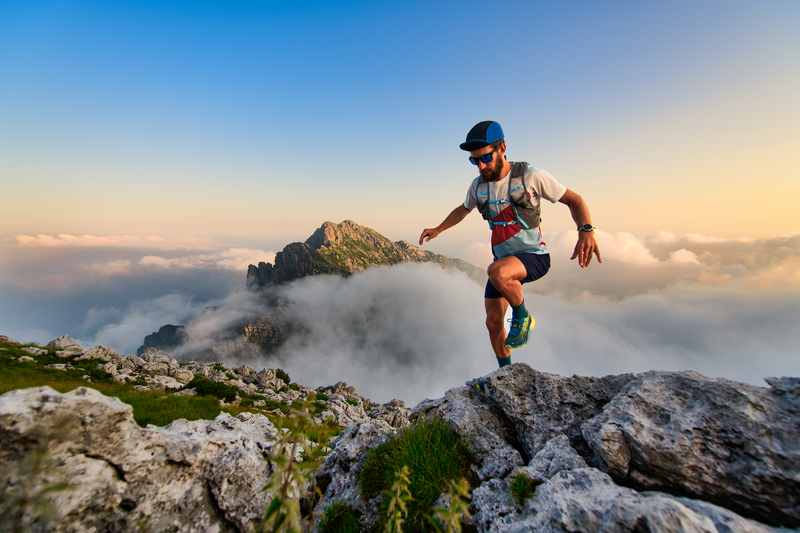
95% of researchers rate our articles as excellent or good
Learn more about the work of our research integrity team to safeguard the quality of each article we publish.
Find out more
REVIEW article
Front. Cardiovasc. Med. , 06 October 2022
Sec. Cardiovascular Genetics and Systems Medicine
Volume 9 - 2022 | https://doi.org/10.3389/fcvm.2022.980718
This article is part of the Research Topic Emerging Roles of miRNAs in Cardiovascular Disease View all 6 articles
Pulmonary arterial hypertension (PAH) is characterized by remodeling of the pulmonary arteries, and defined by elevated pulmonary arterial pressure, measured during right heart catheterization. There are three main challenges to the diagnostic and therapeutic process of patients with PAH. First, it is difficult to differentiate particular PAH etiology. Second, invasive diagnostic is required to precisely determine the severity of PAH, and thus to qualify patients for an appropriate treatment. Third, the results of treatment of PAH are unpredictable and remain unsatisfactory. MicroRNAs (miRNAs) are small non-coding RNAs that regulate post transcriptional gene-expression. Their role as a prognostic, and diagnostic biomarkers in many different diseases have been studied in recent years. MiRNAs are promising novel biomarkers in PAH due to their activity in various molecular pathways and processes underlying PAH. Lack of biomarkers to differentiate between particular PAH etiology and evaluate the severity of PAH, as well as paucity of therapeutic targets in PAH open a new field for the possibility to use miRNAs in these applications. In our article, we discuss the potential of miRNAs use as diagnostic tools, prognostic biomarkers and therapeutic targets in PAH.
PAH is a heterogeneous disease driven most often by pathogenic remodeling of distal pulmonary arterioles and an increase in pulmonary vascular resistance (1). PAH is a rare disease, with the prevalence and incidence of 15–60 subjects per million population and 5–10 cases per million per year (2). Patients with PAH often have severe and progressive disease. Symptoms of PAH are divided by the WHO into four functional classes, graded according to the severity of the symptoms, with class I describing the mildest and class IV the most severe PAH. This classification helps the clinicians to assess patients’ functional status and determine the appropriate treatment.
Recently, the current knowledge regarding the general miRNA expression changes in PAH has been thoroughly summarized (3). In this article, we have focused on three clinical problems in patients with PAH, which could be solved using miRNA-based biomarkers.
Firstly, differentiation of the etiology of PAH is difficult. In registries, about 50% of patients with PAH have idiopathic, heritable or drug-induced PAH. It is important to increase our knowledge in order to minimize the diagnosis of idiopathic PAH. The remaining cases of PAH, described as associated PAH, can be caused by various conditions, such as connective tissue disease (CTD), human immunodeficiency virus (HIV) infection, schistosomiasis or portal hypertension (4). The leading cause in the subgroup of associated PAH is CTD, mainly systemic sclerosis (5). In systemic sclerosis, it is difficult to determine whether increased pressure in pulmonary artery is caused by pulmonary fibrosis (ESC group 3), small vessel disease (ESC group 1 = PAH) or left heart failure associated with left ventricle diastolic dysfunction (ESC group 2). Differentiation of the cause is extremely important due to the different treatment. Congenital heart disease (CHD) can also play a role in the development of PAH. It is estimated, that 10% of adults with CHD may also have PAH (6). Currently, there is no specific blood-based biomarker for PAH, although numerous markers have been tested (7), such as inflammatory markers [growth differentiation factor-15 (8), osteopontin (9), neutrophil to lymphocyte ratio (10), stem cell growth factor β (11)], heart function markers (B-type natriuretic peptide, amino-terminal pro-B type natriuretic peptide or red cell distribution width) or oxidative stress related biomarkers [F2-isoprostanes (12) or oxidized lipids] (9, 13).
Second, it is challenging to determine the severity of PAH, and thus to qualify patients for an appropriate treatment. Although some blood test might help to identify the PAH etiology, they are not useful in diagnosing or assessing the clinical advancement of the disease (7). Hence, the diagnostic workup currently includes repeated RHC, which is an invasive procedure with a risk of complications estimated according at about 1%. Altogether, PAH requires novel tools to evaluate the clinical severity of the disease.
Third, the results of PAH treatment remain unsatisfactory (7). As stated in the guidelines of the European Society of Cardiology, there are three pathways underlying the pathogenesis of PAH: the endothelin, nitric oxide (NO) and prostacyclin (PGI2) pathways (7). Even though treatments targeting each one of them, including endothelin receptor antagonists (ERA), phosphodiesterase type 5 inhibitors (PDE-5) and PGI2 analogs are well established in clinical practice, the functional limitation and survival of patients leaves room for substantial improvement (7). Hence, new therapeutic targets in PAH are urgently needed.
MicroRNAs(miRNAs) are small non-coding RNAs that regulate post transcriptional gene-expression. MiRNAs can induce mRNA degradation and translational repression by interacting with the 3′ untranslated region (3′ UTR) of target mRNAs, but their interactions with other regions of mRNAs have also been reported. In some circumstances, they can also activate translation or regulate transcription. However, miRNA interaction with their target genes is very dynamic and dependent on many factors, such as subcellular location of miRNAs, the abundancy of miRNAs and target mRNAs, and the affinity of miRNA-mRNA interactions (14). MiRNAs are found in all eukaryotic cells across the species. Over the last few years, their role in the progression and development of numerous diseases has been extensively studied, since they hold enormous potential for prognostic, and diagnostic biomarkers as well as therapeutics (15–19). Studies showed that miRNAs are also promising novel biomarkers in PAH due to their activity in various molecular pathways and processes underlying PAH (3, 20). The lack of biomarkers in PAH enabling the differentiation of individual PAH etiologies and the assessment of the severity of PAH as well as the shortage of therapeutic targets in PAH opens a new field for the possibility of using miRNAs in these applications (3).
In this review, we present current challenges in miRNA analysis and interpretation in PAH and summarize the steps which need to be taken before clinical applicability of miRNAs in PAH.
Pathophysiology of PAH may differ depending on the cause of the disease, but in general, it is associated with vascular abnormality. PAH is a progressive disease, causing the narrowing of the blood vessels in the lungs, which results in a resistance to blood flow and an increase in pulmonary artery pressures (21). In addition to that, PAH may be also attributed to lesions occurring in distal muscular-type arteries, ranging in diameter from 500 μm down to even 70 μm in humans (22). The underlying mechanisms involve impaired function of signaling pathways of NO, PGI2, thromboxane A2 (TXA2), and endothelin-1 (ET-1) (23), which leads to a vasoconstrictive effect of an upregulated ET-1, with concurrent impairment of vasodilation caused by reduced PGI2 production and NO synthase function (24). Altogether, these changes lead to an increase in pulmonary vascular resistance (25). The progressive increase in right ventricular (RV) afterload leads to RV hypertrophy and, ultimately, RV failure and death (26).
Moreover, there are differences in the miRNA concentrations between animals with RV hypertrophy and RV failure, which suggests specific changes in the signature of miRNAs during PAH progression (27, 28). The mechanisms underlying the transition from compensated RV hypertrophy to RV failure remain unclear, and further investigations are needed to gain a better understanding of miRNA expression patterns in RV pathological remodeling (28, 29).
Numerous miRNAs participate in the pathogenesis of PAH, some of them being upregulated, and some other downregulated in PAH lung samples compared to normal controls (20). The expression of many miRNAs in patients with PAH, and healthy control subjects in different tissues was examined. Many of these miRNAs were characterized by distinct expression pattern in human PAH lungs and in animal PAH models, although 4 miRNAs turned out to have similar expression pattern among the different models, making them the key miRNAs for the potential use in diagnostic and therapeutic processes. These four highlighted miRNAs were miR-29, miR-124, miR-140, and miR-204 (3).
MiR-204, as a representative of this group, is a muscle-specific miRNA that is highly active in cardiomyocytes and vascular muscle cells (30). MiR-204 has been found to be downregulated in diseased pulmonary arterial smooth muscle cells (PASMCs) in PAH and is linked to signaling molecules crucial to PAH including PPAR-γ, TGF-β1, STAT3, SHP2, BRD4, NFAT, and HIF-1α (20, 30, 31). Mir-204 may also mediate the protective action of mesenchymal stromal cell-derived exosomes (32) and altered levels correlate with more angiogenic and proliferative disease (33) in both the pulmonary and coronary circulation (34).
Moreover, the specific miRNAs grouped into clusters might be associated with particular mechanisms of PAH pathogenesis. MiRNAs associated with PASMCs proliferation, migration and contraction were the miR-17-92 cluster, miR-21, miR-124, miR-143/145 cluster, miR-204, and miR-210. Those associated with pulmonary artery endothelial homeostasis in PAH were the miR-17-92 cluster, miR-21, miR-27a, miR-424, and miR-503. MiRNA reported to regulate fibroblasts was miR-124 (20). Reduced miR-124 causes hyperproliferation and migration of fibroblasts, whereas overexpression of miR-124 inhibits proliferation and migration of fibroblasts (35). A list of dysregulated miRNAs in PAH are presented in Table 1.
Studies suggest that miRNAs can be used as stable blood-based markers for many medical conditions. They were first established as biomarkers for cancer in 2008 when expression levels of tumor-associated miRNAs in serum from diffuse large B-cell lymphoma (DLBCL) patients was compared with healthy controls (36). Since then, their potential use as biomarkers has been repeatedly proposed for various diseases. The advantage of miRNAs as biomarkers also results from the fact, that miRNAs are present in numerous body fluids. The existence of miRNAs in 12 body fluids and urine from patients with various conditions were examined. The results showed the presence of miRNAs in all the body fluid types tested and different compositions in different fluid types. Studies also showed different miRNA patterns in the urine samples from patients with distinct conditions (37), which gives hope that getting to know specific miRNAs expression patterns in particular body fluids can help evaluate crucial information about current condition of the patients, such as severity of the disease or assessing a particular etiology of the disease.
Another advantage of miRNAs is that they are expressed in stable biomolecules, such as extracellular vesicles, RNA-lipid complexes or RNA–protein complexes (38, 39). Plasma miRNAs were found to be remarkably stable even under conditions as harsh as boiling, low or high pH, long-time storage at room temperature, and multiple freeze-thaw cycles (40, 41).
The promising results of the previous studies has encouraged to look for diagnostic roles of miRNAs in PAH. As it turned out, they can be used as biomarkers in two ways: (i) miRNA differentiates the type of PAH; (ii) miRNA differentiates the severity of PAH (31, 42).
Etiology of PAH can be characterized by unique biochemical profile of particular miRNAs expressions. Usually, changes in the concentrations of many miRNAs are observed compared with healthy volunteers. In some etiologies of PAH, the bone morphogenetic protein receptor type II (BMPR2) gene has been found mutated (42). Product of this gene transcription, BMPR2 protein is a serine/threonine receptor kinase, which is the most important mediator of vascular remodeling in PAH. The dysregulation of this receptor kinase has been found in hereditary PAH (43, 44), and downregulated in some other etiologies of PAH (42). The downregulation of BMPR2 may be caused by the action of miRNAs, which appeared to be essential regulators in the pathogenesis of PAH, hence their potential use as biomarkers in this disease as a whole, and also in particular etiologies of PAH. MiRNA was experimentally proven to regulate the expression of BMPR2 (42). An altered expression miR-125a in lung tissue samples of experimental PAH was observed. There is a possibility of evaluating expression levels of miR-125 in order to assess hereditary PAH, since miR-125 takes part in downregulation of BMPR2, which has been seen in this particular PAH etiology. Potential role of miRNA as a regulator of BMPR2 is presented in Figure 1. Importance of BMPR2 signaling pathway in PAH pathogenesis was also proven in a recent study with sotatercept. It is a first-in-class therapeutic fusion protein that targets an imbalance in activin–growth differentiation factor and BMPR2 pathway signaling (45). Twenty four-weeks treatment with sotatercept reduced pulmonary vascular resistance among patients with pulmonary arterial hypertension who were receiving stable background therapy, including prostacyclin infusion therapy. Concordant improvements from baseline in 6-min walk distance (6MWD) and NT-proBNP levels were also observed. 6MWD is a parameter used to assess functional capacity. It is widely used in clinical trials to compare the effects of treatments (46).
Figure 1. Etiologies of pulmonary arterial hypertension (PAH). Low level of bone morphogenetic protein receptor type 2 (BMPR2) is associated with PAH in general, however the association is strongest for the heritable PAH. Created with Biorender.com.
MiRNAs could be used to evaluate the hemodynamic and clinical advancement of PAH. There are studies showing that the concentration of miRNAs in lungs of patients with PAH is different compared with samples from healthy controls (20). Correlation between hemodynamic severity of PAH and plasma concentration of specific miRNAs could be crucial in a diagnostic process and could also provide essential prognostic information (20).
In a recent study, miRNA plasma level in patients with PAH were measured. A microarray screen was performed on plasma RNA from healthy controls and patients with PAH. They identified 58 miRNAs that were dysregulated, with miR-150 being most significantly downregulated in patients with PAH (47). Quantitative polymerase chain reaction confirmed reduced miR-150 concentrations and was then used to measure miR-150 levels. Cox regression analysis confirmed miR-150 levels as a significant predictor of survival (47). This information could potentially be utilized to evaluate the severity of PAH based on plasma levels of specific miRNAs.
The source of circulating miR-150 in PAH is unknown (47). MiR-150 expression levels were significantly reduced in rats with PAH compared with control subjects. This was also accompanied by a significant reduction in KLF2 expression, which is a critical regulator of endothelial gene expression and may reflect endothelial dysfunction and inflammation (47, 48). Importantly, microRNAs such as miR-150 mediate downstream effects of KLF2 in endothelial cells, its expression being promoted by laminar flow and inhibited by turbulent flow and inflammatory cytokines (48).
Altered circulating miR-150 levels in PAH could affect a variety of targets contributing to the dysregulation of pulmonary arterial smooth muscle cells (PASMCs) and pulmonary arterial endothelial cells (PAECs) (47). Altogether miR-150 has a potential to be a marker of PAH severity, but it needs further investigation.
Similar conclusion comes from another study, in which the expression pattern of miR-204 in lung biopsies from individuals with and without PAH was investigated. The results showed that lower concentration of miR-204 correlate with increased PAH severity (31), which could be used in clinical diagnostics in the future. On the other hand, in a study of 47 patients with PAH blood samples were collected during RHC. MiR-204 concentration increased sequentially along the pulmonary vasculature (log fold-change slope = 0.22 [95% CI = 0.06–0.37], P = 0.008) (30). Authors of mentioned study propose that the primary contributor to this finding of disease mediated miR-204 excretion appear to be PASMCs. Such reciprocal directions of intracellular and extracellular miRNAs concentrations are not without precedent, as aerobically exercising skeletal muscle tissue has been shown to release specific miRNAs to allow for a more efficient reduction of miRNA activity in the intracellular space of source tissue (49). The possible types of correlation between the decreased levels of both miR-150 and miR-204 and increased severity of PAH are presented in Figure 2.
Figure 2. Possible correlation between expression level of miR-150 and miR-204 and PAH severity. The colored lines indicate an association between the increased PAH severity and the decrease in plasma expression of both miR-150 and miR-204 levels (31, 47). Created with Biorender.com.
The downregulation of miR-204 accounts for the upregulation of the transcription factors, such as nuclear factor of activated T cells (NFAT) and hypoxia induced factor 1α (HIF-1α) in PAH-PASMCs, which is contributing to their proliferation and resistance to apoptosis (50). In addition to that, PAH-PASMCs produce higher levels of IL-6, which by activating STAT3, contributes to further miR-204 downregulation (50). This closes the vicious circle of PAH development in some patients. Nonetheless, the implication of other pathways cannot be ruled out.
For example, increasing miR-204 level in PAH-PASMCs decreased Src homology 2 domain containing (SHP2), whereas miR-204 inhibition in control PASMCs cells increased SHP2 expression. These results suggest that SHP2 may be the primary target of miR-204 in PAH. SHP2 activation results in activation of STAT3, which is an activator of NFAT. As mentioned before, NFAT is important factor in proliferation and resistance to apoptosis of PAH-PASMCs. The complexity of miR-204 activity gives it the potential to became a biomarker of PAH.
In a study with 12 pediatric PAH patients it was shown that there are correlations of circulating miRNA concentrations with invasive hemodynamic and non-invasive echocardiographic variables. Numerous miRNAs (miR-193a-5p, miR-26a-5p, miR-331-3p, miR-29a-3p, miR-26a-5p, miR590-5p, and miR-200c-3p) positively correlated with the prognostic variables, except for those that decrease with the severity of the disease (tricuspid annular plane systolic excursion and pulmonary flow index) (51). The correlations of the miRNAs which likely exacerbate the disease (miR-423-5p and miR-99a-5p) were in the opposite direction in comparison to the likely beneficial miRNAs (51).
Furthermore, this study provided evidence that sampling site should be taken into account. MiR-193a-5p and miR-423-5p concentrations have differential trans-right-ventricle gradients (PAH patients vs. control) (51). Moreover, these concentration differences correlate with invasive hemodynamic variables. It was the first time that trans-right-ventricle and transpulmonary miRNA gradients in blood plasma were identified. It appears that both the tissue and the specific place of its collection should be taken into account (e.g., the expression of miRNA in blood from the right ventricle differs from that from pulmonary arteries). This observation also should be considered in future tests validation.
MiRNA-based therapy is promising due to characteristic of miRNA, which are short length, highly conserved sequence, and pre-clinical evidence of their efficacy in human disease. Treatment based on miRNA focuses on 2 different approaches: (1) specific artificial elevation by miRmimics; or (2) lowering of selected miRNA levels, which can be achieved by anti-miRNA antisense oligomers (antagomiRs), masking, sponges, and erasers (52). In vitro studies and animal models, though, have produced promising results with potential for a future clinical application (53–55).
The important therapeutic targets in PAH are the PAECs, PASMCs and pulmonary adventitial fibroblasts, which excessive proliferation and resistance to apoptosis is a cornerstone in the pathogenesis of PAH (56). Because miRNAs play an important role in regulating the proliferation, differentiation and metabolism of PASMCs, they are being an promising targets in preventing these mechanisms (57). Reduction of miR-204 expression was observed in PASMCs of patients with PAH vs. healthy controls (31). It was caused by primary signal transducer and activator of transcription (STAT3) activation by circulating pro-PAH factors such as endothelin-1, platelet-derived growth factor (PDGF), and angiotensin II (which all increase at the onset of PAH) (58). It results in the sustained pro-proliferative and antiapoptotic phenotype of cultured PAH-PASMCs (31). MiR-204 mimics can reverse this effect in rats (31). It was reported that increased lung levels of miR-204 inhibited vascular remodeling by suppressing STAT3 signaling (32). As a result, the use of miR-204 mimics in rats indicated a decrease in pulmonary pressure, a decrease in the thickness of the right ventricular wall and a decrease in the thickness of the walls of the pulmonary vessels (31).
Moreover, the effectiveness of antagomirs’ therapy has been proven in animal models with the use of antagomir to miR-17 (59). Its application in PAH mice model resulted in a reduction of pulmonary pressure and right heart hypertrophy (59). It has been suggested that these therapies have some advantages over currently available drug therapies. They are based on the action of single miRNA on multiple pathways, which cause PAH simultaneously. Therefore, they may be associated with better treatment outcomes and a lower risk of drug resistance over time (31). MiRNAs involved in the various mechanisms of PAH development, which might be targets of future PAH therapy, are presented in Figure 3.
Figure 3. Pathophysiological mechanisms involved in the development and progression of pulmonary arterial hypertension, associated with miRNA expression changes. Modification of levels and function of the presented miRNAs might create novel therapeutic options in PAH. Created with Biorender.com.
The huge development in the use of miRNAs as PAH biomarkers is not without limitations. In the last 15 years, as the new miRNAs were discovered in animal and human PAH models, there have also been comments on the challenges associated with these studies and the use of miRNAs in the analysis and interpretation of PAH. Such limitations include: (i.) various mechanisms affecting mRNA expression; (ii.) miRNA differences between experimental animal models and humans; (iii.) differences in the pathogenesis of PAH in humans (3, 60, 61).
In the perfect situation, level of mRNA is constant and the level of the protein produced by it is lowered or increased due to a disturbed level of miRNA (61). However, various mechanisms influence mRNA expression. The long non-coding RNAs (lncRNA), which plays a role not only in regulating post-transcriptional mechanisms, such as miRNA, but also transcriptional mechanisms, may be also responsible for changes in mRNA expression (3, 16, 62, 63). The role of lncRNA has been demonstrated, inter alia, in the pathogenesis of PAH resulting from PASMC and PAEC dysfunction (3, 61). Hence, in cases of PAH where the mRNA level is normal and the level of the protein produced from this mRNA is abnormal, the miRNA biomarkers may be partially insensitive due to overlapping factors, such as lncRNA (64). LncRNAs are >200 base pairs in length and are abundantly present in the genome. Almost 27,000 lncRNAs have been identified in the human genome already (65). They are differentially expressed in key pulmonary vascular cells such as PAECs and PASMCs, which regulate a range of cellular and biological processes and contribute to pulmonary vascular remodeling and PAH pathogenesis (64). Summary of lncRNA involved in PAH is presented in Table 2.
One of the challenges regarding the use of miRNAs as biomarkers of human diseases is deriving conclusions from studies conducted in animal models (60). Although these models show similar miRNA expression for healthy animals as healthy humans, miRNA production for diseased individuals differs in animals and humans (60). These differences are due to different pathophysiological mechanisms of PAH in animals, which are also phenotypically different from human PAH in many aspects (60). However, animal studies are necessary, because the small number of PAH patients makes it difficult to develop cohort studies. In many cases, there is also a need to perform invasive procedures (60). The triggers of PAH used in animal models include, but are not only limited to, administration of monocrotaline or chronic hypoxia (60). The challenge is therefore to find the PAH trigger that most closely matches the cellular and molecular changes present in human PAH.
Another challenge for the use of miRNA as a biomarker is the likely existence of multiple PAH etiologies with different miRNA expression in one patient (3, 66). These etiologies differ in the number of inflammatory and hypoxic stimuli leading to the formation of PAH and causing different expression of miRNAs (42). A suggestion for the existence of different etiologies of PAH in patients is that the animal models of PAH which are otherwise induced, show different miRNA expression (3, 27). Thus, the usefulness of many biomarkers may depend on the etiological factors of PAH.
Furthermore, miRNA expression may also depend on the degree of progression of PAH in the patient. There are evidences, that it depends on the development of right ventricular (RV) hypertrophy or RV failure (3). Some studies suggested that different miRNAs may be detected if there is the presence of RV hypertrophy or failure (28, 29). Thus, the detection of miRNAs, which are produced by dysfunctional heart muscle, should take this phenomenon into account.
There are numerous factors influencing the accuracy of miRNA analyzes. A critical evaluation of such factors is of paramount importance in order to avoid misleading results and to allow the comparability of different analyzes. For example, in RNA isolation methods, maximum efficiency, reproducibility and reliability are of great importance, because, e.g., even minimal co-extraction of inhibitory factors can significantly affect the measurement results. Various detection methods (high-throughput sequencing, real-time PCR, microarrays) are used to quantify miRNA levels. Their application should be determined for specific indications to minimize variation between studies. Standardization method should be standardized and reproducible isolation and detection protocols applied; otherwise, comparability is much more difficult (67). In addition to these analytical aspects, there are parameters influencing the levels of miRNAs in the analyzed biomaterial. Drugs such as statins, antiplatelet drugs and heparin can have a confusing effect on miRNA measurements. For example, antiplatelet drugs reduce the amount of freely circulating thrombocyte-derived miRNAs, and heparin influences the polymerase chain reaction (68). Finally, there are differences in the results of miRNA quantification between cellular and extracellular sources of biomaterial (69).
In a study of 2,391 individuals expression of miRNAs measured by high-throughput RT-qPCR isolated from both whole blood and were compared. There was only modest association between cellular and extracellular miRNA expression in this large study sample (69). These results demonstrate that miRNA expression from cellular vs. acellular sources may be divergent. MiRNA concentration from different human sources (e.g., whole blood, plasma) should not be used interchangeably as biomarkers of disease (69).
Together with the first discoveries in animals related to the role of miRNA in the development of PAH, it was known that these findings should be put into clinical practice as soon as possible. At this point, two possibilities for the future use of miRNAs in PAH patients emerge: (i) miRNAs as biomarkers and (ii) miRNAs as therapeutic targets (3).
The first option is to use miRNA as a diagnostic marker (3, 20). So far, it has not been possible to find an ideal biomarker for PAH, i.e., one that is non-invasive, easy of measure, cost-effective, and has high sensitivity and specificity (70, 71). There is a great hope in finding such a marker among the miRNAs (56). For this purpose, the problem of different fragments and levels of miRNAs in experimental studies caused by the lack of their standardization should be solved (3, 56). It is caused by the lack of systematization in the procedure of taking, storing and processing samples; lack of standardization in the methods of miRNAs diagnosing and lack of indications for their use; lack of standardization in miRNA quantification protocols; rare sampling in the studies and lack of described concomitant disease symptoms (3, 56, 70, 72). After solving the above problems, it would be possible to summarize the results of many different studies and use the obtained results to create studies on a large samples (56). These studies would justify the use of specific miRNAs as biomarkers. Machine learning might be useful for selection of the most promising miRNAs. Machine learning as a field has progressively improved our ability to find relevant features in large and high-dimensional data sets collected from genomic studies (73). Supervised machine learning methods have been used successfully to develop classifiers for disease diagnosis, as well as to identify potential disease biomarkers (74). In a recently published study it was shown that using a consensus of four different supervised machine learning feature selection techniques can help us to identify miRNAs associated with PAH (72). MiR-187-5p and miR-636 were selected as a candidate biomarkers that might be associated with PAH progression, however this method requires further studies and validation (72).
As mentioned earlier, miRNA concentrations in RV hypertrophy and RV failure vary (27, 28). This suggests that during the progression of RV overload caused by, inter alia, PAH there are some changes in miRNA expression. More research is needed to see if we can predict PAH progression from changes in miRNA levels even before structural changes occur, especially in patients with high risk of developing PAH.
The final step of identification of perfect biomarker would be to perform a cost-effectiveness analysis to determine whether the ratio of new information obtained from such a research and its price is satisfactory to use such a miRNA for the diagnosis of PAH (56).
The future prospects of the miRNA are also apparent in their use in the treatment of PAH. Mainly, such therapy would be based on the use of miRNA analogs to increase the amount of miRNA that is decreased in PAH, or miRNA inhibitors to decrease the amount of miRNA whose expression is increased (20, 56). So far, the experiments in animal models show promising results (3). Before they can be used clinically, however, some problems have to be solved. These include: low drug stability, off-target effect and drug delivery to the selected tissue (3, 20, 56, 75).
MiRNAs are small particles, which are degraded in vivo by nucleases. Apart from chemical modifications to improve their stability, miRNA based therapeutics are encoded as prodrugs, in order to protect the active substance from the degradation mechanisms of exogenous genetic material that exist in eukaryotic cells (76). To improve stability, resist degradation by RNA nucleases and slow their removal in vivo by the liver there are needed some structural modifications. The most common chemical modifications alter the internucleotide connections or replace backbone phosphodiester for phosphorothioate, boranophosphate or peptide bonds (77).
MiRNAs uptake can be also improved by decorating miRNAs with receptor-mediated endocytosis pathways. On the other hand, cell uptake can be improved by incorporating, at the 3′ end, lipids or cholesterol which increases the permeability through the cellular lipid bilayer (77). The strategies to chemically modify oligonucleotides have been the subject of many recent reviews and debates about their influence on the pharmacological properties or miRNA-based therapies since often they reduce their efficiency (78, 79).
The off-target effect is associated with the miRNA effect on various tissues, including healthy ones, disrupting cellular pathways unrelated to disease pathogenesis, and inducing an immune response, resulting in the toxic effects of these drugs (3, 20). To reduce the off-target effect it is crucial to choose optimal delivery method. In rats, intranasal aerosolization (IN-A), intratracheal aerosolization with (IT-AV) or without ventilator assistance (IT-A), and intratracheal liquid instillation (IT-L) were compared (80). Intravenous (IV; via jugular vein), intraperitoneal (IP) and subcutaneous (SC) delivery served as controls. Relative levels of cellular miR-39 were quantified by RT-qPCR. At 2 h post-delivery, IT-L showed the highest lung mimic level, which was significantly higher than levels achieved by all other methods (from ∼10- to 10,000-fold, p < 0.05) (80). All lung-targeted strategies showed lung-selective mimic uptake, with mimic levels 10- to 100-fold lower in heart and 100- to 10,000-fold lower in liver, kidney and spleen (80). In contrast, IV, SC, and IP routes showed comparable or higher mimic levels in non-pulmonary tissues. Altogether the choice of delivery strategy could have a significant impact on potential therapeutic outcomes of miRNA-based drug candidates. To sum up, intratracheal administration of a liquid formulation of miRNA mimic provided the highest lung-specific delivery and appears to be the safest therapeutic delivery method in terms of off-target effect.
MiRNA-based therapy suffer from low stability and efficiency (81). To prevent these adversities they require chemical modifications (82, 83) or conjugation/encapsulation in different kinds of nanovectors such as lentivirus, polymeric nanoparticles, or exosomes (84–86).
Virus-like nanovectors (e.g., adenovirus, lentivirus) are used for delivering miRNAs because they dispense miRNAs more efficiently than other carriers (87, 88). On the other hand they can induce mutagenic effects causing cancer and undesired immune responses. These undesired effects limits their clinical application, so their real translation into clinics is controversial (89). However, their exact composition is uncontrolled. They contain active molecules with unknown effects for the organism.
In contrast, extracellular vesicles (EVs) as drug delivery carriers are in ongoing clinical trials aiming to translate them from basic research to clinics (77). They are lipidic bilayer vesicles naturally released by cells which size varies from 10 to 10,000 nm. The main advantage of these nanovectors is that they can be obtained from patients avoiding toxicity (90). EVs are often polydispersed in size and can be further functionalized with nanoparticles, for example for therapy (91). They are currently being used as successful drug delivery systems for miRNAs into particular cells, such as hepatocytes or macrophages (92).
Another possible vector are synthetic nanovectors. They can be finely designed in the nanoscale and manufactured in a large scale (93). Encapsulation of miRNAs has been mainly carried out using polymers and lipids (94, 95). Often the driving force for the entrapment of negatively charged miRNAs is the electrostatic attraction with cationic lipids or polymers (77). Physical caging is also another efficient way to entrap miRNAs in empty cavities (77). Lipofectamine is considered the “gold standard” for nucleic acid transfection. It is a lipid-based nanovector composed of positively charged cationic lipids. Often it is called a “liposome” (77). However, the use of permanently charged cationic lipids can induce cellular and inflammatory toxicity, fast plasma clearance, aggregation, and accumulation in lungs, liver, and spleen (77). In this review, we only give a brief summary of possible vectors for miRNA-based therapy.
Studies of the miRNAs and their therapeutic role would be facilitated by the discovery of animals models, that phenotypically copy PAH in humans, and by finding an antidote to the potential adverse effects of these drugs (3, 56). After resolving the above-mentioned issues, the next steps should be taken in regard to the risk of miRNA and other drugs interferences, standardizing the drugs used and their doses in relation to comorbidities, age and sex of patients, as well as performing studies on a larger number of model animals, and clinical phase 1, 2, and 3 studies, which so far have not been undertaken for miRNAs in PAH (3, 56).
The role of miRNAs in the pathogenesis of PAH cannot be overlooked. With the still developing biotechnology and knowledge about PAH, new miRNAs involved in the pathogenesis of this disease are found every day. From this reason, the range of miRNAs that may be useful in the diagnosis and treatment of PAH is constantly extending. So far, miR-29, miR-124, miR-140, and miR-204 are the most promising miRNA-bases biomarkers and/or therapeutic targets in patients with PAH. To take a step forward in the clinical application of these miRNAs, both more experimental studies and clinical trials with bigger cohorts of are needed to evaluate these miRNAs as biomarkers and therapeutic targets. However, it should be considered whether research concentrated on quantifying several miRNAs at the same time as cluster-biomarker and therapy aimed at group of miRNAs at the same time would not be a better solution in cost-quality ratio than trial on single miRNA. In addition, the difficulties associated with studies on miRNAs and using miRNAs in clinic should be addressed when designing miRNA studies.
SR, BP, NC, and AG: conceptualization and writing—original draft preparation. SR, BP, NC, and CE: methodology. CE, MP, ŁS, KF, MJ, TM, MG, and MK: writing—review and editing. AG: visualization and supervision. SR and AG: project administration. All authors have read and agreed to the published version of the manuscript.
The authors declare that the research was conducted in the absence of any commercial or financial relationships that could be construed as a potential conflict of interest.
All claims expressed in this article are solely those of the authors and do not necessarily represent those of their affiliated organizations, or those of the publisher, the editors and the reviewers. Any product that may be evaluated in this article, or claim that may be made by its manufacturer, is not guaranteed or endorsed by the publisher.
1. Maron BA, Abman SH, Elliott CG, Frantz RP, Hopper RK, Horn EM, et al. Pulmonary arterial hypertension: diagnosis, treatment, and novel advances. Am J Respir Crit Care Med. (2021) 203:1472–87. doi: 10.1164/rccm.202012-4317SO
2. Peacock AJ, Murphy NF, McMurray JJV, Caballero L, Stewart S. An epidemiological study of pulmonary arterial hypertension. Eur Respir J. (2007) 30:104–9. doi: 10.1183/09031936.00092306
3. Santos-Ferreira CA, Abreu MT, Marques CI, Gonçalves LM, Baptista R, Girão HM. Micro-RNA analysis in pulmonary arterial hypertension: current knowledge and challenges. JACC Basic Transl Sci. (2020) 5:1149–62. doi: 10.1016/j.jacbts.2020.07.008
4. Simonneau G, Gatzoulis MA, Adatia I, Celermajer D, Denton C, Ghofrani A, et al. Updated clinical classification of pulmonary hypertension. J Am Coll Cardiol. (2013) 62:D34–D41. doi: 10.1016/j.jacc.2013.10.029
5. Humbert M, Sitbon O, Chaouat A, Bertocchi M, Habib G, Gressin V, et al. Pulmonary arterial hypertension in France: results from a national registry. Am J Respir Crit Care Med. (2006) 173:1023–30. doi: 10.1164/rccm.200510-1668OC
6. Engelfriet PM, Duffels MGJ, Møller T, Boersma E, Tijssen JGP, Thaulow E, et al. Pulmonary arterial hypertension in adults born with a heart septal defect: the Euro Heart Survey on adult congenital heart disease. Heart. (2007) 93:682–7. doi: 10.1136/hrt.2006.098848
7. Galiè N, Humbert M, Vachiery J-L, Gibbs S, Lang I, Torbicki A, et al. 2015 ESC/ERS guidelines for the diagnosis and treatment of pulmonary hypertension: the joint task force for the diagnosis and treatment of pulmonary hypertension of the European Society of Cardiology (ESC) and the European Respiratory Society (ERS): endor. Eur Heart J. (2016) 37:67–119. doi: 10.1093/eurheartj/ehv317
8. Nickel N, Kempf T, Tapken H, Tongers J, Laenger F, Lehmann U, et al. Growth differentiation factor-15 in idiopathic pulmonary arterial hypertension. Am J Respir Crit Care Med. (2008) 178:534–41. doi: 10.1164/rccm.200802-235OC
9. Anwar A, Ruffenach G, Mahajan A, Eghbali M, Umar S. Novel biomarkers for pulmonary arterial hypertension. Respir Res. (2016) 17:88. doi: 10.1186/s12931-016-0396-6
10. Özpelit E, Akdeniz B, Özpelit ME, Tas S, Bozkurt S, Tertemiz KC, et al. Prognostic value of neutrophil-to-lymphocyte ratio in pulmonary arterial hypertension. J Int Med Res. (2015) 43:661–71. doi: 10.1177/0300060515589394
11. Stefanantoni K, Sciarra I, Vasile M, Badagliacca R, Poscia R, Pendolino M, et al. Elevated serum levels of macrophage migration inhibitory factor and stem cell growth factor $β$ in patients with idiopathic and systemic sclerosis associated pulmonary arterial hypertension. Reumatismo. (2014) 66:270–6. doi: 10.4081/reumatismo.2014.774
12. Cracowski J-L, Degano B, Chabot F, Labarère J, Schwedhelm E, Monneret D, et al. Independent association of urinary F2-isoprostanes with survival in pulmonary arterial hypertension. Chest. (2012) 142:869–76. doi: 10.1378/chest.11-1267
13. Banaszkiewicz M, Gąsecka A, Darocha S, Florczyk M, Pietrasik A, Kędzierski P, et al. Circulating blood-based biomarkers in pulmonary hypertension. J Clin Med. (2022) 11:383. doi: 10.3390/jcm11020383
14. O’Brien J, Hayder H, Zayed Y, Peng C. Overview of MicroRNA biogenesis, mechanisms of actions, and circulation. Front Endocrinol. (2018) 9:402. doi: 10.3389/fendo.2018.00402
15. Vishnoi A, Rani S. MiRNA biogenesis and regulation of diseases: an overview. Methods Mol. Biol. (2017) 1509:1–10. doi: 10.1007/978-1-4939-6524-3_1
16. Jakubik D, Fitas A, Eyileten C, Jarosz-Popek J, Nowak A, Czajka P, et al. MicroRNAs and long non-coding RNAs in the pathophysiological processes of diabetic cardiomyopathy: emerging biomarkers and potential therapeutics. Cardiovasc Diabetol. (2021) 20:55. doi: 10.1186/s12933-021-01245-2
17. Zareba L, Fitas A, Wolska M, Junger E, Eyileten C, Wicik Z, et al. MicroRNAs and long noncoding RNAs in coronary artery disease: new and potential therapeutic targets. Cardiol Clin. (2020) 38:601–17. doi: 10.1016/j.ccl.2020.07.005
18. Eyileten C, Wicik Z, De Rosa S, Mirowska-Guzel D, Soplinska A, Indolfi C, et al. MicroRNAs as diagnostic and prognostic biomarkers in ischemic stroke—a comprehensive review and bioinformatic analysis. Cells. (2018) 7:249. doi: 10.3390/cells7120249
19. Pordzik J, Eyileten-Postuła C, Jakubik D, Czajka P, Nowak A, De Rosa S, et al. Mir-126 is an independent predictor of long-term all-cause mortality in patients with type 2 diabetes mellitus. J Clin Med. (2021) 10:2371. doi: 10.3390/jcm10112371
20. Zhou G, Chen T, Raj JU. MicroRNAs in pulmonary arterial hypertension. Am J Respir Cell Mol Biol. (2015) 52:139–51. doi: 10.1165/rcmb.2014-0166TR
21. Lai Y-C, Potoka KC, Champion HC, Mora AL, Gladwin MT. Pulmonary arterial hypertension: the clinical syndrome. Circ Res. (2014) 115:115–30. doi: 10.1161/CIRCRESAHA.115.301146
22. Humbert M, Guignabert C, Bonnet S, Dorfmüller P, Klinger JR, Nicolls MR, et al. Pathology and pathobiology of pulmonary hypertension: state of the art and research perspectives. Eur Respir J. (2019) 53:1801887. doi: 10.1183/13993003.01887-2018
23. Budhiraja R, Tuder RM, Hassoun PM. Current perspective. Circulation. (2004) 109:159–65. doi: 10.1161/01.CIR.0000102381.57477.50
24. Lan NSH, Massam BD, Kulkarni SS, Lang CC. Pulmonary arterial hypertension: pathophysiology and treatment. Diseases. (2018) 6:38. doi: 10.3390/diseases6020038
25. Heath D, Edwards JE. The pathology of hypertensive pulmonary vascular disease: a description of six grades of structural changes in the pulmonary arteries with special reference to congenital cardiac septal defects. Circulation. (1958) 18:533–47. doi: 10.1161/01.CIR.18.4.533
26. Badano LP, Ginghina C, Easaw J, Muraru D, Grillo MT, Lancellotti P, et al. Right ventricle in pulmonary arterial hypertension: haemodynamics, structural changes, imaging, and proposal of a study protocol aimed to assess remodelling and treatment effects. Eur J Echocardiogr. (2010) 11:27–37. doi: 10.1093/ejechocard/jep152
27. Caruso P, MacLean MR, Khanin R, McClure J, Soon E, Southgate M, et al. Dynamic changes in lung microRNA profiles during the development of pulmonary hypertension due to chronic hypoxia and monocrotaline. Arterioscler Thromb Vasc Biol. (2010) 30:716–23. doi: 10.1161/ATVBAHA.109.202028
28. Thum T, Batkai S. MicroRNAs in right ventricular (dys) function (2013 Grover Conference series). Pulm Circ. (2014) 4:185–90. doi: 10.1086/675981
29. Drake JI, Bogaard HJ, Mizuno S, Clifton B, Xie B, Gao Y, et al. Molecular signature of a right heart failure program in chronic severe pulmonary hypertension. Am J Respir Cell Mol Biol. (2011) 45:1239–47. doi: 10.1165/rcmb.2010-0412OC
30. Estephan LE, Genuardi MV, Kosanovich CM, Risbano MG, Zhang Y, Petro N, et al. Distinct plasma gradients of microRNA-204 in the pulmonary circulation of patients suffering from WHO Groups I and II pulmonary hypertension. Pulm Circ. (2019) 9:2045894019840646. doi: 10.1177/2045894019840646
31. Courboulin A, Paulin R, Giguère NJ, Saksouk N, Perreault T, Meloche J, et al. Role for miR-204 in human pulmonary arterial hypertension. J Exp Med. (2011) 208:535–48. doi: 10.1084/jem.20101812
32. Lee C, Mitsialis SA, Aslam M, Vitali SH, Vergadi E, Konstantinou G, et al. Exosomes mediate the cytoprotective action of mesenchymal stromal cells on hypoxia-induced pulmonary hypertension. Circulation. (2012) 126:2601–11. doi: 10.1161/CIRCULATIONAHA.112.114173
33. Bockmeyer CL, Maegel L, Janciauskiene S, Rische J, Lehmann U, Maus UA, et al. Plexiform vasculopathy of severe pulmonary arterial hypertension and microRNA expression. J Hear Lung Transplant. (2012) 31:764–72. doi: 10.1016/j.healun.2012.03.010
34. Meloche J, Lampron M-C, Nadeau V, Maltais M, Potus F, Lambert C, et al. Implication of inflammation and epigenetic readers in coronary artery remodeling in patients with pulmonary arterial hypertension. Arterioscler Thromb Vasc Biol. (2017) 37:1513–23. doi: 10.1161/ATVBAHA.117.309156
35. Wang D, Zhang H, Li M, Frid MG, Flockton AR, McKeon BA, et al. MicroRNA-124 controls the proliferative, migratory, and inflammatory phenotype of pulmonary vascular fibroblasts. Circ Res. (2014) 114:67–78. doi: 10.1161/CIRCRESAHA.114.301633
36. Lawrie CH, Gal S, Dunlop HM, Pushkaran B, Liggins AP, Pulford K, et al. Detection of elevated levels of tumour-associated microRNAs in serum of patients with diffuse large B-cell lymphoma. Br J Haematol. (2008) 141:672–5. doi: 10.1111/j.1365-2141.2008.07077.x
37. Weber JA, Baxter DH, Zhang S, Huang DY, How Huang K, Jen Lee M, et al. The microRNA spectrum in 12 body fluids. Clin Chem. (2010) 56:1733–41. doi: 10.1373/clinchem.2010.147405
38. Creemers EE, Tijsen AJ, Pinto YM. Circulating microRNAs: novel biomarkers and extracellular communicators in cardiovascular disease? Circ Res. (2012) 110:483–95. doi: 10.1161/CIRCRESAHA.111.247452
39. Chen J, Hu C, Pan P. Extracellular vesicle microRNA transfer in lung diseases. Front Physiol. (2017) 8:1028. doi: 10.3389/fphys.2017.01028
40. Mitchell PS, Parkin RK, Kroh EM, Fritz BR, Wyman SK, Pogosova-Agadjanyan EL, et al. Circulating microRNAs as stable blood-based markers for cancer detection. Proc Natl Acad Sci USA. (2008) 105:10513–8. doi: 10.1073/pnas.0804549105
41. Chen X, Ba Y, Ma L, Cai X, Yin Y, Wang K, et al. Characterization of microRNAs in serum: a novel class of biomarkers for diagnosis of cancer and other diseases. Cell Res. (2008) 18:997–1006. doi: 10.1038/cr.2008.282
42. Huber LC, Ulrich S, Leuenberger C, Gassmann M, Vogel J, von Blotzheim LG, et al. Featured article: microRNA-125a in pulmonary hypertension: regulator of a proliferative phenotype of endothelial cells. Exp Biol Med. (2015) 240:1580–9. doi: 10.1177/1535370215579018
43. Lane KB, Machado RD, Pauciulo MW, Thomson JR, Phillips JA, Loyd JE, et al. Heterozygous germline mutations in BMPR2, encoding a TGF-$β$ receptor, cause familial primary pulmonary hypertension. Nat Genet. (2000) 26:81–4. doi: 10.1038/79226
44. Machado RD, Eickelberg O, Elliott CG, Geraci MW, Hanaoka M, Loyd JE, et al. Genetics and genomics of pulmonary arterial hypertension. J Am Coll Cardiol. (2009) 54(1 Suppl.):S32–42. doi: 10.1016/j.jacc.2009.04.015
45. Humbert M, McLaughlin V, Gibbs JSR, Gomberg-Maitland M, Hoeper MM, Preston IR, et al. Sotatercept for the treatment of pulmonary arterial hypertension. N Engl J Med. (2021) 384:1204–15. doi: 10.1056/NEJMoa2024277
46. Thenappan T, Ormiston ML, Ryan JJ, Archer SL. Pulmonary arterial hypertension: pathogenesis and clinical management. BMJ. (2018) 360:j5492. doi: 10.1136/bmj.j5492
47. Rhodes CJ, Wharton J, Boon RA, Roexe T, Tsang H, Wojciak-Stothard B, et al. Reduced microRNA-150 is associated with poor survival in pulmonary arterial hypertension. Am J Respir Crit Care Med. (2013) 187:294–302. doi: 10.1164/rccm.201205-0839OC
48. Hergenreider E, Heydt S, Tréguer K, Boettger T, Horrevoets AJG, Zeiher AM, et al. Atheroprotective communication between endothelial cells and smooth muscle cells through miRNAs. Nat Cell Biol. (2012) 14:249–56. doi: 10.1038/ncb2441
49. Ramos AE, Lo C, Estephan LE, Tai Y-Y, Tang Y, Zhao J, et al. Specific circulating microRNAs display dose-dependent responses to variable intensity and duration of endurance exercise. Am J Physiol Circ Physiol. (2018) 315:H273–H283. doi: 10.1152/ajpheart.00741.2017
50. Meloche J, Pflieger A, Vaillancourt M, Paulin R, Potus F, Zervopoulos S, et al. Role for DNA damage signaling in pulmonary arterial hypertension. Circulation. (2014) 129:786–97. doi: 10.1161/CIRCULATIONAHA.113.006167
51. Chouvarine P, Geldner J, Giagnorio R, Legchenko E, Bertram H, Hansmann G. Trans-right–ventricle and transpulmonary MicroRNA gradients in human pulmonary arterial hypertension. Pediatr Crit Care Med. (2020) 21:340–9. doi: 10.1097/PCC.0000000000002207
52. Schulte C, Karakas M, Zeller T. microRNAs in cardiovascular disease–clinical application. Clin Chem Lab Med. (2017) 55:687–704. doi: 10.1515/cclm-2016-0576
53. Karakikes I, Chaanine AH, Kang S, Mukete BN, Jeong D, Zhang S, et al. Therapeutic cardiac-targeted delivery of miR-1 reverses pressure overload–induced cardiac hypertrophy and attenuates pathological remodeling. J Am Heart Assoc. (2013) 2:e000078. doi: 10.1161/JAHA.113.000078
54. Pan Z, Sun X, Shan H, Wang N, Wang J, Ren J, et al. MicroRNA-101 inhibited postinfarct cardiac fibrosis and improved left ventricular compliance via the FBJ osteosarcoma oncogene/transforming growth factor-$β$1 pathway. Circulation. (2012) 126:840–50. doi: 10.1161/CIRCULATIONAHA.112.094524
55. Huang F, Li M-L, Fang Z-F, Hu X-Q, Liu Q-M, Liu Z-J, et al. Overexpression of MicroRNA-1 improves the efficacy of mesenchymal stem cell transplantation after myocardial infarction. Cardiology. (2013) 125:18–30. doi: 10.1159/000347081
56. Bienertova-Vasku J, Novak J, Vasku A. MicroRNAs in pulmonary arterial hypertension: pathogenesis, diagnosis and treatment. J Am Soc Hypertens. (2015) 9:221–34. doi: 10.1016/j.jash.2014.12.011
57. Yuan K, Orcholski M, Tian X, Liao X, de Jesus Perez VA. MicroRNAs: promising therapeutic targets for the treatment of pulmonary arterial hypertension. Expert Opin Ther Targets. (2013) 17:557–64. doi: 10.1517/14728222.2013.765863
58. Archer S, Rich S. Primary pulmonary hypertension: a vascular biology and translational research “Work in progress.”. Circulation. (2000) 102:2781–91. doi: 10.1161/01.CIR.102.22.2781
59. Pullamsetti SS, Doebele C, Fischer A, Savai R, Kojonazarov B, Dahal BK, et al. Inhibition of microRNA-17 improves lung and heart function in experimental pulmonary hypertension. Am J Respir Crit Care Med. (2012) 185:409–19. doi: 10.1164/rccm.201106-1093OC
60. Schlosser K, Taha M, Deng Y, Jiang B, Stewart DJ. Discordant regulation of microRNA between multiple experimental models and human pulmonary hypertension. Chest. (2015) 148:481–90. doi: 10.1378/chest.14-2169
61. Zahid KR, Raza U, Chen J, Raj UJ, Gou D. Pathobiology of pulmonary artery hypertension: role of long non-coding RNAs. Cardiovasc Res. (2020) 116:1937–47. doi: 10.1093/cvr/cvaa050
62. Wolska M, Jarosz-Popek J, Junger E, Wicik Z, Porshoor T, Sharif L, et al. Long non-coding RNAs as promising therapeutic approach in ischemic stroke: a comprehensive review. Mol Neurobiol. (2021) 58:1664–82. doi: 10.1007/s12035-020-02206-8
63. Jarosz-Popek J, Wolska M, Gasecka A, Czajka P, Jakubik D, Sharif L, et al. The importance of non-coding RNAs in neurodegenerative processes of diabetes-related molecular pathways. J Clin Med. (2020) 10:9. doi: 10.3390/jcm10010009
64. Han Y, Ali MK, Dua K, Spiekerkoetter E, Mao Y. Role of long non-coding RNAs in pulmonary arterial hypertension. Cells. (2021) 10:1892. doi: 10.3390/cells10081892
65. Hon C-C, Ramilowski JA, Harshbarger J, Bertin N, Rackham OJL, Gough J, et al. An atlas of human long non-coding RNAs with accurate 5′ ends. Nature. (2017) 543:199–204. doi: 10.1038/nature21374
66. Boucherat O, Potus F, Bonnet S. microRNA and pulmonary hypertension. Adv Exp Med Biol. (2015) 888:237–52. doi: 10.1007/978-3-319-22671-2_12
67. Hardikar AA, Farr RJ, Joglekar MV. Circulating microRNAs: understanding the limits for quantitative measurement by real-time PCR. J Am Heart Assoc. (2014) 3:e000792. doi: 10.1161/JAHA.113.000792
68. Kaudewitz D, Zampetaki A, Mayr M. MicroRNA biomarkers for coronary artery disease? Curr Atheroscler Rep. (2015) 17:70. doi: 10.1007/s11883-015-0548-z
69. Shah R, Tanriverdi K, Levy D, Larson M, Gerstein M, Mick E, et al. Discordant expression of circulating microRNA from cellular and extracellular sources. PLoS One. (2016) 11:e0153691. doi: 10.1371/journal.pone.0153691
70. Li H, Yang Z, Gao F, Zhang Y, Meng W, Rong S. MicroRNA-17 as a potential diagnostic biomarker in pulmonary arterial hypertension. J Int Med Res. (2020) 48:0300060520920430. doi: 10.1177/0300060520920430
71. Pezzuto B, Badagliacca R, Poscia R, Ghio S, D’Alto M, Vitulo P, et al. Circulating biomarkers in pulmonary arterial hypertension: update and future direction. J Hear Lung Transplant. (2015) 34:282–305. doi: 10.1016/j.healun.2014.12.005
72. Errington N, Iremonger J, Pickworth JA, Kariotis S, Rhodes CJ, Rothman AMK, et al. A diagnostic miRNA signature for pulmonary arterial hypertension using a consensus machine learning approach. EBioMedicine. (2021) 69:103444. doi: 10.1016/j.ebiom.2021.103444
73. Toh TS, Dondelinger F, Wang D. Looking beyond the hype: applied AI and machine learning in translational medicine. EBioMedicine. (2019) 47:607–15. doi: 10.1016/j.ebiom.2019.08.027
74. Hira ZM, Gillies DF. A review of feature selection and feature extraction methods applied on microarray data. Adv Bioinformatics. (2015) 2015:198363. doi: 10.1155/2015/198363
75. Borges LGA, Savi A, Teixeira C, de Oliveira RP, De Camillis MLF, Wickert R, et al. Mechanical ventilation weaning protocol improves medical adherence and results. J Crit Care. (2017) 41:296–302. doi: 10.1016/j.jcrc.2017.07.014
76. Scherr M, Eder M. Gene silencing by small regulatory RNAs in mammalian cells. Cell Cycle. (2007) 6:444–9. doi: 10.4161/cc.6.4.3807
77. Carregal-Romero S, Fadón L, Berra E, Ruíz-Cabello J. MicroRNA nanotherapeutics for lung targeting. Insights into pulmonary hypertension. Int J Mol Sci. (2020) 21:3253. doi: 10.3390/ijms21093253
78. Shen X, Corey DR. Chemistry, mechanism and clinical status of antisense oligonucleotides and duplex RNAs. Nucleic Acids Res. (2018) 46:1584–600. doi: 10.1093/nar/gkx1239
79. Yu A-M, Jian C, Allan HY, Tu M-J. RNA therapy: are we using the right molecules? Pharmacol Ther. (2019) 196:91–104. doi: 10.1016/j.pharmthera.2018.11.011
80. Schlosser K, Taha M, Stewart DJ. Systematic assessment of strategies for lung-targeted delivery of microRNA mimics. Theranostics. (2018) 8:1213. doi: 10.7150/thno.22912
81. Haas G, Cetin S, Messmer M, Chane-Woon-Ming B, Terenzi O, Chicher J, et al. Identification of factors involved in target RNA-directed microRNA degradation. Nucleic Acids Res. (2016) 44:2873–87. doi: 10.1093/nar/gkw040
82. Lennox KA, Behlke MA. Chemical modification and design of anti-miRNA oligonucleotides. Gene Ther. (2011) 18:1111–20. doi: 10.1038/gt.2011.100
83. Duygu B, Juni R, Ottaviani L, Bitsch N, Wit JBM, de Windt LJ, et al. Comparison of different chemically modified inhibitors of miR-199b in vivo. Biochem Pharmacol. (2019) 159:106–15. doi: 10.1016/j.bcp.2018.11.013
84. Wu H, Fan H, Shou Z, Xu M, Chen Q, Ai C, et al. Extracellular vesicles containing miR-146a attenuate experimental colitis by targeting TRAF6 and IRAK1. Int Immunopharmacol. (2019) 68:204–12. doi: 10.1016/j.intimp.2018.12.043
85. Thomas M, Lange-Grünweller K, Dayyoub E, Bakowsky U, Weirauch U, Aigner A, et al. PEI-complexed LNA antiseeds as miRNA inhibitors. RNA Biol. (2012) 9:1088–98. doi: 10.4161/rna.21165
86. Naseri Z, Oskuee RK, Jaafari MR, Moghadam MF. Exosome-mediated delivery of functionally active miRNA-142-3p inhibitor reduces tumorigenicity of breast cancer in vitro and in vivo. Int J Nanomedicine. (2018) 13:7727. doi: 10.2147/IJN.S182384
87. Yang J, Fan Z, Yang J, Ding J, Yang C, Chen L. MicroRNA-24 attenuates neointimal hyperplasia in the diabetic rat carotid artery injury model by inhibiting Wnt4 signaling pathway. Int J Mol Sci. (2016) 17:765. doi: 10.3390/ijms17060765
88. Lee HK, Finniss S, Cazacu S, Xiang C, Brodie C. Mesenchymal stem cells deliver exogenous miRNAs to neural cells and induce their differentiation and glutamate transporter expression. Stem Cells Dev. (2014) 23:2851–61. doi: 10.1089/scd.2014.0146
89. Couto LB, High KA. Viral vector-mediated RNA interference. Curr Opin Pharmacol. (2010) 10:534–42. doi: 10.1016/j.coph.2010.06.007
90. Ingato D, Lee JU, Sim SJ, Kwon YJ. Good things come in small packages: overcoming challenges to harness extracellular vesicles for therapeutic delivery. J Control Release. (2016) 241:174–85. doi: 10.1016/j.jconrel.2016.09.016
91. Sancho-Albero M, Rubio-Ruiz B, Pérez-López AM, Sebastián V, Martín-Duque P, Arruebo M, et al. Cancer-derived exosomes loaded with ultrathin palladium nanosheets for targeted bioorthogonal catalysis. Nat Catal. (2019) 2:864–72. doi: 10.1038/s41929-019-0333-4
92. Momen-Heravi F, Bala S, Bukong T, Szabo G. Exosome-mediated delivery of functionally active miRNA-155 inhibitor to macrophages. Nanomedicine. (2014) 10:1517–27. doi: 10.1016/j.nano.2014.03.014
93. Bai Z, Wei J, Yu C, Han X, Qin X, Zhang C, et al. Non-viral nanocarriers for intracellular delivery of microRNA therapeutics. J Mater Chem B. (2019) 7:1209–25. doi: 10.1039/C8TB02946F
94. Yang C, Yin M, Xu G, Lin W-J, Chen J, Zhang Y, et al. Biodegradable polymers as a noncoding miRNA nanocarrier for multiple targeting therapy of human hepatocellular carcinoma. Adv Healthc Mater. (2019) 8:1801318. doi: 10.1002/adhm.201801318
95. Lin C-W, Jan M-S, Kuo J-HS. The vector-related influences of autophagic microRNA delivery by Lipofectamine 2000 and polyethylenimine 25K on mouse embryonic fibroblast cells. Eur J Pharm Sci. (2017) 101:11–21. doi: 10.1016/j.ejps.2017.01.031
96. Cheng Y, Liu X, Yang J, Lin Y, Xu D-Z, Lu Q, et al. MicroRNA-145, a novel smooth muscle cell phenotypic marker and modulator, controls vascular neointimal lesion formation. Circ Res. (2009) 105:158–66. doi: 10.1161/CIRCRESAHA.109.197517
97. Zhu B, Gong Y, Yan G, Wang D, Qiao Y, Wang Q, et al. Down-regulation of lncRNA MEG3 promotes hypoxia-induced human pulmonary artery smooth muscle cell proliferation and migration via repressing PTEN by sponging miR-21. Biochem Biophys Res Commun. (2018) 495:2125–32. doi: 10.1016/j.bbrc.2017.11.185
98. Parikh VN, Jin RC, Rabello S, Gulbahce N, White K, Hale A, et al. MicroRNA-21 integrates pathogenic signaling to control pulmonary hypertension: results of a network bioinformatics approach. Circulation. (2012) 125:1520–32. doi: 10.1161/CIRCULATIONAHA.111.060269
99. Liu H, Kohane IS. Tissue and process specific microRNA–mRNA co-expression in mammalian development and malignancy. PLoS One. (2009) 4:e5436. doi: 10.1371/journal.pone.0005436
100. Baptista R, Marques C, Catarino S, Enguita FJ, Costa MC, Matafome P, et al. MicroRNA-424 (322) as a new marker of disease progression in pulmonary arterial hypertension and its role in right ventricular hypertrophy by targeting SMURF1. Cardiovasc Res. (2018) 114:53–64. doi: 10.1093/cvr/cvx187
101. Drake KM, Zygmunt D, Mavrakis L, Harbor P, Wang L, Comhair SA, et al. Altered MicroRNA processing in heritable pulmonary arterial hypertension: an important role for Smad-8. Am J Respir Crit Care Med. (2011) 184:1400–8. doi: 10.1164/rccm.201106-1130OC
102. Luo Y, Dong H-Y, Zhang B, Feng Z, Liu Y, Gao Y-Q, et al. miR-29a-3p attenuates hypoxic pulmonary hypertension by inhibiting pulmonary adventitial fibroblast activation. Hypertension. (2015) 65:414–20. doi: 10.1161/HYPERTENSIONAHA.114.04600
103. Zhang Y, Xu J. MiR-140-5p regulates hypoxia-mediated human pulmonary artery smooth muscle cell proliferation, apoptosis and differentiation by targeting Dnmt1 and promoting SOD2 expression. Biochem Biophys Res Commun. (2016) 473:342–8. doi: 10.1016/j.bbrc.2016.03.116
104. Gou D, Ramchandran R, Peng X, Yao L, Kang K, Sarkar J, et al. miR-210 has an antiapoptotic effect in pulmonary artery smooth muscle cells during hypoxia. Am J Physiol Cell Mol Physiol. (2012) 303:L682–L691. doi: 10.1152/ajplung.00344.2011
105. Zehendner CM, Valasarajan C, Werner A, Boeckel J-N, Bischoff FC, John D, et al. Long noncoding RNA TYKRIL plays a role in pulmonary hypertension via the p53-mediated regulation of PDGFR. Am J Respir Crit Care Med. (2020) 202:1445–57. doi: 10.1164/rccm.201910-2041OC
106. Jandl K, Thekkekara Puthenparampil H, Marsh LM, Hoffmann J, Wilhelm J, Veith C, et al. Long non-coding RNAs influence the transcriptome in pulmonary arterial hypertension: the role of PAXIP1-AS1. J Pathol. (2019) 247:357–70. doi: 10.1002/path.5195
107. Zhang H, Liu Y, Yan L, Wang S, Zhang M, Ma C, et al. Long noncoding RNA Hoxaas3 contributes to hypoxia-induced pulmonary artery smooth muscle cell proliferation. Cardiovasc Res. (2019) 115:647–57. doi: 10.1093/cvr/cvy250
108. Wang D, Xu H, Wu B, Jiang S, Pan H, Wang R, et al. Long non-coding RNA MALAT1 sponges miR-124-3p. 1/KLF5 to promote pulmonary vascular remodeling and cell cycle progression of pulmonary artery hypertension. Int J Mol Med. (2019) 44:871–84. doi: 10.3892/ijmm.2019.4256
109. He M, Shen J, Zhang C, Chen Y, Wang W, Tao K. Long-chain non-coding RNA metastasis-related lung adenocarcinoma transcript 1 (MALAT1) promotes the proliferation and migration of human pulmonary artery smooth muscle cells (hPASMCs) by regulating the microRNA-503 (miR-503)/toll-like receptor 4 (TLR4) si. Med Sci Monit Int Med J Exp Clin Res. (2020) 26:e923123. doi: 10.12659/MSM.923123
110. Zhu T-T, Sun R-L, Yin Y-L, Quan J-P, Song P, Xu J, et al. Long noncoding RNA UCA1 promotes the proliferation of hypoxic human pulmonary artery smooth muscle cells. Pflügers Arch J Physiol. (2019) 471:347–55. doi: 10.1007/s00424-018-2219-8
111. Cheng G, He L, Zhang Y. LincRNA-Cox2 promotes pulmonary arterial hypertension by regulating the let-7a-mediated STAT3 signaling pathway. Mol Cell Biochem. (2020) 475:239–47. doi: 10.1007/s11010-020-03877-6
Keywords: microRNA, miRNA, biomarker, pulmonary arterial hypertension, PAH
Citation: Rogula S, Pomirski B, Czyżak N, Eyileten C, Postuła M, Szarpak Ł, Filipiak KJ, Kurzyna M, Jaguszewski M, Mazurek T, Grabowski M and Gąsecka A (2022) Biomarker-based approach to determine etiology and severity of pulmonary hypertension: Focus on microRNA. Front. Cardiovasc. Med. 9:980718. doi: 10.3389/fcvm.2022.980718
Received: 28 June 2022; Accepted: 12 September 2022;
Published: 06 October 2022.
Edited by:
Joanne Tan, South Australian Health and Medical Research Institute (SAHMRI), AustraliaReviewed by:
Daryl Owen Schwenke, University of Otago, New ZealandCopyright © 2022 Rogula, Pomirski, Czyżak, Eyileten, Postuła, Szarpak, Filipiak, Kurzyna, Jaguszewski, Mazurek, Grabowski and Gąsecka. This is an open-access article distributed under the terms of the Creative Commons Attribution License (CC BY). The use, distribution or reproduction in other forums is permitted, provided the original author(s) and the copyright owner(s) are credited and that the original publication in this journal is cited, in accordance with accepted academic practice. No use, distribution or reproduction is permitted which does not comply with these terms.
*Correspondence: Sylwester Rogula, c3lsd2VzdGVyLnJvZ3VsYUB3dW0uZWR1LnBs
Disclaimer: All claims expressed in this article are solely those of the authors and do not necessarily represent those of their affiliated organizations, or those of the publisher, the editors and the reviewers. Any product that may be evaluated in this article or claim that may be made by its manufacturer is not guaranteed or endorsed by the publisher.
Research integrity at Frontiers
Learn more about the work of our research integrity team to safeguard the quality of each article we publish.