- Department of Internal Medicine, The Fourth People's Hospital of Nanning, Nanning, China
The human immunodeficiency virus (HIV) infection can cause damage to multiple systems within the body, and the interaction among these various organ systems means that pathological changes in one system can have repercussions on the functions of other systems. However, the current focus of treatment and research on HIV predominantly centers around individual systems without considering the comprehensive relationship among them. The central nervous system (CNS) and cardiovascular system play crucial roles in supporting human life, and their functions are closely intertwined. In this review, we examine the effects of HIV on the CNS, the resulting impact on the cardiovascular system, and the direct damage caused by HIV to the cardiovascular system to provide new perspectives on HIV treatment.
1. Introduction
Acquired immune deficiency syndrome (AIDS) is a condition characterized by systemic immune deficiency caused by infection with the human immunodeficiency virus (HIV). HIV could evade the innate immune detection in the eclipse phase that follows virus inoculation and establish a foothold in host cells, which lead to massive virus propagation in T cells and significant T cell death (1). HIV primarily targets cells that possess CD4 receptors, particularly CD4+ T cells, leading to immune system dysfunction, damage to multiple systems within the body, and severe consequences (2–6). Each year, an estimated 2.5 million individuals become infected with HIV, and HIV-related illnesses contribute to around 2.1 million deaths (7). Towards the end of the previous century, highly active antiretroviral therapy (ART) was introduced in clinical practice due to its effectiveness in suppressing the viral load of HIV throughout the body, reducing mortality rates, and lowering the incidence of opportunistic infections in individuals with AIDS (8). Consequently, AIDS transformed from a fatal disease to a chronic condition, with the life expectancy of people living with HIV becoming comparable to that of the general population, primarily due to the widespread use of ART (9). However, it's important to note that HIV can impact multiple systems, in addition to the immune system (10–15), such as the central nervous system (CNS), cardiovascular system (CS), respiratory system, digestive system, urinary system, and endocrine system. Compared to individuals without HIV, those infected with HIV have a higher risk of developing neurological and cardiovascular diseases, which tend to occur at an earlier stage, significantly impacting their quality of life (9). The interconnectedness of various organ systems implies that pathological changes in one system can affect the functioning of other systems (16). As a result, even if HIV primarily affects a specific system, its interaction with other systems can disrupt their normal physiological functions, exacerbating the disease in patients. Despite this complexity, most HIV treatment and research remain focused on individual systems, often overlooking the comprehensive interplay among different systems.
The regulatory mechanism of the CNS plays a significant role in the functioning of CS (11). A complex interaction exists between the CNS and CS, where the brain acts as the higher regulatory center, and the autonomic nervous system (ANS) is the final effector in regulating cardiovascular activities. Various brain regions, including the prefrontal cortex (PFC), insular cortex, amygdaloid nucleus, cingulate cortex, hypothalamus and brainstem, form intricate networks within the nervous system to regulate the ANS. Ultimately, the ANS controls CS, establishing the brain-heart axis (17, 18). In this review, our primary focus is to discuss the effects of HIV on the CNS and its subsequent impact on the CS. We also discuss the direct damage caused by HIV to the CS itself, and it is important to note that our discussion specifically centers on the damage caused by HIV and does not cover opportunistic infections resulting from HIV. The references were obtained from PubMed (https://pubmed.ncbi.nlm.nih.gov/). The main search keywords were “HIV”, “AIDS”, “brain”, “central nervous system”, “heart”, “cardiovascular”, “microglia”, “astrocyte”, “neuron”, “prefrontal cortex”, “insular cortex”, “amygdaloid nucleus”, “cingulate” “cortex”, “hypothalamus”, “brainstem”, “heart muscle”, “endocardium”, “pericardium”, “artery” and “Arrhythmia” and so on. The years of search was from 1949 to 2023.
2. Effects of HIV on CNS
The entry of HIV into a target host cell involves the interaction between the viral envelope glycoprotein gp160 and the host receptor. The gp160 is transported through the cellular secretory pathway to the plasma membrane (19). During this process, it undergoes extensive glycosylation, oligomerization into trimers, and proteolytic maturation mediated by a cellular furintype protease that cleaves it into the mature gp120 and gp41 Env subunits, which interact with the CD4 receptor, CC chemokine receptor 5 (CCR5), or C-X-C chemokine receptor 4 (CXCR4) on the surface of the host cell (19, 20). Consequently, HIV has a propensity to invade cells that express CD4 receptors on their cell membranes, including microglia, T lymphocytes, mononuclear/macrophages, and dendritic cells (2–5). Although ART can effectively reduce viral load and the occurrence of opportunistic infections, it is unable to completely eradicate the dormant virus within the host. HIV is known as a neurotropic virus that can cause damage to the nervous system (21). Therefore, to properly diagnose and treat HIV-related neurological damage, it is crucial to understand the relationship between HIV and various cells within the CNS, identify the susceptible sites of the CNS, and comprehend the mechanisms by which HIV induces damage to the CNS.
2.1. Cells in CNS Infected by HIV
In peripheral system, the human leukocyte antigen (HLA) and cytotoxic T lymphocyte (CTL) play the key roles in cell-mediated adaptive immune response (22, 23). The primary function of HLA is to present endogenous and exogenous antigens to T lymphocytes for recognition and response (24). The HLA molecules are cell surface glycoproteins, including two main classes: HLA class I and HLA class II molecules. HLA class I molecules are predominantly involved in the immune defense of intracellular pathogens, and HLA class II play an essential role in displaying peptides from extracellular pathogens (25). Lazaryan et.al suggested that a higher frequency of HLA genotypic supertypes correlated with a higher mean viral load and lower mean CD4 count (26).The CTL delivers a cocktail of cytotoxic substances from secretory lysosomes (cytolytic granules) to destroy the target (23). Madrid-Elena et.al considered that CTLs are suppressed after HIV infection, which may be related to miRNA((hsa-miR-10a-5p) (27). However, the effects of HIV on CNS various cells are more complex.
The precise mechanism by which HIV enters the nervous system is not yet fully understood, but several hypotheses have been put forward. One such hypothesis is the “Trojan horse” theory, suggesting that the virus gains access to the CNS using monocytes or infected CD4+ T lymphocytes as a means of transport (6). Another hypothesis, proposed by Ruifen Xu et al., suggests that HIV can disrupt the integrity of the blood-brain barrier (BBB) by affecting the tight connections between endothelial cells that form the blood vessels, thereby allowing entry into the brain (28). Banks et al. proposed that HIV crosses the BBB through cellular transfer facilitated by the viral envelope protein gp120 (29). Additionally, Kamerman suggested that HIV may directly infect peripheral nerve fibers and subsequently travel in a retrograde manner to reach the CNS (30). Once inside the CNS, HIV invades various cell types, including astrocytes, microglia, oligodendrocytes and neurons Table 1 and Figure 1 (31).
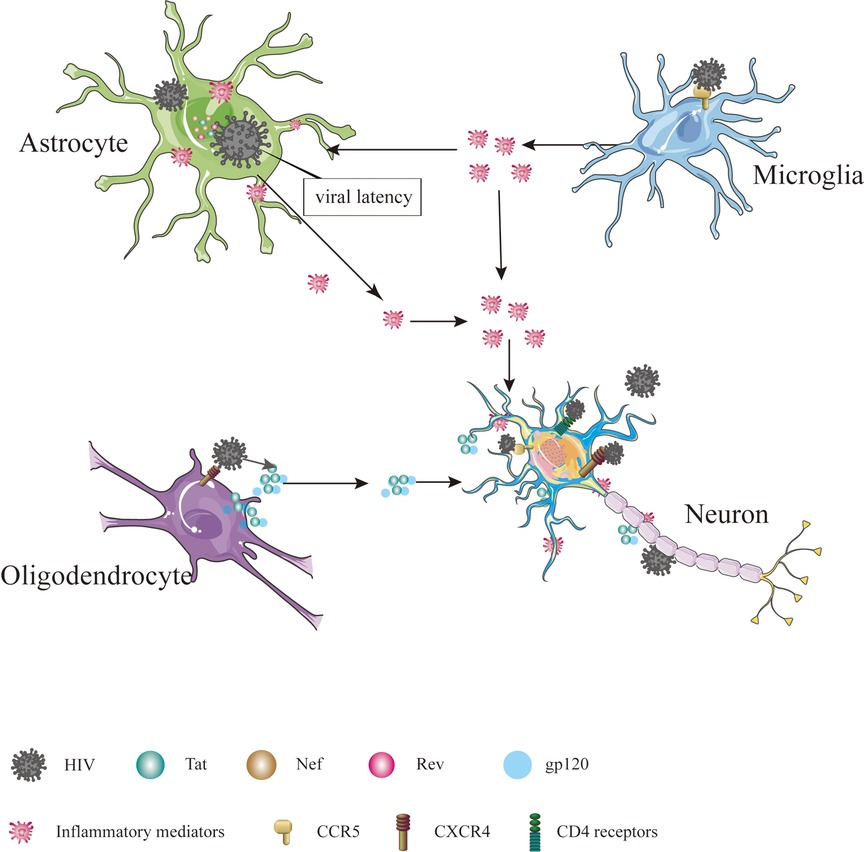
Figure 1. Cells in CNS infected by HIV. The cells in CNS, including astrocyte, microglial, oligodendrocyte and neuron, are affected directly or indirectly by HIV, ultimately leading to dysfunction, injury and death.
2.1.1. Astrocyte
Interestingly, HIV infectious virions and viral proteins have been detected in astrocytes, despite these cells lacking CD4 receptors and CCR5 (32). Astrocytes can internalize HIV through endocytosis and maintain viral latency by suppressing viral replication (33–35). Although HIV cannot replicate, it can interact with astrocytes, leading to the production and release of viral proteins such as Tat, Nef and Rev, contributing to inflammation and nerve damage (36). Inflammation in the nervous system can disrupt the energy metabolism of astrocytes (37). Given that astrocytes provide vital energy support to neurons, any disturbance in their energy metabolism can lead to neuronal energy metabolism disorders (38–40). In addition, inflammation can stimulate the proliferation and differentiation of astrocytes, resulting in the formation of glial scars, which impede axon regeneration and cell migration and directly affect neuronal growth (41, 42). More importantly, as a significant component of BBB, astrocytes may influence the function and structure of the BBB when affected by HIV (43).
2.1.2. Microglia
Microglia, a component of the innate immune system, are often referred to as the “macrophages” of the CNS (44). Excessive activation of microglia can contribute to the development of various neurological disorders, including Alzheimer's disease (45), Parkinson's disease (46) and HIV-associated neurocognitive disorder (HAND) (47). Microglia more susceptible to HIV infection due to CCR5 is expressed on the cell surface (48). Prolonged microglia activation can increase inflammatory chemokines and cytokines, adversely affecting astrocytes and neurons and increasing neurotoxic substances that induce neuronal apoptosis, ultimately contributing to the development of HAND (49, 50).
2.1.3. Oligodendrocytes
Oligodendrocytes, which form the myelin sheath of the axon of the brain, do not touch the axon directly but repeatedly wrap the extension of axons and then gather the myelin sheath of multiple helical structures (51). The formation of the myelin sheath increases the speed and energy efficiency of nerve conduction by facilitating the jump in signal transmission (52), which is essential for cognitive functions (53). The involvement of HIV infection in oligodendrocytes, which specifically express the CXCR4 receptor, remains a topic of debate (54). However, research conducted by Zou et al. indicates that damage to oligodendrocytes is caused by the release of the Tat protein by HIV, resulting in the inhibition of cell growth, developmental retardation, and even cell death (55). In addition to Tat, the gp120 protein has been implicated in oligodendrocyte injury, as it inhibits myelination, induces dysfunction and triggers apoptosis of oligodendrocytes (56, 57).
2.1.4. Neuron
Neurons are the fundamental functional units of the nervous system and are considered non-regenerative cells (58). The ability of HIV to infect neurons is still a matter of debate. Previous studies have shown that adult neurons express CXCR4, CCR5 and CCR3 on their surface and that CD4 receptors may also be present in certain neurons located in the cerebellum, thalamus, pons and hippocampus (59–61). Nath et al. proposed that proteins such as Tat and gp120 can disrupt the Ca2+ homeostasis of neurons and normal neuronal functions, leading to neuronal dysfunction, injury and death (62). Exposure of neurons to Tat and gp120 has been shown to increase the levels of glutamate (Glu) and the expression of N-methyl-D-aspartic acid (NMDA) receptors (63, 64). Furthermore, gp120 can enhance the inhibitory function of neurons by increasing the expression of gamma-aminobutyric acid (GABA) type A receptors (65).
2.2. The areas of CNS vulnerable to HIV
HIV can have wide-ranging effects on the metabolism, neural network structure, and volume of the brain (66–68). Certain cortical and subcortical regions of the brain, including the medial prefrontal cortex (MPFC), basal ganglia, and hippocampus, which play crucial roles in cognition and emotion, are susceptible to the impact of HIV and HIV-related proteins, leading to functional and structural alterations within these regions (69, 70). Jason J. Paris et al. has shown that the Tat protein can induce microglia activation in the MPFC, anterior cingulate cortex (ACC), amygdala, nucleus accumbens, and dentate gyrus, suggesting that these regions are also vulnerable to the effects of HIV (71). Furthermore, Sara R. Nass et al. demonstrated that HIV Tat protein not only affects the PFC and amygdala but also reduces oxytocin levels in the hypothalamus (72). Theresa K. Smit et al. dissected the brains of HIV-infected individuals and identified the presence of HIV in the frontal, parietal, and occipital lobes (73). Jing Sui et al. utilized head MRI scans and observed abnormal changes in gray matter volume in the thalamus, prefrontal lobe, precuneus, posterior parietal lobe, and occipital lobe of HIV-infected patients (68). Additionally, Eleni Giatsou et al. demonstrated continuous HIV replication in multiple brain regions, including the cerebellum, bulbar region, temporal lobe, substantia nigra, and caudate nucleus (74).
2.3. HIV-induced CNS damage
The acute HIV infection period is brief and peak viremia predicts a viral set point that occurs 4–5 weeks following infection (75). Following the detection of HIV RNA, highly activated CD8+ T cells expand and peak approximately 2 weeks following peak viral load (76). Early in HIV infection, the count of CD4+ T cells is greater than 500 cells/ml (77). Days 8 to 30 after infection are characterized by massive viral replication and the death of large numbers of CD4+ T cells (78). Bolzenius et al. indicated that brain volume alterations may occur in acute infection, with the most prominent differences evident in the later stages of acute HIV infection (79). Nevertheless, Hellmuth et.al suggested that no structural neuroimaging abnormalities were observed in acute HIV infection (80). With the success of ART, HIV-seropositive patients can now live for many years despite chronic viral infection. The CD4+ count of chronic HIV-infected patients receiving ART remain above 250 cells/ml (81). Chronic HIV infection causes persistent low-grade inflammation that induces premature aging of the immune system including senescence of memory and effector CD8+ T cells. CD8+ T cell dysfunctions associated with chronic HIV infection may lead to chronic disturbances in the ability of these cells to properly engage with infected target cells (82). In the chronic phase of HIV infection, the HIV-related damage to the CNS has shifted towards chronic lesions, leading to conditions such as cognitive impairment, behavioral disorders, and motor dysfunction collectively known as HAND (83). However, the diagnosis and treatment of HIV-related HAND face significant challenges because the incidence of HAND does not show a significant correlation with the concentration of HIV-RNA (viral load) or the count of CD4 cells, which are commonly used markers to monitor HIV infection. Instead, the risk of developing HAND tends to increase as the duration of HIV infection progresses (84–87). Previous studies have identified two stages of HIV-induced CNS damage. The first stage involves metabolic disorders (88–90), while the second involves structural lesions (91).
2.3.1. Metabolic dysfunction of CNS
In the early stages of HAND, patients may experience mild difficulties with concentration, motor symptoms, and focal cortical deficits such as apraxia, agnosia, or aphasia (92), although there is no significant observable loss of neurons or other substantial pathological changes detected in HIV patients during this stage (93). In addition, although neuroimaging studies also do not typically reveal significant lesions or structural changes, alterations in certain metabolites have been observed Figure 2 (94). The metabolism of the nervous system is a complex physiological process involving the production of sufficient ATP for energy and the synthesis of neurotransmitters essential for maintaining normal physiological brain functions (95). Upon entering the nervous system, glucose in the blood undergoes a series of enzymatic reactions catalyzed by key enzymes like hexokinase, 1,6-diphosphofructokinase-1 and pyruvate kinase, which can convert glucose into pyruvic acid (Pyr), and subsequently, the tricarboxylic acid cycle generates ATP, providing energy for neuronal functions (96). Neuron-astrocyte interaction is another important mechanism for energy supply in the brain (39, 97). Glucose in astrocytes is converted into glycogen under the influence of glycogen synthase (GYS), which is then gradually broken down into pyruvate (98). Some of these pyruvates are converted into lactate by the enzyme lactate dehydrogenase 5 (LDH5), which is then transported to neurons and converted back into pyruvate by lactate dehydrogenase 1 (LDH1), entering the tricarboxylic acid cycle pathway (99). During this cycle, α-ketoglutaric acid in neurons can react with NH3 to form Glu (100, 101), which can be further converted into gamma-aminobutyric acid (GABA) through the catalytic action of glutamic acid decarboxylase (GAD) (102, 103).
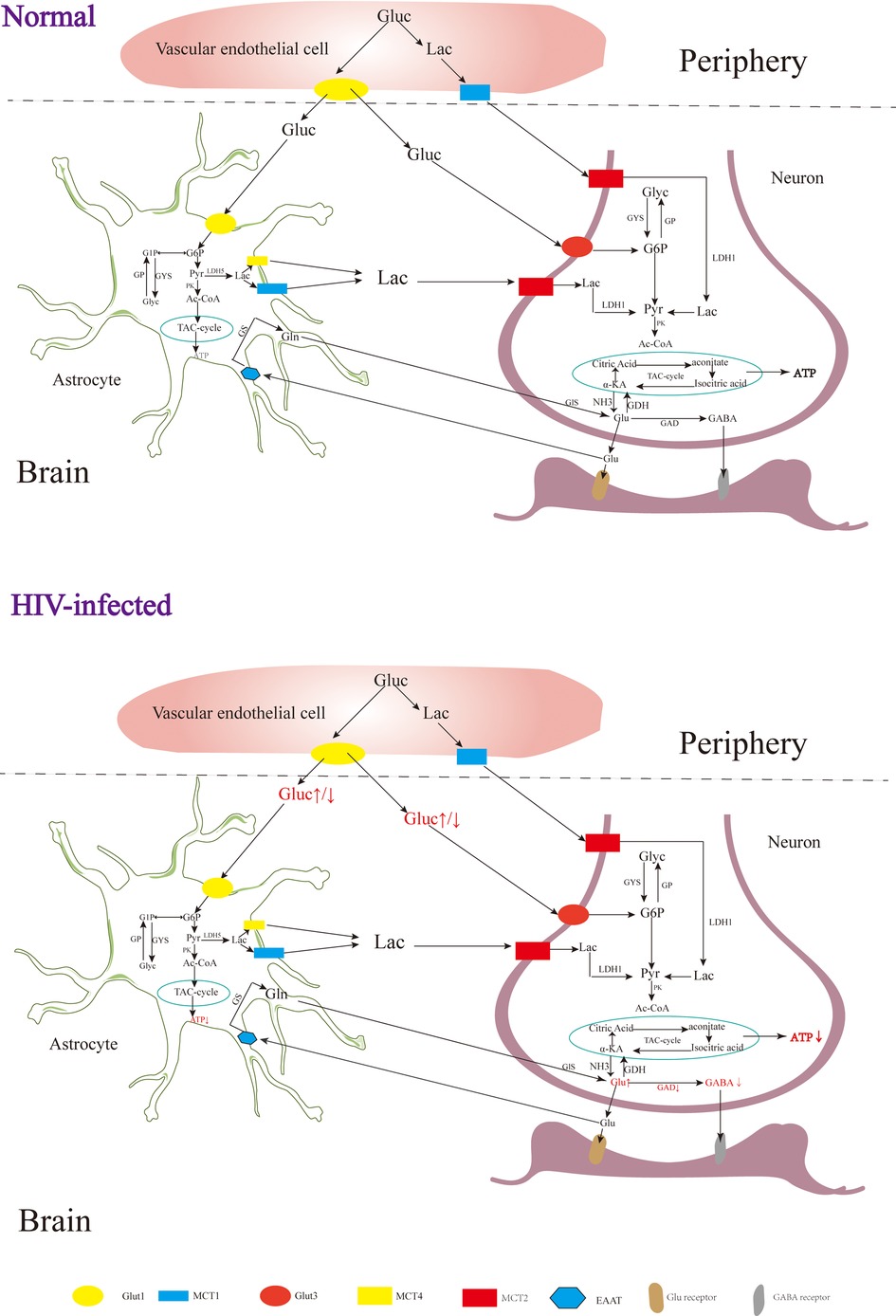
Figure 2. The metabolism of the nervous system. Gluc, glucose; G6P, glucose 6 phosphate; G1P, glucose 1 phosphate; GP, glycogen; Pyr, pyruvate; Lac, lactate; Ac-COA, acetyl coenzyme A; α-KA, α-ketoglutaric acid; Suc-CoA, succinic acid coenzyme A; Suc, succinic acid; Suc-sem, succinic acid semialdehyde; GYS, glycogen synthase; GP, glycogen phosphorylase; PK, pyruvate kinase; LDH, lactate dehydrogenase; GDH, glutamate dehydrogenase; GDA, glutamic acid decarboxylase; GS, glutamine synthetase; Gls, glutaminase; GABA-T, GABA transaminase; SSADH, succinic semialdehyde; Glut, glucose transporter; MCT, monocarboxylic acid transporter; EAAT, excitatory amino acid transporter.
Rottenberg D et al. observed glucose hypermetabolism in the thalamus and basal ganglia, along with hypometabolism in the cortex and subcortical gray matter during the early stages of HIV-associated HAND (104). Zeping Wang et al. found that after receiving ART, glucose metabolism in the frontal cortex of HIV-infected individuals was enhanced, suggesting that the damage to the frontal cortex at this stage is potentially reversible (105). After HIV infection, the level of ATP in the brain is decreased, and the level of ATP in the peripheral blood is increased (106, 107). Tat, an HIV protein, has been implicated in increasing Glu levels and decreasing GABA levels in the brain (108), which may be related to the inhibition of GAD by HIV (109). In the early stages of HIV infection, increased levels of Glu in the brain can contribute to excitatory neurotoxicity, leading to clinical symptoms such as reduced attention (110, 111). As the disease progresses, individuals with HIV and cognitive deficits tend to have lower Glu levels in the parietal gray matter, indicating that decreased Glu levels may contribute to cognitive impairment (112). In addition to HAND, HIV-associated epilepsy is also a significant challenge, with reported incidence rates ranging from 6.1% to 83.75% (113, 114). Although the underlying mechanisms of HIV-related epilepsy are not yet fully understood, it is believed to involve an imbalance of Glu and GABA (108, 115).
2.3.2. Structural damage of CNS
As the disease advances, HIV patients often experience worsening motor dysfunction and cognitive impairments, which become increasingly noticeable and drastically hinder their daily activities. Completing complex tasks often takes them longer, and they may sometimes become unable to accomplish them adequately. Specific motor dysfunctions may include slower, less precise movements, clumsiness, an unstable gait, and a loss of balance (86). Simioni et al. discovered that these patients exhibited significant signs of frontal lobe release, cramps and hyper-reflexes, particularly in the legs (86). At this stage, neuroimaging studies have identified white matter signal abnormalities as the most prevalent (116, 117). White matter (WM) consists of the aggregation of nerve fibers in the central nervous system, predominantly composed of axons and oligodendrocytes of neurons, making it a vital component of the neural network (118). The release of Tat protein and gp120 by HIV can result in the injury and apoptosis of oligodendrocytes (55–57). Consequently, the damage and apoptosis of oligodendrocytes impair the formation of effective myelin sheaths, leading to reduced nerve conduction efficiency and cognitive dysfunction in the brain (52, 53). HIV can also have an impact on the axons of neurons, leading to abnormal neural network connections (119). In advanced cases of HAND with severe cognitive dysfunction and motor symptoms, there may be a connection to white matter injury, whereby white matter injury can disrupt the limbic circuit and damage cortico-cortical connections, thereby affecting intelligence and cognitive function (120). The integrity of nerve fiber connections in various regions such as the corpus callosum, cingulate gyrus, anterior limb of the internal capsule, cerebral foot, anterior crown, and frontal-occipital lobe tract is closely related to balance and gait function (121). HIV-induced damage to the brain's white matter can interfere with nerve fiber connections, leading to abnormal gait in patients with HAND (121). Falls have been associated with injuries to the total white matter, periventricular white matter, and deep white matter (122). Blahak et al. suggested that white matter injury can disrupt the prefrontal subcortical motor circuit, resulting in impaired balance and an increased risk of falls (123). In addition to white matter injury, advanced HAND patients may exhibit decreased subcortical volume, caudate nucleus volume, cerebral malacia foci, and brain atrophy (66, 124, 125). HIV can also contribute to other acute central nervous system diseases, such as stroke. Bertrand et al. revealed a higher incidence of stroke in HIV patients compared to the same age group (126). While men generally have a higher risk of cerebrovascular disease, HIV-infected women aged 25–29 years have a relatively higher risk of ischemic stroke (127), possibly due to HIV-related changes in the production of female endogenous sex hormones, including estrogen deficiency (127, 128). In addition, epigenetics may be involved in brain structural damage.
Epigenetic mechanisms play an important role during the infection with retroviruses, including HIV which mediate the integration of the virus into the host genom (129). The change of epigenetic may be related to regional alterations of brain volumes, cortical thickness, cortical surface areas and neuronal microstructure (130). Persistence of latent HIV infection in the CNS was associated with increased levels of chromatin modifiers, which might result in abnormal transcriptomes, leading to inflammation, neurodegeneration, and neurocognitive impairment (131). Paula Desplats et.al detected changes in the expression of DNMT1, at mRNA and protein levels, that resulted in the increase of global DNA methylation. Moreover, Genome-wide profiling of DNA methylation showed differential methylation on genes related to neurodegeneration; dopamine metabolism and transport; and oxidative phosphorylation (132). Long-term HIV-Tat expression led to poorer short-and long-term memory, lower locomotor activity and impaired coordination and balance ability, increased astrocyte activation and compromised neuronal integrity, and decreased global genomic DNA methylation (133). N-terminal acetylation changes induced by viral infection might play a critical role in virus-host interactions in HIV infection (134). The epigenetic targets that might aid in understanding the aggravated neurodegenerative, cognitive, motor and behavioral symptoms observed in persons living with HIV and addictions Figure 3.
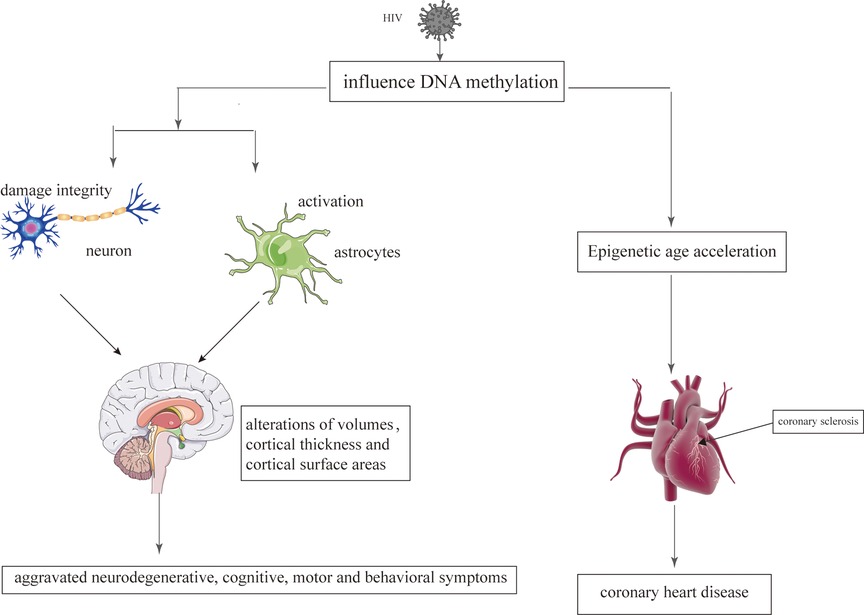
Figure 3. The epigenetic mechanisms modified by HIV on the brain and heart. HIV damages the brain and heart primarily by affecting DNA methylation.
3. Indirect cardiovascular effects of HIV: effects of HIV on brain-heart regulation
The effects of HIV on the CNS encompass both metabolic dysfunction and structural damage. However, it is important to note that these two pathological states have distinct impacts on CS, both in terms of pathogenesis and clinical characteristics Table 2.
3.1. CS alterations induced by metabolic dysfunction of CNS
In the early stages of HIV infection, structural lesions in the brain may not be apparent. However, various metabolic disorders can occur, affecting neurotransmitters such as Noradrenaline (NA), Acetylcholine (Ach), catecholamines (CA), Glu, GABA and neuropeptides, whose abnormal levels may disrupt the regulatory network involving the brain, autonomic nervous system and CS, leading to cardiovascular abnormalities Figure 4 (17, 18, 88–90). While the concentration of NA in the peripheral blood of HIV patients may not show significant changes, there are notable alterations in the concentration of NA in the brain, which can contribute to abnormalities in cardiovascular activity (135, 136). The gp41 and gp120 proteins of HIV can stimulate the release of NA by regulating NMDA receptors in the brain, which activates the sympathetic nervous system, leading to tachycardia and elevated blood pressure (136–139). HIV-Tat protein can induce Ca2+-dependent Ach release through discrete amino acid sequences binding to different acceptance sites in the cortical cholinergic terminals, resulting in decreased heart rate and blood pressure (140, 141).
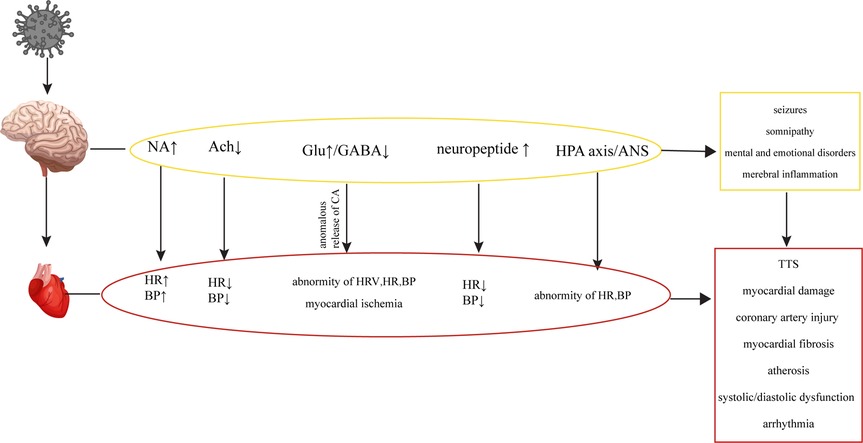
Figure 4. Cardiovascular diseases are caused by metabolic disorders of the CNS. HIV causes CNS metabolic disorders, leading to a series of changes in the cardiovascular system.
HIV infection can induce seizures by disrupting the balance between excitation and inhibition in the brain, known as an imbalance of excitation and inhibition (E-I imbalance), characterized by an increase in Glu levels and a decrease in GABA levels (109, 110, 142). The E-I imbalance in the brain can affect the normal regulatory mechanisms of the CS, leading to alterations in heart rate variability (HRV), changes in heart rate and blood pressure, and potentially causing transient myocardial ischemia (195). The E-I imbalance can also affect catecholamine secretion, leading to repetitive myocardial injury, chronic damage to the heart and coronary arteries, myocardial fibrosis, atherosclerosis, cardiac systolic and diastolic dysfunction, and arrhythmias within the CS (143, 144). In addition to its potential to induce epilepsy, the E-I imbalance in the brain can also impact the sleep patterns of HIV patients, contributing to abnormal functional and structural changes in the heart, leading to an increased risk of myocardial infarction (114, 145, 146). HIV patients frequently experience mental and emotional abnormalities due to the psychological stress associated with the disease itself and the effects of the virus on neurotransmitters in the brain (108, 147). Patients infected with HIV may experience Takotsubo syndrome (TTS) when subjected to both psychological and physical stress (148, 149). TTS is a notable condition highlighting the connection between mental stress, cortical activation, and cardiac disease. It is characterized by clinical symptoms resembling a myocardial infarction, accompanied by acute systolic apical left ventricular dysfunction triggered by physical or emotional stress (150, 151). TTS typically manifests with sudden onset chest pain, dyspnea and electrocardiogram changes similar to acute coronary syndrome, slightly elevated levels of myocardial enzymes, and transient abnormalities in ventricular wall movement unrelated to specific coronary perfusion area (152). Previous studies have demonstrated that HIV can cause dysfunction of the hypothalamic-pituitary-adrenal (HPA) axis and ANS, leading to abnormal levels of peripheral adrenaline and impaired regulation of the CS (135, 153, 154). Additionally, the levels of neuropeptides, which can directly impact the CS by increasing blood pressure and inhibiting sympathetic activity through their actions on the hypothalamus, have been found elevated in the brain (155–157). HIV could cause oxidative stress in the CNS and eventually result in abnormal baroreceptor and chemoreceptor signals transmitted by cardiac afferent fibers to the solitary tract nucleus, paraventricular nucleus and rostral ventrolateral medulla (RVLM), leading to sympathetic dysfunction, fatal arrhythmia, heart failure and myocardial ischemia (158–161).
3.2. CS injury in brain structural damage
Structural damage to the brain resulting from HIV infection can have more severe consequences, and the specific regions affected can influence the extent of cardiovascular damage. In particular, injury to the prefrontal cortical-insula-amygdala-cingulate cortical-hypothalamic-brain stem network is more likely to cause CS impairments Figure 5.
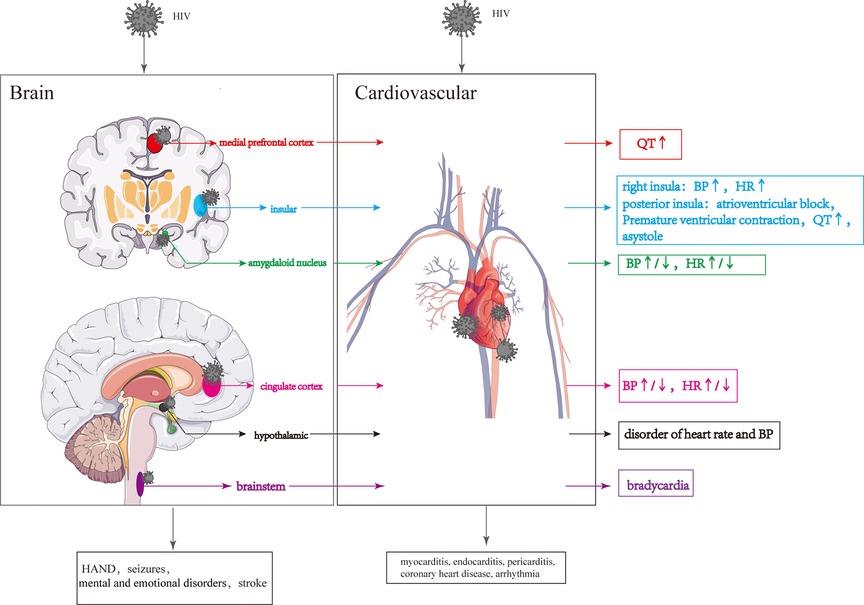
Figure 5. Cardiovascular lesions are caused by structural CNS damage and those directly caused by HIV. HIV invasion of the CNS causes changes in the different brain regions, resulting in changes in the cardiovascular system. HIV directly invades the cardiovascular system leading to various cardiovascular diseases.
3.2.1. Prefrontal cortex
The prefrontal cortex (PFC) can be divided into two regions based on functional, morphological, and evolutionary differences: the lateral PFC (LPFC) and the medial PFC (MPFC) (162). The ventral portion of medial prefrontal cortex (vMPFC), a portion of the MPFC, plays a crucial role in regulating the CS (163). Upon stimulation, the vMPFC activates the sympathetic nerve, increasing mean arterial pressure (MAP) (164). Notably, it has been reported that lower CD4 levels in HIV patients are associated with greater connectivity between the right vMPFC and right posterior insula and longer QT interval (165).
3.2.2. Insular
The insula is involved in various physiological functions, including visceral sensation and visceral movement (166–168). The posterior insula receives and integrates signals related to visceral sensations, which are then projected to the anterior insula (169). After receiving visceral sensory signals from the posterior insular lobe, the anterior insular lobe sends out visceral movement signals, regulating visceral movement, including the CS (170). In HIV-infected individuals, damage to the gray matter of the right insular lobe can result in parasympathetic inhibition and sympathetic excitation, leading to increased heart rate and blood pressure (125, 171, 172). Overstimulation of the posterior insula can contribute to the development of conditions such as atrioventricular block, premature ventricular contractions, prolonged QT interval, and even asystole (173). The insula consists of two different types of neurons simultaneously: 27 percent responsible for sympathetic excitation and 9 percent responsible for sympathetic inhibition (174). Furthermore, the left and right insula have distinct roles in regulating the CS, with the left insula influencing sympathetic activity by regulating peripheral blood and cardiac adrenaline levels, while the right insula exerts the opposite effect by activating the parasympathetic nervous system (171, 172). Due to the insula's complex and multifaceted physiological functions, CS abnormalities resulting from insula damage can be subject to various uncertainties.
3.2.3. Amygdaloid nucleus
The cortical nuclei, including the basolateral nucleus, cortical-like nuclei and central-medial nuclei, are situated in the medial temporal lobe (175). The amygdaloid nucleus, particularly the basolateral nucleus, receives and integrates signals from the cortex, brainstem and thalamus to regulate cardiovascular activity. These signals are then transmitted to the basal ganglia and subsequently reach the central part of the central medial nucleus (CeA). The CeA sends signals to the brainstem, hypothalamus, and other areas to influence blood pressure and heart rate (175–178). Glu secreted by the CeA increases heart rate and blood pressure by activating the sympathetic nervous system, while GABA secreted by the CeA reduces heart rate and blood pressure by activating the parasympathetic nervous system (179–181). In HIV-infected individuals, degeneration or volume loss in the amygdala may impair the transmission of cardiovascular regulatory signals to areas such as the brainstem and hypothalamus, leading to changes in blood pressure and heart rate (182, 183).
3.2.4. Cingulate cortex
The cingulate cortex plays a role in receiving and integrating visceral sensory information, including changes in blood pressure, and it outputs signals that regulate autonomic, neuroendocrine, and cardiovascular responses (184). The posterior cingulate cortex can be further divided into the anterior cingulate cortex (ACC) and posterior cingulate cortex (PCC) (185). Activation of the ACC has been shown to reduce vagal tone and increase heart rate (186, 187), while inactivation of the ACC enhances the vagal tone and increases heart rate variability (188). HIV may cause impairment of ACC, which could change the heart rate and blood pressure. However, cardiovascular lesions caused by HIV injury to the cingulate gyrus are very complicated due to the presence of both the hypertension and hypotensive regions in the cingulate gyrus (165, 189).
3.2.5. Hypothalamic
The hypothalamus, acting as a central hub for autonomic nerve outflow and the hypothalamic-pituitary-adrenal (HPA) axis, plays a vital role in regulating cardiovascular functions (190, 191). The hypothalamic paraventricular nucleus (PVN) releases corticotropin-releasing hormone (CRH), which then activates the HPA axis, leading to the release of adrenocorticotropic hormone (ACTH) from the pituitary gland. ACTH, in turn, stimulates the release of glucocorticoids from the adrenal gland, resulting in an increase in blood pressure (192). The activity level of CRH neurons located in the hypothalamic PVN can influence changes in blood pressure (193). Increased activity and/or number of CRH neurons can enhance CRH synthesis, transport and release, ultimately leading to elevated blood pressure (194). CRH neurons in the PVN receive catecholamine signals from brain regions such as the locus coeruleus (LC), nucleus of the solitary tract (NTS) and parabrachial nucleus (195–198), following which CRH neurons are activated, leading to an increased concentration of NA in the PVN, causing hypertension and an elevated heart rate (199, 200). Blood pressure regulation is also influenced by oxytocin (OXY) and arginine vasopressin (AVP), which are released from the supraoptic nucleus (SON), paraventricular nucleus (PVN) and suprachiasmatic nucleus (SCN) of the hypothalamus (201). Pro-oxytocin and pro-vasopressin, synthesized in the SON, PVN, and SCN, are transported, stored in the posterior pituitary, and released into the peripheral circulation to regulate the CS (202). In addition, OXY and AVP signals can project to various brain regions, including the olfactory bulb, orbitofrontal cortex, cingulate gyrus, amygdala, striatum, hippocampus, bed nucleus terminalis, suprachiasmatic nucleus, and autonomic ganglia (203). HIV-gp120 can invade the hypothalamus and directly stimulate the hypothalamic axis to induce cortisol secretion (204). HIV-Vpr and HIV-Tat can enhance glucocorticoid action by increasing the sensitivity of target tissues to glucocorticoids and stimulate the inflammatory cytokine HPA activity, resulting in cortisol secretion (205). In addition, Furthermore, HIV infection may reduce levels of oxytocin, which can disrupt the regulation of blood pressure (72).
3.2.6. Brainstem
The brainstem consists of the midbrain, pons, and medulla oblongata, with the medulla oblongata particularly involved in cardiovascular regulation (206). When blood pressure increases, the nucleus tractus solitarius receives and integrates signals from baroreceptors, and then transmits these signals to neurons in the lateral caudal ventrolateral medulla (CVLM) (207), providing inhibitory signals to neurons in the rostral ventrolateral medulla (RVLM) (207). Subsequently, the inhibitory neural signals from RVLM neurons reduce the output of sympathetic signals from neurons located in the intermediolateral columns of cells in the spinal cord to peripheral organs, ultimately inhibiting cardiovascular activity (207). Furthermore, cardiac parasympathetic fibers originating from the dorsal vagus and hypochondriac nuclei of the medulla oblongata play a role in inhibiting cardiovascular activity. Brailoiu et al. suggested that HIV-Tat can stimulate the vagus nucleus, increasing parasympathetic activity in the heart and resulting in persistent bradycardia (208). Nagamitsu et al. also noted that structural changes in the medulla oblongata might occur in the later stages of HIV infection, which could potentially impact cardiovascular activity (209).
4. Direct effects of HIV on the CS
In AIDS patients, HIV infection is a significant contributor to the development of acquired heart disease, particularly symptomatic heart failure (210). Cardiovascular complications associated with HIV infection, such as subclinical electrocardiogram (ECG) changes, cardiomyopathy, and sudden cardiac death, are often observed in the later stages of AIDS (211). A model based on the AIDS Treatment Evaluation Cohort in the Netherlands revealed that 78% of HIV-infected individuals receiving ART were still diagnosed with cardiovascular disease, despite living longer (212). HIV infection increases the risk of various cardiac abnormalities, including those affecting the heart muscle, pericardium, heart valves, arterial vessels, and conduction system Table 3 (213).
4.1. Damage of HIV on the heart muscle
HIV-positive individuals are prone to myocarditis, with specific myocarditis accounting for approximately 50% of cases (214). The invasion of cardiomyocytes by HIV is a critical factor in developing specific myocarditis (215). Shaboodien et al. identified histological evidence of myocarditis in 44% of HIV patients using myocardial biopsy, suggesting that HIV can directly induce changes in myocardial inflammation (216). In addition, HIV can indirectly damage heart muscle cells, contributing to cardiac complications. Monsuez et al. demonstrated that HIV induces abnormal inflammation by infecting cardiac dendritic cells and endothelial cells, which mediate chronic inflammation by stimulating the production of tumor necrosis factor-α, interleukin-1 and omega-6, as well as other pro-inflammatory cytokines, leading to myocardial damage and dysfunction (217). HIV-induced cardiomyopathy occurs through mechanisms involving the invasion of cardiomyocytes by the virus and the apoptotic signaling triggered by HIV proteins like gp120, Tat and cytokines (218). Cheryl et al. showed that cardiomyocytes undergo apoptosis through both mitochondrion- and death receptor-controlled apoptotic pathways in HIV patients, which may be associated with gp120-induced apoptosis (219). Additionally, autoimmune mechanisms can play a significant role in myocarditis. HIV-associated B cells are stimulated to produce autoantibodies targeting the heart muscle, resulting in myocardial damage and systolic dysfunction (220).
The pathological features of HIV-associated cardiomyopathy are similar to those seen in patients without HIV infection, which primarily include ventricular dilation, a rounded apex, altered heart shape, and increased heart weight due to fibrosis and hypertrophy of cardiomyocytes (221, 222). Histologically, increased collagen in interstitial and endocardial fibers is a prominent characteristic of HIV-associated cardiomyopathy, along with myocardial cell hypertrophy, cardiomyocyte degeneration, myofibrillar loss, and intramuscular hydropic degeneration (221, 222). Before the widespread use of ART, symptomatic dilated cardiomyopathy with decreased ejection fraction was commonly observed in HIV-related cardiomyopathy. However, with the advent of ART, asymptomatic cardiomyopathy with or without abnormal ejection fraction has become more predominant (223, 224). Among asymptomatic HIV-infected patients, approximately 43.4% have diastolic dysfunction, while 8.3% have systolic dysfunction (225). The phenotype of HIV-associated dilated cardiomyopathy corresponds to the severity of immunosuppression, with a worse prognosis observed in patients with more severe immunosuppression (226). Patients with HIV-associated dilated cardiomyopathy may exhibit acute left heart failure symptoms (227).
4.2. Damage of HIV on the endocardium
In HIV-infected patients, the incidence of endocardial damage, often characterized by valve regurgitation, can be as high as 77% (228). Individuals with HIV infection who have a CD4 count of less than 50 cells/mm3 and a high viral load (greater than 100,000 copies/ml) are at a four-fold increased risk of developing infective endocarditis (IE), with staphylococcus aureus being a common pathogenic organism associated with this condition (229).
4.3. Damage of HIV on the pericardium
Before the widespread use of ART, the prevalence of pericardial effusion in HIV patients was reported to be as high as 25% (230) but has decreased since the widespread use of ART (231). HIV-associated pericardial effusion is often associated with opportunistic infections due to the patient's compromised immune function (232). In addition to opportunistic infections, pericardial effusion can also be caused by capillary leakage resulting from cytokine activation late in HIV infection (232, 233). Moreover, direct invasion of the pericarditis by HIV is also a direct cause of pericarditis (234).
4.4. Damage of HIV on artery
HIV-related arterial vessel injury is a distinct condition characterized by the infiltration of inflammatory cells and the obstruction of blood vessels, which can weaken the walls of the vessels and lead to the formation of aneurysms (235). Following HIV infection, viral replication, immune system disruption, and intestinal microbial translocation can trigger chronic systemic inflammation, leading to pathological manifestations such as dyslipidemia, thrombosis, chronic inflammation of vascular endothelial cells, and ultimately the development of conditions such as coronary heart disease(CHD) (236).
CHD is also considered to be an age-related heart disease (237). Compared to the individuals without HIV, HIV-infected patients have a higher risk of developing CHD, which tend to occur at an earlier stage (9). With regard to CHD that occurs at an earlier stage, Huang et al. believe that it is related to epigenetic changes caused by HIV (238). Epigenetic age acceleration is verified in HIV-infected patients, which may be related to HIV causing DNA methylation (238). DNA methylation is one of the most studied epigenetic markers, which involves changes in the DNA that are influenced by environmental factors (239). DNA methylation is the common HIV-induced epigenetic changes (240). Therefore, HIV interferes with the genome of the cells by affecting the methylation of the DNA, which could promote the aging process and increase the risk of CHD Figure 3 (241, 242).
4.5. Arrhythmia caused by HIV
ECG abnormalities commonly observed in HIV patients include ventricular conduction defects, such as isolated ST-T abnormalities and prolonged QT interval (243). Apart from the effects of medications, electrolyte imbalances and ANS dysregulation, arrhythmias in HIV-infected patients are also associated with the impact of HIV on the cardiac conduction system (244). Nef, the constituent gene of HIV, is also a major determinant of HIV pathogenicity, leading to the entire electrophysiological recombination of the heart (244). Judith Brouillette et al. suggested that Nef could lead to a 50% reduction in the outward potassium current of repolarization in ventricular myocytes, thereby prolonging the duration of ventricular action potential (245). The Nef genome can also result in a 30% reduction in depolarization of sodium current (246). HIV-Tat prolongs the QTc interval by increasing ROS production and decreasing hERG current in cardiomyocytes (247). In addition, the atrial fibrillation risk (AF) of HIV patients, which is related to the lower CD4 count or (and) and the higher viral load, is higher than that of the non-HIV population (248).
5. Overcome the effects of HIV on the brain-heart axis
The dysfunction of the CS is considered as a fatal disease in HIV-infected patients. Diagnosis and therapeutics for the damage of brain and heart are important methods and approaches to overcome the effects of HIV on the brain-heart axis. Through pharmacological and non-pharmacological interventions, as well as emerging technologies, researchers are making significant strides in improving outcomes for individuals affected by brain and heart-related conditions.
5.1. Diagnosis for brain damage
5.1.1. Clinical manifestations
In the early stages of brain damage,the clinical manifestations are characterized by mild difficulties with concentration, motor symptoms and focal cortical deficits (92). As the disease advances, the clinical manifestations are characterized by significant motor dysfunctions, signs of frontal lobe release, cramps and hyper-reflexes, cognitive dysfunction, neuropathic pain, paresthesia, and abnormal gait (86, 120, 121, 249).
5.1.2. Cerebrospinal fluid
Detection of viral load in cerebrospinal fluid (CSF) is an important means to assess whether HIV has invaded the CNS (250). However, viral load levels of HIV in CSF and the count of CD4+ T cells in blood are not necessarily positively correlated with the severity of brain damage (84–87, 251). Some biochemical substances(including glucose, ATP, lactate, Glu, GABA, NA, and catecholamine, et al.) and biomarkers(t-tau, p-tau, β-amiloid42, neopterin, and S100β,et al.) of CSF are utilized to assess metabolic dysfunction and Structural damage of CNS (104, 106–108, 136, 137, 143, 144, 252–255). In addition, integrated brain on a chip and automated organ-on-chips systems,as well as extracellular vesicles, may be employed to evaluate various neurological damage caused by HIV (256–258).
5.1.3. Electroencephalograph
Electroencephalograph (EEG) is commonly utilized for assessing electrophysiological function in the brain. Tinuper et al. found that abnormal EEGs are correlated with CNS involvement and borderline EEGs may be correlated with asymptomatic patients (259). Barco et al.observed a statistically significant correlation between working memory test Trail Making B and delta waves in patients infected with HIV (255). For functional connectivity, connectivity in alpha, beta, theta, and delta band was decreased in the HIV/AIDS patients (255). Compared to the healthy people, the smaller late positive potential and larger P200 amplitudes were observed in HIV/AIDS patients (260).
5.1.4. Magnetic resonance
Magnetic resonance (MRI), a widely used neuroimaging test, is considered to play an important role in the diagnosis of HAND (261). MRI can be employed to observe structural changes in the brain. In addition, some functional MRI (fMRI) tests, including susceptibility weighted imaging (SWI), diffusion tensor imaging (DTI), magnetic resonance spectroscopy (MRS), are utilized to verify the metabolic dysfunction and Structural damage of CNS caused by HIV, as well as CNS metabolic disorders (262–264).
5.2. Therapeutics for brain damage
ART has reduced the prevalence of HIV-associated dementia, but the persistent brain damage caused by HIV is an unmet challenge. Improving ART effectiveness in suppressing HIV replication in the CNS by either increasing penetration into the CNS is a strategy for protecting CNS. Reducing HIV replication in the central nervous system can inhibit the release of neurotoxins from activated microglia and astrocytes (265). As ART regimens are developed, the adjunctive neuroprotective therapies must accelerate. Anti-excitotoxicity, calcium channel blockers, antioxidants, and anti-inflammatory drugs are considered as adjunctive neuroprotective therapies for HIV-infected patients (265, 266). The neurotrophic factors are also applied to protect CNS (267).
5.3. Diagnosis and therapeutics for heart diseases
Diagnosis for HIV-associated heart diseases is similar to those observed in HIV-uninfected patients (223, 226, 230). EEG, ultrasonic cardiogram, coronal artery angiography, left ventriculography, myocardial enzyme, and markers of heart failure are often used to identify HIV-associated heart diseases (211, 268–270). Except for the need for ART, the therapeutics for HIV-associated heart diseases is similar to HIV-uninfected patients (225, 230, 236). Moreover, the nanomedicines which have been used in the treatment of cancer, may be applied to treat HIV associated heart diseases (271–273).
6. Limitations and future directions
Diseases of the cardiovascular system caused by the CNS are more complex and deadly (268). The clinicians and researchers get a new theoretical basis from the concept of the brain-heart axis (274, 275). They think about the role of the brain-heart axis in the face of diseases of the CNS and(or) cardiovascular system, such as stroke (276), seizures (277) and TTS (278). However, the limitations of this review should be acknowledged. No sufficient evidences about the effects of HIV on brain-heart axis were found. We only infer from the current study that HIV may have an impact on the brain-heart axis. There are two main future directions regarding the effects of HIV on the brain axis. On the one hand, a large amount of clinical data, especially CNS data in HIV-infected patients with cardiovascular disease, should be collected. Through these data, we could further understand CNS function in HIV-infected patients with cardiovascular disease. On the other hand, in vitro and in vivo models of HIV-infected CNS should be established to research the changes in the cardiovascular system.
7. Conclusion
In summary, the nervous system is crucial in regulating the CS through a complex neural network. However, HIV infection can cause functional and structural damage to the nervous system, thereby affecting the neural network's regulation of the CS and ultimately leading to abnormalities in the CS of patients, significantly impacting their quality of life. Thus, it is important to closely monitor changes in their nervous system and take a comprehensive approach to address the alterations in their CS when diagnosing and treating HIV patients.
Author contributions
HS: conceived and designed the review. SL: collected the literatures. HS and SL: wrote the manuscript and reviewed and edited the manuscript. HS and SL: revised the manuscript and the language. HS and SL contributed to the article and approved the submitted version. All authors contributed to the article and approved the submitted version.
Acknowledgments
We thank Home for Researchers editorial team (www.home-forresearchers.com) for language editing service.
Conflict of interest
The authors declare that the research was conducted in the absence of any commercial or financial relationships that could be construed as a potential conflict of interest.
Publisher's note
All claims expressed in this article are solely those of the authors and do not necessarily represent those of their affiliated organizations, or those of the publisher, the editors and the reviewers. Any product that may be evaluated in this article, or claim that may be made by its manufacturer, is not guaranteed or endorsed by the publisher.
References
1. Towers GJ, Noursadeghi M. Interactions between HIV-1 and the cell-autonomous innate immune system. Cell Host Microbe. (2014) 16(1):10–8. doi: 10.1016/j.chom.2014.06.009
2. Adamson CS, Freed EO. The role of mononuclear phagocytes in HTLV-III:LAV infection. Science. (1986) 233(4760):215–9. doi: 10.1126/science.3014648
3. Koenig S, Gendelman HE, Orenstein JM, Canto MCD, Pezeshkpour GH, Yungbluth M, et al. Detection of AIDS virus in macrophages in brain tissue from AIDS patients with encephalopathy. Science. (1986) 233(4768):1089–93. doi: 10.1126/science.3016903
4. Pope M, Betjes MG, Romani N, Hirmand H, Cameron PU, Hoffman L, et al. Conjugates of dendritic cells and memory T lymphocytes from skin facilitate productive infection with HIV-1. Cell. (1994) 78(3):389–98. doi: 10.1016/0092-8674(94)90418-9
5. Weissman D, Li Y, Orenstein JM, Fauci AS. Both a precursor and a mature population of dendritic cells can bind HIV. However, only the mature population that expresses CD80 can pass infection to unstimulated CD4+ T cells. J Immunol. (1995) 155(8):4111–7. doi: 10.4049/jimmunol.155.8.4111
6. Spudich S, Gonzalez-Scarano F. HIV-1-related central nervous system disease: current issues in pathogenesis, diagnosis, and treatment. Cold Spring Harb Perspect Med. (2012) 2(6):a007120. doi: 10.1101/cshperspect.a007120
7. Kaushik-Basu N, Basu A, Harris D. Peptide inhibition of hiv 1 current status and future. BioDrugs. (2008) 22(3):161–75. doi: 10.2165/00063030-200822030-00003
8. Xia C, Luo D, Yu X, Jiang S, Liu S. HIV-associated dementia in the era of highly active antiretroviral therapy (HAART). Microbes Infect. (2011) 13(5):419–25. doi: 10.1016/j.micinf.2011.01.004
9. Pathai S, Bajillan H, Landay AL, High KP. Is HIV a model of accelerated or accentuated aging? J Gerontol A Biol Sci Med Sci. (2014) 69(7):833–42. doi: 10.1093/gerona/glt168
10. Wallace DR, Dodson S, Nath A, Booze RM. Estrogen attenuates gp120- and tat1-72-induced oxidative stress and prevents loss of dopamine transporter function. Synapse. (2006) 59(1):51–60. doi: 10.1002/syn.20214
11. So-Armah K, Benjamin LA, Bloomfield GS, Feinstein MJ, Hsue P, Njuguna B, et al. HIV And cardiovascular disease. Lancet HIV. (2020) 7(4):e279–93. doi: 10.1016/S2352-3018(20)30036-9
12. Cribbs SK, Crothers K, Morris A. Pathogenesis of HIV-related lung disease: immunity, infection, and inflammation. Physiol Rev. (2020) 100(2):603–32. doi: 10.1152/physrev.00039.2018
13. Thrift AP, Kramer JR, Hartman CM, Royse K, Richardson P, Dong Y, et al. Risk and predictors of esophageal and stomach cancers in HIV-infected veterans: a matched cohort study. J Acquir Immune Defic Syndr. (2019) 81(3):e65–72. doi: 10.1097/QAI.0000000000002038
14. Ascher SB, Scherzer R, Peralta CA, Tien PC, Grunfeld C, Estrella MM, et al. Association of kidney function and early kidney injury with incident hypertension in HIV-infected women. Hypertension. (2017) 69(2):304–13. doi: 10.1161/HYPERTENSIONAHA.116.08258
15. Bricaire F, Marche C, Zoubi D, Saimot AG, Regnier B. HIV And the pancreas. Lancet. (1988) 1(8575-6):65–6. doi: 10.1016/s0140-6736(88)91052-5
16. Wager TD, Waugh CE, Lindquist M, Noll DC, Fredrickson BL, Taylor SF. Brain mediators of cardiovascular responses to social threat: part I: reciprocal dorsal and ventral sub-regions of the medial prefrontal cortex and heart-rate reactivity. Neuroimage. (2009) 47(3):821–35. doi: 10.1016/j.neuroimage.2009.05.043
17. Tahsili-Fahadan P, Geocadin RG. Heart-brain axis: effects of neurologic injury on cardiovascular function. Circ Res. (2017) 120(3):559–72. doi: 10.1161/CIRCRESAHA.116.308446
18. Taggart P, Critchley H, Lambiase PD. Heart-brain interactions in cardiac arrhythmia. Heart. (2011) 97(9):698–708. doi: 10.1136/hrt.2010.209304
19. Freed EO, Martin MA. The role of human immunodeficiency virus type 1 envelope glycoproteins in virus infection. J Biol Chem. (1995) 270(41):23883–6. doi: 10.1074/jbc.270.41.23883
20. Adamson CS, Freed EO. Human immunodeficiency virus type 1 assembly, release, and maturation. Adv Pharmacol. (2007) 55:347–87. doi: 10.1016/S1054-3589(07)55010-6
21. Wallace DR. HIV-associated neurotoxicity and cognitive decline: therapeutic implications. Pharmacol Ther. (2022) 234:108047. doi: 10.1016/j.pharmthera.2021.108047
22. Wamala D, Buteme HK, Kirimunda S, Kallenius G, Joloba M. Association between human leukocyte antigen class II and pulmonary tuberculosis due to mycobacterium tuberculosis in Uganda. BMC Infect Dis. (2016) 16:23. doi: 10.1186/s12879-016-1346-0
23. Dieckmann NM, Frazer GL, Asano Y, Stinchcombe JC, Griffiths GM. The cytotoxic T lymphocyte immune synapse at a glance. J Cell Sci. (2016) 129(15):2881–6. doi: 10.1242/jcs.186205
24. Ryan SO, Cobb BA. Roles for major histocompatibility complex glycosylation in immune function. Semin Immunopathol. (2012) 34(3):425–41. doi: 10.1007/s00281-012-0309-9
25. Li D, Sun K, Zhao Y, Lin A, Li S, Jiang Y, et al. Polymorphism in the major histocompatibility complex (MHC class II B) genes of the Rufous-backed Bunting (Emberiza jankowskii). PeerJ. (2017) 5:e2917. doi: 10.7717/peerj.2917
26. Lazaryan A, Song W, Lobashevsky E, Tang J, Shrestha S, Zhang K, et al. Human leukocyte antigen class I supertypes and HIV-1 control in African Americans. J Virol. (2010) 84(5):2610–7. doi: 10.1128/JVI.01962-09
27. Madrid-Elena N, Serrano-Villar S, Gutierrez C, Sastre B, Morin M, Luna L, et al. Selective miRNA inhibition in CD8(+) cytotoxic T lymphocytes enhances HIV-1 specific cytotoxic responses. Front Immunol. (2022) 13:998368. doi: 10.3389/fimmu.2022.998368
28. Xu R, Feng X, Xie X, Zhang J, Wu D, Xu L. HIV-1 Tat protein increases the permeability of brain endothelial cells by both inhibiting occludin expression and cleaving occludin via matrix metalloproteinase-9. Brain Res. (2012) 1436:13–9. doi: 10.1016/j.brainres.2011.11.052
29. Banks WA, Freed EO, Wolf KM, Robinson SM, Franko M, Kumar VB. Transport of human immunodeficiency virus type 1 pseudoviruses across the blood-brain barrier: role of envelope proteins and adsorptive endocytosis. J Virol. (2001) 75(10):4681–91. doi: 10.1128/JVI.75.10.4681-4691.2001
30. Kamerman PR, Moss PJ, Weber J, Wallace VCJ, Rice ASC, Huang W. Pathogenesis of HIV-associated sensory neuropathy: evidence from in vivo and in vitro experimental models. J Peripher Nerv Syst. (2012) 17(1):19–31. doi: 10.1111/j.1529-8027.2012.00373.x
31. Vitkovic L, Tardieu M. Neuropathogenesis of HIV-1 infection. Outstanding questions. C R Acad Sci III. (1998) 321(12):1015–21. doi: 10.1016/s0764-4469(99)80057-2
32. Churchill MJ, Wesselingh SL, Cowley D, Pardo CA, McArthur JC, Brew BJ, et al. Extensive astrocyte infection is prominent in human immunodeficiency virus-associated dementia. Ann Neurol. (2009) 66(2):253–8. doi: 10.1002/ana.21697
33. Russell RA, Chojnacki J, Jones DM, Johnson E, Do T, Eggeling C, et al. Astrocytes resist HIV-1 fusion but engulf infected macrophage material. Cell Rep. (2017) 18(6):1473–83. doi: 10.1016/j.celrep.2017.01.027
34. Churchill M, Nath A. Where does HIV hide? A focus on the central nervous system. Curr Opin HIV AIDS. (2013) 8(3):165–9. doi: 10.1097/COH.0b013e32835fc601
35. Narasipura SD, Kim S, Al-Harthi L. Epigenetic regulation of HIV-1 latency in astrocytes. J Virol. (2014) 88(5):3031–8. doi: 10.1128/JVI.03333-13
36. Pu H, Tian J, Flora G, Lee YW, Nath A, Hennig B, et al. HIV-1 Tat protein upregulates inflammatory mediators and induces monocyte invasion into the brain. Mol Cell Neurosci. (2003) 24(1):224–37. doi: 10.1016/s1044-7431(03)00171-4
37. Zhou BY, Liu Y, Kim B, Xiao Y, He JJ. Astrocyte activation and dysfunction and neuron death by HIV-1 Tat expression in astrocytes. Mol Cell Neurosci. (2004) 27(3):296–305. doi: 10.1016/j.mcn.2004.07.003
38. Gerhart DZ, Broderius MA, Borson ND, Drewes LR. Neurons and microvessels express the brain glucose transporter protein GLUT3. Proc Natl Acad Sci U S A. (1992) 89(2):733–7. doi: 10.1073/pnas.89.2.733
39. Chler PM. Lactate receptor sites link neurotransmission, neurovascular coupling, and brain energy metabolism. Cell Metab. (2016) 23(1):94–102. doi: 10.1016/j.cmet.2015.10.010
40. Tang BL. Glucose, glycolysis, and neurodegenerative diseases. J Cell Physiol. (2020) 235(11):7653–62. doi: 10.1002/jcp.29682
41. Gonzalez-Scarano F, Martin-Garcia J. The neuropathogenesis of AIDS. Nat Rev Immunol. (2005) 5(1):69–81. doi: 10.1038/nri1527
42. Bansal AK, Mactutus CF, Nath A, Maragos W, Hauser KF, Booze RM. Neurotoxicity of HIV-1 proteins gp120 and Tat in the rat striatum. Brain Res. (2000) 879(1-2):42–9. doi: 10.1016/s0006-8993(00)02725-6
43. Eugenin EA, Clements JE, Zink MC, Berman JW. Human immunodeficiency virus infection of human astrocytes disrupts blood-brain barrier integrity by a gap junction-dependent mechanism. J Neurosci. (2011) 31(26):9456–65. doi: 10.1523/JNEUROSCI.1460-11.2011
44. Cui Y, Zhang Z, Zhou X, Zhao Z, Zhao R, Xu X, et al. Microglia and macrophage exhibit attenuated inflammatory response and ferroptosis resistance after RSL3 stimulation via increasing Nrf2 expression. J Neuroinflammation. (2021) 18(1):249. doi: 10.1186/s12974-021-02231-x
45. Olah M, Menon V, Habib N, Taga MF, Ma Y, Yung CJ, et al. Single cell RNA sequencing of human microglia uncovers a subset associated with Alzheimer’s disease. Nat Commun. (2020) 11(1):6129. doi: 10.1038/s41467-020-19737-2
46. Yun SP, Kam TI, Panicker N, Kim S, Oh Y, Park JS, et al. Block of A1 astrocyte conversion by microglia is neuroprotective in models of Parkinson’s disease. Nat Med. (2018) 24(7):931–38. doi: 10.1038/s41591-018-0051-5
47. Huang Y, Zhao L, Jia B, Wu L, Li Y, Curthoys N, et al. Glutaminase dysregulation in HIV-1-infected human microglia mediates neurotoxicity: relevant to HIV-1-associated neurocognitive disorders. J Neurosci. (2011) 31(42):15195–204. doi: 10.1523/JNEUROSCI.2051-11.2011
48. He J, Chen Y, Farzan M, Choe H, Ohagen A, Gartner S, et al. CCR3 And CCR5 are co-receptors for HIV-1 infection of microglia. Nature. (1997) 385(6617):645–9. doi: 10.1038/385645a0
49. Adle-Biassette H, Levy Y, Colombel M, Poron F, Natchev S, Keohane C, et al. Neuronal apoptosis in HIV infection in adults. Neuropathol Appl Neurobiol. (1995) 21(3):218–27. doi: 10.1111/j.1365-2990.1995.tb01053.x
50. Wesselingh SL, Takahashi K, Glass JD, McArthur JC, Griffi JW, Griffin DE. Cellular localization of tumor necrosis factor mRNA in neurological tissue from HIV-infected patients by combined reverse transcriptase/polymerase chain reaction in situ hybridization and immunohistochemistry. J Neuroimmunol. (1997) 74(1–2):1–8. doi: 10.1016/s0165-5728(96)00160-9
51. Hildebrand C, Remahl S, Persson H, Bjartmar C. Myelinated nerve fibres in the CNS. Prog Neurobiol. (1993) 40(3):319–84. doi: 10.1016/0301-0082(93)90015-K
52. Liang H. Aldehyde dehydrogenases 1A2 expression and distribution are potentially associated with neuron death in spinal cord of Tg(SOD1*G93A)1Gur mice. Int J Biol Sci. (2017) 13(5):574–87. doi: 10.7150/ijbs.19150
53. Fields RD. White matter in learning, cognition and psychiatric disorders. Trends Neurosci. (2008) 31(7):361–70. doi: 10.1016/j.tins.2008.04.001
54. Albright AV, Strizki J, Harouse JM, Lavi E, O’Connor M, González-Scarano F. HIV-1 infection of cultured human adult oligodendrocytes. Virology. (1996) 217(1):211–9. doi: 10.1006/viro.1996.0108
55. Zou S, Fuss B, Fitting S, Hahn YK, Hauser KF, Knapp PE. Oligodendrocytes are targets of HIV-1 tat: NMDA and AMPA receptor-mediated effects on survival and development. J Neurosci. (2015) 35(32):11384–98. doi: 10.1523/JNEUROSCI.4740-14.2015
56. Bernardo A, Agresti C, Levi G. HIV-gp120 affects the functional activity of oligodendrocytes and their susceptibility to complement. J Neurosci Res. (1997) 50(6):946–57. doi: 10.1002/(SICI)1097-4547(19971215)50:6%3C946::AID-JNR5%3E3.0.CO;2-D
57. Kimura-Kuroda J, Nagashima K, Yasui K. Inhibition of myelin formation by HIV-1 gpl20 in rat cerebral cortex culture. Arch Virol. (1994) 137(1–2):81–99. doi: 10.1007/BF01311175
58. Sorrells SF, Paredes MF, Cebrian-Silla A, Sandoval K, Qi D, Kelley KW, et al. Human hippocampal neurogenesis drops sharply in children to undetectable levels in adults. Nature. (2018) 555(7696):377–81. doi: 10.1038/nature25975
59. Rottman JB, Ganley KP, Williams K, Wu L, Mackay CR, Ringler DJ. Cellular localization of the chemokine receptor CCR5. Correlation to cellular targets of HIV-1 infection. Am J Pathol. (1997) 151(5):1341–51.9358760
60. Sanders VJ, Pittman CA, White MG, Wang G, Wiley CA, Achim CL. Chemokines and receptors in HIV encephalitis. AIDS. (1998) 12(9):1021–6. doi: 10.1097/00002030-199809000-00009
61. Funke I, Hahn A, Rieber EP, Weiss E, Riethmüller G. The cellular receptor (CD4) of the human immunodeficiency virus is expressed on neurons and glial cells in human brain. J Exp Med. (1987) 165(4):1230–5. doi: 10.1084/jem.165.4.1230
62. Nath A, Haughey NJ, Jones M, Anderson C, Bell JE, Geiger JD. Synergistic neurotoxicity by human immunodeficiency virus proteins Tat and gp120: protection by memantine. Ann Neurol. (2000) 47(2):186–94. doi: 10.1002/1531-8249(200002)47:2%3C186::AID-ANA8%3E3.0.CO;2-3
63. Eugenin EA, D’Aversa TG, Lopez L, Calderon TM, Berman JW. MCP-1 (CCL2) protects human neurons and astrocytes from NMDA or HIV-tat-induced apoptosis. J Neurochem. (2003) 85(5):1299–311. doi: 10.1046/j.1471-4159.2003.01775.x
64. Eugenin EA, King JE, Hazleton JE, Major EO, Bennett MV, Zukin RS, et al. Differences in NMDA receptor expression during human development determine the response of neurons to HIV-tat-mediated neurotoxicity. Neurotox Res. (2011) 19(1):138–48. doi: 10.1007/s12640-010-9150-x
65. Green MV, Thayer SA. HIV Gp120 upregulates tonic inhibition through alpha5-containing GABA(A)Rs. Neuropharmacology. (2019) 149:161–68. doi: 10.1016/j.neuropharm.2019.02.024
66. Bernard C, Dilharreguy B, Font H, Diop AN, Tine JM, Diakhate IC, et al. Cerebral alterations in West African HIV and non-HIV adults aged ≥50: an MRI study. Int J Infect Dis. (2021) 103:457–63. doi: 10.1016/j.ijid.2020.12.016
67. Hammoud DA, Sinharay S, Steinbach S, Wakim PG, Geannopoulos K, Traino K, et al. Global and regional brain hypometabolism on FDG-PET in treated HIV-infected individuals. Neurology. (2018) 91(17):e1591–601. doi: 10.1212/WNL.0000000000006398
68. Sui J, Li X, Bell RP, Towe SL, Gadde S, Chen NK, et al. Structural and functional brain abnormalities in human immunodeficiency virus disease revealed by multimodal magnetic resonance imaging fusion: association with cognitive function. Clin Infect Dis. (2021) 73(7):e2287–93. doi: 10.1093/cid/ciaa1415
69. Hu X-T. HIV-1 Tat-mediated calcium dysregulation and neuronal dysfunction in vulnerable brain regions. Curr Drug Targets. (2016) 2016(1):4–14. doi: 10.2174/1389450116666150531162212
70. Chen Y, An H, Zhu H, Stone T, Smith JK, Hall C, et al. White matter abnormalities revealed by diffusion tensor imaging in non-demented and demented HIV+ patients. NeuroImage. (2009) 47(4):1154–62. doi: 10.1016/j.neuroimage.2009.04.030
71. Paris JJ, Singh HD, Carey AN, McLaughlin JP. Exposure to HIV-1 Tat in brain impairs sensorimotor gating and activates microglia in limbic and extralimbic brain regions of male mice. Behav Brain Res. (2015) 291:209–18. doi: 10.1016/j.bbr.2015.05.021
72. Nass SR, Lark ARS, Hahn YK, McLane VD, Ihrig TM, Contois L, et al. HIV-1 Tat and morphine decrease murine inter-male social interactions and associated oxytocin levels in the prefrontal cortex, amygdala, and hypothalamic paraventricular nucleus. Horm Behav. (2021) 133:105008. doi: 10.1016/j.yhbeh.2021.105008
73. Smit TK, Wang B, Ng T, Osborne R, Brew B, Saksena NK. Varied tropism of HIV-1 isolates derived from different regions of adult brain cortex discriminate between patients with and without AIDS dementia complex (ADC): evidence for neurotropic HIV variants. Virology. (2001) 279(2):509–26. doi: 10.1006/viro.2000.0681
74. Giatsou E, Abdi B, Plu I, Desire N, Palich R, Calvez V, et al. Ultradeep sequencing reveals HIV-1 diversity and resistance compartmentalization during HIV-encephalopathy. AIDS. (2020) 34(11):1609–14. doi: 10.1097/QAD.0000000000002616
75. Robb ML, Ananworanich J. Lessons from acute HIV infection. Curr Opin HIV AIDS. (2016) 11(6):555–60. doi: 10.1097/COH.0000000000000316
76. Krebs SJ, Ananworanich J. Immune activation during acute HIV infection and the impact of early antiretroviral therapy. Curr Opin HIV AIDS. (2016) 11(2):163–72. doi: 10.1097/COH.0000000000000228
77. Vergis EN, Mellors JW. Natural history of HIV-1 infection. Infect Dis Clin North Am. (2000) 14(4):809–25. doi: 10.1016/s0891-5520(05)70135-5
78. Self WH. Acute HIV infection: diagnosis and management in the emergency department. Emerg Med Clin North Am. (2010) 28(2):381–92. doi: 10.1016/j.emc.2010.01.002
79. Bolzenius J, Sacdalan C, Ndhlovu LC, Sailasuta N, Trautmann L, Tipsuk S, et al. Brain volumetrics differ by Fiebig stage in acute HIV infection. AIDS. (2023) 37(6):861–69. doi: 10.1097/QAD.0000000000003496
80. Hellmuth J, Fletcher JLK, Valcour V, Kroon E, Ananworanich J. Neurologic signs and symptoms frequently manifest in acute HIV infection. Neurology. (2016) 87(2):148–54. doi: 10.1212/WNL.0000000000002837
81. El-Sadr WM, Lundgren JD, Neaton JD, Gordin F, Abrams D. CD4+ count-guided interruption of antiretroviral treatment. N Engl J Med. (2006) 355(22):2283–96. doi: 10.1056/NEJMoa062360
82. Anikeeva N, Steblyanko M, Kuri-Cervantes L, Buggert M, Betts MR, Sykulev Y. The immune synapses reveal aberrant functions of CD8 T cells during chronic HIV infection. Nat Commun. (2022) 13(1):6436. doi: 10.1038/s41467-022-34157-0
83. Eggers C, Arendt G, Hahn K, Husstedt IW, Maschke M, Neuen-Jacob E, et al. HIV-1-associated neurocognitive disorder: epidemiology, pathogenesis, diagnosis, and treatment. J Neurol. (2017) 264(8):1715–27. doi: 10.1007/s00415-017-8503-2
84. Heaton RK, Franklin DR, Ellis RJ, McCutchan JA, Letendre SL, Leblanc S, et al. HIV-associated neurocognitive disorders before and during the era of combination antiretroviral therapy: differences in rates, nature, and predictors. J Neurovirol. (2011) 17(1):3–16. doi: 10.1007/s13365-010-0006-1
85. Sacktor N, Skolasky RL, Seaberg E, Munro C, Becker JT, Martin E, et al. Prevalence of HIV-associated neurocognitive disorders in the multicenter AIDS cohort study. Neurology. (2016) 86(4):334–40. doi: 10.1212/WNL.0000000000002277
86. Simioni S, Cavassini M, Annoni JM, Rimbault Abraham A, Bourquin I, Schiffer V, et al. Cognitive dysfunction in HIV patients despite long-standing suppression of viremia. AIDS. (2010) 24(9):1243–50. doi: 10.1097/QAD.0b013e3283354a7b
87. Hanna KF, Sayles HR, O’Neill J, White ML, Wilson TW, Swindells S. Incidental findings on brain MRI in people with HIV infection. Sci Rep. (2020) 10(1):9474. doi: 10.1038/s41598-020-66443-6
88. Simpson IA. Decreased concentrations of GLUT1 and GLUT3 glucose transporters in the brains of patients with Alzheimer’s disease. Ann Neurol. (1994) 32:546–51. doi: 10.1002/ana.410350507
89. Alves VS, Leite-Aguiar R, Silva J, Coutinho-Silva R, Savio LEB. Purinergic signaling in infectious diseases of the central nervous system. Brain Behav Immun. (2020) 89:480–90. doi: 10.1016/j.bbi.2020.07.026
90. Niccoli T, Cabecinha M, Tillmann A, Kerr F, Wong CT, Cardenes D, et al. Increased glucose transport into neurons rescues abeta toxicity in Drosophila. Curr Biol. (2016) 26(17):2291–300. doi: 10.1016/j.cub.2016.07.017
91. Tater P, Pandey S. Post-stroke movement disorders: clinical spectrum, pathogenesis, and management. Neurol India. (2021) 69(2):272–83. doi: 10.4103/0028-3886.314574
92. Kaku M, Simpson DM. HIV Neuropathy. Curr Opin HIV AIDS. (2014) 9(6):521–26. doi: 10.1097/coh.0000000000000103
93. Seilhean D, Duyckaerts C, Vazeux R, Bolgert F, Brunet P, Katlama C, et al. HIV-1-associated cognitive/motor complex: absence of neuronal loss in the cerebral neocortex. Neurology. (1993) 43(8):1492–9. doi: 10.1212/wnl.43.8.1492
94. Samboju V, Philippi CL, Chan P, Cobigo Y, Fletcher JLK, Robb M, et al. Structural and functional brain imaging in acute HIV. Neuroimage Clin. (2018) 20:327–35. doi: 10.1016/j.nicl.2018.07.024
95. Håberg A, Qu H, Saether O, Unsgård G, Haraldseth O, Sonnewald U. Differences in neurotransmitter synthesis and intermediary metabolism between glutamatergic and GABAergic neurons during 4 h of middle cerebral artery occlusion in the rat: the role of astrocytes in neuronal survival. J Cereb Blood Flow Metab. (2001) 21(12):1451–63. doi: 10.1097/00004647-200112000-00010
96. Brekke E. Glucose and intermediary metabolism and astrocyte-neuron interactions following neonatal hypoxia-ischemia in rat. Neurochem Res. (2017) 42(1):115–32. doi: 10.1007/s11064-016-2149-9
97. Smith D. Lactate: A preferred fuel for human brain metabolism in vivo. J Cereb Blood Flow Metab. (2003) 23(6):658–64. doi: 10.1097/01.WCB.0000063991.19746.11
98. Duran J. Impairment in long-term memory formation and learning-dependent synaptic plasticity in mice lacking glycogen synthase in the brain. Cereb Blood Flow Metab. (2013) 33(4):550–6. doi: 10.1038/jcbfm.2012.200
99. Sada N. Targeting LDH enzymes with a stiripentol analog to treat epilepsy. Science. (2015) 347(6228):1362–67. doi: 10.1126/science.aaa1299
100. Marcagg P. Ammonium in nervous tissue transport across cell membranes fluxes from neurons to glial cells, and role in signalling. Prog Neurobiol. (2001) 64:157–83. doi: 10.1016/S0301-0082(00)00043-5
101. Kim AY, Baik EJ. Glutamate dehydrogenase as a neuroprotective target against neurodegeneration. Neurochem Res. (2019) 44(1):147–53. doi: 10.1007/s11064-018-2467-1
102. Treiman DM. GABAergic mechanisms in epilepsy. Epilepsia. (2001) 42(3):8–12. doi: 10.1046/j.1528-1157.2001.042suppl.3008.x
103. Feldblum S, Ackermann RF, Tobin AJ. Long-term increase of glutamate decarboxylase mRNA in a rat model of temporal lobe epilepsy. Neuron. (1990) 5(3):361–71. doi: 10.1016/0896-6273(90)90172-C
104. Rottenberg DA, Moeller JR, Strother SC, Sidtis JJ, Navia BA, Dhawan V, et al. The metabolic pathology of the AIDS dementia complex. Ann Neurol. (1987) 22(6):700–6. doi: 10.1002/ana.410220605
105. Wang Z, Manion MM, Laidlaw E, Rupert A, Lau CY, Smith BR, et al. Redistribution of brain glucose metabolism in people with HIV after antiretroviral therapy initiation. AIDS. (2021) 35(8):1209–19. doi: 10.1097/QAD.0000000000002875
106. Ferrucci A, Nonnemacher MR, Cohen EA, Wigdahl B. Extracellular human immunodeficiency virus type 1 viral protein R causes reductions in astrocytic ATP and glutathione levels compromising the antioxidant reservoir. Virus Res. (2012) 167(2):358–69. doi: 10.1016/j.virusres.2012.06.002
107. Velasquez S, Prevedel L, Valdebenito S, Gorska AM, Golovko M, Khan N, et al. Circulating levels of ATP is a biomarker of HIV cognitive impairment. EBioMedicine. (2020) 51:102503. doi: 10.1016/j.ebiom.2019.10.029
108. Musante V, Summa M, Neri E, Puliti A, Godowicz TT, Severi P, et al. The HIV-1 viral protein Tat increases glutamate and decreases GABA exocytosis from human and mouse neocortical nerve endings. Cereb Cortex. (2010) 20(8):1974–84. doi: 10.1093/cercor/bhp274
109. Koirala TR, Nakagaki K, Ishida T, Nonaka S, Morikawa S, Tabira T. Decreased expression of MAP-2 and GAD in the brain of cats infected with feline immunodeficiency virus. Tohoku J Exp Med. (2001) 195(3):3. doi: 10.1620/tjem.195.141
110. Young AC, Yiannoutsos CT, Hegde M, Lee E. Cerebral metabolite changes prior to and after antiretroviral therapy in primary HIV infection. Neurology. (2014) 83(18):1592–600. doi: 10.1212/WNL.0000000000000932
111. Nagarajan R, Sarma MK, Thomas MA, Chang L, Natha U, Wright M, et al. Neuropsychological function and cerebral metabolites in HIV-infected youth. J Neuroimmune Pharmacol. (2012) 7(4):981–90. doi: 10.1007/s11481-012-9407-7
112. Ernst T, Jiang CS, Nakama H, Buchthal S, Chang L. Lower brain glutamate is associated with cognitive deficits in HIV patients: a new mechanism for HIV-associated neurocognitive disorder. J Magn Reson Imaging. (2010) 32(5):1045–53. doi: 10.1002/jmri.22366
113. Kellinghaus C, Engbring C, Kovac S, Moddel G, Boesebeck F, Fischera M, et al. Frequency of seizures and epilepsy in neurological HIV-infected patients. Seizure. (2008) 17(1):27–33. doi: 10.1016/j.seizure.2007.05.017
114. Sikazwe I, Elafros MA, Bositis CM, Siddiqi OK, Koralnik IJ, Kalungwana L, et al. HIV And new onset seizures: slipping through the cracks in HIV care and treatment. HIV Med. (2016) 17(2):118–23. doi: 10.1111/hiv.12283
115. MacMullin P, Hodgson N, Damar U, Lee HHC, Hameed MQ, Dhamne SC, et al. Increase in seizure susceptibility after repetitive concussion results from oxidative stress, parvalbumin-positive interneuron dysfunction and biphasic increases in glutamate/GABA ratio. Cereb Cortex. (2020) 30(12):6108–20. doi: 10.1093/cercor/bhaa157
116. Wu M, Fatukasi O, Yang S, Alger J, Barker PB, Hetherington H, et al. HIV Disease and diabetes interact to affect brain white matter hyperintensities and cognition. AIDS. (2018) 32(13):1803–10. doi: 10.1097/QAD.0000000000001891
117. Saloner R, Heaton RK, Campbell LM, Chen A, Franklin D Jr, Ellis RJ, et al. Effects of comorbidity burden and age on brain integrity in HIV. AIDS. (2019) 33(7):1175–85. doi: 10.1097/QAD.0000000000002192
118. Sherman DL, Brophy PJ. Mechanisms of axon ensheathment and myelin growth. Nat Rev Neurosci. (2005) 6(9):683–90. doi: 10.1038/nrn1743
119. Adle-Biassette H, Chrétien F, Wingertsmann L, Héry C, Ereau T, Scaravilli F, et al. Neuronal apoptosis does not correlate with dementia in HIV infection but is related to microglial activation and axonal damage. Neuropathol Appl Neurobiol. (1999) 25(2):123–33. doi: 10.1046/j.1365-2990.1999.00167.x
120. Vogt BA. Cingulate cortex in the three limbic subsystems. Handb Clin Neurol. (2019) 166:39–51. doi: 10.1016/B978-0-444-64196-0.00003-0
121. Zhang W, Shen H, Yao X, Liu F, Wang S, Yan Y, et al. Clinical and diffusion tensor imaging to evaluate falls, balance and gait dysfunction in leukoaraiosis: an observational, prospective cohort study. J Geriatr Psychiatry Neurol. (2020) 33(4):223–30. doi: 10.1177/0891988719874132
122. Taylor ME. White matter hyperintensities are associated with falls in older people with dementia. Brain Imaging Behav. (2019) 13(5):1265–72. doi: 10.1007/s11682-018-9943-8
123. Blahak C. Deep frontal and periventricular age related white matter changes but not basal ganglia and infratentorial hyperintensities are associated with falls: cross sectional results from the LADIS study. J Neurol Neurosurg Psychiatry. (2009) 80(6):608–13. doi: 10.1136/jnnp.2008.154633
124. Jakabek D, Rae CD, Brew BJ, Cysique LA. Brain aging and cardiovascular factors in HIV: a longitudinal volume and shape MRI study. AIDS. (2022) 36(6):785–94. doi: 10.1097/QAD.0000000000003165
125. Herold CJ, Kong L, Ceballos ME, Schroder J, Toro P. Neurological soft signs and brain morphology in people living with HIV. J Neurovirol. (2022) 28(2):236–47. doi: 10.1007/s13365-022-01071-6
126. Bertrand L, Meroth F, Tournebize M, Leda AR, Sun E, Toborek M. Targeting the HIV-infected brain to improve ischemic stroke outcome. Nat Commun. (2019) 10(1):2009. doi: 10.1038/s41467-019-10046-x
127. Chow FC, Wilson MR, Wu K, Ellis RJ, Bosch RJ, Linas BP. Stroke incidence is highest in women and non-hispanic blacks living with HIV in the AIDS clinical trials group longitudinal linked randomized trials cohort. AIDS. (2018) 32(9):1125–35. doi: 10.1097/QAD.0000000000001799
128. Stone L, Looby SE, Zanni MV. Cardiovascular disease risk among women living with HIV in North America and Europe. Curr Opin HIV AIDS. (2017) 12(6):585–93. doi: 10.1097/COH.0000000000000413
129. Marban C, Suzanne S, Dequiedt F, de Walque S, Redel L, Van Lint C, et al. Recruitment of chromatin-modifying enzymes by CTIP2 promotes HIV-1 transcriptional silencing. EMBO J. (2007) 26(2):412–23. doi: 10.1038/sj.emboj.7601516
130. Hoare J, Stein DJ, Heany SJ, Fouche JP, Phillips N, Er S, et al. Accelerated epigenetic aging in adolescents living with HIV is associated with altered development of brain structures. J Neurovirol. (2022) 28(2):208–16. doi: 10.1007/s13365-021-00947-3
131. Desplats P, Dumaop W, Smith D, Adame A, Everall I, Letendre S, et al. Molecular and pathologic insights from latent HIV-1 infection in the human brain. Neurology. (2013) 80(15):1415–23. doi: 10.1212/WNL.0b013e31828c2e9e
132. Desplats P, Dumaop W, Cronin P, Gianella S, Woods S, Letendre S, et al. Epigenetic alterations in the brain associated with HIV-1 infection and methamphetamine dependence. PLoS One. (2014) 9(7):e102555. doi: 10.1371/journal.pone.0102555
133. Johnny J,H, Nathalie S, Jessica M,W, Philip H,V, Yan F, Xiaojie Z. Long-term HIV-1 Tat expression in the brain led to neurobehavioral, pathological, and epigenetic changes reminiscent of accelerated aging. Aging Dis. (2020) 11(1):93–107. doi: 10.14336/ad.2019.0323
134. Sundar V, McLaughlin JP, Samikkannu T. Epigenetic signature of N-terminal acetyltransferases: a probable mediator of immune and neuropathogenesis in HIV infection. Mol Brain. (2022) 15(1):69. doi: 10.1186/s13041-022-00946-3
135. Chittiprol S, Kumar AM, Satishchandra P, Taranath Shetty K, Bhimasena Rao RS, Subbakrishna DK, et al. Progressive dysregulation of autonomic and HPA axis functions in HIV-1 clade C infection in South India. Psychoneuroendocrinology. (2008) 33(1):30–40. doi: 10.1016/j.psyneuen.2007.09.006
136. Pittaluga A, Pattarini R, Severi P, Raiteri M. Human brain N-methyl-D-aspartate receptors regulating noradrenaline release are positively modulated by HIV-1 coat protein gp120. AIDS. (1996) 10(5):463–8. doi: 10.1097/00002030-199605000-00003
137. Wang YS, White TD. The HIV glycoproteins gp41 and gp120 cause rapid excitation in rat cortical slices. Neurosci Lett. (2000) 291(1):13–6. doi: 10.1016/s0304-3940(00)01385-9
138. Berntson GG, Sarter M, Cacioppo JT. Anxiety and cardiovascular reactivity: the basal forebrain cholinergic link. Behav Brain Res. (1998) 94(2):225–48. doi: 10.1016/s0166-4328(98)00041-2
139. Mingote S, de Bruin JP, Feenstra MG. Noradrenaline and dopamine efflux in the prefrontal cortex in relation to appetitive classical conditioning. J Neurosci. (2004) 24(10):2475–80. doi: 10.1523/JNEUROSCI.4547-03.2004
140. Feligioni M, Raiteri L, Pattarini R, Grilli M, Bruzzone S, Cavazzani P, et al. The human immunodeficiency virus-1 protein Tat and its discrete fragments evoke selective release of acetylcholine from human and rat cerebrocortical terminals through species-specific mechanisms. J Neurosci. (2003) 23(17):6810–8. doi: 10.1523/JNEUROSCI.23-17-06
141. Crippa GE, Lewis SJ, Johnson AK, Corrêa FM. Medial prefrontal cortex acetylcholine injection-induced hypotension: the role of hindlimb vasodilation. J Auton Nerv Syst. (2000) 79(1):1–7. doi: 10.1016/s0165-1838(99)00091-0
142. Boison D, Steinhäuser C. Epilepsy and astrocyte energy metabolism. Glia. (2018) 66(6):1235–43. doi: 10.1002/glia.23247
143. Fialho GL, Wolf P, Walz R, Lin K. Epilepsy and ultra-structural heart changes: the role of catecholaminergic toxicity and myocardial fibrosis. What can we learn from cardiology? Seizure. (2019) 71:105–09. doi: 10.1016/j.seizure.2019.07.002
144. Verrier RL, Pang TD, Nearing BD, Schachter SC. The epileptic heart: concept and clinical evidence. Epilepsy Behav. (2020) 105:106946. doi: 10.1016/j.yebeh.2020.106946
145. Janszky I, Hallqvist J, Tomson T, Ahlbom A, Mukamal KJ, Ahnve S. Increased risk and worse prognosis of myocardial infarction in patients with prior hospitalization for epilepsy--the Stockholm heart epidemiology program. Brain. (2009) 132(Pt 10):2798–804. doi: 10.1093/brain/awp216
146. Luu BR, Nance RM, Delaney JAC, Ruderman SA, Heckbert SR. Brief report: insomnia and risk of myocardial infarction among people with HIV. J Acquir Immune Defic Syndr. (2022) 90(1):50–5. doi: 10.1097/QAI.0000000000002910
147. Rubtsova AA, Marquine MJ, Depp C, Holstad M, Ellis RJ, Letendre S, et al. Psychosocial correlates of frailty among HIV-infected and HIV-uninfected adults. Behav Med. (2019) 45(3):210–20. doi: 10.1080/08964289.2018.1509053
148. Campos CM, Albanez RL. HIV and Takotsubo cardiomyopathy: a deadly combination that could not be explained by the viral infection in isolation. Cardiovasc Revasc Med. (2021) 29:59–60. doi: 10.1016/j.carrev.2021.06.013
149. Barbaro G, Pellicelli A, Barbarini G, Akashi YJ. Takotsubo-like left ventricular dysfunction in an HIV-infected patient. Curr HIV Res. (2006) 4(2):239–41. doi: 10.2174/157016206776055101
150. Stollberger C, Wegner C, Finsterer J. Seizure-associated Takotsubo cardiomyopathy. Epilepsia. (2011) 52(11):e160–7. doi: 10.1111/j.1528-1167.2011.03185.x
151. Costagliola G, Orsini A, Coll M, Brugada R, Parisi P, Striano P. The brain-heart interaction in epilepsy: implications for diagnosis, therapy, and SUDEP prevention. Ann Clin Transl Neurol. (2021) 8(7):1557–68. doi: 10.1002/acn3.51382
152. Primetshofer D, Agladze R, Kratzer H, Reisinger J, Siostrzonek P. Tako-Tsubo syndrome: an important differential diagnosis in patients with acute chest pain. Wien Klin Wochenschr. (2010) 122(1–2):37–44. doi: 10.1007/s00508-009-1275-7
153. Kumar M, Kumar AM, Waldrop D, Antoni MH, Schneiderman N, Eisdorfer C. The HPA axis in HIV-1 infection. J Acquir Immune Defic Syndr. (2002) 31:S89–93. doi: 10.1097/00126334-200210012-00010
154. Panagiotakopoulos L, Kelly S, Neigh GN. HIV-1 proteins accelerate HPA axis habituation in female rats. Physiol Behav. (2015) 150:8–15. doi: 10.1016/j.physbeh.2015.02.011
155. Malessa R, Heimbach M, Brockmeyer NH, Hengge U, Rascher W, Michel MC. Increased neuropeptide Y-like immunoreactivity in cerebrospinal fluid and plasma of human immunodeficiency virus-infected patients: relationship to HIV encephalopathy. J Neurol Sci. (1996) 136(1–2):154–8. doi: 10.1016/0022-510x(95)00305-l
156. Waeber B, Burnier M, Nussberger J, Brunner HR. Role of atrial natriuretic peptides and neuropeptide y in blood pressure regulation. Horm Res. (1990) 34(3–4):161–5. doi: 10.1159/000181817
157. Shi Z, Bonillas AC, Wong J, Padilla SL, Brooks VL. Neuropeptide Y suppresses thermogenic and cardiovascular sympathetic nerve activity via Y1 receptors in the paraventricular nucleus and dorsomedial hypothalamus. J Neuroendocrinol. (2021) 33(8):e13006. doi: 10.1111/jne.13006
158. Zucker IH. Novel mechanisms of sympathetic regulation in chronic heart failure. Hypertension. (2006) 48(6):1005–11. doi: 10.1161/01.HYP.0000246614.47231.25
159. Fukuda K, Kanazawa H, Aizawa Y, Ardell JL, Shivkumar K. Cardiac innervation and sudden cardiac death. Circ Res. (2015) 116(12):2005–19. doi: 10.1161/CIRCRESAHA.116.304679
160. Zucker IH, Patel KP, Schultz HD. Neurohumoral stimulation. Heart Fail Clin. (2012) 8(1):87–99. doi: 10.1016/j.hfc.2011.08.007
161. Louboutin JP, Strayer D. Role of oxidative stress in HIV-1-associated neurocognitive disorder and protection by gene delivery of antioxidant enzymes. Antioxidants (Basel). (2014) 3(4):770–97. doi: 10.3390/antiox3040770
162. Yan Z, Rein B. Mechanisms of synaptic transmission dysregulation in the prefrontal cortex: pathophysiological implications. Mol Psychiatry. (2021) 27(1):445–65. doi: 10.1038/s41380-021-01092-3
163. Lagatta DC, Fassini A, Terzian AL, Correa FMA, Resstel LBM. The medial prefrontal cortex and the cardiac baroreflex activity: physiological and pathological implications. Pflugers Arch. (2023) 475(3):291–307. doi: 10.1007/s00424-022-02786-5
164. Tavares RF, Antunes-Rodrigues J, de Aguiar Correa FM. Pressor effects of electrical stimulation of medial prefrontal cortex in unanesthetized rats. J Neurosci Res. (2004) 77(4):613–20. doi: 10.1002/jnr.20195
165. McIntosh RC, Chow DC, Lum CJ, Hidalgo M, Shikuma CM, Kallianpur KJ. Reduced functional connectivity between ventromedial prefrontal cortex and insula relates to longer corrected QT interval in HIV+ and HIV- individuals. Clin Neurophysiol. (2017) 128(10):1839–50. doi: 10.1016/j.clinph.2017.07.398
166. Bamiou DE, Musiek FE, Luxon LM. The insula (Island of Reil) and its role in auditory processing. Literature review. Brain Res Brain Res Rev. (2003) 42(2):143–54. doi: 10.1016/s0165-0173(03)00172-3
167. Fasold O, von Brevern M, Kuhberg M, Ploner CJ, Villringer A, Lempert T, et al. Human vestibular cortex as identified with caloric stimulation in functional magnetic resonance imaging. Neuroimage. (2002) 17(3):1384–93. doi: 10.1006/nimg.2002.1241
168. Nieuwenhuys R. The insular cortex: a review. Prog Brain Res. (2012) 195:123–63. doi: 10.1016/B978-0-444-53860-4.00007-6
169. Zhang ZH, Oppenheimer SM. Baroreceptive and somatosensory convergent thalamic neurons project to the posterior insular cortex in the rat. Brain Res. (2000) 861(2):241–56. doi: 10.1016/s0006-8993(00)01990-9
170. Schulz SM. Neural correlates of heart-focused interoception: a functional magnetic resonance imaging meta-analysis. Philos Trans R Soc Lond B Biol Sci. (2016) 371(1708):20160018. doi: 10.1098/rstb.2016.0018
171. Min J, Farooq MU, Greenberg E, Aloka F, Bhatt A, Kassab M, et al. Cardiac dysfunction after left permanent cerebral focal ischemia. Stroke. (2009) 40(7):2560–63. doi: 10.1161/strokeaha.108.536086
172. Oppenheimer SM, Gelb A, Girvin JP, Hachinski VC. Cardiovascular effects of human insular cortex stimulation. Neurology. (1992) 42(9):1727–32. doi: 10.1212/wnl.42.9.1727
173. Oppenheimer SM, Wilson JX, Guiraudon C, Cechetto DF. Insular cortex stimulation produces lethal cardiac arrhythmias: a mechanism of sudden death? Brain Res. (1991) 550(1):115–21. doi: 10.1016/0006-8993(91)90412-o
174. Zhang ZH, Dougherty PM, Oppenheimer SM. Characterization of baroreceptor-related neurons in the monkey insular cortex. Brain Res. (1998) 796(1–2):303–6. doi: 10.1016/s0006-8993(98)00268-6
175. Sah P, Faber ESL, Armentia MLD, Power J. The amygdaloid complex: anatomy and physiology. Physiol Rev. (2003) 83(3):803–34. doi: 10.1152/physrev.00002.2003
176. Baklavadzhyan OG, Pogosyan NL, Arshakyan AV, Darbinyan AG, Khachatryan AV, Nikogosyan TG. Studies of the role of the central nucleus of the amygdala in controlling cardiovascular functions. Neurosci Behav Physiol. (2000) 30(2):231–6. doi: 10.1007/BF02463163
177. Saha S. Role of the central nucleus of the amygdala in the control of blood pressure: descending pathways to medullary cardiovascular nuclei. Clin Exp Pharmacol Physiol. (2005) 32(5–6):450–6. doi: 10.1111/j.1440-1681.2005.04210.x
178. Tsukioka K, Yamanaka K, Waki H. Implication of the central nucleus of the amygdala in cardiovascular regulation and limiting Maximum exercise performance during high-intensity exercise in rats. Neuroscience. (2022) 496:52–63. doi: 10.1016/j.neuroscience.2022.06.005
179. Gören Z, Aslan N, Berkman K, Oktay S, Onat F. The role of amygdala and hypothalamus in GABAA antagonist bicuculline-induced cardiovascular responses in conscious rats. Brain Res. (1996) 722(1–2):118–24. doi: 10.1016/0006-8993(96)00201-6
180. Gelsema AJ, McKitrick DJ, Calaresu FR. Cardiovascular responses to chemical and electrical stimulation of amygdala in rats. Am J Physiol. (1987) 253(5):R712–8. doi: 10.1152/ajpregu.1987.253.5.R712
181. Neckel H, Quagliotto E, Casali KR, Montano N, Dal Lago P, Rasia-Filho AA. Glutamate and GABA in the medial amygdala induce selective central sympathetic/parasympathetic cardiovascular responses. Can J Physiol Pharmacol. (2012) 90(5):525–36. doi: 10.1139/y2012-024
182. Ances BM, Ortega M, Vaida F, Heaps J, Paul R. Independent effects of HIV, aging, and HAART on brain volumetric measures. Clin Sci. (2012) 59(5):469–77. doi: 10.1097/QAI.0b013e318249db17
183. Nass SR, Ohene-Nyako M, Hahn YK, Knapp PE, Hauser KF. Neurodegeneration within the amygdala is differentially induced by opioid and HIV-1 tat exposure. Front Neurosci. (2022) 16:804774. doi: 10.3389/fnins.2022.804774
184. Critchley HD, Mathias CJ, Josephs O, O’Doherty J, Zanini S, Dewar BK, et al. Human cingulate cortex and autonomic control: converging neuroimaging and clinical evidence. Brain. (2003) 126(Pt 10):2139–52. doi: 10.1093/brain/awg216
185. Vogt BA, Finch DM, Olson CR. Functional heterogeneity in cingulate cortex: the anterior executive and posterior evaluative regions. Cereb Cortex. (1992) 2(6):435–43. doi: 10.1093/cercor/2.6.435-a
186. Pool JL, Ransohoff J. Autonomic effects on stimulating rostral portion of cingulate gyri in man. J Neurophysiol. (1949) 12(6):385–92. doi: 10.1152/jn.1949.12.6.385
187. Alexander L, Wood CM, Gaskin PLR, Sawiak SJ, Fryer TD, Hong YT, et al. Over-activation of primate subgenual cingulate cortex enhances the cardiovascular, behavioral and neural responses to threat. Nat Commun. (2020) 11(1):5386. doi: 10.1038/s41467-020-19167-0
188. Wallis CU, Cardinal RN, Alexander L, Roberts AC, Clarke HF. Opposing roles of primate areas 25 and 32 and their putative rodent homologs in the regulation of negative emotion. Proc Natl Acad Sci U S A. (2017) 114(20):E4075–84. doi: 10.1073/pnas.1620115114
189. Fisk GD, Wyss JM. Pressor and depressor sites are intermingled in the cingulate cortex of the rat. Brain Res. (1997) 754(1–2):204–12. doi: 10.1016/s0006-8993(97)00076-0
190. Burford NG, Webster NA, Cruz-Topete D. Hypothalamic-pituitary-adrenal axis modulation of glucocorticoids in the cardiovascular system. Int J Mol Sci. (2017) 18(10):2150. doi: 10.3390/ijms18102150
191. Eilam R, Malach R, Bergmann F, Segal M. Hypertension induced by hypothalamic transplantation from genetically hypertensive to normotensive rats. J Neurosci. (1991) 11(2):401–11. doi: 10.1523/JNEUROSCI.11-02-00401.1991
192. Hunter RW, Bailey MA. Glucocorticoids and 11beta-hydroxysteroid dehydrogenases: mechanisms for hypertension. Curr Opin Pharmacol. (2015) 21:105–14. doi: 10.1016/j.coph.2015.01.005
193. Herman JP, Morrison DG. Immunoautoradiographic and in situ hybridization analysis of corticotropin-releasing hormone biosynthesis in the hypothalamic paraventricular nucleus. J Chem Neuroanat. (1996) 11(1):49–56. doi: 10.1016/0891-0618(96)00124-x
194. Goncharuk VD, Van Heerikhuize J, Swaab DF, Buijs RM. Paraventricular nucleus of the human hypothalamus in primary hypertension: activation of corticotropin-releasing hormone neurons. J Comp Neurol. (2002) 443(4):321–31. doi: 10.1002/cne.10124
195. Svensson TH, Thorén P. Brain noradrenergic neurons in the locus coeruleus: inhibition by blood volume load through vagal afferents. Brain Res. (1979) 172(1):174–8. doi: 10.1016/0006-8993(79)90908-9
196. Swanson LW, Hartman BK. Biochemical specificity in central pathways related to peripheral and intracerebral homeostatic functions. Neurosci Lett. (1980) 16(1): 55-60. doi: 10.1016/0304-3940(80)90100-7
197. Sawchenko PE, Swanson LW. Central noradrenergic pathways for the integration of hypothalamic neuroendocrine and autonomic responses. Science. (1981) 214(4521):685–7. doi: 10.1126/science.7292008
198. Petrov T, Krukoff TL, Jhamandas JH. Branching projections of catecholaminergic brainstem neurons to the paraventricular hypothalamic nucleus and the central nucleus of the amygdala in the rat. Brain Res. (1993) 722(1–2):81–92. doi: 10.1016/0006-8993(93)90858-k
199. Mermet CC, Gonon FG. Ether stress stimulates noradrenaline release in the hypothalamic paraventricular nucleus. Neuroendocrinology. (1988) 47(1):75–82. doi: 10.1159/000124894
200. Gurtu S, Pant KK, Sinha JN, Bhargava KP. An investigation into the mechanism of cardiovascular responses elicited by electrical stimulation of locus coeruleus and subcoeruleus in the cat. Brain Res. (1984) 301(1):59–64. doi: 10.1016/0006-8993(84)90402-5
201. Szczepanska-Sadowska E, Wsol A, Cudnoch-Jedrzejewska A, Zera T. Complementary role of oxytocin and vasopressin in cardiovascular regulation. Int J Mol Sci. (2021) 22(21):11465. doi: 10.3390/ijms222111465
202. Gruber CW. Physiology of invertebrate oxytocin and vasopressin neuropeptides. Exp Physiol. (2014) 99(1):55–61. doi: 10.1113/expphysiol.2013.072561
203. Bichet DG. Vasopressin at central levels and consequences of dehydration. Ann Nutr Metab. (2016) 68(Suppl 2):19–23. doi: 10.1159/000446200
204. Raber J, Toggas SM, Lee S, Bloom FE, Epstein CJ, Mucke L. Central nervous system expression of HIV-1 Gp120 activates the hypothalamic-pituitary-adrenal axis: evidence for involvement of NMDA receptors and nitric oxide synthase. Virology. (1996) 226(2):362–73. doi: 10.1006/viro.1996.0664
205. Chrousos GP, Zapanti ED. Hypothalamic-pituitary-adrenal axis in HIV infection and disease. Endocrinol Metab Clin North Am. (2014) 43(3):791–806. doi: 10.1016/j.ecl.2014.06.002
206. Millhorn DE, Eldridge FL. Role of ventrolateral medulla in regulation of respiratory and cardiovascular systems. J Appl Physiol. (1986) 61(4):1249–63. doi: 10.1152/jappl.1986.61.4.1249
207. Kapoor K, Bhandare AM, Nedoboy PE, Mohammed S, Farnham MM, Pilowsky PM. Dynamic changes in the relationship of microglia to cardiovascular neurons in response to increases and decreases in blood pressure. Neuroscience. (2016) 329:12–29. doi: 10.1016/j.neuroscience.2016.04.044
208. Brailoiu E, Deliu E, Sporici RA, Benamar K, Brailoiu GC. HIV-1-Tat excites cardiac parasympathetic neurons of nucleus ambiguus and triggers prolonged bradycardia in conscious rats. Am J Physiol Regul Integr Comp Physiol. (2014) 306(11):R814–22. doi: 10.1152/ajpregu.00529.2013
209. Nagamitsu S, Okabayashi S, Dai S, Morimitsu Y, Murakami T, Matsuishi T, et al. Neuroimaging and neuropathologic findings in AIDS patient with cytomegalovirus infection. Intern Med. (1994) 33(3):158–62. doi: 10.2169/internalmedicine.33.158
210. Starc TJ, Lipshultz SE, Easley KA, Kaplan S, Bricker JT, Colan SD, et al. Incidence of cardiac abnormalities in children with human immunodeficiency virus infection: the prospective P2 C2 HIV study. J Pediatr. (2002) 141(3):327–35. doi: 10.1067/mpd.2002.126301
211. Fisher SD, Bowles NE, Towbin JA, Lipshultz SE. Mediators in HIV-associated cardiovascular disease: a focus on cytokines and genes. AIDS. (2003) Suppl 1:S29–35. doi: 10.1097/00002030-200304001-00005
212. Smit M, Brinkman K, Geerlings S, Smit C, Thyagarajan K, Sighem A, et al. Future challenges for clinical care of an ageing population infected with HIV: a modelling study. Lancet Infect Dis. (2015) 15(7):810–8. doi: 10.1016/S1473-3099(15)00056-0
213. Manga P, McCutcheon K, Tsabedze N, Vachiat A, Zachariah D. HIV and nonischemic heart disease. J Am Coll Cardiol. (2017) 69(1):83–91. doi: 10.1016/j.jacc.2016.09.977
214. Barbeau P, Pellegrin JL, Labouyrie E, Brossard G, Rispal P, Lasseur C, et al. Value and limitations of the endomyocardial biopsy in HIV infection. Rev Med Interne. (1993) 14(10):1032. doi: 10.1016/s0248-8663(05)80149-3
215. Grody WW, Cheng L, Lewis W. Infection of the heart by the human immunodeficiency virus. Am J Cardiol. (1990) 66(2):203–06. doi: 10.1016/0002-9149(90)90589-s
216. Shaboodien G, Maske C, Wainwright H, Smuts H, Ntsekhe M, Commerford PJ, et al. Prevalence of myocarditis and cardiotropic virus infection in Africans with HIV-associated cardiomyopathy, idiopathic dilated cardiomyopathy and heart transplant recipients: a pilot study: cardiovascular topic. Cardiovasc J Afr. (2013) 24(6):218–23. doi: 10.5830/cvja-2013-039
217. Monsuez JJ, Escaut L, Teicher E, Charniot JC, Vittecoq D. Cytokines in HIV-associated cardiomyopathy. Int J Cardiol. (2007) 120(2):150–7. doi: 10.1016/j.ijcard.2006.11.143
218. Barbaro G. HIV-associated cardiomyopathy etiopathogenesis and clinical aspects. Herz. (2005) 30(6):486–92. doi: 10.1007/s00059-005-2728-z
219. Twu C, Liu NQ, Popik W. Cardiomyocytes undergo apoptosis in human immunodeficiency virus cardiomyopathy through mitochondrion- and death receptor-controlled pathways. Proc Natl Acad Sci U S A. (2002) 99(22):14386–91. doi: 10.1073/pnas.212327899
220. Herskowitz A, Willoughby S, Wu TC, Beschorner WE, Neumann DA, Rose NR, et al. Immunopathogenesis of HIV-1-associated cardiomyopathy. Clin Immunol Immunopathol. (1993) 68(2):234–41. doi: 10.1006/clin.1993.1124
221. Goldblum N, Daefler S, Llana T, Ablashi D, Josephs S, Salahuddin Z. Susceptibility to HIV-1 infection of a human B-lymphoblastoid cell line, DG75, transfected with subgenomic DNA fragments of Epstein-Barr virus. Dev Biol Stand. (1990) 72:309–13.2178126
222. Herskowitz A, Wu TC, Willoughby SB, Vlahov D, Ansari AA, Beschorner WE, et al. Myocarditis and cardiotropic viral infection associated with severe left ventricular dysfunction in late-stage infection with human immunodeficiency virus. J Am Coll Cardiol. (1994) 24(4):1025–32. doi: 10.1016/0735-1097(94)90865-6
223. Remick J, Georgiopoulou V, Marti C, Ofotokun I, Kalogeropoulos A, Lewis W, et al. Heart failure in patients with human immunodeficiency virus infection: epidemiology, pathophysiology, treatment, and future research. Circulation. (2014) 129(17):1781–9. doi: 10.1161/CIRCULATIONAHA.113.004574
224. Hsue PY, Hunt PW, Ho JE, Farah HH, Schnell A, Hoh R, et al. Impact of HIV infection on diastolic function and left ventricular mass. Circ Heart Fail. (2010) 3(1):132–9. doi: 10.1161/CIRCHEARTFAILURE.109.854943
225. Cerrato E, D’Ascenzo F, Biondi-Zoccai G, Calcagno A, Frea S, Grosso Marra W, et al. Cardiac dysfunction in pauci symptomatic human immunodeficiency virus patients: a meta-analysis in the highly active antiretroviral therapy era. Eur Heart J. (2013) 34(19):1432–6. doi: 10.1093/eurheartj/ehs471
226. Magula NP, Mayosi BM. Cardiac involvement in HIV-infected people living in Africa: a review. Cardiovasc J S Afr. (2003) 14(5):231–7.14610610
227. Hakim JG, Matenga JA, Siziya S. Myocardial dysfunction in human immunodeficiency virus infection: an echocardiographic study of 157 patients in hospital in Zimbabwe. Heart. (1996) 76(2):161–5. doi: 10.1136/hrt.76.2.161
228. Reinsch N, Esser S, Gelbrich G, Brockmeyer N, Potthoff A, Schadendorf D, et al. Valvular manifestations of human immunodeficiency virus infection--results from the prospective, multicenter HIV-HEART study. J Cardiovasc Med (Hagerstown). (2013) 14(10):733–9. doi: 10.2459/JCM.0b013e32835dc953
229. Gebo KA, Burkey MD, Lucas GM, Moore RD, Wilson LE. Incidence of, risk factors for, clinical presentation, and 1-year outcomes of infective endocarditis in an urban HIV cohort. J Acquir Immune Defic Syndr. (2006) 43(4):426–32. doi: 10.1097/01.qai.0000243120.67529.78
230. Sliwa K, Carrington MJ, Becker A, Thienemann F, Ntsekhe M, Stewart S. Contribution of the human immunodeficiency virus/acquired immunodeficiency syndrome epidemic to de novo presentations of heart disease in the heart of soweto study cohort. Eur Heart J. (2012) 33(7):866–74. doi: 10.1093/eurheartj/ehr398
231. Lind A, Reinsch N, Neuhaus K. Pericardial effusion of HIV-infected patients? Results of a prospective multicenter cohort study in the era of antiretroviral therapy. Eur J Med Res. (2011) 16(11):480–3. doi: 10.1186/2047-783x-16-11-480
232. Heidenreich PA, Eisenberg MJ, Kee LL, Somelofski CA, Hollander H, Schiller NB, et al. Pericardial effusion in AIDS: incidence and survival. Circulation. (1995) 92(11):3229–34. doi: 10.1161/01.cir.92.11.3229
233. Buhary TM, Gayed SL, Hafeez I. Pericardial effusion with Mycobacterium avium complex in HIV-infected patients. BMJ Case Rep. (2016) 2016: bcr2016215686. doi: 10.1136/bcr-2016-215686
234. Calabrese LH, Proffitt MR, Yen-Lieberman B. Congestive cardiomyopathy and illness related to the acquired immunodeficiency syndrome (AIDS) associated with isolation of retrovirus from myocardium. Ann Intern Med. (1987) 107(5):691–2. doi: 10.7326/0003-4819-107-5-691
235. Chetty R, Batitang S, Nair R. Large artery vasculopathy in HIV-positive patients: another vasculitic enigma. Hum Pathol. (2000) 31(3):374–9. doi: 10.1053/hp.2000.5219
236. Hsue PY, Waters DD. HIV Infection and coronary heart disease: mechanisms and management. Nat Rev Cardiol. (2019) 16(12):745–59. doi: 10.1038/s41569-019-0219-9
237. Zheng J, Huang M, Huang Q, Chen Q, Chen Z. The relationship between fetuin-A and coronary atherosclerotic heart disease (CHD) and CHD-related risk factors. Medicine (Baltimore). (2021) 100(43):e27481. doi: 10.1097/md.0000000000027481
238. Huang RC, Lillycrop KA, Beilin LJ, Godfrey KM, Anderson D, Mori TA, et al. Epigenetic age acceleration in adolescence associates with BMI, inflammation, and risk score for middle age cardiovascular disease. J Clin Endocrinol Metab. (2019) 104(7):3012–24. doi: 10.1210/jc.2018-02076
239. Ebner BF, Chueng T, Martinez CA. Epigenetics, HIV, and cardiovascular disease risk. Curr Probl Cardiol. (2021) 46(3):100615. doi: 10.1016/j.cpcardiol.2020.100615
240. Esteban-Cantos A, Rodriguez-Centeno J, Silla JC, Barruz P, Sanchez-Cabo F, Saiz-Medrano G, et al. Effect of HIV infection and antiretroviral therapy initiation on genome-wide DNA methylation patterns. EBioMedicine. (2023) 88:104434. doi: 10.1016/j.ebiom.2022.104434
241. Gross AM, Jaeger PA, Kreisberg JF, Licon K, Jepsen KL, Khosroheidari M, et al. Methylome-wide analysis of chronic HIV infection reveals five-year increase in biological age and epigenetic targeting of HLA. Mol Cell. (2016) 62(2):157–68. doi: 10.1016/j.molcel.2016.03.019
242. Costantino S, Camici GG, Mohammed SA, Volpe M, Luscher TF, Paneni F. Epigenetics and cardiovascular regenerative medicine in the elderly. Int J Cardiol. (2018) 250:207–14. doi: 10.1016/j.ijcard.2017.09.188
243. Soliman EZ, Prineas RJ, Roediger MP, Duprez DA, Boccara F, Boesecke C, et al. Prevalence and prognostic significance of ECG abnormalities in HIV-infected patients: results from the strategies for management of antiretroviral therapy study. J Electrocardiol. (2011) 44(6):779–85. doi: 10.1016/j.jelectrocard.2010.10.027
244. Brouillette J, Cyr S, Fiset C. Mechanisms of arrhythmia and sudden cardiac death in patients with HIV infection. Can J Cardiol. (2019) 35(3):310–19. doi: 10.1016/j.cjca.2018.12.015
245. Brouillette J, Grandy SA, Jolicoeur P, Fiset C. Cardiac repolarization is prolonged in CD4C/HIV transgenic mice. J Mol Cell Cardiol. (2007) 43(2):159–67. doi: 10.1016/j.yjmcc.2007.05.007
246. Grandy SA, Brouillette J, Fiset C. Reduction of ventricular sodium current in a mouse model of HIV. J Cardiovasc Electrophysiol. (2010) 21(8):916–22. doi: 10.1111/j.1540-8167.2009.01713.x
247. Bai YL, Liu HB, Sun B, Zhang Y, Li Q, Hu CW, et al. HIV Tat protein inhibits hERG K+ channels: a potential mechanism of HIV infection induced LQTs. J Mol Cell Cardiol. (2011) 51(5):876–80. doi: 10.1016/j.yjmcc.2011.07.017
248. Park DY, An S, Romero ME, Kaur A, Ravi V, Huang HD, et al. Incidence and risk factors of atrial fibrillation and atrial arrhythmias in people living with HIV: a systematic review and meta-analysis. J Interv Card Electrophysiol. (2022) 65(1):183–91. doi: 10.1007/s10840-022-01233-w
249. Lu HJ, Fu YY, Wei QQ, Zhang ZJ. Neuroinflammation in HIV-related neuropathic pain. Front Pharmacol. (2021) 12:653852. doi: 10.3389/fphar.2021.653852
250. Tyler KL, McArthur JC. Through a glass, darkly: cerebrospinal fluid viral load measurements and the pathogenesis of human immunodeficiency virus infection of the central nervous system. Arch Neurol. (2002) 59(6):909–12. doi: 10.1001/archneur.59.6.909
251. Price RW. The two faces of HIV infection of cerebrospinal fluid. Trends Microbiol. (2000) 8(9):387–91. doi: 10.1016/s0966-842x(00)01821-7
252. Nuwagira E, Hullsiek KH, Jjunju S. Diagnostic and prognostic value of cerebrospinal fluid lactate and glucose in HIV-associated Tuberculosis meningitis. Microbiol Spectr. (2022) 10(4):e0161822. doi: 10.1128/spectrum.01618-22
253. Yilmaz A, Fuchs D, Price RW, Spudich S, Blennow K, Zetterberg H, et al. Cerebrospinal fluid concentrations of the synaptic marker neurogranin in neuro-HIV and other neurological disorders. Curr HIV/AIDS Rep. (2019) 16(1):76–81. doi: 10.1007/s11904-019-00420-1
254. Price RW, Peterson J, Fuchs D, Angel TE, Zetterberg H, Hagberg L, et al. Approach to cerebrospinal fluid (CSF) biomarker discovery and evaluation in HIV infection. J Neuroimmune Pharmacol. (2013) 8(5):1147–58. doi: 10.1007/s11481-013-9491-3
255. Barco A, Orlando S, Stroffolini G, Pirriatore V, Lazzaro A, Vai D, et al. Correlations between cerebrospinal fluid biomarkers, neurocognitive tests, and resting-state electroencephalography (rsEEG) in patients with HIV-associated neurocognitive disorders. J Neurovirol. (2022) 28(2):226–35. doi: 10.1007/s13365-021-01047-y
256. Li Z, Zhao Y, Lv X, Deng Y. Integrated brain on a chip and automated organ-on-chips systems. J Interdiscip Med. (2022) 1(1): e20220002. doi: 10.1002/inmd.20220002
257. Ruan Z, Pathak D, Venkatesan Kalavai S, Yoshii-Kitahara A, Muraoka S, Bhatt N, et al. Alzheimer’s disease brain-derived extracellular vesicles spread tau pathology in interneurons. Brain. (2021) 144(1):288–309. doi: 10.1093/brain/awaa376
258. Dabrowska S, Andrzejewska A, Lukomska B, Janowski M. Neuroinflammation as a target for treatment of stroke using mesenchymal stem cells and extracellular vesicles. J Neuroinflammation. (2019) 16(1):178. doi: 10.1186/s12974-019-1571-8
259. Tinuper P, Carolis PD, Galeotti M, Baldrati A, Gritti FM, Sacquegna T. Electroencephalogram and HIV infection: a prospective study in 100 patients. Clin Electroencephalogr. (1990) 21(3):145–50. doi: 10.1177/155005949002100310
260. Meghdadi AH, Berka C, Richard C, Rupp G, Smith S, Stevanovic Karic M, et al. EEG Event related potentials in sustained, focused and divided attention tasks: potential biomarkers for cognitive impairment in HIV patients. Clin Neurophysiol. (2021) 132(2):598–611. doi: 10.1016/j.clinph.2020.11.026
261. Boerwinkle AH, Meeker KL, Luckett P, Ances BM. Neuroimaging the neuropathogenesis of HIV. Curr HIV/AIDS Rep. (2021) 18(3):221–28. doi: 10.1007/s11904-021-00548-z
262. Thurnher MM, Boban J, Rieger A, Gelpi E. Susceptibility-weighted MR imaging hypointense rim in progressive multifocal leukoencephalopathy: the end point of neuroinflammation and a potential outcome predictor. AJNR Am J Neuroradiol. (2019) 40(6):994–1000. doi: 10.3174/ajnr.A6072
263. Ackermann C, Andronikou S, Saleh MG, Kidd M, Cotton MF, Meintjes EM, et al. Diffusion tensor imaging point to ongoing functional impairment in HIV-infected children at age 5, undetectable using standard neurodevelopmental assessments. AIDS Res Ther. (2020) 17(1):20. doi: 10.1186/s12981-020-00278-z
264. Morgello S, Buyukturkoglu K, Murray J, Veenstra M, Berman JW, Byrd D, et al. MR Spectroscopy and diffusion imaging in people with human immunodeficiency virus: relationships to clinical and immunologic findings. J Neuroimaging. (2022) 32(1):158–70. doi: 10.1111/jon.12931
265. Kolson DL. Developments in neuroprotection for HIV-associated neurocognitive disorders (HAND). Curr HIV/AIDS Rep. (2022) 19(5):344–57. doi: 10.1007/s11904-022-00612-2
266. Lindl KA, Marks DR, Kolson DL, Jordan-Sciutto KL. HIV-associated neurocognitive disorder: pathogenesis and therapeutic opportunities. J Neuroimmune Pharmacol. (2010) 5(3):294–309. doi: 10.1007/s11481-010-9205-z
267. Senecal V, Barat C, Tremblay MJ. The delicate balance between neurotoxicity and neuroprotection in the context of HIV-1 infection. Glia. (2021) 69(2):255–80. doi: 10.1002/glia.23904
268. Gupta S, Gupta MM. Takotsubo syndrome. Indian Heart J. (2018) 70(1):165–74. doi: 10.1016/j.ihj.2017.09.005
269. Akashi YJ, Nef HM, Lyon AR. Epidemiology and pathophysiology of Takotsubo syndrome. Nat Rev Cardiol. (2015) 12(7):387–97. doi: 10.1038/nrcardio.2015.39
270. Ali L, Ghazzal A, Radwan S, Desale S, Garcia-Garcia HM. Impact of human immunodeficiency virus infection on Takotsubo cardiomyopathy outcomes in a large nationwide sample. Cardiovasc Revasc Med. (2021) 29:54–8. doi: 10.1016/j.carrev.2021.05.014
271. Chen M, Sun Y, Liu H. Cell membrane biomimetic nanomedicines for cancer phototherapy. Interdiscip Med. (2023) 1(2):e20220012. doi: 10.1002/inmd.20220012
272. Andre M, Nair M, Raymond AD. HIV Latency and nanomedicine strategies for anti-HIV treatment and eradication. Biomedicines. (2023) 11(2):617. doi: 10.3390/biomedicines11020617
273. Zhang Z, Dalan R, Hu Z, Wang JW, Chew NW, Poh KK, et al. Reactive oxygen species scavenging nanomedicine for the treatment of ischemic heart disease. Adv Mater. (2022) 34(35):e2202169. doi: 10.1002/adma.202202169
274. Samuels MA. The brain-heart connection. Circulation. (2007) 116(1):77–84. doi: 10.1161/CIRCULATIONAHA.106.678995
275. Wood SK, Valentino RJ. The brain norepinephrine system, stress and cardiovascular vulnerability. Neurosci Biobehav Rev. (2017) 74(Pt B):393–400. doi: 10.1016/j.neubiorev.2016.04.018
276. Chen Z, Venkat P, Seyfried D, Chopp M, Yan T, Chen J. Brain-Heart interaction: cardiac complications after stroke. Circ Res. (2017) 121(4):451–68. doi: 10.1161/CIRCRESAHA.117.311170
277. Shmuely S, van der Lende M, Lamberts RJ, Sander JW, Thijs RD. The heart of epilepsy: current views and future concepts. Seizure. (2017) 44:176–83. doi: 10.1016/j.seizure.2016.10.001
Keywords: human immunodeficiency virus, central nervous system, cardiovascular system, brain, heart
Citation: Shao H and Li S (2023) A new perspective on HIV: effects of HIV on brain-heart axis. Front. Cardiovasc. Med. 10:1226782. doi: 10.3389/fcvm.2023.1226782
Received: 22 May 2023; Accepted: 25 July 2023;
Published: 4 August 2023.
Edited by:
Leonardo Roever, Federal University of Uberlandia, BrazilReviewed by:
Hang Zou, Southern Medical University, ChinaAlex Cleber Improta Caria, University of São Paulo, Brazil
Vikas Pandey, Amity University, India
© 2023 Shao and Li. This is an open-access article distributed under the terms of the Creative Commons Attribution License (CC BY). The use, distribution or reproduction in other forums is permitted, provided the original author(s) and the copyright owner(s) are credited and that the original publication in this journal is cited, in accordance with accepted academic practice. No use, distribution or reproduction is permitted which does not comply with these terms.
*Correspondence: Sijun Li dGFua2V5Ym9nZTAyMDRAMTYzLmNvbQ==
Abbreviations AIDS, acquired immune deficiency syndrome; HIV, human immunodeficiency virus; ART, antiretroviral therapy; CNS, central nervous system; CS, cardiovascular system; ANS, autonomic nervous system; PFC, prefrontal cortex; CCR5, CC chemokine receptor 5; CXCR4, C-X-C chemokine receptor 4; BBB, blood-brain barrier; HAND, HIV-associated neurocognitive disorder; HLA, human leukocyte antigen; Glu, Glutamate; NMDA, N-methyl-D-aspartic acid; GABA, gamma-aminobutyric acid; MPFC, medial prefrontal cortex; ACC, anterior cingulate cortex; Pyr, pyruvic acid; LDH5, lactate dehydrogenase 5; LDH1, lactate dehydrogenase 1; NA, Noradrenaline; Ach, Acetylcholine; CA, Catecholamines; HRV, heart rate variability; TTS, Takotsubo syndrome; HPA, hypothalamic-pituitary-adrenal; RVLM, rostral ventrolateral medulla; PFC, prefrontal cortex; LPFC, lateral PFC; MAP, arterial pressure; vMPFC, ventral portion of medial prefrontal cortex; PVN, hypothalamic paraventricular nucleus; CTL, cytotoxic T lymphocyte; CRH, corticotropin-releasing hormone; ACTH, adrenocorticotropic hormone; LC, locus coeruleus; NTS, nucleus of the solitary tract; OXY, oxytocin; AVP, arginine vasopressin; SON, supraoptic nucleus; PVN, paraventricular nucleus; SCN, suprachiasmatic nucleus; CVLM, caudal ventrolateral medulla; RVLM, rostral ventrolateral medulla; ECG, electrocardiogram.