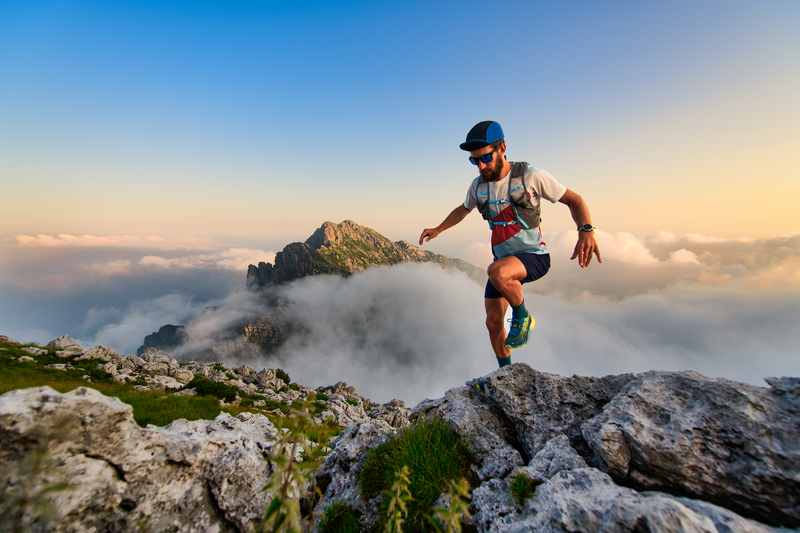
94% of researchers rate our articles as excellent or good
Learn more about the work of our research integrity team to safeguard the quality of each article we publish.
Find out more
MINI REVIEW article
Front. Cardiovasc. Med. , 03 April 2023
Sec. Cardiovascular Biologics and Regenerative Medicine
Volume 10 - 2023 | https://doi.org/10.3389/fcvm.2023.1155444
This article is part of the Research Topic Cellular and Molecular Mechanisms of Cardiovascular and Metabolic Diseases View all 5 articles
Atherosclerosis is a major risk factor for cardiovascular diseases. Hypercholesterolemia has been both clinically and experimentally linked to cardiovascular disease and is involved in the initiation of atherosclerosis. Heat shock factor 1 (HSF1) is involved in the control of atherosclerosis. HSF1 is a critical transcriptional factor of the proteotoxic stress response that regulates the production of heat shock proteins (HSPs) and other important activities such as lipid metabolism. Recently, HSF1 is reported to directly interact with and inhibit AMP-activated protein kinase (AMPK) to promote lipogenesis and cholesterol synthesis. This review highlights roles of HSF1 and HSPs in critical metabolic pathways of atherosclerosis, including lipogenesis and proteome homeostasis.
Atherosclerosis is the leading cause of cardiovascular disease, it is a pathological condition that leads to coronary heart disease, stroke, and peripheral arterial diseases by narrowing the lining of the coronary arteries, cerebral arteries, iliac arteries, femoral arteries, and aorta (1–3). Atherosclerosis typically develops at branch points and curvatures in large arteries where disturbed blood flow creates a pro-atherogenic environment that leads to macrophage accumulation and foam cell formation with the deposition of primarily cholesterol and its esters in the intimal macrophage at early stage of the disease (4). Increased lipid deposition at certain places and the subsequent formation of a cap of smooth muscle and fibrous tissue around a core of lipid and necrotic debris are both seen in atherosclerotic patients (2). These alterations lead to the development of raised lesions termed fibrous plaques, which extend into the lumen and start to disrupt blood flow.
Maintaining a healthy level of cholesterol in the body is essential to prevent atherosclerosis. Homeostasis of cholesterol includes cholesterol absorption, trafficking, and efflux (5). Macrophages are responsible for the absorption of oxidized low-density lipoprotein (oxLDL) and the accumulation of the lipid in the cells (4). If cholesterol is accumulated excessively in macrophages and smooth muscle cells (SMCs), it would contribute to the formation of foam cells and cause atherosclerosis (3). Understanding the modulation of intracellular lipid homeostasis is important to comprehend atherosclerosis.
The intracellular protein homeostasis is required for lipid homeostasis (6, 7). The proteotoxic stress response (PSR) is important to keep the intracellular protein homeostasis stable and has been shown to play an important role in the development of atherosclerosis. Heat shock factor 1 (HSF1) is a transcriptional factor for the expression of heat shock proteins (HSPs) to maintain proteome homeostasis, it also participates in the regulation of the intracellular lipid homeostasis (8). However, roles that HSF1 and HSPs play in the development of atherosclerosis are not completely known. Importantly, HSPs either promote or inhibit atherosclerosis at various stages of atherosclerosis development (9–13). A comprehensive understanding of the relationship between HSF1/HSPs and atherosclerosis is therefore essential for the development of effective treatment approaches or the identification of clinically useful markers for atherosclerosis. This review will focus on discussing influences that HSF1 and HSPs have on atherosclerosis and focus on macrophage foam cells due to space limitation.
Atherosclerosis is caused by a number of physiological and pathological conditions, including chronic inflammation, dysregulation of lipid metabolism, oxidative stress, and incorrect immunological responses (3, 14, 15). Despite advancements in the study of atherosclerosis, the field lacks a systemic understanding of mechanisms of the disease, particularly in molecular regulators with significant pathophysiological commonalities between intracellular proteome homeostasis and atherosclerosis. Growing evidence supports the concept that aberrations in the metabolic programming contribute to the development of atherosclerosis (16, 17). Chronic inflammation induced by metabolic perturbance is also associated with disordered proteomic stability in atherosclerosis (18). Therefore, investigating how cellular metabolism responds to the PSR in cardiovascular disease has immense potential to answer these mechanistic questions and suggest new areas for further basic and translation investigations.
The formation of macrophage foam cells is a critical step in the pathogenesis of atherosclerosis. Early in the development of atherosclerosis, the endothelial dysfunction leads to macrophage cell infiltration and macrophage-derived foam cells proliferate and aggregate in the subendothelial space of a damaged artery (1–4). The processing and metabolism of cholesterol inside macrophages, which involve uptake modified lipoproteins, such as oxLDL, esterification, and outflow that culminates in cholesterol equilibrium, are among the most recognized crucial stages in the creation of foam cells. Dysregulations of the cholesterol equilibrium in macrophages lead to the accumulation of intracellular lipid droplets and the formation of characteristic foam-like structures (19, 20). Later stages of atherosclerosis are characterized by the presence of other cell types, including SMCs and SMC-derived cells (19, 21). SMCs can differentiate into foam cells themselves. SMCs can also contribute to the progression of atherosclerosis by phenotypic switching to a more synthetic state, leading to the formation of a fibrous cap that is prone to rupture (21–24). Several key molecular pathways and transcription factors that regulate SMC phenotypes and foam cell formation, including KLF4 that may be a potential therapeutic target for atherosclerosis, have been identified (25, 26). During the development of the atherosclerotic plaque, there is also a significant infiltration of other cell types that contributes to the progression of atherosclerosis, such as T lymphocytes, dendritic cells, and mast cells, into the intima (1, 15, 27, 28).
Cellular proteome homeostasis (proteostasis) refers to the processes that mediate protein synthesis, folding, trafficking, complex assembly, and destruction inside cells (29). Proteotoxic stressors, such as heat, heavy metals, UV radiation, and hypoxia, perturb proteostasis and cause cellular protein damages or conformational changes, which lead to protein misfolding and can trigger diseases (30, 31). For example, heat stress dramatically alters cell physiology and might cause cell death, the outcome depends on the intensity of the stress (32). These proteotoxic stressors also trigger the PSR, which involves well-characterized cytoprotective mechanisms that produce HSPs (33). HSPs are molecular chaperones. The main purpose of molecular chaperons is to protect the proteome from damaging protein misfoldings and to reduce protein aggregation by regulating protein folding, trafficking, assembly, ubiquination, and proteasomal degradation (33). The PSR is critical for the survival of cells under proteotoxic stress and contributes to the pathophysiology of common human diseases. As a cytoprotective chaperon mechanism, the PSR is induced by diverse environmental stressors and results in several transcriptional changes (34). PSR can trigger HSF1, a transcription factor, to regulate the expression of HSPs (34).
HSF1 and HSPs confer protection against cardiovascular diseases, including atrial fibrillation, cardiac hypertrophy, and cardiomyopathy (35–37). HSPs have been shown to play crucial roles in atherosclerosis but they can both positively and negatively affect the development of atherosclerosis (9, 38). HSF1 has also been linked to atherosclerosis-related cardiovascular disorders, and evidence is mounting that HSF1 plays a crucial role in these conditions (39). PSR, therefore, is now increasingly appreciated to be a key regulator of atherosclerosis but its role in mediating cardiovascular complications of atherosclerosis remains to be further elucidated. Below, we will discuss recent results connecting HSPs (mainly HSP72 and HSP27 in this article) and the perspective role of HSF1 to atherosclerotic cardiovascular disease.
HSPs, including HSP27, HSP72, HSP90, and HSP100, safeguard cells against stress, mainly by serving as molecular chaperones to repair denatured proteins by re-forming their native three-dimensional structures in order to maintain the proteostasis (40). Ample evidence supports that HSPs are involved in atherosclerosis (Figure 1) (9–11). However, it is still unclear how expressions of HSPs affect the development of atherosclerosis.
Figure 1. Roles of HSF1 in atherosclerosis. Macrophages uptake oxLDL through lipid uptake transporters and accumulate lipids intracellularly. Macrophages remove excessive intracellular lipids through lipid efflux transporters. Unbalanced lipid homeostasis leads macrophages to form foam cells, leading to the formation of atherosclerosis plaques. Chronic inflammation, PSR, and ROS induce the transcriptional function of HSF1 to express HSPs, such as HSP27 and HSP72. Intercellular HSPs (iHSPs) promote cholesterol efflux and inhibit lipid uptake. Secreted extracellular HSPs (eHSPs) play dual roles in atherosclerosis. HSPs inhibit atherogenesis during the early stage of atherosclerosis but promote atherosclerosis during the late stage of atherosclerosis. HSF1 non-transcriptionally promotes lipogenesis and cholesterol synthesis via SREBP1/2 by interacting with and inhibiting AMPK. ABCA1, ATP binding cassette subfamily A member 1; ABCG1, ATP binding cassette subfamily G member 1; AMPK, AMP-activated protein kinase; CD36, cluster of differentiation 36; eHSPs, extracellular heat shock proteins; HSE, heat shock element; HSF1, heat shock factor 1; HSPs, heat shock proteins; iHSPs, intracellular heat shock proteins; LDLR, low-density lipoprotein receptor; oxLDL, oxidized low-density lipoprotein; p, phosphorylation; PSR, proteotoxic stress response; ROS, reactive oxygen species; SR-A, scavenger receptor type A; SR-BI, scavenger receptor class B type I; SREBP1/2, sterol regulatory element binding protein 1/2. Images were created with BioRender.com.
Foam cell formation is a hallmark of early atherosclerosis, mainly from macrophage at the earlier stage and from SMCs at late stage (27). Foam cell formation from macrophages is through lipid absorption, efflux, and cholesterol esterification. Inadequate cholesterol efflux may result in the accumulation of lipids and cholesterol esters in macrophages (41). This critical step of cholesterol efflux is carried out by ATP binding cassette subfamily A member 1 (ABCA1), ABCG1, and scavenger receptor (SR)-BI. ABCA1 and ABCG1 transport lipid-deficient Apolipoprotein (apo) A-I free cholesterol to high-density lipoprotein (HDL) particles (5, 42). HDL is believed to play a protective role in atherosclerosis but raising the HDL level by inhibiting cholesteryl ester transfer protein did not reduce cardiovascular risk in several studies (20, 43). The major cholesterol uptake mechanism involves CD36 and SR-A (44). 75%–90% of modified lipoproteins are transported through CD36 and SR-A (Figure 1). Endoplasmic reticulum acetyl-CoA acetyltransferases (ACAT) generate cholesterol ester (45). HSPs are recognized as lipid metabolism regulators because they affect the expression of genes involved in cholesterol absorption (such as CD36 and LOX1) (46, 47) and efflux (ABCA1, ABCG1) (48, 49). Furthermore, HSPs have the capacity to control inflammatory pathways, produce cytokines, and aid in the death of foam cells (50).
HSP72 isoforms may function by themselves or in concert with HSP90, where they are crucial in the substrate recruitment (51). In addition to acting as chaperones, HSP72 isoforms promote cell survival by inhibiting apoptosis at several processes across intrinsic and extrinsic cell death pathways (52, 53). Furthermore, HSP72 induces a strong transcriptional re-programming, including the overexpression of critical targets of liver X receptors (LXR), master regulators of systemic cholesterol elimination (54, 55). As a result of HSP72's interaction with the macrophage LXRα promoter, the expression of LXRα and LXRα’s target genes, including ABCA1 and ABCG1, are increased and, consequently, their protein abundances are enhanced.
On the other hand, HSP72 increases the amount of lipids accumulated in arteries and contributes to the development of atherosclerotic lesions. In mice lacking ApoE, HSP72 accelerates the development of atherosclerosis by inhibiting the production of ABCA1 and ABCG1 in peritoneal macrophages and the aorta through the JNK/Elk-1 pathway (12). Moreover, a positive correlation between the expression of HSP72 and the stability of advanced human atherosclerotic plaques has been shown. The formation of atherosclerotic lesions is also linked to the inhibition of the expression and the activity of Sirtuin 1 (SIRT1), which, in turn, underlies both the expression of HSF1 and HSPs and the ability of HSF1 to transcribe genes (56).
HSP27 has been proposed as a biomarker and therapeutic target in atherosclerosis. Through the PI3K/PKC/Sp1 pathway, HSP27 stimulates ABCA1 expression and cholesterol efflux in THP-1 macrophage-derived foam cells (48). Through a mechanism involving NF-kB signaling, HSP27 suppresses SR-A expression, hence reducing foam cell production and atherogenesis. HSP27 is atherosclerosis protective when it is overexpressed and its level in circulation is elevated, this HSP27-mediated protection in vivo requires SR-A as a cofactor (50).
Mouse Hsp25, the ortholog of human HSP27, has been administered as a vaccine to inhibit atherogenesis in mice, it upregulates low-density lipoprotein receptor (LDLR) expression, reduces proprotein convertase subtilisin/kexin type 9 (PCSK9) levels, and suppresses inflammation (57). Cholesterol levels may be also reduced by Hsp25 immunotherapy, Hsp25 reduces plaque cholesterol by 30% and prevents experimental atherosclerosis in mice, which requires GM-CSF-regulated ABCA1 and ABCG1 expression (49).
The role of HSPs in atherosclerosis is contentious with some studies suggesting a protective role by preventing protein accumulation and inhibiting inflammation while others suggesting a disease progression promoting role by increasing cell migration and proliferation and activating inflammatory pathways (58). A study shows that healthy people have lower levels of extracellular HSP72 than patients with cerebrovascular atherosclerosis, indicating that extracellular HSP72 may be protective against the development of this illness (59). However, high levels of extracellular HSP72 are detected in individuals with severe arterial calcification and are correlated with an increased risk for the development of arterial calcification (60). It is reported that the anti-inflammatory pathway is suppressed in atherosclerosis (61). Differences in the types of HSPs studied and experimental models used may contribute to these conflicting results, further research is needed to clarify the roles of HSPs in atherosclerosis.
HSPs have different effects on atherosclerosis depending on the stage of aortic atherosclerosis (11). In early stages of atherosclerosis, induction of HSPs protects against aortic atherosclerosis via lowering adhesion molecule expression. On the other hand, induction of HSPs promotes aortic atherosclerosis by increasing the production of inflammatory cytokines and activating macrophage activity as atherosclerosis progresses (Figure 1). Thus, HSPs induction and inhibition should be investigated for the prevention and treatment of atherosclerosis, respectively (11).
In order to better understand HSPs' function in atherosclerosis, it is important to understand their upstream regulation. Among multiple signaling pathways that regulate HSPs expression in atherosclerosis, the HSF1 pathway is a dominant mechanism in response to stresses (34). During physiological and pathological processes, inflammatory cytokines and reactive oxygen species may also increase HSF1 activation (62). In atherosclerotic lesions, HSF1 is activated and cytokine stimulation causes HSF1 nuclear translocation, DNA binding, and to increase HSP72 expression (39). Therefore, HSF1 could be a novel target on atherosclerosis.
There are nine HSF family members in mammalians, including HSF1, 2, 3, 4, 5, X1, X2, Y1, and Y2. These nine members have a variety of distinct and overlapping roles and characteristics (34, 63). The N-terminus of HSF proteins contains a helix-turn-helix DNA-binding domain (DBD). However, this helix-turn-helix domain of HSF1 does not interact directly with DNA; rather, it stabilizes the HSF1 trimer that is bound on DNA (64, 65). Under heat shock, inactive monomeric HSF proteins form homotrimer, translocate to the nucleus, interact with heat-shock elements (HSEs) in the genome, and initiate the transcription of their target genes. HSEs are comprised of several inverted repetitions of the pentanucleotide motif nGAAn, different HSF family members have preferences for different HSEs, resulting in the transcription of a wide variety of target genes, including those encoding HSPs (Figure 1). Upon the resolution of the stress or after prolonged stress, HSF proteins revert to a monomeric state and their transcriptional activities decrease (34, 66).
HSF1 controls the chaperon protein production in order to change the misfolded proteins when cells are exposed to a stressor, such as increased temperature (67, 68). HSF1 is the most important transcription factor for the transcription of HSP genes in eukaryotes (69, 70). For example, fibroblasts from mice lacking HSF1 lack stress-induced transcription of HSP genes, indicating that HSF1 is sufficient for HSP production (71). Thus, HSF1 is the major factor determining cellular response to proteotoxic stimuli. HSF1 comprises several functional domains (Figure 2). The oligomerization domain of HSF1 is composed of leucine-zipper-like heptad repeat region A (HR-A) and HR-B, which together establish an all-parallel structure to control HSF1 trimerization. The HR-C region is believed to maintain HSF1 at the inactive state by inhibiting HSF1 trimerization (72, 73). The activation domain (AD) of HSF1 promotes the transcriptional activation of HSF1's target genes and controls HSF1's activity (74, 75). The regulatory domain (RD), situated between HR-A/B and HR-C regions, is important for inhibiting HSF1 function in the absence of stress. In addition, the RD of HSF1 is believed to contain a heat shock sensor and functions as the target for several induced post-translational modifications (76, 77).
Figure 2. HSF1 regulatory domains and post-translational modifications. HSF1 is composed of several functional domains, including a DNA-binding domain (DBD), a regulatory domain (RD), a heptad repeat (HR), and a transactivating domain (AD). Specific sites for serine/threonine phosphorylation (P), acetylation (AC), and SUMOylation (S) that activate or inhibit HSF1's activity are shown. These post-translational modifications are mediated by a variety of kinases, de-acetylases, and SUMOylases. AMPK, AMP-activated protein kinase, Akt, protein kinase B; CaMKII, calcium/calmodulin-dependent protein kinase II; CK2, casein kinase 2; ERK, extracellular signal-regulated kinase; GSK3, glycogen synthase kinase 3; JNK, c-Jun N-terminal kinase; mTOR, mammalian target of rapamycin; MEK, mitogen-activated protein kinase; PKA, protein kinase A; PKC, protein kinase C; PLK1, polo-like kinase 1; SIRT1, sirtuin 1; UBC9, ubiquitin-conjugating enzyme E2I. Images were created with BioRender.com.
Protein-protein interactions and post-translational modifications (PTMs), such as phosphorylation, SUMOylation, and acetylation, influence the activation of HSF1 (62, 78). Figure 2 illustrates PTMs on HSF1 that modulate HSF1's activity and enzymes that are reported to be responsible for these PTMs. Acetylation of HSF1 inhibits HSF1 DNA binding. After exposure to heat, HSF1 trimers bind to HSE and are phosphorylated and SUMOylated at many sites to control its activity. For example, at least 12 phosphorylation sites of HSF1 have been identified, some of which activate whereas others inhibit HSF1 (34, 69, 79). Among these, phosphorylation of HSF1 at S326 in response to stress stimulates HSF1 activation whereas phosphorylation of HSF1 at S303/307 inhibits HSF1's activity in the absence of stress (80, 81). In addition, in HEK293 cells, metformin treatment promotes the phosphorylation of HSF1 at S121 and decreases HSF1's DNA-binding activity (82). Accordingly, ectopically expressed HSF1 S121A mutant is insensitive to the metformin-induced decrease in DNA-binding activity in cells with their endogenous wild type HSF1 being knockdown (82). HSF1 phosphorylation therefore may play a role in atherosclerosis. For example, HSF1 phosphorylation at Ser326 activates HSF1 to upregulate HSPs expressions, which can protect against atherosclerosis. It is possible that different HSF1 phosphorylations may have different effects on HSPs expressions and atherosclerosis. Moreover, kinases and signaling pathways that regulate HSF1 phosphorylation in atherosclerosis have not been fully characterized. Further research is needed to elucidate the precise role of HSF1 phosphorylation in atherosclerosis and mechanisms by which HSF1 phosphorylation affects atherosclerosis and to determine whether targeting HSF1 phosphorylation could be a viable therapeutic strategy for the prevention or treatment of this disease.
AMP-activated protein kinase (AMPK) is the primary regulator of cellular and body energy homeostasis, including glucose and lipid metabolism (83, 84). Animal model studies show that increasing oxidative metabolism improves insulin resistance. For example, activation of AMPK or SIRT1 increases fatty acid oxidation, which decreases lipid esterification and improves insulin resistance (85, 86). Furthermore, AMPK is an important sensor of cellular energy status, its activity is dramatically reduced in insulin resistance whereas activating AMPK promotes insulin sensitivity (87). Furthermore, Cushing's syndrome is defined by reduced activation of AMPK in conjunction with insulin resistance in adipose tissue (88). AMPK activity is reduced in the adipose tissue of highly obese people (89). AMPK-regulated lipid metabolism is particularly crucial in the control of cardiovascular disorders such as atherosclerosis and endothelial-related vascular diseases (90).
In addition to its transcriptional function, HSF1 also non-transcriptionally regulates lipogenesis and cholesterol synthesis. HSF1 stimulates lipid synthesis through many signaling pathways, including suppressing AMPK to induce lipogenesis (8). Knockdown HSF1 reduced lipid droplet (8). In human melanoma cells, inducible HSF1 knockdown results in increased sterol regulatory element-binding protein 1c (SREBP1c) Ser372 phosphorylation, decreased levels of acetyl-CoA carboxylase (ACC1), fatty acid synthase (FASN), LDLR, and 3-Hydroxy-3-Methylglutaryl-CoA Reductase (HMGCR) mRNAs, and reduced SREBP1's nuclear translocation and binding to genomic DNA (8). Primarily via inhibiting the AMPK activity, HSF1 is responsible for the transcriptional activation of SREBP1 (8, 91, 92). Interestingly, HSF1 peptides reduce AMPK's function by preventing AMPK binding to ATP and AMP, promoting phosphatase engagement with AMPK, and eventually leading to AMPK conformational shift, which limits AMPK's activity (8). Thus, HSF1 reduces AMPK's activation by directly interacting with it (Figure 1).
HSF1 also mediates mevalonate and cholesterol biosynthesis. Inhibition of HSF1 by using its inhibitor KRIBB11 or shRNA reduces H-RasV12-induced cholesterol production (91). How HSF1 affects intracellular cholesterol homeostasis is unknown. However, because HSF1 deficiency-induced cellular cholesterol reduction is counteracted by AMPK knockdown, this regulation appears to involve AMPK (8). Statins stabilize HMGCR and SREBP2-mediated cholesterol production, reducing their heart disease therapy effectiveness. These findings suggest that suppressing HSF1 may enhance statin-based cholesterol reduction (91). Inhibiting HSF1 by KRIBB11 significantly reduces the expression of lipogenic enzymes, including ACC and FAS, and increases β-oxidation-associated enzyme palmitoyltransterase-1 (93). These findings indicate that HSF1 plays a crucial role in mediating intracellular lipid metabolism. The HSF1-AMPK signaling may be a promising therapeutic target for lipid dysregulation.
HSF1 has been reported to have a protective role in endothelial cells, HSF1 activation promotes the expression of HSPs and protects against oxidative stress-induced vascular injury and induces angiogenesis (94, 95). HSF1 activation induces the expression of the endothelial nitric oxide synthase to maintain the endothelial function via the nitric oxide (NO) production (94). Besides, low level of NO induces HSP70 expression in vascular SMCs through the induction of HSF1 activity (96). HSF1-induced HSPs expression also protects vascular SMCs from calcification (97). In addition, it has been reported that HSF1 suppresses the lung injury via suppressing macrophage infiltration (98). Nevertheless, the evidence of HSF1 regulation in macrophage-foam cell in atherosclerosis is limited.
HSF1 might be a nice marker in obesity-related disorders, including atherosclerosis (99). HSF1 is reported to be activated in atherosclerosis (39). Furthermore, HSF1 deficiency increases the expression of cholesterol 7-hydroxylase (CYP7A1) and multidrug transporter (MDR1) genes, hence reducing atherosclerosis (100). HSF1 deficiency also significantly represses PPAR-γ2, a nuclear receptor that controls the vast majority of genes involved in the lipid metabolism (100). HSF1 inhibition improves the lipidemic profile through enhancing cholesterol clearance and lowers atherosclerotic lesion load, making it a plausible target in atherosclerosis management. These results indicate that HSF1 has pleotropic roles in atherosclerosis. Nonetheless, the detailed mechanism for how HSF1 regulates atherosclerosis through mediating lipid homeostasis requires further investigated.
This review discusses an intimate relationship between atherosclerosis and PSR, focusing on HSF1 and HSPs in atherosclerotic lipid homeostasis. HSF1 and HSPs serve crucial roles in the quality control of proteins, particularly in regulating protein misfolding, aggregation, and refolding. Atherosclerosis is caused by imbalanced inflammation, lipid composition, as well as lipid uptake and efflux. These stresses induce the activation of multiple responses, including HSPs expression, at various stages of atherosclerotic pathogenic progression. HSF1 and its functionally associated kinases, such as AMPK, control both proteotoxic stress and lipid metabolism, albeit via distinct mechanisms. Notably, HSPs expressions at different stages of atherosclerotic lesions have opposing effects in the progression of atherosclerosis. Therefore, therapeutic approaches targeting HSPs at various stages of atherosclerosis should be administered with caution. Finally, we infer that the mechanism of HSF1-mediated lipid metabolism may present novel therapeutic targets for cardiovascular disorders linked with atherosclerosis and that the activation of HSF1 and HSPs may serve as diagnostic indicators for atherosclerosis.
KS drafted the manuscript, SG drafted the image, SG and AY contributed with the literature search, and KS reviewing and editing the manuscript. All authors contributed to the article and approved the submitted version.
This study was supported by NIH/NCI (K22CA248616) and The University of Toledo Startup funds (#110917).
This manuscript was edited at Life Science Editors. The images were created with BioRender.com.
The authors declare that the research was conducted in the absence of any commercial or financial relationships that could be construed as a potential conflict of interest.
All claims expressed in this article are solely those of the authors and do not necessarily represent those of their affiliated organizations, or those of the publisher, the editors and the reviewers. Any product that may be evaluated in this article, or claim that may be made by its manufacturer, is not guaranteed or endorsed by the publisher.
1. Libby P, Buring JE, Badimon L, Hansson GK, Deanfield J, Bittencourt MS, et al. Atherosclerosis. Nat Rev Dis Primers. (2019) 5:56. doi: 10.1038/s41572-019-0106-z
2. Bjorkegren JLM, Lusis AJ. Atherosclerosis: recent developments. Cell. (2022) 185:1630–45. doi: 10.1016/j.cell.2022.04.004
3. Jebari-Benslaiman S, Galicia-Garcia U, Larrea-Sebal A, Olaetxea JR, Alloza I, Vandenbroeck K, et al. Pathophysiology of atherosclerosis. Int J Mol Sci. (2022) 23. doi: 10.3390/ijms23063346
4. Gui Y, Zheng H, Cao RY. Foam cells in atherosclerosis: novel insights into its origins, consequences, and molecular mechanisms. Front Cardiovasc Med. (2022) 9:845942. doi: 10.3389/fcvm.2022.845942
5. Yu XH, Tang CK. ABCA1, ABCG1, and cholesterol homeostasis. Adv Exp Med Biol. (2022) 1377:95–107. doi: 10.1007/978-981-19-1592-5_7
6. Kuan YC, Hashidume T, Shibata T, Uchida K, Shimizu M, Inoue J, et al. Heat shock protein 90 modulates lipid homeostasis by regulating the stability and function of sterol regulatory element-binding protein (SREBP) and SREBP cleavage-activating protein. J Biol Chem. (2017) 292:3016–28. doi: 10.1074/jbc.M116.767277
7. Xu J, Taubert S. Beyond proteostasis: lipid metabolism as a new player in ER homeostasis. Metabolites. (2021) 11. doi: 10.3390/metabo11010052
8. Su KH, Dai S, Tang Z, Xu M, Dai C. Heat shock factor 1 is a direct antagonist of AMP-activated protein kinase. Mol Cell. (2019) 76:546–61.e8. doi: 10.1016/j.molcel.2019.08.021
9. Wick G, Jakic B, Buszko M, Wick MC, Grundtman C. The role of heat shock proteins in atherosclerosis. Nat Rev Cardiol. (2014) 11:516–29. doi: 10.1038/nrcardio.2014.91
10. Xu Q, Metzler B, Jahangiri M, Mandal K. Molecular chaperones and heat shock proteins in atherosclerosis. Am J Physiol Heart Circ Physiol. (2012) 302:H506–14. doi: 10.1152/ajpheart.00646.2011
11. Hashikawa N, Ido M, Morita Y, Hashikawa-Hobara N. Effects from the induction of heat shock proteins in a murine model due to progression of aortic atherosclerosis. Sci Rep. (2021) 11:7025. doi: 10.1038/s41598-021-86601-8
12. Zhao ZW, Zhang M, Chen LY, Gong D, Xia XD, Yu XH, et al. Heat shock protein 70 accelerates atherosclerosis by downregulating the expression of ABCA1 and ABCG1 through the JNK/Elk-1 pathway. Biochim Biophys Acta Mol Cell Biol Lipids. (2018) 1863:806–22. doi: 10.1016/j.bbalip.2018.04.011
13. Xie F, Zhan R, Yan LC, Gong JB, Zhao Y, Ma J, et al. Diet-induced elevation of circulating HSP70 may trigger cell adhesion and promote the development of atherosclerosis in rats. Cell Stress Chaperones. (2016) 21:907–14. doi: 10.1007/s12192-016-0716-2
14. Berliner JA, Navab M, Fogelman AM, Frank JS, Demer LL, Edwards PA, et al. Atherosclerosis: basic mechanisms. Oxidation, inflammation, and genetics. Circulation. (1995) 91:2488–96. doi: 10.1161/01.cir.91.9.2488
15. Libby P. Inflammation in atherosclerosis. Arterioscler Thromb Vasc Biol. (2012) 32:2045–51. doi: 10.1161/ATVBAHA.108.179705
16. Yvan-Charvet L, Ivanov S. Metabolic reprogramming of macrophages in atherosclerosis: is it all about cholesterol? J Lipid Atheroscler. (2020) 9:231–42. doi: 10.12997/jla.2020.9.2.231
17. Ma Y, Li D, Liu W, Liu X, Xu Y, Zhong X, et al. Resveratrol on the metabolic reprogramming in liver: implications for advanced atherosclerosis. Front Pharmacol. (2021) 12:747625. doi: 10.3389/fphar.2021.747625
18. Langer HF. Chronic inflammation in atherosclerosis-the CD40L/CD40 axis belongs to dendritic cells and T cells, not platelets. J Thromb Haemost. (2022) 20:3–5. doi: 10.1111/jth.15591
19. Soehnlein O, Libby P. Targeting inflammation in atherosclerosis—from experimental insights to the clinic. Nat Rev Drug Discov. (2021) 20:589–610. doi: 10.1038/s41573-021-00198-1
20. Libby P. The changing landscape of atherosclerosis. Nature. (2021) 592:524–33. doi: 10.1038/s41586-021-03392-8
21. Bennett MR, Sinha S, Owens GK. Vascular smooth muscle cells in atherosclerosis. Circ Res. (2016) 118:692–702. doi: 10.1161/CIRCRESAHA.115.306361
22. Gomez D, Owens GK. Smooth muscle cell phenotypic switching in atherosclerosis. Cardiovasc Res. (2012) 95:156–64. doi: 10.1093/cvr/cvs115
23. Koga J, Aikawa M. Crosstalk between macrophages and smooth muscle cells in atherosclerotic vascular diseases. Vascul Pharmacol. (2012) 57:24–8. doi: 10.1016/j.vph.2012.02.011
24. Harman JL, Jorgensen HF. The role of smooth muscle cells in plaque stability: therapeutic targeting potential. Br J Pharmacol. (2019) 176:3741–53. doi: 10.1111/bph.14779
25. Shankman LS, Gomez D, Cherepanova OA, Salmon M, Alencar GF, Haskins RM, et al. KLF4-dependent phenotypic modulation of smooth muscle cells has a key role in atherosclerotic plaque pathogenesis. Nat Med. (2015) 21:628–37. doi: 10.1038/nm.3866
26. Alencar GF, Owsiany KM, Karnewar S, Sukhavasi K, Mocci G, Nguyen AT, et al. Stem cell pluripotency genes Klf4 and Oct4 regulate complex SMC phenotypic changes critical in late-stage atherosclerotic lesion pathogenesis. Circulation. (2020) 142:2045–59. doi: 10.1161/CIRCULATIONAHA.120.046672
27. Weber C, Noels H. Atherosclerosis: current pathogenesis and therapeutic options. Nat Med. (2011) 17:1410–22. doi: 10.1038/nm.2538
28. Hansson GK. Inflammation, atherosclerosis, and coronary artery disease. N Engl J Med. (2005) 352:1685–95. doi: 10.1056/NEJMra043430
29. Dai C, Sampson SB. HSF1: guardian of proteostasis in cancer. Trends Cell Biol. (2016) 26:17–28. doi: 10.1016/j.tcb.2015.10.011
30. Lindquist SL, Kelly JW. Chemical and biological approaches for adapting proteostasis to ameliorate protein misfolding and aggregation diseases: progress and prognosis. Cold Spring Harb Perspect Biol. (2011) 3. doi: 10.1101/cshperspect.a004507
31. Dai C, Dai S, Cao J. Proteotoxic stress of cancer: implication of the heat-shock response in oncogenesis. J Cell Physiol. (2012) 227:2982–7. doi: 10.1002/jcp.24017
32. Pirkkala L, Nykanen P, Sistonen L. Roles of the heat shock transcription factors in regulation of the heat shock response and beyond. FASEB J. (2001) 15:1118–31. doi: 10.1096/fj00-0294rev
33. Fujimoto M, Nakai A. The heat shock factor family and adaptation to proteotoxic stress. FEBS J. (2010) 277:4112–25. doi: 10.1111/j.1742-4658.2010.07827.x
34. Vihervaara A, Sistonen L. HSF1 at a glance. J Cell Sci. (2014) 127:261–6. doi: 10.1242/jcs.132605
35. Liu D, Han X, Zhang Z, Tse G, Shao Q, Liu T. Role of heat shock proteins in atrial fibrillation: from molecular mechanisms to diagnostic and therapeutic opportunities. Cells. (2022) 12. doi: 10.3390/cells12010151
36. Eschricht S, Jarr KU, Kuhn C, Lehmann L, Kreusser M, Katus HA, et al. Heat-shock-protein 90 protects from downregulation of HIF-1alpha in calcineurin-induced myocardial hypertrophy. J Mol Cell Cardiol. (2015) 85:117–26. doi: 10.1016/j.yjmcc.2015.05.018
37. Chakafana G, Spracklen TF, Kamuli S, Zininga T, Shonhai A, Ntusi NAB, et al. Heat shock proteins: potential modulators and candidate biomarkers of peripartum cardiomyopathy. Front Cardiovasc Med. (2021) 8:633013. doi: 10.3389/fcvm.2021.633013
38. Amirfakhryan H. Vaccination against atherosclerosis: an overview. Hellenic J Cardiol. (2020) 61:78–91. doi: 10.1016/j.hjc.2019.07.003
39. Metzler B, Abia R, Ahmad M, Wernig F, Pachinger O, Hu Y, et al. Activation of heat shock transcription factor 1 in atherosclerosis. Am J Pathol. (2003) 162:1669–76. doi: 10.1016/S0002-9440(10)64301-5
40. Hu C, Yang J, Qi Z, Wu H, Wang B, Zou F, et al. Heat shock proteins: biological functions, pathological roles, and therapeutic opportunities. MedComm (2020). (2022) 3:e161. doi: 10.1002/mco2.161
41. van Eijk M, Aerts J. The unique phenotype of lipid-laden macrophages. Int J Mol Sci. (2021) 22. doi: 10.3390/ijms22084039
42. Pownall HJ, Rosales C, Gillard BK, Gotto AM Jr. High-density lipoproteins, reverse cholesterol transport and atherogenesis. Nat Rev Cardiol. (2021) 18:712–23. doi: 10.1038/s41569-021-00538-z
43. Libby P. The changing nature of atherosclerosis: what we thought we knew, what we think we know, and what we have to learn. Eur Heart J. (2021) 42:4781–2. doi: 10.1093/eurheartj/ehab438
44. Shen WJ, Azhar S, Kraemer FB. SR-B1: a unique multifunctional receptor for cholesterol influx and efflux. Annu Rev Physiol. (2018) 80:95–116. doi: 10.1146/annurev-physiol-021317-121550
45. Chang TY, Li BL, Chang CC, Urano Y. Acyl-coenzyme A: cholesterol acyltransferases. Am J Physiol Endocrinol Metab. (2009) 297:E1–9. doi: 10.1152/ajpendo.90926.2008
46. Shi C, Deng J, Chiu M, Chen YX, O'Brien ER. Heat shock protein 27 immune complex altered signaling and transport (ICAST): novel mechanisms of attenuating inflammation. FASEB J. (2020) 34:14287–301. doi: 10.1096/fj.202001389RR
47. Liu B, Li S, Xiu B, Zhang Y, Zhou Y, Yang Q, et al. C-terminus of heat shock protein 60 can activate macrophages by lectin-like oxidized low-density lipoprotein receptor 1. Biochem Biophys Res Commun. (2019) 508:1113–9. doi: 10.1016/j.bbrc.2018.12.008
48. Kuang HJ, Zhao GJ, Chen WJ, Zhang M, Zeng GF, Zheng XL, et al. Hsp27 promotes ABCA1 expression and cholesterol efflux through the PI3K/PKCzeta/Sp1 pathway in THP-1 macrophages. Eur J Pharmacol. (2017) 810:57–62. doi: 10.1016/j.ejphar.2017.06.015
49. Pulakazhi Venu VK, Adijiang A, Seibert T, Chen YX, Shi C, Batulan Z, et al. Heat shock protein 27-derived atheroprotection involves reverse cholesterol transport that is dependent on GM-CSF to maintain ABCA1 and ABCG1 expression in ApoE(−/−) mice. FASEB J. (2017) 31:2364–79. doi: 10.1096/fj.201601188R
50. Raizman JE, Chen YX, Seibert T, Hibbert B, Cuerrier CM, Salari S, et al. Heat shock protein-27 attenuates foam cell formation and atherogenesis by down-regulating scavenger receptor-A expression via NF-kappaB signaling. Biochim Biophys Acta. (2013) 1831:1721–8. doi: 10.1016/j.bbalip.2013.07.015
51. Lang BJ, Guerrero-Gimenez ME, Prince TL, Ackerman A, Bonorino C, Calderwood SK. Heat shock proteins are essential components in transformation and tumor progression: cancer cell intrinsic pathways and beyond. Int J Mol Sci. (2019) 20. doi: 10.3390/ijms20184507
52. Lanneau D, Brunet M, Frisan E, Solary E, Fontenay M, Garrido C. Heat shock proteins: essential proteins for apoptosis regulation. J Cell Mol Med. (2008) 12:743–61. doi: 10.1111/j.1582-4934.2008.00273.x
53. Wang X, Chen M, Zhou J, Zhang X. HSP27, 70 and 90, anti-apoptotic proteins, in clinical cancer therapy (review). Int J Oncol. (2014) 45:18–30. doi: 10.3892/ijo.2014.2399
54. Gungor B, Vanharanta L, Holtta-Vuori M, Pirhonen J, Petersen NHT, Gramolelli S, et al. HSP70 induces liver X receptor pathway activation and cholesterol reduction in vitro and in vivo. Mol Metab. (2019) 28:135–43. doi: 10.1016/j.molmet.2019.07.005
55. Savla SR, Prabhavalkar KS, Bhatt LK. Liver X receptor: a potential target in the treatment of atherosclerosis. Expert Opin Ther Targets. (2022) 26:645–58. doi: 10.1080/14728222.2022.2117610
56. Westerheide SD, Anckar J, Stevens SM Jr, Sistonen L, Morimoto RI. Stress-inducible regulation of heat shock factor 1 by the deacetylase SIRT1. Science. (2009) 323:1063–6. doi: 10.1126/science.1165946
57. Chen YX, Shi C, Deng J, Diao C, Maarouf N, Rosin M, et al. HSP25 vaccination attenuates atherogenesis via upregulation of LDLR expression, lowering of PCSK9 levels and curbing of inflammation. Arterioscler Thromb Vasc Biol. (2021) 41:e338–e53. doi: 10.1161/ATVBAHA.121.315933
58. Kilic A, Mandal K. Heat shock proteins: pathogenic role in atherosclerosis and potential therapeutic implications. Autoimmune Dis. (2012) 2012:502813. doi: 10.1155/2012/502813
59. Galovic R, Flegar-Mestric Z, Vidjak V, Matokanovic M, Barisic K. Heat shock protein 70 and antibodies to heat shock protein 60 are associated with cerebrovascular atherosclerosis. Clin Biochem. (2016) 49:66–9. doi: 10.1016/j.clinbiochem.2015.10.006
60. Krepuska M, Szeberin Z, Sotonyi P, Sarkadi H, Fehervari M, Apor A, et al. Serum level of soluble Hsp70 is associated with vascular calcification. Cell Stress Chaperones. (2011) 16:257–65. doi: 10.1007/s12192-010-0237-3
61. Bruxel MA, Tavares AMV, Zavarize Neto LD, de Souza Borges V, Schroeder HT, Bock PM, et al. Chronic whole-body heat treatment relieves atherosclerotic lesions, cardiovascular and metabolic abnormalities, and enhances survival time restoring the anti-inflammatory and anti-senescent heat shock response in mice. Biochimie. (2019) 156:33–46. doi: 10.1016/j.biochi.2018.09.011
62. Dai C. The heat-shock, or HSF1-mediated proteotoxic stress, response in cancer: from proteomic stability to oncogenesis. Philos Trans R Soc Lond B Biol Sci. (2018) 373. doi: 10.1098/rstb.2016.0525
63. Su KH, Dai C. Metabolic control of the proteotoxic stress response: implications in diabetes mellitus and neurodegenerative disorders. Cell Mol Life Sci. (2016) 73:4231–48. doi: 10.1007/s00018-016-2291-1
64. Vuister GW, Kim SJ, Orosz A, Marquardt J, Wu C, Bax A. Solution structure of the DNA-binding domain of Drosophila heat shock transcription factor. Nat Struct Biol. (1994) 1:605–14. doi: 10.1038/nsb0994-605
65. Littlefield O, Nelson HC. A new use for the ‘wing’ of the ‘winged’ helix-turn-helix motif in the HSF-DNA cocrystal. Nat Struct Biol. (1999) 6:464–70. doi: 10.1038/8269
66. Shi Y, Mosser DD, Morimoto RI. Molecular chaperones as HSF1-specific transcriptional repressors. Genes Dev. (1998) 12:654–66. doi: 10.1101/gad.12.5.654
67. Santoro MG. Heat shock factors and the control of the stress response. Biochem Pharmacol. (2000) 59:55–63. doi: 10.1016/s0006-2952(99)00299-3
68. Lindquist S. The heat-shock response. Annu Rev Biochem. (1986) 55:1151–91. doi: 10.1146/annurev.bi.55.070186.005443
69. Anckar J, Sistonen L. Regulation of HSF1 function in the heat stress response: implications in aging and disease. Annu Rev Biochem. (2011) 80:1089–115. doi: 10.1146/annurev-biochem-060809-095203
70. Mendillo ML, Santagata S, Koeva M, Bell GW, Hu R, Tamimi RM, et al. HSF1 drives a transcriptional program distinct from heat shock to support highly malignant human cancers. Cell. (2012) 150:549–62. doi: 10.1016/j.cell.2012.06.031
71. McMillan DR, Xiao X, Shao L, Graves K, Benjamin IJ. Targeted disruption of heat shock transcription factor 1 abolishes thermotolerance and protection against heat-inducible apoptosis. J Biol Chem. (1998) 273:7523–8. doi: 10.1074/jbc.273.13.7523
72. Rabindran SK, Haroun RI, Clos J, Wisniewski J, Wu C. Regulation of heat shock factor trimer formation: role of a conserved leucine zipper. Science. (1993) 259:230–4. doi: 10.1126/science.8421783
73. Peteranderl R, Rabenstein M, Shin YK, Liu CW, Wemmer DE, King DS, et al. Biochemical and biophysical characterization of the trimerization domain from the heat shock transcription factor. Biochemistry. (1999) 38:3559–69. doi: 10.1021/bi981774j
74. Chen Y, Barlev NA, Westergaard O, Jakobsen BK. Identification of the C-terminal activator domain in yeast heat shock factor: independent control of transient and sustained transcriptional activity. EMBO J. (1993) 12:5007–18. doi: 10.1002/j.1460-2075.1993.tb06194.x
75. Wisniewski J, Orosz A, Allada R, Wu C. The C-terminal region of Drosophila heat shock factor (HSF) contains a constitutively functional transactivation domain. Nucleic Acids Res. (1996) 24:367–74. doi: 10.1093/nar/24.2.367
76. Green M, Schuetz TJ, Sullivan EK, Kingston RE. A heat shock-responsive domain of human HSF1 that regulates transcription activation domain function. Mol Cell Biol. (1995) 15:3354–62. doi: 10.1128/MCB.15.6.3354
77. Guo Y, Guettouche T, Fenna M, Boellmann F, Pratt WB, Toft DO, et al. Evidence for a mechanism of repression of heat shock factor 1 transcriptional activity by a multichaperone complex. J Biol Chem. (2001) 276:45791–9. doi: 10.1074/jbc.M105931200
78. Dayalan Naidu S, Dinkova-Kostova AT. Regulation of the mammalian heat shock factor 1. FEBS J. (2017) 284:1606–27. doi: 10.1111/febs.13999
79. Lazaro-Pena MI, Ward ZC, Yang S, Strohm A, Merrill AK, Soto CA, et al. HSF-1: guardian of the proteome through integration of longevity signals to the proteostatic network. Front Aging. (2022) 3:861686. doi: 10.3389/fragi.2022.861686
80. Guettouche T, Boellmann F, Lane WS, Voellmy R. Analysis of phosphorylation of human heat shock factor 1 in cells experiencing a stress. BMC Biochem. (2005) 6:4. doi: 10.1186/1471-2091-6-4
81. Knauf U, Newton EM, Kyriakis J, Kingston RE. Repression of human heat shock factor 1 activity at control temperature by phosphorylation. Genes Dev. (1996) 10:2782–93. doi: 10.1101/gad.10.21.2782
82. Dai S, Tang Z, Cao J, Zhou W, Li H, Sampson S, et al. Suppression of the HSF1-mediated proteotoxic stress response by the metabolic stress sensor AMPK. EMBO J. (2015) 34:275–93. doi: 10.15252/embj.201489062
83. Hardie DG. Role of AMP-activated protein kinase in the metabolic syndrome and in heart disease. FEBS Lett. (2008) 582:81–9. doi: 10.1016/j.febslet.2007.11.018
84. Lage R, Dieguez C, Vidal-Puig A, Lopez M. AMPK: a metabolic gauge regulating whole-body energy homeostasis. Trends Mol Med. (2008) 14:539–49. doi: 10.1016/j.molmed.2008.09.007
85. Zhou JY, Poudel A, Welchko R, Mekala N, Chandramani-Shivalingappa P, Rosca MG, et al. Liraglutide improves insulin sensitivity in high fat diet induced diabetic mice through multiple pathways. Eur J Pharmacol. (2019) 861:172594. doi: 10.1016/j.ejphar.2019.172594
86. Wu SY, Liang J, Yang BC, Leung PS. SIRT1 activation promotes beta-cell regeneration by activating endocrine progenitor cells via AMPK signaling-mediated fatty acid oxidation. Stem Cells. (2019) 37:1416–28. doi: 10.1002/stem.3073
87. Hardie DG. Sensing of energy and nutrients by AMP-activated protein kinase. Am J Clin Nutr. (2011) 93:891S–6. doi: 10.3945/ajcn.110.001925
88. Puthanveetil P, Rodrigues B. Glucocorticoid excess induces accumulation of cardiac glycogen and triglyceride: suggested role for AMPK. Curr Pharm Des. (2013) 19:4818–30. doi: 10.2174/13816128113199990340
89. Gauthier MS, O'Brien EL, Bigornia S, Mott M, Cacicedo JM, Xu XJ, et al. Decreased AMP-activated protein kinase activity is associated with increased inflammation in visceral adipose tissue and with whole-body insulin resistance in morbidly obese humans. Biochem Biophys Res Commun. (2011) 404:382–7. doi: 10.1016/j.bbrc.2010.11.127
90. Ewart MA, Kennedy S. AMPK and vasculoprotection. Pharmacol Ther. (2011) 131:242–53. doi: 10.1016/j.pharmthera.2010.11.002
91. Kang H, Oh T, Bahk YY, Kim GH, Kan SY, Shin DH, et al. HSF1 regulates mevalonate and cholesterol biosynthesis pathways. Cancers (Basel). (2019) 11. doi: 10.3390/cancers11091363
92. Jin X, Moskophidis D, Mivechi NF. Heat shock transcription factor 1 is a key determinant of HCC development by regulating hepatic steatosis and metabolic syndrome. Cell Metab. (2011) 14:91–103. doi: 10.1016/j.cmet.2011.03.025
93. Li M, Hu J, Jin R, Cheng H, Chen H, Li L, et al. Effects of LRP1B regulated by HSF1 on lipid metabolism in hepatocellular carcinoma. J Hepatocell Carcinoma. (2020) 7:361–76. doi: 10.2147/JHC.S279123
94. Uchiyama T, Atsuta H, Utsugi T, Oguri M, Hasegawa A, Nakamura T, et al. HSF1 and constitutively active HSF1 improve vascular endothelial function (heat shock proteins improve vascular endothelial function). Atherosclerosis. (2007) 190:321–9. doi: 10.1016/j.atherosclerosis.2006.03.037
95. Tian X, Zhou N, Yuan J, Lu L, Zhang Q, Wei M, et al. Heat shock transcription factor 1 regulates exercise-induced myocardial angiogenesis after pressure overload via HIF-1alpha/VEGF pathway. J Cell Mol Med. (2020) 24:2178–88. doi: 10.1111/jcmm.14872
96. Xu Q, Hu Y, Kleindienst R, Wick G. Nitric oxide induces heat-shock protein 70 expression in vascular smooth muscle cells via activation of heat shock factor 1. J Clin Invest. (1997) 100:1089–97. doi: 10.1172/JCI119619
97. Shen Q, Chen Q, Liu Y, Xue X, Shen X, He Q, et al. Aspirin relieves the calcification of aortic smooth muscle cells by enhancing the heat shock response. Pharm Biol. (2022) 60:17–24. doi: 10.1080/13880209.2021.2007268
98. Li T, Xiao G, Tan S, Shi X, Yin L, Tan C, et al. HSF1 attenuates LPS-induced acute lung injury in mice by suppressing macrophage infiltration. Oxid Med Cell Longev. (2020) 2020:1936580. doi: 10.1155/2020/1936580
99. Akoumianakis I, Antoniades C. Is stress response a new link between adipose tissue and atherogenesis? The role of HSPs/HSF1. Cardiovasc Res. (2016) 111:10–2. doi: 10.1093/cvr/cvw099
Keywords: heat shock proteins, heat shock factor 1, foam cells, atherosclerosis, lipid metabolism
Citation: Ghai S, Young A and Su K-H (2023) Proteotoxic stress response in atherosclerotic cardiovascular disease: Emerging role of heat shock factor 1. Front. Cardiovasc. Med. 10:1155444. doi: 10.3389/fcvm.2023.1155444
Received: 31 January 2023; Accepted: 15 March 2023;
Published: 3 April 2023.
Edited by:
Chia-Feng Liu, Cleveland Clinic, United StatesReviewed by:
Lin Chang, University of Michigan, United States© 2023 Ghai, Young and Su. This is an open-access article distributed under the terms of the Creative Commons Attribution License (CC BY). The use, distribution or reproduction in other forums is permitted, provided the original author(s) and the copyright owner(s) are credited and that the original publication in this journal is cited, in accordance with accepted academic practice. No use, distribution or reproduction is permitted which does not comply with these terms.
*Correspondence: Kuo-Hui Su a3VvLWh1aS5zdUB1dG9sZWRvLmVkdQ==
Specialty Section: This article was submitted to Cardiovascular Biologics and Regenerative Medicine, a section of the journal Frontiers in Cardiovascular Medicine
Disclaimer: All claims expressed in this article are solely those of the authors and do not necessarily represent those of their affiliated organizations, or those of the publisher, the editors and the reviewers. Any product that may be evaluated in this article or claim that may be made by its manufacturer is not guaranteed or endorsed by the publisher.
Research integrity at Frontiers
Learn more about the work of our research integrity team to safeguard the quality of each article we publish.