- Department of Vascular Surgery, Zhongshan Hospital, Fudan University, Shanghai, China
Thrombotic complications pose serious health risks worldwide. A significant change in our understanding of the pathophysiology of thrombosis has occurred since the discovery of extracellular traps (ETs) and their prothrombotic properties. As a result of immune cells decondensing chromatin into extracellular fibers, ETs promote thrombus formation by acting as a scaffold that activates platelets and coagulates them. The involvement of ETs in thrombosis has been reported in various thrombotic conditions including deep vein thrombosis (DVT), pulmonary emboli, acute myocardial infarction, aucte ischemic stroke, and abdominal aortic aneurysms. This review summarizes the existing evidence of ETs in human and animal model thrombi. The authors described studies showing the existence of ETs in venous or arterial thrombi. In addition, we studied potential novel therapeutic opportunities related to the resolution or prevention of thrombosis by targeting ETs.
Introduction
Thrombosis is a major contributor to the global disease burden because one in four people die from thrombotic conditions (1). As a result of blood clots impeding normal blood flow in the arteries or veins, thrombosis causes conditions such as ischemic stroke, ischemic heart disease, and venous thromboembolism (VTE). Previously, thrombosis was only considered as a vessel or blood disease. The discovery of extracellular traps (ETs) has significantly changed our understanding of thrombosis. ETs, net-like structures formed from DNA and proteins, studded with histones and cellular proteins, are released by many types of immune cells (2–4). Up to now, much more immune cells have been confirmed to have the ability to form ETs, including mast cells (5), eosinophils (6), monocytes (7) and macrophages (8) etc. After activating stimuli including exogenous microorganisms (bacteria, virus, fungi, and parasites), the immune complexes are released, these cells will undergo a new type of programmed cell death “ETosis” and then release ETs (2, 9–11).
The first evidence for neutrophil extracellular traps (NETs) appeared in 2004 (12). ETs ensnare both gram-positive and gram-negative bacteria, and myeloperoxidase (MPO) or neutrophil elastase (NE) degrades bacterial virulence factors (12). ETs, recently considered as a double-edged sword, were initially found to be capable of immobilizing and killing microorganisms and are also involved in the pathology of many diseases, such as autoimmune diseases (13), occlusions (14), aseptic inflammation (15), and even in coronavirus disease 19 (COVID-19) thrombosis (16). Growing evidence indicated that ETs were present in thrombi from animal models or in patients with thrombosis and played an important role in thrombotic diseases (17–20). There are three forms of ETs: aggregated ETs (aggETs), full-size ETs, and ET remnants. However, the main prothrombotic inducer remains debatable (21). Nevertheless, many important functions of these different types of ETs, such as NETs, macrophage/monocyte extracellular traps (METs), and mast cell extracellular traps (MCETs), remain incompletely understood. For better prevention, diagnosis, and treatment of thrombosis, a deeper understanding of its underlying mechanisms is crucial. This article aimed to describe the ETs formation process, review the roles of ETs in thrombosis, and identify possible drugs that could antagonize ETs.
What is ETosis and ETosis stimuli
The first evidence for the ETosis mechanism of neutrophils was reported in 1996, in which cell death of neutrophils could be induced by phorbol myristate acetate (PMA) for as little as 10 min (22). However, later studies have suggested that ETosis could take several hours (9, 12). The ETosis process includes flattening of isolated neutrophils, formation of intracellular vacuoles, chromatin decondensation, histone citrullination, loss of the nuclear envelope, cytoplasmic granular proteins mixed with nuclear contents, destruction of membrane integrity, and finally release of ETs (23). “NETs” are used as an example here (Figure 1). It’s worth noting that a study reported that not all ETosis pathways ended in cell death (24). Some evidence also suggests that the formation of NETs was not a direct cause of cell death (25, 26). Thereby, ETosis was considered as a new active process which different from apoptosis or necrosis and resulted in substantial morphological changes including signs of chromatin decondensation, mixing of nuclear, granular, and cytoplasmic components (9, 22).
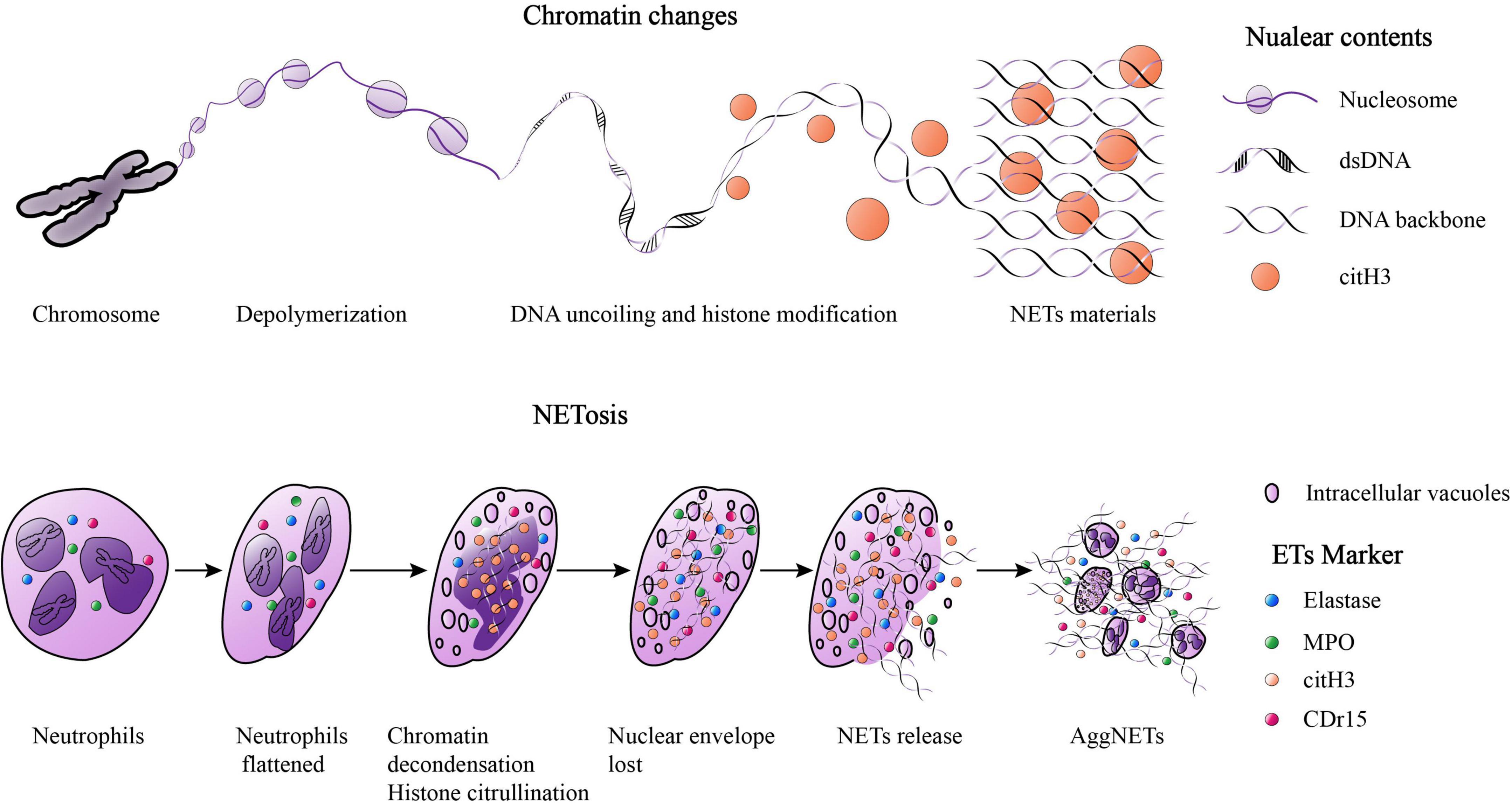
Figure 1. The Chromatin changes when NETosis and the process of NETosis. When “NETosis” occur, the chromosomes in nuclear will experience the following phases: chromatin depolymerization, DNA uncoiling and histone modification, finally forming net-like structures. The NETosis process include: isolated neutrophils flattened, the formation of intracellular vacuoles, chromatin decondensation, histone citrullination, the loss of the nuclear envelope, the mixing of cytoplasmic granular proteins and nuclear contents, membrane integrity destroyed, and ultimately, NETs were released. A large amount of NETs gathered and formed the “aggNETs.”
Even numerous triggers were reported, the pathways of the formation of NETs are still under debate and the key molecules essential are also difficult to clearly explain the specific mechanism of NETosis. In addition to PMA, interleukin (IL)-8, and lipopolysaccharide (LPS) were also found to be capable of activating NETosis, and extracellular structures identified as fragile fibers of decondensed DNA decorated with granule proteins and histones could be observed (12, 27, 28). IL-1 receptor antagonist significant decreased NETs release from neutrophils in vitro and the recombinant IL-1 treatment induced NETs formation again (29). IL-6, was indicated to be an inducer of the formation of energy dependent NETs (30). IFN-r, was verified to be a NETs formation modulator (31). Fewer NETs were observed in the bronchoalveolar lavage fluid of IFN-r–/– mice vs. wild-type mice (32). Neutrophils were still viable when the nuclear lobular shape was lost and chromatin expanded in the cytoplasm; before rupture of the plasma membrane, calcein blue was still present but negative for annexin V (9). Reactive oxygen species (ROS) are reported to be an important prerequisite for NETs formation (33). NETs cannot be produced by neutrophils with mutations in the NADPH oxidase of phagocytes when stimulated by PMA, but can be restored after hydrogen peroxide is added. This study indicated that the activation of NADPH oxidase is essential for NETosis (33). Hakkim et al. reported that protein kinase C (PKC) signaling via the RafMEK-ERK pathway can also activate NETosis (33). Both ROS and MPO are essential for NE activation (34). NE can degrade core histones and facilitate the decondensation of chromatin and citrullination of histones in synergy with the calcium-dependent enzyme peptidyl arginine deiminase 4 (PAD-4) (35). These results indicate that ROS, MPO, NE, and PAD-4 play important roles in NETosis. A previous study showed that PAD-4 activity is necessary for NETs formation (36). After PAD-4 is knocked out or inhibited, the formation of human or mouse NETs is disrupted (37–39). Notably, excessive NETs were observed in influenza A and COVID-19 virus-induced lung inflammation (40–43). This indicated virus might be a stimulus for NETs formation. However, several studies have observed that under the same stimulus, the formation can be independent of the activity of PAD-4 (44, 45).
Neutrophils formed neutrophil extracellular traps in acute inflammation
Neutrophils are the first responders and critical fighters of the innate immune system, and are heterogeneous and abundant leukocytes that participate in acute inflammation, including infection and injury (46). Approximately 60% of leukocytes are circulating neutrophils, released from the bone marrow and with a short lifespan (47, 48).
In 2004, a novel substance NETs produced by neutrophils that underwent NETosis was discovered in vitro (12). NETs are composed of filamentous DNA scaffolds, granular proteins and histones (12). Many substances have been explored to determine whether they can promote or inhibit NET formation. PMA has been verified many times and has been used to induce NETs formation (49). Andzinski et al. reported that type I interferons (IFNs) display a proinflammatory subset of neutrophils and enhance NETs formation (50). Boufenzer et al. (23) demonstrated that the activation of TREM-1 increases the formation and release of NETs, promotes vascular dysfunction, and activates endothelial cells. The direct interaction between platelets and neutrophils during platelet-driven NETosis is mediated mainly by P-selectin/PSGL-1 and GPIbα/Mac-1. Soluble mediators released by platelets, including high mobility group box 1 (HMGB1), regulated on activation normal T-cell expressed and secreted (RANTES) and platelet factor 4 (PF4), further stimulated NETs formation (51–53). Previous studies demonstrated that platelets had the ability to directly stimulate the production of NETs (52, 54). Following the above findings, platelet-derived exosomes in patients with sepsis were shown to promote excessive formation of NETs and subsequently result in organ injury (55). Kono et al. (56) reported that deferasirox (DFS) is an iron chelator that can inhibit ROS production and NETs formation. Several studies on the intracellular regulatory mechanism of NETs formation demonstrated that a lack of miR-146a targeting the TLR4 signaling pathway in mice could effectively prevent the formation of NETs (57, 58). As a result, platelet TLR4 detected TLR4 ligands in the blood and activated neutrophils, forming NETs (59). Although NETs have received increasing attention, most relevant studies have only focused on animal and in vitro experiments (60). The clinical value of NETs in thrombosis requires further exploration.
Macrophages, monocytes and mast cells can also form extracellular traps
The tissue macrophage differs from the circulating blood monocyte, which is derived from bone marrow or originates from tissue-based embryonic precursors and is maintained independently of bone marrow progenitor cells (61–63). Macrophages are involved in many functions, such as maintaining homeostasis, tissue repair, and immune regulation (64–66).
Current studies have reported that macrophages that undergo METosis could also produce ETs, which were named METs (2). METosis occur rapidly within 30 min (67, 68). The METs are produced by macrophages when they encounter microorganisms and are similar to NETs, which are also composed of DNA met-like structures. METs can immobilize and kill microorganisms, but may also contribute to disease pathology. In 2008, a study first reported that mast cells in vitro could produce NETs like extracellular structures which were named MCETs with antimicrobial activity (3). The formation of MCETs in mast cells was later reported when exposed to other GAS strains (69) or to other extracellular bacteria. For instance, BMMCs or HMC-1 infected with S. aureus (70), HMC-1 in contact with Pseudomonas aeruginosa (3), or BMMCs co-cultured with Enterococcus faecalis (71). The bacteria trapped in MCETs are killed (70, 71). Nakazawa et al. (72) demonstrated that macrophage polarization may affect METosis. The M1 activated state is more prone to METosis when exposed to NETs materials.
With the exception of live bacterial cells, Wong et al. (73) reported that METs were released from bacteria when they were exposed to specific virulence factors, such as ESX-1 and the secretion system of Mycobacterium tuberculosis. It was also demonstrated that mouse RAW264.7 and J774A.1 macrophage-like cells incubated at 42°C for 1.5 h and followed by recovery at 37°C were more prone to form METs co-cultivated with Streptococcus agalactiae (57). Halder et al. (67) reported that METs released from monocytes have an important host defense function which is capable of inhibiting fungal growth. Previous studies have shown that METs can be formed if proinflammatory mediators induce the production of ROS, although these findings were not consistent across all experimental conditions and cell types (74, 75). However, why some macrophages develop METosis while others do not remains controversial. To date, an increasing number of types of immune cells have been demonstrated to have the ability to form ETs; however, the specific mechanisms and functions of different types of ETs remain unclear.
Specific markers for identifying extracellular traps and several methods for detecting extracellular traps formation
To clarify the distribution of ETs in tissues and detect ETs content, it is necessary to search for specific markers for subsequent research. Several known ET components, including citrullinated histones, elastase, or MPO, are stained in the additional verification proposal. Although the circulating concentrations of these markers were easily influenced, they were still the most commonly used in many studies (11, 76). Citrullinated histone H3 (citH3) has been identified as one of the most specific markers for ETs (77–79). Elastase was originally considered a neutrophil-specific marker, but has also been discovered in human macrophage-like cells and peripheral blood monocytes (67). In addition, MPO is also found in METs produced from a variety of macrophage populations, including glomerular macrophages, monocytes, J774A.1 macrophage-like cells, and caprine monocytes (67, 68, 80, 81). A recent study reported that CDr15 in the plasma could be a new marker for detecting NETs (82). The surrogate markers for ETs are plasma cell-free DNA, including double-stranded DNA (dsDNA), histone-DNA complexes, and chromatin, which have often been estimated in clinical trials in recent years (83–86). Recently, there were also studies reported that MPO-DNA complexes and cit-H3-DNA complexes in serum samples could be treated as a useful biomarker of serum NETs level (87).
ELISA is the screening test that is commonly used for detection of serum markers. However, there has been a study indicating that ELISAs for MPO, NE and nucleosomes do not specifically determine the formation of NETs, and the standardization of tests for MPO-DNA and citH3 is problematic (88). Recently, flow cytometry analysis has gained increasing interest for NETs identification and quantification. There is no potential for observer bias in this new methodology since it allows rapid and robust assessment of several thousand cells per sample, and it is independent from potential observer bias. To assess the formation of NETs in vivo, detecting NETs components on extracellular vesicles (EVs) (89) and cells (90, 91) by using flow cytometric assays may be an alternative method. Otherwise, for assessing and visualizing the formation of NETs, live cell imaging, conventional microscopy, intravital microscopy, and scanning or transmission electron microscopy are commonly used imaging-based applications (9, 88, 92). The drawback of such approaches is that they are difficult to precisely distinguish if DNA-protein complex from NETs other than another kind of cell death (93–95).
Extracellular traps in venous thromboembolism
VTE, which mainly includes deep vein thrombosis (DVT) and pulmonary embolism (PE) (96), ranks third among all causes of acute cardiovascular syndrome (97). DVT causes post-thrombotic syndrome, whereas PE causes chronic pulmonary hypertension. Both these conditions affect quality of life. Recently, the role of ETs in VTE has gained increasing attention, and the following describes DVT and PE.
Extracellular traps are abundance present in deep vein thrombosis
DVT is a prevalent disease worldwide with severe major complications accompanied by high morbidity and mortality. The Virchow’s triad, including hypercoagulability, vascular dysfunction, and stasis, is considered an excellent guide to understand thrombotic risk factors (98). Recently, dysregulation of the immune system was suggested to be considered in the process of thrombosis (99).
In human venous thrombosis, NETs act as fibrous scaffolds for von Willebrand factor (vWF), fibrin, and platelets. This is the first report to confirm that NETs have a prothrombotic (11). A case report first confirmed that NETs were abundant in human thrombus samples, especially in patients with DVT and microscopic polyangiitis (100). Savchenko et al. (96) collected 16 thrombi obtained from 11 patients during autopsy or surgery. Histological analysis showed that a large number of DNA web structures and citH3 were concentrated in the thrombi organizing parts, with only a few in already organized sections. These results indicate that thrombi development is associated with NETs. In some animal experiments, researchers have obtained similar findings. Brill et al. (101) collected samples from patients with chronic thromboembolic pulmonary hypertension patients and a vena cava ligation mouse model. The NET-specific marker citH3 was abundant and co-localized with vWF in thrombi, especially in the fresh parts. Similarly, large amounts of NET-like structures were observed in thrombi in a mouse DVT model (102). Therefore, dissolution of NETs may facilitate thrombolysis. Based on these findings, plasma DNA levels are clinically used in the diagnosis of DVT with a rather high sensitivity of 81%, and the DNA in thrombi is positively correlated with vWF activity, D-dimer level, neutrophil activation, and clinical Wells score (103, 104).
Extracellular traps have prothrombotic effect in pulmonary embolism
PE is a severe complication of DVT (105). The level of nuclear DNA in circulation is significantly elevated in patients with PE, and there is a clear positive correlation with mortality (106, 107). In patients with chronic thromboembolic pulmonary hypertension, neutrophils were found to exist on the surface of thrombi with high reactivity, and soluble NETs surrogates were significantly increased compared to healthy controls (108). Furthermore, a plasma test in patients with diabetes mellitus verified that NETs were mainly formed in the early stages of thrombosis, especially in the acute stage, which was different from ETs produced by other immune cells (109). Using a murine orthotopic 4T1 breast cancer model, Cao et al. (110) demonstrated that dunnione (a strong substrate of NADPH quinone oxidoreductase 1) could inhibit NETs formation and the subsequent occurrence of PE by decreasing cellular NAD levels. These findings confirm that ETs are involved in PE, especially in the progression of thrombosis. Additionally, controversial studies have suggested that ETs may be associated with outcomes in patients with PE. A clinical study that enrolled 25 healthy controls and 126 normotensive acute PE patients revealed that high circulating citH3 levels enhanced NETs formation and were associated with an increased risk of acute PE-related death (111). In recent years, the coronavirus disease (COVID-19) epidemic has spread globally. Several researchers found that thrombotic complications are associated with severe COVID-19 morbidity and mortality (112, 113). However, Prevel et al. (114) reported that in 50 hospitalized COVID-19-related ARDS patients, plasma markers such as cell-free DNA, MPO–DNA complexes, and citH3 were associated with survival but not with PE. As there is a lack of research on ETs in COVID-19 and limited by sample size, further in-depth studies are needed. Above all, these findings prove that ETs contribute to the progression of thrombosis and may be potential therapeutic targets for preventing PE.
Extracellular traps in arterial thrombosis
Clinical challenges remain in the prevention and treatment of arterial thrombosis. Understanding the relevant molecular mechanisms can help identify new targets and therapeutic approaches that can improve protection against severe thrombotic events. The role of ETs has mostly been studied in the following common arterial thrombosis diseases: acute myocardial infarction (MI), acute ischemic stroke, abdominal aortic aneurysm (AAA), peripheral artery disease (PAD), and thrombotic microangiopathies (TMAs).
Extracellular traps contribute to the pathological progression of acute myocardial infarction
Abundant neutrophils and NETs have been discovered in fresh culprit site thrombi in patients who have died from MI (115–117). NETs combined with pro-inflammatory IL-17 drive the accumulation of neutrophils (118) and have been suggested to play an important role in the pathogenesis of MI (119). A substantial burden of NETs has also been observed in thrombectomy samples from patients with stent thrombosis after percutaneous coronary intervention (120). Several clinical studies have indicated that NETs can promote thrombosis of the coronary microvasculature and damage heart function (121–123). Abo-Aly et al. (124) enrolled 22 ST-segment elevation MI patients who underwent percutaneous coronary intervention in an open-label prospective randomized study. Patients’ total elastase, MPO-elastase complexes in plasma, and thrombolysis in myocardial infarction flow scores were demonstrated to be significantly reduced after receiving a dose of 30 mcg/kg bolus cangrelor followed by a 4 mcg/kg/min intravenous infusion from the start of intervention to 2 hours later (124). Previous studies have revealed the presence of ETs in the thrombus and plasma of patients with MI, and found possible relationships between ETs and adverse events. Whether monitoring of these ETs markers could help prevent adverse events need further exploration.
In addition, if ETs play an important role in the pathological progression of MI, it might be a selective therapeutic target for developing new drugs to prevent MI-relevant thrombosis events. However, whether ETs are simply a marker of adverse prognosis is still unclear (125). A clinical study confirmed that NETs were present at the culprit site of acute MI thrombus, and the formation of NETs was induced by HMGB1, which is an important risk-related molecular pattern (126). Compared with venous thrombi, coronary artery thrombi had a significantly higher NETs burden and were positively correlated with infarct size (127), as well as in patients infected with COVID-9 (128). Recently, the dsDNA level measured 1 d after MI was also found to be related to the myocardial salvage index, left ventricular ejection fraction, and microvascular obstruction during follow-up (129). In vitro, neutrophils collected from the culprit lesion site showed a greater tendency to develop NETosis compared to neutrophils from non-infarcted coronary arteries (130). By activating and differentiating fibrocytes, NETs also contribute to myocardial fibrosis at the culprit site (77). Notably, several previous studies had already confirmed that neutrophil:lymphocyte ratios, peripheral neutrophil count, and NETs-related markers correlated with adverse cardiovascular outcome (131, 132). And meanwhile, there are also evidence demonstrate that markers of ETs, neutrophil activation and DNase activity are related to inflammatory indicators, such as interleukin-6 and C-reactive protein (133). Above all, MI is a complex progression involving numerous inflammatory factors, if ETs played a major role is still debatable. Otherwise, a considerable proportion of patients with MI have a history of chronic coronary heart disease (CHD). Many studies have focused on NETs in the acute stage, and there is still a lack of research on the role of other types of ETs in the long-term progression of MI.
Extracellular traps-induced thrombosis may be the cause of acute ischemic stroke
Strokes caused by thromboembolic occlusions of the middle cerebral artery (MCA) and/or internal carotid artery (ICA) are the second leading cause of death and morbidity worldwide (134). Abundant NETs were discovered in thrombus samples taken from patients with ischemic stroke, and a large number of neutrophils were positive for citH3 in these thrombectomy samples (135, 136). Deng et al. (137) also verified that NETs were present in thrombi obtained from patients with acute ischemic stroke. Inhibition of NETs formation could decrease infarct size in a mouse permanent middle cerebral artery model. There is increasing evidence that NETs can antagonize tissue plasminogen activators by stabilizing clot-induced thrombus formation and promoting coagulation, a frequently encountered problem in the treatment of stroke patients (138–140). This demonstrates that NETs are involved in the pathogenesis of cerebral occlusion. Otherwise, a high level of plasma DNA indicated that patients were at risk of death at follow-up (141, 142), whereas nucleosomes were related to infarction volume and neurological dysfunction (143). Tsai et al. performed a clinical control trial that included 50 patients with acute ischemic stroke and 50 at-risk controls. The results demonstrated that plasma DNA significantly increases after stroke (144). Vallés et al. (145) conducted a clinical study of 243 patients with acute ischemic stroke. They determined NETs markers, including citH3, nucleosomes, and cell-free DNA in plasma. The 12 months follow-up results showed that patients with high citH3 levels had an increased all-cause mortality. Prognosis and outcome were associated with soluble NETs markers, which are indicators of stroke severity based on the National Institutes of Health Stroke Scale (NIHSS). These findings show that close monitoring of plasma ETs markers is essential, and effective treatment of ETs might prevent the occurrence of acute ischemic stroke.
Extracellular trap is a key factor in thrombosis in abdominal aortic aneurysm
AAA, an arterial disease related to thrombosis with potentially fatal consequences of aortic rupture, is characterized by multilayer intraluminal thrombosis and vessel dilation (146). An intraluminal thrombus, whose growth is accelerated by turbulent blood flow, damages aneurysmal walls by immune-inflammatory pathways (147). In human AAA, Delbosc et al. (148) revealed that neutrophil activation resulted in NETs formation in the intraluminal thrombus, leading to cell-free DNA release. Consistent results were obtained in the rat AAA model. Meher et al. (149) performed further clinical research on human AAA thrombi. NETs were found to co-localize with IL-1β, a pro-inflammatory mediator that drives the process of AAA and accelerates the formation of NETs. Johnston et al. (150) verified a similar result and explored the mechanism in an elastase perfusion mouse AAA model. This proved that genetic deletion or receptor antagonism of IL-1β reduced NETs formation and AAA initiation and progression. Fernández-Ruiz (151) also confirmed that NETs stimulated by IL-1β aggravate the formation of AAA in a mouse AAA model induced by elastase perfusion. Despite the association between AAA and thrombotic disease, most clinicians and researchers focus only on the etiological mechanisms of arterial dilation. Other pathological evidence related to NETs in aneurysm thrombosis is scarce and requires further investigation.
Extracellular traps aggravate peripheral artery disease and thrombotic microangiopathies
In general, PAD refers to diseases of the non-coronary vasculature which can be aneurysmal, atherosclerotic, inflammatory, or a combination of pathologies (152). Farkas et al. (153) demonstrated that NETs levels in PAD thrombi were similar to those in the coronary arteries and stroke thrombi. On comparing plasma samples from patients with PAD and DVT, neutrophil elastase alpha1 anti-trypsin complex, showed a significant increase in PAD (154).
TMAs are a group of rare, but serious disorders can be caused by a variety of clinical events, such as disseminated intravascular coagulation, hemolytic uremic syndrome, etc. (155). Fuchs et al. (156) conducted a cohort study of plasma samples from 10 healthy controls and 29 acute TMAs patients. Research has shown that increased plasma extracellular DNA and histones are associated with the activity. Thrombosis in COVID-19 patients affected both arterial and venous circulation, resulting in stroke, acute coronary syndrome, DVT, PE, and TMAs (157, 158). A prospective cohort study compared plasma citH3, cell-free DNA, and MPO-DNA complexes in patients with healthy controls (43). The authors indicated that MPO-DNA complexes increased in COVID-19 and correlated directly with illness severity. NETs have prothrombotic clinical presentations and aggregate occluded small pulmonary vessels widely in the lungs of patients with COVID-19-related acute respiratory distress syndrome (43). To date, there is still a lack of research on ETs in PAD and TMAs; however, it has been verified that ETs are involved in the progression of such thrombotic diseases.
Extracellular traps as a potential therapeutic target
It is essential to find therapeutic compounds that inhibit ETs and block their detrimental effects since ETs play such a crucial role in thrombotic diseases. There have been several pathways proposed to target different ET components. Therefore, the mechanism of ETs formation must be explored for every single condition, and specific therapeutic strategies must be developed for every single condition. Except for DNA bone structure, the histones derived from NETs should also be noted. Previous studies demonstrated that histones within NETs played a cytotoxic role in the pathogenesis of endothelium damage (59, 159). Excessive NETs formation can be suppressed by treating NE, MPO, PAD4, and extracellular DNA (160, 161). Developing drugs, inhibiting PAD4, and treating with Deoxyribonuclease (DNase) 1 are the most promising treatments for NETs-induced occlusions (162).
The inhibition of PAD4 decrease the formation of extracellular traps
A family of enzymes called peptidylarginine deiminases (PADIs) is involved in post-transcriptional deamination/citrullination. A positively charged arginine molecule is converted to an uncharged citrulline molecule in this process. Among the five members of PADI, PAD4 is unique. By decondensing chromatin, it contributes to NET formation, as evidenced in the in vitro studies of peripheral blood neutrophils (163). This makes PAD4 an attractive therapeutic target for treating occlusive NET-related diseases. A synthetic inhibitor of PAD4 called Cl-amidine has been used in the treatment of rheumatoid arthritis in mammals for decades (164, 165). Cl-amidine effectively blocked the synthesis of NETs based on its irreversible blocking properties in both in vitro neutrophils and murine lupus models in vivo (163, 166, 167), and disease severity was effectively attenuated in mouse models of various diseases, such as cardiovascular events (166), sepsis (168), and collagen-induced arthritis (169). Administration of Cl-amidine prevented thrombotic occlusion in a mouse model of ischemic stroke by promoting NET lysis (170). Although conflicting results exist, there is a certain dependence of NETosis on enzymatic PAD4 activity, making PAD4 inhibition a potentially promising treatment for human thrombotic disease.
Deoxyribonuclease can degrade extracellular traps
The Deoxyribonuclease (DNase) family, including DNase1 and DNase1-like 3 (DNase1L3), is a class of enzymes that can degrade ETs (170). Non-hematopoietic tissues express DNase1 and it preferentially degrades protein-free DNA (4, 171). Immune cells secrete DNase1L3, also called DNase gamma, which cleaves protein-DNA complexes (4, 172). DNase degrades NETs by hydrolyzing the DNA backbone (11) and reduces the size and amount of aggNETs (173). Lack of dual host protector DNases in vivo cannot remove ETs efficiently, and aggETs can occlude vessels (21). The homeostatic balance between NET formation and degradation is dependent on adequate DNase activity (174). The mice were protected from vascular occlusion in either reconstitution with or the presence of only one functional type of DNase (170). It has been demonstrated that targeting chromatin and NETs with DNase 1 in several mouse or rat disease models is beneficial for experimental DVT (101, 102) and ischemic injury, including testicular torsion (175) and ischemic stroke (174). Shrestha et al. (176) demonstrated that NETs exert antitumor effects in a murine tumor model. Retinoic acid can induce hypersegmented neutrophils which enhances NETs formation and cytotoxicity against tumor cells in a murine tumor model. However, the addition of DNase reversed this antitumor effect because of NET degradation. The results of this study demonstrated that DNase could effectively remove NETs and that the balance between DNase and NETs plays an important role in cancer diseases. Ge et al. (177) reported that the use of DNase 1 alone could reduce neutrophil infiltration in a rat model of ischemia/reperfusion, and that cardiac function was improved by the co-administration of tissue plasminogen activator (tPA). In contrast, another study indicated that in mice with coronary artery ligations, DNase 1 treatment improved cardiac function without reducing neutrophil infiltration into ischemic myocardium (178). It is possible that these apparently contradictory results are due to variations in the ligation methodology, the duration of ischemia, and the timing of the DNase treatment. In vitro, DNase 1 accelerated thrombolysis mediated by tPA in human coronary (127) and cerebral (135) thrombi compared with tPA alone. Furthermore, patients with MI experiencing low DNase activity typically have a larger infarct size (127). Taking into account the growing evidence for the benefits of applying DNase in well-established models of disease. Patients with neutrophil-driven disease, in which NETs play a pathogenic role, may benefit from DNase 1 treatment.
Heparin has the effect of antagonizing neutrophil extracellular traps
Historically, unfractionated heparin and low-molecular-weight heparins have been recognized as the cornerstones of treatment for DVT (179) and MI (180). Due to the inflammatory nature of thrombotic diseases, it is intriguing that heparin has anti-inflammatory effects (181, 182). Heparins can not only hinder NETs formation as shown by treatment of in vitro human neutrophils and in vivo healthy volunteers (183), but can also target existing NETs in vitro in various ways. In NETs, two major enzymes, neutrophil elastase and cathepsin G, were shown to be inhibited by heparin (184, 185). By binding and disassembling histones, heparin inhibits NETs’ pro-thrombotic properties in vitro (11) and in vivo in mice (102, 186). Heparin’s antithrombotic properties in human plasma have been counteracted by excessive release of histones during cell death and NETosis, according to an in vitro study (187). Sevuparin, a low-anticoagulant heparin analog, inhibits NE and histone H4 proteins associated with NETs secreted by human neutrophils in vitro (188). Another study showed that non-anticoagulant heparin prevented histone-mediated cytotoxicity in a murine sepsis model and improved survival (189). There are many types of heparin, which are commonly used in clinics. Thus, an appropriate dose of heparin under close monitoring might be an important adjunct therapy to reduce NET burdens without increasing bleeding complications. Despite this, there are few reports on heparin-targeted treatment of ET-related diseases, and further research is needed.
Acetylsalicylic acid (aspirin), a non-steroidal drug with an antithrombotic effect
Aspirin, an antiplatelet drug, is used for prevention of arterial thromboses for many years. Previous studies reported that platelets could activate NETosis via the platelet-neutrophil interaction and facilitate innate immunity (51, 88). During endotoxemia and in septic conditions, the binding of glycoprotein 1bα (GP1bα) on platelets and αMβ2 (MAC-1) on neutrophils could activate NETosis and promote the release of NETs in vitro (190, 191). Otherwise, platelet activation by TLR2 and TLR4 results in the release of Pselectin, which binds to the neutrophil receptor (PSGL-1), leading to the development of NETosis as demonstrated in mice (59, 192, 193). When exposed to LPS, platelets could also be activated by arachidonic acid and thrombin, and the formation of NETs increased (51, 194). As platelet and neutrophil interactions mediate NETosis, inhibiting these interactions with antiplatelet therapy can prevent NETs formation (195, 196). The activation of platelet could be inhibited by aspirin had been confirmed in primary graft dysfunction murine models (197). Aspirin pretreatment of mice with endotoxin-triggered acute lung injury decreased the formation of NETs and the severity of lung injury (194, 196). In a vitro study of neutrophils, Lapponi et al. demonstrated that NETs formation was inhibited after the intervention with aspirin (198). Based on these results, aspirin may be useful in treating pathologic NETosis induced by platelets. However, aspirin predisposes patients to stomach ulcers, so more studies are needed to determine what conditions could benefit from taking aspirin without experiencing too many side effects.
Other substances potential inhibit NETosis
In addition to the above-mentioned widely used drugs, there were also other substances reported recently in a few studies that might inhibit NETosis, such as cyclosporine A (199), prostaglandins E2 (200, 201), recombinant human thrombomodulin (202), activated protein C (203), metformin (204), and Vitamin D (205). Although uncertainties still remain in these findings, they represented different directions for future studies.
Conclusions and perspectives
Although the formation and aggregation of ETs actively limits the spread of inflammatory mediators and pathogens, excessive accumulation of ETs is also a cause of thrombotic diseases. A summary of these studies highlighted and discussed above, ETs clearly play an important role in the initiation and progression of thrombosis (Figure 2). Based on these findings, ETs have been considered new potential therapeutic targets. Until now, the digestion of established ETs and prevention of new ETs formation to alleviate the severity of thrombosis remains controversial. Although in vitro and in vivo data are promising, more in-depth studies are needed to evaluate the clinical safety and benefits of anti-ETotic regimens.
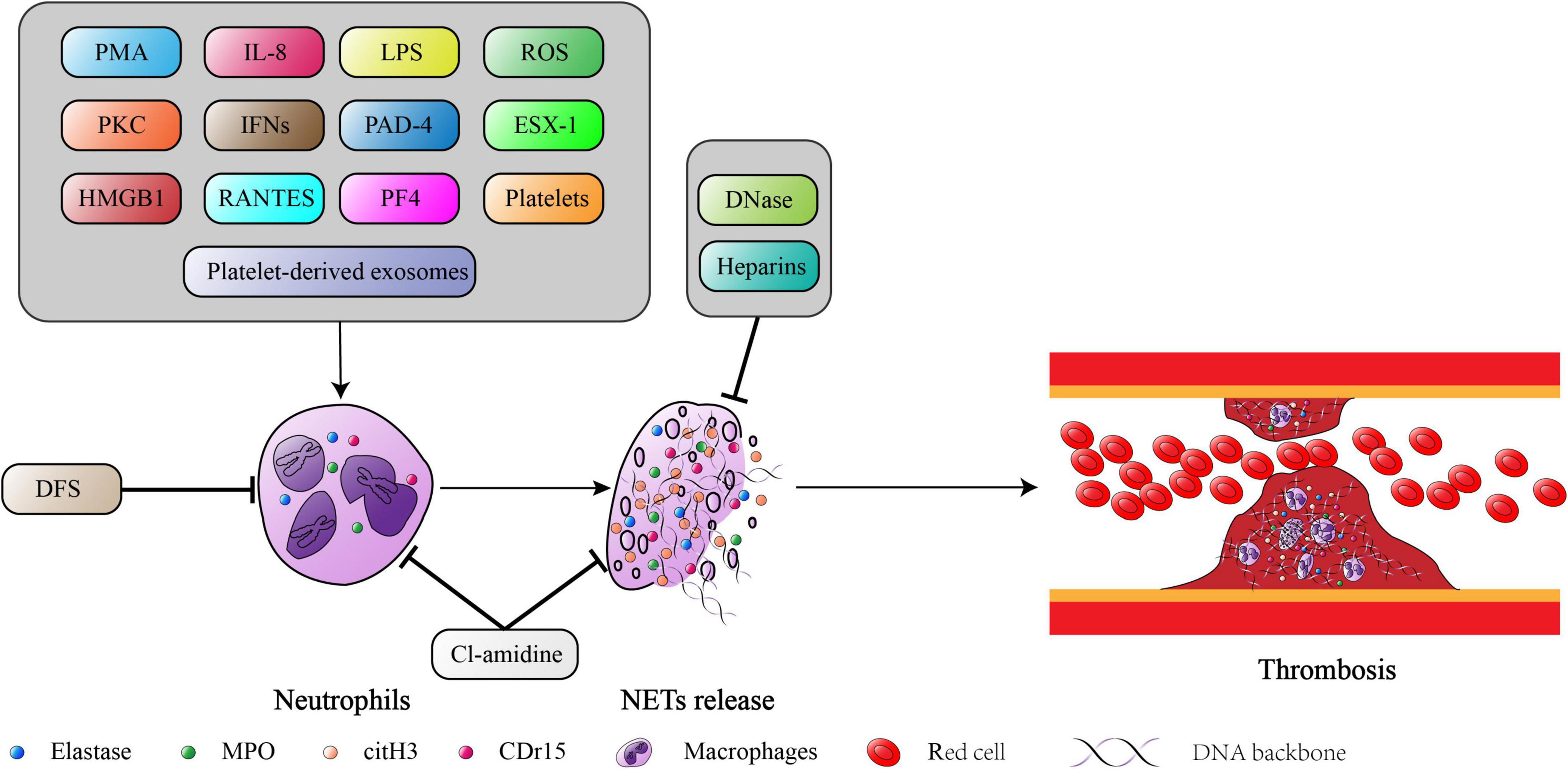
Figure 2. The summarized mechanism of NETs formation and its consequences in thrombosis. The figure was constructed by Adobe Illustrator.
Author contributions
TH, XT, and DG conceived the review. TH, HT, CL, YS, and DY wrote the manuscript and designed the figures. All authors contributed to the article and approved the submitted version.
Funding
This study was supported by the National Natural Science Foundation of China (81970408 and 82100516) and the Science and Technology Commission of Shanghai Municipality (19411966900).
Acknowledgments
All figures were created with Adobe Illustrator CC.
Conflict of interest
The authors declare that the research was conducted in the absence of any commercial or financial relationships that could be construed as a potential conflict of interest.
Publisher’s note
All claims expressed in this article are solely those of the authors and do not necessarily represent those of their affiliated organizations, or those of the publisher, the editors and the reviewers. Any product that may be evaluated in this article, or claim that may be made by its manufacturer, is not guaranteed or endorsed by the publisher.
Abbreviations
AAA, Abdominal aortic aneurysm; aggETs, aggregated ETs; citH3, Citrullinated histone H3; COVID-19, Coronavirus disease 19; DFS, Deferasirox; DNase, Deoxyribonuclease; dsDNA, Double-stranded DNA; DVT, Deep vein thrombosis; ET, Extracellular traps; HMGB1, High mobility group box 1; ICA, Internal carotid artery; IFNs, Type I interferons; IL, Interleukin; LPS, Lipopolysaccharide; MCA, Middle cerebral artery; MI, Myocardial infarction; MPO, Myeloperoxidase; NE, Neutrophil elastase; NETs, Neutrophil extracellular traps; METs, Macrophage extracellular traps; MCETs, Mast cell extracellular traps; PAD, Peripheral artery disease; PAD-4, Peptidylarginine deiminase 4; PE, Pulmonary embolism; PF4, Platelet factor 4; PKC, Protein kinase C; PMA, Phorbol myristate acetate; RANTES, Regulated on activation normal t-cell expressed and secrected; ROS, Reactive oxygen species; VTE, Venous thromboembolism; vWF, von Willebrand factor.
References
1. Lozano R, Naghavi M, Foreman K, Lim S, Shibuya K, Aboyans V, et al. Global and regional mortality from 235 causes of death for 20 age groups in 1990 and 2010: A systematic analysis for the global burden of disease study 2010. Lancet. (2012) 380:2095–128. doi: 10.1016/s0140-6736(12)61728-0
2. Pertiwi KR, de Boer OJ, Mackaaij C, Pabittei DR, de Winter RJ, Li X, et al. Extracellular traps derived from macrophages, mast cells, eosinophils and neutrophils are generated in a time-dependent manner during atherothrombosis. J Pathol. (2019) 247:505–12. doi: 10.1002/path.5212
3. von Köckritz-Blickwede M, Goldmann O, Thulin P, Heinemann K, Norrby-Teglund A, Rohde M, et al. Phagocytosis-independent antimicrobial activity of mast cells by means of extracellular trap formation. Blood. (2008) 111:3070–80. doi: 10.1182/blood-2007-07-104018
4. Napirei M, Ludwig S, Mezrhab J, Klöckl T, Mannherz HG. Murine serum nucleases–contrasting effects of plasmin and heparin on the activities of DNase1 and DNase1-like 3 (DNase1l3). FEBS J. (2009) 276:1059–73. doi: 10.1111/j.1742-4658.2008.06849.x
5. Campillo-Navarro M, Leyva-Paredes K, Donis-Maturano L, González-Jiménez M, Paredes-Vivas Y, Cerbulo-Vázquez A, et al. Listeria monocytogenes induces mast cell extracellular traps. Immunobiology. (2017) 222:432–9. doi: 10.1016/j.imbio.2016.08.006
6. Uribe Echevarría L, Leimgruber C, García González J, Nevado A, Álvarez R, García LN, et al. Evidence of eosinophil extracellular trap cell death in COPD: Does it represent the trigger that switches on the disease? Int J Chron Obstruct Pulmon Dis. (2017) 12:885–96. doi: 10.2147/copd.S115969
7. Granger V, Faille D, Marani V, Noël B, Gallais Y, Szely N, et al. Human blood monocytes are able to form extracellular traps. J Leukoc Biol. (2017) 102:775–81. doi: 10.1189/jlb.3MA0916-411R
8. Seifert A, Tylek T, Blum C, Hemmelmann N, Böttcher B, Gbureck U, et al. Calcium phosphate-based biomaterials trigger human macrophages to release extracellular traps. Biomaterials. (2022) 285:121521. doi: 10.1016/j.biomaterials.2022.121521
9. Fuchs TA, Abed U, Goosmann C, Hurwitz R, Schulze I, Wahn V, et al. Novel cell death program leads to neutrophil extracellular traps. J Cell Biol. (2007) 176:231–41. doi: 10.1083/jcb.200606027
10. Mansour A, Bachelot-Loza C, Nesseler N, Gaussem P, Gouin-Thibault I. P2Y(12) inhibition beyond thrombosis: Effects on inflammation. Int J Mol Sci. (2020) 21:1391. doi: 10.3390/ijms21041391
11. Fuchs TA, Brill A, Duerschmied D, Schatzberg D, Monestier M, Myers DD, et al. Extracellular DNA traps promote thrombosis. Proc Natl Acad Sci U.S.A. (2010) 107:15880–5. doi: 10.1073/pnas.1005743107
12. Brinkmann V, Reichard U, Goosmann C, Fauler B, Uhlemann Y, Weiss DS, et al. Neutrophil extracellular traps kill bacteria. Science. (2004) 303:1532–5. doi: 10.1126/science.1092385
13. Domerecka W, Homa-Mlak I, Mlak R, Michalak A, Wilińska A, Kowalska-Kêpczyńska A, et al. Indicator of inflammation and NETosis-low-density granulocytes as a biomarker of autoimmune hepatitis. J Clin Med. (2022) 11:2174. doi: 10.3390/jcm11082174
14. Sharma S, Hofbauer TM, Ondracek AS, Chausheva S, Alimohammadi A, Artner T, et al. Neutrophil extracellular traps promote fibrous vascular occlusions in chronic thrombosis. Blood. (2021) 137:1104–16. doi: 10.1182/blood.2020005861
15. Nakazawa D, Masuda S, Tomaru U, Ishizu A. Pathogenesis and therapeutic interventions for ANCA-associated vasculitis. Nat Rev Rheumatol. (2019) 15:91–101. doi: 10.1038/s41584-018-0145-y
16. Skendros P, Mitsios A, Chrysanthopoulou A, Mastellos DC, Metallidis S, Rafailidis P, et al. Complement and tissue factor-enriched neutrophil extracellular traps are key drivers in COVID-19 immunothrombosis. J Clin Invest. (2020) 130:6151–7. doi: 10.1172/jci141374
17. Xing Y, Jiang Y, Xing S, Mao T, Guan G, Niu Q, et al. Neutrophil extracellular traps are associated with enhanced procoagulant activity in liver cirrhosis patients with portal vein thrombosis. J Clin Lab Anal. (2022) 36:e24433. doi: 10.1002/jcla.24433
18. Pei S, Xu C, Pei J, Bai R, Peng R, Li T, et al. Lysophosphatidic acid receptor 3 suppress neutrophil extracellular traps production and thrombosis during sepsis. Front Immunol. (2022) 13:844781. doi: 10.3389/fimmu.2022.844781
19. Kawakami T, Yokoyama K, Ikeda T, Nishibata Y, Masuda S, Tomaru U, et al. Presence of neutrophil extracellular traps in superficial venous thrombosis of Behçet’s disease. J Dermatol. (2022) 49:741–5. doi: 10.1111/1346-8138.16391
20. Chen Y, Li X, Lin X, Liang H, Liu X, Zhang X, et al. Complement C5a induces the generation of neutrophil extracellular traps by inhibiting mitochondrial STAT3 to promote the development of arterial thrombosis. Thromb J. (2022) 20:24. doi: 10.1186/s12959-022-00384-0
21. Yaykasli KO, Schauer C, Muñoz LE, Mahajan A, Knopf J, Schett G, et al. Neutrophil extracellular trap-driven occlusive diseases. Cells. (2021) 10:2208. doi: 10.3390/cells10092208
22. Takei H, Araki A, Watanabe H, Ichinose A, Sendo F. Rapid killing of human neutrophils by the potent activator phorbol 12-myristate 13-acetate (PMA) accompanied by changes different from typical apoptosis or necrosis. J Leukoc Biol. (1996) 59:229–40. doi: 10.1002/jlb.59.2.229
23. Boufenzer A, Carrasco K, Jolly L, Brustolin B, Di-Pillo E, Derive M, et al. Potentiation of NETs release is novel characteristic of TREM-1 activation and the pharmacological inhibition of TREM-1 could prevent from the deleterious consequences of NETs release in sepsis. Cell Mol Immunol. (2021) 18:452–60. doi: 10.1038/s41423-020-00591-7
24. Yousefi S, Mihalache C, Kozlowski E, Schmid I, Simon HU. Viable neutrophils release mitochondrial DNA to form neutrophil extracellular traps. Cell Death Dif. (2009) 16:1438–44. doi: 10.1038/cdd.2009.96
25. Madhusoodanan J. Core concept: Role player or cellular rubbish? Biologists debate the function of neutrophil extracellular traps. Proc Nat Acad Sci U.S.A. (2017) 114:13309–11. doi: 10.1073/pnas.1719978115
26. Desai J, Mulay SR, Nakazawa D, Anders HJ. Matters of life and death. How neutrophils die or survive along NET release and is “NETosis” = necroptosis? Cell Mol Life Sci. (2016) 73:2211–9. doi: 10.1007/s00018-016-2195-0
27. Abrams ST, Morton B, Alhamdi Y, Alsabani M, Lane S, Welters ID, et al. A novel assay for neutrophil extracellular trap formation independently predicts disseminated intravascular coagulation and mortality in critically Ill patients. Am J Respir Crit Care Med. (2019) 200:869–80. doi: 10.1164/rccm.201811-2111OC
28. Liu S, Su X, Pan P, Zhang L, Hu Y, Tan H, et al. Neutrophil extracellular traps are indirectly triggered by lipopolysaccharide and contribute to acute lung injury. Sci Rep. (2016) 6:37252. doi: 10.1038/srep37252
29. Mitroulis I, Kambas K, Chrysanthopoulou A, Skendros P, Apostolidou E, Kourtzelis I, et al. Neutrophil extracellular trap formation is associated with IL-1β and autophagy-related signaling in gout. PLoS One. (2011) 6:e29318. doi: 10.1371/journal.pone.0029318
30. Joshi MB, Lad A, Bharath Prasad AS, Balakrishnan A, Ramachandra L, Satyamoorthy K. High glucose modulates IL-6 mediated immune homeostasis through impeding neutrophil extracellular trap formation. FEBS Lett. (2013) 587:2241–6. doi: 10.1016/j.febslet.2013.05.053
31. Martinelli S, Urosevic M, Daryadel A, Oberholzer PA, Baumann C, Fey MF, et al. Induction of genes mediating interferon-dependent extracellular trap formation during neutrophil differentiation. J Biol Chem. (2004) 279:44123–32. doi: 10.1074/jbc.M405883200
32. Yamada M, Gomez JC, Chugh PE, Lowell CA, Dinauer MC, Dittmer DP, et al. Interferon-gamma production by neutrophils during bacterial pneumonia in mice. Am J Respir Crit Care Med. (2011) 183:1391–401. doi: 10.1164/rccm.201004-0592OC
33. Hakkim A, Fuchs TA, Martinez NE, Hess S, Prinz H, Zychlinsky A, et al. Activation of the Raf-MEK-ERK pathway is required for neutrophil extracellular trap formation. Nat Chem Biol. (2011) 7:75–7. doi: 10.1038/nchembio.496
34. Metzler KD, Goosmann C, Lubojemska A, Zychlinsky A, Papayannopoulos V. A myeloperoxidase-containing complex regulates neutrophil elastase release and actin dynamics during NETosis. Cell Rep. (2014) 8:883–96. doi: 10.1016/j.celrep.2014.06.044
35. Wang Y, Wysocka J, Sayegh J, Lee YH, Perlin JR, Leonelli L, et al. Human PAD4 regulates histone arginine methylation levels via demethylimination. Science. (2004) 306:279–83. doi: 10.1126/science.1101400
36. Molinaro R, Yu M, Sausen G, Bichsel CA, Corbo C, Folco EJ, et al. Targeted delivery of protein arginine deiminase-4 inhibitors to limit arterial intimal NETosis and preserve endothelial integrity. Cardiovasc Res. (2021) 117:2652–63. doi: 10.1093/cvr/cvab074
37. Lewis HD, Liddle J, Coote JE, Atkinson SJ, Barker MD, Bax BD, et al. Inhibition of PAD4 activity is sufficient to disrupt mouse and human NET formation. Nat Chem Biol. (2015) 11:189–91. doi: 10.1038/nchembio.1735
38. Li P, Li M, Lindberg MR, Kennett MJ, Xiong N, Wang Y. PAD4 is essential for antibacterial innate immunity mediated by neutrophil extracellular traps. J Exp Med. (2010) 207:1853–62. doi: 10.1084/jem.20100239
39. Martinod K, Demers M, Fuchs TA, Wong SL, Brill A, Gallant M, et al. Neutrophil histone modification by peptidylarginine deiminase 4 is critical for deep vein thrombosis in mice. Proc Natl Acad Sci U.S.A. (2013) 110:8674–9. doi: 10.1073/pnas.1301059110
40. Vlahos R, Stambas J, Bozinovski S, Broughton BR, Drummond GR, Selemidis S. Inhibition of Nox2 oxidase activity ameliorates influenza A virus-induced lung inflammation. PLoS Pathog. (2011) 7:e1001271. doi: 10.1371/journal.ppat.1001271
41. Zuo Y, Yalavarthi S, Shi H, Gockman K, Zuo M, Madison JA, et al. Neutrophil extracellular traps in COVID-19. JCI Insight. (2020) 5:e138999. doi: 10.1172/jci.insight.138999
42. Barnes BJ, Adrover JM, Baxter-Stoltzfus A, Borczuk A, Cools-Lartigue J, Crawford JM, et al. Targeting potential drivers of COVID-19: Neutrophil extracellular traps. J Exp Med. (2020) 217:e20200652. doi: 10.1084/jem.20200652
43. Middleton EA, He XY, Denorme F, Campbell RA, Ng D, Salvatore SP, et al. Neutrophil extracellular traps contribute to immunothrombosis in COVID-19 acute respiratory distress syndrome. Blood. (2020) 136:1169–79. doi: 10.1182/blood.2020007008
44. Claushuis TAM, van der Donk LEH, Luitse AL, van Veen HA, van der Wel NN, van Vught LA, et al. Role of peptidylarginine deiminase 4 in neutrophil extracellular trap formation and host defense during Klebsiella pneumoniae-induced pneumonia-derived sepsis. J Immunol. (2018) 201:1241–52. doi: 10.4049/jimmunol.1800314
45. Kenny EF, Herzig A, Krüger R, Muth A, Mondal S, Thompson PR, et al. Diverse stimuli engage different neutrophil extracellular trap pathways. eLife. (2017) 6:e24437. doi: 10.7554/eLife.24437
46. Chen Q, Zhang L, Li X, Zhuo W. Neutrophil extracellular traps in tumor metastasis: Pathological functions and clinical applications. Cancers. (2021) 13:2832. doi: 10.3390/cancers13112832
47. Bainton DF, Ullyot JL, Farquhar MG. The development of neutrophilic polymorphonuclear leukocytes in human bone marrow. J Exp Med. (1971) 134:907–34. doi: 10.1084/jem.134.4.907
48. Ley K, Hoffman HM, Kubes P, Cassatella MA, Zychlinsky A, Hedrick CC, et al. Neutrophils: New insights and open questions. Sci Immunol. (2018) 3:eaat4579. doi: 10.1126/sciimmunol.aat4579
49. Jing Y, Ding M, Fu J, Xiao Y, Chen X, Zhang Q. Neutrophil extracellular trap from Kawasaki disease alter the biologic responses of PBMC. Biosci Rep. (2020) 40:BSR20200928. doi: 10.1042/bsr20200928
50. Andzinski L, Kasnitz N, Stahnke S, Wu CF, Gereke M, von Köckritz-Blickwede M, et al. Type I IFNs induce anti-tumor polarization of tumor associated neutrophils in mice and human. Int J Cancer. (2016) 138:1982–93. doi: 10.1002/ijc.29945
51. Carestia A, Kaufman T, Schattner M. Platelets: New Bricks in the building of neutrophil extracellular traps. Front Immunol. (2016) 7:271. doi: 10.3389/fimmu.2016.00271
52. Zucoloto AZ, Jenne CN. Platelet-neutrophil interplay: Insights into neutrophil extracellular trap (NET)-driven coagulation in infection. Front Cardiovasc Med. (2019) 6:85. doi: 10.3389/fcvm.2019.00085
53. Kazzaz NM, Sule G, Knight JS. Intercellular interactions as regulators of NETosis. Front Immunol. (2016) 7:453. doi: 10.3389/fimmu.2016.00453
54. Jung CJ, Yeh CY, Hsu RB, Lee CM, Shun CT, Chia JS. Endocarditis pathogen promotes vegetation formation by inducing intravascular neutrophil extracellular traps through activated platelets. Circulation. (2015) 131:571–81. doi: 10.1161/circulationaha.114.011432
55. Jiao Y, Li W, Wang W, Tong X, Xia R, Fan J, et al. Platelet-derived exosomes promote neutrophil extracellular trap formation during septic shock. Crit Care. (2020) 24:380. doi: 10.1186/s13054-020-03082-3
56. Kono M, Matsuhiroya S, Obuchi A, Takahashi T, Imoto S, Kawano S, et al. Deferasirox, an iron-chelating agent, alleviates acute lung inflammation by inhibiting neutrophil activation and extracellular trap formation. J Int Med Res. (2020) 48:300060520951015. doi: 10.1177/0300060520951015
57. Arroyo AB, Fernández-Pérez MP, Del Monte A, Águila S, Méndez R, Hernández-Antolín R, et al. MiR-146a is a pivotal regulator of neutrophil extracellular trap formation promoting thrombosis. Haematologica. (2021) 106:1636–46. doi: 10.3324/haematol.2019.240226
58. Arroyo AB, de Los Reyes-García AM, Rivera-Caravaca JM, Valledor P, García-Barberá N, Roldán V, et al. MiR-146a regulates neutrophil extracellular trap formation that predicts adverse cardiovascular events in patients with atrial fibrillation. Arterioscler Thromb Vasc Biol. (2018) 38:892–902. doi: 10.1161/atvbaha.117.310597
59. Clark SR, Ma AC, Tavener SA, McDonald B, Goodarzi Z, Kelly MM, et al. Platelet TLR4 activates neutrophil extracellular traps to ensnare bacteria in septic blood. Nat Med. (2007) 13:463–9. doi: 10.1038/nm1565
60. Zhou Y, Tao W, Shen F, Du W, Xu Z, Liu Z. The Emerging role of neutrophil extracellular traps in arterial, venous and cancer-associated thrombosis. Front Cardiovasc Med. (2021) 8:786387. doi: 10.3389/fcvm.2021.786387
61. Gautier EL, Shay T, Miller J, Greter M, Jakubzick C, Ivanov S, et al. Gene-expression profiles and transcriptional regulatory pathways that underlie the identity and diversity of mouse tissue macrophages. Nat Immunol. (2012) 13:1118–28. doi: 10.1038/ni.2419
62. van Furth R, Cohn ZA, Hirsch JG, Humphrey JH, Spector WG, Langevoort HL. The mononuclear phagocyte system: A new classification of macrophages, monocytes, and their precursor cells. Bull World Health Organ. (1972) 46:845.–852.
63. Jenkins SJ, Ruckerl D, Cook PC, Jones LH, Finkelman FD, van Rooijen N, et al. Local macrophage proliferation, rather than recruitment from the blood, is a signature of TH2 inflammation. Science. (2011) 332:1284–8. doi: 10.1126/science.1204351
64. Lee C, Ginhoux FJD. Biology of resident tissue macrophages. Development. (2022) 149:dev200270. doi: 10.1242/dev.200270
65. García-Fojeda B, Minutti C, Montero-Fernández C, Stamme C, Casals C. Signaling pathways that mediate alveolar macrophage activation by surfactant protein A and IL-4. Front Physiol. (2022) 13:860262. doi: 10.3389/fimmu.2022.860262
66. Li Q, Huang Z, Wang Q, Gao J, Chen J, Tan H, et al. Targeted immunomodulation therapy for cardiac repair by platelet membrane engineering extracellular vesicles via hitching peripheral monocytes. Biomaterials. (2022) 284:121529. doi: 10.1016/j.biomaterials.2022.121529
67. Halder LD, Abdelfatah MA, Jo EA, Jacobsen ID, Westermann M, Beyersdorf N, et al. Factor H binds to extracellular DNA traps released from human blood monocytes in response to Candida albicans. Front Immunol. (2016) 7:671. doi: 10.3389/fimmu.2016.00671
68. Muñoz-Caro T, Silva LM, Ritter C, Taubert A, Hermosilla C. Besnoitia besnoiti tachyzoites induce monocyte extracellular trap formation. Parasitol Res. (2014) 113:4189–97. doi: 10.1007/s00436-014-4094-3
69. Clark M, Kim J, Etesami N, Shimamoto J, Whalen RV, Martin G, et al. Group a streptococcus prevents mast cell degranulation to promote extracellular trap formation. Frontn Immunol. (2018) 9:327. doi: 10.3389/fimmu.2018.00327
70. Abel J, Goldmann O, Ziegler C, Höltje C, Smeltzer MS, Cheung AL, et al. Staphylococcus aureus evades the extracellular antimicrobial activity of mast cells by promoting its own uptake. J Innate Immun. (2011) 3:495–507. doi: 10.1159/000327714
71. Scheb-Wetzel M, Rohde M, Bravo A, Goldmann O. New insights into the antimicrobial effect of mast cells against Enterococcus faecalis. Infect Immunity. (2014) 82:4496–507. doi: 10.1128/iai.02114-14
72. Nakazawa D, Shida H, Kusunoki Y, Miyoshi A, Nishio S, Tomaru U, et al. The responses of macrophages in interaction with neutrophils that undergo NETosis. J Autoimmunity. (2016) 67:19–28. doi: 10.1016/j.jaut.2015.08.018
73. Wong KW, Jacobs WR Jr. Mycobacterium tuberculosis exploits human interferon γ to stimulate macrophage extracellular trap formation and necrosis. J Infect Dis. (2013) 208:109–19. doi: 10.1093/infdis/jit097
74. Aulik NA, Hellenbrand KM, Czuprynski CJ. Mannheimia haemolytica and its leukotoxin cause macrophage extracellular trap formation by bovine macrophages. Infect Immunity. (2012) 80:1923–33. doi: 10.1128/iai.06120-11
75. Chow OA, von Köckritz-Blickwede M, Bright AT, Hensler ME, Zinkernagel AS, Cogen AL, et al. Statins enhance formation of phagocyte extracellular traps. Cell Host Microbe. (2010) 8:445–54. doi: 10.1016/j.chom.2010.10.005
76. Abrams ST, Zhang N, Manson J, Liu T, Dart C, Baluwa F, et al. Circulating histones are mediators of trauma-associated lung injury. Am J Respir Crit Care Med. (2013) 187:160–9. doi: 10.1164/rccm.201206-1037OC
77. Hofbauer TM, Mangold A, Scherz T, Seidl V, Panzenböck A, Ondracek AS, et al. Neutrophil extracellular traps and fibrocytes in ST-segment elevation myocardial infarction. Basic Res Cardiol. (2019) 114:33. doi: 10.1007/s00395-019-0740-3
78. Hofbauer T, Mangold A, Ondracek A, Panzenböck A, Scherz T, Müller J, et al. Deoxyribonuclease 1 Q222R single nucleotide polymorphism and long-term mortality after acute myocardial infarction. Basic Res Cardiol. (2021) 116:29. doi: 10.1007/s00395-021-00864-w
79. Hirose T, Hamaguchi S, Matsumoto N, Irisawa T, Seki M, Tasaki O, et al. Presence of neutrophil extracellular traps and citrullinated histone H3 in the bloodstream of critically ill patients. PLoS One. (2014) 9:e111755. doi: 10.1371/journal.pone.0111755
80. O’Sullivan KM, Lo CY, Summers SA, Elgass KD, McMillan PJ, Longano A, et al. Renal participation of myeloperoxidase in antineutrophil cytoplasmic antibody (ANCA)-associated glomerulonephritis. Kidney Int. (2015) 88:1030–46. doi: 10.1038/ki.2015.202
81. Pérez D, Muñoz MC, Molina JM, Muñoz-Caro T, Silva LM, Taubert A, et al. Eimeria ninakohlyakimovae induces NADPH oxidase-dependent monocyte extracellular trap formation and upregulates IL-12 and TNF-α, IL-6 and CCL2 gene transcription. Vet Parasitol. (2016) 227:143–50. doi: 10.1016/j.vetpar.2016.07.028
82. Kim SJ, Kim J, Kim B, Lee WW, Liu X, Chang YT, et al. Validation of CDr15 as a new dye for detecting neutrophil extracellular trap. Biochem Biophys Res Commun. (2020) 527:646–53. doi: 10.1016/j.bbrc.2020.04.153
83. Wei M, Wang X, Song Y, Zhu D, Qi D, Jiao S, et al. Inhibition of Peptidyl arginine deiminase 4-dependent neutrophil extracellular trap formation reduces angiotensin II-induced abdominal aortic aneurysm rupture in mice. Front Cardiovasc Med. (2021) 8:676612. doi: 10.3389/fcvm.2021.676612
84. Demyanets S, Stojkovic S, Mauracher L, Kopp C, Wojta J, Thaler J, et al. Surrogate markers of neutrophil extracellular trap formation are associated with ischemic outcomes and platelet activation after peripheral angioplasty and stenting. J Clin Med. (2020) 9:304. doi: 10.3390/jcm9020304
85. Kim T, Gu J, Jung H, Koh Y, Kim I, Kim H, et al. Elevated extracellular trap formation and contact system activation in acute leukemia. J Thromb Thromb. (2018) 46:379–85. doi: 10.1007/s11239-018-1713-3
86. Qaddoori Y, Abrams ST, Mould P, Alhamdi Y, Christmas SE, Wang G, et al. Extracellular histones inhibit complement activation through interacting with complement component 4. J Immunol. (2018) 200:4125–33. doi: 10.4049/jimmunol.1700779
87. Wang H, Sha LL, Ma TT, Zhang LX, Chen M, Zhao MH. Circulating level of neutrophil extracellular traps is not a useful biomarker for assessing disease activity in antineutrophil cytoplasmic antibody-associated vasculitis. PLoS One. (2016) 11:e0148197. doi: 10.1371/journal.pone.0148197
88. Thålin C, Hisada Y, Lundström S, Mackman N, Wallén H. Neutrophil extracellular traps: Villains and targets in arterial, venous, and cancer-associated thrombosis. Arterioscler Thromb Vasc Biol. (2019) 39:1724–38. doi: 10.1161/atvbaha.119.312463
89. Paues Göranson S, Thålin C, Lundström A, Hållström L, Lasselin J, Wallén H, et al. Circulating H3Cit is elevated in a human model of endotoxemia and can be detected bound to microvesicles. Sci Rep. (2018) 8:12641. doi: 10.1038/s41598-018-31013-4
90. Gavillet M, Martinod K, Renella R, Harris C, Shapiro NI, Wagner DD, et al. Flow cytometric assay for direct quantification of neutrophil extracellular traps in blood samples. Am J Hematol. (2015) 90:1155–8. doi: 10.1002/ajh.24185
91. Lee KH, Cavanaugh L, Leung H, Yan F, Ahmadi Z, Chong BH, et al. Quantification of NETs-associated markers by flow cytometry and serum assays in patients with thrombosis and sepsis. Int J Lab Hematol. (2018) 40:392–9. doi: 10.1111/ijlh.12800
92. Gupta S, Chan DW, Zaal KJ, Kaplan MJA. High-throughput real-time imaging technique to quantify netosis and distinguish mechanisms of cell death in human neutrophils. J Immunol. (2018) 200:869–79. doi: 10.4049/jimmunol.1700905
93. Stroun M, Lyautey J, Lederrey C, Olson-Sand A, Anker P. About the possible origin and mechanism of circulating DNA apoptosis and active DNA release. Clin Chim Acta. (2001) 313:139–42. doi: 10.1016/s0009-8981(01)00665-9
94. Korkmaz B, Horwitz MS, Jenne DE, Gauthier F. Neutrophil elastase, proteinase 3, and cathepsin G as therapeutic targets in human diseases. Pharmacol Rev. (2010) 62:726–59. doi: 10.1124/pr.110.002733
95. van der Meer AJ, Kroeze A, Hoogendijk AJ, Soussan AA, Ellen van der Schoot C, Wuillemin WA, et al. Systemic inflammation induces release of cell-free DNA from hematopoietic and parenchymal cells in mice and humans. Blood Adv. (2019) 3:724–8. doi: 10.1182/bloodadvances.2018018895
96. Savchenko AS, Martinod K, Seidman MA, Wong SL, Borissoff JI, Piazza G, et al. Neutrophil extracellular traps form predominantly during the organizing stage of human venous thromboembolism development. J Throm Haemost. (2014) 12:860–70. doi: 10.1111/jth.12571
97. Sobrero M, Montecucco F, Carbone F. Circulating MicroRNAs for Diagnosis of Acute Pulmonary Embolism: Still a Long Way to Go. Biomed Res Int. (2022) 2022:4180215. doi: 10.1155/2022/4180215
98. Kumar DR, Hanlin E, Glurich I, Mazza JJ, Yale SH. Virchow’s contribution to the understanding of thrombosis and cellular biology. Clin Med Res. (2010) 8:168–72. doi: 10.3121/cmr.2009.866
99. Kapoor S, Opneja A, Nayak L. The role of neutrophils in thrombosis. Thromb Res. (2018) 170:87–96. doi: 10.1016/j.thromres.2018.08.005
100. Nakazawa D, Tomaru U, Yamamoto C, Jodo S, Ishizu A. Abundant neutrophil extracellular traps in thrombus of patient with microscopic polyangiitis. Front Immunol. (2012) 3:333. doi: 10.3389/fimmu.2012.00333
101. Brill A, Fuchs TA, Savchenko AS, Thomas GM, Martinod K, De Meyer SF, et al. Neutrophil extracellular traps promote deep vein thrombosis in mice. J Thromb Haemost. (2012) 10:136–44. doi: 10.1111/j.1538-7836.2011.04544.x
102. von Brühl ML, Stark K, Steinhart A, Chandraratne S, Konrad I, Lorenz M, et al. Monocytes, neutrophils, and platelets cooperate to initiate and propagate venous thrombosis in mice in vivo. J Exp Med. (2012) 209:819–35. doi: 10.1084/jem.20112322
103. Diaz JA, Fuchs TA, Jackson TO, Kremer Hovinga JA, Lämmle B, Henke PK, et al. Plasma DNA is Elevated in Patients with Deep Vein Thrombosis. J Vasc Surg Venous Lymphat Disord. (2013) 1:341.e–8.e. doi: 10.1016/j.jvsv.2012.12.002
104. van Montfoort ML, Stephan F, Lauw MN, Hutten BA, Van Mierlo GJ, Solati S, et al. Circulating nucleosomes and neutrophil activation as risk factors for deep vein thrombosis. Arterioscler Thromb Vasc Biol. (2013) 33:147–51. doi: 10.1161/atvbaha.112.300498
105. Di Nisio M, van Es N, Büller HR. Deep vein thrombosis and pulmonary embolism. Lancet. (2016) 388:3060–73. doi: 10.1016/s0140-6736(16)30514-1
106. Arnalich F, Maldifassi MC, Ciria E, Codoceo R, Renart J, Fernández-Capitán C, et al. Plasma levels of mitochondrial and nuclear DNA in patients with massive pulmonary embolism in the emergency department: A prospective cohort study. Crit Care. (2013) 17:R90. doi: 10.1186/cc12735
107. Ząbczyk M, Natorska J, Janion-Sadowska A, Malinowski KP, Janion M, Undas A. Elevated lactate levels in acute pulmonary embolism are associated with prothrombotic fibrin clot properties: Contribution of NETs formation. J Clin Med. (2020) 9:953. doi: 10.3390/jcm9040953
108. Rose F, Hattar K, Gakisch S, Grimminger F, Olschewski H, Seeger W, et al. Increased neutrophil mediator release in patients with pulmonary hypertension–suppression by inhaled iloprost. Thromb Haemost. (2003) 90:1141–9. doi: 10.1160/th03-03-0173
109. de Vries JJ, Hoppenbrouwers T, Martinez-Torres C, Majied R, Özcan B, van Hoek M, et al. Effects of diabetes mellitus on fibrin clot structure and mechanics in a model of acute neutrophil extracellular traps (NETs) formation. Int J Mol Sci. (2020) 21:7107. doi: 10.3390/ijms21197107
110. Cao W, Zhu M, Lee S, Lee S, Kim H, Park B, et al. Modulation of cellular NAD attenuates cancer-associated hypercoagulability and thrombosis via the inhibition of tissue factor and formation of neutrophil extracellular traps. Int J Mol Sci. (2021) 22:12085. doi: 10.3390/ijms222112085
111. Ząbczyk M, Natorska J, Janion-Sadowska A, Metzgier-Gumiela A, Polak M, Plens K, et al. Prothrombotic fibrin clot properties associated with NETs formation characterize acute pulmonary embolism patients with higher mortality risk. Sci Rep. (2020) 10:11433. doi: 10.1038/s41598-020-68375-7
112. Levi M, Thachil J, Iba T, Levy JH. Coagulation abnormalities and thrombosis in patients with COVID-19. Lancet Haematol. (2020) 7:e438–40. doi: 10.1016/s2352-3026(20)30145-9
113. Seitz R, Gürtler L, Schramm W. Thromboinflammation in COVID-19: Can α(2) -macroglobulin help to control the fire? J Thromb Haemost. (2021) 19:351–4. doi: 10.1111/jth.15190
114. Prevel R, Dupont A, Labrouche-Colomer S, Garcia G, Dewitte A, Rauch A, et al. Plasma markers of neutrophil extracellular trap are linked to survival but not to pulmonary embolism in COVID-19-related ARDS patients. Front Immunol. (2022) 13:851497. doi: 10.3389/fimmu.2022.851497
115. Naruko T, Ueda M, Haze K, van der Wal AC, van der Loos CM, Itoh A, et al. Neutrophil infiltration of culprit lesions in acute coronary syndromes. Circulation. (2002) 106:2894–900. doi: 10.1161/01.cir.0000042674.89762.20
116. Rittersma SZ, van der Wal AC, Koch KT, Piek JJ, Henriques JP, Mulder KJ, et al. Plaque instability frequently occurs days or weeks before occlusive coronary thrombosis: A pathological thrombectomy study in primary percutaneous coronary intervention. Circulation. (2005) 111:1160–5. doi: 10.1161/01.Cir.0000157141.00778.Ac
117. de Boer OJ, Li X, Teeling P, Mackaay C, Ploegmakers HJ, van der Loos CM, et al. Neutrophils, neutrophil extracellular traps and interleukin-17 associate with the organisation of thrombi in acute myocardial infarction. Thromb Haemost. (2013) 109:290–7. doi: 10.1160/th12-06-0425
118. Liao YH, Xia N, Zhou SF, Tang TT, Yan XX, Lv BJ, et al. Interleukin-17A contributes to myocardial ischemia/reperfusion injury by regulating cardiomyocyte apoptosis and neutrophil infiltration. J Am Coll Cardiol. (2012) 59:420–9. doi: 10.1016/j.jacc.2011.10.863
119. Mora-Ruíz MD, Blanco-Favela F, Chávez Rueda AK, Legorreta-Haquet MV, Chávez-Sánchez L. Role of interleukin-17 in acute myocardial infarction. Mol Immunol. (2019) 107:71–8. doi: 10.1016/j.molimm.2019.01.008
120. Riegger J, Byrne RA, Joner M, Chandraratne S, Gershlick AH, Ten Berg JM, et al. Histopathological evaluation of thrombus in patients presenting with stent thrombosis. A multicenter European study: A report of the prevention of late stent thrombosis by an interdisciplinary global European effort consortium. Eur Heart J. (2016) 37:1538–49. doi: 10.1093/eurheartj/ehv419
121. La Rocca G, Di Stefano A, Eleuteri E, Anzalone R, Magno F, Corrao S, et al. Oxidative stress induces myeloperoxidase expression in endocardial endothelial cells from patients with chronic heart failure. Basic Res Cardiol. (2009) 104:307–20. doi: 10.1007/s00395-008-0761-9
122. Wang W, Peng W, Ning X. Increased levels of neutrophil extracellular trap remnants in the serum of patients with rheumatoid arthritis. Int J Rheum Dis. (2018) 21:415–21. doi: 10.1111/1756-185x.13226
123. Yazdani HO, Chen HW, Tohme S, Tai S, van der Windt DJ, Loughran P, et al. IL-33 exacerbates liver sterile inflammation by amplifying neutrophil extracellular trap formation. J Hepatol. (2017) 0168-8278:32291–2. doi: 10.1016/j.jhep.2017.09.010
124. Abo-Aly M, George B, Shokri E, Chelvarajan L, El-Helw M, Smyth S, et al. Cangrelor in addition to standard therapy reduces cardiac damage and inflammatory markers in patients with ST-segment elevation myocardial infarction. J Thromb Thromb. (2021) 52:934–40. doi: 10.1007/s11239-020-02345-8
125. Gorog DA, Massberg S. Neutrophil extracellular traps in the infarct-related coronary Artery-a marker or mediator of adverse outcome? Thromb Haemost. (2022). doi: 10.1055/a-1733-9217 [Epub ahead of print].
126. Maugeri N, Campana L, Gavina M, Covino C, De Metrio M, Panciroli C, et al. Activated platelets present high mobility group box 1 to neutrophils, inducing autophagy and promoting the extrusion of neutrophil extracellular traps. J Thromb Haemost. (2014) 12:2074–88. doi: 10.1111/jth.12710
127. Mangold A, Alias S, Scherz T, Hofbauer T, Jakowitsch J, Panzenbock A, et al. Coronary neutrophil extracellular trap burden and deoxyribonuclease activity in ST-elevation acute coronary syndrome are predictors of ST-segment resolution and infarct size. Circ Res. (2015) 116:1182–92. doi: 10.1161/circresaha.116.304944
128. Blasco A, Coronado MJ, Hernández-Terciado F, Martín P, Royuela A, Ramil E, et al. Assessment of neutrophil extracellular traps in coronary thrombus of a case series of patients with COVID-19 and myocardial infarction. JAMA Cardiol. (2020) 6:1–6. doi: 10.1001/jamacardio.2020.7308
129. Helseth R, Shetelig C, Andersen G, Langseth MS, Limalanathan S, Opstad TB, et al. Neutrophil extracellular trap components associate with infarct size, ventricular function, and clinical outcome in STEMI. Med Inflamm. (2019) 2019:7816491. doi: 10.1155/2019/7816491
130. Stakos DA, Kambas K, Konstantinidis T, Mitroulis I, Apostolidou E, Arelaki S, et al. Expression of functional tissue factor by neutrophil extracellular traps in culprit artery of acute myocardial infarction. Eur Heart J. (2015) 36:1405–14. doi: 10.1093/eurheartj/ehv007
131. Kounis NG, Soufras GD, Tsigkas G, Hahalis G. White blood cell counts, leukocyte ratios, and eosinophils as inflammatory markers in patients with coronary artery disease. Clin Appl Thromb Hemost. (2015) 21:139–43. doi: 10.1177/1076029614531449
132. Ramachandra CJA, Ja K, Chua J, Cong S, Shim W, Hausenloy DJ. Myeloperoxidase As a multifaceted target for cardiovascular protection. Antioxid Redox Signal. (2020) 32:1135–49. doi: 10.1089/ars.2019.7971
133. Roeper J. Commentary on “Immler et al. (2021) The voltage-gated potassium channel Kv1.3 regulates neutrophil recruitment during inflammation” Cardiovasc Res 2021 (: Roll over-Kv1.3 ! The function of a voltage-gated potassium channel in leucocytes revealed. Pflugers Arch. (2021) 473:1587–8. doi: 10.1093/cvr/cvab133)
134. Cao Z, Xu J, Song B, Chen L, Sun T, He Y, et al. Deep learning derived automated ASPECTS on non-contrast CT scans of acute ischemic stroke patients. Hum Brain Mapp. (2022) 43:3023–36. doi: 10.1002/hbm.25845
135. Laridan E, Denorme F, Desender L, François O, Andersson T, Deckmyn H, et al. Neutrophil extracellular traps in ischemic stroke thrombi. Ann Neurol. (2017) 82:223–32. doi: 10.1002/ana.24993
136. Essig F, Kollikowski A, Pham M, Solymosi L, Stoll G, Haeusler K, et al. Immunohistological Analysis of neutrophils and neutrophil extracellular traps in human thrombemboli causing acute ischemic stroke. Int J Mol Sci. (2020) 21:7387. doi: 10.3390/ijms21197387
137. Deng J, Zhao F, Zhang Y, Zhou Y, Xu X, Zhang X, et al. Neutrophil extracellular traps increased by hyperglycemia exacerbate ischemic brain damage. Neurosci Lett. (2020) 738:135383. doi: 10.1016/j.neulet.2020.135383
138. Locke M, Longstaff CJT. Extracellular histones inhibit fibrinolysis through noncovalent and covalent interactions with fibrin. Thromb Haemost. (2021) 121:464–76. doi: 10.1055/s-0040-1718760
139. Kim S, Lee JJC. Role of HMGB1 in the interplay between NETosis and thrombosis in ischemic stroke: A review. Cells. (2020) 9:1794. doi: 10.3390/cells9081794
140. Heo J, Nam H, Kim Y, Choi J, Kim B, Kim D, et al. Pathophysiologic and Therapeutic Perspectives Based on Thrombus Histology in Stroke. J Stroke. (2020) 22:64–75. doi: 10.5853/jos.2019.03440
141. Rainer TH, Wong LK, Lam W, Yuen E, Lam NY, Metreweli C, et al. Prognostic use of circulating plasma nucleic acid concentrations in patients with acute stroke. Clin Chem. (2003) 49:562–9. doi: 10.1373/49.4.562
142. Zhou P, Li T, Jin J, Liu Y, Li B, Sun Q, et al. Interactions between neutrophil extracellular traps and activated platelets enhance procoagulant activity in acute stroke patients with ICA occlusion. EBioMedicine. (2020) 53:102671. doi: 10.1016/j.ebiom.2020.102671
143. Geiger S, Holdenrieder S, Stieber P, Hamann GF, Bruening R, Ma J, et al. Nucleosomes in serum of patients with early cerebral stroke. Cerebrovasc Dis. (2006) 21:32–7. doi: 10.1159/000089591
144. Tsai NW, Lin TK, Chen SD, Chang WN, Wang HC, Yang TM, et al. The value of serial plasma nuclear and mitochondrial DNA levels in patients with acute ischemic stroke. Clin Chim Acta. (2011) 412:476–9. doi: 10.1016/j.cca.2010.11.036
145. Vallés J, Lago A, Santos MT, Latorre AM, Tembl JI, Salom JB, et al. Neutrophil extracellular traps are increased in patients with acute ischemic stroke: Prognostic significance. Thromb Haemost. (2017) 117:1919–29. doi: 10.1160/th17-02-0130
146. Cai D, Sun C, Zhang G, Que X, Fujise K, Weintraub NL, et al. A novel mechanism underlying inflammatory smooth muscle phenotype in abdominal aortic aneurysm. Circ Res. (2021) 129:e202–14. doi: 10.1161/circresaha.121.319374
147. Manenti A, Farinetti A, Manco G, Mattioli A. Intraluminal Thrombus and Abdominal Aortic Aneurysm Complications. Ann Vascular Surg. (2022) 83:e11–2. doi: 10.1016/j.avsg.2022.03.007
148. Delbosc S, Alsac JM, Journe C, Louedec L, Castier Y, Bonnaure-Mallet M, et al. Porphyromonas gingivalis participates in pathogenesis of human abdominal aortic aneurysm by neutrophil activation. Proof of concept in rats. PLoS One. (2011) 6:e18679. doi: 10.1371/journal.pone.0018679
149. Meher AK, Spinosa M, Davis JP, Pope N, Laubach VE, Su G, et al. Novel role of IL (Interleukin)-1β in neutrophil extracellular trap formation and abdominal aortic aneurysms. Arterioscler Thromb Vasc Biol. (2018) 38:843–53. doi: 10.1161/atvbaha.117.309897
150. Johnston WF, Salmon M, Su G, Lu G, Stone ML, Zhao Y, et al. Genetic and pharmacologic disruption of interleukin-1β signaling inhibits experimental aortic aneurysm formation. Arterioscler Thromb Vasc Biol. (2013) 33:294–304. doi: 10.1161/atvbaha.112.300432
151. Fernández-Ruiz I. Inflammation: NETs are involved in AAA. Nat Rev Cardiol. (2018) 15:257. doi: 10.1038/nrcardio.2018.28
152. Pabon M, Cheng S, Altin S, Sethi S, Nelson M, Moreau K, et al. Sex differences in peripheral artery disease. Circ Res. (2022) 130(4):496–511. doi: 10.1161/circresaha.121.320702
153. Farkas ÁZ, Farkas VJ, Gubucz I, Szabó L, Bálint K, Tenekedjiev K, et al. Neutrophil extracellular traps in thrombi retrieved during interventional treatment of ischemic arterial diseases. Thromb Res. (2019) 175:46–52. doi: 10.1016/j.thromres.2019.01.006
154. Kremers BMM, Birocchi S, van Oerle R, Zeerleder S, Spronk HMH, Mees BME, et al. Searching for a common thrombo-inflammatory basis in patients with deep vein thrombosis or peripheral artery disease. Front Cardiovasc Med. (2019) 6:33. doi: 10.3389/fcvm.2019.00033
155. Bendapudi P, Hurwitz S, Fry A, Marques M, Waldo S, Li A, et al. Derivation and external validation of the PLASMIC score for rapid assessment of adults with thrombotic microangiopathies: A cohort study. Lancet Haematol. (2017) 4:e157–64. doi: 10.1016/s2352-3026(17)30026-1
156. Fuchs TA, Kremer Hovinga JA, Schatzberg D, Wagner DD, Lämmle B. Circulating DNA and myeloperoxidase indicate disease activity in patients with thrombotic microangiopathies. Blood. (2012) 120:1157–64. doi: 10.1182/blood-2012-02-412197
157. Shahjouei S, Naderi S, Li J, Khan A, Chaudhary D, Farahmand G, et al. Risk of stroke in hospitalized SARS-CoV-2 infected patients: A multinational study. EBioMedicine. (2020) 59:102939. doi: 10.1016/j.ebiom.2020.102939
158. Ackermann M, Verleden SE, Kuehnel M, Haverich A, Welte T, Laenger F, et al. Pulmonary vascular endothelialitis, thrombosis, and angiogenesis in Covid-19. N Engl J Med. (2020) 383:120–8. doi: 10.1056/NEJMoa2015432
159. Kolaczkowska E, Jenne CN, Surewaard BG, Thanabalasuriar A, Lee WY, Sanz MJ, et al. Molecular mechanisms of NET formation and degradation revealed by intravital imaging in the liver vasculature. Nat Commun. (2015) 6:6673. doi: 10.1038/ncomms7673
160. Blanch-Ruiz MA, Ortega-Luna R, Martínez-Cuesta M, Álvarez Á. The Neutrophil secretome as a crucial link between inflammation and thrombosis. Int J Mol Sci. (2021) 22:4170. doi: 10.3390/ijms22084170
161. Ackermann M, Anders HJ, Bilyy R, Bowlin GL, Daniel C, De Lorenzo R, et al. Patients with COVID-19: In the dark-NETs of neutrophils. Cell Death Dif. (2021) 28:3125–39. doi: 10.1038/s41418-021-00805-z
162. Wang J, Zhou Y, Ren B, Zou L, He B, Li M. The role of neutrophil extracellular traps in periodontitis. Front Cell Infect Microbiol. (2021) 11:639144. doi: 10.3389/fcimb.2021.639144
163. Wang Y, Li M, Stadler S, Correll S, Li P, Wang D, et al. Histone hypercitrullination mediates chromatin decondensation and neutrophil extracellular trap formation. J Cell Biol. (2009) 184:205–13. doi: 10.1083/jcb.200806072
164. Kearney PL, Bhatia M, Jones NG, Yuan L, Glascock MC, Catchings KL, et al. Kinetic characterization of protein arginine deiminase 4: A transcriptional corepressor implicated in the onset and progression of rheumatoid arthritis. Biochemistry. (2005) 44:10570–82. doi: 10.1021/bi050292m
165. Luo Y, Knuckley B, Lee YH, Stallcup MR, Thompson PR. A fluoroacetamidine-based inactivator of protein arginine deiminase 4: Design, synthesis, and in vitro and in vivo evaluation. J Am Chem Soc. (2006) 128:1092–3. doi: 10.1021/ja0576233
166. Knight JS, Zhao W, Luo W, Subramanian V, O’Dell AA, Yalavarthi S, et al. Peptidylarginine deiminase inhibition is immunomodulatory and vasculoprotective in murine lupus. J Clin Invest. (2013) 123:2981–93. doi: 10.1172/jci67390
167. Suzuki M, Ikari J, Anazawa R, Tanaka N, Katsumata Y, Shimada A, et al. PAD4 deficiency improves bleomycin-induced neutrophil extracellular traps and fibrosis in mouse lung. Am J Respir Cell Mol Biol. (2020) 63:806–18. doi: 10.1165/rcmb.2019-0433OC
168. Biron BM, Chung CS, O’Brien XM, Chen Y, Reichner JS, Ayala A. Cl-Amidine prevents histone 3 citrullination and neutrophil extracellular trap formation, and improves survival in a murine sepsis model. J Innate Immun. (2017) 9:22–32. doi: 10.1159/000448808
169. Willis VC, Gizinski AM, Banda NK, Causey CP, Knuckley B, Cordova KN, et al. N-α-benzoyl-N5-(2-chloro-1-iminoethyl)-L-ornithine amide, a protein arginine deiminase inhibitor, reduces the severity of murine collagen-induced arthritis. J Immunol. (2011) 186:4396–404. doi: 10.4049/jimmunol.1001620
170. Jiménez-Alcázar M, Rangaswamy C, Panda R, Bitterling J, Simsek YJ, Long AT, et al. Host DNases prevent vascular occlusion by neutrophil extracellular traps. Science. (2017) 358:1202–6. doi: 10.1126/science.aam8897
171. Napirei M, Ricken A, Eulitz D, Knoop H, Mannherz HG. Expression pattern of the deoxyribonuclease 1 gene: Lessons from the Dnase1 knockout mouse. Biochem J. (2004) 380:929–37. doi: 10.1042/bj20040046
172. Sisirak V, Sally B, D’Agati V, Martinez-Ortiz W, Ozcakar ZB, David J, et al. Digestion of chromatin in apoptotic cell microparticles prevents autoimmunity. Cell. (2016) 166:88–101. doi: 10.1016/j.cell.2016.05.034
173. Podolska MJ, Mahajan A, Hahn J, Knopf J, Maueröder C, Petru L, et al. Treatment with DNases rescues hidden neutrophil elastase from aggregated NETs. J Leukoc Biol. (2019) 106:1359–66. doi: 10.1002/jlb.3ab0918-370r
174. Peña-Martínez C, Durán-Laforet V, García-Culebras A, Ostos F, Hernández-Jiménez M, Bravo-Ferrer I, et al. Pharmacological modulation of neutrophil extracellular traps reverses thrombotic stroke tPA (Tissue-Type Plasminogen Activator) resistance. Stroke. (2019) 50:3228–37. doi: 10.1161/strokeaha.119.026848
175. Boettcher M, Meier D, Jiménez-Alcázar M, Eschenburg G, Mietzsch S, Vincent D, et al. Degradation of extracellular DNA by DNase1 significantly reduces testicular damage after testicular torsion in rats. Urology. (2017) 109:.e1–223. doi: 10.1016/j.urology.2017.07.031
176. Shrestha S, Kim SY, Yun YJ, Kim JK, Lee JM, Shin M, et al. Retinoic acid induces hypersegmentation and enhances cytotoxicity of neutrophils against cancer cells. Immunol Lett. (2017) 182:24–9. doi: 10.1016/j.imlet.2017.01.001
177. Ge L, Zhou X, Ji WJ, Lu RY, Zhang Y, Zhang YD, et al. Neutrophil extracellular traps in ischemia-reperfusion injury-induced myocardial no-reflow: Therapeutic potential of DNase-based reperfusion strategy. Am J Physiol Heart Circ Physiol. (2015) 308(5):H500–9. doi: 10.1152/ajpheart.00381.2014
178. Vogel B, Shinagawa H, Hofmann U, Ertl G, Frantz S. Acute DNase1 treatment improves left ventricular remodeling after myocardial infarction by disruption of free chromatin. Basic Res Cardiol. (2015) 110:15. doi: 10.1007/s00395-015-0472-y
179. Mazzolai L, Aboyans V, Ageno W, Agnelli G, Alatri A, Bauersachs R, et al. Diagnosis and management of acute deep vein thrombosis: A joint consensus document from the European society of cardiology working groups of aorta and peripheral vascular diseases and pulmonary circulation and right ventricular function. Eur Heart J. (2018) 39:4208–18. doi: 10.1093/eurheartj/ehx003
180. Neumann FJ, Sousa-Uva M, Ahlsson A, Alfonso F, Banning AP, Benedetto U, et al. 2018 ESC/EACTS Guidelines on myocardial revascularization. Eur Heart J. (2019) 40:87–165. doi: 10.1093/eurheartj/ehy394
181. Mulloy B, Hogwood J, Gray E, Lever R, Page CP. Pharmacology of heparin and related drugs. Pharmacol Rev. (2016) 68:76–141. doi: 10.1124/pr.115.011247
182. Rao NV, Argyle B, Xu X, Reynolds PR, Walenga JM, Prechel M, et al. Low anticoagulant heparin targets multiple sites of inflammation, suppresses heparin-induced thrombocytopenia, and inhibits interaction of RAGE with its ligands. Am J Physiol Cell Physiol. (2010) 299:C97–110. doi: 10.1152/ajpcell.00009.2010
183. Manfredi AA, Rovere-Querini P, D’Angelo A, Maugeri N. Low molecular weight heparins prevent the induction of autophagy of activated neutrophils and the formation of neutrophil extracellular traps. Pharmacol Res. (2017) 123:146–56. doi: 10.1016/j.phrs.2016.08.008
184. Fryer A, Huang YC, Rao G, Jacoby D, Mancilla E, Whorton R, et al. Selective O-desulfation produces nonanticoagulant heparin that retains pharmacological activity in the lung. J Pharmacol Exp Ther. (1997) 282:208–19.
185. Folco EJ, Mawson TL, Vromman A, Bernardes-Souza B, Franck G, Persson O, et al. Neutrophil Extracellular traps induce endothelial cell activation and tissue factor production through interleukin-1α and cathepsin G. Arterioscler Thromb Vasc Biol. (2018) 38:1901–12. doi: 10.1161/atvbaha.118.311150
186. Cheng Z, Abrams ST, Alhamdi Y, Toh J, Yu W, Wang G, et al. circulating histones are major mediators of multiple organ dysfunction syndrome in acute critical illnesses. Crit Care Med. (2019) 47:e677–84. doi: 10.1097/ccm.0000000000003839
187. Longstaff C, Hogwood J, Gray E, Komorowicz E, Varjú I, Varga Z, et al. Neutralisation of the anti-coagulant effects of heparin by histones in blood plasma and purified systems. Thromb Haemost. (2016) 115:591–9. doi: 10.1160/th15-03-0214
188. Rasmuson J, Kenne E, Wahlgren M, Soehnlein O, Lindbom L. Heparinoid sevuparin inhibits Streptococcus-induced vascular leak through neutralizing neutrophil-derived proteins. FASEB J. (2019) 33:10443–52. doi: 10.1096/fj.201900627R
189. Wildhagen KC, García de Frutos P, Reutelingsperger CP, Schrijver R, Aresté C, Ortega-Gómez A, et al. Nonanticoagulant heparin prevents histone-mediated cytotoxicity in vitro and improves survival in sepsis. Blood. (2014) 123:1098–101. doi: 10.1182/blood-2013-07-514984
190. Merza M, Rahman M, Zhang S, Hwaiz R, Regner S, Schmidtchen A, et al. Human thrombin-derived host defense peptides inhibit neutrophil recruitment and tissue injury in severe acute pancreatitis. Am J Physiol Gastrointest Liver Physiol. (2014) 307:G914–21. doi: 10.1152/ajpgi.00237.2014
191. Jenne CN, Wong CH, Zemp FJ, McDonald B, Rahman MM, Forsyth PA, et al. Neutrophils recruited to sites of infection protect from virus challenge by releasing neutrophil extracellular traps. Cell Host Microbe. (2013) 13:169–80. doi: 10.1016/j.chom.2013.01.005
192. Etulain J, Martinod K, Wong SL, Cifuni SM, Schattner M, Wagner DD. P-selectin promotes neutrophil extracellular trap formation in mice. Blood. (2015) 126:242–6. doi: 10.1182/blood-2015-01-624023
193. Caudrillier A, Kessenbrock K, Gilliss BM, Nguyen JX, Marques MB, Monestier M, et al. Platelets induce neutrophil extracellular traps in transfusion-related acute lung injury. J Clin Invest. (2012) 122:2661–71. doi: 10.1172/jci61303
194. Ortiz-Muñoz G, Mallavia B, Bins A, Headley M, Krummel MF, Looney MR. Aspirin-triggered 15-epi-lipoxin A4 regulates neutrophil-platelet aggregation and attenuates acute lung injury in mice. Blood. (2014) 124:2625–34. doi: 10.1182/blood-2014-03-562876
195. Chen J, Shetty S, Zhang P, Gao R, Hu Y, Wang S, et al. Aspirin-triggered resolvin D1 down-regulates inflammatory responses and protects against endotoxin-induced acute kidney injury. Toxicol Appl Pharmacol. (2014) 277:118–23. doi: 10.1016/j.taap.2014.03.017
196. Tilgner J, von Trotha KT, Gombert A, Jacobs MJ, Drechsler M, Döring Y, et al. Aspirin, but not tirofiban displays protective effects in endotoxin induced lung injury. PLoS One. (2016) 11:e0161218. doi: 10.1371/journal.pone.0161218
197. Sayah DM, Mallavia B, Liu F, Ortiz-Muñoz G, Caudrillier A, DerHovanessian A, et al. Neutrophil extracellular traps are pathogenic in primary graft dysfunction after lung transplantation. Am J Respir Crit Care Med. (2015) 191:455–63. doi: 10.1164/rccm.201406-1086OC
198. Lapponi MJ, Carestia A, Landoni VI, Rivadeneyra L, Etulain J, Negrotto S, et al. Regulation of neutrophil extracellular trap formation by anti-inflammatory drugs. J Pharmacol Exp Ther. (2013) 345:430–7. doi: 10.1124/jpet.112.202879
199. Gupta AK, Giaglis S, Hasler P, Hahn S. Efficient neutrophil extracellular trap induction requires mobilization of both intracellular and extracellular calcium pools and is modulated by cyclosporine A. PLoS One. (2014) 9:e97088. doi: 10.1371/journal.pone.0097088
200. Shishikura K, Horiuchi T, Sakata N, Trinh DA, Shirakawa R, Kimura T, et al. Prostaglandin E2 inhibits neutrophil extracellular trap formation through production of cyclic AMP. Br J Pharmacol. (2016) 173:319–31. doi: 10.1111/bph.13373
201. Domingo-Gonzalez R, Martínez-Colón GJ, Smith AJ, Smith CK, Ballinger MN, Xia M, et al. Inhibition of neutrophil extracellular trap formation after stem cell transplant by prostaglandin E2. Am J Respir Crit Care Med. (2016) 193:186–97. doi: 10.1164/rccm.201501-0161OC
202. Helms J, Clere-Jehl R, Bianchini E, Le Borgne P, Burban M, Zobairi F, et al. Thrombomodulin favors leukocyte microvesicle fibrinolytic activity, reduces NETosis and prevents septic shock-induced coagulopathy in rats. Ann Intensive Care. (2017) 7:118. doi: 10.1186/s13613-017-0340-z
203. Healy LD, Puy C, Fernández JA, Mitrugno A, Keshari RS, Taku NA, et al. Activated protein C inhibits neutrophil extracellular trap formation in vitro and activation in vivo. J Biol Chem. (2017) 292:8616–29. doi: 10.1074/jbc.M116.768309
204. Menegazzo L, Scattolini V, Cappellari R, Bonora BM, Albiero M, Bortolozzi M, et al. The antidiabetic drug metformin blunts NETosis in vitro and reduces circulating NETosis biomarkers in vivo. Acta Diabetol. (2018) 55:593–601. doi: 10.1007/s00592-018-1129-8
Keywords: extracellular traps, neutrophil, macrophage, thrombosis, venous
Citation: Han T, Tang H, Lin C, Shen Y, Yan D, Tang X and Guo D (2022) Extracellular traps and the role in thrombosis. Front. Cardiovasc. Med. 9:951670. doi: 10.3389/fcvm.2022.951670
Received: 24 May 2022; Accepted: 05 August 2022;
Published: 25 August 2022.
Edited by:
Nicola J. Mutch, University of Aberdeen, United KingdomReviewed by:
Diana Gorog, Imperial College London, United KingdomSimon Timothy Abrams, University of Liverpool, United Kingdom
Copyright © 2022 Han, Tang, Lin, Shen, Yan, Tang and Guo. This is an open-access article distributed under the terms of the Creative Commons Attribution License (CC BY). The use, distribution or reproduction in other forums is permitted, provided the original author(s) and the copyright owner(s) are credited and that the original publication in this journal is cited, in accordance with accepted academic practice. No use, distribution or reproduction is permitted which does not comply with these terms.
*Correspondence: Xiao Tang, dGFuZ194aWFvQGFsaXl1bi5jb20=; Daqiao Guo, Z3VvLmRhcWlhb0B6cy1ob3NwaXRhbC5zaC5jbg==
†These authors have contributed equally to this work