- 1Institute for Clinical Chemistry, University of Zurich and University Hospital Zurich, Zurich, Switzerland
- 2Department of Cardiology, Heart Center, University Hospital Zurich, Zurich, Switzerland
Atherosclerotic cardiovascular disease is the leading cause of death worldwide. Intense research in vascular biology has advanced our knowledge of molecular mechanisms of its onset and progression until complications; however, several aspects of the patho-physiology of atherosclerosis remain to be further elucidated. Endothelial cell homeostasis is fundamental to prevent atherosclerosis as the appearance of endothelial cell dysfunction is considered the first pro-atherosclerotic vascular modification. Physiologically, high density lipoproteins (HDLs) exert protective actions for vessels and in particular for ECs. Indeed, HDLs promote endothelial-dependent vasorelaxation, contribute to the regulation of vascular lipid metabolism, and have immune-modulatory, anti-inflammatory and anti-oxidative properties. Sex- and gender-dependent differences are increasingly recognized as important, although not fully elucidated, factors in cardiovascular health and disease patho-physiology. In this review, we highlight the importance of sex hormones and sex-specific gene expression in the regulation of HDL and EC cross-talk and their contribution to cardiovascular disease.
Introduction
The relationship between high-density lipoproteins (HDLs) and cardiovascular disease (CVD) is a topic of intense investigation since decades (1).
Epidemiological studies have shown a correlation between low levels of HDL-cholesterol (HDL-C) and increased incidence of CVD (2). Indeed, a U-shape correlation has been recently reported whereby both low (< 50 mg/dl in women and < 40 mg/dl in men; 0.8 and 1.3 mmol/L, respectively) and high (>80 to 90 mg/dl; >2.3 mmol/L) levels of HDL have been associated to increased all-cause and CV mortality in both men and women without previous CVD (3–5).
Increasing evidence suggests that rather than cholesterol levels present on HDL, HDL particle number, lipid and protein composition play a key protective role in reducing CVD risk (6–8). HDL particle composition directly influences HDL vaso-protective functions (i.e. reverse cholesterol transport (RCT), nitric oxide (NO) production from endothelial cells (ECs), anti-oxidative and anti-inflammatory properties).
ECs are a physical barrier between blood and body tissues, which act as gatekeepers of cardiovascular homeostasis. Indeed, EC-released vasoactive substances (in particular NO) regulate hemostasis, control vascular permeability and modulate both acute and chronic immune responses to injuries (9). In light of its strong vasodilatory, anti-inflammatory and anti-oxidative properties, NO plays a central role in the maintenance of vascular health (10). Reduction in NO bioavailability is the hallmark of endothelial cell dysfunction (ECD), which in turn favors atherosclerosis (11).
Sex-related inter-individual variability (hormonal levels, hormone therapies, gene expression profiles etc.) can influence CVD risk by acting on both HDLs and ECs (12–14).
Increasing evidence suggests that sexual hormone levels—in particular testosterone and estradiol—and sex-specific cellular gene expression profile can influence not only HDL-C levels but also HDL subclasses and function. Indeed, men display reduced levels of HDL-C and a more pro-atherogenic phenotype compared to women (15–17).
Furthermore, estrogens are well-recognized EC protective molecules, able to stimulate NO production, EC growth and wound healing mechanisms (18, 19). Of note, differences in gene expression profile between female and male ECs appear to influence EC susceptibility to insults, with the activation in female ECs of more efficient stress-response mechanisms compared to male ECs (20, 21). These differences could explain, at least in part, why pre-menopausal women have lesser CVD risk than age-matched men and could give useful hints for personalized therapy development.
In this Review, we mainly focused on the influence of sex-specific factors on both HDL and EC function and how sex-dependent differences modulating HDL-EC cross- talk may contribute to the CV protection of pre-menopausal women compared to age-matched men (22–24). Indeed, sex closely interacts with gender in the development of atherosclerosis therefore, although not systematically addressed, some gender-specific aspects (i.e., pertaining to the socio-economic and cultural sphere) have been also mentioned in case of their known influence on HDL and EC function and potential CV patho-physiological impact (23–26).
HDL-targeting drugs: The failure of cholesteryl ester transfer protein inhibitors
The concept that HDL is the “good cholesterol” first originated from the Framingham Heart Study, which showed strong inverse association between HDL-C and coronary heart disease (CHD) (27). However, this concept has been challenged by results of following clinical trials in which cholesteryl ester transfer protein (CETP) inhibitors, despite raising HDL-C levels, failed to reduce CV morbidity and mortality. These results suggested that beyond the simple increase of HDL-C plasma levels, the modulation of HDL composition could be more important to achieve cardiovascular benefits (28–30). CETP is a plasma protein that transfers cholesteryl ester from HDL to apolipoprotein B (ApoB)-containing lipoproteins in exchange for triglyceride (TG). The inhibition of CETP leads to higher cholesterol levels in HDLs. Indeed, species lacking CETP and patients with CETP deficiency are characterized by increased HDL-C levels and reduced risk for CVD (31–34). In the Investigation of Lipid Level Management to Understand its Impact in Atherosclerotic Events (ILLUMINATE) trial, the CETP inhibitor Torcetrapib increased HDL-C levels as expected, but this increase was not paralleled by decreased CHD and the trial was stopped due to elevated risk of cardiac and death events (35).
In line with the notion that HDL-C alone may not be a reliable marker of the cardio-protective quality of HDLs, it has been recently shown in a sex-mixed pool of patients that CETP inhibitors, Torcetrapib and Evacetrapib, not only increased HDL-C but also enhanced the concomitant content of apoC3/apoE in HDLs. These two proteins rendered HDLs dysfunctional and were associated with higher CHD (36). Different CETP inhibitors, such as Dalcetrapib and Anacetrapib slightly reduced CHD risk, although this effect could have been influenced by the concomitant reduction in non-HDL-C in treated patients (37–42).
Genetic polymorphisms associated with increased HDL-C levels also did not influence the risk score for myocardial infarction (1, 43). Population studies carried out in Copenhagen highlighted a dramatic enhancement of CHD risk in women with CETP deficiency, in spite of the elevated HDL-C levels (44). Furthermore, as result of rare genetic variants on scavenger receptor BI (SR-BI) gene and reduced ability of HDLs to deliver cholesterol to the liver, the consequent increased HDL-C levels were linked to higher rather than lower risk of CHD risk in both men and women (45, 46). Indeed, the increased cholesterol in HDL in these specific circumstances was linked to an impaired HDL-mediated RCT. Taken together; this evidence questioned the rationale of using CETP inhibitors as treatment for CVD and highlighted the need for a better characterization of the complexity of HDL, in particular focusing on HDL composition as key determinant of function in health and disease.
Sex- and age-related differences in HDL measurements
HDL-C is commonly used as a predictor marker for CVD risk, as reported in SCORE (Systematic Coronary Risk Evaluation) risk charts and the ASCVD Pooled Cohort Equations (47, 48). So far, reference values for lipid profiles, including HDL, used in clinical practice are the same for both men and women, despite growing evidence on the influence of sex differences in the discriminative performance of CVD risk scores. Indeed, pre-menopausal women have higher HDL-C levels and lower risk of CVD compared to men (Figure 1) (12, 49–51). Phases of menstrual cycle may influence lipid profile. While post-prandial serum TG were higher in women during the follicular compared to the luteal phase of menstrual cycle, HDL and ApoB levels were stable in both phases (54). In another study, a significant decrease in the mean levels of TC, LDL-C, TC/HDL-C, LDL/HDL and TG/HDL was observed in the luteal compared to the follicular phase of menstrual cycle (55). Some studies suggested assessing female parameters during the follicular phase of menstrual cycle could help to minimize differences due to sexual hormones fluctuations (55, 56).
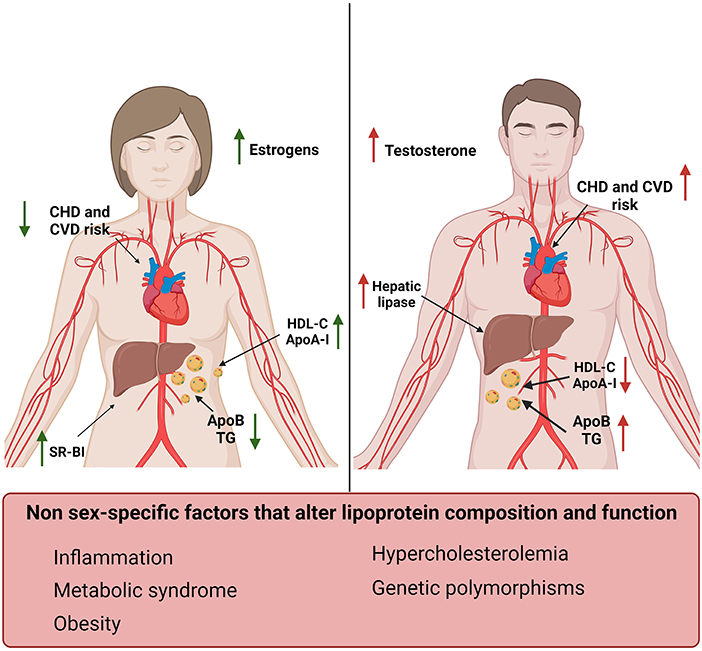
Figure 1. Sex-specific differences in lipoproteins and their effect on cardiovascular risk factors. Women (left) display increased levels of HDL-C and ApoA-I and reduced levels of TG and ApoB compared to men (right) (12, 49–52). Men also display increased levels of hepatic lipase compared to women, which in turn have higher SR-BI expression levels. These differences correlate with estrogens and testosterone levels and result in a reduced risk for CVD and CHD in women compared to men (53). Sex-independent factors associated with increased risk for CVD and changes in lipid profile have also been reported in this figure (bottom).
Patient age is another important factor influencing sex-dependent differences, since HDL-C levels can vary during individual lifetime. Healthy pre-pubertal children had high levels of HDL-C independently from their sex (56). HDL-C levels then drastically decreased in boys after puberty (~45 mg/dL/1.16 mmol/L), while remaining higher in girls (~55 mg/dL/1.42 mmol/L) (57). These differences were lost in post- menopausal women, independently from menopausal age (58, 59).
Hormonal therapies can also alter lipid parameters. Transgender men (i.e., female to male) displayed a clear reduction in HDL compared to women, but higher levels than cis-gender men (25, 60).
These sex- and age-dependent differences need to be taken into account when HDL-C levels are used as a CVD prognostic marker. Moreover, when considering the relationship between high HDL-C levels and increased all cause and CV mortality, relevant factors to be evaluated are sex differences together with the presence of CVD and other comorbidities. In fact, the increased cardiovascular risk associated with high HDL-C initially reported in a sex-mixed pool of patients without previous cardiovascular conditions by the CANHEART Study and others (3, 5) has not been confirmed afterwards in women with hypertension (61). Another study analyzed six community-based cohorts and showed that in men the inverse linear association between HDL-C and CHD events has a broader span compared to women. For HDL-C values >90 mg/dL (>2.33 mmol/L) in men and HDL-C values >75 mg/dL (>1.94 mmol/L) in women, the association between HDL-C and CHD events reached a plateau with no further reductions in CHD risk (62).
All-cause mortality in healthy, smoking, middle-aged (50–59 years) and older (>60 years) Finnish men was positively associated with HDL-C in the middle-aged group, while there was a U-shaped pattern in older men. Of note, the middle-aged group had a higher reported alcohol intake than the older individuals. Moreover, alcohol- and violence-related mortality was strongly positively associated with HDL-C specifically in the middle age group (63). Thus, alcohol may have influenced the association of HDL-C and mortality through its HDL raising effect and being a risk factor for behavioral-related non-natural as well as alcohol-related deaths beyond coronary disease, such as cancer, cardiomyopathy, stroke (5, 63).
Insights into sex-dependent and independent differences in HDL structure and composition
HDLs are heterogeneous lipoproteins formed by a cholesterol ester and TG enriched hydrophobic core and a surface lipid bilayer containing mainly free cholesterol, phospholipids and various proteins (6, 64). The biogenesis of HDLs starts from the synthesis and secretion of apolipoprotein- AI (ApoA-I) in the liver and intestine (65). The interaction between secreted ApoA-I and cell membrane protein ATP-binding cassette transporter A1 (ABCA1), expressed by hepatocytes and enterocytes (66), allows the acquisition of lipids and formation of nascent HDLs. Nascent HDLs are converted into mature particles via cholesterol esterification performed by lecithin-cholesterol-acyl transferase (LCAT) (67). Endothelial lipase and hepatic lipase are involved in the lipolysis of phospholipids and TGs in HDLs, leading to smaller HDL particles (68). Phospholipid transfer protein further exchanges lipids between HDLs (69). HDL clearance is orchestrated by SR-BI and CETP, which regulate the transfer of cholesteryl-ester from HDLs to the liver and the exchange of ApoB-containing lipoproteins with TGs (70).
Women have increased HDL-C and ApoA-I levels and lower ApoB compared to men. These sex-related differences in plasma lipoproteins start to be evident during puberty, in concomitance with the increase in testosterone in males and estrogens in females (Figure 1) (52).
Estrogens increased ApoA-I expression in the liver and HDL-C levels in pre-menopausal women by modulating the expression of SR-BI and hepatic lipase (71–73). On the contrary, testosterone administration enhanced hepatic lipase activity, increasing HDL catabolism (Figure 1) (52). Androgen therapy was also associated with an unfavorable shift toward an atherogenic lipid profile characterized by reduced ApoA-I and increased apo-B levels in men (74). Suppression of androgens in men, in fact, leaded to an increase in HDL-C, ApoA-I and reduced ApoB levels (75). It has also been shown that hyperandrogenism, which is a common feature of polycystic ovary syndrome, was associated with lower HDL-C levels and dyslipidemia (76, 77).
Differences in lipid profile have also been associated with sex-specific gene expression profile. The KDM6A gene encodes for a histone-demethylase protein highly expressed in the female liver and its expression levels positively correlated with HDL-C (78, 79). In turn, KDM6A silencing in hepatocytes lead to downregulation of genes regulating HDL-C levels (13).
Single nucleotide polymorphisms (SNPs) on the CETP gene have been associated with higher HDL-C and ApoA-I levels (80, 81). TaqIB is the most common SNP variant of the CETP gene and the TaqIB genotype can be expressed as either dominant B1B1 homozygote, B1B2 heterozygote or recessive B2B2. In particular, B2B2 carriers had higher HDL-C plasma levels and 20% lower risk of CHD vs. the B1B1 carriers (82). Of note, the increase in HDL-C levels in CETP-TaqIB, B2B2 carriers seemed to be independent from sexual hormones (81) and was lost in obesity and type 2 diabetes (T2D). Indeed, other CETP SNP variants in both sexes were not associated with HDL-C levels nor with metabolic syndrome and obesity (83). A 16% increase in HDL-C levels has been reported in men with B2 TaqIB variant affected by T2D compared with those homozygous for the B1 allele (83).
ApoE, encoded by APOE gene, is the major ligand for clearance of TG-rich lipoproteins and has anti-atherogenic function (84, 85). APOE-e2 polymorphism has a sex-specific effect on lipid profile and has been associated with high HDL-C levels in woman and increased TG levels in men (86). There are no sex-differences reported for ApoE isoform 4 in the context of CVD risk, while the ApoE4 allele seems to confer a memory advantage in midlife men and an increased risk of Alzheimer in women (87, 88).
HDL-associated LCAT increased mass concentration and higher LCAT activity have been correlated with CHD risk in women but not in men (89, 90). However, mechanisms of the sex-specific association of LCAT and CV risk need further investigation given the conflicting results so far available, for instance in patients with sickle cell anemia and proteinuria where a less pronounced reduction of LCAT activity in women compared to men has been considered protective against accelerated kidney disease progression in this patient population (91). Moreover, LCAT deficiency led to the development of spontaneous atherosclerotic lesions similarly in aged male and female mice (92) and a female specific protection against diet-induced obesity and insulin resistance has been described in mice with combined LCAT and LDL receptor deficiency (93).
Inflammation decreases HDL-C levels and altered HDL composition in a sex-independent manner (Figure 1) (94, 95). Changes in the HDL-associated lipids include a decrease in cholesterol ester and an increase in free cholesterol, TG, free fatty acids and ceramide-enriched lipoproteins. Dysfunctional HDLs show marked alterations in protein composition and become pro-atherogenic. These changes include an increase of serum amyloid A (SAA), a decrease apoA-I but also variations in enzymes and transfer proteins, such as LCAT, CETP, PON-1, and apolipoprotein-M (apoM) (94).
Central adiposity directly correlates with CVD risk (96, 97). Increase in central adiposity was able to alter HDL subclasses distribution, but overall HDL-C levels seemed not affected by this parameter (98). Obesity also affects HDL composition, function and subclasses distribution (99, 100). Obesity induces, most prominently in women compared to men, a pro-atherogenic dyslipidemia characterized by increased LDL and TG and reduced HDL-C, ApoA-I and ApoA-II levels. We and others showed that in morbidly severe obese patients bariatric surgery restores HDL endothelial-protective properties by modulating HDL composition (101–104). Bariatric surgery improves CV morbidity and mortality regardless of sex and gender (105, 106). Indeed, in a small patient cohort, we also showed after Roux-en-Y gastric bypass similar benefits on HDL endothelial protective function for both sexes (103). Circulating HDL-C levels increased in our patients after RYGB in agreement with other studies (107) however concentrations usually remains well below cut offs (80–90 mg/dL; 2.06–2.33 mmol/L) that are associated with higher CVD risk (53, 108). Finally, it is worth to consider that, in the context of obesity and bariatric surgery, gender-dependent differences (e.g., differences between women and men in the perception of their body weight in relation to esthetic, health and therapeutic perspective) are very important and need to be appraised when evaluating study results and identifying gaps of existing knowledge (106).
Insight into sex-dependent regulation of EC function
Women before menopause have lower risk of developing CHD and endothelial-protective properties of estrogens can be important contributors (Figure 1) (109). Aging-driven reduction of flow-mediated dilation appears at the age of menopause, more than a decade later than in men, in concomitance with the loss of circulating estrogens (110). Chronic treatment with estrogens improved endothelial-dependent vasodilation in both ovariectomized animal models and post-menopausal women (111–113). Moreover, clinical studies suggested that estradiol treatment was able to revert endothelial dysfunction in post-menopausal women with atherosclerotic, non-stenotic arteries by preventing acetylcholine-induced coronary vasospasm (114). At present however, controversies still exist on the cardio-protective effect of hormonal replacement therapy in post-menopausal women (115).
At the molecular level, estrogens can stimulate ECs primarily through estrogen receptors (ERα and Erβ, GPER1) on EC surface. Activation of ERs increases eNOS activity and NO production, thus promoting EC-mediated vasodilation (Figure 2) (116, 123). EC glycocalyx protects ECs from second insults after trauma hemorrhagic shock (T/SH) (117). Recently, it has been shown that estrogen administration after T/SH protects the EC glycocalyx from degradation by regulating tPA and PAI-1 levels, making ECs more resistant to additional damages (124). Furthermore, estradiol signaling attenuated endothelial inflammatory response by reducing cytokine and chemokine release (such as monocytes chemoattractant protein 1 (MCP-1) and IL-8) as well as EC adhesion molecule expression (125, 126). Prolonged exposure to estradiol also created a new homeostatic status in which immune cells were potentiated and ECs were less sensible to pro-inflammatory stimuli and apoptosis (Figure 2) (109).
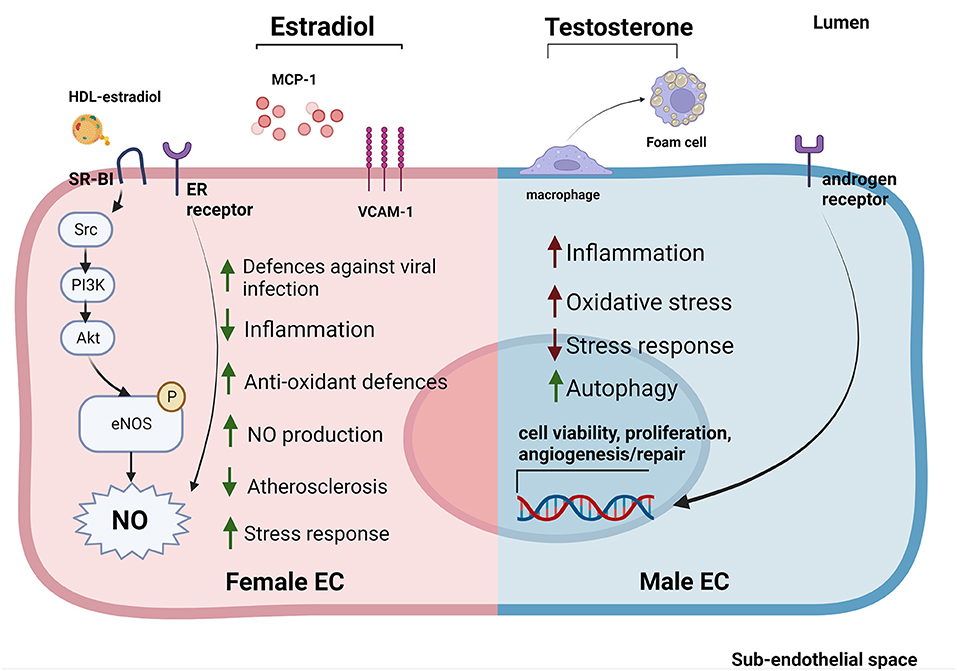
Figure 2. Sex-specific gene expression patterns and sexual hormones influence EC response to stimuli and EC-HDL cross talk. ECs derived from female donors (left) display reduced sensibility to stress and inflammation thanks to the activation of sex-specific pathways involved in stress response. Estradiol is able to reduce inflammation by reducing MCP-1 release and VCAM-1 expression. Furthermore, estradiol bound to HDLs is able to strongly activate SR-BI pathway increasing the NO production (53, 114, 116, 117). On the contrary, ECs derived from male donors (right) display a stronger susceptibility to inflammation, increased levels of oxidative stress and autophagy (118–120). Testosterone also contributes to create a pro-inflammatory environment by favoring the transformation of macrophages into foam cells (121). Estrogens stimulate NO production in both sexes through the activation of ER receptors (114). In contrast, androgen receptors are able to increase the expression of genes involved in cell viability, proliferation and angiogenesis/repair both in men and women ECs (122).
While the EC protective role of estrogens have been well established, the effect of androgens on ECs is still under debate. Monocytes binding to aortic ECs seemed to be higher in male than female rabbits with hypercholesterolemia, suggesting a correlation between androgen levels and EC inflammation. However, this sex-dependent difference was also evident in non-hypercholesterolemic rabbits (127). Testosterone has also been associated with impaired vascular function in women (122, 128). This could be due to the fact that, although testosterone production is 10 times higher in men compared to women, women may be more sensitive to this hormone (129). Indeed, several studies pointed out an important sex-independent role of androgen receptors in regulating EC viability, proliferation, and angiogenesis/repair likely via upregulation of the VEGF-A, cyclin A, and cyclin D1 expression (Figure 2) (121). Of note, abundance of testosterone in male mice may favor its conversion into estradiol mediated by aromatase P450, causing hyper-activation of ERs promoting atherosclerosis (130, 131).
An overall marker of early atherosclerosis is the transformation of macrophages into foam cells through intracellular lipid accumulation. Treatment with testosterone promoted foam cell formation in men (but not in women) by increasing lipid loading, thus contributing to the development of atherosclerosis (Figure 2) (132).
Interestingly, transgender men treated with testosterone for 12 weeks displayed increased leukocytes-endothelium interactions, expression of adhesion molecules on EC surface, pro-inflammatory cytokine release, decreased HDL-C levels and dyslipidemia (23, 26). Furthermore, the levels of polymorphonucleate adhesion to ECs in transgender men were similar to diabetic men with silent myocardial ischemia, which highlight the need of a closer monitoring of cardiovascular risk in these patients (26). Progesterone, instead, protected ECs after cerebrovascular occlusion in male rats (133) and has been associated with increased NO production in women (134). However, administration of synthetic progesterone analogs, such as medroxyprogesterone, correlated with increased risk of coronary disease and stroke in women under hormonal replacement therapy (115, 135). Indeed, it has been shown that estrogen or progesterone and its synthetic analogs differently affect plasma lipoproteins, in particular, estrogen increases whereas progesterone and its synthetic analogs decrease HDL-C concentrations (136). Accordingly, while 17beta-estradiol had no effects, progesterone and three synthetic analogs suppressed ApoA-I-mediated cellular cholesterol release from human fibroblasts resulting in generation of less HDL particles (137).
Sex is a key variable in vascular biology and in particular, EC function is influenced by sexual hormones, but also by chromosomes resulting in sex-specific differences in gene expression profile. Human umbilical vein endothelial cells (HUVECs) derived from females and males were found intrinsically different independently from their exposure to sexual hormones, implicating a role for genomic sexual dimorphisms in CV system (14, 138). Transcriptomic performed in HUVECs in boy-girl twins or in non-twin adult ECs showed that sex-differences were present either at birth and maintained throughout life or acquired over life (118). As expected, sex differences in adult EC transcriptome involved many genes influenced by estrogens or androgens. Interestingly, androgen and estrogen receptors were not differentially expressed in adult ECs. Intriguingly, half of the genes showing sex-specific differences in HUVECs were sex chromosomal genes. Moreover, coronary artery disease targets (derived using multiple genome-wide association studies) were also enriched in the gene set showing sex difference in HUVECs, making possible to speculate about sex differences in CAD rooted in differential gene expression in ECs already at birth (118). Gene hallmark analysis showed increased expression of genes involved in endothelial to mesenchymal transition, NF-kB pathway and hypoxia in females, while increased expression in MYC targets, oxidative phosphorylation and mTOR pathway were reported in males (118). Other studies reported target-specific differences comparing male and female non-twin HUVECs, which may contribute to sex differences between males and females in endothelial function. Higher cell proliferation, migratory properties and endothelial NO synthase expression were observed in female HUVECs, while in the male cells beclin-1 and the LC3-II/LC3-I ratio, two widely accepted markers of autophagy, were higher (119). Notably, cellular size, shape as well as mRNA and protein expression of estrogen and androgen receptors were similar among sexes (119). Proteomic analysis of the secretome of serum-deprived HUVECs isolated from healthy female and male newborns revealed higher expression of proteins involved in cellular response to stress (e.g., several members of Annexin and Heat Shock Protein families) and apoptosis (e.g., PTX3) in male cells (120). These results are in agreement with reports obtained in different cells (e.g., neurons or cardiomyocytes) challenged with stressor stimuli and overall suggest lower resistance to oxidative stress and higher propensity of male cells to undergo apoptosis. On the contrary, female neurons/cardiomyocytes may be more resistant to oxidative stress with a pro-autophagy predisposition (139, 140); the latter characteristic will need to be further investigated in ECs as the above mentioned study on HUVEC transcriptome in boy-girls twins shows a male and not a female pro-autophagy gene signature (118).
Female HUVECs showed a stronger transcriptional response after shear stress exposure compared to male cells involving, for instance, upregulation of genes such as eNOS, heme-oxygenase 1 (HO-1) downregulation of NADPH oxidase 4 (Nox 4), endothelin-1 (ET-1) or vascular cell adhesion molecule 1 (VCAM-1), the latter downregulated by 22.2-fold in female vs only 3.5-fold in males (141).
Regarding EC energy supply, similar baseline ratios of glycolysis vs. mitochondrial respiration were observed in HUVECs obtained from male/female twins, but female cells performed better under starvation or under VEGF stimulation with higher ATP and metabolite levels compared to male cells, suggesting a more flexible modulation of energy production in females (120, 142).
Further studies will need to elucidate whether the described higher adaptability of female ECs to stress may confer them protection against CVD risk. Conversely, a stronger transcriptional response in female ECs might, in specific cases, favor disease onset and progression (e.g., in the context of the higher prevalence of autoimmune diseases in the female population) (143–145).
Collectively, increasing evidence highlights the presence of sex dependent differences in ECs at different stages of life. However, there are very few studies in adult ECs (i.e., HAECs) compared to the studies in HUVECs, which makes difficult to adequately investigate or compare changes in EC gene expression acquired later in life. Moreover, it is important to consider sex as a crucial biological variable not only in cardiovascular clinical research but also in experimental studies on EC biology to increase the quality and translational value of results.
Insights into sex-dependent differences in HDL and EC crosstalk
Lifestyle and CVD: Sex-dependent differences
Smoking, alcohol consumption, diet and exercise are modifiable CVD risk factors (Table 1).
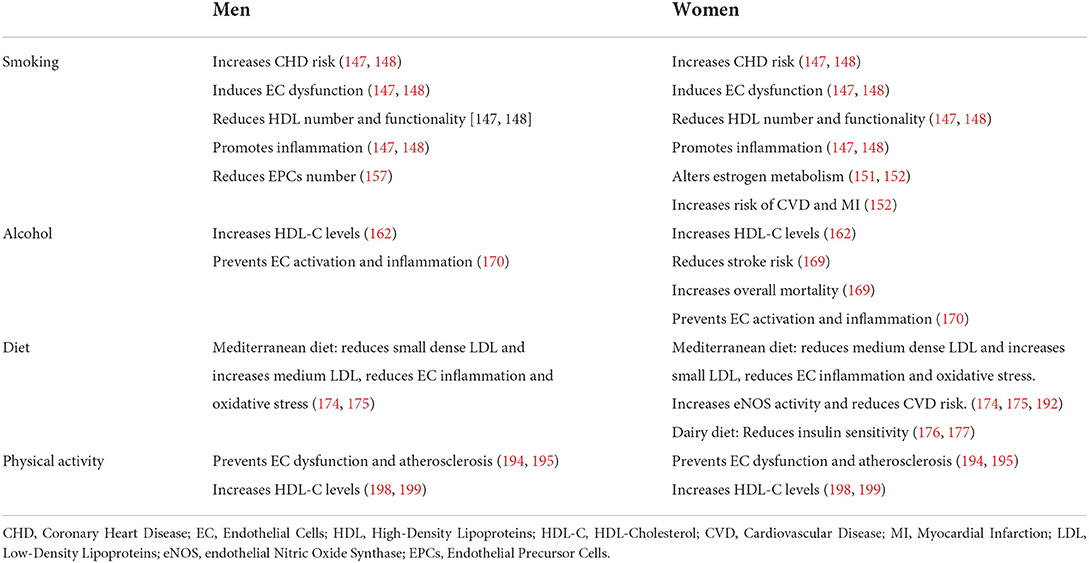
Table 1. Comparative description of the effect of lifestyle habits on HDLs and ECs in men and women.
The number of smoked cigarettes positively correlated with increased CHD risk in both sexes. In fact, smoking induced endothelial dysfunction and damage, increasing lipid oxidation, decreasing HDL, and promoting inflammation, and a pro-thrombotic state (146, 147). Furthermore, a worse lipid profile characterized by increased ApoB and reduced ApoA-I and ApoA-II was reported in smokers compared to non-smokers independently from their sex (148). Interestingly, smoking was reported as a stronger risk factor for CVD in women than in men accordingly to the Finnmark Study (149). This could be partially attributed to the ability of smoking to alter estradiol metabolism leading to the formation of inactive catechols (150, 151), thus inhibiting estradiol vaso-protective properties. Moreover, exposure to passive smoking from birth was associated with reduced HDL-C levels in adolescent girls but not in boys (152). The anti-estrogenic effect of smoking positively correlates with increased CHD risk and strong reduction in HDL-C levels in young compared to older women and men (150, 153). The evidence that ex-smokers had higher HDL-C levels compared to smokers of both sexes further confirm these results (154). Furthermore, the Copenhagen City Heart Study reported that smoking women had 9.4 higher risk of myocardial infarction compared to non-smoking women, while the risk score was only 2.9 times higher in smoking men compared to non-smokers (155). On the contrary, reduced levels of endothelial progenitor cells (EPCs) have been reported in men compared to women. Smoking further decreased EPCs in men, while no difference was found between smoking and non-smoking women. In this case, sex-differences on the effect of smoking were mostly attributed to a protective effect of estradiol on EPCs (156).
Low to moderate alcohol consumption did not affect CVD risk in both sexes (157). The CoLaus Study reported no differences in expression of HDL-related genes (i.e., ABCA1, APOE5, CETP, hepatic lipase and lipoprotein lipase) based on alcohol consume in a sex-mixed pool of Caucasian patients (158). CHD risk could perhaps vary depending on the ethnicity of the patients. The Atherosclerosis Risk in Communities (ARIC) study reported reduced CHD in whites but increased disease in black alcohol consumer men independently from levels of alcohol consumed (159). This was partially attributed to different hepatic gene variants and expression levels (i.e., CETP, hepatic lipase, LPL, and PON1) between these two ethnicities (159, 160). Meta-analysis data suggested that HDL-C levels increased an average of 0.06 mmol/L per 23 g/day of alcohol consumed (161). The increase of HDL-C levels in alcohol consumers have been attributed to enhanced HDL production (hepatic and extra-hepatic), decreased CETP activity and lower HDL-C clearance (162). However, this increase is strongly influenced by alcohol-gene interactions (163). As an example, men and post-menopausal women carrying the homodimeric γ2 variant of the ADHIC gene had a slower rate of alcohol clearance, which was associated with elevated HDL-C levels (164, 165). It is worth specifying that sex differences in alcohol consumption are difficult to detect, since generally women can tolerate lesser amount of alcohol due to their sex-specific absorption, body fat/water ratio, reduced levels of enzymes involved in alcohol metabolism and glomerular filtration rate (166). Furthermore, studies in the general population indicated that among all alcohol consumers/abusers, only 1/3 were women (167). Nevertheless, moderate alcohol consumption was associated with lower risk of stroke in women compare to men but also with a 10% increased risk of overall mortality (168).
Alcohol has also a direct effect on ECs. Indeed, moderate levels of alcohol were able to prevent endothelial activation and pro-inflammatory cytokine release in human coronary artery ECs stimulated with the pro-inflammatory SAA (169). Furthermore, a reduction in monocyte adhesion to TNF-α-stimulated ECs was reported in moderate alcohol consumer men compared to non-consumers (170). On the other hand, heavy alcohol consumption (both measured and self-reported) was associated with increased circulating vascular adhesion molecules (i.e., E-selectin, intracellular adhesion molecule 1 (ICAM-1) and VCAM-1) and reduced flow-mediated dilation in sex-mixed cohorts of patients, independently of alcoholic liver disease (171, 172).
Diet also affects women and men in a different manner. Mediterranean diet was able to reduce total cholesterol, LDL-C, ApoB and ApoA-1 plasma levels in both sexes. However, men under Mediterranean diet experienced a reduction in small dense LDL and an increase in medium LDL, while the opposite trend was observed in women (173, 174). Furthermore, a comparison between red meat and dairy diet highlighted a reduction in insulin sensitivity in women following the dairy diet. Instead, no differences between the two diets were reported in men (175, 176). ABCA1 is one of the most sex-influenced gene in the liver and its expression is higher in females (177). Indeed, estrogen levels and dietary components were able to regulate ABCA1 expression in macrophages, leukocytes and liver in human and rodents, increasing ApoE-positive HDL particles and improving cholesterol efflux (178–181). Among all the genetic variants, ABCA1/R230C was associated with low HDL-C (182). It has been shown that dietary macronutrient proportions regulated the effect of ABCA1/R230C in premenopausal women by directly interacting with ABCA1 gene (183). In particular, metabolically unfavorable pattern was found in ABCA1/R230C premenopausal women following high carbohydrates and low fat diet, while the opposite pattern was found in women following high fat and low carbohydrates diet (183).
On the contrary, lowering dietary fat intake was able to restore HDL functionality (in particular HDL-CEC) in hypercholesterolemic female pigs by reducing cholesterol plasma levels (184).
There is some evidence that diet could directly affect EC function. Transitory disruption of endothelial function and reduction in vasorelaxation have been reported after acute administration of high-fat meal, in concomitance with increased triglyceride-rich lipoproteins in plasma (185, 186). On the contrary, chronic consumption of low-fat diets (i.e., Mediterranean diet) was associated with improved endothelial function and reduced markers of endothelial activation in plasma in men (187–189), most likely through changes in cholesterol metabolism and the presence of oleate and decosahexanoic acid, which were able to reduce pro-inflammatory molecule expression and monocyte adhesion in ECs in vitro (190). Same results were shown also in women. Indeed, a pilot study demonstrated that specific components of Mediterranean diet (i.e., legumes, red meat, and overall proteins) were associated with reduced inflammation and oxidative stress, increased eNOS activity and reduced CVD risk in a cohort of ethnically mixed women (191).
Physical activity is well known as protective factor against CVD (192). Evidence showed that physical activity was able to slow down EC dysfunction and atherosclerosis progression in both sexes (193, 194). However, how physical activity differently influence CVD risk in women and men is still under debate. A systematic review focused on physical activity and stroke incidence claiming that, among all the analyzed studies, 35% of them reported a strong association between physical activity and stroke incidence in women, while the same correlation in men was evident only in few studies (195). Even so, the Framingham Study reported that physical activity conferred protection against stroke in men, but not in women (196).
The correlation between physical activity and reduction in CVD risk could be partially attributed to increased HDL-C levels in physically active individuals compared to sedentary ones. However, the increase in HDL-C seemed to be significant only when a threshold volume of physical activity was reached (197, 198). Even if the threshold level has not been accurately and systematically estimated yet, epidemiological and cross-sectional studies suggested that the threshold value was 1,500 kcal/week in men and 1,200 kcal/week in women independently from their menopausal status (199–201).
Reverse cholesterol transport
The best-known property of HDL is RCT, which consists in the ability of HDL to accept excess cholesterol from peripheral cells, in particular macrophages, and transport it to the liver for excretion or re-utilization. RCT is considered the most important anti-atherogenic function of HDLs. Components of cholesterol efflux include the passive diffusion of cholesterol from cells as well as the active cellular cholesterol transfer by ABCA1, ABCG1, and SR-BI. In this context, ECs may represent a potential barrier to HDL in reaching macrophages within the vessel wall. However, HDL and lipid-free ApoA-I are able to cross intact aortic EC monolayers from the apical to the basolateral compartment in a transcytosis process, involving ABCG1 and SR-BI (202). Contrary to other cells that form the atherosclerotic plaque (smooth muscle cells and macrophages), ECs do not accumulate cholesterol (203) and have a strong ability to efflux cellular cholesterol to HDLs independently of ABCA1, ABCG1, and SR-BI expression or activity (204). On the other hand, it has been shown that in conditions of hyperlipidemia ECs can metabolize LDLs into cholesterol crystals, which accumulate intracellularly and confer a foam cell-like morphology to ECs (205).
HDLs derived from healthy normolipidemic men and women had different RCT capacities due to the activation of sex-specific mechanisms for cholesterol efflux (206). The rs1799837 (APOA1) and rs1800588 (LIPC) SNP variants represented the major determinants of HDL cholesterol efflux capacity in women, while rs2230806 (ABCA1) and rs5082 (APOAII) variants were key determinants in men (207). Furthermore, serum isolated from women displayed an enrichment in large-HDL-particles (L-HDL-P) and increased capacity to mediate cholesterol efflux through SR-BI receptors. On the other hand, serum isolated from men showed increased preβ-HDL particles and cholesterol efflux through ABCA1 receptors (206). Both low and high HDL-C levels were associated with reduced free cholesterol transfer on HDLs in both sexes, especially in women, in patients with acute myocardial infarction and Tangier disease (208).
These findings not only suggest that HDL composition differs in men compared to women, but also that these differences in HDL pool may have an impact in their ability to stimulate cholesterol efflux. Estradiol levels, in fact, positively correlated with HDL cholesterol efflux capacity (HDL-CEC) from macrophages in pre-menopausal women and were associated with increased concentration of L-HDL-P and lower concentration of small-HDL-particles (S-HDL-P) (209). However, HDL-CEC decreased in transgender women (men to women) under estradiol hormone therapy, suggesting that reduction of testosterone and increase in estradiol may act synergistically in reducing HDL-CEC (24). This hypothesis is in line with the evidence that testosterone deprivation in men increased HDL-C levels but not HDL-CEC, while estradiol treatment had the opposite effect (210). The correlation between androgen levels and CVD in men is controversial. Low levels of androgens were associated to increased CVD in older men (211). On the other hand, testosterone administration in hypogonadal men can blunt EC-mediated vasorelaxation (212, 213). Thus, age and type of androgen used are important factors to be considered.
Inflammation
Increased expression levels of specific adhesion molecules—such as VCAM-1 and ICAM-1 and E-selectin—are a well-recognized marker of EC inflammation and oxidative stress. HDL particle concentration is inversely correlated with the expression of cellular adhesion molecules, as well as inflammatory mediators C-reactive protein (CRP) and TNF-α. The underlying mechanism can be partly attributed to HDL-associated sphingosine 1 phosphate (S1P). S1P signaling through S1P receptor has been shown to protect against TNF-α-induced monocyte binding to ECs preventing the activation of NF-kB and c-Jun pathways as well as reducing the secretion of pro-inflammatory chemokines (214).
OxLDLs can induce MCP-1, which is involved in the recruitment of monocytes into the sub-endothelial space and their differentiation into foam cells. It is an important inflammatory process in the initial stages of atherosclerosis (215). In vitro and in vivo experimental studies suggested that HDL associated-PON1 inhibited LDL-oxidation by catalyzing the breakdown of oxidized phospholipids, thus abolishing the production of pro-inflammatory cytokines (MCP-1, IL-8 and macrophage colony stimulating factor) from ECs (216–220).
HDLs are also able to transfer microRNA to ECs (221). It was shown that HDL-transferred microRNA-223 directly targeted ICAM-1 gene at 3'UTR sites suppressing gene expression and function in HUVECs stimulated with TNF-α thus reducing leukocyte adhesion. Regulation of TNFα-induced ICAM-1 expression by HDLs was not found in fibroblasts, suggesting a specific miR-223 delivery on ECs (222–224).
HDL-induced NO production also plays an important role in the reduction of EC inflammation. The induction of PI3K-Akt-eNOS signaling mediated by the binding of ApoA-1 and SR-B1 up-regulates cyclooxygenase (COX-2) expression and prostaglandin I2 (PGI2) release in ECs (225). PGI2 is a potent inhibitor of inflammation, which limits immune cell proliferation as well as inhibits platelet aggregation, thus affecting smooth muscle relaxation and vasodilation (226).
HDL-C levels also correlated with risk of infection. Similarly to what was showed regarding all-cause mortality risk (3, 4), both low and high levels of HDL-C were associated with increased risk of infection (227). The increased risk of infection associated with low levels of HDL-C could be in part due to the loss of leucopoietic control and immune cell modulation from HDLs (228, 229). The mechanism in case of high levels of HDL-C is less clear, but particular genetic mutations associated with increased HDL levels may also affect disease susceptibility. For instance, in case of LIPC and SCARB1 (encoding for hepatic lipase and SR-BI, respectively), whose mutations were associated with increased risk of CAD (46, 230). Interestingly, several SNP variants located in the promoter and intron 1 of LIPC gene were associated with changes in HDL-C levels in women but not in men (231). SCARB1 rs5888 SNP variant, instead, has been associated with a greater reduction in total cholesterol, LDL-C and ApoB in women treated with atorvastatin compared to men in patients with acute coronary syndrome (232).
Peri-menopausal and menopausal women had increased levels of TNF-α, CRP, TG and LDL compared to their pre-menopausal counterparts, which may underline a reduction on HDL functionality driven by the collapse in estrogen levels (233).
HDL anti-inflammatory properties are impaired or lost in chronic inflammatory conditions. Concomitantly with a decrease of HDL particle levels also changes in the structure and function of HDLs are observed. Patients with chronic inflammatory disorders, including rheumatoid arthritis, systemic lupus erythematosus, and psoriasis, which are associated with an increased risk of atherosclerotic CVD, exhibit a consistent decrease in HDL particles and ApoA-1 levels and HDL vaso-protective properties in a sex-independent manner (234–236). However, in other diseases as in the antiphospholipid syndrome, studies reporting a strong reduction in HDL functionality were conducted comparing only affected and healthy women (237). Thus, it will be important to further elucidate with sex-matched comparisons whether or not men and women HDLs are similarly affected also in those immune-inflammatory diseases.
Interestingly, it has been recently shown that HDL-C and ApoA-I levels measured before SARS-CoV-2 infection negatively correlated with COVID-19 mortality and hospitalization, independently of age, sex, comorbidities, or statin treatment (238–240). Furthermore, HDL cargo was profoundly altered in severe COVID-19 patients, with increased abundance of SAA-1 and−2, SFTPB, ApoF, and inter-alpha-trypsin inhibitor heavy chain H4 (241). These findings are corroborated by the evidence that treatment with reconstituted HDLs in COVID-19 patients reduced SAA-1, SFTPB, and ApoF in HDLs (242). Of note, men with COVID-19 were more prone to develop into the severe condition and die compared to women (Figure 2) (243, 244). Indeed, no striking differences were found between pre- and post-menopausal women, suggesting that reduction in female mortality may be independent from estrogen levels (243, 244). In vitro experiments conducted on ECs exposed to SARS-CoV-2 S1 spike protein showed a significant increase in the overall inflammatory status in cells treated with androgens (245). Recent findings showed that male sex clinical-biological characteristics, rather than male gender-related differences (i.e., pertaining to the socio-economic sphere, such as education) were independently associated with intensive care unit admission, invasive ventilation, and/or death in COVID-19 (246).
Anti-apoptotic and anti-oxidative properties
EC homeostasis relies on the balance between pro- and anti-apoptotic stimuli coming from bloodstream and neighboring cells (247, 248). Once the balance is disrupted, (pro)-apoptotic ECs favor platelet aggregation and coagulation, creating a pro-atherogenic environment (249, 250). OxLDL can promote EC apoptosis by increasing intracellular Ca2+ levels (251–253), favoring the onset and progression of CVD (114). In contrast to LDLs, HDLs protect ECs from apoptosis by preserving mitochondrial integrity and inhibiting the activation of the caspase-downstream cascade (254–256). Indeed, HDLs isolated from a mixed sex pool of healthy donors was able to reduce EC apoptosis both in vivo and in vitro, while sex-matched HDLs isolated from CAD patients showed opposite results (257).
In particular, HDL particles containing ApoA-I seemed to be more cytoprotective than other HDL subclasses (258, 259).
Moreover EPCs can quickly differentiate into mature ECs to rescue vascular integrity in conditions of high cell turnover (260). HDLs can promote endothelial repair by increasing EPC number and function in male mice (261, 262).
In addition to their anti-apoptotic properties, HDLs can also reduce oxidative stress. PON-1 is an accessory protein of HDL that, in coordination with ApoA-I, protect lipoproteins, ECs and intimal macrophages from oxidative insults by hydrolyzing lipo-lactones (263–266). High levels of oxidative stress can increase HDL lipid peroxide loading, decreasing their protective activity against LDL oxidation (267, 268). Significant differences in HDL peroxide levels between men and women have been reported as a readout of sex-specific reduction in HDL anti-oxidative functions in men (269).
Decreased HDL anti-oxidative properties driven by high glycemic burden have also been reported in both type 1 and type 2 diabetic men and post-menopausal women (270, 271). In this context, it has been shown that PON-1 activity was more strongly impaired in T2D women compared to men (272).
Furthermore, women with hypertension, metabolic syndrome or peri-menopause not only had higher levels of oxLDLs compared to healthy middle-age or pre-menopausal women, but also reduced defenses against oxLDLs. However, the contribution of estrogens in this context is still unclear (273–275).
HDLs can serve as carriers for other molecules, for instance estrogens. It has been shown that the binding of estrogens with HDLs increases their anti-oxidative properties due to estrogen esterification performed by LCAT (276). Indeed, LCAT was able to esterify HDL-bounded E2. Esterified E2 was then transferred from HDL to LDL thanks to CETP (276). Incubation experiments demonstrated that E2 esterification and further association with LDL was able to increase LDL resistance to oxidation (276, 277). On the contrary, hyperandrogenism is associated with increased oxidative stress and reduced HDL anti-oxidative functionality in women (278, 279). To the best of our knowledge HDLs have not been reported as carriers for androgens.
HDL-mediated endothelial NO-production
Several mechanisms account for the endothelial NO-stimulating capacity of HDLs. In cultured ECs, HDLs directly activate the production and release of NO by binding of ApoA-I to SR-BI, leading to increased intracellular ceramide levels and phosphorylation of endothelial NO-synthase (eNOS) (280). Cholesterol efflux from ECs to HDLs via ABCA1 also promotes NO synthesis by modulating cholesterol-binding protein caveolin-1 and eNOS (281). Mechanistically, the binding of HDLs to SR-B1 leads to tyrosine kinase Src-mediated activation of phosphoinositide 3-kinase (PI3K), which in turn stimulates Akt and Erk pathways. The activation of Akt directly stimulates eNOS by phosphorylation at Ser-1179 (282) (see Figure 2).
Estradiol is able to induce rapid arterial vasodilation by stimulating eNOS activity by acting via ERs (18). HDLs isolated from female mice and healthy women (but not male mice or men) were able to enhance NO production. In addition to ERs, estradiol bound to HDLs was also able to enhance eNOS activity through the activation of SR-BI receptors (Figure 2) (283).
Specific lipid and protein components of HDLs have a strong impact on NO production. S1P is a lipid carried mainly on apoM-containing HDLs (>50% of circulating S1P). S1P-apoM HDLs are involved in persistent activation of Akt/eNOS pathway, thus leading to NO dependent vasodilation through S1P receptor stimulation (284–286). Decreased levels of ApoM/HDL correlated with increased CVD risk and have been reported in type 2 diabetes in both sexes and in women (but not in men) with type 1 diabetes (287–289).
Reduced HDL-associated PON-1 activity leads to the activation of endothelial lectin-like oxidized LDL receptor (LOX-1) and PKCαII, thus inhibiting the activity of eNOS (290). Inflammation and metabolic syndromes can also drastically affect the ability of HDLs to stimulate NO production (291, 292), thus promoting atherosclerosis (293).
Conclusions
Sex hormones and sex-specific gene expression are important although still incompletely understood determinants in the regulation of HDL and EC cross talk and their contribution to cardiovascular health and disease. Despite increasing evidence pointing out that sex-origin of cultured cells and in particular ECs can strongly affect scientific results (294–296), most of the articles do not report any information about the sex of the cells or of the animals used in their experiments. The use of sex-mixed cohorts of patients or imbalanced number of women and men during clinical trials also represents a potential source of bias. As highlighted in this Review, it is of foremost importance to always consider the influence of sex as biological variable in each step of research and to clearly report and analyze this information.
Author contributions
ED, AJ, and EO wrote the manuscript. ED designed the figures under BioRender common creative license. All authors contributed to the article and approved the submitted version.
Funding
This research was funded by the Swiss National Science Foundation (SNSF), grant number PRIMA: PR00P3_179861/1 and the Swiss Life Foundation, the Alfred and Annemarie von Sick Grants for Translational and Clinical Research Cardiology and Oncology, the Heubergstiftung and the Swiss Heart Foundation, Switzerland all to EO.
Conflict of interest
The authors declare that the research was conducted in the absence of any commercial or financial relationships that could be construed as a potential conflict of interest.
Publisher's note
All claims expressed in this article are solely those of the authors and do not necessarily represent those of their affiliated organizations, or those of the publisher, the editors and the reviewers. Any product that may be evaluated in this article, or claim that may be made by its manufacturer, is not guaranteed or endorsed by the publisher.
References
1. Voight BF, Peloso GM, Orho-Melander M, Frikke-Schmidt R, Barbalic M, Jensen MK, et al. Plasma HDL cholesterol and risk of myocardial infarction: a mendelian randomisation study. Lancet. (2012) 380:572–80. doi: 10.1016/S0140-6736(12)60312-2
2. Casula M, Colpani O, Xie S, Catapano AL, Baragetti A. HDL in atherosclerotic cardiovascular disease: in search of a role. Cells. (2021) 10:1869. doi: 10.3390/cells10081869
3. Madsen CM, Varbo A, Nordestgaard BG. Extreme high high-density lipoprotein cholesterol is paradoxically associated with high mortality in men and women: two prospective cohort studies. Eur Heart J. (2017) 38:2478–86. doi: 10.1093/eurheartj/ehx163
4. Yu Y, Li M, Huang X, Zhou W, Wang T, Zhu L, et al. A U-shaped association between the LDL-cholesterol to HDL-cholesterol ratio and all-cause mortality in elderly hypertensive patients: a prospective cohort study. Lipids Health Dis. (2020) 19:238. doi: 10.1186/s12944-020-01413-5
5. Ko DT, Alter DA, Guo H, Koh M, Lau G, Austin PC, et al. High-density lipoprotein cholesterol and cause-specific mortality in individuals without previous cardiovascular conditions: the CANHEART study. J Am Coll Cardiol. (2016) 68:2073–83. doi: 10.1016/j.jacc.2016.08.038
6. Kontush A, Lhomme M, Chapman MJ. Unraveling the complexities of the HDL lipidome. J Lipid Res. (2013) 54:2950–63. doi: 10.1194/jlr.R036095
7. Birner-Gruenberger R, Schittmayer M, Holzer M, Marsche G. Understanding high-density lipoprotein function in disease: recent advances in proteomics unravel the complexity of its composition and biology. Prog Lipid Res. (2014) 56:36–46. doi: 10.1016/j.plipres.2014.07.003
8. Kontush A, Lhomme M, Calabresi L, Chapman MJ, Davidson WS, Davidson WS. Structure of HDL: particle subclasses and molecular components. Handb Exp Pharmacol. (2015) 224:3–15. doi: 10.1007/978-3-319-09665-0_1
9. Sturtzel C. Endothelial cells. Adv Exp Med Biol. (2017) 1003:71–91. doi: 10.1007/978-3-319-57613-8_4
10. Cyr AR, Huckaby LV, Shiva SS, Zuckerbraun BS. Nitric oxide and endothelial dysfunction. Crit Care Clin. (2020) 36:307–21. doi: 10.1016/j.ccc.2019.12.009
11. Gimbrone MA, García-Cardeña G. Endothelial cell dysfunction and the pathobiology of atherosclerosis. Circ Res. (2016) 118:620–36. doi: 10.1161/CIRCRESAHA.115.306301
12. Davis CE, Williams DH, Oganov RG, Tao SC, Rywik SL, Stein Y, et al. Sex difference in high density lipoprotein cholesterol in six countries. Am J Epidemiol. (1996) 143:1100–6. doi: 10.1093/oxfordjournals.aje.a008686
13. García-Calzón S, Perfilyev A, de Mello VD, Pihlajamäki J, Ling C. Sex differences in the methylome and transcriptome of the human liver and circulating HDL-cholesterol levels. J Clin Endocrinol Metab. (2018) 103:4395–408. doi: 10.1210/jc.2018-00423
14. Huxley VH, Kemp SS, Schramm C, Sieveking S, Bingaman S, Yu Y, et al. Sex differences influencing micro- and macrovascular endothelial phenotype in vitro. J Physiol. (2018) 596:3929–49. doi: 10.1113/JP276048
15. Agirbasli M, Agaoglu NB, Orak N, Caglioz H, Ocek T, Karabag T, et al. Sex hormones, insulin resistance and high-density lipoprotein cholesterol levels in children. Horm Res Paediatr. (2010) 73:166–74. doi: 10.1159/000284357
16. Morrison JA, Barton BA, Biro FM, Sprecher DL. Sex hormones and the changes in adolescent male lipids: longitudinal studies in a biracial cohort. J Pediatr. (2003) 142:637–42. doi: 10.1067/mpd.2003.246
17. El Khoudary SR, Brooks MM, Thurston RC, Matthews KA. Lipoprotein subclasses and endogenous sex hormones in women at midlife. J Lipid Res. (2014) 55:1498–504. doi: 10.1194/jlr.P049064
18. Mendelsohn ME. Protective effects of estrogen on the cardiovascular system. Am J Cardiol. (2002) 89:12E−7E. doi: 10.1016/S0002-9149(02)02405-0
19. Mendelsohn ME. Mechanisms of estrogen action in the cardiovascular system. J Steroid Biochem Mol Biol. (2000) 74:337–43. doi: 10.1016/S0960-0760(00)00110-2
20. James BD, Allen JB. Sex-specific response to combinations of shear stress and substrate stiffness by endothelial cells in vitro. Adv Healthc Mater. (2021) 10:e2100735. doi: 10.1002/adhm.202100735
21. Boscaro C, Trenti A, Baggio C, Scapin C, Trevisi L, Cignarella A, et al. Sex differences in the pro-angiogenic response of human endothelial cells: focus on PFKFB3 and FAK activation. Front Pharmacol. (2020) 11:587221. doi: 10.3389/fphar.2020.587221
22. Polderman TJC, Kreukels BPC, Irwig MS, Beach L, Chan YM, Derks EM, et al. The biological contributions to gender identity and gender diversity: bringing data to the table. Behav Genet. (2018) 48:95–108. doi: 10.1007/s10519-018-9889-z
23. Maraka S, Singh Ospina N, Rodriguez-Gutierrez R, Davidge-Pitts CJ, Nippoldt TB, Prokop LJ, et al. Sex steroids and cardiovascular outcomes in transgender individuals: a systematic review and meta-analysis. J Clin Endocrinol Metab. (2017) 102:3914–23. doi: 10.1210/jc.2017-01643
24. van Velzen DM, Adorni MP, Zimetti F, Strazzella A, Simsek S, Sirtori CR, et al. The effect of transgender hormonal treatment on high density lipoprotein cholesterol efflux capacity. Atherosclerosis. (2021) 323:44–53. doi: 10.1016/j.atherosclerosis.2021.03.008
25. Humble RM, Greene DN, Schmidt RL, Winston McPherson G, Rongitsch J, Imborek KL, et al. Reference intervals for clinical chemistry analytes for transgender men and women on stable hormone therapy. J Appl Lab Med. (2022). doi: 10.1093/jalm/jfac025
26. Iannantuoni F, Salazar JD, Martinez de. Marañon A, Bañuls C, López-Domènech S, Rocha M, et al. Testosterone administration increases leukocyte-endothelium interactions and inflammation in transgender men. Fertil Steril. (2021) 115:483–9. doi: 10.1016/j.fertnstert.2020.08.002
27. Kannel WB, Dawber TR, Friedman GD, Glennon WE, McNamara. PMRISK factors in coronary heart diseasean evaluation of several serum lipids as predictors of coronary heart disease, the framingham. study. Ann Intern Med. (1964) 61:888–99. doi: 10.7326/0003-4819-61-5-888
28. Cheung MC, Brown BG, Wolf AC, Albers JJ. Altered particle size distribution of apolipoprotein A-I-containing lipoproteins in subjects with coronary artery disease. J Lipid Res. (1991) 32:383–94. doi: 10.1016/S0022-2275(20)42061-9
29. Robert J, Osto E, von Eckardstein A. The endothelium is both a target and a barrier of HDL's protective functions. Cells. (2021) 10:1041. doi: 10.3390/cells10051041
30. Asztalos BF, Cupples LA, Demissie S, Horvath KV, Cox CE, Batista MC, et al. High-density lipoprotein subpopulation profile and coronary heart disease prevalence in male participants of the Framingham Offspring Study. Arterioscler Thromb Vasc Biol. (2004) 24:2181–7. doi: 10.1161/01.ATV.0000146325.93749.a8
31. Smith JD, Breslow JL. The emergence of mouse models of atherosclerosis and their relevance to clinical research. J Intern Med. (1997) 242:99–109. doi: 10.1046/j.1365-2796.1997.00197.x
32. Calabresi L, Gomaraschi M, Franceschini G. Endothelial protection by high-density lipoproteins: from bench to bedside. Arterioscler Thromb Vasc Biol. (2003) 23:1724–31. doi: 10.1161/01.ATV.0000094961.74697.54
33. Inazu A, Brown ML, Hesler CB, Agellon LB, Koizumi J, Takata K, et al. Increased high-density lipoprotein levels caused by a common cholesteryl-ester transfer protein gene mutation. N Engl J Med. (1990) 323:1234–8. doi: 10.1056/NEJM199011013231803
34. Barter PJ, Brewer HB. Jr, Chapman MJ, Hennekens CH, Rader DJ, Tall AR Cholesteryl ester transfer protein: a novel target for raising HDL and inhibiting atherosclerosis Arterioscler. Thromb Vasc Biol. (2003) 23:160–7. doi: 10.1161/01.ATV.0000054658.91146.64
35. Barter PJ, Caulfield M, Eriksson M, Grundy SM, Kastelein JJ, Komajda M, et al. Effects of torcetrapib in patients at high risk for coronary events. N Engl J Med. (2007) 357:2109–22. doi: 10.1056/NEJMoa0706628
36. Furtado JD, Ruotolo G, Nicholls SJ, Dullea R, Carvajal-Gonzalez S, Sacks FM. Pharmacological inhibition of CETP (Cholesteryl Ester Transfer Protein) increases HDL (High-Density Lipoprotein) that contains ApoC3 and other HDL subspecies associated with higher risk of coronary heart disease. Arterioscler Thromb Vasc Biol. (2022) 42:227–37. doi: 10.1161/ATVBAHA.121.317181
37. Schwartz GG, Olsson AG, Barter PJ. Dalcetrapib in patients with an acute coronary syndrome. N Engl J Med. (2013) 368:869–70. doi: 10.1056/NEJMc1300057
38. Tardif JC, Rhéaume E, Lemieux Perreault LP, Grégoire JC, Feroz Zada Y, Asselin G, et al. Pharmacogenomic determinants of the cardiovascular effects of dalcetrapib. Circ Cardiovasc Genet. (2015) 8:372–82. doi: 10.1161/CIRCGENETICS.114.000663
39. Miyares MA. Anacetrapib and dalcetrapib: two novel cholesteryl ester transfer protein inhibitors. Ann Pharmacother. (2011) 45:84–94. doi: 10.1345/aph.1P446
40. Bowman L, Chen F, Sammons E, Hopewell JC, Wallendszus K, Stevens W, et al. Randomized Evaluation of the Effects of Anacetrapib through Lipid-modification (REVEAL)-A large-scale, randomized, placebo-controlled trial of the clinical effects of anacetrapib among people with established vascular disease: Trial design, recruitment, and baseline characteristics. Am Heart J. (2017) 187:182–90. doi: 10.1016/j.ahj.2017.02.021
41. Group THT-RC, Committee W, Sammons E, Hopewell JC, Chen F, Stevens W, et al. Long-term safety and efficacy of anacetrapib in patients with atherosclerotic vascular disease. Eur Heart J. (2021) 43:1416–24.
42. Krishna R, Gheyas F, Liu Y, Hagen DR, Walker B, Chawla A, et al. Chronic administration of anacetrapib is associated with accumulation in adipose and slow elimination. Clin Pharmacol Ther. (2017) 102:832–40. doi: 10.1002/cpt.700
43. Palmer TM, Lawlor DA, Harbord RM, Sheehan NA, Tobias JH, Timpson NJ, et al. Using multiple genetic variants as instrumental variables for modifiable risk factors. Stat Methods Med Res. (2012) 21:223–42. doi: 10.1177/0962280210394459
44. Agerholm-Larsen B, Tybjaerg-Hansen A, Schnohr P, Steffensen R, Nordestgaard BG. Common cholesteryl ester transfer protein mutations, decreased HDL cholesterol, and possible decreased risk of ischemic heart disease: The Copenhagen City Heart Study. Circulation. (2000) 102:2197–203. doi: 10.1161/01.CIR.102.18.2197
45. Vergeer M, Korporaal SJ, Franssen R, Meurs I, Out R, Hovingh GK, et al. Genetic variant of the scavenger receptor BI in humans. N Engl J Med. (2011) 364:136–45. doi: 10.1056/NEJMoa0907687
46. Zanoni P, Khetarpal SA, Larach DB, Hancock-Cerutti WF, Millar JS, Cuchel M, et al. Rare variant in scavenger receptor BI raises HDL cholesterol and increases risk of coronary heart disease. Science. (2016) 351:1166–71. doi: 10.1126/science.aad3517
47. Arnett DK, Blumenthal RS, Albert MA, Buroker AB, Goldberger ZD, Hahn EJ, et al. (2019). ACC/AHA guideline on the primary prevention of cardiovascular disease: executive summary: a report of the american college of cardiology/american heart association task force on clinical practice guidelines. J Am Coll Cardiol. (2019) 74:1376–414.
48. Conroy RM, Pyörälä K, Fitzgerald AP, Sans S, Menotti A, De Backer G, et al. Estimation of ten-year risk of fatal cardiovascular disease in Europe: the SCORE project. Eur Heart J. (2003) 24:987–1003. doi: 10.1016/S0195-668X(03)00114-3
49. Kim HJ, Park HA, Cho YG, Kang JH, Kim KW, Kang JH, et al. Gender difference in the level of HDL cholesterol in Korean adults. Korean J Fam Med. (2011) 32:173–81. doi: 10.4082/kjfm.2011.32.3.173
50. Klingel SL, Roke K, Hidalgo B, Aslibekyan S, Straka RJ, An P, et al. Sex differences in blood HDL-c, the total cholesterol/HDL-c ratio, and palmitoleic acid are not associated with variants in common candidate genes. Lipids. (2017) 52:969–80. doi: 10.1007/s11745-017-4307-5
51. Yi S-W, Park H-B, Jung M-H, Yi J-J, Ohrr H. High-density lipoprotein cholesterol and cardiovascular mortality: a prospective cohort study among 15.8 million adults. Eur J Prev Cardiol. (2021) 29:844–54. doi: 10.1093/eurjpc/zwab230
52. Bagatell CJ, Bremner WJ. Androgen and progestagen effects on plasma lipids. Prog Cardiovasc Dis. (1995) 38:255–71. doi: 10.1016/S0033-0620(95)80016-6
53. Jomard A, Liberale L, Doytcheva P, Reiner MF, Müller D, Visentin M, et al. Effects of acute administration of trimethylamine N-oxide on endothelial function: a translational study. Sci Rep. (2022) 12:8664. doi: 10.1038/s41598-022-12720-5
54. Tzeravini E, Tentolouris A, Eleftheriadou I, Chaviaras N, Kolovou G, Apostolidou-Kiouti F, et al. Comparison of postprandial serum triglyceride and apolipoprotein B concentrations between the two phases of menstrual cycle in healthy women. Curr Vasc Pharmacol. (2021) 19:411–22. doi: 10.2174/1573406416666200611105113
55. Vashishta S, Gahlot S, Goyal R. Effect of menstrual cycle phases on plasma lipid and lipoprotein levels in regularly menstruating women. J Clin Diagn Res. (2017) 11:Cc05–cc7. doi: 10.7860/JCDR/2017/26031.9799
56. López-Simón L, de Oya M, Lasunción MA, Riestra P, Benavente M, de Oya I, et al. Genetic determinants of plasma HDL-cholesterol levels in prepubertal children. Clin Chim Acta. (2009) 403:203–6. doi: 10.1016/j.cca.2009.03.002
57. Cho KH, Kim JR. Rapid decrease in HDL-C in the puberty period of boys associated with an elevation of blood pressure and dyslipidemia in Korean teenagers: an explanation of why and when men have lower HDL-C levels than women. Med Sci (Basel). (2021) 9:35. doi: 10.3390/medsci9020035
58. Inaraja V, Thuissard I, Andreu-Vazquez C, Jodar E. Lipid profile changes during the menopausal transition. Menopause. (2020) 27:780–7. doi: 10.1097/GME.0000000000001532
59. Collins P, HDL-C. in post-menopausal women: an important therapeutic target. Int J Cardiol. (2008) 124:275–82. doi: 10.1016/j.ijcard.2007.06.009
60. Jacobson TA, Ito MK, Maki KC, Orringer CE, Bays HE, Jones PH, et al. National Lipid Association recommendations for patient-centered management of dyslipidemia: part 1 - executive summary. J Clin Lipidol. (2014) 8:473–88. doi: 10.1016/j.jacl.2014.07.007
61. Trimarco V, Izzo R, Morisco C, Mone P, Maria Virginia M, Falco A, et al. High HDL (high-density lipoprotein) cholesterol increases cardiovascular risk in hypertensive patients. Hypertension. 2022:101161hypertensionaha12219912. doi: 10.1161/HYPERTENSIONAHA.122.19912
62. Wilkins JT, Ning H, Stone NJ, Criqui MH, Zhao L, Greenland P, et al. Coronary heart disease risks associated with high levels of HDL cholesterol. J Am Heart Assoc. (2014) 3:e000519. doi: 10.1161/JAHA.113.000519
63. Paunio M, Heinonen OP, Virtamo J, Klag MJ, Manninen V, Albanes D, et al. HDL cholesterol and mortality in Finnish men with special reference to alcohol intake. Circulation. (1994) 90:2909–18. doi: 10.1161/01.CIR.90.6.2909
64. Rached FH, Chapman MJ, Kontush A. HDL particle subpopulations: focus on biological function. Biofactors. (2015) 41:67–77. doi: 10.1002/biof.1202
65. Zannis VI, Fotakis P, Koukos G, Kardassis D, Ehnholm C, Jauhiainen M, et al. HDL biogenesis, remodeling, and catabolism. Handb Exp Pharmacol. (2015) 224:53–111. doi: 10.1007/978-3-319-09665-0_2
66. Parks JS, Chung S Fau - Shelness GS, Shelness GS. Hepatic ABC transporters and triglyceride metabolism. Curr Opin Lipidol. (2012) 23:1473-6535. doi: 10.1097/MOL.0b013e328352dd1a
67. Norum KR, Remaley AT, Miettinen HE, Strøm EH, Balbo BEP, Sampaio C, et al. Lecithin:cholesterol acyltransferase: symposium on 50 years of biomedical research from its discovery to latest findings. J Lipid Res. (2020). 61:1539-7262. doi: 10.1194/jlr.S120000720
68. Duong M, Psaltis M, Rader DJ, Marchadier D, Barter PJ, Rye K-A. Evidence that hepatic lipase and endothelial lipase have different substrate specificities for high-density lipoprotein phospholipids. Biochemistry. (2003) 42:13778–85. doi: 10.1021/bi034990n
69. Albers JJ, Vuletic S, Cheung MC. Role of plasma phospholipid transfer protein in lipid and lipoprotein metabolism. Biochim Biophys Acta Mol Cell Biol Lipids. (2012) 1821:345–57. doi: 10.1016/j.bbalip.2011.06.013
70. Schaefer EJ, Anthanont P, Asztalos BF. High-density lipoprotein metabolism, composition, function, and deficiency. Curr Opin Lipidol. (2014) 25:194–9. doi: 10.1097/MOL.0000000000000074
71. Lamon-Fava S, Micherone D. Regulation of apoA-I gene expression: mechanism of action of estrogen and genistein. J Lipid Res. (2004) 45:106–12. doi: 10.1194/jlr.M300179-JLR200
72. Lopez D, Sanchez MD, Shea-Eaton W, McLean MP. Estrogen activates the high-density lipoprotein receptor gene via binding to estrogen response elements and interaction with sterol regulatory element binding protein-1A. Endocrinology. (2002) 143:2155–68. doi: 10.1210/endo.143.6.8855
73. Jones DR, Schmidt RJ, Pickard RT, Foxworthy PS, Eacho PI. Estrogen receptor-mediated repression of human hepatic lipase gene transcription. J Lipid Res. (2002) 43:383–91. doi: 10.1016/S0022-2275(20)30144-9
74. Hartgens F, Rietjens G, Keizer HA, Kuipers H, Wolffenbuttel BH. Effects of androgenic-anabolic steroids on apolipoproteins and lipoprotein (a). Br J Sports Med. (2004) 38:253–9. doi: 10.1136/bjsm.2003.000199
75. Goldberg RB, Rabin D, Alexander AN, Doelle GC, Getz GS. Suppression of plasma testosterone leads to an increase in serum total and high density lipoprotein cholesterol and apoproteins A-I and B. J Clin Endocrinol Metab. (1985) 60:203–7. doi: 10.1210/jcem-60-1-203
76. Wekker V, van Dammen L, Koning A, Heida KY, Painter RC, Limpens J, et al. Long-term cardiometabolic disease risk in women with PCOS: a systematic review and meta-analysis. Hum Reprod Update. (2020) 26:942–60. doi: 10.1093/humupd/dmaa029
77. Spałkowska M, Mrozińska S, Gałuszka-Bednarczyk A, Gosztyła K, Przywara A, Guzik J, et al. The PCOS patients differ in lipid profile according to their phenotypes. Exp Clin Endocrinol Diabetes. (2018) 126:437–44. doi: 10.1055/s-0043-121264
78. Hall E, Volkov P, Dayeh T, Esguerra JL, Salö S, Eliasson L, et al. Sex differences in the genome-wide DNA methylation pattern and impact on gene expression, microRNA levels and insulin secretion in human pancreatic islets. Genome Biol. (2014) 15:522. doi: 10.1186/s13059-014-0522-z
79. Mayne BT, Bianco-Miotto T, Buckberry S, Breen J, Clifton V, Shoubridge C, et al. Large scale gene expression meta-analysis reveals tissue-specific, sex-biased gene expression in humans. Front Genet. (2016) 7:183. doi: 10.3389/fgene.2016.00183
80. Russo GT, Horvath KV, Di Benedetto A, Giandalia A, Cucinotta D, Asztalos B. Influence of menopause and cholesteryl ester transfer protein (CETP) TaqIB polymorphism on lipid profile and HDL subpopulations distribution in women with and without type 2 diabetes. Atherosclerosis. (2010) 210:294–301. doi: 10.1016/j.atherosclerosis.2009.11.011
81. Lamon-Fava S, Asztalos BF, Howard TD, Reboussin DM, Horvath KV, Schaefer EJ, et al. Association of polymorphisms in genes involved in lipoprotein metabolism with plasma concentrations of remnant lipoproteins and HDL subpopulations before and after hormone therapy in postmenopausal women. Clin Endocrinol (Oxf). (2010) 72:169–75. doi: 10.1111/j.1365-2265.2009.03644.x
82. Boekholdt SM, Sacks FM, Jukema JW, Shepherd J, Freeman DJ, McMahon AD, et al. Cholesteryl ester transfer protein TaqIB variant, high-density lipoprotein cholesterol levels, cardiovascular risk, and efficacy of pravastatin treatment: individual patient meta-analysis of 13,677 subjects. Circulation. (2005) 111:278–87. doi: 10.1161/01.CIR.0000153341.46271.40
83. Heilbronn LK, Noakes M, Clifton PM. Association between HDL-cholesterol and the Taq1B polymorphism in the cholesterol ester transfer protein gene in obese women. Atherosclerosis. (2002) 162:419–24. doi: 10.1016/S0021-9150(01)00733-X
84. Rader DJ. Molecular regulation of HDL metabolism and function: implications for novel therapies. J Clin Invest. (2006) 116:3090–100. doi: 10.1172/JCI30163
85. Reilly M, Rader DJ. Apolipoprotein E and coronary disease: a puzzling paradox. PLoS Med. (2006) 3:e258. doi: 10.1371/journal.pmed.0030258
86. Mosher MJ, Lange LA, Howard BV, Lee ET, Best LG, Fabsitz RR, et al. Sex-specific interaction between APOE genotype and carbohydrate intake affects plasma HDL-C levels: the Strong Heart Family Study. Genes Nutr. (2008) 3:87–97. doi: 10.1007/s12263-008-0075-4
87. Zokaei N, Giehl K, Sillence A, Neville MJ, Karpe F, Nobre AC, et al. Sex and APOE: A memory advantage in male APOE ε4 carriers in midlife. Cortex. (2017) 88:98–105. doi: 10.1016/j.cortex.2016.12.016
88. Altmann A, Tian L, Henderson VW, Greicius MD. Sex modifies the APOE-related risk of developing Alzheimer disease. Ann Neurol. (2014) 75:563–73. doi: 10.1002/ana.24135
89. Tanaka S, Yasuda T, Ishida T, Fujioka Y, Tsujino T, Miki T, et al. Increased serum cholesterol esterification rates predict coronary heart disease and sudden death in a general population. Arterioscler Thromb Vasc Biol. (2013) 33:1098–104. doi: 10.1161/ATVBAHA.113.301297
90. Holleboom AG, Kuivenhoven JA, Vergeer M, Hovingh GK, van Miert JN, Wareham NJ, et al. Plasma levels of lecithin:cholesterol acyltransferase and risk of future coronary artery disease in apparently healthy men and women: a prospective case-control analysis nested in the EPIC-Norfolk population study. J Lipid Res. (2010) 51:416–21. doi: 10.1194/P900038-JLR200
91. Emokpae MA, Uwumarongie OH, Osadolor HB. Sex dimorphism in serum lecithin: cholesterol acyltransferase and lipoprotein lipase activities in adult sickle cell anaemia patients with proteinuria. Indian J Clin Biochem. (2011) 26:57–61. doi: 10.1007/s12291-010-0096-9
92. Guo M, Liu Z, Xu Y, Ma P, Huang W, Gao M, et al. Spontaneous atherosclerosis in aged LCAT-deficient hamsters with enhanced oxidative stress-brief report. Arterioscler Thromb Vasc Biol. (2020) 40:2829–36. doi: 10.1161/ATVBAHA.120.315265
93. Li L, Hossain MA, Sadat S, Hager L, Liu L, Tam L, et al. Lecithin cholesterol acyltransferase null mice are protected from diet-induced obesity and insulin resistance in a gender-specific manner through multiple pathways. J Biol Chem. (2011) 286:17809–20. doi: 10.1074/jbc.M110.180893
94. Khovidhunkit WKMM, Memon RR, Shigenaga JK, Moser AH, Feingold KR, Grunfeld C. Effects of infection and inflammation on lipid and lipoprotein metabolism: mechanisms and consequences to the host. J Lipid Res. (2004) 45:1169–96. doi: 10.1194/jlr.R300019-JLR200
95. Memon RA, Holleran WM, Moser AH, Seki T, Uchida Y, Fuller J, et al. Endotoxin and cytokines increase hepatic sphingolipid biosynthesis and produce lipoproteins enriched in ceramides and sphingomyelin. Arterioscler Thromb Vasc Biol. (1998) 18:1257–65. doi: 10.1161/01.ATV.18.8.1257
96. Prasad DS, Kabir Z, Dash AK, Das BC. Abdominal obesity, an independent cardiovascular risk factor in Indian subcontinent: a clinico epidemiological evidence summary. J Cardiovasc Dis Res. (2011) 2:199–205. doi: 10.4103/0975-3583.89803
97. Selvaraj S, Martinez EE, Aguilar FG, Kim KY, Peng J, Sha J, et al. Association of central adiposity with adverse cardiac mechanics: findings from the hypertension genetic epidemiology network study. Circ Cardiovasc Imaging. (2016) 9:e004396. doi: 10.1161/CIRCIMAGING.115.004396
98. Woudberg NJ, Lecour S, Goedecke JH. HDL subclass distribution shifts with increasing central adiposity. J Obes. (2019) 2019:2107178. doi: 10.1155/2019/2107178
99. Stadler JT, Lackner S, Mörkl S, Trakaki A, Scharnagl H, Borenich A, et al. Obesity affects HDL metabolism, composition and subclass distribution. Biomedicines. (2021) 9. doi: 10.3390/biomedicines9030242
100. Talbot CPJ, Plat J, Joris PJ, Konings M, Kusters Y, Schalkwijk CG, et al. HDL cholesterol efflux capacity and cholesteryl ester transfer are associated with body mass, but are not changed by diet-induced weight loss: A randomized trial in abdominally obese men. Atherosclerosis. (2018) 274:23–8. doi: 10.1016/j.atherosclerosis.2018.04.029
101. Lorkowski SW, Brubaker G, Rotroff DM, Kashyap SR, Bhatt DL, Nissen SE, et al. Bariatric surgery improves HDL function examined by apoa1 exchange rate and cholesterol efflux capacity in patients with obesity and type 2 diabetes. Biomolecules. (2020) 10:551. doi: 10.3390/biom10040551
102. Thakkar H, Vincent V, Shukla S, Sra M, Kanga U, Aggarwal S, et al. Improvements in cholesterol efflux capacity of HDL and adiponectin contribute to mitigation in cardiovascular disease risk after bariatric surgery in a cohort with morbid obesity. Diabetol Metab Syndr. (2021) 13:46. doi: 10.1186/s13098-021-00662-3
103. Osto E, Doytcheva P, Corteville C, Bueter M, Dörig C, Stivala S, et al. Rapid and body weight-independent improvement of endothelial and high-density lipoprotein function after Roux-en-Y gastric bypass: role of glucagon-like peptide-1. Circulation. (2015) 131:871–81. doi: 10.1161/CIRCULATIONAHA.114.011791
104. Asztalos BF, Swarbrick MM, Schaefer EJ, Dallal GE, Horvath KV Ai M, et al. Effects of weight loss, induced by gastric bypass surgery, on HDL remodeling in obese women. J Lipid Res. (2010) 51:2405–12. doi: 10.1194/jlr.P900015-JLR200
105. van Veldhuisen SL, Gorter TM, van Woerden G, de Boer RA, Rienstra M, Hazebroek EJ, et al. Bariatric surgery and cardiovascular disease: a systematic review and meta-analysis. Eur Heart J. (2022) 43:1955–69. doi: 10.1093/eurheartj/ehac071
106. Aly S, Hachey K, Pernar LIM. Gender disparities in weight loss surgery. Mini-invasive Surgery. (2020) 4:21. doi: 10.20517/2574-1225.2019.57
107. Aminian A, Zelisko A, Kirwan JP, Brethauer SA, Schauer PR. Exploring the impact of bariatric surgery on high density lipoprotein. Surg Obes Relat Dis. (2015) 11:238–47. doi: 10.1016/j.soard.2014.07.017
108. Genua I, Ramos A, Caimari F, Balagué C, Sánchez-Quesada JL, Pérez A, et al. Effects of bariatric surgery on HDL cholesterol. Obes Surg. (2020) 30:1793–8. doi: 10.1007/s11695-020-04385-8
109. Arnal JF, Fontaine C, Billon-Galés A, Favre J, Laurell H, Lenfant F, et al. Estrogen receptors and endothelium. Arterioscler Thromb Vasc Biol. (2010) 30:1506–12. doi: 10.1161/ATVBAHA.109.191221
110. Celermajer DS, Sorensen KE, Spiegelhalter DJ, Georgakopoulos D, Robinson J, Deanfield JE. Aging is associated with endothelial dysfunction in healthy men years before the age-related decline in women. J Am Coll Cardiol. (1994) 24:471–6. doi: 10.1016/0735-1097(94)90305-0
111. Sader MA, Celermajer DS. Endothelial function, vascular reactivity and gender differences in the cardiovascular system. Cardiovasc Res. (2002) 53:597–604. doi: 10.1016/S0008-6363(01)00473-4
112. Simoncini T. Mechanisms of action of estrogen receptors in vascular cells: relevance for menopause and aging. Climacteric. (2009) 12 (Suppl 1):6–11. doi: 10.1080/13697130902986385
113. Mendelsohn ME, Karas RH. Molecular and cellular basis of cardiovascular gender differences. Science. (2005) 308:1583–7. doi: 10.1126/science.1112062
114. Collins P, Rosano GM, Sarrel PM, Ulrich L, Adamopoulos S, Beale CM, et al. 17 beta-Estradiol attenuates acetylcholine-induced coronary arterial constriction in women but not men with coronary heart disease. Circulation. (1995) 92:24–30. doi: 10.1161/01.CIR.92.1.24
115. Rossouw JE, Anderson GL, Prentice RL, LaCroix AZ, Kooperberg C, Stefanick ML, et al. Risks and benefits of estrogen plus progestin in healthy postmenopausal women: principal results From the Women's Health Initiative randomized controlled trial. JAMA. (2002) 288:321–33. doi: 10.1001/jama.288.3.321
116. Fredette NC, Meyer MR, Prossnitz ER. Role of GPER in estrogen-dependent nitric oxide formation and vasodilation. J Steroid Biochem Mol Biol. (2018) 176:65–72. doi: 10.1016/j.jsbmb.2017.05.006
117. Tuma M, Canestrini S, Alwahab Z, Marshall J. Trauma and endothelial glycocalyx: the microcirculation helmet? Shock. (2016) 46:352–7. doi: 10.1097/SHK.0000000000000635
118. Hartman RJG, Kapteijn DMC, Haitjema S, Bekker MN, Mokry M, Pasterkamp G, et al. Intrinsic transcriptomic sex differences in human endothelial cells at birth and in adults are associated with coronary artery disease targets. Sci Rep. (2020) 10:12367. doi: 10.1038/s41598-020-69451-8
119. Addis R, Campesi I, Fois M, Capobianco G, Dessole S, Fenu G, et al. Human umbilical endothelial cells (HUVECs) have a sex: characterisation of the phenotype of male and female cells. Biol Sex Differ. (2014) 5:18. doi: 10.1186/s13293-014-0018-2
120. Cattaneo MG, Banfi C, Brioschi M, Lattuada D, Vicentini LM. Sex-dependent differences in the secretome of human endothelial cells. Biol Sex Differ. (2021) 12:7. doi: 10.1186/s13293-020-00350-3
121. Torres-Estay V, Carreño DV, San Francisco IF, Sotomayor P, Godoy AS, Smith GJ. Androgen receptor in human endothelial cells. J Endocrinol. (2015) 224:R131–7. doi: 10.1530/JOE-14-0611
122. Liu PY, Death AK, Handelsman DJ. Androgens and cardiovascular disease. Endocr Rev. (2003) 24:313–40. doi: 10.1210/er.2003-0005
123. Kim KH, Bender JR. Membrane-initiated actions of estrogen on the endothelium. Mol Cell Endocrinol. (2009) 308:3–8. doi: 10.1016/j.mce.2009.03.025
124. Diebel LN, Wheaton M, Liberati DM. The protective role of estrogen on endothelial and glycocalyx barriers after shock conditions: a microfluidic study. Surgery. (2021) 169:678–85. doi: 10.1016/j.surg.2020.08.006
125. Rodríguez E, López R, Paez A, Massó F, Montaño LF. 17Beta-estradiol inhibits the adhesion of leukocytes in TNF-alpha stimulated human endothelial cells by blocking IL-8 and MCP-1 secretion, but not its transcription. Life Sci. (2002) 71:2181–93. doi: 10.1016/S0024-3205(02)01999-9
126. Caulin-Glaser T, Watson CA, Pardi R, Bender JR. Effects of 17beta-estradiol on cytokine-induced endothelial cell adhesion molecule expression. J Clin Invest. (1996) 98:36–42. doi: 10.1172/JCI118774
127. Holm P, Andersen HL, Arrøe G, Stender S. Gender gap in aortic cholesterol accumulation in cholesterol-clamped rabbits: role of the endothelium and mononuclear-endothelial cell interaction. Circulation. (1998) 98:2731–7. doi: 10.1161/01.CIR.98.24.2731
128. Usselman CW, Yarovinsky TO, Steele FE, Leone CA, Taylor HS, Bender JR, et al. Androgens drive microvascular endothelial dysfunction in women with polycystic ovary syndrome: role of the endothelin B receptor. J Physiol. (2019) 597:2853–65. doi: 10.1113/JP277756
129. Lopes RA, Neves KB, Carneiro FS, Tostes RC. Testosterone and vascular function in aging. Front Physiol. (2012) 3:89. doi: 10.3389/fphys.2012.00089
130. Villablanca A, Lubahn D, Shelby L, Lloyd K, Barthold S. Susceptibility to early atherosclerosis in male mice is mediated by estrogen receptor. Arterioscler Thromb Vasc Biol. (2004) 24:1055–61. doi: 10.1161/01.ATV.0000130467.65290.d4
131. Villablanca AC, Tetali S, Altman R, Ng KF, Rutledge JC. Testosterone-derived estradiol production by male endothelium is robust and dependent on p450 aromatase via estrogen receptor alpha. Springerplus. (2013) 2:214. doi: 10.1186/2193-1801-2-214
132. McCrohon JA, Death AK, Nakhla S, Jessup W, Handelsman DJ, Stanley KK, et al. Androgen receptor expression is greater in macrophages from male than from female donors. A sex difference with implications for atherogenesis. Circulation. (2000) 101:224–6. doi: 10.1161/01.CIR.101.3.224
133. Remus EW, Sayeed I, Won S, Lyle AN, Stein DG. Progesterone protects endothelial cells after cerebrovascular occlusion by decreasing MCP-1- and CXCL1-mediated macrophage infiltration. Exp Neurol. (2015) 271:401–8. doi: 10.1016/j.expneurol.2015.07.010
134. You Y, Tan W, Guo Y, Luo M, Shang FF, Xia Y, et al. Progesterone promotes endothelial nitric oxide synthase expression through enhancing nuclear progesterone receptor-SP-1 formation. Am J Physiol Heart Circ Physiol. (2020) 319:H341–h8. doi: 10.1152/ajpheart.00206.2020
135. Grady D, Herrington D, Bittner V, Blumenthal R, Davidson M, Hlatky M, et al. Cardiovascular disease outcomes during 6.8 years of hormone therapy: heart and estrogen/progestin replacement study follow-up (HERS II). JAMA. (2002) 288:49–57. doi: 10.1001/jama.288.1.49
136. Jiang Y, Tian W. The effects of progesterones on blood lipids in hormone replacement therapy. Lipids Health Dis. (2017) 16:219. doi: 10.1186/s12944-017-0612-5
137. Kojima K, Abe-Dohmae S, Arakawa R, Murakami I, Suzumori K, Yokoyama S. Progesterone inhibits apolipoprotein-mediated cellular lipid release: a putative mechanism for the decrease of high-density lipoprotein. Biochim Biophys Acta. (2001) 1532:173–84. doi: 10.1016/S1388-1981(01)00124-X
138. Wang J, Bingaman S, Huxley VH. Intrinsic sex-specific differences in microvascular endothelial cell phosphodiesterases. Am J Physiol Heart Circ Physiol. (2010) 298:H1146–54. doi: 10.1152/ajpheart.00252.2009
139. Du L, Bayir H, Lai Y, Zhang X, Kochanek PM, Watkins SC, et al. Innate gender-based proclivity in response to cytotoxicity and programmed cell death pathway. J Biol Chem. (2004) 279:38563–70. doi: 10.1074/jbc.M405461200
140. Du L, Hickey RW, Bayir H, Watkins SC, Tyurin VA, Guo F, et al. Starving neurons show sex difference in autophagy. J Biol Chem. (2009) 284:2383–96. doi: 10.1074/jbc.M804396200
141. Lorenz M, Koschate J, Kaufmann K, Kreye C, Mertens M, Kuebler WM, et al. Does cellular sex matter? Dimorphic transcriptional differences between female and male endothelial cells. Atherosclerosis. (2015) 240:61–72. doi: 10.1016/j.atherosclerosis.2015.02.018
142. Lorenz M, Blaschke B, Benn A, Hammer E, Witt E, Kirwan J, et al. Sex-specific metabolic and functional differences in human umbilical vein endothelial cells from twin pairs. Atherosclerosis. (2019) 291:99–106. doi: 10.1016/j.atherosclerosis.2019.10.007
143. McDonald G, Cabal N, Vannier A, Umiker B, Yin RH, Orjalo AV, et al. Female bias in systemic lupus erythematosus is associated with the differential expression of X-linked toll-like receptor 8. Front Immunol. (2015) 6:457. doi: 10.3389/fimmu.2015.00457
144. Khan D, Ansar Ahmed S. The immune system is a natural target for estrogen action: opposing effects of estrogen in two prototypical autoimmune diseases. Front Immunol. (2015) 6:635. doi: 10.3389/fimmu.2015.00635
145. Keselman A, Heller N. Estrogen signaling modulates allergic inflammation and contributes to sex differences in asthma. Front Immunol. (2015) 6:568. doi: 10.3389/fimmu.2015.00568
146. Messner B, Bernhard D. Smoking and cardiovascular disease: mechanisms of endothelial dysfunction and early atherogenesis. Arterioscler Thromb Vasc Biol. (2014) 34:509–15. doi: 10.1161/ATVBAHA.113.300156
147. He BM, Zhao SP, Peng ZY. Effects of cigarette smoking on HDL quantity and function: implications for atherosclerosis. J Cell Biochem. (2013) 114:2431–6. doi: 10.1002/jcb.24581
148. Kauss AR, Antunes M, de La Bourdonnaye G, Pouly S, Hankins M, Heremans A, et al. Smoking and apolipoprotein levels: a meta-analysis of published data. Toxicology Reports. (2022) 9:1150–71. doi: 10.1016/j.toxrep.2022.05.009
149. Njølstad I, Arnesen E, Lund-Larsen PG. Smoking, serum lipids, blood pressure, and sex differences in myocardial infarction. A 12-year follow-up of the Finnmark Study. Circulation. (1996) 93:450–6. doi: 10.1161/01.CIR.93.3.450
150. Bolego C, Poli A, Paoletti R. Smoking and gender. Cardiovasc Res. (2002) 53:568–76. doi: 10.1016/S0008-6363(01)00520-X
151. Baron JA, La Vecchia C, Levi F. The antiestrogenic effect of cigarette smoking in women. Am J Obstet Gynecol. (1990) 162:502–14. doi: 10.1016/0002-9378(90)90420-C
152. Le-Ha C, Beilin LJ, Burrows S, Huang R-C, Oddy WH, Hands B, et al. Gender difference in the relationship between passive smoking exposure and HDL-cholesterol levels in late adolescence. J Clin Endocr. (2013) 98:2126–35. doi: 10.1210/jc.2013-1016
153. Criqui MH, Wallace RB, Heiss G, Mishkel M, Schonfeld G, Jones GT. Cigarette smoking and plasma high-density lipoprotein cholesterol. The lipid research clinics program prevalence study. Circulation. (1980) 62:Iv70–6.
154. Forey BA, Fry JS, Lee PN, Thornton AJ, Coombs KJ. The effect of quitting smoking on HDL-cholesterol - a review based on within-subject changes. Biomark Res. (2013) 1:26. doi: 10.1186/2050-7771-1-26
155. Nyboe J, Jensen G, Appleyard M, Schnohr P. Smoking and the risk of first acute myocardial infarction. Am Heart J. (1991) 122:438–47. doi: 10.1016/0002-8703(91)90997-V
156. Shao Y, Luo L, Ren Z, Guo J, Xiao X, Huang J, et al. Smoking-induced inhibition of number and activity of endothelial progenitor cells and nitric oxide in males were reversed by estradiol in premenopausal females. Cardiol Res Pract. (2020) 2020:9352518. doi: 10.1155/2020/9352518
157. Piano MR, Thur LA, Hwang CL, Phillips SA. Effects of alcohol on the cardiovascular system in women. Alcohol Res. (2020) 40:12. doi: 10.35946/arcr.v40.2.12
158. Marques-Vidal P, Bochud M, Paccaud F, Waterworth D, Bergmann S, Preisig M, et al. No interaction between alcohol consumption and HDL-related genes on HDL cholesterol levels. Atherosclerosis. (2010) 211:551–7. doi: 10.1016/j.atherosclerosis.2010.04.001
159. Fuchs FD, Chambless LE, Folsom AR, Eigenbrodt ML, Duncan BB, Gilbert A, et al. Association between alcoholic beverage consumption and incidence of coronary heart disease in whites and blacks: the Atherosclerosis Risk in Communities Study. Am J Epidemiol. (2004) 160:466–74. doi: 10.1093/aje/kwh229
160. Volcik K, Ballantyne CM, Pownall HJ, Sharrett AR, Boerwinkle E. Interaction effects of high-density lipoprotein metabolism gene variation and alcohol consumption on coronary heart disease risk: the atherosclerosis risk in communities study. J Stud Alcohol Drugs. (2007) 68:485–92. doi: 10.15288/jsad.2007.68.485
161. Hata Y, Nakajima K. Life-style and serum lipids and lipoproteins. J Atheroscler Thromb. (2000) 7:177–97. doi: 10.5551/jat1994.7.177
162. Hannuksela M, Marcel YL, Kesäniemi YA, Savolainen MJ. Reduction in the concentration and activity of plasma cholesteryl ester transfer protein by alcohol. J Lipid Res. (1992) 33:737–44. doi: 10.1016/S0022-2275(20)41437-3
163. Williams PT. Quantile-dependent expressivity and gene-lifestyle interactions involving high-density lipoprotein cholesterol. Lifestyle Genom. (2021) 14:1–19. doi: 10.1159/000511421
164. Hines LM, Hunter DJ, Stampfer MJ, Spiegelman D, Chu NF, Rifai N, et al. Alcohol consumption and high-density lipoprotein levels: the effect of ADH1C genotype, gender and menopausal status. Atherosclerosis. (2005) 182:293–300. doi: 10.1016/j.atherosclerosis.2005.02.005
165. Hines LM, Stampfer MJ, Ma J, Gaziano JM, Ridker PM, Hankinson SE, et al. Genetic variation in alcohol dehydrogenase and the beneficial effect of moderate alcohol consumption on myocardial infarction. N Engl J Med. (2001) 344:549–55. doi: 10.1056/NEJM200102223440802
166. Greaves L, Poole N, Brabete AC. Sex, gender, and alcohol use: implications for women and low-risk drinking guidelines. Int J Environ Res Public Health. (2022) 19:4523. doi: 10.3390/ijerph19084523
167. Williams GD, Grant BF, Harford TC, Noble J. Population projections using DSM-III criteria: alcohol abuse and dependence, 190-2000. Alcohol Res Health. (1989) 1989:366.
168. Zheng YL, Lian F, Shi Q, Zhang C, Chen YW, Zhou YH, et al. Alcohol intake and associated risk of major cardiovascular outcomes in women compared with men: a systematic review and meta-analysis of prospective observational studies. BMC Public Health. (2015) 15:773. doi: 10.1186/s12889-015-2081-y
169. Rajendran NK, Liu W, Chu CC, Cahill PA, Redmond EM. Moderate dose alcohol protects against serum amyloid protein A1-induced endothelial dysfunction via both notch-dependent and notch-independent pathways. Alcohol Clin Exp Res. (2021) 45:2217–30. doi: 10.1111/acer.14706
170. Badía E, Sacanella E, Fernández-Solá J, Nicolás JM, Antúnez E, Rotilio D, et al. Decreased tumor necrosis factor-induced adhesion of human monocytes to endothelial cells after moderate alcohol consumption. Am J Clin Nutr. (2004) 80:225–30. doi: 10.1093/ajcn/80.1.225
171. Tanaka A, Cui R, Kitamura A, Liu K, Imano H, Yamagishi K, et al. Heavy alcohol consumption is associated with impaired endothelial function. J Atheroscler Thromb. (2016) 23:1047–54. doi: 10.5551/jat.31641
172. Sacanella E, Estruch R, Badía E, Fernández-Sola J, Nicolás JM, Urbano-Márquez A. Chronic alcohol consumption increases serum levels of circulating endothelial cell/leucocyte adhesion molecules E-selectin and ICAM-1. Alcohol Alcohol. (1999) 34:678–84. doi: 10.1093/alcalc/34.5.678
173. Bédard A, Riverin M, Dodin S, Corneau L, Lemieux S. Sex differences in the impact of the Mediterranean diet on cardiovascular risk profile. Br J Nutr. (2012) 108:1428–34. doi: 10.1017/S0007114511006969
174. Bédard A, Corneau L, Lamarche B, Dodin S, Lemieux S. Sex differences in the impact of the mediterranean diet on LDL particle size distribution and oxidation. Nutrients. (2015) 7:3705–23. doi: 10.3390/nu7053705
175. Turner KM, Keogh JB, Clifton PM. Red meat, dairy, and insulin sensitivity: a randomized crossover intervention study. Am J Clin Nutr. (2015) 101:1173–9. doi: 10.3945/ajcn.114.104976
176. Brennan L, Gibbons H. Sex matters: a focus on the impact of biological sex on metabolomic profiles and dietary interventions. Proc Nutr Soc. (2020) 79:205–9. doi: 10.1017/S002966511900106X
177. Zhang Y, Klein K, Sugathan A, Nassery N, Dombkowski A, Zanger UM, et al. Transcriptional profiling of human liver identifies sex-biased genes associated with polygenic dyslipidemia and coronary artery disease. PLoS ONE. (2011) 6:e23506. doi: 10.1371/journal.pone.0023506
178. Darabi M, Rabbani M, Ani M, Zarean E, Panjehpour M, Movahedian A. Increased leukocyte ABCA1 gene expression in post-menopausal women on hormone replacement therapy. Gynecol Endocrinol. (2011) 27:701–5. doi: 10.3109/09513590.2010.507826
179. Shinohata R, Shibakura M, Arao Y, Watanabe S, Hirohata S, Usui S, et al. high-fat/high-cholesterol diet, but not high-cholesterol alone, increases free cholesterol and apoE-rich HDL serum levels in rats and upregulates hepatic ABCA1 expression. Biochimie. (2022) 197:49–58. doi: 10.1016/j.biochi.2022.01.011
180. Mauerer R, Ebert S, Langmann T. High glucose, unsaturated and saturated fatty acids differentially regulate expression of ATP-binding cassette transporters ABCA1 and ABCG1 in human macrophages. Exp Mol Med. (2009) 41:126–32. doi: 10.3858/emm.2009.41.2.015
181. Singaraja RR, James ER, Crim J, Visscher H, Chatterjee A, Hayden MR. Alternate transcripts expressed in response to diet reflect tissue-specific regulation of ABCA1. J Lipid Res. (2005) 46:2061–71. doi: 10.1194/jlr.M500133-JLR200
182. Villarreal-Molina MT, Aguilar-Salinas CA, Rodríguez-Cruz M, Riaño D, Villalobos-Comparan M, Coral-Vazquez R, et al. The ATP-binding cassette transporter A1 R230C variant affects HDL cholesterol levels and BMI in the Mexican population: association with obesity and obesity-related comorbidities. Diabetes. (2007) 56:1881–7. doi: 10.2337/db06-0905
183. Jacobo-Albavera L, Posadas-Romero C, Vargas-Alarcón G, Romero-Hidalgo S, Posadas-Sánchez R, González-Salazar Mdel C, et al. Dietary fat and carbohydrate modulate the effect of the ATP-binding cassette A1 (ABCA1) R230C variant on metabolic risk parameters in premenopausal women from the Genetics of Atherosclerotic Disease (GEA) Study. Nutr Metab (Lond). (2015) 12:45. doi: 10.1186/s12986-015-0040-3
184. Schoch L, Sutelman P, Suades R, Casani L, Padro T, Badimon L, et al. Hypercholesterolemia-induced HDL dysfunction can be reversed: the impact of diet and statin treatment in a preclinical animal model. Int J Mol Sci. (2022) 23:8596. doi: 10.3390/ijms23158596
185. Vogel RA, Corretti MC, Plotnick GD. Effect of a single high-fat meal on endothelial function in healthy subjects. Am J Cardiol. (1997) 79:350–4. doi: 10.1016/S0002-9149(96)00760-6
186. Ong PJ, Dean TS, Hayward CS, Della Monica PL, Sanders TA, Collins P. Effect of fat and carbohydrate consumption on endothelial function. Lancet. (1999) 354:2134. doi: 10.1016/S0140-6736(99)03374-7
187. Pérez-Jiménez F, Castro P, López-Miranda J, Paz-Rojas E, Blanco A, López-Segura F, et al. Circulating levels of endothelial function are modulated by dietary monounsaturated fat. Atherosclerosis. (1999) 145:351–8. doi: 10.1016/S0021-9150(99)00116-1
188. Fuentes F, López-Miranda J, Sánchez E, Sánchez F, Paez J, Paz-Rojas E, et al. Mediterranean and low-fat diets improve endothelial function in hypercholesterolemic men. Ann Intern Med. (2001) 134:1115–9. doi: 10.7326/0003-4819-134-12-200106190-00011
189. Cuevas AM, Germain AM. Diet and endothelial function. Biol Res. (2004) 37:225–30. doi: 10.4067/S0716-97602004000200008
190. Mata P, Alonso R, Lopez-Farre A, Ordovas JM, Lahoz C, Garces C, et al. Effect of dietary fat saturation on LDL oxidation and monocyte adhesion to human endothelial cells in vitro. Arterioscler Thromb Vasc Biol. (1996) 16:1347–55. doi: 10.1161/01.ATV.16.11.1347
191. Shah R, Makarem N, Emin M, Liao M, Jelic S, Aggarwal B. Mediterranean diet components are linked to greater endothelial function and lower inflammation in a pilot study of ethnically diverse women. Nutr Res. (2020) 75:77–84. doi: 10.1016/j.nutres.2020.01.004
192. Yusuf S, Joseph P, Rangarajan S, Islam S, Mente A, Hystad P, et al. Modifiable risk factors, cardiovascular disease, and mortality in 155 722 individuals from 21 high-income, middle-income, and low-income countries (PURE): a prospective cohort study. Lancet. (2020) 395:795–808. doi: 10.1016/S0140-6736(19)32008-2
193. Gambardella J, Morelli MB, Wang XJ, Santulli G. Pathophysiological mechanisms underlying the beneficial effects of physical activity in hypertension. J Clin Hypertens (Greenwich). (2020) 22:291–5. doi: 10.1111/jch.13804
194. Alevizos A, Lentzas J, Kokkoris S, Mariolis A, Korantzopoulos P. Physical activity and stroke risk. Int J Clin Pract. (2005) 59:922–30. doi: 10.1111/j.1742-1241.2005.00536.x
195. Madsen TE, Samaei M, Pikula A, Yu AYX, Carcel C, Millsaps E, et al. Sex differences in physical activity and incident stroke: a systematic review. Clin Ther. (2022) 44:586–611. doi: 10.1016/j.clinthera.2022.02.006
196. Kiely DK, Wolf PA, Cupples LA, Beiser AS, Kannel WB. Physical activity and stroke risk: the Framingham Study. Am J Epidemiol. (1994) 140:608–20. doi: 10.1093/oxfordjournals.aje.a117298
197. Superko HR, Haskell WH. The role of exercise training in the therapy of hyperlipoproteinemia. Cardiol Clin. (1987) 5:285–310. doi: 10.1016/S0733-8651(18)30552-6
198. Kokkinos PF, Fernhall B. Physical activity and high density lipoprotein cholesterol levels: what is the relationship? Sports Med. (1999) 28:307–14. doi: 10.2165/00007256-199928050-00002
199. Drygas W, Jegler A, Kunski H. Study on threshold dose of physical activity in coronary heart disease prevention. Part I relationship between leisure time physical activity and coronary risk factors. Int J Sports Med. (1988) 9:275–8. doi: 10.1055/s-2007-1025021
200. Kokkinos PF, Holland JC, Narayan P, Colleran JA, Dotson CO, Papademetriou V. Miles run per week and high-density lipoprotein cholesterol levels in healthy, middle-aged men. A dose-response relationship. Arch Intern Med. (1995) 155:415–20. doi: 10.1001/archinte.1995.00430040091011
201. Durstine JL, Haskell WL. Effects of exercise training on plasma lipids and lipoproteins. Exerc Sport Sci Rev. (1994) 22:477–521. doi: 10.1249/00003677-199401000-00017
202. Rohrer L, Ohnsorg Pascale M, Lehner M, Landolt F, Rinninger F, von Eckardstein A. High-density lipoprotein transport through aortic endothelial cells involves scavenger receptor BI and ATP-binding cassette transporter G1. Circ Res. (2009) 104:1142–50. doi: 10.1161/CIRCRESAHA.108.190587
203. Hassan HH, Denis M, Krimbou L, Marcil M, Genest J. Cellular cholesterol homeostasis in vascular endothelial cells. Can J Cardiol. (2006) 22 (Suppl B):35b−40b. doi: 10.1016/S0828-282X(06)70985-0
204. O'Connell Brian J, Denis M, Genest J. Cellular physiology of cholesterol efflux in vascular endothelial cells. Circulation. (2004) 110:2881–8. doi: 10.1161/01.CIR.0000146333.20727.2B
205. Baumer Y, McCurdy S, Weatherby TM, Mehta NN, Halbherr S, Halbherr P, et al. Hyperlipidemia-induced cholesterol crystal production by endothelial cells promotes atherogenesis. Nat Commun. (2017) 8:1129. doi: 10.1038/s41467-017-01186-z
206. Catalano G, Duchene E, Julia Z, Le Goff W, Bruckert E, Chapman MJ, et al. Cellular SR-BI and ABCA1-mediated cholesterol efflux are gender-specific in healthy subjects. J Lipid Res. (2008) 49:635–43. doi: 10.1194/jlr.M700510-JLR200
207. Villard EF. P EIK, Frisdal E, Bruckert E, Clement K, Bonnefont-Rousselot D, et al. Genetic determination of plasma cholesterol efflux capacity is gender-specific and independent of HDL-cholesterol levels. Arterioscler Thromb Vasc Biol. (2013) 33:822–8. doi: 10.1161/ATVBAHA.112.300979
208. Ma F, Darabi M, Lhomme M, Tubeuf E, Canicio A, Brerault J, et al. Phospholipid transfer to high-density lipoprotein (HDL) upon triglyceride lipolysis is directly correlated with HDL-cholesterol levels and is not associated with cardiovascular risk. Atherosclerosis. (2021) 324:1–8. doi: 10.1016/j.atherosclerosis.2021.03.002
209. El Khoudary SR, Hutchins PM, Matthews KA, Brooks MM, Orchard TJ, Ronsein GE, et al. Cholesterol efflux capacity and subclasses of HDL particles in healthy women transitioning through menopause. J Clin Endocrinol Metab. (2016) 101:3419–28. doi: 10.1210/jc.2016-2144
210. Rubinow KB, Vaisar T, Chao JH, Heinecke JW, Page ST. Sex steroids mediate discrete effects on HDL cholesterol efflux capacity and particle concentration in healthy men. J Clin Lipidol. (2018) 12:1072–82. doi: 10.1016/j.jacl.2018.04.013
211. Holmboe SA, Vradi E, Jensen TK, Linneberg A, Husemoen LL, Scheike T, et al. The association of reproductive hormone levels and all-cause, cancer, and cardiovascular disease mortality in men. J Clin Endocrinol Metab. (2015) 100:4472–80. doi: 10.1210/jc.2015-2460
212. Bernini G, Versari D, Moretti A, Virdis A, Ghiadoni L, Bardini M, et al. Vascular reactivity in congenital hypogonadal men before and after testosterone replacement therapy. J Clin Endocrinol Metab. (2006) 91:1691–7. doi: 10.1210/jc.2005-1398
213. Stanhewicz AE, Wenner MM, Stachenfeld NS. Sex differences in endothelial function important to vascular health and overall cardiovascular disease risk across the lifespan. Am J Physiol Heart Circ Physiol. (2018) 315:H1569–h88. doi: 10.1152/ajpheart.00396.2018
214. Bolick David T, Srinivasan S, Kim Kyu W, Hatley Melissa E, Clemens Jeremy J, Whetzel A, et al. Sphingosine-1-phosphate prevents tumor necrosis factor-α-mediated monocyte adhesion to aortic endothelium in mice. Arterioscler Thromb Vasc Biol. (2005) 25:976–81. doi: 10.1161/01.ATV.0000162171.30089.f6
215. Ross R. The pathogenesis of atherosclerosis: a perspective for the (1990s). Nature. (1993) 362:801–9. doi: 10.1038/362801a0
216. Navab M, Berliner Judith A, Subbanagounder G, Hama S, Lusis Aldons J, Castellani Lawrence W, et al. HDL and the inflammatory response induced by LDL-derived oxidized phospholipids. Arterioscler Thromb Vasc Biol. (2001) 21:481–8. doi: 10.1161/01.ATV.21.4.481
217. Mackness B, Durrington P, McElduff P, Yarnell J, Azam N, Watt M, et al. Low paraoxonase activity predicts coronary events in the Caerphilly Prospective Study. Circulation. (2003) 107:2775–9. doi: 10.1161/01.CIR.0000070954.00271.13
218. Mackness B, Hine D, Liu Y, Mastorikou M, Mackness M. Paraoxonase-1 inhibits oxidised LDL-induced MCP-1 production by endothelial cells. Biochem Biophys Res Commun. (2004) 318:680–3. doi: 10.1016/j.bbrc.2004.04.056
219. Gharavi NM, Gargalovic PS, Chang I, Araujo JA, Clark MJ, Szeto WL. et al. High-density lipoprotein modulates oxidized phospholipid signaling in human endothelial cells from proinflammatory to anti-inflammatory. Arterioscler Thromb Vasc Biol. (2007) 27:1346–53. doi: 10.1161/ATVBAHA.107.141283
220. Nicholls SJ, Dusting GJ, Cutri B, Bao S, Drummond GR, Rye K-A, et al. Reconstituted high-density lipoproteins inhibit the acute pro-oxidant and proinflammatory vascular changes induced by a periarterial collar in normocholesterolemic rabbits. Circulation. (2005) 111:1543–50. doi: 10.1161/01.CIR.0000159351.95399.50
221. Vickers KC, Palmisano BT, Shoucri BM, Shamburek RD, Remaley AT. MicroRNAs are transported in plasma and delivered to recipient cells by high-density lipoproteins. Nat Cell Biol. (2011) 13:423–33. doi: 10.1038/ncb2210
222. Michell DL, Vickers KC. Lipoprotein carriers of microRNAs. Biochimica et biophysica acta. (2016) 1861:2069–74. doi: 10.1016/j.bbalip.2016.01.011
223. Cockerill Gillian W, Rye K-A, Gamble Jennifer R, Vadas Mathew A, Barter Philip J. High-density lipoproteins inhibit cytokine-induced expression of endothelial cell adhesion molecules. Arterioscler Thromb Vasc Biol. (1995) 15:1987–94. doi: 10.1161/01.ATV.15.11.1987
224. Tabet F, Vickers KC, Cuesta Torres LF, Wiese CB, Shoucri BM, Lambert G, et al. HDL-transferred microRNA-223 regulates ICAM-1 expression in endothelial cells. Nat Commun. (2014) 5:3292. doi: 10.1038/ncomms4292
225. Zhang Q-H, Zu X-Y, Cao R-X, Liu J-H, Mo Z-C, Zeng Y, et al. An involvement of SR-B1 mediated PI3K–Akt–eNOS signaling in HDL-induced cyclooxygenase 2 expression and prostacyclin production in endothelial cells. Biochem Biophys Res Commun. (2012) 420:17–23. doi: 10.1016/j.bbrc.2012.02.103
226. Dorris SL, Peebles RS. PGI2 as a regulator of inflammatory diseases. Mediators Inflamm. (2012) 2012:926968. doi: 10.1155/2012/926968
227. Madsen CM, Varbo A, Tybjærg-Hansen A, Frikke-Schmidt R, Nordestgaard BG. U-shaped relationship of HDL and risk of infectious disease: two prospective population-based cohort studies. Eur Heart J. (2017) 39:1181–90. doi: 10.1093/eurheartj/ehx665
228. Catapano AL, Pirillo A, Bonacina F, Norata GD, HDL. in innate and adaptive immunity. Cardiovasc Res. (2014) 103:372–83. doi: 10.1093/cvr/cvu150
229. Shor R, Wainstein J, Oz D, Boaz M, Matas Z, Fux A, et al. Low HDL levels and the risk of death, sepsis and malignancy. Clin Res Cardiol. (2008) 97:227–33. doi: 10.1007/s00392-007-0611-z
230. Andersen RV, Wittrup HH, Tybjaerg-Hansen A, Steffensen R, Schnohr P, Nordestgaard BG. Hepatic lipase mutations,elevated high-density lipoprotein cholesterol, and increased risk of ischemic heart disease: the Copenhagen City Heart Study. J Am Coll Cardiol. (2003) 41:1972–82. doi: 10.1016/S0735-1097(03)00407-8
231. Feitosa MF, Myers RH, Pankow JS, Province MA, Borecki IB, LIPC. variants in the promoter and intron 1 modify HDL-C levels in a sex-specific fashion. Atherosclerosis. (2009) 204:171–7. doi: 10.1016/j.atherosclerosis.2008.09.007
232. Wu DF, Lin D, Lu F, Liao QC, Wu YJ, Wang Z, et al. Sex-specific influence of the SCARB1 Rs5888 SNP on the serum lipid response to atorvastatin in patients with acute coronary syndrome undergoing percutaneous coronary intervention. Pharmgenomics Pers Med. (2020) 13:553–61. doi: 10.2147/PGPM.S273346
233. Taleb-Belkadi O, Chaib H, Zemour L, Fatah A, Chafi B, Mekki K. Lipid profile, inflammation, and oxidative status in peri- and postmenopausal women. Gynecol Endocrinol. (2016) 32:982–5. doi: 10.1080/09513590.2016.1214257
234. Feingold KR, Grunfeld C. Effect of inflammation on HDL structure and function. Curr Opin Lipidol. (2016) 27:521–30. doi: 10.1097/MOL.0000000000000333
235. Chung CP, Oeser A, Raggi P, Sokka T, Pincus T, Solus JF, et al. Lipoprotein subclasses determined by nuclear magnetic resonance spectroscopy and coronary atherosclerosis in patients with rheumatoid arthritis. J Rheumatol. (2010) 37:1633–8. doi: 10.3899/jrheum.090639
236. Schulte DM, Paulsen K, Türk K, Brandt B, Freitag-Wolf S, Hagen I, et al. Small dense LDL cholesterol in human subjects with different chronic inflammatory diseases. Nutr Metab Cardiovasc Dis. (2018) 28:1100–5. doi: 10.1016/j.numecd.2018.06.022
237. Charakida M, Besler C, Batuca JR, Sangle S, Marques S, Sousa M, et al. Vascular abnormalities, paraoxonase activity, and dysfunctional HDL in primary antiphospholipid syndrome. JAMA. (2009) 302:1210–7. doi: 10.1001/jama.2009.1346
238. Mostaza JM, Salinero-Fort MA, Cardenas-Valladolid J, Rodriguez-Artalejo F, Díaz-Almiron M, Vich-Pérez P, et al. Pre-infection HDL-cholesterol levels and mortality among elderly patients infected with SARS-CoV-2. Atherosclerosis. (2022) 341:13–9. doi: 10.1016/j.atherosclerosis.2021.12.009
239. Hilser JR, Han Y, Biswas S, Gukasyan J, Cai Z, Zhu R, et al. Association of serum HDL-cholesterol and apolipoprotein A1 levels with risk of severe SARS-CoV-2 infection. J Lipid Res. (2021) 62:100061. doi: 10.1016/j.jlr.2021.100061
240. Peng F, Lei S, Zhang Q, Zhong Y, Wu S. Triglyceride/high-density lipoprotein cholesterol ratio is associated with the mortality of COVID-19: a retrospective study in China. Int J Gen Med. (2022) 15:985–96. doi: 10.2147/IJGM.S346690
241. Souza Junior DR, Silva ARM, Rosa-Fernandes L, Reis LR, Alexandria G, Bhosale SD, et al. HDL proteome remodeling associates with COVID-19 severity. J Clin Lipidol. (2021) 15:796–804. doi: 10.1016/j.jacl.2021.10.005
242. Tanaka S, Begue F, Veeren B, Tran-Dinh A, Robert T, Tashk P, et al. First recombinant high-density lipoprotein particles administration in a severe ICU COVID-19 patient, a multi-omics exploratory investigation. Biomedicines. (2022) 10:754. doi: 10.3390/biomedicines10040754
243. Sha J, Qie G, Yao Q, Sun W, Wang C, Zhang Z, et al. Sex differences on clinical characteristics, severity, and mortality in adult patients with COVID-19: a multicentre retrospective study. Front Med (Lausanne). (2021) 8:607059. doi: 10.3389/fmed.2021.607059
244. Gebhard C, Regitz-Zagrosek V, Neuhauser HK, Morgan R, Klein SL. Impact of sex and gender on COVID-19 outcomes in Europe. Biol Sex Differ. (2020) 11:29. doi: 10.1186/s13293-020-00304-9
245. Kumar N, Zuo Y, Yalavarthi S, Hunker KL, Knight JS, Kanthi Y, et al. SARS-CoV-2 spike protein S1-mediated endothelial injury and pro-inflammatory state is amplified by dihydrotestosterone and prevented by mineralocorticoid antagonism. Viruses. (2021) 13:2209. doi: 10.3390/v13112209
246. Gebhard CE, Hamouda N, Gebert P, Regitz-Zagrosek V, Gebhard C, Bengs S, et al. Sex versus gender-related characteristics: which predicts clinical outcomes of acute COVID-19? Intensive Care Med. (2022). doi: 10.1007/s00134-022-06836-5
247. Mallat Z, Tedgui A. Apoptosis in the vasculature: mechanisms and functional importance. Br J Pharmacol. (2000) 130:947–62. doi: 10.1038/sj.bjp.0703407
248. Winn RK, Harlan JM. The role of endothelial cell apoptosis in inflammatory and immune diseases. J Thromb Haemost. (2005) 3:1815–24. doi: 10.1111/j.1538-7836.2005.01378.x
249. Bombeli T, Schwartz BR, Harlan JM. Endothelial cells undergoing apoptosis become proadhesive for nonactivated platelets. Blood. (1999) 93:3831–8. doi: 10.1182/blood.V93.11.3831
250. Bombeli T, Karsan A, Tait JF, Harlan JM. Apoptotic vascular endothelial cells become procoagulant. Blood. (1997) 89:2429–42. doi: 10.1182/blood.V89.7.2429
251. Escargueil-Blanc I, Salvayre R, Nègre-Salvayre A. Necrosis and apoptosis induced by oxidized low density lipoproteins occur through two calcium-dependent pathways in lymphoblastoid cells. FASEB J. (1994) 8:1075–80. doi: 10.1096/fasebj.8.13.7926374
252. Hessler JR, Morel DW, Lewis LJ, Chisolm GM. Lipoprotein oxidation and lipoprotein-induced cytotoxicity. Arteriosclerosis. (1983) 3:215–22. doi: 10.1161/01.ATV.3.3.215
253. Escargueil-Blanc I, Meilhac O, Pieraggi MT, Arnal JF, Salvayre R, Nègre-Salvayre A. Oxidized LDLs induce massive apoptosis of cultured human endothelial cells through a calcium-dependent pathway. Prevention by aurintricarboxylic acid. Arterioscler Thromb Vasc Biol. (1997) 17:331–9. doi: 10.1161/01.ATV.17.2.331
254. Nofer JR, Levkau B, Wolinska I, Junker R, Fobker M, von Eckardstein A, et al. Suppression of endothelial cell apoptosis by high density lipoproteins (HDL) and HDL-associated lysosphingolipids. J Biol Chem. (2001) 276:34480–5. doi: 10.1074/jbc.M103782200
255. Walter M. Interrelationships among HDL metabolism, aging, and atherosclerosis. Arterioscler Thromb Vasc Biol. (2009) 29:1244–50. doi: 10.1161/ATVBAHA.108.181438
256. Nofer JR, Kehrel B, Fobker M, Levkau B, Assmann G, von Eckardstein A, et al. and arteriosclerosis: beyond reverse cholesterol transport. Atherosclerosis. (2002) 161:1–16. doi: 10.1016/S0021-9150(01)00651-7
257. Riwanto M, Rohrer L, Roschitzki B, Besler C, Mocharla P, Mueller M, et al. Altered activation of endothelial anti- and proapoptotic pathways by high-density lipoprotein from patients with coronary artery disease: role of high-density lipoprotein-proteome remodeling. Circulation. (2013) 127:891–904. doi: 10.1161/CIRCULATIONAHA.112.108753
258. Suc I, Escargueil-Blanc I, Troly M, Salvayre R, Nègre-Salvayre A, HDL. and ApoA prevent cell death of endothelial cells induced by oxidized LDL. Arterioscler Thromb Vasc Biol. (1997) 17:2158–66. doi: 10.1161/01.ATV.17.10.2158
259. de Souza JA, Vindis C, Nègre-Salvayre A, Rye KA, Couturier M, Therond P, et al. Small, dense HDL 3 particles attenuate apoptosis in endothelial cells: pivotal role of apolipoprotein A-I. J Cell Mol Med. (2010) 14:608–20. doi: 10.1111/j.1582-4934.2009.00713.x
260. Op den. Buijs J, Musters M, Verrips T, Post JA, Braam B, van Riel N. Mathematical modeling of vascular endothelial layer maintenance: the role of endothelial cell division, progenitor cell homing, and telomere shortening. Am J Physiol Heart Circ Physiol. (2004) 287:H2651–8. doi: 10.1152/ajpheart.00332.2004
261. Tso C, Martinic G, Fan WH, Rogers C, Rye KA, Barter PJ. High-density lipoproteins enhance progenitor-mediated endothelium repair in mice. Arterioscler Thromb Vasc Biol. (2006) 26:1144–9. doi: 10.1161/01.ATV.0000216600.37436.cf
262. Pu DR, Liu L, HDL. slowing down endothelial progenitor cells senescence: a novel anti-atherogenic property of HDL. Med Hypotheses. (2008) 70:338–42. doi: 10.1016/j.mehy.2007.05.025
263. Cervellati C, Vigna GB, Trentini A, Sanz JM, Zimetti F, Dalla Nora E, et al. Paraoxonase-1 activities in individuals with different HDL circulating levels: Implication in reverse cholesterol transport and early vascular damage. Atherosclerosis. (2019) 285:64–70. doi: 10.1016/j.atherosclerosis.2019.04.218
264. Furlong CE, Marsillach J, Jarvik GP, Costa LG. Paraoxonases-1,−2 and−3: what are their functions? Chem Biol Interact. (2016) 259:51–62. doi: 10.1016/j.cbi.2016.05.036
265. Rosenblat M, Karry R, Aviram M. Paraoxonase 1 (PON1) is a more potent antioxidant and stimulant of macrophage cholesterol efflux, when present in HDL than in lipoprotein-deficient serum: relevance to diabetes. Atherosclerosis. (2006) 187:74–81. doi: 10.1016/j.atherosclerosis.2005.08.026
266. Tavori H, Khatib S, Aviram M, Vaya J. Characterization of the PON1 active site using modeling simulation, in relation to PON1 lactonase activity. Bioorg Med Chem. (2008) 16:7504–9. doi: 10.1016/j.bmc.2008.06.008
267. Kelesidis T, Roberts CK, Huynh D, Martínez-Maza O, Currier JS, Reddy ST, et al. A high throughput biochemical fluorometric method for measuring lipid peroxidation in HDL. PLoS ONE. (2014) 9:e111716. doi: 10.1371/journal.pone.0111716
268. Farbstein D, Levy AP, HDL. dysfunction in diabetes: causes and possible treatments. Expert Rev Cardiovasc Ther. (2012) 10:353–61. doi: 10.1586/erc.11.182
269. Flaherty SM, Wood EK, Ryff CD, Love GD, Kelesidis T, Berkowitz L, et al. Race and sex differences in HDL peroxide content among American adults with and without type 2 diabetes. Lipids Health Dis. (2022) 21:18. doi: 10.1186/s12944-021-01608-4
270. Gomez Rosso L, Lhomme M, Meroño T, Dellepiane A, Sorroche P, Hedjazi L, et al. Poor glycemic control in type 2 diabetes enhances functional and compositional alterations of small, dense HDL3c. Biochim Biophys Acta Mol Cell Biol Lipids. (2017) 1862:188–95. doi: 10.1016/j.bbalip.2016.10.014
271. Chapman MJ, HDL. functionality in type 1 and type 2 diabetes: new insights. Curr Opin Endocrinol Diabetes Obes. (2022) 29:112–23. doi: 10.1097/MED.0000000000000705
272. Rosta V, Trentini A, Passaro A, Zuliani G, Sanz JM, Bosi C, et al. Sex difference impacts on the relationship between paraoxonase-1 (PON1) and type 2 diabetes. Antioxidants (Basel). (2020) 9:683. doi: 10.3390/antiox9080683
273. Garrido-Sánchez L, García-Fuentes E, Cardona F, Rojo-Martínez G, Soriguer F, Tinahones FJ. Anti-oxidized LDL antibody levels are reduced in women with hypertension. Eur J Clin Invest. (2009) 39:800–6. doi: 10.1111/j.1365-2362.2009.02156.x
274. Lapointe A, Couillard C, Piché ME, Weisnagel SJ, Bergeron J, Nadeau A, et al. Circulating oxidized LDL is associated with parameters of the metabolic syndrome in postmenopausal women. Atherosclerosis. (2007) 191:362–8. doi: 10.1016/j.atherosclerosis.2006.03.036
275. Chae JS, Kim OY, Paik JK, Kang R, Seo WJ, Jeong TS, et al. Association of Lp-PLA(2) activity and LDL size with interleukin-6, an inflammatory cytokine and oxidized LDL, a marker of oxidative stress, in women with metabolic syndrome. Atherosclerosis. (2011) 218:499–506. doi: 10.1016/j.atherosclerosis.2011.06.036
276. Tikkanen MJ, Vihma V, Jauhiainen M, Höckerstedt A, Helisten H, Kaamanen M. Lipoprotein-associated estrogens. Cardiovasc Res. (2002) 56:184–8. doi: 10.1016/S0008-6363(02)00535-7
277. Shwaery GT, Vita JA, Keaney JF. Antioxidant protection of LDL by physiologic concentrations of estrogens is specific for 17-beta-estradiol. Atherosclerosis. (1998) 138:255–62. doi: 10.1016/S0021-9150(98)00020-3
278. González F, Nair KS, Daniels JK, Basal E, Schimke JM, Blair HE. Hyperandrogenism sensitizes leukocytes to hyperglycemia to promote oxidative stress in lean reproductive-age women. J Clin Endocrinol Metab. (2012) 97:2836–43. doi: 10.1210/jc.2012-1259
279. Sun Y, Li S, Liu H, Bai H, Hu K, Zhang R, et al. Oxidative stress promotes hyperandrogenism by reducing sex hormone-binding globulin in polycystic ovary syndrome. Fertil Steril. (2021) 116:1641–50. doi: 10.1016/j.fertnstert.2021.07.1203
280. Li X-A, Titlow WB, Jackson BA, Giltiay N, Nikolova-Karakashian M, Uittenbogaard A, et al. High density lipoprotein binding to scavenger receptor, class b, type I activates endothelial nitric-oxide synthase in a ceramide-dependent manner*. J Biol Chem. (2002) 277:11058–63. doi: 10.1074/jbc.M110985200
281. Terasaka N, Westerterp M, Koetsveld J, Fernández-Hernando C, Yvan-Charvet L, Wang N, et al. ATP-binding cassette transporter G1 and high-density lipoprotein promote endothelial NO synthesis through a decrease in the interaction of caveolin-1 and endothelial NO synthase. Arterioscler Thromb Vasc Biol. (2010) 30:2219–25. doi: 10.1161/ATVBAHA.110.213215
282. Mineo C, Yuhanna IS, Quon MJ, Shaul PW. High density lipoprotein-induced endothelial nitric-oxide synthase activation is mediated by Akt and MAP Kinases*. J Biol Chem. (2003) 278:9142–9. doi: 10.1074/jbc.M211394200
283. Gong M, Wilson M, Kelly T, Su W, Dressman J, Kincer J, et al. HDL-associated estradiol stimulates endothelial NO synthase and vasodilation in an SR-BI-dependent manner. J Clin Invest. (2003) 111:1579–87. doi: 10.1172/JCI16777
284. Ruiz M, Okada H, Dahlbäck B. HDL-associated ApoM is anti-apoptotic by delivering sphingosine 1-phosphate to S1P1 & S1P3 receptors on vascular endothelium. Lipids Health Dis. (2017) 16:36. doi: 10.1186/s12944-017-0429-2
285. Christoffersen C, Obinata H, Kumaraswamy SB, Galvani S, Ahnström J, Sevvana M, et al. Endothelium-protective sphingosine-1-phosphate provided by HDL-associated apolipoprotein M. Proc Nat Acad Sci. (2011) 108:9613. doi: 10.1073/pnas.1103187108
286. Wilkerson BA, Grass GD, Wing SB, Argraves WS, Argraves KM. Sphingosine 1-phosphate (S1P) carrier-dependent regulation of endothelial barrier: high density lipoprotein (HDL)-s1p prolongs endothelial barrier enhancement as compared with albumin-s1p via effects on levels, trafficking, and signaling of S1p1*. J Biol Chem. (2012) 287:44645–53. doi: 10.1074/jbc.M112.423426
287. Memon AA, Bennet L, Zöller B, Wang X, Palmér K, Dahlbäck B, et al. The association between apolipoprotein M and insulin resistance varies with country of birth. Nutr Metab Cardiovasc Dis. (2014) 24:1174–80. doi: 10.1016/j.numecd.2014.05.007
288. Brinck JW, Thomas A, Lauer E, Jornayvaz FR, Brulhart-Meynet MC, Prost JC, et al. Diabetes mellitus is associated with reduced high-density lipoprotein sphingosine-1-phosphate content and impaired high-density lipoprotein cardiac cell protection. Arterioscler Thromb Vasc Biol. (2016) 36:817–24. doi: 10.1161/ATVBAHA.115.307049
289. Frej C, Mendez AJ, Ruiz M, Castillo M, Hughes TA, Dahlbäck B, et al. A Shift in ApoM/S1P between HDL-particles in women with type 1 diabetes mellitus is associated with impaired anti-inflammatory effects of the ApoM/S1P complex. Arterioscler Thromb Vasc Biol. (2017) 37:1194–205. doi: 10.1161/ATVBAHA.117.309275
290. Besler C, Heinrich K, Rohrer L, Doerries C, Riwanto M, Shih DM, et al. Mechanisms underlying adverse effects of HDL on eNOS-activating pathways in patients with coronary artery disease. J Clin Invest. (2011) 121:2693–708. doi: 10.1172/JCI42946
291. Denimal D, Monier S, Brindisi MC, Petit JM, Bouillet B, Nguyen A, et al. Impairment of the ability of HDL from patients with metabolic syndrome but without diabetes mellitus to activate eNOS: correction by S1P enrichment. Arterioscler Thromb Vasc Biol. (2017) 37:804–11. doi: 10.1161/ATVBAHA.117.309287
292. Gomaraschi M, Ossoli A, Favari E, Adorni MP, Sinagra G, Cattin L, et al. Inflammation impairs eNOS activation by HDL in patients with acute coronary syndrome. Cardiovasc Res. (2013) 100:36–43. doi: 10.1093/cvr/cvt169
293. Eren E, Yilmaz N, Aydin O. Functionally defective high-density lipoprotein and paraoxonase: a couple for endothelial dysfunction in atherosclerosis. Cholesterol. (2013) 2013:792090. doi: 10.1155/2013/792090
294. Shah K, McCormack CE, Bradbury NA. Do you know the sex of your cells? Am J Physiol Cell Physiol. (2014) 306:C3–18. doi: 10.1152/ajpcell.00281.2013
295. Taylor KE, Vallejo-Giraldo C, Schaible NS, Zakeri R, Miller VM. Reporting of sex as a variable in cardiovascular studies using cultured cells. Biol Sex Differ. (2011) 2:11. doi: 10.1186/2042-6410-2-11
Keywords: HDL, endothelial cells, sex differences, cardiovascular disease, HDL-endothelial crosstalk
Citation: Dietrich E, Jomard A and Osto E (2022) Crosstalk between high-density lipoproteins and endothelial cells in health and disease: Insights into sex-dependent modulation. Front. Cardiovasc. Med. 9:989428. doi: 10.3389/fcvm.2022.989428
Received: 08 July 2022; Accepted: 16 September 2022;
Published: 06 October 2022.
Edited by:
Tatsuya Sawamura, Shinshu University, JapanReviewed by:
Masatsune Ogura, Eastern Chiba Medical Center, JapanFabrizia Bonacina, University of Milan, Italy
Copyright © 2022 Dietrich, Jomard and Osto. This is an open-access article distributed under the terms of the Creative Commons Attribution License (CC BY). The use, distribution or reproduction in other forums is permitted, provided the original author(s) and the copyright owner(s) are credited and that the original publication in this journal is cited, in accordance with accepted academic practice. No use, distribution or reproduction is permitted which does not comply with these terms.
*Correspondence: Elena Osto, ZWxlbmEub3N0byYjeDAwMDQwO3V6aC5jaA==
†ORCID: Elisa Dietrich orcid.org/0000-0002-3733-2872
Anne Jomard orcid.org/0000-0002-9733-7958
Elena Osto orcid.org/0000-0001-8196-5696