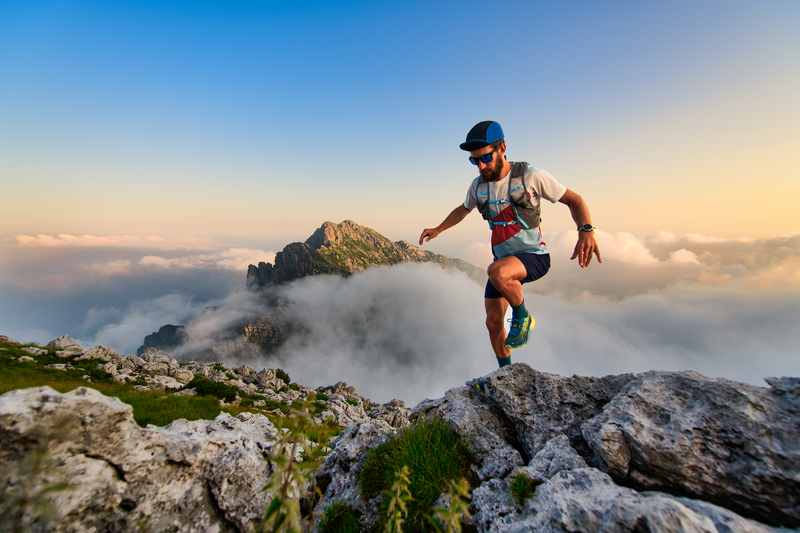
95% of researchers rate our articles as excellent or good
Learn more about the work of our research integrity team to safeguard the quality of each article we publish.
Find out more
REVIEW article
Front. Cardiovasc. Med. , 24 May 2023
Sec. Clinical and Translational Cardiovascular Medicine
Volume 10 - 2023 | https://doi.org/10.3389/fcvm.2023.1174816
This article is part of the Research Topic Cardiac Energetic Efficiency and Cardiometabolic Diseases View all 7 articles
Polyphenols are abundant in regular diets and possess antioxidant, anti-inflammatory, anti-cancer, neuroprotective, and cardioprotective effects. Regarding the inadequacy of the current treatments in preventing cardiac remodeling following cardiovascular diseases, attention has been focused on improving cardiac function with potential alternatives such as polyphenols. The following online databases were searched for relevant orginial published from 2000 to 2023: EMBASE, MEDLINE, and Web of Science databases. The search strategy aimed to assess the effects of polyphenols on heart failure and keywords were “heart failure” and “polyphenols” and “cardiac hypertrophy” and “molecular mechanisms”. Our results indicated polyphenols are repeatedly indicated to regulate various heart failure-related vital molecules and signaling pathways, such as inactivating fibrotic and hypertrophic factors, preventing mitochondrial dysfunction and free radical production, the underlying causes of apoptosis, and also improving lipid profile and cellular metabolism. In the current study, we aimed to review the most recent literature and investigations on the underlying mechanism of actions of different polyphenols subclasses in cardiac hypertrophy and heart failure to provide deep insight into novel mechanistic treatments and direct future studies in this context. Moreover, due to polyphenols' low bioavailability from conventional oral and intravenous administration routes, in this study, we have also investigated the currently accessible nano-drug delivery methods to optimize the treatment outcomes by providing sufficient drug delivery, targeted therapy, and less off-target effects, as desired by precision medicine standards.
Natural compounds derived from plants, marine organisms, and animals have attracted considerable attention due to their benefits for the health of the human body. It has been shown that natural products have bioactive properties and can improve various chronic diseases at the molecular level (1). Polyphenols contain one or more phenolic rings and are abundant in red fruits, vegetables, nuts, cocoa, wine, grapes, and tea (2, 3). These natural compounds have received wide attention recently regarding the well-known therapeutic effects and potential applications of polyphenols (4, 5). Many studies have highlighted the benefits of polyphenols in the prevention of various diseases such as cardiovascular diseases (CVDs), neurodegenerative diseases, diabetes, and cancer, which are mainly due to their ability to reduce reactive oxygen and nitrogen species, activate antioxidant enzymes, attenuate oxidative stress and inflammatory response (6–8).
CVDs describe a range of pathological conditions that affect the structure and function of the heart and blood vessels, which are responsible for the highest mortality worldwide, with an estimated 17.9 million deaths annually (9). Most CVDs could eventually lead to heart failure (HF), a clinical syndrome in which the heart cannot pump enough blood to meet the body's metabolic needs (10). HF is a critical public health problem with noticeable morbidity and mortality (11). In several conditions, the onset of HF is accompanied by cardiac hypertrophy (CH), defined as an adaptive reaction in response to chronic cardiac stress, such as hypertension, myocardial infarction (MI), valvular heart disease, as well as hereditary diseases that cause enlargement of cardiomyocytes and thickening of cardiac muscle fibers. At first, such growth maintains the function of the heart. However, chronic and persistent stress conditions lead to dilation of the ventricles, reduced contractile function, and eventual progression to HF (10, 12). Although drug therapies have partly successfully reduced HF mortality, most patients experience a downhill phase; therefore, more therapeutic measures are necessary (13).
Currently, pharmacological products derived from natural compounds, possessing unique structural and functional diversity, are regarded as promising adjutant approaches to prevent and treat CVD and HF (14, 15). Polyphenols are one of the natural compounds that can treat and prevent the progression of HF and CVD due to their various therapeutic properties, including anti-inflammatory, antioxidant, antiapoptotic, antiatherogenic, and antihypertension effects. Clinical and animal studies have reported that polyphenols reduce reactive oxygen species (ROS) and malondialdehyde (MDA) in the heart (16) and induce the expression of enzymes involved in the detoxification system, such as superoxide dismutase (SOD), catalase (CAT), and glutathione (GSH) peroxidase (17). In addition, polyphenols are indicated to inhibit the oxidation of low-density lipoproteins (LDL) and reduce the cytotoxicity caused by oxidized LDL (ox-LDL) in endothelial cells; therefore, polyphenols could prevent the onset and progression of atherosclerosis (18). On the other hand, studies conducted on both ischemic and non-ischemic HF animal models have demonstrated that resveratrol (RES), a principal polyphenol compound, improved systolic/diastolic ventricular function (19, 20), cardiac hemodynamic (21), and survival, as well as reduced left ventricular remodeling (22); all of which effects reduce the risk of HF.
Without a doubt, clearing the role of polyphenols will enhance knowledge of HF and CH and provide novel opportunities to extend more effective therapeutic approaches. Therefore, in this study, we aimed to summarize the findings regarding the potential positive effects of polyphenol compounds and signaling pathways and target molecules affected by several of these unique structures in HF and CH. We also highlighted the clinical roles of polyphenols that may provide promising therapeutic strategies in these pathological settings.
HF occurs under different pathological circumstances, including MI (23), valvular inadequacy (24), hypertension (25), cardiomyopathy (26), diabetes (27), and autoimmune diseases (28). Heart loss of function is primarily due to abnormal remodeling after the defects in cardiomyocytes' cellular metabolisms (29–31). Following hemodynamic alterations, compensatory mechanisms help maintain the heart's function, like the renin-angiotensin process, the sympathetic nervous system, and other neurohormonal mechanisms (32). Elevating the vasoconstrictors and neural hormones increases the cardiac muscle mass and empowers contractility, resulting in cardiac hypertrophy (33).
Physiological hypertrophy enhances heart performance by adding sarcomere units in parallel, which leads to a higher contractility; however, if the stress continues chronically, especially in the left ventricle, it eventually leads to pathologic hypertrophy and HF (12). Cardiac hypertrophy (CH) is the result of cardiomyocytes’ metabolism and calcium handling alterations, cardiac inflammation, cell death (e.g., apoptosis and autophagy), extracellular matrix (ECM) deposition, fibrosis, and angiogenesis (34). Sub-cellular changes in the heart, including oxidative stress, the increased entrance of Ca2+ in cells, and stimulant of proteases, combined with inflammation, lead to cardiac remodeling, pathological hypertrophy, and eventually, HF (35).
The metabolic changes in pathological hypertrophy led to using glucose instead of fatty acid oxidation to produce ATP as the primary energy source (36). In hypertrophic conditions, using less oxygen to produce ATP is more desirable (37). As the energy demand exceeds the supply, myocardial ATP levels decrease, and contractility becomes compromised, leading to HF development (33). Maintaining balanced ATP levels and Ca2+ concentration is imperative for healthy cardiac contractility. Intracellular levels of calcium increase in favor of improving contractile function during hypertrophy.
On the other hand, under metabolic alterations, sarco/endoplasmic reticulum Ca2+-ATPase (SERCA2a), an organelle that pumps calcium back into the sarcoplasmic reticulum, does not work correctly, which leads to the sizeable intracellular concentration of Ca2+ (38). Overload of Ca2+ enrolls pathways such as proteolytic enzymes, which promote HF (33, 35). The steady high amount of vasoactive hormones leads to the dominance of oxyradicals against antioxidants, boost redox-sensitive signals, and results in oxidative stress (39) and the formation of inflammatory cytokines, such as TNF-α, IL-6, and IL-1 (40). Besides, the inflammatory response activates macrophages and T immune cells (CD3+, CD8+, CD4+), which provoke abnormal heart remodeling and dysfunction of the left ventricle (41).
Fibroblasts are associated with maintaining cardiac contraction and signal pathways through forming the ECM. These cells develop ECM by secretion of humoral mediators, produce collagen type I and III, fibronectin, matrix metalloproteinases (MMPs), and tissue inhibitor of matrix metalloproteinases (TIMPs) (42). In the process of cardiac remodeling by inflammation, fibroblasts change into myofibroblasts. Myofibroblasts' constant activation and proliferation lead to the abnormal deposition (interstitial/replacement) and following accumulation of collagen in the heart that cause defective electrical conduction and inability to contractile function, which leads to cardiac dysfunction and HF (34, 42, 43). Loss of cardiomyocytes is a consequence of former apoptosis, fibrosis, and necrosis mechanisms (44, 45).
Polyphenols are secondary metabolites in plants with more than one phenolic group and are synthesized from pentose phosphate, phenylpropanoid, and shikimate pathways (4). Based on the number of rings and the elements that connect these rings, phenolic compounds are divided into subclasses, including flavonoids, phenolic acids, stilbenes, and lignans (46). Flavonoids are the most common phenolic metabolites found abundantly in vegetables and fruits (47). They have a typical basic structure consisting of two aromatic rings, A and B, connected by an oxygen-containing heterocyclic ring, the C ring. Based on their C ring structure, flavonoid compounds can be divided into six categories: flavanols, isoflavones, flavones, flavanones, and anthocyanins, each of which has a different hydroxylation and methylation pattern of A and B rings (48). Quercetin, myricetin, hesperetin, naringenin, nobiletin, eriodicytol, and catechins are the most common flavonoids in the human diet (49). Flavonoids possess antioxidant activities owning to having several phenolic hydroxyl groups that neutralize active hydrogen atoms and prevent the auto-oxidation of lipids (50). In addition, many flavonoids exhibit various medicinal effects other than antioxidative effects. For instance, pinocembrin, quercetin, rutin, and catechin have anti-inflammatory, anti-tumor, anti-apoptotic, anti-microbial, anti-hyperlipidaemic, and anti-diabetic properties that make them suitable therapeutic candidates in various pathological conditions (51–55).
Phenolic acids are another critical group of polyphenols, constituting approximately one-third of the polyphenolic compounds in the human diet. This group is found in all plant materials, especially in fruits with an acidic taste (7). Hydroxybenzoic acid and hydroxycinnamic acid derivatives are the two major groups of phenolic acids. Hydroxycinnamic acids are characterized by a saturated tail containing a carboxyl group (C6H5CHCHCOOH) and include compounds such as ρ-hydroxycinnamic, ρ-coumaric, caffeic, and ferulic acids. The compounds derived from hydroxybenzoic acid only have carboxyl groups (COOH), and the most common ones are ρ-hydroxybenzoic, gallic, protocatechuic, and vanillic acids (53). Like structural diversity, the biological potential of phenolic acids has a broad spectrum, such as anti-inflammatory, antioxidative stress, anti-hyperglycemic, neutralizing free radicals, and anti-cancer effects (56).
Lignans are phenolic compounds consisting of two phenylpropane units (57). Secoisolariciresinol, matairesinol and lariciresinol are common lignans. These compounds are abundant in flaxseed; however, grains, nuts, and vegetables also contain small amounts of lignans (58). In the human body, lignans are metabolized by intestinal microflora into enterodiol and enterolactone, which have estrogen-like structures; therefore, lignans are being investigated for possible use in ameliorating cancer, especially breast cancer (59). In addition, many studies have shown that consuming foods rich in lignan has favorable effects in preventing and improving diseases such as hypertension, type 2 diabetes, metabolic syndrome, and CVDs (60–63). The last class of primary polyphenols is stilbenes, which consist of two phenyl groups connected by a two-carbon methylene bridge (64). One of the widely studied stilbene polyphenols is RES, which is abundant in the skin of red grapes; however, it is also present in lower concentrations in blueberries, raspberries, mulberries, and peanuts (65). RES has many biological properties, including antioxidant, anti-inflammatory, cardioprotective, neuroprotective, and anti-tumoral activities (66).
Polyphenols have many potential effects that can modify the involved processes in HF and cardiac CH via multiple mechanisms. Figure 1 represents several familiar and vital underlying mechanisms of polyphenols in preventing and treating HF. Excessive cardiac oxidative stress caused by upregulated nicotinamide adenine dinucleotide phosphate (NADPH)-oxidases (Nox) and increased ROS production in mitochondria are the main inducers of HF (67, 68). In addition, in conditions of severe oxidative stress, the antioxidant system is overwhelmed due to the inhibition of nuclear factor erythroid 2-related factor 2 (Nrf2) activity by nuclear factor kappa-light-chain-enhancer of activated B cells (NF-κB). Nrf2 and NF-κB are crucial pathways modulating the adequate balance of the status of cellular redox and react to inflammation and stress. The interplay between such signaling molecules controls via various elaborate molecular interactions and mostly is determined by the type of cells and tissues. These interactions act through transcriptional and post-transcriptional machinery leading to the adjustment of dynamic reactions to ever-altering environmental signals (69). Pharmacological and genetic investigations have confirmed the functional interplay between Nrf2 and NF-κB. The lack of Nrf2 aggravates NF-κB activity resulting in augmented cytokine production, whiles NF-κB regulates the transcription and activity of Nrf2, owning both positive and negative impacts on the expression of target genes. Both Nrf2 and NF-κB are modulated by elements sensitive to redox. The deficiency of Nrf2 is correlated with incremented oxidative and nitrosative stress resulting in augmentation of cytokine release since NF-κB is easily activated in oxidative conditions (70). Cells mostly counteract oxidative stress by activating antioxidant enzymes (such as catalase, glutathione peroxidase, and superoxide dismutase) and antioxidant compounds. The antioxidant response element (ARE) is the DNA sequence accountable for regulating the antioxidative and cytoprotective responses of the cells, while Nrf2 is the main modulator of the xenobiotic-activated receptor (XAR) responsible for triggering the ARE pathway. In this regard, the Nrf2-ARE signaling is a key cytoprotective system to protect cells against oxidative stress and provides an acceptable redox balance in cells (69). NF-κB is a redox-modulated transcription factor involved in the regulation of cell growth, apoptosis, and inflammation. Oxidative stress triggers the activation of IκB kinase (IKK) mediating the phosphorylation of IκB which finally elevates NF-κB release. NF-κB stimulates the transcription of proinflammatory agents such as cytokines, cyclooxygenase-2, iNOS, and vascular adhesion molecules. Nrf2 restricts the activation of NF-κB signaling via neutralizing ROS by antioxidants, therefore inhibiting activation of NF-κB via ROS mediation (71, 72). As a result, NF-κB is the main molecular target to improve the antioxidant system (70). It has been shown that some polyphenols prevent the degradation of IκB, the major NF-κB inhibitor protein, by inhibiting the phosphorylation or ubiquitination of IκB kinase, thus blocking the entry of the NF-kB p65 subunit into the nucleus. In addition, polyphenols interfere with the binding of NF-κB to DNA (73, 74).
Figure 1. Impact of polyphenols on modulation of heart failure and cardiac hypertrophy: clinical effects and molecular mechanisms.
Sirtuins are a group of NAD+-dependent deacetylases located in mitochondria, nucleus, and cytoplasm, with seven subgroups. Among them, sirtuin1 (SIRT1) inhibits NF-κB-induced inflammatory signaling (75). SIRT1, a redox-sensitive enzyme, is abundantly expressed in embryo rat hearts, and suppressing its expression leads to developmental disorders in the heart (76). Sirt1 is upregulated by polyphenols, such as RES, which was also found to induce the deacetylation of NF-κB in Sirt1 dependent manner, suppressing NF-κB activity (77). On the other hand, a study reported that gallic acid could attenuate oxidative stress by directly reducing the expression of Noxs such as Nox1, Nox2, and Nox4 in the cardiac tissue of hypertensive rat models (78).
In cardiomyocytes, mitochondria constitute approximately one-third of the cell volume, reflecting the high energy requirements of these cells (79). Mitochondrial dysfunction is another cause of HF and is characterized by excessive leakage of ROS that results from complexes I and III of the electron transport chain (ETC). This process leads to membrane depolarization and reduced ATP synthesis, followed by the release of cytochrome c (Cyt c) from mitochondria, ultimately triggering apoptosis (80). Anthocyanins, a subclass of flavonoids, have a high antioxidant capacity. A study showed that they act as Cyt c reductase and thus prevent the activation of caspases and inhibit apoptosis (81). Further investigations revealed that anthocyanins could act as electron acceptors in complex I of the mitochondrial respiratory chain, leading to increased ATP production after ischemia in rats (82).
Atherosclerosis is a chronic inflammatory condition characterized by the deposition of extracellular lipids and, eventually, the narrowing of the arteries (83). Atherosclerosis is also a significant cause of CVDs, such as coronary artery disease, the most common etiology of HF (84). Improving the lipid profile can be beneficial by considering the role of lipids, especially LDL, in the atherosclerotic process. The effectiveness of polyphenols on the lipid profile has been widely investigated. Many of these studies have shown that polyphenols could improve lipid disorders by reducing plasma levels of triglycerides and LDL-cholesterol and increasing high-density lipoprotein (HDL)-cholesterol (85, 86). In addition, it has been reported that RES increased the expression of LDL receptors on hepatocytes in vitro, thus helping to reduce blood LDL cholesterol levels further (87). On the other hand, it has been shown that polyphenols reduced ox-LDL by inducing the expression of the antioxidant system (88).
Red wine polyphenols improve hypertension, a leading cause of cardiac hypertrophy, by increasing the activity of nitric oxide synthase (NOS) and reducing end-organ damage such as myocardial fibrosis (89). Moreover, several preclinical and clinical studies have shown the antihypertensive properties of RES (90–92). Cumulatively, polyphenols present a potentially effective treatment for patients with HF and CH using a plant-based diet.
Resveratrol (3, 5, 4′-trihydroxystilbene) is a phytoalexin with a polyphenol structure, and various plants naturally produce it as a defense mechanism against stress, such as infection and UV radiation. Grapes, peanuts, and peanut sprouts are considered primary dietary sources of RES (93–95). Several studies have reported that RES exerts multiple functions in the biological processes, such as anti-inflammatory, antioxidant, anticancer, neuroprotective, and cardioprotective (96, 97). The safety of RES has been evaluated in healthy individuals and it has been disclosed to regulate different indicators of various diseases. It has been reported in clinical trials that RES is safe up to 5 g/day and known as a safe phytochemical (98) According to its properties, RES has various beneficial effects on cardiovascular disorders, such as improving cardiac remodeling, function, fibrosis, and regulating cardiac metabolism and blood lipid (19, 99). A low incidence of CVDs in French people, despite their high-risk diet, could be associated with long-term red wine intake, which contains RES, and this is called the “French paradox” (100) (Table 1).
The dysregulated metabolic state contributes significantly to the progression of HF, leading to metabolism disorders. AMP-activated protein kinase (AMPK) is an energy regulator that controls cells’ metabolic function and balances ATP generation and consumption. AMPK is activated by an increased cellular AMP-to-ATP ratio, shifting cell metabolism towards the ATP-generation pathway to restore cellular ATP levels (145, 146). Previous studies have shown that AMPK activation can decelerate HF progression (147). Some in vitro and in vivo studies have shown that Sirt1 exerts antioxidative effects by activating the AMPK pathway. GU et al. found that RES (2.5 mg/kg/day, 16 weeks) benefits HF mice's survival rate by upregulating AMPK through Sirt1 activation (104). Low-dose treatment with RES was demonstrated to alleviate cardiac remodeling in MI-induced HF rats. Matsumura et al. showed that RES (0.2 g/kg/day, two weeks) restored levels of fatty acid oxidation and enhanced cardiac energy metabolism in Male Sprague-Dawley (SD) rats. Inhibition of the activity of cytochrome P450 1B1 (CYP1B1), resulting in decreased expression of its metabolites, especially mid-chain Hydroxyeicosatetraenoic acid (HETE) metabolites, has been suggested as a possible pathway of cardioprotective effects of RES (22). HETEs is a cardiotoxic metabolite of arachidonic acid (AA) catalyzed by CYP1B1, which induces cardiac fibrosis, systolic dysfunction, and vasoconstriction (148, 149). Additionally, RES suppressed the development and progression of cardiac remodeling and dysfunction in pressure-overload (PO)-induced hypertrophy. RES treatment (2.5 mg/kg/day, 28 days) alleviated lipid peroxidation (LPO) in Male Sprague-Dawley rats, indicating that improving effects of RES on CH is associated with its direct antioxidant effect on the heart (108).
In a study by Rimbaud et al., RES treatment (18 mg/kg/day, 8 weeks) in hypertensive Dahl salt-sensitive rat models was associated with preventing cardiac hypertrophy, remodeling, and dysfunction. RES Improved the cholesterol profile and prevented reduction in Mfn1 and OPA1 expression (necessary for mitochondrial fusion), cardiomyocyte oxidative capacity, and citrate synthase. In addition, RES protects the heart tissue against impaired fatty acid oxidation (FAO), which is dysregulated in HF. In contrast to untreated hypertensive models, RES-treated rats showed no decreased expression of PPARα (a gene that regulates FAO), CPT-1b (a gene involved in fatty acid transport), and utilization of carbohydrates (PDK4) (21). Similarly, the results of Sung et al. determined that RES (320 mg/kg/day, 2 weeks) could exert its effects on myocardial metabolism in C57Bl/6 mice by enhancing antioxidant defense enzymes, shifting heart metabolism from FAO towards glucose oxidation by activating AMPK, and increasing insulin sensitivity. Besides, collagen density, transcription of genes participating in cardiac fibrosis, and expression of MMP2 were reduced after treatment with RES, suppressing cardiac fibrosis and improving diastolic dysfunction (19). Moreover, the benefits of RES on the improvement of diastolic function and cardiac energy have been shown in mice metabolism with transverse aortic constriction (TAC)-induced HF. Treatment with RES decreased the expression of gene-related CH, such as anf, bnp, β-mhc, and ska, to the near-normal level, suggesting its positive effects on cardiac remodeling and HF on the transcriptional level (19).
Inflammatory processes mediated by cytokines promote the development and progression of HF (150). Previous studies revealed that CX3CL1, also known as fractalkine (FKN), is a transmembrane cytokine primarily expressed in endothelial and epithelial cells, and its expression is induced by stress. FKN is one of the most overexpressed cytokines in HF (151). RES has been shown to regulate the expression of FKN and suppress FKN-mediated inflammatory response (152). In this regard, Xuan et al. revealed that FKN is associated with MI- or PO-induced cardiac remodeling and HF. Based on their results, injecting RES (20 mg/kg/day, 42 days) into mice's hearts with Transverse aortic constriction (TAC) was correlated with acute phase amelioration in MI-induced HF and improved survival rate. Expression of atrial natriuretic peptide (ANP), intercellular adhesion molecule 1 (ICAM-1), and MMP-9, which participate in cardiac fibrosis, was increased in HF mice, and RES treatment reversed these changes. Further investigation showed that RES (20 mg/kg/day, 42 days) suppresses FKN and FKN-mediated inflammation to improve HF in mice models (103, 153). Aligned with previous studies, transfecting FKN into cardiomyocytes, fibroblasts, and endothelial cells, led to increased expression of ANP, ICAM-1, MMP-9, TGF-β, procollagens I and III in cells in vitro while treating with RES reversed all these results. Exposing neonatal rat cardiomyocytes to RES led to increased autophagosomes and overexpression of LC3-II and Atg5 proteins (participate in autophagy) while treating these cells with FKN reversed the effects of RES, indicating contrary effects of FKN on HF. This study suggests that RES collectively improves HF conditions and complications by antagonizing the effects of FKT (103).
Moreover, RES treatment (10 mg/kg/day, 4 weeks) in the murine model of heart failure decreased ROS levels and increased CAT, SOD, and GSH, indicating the role of RES in reducing oxidative stress. Moreover, the expression of collagen-I and -III and TGF-β (cardiac fibrosis factors) decreased after RES treatment, and the expression of phosphorylated eNOS (a marker for cardiac stiffness) was increased after treatment with RES, indicating that RES reduces cardiac fibrosis and stiffness (101). Silent information regulator 1 (SIRT1) is a class III histone deacetylase that protects the cardiovascular system against oxidative stress by boosting antioxidant defense and inhibiting apoptosis, inflammation, and autophagy (154–156). RES is an activator of SIRT1, which exerts cardioprotective effects by enhancing its expression. It has been indicated that RES reduces CH and cardiac fibrosis by activating SIRT1, leading to suppression of the TGF-β1/p-Smad3 signaling pathway (157). The TGF-β/Smad3 signaling pathway is an essential regulator of fibrosis in various organs, including the heart (158, 159). This study suggested inhibition of the TGF-β/Smad3 signaling pathway as the RES mechanism in suppressing cardiac fibrosis (101). Sirt1 is a primary regulator of cell metabolism, and recent studies revealed its role in oxidative stress and fibrosis (160, 161). Previous studies have shown that increased activity of Sirt1 reduces cardiac fibrosis by negatively targeting Smad3 and decreasing its acetylation and transcription (160, 162, 163). In this way, RES was identified to ameliorate cardiac fibrosis by suppressing the TGF-β/Smad3 pathway by reducing Smad3 acetylation, which was mediated by promoting the activity of Sirt1 (101).
Autophagy and apoptosis are two crucial CH processes (164, 165). Various signaling pathways regulate these processes, and PI3K/AKT/mTOR pathway is one of them. Guan et al. investigated the preventive effects of RES treatment (30 mg/kg/day, 5 weeks) in male SD rats on autophagy and apoptosis in chronic intermittent hypoxia (CIH)-induced CH (110), which is mediated by decreasing Bax/Bcl-2 ratio, CIH-induced apoptosis, and oxidative stress, as well as increasing autophagy in rat models. Mechanistically, RES exerts its effects by suppressing PI3K/AKT/mTOR signaling pathway (110). Furthermore, PI3K/AKT/mTOR signaling pathway is suppressed by PTEN, while immunoproteasomes degrade PTEN. In this regard, RES (25 and 50 mg/kg/day, 2 weeks) was shown to partly protect cells from oxidative stress by inhibiting immunoproteasomes from degrading PTEN and eventually improving PO-induced CH in Male wild-type (WT) C57BL/6 mice (109). Immunoproteasomes are proteins involved in regulating immune responses, cellular metabolism, growth, and survival and consist of three immune subunits: β1i (LMP2/PSMB9), β2i (MECL-1/PSMΒ10), and β5i (LMP7/PSMB8). These subunits are associated with cardiac disorders (166, 167). A study by Chen et al. demonstrated that RES inhibited PTEN degradation by reducing the expression of immune subunits, which led to the activation of AMPK and inhibition of ATK/mTOR. In this study, RES treatment significantly alleviated cardiac hypertrophy and dysfunction. Treating Ang II-induced cardiomyocytes in-vitro with RES also decreased proteasome caspase-like, trypsin-like, and chymotrypsin-like activities, increased expression of PTEN, and decreased expression of cardiomyocyte size (109).
Oxidative stress inhibits LKB1 activity, leading to AMPK suppression (168). AMPK improves vascular function by activating endothelial NO synthase (eNOS) and producing nitric oxide (NO) (169, 170). In this light, RES was found to activate AMPK by enhancing the expression of LKB1, leading to increase NO production and improved vascular function. Indeed, Dolinsky et al. showed that RES (320 mg/kg/day, 5 weeks) prevents hypertension-induced hypertrophy by promoting the LKB1/AMPK/eNOS pathway and suppressing the p70S6k pathway in SHRs and C57BL/6 mice (90). Consistent with this study, Thandapilly et al. reported that RES (2.5 mg/kg/day, 10 weeks) improves CH and myocardial contractibility by exerting direct antioxidant effects on the heart in spontaneously hypertensive rats (SHRs) models (113). Inflammation, oxidative stress, and coronary artery endothelial dysfunction are essential in developing cardiac fibrosis (171). In a study by Zhang et al., treating HF mice with RES (10 mg/kg/day, 4 weeks) significantly reduced the cardiomyocytes' size and the heart weight-to-body weight (HW/BW) ratio, indicating that RES decreased CH. Functionally, RES suppressed cardiac inflammatory response by downregulating IL-1β, IL-6, and TNF-α and reducing inflammatory cell infiltration in cardiac tissue (101). Based on IL-10 production by macrophages involved in inducing myocardial fibrosis (172), the role of RES on cardiac macrophage activity was investigated. They found that the expression of M1 markers increased in the HF group. However, treatment with RES shifted macrophage polarization to the M2 phenotype, which has tissue repair effects (101). Atorvastatin is a lipid-lowering drug with anti-inflammatory, antioxidant, and antiproliferative properties (173, 174). Besides lowering cholesterol levels, atorvastatin has many beneficial effects on the cardiovascular system (175). Chakraborty et al. demonstrated that combination therapy with RES and atorvastatin (10 + 10 mg/kg/day, 25 days) in Wister Albino rats exerts more potent cardioprotective effects, compared with alone RES (20 mg/kg/day, 25 days) treatment, in terms of improved CH as well as decreased cardiac inflammation and oxidative stress (107).
CH is the pathological growth of the heart due to pressure or volume overload, loss of contractibility, chemical toxicity, and congenital disorders (176). CH is a beneficial adaptive response that removes the effect of extrinsic or intrinsic stress to preserve cardiac function. However, excessive CH is deleterious and leads to adverse complications such as HF (177). Cellularly, various transcription factors and signaling pathways, such as calcineurin-NFAT, PI3K/Akt/GSK-3, MAPK, and Gp130/STAT3 signaling pathways, participate in CH pathogenesis (178, 179). RES suppresses CH by regulating diverse signaling pathways in cardiomyocytes. Calcineurin is a calcium/calmodulin-activated serine-threonine phosphatase that plays a vital role in the development and progression of CH by regulating the nuclear factor of activated T cells (NFAT) phosphorylation. NFAT is a transcriptional factor that activates GATA4, which induces cell hypertrophy (180). The benefits of calcineurin inhibitors have been shown in terms of preventing CH (181). The study by Chan et al. showed that RES (0–100 µM, 24 h) inhibited NFAT transcriptional activity more efficiently than calcineurin inhibitors in neonatal rat cardiac myocyte cells, indicating an additional mechanism involved in the inhibitory effect of RES. Further examination revealed that RES inhibited CH processes by activating AMP-AMPK through upregulating liver kinase B1 (LKB1), the activating kinase of AMPK, leading to inhibition of p70S6K, eEF2, and NFAT signaling pathways (105). Also, AMPK could inhibit CH through NFAT, NF-κB, and MAPK signal pathways (182).
RES could exert beneficial effects by regulating micro RNAs (miRNAs), a central subgroup of non-coding RNAs, as an alternative molecular mechanism. Fan et al. showed that RES (150 mg/kg, 4 weeks) improves cardiac function and ameliorates CH by inhibiting the expression of miR-155 through upregulating BRCA1 in mice models of cardiac hypertrophy. They showed that miR-155 induces cardiomyocyte hypertrophy and disrupts cardiac function (111). BRCA-1 is an essential gene for heart development and exerts cardioprotective effects in adult hearts (183). Angiotensin II is one of the renin-angiotensin system hormones with a vital role in the progression and development of several CVDs, such as hypertension, CH, and HF (184). Mashhadi et al. showed that expression of AT1a (Ang receptor) was increased in the hypertrophic rat heart, indicating that the Ang receptor plays a vital role in the development of PO-induced cell hypertrophy. It has been demonstrated that RES (1 mg/kg/day, 35 days) decreased the expression of AT1a mRNA in the hypertension group of Male Wistar rats, indicating that RES prevents PO-induced CH by decreasing the expression of AT1a (112). Moreover, it was observed that treatment of RES (10–50 mg/kg/day, 4 weeks) in the partially nephrectomized rat models was effective in nephrectomy-induced hypertension and the subsequent CH by reducing NO, AngII, and ET-1 concentrations, which are hypertrophic cell agents (91).
Exercise intolerance is an essential feature of chronic HF, and identifying its underlying mechanism may help improve the quality of life of patients with HF (185). Recent studies have shown that impaired skeletal muscle function, structure, and metabolism play an important role in exercise intolerance in HF patients (186). In this way, some studies have shown that RES can improve rodents' skeletal muscle biogenesis, isometric force, and metabolism (187, 188). Sung et al. in 2015 showed that RES (320 mg/kg/day, 2 weeks) improved the exercise capacity of HF C57Bl/6N mice by increasing the flow-mediated vasodilatation and vascular function (19). A further study investigated the treatment effect of 450 mg/kg/day RES for two weeks on improving exercise intolerance of C57Bl/6N mice with pressure overload-induced HF. A significant improvement in HF mice's respiratory exchange ratio (RER) was observed. Decreased RER in HF mice indicates more use of fatty acids than glucose in metabolism, producing more ROS and increasing oxidative stress. Treatment with RES elevated RER level, indicating that substrate utilization in the metabolic cycle shifted towards glucose; also, the total metabolic rate was increased after RES treatment (102). Mechanistically, RES treatment increased phosphorylation and expression of IRS-1, Akt, and AMPKα, which were reduced in HF and enhanced insulin sensitivity of skeletal muscles. This study also indicated that RES improved metabolism and insulin resistance in HF mice by altering the gut microbial community, which is associated with systemic metabolic rate and insulin sensitivity. Sung et al. showed that RES enhanced the cecum bacterial profile, increasing glucose homeostasis and carbohydrate metabolism (102).
Curcumin [(1E,6E)-1,7-bis(4-hydroxy-3-methoxyphenyl) hepta-1,6-diene-3,5-dione] is a natural polyphenol substance extracted from turmeric that plays substantial roles in cellular function by regulating various signaling pathway. Curcumin benefits various pathologic conditions and has anti-inflammatory and antioxidant effects (189). Various clinical and pre-clinical investigations have revealed Curcumin is a safe natural polyphenol compound without any severe adverse effects in a dose of 12 g/day for human subjects (190). Moreover, EFSA stated acceptable daily intake of curcumin is about 3 mg/kg in healthy individuals (191). According to these features, curcumin can be used as a therapeutic element in various diseases (192). Several studies have reported the positive role of curcumin in attenuating CVDs, such as atherosclerosis, MI, and HF (193, 194). Tang et al. studied the effects of curcumin (100 mg/kg/day, 8 weeks) on the cardiac function and structure of rabbits with chronic heart failure. The results demonstrated the ameliorating of curcumin's role in cardiac remodeling and HF's diastolic dysfunction. Also, curcumin significantly reduced collagen deposition in heart tissue (114) (Table 1).
The p300, a histone acetyltransferase (HAT) enzyme, regulates the expression of multiple genes by chromatin remodeling. P300 can also coactivate other transcriptional factors, such as GATA4 (195, 196), a member of the zinc-finger transcription factor family with high expression in cardiomyocytes and plays a critical role in cardiomyocyte differentiation (197). GATA4 regulates the transcription of ANP, α- and β-myosin heavy chain (α-MHC and β-MHC), which play a role in cardiac remodeling (198). Therefore, it seems that overexpression of p300 induces cardiac remodeling by increasing the activation of GATA4 (199). Several studies have reported curcumin as a natural p300-specific HAT inhibitor that can improve cardiac remodeling in HF. Morimoto et al. in vitro and in vivo studies exhibited the promising effect of curcumin (50 mg/kg/day, 7 weeks) as a p300 inhibitor on preventing cardiac remodeling in both MI-induced HF and hypertensive rats with HF (116). Mechanistically, curcumin reduced GATA4 and p300 binding but did not change their expression. Indeed, curcumin reverses cardiac remodeling and hypertrophy by inhibiting p300 histone acetyltransferase activity and decreasing the acetylated form of GATA4 and p300/GATA4 complex (116).
Dickkopf-related protein 3 (DKK-3), a member of dickkopf glycoprotein, regulates cell proliferation. Also, DKK-3 could modulate the immune system by acting as a cytokine-like protein (200, 201). DDK-3 is involved in cardiac remodeling and vascular smooth muscle differentiation, and several studies demonstrated the cardioprotective effects of DDK-3 (202). Zhang et al. showed that suppressing the expression of DDK-3 increases cardiac dysfunction and remodeling while augmenting the expression of DDK-3 improves cardiac function in an animal model of HFrEF (203). Moreover, DDK-3 improves chronic HF conditions by suppressing c-Jun N-terminal kinase (JNK) signaling pathways by inhibiting p38 mitogen-activated protein kinase (p38) (204). It has been found that P38 and JNK signaling pathways are highly activated in HF and participate in cardiac remodeling (205, 206). In an intriguing study, treating HF mice with curcumin for ten weeks significantly improved cardiac function, remodeling, and decreased expression of biomarkers related to them_ ROS, TNF-α, MMP-2, and MMP-9. In addition, expression of DDK-3 increased in curcumin-treated mice, compared with untreated mice with HF. Curcumin treatment (100 mg/day/kg, 10 weeks) in rabbits with chronic heart failure was associated with decreased p38/JNK signaling pathway activation and expression of ASK1, one of the upstream components of these pathways. So, these results indicate that curcumin exerts cardioprotective effects by increasing DDK-3 and ASK1 expression, suppressing the p38/JNK signaling pathway (115).
Liu et al. studied the effects of curcumin on isoproterenol (ISO)-induced CH and the molecular mechanism behind it. The result showed that curcumin (200 mg/kg/day, 4 weeks) prevented ISO-induced CH in Male Sprague-Dawley rats and cardiac fibrosis by decreasing autophagy through positively modulating mTOR (117). It has been found that the mTOR signaling pathway exerted cardioprotective effects by decreasing autophagy (207). Consistent with these studies, Bai et al. also showed that curcumin treatment (50 mg/kg/day, 9 weeks) prevented CH and improved cardiac function in male Wistar rats. Also, curcumin improved vascular relaxation by increasing endothelium response to acetylcholine and increasing expression of the Na+/Ca2+ exchanger (NCX) and eNOS in the myocardium and vascular endothelium. Treatment with NCX inhibitor KB-R7943 reversed the protective effects of curcumin on the myocardium and vessels. These results indicate that curcumin exerts cardioprotective effects by upregulating NCX expression in response to increased afterload (119).
Quercetin (QCT) is a natural flavonoid polyphenol widely found in fruits and vegetables such as onions, peppers, plums, mangos, and berries and is a potent anti-inflammatory, antioxidant, and anti-cancer component (208–211). Quercetin regulates several molecular pathways in cellular processes and exerts many beneficial effects on the cardiovascular system, such as anti-hypertensive and cardioprotective effects, by suppressing inflammation, oxidative stress, and apoptosis in the heart (212, 213). Several studies investigated the mechanisms behind the cardioprotective effects of QCT. Liu et al. showed that QCT inhibits the NF-κB pathway by activating peroxisome proliferator-activated receptor-γ (PPARγ) protein and preventing cardiac damage (214). Moreover, a recent study showed that QCT protects cardiomyocytes against oxidative stress by regulating mitophagy and endoplasmic reticulum stress by suppressing the SIRT1/TMBIM6 pathway (215).
For further investigation, Chang et al. administered QCT to improve cardiac function and hypertrophy in rats with TAC-induced HF. QCT decreased cardiac fibrosis by modulating TGF-β and MMPs, inflammatory cytokines (TNF-α, IL-13, IL-18), and production of ROS by activating the expression of SIRT5 and IDH2 desuccinylation. Succinylation regulates mitochondrial metabolism and function; impaired succinylation and mitochondrial function lead to oxidative stress and inflammation. This study showed that QCT regulates mitochondrial energy metabolism and improves oxidative stress by increasing succinylation through increasing expression of SIRT5 (216). Anti-hypertrophic properties of quercetin have been reported in several studies (217–219). QCT can prevent cardiac remodeling in mice. Wang et al. showed that QCT decreased cardiomyocyte Ca2+ oscillation frequency, resulting in regulated excitation-contraction of the myocardium and anti-arrhythmia effects. Also, quercetin attenuated ventricular wall thickness in mice, indicating the anti-hypertrophic effects of QCT (219). Additionally, QCT treatment (20 mg/kg/day, 8–12 weeks) in Spontaneous hypertensive rats improved CH and cardiac fibrosis by preventing mitochondrial dysfunction. SIRT3/PARP-1 was this study's suggested target pathway of QCT (120). SIRT3 is a type III histone deacetylase with anti-hypertrophic effects on the heart (220). The expression of SIRT3 was increased by QCT, indicating that the anti-hypertrophic effects of QCT are related to the SIRT3-mediated signaling pathways. PARP-1 is an up-regulated enzyme in hypertrophic hearts, and overexpression of SIRT3 inhibits its function (220). Moreover, In vitro investigation showed that treating Ang II-induced H9c2 cells with QCT prevents hypertrophic response, restores impaired mitochondrial structure and function, reduces ROS generation, and decreases oxidative stress (120).
In another study, QCT treatment (10 mg/kg/day, 4 days) reversed ISO-induced CH in male swiss mice by conducting antioxidant effects and improving mitochondrial dysfunction (121). Oxidative stress and ROS play essential roles in the development of CH. In this study, QCT treatment restored endogenous antioxidant enzyme (CAT and SOD) activity, increased sulfhydryl protein levels, and decreased H2O2 (a major ROS). Ca2+-induced swelling of mitochondria in ISO rats reduced after treatment with QCT; activating the mitochondrial SOD enzyme and increasing resistance toward Ca2+-induced swelling are the possible mechanisms. Altogether this study revealed that QCT attenuates pre-existing CH by balancing oxidation and protecting mitochondria (121). QCT (130 mg/kg/day, 7 days) also reduced mean arterial blood pressure and aortic medial wall thickening, improved cardiac function, and prevented cardiac hypertrophy in PO-induced male Sprague-Dawley rats by abdominal aortic constriction (AAC) (221). Protein kinase C (PKC), extracellular regulated kinase 1/2 (ERK1/2), and Akt are contributing signaling pathways in CH pathogenesis. In this study, treatment with QCT normalized PKCβII translocation and inhibited PKC and ERK1/2 pathways in AAC rats. QCT also reduced oxidative stress in the heart tissue of AAC rats. This study showed that rats with a QCT-supplemented diet have lower blood pressure and CH in pressure-overload stress conditions (221) (Table 1).
Gallic acid (3, 4, 5-trihydroxy benzoic acid) is a polyphenol component in green tea, blackberry, grapes, wine, mangoes, and walnuts. It can be an anti-cancer, anti-allergic, anti-microbial, antioxidant, and anti-inflammatory factor (222, 223). Gallic acid (GA) impacts the cardiovascular system, such as attenuating cardiac fibrosis, hypertension, oxidative stress, and eventually HF. The antioxidative effects of gallic acid suppress cardiac hypertrophy and protect cardiomyocytes against damage (127, 224, 225). An in vitro investigation conducted by Yan et al. showed that GA suppresses angiotensin II (Ang II)-induced cardiomyocytes hypertrophy by inhibiting Ang II-related pathways such as JAK2, p-STAT3, p-ERK1/2, p-AKT, and NFATc1 and reducing expression of Ang II downstream products (GFR, gp130, and CaNA). In this experiment, GA increased cardiomyocyte autophagy by inhibiting ULK1 phosphorylation (autophagy regulator), which leads to the degradation of unnecessary products and improved cardiac remodeling. GA at 1–200 μM had no significant toxic effect on cardiomyocytes. On the other hand, in vivo study revealed that treated TAC-induced HF C57BL/6 mice with GA (5 or 20 mg/kg, 8 weeks) led to downregulating the expression of ANP, BNP, and β-MHC, thereby decreasing CH. GA decreased inflammatory markers, myocardial superoxide products, and fibrosis factors in the heart of mice with HF. Collectively, GA exerts its cardioprotective effects and improves cardiac remodeling by activating autophagy through various pathways (126). In another study, GA (100 mg/kg/day, 2 weeks) significantly improved cardiac size and cardiac function in TAC-induced HF CD-1 male mice models, compared to conventional drugs of HF. The levels of HF markers, such as ANP, BNP, and β-MHC, significantly decreased after GA treatment. Further investigation revealed that GA reduced cardiac fibrosis by decreasing the phosphorylation of Smad3 protein and inhibiting the TGF-β1/Smad signaling pathway (127).
Pulmonary fibrosis is a severe complication of HF due to increased left atrial pressure, pulmonary edema, and fibrosis formation (226). According to the anti-fibrotic effects of gallic acid in other studies, Jin et al. found that treatment of TAC-induced HF CD-1 male mice with GA (100 mg/kg/day, 2 weeks) reduced pulmonary fibrosis by downregulating the expression of collagen type I, fibronectin, and connective tissue growth factor (CTGF). Expression of epithelial-mesenchymal transition (EMT) markers, including N-cadherin and SNAI1, was increased in the TAC group, and treatment with GA reduced their expression, suggesting the inhibitory role of GA in HF-induced pulmonary fibrosis by inhibiting the EMT process (128). EMT participates in tissue repair and induces pulmonary fibrosis (227). According to the well-established effects of GA on CVDs, Jin et al. investigated mechanisms of GA affecting blood pressure and CH. Treatment with GA (320 mg/kg/day, 16 weeks) lowered systolic blood pressure and suppressed the RAAS system by decreasing aortic ACE1 and AT1 receptor levels in Wistar-Kyoto rats owning hypertension-induced cardiac hypertrophy. Arterial remodeling and vascular contractibility were also reduced by GA treatment, indicating the regulatory effect of GA on vascular smooth muscle. Collectively, GA alleviated hypertension and CH by suppressing Nox2 activity and Nox2-induced oxidative stress via inhibiting GATA4 expression (78).
Additionally, GA prevented ISO-induced CH and improved cardiac dysfunction by decreasing the expression of hypertrophy-related and fibrosis-related genes. GA (100 mg/kg/day, 3 weeks) suppressed the JNK2/ERK1/2 signaling axis (members of the MAPK pathway) in Male CD-1(ICR) mice with ISO-induced CH. To investigate the role of JNK2 in cardiac fibrosis and hypertrophy, they transfected JNK2 into cardiomyocytes which led to increased Smad3 and collagen type I protein levels, inducing cell hypertrophy and fibrosis. Consequently, this study showed that GA prevents ISO-induced cardiac hypertrophy by regulating JNK2 signaling and Smad3 binding activity (129) (Table 1).
Estrogen has shown an influential role in protecting the cardiovascular system, especially in improving the pulmonary and cardiac function of pulmonary hypertension (PH) cases, by binding to estrogen receptor-β (ERβ) (228, 229). Genistein, an isoflavone derived from soybean, functions as a natural estrogen, can bind to ERβ stronger than estrogen, and can exert anti-inflammatory and anti-cancer effects (230–232). According to the unwanted side effects of estrogen as a drug, genistein can be used instead of the pharmacological form of estrogen. Also, several studies have shown that genistein intake can ameliorate cardiovascular risk factors such as hypertension and lipid profile (233). Matori et al. showed that treating the PH Male Sprague-Dawley rats models with 1 mg/kg/day genistein attenuated RV remodeling, PH-induced RHF, lung remodeling, and pulmonary fibrosis. An in vitro examination demonstrated that genistein (1 μmol/l, 48 h) suppressed the proliferation of human pulmonary artery smooth muscle cells and inhibited cardiomyocyte hypertrophy by binding to the ER-β receptor. This study confirms the beneficial cardiopulmonary effects of genistein in rats with PH (130).
Genistein has shown anti-hypertrophic effects in several studies. Meng et al. reported that genistein (40 mg/kg/day, 7 weeks) attenuates PO-induced CH in C57/BL6 male mice and improves cardiac function by directly decreasing phosphorylation JNK1/2, thereby blocking this signaling pathway (131). Nitric oxide synthases (NOS) catalyze NO production from L-arginine. Neuronal nitric oxide synthase (nNOS) and endothelial nitric oxide synthase (eNOS) have anti-hypertrophic effects on the heart, but inducible nitric oxide synthase (iNOS) induces hypertrophy (234). Maulik et al. investigated the effects of genistein (0.1 and 0.2 mg/kg/day, 14 days) on ISO-induced CH in male Wistar rats and investigated the role of NOS enzymes in developing cardiac hypertrophy. Genistein exerts anti-hypertrophic effects via eNOS, nNOS, and antioxidant effects in ISO-induced CH rat models (132). Recent studies have shown that genistein exerts parts of its effects by regulating the expression of miRNAs (235). Gan et al. showed that treatment of female ICR mice with genistein (100 mg/kg/day, 21 days) upregulated miR-451, suggesting that genistein exerts its anti-hypertrophic effects via enhancing expression of miR-451 and miR-451 suppresses cardiac hypertrophy by directly inhibiting TIMP2 expression. Expression of the TIMP2 gene was increased in the ISO-induced CH heart, indicating pro-hypertrophic effects of TIMP2 (133) (Table 1).
Pterostilbene [4-(3,5dimethoxystyryl) phenol] is a polyphenol structurally similar to RES and has potential pharmacological impacts such as anti-cancer, anti-inflammatory, antioxidant, and anti-apoptosis (236–238). Similar to RES, Pterostilbene (PTS) also exerts cardioprotective effects by suppressing oxidative stress and apoptosis by activating the AMPK pathway (239) or by upregulating PGC1α through activating AMPK/SIRT1 pathway (240). Furthermore, Lacerda et al. revealed that administration of PTS-HPβCD complex (25, 50, or 100 mg/kg/day, 2 weeks) prevents right ventricular (RV) remodeling and improves RV function in pulmonary hypertension-induced right-sided HF rat models. Enhancing the glutathione redox cycle was suggested as an underlying mechanism of the antioxidative effects of PTS (134). The glutathione redox cycle protects the cell membrane from LPO and oxidative stress (241). Also, expression of total phospholamban (PLB) and SERCA (sarco/endoplasmic reticulum Ca2+-ATPase) was increased after treatment with PTS-HPβCD complex, indicating that PTS improves cardiac contractibility and function by improving the calcium handling process in the heart (134). Phospholamban is a protein that regulates calcium channels in the heart, and SERCA is a calcium transporter in the cell. Both these proteins play a significant role in calcium transportation and cardiac muscle contractility (242, 243) (Table 1).
Kaempferol (3,4′,5,7-tetrahydroxyflavone) (KF) is a type of flavonoid found in grapes, tomatoes, aloe Vera, coccinia grandis, and moringa oleifera (244). Kaempferol can protect cells against oxidative stress and inflammation and exerts various pharmacological effects such as anti-microbial, anti-diabetic, and anti-cancer (245). Kaempferol exerts pleiotropic beneficial effects on the cardiovascular system, including ameliorating cardiac fibrosis, preventing HF, improving myocardial damage repair, and decreasing atherosclerosis (135, 246, 247). Zhou et al. showed that KF (15 mmol/l, 10 min) improves myocardial ischemia by exerting antioxidant activity and inhibiting glycogen synthase kinase-3 beta (GSK-3β) activity in adult male Sprague-Dawley rats with myocardial ischemia/reperfusion (I/R) injury (247). Diabetes is one of the significant risk factors for HF development (248); thus, according to the positive effects of KF on both the cardiovascular system and diabetes, Zhang et al. investigated the effect of KF (20 mg/kg/day, 42 days) in diabetic adult male Wistar rats with HF. Results of this study demonstrated that treatment with KF exerts significant cardioprotective effects in diabetic rats as it suppresses inflammation, oxidative stress, and apoptosis and alleviates blood glucose levels and serum cardiac markers (135).
Regarding the underlying mechanism, KF modulated the nuclear factor erythroid 2-related factor 2 (Nrf2) signaling pathway, which protects cells against ROS, and the nuclear factor-light-chain-enhancer of activated B cells (NF-κβ) signaling pathway, which has a vital role in fibrosis. The activity of antioxidant enzymes was increased, while the LPO level was decreased after treatment with KF. Expression of inflammatory factors, including NF-κβ p65, TNF-α, IL-6, IL-1β, p-IKKβ, and COX-2, significantly decreased. KF enhanced AKT/GSK-3β singling pathway by increasing the phosphorylation of AKT and GSK-3β. Decreased phosphorylation of p-p38 MAPK and decreased expression of PI3K in the KF group indicates the inhibitory role of KF on ERK/p38 MAPK and PI3K signaling pathways. Cardiac apoptosis markers (caspase-3 and Bax) were decreased, while the anti-apoptotic marker (Bcl-2) was increased in cardiac tissue after KF treatment. The histopathological investigation revealed decreased destruction of myofibrils and lesser TUNEL-positive cells in the cardiac tissue of rats treated with KF (135).
Feng et al. investigated the role of KF in CH and the molecular mechanism behind it. Treatment with KF (100 mg/kg/day, 6 weeks) improved cardiac function and prevented CH in mice that underwent aorta banding surgery. Interstitial fibrosis, oxidative stress, and apoptosis were also reduced after KF administration (141). ASK1/MAPK and their downstream targets, p38, and JNK were upregulated in the PO-induced CH condition. The stimulating activity of ASK1/MAPK signaling pathways in H9c2 cardiomyocytes in vitro enhanced cardiomyocyte growth and enlargement, whereas treatment with KF reversed these results. Therefore, it could be speculated that KF functioned by reducing oxidative stress and suppressing apoptosis by inhibiting ASK1/MAPK signaling pathway (141). ASK1/MAPK signaling pathway plays a pivotal role in response to stress, especially oxidative stress, and induces apoptosis (249). P38 and JNK are subfamilies of MAPKs and regulate cell apoptosis (250) (Table 1).
Luteolin (3′,4′,5′,7′-tetrahydroxyflavone) (LUT) is a type of flavonoid found in carrot, cabbage, tea, celery, and apple that possess various beneficial effects such as antioxidant, anti-inflammatory, and anti-apoptotic (251). Recent studies have reported that LUT exerts cardioprotective effects against myocardial ischemia, coronary artery disease, and HF (136, 251). Hu et al. reported that treating HF Sprague-Dawley rats with LUT (10 ug/kg/day, 10 days) improves cardiomyocyte contractibility and heart function and decreases apoptosis and cardiac fibrosis. LUT increases cardiac contractibility by increasing intracellular Ca2+ (136). Sarcoplasmic reticulum Ca2+-ATPase 2a (SERCA2a) regulates excitation/contraction coupling in the heart by regulating the concentration of Ca2+ and has been shown to play an essential role in HF pathogenesis as its expression decrease in HF (252, 253). PLB is a SERCA2a inhibitor whose phosphorylation suppresses activity (254). Hu et al. showed that LUT increased the expression of SERCA2a and Sp1, a transcription factor, by activating the PI3K/Akt pathway and increasing PLB phosphorylation. This study verified the enhancing role of LUT on cardiac contractibility in HF (136) (Table 1).
Epigallocatechin gallate (EGCG) is a natural polyphenol flavonoid abundant in green tea and is known for its antioxidant properties. Several studies have shown that EGCG is vital in decreasing oxidative stress in the cardiovascular system (255). For instance, a study by Sheng et al. showed that EGCG attenuates PO-induced CH by suppressing apoptosis and oxidative stress in rats (256). Another study investigated the possible mechanism of EGCG (50, 100 mg/kg/day, 6 weeks) in attenuating PO-induced CH in Male Sprague–Dawley rats. The findings implied the role of EGCG in reducing oxidative stress and apoptosis; EGCG increased the expression of Bcl-2 and telomere repeat-binding factor 2 (TRF2), which are anti-apoptotic agents. In addition, EGCG reduces oxidative stress in the heart by decreasing the level of MDA and increasing SOD activity (140). According to the cardioprotective effects of EGCG, Muhammad et al. showed the beneficial effects of EGCG (200 mg/kg/day, 30 days) in preventing aging-mediated CH, fibrosis, and remodeling, as well as in improving cardiac function in aged Wistar albino rats. Mechanistically, EGCG decreased the production of ROS, improved the antioxidant defense system, and reduced apoptosis via suppressing TGFβ/TNFα/NF-κB pathway in (139) (Table 1).
Apigenin (4′,5,7-trihydroxyflavone) is a flavonoid component distributed in various plants like parsley, oranges, and garlic and has different beneficial pharmacological effects, including antioxidant and anti-inflammatory effects (257). In this regard, Gao et al. investigated the effect of apigenin treatment on improving hypertension and hypertension-induced CH in Wistar-Kyoto rats. They showed that apigenin (20 μg/h, 4 weeks) prevents cardiac hypertrophy via suppressing NADPH oxidase-dependent ROS (137). Previous studies have reported that hypoxia-inducible factor (HIF)-1α mediates CH through its effect on HIMF (hypoxia-induced mitogenic factor) (38, 258). Apigenin can exert inhibitory effects on HIF-1α; therefore, Zhu et al. investigated the anti-hypertrophic effects of apigenin by inhibiting HIF-1α. They treated cardiac hypertrophy induced by renovascular hypertension in rats with apigenin (50–100 mg/kg/day, 4 weeks). The result showed that apigenin decreased blood pressure, heart weight, and serum angiotensin II, indicating a positive role of apigenin in hypertension and CH. Levels of serum and myocardial-free fatty acids also decreased after apigenin treatment. HIF-1α deregulates cardiac metabolism by inhibiting the expression of mitochondrial proteins involved in metabolisms, such as PPARα, CPT-1, and PDK-4. Apigenin improved abnormal myocardial glucolipid metabolism by upregulating PPARα, CPT-1, and PDK-4 via suppressing HIF-1α. This study revealed that apigenin's anti-hypertrophic effects are associated with its effect on HIF-1α (138) (Table 1).
Caffeic acid (CA) is a natural flavonoid found in various foods and herbs, such as coffee, red wine, thyme, sage, and spearmint (259). CA has a wide range of beneficial effects on cellular processes, such as modulating cell growth, proliferation, and anti-inflammatory effects; hence, this molecule could positively affect the cardiovascular system. It is reported that intravenous administration of caffeic acid decreases blood pressure and improves cardiac function (260). It also exerts cardioprotective effects by reducing MI-related oxidative stress (261). According to the association of CA with the MAPK pathway and the role of this pathway in CH, Ren et al. indicated inhibitory effects of CA on PO-induced CH. In addition, the treatment improved cardiac function and decreased cardiac fibrosis. CA treatment (100 mg/kg/day, 6 weeks) in the C57 male mice with induced cardiac hypertrophy downregulated the MAPK pathway by decreasing phosphorylation of ERK1/2, indicating that MAPK suppression mediates CA anti-hypertrophic effects. Consistent with these results, CA suppressed PE-induced hypertrophy in vitro by downregulating MEK/ERK signaling pathway (143) (Table 1).
Delphinidin (3,3′,4′,5,5′,7-hexahydroxyflavylium) is a flavonoid anthocyanidin found in pigmented fruits and vegetables, such as blueberry. The anti-inflammatory and antioxidant effects of delphinidin were well described (262). ROS is a significant mediator of oxidative stress and has a vital role in the development of CH by regulating various signaling pathways and protein kinases (263). NADPH oxidase (NOX) is an oxidoreductase enzyme and the leading producer of ROS in cardiac myocytes (264, 265). Previous studies have shown that delphinidin exerts parts of its function by regulating NOX activity. Chen et al. showed that treatment with a high dosage of delphinidin ameliorated CH and cardiac dysfunction and reduced oxidative stress and cardiac fibrosis in the heart. In vitro, delphinidin (50 μM, 24 h) decreased the increased myocardial NOX activity after Ang II induction; reduced ROS production; and prevented cardiomyocyte hypertrophy in Neonatal rat cardiomyocytes, with no cytotoxic effects (142). Treatment with delphinidin prevented the increased expression of NOX by activating AMPK, a NOX inhibitor, in response to hypertrophic stimulators. Furthermore, high-dosage delphinidin treatment increased the expression of Erk1/2, Jnk1/2, and p38 (kinases of MAPK signaling pathway), indicating the role of the MAPK signaling pathway in the anti-hypertrophy effect of delphinidin. Exploring the effects of delphinidin (15 mg/kg/day, 8 weeks) on aged-mediated cardiac remodeling showed that delphinidin reversed CH by suppressing the phosphorylation of AMPK and the activity of NOX in C57BL/6 mice with the aging-related cardiac hypertrophy (142) (Table 1).
Hesperidin (3,5,7-trihydroxyflavanone 7-rhamnoglucoside) (HES) is a natural flavonoid with comprehensive pharmacological properties such as anti-inflammatory and antioxidant (266). HES has beneficial cardioprotective effects, especially in attenuating CH (267). A recent study revealed that HES (200 mg/kg/day, 28 days) alleviates ISO-induced CH by suppressing oxidative stress, apoptosis, and inflammation in CH-induced male albino Wistar rats. It was indicated that treatment with HES improved hemodynamic state and attenuated left ventricular function. HES preserved the function and structure of mitochondria, myofibril, and myocyte. Cardiac injury, apoptosis, and inflammatory markers were decreased, and oxidative stress was reduced in heart tissue by HES treatment. PPAR-γ is a metabolism regulator which suppresses CH. Bhargava et al. showed that HES upregulated the expression of PPAR-γ, indicating that HES anti-hypertrophic effects arise from enhancing PPAR-γ expression (144) (Table 1).
Bioavailability is an essential issue regarding the clinical application of pharmacological substances. Although RES is reported as a safe component with no adverse effects on humans, its low bioavailability (about 20%) is an essential drawback of using it as a drug. The concentration of RES is dose-depended, and the maximal concentration of 2.4 µM results from the administration of 5 g RES. Drug delivery systems and reformed formulation are novel ways to improve the bioavailability of RES in humans. RES has been shown to improve myocardial reperfusion, enhancing re-endothelialization and reducing inflammation. Also, RES consumption before MI reduced infarct size and cardiac arrhythmia and relieved myocardial injury faster. RES can increase the viability and proliferation of cardiac stem cells, therefor transplanting RES-treated stem cells into an ischemic heart improved cardiac injury in the peri-infarct zone (268).
While curcumin could be administered as a drug, pure curcumin has low oral bioavailability due to its low absorption and rapid metabolism. In this regard, Ray et al. increased curcumin bioavailability by designing a novel delivery system; they encapsulated curcumin within the carboxymethyl chitosan (CMC) nanoparticle and conjugated it to a myocyte-specific homing peptide (CMC/peptide). This agent's 5 mg/kg showed better bioavailability than 35 mg/kg of pure curcumin. Treatment with low dose CMC/peptide downregulated expression of hypertrophic markers, apoptotic markers, and mediators. Collectively, Ray et al. showed that an efficiently targeted delivery regimen for curcumin enhances its therapeutic effects, reduces CH, and improves cardiac function (269).
Additionally, Sungawa et al. investigated the effectiveness of a novel surfactant-soluble oral drug delivery system (DDS) for curcumin to develop a therapeutic aid for patients with MI-induced HF. This DDS significantly enhanced gastrointestinal absorption and effectiveness of curcumin, as administration of 0.5 mg/kg of DDS curcumin exerted the same effect as 50 mg/kg of pure curcumin. In the meantime, no significant side effects were detected regarding DDS curcumin administration. The histopathologic examination showed that DDS curcumin suppressed myocardial cell hypertrophy and perivascular fibrosis. However, the exact dosage of pure oral curcumin did not apply the same effects (270).
Creating a synthetic analog for curcumin is another way to increase its bioavailability to use it as an efficient therapeutic drug. Shimizu et al. examined the role of five different curcumin analogs in inhibiting p300-HAT. Among these five analogues, GO-Y030 ((1E, 4E)-1,5-bis[3,5-bis(methoxymethoxy)phenyl]-1,4-pentadiene-3-one) inhibited p300-HAT and improved HF more efficiently than curcumin. Treating epinephrin-induced cardiomyocyte with GO-Y030 at 1/10th of the curcumin dose (1 μM vs. 10 µM) suppresses the interaction between p300 and GATA4. The in-vivo investigation conducted by Shimizu et al. revealed that administration of 0.5 mg/kg of GO-Y030 prevented TAC-induced HF, CH, and cardiac fibrosis significantly, to the same extent as 50 mg/kg/day curcumin. GO-Y030, at a dose 1/100th that of curcumin, collectively improves HF and can be effective clinically in treating HF without notable liver and renal toxicity (271).
HF treatment is based on improving symptoms and preventing further complications. Although these treatments effectively relieve symptoms, the survival rate has not met the desirable goal yet. Therefore, recent studies and clinical trials have focused on targeting the pathogenic mechanism of HF (such as myocardial contractibility and metabolism, inflammation, oxidative stress, etc.) with new drugs (272, 273). Therefore, Gal et al. investigated the role of RES on left ventricular function and cardiac inflammation in patients with HF. In this randomized, double-blind study, 3-month treatment with 100 mg/day RES significantly reduced levels of total cholesterol, LDL-cholesterol, and inflammatory cytokines (IL -1 and IL -6).
Moreover, the levels of NT-proBNP (a marker of the severity of HF) and galectin-3 were significantly lower in the RES-treated group compared to the placebo group. Echocardiography results showed that treatment with RES significantly increased ejection fraction, left ventricular stroke volume, left ventricular end-systolic volume, and ventricular dilation, indicating positive effects of RES on cardiac remodeling. The results showed that RES suppressed the expression of ATP synthesis-related genes via oxidative phosphorylation in leukocytes; however, this did not result in mitochondrial dysfunction. This clinical study demonstrated that RES has anti-inflammatory effects and improves the quality of life, physical performance, cardiac function, and remodeling in patients with MI-induced HF (99).
In another similar study, Gal et al. evaluated the effects of RES on hemorheological parameters in HF patients. They found that RES could significantly improve impaired red blood cell (RBC) aggregation resulting from HF, which may be attributed to the antioxidative effects of RES. Also, the result showed that RES significantly improved the results of the 6-minute walk test of HF patients (274). Various cardiovascular disorders are associated with increased RBC aggregation, such as ischemic heart disease, diabetes, venous thrombosis, and HF (275). Increased levels of inflammatory cytokines and oxidants in the blood due to HF are significant factors in RBC aggregation and hemorheological disturbances (276, 277). Unstable angina, a subtype of acute coronary syndrome (ACS), leads to cardiac complications, such as arrhythmia, MI, and eventually HF. Inflammation is a critical factor in the progression of ACS, leading to further complications. According to the anti-inflammatory effects of curcumin, it can be used as a therapeutic drug in ACS, preventing complications such as HF. Dastani et al. investigated the effects of administrating 80 mg/day of curcumin to patients with unstable angina in a randomized, double-blind clinical trial. This novel clinical trial showed no significant effect of curcumin on preventing cardiovascular complications of unstable angina, such as HF (278). In a double-blind, placebo-controlled, randomized clinical trial, the effect of high-dose of curcumin (a 90 mg curcumin capsule, twice a day for 24 weeks) on the prevention of hypertensive heart disease was investigated. The results indicated that high curcumin absorption had no significant effects on left ventricular diastolic function but significantly suppressed increment in the plasma BNP levels (279). In another double-blind, placebo-controlled, randomized clinical study, the efficiency of nano-curcumin (80 mg/day) consumption on cardiovascular risk factors in patients with type diabetics as well as mild to moderate coronary artery disease. The result revealed that nano-curcumin reduced atherosclerosis and hs-CRP levels as an inflammation indicator in diabetic heart patients (280). However, future studies still need to investigate the theory more widely.
As reviewed earlier, polyphenols can play a critical role in attenuating HF and CH, as they can prevent cardiac remodeling mechanistically by blocking oxidative, inflammatory, apoptotic, and fibrotic-related pathways. Moreover, polyphenols are easily accessible and enriched within various natural plant-based sources and could be considered novel promising therapeutic approaches for HF. Additionally, the conventional HF treatments' low survival rates have forced studies to search for other viable options as CVDs are growing globally. However, these studies are still in their infancy, and limited clinical data regarding the efficacy, side effects, and administration routes of polyphenol-based drugs for treating cardiovascular diseases such as HF and CH are available.
Despite the abundant presence of polyphenols in a human's regular nutritional diet, the poor solubility, low stability, rapid metabolism and elimination, and eventually, weak bioavailability are the rate-limiting factors for inhibiting these compounds from exerting their full cardioprotective effects (281). Therefore, some recent studies have focused on enhancing the polyphenols' bioavailability, especially RES and curcumin, by employing nanoformulations, such as lipid-based nanoparticles (liposomes), protein-based nanoformulations, polymers, micelles, and metal nanoparticles for drug delivery (281). For instance, RES and curcumin co-delivery by polymeric micelles to doxorubicin-treated cardiomyocyte cell lines resulted in higher drug solubility and efficacy than non-polymeric forms and single-drug treatment (282). Polymeric micelles used in these studies are biocompatible and FDA-approved. Moreover, polymer-based drug delivery vehicles such as Poly (D,L-lactic-co-glycolic acid) (PLGA) nanoparticles demonstrated superior anti-atherosclerotic effects of curcumin-bioperine treatment compared to non-coated ones. Pillai et al. reported that curcumin-bioperine coated with PLGA nanoparticles significantly reduced ox-LDL levels, downregulating the atherosclerotic plaque-related gene expression in vitro (283). The efficacy of PLGA-encapsulated curcumin was also illustrated in vivo in later studies, where gold-capped curcumin encapsulated within PLGA demonstrated enhanced solubility, bioavailability, and eventually, improved anti-hypertrophic characteristics in hypertrophic Wistar rat models (284).
Aside from polymeric-based drug delivery, extensive studies were carried out on the efficacy of liposome-targeted therapy (285–287). Liposomes are phospholipid-based nanocarriers designed for both hydrophilic and hydrophobic drug delivery. Liposome preparation progress in recent years has introduced the ligand surface-engineered and long-circulating liposomes, allowing stability and sustained drug release in target tissues and enhanced bioavailability (288). The use of liposomes in CVDs has become a promising candidate for future safe drug delivery without cytotoxicity. RES-contained liposomes were demonstrated to enhance the respiratory capacity of the cardiomyocytes in vitro (289). Moreover, the transplantation of mitochondrial-activated cardiac progenitor cells by liposomal RES into the heart of the mouse models with cardiomyopathy resulted in reduced oxidative stress and apoptotic activities of the cardiomyocytes (290).
Considering their minimal side effects and significant therapeutic features, polyphenols have introduced themselves as intriguing options for future HF treatment. However, due to the low bioavailability and stability of polyphenols in the circulating system, limited clinical trials have been conducted evaluating their impact on human HF, and most of the studies are in vitro and in vivo animal models. However, with recent advances in novel drug delivery systems, there is rising hope for translating nanoparticle delivery of these compounds to the clinical setting soon.
In conclusion, this study predominantly reviewed the therapeutic effects of polyphenol compounds and their underlying mechanisms in improving pathologic cardiac remodeling, leading to heart failure. Also, this study discusses the most recent advances and challenges in translating the antioxidative, anti-inflammatory, anti-apoptotic, and antifibrotic characteristics of these nutraceuticals into clinical settings. The potential application of nano-drug delivery systems was also investigated regarding the limited solubility, stability, and bioavailability of the polyphenols in humans. Finally, further in-depth surveys are needed to understand better the involved molecular pathways of polyphenols in modulating cardiac remodeling and HF. Also, there is a rising demand for viable strategies to better translate the in vitro and animal study results into the clinic.
NH, AY, MS, YG, FA, RE, M-HA, MH, ZA, HM, MHJ, AM, and MR took part in creating the idea, design, and composing of the manuscript. All authors contributed to the article and approved the submitted version.
The authors declare that the research was conducted in the absence of any commercial or financial relationships that could be construed as a potential conflict of interest.
All claims expressed in this article are solely those of the authors and do not necessarily represent those of their affiliated organizations, or those of the publisher, the editors and the reviewers. Any product that may be evaluated in this article, or claim that may be made by its manufacturer, is not guaranteed or endorsed by the publisher.
1. Ooi BK, Chan K-G, Goh BH, Yap WH. The role of natural products in targeting cardiovascular diseases via Nrf2 pathway: novel molecular mechanisms and therapeutic approaches. Front Pharmacol. (2018) 9:1308. doi: 10.3389/fphar.2018.01308
2. Rana A, Samtiya M, Dhewa T, Mishra V, Aluko RE. Health benefits of polyphenols: a concise review. J Food Biochem. (2022) 46(10):e14264. doi: 10.1111/jfbc.14264
3. Tangney CC, Rasmussen HE. Polyphenols, inflammation, and cardiovascular disease. Curr Atheroscler Rep. (2013) 15:1–10. doi: 10.1007/s11883-013-0324-x
4. de Paulo Farias D, Neri-Numa IA, de Araujo FF, Pastore GM. A critical review of some fruit trees from the Myrtaceae family as promising sources for food applications with functional claims. Food Chem. (2020) 306:125630. doi: 10.1016/j.foodchem.2019.125630
5. Zhang Z, Qiu C, Li X, McClements DJ, Jiao A, Wang J, et al. Advances in research on interactions between polyphenols and biology-based nano-delivery systems and their applications in improving the bioavailability of polyphenols. Trends Food Sci Technol. (2021) 116:492–500. doi: 10.1016/j.tifs.2021.08.009
6. Chen G-L, Fan M-X, Wu J-L, Li N, Guo M-Q. Antioxidant and anti-inflammatory properties of flavonoids from lotus plumule. Food Chem. (2019) 277:706–12. doi: 10.1016/j.foodchem.2018.11.040
7. Pandey KB, Rizvi SI. Plant polyphenols as dietary antioxidants in human health and disease. Oxid Med Cell Longevity. (2009) 2(5):270–8. doi: 10.4161/oxim.2.5.9498
8. Sarker U, Oba S. Polyphenol and flavonoid profiles and radical scavenging activity in leafy vegetable Amaranthus gangeticus. BMC Plant Biol. (2020) 20(1):1–12. doi: 10.1186/s12870-019-2170-7
9. Bharti R, Khamparia A, Shabaz M, Dhiman G, Pande S, Singh P. Prediction of heart disease using a combination of machine learning and deep learning. Comput Intell Neurosci. (2021) 2021. doi: 10.1155/2021/8387680
10. Tham YK, Bernardo BC, Ooi JY, Weeks KL, McMullen JR. Pathophysiology of cardiac hypertrophy and heart failure: signaling pathways and novel therapeutic targets. Arch Toxicol. (2015) 89(9):1401–38. doi: 10.1007/s00204-015-1477-x
11. Bragazzi NL, Zhong W, Shu J, Abu Much A, Lotan D, Grupper A, et al. Burden of heart failure and underlying causes in 195 countries and territories from 1990 to 2017. Eur J Prev Cardiol. (2021) 28(15):1682–90. doi: 10.1093/eurjpc/zwaa147
12. Gallo S, Vitacolonna A, Bonzano A, Comoglio P, Crepaldi T. ERK: a key player in the pathophysiology of cardiac hypertrophy. Int J Mol Sci. (2019) 20(9):2164. doi: 10.3390/ijms20092164
13. McCarty MF. Nutraceutical, dietary, and lifestyle options for prevention and treatment of ventricular hypertrophy and heart failure. Int J Mol Sci. (2021) 22(7):3321. doi: 10.3390/ijms22073321
14. Chang X, Zhang T, Zhang W, Zhao Z, Sun J. Natural drugs as a treatment strategy for cardiovascular disease through the regulation of oxidative stress. Oxid Med Cell Longevity. (2020) 2020.
15. Mollace V, Rosano GM, Anker SD, Coats AJ, Seferovic P, Mollace R, et al. Pathophysiological basis for nutraceutical supplementation in heart failure: a comprehensive review. Nutrients. (2021) 13(1):257. doi: 10.3390/nu13010257
16. Kutan Fenercioglu A, Saler T, Genc E, Sabuncu H, Altuntas Y. The effects of polyphenol-containing antioxidants on oxidative stress and lipid peroxidation in type 2 diabetes mellitus without complications. J Endocrinol Investig. (2010) 33(2):118–24. doi: 10.1007/BF03346565
17. Cheng Y-C, Sheen J-M, Hu WL, Hung Y-C. Polyphenols and oxidative stress in atherosclerosis-related ischemic heart disease and stroke. Oxid Med Cell Longevity. (2017) 2017.
18. Brito PM, Devillard R, Nègre-Salvayre A, Almeida LM, Dinis TC, Salvayre R, et al. Resveratrol inhibits the mTOR mitogenic signaling evoked by oxidized LDL in smooth muscle cells. Atherosclerosis. (2009) 205(1):126–34. doi: 10.1016/j.atherosclerosis.2008.11.011
19. Sung MM, Das SK, Levasseur J, Byrne NJ, Fung D, Kim TT, et al. Resveratrol treatment of mice with pressure-overloadinduced heart failure improves diastolic function and cardiac energy metabolism. Circ Heart Fail. (2015) 8(1):128–37. doi: 10.1161/CIRCHEARTFAILURE.114.001677
20. Ahmet I, Tae H-J, Lakatta EG, Talan M. Long-term low dose dietary resveratrol supplement reduces cardiovascular structural and functional deterioration in chronic heart failure in rats. Can J Physiol Pharmacol. (2017) 95(3):268–74. doi: 10.1139/cjpp-2016-0512
21. Rimbaud S, Ruiz M, Piquereau J, Mateo P, Fortin D, Veksler V, et al. Resveratrol improves survival, hemodynamics and energetics in a rat model of hypertension leading to heart failure. PLoS One. (2011) 6(10):e26391. doi: 10.1371/journal.pone.0026391
22. Matsumura N, Takahara S, Maayah ZH, Parajuli N, Byrne NJ, Shoieb SM, et al. Resveratrol improves cardiac function and exercise performance in MI-induced heart failure through the inhibition of cardiotoxic HETE metabolites. J Mol Cell Cardiol. (2018) 125:162–73. doi: 10.1016/j.yjmcc.2018.10.023
23. Bahit MC, Kochar A, Granger CB. Post-myocardial infarction heart failure. JACC Heart Fail. (2018) 6(3):179–86. doi: 10.1016/j.jchf.2017.09.015
24. Martin AC, Bories MC, Tence N, Baudinaud P, Pechmajou L, Puscas T, et al. Epidemiology, pathophysiology, and management of native atrioventricular valve regurgitation in heart failure patients. Front Cardiovasc Med. (2021) 8:713658. doi: 10.3389/fcvm.2021.713658
25. Slivnick J, Lampert BC. Hypertension and heart failure. Heart Fail Clin. (2019) 15(4):531–41. doi: 10.1016/j.hfc.2019.06.007
26. Rosenbaum AN, Agre KE, Pereira NL. Genetics of dilated cardiomyopathy: practical implications for heart failure management. Nat Rev Cardiol. (2020) 17(5):286–97. doi: 10.1038/s41569-019-0284-0
27. Lehrke M, Marx N. Diabetes mellitus and heart failure. Am J Cardiol. (2017) 120(1):S37–47. doi: 10.1016/j.amjcard.2017.05.014
28. Bracamonte-Baran W, Čiháková D. Cardiac autoimmunity: myocarditis. Adv Exp Med Biol. (2017) 1003:187–221. doi: 10.1007/978-3-319-57613-8_10
29. Lyon RC, Zanella F, Omens JH, Sheikh F. Mechanotransduction in cardiac hypertrophy and failure. Circ Res. (2015) 116(8):1462–76. doi: 10.1161/CIRCRESAHA.116.304937
30. Dhalla NS, Saini-Chohan HK, Rodriguez-Leyva D, Elimban V, Dent MR, Tappia PS. Subcellular remodelling may induce cardiac dysfunction in congestive heart failure. Cardiovasc Res. (2009) 81(3):429–38. doi: 10.1093/cvr/cvn281
31. Lin YT, Lin YH, Wu XM, Ko CL, Yen RF, Chen YH, et al. The relationship between serum fibrosis markers and restrictive ventricular filling in patients with heart failure with reduced ejection fraction: a technetium-99m radionuclide ventriculography study. Oncotarget. (2017) 8(2):2381–90. doi: 10.18632/oncotarget.13795
32. Hartupee J, Mann DL. Neurohormonal activation in heart failure with reduced ejection fraction. Nat Rev Cardiol. (2017) 14(1):30–8. doi: 10.1038/nrcardio.2016.163
33. Nakamura M, Sadoshima J. Mechanisms of physiological and pathological cardiac hypertrophy. Nat Rev Cardiol. (2018) 15(7):387–407. doi: 10.1038/s41569-018-0007-y
34. He J, Luo Y, Song J, Tan T, Zhu H. Non-coding RNAs and pathological cardiac hypertrophy. Adv Exp Med Biol. (2020) 1229:231–45. doi: 10.1007/978-981-15-1671-9_13
35. Shah AK, Bhullar SK, Elimban V, Dhalla NS. Oxidative stress as a mechanism for functional alterations in cardiac hypertrophy and heart failure. Antioxidants (Basel). (2021) 10(6).
36. Shimizu I, Minamino T. Physiological and pathological cardiac hypertrophy. J Mol Cell Cardiol. (2016) 97:245–62. doi: 10.1016/j.yjmcc.2016.06.001
37. van Berlo JH, Maillet M, Molkentin JD. Signaling effectors underlying pathologic growth and remodeling of the heart. J Clin Invest. (2013) 123(1):37–45. doi: 10.1172/JCI62839
38. Kumar S, Wang G, Liu W, Ding W, Dong M, Zheng N, et al. Hypoxia-induced mitogenic factor promotes cardiac hypertrophy via calcium-dependent and hypoxia-inducible factor-1α mechanisms. Hypertension. (2018) 72(2):331–42. doi: 10.1161/HYPERTENSIONAHA.118.10845
39. Senoner T, Dichtl W. Oxidative stress in cardiovascular diseases: still a therapeutic target? Nutrients. (2019) 11(9). doi: 10.3390/nu11092090
40. Strassheim D, Dempsey EC, Gerasimovskaya E, Stenmark K, Karoor V. Role of inflammatory cell subtypes in heart failure. J Immunol Res. (2019) 2019:2164017. doi: 10.1155/2019/2164017
41. Adamo L, Rocha-Resende C, Prabhu SD, Mann DL. Reappraising the role of inflammation in heart failure. Nat Rev Cardiol. (2020) 17(5):269–85. doi: 10.1038/s41569-019-0315-x
42. Hall C, Gehmlich K, Denning C, Pavlovic D. Complex relationship between cardiac fibroblasts and cardiomyocytes in health and disease. J Am Heart Assoc. (2021) 10(5):e019338. doi: 10.1161/JAHA.120.019338
43. Kanagala P, Cheng ASH, Singh A, Khan JN, Gulsin GS, Patel P, et al. Relationship between focal and diffuse fibrosis assessed by CMR and clinical outcomes in heart failure with preserved ejection fraction. JACC Cardiovasc Imaging. (2019) 12(11 Pt 2):2291–301. doi: 10.1016/j.jcmg.2018.11.031
44. Milinković I, Polovina M, Simeunović DS, Ašanin M, Seferović PM. Oxidative stress and inflammation in heart failure: the best is yet to come. Eur J Prev Cardiol. (2020) 27(5):490–3. doi: 10.1177/2047487319900294
45. Wu S, Lan J, Li L, Wang X, Tong M, Fu L, et al. Sirt6 protects cardiomyocytes against doxorubicin-induced cardiotoxicity by inhibiting P53/fas-dependent cell death and augmenting endogenous antioxidant defense mechanisms. Cell Biol Toxicol. (2023) 39:237258. doi: 10.1007/s10565-020-09576-8
46. Juhasz B, Varga B, Gesztelyi R, Kemeny-Beke A, Zsuga J, Tosaki A. Resveratrol: a multifunctional cytoprotective molecule. Curr Pharm Biotechnol. (2010) 11(8):810–8. doi: 10.2174/138920110793262079
47. Vuolo MM, Lima VS, Junior MRM. Phenolic compounds: structure, classification, and antioxidant power. In: Bioactive compounds. Amesterdam, Netherlands: Elsevier (2019). p. 33–50.
48. Gómez-Caravaca AM, Verardo V, Segura-Carretero A, Fernández-Gutiérrez A, Caboni MF. Phenolic compounds and saponins in plants grown under different irrigation regimes. In: Polyphenols in plants. Amesterdam, Netherlands: Elsevier (2014). p. 37–52.
49. Behl T, Bungau S, Kumar K, Zengin G, Khan F, Kumar A, et al. Pleotropic effects of polyphenols in cardiovascular system. Biomed Pharmacother. (2020) 130:110714. doi: 10.1016/j.biopha.2020.110714
50. Zeb A, Zeb A. Concept of antioxidants in foods. In: Phenolic antioxidants in foods: Chemistry, biochemistry and analysis Amesterdam, Netherlands: Springer (2021). p. 3–23.
51. Rasul A, Millimouno FM, Ali Eltayb W, Ali M, Li J, Li X. Pinocembrin: a novel natural compound with versatile pharmacological and biological activities. BioMed Res Int. (2013) 2013. doi: 10.1155/2013/379850
52. Mihanfar A, Nouri M, Roshangar L, Khadem-Ansari MH. Polyphenols: natural compounds with promising potential in treating polycystic ovary syndrome. Reprod Biol. (2021) 21(2):100500. doi: 10.1016/j.repbio.2021.100500
53. Salami M, Salami R, Mafi A, Aarabi MH, Vakili O, Asemi Z. Therapeutic potential of resveratrol in diabetic nephropathy according to molecular signaling. Current Molecular Pharmacology. (2022) 15(5):716–35.
54. Ahmadi A, Mazloomnejad R, Kasravi M, Gholamine B, Bahrami S, Sarzaeem MM, et al. Recent advances on small molecules in osteogenic differentiation of stem cells and the underlying signaling pathways. Stem Cell Research & Therapy. (2022) 3(1):1–22.
55. Pathak S, Kesavan P, Banerjee A, Banerjee A, Celep GS, Bissi L, et al. Metabolism of dietary polyphenols by human gut microbiota and their health benefits. In: Polyphenols: Mechanisms of action in human health and disease. Elsevier (2018). p. 347–59.
56. de Araْjo FF, de Paulo Farias D, Neri-Numa IA, Pastore GM. Polyphenols and their applications: an approach in food chemistry and innovation potential. Food Chem. (2021) 338:127535. doi: 10.1016/j.foodchem.2020.127535
57. Cui Q, Du R, Liu M, Rong L. Lignans and their derivatives from plants as antivirals. Molecules. (2020) 25(1):183. doi: 10.3390/molecules25010183
58. Rodríguez-García C, Sánchez-Quesada C, Toledo E, Delgado-Rodríguez M, Gaforio JJ. Naturally lignan-rich foods: a dietary tool for health promotion? Molecules. (2019) 24(5):917. doi: 10.3390/molecules24050917
59. Calado A, Neves PM, Santos T, Ravasco P. The effect of flaxseed in breast cancer: a literature review. Front Nutr. (2018) 5:4. doi: 10.3389/fnut.2018.00004
60. Liu W, Lu Y, Chu S, Jiang M, Bai G. Phillygenin, a lignan compound, inhibits hypertension by reducing PLCβ3-dependent Ca2+ oscillation. J Funct Foods. (2019) 60:103432. doi: 10.1016/j.jff.2019.103432
61. Hu Y, Li Y, Sampson L, Wang M, Manson JE, Rimm E, et al. Lignan intake and risk of coronary heart disease. J Am Coll Cardiol. (2021) 78(7):666–78. doi: 10.1016/j.jacc.2021.05.049
62. Akrami A, Nikaein F, Babajafari S, Faghih S, Yarmohammadi H. Comparison of the effects of flaxseed oil and sunflower seed oil consumption on serum glucose, lipid profile, blood pressure, and lipid peroxidation in patients with metabolic syndrome. J Clin Lipidol. (2018) 12(1):70–7. doi: 10.1016/j.jacl.2017.11.004
63. Hasaniani N, Rahimlou M, Ahmadi AR, Khalifani AM, Alizadeh M. The effect of flaxseed enriched yogurt on the glycemic status and cardiovascular risk factors in patients with type 2 diabetes mellitus: randomized, open-labeled, controlled study. Clin Nutr Res. (2019) 8(4):284–95. doi: 10.7762/cnr.2019.8.4.284
64. Câmara JS, Albuquerque BR, Aguiar J, Corrêa RC, Gonçalves JL, Granato D, et al. Food bioactive compounds and emerging techniques for their extraction: polyphenols as a case study. Foods. (2020) 10(1):37. doi: 10.3390/foods10010037
65. Zhang L-X, Li C-X, Kakar MU, Khan MS, Wu P-F, Amir RM, et al. Resveratrol (RV): a pharmacological review and call for further research. Biomed Pharmacother. (2021) 143:112164. doi: 10.1016/j.biopha.2021.112164
66. Salehi B, Mishra AP, Nigam M, Sener B, Kilic M, Sharifi-Rad M, et al. Resveratrol: a double-edged sword in health benefits. Biomedicines. (2018) 6(3):91. doi: 10.3390/biomedicines6030091
67. Nabeebaccus A, Zhang M, Shah AM. NADPH oxidases and cardiac remodelling. Heart Fail Rev. (2011) 16(1):5–12. doi: 10.1007/s10741-010-9186-2
68. Peoples JN, Saraf A, Ghazal N, Pham TT, Kwong JQ. Mitochondrial dysfunction and oxidative stress in heart disease. Exp Mol Med. (2019) 51(12):1–13. doi: 10.1038/s12276-019-0355-7
69. Sivandzade F, Prasad S, Bhalerao A, Cucullo L. NRF2 and NF-κB interplay in cerebrovascular and neurodegenerative disorders: molecular mechanisms and possible therapeutic approaches. Redox Biol. (2019) 21:101059. doi: 10.1016/j.redox.2018.11.017
70. Wardyn JD, Ponsford AH, Sanderson CM. Dissecting molecular cross-talk between Nrf2 and NF-κB response pathways. Biochem Soc Trans. (2015) 43(4):621–6. doi: 10.1042/BST20150014
71. Saha S, Buttari B, Panieri E, Profumo E, Saso L. An overview of Nrf2 signaling pathway and its role in inflammation. Molecules. (2020) 25(22):5474. doi: 10.3390/molecules25225474
72. Buelna-Chontal M, Zazueta C. Redox activation of Nrf2 & NF-κB: a double end sword? Cell Signal. (2013) 25(12):2548–57. doi: 10.1016/j.cellsig.2013.08.007
73. Chauhan A, Islam AU, Prakash H, Singh S. Phytochemicals targeting NF-κB signaling: potential anti-cancer interventions. J Pharm Anal. (2022) 12(3):394–405. doi: 10.1016/j.jpha.2021.07.002
74. Khan H, Ullah H, Castilho PCMF, Gomila AS, D'Onofrio G, Filosa R, et al. Targeting NF-κB signaling pathway in cancer by dietary polyphenols. Crit Rev Food Sci Nutr. (2020) 60(16):2790–800. doi: 10.1080/10408398.2019.1661827
75. Chen M, Chen Z, Huang D, Sun C, Xie J, Chen T, et al. Myricetin inhibits TNF-α-induced inflammation in A549 cells via the SIRT1/NF-κB pathway. Pulm Pharmacol Ther. (2020) 65:102000. doi: 10.1016/j.pupt.2021.102000
76. Marino A, Hausenloy DJ, Andreadou I, Horman S, Bertrand L, Beauloye C. AMP-activated protein kinase: a remarkable contributor to preserve a healthy heart against ROS injury. Free Radic Biol Med. (2021) 166:238–54. doi: 10.1016/j.freeradbiomed.2021.02.047
77. Najjar RS, Feresin RG. Protective role of polyphenols in heart failure: molecular targets and cellular mechanisms underlying their therapeutic potential. Int J Mol Sci. (2021) 22(4):1668. doi: 10.3390/ijms22041668
78. Jin L, Piao ZH, Sun S, Liu B, Kim GR, Seok YM, et al. Gallic acid reduces blood pressure and attenuates oxidative stress and cardiac hypertrophy in spontaneously hypertensive rats. Sci Rep. (2017) 7(1):1–14. doi: 10.1038/s41598-016-0028-x
79. Murphy E, Ardehali H, Balaban RS, DiLisa F, Dorn GW, Kitsis RN, et al. Mitochondrial function, biology, and role in disease: a scientific statement from the American heart association. Circ Res. (2016) 118(12):1960–91. doi: 10.1161/RES.0000000000000104
80. Zhou B, Tian R. Mitochondrial dysfunction in pathophysiology of heart failure. J Clin Invest. (2018) 128(9):3716–26. doi: 10.1172/JCI120849
81. Skemiene K, Rakauskaite G, Trumbeckaite S, Liobikas J, Brown GC, Borutaite V. Anthocyanins block ischemia-induced apoptosis in the perfused heart and support mitochondrial respiration potentially by reducing cytosolic cytochrome c. Int J Biochem Cell Biol. (2013) 45(1):23–9. doi: 10.1016/j.biocel.2012.07.022
82. Skemiene K, Liobikas J, Borutaite V. Anthocyanins as substrates for mitochondrial complex iprotective effect against heart ischemic injury. FEBS J. (2015) 282(5):963–71. doi: 10.1111/febs.13195
83. Libby P. Inflammation during the life cycle of the atherosclerotic plaque. Cardiovasc Res. (2021) 117(13):2525–36.34550337
84. Lala A, Desai AS. The role of coronary artery disease in heart failure. Heart Fail Clin. (2014) 10(2):353–65. doi: 10.1016/j.hfc.2013.10.002
85. Göçmen AY, Burgucu D, Gümüşlü S. Effect of resveratrol on platelet activation in hypercholesterolemic rats: CD40CD40l system as a potential target. Appl Physiol Nutr Metab. (2011) 36(3):323–30. doi: 10.1139/h11-022
86. Ricketts M-L, Ferguson BS. Polyphenols: novel signaling pathways. Curr Pharm Des. (2018) 24(2):158–70. doi: 10.2174/1381612824666171129204054
87. Yashiro T, Nanmoku M, Shimizu M, Inoue J, Sato R. Resveratrol increases the expression and activity of the low density lipoprotein receptor in hepatocytes by the proteolytic activation of the sterol regulatory element-binding proteins. Atherosclerosis. (2012) 220(2):369–74. doi: 10.1016/j.atherosclerosis.2011.11.006
88. Berrougui H, Grenier G, Loued S, Drouin G, Khalil A. A new insight into resveratrol as an atheroprotective compound: inhibition of lipid peroxidation and enhancement of cholesterol efflux. Atherosclerosis. (2009) 207(2):420–7. doi: 10.1016/j.atherosclerosis.2009.05.017
89. Andriantsitohaina R, Auger C, Chataigneau T, Étienne-Selloum N, Li H, Martínez MC, et al. Molecular mechanisms of the cardiovascular protective effects of polyphenols. Br J Nutr. (2012) 108(9):1532–49. doi: 10.1017/S0007114512003406
90. Dolinsky VW, Chakrabarti S, Pereira TJ, Oka T, Levasseur J, Beker D, et al. Resveratrol prevents hypertension and cardiac hypertrophy in hypertensive rats and mice. Biochim Biophys Acta Mol Bas Dis. (2013) 1832(10):1723–33. doi: 10.1016/j.bbadis.2013.05.018
91. Liu Z, Song Y, Zhang X, Liu Z, Zhang W, Mao W, et al. Effects of trans-resveratrol on hypertension-induced cardiac hypertrophy using the partially nephrectomized rat model. Clin Exp Pharmacol Physiol. (2005) 32(12):1049–54.16445570
92. Zordoky BN, Robertson IM, Dyck JR. Preclinical and clinical evidence for the role of resveratrol in the treatment of cardiovascular diseases. Biochim Biophys Acta Mol Bas Dis. (2015) 1852(6):1155–77. doi: 10.1016/j.bbadis.2014.10.016
93. Abo-Kadoum M, Abouelela ME, Al Mousa AA, Abo-Dahab NF, Mosa MA, Helmy YA, et al. Resveratrol biosynthesis, optimization, induction, bio-transformation and bio-degradation in mycoendophytes. Front Microbiol. (2022) 13. doi: 10.3389/fmicb.2022.1010332
94. Hasan MM, Bae H. An overview of stress-induced resveratrol synthesis in grapes: perspectives for resveratrol-enriched grape products. Molecules. (2017) 22(2):294. doi: 10.3390/molecules22020294
95. Sales JM, Resurreccion AV. Resveratrol in peanuts. Crit Rev Food Sci Nutr. (2014) 54(6):734–70. doi: 10.1080/10408398.2011.606928
96. An L, Liu Y, Wu A, Guan Y. microRNA-124 inhibits migration and invasion by down-regulating ROCK1 in glioma. PLoS One. (2013) 8(7):e69478. doi: 10.1371/journal.pone.0069478
97. Shukla Y, Singh R. Resveratrol and cellular mechanisms of cancer prevention. Ann N Y Acad Sci. (2011) 1215(1):1–8. doi: 10.1111/j.1749-6632.2010.05870.x
98. Singh AP, Singh R, Verma SS, Rai V, Kaschula CH, Maiti P, et al. Health benefits of resveratrol: evidence from clinical studies. Med Res Rev. (2019) 39(5):1851–91. doi: 10.1002/med.21565
99. Gal R, Deres L, Horvath O, Eros K, Sandor B, Urban P, et al. Resveratrol improves heart function by moderating inflammatory processes in patients with systolic heart failure. Antioxidants. (2020) 9(11):1108. doi: 10.3390/antiox9111108
100. Bonnefont-Rousselot D. Resveratrol and cardiovascular diseases. Nutrients. (2016) 8(5):250. doi: 10.3390/nu8050250
101. Zhang L, Chen J, Yan L, He Q, Xie H, Chen M. Resveratrol ameliorates cardiac remodeling in a murine model of heart failure with preserved ejection fraction. Front Pharmacol. (2021) 12:1356.
102. Sung MM, Byrne NJ, Robertson IM, Kim TT, Samokhvalov V, Levasseur J, et al. Resveratrol improves exercise performance and skeletal muscle oxidative capacity in heart failure. Am J Physiol Heart Circ Physiol. (2017) 312(4):H842–53. doi: 10.1152/ajpheart.00455.2016
103. Xuan W, Wu B, Chen C, Chen B, Zhang W, Xu D, et al. Resveratrol improves myocardial ischemia and ischemic heart failure in mice by antagonizing the detrimental effects of fractalkine. Crit Care Med. (2012) 40(11):3026–33. doi: 10.1097/CCM.0b013e31825fd7da
104. Gu X, Wang Z, Ye Z, Lei J, Li L, Su D, et al. Resveratrol, an activator of SIRT1, upregulates AMPK and improves cardiac function in heart failure. Genet Mol Res. (2014) 13(1):323–35. doi: 10.4238/2014.January.17.17
105. Chan AY, Dolinsky VW, Soltys C-LM, Viollet B, Baksh S, Light PE, et al. Resveratrol inhibits cardiac hypertrophy via AMP-activated protein kinase and Akt. J Biol Chem. (2008) 283(35):24194–201. doi: 10.1074/jbc.M802869200
106. Ren S, Shen L, Lin S, Xiao D, Xiao W, Yan P-M, et al. Mechanistic analysis of resveratrol in cardiac hypertrophy by network pharmacology and animal experiments. Mol Med Rep. (2022) 26(5):1–11.
107. Chakraborty S, Pujani M, Haque SE. Combinational effect of resveratrol and atorvastatin on isoproterenol-induced cardiac hypertrophy in rats. J Pharm Bioallied Sci. (2015) 7(3):233. doi: 10.4103/0975-7406.160037
108. Wojciechowski P, Juric D, Louis XL, Thandapilly SJ, Yu L, Taylor C, et al. Resveratrol arrests and regresses the development of pressure overload-but not volume overload-induced cardiac hypertrophy in rats. J Nutr. (2010) 140(5):962–8. doi: 10.3945/jn.109.115006
109. Chen C, Zou L-X, Lin Q-Y, Yan X, Bi H-L, Xie X, et al. Resveratrol as a new inhibitor of immunoproteasome prevents PTEN degradation and attenuates cardiac hypertrophy after pressure overload. Redox Biol. (2019) 20:390–401. doi: 10.1016/j.redox.2018.10.021
110. Guan P, Sun Z-M, Wang N, Zhou J, Luo L-F, Zhao Y-S, et al. Resveratrol prevents chronic intermittent hypoxia-induced cardiac hypertrophy by targeting the PI3K/AKT/mTOR pathway. Life Sci. (2019) 233:116748. doi: 10.1016/j.lfs.2019.116748
111. Fan Y, Liu L, Fang K, Huang T, Wan L, Liu Y, et al. Resveratrol ameliorates cardiac hypertrophy by down-regulation of miR-155 through activation of breast cancer type 1 susceptibility protein. J Am Heart Assoc. (2016) 5(4):e002648.27107135
112. Dorri Mashhadi F, Zavvar Reza Z, Jamhiri M, Hafizi Z, Zare Mehrjardi F, et al. The effect of resveratrol on angiotensin II levels and the rate of transcription of its receptors in the rat cardiac hypertrophy model. J Physiol Sci. (2017) 67(2):303–9. doi: 10.1007/s12576-016-0465-0
113. Thandapilly SJ, Wojciechowski P, Behbahani J, Louis XL, Yu L, Juric D, et al. Resveratrol prevents the development of pathological cardiac hypertrophy and contractile dysfunction in the SHR without lowering blood pressure. Am J Hypertens. (2010) 23(2):192–6. doi: 10.1038/ajh.2009.228
114. Tang Y-H, Bao M-W, Yang B, Zhang Y, Zhang B-S, Zhou Q, et al. Curcumin attenuates left ventricular dysfunction and remodeling in rabbits with chronic heart failure. Zhonghua Xin Xue Guan Bing Za Zhi. (2009) 37(3):262–7.19781154
115. Cao Q, Zhang J, Gao L, Zhang Y, Dai M, Bao M. Dickkopf-3 upregulation mediates the cardioprotective effects of curcumin on chronic heart failure. Mol Med Rep. (2018) 17(5):7249–57.29568962
116. Morimoto T, Sunagawa Y, Kawamura T, Takaya T, Wada H, Nagasawa A, et al. The dietary compound curcumin inhibits p300 histone acetyltransferase activity and prevents heart failure in rats. J Clin Invest. (2008) 118(3):868–78.18292809
117. Liu R, Zhang H, Yang J, Wang J, Liu J, Li C. Curcumin alleviates isoproterenol-induced cardiac hypertrophy and fibrosis through inhibition of autophagy and activation of mTOR. Eur Rev Med Pharmacol Sci. (2018) 22(21):7500–8.30468499
118. Chowdhury R, Nimmanapalli R, Graham T, Reddy G. Curcumin attenuation of lipopolysaccharide induced cardiac hypertrophy in rodents. Int Sch Res Notices. (2013) 2013.
119. Bai X-J, Hao J-T, Wang J, Zhang W-F, Yan C-P, Zhao J-H, et al. Curcumin inhibits cardiac hypertrophy and improves cardiovascular function via enhanced Na+/Ca2+ exchanger expression after transverse abdominal aortic constriction in rats. Pharmacol Rep. (2018) 70(1):60–8. doi: 10.1016/j.pharep.2017.07.014
120. Chen W-J, Cheng Y, Li W, Dong X-K, Wei J-L, Yang C-H, et al. Quercetin attenuates cardiac hypertrophy by inhibiting mitochondrial dysfunction through SIRT3/PARP-1 pathway. Front Pharmacol. (2021) 9: 3043.
121. de Lacerda Alexandre JV, Viana YIP, David CEB, Cunha PLO, Albuquerque AC, Varela ALN, et al. Quercetin treatment increases H2O2 removal by restoration of endogenous antioxidant activity and blocks isoproterenol-induced cardiac hypertrophy. Naunyn Schmiedebergs Arch Pharmacol. (2021) 394(2):217–26. doi: 10.1007/s00210-020-01953-8
122. Chen K, Rekep M, Wei W, Wu Q, Xue Q, Li S, et al. Quercetin prevents in vivo and in vitro myocardial hypertrophy through the proteasome-GSK-3 pathway. Cardiovasc Drugs Ther. (2018) 32(1):5–21. doi: 10.1007/s10557-018-6771-4
123. Wang L, Tan A, An X, Xia Y, Xie Y. Quercetin dihydrate inhibition of cardiac fibrosis induced by angiotensin II in vivo and in vitro. Biomed Pharmacother. (2020) 127:110205. doi: 10.1016/j.biopha.2020.110205
124. Han J-J, Hao J, Kim C-H, Hong JS, Ahn H-Y, Lee Y-S. Quercetin prevents cardiac hypertrophy induced by pressure overload in rats. J Vet Med Sci. (2009) 71(6):737–43. doi: 10.1292/jvms.71.737
125. Jalili T, Carlstrom J, Kim S, Freeman D, Jin H, Wu T-C, et al. Quercetin-supplemented diets lower blood pressure and attenuate cardiac hypertrophy in rats with aortic constriction. J Cardiovasc Pharmacol. (2006) 47(4):531–41. doi: 10.1097/01.fjc.0000211746.78454.50
126. Yan X, Zhang YL, Zhang L, Zou LX, Chen C, Liu Y, et al. Gallic acid suppresses cardiac hypertrophic remodeling and heart failure. Mol Nutr Food Res. (2019) 63(5):1800807. doi: 10.1002/mnfr.201800807
127. Jin L, Sun S, Ryu Y, Piao ZH, Liu B, Choi SY, et al. Gallic acid improves cardiac dysfunction and fibrosis in pressure overload-induced heart failure. Sci Rep. (2018) 8(1):1–11.29311619
128. Jin L, Piao ZH, Sun S, Liu B, Ryu Y, Choi SY, et al. Gallic acid attenuates pulmonary fibrosis in a mouse model of transverse aortic contraction-induced heart failure. Vasc Pharmacol. (2017) 99:74–82. doi: 10.1016/j.vph.2017.10.007
129. Ryu Y, Jin L, Kee HJ, Piao ZH, Cho JY, Kim GR, et al. Gallic acid prevents isoproterenol-induced cardiac hypertrophy and fibrosis through regulation of JNK2 signaling and Smad3 binding activity. Sci Rep. (2016) 6(1):1–14. doi: 10.1038/s41598-016-0001-8
130. Matori H, Umar S, Nadadur RD, Sharma S, Partow-Navid R, Afkhami M, et al. Genistein, a soy phytoestrogen, reverses severe pulmonary hypertension and prevents right heart failure in rats. Hypertension. (2012) 60(2):425–30. doi: 10.1161/HYPERTENSIONAHA.112.191445
131. Meng Y, Zhang Y, Ma Z, Zhou H, Ni J, Liao H, et al. Genistein attenuates pathological cardiac hypertrophy in vivo and in vitro. Herz. (2019) 44(3):247–56. doi: 10.1007/s00059-017-4635-5
132. Maulik SK, Prabhakar P, Dinda AK, Seth S. Genistein prevents isoproterenol-induced cardiac hypertrophy in rats. Can J Physiol Pharmacol. (2012) 90(8):1117–25. doi: 10.1139/y2012-068
133. Gan M, Zheng T, Shen L, Tan Y, Fan Y, Shuai S, et al. Genistein reverses isoproterenol-induced cardiac hypertrophy by regulating miR-451/TIMP2. Biomed Pharmacother. (2019) 112:108618. doi: 10.1016/j.biopha.2019.108618
134. Lacerda D, Türck P, Campos-Carraro C, Hickmann A, Ortiz V, Bianchi S, et al. Pterostilbene improves cardiac function in a rat model of right heart failure through modulation of calcium handling proteins and oxidative stress. Appl Physiol Nutr Metab. (2020) 45(9):987–95.
135. Zhang L, Guo Z, Wang Y, Geng J, Han S. The protective effect of kaempferol on heart via the regulation of Nrf2, NF-κβ, and PI3K/Akt/GSK-3β signaling pathways in isoproterenol-induced heart failure in diabetic rats. Drug Dev Res. (2019) 80(3):294–309. doi: 10.1002/ddr.21495
136. Hu W, Xu T, Wu P, Pan D, Chen J, Chen J, et al. Luteolin improves cardiac dysfunction in heart failure rats by regulating sarcoplasmic reticulum Ca2+-ATPase 2a. Sci Rep. (2017) 7(1):1–16. doi: 10.1038/s41598-016-0028-x
137. Gao H-L, Yu X-J, Hu H-B, Yang Q-W, Liu K-L, Chen Y-M, et al. Apigenin improves hypertension and cardiac hypertrophy through modulating NADPH oxidase-dependent ROS generation and cytokines in hypothalamic paraventricular nucleus. Cardiovasc Toxicol. (2021) 21(9):721–36. doi: 10.1007/s12012-021-09662-1
138. Zhu Z-Y, Gao T, Huang Y, Xue J, Xie M-L. Apigenin ameliorates hypertension-induced cardiac hypertrophy and down-regulates cardiac hypoxia inducible factor-lα in rats. Food Funct. (2016) 7(4):1992–8. doi: 10.1039/C5FO01464F
139. Muhammed I, Sankar S, Govindaraj S. Ameliorative effect of epigallocatechin gallate on cardiac hypertrophy and fibrosis in aged rats. J Cardiovasc Pharmacol. (2018) 71(2):65–75. doi: 10.1097/FJC.0000000000000545
140. Sheng R, Gu Z-L, Xie M-L. Epigallocatechin gallate, the major component of polyphenols in green tea, inhibits telomere attrition mediated cardiomyocyte apoptosis in cardiac hypertrophy. Int J Cardiol. (2013) 162(3):199–209. doi: 10.1016/j.ijcard.2011.07.083
141. Feng H, Cao J, Zhang G, Wang Y. Kaempferol attenuates cardiac hypertrophy via regulation of ASK1/MAPK signaling pathway and oxidative stress. Planta Med. (2017) 83(10):837–45. doi: 10.1055/s-0043-103415
142. Chen Y, Ge Z, Huang S, Zhou L, Zhai C, Chen Y, et al. Delphinidin attenuates pathological cardiac hypertrophy via the AMPK/NOX/MAPK signaling pathway. Aging (Albany, NY). (2020) 12(6):5362. doi: 10.18632/aging.102956
143. Ren J, Zhang N, Liao H, Chen S, Xu L, Li J, et al. Caffeic acid phenethyl ester attenuates pathological cardiac hypertrophy by regulation of MEK/ERK signaling pathway in vivo and vitro. Life Sci. (2017) 181:53–61. doi: 10.1016/j.lfs.2017.04.016
144. Bhargava P, Verma VK, Malik S, Khan SI, Bhatia J, Arya DS. Hesperidin regresses cardiac hypertrophy by virtue of PPAR-γ agonistic, anti-inflammatory, antiapoptotic, and antioxidant properties. J Biochem Mol Toxicol. (2019) 33(5):e22283. doi: 10.1002/jbt.22283
145. Mihaylova MM, Shaw RJ. The AMPK signalling pathway coordinates cell growth, autophagy and metabolism. Nat Cell Biol. (2011) 13(9):1016–23. doi: 10.1038/ncb2329
146. Steinberg GR, Hardie DG. New insights into activation and function of the AMPK. Nat Rev Mol Cell Biol. (2022):1–18.
147. Beauloye C, Bertrand L, Horman S, Hue L. AMPK activation, a preventive therapeutic target in the transition from cardiac injury to heart failure. Cardiovasc Res. (2011) 90(2):224–33. doi: 10.1093/cvr/cvr034
148. Alammari AH, Shoieb SM, Maayah ZH, El-Kadi AO. Fluconazole represses cytochrome P450 1B1 and its associated arachidonic acid metabolites in the heart and protects against angiotensin II-induced cardiac hypertrophy. J Pharm Sci. (2020) 109(7):2321–35. doi: 10.1016/j.xphs.2020.03.016
149. El-Sherbeni AA, El-Kadi AO. Alterations in cytochrome P450-derived arachidonic acid metabolism during pressure overload-induced cardiac hypertrophy. Biochem Pharmacol. (2014) 87(3):456–66. doi: 10.1016/j.bcp.2013.11.015
150. Escher F, Vetter R, Kühl U, Westermann D, Schultheiss H, Tschِpe C. Fractalkine in human inflammatory cardiomyopathy. Heart. (2011) 97(9):733–9. doi: 10.1136/hrt.2010.205716
151. Ruchaya P, Antunes VR, Paton J, Murphy D, Yao S. The cardiovascular actions of fractalkine/CX3CL1 in the hypothalamic paraventricular nucleus are attenuated in rats with heart failure. Exp Physiol. (2014) 99(1):111–22. doi: 10.1113/expphysiol.2013.075432
152. Fan S, Hu Y, You Y, Xue W, Chai R, Zhang X, et al. Role of resveratrol in inhibiting pathological cardiac remodeling. Front Pharmacol. (2022) 73: 3452.
153. Xuan W, Liao Y, Chen B, Huang Q, Xu D, Liu Y, et al. Detrimental effect of fractalkine on myocardial ischaemia and heart failure. Cardiovasc Res. (2011) 92(3):385–93. doi: 10.1093/cvr/cvr221
154. Rodriguez F, Seta F. The role of sirtuin-1 in cardiovascular and renal pathophysiology. Front Physiol. (2021) 590: 1830.
155. Singh V, Ubaid S. Role of silent information regulator 1 (SIRT1) in regulating oxidative stress and inflammation. Inflammation. (2020) 43(5):1589–98. doi: 10.1007/s10753-020-01242-9
156. Hsu C-P, Zhai P, Yamamoto T, Maejima Y, Matsushima S, Hariharan N, et al. Silent information regulator 1 protects the heart from ischemia/reperfusion. Circulation. (2010) 122(21):2170–82. doi: 10.1161/CIRCULATIONAHA.110.958033
157. Chen Y, He T, Zhang Z, Zhang J. Activation of SIRT1 by resveratrol alleviates pressure overload-induced cardiac hypertrophy via suppression of TGF-β1 signaling. Pharmacology. (2021) 106(9–10):1–15. doi: 10.1159/000516631
158. Qin W, Chung AC, Huang XR, Meng X-M, Hui DS, Yu C-M, et al. TGF-β/Smad3 signaling promotes renal fibrosis by inhibiting miR-29. J Am Soc Nephrol. (2011) 22(8):1462–74. doi: 10.1681/ASN.2010121308
159. Zhang J, Wang Q-Z, Zhao S-H, Ji X, Qiu J, Wang J, et al. Astaxanthin attenuated pressure overload-induced cardiac dysfunction and myocardial fibrosis: partially by activating SIRT1. Biochim Biophys Acta Gen Subj. (2017) 1861(7):1715–28. doi: 10.1016/j.bbagen.2017.03.007
160. Li N, Zhou H, Ma Z-G, Zhu J-X, Liu C, Song P, et al. Geniposide alleviates isoproterenol-induced cardiac fibrosis partially via SIRT1 activation in vivo and in vitro. Front Pharmacol. (2018) 9:854. doi: 10.3389/fphar.2018.00854
161. Ma S, Feng J, Zhang R, Chen J, Han D, Li X, et al. SIRT1 activation by resveratrol alleviates cardiac dysfunction via mitochondrial regulation in diabetic cardiomyopathy mice. Oxid Med Cell Longevity. (2017) 2017.
162. Cappetta D, Esposito G, Piegari E, Russo R, Ciuffreda LP, Rivellino A, et al. SIRT1 activation attenuates diastolic dysfunction by reducing cardiac fibrosis in a model of anthracycline cardiomyopathy. Int J Cardiol. (2016) 205:99–110. doi: 10.1016/j.ijcard.2015.12.008
163. Liu Z-H, Zhang Y, Wang X, Fan X-F, Zhang Y, Li X, et al. SIRT1 activation attenuates cardiac fibrosis by endothelial-to-mesenchymal transition. Biomed Pharmacother. (2019) 118:109227. doi: 10.1016/j.biopha.2019.109227
164. Fan W, Zhang B, Wu C, Wu H, Wu J, Wu S, et al. Plantago asiatica L. Seeds extract protects against cardiomyocyte injury in isoproterenol-induced cardiac hypertrophy by inhibiting excessive autophagy and apoptosis in mice. Phytomedicine. (2021) 91:153681. doi: 10.1016/j.phymed.2021.153681
165. Zaglia T, Milan G, Ruhs A, Franzoso M, Bertaggia E, Pianca N, et al. Atrogin-1 deficiency promotes cardiomyopathy and premature death via impaired autophagy. J Clin Invest. (2014) 124(6):2410–24. doi: 10.1172/JCI66339
166. Xie X, Bi H-L, Lai S, Zhang Y-L, Li N, Cao H-J, et al. The immunoproteasome catalytic β5i subunit regulates cardiac hypertrophy by targeting the autophagy protein ATG5 for degradation. Sci Adv. (2019) 5(5):eaau0495.31086810
167. Yan W, Bi H-L, Liu L-X, Li N-N, Liu Y, Du J, et al. Knockout of immunoproteasome subunit β2i ameliorates cardiac fibrosis and inflammation in DOCA/salt hypertensive mice. Biochem Biophys Res Commun. (2017) 490(2):84–90. doi: 10.1016/j.bbrc.2017.05.011
168. Alexander A, Walker CL. The role of LKB1 and AMPK in cellular responses to stress and damage. FEBS Lett. (2011) 585(7):952–7. doi: 10.1016/j.febslet.2011.03.010
169. Calamaras TD, Lee C, Lan F, Ido Y, Siwik DA, Colucci WS. Post-translational modification of serine/threonine kinase LKB1 via adduction of the reactive lipid species 4-hydroxy-trans-2-nonenal (HNE) at lysine residue 97 directly inhibits kinase activity. J Biol Chem. (2012) 287(50):42400–6. doi: 10.1074/jbc.M112.385831
170. Rodríguez C, Muñoz M, Contreras C, Prieto D. AMPK, metabolism, and vascular function. FEBS J. (2021) 288(12):3746–71. doi: 10.1111/febs.15863
171. Espino J, Pariente JA, Rodríguez AB. Role of melatonin on diabetes-related metabolic disorders. World J Diabetes. (2011) 2(6):82. doi: 10.4239/wjd.v2.i6.82
172. Hulsmans M, Sager HB, Roh JD, Valero-Muñoz M, Houstis NE, Iwamoto Y, et al. Cardiac macrophages promote diastolic dysfunction. J Exp Med. (2018) 215(2):423–40. doi: 10.1084/jem.20171274
173. Koushki K, Shahbaz SK, Mashayekhi K, Sadeghi M, Zayeri ZD, Taba MY, et al. Anti-inflammatory action of statins in cardiovascular disease: the role of inflammasome and toll-like receptor pathways. Clin Rev Allergy Immunol. (2021) 60:175–99. doi: 10.1007/s12016-020-08791-9
174. Tulbah AS. The potential of atorvastatin for chronic lung diseases therapy. Saudi Pharm J. (2020) 28(11):1353–63. doi: 10.1016/j.jsps.2020.08.025
175. Margaritis M, Sanna F, Antoniades C. Statins and oxidative stress in the cardiovascular system. Curr Pharm Des. (2017) 23(46):7040–7. doi: 10.2174/1381612823666170926130338
176. Tham YK, Bernardo BC, Ooi JY, Weeks KL, McMullen JR. Pathophysiology of cardiac hypertrophy and heart failure: signaling pathways and novel therapeutic targets. Arch Toxicol. (2015) 89:1401–38. doi: 10.1007/s00204-015-1477-x
177. Saheera S, Krishnamurthy P. Cardiovascular changes associated with hypertensive heart disease and aging. Cell Transplant. (2020) 29:0963689720920830. doi: 10.1177/0963689720920830
178. Haque ZK, Wang D-Z. How cardiomyocytes sense pathophysiological stresses for cardiac remodeling. Cell Mol Life Sci. (2017) 74:983–1000. doi: 10.1007/s00018-016-2373-0
179. Skoumal R, Tóth M, Serpi R, Rysä J, Leskinen H, Ulvila J, et al. Parthenolide inhibits STAT3 signaling and attenuates angiotensin II-induced left ventricular hypertrophy via modulation of fibroblast activity. J Mol Cell Cardiol. (2011) 50(4):634–41. doi: 10.1016/j.yjmcc.2011.01.001
180. Stansfield WE, Ranek M, Pendse A, Schisler JC, Wang S, Pulinilkunnil T, et al. The pathophysiology of cardiac hypertrophy and heart failure. In: Cellular and molecular pathobiology of cardiovascular disease. Amesterdam, Netherlands: Elsevier (2014). p. 51–78.
181. Samak M, Fatullayev J, Sabashnikov A, Zeriouh M, Schmack B, Farag M, et al. Cardiac hypertrophy: an introduction to molecular and cellular basis. Med Sci Monit Basic Res. (2016) 22:75. doi: 10.12659/MSMBR.900437
182. Nakamura M, Sadoshima J. Mechanisms of physiological and pathological cardiac hypertrophy. Nat Rev Cardiol. (2018) 15(7):387–407. doi: 10.1038/s41569-018-0007-y
183. Shukla PC, Singh KK, Quan A, Al-Omran M, Teoh H, Lovren F, et al. BRCA1 is an essential regulator of heart function and survival following myocardial infarction. Nat Commun. (2011) 2(1):1–11. doi: 10.1038/ncomms1601
184. Paz Ocaranza M, Riquelme JA, Garcيa L, Jalil JE, Chiong M, Santos RA, et al. Counter-regulatory reninangiotensin system in cardiovascular disease. Nat Rev Cardiol. (2020) 17(2):116–29. doi: 10.1038/s41569-019-0244-8
185. Del Buono MG, Arena R, Borlaug BA, Carbone S, Canada JM, Kirkman DL, et al. Exercise intolerance in patients with heart failure: JACC state-of-the-art review. J Am Coll Cardiol. (2019) 73(17):2209–25. doi: 10.1016/j.jacc.2019.01.072
186. Tucker WJ, Haykowsky MJ, Seo Y, Stehling E, Forman DE. Impaired exercise tolerance in heart failure: role of skeletal muscle morphology and function. Curr Heart Fail Rep. (2018) 15:323–31. doi: 10.1007/s11897-018-0408-6
187. Dolinsky VW, Jones KE, Sidhu RS, Haykowsky M, Czubryt MP, Gordon T, et al. Improvements in skeletal muscle strength and cardiac function induced by resveratrol during exercise training contribute to enhanced exercise performance in rats. J Physiol (Lond). (2012) 590(11):2783–99. doi: 10.1113/jphysiol.2012.230490
188. Toniolo L, Fusco P, Formoso L, Mazzi A, Canato M, Reggiani C, et al. Resveratrol treatment reduces the appearance of tubular aggregates and improves the resistance to fatigue in aging mice skeletal muscles. Exp Gerontol. (2018) 111:170–9. doi: 10.1016/j.exger.2018.07.012
189. Hewlings SJ, Kalman DS. Curcumin: a review of its effects on human health. Foods. (2017) 6(10):92. doi: 10.3390/foods6100092
190. Mirzabeigi P, Mohammadpour AH, Salarifar M, Gholami K, Mojtahedzadeh M, Javadi MR. The effect of curcumin on some of traditional and non-traditional cardiovascular risk factors: a pilot randomized, double-blind, placebo-controlled trial. Iran J Pharm Res. (2015) 14(2):479.25901155
191. Patel SS, Acharya A, Ray R, Agrawal R, Raghuwanshi R, Jain P. Cellular and molecular mechanisms of curcumin in prevention and treatment of disease. Crit Rev Food Sci Nutr. (2020) 60(6):887–939. doi: 10.1080/10408398.2018.1552244
192. Gupta SC, Patchva S, Aggarwal BB. Therapeutic roles of curcumin: lessons learned from clinical trials. AAPS J. (2013) 15(1):195–218. doi: 10.1208/s12248-012-9432-8
193. Wang NP, Wang ZF, Tootle S, Philip T, Zhao ZQ. Curcumin promotes cardiac repair and ameliorates cardiac dysfunction following myocardial infarction. Br J Pharmacol. (2012) 167(7):1550–62. doi: 10.1111/j.1476-5381.2012.02109.x
194. Yu W, Wu J, Cai F, Xiang J, Zha W, Fan D, et al. Curcumin alleviates diabetic cardiomyopathy in experimental diabetic rats. PLoS One. (2012) 7(12):e52013. doi: 10.1371/journal.pone.0052013
195. Zhou P, He A, Pu WT. Regulation of GATA4 transcriptional activity in cardiovascular development and disease. Curr Top Dev Biol. (2012) 100:143–69. doi: 10.1016/B978-0-12-387786-4.00005-1
196. Han P, Hang CT, Yang J, Chang C-P. Chromatin remodeling in cardiovascular development and physiology. Circ Res. (2011) 108(3):378–96. doi: 10.1161/CIRCRESAHA.110.224287
197. Whitcomb J, Gharibeh L, Nemer M. From embryogenesis to adulthood: critical role for GATA factors in heart development and function. Iubmb Life. (2020) 72(1):53–67. doi: 10.1002/iub.2163
198. Kuwahara K, Nishikimi T, Nakao K. Transcriptional regulation of the fetal cardiac gene program. J Pharmacol Sci. (2012) 119(3):198–203. doi: 10.1254/jphs.12R04CP
199. Yoshida Y, Morimoto T, Takaya T, Kawamura T, Sunagawa Y, Wada H, et al. Aldosterone signaling associates with p300/GATA4 transcriptional pathway during the hypertrophic response of cardiomyocytes. Circ J. (2010) 74(1):156–62. doi: 10.1253/circj.CJ-09-0050
200. Ochiai K, Watanabe M, Ueki H, Huang P, Fujii Y, Nasu Y, et al. Tumor suppressor REIC/dkk-3 interacts with the dynein light chain, tctex-1. Biochem Biophys Res Commun. (2011) 412(2):391–5. doi: 10.1016/j.bbrc.2011.07.109
201. Yu B, Kiechl S, Qi D, Wang X, Song Y, Weger S, et al. A cytokine-like protein dickkopf-related protein 3 is atheroprotective. Circulation. (2017) 136(11):1022–36. doi: 10.1161/CIRCULATIONAHA.117.027690
202. Piek A, Smit L, Suthahar N, Bakker SJ, de Boer RA, Silljé HH. The emerging plasma biomarker dickkopf-3 (DKK3) and its association with renal and cardiovascular disease in the general population. Sci Rep. (2021) 11(1):1–8. doi: 10.1038/s41598-021-88107-9
203. Zhang Y, Liu Y, Zhu X-H, Zhang X-D, Jiang D-S, Bian Z-Y, et al. Dickkopf-3 attenuates pressure overload-induced cardiac remodelling. Cardiovasc Res. (2014) 102(1):35–45. doi: 10.1093/cvr/cvu004
204. Bao M-W, Cai Z, Zhang X-J, Li L, Liu X, Wan N, et al. Dickkopf-3 protects against cardiac dysfunction and ventricular remodelling following myocardial infarction. Basic Res Cardiol. (2015) 110(3):1–17.25589055
205. Romero-Becerra R, Santamans AM, Folgueira C, Sabio G. P38 MAPK pathway in the heart: new insights in health and disease. Int J Mol Sci. (2020) 21(19):7412. doi: 10.3390/ijms21197412
206. Shvedova M, Anfinogenova Y, Atochina-Vasserman EN, Schepetkin IA, Atochin DN. c-Jun N-terminal kinases (JNKs) in myocardial and cerebral ischemia/reperfusion injury. Front Pharmacol. (2018) 9:715. doi: 10.3389/fphar.2018.00715
207. Fan G, Yu J, Asare PF, Wang L, Zhang H, Zhang B, et al. Danshensu alleviates cardiac ischaemia/reperfusion injury by inhibiting autophagy and apoptosis via activation of mTOR signalling. J Cell Mol Med. (2016) 20(10):1908–19. doi: 10.1111/jcmm.12883
208. Panche AN, Diwan AD, Chandra SR. Flavonoids: an overview. J Nutr Sci. (2016) 5. doi: 10.1017/jns.2016.41
209. Karuppagounder V, Arumugam S, Thandavarayan RA, Sreedhar R, Giridharan VV, Watanabe K. Molecular targets of quercetin with anti-inflammatory properties in atopic dermatitis. Drug Discov Today. (2016) 21(4):632–9. doi: 10.1016/j.drudis.2016.02.011
210. Reyes-Farias M, Carrasco-Pozo C. The anti-cancer effect of quercetin: molecular implications in cancer metabolism. Int J Mol Sci. (2019) 20(13):3177. doi: 10.3390/ijms20133177
211. Xu D, Hu M-J, Wang Y-Q, Cui Y-L. Antioxidant activities of quercetin and its complexes for medicinal application. Molecules. (2019) 24(6):1123. doi: 10.3390/molecules24061123
212. Perez-Vizcaino F, Duarte J, Jimenez R, Santos-Buelga C, Osuna A. Antihypertensive effects of the flavonoid quercetin. Pharmacol Rep. (2009) 61(1):67–75. doi: 10.1016/S1734-1140(09)70008-8
213. Roslan J, Giribabu N, Karim K, Salleh N. Quercetin ameliorates oxidative stress, inflammation and apoptosis in the heart of streptozotocin-nicotinamide-induced adult male diabetic rats. Biomed Pharmacother. (2017) 86:570–82. doi: 10.1016/j.biopha.2016.12.044
214. Liu X, Yu Z, Huang X, Gao Y, Wang X, Gu J, et al. Peroxisome proliferator-activated receptor γ (PPARγ) mediates the protective effect of quercetin against myocardial ischemia-reperfusion injury via suppressing the NF-κB pathway. Am J Transl Res. (2016) 8(12):5169.28077993
215. Chang X, Zhang T, Meng Q, Yan P, Wang X, Luo D, et al. Quercetin improves cardiomyocyte vulnerability to hypoxia by regulating SIRT1/TMBIM6-related mitophagy and endoplasmic reticulum stress. Oxid Med Cell Longevity. (2021) 2021.
216. Chang X, Zhang T, Wang J, Liu Y, Yan P, Meng Q, et al. SIRT5-related Desuccinylation modification contributes to quercetin-induced protection against heart failure and high-glucose-prompted cardiomyocytes injured through regulation of mitochondrial quality surveillance. Oxid Med Cell Longevity. (2021) 2021.
217. Chen K, Rekep M, Wei W, Wu Q, Xue Q, Li S, et al. Quercetin prevents in vivo and in vitro myocardial hypertrophy through the proteasome-GSK-3 pathway. Cardiovasc Drugs Ther. (2018) 32(1):5–21. doi: 10.1007/s10557-018-6771-4
218. Wang L, Tan A, An X, Xia Y, Xie Y. Quercetin dihydrate inhibition of cardiac fibrosis induced by angiotensin II in vivo and in vitro. Biomed Pharmacother. (2020) 127:110205. doi: 10.1016/j.biopha.2020.110205
219. Han J-J, Hao J, Kim C-H, Hong J-S, Ahn H-Y, Lee Y-S. Quercetin prevents cardiac hypertrophy induced by pressure overload in rats. J Vet Med Sci. (2009) 71(6):737–43. doi: 10.1292/jvms.71.737
220. Feng X, Wang Y, Chen W, Xu S, Li L, Geng Y, et al. SIRT3 Inhibits cardiac hypertrophy by regulating PARP-1 activity. Aging (Albany NY). (2020) 12(5):4178.32139662
221. Jalili T, Carlstrom J, Kim S, Freeman D, Jin H, Wu T-C, et al. Quercetin-supplemented diets lower blood pressure and attenuate cardiac hypertrophy in rats with aortic constriction. J Cardiovasc Pharmacol. (2006) 47(4):531–41. doi: 10.1097/01.fjc.0000211746.78454.50
222. Akbari G. Molecular mechanisms underlying gallic acid effects against cardiovascular diseases: an update review. Avicenna J Phytomed. (2020) 10(1):11.31921604
223. Choubey S, Varughese LR, Kumar V, Beniwal V. Medicinal importance of gallic acid and its ester derivatives: a patent review. Pharm Pat Anal. (2015) 4(4):305–15. doi: 10.4155/ppa.15.14
224. Jin L, Lin MQ, Piao ZH, Cho JY, Kim GR, Choi SY, et al. Gallic acid attenuates hypertension, cardiac remodeling, and fibrosis in mice with NG-nitro-L-arginine methyl ester-induced hypertension via regulation of histone deacetylase 1 or histone deacetylase 2. J Hypertens. (2017) 35(7):1502–12. doi: 10.1097/HJH.0000000000001327
225. Shackebaei D, Hesari M, Ramezani-Aliakbari S, Hoseinkhani Z, Ramezani-Aliakbari F. Gallic acid protects against isoproterenol-induced cardiotoxicity in rats. Hum Exp Toxicol. (2022) 41:09603271211064532. doi: 10.1177/09603271211064532
226. Uray KS, Peng Z, Cattano D, Eltzschig HK, Doursout M-F. Development of pulmonary fibrosis after heart failure induced by elevated left atrial pressure. Am J Transl Res. (2020) 12(8):4639.32913537
227. Rout-Pitt N, Farrow N, Parsons D, Donnelley M. Epithelial mesenchymal transition (EMT): a universal process in lung diseases with implications for cystic fibrosis pathophysiology. Respir Res. (2018) 19(1):1–10. doi: 10.1186/s12931-018-0834-8
228. Aryan L, Younessi D, Zargari M, Banerjee S, Agopian J, Rahman S, et al. The role of estrogen receptors in cardiovascular disease. Int J Mol Sci. (2020) 21(12):4314. doi: 10.3390/ijms21124314
229. Umar S, Iorga A, Matori H, Nadadur RD, Li J, Maltese F, et al. Estrogen rescues preexisting severe pulmonary hypertension in rats. Am J Respir Crit Care Med. (2011) 184(6):715–23. doi: 10.1164/rccm.201101-0078OC
230. Kim I-S. Current perspectives on the beneficial effects of soybean isoflavones and their metabolites for humans. Antioxidants. (2021) 10(7):1064. doi: 10.3390/antiox10071064
231. Mazumder MAR, Hongsprabhas P. Genistein as antioxidant and antibrowning agents in in vivo and in vitro: a review. Biomed Pharmacother. (2016) 82:379–92. doi: 10.1016/j.biopha.2016.05.023
232. Nazari-Khanamiri F, Ghasemnejad-Berenji M. Cellular and molecular mechanisms of genistein in prevention and treatment of diseases: an overview. J Food Biochem. (2021) 45(11):e13972. doi: 10.1111/jfbc.13972
233. Amerizadeh A, Asgary S, Vaseghi G, Farajzadegan Z. Effect of genistein intake on some cardiovascular risk factors: an updated systematic review and meta-analysis. Curr Probl Cardiol. (2021) 100902.
234. Loyer X, Heymes C, Samuel JL. Constitutive nitric oxide synthases in the heart from hypertrophy to failure. Clin Exp Pharmacol Physiol. (2008) 35(4):483–8. doi: 10.1111/j.1440-1681.2008.04901.x
235. Gan M, Yang D, Fan Y, Du J, Shen L, Li Q, et al. Bidirectional regulation of genistein on the proliferation and differentiation of C2C12 myoblasts. Xenobiotica. (2020) 50(11):1352–8. doi: 10.1080/00498254.2017.1409917
236. Guo Y, Zhang L, Li F, Hu C-P, Zhang Z. Restoration of sirt1 function by pterostilbene attenuates hypoxia-reoxygenation injury in cardiomyocytes. Eur J Pharmacol. (2016) 776:26–33. doi: 10.1016/j.ejphar.2016.02.052
237. Li Q, Li X, Tian B, Chen L. Protective effect of pterostilbene in a streptozotocin-induced mouse model of Alzheimer’s disease by targeting monoamine oxidase B. J Appl Toxicol. (2022) 42(11):1777–86. doi: 10.1002/jat.4355
238. Liu K-F, Li C-X, Dai L, Liu J, Wang L-Y, Guo L-Q. Design, synthesis and in vivo antitumor efficacy of novel eight-arm-polyethylene glycolpterostilbene prodrugs. RSC Adv. (2015) 5(64):51592–9. doi: 10.1039/C5RA06253E
239. Kosuru R, Cai Y, Kandula V, Yan D, Wang C, Zheng H, et al. AMPK contributes to cardioprotective effects of pterostilbene against myocardial ischemia-reperfusion injury in diabetic rats by suppressing cardiac oxidative stress and apoptosis. Cell Physiol Biochem. (2018) 46(4):1381–97. doi: 10.1159/000489154
240. Liu D, Ma Z, Xu L, Zhang X, Qiao S, Yuan J. PGC1α activation by pterostilbene ameliorates acute doxorubicin cardiotoxicity by reducing oxidative stress via enhancing AMPK and SIRT1 cascades. Aging (Albany NY). (2019) 11(22):10061.31733141
241. Angelova PR, Esteras N, Abramov AY. Mitochondria and lipid peroxidation in the mechanism of neurodegeneration: finding ways for prevention. Med Res Rev. (2021) 41(2):770–84. doi: 10.1002/med.21712
242. Shaikh SA, Sahoo SK, Periasamy M. Phospholamban and sarcolipin: are they functionally redundant or distinct regulators of the sarco (endo) plasmic Reticulum calcium ATPase? J Mol Cell Cardiol. (2016) 91:81–91. doi: 10.1016/j.yjmcc.2015.12.030
243. Lipskaia L, Chemaly ER, Hadri L, Lompre A-M, Hajjar RJ. Sarcoplasmic reticulum Ca2+ ATPase as a therapeutic target for heart failure. Expert Opin Biol Ther. (2010) 10(1):29–41. doi: 10.1517/14712590903321462
244. Calderon-Montano JM, Burgos-Morón E, Pérez-Guerrero C, López-Lázaro M. A review on the dietary flavonoid kaempferol. Mini Rev Med Chem. (2011) 11(4):298–344. doi: 10.2174/138955711795305335
245. Shields M. Chemotherapeutics. In: Pharmacognosy. Amesterdam, Netherlands: Elsevier (2017). p. 295–313.
246. Chen M, Xiao J, El-Seedi HR, Woźniak KS, Daglia M, Little PJ, et al. Kaempferol and atherosclerosis: from mechanism to medicine. Crit Rev Food Sci Nutr. (2022) 9:1–19.
247. Zhou M, Ren H, Han J, Wang W, Zheng Q, Wang D. Protective effects of kaempferol against myocardial ischemia/reperfusion injury in isolated rat heart via antioxidant activity and inhibition of glycogen synthase kinase-3. Oxid Med Cell Longevity. (2015) 2015.
248. Low Wang CC, Hess CN, Hiatt WR, Goldfine AB. Clinical update: cardiovascular disease in diabetes mellitus: atherosclerotic cardiovascular disease and heart failure in type 2 diabetes mellitusmechanisms, management, and clinical considerations. Circulation. (2016) 133(24):2459–502. doi: 10.1161/CIRCULATIONAHA.116.022194
249. Fujisawa T, Ichijo H. ASK1-MAP kinase signaling pathway as a therapeutic target for human diseases. Nippon Rinsho. (2014) 72(5):957–65.24912301
250. Lu M, Wang Y, Zhan X. The MAPK pathway-based drug therapeutic targets in pituitary adenomas. Front Endocrinol (Lausanne). (2019) 10:330. doi: 10.3389/fendo.2019.00330
251. Luo Y, Shang P, Li D. Luteolin: a flavonoid that has multiple cardio-protective effects and its molecular mechanisms. Front Pharmacol. (2017) 8:692. doi: 10.3389/fphar.2017.00692
252. Kranias EG, Hajjar RJ. Modulation of cardiac contractility by the phopholamban/SERCA2a regulatome. Circ Res. (2012) 110(12):1646–60. doi: 10.1161/CIRCRESAHA.111.259754
253. Kho C, Lee A, Jeong D, Oh JG, Chaanine AH, Kizana E, et al. SUMO1-dependent modulation of SERCA2a in heart failure. Nature. (2011) 477(7366):601–605.21900893
254. Shanmugam M, Gao S, Hong C, Fefelova N, Nowycky MC, Xie L-H, et al. Ablation of phospholamban and sarcolipin results in cardiac hypertrophy and decreased cardiac contractility. Cardiovasc Res. (2011) 89(2):353–61. doi: 10.1093/cvr/cvq294
255. Cui Y, Wang Y, Liu G. Epigallocatechin gallate (EGCG) attenuates myocardial hypertrophy and fibrosis induced by transverse aortic constriction via inhibiting the Akt/mTOR pathway. Pharm Biol. (2021) 59(1):1303–11. doi: 10.1080/13880209.2021.1972124
256. Sheng R, Gu ZL, Xie ML, Zhou WX, Guo CY. EGCG inhibits cardiomyocyte apoptosis in pressure overload-induced cardiac hypertrophy and protects cardiomyocytes from oxidative stress in rats 1. Acta Pharmacol Sin. (2007) 28(2):191–201. doi: 10.1111/j.1745-7254.2007.00495.x
257. Xu Y, Li X, Wang H. Protective roles of apigenin against cardiometabolic diseases: a systematic review. Front Nutr. (2022) 9.
258. Liu M, Galli G, Wang Y, Fan Q, Wang Z, Wang X, et al. Novel therapeutic targets for hypoxia-related cardiovascular diseases: the role of HIF-1. Front Physiol. (2020) 11:774. doi: 10.3389/fphys.2020.00774
259. Magnani C, Isaac VLB, Correa MA, Salgado HRN. Caffeic acid: a review of its potential use in medications and cosmetics. Anal Methods. (2014) 6(10):3203–10. doi: 10.1039/C3AY41807C
260. Silva H, Lopes NMF. Cardiovascular effects of caffeic acid and its derivatives: a comprehensive review. Front Physiol. (2020) 11:595516. doi: 10.3389/fphys.2020.595516
261. Ince H, Kandemir E, Bagci C, Gulec M, Akyol O. The effect of caffeic acid phenethyl ester on short-term acute myocardial ischemia. Med Sci Monit. (2006) 12(5):BR187-93.16641873
262. Bonesi M, Loizzo MR, Menichini F, Tundis R. Flavonoids in treating psoriasis. In: Immunity and inflammation in health and disease. Amesterdam, Netherlands: Elsevier (2018). p. 281–94.
263. Maulik SK, Kumar S. Oxidative stress and cardiac hypertrophy: a review. Toxicol Mech Methods. (2012) 22(5):359–66. doi: 10.3109/15376516.2012.666650
264. Shah AM. Parsing the role of NADPH oxidase enzymes and reactive oxygen species in heart failure. Am Heart Assoc. (2015):602–4.
265. Wen Y, Liu R, Lin N, Luo H, Tang J, Huang Q, et al. NADPH oxidase hyperactivity contributes to cardiac dysfunction and apoptosis in rats with severe experimental pancreatitis through ROS-mediated MAPK signaling pathway. Oxid Med Cell Longevity. (2019) 2019.
266. Ekinci Akdemir FN, Gülçin İ, Karagöz B, Soslu R, Alwasel SH. A comparative study on the antioxidant effects of hesperidin and ellagic acid against skeletal muscle ischemia/reperfusion injury. J Enzyme Inhib Med Chem. (2016) 31(Suppl 4):114–8. doi: 10.1080/14756366.2016.1220378
267. Bhargava P, Arya D, Bhatia J. Cardioprotective effect of hesperidin in an experimental model of cardiac hypertrophy. J Hypertens. (2019) 37:e183–4. doi: 10.1097/01.hjh.0000572356.32275.f4
268. Raj P, Thandapilly SJ, Wigle J, Zieroth S, Netticadan T. A comprehensive analysis of the efficacy of resveratrol in atherosclerotic cardiovascular disease, myocardial infarction and heart failure. Molecules. (2021) 26(21):6600. doi: 10.3390/molecules26216600
269. Ray A, Rana S, Banerjee D, Mitra A, Datta R, Naskar S, et al. Improved bioavailability of targeted curcumin delivery efficiently regressed cardiac hypertrophy by modulating apoptotic load within cardiac microenvironment. Toxicol Appl Pharmacol. (2016) 290:54–65. doi: 10.1016/j.taap.2015.11.011
270. Sunagawa Y, Wada H, Suzuki H, Sasaki H, Imaizumi A, Fukuda H, et al. A novel drug delivery system of oral curcumin markedly improves efficacy of treatment for heart failure after myocardial infarction in rats. Biol Pharm Bull. (2012) 35(2):139–44. doi: 10.1248/bpb.35.139
271. Shimizu K, Sunagawa Y, Funamoto M, Wakabayashi H, Genpei M, Miyazaki Y, et al. The synthetic curcumin analogue GO-Y030 effectively suppresses the development of pressure overload-induced heart failure in mice. Sci Rep. (2020) 10(1):1–12. doi: 10.1038/s41598-019-56847-4
272. Kim DH, Chien F-J, Eisen HJ. Pharmacologic management for heart failure and emerging therapies. Curr Cardiol Rep. (2017) 19(10):1–6.28084596
273. Patel C, Deoghare S. Heart failure: novel therapeutic approaches. J Postgrad Med. (2015) 61(2):101. doi: 10.4103/0022-3859.153104
274. Gal R, Praksch D, Kenyeres P, Rabai M, Toth K, Halmosi R, et al. Hemorheological alterations in patients with heart failure with reduced ejection fraction treated by resveratrol. Cardiovasc Ther. (2020) 2020.32695229
275. Biro K, Sandor B, Kovacs D, Csiszar B, Vekasi J, Totsimon K, et al. Lower limb ischemia and microrheological alterations in patients with diabetic retinopathy. Clin Hemorheol Microcirc. (2018) 69(1–2):23–35. doi: 10.3233/CH-189103
276. Holsworth R Jr, Cho Y, Weidman J, Sloop G, Cyr JS. Cardiovascular benefits of phlebotomy: relationship to changes in hemorheological variables. Perfusion. (2014) 29(2):102–16. doi: 10.1177/0267659113505637
277. Tsutsui H, Kinugawa S, Matsushima S. Oxidative stress and heart failure. Am J Physiol Heart Circ Physiol. (2011) 13.
278. Dastani M, Bigdelu L, Hoseinzadeh M, Rahimi HR, Karimani A, Mohammadpour AH, et al. The effects of curcumin on the prevention of atrial and ventricular arrhythmias and heart failure in patients with unstable angina: a randomized clinical trial. Avicenna J Phytomed. (2019) 9(1):1.30788273
279. Funamoto M, Sunagawa Y, Katanasaka Y, Kato T, Funada J, Ajiro Y, et al. Effects of high-absorption curcumin for the prevention of hypertensive heart disease: a double-blind, placebo-controlled, randomized clinical study. Eur Heart J Open. (2022) 2(5):oeac057. doi: 10.1093/ehjopen/oeac057
280. Dastani M, Rahimi HR, Askari VR, Jaafari MR, Jarahi L, Yadollahi A, et al. Three months of combination therapy with nano-curcumin reduces the inflammation and lipoprotein (a) in type 2 diabetic patients with mild to moderate coronary artery disease: evidence of a randomized, double-blinded, placebo-controlled clinical trial. Biofactors. (2023) 49(1):108–18. doi: 10.1002/biof.1874
281. Yang B, Dong Y, Wang F, Zhang Y. Nanoformulations to enhance the bioavailability and physiological functions of polyphenols. Molecules. (2020) 25(20).
282. Carlson LJ, Cote B, Alani AW, Rao DA. Polymeric micellar co-delivery of resveratrol and curcumin to mitigate in vitro doxorubicin-induced cardiotoxicity. J Pharm Sci. (2014) 103(8):2315–22. doi: 10.1002/jps.24042
283. Pillai SC, Borah A, Le MNT, Kawano H, Hasegawa K, Kumar DS. Co-delivery of curcumin and bioperine via PLGA nanoparticles to prevent atherosclerotic foam cell formation. Pharmaceutics. (2021) 13(9). doi: 10.3390/pharmaceutics13091420
284. Liu Y, Liu Q, Liu Y, Ju F, Ma Q, He Q. In vivo evaluation of enhanced drug carrier efficiency and cardiac anti-hypertrophy therapeutic potential of nano-curcumin encapsulated photo-plasmonic nanoparticles combined polymerized nano-vesicles: a novel strategy. J Photochem Photobiol B. (2019) 199:111619. doi: 10.1016/j.jphotobiol.2019.111619
285. Zheng B, McClements DJ. Formulation of more efficacious curcumin delivery systems using colloid science: enhanced solubility, stability, and bioavailability. Molecules. (2020) 25(12). doi: 10.3390/molecules25122791
286. Huang M, Liang C, Tan C, Huang S, Ying R, Wang Y, et al. Liposome co-encapsulation as a strategy for the delivery of curcumin and resveratrol. Food Funct. (2019) 10(10):6447–58. doi: 10.1039/C9FO01338E
287. Vanaja K, Wahl MA, Bukarica L, Heinle H. Liposomes as carriers of the lipid soluble antioxidant resveratrol: evaluation of amelioration of oxidative stress by additional antioxidant vitamin. Life Sci. (2013) 93(24):917–23. doi: 10.1016/j.lfs.2013.10.019
288. Feng T, Wei Y, Lee RJ, Zhao L. Liposomal curcumin and its application in cancer. Int J Nanomed. (2017) 12:6027–44. doi: 10.2147/IJN.S132434
289. Tsujioka T, Sasaki D, Takeda A, Harashima H, Yamada Y. Resveratrol-encapsulated mitochondria-targeting liposome enhances mitochondrial respiratory capacity in myocardial cells. Int J Mol Sci. (2021) 23(1). doi: 10.3390/ijms23010112
290. Abe J, Yamada Y, Takeda A, Harashima H. Cardiac progenitor cells activated by mitochondrial delivery of resveratrol enhance the survival of a doxorubicin-induced cardiomyopathy mouse model via the mitochondrial activation of a damaged myocardium. J Control Release. (2018) 269:177–88. doi: 10.1016/j.jconrel.2017.11.024
Keywords: polyphenols, oxidative stress, inflammation, heart failure, targeted therapy
Citation: Hedayati N, Yaghoobi A, Salami M, Gholinezhad Y, Aghadavood F, Eshraghi R, Aarabi M-H, Homayoonfal M, Asemi Z, Mirzaei H, Hajijafari M, Mafi A and Rezaee M (2023) Impact of polyphenols on heart failure and cardiac hypertrophy: clinical effects and molecular mechanisms. Front. Cardiovasc. Med. 10:1174816. doi: 10.3389/fcvm.2023.1174816
Received: 27 February 2023; Accepted: 2 May 2023;
Published: 24 May 2023.
Edited by:
Speranza Rubattu, Sapienza University of Rome, ItalyReviewed by:
Albino Carrizzo, University of Salerno, Italy© 2023 Hedayati, Yaghoobi, Salami, Gholinezhad, Aghadavood, Eshraghi, Aarabi, Homayoonfal, Asemi, Mirzaei, Hajijafari, Mafi and Rezaee. This is an open-access article distributed under the terms of the Creative Commons Attribution License (CC BY). The use, distribution or reproduction in other forums is permitted, provided the original author(s) and the copyright owner(s) are credited and that the original publication in this journal is cited, in accordance with accepted academic practice. No use, distribution or reproduction is permitted which does not comply with these terms.
*Correspondence: Hamed Mirzaei aC5taXJ6YWVpMjAwMkBnbWFpbC5jb20= Mohammad Hajijafari ZHJfaGFqaWphZmFyaUB5YWhvby5jb20= Alireza Mafi YXJtYWZpLm1AZ21haWwuY29t Malihe Rezaee bWFsaWhlcmV6YWVlODU1QGdtYWlsLmNvbQ==
†These authors have contributed equally to this work and share first authorship
‡ORCID Alireza Mafi orcid.org/0000-0002-9485-0326
Disclaimer: All claims expressed in this article are solely those of the authors and do not necessarily represent those of their affiliated organizations, or those of the publisher, the editors and the reviewers. Any product that may be evaluated in this article or claim that may be made by its manufacturer is not guaranteed or endorsed by the publisher.
Research integrity at Frontiers
Learn more about the work of our research integrity team to safeguard the quality of each article we publish.