- 1Laboratory of Experimental and Applied Exercise Physiology, Biomedical Institute, Department of Physiology and Pharmacology, Fluminense Federal University (UFF), Niterói, Brazil
- 2Department of Clinical Sciences and Community Health, University of Milan, Milan, Italy
- 3Department of Physiology, Paulista School of Medicine, Federal University of São Paulo (UNIFESP), São Paulo, Brazil
Introduction: Males and females differ anatomically and functionally in cardiorespiratory regulation, with males tending to experience greater oxygen desaturation under hypoxia. Therefore, sex might moderate cardiorespiratory responses to acute hypoxia exposure. Accordingly, we hypothesized that sex differences in cardiovascular and ventilatory responses would be more pronounced with equal hypoxia duration (iso-time) but less pronounced at similar oxygen desaturation levels (iso-saturation).
Methods: Twenty-two (12 females) healthy individuals were exposed to normoxia (10 min at FiO2 = 0.21) and hypoxia (10 min at FiO2 = 0.115), respectively. Pulse oxygen saturation (SpO2), R-R intervals, cardiac output, blood pressure (BP), and ventilatory data were continuously recorded during spontaneous breathing. Spectral analysis of R-R intervals and systolic BP revealed cardiovascular autonomic modulation in the low- (LF; 0.04–0.15 Hz) and high-frequency (HF; 0.15–0.40 Hz) bands and alpha-index (α–LF) assessed spontaneous baroreflex sensitivity (BRS). Sex differences were compared in iso-saturation and iso-time analyses.
Results: At 10 min of hypoxia (iso-time), males desaturated more than females (interaction: p = 0.004), and hypoxia-induced tachycardia in both groups (p < 0.001), but no “sex-time” interaction was found for cardiovascular data. In contrast, only males responded with ventilatory responses during iso-time hypoxia, decreasing breathing frequency (interaction: p = 0.022) and increasing tidal volume (Vt) (interaction: p = 0.036). Otherwise, during iso-saturation (SpO2-matched ∼91%), heart rate and LF of R-R intervals increased more in females than in males (interaction: p = 0.049). However, only males increased Vt (interaction; p = 0.037).
Conclusion: Our data indicate that females counterbalance hypoxia mainly by systemic circulatory adjustments, while males use both, circulatory and ventilatory adjustments.
1 Introduction
High-altitude environments are well known to induce systemic hypoxia, a physiological stress that is not well tolerated by all humans (1–3). For a given inspired hypoxic level, individuals present a widely scattered oxygen saturation response (4, 5), and probably lower oxygen saturation contributes to hypoxic intolerance. A plausible factor related to inter-individual differences in the responses to hypoxia may be sex, as suggested by previous studies showing that females present more incidence of symptoms high altitude-related than males (6). Whether sex is a relevant factor in the inter-individual responsiveness to hypoxia, a further deep investigation into sex-related physiological differences to hypoxia is needed.
In normoxia, sex differences are notable in the lung's shape and size (7) and in the luminal area of large conducting airways, which are smaller in females than males (8). In the cardiovascular system, sex differences are present in anatomy, morphology, and regulation. For instance, compared to males, females have smaller hearts, higher heart rates, lower blood pressure (9), and greater peripheral vasodilation (10). However, in hypoxia, cardiorespiratory regulation remains controversial. Some studies have shown similar oxygen desaturation between sexes, with females presenting a higher heart rate and sympathetic activity (11). In contrast, although Boos et al. (12) also found a greater increase in heart rate in females than in males under hypoxia, oxygen saturation in females tended to be higher than in males. Higher oxygen saturation levels in females have been observed under hypoxia compared to males (13, 14). The reasons behind the controversial results involving sex differences in oxygen desaturation and cardiac responses remain unclear.
Overall, sex may be a relevant factor for the well-known inter-individual differences under hypoxia. The reasons for investigating sex differences are based on multiple anatomical and physiological aspects, especially under hypoxia since sex differences in chemoreflex have been widely discussed (15, 16). Also, inconclusive results on autonomic responses to hypoxia may stem from diverse methodological approaches, as exposure time and desaturation levels have not been considered confounding factors that could mask potential sex differences. Thus, we investigated whether sex differences in cardiorespiratory responses to hypoxia are due to distinct desaturation levels or different hypoxic responsiveness between males and females at the same desaturation level, focusing on iso-time and iso-saturation analyses to emphasize the roles of exposure time and desaturation in cardiorespiratory responses.
Considering that males tend to experience greater oxygen desaturation to short-term hypoxia (14), we tested the hypothesis that males would exhibit greater cardiovascular and ventilatory responses to hypoxia of equal duration (iso-time) due to greater desaturation compared to females. Additionally, at equal desaturation (iso-saturation), the sex differences in hypoxic responsiveness would be less pronounced but still present. Thus, we aimed to investigate the potential sex differences in cardiovascular autonomic modulation and ventilatory response at equivalent levels of oxygen desaturation (iso-saturation) and hypoxic exposure time (iso-time). This approach makes it possible to clarify if sex differences are related to oxygen desaturation or hypoxic responsiveness.
2 Methods
2.1 Participants
Twelve females and ten males, matched by age and BMI, participated as volunteers in this study (Table 1) after undergoing the anamnesis and electrocardiogram screening. The females who engaged in this study had not used contraceptives for at least 6 months. The group of females was assessed during the early follicular phase (i.e., the first week) of the menstrual cycle. The current study included healthy volunteers, lowlanders residents, and non-smokers who did not travel to high altitudes for at least 6-months previous to the present study. Individuals with any known cardiopulmonary or metabolic diseases were not included in the present investigation. The study was approved (number: 6.506.154) by the institutional ethics committee, registered in the public database Plataforma Brasil (CAAE: 28151519.0.0000.5243; National Council of Health, Ministry of Health, Brazil), and followed the Declaration of Helsinki. All participants signed the informed consent form.
2.2 Experimental protocol
The experiments were conducted in the afternoon, between 2:00 and 4:00 p.m., in an air-conditioned laboratory room, with 50%–60% relative air humidity and temperatures between 20°C and 22°C (Kestrel 4500, Minneapolis, MN, USA). Upon arriving at the laboratory, participants rested for 20 min in a sitting position. During this time, beat–to–beat hemodynamic variables such as heart rate (HR), stroke volume (SV), cardiac output (CO), and blood pressure (BP) were recorded. Participants were also instrumented using a mask coupled to the spirometer flow head to record breathing frequency (BF), tidal volume (Vt), minute ventilation (VE), and a pulse oximeter for SpO2 monitoring. Thereafter, 10 min in NOR (FiO2 = 0.21 ∼sea level) and 10 min in HYP (FiO2 = 0.115 ∼4,800 m) (Figure 1).
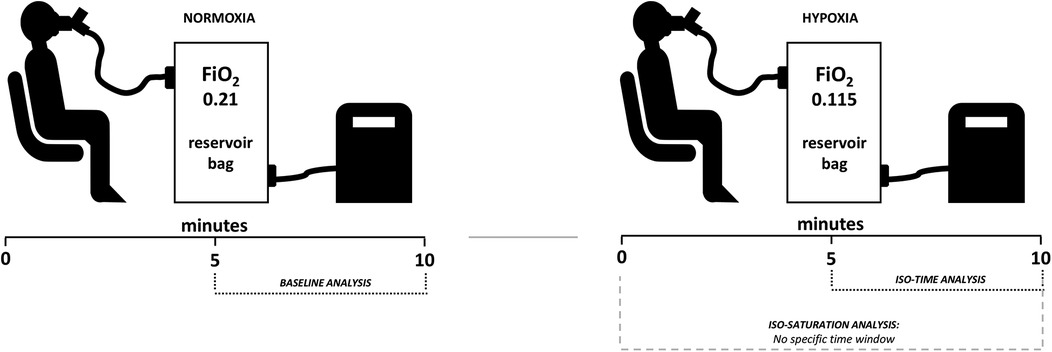
Figure 1. Illustration of the experimental design. As described in the methods, males and females underwent baseline normoxia followed by hypoxia, with both conditions lasting 10 min. Iso-time analysis was conducted at a fixed time, while iso-saturation analysis had no specific time window, aiming to analyze sexes at matched oxygen desaturation.
2.3 Simulated altitude
The hypoxic condition was produced through an oxygen dilution system (Everest Summit II—Hypoxico®, NY, USA). Two hypoxic systems were coupled with two 200-L non-diffusing gas reservoirs, with one reservoir supplied with FiO2 of 0.21 (i.e., normoxia, sea-level equivalent) and the other with FiO2 of 0.115 (i.e., hypoxia∼4,800 m). The reservoirs contained an O2 monitor at their outputs to ensure accurate control of the FiO2 offered. In addition, the reservoirs were essential to ensure that the flow promoted by the hypoxic generators did not interfere with the ventilatory data recorded by the spirometer.
2.4 Experimental procedures
Heart rate, stroke volume, and cardiac output recordings were done through a noninvasive trans-thoracic bioimpedance device (17) (PhysioFlow®—Manatec Biomedical, FRA). The R-R intervals were recorded by an electrocardiogram signal (ECG Module®—FMS, NL), and the continuous blood pressure data were recorded by infrared photoplethysmography (Finometer® PRO—FMS, NL). The data acquisition frequency was sampled at 1 kHz. Minute ventilation (VE) was calculated as the product of breath-by-breath volume tidal (Vt; determined from the integral of the flow signal) and breathing frequency (BF; determined by the number of events per minute in the flow signal) recorded by the spirometer (Spirometer FE141—ADInstruments, NZL). All the devices were connected to a digital signal integrator (PowerLab®—ADInstruments, NZL) to allow the recordings would be synchronized through a specific software (LabChart 8.1 PRO—ADInstruments, NZL). A bidirectional valve (Y730—Hans Rudolph, USA) was connected at the outlet of the spirometer flow-head, allowing inhalation of gases with FiO2 of 0.21 or 0.115 from the reservoirs, and exhalation to room air. Before the start of the recordings, the spirometer was calibrated with a known volume (i.e., 3-L) through an appropriate syringe. Oxygen saturation data were assessed by a pulse oximeter (Oxi–Go® QuickCheck PRO—Oximeter Plus, USA).
2.5 Data analysis
For the heart rate variability (HRV) analysis, beat-to-beat time series of the R-R intervals were analyzed using a time window of 300 beats, focusing on the last 5 min for normoxia and iso-time hypoxia. In the case of iso-saturation hypoxia, the HRV segment was adjusted to match the period of equal desaturation. Spectral analysis was performed using Fast Fourier Transform (FFT) using Welch's method and a Hanning window (50% overlap), obtaining indexes in the frequency-domain as the total power (TP), the low-frequency component (LF, 0.04–0.15 Hz; often associated with sympathetic modulation), the high-frequency component (HF, 0.15–0.40 Hz; a marker of vagal modulation and synchronous with respiration), and the sympatho-vagal (LF/HF) balance. The LF and HF spectral powers were normalized by dividing the LF and HF values expressed in absolute units by the total power minus the VLF spectral power. Spontaneous cardiac baroreflex sensitivity (SBR) was analyzed by the alpha coefficient for the low component (α–LF) through the square root of the ratio of LFR-R and LF from systolic blood pressure (LFSBP) powers (18). All HRV and BRS data were analyzed through ad hoc software (HeartScope II; A.M.P.S. LLC version 1.4.3.0., ITA).
Total vascular conductance (TVC) was calculated through the ratio between CO and mean blood pressure. Given the difference between males and females in body size, we normalized SV, CO and Vt by body surface area (BSA; in m2) = [0.20247 × height (in m) 0.725 × weight (in kg) 0.425] (19). Thus, stroke volume (SV), cardiac output (CO), and tidal volume (Vt) were normalized to body surface area, resulting in the following indexes: SVi, CI, and Vt/BSA, respectively.
2.6 Statistical analysis
The sample size was calculated for the following variables (iso-time: SpO2), and (iso-saturation: HR and Vt) considering a β of 0.80 and an α of 0.05, using an ad hoc software (G*Power 3.1.9.7; Heinrich-Heine-Universität, Düsseldorf, Germany). Data normality was tested using the Shapiro-Wilk test (see Supplementary Tables S1, S2). To compare the characteristics of the participants between males and females, an unpaired Student's t-test was carried out. The physiological changes provided by hypoxia were calculated by delta (Δ = hypoxia—normoxia) for both groups. The same test or the Mann-Whitney U test was used to compare the differences between deltas when appropriate. After that, the analysis of variance (ANOVA) for two factors (time and group) was done to evaluate hypoxia over time in both sexes. Iso-saturation and iso-time analyses were conducted separately using a mixed two-way ANOVA (group and condition), with repeated measures for the gas condition, to assess the main effects of hypoxia and sex differences and their interactions. Multiple comparisons were performed using the Bonferroni post-hoc test to find where the differences are. Graphics and statistical analysis were carried out through GraphPad (GraphPad Prism v 8.0.2, Inc., CA, USA). All data are presented as mean ± SD, and significance was set at p ≤ 0.05.
3 Results
The power analysis revealed large effect sizes (f) of 0.68 for SpO2, and 0.61 and 0.52 for HR and Vt, respectively, and that a sample size of 8–10 participants would be required for accurate data. Therefore, the sample size in this study meets the power analysis requirements. The results of normality tests may be seen in the supplementary data (Supplementary Tables S1, S2). The characteristic data of both groups were compared, showing that males and females were matched in age and body mass index, but, as expected, differed significantly in height, weight, and body surface (Table 1).
Regarding the time effect, an interaction between sex and time was observed only for oxygen desaturation (Figure 2). The ANOVA showed that the time effect of hypoxia was similar for both groups, increasing heart rate, cardiac output, and total vascular conductance while decreasing mean blood pressure. However, the ventilatory response was higher in males than in females, and was not influenced by time (Figure 2). Hemodynamic, autonomic, and ventilatory data for all participants are shown in Table 2, which presents sex-related comparisons for iso-saturation analysis, and in Table 3, which presents sex-related comparisons for iso-time hypoxia analysis.
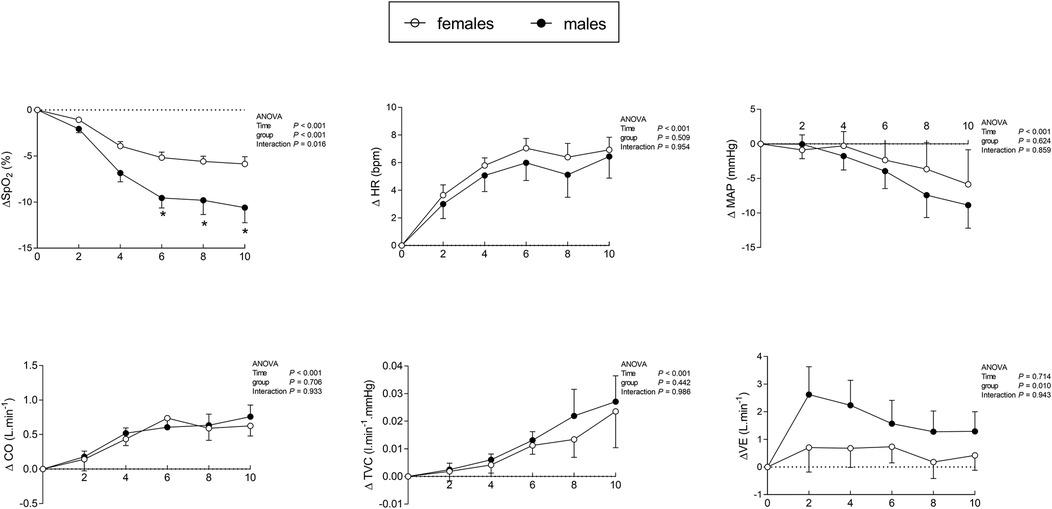
Figure 2. Cardiorespiratory responses in males and females averaged over each 2-minute interval during 10 min of hypoxia. Δ, difference (hypoxia—normoxia); ΔSpO2, oxygen desaturation; ΔHR, heart rate response; ΔMAP, mean arterial pressure; ΔCO, cardiac output; ΔTVC, total vascular conductance; ΔVE, minute ventilation. *p < 0.05 for interaction (time × group). Results are described as mean ± SE.
Iso-saturation period analysis (i.e., matched SpO2; see Table 2) was confirmed by the absence of differences in SpO2 levels between males and females under hypoxia in this analysis segment. Herein, females showed an increased heart rate and low-frequency component of R-R intervals and a trend to reduce baroreflex sensitivity (α–LF) during iso-saturation hypoxia compared to males. On the other hand, ventilatory data remained unchanged in females, while only males increased tidal volume under iso-saturation, as evidenced by the sex-condition interaction. The other physiological data presented only the main effects of ANOVA, without interactions, such as those related to hypoxia showed increases in heart rate, cardiac output, and low-frequency bands of R-R intervals and blood pressure, as well as a decrease in baroreflex sensitivity, total power, and the high-frequency band of R-R intervals, and increases in tidal volume and ventilation in response to iso-saturation hypoxia (Table 2). Additionally, other main effects such as those related to sex as a factor, showed that females had a higher heart rate and cardiac output index but lower tidal volume, ventilation, and low-frequency band of blood pressure (Table 2). Sex differences are also found for the relative tidal volume (i.e., indexed by body surface area) in response to hypoxia when comparing this variable between sexes by delta (ΔVt/BSA; males: 0.062 ± 0.077 vs. females: 0.007 ± 0.053 L/m2; p = 0.03).
During iso-time analysis (i.e., matched timeframes; see Table 3), an interaction effect was observed for oxygen saturation, showing that males desaturated more than females. Other interactions from ANOVA revealed that only males increased tidal volume and decreased breathing frequency. Further main effects related to iso-time hypoxia included increases in heart rate, cardiac output, the low-frequency band of R-R intervals and blood pressure, and vascular conductance, as well as decreases in the high-frequency band of R-R intervals and baroreflex sensitivity. Sex-related main effects from the ANOVA revealed that females had higher heart rates, cardiac output, and total vascular conductance, but lower tidal volume, ventilation, and the low-frequency band of blood pressure (Table 3). In addition, males showed a greater response of relative tidal volume to iso-time hypoxia than females, as shown by delta (ΔVt/BSA; males: 0.064 ± 0.082 vs. females: 0.007 ± 0.053 L/m2; p = 0.03).
4 Discussion
The present study investigated sex differences in cardiorespiratory responses to hypoxia, focusing on oxygen saturation levels and time under hypoxia. The main findings in this investigation were: (1) under hypoxic iso-saturation, females had higher heart rates and greater low-frequency band response of HRV compared to males. Whereas, males presented a higher tidal volume in response to hypoxic iso-saturation than females. (2) The iso-time hypoxic analyses revealed that females defended oxygen saturation over time better compared to males. Despite different levels of desaturation, there were no sex differences in cardiovascular responses to iso-time hypoxia, but males needed ventilatory compensation by presenting greater tidal volume under iso-time hypoxia, whereas females did not. Collectively, these findings suggest that in short-term normobaric hypoxia, females preserve oxygen saturation and counterbalance hypoxemia mainly through tachycardia. Differently, males achieve lower saturation levels and even tachycardia and higher tidal volume fail to maintain preserved oxygen saturation. These results provide evidence that males and females exhibit distinct modulation of cardiorespiratory responses to acute hypoxia.
4.1 Sex differences and oxygen desaturation
Oxygen desaturation diverges between sexes over time, as shown by some studies (12–14, 20), although this finding is not reported in all studies (21–23). Boos and colleagues (12) in a study involving 14 individuals (seven females), found that females tend to remain with higher oxygen saturation than males after 150 min of normobaric hypoxia. It is well known that pulmonary vessels when exposed to hypoxia can lead to blood flow diversion from hypoxic to non-hypoxic lung areas (i.e., hypoxic pulmonary vasoconstriction) preventing at least in part the arterial deoxygenation (24). Despite Boos and colleagues (12) having shown that pulmonary vascular resistance was increased by hypoxia, the effect occurred similarly in both sexes (12). In our study, pulmonary circulation was not evaluated, but in females, the absence of ventilatory responses to hypoxia (Tables 2, 3) might have provided a lower rate of alveolar gas turnover between the hypoxic gas from the mask to the alveoli. Concomitantly, even with a slight desaturation (Figure 2), females presented tachycardia, increasing cardiac output (Figure 2), which perhaps may contribute to an augment in blood flow velocity in alveolar-capillary units leading to a lower time to hematosis. Importantly, in normoxia, adult females may also present higher oxygen saturation than males, which is not evident in newborns (25) and prepubertal children (26), remaining unknown the contribution of female hormones in oxyhemoglobin affinity. However, sex differences in oxygen saturation in normoxia were not found in the present study.
In humans, previous studies have shown an inverse relation between hypoxic ventilatory response and hypoxic pulmonary vasoconstriction (27), which means that less ventilatory response, more pulmonary vasoconstriction under hypoxia. In a certain way, this mechanism could offer a short-term protection to desaturation, but if this condition continues for more time, it could lead to pulmonary hypertension, right heart failure, and edema. However, the defense of oxygen saturation in females might be related to other mechanisms, because in contrast, female hormones, such as estrogen, seem to contribute to pulmonary vasodilation, as shown in animal models, attenuating hypoxic pulmonary vasocontriction (24). Another possibility would be the oxygen affinity to hemoglobin, however, previous studies showed that females have lower oxygen affinity to hemoglobin than males, which is attributed to higher 2,3-diphosphoglycerate levels (28). Nevertheless, blood gases and hematocrit were not measured in the current study, which limits our interpretation. Therefore, the mechanisms behind a higher saturation under hypoxia in females deserve further investigation.
4.2 Sex-related differences in cardiac autonomic control in normoxia
Our results revealed that females had higher heart rates and lower total R-R variability compared to males under baseline normoxic conditions (Table 2), partially corroborating with the literature that typically reports the same result in females, but paradoxically also reports a relative vagal dominance in females, while in males reports lower heart rates with a relative sympathetic dominance (29). In our data, no sex differences were found for sympatho-vagal balance in normoxia, despite females having a lower baseline mean of LF/HF (Table 2). Additionally, in normoxia, females had lower sympathetic vascular modulation (i.e., estimated by LF spectral component of systolic blood pressure), but similar spontaneous cardiac baroreflex sensitivity compared to males (Table 2). An in-depth discussion of sex-specific cardiovascular autonomic modulation is beyond the scope of this investigation. Briefly, these sex differences may be partially attributed to the effects of estrogen, oxytocin-stimulated neurons affecting vagal tone, amygdala activity, sex-related differences in brain structures (29), and the predominance of beta-adrenergic vasodilation in young females (30). Notably, autonomic activity may be influenced by several other factors, and in the context of biological sex differences, intra-individual variability should be considered, particularly in females due to potential differences in autonomic activity across different phases of the menstrual cycle (16).
4.3 Sex-related differences in cardiac autonomic control in hypoxia
During iso-time analysis, our results demonstrated increased heart rate in both, males and females under hypoxia, characterized by vagal withdrawal and augmented sympathetic modulation, shifting the sympatho-vagal balance towards sympathetic dominance (Table 3). Additionally, we observed a reduction in spontaneous baroreflex sensitivity in both sexes under iso-time hypoxia. Importantly, these cardiovascular autonomic responses were consistent in females, but were only observed in males when they exhibited lower oxygen saturation (Table 3). Previous findings indicate that the augmented heart rate under hypoxia is often a result of vagal withdrawal, as triggered by the aortic bodies (31, 32). Cardiac vagal withdrawal under hypoxia was also reported by other investigations (4, 21, 33), and when sex was compared no differences were noted in cardiac vagal modulation (21).
During iso-saturation (SpO2-matched) analysis, we found that females exhibited higher heart rates, increased cardiac sympathetic modulation, and a tendency towards lower spontaneous baroreflex sensitivity (Table 2). Our findings contrast with those of Botek and colleagues (21), who showed that males exhibited relatively higher cardiac sympathetic responses compared to females under short-term severe hypoxia with similar oxygen desaturation levels. The hypoxic level used by Botek et al. (21) was considerably more severe (FiO2 = 0.096) than in the current investigation (FiO2 = 0.115). Overall, the average oxygen saturation achieved by males and females in Botek et al.'s study (21) was near 70%, with considerable dispersion, whereas in the current study, iso-saturation analysis was near SpO2 91%, and in the iso-time analysis, only males reached an average saturation near 87%, with more discrete dispersion. These differences are important because a stronger hypoxic stimulus contributes to greater oxygen desaturation dispersion among participants but might overshadow potential differences in cardiovascular autonomic sensitivity that could be observed under moderate levels of hypoxemia.
It is known that hypoxia increases sympathoexcitation, but the results are inconclusive when sex differences are compared. A study that measured peripheral sympathetic activity directly showed that females may exhibit faster sympathoexcitation (11), while another study found that the sympathetic response of females to isocapnic hypoxia is similar to that of males (15). These studies measured peripheral sympathetic activity directly, so it is not appropriate to compare their results directly with ours, even though the normalized low-frequency band of the R-R intervals has been correlated with sympathetic activity (34, 35). Herein, although the normalized LF of the R-R interval increased more in females during iso-saturation hypoxia, suggesting a possible greater sympathetic predominance over heart rate compared to males, this phenomenon needs to be further investigated using a similar protocol that integrates HRV markers and direct sympathetic measurements to explore the underlying mechanisms in sex differences in cardiovascular rhythms to hypoxia.
4.4 Sex differences and ventilatory response
In contrast with males, females did not change ventilation in response to hypoxia in both segment analyses (iso-saturation and iso-time), which was intriguing. The absence of ventilatory response to hypoxia in females contrasts with previous studies (14), and it likely may not be explained by higher oxygen saturation levels because males increased the tidal volume in SpO2-matched analysis. In a longer exposure to moderate normobaric hypoxia for 7 h, Camacho-Cardenosa and co-authors (36) showed a robust increase in ventilation in males and females, however, comparing the sexes, it was significantly greater in males. In our study, ventilation did not differ between sexes, but males tend to present greater averages. Conversely, tidal volume markedly increased in response to short-term hypoxia in males. Additionally, only males reduced breathing frequency in iso-time hypoxia (Table 3), which supports previous investigations in humans suggesting that sex influences breathing frequency response to hypoxia (14). The mechanisms underlying sex differences in hypoxic ventilatory response in humans remain largely unknown. Previous studies suggest that increased circulating female hormones play a role in an enhanced hypoxic ventilatory response (37, 38). However, in our study, females were assessed during the low-hormone phase (i.e., the early follicular phase) of the menstrual cycle, thereby reducing the potential effect on ventilatory response. Sex hormones appear to act directly on the peripheral chemoreflex, as experiments in rats have shown that ovarian steroids can influence breathing by decreasing dopaminergic inhibitory activity in the carotid bodies (39). However, the differences in hypoxic response may extend beyond sex, as there are species variations in the mechanisms underlying the peripheral chemoreflex (40), which makes studying this in humans essential.
A matter of future debate should address additional factors contributing to inter- and intra-individual differences in physiological responses to hypoxia. These factors include a detailed investigation of anatomical and functional differences between sexes, the influence of different phases of the menstrual cycle, and follow-up studies to assess the impact of aging and various clinical populations.
4.5 Limitations
This study has some limitations that should be acknowledged. First, females were evaluated during the follicular phase of the menstrual cycle, but this was self-reported based on their cycle calendars. Therefore, estrogen and progesterone levels were not measured in this investigation. Second, health status was assessed through anamnesis and electrocardiogram, with participants self-reporting as healthy. However, the participants did not show any signs of cardiorespiratory alterations that could be considered abnormal before or during the tests. Third, the lack of end-tidal gas recordings (i.e., PETO2 and PETCO2) and the absence of carbon dioxide supplementation to maintain isocapnia represent significant limitations. However, the model of hypoxia used here (i.e., poikilocapnic hypoxia, or no CO2 addition) has shown results consistent with isocapnic hypoxia with regard to cardiovascular responsiveness (41, 42). Additionally, the hypoxic condition in this study has higher external validity, as in natural hypoxic environments, carbon dioxide is not artificially added to the hypoxic gas to maintain isocapnia. Fourth, venous and arterial blood samples were not collected, limiting interpretations as hematocrit and arterial gases (PaO2, PaCO2) would provide valuable data. However, for our objectives, only oxygen saturation offers information in real time, making it possible to select the correct time window analysis for iso-time and iso-hypoxemia. Lastly, the current findings on sex differences should not be extrapolated to clinical contexts, taking into account that further investigations involving specific diseases, considering factors beyond sex such as age, obesity, and comorbidities, are needed.
5 Conclusion
In conclusion, our data indicate that females primarily counterbalance hypoxia through systemic circulatory adjustments, preserving oxygen saturation, while males rely on both circulatory and ventilatory adjustments but experience greater oxygen desaturation. Therefore, sex differences should be considered a significant factor that may considerably influence cardiorespiratory adjustments during hypoxia.
Data availability statement
The original contributions presented in the study are included in the article/Supplementary Material, further inquiries can be directed to the corresponding author.
Ethics statement
The studies involving humans were approved by University Hospital Antônio Pedro/Fluminense Federal University. The studies were conducted in accordance with the local legislation and institutional requirements. The participants provided their written informed consent to participate in this study.
Author contributions
AO: Conceptualization, Data curation, Formal Analysis, Investigation, Methodology, Writing – original draft, Writing – review & editing. GR: Conceptualization, Formal Analysis, Supervision, Writing – original draft, Writing – review & editing. BS: Conceptualization, Writing – original draft, Writing – review & editing. PR: Formal Analysis, Investigation, Writing – original draft, Writing – review & editing. PS: Conceptualization, Formal Analysis, Funding acquisition, Methodology, Project administration, Resources, Supervision, Writing – original draft, Writing – review & editing.
Funding
The author(s) declare financial support was received for the research, authorship, and/or publication of this article. This research was funded by the Rio de Janeiro Research Foundation (FAPERJ), grant number: E-26/110.166/2014 and E-26/200.477/2023 received by PS. National Council for Scientific and Technological Development (CNPq), grant number: 311049/2022-2 received by PS. AO received a scholarship from the Coordination for the Improvement of Higher Education Personnel (CAPES). AO is grateful for his current postdoctoral fellowship from FAPERJ, grant number: E-26/201.561/2024.
Acknowledgments
We greatly appreciate all participants' time expended during data collection in this study.
Conflict of interest
The authors declare that the research was conducted in the absence of any commercial or financial relationships that could be construed as a potential conflict of interest.
Publisher's note
All claims expressed in this article are solely those of the authors and do not necessarily represent those of their affiliated organizations, or those of the publisher, the editors and the reviewers. Any product that may be evaluated in this article, or claim that may be made by its manufacturer, is not guaranteed or endorsed by the publisher.
Supplementary material
The Supplementary Material for this article can be found online at: https://www.frontiersin.org/articles/10.3389/fcvm.2025.1473910/full#supplementary-material
References
1. Karinen HM, Peltonen JE, Kähönen M, Tikkanen HO. Prediction of acute mountain sickness by monitoring arterial oxygen saturation during ascent. High Alt Med Biol. (2010) 11(4):325–32. doi: 10.1089/ham.2009.1060
2. Karinen HM, Uusitalo A, Vähä-Ypyä H, Kähönen M, Peltonen JE, Stein PK, et al. Heart rate variability changes at 2400 m altitude predicts acute mountain sickness on further ascent at 3000-4300 m altitudes. Front Physiol. (2012) 3:336. doi: 10.3389/fphys.2012.00336
3. Mairer K, Wille M, Grander W, Burtscher M. Effects of exercise and hypoxia on heart rate variability and acute mountain sickness. Int J Sports Med. (2013) 34(8):700–6. doi: 10.1055/s-0032-1327577
4. Botek M, Krejčí J, De Smet S, Gába A, McKune AJ. Heart rate variability and arterial oxygen saturation response during extreme normobaric hypoxia. Auton Neurosci. (2015) 190:40–5. doi: 10.1016/j.autneu.2015.04.001
5. Krejčí J, Botek M, McKune AJ. Dynamics of the heart rate variability and oxygen saturation response to acute normobaric hypoxia within the first 10 min of exposure. Clin Physiol Funct Imaging. (2018) 38(1):56–62. doi: 10.1111/cpf.12381
6. MacInnis MJ, Carter EA, Freeman MG, Pandit BP, Siwakoti A, Subedi A, et al. A prospective epidemiological study of acute mountain sickness in Nepalese pilgrims ascending to high altitude (4380 m). PLoS One. (2013) 8(10):e75644. doi: 10.1371/journal.pone.0075644
7. Torres-Tamayo N, García-Martínez D, Lois Zlolniski S, Torres-Sánchez I, García-Río F, Bastir M. 3D analysis of sexual dimorphism in size, shape and breathing kinematics of human lungs. J Anat. (2018) 232(2):227–37. doi: 10.1111/joa.12743
8. Dominelli PB, Ripoll JG, Cross TJ, Baker SE, Wiggins CC, Welch BT, et al. Sex differences in large conducting airway anatomy. J Appl Physiol. (2018) 125(3):960–5. doi: 10.1152/japplphysiol.00440.2018
9. Prabhavathi K, Selvi KT, Poornima KN, Sarvanan A. Role of biological sex in normal cardiac function and in its disease outcome - a review. J Clin Diagn Res. (2014) 8(8):BE01–4. doi: 10.7860/JCDR/2014/9635.4771
10. Barnes JN. Sex-specific factors regulating pressure and flow. Exp Physiol. (2017) 102(11):1385–92. doi: 10.1113/EP086531
11. Jones PP, Davy KP, Seals DR. Influence of gender on the sympathetic neural adjustments to alterations in systemic oxygen levels in humans. Clin Physiol. (1999) 19(2):153–60. doi: 10.1046/j.1365-2281.1999.00158.x
12. Boos CJ, Mellor A, O'Hara JP, Tsakirides C, Woods DR. The effects of sex on cardiopulmonary responses to acute normobaric hypoxia. High Alt Med Biol. (2016) 17(2):108–15. doi: 10.1089/ham.2015.0114
13. Riveros-Rivera A, Penzel T, Gunga HC, Opatz O, Paul F, Klug L, et al. Hypoxia differentially affects healthy men and women during a daytime nap with a dose-response relationship: a randomized, cross-over pilot study. Front Physiol. (2022) 13:899636. doi: 10.3389/fphys.2022.899636
14. Rispen L, Marks D, Green S. Dynamic ventilatory responses of females and males to acute isocapnic and poikilocapnic hypoxia. Respir Physiol Neurobiol. (2017) 245:57–64. doi: 10.1016/j.resp.2017.05.005
15. Sayegh ALC, Fan JL, Vianna LC, Dawes M, Paton JFR, Fisher JP. Sex differences in the sympathetic neurocirculatory responses to chemoreflex activation. J Physiol. (2022) 600(11):2669–89. doi: 10.1113/JP282327
16. Usselman CW, Gimon TI, Nielson CA, Luchyshyn TA, Coverdale NS, Van Uum SH, et al. Menstrual cycle and sex effects on sympathetic responses to acute chemoreflex stress. Am J Physiol Heart Circ Physiol. (2015) 308(6):H664–71. doi: 10.1152/ajpheart.00345.2014
17. Siebenmann C, Rasmussen P, Sørensen H, Zaar M, Hvidtfeldt M, Pichon A, et al. Cardiac output during exercise: a comparison of four methods. Scand J Med Sci Sports. (2015) 25(1):e20–7. doi: 10.1111/sms.12201
18. Parati G, Di Rienzo M, Mancia G. How to measure baroreflex sensitivity: from the cardiovascular laboratory to daily life. J Hypertens. (2000) 18(1):7–19. doi: 10.1097/00004872-200018010-00003
19. Du Bois D, Du Bois EF. A formula to estimate the approximate surface area if height and weight be known. 1916. Nutrition. (1989) 5(5):303–11; discussion 312–3.2520314
20. Nishimura T, Ugarte J, Ohnishi M, Nishihara M, Alvarez G, Yasukochi Y, et al. Individual variations and sex differences in hemodynamics with percutaneous arterial oxygen saturation (SpO2) in young Andean highlanders in Bolivia. J Physiol Anthropol. (2020) 39(1):31. doi: 10.1186/s40101-020-00240-y
21. Botek M, Krejčí J, McKune A. Sex differences in autonomic cardiac control and oxygen saturation response to short-term normobaric hypoxia and following recovery: effect of aerobic fitness. Front Endocrinol. (2018) 9:697. doi: 10.3389/fendo.2018.00697
22. Nishimura T, Arima H, Koirala S, Ito H, Yamamoto T. Individual variations and sex differences in hemodynamics and percutaneous arterial oxygen saturation (SpO2) in Tibetan highlanders of Tsarang in the Mustang district of Nepal. J Physiol Anthropol. (2022) 41(1):9. doi: 10.1186/s40101-022-00282-4
23. Miller AJ, Cui J, Luck JC, Sinoway LI, Muller MD. Age and sex differences in sympathetic and hemodynamic responses to hypoxia and cold pressor test. Physiol Rep. (2019) 7(2):e13988. doi: 10.14814/phy2.13988
24. Sylvester JT, Shimoda LA, Aaronson PI, Ward JPT. Hypoxic pulmonary vasoconstriction. Physiol Rev. (2012) 92:367–520. doi: 10.1152/physrev.00041.2010
25. Levental S, Picard E, Mimouni F, Joseph L, Samuel TY, Bromiker R, et al. Sex-linked difference in blood oxygen saturation. Clin Respir J. (2018) 12(5):1900–4. doi: 10.1111/crj.12753
26. Frimer Z, Goldberg S, Joseph L, Picard E. Are there gender differences in blood oxygen saturation in prepubertal children? Clin Respir J. (2021) 15(6):657–60. doi: 10.1111/crj.13340
27. Albert TJ, Swenson ER. Peripheral chemoreceptor responsiveness and hypoxic pulmonary vasoconstriction in humans. High Alt Med Biol. (2014) 15(1):15–20. doi: 10.1089/ham.2013.1072
28. Balcerek B, Steinach M, Lichti J, Maggioni MA, Becker PN, Labes R, et al. A broad diversity in oxygen affinity to haemoglobin. Sci Rep. (2020) 10(1):16920. doi: 10.1038/s41598-020-73560-9
29. Koenig J, Thayer JF. Sex differences in healthy human heart rate variability: a meta-analysis. Neurosci Biobehav Rev. (2016) 64:288–310. doi: 10.1016/j.neubiorev.2016.03.007
30. Joyner MJ, Wallin BG, Charkoudian N. Sex differences and blood pressure regulation in humans. Exp Physiol. (2016) 101(3):349–55. doi: 10.1113/EP085146
31. Tubek S, Niewinski P, Reczuch K, Janczak D, Rucinski A, Paleczny B, et al. Effects of selective carotid body stimulation with adenosine in conscious humans. J Physiol. (2016) 594:6225–40. doi: 10.1113/JP272109
32. Siebenmann C, Ryrsø CK, Oberholzer L, Fisher JP, Hilsted LM, Rasmussen P, et al. Hypoxia-induced vagal withdrawal is independent of the hypoxic ventilatory response in men. J Appl Physiol. (2019) 126(1):124–31. doi: 10.1152/japplphysiol.00701.2018
33. Buchheit M, Richard R, Doutreleau S, Lonsdorfer-Wolf E, Brandenberger G, Simon C. Effect of acute hypoxia on heart rate variability at rest and during exercise. Int J Sports Med. (2004) 25(4):264–9. doi: 10.1055/s-2004-819938
34. DeBeck LD, Petersen SR, Jones KE, Stickland MK. Heart rate variability and muscle sympathetic nerve activity response to acute stress: the effect of breathing. Am J Physiol Regul Integr Comp Physiol. (2010) 299(1):R80–91. doi: 10.1152/ajpregu.00246.2009
35. Pagani M, Montano N, Porta A, Malliani A, Abboud FM, Birkett C, et al. Relationship between spectral components of cardiovascular variabilities and direct measures of muscle sympathetic nerve activity in humans. Circulation. (1997) 95(6):1441–8. doi: 10.1161/01.CIR.95.6.1441
36. Camacho-Cardenosa A, Camacho-Cardenosa M, Tomas-Carus P, Timón R, Olcina G, Burtscher M. Acute physiological response to a normobaric hypoxic exposure: sex differences. Int J Biometeorol. (2022) 66(7):1495–504. doi: 10.1007/s00484-022-02298-y
37. Moore LG, McCullough RE, Weil JV. Increased HVR in pregnancy: relationship to hormonal and metabolic changes. J Appl Physiol. (1987) 62:158–63. doi: 10.1152/jappl.1987.62.1.158
38. Regensteiner JG, Woodard WD, Hagerman DD, Weil JV, Pickett CK, Bender PR, et al. Combined effects of female hormones and metabolic rate on ventilatory drives in women. J Appl Physiol. (1989) 66(2):808–13. doi: 10.1152/jappl.1989.66.2.808
39. Joseph V, Soliz J, Soria R, Pequignot J, Favier R, Spielvogel H, et al. Dopaminergic metabolism in carotid bodies and high-altitude acclimatization in female rats. Am J Physiol Regul Integr Comp Physiol. (2002) 282(3):R765–73. doi: 10.1152/ajpregu.00398.2001
40. Zera T, Moraes DJA, da Silva MP, Fisher JP, Paton JFR. The logic of carotid body connectivity to the brain. Physiology. (2019) 34(4):264–82. doi: 10.1152/physiol.00057.2018
41. Halliwill JR, Minson CT. Effect of hypoxia on arterial baroreflex control of heart rate and muscle sympathetic nerve activity in humans. J Appl Physiol. (2002) 93(3):857–64. doi: 10.1152/japplphysiol.01103.2001
Keywords: autonomic nervous system, heart rate variability, hypoxemia, breathing, sex differences
Citation: Oliveira ALMB, Rodrigues GD, Silva BM, Rohan PdA and Soares PPS (2025) Sex differences in cardiorespiratory control under hypoxia: the roles of oxygen desaturation and hypoxic exposure time. Front. Cardiovasc. Med. 12:1473910. doi: 10.3389/fcvm.2025.1473910
Received: 31 July 2024; Accepted: 16 January 2025;
Published: 31 January 2025.
Edited by:
Rubens Fazan Jr, University of São Paulo, BrazilReviewed by:
Jui-Lin Fan, The University of Auckland, New ZealandSascha Treskatsch, Charité University Medicine Berlin, Germany
Copyright: © 2025 Oliveira, Rodrigues, Silva, Rohan and Soares. This is an open-access article distributed under the terms of the Creative Commons Attribution License (CC BY). The use, distribution or reproduction in other forums is permitted, provided the original author(s) and the copyright owner(s) are credited and that the original publication in this journal is cited, in accordance with accepted academic practice. No use, distribution or reproduction is permitted which does not comply with these terms.
*Correspondence: Pedro Paulo da Silva Soares, cHBzc29hcmVzQGlkLnVmZi5icg==