- 1School of Basic Medical Sciences, Chengdu Medical College, Chengdu, China
- 2Department of Cardiology, The Third Affiliated Hospital of Chengdu Medical College, Chengdu, China
- 3School of Health Management, Xihua University, Chengdu, China
- 4Cardiology and Vascular Health Research Center, Chengdu Medical College, Chengdu, China
- 5Key Laboratory for Biorheological Science and Technology of Ministry of Education, State and Local Joint Engineering Laboratory for Vascular Implants, Bioengineering College of Chongqing University, Chongqing, China
- 6School of Biosciences and Technology, Chengdu Medical College, Chengdu, China
Biomechanical forces, including vascular shear stress, cyclic stretching, and extracellular matrix stiffness, which influence mechanosensitive channels in the plasma membrane, determine cell function in atherosclerosis. Being highly associated with the formation of atherosclerotic plaques, endocytosis is the key point in molecule and macromolecule trafficking, which plays an important role in lipid transportation. The process of endocytosis relies on the mobility and tension of the plasma membrane, which is sensitive to biomechanical forces. Several studies have advanced the signal transduction between endocytosis and biomechanics to elaborate the developmental role of atherosclerosis. Meanwhile, increased plaque growth also results in changes in the structure, composition and morphology of the coronary artery that contribute to the alteration of arterial biomechanics. These cross-links of biomechanics and endocytosis in atherosclerotic plaques play an important role in cell function, such as cell phenotype switching, foam cell formation, and lipoprotein transportation. We propose that biomechanical force activates the endocytosis of vascular cells and plays an important role in the development of atherosclerosis.
1 Introduction
Atherosclerosis, as the underlying mechanism of cardiovascular and cerebrovascular diseases, is caused by many complicated risk factors, such as lipid accumulation and abnormal biomechanics (1, 2). These disordered microenvironments in the vasculature result in vascular inflammation, excessive proliferation and migration, fibrosis, and extensive necrosis, which contribute to atherogenesis and the formation of vulnerable plaques (3). Biomechanics, as an emerging field of cell and developmental biology, is considered as a regulator of atherosclerosis through mechanosensitive channels. A recent study used the computed tomography angiography of human circumflex coronary artery to construct computational simulation model and revealed a causal link between low-density lipoprotein (LDL) transportation and wall shear stress in the coronary artery, in which flow patterns altered the influx and efflux of cholesterol (4). Moreover, endocytosis regulates the transportation and degradation of lipids and apoptotic cell debris in atherosclerotic plaques, which controls the interaction between cells and their microenvironment (5). Due to the important role of the mechanical environment and membrane trafficking in vascular dysfunction and atherosclerosis, it is important to investigate the changes in endocytosis induced by abnormal biomechanics.
Endocytosis-mediated intracellular transport and the positive regulation of signalling cascades are the key regulators of cholesterol homeostasis, which play a casual role in atherogenesis (6). Endocytosis and the subsequent intracellular itinerary are based on the encapsulation of the fluid plasma membrane, selective receptors and vesicle-associated proteins. This selective route allows endothelial cells (ECs) to act as a vascular barrier to regulate the transport of hydrophilic and hydrophobic substances in blood and prevent harmful substances in tissues (7, 8). Meanwhile, the influx and efflux of cholesterol are specifically controlled by endocytosis with lipoprotein receptors including low-density lipoprotein receptor family and scavenger receptor family (Table 1) (6, 9). Recent studies have revealed that the scavenger receptors-mediated transcytosis, which rely on caveolae-based intracellular vesicles, play an important role in the delivery and accumulation of LDL in artery (10, 11). The accumulation of LDL in the sub-endothelial area will further stimulate excessive uptake and exhausted metabolism of lipid in vascular smooth muscle cells (VSMCs) and macrophages, which will result in unexpected inflammation, polarization/phenotype transformation, autophagy and the formation of foam cells (12, 13).
During the development of atherosclerosis, biomechanics, including shear stress, tensile force, and stiffness, play an important role in vascular inflammation, oxidative stress and lipid transportation (23, 24). Low shear stress (LSS) and oscillatory shear stress (OSS) generated by disturbed flow have been identified as hazard factors of atherosclerosis in hypercholesterolemic mini-pigs that correlate plaque growth with vulnerable features (25). These LSS and OSS will result in unexpected uptake of LDL and promote the development of atherosclerosis (26). Meanwhile, studies have also revealed that tensile force activation of stretch-related ion channels clinically contributes to arterial remodeling and relevant vascular dysfunction (27, 28). Moreover, recent research has also suggested that arterial stiffness is associated with artery atherosclerosis stroke in human with GWAS analysis that comprises 127,121 individuals of European ancestry (29). Thus, it is important to discuss the relationship between biomechanics and endocytosis in atherosclerosis.
In this review, we summarized the major types of endocytosis in atherosclerosis, including clathrin-mediated endocytosis, caveolae-mediated endocytosis, phagocytosis and micropinocytosis. We then focused on the role of endocytosis in responding to biomechanical forces and describe the signal transduction involved in lipid transportation in the biomechanical microenvironment. The integration of biomechanics and endocytosis might improve our understanding of the transportation of high-risk lipoprotein and contribute to a better understanding of the formation of atherosclerotic plaques.
2 General mechanisms of endocytosis in atherosclerosis
Endocytosis, as an important part of the cellular trafficking system, is a conserved channel for internalizing molecules and macromolecules through membrane deformation (30). The typical process of endocytosis contains four fundamental steps: (1) molecules specifically bind to receptors or the cell membrane; (2) coating proteins, cytoskeleton and membrane fusion proteins are rearranged to drive cell membrane-encapsulating molecules; (3) molecules are encapsulated in trafficking vesicles, such as endosomes and phagosomes; and (4) vesicles are transported to subcellular organelles (Figure 1). Due to the entry mechanism, endocytosis can be classified into pinocytosis and phagocytosis. The pinocytosis can further be classified into clathrin-mediated endocytosis and clathrin-independent endocytosis, such as caveolae-mediated endocytosis, caveolae- and clathrin- independent endocytosis and macropinocytosis, which can form differently sized vesicles (31) (Figure 1). Meanwhile, some specific inhibitors are also widely used to detect the influence of endocytosis to cell functions (Table 2). These engulfment processes drive lipid transportation and apoptotic cell clearance in the vasculature, which are inexorable events in atherosclerosis (37, 38). Here, we briefly discussed the role of different endocytosis mechanisms in atherosclerosis.
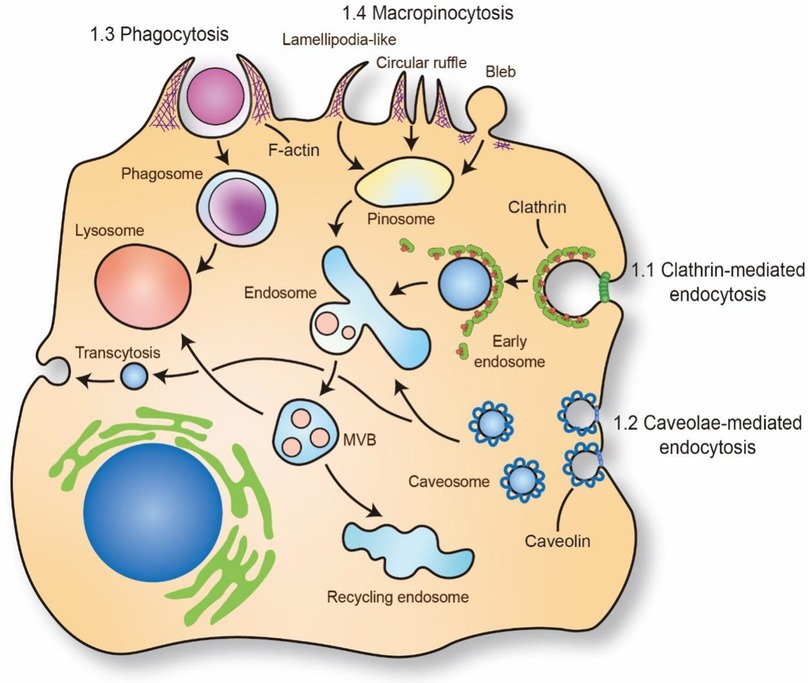
Figure 1. Mechanism of molecules and macromolecules trafficking through endocytosis. The putative endocytic portals in atherosclerosis mainly includes phagocytosis, micropinocytosis, clathrin-mediated endocytosis, caveolae-mediated endocytosis. The phagocytosis and micropinocytosis are driven by the reorganization of cortical cytoskeleton with the formation of elaborate membrane protrusions. Clathrin-mediated endocytosis and caveolae-mediated endocytosis require the recruitment of clathrin and caveolin on plasma membrane to form “coated pit” and vesicle and internalize cargo. The trafficking cargo will transport to endosome and lysosome. Caveolae-mediated endocytosis also regulate transcytosis in ECs which is important for maintaining the barrier of vascular. MVB: multivesicular bodies. The schematic diagram was made by the authors using Adobe Illustrator 2021.
2.1 Clathrin-mediated endocytosis
Clathrin-mediated endocytosis that molecules are packaged by a clathrin coat by clathrin triskelia based polymerization, can internalize and transport proteins, lipids, hormones and metabolites (39). The formation of clathrin-based vesicles requires the recruitment of adaptor proteins (APs) on the plasma membrane to initiate and construct clathrin-coated pits (40). Meanwhile, this binding process of APs is mediated by transporting receptors, plasma membrane-specific lipid phosphatidylinositol-4,5-bisphosphate, EGFR pathway substrate 15, and epsin proteins, which supports the assembly of clathrin-coated vesicles (41–44). Moreover, clathrin-coated pits are scissored via the enzyme dynamin recruited by BAR domain-containing proteins and form individual clathrin-coated vesicles (45, 46). Finally, the clathrin coat is disassembled and recycled from vesicles to allow the vesicle to fuse with endosomes (Figure 1) (47).
AP1 binds membranes enriched for phosphatidylinositol 4-phosphate, such as the trans Golgi network, while AP2 associates with phosphatidylinositol 4,5-bisphosphate of the plasma membrane. At their respective membranes, AP1 and AP2 bind the cytoplasmic tails of transmembrane protein cargo and clathrin triskelions, thereby coupling cargo recruitment to coat polymerization (48). Under physiological conditions, EGFR is internalized mostly by clathrin-mediated endocytosis. Growth factor binding to EGFR accelerates its internalization through clathrin-coated pits which is followed by the efficient lysosomal targeting of internalized receptors and results in receptor down-regulation. A recent study revealed that clathrin-mediated endocytosis was involved in the uptake of oxidized low-density lipoprotein (oxLDL) in macrophages through protease-activated receptor trafficking (49). Moreover, knockout of espins, which are important in clathrin-mediated endocytosis in myeloid cells, reduced the uptake of oxLDL through LRP1 and reduced the process of atherosclerosis (50). These results suggested that clathrin-mediated endocytosis, as an important cargo transporter, was involved in the progression of atherosclerosis.
2.2 Caveolae-mediated endocytosis
Caveolae, as the most abundant features in lipid rafts that invaginate to initialize bulb-shaped caveolar pit and form 60–80 nm specialized vesicles, are considered to participate in many biological functions, such as lipid regulation, material transportation, and signal transduction (51). The start and maintenance of caveolae-mediated endocytosis rely on caveolins, which are coated on the membrane surface of vesicles for structural formation (52). Generically, caveolin-1 (Cav1) and caveolin-2 are expressed in non-muscle cells, and caveolin-3 is expressed in some smooth muscle cells, which are anchored to the cell membrane and compose caveolae (53). Moreover, the caveolar structure contains approximately 144 molecules of caveolin that can bud off to construct endocytic caveolar vesicles and fuse with the caveosome and endosome, which can transport various cargo, such as lipid droplets and fatty acids (52, 54, 55). Recent studies have found that caveolae-based endocytosis is contributed to internalize LDL and VLDL in EC and macrophages. Silence the structural protein Cav1 of caveolae in Ldlr−/− mice will dramatically decrease the accumulation of lipids in the vasculature and reduce atherosclerotic plaque (56, 57). Cav1 is also responded shear stress and increases flow velocity in artery and reduces vascular inflammation and macrophage infiltration in disturbed flow area (56). Meanwhile, the loss of Cav1 in ECs reduces the endocytosis and transcytosis of LDL and disturbs autophagy. However, the relationship between autophagy and caveolae-mediated endocytosis is mutually regulated, and the loss of one of them will affect the functional process of the other (58, 59). Recent study has also revealed that LDL particles are colocalized with endothelial SR-B1 and cross endothelial cell barrier through caveolae-mediated endocytosis (11). These results suggested that caveolae-mediated endocytosis in vascular is closely related to the transport of LDL in ECs and accelerating atherosclerosis.
2.3 Phagocytosis
Phagocytosis, defined as the uptake of particles larger than 0.5 μm through plasma membrane encapsulation, is important in eliminating apoptotic cells, bacteria and other foreign materials in macrophages and other phagocytes (60). Typically, phagocytosis occurs in immune cells such as macrophages, monocytes, microglia and neutrophils and is activated by specific receptors in the cell membrane to recognize particles. Particles are then encapsulated by a cup-shaped membrane and transported in phagosomes, resulting in progressive degradation of cargo. Meanwhile, non-professional phagocytes (such as epithelial cells and ECs) also participate in the clearance of apoptotic cells and particles through phagocytosis and are associated with the recruiting role of macrophages (61, 62). The progression of atherosclerosis is associated with the excessive accumulation of apoptotic cells, including macrophages and VSMCs, and sufficient phagocytosis, resulting in the formation of a necrotic core and rupture plaque (63). Macrophages deactivate efferocytosis-related signalling such as ERK5, Rac2 that involve in cytoskeleton remodeling will lead to losing phagocytic capacity and accelerate atherosclerotic plaque formation (64, 65). Meanwhile, lack of phagocytosis will further promote the transition of VSMCs to macrophage-like cells through activating KLF4 and exacerbating the instability of atherosclerotic plaque (66). Yoko et al. found that blocking the “don’t eat me” molecule CD47, which is activated by TNF-α via NFKB1 and important for avoiding phagocytosis from phagocyte, can help macrophages to recognize foam or apoptotic cell and stimulate efferocytosis to promote phagocytic clearance and reduce atherosclerotic plaque (38). Furthermore, recent study has revealed that the enhancing phagocytosis of macrophages by CD47 relies on LRP1 to internalize during atherosclerosis. Loss LRP1 in macrophages will decrease the blocking effect of CD47 on efferocytosis and promote the formation of necrotic core in atherosclerotic plaque (67).
2.4 Macropinocytosis
Macropinocytosis is a non-selectively endocytic process for engulfing fluids and particles by forming vacuole-like extensions of the plasma membrane, which is an important route for the degradation of lipids, proteins in the extracellular matrix and dead cells (68). Macropincytosis, as an actin-dependent endocytic process, starts with ruffing across and encapsulating the plasma membrane, including protrusion, folding, and closure on the cell surface (69). In atherosclerosis, recent studies have revealed that macropinocytosis is responsible for engulfing LDL in macrophages and VSMCs and contributes to the formation of foam cells (36, 70). Meanwhile the engulfing enzyme-modified LDL but not acetylated or oxidized LDL through calcium dependent macropinocytosis is a potent role in forming VSMC derived foam cell (36). These lipid-overlapping foam cells in the vascular wall play an important role in the development of the necrotic core and late-stage atherosclerosis.
3 Shear stress on endocytosis
Shear stress is a blood flow-generating frictional force that is closely associated with vascular dysfunction and atherosclerosis. Several studies have demonstrated that atherosclerotic plaque usually emerge in near branches and bends of arteries that are exposed to disturbed flow, generating LSS or OSS (71, 72). These blood flow-induced vascular dysfunctions are linked to the changes of several signalling pathways, such as Klf2/4, Hippo–Yap–Taz, and Wnt/β-catenin, which participate in maintaining vascular integrity and tissue homeostasis (72–74). A recent study has revealed that shear stress not only mediates signal transduction but also increases LDL coverage on the endothelial glycocalyx, which controls the transportation of LDL across the vascular wall (75). Thus, it is essential to discuss the influence of shear stress on cell endocytosis during atherosclerosis (Figure 2).
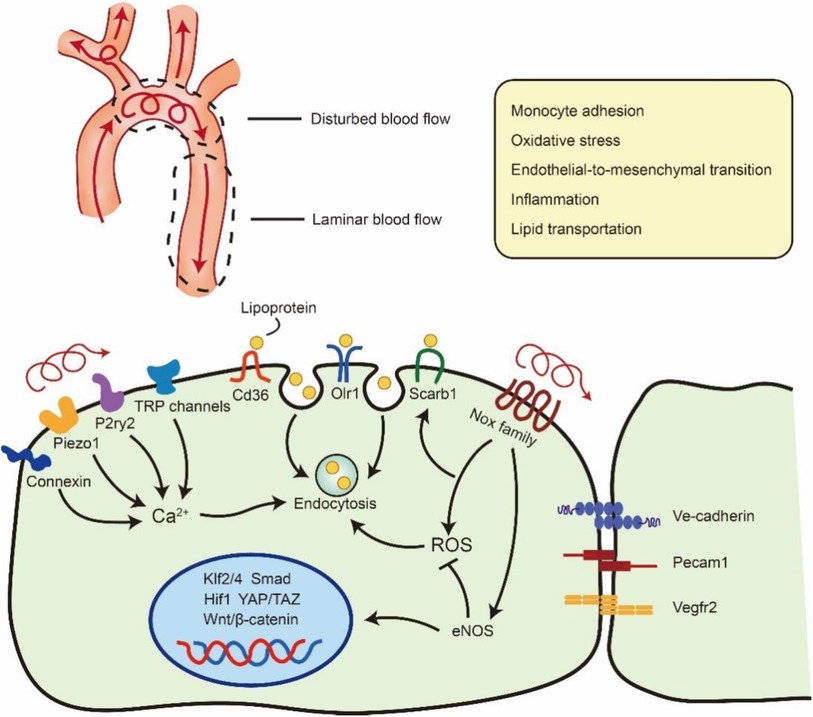
Figure 2. Flow regulation of lipoprotein uptake via endocytosis in ECs. Blood flow at branches and bends of arteries will be disturbed and generate LSS or OSS. The unexpected OSS in artery will result vascular inflammation, oxidative stress and so on. OSS also can stimulate Ca2+ channel and Nox family to promote Lipoprotein endocytosis in atheroprone area. Meanwhile the disturbed flow will down-regulate the expression of eNOS and further influence the transseptal manner of ECs such as Klf2/4, Smad, Hif1, YAP/TAZ, Wntβ/-catenin. ROS: reactive oxygen species. eNOS: endothelial nitric oxide synthase. The schematic diagram was made by the authors using Adobe Illustrator 2021.
ECs, as the barrier of vessel and blood flow, are equipped with various mechanosensitive channels that transfer biomechanical signals to modulate cellular function and behaviour, such as inflammation, endothelial-to-mesenchymal transition, and endocytosis (2). These shear stress-sensitive signals are mostly membrane receptors, cell‒cell junctional proteins and cell-matrix adhesion proteins (76). Moreover, Exposure of ECs on 10 dynes/cm2 shear stress will result an increased endocytosis and can be reduced by inhibiting reactive oxygen species (ROS) (77). Meanwhile, the accumulation of NO and ROS in LSS or OSS areas will result in the increased stabilization and expression of Cav1, which may enhance Cav1-mediated transcytosis (78). The surface density of caveolae in the cell membrane, which has been implicated in haemodynamic forces, is necessary for mechanotransduction and arterial remodelling (79). Ramírez et al. found that Cav1 is highly expressed in the “athero-prone” area and controls the transcytosis of LDL in atherosclerosis. Moreover, mice lacking Cav1 expression show less accumulation of lipids in athero-prone areas and atherosclerotic plaques (56). Our laboratory's recent study has revealed that LSS- and OSS-induced ROS can promote the internalization of extracellular vesicles in vascular ECs. We also observed that extracellular vesicles accumulated in the artery arch that underwent LSS and OSS (80). Abnormal shear stress can also activate multiple Ca2+ channels, including Piezo1, P2ry2, connexin and transient receptor potential (TRP) channels, which are considered mechanosensors and are significantly associated with inflammation in ECs during atherosclerosis (72, 81, 82). Meanwhile, Ca2+ influx can initiate activity-dependent bulk endocytosis and participate in the engulfment of LDL (83, 84).
Ongoing studies of OSS and LSS in ECs also report the role of mechano-transduction in endocytic receptors. Due to numerous researchers have performed transcriptome data under different shear stress, these analyses reveal that shear stress can mediate multiple transcriptional processes of endocytic receptors, such as Cd36 and Scarb1 (85). The overexpression of these lipoprotein receptors in arteries promotes the uptake of macromolecules into cells, which participates in the formation of atherosclerosis (6). Meanwhile, shear stress also plays an important role in the reorganization of Cdc42-dependent actin polymerization, which is important for LDL endocytosis (86, 87). Macrophage cortical F-actin depolymerization is required for actin polymerization to form a hydrolytic compartment-the lysosomal synapse, which digests aggregated LDL via Cdc42 Rho GTPase and GEF pathway. In summary, shear stress regulates multiple processes of endocytosis that control the accumulation of LDL and other macromolecules in the vasculature, thereby critically contributing to the development of atherosclerosis near branches and bends of arteries.
4 Stretch and tension force on endocytosis
ECs and VSMCs are sensitive to changes in physiological mechanical stretch and tension force that contain circumferential and axial stresses of approximately 100 kPa (1 × 106 dyne/cm2) from periodical distention and relaxation in arteries (88). Arteries under hypertension also inherit axial stress, which is associated with blood pressure and periodic distention and relaxation of arteries (89) (Figure 3). Meanwhile, VSMCs can be the engine of artery showing stronger contractile ability to generate and sustain stress on artery that is important in maintaining vessel tone and blood pressure (102). Elastic lamellae comprising elastin and collagens, which attaches and sandwiches VSMCs in the middle through focal adhesion complex, affects VSMCs contraction and relaxation (103, 104). Furthermore, ECs and VSMCs subjected to cyclic stretch display an elongated spindle morphology and show reorganization of the cytoskeleton. Studies have defined that 5%–10% strain is the physiological stretch, while excessive strain (15%–20%) is the pathological stretch in artery (105, 106). The unexpected stretch and tension force on vascular cells including ECs and VSMCs will activate multiple signalling and result excessive proliferation, migration and apoptosis (Figure 3) (107, 108). The VSMCs response to cycle stretch can express integrin αVβ3, which can inhibit ox-LDL-induced apoptosis although PINCH-1 in the progression of atherosclerosis (109, 110). Moreover, the cardiovascular cells response to cyclic stretch increase the accumulation and update of extracellular matrix (111). These studies suggest that normal physiological stretch and tension are important signals for cardiovascular function and contribute to the homeostasis and development of vessels.
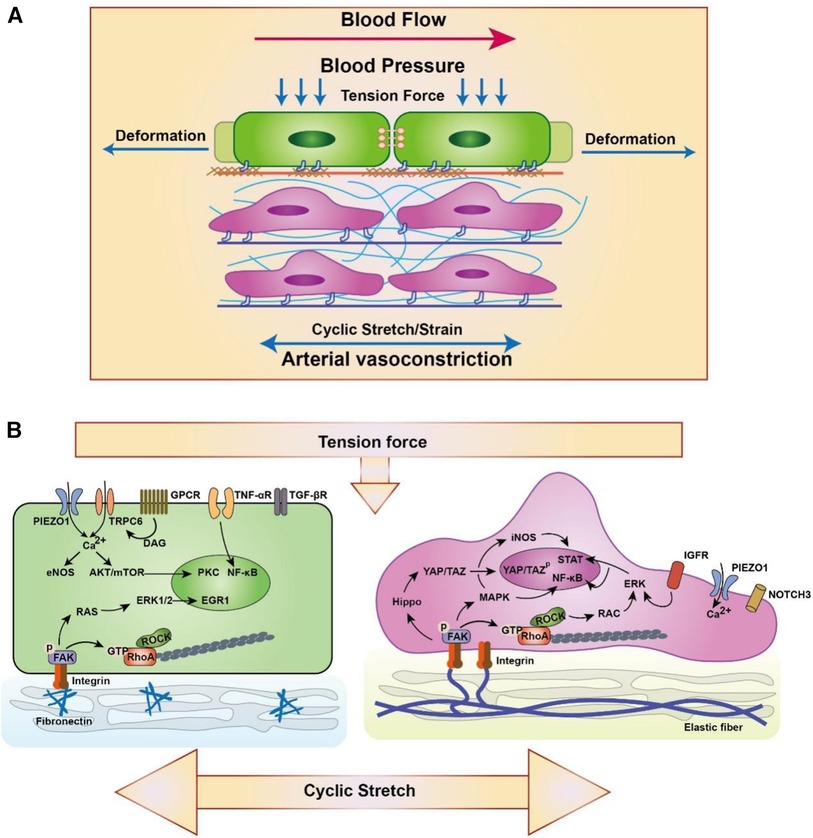
Figure 3. Schematic diagram of EC (left) and SMC (right) responding to the high strain/stretch and hypertension. (A) ECs are from the intima and VSMCs are form the media and adventitia. Blood pressure is perpendicular to the artery, which results in circumferential stretching and deformation of artery. Meanwhile, the physiological extension or arterial vasoconstriction derived from VSMCs also play an important role in the circumferential stretching of artery. (B) Biomechanics are sensed by varieties of membrane mechanoreceptors in artery such as PIEZO1 (28, 90), TRPC6 (90), GPCR (90), TNF-αR (91), TGF-βR (92), IGFR (93) and NOTCH3 (94). Meanwhile, ECs and VSCMC can connect with the extracellular matrix through focal adhesion complex to response and sustain stress (95). Focal adhesion complex contain integrin and FAK bind to ECM and is associated with multiple signaling transduction including ERK/MAPK (96–98), Hippo/YAP (99), RhoA (100) and so on (88). Furthermore, Ca2+ and other ionic signaling also participate in the mechano-transduction and involves in the transcriptional regulation (101). The schematic diagram was made by the authors using Adobe Illustrator 2021.
Pressure-derived stretch and tension force, which derived from the vascular deformation caused by blood pressure on the vessel wall, can influence the transportation of albumin and LDL. Studies have used 4 mm, 5 mm and 6 mm sleeves to restrict vessel from in New Zealand White rabbit and pressurized at 70, 120, or 160 mm Hg blood pressure, which control the deformation of vessel. The results showed that the accumulation of LDL in vessel was increased between 120 and 160 mm Hg with 5 mm sleeves, which explains the relationship between pressure-derived stretch and atherosclerosis (112). Stretch force also promotes the oxidation of LDL and accelerates the accumulation of ox-LDL in VSMCs (113). Meanwhile, cells stimulated with cyclical tensile stretch highly express the ox-LDL receptor Lox1, which is essential for internalizing ox-LDL (114). Moreover, membrane tension is also an important regulator of clathrin-mediated endocytosis through controlling the formation of clathrin-coated pits. Joseph et al. found that high tension interrupts the process of flat membrane-to-clathrin-coated structure transition by inhibiting the recruitment of epsin to the plasma membrane (44). Moreover, a recent study found that Torc2, a rapamycin-mediated protein kinase, can regulate plasma membrane tension to affect the reorganization of the actin cytoskeleton and vesicle fission to endocytosis sites (115). These results suggest that stretch and tension forces are essential for endocytic processes that regulate the transportation of multiple lipids and proteins in the vasculature (Figure 4).
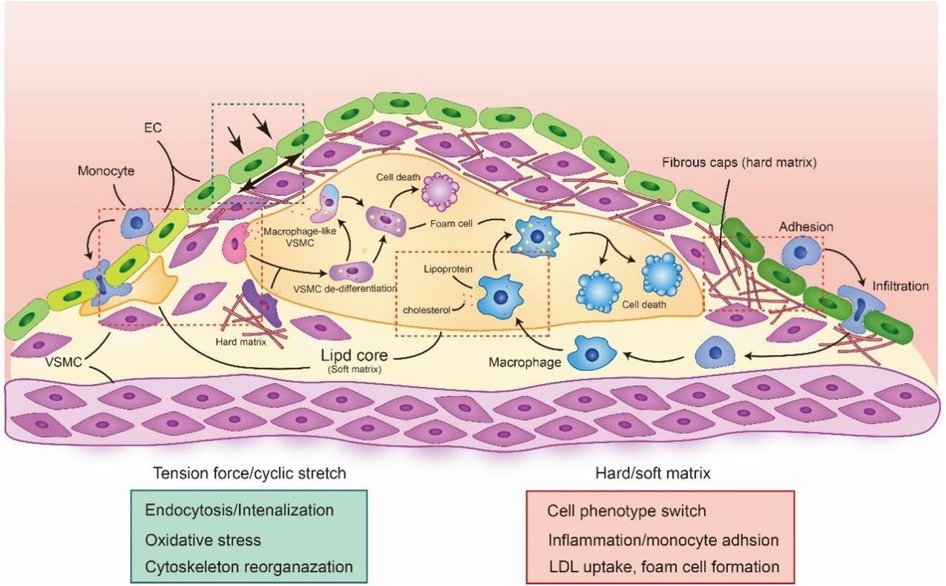
Figure 4. The biomechanical forces in atherosclerotic plaque. The alteration of biomechanical forces in atherosclerotic is important in plaque development and progression. The cyclic stretch and tension force will be induced by the changes of atherosclerotic plaque, which regulate multiple cell function. Meanwhile the changes in plaque composition and architecture also result the alteration of cell stiffness, making cell phenotype switching, inflammation and foam cell formation. The red rectangle is highlighted the biological process under hard or soft matrix and green rectangle is highlighted the biological process under tension force or cyclic stretch. The schematic diagram was made by the authors using Adobe Illustrator 2021.
5 Stiffness on endocytosis
Stiffness, also known as elasticity, is the mechanical force to resist deformation and is important for cell proliferation, migration and signal transduction. Arterial stiffness is closely associated with degenerative and remodelling changes in the extracellular matrix, resulting in vascular calcification, hypertension, and atherosclerosis (116). A recent Multi-Ethnic study with 6,814 men and women aged 45–84 years found that high load-dependent carotid artery stiffness is associated with a higher incidence of subclinical atherosclerosis and thus contributes to multiple cardiovascular events, such as stroke and hypertension (117). The stiffness of the artery is determined by their physiological conditions during atherosclerosis, such as the lipid core/necrotic core (1 kPa), fibrous plaque (35.5–54 kPa) and calcification (80–300 kPa), compared with normal artery (10–50 kPa) (118, 119). Meanwhile, the changing matrix stiffness also mediates the mechanical signal transduction and phenotypic transformation of vascular cells, which is closely related to cell fate and vascular function (118, 120). Recent studies have revealed that changing matrix stiffness in the vasculature promotes the adhesion of monocytes on ECs by enhancing the expression of miR-126 (targeting VCAM-1) and miR-222 (targeting ICAM-1) (121). Meanwhile, 1 kPa (soft) and 100 kPa (hard) substrate stiffnesses also increase cholesterol efflux in VSMCs and increase the expression of the macrophage marker CD68, suggesting that substrate stiffness can regulate the phenotypic switching of VSMCs (Figure 3) (122).
Endocytosis, as an important route of lipid metabolism, can also be regulated by cell and matrix stiffness. The surface topography of substrates regulates cell stiffness by activating mechanical signalling pathways. Li et al. found that a nanostructure stiff substrate can alter cell stiffness and behaviour to enhance clathrin-dependent endocytosis through its nano-topographical effect on integrin receptors. It has also been revealed that cells on a glass-based nanostructure stiff substrate respond similarly to 1 kPa soft hydrogels, which can reduce cell stiffness and membrane tension force (123). Furthermore, the cytoplasmic stiffness that influences deformability and membrane invagination can modulate the endocytic ability of cells. The high cortex stiffness of the subcellular structure will affect cell deformability, resulting in less phagocytic ability on macrophages (124). Because the stiffness of the necrotic core and lipid core in atherosclerotic plaques are nearly 1 kPa, we suspect that cells in atherosclerotic plaques will engulf more lipoprotein and apoptotic cells, resulting in the formation of foam cells.
A recent study revealed that macrophages prefer to take up native LDL and ox-LDL on 1 kPa soft substrates but with no difference in proliferative activity on soft and stiff substrates in an inflammatory microenvironment (125). However, Li et al. found that macrophages on soft 4 kPa PA hydrogels promote cell apoptosis and have less ox-LDL phagocytosis than those on 30 kPa substrates (126). Moreover, TRPV4 calcium-permeable channels that respond to matrix stiffness promote the transmembrane transport of Ca2+ and regulate the endocytosis of ox-LDL although CD36 (127). They also found that TRPV4, known as a mechano-sensor, plays an important role in regulating macrophage foam cell formation (127). Furthermore, Cav1 as the important part of caveolae will stimulate by soft substrates to modulate YAP activity through controlling actin polymerization and mediate caveolae internalization (128). These mechanotransducting property of Cav1 is also associated with cell stiffness and caveolae-based endocytosis. Le et al. discovered that disturber flow will increase the elastic modulus of ECs by increasing the expression of Cav1, which is positive correlation with the uptake of oxLDL (129). Recent studies have also revealed that the membrane tension force is important for Cav1-based vesicular trafficking, which is regulated by the stiffness of the extracellular matrix and depends on the mechano-transduction of the integrin/RhoA axis to stimulate a Cav1-dependent manner (130). These studies suggest that matrix and cell stiffness may regulate the transportation of lipids and proteins, which may contribute to the progression of atherosclerosis (Table 3).
6 Endocytosis for nanomedicine in atherosclerosis
Nanomedicine have widely applied in detecting and treating atherosclerosis with designing multiple responded or targeting molecule. Whereas, there are few researches focusing on the transporting route of nanoparticles into cells during treatment. Researchers have considered that nanoparticles firstly will closely contact with targeted cells and induce cell membrane to generate forces. Then, cell membrane will further encapsule nanoparticles through endocytic route and internalize nanoparticles (131). Thus, according to the endocytic route, we can design engineered nanoparticles to target diseased cells or even loading nano-drugs into living cells to assist drug delivery (132). Hu et al. design a tetrapod needle-like PdH nanozyme that can be internalized and storage into macrophages. By using the inflammatory response property, macrophages as the vesicles can deliver the nanozyme to atherosclerotic area and inhibit ROS (133). Furthermore, the biomechanics also can influence the endocytic process. Qin et al. have found that disturbed flow in artery will accelerate the internalized process of nano-sized extracellular vesicles through activating ROS in ECs (80). Due to the important role in avoiding the phagocytosis from immunity, researchers have used macrophages-membrane to encapsule nanoparticles and can efficiently and safely inhibit the progression of atherosclerosis (134). Thus it is important to develop nanomedicine based on the mechanism of intracellular endocytosis.
7 Discussion
The mechano-environments of atherosclerotic plaques are complex and involve multiple factors for vascular homeostasis that control cell transcription and biological function. Endocytosis is a fundamental process in which vascular cells internalize nutrients, lipids and other molecules. However, the unexpected endocytosis of lipids and inflammatory molecules accelerates atherosclerosis via lipid accumulation and the formation of foam cells (56, 135). It is urgent to fully elucidate the interactions between biomechanical force and vascular endocytosis. Recent studies of the single-cell transcriptome and functional screening of membrane receptors have already revealed the role of biomechanics in vascular cell endocytosis in atherosclerosis. They presented a comprehensive single-cell atlas of all cells in the carotid artery under d-flow, identified previously unrecognized cell subpopulations and gene expression signatures. Long-term exposure of ECs to low laminar shear stress leads to enhanced Endoglin expression and endocytosis of Endoglin in Cav1-positive early endosomes, highlighting Cav-1 vesicles as a SMAD signaling compartment in cells exposed to low atheroprone laminar shear stress (85, 136). However, a challenge remains regarding how the signal transduction of biomechanical force to endocytosis in atherosclerosis is regulated via different pathways, responsive molecules and endocytosis-related proteins. Nevertheless, some researchers have explored whether biomechanical force regulates multiple pathways that are associated with mechanosensitive transcription factors that typically participate in the transcription of lipoprotein-transported proteins (137, 138). Moreover, cells are prefer to engulfing stiff nanoparticles which can easily achieve full wrapping (131). The size and stiffness of LDL also will decrease in acidic condition or oxidation which suggests cells may hard to engulf LDL particles (139, 140). Therefore, it is important to identify novel mechanosensitive pathways and dynamic processes of endocytosis in arteries.
The material transported by endocytosis is also essential for nanomedicine, which provides a targeted route for nanoparticles to enter and deliver cargo in cells. Due to the intracellular delivery of nanoparticles relying on vascular physiology, microenvironment and cell phenotype, it is important to select and design suitable nanomaterials to deliver drugs. Several studies of nanomedicine have found that the accumulation of nanoparticles in cells is influenced by the biomechanical environment in the vasculature. The engulfment of nanoparticles is associated with the shear stress of the vessels, and physiological changes in ECs under disturbed flow increase the accumulation of nanoparticles (141). Meanwhile, disturbed flow inducing oxidative stress can also promote the uptake of nanoscale materials (80). This flow-dependent accumulation is important for drug delivery during atherosclerosis, which is usually present at the vascular branch and downstream of curved regions. Researchers have also found that physiological cyclic stretch promotes the internalization of silica nanoparticles, which is related to cell stress and exocytotic events (142). Moreover, the changes in plasma membrane morphology by cyclic stretch will affect the distribution of actin and reduce the internalization of nanoparticles in VSMCs (143). Thus, understanding the role of nanoscale materials in endocytosis is important for investigating intracellular delivery and providing more targeted points for nanomedicine.
Overall, biomechanical force is an essential feature to regulate endocytosis that accommodates molecule and macromolecule trafficking and lipid metabolism in atherosclerosis. Endocytosis is not only important for nutrient uptake but also a primary route by which lipid particles enter cells. Here, we summarized the main endocytic process in atherosclerosis and described the interaction role of biomechanics and endocytosis in atherosclerosis. A consistent conclusion from these studies is that the changing biomechanics of the vasculature will result in disordered endocytosis, which is typically associated with lipoprotein transportation. Therefore, further analysis of pathophysiological endocytosis under biomechanical force will improve our understanding of the development of atherosclerosis and lead to the discovery of new therapeutic drugs and targets in atherosclerosis.
Author contributions
JW: Writing – original draft, Writing – review & editing, Investigation, Methodology. JX: Writing – original draft, Writing – review & editing, Investigation, Methodology. TL: Investigation, Project administration, Writing – review & editing. CY: Resources, Supervision, Writing – review & editing. FX: Investigation, Project administration, Writing – review & editing. GW: Resources, Writing – review & editing. SL: Methodology, Resources, Writing – review & editing. XD: Writing – original draft, Writing – review & editing.
Funding
The author(s) declare that financial support was received for the research, authorship, and/or publication of this article.
This work was supported by the China Postdoctoral Science Foundation (2023MD734198) to JW, the National Natural Science Foundation of China (82170420), Project of Central Guiding Local Scientific and Technological Development in Sichuan (2023ZYD0058) and Disciplinary Construction Innovation Team Foundation of Chengdu Medical College (CMCXK-2101) to XD, the Foundation of Chengdu Medical College and Chengdu Seventh Hospital Clinical Science Research innovation team (2022LHTD-04) to SL.
Conflict of interest
The authors declare that the research was conducted in the absence of any commercial or financial relationships that could be construed as a potential conflict of interest.
Publisher's note
All claims expressed in this article are solely those of the authors and do not necessarily represent those of their affiliated organizations, or those of the publisher, the editors and the reviewers. Any product that may be evaluated in this article, or claim that may be made by its manufacturer, is not guaranteed or endorsed by the publisher.
References
1. Libby P. The changing landscape of atherosclerosis. Nature. (2021) 592(7855):524–33. doi: 10.1038/s41586-021-03392-8
2. Souilhol C, Serbanovic-Canic J, Fragiadaki M, Chico TJ, Ridger V, Roddie H, et al. Endothelial responses to shear stress in atherosclerosis: a novel role for developmental genes. Nat Rev Cardiol. (2020) 17(1):52–63. doi: 10.1038/s41569-019-0239-5
3. Wu MY, Li CJ, Hou MF, Chu PY. New insights into the role of inflammation in the pathogenesis of atherosclerosis. Int J Mol Sci. (2017) 18(10):2034. doi: 10.3390/ijms18102034
4. Kenjereš S, van der Krieke JP, Li C. Endothelium resolving simulations of wall shear-stress dependent mass transfer of LDL in diseased coronary arteries. Comput Biol Med. (2019) 114:103453. doi: 10.1016/j.compbiomed.2019.103453
5. Manta CP, Leibing T, Friedrich M, Nolte H, Adrian M, Schledzewski K, et al. Targeting of scavenger receptors stabilin-1 and stabilin-2 ameliorates atherosclerosis by a plasma proteome switch mediating monocyte/macrophage suppression. Circulation. (2022) 146(23):1783–99. doi: 10.1161/CIRCULATIONAHA.121.058615
6. Mineo C. Lipoprotein receptor signalling in atherosclerosis. Cardiovasc Res. (2020) 116(7):1254–74. doi: 10.1093/cvr/cvz338
7. Andreone BJ, Chow BW, Tata A, Lacoste B, Ben-Zvi A, Bullock K, et al. Blood-brain barrier permeability is regulated by lipid transport-dependent suppression of caveolae-mediated transcytosis. Neuron. (2017) 94(3):581–94.e5. doi: 10.1016/j.neuron.2017.03.043
8. Doherty GJ, McMahon HT. Mechanisms of endocytosis. Annu Rev Biochem. (2009) 78:857–902. doi: 10.1146/annurev.biochem.78.081307.110540
9. Zanoni P, Velagapudi S, Yalcinkaya M, Rohrer L, von Eckardstein A. Endocytosis of lipoproteins. Atherosclerosis. (2018) 275:273–95. doi: 10.1016/j.atherosclerosis.2018.06.881
10. Apostolov EO, Shah SV, Ray D, Basnakian AG. Scavenger receptors of endothelial cells mediate the uptake and cellular proatherogenic effects of carbamylated LDL. Arterioscler Thromb Vasc Biol. (2009) 29(10):1622–30. doi: 10.1161/ATVBAHA.109.189795
11. Huang L, Chambliss KL, Gao X, Yuhanna IS, Behling-Kelly E, Bergaya S, et al. SR-B1 drives endothelial cell LDL transcytosis via DOCK4 to promote atherosclerosis. Nature. (2019) 569(7757):565–9. doi: 10.1038/s41586-019-1140-4
12. Grootaert MOJ, Moulis M, Roth L, Martinet W, Vindis C, Bennett MR, et al. Vascular smooth muscle cell death, autophagy and senescence in atherosclerosis. Cardiovasc Res. (2018) 114(4):622–34. doi: 10.1093/cvr/cvy007
13. Moore KJ, Tabas I. Macrophages in the pathogenesis of atherosclerosis. Cell. (2011) 145(3):341–55. doi: 10.1016/j.cell.2011.04.005
14. Abifadel M, Varret M, Rabès JP, Allard D, Ouguerram K, Devillers M, et al. Mutations in PCSK9 cause autosomal dominant hypercholesterolemia. Nat Genet. (2003) 34(2):154–6. doi: 10.1038/ng1161
15. Llorente-Cortés V, Royo T, Otero-Viñas M, Berrozpe M, Badimon L. Sterol regulatory element binding proteins downregulate LDL receptor-related protein (LRP1) expression and LRP1-mediated aggregated LDL uptake by human macrophages. Cardiovasc Res. (2007) 74(3):526–36. doi: 10.1016/j.cardiores.2007.02.020
16. Badimon L, Luquero A, Crespo J, Peña E, Borrell-Pages M. PCSK9 and LRP5 in macrophage lipid internalization and inflammation. Cardiovasc Res. (2021) 117(9):2054–67. doi: 10.1093/cvr/cvaa254
17. Tacken PJ, de Beer F, van Vark LC, Havekes LM, Hofker MH, van Dijk KW. Very-low-density lipoprotein binding to the apolipoprotein E receptor 2 is enhanced by lipoprotein lipase, and does not require apolipoprotein E. Biochem J. (2000) 347:357–61. doi: 10.1042/bj3470357
18. Qu S, Feng N, Liu Z, Zhou H, Deng Y, Feng Z. The binding ability analysis of the normal VLDL receptor and its mutant. J Tongji Med Univ. (2001) 21(3):177–80. doi: 10.1007/BF02886422
19. Calvo D, Gómez-Coronado D, Suárez Y, Lasunción MA, Vega MA. Human CD36 is a high affinity receptor for the native lipoproteins HDL, LDL, and VLDL. J Lipid Res. (1998) 39(4):777–88. doi: 10.1016/S0022-2275(20)32566-9
20. Han J, Hajjar DP, Febbraio M, Nicholson AC. Native and modified low density lipoproteins increase the functional expression of the macrophage class B scavenger receptor, CD36. J Biol Chem. (1997) 272(34):21654–9. doi: 10.1074/jbc.272.34.21654
21. Zhou X, Chen X, Zhang L, Yuan J, Lin H, Zhu M, et al. Mannose-binding lectin reduces oxidized low-density lipoprotein induced vascular endothelial cells injury by inhibiting LOX1-ox-LDL binding and modulating autophagy. Biomedicines. (2023) 11(6):1743. doi: 10.3390/biomedicines11061743
22. Gan C, Wang K, Tang Q, Chen Y. Comparative investigation on the sizes and scavenger receptor binding of human native and modified lipoprotein particles with atomic force microscopy. J Nanobiotechnology. (2018) 16(1):25. doi: 10.1186/s12951-018-0352-3
23. Mahmoud MM, Kim HR, Xing R, Hsiao S, Mammoto A, Chen J, et al. TWIST1 integrates endothelial responses to flow in vascular dysfunction and atherosclerosis. Circ Res. (2016) 119(3):450–62. doi: 10.1161/CIRCRESAHA.116.308870
24. Pyle AL, Young PP. Atheromas feel the pressure: biomechanical stress and atherosclerosis. Am J Pathol. (2010) 177(1):4–9. doi: 10.2353/ajpath.2010.090615
25. Pedrigi RM, Poulsen CB, Mehta VV, Ramsing Holm N, Pareek N, Post AL, et al. Inducing persistent flow disturbances accelerates atherogenesis and promotes thin cap fibroatheroma development in D374Y-PCSK9 hypercholesterolemic minipigs. Circulation. (2015) 132(11):1003–12. doi: 10.1161/CIRCULATIONAHA.115.016270
26. Zhang K, Chen Y, Zhang T, Huang L, Wang Y, Yin T, et al. A novel role of Id1 in regulating oscillatory shear stress-mediated lipid uptake in endothelial cells. Ann Biomed Eng. (2018) 46(6):849–63. doi: 10.1007/s10439-018-2000-3
27. Douguet D, Patel A, Xu A, Vanhoutte PM, Honoré E. Piezo ion channels in cardiovascular mechanobiology. Trends Pharmacol Sci. (2019) 40(12):956–70. doi: 10.1016/j.tips.2019.10.002
28. Retailleau K, Duprat F, Arhatte M, Ranade SS, Peyronnet R, Martins JR, et al. Piezo1 in smooth muscle cells is involved in hypertension-dependent arterial remodeling. Cell Rep. (2015) 13(6):1161–71. doi: 10.1016/j.celrep.2015.09.072
29. Liu W, Zhang L, Gao Y, Liu K, Li Y, Liu C, et al. The association of arterial stiffness index with cerebrovascular and cardiometabolic disease: a Mendelian randomization study. Int J Stroke. (2022) 17(10):1145–50. doi: 10.1177/17474930211066432
30. Baranov MV, Olea RA, van den Bogaart G. Chasing uptake: super-resolution microscopy in endocytosis and phagocytosis. Trends Cell Biol. (2019) 29(9):727–39. doi: 10.1016/j.tcb.2019.05.006
31. Sahay G, Alakhova DY, Kabanov AV. Endocytosis of nanomedicines. J Control Release. (2010) 145(3):182–95. doi: 10.1016/j.jconrel.2010.01.036
32. McCluskey A, Daniel JA, Hadzic G, Chau N, Clayton EL, Mariana A, et al. Building a better dynasore: the dyngo compounds potently inhibit dynamin and endocytosis. Traffic. (2013) 14(12):1272–89. doi: 10.1111/tra.12119
33. Wang X, Yang Y, Zhang G, Tang CY, Law WC, Yu C, et al. NIR-cleavable and pH-responsive polymeric yolk-shell nanoparticles for controlled drug release. Biomacromolecules. (2023) 24(5):2009–21. doi: 10.1021/acs.biomac.2c01404
34. Huang JL, Jiang G, Song QX, Gu X, Hu M, Wang XL, et al. Lipoprotein-biomimetic nanostructure enables efficient targeting delivery of siRNA to ras-activated glioblastoma cells via macropinocytosis. Nat Commun. (2017) 8:15144. doi: 10.1038/ncomms15144
35. Smith CM, Haucke V, McCluskey A, Robinson PJ, Chircop M. Inhibition of clathrin by pitstop 2 activates the spindle assembly checkpoint and induces cell death in dividing HeLa cancer cells. Mol Cancer. (2013) 12:4. doi: 10.1186/1476-4598-12-4
36. Chellan B, Reardon CA, Getz GS, Hofmann Bowman MA. Enzymatically modified low-density lipoprotein promotes foam cell formation in smooth muscle cells via macropinocytosis and enhances receptor-mediated uptake of oxidized low-density lipoprotein. Arterioscler Thromb Vasc Biol. (2016) 36(6):1101–13. doi: 10.1161/ATVBAHA.116.307306
37. Tsimikas S. A test in context: lipoprotein(a): diagnosis, prognosis, controversies, and emerging therapies. J Am Coll Cardiol. (2017) 69(6):692–711. doi: 10.1016/j.jacc.2016.11.042
38. Kojima Y, Volkmer JP, McKenna K, Civelek M, Lusis AJ, Miller CL, et al. CD47-blocking antibodies restore phagocytosis and prevent atherosclerosis. Nature. (2016) 536(7614):86–90. doi: 10.1038/nature18935
39. McMahon HT, Boucrot E. Molecular mechanism and physiological functions of clathrin-mediated endocytosis. Nat Rev Mol Cell Biol. (2011) 12(8):517–33. doi: 10.1038/nrm3151
40. Brodsky FM, Chen CY, Knuehl C, Towler MC, Wakeham DE. Biological basket weaving: formation and function of clathrin-coated vesicles. Annu Rev Cell Dev Biol. (2001) 17:517–68. doi: 10.1146/annurev.cellbio.17.1.517
41. Höning S, Ricotta D, Krauss M, Späte K, Spolaore B, Motley A, et al. Phosphatidylinositol-(4,5)-bisphosphate regulates sorting signal recognition by the clathrin-associated adaptor complex AP2. Mol Cell. (2005) 18(5):519–31. doi: 10.1016/j.molcel.2005.04.019
42. Zhao Z, Pompey S, Dong H, Weng J, Garuti R, Michaely P. S-nitrosylation of ARH is required for LDL uptake by the LDL receptor. J Lipid Res. (2013) 54(6):1550–9. doi: 10.1194/jlr.M033167
43. Tebar F, Sorkina T, Sorkin A, Ericsson M, Kirchhausen T. Eps15 is a component of clathrin-coated pits and vesicles and is located at the rim of coated pits. J Biol Chem. (1996) 271(46):28727–30. doi: 10.1074/jbc.271.46.28727
44. Joseph JG, Osorio C, Yee V, Agrawal A, Liu AP. Complimentary action of structured and unstructured domains of epsin supports clathrin-mediated endocytosis at high tension. Commun Biol. (2020) 3(1):743. doi: 10.1038/s42003-020-01471-6
45. van der Bliek AM, Redelmeier TE, Damke H, Tisdale EJ, Meyerowitz EM, Schmid SL. Mutations in human dynamin block an intermediate stage in coated vesicle formation. J Cell Biol. (1993) 122(3):553–63. doi: 10.1083/jcb.122.3.553
46. Sathe M, Muthukrishnan G, Rae J, Disanza A, Thattai M, Scita G, et al. Small GTPases and BAR domain proteins regulate branched actin polymerisation for clathrin and dynamin-independent endocytosis. Nat Commun. (2018) 9(1):1835. doi: 10.1038/s41467-018-03955-w
47. Wu M, Wu X. A kinetic view of clathrin assembly and endocytic cargo sorting. Curr Opin Cell Biol. (2021) 71:130–8. doi: 10.1016/j.ceb.2021.02.010
48. Beacham GM, Partlow EA, Hollopeter G. Conformational regulation of AP1 and AP2 clathrin adaptor complexes. Traffic. (2019) 20(10):741–51. doi: 10.1111/tra.12677
49. Izem L, Bialkowska K, Pluskota E, Das M, Das R, Nieman MT, et al. Plasminogen-induced foam cell formation by macrophages occurs through a histone 2B (H2B)-PAR1 pathway and requires integrity of clathrin-coated pits. J Thromb Haemost. (2021) 19(4):941–53. doi: 10.1111/jth.15253
50. Brophy ML, Dong Y, Tao H, Yancey PG, Song K, Zhang K, et al. Myeloid-specific deletion of epsins 1 and 2 reduces atherosclerosis by preventing LRP-1 downregulation. Circ Res. (2019) 124(4):e6–19. doi: 10.1161/CIRCRESAHA.118.313028
51. Potje SR, Paula TD, Paulo M, Bendhack LM. The role of glycocalyx and caveolae in vascular homeostasis and diseases. Front Physiol. (2020) 11:620840. doi: 10.3389/fphys.2020.620840
52. Pelkmans L, Zerial M. Kinase-regulated quantal assemblies and kiss-and-run recycling of caveolae. Nature. (2005) 436(7047):128–33. doi: 10.1038/nature03866
53. Parton RG, Simons K. The multiple faces of caveolae. Nat Rev Mol Cell Biol. (2007) 8(3):185–94. doi: 10.1038/nrm2122
54. Hao JW, Wang J, Guo H, Zhao YY, Sun HH, Li YF, et al. CD36 facilitates fatty acid uptake by dynamic palmitoylation-regulated endocytosis. Nat Commun. (2020) 11(1):4765. doi: 10.1038/s41467-020-18565-8
55. Hubert M, Larsson E, Vegesna NVG, Ahnlund M, Johansson AI, Moodie LW, et al. Lipid accumulation controls the balance between surface connection and scission of caveolae. Elife. (2020) 9:e55038. doi: 10.7554/eLife.55038
56. Ramírez CM, Zhang X, Bandyopadhyay C, Rotllan N, Sugiyama MG, Aryal B, et al. Caveolin-1 regulates atherogenesis by attenuating low-density lipoprotein transcytosis and vascular inflammation independently of endothelial nitric oxide synthase activation. Circulation. (2019) 140(3):225–39. doi: 10.1161/CIRCULATIONAHA.118.038571
57. Deng L, Vrieling F, Stienstra R, Hooiveld GJ, Feitsma AL, Kersten S. Macrophages take up VLDL-sized emulsion particles through caveolae-mediated endocytosis and excrete part of the internalized triglycerides as fatty acids. PLoS Biol. (2022) 20(8):e3001516. doi: 10.1371/journal.pbio.3001516
58. Bai X, Yang X, Jia X, Rong Y, Chen L, Zeng T, et al. CAV1-CAVIN1-LC3B-mediated autophagy regulates high glucose-stimulated LDL transcytosis. Autophagy. (2020) 16(6):1111–29. doi: 10.1080/15548627.2019.1659613
59. Zhang X, Ramírez CM, Aryal B, Madrigal-Matute J, Liu X, Diaz A, et al. Cav-1 (caveolin-1) deficiency increases autophagy in the endothelium and attenuates vascular inflammation and atherosclerosis. Arterioscler Thromb Vasc Biol. (2020) 40(6):1510–22. doi: 10.1161/ATVBAHA.120.314291
60. Gordon S. Phagocytosis: an immunobiologic process. Immunity. (2016) 44(3):463–75. doi: 10.1016/j.immuni.2016.02.026
61. Han CZ, Juncadella IJ, Kinchen JM, Buckley MW, Klibanov AL, Dryden K, et al. Macrophages redirect phagocytosis by non-professional phagocytes and influence inflammation. Nature. (2016) 539(7630):570–4. doi: 10.1038/nature20141
62. Zhou T, Zheng Y, Sun L, Badea SR, Jin Y, Liu Y, et al. Microvascular endothelial cells engulf myelin debris and promote macrophage recruitment and fibrosis after neural injury. Nat Neurosci. (2019) 22(3):421–35. doi: 10.1038/s41593-018-0324-9
63. Van Vré EA, Ait-Oufella H, Tedgui A, Mallat Z. Apoptotic cell death and efferocytosis in atherosclerosis. Arterioscler Thromb Vasc Biol. (2012) 32(4):887–93. doi: 10.1161/ATVBAHA.111.224873
64. Heo KS, Cushman HJ, Akaike M, Woo CH, Wang X, Qiu X, et al. ERK5 activation in macrophages promotes efferocytosis and inhibits atherosclerosis. Circulation. (2014) 130(2):180–91. doi: 10.1161/CIRCULATIONAHA.113.005991
65. Zhang J, Zhao X, Guo Y, Liu Z, Wei S, Yuan Q, et al. Macrophage ALDH2 (aldehyde dehydrogenase 2) stabilizing Rac2 is required for efferocytosis internalization and reduction of atherosclerosis development. Arterioscler Thromb Vasc Biol. (2022) 42(6):700–16. doi: 10.1161/ATVBAHA.121.317204
66. Shankman LS, Gomez D, Cherepanova OA, Salmon M, Alencar GF, Haskins RM, et al. KLF4-dependent phenotypic modulation of smooth muscle cells has a key role in atherosclerotic plaque pathogenesis. Nat Med. (2015) 21(6):628–37. doi: 10.1038/nm.3866
67. Mueller PA, Kojima Y, Huynh KT, Maldonado RA, Ye J, Tavori H, et al. Macrophage LRP1 (low-density lipoprotein receptor-related protein 1) is required for the effect of CD47 blockade on efferocytosis and atherogenesis-brief report. Arterioscler Thromb Vasc Biol. (2022) 42(1):e1–9. doi: 10.1161/ATVBAHA.121.316854
68. Stow JL, Hung Y, Wall AA. Macropinocytosis: insights from immunology and cancer. Curr Opin Cell Biol. (2020) 65:131–40. doi: 10.1016/j.ceb.2020.06.005
69. Mercer J, Helenius A. Virus entry by macropinocytosis. Nat Cell Biol. (2009) 11(5):510–20. doi: 10.1038/ncb0509-510
70. Chaves LD, Abyad S, Honan AM, Bryniarski MA, McSkimming DI, Stahura CM, et al. Unconjugated p-cresol activates macrophage macropinocytosis leading to increased LDL uptake. JCI Insight. (2021) 6(11):e144410. doi: 10.1172/jci.insight.144410
71. Malik J, Novakova L, Valerianova A, Chytilova E, Lejsek V, Buryskova Salajova K, et al. Wall shear stress alteration: a local risk factor of atherosclerosis. Curr Atheroscler Rep. (2022) 24(3):143–51. doi: 10.1007/s11883-022-00993-0
72. Albarrán-Juárez J, Iring A, Wang S, Joseph S, Grimm M, Strilic B, et al. Piezo1 and G(q)/G(11) promote endothelial inflammation depending on flow pattern and integrin activation. J Exp Med. (2018) 215(10):2655–72. doi: 10.1084/jem.20180483
73. Sangwung P, Zhou G, Nayak L, Chan ER, Kumar S, Kang DW, et al. KLF2 and KLF4 control endothelial identity and vascular integrity. JCI Insight. (2017) 2(4):e91700. doi: 10.1172/jci.insight.91700
74. Wang KC, Yeh YT, Nguyen P, Limqueco E, Lopez J, Thorossian S, et al. Flow-dependent YAP/TAZ activities regulate endothelial phenotypes and atherosclerosis. Proc Natl Acad Sci U S A. (2016) 113(41):11525–30. doi: 10.1073/pnas.1613121113
75. Kang H, Yang J, Zhang W, Lu J, Ma X, Sun A, et al. Effect of endothelial glycocalyx on water and LDL transport through the rat abdominal aorta. Am J Physiol Heart Circ Physiol. (2021) 320(4):H1724–37. doi: 10.1152/ajpheart.00861.2020
76. He L, Zhang CL, Chen Q, Wang L, Huang Y. Endothelial shear stress signal transduction and atherogenesis: from mechanisms to therapeutics. Pharmacol Ther. (2022) 235:108152. doi: 10.1016/j.pharmthera.2022.108152
77. Niwa K, Sakai J, Karino T, Aonuma H, Watanabe T, Ohyama T, et al. Reactive oxygen species mediate shear stress-induced fluid-phase endocytosis in vascular endothelial cells. Free Radic Res. (2006) 40(2):167–74. doi: 10.1080/10715760500474287
78. Chen X, Xia Q, Sun N, Zhou H, Xu Z, Yang X, et al. Shear stress enhances anoikis resistance of cancer cells through ROS and NO suppressed degeneration of caveolin-1. Free Radic Biol Med. (2022) 193(Pt 1):95–107. doi: 10.1016/j.freeradbiomed.2022.10.271
79. Yu J, Bergaya S, Murata T, Alp IF, Bauer MP, Lin MI, et al. Direct evidence for the role of caveolin-1 and caveolae in mechanotransduction and remodeling of blood vessels. J Clin Invest. (2006) 116(5):1284–91. doi: 10.1172/JCI27100
80. Qin X, Zhang K, Qiu J, Wang N, Qu K, Cui Y, et al. Uptake of oxidative stress-mediated extracellular vesicles by vascular endothelial cells under low magnitude shear stress. Bioact Mater. (2022) 9:397–410. doi: 10.1016/j.bioactmat.2021.10.038
81. Pfenniger A, Meens MJ, Pedrigi RM, Foglia B, Sutter E, Pelli G, et al. Shear stress-induced atherosclerotic plaque composition in ApoE(-/-) mice is modulated by connexin37. Atherosclerosis. (2015) 243(1):1–10. doi: 10.1016/j.atherosclerosis.2015.08.029
82. Thilo F, Vorderwülbecke BJ, Marki A, Krueger K, Liu Y, Baumunk D, et al. Pulsatile atheroprone shear stress affects the expression of transient receptor potential channels in human endothelial cells. Hypertension. (2012) 59(6):1232–40. doi: 10.1161/HYPERTENSIONAHA.111.183608
83. Liang SJ, Zeng DY, Mai XY, Shang JY, Wu QQ, Yuan JN, et al. Inhibition of orai1 store-operated calcium channel prevents foam cell formation and atherosclerosis. Arterioscler Thromb Vasc Biol. (2016) 36(4):618–28. doi: 10.1161/ATVBAHA.116.307344
84. Yao CK, Liu YT, Lee IC, Wang YT, Wu PY. A Ca2+ channel differentially regulates clathrin-mediated and activity-dependent bulk endocytosis. PLoS Biol. (2017) 15(4):e2000931. doi: 10.1371/journal.pbio.2000931
85. Li FC, Yan KM, Wu LL, Zheng Z, Du Y, Liu ZT, et al. Single-cell RNA-seq reveals cellular heterogeneity of mouse carotid artery under disturbed flow. Cell Death Discov. (2021) 7(1):180. doi: 10.1038/s41420-021-00567-0
86. Singh RK, Haka AS, Bhardwaj P, Zha X, Maxfield FR. Dynamic actin reorganization and vav/Cdc42-dependent actin polymerization promote macrophage aggregated LDL (low-density lipoprotein) uptake and catabolism. Arterioscler Thromb Vasc Biol. (2019) 39(2):137–49. doi: 10.1161/ATVBAHA.118.312087
87. Tzima E. Role of small GTPases in endothelial cytoskeletal dynamics and the shear stress response. Circ Res. (2006) 98(2):176–85. doi: 10.1161/01.RES.0000200162.94463.d7
88. Yamashiro Y, Yanagisawa H. The molecular mechanism of mechanotransduction in vascular homeostasis and disease. Clin Sci (Lond). (2020) 134(17):2399–418. doi: 10.1042/CS20190488
89. Le VP, Knutsen RH, Mecham RP, Wagenseil JE. Decreased aortic diameter and compliance precedes blood pressure increases in postnatal development of elastin-insufficient mice. Am J Physiol Heart Circ Physiol. (2011) 301(1):H221–9. doi: 10.1152/ajpheart.00119.2011
90. Zhao T, Parmisano S, Soroureddin Z, Zhao M, Yung L, Thistlethwaite PA, et al. Mechanosensitive cation currents through TRPC6 and Piezo1 channels in human pulmonary arterial endothelial cells. Am J Physiol Cell Physiol. (2022) 323(4):C959–73. doi: 10.1152/ajpcell.00313.2022
91. Wang BW, Chang H, Lin S, Kuan P, Shyu KG. Induction of matrix metalloproteinases-14 and -2 by cyclical mechanical stretch is mediated by tumor necrosis factor-alpha in cultured human umbilical vein endothelial cells. Cardiovasc Res. (2003) 59(2):460–9. doi: 10.1016/S0008-6363(03)00428-0
92. Vorisek C, Weixler V, Dominguez M, Axt-Fliedner R, Hammer PE, Lin RZ, et al. Mechanical strain triggers endothelial-to-mesenchymal transition of the endocardium in the immature heart. Pediatr Res. (2022) 92(3):721–8. doi: 10.1038/s41390-021-01843-6
93. Liu G, Hitomi H, Hosomi N, Lei B, Nakano D, Deguchi K, et al. Mechanical stretch augments insulin-induced vascular smooth muscle cell proliferation by insulin-like growth factor-1 receptor. Exp Cell Res. (2011) 317(17):2420–8. doi: 10.1016/j.yexcr.2011.07.016
94. Morrow D, Sweeney C, Birney YA, Cummins PM, Walls D, Redmond EM, et al. Cyclic strain inhibits notch receptor signaling in vascular smooth muscle cells in vitro. Circ Res. (2005) 96(5):567–75. doi: 10.1161/01.RES.0000159182.98874.43
95. Sen U, Moshal KS, Singh M, Tyagi N, Tyagi SC. Homocysteine-induced biochemical stress predisposes to cytoskeletal remodeling in stretched endothelial cells. Mol Cell Biochem. (2007) 302(1–2):133–43. doi: 10.1007/s11010-007-9435-4
96. Wung BS, Cheng JJ, Chao YJ, Hsieh HJ, Wang DL. Modulation of ras/raf/extracellular signal-regulated kinase pathway by reactive oxygen species is involved in cyclic strain-induced early growth response-1 gene expression in endothelial cells. Circ Res. (1999) 84(7):804–12. doi: 10.1161/01.RES.84.7.804
97. Zampetaki A, Zhang Z, Hu Y, Xu Q. Biomechanical stress induces IL-6 expression in smooth muscle cells via ras/Rac1-p38 MAPK-NF-kappaB signaling pathways. Am J Physiol Heart Circ Physiol. (2005) 288(6):H2946–54. doi: 10.1152/ajpheart.00919.2004
98. Tang X, Liu Y, Xiao Q, Yao Q, Allen M, Wang Y, et al. Pathological cyclic strain promotes proliferation of vascular smooth muscle cells via the ACTH/ERK/STAT3 pathway. J Cell Biochem. (2018) 119(10):8260–70. doi: 10.1002/jcb.26839
99. Daoud F, Arévalo Martinez M, Holmberg J, Alajbegovic A, Ali N, Rippe C, et al. YAP and TAZ in vascular smooth muscle confer protection against hypertensive vasculopathy. Arterioscler Thromb Vasc Biol. (2022) 42(4):428–43. doi: 10.1161/ATVBAHA.121.317365
100. Abiko H, Fujiwara S, Ohashi K, Hiatari R, Mashiko T, Sakamoto N, et al. Rho guanine nucleotide exchange factors involved in cyclic-stretch-induced reorientation of vascular endothelial cells. J Cell Sci. (2015) 128(9):1683–95. doi: 10.1242/jcs.157503
101. Martinac B, Poole K. Mechanically activated ion channels. Int J Biochem Cell Biol. (2018) 97:104–7. doi: 10.1016/j.biocel.2018.02.011
102. Ratz PH. Mechanics of vascular smooth muscle. Compr Physiol. (2015) 6(1):111–68. doi: 10.1002/cphy.c140072
103. Ribeiro-Silva JC, Miyakawa AA, Krieger JE. Focal adhesion signaling: vascular smooth muscle cell contractility beyond calcium mechanisms. Clin Sci (Lond). (2021) 135(9):1189–207. doi: 10.1042/CS20201528
104. Steucke KE, Tracy PV, Hald ES, Hall JL, Alford PW. Vascular smooth muscle cell functional contractility depends on extracellular mechanical properties. J Biomech. (2015) 48(12):3044–51. doi: 10.1016/j.jbiomech.2015.07.029
105. Zheng TF, Liu XL, Li X, Wang QQ, Zhao YC, Li X, et al. Dickkopf-1 promotes vascular smooth muscle cell proliferation and migration through upregulating UHRF1 during cyclic stretch application. Int J Biol Sci. (2021) 17(5):1234–49. doi: 10.7150/ijbs.56247
106. Zhong HY, Yuan C, Liu XL, Wang QQ, Li X, Zhao YC, et al. Mechanical stretch aggravates vascular smooth muscle cell apoptosis and vascular remodeling by downregulating EZH2. Int J Biochem Cell Biol. (2022) 151:106278. doi: 10.1016/j.biocel.2022.106278
107. James BD, Allen JB. Vascular endothelial cell behavior in complex mechanical microenvironments. ACS Biomater Sci Eng. (2018) 4(11):3818–42. doi: 10.1021/acsbiomaterials.8b00628
108. Lacolley P, Regnault V, Segers P, Laurent S. Vascular smooth muscle cells and arterial stiffening: relevance in development, aging, and disease. Physiol Rev. (2017) 97(4):1555–617. doi: 10.1152/physrev.00003.2017
109. Dartsch PC, Betz E. Response of cultured endothelial cells to mechanical stimulation. Basic Res Cardiol. (1989) 84(3):268–81. doi: 10.1007/BF01907974
110. Cheng J, Zhang J, Merched A, Zhang L, Zhang P, Truong L, et al. Mechanical stretch inhibits oxidized low density lipoprotein-induced apoptosis in vascular smooth muscle cells by up-regulating integrin alphavbeta3 and stablization of PINCH-1. J Biol Chem. (2007) 282(47):34268–75. doi: 10.1074/jbc.M703115200
111. Gupta V, Grande-Allen KJ. Effects of static and cyclic loading in regulating extracellular matrix synthesis by cardiovascular cells. Cardiovasc Res. (2006) 72(3):375–83. doi: 10.1016/j.cardiores.2006.08.017
112. Meyer G, Merval R, Tedgui A. Effects of pressure-induced stretch and convection on low-density lipoprotein and albumin uptake in the rabbit aortic wall. Circ Res. (1996) 79(3):532–40. doi: 10.1161/01.RES.79.3.532
113. Inoue N, Kawashima S, Hirata KI, Rikitake Y, Takeshita S, Yamochi W, et al. Stretch force on vascular smooth muscle cells enhances oxidation of LDL via superoxide production. Am J Physiol. (1998) 274(6):H1928–32. doi: 10.1152/ajpheart.1998.274.6.H1928
114. Akagi M, Nishimura S, Yoshida K, Kakinuma T, Sawamura T, Munakata H, et al. Cyclic tensile stretch load and oxidized low density lipoprotein synergistically induce lectin-like oxidized ldl receptor-1 in cultured bovine chondrocytes, resulting in decreased cell viability and proteoglycan synthesis. J Orthop Res. (2006) 24(8):1782–90. doi: 10.1002/jor.20211
115. Riggi M, Bourgoint C, Macchione M, Matile S, Loewith R, Roux A. TORC2 controls endocytosis through plasma membrane tension. J Cell Biol. (2019) 218(7):2265–76. doi: 10.1083/jcb.201901096
116. Palombo C, Kozakova M. Arterial stiffness, atherosclerosis and cardiovascular risk: pathophysiologic mechanisms and emerging clinical indications. Vascul Pharmacol. (2016) 77:1–7. doi: 10.1016/j.vph.2015.11.083
117. Pewowaruk RJ, Korcarz C, Tedla Y, Burke G, Greenland P, Wu C, et al. Carotid artery stiffness mechanisms associated with cardiovascular disease events and incident hypertension: the multi-ethnic study of atherosclerosis (MESA). Hypertension. (2022) 79(3):659–66. doi: 10.1161/HYPERTENSIONAHA.121.18772
118. Wang Y, Shi R, Zhai R, Yang S, Peng T, Zheng F, et al. Matrix stiffness regulates macrophage polarization in atherosclerosis. Pharmacol Res. (2022) 179:106236. doi: 10.1016/j.phrs.2022.106236
119. Kucherenko MM, Sang P, Yao J, Gransar T, Dhital S, Grune J, et al. Elastin stabilization prevents impaired biomechanics in human pulmonary arteries and pulmonary hypertension in rats with left heart disease. Nat Commun. (2023) 14(1):4416. doi: 10.1038/s41467-023-39934-z
120. Durham AL, Speer MY, Scatena M, Giachelli CM, Shanahan CM. Role of smooth muscle cells in vascular calcification: implications in atherosclerosis and arterial stiffness. Cardiovasc Res. (2018) 114(4):590–600. doi: 10.1093/cvr/cvy010
121. Chen W, Tian B, Liang J, Yu S, Zhou Y, Li S. Matrix stiffness regulates the interactions between endothelial cells and monocytes. Biomaterials. (2019) 221:119362. doi: 10.1016/j.biomaterials.2019.119362
122. Mao X, Tan Y, Wang H, Li S, Zhou Y. Substrate stiffness regulates cholesterol efflux in smooth muscle cells. Front Cell Dev Biol. (2021) 9:648715. doi: 10.3389/fcell.2021.648715
123. Li X, Klausen LH, Zhang W, Jahed Z, Tsai CT, Li TL, et al. Nanoscale surface topography reduces focal adhesions and cell stiffness by enhancing integrin endocytosis. Nano Lett. (2021) 21(19):8518–26. doi: 10.1021/acs.nanolett.1c01934
124. Agarwal M, Biswas P, Bhattacharya A, Sinha DK. Reactive oxygen species-mediated cytoplasmic stiffening impairs the phagocytic ability of the macrophage. J Cell Sci. (2020) 133(5):jcs236471. doi: 10.1242/jcs.236471
125. Ammanamanchi M, Maurer M, Hayenga HN. Inflammation drives stiffness mediated uptake of lipoproteins in primary human macrophages and foam cell proliferation. Ann Biomed Eng. (2021) 49(12):3425–37. doi: 10.1007/s10439-021-02881-1
126. Li J, Wang S, Li Y, Zhang N, Gribskov M, Zhang X, et al. miRNA-mediated macrophage behaviors responding to matrix stiffness and ox-LDL. J Cell Physiol. (2020) 235(9):6139–53. doi: 10.1002/jcp.29543
127. Goswami R, Merth M, Sharma S, Alharbi MO, Aranda-Espinoza H, Zhu X, et al. TRPV4 calcium-permeable channel is a novel regulator of oxidized LDL-induced macrophage foam cell formation. Free Radic Biol Med. (2017) 110:142–50. doi: 10.1016/j.freeradbiomed.2017.06.004
128. Moreno-Vicente R, Pavón DM, Martín-Padura I, Català-Montoro M, Díez-Sánchez A, Quílez-Álvarez A, et al. Caveolin-1 modulates mechanotransduction responses to substrate stiffness through actin-dependent control of YAP. Cell Rep. (2018) 25(6):1622–35.e6. doi: 10.1016/j.celrep.2018.10.024
129. Le Master E, Paul A, Lazarko D, Aguilar V, Ahn SJ, Lee JC, et al. Caveolin-1 is a primary determinant of endothelial stiffening associated with dyslipidemia, disturbed flow, and ageing. Sci Rep. (2022) 12(1):17822. doi: 10.1038/s41598-022-20713-7
130. Lachowski D, Matellan C, Gopal S, Cortes E, Robinson BK, Saiani A, et al. Substrate stiffness-driven membrane tension modulates vesicular trafficking via caveolin-1. ACS Nano. (2022) 16(3):4322–37. doi: 10.1021/acsnano.1c10534
131. Zhang S, Gao H, Bao G. Physical principles of nanoparticle cellular endocytosis. ACS Nano. (2015) 9(9):8655–71. doi: 10.1021/acsnano.5b03184
132. Xu J, Wang J, Chen Y, Hou Y, Hu J, Wang G. Recent advances of natural and bioengineered extracellular vesicles and their application in vascular regeneration. Regen Biomater. (2022) 9:rbac064. doi: 10.1093/rb/rbac064
133. Hu R, Dai C, Dong C, Ding L, Huang H, Chen Y, et al. Living macrophage-delivered tetrapod PdH nanoenzyme for targeted atherosclerosis management by ROS scavenging, hydrogen anti-inflammation, and autophagy activation. ACS Nano. (2022) 16(10):15959–76. doi: 10.1021/acsnano.2c03422
134. Wang Y, Zhang K, Li T, Maruf A, Qin X, Luo L, et al. Macrophage membrane functionalized biomimetic nanoparticles for targeted anti-atherosclerosis applications. Theranostics. (2021) 11(1):164–80. doi: 10.7150/thno.47841
135. Patel KM, Strong A, Tohyama J, Jin X, Morales CR, Billheimer J, et al. Macrophage sortilin promotes LDL uptake, foam cell formation, and atherosclerosis. Circ Res. (2015) 116(5):789–96. doi: 10.1161/CIRCRESAHA.116.305811
136. Mendez PL, Obendorf L, Jatzlau J, Burdzinski W, Reichenbach M, Nageswaran V, et al. Atheroprone fluid shear stress-regulated ALK1-endoglin-SMAD signaling originates from early endosomes. BMC Biol. (2022) 20(1):210. doi: 10.1186/s12915-022-01396-y
137. Chen JL, Lu XJ, Zou KL, Ye K. Krüppel-like factor 2 promotes liver steatosis through upregulation of CD36. J Lipid Res. (2014) 55(1):32–40. doi: 10.1194/jlr.M039453
138. Mwaikambo BR, Yang C, Chemtob S, Hardy P. Hypoxia up-regulates CD36 expression and function via hypoxia-inducible factor-1- and phosphatidylinositol 3-kinase-dependent mechanisms. J Biol Chem. (2009) 284(39):26695–707. doi: 10.1074/jbc.M109.033480
139. Wang K, Li Y, Luo C, Chen Y. Dynamic AFM detection of the oxidation-induced changes in size, stiffness, and stickiness of low-density lipoprotein. J Nanobiotechnology. (2020) 18(1):167. doi: 10.1186/s12951-020-00727-x
140. Wang K, Gan C, Wang H, Ao M, Fan Y, Chen Y. AFM detects the effects of acidic condition on the size and biomechanical properties of native/oxidized low-density lipoprotein. Colloids Surf B Biointerfaces. (2021) 208:112053. doi: 10.1016/j.colsurfb.2021.112053
141. Gomez-Garcia MJ, Doiron AL, Steele RRM, Labouta HI, Vafadar B, Shepherd RD, et al. Nanoparticle localization in blood vessels: dependence on fluid shear stress, flow disturbances, and flow-induced changes in endothelial physiology. Nanoscale. (2018) 10(32):15249–61. doi: 10.1039/C8NR03440K
142. Freese C, Schreiner D, Anspach L, Bantz C, Maskos M, Unger RE, et al. In vitro investigation of silica nanoparticle uptake into human endothelial cells under physiological cyclic stretch. Part Fibre Toxicol. (2014) 11:68. doi: 10.1186/s12989-014-0068-y
Keywords: atherosclerosis, endocytosis, biomechanics, lipid transportation, vascular cells
Citation: Wang J, Xu J, Liu T, Yu C, Xu F, Wang G, Li S and Dai X (2024) Biomechanics-mediated endocytosis in atherosclerosis. Front. Cardiovasc. Med. 11:1337679. doi: 10.3389/fcvm.2024.1337679
Received: 13 November 2023; Accepted: 4 March 2024;
Published: 4 April 2024.
Edited by:
Angeliki Chroni, National Centre of Scientific Research Demokritos, Greece© 2024 Wang, Xu, Liu, Yu, Xu, Wang, Li and Dai. This is an open-access article distributed under the terms of the Creative Commons Attribution License (CC BY). The use, distribution or reproduction in other forums is permitted, provided the original author(s) and the copyright owner(s) are credited and that the original publication in this journal is cited, in accordance with accepted academic practice. No use, distribution or reproduction is permitted which does not comply with these terms.
*Correspondence: Jianxiong Xu eHVqaWFueGlvbmdAbWFpbC54aHUuZWR1LmNu Xiaozhen Dai ZGFpeGlhb3poZW5AY21jLmVkdS5jbg==
Abbreviations LDL, low density lipoprotein; VLDL, very low density lipoprotein; LDLR, low density lipoprotein receptor; ECs, endothelial cells; LSS, Low shear stress; OSS, oscillatory shear stress; APs, adaptor proteins; oxLDL, oxidized low-density lipoprotein; Cav1, caveolin-1; VSMCs, vascular smooth muscle cells; NO, nitric oxide; TRP, transient receptor potential; ROS, reactive oxygen species.