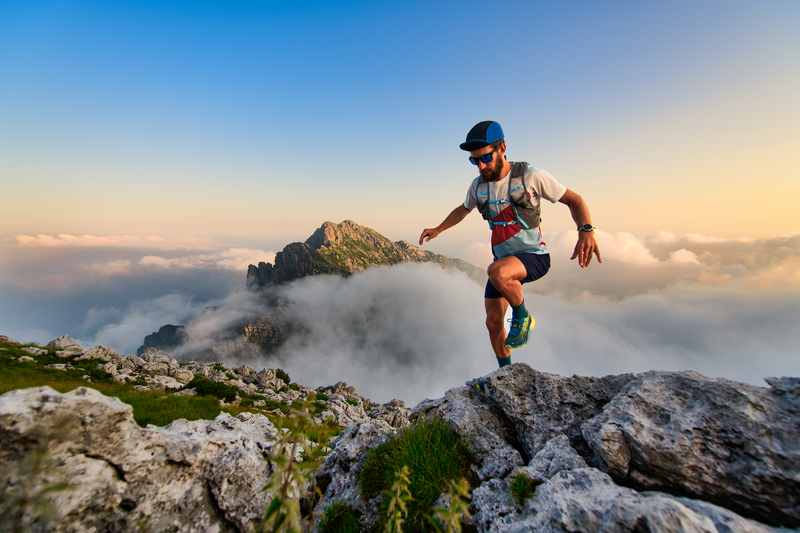
94% of researchers rate our articles as excellent or good
Learn more about the work of our research integrity team to safeguard the quality of each article we publish.
Find out more
ORIGINAL RESEARCH article
Front. Cardiovasc. Med. , 21 March 2024
Sec. Cardio-Oncology
Volume 11 - 2024 | https://doi.org/10.3389/fcvm.2024.1286620
Background: Chemotherapy with anthracyclines can cause cardiotoxicity, possibly leading to stopping treatment in some cancer patients. In cardio-oncology research, preventing and minimizing anthracycline-induced cardiotoxicity (AIC) is a hot issue. For the treatment of AIC, calycosin (CA), an isoflavone component in astragali radix (AR), has become a research focus. However, the elaborate mechanisms of calycosin treating AIC remain to be unrevealed.
Aim of the study: To explore the effects of CA on AIC through multiple dimensions concerning network pharmacology, molecular docking, and experimental evaluations.
Methods: The study evaluated calycosin's potential targets and mechanisms for treating AIC using network pharmacology and molecular docking. The candidate genes/targets of CA and AIC were screened using the online-available database. Protein-protein interactions (PPI) between the common targets were constructed using the STRING platform, and the results were then visualized using Cytoscape. Molecular docking was used to evaluate the strength of the binding force between CA and the common targets. The possible pharmacological mechanisms of CA were explained by pathway enrichment and GSEA. Subsequently, the candidate targets were identified in vitro experiments.
Results: Network pharmacology effectively discovered the CA's multitarget intervention in AIC, including TNF, ABCC1, TOP2A, ABCB1, and XDH. CA binds to the ATP-binding cassette subfamily B member 1(ABCB1) had the highest binding energy (−7.5 kcal/mol) according to the molecular docking analysis and was selected and visualized for subsequent analysis. In vitro experiments showed that ABCB1 exhibited significant time-curve changes under different doses of doxorubicin (DOX) compared with DMSO control experiments. The anti-AIC pharmacological mechanism of CA were revealed by highlighting the biological processes of oxidative stress (OR) and inflammation.
Conclusions: We employed a practicable bioinformatics method to connect network and molecular docking to determine the calycosin's therapeutic mechanism against AIC and identified some bioinformatics results in in vitro experiments. The results presented show that CA may represent an encouraging treatment for AIC.
Anthracycline antineoplastic agents (ANTs), such as doxorubicin (DOX) and epirubicin, have been perceived as one of the most effective anticancer drugs widely employed in solid tumors and hematologic malignancies; however, its dose-dependent cardiotoxicity, which affects the progress of subsequent treatment to a great extent (1–4), limits its clinical practice in a great measure. ANTs-induced cardiotoxicity (AIC), described by heart function or structure changes, can lead to a progressive cardiomyopathy and ultimately lead to heart failure (5). AIC research mainly focuses on oxidative stress (6), mitochondrial dysfunction (7), inflammation (8), autophagy, apoptosis (9, 10), pyroptosis (11), ferroptosis (12), and altered Ca2+ homeostasis (13). The role of oxidative stress, which represents an imbalance between the production of ROS and antioxidant protection effect (14), in the development and progression of AIC is an essential academic area that is currently well-studied (6, 10, 15, 16). Although multiple drugs have been demonstrated to mitigate the adverse effects of DOX (17, 18), dexrazoxane is the only prescription authorized by the U.S. Food and Drug Administration for the treatment of DOX induced cardiotoxicity (19). However, clinics restrict it due to serious side effects that increase the risk of myelodysplastic syndrome and acute myeloid leukemia. Thus, to better prevent and manage this challenge, there is a pressing need for more effective therapeutic agents and strategies.
Natural compounds have a significant role in oncology drug research as well as drug discovery. Researchers have discovered that certain components in traditional Chinese medicine may counteract the harmful effects of chemoradiotherapy on the heart while maintaining the treatment's ability to fight tumors (17). This could significantly raise the survival rates and quality of life for people receiving this treatment. For example, calycosin (C16H12O5, CA; Figure 1), an isoflavonoid that is the major active component present in Radix astragalithe, is growing into a highly valued herb used in traditional Chinese medicine to treat cardiovascular disease (18, 19). CA has been interpreted in numerous bioactivities, including anti-inflammatory, antioxidative, antiapoptotic, anticancer, immunomodulating, and cardiovascular protection (20–26). Yet, there have been few studies addressing the role of calycosin in drug-induced myocardial damage. Following CA therapy in vivo and in vitro, the DOX-induced damage to cardiomyocytes was significantly reduced. Furthermore, the reduction of ROS generation, inflammation, autophagy, apoptosis, and pyroptosis could be responsible for the cardioprotective properties of CA. By preventing the expression of pro-inflammatory chemicals, CA serves to protect cardiomyocytes from DOX-induced injury. This has been mainly shown by a reduction in NLRP3 and systemic inflammation. In regards to one study, CA can reduce DOX-induced cardiotoxicity while also lowering cell apoptosis and inhibiting oxidative stress both in vivo and in vitro by modulating the Sirt1-NLRP3 pathway (20, 27). Through the NLRP3-caspase-1-GSDMD pathway in vitro and in vivo experiments, CA reduces doxorubicin-induced ROS, mitochondrial damage, and cardiomyocyte pyroptosis, a crucial immune response that is linked to pro-inflammatory effects (26). Furthermore, it has been reported that CA modifies Atg7-related autophagy to protect against DOX-induced cardiotoxicity (28). Calycosin improves embryo viability and reduces pericardial edema and morphological changes induced by doxorubicin in a zebrafish model (28). However, the findings are still debatable. It is known that inflammation plays a role in DOX-induced cardiotoxicity. However, inflammation is even worse once cardiotoxicity manifests (29). Preliminary evidence suggests that CA's cardioprotective action is closely related to its claimed bioactivities, although some of this correlation remains controversial. There has been no systematic investigation into the effects and pharmacological actions of calycosin against AIC.
Figure 1. A strategy combining network pharmacology, molecular docking, and experimental evaluations to highlight the core targets and biological functions of calycosin treating AIC. The common targets of CA treating AIC and AIC were screened using network pharmacology. The Molecular docking was the used to evaluate the candidate targets and the candidate targets were subsequently identified in vitro experiments.The possible pharmacological mechanisms of CA were explained by pathway enrichment and GSEA.
Therefore, we proposed the notion that the cotreatment of CA with chemotherapeutic drugs would play an impact boosting and toxicity decreasing role, taking use of the aforementioned features. The cardioprotective effects and potential mechanisms of CA on doxorubicin-DOX-induced cardiotoxicity merit further research given that numerous active ingredients in traditional Chinese medicine exhibit noteworthy protective effects on DOX-induced cardiotoxicity through interactions with multiple targets.
Network pharmacology, analyzing the molecular association between drugs and treatment targets and revealing the systematic pharmacological mechanisms of drugs from a system-level perspective using a network, was first proposed in 2007 (30). Drug development will become more cost-effective owing to network pharmacology-based drug research and the burgeoning fields of bioinformatics and integrated pharmacology. By modelling the interactions between ligands and receptors, molecular docking can also forecast binding capability and conformation accurately (31). The current network pharmacology and molecular docking-based approach identified the potential targets, functions, and signaling pathways of calycosin against AIC. It is presumed that functional regulation of these ideal biological processes and signaling pathways by calycosin could contribute to an anti-AIC effect. Additionally, calycosin’s principal pharmacological biotargets have been assessed.
This study involved bioinformatics analysis only and thus did not require medical ethics approval. The study followed the Declaration of Helsinki (as revised in 2013).
The molecular structure of calycosin was downloaded from PubChem (https://pubchem.ncbi.nlm.nih.gov/) (32). The therapeutic targets of calycosin were collected in the PubMed database, Traditional Chinese Medicine Systems Pharmacology Database and Analysis Platform (TCMSP; https://old.tcmsp-e.com/tcmsp.php), Chemical-Protein Interaction Networks (STITCH; http://stitch.embl.de/), Swiss Target Prediction (https://www.swisstargetprediction.ch/), SuperPred web server (https://prediction.charite.de/), Bioinformatics Analysis Tool for Molecular mechANism of Traditional Chinese Medicine (BATMANTCM; http://bionet.ncpsb.org.cn/batman-tcm/), and ChemMapper (http://www.lilab-ecust.cn/chemmapper). All the datebase used “calycosin” or the molecular structure of calycosin as search keywords, and only collected species limited as “Homo sapiens”.
The ACT-related targets were identified using the query term “anthracycline, cardiotoxicity” from PharmGkb database (https://www.pharmgkb.org/), OMIM database (https://www.omim.org/) (33), GeneCards database (https://www.genecards.org/) (34), The genetic association database (GAD) database (https://geneticassociationdb.nih.gov/) and DISGNET database (https://www.disgenet.org/home/), which offers information about disease targets.
After the genes/targets of calycosin and AIC had been mapped by using R language (R version 4.2.1), the overlapping targets determined by the Venn diagram were selected as the predictive targets of calycosin against AIC.
To clarify the interaction of the genes targeted for therapy, the acquirable targets of calycosin and AIC were connected methodologically to create a calycosin-based anti-AIC network through a tool of the Search Tool for the Retrieval of Interacting Genes/Proteins database (STRING, https://string-db.org/) (35). The common targets obtained by Venn analysis were input into the STRING platform. The species were set to “Homo sapiens,” and the minimum required interaction score was “high confidence.” Furthermore, these screened targets were further analyzed after creating a protein-protein interaction (PPI) network based on the Cytoscape tool. Previous studies have shown that proteins exert biological activities through protein-protein interactions (PPI).
To identify underlying functional processes and signaling pathways of calycosin treating AIC, the Gene Ontology (GO) pathway enrichment analysis was carried out using the R packages ClusterProfiler (version 4.4.4) and AnnotationHub (version 1.60.2). The parameters were as follows: the minimum number of genes was 2, the maximum number of genes was 5,000, and the P value was <0.05. Gene set enrichment analysis (GSEA) was performed using ClusterProfiler (version 4.4.4) to quantify the associations between the target gene and each gene set. The genes were ranked based on the strength of the association between the target gene and the RNA sequencing data, quantified by Pearson's correlation coefficients. Gene sets with a false discovery rate (FDR) <0.25 were considered significant.
In order to investigate the the binding affinities between calycosin molecule and its predicted proteins, molecular docking was performed. Molecular docking using Autodock Vina 1.5.6 software (http://autodock.scripps.edu/) is generally divided into four steps: preparation of protein, preparation of ligand and the standard inhibitor, docking and analysis of results.
The protein structure was downloaded from Protein Data Bank (https://www.rcsb.org/), while the crystal structure of CA and the standard inhibitor were downloaded from PubChem databases (https://pubchem.ncbi.nlm.nih.gov/) (39), then converted to three-dimensional (3D) structure and energy minimized using OpenBabel 2.4.1. The ligands and proteins were prepared through Autodock Vina, including removal of water molecules, addition with hydrogens and so on. The prepared pdb files of the ligand and proteins were then converted into the pdbqt format by assigning charges using Autodock Vina.
The protein file to be docked was selected in macromolecular input of Autodock Vina, and the ligand file to be docked was selected in ligand input. Secondly, the active pocket (the position of the original ligand in the protein crystal, including all residues around the original ligand) on protein structure was determined by GetBox-PyMOL-Plugin (https://github.com/MengwuXiao/GetBox-PyMOL-Plugin). The values of the utilized parameters in Autodock Vina software were presented in Supplementary Table S1.
The docking results showed 9 different conformations, ranked according to the values of predicted binding-energy value, and higher binding energy indicate a greater possibility of the the drug binding to the protein. The docking models with the highest binding-energy were selected and visualized for subsequent analysis. The PyMOL Molecular Graphics System,version 2.5.5 (https://pymol.org/2/) was then used to prepare the figures to visualize the drug-protein interactions.
The human cardiac microtissues, containing 4,000 iPS-derived human cardiomyocytes from a female Caucasian donor and 1,000 cardiac fibroblasts from a male Caucasian donor, were exposed to DOX for 2 weeks. The cardiac microtissues were subjected to incubation with either a therapeutic or toxic dosage. The therapeutic dosage is equivalent to the clinical dosage, while the toxic dosage is based on the IC20 value determined through ATP production (cell viability) after a 7-day exposure (36). The RNA expression data were harvested after 2, 8, 24, 72, 168, 240, and 336 h of DOX exposure with 3 replicates per dose. MaSigPro (37) was used to utilize the time-series data generated by each gene to estimate its temporal response and the importance of the deviation of the treatment-time curve from the respective control curve. The above information is publicly accessible at the Hepatic and Cardiac Toxicity Systems modeling project funded by the European Union Seventh Framework Programme (FP7/2007-2013) (38).
The flowchart of this article is shown in Figure 1.
The study evaluated calycosin's potential targets and mechanisms for treating AIC using network pharmacology and molecular docking. In the present study, 226 drug-related targets of CA and 148 AIC disease-related targets were gathered. Drug-related and disease-related targets were intersected, and 5 central genes/targets of calycosin for AIC were displayed in a Venn diagram (Figure 2). And the central targets are detailed as TNF, ABCC1, TOP2A, ABCB1, and XDH. These interceptive targets in the Venn diagram were used to further build an optimal PPI network in order to investigate the protein interactions and putative mechanism of CA in treating AIC. This network effectively displays the CA's multitarget intervention in AIC.
Figure 2. All candidate biotargets of calycosin and AIC were identified using network pharmacology, and then a PPI network from these biotargets was represented. This network effectively displays the CA's multitarget intervention in AIC. Left: Venn diagram of targets shared by CA and AIC. 226 drug-related targets of CA and 148 AIC disease-related targets were gathered. Right: Common targets screening in the PPI network. Drug-related and disease-related targets were intersected, and 5 central genes/targets of calycosin for AIC were displayed. PPI, protein-protein interaction.
All central targets of calycosin for anti-AIC were enriched to display molecular pathways. As a result, the major targets could be divided into various functional categories by Gene Ontology enrichment analysis, including positive regulation of inflammatory response, response to oxidative stress, and cellular response to oxidative stress (Figure 3).
Figure 3. Calycosin's essential biological and functional roles in combating AIC by gene ontology enrichment analysis. The color represents the size of the P value. Redder colors indicate smaller P values and closer relationships. Bluer colors indicate larger P values and more distant relationships.
Molecular docking analysis was used to evaluate the binding energies of the CA for all central targets. The results indicated that CA (PubChem CID 5280448) binds to ABCB1 (PDB ID, 7A69; resolution, 3.2 Å) (39) had the highest binding energy (−7.5 kcal/mol). According to broad consensus, a docking score of less than 0 kcal/mol means that the component can spontaneously interact to the target, a score of less than −4.25 kcal/mol means that the docking affinity is good, and a score of less than −7 kcal/mol means that the component has strong docking affinity. The standard inhibitor verapamil (PubChem CID 2520) used to compare the docking efficacy, showed −6.0 kcal/mole binding efficacy. The 3D binding diagram, hydrogen bonding diagram and the amino acid residues were displayed in Supplementary Figure S1. The values of binding energies of the CA for other central targets and the utilized parameters in Autodock Vina software were presented in Supplementary Tables S1,S2. Figure 4 displays the 3D binding diagram and hydrogen bonding diagram, along with detailed information on molecular docking. It can be seen that CA has developed many hydrogen bonds with the amino acid residues ARG100, SER-30, GLY-83and ASP-973.
Figure 4. The 3D diagrams of calycosin and its binding to ABCB1 are shown. The yellow image represents CA and yellow hydrogen bonds connect the binding sites. The length of the hydrogen bonds is indicated next to the bond. The protein target that binds to CA is grey, and the amino acid residues is green.
To identify the target genes, human 3D cardiac microtissues subjected to either a therapeutic dose or a toxic dose of DOX were evaluated. By comparing the DOX treatment time profiles with control profiles from time-matched DMSO-treated microtissues, we found that ABCB1 displayed significant changes in time profiles with different doses compared to the DMSO control experiments (Figure 5). Gene set enrichment analysis (GSEA) was used to identify various pathways with ABCB1, and ABCB1 was most significantly connected with HALLMARK_OXIDATIVE_PHOSPHORYLATION, HALLMARK_MYOGENESIS, HALLMARK_PEROXISOME (Supplementary Figure S2).
Figure 5. The dynamic changes of ABCB1 in vitro human 3D cardiac microtissues subjected to either a therapeutic dose or a toxic dose of DOX. The: therapeutic dose, Tox: toxic dose; 002, 008, 024, 072, 168, 240, 336 are corresponding exposure periods in hours; Dox: doxorubicin.
Anthracycline chemotherapeutics, addressed by doxorubicin, play an important role in treating various cancers. Unfortunately, side effects such as cardiotoxicity have severely confined the clinical application of these drugs. AIC's precise mechanism remains unclear. Calycosin, as a multi-target anti-tumor drug, has been widely studied. However, the effectiveness of CA for treating AIC and the underlying molecular mechanism has not yet been clarified. Hopkins first introduced network pharmacology, which has been applied to investigate pharmacological effects or show the synergism function mechanism of multiple component drugs (40). The powerful methodology of joining network pharmacology with molecular docking might assist targets and pathways for calycosin in AIC treatment. Based on the network pharmacology integrated molecular docking, we concluded that the main target of CA in treating AIC might be ABCB1. Additionally, the biological mechanisms of oxidative stress (OR) and inflammation were emphasized, illuminating the pharmacological underpinnings of calycosin's anti-AIC effects.
A total of 5 cross-targets of CA and AIC were obtained by constructing a Venn diagram, and the leading core target was found to be ABCB1 through molecular docking analysis. ABCB1 exhibited the strongest binding affinity with calycosin, suggesting that this gene could serve as a powerful pharmacological target to combat AIC. ABCB1(P-gp, MDR1) (41) is one of the membrane transporters expressed at high levels in cardiomyocytes and cardiac capillary endothelial cells of the mammalian heart. It is responsible for DOX cell influx and efflux and regulates both intra- and extracellular concentrations and bioavailability of the drug and its metabolites. Recent research has revealed that tissue-specific transporter expression is involved in local drug accumulation and drug-drug interactions and that perturbations to these transporters’ functions could determine the vulnerability an individual is to drug-induced toxicity. ABCB1 typically works to extrude drugs or toxic xenobiotic substances from cells or tissues and has been specifically studied with cardiotoxicity (42, 43). It is speculated that ABCB1 transports anthracyclines extracellularly and reduces their intracellular accumulation, resulting in the resistance of cancer cells and the protection of healthy cells. Numerous animal models have demonstrated that inhibiting endogenous ABCB1 increases the risk of doxorubicin-induced cardiotoxicity (44, 45). In the hearts of Abcb1a_/_ mice, despite only a 1.2-fold increase in plasma levels compared to wild-type mice, the retention of doxorubicin and its main metabolite, doxorubicin, is substantially prolonged (45). In the hearts of HSF-1/_ mice heart, the increased expression of ABCB1 enhanced the extrusion of doxorubicin and diminished left ventricular dysfunction (46). Notwithstanding, clinical proof of this proposition remains controversial (47). It is also worth pointing out that ABCB1 transports many cancer drugs very efficiently but still causes shifting levels of cardiotoxicity in patients, an observation that appears to be conflicting with ABCB1 serving a vital protective capability. The link between genetic polymorphisms in the ABCB1 gene and cardiotoxicity was identified (43). The variant allele of ABCB1 3435C>T (rs1045642) had an additive protective effect against cardiotoxicity in breast cancer patients treated with anthracyclines (43), and the results remained nominally significant after adjustment for clinical covariates. According to a meta-analysis, ABCB1 rs1045642 is associated with a lower risk of developing AIC (48). Only a little amount of research was conducted on the targets relating calycosin against AIC.
Our results showed that calycosin ameliorates AIC involved with oxidative stress (OR) and inflammation, which were representatively validated in vivo and in vitro. The most significant molecular mechanisms for the pathophysiology of chemoradiotherapy cardiotoxicity are OR and inflammation. Oxidative stress is brought on by the breakdown of redox equilibrium, which is manifested by an increase in ROS and a decrease in antioxidant enzymes (15, 16). Inflammation is a key component of OR-related cardiotoxicity caused by chemotherapy drugs. Interleukin-1 (IL-1) and tumor necrosis factor (TNF) are two inflammatory factors that DOX increases in the heart, prompting inflammatory and immunological responses and impairing cardiomyocytes (49). NLRP3-related signaling pathways regulate the release of inflammatory factors. The NLRP3 inflammasome is a key mediator of the innate immune system, mediating caspase-1 activation and secretion of the proinflammatory cytokines IL-1β/IL-18 (50). NLRP3 inflammatory vesicles are activated by DOX-induced ROS (11), and calycosin may reduce inflammation and OR by boosting the levels of NLRP3 and associated proteins in cells and mouse hearts (20, 51). Calycosin minimized AIC and increased the viability of rat cardiomyocytes by blocking the NLRP3/caspase-1/GSDMD pathway (26, 52). According to a similar study, CA can reduce DOX-induced cardiotoxicity via controlling the Sirt1-NLRP3 pathway, which also reduces oxidative stress both in vivo and in vitro (20).
This study also has some limitations. Calycosin is a monomer molecule with a definitive chemical structure characterized by low toxicity and a range of biological effects (53). This makes it more conducive to research and applications in pharmacology research. Calycosin and its derivatives have good cardiovascular protective potential. However, existing studies have only addressed the role of calycosin in ischemic disease, myocardial hypertrophy, and viral myocarditis. Fewer studies have examined pathological circumstances such as drug-induced myocardial damage. Robust patient cohorts in clinical trials are needed to confirm the efficacy of calycosin. Furthermore, drug targets involved in cardiotoxicity need larger samples to expand. Thirdly, although the key targets and pathways can be screened in the research, further phytochemical and pharmacological research is needed to determine the exact mechanism. Significantly, calycosin may influence the corresponding signaling pathways under different pathological conditions.
The study established a network pharmacology-integrated molecular docking strategy, and through partial in vitro verification, highlighted the therapeutic targets, functional processes, and molecular mechanisms of calycosin for AIC through partial verification in vitro. Based on the network pharmacology integrated molecular docking, we concluded that the main target of CA in treating AIC might be ABCB1. Additionally, the biological mechanisms of oxidative stress (OR) and inflammation were emphasized, illuminating the pharmacological underpinnings of calycosin's anti-AIC effects. Additional validated experiments will be performed in a preclinical research during subsequent studies. The foundation for the clinical application is laid by this study's effective combination technique for systematically elucidating medication therapeutic mechanisms.
Publicly available datasets were analyzed in this study. This data can be found here: Hepatic and Cardiac Toxicity Systems modeling project funded by the European Union Seventh Framework Programme (FP7/2007-2013). Further inquiries can be directed to the corresponding author.
Ethical approval was not required for the studies on humans in accordance with the local legislation and institutional requirements because only commercially available established cell lines were used.
ZP: Conceptualization, Data curation, Investigation, Methodology, Software, Writing – review & editing, Formal Analysis, Resources, Validation, Visualization, Writing – original draft. RQ: Conceptualization, Formal Analysis, Investigation, Software, Supervision, Validation, Writing – review & editing, Funding acquisition. ZR: Data curation, Software, Visualization, Writing – review & editing. ZL: Formal Analysis, Investigation, Software, Visualization, Writing – original draft. XX: Conceptualization, Investigation, Software, Supervision, Writing – review & editing. ZC: Supervision, Validation, Writing – review & editing. YT: Conceptualization, Data curation, Investigation, Methodology, Project administration, Software, Supervision, Writing – review & editing.
The author(s) declare financial support was received for the research, authorship, and/or publication of this article.
This study was supported by the Wuhan Knowledge Innovation Special (Item Number: 2022020801020532) and the 2022 Molecular Imaging Hubei Provincial Key Laboratory Open Fund.
The authors declare that the research was conducted in the absence of any commercial or financial relationships that could be construed as a potential conflict of interest.
All claims expressed in this article are solely those of the authors and do not necessarily represent those of their affiliated organizations, or those of the publisher, the editors and the reviewers. Any product that may be evaluated in this article, or claim that may be made by its manufacturer, is not guaranteed or endorsed by the publisher.
The Supplementary Material for this article can be found online at: https://www.frontiersin.org/articles/10.3389/fcvm.2024.1286620/full#supplementary-material
Supplementary Figure S1
The 3D diagrams of the standard inhibitor and its binding to ABCB1.
Supplementary Figure S2
The ABCB1 associated pathways based the GSEA analysis.
Supplementary Table S1
The utilized parameters in Autodock Vina software.
Supplementary Table S2
Docking score of CA and the central targets.
1. Bernstein D. Anthracycline cardiotoxicity: worrisome enough to have you quaking? Circ Res. (2018) 2:188–90. doi: 10.1161/CIRCRESAHA.117.312395
2. Albini A, Pennesi G, Donatelli F, Cammarota R, De Flora S, Noonan DM. Cardiotoxicity of anticancer drugs: the need for cardio-oncology and cardio-oncological prevention. J Natl Cancer Inst. (2010) 1:14–25. doi: 10.1093/jnci/djp440
3. Zamorano JL, Lancellotti P, Rodriguez MD, Aboyans V, Asteggiano R, Galderisi M, et al. 2016 ESC position paper on cancer treatments and cardiovascular toxicity developed under the auspices of the ESC committee for practice guidelines: the task force for cancer treatments and cardiovascular toxicity of the European society of cardiology (ESC). Eur Heart J. (2016) 36:2768–801. doi: 10.1093/eurheartj/ehw211
4. Curigliano G, Cardinale D, Dent S, Criscitiello C, Aseyev O, Lenihan D, et al. Cardiotoxicity of anticancer treatments: epidemiology, detection, and management. CA Cancer J Clin. (2016) 4:309–25. doi: 10.3322/caac.21341
5. Banke A, Fosbøl EL, Møller JE, Gislason GH, Andersen M, Bernsdorf M, et al. Long-term effect of epirubicin on incidence of heart failure in women with breast cancer: insight from a randomized clinical trial. Eur J Heart Fail. (2018) 10:1447–53. doi: 10.1002/ejhf.1168
6. Wenningmann N, Knapp M, Ande A, Vaidya TR, Ait-Oudhia S. Insights into doxorubicin-induced cardiotoxicity: molecular mechanisms, preventive strategies, and early monitoring. Mol Pharmacol. (2019) 2:219–32. doi: 10.1124/mol.119.115725
7. Varga ZV, Ferdinandy P, Liaudet L, Pacher P. Drug-induced mitochondrial dysfunction and cardiotoxicity. Am J Physiol Heart Circ Physiol. (2015) 9:H1453–67. doi: 10.1152/ajpheart.00554.2015
8. Wang ZQ, Chen MT, Zhang R, Zhang Y, Li W, Li YG. Docosahexaenoic acid attenuates doxorubicin-induced cytotoxicity and inflammation by suppressing NF-κB/iNOS/NO signaling pathway activation in H9C2 cardiac cells. J Cardiovasc Pharmacol. (2016) 4:283–9. doi: 10.1097/FJC.0000000000000350
9. Prathumsap N, Shinlapawittayatorn K, Chattipakorn SC, Chattipakorn N. Effects of doxorubicin on the heart: from molecular mechanisms to intervention strategies. Eur J Pharmacol. (2020) 866:172818. doi: 10.1016/j.ejphar.2019.172818
10. Mantawy EM, El-Bakly WM, Esmat A, Badr AM, El-Demerdash E. Chrysin alleviates acute doxorubicin cardiotoxicity in rats via suppression of oxidative stress, inflammation and apoptosis. Eur J Pharmacol. (2014) 728:107–18. doi: 10.1016/j.ejphar.2014.01.065
11. Tavakoli DZ, Singla DK. Embryonic stem cell-derived exosomes inhibit doxorubicin-induced TLR4-NLRP3-mediated cell death-pyroptosis. Am J Physiol Heart Circ Physiol. (2019) 2:H460–71. doi: 10.1152/ajpheart.00056.2019
12. Fang X, Wang H, Han D, Xie E, Yang X, Wei J, et al. Ferroptosis as a target for protection against cardiomyopathy. Proc Natl Acad Sci U S A. (2019) 7:2672–80. doi: 10.1073/pnas.1821022116
13. Osataphan N, Phrommintikul A, Chattipakorn SC, Chattipakorn N. Effects of doxorubicin-induced cardiotoxicity on cardiac mitochondrial dynamics and mitochondrial function: insights for future interventions. J Cell Mol Med. (2020) 12:6534–57. doi: 10.1111/jcmm.15305
14. Tian Z, Li X, Ma Y, Chen T, Xu D, Wang B, et al. Quantitatively intrinsic biomimetic catalytic activity of nanocerias as radical scavengers and their ability against H(2)O(2) and doxorubicin-induced oxidative stress. ACS Appl Mater Interfaces. (2017) 28:23342–52. doi: 10.1021/acsami.7b04761
15. Octavia Y, Tocchetti CG, Gabrielson KL, Janssens S, Crijns HJ, Moens AL. Doxorubicin-induced cardiomyopathy: from molecular mechanisms to therapeutic strategies. J Mol Cell Cardiol. (2012) 6:1213–25. doi: 10.1016/j.yjmcc.2012.03.006
16. Kim SY, Kim SJ, Kim BJ, Rah SY, Chung SM, Im MJ, et al. Doxorubicin-induced reactive oxygen species generation and intracellular Ca2+ increase are reciprocally modulated in rat cardiomyocytes. Exp. Mol. Med. (2006) 5:535–45. doi: 10.1038/emm.2006.63
17. Yang X, Liu N, Li X, Yang Y, Wang X, Li L, et al. A review on the effect of traditional Chinese medicine against anthracycline-induced cardiac toxicity. Front Pharmacol. (2018) 9:444. doi: 10.3389/fphar.2018.00444
18. Zhang S, Liu X, Bawa-Khalfe T, Lu LS, Lyu YL, Liu LF, et al. Identification of the molecular basis of doxorubicin-induced cardiotoxicity. Nat Med. (2012) 11:1639–42. doi: 10.1038/nm.2919
19. Jia Z, Wang X, Wang X, Wei P, Li L, Wu P, et al. Calycosin alleviates allergic contact dermatitis by repairing epithelial tight junctions via down-regulating HIF-1α. J Cell Mol Med. (2018) 9:4507–21. doi: 10.1111/jcmm.13763
20. Zhai J, Tao L, Zhang S, Gao H, Zhang Y, Sun J, et al. Calycosin ameliorates doxorubicin-induced cardiotoxicity by suppressing oxidative stress and inflammation via the sirtuin 1-NOD-like receptor protein 3 pathway. Phytother Res. (2020) 3:649–59. doi: 10.1002/ptr.6557
21. Yarmohammadi F, Hayes AW, Karimi G. Natural compounds against cytotoxic drug-induced cardiotoxicity: a review on the involvement of PI3K/akt signaling pathway. J Biochem Mol Toxicol. (2021) 3:e22683. doi: 10.1002/jbt.22683
22. Gao J, Liu ZJ, Chen T, Zhao D. Pharmaceutical properties of calycosin, the major bioactive isoflavonoid in the dry root extract of radix astragali. Pharm Biol. (2014) 9:1217–22. doi: 10.3109/13880209.2013.879188
23. Chen J, Liu L, Hou R, Shao Z, Wu Y, Chen X, et al. Calycosin promotes proliferation of estrogen receptor-positive cells via estrogen receptors and ERK1/2 activation in vitro and in vivo. Cancer Lett. (2011) 2:144–51. doi: 10.1016/j.canlet.2011.04.022
24. Ma R, Yuan F, Wang S, Liu Y, Fan T, Wang F. Calycosin alleviates cerulein-induced acute pancreatitis by inhibiting the inflammatory response and oxidative stress via the p38 MAPK and NF-κB signal pathways in mice. Biomed Pharmacother. (2018) 105:599–605. doi: 10.1016/j.biopha.2018.05.080
25. Deng M, Chen H, Long J, Song J, Xie L, Li X. Calycosin: a review of its pharmacological effects and application prospects. Expert Rev Anti Infect Ther. (2021) 7:911–25. doi: 10.1080/14787210.2021.1863145
26. Zhang L, Fan C, Jiao HC, Zhang Q, Jiang YH, Cui J, et al. Calycosin alleviates doxorubicin-induced cardiotoxicity and pyroptosis by inhibiting NLRP3 inflammasome activation. Oxid Med Cell Longev. (2022) 2022:1733834. doi: 10.1155/2022/1733834
27. Liu B, Zhang J, Liu W, Liu N, Fu X, Kwan H, et al. Calycosin inhibits oxidative stress-induced cardiomyocyte apoptosis via activating estrogen receptor-α/β. Bioorg Med Chem Lett. (2016) 1:181–5. doi: 10.1016/j.bmcl.2015.11.005
28. Lu X, Lu L, Gao L, Wang Y, Wang W. Calycosin attenuates doxorubicin-induced cardiotoxicity via autophagy regulation in zebrafish models. Biomed Pharmacother. (2021) 137:111375. doi: 10.1016/j.biopha.2021.111375
29. Maayah ZH, Takahara S, Dyck J. The beneficial effects of reducing NLRP3 inflammasome activation in the cardiotoxicity and the anti-cancer effects of doxorubicin. Arch Toxicol. (2021) 1:1–9. doi: 10.1007/s00204-020-02876-2
30. Ren L, Zheng X, Liu J, Li W, Fu W, Tang Q, et al. Network pharmacology study of traditional Chinese medicines for stroke treatment and effective constituents screening. J Ethnopharmacol. (2019) 242:112044. doi: 10.1016/j.jep.2019.112044
31. Pinzi L, Rastelli G. Molecular docking: shifting paradigms in drug discovery. Int J Mol Sci. (2019) 18:4331. doi: 10.3390/ijms20184331
32. Kim S. Getting the most out of PubChem for virtual screening. Expert Opin Drug Discov. (2016) 9:843–55. doi: 10.1080/17460441.2016.1216967
33. Amberger JS, Bocchini CA, Schiettecatte F, Scott AF, Hamosh A. OMIM.Org: online mendelian inheritance in man (OMIM®), an online catalog of human genes and genetic disorders. Nucleic Acids Res. (2015) 43(Database issue):D789–98. doi: 10.1093/nar/gku1205
34. Stelzer G, Rosen N, Plaschkes I, Zimmerman S, Twik M, Fishilevich S, et al. The GeneCards suite: from gene data mining to disease genome sequence analyses. Curr Protoc Bioinformatics. (2016) 54:1–30. doi: 10.1002/cpbi.5
35. Szklarczyk D, Morris JH, Cook H, Kuhn M, Wyder S, Simonovic M, et al. The STRING database in 2017: quality-controlled protein-protein association networks, made broadly accessible. Nucleic Acids Res. (2017) 45(D1):D362–8. doi: 10.1093/nar/gkw937
36. Verheijen M, Schrooders Y, Gmuender H, Nudischer R, Clayton O, Hynes J, et al. Bringing in vitro analysis closer to in vivo: studying doxorubicin toxicity and associated mechanisms in 3D human microtissues with PBPK-based dose modelling. Toxicol Lett. (2018) 294:184–92. doi: 10.1016/j.toxlet.2018.05.029
37. Nueda MJ, Tarazona S, Conesa A. Next maSigPro: updating maSigPro bioconductor package for RNA-Seq time series. Bioinformatics. (2014) 18:2598–602. doi: 10.1093/bioinformatics/btu333
38. Verheijen M, Sarkans U, Wolski W, Jennen D, Caiment F, Kleinjans J. Multi-omics HeCaToS dataset of repeated dose toxicity for cardiotoxic & hepatotoxic compounds. Sci Data. (2022) 1:699. doi: 10.1038/s41597-022-01825-1
39. Nosol K, Romane K, Irobalieva RN, Alam A, Kowal J, Fujita N, et al. Cryo-EM structures reveal distinct mechanisms of inhibition of the human multidrug transporter ABCB1. Proc Natl Acad Sci U S A. (2020) 42:26245–53. doi: 10.1073/pnas.2010264117
40. Hopkins AL. Network pharmacology: the next paradigm in drug discovery. Nat Chem Biol. (2008) 11:682–90. doi: 10.1038/nchembio.118
41. Schumacher T, Benndorf RA. ABC transport proteins in cardiovascular disease-a brief summary. Molecules. (2017) 4:589. doi: 10.3390/molecules22040589
42. Huang KM, Hu S, Sparreboom A. Drug transporters and anthracycline-induced cardiotoxicity. Pharmacogenomics. (2018) 11:883–8. doi: 10.2217/pgs-2018-0056
43. Hertz DL, Caram MV, Kidwell KM, Thibert JN, Gersch C, Seewald NJ, et al. Evidence for association of SNPs in ABCB1 and CBR3, but not RAC2, NCF4, SLC28A3 or TOP2B, with chronic cardiotoxicity in a cohort of breast cancer patients treated with anthracyclines. Pharmacogenomics. (2016) 3:231–40. doi: 10.2217/pgs.15.162
44. Durmus S, Naik J, Buil L, Wagenaar E, van Tellingen O, Schinkel AH. In vivo disposition of doxorubicin is affected by mouse Oatp1a/1b and human OATP1A/1B transporters. Int J Cancer. (2014) 7:1700–10. doi: 10.1002/ijc.28797
45. van Asperen J, van Tellingen O, Tijssen F, Schinkel AH, Beijnen JH. Increased accumulation of doxorubicin and doxorubicinol in cardiac tissue of mice lacking mdr1a P-glycoprotein. Br J Cancer. (1999) 1:108–13. doi: 10.1038/sj.bjc.6690019
46. Krishnamurthy K, Vedam K, Kanagasabai R, Druhan LJ, Ilangovan G. Heat shock factor-1 knockout induces multidrug resistance gene, MDR1b, and enhances P-glycoprotein (ABCB1)-based drug extrusion in the heart. Proc Natl Acad Sci U S A. (2012) 23:9023–8. doi: 10.1073/pnas.1200731109
47. Kolitz JE, George SL, Marcucci G, Vij R, Powell BL, Allen SL, et al. P-glycoprotein inhibition using valspodar (PSC-833) does not improve outcomes for patients younger than age 60 years with newly diagnosed acute myeloid leukemia: cancer and leukemia group B study 19808. Blood. (2010) 9:1413–21. doi: 10.1182/blood-2009-07-229492
48. Leong SL, Chaiyakunapruk N, Lee SW. Candidate gene association studies of anthracycline-induced cardiotoxicity: a systematic review and meta-analysis. Sci Rep. (2017) 1:39. doi: 10.1038/s41598-017-00075-1
49. Quagliariello V, Vecchione R, Coppola C, Di Cicco C, De Capua A, Piscopo G, et al. Cardioprotective effects of nanoemulsions loaded with anti-inflammatory nutraceuticals against doxorubicin-induced cardiotoxicity. Nutrients. (2018) 9:1304. doi: 10.3390/nu10091304
50. Kelley N, Jeltema D, Duan Y, He Y. The NLRP3 inflammasome: an overview of mechanisms of activation and regulation. Int J Mol Sci. (2019) 13:3328. doi: 10.3390/ijms20133328
51. Sun Z, Lu W, Lin N, Lin H, Zhang J, Ni T, et al. Dihydromyricetin alleviates doxorubicin-induced cardiotoxicity by inhibiting NLRP3 inflammasome through activation of SIRT1. Biochem Pharmacol. (2020) 175:113888. doi: 10.1016/j.bcp.2020.113888
52. Zhang L, Jiang YH, Fan C, Zhang Q, Jiang YH, Li Y, et al. MCC950 attenuates doxorubicin-induced myocardial injury in vivo and in vitro by inhibiting NLRP3-mediated pyroptosis. Biomed Pharmacother. (2021) 143:112133. doi: 10.1016/j.biopha.2021.112133
53. Yu DH, Bao YM, Wei CL, An LJ. Studies of chemical constituents and their antioxidant activities from Astragalus mongholicus bunge. Biomed Environ Sci. (2005) 5:297–301. https://pubmed.ncbi.nlm.nih.gov/16370311/
Keywords: network pharmacology, molecular docking, anthracycline, cardiotoxicity, calycosin
Citation: Peng Z, Qianqian R, Ruizhi Z, Licai Z, Xiangwen X, Chuansheng Z and Tianhe Y (2024) Exploring the effects of calycosin on anthracycline-induced cardiotoxicity: a network pharmacology, molecular docking, and experimental study. Front. Cardiovasc. Med. 11:1286620. doi: 10.3389/fcvm.2024.1286620
Received: 31 August 2023; Accepted: 11 March 2024;
Published: 21 March 2024.
Edited by:
John David Horowitz, University of Adelaide, AustraliaReviewed by:
Hong Li, Southern Medical University, China© 2024 Peng, Qianqian, Ruizhi, Licai, Xiangwen, Chuansheng and Tianhe. This is an open-access article distributed under the terms of the Creative Commons Attribution License (CC BY). The use, distribution or reproduction in other forums is permitted, provided the original author(s) and the copyright owner(s) are credited and that the original publication in this journal is cited, in accordance with accepted academic practice. No use, distribution or reproduction is permitted which does not comply with these terms.
*Correspondence: Ye Tianhe eWV0aWFuaGVAMTYzLmNvbQ==
Disclaimer: All claims expressed in this article are solely those of the authors and do not necessarily represent those of their affiliated organizations, or those of the publisher, the editors and the reviewers. Any product that may be evaluated in this article or claim that may be made by its manufacturer is not guaranteed or endorsed by the publisher.
Research integrity at Frontiers
Learn more about the work of our research integrity team to safeguard the quality of each article we publish.