- Department of Cardiology, Nanfang Hospital, Southern Medical University, Guangzhou, China
With growing evidence in clinical practice, the understanding of coronary syndromes has gradually evolved out of focusing on the well-established link between stenosis of epicardial coronary artery and myocardial ischemia to the structural and functional abnormalities at the level of coronary microcirculation, known as coronary microvascular dysfunction (CMD). CMD encompasses several pathophysiological mechanisms of coronary microcirculation and is considered as an important cause of myocardial ischemia in patients with angina symptoms without obstructive coronary artery disease (CAD). As a result of growing knowledge of the understanding of CMD assessed by multiple non-invasive modalities, CMD has also been found to be involved in other cardiovascular diseases, including primary cardiomyopathies as well as heart failure with preserved ejection fraction (HFpEF). In the past 2 decades, almost all the imaging modalities have been used to non-invasively quantify myocardial blood flow (MBF) and promote a better understanding of CMD. Myocardial contrast echocardiography (MCE) is a breakthrough as a non-invasive technique, which enables assessment of myocardial perfusion and quantification of MBF, exhibiting promising diagnostic performances that were comparable to other non-invasive techniques. With unique advantages over other non-invasive techniques, MCE has gradually developed into a novel modality for assessment of the coronary microvasculature, which may provide novel insights into the pathophysiological role of CMD in different clinical conditions. Moreover, the sonothrombolysis and the application of artificial intelligence (AI) will offer the opportunity to extend the use of contrast ultrasound theragnostics.
Introduction
It has been gradually recognized that coronary microvascular dysfunction (CMD) is emerging as a major cause of myocardial ischemia in patients with angina symptoms but in the absence of obstructive coronary artery disease (CAD) as well as in other clinical conditions, including obstructive CAD, primary cardiomyopathies, heart failure with preserved ejection fraction (HFpEF) (1, 2). CMD refers to the spectrum of structural and functional abnormalities in coronary microcirculation, resulting in a blunted coronary blood flow (CBF) and coronary flow reserve (CFR), which can be detected by several invasive or non-invasive methods (3). With the advent and development of current imaging modalities, there is growing evidence in diagnosing and treating patients involved with CMD, making it possible to advance the understanding of the role of CMD across different cardiovascular diseases (4–6).
Despite currently positron emission tomography (PET) is the most used tool for quantitative assessment of the coronary microvasculature, many other techniques including cardiac magnetic resonance (CMR), computed tomography angiography (CTA), transthoracic Doppler echocardiography (TTDE), and myocardial contrast echocardiography (MCE), have emerged with great promise for the assessment of CMD (7–11). In the past 2 decades, MCE, a bedside, low cost, radiation-free technique, has gained growing evidence and exhibited promising diagnostic performances that were comparable to PET for determination of microvascular dysfunction in a variety of cardiovascular diseases (12). This review aims at discussing CMD lying in different clinical settings with the use of MCE. We will also briefly discuss the sonothrombolysis of contrast ultrasound and the incorporation of artificial intelligence (AI) into echocardiography.
Normal Physiology of Coronary Blood Perfusion
Myocardial perfusion is mainly governed by dynamic and combined changes in the epicardial coronary vasculature and microcirculation. Under resting conditions, the myocardium extracts roughly 75% of the blood oxygen with minimal reserve for additional oxygen extraction (13). Thus, the regulation of myocardial blood flow (MBF) becomes especially critical to keep balance between oxygen consumption and demand, which mainly resides in the microcirculation of coronary vasculature. Based on the size of the arterial structure, the capacitance and the resistance to MBF, the coronary arterial vasculature is divided into three compartments (Figure 1). The large epicardial coronary arteries (5 mm to 500 μm in diameter) have a capacitance function and normally offer negligible coronary resistance to blood flow. Epicardial coronary arteries can increase their blood content by around 25% and accumulate elastic energy by dilating during systole. The elastic energy is then converted into blood kinetic energy at the beginning of diastole. The pre-arterioles vessels (100–500 μm in diameter) represent the middle compartment, with a measurable drop in pressure. Their main function is to maintain pressure within a narrow range at the origin of the arterioles in response to changes in coronary perfusion pressure or flow. Finally, the distal compartment is made of arterioles (diameter <100 μm), which have the largest pressure drop compared to other compartments, characterized by matching blood supply to myocardial oxygen consumption with the tone influenced by metabolites produced during myocardial metabolism.
Under normal conditions, MBF remains relatively stable across a large range of perfusion pressures as a result of autoregulation, which is achieved by dynamic regulation in the pre-arteriolar and arteriolar microcirculation through flow-mediated dilation and pressure-dependent intrinsic mechanisms (14). For instance, in response to metabolic stimulation, the arterioles dilate correspondingly, resulting in the decrease of resistance in the entire arteriolar network. Subsequently, the pressure in the pre-arterioles also reduces accordingly, causing dilation in the pre-arterioles and the large epicardial coronary (15). However, in the setting of CMD, the disruption of these adaptive mechanisms determines alterations in the blood supply, which can be assessed by various noninvasive methods.
Coronary Microvascular Dysfunction
The term CMD, referring to the structural and functional abnormalities of coronary microvasculature, has gradually been recognized as an additional mechanism of myocardial ischemia in a variety of cardiovascular diseases with elevated risk for adverse outcomes (16). Owing to the growing knowledge of the pathophysiological mechanisms of CMD, this universal phenomenon regarding myocardial ischemia is not only confined to atherosclerosis of coronary microvasculature, but also presents in other clinical conditions including non-ischemic cardiomyopathies, takotsubo syndrome and HFpEF. From the clinical perspective, CMD can be assigned into 4 groups with several pathogenetic mechanisms, which may coexist in the same condition and their importance varies in various clinical settings (Table 1). Any pathogenetic mechanism that may disrupt the coronary microvasculature is responsible for CMD, encompassing coronary spasm, endothelial dysfunction, smooth muscle dysfunction, microvascular inflammation, microvascular rarefaction, and extramural compression (17). These structural and functional abnormalities determine alterations in the coronary blood supply, leading to a blunted MBF and ultimately resulting in myocardial ischemia. Structural abnormalities associated with CMD are mainly manifested as luminal narrowing of the intramural arterioles and capillaries, capillary rarefaction, perivascular fibrosis and microvascular remodeling, often presenting in patients with hypertensive heart disease and hypertrophic cardiomyopathy (HCM) (18). As a consequence of medial wall thickening and different degrees of intimal thickening by smooth cell hypertrophy and increased deposition of collagen in these clinical conditions, coronary physiology and coronary blood flow (CFR) were subsequently impaired. Functional alterations are mainly associated with the presence of an impaired dilatation or an increased constriction of the coronary microvessels (19). The role of impaired microvascular vasodilator capacity is characterized as suboptimal coronary vasodilator response to exercise or pharmacological stress. In addition, increased vasoconstriction can be detected by intracoronary provocative testing with acetylcholine. It should be noted that the functional responsiveness of coronary microcirculation can be affected by some factors, including blood pressure, heart rate and diastolic time.
Although the coronary microvasculature cannot be directly visualized in vivo due to the restriction of current techniques, CMD can be functionally expressed as reduced CFR by several invasive and non-invasive techniques, which is the ratio of the maximum increase in coronary flow to the resting value after pharmacological vasodilatation and represents an integrated measure of epicardial stenosis severity and microcirculation. In the absence of coronary epicardial artery stenosis, reduced CFR is a marker of CMD. Reduced CFR has been described in a certain number of patients with chronic coronary syndromes and those with acute coronary syndromes (ACS) in the absence of coronary epicardial obstruction, and is also associated with a higher risk of major adverse cardiovascular events, including myocardial infarction, progressive heart failure, and sudden death (20, 21). Traditionally, CFR has referred to the invasive measurement of flow reserve, while myocardial perfusion reserve (MPR) is used in this review to focus on the non-invasive measurement methods. Other potential imaging parameters have also been demonstrated to be associated with CMD, including MBF velocity, myocardial blood volume (MBV) and coronary flow velocity reserve (CFVR) (22, 23).
Current Status of CMD Assessment by Non-invasive Imaging Modalities
CMD can be assessed by several invasive and non-invasive modalities (Figure 2). More recently, almost all the non-invasive cardiac imaging modalities have been used to explore the mystery of CMD. Characterizing CMD requires reliable and reproducible measurements which allow for better understanding of its pathophysiological mechanisms. Notably, unlike invasive method, non-invasive techniques only assess the vasodilator capacity of vascular smooth muscle cell, focusing on the identification of impaired vasodilatory capacity of the coronary microcirculation. Along with the advances in current techniques, non-invasive assessment of CMD can now be obtained by several imaging techniques, including PET, CMR, CTA, TTDE and MCE (Table 2).
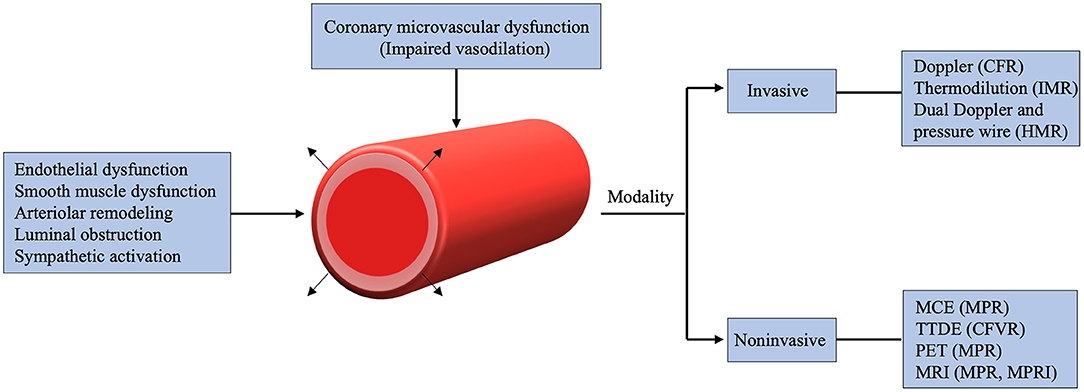
Figure 2. Mechanisms and various imaging modalities of coronary microvascular dysfunction. CFR, coronary flow reserve; CFVR, coronary flow velocity reserve; HMR, hyperemic microvascular resistance; IMR, index of microcirculatory resistance; MCE, myocardial contrast echocardiography; MPR, myocardial perfusion reserve; MPRI, myocardial perfusion reserve index; TTDE, Transthoracic Doppler echocardiography; MRI, magnetic resonance imaging; PET, positron emission tomography.
Nuclear Imaging
PET is the mostly used and well-validated non-invasive technique for the quantification of MBF and is now considered the gold standard for non-invasive assessment of CMD. It involves the utility of post-processing software that performs automated segmentation and arterial input function measurements during dynamic first pass scanning to assess the regional and global rest and stress MBF (24). PET derived MPR showed a good correlation with adverse prognosis in patients with coronary syndromes in several prospective studies (25, 26). The predictive value of reduced MPR for cardiac events has also been described in different subsets of populations, such as in women (27), HCM (28), cardiometabolic disease (29), diabetes mellitus (30), and chronic kidney disease (31). Based on these strong data, MBF and MPR by PET may allow for the risk stratification in some clinical conditions. Furthermore, these parameters have been used as a surrogate marker for coronary vascular health and to monitor therapeutic interventions (4, 32, 33), and this image-guided cardiovascular therapy may have great significance for clinical decision making in the future. Despite well-validated diagnostic and prognostic data by PET, its use is restricted in clinical practice due to some limitations including high radiation exposure, time consuming, limited availability and high cost.
CMR
The utility of MBF and MPR quantification by CMR allows a simultaneous assessment of coronary anatomy, functionality and myocardial perfusion. It exploits the first-pass kinetics of T1-enhancing extracellular gadolinium-based contrast media and the increase in signal intensity is proportional to the perfusion and blood volume as the contrast medium diffuses into the interstitial space from the microvasculature (34). There was a good agreement in global MBF measurements between CMR and PET (r = 0.92, p < 0.001) in patients with stable CAD (35). The predictive value of MBF obtained by stress perfusion CMR for adverse cardiac events has also been validated in specific risk populations such as in patients with cardiovascular risk factors (36), non-obstructive CAD (37), acute myocardial infarction (AMI) after revascularization (38) and HCM (39). Nevertheless, several shortcomings of this technique should be noted, including time-consuming for scan and post-processing, interobserver variability, lack of widespread availability and imaging artifacts. More studies are needed to demonstrate the clinical utility of assessment of CMD by CMR.
Computed Tomography
CTA in combination with CT perfusion (CTP) has the ability to obtain coronary anatomic and myocardial perfusion in one examination (40). Dynamic CTP permits serial myocardial and left ventricular cavity sampling for quantifying blood flow, based on first-pass detection of the maximum slope of the time-attenuation curve in the target tissue, divided by the maximum arterial input function. It provides good diagnostic accuracy for the detection of perfusion defects compared with CMR (41). However, CTA does not have any advantages over other imaging techniques, and its application to assess CMD is restricted in regular clinical practice currently. Further functional information regarding the hemodynamic evaluation of lesion specific ischemia can be obtained by CTA-derived fractional flow reserve (FFR), which involves a 3-dimensional derived coronary model to stimulates maximal hyperemia and quantify MBF (42). It seems to be a promising tool to assess microvascular function in patients with CTA defined intermediate stenosis (43). Despite the potential for identification of CMD, major limitations of this technique include the high effective radiation dose, beam hardening artifacts and restricted use of iodinated contrast agents in renal insufficiency.
TTDE
CFVR by TTDE, is the ratio of coronary flow velocity at stress and rest obtained by the pulsed-wave Doppler sampling of the left anterior descending (LAD) coronary artery in the absence of epicardial artery stenoses (44). CFVR correlates well with flow acquired by an intracoronary Doppler wire (45) and cutoff values ≤ 2–2.5 are commonly used as indicative for impaired coronary microvascular function. CFVR reveals its diagnostic and prognostic capacity in various populations such as in CAD (46, 47), ACS (48), diabetes (49), and HFpEF (50). Advantages of TTDE are low cost, radiation-free, high feasibility, but it is operator dependent, and the image quality affected by artifacts in obese or patients with lung disease needs to be taken into account.
Basic Principles of Myocardial Contrast Echocardiography (MCE) for Assessment of CMD
Ultrasound Contrast Agents
MCE is a non-invasive, bedside and inexpensive technique developed in the past 3 decades, exploiting strong backscatter and non-linear behavior of ultrasound enhancing agents (UEA) to help improve endocardial border definition, detect myocardial perfusion abnormalities and quantify MBF (51). Contrast echocardiography microbubbles are UEA that consist of a hemodynamically inert gaseous core and a stabilizing outer shell, which oscillate under the influence of ultrasound waves. These microbubbles containing the low diffusible and low solubility gas have tiny size (smaller than 10 μm) and similar rheology behavior as red blood cell, which can traverse the smallest human blood vessels, the capillaries, without disrupting the local environment, resulting in opacification of the left ventricle and myocardium. Currently, the most used contrast agents are Sonovue (Bracco, Milan, Italy), Optison (Amersham Health AS, Oslo, Norway), and Definity (Bristol-Myers Squibb, Billerica, Massachusetts). There are some differences in the shell compositions and gas cores among these agents, but all are suitable for MCE. Furthermore, these agents have been evaluated extensively, showing both safe and effective in patients with various cardiovascular diseases as well as in pediatric patients.
Physical Principles
It was discovered that microbubbles were gradually destroyed at a high mechanical index (MI > 0.3) as they transited through the myocardial microcirculation. Notably, with the non-linear oscillation effect of microbubbles under low MI ultrasound, different reflections can appear compared with tissue, providing a better differentiation by imaging techniques. Based on these characteristics of microbubbles, by either triggering ultrasound to one frame every cardiac cycle or by using a very low mechanical index (VLMI) (<0.2) imaging, myocardial contrast enhancement can be visualized by intravenous injections (52). A landmark discovery was made that the triggering technique could be used to quantify MBF, and therefore it provides important bedside information on MBF during stress echocardiography in various clinical conditions (11). Initially, high MI-triggered imaging was used to analyze myocardial perfusion (53), but it has gradually been replaced by VLMI imaging which permits simultaneous assessment of wall motion and myocardial perfusion (54). When a high MI is used, the ultrasound beam destroys the microbubbles, resulting in nearly complete bubble destruction with every pulse. Triggering ultrasound to one frame timed to end systole in the cardiac cycle at a sequence of incrementally longer cardiac cycles allows a replenishment of contrast agent corresponding to flow to the given region during that time sequence. With longer triggering intervals, more microbubbles replenish the capillaries and higher signal intensity appears until finally a plateau phase is reached. Thus, when imaging at a low MI in real time, brief high MI impulses can be used to the imaging plane, after which replenishment can be visualized in real time at the low MI. Calculation of MBF depends on the microvascular cross-sectional area as well as mean velocity of microbubbles during a constant venous infusion of microbubble contrast. When reaching a stable concentration of microbubbles in the blood during continuous infusion, high MI ultrasound is utilized to thoroughly destroy microbubbles and the rate of reappearance of microbubbles gives the mean velocity, whereas the concentration in the myocardium provides the cross-section area (55). Thus, MBF can be quantified by multiplying these 2 variables together. Intensity values “VI” fitted to a monoexponential function: VI = A × (1-e−βt) where VI is the video intensity at pulse interval t, A is the plateau video intensity reflecting the microvascular cross-sectional area and β gives the rate constant that determines the rise of video intensity after bubble destruction representing the velocity of the microbubbles. The product of A and β represents MBF and has shown an excellent relationship with the radiolabeled microsphere-derived MBF in experimental animals (r = 0.96, p < 0.001) (11). When combined with stress technology, MPR can be obtained as well as MBF velocity reserve and MBV reserve. Due to attenuation or some technical reasons, the inhomogeneous contrast enhancement of the myocardium can be adjusted using ratio of myocardial video intensity to the adjacent left ventricular cavity. Different ultrasound contrast imaging techniques, each with relative advantages and pitfalls, are currently applied to utilize the specific reflection features for microbubbles vs. tissue, including pulse inversion, power modulation and coherent contrast imaging. These techniques are multi-pulse schemes that can cancel linear reflections from tissue and enhance non-linear reflections from microbubbles, which allows for high sensitivity contrast imaging with less signal-to-noise ratio.
Qualitative and Quantitative MCE
CMD is diagnosed when there is inadequate visual microvascular refill within 2 s after the destruction of high MI (56). MCE can detect microvascular perfusion abnormalities in 46.8% (range, 41–52%) of patients with no obstructive CAD, which is similar to that obtained invasively in the WISE registry (57). However, visual qualitative MCE fails to detect abnormal FFR in every vessel manifesting abnormal microvascular perfusion (58). With various quantitative parameters, quantitative MCE has the potential to identify pathologic microvascular patterns in patients with CMD. A significantly lower hyperemic MBF and a lower β reserve were found in patients with CMD (56). This phenomenon can also be found in patients with cardiac syndrome X or diabetes by MCE (44, 59). In addition, MCE derived MBF reserve and β reserve were highly correlated with CFVR by TTDE in patients with cardiac syndrome X (44). A recent study also showed that an MBF reserve <2 by MCE classified 37% of patients with chest pain but no obstructive CAD as having CMD (60). Although there is no consensus regarding the diagnostic criteria, data regarding CMD by MCE is growing as the rapid development of MCE. There is an increasing interest in the role of MCE in the evaluation of CMD involved in various kinds of diseases.
Assessment of CMD by MCE in Different Disease Categories
Myocardial Ischemia Without Obstructive CAD
The past paradigm of ischemic heart disease was the well-established link between myocardial ischemia and obstructive atherosclerosis of epicardial coronary arteries. Coronary angiography has been recognized as the gold standard to evaluate the severity and extent of CAD. Nevertheless, growing evidence indicates that angiography frequently fails to detect obstructive CAD in patients with angina symptoms suggestive of myocardial ischemia in clinical practice (61). Thus, this paradigm has been challenged by increasing clinical data, which has led to the gradual recognition that CMD is one of the mechanisms responsible for myocardial ischemia and symptoms in ischemia with non-obstructive coronary artery disease (INOCA) and constitute the clinical picture of primary microvascular angina (MVA). Importantly, contrary to the past understanding, there is a worse prognosis, a poor physical functioning and a reduced quality of life in patients with INOCA based on the data from the National Heart, Lung, and Blood Institute-sponsored WISE (Women's Ischemia Syndrome Evaluation) (16).
On the basis of ischemic cascade, where abnormal perfusion precedes wall motion abnormalities during increased demand induced ischemia, a large number of MCE studies have demonstrated that perfusion analysis provides an incremental benefit for CAD detection over wall motion abnormalities alone in the setting of stress echocardiography (62–64). However, it has been frequently encountered in clinical practice that an inducible defect within a given coronary artery territory does not have a significant obstructive lesion detected at angiography. In a MCE study involving 380 consecutive patients referred for coronary angiography, 91 patients had abnormal myocardial perfusion at peak stress but without a significant epicardial stenosis, implying the potential role of CMD with myocardial ischemia in patients with chest pain. More importantly, these patients appeared to have a 2-fold higher likelihood of adverse events at 4-year follow-up compared with those with negative myocardial perfusion and normal or non-obstructive angiography, indicating that patients with false-positive stress perfusion studies should be followed closely and evaluated for new therapeutic strategies (65). Apart from the qualitative analysis of myocardial perfusion, non-invasive quantification of MPR can also be assessed by MCE, showing a good correlation with Doppler flow wire (r = 0.76, p < 0.001) (66). For the majority of patients with CAD (<85% luminal diameter narrowing of the coronary arteries), resting MBF is normal or even higher as <300-μm arterioles distal to it dilate in order to maintain myocardial oxygen supply. Interestingly, this phenomenon was also presented in patients with INOCA. Since microvessels in these regions have already used some of their reserve to maintain normal resting MBF, MBF cannot increase during stress to the same extent as in other regions with greater microvascular reserve. These findings were further elucidated where the MCE derived MPR was significantly blunted in 18 women with INOCA compared with controls, implying the coronary resistance vessels as the site of microvascular dysfunction (67).
Obstructive CAD: Atherosclerotic Acute Coronary Syndrome
The “no reflow” phenomenon refers to the inability to reperfuse the coronary microcirculation in a previously ischemic region despite opening of the epicardial vessel and thrombolysis in myocardial infarction (TIMI) grade 3 flow on coronary angiography after percutaneous coronary intervention (PCI) in patients with AMI (68). This phenomenon was first described by Ito et al. (69) and subsequently confirmed by multiple MCE studies (70, 71). It is related to the structural and functional abnormalities of coronary microcirculation and extravascular compression. The microvascular obstruction and the damage of tissue and microvasculature during myocardial ischemia were believed to be the main causes of the no reflow phenomenon. Microvascular obstruction may result from distal atherothrombotic embolization as well as myocardial edema and inflammation leading to microvascular compression. The no reflow phenomenon seems to be associated with the duration of ischemia, determining the occurrence and extent of no reflow zone regardless of distal microembolization (72). Despite the wide range of interventional and pharmacologic therapeutic strategies for STEMI management, the no reflow phenomenon may still be present in a significant percentage of patients, especially in patients with myocardial infarction involving the LAD territory (Figure 3), with a poor prognosis including higher incidence of LV remodeling, heart failure (HF) and death. A MCE study showed that >60% of patients with STEMI after successful PCI still had some form of microvascular perfusion abnormality, and the occurrence of the no reflow phenomenon was found to be associated with poor recovery of LV systolic function at 6-month follow-up and was the most significant variable in predicting major adverse cardiac events over the 1-year follow-up period (68). Given the association of the no reflow phenomenon after reopened infarcted arteries with relatively poor clinical outcomes, it has become a treatment target for medical and pharmacological interventions and may be useful for risk stratification. Several studies demonstrated that pharmacological interventions and occlusive protection devices may increase microvascular perfusion or reduce the no reflow area, raising the possibility for individualized intervention which may be beneficial to cardiac outcomes by addressing the no reflow phenomenon (73, 74).
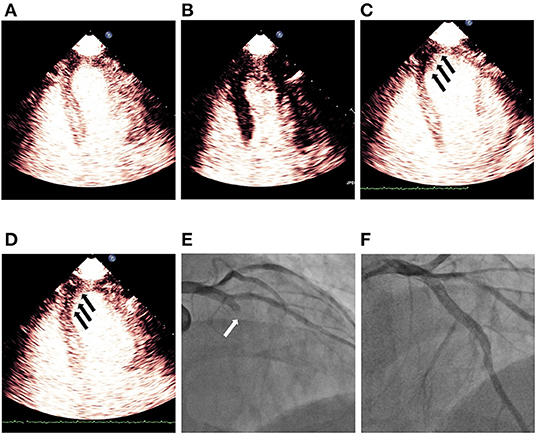
Figure 3. Demonstration of persistent no reflow phenomenon noticed on MCE after successful revascularization of LAD STEMI. (A) Shows myocardial contrast replenishment before the high MI impulse, while (B) indicates complete microbubble destruction immediately after the high MI impulse. (C) Shows a defect (black arrows) in the segments of the infarct zone during replenishment (~4 beats post-high MI impulse), while (D) indicates a persistent defect (black arrows) in LAD territory although a plateau intensity (~10 beats after the high MI impulse) had been reached. (E) Shows blocked LAD (white arrow) before PCI and (F) indicates revascularization of LAD after successful PCI. LAD, left anterior descending; MCE, myocardial contrast echocardiography; PCI, percutaneous coronary intervention; STEMI, ST segment elevated myocardial infarction.
Myocardial Diseases: HFpEF; Hypertrophic Cardiomyopathy; Takotsubo Cardiomyopathy
HFpEF is characterized by the signs and symptoms of HF despite a normal or near-normal EF, which has become a challenge for diagnosis and treatment in clinical practice. With common comorbidities such as hypertension, diabetes mellitus and metabolic syndrome in HFpEF, its pathophysiological mechanisms appear to be complicated and heterogeneous, including arterial stiffness, ventricular-arterial coupling, vascular endothelial cell inflammation and dysfunction, and chronotropic incompetence. The role of CMD has gained growing consent over years in the pathophysiology of HFpEF by an increasing number of studies (75). Recent advances in non-invasive imaging modalities enable accurate quantification of MPR, presenting a good correlation with that of invasive method in many cardiovascular diseases, including HFpEF. Prevalence and correlates of CMD in HFpEF patients were analyzed with echocardiography in the PROMIS-HFpEF study. Seventy-five percentage of HFpEF patients had CMD (defined as CFVR <2.5) in this prospective multi-center study and the degree of CMD was shown to correlate with markers of HF severity (76). Apart from the high prevalence of CMD in HFpEF, the impairment of MPR also directly correlated with excise capacity, which is an independent predictor of reduced exercise tolerance (77, 78). Furthermore, CMD was also found to correlate with markers of systemic endothelial dysfunction, suggesting HFpEF as a systemic disorder associated with endothelial dysfunction and microvascular disease (79). However, it remains to be determined whether CMD is the primary cause of ventricular remodeling and HFpEF, or the myocardial remodeling property of HFpEF may lead to CMD. A follow-up of PROMIS-HFpEF study showed a strong association between the presence of CMD and the risk of adverse cardiovascular outcomes including cardiac death and HF hospitalizations at follow-up, raising the awareness of the urgent need to gain more insight into the pathophysiology and therapeutic advances at improving prognosis in patients with HFpEF (50). Currently, the non-invasive assessment of CMD in HFpEF mainly resides in PET and CMR. However, with the capacity to clearly present endocardial border, MCE is particularly useful in determination of LVEF compared with traditional echocardiography, which is of great importance in the diagnosis in patients with HF and can provide prognostic information. Moreover, unlike other non-invasive techniques, MCE can assess LV function and MPR in a single examination, simultaneously providing structural and functional information for diagnosis and prognostic evaluation. Based on these, MCE may be helpful to address the pathophysiological mechanisms of CMD in patients with HFpEF, which needs to be investigated in future research.
HCM is characterized by unexplained left ventricular asymmetric hypertrophy and the absence of cardiac or systemic triggers. MCE has been proposed as a helpful diagnosis tool in questioned HCM and the precise determination of left ventricular wall thickness by MCE is of great significance in the prognostic assessment of patients with HCM (80). Recently, CMD, representing a predisposing factor for myocardial ischemia, has also been recognized as one of the most important pathophysiological features. The structural abnormalities, characterized by marked wall thickening of intramural coronary arterioles resulting in severe reduction in luminal area, are considered the most relevant substrate of CMD in the presence of increased oxygen demand. In addition to structural changes of small vessel, functional abnormalities have also been described, causing blunted MBF and MPR by several non-invasive imaging techniques (81, 82). The blunted MPR in HCM is mainly due to the failure of MBV to increase during hyperemia, as the affected segments have exhausted myocardial capillary autoregulation by presenting near-maximal capillary vasodilatation at rest. In agreement with previous studies in patients with HCM, these findings of myocardial microcirculation were also clearly shown in a MCE study, demonstrating a good correlation with PET (83). Noteworthy, CMD can be present not only in the hypertrophied myocardium, but also in the non-hypertrophied LV segments and the degree of microvascular dysfunction is related to the extent of LV hypertrophy (84, 85). The degree of CMD, expressed as the perfusion defect size by MCE, was found to correlate with decreased myocardial contractile property, indicating an important role of microvascular dysfunction in the process of HCM (86). Despite a generally benign prognosis in patients with HCM, a certain quantity of patients will progress to LV dysfunction and eventually heart failure, and sudden death owing to ventricular arrhythmia can be the first manifestation of the disease. The degree of CMD, by presenting severely blunted MBF following dipyridamole stress assessed by PET, was a strong, independent predictor of long-term clinical deterioration and death from cardiovascular causes in patients with HCM, suggesting CMD as a underlying target to address the adverse prognosis (87). Two invasive methods, alcohol septal ablation (ASA) and surgical myectomy are used to reduce LV outflow tract gradients in patients with obstructive HCM by relief of extravascular compression, which is also one of the causes of CMD. MCE is widely used to address ASA by precise identification of the coronary artery branch supplying the target septal zone. After relieving these extravascular compression forces with ASA, MCE-derived MPR improved, but did not normalize compared with healthy controls after 6 months (88). This could be partly explained by the residual vascular remolding and fibrosis that results in limiting myocardial perfusion in these patients. In recent years, a novel minimally invasive treatment, echocardiography-guided percutaneous intramyocardial septal radiofrequency ablation has shown promising results and the combination with MCE can be useful in the selection of ablation area and at follow-up in these patients (89).
Takotsubo cardiomyopathy (TC), also known as stress cardiomyopathy or apical ballooning syndrome, was identified in 1990 and is characterized by the sudden onset of chest symptoms, evidence of myocardial ischemia and transient left ventricular dysfunction without substantial angiographic stenosis. A number of pathophysiologic mechanisms have been proposed to explain the apical dysfunction observed in TC, including multivessel coronary vasospasm, spontaneous coronary thrombus lysis, abnormalities in coronary endothelial function and direct catecholaminergic effects on the myocardium (90). The involvement of CMD has also been advocated as a possible pathogenic mechanism underlying the wall motion abnormalities in TC by several invasive and non-invasive methods (91, 92). In the acute phase (1 day) of TC, reduced MBF and velocity were detected in dysfunctional LV segments compared with those with normal wall motion (93). These findings were also described in a prospective MCE study involving 11 patients (mean age, 70.9 ± 17.5 years; 8 women) received diagnoses of TC, suggestive of a potential pathophysiologic role of microvascular dysfunction (94). With uniquely favorable prognosis compared with other causes of ACS, it is often characterized by a rapid improvement of LV wall motion over a period of days or a few weeks. Nine consecutive patients with TC were serially followed by MCE at 1 day, within 1 week and 3–6 months after index admission (95). By 1 week, the relative improvement of LVEF was 26%, whereas myocardial perfusion had improved by nearly 50% and returned to normal by 3–6 months, suggesting a pronounced improvement in microvascular function preceding recovery of wall motion abnormalities. This transient and complete restoration of the myocardial microcirculation was less inclined to the fact that disruption of the microcirculatory architecture was caused by epicardial coronary artery occlusion or vasospasm. To further elucidate the underlying pathophysiological mechanism, Galiuto et al. found a clear perfusion defect within the dysfunctional myocardial area at MCE in 15 consecutive women (68 ± 14 years) with TC, which can be transiently reduced by adenosine and entirely resolved at 1 month follow-up, whereas no changes were observed in anterior STEMI patients (96). These findings were endorsed by other MCE studies, by exhibiting lower quantitative parameters (MBV, MBF and velocity) in the akinetic segments than in normokinetic segments in TC patients, and these parameters were further deteriorated in dysfunctional segments in patients with STEMI (96–98). Thus, the authors speculated that coronary microvascular constriction appears to be a potential mechanism as adenosine vasodilates constricted microvessels. The greater prevalence of TC among post-menopausal women, along with the association to physical or emotional stress, may support the hypothesis of stress-mediated vasoconstriction enhanced by estrogens depletion. However, it remains debated whether CMD is the primary cause, or a secondary phenomenon and further studies are needed to gain insights into the causes, triggers and mechanisms responsible for TC. Of importance, it has been reported that the degree of CMD, by presenting with coronary slow flow on coronary angiography, may have a worse clinical presentation and carry a poor prognosis (99).
Applications of MCE in the Treatment of CMD
Contrast ultrasound has a variety of applications not only in diagnosing and providing prognostic information of cardiovascular disease, but also in the treatment of patients with STEMI through sonoperfusion and sonothrombolysis. When exposed to lower MI, the microbubbles expand and compress steadily (stable cavitation), whereas microbubbles will have greater volumetric changes and eventually collapse at higher MI, as known inertial cavitation (100). Multiple animal studies have shown that high MI impulses of diagnostic ultrasound can successfully dissolve acute intravascular thrombi mainly due to the effects of inertial cavitation, which causes increased shear stress and powerful jetting inducing direct mechanical destruction of the clot (101, 102). Noteworthy, even in the absence of upstream recanalization, diagnostic high MI impulses was demonstrated to have a positive effect on microvascular flow (103). This phenomenon can be partly explained by the role of cavitation-mediated adenosine triphosphate (ATP) release, which can be converted to adenosine for reducing vascular tone and increase nitric oxide (NO) synthesis, finally leading to vasodilatation (104). The first prospective randomized human study by MRUSMI group (Microvascular Reperfusion Utilizing Sonothrombolysis in acute Myocardial Infarction) used short pulse duration high MI pulses to examine the effects in 100 patients with STEMI, with each 50 of whom randomized to pre- and post-PCI sonothrombolysis and to PCI only, respectively (105). Patients in sonothrombolysis group had a higher recanalization rate and TIMI flow before PCI, and reduced infarct size on CMR, resulting in sustained improvements in LVEF and less need for intracardiac defibrillator placement at 6 months follow-up. Furthermore, a substudy showed improved LV global longitudinal strain and decreased perfusion defect size by MCE at follow up, and the only independent predictor of LV remodeling was treatment with sonothrombolysis (106). As contrast ultrasound is portable, this technique was also shown to have a promising feasibility of in-ambulance sonothrombolysis for STEMI (107). These findings have provided more insight into the background of sonothrombolysis by contrast ultrasound and additional multi-center prospective studies are needed to test the clinical effectiveness of this method. It is also very interesting to determine whether the sonothrombolysis will have a positive effect on patients with other cardiovascular diseases involving CMD as it can augment myocardial flow through mechanical and biological mechanisms.
Apart from enhancement of vascular compartments, microbubbles can be designed to carry drugs or genes and used to deliver site-specific therapy to targeted organs in the body. When activated by external ultrasound energy, the acoustic microspheres can serve as catalysts and vehicles of drug or gene packages, providing a direct and transient access to tissues and organs. Targets for molecular imaging, therapy and drug delivery with ultrasound have been described in several clinical conditions, such as in myocardial ischemia, vulnerable plague, vascular remodeling and inflammation. Of note, low dose fibrinolytic therapy combined with targeted microbubbles appears to enhance sonothrombolysis efficacy by localizing and binding cavitation nuclei directly to the clot, which may be beneficial in the settings where primary PCI is not available emergently (103). Moreover, induced by contrast ultrasound, the increased permeability of cells and vessels can be used to delivery genetic material. There have been preclinical trials showing improved blood flow with targeted gene delivery microbubbles in animal models with vascular disease, implying a new interest in treatment strategies for these conditions (108). Nevertheless, it remains challenging for combined microbubbles to be fully incorporated into the clinical practice.
Potential Applications of AI in MCE
Artificial intelligence (AI) has been incorporated into diverse areas of clinical medicine, especially in cardiovascular imaging analysis, which can address observer variability during imaging acquisition and interpretation. Recently, using AI quantification of CMR perfusion mapping involving over 1,000 patients with known or suspected CAD, both reduced MBF and MPR were associated with death and major adverse cardiovascular events independently of other clinical risk markers (109). AI is becoming a hotspot particularly in echocardiography compared to other techniques, which is more affected by interobserver variability and is strongly dependent on the expertise of operators. The applications of AI in echocardiography has shown promising results in view classification, automated analysis of size and function, wall motion abnormality, diagnosis of cardiovascular diseases and event prediction. In the field of MCE, despite its promising diagnostic performance in various clinical conditions, it is limited by time-consuming manual segmentation and requirement of high level of expertise and training. Of note, there have been several studies demonstrating that AI-empowered MCE allows a fully automatic approach for fast and accurate segmentation of myocardium, overcoming the above limitations by exploit different machine learning techniques (110). However, the current AI on MCE is still in its infancy, but it stands a chance of being utilized in routine clinical practice, improving the accuracy of screening of early cardiovascular diseases, and achieving early detection, intervention and treatment for a better prognosis.
Conclusion
CMD is prevalent across a broad spectrum of cardiovascular diseases with a significant impact on prognosis. Current research provides good evidence that MCE is capable of assessing myocardial perfusion and quantifying MBF, addressing and advancing the pathophysiologic role of CMD in heterogeneous clinical conditions. Apart from the promising performance for diagnosis and prognosis in various types of cardiovascular diseases, contrast enhanced ultrasound also reveals therapeutic capacity to dissolve clot and restore microvascular function in the setting of STEMI. With the advent of targeted microbubbles, microbubbles attached drugs or genes may be used to deliver site-specific therapy to targeted organs. Finally, MCE combined with AI possesses a promising application prospect, and may be a feasible method to be used in the future. However, there is still limited knowledge about CMD in all of these conditions, indicating that additional research in this area is warranted to fully implement MCE into the daily workflow in clinical practice.
Author Contributions
All authors participated in writing and critically reviewing this manuscript. All authors have read and agreed to the published version of the manuscript.
Funding
This study was funded by Guangdong Natural Science Funds for Distinguished Young Scholar (Grant 2016A030306028), Guangzhou Science and Technology Program (Grant 201506010021), and Foundation of President of Nanfang Hospital (Grant 2020Z006 and 2018Z018).
Conflict of Interest
The authors declare that the research was conducted in the absence of any commercial or financial relationships that could be construed as a potential conflict of interest.
Publisher's Note
All claims expressed in this article are solely those of the authors and do not necessarily represent those of their affiliated organizations, or those of the publisher, the editors and the reviewers. Any product that may be evaluated in this article, or claim that may be made by its manufacturer, is not guaranteed or endorsed by the publisher.
References
1. Camici PG, Crea F. Coronary microvascular dysfunction. N Engl J Med. (2007) 356:830–40. doi: 10.1056/NEJMra061889
2. Crea F, Camici PG, Bairey Merz CN. Coronary microvascular dysfunction: an update. Eur Heart J. (2014) 35:1101–11. doi: 10.1093/eurheartj/eht513
3. Mathew RC, Bourque JM, Salerno M, Kramer CM. Cardiovascular imaging techniques to assess microvascular dysfunction. JACC Cardiovasc Imaging. (2020) 13:1577–90. doi: 10.1016/j.jcmg.2019.09.006
4. Schindler TH, Schelbert HR, Quercioli A, Dilsizian V. Cardiac PET imaging for the detection and monitoring of coronary artery disease and microvascular health. JACC Cardiovasc Imaging. (2010) 3:623–40. doi: 10.1016/j.jcmg.2010.04.007
5. Ong P, Athanasiadis A, Sechtem U. Pharmacotherapy for coronary microvascular dysfunction. Eur Heart J Cardiovasc Pharmacother. (2015) 1:65–71. doi: 10.1093/ehjcvp/pvu020
6. Del Buono MG, Iannaccone G, Scacciavillani R, Carbone S, Camilli M, Niccoli G, et al. Heart failure with preserved ejection fraction diagnosis and treatment: an updated review of the evidence. Prog Cardiovasc Dis. (2020) 63:570–84. doi: 10.1016/j.pcad.2020.04.011
7. Bengel FM. Leaving relativity behind: quantitative clinical perfusion imaging. J Am Coll Cardiol. (2011) 58:749–51. doi: 10.1016/j.jacc.2011.02.068
8. Liu A, Wijesurendra RS, Liu JM, Forfar JC, Channon KM, Jerosch-Herold M, et al. Diagnosis of microvascular angina using cardiac magnetic resonance. J Am Coll Cardiol. (2018) 71:969–79. doi: 10.1016/j.jcmg.2018.08.028
9. Rossi A, Wragg A, Klotz E, Pirro F, Moon JC, Nieman K, et al. Dynamic computed tomography myocardial perfusion imaging: comparison of clinical analysis methods for the detection of vessel-specific ischemia. Circ Cardiovasc Imaging. (2017) 10:e005505. doi: 10.1161/CIRCIMAGING.116.005505
10. Michelsen MM, Mygind ND, Pena A, Olsen RH, Christensen TE, Ghotbi AA, et al. Transthoracic doppler echocardiography compared with positron emission tomography for assessment of coronary microvascular dysfunction: The iPOWER study. Int J Cardiol. (2017) 228:435–43. doi: 10.1016/j.ijcard.2016.11.004
11. Wei K, Jayaweera AR, Firoozan S, Linka A, Skyba DM, Kaul S. Quantification of myocardial blood flow with ultrasound-induced destruction of microbubbles administered as a constant venous infusion. Circulation. (1998) 97:473–83. doi: 10.1161/01.CIR.97.5.473
12. Vogel R, Indermuhle A, Reinhardt J, Meier P, Siegrist PT, Namdar M, et al. The quantification of absolute myocardial perfusion in humans by contrast echocardiography: algorithm and validation. J Am Coll Cardiol. (2005) 45:754–62. doi: 10.1016/j.jacc.2004.11.044
13. Ong P, Camici PG, Beltrame JF, Crea F, Shimokawa H, Sechtem U, et al. International standardization of diagnostic criteria for microvascular angina. Int J Cardiol. (2018) 250:16–20. doi: 10.1016/j.ijcard.2017.08.068
14. Deussen A, Ohanyan V, Jannasch A, Yin L, Chilian W. Mechanisms of metabolic coronary flow regulation. J Mol Cell Cardiol. (2012) 52:794–801. doi: 10.1016/j.yjmcc.2011.10.001
15. Schelbert HR. Anatomy and physiology of coronary blood flow. J Nucl Cardiol. (2010) 17:545–54. doi: 10.1007/s12350-010-9255-x
16. Pepine CJ, Anderson RD, Sharaf BL, Reis SE, Smith KM, Handberg EM, et al. Coronary microvascular reactivity to adenosine predicts adverse outcome in women evaluated for suspected ischemia results from the national heart, lung and blood institute WISE (Women's Ischemia Syndrome Evaluation) study. J Am Coll Cardiol. (2010) 55:2825–32. doi: 10.1016/j.jacc.2010.01.054
17. Marinescu MA, Löffler AI, Ouellette M, Smith L, Kramer CM, Bourque JM. Coronary microvascular dysfunction, microvascular angina, and treatment strategies. JACC Cardiovasc Imaging. (2015) 8:210–20. doi: 10.1016/j.jcmg.2014.12.008
18. Shome JS, Perera D, Plein S, Chiribiri A. Current perspectives in coronary microvascular dysfunction. Microcirculation. (2017) 24:e12340. doi: 10.1111/micc.12340
19. Camici PG, d'Amati G, Rimoldi O. Coronary microvascular dysfunction: mechanisms and functional assessment. Nat Rev Cardiol. (2015) 12:48–62. doi: 10.1038/nrcardio.2014.160
20. Indorkar R, Kwong RY, Romano S, White BE, Chia RC, Trybula M, et al. Global coronary flow reserve measured during stress cardiac magnetic resonance imaging is an independent predictor of adverse cardiovascular events. JACC Cardiovasc Imaging. (2019) 12:1686–95. doi: 10.1016/j.jcmg.2018.08.018
21. Kato S, Saito N, Nakachi T, Fukui K, Iwasawa T, Taguri M, et al. Stress perfusion coronary flow reserve versus cardiac magnetic resonance for known or suspected CAD. J Am Coll Cardiol. (2017) 70:869–79. doi: 10.1016/j.jacc.2017.06.028
22. McCommis KS, Zhang H, Goldstein TA, Misselwitz B, Abendschein DR, Gropler RJ, et al. Myocardial blood volume is associated with myocardial oxygen consumption: an experimental study with cardiac magnetic resonance in a canine model. JACC Cardiovasc Imaging. (2009) 2:1313–20. doi: 10.1016/j.jcmg.2009.07.010
23. Pena A, Michelsen MM, Mygind ND, Gustafsson I, Høst N, Bech J, et al. Coronary microvascular dysfunction is associated with cardiac time intervals in women with angina and no obstructive coronary artery disease: an iPOWER substudy. Echocardiography. (2019) 36:1110–7. doi: 10.1111/echo.14356
24. Lortie M, Beanlands RS, Yoshinaga K, Klein R, Dasilva JN, DeKemp RA. Quantification of myocardial blood flow with 82Rb dynamic PET imaging. Eur J Nucl Med Mol Imaging. (2007) 34:1765–74. doi: 10.1007/s00259-007-0478-2
25. Gulati M, Cooper-DeHoff RM, McClure C, Johnson BD, Shaw LJ, Handberg EM, et al. Adverse cardiovascular outcomes in women with nonobstructive coronary artery disease: a report from the women's ischemia syndrome evaluation study and the st james women take heart project. Arch Intern Med. (2009) 169:843–50. doi: 10.1001/archinternmed.2009.50
26. Ziadi MC, Dekemp RA, Williams KA, Guo A, Chow BJ, Renaud JM, et al. Impaired myocardial flow reserve on rubidium-82 positron emission tomography imaging predicts adverse outcomes in patients assessed for myocardial ischemia. J Am Coll Cardiol. (2011) 58:740–8. doi: 10.1016/j.jacc.2011.01.065
27. Taqueti VR, Shaw LJ, Cook NR, Murthy VL, Shah NR, Foster CR, et al. Excess cardiovascular risk in women relative to men referred for coronary angiography is associated with severely impaired coronary flow reserve, not obstructive disease. Circulation. (2017) 135:566–77. doi: 10.1161/CIRCULATIONAHA.116.023266
28. Lu DY, Yalçin H, Yalçin F, Zhao M, Sivalokanathan S, Valenta I, et al. Stress myocardial blood flow heterogeneity is a positron emission tomography biomarker of ventricular arrhythmias in patients with hypertrophic cardiomyopathy. Am J Cardiol. (2018) 121:1081–9. doi: 10.1016/j.amjcard.2018.01.022
29. Osborne MT, Bajaj NS, Taqueti VR, Gupta A, Bravo PE, Hainer J, et al. Coronary microvascular dysfunction identifies patients at high risk of adverse events across cardiometabolic diseases. J Am Coll Cardiol. (2017) 70:2835–7. doi: 10.1016/j.jacc.2017.09.1104
30. Murthy VL, Naya M, Foster CR, Gaber M, Hainer J, Klein J, et al. Association between coronary vascular dysfunction and cardiac mortality in patients with and without diabetes mellitus. Circulation. (2012) 126:1858–68. doi: 10.1161/CIRCULATIONAHA.112.120402
31. Murthy VL, Naya M, Foster CR, Hainer J, Gaber M, Dorbala S, et al. Coronary vascular dysfunction and prognosis in patients with chronic kidney disease. JACC Cardiovasc Imaging. (2012) 5:1025–34. doi: 10.1016/j.jcmg.2012.06.007
32. Bairey Merz CN, Handberg EM, Shufelt CL, Mehta PK, Minissian MB, Wei J, et al. A randomized, placebo-controlled trial of late Na current inhibition (ranolazine) in coronary microvascular dysfunction (CMD): impact on angina and myocardial perfusion reserve. Eur Heart J. (2016) 37:1504–13. doi: 10.1093/eurheartj/ehv647
33. Shah NR, Cheezum MK, Veeranna V, Horgan SJ, Taqueti VR, Murthy VL, et al. Ranolazine in symptomatic diabetic patients without obstructive coronary artery disease: impact on microvascular and diastolic function. J Am Heart Assoc. (2017) 6:e005027. doi: 10.1161/JAHA.116.005027
34. Klem I, Heitner JF, Shah DJ, Sketch MH, Behar V, Weinsaft J, et al. Improved detection of coronary artery disease by stress perfusion cardiovascular magnetic resonance with the use of delayed enhancement infarction imaging. J Am Coll Cardiol. (2006) 47:1630–8. doi: 10.1016/j.jacc.2005.10.074
35. Engblom H, Xue H, Akil S, Carlsson M, Hindorf C, Oddstig J, et al. Fully quantitative cardiovascular magnetic resonance myocardial perfusion ready for clinical use: a comparison between cardiovascular magnetic resonance imaging and positron emission tomography. J Cardiovasc Magn Reson. (2017) 19:78. doi: 10.1186/s12968-017-0388-9
36. Lipinski MJ, McVey CM, Berger JS, Kramer CM, Salerno M. Prognostic value of stress cardiac magnetic resonance imaging in patients with known or suspected coronary artery disease: a systematic review and meta-analysis. J Am Coll Cardiol. (2013) 62:826–38. doi: 10.1016/j.jacc.2013.03.080
37. Doyle M, Weinberg N, Pohost GM, Bairey Merz CN, Shaw LJ, Sopko G, et al. Prognostic value of global MR myocardial perfusion imaging in women with suspected myocardial ischemia and no obstructive coronary disease: results from the NHLBI-sponsored WISE (Women's Ischemia Syndrome Evaluation) study. JACC Cardiovasc Imaging. (2010) 3:1030–6. doi: 10.1016/j.jcmg.2010.07.008
38. Larose E, Rodés-Cabau J, Pibarot P, Rinfret S, Proulx G, Nguyen CM, et al. Predicting late myocardial recovery and outcomes in the early hours of ST-segment elevation myocardial infarction traditional measures compared with microvascular obstruction, salvaged myocardium, and necrosis characteristics by cardiovascular magnetic resonance. J Am Coll Cardiol. (2010) 55:2459–69. doi: 10.1016/j.jacc.2010.02.033
39. Karamitsos TD, Dass S, Suttie J, Sever E, Birks J, Holloway CJ, et al. Blunted myocardial oxygenation response during vasodilator stress in patients with hypertrophic cardiomyopathy. J Am Coll Cardiol. (2013) 61:1169–76. doi: 10.1016/j.jacc.2012.12.024
40. Danad I, Szymonifka J, Schulman-Marcus J, Min JK. Static and dynamic assessment of myocardial perfusion by computed tomography. Eur Heart J Cardiovasc Imaging. (2016) 17:836–44. doi: 10.1093/ehjci/jew044
41. Bamberg F, Marcus RP, Becker A, Hildebrandt K, Bauner K, Schwarz F, et al. Dynamic myocardial CT perfusion imaging for evaluation of myocardial ischemia as determined by MR imaging. JACC Cardiovasc Imaging. (2014) 7:267–77. doi: 10.1016/j.jcmg.2013.06.008
42. Taylor CA, Fonte TA, Min JK. Computational fluid dynamics applied to cardiac computed tomography for noninvasive quantification of fractional flow reserve: scientific basis. J Am Coll Cardiol. (2013) 61:2233–41. doi: 10.1016/j.jacc.2012.11.083
43. Nørgaard BL, Leipsic J, Gaur S, Seneviratne S, Ko BS, Ito H, et al. Diagnostic performance of noninvasive fractional flow reserve derived from coronary computed tomography angiography in suspected coronary artery disease: the NXT trial (Analysis of Coronary Blood Flow Using CT Angiography: Next Steps). J Am Coll Cardiol. (2014) 63:1145–55. doi: 10.1016/j.jacc.2013.11.043
44. Galiuto L, Sestito A, Barchetta S, Sgueglia GA, Infusino F, La Rosa C, et al. Noninvasive evaluation of flow reserve in the left anterior descending coronary artery in patients with cardiac syndrome X. Am J Cardiol. (2007) 99:1378–83. doi: 10.1016/j.amjcard.2006.12.070
45. Hozumi T, Yoshida K, Akasaka T, Asami Y, Ogata Y, Takagi T, et al. Noninvasive assessment of coronary flow velocity and coronary flow velocity reserve in the left anterior descending coronary artery by doppler echocardiography: comparison with invasive technique. J Am Coll Cardiol. (1998) 32:1251–9. doi: 10.1016/S0735-1097(98)00389-1
46. Cortigiani L, Rigo F, Gherardi S, Bovenzi F, Picano E, Sicari R. Implication of the continuous prognostic spectrum of doppler echocardiographic derived coronary flow reserve on left anterior descending artery. Am J Cardiol. (2010) 105:158–62. doi: 10.1016/j.amjcard.2009.08.669
47. Sicari R, Rigo F, Cortigiani L, Gherardi S, Galderisi M, Picano E. Additive prognostic value of coronary flow reserve in patients with chest pain syndrome and normal or near-normal coronary arteries. Am J Cardiol. (2009) 103:626–31. doi: 10.1016/j.amjcard.2008.10.033
48. Watanabe N. Noninvasive assessment of coronary blood flow by transthoracic doppler echocardiography: basic to practical use in the emergency room. J Echocardiogr. (2017) 15:49–56. doi: 10.1007/s12574-016-0324-2
49. Cortigiani L, Rigo F, Gherardi S, Galderisi M, Bovenzi F, Sicari R. Prognostic meaning of coronary microvascular disease in type 2 diabetes mellitus: a transthoracic doppler echocardiographic study. J Am Soc Echocardiogr. (2014) 27:742–8. doi: 10.1016/j.echo.2014.02.010
50. Hage C, Svedlund S, Saraste A, Faxén UL, Benson L, Fermer ML, et al. Association of coronary microvascular dysfunction with heart failure hospitalizations and mortality in heart failure with preserved ejection fraction: a follow-up in the PROMIS-HFpEF study. J Card Fail. 26:1016–21. (2020). doi: 10.1016/j.cardfail.2020.08.010
51. Porter TR, Xie F. Visually discernible myocardial echocardiographic contrast after intravenous injection of sonicated dextrose albumin microbubbles containing high molecular weight, less soluble gases. J Am Coll Cardiol. (1995) 25:509–15. doi: 10.1016/0735-1097(94)00376-2
52. Porter TR, Abdelmoneim S, Belcik JT, McCulloch ML, Mulvagh SL, Olson JJ, et al. Guidelines for the cardiac sonographer in the performance of contrast echocardiography: a focused update from the American society of echocardiography. J Am Soc Echocardiogr. (2014) 27:797–810. doi: 10.1016/j.echo.2014.05.011
53. Senior R, Kaul S, Soman P, Lahiri A. Power doppler harmonic imaging: a feasibility study of a new technique for the assessment of myocardial perfusion. Am Heart J. (2000) 139:245–51. doi: 10.1016/S0002-8703(00)90233-3
54. Rafter P, Phillips P, Vannan MA. Imaging technologies and techniques. Cardiol Clin. (2004) 22:181–97. doi: 10.1016/j.ccl.2004.02.002
55. Porter TR, Li S, Kricsfeld D, Armbruster RW. Detection of myocardial perfusion in multiple echocardiographic windows with one intravenous injection of microbubbles using transient response second harmonic imaging. J Am Coll Cardiol. (1997) 29:791–9. doi: 10.1016/S0735-1097(96)00575-X
56. Taqui S, Ferencik M, Davidson BP, Belcik JT, Moccetti F, Layoun M, et al. Coronary microvascular dysfunction by myocardial contrast echocardiography in nonelderly patients referred for computed tomographic coronary angiography. J Am Soc Echocardiogr. (2019) 32:817–25. doi: 10.1016/j.echo.2019.03.001
57. Reis SE, Holubkov R, Conrad Smith AJ, Kelsey SF, Sharaf BL, Reichek N, et al. Coronary microvascular dysfunction is highly prevalent in women with chest pain in the absence of coronary artery disease: results from the NHLBI WISE study. Am Heart J. (2001) 141:735–41. doi: 10.1067/mhj.2001.114198
58. Barton D, Xie F, O'Leary E, Chatzizisis YS, Pavlides G, Porter TR. The relationship of capillary blood flow assessments with real time myocardial perfusion echocardiography to invasively derived microvascular and epicardial assessments. J Am Soc Echocardiogr. (2019) 32:1095–101. doi: 10.1016/j.echo.2019.04.424
59. Abdelmoneim SS, Basu A, Bernier M, Dhoble A, Abdel-Kader SS, Pellikka PA, et al. Detection of myocardial microvascular disease using contrast echocardiography during adenosine stress in type 2 diabetes mellitus: prospective comparison with single-photon emission computed tomography. Diab Vasc Dis Res. (2011) 8:254–61. doi: 10.1177/1479164111419973
60. Lam JH, Quah JX, Davies T, Boos CJ, Nel K, Anstey CM, et al. Relationship between coronary microvascular dysfunction and left ventricular diastolic function in patients with chest pain and unobstructed coronary arteries. Echocardiography. (2020) 37:1199–204. doi: 10.1111/echo.14794
61. Patel MR, Peterson ED, Dai D, Brennan JM, Redberg RF, Anderson HV, et al. Low diagnostic yield of elective coronary angiography. N Engl J Med. (2010) 362:886–95. doi: 10.1056/NEJMoa0907272
62. Xie F, Dodla S, O'Leary E, Porter TR. Detection of subendocardial ischemia in the left anterior descending coronary artery territory with real-time myocardial contrast echocardiography during dobutamine stress echocardiography. JACC Cardiovasc Imaging. (2008) 1:271–8. doi: 10.1016/j.jcmg.2008.02.004
63. Porter TR, Smith LM, Wu J, Thomas D, Haas JT, Mathers DH, et al. Patient outcome following 2 different stress imaging approaches: a prospective randomized comparison. J Am Coll Cardiol. (2013) 61:2446–55. doi: 10.1016/j.jacc.2013.04.019
64. Shah BN, Chahal NS, Bhattacharyya S, Li W, Roussin I, Khattar RS, et al. The feasibility and clinical utility of myocardial contrast echocardiography in clinical practice: results from the incorporation of myocardial perfusion assessment into clinical testing with stress echocardiography study. J Am Soc Echocardiogr. (2014) 27:520–30. doi: 10.1016/j.echo.2014.01.028
65. Kutty S, Bisselou Moukagna KS, Craft M, Shostrom V, Xie F, Porter TR. Clinical outcome of patients with inducible capillary blood flow abnormalities during demand stress in the presence or absence of angiographic coronary disease. Circ Cardiovasc Imaging. (2018) 11:e007483. doi: 10.1161/CIRCIMAGING.117.007483
66. Wei K, Ragosta M, Thorpe J, Coggins M, Moos S, Kaul S. Noninvasive quantification of coronary blood flow reserve in humans using myocardial contrast echocardiography. Circulation. (2001) 103:2560–5. doi: 10.1161/01.CIR.103.21.2560
67. Rinkevich D, Belcik T, Gupta NC, Cannard E, Alkayed NJ, Kaul S. Coronary autoregulation is abnormal in syndrome X: insights using myocardial contrast echocardiography. J Am Soc Echocardiogr. (2013) 26:290–6. doi: 10.1016/j.echo.2012.12.008
68. Aggarwal S, Xie F, High R, Pavlides G, Porter TR. Prevalence and predictive value of microvascular flow abnormalities after successful contemporary percutaneous coronary intervention in acute st-segment elevation myocardial infarction. J Am Soc Echocardiogr. (2018) 31:674–82. doi: 10.1016/j.echo.2018.01.009
69. Ito H, Maruyama A, Iwakura K, Takiuchi S, Masuyama T, Hori M, et al. Clinical implications of the 'no reflow' phenomenon. A predictor of complications and left ventricular remodeling in reperfused anterior wall myocardial infarction. Circulation. (1996) 93:223–8. doi: 10.1161/01.CIR.93.2.223
70. Lepper W, Kamp O, Vanoverschelde JL, Franke A, Sieswerda GT, Pasquet A, et al. Intravenous myocardial contrast echocardiography predicts left ventricular remodeling in patients with acute myocardial infarction. J Am Soc Echocardiogr. (2002) 15:849–56. doi: 10.1067/mje.2002.121277
71. Funaro S, La Torre G, Madonna M, Galiuto L, Scara A, Labbadia A, et al. Incidence, determinants, and prognostic value of reverse left ventricular remodelling after primary percutaneous coronary intervention: results of the acute myocardial infarction contrast imaging (AMICI) multicenter study. Eur Heart J. (2009) 30:566–75. doi: 10.1093/eurheartj/ehn529
72. Kaul S. The “no reflow” phenomenon following acute myocardial infarction: mechanisms and treatment options. J Cardiol. (2014) 64:77–85. doi: 10.1016/j.jjcc.2014.03.008
73. Ito H, Taniyama Y, Iwakura K, Nishikawa N, Masuyama T, Kuzuya T, et al. Intravenous nicorandil can preserve microvascular integrity and myocardial viability in patients with reperfused anterior wall myocardial infarction. J Am Coll Cardiol. (1999) 33:654–60. doi: 10.1016/S0735-1097(98)00604-4
74. Ross AM, Gibbons RJ, Stone GW, Kloner RA, Alexander RW, Investigators A-I. A randomized, double-blinded, placebo-controlled multicenter trial of adenosine as an adjunct to reperfusion in the treatment of acute myocardial infarction (AMISTAD-II). J Am Coll Cardiol. (2005) 45:1775–80. doi: 10.1016/j.jacc.2005.02.061
75. D'Amario D, Migliaro S, Borovac JA, Restivo A, Vergallo R, Galli M, et al. Microvascular dysfunction in heart failure with preserved ejection fraction. Front Physiol. (2019) 10:1347. doi: 10.3389/fphys.2019.01347
76. Shah SJ, Lam CSP, Svedlund S, Saraste A, Hage C, Tan RS, et al. Prevalence and correlates of coronary microvascular dysfunction in heart failure with preserved ejection fraction: PROMIS-HFpEF. Eur Heart J. (2018) 39:3439–50. doi: 10.1093/eurheartj/ehy531
77. Mahfouz RA, Gouda M, Abdelhamid M. Relation of microvascular dysfunction and exercise tolerance in patients with heart failure with preserved ejection fraction. Echocardiography. (2020) 37:1192–8. doi: 10.1111/echo.14799
78. Ahmad A, Corban MT, Toya T, Verbrugge FH, Sara JD, Lerman LO, et al. Coronary microvascular dysfunction is associated with exertional haemodynamic abnormalities in patients with heart failure with preserved ejection fraction. Eur J Heart Fail. (2021) 23:765–72. doi: 10.1002/ejhf.2010
79. Strain WD, Adingupu DD, Shore AC. Microcirculation on a large scale: techniques, tactics and relevance of studying the microcirculation in larger population samples. Microcirculation. (2012) 19:37–46. doi: 10.1111/j.1549-8719.2011.00140.x
80. Urbano-Moral JA, Gonzalez-Gonzalez AM, Maldonado G, Gutierrez-Garcia-Moreno L, Vivancos-Delgado R, De Mora-Martin M, et al. Contrast-enhanced echocardiographic measurement of left ventricular wall thickness in hypertrophic cardiomyopathy: comparison with standard echocardiography and cardiac magnetic resonance. J Am Soc Echocardiogr. (2020) 33:1106–15. doi: 10.1016/j.echo.2020.04.009
81. Cardim N, Galderisi M, Edvardsen T, Plein S, Popescu BA, D'Andrea A, et al. Role of multimodality cardiac imaging in the management of patients with hypertrophic cardiomyopathy: an expert consensus of the European association of cardiovascular imaging endorsed by the saudi heart association. Eur Heart J Cardiovasc Imaging. (2015) 16:280. doi: 10.1093/ehjci/jeu291
82. Bravo PE. Is there a role for cardiac positron emission tomography in hypertrophic cardiomyopathy? J Nucl Cardiol. (2019) 26:1125–34. doi: 10.1007/s12350-018-1298-4
83. Soliman OI, Knaapen P, Geleijnse ML, Dijkmans PA, Anwar AM, Nemes A, et al. Assessment of intravascular and extravascular mechanisms of myocardial perfusion abnormalities in obstructive hypertrophic cardiomyopathy by myocardial contrast echocardiography. Heart. (2007) 93:1204–12. doi: 10.1136/hrt.2006.110460
84. Camici P, Chiriatti G, Lorenzoni R, Bellina RC, Gistri R, Italiani G, et al. Coronary vasodilation is impaired in both hypertrophied and nonhypertrophied myocardium of patients with hypertrophic cardiomyopathy: a study with nitrogen-13 ammonia and positron emission tomography. J Am Coll Cardiol. (1991) 17:879–86. doi: 10.1016/0735-1097(91)90869-B
85. Petersen SE, Jerosch-Herold M, Hudsmith LE, Robson MD, Francis JM, Doll HA, et al. Evidence for microvascular dysfunction in hypertrophic cardiomyopathy: new insights from multiparametric magnetic resonance imaging. Circulation. (2007) 115:2418–25. doi: 10.1161/CIRCULATIONAHA.106.657023
86. Moon J, Cho IJ, Shim CY, Ha JW, Jang Y, Chung N, et al. Abnormal myocardial capillary density in apical hypertrophic cardiomyopathy can be assessed by myocardial contrast echocardiography. Circ J. (2010) 74:2166–72. doi: 10.1253/circj.CJ-10-0241
87. Cecchi F, Olivotto I, Gistri R, Lorenzoni R, Chiriatti G, Camici PG. Coronary microvascular dysfunction and prognosis in hypertrophic cardiomyopathy. N Engl J Med. (2003) 349:1027–35. doi: 10.1056/NEJMoa025050
88. Soliman OI, Geleijnse ML, Michels M, Dijkmans PA, Nemes A, van Dalen BM, et al. Effect of successful alcohol septal ablation on microvascular function in patients with obstructive hypertrophic cardiomyopathy. Am J Cardiol. (2008) 101:1321–7. doi: 10.1016/j.amjcard.2007.12.032
89. Liu L, Li J, Zuo L, Zhang J, Zhou M, Xu B, et al. Percutaneous intramyocardial septal radiofrequency ablation for hypertrophic obstructive cardiomyopathy. J Am Coll Cardiol. (2018) 72:1898–909. doi: 10.1016/j.jacc.2018.07.080
90. Lyon AR, Rees PS, Prasad S, Poole-Wilson PA, Harding SE. Stress (Takotsubo) cardiomyopathy–a novel pathophysiological hypothesis to explain catecholamine-induced acute myocardial stunning. Nat Clin Pract Cardiovasc Med. (2008) 5:22–9. doi: 10.1038/ncpcardio1066
91. Yoshida T, Hibino T, Kako N, Murai S, Oguri M, Kato K, et al. A pathophysiologic study of tako-tsubo cardiomyopathy with F-18 fluorodeoxyglucose positron emission tomography. Eur Heart J. (2007) 28:2598–604. doi: 10.1093/eurheartj/ehm401
92. Meimoun P, Malaquin D, Sayah S, Benali T, Luycx-Bore A, Levy F, et al. The coronary flow reserve is transiently impaired in tako-tsubo cardiomyopathy: a prospective study using serial doppler transthoracic echocardiography. J Am Soc Echocardiogr. (2008) 21:72–7. doi: 10.1016/j.echo.2007.05.024
93. Meimoun P, Malaquin D, Benali T, Boulanger J, Zemir H, Tribouilloy C. Transient impairment of coronary flow reserve in tako-tsubo cardiomyopathy is related to left ventricular systolic parameters. Eur J Echocardiogr. (2009) 10:265–70. doi: 10.1093/ejechocard/jen222
94. Abdelmoneim SS, Mankad SV, Bernier M, Dhoble A, Hagen ME, Ness SA, et al. Microvascular function in Takotsubo cardiomyopathy with contrast echocardiography: prospective evaluation and review of literature. J Am Soc Echocardiogr. (2009) 22:1249–55. doi: 10.1016/j.echo.2009.07.012
95. Jain M, Upadaya S, Zarich SW. Serial evaluation of microcirculatory dysfunction in patients with Takotsubo cardiomyopathy by myocardial contrast echocardiography. Clin Cardiol. (2013) 36:531–4. doi: 10.1002/clc.22154
96. Galiuto L, De Caterina AR, Porfidia A, Paraggio L, Barchetta S, Locorotondo G, et al. Reversible coronary microvascular dysfunction: a common pathogenetic mechanism in apical ballooning or tako-tsubo syndrome. Eur Heart J. (2010) 31:1319–27. doi: 10.1093/eurheartj/ehq039
97. Rigo F, Sicari R, Citro R, Ossena G, Buja P, Picano E. Diffuse, marked, reversible impairment in coronary microcirculation in stress cardiomyopathy: a doppler transthoracic echo study. Ann Med. (2009) 41:462–70. doi: 10.1080/07853890903022793
98. Min SY, Song JM, Shin Y, Sin MJ, Kim DH, Kang DH, et al. Quantitative segmental analysis of myocardial perfusion to differentiate stress cardiomyopathy from acute myocardial infarction: a myocardial contrast echocardiography study. Clin Cardiol. (2017) 40:679–85. doi: 10.1002/clc.22714
99. Montone RA, Galiuto L, Meucci MC, Del Buono MG, Vergni F, Camilli M, et al. Coronary slow flow is associated with a worse clinical outcome in patients with Takotsubo syndrome. Heart. (2020) 106:923–30. doi: 10.1136/heartjnl-2019-315909
100. Bader KB, Gruber MJ, Holland CK. Shaken and stirred: mechanisms of ultrasound-enhanced thrombolysis. Ultrasound Med Biol. (2015) 41:187–96. doi: 10.1016/j.ultrasmedbio.2014.08.018
101. Hitchcock KE, Ivancevich NM, Haworth KJ, Caudell Stamper DN, Vela DC, Sutton JT, et al. Ultrasound-enhanced rt-PA thrombolysis in an ex vivo porcine carotid artery model. Ultrasound Med Biol. (2011) 37:1240–51. doi: 10.1016/j.ultrasmedbio.2011.05.011
102. Brown AT, Flores R, Hamilton E, Roberson PK, Borrelli MJ, Culp WC. Microbubbles improve sonothrombolysis in vitro and decrease hemorrhage in vivo in a rabbit stroke model. Invest Radiol. (2011) 46:202–7. doi: 10.1097/RLI.0b013e318200757a
103. Xie F, Lof J, Matsunaga T, Zutshi R, Porter TR. Diagnostic ultrasound combined with glycoprotein IIb/IIIa-targeted microbubbles improves microvascular recovery after acute coronary thrombotic occlusions. Circulation. (2009) 119:1378–85. doi: 10.1161/CIRCULATIONAHA.108.825067
104. Belcik JT, Mott BH, Xie A, Zhao Y, Kim S, Lindner NJ, et al. Augmentation of limb perfusion and reversal of tissue ischemia produced by ultrasound-mediated microbubble cavitation. Circ Cardiovasc Imaging. (2015) 8:e002979. doi: 10.1161/CIRCIMAGING.114.002979
105. Mathias W Jr., Tsutsui JM, Tavares BG, Fava AM, Aguiar MOD, et al. Sonothrombolysis in ST-Segment elevation myocardial infarction treated with primary percutaneous coronary intervention. J Am Coll Cardiol. (2019) 73:2832–42. doi: 10.1016/j.jacc.2019.03.006
106. Aguiar MOD, Tavares BG, Tsutsui JM, Fava AM, Borges BC, Oliveira MT, et al. Sonothrombolysis improves myocardial dynamics and microvascular obstruction preventing left ventricular remodeling in patients with ST elevation myocardial infarction. Circ Cardiovasc Imaging. (2020) 13:e009536. doi: 10.1161/CIRCIMAGING.119.009536
107. El Kadi S, Porter TR, van Rossum AC, Kamp O. Sonothrombolysis in the ambulance for ST-elevation myocardial infarction: rationale and protocol. Neth Heart J. (2021) 29:330–7. doi: 10.1007/s12471-020-01516-9
108. Fujii H, Sun Z, Li SH, Wu J, Fazel S, Weisel RD, et al. Ultrasound-targeted gene delivery induces angiogenesis after a myocardial infarction in mice. JACC Cardiovasc Imaging. (2009) 2:869–79. doi: 10.1016/j.jcmg.2009.04.008
109. Knott KD, Seraphim A, Augusto JB, Xue H, Chacko L, Aung N, et al. The prognostic significance of quantitative myocardial perfusion: an artificial intelligence-based approach using perfusion mapping. Circulation. (2020) 141:1282–91. doi: 10.1161/CIRCULATIONAHA.119.044666
Keywords: coronary microvascular dysfunction (CMD), myocardial contrast echocardiography (MCE), contrast enhanced ultrasound (CEUS), coronary flow, myocardial ischemia
Citation: Zhan J, Zhong L and Wu J (2022) Assessment and Treatment for Coronary Microvascular Dysfunction by Contrast Enhanced Ultrasound. Front. Cardiovasc. Med. 9:899099. doi: 10.3389/fcvm.2022.899099
Received: 18 March 2022; Accepted: 26 May 2022;
Published: 20 June 2022.
Edited by:
Hai-Ling Margaret Cheng, University of Toronto, CanadaReviewed by:
Francesco Radico, Asl Lanciano Vasto Chieti, ItalyYu-Qing Zhou, University of Toronto, Canada
Copyright © 2022 Zhan, Zhong and Wu. This is an open-access article distributed under the terms of the Creative Commons Attribution License (CC BY). The use, distribution or reproduction in other forums is permitted, provided the original author(s) and the copyright owner(s) are credited and that the original publication in this journal is cited, in accordance with accepted academic practice. No use, distribution or reproduction is permitted which does not comply with these terms.
*Correspondence: Juefei Wu, anVlZmVpd3U4MkBzbXUuZWR1LmNu