- 1Postdoctoral Mobile Station of Shandong University of Traditional Chinese Medicine, Shandong University of Traditional Chinese Medicine, Jinan, China
- 2Department of Cardiovascular Disease, Affiliated Hospital of Shandong University of Traditional Chinese Medicine, Jinan, China
- 3Department of Urinary Surgery, No.3 People's Hospital, Jinan, China
- 4Postdoctoral Mobile Station of Wangjing Hospital, Wangjing Hospital, China Academy of Chinese Medicine Sciences, Beijing, China
- 5Section of Integrated Chinese and Western Medicine, Shandong university of Traditional Chinese Medicine, Jinan, China
- 6Department of Geriatrics, Affiliated Hospital of Shandong University of Traditional Chinese Medicine, Jinan, China
Heart failure (HF) is a common cardiovascular disorder and a major cause of mortality and morbidity in older people. The mechanisms underlying HF are still not fully understood, restricting novel therapeutic target discovery and drug development. Besides, few drugs have been shown to improve the survival of HF patients. Increasing evidence suggests that long non-coding RNAs (lncRNAs) serve as a critical regulator of cardiac physiological and pathological processes, regarded as a new target of treatment for HF. lncRNAs are versatile players in the pathogenesis of HF. They can interact with chromatin, protein, RNA, or DNA, thereby modulating chromatin accessibility, gene expressions, and signaling transduction. In this review, we summarized the current knowledge on how lncRNAs involve in HF and categorized them into four aspects based on their biological functions, namely, cardiomyocyte contractility, cardiac hypertrophy, cardiac apoptosis, and myocardial fibrosis. Along with the extensive laboratory data, RNA-based therapeutics achieved great advances in recent years. These indicate that targeting lncRNAs in the treatment of HF may provide new strategies and address the unmet clinical needs.
Introduction
Heart failure (HF) is one of the most common cardiovascular disorders in the elderly, caused by left (or global) ventricular dysfunction, which is manifested by fatigue and dyspnea, often with signs of volume overload. Its prevalence and cost are still rising. In the guidelines for the diagnosis and treatment of HF issued by the European Society of Cardiology (ESC), HF is divided into three types according to the left ventricular ejection fraction (EF), namely, HF with reduced ejection fraction (HFrEF, EF <40%), HF with preserved ejection fraction (HFpEF, EF >50%), and the newly defined HF with mildly reduced ejection fraction (HFmrEF, 40–49%) (1). The American College of Cardiology Foundation/American Heart Association and Japanese Circulation Society/Japanese Heart Failure Society guidelines introduced a category called “HF with improved EF (HFiEF),” which is defined as patients with previous LVEF <35% and a follow-up measurement of LVEF >40% (2). Past decades have seen great achievements made in the treatment of HFrEF. However, seldom has been clinically demonstrated to improve the survival. New insights in the pathogenesis and treatment of HF are warranted (3).
Our understanding regarding the pathogenesis of HF has changed over decades. In the past, HF has been viewed as a hemodynamic disorder. Gradually, evidence unravels that activation of the renin–angiotensin–aldosterone system (RAAS) is independent of the hemodynamics and further gives birth to the neurohormonal hypothesis (4). Now, the prevailing view is that HF is a multisystemic disorder, which involves various organ pathologies, such as renal, pulmonary, and skeletal muscle diseases, as well as obesity (5). Besides, different types of HF share similarities in proposed cellular and molecular mechanisms, such as mitochondrial dysfunction, oxidative stress, cardiomyocyte and extracellular matrix-based stiffening, reduced NO bioavailability, impaired cation channel homeostasis, inflammation, and endoplasmic reticulum stress (6, 7). In the last decades, increasing evidence suggests that long non-coding RNAs (lncRNAs) serve as a critical regulator of cardiac physiological and pathological processes, regarded as a new target of treatment for heart failure. Long non-coding RNAs are transcripts exceeding 200 nucleotides in length without functional protein-coding potential.
In this study, we summarized the current advances made using the involvement of lncRNA in the pathogenesis of HF, as well as its possible clinical applications in diagnosis and treatments.
Molecular and biological functions of lncRNAs
The Human Genome Project prevailed that the human genome compromises about 3.1 billion base pairs with only about 22,300 protein-coding genes, whereas the remaining was considered “junk DNA” (8). Consistent with this, RNA sequencing technology in recent years revealed only 2% of transcribed genomes eventually translated into proteins (9, 10). Apart from protein-coding messenger RNAs (mRNAs), transfer RNA (tRNAs), and ribosomal RNAs (rRNAs), non-coding RNAs (ncRNAs) are classified into long non-coding RNAs (lncRNAs), circular RNAs (circRNAs), small non-coding RNAs (sncRNAs), and PIWI-interacting RNAs (piRNAs) based on their structural features (11, 12).
Among these, lncRNAs are longer than ~200 nucleotides (nt), and their biogenesis is similar to mRNAs. lncRNAs are transcribed by RNA polymerase II (RNAPII) from intergenic, exonic, or distal protein-coding regions of the genome, and they often undergo 3′-polyadenylating, 5′-methyl-guanosine capping, and splicing (13). lncRNAs are versatile gene expression regulators at different levels, and their functions largely depend on interacting partners.
LncRNAs regulate chromatin structure and accessibility
In the nucleus, lncRNAs with negative charge bind to positively charged histone tails resulting in chromatin de-compaction, which may function as a rapid switch of gene expression (14). In addition, lncRNAs directly bind to DNA forming a hybrid structure, termed triple triplexes or R-loops, which influence chromatin accessibility and DNA repair (15, 16). Recently, it was reported that NEAT1 is responsible for the assembly of paraspeckles, a form of nuclear condensates, through liquid–liquid phase separation (17).
LncRNAs modulate transcriptional activity
lncRNA can recruit or guide chromatin modifiers, thus affecting the transcriptional activity, such as epigenetic regulatory enzymes (18). A widely known example is that HOX antisense intergenic RNA (HOTAIR) regulates chromatin dynamics and induces gene silencing via acting as a guide of histone methylase (PRC2) and histone demethylase (LSD1) to the correct genomic loci (19).
Besides, lncRNA can serve as a scaffold or platform at a specific genomic locus for other regulators. For instance, SPRY4-intronic transcript 1 (SPRY4-IT1) scaffolds enhancer of zeste homolog 2 (EZH2) along with LSD1 or DNA methyltransferase 1 (DNMT1) induces downstream gene silencing (20). In addition, lncRNAs compete for binding sites and prevent gene-modifying proteins from acting on the loci of interest, known as decoys.
LncRNAs involve in translational process
In the cytoplasm, lncRNAs can alter mRNA splicing by interacting with ribosomes, competing with endogenous RNAs (CeRNA), and directly bind or sponge microRNAs (21). lncRNA, the antisense transcript of the gene ZNFX1 (ZFAS1), can interact with 40S subunit of ribosome. It is involved in ribosome production and assembly (22). Regarding CeRNA, the antisense transcript for beta-secretase-1 (BACE1-AS) competes with miR-485-5p for binding within the same region in the open reading frame of the BACE1 mRNA (23).
In addition to these functions, lncRNAs can interact with mitochondria and exosomes. lncRNAs can be encoded by mitochondrial DNA and are specifically located in mitochondria affecting mitochondrial functions and the crosstalk of mitochondria with nuclei (24, 25). A previous study found that in gene imprinting, lncRNA directly acts as a signal molecule without translation to protein, which enables a prompt response to stimulation (26). lncRNAs can also be packed into exosomes and delivered to recipient cells (27).
Roles of lncRNAs in the pathogenesis of HF
LncRNAs modulating cardiomyocyte contractility
A critical way for cardiomyocytes to overcome insufficient blood output is to increase their contractility. From another perspective, pathological insults impairing the cardiac contractility are considered a reason for HF.
Rhythmically, heart pumping largely relies on cellular Ca2+ homeostasis and excitation-contraction coupling (28). Under pathological conditions, cardiomyocytes expand contractility by elevating Ca2+ release from the sarcoplasmic reticulum (SR), a convoluted membrane structure serving as an iron critical storage site, into the cytoplasm (29). Cellular Ca2+ dynamics is controlled by two major regulators. One is the ryanodine receptors (RyRs), mediating the calcium release from the SR. The other is sarcoplasmic/endoplasmic reticulum Ca2+ ATPase 2a (SERCA2a), responsible for Ca2+ retrieval back into SR (30).
Several lines of data have revealed that lncRNAs interact with these key Ca2+ regulators, such as zinc finger antisense 1 (ZFAS1), myocardial infarction (MI) associated transcript (Miat), and zinc finger protein 593 antisense RNA (ZNF593-AS). Mechanistically, myocardial infarction induces the expression of ZFAS1, which is controlled by the nuclear factor of activated T-cells C2 (NFATc2). ZFAS1 binds SERCA2a and restricts its activity, thus leading to intracellular Ca2+ overload, abnormal Ca2+ transient in cardiomyocytes, and finally impaired contractile function (31). Besides, it has been identified that Miat jeopardizes cardiomyocyte calcium handling by interfering pan-RNA splicing, reducing the levels of two important calcium releasing and intaking proteins, namely, SERCA2a and RyR2, thereby contributing to contractility damage (32). Contrary to the mentioned lncRNAs, ZNF593-AS enhances the stability of RYR2 mRNA by recruiting HNRNPC (heterogeneous nuclear ribonucleoprotein C [C1/C2]) to the poly-U tracts of RYR2 mRNA, thereby contributing to the improvement of cardiac Ca2+ handling and contractile function (33). In addition, SR is physically and functionally linked with mitochondria. It controls ATP production by Ca2+ transferring and drives mitochondrial dynamics (34). lncRNA cardiomyocyte-enriched non-coding transcript (Caren) preserves cardiac function during pressure overload by suppressing the ataxia telangiectasia mutated (ATM)/DNA damage response (DDR) pathway, thus maintaining mitochondrial biogenesis and function (35). LncHrt modulates the deacetylase activity of sirtuin 2, which enhances the oxidative phosphorylation (OXPHOS) capacity of mitochondria, thereby preserving cardiac metabolic homeostasis and function (36).
In addition, potassium channels are key regulators in maintaining action potential prolongation and cardiac rhythm (37). Abnormal potassium channel expression results in aberrant cardiac currents and even sudden cardiac death (38). One of the major outward currents responsible for potential repolarization is the delayed rectifier potassium current (IK) and is dominated by Kv1.2, a subunit of the voltage-gated shaker channel family (39). An antisense RNA of Kv1.2, known as Kcna2 AS, has been shown to be elevated in cardiomyocyte hypertrophy. This lncRNA is complementary to Kcna2 mRNA, thus repressing its expression and thereby leading to malignant ventricular arrhythmias and HF (40) (Table 1).
LncRNAs regulating cardiac hypertrophy
Cardiomyocytes are terminally differentiated cells, and therefore they cannot expand stroke work by cell division. When the contractility cannot counteract loading conditions, the cardiomyocytes synthesize excessive contractile proteins and increase in size to serve as a compensatory alternation to maintain wall stress and oxygen consumption, termed as cardiac hypertrophy (41). However, this structural change can lead to impaired subcellular organelles for efficient Ca2+ signaling, contraction/relaxation, and energy metabolism, and it collectively results in cardiomyocyte apoptosis, which further triggers inflammation, fibrosis, and extracellular matrix protein aggregation.
Cardiac hypertrophy has been observed in various heart diseases, such as hypertension, myocardial infarction, and valvular disease. Besides, enlarged cardiomyocytes result in ventricular hypertrophy, which increases the risk of heart failure and malignant arrhythmia. Two different types of cardiac hypertrophy have been reported. One is concentric hypertrophy, characterized by lateral growth of individual cardiomyocytes and parallel addition of sarcomeres, and the other is eccentric hypertrophy, characterized by longitudinal cellular growth and addition of sarcomeres in series (42). Mechanisms involved in pathological hypertrophy have been recognized as metabolic reprogramming, mitochondrial dysfunction, fetal gene program, and impaired Ca2+ homeostasis (43).
lncRNA H19 is becoming a hotspot in recent decades, due to its important role in both early postnatal development and various diseases (44, 45). H19 is a highly conserved lncRNA, transcripted from H19-insulin growth factor 2 (IGF2) locus, and rich in muscle (46). In the heart, it is mainly expressed in endothelial cells and is located in both the nucleus and the cytoplasm. Previous studies revealed its important role in various cardiovascular diseases such as ischemic myocardial injuries (47), diabetic cardiomyopathy (48), and calcific aortic valve disease (49). In heart failure, H19 is reported to be upregulated in a transverse aortic constriction model, and it inhibits cardiomyocyte hypertrophy by encoding miR-675, which targets CaMKIIδ (50). Contrary to that, another line of data finds a low expression of H19 in heart failure mice (51). Mechanistically, suppression of H19 disembarrasses the polycomb repressive complex 2 (PRC2). Then, PRC2 increases H3K27 tri-methylation of Tescalcin, followed by GSK activation and calcineurin inhibition, which finally leads to the expression and activation of NFAT, a well-known hypotrophic-promoting factor (51). Similarly, another epigenetic regulatory lncRNA, cardiac-hypertrophy-associated epigenetic regulator (Chaer), directly binds with PRC2, thereby repressing its genomic targeting ability and reducing H3K27me3 levels at the promoter regions of genes involved in cardiac hypertrophy (52). lncRNA antihypertrophic interrelated transcript (Ahit) also represses the H3K27me3 level of a critical hypertrophy-inducer, myocyte enhancer factor 2a (MEF2A). It acts as a scaffold to guide the suppressor of zeste 12 protein homolog (SUZ12) to the promotor of MEF2A, thereby mitigating hypertrophic modifications (53).
In addition to functions within nucleus, several lncRNAs have been shown to serve as the sponge of miRNA in the cytoplasm in HF. It is reported that lncRNA cardiac hypertrophy-related factor (CHRF) acts as an endogenous sponge, thus downregulating miR-489 and thereby elevating the level of myeloid differentiation primary response gene 88 (Myd88) in cardiomyocytes, and finally it contributes to cardiac hypertrophy (54). Plscr4 negatively regulates cardiac hypertrophy by sponging miR-214, which suppresses the expression of Mitofusin 2 (Mfn2) (55) (Table 2).
LncRNAs involving in cardiac apoptosis
Apoptosis is an evolutionarily conserved programmed cell death that participates in embryonic development, tissue homeostasis, and pathological remodeling (56). Cardiac myocytes sustain apoptosis in response to various insults, such as hypoxia, acidosis, oxidative stress, metabolic crisis, β1-adrenergic agonists, and angiotensin II (57, 58). Cardiac myocyte apoptotic is one of the major pathological processes during heart failure. Analyses from postmortem samples reveal a small portion (<1%) of apoptotic cells in failed hearts, which is 10-to 100-fold higher than that of functional healthy control (59, 60). Apoptotic myocyte has also been shown to present in different heart failure animal models, such as ascending aortic constriction and left coronary ligation (61–63). Besides, conditionally activated procaspase-8 resulting in 0.023% of apoptotic myocardial cells generated a lethal dilated cardiomyopathy (64). Therefore, it is believed that low level, but persistent loss, of the cardiac myocyte apoptosis leads to functional limitation and finally decompensated heart failure.
lncRNA cardiac autophagy inhibitory factor (CAIF) directly binds to p53 protein and hence inhibits the activation and expression of myocardin, which suppresses autophagic apoptosis and cardiac dysfunction (65). Another protective lncRNA, myosin heavy-chain-associated RNA transcripts (MHRT), has been shown to inhibit ROS-induced apoptosis and can be used as a prognostic factor of survival in patients with heart failure (66). In diabetic cardiomyopathy, high glucose induces the expression of myocardial infarction-associated transcript (MIAT) and upregulates the death-associated protein kinase 2 (DAPK2) expression by sponging miR-22-3p, which consequently leads to cardiomyocyte apoptosis (67). It is reported that ischemia induces the elevation of sex-determining region Y-box 2 (SOX2) overlapping transcript (SOX2-OT), and hence a lncRNA sponging miR-455-3p exacerbates apoptosis rate, cell oxidative damage, and inflammatory response (68). Another ischemic-related lncRNA is H19, which has been shown to bind miR-877-3p thereby inhibiting mitochondrial apoptosis (69). Besides, lncRNA small nucleolar RNA host gene 1 (Snhg1) exerts anti-apoptotic effects on cardiomyocyte and myocardial infarction (MI) heart, by activating the PI3K/AKT/c-Myc pathway, which in turn enhances the expression of Snhg1, thus forming a positive feedback loop (70). Apart from these, cardiomyocyte mitochondrial dynamic-related lncRNA 1 (CMDL-1) has been shown to interact with dynamin-related protein 1, thereby regulating mitochondrial fission and apoptosis in cardiomyocytes (71) (Table 3).
LncRNAs participating in cardiac fibrosis
Consistent detrimental stimuli contribute to cardiomyocyte cell death and hypertrophic changes, which in turn prompts an inflammatory circumstance, and excessive accumulation of collagen stiffens the ventricles, which further damages the contraction and relaxation activity of the myocardium. This adverse structural remodeling is termed as cardiac fibrosis and is characterized by collagen type I deposition, as well as cardiac fibroblast activation and differentiation into myofibroblasts.
Several types of myocardial fibrosis have been reported based on etiology and pathological features: (1) reactive interstitial fibrosis, (2) replacement fibrosis, (3) infiltrative interstitial fibrosis, and (4) endomyocardial fibrosis (72, 73).
It has been well established that lncRNAs play an important role in myocardial infarction (MI)-induced cardiac fibrosis and HF, such as lncRNA-Safe (74), LncHrt (36), Wisp2 super-enhancer-associated RNA (Wisper) (75), and cardiac fibroblast-associated transcript (Cfast) (76). All of them are enriched in fibroblasts.
lncRNA-Safe elevates in both MI and TGF-β-induced cardiac fibrosis. It acts in cis by controlling the expression of neighboring gene, secreted frizzled related protein 2 (Sfrp2) (74). Wisper enhances the proliferation and survival of fibroblast and extracellular matrix synthesis, by interacting with T-cell intracellular antigen 1 (TIA1)-related protein to regulate the expression of lysyl hydroxylase 2 (75). Cfast competitively suppresses the coactosin-like 1 (COTL1) interaction with TRAP1 (transforming growth factor-β receptor-associated protein 1) and further transdifferentiation of myofibroblasts into cardiac fibroblasts (76).
However, less is known about how pressure overload-associated lncRNAs involve in the pathogenesis of cardiac fibrosis. A recent study revealed that a chromatin-associated lncRNA, lncRNA maternally expressed gene 3 (MEG3), regulates the transcriptional activity of p53 by direct interaction, which further enhances the expression of Mmp-2 and cardiac remodeling (77) (Table 4).
LncRNAs mediating inflammation in HF
In addition, the inflammatory response has been shown to play a vital role in the pathogenesis of ventricular remodeling after MI. Piles of data from both laboratory and clinical aspects identify an inflammatory state, in the circulation and the heart tissue during MI with or without established HF, and have been well reviewed elsewhere (78–80). The CANTOS trial proved anti-cytokine therapy to be a promising approach toward an improved clinical outcome regarding cardiac function in patients with MI (81). However, the details of how lncRNAs mediate cardiac dysfunction remain largely unknown. One recent study revealed that SOX2-OT drives the onset of HF by sponging miR-455-3p, thereby augmenting the level of TNF receptor-associated factor 6 (TRAF6) and the activation of NF-κb signaling (68).
Therapeutic potential of lncRNAs in heart failure
With the expansion of our understanding in lncRNAs and other non-coding RNAs, and their roles in the pathogenesis of cardiac remodeling and heart failure (Figure 1), efforts are taken to focus on their therapeutic potential. Along with the extensive preclinical data, RNA-based therapeutic development has achieved enormous advances in recent years rapidly in the field of biotherapeutics. RNA therapy is termed as an approach using RNA-based molecules to modulate specific proteins and biological pathways to treat diseases (82). It bears several advantages over traditional drugs. It can target every disease-relevant protein or gene theoretically and can be produced much faster than protein and small molecule drugs at lower costs (83). The first RNA aptamer, Pegaptanib, which is an anti-VEGF agent for the treatment of retinal diseases is licensed by the FDA in 2004 (84). After two decades of development, more antisense oligonucleotide drugs have been approved by the FDA for clinical use, such as fomivirsen, eteplirsen, nusinersen, defibrotide, inotersen, mipomersen, golodirsen, and casimersen. Another example of RNA-based therapeutic is the successful use of two mRNA vaccines, namely, tozinameran and elasomeran, which translate to a modified spike protein from COVID-19, thus facilitating a specific immune response in recipients (85). Although great achievements in RNA therapeutics have been made, the drugs to directly modulate lncRNAs' expression are still in infancy. The challenges are that several drawbacks halt the clinical translation of RNA-based therapeutics, including adverse effects, off-target activity, and aspecific delivery (12).
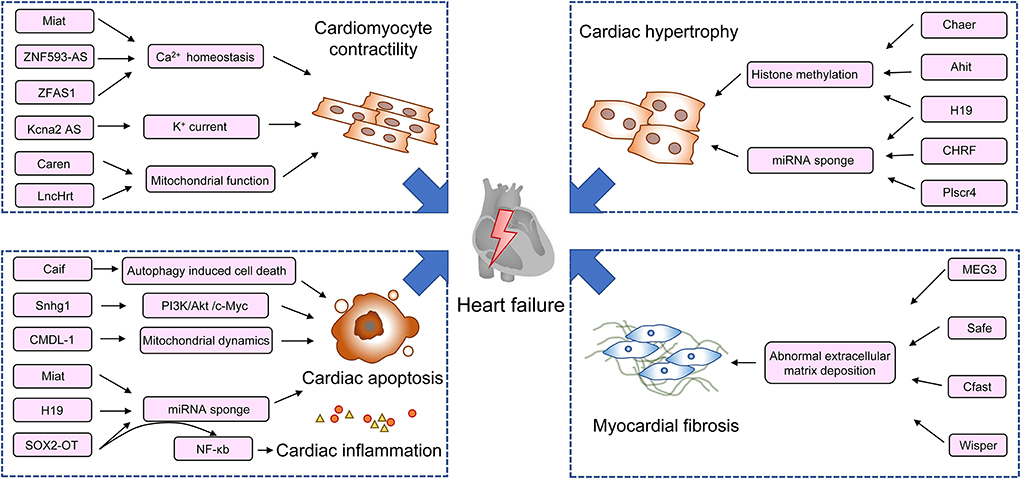
Figure 1. Schematic diagram of lncRNAs in the regulation of pathogenesis of heart failure. Ahit, lncRNA antihypertrophic interrelated transcript; CAIF, lncRNA cardiac autophagy inhibitory factor; Caren, lncRNA cardiomyocyte-enriched non-coding transcript; Cfast, lncRNA cardiac fibroblast-associated transcript; Chaer, cardiac-hypertrophy-associated epigenetic regulator; CHRF, lncRNA cardiac hypertrophy-related factor; CMDL-1, cardiomyocyte mitochondrial dynamic-related lncRNA 1; Drp1, dynamin-related protein 1; DAPK2, death-associated protein kinase 2; Kcna2 AS, potassium voltage-gated channel subfamily A member 2 antisense; MEF2A, myocyte enhancer factor 2a; MEG3, lncRNA maternally expressed gene 3; Miat, lncRNA myocardial infarction-associated transcript; MHRT, lncRNA myosin heavy-chain-associated RNA transcripts; Snhg1, lncRNA small nucleolar RNA host gene 1; SOX2-OT, sex-determining region Y-box 2 overlapping transcript; Wisp2, Wisp2 super-enhancer-associated RNA; ZFAS1, zinc finger antisense 1; ZNF593-AS, zinc finger protein 593 antisense RNA.
The future endeavor would pay on a deeper understanding of lncRNA pathology in heart failure, optimizing specificity and efficiency of the delivery system, and reducing adverse events, so as to fuel the clinical transformation of lncRNAs.
Concluding remarks
Given the versatility of RNA properties, lncRNAs extensively participate in the cardiac physiology and pathological remodeling (Figure 1). Unraveling the details of their regulatory activities will incontestably help seek more potential therapeutic targets to supplement traditional Treatments. In addition, recent years have seen the sprouting up of innovative RNA-based technologies. The successful application of RNA-based therapeutics in the treatment of HF requires interdisciplinary collaboration, which includes chemistry, molecular biology, immunology, and pharmacology. Although challenging, with the development of the state-of-the-art technology, these laboratory efforts will pave the way for the translation of transformative therapies and to achieve accessible and personalized healthcare.
Author contributions
WW, ZC, and LZ participated in the conception of the review. XF and ZZ drafted the initial full manuscript. WW and ZC edited the manuscript. All authors contributed to the article and approved the submitted version.
Funding
This study was supported by the National Key Research and Development Program for Key Research Project of Modernization of Traditional Chinese Medicine (2018YFC1707402) and the Natural Science Foundation of Shandong Province (Grant Number: ZR2021MH410).
Conflict of interest
The authors declare that the research was conducted in the absence of any commercial or financial relationships that could be construed as a potential conflict of interest.
Publisher's note
All claims expressed in this article are solely those of the authors and do not necessarily represent those of their affiliated organizations, or those of the publisher, the editors and the reviewers. Any product that may be evaluated in this article, or claim that may be made by its manufacturer, is not guaranteed or endorsed by the publisher.
References
1. McDonagh TA, Metra M, Adamo M, Gardner RS, Baumbach A, Böhm M, et al. ESC Guidelines for the diagnosis and treatment of acute and chronic heart failure. Eur Heart J. (2021) 42:3599–726. doi: 10.1093/eurheartj/ehab368
2. Gulati G, Udelson JE. Heart failure with improved ejection fraction: is it possible to escape one's past? JACC Heart Fail. (2018) 6:725–33. doi: 10.1016/j.jchf.2018.05.004
3. Van Linthout S, Tschöpe C. Inflammation - cause or consequence of heart failure or both? Curr Heart Fail Rep. (2017) 14:251–65. doi: 10.1007/s11897-017-0337-9
4. Packer M. The neurohormonal hypothesis: a theory to explain the mechanism of disease progression in heart failure. J Am Coll Cardiol. (1992) 20:248–54. doi: 10.1016/0735-1097(92)90167-L
5. Mishra S, Kass DA. Cellular and molecular pathobiology of heart failure with preserved ejection fraction. Nat Rev Cardiol. (2021) 18:400–23. doi: 10.1038/s41569-020-00480-6
6. Jia G, Hill MA, Sowers JR. Diabetic cardiomyopathy: an update of mechanisms contributing to this clinical entity. Circ Res. (2018) 122:624–38. doi: 10.1161/CIRCRESAHA.117.311586
7. Paulus WJ. Unfolding discoveries in heart failure. N Engl J Med. (2020) 382:679–82. doi: 10.1056/NEJMcibr1913825
8. Collins FS, Morgan M, Patrinos A. The human genome project: lessons from large-scale biology. Science. (2003) 300:286–29. doi: 10.1126/science.1084564
9. Hon C, Ramilowski JA, Harshbarger J, Bertin N, Rackham OJ, et al. An atlas of human long non-coding RNAs with accurate 5′ ends. Nature. (2017) 543:199–204. doi: 10.1038/nature21374
10. Zhao L, Wang J, Li Y, Song T, Wu Y, Fang S, et al. NONCODEV6: an updated database dedicated to long non-coding RNA annotation in both animals and plants. Nucleic Acids Res. (2021) 49:D165–71. doi: 10.1093/nar/gkaa1046
11. Anastasiadou E, Jacob LS, Slack FJ. Non-coding RNA networks in cancer. Nat Rev Cancer. (2018) 18:5–18. doi: 10.1038/nrc.2017.99
12. Winkle M, El-Daly SM, Fabbri M, Calin GA. Noncoding RNA therapeutics—challenges and potential solutions. Nature Rev Drug Discovery. (2021) 20:629–51. doi: 10.1038/s41573-021-00219-z
13. Dhanoa JK, Sethi RS, Verma R, Arora JS, Mukhopadhyay CS. Long non-coding RNA: its evolutionary relics and biological implications in mammals: a review. J Anim Sci Technol. (2018) 60:25. doi: 10.1186/s40781-018-0183-7
14. Dueva R, Akopyan K, Pederiva C, Trevisan D, Dhanjal S, Lindqvist A, et al. Neutralization of the positive charges on histone tails by RNA promotes an open chromatin structure. Cell Chem Biol. (2019) 26:1436–49.e1435. doi: 10.1016/j.chembiol.2019.08.002
15. Michelini F, Pitchiaya S, Vitelli V, Sharma S, Gioia U, Pessina F, et al. Damage-induced lncRNAs control the DNA damage response through interaction with DDRNAs at individual double-strand breaks. Nat Cell Biol. (2017) 19:1400–11. doi: 10.1038/ncb3643
16. Niehrs C, Luke B. Regulatory R-loops as facilitators of gene expression and genome stability. Nat Rev Mol Cell Biol. (2020) 21:167–78. doi: 10.1038/s41580-019-0206-3
17. Yamazaki T, Souquere S, Chujo T, Kobelke S, Chong YS, Fox AH, et al. Functional Domains of NEAT1 Architectural lncRNA Induce Paraspeckle Assembly through Phase Separation. Mol Cell. (2018) 70:1038–53.e1037 doi: 10.1016/j.molcel.2018.05.019
18. Morlando M, Fatica A. Alteration of epigenetic regulation by long noncoding RNAs in cancer. Int J Mol Sci. (2018) 19: 570. doi: 10.3390/ijms19020570
19. Bhan A, Mandal SS. LncRNA HOTAIR: a master regulator of chromatin dynamics and cancer. Biochim Biophys Acta. (2015) 1856:151–64. doi: 10.1016/j.bbcan.2015.07.001
20. Xu Y, Yao Y, Jiang X, Zhong X, Wang Z, Li C, et al. SP1.-induced upregulation of lncRNA SPRY4-IT1 exerts oncogenic properties by scaffolding EZH2/LSD1/DNMT1 and sponging miR-101-3p in cholangiocarcinoma. J Exp Clin Cancer Res. (2018) 37:81. doi: 10.1186/s13046-018-0747-x
21. Rashid F, Shah A, Shan G. Long Non-coding RNAs in the Cytoplasm. Genomics Proteomics Bioinformatics. (2016) 14:73–80. doi: 10.1016/j.gpb.2016.03.005
22. Hansji H, Leung EY, Baguley BC, Finlay GJ, Cameron-Smith D, Figueiredo VC, et al. ZFAS1: a long noncoding RNA associated with ribosomes in breast cancer cells. Biol Direct. (2016) 11:62. doi: 10.1186/s13062-016-0165-y
23. Faghihi MA, Zhang M, Huang J, Modarresi F, Van der Brug MP, Nalls MA, et al. Evidence for natural antisense transcript-mediated inhibition of microRNA function. Genome Biol. (2010) 11:R56. doi: 10.1186/gb-2010-11-5-r56
24. Leucci E, Vendramin R, Spinazzi M, Laurette P, Fiers M, Wouters J, et al. Melanoma addiction to the long non-coding RNA SAMMSON. Nature. (2016) 531:518–22. doi: 10.1038/nature17161
25. Vendramin R, Verheyden Y, Ishikawa H, Goedert L, Nicolas E, Saraf K, et al. SAMMSON fosters cancer cell fitness by concertedly enhancing mitochondrial and cytosolic translation. Nat Struct Mol Biol. (2018) 25:1035–46. doi: 10.1038/s41594-018-0143-4
26. Mohammad F, Mondal T, Kanduri C. Epigenetics of imprinted long noncoding RNAs. Epigenetics. (2009) 4:277–86. doi: 10.4161/epi.4.5.9242
27. Dragomir M, Chen B, Calin GA. Exosomal lncRNAs as new players in cell-to-cell communication. Transl Cancer Res. (2018) 7:S243–s252. doi: 10.21037/tcr.2017.10.46
28. Cortassa S, Juhaszova M, Aon MA, Zorov DB, Sollott SJ. Mitochondrial Ca2+, redox environment and ROS emission in heart failure: two sides of the same coin? J Mol Cell Cardiol. (2021) 151:113–25. doi: 10.1016/j.yjmcc.2020.11.013
29. Denniss AL, Dashwood AM, Molenaar P, Beard NA. Sarcoplasmic reticulum calcium mishandling: central tenet in heart failure? Biophys Rev. (2020) 12:865–78. doi: 10.1007/s12551-020-00736-y
30. Rossini M, Filadi R. Sarcoplasmic reticulum-mitochondria kissing in cardiomyocytes: Ca2+, ATP, and undisclosed secrets. Front Cell Dev Biol. (2020) 8:532. doi: 10.3389/fcell.2020.00532
31. Zhang Y, Jiao L, Sun L, Li Y, Gao Y, Xu C, et al. LncRNA ZFAS1 as a SERCA2a inhibitor to cause intracellular Ca2+ overload and contractile dysfunction in a mouse model of myocardial infarction. Circ Res. (2018) 122:1354–68. doi: 10.1161/CIRCRESAHA.117.312117
32. Yang L, Deng J, Ma W, Qiao A, Xu S, Yu Y, et al. Ablation of lncRNA Miat attenuates pathological hypertrophy and heart failure. Theranostics. (2021) 11:7995–8007. doi: 10.7150/thno.50990
33. Fan J, Li H, Xie R, Zhang X, Nie X, Shi X, et al. LncRNA ZNF593-AS alleviates contractile dysfunction in dilated cardiomyopathy. Circ Res. (2021) 128:1708–23. doi: 10.1161/CIRCRESAHA.120.318437
34. Lopez-Crisosto C, Pennanen C, Vasquez-Trincado C, Morales PE, Bravo-Sagua R, Quest AFG, et al. Sarcoplasmic reticulum-mitochondria communication in cardiovascular pathophysiology. Nat Rev Cardiol. (2017) 14:342–60. doi: 10.1038/nrcardio.2017.23
35. Sato M, Kadomatsu T, Miyata K, Warren JS, Tian Z, Zhu S, et al. The lncRNA Caren antagonizes heart failure by inactivating DNA damage response and activating mitochondrial biogenesis. Nat Commun. (2021) 12:2529. doi: 10.1038/s41467-021-22735-7
36. Liu N, Kataoka M, Wang Y, Pu L, Dong X, Fu X, et al. LncRNA LncHrt preserves cardiac metabolic homeostasis and heart function by modulating the LKB1-AMPK signaling pathway. Basic Res Cardiol. (2021) 116:48. doi: 10.1007/s00395-021-00887-3
37. Burg S, Attali B. Targeting of potassium channels in cardiac arrhythmias. Trends Pharmacol Sci. (2021) 42:491–506. doi: 10.1016/j.tips.2021.03.005
38. Walsh KB. Targeting cardiac potassium channels for state-of-the-art drug discovery. Expert Opin Drug Discov. (2015) 10:157–69. doi: 10.1517/17460441.2015.983471
39. Jost N, Papp JG, Varró A. Slow delayed rectifier potassium current (IKs) and the repolarization reserve. Ann Noninvasive Electrocardiol. (2007) 12:64–78. doi: 10.1111/j.1542-474X.2007.00140.x
40. Long QQ, Wang H, Gao W, Fan Y, Li YF, Ma Y, et al. Long noncoding RNA Kcna2 antisense RNA contributes to ventricular arrhythmias via silencing Kcna2 in rats with congestive heart failure. J Am Heart Assoc. (2017) 6:e005965. doi: 10.1161/JAHA.117.005965
41. Shimizu I, Minamino T. Physiological and pathological cardiac hypertrophy. J Mol Cell Cardiol. (2016) 97:245–62. doi: 10.1016/j.yjmcc.2016.06.001
42. Dorn GW, Robbins J, Sugden PH. “Phenotyping hypertrophy: eschew obfuscation” Am Heart Assoc. (2003). doi: 10.1161/01.RES.0000077012.11088.BC
43. Nakamura M, Sadoshima J. Mechanisms of physiological and pathological cardiac hypertrophy. Nature Reviews Cardiol. (2018) 15:387–407. doi: 10.1038/s41569-018-0007-y
44. Lecerf C, Le Bourhis X, Adriaenssens E. The long non-coding RNA H19: an active player with multiple facets to sustain the hallmarks of cancer. Cell Mol Life Sci. (2019) 76:4673–87. doi: 10.1007/s00018-019-03240-z
45. Ghafouri-Fard S, Esmaeili M, Taheri M. H19. lncRNA: Roles in tumorigenesis. Biomed Pharmacother. (2020) 123:109774. doi: 10.1016/j.biopha.2019.109774
46. Ratajczak MZ. Igf2-H19, an imprinted tandem gene, is an important regulator of embryonic development, a guardian of proliferation of adult pluripotent stem cells, a regulator of longevity, and a 'passkey' to cancerogenesis. Folia Histochem Cytobiol. (2012) 50:171–9. doi: 10.5603/FHC.2012.0026
47. Luo H, Wang J, Liu D, Zang S, Ma N, Zhao L, et al. The lncRNA H19/miR-675 axis regulates myocardial ischemic and reperfusion injury by targeting PPARα. Mol Immunol. (2019) 105:46–54. doi: 10.1016/j.molimm.2018.11.011
48. Li X, Wang H, Yao B, Xu W, Chen J, Zhou X, et al. lncRNA H19/miR-675 axis regulates cardiomyocyte apoptosis by targeting VDAC1 in diabetic cardiomyopathy. Sci Rep. (2016) 6:36340. doi: 10.1038/srep36340
49. Hadji F, Boulanger MC, Guay SP, Gaudreault N, Amellah S, Mkannez G, et al. Altered DNA methylation of long noncoding RNA H19 in calcific aortic valve disease promotes mineralization by silencing NOTCH1. Circulation. (2016) 134:1848–62. doi: 10.1161/CIRCULATIONAHA.116.023116
50. Liu L, An X, Li Z, Song Y, Li L, Zuo S, et al. The H19 long noncoding RNA is a novel negative regulator of cardiomyocyte hypertrophy. Cardiovasc Res. (2016) 111:56–65. doi: 10.1093/cvr/cvw078
51. Viereck J, Bührke A, Foinquinos A, Chatterjee S, Kleeberger JA, Xiao K, et al. Targeting muscle-enriched long non-coding RNA H19 reverses pathological cardiac hypertrophy. Eur Heart J. (2020) 41:3462–74. doi: 10.1093/eurheartj/ehaa519
52. Wang Z, Zhang XJ, Ji YX, Zhang P, Deng KQ, Gong J, et al. The long noncoding RNA Chaer defines an epigenetic checkpoint in cardiac hypertrophy. Nat Med. (2016) 22:1131–9. doi: 10.1038/nm.4179
53. Yu J, Yang Y, Xu Z, Lan C, Chen C, Li C, et al. Long Noncoding RNA Ahit protects against cardiac hypertrophy through SUZ12 (suppressor of Zeste 12 protein homolog)-mediated downregulation of MEF2A (myocyte enhancer factor 2A). Circ Heart Fail. (2020) 13:e006525. doi: 10.1161/CIRCHEARTFAILURE.119.006525
54. Wang K, Liu F, Zhou LY, Long B, Yuan SM, Wang Y, et al. The long noncoding RNA CHRF regulates cardiac hypertrophy by targeting miR-489. Circ Res. (2014) 114:1377–88. doi: 10.1161/CIRCRESAHA.114.302476
55. Lv L, Li T, Li X, Xu C, Liu Q, Jiang H, et al. The lncRNA Plscr4 controls cardiac hypertrophy by regulating miR-214. Mol Ther Nucleic Acids. (2018) 10:387–97. doi: 10.1016/j.omtn.2017.12.018
56. D'Arcy MS. Cell death: a review of the major forms of apoptosis, necrosis and autophagy. Cell Biol Int. (2019) 43:582–92. doi: 10.1002/cbin.11137
57. Del Re DP, Amgalan D, Linkermann A, Liu Q, Kitsis RN. Fundamental mechanisms of regulated cell death and implications for heart disease. Physiol Rev. (2019) 99:1765–817. doi: 10.1152/physrev.00022.2018
58. Nair N. Epidemiology and pathogenesis of heart failure with preserved ejection fraction. Rev Cardiovasc Med. (2020) 21:531–40. doi: 10.31083/j.rcm.2020.04.154
59. Olivetti G, Abbi R, Quaini F, Kajstura J, Cheng W, Nitahara JA, et al. Apoptosis in the failing human heart. New EngJ Med. (1997) 336:1131–41. doi: 10.1056/NEJM199704173361603
60. Saraste A, Pulkki K, Kallajoki M, Heikkilä P, Laine P, Mattila S, et al. Cardiomyocyte apoptosis and progression of heart failure to transplantation. Eur J Clin Invest. (1999) 29:380–6. doi: 10.1046/j.1365-2362.1999.00481.x
61. Bae S, Siu PM, Choudhury S, Ke Q, Choi JH, Koh YY, et al. Delayed activation of caspase-independent apoptosis during heart failure in transgenic mice overexpressing caspase inhibitor CrmA. Am J Physiol Heart Circ Physiol. (2010) 299:H1374–81. doi: 10.1152/ajpheart.00168.2010
62. Wang K, Long B, Liu F, Wang JX, Liu CY, Zhao B, et al. A circular RNA protects the heart from pathological hypertrophy and heart failure by targeting miR-223. Eur Heart J. (2016) 37:2602–11. doi: 10.1093/eurheartj/ehv713
63. Yao Y, Lu Q, Hu Z, Yu Y, Chen Q, Wang QK, et al. A non-canonical pathway regulates ER stress signaling and blocks ER stress-induced apoptosis and heart failure. Nat Commun. (2017) 8:133. doi: 10.1038/s41467-017-00171-w
64. Wencker D, Chandra M, Nguyen K, Miao W, Garantziotis S, Factor SM, et al. A mechanistic role for cardiac myocyte apoptosis in heart failure. J Clin Invest. (2003) 111:1497–504. doi: 10.1172/JCI17664
65. Liu CY, Zhang YH, Li RB, Zhou LY, et al. LncRNA CAIF inhibits autophagy and attenuates myocardial infarction by blocking p53-mediated myocardin transcription. Nature commun. (2018) 9:1–12. doi: 10.1038/s41467-017-02280-y
66. Zhang L, Wu YJ, Zhang SL. Circulating lncRNA MHRT predicts survival of patients with chronic heart failure. J Geriatr Cardiol. (2019) 16:818–21. doi: 10.11909/j.issn.1671-5411.2019.11.006
67. Zhou X, Zhang W, Jin M, Chen J, Xu W, Kong X, et al. lncRNA MIAT functions as a competing endogenous RNA to upregulate DAPK2 by sponging miR-22-3p in diabetic cardiomyopathy. Cell Death Dis. (2017) 8:1–8. doi: 10.1038/cddis.2017.321
68. Gu Q, Wang B, Zhao H, Wang W, Wang P, Deng Y, et al. LncRNA promoted inflammatory response in ischemic heart failure through regulation of miR-455-3p/TRAF6 axis. Inflammation Res. (2020) 69:667–81. doi: 10.1007/s00011-020-01348-8
69. Li X, Luo S, Zhang J, Yuan Y, Jiang W, Zhu H, et al. lncRNA H19 alleviated myocardial I/RI via suppressing miR-877-3p/Bcl-2-mediated mitochondrial apoptosis. Molecular Therapy-Nucleic Acids. (2019) 17:297–309. doi: 10.1016/j.omtn.2019.05.031
70. Li M. LncRNA Snhg1-driven self-reinforcing regulatory network promoted cardiac regeneration and repair after myocardial infarction. Theranostics. (2021) 11:9397. doi: 10.7150/thno.57037
71. Chen X, Yu H, Li Z, Ye W, Liu Z, Gao J, et al. (2021). Cardiomyocyte mitochondrial dynamic-related lncRNA 1 (CMDL-1) may serve as a potential therapeutic target in doxorubicin cardiotoxicity. Mol Ther Nucleic Acid. (2021) 25:638–51. doi: 10.1016/j.omtn.2021.08.006
72. Tian J, An X, Niu L. Myocardial fibrosis in congenital and pediatric heart disease. Exp Ther Med. (2017) 13:1660–4. doi: 10.3892/etm.2017.4224
73. Hinderer S, Schenke-Layland K. Cardiac fibrosis - a short review of causes and therapeutic strategies. Adv Drug Deliv Rev. (2019) 146:77–82. doi: 10.1016/j.addr.2019.05.011
74. Hao K, Lei W, Wu H, Wu J, Yang Z, Yan S, et al. LncRNA-Safe contributes to cardiac fibrosis through Safe-Sfrp2-HuR complex in mouse myocardial infarction. Theranostics. (2019) 9:7282–97. doi: 10.7150/thno.33920
75. Micheletti R, Plaisance I, Abraham BJ, Sarre A, Ting CC, Alexanian M, et al. The long noncoding RNA Wisper controls cardiac fibrosis and remodeling. Sci Transl Med. (2017) 9:eaai9118. doi: 10.1126/scitranslmed.aai9118
76. Zhang F, Fu X, Kataoka M, Liu N, Wang Y, Gao F, et al. Long noncoding RNA Cfast regulates cardiac fibrosis. Mol Ther Nucleic Acids. (2021) 23:377–92. doi: 10.1016/j.omtn.2020.11.013
77. Piccoli MT, Gupta SK, Viereck J, Foinquinos A, Samolovac S, Kramer FL, et al. Inhibition of the cardiac fibroblast-enriched lncRNA Meg3 prevents cardiac fibrosis and diastolic dysfunction. Circ Res. (2017) 121:575–83. doi: 10.1161/CIRCRESAHA.117.310624
78. Briasoulis A, Androulakis E, Christophides T, Tousoulis D. The role of inflammation and cell death in the pathogenesis, progression and treatment of heart failure. Heart Fail Rev. (2016) 21:169–76. doi: 10.1007/s10741-016-9533-z
79. Zhang Y, Bauersachs J, Langer HF. Immune mechanisms in heart failure. Eur J Heart Fail. (2017) 19:1379–89. doi: 10.1002/ejhf.942
80. Adamo L, Rocha-Resende C, Prabhu SD, Mann DL. Reappraising the role of inflammation in heart failure. Nat Rev Cardiol. (2020) 17:269–85. doi: 10.1038/s41569-019-0315-x
81. Ridker PM, Everett BM, Thuren T, MacFadyen JG, Chang WH, Ballantyne C, et al. Antiinflammatory therapy with canakinumab for atherosclerotic disease. N Engl J Med. (2017) 377:1119–31. doi: 10.1056/NEJMoa1707914
82. Damase TR, Sukhovershin R, Boada C, Taraballi F, Pettigrew RI, Cooke JP, et al. The limitless future of RNA therapeutics. Front Bioengineering Biotechnol. (2021) 161:628137. doi: 10.3389/fbioe.2021.628137
83. Kaczmarek JC, Kowalski PS, Anderson DG. Advances in the delivery of RNA therapeutics: from concept to clinical reality. Genome Med. (2017) 9:60. doi: 10.1186/s13073-017-0450-0
84. Yu AM, Tu MJ. Deliver the promise: RNAs as a new class of molecular entities for therapy and vaccination. Pharmacol Ther. (2021) 230:107967 doi: 10.1016/j.pharmthera.2021.107967
Keywords: heart failure, long non-coding RNA, pathogenesis, cardiac remodeling, cardiac hypertrophy
Citation: Fan X, Zhang Z, Zheng L, Wei W and Chen Z (2022) Long non-coding RNAs in the pathogenesis of heart failure: A literature review. Front. Cardiovasc. Med. 9:950284. doi: 10.3389/fcvm.2022.950284
Received: 22 May 2022; Accepted: 11 July 2022;
Published: 03 August 2022.
Edited by:
Matteo Pagnesi, ASST Spedali Civili di Brescia, ItalyReviewed by:
Vincenzo Castiglione, Sant'Anna School of Advanced Studies, ItalyEmma Louise Robinson, University of Colorado, United States
Copyright © 2022 Fan, Zhang, Zheng, Wei and Chen. This is an open-access article distributed under the terms of the Creative Commons Attribution License (CC BY). The use, distribution or reproduction in other forums is permitted, provided the original author(s) and the copyright owner(s) are credited and that the original publication in this journal is cited, in accordance with accepted academic practice. No use, distribution or reproduction is permitted which does not comply with these terms.
*Correspondence: Wei Wei, 869641942@qq.com; Zetao Chen, zetaochen2007@126.com