- 1Drexel University College of Medicine, Philadelphia, PA, United States
- 2Division of Endocrinology, University of Missouri School of Medicine, Columbia, MO, United States
- 3Division of Endocrinology, Wayne State University School of Medicine, Detroit, MI, United States
We review the pathways by which arginine vasopressin (AVP) and hydration influence the sequelae of the metabolic syndrome induced by high fructose consumption. AVP and inadequate hydration have been shown to worsen the severity of two phenotypes associated with metabolic syndrome induced by high fructose intake–enhanced lipogenesis and insulin resistance. These findings have implications for those who frequently consume sweeteners such as high fructose corn syrup (HFCS). Patients with metabolic syndrome are at higher risk for microalbuminuria and/or chronic kidney disease; however, it is difficult to discriminate the detrimental renal effects of the metabolic syndrome from those of hypertension, impaired glucose metabolism, and obesity. It is not surprising the prevalence of chronic renal insufficiency is growing hand in hand with obesity, insulin resistance, and metabolic syndrome in those who consume large amounts of fructose. Higher AVP levels and low hydration status worsen the renal insufficiency found in patients with metabolic syndrome. This inter-relationship has public health consequences, especially among underserved populations who perform physical labor in environments that place them at risk for dehydration. MesoAmerican endemic nephropathy is a type of chronic kidney disease highly prevalent in hot ambient climates from southwest Mexico through Latin America. There is growing evidence that this public health crisis is being spurred by greater fructose consumption in the face of dehydration and increased dehydration-dependent vasopressin secretion. Work is needed at unraveling the mechanism(s) by which fructose consumption and increased AVP levels can worsen the renal disease associated with components of the metabolic syndrome.
Introduction
The incidence of obesity and metabolic syndrome related renal disorders has increased in recent years. A recent cross-sectional analysis from 2011 to 2016, found the prevalence of metabolic syndrome in the United States to be 34.7% of adults (1). Meanwhile, from 1986 to 2000, the biopsy incidence of obesity-related glomerulopathy increased 10-fold, from 0.2 to 2% (2). It is believed that the rising prevalence of obesity comes from excess consumption of sugars, and especially fructose (2, 3). It is important to understand the causes of increased fructose consumption in men and women. Fructose, “fruit sugar,” is a hexose monosaccharide with the same molecular formula as glucose (C6H12O6). High fructose corn syrup (HFCS) is a manufactured sweetener that contains a mixture of the monosaccharides, glucose and fructose, in varying ratios (e.g., 45%:55%). In “sweetness,” HFCS is similar to cane sugar (sucrose), a disaccharide first hydrolyzed in the small intestines before systemic absorption; however sucrose digestion yields glucose and fructose in equal parts (i.e., 50%:50%, Figure 1A) (5). The average fructose content of popular beverages sweetened with HFCS is 59% with several major brands approaching 65% (6). Relative to sucrose extracted from cane sugar, high fructose, corn-based syrup is less expensive, and the low cost explains its widespread use. Although excess intake of both HFCS and sucrose from cane sugar is linked to the metabolic syndrome, some have argued that the incrementally greater percentage of fructose in HFCS compared to sucrose puts those at greater risk of metabolic disease when consuming more HFCS relative to sucrose. In either case, an understanding of the role fructose plays in the pathogenesis of metabolic syndrome is needed.
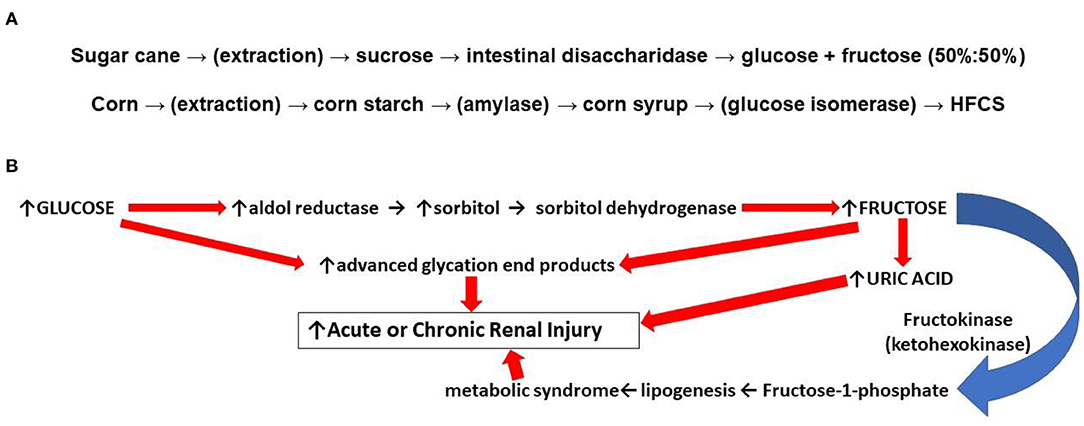
Figure 1. (A) (top): Differences in food sweeteners from sugar and corn. Sucrose is extracted from sugar cane or beets. Ingested sucrose from processed foods is digested in the small intestines by disaccharidase into equal parts glucose and fructose (50%:50%). Absorbed fructose is almost completely taken up by GLUT-1 and GLUT-5 transporters in the liver which accounts for low levels of plasma fructose. Corn starch is a polysaccharide that is enzymatically hydrolyzed to monosaccharides which in the presence of isomerase increases the relative content of fructose yielding a high fructose corn syrup (HFCS). (B) (bottom): Some mechanisms by which fructose can result in renal injury. The endogenous conversion of glucose to fructose through the polyol pathway contributes to formation of uric acid, which promotes mitochondrial oxidative stress and inflammation. Endogenous fructose generation also promotes lipogenesis which contributes to the development of the metabolic syndrome. Glucose and fructose can both react directly with cellular lipids and proteins; oxidized LDL and collagen cross linked by these reactions can induce vascular stiffness, stimulate inflammation, and promote atherogenesis (4).
Several potential mechanisms by which the increased consumption and metabolism of fructose can worsen the phenotypic expression of the metabolic syndrome relative to glucose have been proposed. First, advanced glycation end products (“AGE's”) are irreversible molecular adducts, damaging to cells, that are formed from the nonenzymatic reaction between reducing sugars such as fructose and glucose with proteins or lipids. Whereas, glucose contains an aldehyde, fructose has a ketone group which makes it even more reactive with amines found in proteins (the non-enzymatic “Maillard reaction”) (4). Second, when fructose is administered exogenously, the sugar is taken up by the liver and sequentially metabolized by fructokinase and aldolase with the rapid consumption of adenosine triphosphate (ATP) (7). A transient depletion of ATP and intracellular phosphate shunts adenosine and inosine to purine degradation pathways and the synthesis of uric acid; the increased production of uric acid is accompanied by mitochondrial oxidative stress and inflammation in the liver (8, 9). Furthermore, in multiple tissues, uric acid can promote the metabolism of glucose through the polyol pathway where, in the face of high glucose concentrations, glucose is reduced to sorbitol with its subsequent oxidation to fructose leading to further hepatic steatosis or renal damage (Figure 1B) (10, 11). Uric acid can also inhibit insulin signaling and induce insulin resistance (12).
Arginine Vasopressin, Fructose, and the Metabolic Syndrome
Arginine vasopressin (AVP) is now being implicated in the development of metabolic syndrome. AVP is produced by two groups of cells—neurons of the hypothalamic paraventricular nuclei (PVN) and the supraoptic nuclei (SON). These neurons are divided into either the magnocellular system secreting AVP into the peripheral circulation from axon terminals within the pituitary, and also, the parvocellular system with axons projecting to the median eminence of the hypothalamus where AVP is secreted into the pituitary portal circulation (13, 14). Through the systemic circulation, or alternatively, through the pituitary portal system, AVP activates three distinct vasopressin receptors, V1a, V1b, and V2, encoded by three distinct genes in humans, on chromosomes 12q14.2, 1q32.1, and Xq28, respectively, with variation in the mechanisms of post-receptor signal transduction (Figure 2) (15–17).

Figure 2. There are three subtypes of the G-protein linked vasopressin receptors. V1a receptors are found on vascular smooth muscle and platelets and induce vasoconstriction through increased phosphatidyl inositol (PI) metabolism; V2 receptors are found primarily in on principal cells of the kidney collecting ducts and mediate water reabsorption through aquaporins stimulated by AVP in the systemic circulation; V1b receptors are found primarily in cells of the pituitary, white adipose tissue, and the pancreas. AVP secreted from parvocellular neurons and flowing through the hypophyseal portal circulation stimulates pituitary adrenocorticotropic hormone (ACTH) secretion through interaction with V1b receptors in pituitary corticotrophs by potentiating the stimulatory effects of corticotrophin-releasing hormone on ACTH.
There are several pathways by which increases in circulating AVP can contribute to the development of the metabolic syndrome. Indeed, over 10 years ago, it was recognized that AVP levels significantly correlated with several markers of the metabolic syndrome, i.e., body mass index, fasting plasma glucose and insulin concentrations, insulin resistance, and triglyceride levels. Vasopressin has been shown to increase stress-induced ACTH secretion in a V1b dependent manner, and repetitive stress is associated with the development of the metabolic syndrome (18, 19). Subsequently, it has been shown that copeptin, the stable carboxy-terminal portion of pro-arginine vasopressin secreted in equimolar amounts with AVP, is independently associated with hyperinsulinemia, the development of diabetes mellitus, and the metabolic syndrome (20–22). Because hydration levels mediate central AVP release and plasma AVP concentrations, it is not surprising that hydration levels have also been shown convincingly to correlate with the presence of the metabolic syndrome (23–33).
AVP has been shown to stimulate of hepatic gluconeogenesis and glycogenolysis through the activation of hepatic V1a receptors, and also contributes to the secretion of glucagon or insulin through the activation of pancreatic V1b receptors. These findings are consistent with the observation that sustained AVP infusion in laboratory rodents induced both a time and dose-dependent increase in fasting blood glucose concentrations, whereas lowering endogenous AVP by increasing water intake had no effect. In the same study, the effect of AVP on fasting blood glucose was attenuated by cotreatment with a V1a receptor antagonist. This may help explain the reported observations of elevated plasma AVP (copeptin) levels in patients with diabetes (34). It is likely that these findings, that AVP increases glycemia through the activation of V1a receptors, are mediated by the activation of hepatic V1a receptors and the stimulation of hepatic gluconeogenic processes. However, further study is needed to confirm this is a purely hepatic process. AVP also acts synergistically with central V1b receptors to not only promote ACTH secretion, as noted above, but also catecholamine secretion from the adrenal medulla In one clinical study, 31 healthy adults with high copeptin levels, low 24 h urine volumes, and high urine osmolality were recruited to consume an additional 1.5 L of water daily for 6 weeks. Although the intervention did not significantly affect fasting ACTH, cortisol, fasting glucose, insulin, or glucagon concentrations, changes in copeptin levels were highly associated with changes in ACTH levels. These findings also make tenable that central AVP concentrations in the pituitary portal circulation can contribute to ACTH-mediated stress responses and the development of the metabolic syndrome (35). In summary, there appears to be both metabolic (mediated by V1a receptor-induced gluconeogenesis) and central (mediated by activation of V1b receptors and the stress response) mechanisms by which AVP contributes to hyperglycemia and the metabolic syndrome.
As noted, the V1b receptor plays a critical role in regulating hypothalamic-pituitary-adrenal axis activity by contributing to pituitary ACTH secretion and corticosterone release from the adrenal gland levels under basal and stress conditions (18). Indeed, the normal increase in circulating ACTH levels in response to AVP is impaired in V1b receptor knockout mice, although corticotropin-releasing hormone (CRH)-stimulated ACTH release is not impaired. Vasopressin levels and V1b receptor expression were elevated in rodents with exposure to chronic stress, and the measured increase in ACTH after stress (e.g., after a forced swim test) has been found to be significantly suppressed in V1b receptor null mice (18, 36). Immediately after a stressful event, there is a CRH-mediated suppression of food intake, followed by a CRH/ACTH-dependent, glucocorticoid mediated, stimulation of hunger and eating behavior (37). Accordingly, chronic stress, and the resultant chronic activation of the hypothalamic-pituitary adrenal axis, induces weight gain in rodents and humans where it is known as “emotional eating” or “stress-induced eating” (38–41). In addition to the hyperglycemic effects of AVP described above, it is likely that AVP produces behavioral changes via V1b potentiation of the CRF-ACTH-glucocorticoid axis.
Teleologically, the secretion of AVP has evolved as a mechanism to ensure appropriate hydration status and fluid balance. Because a significant amount of water is endogenously provided through the catabolism of fat, specifically through fatty acid oxidation, it is intriguing to speculate that AVP would play a role at increasing fat storage (42). Indeed, it is one reason posited to explain the evolution of the camel's hump, which is a fat storage depot (43). It is noteworthy that fructose stimulates the secretion of AVP to a greater degree than glucose. In hypothalamic explants, fructose directly stimulates AVP secretion (44). When rodents are slowly infused with a hypertonic 20% solution of either glucose or fructose, AVP levels only rise in response to the administration of fructose. This is likely due to the facilitated uptake of glucose, relative to impermeant fructose, into AVP-releasing neurons sensing osmolality (45).
It has also been shown that the effect of AVP on inducing hepatic lipogenesis and the metabolic syndrome is dependent upon the V1b receptor. In a study utilizing vasopressin receptor knockout mice being fed high fructose diets, V1b receptor null mice were found to have suppressed markers of the metabolic syndrome phenotype, including fat mass, hepatic steatosis, serum triglycerides, transaminases, insulin, and leptin. Interestingly, these same markers were found to be elevated in V1a receptor deficient mice, suggesting that while V1b receptors promote lipogenesis and the development of the metabolic syndrome, activation of V1a receptors may play a protective, counterregulatory role (42). These findings from rodents were corroborated by the study of overweight and obese subjects who consumed glucose- or fructose-sweetened beverages providing 25% of energy requirements for 10 weeks. Although both groups exhibited similar weight gain during the intervention, visceral adipose volume was significantly increased only in subjects consuming fructose, and hepatic de novo lipogenesis was increased specifically during fructose consumption (46). Fructose is a secretagogue for AVP, and AVP promotes lipogenesis, hyperinsulinemia, and many of the pathophysiological intermediaries associated with the metabolic syndrome.
Very little orally ingested fructose hits the cerebral circulation, therefore the effect of endogenous fructose production on AVP secretion should be considered. Exogenous fructose is preferentially taken up by glucose transporters (especially GLUT5) and metabolized in the liver while glucose, transported by GLUT1 and GLUT3, is metabolized primarily in the brain (47). Accordingly, plasma fructose concentrations are typically 0.01% of plasma glucose levels. Meanwhile, glucose concentrations in cerebrospinal fluid (CSF) are roughly 60% of its plasma concentrations whereas CSF levels of fructose are twenty times greater than those levels found in the blood. It is suggested from these observations that because fructose is so rapidly metabolized by the liver, endogenous fructose generation by the brain is physiologically significant (48). Indeed, both the catabolism of fructose by fructokinase and synthesis of fructose by the polyol pathway have been demonstrated in the brain (49). Clearly, endogenous fructose production in the brain can contribute to any AVP-dependent pathway that promotes the metabolic syndrome phenotype despite having low plasma concentrations of fructose.
Arginine Vasopressin, Fructose, and Renal Insufficiency
The potential potentiation of the metabolic syndrome from chronically increased levels of AVP, albeit at low levels, and increased fructose consumption has significant public health implications (50). This is particularly true in hot, arid lands, such as Mesoamerica where high ambient temperatures and physical exertion, and accordingly high levels of AVP, are associated with the growing prevalence of the metabolic syndrome and the development of chronic kidney disease (51, 52). Indeed, Mesoamerican endemic nephropathy is a type of chronic kidney disease of unknown origin that is highly prevalent among manual laborers from southwest Mexico through Latin America (53, 54). In fact, study of manual laborers in Nicaragua revealed 14% of all men had an estimated glomerular filtration rate (eGFR) of <60 mL/min/1.73 m2 (55). Similar findings were yielded in a study of El Salvadorian coastal communities, where 18% of men had an eGFR <60 mL/min/1.73 m2 (56). As a chronic kidney disease of nontraditional origin (CKDnt), the incidence and public health burden of Mesoamerican endemic nephropathy cannot be attributed to classical risk factors such as hypertension or diabetes. The exact cause of CKDnt has yet to be determined, and recurrent heat stress/dehydration and agrochemicals remain among the most widely discussed risk factors (57). The Pan American Health Organization (PANAHO) has reported “the death toll of the epidemic of CKDnt in Mesoamerica runs into the tens of thousands, affecting mostly young men.” Because this deteriorating renal disease is observed mostly in plantation workers exposed to physically stressful work environments and high ambient temperatures, PANAHO has further gone on to recommend a “water-shade-rest” intervention to farm workers (58).
The increased consumption of sweetened beverages is associated with urbanization and economic growth. As such, more intensified policy efforts are needed to ensure that hydration occurs with water, not through sweetened beverages that increase the intake of sugar and, subsequently, increase the global burden of obesity and chronic diseases. There is growing evidence that hydration with beverages sweetened with high fructose corn syrup potentiates the renal insufficiency found in those who must toil in high ambient temperature climates. However, as any traveler to an underdeveloped country has observed, the advertising for sugar sweetened beverages is ubiquitous, equaled only by the fervent pitches for various cell phone carriers. In the United States, it is estimated that the average American consumes roughly 54.7 g of fructose per day and nearly 30 kg of carbohydrate each year from added sweetners (59, 60). In Latin America, average individual sugar intake is estimated at 99.4 g/day, with an average of 65.5 g/day coming from added sugar (61).
In a study with laboratory rodents pair fed a diet containing 60% fructose, 60% dextrose, or standard rat chow, the fructose fed rats had worse outcomes in a reduced renal mass model of chronic kidney disease. Proteinuria was increased and creatinine clearance was diminished in the rodents fed fructose when compared to glucose- and control-fed rodents. Glomerulosclerosis, tubular atrophy, tubular dilatation, and inflammation were all greater in the fructose fed rats (62). The toxic effect of fructose is not limited to injured kidneys as dietary fructose has been shown to produce a similarly pathologic effect on normal rodent kidneys (63). Endogenous fructose generation via the polyol pathway may be particularly pathologic because, in addition to the production of fructose, this pathway may also increase the relative abundance of sorbitol, which plays a role in directly inducing renal injury (64). In a study of the potential role of endogenous fructose production, as opposed to dietary fructose, wild-type mice with streptozotocin-induced diabetes were shown to develop proteinuria, reduced glomerular filtration rate, and renal glomerular and proximal tubular injury. However, unlike control mice, rodents genetically deficient in fructokinase demonstrated significantly less proteinuria, renal dysfunction, renal injury, and inflammation. These studies further support a role for fructokinase and its metabolites as a novel mediator of diabetic nephropathy (65).
Fructose can directly stimulate AVP release or induce the secretion of AVP by the hyperosmolarity generated by increasing sorbitol concentrations via the polyol pathway. Conversely, genetic ablation of fructokinase diminishes AVP secretion induced by dehydration (45). Given this intimate relationship between AVP and fructose, it is necessary to understand what effect fructose has on the physiologic responses to dehydration in men and women if we are to understand the endemic nephropathy of Mesoamerica. In one study, mice were exposed to repetitive heat stress for 5 weeks. Compared to control animals given water, there was a progressive worsening of renal inflammation and fibrosis in mice given rehydration with 10% fructose in water. It was suggested that following heat-induced renal injury, oral fructose ingestion may accelerate kidney damage (66). Another study examining heat-induced renal injury found that compared to water, rehydration with fructose following heat stress resulted in significant decrements in renal blood flow (measured via Doppler ultrasound) and creatinine clearance along with significantly elevated renal vascular resistance. Interestingly, co-administration of conivaptan, a nonselective vasopressin receptor antagonist, prevented these changes, suggesting fructose mediated renal injury following heat stress occurs in an AVP-dependent fashion (67).
Many of these findings have been supported by additional study. Fluid restriction followed by water hydration mildly increased urine osmolality and induced a 15% decrement in creatinine clearance while increasing two markers of tubular damage, urinary N-Acetyl-β-D-glucosaminidase and kidney injury molecule-1. These changes were accompanied by overexpression of V1a and V2 renal receptors, the polyol fructokinase pathway, and increased renal oxidative stress with reduced expression of antioxidant enzymes. Hydration with an unspecified 11% glucose/fructose sweetened beverage significantly amplified those alterations, and it is suggested that current habits of re-hydration with sweetened beverages could be a risk factor in developing kidney damage (68).
The findings in rodents regarding fructose and renal injury have been recapitulated in men and women. In one study of 12 healthy adults, subjects drank 2 liters of a fructose containing soft drink or water during 4 h of exercise. Serum creatinine increased ≥0.30 mg/dl post exercise in 75% of participants in the soft drink trial compared with 8% in water trial, which is consistent with the Kidney Disease Improving Global Outcomes definition of acute kidney injury (69). Changes in serum uric acid from pre-exercise were greater in the soft drink trial than the water trial at post-exercise, and there were greater increases from pre-exercise to post-exercise in serum copeptin in the soft drink trial. It was suggested that consuming a fructose beverage during and following exercise in the heat induces kidney injury through a vasopressin-mediated mechanism, which was consistent with the findings observed in rodents (70). Subsequently,13 healthy subjects who consumed 500 mL of a HFCS-sweetened soft drink had augmented kidney vasoconstriction (measured by Doppler ultrasound) to sympathetic stimulation induced with the cold pressor test when compared to subjects who consumed water. During a separate trial, venous blood samples were obtained in 12 healthy adults before and 30 min after consumption of 500 mL water with artificial sweetener, sucrose, or high fructose corn syrup. Increases in serum uric acid were greater in those who consumed high fructose corn syrup compared to those who consumed artificial sweetener or sucrose.
In summary, it is suggested that high fructose corn syrup acutely increases vascular resistance in the kidneys with simultaneous elevations in circulating uric acid and vasopressin (71). There are consistent suggestions that hypohydration and rehydration with fructose-based sweetened beverages facilitate the secretion of AVP and predispose humans to metabolic syndrome and the development of renal insufficiency. Despite such consistent suggestions, our understanding of this relationship and the mechanisms by which fructose and vasopressin could induce metabolic and renal disease remain incomplete. Further study utilizing selective and nonselective vasopressin agonists and antagonists could prove useful in characterizing the physiological changes produced by AVP, establishing the precise mechanisms by which AVP verses fructose contributes to renal disease, and identifying the utility of AVP receptor blockade to combat diseases such as Mesoamerican endemic nephropathy. Fructose could be a key mediator in this cascade and the mechanisms by which it exerts its toxic effects warrants further exploration. It is worth noting that exogenous AVP is frequently administered, in the absence of fructose, as a means of pressor support in critically ill patients and has been found to protect against renal injury in this setting (72). Finally, further epidemiological study of fructose consumption and renal insufficiency, particularly in Mesoamerica, could help reduce the impact of CKDnt and obesity related kidney disease. Regardless, as evidenced by this review, there is ample evidence to suggest fructose and AVP contribute to the development of metabolic and renal disease. We should not be thirsty for fructose, but instead, thirsty for water.
Author Contributions
All authors listed have made a substantial, direct, and intellectual contribution to the work and approved it for publication.
Funding
This work was supported, in part, by the Department of Defense and Henry M. Jackson Foundation for the Advancement of Military Medicine, HU0001-18-2-0016, HJF Award, 309833-1.00-65546.
Conflict of Interest
The authors declare that the research was conducted in the absence of any commercial or financial relationships that could be construed as a potential conflict of interest.
Publisher's Note
All claims expressed in this article are solely those of the authors and do not necessarily represent those of their affiliated organizations, or those of the publisher, the editors and the reviewers. Any product that may be evaluated in this article, or claim that may be made by its manufacturer, is not guaranteed or endorsed by the publisher.
References
1. Hirode G, Wong RJ. Trends in the prevalence of metabolic syndrome in the United States, 2011-2016. JAMA. (2020) 323:2526–8. doi: 10.1001/jama.2020.4501
2. Locatelli F, Pozzoni P, Del Vecchio L. Renal manifestations in the metabolic syndrome. J Am Soc Nephrol. (2006) 17:S81–S85. doi: 10.1681/ASN.2005121332
3. Zammit AR, Katz MJ, Derby C, Bitzer M, Lipton RB. Chronic kidney disease in non-diabetic older adults: associated roles of the metabolic syndrome, inflammation, and insulin resistance. PLoS ONE. (2015) 10:e0139369. doi: 10.1371/journal.pone.0139369
4. Ruiz HH, Ramasamy R, Schmidt AM. Advanced Glycation End Products: Building on the Concept of the “Common Soil” in Metabolic Disease. Endocrinology. (2020) 161:bqz006. doi: 10.1210/endocr/bqz006
5. Rippe JM. Nutrition Health: Fructose, High Fructose Corn Syrup, Sucrose, Health. Berlin: Springer Publications, Human Press (2014). Available online at: https://doi.org/10.1007/978-1-4899-8077-9
6. Ventura EE, Davis JN, Goran MI. Sugar content of popular sweetened beverages based on objective laboratory analysis: focus on fructose content. Obesity (Silver Spring). (2011) 19:868–74. doi: 10.1038/oby.2010.255
7. Mäenpää PH, Raivio KO, Kekomäki MP. Liver adenine nucleotides: fructose-induced depletion and its effect on protein synthesis. Science. (1968) 161:1253–4. doi: 10.1126/science.161.3847.1253
8. Perheentupa J, Raivio K. Fructose-induced hyperuricemia. Lancet. (1967) 290:528–31. doi: 10.1016/S0140-6736(67)90494-1
9. Zhang C, Li L, Zhang Y, Zeng C. Recent advances in fructose intake and risk of hyperuricemia. Biomed Pharmacother. (2020) 131:110795. doi: 10.1016/j.biopha.2020.110795
10. Sanchez-Lozada LG, Andres-Hernando A, Garcia-Arroyo FE, Cicerchi C, Li N, Kuwabara M, et al. Uric acid activates aldose reductase and the polyol pathway for endogenous fructose and fat production causing development of fatty liver in rats. J Biol Chem. (2019) 294:4272–81. doi: 10.1074/jbc.RA118.006158
11. Lanaspa MA, Sanchez-Lozada LG, Choi YJ, Cicerchi C, Kanbay M, Roncal-Jimenez CA, et al. Uric acid induces hepatic steatosis by generation of mitochondrial oxidative stress: potential role in fructose-dependent and -independent fatty liver. J Biol Chem. (2012) 287:40732–44. doi: 10.1074/jbc.M112.399899
12. Zhu Y, Hu Y, Huang T, Zhang Y, Li Z, Luo C, et al. High uric acid directly inhibits insulin signalling and induces insulin resistance. Biochem Biophys Res Commun. (2014) 447:707–14. doi: 10.1016/j.bbrc.2014.04.080
13. Aguilera G, Subburaju S, Young S, Chen J. The parvocellular vasopressinergic system and responsiveness of the hypothalamic pituitary adrenal axis during chronic stress. Prog Brain Res. (2008) 170:29–39. doi: 10.1016/S0079-6123(08)00403-2
14. Antoni FA. Vasopressinergic control of pituitary adrenocorticotropin secretion comes of age. Front Neuroendocrinol. (1993) 14:76–122. doi: 10.1006/frne.1993.1004
15. Thibonnier M, Graves MK, Wagner MS, Auzan C, Clauser E, Willard HF. Structure, sequence, expression, and chromosomal localization of the human V1a vasopressin receptor gene. Genomics. (1996) 31:327–34. doi: 10.1006/geno.1996.0055
16. Rousseau-Merck MF, René P, Derré J, Bienvenu T, Berger R, de Keyzer Y. Chromosomal localization of the human V3 pituitary vasopressin receptor gene (AVPR3) to 1q32. Genomics. (1995) 30:405–6.
17. Lolait SJ, O'Carroll AM, McBride OW, Konig M, Morel A, Brownstein MJ. Cloning and characterization of a vasopressin V2 receptor and possible link to nephrogenic diabetes insipidus. Nature. (1992) 357:336–9. doi: 10.1038/357336a0
18. Lolait SJ, Stewart LQ, Jessop DS, Young WS. 3rd, O'Carroll AM. The hypothalamic-pituitary-adrenal axis response to stress in mice lacking functional vasopressin V1b receptors. Endocrinology. (2007) 148:849–56. doi: 10.1210/en.2006-1309
19. Räikkönen K, Keltikangas-Järvinen L, Adlercreutz H, Hautanen A. Psychosocial stress and the insulin resistance syndrome. Metabolism. (1996) 45:1533–8. doi: 10.1016/S0026-0495(96)90184-5
20. Saleem U, Khaleghi M, Morgenthaler NG, Bergmann A, Struck J, Mosley TH Jr, et al. Plasma carboxy-terminal provasopressin (copeptin): a novel marker of insulin resistance and metabolic syndrome. J Clin Endocrinol Metab. (2009) 94:2558–64. doi: 10.1210/jc.2008-2278
21. Enhörning S, Wang TJ, Nilsson PM, Almgren P, Hedblad B, Berglund G, et al. Plasma copeptin and the risk of diabetes mellitus. Circulation. (2010) 121:2102–8. doi: 10.1161/CIRCULATIONAHA.109.909663
22. Enhörning S, Struck J, Wirfält E, Hedblad B, Morgenthaler NG, Melander O. Plasma copeptin, a unifying factor behind the metabolic syndrome. J Clin Endocrinol Metab. (2011) 96:E1065–72. doi: 10.1210/jc.2010-2981
23. Jacques PF, Rogers G, Stookey JD, Perrier ET. Water intake and markers of hydration are related to cardiometabolic risk biomarkers in community-dwelling older adults: a cross-sectional analysis. J Nutr. (2021) 151:3205–13. doi: 10.1093/jn/nxab233
24. Sedaghat G, Montazerifar F, Keykhaie MA, Karajibani M, Shourestani S, Dashipour A. Effect of pre-meal water intake on the serum levels of Copeptin, glycemic control, lipid profile and anthropometric indices in patients with type 2 diabetes mellitus: a randomized, controlled trial. J Diabetes Metab Disord. (2021) 20:171–7. doi: 10.1007/s40200-020-00724-9
25. Vanhaecke T, Dolci A, Fulgoni VL, Lieberman HR. Associations between urinary hydration markers and metabolic dysfunction: a cross-sectional analysis of NHANES data, 2008-2010. Eur J Nutr. (2021) 60:4229–41. doi: 10.1007/s00394-021-02575-3
26. Brunkwall L, Ericson U, Nilsson PM, Enhörning S. High water intake and low urine osmolality are associated with favorable metabolic profile at a population level: low vasopressin secretion as a possible explanation. Eur J Nutr. (2020) 59:3715–22. doi: 10.1007/s00394-020-02202-7
27. Barchetta I, Enhörning S, Cimini FA, Capoccia D, Chiappetta C, Cristofano CD, et al. Elevated plasma copeptin levels identify the presence and severity of non-alcoholic fatty liver disease in obesity. BMC Med. (2019) 17:85. doi: 10.1186/s12916-019-1319-4
28. Muscogiuri G, Barrea L, Annunziata G, Vecchiarini M, Orio F, Somma CD, et al. Water intake keeps type 2 diabetes away? Focus on copeptin. Endocrine. (2018) 62:292–8. doi: 10.1007/s12020-018-1680-7
29. Padrão P, Sousa AS, Guerra RS, Alvares L, Santos A, Borges N, et al. A cross-sectional study on the association between 24-h urine osmolality and weight status in older adults. Nutrients. (2017) 9:1272. doi: 10.3390/nu9111272
30. Rosinger AY, Lawman HG, Akinbami LJ, Ogden CL. The role of obesity in the relation between total water intake and urine osmolality in US adults, 2009-2012. Am J Clin Nutr. (2016) 104:1554–61. doi: 10.3945/ajcn.116.137414
31. Chang T, Ravi N, Plegue MA, Sonneville KR, Davis MM. Inadequate Hydration, BMI, and Obesity Among US Adults: NHANES 2009-2012 [published correction appears in Ann Fam Med. (2020 Nov) 18:485]. Ann Fam Med. (2016) 14:320–324. doi: 10.1370/afm.1951
32. Carroll HA, Betts JA, Johnson L. An investigation into the relationship between plain water intake and glycated Hb (HbA1c): a sex-stratified, cross-sectional analysis of the UK National Diet and Nutrition Survey (2008-2012) [published online ahead of print, 2016 Nov 10]. Br J Nutr. (2016) 116:1–11. doi: 10.1017/S0007114516003688
33. Enhörning S, Bankir L, Bouby N, Hedblad B, Persson M, Morgenthaler NG, et al. Copeptin, a marker of vasopressin, in abdominal obesity, diabetes and microalbuminuria: the prospective Malmö Diet and Cancer Study cardiovascular cohort. Int J Obes (Lond). (2013) 37:598–603. doi: 10.1038/ijo.2012.88
34. Taveau C, Chollet C, Bichet DG, Velho G, Guillon G, Corbani M, et al. Acute and chronic hyperglycemic effects of vasopressin in normal rats: involvement of V1A receptors. Am J Physiol Endocrinol Metab. (2017) 312:E127–35. doi: 10.1152/ajpendo.00269.2016
35. Enhörning S, Vanhaecke T, Dolci A, Perrier ET, Melander O. Investigation of possible underlying mechanisms behind water-induced glucose reduction in adults with high copeptin. Sci Rep. (2021) 11:24481. doi: 10.1038/s41598-021-04224-5
36. Tanoue A, Ito S, Honda K, Oshikawa S, Kitagawa Y, Koshimizu TA, et al. The vasopressin V1b receptor critically regulates hypothalamic-pituitary-adrenal axis activity under both stress and resting conditions. J Clin Invest. (2004) 113:302–9. doi: 10.1172/JCI200419656
37. Sominsky L, Spencer SJ. Eating behavior and stress: a pathway to obesity. Front Psychol. (2014) 5:434. doi: 10.3389/fpsyg.2014.00434
38. Klatzkin RR, Nolan LJ, Kissileff HR. Self-reported emotional eaters consume more food under stress if they experience heightened stress reactivity and emotional relief from stress upon eating. Physiol Behav. (2022) 243:113638. doi: 10.1016/j.physbeh.2021.113638
39. Woodman R, Student J, Malcolm T, Miller C, Lockette W. Acetazolamide increases locomotion, exploratory behavior, and weight loss following social stress: A treatment for emotional eating? Metabol Open. (2020) 5:100023. doi: 10.1016/j.metop.2020.100023
40. Torres SJ, Nowson CA. Relationship between stress, eating behavior, and obesity. Nutrition. (2007) 23:887–94. doi: 10.1016/j.nut.2007.08.008
41. Greeno CG, Wing RR. Stress-induced eating. Psychol Bull. (1994) 115:444–64. doi: 10.1037/0033-2909.115.3.444
42. Andres-Hernando A, Jensen TJ, Kuwabara M, Orlicky DJ, Cicerchi C, Li N, et al. Vasopressin mediates fructose-induced metabolic syndrome by activating the V1b receptor. JCI Insight. (2021) 6:e140848. doi: 10.1172/jci.insight.140848
43. Johnson RJ, Stenvinkel P, Andrews P, Sanchez-Lozada LG, Nakagawa T, Gaucher E, et al. Fructose metabolism as a common evolutionary pathway of survival associated with climate change, food shortage and droughts. J Intern Med. (2020) 287:252–62. doi: 10.1111/joim.12993
44. Wolf JP, Nguyen NU, Dumoulin G, Berthelay S. Influence of hypertonic monosaccharide infusions on the release of plasma arginine vasopressin in normal humans. Horm Metab Res. (1992) 24:379–83. doi: 10.1055/s-2007-1003340
45. Song 宋志林 Z, Roncal-Jimenez CA, Lanaspa-Garcia MA, Oppelt SA, Kuwabara M, Jensen T, et al. Role of fructose and fructokinase in acute dehydration-induced vasopressin gene expression and secretion in mice. J Neurophysiol. (2017) 117:646–54. doi: 10.1152/jn.00781.2016
46. Stanhope KL, Schwarz JM, Keim NL, Griffen SC, Bremer AA, Graham JL, et al. Consuming fructose-sweetened, not glucose-sweetened, beverages increases visceral adiposity and lipids and decreases insulin sensitivity in overweight/obese humans. J Clin Invest. (2009) 119:1322–34. doi: 10.1172/JCI37385
47. Benarroch EE. Brain glucose transporters: implications for neurologic disease. Neurology. (2014) 82:1374–9. doi: 10.1212/WNL.0000000000000328
48. Hwang JJ, Johnson A, Cline G, Belfort-DeAguiar R, Snegovshkikh D, Khokhar B, et al. Fructose levels are markedly elevated in cerebrospinal fluid compared to plasma in pregnant women. PLoS ONE. (2015) 10:e0128582. doi: 10.1371/journal.pone.0128582
49. Hwang JJ, Jiang L, Hamza M, Dai F, Belfort-DeAguiar R, Cline G, et al. The human brain produces fructose from glucose. JCI Insight. (2017) 2:e90508. doi: 10.1172/jci.insight.90508
50. Malik VS, Hu FB. The role of sugar-sweetened beverages in the global epidemics of obesity and chronic diseases. Nat Rev Endocrinol. (2022) 18:205–18. doi: 10.1038/s41574-021-00627-6
51. Villamor E, Finan CC, Ramirez-Zea M. Roman AV) Nine Mesoamerican Countries Metabolic Syndrome Study (NiMeCoMeS) Group. Prevalence and sociodemographic correlates of metabolic syndrome in school-aged children and their parents in nine Mesoamerican countries. Public Health Nutr. (2017) 20:255–65. doi: 10.1017/S1368980016002342
52. Rivera-Andrade A, Kroker-Lobos MF, Lazo M, Freedman ND, Smith JW, Torres O, et al. High prevalence of non-alcoholic fatty liver disease and metabolic risk factors in Guatemala: A population-based study. Nutr Metab Cardiovasc Dis. (2019) 29:191–200. doi: 10.1016/j.numecd.2018.10.008
53. Chapman CL, Hess HW, Lucas RAI, Glaser J, Saran R, Bragg-Gresham J, et al. Occupational heat exposure and the risk of chronic kidney disease of nontraditional origin in the United States. Am J Physiol Regul Integr Comp Physiol. (2021) 321:R141–51. doi: 10.1152/ajpregu.00103.2021
54. Hansson E, Mansourian A, Farnaghi M, Petzold M, Jakobsson K. An ecological study of chronic kidney disease in five Mesoamerican countries: associations with crop and heat. BMC Public Health. (2021) 21:840. doi: 10.1186/s12889-021-10822-9
55. Torres C, Aragón A, González M, Lopez I, Jakobsson K, Elinder CG, et al. Decreased kidney function of unknown cause in Nicaragua: a community-based survey. Am J Kidney Dis. (2010) 55:485–96. doi: 10.1053/j.ajkd.2009.12.012
56. Peraza S, Wesseling C, Aragon A, Leiva R, Garcia-Trabanino RA, Torres C, et al. Decreased kidney function among agricultural workers in El Salvador. Am J Kidney Dis. (2012) 59:531–40. doi: 10.1053/j.ajkd.2011.11.039
57. Sanchez Polo V, Garcia-Trabanino R, Rodriguez G, Madero M. Mesoamerican Nephropathy (MeN): What We Know so Far. Int J Nephrol Renovasc Dis. (2020) 13:261–72. doi: 10.2147/IJNRD.S270709
58. Wesseling C, Glaser J, Rodríguez-Guzmán J, Weiss I, Lucas R, Peraza S, et al. Chronic kidney disease of non-traditional origin in Mesoamerica: a disease primarily driven by occupational heat stress. Rev Panam Salud Publica. (2020) 44:e15. doi: 10.26633/RPSP.2020.15
59. Vos MB, Kimmons JE, Gillespie C, Welsh J, Blanck HM. Dietary fructose consumption among US children and adults: the Third National Health and Nutrition Examination Survey. Medscape J Med. (2008) 10:160.
60. Guthrie JF, Morton JF. Food sources of added sweeteners in the diets of Americans. J Am Diet Assoc. (2000) 100:43–50. doi: 10.1016/S0002-8223(00)00018-3
61. Fisberg M, Kovalskys I, Gómez G, Rigotti A, Sanabria LYC, Garcia MCY, et al. Total and added sugar intake: assessment in eight Latin American Countries. Nutrients. (2018) 10:389. doi: 10.3390/nu10040389
62. Gersch MS, Mu W, Cirillo P, Reungjui S, Zhang L, Roncal C, et al. Fructose, but not dextrose, accelerates the progression of chronic kidney disease. Am J Physiol Renal Physiol. (2007) 293:F1256–61. doi: 10.1152/ajprenal.00181.2007
63. Nakayama T, Kosugi T, Gersch M, Connor T, Sanchez-Lozada LG, Lanaspa MA, et al. Dietary fructose causes tubulointerstitial injury in the normal rat kidney. Am J Physiol Renal Physiol. (2010) 298:F712–20. doi: 10.1152/ajprenal.00433.2009
64. Roncal Jimenez CA, Ishimoto T, Lanaspa MA, Rivard CJ, Nakagawa T, Ejaz AA, et al. Fructokinase activity mediates dehydration-induced renal injury. Kidney Int. (2014) 86:294–302. doi: 10.1038/ki.2013.492
65. Lanaspa MA, Ishimoto T, Cicerchi C, Tamura Y, Ronchal-Jimenez CA, Chen W, et al. Endogenous fructose production and fructokinase activation mediate renal injury in diabetic nephropathy. J Am Soc Nephrol. (2014) 25:2526–38. doi: 10.1681/ASN.2013080901
66. Milagres T, García-Arroyo FE, Lanaspa MA, Garcia G, Ishimoto T, Andres-Hernando A, et al. Rehydration with fructose worsens dehydration-induced renal damage. BMC Nephrol. (2018) 19:180. doi: 10.1186/s12882-018-0963-9
67. García-Arroyo FE, Tapia E, Blas-Marron MG, Gonzaga G, Silverio O, Cristobal M et al. Vasopressin mediates the renal damage induced by limited fructose rehydration in recurrently dehydrated rats. Int J Biol Sci. (2017) 13:961–75. doi: 10.7150/ijbs.20074
68. García-Arroyo FE, Tapia E, Muñoz-Jiménez I, Gonzaga-Sanchez G, Arellano-Buendia AS, Osorio-Alonso H, et al. Fluid intake restriction concomitant to sweetened beverages hydration induce kidney damage. Oxid Med Cell Longev. (2020) 2020:8850266. doi: 10.1155/2020/8850266
69. Kellum JA, Lameire N, KDIGO AKI Guideline Work Group. Diagnosis, evaluation, and management of acute kidney injury: a KDIGO summary (Part 1). Crit Care. (2013) 17:204. doi: 10.1186/cc11454
70. Chapman CL, Johnson BD, Sackett JR, Parker MD, Schlader ZJ. Soft drink consumption during and following exercise in the heat elevates biomarkers of acute kidney injury. Am J Physiol Regul Integr Comp Physiol. (2019) 316:R189–98. doi: 10.1152/ajpregu.00351.2018
71. Chapman CL, Grigoryan T, Vargas NT, Reed EL, Kueck PJ, Pietrfesa LD, et al. High-fructose corn syrup-sweetened soft drink consumption increases vascular resistance in the kidneys at rest and during sympathetic activation. Am J Physiol Renal Physiol. (2020) 318:F1053–65. doi: 10.1152/ajprenal.00374.2019
Keywords: fructose, vasopressin (ADH), Mesoamerican nephropathy, metabolic syndrome, high fructose corn syrup (HFCS)
Citation: Student J, Sowers J and Lockette W (2022) THIRSTY FOR FRUCTOSE: Arginine Vasopressin, Fructose, and the Pathogenesis of Metabolic and Renal Disease. Front. Cardiovasc. Med. 9:883365. doi: 10.3389/fcvm.2022.883365
Received: 25 February 2022; Accepted: 19 April 2022;
Published: 17 May 2022.
Edited by:
Sunil K. Panchal, Western Sydney University, AustraliaReviewed by:
Glenda Gobe, The University of Queensland, AustraliaLindsay Brown, Griffith University, Australia
Copyright © 2022 Student, Sowers and Lockette. This is an open-access article distributed under the terms of the Creative Commons Attribution License (CC BY). The use, distribution or reproduction in other forums is permitted, provided the original author(s) and the copyright owner(s) are credited and that the original publication in this journal is cited, in accordance with accepted academic practice. No use, distribution or reproduction is permitted which does not comply with these terms.
*Correspondence: Jeffrey Student, anRzMzc0QGRyZXhlbC5lZHU=