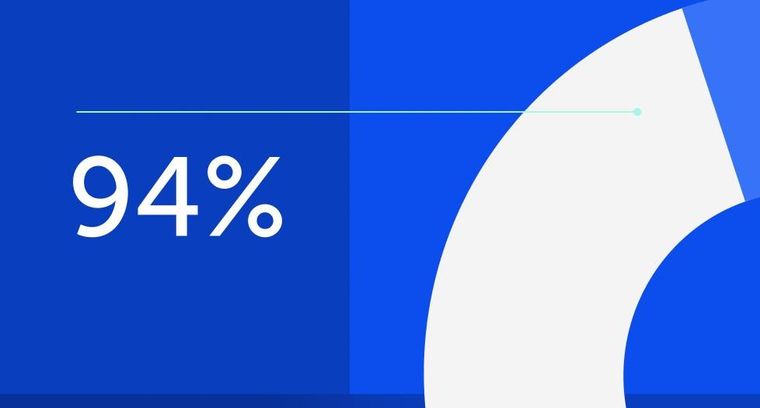
94% of researchers rate our articles as excellent or good
Learn more about the work of our research integrity team to safeguard the quality of each article we publish.
Find out more
REVIEW article
Front. Cardiovasc. Med., 13 March 2024
Sec. Atherosclerosis and Vascular Medicine
Volume 11 - 2024 | https://doi.org/10.3389/fcvm.2024.1356152
This article is part of the Research TopicProteomics in AtherosclerosisView all 8 articles
The occurrence and development of myocardial dysfunction are associated with damage in the cardiac microvascular endothelial cells (CMECs), which can regulate nutrient exchange and oxy-gen-carbon cycling to protect cardiomyocytes. Interventions targeting microRNAs (miRNAs) can effectively mitigate CMEC injury and thus improve cardiovascular diseases. MiRNAs are a class of noncoding single-strand RNA molecules typically 21–23 nucleotides in length that are encoded by endogenous genes. They are critical regulators of organism development, cell differentiation, metabolism, and apoptosis. Current clinical trials on miRNA drugs indicate that patient-specific miRNA levels are now being used as one of the criteria for predicting heart disease. However, the cellular process of various miRNAs in CMECs in cardiovascular diseases has not been fully elucidated. These mechanisms are a field that immediately requires further investigation. Accordingly, this review summarizes the roles and mechanisms of various miRNAs in CMECs in cardiovascular disease and includes the process of CMEC crosstalk between miRNAs and other cell types in the heart. Our study serves as a theoretical basis for the formal introduction of miRNA use into the treatment of cardiovascular diseases in the future.
Cardiovascular disease (CVD) now accounts for one-third of all fatalities globally, and it has recently emerged as one of the most significant variables affecting people's health (1). Coronary artery disease (CAD) is the most common type of CVDs and is caused by atherosclerotic blockage of the coronary arteries (2). Its clinical manifestations are usually myocardial infarction (MI) and ischemic cardiomyopathy (3). CAD is becoming more common every year in developed and developing countries, which is seriously endangering people's lives (3, 4). Inflammation, oxidative stress, autophagy, and apoptosis are the fundamental pathological reactions to CAD. The primary pathological processes are cell damage and excessive cellular autophagy (5). Additionally, CAD has the further pathophysiological features of vascular dysfunction and myocardial dysfunction. Previous research has demonstrated that endothelial damage causes reduced bioavailability of nitric oxide (NO), which throws off the delicate balance between vasoconstriction and diastole. The outcomes are increased endothelial permeability and endothelial secretion disorders, further resulting in vascular dysfunction (6). Cardiovascular dysfunction is an early event of CVDs such as atherosclerosis and heart failure and can be utilized as an early predictor for the assessment of heart failure (7–9). Therefore, cardiac vascular dysfunction has become a major potential target for the treatment of heart disease.
The cardiac microvascular endothelial cell (CMEC) is the first line of defense for tissues and organs and can be used to detect alterations in hemodynamic and blood propagation signals. Together with smooth muscle cells, it regulates blood flow and plays a critical role in regulating angiogenesis and inflammatory reactions (10). The primary cause of coronary microvascular dysfunction (CMD) is endothelial dysfunction occurring in the CMECs (11), and it leads to severe tissue damage. Damaged vascular endothelial cells can have an impact on other cells and aggravate the illness (12). Moreover, the damage to CMECs precedes cardiac myocytes in the development of CVDs (13). In the event of hypoxic or inflammatory injury, among others, CMEC not only regulates nutrient exchange and oxygen-carbon cycling in cardiomyocytes but also protects cardiomyocytes from damage (14). In the heart, multiple capillary-based CMECs tightly encircle the cardiomyocytes to create a perfusion unit. The space between the two cells is extremely narrow and contiguous. For example, NO has an important regulatory role in the cardiovascular system. Decreased NO activity can lead to increased coronary artery constriction and inflammation. Two NO-converting enzymes, constitutive NO synthase (cNOS; type III) and inducible NO synthase (iNOS, type II), are highly expressed in endothelial cells (15). The released NO affects cardiac diastolic function by raising cyclic guanosine monophosphate (cGMP) levels in cardiomyocytes. However, excessive NO can induce myocardial disorders under pathological conditions (16). Several studies have demonstrated that the crosstalk between CMECs and other cells plays a crucial role in cardiac function (17, 18). Together, targeted CMECs have become a significant prospective therapeutic pathway in the management of CVD.
MiRNAs are a class of noncoding single-strand RNA molecules typically 21–23 nucleotides in length that are encoded by endogenous genes. They have been found to play a regulatory role in gene expression (19). qPCR, in situ hybridization, microarrays, and RNA sequencing are some of the current techniques for miRNA detection (20). Typically, the sequence of miRNA is located within an intron or exon of a noncoding RNA (21). Most genes are transcribed by Pol II to pri-miRNA (22). The pri-miRNA is cleaved in the nucleus by Drosha and DiGeorge syndrome critical region gene 8 (DGCR8) into a pre-miRNA of approximately 70 nucleotides long (23). The pre-miRNA is transported into the cytoplasm via XPO5 and ras-associated nuclear protein (RAN), which is cleaved by Dicer into multiple double-strand miRNAs (24). After the original structure is restored in the Argonaute protein, single-strand mature miRNA is generated by eliminating the passenger strand of the miRNA double-strand body (22).
The mode of action of miRNAs is usually through the base pairing of several nucleotide sites of the miRNA with the untranslated region of the target mRNA, which in turn inhibits the expression of the target mRNA. Particularly crucial to the suppression of mRNA expression are nucleotide 2–8 sites (25). Target prediction often relies on the nucleotides in this region of the binding site (25, 26).
A single miRNA can control the expression of multiple target mRNAs, and a single mRNA can also be regulated by multiple miRNAs. Thus, miRNAs are generally considered promising for their potent and diverse therapeutic functional effects as well as their ability to affect multiple target genes (27). MiRNAs are critical regulators of organism development, cell differentiation, metabolism, and apoptosis (24). Clinical therapeutic strategies targeting miRNAs are already being implemented. For example, clinical study has shown that therapeutic measures targeting miRNAs in viral hepatitis C are efficacious and well tolerated (28). Beg et al. found that miRNA-43 mimics showed considerable potential for the treatment of patients with advanced cancer in phase I clinical studies (29).
MiRNAs can regulate necrotic apoptosis (30). Necrotizing apoptosis in cardiac myocytes in patients with heart disease is usually regulated by miRNAs, which in turn increases or decreases infarct size (31). For example, the hearts of pre-miR-223 knock-out mouse are more vulnerable to ischemia reperfusion (IR) injury. MiR-873 can reduce the infarct size of heart in mice with coronary artery occlusion and reperfusion by inhibiting the necrotic death of cardiomyocytes (31, 32). Although few clinical trials focus on miRNAs for CVDs, changes in the levels of particular miRNAs can often be used in the clinic as a marker to evaluate disease severity. MiRNA-133a/b, a myocardial-specific miRNA, maybe a potential target for the therapy of ventricular fibrillation after MI. Several targets associated with miRNA-133 are related to cardiac disease (33). Reduced miRNA-133a/b expression in patients is reportedly a hallmark of the development of ventricular fibrillation after MI in clinical studies (34). Additionally, miRNA-133 plays an important regulatory role in the differentiation of embryonic stem cells to cardiomyocytes (35). Patients with CAD have significantly elevated peripheral blood miRNA-218 levels, which can also be used as one of the criteria to measure the condition. This finding indicates the feasibility of monitoring variations in miRNA levels to measure and predict the progression of disease (36). Meanwhile, miRNA-133 can inhibit angiogenesis in endothelial cells by targeting vascular endothelial growth factor receptor 2 (VEGFR2) and fibroblast growth factor receptor 1 (FGFR1) (37). Cardiac microvascular injury has important implications for cardiac pathological recovery and the maintenance of physiological homeostasis (38). Therefore, this review summarizes the role and mechanism of various miRNAs in CMECs in CVDs. This review can guide the use of miRNA drugs for the clinical treatment of CVDs.
CAD is an atherosclerotic disease with inflammatory properties (3, 39). MI is a CVD caused by the formation of plaques in the interior walls of the arteries, reducing blood flow into the heart and injuring heart muscles because of insufficient oxygen supply (40). MI leads to the apoptosis of cardiac myocytes and nonmyocytes including CMECs (41), which ultimately induces apoptosis and fibrotic scarring with impaired heart function. In patients with CAD, CMD always coexist. CAD-induced reduction in perfusion pressure can trigger functional and structural alterations in microvasculature. Functional alterations include impaired vasodilator function, whereas structural alterations include arteriolar and capillary rarefaction distal to the coronary stenosis. Conversely, CMD-induced impaired vasodilatation and metabolic disturbances can exacerbate CAD (42). Previous studies have shown that CMD in the coronary vasculature is an important contributor (43, 44). The degree of improvement in the coronary microvascular system of patients with MI has a serious impact on morbidity and mortality in a clinic (45). Coronary microcirculation has become a primary target for therapies aimed at mitigating MI injury (46). The ratio of cardiac CMECs to cardiomyocytes is about 3:1, which determines its importance in cardiovascular health (17). Therefore, we summarize the roles and mechanisms of various miRNAs in CAD in CMECs and briefly describe them in Figure 1.
MiRNA-218 is located at the introns of the SLIT2 and SLIT3 genes (47, 48). In rat primary CMECs, miRNA-218 is significantly lower after homocysteine (Hcy) treatment than in the control group. Hcy-induced reductions in NO bioavailability result in impaired endothelium capacity to regulate vascular tone. Hcy-induced oxidative stress causes the oxidative inactivation of NO and endothelial NO synthase (eNOS) uncoupling and contributing to the formation of more reactive oxygen species (ROS) (49, 50). Elevated Hcy levels are considered a risk factor for the development of atherosclerotic vascular injuries in coronary heart disease (51). Therefore, a CAD model is usually built by Hcy (52). Overexpression of miRNA-218 alleviates Hcy-induced apoptosis, pro-inflammatory cytokine release, and improved CMEC migration. When CMEC injury occurs in advanced atherosclerosis, miRNA-218 expression is likewise decreased to increase high mobility group protein B1 (HMGB1) expression. HMGB1 is released from macrophages/monocytes upon stimulation with lipopolysaccharide (LPS), tumor necrosis factor (TNF), interleukin-1beta (IL-1β), and necrotic cells. HMGB1 binds to cell surface receptors and mediates the inflammatory response via the mitogen-activated protein kinase (MAPK) signal pathway. In clinical studies, miRNA-218 is also found to be significantly higher than normal in the peripheral blood of patients with CAD, which can be one of the criteria for diagnosing CAD (36, 53).
MiRNA-26b-5p is a member of the miR-26 family, which is located at CHR2 gene in human. Bidzhekov et al. have demonstrated that miRNA-26b-5p is also associated with atherosclerosis, the main cause of CVDs (54). In the MI model (55), the long non-coding RNA (lncRNA) expression of Malat1 is significantly elevated in injured CMEC after 7 days. LncRNAs are transcripts >200 nucleotides long which modulate transcription, epigenetic modifications, and posttranslational modifications by interacting with DNA and signaling receptors (56). Previous study has shown that Malat1 plays an important regulatory role by sponging miRNAs in CVD and endothelial dysfunction (57). Its specific target miRNA-26b-5p causes a significant increase in the expression of downstream signaling factor mitofusin 1 (MFN1). MFN1 is usually involved in oxidative stress pathways and mitochondrial apoptosis, such as decreasing ROS activity, maintaining mitochondrial homeostasis in CMEC thereby alleviating cardiac microvascular dysfunction. What's more, Malat1 is the competing endogenous RNA (ceRNA) for miR-26b-5p. Knockdown of Malat1 caused an exacerbation of MI, suggesting that endogenous miRNAs are also critical for the development of CVD. However, miRNA-26b-5p expression in circulating blood is significantly lower in heart failure patients than in normal subjects in clinical trials and is positively correlated with ejection fraction (58).
MiRNA-128 is embedded on CHR 2q21.3 and 3p22.3 (59). MiRNA-128 is often considered as a potential therapeutic target to regulate vascular smooth muscle cells (60). The study has shown that overexpression of miRNA-128 promotes mitochondrial dysfunction by directly targeting NADH Dehydrogenase (Ubiquinone) Fe-S Protein 4 (NDUFS4) (61). MiRNA-128 in CMEC targets IRS1 in the Hcy-induced coronary heart disease model (62). Inhibition of IRS1 expression promotes vascular endothelial growth factor (VEGF) expression inhibits oxidative stress, and enhances the anti-apoptotic capacity and migration ability of CMEC.
MiRNA-33a-5p is an intron miRNA that is located inside the intron sequence of the sterol-response-element-binding protein gene 2 (SREBP2) (63). The study has shown that miR-33a-5p promotes apoptosis via targeting nodal modulator1 (NOMO1) and other targets (64). After treatment with Hcy in the CMEC vascular dysfunction model, growth-arrest specific transcript 5 (GAS5) expression is markedly reduced whereas miRNA-33a-5p expression is elevated (65). GAS5 would compete with miRNA-33a-5p to bind the target ATP-binding cassette transporter A1 (ABCA1), while miRNA-33a-5p action on ABCA1 would promote apoptosis and inhibit cell growth (66).
MiRNA-21 is located on CHR 17 in human. The study has shown that miRNA-21 enhances hypoxia inducible factor-1α (HIF-1α) activity via targeting Hsc70-interacting protein (CHIP), playing a role in promoting angiogenesis (67). In a rat MI model simulated by left anterior descending branch ligation (LAD) infarct surgery, miRNA-21 expression was elevated in CMEC. MiRNA-21 has an impact on the downstream target phosphatase and tensin homolog deleted on phosphatase and tensin homolog (PTEN), regulates protein kinase B (AKT) and extracellular regulated kinases (ERK) pathway, promotes VEGF expression, which ameliorates the injury caused by acute infarction. And when miRNA-21 inhibitors are administered, angiogenesis is inhibited to aggravate the disease (68, 69).
LncRNA HULC expression is downregulated in cardiac tissue in a LAD infarct model. HULC can interact directly with miRNA-29b to inhibit inflammatory cytokines such as interleukin-6 (IL-6) and interleukin-8 (IL-8) and promote angiogenesis. And miRNA-29b overexpression in CMEC eliminates the effect of HULC and exacerbates the inflammatory response (70). In primary CMEC isolated from rats with MI, the level of miRNA-223-3p is considerably increased during the migratory and proliferative phases (71), which can directly target the gene recombinant ribosomal protein S6 kinase Beta 1 (RPS6KB1) and participate in the RPS6KB1/HIF-1α signaling pathway. This results in the cell being blocked in the S-phase of the cell cycle, inhibiting migration and proliferation of ischemic CMEC.
Reperfusion is currently the only effective strategy to address cardiac ischemia (72). Thrombolysis, percutaneous coronary intervention, and coronary artery bypass surgery have been applied in clinics (72, 73). However, these techniques inflict secondary damage due to myocardial IR injury (74). IR injury not only leads to necrotic apoptosis and autophagy due to excessive ROS release (75, 76), but also activates senescent cells and exacerbates fibrosis formation (77, 78). Previous studies have shown that endothelial function plays a fundamental role in the protection against IR injury (79). IR is a major cause of the coronary microcirculation injury, inducing endothelial cell dysfunction and increased vascular leakage. Conversely, ischemia alone has a limited effect (80). Thus, understanding the functional role of miRNAs under IR injury is crucial.
MiRNA-206 is a member of the miRNA-1 family, which is located between the interleukin-17 gene and the polycystic kidney and hepatic disease 1 gene in human (chr 6), mouse (chr 1) and rat (chr 9) (81). MiRNA-206 normally regulates the function of skeletal muscle. Once its dysregulation is usually accompanied by skeletal muscle disorders such as duchenne muscular dystrophy (DMD) and amyotrophic lateral sclerosis (ALS) (82). In the hypoxia/reoxygenation (H/R) model, YAP expression is reduced and miRNA-206 expression is downregulated within CMEC. Elevated expression of its direct target gene, programmed cell death 4 (PDCD4), decreases eNOS phosphorylation and inhibits platelet endothelial cell adhesion molecule-1 (PECAM-1) expression with CMEC damage (83). PDCD4 may improve the mitigation of inflammatory responses as well as oxidative stress through the heme oxygenase 1 (HO-1) pathway (84).
MiRNA-126 is located on CHR 9 in human, which is involved in cell apoptosis, inflammation, proliferation, angiogenesis, and other processes by negatively targeting phosphatidylinositol 3-kinase (PI3K), VEGF, vascular cell adhesion molecule-1 (VCAM-1), and low density lipoprotein receptor related protein (LRP6) (85). Yang et al. found that miRNA-126 expression is reduced in CMEC after H/R treatment. MiRNA-126 reduced pro-inflammatory cytokine expression and controlled the inflammatory response by stimulating the PI3K/Akt/eNOS signaling pathway in human CMEC. When miRNA-126 expression is reduced, the anti-inflammatory effects and lumen formation are inhibited after CMEC injury (86).
MiRNA-200a is located on CHR 1p36.33 (87). The study has shown that miRNA-200a is highly sensitive to ROS (88). In the H/R model, the level of ROS increases in both cardiomyocytes and CMEC, and miRNA-200a expression reduced in CMEC. Accordingly, miRNA-200a is usually involved in pathway responses related to oxidative stress. When miRNA-200a is overexpressed in H/R-treated CMEC, apoptosis is ameliorated. The mechanism may be through inhibiting the expression of oxidative stress pathways such as nuclear factor erythroid 2-related factor 2 (NRF2) (89, 90).
In the IR model, miRNA-495 expression is decreased, while pro-inflammatory cytokine expression is increased in CMEC. Excessive miRNA-495 can inhibit pyrin domain-containing 3 (NLRP3), thereby reducing the secretion of pro-inflammatory cytokines such as IL-6 and TNF-α. It alleviates inflammation while enhancing CMEC cell activity as well as migration ability (91). MiRNA-145-5p levels decreases in patients with coronary artery disease, while its overexpression effectively alleviates H/R-induced CMEC injury by inhibiting mothers against decapentaplegic homolog 4 (SMAD4). SMAD4 is a crucial element of the transforming growth factor β (TGFβ) signaling pathway and is involved in cell proliferation, differentiation, migration, and apoptosis (92).
Heart failure is the end stage in the development of various heart diseases, such as MI and myocarditis. Several studies show that endothelium-dependent vasodilation is blunted in patients with heart failure (8, 93, 94). Currently, Nebivolol is often used clinically to treat heart failure. Nebivolol plays a role in the beta (3)-adrenoceptor agonistic effect on endothelial cells that stimulates eNOS, as well as in repairing vascular dysfunction (95, 96). Nebivolol also inhibits the high salt-induced elevated expression of miRNA-320, which is located in CMECs and is involved in IR injury after MI via the antithetical regulation of heat-shock protein 20 (Hsp20) (97–99). Thus, improving vascular dysfunction has become an important direction in the treatment of heart failure in clinics.
MiR-1-3p is encoded by the miR-1-2 gene located on CHR 18q11.2 (100). Wang et al. found that the level of miRNA-1-3p is decreased in CMEC when chronic heart failure occurs (101). When a vast array of miRNA-1-3p is secreted, it could directly inhibit the expression of endothelin-1 (ET-1), improve vascular endothelial dysfunction and cardiac remodeling. Moreover, up-regulated miR-1-3p inhibits cell proliferation and promotes apoptosis by targeting stress-associated endoplasmic reticulum protein 1 (SERP1), which leads to dysfunction of CMECs (102). We summarize the miRNA of CMEC in cardiovascular disease to provide a theoretical basis (Table 1, Figure 1).
Diabetes mellitus (DM), caused either by deficient insulin secretion or damaged of pancreatic β cell consists of type I and type II DM. Type I DM is an autoimmune disease that affects pancreatic cells and reduces insulin production. Type II DM is a disease caused by the dysfunction or defect of pancreatic β cells, which reduces insulin signaling and secretion from the pancreas (103). Diabetic patients have elevated blood glucose due to islet damage. High intracellular glucose levels usually lead to metabolic abnormalities with a variety of complications, including cardiac microangiopathy (104). Endothelial cells are some of the first cell types to be exposed to hyperglycemia (105). Hyperglycaemia-induced mitochondrial dysfunction and endoplasmic reticulum stress are associated with mechanisms, such as the activation of protein kinase C (PKC), polyol, and hexosamine pathways. Hyperglycaemic lesion also induces ROS accumulation that damages proteins or DNA and modulates intracellular signaling pathways, such as mitogen activated protein kinases and redox sensitive transcription factors (106). In hyperglycemic CMECs, the glycolytic reserve is significantly reduced, and the ability to produce ATP via glycolysis is impaired (107). Additionally, higher ROS levels and oxidative stress brought on by hyperglycemia frequently result in endothelial cell apoptosis, lowering the mRNA levels of oxidative phosphorylation (OXPHOS)-related genes. Thus, diabetes-induced hyperglycemia damages CMECs through oxidative stress, as well as inhibits mitochondrial glycolysis and the ability of OXPHOS to generate ATP. In turn, CMEC proliferation is inhibited, apoptosis is promoted (107–109). Moreover, this damaged CMECs activate PKC, and release inflammatory cytokines and adhesion factors (110, 111).
Clinical research is gradually focusing on targeting miRNAs in the blood of diabetic patients. Studies have shown that diabetic patients with high plasma miRNA-19a expression have less vascular circulating tissue factor (TF) expression (112). TF is a procoagulant factor released by vascular endothelial cells, which are suppressed by miRNA-19a and miRNA-126. TF content in the peripheral blood of type II diabetic patients is also significantly negatively correlated with miRNA-181b (113). We summarize the miRNA of CMEC in diabetes (Table 2).
Mature miRNA-92a can be produced by miR-92a-1 and miR-92a-2 manipulation. MiRNA-92a-1 is situated within the third intron of an open reading frame 25 (C13orf25) gene located at chromosome 13q31-q32 (120). MiRNA-92a-2 is encoded in miR-106-363 cluster on the X chromosome, region q26.2 (121), which is of great importance in cardiac development and angiogenesis. According to the study, high glucose induction dramatically increases the level of miRNA-92a in human CMECs, reduces the ability of cells to migrate and proliferate, and increases inflammatory response. MiRNA-92a can inhibit the expression of A disintegrin and metalloproteinase 10 (ADAM10), Krüpple-like factor2 (KLF2) and Krüpple-like factor4 (KLF4). ADAM10 promotes cell migration, KLF2 and KLF4 are important transcriptional regulators of anti-inflammatory and antioxidant activity (114). Therefore, cells in the high glucose group with elevated miRNA-92a expression have a significantly reduced ability to migrate and proliferate, and an increased inflammatory response.
MiRNA-146a is located on CHR 5, which is an important element in the regulation of inflammatory responses (122). Previous study has shown that miRNA-146a inhibits the target gene TGF-β1 to anti-angiogenesis. The high glucose treatment of CMECs induces a decrease in miRNA-146a expression with increasing the expression of tumor necrosis factor receptor associated factor 6 (TRAF6) and interleukin-1 Receptor-Associated Kinase 1 (IRAK1) (119). Promoting the release of pro-inflammatory cytokines, such as IL-6 and IL-1β, increases inflammatory response and promotes fibrosis. Moreover, when normal CMEC's miRNA-146a expression is suppressed, it also causes hyperglycemic lesions.
MiRNA-503 is located on chromosome Xq26.3 and is elevated in the high-glucose treated CMEC model (115, 123). MiRNA-503 inhibits Apelin-12 levels by binding to the promoter region of Apelin gene, which can promote the release of pro-inflammatory cytokines such as TNF-α, IL-6, and IL-1β through c-Jun N-terminal kinase (JNK) and p38 MAPK signaling pathways, further causing vascular dysfunction and the development of heart failure in severe cases (115).
Elevated miR-216b expression in diabetic CMECs inhibits the expression of its downstream target frizzled class receptor 5 (FZD5), while decreased miR-216b level inhibits the proliferative capacity and migratory capacity of CMEC (116). In addition, miR-193-5p expression is increased in CMECs, which interacts with the direct target insulin growth factor 2 (IGF2) to inhibit cell proliferation (117). Feng et al. show that hyperglycemia in diabetic models is expressed by suppressing endothelial cell miRNA-200b (118). MiRNA-200b prevents endothelial mesenchymal transition (EndMT) which is caused by decreased levels of endothelial-specific markers like VEGF and PECAM-1, which impact greatly on heart failure and cardiac fibrosis.
MiRNAs play an important role in cellular crosstalk, especially in cardiovascular aspects. We summarize the crosstalk mechanisms between other cells in the heart and CMEC (Figure 2).
One cardiomyocyte is typically surrounded by multiple CMECs in the heart to create a perfusion unit, and the space between the two cells is incredibly narrow. Consequently, the interaction between CMECs and cardiomyocytes has significant effects on heart function (18).
Hypoxia-treated cardiomyocytes release exosomes containing excessive circHIPK3, which acts as an endogenous miR-29a sponge. Exosomes then enter oxidative stress-injured CMECs to inhibit miRNA-29a activity, drive increased insulin-like growth factor 1 (IGF-1) expression, and reduce ROS and malondialdehyde (MDA) expression (124). In contrast to the above results (125), in the H/R model, injured cardiomyocytes release a large amount of miRNA-499. Subsequently, miRNA-499 translates into CMEC to inhibit the level of α7-nachr, promote an inflammatory response, and exacerbate vascular dysfunction.
CMEC also affects cardiomyocytes. Hypoxia-treated primary CMECs in vitro release miRNA-27b-3p-rich exosomes to prevent cardiomyocyte damage (126). MiRNA-27b-3p-rich exosomes released from hypoxia-treated CMECs injected in advance into the myocardium significantly inhibit H/R-induced MI injury in rats. The mechanism involves the ability of miRNA-27b-3p to significantly inhibit Foxo1 expression and this suppress cardiomyocyte apoptosis. Moreover, rat hypoxia-treated CMECs release miRNA-210-3p-rich exosomes that are delivered to H/R-treated cardiomyocytes. MiRNA-210-3p further targets transferrin receptor (TFR) in cardiomyocytes to alleviate cardiomyocyte ferroptosis (127).
Cardiac telocyte (CT) is a population of cells with a very small gap to cardiac muscle cells (128). When a cardiac infarction occurs, CTs in the area of ischemic infarction die in large numbers. Conversely, CT cell density is gradually restored during infarct healing. When CT is therapeutically transplanted into the damaged myocardial area, it can effectively improve myocardial function and the degree of myocardial fibrosis (129). Additionally, CT can release exosomes and transfer them to hypoxic CMECs, and the most abundant miRNA in the exosomes is miRNA-21-5p (130). MiRNA-21-5p enters CMECs to repress the target gene cell death inducing p53 target 1 (Cdip1), thereby downregulating activated caspase-3 and inhibiting apoptosis.
Mesenchymal stem cells (MSCs) are a group of multipotent precursor cells that can differentiate into multiple cell types and are used clinically to address hematologic and CVDs. MSC can secrete immunomodulatory and angiogenic bioactive factors that promote tissue repair and regeneration (131, 132). Previous studies have shown that MSC is beginning to become the focus of clinical studies for the treatment of AMI and ischemic heart failure (133). Moreover, hMSC can out miR-543-rich exosomes that transfer into hypoxia-treated CMEC to down-regulate recombinant collagen type IV alpha 1 (COL4A1) expression and ameliorate hypoxic injury (134). Ning et al. showed that MSCs protected cardiac endothelial cells from damage by releasing miRNA-153-3p low-expressing exosomes and activating the Angiopoietin-1 (ANGPT1) and VEGF/VEGFR2/PI3K/Akt/eNOS pathways (135).
A single miRNA can have multitargeting effects, so miRNA-targeted therapeutic approaches have recently become a research hotspot with great prospects. For example, miRNA-145 can reduce in-stent restenosis rate via anti-vascular proliferation with no side effects (136). Improving microvascular endothelial dysfunction has also become an important direction for studying CVD treatment. This review summarizes the roles played by numerous significant miRNAs in CMECs and their mechanisms of action, with the aim of providing a theoretical basis for the clinical use of miRNA therapies. This review also elaborates on the role of miRNAs in the crosstalk between CMECs and other cells in the heart and summarizes the mechanisms of action. MiRNAs in diseases are currently being investigated further and are frequently used as diagnostic criteria (36). However, some remaining issues with miRNA therapy need to be resolved, including the requirement for extremely accurate miRNA sequence prediction and a certain number of miRNAs with highly conserved sequences among different organisms. MiRNA-targeted treatment will undoubtedly advance with the improvement in detecting technologies, such as gene sequencing. Furthermore, miRNA therapy has the advantage of multitargeting, which theoretically fits cardiovascular therapies well. This promising future will undoubtedly serve as a catalyst for the development of cardiovascular therapies.
JY: Writing – review & editing, Writing – original draft. XZ: Writing – review & editing, Writing – original draft. YZ: Writing – review & editing, Writing – original draft. XW: Writing – review & editing, Writing – original draft, Supervision.
The author(s) declare that financial support was received for the research, authorship, and/or publication of this article.
This research was funded by National Key R&D Program of China (2022YFC3500300).
The authors declare that the research was conducted in the absence of any commercial or financial relationships that could be construed as a potential conflict of interest.
All claims expressed in this article are solely those of the authors and do not necessarily represent those of their affiliated organizations, or those of the publisher, the editors and the reviewers. Any product that may be evaluated in this article, or claim that may be made by its manufacturer, is not guaranteed or endorsed by the publisher.
1. Goldsborough E 3rd, Osuji N, Blaha MJ. Assessment of cardiovascular disease risk: a 2022 update. Endocrinol Metab Clin North Am. (2022) 51:483–509. doi: 10.1016/j.ecl.2022.02.005
2. Libby P, Theroux P. Pathophysiology of coronary artery disease. Circulation. (2005) 111:3481–8. doi: 10.1161/CIRCULATIONAHA.105.537878
3. Malakar AK, Choudhury D, Halder B, Paul P, Uddin A, Chakraborty S. A review on coronary artery disease, its risk factors, and therapeutics. J Cell Physiol. (2019) 234:16812–23. doi: 10.1002/jcp.28350
4. Khan MA, Hashim MJ, Mustafa H, Baniyas MY, Al Suwaidi S, Alkatheeri R, et al. Global epidemiology of ischemic heart disease: results from the global burden of disease study. Cureus. (2020) 12:e9349. doi: 10.7759/cureus.9349
5. Dong Y, Chen H, Gao J, Liu Y, Li J, Wang J. Molecular machinery and interplay of apoptosis and autophagy in coronary heart disease. J Mol Cell Cardiol. (2019) 136:27–41. doi: 10.1016/j.yjmcc.2019.09.001
6. Davignon J, Ganz P. Role of endothelial dysfunction in atherosclerosis. Circulation. (2004) 109:Iii27–32. doi: 10.1161/01.CIR.0000131515.03336.f8
7. Heitzer T, Baldus S, Von Kodolitsch Y, Rudolph V, Meinertz T. Systemic endothelial dysfunction as an early predictor of adverse outcome in heart failure. Arterioscler Thromb Vasc Biol. (2005) 25:1174–9. doi: 10.1161/01.ATV.0000166516.52477.81
8. Katz SD, Hryniewicz K, Hriljac I, Balidemaj K, Dimayuga C, Hudaihed A, et al. Vascular endothelial dysfunction and mortality risk in patients with chronic heart failure. Circulation. (2005) 111:310–4. doi: 10.1161/01.CIR.0000153349.77489.CF
9. Tousoulis D, Charakida M, Stefanadis C. Inflammation and endothelial dysfunction as therapeutic targets in patients with heart failure. Int J Cardiol. (2005) 100:347–53. doi: 10.1016/j.ijcard.2004.05.030
10. Khaddaj Mallat R, Mathew John C, Kendrick DJ, Braun AP. The vascular endothelium: a regulator of arterial tone and interface for the immune system. Crit Rev Clin Lab Sci. (2017) 54:458–70. doi: 10.1080/10408363.2017.1394267
11. Qin XF, Shan YG, Gao JH, Li FX, Guo YX. E3 ubiquitin ligase mind bomb 1 overexpression reduces apoptosis and inflammation of cardiac microvascular endothelial cells in coronary microvascular dysfunction. Cell Signal. (2022) 91:110223. doi: 10.1016/j.cellsig.2021.110223
12. Scarabelli T, Stephanou A, Rayment N, Pasini E, Comini L, Curello S, et al. Apoptosis of endothelial cells precedes myocyte cell apoptosis in ischemia/reperfusion injury. Circulation. (2001) 104:253–6. doi: 10.1161/01.CIR.104.3.253
13. Krüger-Genge A, Blocki A, Franke RP, Jung F. Vascular endothelial cell biology: an update. Int J Mol Sci. (2019) 20:4411. doi: 10.3390/ijms20184411
14. Alakoski T, Ulvila J, Yrjölä R, Vainio L, Magga J, Szabo Z, et al. Inhibition of cardiomyocyte Sprouty1 protects from cardiac ischemia-reperfusion injury. Basic Res Cardiol. (2019) 114:7. doi: 10.1007/s00395-018-0713-y
15. Tousoulis D, Kampoli AM, Tentolouris C, Papageorgiou N, Stefanadis C. The role of nitric oxide on endothelial function. Curr Vasc Pharmacol. (2012) 10:4–18. doi: 10.2174/157016112798829760
16. Shah AM, Prendergast BD, Grocott-Mason R, Lewis MJ, Paulus WJ. The influence of endothelium-derived nitric oxide on myocardial contractile function. Int J Cardiol. (1995) 50:225–31. doi: 10.1016/0167-5273(95)02381-6
17. Brutsaert DL. Cardiac endothelial-myocardial signaling: its role in cardiac growth, contractile performance, and rhythmicity. Physiol Rev. (2003) 83:59–115. doi: 10.1152/physrev.00017.2002
18. Colliva A, Braga L, Giacca M, Zacchigna S. Endothelial cell-cardiomyocyte crosstalk in heart development and disease. J Physiol. (2020) 598:2923–39. doi: 10.1113/JP276758
19. Mohr AM, Mott JL. Overview of microRNA biology. Semin Liver Dis. (2015) 35:3–11. doi: 10.1055/s-0034-1397344
20. Lu TX, Rothenberg ME. MicroRNA. J Allergy Clin Immunol. (2018) 141:1202–7. doi: 10.1016/j.jaci.2017.08.034
21. Kalla R, Ventham NT, Kennedy NA, Quintana JF, Nimmo ER, Buck AH, et al. MicroRNAs: new players in IBD. Gut. (2015) 64:504–17. doi: 10.1136/gutjnl-2014-307891
23. Nguyen TA, Park J, Dang TL, Choi YG, Kim VN. Microprocessor depends on hemin to recognize the apical loop of primary microRNA. Nucleic Acids Res. (2018) 46:5726–36. doi: 10.1093/nar/gky248
24. Ha M, Kim VN. Regulation of microRNA biogenesis. Nat Rev Mol Cell Biol. (2014) 15:509–24. doi: 10.1038/nrm3838
25. Agarwal V, Bell GW, Nam JW, Bartel DP. Predicting effective microRNA target sites in mammalian mRNAs. Elife. (2015) 4:e05005. doi: 10.7554/eLife.05005
26. Bartel DP. MicroRNAs: target recognition and regulatory functions. Cell. (2009) 136:215–33. doi: 10.1016/j.cell.2009.01.002
27. Cai Y, Yu X, Hu S, Yu J. A brief review on the mechanisms of miRNA regulation. Genomics Proteomics Bioinformatics. (2009) 7:147–54. doi: 10.1016/S1672-0229(08)60044-3
28. Van Der Ree MH, De Vree JM, Stelma F, Willemse S, Van Der Valk M, Rietdijk S, et al. Safety, tolerability, and antiviral effect of RG-101 in patients with chronic hepatitis C: a phase 1B, double-blind, randomised controlled trial. Lancet. (2017) 389:709–17. doi: 10.1016/S0140-6736(16)31715-9
29. Beg MS, Brenner AJ, Sachdev J, Borad M, Kang YK, Stoudemire J, et al. Phase I study of MRX34, a liposomal miR-34a mimic, administered twice weekly in patients with advanced solid tumors. Invest New Drugs. (2017) 35:180–8. doi: 10.1007/s10637-016-0407-y
30. Schober A, Blay RM, Saboor Maleki S, Zahedi F, Winklmaier AE, Kakar MY, et al. MicroRNA-21 controls circadian regulation of apoptosis in atherosclerotic lesions. Circulation. (2021) 144:1059–73. doi: 10.1161/CIRCULATIONAHA.120.051614
31. Maslov LN, Popov SV, Naryzhnaya NV, Mukhomedzyanov AV, Kurbatov BK, Derkachev IA, et al. The regulation of necroptosis and perspectives for the development of new drugs preventing ischemic/reperfusion of cardiac injury. Apoptosis. (2022) 27:697–719. doi: 10.1007/s10495-022-01760-x
32. Wang K, Liu F, Liu CY, An T, Zhang J, Zhou LY, et al. The long noncoding RNA NRF regulates programmed necrosis and myocardial injury during ischemia and reperfusion by targeting miR-873. Cell Death Differ. (2016) 23:1394–405. doi: 10.1038/cdd.2016.28
33. Spengler RM, Zhang X, Cheng C, Mclendon JM, Skeie JM, Johnson FL, et al. Elucidation of transcriptome-wide microRNA binding sites in human cardiac tissues by Ago2 HITS-CLIP. Nucleic Acids Res. (2016) 44:7120–31. doi: 10.1093/nar/gkw640
34. Boštjančič E, Brandner T, Zidar N, Glavač D, Štajer D. Down-regulation of miR-133a/b in patients with myocardial infarction correlates with the presence of ventricular fibrillation. Biomed Pharmacother. (2018) 99:65–71. doi: 10.1016/j.biopha.2018.01.019
35. Porrello ER. MicroRNAs in cardiac development and regeneration. Clin Sci (Lond). (2013) 125:151–66. doi: 10.1042/CS20130011
36. Gao W, Cui H, Li Q, Zhong H, Yu J, Li P, et al. Upregulation of microRNA-218 reduces cardiac microvascular endothelial cells injury induced by coronary artery disease through the inhibition of HMGB1. J Cell Physiol. (2020) 235:3079–95. doi: 10.1002/jcp.29214
37. Xiao Y, Zhao J, Tuazon JP, Borlongan CV, Yu G. MicroRNA-133a and myocardial infarction. Cell Transplant. (2019) 28:831–8. doi: 10.1177/0963689719843806
38. Zhou H, Zhang Y, Hu S, Shi C, Zhu P, Ma Q, et al. Melatonin protects cardiac microvasculature against ischemia/reperfusion injury via suppression of mitochondrial fission-VDAC1-HK2-mPTP-mitophagy axis. J Pineal Res. (2017) 63:e12413. doi: 10.1111/jpi.12413
39. Sossalla S, Vollmann D. Arrhythmia-Induced cardiomyopathy. Dtsch Arztebl Int. (2018) 115:335–41. doi: 10.3238/arztebl.2018.0335
40. Lu L, Liu M, Sun R, Zheng Y, Zhang P. Myocardial infarction: symptoms and treatments. Cell Biochem Biophys. (2015) 72:865–7. doi: 10.1007/s12013-015-0553-4
41. Park M, Shen YT, Gaussin V, Heyndrickx GR, Bartunek J, Resuello RR, et al. Apoptosis predominates in nonmyocytes in heart failure. Am J Physiol Heart Circ Physiol. (2009) 297:H785–91. doi: 10.1152/ajpheart.00310.2009
42. Crea F, Montone RA, Rinaldi R. Pathophysiology of coronary microvascular dysfunction. Circ J. (2022) 86:1319–28. doi: 10.1253/circj.CJ-21-0848
43. Halcox JP, Schenke WH, Zalos G, Mincemoyer R, Prasad A, Waclawiw MA, et al. Prognostic value of coronary vascular endothelial dysfunction. Circulation. (2002) 106:653–8. doi: 10.1161/01.CIR.0000025404.78001.D8
44. Ong P, Athanasiadis A, Borgulya G, Mahrholdt H, Kaski JC, Sechtem U. High prevalence of a pathological response to acetylcholine testing in patients with stable angina pectoris and unobstructed coronary arteries. The ACOVA study (abnormal COronary VAsomotion in patients with stable angina and unobstructed coronary arteries). J Am Coll Cardiol. (2012) 59:655–62. doi: 10.1016/j.jacc.2011.11.015
45. Rush CJ, Berry C, Oldroyd KG, Rocchiccioli JP, Lindsay MM, Touyz RM, et al. Prevalence of coronary artery disease and coronary microvascular dysfunction in patients with heart failure with preserved ejection fraction. JAMA Cardiol. (2021) 6:1130–43. doi: 10.1001/jamacardio.2021.1825
46. Konijnenberg LSF, Damman P, Duncker DJ, Kloner RA, Nijveldt R, Van Geuns RM, et al. Pathophysiology and diagnosis of coronary microvascular dysfunction in ST-elevation myocardial infarction. Cardiovasc Res. (2020) 116:787–805. doi: 10.1093/cvr/cvz301
47. Dwivedi Y, Roy B, Lugli G, Rizavi H, Zhang H, Smalheiser NR. Chronic corticosterone-mediated dysregulation of microRNA network in prefrontal cortex of rats: relevance to depression pathophysiology. Transl Psychiatry. (2015) 5:e682. doi: 10.1038/tp.2015.175
48. Yoshino Y, Roy B, Dwivedi Y. Corticosterone-mediated regulation and functions of miR-218-5p in rat brain. Sci Rep. (2022) 12:194. doi: 10.1038/s41598-021-03863-y
49. Förstermann U, Münzel T. Endothelial nitric oxide synthase in vascular disease: from marvel to menace. Circulation. (2006) 113:1708–14. doi: 10.1161/CIRCULATIONAHA.105.602532
50. Esse R, Barroso M, Tavares De Almeida I, Castro R. The contribution of homocysteine metabolism disruption to endothelial dysfunction: state-of-the-art. Int J Mol Sci. (2019) 20:867. doi: 10.3390/ijms20040867
51. Liu W, Wang T, Sun P, Zhou Y. Expression of Hcy and blood lipid levels in serum of CHD patients and analysis of risk factors for CHD. Exp Ther Med. (2019) 17:1756–60. doi: 10.3892/etm.2018.7111
52. Verdoia M, Nardin M, Gioscia R, Saghir Afifeh AM, Viglione F, Negro F, et al. Association between vitamin D deficiency and serum homocysteine levels and its relationship with coronary artery disease. J Thromb Thrombolysis. (2021) 52:523–31. doi: 10.1007/s11239-021-02391-w
53. Chen G, Ward MF, Sama AE, Wang H. Extracellular HMGB1 as a proinflammatory cytokine. J Interferon Cytokine Res. (2004) 24:329–33. doi: 10.1089/107999004323142187
54. Bidzhekov K, Gan L, Denecke B, Rostalsky A, Hristov M, Koeppel TA, et al. microRNA expression signatures and parallels between monocyte subsets and atherosclerotic plaque in humans. Thromb Haemost. (2012) 107:619–25. doi: 10.1160/TH11-09-0607
55. Chen Y, Li S, Zhang Y, Wang M, Li X, Liu S, et al. The lncRNA Malat1 regulates microvascular function after myocardial infarction in mice via miR-26b-5p/Mfn1 axis-mediated mitochondrial dynamics. Redox Biol. (2021) 41:101910. doi: 10.1016/j.redox.2021.101910
56. Bridges MC, Daulagala AC, Kourtidis A. LNCcation: lncRNA localization and function. J Cell Biol. (2021) 220:e202009045. doi: 10.1083/jcb.202009045
57. Yan Y, Song D, Song X, Song C. The role of lncRNA MALAT1 in cardiovascular disease. IUBMB Life. (2020) 72:334–42. doi: 10.1002/iub.2210
58. Marfella R, Di Filippo C, Potenza N, Sardu C, Rizzo MR, Siniscalchi M, et al. Circulating microRNA changes in heart failure patients treated with cardiac resynchronization therapy: responders vs. non-responders. Eur J Heart Fail. (2013) 15:1277–88. doi: 10.1093/eurjhf/hft088
59. Budi HS, Younus LA, Lafta MH, Parveen S, Mohammad HJ, Al-Qaim ZH, et al. The role of miR-128 in cancer development, prevention, drug resistance, and immunotherapy. Front Oncol. (2022) 12:1067974. doi: 10.3389/fonc.2022.1067974
60. Farina FM, Hall IF, Serio S, Zani S, Climent M, Salvarani N, et al. miR-128-3p is a novel regulator of vascular smooth muscle cell phenotypic switch and vascular diseases. Circ Res. (2020) 126:e120–35. doi: 10.1161/CIRCRESAHA.120.316489
61. Sharma K, Chandra A, Hasija Y, Saini N. MicroRNA-128 inhibits mitochondrial biogenesis and function via targeting PGC1α and NDUFS4. Mitochondrion. (2021) 60:160–9. doi: 10.1016/j.mito.2021.08.008
62. Yan P, Sun C, Ma J, Jin Z, Guo R, Yang B. MicroRNA-128 confers protection against cardiac microvascular endothelial cell injury in coronary heart disease via negative regulation of IRS1. J Cell Physiol. (2019) 234:13452–63. doi: 10.1002/jcp.28025
63. Wang XM, Chen SW, Chen G, Zhou HF, Gan TQ, Zeng JJ, et al. Clinical value and potential mechanism of miRNA-33a-5p in lung squamous cell carcinoma. Anal Cell Pathol (Amst). (2021) 2021:6614331. doi: 10.1155/2021/6614331
64. Xing W, Li T, Wang Y, Qiang Y, Ai C, Tang H. MiR-33a-5p targets NOMO1 to modulate human cardiomyocyte progenitor cells proliferation and differentiation and apoptosis. J Recept Signal Transduct Res. (2021) 41:476–87. doi: 10.1080/10799893.2020.1825492
65. Diao L, Bai L, Jiang X, Li J, Zhang Q. Long-chain noncoding RNA GAS5 mediates oxidative stress in cardiac microvascular endothelial cells injury. J Cell Physiol. (2019) 234:17649–62. doi: 10.1002/jcp.28388
66. Xiong W, Liu D, Chen X, Liu L, Xiao W. lncRNA JPX modulates malignant progress of osteosarcoma through targeting miR-33a-5p and PNMA1 regulatory loop. Transl Oncol. (2022) 25:101504. doi: 10.1016/j.tranon.2022.101504
67. Zhou Y, Zhu Y, Zhang L, Wu T, Wu T, Zhang W, et al. Human stem cells overexpressing miR-21 promote angiogenesis in critical limb ischemia by targeting CHIP to enhance HIF-1α activity. Stem Cells. (2016) 34:924–34. doi: 10.1002/stem.2321
68. Liu LZ, Li C, Chen Q, Jing Y, Carpenter R, Jiang Y, et al. MiR-21 induced angiogenesis through AKT and ERK activation and HIF-1α expression. PLoS One. (2011) 6:e19139. doi: 10.1371/journal.pone.0019139
69. Yang F, Liu W, Yan X, Zhou H, Zhang H, Liu J, et al. Effects of mir-21 on cardiac microvascular endothelial cells after acute myocardial infarction in rats: role of phosphatase and tensin homolog (PTEN)/vascular endothelial growth factor (VEGF) signal pathway. Med Sci Monit. (2016) 22:3562–75. doi: 10.12659/MSM.897773
70. Chen ZL, Chen YX, Zhou J, Li Y, Gong CY, Wang XB. LncRNA HULC alleviates HUVEC inflammation and improves angiogenesis after myocardial infarction through down-regulating miR-29b. Eur Rev Med Pharmacol Sci. (2020) 24:6288–98. doi: 10.26355/eurrev_202006_21527
71. Dai GH, Ma PZ, Song XB, Liu N, Zhang T, Wu B. MicroRNA-223-3p inhibits the angiogenesis of ischemic cardiac microvascular endothelial cells via affecting RPS6KB1/hif-1a signal pathway. PLoS One. (2014) 9:e108468. doi: 10.1371/journal.pone.0108468
72. Pfeffer MA. Left ventricular remodeling after acute myocardial infarction. Annu Rev Med. (1995) 46:455–66. doi: 10.1146/annurev.med.46.1.455
73. Hausenloy DJ, Botker HE, Engstrom T, Erlinge D, Heusch G, Ibanez B, et al. Targeting reperfusion injury in patients with ST-segment elevation myocardial infarction: trials and tribulations. Eur Heart J. (2017) 38:935–41. doi: 10.1093/eurheartj/ehw145
74. Ndrepepa G, Tiroch K, Fusaro M, Keta D, Seyfarth M, Byrne RA, et al. 5-year prognostic value of no-reflow phenomenon after percutaneous coronary intervention in patients with acute myocardial infarction. J Am Coll Cardiol. (2010) 55:2383–9. doi: 10.1016/j.jacc.2009.12.054
75. Li L, Tan J, Miao Y, Lei P, Zhang Q. ROS and autophagy: interactions and molecular regulatory mechanisms. Cell Mol Neurobiol. (2015) 35:615–21. doi: 10.1007/s10571-015-0166-x
76. Li X, Liu M, Sun R, Zeng Y, Chen S, Zhang P. Protective approaches against myocardial ischemia reperfusion injury. Exp Ther Med. (2016) 12:3823–9. doi: 10.3892/etm.2016.3877
77. Zhu F, Li Y, Zhang J, Piao C, Liu T, Li HH, et al. Senescent cardiac fibroblast is critical for cardiac fibrosis after myocardial infarction. PLoS One. (2013) 8:e74535. doi: 10.1371/journal.pone.0074535
78. Shan H, Li T, Zhang L, Yang R, Li Y, Zhang M, et al. Heme oxygenase-1 prevents heart against myocardial infarction by attenuating ischemic injury-induced cardiomyocytes senescence. EBioMedicine. (2019) 39:59–68. doi: 10.1016/j.ebiom.2018.11.056
79. Erkens R, Totzeck M, Brum A, Duse D, Bøtker HE, Rassaf T, et al. Endothelium-dependent remote signaling in ischemia and reperfusion: alterations in the cardiometabolic continuum. Free Radic Biol Med. (2021) 165:265–81. doi: 10.1016/j.freeradbiomed.2021.01.040
80. Hollander MR, De Waard GA, Konijnenberg LS, Meijer-Van Putten RM, Van Den Brom CE, Paauw N, et al. Dissecting the effects of ischemia and reperfusion on the coronary microcirculation in a rat model of acute myocardial infarction. PLoS One. (2016) 11:e0157233. doi: 10.1371/journal.pone.0157233
81. Mccarthy JJ. MicroRNA-206: the skeletal muscle-specific myomiR. Biochim Biophys Acta. (2008) 1779:682–91. doi: 10.1016/j.bbagrm.2008.03.001
82. Ma G, Wang Y, Li Y, Cui L, Zhao Y, Zhao B, et al. MiR-206, a key modulator of skeletal muscle development and disease. Int J Biol Sci. (2015) 11:345–52. doi: 10.7150/ijbs.10921
83. Zhang Q, Cao Y, Liu Y, Huang W, Ren J, Wang P, et al. Shear stress inhibits cardiac microvascular endothelial cells apoptosis to protect against myocardial ischemia reperfusion injury via YAP/miR-206/PDCD4 signaling pathway. Biochem Pharmacol. (2021) 186:114466. doi: 10.1016/j.bcp.2021.114466
84. Wang X, Ma L, Zhang S, Song Q, He X, Wang J. WWP2 ameliorates oxidative stress and inflammation in atherosclerotic mice through regulation of PDCD4/HO-1 pathway. Acta Biochim Biophys Sin (Shanghai). (2022) 54:1057–67. doi: 10.3724/abbs.2022091
85. Yang QY, Yu Q, Zeng WY, Zeng M, Zhang XL, Zhang YL, et al. Killing two birds with one stone: miR-126 involvement in both cancer and atherosclerosis. Eur Rev Med Pharmacol Sci. (2022) 26:6145–68. doi: 10.26355/eurrev_202209_29632
86. Yang HH, Chen Y, Gao CY, Cui ZT, Yao JM. Protective effects of MicroRNA-126 on human cardiac microvascular endothelial cells against hypoxia/reoxygenation-induced injury and inflammatory response by activating PI3K/akt/eNOS signaling pathway. Cell Physiol Biochem. (2017) 42:506–18. doi: 10.1159/000477597
87. Teng Y, Su X, Zhang X, Zhang Y, Li C, Niu W, et al. miRNA-200a/c as potential biomarker in epithelial ovarian cancer (EOC): evidence based on miRNA meta-signature and clinical investigations. Oncotarget. (2016) 7:81621–33. doi: 10.18632/oncotarget.13154
88. Lo WY, Yang WK, Peng CT, Pai WY, Wang HJ. MicroRNA-200a/200b modulate high glucose-induced endothelial inflammation by targeting O-linked N-acetylglucosamine transferase expression. Front Physiol. (2018) 9:355. doi: 10.3389/fphys.2018.00355
89. Sun X, Zuo H, Liu C, Yang Y. Overexpression of miR-200a protects cardiomyocytes against hypoxia-induced apoptosis by modulating the kelch-like ECH-associated protein 1-nuclear factor erythroid 2-related factor 2 signaling axis. Int J Mol Med. (2016) 38:1303–11. doi: 10.3892/ijmm.2016.2719
90. Fu H, Song W, Chen X, Guo T, Duan B, Wang X, et al. MiRNA-200a induce cell apoptosis in renal cell carcinoma by directly targeting SIRT1. Mol Cell Biochem. (2018) 437:143–52. doi: 10.1007/s11010-017-3102-1
91. Zhou T, Xiang DK, Li SN, Yang LH, Gao LF, Feng C. MicroRNA-495 ameliorates cardiac microvascular endothelial cell injury and inflammatory reaction by suppressing the NLRP3 inflammasome signaling pathway. Cell Physiol Biochem. (2018) 49:798–815. doi: 10.1159/000493042
92. Li LL, Mao CD, Wang GP, Wang N, Xue AG. MiR-145-5p alleviates hypoxia/reoxygenation- induced cardiac microvascular endothelial cell injury in coronary heart disease by inhibiting Smad4 expression. Eur Rev Med Pharmacol Sci. (2020) 24:5008–17. doi: 10.26355/eurrev_202005_21192
93. Kubo SH, Rector TS, Bank AJ, Williams RE, Heifetz SM. Endothelium-dependent vasodilation is attenuated in patients with heart failure. Circulation. (1991) 84:1589–96. doi: 10.1161/01.CIR.84.4.1589
94. Ramsey MW, Goodfellow J, Jones CJ, Luddington LA, Lewis MJ, Henderson AH. Endothelial control of arterial distensibility is impaired in chronic heart failure. Circulation. (1995) 92:3212–9. doi: 10.1161/01.CIR.92.11.3212
95. De Groot AA, Mathy MJ, Van Zwieten PA, Peters SL. Involvement of the beta3 adrenoceptor in nebivolol-induced vasorelaxation in the rat aorta. J Cardiovasc Pharmacol. (2003) 42:232–6. doi: 10.1097/00005344-200308000-00012
96. Rozec B, Quang TT, Noireaud J, Gauthier C. Mixed beta3-adrenoceptor agonist and alpha1-adrenoceptor antagonist properties of nebivolol in rat thoracic aorta. Br J Pharmacol. (2006) 147:699–706. doi: 10.1038/sj.bjp.0706648
97. Ren XP, Wu J, Wang X, Sartor MA, Jones K, Qian J, et al. MicroRNA-320 is involved in the regulation of cardiac ischemia/reperfusion injury by targeting heat-shock protein 20. Circulation. (2009) 119:2357–66. doi: 10.1161/CIRCULATIONAHA.108.814145
98. Wang XH, Qian RZ, Zhang W, Chen SF, Jin HM, Hu RM. MicroRNA-320 expression in myocardial microvascular endothelial cells and its relationship with insulin-like growth factor-1 in type 2 diabetic rats. Clin Exp Pharmacol Physiol. (2009) 36:181–8. doi: 10.1111/j.1440-1681.2008.05057.x
99. Ling S, Nanhwan M, Qian J, Kodakandla M, Castillo AC, Thomas B, et al. Modulation of microRNAs in hypertension-induced arterial remodeling through the β1 and β3-adrenoreceptor pathways. J Mol Cell Cardiol. (2013) 65:127–36. doi: 10.1016/j.yjmcc.2013.10.003
100. Dai S, Li F, Xu S, Hu J, Gao L. The important role of miR-1-3p in cancers. J Transl Med. (2023) 21:769. doi: 10.1186/s12967-023-04649-8
101. Wang S, Guo X, Long CL, Li C, Zhang YF, Wang J, et al. SUR2B/Kir6.1 Channel openers correct endothelial dysfunction in chronic heart failure via the miR-1-3p/ET-1 pathway. Biomed Pharmacother. (2019) 110:431–9. doi: 10.1016/j.biopha.2018.11.135
102. Gao M, Yu T, Liu D, Shi Y, Yang P, Zhang J, et al. Sepsis plasma-derived exosomal miR-1-3p induces endothelial cell dysfunction by targeting SERP1. Clin Sci (Lond). (2021) 135:347–65. doi: 10.1042/CS20200573
103. Padhi S, Nayak AK, Behera A. Type II diabetes mellitus: a review on recent drug based therapeutics. Biomed Pharmacother. (2020) 131:110708. doi: 10.1016/j.biopha.2020.110708
104. Li Y, Liu Y, Liu S, Gao M, Wang W, Chen K, et al. Diabetic vascular diseases: molecular mechanisms and therapeutic strategies. Signal Transduct Target Ther. (2023) 8:152. doi: 10.1038/s41392-023-01400-z
105. Yang J, Liu Z. Mechanistic pathogenesis of endothelial dysfunction in diabetic nephropathy and retinopathy. Front Endocrinol (Lausanne). (2022) 13:816400. doi: 10.3389/fendo.2022.816400
106. Fiorentino TV, Prioletta A, Zuo P, Folli F. Hyperglycemia-induced oxidative stress and its role in diabetes mellitus related cardiovascular diseases. Curr Pharm Des. (2013) 19:5695–703. doi: 10.2174/1381612811319320005
107. Zhang H, Shen Y, Kim IM, Weintraub NL, Tang Y. The impaired bioenergetics of diabetic cardiac microvascular endothelial cells. Front Endocrinol (Lausanne). (2021) 12:642857. doi: 10.3389/fendo.2021.642857
108. Reusch JE. Diabetes, microvascular complications, and cardiovascular complications: what is it about glucose? J Clin Invest. (2003) 112:986–8. doi: 10.1172/JCI200319902
109. Park MH, Han JS. Protective effect of padina arborescens extract against high glucose-induced oxidative damage in human umbilical vein endothelial cells. Prev Nutr Food Sci. (2013) 18:11–7. doi: 10.3746/pnf.2013.18.1.011
110. Zorena K, Kula M, Malinowska E, Raczyńska D, Myśliwiec M, Raczyńska K. Threshold serum concentrations of tumour necrosis factor alpha (TNFα) as a potential marker of the presence of microangiopathy in children and adolescents with type 1 diabetes mellitus (T1DM). Hum Immunol. (2013) 74:75–81. doi: 10.1016/j.humimm.2012.10.002
111. Domingueti CP, Dusse LM, Carvalho M, De Sousa LP, Gomes KB, Fernandes AP. Diabetes mellitus: the linkage between oxidative stress, inflammation, hypercoagulability and vascular complications. J Diabetes Complications. (2016) 30:738–45. doi: 10.1016/j.jdiacomp.2015.12.018
112. Witkowski M, Tabaraie T, Steffens D, Friebel J, Dörner A, Skurk C, et al. MicroRNA-19a contributes to the epigenetic regulation of tissue factor in diabetes. Cardiovasc Diabetol. (2018) 17:34. doi: 10.1186/s12933-018-0678-z
113. Witkowski M, Witkowski M, Saffarzadeh M, Friebel J, Tabaraie T, Ta Bao L, et al. Vascular miR-181b controls tissue factor-dependent thrombogenicity and inflammation in type 2 diabetes. Cardiovasc Diabetol. (2020) 19:20. doi: 10.1186/s12933-020-0993-z
114. Samak M, Kaltenborn D, Kues A, Le Noble F, Hinkel R, Germena G. Micro-RNA 92a as a therapeutic target for cardiac microvascular dysfunction in diabetes. Biomedicines. (2021) 10:58. doi: 10.3390/biomedicines10010058
115. Chen K, Zhao XL, Li LB, Huang LY, Tang Z, Luo J, et al. miR-503/Apelin-12 mediates high glucose-induced microvascular endothelial cells injury via JNK and p38MAPK signaling pathway. Regen Ther. (2020) 14:111–8. doi: 10.1016/j.reth.2019.12.002
116. Dai Y, Lu H, Wang S, Chang S, Li C, Huang Z, et al. MicroRNA-216b actively modulates diabetic angiopathy through inverse regulation on FZD5. Gene. (2018) 658:129–35. doi: 10.1016/j.gene.2018.02.050
117. Yi F, Shang Y, Li B, Dai S, Wu W, Cheng L, et al. MicroRNA-193-5p modulates angiogenesis through IGF2 in type 2 diabetic cardiomyopathy. Biochem Biophys Res Commun. (2017) 491:876–82. doi: 10.1016/j.bbrc.2017.07.108
118. Feng B, Cao Y, Chen S, Chu X, Chu Y, Chakrabarti S. miR-200b mediates endothelial-to-mesenchymal transition in diabetic cardiomyopathy. Diabetes. (2016) 65:768–79. doi: 10.2337/db15-1033
119. Feng B, Chen S, Gordon AD, Chakrabarti S. miR-146a mediates inflammatory changes and fibrosis in the heart in diabetes. J Mol Cell Cardiol. (2017) 105:70–6. doi: 10.1016/j.yjmcc.2017.03.002
120. Ota A, Tagawa H, Karnan S, Tsuzuki S, Karpas A, Kira S, et al. Identification and characterization of a novel gene, C13orf25, as a target for 13q31-q32 amplification in malignant lymphoma. Cancer Res. (2004) 64:3087–95. doi: 10.1158/0008-5472.CAN-03-3773
121. Landais S, Landry S, Legault P, Rassart E. Oncogenic potential of the miR-106-363 cluster and its implication in human T-cell leukemia. Cancer Res. (2007) 67:5699–707. doi: 10.1158/0008-5472.CAN-06-4478
122. Lina S, Lihong Q, Di Y, Bo Y, Xiaolin L, Jing M. microRNA-146a and Hey2 form a mutual negative feedback loop to regulate the inflammatory response in chronic apical periodontitis. J Cell Biochem. (2019) 120:645–57. doi: 10.1002/jcb.27422
123. Tang SJ, Fan KH, You GR, Huang SF, Kang CJ, Huang YF, et al. Tumor suppressor miRNA-503 inhibits cell invasion in head and neck cancer through the wnt signaling pathway via the WNT3A/MMP molecular axis. Int J Mol Sci. (2022) 23:15900. doi: 10.3390/ijms232415900
124. Wang Y, Zhao R, Liu W, Wang Z, Rong J, Long X, et al. Exosomal circHIPK3 released from hypoxia-pretreated cardiomyocytes regulates oxidative damage in cardiac microvascular endothelial cells via the miR-29a/IGF-1 pathway. Oxid Med Cell Longev. (2019) 2019:7954657. doi: 10.1155/2019/7954657
125. Zhou R, Huang W, Fan X, Liu F, Luo L, Yuan H, et al. miR-499 released during myocardial infarction causes endothelial injury by targeting α7-nAchR. J Cell Mol Med. (2019) 23:6085–97. doi: 10.1111/jcmm.14474
126. Zhang B, Sun C, Liu Y, Bai F, Tu T, Liu Q. Exosomal miR-27b-3p derived from hypoxic cardiac microvascular endothelial cells alleviates rat myocardial ischemia/reperfusion injury through inhibiting oxidative stress-induced pyroptosis via Foxo1/GSDMD signaling. Oxid Med Cell Longev. (2022) 2022:8215842. doi: 10.1155/2022/8215842
127. Lei D, Li B, Isa Z, Ma X, Zhang B. Hypoxia-elicited cardiac microvascular endothelial cell-derived exosomal miR-210-3p alleviate hypoxia/reoxygenation-induced myocardial cell injury through inhibiting transferrin receptor 1-mediated ferroptosis. Tissue Cell. (2022) 79:101956. doi: 10.1016/j.tice.2022.101956
128. Popescu LM, Faussone-Pellegrini MS. TELOCYTES—a case of serendipity: the winding way from interstitial cells of cajal (ICC), via interstitial cajal-like cells (ICLC) to TELOCYTES. J Cell Mol Med. (2010) 14:729–40. doi: 10.1111/j.1582-4934.2010.01059.x
129. Zhao B, Chen S, Liu J, Yuan Z, Qi X, Qin J, et al. Cardiac telocytes were decreased during myocardial infarction and their therapeutic effects for ischaemic heart in rat. J Cell Mol Med. (2013) 17:123–33. doi: 10.1111/j.1582-4934.2012.01655.x
130. Liao Z, Chen Y, Duan C, Zhu K, Huang R, Zhao H, et al. Cardiac telocytes inhibit cardiac microvascular endothelial cell apoptosis through exosomal miRNA-21-5p-targeted cdip1 silencing to improve angiogenesis following myocardial infarction. Theranostics. (2021) 11:268–91. doi: 10.7150/thno.47021
131. Yong KW, Choi JR, Mohammadi M, Mitha AP, Sanati-Nezhad A, Sen A. Mesenchymal stem cell therapy for ischemic tissues. Stem Cells Int. (2018) 2018:8179075. doi: 10.1155/2018/8179075
132. Mao Q, Liang XL, Zhang CL, Pang YH, Lu YX. LncRNA KLF3-AS1 in human mesenchymal stem cell-derived exosomes ameliorates pyroptosis of cardiomyocytes and myocardial infarction through miR-138-5p/Sirt1 axis. Stem Cell Res Ther. (2019) 10:393. doi: 10.1186/s13287-019-1522-4
133. Liang P, Ye F, Hou CC, Pi L, Chen F. Mesenchymal stem cell therapy for patients with ischemic heart failure- past, present, and future. Curr Stem Cell Res Ther. (2021) 16:608–21. doi: 10.2174/1574888X15666200309144906
134. Yang M, Liu X, Jiang M, Li J, Tang Y, Zhou L. miR-543 in human mesenchymal stem cell-derived exosomes promotes cardiac microvascular endothelial cell angiogenesis after myocardial infarction through COL4A1. IUBMB Life. (2021) 73:927–40. doi: 10.1002/iub.2474
135. Ning W, Li S, Yang W, Yang B, Xin C, Ping X, et al. Blocking exosomal miRNA-153-3p derived from bone marrow mesenchymal stem cells ameliorates hypoxia-induced myocardial and microvascular damage by targeting the ANGPT1-mediated VEGF/PI3k/akt/eNOS pathway. Cell Signal. (2021) 77:109812. doi: 10.1016/j.cellsig.2020.109812
Keywords: cardiac microvascular endothelial cell, miRNA, coronary artery disease, microvascular dysfunction, diabetes, crosstalk
Citation: Yan J, Zhong X, Zhao Y and Wang X (2024) Role and mechanism of miRNA in cardiac microvascular endothelial cells in cardiovascular diseases. Front. Cardiovasc. Med. 11:1356152. doi: 10.3389/fcvm.2024.1356152
Received: 15 December 2023; Accepted: 4 March 2024;
Published: 13 March 2024.
Edited by:
Paul H. A. Quax, Leiden University, NetherlandsReviewed by:
Teresa Padro, Institut de Recerca de l'Hospital de la Santa Creu i Sant Pau, Spain© 2024 Yan, Zhong, Zhao and Wang. This is an open-access article distributed under the terms of the Creative Commons Attribution License (CC BY). The use, distribution or reproduction in other forums is permitted, provided the original author(s) and the copyright owner(s) are credited and that the original publication in this journal is cited, in accordance with accepted academic practice. No use, distribution or reproduction is permitted which does not comply with these terms.
*Correspondence: Xiaoying Wang d3h5QHRqdXRjbS5lZHUuY24=
Disclaimer: All claims expressed in this article are solely those of the authors and do not necessarily represent those of their affiliated organizations, or those of the publisher, the editors and the reviewers. Any product that may be evaluated in this article or claim that may be made by its manufacturer is not guaranteed or endorsed by the publisher.
Research integrity at Frontiers
Learn more about the work of our research integrity team to safeguard the quality of each article we publish.