Korthuis, R. J. (2012). Cell biology of ischemia/reperfusion injury. Int. Rev. Cell Mol. Biol. 298, 229–317. doi:10.1016/B978-0-12-394309-5.00006-7
Kang, R., Gamdzyk, M., Lenahan, C., Tang, J., Tan, S., and Zhang, J. H. (2020). The dual role of microglia in blood-brain barrier dysfunction after stroke. Curr. Neuropharmacol. 18, 1237–1249. doi:10.2174/1570159X18666200529150907
Keaney, J., Walsh, D. M., O'Malley, T., Hudson, N., Crosbie, D. E., Loftus, T., et al. (2015). Autoregulated paracellular clearance of amyloid-beta across the blood-brain barrier. Sci. Adv. 1, e1500472. doi:10.1126/sciadv.1500472
Keiper, T., Santoso, S., Nawroth, P. P., Orlova, V., and Chavakis, T. (2005). The role of junctional adhesion molecules in cell-cell interactions. Histol. Histopathol. 20, 197–203. doi:10.14670/HH-20.197
Kettenmann, H., Hanisch, U. K., Noda, M., and Verkhratsky, A. (2011). Physiology of microglia. Physiol. Rev. 91, 461–553. doi:10.1152/physrev.00011.2010
Kilic, E., Spudich, A., Kilic, U., Rentsch, K. M., Vig, R., Matter, C. M., et al. (2008). ABCC1: a gateway for pharmacological compounds to the ischaemic brain. Brain 131, 2679–2689. doi:10.1093/brain/awn222
Kindrat, I., Tryndyak, V., de Conti, A., Shpyleva, S., Mudalige, T. K., Kobets, T., et al. (2016). MicroRNA-152-mediated dysregulation of hepatic transferrin receptor 1 in liver carcinogenesis. Oncotarget 7, 1276–1287. doi:10.18632/oncotarget.6004
Kirkley, K. S., Popichak, K. A., Afzali, M. F., Legare, M. E., and Tjalkens, R. B. (2017). Microglia amplify inflammatory activation of astrocytes in manganese neurotoxicity. J. Neuroinflammation 14, 99. doi:10.1186/s12974-017-0871-0
Kleinschnitz, C., Blecharz, K., Kahles, T., Schwarz, T., Kraft, P., Gobel, K., et al. (2011). Glucocorticoid insensitivity at the hypoxic blood-brain barrier can be reversed by inhibition of the proteasome. Stroke 42, 1081–1089. doi:10.1161/STROKEAHA.110.592238
Lambertsen, K. L., Biber, K., and Finsen, B. (2012). Inflammatory cytokines in experimental and human stroke. J. Cereb. Blood Flow. Metab. 32, 1677–1698. doi:10.1038/jcbfm.2012.88
Lan, X., Han, X., Li, Q., Yang, Q. W., and Wang, J. (2017). Modulators of microglial activation and polarization after intracerebral haemorrhage. Nat. Rev. Neurol. 13, 420–433. doi:10.1038/nrneurol.2017.69
Larsen, B. R., and MacAulay, N. (2017). Activity-dependent astrocyte swelling is mediated by pH-regulating mechanisms. Glia 65, 1668–1681. doi:10.1002/glia.23187
Li, Y. N., Pan, R., Qin, X. J., Yang, W. L., Qi, Z., Liu, W., et al. (2014). Ischemic neurons activate astrocytes to disrupt endothelial barrier via increasing VEGF expression. J. Neurochem. 129, 120–129. doi:10.1111/jnc.12611
Li, H., Han, G., He, D., Wang, Y., Lin, Y., Zhang, T., et al. (2021). miR-539 targeting SNAI2 regulates MMP9 signaling pathway and affects blood-brain barrier permeability in cerebrovascular occlusive diseases: A study based on head and neck ultrasound and CTA. J. Healthc. Eng. 2021, 1–8. doi:10.1155/2021/5699025
Lian, L., Zhang, Y., Liu, L., Yang, L., Cai, Y., Zhang, J., et al. (2020). Neuroinflammation in ischemic stroke: Focus on MicroRNA-mediated polarization of microglia. Front. Mol. Neurosci. 13, 612439. doi:10.3389/fnmol.2020.612439
Liebner, S., Dijkhuizen, R. M., Reiss, Y., Plate, K. H., Agalliu, D., and Constantin, G. (2018). Functional morphology of the blood-brain barrier in health and disease. Acta Neuropathol. 135, 311–336. doi:10.1007/s00401-018-1815-1
Lin, M., Zhu, L., Wang, J., Xue, Y., and Shang, X. (2019). miR-424-5p maybe regulate blood-brain barrier permeability in a model in vitro with Abeta incubated endothelial cells. Biochem. Biophys. Res. Commun. 517, 525–531. doi:10.1016/j.bbrc.2019.07.075
Lindner, C., Sigruner, A., Walther, F., Bogdahn, U., Couraud, P. O., Schmitz, G., et al. (2012). ATP-binding cassette transporters in immortalised human brain microvascular endothelial cells in normal and hypoxic conditions. Exp. Transl. Stroke Med. 4, 9. doi:10.1186/2040-7378-4-9
Liu da, Z., Jickling, G. C., Ander, B. P., Hull, H., Zhan, X., Cox, C., et al. (2016). Elevating microRNA-122 in blood improves outcomes after temporary middle cerebral artery occlusion in rats. J. Cereb. Blood Flow. Metab. 36, 1374–1383. doi:10.1177/0271678X15610786
Liu, S., Agalliu, D., Yu, C., and Fisher, M. (2012). The role of pericytes in blood-brain barrier function and stroke. Curr. Pharm. Des. 18, 3653–3662. doi:10.2174/138161212802002706
Liu, M., Gao, J., Huang, Q., Jin, Y., and Wei, Z. (2016). Downregulating microRNA-144 mediates a metabolic shift in lung cancer cells by regulating GLUT1 expression. Oncol. Lett. 11, 3772–3776. doi:10.3892/ol.2016.4468
Liu, L. R., Liu, J. C., Bao, J. S., Bai, Q. Q., and Wang, G. Q. (2020). Interaction of microglia and astrocytes in the neurovascular unit. Front. Immunol. 11, 1024. doi:10.3389/fimmu.2020.01024
Loike, J. D., Cao, L., Brett, J., Ogawa, S., Silverstein, S. C., and Stern, D. (1992). Hypoxia induces glucose transporter expression in endothelial cells. Am. J. Physiol. 263, C326–C333. doi:10.1152/ajpcell.1992.263.2.C326
Lopes-Rodrigues, V., Seca, H., Sousa, D., Sousa, E., Lima, R. T., and Vasconcelos, M. H. (2014). The network of P-glycoprotein and microRNAs interactions. Int. J. Cancer 135, 253–263. doi:10.1002/ijc.28500
Loscher, W., and Potschka, H. (2005). Blood-brain barrier active efflux transporters: ATP-binding cassette gene family. NeuroRx 2, 86–98. doi:10.1602/neurorx.2.1.86
Lou, Y. L., Guo, F., Liu, F., Gao, F. L., Zhang, P. Q., Niu, X., et al. (2012). miR-210 activates notch signaling pathway in angiogenesis induced by cerebral ischemia. Mol. Cell. Biochem. 370, 45–51. doi:10.1007/s11010-012-1396-6
Ma, Q., Dasgupta, C., Li, Y., Huang, L., and Zhang, L. (2017). MicroRNA-210 suppresses junction proteins and disrupts blood-brain barrier integrity in neonatal rat hypoxic-ischemic brain injury. Int. J. Mol. Sci. 18, E1356. doi:10.3390/ijms18071356
MacAulay, N., and Zeuthen, T. (2010). Water transport between CNS compartments: contributions of aquaporins and cotransporters. Neuroscience 168, 941–956. doi:10.1016/j.neuroscience.2009.09.016
Manley, G. T., Fujimura, M., Ma, T., Noshita, N., Filiz, F., Bollen, A. W., et al. (2000). Aquaporin-4 deletion in mice reduces brain edema after acute water intoxication and ischemic stroke. Nat. Med. 6, 159–163. doi:10.1038/72256
Mao, S., Zheng, S., Lu, Z., Wang, X., Wang, Y., Zhang, G., et al. (2021). Exosomal miR-375-3p breaks vascular barrier and promotes small cell lung cancer metastasis by targeting claudin-1. Transl. Lung Cancer Res. 10, 3155–3172. doi:10.21037/tlcr-21-356
Matsumoto, J., Dohgu, S., Takata, F., Nishioku, T., Sumi, N., Machida, T., et al. (2012). Lipopolysaccharide-activated microglia lower P-glycoprotein function in brain microvascular endothelial cells. Neurosci. Lett. 524, 45–48. doi:10.1016/j.neulet.2012.07.004
Medina, R. J., O'Neill, C. L., O'Doherty, T. M., Knott, H., Guduric-Fuchs, J., Gardiner, T. A., et al. (2011). Myeloid angiogenic cells act as alternative M2 macrophages and modulate angiogenesis through interleukin-8. Mol. Med. 17, 1045–1055. doi:10.2119/molmed.2011.00129
Miller, D. S. (2015). Regulation of ABC transporters at the blood-brain barrier. Clin. Pharmacol. Ther. 97, 395–403. doi:10.1002/cpt.64
Mitra, B., Rau, T. F., Surendran, N., Brennan, J. H., Thaveenthiran, P., Sorich, E., et al. (2017). Plasma micro-RNA biomarkers for diagnosis and prognosis after traumatic brain injury: A pilot study. J. Clin. Neurosci. 38, 37–42. doi:10.1016/j.jocn.2016.12.009
Miyazawa, M., Bogdan, A. R., Hashimoto, K., and Tsuji, Y. (2018). Regulation of transferrin receptor-1 mRNA by the interplay between IRE-binding proteins and miR-7/miR-141 in the 3'-IRE stem-loops. RNA 24, 468–479. doi:10.1261/rna.063941.117
Moon, J. M., Xu, L., and Giffard, R. G. (2013). Inhibition of microRNA-181 reduces forebrain ischemia-induced neuronal loss. J. Cereb. Blood Flow. Metab. 33, 1976–1982. doi:10.1038/jcbfm.2013.157
Mun-Bryce, S., and Rosenberg, G. A. (1998). Matrix metalloproteinases in cerebrovascular disease. J. Cereb. Blood Flow. Metab. 18, 1163–1172. doi:10.1097/00004647-199811000-00001
Murakami, T., Felinski, E. A., and Antonetti, D. A. (2009). Occludin phosphorylation and ubiquitination regulate tight junction trafficking and vascular endothelial growth factor-induced permeability. J. Biol. Chem. 284, 21036–21046. doi:10.1074/jbc.M109.016766
Nagelhus, E. A., and Ottersen, O. P. (2013). Physiological roles of aquaporin-4 in brain. Physiol. Rev. 93, 1543–1562. doi:10.1152/physrev.00011.2013
Nakagomi, T., Kubo, S., Nakano-Doi, A., Sakuma, R., Lu, S., Narita, A., et al. (2015). Brain vascular pericytes following ischemia have multipotential stem cell activity to differentiate into neural and vascular lineage cells. Stem Cells 33, 1962–1974. doi:10.1002/stem.1977
Neuhaus, W., Burek, M., Djuzenova, C. S., Thal, S. C., Koepsell, H., Roewer, N., et al. (2012). Addition of NMDA-receptor antagonist MK801 during oxygen/glucose deprivation moderately attenuates the upregulation of glucose uptake after subsequent reoxygenation in brain endothelial cells. Neurosci. Lett. 506, 44–49. doi:10.1016/j.neulet.2011.10.045
Neuhaus, W., Gaiser, F., Mahringer, A., Franz, J., Riethmuller, C., and Forster, C. (2014). The pivotal role of astrocytes in an in vitro stroke model of the blood-brain barrier. Front. Cell. Neurosci. 8, 352. doi:10.3389/fncel.2014.00352
Ni, J., Wang, X., Chen, S., Liu, H., Wang, Y., Xu, X., et al. (2015). MicroRNA let-7c-5p protects against cerebral ischemia injury via mechanisms involving the inhibition of microglia activation. Brain Behav. Immun. 49, 75–85. doi:10.1016/j.bbi.2015.04.014
Nilles, K. L., Williams, E. I., Betterton, R. D., Davis, T. P., and Ronaldson, P. T. (2022). Blood-brain barrier transporters: Opportunities for therapeutic development in ischemic stroke. Int. J. Mol. Sci. 23, 1898. doi:10.3390/ijms23031898
Nishioku, T., Matsumoto, J., Dohgu, S., Sumi, N., Miyao, K., Takata, F., et al. (2010). Tumor necrosis factor-alpha mediates the blood-brain barrier dysfunction induced by activated microglia in mouse brain microvascular endothelial cells. J. Pharmacol. Sci. 112, 251–254. doi:10.1254/jphs.09292sc
Obermeier, B., Daneman, R., and Ransohoff, R. M. (2013). Development, maintenance and disruption of the blood-brain barrier. Nat. Med. 19, 1584–1596. doi:10.1038/nm.3407
Ouyang, Y. B., Lu, Y., Yue, S., Xu, L. J., Xiong, X. X., White, R. E., et al. (2012). miR-181 regulates GRP78 and influences outcome from cerebral ischemia in vitro and in vivo. Neurobiol. Dis. 45, 555–563. doi:10.1016/j.nbd.2011.09.012
Ouyang, Y. B., Lu, Y., Yue, S., and Giffard, R. G. (2012). miR-181 targets multiple Bcl-2 family members and influences apoptosis and mitochondrial function in astrocytes. Mitochondrion 12, 213–219. doi:10.1016/j.mito.2011.09.001
Ouyang, Y. B., Xu, L., Lu, Y., Sun, X., Yue, S., Xiong, X. X., et al. (2013). Astrocyte-enriched miR-29a targets PUMA and reduces neuronal vulnerability to forebrain ischemia. Glia 61, 1784–1794. doi:10.1002/glia.22556
Ozen, I., Deierborg, T., Miharada, K., Padel, T., Englund, E., Genove, G., et al. (2014). Brain pericytes acquire a microglial phenotype after stroke. Acta Neuropathol. 128, 381–396. doi:10.1007/s00401-014-1295-x
Oztop-Cakmak, O., Solaroglu, I., and Gursoy-Ozdemir, Y. (2017). The role of pericytes in neurovascular unit: Emphasis on stroke. Curr. Drug Targets 18, 1386–1391. doi:10.2174/1389450117666160613104523
Pan, Q., He, C., Liu, H., Liao, X., Dai, B., Chen, Y., et al. (2016). Microvascular endothelial cells-derived microvesicles imply in ischemic stroke by modulating astrocyte and blood brain barrier function and cerebral blood flow. Mol. Brain 9, 63. doi:10.1186/s13041-016-0243-1
Pardridge, W. M. (2012). Drug transport across the blood-brain barrier. J. Cereb. Blood Flow. Metab. 32, 1959–1972. doi:10.1038/jcbfm.2012.126
Patabendige, A., Singh, A., Jenkins, S., Sen, J., and Chen, R. (2021). Astrocyte activation in neurovascular damage and repair following ischaemic stroke. Int. J. Mol. Sci. 22, 4280. doi:10.3390/ijms22084280
Patak, P., Jin, F., Schafer, S. T., Metzen, E., and Hermann, D. M. (2011). The ATP-binding cassette transporters ABCB1 and ABCC1 are not regulated by hypoxia in immortalised human brain microvascular endothelial cells. Exp. Transl. Stroke Med. 3, 12. doi:10.1186/2040-7378-3-12
Pena-Philippides, J. C., Gardiner, A. S., Caballero-Garrido, E., Pan, R., Zhu, Y., and Roitbak, T. (2018). Inhibition of MicroRNA-155 supports endothelial tight junction integrity following oxygen-glucose deprivation. J. Am. Heart Assoc. 7, e009244. doi:10.1161/JAHA.118.009244
Persidsky, Y., Hill, J., Zhang, M., Dykstra, H., Winfield, M., Reichenbach, N. L., et al. (2016). Dysfunction of brain pericytes in chronic neuroinflammation. J. Cereb. Blood Flow. Metab. 36, 794–807. doi:10.1177/0271678X15606149
Petr, G. T., Sun, Y., Frederick, N. M., Zhou, Y., Dhamne, S. C., Hameed, M. Q., et al. (2015). Conditional deletion of the glutamate transporter GLT-1 reveals that astrocytic GLT-1 protects against fatal epilepsy while neuronal GLT-1 contributes significantly to glutamate uptake into synaptosomes. J. Neurosci. 35, 5187–5201. doi:10.1523/JNEUROSCI.4255-14.2015
Prakash, R., and Carmichael, S. T. (2015). Blood-brain barrier breakdown and neovascularization processes after stroke and traumatic brain injury. Curr. Opin. Neurol. 28, 556–564. doi:10.1097/WCO.0000000000000248
Qiang, J., Zhu, X. W., He, J., Tao, Y. F., Bao, J. W., Zhu, J. H., et al. (2020). miR-34a regulates the activity of HIF-1a and P53 signaling pathways by promoting GLUT1 in genetically improved farmed Tilapia (GIFT, Oreochromis niloticus) under hypoxia stress. Front. Physiol. 11, 670. doi:10.3389/fphys.2020.00670
Rabquer, B. J., Amin, M. A., Teegala, N., Shaheen, M. K., Tsou, P. S., Ruth, J. H., et al. (2010). Junctional adhesion molecule-C is a soluble mediator of angiogenesis. J. Immunol. 185, 1777–1785. doi:10.4049/jimmunol.1000556
Ren, S., Wu, G., Huang, Y., Wang, L., Li, Y., and Zhang, Y. (2021). MiR-18a aggravates intracranial hemorrhage by regulating RUNX1-occludin/ZO-1 Axis to increase BBB permeability. J. Stroke Cerebrovasc. Dis. 30, 105878. doi:10.1016/j.jstrokecerebrovasdis.2021.105878
Rochfort, K. D., and Cummins, P. M. (2015). The blood-brain barrier endothelium: a target for pro-inflammatory cytokines. Biochem. Soc. Trans. 43, 702–706. doi:10.1042/BST20140319
Rodriguez-Gomez, J. A., Kavanagh, E., Engskog-Vlachos, P., Engskog, M. K. R., Herrera, A. J., Espinosa-Oliva, A. M., et al. (2020). Microglia: Agents of the CNS pro-inflammatory response. Cells 9, E1717. doi:10.3390/cells9071717
Rosenberg, P. A., Amin, S., and Leitner, M. (1992). Glutamate uptake disguises neurotoxic potency of glutamate agonists in cerebral cortex in dissociated cell culture. J. Neurosci. 12, 56–61. doi:10.1523/jneurosci.12-01-00056.1992
Rosing, N., Salvador, E., Guntzel, P., Kempe, C., Burek, M., Holzgrabe, U., et al. (2020). Neuroprotective effects of isosteviol sodium in murine brain capillary cerebellar endothelial cells (cerebEND) after hypoxia. Front. Cell. Neurosci. 14, 573950. doi:10.3389/fncel.2020.573950
Rymo, S. F., Gerhardt, H., Wolfhagen Sand, F., Lang, R., Uv, A., and Betsholtz, C. (2011). A two-way communication between microglial cells and angiogenic sprouts regulates angiogenesis in aortic ring cultures. PLoS One 6, e15846. doi:10.1371/journal.pone.0015846
Sakuma, R., Kawahara, M., Nakano-Doi, A., Takahashi, A., Tanaka, Y., Narita, A., et al. (2016). Brain pericytes serve as microglia-generating multipotent vascular stem cells following ischemic stroke. J. Neuroinflammation 13, 57. doi:10.1186/s12974-016-0523-9
Salvador, E., Burek, M., and Forster, C. Y. (2015). Stretch and/or oxygen glucose deprivation (OGD) in an in vitro traumatic brain injury (TBI) model induces calcium alteration and inflammatory cascade. Front. Cell. Neurosci. 9, 323. doi:10.3389/fncel.2015.00323
Schaar, D. G., Medina, D. J., Moore, D. F., Strair, R. K., and Ting, Y. (2009). miR-320 targets transferrin receptor 1 (CD71) and inhibits cell proliferation. Exp. Hematol. 37, 245–255. doi:10.1016/j.exphem.2008.10.002
Schober, A. (2008). Chemokines in vascular dysfunction and remodeling. Arterioscler. Thromb. Vasc. Biol. 28, 1950–1959. doi:10.1161/ATVBAHA.107.161224
Sepramaniam, S., Armugam, A., Lim, K. Y., Karolina, D. S., Swaminathan, P., Tan, J. R., et al. (2010). MicroRNA 320a functions as a novel endogenous modulator of aquaporins 1 and 4 as well as a potential therapeutic target in cerebral ischemia. J. Biol. Chem. 285, 29223–29230. doi:10.1074/jbc.M110.144576
Sepramaniam, S., Ying, L. K., Armugam, A., Wintour, E. M., and Jeyaseelan, K. (2012). MicroRNA-130a represses transcriptional activity of aquaporin 4 M1 promoter. J. Biol. Chem. 287, 12006–12015. doi:10.1074/jbc.M111.280701
Shen, X. Y., Gao, Z. K., Han, Y., Yuan, M., Guo, Y. S., and Bi, X. (2021). Activation and role of astrocytes in ischemic stroke. Front. Cell. Neurosci. 15, 755955. doi:10.3389/fncel.2021.755955
Shi, H., and Liu, K. J. (2007). Cerebral tissue oxygenation and oxidative brain injury during ischemia and reperfusion. Front. Biosci. 12, 1318–1328. doi:10.2741/2150
Sierra, A., Encinas, J. M., Deudero, J. J., Chancey, J. H., Enikolopov, G., Overstreet-Wadiche, L. S., et al. (2010). Microglia shape adult hippocampal neurogenesis through apoptosis-coupled phagocytosis. Cell Stem Cell 7, 483–495. doi:10.1016/j.stem.2010.08.014
Sladojevic, N., Stamatovic, S. M., Johnson, A. M., Choi, J., Hu, A., Dithmer, S., et al. (2019). Claudin-1-Dependent destabilization of the blood-brain barrier in chronic stroke. J. Neurosci. 39, 743–757. doi:10.1523/JNEUROSCI.1432-18.2018
Song, H., and Bu, G. (2009). MicroRNA-205 inhibits tumor cell migration through down-regulating the expression of the LDL receptor-related protein 1. Biochem. Biophys. Res. Commun. 388, 400–405. doi:10.1016/j.bbrc.2009.08.020
Spudich, A., Kilic, E., Xing, H., Kilic, U., Rentsch, K. M., Wunderli-Allenspach, H., et al. (2006). Inhibition of multidrug resistance transporter-1 facilitates neuroprotective therapies after focal cerebral ischemia. Nat. Neurosci. 9, 487–488. doi:10.1038/nn1676
Stamatovic, S. M., Johnson, A. M., Keep, R. F., and Andjelkovic, A. V. (2016). Junctional proteins of the blood-brain barrier: New insights into function and dysfunction. Tissue Barriers 4, e1154641. doi:10.1080/21688370.2016.1154641
Stamatovic, S. M., Johnson, A. M., Sladojevic, N., Keep, R. F., and Andjelkovic, A. V. (2017). Endocytosis of tight junction proteins and the regulation of degradation and recycling. Ann. N. Y. Acad. Sci. 1397, 54–65. doi:10.1111/nyas.13346
Stark, K., Eckart, A., Haidari, S., Tirniceriu, A., Lorenz, M., von Bruhl, M. L., et al. (2013). Capillary and arteriolar pericytes attract innate leukocytes exiting through venules and 'instruct' them with pattern-recognition and motility programs. Nat. Immunol. 14, 41–51. doi:10.1038/ni.2477
Stary, C. M., Xu, L., Li, L., Sun, X., Ouyang, Y. B., Xiong, X., et al. (2017). Inhibition of miR-181a protects female mice from transient focal cerebral ischemia by targeting astrocyte estrogen receptor-α. Mol. Cell. Neurosci. 82, 118–125. doi:10.1016/j.mcn.2017.05.004
Sun, P., Liu, D. Z., Jickling, G. C., Sharp, F. R., and Yin, K. J. (2018). MicroRNA-based therapeutics in central nervous system injuries. J. Cereb. Blood Flow. Metab. 38, 1125–1148. doi:10.1177/0271678X18773871
Sundstrom, J. M., Tash, B. R., Murakami, T., Flanagan, J. M., Bewley, M. C., Stanley, B. A., et al. (2009). Identification and analysis of occludin phosphosites: a combined mass spectrometry and bioinformatics approach. J. Proteome Res. 8, 808–817. doi:10.1021/pr7007913
Sung, H. Y., Choi, E. N., Han, J., Chae, Y. J., Im, S. W., Kim, H. S., et al. (2021). Protective role of ABCA1 in ischemic preconditioning is mediated by downregulation of miR-33-5p and miR-135-5p. Sci. Rep. 11, 12511. doi:10.1038/s41598-021-91982-x
Sweeney, M. D., Zhao, Z., Montagne, A., Nelson, A. R., and Zlokovic, B. V. (2019). Blood-brain barrier: from physiology to disease and back. Physiol. Rev. 99, 21–78. doi:10.1152/physrev.00050.2017
Sykova, E. (2001). Glial diffusion barriers during aging and pathological states. Prog. Brain Res. 132, 339–363. doi:10.1016/S0079-6123(01)32087-3
Tashima, T. (2022). Brain cancer chemotherapy through a delivery system across the blood-brain barrier into the brain based on receptor-mediated transcytosis using monoclonal antibody conjugates. Biomedicines 10, 1597. doi:10.3390/biomedicines10071597
Tian, Y., Zhu, P., Liu, S., Jin, Z., Li, D., Zhao, H., et al. (2019). IL-4-polarized BV2 microglia cells promote angiogenesis by secreting exosomes. Adv. Clin. Exp. Med. 28, 421–430. doi:10.17219/acem/91826
Tiemin, P., Peng, X., Qingfu, L., Yan, W., Junlin, X., Zhefeng, H., et al. (2020). Dysregulation of the miR-148a-GLUT1 axis promotes the progression and chemoresistance of human intrahepatic cholangiocarcinoma. Oncogenesis 9, 19. doi:10.1038/s41389-020-0207-2
Tietz, S., and Engelhardt, B. (2015). Brain barriers: Crosstalk between complex tight junctions and adherens junctions. J. Cell Biol. 209, 493–506. doi:10.1083/jcb.201412147
Tornabene, E., and Brodin, B. (2016). Stroke and drug delivery--in vitro models of the ischemic blood-brain barrier. J. Pharm. Sci. 105, 398–405. doi:10.1016/j.xphs.2015.11.041
Tornabene, E., Helms, H. C. C., Pedersen, S. F., and Brodin, B. (2019). Effects of oxygen-glucose deprivation (OGD) on barrier properties and mRNA transcript levels of selected marker proteins in brain endothelial cells/astrocyte co-cultures. PLoS One 14, e0221103. doi:10.1371/journal.pone.0221103
Tourdias, T., Mori, N., Dragonu, I., Cassagno, N., Boiziau, C., Aussudre, J., et al. (2011). Differential aquaporin 4 expression during edema build-up and resolution phases of brain inflammation. J. Neuroinflammation 8, 143. doi:10.1186/1742-2094-8-143
Vasudeva, K., and Munshi, A. (2020). miRNA dysregulation in ischaemic stroke: Focus on diagnosis, prognosis, therapeutic and protective biomarkers. Eur. J. Neurosci. 52, 3610–3627. doi:10.1111/ejn.14695
Vemula, S., Roder, K. E., Yang, T., Bhat, G. J., Thekkumkara, T. J., and Abbruscato, T. J. (2009). A functional role for sodium-dependent glucose transport across the blood-brain barrier during oxygen glucose deprivation. J. Pharmacol. Exp. Ther. 328, 487–495. doi:10.1124/jpet.108.146589
Wan, Y., Jin, H. J., Zhu, Y. Y., Fang, Z., Mao, L., He, Q., et al. (2018). MicroRNA-149-5p regulates blood-brain barrier permeability after transient middle cerebral artery occlusion in rats by targeting S1PR2 of pericytes. FASEB J. 32, 3133–3148. doi:10.1096/fj.201701121R
Wang, Y., Huang, J., Ma, Y., Tang, G., Liu, Y., Chen, X., et al. (2015). MicroRNA-29b is a therapeutic target in cerebral ischemia associated with aquaporin 4. J. Cereb. Blood Flow. Metab. 35, 1977–1984. doi:10.1038/jcbfm.2015.156
Wang, J., Xu, Z., Chen, X., Li, Y., Chen, C., Wang, C., et al. (2018). MicroRNA-182-5p attenuates cerebral ischemia-reperfusion injury by targeting Toll-like receptor 4. Biochem. Biophys. Res. Commun. 505, 677–684. doi:10.1016/j.bbrc.2018.09.165
Wang, J., Nie, Z., Zhao, H., Gao, K., and Cao, Y. (2020). MiRNA-125a-5p attenuates blood-spinal cord barrier permeability under hypoxia in vitro. Biotechnol. Lett. 42, 25–34. doi:10.1007/s10529-019-02753-8
Wei, W., Zhang, W. Y., Bai, J. B., Zhang, H. X., Zhao, Y. Y., Li, X. Y., et al. (2016). The NF-κB-modulated microRNAs miR-195 and miR-497 inhibit myoblast proliferation by targeting Igf1r, Insr and cyclin genes. J. Cell Sci. 129, 39–50. doi:10.1242/jcs.174235
Willenborg, S., Lucas, T., van Loo, G., Knipper, J. A., Krieg, T., Haase, I., et al. (2012). CCR2 recruits an inflammatory macrophage subpopulation critical for angiogenesis in tissue repair. Blood 120, 613–625. doi:10.1182/blood-2012-01-403386
Wright, E. M., and Turk, E. (2004). The sodium/glucose cotransport family SLC5. Pflugers Arch. 447, 510–518. doi:10.1007/s00424-003-1063-6
Wyss, L., Schafer, J., Liebner, S., Mittelbronn, M., Deutsch, U., Enzmann, G., et al. (2012). Junctional adhesion molecule (JAM)-C deficient C57BL/6 mice develop a severe hydrocephalus. PLoS One 7, e45619. doi:10.1371/journal.pone.0045619
Xiang, B., Zhong, P., Fang, L., Wu, X., Song, Y., and Yuan, H. (2019). miR-183 inhibits microglia activation and expression of inflammatory factors in rats with cerebral ischemia reperfusion via NF-κB signaling pathway. Exp. Ther. Med. 18, 2540–2546. doi:10.3892/etm.2019.7827
Xu, L., Nirwane, A., and Yao, Y. (2019). Basement membrane and blood-brain barrier. Stroke Vasc. Neurol. 4, 78–82. doi:10.1136/svn-2018-000198
Xu, S. Y., Jiang, X. L., Liu, Q., Xu, J., Huang, J., Gan, S. W., et al. (2019). Role of rno-miR-124-3p in regulating MCT1 expression in rat brain after permanent focal cerebral ischemia. Genes Dis. 6, 398–406. doi:10.1016/j.gendis.2019.01.002
Xu, Y., Chai, B., Wang, X., Wu, Z., Gu, Z., Liu, X., et al. (2022). miRNA-199a-5p/SLC2A1 axis regulates glucose metabolism in non-small cell lung cancer. J. Cancer 13, 2352–2361. doi:10.7150/jca.67990
Xue, M., Song, M., Yan, D., Sun, S., Wang, Y., Fu, T., et al. (2022). Effect of SLC16A1 on hepatic glucose metabolism in newborn and post-weaned holstein bulls. Front. Genet. 13, 811849. doi:10.3389/fgene.2022.811849
Yamamoto, M., Ramirez, S. H., Sato, S., Kiyota, T., Cerny, R. L., Kaibuchi, K., et al. (2008). Phosphorylation of claudin-5 and occludin by rho kinase in brain endothelial cells. Am. J. Pathol. 172, 521–533. doi:10.2353/ajpath.2008.070076
Yan, Z., Deng, Y., Zou, Y., Liu, S., Li, K., Yang, J., et al. (2021). Analysis of regulatory effect of miR-149-5p on Sphingosine-1-phosphate receptor 2 of pericytes and its neuroprotective molecular mechanism after acute cerebral ischemia reperfusion in rats. Bioengineered 12, 3348–3357. doi:10.1080/21655979.2021.1947167
Yang, Y., Estrada, E. Y., Thompson, J. F., Liu, W., and Rosenberg, G. A. (2007). Matrix metalloproteinase-mediated disruption of tight junction proteins in cerebral vessels is reversed by synthetic matrix metalloproteinase inhibitor in focal ischemia in rat. J. Cereb. Blood Flow. Metab. 27, 697–709. doi:10.1038/sj.jcbfm.9600375
Yang, Y., Salayandia, V. M., Thompson, J. F., Yang, L. Y., Estrada, E. Y., and Yang, Y. (2015). Attenuation of acute stroke injury in rat brain by minocycline promotes blood-brain barrier remodeling and alternative microglia/macrophage activation during recovery. J. Neuroinflammation 12, 26. doi:10.1186/s12974-015-0245-4
Yang, X., Tang, X., Sun, P., Shi, Y., Liu, K., Hassan, S. H., et al. (2017). MicroRNA-15a/16-1 antagomir ameliorates ischemic brain injury in experimental stroke. Stroke 48, 1941–1947. doi:10.1161/STROKEAHA.117.017284
Yemisci, M., Gursoy-Ozdemir, Y., Vural, A., Can, A., Topalkara, K., and Dalkara, T. (2009). Pericyte contraction induced by oxidative-nitrative stress impairs capillary reflow despite successful opening of an occluded cerebral artery. Nat. Med. 15, 1031–1037. doi:10.1038/nm.2022
Yin, K. J., Hamblin, M., and Chen, Y. E. (2014). Non-coding RNAs in cerebral endothelial pathophysiology: emerging roles in stroke. Neurochem. Int. 77, 9–16. doi:10.1016/j.neuint.2014.03.013
Yu, H., Wu, M., Zhao, P., Huang, Y., Wang, W., and Yin, W. (2015). Neuroprotective effects of viral overexpression of microRNA-22 in rat and cell models of cerebral ischemia-reperfusion injury. J. Cell. Biochem. 116, 233–241. doi:10.1002/jcb.24960
Zajac, E., Schweighofer, B., Kupriyanova, T. A., Juncker-Jensen, A., Minder, P., Quigley, J. P., et al. (2013). Angiogenic capacity of M1- and M2-polarized macrophages is determined by the levels of TIMP-1 complexed with their secreted proMMP-9. Blood 122, 4054–4067. doi:10.1182/blood-2013-05-501494
Zeng, L., He, X., Wang, Y., Tang, Y., Zheng, C., Cai, H., et al. (2014). MicroRNA-210 overexpression induces angiogenesis and neurogenesis in the normal adult mouse brain. Gene Ther. 21, 37–43. doi:10.1038/gt.2013.55
Zhang, F., Vannucci, S. J., Philp, N. J., and Simpson, I. A. (2005). Monocarboxylate transporter expression in the spontaneous hypertensive rat: effect of stroke. J. Neurosci. Res. 79, 139–145. doi:10.1002/jnr.20312
Zhao, H., Wang, J., Gao, L., Wang, R., Liu, X., Gao, Z., et al. (2013). MiRNA-424 protects against permanent focal cerebral ischemia injury in mice involving suppressing microglia activation. Stroke 44, 1706–1713. doi:10.1161/STROKEAHA.111.000504
Zhao, H., Zheng, T., Yang, X., Fan, M., Zhu, L., Liu, S., et al. (2019). Cryptotanshinone attenuates oxygen-glucose deprivation/recovery-induced injury in an in vitro model of neurovascular unit. Front. Neurol. 10, 381. doi:10.3389/fneur.2019.00381
Zheng, Y., Wang, L., Chen, M., Pei, A., Xie, L., and Zhu, S. (2017). Upregulation of miR-130b protects against cerebral ischemic injury by targeting water channel protein aquaporin 4 (AQP4). Am. J. Transl. Res. 9 (7), 3452–3461.
Keywords: blood-brain barrier, microRNA, stroke, traumatic brain injury, tight junctions, transporter
Citation: Sun A, Blecharz-Lang KG, Małecki A, Meybohm P, Nowacka-Chmielewska MM and Burek M (2022) Role of microRNAs in the regulation of blood-brain barrier function in ischemic stroke and under hypoxic conditions in vitro. Front. Drug. Deliv. 2:1027098. doi: 10.3389/fddev.2022.1027098
Received: 24 August 2022; Accepted: 20 October 2022;
Published: 01 November 2022.
Edited by:
Xavier Decleces, Université Paris Cité, FranceReviewed by:
Irina Vazquez Villasenor, The University of Sheffield, United KingdomMabondzo Aloïse, Commissariat à l’Energie Atomique et aux Energies Alternatives (CEA), France
Copyright © 2022 Sun, Blecharz-Lang, Małecki, Meybohm, Nowacka-Chmielewska and Burek. This is an open-access article distributed under the terms of the Creative Commons Attribution License (CC BY). The use, distribution or reproduction in other forums is permitted, provided the original author(s) and the copyright owner(s) are credited and that the original publication in this journal is cited, in accordance with accepted academic practice. No use, distribution or reproduction is permitted which does not comply with these terms.
*Correspondence: Marta M. Nowacka-Chmielewska, bS5ub3dhY2thQGF3Zi5rYXRvd2ljZS5wbA==; Malgorzata Burek, QnVyZWtfTUB1a3cuZGU=
†These authors have contributed equally to this work and share last authorship