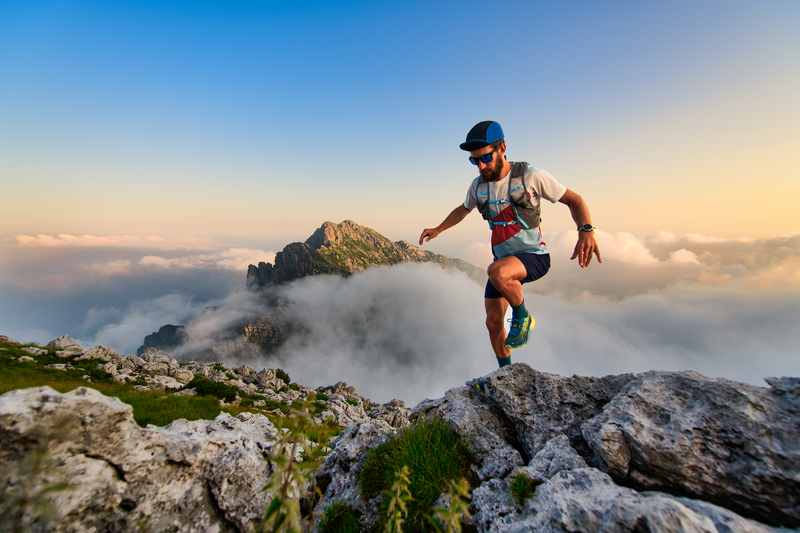
95% of researchers rate our articles as excellent or good
Learn more about the work of our research integrity team to safeguard the quality of each article we publish.
Find out more
ORIGINAL RESEARCH article
Front. Cardiovasc. Med. , 29 August 2024
Sec. Heart Valve Disease
Volume 11 - 2024 | https://doi.org/10.3389/fcvm.2024.1411398
This article is part of the Research Topic Proven and Innovative Methods of ex vivo Examination of Atherosclerotic and Aortic Valve Lesions View all 7 articles
The development of drug therapy for the pathological calcification of the aortic valve is still an open issue due to the lack of effective treatment strategies. Currently, the only option for treating this condition is surgical correction and symptom management. The search for models to study the safety and efficacy of anti-calcifying drugs requires them to not only be as close as possible to in vivo conditions, but also to be flexible with regard to the molecular studies that can be applied to them. The ex vivo model has several advantages, including the ability to study the effect of a drug on human cells while preserving the original structure of the valve. This allows for a better understanding of how different cell types interact within the valve, including non-dividing cells. The aim of this study was to develop a reproducible ex vivo calcification model based on valves from patients with calcific aortic stenosis. We aimed to induce spontaneous calcification in valve tissue fragments under osteogenic conditions, and to demonstrate the possibility of significantly suppressing it using a calcification inhibitor. To validate the model, we tested a Notch inhibitor Crenigacestat (LY3039478), which has been previously shown to have an anti-calcifying effect on interstitial cell of the aortic valve. We demonstrate here an approach to testing calcification inhibitors using an ex vivo model of cultured human aortic valve tissue fragments. Thus, we propose that ex vivo models may warrant further investigation for their utility in studying aortic valve disease and performing pre-clinical assessment of drug efficacy.
Calcifying aortic stenosis is a long-lasting and often asymptomatic condition that manifests itself at the stage of decompensation, when the risk of sudden death increases (1). At the moment, the most effective treatment for this condition is aortic valve replacement. Drug therapy serves as a supportive measure to manage symptoms and prevent complications (2, 3).
The presence of effective strategies to slow down calcium accumulation could potentially lead to the abandonment of surgical treatment for asymptomatic patients with mild to moderate stenosis, provided that there is no negative progression in the degree of calcification and myocardial remodeling (4). In severe stenosis and with hemodynamic disorders, surgery with symptomatic therapy is still the main treatment option. In these cases, anticalcific therapy can be used as a bridge to surgery. Changes in calcification processes after exposure to potential therapeutic targets are being investigated, including lipid and carbohydrate metabolism, the renin-angiotensin-aldosterone system, cyclic guanosine monophosphate signaling, the Notch signaling pathway, and calcium and phosphorus metabolism (4).
Calcifying aortic stenosis is a pathological process characterized by the accumulation of calcium in the aortic valve leaflets, by chronic pressure overload of the left ventricle and by heart failure (5). It has long been believed that the pathogenesis of this condition is based on the gradual mechanical wear and tear of the leaflets over time. However, current research suggests that the development of this pathology may be due to specific molecular processes, which in turn are the result of various factors, including genetics, pressure in the aortic bulb, shear stress, dyslipidemia (abnormal levels of lipids in the blood), inflammation, fibrosis (scarring), and disorders of calcium and phosphorus metabolism (6, 7). Due to the complex and multifaceted nature of the disease, the precise mechanisms underlying aortic valve calcification remain to be fully understood.
Depending on the goals of the researchers, the study of the pathogenesis of aortic stenosis and preclinical testing of anticalcifying drugs (as well as new prosthetics strategies) is carried out using various models. Within the context of research into calcification processes, a diverse range of models can be categorized based on the level of biological organization at which they generate data: molecular, cellular, tissue, system, and organismal. The study of calcification process at the macroorganism level is carried out using biomodels: sheep, since they have the property of spontaneous calcification of valve prostheses (8, 9); pigs (10); alpha-Gal knockout baboons (10). The choice of biological models for calcifying aortic stenosis is limited due to the lack of spontaneous calcification in many of these models. For example, the widely used mouse model does not have a three-layered structure of aortic valves. There are several layers of cells without clearly defined tissue architectonics. Spontaneous calcification does not occur in wild-type mice, but the use of transgenic models solves this problem. Until recently, models knocked out by the genes of the low-density lipoprotein receptor (Ldlr −/−) and apolipoprotein E (ApoE −/−) were widely used (11). It is possible to achieve severe calcification of the aortic valve using this model, however, they did not develop hemodynamically significant stenosis. It can be achieved using the Reversa model, a complex model of hypercholesterolemia with knocked–out Ldlr −/−/apoB 100/100 genes, as well as by introducing a spring guide into the left ventricle that mechanically irritates the valve leaflets, leading to calcification and stenosis (11). An additional factor contributing to hyperlipidemia may be a high-fat diet (12). There are also non-hyperlipidemic calcification models that include MGP modified mice (13, 14), EGFR (11), RBPJk (11), IL1RN (15), but they also lack the development of stenosis. The aortic valves of rats also do not have three-layered structure. The calcification model in rats based on intravenous injections of warfarin does not cause stenosis, and the resulting calcinates differ from those formed in humans (11). Currently, models based on the excessive intake of vitamin D and nicotine are used to induce vascular calcification (16), as well as models involving subtotal nephrectomy and a hyperphosphatemic diet (17). The heart valves of rabbits have a three-layered structure that is similar to those of humans in terms of lipid metabolism. A diet with high cholesterol, in combination with high levels of calcium induced by an excess of vitamin D2, is effective in inducing calcification and stenosis (11). However, the use of the nutrition factor is limited due to the high risk of animal death due to hepatic dysfunction caused by lipid overload (11). Pig valves also have a three-layer construction, and, in contrast to the models mentioned above, pigs are susceptible to the spontaneous development of valve calcium deposits, which can also be exacerbated by the consumption of a high-fat diet (18). Sheep are frequently utilized as models for research on biological prosthetics and homografts in vivo (19, 20).
In vitro cell cultures are used to reproduce calcification processes at the cellular level. This group of models includes primary cell cultures [interstitial valve cells (VICs) (21), endothelial valve cells (VECs)] (22) and induced pluripotent stem cells (iPSCs) (23). In vitro cell culture models are used for both fundamental research and selecting compounds that will be tested later. The advantages of this approach are the relatively short duration of the study and its cost-effectiveness. However, the disadvantage of this model is the need to provide suitable conditions for cell culture (21). In the context of studying calcification of the aortic valve, a structure consisting mainly of an extracellular matrix, this model is not enough to understand the features of interaction between cell populations (including non–dividing ones) and the microenvironment. This is partially compensated by co-cultivation with other cell cultures (5, 24) and the use of 3D cultures and cell culture pump systems (10, 11, 25, 26).
Currently, there is an increasing interest in models that aim to reduce the number of laboratory animals used in research. The “3R” principle—reduction, refinement and replacement—emphasizes the importance of using biomodels more efficiently in experiments (27). There is also a growing demand for bioinformatics models, big data analysis, and machine learning technologies (10, 28, 29). The use of human biological materials in preclinical research is common. These materials are often in the form of cell cultures derived from postoperative specimens and biopsy samples (30–32). The ex vivo aortic valve calcification model, which involves the use of native human valve leaflets, seems to be closer to the conditions in the whole organism because of preserving interactions between cell populations and the extracellular matrix. However, the use of this model requires collaboration with medical institutions that are capable of providing biological material. Additionally, appropriate ethical approvals must be obtained, as well as informed and voluntary patient consent. Ex vivo models using porcine aortic valves are described in the literature, including evaluating the effectiveness of calcification inhibitors (11, 33–35). Due to the potential for using native valves while preserving the fibrous annulus and the integrity of leaflets, it is now possible to investigate the role of hemodynamic factors in the pathogenesis of aortic stenosis using advanced equipment. Currently, such models are developed using porcine valves (36, 37).
The aim of this study was to develop a reproducible ex vivo calcification model based on valves from patients with calcific aortic stenosis. We aimed to induce spontaneous calcification in valve tissue fragments under osteogenic conditions, and to demonstrate the possibility of significantly suppressing it using a calcification inhibitor. To validate the model, we tested the Notch inhibitor Crenigacestat (LY3039478), which has been previously shown to have an anti-calcifying effect on vascular smooth muscle cells (30). We demonstrate here an approach to testing calcification inhibitors using an ex vivo model of cultured human aortic valve tissue fragments.
The aortic valve leaflets were provided by the V. A. Almazov National Medical Research Center. The study was conducted in accordance with the principles of the Helsinki Declaration and approved by the Ethics Committee of the V.A. Almazov National Medical Research Center (Ethical authorization No. 12.26/2014). Aortic valve leaflets were obtained from patients undergoing surgical aortic valve replacement procedures after receiving the permission. This study included 14 patients (7 men and 7 women), whose ages ranged from 41 to 76 years (62.79 ± 9.18). 11 patients had severe aortic stenosis, 4 patients had grade 3 aortic insufficiency with mild (n = 3) and moderate (n = 1) calcification of aortic valve. The peak gradient on the aortic valve in patients with aortic stenosis was 94.9 ± 32.86 mmHg, the average gradient was 54.59 ± 19.5 mmHg, the aortic transvalvular peak velocity was 4.43 ± 1.12 m/s. The final diastolic volume was 162.42 ± 88.43 ml, the final systolic volume was 86.5 ± 71.81 ml, and the Simpson ejection fraction was 52.17% ± 12.48%.
On the day of reception of the entire leaflet (one leaflet from one patient), regions free of calcified plaque were excised for further analysis. The material obtained from a single valve was divided into a number of fragments, which corresponded to the number of experimental groups. The aim of this stage is to achieve maximum uniformity among the fragments and prevent the formation of elevated “calcium background” levels. In order to achieve this goal, we propose visual and microscopic detection of calcium deposits, as well as normalization of fragment sizes to area within a single leaflet. The ways to reduce the “calcium background” by cutting of the calcium deposits is schematically shown in the Figure 1. The cutting was performed within the uncalcified tissues, which was evaluated visually and microscopically. All manipulations were performed under aseptic conditions using sterile equipment. After that, the valve fragments were randomized by experimental groups and placed on 24-well plates (Corning, USA). For further cultivation, the antimyofibroblastic medium was adapted due of the risk of myofibroblast contraction, which can disrupt the penetration of nutrients and the procalcification stimulus (33). A low glucose DMEM medium (Gibco, USA) was used with the addition of 2% fetal bovine serum (FBS, Gibco, USA), 1% insulin-transferrin-selenium (Gibco, USA) and 50 µg/ml gentamicin. The medium was changed twice a week. The fragments were incubated in conditions with a content of 5% CO2 at a temperature of 37 °C. The absence of mycoplasma contamination was verified by quantitative polymerase chain reaction (qPCR) in accordance with Janetzko et al. (38). All experiments were conducted in biological triplicates (cells isolated from at least three donors). Leaflet fragments were cultured for 8 weeks to assess the degree of calcification, as well as for histological assessment. For quantitative polymerase chain reaction (qPCR), the leaflet fragments were cultured for 4 weeks.
Figure 1. Options for cutting valves for cultivation. (A) The sections of a calcified valve available for cutting; (B) an example of cutting a slightly calcified leaflet (without visible calcium overlays); (C) an example of cutting a moderately calcified leaflet (less than 1/2 of the area); (D) an example of cutting a strongly calcified leaflet (1/2 of the area). FE, free edge; AE, anchored edge.
Osteogenic medium includes 50 µM of ascorbic acid, 100 nM of dexamethasone and 10 mM of β-glycerophosphate to the culture medium (Sigma, USA). Other cultivation conditions were the same as for the described ones above; the osteogenic medium was also changed twice a week.
Calcium accumulation was assessed by spectrophotometric method using a kit “Calcium Olvex” (Olvex Diagnosticum, Russia) at the 8th week of cultivation in accordance with the manufacturer's protocol with some changes. The fragments of the leaflets were washed twice with PBS, after that they were homogenized in the distilled water (500 µl per fragment). Homogenization was performed mechanically using 2 stainless steel grinding beads to grind one fragment placed in a 2 ml thick-bottomed centrifuge tube. The oscillation process lasted for 30 min and the homogeneity of the product was checked every 15 min. Next, the homogenate was centrifuged at a speed of 300G for 5 min in order to remove the beads and destroyed tissues. The supernatant was moved to the new centrifuge tube and was re-centrifuged at a speed of 1000G for 5 min. 60 µl of the supernatant was placed in centrifuge tube with 500 µl of the Monoreagent from the Calcium Olvex kit, after which, according to the manufacturer's protocol, the mixture was incubated for 5 min at room temperature. After that, the optical density of experimental, calibration and blank samples was measured at a wavelength of 650 (640–660) nm in a cuvette with an optical path length of 1.0 cm on the multi-mode plate reader CLARIOstar® Plus. To measure the area of the valve fragment (S), photographs of each fragment were utilized, which were taken in order to monitor the dynamic accumulation of calcium (photographs for each fragment were taken). Using the ImageJ program, the area of the fragments on all photos was measured (4 photos for each fragment) and the arithmetic mean among them was calculated {thus, for each fragment had one area value [S(average)]}. Then, using the formula , was obtained a normalized calcium concentration normalized by the area of a particular fragment, that is, the concentration of calcium in mmol/L/cm2. Data processing was carried out using Microsoft Excel and GraphPad Prism.
The gamma secretase inhibitor Crenigacestat (LY3039478; Medchemexpress, USA) was dissolved in DMSO (dimethyl sulfoxide) to a concentration of 10 mM. Dosages were used in studies that showed the minimum number of side effects in patients in clinical trials: 100 nM (Cmax = 46.444 ng/ml), 300 nM (Cmax = 139.332 ng/ml), 500 nM (Cmax = 232.22 ng/ml) (39, 40).
On day 1, 2, 4, 8 weeks, the valves were photographed at a magnification of 30x. The morphology of the leaflets and the qualitative assessment of calcification in dynamics were evaluated.
To isolate RNA, the extraRNA reagent (Eurogen, Russia) was used in accordance with the manufacturer's instructions with some modifications. After placing the fragments of the leaflets in the extraRNA reagent, its homogenization was carried out in 2 ml thick-bottomed centrifuge tubes using stainless steel beads (2 beads per fragment). The oscillation lasted no longer than 10 min, and the homogenization quality was checked every 5 min. Next, the homogenate was centrifuged at a speed of 1000G for 5 min, after which the supernatant was placed in new centrifuge tubes. Next, we followed the manufacturer's protocol. After isolation 1 μg of total RNA, was used for reverse transcription using MMLV reverse transcriptase (Eurogen, Russia).
Real-time PCR was performed using SybrGreen qPCR mastermix “qPCRmix-HS SYBR” (Eurogene, Russia) in the LightCycler 96 system (Roshe, Switzerland) according to the following scheme: (1) denaturation before amplification for 300c at 95 °C; (2) 45 cycles of three-step amplification (15c at 95 °C, 30c at 60 °C, 30c at 70 °C); (3) High resolution melting. Expression of GAPDH, RUNX2, COL1A1, SNAIL, SLUG, ACTA2 was evaluated after 4 weeks of osteogenic conditions. mRNA levels were normalized to GAPDH mRNA. All primer sequences are available on request.
The data obtained by spectrophotometric calcification assessment and qPCR were processed using Microsoft Excel (calculations) and GraphPad Prism (graphs, statistical analysis). The optical density levels were calculated minus the blank sample. The data was analyzed using ANOVA in GraphPad Prism. The Shapiro-Wilk test was used to check the normality. Since not all samples passed the normality test, the statistical significance of the differences between the samples was assessed using the Mann-Whitney test. Those samples that passed the normality test were tested using the t-test. The standard errors of the mean value (SEM) are indicated.
Changes in the expression levels of target genes were calculated using the comparative ΔΔCT method. GAPDH was used as a housekeeping gene. The results are presented as an average of technical duplicates. The standard errors of the mean value (SEM) are indicated. Relative expression levels were compared using the Mann-Whitney test in GraphPad Prism.
Sections of the aortic valve (1–2 µm) were dewaxed, rehydrated in a series of ethanol and water solutions and stained with hematoxylin-eosin. The morphology of the sections was evaluated using a microscope Zeiss Axio Observer Z1 in the program ToupView.
First, we set up the experimental conditions to culture aortic valve fragments (see Materials and methods section and Figure 1 for details). Then we induced osteogenic differentiation of the valve fragments by culturing them in the osteogenic conditions (Figure 2). After 8 weeks we estimated calcium accumulation by spectrophotometric assessment of valves in control and osteogenic medium and detected a significant accumulation of calcium in osteogenic conditions (Figure 2). During the morphological assessment of the valves (Figure 2B), a distinguishable formation of calcium plaques was found in samples under osteogenic conditions.
Figure 2. Assessment of calcium accumulation under ex vivo conditions (A) spectrophotometric assessment of valves in the control, osteogenic medium (OM) and osteogenic medium with the addition of 100 nM, 300 nM, 500 nM crenigacestat (LY3039478). (B) Morphological assessment of calcium accumulation in the control, in an osteogenic medium (OM), an osteogenic medium with the addition of 300 nM crenigacestat (OM + cre). The groups were compared using the t-test, *p = 0.0111; **p = 0.0022, ****p = <0.0001. The groups were compared using t-test.
To evaluate the ability of our ex vivo culture method for valve fragments to detect the anti-calcification effect of a drug, we used crenigacestat, a substance that has been previously shown to inhibit the osteogenic differentiation of human aortic valve interstitial cells. We cultured valve fragments in either a control medium or an osteogenic medium, or an osteogenic medium supplemented with crenigacestat. A significant suppression of calcium accumulation was found in samples with 300 nM and 500 nM of crenigacestat (Figure 2A). Crenigacestat suppressed the expression of the genes associated with osteogenic differentiation. The effect of crenigacestat (LY3039478) on RUNX2 (41) (the main regulatory gene for osteogenic differentiation), COL1A1 (a gene encoding fibrillar protein of the extracellular matrix), ACTA2 (a gene encoding a protein from the actin family used as a marker of myofibroblast formation) and on markers of endothelial-mesenchymal transition (SNAIL, SLUG) (Figure 3) (42, 43).
Figure 3. Crenigacestat suppresses the expression of the genes associated with osteogenic differentiation. The effect of crenigacestat (LY3039478) on RUNX2 (the main regulatory gene for osteogenic differentiation), COL1A1 (a gene encoding fibrillar protein of the extracellular matrix), ACTA2 (a gene encoding a protein from the actin family used as a marker of myofibroblast formation) and on markers of endothelial-mesenchymal transition (SNAIL, SLUG). OM is an osteogenic medium; OM + 300 nM is an osteogenic medium with the addition of 300 nM of crenigacestat. The groups were compared using the Mann-Whitney test, *p < 0.05.
Finally, we assessed the valve fragments by histological staining with hematoxilin-eosin to show calcification in osteogenic medium and its suppression by crenigacestat (Figure 4). Newly formed foci of calcification were identified in all samples under osteogenic conditions without the addition of crenigacestat. In the group in osteogenic medium with the addition of 300 nM crenigacestat new calcification foci were observed in the pars spongiosa. In the control group without osteogenic conditions, no newly formed foci of calcification were observed. Microscopically, we found old foci of calcification formed in vivo as well as foci of fibrosis, hyalinosis, and cartilaginous metaplasia in the samples, without a clear dependence on the experimental conditions.
Figure 4. Staining of fragments of the aortic valve leaflets with hematoxylin-eosin of the control group (C), under osteogenic conditions (OM), under osteogenic conditions with the addition of 300 nM crenigacestat (LY3039478). The formation of new foci of calcification in the group with osteogenic conditions (OM) is observed.
The aim of this study was to develop an ex vivo calcification model using fragments of aortic valve tissue in laboratory conditions. The fragments were exposed to specific experimental procedures for a in order to induce calcification. The main advantages of this model include the preservation of the histological structure of the valve leaflets and the ability to maintain them in osteogenic conditions for an extended period. As seen from the presented data the model allows to evaluate the expression of genes responsible for calcification and biological activity of an inhibitor in the valve.
Addition of 300 nM crenigacestat into an osteogenic medium led to a significant decrease of RUNX2 expression in valve tissue fragments compared to the control samples in osteogenic medium. The expression of other genes that are also associated with osteogenic differentiation, such as COL1A, ACTA2, and SNAIL, decreased when the valve fragments that had been osteogenically induced were treated with crengiacestat. All this demonstrates the potential of this ex vivo model for testing substances for their ability to prevent calcification.
A major limitation of this study is that it uses ex vivo models that use tissues obtained from patients with aortic valve disease. It is difficult to obtain non-diseased tissues with living cells. Difficulties in interpreting the results may be caused by “calcium background” associated with plaques formed in vivo.
We have concluded that addressing this issue depends on selecting patients to participate in the study. Leaflets with more than 50% of the valve area affected by calcification were excluded due to technical difficulties in isolating unaffected areas from macroscopically visible calcifications. Additionally, we used microscopy at a 30× magnification to evaluate visible calcinates that were undetectable to the unaided eye. If technically possible, the calcinates were removed. If not, a fragment of the leaflet was left under dynamic observation. Special attention was paid to minimizing the “calcium background” during the process of assessing calcium accumulation using spectrophotometry. For statistical analysis, it was necessary to compare the degree of calcium accumulation within a single leaflet, due to the individual characteristics of patients, which created a different calcium background despite the measures taken. At the same time, if the previously mentioned measures were insufficient, it can be assumed that we would not have observed statistically significant differences, even with such an analysis design. Another way to interpret the results, even in the presence of significant calcium background, is through the use of non-invasive techniques that allow for assessing the degree of calcification over time. We used microscopy with a 30× magnification, but this technique is not sensitive enough to fully detect dynamic changes. Therefore, for these purposes, more sensitive imaging techniques, such as micro-CT, should be employed (11, 44). The use of these techniques would also allow for the implementation of software programs for calculating the area of calcification, thereby providing not only qualitative but also quantitative data (45).
Therefore, we propose that ex vivo models of aortic valves derived from patients with calcific aortic stenosis may be worth further investigation in order to determine their potential utility in pre-clinical evaluation of drug efficacy. In addition, this model may be useful for future basic research. Since the preservation of the extracellular matrix and cell composition in the samples would allow for the use of histological methods to detect local differences in the expression of specific markers. However, one of the most important factors of aggression leading to aortic stenosis, mechanical stimulation, is still not observed in this study. It is possible to utilize hemodynamic systems that create circulation of a culture medium. However, to create systems that replicate in vivo hemodynamic conditions (i.e., a combination of linear and turbulent flow patterns relative to the ventricular and aortic sides), at minimum, an entire valve with its fibrous ring preserved is required. Such work is carried out with valves of large animals (36). Additionally, a significant limitation of the model is the absence of reliable data regarding whether leukocytes, which are known to play a significant role in remodeling processes, can survive under ex vivo conditions. Further investigation is required to address this issue.
We have developed an ex vivo calcification model using aortic valves from patients with calcified aortic stenosis. This model allowed us to demonstrate the calcification-inhibiting effects of the gamma secretase inhibitor crenigacestat (LY3039478). Therefore, we propose that this model warrants further investigation in order to assess its potential utility for studying calcification processes and preclinical trials.
The original contributions presented in the study are included in the article/Supplementary Material, further inquiries can be directed to the corresponding author.
The studies involving humans were approved by The Local Ethics Committee of the Almazov Federal Medical Research Center (ethical permit number: 12.26/2014). The studies were conducted in accordance with the local legislation and institutional requirements. The participants provided their written informed consent to participate in this study.
OK: Conceptualization, Formal Analysis, Investigation, Methodology, Software, Validation, Visualization, Writing – original draft. NB: Investigation, Methodology, Validation, Writing – original draft. PD: Investigation, Methodology, Validation, Writing – original draft. TS: Investigation, Resources, Writing – original draft. VZ: Investigation, Resources, Writing – original draft. VS: Investigation, Resources, Writing – original draft. VU: Investigation, Resources, Writing – original draft. LM: Investigation, Resources, Supervision, Writing – review & editing. AM: Conceptualization, Formal Analysis, Funding acquisition, Investigation, Methodology, Project administration, Resources, Supervision, Validation, Visualization, Writing – review & editing.
The author(s) declare that financial support was received for the research, authorship, and/or publication of this article. The study was carried out with the financial support of the Ministry of Science and Higher Education of the Russian Federation (Agreement No. 075-15-2022-301 dated 04/20/2022).
The histological assessment was carried out with the support of the Pathology Unit of the Almazov Federal Medical Research Center.
The authors declare that the research was conducted in the absence of any commercial or financial relationships that could be construed as a potential conflict of interest.
All claims expressed in this article are solely those of the authors and do not necessarily represent those of their affiliated organizations, or those of the publisher, the editors and the reviewers. Any product that may be evaluated in this article, or claim that may be made by its manufacturer, is not guaranteed or endorsed by the publisher.
1. Lindman BR, Dweck MR, Lancellotti P, Généreux P, Piérard LA, O’Gara PT, et al. Management of asymptomatic severe aortic stenosis: evolving concepts in timing of valve replacement. JACC Cardiovasc Imaging. (2020) 13(2, Part 1):481–93. doi: 10.1016/j.jcmg.2019.01.036
2. Avvedimento M, Angellotti D, Ilardi F, Leone A, Scalamogna M, Castiello DS, et al. Acute advanced aortic stenosis. Heart Fail Rev. (2023) 28(5):1101–11. doi: 10.1007/s10741-023-10312-7
3. Boskovski MT, Gleason TG. Current therapeutic options in aortic stenosis. Circ Res. (2021) 128(9):1398–417. doi: 10.1161/CIRCRESAHA.121.318040
4. Lindman BR, Sukul D, Dweck MR, Madhavan MV, Arsenault BJ, Coylewright M, et al. Evaluating medical therapy for calcific aortic stenosis: JACC state-of-the-art review. J Am Coll Cardiol. (2021) 78(23):2354–76. doi: 10.1016/j.jacc.2021.09.1367
5. Hutcheson JD, Aikawa E. The history of cardiovascular calcification. In: Aikawa E, Hutcheson JD, editors. Cardiovascular Calcification and Bone Mineralization. Cham: Springer International Publishing (2020). p. 3–11. doi: 10.1007/978-3-030-46725-8_1
6. Zheng KH, Tzolos E, Dweck MR. Pathophysiology of aortic stenosis and future perspectives for medical therapy. Cardiol Clin. (2020) 38(1):1–12. doi: 10.1016/j.ccl.2019.09.010
7. Goody PR, Hosen MR, Christmann D, Niepmann ST, Zietzer A, Adam M, et al. Aortic valve stenosis. Arterioscler Thromb Vasc Biol. (2020) 40(4):885–900. doi: 10.1161/ATVBAHA.119.313067
8. Xue Y, Kossar AP, Abramov A, Frasca A, Sun M, Zyablitskaya M, et al. Age-related enhanced degeneration of bioprosthetic valves due to leaflet calcification, tissue crosslinking, and structural changes. Cardiovasc Res. (2022) 119(1):302–15. doi: 10.1093/cvr/cvac002
9. Niazy N, Barth M, Selig JI, Feichtner S, Shakiba B, Candan A, et al. Degeneration of aortic valves in a bioreactor system with pulsatile flow. Biomedicines. (2021) 9(5):462. doi: 10.3390/biomedicines9050462s
10. van der Velden J, Asselbergs FW, Bakkers J, Batkai S, Bertrand L, Bezzina CR, et al. Animal models and animal-free innovations for cardiovascular research: current status and routes to be explored. Consensus document of the ESC working group on myocardial function and the ESC working group on cellular biology of the heart. Cardiovasc Res. (2022) 118(15):3016–51. doi: 10.1093/cvr/cvab370
11. Bogdanova M, Zabirnyk A, Malashicheva A, Semenova D, Kvitting JPE, Kaljusto ML, et al. Models and techniques to study aortic valve calcification in vitro, ex vivo and in vivo. An overview. Front Pharmacol. (2022) 13:835825. doi: 10.3389/fphar.2022.835825
12. Smit V, de Mol J, Schaftenaar FH, Depuydt MAC, Postel RJ, Smeets D, et al. Single-cell profiling reveals age-associated immunity in atherosclerosis. Cardiovasc Res. (2023) 119(15):2508–21. doi: 10.1093/cvr/cvad099
13. Laurent C, Marano A, Baldit A, Ferrari M, Perrin JC, Perroud O, et al. A preliminary study exploring the mechanical properties of normal and Mgp-deficient mouse femurs during early growth. Proc Inst Mech Eng. (2022) 236(8):1106–17. doi: 10.1177/09544119221109019
14. Parashar A, Gourgas O, Lau K, Li J, Muiznieks L, Sharpe S, et al. Elastin calcification in in vitro models and its prevention by MGP’s N-terminal peptide. J Struct Biol. (2021) 213(1):107637. doi: 10.1016/j.jsb.2020.107637
15. Yoon D, Choi B, Kim JE, Kim EY, Chung SH, Min HJ, et al. Autotaxin inhibition attenuates the aortic valve calcification by suppressing inflammation-driven fibro-calcific remodeling of valvular interstitial cells. BMC Med. (2024) 22(1):122. doi: 10.1186/s12916-024-03342-x
16. Li H, Pan K, Meng Y, Deng J, Zhang P, Song W, et al. Mutual promotions between periodontitis and vascular calcification by rat animal model. J Periodontal Res. (2020) 55(6):810–20. doi: 10.1111/jre.12757
17. Wang L, Tang R, Zhang Y, Liu Z, Chen S, Song K, et al. A rat model with multivalve calcification induced by subtotal nephrectomy and high-phosphorus diet. Kidney Dis Basel Switz. (2020) 6(5):346–54. doi: 10.1159/000506013
18. Sider KL, Zhu C, Kwong AV, Mirzaei Z, de Langé CFM, Simmons CA. Evaluation of a porcine model of early aortic valve sclerosis. Cardiovasc Pathol Off J Soc Cardiovasc Pathol. (2014) 23(5):289–97. doi: 10.1016/j.carpath.2014.05.004
19. van den Heever JJ, Jordaan CJ, Lewies A, Bester D, Goedhals J, Botes L, et al. Comparison of the function and structral integrity of cryopreserved pulmonary homografts versus decellularized pulmonary homografts after 180 days implantation in the juvenile ovine model. Cell Tissue Bank. (2022) 23(2):347–66. doi: 10.1007/s10561-021-09948-2
20. Vafaee T, Walker F, Thomas D, Roderjan JG, Veiga Lopes S, da Costa FD, et al. Repopulation of decellularised porcine pulmonary valves in the right ventricular outflow tract of sheep: role of macrophages. J Tissue Eng. (2022) 13:20417314221102680. doi: 10.1177/20417314221102680
21. Rutkovskiy A, Malashicheva A, Sullivan G, Bogdanova M, Kostareva A, Stensløkken K, et al. Valve interstitial cells: the key to understanding the pathophysiology of heart valve calcification. J Am Heart Assoc. (2017) 6(9):e006339. doi: 10.1161/JAHA.117.006339
22. Hsu CPD, Tchir A, Mirza A, Chaparro D, Herrera RE, Hutcheson JD, et al. Valve endothelial cell exposure to high levels of flow oscillations exacerbates valve interstitial cell calcification. Bioengineering. (2022) 9(8):393. doi: 10.3390/bioengineering9080393
23. Orlova VV, Mummery CL. What endothelial cells from patient iPSCs can tell us about aortic valve disease. Cell Stem Cell. (2015) 16(5):455–7. doi: 10.1016/j.stem.2015.04.006
24. Gendron N, Rosa M, Blandinieres A, Sottejeau Y, Rossi E, Van Belle E, et al. Human aortic valve interstitial cells display proangiogenic properties during calcific aortic valve disease. Arterioscler Thromb Vasc Biol. (2021) 41(1):415–29. doi: 10.1161/ATVBAHA.120.314287
25. Liu MM, Flanagan TC, Jockenhovel S, Black A, Lu CC, French AT, et al. Development and evaluation of a tissue-engineered fibrin-based canine mitral valve three-dimensional cell culture system. J Comp Pathol. (2018) 160:23–33. doi: 10.1016/j.jcpa.2018.02.001
26. Geng B, Chen X, Chi J, Li F, Yim WY, Wang K, et al. Platelet membrane-coated alterbrassicene A nanoparticle inhibits calcification of the aortic valve by suppressing phosphorylation P65 NF-κB. Theranostics. (2023) 13(11):3781–93. doi: 10.7150/thno.85323
27. Robinson NB, Krieger K, Khan FM, Huffman W, Chang M, Naik A, et al. The current state of animal models in research: a review. Int J Surg. (2019) 72:9–13. doi: 10.1016/j.ijsu.2019.10.015
28. Zhu E, Shu X, Xu Z, Peng Y, Xiang Y, Liu Y, et al. Screening of immune-related secretory proteins linking chronic kidney disease with calcific aortic valve disease based on comprehensive bioinformatics analysis and machine learning. J Transl Med. (2023) 21:359. doi: 10.1186/s12967-023-04171-x
29. Theodoris CV, Zhou P, Liu L, Zhang Y, Nishino T, Huang Y, et al. Network-based screen in iPSC-derived cells reveals therapeutic candidate for heart valve disease. Science. (2021) 371(6530):eabd0724. doi: 10.1126/science.abd0724
30. Lobov AA, Boyarskaya NV, Kachanova OS, Gromova ES, Shishkova AA, Zainullina BR, et al. Crenigacestat (LY3039478) inhibits osteogenic differentiation of human valve interstitial cells from patients with aortic valve calcification in vitro. Front Cardiovasc Med. (2022) 9:969096. doi: 10.3389/fcvm.2022.969096
31. Wang Y, Han D, Zhou T, Chen C, Cao H, Zhang JZ, et al. DUSP26 induces aortic valve calcification by antagonizing MDM2-mediated ubiquitination of DPP4 in human valvular interstitial cells. Eur Heart J. (2021) 42(30):2935–51. doi: 10.1093/eurheartj/ehab316
32. Iqbal F, Schlotter F, Becker-Greene D, Lupieri A, Goettsch C, Hutcheson JD, et al. Sortilin enhances fibrosis and calcification in aortic valve disease by inducing interstitial cell heterogeneity. Eur Heart J. (2023) 44(10):885–98. doi: 10.1093/eurheartj/ehac818
33. Zabirnyk A, del Mar Perez M, Blasco M, Stensløkken KO, Ferrer MD, Salcedo C, et al. A novel ex vivo model of aortic valve calcification. A preliminary report. Front Pharmacol. (2020) 11:568764. doi: 10.3389/fphar.2020.568764
34. Avilés-Reyes A, Freires IA, Rosalen PL, Lemos JA, Abranches J. Ex vivo model of human aortic valve bacterial colonization. Bio-Protoc. (2017) 7(11):e2316. doi: 10.21769/BioProtoc.2316
35. Sadat N, Aherrahrou Z, Osterloh A, Erdmann J, Fujita B, Ensminger S. Comparison of different calcification media on gene expression using a porcine aortic valve ex vivo assay. Thorac Cardiovasc Surg. (2023) 71(S 01): S1–S72. doi: 10.1055/s-0043-1761802
36. Reil JC, Saisho H, Jockwer A, Fujita B, Paluszkiewicz L, Reil GH, et al. Impact of heart rate, aortic compliance and stroke volume on the aortic regurgitation fraction studied in an ex vivo pig model. Open Heart. (2023) 10(2):e002319. doi: 10.1136/openhrt-2023-002319
37. Zhu Y, Imbrie-Moore AM, Paulsen MJ, Priromprintr B, Wang H, Lucian HJ, et al. Novel bicuspid aortic valve model with aortic regurgitation for hemodynamic status analysis using an ex vivo simulator. J Thorac Cardiovasc Surg. (2022) 163(2):e161–71. doi: 10.1016/j.jtcvs.2020.06.028
38. Janetzko K, Rink G, Hecker A, Bieback K, Klüter H, Bugert P. A single-tube real-time PCR assay for mycoplasma detection as a routine quality control of cell therapeutics. Transfus Med Hemother. (2014) 41(1):83–9. doi: 10.1159/000357096
39. Yuen E, Posada M, Smith C, Thorn K, Greenwood D, Burgess M, et al. Evaluation of the effects of an oral notch inhibitor, crenigacestat (LY3039478), on QT interval, and bioavailability studies conducted in healthy subjects. Cancer Chemother Pharmacol. (2019) 83(3):483–92. doi: 10.1007/s00280-018-3750-1
40. Azaro A, Baldini C, Rodon J, Soria JC, Yuen E, Lithio A, et al. Phase 1 study of 2 high dose intensity schedules of the pan-Notch inhibitor crenigacestat (LY3039478) in combination with prednisone in patients with advanced or metastatic cancer. Invest New Drugs. (2021) 39(1):193–201. doi: 10.1007/s10637-020-00944-z
41. Kostina A, Lobov A, Semenova D, Kiselev A, Klausen P, Malashicheva A. Context-specific osteogenic potential of mesenchymal stem cells. Biomedicines. (2021) 9(6):673. doi: 10.3390/biomedicines9060673
42. Lu D, Jiang H, Zou T, Jia Y, Zhao Y, Wang Z. Endothelial-to-mesenchymal transition: new insights into vascular calcification. Biochem Pharmacol. (2023) 213:115579. doi: 10.1016/j.bcp.2023.115579
43. Docshin P, Bairqdar A, Malashicheva A. Interplay between BMP2 and notch signaling in endothelial-mesenchymal transition: implications for cardiac fibrosis. Stem Cell Investig. (2023) 10:18. doi: 10.21037/sci-2023-019
44. Pestiaux C, Pyka G, Quirynen L, De Azevedo D, Vanoverschelde JL, Lengelé B, et al. 3D histopathology of stenotic aortic valve cusps using ex vivo microfocus computed tomography. Front Cardiovasc Med. (2023) 10:1129990. doi: 10.3389/fcvm.2023.1129990
Keywords: calcifying aortic stenosis, ex vivo, human, pathological calcification, osteogenic differentiation, whole leaflet
Citation: Kachanova OS, Boyarskaya NV, Docshin PM, Scherbinin TS, Zubkova VG, Saprankov VL, Uspensky VE, Mitrofanova LB and Malashicheva AB (2024) Ex vivo model of pathological calcification of human aortic valve. Front. Cardiovasc. Med. 11:1411398. doi: 10.3389/fcvm.2024.1411398
Received: 5 April 2024; Accepted: 13 August 2024;
Published: 29 August 2024.
Edited by:
Joshua D. Hutcheson, Florida International University, United StatesReviewed by:
Marta Scatena, University of Washington, United StatesCopyright: © 2024 Kachanova, Boyarskaya, Docshin, Scherbinin, Zubkova, Saprankov, Uspensky, Mitrofanova and Malashicheva. This is an open-access article distributed under the terms of the Creative Commons Attribution License (CC BY). The use, distribution or reproduction in other forums is permitted, provided the original author(s) and the copyright owner(s) are credited and that the original publication in this journal is cited, in accordance with accepted academic practice. No use, distribution or reproduction is permitted which does not comply with these terms.
*Correspondence: A. B. Malashicheva, bWFsYXNoaWNoZXZhX2FiQGFsbWF6b3ZjZW50cmUucnU=; YW1hbGFzaGljaGV2YUBnbWFpbC5jb20=
Disclaimer: All claims expressed in this article are solely those of the authors and do not necessarily represent those of their affiliated organizations, or those of the publisher, the editors and the reviewers. Any product that may be evaluated in this article or claim that may be made by its manufacturer is not guaranteed or endorsed by the publisher.
Research integrity at Frontiers
Learn more about the work of our research integrity team to safeguard the quality of each article we publish.