- 1School of Medicine, Kashan University of Medical Sciences, Kashan, Iran
- 2Student Research Committee, Kashan University of Medical Sciences, Kashan, Iran
- 3Advanced Therapy Medicinal Product (ATMP) Department, Breast Cancer Research Center, Motamed Cancer Institute, ACECR, Tehran, Iran
- 4Proteomics Research Center, Shahid Beheshti University of Medical Sciences, Tehran, Iran
- 5Department of Internal Medicine and Endocrinology, Beheshti University of Medical Sciences, Tehran, Iran
- 6Department of Medical Biotechnology, School of Medicine, Mashhad University of Medical Sciences, Mashhad, Iran
- 7Department of Pharmacy, Abdul Wali Khan University, Mardan, Pakistan
- 8Research Center for Biochemistry and Nutrition in Metabolic Diseases, Institute for Basic Sciences, Kashan University of Medical Sciences, Kashan, Iran
- 9Department of Cell Systems and Anatomy, UT Health. Long School of Medicine, San Antonio, TX, United States
- 10Laser Research Centre, Faculty of Health Science, University of Johannesburg, Johannesburg, South Africa
The pineal gland is a neuroendocrine gland which produces melatonin, a neuroendocrine hormone with critical physiological roles in the circadian rhythm and sleep-wake cycle. Melatonin has been shown to possess anti-oxidant activity and neuroprotective properties. Numerous studies have shown that melatonin has significant functions in cardiovascular disease, and may have anti-aging properties. The ability of melatonin to decrease primary hypertension needs to be more extensively evaluated. Melatonin has shown significant benefits in reducing cardiac pathology, and preventing the death of cardiac muscle in response to ischemia-reperfusion in rodent species. Moreover, melatonin may also prevent the hypertrophy of the heart muscle under some circumstances, which in turn would lessen the development of heart failure. Several currently used conventional drugs show cardiotoxicity as an adverse effect. Recent rodent studies have shown that melatonin acts as an anti-oxidant and is effective in suppressing heart damage mediated by pharmacologic drugs. Therefore, melatonin has been shown to have cardioprotective activity in multiple animal and human studies. Herein, we summarize the most established benefits of melatonin in the cardiovascular system with a focus on the molecular mechanisms of action.
Introduction
Cardiovascular disease (CVDs) accounts for the majority of deaths worldwide (1, 2), and is more predominant in older generation (3). According to WHO report, CVDs accounted for 17.9 million deaths in 2019, representing 32% of all global deaths (3–8). Although the mortality rate is now being reduced, the prevalence of CVDs still remains too high (9). The CDC reports that ~610,000 people die due to CVDs each year in the USA (10).
Cardiovascular disease encompasses a group of disorders involving blood vessels or the heart (11), including coronary artery disease, myocardial infarction, angina, hypertensive heart disease, heart failure, cardiomyopathy, arrhythmia, congenital heart disease, valvulopathy, aortic aneurysm, carditis, rheumatic heart disease, venous thrombosis, thromboembolic disease, and peripheral vascular disease (4, 7, 11, 12). Coronary artery disease accounts for the majority of cases, causing ~375,000 deaths per year in the USA (10, 13).
The beneficial effects of melatonin in treating various human diseases has been broadly investigated (14–20). Melatonin is an indoleamine-derived molecule, which is synthesized at night in the pineal gland of the brain under control by the hypothalamic suprachiasmatic nucleus (21–24). The traditional function of melatonin could be exert as an endogenous synchronizer of circadian and seasonal rhythms, which modulates sleep patterns (22, 23). In addition, melatonin exhibits many other biological functions, such as anti-inflammatory, antioxidant, anti-excitatory, immunomodulatory, metabolic, and vasomotor activities (25, 26). In particular, endogenous melatonin plays a significant role in numerous CVDs and metabolic disorders, which can result in the development of heart failure (15, 17, 27–29).
The effects of melatonin in the cardiovascular system have been investigated in several previous studies (30–34). Melatonin has direct interactions with the nervous system, and indirect interactions with blood vessels and the heart (29, 32, 34). Melatonin exerts its direct functions by a receptor-dependent signaling pathway, and its indirect functions as a free radical scavenger (33, 35). The receptors for melatonin are G-protein coupled receptors, such as membrane receptors type 1 (MT1, Mel1A, MTNR1A) and type 2 (MT2, Mel1B, MTNR1B), as well as the retinoid-related orphan nuclear receptors RZR and RORα (26, 33). Upon binding to these receptors, melatonin can exert modulatory effects in the blood vessels and the heart (33). Various signaling pathways have been shown to mediate the downstream effects of melatonin, such as adenylate cyclase, phospholipase C, protein kinase C (PKC), guanylate cyclase, potassium channels, calcium channels, and phospholipase A2. Some of these mediate the anti-adrenergic effects of melatonin (33, 36, 37). Melatonin receptors play an essential role in reducing the risk of heart failure (37–41) and cardiomyopathy (28, 42–44) after myocardial infarction. In this review the authors aim to point out therapeutic potentioals of melatonin in the treatment of CVDs with an emphesis on the molecular mechanisms of action. Moreover, current clinical trials using melatonin in heart disease are discussed.
The Biological Functions of Melatonin as a Neurohormone and Antioxidant
When melatonin binds to MT1 and MT2 receptors, it exerts its regulatory function on the circadian rhythm, sleep-wake cycle, and body temperature cycles (Figure 1) (46–54). Administration of melatonin to humans results in sleepiness, fatigue and reduced sleep latency (55). Impaired circadian rhythms have been associated with poor health and sleep disorders (56). For instance, pediatric populations with various neuropsychiatric, developmental, or health disorders often exhibit a deficiency of melatonin (57). Following the restoration of melatonin levels, circadian rhythms, as well as developmental, mood, behavioral, and health disorders may be improved. Improved intellectual ability and even control of seizures may be obtained (56, 58). It is known that circadian rhythms are critical for the normal development and function of the nervous system, and their disruption eliminates neurogenesis in laboratory animal models (59–62). Melatonin may also play an important role in embryonic development, with direct effects on the placenta and neuroglial structures. Additionally, melatonin has important functions in several stages of ontogenesis, including establishing diurnal rhythms and synchronization of the biological clock in the fetus (63, 64).
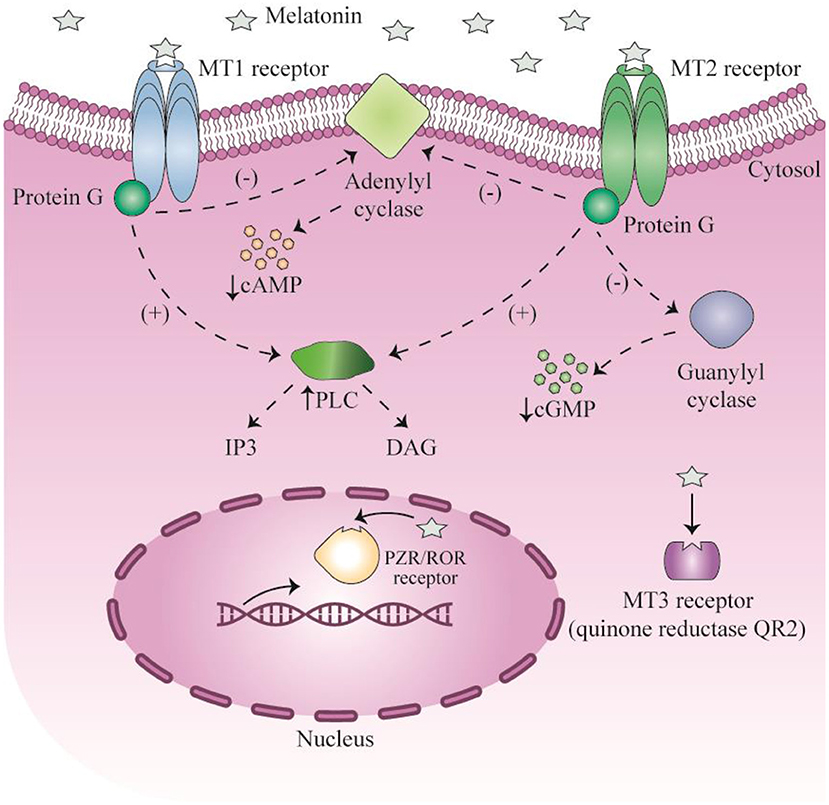
Figure 1. Signaling of melatonin and their receptors. This figure adapted from Millet-Boureima et al. (45).
In addition to the well-established role of melatonin in sleep-wake rhythm modulation, melatonin functions as an endogenous chronobiotic and synchronizing agent, which strengthens oscillations and regulates central biological clock timing in the hypothalamic suprachiasmatic nucleus resulting in stable bodily rhythms (65). Moreover, a study by Pevet and Challet (66) showed that melatonin can serve as both an endogenous-time regulator, and can control the master clock output in complex circadian networks. Melatonin transfers temporal signals to various tissues possessing melatonin receptors, which results in the induction and regulation of circadian rhythms in a number of organs. These organs include the adenohypophysis, and peripheral tissues such as the liver, pancreas, lungs, heart, kidneys, adipose tissue, gastrointestinal tract, as well as the fetal adrenal gland. Circadian rhythms and the circadian clock network enable biological processes to be temporally organized in response to episodic environmental changes, thus providing environmental adaptability (67).
Melatonin exerts its anti-oxidant effects via a cell surface receptor-independent pathway, since the MT3 receptor is a QR2 (quinone oxidoreductase 2) cytosolic enzyme. Melatonin reduces the generation of new free radicals and reactive oxygen species (ROS). The ability of melatonin to scavenge ROS was present in mammalian species from the evolutionary period (68). Melatonin acts as a natural antioxidant and scavenger, and can reduce both reactive nitrogen species and ROS (69). Melatonin binds directly to the cytosolic QR2 catalytic site, and regulates the function of QR2 in order to detoxify or reduce production of ROS (70, 71). The detoxification mediated by melatonin maintains redox homeostasis in cells, and protects cells against damage and oxidative stress (72). The QR2 MT3 receptor is involved in protection against neurodegeneration in brain cells, and reduces ulceration and carcinogenesis in the gastrointestinal tract.
The pineal gland is also involved in the regulation of the immune system responses (73), as shown by the reduced cell-mediated immunity and humoral responses following pharmacological inhibition (administration of propranolol) or functional inhibition (constant light condition) of melatonin synthesis in mouse models. The immune system and the pineal gland display bidirectional interactions, because cytokines, interleukins, and interferon-γ can alter the production and release of melatonin (74). The transcription factor NF-κB plays a crucial role in inflammatory responses. NF-κB activity is typically blocked by binding of a specific protein IκB (inhibitor of NF-κB). In inflammation, the release of pro-inflammatory mediators, ROS generation, and TLR (toll-like receptor) activation stimulates a kinase enzyme (IκB kinase), which phosphorylates IκB and promotes its dissociation from NF-κB, allowing NF-κB to translocate to the nucleus. In the nucleus, NF-κB regulates the transcription of many pro-inflammatory genes. Melatonin suppresses the activation as well as the translocation of NF-κB in various cells, including T cells, neuronal cells and macrophages (75–77). There are two RAR Related Orphan Receptors (RORγ and RORα), which can modulate the inflammatory response. RORγ stimulates Th17 cell lineage differentiation, and modulates the expression of numerous pro-inflammatory mediators (IFN-γ, IL-17F, IL-17, IL-2, and TNF-α) (78, 79). Melatonin binds to ROR/RZR nuclear receptors, and affects gene transcription and thus inflammatory responses (80).
Melatonin has also been shown to have anti-cancer activity in several previous studies, and has the potential to protect against tumorigenesis. Melatonin has shown anti-proliferative effects, anti-oxidant properties, and can activate the anti-tumor immune response. However, there are some studies showing that melatonin can promote tumor formation and growth, particularly when melatonin is given in the morning. These observations suggest that the anti-cancer activity of melatonin may be dependent on the stage of the circadian cycle (81). In metastatic non-small cell lung carcinoma, a controlled clinical trial found that simultaneous administration of cisplatin and etoposide combined with melatonin could improve treatment outcomes in terms of both quality of life and survival rate (82).
Reduced overall production of melatonin and dysregulated nocturnal synthesis of melatonin have been associated with several disorders of the CNS (central nervous system), including schizophrenia, obsessive-compulsive disorder, and stroke (Figure 2) (84). The human brain accounts for only 2% of total body weight, but consumes 20% of the overall intake of oxygen and glucose in the body. Cells in brain tissue produce more ROS in comparison with other tissues. There is a high concentration of ascorbic acid and polyunsaturated fatty acids in brain tissue, which are vulnerable to free radical-mediated injury, when enzymatic antioxidants are inadequate. Melatonin has a neuroprotective effect in several diseases, including amyotrophic lateral sclerosis, epilepsy, Parkinson's disease, Alzheimer's disease, traumatic brain injury, and brain ischemia (85, 86). In the majority of these disorders, there is a progressive loss of neurons, accompanied by mitochondrial dysfunction, glutamate excitotoxicty, and free radical damage (86).
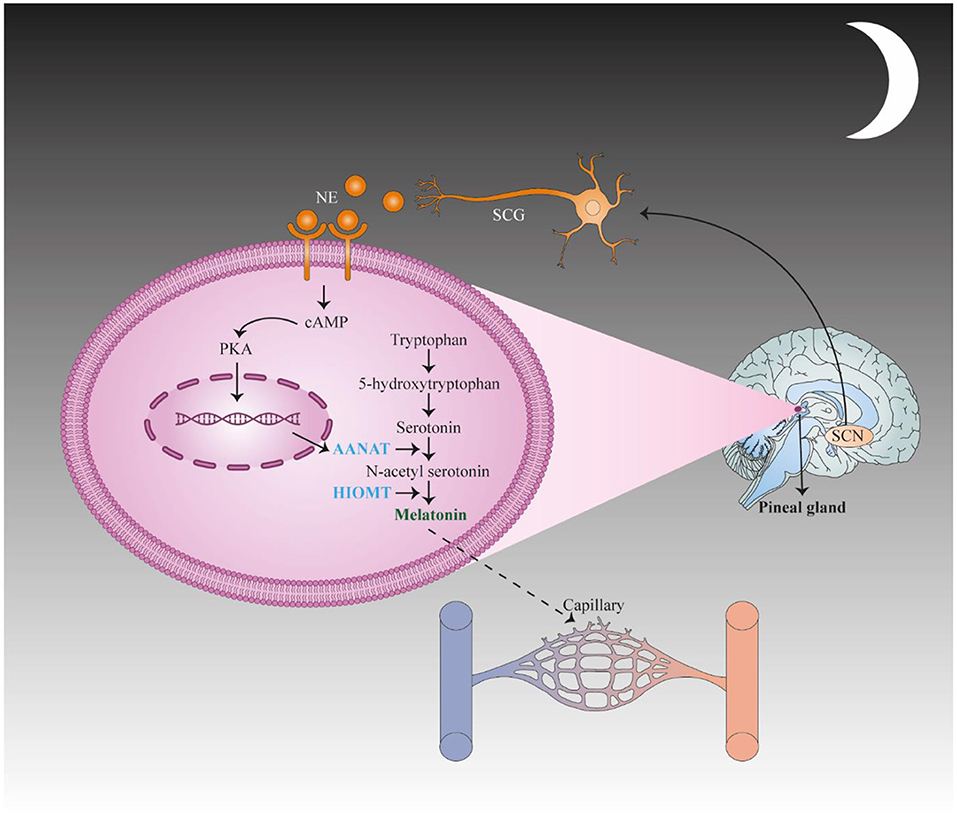
Figure 2. First, the suprachiasmatic nucleus (SCN) translates stimulation to the spinal cord and the superior cervical ganglia (SCG) of the sympathetic nervous system and subsequently activates adrenergic fibers to secrete norepinephrine (NE), which binds to adrenergic receptors in pinealocytes. These changes result in the up regulation of N-acetyltransferase (AANAT), the key enzyme in melatonin synthesis, via activating CAMP signaling. This change causes an increase in the concentration of N-acetyl serotonin, which is converted to melatonin by hydroxyindole-O-methyltransferase (HIOMT). This figure adapted from Song et al. (83).
Melatonin has many beneficial effects on cardiovascular system. In this context, melatonin can regulate heart rate (87), and reduce nocturnal blood pressure in patients with hypertension (88, 89). Moreover, melatonin may serve as a potent protective agent in the cardiovascular system, and diminish the risk of developing reperfusion injury after myocardial infarction (90). The benefits of melatonin are related to its ability to scavenge free radicals, reduce oxidative stress, modulate metabolic activity, regulate production of cytokines, and prevent against apoptosis. These findings have been confirmed by various preclinical and animal studies (91, 92). Nevertheless, a larger number of clinical studies are required to confirm these benefits in humans. Beta-blockers are often administered to patients with hypertensive disorders, and these drugs can block endogenous secretion of melatonin. Consequently, hypertensive patients may experience insomnia as an adverse effect of beta-blockers. Administration of exogenous melatonin supplements can improve the quality and amount of sleep in these patients (93).
Melatonin can affect multiple cardiovascular functions, such as cardiac output, blood pressure, heart rate, and seasonal rhythms. The functions of melatonin are related to the activity of the melatonergic system and the suprachiasmatic nucleus (94). After resection of the pineal gland, the essential source of melatonin circulating in plasma, the blood pressure in rats was elevated, while administration of melatonin to rats with hypertension can reduce arterial pressure, baroreflex response, and heart rate. The mechanism for this effect involves a decrease in CAMP and an increase in hydrolysis of phosphatidylinositol-4,5-bisphosphate (95). It has also been speculated that melatonin can activate endothelial cells via binding to MT2 receptors, leading to the synthesis of nitric oxide which promotes the generation of soluble guanylate cyclase in smooth muscle cells. This leads to an increase in cGMP production and hence to vasodilation. ROS and reactive nitrogen species are known to contribute to the pathogenesis of cardiac ischemic reperfusion injury. Melatonin exerts its ROS scavenging function in mitochondria, with beneficial effects in ischemic heart disease and prevents ischemia reperfusion-mediated myocardial damage. Moreover, melatonin shows therapeutic efficacy in vasculopathy caused by nicotine. Administration of nicotine has been associated with significant endothelial injury and aortic vasoconstriction, which can be counteracted by melatonin (96, 97). The reduced levels of superoxide dismutase and nitric oxide synthase caused by chronic nicotine administration may also be improved by melatonin.
Melatonin Protective Effects Against Drug-Induced Cardiotoxicity
Doxorubicin (Dox) is a commonly used anticancer chemotherapeutic drug in the class of anthracyclines, which has been extensively used in the management of both hematologic and solid tumors (98). However, the therapeutic application of Dox has been limited, because it causes cardiac hypertrophy and heart failure. A study showed that administration of trastuzumab plus anthracyclines in patients led to increased left ventricular afterload and preload, whereas it resulted in reduced strain, heart rate and ejection fraction. In addition, recovery from these effects was not observed even after a 2-year-follow up (99, 100). Dox produces cardiac toxicity via multiple pathways, including a large increase in ROS proudction (101). Myocytes have abundant mitochondria which are needed for production of sufficient ATP for heart contraction, and maintaining cell viability in the myocardium (102, 103). Dox accumulates in the mitochondria, where it damages the mitochondrial membrane, reducing the MMP (mitochondrial membrane potential) and producing ROS, leading to the death of cardiomyocytes (104). In addition, studies have found that Dox can trigger apoptosis by decreasing the anti-apoptotic protein Bcl2, and inceasing pro-apoptotic Bax (105–107).
Yes-associated protein (YAP) is a down-stream component of the Hippo signaling pathway, which affects several cardiac physiological and pathological processes. These include the development of cardiac muscle, formation of new blood vessels, cellular apoptosis, autophagy, hypertrophy, and metabolic homeostasis (108). Suppression of YAP leads to aggravated heart failure after myocardial infarction, and apoptosis and fibrosis in cardiac muscle cells (109). In previous studies, YAP was found to decrease myocardial injury after MI, and increase the survival of cardiomyocytes by increasing the expression of YAP-target genes, which ultimately improves heart performance. Activation of genes involved in the cell cycle are involved in the YAP response (110). Both post-MI heart failure and non-ischemic heart failure are related to the Hippo signaling pathway. Systolic heart failure following MI can be inhibited by suppression of the Hippo pathway (111). Furthermore, YAP can regulate the antioxidant activity within heart cells. Inhibition of YAP expression leads to suppression of the activity of FoxO1 transcription factor and lower expression of anti-oxidant genes, which in turn worsens myocardial ischemia-perfusion injury (112).
Li et al. carried out a study to explore the potential anti-oxidant effects of melatonin, and whether it could protect against apoptosis mediated by Dox in cardiac muscle cells (113). Their study showed that treatment with Dox increased apoptosis and decreased viability of H9c2 cells. They found increased TUNEL positive cells, elevated expression of Bax, and reduced expression of Bcl2. This effect was linked to decreases in MMP, and increases in ROS. Treatment with Dox for 5 weeks was associated with significant LV dysfunction detected by echocardiography in vivo. Although Dox-mediated apoptosis was higher by TUNEL staining, concomitant administration of melatonin and Dox reduced ROS generation in cardiomyocytes, and inhibited apoptosis by increasing the MMP. Moreover, melatonin-Dox combined therapy decreased Dox-induced cardiac damage in vivo. In vivo immunohistochemistry staining, in vitro immunofluorescence, and Western blotting showed that treatment with Dox significantly suppressed expression of YAP, while YAP levels were unchanged following concomitant administration of melatonin plus Dox. The protective effects of melatonin against toxicity in cardiac muscle cells were counteracted by YAP suppression mediated by siRNA, which led to increased ROS and apoptosis. Taken together, melatonin therapy decreased the cardiotoxicity mediated by Dox through maintaining YAP levels, leading to lower apoptosis and oxidative stress (113).
AGO (agomelatine) is a small molecule anti-depressant drug, but also acts as an M1 and M2 melatonergic receptor agonist, and 5-HT2C serotonergic receptor antagonist (114). AGO shows marked affinity for M2 and M1 receptors, and has similar antioxidant properties to melatonin (115). AGO protects against ischemia-reperfusion injury by improving anti-oxidant capacity (116).
Aygun and Gul performed a study to evaluate the cardioprotective properties of AGO, melatonin, and AGO + melatonin combined against Dox-induced cardiotoxicity using electrocardiographic, biochemical, and scintigraphic methods (117). In this study, 49 male Wistar rats were randomly allocated to seven different groups, namely control, AGO, melatonin, Dox, Dox + AGO, Dox+melatonin, and Dox + AGO + melatonin. AGO and melatonin were administered intraperitoneally to rats at a dose of 40 mg/kg/day for 7 days; doxorubicin was administered intraperitoneally at 18 mg/kg/day on days 5–7 to induce cardiotoxicity. They carried out 99mTc PYP (technetium-99m pyrophosphate) scintigraphy and ECG (electrocardiography) on the 8th day of the study, in addition to biochemical measures, like BUN, cardiac troponin T (cTnT), and creatine kinase (CK) in the rats. To define acute cardiotoxicity induced by Dox, the following criteria were used: ECG disturbance (reduced duration of QRS and p, ST-elevation, and increased QT and RR intervals), elevated serum CK, BUN and cTnT1, and enhanced uptake of 99mTc PYP in the heart. Pretreatment with AGO, melatonin, or AGO+melatonin could efficiently restore Dox-induced ECG abnormalities to near normal (p < 0.001). Furthermore, 99mTc PYP uptake and serum biochemical markers showed that pretreatment with AGO, melatonin, or AGO + melatonin showed equal protective benefits against Dox-induced cardiotoxicity (p < 0.001). Their study showed that 99mTc PYP could be an appropriate non-invasive method to monitor Dox-mediated cardiotoxicity (117).
AMPK (adenosine monophosphate activated protein kinase) is a serine/threonine protein kinase (118), which plays a crucial role in regulating endogenous defense mechanisms in cardiomyocytes (119–121). PGC1α controls mitochondrial energy homeostasis by modulating expression of genes, such as UCP2, TFAM, and NRF1 (122, 123). Previous studies have shown that treatment with Dox suppresses the PGC1α and AMPK signaling pathways, leading to exacerbation of myocardial injury (120–122). On the other hand, melatonin activates PGC1α and AMPK signaling pathways, and results in protection of myocardial mitochondrial function (38, 124, 125).
In a study by Liu et al. C57BL/6 mice and H9c2 cells were used to assess the effects of melatonin against acute cardiotoxicity caused by Dox. They studied mitochondrial function, cell viability, apoptosis, oxidative stress, and the AMPK/PGC1α pathway activity (99). Dox caused acute cardiotoxicity in both C57BL/6 mice and H9c2 cells, which was ameliorated by melatonin both in vivo and in vitro. Melatonin suppressed Dox-mediated mitochondrial dysfunction, apoptosis, cellular morphological abnormalities, and oxidative stress, through activating the AMPK/PGC1α axis, and its target genes (UCP2, NRF1, and TFAM). In vitro, either PGC1α siRNA or AMPK siRNA were able to block these effects. In vivo, the AMPK inhibitor compound C also abrogated the benefits of melatonin. They concluded that melatonin improved Dox-induced acute cardiotoxicity by activating the AMPK/PGC1α pathway (99).
Reiter et al. (126) reviewed the high concentration of melatonin in the mitochondria, and its antioxidant effects. Previous studies have suggested that mitochondria originally entered eukaryotic cells by the process of endosymbiosis. Additionally, mitochondria have been found to have the potential to effectively synthesize melatonin (127). Melatonin can feasibly diffuse across biological membranes due to its amphiphilic nature, however the high intra-mitochondrial concentration was found to be related to localized mitochondrial membrane transporters, peptide transporters 1 and 2 (PEPT1/2) (128). Melatonin was suggested to be superior to commonly used mitochondrial antioxidants, since its metabolites including N [1]-acetyl-5-methoxykynuramine (AMK) and N1-acetyl-N2-formyl-5-methoxykynuramine (AFMK) also show antioxidant properties, in addition to melatonin itself. Therefore, melatonin may exert its antioxidant effects in a cascade manner (129, 130). Melatonin serves to promote the antioxidant defense systems of the organism, as both pharmacological and physiological doses of melatonin can promote the expression of genes associated with antioxidant activity (CAT, GPx, SOD, GRd) (131–133). Moreover, melatonin (in contrast with the vast majority of small molecule antioxidants) is not able to carry out redox cycling (134). The majority of antioxidants can also act as pro-oxidants by producing relatively stable free radicals, which can then generate additional free radicals. Considering the electron-rich structure of melatonin, it can covalently bind to free radicals producing stable water-soluble molecules (134). As a result, melatonin is a suicidal/terminal antioxidant (as distinct from other antioxidants) (134).
Furthermore, several studies have assessed the effects of melatonin combined with various chemotherapeutic drugs in patients with advanced stage cancer who have an unfavorable clinical prognosis. According to these studies, melatonin could significantly enhance the efficiency of chemotherapy, and reduce Dox-related cardiotoxicity (135–137). In this context, melatonin has both cardioprotective and anticancer properties. The cardioprotective potential of melatonin against Dox-mediated cardiotoxicity is most likely due to indirect antioxidant activity combined with direct free radical scavenging properties. Comprehensive studies on the effects of melatonin on mitochondrial bioenergetics, mitochondrial fusion and fission, cell death and mitophagy, and mitochondrial sirtuin, would improve our understanding of the protective mechanisms against Dox-induced cardiotoxicity.
Govender et al. demonstrated the effects of melatonin on cell death, mitochondrial fission and fusion, cardiac function, sirtuin and PGC1-α expression, in a rat model of acute Dox-mediated cardiotoxicity in vivo. Moreover, they investigated ATP synthesis and mitochondrial structure in acute Dox-induced cardiotoxicity in vitro. According to their results, administration of melatonin prior to Dox treatment can preserve mitochondrial function in DOX-mediated cardiotoxicity, and improve survival of cardiomyocytes (138). In vitro H9c2 rat cardiomyoblasts received melatonin as pre-treatment (10 μM, 24 h), which was followed by administration of Dox (3 μM, 24 h). Mitochondrial structure and ATP levels in cells were evaluated. Dox caused cell death and fission of mitochondria, both of which were decreased following administration of melatonin. The in vivo study employed Sprague Dawley rats bearing breast cancer tumors (LA7) which received a Dox injection with or without melatonin in their drinking water for 14 days. Rats receiving melatonin in combination with Dox showed higher cardiac output in comparison to rats receiving Dox alone. The mean tumor volume on day 8 was remarkably lower in rats receiving melatonin plus Dox, compared to rats treated with Dox alone. The combined melatonin and Dox treatment was associated with higher intracellular ATP levels, SIRT1, and PGC1-α expression levels, compared to Dox alone. They concluded that melatonin provides a dual anticancer and cardioprotective benefit by increasing mitochondrial and cardiac functions (138).
The anticancer effects of melatonin have been shown in numerous tumor types, such as ER+ breast cancer (139–142). SOD (superoxide dismutase) is an enzyme which produces hydrogen peroxide and oxygen molecules from superoxide radicals, and thus plays a major role in protecting against cellular injury mediated by ROS. GPx (glutathione peroxidase) is another antioxidant enzyme extensively found in vivo. This enzyme serves as an antioxidant, preventing the accumulation of lipid peroxides (LPO) in cellular membrane. LPO are generated from polyunsaturated fatty acids present in cell membranes. Cell membrane oxidative damage has been associated with a wide range of diseases.
Floyd et al. reported that LPO, GPx, and SOD to be involved in Dox induced cardiotoxicity (143). They observed a negative correlation between GPx and SOD and the damage induced by Dox, meanwhile LPO showed a positive correlation. Melatonin has demonstrated a significant role in protection against lipid peroxidation and oxidative membrane damage. This mechanism may explain why melatonin protects against Dox associated myocardial injury (144–147).
In a study by Zhang et al. the cardioprotective properties of melatonin and its role in enhancing the anticancer effects of Dox were investigated. They used a model of rat ER+ breast tumor to study the effects of melatonin (148). After induction of the breast tumor, they randomly distributed the rats between five groups: no treatment control, solvent [dehydrated alcohol: physiological saline (1:9)], melatonin alone, Dox alone, melatonin + Dox (M + D). They measured LPO, GPx, and SOD levels in the myocardium, myocardial tissue was evaluated by electron and light microscopy, and they followed the survival rates of the different groups for a 1-month period. Breast tumor was identified in 116 rats. In comparison to the control group, the group receiving Dox showed high lipid peroxides, whereas GPx and SOD activity were considerably lower. M+D group had higher GPx and SOD activity (P < 0.05), whereas lipid peroxide was lower than Dox alone (P < 0.05). Moreover, the rats group exposed to M + D had less significant myocardial damage compared to Dox alone, and 1-month life expectancy was higher in the group receiving M + D in comparison with Dox alone. Consequently, melatonin can decrease oxidative damage mediated by Dox in myocardial tissue and play a cardioprotective role (148).
Table 1 lists some studies on the effects of melatonin on drug-induced cardiotoxicity and heart damage.
Melatonin and Stem Cell Therapy for Heart Regeneration: Synergistic Effects on Cardiac Progenitor Cells
Myocardial infarction (MI) is the most common cause of morbidity and mortality globally. Despite the success of surgical intervention and pharmacological therapy, which have reduced MI-related mortality, the heart does not possess the ability to naturally regenerate itself, and hence cardiac function is often impaired in the long term after MI (168). Stem cells possess the potential for multi-lineage differentiation, and combined with paracrine signaling, their transplantation offers a potential treatment for the regeneration and repair of injured cardiac and vascular tissue following MI (169). Nonetheless, transplanted stem cells are prone to death by necrosis and/or apoptosis within the ischemic cardiac muscle, and the presence of inflammatory mediators and oxidative stress in the infarcted region can significantly limit the efficiency of stem cell transplantation (170, 171). Several techniques have been proposed for improving stem cell viability after transplantation into infarcted heart tissue (172). For example, the survival rate of stem cells was increased by transferring genes encoding anti-apoptosic proteins, like Bcl-2 (173) or survivin (174). Several small molecule chemical compounds, like melatonin have also been tested for this purpose (175). Moreover, other tissue engineering techniques such as cellular aggregates or cellular sheets, have been tested to improve the survival rate in transplanted stem cells (176, 177). Among the approaches listed above, pre-treatment with a natural supplement (melatonin) may be more practical and economical.
According to previous studies, melatonin pre-treated stem cells show a higher resistance to oxidative stress damage, and several mechanisms have been proposed to explain this observation, including direct ROS detoxification, and indirect stimulation of antioxidant defense enzymes (126, 178–180). However, melatonin does not apprear to exert a prolonged protective effect on transplanted stem cells to ensure their long-term engraftment (181).
Nanoscale drug delivery carriers can regulate the release of drugs from polymeric nanoparticles, to enhance bioavailability and decrease dosage to avoid adverse effects (182). This approach could be used for drug pretreatment before strem cell transplantation. Nonetheless, whether nano drug delivery carriers for melatonin are more effective compared to free melatonin, had not been tested.
Ma et al. (183) described the preparation of melatonin nanoparticles (Mel-NPs) by encapsulating melatonin inside the biodegradable, non-antigenic, and non-toxic polymer, PLGA-mPEG. The protective effects and the underlying mechanisms of the melatonin nanoparticles were investigated. A hypoxia/reperfusion (H/R) model was utilized to reproduce the oxidative stress microenvironment following MI. They evaluated the association between p53-cyclophilin D (CypD) complex, which controls mPTP (mitochondrial permeability transition pore) opening and melatonin. In their study, the protective effects of melatonin nanoparticles (Mel-NPs) on adipose derived stem cells (ADSC) were evaluated and compared to those of melatonin alone in vitro and in vivo. In vitro, Mel-NPs inhibited the p53-cyclophilin D complex, suppressed mPTP opening, and alleviated H/R damage in ADSCs. In addition, Mel-NPs resulted in longer survival rates of ADSCs in infarcted myocardial tissue of rats, compared to free melatonin, and the therapeutic benefits were more pronounced. Taken together, the combined approach of stem cell transplantation and Mel-NPs for treament of MI, may be a novel and efficient strategy (183).
The normal protein PrPC (cellular prion protein) is an ubiquitous glycoprotein anchored to the cell membrane via glycosylphosphatidylinositol, which is conserved across species (184). Despite the fact that the abnormal prion protein (PrP) plays a role in pathogenesis of neurodegenerative disorders and prion diseases (185), accumulating evidence suggests that the normal PrPC plays a major role in the proliferation and self-renewal of stem cells (186–188), and could enhance their protective role against neurodegenerative disorders (189). Several studies have shown that PrPC has a critical function in the differentiation of progenitor and/or stem cells (190, 191), neurogenesis (188, 192), and formation of blood vessels (193). Nevertheless, the underlying mechanism by which PrPC protects transplanted stem cells in various pathophysiological disorders, remains poorly understood.
Lee et al. investigated the beneficial effects of melatonin in improving the biological activity of mesenchymal stem cells in the ischemic myocardium. Their study showed that melatonin could increase the expression of PrPC, which in turn regulated resistance to oxidative stress, proliferation, and the immunomodulatory properties of mesenchymal stem cells. Subsequently, the potential capacity of melatonin activated mesenchymal stem cells to promote neovascularization was evaluated in a mouse model of hind-limb ischemia (194). Administration of melatonin promoted the proliferation of mesenchymal stem cells and self-regeneration by increasing the expression of PrPC. Furthermore, melatonin decreased apoptosis in mesenchymal stem cells during oxidative stress by different mechanisms, such as regulating apoptotic proteins, caspase-3, PARP-1, BCL-2, and BAX in a PrPC-dependent manner. Additionally, melatonin could modulate the immunomodulatory properties of mesenchymal stem cells through the PrPC-IDO (Indoleamine 2,3-dioxygenase) axis. Furthermore, melatonin stimulated stem cells improved limb salvage, blood flow perfusion, and angiogenesis while lowering macrophage infiltration in the model of hind-limb ischemia. The therapeutic effects of melatonin were suppressed by blocking the expression of PrPC. According to their study, melatonin could enhance the performance of mesenchymal stem cells and stimulate angiogenesis in ischemic tissues via increasing the expression of PrPC. Melatonin-mediated PrPC targeting may provide a novel treatment approach in mesenchymal stem cell therapy (194).
As mentioned above, melatonin has been shown to stimulate antioxidant enzymes, like SOD and catalase, which could increase mesenchymal stem cell resistance to apoptosis induced by hydrogen peroxide (195, 196). Regulating the ischemic environment through inhibiting excessive inflammation and oxidative damage could improve the efficacy of mesenchymal stem cell transplantation in ischemic tissues (197, 198).
Han et al. performed a study to assess whether the cardioprotective effect of AD-MSCs (adipose-derived mesenchymal stem cells) could be promoted by melatonin (199). The mechanism of action of melatonin on SIRT1 signaling was evaluated in a cell model of hypoxia/serum deprivation (H/SD) in vitro. SIRT1 or sirtuin 1 (silent mating type information regulation 2 homolog 1) is a deactylating enzyme in the nucleus that activates transcription factors. In vivo, melatonin increased transplanted AD-MSC survival as well as promoting cardiac function following MI. They demonstrated that melatonin could enhance the survival rate of AD-MSCs in the ischemic myocardium and synergistically improve cardiac function in combination with AD-MSCs. Melatonin resulted in less oxidative stress, apoptosis and inflammation in the ischemic tissue in vivo. Mechanistically melatonin may promote SIRT1 signaling, resulting in an increase in Bcl2 and inhibition of Bax, Ac-p53, Ac-NF-κB and Ac-FoxO1. Therefore, melatonin may be a promising treatment strategy to improve MSC therapy in ischemic cardiac disease, by regulating SIRT1 signaling (199).
Table 2 lists some studies on the synergistic effects of melatonin and progenitor cells for cardiac regeneration.
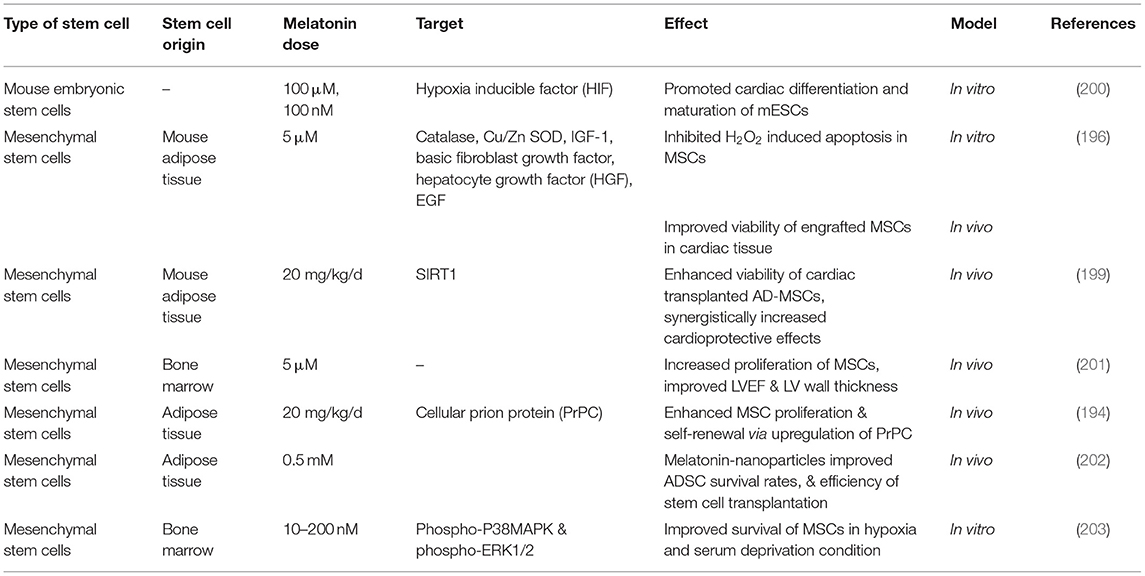
Table 2. Studies on the synergistic effects of melatonin and progenitor cells for cardiac regeneration.
Melatonin for Ischemia-Reperfusion Injury and Preventing Myocardial Damage
Two melatonin receptor subtypes (MT1 and MT2) are present in mammalian cells. Both of these receptors are coupled to Gi/o-type proteins, while MT1 is also coupled to Gq-type proteins (80, 204). Melatonin affects a wide range of physiological processes in mammals, by activating membrane receptors (80, 205). Melatonin membrane receptors in the myocardium have been shown to regulate numerous survival signaling pathways, such as SIRT1 and Hes1 (33, 206, 207). Nevertheless, the exact mechanism by which melatonin exerts its effects in the myocardium is still poorly understood. Melatonin receptors can modulate various signaling pathways within the cells, including cAMP, PKA (protein kinase A), PKG (protein kinase G), cGMP, and PLC (phospholipase C) signaling pathways (80, 204, 205). Amongst the pathways listed above, PKG and cGMP signaling have been shown to act as major mediators of cardiac protection (208). cGMP-PKG may be involved in cardioprotective pathways in MI or reperfusion injury, particularly in patients with diabetes (209–211). Up to now, how exactly melatonin regulates cGMP-PKG remains to be understood. Melatonin may decrease or increase the intracellular levels of cGMP, depending on the pathological or physiological conditions, and on the cell type (212–215). Nrf-2 (nuclear factor-erythroid factor 2-related factor 2) is a transcription factor that modulates several antioxidant genes and protective enzymes, which is ubiquitously expressed in the cardiovascular system (216). In previous studies, Nrf-2 and its target HO-1 (heme oxygenase-1) showed protective effects against MI and reperfusion injury in patients with diabetes (216, 217). In several different organs, an association between Nrf-2/HO-1 and cGMP-PKG activity has been demonstrated (218). Additionally, the cGMP-PKG axis could regulate the MAPK (mitogen-activated protein kinase) cascade in ischemic myocardium. Alterations in JNK, ERK, and p38 kinase, could modulate apoptosis in cardiac muscle cells (219–221). Nevertheless, it is not clear if these signaling pathways play a role in melatonin-related cardioprotection or how they interact with melatonin membrane receptors.
Yu et al. designed a study to evaluate how cGMP-PKG, the Nrf-2-HO-1 axis, and the MAPK cascade were involved in the cardioprotective effects of melatonin (222). They used an in vivo model of Sprague-Dawley rats with diabetes induced by streptozotocin, while in vitro studies used H9c2 cardiomyoblasts incubated in high-glucose medium. Melatonin increased intracellular levels of cGMP, expression of PKGIα, the p-VASP/VASP ratio, as well as regulating the MAPK and Nrf-2-HO-1 signaling pathways in the myocardium. These effects were abrogated by KT5823, which is a selective PKG inhibitor, or by PKGIα siRNA, with the exception of intracellular cGMP levels, which remained unchanged. In addition, their study showed that 4P-PDOT (selective antagonist of MT2 receptor) or luzindole (non-selective antagonist of melatonin receptors) suppressed the protective property of melatonin, and prevented the regulation of cGMP-PKGIα, Nrf-2-HO-1, and MAPK axes in vitro. They concluded that melatonin could ameliorate oxidative stress, reduce apoptosis, and restore cardiac function by regulating MAPK and Nrf-2-HO-1 axes in MI reperfusion injury in diabetic subjects. cGMP-PKGIα signaling coupled with membrane receptors, particularly MT2 receptors, plays a major role in this process (222).
Mitochondrial fission is a process by which the mitochondrial structure is initially fragmented into small particles during MI and/or reperfusion injury (223). Abundant mitochondrial fission results in damage to the mitochondrial DNA. These degraded mitochondria cannot generate adequate mitochondrial respiratory complexes, which results in enhanced synthesis of ROS and reduced oxidative phosphorylation (224). Furthermore, fragmented mitochondria release pro-apoptotic mediators such as cytochrome c into the cytoplasm, which triggers the mitochondrial apoptosis pathway (225). According to previous studies, reducing mitochondrial fission could ameliorate damage after MI and/or reperfusion injury. The opposite process of mitochondrial fusion can stimulate the mitochondria, and allow damaged mitochondria to repair themselves (226). The mitochondrial fusion molecule OPA1 (optic atrophy 1) is a GTPase involved in the repair of mitochondrial DNA, or the disposal of unrepairable mitochondria by the process of mitophagy (227). Maintenance of the OPA1 level interferes with the process of mitochondrial fission, leading to the suppression of reperfusion injury in the brain (228) and the liver (229). Likewise, a cardiac reperfusion model suggested that upregulation of OPA1 could maintain the viability of cardiac muscle cells and provide mitochondrial homeostasis (230). The inhibitory activity of melatonin against mitochondrial fission has been reviewed in prevuious publications (231, 232). Nevertheless, the upstream mediators of OPA1-induced mitochondrial fusion remain poorly understood.
Yap is a main downstream regulator of the Hippo pathway, and has been shown to be involved in cardioprotection in myocardial reperfusion injury (233). High Yap levels suppress the expression of Mst1, which decreases reperfusion-induced apoptosis in cardiac muscle cells (234). The Yap–Hippo pathway can also inhibit mitochondrial fission mediated by Drp1 (dynamin-related protein 1) and thus ameliorate reperfusion injury in the heart or the brain (235). Up-regulation of Yap promotes mitochondrial autophagy and reduces mitochondrial fission (43). The cross-talk between Yap–Hippo signaling and mitochondrial fission has been shown in previous studies (236). Nonetheless, if Yap plays a role in mitochondrial fusion during reperfusion was uncertain.
In a study by Ma and Dong the beneficial effects melatonin on mitochondrial fusion induced by OPA1 were studied in MI and/or reperfusion injury (237). According to their study, melatonin could preserve myocardial function, decrease the infarct area and reduce death of cardiac myocytes in response to cardiac reperfusion stress. Melatonin increased expression of OPA1, which largely restored the mitochondrial fusion, which had been inhibited by MI or reperfusion. Silencing of OPA1 abrogated the protective effects of melatonin on mitochondrial apoptosis and mitochondrial energy metabolism. Furthermore, their study showed that melatonin could regulate expression of OPA1 via the Yap–Hippo pathway, inhibition of which resulted in death of cardiac muscle cells and damage to mitochondria despite the treatment with melatonin (237).
The JAK/STAT axis regulates several biological activities, such as proliferation, differentiation, tumor metastasis, and inflammation. Upregulating the JAK2/STAT3 axis can decrease apoptosis and oxidative stress in response to MI and/or reperfusion. Furthermore, the JAK2/STAT3 signaling pathway could be involved in cardiac protection in ischemia and/or reperfusion injury (238, 239). It still remains unclear whether the JAK2/STAT3 signaling pathway is involved in melatonin-induced cardiac protection in the heart.
The success of heart transplantation is critcally dependent on the satisfactory functioning of the donor heart when removed from the donor after circulatory death (DCD). Lan et al. developed a DCD heart model to identify the effects of melatonin on myocardial function in donor hearts, and investigated whether JAK2/STAT3 signaling was involved in the mechanism of action (240). Donor hearts were obtained from DCD model rats, which had received melatonin pre-treatment or not. They took biopsies 3, 12, and 24 h following heart transplantation. Myocardial edema was measured by the wet/dry ratio and water content, while hematoxylin and eosin staining assessed inflammation. Levels of expression of IL-6, TNF-α, and matrix metalloproteinase-9 were measured. Oxidative stress was quantified by the activity of GPx and SOD, MDA levels, and expression of cytochrome-C, Nrf2, and NQO1. Cellular apoptosis was determined by measuring Bcl-2, Bax, cleaved caspase-3, and total caspase-3. To assess JAK2/STAT3 activity, p-STAT3 and p-JAK2 levels were measured by Western blotting. Melatonin was shown to exert cardiac protection against MI and/or reperfusion by decreasing myocardial inflammation and edema, inhibiting apoptosis and oxidative stress, and activating the JAK2/STAT3 axis. The JAK inhinitor AG490 (tyrphostin) suppressed all of these effects. In conclusion, melatonin may protect cardiac muscle against reperfusion injury caused by ex vivo perfusion in a DCD heart transplant model by activating the JAK2/STAT3 signaling pathway (240).
Mitophagy is a way of eliminating defective mitochondria by destroying them inside lysosomes (241, 242). Two proteins, PINK1 (PTEN-induced kinase 1) and Parkin (E3 ubiquitin ligase) have been shown to control mitophagy (241, 243). Alternative (non-conventional) mitophagy may act to reduce ischemic stress in the heart, as suggested by recent research. A recent study showed that the proteins ULK1, Rab9, Rip1, and Drp-1 were involved in alternative mitophagy in cardiomyocytes under stress. This kind of mitophagy is thought to be the most common type during stressful conditions, and is distinctly different from PINK1-Parkin dependent mitophagy (244).
Melatonin helps in closing the mitochondrial permeability transition pores (MPTPs) (245, 246). When the subcellular distribution of melatonin was measured, it was found that the concentration inside the mitochondria was much higher than that measured in the blood (128, 247). Besides passive diffusion, melatonin is actively transported into mitochondria, which enhances cell tolerance to different insults (128, 248).
Aralkylamine N-acetyltransferase (AANAT) and acetylserotonin o-methyltransferase (ASMT) are two important enzymes involved in melatonin biosynthesis, which were recently found to be expressed in the mitochondrial matrix of mouse brain (249). The outer mitochondrial membrane contains the highly specific MT1 receptor, which has high affinity for melatonin. Adenylate cyclase activity is inhibited by melatonin, as is the release of cytochrome C in response to stressful conditions (250). More research is required to understand the importance of melatonin in the mitochondria, and whether they can take up or synthesize this hormone. Moreover, how melatonin affects mitochondrial dynamics under stressful conditions, and whether it can protect the heart from oxidative stress is uncertain.
Dube et al. (251) investigated how both conventional and non-conventional mitophagy could affect oxidative phosphorylation in rat hearts. The hearts were isolated and perfused for 30 min, then exposed to ischemia for 20 min, and then reperfused for another 30 min. Biopsy samples were used to assess mitochondrial oxygen consumption. Melatonin was administered before ischemia and after reperfusion. Melatonin had a minimal effect on mitochondrial O2 consumption, which was notably decreased after reperfusion. Beclin 1 was shown to be decreased by ischemia and increased after reperfusion, but in both states, PINK1 and Parkin were reduced. Reperfusion increased p62 expression. During myocardial IR, Rab9 activates a surrogate type of mitophagy. Hemorrhage lowered the cytosolic expression of ULK1, while reperfusion enhanced it, which was linked with Rab9 and Drp1 being redistributed from the mitochondria to the cytosol. Melatonin significantly reduced mitochondrial p62 expression in IR injury. Overall, melatonin I increased levels of ULK1, Rab9, and P-ULK1, while decreasing levels of pDrp1 as well as the mitochondrial P/t Drp1 ratio. This suggests that melatonin may be able to inhibit fission of mitochondria. Fusion was also affected, but to a lesser extent compared to the other processes. Although cardioprotection by melatonin is linked to its effects on mitophagy, the relevance of these findings has yet to be established (251).
Cardiac cell degeneration following IR injury significantly involves apoptosis (252, 253). The pan-caspase inhibitorz VAD is able to reduce cellular death triggered by reperfusion (254–257). Apoptosis and necroptosis are the primary modes of death following IR injury, according to these studies. On a molecular level, Ripk3 (receptor-interacting serine/threonine-protein kinase 3) activity can regulate necroptosis (258). Ripk3 stimulates PGAM5 (phosphoglycerate mutase 5) to open MPTPs, disturbs energy generation, and therefore can make the organelles or cells swell in size (259, 260). But how IR and necroptosis are connected at the microvascular level, and how Ripk3 affects MPTPs was unclear. Some clinical trials have used melatonin to reduce the size of the infarct after MI, however, its effect on microvascular protection has not been clearly shown (126, 261–264). How Melatonin could prevent endothelial damage by necroptosis, because earlier studies have shown that it can prolong microvascular blood flow, resulting in decreased endothelial damage during myocardial IR (232, 265).
In a study by Zhou et al. the researchers sought to determine how IR injury and necroptosis were connected at the microvascular level, and whether interactions between Ripk3, PGAM5, and mPTP could be inhibited by melatonin (266). It has been shown that Ripk3 is the primary mediator of microvessel barrier failure, endothelial necrosis, capillary hyperpermeability, and inflammatory response in IR injury. After the genetic deletion of Ripk3, myocardial IR damage, and the endothelial function was improved, and the same benefits were provided by melatonin, which inhibited Ripk3 and gave a pro-survival advantage during IR. Ripk3 upregulates PGAM5, which phosphorylates CypD, and causes the MPTPs to open thus leading the endothelial cells toward necroptosis. Melatonin was able to suppress this process resulting in reduced necroptosis. A novel route for IR-mediated microvascular damage and endothelial necroptosis was demonstrated by these studies, namely the Ripk3-PGAM5-CypD/mPTP axis. Melantoin therapy on the other hand decreased cellular necroptosis by inhibiting the Ripk3/PGAM5/CypD/mPTP cascade, and protecting the endothelial system under IR stress (266).
IR damage is caused by Ca2+ excess, which leads to cardiomyocyte death under these conditions. Melatonin may protect the heart from IR damage by regulating intracellular calcium homeostasis, although this is not completely understood. Myocardial damage caused by prolonged hypoxia may be protected by melatonin, according to Yeung et al. Melatonin is thought to enhance calcium handling in cardiomyocytes by an antioxidant mechanism (267). Ca2+ overload under acute IR stress has not been well-investigated, so the effects of melatonin and the underlying mechanism are not well-understood. Cellular intracellular calcium handling and cell death are mediated by the cardiac proteins IP3R and SERCA2a, both of which are involved in intracellular calcium handling, cell contractility, and cell death (268–271). A recent study has demonstrated that IR activates an anti-apoptotic pro-survival kinase mechanism, e.g., ERK1/2 (extracellular signal-regulated kinase) and p42/p44 (272, 273).
In their study Hu et al. sought to determine if melatonin could protect cardiomyocytes from IR injury by regulating IP3R (inositol triphosphate receptor) and SERCA2a (sarcoplasmic/endoplasmic reticulum Ca2+ ATPase 2a) to decrease calcium overload via the ERK1 pathway (274). H9C2 cells were used in an in vitro study to simulate hypoxia/reoxygenation (H/R). The actin filament organization in cardiomyocytes was assessed by phalloidin staining, while Fura-2/AM was used to assess intracellular Ca2+ levels. Using a myocardial ischemia/ reperfusion (I/R) paradigm in rats, immunofluorescence labeling was used to identify the expression of IP3R and SERCA2a in the myocardium. H/R cardiomyocytes treated with melatonin showed a reduction in calcium overload as well as a decrease in IP3R expression and an increase in SERCA2a expression, mediated via ERK1. These effects could be reversed by PD98059, a small molecule inhibitor of MEK1 activation and MAP kinase signaling. IP3R and SERCA2a can regulate ERK1 to maintain intracellular calcium concentration at a stable level. They concluded that melatonin-induced cardioprotection against IR injury was at least in part due to ERK1 (274).
Table 3 lists some studies on the therapeutic effects of melatonin on ischemia reperfusion injury.
Melatonin and Blood Pressure: Recent Evidence and Signaling Pathways
Nitric oxide (NO) has protective effects on the cardiovascular system, and shows antiproliferative, antifibrotic, and antihypertensive activity (296, 297). Prolonged administration of L-NAME (Nγ-nitro-L-arginine methyl ester) to rats inhibits NO synthesis and release, causing organ damage and hypertension (298–300). Another effect of L-NAME is to weaken the renal artery by affecting RAAS (renin–angiotensin–aldosterone system) and promoting renin release (295, 296).
When rats were subjected to continuous light exposure and experimental pinealectomy, the resulting melatonin deficiency led to myocardial fibrosis and hypertension (301, 302). Melatonin has pleiotropic effects via nuclear receptors as well as membrane receptors (303, 304). Furthermore, it provides cardiovascular protection by ROS scavenging, and endothelial protection via sympatholytic effects (19, 25, 126, 301, 305, 306). However, how melatonin affects neurohumoral pathways, including the RAAS was not clear.
Simko et al. evaluated the structural and hemodynamic effects of L-NAME and its connection with the RAAS, and how melatonin could benefit them (307). Wistar rats were divided into 4 groups. The first group received melatonin, the second group received L-NAME, the third group received l-NAME + melatonin, and the fourth group was an untreated control group. Hypertension and LV fibrosis were quantified by measuring soluble, insoluble, and total collagen levels. Melatonin led to a decrease in the amount of total and insoluble collagen in the left ventricle, and also lowered systolic blood pressure. L-NAME decreased serum angiotensin II (Ang2) and its derivatives, but these they were unaltered by melatonin. The L-NAME group showed elevated serum aldosterone as well as increased aldosterone to Ang2 ratio (AA2-ratio), while the melatonin group showed no change in these two measures. In conclusion, L-NAME exerts its hypertensive effects through lowering Ang II and increasing aldosterone, while melatonin reverses hypertension without modifying the RAAS (307).
The most common treatment for hypertension involves a constant drug dosage, which ignores the daily cycle and rhythm of blood pressure. Hermida et al. showed that antihypertensive medication that takes into account the body's natural rhythms and cycles was more effective compared to traditional blood pressure treatment. This regimen provided a bigger reduction in hemorrhagic and ischemic strokes, fewer myocardial infarctions, and reduced cardiovascular death (308). Because the RAAS is active during sleep, antihypertensive medication during the night has more benefits and reduces cardiovascular complications (308, 309). The results revealed that blood pressure treatment without taking into account the circadian rhythm was not so successful; therefore, physicians should consider antihypertensive chronotherapy. Different types of brain hormones, such as melatonin, must synchronize with the body clock in order to maintain appropriate blood pressure (310, 311). Two additional characteristics of melatonin make this neurohormone suitable for protecting against hypertension, its antioxidant and anti-inflammatory properties (312–314). Pechanova et al. discussed the peripheral and central effects of melatonin on blood pressure regulation, highlighting the fact that melatonin reduced inflammation, oxidative damage, and promoted endothelium function (34). The effects of melatonin on blood pressure may involve the modulation of nitric oxide (NO), angiotensin II (Ang II), and endothelin (ET) (315–317). When human umbilical vein endothelial cells (HUVEC) were treated with 1-palmitoyl-2-(5-oxovaleroyl)-sn-glycero-3-phosphocholine (POVPC), a product of oxidized low-density lipoprotein that acts as a proinflammatory lipid, they developed a deficiency in NO production and eNOS activity, as typically found in atherosclerosis (318). In a HUVEC model, melatonin decreased angiogenesis triggered by VEGF (319).
Shao et al. also used HUVECs to assess how melatonin affected endothelial function, circadian changes in blood pressure and hypertension, and also the molecular mechainism (320). To simulate hypertension, they incubated HUVECs under 25 kPa external pressure, and incubated them with melatonin. They measured vasoactive agents, namely Ang II, endothelin (ET), eNOS, and NO. Melatonin significantly reduced endothelin at 18 and 24 h and angiotansin II at 18 h after treatment, and led to a rise in NO levels and elevated eNOS activity at 6–12–18 and 24 h. Melatonin could regulate genes related to circadian rhythm, cGMP-PKG activity, NO production, and renin/insulin metabolism, possibly explaining its effects on blood pressure. In conclusion, melatonin exerts its circadian protective effects on hypertension by lowering Ag II and ET, and elevating NO and eNOS (320).
Melatonin, in addition to its antioxidant properties, appears to play a role in epigenetic regulation, according to recent research (321). Epigenetic modification mediated by melatonin could prevent cellular programmes that affect hypertension (322, 323). The effects of melatonin on programming complications related to endangered pregnancies have been recognized (324), but how it affects programmed hypertension over long periods is unclear. However, some theories have been suggested, such as corticosteroid effects, oxidative damage, epigenetic modification, RASS modification, and reversing the loss of nephrons in the kidney (325).
Tain et al. investigated if high fructose (HF) consumption by the mother could cause programmed hypertension, and if melatonin was able to protect against this process through epigenetic regulation (326). During pregnancy and lactation, Sprague-Dawley rats were fed ordinary chow, chow + HF, or chow + HF + melatonin. HF comsumption by the mother increased the blood pressure in the 12-week old offspring. Melatonin inhibited this process by increasing kidney levels of NO. Melatonin downregulated SEH (soluble epoxide hydrolase) a gene which is involved in blood pressure regulation. In addition, they found that there were some genes involved in arachidonic acid metabolism that may mediate hypertension triggered by HF, and melatonin could regulate them. Their results suggested that melatonin can increase NO levels in the kidney, inhibit SEH expression, and epigenetically modulate blood pressure-controlling genes (326). eNOS and ADMA (asymmetric dimethylarginine) are able to induce hypertension by inducing oxidative stress (327). In rats born from mothers with malnutrition/diabetes, a defective ADMA-NO axis and a reduction in nephrons were linked to hypertension (328, 329). In addition, a low protein diet may cause hypertension through epigenetic changes affecting RASS (330). HDAC1-3 is widely expressed in nephron precursors, and HDAC enzymes can affect nephron generation (331). In a rat model, melatonin treatment inhibited oxidative stress as well as reducing hypertension (332). It also prevented Ang II induced hypertension (333). New data has clarified how melatonin could epigenetically modulate HDACs (321, 334, 335). Melatonin has a protective role in the rat placenta against oxidative/nitrosative mitochondrial damage and IR injury (336). Melatonin can pass through the placenta during pregnancy where it plays a key role in fetal development, but its epigenetic activity needs more research (324).
Tain et al. assessed the protective effects of melatonin on programmed hypertension triggered by corticosteroids during pregnancy (322). They divided young rats into four groups: (1) control; (2) dexamethasone (DEX); (3) control plus melatonin; (4) DEX plus melatonin. Pregnant rats were administered with the above agents, and hypertension occurred in group 2 at week 16, which was decreased by melatonin in group 4. If the nephrons were reduced, DEX will accumulate in the kidneys, which could be inhibited by melatonin therapy. All groups had equal kidney contents of superoxide and NO. DEX upregulated prorenin and renin receptors, as well as histone deacetylase-1 (HDAC-1) in the kidneys of 4-month old rats. Melatonin increased the weight of the kidneys in group 4, and upregulated HDAC-1, HDAC-2, and HDAC-8 in the kidneys of groups 3 and 4. Melatonin could inhibit hypertension triggered by DEX during pregnancy, by altering RAS components, protecting nephrons, and regulating HDACs (322).
Chronic kidney disease (CKD) is accompanied by high blood pressure when NO production is reduced (337, 338). Endogenous inhibitors of NOS, such as ADMA can lead to a decrease in NO synthesis. CKD and high ADMA levels are often seen in patients with hypertension (327). Many experimental models have been used to produce renal failure leading to hypertension. As spontaneously hypertensive rats (SHR) get older, the onset of CKD is an inevitable consequence (339). Mature SHRs treated with L-NAME, a NOS inhibitor, developed accelerated glomerulosclerosis as well as premature kidney failure (340). It was shown that melatonin produced by the pineal gland prevents the ADMA level from rising, and reduces the blood pressure in young SHRs (332). Melatonin may suppress hypertension by upregulating DDAH (an enzyme that degrades ADMA) in the kidneys of SHRs (332). It has also been shown that a reduction in renal ADMA concentrations may be protective against hypertension (341). However, whether L-NAME could induce nephrosclerosis in mature SHRs, or even in young SHRs was uncertain (327).
Young SHRs were used by Cheng and colleagues to investigate the interactions between L-NAME, ADMA, and melatonin (312). They randomly allocated 4-week old SHR rats into three groups: (1) control; (2) L-NAME; (3) L-NAME + melatonin. Rats were sacrificed at 10 weeks old. L-NAME caused renal dysfunction, glomerular sclerosis, and high blood pressure in young SHRs. L-NAME led to a lower arginine-to-ADMA ratio, but increased the total level of ADMA, but melatonin could reverse these effects. The researchers were able to restore DDAH (dimethylarginine dimethylaminohydrolase) activity with melatonin treatment. Melatonin therefore led to a decrease in ADMA concentration, restored the production of NO, increased the arginne-to-ADMA ratio, and decreased the amount of 8-hydroxydeoxyguanosine immunostaining (a marker of DNA oxidative damage) in SHR kidneys treated with L-NAME (310). They also proved that young SHRs were amenable to L-NAME effects.
Table 4 lists some studies on the effects of melatonin on high blood pressure.
Effects of Melatonin on Atherosclerotic Plaque
Atherosclerosis-related cardiocerebrovascular diseases include, peripheral vascular disease (PVD), stroke, acute coronary syndrome (ACS), and stable angina pectoris (375). Atherosclerosis (AS) is characterized by the subendothelial accumulation of plaques in arteries, consisting of oxidized-LDL, and inflammatory cells like macrophages, T lymphocytes, and DCs (dendritic cells) (377, 378). The pathology starts with gradually progressive endothelial damage, which induces initial vascular lesions, eventually leading to the rupture of vulnerable plaque and the formation of thrombosis (379). Pyroptosis plays a crucial role in the pathophysiology of atherosclerosis, according to recent research (380). Pyroptosis is distinct from apoptotic cell death, instead representing a form of highly inflammatory necrotic cell death, wherein the plasma membrane ruptures and inflammatory factors such as interleukin (IL)-1β and IL-18 are released, along with other components from the cytoplasm (381).
Smoking can cause both inflammation and oxidative stress, thereby damaging the endothelial function (382). However, there has been little research into the relationship between smoking-induced oxidative stress and pyroptosis. A recent study suggested that nicotine could induce endothelial cell pyroptosis via the ROS/NLRP3 axis (383), and that the ROS pathway may interact with the pyroptosis-related pathway in inflammatory signaling.
Nrf2 acts as the major regulator of antioxidant enzymes like HO-1 (384). The Nrf2 pathway is activate under stressful conditions, and it is essential for sensing oxidative stress to protect cells against ROS (385). Nrf2 may have beneficial roles in cardiac IR damage (386), sepsis (387), and neurodegenerative diseases (388). Furthermore, Nrf2 has been shown to attenuate inflammation in smoking-induced chronic obstructive pulmonary disease, emphysema, and asthma (389, 390). However, the involvement of Nrf2 in smoking-induced vascular endothelial injury and its mechanism was unclear.
Zhao et al. investigated the interactions between melatonin and cigarette smoke in vascular injury (391). Cigarette smoke extract (CSE) could cause human aortic endothelial cells (HAECs) to undergo pyroptosis by affecting NLR Family Pyrin Domain Containing 3 (NLRP3). Furthermore, HAECs increased ROS production and Nrf2 activity in response to CSE. Nrf2-specific siRNA as well as an Nrf2 inhibitor were able to prevent CSE from activating the ROS/NLRP3 axis. In addition, Nrf2 increased cell survival and upregulated VEGF and eNOS. Melatonin suppressed intimal hyperplasia in a model of carotid artery injury. Melatonin also upregulated Nrf2, while suppressing the ROS/NLRP3 axis. In conclusion, melatonin could suppress atherosclerosis triggered by cigarette smoke by affecting the Nrf2/ROS/NLRP3 axis (391).
Macrophages are an important contributor to AS (392, 393). In the atheromatous plaque, they phagocytize oxidative LDL (ox-LDL) and form a necrotic core; they also secrete many pro-inflammatory mediators, resulting in degenerative and fibrotic changes, which increases the plaque size while reducing its stability, exposing it to rupture and thrombus formation (392). Melatonin was discovered to suppress ox-LDL modification in vitro, which may translate into less production of atherogenic plaques in vivo. Melatonin may also increase the plaque stability (393, 394). One study has been conducted to investigate whether melatonin could ameliorate vascular endothelial dysfunction, inflammation, and AS by inhibiting the Toll-like receptor 4 (TLR4)/nuclear factor kappa B (NF-κB) pathway in high-fat-fed rabbits (377). In this study rabbits were randomly divided into three groups: a standard diet (control group), a high-cholesterol diet (atherosclerosis group), and a high-cholesterol diet plus 10 mg/kg/day melatonin (melatonin group) for 12 weeks. When compared to the control group, a high-fat diet dramatically elevated serum lipid and inflammatory markers in rabbits with atherosclerosis. Results revealed that melatonin improves lipid metabolism, vascular endothelial dysfunction, and inflammation, as well as slowing the progression of atherosclerosis in high-fat-fed rabbits. Furthermore, it suggests that suppressing the TLR4/NF-κB system in local vasculature with atherosclerotic damage is critical for melatonin's protective effects (377). Endothelial dysfunction is linked to cholesterol feeding. Pita et al. (395) demonstrated that long-term administration of melatonin altered the fatty acid content of rat plasma and reduced fatty infiltration in the intima caused by cholesterol feeding. A research group shown that melatonin administration prevents in vitro smooth muscle cell inflammation and proliferation, as well as atherosclerosis in apolipoprotein E-deficient mice (396). Hepatocyte growth factor (HGF) may also exert beneficial effects in the cardiovascular system (397). Considerable evidence has recently been provided to show that HGF acts as a potent anti-inflammatory agent (377, 396–406). Cardiovascular pathology and AS have been observed to be associated with reduced local amounts of HGF in cardiac and vascular cells (398–400). Several drugs such as ARBs (angiotensin II receptor blockers) (400–402), ACEIs (angiotensin-converting-enzyme inhibitors) (399, 400, 403) and PPAR-γ agonists (404) have been shown to upregulated HGF, resulting in suppression of AS (402, 405). Interestingly, melatonin has also been shown to upregulate HGF expression both in vitro (189, 190) and in vivo (407).
The ability of melatonin to prevent macrophage infiltration and improve plaque stability by activating the HGF/c-Met (HGF receptor) axis was assessed by Hu et al. in rabbits, using USPIO-enhanced MRI to monitor AS plaques (406). They randomly assigned rabbits into three groups: (1) standard diet; (2) high-fat diet; (3) high-fat diet + melatonin. In the atherosclerotic abdominal aorta, melatonin notably reduced the signal voids in 3D-TOF MRI, decreased the standard signal intensity in T2WI MRI, and decreased the aortic luminal area in 2D-TOF MRI. Furthermore, melatonin reduced serum IL-6, intima/media thickness ratio, and CD68+ as well as USPIO-positive regions of the intima. Melatonin increased serum IL-10, HGF, and c-Met, and induced smooth muscle cells and collagen fibers to accumulate in the intima. In conclusion, melatonin notably prevented macrophage infiltration in the plaque, and its increased stability could be partially attributed to the HGF/c-Met axis (406).
The nuclear receptor RORα can regulate circadian rhythm, immune response, and cellular metabolism (408, 409). It has been proposed that some melatonin effects, like its anti-inflammatory activity could be attributed to RORα (410–412). In addition, some cardiovascular benefits of melatonin have been proposed to be mediated by RORα (42, 303, 413).
How melatonin affects atheromatous plaque and whether it was mediated by RORα was investigated by Ding et al. (393). They used ApoE−/− mice with high cholesterol and elevated blood pressure to assess plaque vulnerability to rupture. The rate of plaque rupture was markedly reduced by melatonin. Melatonin suppressed inflammation inside the plaque by preventing plaque macrophages from differentiating into the M1 phenotype, by affecting RORα. Additional evidence has supported the fact that melatonin can modify the macrophage phenotype through RORα and affecting the AMPKα-STATs axis (393).
Collagen metabolism is regulated by the P4H (prolyl 4-hydroxylase) enzyme (407). The P4H α subunit (P4Ha1) converts procollagen into a mature and stable form of collagen (414). P4Ha1 inhibition led to a reduction in mature collagen, which reduced plaque stability (415).
Li et al. used ApoE−/− mice to assess how melatonin affected plaque stabilization (394). Melatonin upregulated P4Hα1 expression in leiomyocytes in vitro by phosphorylating Akt and activating Sp1. This effect was blocked by small molecule inhibitors LY294002 (Akt inhibitor) or MTM (mithramycin a Sp1 inhibitor). Furthermore, melatonin stabilized plaque in vivo by upregulating P4Hα1, and MTM blocked this effect (394).
Table 5 lists some studies on the effects of melatonin on atherosclerosis and intimal hyperplasia.
Melatonin and Cardiac Arrhythmia
Cardiac arrhythmias may lead to many complications including death (421). VT (ventricular tachycardia) and ventricular fibrillation (VF) commonly occur after cardiac IR injury, which may be fatal if untreated. Appropriate antiarrhythmic agents may prevent these outcomes. Oxidative stress occurring during IR injury may explain the onset of cardiac rhythm abnormalities. The “Metabolic sink” hypothesis (422–424) is as follows: superoxide anions pass through the mitochondrial inner membrane which induces a drop in the Δψm (MMP) and a reduction in cellular ATP levels. In response to these effects the sarcolemmal ATP-sensitive potassium current (IKATP) is increased. As voltage-gated potassium channels open to repolarize the membrane, the potassium conductance increases dramatically to bring the membrane potential closer to the equilibrium potential for potassium. ROS may also inhibit the sodium current (INa) to cause rhythm abnormalities (425). Melatonin ameliorates the shortened action potential, and upregulates connexin 43 during ischemia (426), which may explain its antiarrhythmic activity, along with its antioxidant effects (18). However, further resaerch is needed to confirm the relationship between melatonin, oxidative stress and cardiac rhythm abnormalities.
Sedova et al. used an IR injury model to assess how VT and VF, oxidative damage, cardiac electrophysiological parameters, and melatonin may be connected to each other (427). Melatonin reduced the rate of VT and VF, shortened baseline activation times (ATs), as well as activation-repolarization intervals, and also improved recovery of repolarization times (RTs). SOD activity was observed to be elevated in the melatonin group. In vitro, melatonin restored the action and resting membrane potentials even more In conclusion, melatonin affected repolarization through exerting antioxidant effects, while its suppression of arrhythmia could be attributed to its ability to improve ventricular function (427).
The anti-arrhythmic effects of melatonin could be attributed to its effects on action potential length (284, 428). Melatonin may suppress VF (426) by upregulating connexin-43 in the myocardium, which is the major mediator of electrical coupling. The probability that myocardium upregulates connexin-43 in acute hypokalemia is low, however melatonin may enhance the coupling between cells (429, 430).
Prado et al. investigated whether melatonin was able to suppress arrhythmia triggered by hypokalemia (431). A hypokalemic medium was employed, and melatonin and its receptor antagonist, luzindole, were added. They measured the connexin-43 concentration, and how it was dephosphorylated and distributed. Melatonin suppressed VF development, induced a delay in its development, as well as restoring potassium currents and accelerated sinus rhythm. Melatonin prevented the widening of the QRS, accelerated the development of action potentials, and shortened their length. Melatonin also inhibited the dephosphorylation of connexin-43, and normalized its distribution (not lateralized). Luzindole reversed all the above-mentioned effects. In conclusion, melatonin suppressed VF triggered by hypokalemia, prevented the action potential from widening, enhanced the electrical activity of the ventricles, and corrected connexin-43 misdistribution (431).
Diez et al. used both fructose-fed rats (FFR) and SHRs to assess the effects of melatonin on cardiac arrhythmia induced by IR injury (428). Both of these groups of rats exhibited abnormal metabolic features, including decreased ability of the myocardium to protect against oxidative stress, hypertension, reduced eNOS activity, etc. Melatonin suppressed the occurrence of VF in both groups and reduced VT severity. Melatonin affected both the length and amplitude of the action potentials, the length was shortened and the amplitude was restored. Therefore, the anti-arrhythmic effects of melatonin could be observed even in rats with genetic cardiac disease (428).
Table 6 lists some studies on the effects of melatonin on cardiac arrhythmias.
Melatonin and Heart Failure
Heart failure (HF) is a complex clinical disease in the aging population characterized by the heart's inability to pump enough blood to the body as a result of structural and/or functional cardiac abnormalities (435). This syndrome is also associated with inadequate clearance of metabolic end products, leading to cardiovascular loss, followed by reparative fibrotic repair, ventricular remodeling, and eventually HF (39, 436). More than 37.7 million people worldwide are affected by HF, and this prevalence is rising (437). Importantly, despite recent advances in HF medical care and management, novel therapeutic approaches are required to reverse HF and restore heart tissue function. In this context, several experimental studies report the beneficial effects of melatonin treatment in variety HF models, including chronic intermittent hypoxia-induced HF, post-infarction HF caused by the left anterior descending coronary artery ligation, and isoproterenol-induced myocardial infarction (435, 438, 439). In these models, melatonin via its antioxidant properties cures significant pathogenic processes associated with HF including oxidative stress, apoptosis, necrosis, necroptosis, fibrosis, autophagy, inflammation, and pathological remodeling and dysfunction. Melatonin administration normalizes the blood pressure circadian rhythm, reduces cardiomyocyte loss, and improves the left ventricular function in patients with HF with reduced ejection fraction (HFrEF) (440). Melatonin is a molecule with a lot of electrons that may react with free radicals for directly scavenging them as well as up-regulating antioxidant enzymes. It acts as an antioxidant and protects cardiac tissue from ischemia and reperfusion injury caused by free O2 radicals and their byproducts. Melatonin also activates many antioxidative enzymes, such as glutathione peroxidase (GSH), modifies gene expression for various protective enzymes, and lowers lipid peroxidation (436). Melatonin reduces oxidative stress-induced cardiac tissue damage by increasing cardiac Na+/K+ ATPase and Sarco/endoplasmic reticulum calcium ATPase (SERCA) activity and decreasing myeloperoxidase (MPO) activity, caveolin-3, caspase-3 expression, and malondialdehyde (MDA) levels (441). Melatonin's protective impact has been related to its ability to increase antioxidant enzymes such as Cu/Zn superoxide dismutase, stimulate phosphorylated protein kinase B (p-Akt), and block caspase cascade activation, hence decreasing mesenchymal cell death (196, 442). Furthermore, the antioxidant effects of melatonin are most likely due to its inhibitory influence on NOS expression. Nitric oxide generates peroxynitrite and hydroxyl radicals, which cause membrane lipid peroxidation and oxidation of other molecules. Superoxide generation contributes to ventricular remodeling in heart failure, and as an antioxidant, melatonin appears to reduce myocardial remodeling (196).
Simko et al. in their study on rats with isoproterenol-induced heart failure found that melatonin declines the insoluble and total collagen and enhanced survival by modulating remodeling (443). Catecholamines and glucocorticoids, which are stress hormones, are also up-regulated in HF, boosting catabolic state and worsening cardiac failure. Melatonin is thought to lower catecholamine and cortisol levels in animal studies and may reverse their effects via antioxidant capabilities and activation of anabolic signaling pathways (444). Melatonin stimulates GH/IGF-1 signaling via the PI3k/AKT/mTOR pathway, activates AMPK pathway, and controls mitochondrial biogenesis to promote protein synthesis and reduce apoptosis.
Melatonin activates miR-200a-NF-E2-related factor 2 (Nrf2) in cardiomyocytes, causing adipose tissue to secrete C1q/tumor necrosis factor-related protein 3 (CTRP3). Because CTRP3 deficiency is linked to increased oxidative stress and cell death in cardiomyocytes, the influence of melatonin on CTRP3 secretion into the circulatory system and increased cardiac CTRP3 expression may help to prevent obesity in patients with heart failure with preserved ejection fraction (445). According to epidemiological research melatonin levels as a useful biomarker for HF reduced in patients with both acute and chronic HF. In this setting, serum melatonin levels have a negative correlation with N-terminal pro-brain natriuretic peptide (NT-pro-BNP), a well-known HF indicator (446). Surprisingly, it is also linked to reversal remodeling following cardiac resynchronization therapy in HF conditions (447). Melatonin affects homeostasis of extracellular matrix (ECM) in the left ventricular myocardium by improving the balance of MMP-1 and TIM-1 protein expression (445). Moreover, in ovariectomized rats with HF, melatonin prevents apoptosis and restores hypertrophy of contractile cardiomyocytes in the left ventricular myocardium. In one study decreased melatonin levels has been shown in patients admitted to hospital with congestive heart failure, so it is concluded that low melatonin levels exacerbate congestive heart failure (448).
Melatonin's cytoprotective impact is dependent on the timing of delivery. Melatonin, when given early in the course of a myocardial infarction, has been shown to slow the progression of heart failure (444). Thanks to its antioxidant, anti-inflammatory and immunomodulatory properties, melatonin protects the heart against ischemic heart disease with myocardial cell death as well as post-infarction cardiac dysfunction and ischemic HF. Arterial hypertension and pulmonary hypertension induce both cardiac fibrosis and pathological regeneration (cardiomyopathy) with ventricular dysfunction and HF. Melatonin reverses these effects and prevents HF (435). Overall, melatonin seems to be an important, safe, and affordable molecule for the treatment of HF via improving cardiac defense mechanisms, which restores the myocardial function, muscle wasting, and cardiac cachexia. The beneficial effects of melatonin in HF have been illustrated in Figure 3.
Melatonin as a Protective Agent Against Septic Cardiomyopathy
Approximately 60% of patients diagnosed with sepsis suffer the most common complication, namely septic cardiomyopathy. Elderly patients with septic cardiomyopathy have an estimated 30% risk of death, and the lack of effective therapies leads to a poor prognosis. Therefore, there is a need to for more research to clarify the pathogenesis of septic cardiomyopathy to improve the patient prognosis. Molecular studies have revealed that the pathogenesis of septic cardiomyopathy is related to endoplasmic reticulum (ER) stress and mitochondrial damage, because mitochondrial damage disturbs the energy supply of the myocardium, while ER stress inhibits protein turnover (266, 449). BAP31 (B-cell receptor-associated protein 31) regulates the apoptosis mediated by ER stress. Down-regulation of BAP31 suppresses cervical cancer development by altering the mitochondrial apoptosis pathway (450). BAP31 also affects apoptosis in colorectal cancer, and is linked to caspase-12. BAP31 regulates mitochondrial activity by interacting with TOMM40 (translocase of outer mitochondrial membrane 40), which explains the connections between the ER and mitochondria (451). BAP31 also transmits apoptosis signals from the ER to the mitochondria by interacting with CDIP1 (cell death-inducing p53-target protein 1) (452). Furthermore; BAP31 has been shown to be involved in Alzheimer's disease, hepatic steatosis, immunomodulation, and lipid metabolism (450–453). The MAPK/ERK axis increases cell survival through regulating genes connected to apoptosis. Pharmacological stimulation of the ERK pathway can reduce toxicity caused by lipopolysaccharide (LPS) in cardiomyocytes (453, 454). Furthermore, the ERK pathway appears to have a variety of effects on mitochondrial activity as well as ER stress. ERK influences ROS production in mitochondria, mitochondrial energy matabolism and membrane potential (455, 456). The ER has many functions in cells, including calcium homeostasis, protein synthesis, and the caspase-12 apoptotic pathway, which is controlled by the ERK pathway (457–459). Recent studies have revealed a close connection between BAP31 and the ERK pathway (455, 460).
Zhang et al. investigated the dual protective effects of melatonin on both mitochondria and ER, focusing on the BAP31 and ERK pathways. Their study investigated how BAP31 could affect the development of septic cardiomyopathy, and clarified the effect of melatonin on the ERK pathway, ER stress, and mitochondrial function (456). An in vivo septic cardiomyopathy model was created using lipopolysaccharide (LPS). Western blotting, quantitative-PCR and immunofluorescence were used to investigate the pathways. Due to the overwhelming inflammatory response and loss of cardiomyocytes, heart function was significantly disturbed after exposure to LPS. Melatonin treatment might increase cardiomyocyte survival by enhancing mitochondrial activity and decreasing ER stress. LPS suppressed BAP31 transcription, but melatonin restored BAP31 levels, which may be attributed to the MAPK-ERK axis. Inhibition of ERK and BAP31 blocked these effects. Overall, their study showed that melatonin affected the ERK-BAP31 axis to regulate ER-mitochondrial interactions during sepsis to prevent septic cardiomyopathy (456).
Irisin is a polypeptide hormone (112 amino acids) produced by proteolytic cleavage of the membrane bound FNDC5 (fibronectin type III domain-containing protein 5). Irisin and melatonin have both been investigated to see whether they can prevent the development of sepsis-related cardiac damage (457, 458). Irisin and melatonin have beneficial effects by control of ROS production, management of calcium homeostasis, and inhibition of cardiomyocyte apoptosis (458). Melatonin protects mitochondria in various conditions, including brain damage following cerebral hemorrhage, diabetic retinopathy, ARDS, IR injury in the liver, and endothelial cell oxidative dmage (461, 462). Irisin has protective effects on mitochondrial function in ischemia of the lung parenchyma, obesity, cardiac hypertrophy following hypertension, and cardiac IR injury (459, 463). According to numerous recent studies, Mst1 (mammalian STE20-like kinase 1) is a major subunit of the Hippo axis, which appears to be a primary regulator of mitochondrial homeostasis. High levels of Mst1 inhibited BCL2 activity and induced mitochondrial apoptosis. Furthermore, the posttranscriptional Mst1 levels directly regulate oxidative status and mitochondrial respiration (464). However, if the Mst1 level is low, mitochondrial autophagy would be activated to mediate the repair or elimination of mitochondria (464). Mst1 deletion also efficiently inhibited mitochondrial fission, and prevented the reduction in mitochondrial membrane potential and cardiomyocyte apoptosis (464). Despite the fact that Mst1 has been implicated in the regulation of mitochondrial homeostasis in numerous studies, it was unclear if it was involved in septic cardiomyopathy. JNK is a marker of myocardial damage since it is a downstream effector of Mst1. Increased JNK levels might promote Bax-mediated mitochondrial apoptosis by translocating it to the mitochondrial surface. Active JNK may also induce phosphorylation of Drp1 and c-Jun (465). Drp1 has been linked to mitochondrial fission, while c-Jun acts as a transcription factor for a number of genes that favor apoptosis (461, 466).
Ouyang et al. investigated whether melatonin combined with irisin could ameliorate septic cardiomyopathy by affecting the Mst1–JNK axis (462). According to their study, melatonin plus irisin decreased the depressive effect of LPS on the myocardium. LPS strongly activated Mst1, while the melatonin/irisin combination treatment substantially blocked this activation. Mst1 induced JNK activity, which increased oxidative damage, mitochondrial dysfunction, and apoptosis. The Mst1-JNK pathway was efficiently inhibited by melatonin plus irisin co-treatment, promoting myocardial viability and mitochondrial function. Upregulation of Mst-1 inhibited the beneficial effects of irisin and melatonin in the animal model, and also in cell culture, suggesting that irisin plus melatonin co-treatment inhibited septic cardiomyopathy by regulating the Mst1/JNK axis (462).
Autophagy is a process in which injured damaged proteins and dysfunctional organelles are degraded in lysosomes and recycled (463). The LC3-II/LC3-I ratio can measure autophagic activity. By binding to LC3-II, p62 is incorporated into autophagosomes and then destroyed during autophagy. As a result, p62 is negatively correlated with autophagy (467, 468). New studies have shown the involvement of autophagy in cardiac function, and have suggested that induction of autophagy could enhance heart function during sepsis (469, 470). On the other hand, a deficiency in autophagy exacerbated sepsis-induced heart failure (471). Recent research has shown that melatonin treatment could prevent the progress of cardiac dysfunction and reduce mortality and morbidity in sepsis (472, 473). SIRT1 is a histone deacetylase involved in many cellular processes, including senescence, inflammation, apoptosis, and autophagy (474–477). In a murine model of septic cardiac injury, upregulation of SIRT1 enhanced heart function (478). Melatonin has protective effects against cardiac dysfunction, and could also regulate SIRT1 activity in various diseases (206, 472, 473, 479, 480).
Zhang et al. examined the effects of melatonin on heart function in a mouse model of LPS-induced sepsis, and the involvement of SIRT1 (481). They assigned C57BL/6 mice into four groups: (1) control; (2) LPS; (3) LPS + melatonin; (4) LPS + melatonin + EX527 (SIRT1 inhibitor). The heart was examined for myocardial damage biomarkers, cardiac function, cardiomyocyte apoptosis, cardiac histology, autophagosomes, and expression of SIRT1, cleaved caspase-3, LC3-II/LC3-I ratio, and p62. When compared to the LPS group, melatonin reduced heart dysfunction, downregulated CK as well as CK-MB, decreased structural heart damage, prevented apoptosis, and promoted autophagy. Furthermore, melatonin also upregulated SIRT1 in cardiomyocytes, whereas EX527 inhibition of SIRT1 abrogated the cardioprotective effects of melatonin in sepsis. Melatonin rescued mice from septic heart damage by modulating apoptosis and autophagy, and activating SIRT1 (481).
Activation of Ripk3 is involved in the pathological development of inflammation (482). Ripk3 promoted mitochondrial dysfunction, which increased sepsis-induced kidney damage (483). This discovery was further reinforced by the finding that genetic deletion of Ripk3 decreased cardiomyocyte death by increasing mitochondrial homeostasis in a myocardial IR injury model (266). Furthermore, Ripk3 activity and ER stress have been observed to be connected in several diseases (484). Ripk3 appears to be able to reduce damage to mitochondria as well as ER stress (485, 486), and could also affect sepsis-induced cardiac injury. Tryptophan and melatonin both affect the immune system, cell apoptosis, and microvascular vasoconstriction in numerous disease models, including heart IR injury, hepatic steatosis, acute renal injury (ARI), Parkinson's disease (PD), and cancer (14, 232, 487–489). Melatonin has also been extensively studied for the treatment of sepsis, and many protective mechanisms have been postulated. These have included, inflammasome inhibition, PI3K/Akt pathway activation, and antioxidant activity. Researchers reported that melatonin inhibited the activation of Ripk3, and had pro-survival effects in the reperfused heart (266). However, the effect of melatonin on Ripk3 in septic cardiomyopathy had not been shown.
One study explored how Ripk3 levels affected cardiac dysfunction induced by sepsis, and whether melatonin could improve it and the molecular mechanism (458). Following induction of inflammation in the myocardium by LPS, Ripk3 was elevated in heart tissue samples, along with cardiomyocyte death. Septic myocardial damage was reduced after treatment with melatonin, which had the same effect as genetic deletion of Ripk3. Molecular studies have shown that Ripk3 can regulate mitochondrial activity, ER stress, and cytoskeleton organization, resulting in a cardioprotective activity. To summarize, melatonin-mediated inhibition of Ripk3 in cardiomyocytes enhanced bioenergetics and decreased oxidative damage in the mitochondria. Melatonin also alleviated the effects of ER stress in cells and restored calcium recycling. When LPS-treated hearts were infected with an adenovirus expressing Ripk3, melatonin treatment became ineffective due to the overexpression of Ripk3. It was concluded that Ripk3 overexpression was associated with septic cardiomyopathy, which could be suppressed by melatonin, presumably by inhibiting Ripk3 (458).
Table 7 lists some studies on the effects of melatonin on septic cardiomyopathy.
Clinical Trials Using Melatonin for Cardiovascular Diseases
The experimental studies led to clinical trials, in which melatonin was administered in combination with heart disease medications for the prevention and treatment of CVDs.
According to an analysis of the results of numerous clinical trials, melatonin is useful in decreasing nocturnal hypertension when supplied in a controlled-release form, but useless when administered as a fast-release formulation. A patent issued in the United States in December 2011 describes a formulation for the prevention and treatment of hypertension symptoms in people who are resistant to classical hypertension (496). This formula contains an antihypertensive drug (e.g., captopril, diltiazem, etc.) and melatonin, which is expected to lower blood pressure, especially nocturnal hypertension, in patients with nocturnal hypotension. Treatment with this combination can also reduce cortisol production and postpone serum cortisol levels, thereby lowering the risk of morning ischemic attacks. The compound is prescribed in a controlled-release formulation because the patent reports that fast-acting or controlled-release melatonin has different effects on blood pressure, cortisol levels, and mood of patient. In particular, melatonin with controlled-release lowers diastolic and systolic blood pressure throughout the day without having a significant effect on patients with normal blood pressure, but regular formulation of melatonin (5 mg) has been observed to lower blood pressure in individual with normal blood pressure. This patent does not provide any information about the co-administration of melatonin and an antihypertensive drug (496).
Ahsanova et al. have lunched a study to assess the efficacy and safety of melatonin therapy in patients with hypertension based on the evaluation of daily blood pressure monitoring (497). They reported that after melatonin intake, average 24 h brachial systolic (SBP) and diastolic blood pressure (DBP)values decreased significantly from 124.6 ± 12.1 to 121.0 ± 10.2 mmHg and 79.7 ± 8.8 to 77.3 ± 6.5 mmHg, respectively, as well as average day-time SBP and DBP values from 128.2 ± 13.2 to 122.5 ± 9.9 and 82.3 ± 9.7 to 78.5 ± 7.2 mmHg, considerably (497).
In another study, melatonin 5 mg reduced nocturnal blood pressure but increased daytime blood pressure in non-dippers with coronary artery disease (363). However, melatonin 24 mg sustained-release had no effect on nocturnal blood pressure in African Americans with essential hypertension (NCT01114373) (347). Melatonin administration prior to coronary artery bypass grafting increased EF, decreased heart rate, and lowered markers of reperfusion injury in a dose-dependent manner (10 vs. 20 mg, 5 days before surgery), and in another study, 10 mg melatonin administration for 1 month prior to coronary artery bypass grafting increased antioxidant defense. Likewise, 12 weeks of 10 mg melatonin treatment had a positive effect on antioxidant capacity, glycemic management, HDL cholesterol, and blood pressure in diabetic patients with coronary heart disease (348). One study has been conducted in order to compare the efficacy of melatonin monotherapy (MT) and combined treatment (CT) in elderly patients (mean age 64 years) with arterial hypertension (AH) and coronary heart disease (CHD) (498). The results indicate that MT has an antihypertensive effect. In the control groups, CT with melatonin and antihypertensive medications outperformed standard therapy. Melatonin inclusion in CT of CHD produced significant anti-ischemic and anti-anginal benefits, as well as corrected oxidant/antioxidant balance (498). Therefore, melatonin should be considered as an important element of MT and CT of cardiovascular disorders. In recently published paper, evaluation of the effect of melatonin on endothelial function in HF explored that oral melatonin for 24 weeks had a beneficial effect on endothelial function in patients with HF with reduced ejection fraction (HFrEF) (499).
Melatonin supplementation (20 mg for 8 weeks) has been shown in a randomized controlled trial to improve fatigue, appetite, and quality of life in HF patients with cachexia, and the combination of melatonin and branched chain amino acids amplifies these effects (500).
Conclusions
The study of the pineal gland, and the effects of melatonin on a wide range of organs and bodily systems is a growing field of research. It has long been known that the cardiovascular system is affected by circadian rhythms, and this connection has now been realized to have therapeutic significance. Melatonin is a ubiquitous component of the human diet, and is also widely available as a healthfood supplement. While many of the applications of melatonin supplements have been directed to normalizing sleep patterns, treating jet-lag and insomnia, the benefits of melatonin for other conditions are becoming increasingly understood. It is well-known that melatonin is an antioxidant and free redical quencher, but the discovery of high affinity receptors for melatonin expressed in many tissues has widened the scope of mechanistic investigations. Nevertheless, although research into the role of melatonin in CVDs has only recently begun in earnest, the literature on this subject has been expanding to such an extent, that it is difficult to summarize in a single article. Nearly all the studies have reported positive effects of melatonin on cardiovascular physiology, and the prevention of damage to the myocardium after heart attack, IR injury, or sepsis. Melatonin can also help blood pressure and heart arrythmia. Since melatonin is inexpensive and non-toxic if consumed in reasonable quantities, it should be tested in many more extensive clinical trials to assess its efficacy in a variety of cardiovascular disorders. Moreover, some clinical studies indicated that utilization melatonin in CVDs is associated with more inconsistencies regarding its cardioprotective effects (501, 502). “Apart from dosage issues and mode of administration, previous failures could be partially explained by the use of young and healthy animals with eventual lack of various cardiovascular risk factors, comorbidities and comedications which are characteristics of patients suffering an acute myocardial infarction or undergoing cardiovascular surgery (503). Considering the current disappointment, further well-planned preclinical and clinical studies are needed to better delineate the cardiovascular benefits of melatonin” (15).
Author Contributions
MT, SM, FD, AA, HK, MH, ZA, RJR, and HM contributed in conception, design, and drafting of the manuscript. All authors contributed to the article and approved the submitted version.
Conflict of Interest
The authors declare that the research was conducted in the absence of any commercial or financial relationships that could be construed as a potential conflict of interest.
Publisher's Note
All claims expressed in this article are solely those of the authors and do not necessarily represent those of their affiliated organizations, or those of the publisher, the editors and the reviewers. Any product that may be evaluated in this article, or claim that may be made by its manufacturer, is not guaranteed or endorsed by the publisher.
References
1. North BJ, Sinclair DA. The intersection between aging and cardiovascular disease. Circ Res. (2012) 110:1097–108. doi: 10.1161/CIRCRESAHA.111.246876
2. Stenling A, Häggström C, Norberg M, Norström F. Lifetime risk predictions for cardiovascular diseases: competing risks analyses on a population-based cohort in Sweden. Atherosclerosis. (2020) 312:90–8. doi: 10.1016/j.atherosclerosis.2020.08.014
3. Maleki B, Alani B, Zadeh SST, Saadat S, Rajabi A, Ayoubzadeh SMJ, et al. MicroRNAs and exosomes: cardiac stem cells in heart diseases. Pathol Res Pract. (2022) 229:153701. doi: 10.1016/j.prp.2021.153701
4. Mortality GBD, Causes of Death C. Global, regional, and national age-sex specific all-cause and cause-specific mortality for 240 causes of death, 1990-2013: a systematic analysis for the global burden of disease study 2013. Lancet. (2015) 385:117–71. doi: 10.1016/S0140-6736(14)61682-2
5. Mortality GBD, Causes of Death C. Global, regional, and national life expectancy, all-cause mortality, and cause-specific mortality for 249 causes of death, 1980-2015: a systematic analysis for the global burden of disease study 2015. Lancet. (2016) 388:1459–544. doi: 10.1016/S0140-6736(16)31012-1
6. Behjati M, Sabri MR, Far ME, Nejati M. Cardiac complications in inherited mitochondrial diseases. Heart Fail Rev. (2021) 26:391–403. doi: 10.1007/s10741-020-10009-1
7. Khajouei AS, Adibi A, Maghsodi Z, Nejati M, Behjati M. Prognostic value of normal and non-obstructive coronary artery disease based on CT angiography findings. A 12 month follow up study. J Cardiovasc Thorac Res. (2019) 11:318. doi: 10.15171/jcvtr.2019.52
8. Khosravi A, Andalib E, Khaledifar A, Hajizadeh M, Nejati M, Behjati M. Pulmonary thromboembolism presenting with recurrent bradycardia and hypotension. Tanaffos. (2017) 16:248–50.
9. Bhatnagar P, Wickramasinghe K, Wilkins E, Townsend N. Trends in the epidemiology of cardiovascular disease in the UK. Heart. (2016) 102:1945–52. doi: 10.1136/heartjnl-2016-309573
10. Centers for Disease Control and Prevention. Heart Disease Facts. Centers for Disease Control and Prevention (2020). Available online at: https://www.cdc.gov/heartdisease/facts.htm
11. Mendis S, Puska P, Norrving B, World Health Organization. Global Atlas on Cardiovascular Disease Prevention and Control. Geneva: World Health Organization (2011).
12. Khosravi A, Bideh FZ, Roghani F, Saadatnia M, Khorvash F, Nejati M, et al. Carotid arterial stent implantation follow-up and results in 50 patients: preliminary report. Electro Phys. (2018) 10:6400. doi: 10.19082/6400
13. Xu J, Kochanek KD, Murphy SL, Tejada-Vera B. Deaths: Final Data for 2014. Natl Vital Stat Rep. (2015) 64:1–64.
14. Zhou H, Ma Q, Zhu P, Ren J, Reiter RJ, Chen Y. Protective role of melatonin in cardiac ischemia-reperfusion injury: from pathogenesis to targeted therapy. J Pineal Res. (2018) 64:e12471. doi: 10.1111/jpi.12471
15. Jiki Z, Lecour S, Nduhirabandi F. Cardiovascular benefits of dietary melatonin: a myth or a reality? Front Physiol. (2018) 9:528. doi: 10.3389/fphys.2018.00528
16. Pandi-Perumal SR, BaHammam AS, Ojike NI, Akinseye OA, Kendzerska T, Buttoo K, et al. Melatonin and human cardiovascular disease. J Cardiovasc Pharmacol Ther. (2017) 22:122–32. doi: 10.1177/1074248416660622
17. Baker J, Kimpinski K. Role of melatonin in blood pressure regulation: an adjunct anti-hypertensive agent. Clin Exp Pharmacol Physiol. (2018) 45:755–66. doi: 10.1111/1440-1681.12942
18. Lochner A, Marais E, Huisamen B. Melatonin and cardioprotection against ischaemia/reperfusion injury: what's new? A review. J Pineal Res. (2018) 65:e12490. doi: 10.1111/jpi.12490
19. Opie LH, Lecour S. Melatonin has multiorgan effects. Euro Heart J Cardiovasc Pharmacother. (2016) 2:258–65. doi: 10.1093/ehjcvp/pvv037
20. Hausenloy DJ, Garcia-Dorado D, Erik Bøtker H, Davidson SM, Downey J, Engel FB, et al. Melatonin as a cardioprotective therapy following ST-segment elevation myocardial infarction: is it really promising? Reply. Cardiovasc Res. (2017) 113:1418–9. doi: 10.1093/cvr/cvx137
21. Venegas C, García JA, Escames G, Ortiz F, López A, Doerrier C, et al. Extrapineal melatonin: analysis of its subcellular distribution and daily fluctuations. J Pineal Res. (2012) 52:217–27. doi: 10.1111/j.1600-079X.2011.00931.x
22. Acuña-Castroviejo D, Escames G, Venegas C, Díaz-Casado ME, Lima-Cabello E, López LC, et al. Extrapineal melatonin: sources, regulation, and potential functions. Cell Mol Life Sci. (2014) 71:2997–3025. doi: 10.1007/s00018-014-1579-2
23. Zawilska JB, Skene DJ, Arendt J. Physiology and pharmacology of melatonin in relation to biological rhythms. Pharmacol Rep. (2009) 61:383–410. doi: 10.1016/S1734-1140(09)70081-7
24. Carracedo G, Carpena C, Concepción P, Díaz V, García-García M, Jemni N, et al. Presence of melatonin in human tears. J Optom. (2017) 10:3. doi: 10.1016/j.optom.2016.03.002
25. Reiter RJ, Tan DX, Galano A. Melatonin: exceeding expectations. Physiology. (2014) doi: 10.1152/physiol.00011.2014
26. Hardeland R, Cardinali DP, Srinivasan V, Spence DW, Brown GM, Pandi-Perumal SR. Melatonin—A pleiotropic, orchestrating regulator molecule. Prog Neurobiol. (2011) 93:350–84. doi: 10.1016/j.pneurobio.2010.12.004
27. Zhang M, Lin J, Wang S, Cheng Z, Hu J, Wang T, et al. Melatonin protects against diabetic cardiomyopathy through Mst1/Sirt3 signaling. J Pineal Res. (2017) 63:e12418. doi: 10.1111/jpi.12418
28. Maarman G, Blackhurst D, Thienemann F, Blauwet L, Butrous G, Davies N, et al. Melatonin as a preventive and curative therapy against pulmonary hypertension. J Pineal Res. (2015) 59:343–53. doi: 10.1111/jpi.12263
29. Nduhirabandi F, du Toit EF, Lochner A. Melatonin and the metabolic syndrome: a tool for effective therapy in obesity-associated abnormalities? Acta Physiol. (2012) 205:209–23. doi: 10.1111/j.1748-1716.2012.02410.x
30. Mizrak B, Parlakpinar H, Acet A, Turkoz Y. Effects of pinealectomy and exogenous melatonin on rat hearts. Acta Histochem. (2004) 106:29–36. doi: 10.1016/j.acthis.2003.10.003
31. Favero G, Franceschetti L, Buffoli B, Moghadasian MH, Reiter RJ, Rodella LF, et al. Melatonin: protection against age-related cardiac pathology. Ageing Res Rev. (2017) 35:336–49. doi: 10.1016/j.arr.2016.11.007
33. Paulis L, Simko F, Laudon M. Cardiovascular effects of melatonin receptor agonists. Expert Opin Investig Drugs. (2012) 21:1661–78. doi: 10.1517/13543784.2012.714771
34. Pechanova O, Paulis L, Simko F. Peripheral and central effects of melatonin on blood pressure regulation. Int J Mol Sci. (2014) 15:17920–37. doi: 10.3390/ijms151017920
35. Galano A, Reiter RJ. Melatonin and its metabolites vs oxidative stress: from individual actions to collective protection. J Pineal Res. (2018) 65:e12514. doi: 10.1111/jpi.12514
36. Genade S, Genis A, Ytrehus K, Huisamen B, Lochner A. Melatonin receptor-mediated protection against myocardial ischaemia/reperfusion injury: role of its anti-adrenergic actions. J Pineal Res. (2008) 45:449–58. doi: 10.1111/j.1600-079X.2008.00615.x
37. Lochner A, Huisamen B, Nduhirabandi F. Cardioprotective effect of melatonin against ischaemia/reperfusion damage. Front Biosci. (2013) 5:305–15. doi: 10.2741/E617
38. Pei HF, Hou JN, Wei FP, Xue Q, Zhang F, Peng CF, et al. Melatonin attenuates postmyocardial infarction injury via increasing Tom70 expression. J Pineal Res. (2017) 62:e12371. doi: 10.1111/jpi.12371
39. Pei H, Du J, Song X, He L, Zhang Y, Li X, et al. Melatonin prevents adverse myocardial infarction remodeling via Notch1/Mfn2 pathway. Free Radic Biol Med. (2016) 97:408–17. doi: 10.1016/j.freeradbiomed.2016.06.015
40. Stroethoff M, Behmenburg F, Spittler K, Raupach A, Heinen A, Hollmann MW, et al. Activation of melatonin receptors by ramelteon induces cardioprotection by postconditioning in the rat heart. Anesth Analg. (2018) 126:2112–5. doi: 10.1213/ANE.0000000000002625
41. Hu J, Zhang L, Yang Y, Guo Y, Fan Y, Zhang M, et al. Melatonin alleviates postinfarction cardiac remodeling and dysfunction by inhibiting Mst1. J Pineal Res. (2017) 62:e12368. doi: 10.1111/jpi.12368
42. Zhao Y, Xu L, Ding S, Lin N, Ji Q, Gao L, et al. Novel protective role of the circadian nuclear receptor retinoic acid-related orphan receptor-α in diabetic cardiomyopathy. J Pineal Res. (2017) 62:e12378. doi: 10.1111/jpi.12378
43. Zhou H, Yue Y, Wang J, Ma Q, Chen Y. Melatonin therapy for diabetic cardiomyopathy: a mechanism involving Syk-mitochondrial complex I-SERCA pathway. Cell Signal. (2018) 47:88–100. doi: 10.1016/j.cellsig.2018.03.012
44. Uchinaka A, Kawashima Y, Sano Y, Ito S, Sano Y, Nagasawa K, et al. Effects of ramelteon on cardiac injury and adipose tissue pathology in rats with metabolic syndrome. Ann N Y Acad Sci. (2018) 1421:73–87. doi: 10.1111/nyas.13578
45. Millet-Boureima C, Ennis CC, Jamison J, McSweeney S, Park A, Gamberi C. Empowering melatonin therapeutics with drosophila models. Diseases. (2021) 9:67. doi: 10.3390/diseases9040067
46. Axelrod J. The pineal gland: a neurochemical transducer. Science. (1974) 184:1341–8. doi: 10.1126/science.184.4144.1341
47. Liu J, Clough SJ, Hutchinson AJ, Adamah-Biassi EB, Popovska-Gorevski M, Dubocovich ML. MT1 and MT2 melatonin receptors: a therapeutic perspective. Annu Rev Pharmacol Toxicol. (2016) 56:361–83. doi: 10.1146/annurev-pharmtox-010814-124742
48. Zisapel N. Circadian rhythm sleep disorders. CNS Drugs. (2001) 15:311–28. doi: 10.2165/00023210-200115040-00005
49. Ekmekcioglu C. Melatonin receptors in humans: biological role and clinical relevance. Biomed Pharmacother. (2006) 60:97–108. doi: 10.1016/j.biopha.2006.01.002
50. Dahlitz M, Alvarez B, Parkes J, English J, Arendt J, Vignau J. Delayed sleep phase syndrome response to melatonin. Lancet. (1991) 337:1121–4. doi: 10.1016/0140-6736(91)92787-3
51. von Gall C, Stehle JH, Weaver DR. Mammalian melatonin receptors: molecular biology and signal transduction. Cell Tissue Res. (2002) 309:151–62. doi: 10.1007/s00441-002-0581-4
52. Jin X, Von Gall C, Pieschl RL, Gribkoff VK, Stehle JH, Reppert SM, et al. Targeted disruption of the mouse Mel1b melatonin receptor. Mol Cell Biol. (2003) 23:1054–60. doi: 10.1128/MCB.23.3.1054-1060.2003
54. Morgan PJ, Barrett P, Howell HE, Helliwell R. Melatonin receptors: localization, molecular pharmacology and physiological significance. Neurochem Int. (1994) 24:101–46. doi: 10.1016/0197-0186(94)90100-7
55. Zhdanova IV, Lynch HJ, Wurtman RJ. Melatonin: a sleep-promoting hormone. Sleep. (1997) 20:899–907.
56. Arendt J. Melatonin: characteristics, concerns, and prospects. J Biol Rhythms. (2005) 20:291–303. doi: 10.1177/0748730405277492
57. Jan JE, Bax MC, Owens JA, Ipsiroglu OS, Wasdell MB. Neurophysiology of circadian rhythm sleep disorders of children with neurodevelopmental disabilities. Euro J Paediatr Neurol. (2012) 16:403–12. doi: 10.1016/j.ejpn.2012.01.002
58. Mareš J, Stopka P, Nohejlová K, Rokyta R. Oxidative stress induced by epileptic seizure and its attenuation by melatonin. Physiol Res. (2013) 62 (Suppl. 1):S67–74. doi: 10.33549/physiolres.932576
59. Kong X, Li X, Cai Z, Yang N, Liu Y, Shu J, et al. Melatonin regulates the viability and differentiation of rat midbrain neural stem cells. Cell Mol Neurobiol. (2008) 28:569–79. doi: 10.1007/s10571-007-9212-7
60. Moriya T, Horie N, Mitome M, Shinohara K. Melatonin influences the proliferative and differentiative activity of neural stem cells. J Pineal Res. (2007) 42:411–8. doi: 10.1111/j.1600-079X.2007.00435.x
61. Reiter RJ. Neuroendocrine effects of light. Int J Biometeorol. (1991) 35:169–75. doi: 10.1007/BF01049063
62. Guzman-Marin R, Suntsova N, Methippara M, Greiffenstein R, Szymusiak R, McGinty D. Sleep deprivation suppresses neurogenesis in the adult hippocampus of rats. Eur J Neurosci. (2005) 22:2111–6. doi: 10.1111/j.1460-9568.2005.04376.x
63. Iwasaki S, Nakazawa K, Sakai J, Kometani K, Iwashita M, Yoshimura Y, et al. Melatonin as a local regulator of human placental function. J Pineal Res. (2005) 39:261–5. doi: 10.1111/j.1600-079X.2005.00244.x
64. Niles LP, Armstrong KJ, Castro LMR, Dao CV, Sharma R, McMillan CR, et al. Neural stem cells express melatonin receptors and neurotrophic factors: colocalization of the MT 1 receptor with neuronal and glial markers. BMC Neurosci. (2004) 5:42. doi: 10.1186/1471-2202-5-41
65. Pandi-Perumal SR, Srinivasan V, Maestroni G, Cardinali D, Poeggeler B, Hardeland R. Melatonin: nature's most versatile biological signal? FEBS J. (2006) 273:2813–38. doi: 10.1111/j.1742-4658.2006.05322.x
66. Pevet P, Challet E. Melatonin: both master clock output and internal time-giver in the circadian clocks network. J Physiol. (2011) 105:170–82. doi: 10.1016/j.jphysparis.2011.07.001
67. Arendt J. Melatonin and human rhythms. Chronobiol Int. (2006) 23:21–37. doi: 10.1080/07420520500464361
68. Zhao D, Yu Y, Shen Y, Liu Q, Zhao Z, Sharma R, et al. Melatonin synthesis and function: evolutionary history in animals and plants. Front Endocrinol. (2019) 10:249. doi: 10.3389/fendo.2019.00249
69. Amaral FGd, Cipolla-Neto J. A brief review about melatonin, a pineal hormone. Arch Endocrinol Metab. (2018) 62:472–9. doi: 10.20945/2359-3997000000066
70. Boutin JA, Saunier C, Guenin S-P, Berger S, Moulharat N, Gohier A, et al. Studies of the melatonin binding site location onto quinone reductase 2 by directed mutagenesis. Arch Biochem Biophys. (2008) 477:12–9. doi: 10.1016/j.abb.2008.04.040
71. Reybier K, Perio P, Ferry G, Bouajila J, Delagrange P, Boutin JA, et al. Insights into the redox cycle of human quinone reductase 2. Free Radic Res. (2011) 45:1184–95. doi: 10.3109/10715762.2011.605788
72. Tan DX, Manchester LC, Terron MP, Flores LJ, Reiter RJ. One molecule, many derivatives: a never-ending interaction of melatonin with reactive oxygen and nitrogen species? J Pineal Res. (2007) 42:28–42. doi: 10.1111/j.1600-079X.2006.00407.x
73. Guerrero JM, Reiter RJ. Melatonin-immune system relationships. Curr Top Med Chem. (2002) 2:167–79. doi: 10.2174/1568026023394335
74. Withyachumnarnkul B, Nonaka KO, Santana C, Attia AM, Reiter RJ. Interferon-gamma modulates melatonin production in rat pineal glands in organ culture. J Interferon Res. (1990) 10:403–11. doi: 10.1089/jir.1990.10.403
75. Song J. Pineal gland dysfunction in Alzheimer's disease: relationship with the immune-pineal axis, sleep disturbance, and neurogenesis. Mol Neurodegener. (2019) 14:28. doi: 10.1186/s13024-019-0330-8
76. Jung KH, Hong SW, Zheng HM, Lee DH, Hong SS. Melatonin downregulates nuclear erythroid 2-related factor 2 and nuclear factor-kappaB during prevention of oxidative liver injury in a dimethylnitrosamine model. J Pineal Res. (2009) 47:173–83. doi: 10.1111/j.1600-079X.2009.00698.x
77. Huang SH, Cao XJ, Wei W. Melatonin decreases TLR3-mediated inflammatory factor expression via inhibition of NF-kappa B activation in respiratory syncytial virus-infected RAW264.7 macrophages. J Pineal Res. (2008) 45:93–100. doi: 10.1111/j.1600-079X.2008.00560.x
78. Jetten AM. Retinoid-related orphan receptors (RORs): critical roles in development, immunity, circadian rhythm, and cellular metabolism. Nucl Recept Signal. (2009) 7:e003. doi: 10.1621/nrs.07003
79. Ivanov, II, McKenzie BS, Zhou L, Tadokoro CE, Lepelley A, et al. The orphan nuclear receptor RORgammat directs the differentiation program of proinflammatory IL-17+ T helper cells. Cell. (2006) 126:1121–33. doi: 10.1016/j.cell.2006.07.035
80. Jockers R, Delagrange P, Dubocovich ML, Markus RP, Renault N, Tosini G, et al. Update on melatonin receptors: IUPHAR review 20. Br J Pharmacol. (2016) 173:2702–25. doi: 10.1111/bph.13536
81. Bartsch H, Bartsch C. Effect of melatonin on experimental tumors under different photoperiods and times of administration. J Neural Transm. (1981) 52:269–79. doi: 10.1007/BF01256752
82. Lissoni P, Chilelli M, Villa S, Cerizza L, Tancini G. Five years survival in metastatic non-small cell lung cancer patients treated with chemotherapy alone or chemotherapy and melatonin: a randomized trial. J Pineal Res. (2003) 35:12–5. doi: 10.1034/j.1600-079X.2003.00032.x
83. Song YJ, Zhong CB, Wu W. Cardioprotective effects of melatonin: focusing on its roles against diabetic cardiomyopathy. Biomed Pharmacother. (2020) 128:110260. doi: 10.1016/j.biopha.2020.110260
84. Hardeland R, Poeggeler B. Melatonin beyond its classical functions. Open Physiol J. (2008) 1:1–22. doi: 10.2174/1874360900901010001
85. Cardinali DP, Pagano ES, Bernasconi PAS, Reynoso R, Scacchi P. Melatonin and mitochondrial dysfunction in the central nervous system. Horm Behav. (2013) 63:322–30. doi: 10.1016/j.yhbeh.2012.02.020
86. Takuma K, Yan SS, Stern DM, Yamada K. Mitochondrial dysfunction, endoplasmic reticulum stress, and apoptosis in Alzheimer's disease. J Pharmacol Sci. (2005) 97:312–6. doi: 10.1254/jphs.CPJ04006X
87. Vandewalle G, Middleton B, Rajaratnam SM, Stone BM, Thorleifsdottir B, Arendt J, et al. Robust circadian rhythm in heart rate and its variability: influence of exogenous melatonin and photoperiod. J Sleep Res. (2007) 16:148–55. doi: 10.1111/j.1365-2869.2007.00581.x
88. Scheer FA, Van Montfrans GA, van Someren EJ, Mairuhu G, Buijs RM. Daily nighttime melatonin reduces blood pressure in male patients with essential hypertension. Hypertension. (2004) 43:192–7. doi: 10.1161/01.HYP.0000113293.15186.3b
89. Scheer FA. Potential use of melatonin as adjunct antihypertensive therapy. Am J Hypertens. (2005) 18 (12 Pt. 1):1619–20. doi: 10.1016/j.amjhyper.2005.07.013
90. Arendt J, Skene DJ. Melatonin as a chronobiotic. Sleep Med Rev. (2005) 9:25–39. doi: 10.1016/j.smrv.2004.05.002
91. O'Collins VE, Macleod MR, Cox SF, Van Raay L, Aleksoska E, Donnan GA, et al. Preclinical drug evaluation for combination therapy in acute stroke using systematic review, meta-analysis, and subsequent experimental testing. J Cereb Blood Flow Metab. (2011) 31:962–75. doi: 10.1038/jcbfm.2010.184
92. Macleod MR, O'Collins T, Horky LL, Howells DW, Donnan GA. Systematic review and meta-analysis of the efficacy of melatonin in experimental stroke. J Pineal Res. (2005) 38:35–41. doi: 10.1111/j.1600-079X.2004.00172.x
93. Shechter A, Lespérance P, Ng Ying Kin NM, Boivin DB. Nocturnal polysomnographic sleep across the menstrual cycle in premenstrual dysphoric disorder. Sleep Med. (2012) 13:1071–8. doi: 10.1016/j.sleep.2012.05.012
94. Dominguez-Rodriguez A, Abreu-Gonzalez P, Kaski JC. Disruption of normal circadian rhythms and cardiovascular events. Heart Metab. (2009) 44:11–5.
95. Paulis L, Šimko F. Blood pressure modulation and cardiovascular protection by melatonin: potential mechanisms behind. Physiol Res. (2007) 56:671–84. doi: 10.33549/physiolres.931236
96. Rodella LF, Favero G, Rossini C, Foglio E, Reiter RJ, Rezzani R. Endothelin-1 as a potential marker of melatonin's therapeutic effects in smoking-induced vasculopathy. Life Sci. (2010) 87:558–64. doi: 10.1016/j.lfs.2010.09.011
97. Pang CS, Xi SC, Brown GM, Pang SF, Shiu SY. 2 [125I] Iodomelatonin binding and interaction with β-adrenergic signaling in chick heart/coronary artery physiology. J Pineal Res. (2002) 32:243–52. doi: 10.1034/j.1600-079X.2002.01860.x
98. Higgins AY, O'Halloran TD, Chang JD. Chemotherapy-induced cardiomyopathy. Heart Fail Rev. (2015) 20:721–30. doi: 10.1007/s10741-015-9502-y
99. Liu D, Ma Z, Di S, Yang Y, Yang J, Xu L, et al. AMPK/PGC1α activation by melatonin attenuates acute doxorubicin cardiotoxicity via alleviating mitochondrial oxidative damage and apoptosis. Free Radic Biol Med. (2018) 129:59–72. doi: 10.1016/j.freeradbiomed.2018.08.032
100. Tan TC, Bouras S, Sawaya H, Sebag IA, Cohen V, Picard MH, et al. Time trends of left ventricular ejection fraction and myocardial deformation indices in a cohort of women with breast cancer treated with anthracyclines, taxanes, and trastuzumab. J Am Soc Echocardiogr. (2015) 28:509–14. doi: 10.1016/j.echo.2015.02.001
101. Govender J, Loos B, Marais E, Engelbrecht AM. Mitochondrial catastrophe during doxorubicin-induced cardiotoxicity: a review of the protective role of melatonin. J Pineal Res. (2014) 57:367–80. doi: 10.1111/jpi.12176
102. Tokarska-Schlattner M, Zaugg M, Zuppinger C, Wallimann T, Schlattner U. New insights into doxorubicin-induced cardiotoxicity: the critical role of cellular energetics. J Mol Cell Cardiol. (2006) 41:389–405. doi: 10.1016/j.yjmcc.2006.06.009
103. Gottlieb RA, Gustafsson ÅB. Mitochondrial turnover in the heart. Biochim Biophys Acta. (2011) 1813:1295–301. doi: 10.1016/j.bbamcr.2010.11.017
104. Zhang Y-W, Shi J, Li Y-J, Wei L. Cardiomyocyte death in doxorubicin-induced cardiotoxicity. Arch Immunol Ther Exp. (2009) 57:435–45. doi: 10.1007/s00005-009-0051-8
105. Zhang X, Zhu J-X, Ma Z-G, Wu H-M, Xu S-C, Song P, et al. Rosmarinic acid alleviates cardiomyocyte apoptosis via cardiac fibroblast in doxorubicin-induced cardiotoxicity. Int J Biol Sci. (2019) 15:556. doi: 10.7150/ijbs.29907
106. Chakraborti S, Pramanick A, Saha S, Roy SS, Chaudhuri AR, Das M, et al. Atypical G protein β5 promotes cardiac oxidative stress, apoptosis, and fibrotic remodeling in response to multiple cancer chemotherapeutics. Cancer Res. (2018) 78:528–41. doi: 10.1158/0008-5472.CAN-17-1280
107. Tian X-Q, Ni X-W, Xu H-L, Zheng L, ZhuGe D-L, Chen B, et al. Prevention of doxorubicin-induced cardiomyopathy using targeted MaFGF mediated by nanoparticles combined with ultrasound-targeted MB destruction. Int J Nanomed. (2017) 12:7103. doi: 10.2147/IJN.S145799
108. Del Re DP. Hippo signaling in the heart–non-canonical pathways impact growth, survival and function. Circul J. (2016) 80:1504–10. doi: 10.1253/circj.CJ-16-0426
109. Del Re DP, Yang Y, Nakano N, Cho J, Zhai P, Yamamoto T, et al. Yes-associated protein isoform 1 (Yap1) promotes cardiomyocyte survival and growth to protect against myocardial ischemic injury. J Biol Chem. (2013) 288:3977–88. doi: 10.1074/jbc.M112.436311
110. Lin Z, von Gise A, Zhou P, Gu F, Ma Q, Jiang J, et al. Cardiac-specific YAP activation improves cardiac function and survival in an experimental murine MI model. Circ Res. (2014) 115:354–63. doi: 10.1161/CIRCRESAHA.115.303632
111. Leach JP, Heallen T, Zhang M, Rahmani M, Morikawa Y, Hill MC, et al. Hippo pathway deficiency reverses systolic heart failure after infarction. Nature. (2017) 550:260–4. doi: 10.1038/nature24045
112. Shao D, Zhai P, Del Re DP, Sciarretta S, Yabuta N, Nojima H, et al. A functional interaction between Hippo-YAP signalling and FoxO1 mediates the oxidative stress response. Nat Commun. (2014) 5:1–10. doi: 10.1038/ncomms4315
113. Jafari A, Rezaei-Tavirani M, Farhadihosseinabadi B, Taranejoo S, Zali H. HSP90 and co-chaperones: impact on tumor progression and prospects for molecular-targeted cancer therapy. Cancer Invest. (2020) 38:310–28. doi: 10.1080/07357907.2020.1752227
114. Demyttenaere K. Agomelatine: a narrative review. Euro Neuropsychopharmacol. (2011) 21:S703–9. doi: 10.1016/j.euroneuro.2011.07.004
115. Delagrange P, Boutin JA. Therapeutic potential of melatonin ligands. Chronobiol Int. (2006) 23:413–8. doi: 10.1080/07420520500464387
116. Jia P, Liu C, Wu N, Jia D, Sun Y. Agomelatine protects against myocardial ischemia reperfusion injury by inhibiting mitochondrial permeability transition pore opening. Am J Transl Res. (2018) 10:1310–23.
117. Aygun H, Gul SS. Cardioprotective effect of melatonin and agomelatine on doxorubicin-induced cardiotoxicity in a rat model: an electrocardiographic, scintigraphic and biochemical study. Bratisl Lek Listy. (2019) 120:249–55. doi: 10.4149/BLL_2019_045
118. Xu Z, Feng W, Shen Q, Yu N, Yu K, Wang S, et al. Rhizoma coptidis and berberine as a natural drug to combat aging and aging-related diseases via anti-oxidation and AMPK activation. Aging Dis. (2017) 8:760–77. doi: 10.14336/AD.2016.0620
119. Liu D, Xu L, Zhang X, Shi C, Qiao S, Ma Z, et al. Snapshot: implications for mTOR in aging-related ischemia/reperfusion injury. Aging Dis. (2019) 10:116. doi: 10.14336/AD.2018.0501
120. Wang S, Wang Y, Zhang Z, Liu Q, Gu J. Cardioprotective effects of fibroblast growth factor 21 against doxorubicin-induced toxicity via the SIRT1/LKB1/AMPK pathway. Cell Death Dis. (2017) 8:e3018. doi: 10.1038/cddis.2017.410
121. Kawaguchi T, Takemura G, Kanamori H, Takeyama T, Watanabe T, Morishita K, et al. Prior starvation mitigates acute doxorubicin cardiotoxicity through restoration of autophagy in affected cardiomyocytes. Cardiovasc Res. (2012) 96:456–65. doi: 10.1093/cvr/cvs282
122. Yuan H, Zhang Q, Guo J, Zhang T, Zhao J, Li J, et al. A PGC-1α-mediated transcriptional network maintains mitochondrial redox and bioenergetic homeostasis against doxorubicin-induced toxicity in human cardiomyocytes: implementation of TT21C. Toxicol Sci. (2016) 150:400–17. doi: 10.1093/toxsci/kfw006
123. Villena JA. New insights into PGC-1 coactivators: redefining their role in the regulation of mitochondrial function and beyond. FEBS J. (2015) 282:647–72. doi: 10.1111/febs.13175
124. Rahman MM, Kwon HS, Kim MJ, Go HK, Oak MH, Kim DH. Melatonin supplementation plus exercise behavior ameliorate insulin resistance, hypertension and fatigue in a rat model of type 2 diabetes mellitus. Biomed Pharmacother. (2017) 92:606–14. doi: 10.1016/j.biopha.2017.05.035
125. Yu L, Gong B, Duan W, Fan C, Zhang J, Li Z, et al. Melatonin ameliorates myocardial ischemia/reperfusion injury in type 1 diabetic rats by preserving mitochondrial function: role of AMPK-PGC-1α-SIRT3 signaling. Sci Rep. (2017) 7:41337. doi: 10.1038/srep41337
126. Reiter RJ, Mayo JC, Tan DX, Sainz RM, Alatorre-Jimenez M, Qin L. Melatonin as an antioxidant: under promises but over delivers. J Pineal Res. (2016) 61:253–78. doi: 10.1111/jpi.12360
127. Tan DX, Manchester LC, Liu X, Rosales-Corral SA, Acuna-Castroviejo D, Reiter RJ. Mitochondria and chloroplasts as the original sites of melatonin synthesis: a hypothesis related to melatonin's primary function and evolution in eukaryotes. J Pineal Res. (2013) 54:127–38. doi: 10.1111/jpi.12026
128. Tan D-X, Manchester LC, Qin L, Reiter RJ. Melatonin: a mitochondrial targeting molecule involving mitochondrial protection and dynamics. Int J Mol Sci. (2016) 17:2124. doi: 10.3390/ijms17122124
129. Tan DX, Manchester LC, Burkhardt S, Sainz RM, Mayo JC, Kohen R, et al. N1-acetyl-N2-formyl-5-methoxykynuramine, a biogenic amine and melatonin metabolite, functions as a potent antioxidant. FASEB J. (2001) 15:2294–6. doi: 10.1096/fj.01-0309fje
130. Galano A, Tan DX, Reiter RJ. On the free radical scavenging activities of melatonin's metabolites, AFMK and AMK. J Pineal Res. (2013) 54:245–57. doi: 10.1111/jpi.12010
131. Antolín I, Rodríguez C, Sáinz RM, Mayo JC, Uría H, Kotler ML, et al. Neurohormone melatonin prevents cell damage: effect on gene expression for antioxidant enzymes. FASEB J. (1996) 10:882–90. doi: 10.1096/fasebj.10.8.8666165
132. Reiter RJ, Tan D-x, Osuna C, Gitto E. Actions of melatonin in the reduction of oxidative stress. J Biomed Sci. (2000) 7:444–58. doi: 10.1007/BF02253360
133. Fischer TW, Kleszczyński K, Hardkop LH, Kruse N, Zillikens D. Melatonin enhances antioxidative enzyme gene expression (CAT, GPx, SOD), prevents their UVR-induced depletion, and protects against the formation of DNA damage (8-hydroxy-2'-deoxyguanosine) in ex vivo human skin. J Pineal Res. (2013) 54:303–12. doi: 10.1111/jpi.12018
134. Tan D-X, Manchester LC, Reiter RJ, Qi W-B, Karbownik M, Calvo JR. Significance of melatonin in antioxidative defense system: reactions and products. Neurosignals. (2000) 9:137–59. doi: 10.1159/000014635
135. Lissoni P, Rovelli F, Malugani F, Bucovec R, Conti A, Maestroni G. Anti-angiogenic activity of melatonin in advanced cancer patients. Neuroendocrinol Lett. (2001) 22:45–8.
136. Lissoni P, Barni S, Mandalà M, Ardizzoia A, Paolorossi F, Vaghi M, et al. Decreased toxicity and increased efficacy of cancer chemotherapy using the pineal hormone melatonin in metastatic solid tumour patients with poor clinical status. Eur J Cancer. (1999) 35:1688–92. doi: 10.1016/S0959-8049(99)00159-8
137. Kim C, Kim N, Joo H, Youm JB, Park WS, Van Cuong D, et al. Modulation by melatonin of the cardiotoxic and antitumor activities of adriamycin. J Cardiovasc Pharmacol. (2005) 46:200–10. doi: 10.1097/01.fjc.0000171750.97822.a2
138. Govender J, Loos B, Marais E, Engelbrecht AM. Melatonin improves cardiac and mitochondrial function during doxorubicin-induced cardiotoxicity: a possible role for peroxisome proliferator-activated receptor gamma coactivator 1-alpha and sirtuin activity? Toxicol Appl Pharmacol. (2018) 358:86–101. doi: 10.1016/j.taap.2018.06.031
139. Lissoni P. Biochemotherapy with immunomodulating pineal hormones other than melatonin: 5-methoxytryptamine as a new oncostatic pineal agent. Pathol Biol. (2007) 55:198–200. doi: 10.1016/j.patbio.2006.12.008
140. Jawed S, Kim B, Ottenhof T, Brown GM, Werstiuk ES, Niles LP. Human melatonin MT1 receptor induction by valproic acid and its effects in combination with melatonin on MCF-7 breast cancer cell proliferation. Eur J Pharmacol. (2007) 560:17–22. doi: 10.1016/j.ejphar.2007.01.022
141. Lemus-Wilson A, Kelly P, Blask D. Melatonin blocks the stimulatory effects of prolactin on human breast cancer cell growth in culture. Br J Cancer. (1995) 72:1435–40. doi: 10.1038/bjc.1995.526
142. Sánchez-Barceló EJ, Cos S, Mediavilla D, Martínez-Campa C, González A, Alonso-González C. Melatonin–estrogen interactions in breast cancer. J Pineal Res. (2005) 38:217–22. doi: 10.1111/j.1600-079X.2004.00207.x
143. Floyd JD, Nguyen DT, Lobins RL, Bashir Q, Doll DC, Perry MC. Cardiotoxicity of cancer therapy. J Clin Oncol. (2005) 23:7685–96. doi: 10.1200/JCO.2005.08.789
144. Bruck R, Aeed H, Avni Y, Shirin H, Matas Z, Shahmurov M, et al. Melatonin inhibits nuclear factor kappa B activation and oxidative stress and protects against thioacetamide induced liver damage in rats. J Hepatol. (2004) 40:86–93. doi: 10.1016/S0168-8278(03)00504-X
145. Ayanoglu-Dülger G, Hürdag C, Arbak S, Paskaloglu K, Sener-Muratoglu G. Protective effect of famotidine, omeprazole, and melatonin against acetylsalicylic acid-induced gastric damage in rats. Dig Dis Sci. (2001) 46:318–30. doi: 10.1023/a:1005652815921
146. Reiter RJ, Tan DX. Melatonin: a novel protective agent against oxidative injury of the ischemic/reperfused heart. Cardiovasc Res. (2003) 58:10–9. doi: 10.1016/S0008-6363(02)00827-1
147. Sankaran M, Kandhan K, Ganesan D, Shalini M. Melatonin attenuates lipid peroxidation and enhances circulatory antioxidants during mammary carcinogenesis in rats. J Biochem Technol. (2010) 2.
148. Zhang Y, Li L, Xiang C, Ma Z, Ma T, Zhu S. Protective effect of melatonin against adriamycin-induced cardiotoxicity. Exp Ther Med. (2013) 5:1496–500. doi: 10.3892/etm.2013.989
149. Sahna E, Parlakpinar H, Ozer MK, Ozturk F, Ozugurlu F, Acet A. Melatonin protects against myocardial doxorubicin toxicity in rats: role of physiological concentrations. J Pineal Res. (2003) 35:257–61. doi: 10.1034/j.1600-079X.2003.00084.x
150. Öz E, Erbaş D, Sürücü HS, Düzgün E. Prevention of doxorubicin-induced cardiotoxicity by melatonin. Mol Cell Biochem. (2006) 282:31–7. doi: 10.1007/s11010-006-1153-9
151. Dziegiel P, Jethon Z, Suder E, Sopel M, Rabczyński J, Surowiak P, et al. Role of exogenous melatonin in reducing the cardiotoxic effect of daunorubicin and doxorubicin in the rat. Exp Toxicol Pathol. (2002) 53:433–9. doi: 10.1078/0940-2993-00217
152. Agapito MT, Antolín Y, Del Brio MT, López-Burillo S, Pablos MI, Recio JM. Protective effect of melatonin against adriamycin toxicity in the rat. J Pineal Res. (2001) 31:23–30. doi: 10.1034/j.1600-079X.2001.310104.x
153. Li HR, Wang C, Sun P, Liu DD, Du GQ, Tian JW. Melatonin attenuates doxorubicin-induced cardiotoxicity through preservation of YAP expression. J Cell Mol Med. (2020) 24:3634–46. doi: 10.1111/jcmm.15057
154. Ahmed HH, Mannaa F, Elmegeed GA, Doss SH. Cardioprotective activity of melatonin and its novel synthesized derivatives on doxorubicin-induced cardiotoxicity. Bioorg Med Chem. (2005) 13:1847–57. doi: 10.1016/j.bmc.2004.10.066
155. Liu X, Chen Z, Chua CC, Ma Y-S, Youngberg GA, Hamdy R, et al. Melatonin as an effective protector against doxorubicin-induced cardiotoxicity. Am J Physiol Heart Circ Physiol. (2002) 283:H254–63. doi: 10.1152/ajpheart.01023.2001
156. Dzie giel P, Murawska-Ciałowicz E, Jethon Z, Januszewska L, Podhorska-Okołów M, Surowiak P, et al. Melatonin stimulates the activity of protective antioxidative enzymes in myocardial cells of rats in the course of doxorubicin intoxication. J Pineal Res. (2003) 35:183–7. doi: 10.1034/j.1600-079X.2003.00079.x
157. Ozgen ZE, Erdinc M, Akkoc H, Kelle I. Protective effects of melatonin on doxorubicin induced cardiotoxicity in isolated rat heart. East J Med. (2016) 21:119. doi: 10.5505/ejm.2016.19483
158. Guven A, Yavuz O, Cam M, Ercan F, Bukan N, Comunoglu C. Melatonin protects against epirubicin-induced cardiotoxicity. Acta Histochem. (2007) 109:52–60. doi: 10.1016/j.acthis.2006.09.007
159. Morishima I, Matsui H, Mukawa H, Hayashi K, Toki Y, Okumura K, et al. Melatonin, a pineal hormone with antioxidant property, protects against adriamycin cardiomyopathy in rats. Life Sci. (1998) 63:511–21. doi: 10.1016/S0024-3205(98)00302-6
160. Ozturk M, Ozler M, Kurt YG, Ozturk B, Uysal B, Ersoz N, et al. Efficacy of melatonin, mercaptoethylguanidine and 1400W in doxorubicin-and trastuzumab-induced cardiotoxicity. J Pineal Res. (2011) 50:89–96. doi: 10.1111/j.1600-079X.2010.00818.x
161. Alghasham AA. Comparative assessment of melatonin-afforded protection in liver, kidney and heart of male mice against doxorubicin induced toxicity. Pharmacol Pharm. (2013) 4:590. doi: 10.4236/pp.2013.48085
162. Rezzani R, Rodella LF, Bonomini F, Tengattini S, Bianchi R, Reiter RJ. Beneficial effects of melatonin in protecting against cyclosporine A-induced cardiotoxicity are receptor mediated. J Pineal Res. (2006) 41:288–95. doi: 10.1111/j.1600-079X.2006.00368.x
163. Bilginoglu A, Aydin D, Özsoy S, Aygün H. Protective effect of melatonin on adriamycin-induced cardiotoxicity in rats. Arch Turk Soc Cardiol. (2014) 42:265–73. doi: 10.5543/tkda.2014.36089
164. Vazan R, Ravingerova T. Protective effect of melatonin against myocardial injury induced by epinephrine. J Physiol Biochem. (2015) 71:43–9. doi: 10.1007/s13105-014-0377-5
165. Wahab MHA, Akoul E-SE, Abdel-Aziz A-AH. Modulatory effects of melatonin and vitamin E on doxorubicin-induced cardiotoxicity in ehrlich ascites carcinoma-bearing mice. Tumori J. (2000) 86:157–62. doi: 10.1177/030089160008600210
166. Xu MF, Ho S, Qian ZM, Tang PL. Melatonin protects against cardiac toxicity of doxorubicin in rat. J Pineal Res. (2001) 31:301–7. doi: 10.1034/j.1600-079X.2001.310403.x
167. Xu M, Ashraf M. Melatonin protection against lethal myocyte injury induced by doxorubicin as reflected by effects on mitochondrial membrane potential. J Mol Cell Cardiol. (2002) 34:75–9. doi: 10.1006/jmcc.2001.1485
168. Karantalis V, Hare JM. Use of mesenchymal stem cells for therapy of cardiac disease. Circ Res. (2015) 116:1413–30. doi: 10.1161/CIRCRESAHA.116.303614
169. Behbahan IS, Keating A, Gale RP. Bone marrow therapies for chronic heart disease. Stem Cells. (2015) 33:3212–27. doi: 10.1002/stem.2080
170. Fan PC. Review of enterobiasis in Taiwan and offshore islands. J Microbiol Immunol Infect. (1998) 31:203–10.
171. Liu Z, Wang H, Wang Y, Lin Q, Yao A, Cao F, et al. The influence of chitosan hydrogel on stem cell engraftment, survival and homing in the ischemic myocardial microenvironment. Biomaterials. (2012) 33:3093–106. doi: 10.1016/j.biomaterials.2011.12.044
172. Shafiq M, Jung Y, Kim SH. Insight on stem cell preconditioning and instructive biomaterials to enhance cell adhesion, retention, and engraftment for tissue repair. Biomaterials. (2016) 90:85–115. doi: 10.1016/j.biomaterials.2016.03.020
173. Li W, Ma N, Ong LL, Nesselmann C, Klopsch C, Ladilov Y, et al. Bcl-2 engineered MSCs inhibited apoptosis and improved heart function. Stem Cells. (2007) 25:2118–27. doi: 10.1634/stemcells.2006-0771
174. Fan L, Lin C, Zhuo S, Chen L, Liu N, Luo Y, et al. Transplantation with survivin-engineered mesenchymal stem cells results in better prognosis in a rat model of myocardial infarction. Eur J Heart Fail. (2009) 11:1023–30. doi: 10.1093/eurjhf/hfp135
175. Khan I, Ali A, Akhter MA, Naeem N, Chotani MA, Mustafa T, et al. Preconditioning of mesenchymal stem cells with 2,4-dinitrophenol improves cardiac function in infarcted rats. Life Sci. (2016) 162:60–9. doi: 10.1016/j.lfs.2016.08.014
176. Masuda S, Shimizu T, Yamato M, Okano T. Cell sheet engineering for heart tissue repair. Adv Drug Deliv Rev. (2008) 60:277–85. doi: 10.1016/j.addr.2007.08.031
177. Forte G, Pietronave S, Nardone G, Zamperone A, Magnani E, Pagliari S, et al. Human cardiac progenitor cell grafts as unrestricted source of supernumerary cardiac cells in healthy murine hearts. Stem Cells. (2011) 29:2051–61. doi: 10.1002/stem.763
178. Zhou L, Chen X, Liu T, Gong Y, Chen S, Pan G, et al. Melatonin reverses H2 O2 -induced premature senescence in mesenchymal stem cells via the SIRT1-dependent pathway. J Pineal Res. (2015) 59:190–205. doi: 10.1111/jpi.12250
179. Lee SJ, Jung YH, Oh SY, Yun SP, Han HJ. Melatonin enhances the human mesenchymal stem cells motility via melatonin receptor 2 coupling with Gαq in skin wound healing. J Pineal Res. (2014) 57:393–407. doi: 10.1111/jpi.12179
180. Wang FW, Wang Z, Zhang YM, Du ZX, Zhang XL, Liu Q, et al. Protective effect of melatonin on bone marrow mesenchymal stem cells against hydrogen peroxide-induced apoptosis in vitro. J Cell Biochem. (2013) 114:2346–55. doi: 10.1002/jcb.24582
181. Harpsøe NG, Andersen LP, Gögenur I, Rosenberg J. Clinical pharmacokinetics of melatonin: a systematic review. Eur J Clin Pharmacol. (2015) 71:901–9. doi: 10.1007/s00228-015-1873-4
182. Wang F, Chen L, Zhang R, Chen Z, Zhu L. RGD peptide conjugated liposomal drug delivery system for enhance therapeutic efficacy in treating bone metastasis from prostate cancer. J Control Release. (2014) 196:222–33. doi: 10.1016/j.jconrel.2014.10.012
183. Ma Q, Yang J, Huang X, Guo W, Li S, Zhou H, et al. Poly(lactide-co-glycolide)-monomethoxy-poly-(polyethylene glycol) nanoparticles loaded with melatonin protect adipose-derived stem cells transplanted in infarcted heart tissue. Stem Cells. (2018) 36:540–50. doi: 10.1002/stem.2777
184. Caughey B, Baron GS. Prions and their partners in crime. Nature. (2006) 443:803–10. doi: 10.1038/nature05294
185. Aguzzi A, Baumann F, Bremer J. The prion's elusive reason for being. Annu Rev Neurosci. (2008) 31:439–77. doi: 10.1146/annurev.neuro.31.060407.125620
186. Lopes MH, Santos TG. Prion potency in stem cells biology. Prion. (2012) 6:142–6. doi: 10.4161/pri.19035
187. Martin-Lannerée S, Hirsch TZ, Hernandez-Rapp J, Halliez S, Vilotte J-L, Launay J-M, et al. PrP(C) from stem cells to cancer. Front Cell Dev Biol. (2014) 2:55–. doi: 10.3389/fcell.2014.00055
188. Martin-Lannerée S, Halliez S, Hirsch TZ, Hernandez-Rapp J, Passet B, Tomkiewicz C, et al. The cellular prion protein controls notch signaling in neural stem/progenitor cells. Stem Cells. (2017) 35:754–65. doi: 10.1002/stem.2501
189. Black SAG, Stys PK, Zamponi GW, Tsutsui S. Cellular prion protein and NMDA receptor modulation: protecting against excitotoxicity. Front Cell Dev Biol. (2014) 2:45–. doi: 10.3389/fcell.2014.00045
190. Mohanty ST, Cairney CJ, Chantry AD, Madan S, Fernandes JA, Howe SJ, et al. A small molecule modulator of prion protein increases human mesenchymal stem cell lifespan, ex vivo expansion, and engraftment to bone marrow in NOD/SCID mice. Stem Cells. (2012) 30:1134–43. doi: 10.1002/stem.1065
191. Shi F, Yang Y, Wang T, Kouadir M, Zhao D, Hu S. cellular prion protein promotes neuronal differentiation of adipose-derived stem cells by upregulating miRNA-124. J Mol Neurosci. (2016) 59:48–55. doi: 10.1007/s12031-016-0733-8
192. Mehrabian M, Hildebrandt H, Schmitt-Ulms G. NCAM1 polysialylation: the prion protein's elusive reason for being? ASN Neuro. (2016) 8:1759091416679074. doi: 10.1177/1759091416679074
193. Doeppner TR, Kaltwasser B, Schlechter J, Jaschke J, Kilic E, Bähr M, et al. Cellular prion protein promotes post-ischemic neuronal survival, angioneurogenesis and enhances neural progenitor cell homing via proteasome inhibition. Cell Death Dis. (2015) 6:e2024. doi: 10.1038/cddis.2015.365
194. Lee JH, Han YS, Lee SH. Potentiation of biological effects of mesenchymal stem cells in ischemic conditions by melatonin via upregulation of cellular prion protein expression. J Pineal Res. (2017) 62:e12385. doi: 10.1111/jpi.12385
195. Mias C, Trouche E, Seguelas MH, Calcagno F, Dignat-George F, Sabatier F, et al. Ex vivo pretreatment with melatonin improves survival, proangiogenic/mitogenic activity, and efficiency of mesenchymal stem cells injected into ischemic kidney. Stem Cells. (2008) 26:1749–57. doi: 10.1634/stemcells.2007-1000
196. Zhu P, Liu J, Shi J, Zhou Q, Liu J, Zhang X, et al. Melatonin protects ADSCs from ROS and enhances their therapeutic potency in a rat model of myocardial infarction. J Cell Mol Med. (2015) 19:2232–43. doi: 10.1111/jcmm.12610
197. Gurusamy N, Ray D, Lekli I, Das DK. Red wine antioxidant resveratrol-modified cardiac stem cells regenerate infarcted myocardium. J Cell Mol Med. (2010) 14:2235–9. doi: 10.1111/j.1582-4934.2010.01140.x
198. Wang Y, Li C, Cheng K, Zhang R, Narsinh K, Li S, et al. Activation of liver X receptor improves viability of adipose-derived mesenchymal stem cells to attenuate myocardial ischemia injury through TLR4/NF-κB and Keap-1/Nrf-2 signaling pathways. Antioxid Redox Signal. (2014) 21:2543–57. doi: 10.1089/ars.2013.5683
199. Han D, Huang W, Li X, Gao L, Su T, Li X, et al. Melatonin facilitates adipose-derived mesenchymal stem cells to repair the murine infarcted heart via the SIRT1 signaling pathway. J Pineal Res. (2016) 60:178–92. doi: 10.1111/jpi.12299
200. Kudová J, Vašíček O, CíŽ M, Kubala L. Melatonin promotes cardiomyogenesis of embryonic stem cells via inhibition of HIF-1α stabilization. J Pineal Res. (2016) 61:493–503. doi: 10.1111/jpi.12366
201. Mias C, Lairez O, Trouche E, Roncalli J, Calise D, Seguelas MH, et al. Mesenchymal stem cells promote matrix metalloproteinase secretion by cardiac fibroblasts and reduce cardiac ventricular fibrosis after myocardial infarction. Stem Cells. (2009) 27:2734–43. doi: 10.1002/stem.169
202. Masoudi MS, Mehrabian E, Mirzaei H. MiR-21: a key player in glioblastoma pathogenesis. J Cell Biochem. (2018) 119:1285–90. doi: 10.1002/jcb.26300
203. Wang F, Zhou H, Du Z, Chen X, Zhu F, Wang Z, et al. Cytoprotective effect of melatonin against hypoxia/serum deprivation-induced cell death of bone marrow mesenchymal stem cells in vitro. Eur J Pharmacol. (2015) 748:157–65. doi: 10.1016/j.ejphar.2014.09.033
204. Tosini G, Owino S, Guillaume JL, Jockers R. Understanding melatonin receptor pharmacology: latest insights from mouse models, and their relevance to human disease. Bioessays. (2014) 36:778–87. doi: 10.1002/bies.201400017
205. Reiter RJ, Tan DX, Manchester LC, Pilar Terron M, Flores LJ, Koppisepi S. Medical implications of melatonin: receptor-mediated and receptor-independent actions. Adv Med Sci. (2007) 52:11–28.
206. Yu L, Sun Y, Cheng L, Jin Z, Yang Y, Zhai M, et al. Melatonin receptor-mediated protection against myocardial ischemia/reperfusion injury: role of SIRT1. J Pineal Res. (2014) 57:228–38. doi: 10.1111/jpi.12161
207. Yu L, Liang H, Lu Z, Zhao G, Zhai M, Yang Y, et al. Membrane receptor-dependent Notch1/Hes1 activation by melatonin protects against myocardial ischemia-reperfusion injury: in vivo and in vitro studies. J Pineal Res. (2015) 59:420–33. doi: 10.1111/jpi.12272
208. Inserte J, Garcia-Dorado D. The cGMP/PKG pathway as a common mediator of cardioprotection: translatability and mechanism. Br J Pharmacol. (2015) 172:1996–2009. doi: 10.1111/bph.12959
209. Shi Z, Fu F, Yu L, Xing W, Su F, Liang X, et al. Vasonatrin peptide attenuates myocardial ischemia-reperfusion injury in diabetic rats and underlying mechanisms. Am J Physiol Heart Circ Physiol. (2015) 308:H281–90. doi: 10.1152/ajpheart.00666.2014
210. Andreadou I, Iliodromitis EK, Szabo C, Papapetropoulos A. Hydrogen sulfide and PKG in ischemia-reperfusion injury: sources, signaling, accelerators and brakes. Basic Res Cardiol. (2015) 110:510. doi: 10.1007/s00395-015-0510-9
211. Gevi F, Campolo F, Naro F, Zolla L. The cardioprotective effect of sildenafil is mediated by the activation of malate dehydrogenase and an increase in the malate-aspartate shuttle in cardiomyocytes. Biochem Pharmacol. (2017) 127:60–70. doi: 10.1016/j.bcp.2016.12.017
212. Dortch-Carnes J, Tosini G. Melatonin receptor agonist-induced reduction of SNP-released nitric oxide and cGMP production in isolated human non-pigmented ciliary epithelial cells. Exp Eye Res. (2013) 107:1–10. doi: 10.1016/j.exer.2012.11.007
213. Shukla P, Sun C, O'Rourke ST. Melatonin inhibits nitric oxide signaling by increasing PDE5 phosphorylation in coronary arteries. Am J Physiol Heart Circ Physiol. (2012) 303:H1418–25. doi: 10.1152/ajpheart.00211.2012
214. Tavukçu HH, Sener TE, Tinay I, Akbal C, Erşahin M, Cevik O, et al. Melatonin and tadalafil treatment improves erectile dysfunction after spinal cord injury in rats. Clin Exp Pharmacol Physiol. (2014) 41:309–16. doi: 10.1111/1440-1681.12216
215. Tian X, Wang F, Zhang L, He C, Ji P, Wang J, et al. Beneficial effects of melatonin on the in vitro maturation of sheep oocytes and its relation to melatonin receptors. Int J Mol Sci. (2017) 18:834. doi: 10.3390/ijms18040834
216. Wang J, Hu X, Jiang H. The Nrf-2/ARE-HO-1 axis: an important therapeutic approach for attenuating myocardial ischemia and reperfusion injury-induced cardiac remodeling. Int J Cardiol. (2015) 184:263–4. doi: 10.1016/j.ijcard.2015.02.073
217. Yu L, Li S, Tang X, Li Z, Zhang J, Xue X, et al. Diallyl trisulfide ameliorates myocardial ischemia-reperfusion injury by reducing oxidative stress and endoplasmic reticulum stress-mediated apoptosis in type 1 diabetic rats: role of SIRT1 activation. Apoptosis. (2017) 22:942–54. doi: 10.1007/s10495-017-1378-y
218. Manchope MF, Calixto-Campos C, Coelho-Silva L, Zarpelon AC, Pinho-Ribeiro FA, Georgetti SR, et al. Naringenin inhibits superoxide anion-induced inflammatory pain: role of oxidative stress, cytokines, Nrf-2 and the NO-cGMP-PKG-KATP channel signaling pathway. PLoS ONE. (2016) 11:e0153015. doi: 10.1371/journal.pone.0153015
219. Das A, Salloum FN, Xi L, Rao YJ, Kukreja RC. ERK phosphorylation mediates sildenafil-induced myocardial protection against ischemia-reperfusion injury in mice. Am J Physiol Heart Circ Physiol. (2009) 296:H1236–43. doi: 10.1152/ajpheart.00100.2009
220. Das A, Smolenski A, Lohmann SM, Kukreja RC. Cyclic GMP-dependent protein kinase Ialpha attenuates necrosis and apoptosis following ischemia/reoxygenation in adult cardiomyocyte. J Biol Chem. (2006) 281:38644–52. doi: 10.1074/jbc.M606142200
221. Fiedler B, Feil R, Hofmann F, Willenbockel C, Drexler H, Smolenski A, et al. cGMP-dependent protein kinase type I inhibits TAB1-p38 mitogen-activated protein kinase apoptosis signaling in cardiac myocytes. J Biol Chem. (2006) 281:32831–40. doi: 10.1074/jbc.M603416200
222. Yu LM, Di WC, Dong X, Li Z, Zhang Y, Xue XD, et al. Melatonin protects diabetic heart against ischemia-reperfusion injury, role of membrane receptor-dependent cGMP-PKG activation. Biochim Biophys Acta Mol Basis Dis. (2018) 1864:563–78. doi: 10.1016/j.bbadis.2017.11.023
223. Zhou H, Hu S, Jin Q, Shi C, Zhang Y, Zhu P, et al. Mff-Dependent mitochondrial fission contributes to the pathogenesis of cardiac microvasculature ischemia/reperfusion injury via induction of mROS-Mediated cardiolipin oxidation and HK2/VDAC1 disassociation-involved mPTP opening. J Am Heart Assoc. (2017) 6:e005328. doi: 10.1161/JAHA.116.005328
224. Li R, Xin T, Li D, Wang C, Zhu H, Zhou H. Therapeutic effect of sirtuin 3 on ameliorating nonalcoholic fatty liver disease: the role of the ERK-CREB pathway and Bnip3-mediated mitophagy. Redox Biol. (2018) 18:229–43. doi: 10.1016/j.redox.2018.07.011
225. Ackermann M, Kim YO, Wagner WL, Schuppan D, Valenzuela CD, Mentzer SJ, et al. Effects of nintedanib on the microvascular architecture in a lung fibrosis model. Angiogenesis. (2017) 20:359–72. doi: 10.1007/s10456-017-9543-z
226. Tamura H, Kawamoto M, Sato S, Tamura I, Maekawa R, Taketani T, et al. Long-term melatonin treatment delays ovarian aging. J Pineal Res. (2017) 62:e12381. doi: 10.1111/jpi.12381
227. Hambright WS, Fonseca RS, Chen L, Na R, Ran Q. Ablation of ferroptosis regulator glutathione peroxidase 4 in forebrain neurons promotes cognitive impairment and neurodegeneration. Redox Biol. (2017) 12:8–17. doi: 10.1016/j.redox.2017.01.021
228. Ligeza J, Marona P, Gach N, Lipert B, Miekus K, Wilk W, et al. MCPIP1 contributes to clear cell renal cell carcinomas development. Angiogenesis. (2017) 20:325–40. doi: 10.1007/s10456-017-9540-2
229. Gao Y, Xiao X, Zhang C, Yu W, Guo W, Zhang Z, et al. Melatonin synergizes the chemotherapeutic effect of 5-fluorouracil in colon cancer by suppressing PI3K/AKT and NF-κB/iNOS signaling pathways. J Pineal Res. (2017) 62:e12380. doi: 10.1111/jpi.12380
230. Zhou H, Wang S, Hu S, Chen Y, Ren J. ER-Mitochondria microdomains in cardiac ischemia-reperfusion injury: a fresh perspective. Front Physiol. (2018) 9:755. doi: 10.3389/fphys.2018.00755
231. Ding M, Feng N, Tang D, Feng J, Li Z, Jia M, et al. Melatonin prevents Drp1-mediated mitochondrial fission in diabetic hearts through SIRT1-PGC1α pathway. J Pineal Res. (2018) 65:e12491. doi: 10.1111/jpi.12491
232. Zhou H, Zhang Y, Hu S, Shi C, Zhu P, Ma Q, et al. Melatonin protects cardiac microvasculature against ischemia/reperfusion injury via suppression of mitochondrial fission-VDAC1-HK2-mPTP-mitophagy axis. J Pineal Res. (2017) 63:e12413. doi: 10.1111/jpi.12413
233. Ramjee V, Li D, Manderfield LJ, Liu F, Engleka KA, Aghajanian H, et al. Epicardial YAP/TAZ orchestrate an immunosuppressive response following myocardial infarction. J Clin Invest. (2017) 127:899–911. doi: 10.1172/JCI88759
234. Matsuda T, Zhai P, Sciarretta S, Zhang Y, Jeong JI, Ikeda S, et al. NF2 activates hippo signaling and promotes ischemia/reperfusion injury in the heart. Circ Res. (2016) 119:596–606. doi: 10.1161/CIRCRESAHA.116.308586
235. Zhou H, Wang J, Zhu P, Hu S, Ren J. Ripk3 regulates cardiac microvascular reperfusion injury: the role of IP3R-dependent calcium overload, XO-mediated oxidative stress and F-action/filopodia-based cellular migration. Cell Signal. (2018) 45:12–22. doi: 10.1016/j.cellsig.2018.01.020
236. Huang S, Wang X, Wu X, Yu J, Li J, Huang X, et al. Yap regulates mitochondrial structural remodeling during myoblast differentiation. Am J Physiol Cell Physiol. (2018) 315:C474–84. doi: 10.1152/ajpcell.00112.2018
237. Ma S, Dong Z. Melatonin attenuates cardiac reperfusion stress by improving OPA1-related mitochondrial fusion in a yap-hippo pathway-dependent manner. J Cardiovasc Pharmacol. (2019) 73:27–39. doi: 10.1097/FJC.0000000000000626
238. Guo S, Gao C, Xiao W, Zhang J, Qu Y, Li J, et al. Matrine protects cardiomyocytes from ischemia/reperfusion injury by regulating HSP70 expression via activation of the JAK2/STAT3 pathway. Shock. (2018) 50:664–70. doi: 10.1097/SHK.0000000000001108
239. Wang Z, Yu J, Wu J, Qi F, Wang H, Wang Z, et al. Scutellarin protects cardiomyocyte ischemia-reperfusion injury by reducing apoptosis and oxidative stress. Life Sci. (2016) 157:200–7. doi: 10.1016/j.lfs.2016.01.018
240. Lan H, Su Y, Liu Y, Deng C, Wang J, Chen T, et al. Melatonin protects circulatory death heart from ischemia/reperfusion injury via the JAK2/STAT3 signalling pathway. Life Sci. (2019) 228:35–46. doi: 10.1016/j.lfs.2019.04.057
241. Anzell AR, Maizy R, Przyklenk K, Sanderson TH. Mitochondrial quality control and disease: insights into ischemia-reperfusion injury. Mol Neurobiol. (2018) 55:2547–64. doi: 10.1007/s12035-017-0503-9
242. Carreira RS, Lee Y, Ghochani M, Gustafsson Å B, Gottlieb RA. Cyclophilin D is required for mitochondrial removal by autophagy in cardiac cells. Autophagy. (2010) 6:462–72. doi: 10.4161/auto.6.4.11553
243. Kane LA, Lazarou M, Fogel AI, Li Y, Yamano K, Sarraf SA, et al. PINK1 phosphorylates ubiquitin to activate Parkin E3 ubiquitin ligase activity. J Cell Biol. (2014) 205:143–53. doi: 10.1083/jcb.201402104
244. Saito T, Nah J, Oka SI, Mukai R, Monden Y, Maejima Y, et al. An alternative mitophagy pathway mediated by Rab9 protects the heart against ischemia. J Clin Invest. (2019) 129:802–19. doi: 10.1172/JCI122035
245. Reiter RJ, Rosales-Corral S, Tan DX, Jou MJ, Galano A, Xu B. Melatonin as a mitochondria-targeted antioxidant: one of evolution's best ideas. Cell Mol Life Sci. (2017) 74:3863–81. doi: 10.1007/s00018-017-2609-7
246. Petrosillo G, Di Venosa N, Pistolese M, Casanova G, Tiravanti E, Colantuono G, et al. Protective effect of melatonin against mitochondrial dysfunction associated with cardiac ischemia-reperfusion: role of cardiolipin. FASEB J. (2006) 20:269–76. doi: 10.1096/fj.05-4692com
247. Wang Z, Ma C, Meng CJ, Zhu GQ, Sun XB, Huo L, et al. Melatonin activates the Nrf2-ARE pathway when it protects against early brain injury in a subarachnoid hemorrhage model. J Pineal Res. (2012) 53:129–37. doi: 10.1111/j.1600-079X.2012.00978.x
248. Huo X, Wang C, Yu Z, Peng Y, Wang S, Feng S, et al. Human transporters, PEPT1/2, facilitate melatonin transportation into mitochondria of cancer cells: an implication of the therapeutic potential. J Pineal Res. (2017) 62(4). doi: 10.1111/jpi.12390
249. Suofu Y, Li W, Jean-Alphonse FG, Jia J, Khattar NK, Li J, et al. Dual role of mitochondria in producing melatonin and driving GPCR signaling to block cytochrome c release. Proc Natl Acad Sci USA. (2017) 114:E7997–8006. doi: 10.1073/pnas.1705768114
250. Hardeland R. Melatonin and the electron transport chain. Cell Mol Life Sci. (2017) 74:3883–96. doi: 10.1007/s00018-017-2615-9
251. Dube K, Dhanabalan K, Salie R, Blignaut M, Huisamen B, Lochner A. Melatonin has profound effects on mitochondrial dynamics in myocardial ischaemia/reperfusion. Heliyon. (2019) 5:e02659. doi: 10.1016/j.heliyon.2019.e02659
252. Zhou H, Zhu P, Wang J, Zhu H, Ren J, Chen Y. Pathogenesis of cardiac ischemia reperfusion injury is associated with CK2α-disturbed mitochondrial homeostasis via suppression of FUNDC1-related mitophagy. Cell Death Differ. (2018) 25:1080–93. doi: 10.1038/s41418-018-0086-7
253. Fearnhead HO, Vandenabeele P, Vanden Berghe T. How do we fit ferroptosis in the family of regulated cell death? Cell Death Differ. (2017) 24:1991–8. doi: 10.1038/cdd.2017.149
254. de Almagro MC, Goncharov T, Izrael-Tomasevic A, Duttler S, Kist M, Varfolomeev E, et al. Coordinated ubiquitination and phosphorylation of RIP1 regulates necroptotic cell death. Cell Death Differ. (2017) 24:26–37. doi: 10.1038/cdd.2016.78
255. Zhu P, Hu S, Jin Q, Li D, Tian F, Toan S, et al. Ripk3 promotes ER stress-induced necroptosis in cardiac IR injury: a mechanism involving calcium overload/XO/ROS/mPTP pathway. Redox Biol. (2018) 16:157–68. doi: 10.1016/j.redox.2018.02.019
256. Zhou H, Zhu P, Guo J, Hu N, Wang S, Li D, et al. Ripk3 induces mitochondrial apoptosis via inhibition of FUNDC1 mitophagy in cardiac IR injury. Redox Biol. (2017) 13:498–507. doi: 10.1016/j.redox.2017.07.007
257. Wang K, Liu F, Liu CY, An T, Zhang J, Zhou LY, et al. The long noncoding RNA NRF regulates programmed necrosis and myocardial injury during ischemia and reperfusion by targeting miR-873. Cell Death Differ. (2016) 23:1394–405. doi: 10.1038/cdd.2016.28
258. Su Z, Yang Z, Xie L, DeWitt JP, Chen Y. Cancer therapy in the necroptosis era. Cell Death Differ. (2016) 23:748–56. doi: 10.1038/cdd.2016.8
259. Liu Y, Fan C, Zhang Y, Yu X, Wu X, Zhang X, et al. RIP1 kinase activity-dependent roles in embryonic development of fadd-deficient mice. Cell Death Differ. (2017) 24:1459–69. doi: 10.1038/cdd.2017.78
260. Brasacchio D, Alsop AE, Noori T, Lufti M, Iyer S, Simpson KJ, et al. Epigenetic control of mitochondrial cell death through PACS1-mediated regulation of BAX/BAK oligomerization. Cell Death Differ. (2017) 24:961–70. doi: 10.1038/cdd.2016.119
261. Gögenur I, Kücükakin B, Panduro Jensen L, Reiter RJ, Rosenberg J. Melatonin reduces cardiac morbidity and markers of myocardial ischemia after elective abdominal aortic aneurism repair: a randomized, placebo-controlled, clinical trial. J Pineal Res. (2014) 57:10–5. doi: 10.1111/jpi.12138
262. Dwaich KH, Al-Amran FG, Al-Sheibani BI, Al-Aubaidy HA. Melatonin effects on myocardial ischemia-reperfusion injury: impact on the outcome in patients undergoing coronary artery bypass grafting surgery. Int J Cardiol. (2016) 221:977–86. doi: 10.1016/j.ijcard.2016.07.108
263. Ghaeli P, Vejdani S, Ariamanesh A, Hajhossein Talasaz A. Effect of melatonin on cardiac injury after primary percutaneous coronary intervention: a randomized controlled trial. Iran J Pharm Res. (2015) 14:851–5.
264. Dominguez-Rodriguez A, Abreu-Gonzalez P, de la Torre-Hernandez JM, Consuegra-Sanchez L, Piccolo R, Gonzalez-Gonzalez J, et al. Usefulness of early treatment with melatonin to reduce infarct size in patients with ST-segment elevation myocardial infarction receiving percutaneous coronary intervention (from the melatonin adjunct in the acute myocardial infarction treated with angioplasty trial). Am J Cardiol. (2017) 120:522–6. doi: 10.1016/S0735-1097(17)34668-5
265. Zhu H, Jin Q, Li Y, Ma Q, Wang J, Li D, et al. Melatonin protected cardiac microvascular endothelial cells against oxidative stress injury via suppression of IP3R-[Ca(2+)]c/VDAC-[Ca(2+)]m axis by activation of MAPK/ERK signaling pathway. Cell Stress Chaperones. (2018) 23:101–13. doi: 10.1007/s12192-017-0827-4
266. Zhou H, Li D, Zhu P, Ma Q, Toan S, Wang J, et al. Inhibitory effect of melatonin on necroptosis via repressing the Ripk3-PGAM5-CypD-mPTP pathway attenuates cardiac microvascular ischemia-reperfusion injury. J Pineal Res. (2018) 65:e12503. doi: 10.1111/jpi.12503
267. Yeung HM, Hung MW, Fung ML. Melatonin ameliorates calcium homeostasis in myocardial and ischemia-reperfusion injury in chronically hypoxic rats. J Pineal Res. (2008) 45:373–82. doi: 10.1111/j.1600-079X.2008.00601.x
268. Signore S, Sorrentino A, Ferreira-Martins J, Kannappan R, Shafaie M, Del Ben F, et al. Inositol 1, 4, 5-trisphosphate receptors and human left ventricular myocytes. Circulation. (2013) 128:1286–97. doi: 10.1161/CIRCULATIONAHA.113.002764
269. Hayashi T, Su TP. Sigma-1 receptor chaperones at the ER-mitochondrion interface regulate Ca(2+) signaling and cell survival. Cell. (2007) 131:596–610. doi: 10.1016/j.cell.2007.08.036
270. Kho C, Lee A, Jeong D, Oh JG, Gorski PA, Fish K, et al. Small-molecule activation of SERCA2a SUMOylation for the treatment of heart failure. Nat Commun. (2015) 6:7229. doi: 10.1038/ncomms8229
271. Paillard M, Tubbs E, Thiebaut PA, Gomez L, Fauconnier J, Da Silva CC, et al. Depressing mitochondria-reticulum interactions protects cardiomyocytes from lethal hypoxia-reoxygenation injury. Circulation. (2013) 128:1555–65. doi: 10.1161/CIRCULATIONAHA.113.001225
272. Hausenloy DJ, Yellon DM. New directions for protecting the heart against ischaemia-reperfusion injury: targeting the reperfusion injury salvage kinase (RISK)-pathway. Cardiovasc Res. (2004) 61:448–60. doi: 10.1016/j.cardiores.2003.09.024
273. Skyschally A, Gent S, Amanakis G, Schulte C, Kleinbongard P, Heusch G. Across-Species transfer of protection by remote ischemic preconditioning with species-specific myocardial signal transduction by reperfusion injury salvage kinase and survival activating factor enhancement pathways. Circ Res. (2015) 117:279–88. doi: 10.1161/CIRCRESAHA.117.306878
274. Hu S, Zhu P, Zhou H, Zhang Y, Chen Y. Melatonin-Induced protective effects on cardiomyocytes against reperfusion injury partly through modulation of IP3R and SERCA2a via activation of ERK1. Arq Bras Cardiol. (2018) 110:44–51.
275. Szárszoi O, Asemu G, Vaněček J, Ošt'ádal B, Kolár F. Effects of melatonin on ischemia and reperfusion injury of the rat heart. Cardiovasc Drugs Ther. (2001) 15:251–7. doi: 10.1023/A:1011920407691
276. Gul-Kahraman K, Yilmaz-Bozoglan M, Sahna E. Physiological and pharmacological effects of melatonin on remote ischemic perconditioning after myocardial ischemia-reperfusion injury in rats: role of Cybb, Fas, NfκB, Irisin signaling pathway. J Pineal Res. (2019) 67:e12589. doi: 10.1111/jpi.12589
277. Hosseini L, Vafaee MS, Badalzadeh R. Melatonin and nicotinamide mononucleotide attenuate myocardial ischemia/reperfusion injury via modulation of mitochondrial function and hemodynamic parameters in aged rats. J Cardiovasc Pharmacol Ther. (2019) 2019:1074248419882002. doi: 10.1177/1074248419882002
278. Sahna E, Parlakpinar H, Turkoz Y, Acet A. Protective effects of melatonin on myocardial ischemia-reperfusion induced infarct size and oxidative changes. Physiol Res. (2005) 54:491.
279. Liu L, Qin Q, Qian Z, Shi M, Deng Q, Zhu W, et al. Protective effects of melatonin on ischemia-reperfusion induced myocardial damage and hemodynamic recovery in rats. Eur Rev Med Pharmacol Sci. (2014) 18:3681–6.
280. Petrosillo G, Colantuono G, Moro N, Ruggiero FM, Tiravanti E, Di Venosa N, et al. Melatonin protects against heart ischemia-reperfusion injury by inhibiting mitochondrial permeability transition pore opening. Am J Physiol Heart Circ Physiol. (2009) 297:H1487–93. doi: 10.1152/ajpheart.00163.2009
281. Bertuglia S, Reiter RJ. Melatonin reduces ventricular arrhythmias and preserves capillary perfusion during ischemia-reperfusion events in cardiomyopathic hamsters. J Pineal Res. (2007) 42:55–63. doi: 10.1111/j.1600-079X.2006.00383.x
282. Ceyran H, Narin F, Narin N, Akgün H, Ceyran AB, Öztürk F, et al. The effect of high dose melatonin on cardiac ischemia-reperfusion injury. Yonsei Med J. (2008) 49:735–41. doi: 10.3349/ymj.2008.49.5.735
283. Chen WR, Liu HB, Dai Chen Y, Sha Y, Ma Q, Zhu PJ, et al. Melatonin attenuates myocardial ischemia/reperfusion injury by inhibiting autophagy via an AMPK/mTOR signaling pathway. Cell Physiol Biochem. (2018) 47:2067–76. doi: 10.1159/000491474
284. Diez ER, Prados LV, Carrión A, Ponce ZAZ, Miatello RM. A novel electrophysiologic effect of melatonin on ischemia/reperfusion-induced arrhythmias in isolated rat hearts. J Pineal Res. (2009) 46:155–60. doi: 10.1111/j.1600-079X.2008.00643.x
285. Dobsak P, Siegelova J, Eicher J, Jancik J, Svacinova H, Vasku J, et al. Melatonin protects against ischemia-reperfusion injury and inhibits apoptosis in isolated working rat heart. Pathophysiology. (2003) 9:179–87. doi: 10.1016/S0928-4680(02)00080-9
286. Feng J, Chen X, Liu R, Cao C, Zhang W, Zhao Y, et al. Melatonin protects against myocardial ischemia–reperfusion injury by elevating sirtuin3 expression and manganese superoxide dismutase activity. Free Radic Res. (2018) 52:840–9. doi: 10.1080/10715762.2018.1461215
287. Mianehsaz E, Mirzaei HR, Mahjoubin-Tehran M, Rezaee A, Sahebnasagh R, Pourhanifeh MH, et al. Mesenchymal stem cell-derived exosomes: a new therapeutic approach to osteoarthritis? Stem Cell Res Ther. (2019) 10:340. doi: 10.1186/s13287-019-1445-0
288. Yang J-B, Kang Y-M, Zhang C, Yu X-J, Chen W-S. Infusion of melatonin into the paraventricular nucleus ameliorates myocardial ischemia–reperfusion injury by regulating oxidative stress and inflammatory cytokines. J Cardiovasc Pharmacol. (2019) 74:336. doi: 10.1097/FJC.0000000000000711
289. Schaefer M, Gebhard M-M, Gross W. The effect of melatonin on hearts in ischemia/reperfusion experiments without and with HTK cardioplegia. Bioelectrochemistry. (2019) 129:170–8. doi: 10.1016/j.bioelechem.2019.05.017
290. Zhang Y, Wang Y, Xu J, Tian F, Hu S, Chen Y, et al. Melatonin attenuates myocardial ischemia-reperfusion injury via improving mitochondrial fusion/mitophagy and activating the AMPK-OPA1 signaling pathways. J Pineal Res. (2019) 66:e12542. doi: 10.1111/jpi.12542
291. Zhai M, Li B, Duan W, Jing L, Zhang B, Zhang M, et al. Melatonin ameliorates myocardial ischemia reperfusion injury through SIRT 3-dependent regulation of oxidative stress and apoptosis. J Pineal Res. (2017) 63:e12419. doi: 10.1111/jpi.12419
292. Zhou H, Li D, Zhu P, Hu S, Hu N, Ma S, et al. Melatonin suppresses platelet activation and function against cardiac ischemia/reperfusion injury via PPAR γ/FUNDC 1/mitophagy pathways. J Pineal Res. (2017) 63:e12438. doi: 10.1111/jpi.12438
293. Yu L, Li B, Zhang M, Jin Z, Duan W, Zhao G, et al. Melatonin reduces PERK-eIF2α-ATF4-mediated endoplasmic reticulum stress during myocardial ischemia–reperfusion injury: role of RISK and SAFE pathways interaction. Apoptosis. (2016) 21:809–24. doi: 10.1007/s10495-016-1246-1
294. Yu L, Liang H, Dong X, Zhao G, Jin Z, Zhai M, et al. Reduced silent information regulator 1 signaling exacerbates myocardial ischemia–reperfusion injury in type 2 diabetic rats and the protective effect of melatonin. J Pineal Res. (2015) 59:376–90. doi: 10.1111/jpi.12269
295. Yang Y, Duan W, Jin Z, Yi W, Yan J, Zhang S, et al. JAK 2/STAT 3 activation by melatonin attenuates the mitochondrial oxidative damage induced by myocardial ischemia/reperfusion injury. J Pineal Res. (2013) 55:275–86. doi: 10.1111/jpi.12070
296. Simko F, Simko J. The potential role of nitric oxide in the hypertrophic growth of the left ventricle. Physiol Res. (2000) 49:37–46.
297. Šimko F. Is NO the king? Pathophysiological benefit with uncertain clinical impact. Physiol Res. (2007) 56 (Suppl. 2):S1–6. doi: 10.33549/physiolres.931391
298. Bernatova I, Pechanova O, Simko F. Captopril prevents NO-deficient hypertension and left ventricular hypertrophy without affecting nitric oxide synthase activity in rats. Physiol Res. (1996) 45:311–6.
299. Bernátová I, Pelouch V, Šimko F. Regression of chronic L-NAME-treatment-induced left ventricular hypertrophy: effect of captopril. J Mol Cell Cardiol. (2000) 32:177–85. doi: 10.1006/jmcc.1999.1071
300. Pechánová Og, Bernátová I, Pelouch V, Šimko F. Protein remodelling of the heart in NO-deficient hypertension: the effect of captopril. J Mol Cell Cardiol. (1997) 29:3365–74. doi: 10.1006/jmcc.1997.0566
301. Simko F, Pechanova O. Remodelling of the heart and vessels in experimental hypertension: advances in protection. LWW. (2010) 28 (Suppl. 1):S1–6. doi: 10.1097/01.hjh.0000388487.43460.db
302. Simko F, Reiter RJ, Pechanova O, Paulis L. Experimental models of melatonin-deficient hypertension. Front Biosci. (2013) 18:616–25. doi: 10.2741/4125
303. He B, Zhao Y, Xu L, Gao L, Su Y, Lin N, et al. The nuclear melatonin receptor RORα is a novel endogenous defender against myocardial ischemia/reperfusion injury. J Pineal Res. (2016) 60:313–26. doi: 10.1111/jpi.12312
304. Nduhirabandi F, Lamont K, Albertyn Z, Opie LH, Lecour S. Role of toll-like receptor 4 in melatonin-induced cardioprotection. J Pineal Res. (2016) 60:39–47. doi: 10.1111/jpi.12286
305. Simko F, Baka T, Paulis L, Reiter RJ. Elevated heart rate and nondipping heart rate as potential targets for melatonin: a review. J Pineal Res. (2016) 61:127–37. doi: 10.1111/jpi.12348
306. Simko F, Pechanova O. Recent trends in hypertension treatment: perspectives from animal studies. LWW. (2009) 27:S1–4. doi: 10.1097/01.hjh.0000358829.87815.d4
307. Simko F, Baka T, Krajcirovicova K, Repova K, Aziriova S, Zorad S, et al. Effect of melatonin on the renin-angiotensin-aldosterone system in l-NAME-induced hypertension. Molecules. (2018) 23:265. doi: 10.3390/molecules23020265
308. Hermida RC, Ayala DE, Smolensky MH, Fernández JR, Mojón A, Portaluppi F. Chronotherapy with conventional blood pressure medications improves management of hypertension and reduces cardiovascular and stroke risks. Hypertens Res. (2016) 39:277–92. doi: 10.1038/hr.2015.142
309. Hermida RC, Ayala DE, Calvo C, López JE, Mojón A, Fontao MJ, et al. Effects of time of day of treatment on ambulatory blood pressure pattern of patients with resistant hypertension. Hypertension. (2005) 46:1053–9. doi: 10.1161/01.HYP.0000172757.96281.bf
310. Dominguez-Rodriguez A. Melatonin in cardiovascular disease. Expert Opin Investig Drugs. (2012) 21:1593–6. doi: 10.1517/13543784.2012.716037
311. Dominguez-Rodriguez A, Abreu-Gonzalez P, Sanchez-Sanchez JJ, Kaski JC, Reiter RJ. Melatonin and circadian biology in human cardiovascular disease. J Pineal Res. (2010) 49:14–22. doi: 10.1111/j.1600-079X.2010.00773.x
312. Cheng MC, Wu TH, Huang LT, Tain YL. Renoprotective effects of melatonin in young spontaneously hypertensive rats with L-NAME. Pediatr Neonatol. (2014) 55:189–95. doi: 10.1016/j.pedneo.2013.09.005
313. Hung MW, Yeung HM, Lau CF, Poon AMS, Tipoe GL, Fung ML. Melatonin attenuates pulmonary hypertension in chronically hypoxic rats. Int J Mol Sci. (2017) 18:1125. doi: 10.3390/ijms18061125
314. Klimentova J, Cebova M, Barta A, Matuskova Z, Vrankova S, Rehakova R, et al. Effect of melatonin on blood pressure and nitric oxide generation in rats with metabolic syndrome. Physiol Res. (2016) 65 (Suppl 3):S373–80. doi: 10.33549/physiolres.933436
315. Paulis L, Pechanova O, Zicha J, Liskova S, Celec P, Mullerova M, et al. Melatonin improves the restoration of endothelium-derived constricting factor signalling and inner diameter in the rat femoral artery after cessation of L-NAME treatment. J Hypertens. (2010) 28 (Suppl. 1):S19–24. doi: 10.1097/01.hjh.0000388490.28213.de
316. Su H, Li J, Chen T, Li N, Xiao J, Wang S, et al. Melatonin attenuates angiotensin II-induced cardiomyocyte hypertrophy through the CyPA/CD147 signaling pathway. Mol Cell Biochem. (2016) 422:85–95. doi: 10.1007/s11010-016-2808-9
317. Tao J, Lv J, Li W, Zhang P, Mao C, Xu Z. Exogenous melatonin reduced blood pressure in late-term ovine fetus via MT1/MT2 receptor pathways. Reprod Biol. (2016) 16:212–7. doi: 10.1016/j.repbio.2016.06.001
318. Yan FX, Li HM, Li SX, He SH, Dai WP, Li Y, et al. The oxidized phospholipid POVPC impairs endothelial function and vasodilation via uncoupling endothelial nitric oxide synthase. J Mol Cell Cardiol. (2017) 112:40–8. doi: 10.1016/j.yjmcc.2017.08.016
319. Cerezo AB, Hornedo-Ortega R, Álvarez-Fernández MA, Troncoso AM, García-Parrilla MC. Inhibition of VEGF-induced VEGFR-2 activation and HUVEC migration by melatonin and other bioactive indolic compounds. Nutrients. (2017) 9:249. doi: 10.3390/nu9030249
320. Shao G, Zhang S, Nie J, Li J, Tong J. Effects of melatonin on mechanisms involved in hypertension using human umbilical vein endothelial cells. J Toxicol Environ Health A. (2017) 80:1342–8. doi: 10.1080/15287394.2017.1384171
321. Korkmaz A, Rosales-Corral S, Reiter RJ. Gene regulation by melatonin linked to epigenetic phenomena. Gene. (2012) 503:1–11. doi: 10.1016/j.gene.2012.04.040
322. Tain YL, Chen CC, Sheen JM, Yu HR, Tiao MM, Kuo HC, et al. Melatonin attenuates prenatal dexamethasone-induced blood pressure increase in a rat model. J Am Soc Hypertens. (2014) 8:216–26. doi: 10.1016/j.jash.2014.01.009
323. Tain YL, Huang LT, Hsu CN, Lee CT. Melatonin therapy prevents programmed hypertension and nitric oxide deficiency in offspring exposed to maternal caloric restriction. Oxid Med Cell Longev. (2014) 2014:283180. doi: 10.1155/2014/283180
324. Chen YC, Sheen JM, Tiao MM, Tain YL, Huang LT. Roles of melatonin in fetal programming in compromised pregnancies. Int J Mol Sci. (2013) 14:5380–401. doi: 10.3390/ijms14035380
325. Ojeda NB, Grigore D, Alexander BT. Developmental programming of hypertension: insight from animal models of nutritional manipulation. Hypertension. (2008) 52:44–50. doi: 10.1161/HYPERTENSIONAHA.107.092890
326. Tain YL, Leu S, Wu KL, Lee WC, Chan JY. Melatonin prevents maternal fructose intake-induced programmed hypertension in the offspring: roles of nitric oxide and arachidonic acid metabolites. J Pineal Res. (2014) 57:80–9. doi: 10.1111/jpi.12145
327. Tain YL, Huang LT. Asymmetric dimethylarginine: clinical applications in pediatric medicine. J Formos Med Assoc. (2011) 110:70–7. doi: 10.1016/S0929-6646(11)60012-0
328. Tain YL, Hsieh CS, Lin IC, Chen CC, Sheen JM, Huang LT. Effects of maternal L-citrulline supplementation on renal function and blood pressure in offspring exposed to maternal caloric restriction: the impact of nitric oxide pathway. Nitric Oxide. (2010) 23:34–41. doi: 10.1016/j.niox.2010.03.005
329. Tain YL, Lee WC, Hsu CN, Lee WC, Huang LT, Lee CT, et al. Asymmetric dimethylarginine is associated with developmental programming of adult kidney disease and hypertension in offspring of streptozotocin-treated mothers. PLoS ONE. (2013) 8:e55420. doi: 10.1371/journal.pone.0055420
330. Bogdarina I, Welham S, King PJ, Burns SP, Clark AJ. Epigenetic modification of the renin-angiotensin system in the fetal programming of hypertension. Circ Res. (2007) 100:520–6. doi: 10.1161/01.RES.0000258855.60637.58
331. Chen S, Bellew C, Yao X, Stefkova J, Dipp S, Saifudeen Z, et al. Histone deacetylase (HDAC) activity is critical for embryonic kidney gene expression, growth, and differentiation. J Biol Chem. (2011) 286:32775–89. doi: 10.1074/jbc.M111.248278
332. Tain YL, Huang LT, Lin IC, Lau YT, Lin CY. Melatonin prevents hypertension and increased asymmetric dimethylarginine in young spontaneous hypertensive rats. J Pineal Res. (2010) 49:390–8. doi: 10.1111/j.1600-079X.2010.00806.x
333. Erşahin M, Sehirli O, Toklu HZ, Süleymanoglu S, Emekli-Alturfan E, Yarat A, et al. Melatonin improves cardiovascular function and ameliorates renal, cardiac and cerebral damage in rats with renovascular hypertension. J Pineal Res. (2009) 47:97–106. doi: 10.1111/j.1600-079X.2009.00693.x
334. Sharma R, Ottenhof T, Rzeczkowska PA, Niles LP. Epigenetic targets for melatonin: induction of histone H3 hyperacetylation and gene expression in C17.2 neural stem cells. J Pineal Res. (2008) 45:277–84. doi: 10.1111/j.1600-079X.2008.00587.x
335. Jung-Hynes B, Reiter RJ, Ahmad N. Sirtuins, melatonin and circadian rhythms: building a bridge between aging and cancer. J Pineal Res. (2010) 48:9–19. doi: 10.1111/j.1600-079X.2009.00729.x
336. Nagai R, Watanabe K, Wakatsuki A, Hamada F, Shinohara K, Hayashi Y, et al. Melatonin preserves fetal growth in rats by protecting against ischemia/reperfusion-induced oxidative/nitrosative mitochondrial damage in the placenta. J Pineal Res. (2008) 45:271–6. doi: 10.1111/j.1600-079X.2008.00586.x
337. Wilcox CS. Oxidative stress and nitric oxide deficiency in the kidney: a critical link to hypertension? Am J Physiol Regul Integrat Compar Physiol. (2005) 289:R913–35. doi: 10.1152/ajpregu.00250.2005
338. Baylis C. Arginine, arginine analogs and nitric oxide production in chronic kidney disease. Nat Clin Pract Nephrol. (2006) 2:209–20. doi: 10.1038/ncpneph0143
339. Ono H, Ono Y, Frohlich ED. Nitric oxide synthase inhibition in spontaneously hypertensive rats: systemic, renal, and glomerular hemodynamics. Hypertension. (1995) 26:249–55. doi: 10.1161/01.HYP.26.2.249
340. Francischetti A, Ono H, Frohlich ED. Renoprotective effects of felodipine and/or enalapril in spontaneously hypertensive rats with and without L-NAME. Hypertension. (1998) 31:795–801. doi: 10.1161/01.HYP.31.3.795
341. Hsu C-N, Huang L-T, Lau Y-T, Lin C-Y, Tain Y-L. The combined ratios of L-arginine and asymmetric and symmetric dimethylarginine as biomarkers in spontaneously hypertensive rats. Transl Res. (2012) 159:90–8. doi: 10.1016/j.trsl.2011.09.002
342. Sun Y, Wang C, Zhang N, Liu F. Melatonin ameliorates hypertension in hypertensive pregnant mice and suppresses the hypertension-induced decrease in Ca(2+)-activated K(+) channels in uterine arteries. Hypertens Res. (2021) 44:1079–86. doi: 10.1038/s41440-021-00675-5
343. Bazyar H, Zare Javid A, Bavi Behbahani H, Moradi F, Moradi Poode B, Amiri P. Consumption of melatonin supplement improves cardiovascular disease risk factors and anthropometric indices in type 2 diabetes mellitus patients: a double-blind, randomized, placebo-controlled trial. Trials. (2021) 22:231. doi: 10.1186/s13063-021-05174-z
344. Zuo J, Jiang Z. Melatonin attenuates hypertension and oxidative stress in a rat model of L-NAME-induced gestational hypertension. Vasc Med. (2020) 25:295–301. doi: 10.1177/1358863X20919798
345. Bahrami M, Cheraghpour M, Jafarirad S, Alavinejad P, Asadi F, Hekmatdoost A, et al. The effect of melatonin on treatment of patients with non-alcoholic fatty liver disease: a randomized double blind clinical trial. Complement Ther Med. (2020) 52:102452. doi: 10.1016/j.ctim.2020.102452
346. Nishi EE, Almeida VR, Amaral FG, Simon KA, Futuro-Neto HA, Pontes RB, et al. Melatonin attenuates renal sympathetic overactivity and reactive oxygen species in the brain in neurogenic hypertension. Hypertens Res. (2019) 42:1683–91. doi: 10.1038/s41440-019-0301-z
347. Rahbari-Oskoui FF, Abramson JL, Bruckman AM, Chapman AB, Cotsonis GA, Johnson SA, et al. Nighttime administration of high-dose, sustained-release melatonin does not decrease nocturnal blood pressure in African-American patients: results from a preliminary randomized, crossover trial. Complement Ther Med. (2019) 43:157–64. doi: 10.1016/j.ctim.2019.01.026
348. Raygan F, Ostadmohammadi V, Bahmani F, Reiter RJ, Asemi Z. Melatonin administration lowers biomarkers of oxidative stress and cardio-metabolic risk in type 2 diabetic patients with coronary heart disease: a randomized, double-blind, placebo-controlled trial. Clin Nutr. (2019) 38:191–6. doi: 10.1016/j.clnu.2017.12.004
349. Uzun M, Gencer M, Turkon H, Oztopuz RO, Demir U, Ovali MA. Effects of melatonin on blood pressure, oxidative stress and placental expressions of TNFα, IL-6, VEGF and sFlt-1 in RUPP rat model of preeclampsia. Arch Med Res. (2017) 48:592–8. doi: 10.1016/j.arcmed.2017.08.007
350. Simko F, Pechanova O, Repova K, Aziriova S, Krajcirovicova K, Celec P, et al. Lactacystin-Induced model of hypertension in rats: effects of melatonin and captopril. Int J Mol Sci. (2017) 18:1612. doi: 10.3390/ijms18081612
351. Ovali MA, Uzun M. The effects of melatonin administration on KCNQ and KCNH2 gene expressions and QTc interval in pinealectomised rats. Cell Mol Biol. (2017) 63:45–50. doi: 10.14715/cmb/2017.63.3.9
352. Gupta P, Jethava D, Choudhary R, Jethava DD. Role of melatonin in attenuation of haemodynamic responses to laryngoscopy and intubation. Indian J Anaesth. (2016) 60:712–8. doi: 10.4103/0019-5049.191667
353. Ewida SF, Al-Sharaky DR. Implication of renal aquaporin-3 in fructose-induced metabolic syndrome and melatonin protection. J Clin Diagn Res. (2016) 10:Cf06–11. doi: 10.7860/JCDR/2016/18362.7656
354. Gubin DG, Gubin GD, Gapon LI, Weinert D. Daily melatonin administration attenuates age-dependent disturbances of cardiovascular rhythms. Curr Aging Sci. (2016) 9:5–13. doi: 10.2174/1874609809666151130220011
355. Mozdzan M, Mozdzan M, Chałubiński M, Wojdan K, Broncel M. The effect of melatonin on circadian blood pressure in patients with type 2 diabetes and essential hypertension. Arch Med Sci. (2014) 10:669–75. doi: 10.5114/aoms.2014.44858
356. Simko F, Pechanova O, Repova Bednarova K, Krajcirovicova K, Celec P, Kamodyova N, et al. Hypertension and cardiovascular remodelling in rats exposed to continuous light: protection by ACE-inhibition and melatonin. Mediators Inflamm. (2014) 2014:703175. doi: 10.1155/2014/703175
357. Bernasconi PA, Cardoso NP, Reynoso R, Scacchi P, Cardinali DP. Melatonin and diet-induced metabolic syndrome in rats: impact on the hypophysial-testicular axis. Horm Mol Biol Clin Investig. (2013) 16:101–12. doi: 10.1515/hmbci-2013-0005
358. Huang L, Zhang C, Hou Y, Laudon M, She M, Yang S, et al. Blood pressure reducing effects of piromelatine and melatonin in spontaneously hypertensive rats. Eur Rev Med Pharmacol Sci. (2013) 17:2449–56.
359. Hrenák J, Arendášová K, Rajkovičová R, Aziriová S, Repová K, Krajčírovičová K, et al. Protective effect of captopril, olmesartan, melatonin and compound 21 on doxorubicin-induced nephrotoxicity in rats. Physiol Res. (2013) 62 (Suppl. 1):S181–9. doi: 10.33549/physiolres.932614
360. Lee SK, Sirajudeen KN, Sundaram A, Zakaria R, Singh HJ. Effects of antenatal, postpartum and post-weaning melatonin supplementation on blood pressure and renal antioxidant enzyme activities in spontaneously hypertensive rats. J Physiol Biochem. (2011) 67:249–57. doi: 10.1007/s13105-010-0070-2
361. Koziróg M, Poliwczak AR, Duchnowicz P, Koter-Michalak M, Sikora J, Broncel M. Melatonin treatment improves blood pressure, lipid profile, and parameters of oxidative stress in patients with metabolic syndrome. J Pineal Res. (2011) 50:261–6. doi: 10.1111/j.1600-079X.2010.00835.x
362. Paulis L, Pechanova O, Zicha J, Barta A, Gardlik R, Celec P, et al. Melatonin interactions with blood pressure and vascular function during L-NAME-induced hypertension. J Pineal Res. (2010) 48:102–8. doi: 10.1111/j.1600-079X.2009.00732.x
363. Rechciński T, Trzos E, Wierzbowska-Drabik K, Krzemińska-Pakuła M, Kurpesa M. Melatonin for nondippers with coronary artery disease: assessment of blood pressure profile and heart rate variability. Hypertens Res. (2010) 33:56–61. doi: 10.1038/hr.2009.174
364. Simko F, Pechanova O, Pelouch V, Krajcirovicova K, Mullerova M, Bednarova K, et al. Effect of melatonin, captopril, spironolactone and simvastatin on blood pressure and left ventricular remodelling in spontaneously hypertensive rats. J Hypertens. (2009) 27:S5–10. doi: 10.1097/01.hjh.0000358830.95439.e8
365. Li HL, Kang YM, Yu L, Xu HY, Zhao H. Melatonin reduces blood pressure in rats with stress-induced hypertension via GABAA receptors. Clin Exp Pharmacol Physiol. (2009) 36:436–40. doi: 10.1111/j.1440-1681.2008.05080.x
366. Xia CM, Shao CH, Xin L, Wang YR, Ding CN, Wang J, et al. Effects of melatonin on blood pressure in stress-induced hypertension in rats. Clin Exp Pharmacol Physiol. (2008) 35:1258–64. doi: 10.1111/j.1440-1681.2008.05000.x
367. Grossman E, Laudon M, Yalcin R, Zengil H, Peleg E, Sharabi Y, et al. Melatonin reduces night blood pressure in patients with nocturnal hypertension. Am J Med. (2006) 119:898–902. doi: 10.1016/j.amjmed.2006.02.002
368. Deniz E, Sahna E, Aksulu HE. Nitric oxide synthase inhibition in rats: melatonin reduces blood pressure and ischemia/reperfusion-induced infarct size. Scand Cardiovasc J. (2006) 40:248–52. doi: 10.1080/14017430600833116
369. Cagnacci A, Cannoletta M, Renzi A, Baldassari F, Arangino S, Volpe A. Prolonged melatonin administration decreases nocturnal blood pressure in women. Am J Hypertens. (2005) 18(12 Pt 1):1614–8. doi: 10.1016/j.amjhyper.2005.05.008
370. Cavallo A, Daniels SR, Dolan LM, Bean JA, Khoury JC. Blood pressure-lowering effect of melatonin in type 1 diabetes. J Pineal Res. (2004) 36:262–6. doi: 10.1111/j.1600-079X.2004.00126.x
371. Cavallo A, Daniels SR, Dolan LM, Khoury JC, Bean JA. Blood pressure response to melatonin in type 1 diabetes. Pediatr Diabetes. (2004) 5:26–31. doi: 10.1111/j.1399-543X.2004.00031.x
372. Ding CN, Cao YX, Zhou L, Zhu DN, Shen ZY, Fei MY, et al. Effects of microinjection of melatonin and its receptor antagonists into anterior hypothalamic area on blood pressure and heart rate in rats. Acta Pharmacol Sin. (2001) 22:997–1002.
373. Mailliet F, Galloux P, Poisson D. Comparative effects of melatonin, zolpidem and diazepam on sleep, body temperature, blood pressure and heart rate measured by radiotelemetry in wistar rats. Psychopharmacology. (2001) 156:417–26. doi: 10.1007/s002130100769
374. Arangino S, Cagnacci A, Angiolucci M, Vacca AM, Longu G, Volpe A, et al. Effects of melatonin on vascular reactivity, catecholamine levels, and blood pressure in healthy men. Am J Cardiol. (1999) 83:1417–9. doi: 10.1016/S0002-9149(99)00112-5
375. Kulczykowska E. Effects of arginine vasotocin, isotocin and melatonin on blood pressure in the conscious atlantic cod (Gadus morhua): hormonal interactions? Exp Physiol. (1998) 83:809–20. doi: 10.1113/expphysiol.1998.sp004161
376. Lusardi P, Preti P, Savino S, Piazza E, Zoppi A, Fogari R. Effect of bedtime melatonin ingestion on blood pressure of normotensive subjects. Blood Press Monit. (1997) 2:99–103.
377. Hu ZP, Fang XL, Fang N, Wang XB, Qian HY, Cao Z, et al. Melatonin ameliorates vascular endothelial dysfunction, inflammation, and atherosclerosis by suppressing the TLR4/NF-κB system in high-fat-fed rabbits. J Pineal Res. (2013) 55:388–98. doi: 10.1111/jpi.12085
378. Kasikara C, Doran AC, Cai B, Tabas I. The role of non-resolving inflammation in atherosclerosis. J Clin Invest. (2018) 128:2713–23. doi: 10.1172/JCI97950
379. Yakala GK, Cabrera-Fuentes HA, Crespo-Avilan GE, Rattanasopa C, Burlacu A, George BL, et al. FURIN inhibition reduces vascular remodeling and atherosclerotic lesion progression in mice. Arterioscler Thromb Vasc Biol. (2019) 39:387–401. doi: 10.1161/ATVBAHA.118.311903
380. Xu Y-J, Zheng L, Hu Y-W, Wang Q. Pyroptosis and its relationship to atherosclerosis. Clinica Chimica Acta. (2018) 476:28–37. doi: 10.1016/j.cca.2017.11.005
381. Shi J, Gao W, Shao F. Pyroptosis: gasdermin-mediated programmed necrotic cell death. Trends Biochem Sci. (2017) 42:245–54. doi: 10.1016/j.tibs.2016.10.004
382. Cui M, Cui R, Liu K, Dong J-Y, Imano H, Hayama-Terada M, et al. Associations of tobacco smoking with impaired endothelial function: the circulatory risk in communities study (CIRCS). J Atheroscler Thromb. (2018) 2018:42150. doi: 10.5551/jat.42150
383. Wu X, Zhang H, Qi W, Zhang Y, Li J, Li Z, et al. Nicotine promotes atherosclerosis via ROS-NLRP3-mediated endothelial cell pyroptosis. Cell Death Dis. (2018) 9:1–12. doi: 10.1038/s41419-017-0257-3
384. Loboda A, Damulewicz M, Pyza E, Jozkowicz A, Dulak J. Role of Nrf2/HO-1 system in development, oxidative stress response and diseases: an evolutionarily conserved mechanism. Cell Mol Life Sci. (2016) 73:3221–47. doi: 10.1007/s00018-016-2223-0
385. Pogu J, Tzima S, Kollias G, Anegon I, Blancou P, Simon T. Genetic restoration of heme oxygenase-1 expression protects from type 1 diabetes in NOD mice. Int J Mol Sci. (2019) 20:1676. doi: 10.3390/ijms20071676
386. Xu G, Zhao X, Fu J, Wang X. Resveratrol increase myocardial Nrf2 expression in type 2 diabetic rats and alleviate myocardial ischemia/reperfusion injury (MIRI). Ann Palliat Med. (2019) 8:565–75. doi: 10.21037/apm.2019.11.25
387. Ling Y, Li Z-Z, Zhang J-F, Zheng X-W, Lei Z-Q, Chen R-Y, et al. MicroRNA-494 inhibition alleviates acute lung injury through Nrf2 signaling pathway via NQO1 in sepsis-associated acute respiratory distress syndrome. Life Sci. (2018) 210:1–8. doi: 10.1016/j.lfs.2018.08.037
388. Deshmukh P, Unni S, Krishnappa G, Padmanabhan B. The Keap1–Nrf2 pathway: promising therapeutic target to counteract ROS-mediated damage in cancers and neurodegenerative diseases. Biophys Rev. (2017) 9:41–56. doi: 10.1007/s12551-016-0244-4
389. Sakurai H, Morishima Y, Ishii Y, Yoshida K, Nakajima M, Tsunoda Y, et al. Sulforaphane ameliorates steroid insensitivity through an Nrf2-dependent pathway in cigarette smoke-exposed asthmatic mice. Free Radic Biol Med. (2018) 129:473–85. doi: 10.1016/j.freeradbiomed.2018.10.400
390. Cui W, Zhang Z, Zhang P, Qu J, Zheng C, Mo X, et al. Nrf2 attenuates inflammatory response in COPD/emphysema: crosstalk with Wnt3a/β-catenin and AMPK pathways. J Cell Mol Med. (2018) 22:3514–25. doi: 10.1111/jcmm.13628
391. Zhao Z, Wang X, Zhang R, Ma B, Niu S, Di X, et al. Melatonin attenuates smoking-induced atherosclerosis by activating the Nrf2 pathway via NLRP3 inflammasomes in endothelial cells. Aging. (2021) 13:11363–80. doi: 10.18632/aging.202829
392. Park I, Kassiteridi C, Monaco C. Functional diversity of macrophages in vascular biology and disease. Vascul Pharmacol. (2017) 99:13–22. doi: 10.1016/j.vph.2017.10.005
393. Ding S, Lin N, Sheng X, Zhao Y, Su Y, Xu L, et al. Melatonin stabilizes rupture-prone vulnerable plaques via regulating macrophage polarization in a nuclear circadian receptor RORα-dependent manner. J Pineal Res. (2019) 67:e12581. doi: 10.1111/jpi.12581
394. Li H, Li J, Jiang X, Liu S, Liu Y, Chen W, et al. Melatonin enhances atherosclerotic plaque stability by inducing prolyl-4-hydroxylase α1 expression. J Hypertens. (2019) 37:964–71. doi: 10.1097/HJH.0000000000001979
395. Pita ML, Hoyos M, Martin-Lacave I, Osuna C, Fernández-Santos JM, Guerrero JM. Long-term melatonin administration increases polyunsaturated fatty acid percentage in plasma lipids of hypercholesterolemic rats. J Pineal Res. (2002) 32:179–86. doi: 10.1034/j.1600-079x.2002.1o851.x
396. Li H-Y, Leu Y-L, Wu Y-C, Wang S-H. Melatonin inhibits in vitro smooth muscle cell inflammation and proliferation and atherosclerosis in apolipoprotein E-deficient mice. J Agric Food Chem. (2019) 67:1889–901. doi: 10.1021/acs.jafc.8b06217
397. Gallo S, Sala V, Gatti S, Crepaldi T. Cellular and molecular mechanisms of HGF/Met in the cardiovascular system. Clin Sci. (2015) 129:1173–93. doi: 10.1042/CS20150502
398. Matsumoto K, Morishita R, Tomita N, Moriguchi A, Komai N, Aoki M, et al. Improvement of endothelial dysfunction by angiotensin II blockade accompanied by induction of vascular hepatocyte growth factor system in diabetic spontaneously hypertensive rats. Heart Vessels. (2003) 18:18–25. doi: 10.1007/s003800300003
399. Matsumoto K, Morishita R, Moriguchi A, Tomita N, Aoki M, Sakonjo H, et al. Inhibition of neointima by angiotensin-converting enzyme inhibitor in porcine coronary artery balloon-injury model. Hypertension. (2001) 37:270–4. doi: 10.1161/01.HYP.37.2.270
400. Taniyama Y, Morishita R, Nakagami H, Moriguchi A, Sakonjo H, Shokei K, et al. Potential contribution of a novel antifibrotic factor, hepatocyte growth factor, to prevention of myocardial fibrosis by angiotensin II blockade in cardiomyopathic hamsters. Circulation. (2000) 102:246–52. doi: 10.1161/01.CIR.102.2.246
401. Hu ZP, Fang XL, Qian HY, Fang N, Wang BN, Wang Y. Telmisartan prevents angiotensin II-induced endothelial dysfunction in rabbit aorta via activating HGF/Met system and PPARγ pathway. Fundam Clin Pharmacol. (2014) 28:501–11. doi: 10.1111/fcp.12057
402. Nakagami H, Osako MK, Takami Y, Hanayama R, Koriyama H, Mori M, et al. Differential response of vascular hepatocyte growth factor concentration and lipid accumulation between telmisartan and losartan in ApoE-deficient mice. Mol Med Rep. (2008) 1:657–61. doi: 10.3892/mmr_00000008
403. Morishita R, Nakamura S, Hayashi S, Taniyama Y, Moriguchi A, Nagano T, et al. Therapeutic angiogenesis induced by human recombinant hepatocyte growth factor in rabbit hind limb ischemia model as cytokine supplement therapy. Hypertension. (1999) 33:1379–84. doi: 10.1161/01.HYP.33.6.1379
404. Li Y, Wen X, Spataro BC, Hu K, Dai C, Liu Y. hepatocyte growth factor is a downstream effector that mediates the antifibrotic action of peroxisome proliferator-activated receptor-gamma agonists. J Am Soc Nephrol. (2006) 17:54–65. doi: 10.1681/ASN.2005030257
405. Sanada F, Taniyama Y, Iekushi K, Azuma J, Okayama K, Kusunoki H, et al. Negative action of hepatocyte growth factor/c-Met system on angiotensin II signaling via ligand-dependent epithelial growth factor receptor degradation mechanism in vascular smooth muscle cells. Circ Res. (2009) 105:667–75:13 p following 75. doi: 10.1161/CIRCRESAHA.109.202713
406. Hu ZP, Fang XL, Sheng B, Guo Y, Yu YQ. Melatonin inhibits macrophage infiltration and promotes plaque stabilization by upregulating anti-inflammatory HGF/c-Met system in the atherosclerotic rabbit: USPIO-enhanced MRI assessment. Vasc Pharmacol. (2020) 127:106659. doi: 10.1016/j.vph.2020.106659
407. Annunen P, Autio-Harmainen H, Kivirikko KI. The novel type II prolyl 4-hydroxylase is the main enzyme form in chondrocytes and capillary endothelial cells, whereas the type I enzyme predominates in most cells. J Biol Chem. (1998) 273:5989–92. doi: 10.1074/jbc.273.11.5989
408. Cook DN, Kang HS, Jetten AM. Retinoic acid-related orphan receptors (RORs): regulatory functions in immunity, development, circadian rhythm, and metabolism. Nucl Receptor Res. (2015) 2:101185. doi: 10.11131/2015/101185
409. Sato TK, Panda S, Miraglia LJ, Reyes TM, Rudic RD, McNamara P, et al. A functional genomics strategy reveals rora as a component of the mammalian circadian clock. Neuron. (2004) 43:527–37. doi: 10.1016/j.neuron.2004.07.018
410. Peliciari-Garcia RA, Zanquetta MM, Andrade-Silva J, Gomes DA, Barreto-Chaves ML, Cipolla-Neto J. Expression of circadian clock and melatonin receptors within cultured rat cardiomyocytes. Chronobiol Int. (2011) 28:21–30. doi: 10.3109/07420528.2010.525675
411. Carlberg C, Wiesenberg I. The orphan receptor family RZR/ROR, melatonin and 5-lipoxygenase: an unexpected relationship. J Pineal Res. (1995) 18:171–8. doi: 10.1111/j.1600-079X.1995.tb00157.x
412. Shajari S, Laliena A, Heegsma J, Tuñón MJ, Moshage H, Faber KN. Melatonin suppresses activation of hepatic stellate cells through RORα-mediated inhibition of 5-lipoxygenase. J Pineal Res. (2015) 59:391–401. doi: 10.1111/jpi.12271
413. Mamontova A, Séguret-Macé S, Esposito B, Chaniale C, Bouly M, Delhaye-Bouchaud N, et al. Severe atherosclerosis and hypoalphalipoproteinemia in the staggerer mouse, a mutant of the nuclear receptor RORalpha. Circulation. (1998) 98:2738–43. doi: 10.1161/01.CIR.98.24.2738
414. Kivirikko KI, Pihlajaniemi T. Collagen hydroxylases and the protein disulfide isomerase subunit of prolyl 4-hydroxylases. Adv Enzymol Relat Areas Mol Biol. (1998) 72:325–98. doi: 10.1002/9780470123188.ch9
415. Li L, Cai XJ, Feng M, Rong YY, Zhang Y, Zhang M. Effect of adiponectin overexpression on stability of preexisting plaques by inducing prolyl-4-hydroxylase expression. Circ J. (2010) 74:552–9. doi: 10.1253/circj.CJ-09-0304
416. Sezgin D, Aslan G, Sahin K, Tuzcu M, Ilhan N, Sahna E. The effects of melatonin against atherosclerosis-induced endothelial dysfunction and inflammation in hypercholesterolemic rats. Arch Physiol Biochem. (2020) 2020:1838550. doi: 10.1080/13813455.2020.1838550
417. Ma S, Chen J, Feng J, Zhang R, Fan M, Han D, et al. Melatonin ameliorates the progression of atherosclerosis via mitophagy activation and NLRP3 inflammasome inhibition. Oxid Med Cell Longev. (2018) 2018:9286458. doi: 10.1155/2018/9286458
418. Cheng X, Wan Y, Xu Y, Zhou Q, Wang Y, Zhu H. Melatonin alleviates myosin light chain kinase expression and activity via the mitogen-activated protein kinase pathway during atherosclerosis in rabbits. Mol Med Rep. (2015) 11:99–104. doi: 10.3892/mmr.2014.2753
419. Tailleux A, Gozzo A, Torpier G, Martin-Nizard F, Bonnefont-Rousselot D, Lemdani M, et al. Increased susceptibility of low-density lipoprotein to ex vivo oxidation in mice transgenic for human apolipoprotein B treated with 1 melatonin-related compound is not associated with atherosclerosis progression. J Cardiovasc Pharmacol. (2005) 46:241–9. doi: 10.1097/01.fjc.0000175232.11079.7e
420. Tailleux A, Torpier G, Bonnefont-Rousselot D, Lestavel S, Lemdani M, Caudeville B, et al. Daily melatonin supplementation in mice increases atherosclerosis in proximal aorta. Biochem Biophys Res Commun. (2002) 293:1114–23. doi: 10.1016/S0006-291X(02)00336-4
421. Steinberg C, Laksman ZW, Krahn AD. Sudden cardiac death: a reappraisal. Trends Cardiovasc Med. (2016) 26:709–19. doi: 10.1016/j.tcm.2016.05.006
422. Akar FG, O'Rourke B. Mitochondria are sources of metabolic sink and arrhythmias. Pharmacol Ther. (2011) 131:287–94. doi: 10.1016/j.pharmthera.2011.04.005
423. Solhjoo S, O'Rourke B. Mitochondrial instability during regional ischemia–reperfusion underlies arrhythmias in monolayers of cardiomyocytes. J Mol Cell Cardiol. (2015) 78:90–9. doi: 10.1016/j.yjmcc.2014.09.024
424. Brown DA, Aon MA, Frasier CR, Sloan RC, Maloney AH, Anderson EJ, et al. Cardiac arrhythmias induced by glutathione oxidation can be inhibited by preventing mitochondrial depolarization. J Mol Cell Cardiol. (2010) 48:673–9. doi: 10.1016/j.yjmcc.2009.11.011
425. Liu M, Liu H, Dudley SC Jr. Reactive oxygen species originating from mitochondria regulate the cardiac sodium channel. Circ Res. (2010) 107:967–74. doi: 10.1161/CIRCRESAHA.110.220673
426. Benova T, Viczenczova C, Radosinska J, Bacova B, Knezl V, Dosenko V, et al. Melatonin attenuates hypertension-related proarrhythmic myocardial maladaptation of connexin-43 and propensity of the heart to lethal arrhythmias. Can J Physiol Pharmacol. (2013) 91:633–9. doi: 10.1139/cjpp-2012-0393
427. Sedova KA, Bernikova OG, Cuprova JI, Ivanova AD, Kutaeva GA, Pliss MG, et al. Association between antiarrhythmic, electrophysiological, and antioxidative effects of melatonin in ischemia/reperfusion. Int J Mol Sci. (2019) 20:6331. doi: 10.3390/ijms20246331
428. Diez ER, Renna NF, Prado NJ, Lembo C, Ponce Zumino AZ, Vazquez-Prieto M, et al. Melatonin, given at the time of reperfusion, prevents ventricular arrhythmias in isolated hearts from fructose-fed rats and spontaneously hypertensive rats. J Pineal Res. (2013) 55:166–73. doi: 10.1111/jpi.12059
429. Sharkey JT, Puttaramu R, Word RA, Olcese J. Melatonin synergizes with oxytocin to enhance contractility of human myometrial smooth muscle cells. J Clin Endocrinol Metab. (2009) 94:421–7. doi: 10.1210/jc.2008-1723
430. Egan Benova T, Szeiffova Bacova B, Viczenczova C, Diez E, Barancik M, Tribulova N. Protection of cardiac cell-to-cell coupling attenuate myocardial remodeling and proarrhythmia induced by hypertension. Physiol Res. (2016) 65 (Suppl. 1):S29–42. doi: 10.33549/physiolres.933391
431. Prado NJ, Egan Benová T, Diez ER, Knezl V, Lipták B, Ponce Zumino AZ, et al. Melatonin receptor activation protects against low potassium-induced ventricular fibrillation by preserving action potentials and connexin-43 topology in isolated rat hearts. J Pineal Res. (2019) 67:e12605. doi: 10.1111/jpi.12605
432. Vazan R, Pancza D, Béder I, Styk J. Ischemia-reperfusion injury–antiarrhythmic effect of melatonin associated with reduced recovering of contractility. Gen Physiol Biophys. (2005) 24:355–9.
433. Sahna E, Olmez E, Acet A. Effects of physiological and pharmacological concentrations of melatonin on ischemia-reperfusion arrhythmias in rats: can the incidence of sudden cardiac death be reduced? J Pineal Res. (2002) 32:194–8. doi: 10.1034/j.1600-079x.2002.1o853.x
434. Tan DX, Manchester LC, Reiter RJ, Qi W, Kim SJ, El-Sokkary GH. Ischemia/reperfusion-induced arrhythmias in the isolated rat heart: prevention by melatonin. J Pineal Res. (1998) 25:184–91. doi: 10.1111/j.1600-079X.1998.tb00558.x
435. Nduhirabandi F, Maarman GJ. Melatonin in heart failure: a promising therapeutic strategy? Molecules. (2018) 23:1819. doi: 10.3390/molecules23071819
436. Sehirli AÖ, Koyun D, Tetik S, Özsavci D, Yiginer Ö, Çetinel S, et al. Melatonin protects against ischemic heart failure in rats. J Pineal Res. (2013) 55:138–48. doi: 10.1111/jpi.12054
437. Zannad F. Rising incidence of heart failure demands action. Lancet. (2018) 391:518–9. doi: 10.1016/S0140-6736(17)32873-8
438. Mukherjee D, Roy SG, Bandyopadhyay A, Chattopadhyay A, Basu A, Mitra E, et al. Melatonin protects against isoproterenol-induced myocardial injury in the rat: antioxidative mechanisms. J Pineal Res. (2010) 48:251–62. doi: 10.1111/j.1600-079X.2010.00749.x
439. Patel V, Upaganlawar A, Zalawadia R, Balaraman R. Cardioprotective effect of melatonin against isoproterenol induced myocardial infarction in rats: a biochemical, electrocardiographic and histoarchitectural evaluation. Eur J Pharmacol. (2010) 644:160–8. doi: 10.1016/j.ejphar.2010.06.065
440. Garakyaraghi M, Siavash M, Alizadeh MK. Effects of melatonin on left ventricular ejection fraction and functional class of heart failure patients: a randomized, double-blind, placebo-controlled trial. J Res Med Sci. (2012) 17.
441. Kawai Y, Anno K. Mucopolysaccharide-degrading enzymes from the liver of the squid, Ommastrephes sloani pacificus I. hyaluronidase. Biochim Biophys Acta. (1971) 242:428–36. doi: 10.1016/0005-2744(71)90234-8
442. Ozkalayci F, Kocabas U, Altun BU, Pandi-Perumal S, Altun A. Relationship between melatonin and cardiovascular disease. Cureus. (2021) 13:e12935. doi: 10.7759/cureus.12935
443. Simko F, Bednarova KR, Krajcirovicova K, Hrenak J, Celec P, Kamodyova N, et al. Melatonin reduces cardiac remodeling and improves survival in rats with isoproterenol-induced heart failure. J Pineal Res. (2014) 57:177–84. doi: 10.1111/jpi.12154
444. Sadeghi M, Khosrawi S, Heshmat-Ghahdarijani K, Gheisari Y, Roohafza H, Mansoorian M, et al. Effect of melatonin on heart failure: design for a double-blinded randomized clinical trial. ESC Heart Fail. (2020) 7:3142–50. doi: 10.1002/ehf2.12829
445. IuV L, Salikova S, Stadnikov A. Extracellular matrix remodeling myocardium of the left ventricle rats with experimental heart failure after perindopril and melatonin administration. Kardiologiia. (2014) 54:52–6. doi: 10.18565/cardio.2014.9.52-56
446. Dzida G, Prystupa A, Lachowska-Kotowska P, Kardas T, Kamienski P, Kimak E, et al. Alteration in diurnal and nocturnal melatonin serum level in patients with chronic heart failure. Ann Agric Environ Med. (2013) 20.
447. Dominguez-Rodriguez A, Abreu-Gonzalez P, Piccolo R, Galasso G, Reiter RJ. Melatonin is associated with reverse remodeling after cardiac resynchronization therapy in patients with heart failure and ventricular dyssynchrony. Int J Cardiol. (2016) 221:359–63. doi: 10.1016/j.ijcard.2016.07.056
448. Girotti L, Lago M, Ianovsky O, Elizari MV, Dini A, Lloret SP, et al. Low urinary 6-sulfatoxymelatonin levels in patients with severe congestive heart failure. Endocrine. (2003) 22:245–8. doi: 10.1385/ENDO:22:3:245
449. Jin Q, Li R, Hu N, Xin T, Zhu P, Hu S, et al. DUSP1 alleviates cardiac ischemia/reperfusion injury by suppressing the Mff-required mitochondrial fission and Bnip3-related mitophagy via the JNK pathways. Redox Biol. (2018) 14:576–87. doi: 10.1016/j.redox.2017.11.004
450. Wang A, Zhang Y, Cao P. Inhibition of BAP31 expression inhibits cervical cancer progression by suppressing metastasis and inducing intrinsic and extrinsic apoptosis. Biochem Biophys Res Commun. (2019) 508:499–506. doi: 10.1016/j.bbrc.2018.11.017
451. Namba T. BAP31 regulates mitochondrial function via interaction with Tom40 within ER-mitochondria contact sites. Sci Adv. (2019) 5:eaaw1386. doi: 10.1126/sciadv.aaw1386
452. Namba T, Tian F, Chu K, Hwang SY, Yoon KW, Byun S, et al. CDIP1-BAP31 complex transduces apoptotic signals from endoplasmic reticulum to mitochondria under endoplasmic reticulum stress. Cell Rep. (2013) 5:331–9. doi: 10.1016/j.celrep.2013.09.020
453. Han CK, Tien YC, Jine-Yuan Hsieh D, Ho TJ, Lai CH, Yeh YL, et al. Attenuation of the LPS-induced, ERK-mediated upregulation of fibrosis-related factors FGF-2, uPA, MMP-2, and MMP-9 by Carthamus tinctorius L in cardiomyoblasts. Environ Toxicol. (2017) 32:754–63. doi: 10.1002/tox.22275
454. Yu X, Jia B, Wang F, Lv X, Peng X, Wang Y, et al. α1 adrenoceptor activation by norepinephrine inhibits LPS-induced cardiomyocyte TNF-α production via modulating ERK1/2 and NF-κB pathway. J Cell Mol Med. (2014) 18:263–73. doi: 10.1111/jcmm.12184
455. Niu K, Xu J, Cao Y, Hou Y, Shan M, Wang Y, et al. BAP31 is involved in T cell activation through TCR signal pathways. Sci Rep. (2017) 7:44809. doi: 10.1038/srep44809
456. Zhang J, Wang L, Xie W, Hu S, Zhou H, Zhu P, et al. Melatonin attenuates ER stress and mitochondrial damage in septic cardiomyopathy: a new mechanism involving BAP31 upregulation and MAPK-ERK pathway. J Cell Physiol. (2020) 235:2847–56. doi: 10.1002/jcp.29190
457. Tan Y, Ouyang H, Xiao X, Zhong J, Dong M. Irisin ameliorates septic cardiomyopathy via inhibiting DRP1-related mitochondrial fission and normalizing the JNK-LATS2 signaling pathway. Cell Stress Chaperones. (2019) 24:595–608. doi: 10.1007/s12192-019-00992-2
458. Zhong J, Tan Y, Lu J, Liu J, Xiao X, Zhu P, et al. Therapeutic contribution of melatonin to the treatment of septic cardiomyopathy: a novel mechanism linking Ripk3-modified mitochondrial performance and endoplasmic reticulum function. Redox Biol. (2019) 26:101287. doi: 10.1016/j.redox.2019.101287
459. Li RL, Wu SS, Wu Y, Wang XX, Chen HY, Xin JJ, et al. Irisin alleviates pressure overload-induced cardiac hypertrophy by inducing protective autophagy via mTOR-independent activation of the AMPK-ULK1 pathway. J Mol Cell Cardiol. (2018) 121:242–55. doi: 10.1016/j.yjmcc.2018.07.250
460. Burgeiro A, Gajate C, Dakir el H, Villa-Pulgarín JA, Oliveira PJ, Mollinedo F. Involvement of mitochondrial and B-RAF/ERK signaling pathways in berberine-induced apoptosis in human melanoma cells. Anticancer Drugs. (2011) 22:507–18. doi: 10.1097/CAD.0b013e32834438f6
461. Gao GY, Ma J, Lu P, Jiang X, Chang C. Ophiopogonin B induces the autophagy and apoptosis of colon cancer cells by activating JNK/c-Jun signaling pathway. Biomed Pharmacother. (2018) 108:1208–15. doi: 10.1016/j.biopha.2018.06.172
462. Ouyang H, Li Q, Zhong J, Xia F, Zheng S, Lu J, et al. Combination of melatonin and irisin ameliorates lipopolysaccharide-induced cardiac dysfunction through suppressing the Mst1-JNK pathways. J Cell Physiol. (2020) 235:6647–59. doi: 10.1002/jcp.29561
463. Doherty J, Baehrecke EH. Life, death and autophagy. Nat Cell Biol. (2018) 20:1110–7. doi: 10.1038/s41556-018-0201-5
464. Zhou T, Chang L, Luo Y, Zhou Y, Zhang J. Mst1 inhibition attenuates non-alcoholic fatty liver disease via reversing Parkin-related mitophagy. Redox Biol. (2019) 21:101120. doi: 10.1016/j.redox.2019.101120
465. Kim JE, Choi HC, Song HK, Kang TC. Blockade of AMPA receptor regulates mitochondrial dynamics by modulating ERK1/2 and PP1/PP2A-mediated DRP1-S616 phosphorylations in the normal rat hippocampus. Front Cell Neurosci. (2019) 13:179. doi: 10.3389/fncel.2019.00179
466. Chen L, Meng Y, Guo X, Sheng X, Tai G, Zhang F, et al. Gefitinib enhances human colon cancer cells to TRAIL-induced apoptosis of via autophagy- and JNK-mediated death receptors upregulation. Apoptosis. (2016) 21:1291–301. doi: 10.1007/s10495-016-1287-5
467. Bansal M, Moharir SC, Swarup G. Autophagy receptor optineurin promotes autophagosome formation by potentiating LC3-II production and phagophore maturation. Commun Integr Biol. (2018) 11:1–4. doi: 10.1080/19420889.2018.1467189
468. Chen Y, Wang HH, Chang HH, Huang YH, Wang JR, Changchien CY, et al. Guggulsterone induces apoptosis and inhibits lysosomal-dependent migration in human bladder cancer cells. Phytomedicine. (2021) 87:153587. doi: 10.1016/j.phymed.2021.153587
469. Hsieh CH, Pai PY, Hsueh HW, Yuan SS, Hsieh YC. Complete induction of autophagy is essential for cardioprotection in sepsis. Ann Surg. (2011) 253:1190–200. doi: 10.1097/SLA.0b013e318214b67e
470. Ren J, Xu X, Wang Q, Ren SY, Dong M, Zhang Y. Permissive role of AMPK and autophagy in adiponectin deficiency-accentuated myocardial injury and inflammation in endotoxemia. J Mol Cell Cardiol. (2016) 93:18–31. doi: 10.1016/j.yjmcc.2016.02.002
471. Li F, Lang F, Zhang H, Xu L, Wang Y, Hao E. Role of TFEB mediated autophagy, oxidative stress, inflammation, and cell death in endotoxin induced myocardial toxicity of young and aged mice. Oxid Med Cell Longev. (2016) 2016:5380319. doi: 10.1155/2016/5380319
472. An R, Zhao L, Xi C, Li H, Shen G, Liu H, et al. Melatonin attenuates sepsis-induced cardiac dysfunction via a PI3K/Akt-dependent mechanism. Basic Res Cardiol. (2016) 111:8. doi: 10.1007/s00395-015-0526-1
473. Pan P, Zhang H, Su L, Wang X, Liu D. Melatonin balance the autophagy and apoptosis by regulating UCP2 in the LPS-induced cardiomyopathy. Molecules. (2018) 23:675. doi: 10.3390/molecules23030675
474. Arunachalam G, Samuel SM, Marei I, Ding H, Triggle CR. Metformin modulates hyperglycaemia-induced endothelial senescence and apoptosis through SIRT1. Br J Pharmacol. (2014) 171:523–35. doi: 10.1111/bph.12496
475. Hwang JW, Yao H, Caito S, Sundar IK, Rahman I. Redox regulation of SIRT1 in inflammation and cellular senescence. Free Radic Biol Med. (2013) 61:95–110. doi: 10.1016/j.freeradbiomed.2013.03.015
476. Vachharajani VT, Liu T, Brown CM, Wang X, Buechler NL, Wells JD, et al. SIRT1 inhibition during the hypoinflammatory phenotype of sepsis enhances immunity and improves outcome. J Leukoc Biol. (2014) 96:785–96. doi: 10.1189/jlb.3MA0114-034RR
477. Qiu R, Li W, Liu Y. MicroRNA-204 protects H9C2 cells against hypoxia/reoxygenation-induced injury through regulating SIRT1-mediated autophagy. Biomed Pharmacother. (2018) 100:15–9. doi: 10.1016/j.biopha.2018.01.165
478. Han D, Li X, Li S, Su T, Fan L, Fan WS, et al. Reduced silent information regulator 1 signaling exacerbates sepsis-induced myocardial injury and mitigates the protective effect of a liver X receptor agonist. Free Radic Biol Med. (2017) 113:291–303. doi: 10.1016/j.freeradbiomed.2017.10.005
479. Bai XZ, He T, Gao JX, Liu Y, Liu JQ, Han SC, et al. Melatonin prevents acute kidney injury in severely burned rats via the activation of SIRT1. Sci Rep. (2016) 6:32199. doi: 10.1038/srep32199
480. Zhao L, An R, Yang Y, Yang X, Liu H, Yue L, et al. Melatonin alleviates brain injury in mice subjected to cecal ligation and puncture via attenuating inflammation, apoptosis, and oxidative stress: the role of SIRT1 signaling. J Pineal Res. (2015) 59:230–9. doi: 10.1111/jpi.12254
481. Zhang WX, He BM, Wu Y, Qiao JF, Peng ZY. Melatonin protects against sepsis-induced cardiac dysfunction by regulating apoptosis and autophagy via activation of SIRT1 in mice. Life Sci. (2019) 217:8–15. doi: 10.1016/j.lfs.2018.11.055
482. Bagati A, Bianchi-Smiraglia A, Moparthy S, Kolesnikova K, Fink EE, Kolesnikova M, et al. FOXQ1 controls the induced differentiation of melanocytic cells. Cell Death Differ. (2018) 25:1040–9. doi: 10.1038/s41418-018-0066-y
483. Sureshbabu A, Patino E, Ma KC, Laursen K, Finkelsztein EJ, Akchurin O, et al. RIPK3 promotes sepsis-induced acute kidney injury via mitochondrial dysfunction. JCI Insight. (2018) 3:e98411. doi: 10.1172/jci.insight.98411
484. Biernacki M, Ambrozewicz E, Gegotek A, Toczek M, Bielawska K, Skrzydlewska E. Redox system and phospholipid metabolism in the kidney of hypertensive rats after FAAH inhibitor URB597 administration. Redox Biol. (2018) 15:41–50. doi: 10.1016/j.redox.2017.11.022
485. Davidson SM, Arjun S, Basalay MV, Bell RM, Bromage DI, Bøtker HE, et al. The 10th biennial hatter cardiovascular institute workshop: cellular protection-evaluating new directions in the setting of myocardial infarction, ischaemic stroke, and cardio-oncology. Basic Res Cardiol. (2018) 113:43. doi: 10.1007/s00395-018-0704-z
486. Daniel E, Azizoglu DB, Ryan AR, Walji TA, Chaney CP, Sutton GI, et al. Spatiotemporal heterogeneity and patterning of developing renal blood vessels. Angiogenesis. (2018) 21:617–34. doi: 10.1007/s10456-018-9612-y
487. Ding M, Ning J, Feng N, Li Z, Liu Z, Wang Y, et al. Dynamin-related protein 1-mediated mitochondrial fission contributes to post-traumatic cardiac dysfunction in rats and the protective effect of melatonin. J Pineal Res. (2018) 64:e12447. doi: 10.1111/jpi.12447
488. Zhou H, Du W, Li Y, Shi C, Hu N, Ma S, et al. Effects of melatonin on fatty liver disease: the role of NR4A1/DNA-PKcs/p53 pathway, mitochondrial fission, and mitophagy. J Pineal Res. (2018) 64:e12450. doi: 10.1111/jpi.12450
489. Bellomo C, Caja L, Fabregat I, Mikulits W, Kardassis D, Heldin CH, et al. Snail mediates crosstalk between TGFβ and LXRα in hepatocellular carcinoma. Cell Death Differ. (2018) 25:885–903. doi: 10.1038/s41418-017-0021-3
490. Doerrier C, García JA, Volt H, Díaz-Casado ME, Luna-Sánchez M, Fernández-Gil B, et al. Permeabilized myocardial fibers as model to detect mitochondrial dysfunction during sepsis and melatonin effects without disruption of mitochondrial network. Mitochondrion. (2016) 27:56–63. doi: 10.1016/j.mito.2015.12.010
491. Escames G, López LC, Ortiz F, López A, García JA, Ros E, et al. Attenuation of cardiac mitochondrial dysfunction by melatonin in septic mice. FEBS J. (2007) 274:2135–47. doi: 10.1111/j.1742-4658.2007.05755.x
492. Ortiz F, García JA, Acuña-Castroviejo D, Doerrier C, López A, Venegas C, et al. The beneficial effects of melatonin against heart mitochondrial impairment during sepsis: inhibition of i NOS and preservation of n NOS. J Pineal Res. (2014) 56:71–81. doi: 10.1111/jpi.12099
493. Volt H, García JA, Doerrier C, Díaz-Casado ME, Guerra-Librero A, López LC, et al. Same molecule but different expression: aging and sepsis trigger NLRP3 inflammasome activation, a target of melatonin. J Pineal Res. (2016) 60:193–205. doi: 10.1111/jpi.12303
494. Zhang H, Liu D, Wang X, Chen X, Long Y, Chai W, et al. Melatonin improved rat cardiac mitochondria and survival rate in septic heart injury. J Pineal Res. (2013) 55:1–6. doi: 10.1111/jpi.12033
495. Sener G, Toklu H, Kapucu C, Ercan F, Erkanli G, Kaçmaz A, et al. Melatonin protects against oxidative organ injury in a rat model of sepsis. Surg Today. (2005) 35:52–9. doi: 10.1007/s00595-004-2879-1
496. Zisapel N, Laudon M. Method and Formulation for Treating Resistance to Antihypertensives and Related Conditions. Portugal: Google Patents (2011).
497. Ahsanova E, Popov V, Bulanova N, Morozova T. Hypotensive action of melatonin in patients with arterial hypertension. Euro Cardiol Rev. (2020) 15:e43. doi: 10.15420/ecr.2020.15.1.PO20
498. Zaslavskaia R, Shcherban E, Lilitsa G, Logvinenko S. Melatonin in the combined treatment of cardiovascular diseases. Klin Med. (2010) 88:26–30.
499. Hoseini SG, Heshmat-Ghahdarijani K, Khosrawi S, Garakyaraghi M, Shafie D, Roohafza H, et al. Effect of melatonin supplementation on endothelial function in heart failure with reduced ejection fraction: a randomized, double-blinded clinical trial. Clin Cardiol. (2021) 44:1263–71. doi: 10.1002/clc.23682
500. Jafari-Vayghan H, Moludi J, Saleh-Ghadimi S, Enamzadeh E, Seyed-Mohammadzad MH, Alizadeh M. Impact of melatonin and branched-chain amino acids cosupplementation on quality of life, fatigue, and nutritional status in cachectic heart failure patients: a randomized controlled trial. Am J Lifestyle Med. (2022) 16:130–40. doi: 10.1177/1559827619874044
501. Andersen LP, Gögenur I, Rosenberg J, Reiter RJ. The safety of melatonin in humans. Clin Drug Investig. (2016) 36:169–75. doi: 10.1007/s40261-015-0368-5
502. Dominguez-Rodriguez A, Abreu-Gonzalez P, de la Torre-Hernandez JM, Gonzalez-Gonzalez J, Garcia-Camarero T, Consuegra-Sanchez L, et al. Effect of intravenous and intracoronary melatonin as an adjunct to primary percutaneous coronary intervention for acute ST-elevation myocardial infarction: results of the melatonin adjunct in the acute myocaRdial infarction treated with angioplasty trial. J Pineal Res. (2017) 62:e12374. doi: 10.1111/jpi.12374
Keywords: cardiovascular disease, pathophysiology, melatonin, antioxidant, cardiotoxicity
Citation: Tobeiha M, Jafari A, Fadaei S, Mirazimi SMA, Dashti F, Amiri A, Khan H, Asemi Z, Reiter RJ, Hamblin MR and Mirzaei H (2022) Evidence for the Benefits of Melatonin in Cardiovascular Disease. Front. Cardiovasc. Med. 9:888319. doi: 10.3389/fcvm.2022.888319
Received: 02 March 2022; Accepted: 10 May 2022;
Published: 20 June 2022.
Edited by:
Xiaoyue Pan, New York University, United StatesReviewed by:
Paul C. Tang, University of Michigan, United StatesSuowen Xu, University of Science and Technology of China, China
Copyright © 2022 Tobeiha, Jafari, Fadaei, Mirazimi, Dashti, Amiri, Khan, Asemi, Reiter, Hamblin and Mirzaei. This is an open-access article distributed under the terms of the Creative Commons Attribution License (CC BY). The use, distribution or reproduction in other forums is permitted, provided the original author(s) and the copyright owner(s) are credited and that the original publication in this journal is cited, in accordance with accepted academic practice. No use, distribution or reproduction is permitted which does not comply with these terms.
*Correspondence: Michael R. Hamblin, SGFtYmxpbi5sYWJAZ21haWwuY29t; Zatollah Asemi, YXNlbWkuOW84aUBnbWFpbC5jb20=; Hamed Mirzaei, bWlyemFlaS1oQGthdW1zLmFjLmly; aC5taXJ6YWVpMjAwMkBnbWFpbC5jb20=
†These authors have contributed equally to this work