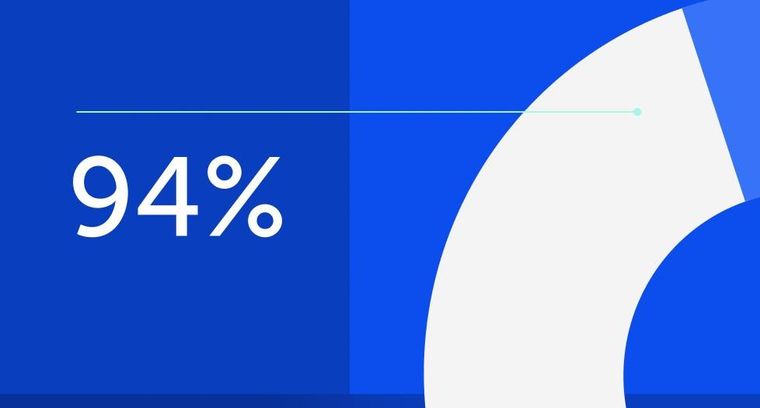
94% of researchers rate our articles as excellent or good
Learn more about the work of our research integrity team to safeguard the quality of each article we publish.
Find out more
REVIEW article
Front. Cardiovasc. Med., 17 February 2023
Sec. Cardio-Oncology
Volume 10 - 2023 | https://doi.org/10.3389/fcvm.2023.1052959
This article is part of the Research TopicInsights in Cardio-Oncology: 2022View all 5 articles
Galectins are carbohydrate-binding proteins that regulate many cellular functions including proliferation, adhesion, migration, and phagocytosis. Increasing experimental and clinical evidence indicates that galectins influence many steps of cancer development by inducing the recruitment of immune cells to the inflammatory sites and modulating the effector function of neutrophils, monocytes, and lymphocytes. Recent studies described that different isoforms of galectins can induce platelet adhesion, aggregation, and granule release through the interaction with platelet-specific glycoproteins and integrins. Patients with cancer and/or deep-venous thrombosis have increased levels of galectins in the vasculature, suggesting that these proteins could be important contributors to cancer-associated inflammation and thrombosis. In this review, we summarize the pathological role of galectins in inflammatory and thrombotic events, influencing tumor progression and metastasis. We also discuss the potential of anti-cancer therapies targeting galectins in the pathological context of cancer-associated inflammation and thrombosis.
Galectins are β-galactoside-binding proteins, belonging to the protein family of lectins that share a common amino acid sequence and the carbohydrate recognition domain (CRD) (1). They are expressed in vertebrates, invertebrates, and unicellular eukaryotic organisms (protists), indicating their fundamental functions during evolution (2). Although galectins are distributed in many tissues, some of the isoforms are more specifically expressed. Galectins regulate the cell cycle, inflammation, immune response, cell adhesion, and cell signaling (1, 3). Galectins are also important players in cancer progression and metastasis by mediating interactions between tumor and tumor microenvironment (1, 3). Galectins are synthesized on free ribosomes with a frequently acetylated carboxy-terminal end (4). Galectins are secreted by an unconventional transport pathway since no signal peptide is detected in these proteins (5). Most studies focused on the extracellular effects of galectins; they can bind plasma membrane proteins and interact with extracellular matrix (ECM) components (6). However, galectins have also important intracellular functions, described in the cytoplasm, nucleus, mitochondria, exosomes, and lysosomes (5, 7). Furthermore, galectins are involved in intracellular trafficking by participating in the apical transport; stabilizing, and sorting of glycoproteins toward their destination (7). Some of the galectin isoforms play a pivotal role in the biogenesis of endocytic vesicles (8). Although most of the extracellular ligands of galectins are glycosylated, the main parts of intracellular ligands are not glycosylated. The gene expression of galectins is regulated in a tissue-specific and developmental stage-dependent manner, including many factors, divalent or multivalent protein complexes, and specific counter-receptors (9). Cancer is one of the pathological conditions that dysregulate gene expression of galectins and these alterations may contribute to common hallmarks of cancers such as neoplastic transformation, resistance to apoptosis, angiogenesis, or tumor metastasis (7). Current research showed that galectins can modulate immune responses and inflammatory effects on tumor cells and may help cancer cells to escape immune surveillance and form metastases (1). Furthermore, galectins bind platelet receptors, and are involved in platelet adhesion and activation, inducing pro-thrombotic events, as observed during atherosclerosis and venous thromboembolism (10–12). In this review, we focus on multiple functions of galectins, which modulate not only tumor cell-autonomous functions but also inflammation and thrombosis, thereby increasing cancer malignancy.
To determine the structure of galectins it is necessary to understand the biochemical properties of galectins which crosslink glycoconjugates on different receptors thereby modulating diverse signaling pathways. Galectins are carbohydrate-binding proteins, characterized by the ability to bind β-galactose-containing glycoconjugates, which is maintained through a carbohydrate recognition domain (CRD) (13, 14). Based on their CRD domain structures, mammalian galectins were classified into proto, chimera, and tandem-repeat isoforms (Figure 1). Prototype of galectins (Galectin −1, −2, −5, −7, −10, −11, −13, −14, and −15) contains one CRD domain per polypeptide and non-covalently linked homodimers (9, 14). Tandem-repeat galectins (Galectin-4,- 6, −8, −9, and −12) are composed of two CRDs that are connected by an unstructured linker peptide (9, 14). Galectin-5 and galectin-6 are expressed in rodents, but not in humans, whereas galectin-11 is found in sheep and galectin-15 was detected in sheep and goats (13, 15). Galectin-3 is a unique member of chimeric isoforms in mammalians, consisting of one CRD domain on the C-terminus, fused to a non-lectin N-terminus which represents two phosphorylation sites (Ser6, Ser12). The phosphorylation status of galectin-3 regulates subcellular localization and translocation of the protein (16). The non-lectin tail also codes a Gly-Pro-Tyr-rich domain with a repetitive “PGAY” motif, assembling a collagen-like structure. The CRD domain forms a β-sandwich to bind complexes with oligosaccharides, poly-N-acetyllactosamine, galactomannan, and polymannan (17, 18).
Figure 1. Galectins bind to β-galactose-containing glycoconjugates through a carbohydrate recognition domain (CRD). Based on their CRD domain structures, mammalian galectins were classified into proto, chimera, and tandem-repeat isoforms. Prototype galectins (Galectin −1, −2, −5, −7, −10, −11, −13, −14, and −15) contain an individual CRD domain per polypeptide and form non-covalently linked homodimers. Tandem-repeat galectins (Galectin −4,− 6, −8, −9, and −12) are composed of two CRDs connected by an unstructured linker peptide that allows for crosslinking of galectins on the cell surface. The chimera-type galectin-3 consists of one CRD domain on the C-terminus and a non-lectin N-terminus which represents two phosphorylation sites crucial for subcellular trans-localization. The N-terminus repetitive “PGAY” motif allows for N-type polymerization to pentamers, whereas multiple CRD domains can form β-sandwich structures.
Dimerization and alternative splicing of galectins can modify the biochemical properties of these proteins. Galectin-1 can form non-covalently linked homodimers through hydrophobic N- and C-terminal residues of two subunits interacting and associating by a 2-fold rotation axis vertical to the β-sheet plane. Although it was experimentally proven that only the dimeric form of galectin-1 is a biologically active form, monomers may also act in a limited fashion in certain signaling pathways as well (17, 18). Galectin-3 forms dimer and oligomer (pentamer) by using a repetitive PGAY motif (17). Galectin-7 forms a “symmetric” sandwich dimer by electrostatic interactions between the F-faces of two monomers. Galectin-7 and Galectin-2 can form “non-symmetric” dimers by electrostatic interactions between β-strand subunits (β1 and β6) of two monomers (4). Galectin-10 recognizes mannose residues rather than β-galactosides (19). Galectin-10 forms dimers between S-faces of two CRD domains of monomers, and mutation of Trp127 in this region could abolish dimerization (20). S-face to S-face dimer formation is unique among galectins, suggesting that galectin-10 may bind different ligands than other galectin isoforms (4, 20). Galectin-10 shares 6 of 7 residues involved in the recognition of glycans and its three-dimensional structure remains identical to other galectins (21). Galectin-13 forms dimers with a disulfide bridge between Cys 136 and Cys138 amino acid residue, and mutation of these two amino acids to serine could abolish dimerization (9, 22). Additionally, six hydrogen bonds, including amino acids of Val135, Val137, and Gln139 could stabilize dimer formation (23). Galectin-4, −6, −8, −9, and −12 form the group of tandem-repeat type galectins, in which CRD domains are connected by a linker peptide, whose length may vary. This enables galectins to crosslink glycan ligands to self-associate as dimers or oligomers (4).
Inflammation is closely associated with the progression of cancer and plays a pivotal role in tumor growth, metastasis, and drug resistance. Therefore, targeting inflammatory processes in cancer may represent an alternative therapeutic strategy (24). Tumor-extrinsic inflammation is triggered by many factors, including infection, obesity, autoimmune disorders, and exposure to tobacco and toxic substances (25). However, inflammation can also be induced by genomic mutation or genome instability of cancer cells, −called tumor-intrinsic inflammation-, that fuel immunosuppressive or tumor-promoting traits, leading to the recruitment and activation of inflammatory cells (25).
Glycosylation of cell surface proteins on cancer and immune cells is a dynamic process that affects cancer growth and inflammatory processes (26). The glycans decorate the cell surface of all mammalian cells and their structures can be altered during the course of cancer-induced inflammation. Multiple enzymatic processes generate glycosidic linkages of saccharides to other saccharides (26). Several factors and enzymes regulate the pathological modifications of these sugar trees during cancer cell differentiation and activation and are also influenced by inflammatory insults (26). Many of these processes are regulated by glycan-modifying proteins such as glycosyltransferases and glycosidases. The expression and enzymatic activity of these proteins depend on the cancer cell type, tumor microenvironment, and developmental stage of cancer. Several modifications are associated with tumor-mediated inflammatory responses such as the secretion and release of chemokines, cytokines, and growth factors (27). Galectins are the key players to regulate tissue homeostasis in cancer, thereby contributing to the progression of chronic inflammation in the tumor microenvironment and vasculature (Figure 2).
Figure 2. Galectins regulate tumor progression and metastasis by responding to inflammatory cues. Galectins can also prime tumor cells and tumor microenvironment to enhance tumor growth and angiogenesis by regulating cell adhesion, migration, and cytokine and chemokine release. The majority of galectins display pro-tumorigenic and metastatic functions, which promote immunosuppressive functions in immune cells and consequently inhibit anti-tumor immunity. TME: tumor microenvironment.
Galectin-1 is synthesized by different cell types in the tumor microenvironment, including macrophages, regulatory T cells, dendritic cells, myeloid-derived suppressor cells, cancer cells, endothelial cells, and fibroblasts (28, 29). The contribution of galectin-1 to inflammation was initially described as a suppressor of cancer-associated inflammation. Glycans are found on many proteins on the immune cell surface such as CD43, CD45, CD69, pre-B cell receptor (BCR), and vascular endothelial growth factor 2 (VEGFR2), and changes in glycosylation modify immune cell responses. Galectin-1 recognizes lactosamines on N- and O-linked glycans and this interaction frequently induces T-cell apoptosis, which could be prevented by α2,6-linked sialic acid treatment (30). Galectin-1 is proposed as a negative regulator of the immune response since it can inhibit T cell-extracellular matrix (fibronectin and laminin) interactions thereby inhibiting the production of pro-inflammatory cytokines, released by activated T cells (31). Galectin-1 treatment can induce a shift from a Th1 towards a Th2-polarized immune response, characterized by decreased interferon gamma (IFNγ) and interleukin 2 (IL-2) secretion and increased IL-5 production, generated by lymph node cells (32–38). The inflammation-suppressing effect of galectin-1 was explained by the depletion of antigen-specific activated T cells through apoptosis (39). In line with these results, selective blockade of galectin-1 in tumor tissues resulted in increased Th1-mediated anti-tumor responses, suggesting potential involvement of this protein in tumor-immune escape (38). Furthermore, galectin-1 can mediate anti-tumor immunity by reducing transendothelial T cell migration (40). Galectin-1 function is associated with the presence of carcinoma-associated fibroblasts (CAFs) in solid tumors. In hepatocellular carcinoma (HCC) which is associated with chronic inflammation and fibrosis, galectin-1 deficiency with increased osteopontin and S100A4 expressions could amplify inflammatory processes, thereby exacerbating the liver injury and fibrosis in a mouse model of HCC (41). In galectin-1-deficient mice, high expression levels of chemokine ligand 5 (CCL5), C-C chemokine receptor 1 (CCR1), chemokine (C-X-C motif) ligand 2 (CXCL2), lymphotoxin beta receptor (LTBR), and serum amyloid A1 (SAA1) were detected with a robust infiltration of neutrophils and macrophages in the liver. Interestingly, galectin-1 effectively protects mice from acute liver inflammation in the C57BL/6 genetic background, but this was not observed in the FVB/NJ mouse strain implicating independent genetic factors (41). Although increased galectin-1 expression in HCC might inhibit fibrosis and inflammation, secreted galectin-1 promotes liver stellate cell activation and migration through clustering neuropilin-1/platelet-derived growth factor beta (NRP1/PDGFRβ) and NRP1/transforming growth factor beta receptor II (TGF-βRII) complexes (42). Therefore, disrupting glycosylation-dependent galectin-1/NRP1 interactions may provide new implications for the development of liver fibrosis therapy (42).
Activated stellate cells and cancer-associated fibroblasts, which represent most of the stroma cells in pancreatic ductal adenocarcinoma (PDAC), could develop an immunosuppressive microenvironment with increased numbers of regulatory T cells, but lack of effector CD4+/CD8+ T cells (43). Galectin-1 is mostly detected in the stroma of the pancreas, thereby promoting tumor-stroma crosstalk in PDAC. In pancreatic stellate cells and CAFs, knock-down of galectin-1 expression could impair fibroblast activation, cancer cell migration and invasion (44). Conversely, treatment of RWP1 pancreatic cells with recombinant galectin-1 could enhance the expression of several pro-inflammatory mediators, such as IL-1α, metalloproteinase 1 (MMP1), S100 calcium-binding protein A7 (S100A7) and ankyrin 3 (ANK3), thereby inducing metastatic potential (44).
In ovarian and breast cancers, IL-6-induced systemic inflammation enhances the mobilization of myeloid-derived suppressor cells which primed ɣδ T cells to produce galectin-1, thereby abolishing anti-tumor immunity (45). Galectin-1-mediated anti-tumor immunity is also regulated through its direct interactions with CD43, CD45, and CD7 receptors expressed on the surface of T cells, leading to the redistribution of glycoreceptors into segregated microdomains (46–48). Besides these mechanisms, glycosyltransferases can create N-acetyllactosamine ligands during cancer progression, which determine the susceptibility of T cells to cell death by galectin-1 (49). Galectin-1-induced apoptosis is regulated by several intracellular mediators, including transcription factors, caspases, cytochrome-C, and ceramide-associated signaling pathways in primary T cells. The pathological impact of galectin-1-induced T cell death was established using different disease mouse models. At lower concentrations, galectin-1 could effectively block T cell adhesion to the ECM, thereby abolishing pro-inflammatory cytokine secretions, such as tumor necrosis factor alpha (TNFα) and IFNγ, while at higher concentrations galectin-1 could induce T cell apoptosis (31). Blockade of immunosuppressive functions of galectin-1 induced tumor rejection, stimulating the generation of tumor-specific T-cell-associated response (31). Galectin-1 can also promote IL-10 production in T cells, suppressing Th1 responses (50). Galectin-1 also has a prominent expression in an immunosuppressive subset of dendritic cells, Zbtb46+, that infiltrate ovarian tumors (51). In melanoma cancer, galectin-1 had a substantial contribution to the immunosuppressive microenvironment, inducing apoptosis of cytolytic T cells and modulating the Th1-Th2 cytokine balance (38). In colon carcinoma, both tumor cell and stroma-resident galectin-1 could influence tumor growth by controlling the frequency and suppressive activity of CD8+ Treg cells (52). In line with this, high galectin-1 expression in colorectal adenocarcinoma patients was correlated to an elevated CD8+ Treg score and poor prognosis (52). In human chronic lymphocytic leukemia, galectin-1 is also expressed in nurse-like myeloid cells and macrophages, which induce the establishment of tumorigenic niches (53).
High levels of galectin-1 were found in the vascular endothelium of primary tumors in the lung, colon, head and neck, and oral cancers, and this high expression was usually associated with tumor-induced hypoxia, and angiogenesis (54). Consistently, knock-out of the galectin-1 gene in zebrafish and mouse models strongly impaired vascular guidance, tumor growth and angiogenesis (55). Knock-down of galectin-1 expression in hs683 glioblastoma cancer cells also inhibited angiogenesis by attenuating endoplasmic stress response and modulating expression of hypoxia-related genes such as CTGF, ATF3, PPP1R15A, HSPA5, TRA1, and CYR61 (56). Galectin-1 is upregulated on the endothelial cell surface after lipopolysaccharide (LPS) or cytokine treatment in vitro and increased galectin-1 expression of endothelial cells was found in inflamed lymph nodes (57). Galectin-1 expression is also enhanced in stromal and endothelial cells, which are treated by conditioned media derived from ovarian and prostate cancer cells (58, 59), indicating the role of galectin-1 in the crosstalk of tumor cells with inflamed vasculature.
Similar to galectin-1, galectin-2 can also bind T cells in a β-galactoside-specific manner and induce apoptosis (60). Elevated serum galectin-2 levels were detected in patients with colorectal and breast cancers. The pathological consequence of higher galectin-2 concentrations is to induce granulocyte colony-stimulating factor (G-CSF), IL-6, monocyte chemoattractant protein-1 (MCP-1) and growth-related oncogene alpha (GROα) secretion from endothelial cells, thereby increasing the expression of endothelial cell surface adhesion molecules, and enhancing cancer cell-endothelial cell interactions, angiogenesis and tumor metastasis (61). Higher galectin-2 levels are also a driving force of pro-inflammatory M1 phenotype in human monocytes by inducing toll-like receptor 4 (TLR4) signaling through CD14 interaction (62).
The role of galectin-2 in T cell apoptosis was studied in mice in which galectin-2 levels were inversely correlated to the occurrence of colitis. Mouse treatment with recombinant galectin-2 strongly reduced the rate of colitis by inducing T-cell apoptosis, located in the mucous membranes (63). In other studies, galectin-2 could interact with a pro-inflammatory cytokine lymphotoxin-α in smooth muscle cells and macrophages (64). Although several studies pointed out the pro-inflammatory and pro-angiogenic functions of galectin-2, experimental evidence is still missing to conclude the exact role of galectin-2 during cancer progression and metastasis.
Galectin-3 expression is upregulated in many types of solid tumors and increased protein levels correlate with the degree of malignancy (65). Galectin-3 levels in the blood are also increased in patients with breast, colon, and lung cancers (66). Interestingly, higher concentrations of galectin-3 were detected in metastatic tumors than in primary tumors (67). Galectin-3 is detected nearly in all the stages of tumor development (65, 67). Galectin-3 inhibits apoptosis by competing for a conserved structure with B-cell lymphoma 2 (Bcl-2) inhibiting the function of cell cycle inhibitors (68). Galectin-3 also enhances cell survival and proliferation by regulating phosphatidylinositol 3-kinase (PI3K)/protein kinase B (AKT) signaling and nuclear factor kappa B (NF-κB) pathways (69).
Galectin-3 induces secretion of IL-6, G-CSF, soluble intercellular adhesion molecule-1 (sICAM-1), and granulocyte-macrophage colony-stimulating factor (GM-CSF) in endothelial cells (70). These cytokines interact with the vascular endothelium and trigger diverse signaling pathways, thereby increasing the expression of endothelial cell surface markers and integrins, such as E-selectin, ICAM-1, and vascular intercellular adhesion molecule (VCAM-1) and αvβ1 integrin (70). In patients with metastatic colon cancer, higher serum levels of galectin-3 correlated with increased serum levels of G-CSF, IL-6, and sICAM1. In breast and colon cancer patients, increased serum levels of galectin-1 and galectin-3 also correlated with enhanced expression of G-CSF, and IL-6 (70), suggesting a modulatory role of galectin-3 in inflammation. Furthermore, neutralizing antibodies against pro-inflammatory mediators could prevent the adhesion of mucin-1 (MUC-1)-negative melanoma cells to the HMVECs endothelial cells, indicating that galectin-3-mediated secretion is regulated by inflammatory cytokines induces endothelium-tumor cell interaction (71).
Galectin-3 can directly interact with adhesion molecules. Galectin-3 binds the CD146 receptor on the surface of endothelial cells and consequently induces inflammatory cytokine secretion (72). Galectin-3-CD146 interactions could enhance endothelial cell migration (72). The oncofetal Thomsen-Friedenreich carbohydrate antigen (TF-antigen), Galβ1-3GalNAcα, is a pan-carcinoma antigen and highly expressed in almost all human carcinomas due to the aberrant glycosylation. Galectin-3 binds TF-antigen and this interaction induces diverse pathological processes such as tumor cell aggregation, cancer metastasis, and T cell apoptosis (73). Galectin-3 interacts with TF-antigen on the surface of the transmembrane mucin protein MUC-1 in cancer cells. The protein complex of Galectin-3-TF/MUC-1 induces MUC-1 cell surface polarization leading to the exposure of cell adhesion molecules, thus inducing tumor cell adhesion to the vascular endothelium which increases the number of cancer cell aggregates in the circulation (74). In contrast, O-glycan-modifying sialyltransferase ST6GalNAcs reduces the binding affinity of galectins (galectin-1 and galectin-3) to the tumor cell surface, thereby inhibiting intravascular aggregation of tumor cells and consequent metastasis (75, 76). Interestingly, liver macrophage-resident galectin-3 enhanced the liver retention of cancer cells that expressed high levels of sialyltransferase ST6GalNAc4, but low levels of glucosaminyltransferase GCNT3 (77).
Cancer stemness is a stem-cell-like phenotype of cancer cells and is involved in the reconstitution and propagation of tumor formation. Galectin-3 is upregulated in human renal cell carcinoma and associated with higher expression levels of stemness-related genes, such as Oct4, Sox2, and Nanog (78). In renal cell carcinoma, downregulation of galectin-3 could strongly inhibit cancer cell invasion, colony formation, sphere-forming ability and stemness-associated gene expression (78). In galectin-3 knock-down cells, CXCL6, CXCL7, and CXC chemokine receptor 2 (CXCR2) expressions were downregulated and overexpression of CXCR2 could restore the ability of galectin-3 knock-down cells to form spheres (78). Altogether, these results suggest that galectin-3 regulates cancer stemness, and induces cancer-cell intrinsic inflammation by upregulating CXCR2, thereby increasing tumor progression.
The function of Galectin-3 is also associated with RAS signaling in cancer since the CRD domain binds and stabilizes the active conformation of KRAS (79, 80). Galectin-3 with KRAS can induce PI3K activation as well as constitutive activation of the RAF/MEK/ERK signaling cascade, thereby regulating tumor cell functions. Moreover, in glioblastoma cancers, galectin-3 favors tumor invasiveness through a micropinocytosis-mediated uptake mechanism (80). Upregulation of galectin-3 expression was correlated with increased anchorage-independent growth and organ colonization. Galectin-3 potentiates cell migration and metastasis through activation of the K-RAS–RAF-Erk1/2 pathway in colon cancer. Galectin-3 also regulates pancreatic cancer metastasis through the activation of RAS–ERK/AKT and Rel-A signaling pathways, thereby increasing cell migration, and survival (81).
Downregulation of galectin-3 could effectively inhibit tumor cell migration, invasion, cell proliferation and metastasis in osteosarcoma, thyroid, and gastric cancer (82–84). Interestingly, galectin-3 knock-down studies published by Bresalier et al. showed direct evidence of its role in tumor invasion and metastasis. The authors observed reduced liver colonization and spontaneous metastasis of galectin-3 knock-down LSLiM6 and HM7 cells, two derivatives of the colonic adenocarcinoma LS174T cells with high liver-metastasizing potential (85). Consistently, knock-down of the galectin-3 gene was associated with the inhibition of cell migration, invasion, cell proliferation, colony formation and tumor growth in nude mice (85). Galectin-3 expression in PC3 human cancer cells resulted in cell cycle arrest at the G1 phase; upregulation of p21 levels in nuclei, and hypophosphorylation of the tumor suppressor protein pRb (86). In mouse models of breast and prostate cancers, galectin-3 was cleaved by MMP2 and MMP9 and this was associated with enhanced tumor growth and angiogenesis (87, 88). In line with this result, the cleavage products of galectin-3 were detected in the blood serum of prostate cancer patients with advanced or metastatic tumors (86, 89).
Galectin-3 is also involved in tumor immunity to regulate natural killer (NK) and T cell functions. Galectin-3 could inhibit the interaction of NK cells with cancer cells, thereby evading the ability of cytotoxic effects of NK cells to kill them (90). Extracellular galectin-3 binds several glycoproteins on the T cell surface and induces apoptosis (91). Galectin-3 also suppresses CD8 T cell function in melanoma cancer, possibly influencing lymphocyte activation gene-3 (LAG-3) function on the immune cell surface (92). Furthermore, increased galectin-3 expression is associated with the loss of T cell receptor (TCR) and CD8 marker localization and loss of effector T cell function (93, 94). Galectin-3 could induce a robust production of pro-inflammatory cytokines in different immune cells (95). In line with this, in vivo depletion of galectin-3 was shown to increase both the number of functional CD8+ T cells and the consequent expression of pro-inflammatory cytokines, thereby inducing tumor rejection in galectin-3-deficient mice (92, 94).
Galectin-3-mediated ligand clustering triggers neutrophils to phagocytose, produce reactive oxygen species (ROS), release proteases, and secrete IL-8 (96–98). Galectin-3 induces degranulation in mast cells. Recent studies revealed the critical roles of galectin-3 in this cell type since galectin-3-deficient mast cells show reduced histamine release and IL-4 secretion (99).
Downregulation of galectin-4 was observed in acute myeloid leukemia and colon cancer (100, 101). Galectin-4 was proposed as a tumor suppressor inhibiting cell proliferation by down-regulating wingless/integrated (Wnt) and IL-6/NF-κB/Signal transducer and activator of transcription 3 (STAT3) signaling that balance epithelial cell homeostasis in the intestine (102). Galectin-4 also inhibits cell migration and metastasis in PDAC (103). In this pathological condition, galectin-4 markedly reduced cytoplasmic β-catenin levels, counteracted Wnt signaling function, and rendered pancreatic cancer cells sensitive to Wnt inhibitors (103). Consequently, the galectin-4 deficiency was associated with early cancer recurrence and death, defined as occurring twelve months after curative surgery. Under inflammatory conditions, galectin-4 suppresses tumor growth by stimulating memory CD4+ T cell expansion (104, 105). This process involves the interaction of galectin-4 with immature core-1-expressing O-glycans generated by downregulation of the core 2-β1,6-N-acetylglucosaminyltransferase-1 (104). Consequently, ectopic expression of core 2-β1,6-N-acetylglucosaminyltransferase-1 could reduce tumor growth (104).
In sharp contrast with these studies, the galectin-4 function was also proposed to enhance tumor angiogenesis and metastasis (61, 100, 106). Upregulation of galectin-4 was observed in patients with advanced liver cancer, intraductal breast cancer, and gastric and colorectal cancers. Elevated galectin-4 expression was associated with venous but not lymphatic invasion of lung adenoma cancer cells (107). Therefore, quantification of galectin-4 expression levels in the blood could serve as an oncogenic biomarker. Galectin-4 enhanced cancer cell adhesion to the endothelial cells through binding with TF-antigen on cancer-associated MUC-1 (108).
Furthermore, galectin-4 regulates the pathogenesis of inflammatory bowel diseases and colitis. Galectin-4 activates CD4+ T cells and IL-6 secretion that contributes to the progression of colitis in mice. This disease was cured by injecting anti-galectin-4 blocking antibodies into mice (109). Galectin-4 directly interacts with the immunological synapse of CD4 + T cells thereby activating protein kinase C (PKC) signaling which further stimulates IL-6 production, thereby exacerbating inflammation in the intestine (109).
Galectin-7 was described as an epithelial differentiation marker in different organs (110). High expression levels of galectin-7 were found in aggressive subtypes of breast cancer, frequently with a basal-like phenotype and estrogen receptor-negative tumors (111). Galectin-7 also enhances spontaneous metastasis in both human epidermal growth factor receptor 2 (HER2) overexpressed and basal-like lineages of breast cancer (112). Upregulation of galectin-7 was sufficient to induce a metastatic feature of tumor cells and rendered them resistant to cell death (112). Galectin-7 was detected in the cytoplasm, but it can translocate into the nuclei after cellular stimuli or also be secreted into the extracellular place (113). Galectin-7 gene expression is modulated by several cytokines, transcription and growth factors. P53-induced galectin-7 expression in breast cancer cells correlated with high NF-κB activity, suggesting that induction of galectin-7 expression by P53 is dependent on NF-κB (114). In addition, NF-κB can bind galectin-7 promoter, indicating that galectin-7 and P53 may regulate cancer metastasis through common mechanisms (114). In galectin-7-transfected urothelial cancer cells, increased ROS production and jun N-terminal kinase JNK/ BCL2-associated x protein (Bax) signaling were detected (115). Galectin-7 expression was strongly enhanced by insulin growth factor 1 (IGF-1) and cyclooxygenase-2 (COX-2) in melanoma (116, 117). Although galectin-7 rendered B16F1 melanoma cells resistant to apoptosis but also inhibited cancer cell motility through increased expression of early growth response protein 1 (EGR-1) (118). It was proposed that the upregulation of galectin-7 expression contributes to cell survival by enhancing extracellular signal-regulated kinase (ERK) and Jnk signaling pathways (119). Pro-invasive and pro-metastatic functions of galectin-7 were associated with increased expression of MMP2 and MMP9 in oral squamous cells and ovarian carcinoma (119). Galectin-7 binds human tumorous imaginal disc (Tid1) heat shock protein 40 (Hsp40) and this interaction attenuates tumorigenicity and metastasis of head and neck squamous sarcoma cancer cells (120). Interestingly, N-linked glycosylation of Tid1 is required to interact with galectin-7, which induces protein degradation of galectin-7, explaining the protective effect of this molecular interaction (120).
Galectin-7 is secreted by ovarian cancer cells and is involved in the regulation of tumor invasiveness. Recombinant human galectin-7 killed Jurkat T cells and human peripheral T cells, suggesting that galectin-7 has strong immunosuppressive properties (121). Constitutive expression of galectin-7 was found in aggressive metastatic lymphoma. Transfection of T-cell lymphoma cells with galectin-7 also increases tumor growth in mice (122). The histopathological analysis of mice showed large metastatic tumors with increased invasive and infiltration rates (122). Although galectin-7 can act as an oncogene, the opposite roles have been also shown in gastrointestinal cancers. Ectopic expression of galectin-7 in colon cancer cells rendered them more sensitive to apoptosis after treatment with actinomycin D, cobalt chloride, hydrogen peroxide or under hypoxia (123). Consequently, overexpression of galectin-7 strongly reduced gastric cancer cell proliferation, migration, and invasion. Interestingly, lower galectin-7 expression was detected in patients with gastric cancer compared to controls and the expression levels were significantly associated with tumor grade, stage, and better survival of patients with gastric cancer (123). Galectin-7 also reduces the invasiveness of prostate cancer cells by inhibiting cell motility and rendering cancer cells sensitive to apoptosis in response to chemotherapeutic agents (124). Interestingly, the CRD-defective mutant form of galectin-7 (R7S) can modulate apoptosis or translocate to the mitochondria and nucleus, indicating functional independency from its CRD domain (124). However, the CRD domain was necessary to inhibit the invasive behavior of cancer cells thereby potentiating tumor growth.
Galectin-8 binds different types of integrins (α1β1, α3β1, α5β1 and α6β1) with similar kinetics to fibronectin, but not interacts with α2β1 or α4β1 integrins, suggesting an important but selective function in cell spreading and adhesion (125). Galectin-8 and integrin interaction creates a complex structure, involving sugar/protein interactions, which regulates actin cytoskeletal rearrangements. After integrin activation with galectin-8, phosphorylation of paxillin, focal adhesion kinases, and activation of PI3K, Rac family small GTPase 1 (Rac-1) and ERK1/2 signaling was detected (126, 127). Galectin-8 can enhance tumor metastasis by regulating the rearrangement of the cytoskeleton and E cadherin expression, inhibiting anoikis and homotypic aggregation of cancer cells (128). Galectin-8 is highly expressed in breast, prostate, and lung cancer tissues and elevated serum levels of galectin-8 promote cellular interactions between cancer cells and vascular endothelium (129). The treatment of endothelial cells with galectin-8 induces the production of several inflammatory cytokines such as IL-8, RANTES/CCL5, GRO-α, GRO-γ, M-CSF, IL-6, and MCP1 (130). Galectin-8 also stimulates cytokine expression in other cell types such as liver, lung, spleen, osteoclasts, and bone marrow-derived dendritic cells (129). Interestingly, metastasis-promoting effects of galectin-8 are independent of endothelial cell interaction. Galectin-8-linked tumor invasion and metastatic tumor cell spread are also regulated by immunomodulatory cytokines and chemokines (129).
Galectin-9 is constitutively expressed in antigen-presenting cells and its expression is upregulated by interferons in cancer cells (131). The function of galectin-9 is strongly associated with tumor-immune microenvironment and immunosuppression in different cancer types. Galectin-9 binds to Ig and mucin domain-containing molecule 3 (Tim-3) on the surface of T cells, thereby inducing cell death (132–134). Besides, galectin-9 also regulates immunosuppressive immune cell function by promoting regulatory T-cell differentiation and expansion through binding with a cluster of differentiation 44 (CD44) (132). Although the galectin-9 expression is associated with a good prognosis in some cancers, it is associated with unfavorable outcomes in other tumor types (135). Galectin-9 is strongly upregulated in the most invasive type of multiple glioblastomas and is correlated with poor patient survival (136). It was proposed that the blockade of galectin-9-mediated Tim3 signaling is effective to impair glioma progression by inhibiting macrophage M2 polarization and tumor angiogenesis (137). Interestingly, endothelial cell activation and angiogenesis can regulate the alternative splicing of galectin-9 gene, indicating that the pathophysiological change of the tumor microenvironment could induce epigenetic factors, thereby changing the alternative splicing mechanism, protein structure and function of galectin-9 variants (138).
Upregulation of galectin-9 expression was found in immune cells, tumor cells, and blood plasma of PDAC and melanoma patients (139, 140). Furthermore, elevated galectin-9 levels were detected in tumor-infiltrating T lymphocytes from non-responders to anti-programmed death-1 (PD-1) therapy compared with those from the responders (131). In a mouse model of PDAC, blockade of galectin-9 function could effectively retard tumor progression and prolonged survival by inducing maturation and expansion of macrophages and dendritic cells, and modulating the immune response of NK and tumor-associated CD8+ T cells (141–143). In the mouse models of triple-negative breast cancer, 4-1BB, a galectin-9-neutralizing antibody in combination with an agonist antibody to the tumor necrosis factor receptor (TNFR) synergistically inhibited tumor growth and prolonged mouse survival (144).
Galectin-9 interacts with cell surface adhesive molecule CD44 and this interaction inhibits the complex formation of CD44 with hyaluronic acid, which consequently attenuates metastatic dissemination of melanoma and colon cancer cells (145). Similarly, galectin-9 also acts as a competitive inhibitor by blocking the VCAM1-α4β1 interactions, thereby reducing tumor cell adhesion to the vascular endothelium and consequent tumor cell extravasation and metastasis (145). Furthermore, galectin-9 can inhibit melanoma cancer metastasis by triggering the aggregation of cancer cells, which impairs cell detachment and escape from the primary tumor (146). Galectin-9 is also involved in epithelial cell polarity through binding with apically residing glycolipid (Forssman antigen) (147). The pro-apoptotic function of galectin-9 has been described in ovarian cancer, leukemia, and myeloma cell culture models (148–150). Galectin-9 inhibited the growth of leukemia and myeloma cancer cells through activating transcription factor-ATF-Noxa, JNK, P38 mitogen-activated protein kinase (MAPK) and caspase-3 pathways (149, 150). Altogether, these studies suggested that depending on the disease context, galectin-9 can serve as a biomarker and therapeutic target in cancer.
Galectin-10 is highly expressed in human eosinophilic and basophilic granulocytes, but not in mouse granulocytes (151–153). Interestingly, a subgroup of eosinophils was identified with high levels of galectin-10 and CD16 expression, and these eosinophils are more potent T-cell suppressors than conventional eosinophils (154). Proteomic analysis of human CD4+ and CD25+ regulatory T cells identified galectin-10 as a novel marker that distinguishes this population from resting and activated CD4+ T cells (155). Inhibition of galectin-10 restored the proliferative capacity of human Tregs and abolished their immunosuppressive function (155).
Galectin-12 deficiency leads to M2 macrophage polarization that consequently results in reduced foam cell formation and pro-inflammatory cytokine production (156). Galectin-12 also affects myeloid differentiation, which is associated with chemotherapy resistance (157, 158). Overexpression of galectin-12 induces cell cycle arrest at the G1 phase in cancer cells, thereby suppressing cell proliferation (159). A positive association between cell differentiation and galectin-12 expression was described in human colorectal cancer cell lines (160).
Galectin-13 is predominantly detected in the placenta (161). It is also expressed in the kidney, bladder, and spleen and also in neurogenic tumors, liver adenocarcinoma, and melanoma (162). Placental galectin-13 was shown to enhance the apoptosis of T cells, by inducing the synthesis of IL-8 (163). Furthermore, T cells produce a high amount of chemotactic molecules, thereby inducing neutrophil extravasation in the endometrial decidua (164). Interestingly, galectin-13 can also trigger necrosis to induce the recruitment of immune cells, thereby allowing trophoblast invasion and vessel remodeling (165). Galectin-13 also polarizes placental neutrophils toward an immune regulatory phenotype by triggering the production of ROS, hepatocyte growth factor (HGF), and MMP9 and upregulating the expression of programmed death-ligand 1 (PD-L1) (166). Future studies are required to study the role of galectin-13 in the regulation of cancer-associated immunosuppressive and inflammatory phenotype.
Galectin-14 is expressed in the placenta. It was proposed that galectin-14 may regulate immune tolerance at the maternal-fetal interface by inducing apoptosis of leukocytes and T cells (163). Galectin-14 also facilitates migration and invasion by promoting an epithelial-mesenchymal transition (EMT)-like phenotype in trophoblasts (167). Galectin-14 is expressed at a high level in several cancer types, including liver, breast, uterine, and ovarian cancer (168). Furthermore, increased expression of galectin-14 has been reported to correlate with shorter survival in high-grade serous adenocarcinoma ovarian cancer (168).
Besides the regulation of the immune system, galectins also regulate hemostasis and thrombosis through interplay with platelets, endothelium, and the coagulation system. Upon vessel injury, subendothelial matrix proteins, such as collagen are exposed to the blood flow, anchoring von-Willebrand-Factor (vWF) and initiating platelet glycoprotein (GP)Ibα–vWF interaction and subsequent GPVI–collagen interaction, a crucial step in platelet activation (169). Activated platelets express several integrins on the surface. The outside-in activation of integrins with their extracellular ligands also induces platelet adhesion to the injured vessel wall. In addition, activated platelets release many bioactive molecules and secondary mediators, such as fibrinogen (FGN), vWF, adenosine diphosphate/adenosine triphosphate (ADP/ATP), and serotonin from their alpha (α) and dense delta (δ) granules further enhancing the pro-thrombotic process to induce the recruitment of circulating platelets to the growing thrombi. After platelet accumulation, the blood coagulation pathway induces the second wave of hemostasis, generating thrombin through extrinsic and intrinsic pathways. In turn, thrombin converts soluble fibrinogen to fibrin, resulting in enhanced platelet activation (169, 170). Activated platelets expose phosphatidylserine (PS) on their surface, which facilitates the binding of coagulation factors that stimulate the generation of thrombin in other circulating and vascular cells. These processes can also occur in diseased vessels, such as atherosclerotic inflamed vessels as well as when cancer cells grow and or propagate to the organism, forming metastases (171).
The simultaneous activation of thrombotic and inflammatory responses occurs in various diseases and has been referred to as thromboinflammation (172, 173). Platelets and the intravascular thrombus formation represent a scaffold for the cells of the innate immune system to facilitate the recognition, containment, and destruction of pathogens (174, 175). Innate immune cells and platelets interact and reciprocally influence the thrombotic potential and immune reaction. Neutrophils interact with P-selectin expressed on the surface of activated platelets via P-selectin glycoprotein ligand-1 (PSGL-1) (176). Platelet interaction enhances downstream MAPK activation in neutrophils, IL-8 production, and neutrophil adhesion to fibrinogen by Src kinase-dependent activation of β2 integrin, thereby facilitating increased immunogenic potential (177). Platelet GPIbα binds myeloid cells via various expressed receptors like integrin αMβ2 or complement receptor 3 (CR3), promoting leukocyte recruitment and activation, as well as integrin-mediated adhesion and diapedesis, that result in the infiltration of inflammatory cells into the tissues (178). Galectin levels are dramatically increased in cardiovascular diseases, including atherosclerosis, diabetes mellitus, ischemic stroke, and venous thromboembolism (Figure 3), suggesting a pathological link between galectin function and thromboinflammation (10).
Figure 3. Galectins are involved in thrombosis and thromboinflammation through interplay with vascular endothelium, immune cells, and blood platelets. Galectins can promote platelet adhesion activation and regulate coagulation pathways, through interactions with glycoproteins, integrins, and coagulation factors. Galectins have multiple roles in thromboinflammatory diseases, such as atherosclerosis, myocardial infarction, deep vein thrombosis, or ischemic stroke. The regulatory role of galectins strongly depends on the pro-thrombotic, pro-inflammatory, and immunosuppressive landscape of the vasculature and surrounding environment. Therefore, the effects of galectins may vary depending on the stage and pathogenesis of the disease.
Two isoforms, galectin-1 and galectin-3 can bind N- and O-glycans on the surface of coagulation factor VIII (FVIII), indicating an important role of these interactions in the regulation of the coagulation cascade (179). In normal physiological conditions, plasma contains 1–10 μg/ml galectin-1 and galectin-3 and their levels are stabilized through binding to vWF (179, 180). In the absence of galectin-1 and galectin-3, a strong accumulation of platelet-decorated vWF strings was observed on the surface of endothelial cells, subsequently enhancing the formation of arterial thrombi (181). Galectin-1 also regulates fibrinolysis through binding to tissue-plasminogen activator (t-PA) and increasing its catalytic activity (182). Galectin-1 can directly activate platelets. Pre-incubation of human platelets with exogenous galectin-1 enhanced integrin αIIbβ3 activation, P-selectin exposure, and microparticle shedding (183). Galectin-1-deficient (Lgals1−/−) mice displayed normal platelet count, but increased bleeding time, indicating altered hemostasis (183). Biochemical analysis using integrin αIIbβ3-deficient platelets, isolated from patients with Glanzmann’s thrombasthenia, identified galectin-1 as a functional binding partner of integrin αIIbβ3 (183). Although normal levels of integrin αIIbβ3 were observed in Lgals1−/− platelets, impaired spreading on fibrinogen and reduced clot retraction were observed, indicating a failure in the “outside-in” activation of αIIbβ3 signaling. Galectin-1 was proposed as a prominent factor linking thrombosis and inflammation (183). Galectin-1-stimulated platelets expose P-selectin which interacts with PSGL on leukocytes, thereby inducing the formation of heterotypic aggregates (183).
Although galectin-1 is detected in normal heart tissues including cardiomyocytes, its level is strongly increased during acute myocardial infarction (184). It was hypothesized that early upregulation of galectin-1 can serve as a safeguard mechanism to prevent dysregulated inflammatory responses (184). Elevated galectin-1 expression influences the resolution of inflammation at later steps of acute myocardial infarction by restoring tissue homeostasis. In line with these assumptions, Lgals1−/− mice displayed adverse ventricular remodeling after acute myocardial infarction associated with excessive inflammation (184). Galectin-1 deficiency increased cardiac infiltration of T cells, macrophages, and NK cells, but reduced recruitment of anti-inflammatory Treg cells, indicating that galectin-1 regulates Treg-mediated protection during acute myocardial infarction (184). Protective effects of galectin-1 were also observed in the early phase of ischemia–reperfusion-induced kidney injury, improving renal function and inhibiting the release of pro-inflammatory mediators and leukocyte transmigration (185).
Galectin-1 is expressed in neurons and glial cells. Galectin-1 stimulates astrocyte differentiation and secretion of brain-derived neurotrophic factor (186). The function of galectin-1 was studied in an experimental stroke model and elevated galectin-1 expression was detected in the infarcted and penumbra area (187). After ischemic insult in stroke, galectin-1 treatment sustained neurogenesis in a carbohydrate-dependent manner and this process was inhibited by an anti-galectin-1 blocking antibody (187). The transfer of galectin-1-overexpressing stem cells into the mice strongly reduced infarct volume upon ischemic stroke. Galectin-1 treatment also showed neuroprotective effects by inhibiting central nervous system inflammation and preventing inflammation-induced neurodegeneration (186). Proposed mechanisms involved galectin-1 binding to M1-type microglia, sloping the balance toward the M2-type anti-inflammatory phenotype (186). Furthermore, galectin-1 protected neurons by modulating the expression of the glutamate receptor NMDA, which prevented the neurotoxicity of glutamate during the stroke (188). Although increased serum galectin-1 levels were observed after ischemic stroke, no association has been observed between galectin-1 levels and post-stroke recovery (189).
Macrophages and smooth muscle cells of atherosclerotic lesions express both galectin-2 and lymphotoxin-α, indicating the involvement of galectin-2-mediated pro-inflammatory cytokine responses in this process (64). Galectin-2 acts as a pro-inflammatory factor by transforming human macrophages toward an M1 phenotype through binding to CD14 and activating the TLR4 pathway, thereby inhibiting arteriogenesis (62). Studies on the Japanese population showed the existence of a functional single-nucleotide polymorphism (SNP) rs7291467 (C3279C-T) in the galectin-2 coding gene that was strongly associated with myocardial infarction (64). C3279C-T genetic substitution affected the transcriptional levels of galectin-2 in vitro, altering the secretion of lymphotoxin-α and inflammation (64), indicating on the link between galectin-2 function and pathogenesis of myocardial infarction.
Atherosclerotic plaques express high levels of galectin-3 and correlate with the degree of atherosclerosis and inflammation (190). Galectin-3 exacerbates vascular inflammation by stimulating macrophages to produce a range of pro-inflammatory mediators (191). Galectin-3 is also associated with macrophage differentiation absorbing oxidized low-density lipoprotein (ox-LDL) and transforming macrophages into foam cells, thereby accelerating atherosclerosis (192). Galectin-3 aggravates the ox-LDL-mediated endothelial injury by stimulating inflammation through the activation of integrin β1-RhoA-JNK signaling (193). Galectin-3 inhibition was shown to reduce the pathological consequences of a high-fat diet-induced cardiac lipotoxicity in mice (194). Galectin-3 also activates fibroblasts and contributes to the synthesis of collagen I, additionally modulating the ECM degradation through tissue inhibitor of metalloproteinase (TIMP) and MMP activation (195–197). Galectin-3 strongly binds the immunoglobulin E (IgE) receptor and this interaction triggers basophil and mast cell activation, thereby enhancing inflammatory processes (198).
Using mouse stasis models of venous thrombosis, galectin-3 and its binding protein (Gal3-BP) were detected on vein walls, red blood cells, platelets, and microparticles, contributing galectin-3 functions to venous thrombosis (180). In prospective epidemiological studies, participants with plasma galectin-3 concentrations in the highest quintile had a higher risk of venous thromboembolism than did those in the lowest quintile. Gal3-BP was also detected on leukocyte-derived microparticles during deep venous thrombosis (180). Consistently, galectin-3 and Gal3-BP-deficient mice showed significantly reduced deep venous thrombosis. Interestingly, a similar phenotype was observed in IL-6-deficient mice (180). Although the galectin-3 treatment could restore thrombus formation in galectin-3-deficient mice, it had no effect in IL-6-deficient mice, suggesting that galectin-3 may promote deep venous thrombosis through upstream signaling of IL-6 pathway (180). A direct correlation between blood plasma levels of galectin-3/Gal3-BP and pro-inflammatory cytokines and chemokines was observed in patients with deep venous thrombosis (180). Galectin-3 treatment in mice or cell cultures could induce IL-6, CCL2, and TNF-α secretion in a dose-dependent manner (180).
During venous thrombosis, red blood cells promote thrombus growth by enhancing platelet recruitment, subsequent blood coagulation, and fibrin formation (174). Platelet GPVI contributes to deep venous thrombosis by interacting with fibrin (199). Galectin-3 is highly expressed in red blood cells, vessel walls, and leukocytes (180), and can interact with platelet GPVI, indicating that this interaction may regulate both thrombosis and thromboinflammation in different vascular territories. Recently we showed that galectin-3 is a binding partner of platelet GPVI and that interaction induces platelet activation, shape change, degranulation, and ATP release, thereby inducing tumor metastasis (200).
High plasma galectin-3 levels participate in cardiovascular disease by directly enhancing endothelial cell injury, platelet activation, and immune cell migration. High serum and plasmatic galectin-3 levels are also associated with the severity of coronary stenosis in patients with coronary artery disease (201, 202). Accumulated galectin-3 directly activates the dectin-1 receptor, thereby potentiating platelet aggregation, and ATP release in the blood, which contributes to the development of thrombosis and myocardial infarction (201). Galectin-3 has a pivotal role in the regulation of immunological functions in dendritic cells, monocytes, and macrophages (95). Galectin-3 stimulates the expression of pro-inflammatory chemokines to attract monocytes and macrophages to the vascular injury sites (191). Galectin-3 can also bind mucins and integrins at the cell surface thereby promoting adhesion between granulocytes and vascular endothelium (203). Inhibition of galectin-3 function strongly reduces expression of pro-inflammatory mediators, such as IL-6, IL-1β, IL-23, and P19, and upregulates IL-10, IL-12, TLR/NLR-pathways in dendritic cells and monocytes, thereby inhibiting the development of Th17/T2 cells and innate immunity (204). Plasma galectin-3 levels are increased in patients with peripheral artery disease and are positively correlated with oxidative stress markers, such as F2-isoprostanes (205). Interestingly, oxidative stress increases galectin-3 levels in monocytes (206). In activated neutrophils, galectin-3 enhances the secretion of hyperoxides (205). Oxidative stress-promoting effects of galectin-3 were also observed in mast cells, this was blocked by an antioxidant superoxide dismutase. High galectin-3 expression was detected in M1 macrophages in pancreatic inflammatory infiltrates in a model of type 2 diabetes (207). Galectin-3 also acts as an endogenous paracrine ligand of TLR4 on microglia cells, inducing M1-type phenotype exacerbating inflammation (208).
The role of galectin-3 in ischemic stroke remains controversial. In the rat middle cerebral artery occlusion (MCAO) model of ischemic stroke, galectin-3 promotes neuronal cell viability during oxygen–glucose deprivation conditions by increased phosphorylation of AKT, decreased phosphorylation of ERK1/2 and impaired activation of caspase-3 (209). Galectin-3 supports neuro-vascular protection and functional post-stroke recovery by modulating angiogenic and apoptotic pathways in the brain (209). In mouse models of hypoxic–ischemic brain injury, galectin-3 deficiency reduces oxidative stress, MMP activity, and overall brain injury. Recently the studies by Zhuang et al., which included 288 stroke patients showed that higher serum levels of galectin-3 were associated with stroke severity at admission and stroke prognosis at the discharge of ischemic stroke (210). In another study, higher levels of serum galectin-3 were independently associated with an increased risk of death or major disability after stroke onset (210).
Obesity is strongly associated with the progression of cardiovascular diseases and diabetes. Elevated galectin-4 levels were associated with a higher probability of obesity-associated hospitalization in diabetic patients (211). Thus, galectin-4 could be a risk factor in the pathogenesis of diabetes mellitus.
Galectin-7 is predominantly expressed in stratified skin epithelium and upregulated during skin injury (113). Galectin-7-deficient mice showed delayed wound healing which is caused by impaired cicatrization (113). Interestingly, the process of wound healing was attenuated by lactose, but not sucrose, indicating a selective, lactose-dependent involvement of galectin-7 in this process (113). Upregulation of galectin-7 was observed during placenta formation in mice that induce systemic preeclampsia via impaired placental formation and enhanced release of inflammatory cytokines (212). These studies highlighted galectin-7 as a sentinel molecule, constantly sensing the integrity of the tissues under a steady state while contributing to posttraumatic tissue generation. Disrupted integrity of epithelium and elevated galectin-7 levels were usually observed in asthma and chronic obstructive pulmonary disease. In these pathological conditions, silencing of galectin-7 expression could inhibit TGF-β-induced apoptosis in airway epithelium through alteration of the JNK-pathway (213, 214).
Galectin-7 can bind to red blood cells, thereby recognizing blood group-containing antigens (215). Diverse microorganisms such as Escherichia coli, Klebsiella pneumoniae, Providencia alcalifaciens, and Streptococcus pneumoniae express blood group-like antigens, allowing them to act as invading pathogens and induce injury in host cells (215). Interestingly, galectin-7 can recognize these pathogens, each of which uses molecular mimicry while failing to induce host cell injury, thereby providing an innate form of immunity (215). Galectin-7 also regulates CD4+ T cell immunity, by promoting the proliferation and polarization of Th1/2 cells, balancing toward the Th1 phenotype, which can potentially induce autoimmune diseases (216).
Platelets present two splice variants of galectin-8 with variable linker regions that are exposed on the platelet surface after thrombin stimulation (217). Using mass spectrometric and biochemical analysis it has been shown that galectin-8 binds αIIbβ3 integrin on the platelet surface, indicating an important function in platelet adhesion and spreading (217). Soluble galectin-8 binding to integrin modulates the conformational change of integrin to a high-affinity state, thereby opening the molecular surface for fibrinogen binding (217). Using platelets isolated from Glanzmann’s thrombasthenia patients with αIIbβ3 integrin deficiency, functional studies showed a reduction of galectin-8 binding on the platelet surface (217). Moreover, galectin-8 could activate platelets through Src, PI3K/Akt, and PLCγ2 signaling pathway (217).
Factor V (FV) is not synthetized in megakaryocytes or platelets, rather it is taken up from the blood plasma and stored in α-granules, although the exact endocytic pathway is not fully understood. Interestingly, on the cell surface of megakaryocytes, galectin-8 directly binds FV in a carbohydrate-dependent manner, and this interaction is most likely mediated by several N-linked glycans located on FV (218). In line with this result, genetic or pharmacological blockade of galectin-8 function could strongly inhibit FV uptake. Thrombopoietin (TPO) treatment in megakaryocytes could inhibit galectin-8 expression on the cell surface, and consequently, FV uptake was also inhibited, indicating that this molecular mechanism only exists in premature megakaryocytes (218).
Galectin-8 exposure was observed upon activation of vascular cells, suggesting that galectin-8 could potentially link both processes of platelet activation and inflammation on the surface of endothelial cells. vWF is stored in the Weibel-Palade bodies of endothelial cells and exposed on the cell surface under thrombotic and inflammatory conditions, responsible for linking platelet GPIb-GPV-GPIX complex to the activated endothelium (181, 219). Galectin-8 can activate endothelial cells, thereby increasing vWF secretion and enhancing platelet adhesion. Interestingly, both galectin-1 and galectin-3 can bind vWF in endothelial cells, thereby modulating vWF-mediated thrombus formation (181). Furthermore, galectin-8 increases the expression of pro-inflammatory molecules, including chemokines and interleukins, thereby mediating the interactions among leukocytes, blood platelets, and inflamed vascular endothelium. Galectin-8 enhanced the release of inflammatory cytokines and chemokines, namely CCL2, CXCL3, CXCL8, CXCL1, GM-CSF, IL-6, and CCL5, thereby influencing angiogenic response and recruitment of immune cells (130).
Inflammatory mediators can modulate the expression of galectin-9. Phorbol 12-myristate 13-acetate (PMA), IFN-γ and LPS can upregulate galectin-9 levels, indicating an important role of galectin-9 in inflammatory diseases (220–222). Elevated blood galectin-9 levels were found in patients with stroke after 6 days of ischemic insult (223). In the rat model of ischemic stroke, galectin-9 expression is also increased, indicating that blocking galectin-9 function may have protective effects in stroke (38). Increased serum galectin-9 levels were also observed in patients with stable coronary artery disease, suggesting an important role of galectin-9 in cardiovascular pathology (224).
Disruption of the blood–brain barrier is responsible for the development of hemorrhagic and thromboinflammatory diseases, including stroke and cerebral malaria (173, 225). Interactions between galectin-9 and CD146 facilitate the sequestration of infected red blood cells and pro-inflammatory lymphocytes in the vasculature of the central nervous system, thereby promoting the disruption of the blood–brain barrier (226). Galectin-9 also acts as an astrocyte-microglia communication signal by potentiating the release of TNFα from microglial cells (227). Endothelial cells are also major sources of galectin-9, which is exposed on the cell surface upon inflammation and endothelial cell activation (228). Therefore, galectin-9 has been proposed as a mediator of thromboinflammation by inducing the capture of immune cells and blood platelets to the activated endothelium. Galectin-9 was recently discovered as a novel platelet agonist that induced platelet adhesion, spreading, aggregation and degranulation through interactions with platelet hem immunoreceptor tyrosine-based activation motif (ITAM) receptors GPVI and C-type lectin-like receptor 2 (CLEC2), suggesting that galectin-9 regulates thrombus formation (229). Although lactose-dependent blockade of galectin-9 function was observed in platelet aggregation, and this suggests a carbohydrate-dependent action on (hem) ITAM signaling in platelets (229), further investigation is necessary to identify the exact molecular mechanisms of how galectin-9 may bind (hem) ITAM receptors.
Thrombotic complications are frequently observed in severe acute respiratory syndrome coronavirus 2 (SARS-CoV-2) syndrome patients. A recent study reported a strong elevation of blood galectin-9 levels in patients with SARS-CoV-2 infection, suggesting a modulatory effect of galectin-9 in platelets, immune cells, and endothelium (230), which may accelerate thromboinflammation in the infected lung tissues. Galectin-9 levels were higher in the plasma of hospitalized SARS-CoV-2 patients who died, compared to survivors (230). Although these studies highlighted galectin-9 function in thromboinflammation, the exact molecular mechanism, regulated by galectin-9 in infected patients awaits further investigations.
During atherosclerosis, galectin-9 modulates inflammation through the activation of T cells, monocytes, and macrophages (231). The imbalanced immune functions mediated by Th1 and Th2 cells are important hallmarks of the pathogenesis of atherosclerosis. Th1-induced immune responses enhance the development of atherosclerosis, while Th2-mediated responses attenuate the atherosclerosis-enhancing effects of Th1 cells and provide protection (232). Immunologic protective effects in atherosclerosis are also contributed by other subsets of CD4+ T and Treg cells. Although galectin-9 induces apoptosis in CD4+ Th1 cells, on the other hand, galectin-9 can activate T cells, thereby expanding Th1 cells and central memory cells. Furthermore, galectin-9 induces the differentiation of naïve T cells to Treg cells. Consistently, the numbers of Treg cells are reduced in Lgals9−/− mice (233). The blockade of galectin-9-Tim-3 interactions increases macrophage infiltration in atherosclerotic plaques (231). In an experimental model of preeclampsia, characterized by abnormal macrophage polarization, decreased Tim-3 levels were also correlated with the pathogenesis of the disease (234). Interestingly, galectin-9 treatment reversed inflammation-associated damages by activating and upregulating Tim-3 expression in immunosuppressive macrophages (234). Besides, the expression of human galectin-9 in transgenic mice also induced differentiation and activation of immunosuppressive CD11b1-Ly-6G-positive granulocytes (235). Recent studies by Zhu et al. demonstrated that the serum level of galectin-9 was reduced significantly in patients with coronary artery disease (224). Application of exogenous galectin-9 increased the number of Tregs cells and inhibited Th17 cell function, thereby increasing the production of TGFβ and decreasing the synthesis of IL-17 (236, 237). Patients with the acute coronary syndrome also displayed decreased mRNA levels of galectin-9, TIM-3, and forkhead box P3 (Foxp3) in peripheral blood mononuclear cells (238). Altogether these data indicate that upregulation of the galectin-9-Tim-3 signaling pathway attenuates atherosclerotic plaque development by inhibiting the recruitment of monocytes/macrophages and effector T cells and by enhancing the expansion of Tregs. Under flow conditions, galectin-9 promotes the adhesion of B cells to the vascular endothelium by decelerating their transendothelial migration (239). Galectin-9 also acts as an eosinophil and monocyte chemoattractant, inducing cell activation, differentiation, degranulation, and ROS production (240–242). Galectin-9 also mediates neutrophil chemotaxis, thereby inducing neutrophil activation and migration (243, 244).
Stimulation of monocytic cells with LPS induced nuclear translocation of galectin-9. Only intracellular galectin-9 was shown to regulate promoter activity of pro-inflammatory genes such as IL-1α, IL-1β, and IFNγ (245). Furthermore, intracellular galectin-9 could activate transcription factors such as nuclear factor (NF)-IL-6 and AP-1 but did not activate the NF-κB pathway (245). Interestingly, extracellular galectin-9 does not stimulate these processes (245).
Eosinophilic granulomatosis with polyangiitis (EGPA) is a rare chronic inflammatory systemic disease that occurs in patients with bronchial asthma and is associated with significant blood and tissue eosinophilia (246). Galectin-10 functions have been studied primarily in the context of this disease. Eosinophils of patients with EGPA are primed to generate cytolytic eosinophil extracellular traps (247). This process was shown to rely on the production of ROS and histone-citrullination resulting in the release of free galectin-10 and membrane-bound intact eosinophil granules, thereby increasing serum IL-10 levels (247). In ruptured atherosclerotic plaques and arterial thrombi, eosinophil extracellular trap formation was also detected (248, 249). Whether galectin-10 may induce extracellular trap formation of eosinophils or other immune cells in a pro-thrombotic environment is still unknown.
Galectin-12 is highly expressed in adipose tissues and regulates the differentiation and expansion of preadipocytes (250). Lgals12−/− mice have increased insulin sensitivity and glucose tolerance, contributing galectin-12 function to diabetes and metabolic syndrome, and indicating the potential involvement in cardiovascular diseases (251). Ablation of galectin-12 decreased the secretion of MCP-1, TNF-α, and IL-6 in LPS- and saturated fatty-acid-stimulated macrophages, suggesting the involvement in the pathogenesis of atherosclerosis (156). The ablation of galectin-12 also results in the polarization of macrophages into the M2 subset. The negative regulation of M2 macrophage polarization by galectin-12 causes enhanced inflammation and decreases insulin sensitivity in adipocytes (252). Galectin-12 deficiency in mice leads to M2 macrophage polarization that consequently reduced foam cell formation and pro-inflammatory cytokine production thereby reducing atheroma formation in an atherosclerosis animal model (156). Based on these results, it was proposed that inhibition of galectin-12 may be a novel therapeutic strategy to slow down the progression of atherosclerosis.
High levels of galectin-13 are detected in the blood of pregnant women at the early stage of pregnancy. In the placenta, galectin-13 is expressed in syncytiotrophoblasts and blood vessels. Reduced levels of galectin-13 caused by genetic mutations are associated with a high risk of preeclampsia. In parallel to its decreased placental expression, an enhanced galectin-13 shedding from the cell surface was shown to contribute to the elevated levels of serum galectin-13 during the third trimester of pregnancy in women with preterm preeclampsia. The release of galectin-13-positive microvesicles was proposed to aggravate placental ischemic stress during preeclampsia. Preeclampsia and hypertension during pregnancy are associated with an elevated risk of arterial thrombosis. The similar pathological observation was described in the blood of rare liver and blood clotting disorder (HELLP syndrome).
The dysregulation of galectin-14 is also involved in hypertension and preeclampsia (253). However, the studies focusing on galectin-14-mediated regulatory mechanisms are limited.
Thrombotic complications, such as a deep vein or arterial thrombosis and pulmonary embolism are frequent pathogenic events in patients with cancer (171, 254). Elevated platelet count and a pro-coagulant tumor microenvironment are indicators of a poor prognosis, implying a high risk of thromboembolic events and resistance to anti-cancer therapies (171). In mouse models of melanoma, breast, and colon cancer, platelets interact with circulating tumor cells, thereby protecting them from shear stress and NK-cell-induced destruction and enhancing tumor metastasis (255, 256). Platelets also induce EMT and recruitment of granulocytes to the metastatic niches (171, 172, 257). Platelet membrane receptors, integrins, and glycoproteins actively contribute to complex interactions between blood platelets, tumor cells, vascular endothelium, and fibrin clots, thereby promoting tumor growth and metastasis (171, 200, 257, 258). Tumor cells can directly trigger platelet activation through binding to the platelet-resident receptors, thereby amplifying pro-thrombotic and pro-coagulant phenotypes (171). Cancer cells can also trigger indirect platelet activation by inducing the release of tissue factors and ECM proteins from activated vascular endothelium, which create an active surface for platelet adhesion and thrombosis (171).
Abnormal O- and N-glycosylation and glycan binding, triggered by the tumor microenvironment, could potentially modify galectin-mediated responses, thereby influencing thrombotic and hemostatic events in cancer patients (170, 259, 260). Recently, we showed that platelet GPVI interacts with a tumor-resident galectin-3 through the binding to its collagen-like domain, expressed on the surface of breast and colon cancer cells (200). This interaction could induce platelet activation and granule release, thereby enhancing tumor cell extravasation and lung metastasis (200). More recently, galectin-3 has also been proposed as a ligand of platelet GPVI on ovarian cancer cells (261).
During thrombotic events, red blood cells enhance platelet interactions with thrombi by increasing the viscosity of the blood and margination of platelets toward endothelium. Galectin-4 can form a bridge between cancer cells, vascular endothelium, and red blood cells. The CRD domain of galectin-4 interacts with human blood group antigens (ABH) (262). The tumor cell-resident galectin-4 possibly mediates metastasis through interactions with ABH antigen-binding glycoproteins present on the surface of red blood cells (263) (Figure 4).
Figure 4. Invasive cancer cells enter into the blood circulation where they can build physical and functional interactions with platelets, red blood cells, and vascular endothelium. These interactions support the metastatic spread of cancer cells, thereby inducing their survival and proliferation at distant organs. Hematogenous metastasis is strongly influenced by tumor-resident galectins (galectin-3 and galectin-4) that interact with glycoproteins on the surface of platelets and red blood cells. Galectin-3 promotes platelet adhesion and activation through binding to platelet GPVI, thereby enhancing tumor cell extravasation and subsequent metastasis. These interactions also contribute to the epithelial-mesenchymal transition and pro-inflammatory phenotype, further sustaining the invasive and metastatic potential of cancer cells.
Galectins have multiple effects on cancer and many cell types involved in thromboinflammation, therefore therapeutic strategies have been applied to target galectin-mediated signaling in immune and cancer cells. However, the functional blockade of platelet-resident galectins has been out of focus in these studies. During the last years, several blocking antibodies, peptides, competitive carbohydrates, and small non-carbohydrates have been developed to selectively inhibit galectin isoforms. Some of the galectin blockers are currently in clinical studies to inhibit certain pathogenic functions in patients with solid cancers. In this chapter, we summarize the results of preclinical studies using different mouse models (Table 1), and we also discuss important clinical trials.
Table 1. Preclinical and clinical applications of galectin inhibitors in thromboinflammation and cancer.
Thiodigalactoside (TDG) is a synthetic disaccharide with a high affinity for galectin-1, thereby blocking galectin-1-mediated tumor angiogenesis and immune response, and preventing oxidative stress in a non-selective manner. In mouse models of breast and colon cancers, TDG reduced the number of CD31-positive endothelial cells, vessel formation, and the number and size of lung metastases. TDG could also prevent galectin-1 binding to CD44 and CD326 receptors on the surface of cancer stem cells (277, 278, 311). Although it was considered that TDG is a non-selective blocker for galectin-1, TDG treatment did not induce any additional tumor-suppressive effects in Lgals1−/− mice (311).
Anginex (β Pep-25) is an anti-angiogenic peptide, which contains anti-angiogenic factor motifs to bind the CRD domain of galectin-1. Anginex also inhibits galectin-1 uptake in endothelial cells, thereby inhibiting Raf/MEK/ERK kinase signaling (264). Anginex rendered tumor-associated endothelial cells sensitive to radiotherapy and displayed a synergistic effect with a suboptimal dose of carboplatin, triggering radiation-induced tumor regression (312). Nanoparticles of Anginex were used in a galectin-1-overexpressing breast cancer mouse model, showing a robust reduction in tumor growth, and similar results were obtained with Anginex analogues (6DBF7, DB16, DB21) (265). However, Anginex treatment has limitations, as reduced stability and half-life were observed with limited effects on endothelial cells to radiotherapy in neovascularized tumors, but not on cancer cells. Anginex also interacts with other galectins, such as galectin-2, 7, 8 N, and 9 N, possibly blocking their biological activities as well (266).
Galectin-1-VEGFR-2 interaction induces tumor outgrowth and limits the efficacy of anti-VEGF therapy on the endothelium (313). Therefore, a monoclonal anti-galectin-1 antibody, F8.G7, was designed to target galectin-1-associated VEGF signaling (267, 314). Kaposi sarcoma treatment with F8.G7 antibody in mice strongly inhibited tumor growth, angiogenesis, and VEGFR2-induced signaling pathway (267). F8.G7 treatment could also increase anti-tumor immunity by increasing the infiltration of T lymphocytes, and the release of IFNγ and IL-17 (267). Although non-canonical VEGF signaling was targeted by F8.G7 treatment, the canonical VEGF signaling still induced tumor angiogenesis, limiting the effectiveness of this treatment.
OTX008 is a calixarene derivative designed to target galectin-1 at a distant site of CRD, compared to Anginex (269). OTX008 could inhibit the invasion of laryngeal squamous cell carcinoma and ovarian endometrioid adenocarcinoma cells by inhibiting ERK1/2 and AKT-dependent cell survival signaling, CDK1-mediated G2/M cell cycle arrest and angiogenesis (270, 315). OTX008 could inhibit tumor growth with a strong reduction of galectin-1, Ki-67, and VEGFR-2 expressions in vivo (270). OTX008 has synergistic effects with immuno- and chemotherapeutic agents, such as everolimus, regorafenib, sunitinib, 5-FU, docetaxel, oxaliplatin, and cisplatin (316). The potential effects of OTX008 on thrombosis and hemostasis have not been investigated; therefore, we only speculate that OTX008 may represent a potential therapeutic approach to target galectin-1-mediated platelet adhesion and platelet–neutrophil interactions in cancer. However, these protective effects appear to depend on the pathogenesis of the thrombotic disease as an opposite therapeutic effect of galectin-1 treatment was observed in a mouse model of acute myocardial infarction (184). Treated mice with recombinant galectin-1 could improve cardiac homeostasis and post-infarction remodeling by attenuating tissue damage and preventing cardiac inflammation (184).
Targeting galectin-2 function in triple-negative breast cancer was proposed to be effective in reverting immunosuppression induced by M2-like macrophages (280). Injection of 4 T1 cells into the mouse mammary fat pad, followed by injection of therapeutic antibody against galectin-2 function, arrested tumor growth and successfully reversed the immunosuppressive phenotype (280). Although validated results are limited, it was proposed that blocking galectin-2 with selective antibodies or nanobody clones would be an excellent strategy to target galectin-2-mediated collateral arteriogenesis, myocardial infarction, immune cell adhesion, inflammation, and T-cell apoptosis in atherosclerosis (317). Therefore, it would be necessary to investigate further whether galectin-2 blockade may inhibit several routes of galectin-2-mediated malignancy, including tumor growth, immunosuppression, and thromboinflammation.
Several galectin-3 inhibitors have been developed and tested in preclinical mouse models. RN1 is a polysaccharide isolated from flowers of Panax pseudo-ginsieng, which binds and downregulates galectin-3-associated signaling pathways, thereby reducing the proliferation rate of pancreatic cancer cells and the growth of patient-derived xenografts both in vitro and in vivo conditions (292).
TFD10 is a glycopeptide isolated from polar cod fish that displayed inhibitory effects in prostate cancer metastasis by blocking galectin-3-mediated angiogenesis and tumor cell-endothelial cell interactions and inhibiting the apoptosis of activated T cells (291).
A dominant negative form of galectin-3 (Galectin-3C) was developed to block endogenous galectin-3 functions (290). Galectin-3C strongly reduced the proliferation, migration, invasion and angiogenic potential of human and mouse ovarian cancer cells (290). Galectin-3C could increase the anticancer activity of bortezomib in human multiple myeloma cells (290).
G3-C12 oligopeptide is a frequently used galectin-3-CRD domain blocker, which inhibited lung metastasis in a mouse model of breast cancer (288). The protective effects of G3-C12 were improved in combined therapy with N-(2-hydroxypropyl) methacrylamide (HPMA) compound and 5-FU. G3-G12-HPMA complex strongly improved the anti-tumor activity of 5-FU in nude mice bearing tumor of human PC-3 cancer cells (289).
GM-CT-01 (DAVANAT) is a modified vegetal galactomannan oligomer that strongly binds the dimer interface of galectin-1 and galectin-3 (318). GM-CT-01 enhances the infiltration of T cells into the tumor microenvironment and induces IFNγ synthesis (319). GM-CT-01 was administered in combination with 5-FU in patients with metastatic solid cancers that failed initial therapy (NCT00388700). In clinical trial phase II, 70% of the patients were stabilized at the highest DAVANAT dose level, 46% of patients experienced an increase in life expectancy, and merely 41% of patients developed serious adverse effects, compared to the best standard of care. Food Drug Administration (FDA) approval was given to progress to clinical trial phase III studies, although this was not followed up as of now (320). Multiple factors led to the withdrawal of two further clinical trial phase II studies using GM-CT-01 therapy (NCT00388700 and NCT00386516). The vaccine form of GM-CT-01 is currently in clinical trial phase II, injecting patients with diffuse melanoma (NCT01723813).
GR-MD-02 (Belapectin) binds galectin-3 and preclinical settings could improve liver function by reducing collagen deposition, fibrosis in non-alcoholic steatohepatitis (NASH), and cirrhosis (321). GR-MD-02 increased the efficacy of immunotherapeutic agents such as the checkpoint inhibitor pembrolizumab (322). Recently, GR-MD-02 in combination with ipilimumab or pembrolizumab is being investigated in melanoma, non-small cell lung cancer, and squamous cell head and neck cancer in a clinical trial phase I without published results and still ongoing studies (NCT02575404 and NCT02117362).
Modified citrus pectin (MCP) is originally a β-galactoside nutritional supplement, which inhibits galectin-3 function through multiple mechanisms. Indeed, MCP could inhibit cell adhesion in galectin-3-overexpressing tumor cells, reducing tumor cell aggregation and adhesion to the endothelial cells, thereby inhibiting the metastatic potential of cancer cells (323, 324). In prostate cancer cells, MCP enhanced anoikis by inhibiting cyclin B and cdc function, which subsequently induced G2/M cell cycle blockade and apoptosis (325). Furthermore, MCP function was also studied in cardiovascular and renal diseases. MCP could inhibit aldosterone-induced cardiac and renal fibrosis and improve cardio-renal dysfunction (326). MCP could prevent fibrosis in rodents with heart failure and hyperaldosteronism and inhibit obesity-associated adipose tissue inflammation and differentiation of adipocytes and atherosclerotic plaque progression (327–329). Additionally, MCP reduced myocardial injury after ischemic reperfusion (330).
A derivative of MCP, PectaSol-MCP was evaluated in clinical trial phase II (NCT01681823) regarding its capability to improve prostate-specific antigen (PSA) kinetics in men with increased PSA levels and biochemically relapsed prostate cancer, PSA progression was inhibited in 58% of patients. PSA doubling time, a prognostic marker predicting the development of metastasis, lengthened in 75% of patients versus baseline indicating a reduced risk of metastatic spread (331). However, the major limitation of this study was the lack of a placebo arm, which, given the perceived positivity of PectaSol-MCP, was not conducted.
GCS-100 is an MCP-derived polysaccharide and was used to study galectin-3-mediated pro-tumorigenic effects. GCS-100 could induce apoptosis in multiple myeloma cells, which displayed strong resistance to doxorubicin, melafalan, bortezomib, and dexamethasone (295). GCS-100 treatment together with a BH3-mimetic also induced apoptosis in acute myeloma cells and interestingly these effects were associated with the induction of P53 (296). Although GCS-100 was well tolerated by patients in clinical trial phase II, partial response and stable disease were observed in patients with recurrent chronic lymphocytic leukemia (NCT00514696).
TD139 binds the CRD domain of galectin-3 with a strong anti-inflammatory and anti-fibrotic potential in mouse models of lung injury and fibrosis (332, 333). TD139 downregulates galectin-3 expression on broncho-alveolar lavage macrophages inhibits fibroblast activation and reduces the levels of plasma biomarkers associated with idiopathic fibrosis progression (334, 335). TD139 treatment also inhibits pro-tumorigenic effects in thyroid cancer cells by attenuating AKT phosphorylation and reducing the expression of MMP2 and β-catenin, which leads to the suppression of anoikis resistance, motility and invasion (336). The β-glycan receptor dectin-1 is a potential biomarker of lung fibrosis (337). Dectin-1 is highly expressed in bone-marrow-derived myeloid cells, including monocytes, macrophages, and neutrophils (338). Interaction between dectin-1 and galectin-3 is required for pathogen recognition and TNF-α response in bone marrow-derived myeloid cells (339). It was proposed that this interaction is involved in platelet aggregation and thrombosis, thereby enhancing atherothrombosis and myocardial infarction (201). Plasma galectin-3 levels are increased in patients with coronary artery diseases and atherosclerosis (201, 340). In ApoE−/− atherosclerotic mice, elevated plasma galectin-3 levels were detected and TD139 could effectively inhibit galectin-3-mediated platelet activation and thrombosis (201). Furthermore, TD139 also inhibited microvascular thrombosis and prevented myocardial infarction. Therefore, TD139 was proposed as a potent galectin-3 inhibitor improving atherothrombosis and myocardial infarction (201).
Interestingly, the collagen-like domain of galectin-3 binds GPVI on the platelet surface, thereby inducing platelet activation and adhesion (200, 261). Blockade of GPVI was proposed as an alternative therapeutic approach, which inhibits galectin-3-mediated platelet signaling and platelet-mediated pro-tumorigenic effects (171). Recently, we showed that functional inhibition of GPVI with F (ab′)2 fragments of Jaq1 antibody could increase the intratumoral efficacy of chemotherapeutic drugs (341). Jaq1 F(ab′)2 antibody also blocked platelet adhesion to galectin-3-positive cancer cells, thereby inhibiting platelet-tumor cell interaction and consequent tumor metastasis (200). Soluble dimeric GPVI - Revacept is a clinically relevant competitive inhibitor of GPVI, which directly binds collagen thereby removing collagen fibers from the platelet surface (171, 342). Similar to the Jaq1 F(ab′)2 antibody, Revacept and other soluble dimeric-like GPVI blockers also inhibited galectin-3-mediated platelet-cancer cell interaction and lung metastasis (200, 261). Using galectin-3-positive HT29 human colon cancer cells, Dovizio et al. showed that Revacept had an inhibitory effect on COX-2 and platelet-mediated EMT in vitro (343). In ovarian cancer, galectin-3 is a ligand of platelet GPVI, and this interaction regulates platelet-dependent tumor cell extravasation and pro-inflammatory signature in cancer cells (261). In human ovarian cancer on a chip model, Revacept treatment also inhibited galectin-3-GPVI-mediated platelet-cancer cell interactions and pro-inflammatory phenotype, thereby attenuating the invasive potential of cancer cells (261).
Intracellular galectin-4 interacts with β-catenin, leading to the inhibition of the Wnt pathway-associated cell proliferation and cancer growth (102). Galectin-4 is also released to extracellular space and high galectin-4 levels in the blood were observed in patients with metastatic colon, hepatocellular, and breast cancers (108, 344–346). Extracellular galectin-4 inhibited the secretion of IL-17 from activated T cells in the colon, thereby inducing apoptosis and preventing inflammation (347). Interestingly, extracellular galectin-4 could induce apoptosis in galectin-4-deficient colorectal cancer cells, while in galectin-4-positive colorectal cancer cells, inflammatory chemokine gene expression and secretion were downregulated (348). In line with this result, an anti-galectin-4 antibody that inhibits extracellular galectin-4 function could increase cell proliferation with the concomitant secretion of chemokines (348). In other studies, lower expression of galectin-4 was associated with an advanced form of colorectal cancer, and galectin-4 overexpression could improve the efficacy of camptothecin treatment (105, 345). Galectin-4 binds carbohydrate groups on red blood cell group antigens and cholesterol 3-sulfate and sulfatides within lipid raft membranes through its CRD domains (263, 349). Further studies are required to design blocking approaches to interfere with these binding sites.
Based on the crystal structure of galectin-7A, 2-O-galactoside benzyl-phosphorane was synthesized which displayed a 60-fold increased affinity for this galectin isoform, as compared to galactoside (350). Recombinant human galectin-7 protein could inhibit apoptosis in Jurkat T cells, indicating on immunosuppressive function (351). This proapoptotic effect was inhibited by targeting the dimer interface of galectin-7, thereby disrupting homodimerization (352). Galectin-7 also regulates immune response to transplantation by promoting Th1/Th2 differentiation toward Th1, which results in increased rejection of grafted hearts (216, 353). Recently, gene therapy was developed based on galectin-7-siRNA knock-down with ultrasound-targeted microbubble destruction that could prevent acute rejection during allograft heart transplantation (353).
The inhibition of one CRD domain of galectin-8 was sufficient to inhibit the biological function of this isoform. D-galactal-benzimidazole hybrid was designed to block galectin-8 function through binding to its N-terminal domain (304). Although the D-galactal-benzimidazole hybrid showed no effect on the proliferation of human breast cancer cells, the secretion of pro-inflammatory cytokines, IL-6 and IL-8, was reduced in a dose-dependent manner (304). The CRD of galectin-8 and the dominant negative version of galectin-8 (Gal-8 N) were both blocked by 3′-sialyl lactose in a mouse model of corneal allogeneic transplantation, resulting in severely inhibited angiogenesis (351).
Galectin-9 inhibition in hepatocellular carcinoma cells showed increased cell proliferation and migration in vitro (354). Patients with higher galectin-9 levels had better survival than those who were negative for galectin-9 expression (135). Adding recombinant galectin-9 into cell culture promoted cell death in colorectal carcinoma with KRAS mutation (355). This therapeutic approach could also potentiate rapid endocytosis and accumulation of rsGal-9 in lysosomes, resulting in lysosome swelling and accumulation of autophagosomes and cell death (356). Treatment of chronic myeloid leukemia cells with human galectin-9 rendered the cells sensitive to tyrosine kinase inhibitors, thereby inducing apoptosis through modulation of activating transcription factor 3-Noxa proapoptotic pathway (305).
Galectin-9 regulates anti-tumor immunity through modulating Tim-3/galectin-9, PD-1/PD-L1, and dectin-associated immune checkpoints. Interestingly, Galectin-9 binds PD-1 which is distinct from the PD-L1-binding site (131). Anti-Tim-3 antibodies have been generated to block the interactions with galectin-9 and also other ligands such as CEA cell adhesion molecule 1 (CEACAM1) and high-mobility group protein B1 (HMGB1) (306). These antibodies could strongly prevent T cell inhibition and enhance anti-tumor immunity. A humanized anti-Tim-3 antibody, TSR-022 has been evaluated in clinical trial phases I and II and resulted in stable diseases in patients with progressed solid tumors (307). TRS-022 therapy was also combined with an anti-PD1 antibody (TSR-042) (307). The first results showed good tolerance of TRS-022/TSR-042 in melanoma and non-small cell lung cancer (NSCLC) (308). Another anti-Tim-3 antibody, LY3321367 also presented a safety profile and modest anti-tumor activity or in combination with LY300054, an anti-PD-L1 antibody (309). Similar combinations were also developed using MBG453, anti-Tim-3 antibody and spartalizumab, an anti-PD1 antibody, which presented better outcome and antitumor activity as compared to monotherapy (310).
LYT-200 is an IgG4 monoclonal antibody, designed to inhibit the activity of galectin-9. It was given orphan drug designation by the FDA and it is tested in an open-label, uncontrolled, multicenter phase 1/2 study with a dose escalation phase and a cohort expansion phase in patients with relapsed/refractory metastatic solid tumors, including PDAC, colorectal cancer, and cholangiocarcinoma (NCT04666688). The study was posted in 2020 and is currently in its recruiting stage. Galectin-9-CD146 interaction contributes to cerebral malaria, inducing the adhesion of red blood cells, and T cells, and the rupture of the blood–brain barrier (226). Blockade of CD146 counter receptor using AA498 could block galectin-9-mediated cell adhesion and inflammation and disease pathogenesis in cerebral malaria (226). CD146 can also bind to galectin-1 and galectin-3 (226). Whether such an approach interferes with interactions of other galectins requires further investigation.
Galectin-9 can also support platelet adhesion and activation through interactions with hemi-ITAM receptors GPVI and CLEC2 (229). In vitro blockade of galectin-9-mediated platelet adhesion using an anti-GPVI Jaq1 Fab fragment and anti-CLEC2 AYP1 antibody could inhibit galectin-9-mediated platelet adhesion and activation (229). Similar inhibition was observed using a pan galectin inhibitor, lactose (229). Thrombosis occurs as a consequence of endothelial disruption or rupture of atherosclerotic plaques (174). One would expect beneficial effects of galectin-9 inhibition in atherosclerosis. However, the blockade of galectin-9 function was shown to increase atherosclerotic plaque formation (231). Therefore, we conclude that the effect of galectin-9 targeting therapies may depend on the stage and progression of the disease. Future studies are needed to test the exact functions of galectin-9 under disease conditions, including deep venous thrombosis, cancer-associated thromboembolism, and thromboinflammation.
Therapeutic strategies disrupting multiple interactions between tumor cells, immune cells, vasculature, and platelets may provide advantages limiting tumor progression and metastasis. However, the biological effects that galectin isoforms are contributed to these processes are variable. Some of the galectin isoforms are strong mediators of inflammation and thrombosis; others are not contributed or not investigated so far. Nevertheless, published results and clinical trials suggest that targeting galectin functions may offer a powerful therapeutic strategy to inhibit not only tumor cell-autonomous attributes but also thrombotic and pro-inflammatory events in the cancer environment. Systematic analysis of tumor stage, platelet and inflammatory markers, and galectin levels in the blood may help to develop therapeutic strategies to inhibit malignancy of tumors, inflammation, and thrombosis.
Studying galectin functions in cancer is surrounded by challenges due to their complex roles in cancer cells and their microenvironment. Some of these challenges are related to the fact that galectin isoforms may have multiple functions in different tumors and depending on the developmental stage and tumor type, also opposite observed functions in tumor progression. Galectins may have pro- or anti-tumor functions. The explanation of these biphasic effects of galectin function is still the subject of many research studies. One interpretation is that the diversity of galectin binding partners or intracellular/extracellular localization of galectins may modify their biological functions during tumor progression. Thus, galectins can act as negative or positive regulators of tumor growth and metastasis in mice and humans.
LK wrote the manuscript and drafted the figures. AB and EC contributed to the writing. TG critically reviewed the manuscript and contributed to the writing. EM-B conceptualized and wrote the manuscript. All authors contributed to the article and approved the submitted version.
This work was supported by the Bayersiches Landesamt für Gesundheit und Lebensmittelsicherheit (BLGL; project number 15–25), Deutsche Forschungsgemeinschaft, CRC TRR152/P15. LK was the recipient of a fellowship from Förderprogramm für Forschung und Lehre (FöFoLe), LMU, Munich, Germany.
The authors declare that the research was conducted in the absence of any commercial or financial relationships that could be construed as a potential conflict of interest.
All claims expressed in this article are solely those of the authors and do not necessarily represent those of their affiliated organizations, or those of the publisher, the editors and the reviewers. Any product that may be evaluated in this article, or claim that may be made by its manufacturer, is not guaranteed or endorsed by the publisher.
1. Girotti, MR, Salatino, M, Dalotto-Moreno, T, and Rabinovich, GA. Sweetening the hallmarks of cancer: Galectins as multifunctional mediators of tumor progression. J Exp Med. (2020) 217:e20182041. doi: 10.1084/jem.20182041
2. Vasta, GR, Feng, C, Bianchet, MA, Bachvaroff, TR, and Tasumi, S. Structural, functional, and evolutionary aspects of galectins in aquatic mollusks: From a sweet tooth to the Trojan horse. Fish Shellfish Immunol. (2015) 46:94–106. doi: 10.1016/j.fsi.2015.05.012
3. Johannes, L, Jacob, R, and Leffler, H. Galectins at a glance. J Cell Sci. (2018) 131:cs208884. doi: 10.1242/jcs.208884
4. Dings, RPM, Miller, MC, Griffin, RJ, and Mayo, KH. Galectins as Molecular Targets for Therapeutic Intervention. Int J Mol Sci. (2018) 19:905. doi: 10.3390/ijms19030905
5. Popa, SJ, Stewart, SE, and Moreau, K. Unconventional secretion of annexins and galectins. Semin Cell Dev Biol. (2018) 83:42–50. doi: 10.1016/j.semcdb.2018.02.022
6. Elola, MT, Wolfenstein-Todel, C, Troncoso, MF, Vasta, GR, and Rabinovich, GA. Galectins: matricellular glycan-binding proteins linking cell adhesion, migration, and survival. Cell Mol Life Sci. (2007) 64:1679–700. doi: 10.1007/s00018-007-7044-8
7. Delacour, D, Koch, A, and Jacob, R. The role of galectins in protein trafficking. Traffic. (2009) 10:1405–13. doi: 10.1111/j.1600-0854.2009.00960.x
8. Banfer, S, and Jacob, R. Galectins in Intra- and Extracellular Vesicles. Biomol Ther. (2020) 10:1232. doi: 10.3390/biom10091232
9. Di Lella, S, Sundblad, V, Cerliani, JP, Guardia, CM, Estrin, DA, Vasta, GR, et al. When galectins recognize glycans: from biochemistry to physiology and back again. Biochemistry. (2011) 50:7842–57. doi: 10.1021/bi201121m
10. Schattner, M. Platelets and galectins. Ann Transl Med. (2014) 2:85. doi: 10.3978/j.issn.2305-5839.2014.09.02
11. Schattner, M, and Rabinovich, GA. Galectins: new agonists of platelet activation. Biol Chem. (2013) 394:857–63. doi: 10.1515/hsz-2013-0108
12. Diaz, JA, Ramacciotti, E, and Wakefield, TW. Do galectins play a role in venous thrombosis? a review. Thromb Res. (2010) 125:373–6. doi: 10.1016/j.thromres.2009.11.011
13. Cummings, RD, Liu, FT, and Vasta, GR. Galectins. In: rd, A Varki, RD Cummings, JD Esko, P Stanley, and GW Hart, et al., editors. Essentials of Glycobiology. Cold Spring Harbor (NY) (2015). p. 469–480.
14. Cummings, RD, Liu, FT, Rabinovich, GA, Stowell, SR, and Vasta, GR. Galectins. In: th, A Varki, RD Cummings, JD Esko, P Stanley, and GW Hart, et al., editors. Essentials of Glycobiology. Cold Spring Harbor (NY) (2022). p. 491–504.
15. Lewis, SK, Farmer, JL, Burghardt, RC, Newton, GR, Johnson, GA, Adelson, DL, et al. Galectin 15 (LGALS15): a gene uniquely expressed in the uteri of sheep and goats that functions in trophoblast attachment. Biol Reprod. (2007) 77:1027–36. doi: 10.1095/biolreprod.107.063594
16. Takenaka, Y, Fukumori, T, Yoshii, T, Oka, N, Inohara, H, Kim, HR, et al. Nuclear export of phosphorylated galectin-3 regulates its antiapoptotic activity in response to chemotherapeutic drugs. Mol Cell Biol. (2004) 24:4395–406. doi: 10.1128/MCB.24.10.4395-4406.2004
17. Ippel, H, Miller, MC, Vértesy, S, Zheng, Y, Cañada, FJ, Suylen, D, et al. Intra- and intermolecular interactions of human galectin-3: assessment by full-assignment-based NMR. Glycobiology. (2016) 26:888–903. doi: 10.1093/glycob/cww021
18. Flores-Ibarra, A, Vértesy, S, Medrano, FJ, Gabius, H-J, and Romero, A. Crystallization of a human galectin-3 variant with two ordered segments in the shortened N-terminal tail. Sci Rep. (2018) 8:9835. doi: 10.1038/s41598-018-28235-x
19. Leonidas, DD, Elbert, BL, Zhou, Z, Leffler, H, Ackerman, SJ, and Acharya, KR. Crystal structure of human Charcot-Leyden crystal protein, an eosinophil lysophospholipase, identifies it as a new member of the carbohydrate-binding family of galectins. Structure. (1995) 3:1379–93. doi: 10.1016/S0969-2126(01)00275-1
20. Su, J, Song, C, Si, Y, Cui, L, Yang, T, Li, Y, et al. Identification of key amino acid residues determining ligand binding specificity, homodimerization and cellular distribution of human galectin-10. Glycobiology. (2019) 29:85–93. doi: 10.1093/glycob/cwy087
21. Itoh, A, Nonaka, Y, Nakakita, S-i, Yoshida, H, Nishi, N, Nakamura, T, et al. Structures of human galectin-10/monosaccharide complexes demonstrate potential of monosaccharides as effectors in forming Charcot-Leyden crystals. Biochem Biophys Res Commun. (2020) 525:87–93. doi: 10.1016/j.bbrc.2020.02.037
22. Su, J, Gao, J, Si, Y, Cui, L, Song, C, Wang, Y, et al. Galectin-10: a new structural type of prototype galectin dimer and effects on saccharide ligand binding. Glycobiology. (2018) 28:159–68. doi: 10.1093/glycob/cwx107
23. Su, J, Wang, Y, Si, Y, Gao, J, Song, C, Cui, L, et al. Galectin-13, a different prototype galectin, does not bind beta-galacto-sides and forms dimers via intermolecular disulfide bridges between Cys-136 and Cys-138. Sci Rep. (2018) 8:980. doi: 10.1038/s41598-018-19465-0
24. Greten, FR, and Grivennikov, SI. Inflammation and Cancer: Triggers, Mechanisms, and Consequences. Immunity. (2019) 51:27–41. doi: 10.1016/j.immuni.2019.06.025
25. Singh, N, Baby, D, Rajguru, JP, Patil, PB, Thakkannavar, SS, and Pujari, VB. Inflammation and cancer. Ann Afr Med. (2019) 18:121–6. doi: 10.4103/aam.aam_56_18
26. Radovani, B, and Gudelj, I. N-Glycosylation and Inflammation; the Not-So-Sweet Relation. Front Immunol. (2022) 13:893365. doi: 10.3389/fimmu.2022.893365
27. Borsig, L, Wolf, MJ, Roblek, M, Lorentzen, A, and Heikenwalder, M. Inflammatory chemokines and metastasis—tracing the accessory. Oncogene. (2014) 33:3217–24. doi: 10.1038/onc.2013.272
28. Sundblad, V, Morosi, LG, Geffner, JR, and Rabinovich, GA. Galectin-1: A Jack-of-All-Trades in the Resolution of Acute and Chronic Inflammation. J Immunol. (2017) 199:3721–30. doi: 10.4049/jimmunol.1701172
29. Thijssen, VL, Hulsmans, S, and Griffioen, AW. The galectin profile of the endothelium: altered expression and localization in activated and tumor endothelial cells. Am J Pathol. (2008) 172:545–53. doi: 10.2353/ajpath.2008.070938
30. Earl, LA, Bi, S, and Baum, LG. N- and O-glycans modulate galectin-1 binding, CD45 signaling, and T cell death. J Biol Chem. (2010) 285:2232–44. doi: 10.1074/jbc.M109.066191
31. Rabinovich, GA, Ariel, A, Hershkoviz, R, Hirabayashi, J, Kasai, KI, and Lider, O. Specific inhibition of T-cell adhesion to extracellular matrix and proinflammatory cytokine secretion by human recombinant galectin-1. Immunology. (1999) 97:100–6. doi: 10.1046/j.1365-2567.1999.00746.x
32. Rabinovich, GA, Daly, G, Dreja, H, Tailor, H, Riera, CM, Hirabayashi, J, et al. Recombinant galectin-1 and its genetic delivery suppress collagen-induced arthritis via T cell apoptosis. J Exp Med. (1999) 190:385–98. doi: 10.1084/jem.190.3.385
33. Santucci, L, Fiorucci, S, Cammilleri, F, Servillo, G, Federici, B, and Morelli, A. Galectin-1 exerts immunomodulatory and protective effects on concanavalin A-induced hepatitis in mice. Hepatology. (2000) 31:399–406. doi: 10.1002/hep.510310220
34. Santucci, L, Fiorucci, S, Rubinstein, N, Mencarelli, A, Palazzetti, B, Federici, B, et al. Galectin-1 suppresses experimental colitis in mice. Gastroenterology. (2003) 124:1381–94. doi: 10.1016/S0016-5085(03)00267-1
35. Toscano, MA, Commodaro, AG, Ilarregui, JM, Bianco, GA, Liberman, A, Serra, HM, et al. Galectin-1 suppresses autoimmune retinal disease by promoting concomitant Th2- and T regulatory-mediated anti-inflammatory responses. J Immunol. (2006) 176:6323–32. doi: 10.4049/jimmunol.176.10.6323
36. Perone, MJ, Bertera, S, Tawadrous, ZS, Shufesky, WJ, Piganelli, JD, Baum, LG, et al. Dendritic cells expressing transgenic galectin-1 delay onset of autoimmune diabetes in mice. J Immunol. (2006) 177:5278–89. doi: 10.4049/jimmunol.177.8.5278
37. Baum, LG, Blackall, DP, Arias-Magallano, S, Nanigian, D, Uh, SY, Browne, JM, et al. Amelioration of graft versus host disease by galectin-1. Clin Immunol. (2003) 109:295–307. doi: 10.1016/j.clim.2003.08.003
38. Rubinstein, N, Alvarez, M, Zwirner, NW, Toscano, MA, Ilarregui, JM, Bravo, A, et al. Targeted inhibition of galectin-1 gene expression in tumor cells results in heightened T cell-mediated rejection; A potential mechanism of tumor-immune privilege. Cancer Cell. (2004) 5:241–51. doi: 10.1016/S1535-6108(04)00024-8
39. Rabinovich, GA, Toscano, MA, Ilarregui, JM, and Rubinstein, N. Shedding light on the immunomodulatory properties of galectins: novel regulators of innate and adaptive immune responses. Glycoconj J. (2002) 19:565–73. doi: 10.1023/B:GLYC.0000014087.41914.72
40. He, J, and Baum, LG. Endothelial cell expression of galectin-1 induced by prostate cancer cells inhibits T-cell transendothelial migration. Lab Investig. (2006) 86:578–90. doi: 10.1038/labinvest.3700420
41. Potikha, T, Pappo, O, Mizrahi, L, Olam, D, Maller, SM, Rabinovich, GA, et al. Lack of galectin-1 exacerbates chronic hepatitis, liver fibrosis, and carcinogenesis in murine hepatocellular carcinoma model. FASEB J. (2019) 33:7995–8007. doi: 10.1096/fj.201900017R
42. Wu, MH, Chen, YL, Lee, KH, Chang, CC, Cheng, TM, Wu, SY, et al. Glycosylation-dependent galectin-1/neuropilin-1 interactions promote liver fibrosis through activation of TGF-beta- and PDGF-like signals in hepatic stellate cells. Sci Rep. (2017) 7:11006. doi: 10.1038/s41598-017-11212-1
43. Ene-Obong, A, Clear, AJ, Watt, J, Wang, J, Fatah, R, Riches, JC, et al. Activated pancreatic stellate cells sequester CD8+ T cells to reduce their infiltration of the juxtatumoral compartment of pancreatic ductal adenocarcinoma. Gastroenterology. (2013) 145:1121–32. doi: 10.1053/j.gastro.2013.07.025
44. Orozco, CA, Martinez-Bosch, N, Guerrero, PE, Vinaixa, J, Dalotto-Moreno, T, Iglesias, M, et al. Targeting galectin-1 inhibits pancreatic cancer progression by modulating tumor-stroma crosstalk. Proc Natl Acad Sci U S A. (2018) 115:E3769–78. doi: 10.1073/pnas.1722434115
45. Rutkowski, MR, Stephen, TL, Svoronos, N, Allegrezza, MJ, Tesone, AJ, Perales-Puchalt, A, et al. Microbially driven TLR5-dependent signaling governs distal malignant progression through tumor-promoting inflammation. Cancer Cell. (2015) 27:27–40. doi: 10.1016/j.ccell.2014.11.009
46. Pace, KE, Lee, C, Stewart, PL, and Baum, LG. Restricted receptor segregation into membrane microdomains occurs on human T cells during apoptosis induced by galectin-1. J Immunol. (1999) 163:3801–11. doi: 10.4049/jimmunol.163.7.3801
47. Pace, KE, Hahn, HP, Pang, M, Nguyen, JT, and Baum, LG. CD7 delivers a pro-apoptotic signal during galectin-1-induced T cell death. J Immunol. (2000) 165:2331–4. doi: 10.4049/jimmunol.165.5.2331
48. Hahn, HP, Pang, M, He, J, Hernandez, JD, Yang, RY, Li, LY, et al. Galectin-1 induces nuclear translocation of endonuclease G in caspase- and cytochrome c-independent T cell death. Cell Death Differ. (2004) 11:1277–86. doi: 10.1038/sj.cdd.4401485
49. Galvan, M, Tsuboi, S, Fukuda, M, and Baum, LG. Expression of a specific glycosyltransferase enzyme regulates T cell death mediated by galectin-1. J Biol Chem. (2000) 275:16730–7. doi: 10.1074/jbc.M001117200
50. Cedeno-Laurent, F, Opperman, M, Barthel, SR, Kuchroo, VK, and Dimitroff, CJ. Galectin-1 triggers an immunoregulatory signature in Th cells functionally defined by IL-10 expression. J Immunol. (2012) 188:3127–37. doi: 10.4049/jimmunol.1103433
51. Tesone, AJ, Rutkowski, MR, Brencicova, E, Svoronos, N, Perales-Puchalt, A, Stephen, TL, et al. Satb1 Overexpression Drives Tumor-Promoting Activities in Cancer-Associated Dendritic Cells. Cell Rep. (2016) 14:1774–86. doi: 10.1016/j.celrep.2016.01.056
52. Cagnoni, AJ, Giribaldi, ML, Blidner, AG, Cutine, AM, Gatto, SG, Morales, RM, et al. Galectin-1 fosters an immunosuppressive microenvironment in colorectal cancer by reprogramming CD8(+) regulatory T cells. Proc Natl Acad Sci U S A. (2021) 118:e2102950118. doi: 10.1073/pnas.2102950118
53. Croci, DO, Morande, PE, Dergan-Dylon, S, Borge, M, Toscano, MA, Stupirski, JC, et al. Nurse-like cells control the activity of chronic lymphocytic leukemia B cells via galectin-1. Leukemia. (2013) 27:1413–6. doi: 10.1038/leu.2012.315
54. Griffioen, AW, and Thijssen, VL. Galectins in tumor angiogenesis. Ann Transl Med. (2014) 2:90. doi: 10.3978/j.issn.2305-5839.2014.09.01
55. Thijssen, VL, Postel, R, Brandwijk, RJ, Dings, RP, Nesmelova, I, Satijn, S, et al. Galectin-1 is essential in tumor angiogenesis and is a target for antiangiogenesis therapy. Proc Natl Acad Sci U S A. (2006) 103:15975–80. doi: 10.1073/pnas.0603883103
56. Le Mercier, M, Mathieu, V, Haibe-Kains, B, Bontempi, G, Mijatovic, T, Decaestecker, C, et al. Knocking down galectin 1 in human hs683 glioblastoma cells impairs both angiogenesis and endoplasmic reticulum stress responses. J Neuropathol Exp Neurol. (2008) 67:456–69. doi: 10.1097/NEN.0b013e318170f892
57. Baum, LG, Seilhamer, JJ, Pang, M, Levine, WB, Beynon, D, and Berliner, JA. Synthesis of an endogeneous lectin, galectin-1, by human endothelial cells is up-regulated by endothelial cell activation. Glycoconj J. (1995) 12:63–8. doi: 10.1007/BF00731870
58. van den Brule, F, Califice, S, Garnier, F, Fernandez, PL, Berchuck, A, and Castronovo, V. Galectin-1 accumulation in the ovary carcinoma peritumoral stroma is induced by ovary carcinoma cells and affects both cancer cell proliferation and adhesion to laminin-1 and fibronectin. Lab Investig. (2003) 83:377–86. doi: 10.1097/01.LAB.0000059949.01480.40
59. Clausse, N, van den Brule, F, Waltregny, D, Garnier, F, and Castronovo, V. Galectin-1 expression in prostate tumor-associated capillary endothelial cells is increased by prostate carcinoma cells and modulates heterotypic cell-cell adhesion. Angiogenesis. (1999) 3:317–25. doi: 10.1023/A:1026584523789
60. Sturm, A, Lensch, M, Andre, S, Kaltner, H, Wiedenmann, B, Rosewicz, S, et al. Human galectin-2: novel inducer of T cell apoptosis with distinct profile of caspase activation. J Immunol. (2004) 173:3825–37. doi: 10.4049/jimmunol.173.6.3825
61. Chen, C, Duckworth, CA, Fu, B, Pritchard, DM, Rhodes, JM, and Yu, LG. Circulating galectins −2, −4 and −8 in cancer patients make important contributions to the increased circulation of several cytokines and chemokines that promote angiogenesis and metastasis. Br J Cancer. (2014) 110:741–52. doi: 10.1038/bjc.2013.793
62. Yildirim, C, Vogel, DY, Hollander, MR, Baggen, JM, Fontijn, RD, Nieuwenhuis, S, et al. Galectin-2 induces a proinflammatory, anti-arteriogenic phenotype in monocytes and macrophages. PLoS One. (2015) 10:e0124347. doi: 10.1371/journal.pone.0124347
63. Paclik, D, Berndt, U, Guzy, C, Dankof, A, Danese, S, Holzloehner, P, et al. Galectin-2 induces apoptosis of lamina propria T lymphocytes and ameliorates acute and chronic experimental colitis in mice. J Mol Med (Berl). (2008) 86:1395–406. doi: 10.1007/s00109-007-0290-2
64. Ozaki, K, Inoue, K, Sato, H, Iida, A, Ohnishi, Y, Sekine, A, et al. Functional variation in LGALS2 confers risk of myocardial infarction and regulates lymphotoxin-alpha secretion in vitro. Nature. (2004) 429:72–5. doi: 10.1038/nature02502
65. Farhad, M, Rolig, AS, and Redmond, WL. The role of Galectin-3 in modulating tumor growth and immunosuppression within the tumor microenvironment. Onco Targets Ther. (2018) 7:e1434467. doi: 10.1080/2162402X.2018.1434467
66. Blair, BB, Funkhouser, AT, Goodwin, JL, Strigenz, AM, Chaballout, BH, Martin, JC, et al. Increased Circulating Levels of Galectin Proteins in Patients with Breast, Colon, and Lung Cancer. Cancers (Basel). (2021) 13:4819. doi: 10.3390/cancers13194819
67. Ahmed, H, and AlSadek, DM. Galectin-3 as a Potential Target to Prevent Cancer Metastasis. Clin Med Insights Oncol. (2015) 9:113–21. doi: 10.4137/CMO.S29462
68. Yang, RY, Hsu, DK, and Liu, FT. Expression of galectin-3 modulates T-cell growth and apoptosis. Proc Natl Acad Sci U S A. (1996) 93:6737–42. doi: 10.1073/pnas.93.13.6737
69. Song, S, Mazurek, N, Liu, C, Sun, Y, Ding, QQ, Liu, K, et al. Galectin-3 mediates nuclear beta-catenin accumulation and Wnt signaling in human colon cancer cells by regulation of glycogen synthase kinase-3beta activity. Cancer Res. (2009) 69:1343–9. doi: 10.1158/0008-5472.CAN-08-4153
70. Chen, C, Duckworth, CA, Zhao, Q, Pritchard, DM, Rhodes, JM, and Yu, LG. Increased circulation of galectin-3 in cancer induces secretion of metastasis-promoting cytokines from blood vascular endothelium. Clin Cancer Res. (2013) 19:1693–704. doi: 10.1158/1078-0432.CCR-12-2940
71. Zhao, Q, Guo, X, Nash, GB, Stone, PC, Hilkens, J, Rhodes, JM, et al. Circulating galectin-3 promotes metastasis by modifying MUC1 localization on cancer cell surface. Cancer Res. (2009) 69:6799–806. doi: 10.1158/0008-5472.CAN-09-1096
72. Colomb, F, Wang, W, Simpson, D, Zafar, M, Beynon, R, Rhodes, JM, et al. Galectin-3 interacts with the cell-surface glycoprotein CD146 (MCAM, MUC18) and induces secretion of metastasis-promoting cytokines from vascular endothelial cells. J Biol Chem. (2017) 292:8381–9. doi: 10.1074/jbc.M117.783431
73. Yu, LG. The oncofetal Thomsen-Friedenreich carbohydrate antigen in cancer progression. Glycoconj J. (2007) 24:411–20. doi: 10.1007/s10719-007-9034-3
74. Yu, LG, Andrews, N, Zhao, Q, McKean, D, Williams, JF, Connor, LJ, et al. Galectin-3 interaction with Thomsen-Friedenreich disaccharide on cancer-associated MUC1 causes increased cancer cell endothelial adhesion. J Biol Chem. (2007) 282:773–81. doi: 10.1074/jbc.M606862200
75. Murugaesu, N, Iravani, M, van Weverwijk, A, Ivetic, A, Johnson, DA, Antonopoulos, A, et al. An in vivo functional screen identifies ST6GalNAc2 sialyltransferase as a breast cancer metastasis suppressor. Cancer Discov. (2014) 4:304–17. doi: 10.1158/2159-8290.CD-13-0287
76. Yazawa, EM, Geddes-Sweeney, JE, Cedeno-Laurent, F, Walley, KC, Barthel, SR, Opperman, MJ, et al. Melanoma Cell Galectin-1 Ligands Functionally Correlate with Malignant Potential. J Invest Dermatol. (2015) 135:1849–62. doi: 10.1038/jid.2015.95
77. Reticker-Flynn, NE, and Bhatia, SN. Aberrant glycosylation promotes lung cancer metastasis through adhesion to galectins in the metastatic niche. Cancer Discov. (2015) 5:168–81. doi: 10.1158/2159-8290.CD-13-0760
78. Huang, CS, Tang, SJ, Lee, MH, Chang Wang, CC, Sun, GH, and Sun, KH. Galectin-3 promotes CXCR2 to augment the stem-like property of renal cell carcinoma. J Cell Mol Med. (2018) 22:5909–18. doi: 10.1111/jcmm.13860
79. Seguin, L, Camargo, MF, Wettersten, HI, Kato, S, Desgrosellier, JS, von Schalscha, T, et al. Galectin-3, a Druggable Vulnerability for KRAS-Addicted Cancers. Cancer Discov. (2017) 7:1464–79. doi: 10.1158/2159-8290.CD-17-0539
80. Seguin, L, Odouard, S, Corlazzoli, F, Haddad, SA, Moindrot, L, Calvo Tardon, M, et al. Macropinocytosis requires Gal-3 in a subset of patient-derived glioblastoma stem cells. Commun Biol. (2021) 4:718. doi: 10.1038/s42003-021-02258-z
81. Song, S, Ji, B, Ramachandran, V, Wang, H, Hafley, M, Logsdon, C, et al. Overexpressed galectin-3 in pancreatic cancer induces cell proliferation and invasion by binding Ras and activating Ras signaling. PLoS One. (2012) 7:e42699. doi: 10.1371/journal.pone.0042699
82. Zheng, J, Lu, W, Wang, C, Xing, Y, Chen, X, and Ai, Z. Galectin-3 induced by hypoxia promotes cell migration in thyroid cancer cells. Oncotarget. (2017) 8:101475–88. doi: 10.18632/oncotarget.21135
83. Park, GB, Kim, DJ, Kim, YS, Lee, HK, Kim, CW, and Hur, DY. Silencing of galectin-3 represses osteosarcoma cell migration and invasion through inhibition of FAK/Src/Lyn activation and beta-catenin expression and increases susceptibility to chemotherapeutic agents. Int J Oncol. (2015) 46:185–94. doi: 10.3892/ijo.2014.2721
84. Kim, SJ, Choi, IJ, Cheong, TC, Lee, SJ, Lotan, R, Park, SH, et al. Galectin-3 increases gastric cancer cell motility by up-regulating fascin-1 expression. Gastroenterology. (2010) 138:1035–45 e1-2. doi: 10.1053/j.gastro.2009.09.061
85. Bresalier, RS, Mazurek, N, Sternberg, LR, Byrd, JC, Yunker, CK, Nangia-Makker, P, et al. Metastasis of human colon cancer is altered by modifying expression of the beta-galactoside-binding protein galectin 3. Gastroenterology. (1998) 115:287–96. doi: 10.1016/S0016-5085(98)70195-7
86. Ebrahim, AH, Alalawi, Z, Mirandola, L, Rakhshanda, R, Dahlbeck, S, Nguyen, D, et al. Galectins in cancer: carcinogenesis, diagnosis and therapy. Ann Transl Med. (2014) 2:88. doi: 10.3978/j.issn.2305-5839.2014.09.12
87. Knapp, JS, Lokeshwar, SD, Vogel, U, Hennenlotter, J, Schwentner, C, Kramer, MW, et al. Galectin-3 expression in prostate cancer and benign prostate tissues: correlation with biochemical recurrence. World J Urol. (2013) 31:351–8. doi: 10.1007/s00345-012-0925-y
88. Compagno, D, Gentilini, LD, Jaworski, FM, Perez, IG, Contrufo, G, and Laderach, DJ. Glycans and galectins in prostate cancer biology, angiogenesis and metastasis. Glycobiology. (2014) 24:899–906. doi: 10.1093/glycob/cwu055
89. Balan, V, Nangia-Makker, P, Schwartz, AG, Jung, YS, Tait, L, Hogan, V, et al. Racial disparity in breast cancer and functional germ line mutation in galectin-3 (rs4644): a pilot study. Cancer Res. (2008) 68:10045–50. doi: 10.1158/0008-5472.CAN-08-3224
90. Wang, W, Guo, H, Geng, J, Zheng, X, Wei, H, Sun, R, et al. Tumor-released Galectin-3, a soluble inhibitory ligand of human NKp30, plays an important role in tumor escape from NK cell attack. J Biol Chem. (2014) 289:33311–9. doi: 10.1074/jbc.M114.603464
91. Fukumori, T, Takenaka, Y, Yoshii, T, Kim, HR, Hogan, V, Inohara, H, et al. CD29 and CD7 mediate galectin-3-induced type II T-cell apoptosis. Cancer Res. (2003) 63:8302–11.
92. Kouo, T, Huang, L, Pucsek, AB, Cao, M, Solt, S, Armstrong, T, et al. Galectin-3 Shapes Antitumor Immune Responses by Suppressing CD8+ T Cells via LAG-3 and Inhibiting Expansion of Plasmacytoid Dendritic Cells. Cancer Immunol Res. (2015) 3:412–23. doi: 10.1158/2326-6066.CIR-14-0150
93. Demotte, N, Stroobant, V, Courtoy, PJ, Van Der Smissen, P, Colau, D, Luescher, IF, et al. Restoring the association of the T cell receptor with CD8 reverses anergy in human tumor-infiltrating lymphocytes. Immunity. (2008) 28:414–24. doi: 10.1016/j.immuni.2008.01.011
94. Demotte, N, Wieers, G, Van Der Smissen, P, Moser, M, Schmidt, C, Thielemans, K, et al. A galectin-3 ligand corrects the impaired function of human CD4 and CD8 tumor-infiltrating lymphocytes and favors tumor rejection in mice. Cancer Res. (2010) 70:7476–88. doi: 10.1158/0008-5472.CAN-10-0761
95. Diaz-Alvarez, L, and Ortega, E. The Many Roles of Galectin-3, a Multifaceted Molecule, in Innate Immune Responses against Pathogens. Mediat Inflamm. (2017) 2017:9247574. doi: 10.1155/2017/9247574
96. Nieminen, J, Kuno, A, Hirabayashi, J, and Sato, S. Visualization of galectin-3 oligomerization on the surface of neutrophils and endothelial cells using fluorescence resonance energy transfer. J Biol Chem. (2007) 282:1374–83. doi: 10.1074/jbc.M604506200
97. Nieminen, J, St-Pierre, C, and Sato, S. Galectin-3 interacts with naive and primed neutrophils, inducing innate immune responses. J Leukoc Biol. (2005) 78:1127–35. doi: 10.1189/jlb.1204702
98. Fernandez, GC, Ilarregui, JM, Rubel, CJ, Toscano, MA, Gomez, SA, Beigier Bompadre, M, et al. Galectin-3 and soluble fibrinogen act in concert to modulate neutrophil activation and survival: involvement of alternative MAPK pathways. Glycobiology. (2005) 15:519–27. doi: 10.1093/glycob/cwi026
99. Chen, HY, Sharma, BB, Yu, L, Zuberi, R, Weng, IC, Kawakami, Y, et al. Role of galectin-3 in mast cell functions: galectin-3-deficient mast cells exhibit impaired mediator release and defective JNK expression. J Immunol. (2006) 177:4991–7. doi: 10.4049/jimmunol.177.8.4991
100. El Leithy, AA, Helwa, R, Assem, MM, and Hassan, NH. Expression profiling of cancer-related galectins in acute myeloid leukemia. Tumour Biol. (2015) 36:7929–39. doi: 10.1007/s13277-015-3513-0
101. Rechreche, H, Mallo, GV, Montalto, G, Dagorn, JC, and Iovanna, JL. Cloning and expression of the mRNA of human galectin-4, an S-type lectin down-regulated in colorectal cancer. Eur J Biochem. (1997) 248:225–30. doi: 10.1111/j.1432-1033.1997.00225.x
102. Maftouh, M, Belo, AI, Avan, A, Funel, N, Peters, GJ, Giovannetti, E, et al. Galectin-4 expression is associated with reduced lymph node metastasis and modulation of Wnt/beta-catenin signalling in pancreatic adenocarcinoma. Oncotarget. (2014) 5:5335–49. doi: 10.18632/oncotarget.2104
103. Belo, AI, van der Sar, AM, Tefsen, B, and van Die, I. Galectin-4 Reduces Migration and Metastasis Formation of Pancreatic Cancer Cells. PLoS One. (2013) 8:e65957. doi: 10.1371/journal.pone.0065957
104. Nishida, A, Nagahama, K, Imaeda, H, Ogawa, A, Lau, CW, Kobayashi, T, et al. Inducible colitis-associated glycome capable of stimulating the proliferation of memory CD4+ T cells. J Exp Med. (2012) 209:2383–94. doi: 10.1084/jem.20112631
105. Satelli, A, Rao, PS, Thirumala, S, and Rao, US. Galectin-4 functions as a tumor suppressor of human colorectal cancer. Int J Cancer. (2011) 129:799–809. doi: 10.1002/ijc.25750
106. Watanabe, M, Takemasa, I, Kaneko, N, Yokoyama, Y, Matsuo, E, Iwasa, S, et al. Clinical significance of circulating galectins as colorectal cancer markers. Oncol Rep. (2011) 25:1217–26. doi: 10.3892/or.2011.1198
107. Hayashi, T, Saito, T, Fujimura, T, Hara, K, Takamochi, K, Mitani, K, et al. Galectin-4, a Novel Predictor for Lymph Node Metastasis in Lung Adenocarcinoma. PLoS One. (2013) 8:e81883. doi: 10.1371/journal.pone.0081883
108. Barrow, H, Guo, X, Wandall, HH, Pedersen, JW, Fu, B, Zhao, Q, et al. Serum galectin-2, −4, and −8 are greatly increased in colon and breast cancer patients and promote cancer cell adhesion to blood vascular endothelium. Clin Cancer Res. (2011) 17:7035–46. doi: 10.1158/1078-0432.CCR-11-1462
109. Hokama, A, Mizoguchi, E, Sugimoto, K, Shimomura, Y, Tanaka, Y, Yoshida, M, et al. Induced reactivity of intestinal CD4(+) T cells with an epithelial cell lectin, galectin-4, contributes to exacerbation of intestinal inflammation. Immunity. (2004) 20:681–93. doi: 10.1016/j.immuni.2004.05.009
110. Sewgobind, NV, Albers, S, and Pieters, RJ. Functions and Inhibition of Galectin-7, an Emerging Target in Cellular Pathophysiology. Biomol Ther. (2021) 11:1720. doi: 10.3390/biom11111720
111. Perou, CM, Sorlie, T, Eisen, MB, van de Rijn, M, Jeffrey, SS, Rees, CA, et al. Molecular portraits of human breast tumours. Nature. (2000) 406:747–52. doi: 10.1038/35021093
112. Demers, M, Rose, AA, Grosset, AA, Biron-Pain, K, Gaboury, L, Siegel, PM, et al. Overexpression of galectin-7, a myoepithelial cell marker, enhances spontaneous metastasis of breast cancer cells. Am J Pathol. (2010) 176:3023–31. doi: 10.2353/ajpath.2010.090876
113. Gendronneau, G, Sidhu, SS, Delacour, D, Dang, T, Calonne, C, Houzelstein, D, et al. Galectin-7 in the control of epidermal homeostasis after injury. Mol Biol Cell. (2008) 19:5541–9. doi: 10.1091/mbc.e08-02-0166
114. St-Pierre, Y. Towards a Better Understanding of the Relationships between Galectin-7, p53 and MMP-9 during Cancer Progression. Biomolecules. (2021) 11:879. doi: 10.3390/biom11060879
115. Matsui, Y, Ueda, S, Watanabe, J, Kuwabara, I, Ogawa, O, and Nishiyama, H. Sensitizing effect of galectin-7 in urothelial cancer to cisplatin through the accumulation of intracellular reactive oxygen species. Cancer Res. (2007) 67:1212–20. doi: 10.1158/0008-5472.CAN-06-3283
116. Shen, J, Riggs, PK, Hensley, SC, Schroeder, LJ, Traner, AR, Kochan, KJ, et al. Differential expression of multiple anti-apoptotic proteins in epidermis of IGF-1 transgenic mice as revealed by 2-dimensional gel electrophoresis/mass spectrometry analysis. Mol Carcinog. (2007) 46:331–40. doi: 10.1002/mc.20256
117. Shen, J, Pavone, A, Mikulec, C, Hensley, SC, Traner, A, Chang, TK, et al. Protein expression profiles in the epidermis of cyclooxygenase-2 transgenic mice by 2-dimensional gel electrophoresis and mass spectrometry. J Proteome Res. (2007) 6:273–86. doi: 10.1021/pr060418h
118. Biron-Pain, K, Grosset, AA, Poirier, F, Gaboury, L, and St-Pierre, Y. Expression and functions of galectin-7 in human and murine melanomas. PLoS One. (2013) 8:e63307. doi: 10.1371/journal.pone.0063307
119. Guo, JP, and Li, XG. Galectin-7 promotes the invasiveness of human oral squamous cell carcinoma cells via activation of ERK and JNK signaling. Oncol Lett. (2017) 13:1919–24. doi: 10.3892/ol.2017.5649
120. Chen, YS, Chang, CW, Tsay, YG, Huang, LY, Wu, YC, Cheng, LH, et al. HSP40 co-chaperone protein Tid1 suppresses metastasis of head and neck cancer by inhibiting Galectin-7-TCF3-MMP9 axis signaling. Theranostics. (2018) 8:3841–55. doi: 10.7150/thno.25784
121. Shimada, C, Xu, R, Al-Alem, L, Stasenko, M, Spriggs, DR, and Rueda, BR. Galectins and Ovarian Cancer. Cancers (Basel) (2020) 12:1421. doi: 10.3390/cancers12061421
122. Demers, M, Biron-Pain, K, Hebert, J, Lamarre, A, Magnaldo, T, and St-Pierre, Y. Galectin-7 in lymphoma: elevated expression in human lymphoid malignancies and decreased lymphoma dissemination by antisense strategies in experimental model. Cancer Res. (2007) 67:2824–9. doi: 10.1158/0008-5472.CAN-06-3891
123. Ueda, S, Kuwabara, I, and Liu, FT. Suppression of tumor growth by galectin-7 gene transfer. Cancer Res. (2004) 64:5672–6. doi: 10.1158/0008-5472.CAN-04-0985
124. Advedissian, T, Deshayes, F, and Viguier, M. Galectin-7 in epithelial homeostasis and carcinomas. Int J Mol Sci. (2017) 18:2760. doi: 10.3390/ijms18122760
125. Le Mercier, M, Fortin, S, Mathieu, V, Kiss, R, and Lefranc, F. Galectins and Gliomas. Brain Pathol. (2010) 20:17–27. doi: 10.1111/j.1750-3639.2009.00270.x
126. Bidon, N, Brichory, F, Bourguet, P, Le Pennec, P, and Dazord, L. Galectin-8: a complex sub-family of galectins (Review). Int J Mol Med. (2001) 8:245–50. doi: 10.3892/ijmm.8.3.245
127. Carcamo, C, Pardo, E, Oyanadel, C, Bravo-Zehnder, M, Bull, P, Caceres, M, et al. Galectin-8 binds specific beta1 integrins and induces polarized spreading highlighted by asymmetric lamellipodia in Jurkat T cells. Exp Cell Res. (2006) 312:374–86. doi: 10.1016/j.yexcr.2005.10.025
128. Gentilini, LD, Jaworski, FM, Tiraboschi, C, Perez, IG, Kotler, ML, Chauchereau, A, et al. Stable and high expression of Galectin-8 tightly controls metastatic progression of prostate cancer. Oncotarget. (2017) 8:44654–68. doi: 10.18632/oncotarget.17963
129. Vinik, Y, Shatz-Azoulay, H, and Zick, Y. Molecular mechanisms underlying the role of galectin-8 as a regulator of cancer growth and metastasis. Trends Glycosci Glycotechnol. (2018) 30:SE119–28. doi: 10.4052/tigg.1742.1SE
130. Cattaneo, V, Tribulatti, MV, Carabelli, J, Carestia, A, Schattner, M, and Campetella, O. Galectin-8 elicits pro-inflammatory activities in the endothelium. Glycobiology. (2014) 24:966–73. doi: 10.1093/glycob/cwu060
131. Yang, R, Sun, L, Li, C-F, Wang, Y-H, Yao, J, Li, H, et al. Galectin-9 interacts with PD-1 and TIM-3 to regulate T cell death and is a target for cancer immunotherapy. Nat Commun. (2021) 12:832. doi: 10.1038/s41467-021-21099-2
132. Li, H, van der Leun, AM, Yofe, I, Lubling, Y, Gelbard-Solodkin, D, van Akkooi, ACJ, et al. Dysfunctional CD8 T Cells Form a Proliferative, Dynamically Regulated Compartment within Human Melanoma. Cells. (2019) 176:775–89.e18. doi: 10.1016/j.cell.2018.11.043
133. Thommen, DS, Koelzer, VH, Herzig, P, Roller, A, Trefny, M, Dimeloe, S, et al. A transcriptionally and functionally distinct PD-1(+) CD8(+) T cell pool with predictive potential in non-small-cell lung cancer treated with PD-1 blockade. Nat Med. (2018) 24:994–1004. doi: 10.1038/s41591-018-0057-z
134. Sakuishi, K, Apetoh, L, Sullivan, JM, Blazar, BR, Kuchroo, VK, and Anderson, AC. Targeting Tim-3 and PD-1 pathways to reverse T cell exhaustion and restore anti-tumor immunity. J Exp Med. (2010) 207:2187–94. doi: 10.1084/jem.20100643
135. Zhou, X, Sun, L, Jing, D, Xu, G, Zhang, J, Lin, L, et al. Galectin-9 Expression Predicts Favorable Clinical Outcome in Solid Tumors: A Systematic Review and Meta-Analysis. Front Physiol. (2018) 9:452. doi: 10.3389/fphys.2018.00452
136. Yuan, F, Ming, H, Wang, Y, Yang, Y, Yi, L, Li, T, et al. Molecular and clinical characterization of Galectin-9 in glioma through 1,027 samples. J Cell Physiol. (2020) 235:4326–34. doi: 10.1002/jcp.29309
137. Ni, X, Wu, W, Sun, X, Ma, J, Yu, Z, He, X, et al. Interrogating glioma-M2 macrophage interactions identifies Gal-9/Tim-3 as a viable target against PTEN-null glioblastoma. Sci Adv. (2022) 8:eabl5165. doi: 10.1126/sciadv.abl5165
138. Aanhane, E, Schulkens, IA, Heusschen, R, Castricum, K, Leffler, H, Griffioen, AW, et al. Different angioregulatory activity of monovalent galectin-9 isoforms. Angiogenesis. (2018) 21:545–55. doi: 10.1007/s10456-018-9607-8
139. Enninga, EA, Nevala, WK, Holtan, SG, Leontovich, AA, and Markovic, SN. Galectin-9 modulates immunity by promoting Th2/M2 differentiation and impacts survival in patients with metastatic melanoma. Melanoma Res. (2016) 26:429–41. doi: 10.1097/CMR.0000000000000281
140. Seifert, AM, Reiche, C, Heiduk, M, Tannert, A, Meinecke, A-C, Baier, S, et al. Detection of pancreatic ductal adenocarcinoma with galectin-9 serum levels. Oncogene. (2020) 39:3102–13. doi: 10.1038/s41388-020-1186-7
141. Daley, D, Mani, VR, Mohan, N, Akkad, N, Ochi, A, Heindel, DW, et al. Dectin 1 activation on macrophages by galectin 9 promotes pancreatic carcinoma and peritumoral immune tolerance. Nat Med. (2017) 23:556–67. doi: 10.1038/nm.4314
142. Okoye, I, Xu, L, Motamedi, M, Parashar, P, Walker, JW, and Elahi, S. Galectin-9 expression defines exhausted T cells and impaired cytotoxic NK cells in patients with virus-associated solid tumors. J Immunother Cancer. (2020) 8:e001849. doi: 10.1136/jitc-2020-001849
143. Daley, D, Zambirinis, CP, Seifert, L, Akkad, N, Mohan, N, Werba, G, et al. γδ T Cells Support Pancreatic Oncogenesis by Restraining αβ T Cell Activation. Cells. (2016) 166:1485–99.e15. doi: 10.1016/j.cell.2016.07.046
144. Harao, M, Forget, M-A, Roszik, J, Gao, H, Babiera, GV, Krishnamurthy, S, et al. 4-1BB–Enhanced Expansion of CD8+ TIL from Triple-Negative Breast Cancer Unveils Mutation-Specific CD8+ T Cells. Cancer Immunol Res. (2017) 5:439–45. doi: 10.1158/2326-6066.CIR-16-0364
145. Nobumoto, A, Nagahara, K, Oomizu, S, Katoh, S, Nishi, N, Takeshita, K, et al. Galectin-9 suppresses tumor metastasis by blocking adhesion to endothelium and extracellular matrices. Glycobiology. (2008) 18:735–44. doi: 10.1093/glycob/cwn062
146. Kageshita, T, Kashio, Y, Yamauchi, A, Seki, M, Abedin, MJ, Nishi, N, et al. Possible role of galectin-9 in cell aggregation and apoptosis of human melanoma cell lines and its clinical significance. Int J Cancer. (2002) 99:809–16. doi: 10.1002/ijc.10436
147. Mishra, R, Grzybek, M, Niki, T, Hirashima, M, and Simons, K. Galectin-9 trafficking regulates apical-basal polarity in Madin-Darby canine kidney epithelial cells. Proc Natl Acad Sci U S A. (2010) 107:17633–8. doi: 10.1073/pnas.1012424107
148. Jafari, SM, Nazri, A, Shabani, M, Balajam, NZ, and Aghaei, M. Galectin-9 induces apoptosis in OVCAR-3 ovarian cancer cell through mitochondrial pathway. Res Pharm Sci. (2018) 13:557–65. doi: 10.4103/1735-5362.245967
149. Kobayashi, T, Kuroda, J, Ashihara, E, Oomizu, S, Terui, Y, Taniyama, A, et al. Galectin-9 exhibits anti-myeloma activity through JNK and p38 MAP kinase pathways. Leukemia. (2010) 24:843–50. doi: 10.1038/leu.2010.25
150. Zargar Balajam, N, Shabani, M, and Aghaei, M. Galectin-9 inhibits cell proliferation and induces apoptosis in Jurkat and KE-37 acute lymphoblastic leukemia cell lines via caspase-3 activation. Res Pharm Sci. (2021) 16:612–22. doi: 10.4103/1735-5362.327507
151. Grozdanovic, MM, Doyle, CB, Liu, L, Maybruck, BT, Kwatia, MA, Thiyagarajan, N, et al. Charcot-Leyden crystal protein/galectin-10 interacts with cationic ribonucleases and is required for eosinophil granulogenesis. J Allergy Clin Immunol. (2020) 146:377–389.e10. doi: 10.1016/j.jaci.2020.01.013
152. Ackerman, SJ, Corrette, SE, Rosenberg, HF, Bennett, JC, Mastrianni, DM, Nicholson-Weller, A, et al. Molecular cloning and characterization of human eosinophil Charcot-Leyden crystal protein (lysophospholipase). Similarities to IgE binding proteins and the S-type animal lectin superfamily. J Immunol. (1993) 150:456–68. doi: 10.4049/jimmunol.150.2.456
153. Ackerman, SJ, Weil, GJ, and Gleich, GJ. Formation of Charcot-Leyden crystals by human basophils. J Exp Med. (1982) 155:1597–609. doi: 10.1084/jem.155.6.1597
154. Lingblom, C, Andersson, K, and Wenneras, C. Kinetic studies of galectin-10 release from eosinophils exposed to proliferating T cells. Clin Exp Immunol. (2021) 203:230–43. doi: 10.1111/cei.13540
155. Tomizawa, H, Yamada, Y, Arima, M, Miyabe, Y, Fukuchi, M, Hikichi, H, et al. Galectin-10 as a potential biomarker for eosinophilic diseases. Biomolecules. (2022) 12:1385. doi: 10.3390/biom12101385
156. Lin, ES, Hsu, YA, Chang, CY, Lin, HJ, Chen, CS, and Wan, L. Ablation of Galectin-12 Inhibits Atherosclerosis through Enhancement of M2 Macrophage Polarization. Int J Mol Sci. (2020) 21:5511. doi: 10.3390/ijms21155511
157. Xue, H, Yang, RY, Tai, G, and Liu, FT. Galectin-12 inhibits granulocytic differentiation of human NB4 promyelocytic leukemia cells while promoting lipogenesis. J Leukoc Biol. (2016) 100:657–64. doi: 10.1189/jlb.1HI0316-134R
158. Helbawi, F, Ahmed, S, Mahmoud, M, Assem, M, Kamal, T, and Abdou, MA. Methylation status of galectin-12 gene promoter in acute myeloid leukemia. The Egyptian Journal of Haematology. (2021) 46:1–6. doi: 10.4103/ejh.ejh_47_20
159. Yang, RY, Hsu, DK, Yu, L, Ni, J, and Liu, FT. Cell cycle regulation by galectin-12, a new member of the galectin superfamily. J Biol Chem. (2001) 276:20252–60. doi: 10.1074/jbc.M010914200
160. Katzenmaier, EM, Kloor, M, Gabius, HJ, Gebert, J, and Kopitz, J. Analyzing epigenetic control of galectin expression indicates silencing of galectin-12 by promoter methylation in colorectal cancer. IUBMB Life. (2017) 69:962–70. doi: 10.1002/iub.1690
161. Than, NG, Pick, E, Bellyei, S, Szigeti, A, Burger, O, Berente, Z, et al. Functional analyses of placental protein 13/galectin-13. Eur J Biochem. (2004) 271:1065–78. doi: 10.1111/j.1432-1033.2004.04004.x
162. Than, NG, Sumegi, B, Than, GN, Berente, Z, and Bohn, H. Isolation and sequence analysis of a cDNA encoding human placental tissue protein 13 (PP13), a new lysophospholipase, homologue of human eosinophil Charcot-Leyden Crystal protein. Placenta. (1999) 20:703–10. doi: 10.1053/plac.1999.0436
163. Balogh, A, Toth, E, Romero, R, Parej, K, Csala, D, Szenasi, NL, et al. Placental Galectins Are Key Players in Regulating the Maternal Adaptive Immune Response. Front Immunol. (2019) 10:1240. doi: 10.3389/fimmu.2019.01240
164. Nadkarni, S, Smith, J, Sferruzzi-Perri, AN, Ledwozyw, A, Kishore, M, Haas, R, et al. Neutrophils induce proangiogenic T cells with a regulatory phenotype in pregnancy. Proc Natl Acad Sci U S A. (2016) 113:E8415–24. doi: 10.1073/pnas.1611944114
165. Kliman, HJ, Sammar, M, Grimpel, YI, Lynch, SK, Milano, KM, Pick, E, et al. Placental protein 13 and decidual zones of necrosis: an immunologic diversion that may be linked to preeclampsia. Reprod Sci. (2012) 19:16–30. doi: 10.1177/1933719111424445
166. Vokalova, L, Balogh, A, Toth, E, Van Breda, SV, Schafer, G, Hoesli, I, et al. Placental Protein 13 (Galectin-13) Polarizes Neutrophils Toward an Immune Regulatory Phenotype. Front Immunol. (2020) 11:145. doi: 10.3389/fimmu.2020.00145
167. Wang, M, Xu, Y, Wang, P, Xu, Y, Jin, P, Wu, Z, et al. Galectin-14 Promotes Trophoblast Migration and Invasion by Upregulating the Expression of MMP-9 and N-Cadherin. Front Cell Dev Biol. (2021) 9:645658. doi: 10.3389/fcell.2021.645658
169. Scharf, RE. Platelet Signaling in Primary Haemostasis and Arterial Thrombus Formation: Part 1. Hamostaseologie. (2018) 38:203–10. doi: 10.1055/s-0038-1675144
170. Mammadova-Bach, E, Jaeken, J, Gudermann, T, and Braun, A. Platelets and Defective N-Glycosylation. Int J Mol Sci. (2020) 21:5630. doi: 10.3390/ijms21165630
171. Braun, A, Anders, HJ, Gudermann, T, and Mammadova-Bach, E. Platelet-Cancer Interplay: Molecular Mechanisms and New Therapeutic Avenues. Front Oncol. (2021) 11:665534. doi: 10.3389/fonc.2021.665534
172. Mammadova-Bach, E, Nagy, M, Heemskerk, JWM, Nieswandt, B, and Braun, A. Store-operated calcium entry in thrombosis and thrombo-inflammation. Cell Calcium. (2019) 77:39–48. doi: 10.1016/j.ceca.2018.11.005
173. Kazandzhieva, K, Mammadova-Bach, E, Dietrich, A, Gudermann, T, and Braun, A. TRP channel function in platelets and megakaryocytes: basic mechanisms and pathophysiological impact. Pharmacol Ther. (2022) 237:108164. doi: 10.1016/j.pharmthera.2022.108164
174. Stark, K, and Massberg, S. Interplay between inflammation and thrombosis in cardiovascular pathology. Nat Rev Cardiol. (2021) 18:666–82. doi: 10.1038/s41569-021-00552-1
175. Engelmann, B, and Massberg, S. Thrombosis as an intravascular effector of innate immunity. Nat Rev Immunol. (2013) 13:34–45. doi: 10.1038/nri3345
176. Doré, M. Platelet-leukocyte interactions. Am Heart J. (1998) 135:S146–51. doi: 10.1016/S0002-8703(98)70242-X
177. Hidari, KI, Weyrich, AS, Zimmerman, GA, and McEver, RP. Engagement of P-selectin glycoprotein ligand-1 enhances tyrosine phosphorylation and activates mitogen-activated protein kinases in human neutrophils. J Biol Chem. (1997) 272:28750–6. doi: 10.1074/jbc.272.45.28750
178. Mezu-Ndubuisi, OJ, and Maheshwari, A. The role of integrins in inflammation and angiogenesis. Pediatr Res. (2021) 89:1619–26. doi: 10.1038/s41390-020-01177-9
179. O'Sullivan, JM, Jenkins, PV, Rawley, O, Gegenbauer, K, Chion, A, Lavin, M, et al. Galectin-1 and Galectin-3 Constitute Novel-Binding Partners for Factor VIII. Arterioscler Thromb Vasc Biol. (2016) 36:855–63. doi: 10.1161/ATVBAHA.115.306915
180. DeRoo, EP, Wrobleski, SK, Shea, EM, Al-Khalil, RK, Hawley, AE, Henke, PK, et al. The role of galectin-3 and galectin-3-binding protein in venous thrombosis. Blood. (2015) 125:1813–21. doi: 10.1182/blood-2014-04-569939
181. Saint-Lu, N, Oortwijn, BD, Pegon, JN, Odouard, S, Christophe, OD, de Groot, PG, et al. Identification of galectin-1 and galectin-3 as novel partners for von Willebrand factor. Arterioscler Thromb Vasc Biol. (2012) 32:894–901. doi: 10.1161/ATVBAHA.111.240309
182. Roda, O, Ortiz-Zapater, E, Martinez-Bosch, N, Gutierrez-Gallego, R, Vila-Perello, M, Ampurdanes, C, et al. Galectin-1 is a novel functional receptor for tissue plasminogen activator in pancreatic cancer. Gastroenterology. (2009) 136:1379–1390.e5. doi: 10.1053/j.gastro.2008.12.039
183. Romaniuk, MA, Croci, DO, Lapponi, MJ, Tribulatti, MV, Negrotto, S, Poirier, F, et al. Binding of galectin-1 to alphaIIbbeta(3) integrin triggers "outside-in" signals, stimulates platelet activation, and controls primary hemostasis. FASEB J. (2012) 26:2788–98. doi: 10.1096/fj.11-197541
184. Seropian, IM, Cerliani, JP, Toldo, S, Van Tassell, BW, Ilarregui, JM, Gonzalez, GE, et al. Galectin-1 controls cardiac inflammation and ventricular remodeling during acute myocardial infarction. Am J Pathol. (2013) 182:29–40. doi: 10.1016/j.ajpath.2012.09.022
185. Carlos, CP, Silva, AA, Gil, CD, and Oliani, SM. Pharmacological treatment with galectin-1 protects against renal ischaemia-reperfusion injury. Sci Rep. (2018) 8:9568. doi: 10.1038/s41598-018-27907-y
186. Starossom, SC, Mascanfroni, ID, Imitola, J, Cao, L, Raddassi, K, Hernandez, SF, et al. Galectin-1 deactivates classically activated microglia and protects from inflammation-induced neurodegeneration. Immunity. (2012) 37:249–63. doi: 10.1016/j.immuni.2012.05.023
187. Seropian, IM, Gonzalez, GE, Maller, SM, Berrocal, DH, Abbate, A, and Rabinovich, GA. Galectin-1 as an Emerging Mediator of Cardiovascular Inflammation: Mechanisms and Therapeutic Opportunities. Mediat Inflamm. (2018) 2018:8696543. doi: 10.1155/2018/8696543
188. Lekishvili, T, Hesketh, S, Brazier, MW, and Brown, DR. Mouse galectin-1 inhibits the toxicity of glutamate by modifying NR1 NMDA receptor expression. Eur J Neurosci. (2006) 24:3017–25. doi: 10.1111/j.1460-9568.2006.05207.x
189. Dong, H, Wang, ZH, Zhang, N, Liu, SD, Zhao, JJ, and Liu, SY. Serum Galectin-3 level, not Galectin-1, is associated with the clinical feature and outcome in patients with acute ischemic stroke. Oncotarget. (2017) 8:109752–61. doi: 10.18632/oncotarget.18211
190. Gao, Z, Liu, Z, Wang, R, Zheng, Y, Li, H, and Yang, L. Galectin-3 Is a Potential Mediator for Atherosclerosis. J Immunol Res. (2020) 2020:5284728. doi: 10.1155/2020/5284728
191. Papaspyridonos, M, McNeill, E, de Bono, JP, Smith, A, Burnand, KG, Channon, KM, et al. Galectin-3 is an amplifier of inflammation in atherosclerotic plaque progression through macrophage activation and monocyte chemoattraction. Arterioscler Thromb Vasc Biol. (2008) 28:433–40. doi: 10.1161/ATVBAHA.107.159160
192. Lee, YJ, Koh, YS, Park, HE, Lee, HJ, Hwang, BH, Kang, MK, et al. Spatial and temporal expression, and statin responsiveness of galectin-1 and galectin-3 in murine atherosclerosis. Korean Circ J. (2013) 43:223–30. doi: 10.4070/kcj.2013.43.4.223
193. Chen, X, Lin, J, Hu, T, Ren, Z, Li, L, Hameed, I, et al. Galectin-3 exacerbates ox-LDL-mediated endothelial injury by inducing inflammation via integrin beta1-RhoA-JNK signaling activation. J Cell Physiol. (2019) 234:10990–1000. doi: 10.1002/jcp.27910
194. Marin-Royo, G, Gallardo, I, Martinez-Martinez, E, Gutierrez, B, Jurado-Lopez, R, Lopez-Andres, N, et al. Inhibition of galectin-3 ameliorates the consequences of cardiac lipotoxicity in a rat model of diet-induced obesity. Dis Model Mech. (2018) 11:dmm032086. doi: 10.1242/dmm.032086
195. He, J, Li, X, Luo, H, Li, T, Zhao, L, Qi, Q, et al. Galectin-3 mediates the pulmonary arterial hypertension-induced right ventricular remodeling through interacting with NADPH oxidase 4. J Am Soc Hypertens. (2017) 11:275–289.e2. doi: 10.1016/j.jash.2017.03.008
196. Mauris, J, Woodward, AM, Cao, Z, Panjwani, N, and Argueso, P. Molecular basis for MMP9 induction and disruption of epithelial cell-cell contacts by galectin-3. J Cell Sci. (2014) 127:3141–8. doi: 10.1242/jcs.148510
197. Okamura, DM, Pasichnyk, K, Lopez-Guisa, JM, Collins, S, Hsu, DK, Liu, FT, et al. Galectin-3 preserves renal tubules and modulates extracellular matrix remodeling in progressive fibrosis. Am J Physiol Renal Physiol. (2011) 300:F245–53. doi: 10.1152/ajprenal.00326.2010
198. Bambouskova, M, Polakovicova, I, Halova, I, Goel, G, Draberova, L, Bugajev, V, et al. New Regulatory Roles of Galectin-3 in High-Affinity IgE Receptor Signaling. Mol Cell Biol. (2016) 36:1366–82. doi: 10.1128/MCB.00064-16
199. Lehmann, M, Schoeman, RM, Krohl, PJ, Wallbank, AM, Samaniuk, JR, Jandrot-Perrus, M, et al. Platelets Drive Thrombus Propagation in a Hematocrit and Glycoprotein VI-Dependent Manner in an In Vitro Venous Thrombosis Model. Arterioscler Thromb Vasc Biol. (2018) 38:1052–62. doi: 10.1161/ATVBAHA.118.310731
200. Mammadova-Bach, E, Gil-Pulido, J, Sarukhanyan, E, Burkard, P, Shityakov, S, Schonhart, C, et al. Platelet glycoprotein VI promotes metastasis through interaction with cancer cell-derived galectin-3. Blood. (2020) 135:1146–60. doi: 10.1182/blood.2019002649
201. Chen, Y, Fu, W, Zheng, Y, Yang, J, Liu, Y, Qi, Z, et al. Galectin 3 enhances platelet aggregation and thrombosis via Dectin-1 activation: a translational study. Eur Heart J. (2022) 43:3556–74. doi: 10.1093/eurheartj/ehac034
202. Li, M, Guo, K, Huang, X, Feng, L, Yuan, Y, Li, J, et al. Association Between Serum Galectin-3 Levels and Coronary Stenosis Severity in Patients With Coronary Artery Disease. Front Cardiovasc Med. (2022) 9:818162. doi: 10.3389/fcvm.2022.1077332
203. Sato, S, Ouellet, N, Pelletier, I, Simard, M, Rancourt, A, and Bergeron, MG. Role of galectin-3 as an adhesion molecule for neutrophil extravasation during streptococcal pneumonia. J Immunol. (2002) 168:1813–22. doi: 10.4049/jimmunol.168.4.1813
204. Chen, SS, Sun, LW, Brickner, H, and Sun, PQ. Downregulating galectin-3 inhibits proinflammatory cytokine production by human monocyte-derived dendritic cells via RNA interference. Cell Immunol. (2015) 294:44–53. doi: 10.1016/j.cellimm.2015.01.017
205. Fort-Gallifa, I, Hernandez-Aguilera, A, Garcia-Heredia, A, Cabre, N, Luciano-Mateo, F, Simo, JM, et al. Galectin-3 in Peripheral Artery Disease. Relationships with Markers of Oxidative Stress and Inflammation. Int J Mol Sci. (2017) 18:973. doi: 10.3390/ijms18050973
206. Madrigal-Matute, J, Lindholt, JS, Fernandez-Garcia, CE, Benito-Martin, A, Burillo, E, Zalba, G, et al. Galectin-3, a biomarker linking oxidative stress and inflammation with the clinical outcomes of patients with atherothrombosis. J Am Heart Assoc. (2014) 3:e000785. doi: 10.1161/JAHA.114.000785
207. Petrovic, I, Pejnovic, N, Ljujic, B, Pavlovic, S, Miletic Kovacevic, M, Jeftic, I, et al. Overexpression of Galectin 3 in Pancreatic beta Cells Amplifies beta-Cell Apoptosis and Islet Inflammation in Type-2 Diabetes in Mice. Front Endocrinol (Lausanne). (2020) 11:30. doi: 10.3389/fendo.2020.00030
208. Burguillos, MA, Svensson, M, Schulte, T, Boza-Serrano, A, Garcia-Quintanilla, A, Kavanagh, E, et al. Microglia-Secreted Galectin-3 Acts as a Toll-like Receptor 4 Ligand and Contributes to Microglial Activation. Cell Rep. (2015) 10:1626–38. doi: 10.1016/j.celrep.2015.02.012
209. Wesley, UV, Sutton, IC, Cunningham, K, Jaeger, JW, Phan, AQ, Hatcher, JF, et al. Galectin-3 protects against ischemic stroke by promoting neuro-angiogenesis via apoptosis inhibition and Akt/Caspase regulation. J Cereb Blood Flow Metab. (2021) 41:857–73. doi: 10.1177/0271678X20931137
210. Zhuang, JJ, Zhou, L, Zheng, YH, and Ding, YS. The serum galectin-3 levels are associated with the severity and prognosis of ischemic stroke. Aging (Albany NY). (2021) 13:7454–64. doi: 10.18632/aging.202610
211. Korduner, J, Holm, H, Jujic, A, Melander, O, Pareek, M, Molvin, J, et al. Galectin-4 levels in hospitalized versus non-hospitalized subjects with obesity: the Malmo Preventive Project. Cardiovasc Diabetol. (2022) 21:125. doi: 10.1186/s12933-022-01559-9
212. Menkhorst, E, Zhou, W, Santos, LL, Delforce, S, So, T, Rainczuk, K, et al. Galectin-7 Impairs Placentation and Causes Preeclampsia Features in Mice. Hypertension. (2020) 76:1185–94. doi: 10.1161/HYPERTENSIONAHA.120.15313
213. Tian, J, He, R, Fan, Y, Zhang, Q, Tian, B, Zhou, C, et al. Galectin-7 overexpression destroys airway epithelial barrier in transgenic mice. Integr Zool. (2021) 16:270–9. doi: 10.1111/1749-4877.12463
214. Sun, X, and Zhang, W. Silencing of Gal-7 inhibits TGF-beta1-induced apoptosis of human airway epithelial cells through JNK signaling pathway. Exp Cell Res. (2019) 375:100–5. doi: 10.1016/j.yexcr.2018.12.017
215. Wu, SC, Kamili, NA, Dias-Baruffi, M, Josephson, CD, Rathgeber, MF, Yeung, MY, et al. Innate immune Galectin-7 specifically targets microbes that decorate themselves in blood group-like antigens. iScience. (2022) 25:104482. doi: 10.1016/j.isci.2022.104482
216. Luo, Z, Ji, Y, Tian, D, Zhang, Y, Chang, S, Yang, C, et al. Galectin-7 promotes proliferation and Th1/2 cells polarization toward Th1 in activated CD4+ T cells by inhibiting The TGFbeta/Smad3 pathway. Mol Immunol. (2018) 101:80–5. doi: 10.1016/j.molimm.2018.06.003
217. Romaniuk, MA, Tribulatti, MV, Cattaneo, V, Lapponi, MJ, Molinas, FC, Campetella, O, et al. Human platelets express and are activated by galectin-8. Biochem J. (2010) 432:535–47. doi: 10.1042/BJ20100538
218. Zappelli, C, van der Zwaan, C, Thijssen-Timmer, DC, Mertens, K, and Meijer, AB. Novel role for galectin-8 protein as mediator of coagulation factor V endocytosis by megakaryocytes. J Biol Chem. (2012) 287:8327–35. doi: 10.1074/jbc.M111.305151
219. Padilla, A, Moake, JL, Bernardo, A, Ball, C, Wang, Y, Arya, M, et al. P-selectin anchors newly released ultralarge von Willebrand factor multimers to the endothelial cell surface. Blood. (2004) 103:2150–6. doi: 10.1182/blood-2003-08-2956
220. Chabot, S, Kashio, Y, Seki, M, Shirato, Y, Nakamura, K, Nishi, N, et al. Regulation of galectin-9 expression and release in Jurkat T cell line cells. Glycobiology. (2002) 12:111–8. doi: 10.1093/glycob/12.2.111
221. Kasamatsu, A, Uzawa, K, Shimada, K, Shiiba, M, Otsuka, Y, Seki, N, et al. Elevation of galectin-9 as an inflammatory response in the periodontal ligament cells exposed to Porphylomonas gingivalis lipopolysaccharide in vitro and in vivo. Int J Biochem Cell Biol. (2005) 37:397–408. doi: 10.1016/j.biocel.2004.07.014
222. Imaizumi, T, Kumagai, M, Sasaki, N, Kurotaki, H, Mori, F, Seki, M, et al. Interferon-gamma stimulates the expression of galectin-9 in cultured human endothelial cells. J Leukoc Biol. (2002) 72:486–91. doi: 10.1189/jlb.72.3.486
223. He, XW, Li, WL, Li, C, Liu, P, Shen, YG, Zhu, M, et al. Serum levels of galectin-1, galectin-3, and galectin-9 are associated with large artery atherosclerotic stroke. Sci Rep. (2017) 7:40994. doi: 10.1038/srep40994
224. Zhu, R, Liu, C, Tang, H, Zeng, Q, Wang, X, Zhu, Z, et al. Serum Galectin-9 Levels Are Associated with Coronary Artery Disease in Chinese Individuals. Mediat Inflamm. (2015) 2015:457167. doi: 10.1155/2015/457167
225. Nishanth, G, and Schluter, D. Blood-Brain Barrier in Cerebral Malaria: Pathogenesis and Therapeutic Intervention. Trends Parasitol. (2019) 35:516–28. doi: 10.1016/j.pt.2019.04.010
226. Duan, H, Zhao, S, Xiang, J, Ju, C, Chen, X, Gramaglia, I, et al. Targeting the CD146/Galectin-9 axis protects the integrity of the blood-brain barrier in experimental cerebral malaria. Cell Mol Immunol. (2021) 18:2443–54. doi: 10.1038/s41423-020-00582-8
227. Steelman, AJ, and Li, J. Astrocyte galectin-9 potentiates microglial TNF secretion. J Neuroinflammation. (2014) 11:144. doi: 10.1186/s12974-014-0144-0
228. O'Brien, MJ, Shu, Q, Stinson, WA, Tsou, PS, Ruth, JH, Isozaki, T, et al. A unique role for galectin-9 in angiogenesis and inflammatory arthritis. Arthritis Res Ther. (2018) 20:31. doi: 10.1186/s13075-018-1519-x
229. Zhi, Z, Jooss, NJ, Sun, Y, Colicchia, M, Slater, A, Moran, LA, et al. Galectin-9 activates platelet ITAM receptors glycoprotein VI and C-type lectin-like receptor-2. J Thromb Haemost. (2022) 20:936–50. doi: 10.1111/jth.15625
230. Iwasaki-Hozumi, H, Chagan-Yasutan, H, Ashino, Y, and Hattori, T. Blood Levels of Galectin-9, an Immuno-Regulating Molecule, Reflect the Severity for the Acute and Chronic Infectious Diseases. Biomol Ther. (2021) 11:430. doi: 10.3390/biom11030430
231. Foks, AC, Ran, IA, Wasserman, L, Frodermann, V, Ter Borg, MN, de Jager, SC, et al. T-cell immunoglobulin and mucin domain 3 acts as a negative regulator of atherosclerosis. Arterioscler Thromb Vasc Biol. (2013) 33:2558–65. doi: 10.1161/ATVBAHA.113.301879
232. Ilhan, F, and Kalkanli, ST. Atherosclerosis and the role of immune cells. World J Clin Cases. (2015) 3:345–52. doi: 10.12998/wjcc.v3.i4.345
233. Seki, M, Oomizu, S, Sakata, K-m, Sakata, A, Arikawa, T, Watanabe, K, et al. Galectin-9 suppresses the generation of Th17, promotes the induction of regulatory T cells, and regulates experimental autoimmune arthritis. Clin Immunol. (2008) 127:78–88. doi: 10.1016/j.clim.2008.01.006
234. Li, ZH, Wang, LL, Liu, H, Muyayalo, KP, Huang, XB, Mor, G, et al. Galectin-9 Alleviates LPS-Induced Preeclampsia-Like Impairment in Rats via Switching Decidual Macrophage Polarization to M2 Subtype. Front Immunol. (2018) 9:3142. doi: 10.3389/fimmu.2018.03142
235. Dardalhon, V, Anderson, AC, Karman, J, Apetoh, L, Chandwaskar, R, Lee, DH, et al. Tim-3/galectin-9 pathway: regulation of Th1 immunity through promotion of CD11b+Ly-6G+ myeloid cells. J Immunol. (2010) 185:1383–92. doi: 10.4049/jimmunol.0903275
236. Oomizu, S, Arikawa, T, Niki, T, Kadowaki, T, Ueno, M, Nishi, N, et al. Cell surface galectin-9 expressing Th cells regulate Th17 and Foxp3+ Treg development by galectin-9 secretion. PLoS One. (2012) 7:e48574. doi: 10.1371/journal.pone.0048574
237. Lhuillier, C, Barjon, C, Niki, T, Gelin, A, Praz, F, Morales, O, et al. Impact of Exogenous Galectin-9 on Human T Cells: contribution of the T cell receptor complex to antigen-independent activation but not to apoptosis induction. J Biol Chem. (2015) 290:16797–811. doi: 10.1074/jbc.M115.661272
238. Xie, JH, Zhu, RR, Zhao, L, Zhong, YC, and Zeng, QT. Down-regulation and Clinical Implication of Galectin-9 Levels in Patients with Acute Coronary Syndrome and Chronic Kidney Disease. Curr Med Sci. (2020) 40:662–70. doi: 10.1007/s11596-020-2238-5
239. Chakraborty, A, Staudinger, C, King, SL, Erickson, FC, Lau, LS, Bernasconi, A, et al. Galectin-9 bridges human B cells to vascular endothelium while programming regulatory pathways. J Autoimmun. (2021) 117:102575. doi: 10.1016/j.jaut.2020.102575
240. Matsumoto, R, Matsumoto, H, Seki, M, Hata, M, Asano, Y, Kanegasaki, S, et al. Human ecalectin, a variant of human galectin-9, is a novel eosinophil chemoattractant produced by T lymphocytes. J Biol Chem. (1998) 273:16976–84. doi: 10.1074/jbc.273.27.16976
241. Hirashima, M. Ecalectin/galectin-9, a novel eosinophil chemoattractant: its function and production. Int Arch Allergy Immunol. (2000) 122:6–9. doi: 10.1159/000053623
242. Dai, SY, Nakagawa, R, Itoh, A, Murakami, H, Kashio, Y, Abe, H, et al. Galectin-9 induces maturation of human monocyte-derived dendritic cells. J Immunol. (2005) 175:2974–81. doi: 10.4049/jimmunol.175.5.2974
243. Iqbal, AJ, Krautter, F, Blacksell, IA, Wright, RD, Austin-Williams, SN, Voisin, MB, et al. Galectin-9 mediates neutrophil capture and adhesion in a CD44 and beta2 integrin-dependent manner. FASEB J. (2022) 36:e22065. doi: 10.1096/fj.202100832R
244. Steichen, AL, Simonson, TJ, Salmon, SL, Metzger, DW, Mishra, BB, and Sharma, J. Alarmin function of galectin-9 in murine respiratory tularemia. PLoS One. (2015) 10:e0123573. doi: 10.1371/journal.pone.0123573
245. Matsuura, A, Tsukada, J, Mizobe, T, Higashi, T, Mouri, F, Tanikawa, R, et al. Intracellular galectin-9 activates inflammatory cytokines in monocytes. Genes Cells. (2009) 14:511–21. doi: 10.1111/j.1365-2443.2009.01287.x
246. Hellmich, B, Holle, J, and Moosig, F. Eosinophilic granulomatosis with polyangiitis: Update on classification and management. Z Rheumatol. (2022) 81:286–99. doi: 10.1007/s00393-021-01153-6
247. Fukuchi, M, Kamide, Y, Ueki, S, Miyabe, Y, Konno, Y, Oka, N, et al. Eosinophil ETosis-Mediated Release of Galectin-10 in Eosinophilic Granulomatosis With Polyangiitis. Arthritis Rheumatol. (2021) 73:1683–93. doi: 10.1002/art.41727
248. Marx, C, Novotny, J, Salbeck, D, Zellner, KR, Nicolai, L, Pekayvaz, K, et al. Eosinophil-platelet interactions promote atherosclerosis and stabilize thrombosis with eosinophil extracellular traps. Blood. (2019) 134:1859–72. doi: 10.1182/blood.2019000518
249. Pertiwi, KR, de Boer, OJ, Mackaaij, C, Pabittei, DR, de Winter, RJ, Li, X, et al. Extracellular traps derived from macrophages, mast cells, eosinophils and neutrophils are generated in a time-dependent manner during atherothrombosis. J Pathol. (2007) 247:505–12. doi: 10.1002/path.5212
250. Yang, RY, Hsu, DK, Yu, L, Chen, HY, and Liu, FT. Galectin-12 is required for adipogenic signaling and adipocyte differentiation. J Biol Chem. (2004) 279:29761–6. doi: 10.1074/jbc.M401303200
251. Yang, RY, Yu, L, Graham, JL, Hsu, DK, Lloyd, KC, Havel, PJ, et al. Ablation of a galectin preferentially expressed in adipocytes increases lipolysis, reduces adiposity, and improves insulin sensitivity in mice. Proc Natl Acad Sci U S A. (2011) 108:18696–701. doi: 10.1073/pnas.1109065108
252. Wan, L, Lin, HJ, Huang, CC, Chen, YC, Hsu, YA, Lin, CH, et al. Galectin-12 enhances inflammation by promoting M1 polarization of macrophages and reduces insulin sensitivity in adipocytes. Glycobiology. (2016) 26:732–44. doi: 10.1093/glycob/cww013
253. Jovanovic Krivokuca, M, Vilotic, A, Nacka-Aleksic, M, Pirkovic, A, Cujic, D, Legner, J, et al. Galectins in Early Pregnancy and Pregnancy-Associated Pathologies. Int J Mol Sci. (2021) 23:69. doi: 10.3390/ijms23010069
254. Abdel-Razeq, H, Mansour, A, Saadeh, SS, Abu-Nasser, M, Makoseh, M, Salam, M, et al. The Application of Current Proposed Venous Thromboembolism Risk Assessment Model for Ambulatory Patients With Cancer. Clin Appl Thromb Hemost. (2018) 24:429–33. doi: 10.1177/1076029617692880
255. Menter, DG, Kopetz, S, Hawk, E, Sood, AK, Loree, JM, Gresele, P, et al. Platelet "first responders" in wound response, cancer, and metastasis. Cancer Metastasis Rev. (2017) 36:199–213. doi: 10.1007/s10555-017-9682-0
256. van Es, N, Sturk, A, Middeldorp, S, and Nieuwland, R. Effects of cancer on platelets. Semin Oncol. (2014) 41:311–8. doi: 10.1053/j.seminoncol.2014.04.015
257. Mammadova-Bach, E, Mangin, P, Lanza, F, and Gachet, C. Platelets in cancer. From basic research to therapeutic implications. Hamostaseologie. (2015) 35:325–36. doi: 10.5482/hamo-14-11-0065
258. Mammadova-Bach, E, Zigrino, P, Brucker, C, Bourdon, C, Freund, M, De Arcangelis, A, et al. Platelet integrin alpha6beta1 controls lung metastasis through direct binding to cancer cell-derived ADAM9. JCI Insight. (2016) 1:e88245. doi: 10.1172/jci.insight.88245
259. Nielsen, MI, Stegmayr, J, Grant, OC, Yang, Z, Nilsson, UJ, Boos, I, et al. Galectin binding to cells and glycoproteins with genetically modified glycosylation reveals galectin-glycan specificities in a natural context. J Biol Chem. (2018) 293:20249–62. doi: 10.1074/jbc.RA118.004636
260. Dimitroff, CJ. Galectin-Binding O-Glycosylations as Regulators of Malignancy. Cancer Res. (2015) 75:3195–202. doi: 10.1158/0008-5472.CAN-15-0834
261. Saha, B, Mathur, T, Tronolone, JJ, Chokshi, M, Lokhande, GK, Selahi, A, et al. Human tumor microenvironment chip evaluates the consequences of platelet extravasation and combinatorial antitumor-antiplatelet therapy in ovarian cancer. Sci Adv. (2021) 7:eabg5283. doi: 10.1126/sciadv.abg5283
262. Bum-Erdene, K, Leffler, H, Nilsson, UJ, and Blanchard, H. Structural characterization of human galectin-4 C-terminal domain: elucidating the molecular basis for recognition of glycosphingolipids, sulfated saccharides and blood group antigens. FEBS J. (2015) 282:3348–67. doi: 10.1111/febs.13348
263. Helwa, R, Heller, A, Knappskog, S, and Bauer, AS. Tumor cells interact with red blood cells via galectin-4 - a short report. Cell Oncol (Dordr). (2017) 40:401–9. doi: 10.1007/s13402-017-0317-9
264. Thijssen, VL, Barkan, B, Shoji, H, Aries, IM, Mathieu, V, Deltour, L, et al. Tumor Cells Secrete Galectin-1 to Enhance Endothelial Cell Activity. Cancer Res. (2010) 70:6216–24. doi: 10.1158/0008-5472.CAN-09-4150
265. Dings, RPM, Kumar, N, Miller, MC, Loren, M, Rangwala, H, Hoye, TR, et al. Structure-Based Optimization of Angiostatic Agent 6DBF7, an Allosteric Antagonist of Galectin-1. J Pharmacol Exp Ther. (2013) 344:589–99. doi: 10.1124/jpet.112.199646
266. Salomonsson, E, Thijssen, VL, Griffioen, AW, Nilsson, UJ, and Leffler, H. The anti-angiogenic peptide anginex greatly enhances galectin-1 binding affinity for glycoproteins. J Biol Chem. (2011) 286:13801–4. doi: 10.1074/jbc.C111.229096
267. Croci, DO, Salatino, M, Rubinstein, N, Cerliani, JP, Cavallin, LE, Leung, HJ, et al. Disrupting galectin-1 interactions with N-glycans suppresses hypoxia-driven angiogenesis and tumorigenesis in Kaposi's sarcoma. J Exp Med. (2012) 209:1985–2000. doi: 10.1084/jem.20111665
268. Ouyang, J, Juszczynski, P, Rodig, SJ, Green, MR, O'Donnell, E, Currie, T, et al. Viral induction and targeted inhibition of galectin-1 in EBV+ posttransplant lymphoproliferative disorders. Blood. (2011) 117:4315–22. doi: 10.1182/blood-2010-11-320481
269. Dings, RP, Miller, MC, Nesmelova, I, Astorgues-Xerri, L, Kumar, N, Serova, M, et al. Antitumor agent calixarene 0118 targets human galectin-1 as an allosteric inhibitor of carbohydrate binding. J Med Chem. (2012) 55:5121–9. doi: 10.1021/jm300014q
270. Astorgues-Xerri, L, Riveiro, ME, Tijeras-Raballand, A, Serova, M, Rabinovich, GA, Bieche, I, et al. OTX008, a selective small-molecule inhibitor of galectin-1, downregulates cancer cell proliferation, invasion and tumour angiogenesis. Eur J Cancer. (2014) 50:2463–77. doi: 10.1016/j.ejca.2014.06.015
271. Paz, H, Joo, EJ, Chou, CH, Fei, F, Mayo, KH, Abdel-Azim, H, et al. Treatment of B-cell precursor acute lymphoblastic leukemia with the Galectin-1 inhibitor PTX008. J Exp Clin Cancer Res. (2018) 37:67. doi: 10.1186/s13046-018-0721-7
272. Koonce, NA, Griffin, RJ, and Dings, RPM. Galectin-1 Inhibitor OTX008 Induces Tumor Vessel Normalization and Tumor Growth Inhibition in Human Head and Neck Squamous Cell Carcinoma Models. Int J Mol Sci. (2017) 18:2671. doi: 10.3390/ijms18122671
273. Zucchetti, M, Bonezzi, K, Frapolli, R, Sala, F, Borsotti, P, Zangarini, M, et al. Pharmacokinetics and antineoplastic activity of galectin-1-targeting OTX008 in combination with sunitinib. Cancer Chemother Pharmacol. (2013) 72:879–87. doi: 10.1007/s00280-013-2270-2
274. Leung, Z, Ko, FCF, Tey, SK, Kwong, EML, Mao, X, Liu, BHM, et al. Galectin-1 promotes hepatocellular carcinoma and the combined therapeutic effect of OTX008 galectin-1 inhibitor and sorafenib in tumor cells. J Exp Clin Cancer Res. (2019) 38:423. doi: 10.1186/s13046-019-1402-x
275. Delord, J-P, Awada, A, Raymond, E, Lokiec, F, Herait, P, Rezai, K, et al. Abstract A72: A first-in-man Phase I study of the galectin-1 (gal-1) inhibitor OTX008 given subcutaneously as a single agent to patients with advanced solid tumors. Mol Cancer Ther. (2013) 12:A72. doi: 10.1158/1535-7163.TARG-13-A72
276. Rezai, K, Durand, S, Lachaux, N, Raymond, E, Herait, P, and Lokiec, F. Abstract 33: OTX008 pharmacokinetics (PK) during the first-in-man phase I study in patients with advanced solid tumors. Cancer Res. (2013) 73:33. doi: 10.1158/1538-7445.AM2013-33
277. Ito, K, and Ralph, SJ. Inhibiting galectin-1 reduces murine lung metastasis with increased CD4(+) and CD8 (+) T cells and reduced cancer cell adherence. Clin Exp Metastasis. (2012) 29:561–72. doi: 10.1007/s10585-012-9471-7
278. Ito, K, Scott, SA, Cutler, S, Dong, LF, Neuzil, J, Blanchard, H, et al. Thiodigalactoside inhibits murine cancers by concurrently blocking effects of galectin-1 on immune dysregulation, angiogenesis and protection against oxidative stress. Angiogenesis. (2011) 14:293–307. doi: 10.1007/s10456-011-9213-5
279. Giguere, D, Sato, S, St-Pierre, C, Sirois, S, and Roy, R. Aryl O- and S-galactosides and lactosides as specific inhibitors of human galectins-1 and -3: role of electrostatic potential at O-3. Bioorg Med Chem Lett. (2006) 16:1668–72. doi: 10.1016/j.bmcl.2005.12.010
280. Ji, P, Gong, Y, Jin, ML, Wu, HL, Guo, LW, Pei, YC, et al. In vivo multidimensional CRISPR screens identify Lgals2 as an immunotherapy target in triple-negative breast cancer. Sci Adv. (2022) 8:eabl8247. doi: 10.1126/sciadv.abl8247
281. Demotte, N, Bigirimana, R, Wieërs, G, Stroobant, V, Squifflet, J-L, Carrasco, J, et al. A Short Treatment with Galactomannan GM-CT-01 Corrects the Functions of Freshly Isolated Human Tumor–Infiltrating Lymphocytes. Clin Cancer Res. (2014) 20:1823–33. doi: 10.1158/1078-0432.CCR-13-2459
282. Klyosov, A, Zomer, E, and Platt, D. DAVANAT® (GM-CT-01) and colon cancer: preclinical and clinical (phase I and II) studies. Glycobiology and drug design. American Chemical Society; Washington, DC, USA: ACS Publications; (2012) 89–130.
283. Louvain CuS-L-UCd (2012). Peptide Vaccinations Plus GM-CT-01 in Melanoma. Available at: https://ClinicalTrials.gov/show/NCT01723813 [Accessed April].
284. Galectin Therapeutics Inc. (2003). Safety of GM-CT-01 With and Without 5-Fluorouracil in Patients With Solid Tumors. Available at: https://ClinicalTrials.gov/show/NCT00054977 [Accessed February].
285. Goud, NS, Soukya, PSL, Ghouse, M, Komal, D, Alvala, R, and Alvala, M. Human Galectin-1 and Its Inhibitors: Privileged Target for Cancer and HIV. Mini Rev Med Chem. (2019) 19:1369–78. doi: 10.2174/1389557519666190304120821
286. Rabinovich, GA, Cumashi, A, Bianco, GA, Ciavardelli, D, Iurisci, I, D’Egidio, M, et al. Synthetic lactulose amines: novel class of anticancer agents that induce tumor-cell apoptosis and inhibit galectin-mediated homotypic cell aggregation and endothelial cell morphogenesis. Glycobiology. (2006) 16:210–20. doi: 10.1093/glycob/cwj056
287. Delaine, T, Cumpstey, I, Ingrassia, L, Le Mercier, M, Okechukwu, P, Leffler, H, et al. Galectin-inhibitory thiodigalactoside ester derivatives have antimigratory effects in cultured lung and prostate cancer cells. J Med Chem. (2008) 51:8109–14. doi: 10.1021/jm801077j
288. Newton-Northup, JR, Dickerson, MT, Ma, L, Besch-Williford, CL, and Deutscher, SL. Inhibition of metastatic tumor formation in vivo by a bacteriophage display-derived galectin-3 targeting peptide. Clin Exp Metastasis. (2013) 30:119–32. doi: 10.1007/s10585-012-9516-y
289. Yang, Y, Zhou, Z, He, S, Fan, T, Jin, Y, Zhu, X, et al. Treatment of prostate carcinoma with (galectin-3)-targeted HPMA copolymer-(G3-C12)-5-Fluorouracil conjugates. Biomaterials. (2012) 33:2260–71. doi: 10.1016/j.biomaterials.2011.12.007
290. Mirandola, L, Yu, Y, Chui, K, Jenkins, MR, Cobos, E, John, CM, et al. Galectin-3C inhibits tumor growth and increases the anticancer activity of bortezomib in a murine model of human multiple myeloma. PLoS One. (2011) 6:e21811. doi: 10.1371/journal.pone.0021811
291. Guha, P, Kaptan, E, Bandyopadhyaya, G, Kaczanowska, S, Davila, E, Thompson, K, et al. Cod glycopeptide with picomolar affinity to galectin-3 suppresses T-cell apoptosis and prostate cancer metastasis. Proc Natl Acad Sci U S A. (2013) 110:5052–7. doi: 10.1073/pnas.1202653110
292. Wang, P, Zhang, L, Yao, J, Shi, Y, Li, P, and Ding, K. An arabinogalactan from flowers of Panax notoginseng inhibits angiogenesis by BMP2/Smad/Id1 signaling. Carbohydr Polym. (2015) 121:328–35. doi: 10.1016/j.carbpol.2014.11.073
293. Galecto Biotech AB (2019). A First in Human Study to Evaluate the Safety, Tolerability and PK of GB1211 in Healthy Subjects. Available at: https://ClinicalTrials.gov/show/NCT03809052 [Accessed January 14].
294. Galecto Biotech AB, Linical Europe GmbHRoche, Hoffmann-La. (2022). A Study to Investigate the Safety and Efficacy of GB1211 (a Galectin-3 Inhibitor) in Combination With Atezolizumab in Patients With Non-Small Cell Lung Cancer (NSCLC). Available at: https://ClinicalTrials.gov/show/NCT05240131 [Accessed March 15].
295. Chauhan, D, Li, G, Podar, K, Hideshima, T, Neri, P, He, D, et al. A novel carbohydrate-based therapeutic GCS-100 overcomes bortezomib resistance and enhances dexamethasone-induced apoptosis in multiple myeloma cells. Cancer Res. (2005) 65:8350–8. doi: 10.1158/0008-5472.CAN-05-0163
296. Ruvolo, PP, Ruvolo, VR, Benton, CB, AlRawi, A, Burks, JK, Schober, W, et al. Combination of galectin inhibitor GCS-100 and BH3 mimetics eliminates both p53 wild type and p53 null AML cells. Biochim Biophys Acta. (2016) 1863:562–71. doi: 10.1016/j.bbamcr.2015.12.008
297. Streetly, MJ, Maharaj, L, Joel, S, Schey, SA, Gribben, JG, and Cotter, FE. GCS-100, a novel galectin-3 antagonist, modulates MCL-1, NOXA, and cell cycle to induce myeloma cell death. Blood. (2010) 115:3939–48. doi: 10.1182/blood-2009-10-251660
298. Lee, J-J, Hsu, Y-C, Li, Y-S, and Cheng, S-P. Galectin-3 Inhibitors Suppress Anoikis Resistance and Invasive Capacity in Thyroid Cancer Cells. Int J Endocrinol. (2021) 2021:5583491. doi: 10.1155/2021/5583491
299. Galecto Biotech AB (2014). RCT (Randomized Control Trial) of TD139 vs Placebo in HV's (Human Volunteers) and IPF Patients. Available at: https://ClinicalTrials.gov/show/NCT02257177 [Accessed September].
300. Vuong, L, Kouverianou, E, Rooney, CM, McHugh, BJ, Howie, SEM, Gregory, CD, et al. An Orally Active Galectin-3 Antagonist Inhibits Lung Adenocarcinoma Growth and Augments Response to PD-L1 Blockade. Cancer Res. (2019) 79:1480–92. doi: 10.1158/0008-5472.CAN-18-2244
301. Hossein, G, Halvaei, S, Heidarian, Y, Dehghani-Ghobadi, Z, Hassani, M, Hosseini, H, et al. Pectasol-C Modified Citrus Pectin targets Galectin-3-induced STAT3 activation and synergize paclitaxel cytotoxic effect on ovarian cancer spheroids. Cancer Med. (2019) 8:4315–29. doi: 10.1002/cam4.2334
302. Nangia-Makker, P, Hogan, V, Honjo, Y, Baccarini, S, Tait, L, Bresalier, R, et al. Inhibition of human cancer cell growth and metastasis in nude mice by oral intake of modified citrus pectin. J Natl Cancer Inst. (2002) 94:1854–62. doi: 10.1093/jnci/94.24.1854
303. Tehranian, N, Sepehri, H, Mehdipour, P, Biramijamal, F, Hossein-Nezhad, A, Sarrafnejad, A, et al. Combination effect of PectaSol and Doxorubicin on viability, cell cycle arrest and apoptosis in DU-145 and LNCaP prostate cancer cell lines. Cell Biol Int. (2012) 36:601–10. doi: 10.1042/CBI20110309
304. Hassan, M, Baussiere, F, Guzelj, S, Sundin, AP, Hakansson, M, Kovacic, R, et al. Structure-Guided Design of d-Galactal Derivatives with High Affinity and Selectivity for the Galectin-8 N-Terminal Domain. ACS Med Chem Lett. (2021) 12:1745–52. doi: 10.1021/acsmedchemlett.1c00371
305. Kuroda, J, Yamamoto, M, Nagoshi, H, Kobayashi, T, Sasaki, N, Shimura, Y, et al. Targeting activating transcription factor 3 by Galectin-9 induces apoptosis and overcomes various types of treatment resistance in chronic myelogenous leukemia. Mol Cancer Res. (2010) 8:994–1001. doi: 10.1158/1541-7786.MCR-10-0040
306. Wolf, Y, Anderson, AC, and Kuchroo, VK. TIM3 comes of age as an inhibitory receptor. Nat Rev Immunol. (2020) 20:173–85. doi: 10.1038/s41577-019-0224-6
307. Acharya, N, Sabatos-Peyton, C, and Anderson, AC. Tim-3 finds its place in the cancer immunotherapy landscape. J Immunother Cancer. (2020) 8:e000911. doi: 10.1136/jitc-2020-000911
308. Ryser, S, Estellés, A, Tenorio, E, Kauvar, LM, and Gishizky, ML. High affinity anti-TIM-3 and anti-KIR monoclonal antibodies cloned from healthy human individuals. PLoS One. (2017) 12:e0181464. doi: 10.1371/journal.pone.0181464
309. Harding, JJ, Moreno, V, Bang, YJ, Hong, MH, Patnaik, A, Trigo, J, et al. Blocking TIM-3 in Treatment-refractory Advanced Solid Tumors: A Phase Ia/b Study of LY3321367 with or without an Anti-PD-L1 Antibody. Clin Cancer Res. (2021) 27:2168–78. doi: 10.1158/1078-0432.CCR-20-4405
310. Curigliano, G, Gelderblom, H, Mach, N, Doi, T, Tai, D, Forde, PM, et al. Phase I/Ib Clinical Trial of Sabatolimab, an Anti-TIM-3 Antibody, Alone and in Combination with Spartalizumab, an Anti-PD-1 Antibody, in Advanced Solid Tumors. Clin Cancer Res. (2021) 27:3620–9. doi: 10.1158/1078-0432.CCR-20-4746
311. Le, QT, Shi, G, Cao, H, Nelson, DW, Wang, Y, Chen, EY, et al. Galectin-1: a link between tumor hypoxia and tumor immune privilege. J Clin Oncol. (2005) 23:8932–41. doi: 10.1200/JCO.2005.02.0206
312. Amano, M, Suzuki, M, Andoh, S, Monzen, H, Terai, K, Williams, B, et al. Antiangiogenesis therapy using a novel angiogenesis inhibitor, anginex, following radiation causes tumor growth delay. Int J Clin Oncol. (2007) 12:42–7. doi: 10.1007/s10147-006-0625-y
313. Croci, DO, and Rabinovich, GA. Linking tumor hypoxia with VEGFR2 signaling and compensatory angiogenesis: Glycans make the difference. Onco Targets Ther. (2014) 3:e29380. doi: 10.4161/onci.29380
314. Lappchen, T, Dings, RP, Rossin, R, Simon, JF, Visser, TJ, Bakker, M, et al. Novel analogs of antitumor agent calixarene 0118: Synthesis, cytotoxicity, click labeling with 2-[(18)F]fluoroethylazide, and in vivo evaluation. Eur J Med Chem. (2015) 89:279–95. doi: 10.1016/j.ejmech.2014.10.048
315. Ying, X, Che, X, Wang, J, Zou, G, Yu, Q, and Zhang, X. CDK1 serves as a novel therapeutic target for endometrioid endometrial cancer. J Cancer. (2021) 12:2206–15. doi: 10.7150/jca.51139
316. Brandwijk, RJ, Nesmelova, I, Dings, RP, Mayo, KH, Thijssen, VL, and Griffioen, AW. Cloning an artificial gene encoding angiostatic anginex: From designed peptide to functional recombinant protein. Biochem Biophys Res Commun. (2005) 333:1261–8. doi: 10.1016/j.bbrc.2005.06.029
317. Kane, J, Jansen, M, Hendrix, S, Bosmans, LA, Beckers, L, Tiel, CV, et al. Anti-Galectin-2 Antibody Treatment Reduces Atherosclerotic Plaque Size and Alters Macrophage Polarity. Thromb Haemost. (2022) 122:1047–57. doi: 10.1055/a-1711-1055
318. Miller, MC, Klyosov, A, and Mayo, KH. The alpha-galactomannan Davanat binds galectin-1 at a site different from the conventional galectin carbohydrate binding domain. Glycobiology. (2009) 19:1034–45. doi: 10.1093/glycob/cwp084
319. Demotte, N, Bigirimana, R, Wieers, G, Stroobant, V, Squifflet, JL, Carrasco, J, et al. A short treatment with galactomannan GM-CT-01 corrects the functions of freshly isolated human tumor-infiltrating lymphocytes. Clin Cancer Res. (2014) 20:1823–33. doi: 10.1158/1078-0432.CCR-13-2459
320. Klyosov, AA, and Traber, PG. (2012). Galectins in Disease and Potential Therapeutic Approaches. Galectins and Disease Implications for Targeted Therapeutics. ACS Symposium Series. 1115: American Chemical Society. p. 3–43.
321. Traber, PG, and Zomer, E. Therapy of experimental NASH and fibrosis with galectin inhibitors. PLoS One. (2013) 8:e83481. doi: 10.1371/journal.pone.0083481
322. Curti, BD, Koguchi, Y, Leidner, RS, Rolig, AS, Sturgill, ER, Sun, Z, et al. Enhancing clinical and immunological effects of anti-PD-1 with belapectin, a galectin-3 inhibitor. J Immunother Cancer. (2021) 9:e002371. doi: 10.1136/jitc-2021-002371
323. Glinskii, OV, Huxley, VH, Glinsky, GV, Pienta, KJ, Raz, A, and Glinsky, VV. Mechanical entrapment is insufficient and intercellular adhesion is essential for metastatic cell arrest in distant organs. Neoplasia. (2005) 7:522–7. doi: 10.1593/neo.04646
324. Pienta, KJ, Naik, H, Akhtar, A, Yamazaki, K, Replogle, TS, Lehr, J, et al. Inhibition of spontaneous metastasis in a rat prostate cancer model by oral administration of modified citrus pectin. J Natl Cancer Inst. (1995) 87:348–53. doi: 10.1093/jnci/87.5.348
325. Glinsky, VV, and Raz, A. Modified citrus pectin anti-metastatic properties: one bullet, multiple targets. Carbohydr Res. (2009) 344:1788–91. doi: 10.1016/j.carres.2008.08.038
326. Calvier, L, Martinez-Martinez, E, Miana, M, Cachofeiro, V, Rousseau, E, Sadaba, JR, et al. The impact of galectin-3 inhibition on aldosterone-induced cardiac and renal injuries. JACC Heart Fail. (2015) 3:59–67. doi: 10.1016/j.jchf.2014.08.002
327. Martinez-Martinez, E, Calvier, L, Rossignol, P, Rousseau, E, Fernandez-Celis, A, Jurado-Lopez, R, et al. Galectin-3 inhibition prevents adipose tissue remodelling in obesity. Int J Obes. (2016) 40:1034–8. doi: 10.1038/ijo.2016.19
328. Vergaro, G, Prud'homme, M, Fazal, L, Merval, R, Passino, C, Emdin, M, et al. Inhibition of Galectin-3 Pathway Prevents Isoproterenol-Induced Left Ventricular Dysfunction and Fibrosis in Mice. Hypertension. (2016) 67:606–12. doi: 10.1161/HYPERTENSIONAHA.115.06161
329. Lu, Y, Zhang, M, Zhao, P, Jia, M, Liu, B, Jia, Q, et al. Modified citrus pectin inhibits galectin-3 function to reduce atherosclerotic lesions in apoE-deficient mice. Mol Med Rep. (2017) 16:647–53. doi: 10.3892/mmr.2017.6646
330. Ibarrola, J, Matilla, L, Martinez-Martinez, E, Gueret, A, Fernandez-Celis, A, Henry, JP, et al. Myocardial Injury After Ischemia/Reperfusion Is Attenuated By Pharmacological Galectin-3 Inhibition. Sci Rep. (2019) 9:9607. doi: 10.1038/s41598-019-46119-6
331. Milligan, K, Deng, X, Ali-Adeeb, R, Shreeves, P, Punch, S, Costie, N, et al. Prediction of disease progression indicators in prostate cancer patients receiving HDR-brachytherapy using Raman spectroscopy and semi-supervised learning: a pilot study. Sci Rep. (2022) 12:15104. doi: 10.1038/s41598-022-19446-4
332. Mackinnon, AC, Gibbons, MA, Farnworth, SL, Leffler, H, Nilsson, UJ, Delaine, T, et al. Regulation of transforming growth factor-beta1-driven lung fibrosis by galectin-3. Am J Respir Crit Care Med. (2012) 185:537–46. doi: 10.1164/rccm.201106-0965OC
333. Humphries, DC, Mills, R, Boz, C, McHugh, BJ, Hirani, N, Rossi, AG, et al. Galectin-3 inhibitor GB0139 protects against acute lung injury by inhibiting neutrophil recruitment and activation. Front Pharmacol. (2022) 13:949264. doi: 10.3389/fphar.2022.949264
334. Hirani, N, MacKinnon, AC, Nicol, L, Ford, P, Schambye, H, Pedersen, A, et al. Target inhibition of galectin-3 by inhaled TD139 in patients with idiopathic pulmonary fibrosis. Eur Respir J. (2022) 57:2002559. doi: 10.1183/13993003.02559-2020
335. Hirani, N, MacKinnon, AC, Nicol, L, Ford, P, Schambye, H, Pedersen, A, et al. Target inhibition of galectin-3 by inhaled TD139 in patients with idiopathic pulmonary fibrosis. Eur Respir J. (2021) 57:2002559. doi: 10.1183/13993003.02559-2020
336. Lee, JJ, Hsu, YC, Li, YS, and Cheng, SP. Galectin-3 Inhibitors Suppress Anoikis Resistance and Invasive Capacity in Thyroid Cancer Cells. Int J Endocrinol. (2021) 2021:5583491. doi: 10.1155/2021/5583491
337. Effendi, WI, Nagano, T, Hasan, H, and Yudhawati, R. Immunoregulatory Property of C-Type Lectin-Like Receptors in Fibrosing Interstitial Lung Diseases. Int J Mol Sci. (2020) 21:3665. doi: 10.3390/ijms21103665
338. Taylor, PR, Brown, GD, Reid, DM, Willment, JA, Martinez-Pomares, L, Gordon, S, et al. The beta-glucan receptor, dectin-1, is predominantly expressed on the surface of cells of the monocyte/macrophage and neutrophil lineages. J Immunol. (2002) 169:3876–82. doi: 10.4049/jimmunol.169.7.3876
339. Esteban, A, Popp, MW, Vyas, VK, Strijbis, K, Ploegh, HL, and Fink, GR. Fungal recognition is mediated by the association of dectin-1 and galectin-3 in macrophages. Proc Natl Acad Sci U S A. (2011) 108:14270–5. doi: 10.1073/pnas.1111415108
340. Maiolino, G, Rossitto, G, Pedon, L, Cesari, M, Frigo, AC, Azzolini, M, et al. Galectin-3 predicts long-term cardiovascular death in high-risk patients with coronary artery disease. Arterioscler Thromb Vasc Biol. (2015) 35:725–32. doi: 10.1161/ATVBAHA.114.304964
341. Volz, J, Mammadova-Bach, E, Gil-Pulido, J, Nandigama, R, Remer, K, Sorokin, L, et al. Inhibition of platelet GPVI induces intratumor hemorrhage and increases efficacy of chemotherapy in mice. Blood. (2019) 133:2696–706. doi: 10.1182/blood.2018877043
342. Ungerer, M, Rosport, K, Bultmann, A, Piechatzek, R, Uhland, K, Schlieper, P, et al. Novel antiplatelet drug revacept (Dimeric Glycoprotein VI-Fc) specifically and efficiently inhibited collagen-induced platelet aggregation without affecting general hemostasis in humans. Circulation. (2011) 123:1891–9. doi: 10.1161/CIRCULATIONAHA.110.980623
343. Dovizio, M, Maier, TJ, Alberti, S, Di Francesco, L, Marcantoni, E, Munch, G, et al. Pharmacological inhibition of platelet-tumor cell cross-talk prevents platelet-induced overexpression of cyclooxygenase-2 in HT29 human colon carcinoma cells. Mol Pharmacol. (2013) 84:25–40. doi: 10.1124/mol.113.084988
344. Barrow, H, Rhodes, JM, and Yu, LG. Simultaneous determination of serum galectin-3 and -4 levels detects metastases in colorectal cancer patients. Cell Oncol (Dordr). (2013) 36:9–13. doi: 10.1007/s13402-012-0109-1
345. Kim, SW, Park, KC, Jeon, SM, Ohn, TB, Kim, TI, Kim, WH, et al. Abrogation of galectin-4 expression promotes tumorigenesis in colorectal cancer. Cell Oncol (Dordr). (2013) 36:169–78. doi: 10.1007/s13402-013-0124-x
346. Cai, Z, Zeng, Y, Xu, B, Gao, Y, Wang, S, Zeng, J, et al. Galectin-4 serves as a prognostic biomarker for the early recurrence / metastasis of hepatocellular carcinoma. Cancer Sci. (2014) 105:1510–7. doi: 10.1111/cas.12536
347. Paclik, D, Danese, S, Berndt, U, Wiedenmann, B, Dignass, A, and Sturm, A. Galectin-4 controls intestinal inflammation by selective regulation of peripheral and mucosal T cell apoptosis and cell cycle. PLoS One. (2008) 3:e2629. doi: 10.1371/journal.pone.0002629
348. Rao, US, and Rao, PS. Surface-bound galectin-4 regulates gene transcription and secretion of chemokines in human colorectal cancer cell lines. Tumour Biol. (2017) 39:1010428317691687. doi: 10.1177/1010428317691687
349. Ideo, H, Seko, A, and Yamashita, K. Recognition mechanism of galectin-4 for cholesterol 3-sulfate. J Biol Chem. (2007) 282:21081–9. doi: 10.1074/jbc.M703770200
350. Masuyer, G, Jabeen, T, Oberg, CT, Leffler, H, Nilsson, UJ, and Acharya, KR. Inhibition mechanism of human galectin-7 by a novel galactose-benzylphosphate inhibitor. FEBS J. (2012) 279:193–202. doi: 10.1111/j.1742-4658.2011.08414.x
351. Sewgobind, NV, Albers, S, and Pieters, RJ. Functions and Inhibition of Galectin-7, an Emerging Target in Cellular Pathophysiology. Biomolecules. (2021) 11:1720. doi: 10.3390/biom11111720
352. Pham, NTH, Létourneau, M, Fortier, M, Bégin, G, Al-Abdul-Wahid, MS, Pucci, F, et al. Perturbing dimer interactions and allosteric communication modulates the immunosuppressive activity of human galectin-7. J Biol Chem. (2021) 297:101308. doi: 10.1016/j.jbc.2021.101308
353. Wang, Z, Jiang, S, Li, S, Yu, W, Chen, J, Yu, D, et al. Targeted galectin-7 inhibition with ultrasound microbubble targeted gene therapy as a sole therapy to prevent acute rejection following heart transplantation in a Rodent model. Biomaterials. (2020) 263:120366. doi: 10.1016/j.biomaterials.2020.120366
354. Fujita, K, Iwama, H, Sakamoto, T, Okura, R, Kobayashi, K, Takano, J, et al. Galectin-9 suppresses the growth of hepatocellular carcinoma via apoptosis in vitro and in vivo. Int J Oncol. (2015) 46:2419–30. doi: 10.3892/ijo.2015.2941
355. Wiersma, VR, de Bruyn, M, Wei, Y, van Ginkel, RJ, Hirashima, M, Niki, T, et al. The epithelial polarity regulator LGALS9/galectin-9 induces fatal frustrated autophagy in KRAS mutant colon carcinoma that depends on elevated basal autophagic flux. Autophagy. (2015) 11:1373–88. doi: 10.1080/15548627.2015.1063767
Keywords: galectins, cancer, inflammation, thrombosis, anti-cancer therapies
Citation: Kruk L, Braun A, Cosset E, Gudermann T and Mammadova-Bach E (2023) Galectin functions in cancer-associated inflammation and thrombosis. Front. Cardiovasc. Med. 10:1052959. doi: 10.3389/fcvm.2023.1052959
Received: 24 September 2022; Accepted: 12 January 2023;
Published: 17 February 2023.
Edited by:
John David Horowitz, University of Adelaide, AustraliaReviewed by:
Yixuan (Axe) Xie, Washington University in St. Louis, United StatesCopyright © 2023 Kruk, Braun, Cosset, Gudermann and Mammadova-Bach. This is an open-access article distributed under the terms of the Creative Commons Attribution License (CC BY). The use, distribution or reproduction in other forums is permitted, provided the original author(s) and the copyright owner(s) are credited and that the original publication in this journal is cited, in accordance with accepted academic practice. No use, distribution or reproduction is permitted which does not comply with these terms.
*Correspondence: Elmina Mammadova-Bach, ✉ ZS5iYWNoQGxyei51bmktbXVlbmNoZW4uZGU=
Disclaimer: All claims expressed in this article are solely those of the authors and do not necessarily represent those of their affiliated organizations, or those of the publisher, the editors and the reviewers. Any product that may be evaluated in this article or claim that may be made by its manufacturer is not guaranteed or endorsed by the publisher.
Research integrity at Frontiers
Learn more about the work of our research integrity team to safeguard the quality of each article we publish.