- 1Department of Cardiovascular Sciences, Lemole Center for Integrated Lymphatics and Vascular Research, Lewis Katz School of Medicine at Temple University, Philadelphia, PA, United States
- 2Department of Biochemistry and Immunology, Institute of Biological Sciences, Federal University of Minas Gerais, Belo Horizonte, Brazil
- 3Department of Cardiovascular Sciences, Metabolic Disease Research and Thrombosis Research Center, Lewis Katz School of Medicine at Temple University, Philadelphia, PA, United States
Pathological transdifferentiation, where differentiated cells aberrantly transform into other cell types that exacerbate disease rather than promote healing, represents a novel and significant concept. This perspective discusses its role and potential targeting in cardiovascular diseases and chronic inflammation. Current therapies mainly focus on mitigating early inflammatory response through proinflammatory cytokines and pathways targeting, including corticosteroids, TNF-α inhibitors, IL-1β monoclonal antibodies and blockers, IL-6 blockers, and nonsteroidal anti-inflammatory drugs (NSAIDs), along with modulating innate immune memory (trained immunity). However, these approaches often fail to address long-term tissue damage and functional regeneration. For instance, fibroblasts can transdifferentiate into myofibroblasts in cardiac fibrosis, and endothelial cells may undergo endothelial to mesenchymal transition (EndMT) in vascular remodeling, resulting in fibrosis and impaired tissue function. Targeting pathological transdifferentiation represents a promising therapeutic avenue by focusing on key signaling pathways that drive these aberrant cellular phenotypic and transcriptomic transitions. This approach seeks to inhibit these pathways or modulate cellular plasticity to promote effective tissue regeneration and prevent fibrosis. Such strategies have the potential to address inflammation, cell death, and the resulting tissue damage, providing a more comprehensive and sustainable treatment solution. Future research should focus on understanding the mechanisms behind pathological transdifferentiation, identifying relevant biomarkers and master regulators, and developing novel therapies through preclinical and clinical trials. Integrating these new therapies with existing anti-inflammatory treatments could enhance efficacy and improve patient outcomes. Highlighting pathological transdifferentiation as a therapeutic target could transform treatment paradigms, leading to better management and functional recovery of cardiovascular tissues in diseases and chronic inflammation.
Introduction
Chronic inflammation, while contributing to many chronic and degenerative diseases as pathological process, it also plays a role in mitigating infections, clearing damaged cells, and initiating tissue repair. Recent advances have provided insights into the cellular and molecular mechanisms by which traditional inflammatory cytokines (1) and Wnt/b-catenin factors (2, 3) control tissue repair and regeneration, suggesting that reparative inflammation drives tissue regeneration (4). It has long been recognized that chronic tissue damages leads to structural destruction, cell death, and fibrosis, as seen in occupational respiratory diseases like coal workers' pneumoconiosis and silicosis (5), pulmonary artery erosion, Rasmussen aneurysm (6), extensive scarring in the upper lobes of the lungs from tuberculosis (7), burn scars (8), and myocardial scaring after myocardial infarction (9).
Increasing tissue differentiation in damaged tissue has been considered a therapeutic approach for tissue regeneration. In the differentiation process, a pluripotent stem cell undergoes proliferation and progresses through intermediate and progenitor stages before losing its multipotent capacities (10, 11) and differentiating into specialized, mature cells that form organs or tissues (10, 12). Simple combinations of lineage-determining transcription factors (TFs) activate the cis-regulatory elements necessary for establishing macrophage and B cell identities (13). While the B cell lineage is highly resistant to most differentiation factors, the loss of a single B lineage-specific TF or the enforced expression of TFs from non-B cell lineages can interfere with B cell maintenance, allowing for dedifferentiation or trans-differentiation into other different lineages (14). Promoters driven by lineage-determining TF control the expression of macrophage-specific genes in a cell type dependent manner (15). Ng A. et al. introduced the Human TFome, an extensive library containing 1,564 TF genes and 1,732 TF splice isoforms. By screening this library across three human pluripotent stem cell (hPSC) lines, they identified 290 TFs, 241 of which were previously unknown, that trigger differentiation within just four days, without changing external soluble factors or biomechanical signals (16). The temporal regulation of lineage-specific TFs driven by super-enhancer (SE) is fundamental to normal developmental programs (17). Two additional processes related to differentiation are de-differentiation, where a differentiated cell returns to a less-differentiated state to regain its proliferative capacity, and trans-differentiation, where one differentiated cell type converts directly into another without returning to a pluripotent state. As a result, transdifferentiation is commonly referred to as direct cell reprogramming (18). Both dedifferentiation and transdifferentiation can occur spontaneously in natural biological processes (19).
Research has shown that pathological transdifferentiation is often associated with the development of cardiovascular diseases. For example, after myocardial infarction (MI), adult cardiomyocytes have a limited ability to regenerate. As a result, cardiac fibroblasts (CFs) derived from resident and/or other cell types produce the extracellular matrix, resulting in fibrosis, which contributes to cardiac dysfunction and heart failure (20). As Dr. Fu et al. highlighted, the signaling pathways that regulate the various differentiation potentials of cardiac fibroblasts may warrant greater focus, given the growing evidence of these differentiations in diseased hearts (21). In addition, while the cause of aortic aneurysms is not well understood, it is linked to hypercholesterolemia, atherosclerosis, and abnormal signaling of transforming growth factor-β (TGF-β) in vascular smooth muscle cells (VSMCs) (22). This combination of medial VSMC loss with increased non-VSMC aortic cell mass results in excessive growth and dilation of the aorta, along with calcification and ossification of the aortic wall, and inflammation, ultimately leading to aneurysm formation (1, 22–24).
Epithelial to mesenchymal transition (EMT) is a biological process in which epithelial cells shed their defining characteristics, such as tight junctions and polarity, and gain mesenchymal properties, like increased motility and invasiveness (25). This transition is crucial in various physiological processes, including embryogenesis, wound healing (26), tissue fibrosis, and cancer (27, 28). As our knowledge of EMT regulation grows, it is becoming increasingly apparent that EMT exists on a spectrum, with a range of intermediate cellular states between the fully epithelial and fully mesenchymal phenotypes. Rather than existing in distinct, “pure” epithelial or mesenchymal forms, cells can adopt intermediate or hybrid states, exhibiting a combination of traits from both epithelial and mesenchymal characteristics indicating that cells undergo partial EMT (29–31). In the context of cardiovascular diseases, EMT is a crucial factor in various pathological conditions (32), including atherosclerosis, vascular remodeling, cardiac fibrosis, heart valve diseases, and myocardial infarction, involving different signaling pathways and molecular mechanisms such as TGF-β, Notch signaling, Wnt/β-catenin, and inflammatory cytokines (33–35). Endothelial to mesenchymal transition (EndMT) is the process through which endothelial cells lose their specific markers and acquire mesenchymal phenotypes, potentially through metabolic reprogramming (36). This underlies regulatory networks involved in cardiovascular disease (CVD) (37), pulmonary vascular diseases (38), fibrotic disorders (39, 40) such as pulmonary fibrosis (41), and ischemic stroke (42). In addition to a complete EndMT, recent studies indicate that ECs can undergo a partial or incomplete EndMT, leading to the transient and reversible formation of intermediate cells that display a combination of both endothelial and mesenchymal features (40). This partial EndMT allows for flexible cellular states process is transient and reversible, playing a crucial role in developmental angiogenesis, pathological vascular remodeling. The ability of ECs to adopt this intermediate state allows for flexible adaptation in response to various physiological and pathological conditions, contributing to tissue regeneration, wound healing, and disease progression in atherosclerotic cardiovascular diseases (CVDs), metabolic CVDs, vascular fibrosis, and cancer (40, 43). In patients with acute coronary syndrome, thrombus leukocytes show a higher expression of endothelial cell-specific angiogenic markers compared to peripheral blood leukocytes, suggesting transdifferentiation from leukocytes into angiogenic endothelial cells (44). Under the influence of proinflammatory cytokines like tumor necrosis factor (TNF)-α and CD4+ T helper cell 1 (Th1) cytokine interferon-γ (IFNγ) (45), human liver sinusoidal endothelial cells upregulate six immune checkpoints (46) and transdifferentiate into immune tolerogenic cells (47).
In this prospective, we propose pathological transdifferentiation as a new concept and novel therapeutic target for functional tissue regeneration following tissue damage related to cardiovascular diseases and chronic inflammation. Unlike current therapies targeting the proinflammatory cytokine-driven early phase of cardiovascular inflammation and innate immune memory (also known as trained immunity 48–50), our focus here is on targeting pathological transdifferentiation after tissue damage. Further understanding of pathological transdifferentiation in cardiovascular diseases and chronic inflammation will significantly advance the development of new therapies for these conditions.
Pathological transdifferentiation: a key contributor to weakened tissue function in cardiovascular diseases and chronic inflammation
Impaired tissue function in cardiovascular diseases results from persistent cellular damage and maladaptive responses that disturb normal tissue homeostasis and weaken physiological functions (51). In cardiovascular diseases, factors such as atherosclerosis, ischemia, diabetes, and metabolic disorders can lead to endothelial cell dysfunction, fibrosis, and vascular remodeling, impairing blood vessel function and contributing to heart failure (52). Chronic inflammation, driven by immune system dysregulation, leads to prolonged activation of inflammatory pathways, causing tissue damage, pathological cell transdifferentiation, and the loss of regenerative capacity (53). To improve our understanding of chronic cardiovascular inflammation, we and others have demonstrated the following: (1) Endogenous metabolite-derived conditional danger-associated molecular patterns (54, 55), such as lysophospholipids (56), trigger the innate immune transdifferentiation of endothelial cells, leading to sustained endothelial activation (57–59). This supports our working model that endothelial cells, angiogenic endothelial cells (60), and angiogenic progenitor cells (61, 62) function as innate immune cells (50, 58, 59); (2) Similar to other CVD risk factors, including hyperlipidemia, diabetes (63), obesity (64–66), hypertension, and cigarette smoke (67), Dr. Wang's team reported that severe hyperhomocysteinemia (HHcy) (68) promotes the differentiation of both bone marrow–derived and resident inflammatory monocytes, which in turn accelerates the development of atherosclerosis in mice lacking low-density lipoprotein receptor (LDLR) and cystathionine β-synthase (CBS) (69–71). Additionally, advanced single-cell analysis of human peripheral blood mononuclear cells (72) reveals distinct intermediate monocyte subsets that correlate with gender variation in coronary artery disease (73); (3) Pathological conditions re-shape physiological CD4+ Foxp3+ regulatory T cells (Tregs) (74) into pathological Tregs (75). We have extensively published data supporting this concept (11, 76–78). Transcription factors such as ATA binding protein 3 (GATA3), BCL6 transcription repressor (BCL6), and histone deacetylase 6 (HDAC6) regulate Treg trandifferentiation (11, 74–77) into antigen-presenting cell (APC)-like Tregs or Th1-Tregs (45). Additionally, immune checkpoints regulate T cell and Treg transdifferentiation and immune tolerance (46, 47, 79). Cigarette smoke combined with morphine reprograms Treg transcriptomes, transdifferentiating anti-inflammatory/immunosuppressive Tregs into proinflammatory/pathogenic CD4+ T helper cell 17 (Th17) cells (80–82). Moreover, 67 different Treg subsets have been identified in human diseases, with 44 subsets identified in cancers including 7 Treg subsets in colorectal cancer, 10 Treg subsets in lung cancer, 9 Treg subsets in liver cancer, 4 Treg subsets in gastric cancer, 2 Treg subsets in breast cancer, 3 Treg subsets in neck carcinoma, 1 Treg subset in cutaneous carcinoma, 2 Treg subsets in cervical cancer, 2 Treg subsets in ovarian cancer, 2 Treg subsets in melanoma, 1 Treg subset in bladder cancer, and 1 Treg subset in follicular lymphoma (77, 83). In addition, 23 different subsets of Treg have been identified in relation to human autoimmune diseases (84), transplantation, and pregnancy, including 4 Treg subsets in systemic lupus erythematosus, 4 Treg subsets in multiple sclerosis, 3 Treg subsets in rheumatoid arthritis, 2 Treg subsets in type 1 diabetes, 2 Treg subsets in organ transplantation, 5 Treg subsets in graft-vs.-host disease, and 3 Treg subsets in pregnancy (11, 85); (4) The aorta, under pathological conditions, functions as an immune organ, upregulating secretomes and providing a microenvironment that support the activation, differentiation, and trans-differentiation of immune and vascular cells. In the early stages, these secretomes may play a pivotal role in driving trained immunity (innate immune memory for inflammation enhancement) (1), as we recently identified IL-1β as a second-tier driver of trained immunity (86); (5) VSMCs have previously been categorized into two types: contractile and de-differentiated (synthetic), characterized by the upregulation of cell proliferation genes (87). However, research using single-cell RNA sequencing (scRNA-Seq) has shown that de-differentiated arterial VSMCs exhibit significant diversity, including mesenchymal (we propose to newly term VSMC to mesenchymal transition here), fibroblast, myofibroblast, osteogenic, osteochondrogenic, macrophage (88), foam cell, adipocyte, and mesenchymal stem cell-like phenotypes (89, 90). Currently, there is no consensus on the numbers of VSMC phenotypes or transcriptomic types (91). Using knowledge-based transcriptomic analysis (92), we reported that the innate immune function of VSMCs plays a role in two-wave inflammation associated with atherosclerosis and twin-peak inflammation seen in aortic aneurysms, and their potential to trans-differentiate into 25 distinct cell types (24); (6) Angiotensin II (Ang II) was found to induce abdominal aortic aneurysm (AAA) and thoracic aortic aneurysm (TAA) in apolipoprotein E-deficient (ApoE−/−) hyperlipidemic mice, leading to the upregulation of 73 and 68 cytokines, respectively. scRNA-Seq identified specific markers associated with macrophages, immune cells, regulators of cell death, and transdifferentiation indicators of neuronal, glial, and squamous epithelial cells in different segments of the aortic, demonstrating that cell transdifferentiation varies across different aortic pathologies (22); (7) Contrary to the traditional model of proinflammatory M1 macrophages and anti-inflammatory M2 macrophages, 32 macrophage pathways/subsets. This includes 20 novel disease-specific pathways and 12 new shared pathways, which has been observed across eight groups of 34 diseases, encompassing 24 inflammatory organ diseases and 10 different types of tumors (93); (8) Chronic kidney disease (72) has been shown to transdifferentiates veins into specialized immune-endocrine organs, characterized by increased signaling of the MYCN proto-oncogene and BHLH transcription factor (MYCN)- activator protein 1 (AP1) signaling (94); and (9) Dr. Vazquez-Padron's team reported that arteries and veins develop distinct types of occlusive diseases and exhibit different responses to injury. Cellular atlases from six veins and arteries reveal that arteries have a 7.8-fold higher proportion of contractile VSMCs and trend to show more modulated VSMCs. In contrast, veins have a greater abundance of endothelial cells, macrophages, and pericytes, along with a rising trend in fibroblast numbers. While activated fibroblasts are found in similar proportions in both vessel types, there are significant differences in their gene expression. Modulated VSMCs (87) and activated fibroblasts are marked by the upregulation of genes such as myosin heavy chain 10 (MYH10), Fibronectin 1 (FN1), collagen type VIII alpha 1 chain (COL8A1), and integrin subunit alpha 10 (ITGA10). Activated fibroblasts also express coagulation factor II thrombin receptor (F2R), periostin (POSTN), and cartilage oligomeric matrix protein (COMP), which have been confirmed through F2R/CD90 flow cytometry. Among fibroblast populations from both arteries and veins, activated fibroblasts from veins are the highest producers of collagens. These venous fibroblasts also exhibit high levels of angiogenesis, proinflammatory activity, and a heightened response to reactive oxygen species (ROS) (95). In arteries, fibroblasts are predominantly located outside the external elastic lamina, whereas in veins, they are more widely distributed throughout the entire venous wall. Consistent with this observation, extracellular matrix (ECM)-targeted proteomics has revealed a greater abundance of fibrillar collagens in veins, while arteries show a higher concentration of basement membrane ECM components (96). However, molecular mechanisms underlying chronic inflammation-induced transdifferentiation remain poorly characterized.
Pathological transdifferentiation pathways are new therapeutic targets
Cancer heterogeneity, rather than oligomeric or clonal expansion of cancer cells, has emerged as a key factor influencing therapy outcomes. As a result, many new cancer treatments now target these heterogeneous cell populations. Although short-term goals have been achieved with treatments like chemotherapy, radiotherapy, anti-angiogenesis therapy, and immunotherapy, long-term cancer regression remains challenging. Consequently, researchers are exploring cellular reprogramming in cancer, suggesting that cancer is a dynamic process rather than a static cellular state, as highlighted by the classic hallmark of cancer (97). Neuroblastoma (NB), for example, arises from the failure of sympathoadrenal progenitor cells to exit their self-renewal phase. Banerjee, D. et al. demonstrated that all-trans retinoic acid (ATRA) can induce growth arrest and differentiation in NB cells, identifying super-enhancers (SEs) that drive TF regulators (24). Time-course histone 3 lysine 27 acetylation (H3K27ac) (98) chromatin immunoprecipitation-DNA sequencing (ChIP-Seq) (99) and RNA-Seq revealed ATRA-coordinated SE waves. CRISPR-Cas9 and siRNA experiments further confirmed that the stem cell development TFs, including SOX11, MYCN, and GATA3, are crucial for this process both in vitro and in vivo. Additional TFs involved in neural development, such as GATA2 and SOX4, were activated in distinct waves at 2, 4, and 8 days of ATRA treatment, identifying oncogenic lineage drivers that sustain NB self-renewal and are essential for the differentiation process (17).
Moreover, chronic inflammation driven by immune dysregulation plays a key role in promoting tumor development. The inflammatory microenvironment influences the fate of lymphocytes through mediators such as cytokines and chemokines. For example, tumor cells secrete immunosuppressive cytokines like vascular endothelial growth factor (VEGF) and TGF-β (100), which facilitate the conversion of effector T cells into CD4+ Foxp3+ Tregs. These Tregs then secrete anti-inflammatory cytokines, including IL-10 and IL-35 (76, 99–102), as well as exosomes (74) and express two prototypic immune checkpoints (46, 47, 77) such as cytotoxic T-lymphocyte associated protein 4 (CTLA4) and programed cell death 1 ligand 1 (PD-L1), suppressing cytotoxic T lymphocyte (CTL) functions (46, 47, 77, 103, 104).
A recent report using single-nucleus RNA-sequencing (snRNA-Seq) on forty-seven liver biopsies collected from patients at various stages of metabolic dysfunction-associated steatotic liver disease established a detailed cellular map of liver changes throughout disease progression. Combining this single-cell data with advanced 3D imaging techniques, Gribben C. et al. revealed profound changes in liver architecture, including the loss of hepatocytes zonation and significant reorganization of the biliary tree. Remarkably, transdifferentiation events between hepatocytes and cholangiocytes were observed, occurring independently of adult stem cells or the activation of developmental progenitors (105). Functional analyses of cholangiocyte organoids confirmed that the PI3K–protein kinase B (AKT)– mammalian target of rapamycin (mTOR) pathway plays a critical role in plasticity and its connection to insulin signaling (106). These findings demonstrate that chronic diseases and injuries induce cellular plasticity in human organs, potentially opening new therapeutic avenues for managing chronic diseases (107).
Cell transdifferentiation and reprogramming have opened possibilities for manipulating cell fate through various external, pharmacological, and therapeutic interventions. Small molecules and elements of the transcription regulatory machinery are emerging as valuable tools for executing cell transdifferentiation, providing new opportunities for disease modeling and drug discovery (108). At more advanced stages, these methods could lead to the development of more efficient and precise gene therapies, as well as enhanced regenerative medicine approaches (109). To address the challenge of detecting low or absent expression of many disease-related genes/transcripts in clinically accessible samples, Li, S., and colleagues devised a diagnostic method that enhances the identification and analysis of tissue-specific genes/transcripts by employing fibroblast to neuron transdifferentiation. They generated induced neurons (iNeurons) from seventy-one patients with primary neurological symptoms enrolled in the Undiagnosed Diseases Network, achieving a 25.4% diagnostic success rate. More than a quarter of these diagnoses were made possibly by transdifferentiation, which would have been missed using fibroblast RNA-seq alone. This iNeuron transdifferentiation-transcriptomic approach can be seamlessly incorporated into whole transcriptome evaluations for genetic disorders (110). In 2015, Fu, Y. and collaborators announced the successful generation of spontaneously beating cardiomyocyte-like cells derived from mouse fibroblasts, achieved solely using chemical cocktails. These chemically induced cardiomyocyte-like cells (CiCMs) expressed markers specific to cardiomyocytes, showed organized sarcomeric structures, and displayed typical cardiac calcium flux and electrophysiological characteristic. Genetic lineage tracing confirmed that these CiCMs originated from fibroblasts. Furthermore, the generation of CiCMs involved a cardiac progenitor stage rather than reverting to a pluripotent state. This discovery, which eliminate the need of viral factors, establishes a foundation for in vivo cardiac transdifferentiation using pharmacological agents, presenting a potentially safer approach for treating heart failure (111). In addition, advanced cell and gene therapies in cardiology are among the new therapies for pathological transdifferentiation (112).
Role of reactive oxygen species (ROS) in pathological transdifferentiation: impact of stimuli intensity, cellular context, and metabolic status
Reactive oxygen species (ROS) are vital contributors to fundamental cellular functions, including cell proliferation, survival, and the sensing of metabolic reprogramming (95). Additionally, ROS play a key role in innate immunity in endothelial cells (58, 59, 113) and VSMCs (22, 24), as well as in stem cell (10) maintenance and neuronal differentiation. In a model of human embryonic stem cell (hESC) using Ntera2 (NT2) cells (114), assays using CM-H2DCFDA (a general indicator of oxidative stress) and dihydroethidium (DHE) verified that the oxidizing agent paraquat induces high levels of ROS in NT2 cells. Paraquat-induced oxidative stress suppresses the expression of stemness markers, such as POU class 5 homeobox 1 (POU5F1, also known as OCT4), nanog homeobox (NANOG), and Cripto (a member of the epidermal growth factor-Cripto-1/FRL-1/Cryptic (EGF-CFC) family, while enhancing the spontaneous expression of neuronal differentiation markers, including paired box 6 (PAX6), neuronal differentiation 1 (NEUROD1), homeobox A1 (HOXA1), neural cell adhesion molecule 1 (NCAM), GDNF family receptor alpha 1 (GFRA1) and class III beta-tubulin (TUJ1). Additionally, the treated cells displayed a markedly different morphology compared to the control cells, characterized by the extension of long neurite-like processes.
The neurogenic effect of ROS on stem cell behavior is suppressed by an antioxidant, but this effect is further intensified by the knockdown of NFE2 like BZIP transcription factor 2 (Nrf2), which is a crucial transcription factor involved in antioxidant signaling (113). Furthermore, paraquat activates the neurogenic mitogen-activated protein kinase (MAPK) pathways, specifically the extracellular signal-regulated kinase 1/2 (ERK1/2) pathways, in a dose-dependent manner (115, 116). This activation can be counteracted by the MEK1/2 inhibitor SL327. These findings indicate that excessive intracellular ROS can lead to a transition away from the stem cell state, promoting the neuronal differentiation of hESCs, with MAPK-ERK1/2 signaling playing a significant role in ROS-induced neuronal differentiation (114). Additionally, nicotinamide adenine dinucleotide phosphate (NADPH) oxidase 4 (NOX4) (117) generates ROS in CD34+ cells during the vascular differentiation of hESCs, suggesting that and modulation of ROS levels with antioxidants, such as selenium, could provide a novel strategy to enhance vascular differentiation efficiency in hESCs (118).
ROS have been linked to mechanisms of heart development and regenerative therapies, particularly in the context of pluripotent stem cell applications. The role of ROS in mediating cell fate depends on several factors, including the strength of stimuli, the cellular context, and the metabolic state of the cells. ROS exert their effects through various targets, such as kinases and transcription factors, playing diverse roles at distinct phases of cardiac differentiation, proliferation, and maturation. The detrimental effects of the host's ROS on graft (donor) cells have been reported in a paracrine manner during stem cell implantation. Therefore, it is crucial to carefully regulate the timing and levels of ROS production following myocardial injury to maximize the effectiveness of regenerative therapies while minimizing potential harm (119).
Recently, we investigated the role of innate immunity in VSMCs and related aortic pathologies through transcriptome analyses of aortas from proatherogenic apolipoprotein E deficient (Ape−/−) mice during both angiotensin II-induced aortic abdominal aneurysm (AAA) and an Ape−/− atherosclerosis over time. Additionally, we analyzed VSMCs stimulated with conditional danger-associated molecular patterns (DAMPs) (54, 55). The DAMPs-stimulated VSMCs exhibited an enhanced potential for trans-differentiation, indicated by upregulation not only some of the 82 markers of seven VSMC-plastic cell types (91), including mesenchymal cells, macrophages, adipocytes, fibroblasts, myofibroblasts, osteogenic cells, and foam cells) but also markers of 18 novel cell types (from 79 human cell types with 8,065 markers identified in the Human Protein Atlas database https://www.proteinatlas.org/) (24). Further analysis of the transcriptomes from gene-deficient models revealed that the antioxidant transcription factor NRF2 plays a role in suppressing inflammation. In contrast, five other inflammatory transcription factors and master regulators —namely the aryl hydrocarbon receptor (AHR, which integrates environmental, dietary, microbial, and metabolic signals to control complex transcriptional programs in a ligand-specific, cell-type-specific and context-specific manner 120) (82), NF-KB, NADPH Oxidases (117) (NOX, enzyme responsible for ROS production), eukaryotic translation initiation factor 2 alpha kinase 3 [EIF2AK3, also known as PERK, an endoplasmic reticulum (ER) stress kinase] (121, 122), and SET domain containing 7 (SET7), a histone lysine methyltransferase associated with trained immunity (123) —were found to drive the upregulation of twelve lists of innate immune genes in the context of atherosclerosis, AAA, and DAMP-stimulated VSMCs (24).
Metabolic reprogramming modulates cell-type specific gene expression through histone acylation
Liver fibrosis is characterized by the excessive and unbalanced accumulation of fibrous extracellular matrix (ECM) in response to hepatic wound healing. This is a common mechanism impairing liver function in many chronic liver diseases. Despite ongoing efforts, effective therapy for fibrosis have yet to be established. Alarmingly, the incidence of fibrosis is increasing as a result of the expanding obesity pandemic. In this review, we outlined the key components and mechanisms driving liver fibrosis, emphasizing the metabolic control of key fibrogenesis regulators, particularly hepatic stellate cells (HSCs), and their involvement in disease advancement. It underscores how the metabolic reprogramming of hepatic cells necessitates a finely tuned cellular response to fulfill energy demands without compromising cellular integrity. This review also highlights the role of RNA-binding proteins (RBPs), which, due to their context- and stimuli-dependent nature, are well-suited to respond to fibrotic conditions. This review also summarize current research on the metabolic regulation of HSCs in liver fibrosis and the role of RBPs in post-transcriptionally controlling the metabolic shift that drives fibrosis and chronic liver disease progression (124).
Chen, X et al. reviewed the manipulation of metabolic enzymes such as solute carrier family 2 member 1 (SLC2A1, Glut1), pyruvate kinase M1/2 (PKM, PKM2), pyruvate dehydrogenase kinase 4 (PDK4), lactate dehydrogenase A (LDHA), succinate dehydrogenase (SDH), carnitine palmitoyl transferase 1B (CPT1b), and 3-hydroxy-3-methylglutaryl-CoA synthase 2 (HMGCS2) to inhibit fatty acid oxidation or promote glycolysis, which is sufficient to promote cardiomyocyte proliferation, though not transdifferentiation (125). Cardiomyocytes possess distinct metabolic features that set them apart from cardiac fibroblasts and pluripotent stem cells (PSCs), with various overlapping molecular mechanisms driving metabolic reprogramming during cardiomyogenesis. Significant metabolic alterations observed during the differentiation of cardiomyocytes from PSCs and cardiac fibroblasts suggest that metabolic reprogramming may play a crucial role in promoting cardiomyogenesis. Sadahiro and Leda proposed in vivo reprogramming as a novel approach to cardiac regeneration therapy (126). Direct reprogramming or transdifferentiation into cardiomyocytes was first demonstrated by Leda et al., who screened 14 transcription factors involved in cardiac development and identified the core cardiac reprogramming transcription factors: GATA binding protein 4 (GATA4), Myocyte enhancer factor 2C (MEF2C), and T-Box Transcription Factor 5 (TBX5), collectively referred to as GMT (127, 128).
In inflammatory vascular endothelial cells (VECs), metabolic changes are characterized by increased glycolysis. Mechanical low shear stress activates hypoxia-inducible factor 1α (HIF-1α) in cultured VECs through the nuclear factor-kB (NF-kB) pathway, leading to the upregulation of OTU deubiquitinating enzyme 7B (Cezanne). HIF-1α increases inflammatory factor production in VECs by upregulating the expression of glycolysis-related regulators, including hexokinase 2 (HK2), glucose transporter 1 (GLUT1), fructose-2,6-biphosphatase 3 (PFKFB3), enolase 2 (ENO2), and the extracellular acidification rate (ECAR), which serves as a direct indicator of glycolytic activity) (36, 129). Metabolic reprogramming appears to exhibit disease specificity: (1) In atherosclerosis, glycolysis and glutaminolysis are increased, while fatty acid beta-oxidation (FAO) and nitric oxide are decreased, leading to EndMT and VEC inflammation; (2) In diabetic angiopathy, glycolysis decreases, resulting in an accumulation of glycolysis intermediates; (3) In tumor neovascularization, both glycolysis and oxidative phosphorylation increase, leading to VEC hyperproliferation; (4) In pulmonary arterial hypertension, glycolysis increases while FAO and nitric oxide decrease, resulting in VEC hyperproliferation and dysfunction; and (5) In hypertension, xanthine oxidase increases, while nitric oxide decreases, causing oxidative stress (36). However, it remains unclear whether these pathological metabolic reprogramming events lead to pathological transdifferentiation. Several metabolic pathways involved in VSMCs transition from contractile to proliferative states in coronary artery disease have been identified, including nitrogen compound metabolism, cellular macromolecule biosynthesis, primary metabolic processes, and the positive regulation of nitrogen compound metabolism (130).
Protein and histone acylation, a crucial type of post-translational modifications, plays a role in various physiological processes (98), including cell differentiation and energy metabolism (131). Recently, fifteen types of short-chain lysine (Lys) acylation have been identified on histones. These include Lys propionylation, Lys butyrylation, Lys 2-hydroxyisobutyrylation, Lys succinylation, Lys malonylation, Lys glutarylation, Lys crotonylation, Lys β-hydroxybutyrylation (132), Lysine acetylation, Lys lactylation, and 2-hydroxyisobutyrylation (133), formylation, palmitoylation, myristoylation, and benzoylation (131). Research indicates that these modifications influence gene expression and are both structurally and functionally distinct from the well-explored histone Lysine methylation, phosphorylation, small ubiquitin-related modifier (SUMO)ylation, and ubiquitinylation as we reported for chronic metabolic disease-driven 164 histone modification enzyme expressions (98) including regulatory enzymes (writers, readers, and erasers) (131). Similar to our findings on trained immunity (innate immune memory and inflammation enhancement) (50, 80, 86, 134, 135), increased glycolysis (48, 49, 102), increased acetyl-CoA generation and increased mevalonate-cholesterol biosynthesis (49, 102, 136), increased S-adenosylhomocysteine (SAH) (136), and increased bioenergistic activities (137), metabolites-facilitated histone modifications underlie epigenetic memory (138) for pathological transdifferentiation. In chronic inflammation, metabolites-modified histones regulate gene expression, while oncometabolites promote tumorigenesis by increasing protein acylation, which results in chromosomal remodeling and modifications of non-histone protein (133).
Tan, Y et al. demonstrate the therapeutic benefits of metabolic reprogramming in improving cancer chemotherapy. Utilizing high-throughput stimulated Raman scattering imaging and single-cell analysis, they found that cells resistant to cisplatin show heightened uptake of fatty acids (FA), while exhibiting reduced glucose uptake and lipogenesis. This suggests a metabolic reprogramming from glucose-based to FA-dependent anabolic and energy metabolism. Such a shift supports cancer cell survival in the face of oxidative stress induced by cisplatin, priming by boosting fatty acid beta-oxidation (FAO) (139). Inhibiting FAO with a small molecule blocker, when combined with cisplatin or carboplatin, works synergistically to reduce ovarian cancer cell proliferation in vitro and to inhibit the growth of patient-derived xenografts in vivo. This work supports a method for rapidly detecting cisplatin-resistance at the single-cell level, as well as a strategy for treating tumors that are resistant to cisplatin (140). Mitochondria, long viewed as the cell's “powerhouses” for their essential role in energy production, have now been recognized as multifunctional organelles that connect bioenergetics, immunity, and metabolic signaling. We recently introduced the innovative concept of intracellular immunity and proposed that mitochondria as the leading immune organelles, emphasizing the role of mitochondria as crucial hubs for immune signaling (138). Metabolic reprogramming-offers a promising model for developing new cardiovascular disease therapies not only to inhibit trained immunity but also to suppress pathological transdifferentiation.
Conclusion
In this perspective, we have analyzed recent progress in characterizing pathological transdifferentiation across various cardiovascular diseases, highlighting the underlying molecular mechanisms and exploring potential new therapeutics targeting this process. Of note, it has been reported that nonsteroidal anti-inflammatory drugs have cardiovascular risk (141) and anti-inflammatory drug glucocorticoids are also found with increasing cardiovascular risk (142). Current therapies for cardiovascular diseases tend to focus on single molecular target (143) (Figure 1). For example, the cholesterol-lowering drug statins inhibit 3-hydroxy-3-methylglutaryl-CoA (HMG-CoA) reductase (144); the anti-inflammatory and anti-atherosclerosis monoclonal antibody (Mab) drug Canakinumab targets interleukin-1β (IL-1β) (86, 137, 145); and the fully human anti-PD-1 monoclonal antibody MEDI0680 inhibits the prototypic immune checkpoint programmed cell death protein 1 (PD-1) (146). Pathological transdifferentiation represents a new therapeutic target that could prevent chronic inflammation and cardiovascular disease-induced cell death (113), block tissue injury-triggered pathological transdifferentiation and restore cellular and tissue functions. Tissue remodeling is indeed a complex and sometimes poorly defined therapeutic target in various diseases due to the complexity of the remodeling process, lack of biomarkers, and the variability across diseases (147–149). Pathological transdifferentiation provides a more defined and targeted approach compared to the broader and more complex concept of tissue remodeling. With ongoing research and advancements in technology, regenerative transdifferentiation (restore tissue function) holds significant promise for regenerative medicine and therapeutic interventions across various diseases.
To address this perspective, we propose a new working model (Figure 2): (1) cardiovascular diseases such as myocardial diseases, ischemic heart diseases, and peripheral arterial diseases (150) can induce tissue damage and fibrosis leading to pathological trandifferentiation. Chronic inflammation and cardiovascular disease risk factors generate a variety of DAMPs, PAMPs, cytokines and chemokines, secretomes, and cell death receptors. These pathological signals activate intracellular signaling cascades that lead to the activation of cell lineage-specific differentiation and transdifferentiation transcription factors, including oncogenic transcription factors (oncogenesis, outside the scope of this perspective). This process drives transcriptomic reprogramming, which underpins pathological transdifferentiation. To establish the pathological transdifferentiation program, these signals trigger different forms of metabolic reprogramming, including disease-specific, disease stage-specific, pathological cell type-specific, cell origin-specific, and tissue specific metabolic reprogramming. These metabolic reprogramming generate unique sets of metabolites or combinations of metabolites, which in turn drive specific epigenetic and histone modifications. These modifications help to stabilize and epigenetically memorizing the pathological transdifferentiation processes driven by the specific transcription factors. The concept of metabolic reprogramming leading to epigenetic memory has also been reported in studies of trained immunity (also known as innate immune memory) and trained tolerance (22, 24, 48–50, 82, 102, 134, 135, 151). This new working model enhances our understanding of chronic inflammation- and cardiovascular disease-induced pathological transdifferentiation and identifies new therapeutic targets for treating these diseases, as well as for regenerative medicine.
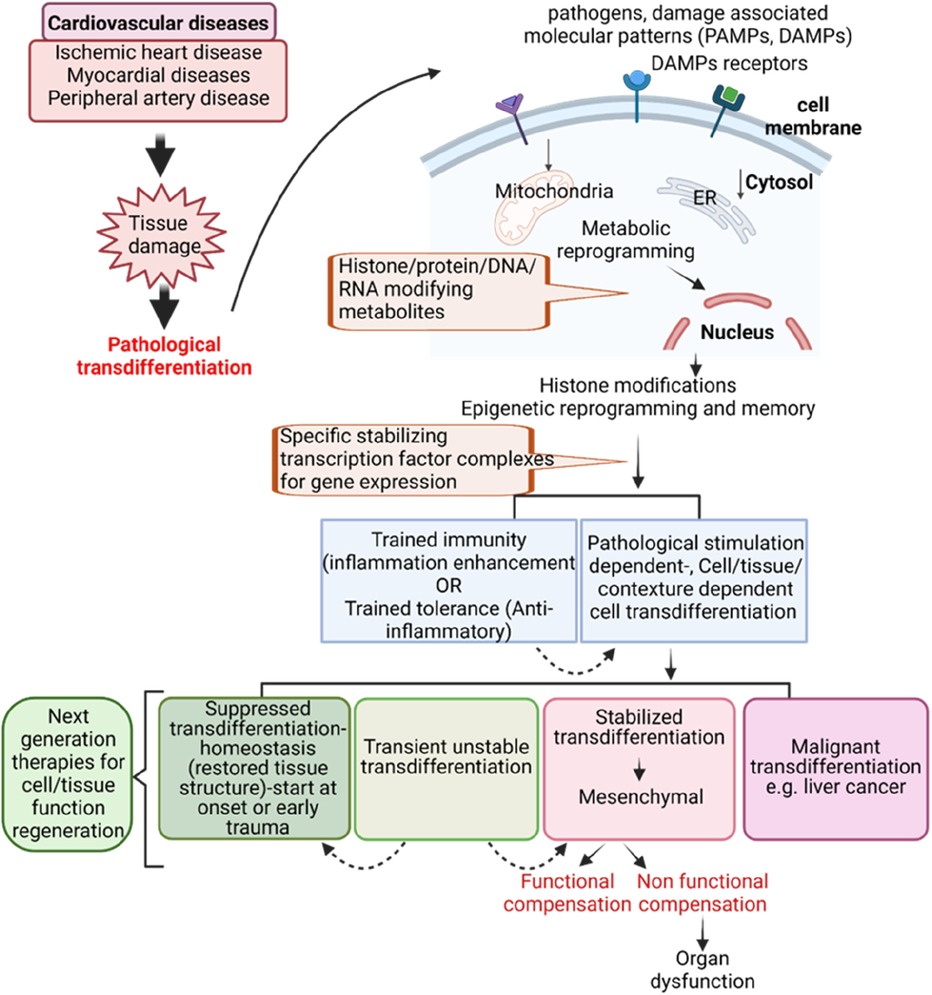
Figure 2. Innovative model for targeting pathological transdifferentiation in cardiovascular disease treatment.
Data availability statement
The original contributions presented in the study are included in the article/Supplementary Material, further inquiries can be directed to the corresponding author.
Author contributions
WY: Conceptualization, Writing – original draft, Writing – review & editing. MB: Conceptualization, Writing – original draft, Writing – review & editing. FS: Conceptualization, Methodology, Supervision, Writing – original draft, Writing – review & editing. KX: Conceptualization, Writing – review & editing. YS: Conceptualization, Writing – review & editing. YL: Conceptualization, Writing – review & editing. WD: Conceptualization, Writing – review & editing. RC: Conceptualization, Writing – review & editing. XJ: Conceptualization, Writing – review & editing. HW: Conceptualization, Supervision, Writing – review & editing. XY: Conceptualization, Funding acquisition, Investigation, Project administration, Resources, Supervision, Visualization, Writing – original draft, Writing – review & editing.
Funding
The author(s) declare financial support was received for the research, authorship, and/or publication of this article. This study was supported by research grants from National Institute of Health to XY (R01 HL163570-01A1 and 1R01HL147565-01), and HW (DK113775) and American Heart Association Postdoctoral fellowship to KX (24POST1196349).
Conflict of interest
The authors declare that the research was conducted in the absence of any commercial or financial relationships that could be construed as a potential conflict of interest.
The author(s) declared that they were an editorial board member of Frontiers, at the time of submission. This had no impact on the peer review process and the final decision.
Publisher's note
All claims expressed in this article are solely those of the authors and do not necessarily represent those of their affiliated organizations, or those of the publisher, the editors and the reviewers. Any product that may be evaluated in this article, or claim that may be made by its manufacturer, is not guaranteed or endorsed by the publisher.
References
1. Lu Y, Sun Y, Xu K, Saaoud F, Shao Y, Drummer C, et al. Aorta in pathologies may function as an immune organ by upregulating secretomes for immune and vascular cell activation, differentiation and trans-differentiation-early secretomes may serve as drivers for trained immunity. Front Immunol. (2022) 13:858256. doi: 10.3389/fimmu.2022.858256
2. Holzem M, Boutros M, Holstein TW. The origin and evolution of Wnt signalling. Nat Rev Genet. (2024) 25:500–12. doi: 10.1038/s41576-024-00699-w
3. Liu J, Xiao Q, Xiao J, Niu C, Li Y, Zhang X, et al. Wnt/beta-catenin signalling: function, biological mechanisms, and therapeutic opportunities. Signal Transduct Target Ther. (2022) 7:3. doi: 10.1038/s41392-021-00762-6
4. Karin M, Clevers H. Reparative inflammation takes charge of tissue regeneration. Nature. (2016) 529:307–15. doi: 10.1038/nature17039
5. Vanka KS, Shukla S, Gomez HM, James C, Palanisami T, Williams K, et al. Understanding the pathogenesis of occupational coal and silica dust-associated lung disease. Eur Respir Rev. (2022) 31(165):210250. doi: 10.1183/16000617.0250-2021
6. Halezeroglu S, Okur E. Thoracic surgery for haemoptysis in the context of tuberculosis: what is the best management approach? J Thorac Dis. (2014) 6:182–5. doi: 10.3978/j.issn.2072-1439.2013.12.25
7. Bansal A, Yanamaladoddi VR, Sarvepalli SS, Vemula SL, Aramadaka S, Mannam R, et al. Surviving pulmonary Tuberculosis: navigating the long term respiratory effects. Cureus. (2023) 15:e38811. doi: 10.7759/cureus.38811
8. Goei H, van der Vlies CH, Hop MJ, Tuinebreijer WE, Nieuwenhuis MK, Middelkoop E, et al. Long-term scar quality in burns with three distinct healing potentials: a multicenter prospective cohort study. Wound Repair Regen. (2016) 24:721–30. doi: 10.1111/wrr.12438
9. Richardson WJ, Clarke SA, Quinn TA, Holmes JW. Physiological implications of myocardial scar structure. Compr Physiol. (2015) 5:1877–909. doi: 10.1002/cphy.c140067
10. Yang XF. Immunology of stem cells and cancer stem cells. Cell Mol Immunol. (2007) 4:161–71.17601370
11. Zhang R, Xu K, Shao Y, Sun Y, Saredy J, Cutler E, et al. Tissue treg secretomes and transcription factors shared with stem cells contribute to a treg niche to maintain treg-ness with 80% innate immune pathways, and functions of immunosuppression and tissue repair. Front Immunol. (2020) 11:632239. doi: 10.3389/fimmu.2020.632239
12. Jopling C, Boue S, Izpisua Belmonte JC. Dedifferentiation, transdifferentiation and reprogramming: three routes to regeneration. Nat Rev Mol Cell Biol. (2011) 12:79–89. doi: 10.1038/nrm3043
13. Heinz S, Benner C, Spann N, Bertolino E, Lin YC, Laslo P, et al. Simple combinations of lineage-determining transcription factors prime cis-regulatory elements required for macrophage and B cell identities. Mol Cell. (2010) 38:576–89. doi: 10.1016/j.molcel.2010.05.004
14. Belcheva KT, Chaudhuri J. Maintenance of lineage identity: lessons from a B cell. J Immunol. (2022) 209:2073–81. doi: 10.4049/jimmunol.2200497
15. Nagy G, Bojcsuk D, Tzerpos P, Cseh T, Nagy L. Lineage-determining transcription factor-driven promoters regulate cell type-specific macrophage gene expression. Nucleic Acids Res. (2024) 52:4234–56. doi: 10.1093/nar/gkae088
16. Ng AHM, Khoshakhlagh P, Rojo Arias JE, Pasquini G, Wang K, Swiersy A, et al. A comprehensive library of human transcription factors for cell fate engineering. Nat Biotechnol. (2021) 39:510–9. doi: 10.1038/s41587-020-0742-6
17. Banerjee D, Bagchi S, Liu Z, Chou HC, Xu M, Sun M, et al. Lineage specific transcription factor waves reprogram neuroblastoma from self-renewal to differentiation. Nat Commun. (2024) 15:3432. doi: 10.1038/s41467-024-47166-y
18. Roccio M. Directed differentiation and direct reprogramming: applying stem cell technologies to hearing research. Stem Cells. (2021) 39:375–88. doi: 10.1002/stem.3315
19. Slack JM. Metaplasia and transdifferentiation: from pure biology to the clinic. Nat Rev Mol Cell Biol. (2007) 8:369–78. doi: 10.1038/nrm2146
20. Tani H, Sadahiro T, Yamada Y, Isomi M, Yamakawa H, Fujita R, et al. Direct reprogramming improves cardiac function and reverses fibrosis in chronic myocardial infarction. Circulation. (2023) 147:223–38. doi: 10.1161/CIRCULATIONAHA.121.058655
21. Fu X, Liu Q, Li C, Li Y, Wang L. Cardiac fibrosis and cardiac fibroblast lineage-tracing: recent advances. Front Physiol. (2020) 11:416. doi: 10.3389/fphys.2020.00416
22. Lu Y, Sun Y, Saaoud F, Shao Y, Xu K, Jiang X, et al. ER stress mediates angiotensin II-augmented innate immunity memory and facilitates distinct susceptibilities of thoracic from abdominal aorta to aneurysm development. Front Immunol. (2023) 14:1268916. doi: 10.3389/fimmu.2023.1268916
23. Chen PY, Qin L, Li G, Malagon-Lopez J, Wang Z, Bergaya S, et al. Smooth muscle cell reprogramming in aortic aneurysms. Cell Stem Cell. (2020) 26:542–57.e511. doi: 10.1016/j.stem.2020.02.013
24. Yang Q, Saaoud F, Lu Y, Pu Y, Xu K, Shao Y, et al. Innate immunity of vascular smooth muscle cells contributes to two-wave inflammation in atherosclerosis, twin-peak inflammation in aortic aneurysms and trans-differentiation potential into 25 cell types. Front Immunol. (2023) 14:1348238. doi: 10.3389/fimmu.2023.1348238
25. Seo J, Ha J, Kang E, Cho S. The role of epithelial-mesenchymal transition-regulating transcription factors in anti-cancer drug resistance. Arch Pharm Res. (2021) 44:281–92. doi: 10.1007/s12272-021-01321-x
26. Haensel D, Dai X. Epithelial-to-mesenchymal transition in cutaneous wound healing: where we are and where we are heading. Dev Dyn. (2018) 247:473–80. doi: 10.1002/dvdy.24561
27. Lamouille S, Xu J, Derynck R. Molecular mechanisms of epithelial-mesenchymal transition. Nat Rev Mol Cell Biol. (2014) 15:178–96. doi: 10.1038/nrm3758
28. Marconi GD, Fonticoli L, Rajan TS, Pierdomenico SD, Trubiani O, Pizzicannella J, et al. Epithelial-mesenchymal transition (EMT): the type-2 EMT in wound healing, tissue regeneration and organ fibrosis. Cells. (2021) 10(7):1587. doi: 10.3390/cells10071587
29. Nieto MA, Huang RY, Jackson RA, Thiery JP. EMT: 2016. Cell. (2016) 166:21–45. doi: 10.1016/j.cell.2016.06.028
30. Dongre A, Weinberg RA. New insights into the mechanisms of epithelial-mesenchymal transition and implications for cancer. Nat Rev Mol Cell Biol. (2019) 20:69–84. doi: 10.1038/s41580-018-0080-4
31. Guo Y, Jiang Y, Rose JB, Nagaraju GP, Jaskula-Sztul R, Hjelmeland AB, et al. Protein kinase D1 signaling in cancer stem cells with epithelial-mesenchymal plasticity. Cells. (2022) 11(23):3885. doi: 10.3390/cells11233885
32. Kardooni A, Bahrampour A, Golmohammadi S, Jalili A, Alishahi MM. The role of epithelial mesenchymal transition (EMT) in pathogenesis of cardiotoxicity: diagnostic & prognostic approach. Mol Biotechnol. (2023) 65:1403–13. doi: 10.1007/s12033-023-00697-z
33. Kovacic JC, Mercader N, Torres M, Boehm M, Fuster V. Epithelial-to-mesenchymal and endothelial-to-mesenchymal transition: from cardiovascular development to disease. Circulation. (2012) 125:1795–808. doi: 10.1161/CIRCULATIONAHA.111.040352
34. Jayachandran J, Srinivasan H, Mani KP. Molecular mechanism involved in epithelial to mesenchymal transition. Arch Biochem Biophys. (2021) 710:108984. doi: 10.1016/j.abb.2021.108984
35. Wesseling M, Sakkers TR, de Jager SCA, Pasterkamp G, Goumans MJ. The morphological and molecular mechanisms of epithelial/endothelial-to-mesenchymal transition and its involvement in atherosclerosis. Vasc Pharmacol. (2018) 106:1–8. doi: 10.1016/j.vph.2018.02.006
36. Peng H, Wang X, Du J, Cui Q, Huang Y, Jin H. Metabolic reprogramming of vascular endothelial cells: basic research and clinical applications. Front Cell Dev Biol. (2021) 9:626047. doi: 10.3389/fcell.2021.626047
37. Peng Q, Shan D, Cui K, Li K, Zhu B, Wu H, et al. The role of endothelial-to-mesenchymal transition in cardiovascular disease. Cells. (2022) 11(11):1834. doi: 10.3390/cells11111834
38. Yun E, Kook Y, Yoo KH, Kim KI, Lee MS, Kim J, et al. Endothelial to mesenchymal transition in pulmonary vascular diseases. Biomedicines. (2020) 8(12):639. doi: 10.3390/biomedicines8120639
39. Piera-Velazquez S, Li Z, Jimenez SA. Role of endothelial-mesenchymal transition (EndoMT) in the pathogenesis of fibrotic disorders. Am J Pathol. (2011) 179:1074–80. doi: 10.1016/j.ajpath.2011.06.001
40. Piera-Velazquez S, Jimenez SA. Endothelial to mesenchymal transition: role in physiology and in the pathogenesis of human diseases. Physiol Rev. (2019) 99:1281–324. doi: 10.1152/physrev.00021.2018
41. Jia W, Wang Z, Gao C, Wu J, Wu Q. Trajectory modeling of endothelial-to-mesenchymal transition reveals galectin-3 as a mediator in pulmonary fibrosis. Cell Death Dis. (2021) 12:327. doi: 10.1038/s41419-021-03603-0
42. Chen D, Li L, Wang Y, Xu R, Peng S, Zhou L, et al. Ischemia-reperfusion injury of brain induces endothelial-mesenchymal transition and vascular fibrosis via activating let-7i/TGF-βR1 double-negative feedback loop. FASEB J. (2020) 34:7178–91. doi: 10.1096/fj.202000201R
43. Welch-Reardon KM, Wu N, Hughes CC. A role for partial endothelial-mesenchymal transitions in angiogenesis? Arterioscler Thromb Vasc Biol. (2015) 35:303–8. doi: 10.1161/ATVBAHA.114.303220
44. Fu H, Vadalia N, Xue ER, Johnson C, Wang L, Yang WY, et al. Thrombus leukocytes exhibit more endothelial cell-specific angiogenic markers than peripheral blood leukocytes do in acute coronary syndrome patients, suggesting a possibility of trans-differentiation: a comprehensive database mining study. J Hematol Oncol. (2017) 10:74. doi: 10.1186/s13045-017-0440-0
45. Xu K, Yang WY, Nanayakkara GK, Shao Y, Yang F, Hu W, et al. GATA3, HDAC6, and BCL6 regulate FOXP3+ treg plasticity and determine treg conversion into either novel antigen-presenting cell-like treg or Th1-treg. Front Immunol. (2018) 9:45. doi: 10.3389/fimmu.2018.00045
46. Shao Y, Saaoud F, Xu K, Lu Y, Jiang X, Wang H, et al. Cardiovascular disease risk factors, immune checkpoints and tregs. In: Agrawal DK, Djuric DM, editors. Environmental Factors in the Pathogenesis of Cardiovascular Diseases. Switzerland AG: Springer Nature (2024). p. 51–93.
47. Shen H, Wu N, Nanayakkara G, Fu H, Yang Q, Yang WY, et al. Co-signaling receptors regulate T-cell plasticity and immune tolerance. Front Biosci (Landmark Ed). (2019) 24:96–132. doi: 10.2741/4710
48. Saaoud F, Liu L, Xu K, Cueto R, Shao Y, Lu Y, et al. Aorta- and liver-generated TMAO enhances trained immunity for increased inflammation via ER stress/mitochondrial ROS/glycolysis pathways. JCI Insight. (2023) 8(1):e158183. doi: 10.1172/jci.insight.158183
49. Lu Y, Sun Y, Drummer C, Nanayakkara GK, Shao Y, Saaoud F, et al. Increased acetylation of H3K14 in the genomic regions that encode trained immunity enzymes in lysophosphatidylcholine-activated human aortic endothelial cells—novel qualification markers for chronic disease risk factors and conditional DAMPs. Redox Biol. (2019) 24:101221. doi: 10.1016/j.redox.2019.101221
50. Drummer C, Saaoud F, Shao Y, Sun Y, Xu K, Lu Y, et al. Trained immunity and reactivity of macrophages and endothelial cells. Arterioscler Thromb Vasc Biol. (2021) 41:1032–46. doi: 10.1161/ATVBAHA.120.315452
51. Alfaddagh A, Martin SS, Leucker TM, Michos ED, Blaha MJ, Lowenstein CJ, et al. Inflammation and cardiovascular disease: from mechanisms to therapeutics. Am J Prev Cardiol. (2020) 4:100130. doi: 10.1016/j.ajpc.2020.100130
52. Gimbrone MA Jr., García-Cardeña G. Endothelial cell dysfunction and the pathobiology of atherosclerosis. Circ Res. (2016) 118:620–36. doi: 10.1161/CIRCRESAHA.115.306301
53. Furman D, Campisi J, Verdin E, Carrera-Bastos P, Targ S, Franceschi C, et al. Chronic inflammation in the etiology of disease across the life span. Nat Med. (2019) 25:1822–32. doi: 10.1038/s41591-019-0675-0
54. Wang X, Li YF, Nanayakkara G, Shao Y, Liang B, Cole L, et al. Lysophospholipid receptors, as novel conditional danger receptors and homeostatic receptors modulate inflammation-novel paradigm and therapeutic potential. J Cardiovasc Transl Res. (2016) 9:343–59. doi: 10.1007/s12265-016-9700-6
55. Shao Y, Nanayakkara G, Cheng J, Cueto R, Yang WY, Park JY, et al. Lysophospholipids and their receptors serve as conditional DAMPs and DAMP receptors in tissue oxidative and inflammatory injury. Antioxid Redox Signal. (2018) 28:973–86. doi: 10.1089/ars.2017.7069
56. Li YF, Li RS, Samuel SB, Cueto R, Li XY, Wang H, et al. Lysophospholipids and their G protein-coupled receptors in atherosclerosis. Front Biosci (Landmark Ed). (2016) 21:70–88. doi: 10.2741/4377
57. Li X, Wang L, Fang P, Sun Y, Jiang X, Wang H, et al. Lysophospholipids induce innate immune transdifferentiation of endothelial cells, resulting in prolonged endothelial activation. J Biol Chem. (2018) 293(28):11033–45. doi: 10.1074/jbc.RA118.002752
58. Shao Y, Saredy J, Yang WY, Sun Y, Lu Y, Saaoud F, et al. Vascular endothelial cells and innate immunity. Arterioscler Thromb Vasc Biol. (2020) 40:e138–52. doi: 10.1161/ATVBAHA.120.314330
59. Mai J, Virtue A, Shen J, Wang H, Yang XF. An evolving new paradigm: endothelial cells–conditional innate immune cells. J Hematol Oncol. (2013) 6:61. doi: 10.1186/1756-8722-6-61
60. Lopez-Pastrana J, Ferrer L, Li YF, Xiong X, Xi H, Cueto R, et al. Inhibition of caspase-1 activation in endothelial cells improves angiogenesis: a novel therapeutic potential for ischemia. J Biol Chem. (2015) 290(28):17485–94. doi: 10.1074/jbc.M115.641191
61. Li Y-F, Huang X, Li X, Gong R, Yin Y, Nelson J, et al. Caspase-1 mediates hyperlipidemia-weakened progenitor cell vessel repair. Frontiers in Bioscience (Landmark Edition). (2015) 20:1. doi: 10.2741/4297
62. Li YF, Huang X, Li X, Gong R, Yin Y, Nelson J, et al. Caspase-1 mediates hyperlipidemia-weakened progenitor cell vessel repair. Front Biosci (Landmark Ed). (2016) 21:178–91. doi: 10.2741/4383
63. Yan Y, Xiong Z, Zhang S, Song J, Huang Y, Thornton AM, et al. CD25high T cells with a prolonged survival inhibit development of diabetes. Int J Immunopathol Pharmacol. (2008) 21:767–80. doi: 10.1177/039463200802100401
64. Virtue A, Johnson C, Lopez-Pastrana J, Shao Y, Fu H, Li X, et al. MicroRNA-155 deficiency leads to decreased atherosclerosis, increased white adipose tissue obesity, and non-alcoholic fatty liver disease: a NOVEL MOUSE MODEL OF OBESITY PARADOX. J Biol Chem. (2017) 292:1267–87. doi: 10.1074/jbc.M116.739839
65. Johnson C, Drummer C, Virtue A, Gao T, Wu S, Hernandez M, et al. Increased expression of resistin in MicroRNA-155-deficient white adipose tissues may be a possible driver of metabolically healthy obesity transition to classical obesity. Front Physiol. (2018) 9:1297. doi: 10.3389/fphys.2018.01297
66. Johnson C, Drummer Iv C, Shan H, Shao Y, Sun Y, Lu Y, et al. A novel subset of CD95(+) pro-inflammatory macrophages overcome miR155 deficiency and may serve as a switch from metabolically healthy obesity to metabolically unhealthy obesity. Front Immunol. (2020) 11:619951. doi: 10.3389/fimmu.2020.619951
67. Yang XF, Yin Y, Wang H. Vascular inflammation and atherogenesis are activated via receptors for pamps and suppressed by regulatory t cells. Drug Discov Today Ther Strateg. (2008) 5:125–42. doi: 10.1016/j.ddstr.2008.11.003
68. Shen W, Gao C, Cueto R, Liu L, Fu H, Shao Y, et al. Homocysteine-methionine cycle is a metabolic sensor system controlling methylation-regulated pathological signaling. Redox Biol. (2020) 28:101322. doi: 10.1016/j.redox.2019.101322
69. Wang H, Jiang X, Yang F, Gaubatz JW, Ma L, Magera MJ, et al. Hyperhomocysteinemia accelerates atherosclerosis in cystathionine beta-synthase and apolipoprotein E double knock-out mice with and without dietary perturbation. Blood. (2003) 101:3901–7. doi: 10.1182/blood-2002-08-2606
70. Zhang D, Fang P, Jiang X, Nelson J, Moore JK, Kruger WD, et al. Severe hyperhomocysteinemia promotes bone marrow-derived and resident inflammatory monocyte differentiation and atherosclerosis in LDLr/CBS-deficient mice. Circ Res. (2012) 111:37–49. doi: 10.1161/CIRCRESAHA.112.269472
71. Zhang D, Jiang X, Fang P, Yan Y, Song J, Gupta S, et al. Hyperhomocysteinemia promotes inflammatory monocyte generation and accelerates atherosclerosis in transgenic cystathionine beta-synthase-deficient mice. Circulation. (2009) 120:1893–902. doi: 10.1161/CIRCULATIONAHA.109.866889
72. Zhang R, Saredy J, Shao Y, Yao T, Liu L, Saaoud F, et al. End-stage renal disease is different from chronic kidney disease in upregulating ROS-modulated proinflammatory secretome in PBMCs—a novel multiple-hit model for disease progression. Redox Biol. (2020) 34:101460. doi: 10.1016/j.redox.2020.101460
73. Chatterjee N, Komaravolu RK, Durant CP, Wu R, McSkimming C, Drago F, et al. Single cell high dimensional analysis of human peripheral blood mononuclear cells reveals unique intermediate monocyte subsets associated with sex differences in coronary artery disease. Int J Mol Sci. (2024) 25(5):2894. doi: 10.3390/ijms25052894
74. Yang Q, Nanayakkara GK, Drummer C, Sun Y, Johnson C, Cueto R, et al. Low-Intensity ultrasound-induced anti-inflammatory effects are mediated by several new mechanisms including gene induction, immunosuppressor cell promotion, and enhancement of exosome biogenesis and docking. Front Physiol. (2017) 8:818. doi: 10.3389/fphys.2017.00818
75. Yang WY, Shao Y, Lopez-Pastrana J, Mai J, Wang H, Yang X-f. Pathological conditions re-shape physiological tregs into pathological tregs. Burns Trauma. (2015) 3:1–11. doi: 10.1186/s41038-015-0001-0
76. Shao Y, Yang WY, Saaoud F, Drummer C, Sun Y, Xu K, et al. IL-35 promotes CD4+Foxp3+ tregs and inhibits atherosclerosis via maintaining CCR5-amplified treg-suppressive mechanisms. JCI Insight. (2021) 6(19):e152511. doi: 10.1172/jci.insight.152511
77. Ni D, Tang T, Lu Y, Xu K, Shao Y, Saaoud F, et al. Canonical secretomes, innate immune caspase-1-, 4/11-gasdermin D non-canonical secretomes and exosomes may contribute to maintain treg-ness for treg immunosuppression, tissue repair and modulate anti-tumor immunity via ROS pathways. Front Immunol. (2021) 12:678201. doi: 10.3389/fimmu.2021.678201
78. Liu M, Wu N, Xu K, Saaoud F, Vasilopoulos E, Shao Y, et al. Organelle crosstalk regulators are regulated in diseases, tumors, and regulatory T cells: novel classification of organelle crosstalk regulators. Front Cardiovasc Med. (2021) 8:713170. doi: 10.3389/fcvm.2021.713170
79. Sakaguchi S, Mikami N, Wing JB, Tanaka A, Ichiyama K, Ohkura N. Regulatory T cells and human disease. Annu Rev Immunol. (2020) 38:541–66. doi: 10.1146/annurev-immunol-042718-041717
80. Shao Y, Saaoud F, Cornwell W, Xu K, Kirchhoff A, Lu Y, et al. Cigarette smoke and morphine promote treg plasticity to Th17 via enhancing trained immunity. Cells. (2022) 11(18):2810. doi: 10.3390/cells11182810
81. Shao Y, Cornwell W, Xu K, Kirchhoff A, Saasoud F, Lu Y, et al. Chronic exposure to the combination of cigarette smoke and morphine decreases CD4(+) regulatory T cell numbers by reprogramming the treg cell transcriptome. Front Immunol. (2022) 13:887681. doi: 10.3389/fimmu.2022.887681
82. Saaoud F, Shao Y, Cornwell W, Wang H, Rogers T, Yang X. Cigarette smoke modulates inflammation and immunity via ROS-regulated trained immunity and trained tolerance mechanisms. Antioxid Redox Signal. (2022) 38(13-15):1041–69. doi: 10.1089/ars.2022.0087
83. Ke X, Wang J, Li L, Chen IH, Wang H, Yang XF. Roles of CD4+CD25(high) FOXP3+ tregs in lymphomas and tumors are complex. Front Biosci. (2008) 13:3986–4001. doi: 10.2741/2986
84. Ng B, Yang F, Huston DP, Yan Y, Yang Y, Xiong Z, et al. Increased noncanonical splicing of autoantigen transcripts provides the structural basis for expression of untolerized epitopes. J Allergy Clin Immunol. (2004) 114:1463–70. doi: 10.1016/j.jaci.2004.09.006
85. Jiang Z, Zhu H, Wang P, Que W, Zhong L, Li XK, et al. Different subpopulations of regulatory T cells in human autoimmune disease, transplantation, and tumor immunity. MedComm (2020). (2022) 3:e137. doi: 10.1002/mco2.137
86. Sun Y, Lu Y, Liu L, Saaoud F, Shao Y, Xu K, et al. Caspase-4/11 promotes hyperlipidemia and chronic kidney disease-accelerated vascular inflammation by enhancing trained immunity. JCI Insight. (2024) 9(16):e177229. doi: 10.1172/jci.insight.177229
87. Monroy MA, Fang J, Li S, Ferrer L, Birkenbach MP, Lee IJ, et al. Chronic kidney disease alters vascular smooth muscle cell phenotype. Front Biosci (Landmark Ed). (2015) 20:784–95. doi: 10.2741/4337
88. Bennett MR, Sinha S, Owens GK. Vascular smooth muscle cells in atherosclerosis. Circ Res. (2016) 118:692–702. doi: 10.1161/CIRCRESAHA.115.306361
89. Grootaert MOJ, Bennett MR. Vascular smooth muscle cells in atherosclerosis: time for a re-assessment. Cardiovasc Res. (2021) 117:2326–39. doi: 10.1093/cvr/cvab046
90. Chen R, McVey DG, Shen D, Huang X, Ye S. Phenotypic switching of vascular smooth muscle cells in atherosclerosis. J Am Heart Assoc. (2023) 12:e031121. doi: 10.1161/jaha.123.031121
91. Yap C, Mieremet A, de Vries CJM, Micha D, de Waard V. Six shades of vascular smooth muscle cells illuminated by KLF4 (Kruppel-like factor 4). Arterioscler Thromb Vasc Biol. (2021) 41:2693–707. doi: 10.1161/ATVBAHA.121.316600
92. Xu K, Shao Y, Saaoud F, Gillespie A, Drummer C, Liu L, et al. Novel knowledge-based transcriptomic profiling of lipid lysophosphatidylinositol-induced endothelial cell activation. Front Cardiovasc Med. (2021) 8:773473. doi: 10.3389/fcvm.2021.773473
93. Lai B, Wang J, Fagenson A, Sun Y, Saredy J, Lu Y, et al. Twenty novel disease group-specific and 12 new shared macrophage pathways in eight groups of 34 diseases including 24 inflammatory organ diseases and 10 types of tumors. Front Immunol. (2019) 10:2612. doi: 10.3389/fimmu.2019.02612
94. Saaoud F, Martinez L, Lu Y, Xu K, Shao Y, Zhuo JL, et al. Chronic kidney disease transdifferentiates veins into a specialized immune-endocrine organ with increased MYCN-AP1 signaling. Cells. (2023) 12(11):1482. doi: 10.3390/cells12111482
95. Sun Y, Lu Y, Saredy J, Wang X, Drummer Iv C, Shao Y, et al. ROS systems are a new integrated network for sensing homeostasis and alarming stresses in organelle metabolic processes. Redox Biol. (2020) 37:101696. doi: 10.1016/j.redox.2020.101696
96. Rojas MG, Pereira-Simon S, Zigmond ZM, Varona Santos J, Perla M, Santos Falcon N, et al. Single-cell analyses offer insights into the different remodeling programs of arteries and veins. Cells. (2024) 13(10):793. doi: 10.3390/cells13100793
97. Xiong S, Feng Y, Cheng L. Cellular reprogramming as a therapeutic target in cancer. Trends Cell Biol. (2019) 29:623–34. doi: 10.1016/j.tcb.2019.05.001
98. Shao Y, Chernaya V, Johnson C, Yang WY, Cueto R, Sha X, et al. Metabolic diseases downregulate the majority of histone modification enzymes, making a few upregulated enzymes novel therapeutic targets–“sand out and gold stays”. J Cardiovasc Transl Res. (2016) 9:49–66. doi: 10.1007/s12265-015-9664-y
99. Li X, Shao Y, Sha X, Fang P, Kuo YM, Andrews AJ, et al. IL-35 (Interleukin-35) suppresses endothelial cell activation by inhibiting mitochondrial reactive oxygen species-mediated site-specific acetylation of H3K14 (histone 3 lysine 14). Arterioscler Thromb Vasc Biol. (2018) 38:599–609. doi: 10.1161/ATVBAHA.117.310626
100. Li X, Mai J, Virtue A, Yin Y, Gong R, Sha X, et al. IL-35 is a novel responsive anti-inflammatory cytokine–a new system of categorizing anti-inflammatory cytokines. PLoS One. (2012) 7:e33628. doi: 10.1371/journal.pone.0033628
101. Li X, Fang P, Yang WY, Wang H, Yang X. IL-35, as a newly proposed homeostasis-associated molecular pattern, plays three major functions including anti-inflammatory initiator, effector, and blocker in cardiovascular diseases. Cytokine. (2019) 122:154076. doi: 10.1016/j.cyto.2017.06.003
102. Li X, Fang P, Sun Y, Shao Y, Yang WY, Jiang X, et al. Anti-inflammatory cytokines IL-35 and IL-10 block atherogenic lysophosphatidylcholine-induced, mitochondrial ROS-mediated innate immune activation, but spare innate immune memory signature in endothelial cells. Redox Biol. (2020) 28:101373. doi: 10.1016/j.redox.2019.101373
103. Wang S, Qu Y, Xia P, Chen Y, Zhu X, Zhang J, et al. Transdifferentiation of tumor infiltrating innate lymphoid cells during progression of colorectal cancer. Cell Res. (2020) 30:610–22. doi: 10.1038/s41422-020-0312-y
104. Zou W. Regulatory T cells, tumour immunity and immunotherapy. Nat Rev Immunol. (2006) 6:295–307. doi: 10.1038/nri1806
105. Li YF, Ren LN, Guo G, Cannella LA, Chernaya V, Samuel S, et al. Endothelial progenitor cells in ischemic stroke: an exploration from hypothesis to therapy. J Hematol Oncol. (2015) 8:33. doi: 10.1186/s13045-015-0130-8
106. Pansuria M, Xi H, Li L, Yang XF, Wang H. Insulin resistance, metabolic stress, and atherosclerosis. Front Biosci (Schol Ed). (2012) 4:916–31. doi: 10.2741/s308
107. Gribben C, Galanakis V, Calderwood A, Williams EC, Chazarra-Gil R, Larraz M, et al. Acquisition of epithelial plasticity in human chronic liver disease. Nature. (2024) 630:166–73. doi: 10.1038/s41586-024-07465-2
108. Mollinari C, Zhao J, Lupacchini L, Garaci E, Merlo D, Pei G. Transdifferentiation: a new promise for neurodegenerative diseases. Cell Death Dis. (2018) 9:830. doi: 10.1038/s41419-018-0891-4
109. Kalra RS, Dhanjal JK, Das M, Singh B, Naithani R. Cell transdifferentiation and reprogramming in disease modeling: insights into the neuronal and cardiac disease models and current translational strategies. Cells. (2021) 10(10):2558. doi: 10.3390/cells10102558
110. Li S, Zhao S, Sinson JC, Bajic A, Rosenfeld JA, Neeley MB, et al. The clinical utility and diagnostic implementation of human subject cell transdifferentiation followed by RNA sequencing. Am J Hum Genet. (2024) 111:841–62. doi: 10.1016/j.ajhg.2024.03.007
111. Fu Y, Huang C, Xu X, Gu H, Ye Y, Jiang C, et al. Direct reprogramming of mouse fibroblasts into cardiomyocytes with chemical cocktails. Cell Res. (2015) 25:1013–24. doi: 10.1038/cr.2015.99
112. Carvalho AB, Kasai-Brunswick TH, Campos de Carvalho AC. Advanced cell and gene therapies in cardiology. eBioMedicine. (2024) 103:105125. doi: 10.1016/j.ebiom.2024.105125
113. Shao Y, Saredy J, Xu K, Sun Y, Saaoud F, Drummer C, et al. Endothelial immunity trained by coronavirus infections, DAMP stimulations and regulated by anti-oxidant NRF2 may contribute to inflammations, myelopoiesis, COVID-19 cytokine storms and thromboembolism. Front Immunol. (2021) 12:653110. doi: 10.3389/fimmu.2021.653110
114. Hu Q, Khanna P, Ee Wong BS, Lin Heng ZS, Subhramanyam CS, Thanga LZ, et al. Oxidative stress promotes exit from the stem cell state and spontaneous neuronal differentiation. Oncotarget. (2018) 9:4223–38. doi: 10.18632/oncotarget.23786
115. Sha X, Meng S, Li X, Xi H, Maddaloni M, Pascual DW, et al. Interleukin-35 inhibits endothelial cell activation by suppressing MAPK-AP-1 pathway. J Biol Chem. (2015) 290(31):19307–18. doi: 10.1074/jbc.M115.663286
116. Mai J, Nanayakkara G, Lopez-Pastrana J, Li X, Li YF, Wang X, et al. Interleukin-17A promotes aortic endothelial cell activation via transcriptionally and post-translationally activating p38 mitogen-activated protein kinase (MAPK) pathway. J Biol Chem. (2016) 291(10):4939–54. doi: 10.1074/jbc.M115.690081
117. Vermot A, Petit-Hartlein I, Smith SME, Fieschi F. NADPH Oxidases (NOX): an overview from discovery, molecular mechanisms to physiology and pathology. Antioxidants (Basel). (2021) 10(6):890. doi: 10.3390/antiox10060890
118. Song SH, Kim K, Park JJ, Min KH, Suh W. Reactive oxygen species regulate the quiescence of CD34-positive cells derived from human embryonic stem cells. Cardiovasc Res. (2014) 103:147–55. doi: 10.1093/cvr/cvu106
119. Liang J, Wu M, Chen C, Mai M, Huang J, Zhu P. Roles of reactive oxygen Species in cardiac differentiation, reprogramming, and regenerative therapies. Oxid Med Cell Longev. (2020) 2020:2102841. doi: 10.1155/2020/2102841
120. Rothhammer V, Quintana FJ. The aryl hydrocarbon receptor: an environmental sensor integrating immune responses in health and disease. Nat Rev Immunol. (2019) 19:184–97. doi: 10.1038/s41577-019-0125-8
121. Chen S, Henderson A, Petriello MC, Romano KA, Gearing M, Miao J, et al. Trimethylamine N-oxide binds and activates PERK to promote metabolic dysfunction. Cell Metab. (2019) 30:1141–51.e1145. doi: 10.1016/j.cmet.2019.08.021
122. Saaoud F, Lu Y, Xu K, Shao Y, Pratico D, Vazquez-Padron RI, et al. Protein-rich foods, sea foods, and gut microbiota amplify immune responses in chronic diseases and cancers – Targeting PERK as a novel therapeutic strategy for chronic inflammatory diseases, neurodegenerative disorders, and cancer. Pharmacol Ther. (2024):108604. doi: 10.1016/j.pharmthera.2024.108604
123. Keating ST, Groh L, van der Heijden C, Rodriguez H, Dos Santos JC, Fanucchi S, et al. The Set7 lysine methyltransferase regulates plasticity in oxidative phosphorylation necessary for trained immunity induced by beta-glucan. Cell Rep. (2020) 31:107548. doi: 10.1016/j.celrep.2020.107548
124. Delgado ME, Cardenas BI, Farran N, Fernandez M. Metabolic reprogramming of liver fibrosis. Cells. (2021) 10(12):3604. doi: 10.3390/cells10123604
125. Chen X, Wu H, Liu Y, Liu L, Houser SR, Wang WE. Metabolic reprogramming: a byproduct or a driver of cardiomyocyte proliferation? Circulation. (2024) 149:1598–610. doi: 10.1161/CIRCULATIONAHA.123.065880
126. Sadahiro T, Ieda M. In vivo reprogramming as a new approach to cardiac regenerative therapy. Semin Cell Dev Biol. (2022) 122:21–7. doi: 10.1016/j.semcdb.2021.06.019
127. Jahng JWS, Zhang M, Wu JC. The role of metabolism in directed differentiation versus trans-differentiation of cardiomyocytes. Semin Cell Dev Biol. (2022) 122:56–65. doi: 10.1016/j.semcdb.2021.05.018
128. Ieda M, Fu JD, Delgado-Olguin P, Vedantham V, Hayashi Y, Bruneau BG, et al. Direct reprogramming of fibroblasts into functional cardiomyocytes by defined factors. Cell. (2010) 142:375–86. doi: 10.1016/j.cell.2010.07.002
129. Feng S, Bowden N, Fragiadaki M, Souilhol C, Hsiao S, Mahmoud M, et al. Mechanical activation of hypoxia-inducible factor 1alpha drives endothelial dysfunction at atheroprone sites. Arterioscler Thromb Vasc Biol. (2017) 37:2087–101. doi: 10.1161/ATVBAHA.117.309249
130. Perry RN, Albarracin D, Aherrahrou R, Civelek M. Network preservation analysis reveals dysregulated metabolic pathways in human vascular smooth muscle cell phenotypic switching. Circ Genom Precis Med. (2023) 16:372–81. doi: 10.1161/CIRCGEN.122.003781
131. Xu Y, Shi Z, Bao L. An expanding repertoire of protein acylations. Mol Cell Proteomics. (2022) 21:100193. doi: 10.1016/j.mcpro.2022.100193
132. Sabari BR, Zhang D, Allis CD, Zhao Y. Metabolic regulation of gene expression through histone acylations. Nat Rev Mol Cell Biol. (2017) 18:90–101. doi: 10.1038/nrm.2016.140
133. Fu Y, Yu J, Li F, Ge S. Oncometabolites drive tumorigenesis by enhancing protein acylation: from chromosomal remodelling to nonhistone modification. J Exp Clin Cancer Res. (2022) 41:144. doi: 10.1186/s13046-022-02338-w
134. Zhong C, Yang X, Feng Y, Yu J. Trained immunity: an underlying driver of inflammatory atherosclerosis. Front Immunol. (2020) 11:284. doi: 10.3389/fimmu.2020.00284
135. Fagenson AM, Xu K, Saaoud F, Nanayakkara G, Jhala NC, Liu L, et al. Liver ischemia reperfusion injury, enhanced by trained immunity, is attenuated in caspase 1/caspase 11 double gene knockout mice. Pathogens. (2020) 9(11):879. doi: 10.3390/pathogens9110879
136. Xu K, Saaoud F, Shao Y, Lu Y, Wu S, Zhao H, et al. Early hyperlipidemia triggers metabolomic reprogramming with increased SAH, increased acetyl-CoA-cholesterol synthesis, and decreased glycolysis. Redox Biol. (2023) 64:102771. doi: 10.1016/j.redox.2023.102771
137. Drummer C, Saaoud F, Jhala NC, Cueto R, Sun Y, Xu K, et al. Caspase-11 promotes high-fat diet-induced NAFLD by increasing glycolysis, OXPHOS, and pyroptosis in macrophages. Front Immunol. (2023) 14:1113883. doi: 10.3389/fimmu.2023.1113883
138. Xu K, Saaoud F, Shao Y, Lu Y, Yang Q, Jiang X, et al. A new paradigm in intracellular immunology: mitochondria emerging as leading immune organelles. Redox Biol. (2024) 76:103331. doi: 10.1016/j.redox.2024.103331
139. Xiong J. Fatty acid oxidation in cell fate determination. Trends Biochem Sci. (2018) 43:854–7. doi: 10.1016/j.tibs.2018.04.006
140. Tan Y, Li J, Zhao G, Huang KC, Cardenas H, Wang Y, et al. Metabolic reprogramming from glycolysis to fatty acid uptake and beta-oxidation in platinum-resistant cancer cells. Nat Commun. (2022) 13:4554. doi: 10.1038/s41467-022-32101-w
141. Varga Z, Sabzwari SRA, Vargova V. Cardiovascular risk of nonsteroidal anti-inflammatory drugs: an under-recognized public health issue. Cureus. (2017) 9:e1144. doi: 10.7759/cureus.1144
142. Deng HW, Mei WY, Xu Q, Zhai YS, Lin XX, Li J, et al. The role of glucocorticoids in increasing cardiovascular risk. Front Cardiovasc Med. (2023) 10:1187100. doi: 10.3389/fcvm.2023.1187100
143. Katzung BG, Kruidering-Hall M, Tuan RL, Vanderah TW, Trevor AJ. Katzung & Trevor’s Pharmacology: Examination & Board Review. New York, NY: McGraw-Hill Education (2021). p. 13e.
144. Murphy AJ, Febbraio MA. Immune-based therapies in cardiovascular and metabolic diseases: past, present and future. Nat Rev Immunol. (2021) 21:669–79. doi: 10.1038/s41577-021-00580-5
145. Ridker PM, Everett BM, Thuren T, MacFadyen JG, Chang WH, Ballantyne C, et al. Antiinflammatory therapy with canakinumab for atherosclerotic disease. N Engl J Med. (2017) 377:1119–31. doi: 10.1056/NEJMoa1707914
146. Naing A, Infante J, Goel S, Burris H, Black C, Marshall S, et al. Anti-PD-1 monoclonal antibody MEDI0680 in a phase I study of patients with advanced solid malignancies. J Immunother Cancer. (2019) 7:225. doi: 10.1186/s40425-019-0665-2
147. Liu G, Philp AM, Corte T, Travis MA, Schilter H, Hansbro NG, et al. Therapeutic targets in lung tissue remodelling and fibrosis. Pharmacol Ther. (2021) 225:107839. doi: 10.1016/j.pharmthera.2021.107839
148. Suthahar N, Meijers WC, Silljé HHW, de Boer RA. From inflammation to fibrosis-molecular and cellular mechanisms of myocardial tissue remodelling and perspectives on differential treatment opportunities. Curr Heart Fail Rep. (2017) 14:235–50. doi: 10.1007/s11897-017-0343-y
149. Fraccarollo D, Galuppo P, Bauersachs J. Novel therapeutic approaches to post-infarction remodelling. Cardiovasc Res. (2012) 94:293–303. doi: 10.1093/cvr/cvs109
Keywords: pathological transdifferentiation, chronic inflammation, cardiovascular diseases, epithelial to mesenchymal transition, endothelial to mesenchymal transition, vascular smooth muscle to mesenchymal transition
Citation: Yang WY, Ben Issa M, Saaoud F, Xu K, Shao Y, Lu Y, Dornas W, Cueto R, Jiang X, Wang H and Yang X (2024) Perspective: Pathological transdifferentiation—a novel therapeutic target for cardiovascular diseases and chronic inflammation. Front. Cardiovasc. Med. 11:1500775. doi: 10.3389/fcvm.2024.1500775
Received: 23 September 2024; Accepted: 11 November 2024;
Published: 26 November 2024.
Edited by:
Yiliang Chen, Medical College of Wisconsin, United StatesReviewed by:
Bin Ren, University of Alabama at Birmingham, United StatesCopyright: © 2024 Yang, Ben Issa, Saaoud, Xu, Shao, Lu, Dornas, Cueto, Jiang, Wang and Yang. This is an open-access article distributed under the terms of the Creative Commons Attribution License (CC BY). The use, distribution or reproduction in other forums is permitted, provided the original author(s) and the copyright owner(s) are credited and that the original publication in this journal is cited, in accordance with accepted academic practice. No use, distribution or reproduction is permitted which does not comply with these terms.
*Correspondence: Xiaofeng Yang, eGZ5YW5nQHRlbXBsZS5lZHU=
†These authors have contributed equally to this work