- 1Division of Cardiology, Department of Medicine, Faculty of Medicine and Health Sciences, Stellenbosch University, Cape Town, South Africa
- 2Department of Health Sciences, Central University of Technology, Bloemfontein, South Africa
- 3Department of Cardiothoracic Surgery, Robert W.M. Frater Cardiovascular Research Centre, University of the Free State, Bloemfontein, South Africa
Introduction: Patients from developing countries who require heart valve surgery are younger and have less access to open heart surgery than those from developed countries. Transcatheter heart valves (THVs) may be an alternative but are currently unsuitable for young patients because of their inadequate durability. We developed and tested a THV utilizing two new types of decellularized bovine pericardial leaflets in an ovine model.
Methods: The two decellularized tissues [one with a very low dose (0.05%) of monomeric glutaraldehyde (GA) fixation and detoxification (DF) and the other without glutaraldehyde (DE)] were compared to an industry standard [Glycar—fixed with the standard dose (0.625%) of glutaraldehyde]. THVs were manufactured with the three tissue types and implanted in the pulmonary position of nine juvenile sheep for 180 days. Baseline and post-explantation evaluations were performed to determine the hemodynamic performance of the valves and their dynamic strength, structure, biological interaction, and calcification.
Results: Heart failure occurred in one animal due to incompetence of its Glycar valve, and the animal was euthanized at 158 days. The gradients over the Glycar valves were higher at the explant than at the implant, but the DE and DF valves maintained normal hemodynamic performance throughout the study. The DF and DE tissues performed well during the mechanical testing of explanted leaflets. Glycar tissue developed thick pannus and calcification. Compared to Glycar, the DF tissue exhibited reduced pannus overgrowth and calcification and the DE tissue exhibited no pannus formation and calcification. All tissues were endothelialized adequately. There was a striking absence of host ingrowth in the DE tissue leaflets, yet these leaflets maintained integrity and mechanical function.
Conclusion: In the juvenile sheep THV model, Glycar tissue developed significant pannus, calcification, and hemodynamic deterioration. Using a very low dose of monomeric GA to fix the decellularized bovine pericardium yielded less pannus formation, less calcification, and better hemodynamic function. We postulate that the limited pannus formation in the DF group results from GA. Bovine pericardium decellularized with our proprietary method resulted in inert tissue, which is a unique finding. These results justify further development and evaluation of the two decellularized tissue types in THVs for use in younger patients.
Introduction
Transcatheter aortic valve implants (TAVIs) have revolutionized the management of elderly patients with severe aortic stenosis (AS) and have now surpassed surgical aortic valve replacement (SAVR) as the preferred treatment modality in First World countries (1). They provide a less invasive and more durable alternative for patients who may well otherwise have struggled to cope with the trauma of SAVR. TAVI, as an alternative procedure to SAVR, has proven to be safe and efficient for the whole risk spectrum of patients, including patients deemed to be inoperable (2), high risk (3, 4), intermediate risk (5, 6), or even low risk (7, 8). All TAVIs and other transcatheter heart valves (THVs) are biological valves of either porcine or bovine origin. These materials have been shown to deteriorate and calcify in younger patients in large surgical series (9–11). Hence, a crucial caveat in all these randomized TAVI trials was that the participants were elderly, with mean age ranging from 74 to 83 years. In view of this, the European Society of Cardiology (ESC) guidelines recommend that TAVIs be reserved for patients ≥75 years or with high surgical risk and mechanical valves should be used in patients younger than 60 years when SAVR is performed (12). In the United States, only 18% of SAVR procedures and 30% of mitral valve replacements utilize mechanical valves, as the mean age of surgical valve recipients is 67 years (13, 14). This is in stark contrast to studies from Africa, where the average age of heart valve surgery candidates was 19 years in Ethiopia (15) and Uganda (16), 26 years in the Côte d'Ivoire (17), 27 years in Nigeria (18), and 42 years in South Africa (19). In these age groups, the recommendation is that all patients receive mechanical prostheses, but the problems associated with lifelong anticoagulation treatment are well documented (20–22). If one adds to this the fact that rheumatic heart disease (RHD) remains the most prevalent cause of significant heart valve disease in the world (23) and that there is a huge unmet need for surgery for these patients (24), there is a lot of potential for improved THVs to address these needs (23, 24). In this context, a durable bioprosthetic transcatheter valve with reliable and reproducible deployment is an attractive future alternative. Although transcatheter valve implantation in younger patients faces design, deployment, and structural challenges, the durability of bioprosthetic or tissue-engineered leaflet alternatives remains the Achilles’ heel of this concept. The durability of bioprosthetic valves in patients under 40 years is poor (25–27) and therefore makes this type of valve inappropriate for the largest group of potential recipients of heart valves in the world (23). Improved durability of bioprosthetic material is therefore imperative. Since the very first valve replacements (28, 29), the only landmark improvement in bioprosthetic tissue fixation has been the switch from formaldehyde to glutaraldehyde (GA) (30). However, GA fixation has been plagued with valve deterioration and calcification, both as a result of ongoing immune processes and toxicity associated with the aldehydes. Various anti-calcification techniques have been investigated (31–33), but an ideal process for producing durable biological valves remains elusive. The process of biological valve deterioration is complex and includes remnant tissue immunogenicity, inflammatory cell infiltration, toxicity of GA fixation, mechanical damage, calcification, lack of repair, and pannus overgrowth (34). Therefore, the development of tissue-engineered alternatives and biological or artificial scaffolds has become a major research focus (35).
The Robert W M Frater Cardiovascular Research Centre (Frater Centre) at the University of the Free State (UFS) has been involved in the development of a biological tissue scaffold with reduced immune response from the host while maintaining sufficient dynamic strength to survive in the harsh mechanical stress environment to which heart valves are exposed. These processes can be broadly divided into decellularization, fixation, and detoxification. After evaluating the contributions of various decellularization steps and combinations, we have developed a unique method to decellularize bovine pericardium (BP) without significantly altering its strength and collagen structure (36, 37). This decellularized (DE) tissue performed well in 6-month implants in ovine aortas and pulmonary arteries, with less pannus formation, limited calcification, and adequate strength (38). Despite these results, it is not known whether the DE tissue would be strong enough to function in a high mechanical stress environment such as a THV. Therefore, additional cross-linking with GA may be required, and we have developed an additional decellularized, fixed, and detoxified (DF) tissue. The hypothesis is that GA has detrimental effects on tissue and should be limited as much as possible. This tissue was therefore fixed with a very low dose of GA, which was combined with a proprietary amino acid detoxification process, which in turn indicated no toxicity, excellent cross-linking, resistance to calcification, and porosity, allowing host cell recellularization in a subcutaneous rat model (39). To improve cross-linking, we utilized monomeric GA, which has been shown to produce less calcification (40).
We have developed a balloon expandable THV designed to be manufactured with bovine pericardial leaflets (41–45). This THV provided the platform on which we tested the different pericardial leaflets.
This study aimed to test DE, DF, and an industry standard bovine pericardium (Glycar, Glycar Pty Ltd, Irene, South Africa) in THVs implanted in the right ventricular outflow tract (RVOT) of juvenile sheep for 180 days. We compared the hemodynamic performance, structural degeneration and tissue strength, host tissue repopulation, inflammatory response, and calcification.
Materials and methods
Study design
The study was conducted as a prospective analytical cohort experimental study. Baseline and post-explantation tissue data were documented and compared between groups. Three THVs per group were constructed from bovine pericardium: either decellularized (DE) according to our proprietary method (n = 3) (37, 38) or decellularized, fixed, and detoxified (DF) (n = 3) or an industry standard (Glycar) (n = 3). The valves were then implanted as interposition grafts in the main pulmonary artery (MPA) of juvenile Merino sheep (n = 3 per group) for 180 days. Echocardiography was performed at implantation and after 3 and 6 months to monitor valve function. Valve leaflet tissue at the explant was evaluated macroscopically and radiographically. Dynamic strength testing was performed using tensile strength (TS) and flexibility [Young's modulus (YM)] analyses, and morphological evaluation included hematoxylin and eosin (H&E) staining, von Kossa staining, Verhoeff–Van Gieson elastic staining (EVG), scanning electron microscopy (SEM), and transmission electron microscopy (TEM). A schematic representation of the study design is presented in Figure 1.
The Animal Ethics Committees of the University of the Free State (UFS-AED2016/0008/2020/21) and the University of Stellenbosch (SU-ACUD15-00120) approved the project.
Experimental animals
The juvenile ovine model was selected for testing the valve in vivo because of the similarity of the animal's anatomy and hemodynamics to humans (46, 47) and because the model is approved by both the FDA and CE Mark (48). The exact definition of what constitutes a juvenile sheep is lacking, and in line with others (49–51), we elected to use animals that were 6 months of age. This negates the excessive growth of younger animals while still providing an accelerated calcification model for the valves. Furthermore, they are considered the ideal valve calcification model (52). Nine juvenile Merino sheep of 6 months of age and weighing between 40 and 45 kg were used to achieve a satisfactory match between valve size and pulmonary artery size. All animals were vaccinated, treated against ecto- and endoparasites, and subjected to a complete blood count prior acceptance for surgery. All animal experiments and surgical procedures were performed in compliance with the Guide for the Care and Use of Laboratory Animals published by the US National Institutes of Health (53) and guided by the South African National Standard 10386:2021 for the care and use of animals for scientific purposes.
Tissue processing
Three groups of bovine pericardial leaflet constructs were used in the study:
(i) Glycar bovine pericardial tissue: Using EnCap technology, Glycar bovine pericardial tissue was selected as the industry standard since it has an established track record in the field (54, 55). Furthermore, we have used Glycar as a comparator in our previous work (37, 38) and deemed it the most suitable tissue available for use as a control. This tissue was GA-tanned (0.625%), formaldehyde (4%)-sterilized, detoxified with propylene glycol (100%), and stored in propylene oxide (2%).
(ii) Decellularized (DE) tissue: A decellularized bovine pericardial scaffold was prepared by decellularizing fresh bovine pericardium using a proprietary process developed by our group. This process combines the use of osmotic shock, a multi-detergent solution, delipidation with ethanol, and sterilization in an antibiotic and anti-mycotic solution according to a patented method (Patent: 16702008.0-1455, EU, 2017) (38).
(iii) Decellularized-fixed (DF) tissue: Bovine pericardium decellularized as in (ii), then fixed with 0.05% monomeric GA (Polysciences, Inc., USA), and detoxified with an amino acid solution containing 0.1 M glycine (39).
Decellularization was evaluated by measuring the DNA content and inspecting H&E staining to confirm acellularity. DNA was extracted from the tissue using a Quick-DNATM Miniprep Plus Kit (Zymo Research, USA), and the complete decellularization of the tissue was confirmed by measuring the DNA content (ng DNA/mg tissue) using a BioDrop spectrophotometer (Biochrom Ltd., Cambridge, UK). After processing, the tissue was confirmed to be culture-negative (anaerobic and aerobic bacteria, fungi, and yeast) by a registered pathology laboratory (PathCare Veterinary Laboratory Bloemfontein, South Africa).
Valve construction
A stainless-steel stent platform for the THV prosthesis was developed and tested in vitro and virtually to optimize the design and geometry of the leaflets (41–45). The valves were constructed in an ISO 14385-compliant clean room (Next Biosciences, Johannesburg, South Africa). A hemostatic cuff made from braided PTFE (Bard, Tempe, AZ, USA) was sutured onto the inside of the stent with individual self-locking sutures using CV-7 monofilament Gore-Tex (W.L. Gore & Associates, DE, USA). Three sets of valves were prepared for comparison using leaflet tissue supplied by Glycar and DE and DF pericardium scaffolds supplied by the Frater Centre. A leaflet template was manufactured to cut three identical-sized leaflets from a single sheet of pericardium, and the leaflets were then sutured with CV-7 Gore-Tex sutures onto the stent frame. A 22-mm THV valve was constructed according to the design parameters developed by our group (Figure 2).
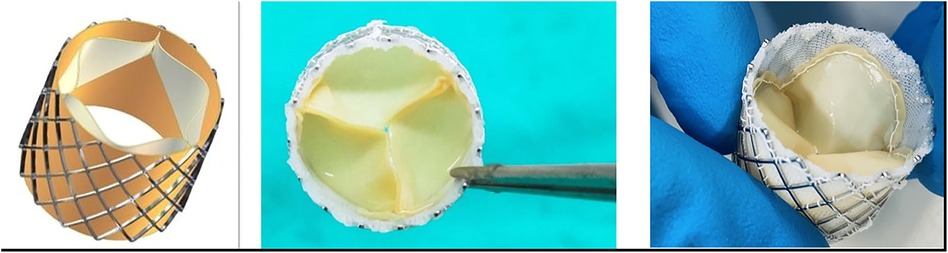
Figure 2. THV. On the left is a computer design of the valve that was developed using finite-element analysis (41–44). Note the balloon expandable stent frame with pericardial leaflets and a braided PTFE hemostatic cuff on the inside of the stent. On the right are two photographs of a manufactured valve.
Swabs for microbiology testing were taken from the covered stent and the completed valve before final storage in 2% propylene oxide (Glycar leaflets) or an 85% glycerol/15% ethanol solution (decellularized leaflets), as well as a tissue sample from the pericardial tissue used for the construction of the valve leaflets.
Surgical implantation of transcatheter heart valves
The THV prosthesis was aseptically removed from the storage solution in the theater and rinsed in sterile 0.9% saline solution for 3–5 min. To expose the tissue to the mechanical forces of a transcatheter implant, it was crimped onto a 23-mm Z-Med balloon (Numed, NY, USA) using a commercially available crimping tool. The crimped diameter was confirmed to be small enough to fit through an 18-French sheath by inserting it into a dedicated Perspex tube with a 6 mm inner diameter. The crimped valve was then fully deployed with balloon inflation within a 22-mm Jotec FlowWeave Bioseal woven polyester vascular graft (JOTEC GmbH, Germany), secured with three polypropylene sutures at each end to prevent migration. The vascular graft was 3 mm longer than the THV on either side (total length ± 24 mm) to enable suturing and was then implanted as an RVOT conduit.
Recipient sheep were sedated (morphine sulfate 1 mg/ml, 0.2–0.5 mg/kg IM; ketamine hydrochloride 100 mg/ml, 5–15 mg/kg IM; Robinul 4 mg/2 ml, 0.005–0.01 mg/kg IM), anesthetized (Propofol 10 mg/ml, 2–6 mg/kg IV, Esmeron 50 mg/5 ml, 0.5 mg/kg IV), intubated, and ventilated (Isofor 2.5%, 1%–3% inhalation). A central venous line (CVP) was inserted in the left jugular vein, an arterial line was placed in the left carotid artery for hemodynamic monitoring, and the left carotid artery was cannulated for bypass. A left thoracotomy was performed, and the fourth rib was removed. Removal of the rib allows for a wider field of view during surgery and better echo windows at the follow-up. The right atrium was cannulated to achieve cardiopulmonary bypass (CPB), allowing the surgical procedure to be performed on a beating heart. The main pulmonary artery was clamped and transected, native pulmonary valve leaflets were removed, and the THV conduit was implanted as an interposition graft using continuous 4/0 prolene sutures in the pulmonary artery. The animal was weaned off CPB, hemostasis was secured, and an intrathoracic sonar was performed to confirm adequate valve functioning. The pressure gradient across the conduit was measured through needle insertion on either side of the conduit. A single chest drain was inserted on the left side, and all incisions were closed. The animal was extubated when awake and breathing on its own, and the chest drain and monitoring lines were removed soon after. The sheep were moved back to the indoor holding pens with companion sheep in adjacent pens. Precisely, 5 ml of Depomycin (MSD Intervet) as a broad-spectrum antibiotic and 0.1–0.2 mg/kg morphine sulfate (Fresenius Medical Care, South Africa) as pain medication were administered intramuscularly twice daily for 5 days after surgery, and the animals were followed up and monitored daily for 6 months until they were killed; the valves were explanted for further analysis of the leaflet tissue. A piece of the adjacent pulmonary artery was excised and sent for microscopy and culture to exclude infection (negative in all cases).
Clinical performance of implanted valves
Echocardiography was performed just after implanting the conduit, at days 30 and 90 post-surgery, and just before killing them using a GE Vivid-Q portable sonar machine. Over the study period, implanted valves were evaluated for transvalvular gradients, valve regurgitation, calcification, and possible aneurysm formation.
At the end of the study period, the animals were again anesthetized as described above and analgesia was administered (morphine sulfate 0.1–0.2 mg/kg); the chest was opened, hemodynamic pressures were measured over the valve by needle insertion, and intrathoracic echocardiography was performed. Animals were then killed by injecting a bolus of 10 ml of 15% hyperosmolar potassium chloride directly into the right atrium to cause cardiac arrest and then exsanguinated, and the valves were removed for further analyses.
Tissue analysis
Mechanical properties, tissue histology, and ultrastructure of a representative piece of pericardium used to manufacture each valve were analyzed (baseline analysis) and compared to results of similar analyses on the tissue leaflets after 180 days in the sheep (explant analysis).
Mechanical properties
Pericardial thickness was measured prior to surgical implantation and repeated after explantation using an ElectroPhysik MiniTest thickness gauge (Cologne, Germany). Samples were cut into 5-mm-wide strips, three to five thickness measurements were taken over the central section of the test strip, and then the average was calculated.
The dynamic strength test (tensile strength and Young’s modulus analyses) of the bovine pericardium tissue was uniaxially performed at room temperature by an automated and computerized TS testing apparatus (Lloyds LS100 Plus, IMP, South Africa). Preimplantation tissue (5 cm × 5 mm) and tissue at the explant (5 mm wide) were cut. The average thickness was then measured, and the tissue sample was gradually stretched between two grips until the breaking point was achieved. Force was calculated using a 500-N load cell. The tensile strength (MPa) and Young's modulus (MPa) were calculated from the stress–strain curve using Nexygen Plus 3 software (Lloyd Instruments, IMP, Johannesburg, South Africa).
Histology
A tissue sample from each pericardial patch used for leaflet construction was collected in 4% buffered formalin, dehydrated in graded alcohol steps, cleared in xylene, embedded in a paraffin wax block, sectioned, and stained according to standard protocols for H&E, von Kossa, and Verhoeff–Van Gieson staining for histological evaluation (56). The H&E-stained samples were evaluated for pannus formation, which was classified into three categories: none, non-confluent, and confluent pannus (covering the whole segment evaluated and both sides of the leaflet).
Pericardial tissue samples for electron microscopy evaluation were collected in 3% buffered GA and processed according to standard protocols for SEM and TEM evaluations (57).
Statistical analysis
Due to the limited sample size, non-parametric analysis was used. Statistical analyses were performed using GraphPad Prism version 9.3.1. Continuous values were subjected to a Kruskal–Wallis (KW) multiple comparison analysis with Dunn's post-hoc test to compare individual groups. For two group comparisons, the Mann–Whitney U-test was used to measure differences between independent groups, and the Wilcoxon singed-rank test was used to measure differences between dependent groups.
Results
Adequate decellularization was confirmed for both DE and DF tissues prior to implantation (Figure 3).
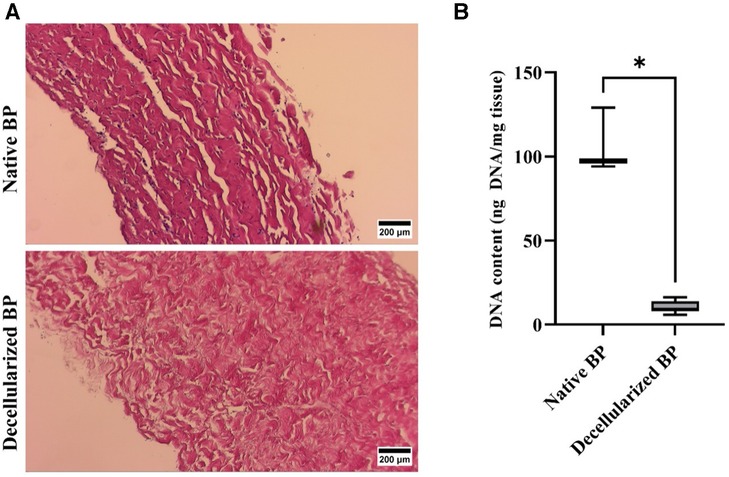
Figure 3. Confirmation of decellularization of bovine pericardium. (A) Representative light microscope images for H&E-stained tissue, indicating the presence of cells in the native BP and the complete removal of cells following decellularization (magnification 100×, scale 200 µM). (B) DNA content of the native and decellularized bovine pericardium (n = 6). * p < 0.05. The DNA content was well below the required 50 ng/mg tissue (71).
Valves were successfully implanted into 10 animals. One animal developed acute respiratory decompensation immediately post-operatively and was sacrificed. The other nine animals (three per tissue type) survived the procedure. All animals survived for 180 days except for one sheep with the Glycar valve, which developed heart failure and was euthanized at 158 days. The heart failure was due to a calcified prosthetic pulmonary valve with one of its leaflets overgrown by pannus and fixed in the open position (Figure 4). Endocarditis was excluded with blood cultures, c-reactive protein (CRP), and histology of this valve. The other valves were found to function adequately and competently, as observed by echocardiography (Supplementary Video S1). Obtaining accurate transvalvular gradients on echocardiography proved unreliable, and this parameter was obtained with direct invasive measurement prior to euthanasia. As can be observed in Figure 5, the gradients remained unchanged from implantation to explantation except for the Glycar valves, where the gradient increased numerically from a mean of 12 to 37 mmHg, although this was not statistically significant.
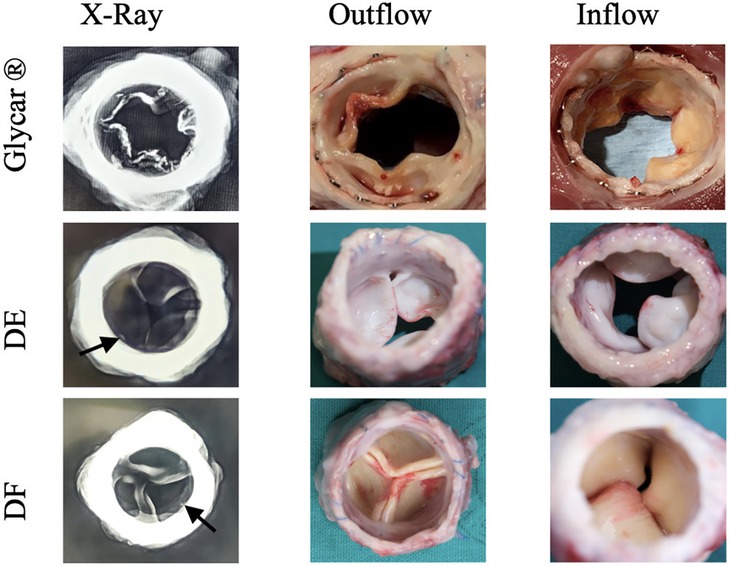
Figure 4. Representative X-ray images and photographs of the three differently processed valves at explantation. Note the calcification visible on the X-ray in the Glycar valve. The outflow view of this valve shows extensive pannus overgrowth, which has fixed one leaflet in the open position. Calcified nodules are visible on the inferior leaflet in the image. The decellularized (DE) and decellularized, low-dose glutaraldehyde-fixed, and detoxified (DF) bovine pericardium leaflets show no overt calcification, although there are possibly punctuated calcium spicules near the sewing margin (arrows), which may represent suture trauma. Glycar is the commercially available Glycar bovine pericardium fixed with conventional high-dose GA.
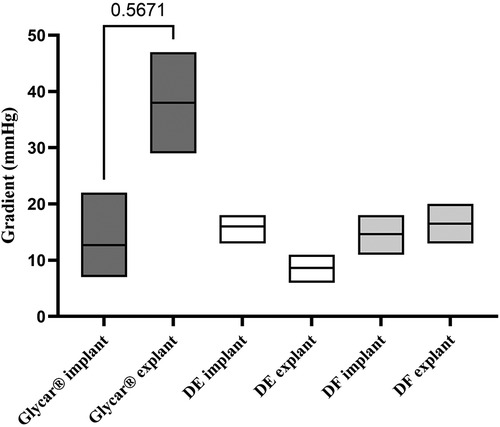
Figure 5. Invasively measured gradients (means and ranges) across the valves at the time of implantation compared to just prior to explantation. Note the unchanged gradients in DF and DE tissue valves. The change in gradients over the Glycar tissue valves was not statistically significant (p = 0.5671). DE denotes decellularized bovine pericardium; DF denotes decellularized, low-dose glutaraldehyde-fixed, and detoxified bovine pericardium; Glycar is the commercially available Glycar bovine pericardium fixed with conventional high-dose GA.
Macroscopic evaluation of the valves indicated that the stents remained structurally unchanged from implantation. Glycar leaflets were rigid with nodules of calcifications and thickened by pannus overgrowth. DE leaflets were soft, pliable, and non-calcified. DF leaflets were pliable, non-calcified, and of the same thickness as at implantation. An X-ray of the explanted valves showed calcification of the Glycar leaflets. The DE and DF leaflets were generally free from calcification, although there were a few spots next to the stent frame that could represent calcification due to suture trauma (Figure 4).
There was no difference in thickness, tensile strength, and Young's modulus from baseline to explantation in either of the two decellularized tissues (DE and DF). The Glycar tissue presented a significant reduction in tensile strength. This was caused by one of the tissues of the Glycar valve that fractured at low traction forces (Figure 6).
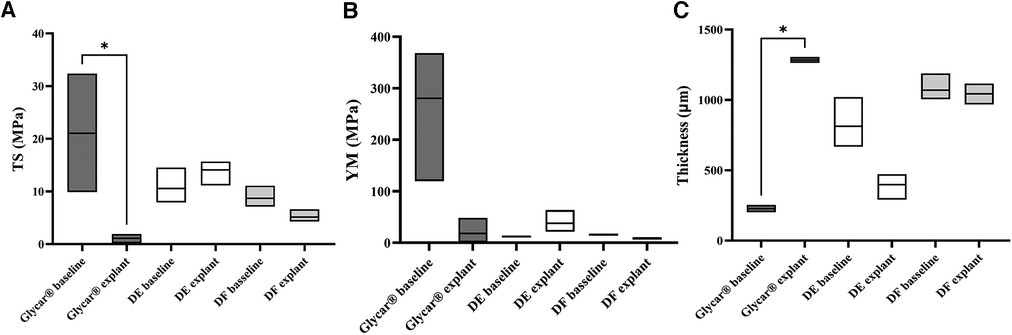
Figure 6. Physical properties of the tissue expressed as medians and ranges. (A) TS. Note the significant (*p = 0.033) difference between the Glycar baseline and at explantation. This is due to a single Glycar sample that was severely calcified and fractured with minimal traction. The other tissues all had non-significant differences from baseline to explantation. (B) YM analysis with no differences between baseline and explantation. (C) Tissue thickness, with only the Glycar tissue showing a significant (*p = 0.0249) change from baseline to explantation. As detailed in the Materials and Methods section, the thickness was measured (physically with calipers) in four places on the leaflet; therefore, a more representative of actual thickness than the single histology slices is shown in Figures 7, 9, and 11. DE denotes decellularized bovine pericardium; DF denotes decellularized, low-dose glutaraldehyde-fixed, and detoxified bovine pericardium; Glycar is the commercially available Glycar bovine pericardium fixed with conventional high-dose GA.
H&E staining demonstrated pronounced pannus overgrowth of the Glycar leaflet tissue. Of seven sections evaluated, six (86%) had confluent pannus and one (14%) had non-confluent pannus. No pannus was observed in the DE group and the DF group; of four sections, three (75%) had non-confluent pannus and one (25%) had none. In DE explants, no inflammatory process could be identified. There were no inflammatory infiltrates in DF explants, apart from the pannus mentioned previously. In fact, apart from the endothelium and the non-confluent pannus in the DF tissue, these explants were acellular. All tissues had good endothelial coverage, although the endothelium covered the pannus in the Glycar leaflets (Figures 7, 8) and in some portions of the DF leaflets. Scanning electron microscopy confirmed good reendothelialization of all three tissue types at the explant (Figure 8).
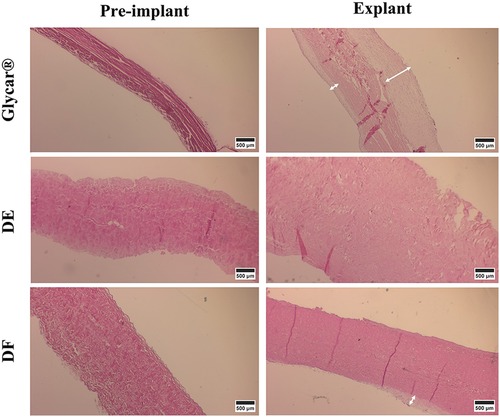
Figure 7. Results of H&E staining of the pericardial tissue at baseline (left) and explantation (right). At the explant, the Glycar tissue has a thick layer of pannus (white arrows), the DF has minimal pannus, and the DE tissue has no pannus. DE denotes decellularized bovine pericardium; DF denotes decellularized, low-dose glutaraldehyde-fixed, and detoxified bovine pericardium; Glycar is the commercially available Glycar bovine pericardium fixed with conventional high-dose GA.
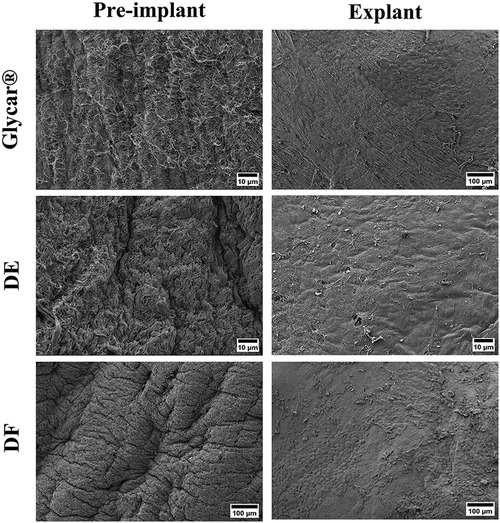
Figure 8. Results of scanning electron microscopy of the pericardial tissue at baseline (left) and explantation (right). All three tissue types were well covered with endothelium at the explant. DE denotes decellularized bovine pericardium; DF denotes decellularized, low-dose glutaraldehyde-fixed, and detoxified bovine pericardium; Glycar is the commercially available Glycar bovine pericardium fixed with conventional high-dose GA.
Evaluation of the elastin content of the tissues with Verhoeff–Van Gieson staining revealed a dense presence in the Glycar tissue pre-implant while limited presence in the DE and DF tissues. All tissues showed little or no elastin upon explantation (Figure 9). Transmission electron microscopy demonstrated a well-preserved collagen framework in all tissues, closely resembling preimplantation (Figure 10).
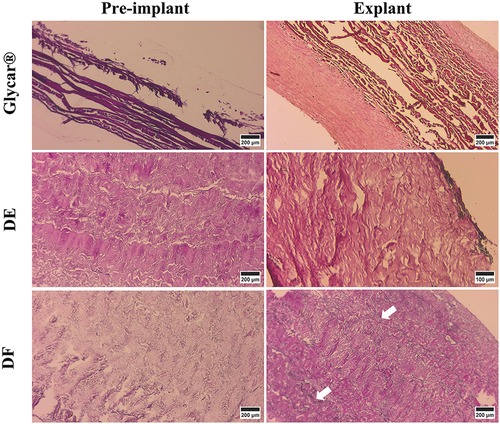
Figure 9. Results of EVG (highlighting elastin) of pericardial tissue at baseline (left) and explantation (right). All tissues had elastin at baseline, but at the explant, DE and Glycar had none and DF had minimal elastin (white arrows). DE denotes decellularized bovine pericardium; DF denotes decellularized, low-dose glutaraldehyde-fixed, and detoxified bovine pericardium; Glycar is the commercially available Glycar bovine pericardium fixed with conventional high-dose GA.
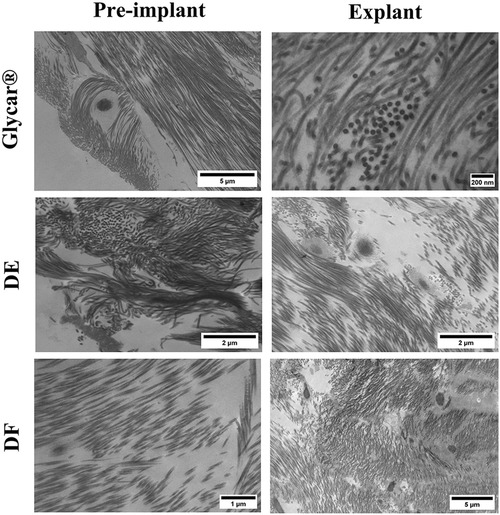
Figure 10. Results of transmission electron microscopy of pericardial tissue at baseline (left) and explantation (right). Note the preservation of collagen structure in all tissues at explantation. DE denotes decellularized bovine pericardium; DF denotes decellularized, low-dose glutaraldehyde-fixed, and detoxified bovine pericardium; Glycar is the commercially available Glycar bovine pericardium fixed with conventional high-dose GA.
The Von Kossa staining results demonstrated marked calcification in the Glycar tissue and none in the DE and DF groups (Figure 11).
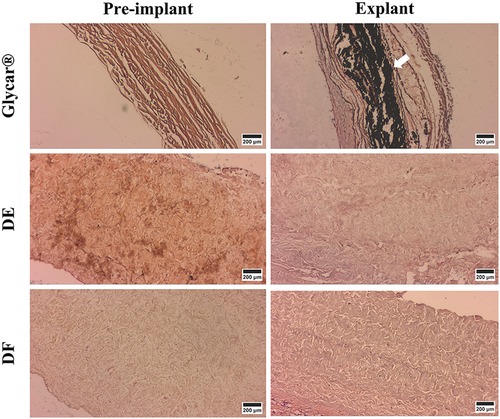
Figure 11. Representative von Kossa-stained samples (indicating calcification in black) of pericardial tissue at baseline (left) and explantation (right). Note the dense calcification in the Glycar tissue (white arrow) at the explant and none in the decellularized tissues. DE denotes decellularized bovine pericardium; DF denotes decellularized, low-dose glutaraldehyde-fixed, and detoxified bovine pericardium; Glycar is the commercially available Glycar bovine pericardium fixed with conventional high-dose GA.
Discussion
We aimed to develop bioprosthetic THV leaflets with improved durability and potential use in younger patients. Both the decellularized tissues (DE and DF) showed promising results when implanted for 180 days in juvenile sheep. The structure of the DE tissue remained intact, elicited no detectable inflammatory response, and showed no calcification. When a very low dose of GA was added, the DF tissue developed small non-confluent areas of pannus. No calcification was seen during histological examination of the DF valve, and the small spots of calcification seen on an X-ray were near the stent frame and interpreted as a consequence of suture trauma. Elastin, as expected from previous work by others (58), was largely absent from all tissues at the explant.
During preparation of bovine pericardial leaflets, we focused on three aspects that are deemed important in the search for more durable tissues for bioprosthetic THVs in younger patients, namely, lower-dose GA exposure, detoxification, and decellularization.
GA has been used in the fixation of pericardium for bioprosthetic heart valves since the 1970s (59) and has remained a crucial step in the preparation of tissues for heart valves. Currently, bovine pericardium is fixed with GA to provide greater mechanical stability, improve tissue handling, and reduce antigenicity, with a standard concentration of 0.625% used (60). Despite the beneficial effects of GA fixation, it is also implicated in various detrimental effects on the durability of the tissue, including reduced endothelial coverage (61), increased calcium influx into cells (62), inflammatory cell infiltration into tissues (61), and pannus formation (38). We hypothesized that a reduced concentration and the use of monomeric GA will at least partially mitigate some of these effects while providing adequate cross-linking. During the development of our proprietary fixation technique, it was found that the degree of cross-linking reduced with reducing doses of GA. Cross-linking was calculated as the ratio of the bound amino groups in the cross-linked (fixed) samples to the free amino groups from unfixed tissues and was determined using the ninhydrin assay (63). However, when the tissue was exposed to H2O2, the cross-linking with a very low dose of monomeric GA (0.05%) was similar to that with standard 0.625% GA fixation. Further support that our cross-linking process was adequate can be deducted from the fact that the tensile strength of the DF tissue remained unchanged from baseline to explantation. We have previously shown that Glycar tissue developed pannus in the ovine aorta and ovine pulmonary artery, but in these vascular locations, the Glycar tissue did not calcify excessively (38). Although Glycar tissue is suitable for use in vascular and valvular repair and congenital repair, the current study suggests that it is not suitable for constructing leaflets for a THV. The major differences that the tissues are exposed to in the two locations include the initial potential injury caused by the crimping and balloon expansion of the THV and the repetitive motion and associated mechanical strain experienced by the valvular leaflets, forces that do not occur in vascular grafts. Furthermore, the pannus observed in the Glycar tissue may also have reduced the flexibility of the leaflets, thereby placing more mechanical stress on them, leading to calcification. The lack of calcification in the DF and DE tissues, where minimal or no pannus developed, is in keeping with this interpretation. Although the exact mechanism of calcification in the Glycar tissue remains speculative, this study supports the notion that reducing the exposure to GA limits calcification, particularly when the tissue is exposed to high mechanical stresses such as crimping and expansion of a THV and functioning as a valve leaflet in vivo.
Inflammation is widely viewed as an important contributor to bioprosthetic heart valve degeneration (64–67). Pannus tissue contains a variety of chronic inflammatory cells (lymphocytes, plasma cells, macrophages, and foreign body giant cells) and represents a host reaction against a foreign material (67). We did not see an acute inflammatory response to any of the tissues, but the extent of pannus formation was significant in Glycar (with high-dose GA exposure), very little in DF tissue (with a very low dose of GA exposure), and absent in DE (with no GA exposure). Since there were other differences in tissue preparation, we cannot conclude that GA exposure was the sole explanation for this finding. The results would however suggest that any use of GA might have to be avoided, or alternatively, the fixation technique requires further refinement to avoid pannus formation, which could alter hemodynamics and ultimately lead to valve degeneration and calcification. Another factor that has been shown to contribute to the immunogenic response to implanted bioprosthetic leaflets is cellular remnants of bacteria on the tissue (65). However, we did not test for this prior to implantation.
Decellularization in bioprosthetic tissues has been utilized and evaluated with the potential benefit of removal of immunogenic components and reducing stimulus for calcium influx into the tissue (68–72). Decellularized allografts (without GA fixation) have been evaluated in humans (73) with good midterm results. In this cohort of younger patients, one patient required a reoperation after 18 months for another indication and a small portion of the allograft tissue was removed at the time. Histology revealed well-preserved collagen fibers in the media and intimal hyperplasia of moderate intensity. There were a limited number of fibroblasts in the media and minimal inflammatory cells. The concept of utilizing decellularization without fixation is therefore not new, but its utility for xenografts is less well established, with some discouraging results described (74). Our two decellularized tissues showed very encouraging results, but because of the proprietary preparation process, these results should not be generalized to other decellularization protocols or other implantation techniques and animal models. Decellularization can be obtained through various methods (physical, enzymatic, or chemical) but needs to be tailored for each tissue type depending on cellularity, density, lipid content, and thickness (75). Each step of the process has different effects on the outcome (36), and related techniques may yield different results depending on small variations in the technique. Our decellularization methodology has been developed over various iterations, and the results reported here are from tissues with encouraging performance in the subcutaneous rat model and aortic and pulmonary artery ovine implants (38). However, the durability of the unfixed DE tissue after 6 months in the high mechanical stress environment of a THV in sheep is somewhat surprising. No host fibroblast ingrowth was seen in this tissue, which implies that the collagen structure from the implant was still sufficiently stable, as seen from the TEM images, and remained functional, as demonstrated by the tensile strength and Young's modulus evaluation. This is contrary to the current view that regenerative tissue engineering supplies a scaffold for the host tissue to infiltrate, produce new structural elements, and eventually take over the functioning of the original xenograft (76). Although recellularization is an accepted endpoint in most tissue-engineered scaffolds, no one has been able to stimulate pericardial tissue to regenerate into the complex and specialized three-layered structure of native aortic valve leaflets. Although researchers have been able to populate scaffolds with the appropriate cells (in vivo and in vitro), they have been unable to stimulate them to produce a new extracellular matrix with mature composition, distribution, and conformation (76). Our decellularized tissue, conversely, was essentially inert despite 6 months of exposure to an accelerated calcification model (juvenile sheep). This finding is unique and challenges conventional dogma. Further research is required to fully understand this finding and its potential impact on future transcatheter valves. Each tissue was tested on three animals only, which makes the data preliminary and exploratory at best. However, because of the encouraging results, they justify expanding it to a larger cohort. The results were obtained in the lower-pressure environment and must be validated with aortic implants. The encouraging results in the RVOT, however, raise the question of whether the tissue is suitable for pulmonary THVs in younger patients. To evaluate long-term mechanical integrity of the tissue, our valves are currently being fatigue-tested to 200 million cycles per ISO 5840 standards. Longer-term implants will be required to prove durability, especially for the unfixed DE tissue. Finally, the response of the tissue to anti-Gal antibodies was not tested, and primate implants or in vitro exposure to human tissue must be performed.
Another feature of our work that is relatively unique is that valves were implanted in a synthetic tube and therefore (although exposed to host blood) not in direct contact with host tissue. This may be part of the explanation for the lack of host cell infiltration in the decellularized tissues, although it did not protect the Glycar tissue from pannus formation nor the DF group from limited non-confluent pannus formation. Applying this concept of limiting host tissue contact to aortic valves will be difficult because of the coronary ostia, but it may have utility in pulmonary valve implants where the recipients also tend to be younger.
Conclusions
In the juvenile sheep THV model, Glycar tissue (with high-dose GA fixation) developed significant pannus, calcification, and hemodynamic deterioration. Using a very low dose of monomeric GA to fix decellularized bovine pericardium yielded less pannus formation, less calcification, and better hemodynamic functioning. We postulate that the limited pannus formation in the DF group results from GA, as no cellular response or pannus formation was demonstrated when GA was omitted in the similarly decellularized DE tissue. Bovine pericardium decellularized with our proprietary method resulted in essentially inert tissue, which is a unique finding. These results justify further development and evaluation of the two decellularized tissue types in THVs for use in younger patients.
Data availability statement
The original contributions presented in the study are included in the article/Supplementary Material, further inquiries can be directed to the corresponding author.
Ethics statement
The Animal Ethics Committees of the University of the Free State (UFS-AED2016/0008/2020/21) as well as the University of Stellenbosch (SU-ACUD15-00120) approved the project.
Author contributions
HW: Conceptualization, Data curation, Investigation, Methodology, Writing – original draft. LB: Data curation, Investigation, Writing – review & editing. AD: Methodology, Writing – review & editing. JJ: Investigation, Methodology. AL: Conceptualization, Data curation, Investigation, Methodology, Software, Writing – review & editing. PM: Investigation, Methodology, Data curation. JH: Conceptualization, Data curation, Investigation, Methodology, Writing – review & editing. FS: Conceptualization, Data curation, Formal Analysis, Funding acquisition, Investigation, Methodology, Project administration, Supervision, Writing – review & editing.
Funding
The authors declare financial support was received for the research, authorship, and/or publication of this article.
This study received support from the research funds of the University of the Free State and the University of Stellenbosch.
Acknowledgments
The authors thank Reynaldo Rodrigues (DeltaV Aerospace) for his invaluable help with developing the THV prosthesis.
Conflict of interest
The authors declare that the research was conducted in the absence of any commercial or financial relationships that could be construed as a potential conflict of interest.
Publisher's note
All claims expressed in this article are solely those of the authors and do not necessarily represent those of their affiliated organizations, or those of the publisher, the editors and the reviewers. Any product that may be evaluated in this article, or claim that may be made by its manufacturer, is not guaranteed or endorsed by the publisher.
Supplementary material
The Supplementary Material for this article can be found online at: https://www.frontiersin.org/articles/10.3389/fcvm.2023.1270496/full#supplementary-material
Supplementary Video S1
Representative echocardiogram [in a view comparable to a parasternal short axis view] showing color Doppler over the right ventricular outflow and pulmonary artery with no regurgitation of the valve in situ.
References
1. Carroll JD, Mack MJ, Vemulapalli S, Herrmann HC, Gleason TG, Hanzel G, et al. STS-ACC TVT registry of transcatheter aortic valve replacement. J Am Coll Cardiol. (2020) 76(21):2492–516. doi: 10.1016/j.jacc.2020.09.595
2. Makkar RR, Fontana GP, Jilaihawi H, Kapadia S, Pichard AD, Douglas PS, et al. Transcatheter aortic-valve replacement for inoperable severe aortic stenosis. N Engl J Med. (2012) 366(18):1696–704. doi: 10.1056/NEJMoa1202277
3. Adams DH, Popma JJ, Reardon MJ, Yakubov SJ, Coselli JS, Deeb GM, et al. Transcatheter aortic-valve replacement with a self-expanding prosthesis. N Engl J Med. (2014) 370(19):1790–8. doi: 10.1056/NEJMoa1400590
4. Smith CR, Leon MB, Mack MJ, Miller DC, Moses JW, Svensson LG, et al. Transcatheter versus surgical aortic-valve replacement in high-risk patients. N Engl J Med. (2011) 364(23):2187–98. doi: 10.1056/NEJMoa1103510
5. Leon MB, Smith CR, Mack MJ, Makkar RR, Svensson LG, Kodali SK, et al. Transcatheter or surgical aortic-valve replacement in intermediate-risk patients. N Engl J Med. (2016) 374(17):1609–20. doi: 10.1056/NEJMoa1514616
6. Reardon MJ, Van Mieghem NM, Popma JJ, Kleiman NS, Søndergaard L, Mumtaz M, et al. Surgical or transcatheter aortic-valve replacement in intermediate-risk patients. N Engl J Med. (2017) 376(14):1321–31. doi: 10.1056/NEJMoa1700456
7. Popma JJ, Deeb GM, Yakubov SJ, Mumtaz M, Gada H, O’Hair D, et al. Transcatheter aortic-valve replacement with a self-expanding valve in low-risk patients. N Engl J Med. (2019) 380(18):1706–15. doi: 10.1056/NEJMoa1816885
8. Mack MJ, Leon MB, Thourani VH, Makkar R, Kodali SK, Russo M, et al. Transcatheter aortic-valve replacement with a balloon-expandable valve in low-risk patients. N Engl J Med. (2019) 380(18):1695–705. doi: 10.1056/NEJMoa1814052
9. Glaser N, Jackson V, Holzmann MJ, Franco-Cereceda A, Sartipy U. Aortic valve replacement with mechanical vs. biological prostheses in patients aged 50–69 years. Eur Heart J. (2016) 37(34):2658–67. doi: 10.1093/eurheartj/ehv580
10. Briffa N, Chambers JB. Biological valves in younger patients undergoing aortic valve replacement: a word of caution. Circulation. (2017) 135(12):1101–3. doi: 10.1161/CIRCULATIONAHA.116.026385
11. Sotade OT, Falster M, Girardi LN, Pearson SA, Jorm LR. Age-stratified outcomes of bioprosthetic and mechanical aortic valve replacements in an Australian cohort of 13 377 patients. BMJ Surg Interv Health Technol. (2020) 2(1):e000036. doi: 10.1136/bmjsit-2020-000036
12. Vahanian A, Beyersdorf F, Praz F, Milojevic M, Baldus S, Bauersachs J, et al. 2021 ESC/EACTS guidelines for the management of valvular heart disease developed by the task force for the management of valvular heart disease of the European Society of Cardiology (ESC) and the European Association for Cardio-Thoracic Surgery (EACTS). Eur Heart J. (2022) 43(7):561–632. doi: 10.1093/eurheartj/ehab395
13. Tam DY, Rocha R V, Wijeysundera HC, Austin PC, Dvir D, Fremes SE. Surgical valve selection in the era of transcatheter aortic valve replacement in the Society of Thoracic Surgeons database. J Thorac Cardiovasc Surg. (2020) 159(2):416–27. doi: 10.1016/j.jtcvs.2019.05.081
14. Gammie JS, Chikwe J, Badhwar V, Thibault DP, Vemulapalli S, Thourani VH, et al. Isolated mitral valve surgery: the Society of Thoracic Surgeons adult cardiac surgery database analysis. Ann Thorac Surg. (2018) 106(3):716–27. doi: 10.1016/j.athoracsur.2018.03.086
15. Mazine A, Tamirat S, Stevens LM, Agwar F, Dejene K, Bedru M, et al. Contemporary outcomes of aortic and mitral valve surgery for rheumatic heart disease in sub-Saharan Africa. Struct Heart. (2020) 4(S1):138–9. doi: 10.1080/24748706.2020.1715148
16. Grimaldi A, Ammirati E, Karam N, Vermi AC, De Concilio A, Trucco G, et al. Cardiac surgery for patients with heart failure due to structural heart disease in Uganda: access to surgery and outcomes. Cardiovasc J Afr. (2014) 25(5):204. doi: 10.5830/CVJA-2014-034
17. Yangni-Angate KH, Meneas C, Diby F, Diomande M, Adoubi A, Tanauh Y. Cardiac surgery in Africa: a thirty-five year experience on open heart surgery in Cote d’Ivoire. Cardiovasc Diagn Ther. (2016) 6(Suppl 1):S44. doi: 10.21037/cdt.2016.10.06
18. Nwiloh JO, Oludara MA, Adebola PA, Edaigbini SA, Danbauchi S, Sowunmi AC, et al. Experience with prosthetic valve replacement in indigents with rheumatic heart disease in Nigeria: 10-year follow-up. World J Cardiovasc Surg. (2015) 5(8):75–81. doi: 10.4236/wjcs.2015.58013
19. Mokitimi N, van der Donck K, Moutlana H, Chakane PM. Profile of adult patients presenting for rheumatic mitral valve surgery at a tertiary academic hospital. Cardiovasc J Afr. (2021) 32(5):261–6. doi: 10.5830/CVJA-2021-024
20. Zühlke L, Karthikeyan G, Engel ME, Rangarajan S, Mackie P, Cupido-Katya Mauff B, et al. Clinical outcomes in 3343 children and adults with rheumatic heart disease from 14 low-and middle-income countries: two-year follow-up of the global rheumatic heart disease registry (the REMEDY study). Circulation. (2016) 134(19):1456–66. doi: 10.1161/CIRCULATIONAHA.116.024769
21. Scherman J, Manganyi R, Human P, Pennel T, Brooks A, Brink J, et al. Isolated mechanical aortic valve replacement in rheumatic patients in a low- to middle-income country. J Thorac Cardiovasc Surg. (2019) 157(3):886–93. doi: 10.1016/j.jtcvs.2018.06.083
22. Kistan D, Booysen M, Alexander G, Madiba TE. A South Africa tertiary centre experience with redo mitral valve replacement. S Afr J Surg. (2022) 60(1):44–8. doi: 10.17159/2078-5151/2022/v60n1a3192
23. Watkins DA, Johnson CO, Colquhoun SM, Karthikeyan G, Beaton A, Bukhman G, et al. Global, regional, and national burden of rheumatic heart disease, 1990–2015. N Engl J Med. (2017) 377(8):713–22. doi: 10.1056/NEJMoa1603693
24. Zilla P, Yacoub M, Zühlke L, Beyersdorf F, Sliwa K, Khubulava G, et al. Global unmet needs in cardiac surgery. Glob Heart. (2018) 13(4):293–303. doi: 10.1016/j.gheart.2018.08.002
25. Une D, Ruel M, David TE. Twenty-year durability of the aortic Hancock II bioprosthesis in young patients: is it durable enough? Eur J Cardiothorac Surg. (2014) 46(5):825–30. doi: 10.1093/ejcts/ezu014
26. Eric Jamieson WR, Munro AI, Miyagishima RT, Allen P, Burr LH, Tyers GFO. Carpentier-Edwards standard porcine bioprosthesis: clinical performance to seventeen years. Ann Thorac Surg. (1995) 60(4):999–1007. doi: 10.1016/0003-4975(95)00692-E
27. Jamieson WRE, Ling H, Burr LH, Fradet GJ, Miyagishima RT, Janusz MT, et al. Carpentier-Edwards supraannular porcine bioprosthesis evaluation over 15 years. Ann Thorac Surg. (1998) 66(6 Suppl):S49–52. doi: 10.1016/S0003-4975(98)01127-8
28. Harken DE, Soroff HS, Taylor WJ. Partial and complete prostheses in aortic insufficiency. J Thorac Cardiovasc Surg. (1960) 40:744–62. doi: 10.1016/S0022-5223(19)32572-3
29. Braunwald N. Complete replacement of the mitral valve. Successful clinical application of a flexible polyurethane prosthesis—PubMed. J Thorac Cardiovasc Surg. (1960) 40(1):1–11. doi: 10.1016/S0022-5223(19)32638-8
30. Carpentier A. Biological factors affecting long-term results of valvular heterografts. J Thorac Cardiovasc Surg. (1969) 58(4):467–89. doi: 10.1016/S0022-5223(19)42561-0
31. Gott JP, Girardot MN, Girardot JMD, Hall JD, Whitlark JD, Horsley WS, et al. Refinement of the alpha aminooleic acid bioprosthetic valve anticalcification technique. Ann Thorac Surg. (1997) 64(1):50–8. doi: 10.1016/S0003-4975(97)00118-5
32. Ogle MF, Kelly SJ, Bianco RW, Levy RJ. Calcification resistance with aluminum-ethanol treated porcine aortic valve bioprostheses in juvenile sheep. Ann Thorac Surg. (2003) 75(4):1267–73. doi: 10.1016/S0003-4975(02)04489-2
33. Isenburg JC, Simionescu DT, Vyavahare NR. Tannic acid treatment enhances biostability and reduces calcification of glutaraldehyde fixed aortic wall. Biomaterials. (2005) 26(11):1237–45. doi: 10.1016/j.biomaterials.2004.04.034
34. Zilla P, Brink J, Human P, Bezuidenhout D. Leading opinion prosthetic heart valves: catering for the few. Biomaterials. (2008) 29:385–406. doi: 10.1016/j.biomaterials.2007.09.033
35. Weber B, Dijkman PE, Scherman J, Sanders B, Emmert MY, Grünenfelder J, et al. Off-the-shelf human decellularized tissue-engineered heart valves in a non-human primate model. Biomaterials. (2013) 34(30):7269–80. doi: 10.1016/j.biomaterials.2013.04.059
36. Laker L, Dohmen PM, Smit FE. The sequential effects of a multifactorial detergent based decellularization process on bovine pericardium. Biomed Phys Eng Express. (2020) 6(6):065011. doi: 10.1088/2057-1976/abb5e9
37. Laker L, Dohmen PM, Smit FE. Synergy in a detergent combination results in superior decellularized bovine pericardial extracellular matrix scaffolds. J Biomed Mater Res B Appl Biomater. (2020) 108(6):2571–8. doi: 10.1002/jbm.b.34588
38. Botes L, Laker L, Dohmen PM, van den Heever JJ, Jordaan CJ, Lewies A, et al. Advantages of decellularized bovine pericardial scaffolds compared to glutaraldehyde fixed bovine pericardial patches demonstrated in a 180-day implant ovine study. Cell Tissue Bank. (2022) 23(4):791–805. doi: 10.1007/s10561-021-09988-8
39. Lewies A, Botes L, van den JJ, Dohmen PM, Smit FE. Monomeric glutaraldehyde fixation and amino acid detoxification of decellularized bovine pericardium for production of biocompatible tissue with tissue-guided regenerative potential. Heliyon. (2023) 0(0):e19712. doi: 10.1016/j.heliyon.2023.e19712
40. Neethling W, Yadav S, Hodge A, Glancy R. Enhanced biostability and biocompatibility of decellularized bovine pericardium, crosslinked with an ultra-low concentration monomeric aldehyde and treated with ADAPT. J Heart Valve Dis. (2008) 17(4):456–63.18751476
41. Dellimore K, Kemp I, Scheffer C, Weich H, Doubell A. The influence of leaflet skin friction and stiffness on the performance of bioprosthetic aortic valves. Australas Phys Eng Sci Med. (2013) 36(4):473–86. doi: 10.1007/s13246-013-0230-0
42. Van Aswegen KHJ, Smuts AN, Scheffer C, Weich HSV, Doubell AF. Investigation of leaflet geometry in a percutaneous aortic valve with the use of fluid-structure interaction simulation. J Mech Med Biol. (2012) 12(01):1250003. doi: 10.1142/S0219519411004538
43. Kemp I, Dellimore K, Rodriguez R, Scheffer C, Blaine D, Weich H, et al. Experimental validation of the fluid-structure interaction simulation of a bioprosthetic aortic heart valve. Australas Phys Eng Sci Med. (2013) 36(3):363–73. doi: 10.1007/s13246-013-0213-1
44. Esterhuyse A, Van Der Westhuizen K, Doubell A, Weich H, Scheffer C, Dellimore K. Application of the finite element method in the fatigue life prediction of a stent for a percutaneous heart valve. J Mech Med Biol. (2012) 12(1). doi: 10.1142/S021951941200448X
45. Smuts AN, Blaine DC, Scheffer C, Weich H, Doubell AF, Dellimore KHH. Application of finite element analysis to the design of tissue leaflets for a percutaneous aortic valve. J Mech Behav Biomed Mater. (2011) 4(1):85–98. doi: 10.1016/j.jmbbm.2010.09.009
46. Modine T, Ben Ali W, Perrin N. Large animal models for transcatheter heart valve prosthesis development: making sheep's eyes at supra-annular banding. Basic Transl Sci. (2022) 7(5):496–7. doi: 10.1016/j.jacbts.2022.03.010
47. Lossi L. Anatomical features for an adequate choice of the experimental animal model in biomedicine: III. Ferret, goat, sheep, and horse. Ann Anat. (2022) 244. doi: 10.1016/j.aanat.2022.151978
48. Iaizzo PA, Bianco RW, Hill AJ, St. Louis JD editors. Heart valves: from design to clinical implantation. Berlin, Germany: Springer US (2013). Vol. 4. p. 1–429.
49. Rey NA, Moreira LFP, Cheung DT, Casagrande ISJ, Benvenuti LA, Stolf NAG. Comparative experimental study between L-hydro treated pulmonary homograft and fresh pulmonary homograft. Rev Bras Cir Cardiovasc. (2011) 26(2):282–90. doi: 10.1590/S0102-76382011000200020
50. Ozaki S, Flameng W. A new model to test the calcification characteristics of bioprosthetic heart. Ann Thorac Cardiovasc Surg. (2004) 2:23–8.
51. Flameng W, Meuris B, Yperman J, De VG, Herijgers P, Verbeken E. Factors influencing calcification of cardiac bioprostheses in adolescent sheep. J Thorac Cardiovasc Surg. (2006) 132(1):89–98. doi: 10.1016/j.jtcvs.2006.02.036
52. Kheradvar A, Zareian R, Kawauchi S, Goodwin RL, Rugonyi S. Animal models for heart valve research and development. Drug Discov Today Dis Models. (2017) Summer 24:55–62. doi: 10.1016/j.ddmod.2018.04.001
53. Animals NRC (US). Guide for the care and use of laboratory animals (2011). Available at: https://www.ncbi.nlm.nih.gov/books/NBK54050/ (Accessed July 31, 2021).
54. Frater W, Sussman M, Mohr FW. Quattro valve trial at mid-term: December 1996 to November 2004. J Heart Valve Dis. (2006) 15(2):230–7.16607906
55. Bjørnstad K, Duran RM, Nassau KG, Gometza B, Hatle LK, Duran CMG. Clinical and echocardiographic follow-up after aortic valve reconstruction with bovine or autologous pericardium. Am Heart J. (1996) 132(6):1173–8. doi: 10.1016/S0002-8703(96)90460-3
56. Mepham BL. Theory and practice of histological techniques. 3rd ed. Bancroft J. D., Stevens A., editors. Edinburgh: Churchill Livingstone (1990). p. 740.
57. Spurr AR. A low-viscosity epoxy resin embedding medium for electron microscopy. J Ultrastruct Res. (1969) 26(1–2):31–43. doi: 10.1016/S0022-5320(69)90033-1
58. Tedder M, Liao J, Weed B, Stabler C, Zhang H. Stabilized collagen scaffolds for heart valve tissue engineering. Tissue Eng. (2009) 15(6):1257–68. doi: 10.1089/ten.tea.2008.0263
59. Ionescu MI, Pakrashi BC, Holden MP, Mary DA, Wooler GH. Results of aortic valve replacement with frame-supported fascia lata and pericardial grafts. J Thorac Cardiovasc Surg. (1972) 64(3):340–53. doi: 10.1016/S0022-5223(19)39830-7
60. Sinha P, Zurakowski D, Susheel Kumar TK, He D, Rossi C, Jonas RA. Effects of glutaraldehyde concentration, pretreatment time, and type of tissue (porcine versus bovine) on postimplantation calcification. J Thorac Cardiovasc Surg. (2012) 143(1):224–7. doi: 10.1016/j.jtcvs.2011.09.043
61. Manji RA, Lee W, Cooper DKC. Xenograft bioprosthetic heart valves: past, present and future. Int J Surg. (2015) 23(Pt B):280–4. doi: 10.1016/j.ijsu.2015.07.009
62. Kim KM, Herrera GA, Battarbee HD. Role of glutaraldehyde in calcification of porcine aortic valve fibroblasts. Am J Pathol. (1999) 154(3):843–52. doi: 10.1016/S0002-9440(10)65331-X
63. Cui L, Jia J, Guo Y, Liu Y, Zhu P. Preparation and characterization of IPN hydrogels composed of chitosan and gelatin cross-linked by genipin. Carbohydr Polym. (2014) 99:31–8. doi: 10.1016/j.carbpol.2013.08.048
64. Shetty R, Pibarot P, Audet A, Janvier R, Dagenais F, Perron J, et al. Lipid-mediated inflammation and degeneration of bioprosthetic heart valves. Eur J Clin Invest. (2009) 39(6):471–80. doi: 10.1111/j.1365-2362.2009.02132.x
65. Human P, Bezuidenhout D, Aikawa E, Zilla P. Residual bioprosthetic valve immunogenicity: forgotten, not lost. Front Cardiovasc Med. (2022) 8:760635. doi: 10.3389/fcvm.2021.760635
66. Kostyunin AE, Yuzhalin AE, Rezvova MA, Ovcharenko EA, Glushkova TV, Kutikhin AG. Degeneration of bioprosthetic heart valves: update 2020. J Am Heart Assoc. (2020) 9(19). doi: 10.1161/JAHA.120.018506
67. Karakoyen S, Ozan Gursoy M, Yesin M. Histopathological and immunohistochemical evaluation of pannus tissue in patients with prosthetic valve dysfunction. J. Heart Valve Dis. (2016) 25(1):104–11.27989094
68. Gilbert T, Sellaro T, Badylak SF. Decellularization of tissues and organs. Biomaterials. (2006) 27(19):3675–83. doi: 10.1016/j.biomaterials.2006.02.014
69. Mendoza-Novelo B, Valerio J. Decellularization, stabilization and functionalization of collagenous tissues used as cardiovascular biomaterials. In: Pignatello R, editor. Biomaterials—physics and chemistry. London, England: InTech (2011).
70. Umashankar PR, Mohanan P V, Kumari T V. Glutaraldehyde treatment elicits toxic response compared to decellularization in bovine pericardium. Toxicol Int. (2012) 19(1):51. doi: 10.4103/0971-6580.94513
71. Li N, Li Y, Gong D, Xia C, Liu X, Xu Z. Efficient decellularization for bovine pericardium with extracellular matrix preservation and good biocompatibility. Interact Cardiovasc Thorac Surg. (2018) 26(5):768–76. doi: 10.1093/icvts/ivx416
72. Mendoza-Novelo B, Avila EE, Cauich-Rodríguez JV, Jorge-Herrero E, Rojo FJ, Guinea G V, et al. Decellularization of pericardial tissue and its impact on tensile viscoelasticity and glycosaminoglycan content. Acta Biomater. (2011) 7(3):1241–8. doi: 10.1016/j.actbio.2010.11.017
73. Da Costa FDA, Costa ACBA, Prestes R, Domanski AC, Balbi EM, Ferreira ADA, et al. The early and midterm function of decellularized aortic valve allografts. Ann Thorac Surg. (2010) 90(6):1854–60. doi: 10.1016/j.athoracsur.2010.08.022
74. Simon P, Kasimir MT, Seebacher G, Weigel G, Ullrich R, Salzer-Muhar U, et al. Early failure of the tissue engineered porcine heart valve SYNERGRAFT® in pediatric patients. Eur J Cardiothorac Surg. (2003) 23(6):1002–6. doi: 10.1016/S1010-7940(03)00094-0
75. Crapo PM, Gilbert TW, Badylak SF. An overview of tissue and whole organ decellularization processes. Biomaterials. (2011) 32(12):3233–43. doi: 10.1016/j.biomaterials.2011.01.057
Keywords: TAVI, transcatheter heart valve, decellularized pericardium, low-dose glutaraldehyde, calcification, ovine model
Citation: Weich H, Botes L, Doubell A, Jordaan J, Lewies A, Marimuthu P, van den Heever J and Smit F (2023) Development and testing of a transcatheter heart valve with reduced calcification potential. Front. Cardiovasc. Med. 10:1270496. doi: 10.3389/fcvm.2023.1270496
Received: 31 July 2023; Accepted: 3 November 2023;
Published: 6 December 2023.
Edited by:
Nalini Marie Rajamannan, Mayo Clinic, United StatesReviewed by:
Paul Human, University of Cape Town, South AfricaAndrea Colli, University of Pisa, Italy
© 2023 Weich, Botes, Doubell, Jordaan, Lewies, Marimuthu, van den Heever and Smit. This is an open-access article distributed under the terms of the Creative Commons Attribution License (CC BY). The use, distribution or reproduction in other forums is permitted, provided the original author(s) and the copyright owner(s) are credited and that the original publication in this journal is cited, in accordance with accepted academic practice. No use, distribution or reproduction is permitted which does not comply with these terms.
*Correspondence: Hellmuth Weich aHdlaWNoQHN1bi5hYy56YQ==