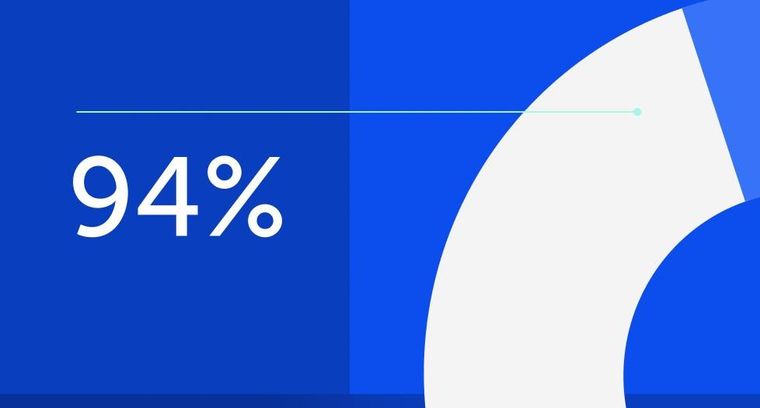
94% of researchers rate our articles as excellent or good
Learn more about the work of our research integrity team to safeguard the quality of each article we publish.
Find out more
REVIEW article
Front. Cardiovasc. Med., 16 March 2022
Sec. General Cardiovascular Medicine
Volume 9 - 2022 | https://doi.org/10.3389/fcvm.2022.856901
This article is part of the Research TopicHuman Microbiota: A key player in the etiology and pathophysiology of cardiovascular and metabolic diseasesView all 9 articles
The human microbiota contains microorganisms found on the skin, mucosal surfaces and in other tissues. The major component, the gut microbiota, can be influenced by diet, genetics, and environmental factors. Any change in its composition results in pathophysiological changes that can further influence the evolution of different conditions, including cardiovascular diseases (CVDs). The microbiome is a complex ecosystem and can be considered the metagenome of the microbiota. MicroRNAs (miRNAs) are speculated to interact with the intestinal microbiota for modulating gene expressions of the host. miRNAs represent a category of small non-coding RNAs, consisting of approximately 22 nucleotides, which can regulate gene expression at post-transcriptional level, by influencing the degradation of mRNA and modifying protein amounts. miRNAs display a multitude of roles, being able to influence the pathogenesis and progression of various diseases. Circulating miRNAs are stable against degradation, due to their enclosure into extracellular vesicles (EVs). This review aims to assess the current knowledge of the possible interactions between gut microbiota, miRNAs, and CVDs. As more scientific research is conducted, it can be speculated that personalized patient care in the future may include the management of gut microbiota composition and the targeted treatment against certain expression of miRNAs.
Gut microbiota, represented by bacteria, viruses, fungi, archaea and protozoa, accounts for approximately 95% of the human microbiota (1, 2). The structure of the gut microbiota is influenced by host genetics and environmental factors. Recent studies have shown that gut microbiota is not just a passive participant in our health, but it can also actively regulate our functions via genes, proteins and metabolites (2–4). Perturbation of the gut microbiota, dysbiosis, can lead to several diseases, not only gastrointestinal conditions, but also disorders of the lung, brain, heart and immune system (5–7). Dysbiosis has an important role in CVDs, especially by activating a proinflammatory status in the body and favoring the atherosclerotic process (8, 9). Gut microbiota can metabolize L-carnitine, choline and phosphatidylcholine and produce vasculotoxic metabolites, such as trimethylamine-N-oxide (TMAO), which is associated with the atherosclerotic process (10). A continuous low-grade inflammation status in the gut can facilitate the transit of bacteria and their metabolites into the bloodstream, thus maintaining chronic inflammation and favoring the development of atherosclerotic plaques, increasing the risk of coronary heart disease, stroke, and other acute complications (9, 11).
miRNAs are regulators in the host gene expression processes and are linked to specific bacteria (12). They enter bacterial cells through endocytosis, influencing gut microbiota (13) by stimulating or inhibiting the expansion of certain bacterial species (14, 15). In the miRNAs-gut microbiota connection, miRNAs have effects on proliferation and differentiation of intestinal cells, architecture and barrier function of the gut (16). Some studies have shown that the gut microbiota can have an impact on miRNAs, by modulating the expression of genes in the host (12, 17). Moreover, the link between miRNAs and gut microbiota has been underlined by studies showing that EVs produced by gut microbiota include various RNA species, including miRNAs, which may influence gene expression of the host cells (13, 18, 19).
In recent years, the association between circulating miRNAs and CVDs has been widely investigated to discover new possible diagnostic markers (20, 21). In CVDs, miRNAs-gut microbiota relationship is involved especially in the regulation of epithelial dysfunction (7). Gut microbiota can influence RNA genes affiliated with miRNAs classes, like vascular miR-204, miR-10B or miR-181 (22), lowering or increasing the risk of developing atherosclerosis (23–25). Due to recent discoveries about the involvement of miRNAs-gut microbiota association in CVDs and the characterization of miRNAs as new possible diagnostic markers, studies have highlighted the importance of new therapeutic strategies (26).
Dietary interventions represent an important therapy, especially the use of plant-derived miRNAs to modify the gut microbiota (27). For example, studies on mice have shown that ginger-derived exosomes contain miRNAs that can influence the gut microbiota by increasing the metabolism of fatty acids. Wang et al. observed an increase in species of the Bifidobacterium genus and SCFA-producing bacteria, reducing body weight, liver steatosis, low-grade inflammation and insulin resistance. This study underlined the possibility of using ginger supplementation as a dietary intervention in CVD (27, 28). The use of probiotics has increased in recent years, aiming to restore the balance of gut microbiota for the benefit of the host (29). The role of miRNAs in modulating gut microbiota underlined their possible implication in the molecular mechanism of action of probiotics (13, 14). This review summarizes the most important current knowledge about the interplay between gut microbiota and miRNAs in development of CVDs. Firstly, the importance of miRNAs-gut microbiota relationship is highlighted. Moreover, the role of the gut microbiota and miRNAs in CVDs was discussed in detail. The current therapeutic strategies and the importance of discovering new treatment options for maintaining the balance of gut microbiota and, therefore, the health of the host are also underlined.
miRNAs are small non-coding RNAs that consist of 18–23 nucleotides in length. RNA polymerase II transcribes miRNA in the nucleus, producing pre-miRNA. It is then carried to the cytoplasm, processed into mature forms and loaded onto the mi-RNA-induced silencing complex (miRISC). By hybridizing with the 3′ untranslated region of the target gene, it promotes messenger ribonucleic acid (mRNA) degradation or inhibit translation (12, 30, 31).
Many studies have highlighted the connection between miRNAs and gut microbiota, especially in case of dysbiosis, which is leading to dysfunction of the intestinal epithelial barrier and inflammatory response (32). miRNAs are particularly involved in the regulation of the intestinal epithelial cells (IEC) (33).
It has been shown that expression of miRNAs is different between small and large IECs (34) and that miRNA-microbiota abundance has an inverse correlation (13). IECs can produce fecal miRNAs that target bacterial mRNA, controlling the destruction or dysregulation of their expression (13). In the dendritic cells of mice suffering from colitis, commensal bacteria can down-regulate the expression of miRNAs, for example miR-10a (13). Studies on mice have shown that expression of miRNAs is also different between colonized mice and germ-free mice (35), influencing intestinal-related diseases. Some studies (36–38) have shown that fecal miRNAs have the ability to influence the gut microbiota composition.
miR-375 has an important role in the production of the mucus layer in epithelial cells and influences the proliferation of stem cells in the intestinal epithelium (39, 40). The suppression of miR-381-3p is leading to proliferation of the IECs mediated by nuclear receptor-related protein, while the intestinal barrier function is being enhanced (41).
Gut microbiota is represented by the multitude of microbes within the human intestinal tract, while gut microbiome consists of the totality of their genetic properties. The gut microbiota can produce biologically active metabolites, which can further influence host physiological processes. Alterations in the microbial population can lead to multiple diseases, including CVDs (42, 43).
Dysbiosis, intestinal inflammation and altered gut barrier can result in high concentrations of bacterial structural elements and microbial metabolites in the circulation, including trimethylamine N-oxide (TMAO) and short-chain fatty acids, which can further favor the evolution of CVDs (44). Low concentrations of microbes which produce butyrate have been associated with heart failure and coronary artery disease (45).
Blood levels of TMAO and phenylacetylglutamine, gut microbiota-dependent metabolites, have been linked to incident CVDs risks after several clinical studies. Phenylacetylglutamine has the potential to favor negative cardiovascular phenotypes in hosts, by acting on adrenergic receptors. TMAO is a metabolite frequently found in Western diets, rich in lecithin, choline (46), phosphatidylcholine (46, 47) and carnitine (48), which predicted the cardiovascular risk in some clinical studies and amplified the atherosclerotic process in animal models (43, 46). In many studies, a plasma value of TMAO over 6 μM anticipated a high risk of adverse cardiac events (49). The TMAO pathway and its effect on CVDs are illustrated in Figure 1.
Figure 1. TMAO pathway. Dietary precursors, like carnitine, choline and phosphatidylcholine are metabolized by gut microbiota into trimethylamine (TMA), which will interact with flavin monooxygenases (FMOs) in the liver, producing trimethylamine N-oxide (TMAO), an important factor in the pathogenesis of CVD. Created with BioRender.com (Last accessed on 16 January 2022).
Circulating TMAO levels are influenced by diet, gut microbial composition and renal function. Several studies indicate a dose-dependent association between meat ingestion and cardiovascular risk and mortality (50). Circulating TMAO concentrations have been linked to CVDs and anticipated prognosis in peripheral artery disease (51), coronary artery disease (52), acute coronary syndromes (52–54) and heart failure (55–59).
The human gut hosts about 1,000 microbial species (60), with two important phyla, Bacteroidetes and Firmicutes, representing the majority, along with Actinobacteria, Cyanobacteria, Fusobacteria, Proteobacteria and Verrucomicrobia (60–62). When disturbances of the immune system occur, levels of facultative anaerobes rise and change the ratio of Bacteroidetes to Firmicutes phyla, with a significant decline in Bifidobacteria (63).
Liu et al. (38) found that a specific subtype of miRNA has a specific role on gut microbiota: miR-515-5p can influence the growth of Fusobacterium nucleatum and miR-1226-5p can stimulate the growth of Escherichia coli. Other studies have underlined that gut microbiota and pathogenic microorganisms have different effects on different types of miRNAs, for example miR-203, miR-483-3p and miR-595 are inhibited by enteropathogenic Escherichia Coli, leading to an intestinal inflammatory status by disrupting the tight junction proteins of the intestinal epithelium (64). miR-182, miR-503 and miR-17-92 can increase the risk of malignancy in correlation with the existence of several taxa, like Proteobacteria, Firmicutes and Bacteroidetes (13, 65). Bitar et al. found miR-155 and miR-144a in IECs of patients with Vibrio cholerae infection in the acute stage, inhibiting the immune system at intestinal level and lowering cytokine secretion (39). Li X et al. have shown that miR-301b can inhibit the secretion of anti-inflammatory cytokines IL-4 and TGF-β1 in patients with Pseudomonas aeruginosa infection (66). miR-143-3p increases the risk of gastric malignancy in Helicobacter pylori positive patients by inhibiting AKT2, a gene that stimulates cell apoptosis and inhibits tumor growth, migration, and invasion (67). Also, pathogenic microorganisms have the ability to influence miRNAs in hosts. For example, miR-23a-5p can inhibit Mycobacterium tuberculosis-induced autophagy in macrophages, assuring bacterial survival (68).
Recently, studies have underlined the involvement of miRNAs in intercellular communication through a RNA delivery system controlled by EVs (13). Gut microbiota can produce and secrete EVs, especially exosomes, which carry genetic material. miRNAs derived from exosomes are involved especially in the communication between dendritic cells, influencing gene expression (19, 69, 70). Dai et al. showed that the downregulation of some bacterial carriers, via miR-193a-3p, is reducing colonic inflammation (71).
By influencing expression of miRNAs in other organs, inflammation of the gut can lead to many diseases, including CVDs (26). For example, gut microbiota can stimulate miR-204, via the signal transducer and activator of transcription three signaling pathway, inhibiting its target gene, Sirtuin 1. This will lead to a lower endothelium-dependent vasorelaxation and atherosclerosis (23). Moreover, studies have shown that anthocyanins reduce atherosclerosis and stimulate cholesterol efflux from macrophages. Gut microbiota can metabolize cyanidin-3 to 0-β-glucoside and produce protocatechuic acid (PCA), the most important constituent of anthocyanins. PCA can inhibit the expression of miR-10b in macrophages and reduce the atherosclerotic process, by stimulating a reverse cholesterol transport (22, 24).
Since their discovery in 1993 by Lee et al. (72, 73), miRNAs have been known as important regulators of various biological functions, also being involved in the pathogenesis of CVDs (74, 75). miRNAs are found in cardiac tissue through all phases of organ development. miR-21, miR-29a, miR-129, miR-210, miR-211, miR-320, miR-423 and let-7c have been identified in fetal heart tissue (74). The most abundant miRNAs in cardiac tissue are miR-1, let-7, miR-133, miR-126-3p, miR-30c and miR-26a (76), while in arteries the most frequent miRNAs are miR-145, let-7, miR-125b, miR-125a, miR-23 and miR-143 (77). In the human heart, several other miRNAs have been often identified: miR-16, miR-100, miR-125 a/b, miR-145, miR-195, miR-199*, miR-20a/b, miR-21, miR-24, miR-23, miR-29a/b, miR-27a/b, miR-30a/b/c, miR-92a/b and miR-99 (78, 79).
Gut microbiota and its metabolites influence many cardiovascular phenotypes, such as atherosclerosis, platelet reactivity, thrombosis, hypertension, lipid and glucose metabolism and vascular inflammation, as we summarized in Figure 2.
Figure 2. Gut microbiota, its metabolites and their effect on CVDs. TMA, trimethylamine; TMAO, trimethylamine N-oxide; PAG, phenylacetylglutamine; SCFAs, short-chain fatty acid; LPS, lipopolysaccharide. Created with BioRender.com (Last accessed on 16 January 2022).
Atherosclerosis is a complex progressive process, involving dysfunction of endothelial cells, vascular smooth muscle cells differentiation, infiltration with inflammatory cells and subendothelial accumulation of lipids (74, 80).
miR-126-5p maintains a proliferative reserve in endothelial cells by inhibiting the Notch1 inhibitor delta-like homolog 1. Decreased levels of miR-126-5p lower the capacity of proliferation of endothelial cells, losing the ability to protect against the formation of atherosclerotic lesions. A novel possible curative perspective could be the administration of miR-126-5p, which improved the proliferation of endothelial cells in certain locations and restricted atherosclerosis, according to Schober et al. (81).
miR-155, a possible prognostic factor and therapeutic target, represses anti-inflammatory signaling in macrophages. Low concentrations of miR-155 are found during the reversion of atherosclerosis in vivo. Furthermore, miR-155 is found in high concentrations in urinary EVs in patients with unstable coronary artery disease (82). Moreover, Zhao et al. have shown that in ovariectomized mice with consecutive estrogen related metabolic syndrome, changes of lumen diameters in common carotid artery, left ventricle ejection fraction and fractional shortening was significantly lower than in sham-operated group, but they registered higher common carotid artery thickness. They observed that miR-155 and let-7g were upregulated in the intestinal epithelium and feces and endocytosed by bacteria, modulating the microbiota and cardiovascular function in the same way as in ovariectomized mice (15).
Another important step in atherosclerosis is vascular smooth muscle cells migration from the media to the intima as a reaction to infiltration with inflammatory cells (74). Some miRNAs are involved in this step of the process. miR-145 is vastly expressed in vascular smooth muscle cells (83). miR-143/145 complex has an important role in influencing the differentiation and plasticity of vascular smooth muscle cells (74, 83, 84). The development of the atherosclerotic plaques was diminished, while the plaque stability was higher in ApoE knockout mice that received vascular smooth muscle cells-targeted miR-145 therapy (83). In Figure 3 are illustrated the most important processes involved in the evolution of atherosclerosis and their association with different subtypes of miRNAs.
Figure 3. miRNAs association with fundamental processes involved in the evolution of atherosclerosis. Created with BioRender.com (Last accessed on 16 January 2022).
Any infectious status will impact atherosclerosis, as it is an inflammatory condition. There are two major mechanisms involved: direct infection of the blood vessel wall or through an indirect mechanism, via infections of other sites, generating proinflammatory mediators within a systemic immune response, which will further influence the growth of the atherosclerotic plaque (85). A high risk of CVDs has been associated with the presence of Chlamydophila pneumoniae, Porphyromonas gingivalis, Helicobacter pylori, Influenza A virus, Hepatitis C virus, cytomegalovirus and human immunodeficiency virus (86).
TMAO is an intestinal metabolite of choline and phosphatidylcholine. Flavin monooxygenases 3 is a regulator of TMAO synthesis, being adjusted by farnesoid X receptor (FXR), whose levels are increased by bile acids. TMAO contributes to atherogenesis by blocking reverse cholesterol transport, leading to low cholesterol clearance from peripheral macrophages. TMAO also alters the protective properties of high-density lipoproteins (44). TMAO increases platelet hyperreactivity by amplifying stimulus-dependent release of calcium from intracellular sites, elevating the risk of thrombosis (87).
Bile acids influence the cardiac function through different mechanisms. They interact with plasma membrane G-protein-coupled receptors and nuclear receptors (FXR) and can modify the contractility of cardiomyocytes and the electrical properties (reducing heart rate by influencing channel conduction and calcium transport). Bile acids also play important roles in lipid metabolism, plaque formation, vasodilation and neovascularization of wounded organs. Conditions associated with high serum concentrations of bile acids, such as advanced liver disease, obstructive jaundice, intrahepatic cholestasis, are associated with abnormal vascular dynamics (88).
The role of miRNAs in hypertension was underlined by several studies and experimental results, especially by interfering with the renin-angiotensin-aldosterone system (89).
miRNAs can regulate renin expression, according to Marques et al. Several miRNAs found in human hypertensive kidneys (hsa-let-7c, hsa-miR-181a, hsa-miR-663) bind to the 3′ untranslated region and regulate AIFM1 (apoptosis-inducing factor mitochondrion-associated 1), APOE (apolipoprotein E), REN (renin) and NR4A2 (nuclear receptor subfamily four group A member 2) mRNA (90). Low levels of hsa-miR-181a and hsa-miR-663 in hypertensive kidneys can be an explanation of higher renin mRNA (91). REN mRNA overexpression and the downregulation of two miRNAs (hsa-miR-181 and hsa-miR-663) which influence REN mRNA levels indicate the involvement of renin in the etiology of hypertension (91).
In the renal medulla, SIK1 (salt-inducible kinase 1) can elevate blood pressure by intensifying active cell sodium transport, as a response to angiotensin II. In hypertensive animal subjects, the activity of SIK1 is increased in the cells from the proximal tubule. As a response to angiotensin II, SIK1 can activate NR4A2 (from the nuclear transcription factor family of NR4A), which has a role in cytokine modulation and may therefore lead to inflammation in the renal medulla in hypertensive subjects (91).
miR-155 targets hypertension-associated SNP (rs5186) in the angiotensin II type 1 receptor 3′ untranslated region. High levels of angiotensin II type 1 receptor in young hypertensive homozygous subjects for the rs5186 C allele correlate positively with blood pressure and negatively with hsa-miR-155 expression (91).
miRNAs regulate gene expressions in cellular responses to vasopressors, such as angiotensin II. miR-132 and miR-212 are transcribed together, regulated by cAMP response element binding protein, which is an angiotensin II-controlled gene (91).
miR-132 and miR-212 were found to be up-regulated in the left ventricle, aorta and kidneys of animals who received infusion with angiotensin-II for a period of 10 days and down-regulated at the level of the internal mammary artery of patients who received angiotensin-II receptor type 1 blockers. This indicates that miR-132 and miR-212 are associated with angiotensin II-induced Gαq-signaling pathway, generating hypertension (74, 92). Eskildsen et al. concluded that miR-132 and miR-212, found in human arteries from patients who underwent bypass surgeries and who were treated with angiotensin II receptor 1 blockers, were in low concentrations, compared to patients treated with beta blockers, which had no effect (91). As a conclusion, miR-132 and miR-212 are involved in angiotensin II induced hypertension (91).
miR-130 and miR-195 in the blood were correlated with hypertension in a study conducted by Karolina et al. (92). miR-197, miR-23a and miR-509-5p contribute to dyslipidemia in metabolic syndrome. miR-150, miR-192, miR-27a, miR-320a and miR-375 were up-regulated in subjects with metabolic syndrome and type 2 diabetes (93).
Santovito et al. concluded that miR-145 was overexpressed in atherosclerotic plaques of hypertensive patients compared to the control group. Downregulation of miR-145 was correlated with vascular smooth muscle cell dedifferentiation, essential for atherosclerosis. Subjects treated with angiotensin II receptor blockers were associated with a maximum elevation of miR-145 concentrations, but this concept did not have statistical significance due to the small sample size (93).
Cardiac structural modifications allow the heart to adapt to various hemodynamic conditions. Chronic triggering of cardiac remodeling (myocyte hypertrophy, fibrosis) can lead to cardiomyopathies and heart failure (74).
miR-1 and miR-133, muscle-specific miRNAs, expressed in cardiac and skeletal muscles, are able to influence the differentiation and proliferation of muscle cells. They are the most frequent miRNAs in the cardiac tissue (76, 78, 94).
The role of miRNAs in the physiological functioning of the adult heart has been validated with Dicer (RNase enzyme involved in miRNA processing, cleaving pre-miRNAs into miRNAs duplexes) deletion. This deletion led to dilation and fibrosis of both ventricles, hypertrophy of cardiac myocytes, initiation of fetal transcriptome programme and sudden death, underlining the influence of miRNAs on cardiac remodeling (miR-133, miR-1) (74).
In both animal and human models with cardiac hypertrophy, low levels of miR-133 and miR-1, that are included in the same transcriptional unit, were noticed. They are considered key regulators of cardiac hypertrophy. In vitro overexpression of miR-133 and miR-1 suppressed cardiac hypertrophy, according to Carè et al. In vivo repression of miR-133 by using antagomir (miRNA antagonist) infusion led to important and prolonged cardiac hypertrophy. Specific targets of miR-133 are: RhoA, a GDP-GTP exchange protein controlling cardiac hypertrophy; Cdc42, a signal transduction kinase involved in hypertrophy, and Nelf-A/WHSC2, a nuclear factor with role in cardiogenesis (95).
miRNAs as new potential therapeutic targets started to be investigated since miRNAs that inhibit contractility are upregulated in heart failure patients (96). High levels of endogenous miR-25 reduced the cardiac function, while antagomiRs targeting miR-25 restored it, with consequential enhanced survival, compared to the control group in an animal model study. Altered calcium uptake during excitation-contraction coupling in cardiomyocytes comes from low expression and activity of SERCA2a (a ATPase involved in calcium transport), which is a feature of heart failure pathophysiological events (96, 97).
According to Gurha et al., a targeted deletion of miRNA-22 favored stress-induced cardiac dilation and contractile impairment. miR-22-deficient animal models had altered inotropic and lusitropic responses to acute stress tests with dobutamine. Genetic ablation of miR-22 resulted in low cardiac expression of SERCA2a. Muscle-restricted genes encrypting proteins in the proximity of the cardiac Z disk/titin cytoskeleton were also a result of the ablation of miR-22. In the absence of miR-22, there was an elevated expression of transcriptional/translational repressor purine-rich element binding protein B, which withstands control of sarcomeric/cardiac expression by serum response factor (98).
However, miRNAs can be regarded as paracrine signaling mediators. Cardiac fibroblasts secrete exosomes which contain miRNAs, “star” miRNAs (miRNA passenger strands, strands that are usually broken down within a cell) more precisely (99). Fibroblast-derived miR-21 can function as an essential paracrine signaling mediator in cardiomyocyte hypertrophy, making miR-21 a new possible target in heart failure treatment (99). Moreover, Thum et al. stated that miRNA-21 can contribute to myocardial disease by stimulating the MAP kinase signaling in fibroblasts, with effects on global cardiac structure and function. In the fibroblasts of failing hearts, miR-21 levels are higher, amplifying the ERK-MAP kinase activity by blocking sprouty homolog 1 (Spry 1) and modulating fibroblast survival and growth factor secretion (with impact on interstitial fibrosis and cardiac hypertrophy). Suppressing miR-21 using targeted antagomiR in animal models with induced pressure overload disease diminished the activity of cardiac ERK-MAP kinase, suppressed interstitial fibrosis and decreased cardiac dysfunction (100).
High levels of miRNAs that suppress SERCA2a can be found in heart failure cases, which can further lead to weakened cardiac function. In conclusion, miRNAs that downregulate SERCA2a can be in elevated levels in heart failure and consequently damage cardiac function (96).
In patients with heart failure, the gut microbiome is characterized by low levels of Ruminococcaceae family, of Blautia (Lachnospiracea family) (101) and of Faecalibacterium prausnitzii (102). Relative diminished populations of Eubacterium rectale and Dorea longicatena (Lachnospiraceae family) and Faecalibacterium (Ruminococcaceae family) were also observed in older patients (103). These results indicate decreased levels of butyrate-producing microorganisms (belonging to Lachnospiraceae or Ruminococcaceae families) in heart failure patients (45). Circulating TMAO levels have predicted prognosis in patients with peripheral artery disease (51), coronary artery disease (52), acute coronary syndrome (52–54) and heart failure (55–59).
In cardiac rhythm abnormalities, several miRNAs were noted to be involved (104).
miRNAs can influence the characteristics of cardiac physiology and excitability (104). The focus of several studies was on miR-1 and miR-133, as they are some of the most frequently expressed miRNAs in cardiac tissue (79, 94).
For instance, in patients with atrial fibrillation, miR-1 concentrations are severely low in the atrial tissue. Overexpression of miR-328 amplified the vulnerability to develop atrial fibrillation, decreased L-type calcium current and reduced the atrial action potential duration (105). The use of antagomiR for miR-328 overturned these conditions. miR-1 has been involved in the adjustment of various calcium-handling proteins (calmodulin, phospholamban, Na+/Ca2+ exchanger, sorcin, junction, triadin), leading to shorter refractory period of sarcoplasmic reticulum Ca2+ release (104) and has been connected to ventricular arrhythmias (74).
There are several miRNAs involved in the electrical and structural changes in atrial fibrillation (Table 1).
A new possible therapeutic target for ischemic heart disease was suggested by the results communicated by Ren et al. Overexpression of miR-320 promoted the apoptosis of cardiac cells and expanded the infarction size in ischemia-reperfusion cases in vivo and ex vivo, compared to wild-type controls. The heat-shock protein 20 (Hsp20), a cardioprotective protein, is a significant target for miR-320. miR-320 acts as a negative regulator of Hsp20 translation. During the ischemia-reperfusion in vivo and ex vivo, the expression of Hsp20 was notably higher after 1 h from reperfusion and 24 h from reperfusion (in vivo). This was negatively correlated with low expression of miR-320 in vivo and ex vivo (in cardiomyocytes). To sum up, expression of miR-320 can be correlated with Hsp20 levels in the cardiac tissue, while miR-320 can reliably suppress expression of Hsp20 in vivo and ex vivo (122).
As miRNAs can be found in the bloodstream, they can be regarded as new biomarkers, especially for myocardial infarction and heart failure (74). The role of cardiac troponins in the diagnosis of acute coronary syndromes is not likely to be outperformed, however miRNAs may be relevant in completing existing prediction patterns and as prognostic markers after acute coronary syndromes (74). Devaux et al. investigated the concentrations of some miRNAs in a large group of patients with acute chest pain. Levels of miR-208b, miR-499 and miR-320a were significantly elevated in patients with acute myocardial infarction in comparison with other diagnoses. However, no miRNA was able to surpass high sensitive troponin T or amplify their diagnostic capacity. Moreover, none of the miRNAs had an important prognosis accuracy (74, 123).
Circulating miRNAs were assessed for the potential role as circulating biomarkers. miR-126 had a positive association with incident myocardial infarction, while miR-223 and miR-197 were inversely related to the risk of myocardial infarction (124).
As a conclusion, we listed the miRNAs involved in different CVDs in Table 2 and illustrated the relationship between gut microbiota, miRNAs and CVDs in Figure 4.
Figure 4. The relationship between gut microbiota, miRNAs, and CVDs. Different types of miRNAs and gut microbiota metabolites can influence each other, influencing the regulation of epithelial dysfunction and leading to many CVDs.
The concept of therapies based on miRNAs is gaining more and more attention, as synthetic antagonists of miRNAs are studied already in the treatment of various diseases, such as hepatitis C virus infection, dyslipidemia or heart failure (74). Cardiovascular afflictions are no exception, progress and research being made in this field as well, for example for dyslipidemia or heart failure (74).
Targeting the gut microbiome in patients with CVDs may improve clinical outcomes and prognosis. Proper fiber intake may reduce low-density lipoprotein (LDL) concentrations, lowering the risk of CVDs and coronary heart disease. Water soluble fibers, such as beta-glucan, psyllium, pectin, guar gum were the most successful in lowering serum LDL cholesterol levels, while not altering high density lipoprotein (HDL) concentrations (128). Whole grain consumption has been inversely associated with metabolic syndrome (p = 0.005) and mortality from cardiovascular conditions (p = 0.04) (129).
The influence of diet modifications on miRNAs is illustrated in Figure 5.
Figure 5. Dietary influence on miRNAs. Dietary food components can influence gene expression, modifying the pathogenesis of different diseases, like cancer, obesity, and CVDs. Created with BioRender.com (Last accessed on 12 January 2022).
Many studies have underlined the positive effects of the probiotics on gut homeostasis. As we mentioned above, miRNAs also influence the gut microbiota. Considering that, recent studies have tried to understand and demonstrate the hypothesis that miRNAs could have an important role in how probiotics regulate gut homeostasis and influence gastrointestinal conditions or extra-intestinal disorders, including CVDs (27).
The beneficial effects of the probiotics are well known, as they produce bacteriocins, lower pH and compete with pathogenic microorganisms, in order to restore and stabilize the gut microbiota (130, 131). For example, organic acids (produced by Lactobacillus and Bifidobacteria probiotic strains) and bacteriocins can lower intestinal pH and inhibit the growth of the pathogenic bacteria (132). Also, the same probiotic bacteria mentioned above can improve the intestinal barrier function, by upregulating the genes that are controlling the mucus secretion (133, 134) and can influence the IECs proliferation (135).
Due to recent discoveries, it is now clear that gut microbiota is very responsive to the influence of probiotics, regulating general health and organic disorders, including CVDs, especially atherosclerosis and its acute consequences (coronary heart disease and stroke) (9) and hypertension (26). Some studies have shown that probiotics can modify the levels of metabolites, DNA, and components of the immune system, can activate immune cells, stimulate the production of immunoglobulins and regulate cytokines, demonstrating anti-inflammatory and immune effects in the host (134, 136). However, the connection between probiotics, miRNA, gut microbiota and organic diseases is still not well known, being a relatively novel field of research that needs further investigations.
Another therapeutic option, which seems to be more and more studied recently, is represented by fecal miRNAs and their influence on gut microbiota. According to Liu et al., fecal miRNAs produced by IECs and Hopx+ cells can cross the bacterial wall and control specific gene transcripts, influencing their growth. Mice with lower levels of IEC-derived miRNAs develop dysbiosis and fecal miRNAs transplantation can reestablish the microbiota composition in these cases (26, 38).
Cardiovascular diseases are the leading cause of mortality worldwide. There is constant emerging evidence regarding new possible interactions between miRNAs, gut microbiota and cardiovascular diseases. miRNAs may represent important diagnostic and/or prognostic markers, nevertheless large studies are needed to determine the suitable target population and to identify reliable, standardized and consistent methods for the quantification of circulating miRNAs. miRNA-based therapies in cardiovascular medicine need to assure a safe and targeted delivery in patients, constituting attractive new treatment options. Besides dietary interventions and probiotics, new possible therapeutic methods that may target the composition of gut microbiota for the treatment or prevention of cardiovascular diseases constitute a promising direction of investigation.
All authors listed have made a substantial, direct, and intellectual contribution to the work and approved it for publication.
The authors declare that the research was conducted in the absence of any commercial or financial relationships that could be construed as a potential conflict of interest.
All claims expressed in this article are solely those of the authors and do not necessarily represent those of their affiliated organizations, or those of the publisher, the editors and the reviewers. Any product that may be evaluated in this article, or claim that may be made by its manufacturer, is not guaranteed or endorsed by the publisher.
1. Friedland RP, Chapman MR. The role of microbial amyloid in neurodegeneration. PLoS Pathog. (2017) 13:e1006654. doi: 10.1371/journal.ppat.1006654
2. Shukla SD, Budden KF, Neal R, Hansbro PM. Microbiome effects on immunity, health and disease in the lung. Clin Transl Immunology. (2017) 6:e133. doi: 10.1038/cti.2017.6
3. Van de Wiele T, Van Praet JT, Marzorati M, Drennan MB, Elewaut D. How the microbiota shapes rheumatic diseases. Nat Rev Rheumatol. (2016) 12:398–411. doi: 10.1038/nrrheum.2016.85
4. Lynch JB, Hsiao EY. Microbiomes as sources of emergent host phenotypes. Science. (2019) 365:1405–9. doi: 10.1126/science.aay0240
5. Chang CS, Kao CY. Current understanding of the gut microbiota shaping mechanisms. J Biomed Sci. (2019) 26:59. doi: 10.1186/s12929-019-0554-5
6. Qin Y, Wade PA. Crosstalk between the microbiome and epigenome: messages from bugs. J Biochem. (2018) 163:105–12. doi: 10.1093/jb/mvx080
7. Li M, Chen WD, Wang YD. The roles of the gut microbiota-miRNA interaction in the host pathophysiology. Mol Med. (2020) 26:101. doi: 10.1186/s10020-020-00234-7
8. Ionescu RF, Boroghina SC, Cretoiu SM. Is there a link between the gut microbiome and arterial hypertension? J Hypertens Res. (2021) 7:12–7. Available online at: http://www.hypertens.org/images/202103/jhr-202103-070103.pdf
9. Cretoiu D, Ionescu RF, Enache RM, Cretoiu SM, Voinea SC. Gut microbiome, functional food, atherosclerosis, and vascular calcifications-is there a missing link? Microorganisms. (2021) 9:1913. doi: 10.3390/microorganisms9091913
10. Ding L, Chang M, Guo Y, Zhang L, Xue C, Yanagita T, et al. Trimethylamine-N-oxide (TMAO)-induced atherosclerosis is associated with bile acid metabolism. Lipids Health Dis. (2018) 17:286. doi: 10.1186/s12944-018-0939-6
11. Komaroff AL. The microbiome and risk for atherosclerosis. JAMA. (2018) 319:2381. doi: 10.1001/jama.2018.5240
12. Hasan N, Yang H. Factors affecting the composition of the gut microbiota, and its modulation. PeerJ. (2019) 7:e7502. doi: 10.7717/peerj.7502
13. Behrouzi A, Ashrafian F, Mazaheri H, Lari A, Nouri M, Rad FR, et al. The importance of interaction between MicroRNAs and gut microbiota in several pathways. Microb Pathog. (2020) 144:104200. doi: 10.1016/j.micpath.2020.104200
14. Spinler JK, Karri V, Hirschi KD. Planting the microbiome. Trends Microbiol. (2019) 27:90–3. doi: 10.1016/j.tim.2018.12.001
15. Zhao L, Zhou X, Cai W, Shi R, Yang G, Yuan L. Host intestinal epithelium derived MIRNAS shape the microbiota and its implication in cardiovascular diseases. J Am Coll Cardiol. (2017) 69:1075. doi: 10.1016/S0735-1097(17)34464-9
16. Ding S, Liu G, Jiang H, Fang J. MicroRNA determines the fate of intestinal epithelial cell differentiation and regulates intestinal diseases. Curr Protein Pept Sci. (2019) 20:666–73. doi: 10.2174/1389203720666190125110626
17. Watson AJM, Hall LJ. Regulation of host gene expression by gut microbiota. Gastroenterology. (2013) 144:841–4. doi: 10.1053/j.gastro.2013.02.028
18. Sarshar M, Scribano D, Ambrosi C, Palamara AT, Masotti A. Fecal microRNAs as innovative biomarkers of intestinal diseases and effective players in host-microbiome interactions. Cancers. (2020) 12:2174. doi: 10.3390/cancers12082174
19. Gaspar BS, Ionescu RF, Enache RM, Dobrica EC, Cretoiu SM, Cretoiu D, et al. Extracellular Vesicles as Intercellular Communication Vehicles in Regenerative Medicine. Available online at: https://www.intechopen.com/online-first/79775 (accessed January 10, 2022.
20. Chen X, Ba Y, Ma L, Cai X, Yin Y, Wang K, et al. Characterization of microRNAs in serum: a novel class of biomarkers for diagnosis of cancer and other diseases. Cell Res. (2008) 18:997–1006. doi: 10.1038/cr.2008.282
21. Creemers EE, Tijsen AJ, Pinto YM. Circulating microRNAs: novel biomarkers and extracellular communicators in cardiovascular disease? Circ Res. (2012) 110:483–95. doi: 10.1161/CIRCRESAHA.111.247452
22. Genecards.org (2022). Available online at: https://www.genecards.org/cgi-bin/carddisp.pl?gene=MIR10B (accessed January 16, 2022).
23. Davignon J, Ganz P. Role of endothelial dysfunction in atherosclerosis. Circulation. (2004) 109:III27–32. doi: 10.1161/01.CIR.0000131515.03336.f8
24. Wang D, Xia M, Yan X, Li D, Wang L, Xu Y, et al. Gut microbiota metabolism of anthocyanin promotes reverse cholesterol transport in mice via repressing miRNA-10b. Circ Res. (2012) 111:967–81. doi: 10.1161/CIRCRESAHA.112.266502
25. Bodogai M, O'Connell J, Kim K, Kim Y, Moritoh K, Chen C, et al. Commensal bacteria contribute to insulin resistance in aging by activating innate B1a cells. Sci Transl Med. (2018) 10:eaat4271. doi: 10.1126/scitranslmed.aat4271
26. Zhao Y, Zeng Y, Zeng D, Wang H, Zhou M, Sun N, et al. Probiotics and microRNA: their roles in the host-microbe interactions. Front Microbiol. (2020) 11:604462. doi: 10.3389/fmicb.2020.604462
27. Nagai M, Obata Y, Takahashi D, Hase K. Fine-tuning of the mucosal barrier and metabolic systems using the diet-microbial metabolite axis. Int Immunopharmacol. (2016) 37:79–86. doi: 10.1016/j.intimp.2016.04.001
28. Wang J, Wang P, Li D, Hu X, Chen F. Beneficial effects of ginger on prevention of obesity through modulation of gut microbiota in mice. Eur J Nutr. (2020) 59:699–718. doi: 10.1007/s00394-019-01938-1
29. Hill C, Guarner F, Reid G, Gibson GR, Merenstein DJ, Pot B, et al. Expert consensus document. The International Scientific Association for Probiotics and Prebiotics consensus statement on the scope and appropriate use of the term probiotic: expert consensus document. Nat Rev Gastroenterol Hepatol. (2014) 11:506–14. doi: 10.1038/nrgastro.2014.66
30. Djuranovic S, Nahvi A, Green R. miRNA-mediated gene silencing by translational repression followed by mRNA deadenylation and decay. Science. (2012) 336:237–40. doi: 10.1126/science.1215691
32. DeGruttola AK, Low D, Mizoguchi A, Mizoguchi E. Current understanding of dysbiosis in disease in human and animal models. Inflamm Bowel Dis. (2016) 22:1137–50. doi: 10.1097/MIB.0000000000000750
33. Ohland CL, Macnaughton WK. Probiotic bacteria and intestinal epithelial barrier function. Am J Physiol Gastrointest Liver Physiol. (2010) 298:G807–19. doi: 10.1152/ajpgi.00243.2009
34. Lee J, Park EJ, Yuki Y, Ahmad S, Mizuguchi K, Ishii KJ, et al. Profiles of microRNA networks in intestinal epithelial cells in a mouse model of colitis. Sci Rep. (2015) 5:18174. doi: 10.1038/srep18174
35. Singh N, Shirdel EA, Waldron L, Zhang RH, Jurisica I, Comelli EM. The murine caecal microRNA signature depends on the presence of the endogenous microbiota. Int J Biol Sci. (2012) 8:171–86. doi: 10.7150/ijbs.8.171
36. Ahmed FE, Jeffries CD, Vos PW, Flake G, Nuovo GJ, Sinar DR, et al. Diagnostic microRNA markers for screening sporadic human colon cancer and active ulcerative colitis in stool and tissue. Cancer Genomics Proteomics. (2009) 6:281–95.
37. Link A, Becker V, Goel A, Wex T, Malfertheiner P. Feasibility of fecal microRNAs as novel biomarkers for pancreatic cancer. PLoS ONE. (2012) 7:e42933. doi: 10.1371/journal.pone.0042933
38. Liu S, da Cunha AP, Rezende RM, Cialic R, Wei Z, Bry L, et al. The host shapes the gut microbiota via fecal microRNA. Cell Host Microbe. (2016) 19:32–43. doi: 10.1016/j.chom.2015.12.005
39. Bitar A, Aung KM, Wai SN, Hammarström ML. Vibrio cholerae derived outer membrane vesicles modulate the inflammatory response of human intestinal epithelial cells by inducing microRNA-146a. Sci Rep. (2019) 9:7212. doi: 10.1038/s41598-019-43691-9
40. Abraham KJ, Zhang X, Vidal R, Paré GC, Feilotter HE, Tron VA. Roles for miR-375 in neuroendocrine differentiation and tumor suppression via Notch pathway suppression in Merkel cell carcinoma. Am J Pathol. (2016) 186:1025–35. doi: 10.1016/j.ajpath.2015.11.020
41. Liu L, Yao J, Li Z, Zu G, Feng D, Li Y, et al. miR-381-3p knockdown improves intestinal epithelial proliferation and barrier function after intestinal ischemia/reperfusion injury by targeting nurr1. Cell Death Dis. (2018) 9:1–14. doi: 10.1038/s41419-018-0450-z
42. Brown JM, Hazen SL. The gut microbial endocrine organ: bacterially derived signals driving cardiometabolic diseases. Annu Rev Med. (2015) 66:343–59. doi: 10.1146/annurev-med-060513-093205
43. Witkowski M, Weeks TL, Hazen SL. Gut microbiota and cardiovascular disease. Circ Res. (2020) 127:553–70. doi: 10.1161/CIRCRESAHA.120.316242
44. Novakovic M, Rout A, Kingsley T, Kirchoff R, Singh A, Verma V, et al. Role of gut microbiota in cardiovascular diseases. World J Cardiol. (2020) 12:110–22. doi: 10.4330/wjc.v12.i4.110
45. Trøseid M, Andersen GØ, Broch K, Hov JR. The gut microbiome in coronary artery disease and heart failure: current knowledge and future directions. EBioMedicine. (2020) 52:102649. doi: 10.1016/j.ebiom.2020.102649
46. Wang Z, Klipfell E, Bennett BJ, Koeth R, Levison BS, Dugar B, et al. Gut flora metabolism of phosphatidylcholine promotes cardiovascular disease. Nature. (2011) 472:57–63. doi: 10.1038/nature09922
47. Tang WH, Wang Z, Levison BS, Koeth RA, Britt EB, Fu X, et al. Intestinal microbial metabolism of phosphatidylcholine and cardiovascular risk. N Engl J Med. (2013) 368:1575–84. doi: 10.1056/NEJMoa1109400
48. Koeth RA, Wang Z, Levison BS, Buffa JA, Org E, Sheehy BT, et al. Intestinal microbiota metabolism of L-carnitine, a nutrient in red meat, promotes atherosclerosis. Nat Med. (2013) 19:576–85. doi: 10.1038/nm.3145
49. Tang WHW, Bäckhed F, Landmesser U, Hazen SL. Intestinal Microbiota in cardiovascular health and disease: JACC state-of-the-art review. J Am Coll Cardiol. (2019) 73:2089–105. doi: 10.1016/j.jacc.2019.03.024
50. Abete I, Romaguera D, Vieira AR, Lopez de Munain A, Norat T. Association between total, processed, red and white meat consumption and all-cause, CVD and IHD mortality: a meta-analysis of cohort studies. Br J Nutr. (2014) 112:762–75. doi: 10.1017/S000711451400124X
51. Senthong V, Wang Z, Fan Y, Wu Y, Hazen SL, Tang WHW. Trimethylamine N-oxide and mortality risk in patients with peripheral artery disease. J Am Heart Assoc. (2016) 5:e004237. doi: 10.1161/JAHA.116.004237
52. Senthong V, Li XS, Hudec T, Coughlin J, Wu Y, Levison B, et al. Plasma trimethylamine N -oxide, a gut microbe–generated phosphatidylcholine metabolite, is associated with atherosclerotic burden. J Am Coll Cardiol. (2016) 67:2620–8. doi: 10.1016/j.jacc.2016.03.546
53. Tan Y, Sheng Z, Zhou P, Liu C, Zhao H, Song L, et al. Plasma trimethylamine N-oxide as a novel biomarker for plaque rupture in patients with ST-segment-elevation myocardial infarction. Circ Cardiovasc Interv. (2019) 12:e007281. doi: 10.1161/CIRCINTERVENTIONS.118.007281
54. Li XS, Obeid S, Klingenberg R, Gencer B, Mach F, Räber L, et al. Gut microbiota-dependent trimethylamine N-oxide in acute coronary syndromes: a prognostic marker for incident cardiovascular events beyond traditional risk factors. Eur Heart J. (2017) 38:814–24. doi: 10.1093/eurheartj/ehw582
55. Lever M, George PM, Slow S, Bellamy D, Young JM, Ho M, et al. Betaine and trimethylamine-N-oxide as predictors of cardiovascular outcomes show different patterns in diabetes mellitus: an observational study. PLoS ONE. (2014) 9:e114969. doi: 10.1371/journal.pone.0114969
56. Suzuki T, Heaney LM, Bhandari SS, Jones DJL, Ng LL. Trimethylamine N-oxide and prognosis in acute heart failure. Heart. (2016) 102:841–8. doi: 10.1136/heartjnl-2015-308826
57. Trøseid M, Ueland T, Hov JR, Svardal A, Gregersen I, Dahl CP, et al. Microbiota-dependent metabolite trimethylamine-N-oxide is associated with disease severity and survival of patients with chronic heart failure. J Intern Med. (2015) 277:717–26. doi: 10.1111/joim.12328
58. Tang WH, Wang Z, Shrestha K, Borowski AG, Wu Y, Troughton RW, et al. Intestinal microbiota-dependent phosphatidylcholine metabolites, diastolic dysfunction, and adverse clinical outcomes in chronic systolic heart failure. J Card Fail. (2015) 21:91–6. doi: 10.1016/j.cardfail.2014.11.006
59. Tang WH, Wang Z, Fan Y, Levison B, Hazen JE, Donahue LM, et al. Prognostic value of elevated levels of intestinal microbe-generated metabolite trimethylamine-N-oxide in patients with heart failure: refining the gut hypothesis. J Am Coll Cardiol. (2014) 64:1908–14. doi: 10.1016/j.jacc.2014.02.617
60. Qin J, Li R, Raes J, Arumugam M, Burgdorf KS, Manichanh C, et al. A human gut microbial gene catalogue established by metagenomic sequencing. Nature. (2010) 464:59–65. doi: 10.1038/nature08821
61. Turnbaugh PJ, Ley RE, Hamady M, Fraser-Liggett CM, Knight R, Gordon JI. The human microbiome project. Nature. (2007) 449:804–10. doi: 10.1038/nature06244
62. Human Microbiome Project Consortium. Structure, function and diversity of the healthy human microbiome. Nature. (2012) 486:207–14. doi: 10.1038/nature11234
63. Mariat D, Firmesse O, Levenez F, Guimarăes VD, Sokol H, Doré J, et al. The Firmicutes/Bacteroidetes ratio of the human microbiota changes with age. BMC Microbiol. (2009) 9:123. doi: 10.1186/1471-2180-9-123
64. Veltman K, Hummel S, Cichon C, Sonnenborn U, Schmidt MA. Identification of specific miRNAs targeting proteins of the apical junctional complex that simulate the probiotic effect of E. coli Nissle 1917 on T84 epithelial cells. Int J Biochem Cell Biol. (2012) 44:341–9. doi: 10.1016/j.biocel.2011.11.006
65. Yuan C, Burns MB, Subramanian S, Blekhman R. Interaction between host microRNAs and the gut Microbiota in colorectal cancer. mSystems. (2018) 3:e00205–17. doi: 10.1128/mSystems.00205-17
66. Li X, He S, Li R, Zhou X, Zhang S, Yu M, et al. Pseudomonas aeruginosa infection augments inflammation through miR-301b repression of c-Myb-mediated immune activation and infiltration. Nat Microbiol. (2016) 1:16132. doi: 10.1038/nmicrobiol.2016.132
67. Wang F, Liu J, Zou Y, Jiao Y, Huang Y, Fan L, et al. MicroRNA-143-3p, up-regulated in H. pylori-positive gastric cancer, suppresses tumor growth, migration and invasion by directly targeting AKT2. Oncotarget. (2017) 8:28711–24. doi: 10.18632/oncotarget.15646
68. Gu X, Gao Y, Mu DG, Fu EQ. MiR-23a-5p modulates mycobacterial survival and autophagy during mycobacterium tuberculosis infection through TLR2/MyD88/NF-κB pathway by targeting TLR2. Exp Cell Res. (2017) 354:71–7. doi: 10.1016/j.yexcr.2017.03.039
69. Nejman-Faleńczyk B, Bloch S, Licznerska K, Dydecka A, Felczykowska A, Topka G, et al. A small, microRNA-size, ribonucleic acid regulating gene expression and development of Shiga toxin-converting bacteriophage Φ24?. Sci Rep. (2015) 5:10080. doi: 10.1038/srep10080
70. Berleman J, Auer M. The role of bacterial outer membrane vesicles for intra- and interspecies delivery: delivery of membrane vesicles. Environ Microbiol. (2013) 15:347–54. doi: 10.1111/1462-2920.12048
71. Dai X, Chen X, Chen Q, Shi L, Liang H, Zhou Z, et al. MicroRNA-193a-3p reduces intestinal inflammation in response to Microbiota via down-regulation of colonic PepT1. J Biol Chem. (2015) 290:16099–115. doi: 10.1074/jbc.M115.659318
72. Bhaskaran M, Mohan M. MicroRNAs: history, biogenesis, and their evolving role in animal development and disease: history, biogenesis, and their evolving role in animal development and disease. Vet Pathol. (2014) 51:759–74. doi: 10.1177/0300985813502820
73. Lee RC, Feinbaum RL, Ambros V. The C. elegans heterochronic gene lin-4 encodes small RNAs with antisense complementarity to lin-14. Cell. (1993) 75:843–54. doi: 10.1016/0092-8674(93)90529-Y
74. Romaine SPR, Tomaszewski M, Condorelli G, Samani NJ. MicroRNAs in cardiovascular disease: an introduction for clinicians. Heart. (2015) 101:921–8. doi: 10.1136/heartjnl-2013-305402
75. Ionescu RF, Cretoiu SM. MicroRNAs as monitoring markers for right-sided heart failure and congestive hepatopathy. J Med Life. (2021) 14:142–7. doi: 10.25122/jml-2021-0071
76. Lagos-Quintana M, Rauhut R, Yalcin A, Meyer J, Lendeckel W, Tuschl T. Identification of tissue-specific microRNAs from mouse. Curr Biol. (2002) 12:735–9. doi: 10.1016/S0960-9822(02)00809-6
77. Ji R, Cheng Y, Yue J, Yang J, Liu X, Chen H, et al. MicroRNA expression signature and antisense-mediated depletion reveal an essential role of microRNA in vascular neointimal lesion formation. Circ Res. (2007) 100:1579–88. doi: 10.1161/CIRCRESAHA.106.141986
78. Luo X, Zhang H, Xiao J, Wang Z. Regulation of human cardiac ion channel genes by microRNAs: theoretical perspective and pathophysiological implications. Cell Physiol Biochem. (2010) 25:571–86. doi: 10.1159/000315076
79. Wang Z, Lu Y, Yang B. MicroRNAs and atrial fibrillation: new fundamentals. Cardiovasc Res. (2011) 89:710–21. doi: 10.1093/cvr/cvq350
81. Schober A, Nazari-Jahantigh M, Wei Y, Bidzhekov K, Gremse F, Grommes J, et al. MicroRNA-126-5p promotes endothelial proliferation and limits atherosclerosis by suppressing Dlk1. Nat Med. (2014) 20:368–76. doi: 10.1038/nm.3487
82. Fitzsimons S, Oggero S, Bruen R, McCarthy C, Strowitzki MJ, Mahon NG, et al. MicroRNA-155 is decreased during atherosclerosis regression and is increased in urinary extracellular vesicles during atherosclerosis progression. Front Immunol. (2020) 11:576516. doi: 10.3389/fimmu.2020.576516
83. Lovren F, Pan Y, Quan A, Singh KK, Shukla PC, Gupta N, et al. MicroRNA-145 targeted therapy reduces atherosclerosis. Circulation. (2012) 126:S81–90. doi: 10.1161/CIRCULATIONAHA.111.084186
84. Cordes KR, Sheehy NT, White MP, Berry EC, Morton SU, Muth AN, et al. miR-145 and miR-143 regulate smooth muscle cell fate and plasticity. Nature. (2009) 460:705–10. doi: 10.1038/nature08195
85. Jonsson AL, Bäckhed F. Role of gut microbiota in atherosclerosis. Nat Rev Cardiol. (2017) 14:79–87. doi: 10.1038/nrcardio.2016.183
86. Rosenfeld ME, Campbell LA. Pathogens and atherosclerosis: update on the potential contribution of multiple infectious organisms to the pathogenesis of atherosclerosis. Thromb Haemost. (2011) 106:858–67. doi: 10.1160/TH11-06-0392
87. Zhu W, Gregory JC, Org E, Buffa JA, Gupta N, Wang Z, et al. Gut microbial metabolite TMAO enhances platelet hyperreactivity and thrombosis risk. Cell. (2016) 165:111–24. doi: 10.1016/j.cell.2016.02.011
88. Khurana S, Raufman JP, Pallone TL. Bile acids regulate cardiovascular function. Clin Transl Sci. (2011) 4:210–8. doi: 10.1111/j.1752-8062.2011.00272.x
89. Marques FZ, Booth SA, Charchar FJ. The emerging role of non-coding RNA in essential hypertension and blood pressure regulation. J Hum Hypertens. (2015) 29:459–67. doi: 10.1038/jhh.2014.99
90. Marques FZ, Campain AE, Tomaszewski M, Zukowska-Szczechowska E, Yang YH, Charchar FJ, et al. Gene expression profiling reveals renin mRNA overexpression in human hypertensive kidneys and a role for microRNAs. Hypertension. (2011) 58:1093–8. doi: 10.1161/HYPERTENSIONAHA.111.180729
91. Eskildsen TV, Jeppesen PL, Schneider M, Nossent AY, Sandberg MB, Hansen PB, et al. Angiotensin II regulates microRNA-132/-212 in hypertensive rats and humans. Int J Mol Sci. (2013) 14:11190–207. doi: 10.3390/ijms140611190
92. Karolina DS, Tavintharan S, Armugam A, Sepramaniam S, Pek SL, Wong MT, et al. Circulating miRNA profiles in patients with metabolic syndrome. J Clin Endocrinol Metab. (2012) 97:E2271–6. doi: 10.1210/jc.2012-1996
93. Santovito D, Mandolini C, Marcantonio P, De Nardis V, Bucci M, Paganelli C, et al. Overexpression of microRNA-145 in atherosclerotic plaques from hypertensive patients. Expert Opin Ther Targets. (2013) 17:217–23. doi: 10.1517/14728222.2013.745512
94. Liang Y, Ridzon D, Wong L, Chen C. Characterization of microRNA expression profiles in normal human tissues. BMC Genomics. (2007) 8:166. doi: 10.1186/1471-2164-8-166
95. Carè A, Catalucci D, Felicetti F, Bonci D, Addario A, Gallo P, et al. MicroRNA-133 controls cardiac hypertrophy. Nat Med. (2007) 13:613–8. doi: 10.1038/nm1582
96. Wahlquist C, Jeong D, Rojas-Muñoz A, Kho C, Lee A, Mitsuyama S, et al. Inhibition of miR-25 improves cardiac contractility in the failing heart. Nature. (2014) 508:531–5. doi: 10.1038/nature13073
97. Meyer M, Schillinger W, Pieske B, Holubarsch C, Heilmann C, Posival H, et al. Alterations of sarcoplasmic reticulum proteins in failing human dilated cardiomyopathy. Circulation. (1995) 92:778–84. doi: 10.1161/01.CIR.92.4.778
98. Gurha P, Abreu-Goodger C, Wang T, Ramirez MO, Drumond AL, van Dongen S, et al. Targeted deletion of microRNA-22 promotes stress-induced cardiac dilation and contractile dysfunction. Circulation. (2012) 125:2751–61. doi: 10.1161/CIRCULATIONAHA.111.044354
99. Bang C, Batkai S, Dangwal S, Gupta SK, Foinquinos A, Holzmann A, et al. Cardiac fibroblast-derived microRNA passenger strand-enriched exosomes mediate cardiomyocyte hypertrophy. J Clin Invest. (2014) 124:2136–46. doi: 10.1172/JCI70577
100. Thum T, Gross C, Fiedler J, Fischer T, Kissler S, Bussen M, et al. MicroRNA-21 contributes to myocardial disease by stimulating MAP kinase signalling in fibroblasts. Nature. (2008) 456:980–4. doi: 10.1038/nature07511
101. Luedde M, Winkler T, Heinsen FA, Rühlemann MC, Spehlmann ME, Bajrovic A, et al. Heart failure is associated with depletion of core intestinal microbiota. ESC Heart Fail. (2017) 4:282–90. doi: 10.1002/ehf2.12155
102. Cui X, Ye L, Li J, Jin L, Wang W, Li S, et al. Metagenomic and metabolomic analyses unveil dysbiosis of gut microbiota in chronic heart failure patients. Sci Rep. (2018) 8:635. doi: 10.1038/s41598-017-18756-2
103. Kamo T, Akazawa H, Suda W, Saga-Kamo A, Shimizu Y, Yagi H, et al. Dysbiosis and compositional alterations with aging in the gut microbiota of patients with heart failure. PLoS ONE. (2017) 12:e0174099. doi: 10.1371/journal.pone.0174099
104. Santulli G, Iaccarino G, De Luca N, Trimarco B, Condorelli G. Atrial fibrillation and microRNAs. Front Physiol. (2014) 5:15. doi: 10.3389/fphys.2014.00015
105. Lu Y, Zhang Y, Wang N, Pan Z, Gao X, Zhang F, et al. MicroRNA-328 contributes to adverse electrical remodeling in atrial fibrillation. Circulation. (2010) 122:2378–87. doi: 10.1161/CIRCULATIONAHA.110.958967
106. Zhao Y, Ransom JF, Li A, Vedantham V, von Drehle M, Muth AN, et al. Dysregulation of cardiogenesis, cardiac conduction, and cell cycle in mice lacking miRNA-1-2. Cell. (2007) 129:303–17. doi: 10.1016/j.cell.2007.03.030
107. Girmatsion Z, Biliczki P, Bonauer A, Wimmer-Greinecker G, Scherer M, Moritz A, et al. Changes in microRNA-1 expression and IK1 up-regulation in human atrial fibrillation. Heart Rhythm. (2009) 6:1802–9. doi: 10.1016/j.hrthm.2009.08.035
108. Biel M, Schneider A, Wahl C. Cardiac HCN channels: structure, function, and modulation. Trends Cardiovasc Med. (2002) 12:206–12. doi: 10.1016/S1050-1738(02)00162-7
109. Hoppe UC, Jansen E, Südkamp M, Beuckelmann DJ. Hyperpolarization-activated inward current in ventricular myocytes from normal and failing human hearts. Circulation. (1998) 97:55–65. doi: 10.1161/01.CIR.97.1.55
110. Duisters RF, Tijsen AJ, Schroen B, Leenders JJ, Lentink V, van der Made I, et al. miR-133 and miR-30 regulate connective tissue growth factor: implications for a role of microRNAs in myocardial matrix remodeling: implications for a role of MicroRNAs in myocardial matrix remodeling. Circ Res. (2009) 104:170–8. doi: 10.1161/CIRCRESAHA.108.182535
111. Adam O, Löhfelm B, Thum T, Gupta SK, Puhl SL, Schäfers HJ, et al. Role of miR-21 in the pathogenesis of atrial fibrosis. Basic Res Cardiol. (2012) 107:278. doi: 10.1007/s00395-012-0278-0
112. Lu Y, Xiao J, Lin H, Bai Y, Luo X, Wang Z, et al. A single anti-microRNA antisense oligodeoxyribonucleotide (AMO) targeting multiple microRNAs offers an improved approach for microRNA interference. Nucleic Acids Res. (2009) 37:e24. doi: 10.1093/nar/gkn1053
113. Luo X, Pan Z, Shan H, Xiao J, Sun X, Wang N, et al. MicroRNA-26 governs profibrillatory inward-rectifier potassium current changes in atrial fibrillation. J Clin Invest. (2013) 123:1939–51. doi: 10.1172/JCI62185
114. Voigt N, Trausch A, Knaut M, Matschke K, Varró A, Van Wagoner DR, et al. Left-to-right atrial inward rectifier potassium current gradients in patients with paroxysmal versus chronic atrial fibrillation. Circ Arrhythm Electrophysiol. (2010) 3:472–80. doi: 10.1161/CIRCEP.110.954636
115. Dawson K, Wakili R, Ordög B, Clauss S, Chen Y, Iwasaki Y, et al. MicroRNA29: a mechanistic contributor and potential biomarker in atrial fibrillation: a mechanistic contributor and potential biomarker in atrial fibrillation. Circulation. (2013) 127:1466–75. doi: 10.1161/CIRCULATIONAHA.112.001207
116. Roy S, Khanna S, Hussain SRA, Biswas S, Azad A, Rink C, et al. MicroRNA expression in response to murine myocardial infarction: miR-21 regulates fibroblast metalloprotease-2 via phosphatase and tensin homologue. Cardiovasc Res. (2009) 82:21–9. doi: 10.1093/cvr/cvp015
117. Cooley N, Cowley MJ, Lin RCY, Marasco S, Wong C, Kaye DM, et al. Influence of atrial fibrillation on microRNA expression profiles in left and right atria from patients with valvular heart disease. Physiol Genomics. (2012) 44:211–9. doi: 10.1152/physiolgenomics.00111.2011
118. Shan H, Zhang Y, Lu Y, Zhang Y, Pan Z, Cai B, et al. Downregulation of miR-133 and miR-590 contributes to nicotine-induced atrial remodelling in canines. Cardiovasc Res. (2009) 83:465–72. doi: 10.1093/cvr/cvp130
119. Luo X, Lin H, Pan Z, Xiao J, Zhang Y, Lu Y, et al. Down-regulation of miR-1/miR-133 contributes to re-expression of pacemaker channel genes HCN2 and HCN4 in hypertrophic heart. J Biol Chem. (2008) 283:20045–52. doi: 10.1074/jbc.M801035200
120. Dobrev D, Ravens U. Remodeling of cardiomyocyte ion channels in human atrial fibrillation. Basic Res Cardiol. (2003) 98:137–48. doi: 10.1007/s00395-003-0409-8
121. Ling TY, Wang XL, Chai Q, Lau TW, Koestler CM, Park SJ, et al. Regulation of the SK3 channel by microRNA-499–potential role in atrial fibrillation. Heart Rhythm. (2013) 10:1001–9. doi: 10.1016/j.hrthm.2013.03.005
122. Ren XP, Wu J, Wang X, Sartor MA, Jones K, Qian J, et al. MicroRNA-320 is involved in the regulation of cardiac ischemia/reperfusion injury by targeting heat-shock protein 20. Circulation. (2009) 119:2357–66. doi: 10.1161/CIRCULATIONAHA.108.814145
123. Devaux Y, Mueller M, Haaf P, Goretti E, Twerenbold R, Zangrando J, et al. Diagnostic and prognostic value of circulating microRNAs in patients with acute chest pain. J Intern Med. (2015) 277:260–71. doi: 10.1111/joim.12183
124. Zampetaki A, Willeit P, Tilling L, Drozdov I, Prokopi M, Renard JM, et al. Prospective study on circulating MicroRNAs and risk of myocardial infarction. J Am Coll Cardiol. (2012) 60:290–9. doi: 10.1016/j.jacc.2012.03.056
125. Kaur S, Kumar S. Crosstalk between food components and microRNAs: role in metabolism, nutrition, health and diseases. Integr Food Nutr Metab. (2020) 7:1–11. doi: 10.15761/IFNM.1000293
126. van Rooij E, Sutherland LB, Liu N, Williams AH, McAnally J, Gerard RD, et al. A signature pattern of stress-responsive microRNAs that can evoke cardiac hypertrophy and heart failure. Proc Natl Acad Sci U S A. (2006) 103:18255–60. doi: 10.1073/pnas.0608791103
127. Wang K, Long B, Zhou J, Li PF. miR-9 and NFATc3 regulate myocardin in cardiac hypertrophy. J Biol Chem. (2010) 285:11903–12. doi: 10.1074/jbc.M109.098004
128. Slavin J. Fiber and prebiotics: mechanisms and health benefits. Nutrients. (2013) 5:1417–35. doi: 10.3390/nu5041417
129. Sahyoun NR, Jacques PF, Zhang XL, Juan W, McKeown NM. Whole-grain intake is inversely associated with the metabolic syndrome and mortality in older adults. Am J Clin Nutr. (2006) 83:124–31. doi: 10.1093/ajcn/83.1.124
130. Cremon C, Barbaro MR, Ventura M, Barbara G. Pre- and probiotic overview. Curr Opin Pharmacol. (2018) 43:87–92. doi: 10.1016/j.coph.2018.08.010
131. Suez J, Zmora N, Segal E, Elinav E. The pros, cons, and many unknowns of probiotics. Nat Med. (2019) 25:716–29. doi: 10.1038/s41591-019-0439-x
132. Dobson A, Cotter PD, Ross RP, Hill C. Bacteriocin production: a probiotic trait? Appl Environ Microbiol. (2012) 78:1–6. doi: 10.1128/AEM.05576-11
133. Sanders ME, Merenstein DJ, Reid G, Gibson GR, Rastall RA. Probiotics and prebiotics in intestinal health and disease: from biology to the clinic. Nat Rev Gastroenterol Hepatol. (2019) 16:605–16. doi: 10.1038/s41575-019-0173-3
134. Yan F, Polk DB. Probiotics and probiotic-derived functional factors-mechanistic insights into applications for intestinal homeostasis. Front Immunol. (2020) 11:1428. doi: 10.3389/fimmu.2020.01428
135. Matsuki T, Pédron T, Regnault B, Mulet C, Hara T, Sansonetti PJ. Epithelial cell proliferation arrest induced by lactate and acetate from Lactobacillus casei and Bifidobacterium breve. PLoS ONE. (2013) 8:e63053. doi: 10.1371/journal.pone.0063053
Keywords: gut microbiota, miRNA, gut microbiome, trimethylamine oxide (TMAO), cardiovascular diseases
Citation: Ionescu RF, Enache RM, Cretoiu SM and Cretoiu D (2022) The Interplay Between Gut Microbiota and miRNAs in Cardiovascular Diseases. Front. Cardiovasc. Med. 9:856901. doi: 10.3389/fcvm.2022.856901
Received: 17 January 2022; Accepted: 16 February 2022;
Published: 16 March 2022.
Edited by:
Jinwei Tian, The Second Affiliated Hospital of Harbin Medical University, ChinaReviewed by:
Moumita Roy Chowdhury, Indian Institute of Technology Kharagpur, IndiaCopyright © 2022 Ionescu, Enache, Cretoiu and Cretoiu. This is an open-access article distributed under the terms of the Creative Commons Attribution License (CC BY). The use, distribution or reproduction in other forums is permitted, provided the original author(s) and the copyright owner(s) are credited and that the original publication in this journal is cited, in accordance with accepted academic practice. No use, distribution or reproduction is permitted which does not comply with these terms.
*Correspondence: Sanda Maria Cretoiu, c2FuZGFAY3JldG9pdS5ybw==; c2FuZGEuY3JldG9pdUB1bWZjZC5ybw==
†These authors have contributed equally to this work and share first authorship
Disclaimer: All claims expressed in this article are solely those of the authors and do not necessarily represent those of their affiliated organizations, or those of the publisher, the editors and the reviewers. Any product that may be evaluated in this article or claim that may be made by its manufacturer is not guaranteed or endorsed by the publisher.
Research integrity at Frontiers
Learn more about the work of our research integrity team to safeguard the quality of each article we publish.