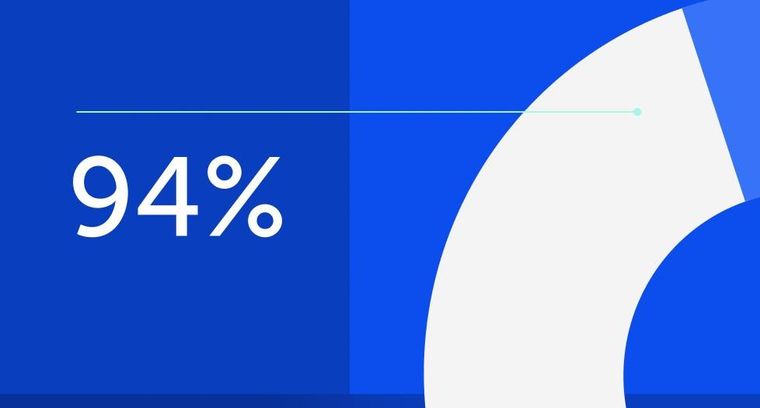
94% of researchers rate our articles as excellent or good
Learn more about the work of our research integrity team to safeguard the quality of each article we publish.
Find out more
REVIEW article
Front. Cardiovasc. Med., 10 February 2022
Sec. General Cardiovascular Medicine
Volume 9 - 2022 | https://doi.org/10.3389/fcvm.2022.845070
This article is part of the Research TopicInsights in General Cardiovascular Medicine: 2021View all 22 articles
Vascular repair upon vessel injury is essential for the maintenance of arterial homeostasis and function. Stem/progenitor cells were demonstrated to play a crucial role in regeneration and replenishment of damaged vascular cells during vascular repair. Previous studies revealed that myeloid stem/progenitor cells were the main sources of tissue regeneration after vascular injury. However, accumulating evidences from developing lineage tracing studies indicate that various populations of vessel-resident stem/progenitor cells play specific roles in different process of vessel injury and repair. In response to shear stress, inflammation, or other risk factors-induced vascular injury, these vascular stem/progenitor cells can be activated and consequently differentiate into different types of vascular wall cells to participate in vascular repair. In this review, mechanisms that contribute to stem/progenitor cell differentiation and vascular repair are described. Targeting these mechanisms has potential to improve outcome of diseases that are characterized by vascular injury, such as atherosclerosis, hypertension, restenosis, and aortic aneurysm/dissection. Future studies on potential stem cell-based therapy are also highlighted.
Vascular injury and repair process has been found to be associated with a variety of cardiovascular diseases, including atherosclerosis, restenosis, hypertension, and arterial aneurysm. They are mainly caused by vascular wall thickening and lumen narrowing. The main causes of the diseases are endothelial injury, vascular smooth muscle cell (VSMC) proliferation, matrix deposition induced abnormal vascular injury and repair (1). The artery wall has three layers as shown in Figure 1: intima, media and adventitia. The intima, which has direct access to blood flow, is mainly made up of endothelial cells (ECs) (2). The media is mainly composed of VSMCs, as well as collagen and elastic fibers. The adventitia is rich in collagen, containing a variety of cells, as well as nerves and blood vessels (3). Previous studies have shown that the occurrence and development of these diseases is due to the phenotypic transformation of VSMCs induced by a variety of factors (4, 5). But there are still unresolved disputations on the reversibility of phenotypic transformation. Recent studies suggested that VSMCs participate in pathological processes, not only through phenotypic transformation, but also from stem/progenitor cells differentiation (6). And it also found that VSMCs derived from stem/progenitor cells participate in atherosclerosis, while maintaining a contractile phenotype without phenotypic transformation (7, 8).
Figure 1. The distribution of cells in vascular wall. The vascular wall consists of three layers: intima, media, and adventitia. The intima consists of ECs and some vascular stem cells such as c-kit+ cells. The media is composed of SMCs. The adventitia is composed of vascular stem cells (Sca-1+ cells, c-kit+ cells, CD34+ cells, pericytes, etc.) and fibroblasts.
In recent years, studies have demonstrated that resident or circulating stem/progenitor cells, such as endothelial progenitor cells (EPCs), smooth muscle progenitor cells (SMPCs), mesenchymal stem cells (MSCs), and pericytes, can differentiate into several types of vascular cells and form neointima during vascular repair (9). Vascular stem cells (VSCs) residing in blood vessels play a key role in vascular remodeling and closely related to the occurrence of vascular remodeling diseases. During embryonic development, vascular networks depend on vasculogenesis and angiogenesis. Adult VSCs are almost dormant in their niches, once blood vessels are injured, these cells can be activated to initiate neointima, with inflammatory cells infiltration and extracellular matrix (ECM) deposition (10). Perivascular tissues, including the adipose layer is also thought to be crucial in vascular development and disease progression (11).
In this review, we describe several stem/progenitor cells mainly in adventitia and their functions in development of atherosclerosis, restenosis, hypertension, and aortic aneurysm/dissection. At present, there are few studies on VSCs, and the sources of various regenerative ECs and VSMCs involved in the pathological process of vascular remodeling diseases have not been fully studied. Therefore, the mechanism of the participation of VSCs in various vascular remodeling diseases needs to be further studied. In this paper, several types of VSCs and their roles in vascular remodeling-related diseases are reviewed, including various vascular stem/progenitor cells in the process of vascular injury and repair as well as the key elements and important signaling pathways in the occurrence and progression of diseases. New potential targets for clinical treatment of vascular diseases are proposed.
During vascular injury, the pathological process of vascular wall changes includes ECs dysfunction, VSMCs proliferation, and inflammatory response. Many studies ‘have demonstrated that vascular stem/progenitor cells can be mobilized in response to various pathological stimuli and play a key role in the vascular repair (12–15). Here we will mainly review studies on EPCs, SMPCs, MSCs, and pericytes (Table 1).
Earlier studies suggested that EPCs are a group of cells mobilized from bone marrow that participate in endothelium repair after injury (35). In fact, EPCs have a variety of tissue sources, including bone marrow, spleen, blood vessel wall, lipid, and placenta (12). At present, EPCs are defined as the cell population that has the typical clonal proliferation ability and characteristics of stem cells and can differentiate into mature ECs (36).
EPCs actually consist of different cell populations, making up “early EPCs” and “late EPCs” (10). The early stage EPCs appears in early culture stage (4–7 days), its survival time is short and will not differentiate into ECs. EPCs can activate adjacent cells by releasing SDF-1 and VEGF to promote vascular growth (37). It's classical immunophenotypes are CD45+,CD14+, and CD31+ and CD146−,CD133−, and Tie2− (10). The late stage EPCs can be known as endothelial colony-forming cells (ECFCs), derived from blood vessel wall, human placenta, and white adipose tissue (38). Compared with the early stage EPCs, ECFCs has a higher degree of proliferation and longer survival time, and can differentiate into mature functional ECs to participate in vascular repair (39). It's typical immunophenotypes are CD31+, vWF+, VE-cadherin+, CD146+, VEGFR2+, and CD45−, CD14−.
By studying HUVECs and HAECs, it was found that the renewal of vascular wall ECs could be attributed to ECFCs (40). EPCs residing in blood vessels possess multiple abilities such as self-renewal, multi-differentiation potential, and robust proliferation (16). The research pointed out the vital role of Sox18/SoxF transcription factor in the differentiation process. The resident CD157+EPCs in vascular wall of large arteries and veins showed regenerative potential of endothelial cells and vasculature (41). Animal studies have proved that EPCs can reduce the formation of neointima after artery injury and restore endothelial function (2). EPCs homing play a central role in vascular remodeling (42). Then neurotrophic factor-3 (NT-3) was proved to be able to accelerate rapid re-endothelialization of damaged carotid arteries by promoting EPCs mobilization and homing (42). It is also regulated by angiogenic chemokines (CXCL1, CXCL7, CXCL12, and CCL2), through their corresponding receptors (CXCR2, CXCR4, and CCR2) (43).
One of the characteristics of SMPCs is the heterogeneity of their origin (44). It is believed that the regenerative VSMCs after vascular injury have several different sources (28). The main functions are contracting blood vessels, regulating angiotasis, and blood pressure (45). There are different sources of VSMCs in different segments of the aorta, expressing different molecular markers and having different functions (2).
Studies have found that if blood vessels are injured, VSMCs will migrate to the intima, changing from a higher differentiated contractile phenotype to a secretory one to initiate cell proliferation (46). It is characterized by increased proliferation, migration, ECM synthesis, along with decreased expression of contraction markers (47). The traditional view holds that imbalance of VSMC plasticity leads to maladaptive phenotypic transformation which leads to the progression of VSMC-driving vascular remodeling diseases (48). But now we found that in vascular injury and repair, sometimes only contractile VSMCs are involved in vascular remodeling process without dedifferentiating to a secretory phenotype (4). Other studies have demonstrated that VSMCs can participate in vascular remodeling process without phenotypic transition (4, 49). From the perspective of stem cell research, recent results have confirmed that VSMCs derived from VSCs can drive vascular remodeling and take part in vascular repair upon injury.
In 2004, Hu et al. (7) first confirmed the presence of Sca-1+, c-Kit+, CD34+, and Flk1+ progenitors in the aortic root of Apoe−/− mice (36). They found that Sca-1+ resident adventitial progenitor cells can migrate and differentiate into VSMCs, playing an important role in atherosclerosis. Later, another group also reported that Sca-1+, CD34+, and PDGFRβ+ cells residented in the adventitia can differentiate into VSMCs in vitro. They also reporterd that the process is mediated by acoustic hedgehog (Shh) signaling pathway (50). Shh signaling is limited to the adventitia of the artery and may play a part in the maintenance of VPCs mainly in adventitia (50). Peripheral vascular cells (VSMC and pericytes) may share a common FlK1+ progenitor cell with ECs. FlK1 is a key regulator of phenotypic transformation in VSMC which inhibits VSMC differentiation and maintains the Sca-1+ progenitor cell phenotype, facilitating multidirectional differentiation and preventing pathological vascular remodeling (47). Based on lineage tracing study, Gli1+ cells were also proved to be progenitor cells in the adventitia, expressing CD34, Sca-1, and PDGFRβ and contributing to vascular repair and related diseases (51).
MSCs are pluripotent stromal cells which have proliferative and immunomodulatory effects (52). MSCs from different tissues share the same triadic differentiation potential, that is, the ability to differentiate into osteoblasts, chondrocytes, and adipocytes. The cells both express typical markers CD13, CD73, and CD90 in intima as well as CD29, CD44, and CD105 in adventitia and intima. However, the differentiation tendency of MSCs from different sources also differ (53). Bone marrow-derived MSCs express SH2, SH3, CD29, CD44, CD71, CD90, CD106, CD120a, CD124, and easily differentiate into osteoblasts. Adipose-derived MSCs express CD34, CD13, CD45, CD14, CD144, CD31, and easily differentiate into adipocytes (14, 54). Studies found that adipose tissue stem cells are easier to isolate and more abundant compared with other types of MSCs, they can sidestep ethical issues so are widely used in stem cell studies (55).
Some research groups (56) have isolated a population of CD34+CD31− cells expressing pericyte and mesenchymal antigens showing high proliferation and multiple-directional differentiation ability. It can have bidirectional interaction with ECs and take part in angiogenesis. It has been proved that co-culture with ECs on electrostatic spinning scaffolds can promote the differentiation of MSCs into osteoblasts (57). In the mouse model of hind limb ischemia, the researchers also found that EC-like cells derived from MSCs perform strong angiogenesis ability in vitro (58). Bone marrow mesenchymal stem cells (BMMSCs) can differentiate into ECs and VSMCs in vitro by means of growth factors modulation (59). MSCs are present in the perivascular niche of many organs, including kidneys, lungs, liver, and heart. The vascular-resident Gli1+ MSCs are the main cellular source of injury-induced organ fibrosis attributed to their colony-forming activity and differentiation capacity into fibroblasts (60). The effects of these cells in vascular repair depend on their metabolic reprogramming and SMC differentiation ability via mir-378a-3p/TGF-β1 signaling pathway (61).
Pericytes are high density parietal cells located in terminal arterioles and capillaries (62, 63). Pericytes are characterized by direct contact with the underlying ECs. It can regulate capillary permeability, endothelial stability, and micro-vasoconstriction (64). In fact, there are still no specific molecular markers for pericytes. The pericyte markers currently known, such as NG2, CD146, PDGFR-B in media and adventitia, are also expressed in SMPCs and MSCs (64).
In 1992, pericyte was first reported for its osteogenic potential, it has been shown that pericytes reside in capillary and microvessels may be osteoblast progenitors (65, 66). Pericytes and MSCs derived from various human tissues are similar in their function of maintenance vascular homeostasis. Pericytes can help maintain tissue homeostasis by promoting local MSC proliferation and differentiation through the paracrine capability (67). In a systematic investigation, the researchers found the angiogenesis and multilineage differentiation potential of pericytes and MSCs from different human tissues in vitro, and enriched CD34−CD146+ pericytes in adipocytes and bone marrow by magnetic activated cell sorting (15). Only bone marrow-derived cells showed triadic differentiation potential, while adipocyte-derived cells exhibited poor chondrogenic differentiation under TGF-β1 stimulation. These results indicate that the regenerative potential of pericytes as stem cells depends on their tissue origins (15). Recent lineage tracing experiments using the induced Tbx18-CreERT2 cell line showed that pericytes maintained the identity under various pathological conditions such as vascular senescence and will not differentiate into other cells. This discovery challenged the view of pericytes as stem cells (68).
As mentioned above, large amount of vascular stem/progenitor cells participate in the regeneration of damaged ECs and VSMCs during vascular repair upon injury. Numerous studies have confirmed the importance of VPCs in vascular remodeling diseases. Below, the roles of vascular stem/progenitor cells in atherosclerosis, restenosis, hypertension, and aneurysm/dissection, as well as their potential application in regenerative medicine will be reviewed (Table 2).
Atherosclerosis is characterized by endothelial damage, VSMC proliferation, and collagen deposition, as well as local thickening of the arterial wall caused by lipid deposition (78). Blood flow in vulnerable areas such as arterial branches has a high oscillation index and a low shear stress (79). This fluid mechanical force can directly act on ECs changing cell morphology, cytoskeleton and intercellular conjunctions, and increasing oxidative stress (80). Low density lipoprotein causes cholesterol storage when attached to the intima of the artery (81). Monocytes enter the intima from the blood, adhere to ECs and turn into macrophage. After phagocytic and ingestion of cholesterol, they form “foam cells” resulting in necrotic nuclei of atherosclerotic plaques with VSMCs (82). Fibrous cap inflammation can determine plaque stability (81). The death of VSMCs, degradation of collagen, and ECM lead to thinning of the vascular cap, resulting in increased plaque rupture with serious consequences. In fact, lumen stenosis often results from repeated ruptures and repair of complex plaques (83).
Decrease in EPCs predicts an increased risk of atherosclerosis. Fluid mechanical forces in arterial branches can directly affect ECs, change cell morphology and increase oxidative stress, inducing renewal of ECs (80). Shear stress is the basic driving factor of EPCs which can promote circulating EPCs homing to the injury site, inducing the anti-atherosclerotic phenotype of EPCs and promoting the differentiation process into mature ECs (84). These effects are mediated by VEGFR2, Tie2, Notch and β 1/3 integrin signaling. In 2020, a new population of EPCs have been identified in vascular walls by single-cell sequencing and lineage tracing techniques (69). The cells can differentiate into luminal and microvascular ECs and participate in endothelial homeostasis in graft sclerosis, with AKT/mTOR dependent glycolysis playing a key role in this process. SDF-1 can mobilize EPCs from bone marrow to the periphery, promote EPC regeneration and prevente cell death under pathological conditions (85). This pathway as mentioned in Figure 2 can provide a potential target for improving the efficacy of progenitor cells in the treatment of vascular diseases (85).
Figure 2. The differentiation signaling pathway of vascular progenitor cells. Vascular development and remodeling depend on many genes and are usually initiated by fluid shear stress which acts on the Notch signaling and closely associated with the vascular remodeling diseases. DKK3 induces ECs migration through Wnt-PCP signaling pathway, accelerating re-endothelialization, and reducing neointima formation. DKK3 can induce the differentiation of Sca-1+ progenitor cells and fibroblasts into VSMC by activating TGF-β/ATF6 signaling pathways.
In recent years, the roles of SMPCs in cardiovascular diseases have been confirmed in several studies (2, 5). The adventitia progenitor cells expressing Sca-1 markers have been studied in a widespread way. In addition to regulating vascular homeostasis and pathological remodeling, these progenitor cells are also closely related to acute vascular injury in atherosclerosis (86). Initially, the Sca-1+ and c-kit+ adventitia progenitor cells were found to be capable of differentiating into VSMCs and participate in the formation of venous graft injury. Through the β-Gal-labeled Sca-1+ progenitor cells to the adventitia side of vein grafts in Apoe−/− mice, the researchers found β-galactose expression in 20% of atherosclerotic lesions (7). Later in 2018, a study implanted GFP-Sca-1+ progenitor cells into the adventitia of mice after ligation surgery and found that it could significantly reduce plaque bleeding (87). DKK3 can induce Sca-1+ progenitor cells to differentiate into VSMCs by activating the Wnt signaling pathway, and maintain plaque stability (87). It can also induce the differentiation of Sca-1+ progenitor cells and fibroblasts into VSMC by activating TGF-β/ATF6 and Wnt signaling pathways (87) (Figure 2).
During atherosclerosis in Apoe intima; these are MSC-like cells expressing Gli1 marker (51). Through acute femoral artery injury repair model, a study confirmed that Gli1+MSC-like cells in the adventitia can differentiate into VSMCs. The team also found that Gli1+cells can migrate to the media and intima plaques and when calcification occurs in atherosclerosis, Gli1+ cells differentiate into osteoblasts (51). In addition, laminar shear stress can activate the Wnt signaling pathway of MSCs, promote β-catenin nuclear transport and activate paracrine factors under laminar shear stress (88).
Many experimental models confirmed the protective effects of MSC therapy on atherosclerosis. The production of Tsg-6, IL-10, NF-κB, and MMP from MSC can inhibit atherosclerotic plaque formation, modulate plaque cellular components and repair endothelial injury, all of which can effectively promote plaque stability (89, 90).
The functions of VPCs in vascular remodeling makes them of great significance in clinical treatment. As EPCs can induce re-endothelialization and angiogenesis of damaged arteries and have a role in regeneration of biological joint structures, they may be a promising clinical therapeutic target (79). Studies have shown a correlation between EPCs dysfunction and reduced angiogenesis in patients with coronary artery disease. As mentioned above, the c-kit+ stem/progenitor cells can differentiate into vascular cells to promote post-injury repair. But a recent study shows that most of c-kit+ cells differentiate into macrophages and granulocytes to reduce vascular immune inflammatory response to endothelial injury (70). This feature provides a theoretical basis for clinical improvement of vascular disease treatment. In vitro, high lipid levels induce MSC migration to intima, initiating the occurrence of atherosclerosis (91). Now MSC transplantation can also be a treatment direction for atherosclerosis (92).
Percutaneous coronary intervention is an effective treatment for patients with ischemic heart disease. Restenosis is the main complication of percutaneous coronary intervention (93).The main mechanisms are vascular endothelial dissection and subintimal hemorrhage caused by vascular injury after stent implantation (94, 95). Endothelial injury and other irritation can lead to a wound-healing response, while subintimal bleeding can lead to thrombosis and inflammatory cell infiltration (96). Inflammatory factors IL-1, TNF-α, and IL-6 can stimulate the proliferation of VSMCs to form neointima, lead to excessive healing of vascular wall and cause restenosis (97). Neointima is part of the injury repair response, and its formation involves inflammatory cell infiltration, matrix degradation, thrombosis, the proliferation of VSMC, and collagen secretion (98). And the proliferation of VSMCs is the main factor leading to neointima formation and restenosis (99).
The increased secretion of CCL2 and CXCL1 by VSMCs can promote Sca-1+ progenitor cell migrate from adventitia to neointima in the injured area. Through the guide wire injury test of mouse femoral artery, the GTPase Rac1/P38 signaling pathway was found to play a key role in this process (100). A study about leptin receptor (OBR) in Sca-1+ progenitor cells has found that the OBR-STAT3-MAPK and Rho-GTPase pathways are activated in response to leptin stimulation, inducing progenitor cell migration (72).
The regulatory role of mir-22 in the differentiation of SMPCs has been found, providing a new target for disease treatment of neointimal formation and restenosis (99). A recent study reported that EGFP+ MSC in mice expressed endothelial markers and showed the ability of tube formation (58). Meanwhile, these mice showed stronger ability for blood perfusion recovery, vascular density, and improved function of ischemic limbs, providing evidence for its role in angiogenesis. These results suggest that the induction of BMMSCs may be a promising option for the treatment of ischemic diseases (58). Vascular remodeling is known to delay the progression of blood flow restriction during stenosis. However, when restenosis occurs, intimal hyperplasia can lead to lumen narrowing, with adverse effects on the body (101). After bare-metal stent implantation in mice, circulating CD34+ cells have a significant increase, which also applies to patients with in-stent restenosis (73). The CD34+ cells differentiated into CD31+ cells and αSMA+ cells after scaffold implantation, which may contribute to the formation of stenosis. Recently, a study found that the elimination of non-bone marrow CD34+ cells could reduce the vascular lumen area and increase intima thickness (23). The non-bone marrow CD34+ cells could differentiate into ECs after femoral artery injury, maintaining vascular integrity, and preventing the formation of neointima. A regulator CircSOD2, may become a new clinical drug target for inhibiting the development of hyperplastic vascular diseases such as restenosis, because its knockdown can attenuate injury-induced neointima formation and reduce VSMCs proliferation (102). The role of VPCs in vascular remodeling is crucial for improving tissue-engineered vascular grafts and designing drug-targeted therapies for angiogenesis. Dkk3 increases Sca-1+ progenitor cell migration and contributes to VSMCs regeneration through CXCR7 activation (103). The DKK3-CXCR7 axis can mediate Sca-1+ cell migration and in vivo regeneration of transplanted cells, which is of great significance in the study of artificial vascular therapy for restenosis. ETV2-mediated differentiation of exogenous Sca-1+ cells into ECs to improve vascular remodeling and reduce neointima after injury (104). Therefore, inducing the differentiation of Sca-1+ cells into endothelial lineages might be a therapeutic strategy for vascular diseases as summarized in Figure 3.
Figure 3. The different roles in vascular remodeling diseases of vascular progenitor cells. c-Kit+ cells can differentiate into ECs and participate in endothelial homeostasis. Sca-1+ progenitor cells in adventitia can enhance the formation of neointima. Non-bone marrow CD34+ cells could differentiate into ECs after femoral artery injury, maintaining vascular integrity and preventing the formation of neointima. Resident NG2+ progenitor cells can differentiate into VSMCs after aortic injury during aortic aneurysm and dissection.
Essential hypertension is defined as an unexplained increase in blood pressure that increases the risk of cardiac, brain, or kidney events. Essential hypertension is often associated with hypercholesterolemia, obesity, and diabetes. These risk factors are also present in cardiovascular disease and hypertension can worsen the symptoms (105).The pathogenesis of hypertension includes endothelial dysfunction, sympathetic nervous system activation, inflammation, oxidative stress, etc. Aldosterone can promote the polarization of macrophages to the pro-inflammatory M1 phenotype, leading to vascular dysfunction and aggravating hypertension (106). In hypertension, collagen deposition is the main factor resulting in vascular fibrosis (107). VPCs participate in the occurrence and development of hypertension and vascular fibrosis is related to a variety of signaling pathways.
In the aorta of hypertensive mice induced by Ang II, the number of Sca-1 + progenitor cells increased and the EGFP-Sca-1+ progenitor cells co-located with the deposition areas of collagen I, III, and V in the adventitia, suggesting that Sca-1+ progenitor cells may be the source of hypertensive vascular fibrosis (76). Lineage tracing technique displayed Adv-Sca-1 cells can differentiate into fibroblasts in the case of vascular injury, leading to vascular remodeling and sclerosis, while KLF4 may maintain the phenotype and prevent pathological vascular remodeling (13). SMPCs express PW1 in the adventitia and participate in pulmonary hypertension-related vascular remodeling (20). The number of PW1+ progenitor cells increased significantly in the mouse pulmonary hypertension model under hypoxia and were able to differentiate into VSMCs through the CXCR4 pathway (20). Dysfunctional ECs can induce FoxM1 expression in VSMCs and activate FoxM1-dependent VSMC proliferation, confirming ECs and VSMCs interaction through FoxM1 signaling in vascular remodeling and promoting hypertension and fibrosis (74). Recently, a study induced perivascular fibrosis through Ang II, and found that vascular remodeling during the process was not dependent on blood pressure regulation but through ADAM17 activating PDGFR then acting on ECs (108). ADAM17 may be a new clinical target for preventing hypertension complications (109).
Aortic aneurysm refers to local dilation of the aortic lumen ≥50% of its original diameter and structural degeneration of different segments of the aorta. Endothelial injury, VSMCs loss and ECM degradation lead to abnormal remodeling of the aortic wall resulting in aortic aneurysm and separation of layers of the aortic wall (aortic dissection) (110, 111). The composition and integrity of ECM are key determinants of the physical properties of the aortic wall (112). ECM undergoes continuous physiological remodeling and the original proteins degraded and replaced by the newly synthesized proteins. MMP plays an important role in this process. Therefore, MMPs are closely related to the pathogenesis of aortic aneurysm (113). Aortic aneurysms can progress gradually, leading to tears in the intima of the aorta or bleeding in the wall of the aorta, further leading to the formation of aortic dissection, which can be life threatening.
Aortic aneurysm formed in two main sites, thoracic aorta and abdominal aorta (114). Abdominal aortic aneurysm (AAA) is most associated with atherosclerosis, they share many common risk factors. Thoracic aortic aneurysms (TAA), located in the aortic root and ascending aorta, are more related with genetic syndromes such as Marfan syndrome (115). AAAs are marked by media and elastic fiber degradation, leading to aortic dissection (116). The pathogenesis of AAA includes VSMCs apoptosis, MMPs degradation of elastin, collagen and glycosaminoglycan, inflammatory reactions and the role of reactive oxygen species (117). Among them, apoptosis of VSMCs and degeneration of aortic media are the hallmark pathological changes of AAA. Studies have found that Il-18 receptor defect, chemokine netrin-1 deficiency, and activated transcription factor EB can reduce apoptosis of VSMCs, decrease the activity of MMP-2/9 and elastin degradation, inhibiting the occurrence and progression of AAA (118). The pathogenesis of TAA includes changes of ECM, apoptosis of VSMCs, MMPs and reactive oxygen species. Mutations of TGFBR1, TGFBR2, SMAD3, TGFB2, COL3A1, FBN1, and other genes can cause the occurrence of TAAs (119). For example, firillin-1 is the major subunit of microfibers that constitute the ECM of the thoracic aortic wall. Changes in Firillin-1 result in reduced adhesion between elastic fibers and VSMCs, thereby damaging the biomechanical integrity of the aortic wall and ultimately leading to Marfan syndrome (120). Fibrllin-1 is highly homologous to potential TGFβ binding proteins, and TGFβ gene defects are found not only in Marfan syndrome but also in Patients with Loeys-Dietz aortic aneurysm syndrome (121). A recent discovery found MFAP4 glycosylation was enhanced in MFS patients, and its expression was further enhanced in the advanced aneurysm stage (122). They also induced pluripotent stem cells to differentiate into VSMC in MFS patients and found that there was no elastin expression and the expression level of MFAP4 was unchanged.
VSMC reprogramming to a MSC-like state may play a key role in the progression of AAAs (123). During the pathogenesis of AAA, the TGF-β signaling pathway of VSMCs was inhibited. The decreased expression of TGF-βR2 could lead to reduced binding of Smad2/3 to transcription factor KLF4, leading the transformation of contractile VSMCs into MSCs-like cells. MSCs possess high plasticity and potential to differentiate into several different cell types, such as osteoblasts, chondrocytes, adipocytes, and macrophages. This characteristic has implications for aortic dilation, aortic dilation, calcification of the aortic wall, and inflammation, thereby promoting the development of aneurysms (123). Another research found Smad3 gene mutations in the second cardiogenic SMPCs and neural crest SMPCs prevented their differentiation into VSMCs and reduced the level of proelastase. This suggests that CPC-SMPCs and NCSC-SMPCs can differentiate into VSMCs through the SMAD3-dependent TGF-β signaling pathway (124). ECs differentiation was reduced in patients with AAAs compared with healthy human MSCs, confirming that the aging MSCs impaired its original vascular repair ability, initiating the occurrence and progress of AAAs (125). Some studies have confirmed that MSCs from calcified and inflammatory aorta have high osteogenic potential and pathological angiogenesis ability under appropriate stimulation (126). Runx2 can enhance the proliferation of MSCs and induce them differentiate into osteoblast lineage cells by regulating Shh, Fgfr2/3, Wnt, and Pthlh signaling pathways (55). Runx2-mediated micro-calcification is a new pathological feature of AAA. It may be a promising strategy for clinical improvement of disease treatment regimens (127). Resident NG2+ progenitor cells can differentiate into VSMCs after aortic injury during aortic aneurysm and dissection, producing growth factors that promote endothelial survival and vascular repair (77) (Figure 3).
In this review, we provide the latest research progresses on VSCs in vascular repair upon injury. VSCs exist in the three-layers of vascular wall and play important roles in the occurrence and progression of vascular remodeling diseases such as atherosclerosis, restenosis, hypertension, aortic aneurysm, and dissection. After vascular injury, VSCs can migrate and differentiate into ECs or VSMCs, resulting in the occurrence or aggravation of vascular remodeling-related diseases. Current studies have shown that VSCs can express stem cell markers such as Sca-1 and CD34, but these markers are not specific and their roles in various diseases need to be distinguished from BMSCs or adipose stem cells. The functions of stem cells and their mechanisms in cardiovascular diseases mentioned in the text have not yet been sufficient studied, which is also the direction of further researches. Most groups are now focused on single cell sequencing and cell lineage tracing and other advanced technology to trace the source of all kinds of cells in vascular remodeling associated diseases, in order to better understand the specific role of distinct population of various vascular stem/progenitor cells in vascular remodeling which offers new potential therapeutic targets.
BY and AQ conceived and supervised this study. JT, XC, BY, and AQ wrote and revised the manuscript. JT and XC draw the figures and tables. All authors contributed to the article and approved the submitted version.
This study was supported by the Key Science and Technology Project of Beijing Municipal Institutions (KZ201910025027 and KZ202010025032); the National Natural Science Foundation of China (81870186 and 82070474); the Importation and Development of High-Caliber Talents Project of Beijing Municipal Institutions (CIT&TCD201904090; CIT&TCD20190332).
The authors declare that the research was conducted in the absence of any commercial or financial relationships that could be construed as a potential conflict of interest.
All claims expressed in this article are solely those of the authors and do not necessarily represent those of their affiliated organizations, or those of the publisher, the editors and the reviewers. Any product that may be evaluated in this article, or claim that may be made by its manufacturer, is not guaranteed or endorsed by the publisher.
We thank Dr. Qingbo Xu (Department of Cardiology, The First Affiliated Hospital of Zhejiang University School of Medicine, Hangzhou, China) and Frank J. Gonzalez (Laboratory of Metabolism, Center for Cancer Research, National Cancer Institute, National Institutes of Health, Bethesda, Maryland, USA) for the critical reading of this manuscript.
AAA, abdominal aortic aneurysm; ADAM17, a disintigrin and metalloproteinase 17; ATF6, activating transcription factor 6; BMMSC, bone marrow mesenchymal stem cells; CCL, Chemokine (C-C motif) ligand; CXCL, Chemokine (C-X-C motif) ligand; CXCR, Chemokine (C-X-C motif) receptor; DKK3, dickkopf 3; EC, endothelial cell; ECFC, endothelial colony-forming cell; ECM, extracellular matrix; EPC, endothelial progenitor cell; Flk-1, fetal liver kinase-1; FoxM1, Forkhead box protein M1; HAECs, human aortic endothelial cells; HUVECs, human umbilical vein endothelial cells; KLF4, krüppel-like factor 4; MACs, myeloid angiogenic cells; MFAP4, microfibrillar-associated protein 4; MFS, marfan syndrome; MMP, matrix metalloproteinase; MSCs, mesenchymal stem cells; NF-κB, nuclear factor-kappa B; PDGF beta, platelets derive growth factors beta; PDGFR, platelet-derived growth factor receptor; SDF-1, stromal cell-derived factor-1; Shh, sonic hedgehog; SMPC, smooth muscle progenitor cell; STAT3, signal transducer and activator of transcription 3; TAA, thoracic aortic aneurysm; TGFβ, transforming growth factor; TNFα, tumor necrosis factor; Tsg6, TNFα-stimulated gene 6; VE-cadherin, vascular endothelial cadherin; VEGF, vascular endothelial growth factor; VSC, vascular progenitor cell; VSMCs, vascular smooth muscle cells; vWF, von willebrand factor.
1. Martínez-González J, Cañes L, Alonso J, Ballester-Servera C, Rodríguez-Sinovas A, Corrales I, et al. NR4A3: a key nuclear receptor in vascular biology, cardiovascular remodeling, and beyond. Int J Mol Sci. (2021) 22:11371. doi: 10.3390/ijms222111371
2. Zhang L, Issa Bhaloo S, Chen T, Zhou B, Xu Q. Role of resident stem cells in vessel formation and arteriosclerosis. Circ Res. (2018) 122:1608–24. doi: 10.1161/CIRCRESAHA.118.313058
3. Wang D, Li LK, Dai T, Wang A, Li S. Adult stem cells in vascular remodeling. Theranostics. (2018) 8:815–29. doi: 10.7150/thno.19577
4. Bkaily G, Abou Abdallah N, Simon Y, Jazzar A, Jacques D. Vascular smooth muscle remodeling in health and disease. Can J Physiol Pharmacol. (2021) 99:171–8. doi: 10.1139/cjpp-2020-0399
5. Owens GK, Kumar MS, Wamhoff BR. Molecular regulation of vascular smooth muscle cell differentiation in development and disease. Physiol Rev. (2004) 84:767–801. doi: 10.1152/physrev.00041.2003
6. Zhang Q, Chen T, Zhang Y, Lyu L, Zhang B, Huang C, et al. MiR-30c-5p regulates adventitial progenitor cells differentiation to vascular smooth muscle cells through targeting OPG. Stem Cell Res Ther. (2021) 12:67. doi: 10.1186/s13287-020-02127-2
7. Hu Y, Zhang Z, Torsney E, Afzal AR, Davison F, Metzler B, et al. Abundant progenitor cells in the adventitia contribute to atherosclerosis of vein grafts in ApoE-deficient mice. J Clin Invest. (2004) 113:1258–65. doi: 10.1172/JCI19628
8. Wang H, Zhao H, Zhu H, Li Y, Tang J, Li Y, et al. Sca-1(+) cells minimally contribute to smooth muscle cells in atherosclerosis. Circ Res. (2021) 128:133–5. doi: 10.1161/CIRCRESAHA.120.317972
9. Liu M, Gomez D. Smooth muscle cell phenotypic diversity. Arterioscler Thromb Vasc Biol. (2019) 39:1715–23. doi: 10.1161/ATVBAHA.119.312131
10. Wang X, Wang R, Jiang L, Xu Q, Guo X. Endothelial repair by stem and progenitor cells. J Mol Cell Cardiol. (2021) 163:133–46. doi: 10.1016/j.yjmcc.2021.10.009
11. Wu H, Zhou X, Gong H, Ni Z, Xu Q. Perivascular tissue stem cells are crucial players in vascular disease. Free Radic Biol Med. (2021) 165:324–33. doi: 10.1016/j.freeradbiomed.2021.02.005
12. Bianconi V, Sahebkar A, Kovanen P, Bagaglia F, Ricciuti B, Calabro P, et al. Endothelial and cardiac progenitor cells for cardiovascular repair: a controversial paradigm in cell therapy. Pharmacol Ther. (2018) 181:156–68. doi: 10.1016/j.pharmthera.2017.08.004
13. Majesky MW, Horita H, Ostriker A, Lu S, Regan JN, Bagchi A, et al. Differentiated smooth muscle cells generate a subpopulation of resident vascular progenitor cells in the adventitia regulated by Klf4. Circ Res. (2017) 120:296–311. doi: 10.1161/CIRCRESAHA.116.309322
14. Al-Nbaheen M, Vishnubalaji R, Ali D, Bouslimi A, Al-Jassir F, Megges M, et al. Human stromal (mesenchymal) stem cells from bone marrow, adipose tissue and skin exhibit differences in molecular phenotype and differentiation potential. Stem Cell Rev Rep. (2013) 9:32–43. doi: 10.1007/s12015-012-9365-8
15. Herrmann M, Bara JJ, Sprecher CM, Menzel U, Jalowiec JM, Osinga R, et al. Pericyte plasticity - comparative investigation of the angiogenic and multilineage potential of pericytes from different human tissues. Eur Cell Mater. (2016) 31:236–49. doi: 10.22203/eCM.v031a16
16. Patel J, Seppanen EJ, Rodero MP, Wong HY, Donovan P, Neufeld Z, et al. Functional definition of progenitors versus mature endothelial cells reveals key SoxF-dependent differentiation process. Circulation. (2017) 135:786–805. doi: 10.1161/CIRCULATIONAHA.116.024754
17. Li X, Chen C, Wei L, Li Q, Niu X, Xu Y, et al. Exosomes derived from endothelial progenitor cells attenuate vascular repair and accelerate reendothelialization by enhancing endothelial function. Cytotherapy. (2016) 18:253–62. doi: 10.1016/j.jcyt.2015.11.009
18. Mund JA, Estes ML, Yoder MC, Ingram DA, Case J. Flow cytometric identification and functional characterization of immature and mature circulating endothelial cells. Arterioscler Thromb Vasc Biol. (2012) 32:1045–53. doi: 10.1161/ATVBAHA.111.244210
19. Hassanpour M, Rezaie J, Darabi M, Hiradfar A, Rahbarghazi R, Nouri M. Autophagy modulation altered differentiation capacity of CD146(+) cells toward endothelial cells, pericytes, and cardiomyocytes. Stem Cell Res Ther. (2020) 11:139. doi: 10.1186/s13287-020-01656-0
20. Dierick F, Héry T, Hoareau-Coudert B, Mougenot N, Monceau V, Claude C, et al. Resident PW1+ progenitor cells participate in vascular remodeling during pulmonary arterial hypertension. Circ Res. (2016) 118:822–33. doi: 10.1161/CIRCRESAHA.115.307035
21. Bearzi C, Leri A, Lo Monaco F, Rota M, Gonzalez A, Hosoda T, et al. Identification of a coronary vascular progenitor cell in the human heart. Proc Natl Acad Sci U S A. (2009) 106:15885–90. doi: 10.1073/pnas.0907622106
22. Fang S, Wei J, Pentinmikko N, Leinonen H, Salven P. Generation of functional blood vessels from a single c-kit+ adult vascular endothelial stem cell. PLoS Biol. (2012) 10:e1001407. doi: 10.1371/journal.pbio.1001407
23. Jiang L, Chen T, Sun S, Wang R, Deng J, Lyu L, et al. Nonbone marrow CD34(+) cells are crucial for endothelial repair of injured artery. Circ Res. (2021) 129:e146–e65. doi: 10.1161/CIRCRESAHA.121.319494
24. Skrzypkowska MW, Gutknecht PG, Ryba-Stanisławowska ME, Słomiński B, Siebert J, Myśliwska JM. CD34+ and CD34+VEGFR2+ cells in poorly controlled hypertensive patients. J Hum Hypertens. (2019) 33:863–72. doi: 10.1038/s41371-018-0145-z
25. Wu W, Zhang J, Shao L, Huang H, Meng Q, Shen Z, et al. Evaluation of circulating endothelial progenitor cells in abdominal aortic aneurysms after endovascular aneurysm repair. Int J Stem Cells. (2021). doi: 10.15283/ijsc21027. [Epub ahead of print].
26. Shen Y, Wu Y, Zheng Y, Ao F, Kang K, Wan Y, et al. Responses of adventitial CD34(+) vascular wall-resident stem/progenitor cells and medial smooth muscle cells to carotid injury in rats. Exp Mol Pathol. (2016) 101:332–40. doi: 10.1016/j.yexmp.2016.11.004
27. Lin R-Z, Moreno-Luna R, Li D, Jaminet S-C, Greene AK, Melero-Martin JM. Human endothelial colony-forming cells serve as trophic mediators for mesenchymal stem cell engraftment via paracrine signaling. Proc Natl Acad Sci U S A. (2014) 111:10137–42. doi: 10.1073/pnas.1405388111
28. Tang J, Wang H, Huang X, Li F, Zhu H, Li Y, et al. Arterial Sca-1(+) vascular stem cells generate de novo smooth muscle for artery repair and regeneration. Cell Stem Cell. (2020) 26:81–96.e4. doi: 10.1016/j.stem.2019.11.010
29. Ni Z, Deng J, Potter CMF, Nowak WN, Gu W, Zhang Z, et al. Recipient c-Kit lineage cells repopulate smooth muscle cells of transplant arteriosclerosis in mouse models. Circ Res. (2019) 125:223–41. doi: 10.1161/CIRCRESAHA.119.314855
30. Sainz J, Al Haj Zen A, Caligiuri G, Demerens C, Urbain D, Lemitre M, et al. Isolation of “side population” progenitor cells from healthy arteries of adult mice. Arterioscler Thromb Vasc Biol. (2006) 26:281–6. doi: 10.1161/01.ATV.0000197793.83391.91
31. Zorzi P, Aplin AC, Smith KD, Nicosia RF. Technical advance: the rat aorta contains resident mononuclear phagocytes with proliferative capacity and proangiogenic properties. J Leukoc Biol. (2010) 88:1051–9. doi: 10.1189/jlb.0310178
32. Guimarães-Camboa N, Evans SM. Are perivascular adipocyte progenitors mural cells or adventitial fibroblasts? Cell Stem Cell. (2017) 20:587–9. doi: 10.1016/j.stem.2017.04.010
33. Teichert M, Milde L, Holm A, Stanicek L, Gengenbacher N, Savant S, et al. Pericyte-expressed Tie2 controls angiogenesis and vessel maturation. Nat Commun. (2017) 8:16106. doi: 10.1038/ncomms16106
34. Hesp ZC, Yoseph RY, Suzuki R, Jukkola P, Wilson C, Nishiyama A, et al. Proliferating NG2-cell-dependent angiogenesis and scar formation alter axon growth and functional recovery after spinal cord injury in mice. J Neurosci. (2018) 38:1366–82. doi: 10.1523/JNEUROSCI.3953-16.2017
35. Asahara T, Murohara T, Sullivan A, Silver M, van der Zee R, Li T, et al. Isolation of putative progenitor endothelial cells for angiogenesis. Science. (1997) 275:964–7. doi: 10.1126/science.275.5302.964
36. Psaltis PJ, Simari RD. Vascular wall progenitor cells in health and disease. Circ Res. (2015) 116:1392–412. doi: 10.1161/CIRCRESAHA.116.305368
37. Luo S, Xia W, Chen C, Robinson EA, Tao J. Endothelial progenitor cells and hypertension: current concepts and future implications. Clin Sci (Lond). (2016) 130:2029–42. doi: 10.1042/CS20160587
38. Medina RJ, Barber CL, Sabatier F, Dignat-George F, Melero-Martin JM, Khosrotehrani K, et al. Endothelial progenitors: a consensus statement on nomenclature. Stem Cells Transl Med. (2017) 6:1316–20. doi: 10.1002/sctm.16-0360
39. Keighron C, Lyons CJ, Creane M, O'Brien T, Liew A. Recent advances in endothelial progenitor cells toward their use in clinical translation. Front Med (Lausanne). (2018) 5:354. doi: 10.3389/fmed.2018.00354
40. Ingram DA, Mead LE, Moore DB, Woodard W, Fenoglio A, Yoder MC. Vessel wall-derived endothelial cells rapidly proliferate because they contain a complete hierarchy of endothelial progenitor cells. Blood. (2005) 105:2783–6. doi: 10.1182/blood-2004-08-3057
41. Wakabayashi T, Naito H, Suehiro JI, Lin Y, Kawaji H, Iba T, et al. CD157 marks tissue-resident endothelial stem cells with homeostatic and regenerative properties. Cell Stem Cell. (2018) 22:384–97.e6. doi: 10.1016/j.stem.2018.01.010
42. Chen Y, Cao J, Peng W, Chen W. Neurotrophin-3 accelerates reendothelialization through inducing EPC mobilization and homing. Open Life Sci. (2020) 15:241–50. doi: 10.1515/biol-2020-0028
43. Yang JX, Pan YY, Wang XX, Qiu YG, Mao W. Endothelial progenitor cells in age-related vascular remodeling. Cell Transplant. (2018) 27:786–95. doi: 10.1177/0963689718779345
44. Alencar GF, Owsiany KM, Karnewar S, Sukhavasi K, Mocci G, Nguyen AT, et al. Stem cell pluripotency genes Klf4 and Oct4 regulate complex SMC phenotypic changes critical in late-stage atherosclerotic lesion pathogenesis. Circulation. (2020) 142:2045–59. doi: 10.1161/CIRCULATIONAHA.120.046672
45. Owens GK. Regulation of differentiation of vascular smooth muscle cells. Physiol Rev. (1995) 75:487–517. doi: 10.1152/physrev.1995.75.3.487
46. Iyemere VP, Proudfoot D, Weissberg PL, Shanahan CM. Vascular smooth muscle cell phenotypic plasticity and the regulation of vascular calcification. J Intern Med. (2006) 260:192–210. doi: 10.1111/j.1365-2796.2006.01692.x
47. Frismantiene A, Philippova M, Erne P, Resink TJ. Smooth muscle cell-driven vascular diseases and molecular mechanisms of VSMC plasticity. Cell Signal. (2018) 52:48–64. doi: 10.1016/j.cellsig.2018.08.019
48. Wang G, Jacquet L, Karamariti E, Xu Q. Origin and differentiation of vascular smooth muscle cells. J Physiol. (2015) 593:3013–30. doi: 10.1113/JP270033
49. Burggren WW. Phenotypic switching resulting from developmental plasticity: fixed or reversible? Front Physiol. (2019) 10:1634. doi: 10.3389/fphys.2019.01634
50. Passman JN, Dong XR, Wu SP, Maguire CT, Hogan KA, Bautch VL, et al. A sonic hedgehog signaling domain in the arterial adventitia supports resident Sca-1+ smooth muscle progenitor cells. Proc Natl Acad Sci U S A. (2008) 105:9349–54. doi: 10.1073/pnas.0711382105
51. Kramann R, Goettsch C, Wongboonsin J, Iwata H, Schneider RK, Kuppe C, et al. Adventitial MSC-like cells are progenitors of vascular smooth muscle cells and drive vascular calcification in chronic kidney disease. Cell Stem Cell. (2016) 19:628–42. doi: 10.1016/j.stem.2016.08.001
52. Abedin M, Tintut Y, Demer LL. Mesenchymal stem cells and the artery wall. Circ Res. (2004) 95:671–6. doi: 10.1161/01.RES.0000143421.27684.12
53. Pittenger MF, Mackay AM, Beck SC, Jaiswal RK, Douglas R, Mosca JD, et al. Multilineage potential of adult human mesenchymal stem cells. Science. (1999) 284:143–7. doi: 10.1126/science.284.5411.143
54. Ding DC, Shyu WC, Lin SZ. Mesenchymal stem cells. Cell Transplant. (2011) 20:5–14. doi: 10.3727/096368910X
55. Komori T. Regulation of proliferation, differentiation and functions of osteoblasts by Runx2. Int J Mol Sci. (2019) 20:1694. doi: 10.3390/ijms20071694
56. Campagnolo P, Cesselli D, Al Haj Zen A, Beltrami AP, Krankel N, Katare R, et al. Human adult vena saphena contains perivascular progenitor cells endowed with clonogenic and proangiogenic potential. Circulation. (2010) 121:1735–45. doi: 10.1161/CIRCULATIONAHA.109.899252
57. Yao T, Chen H, Baker MB, Moroni L. Effects of fiber alignment and coculture with endothelial cells on osteogenic differentiation of mesenchymal stromal cells. Tissue Eng Part C Methods. (2020) 26:11–22. doi: 10.1089/ten.tec.2019.0232
58. Yao Z, Liu H, Yang M, Bai Y, Zhang B, Wang C, et al. Bone marrow mesenchymal stem cell-derived endothelial cells increase capillary density and accelerate angiogenesis in mouse hindlimb ischemia model. Stem Cell Res Ther. (2020) 11:221. doi: 10.1186/s13287-020-01710-x
59. Wang C, Li Y, Yang M, Zou Y, Liu H, Liang Z, et al. Efficient differentiation of bone marrow mesenchymal stem cells into endothelial cells in vitro. Eur J Vasc Endovasc Surg. (2018) 55:257–65. doi: 10.1016/j.ejvs.2017.10.012
60. Kramann R, Schneider RK, DiRocco DP, Machado F, Fleig S, Bondzie PA, et al. Perivascular Gli1+ progenitors are key contributors to injury-induced organ fibrosis. Cell Stem Cell. (2015) 16:51–66. doi: 10.1016/j.stem.2014.11.004
61. Gu W, Nowak WN, Xie Y, Le Bras A, Hu Y, Deng J, et al. Single-cell RNA-sequencing and metabolomics analyses reveal the contribution of perivascular adipose tissue stem cells to vascular remodeling. Arterioscler Thromb Vasc Biol. (2019) 39:2049–66. doi: 10.1161/ATVBAHA.119.312732
62. Armulik A, Genove G, Mae M, Nisancioglu MH, Wallgard E, Niaudet C, et al. Pericytes regulate the blood-brain barrier. Nature. (2010) 468:557–61. doi: 10.1038/nature09522
63. Korn J, Christ B, Kurz H. Neuroectodermal origin of brain pericytes and vascular smooth muscle cells. J Comp Neurol. (2002) 442:78–88. doi: 10.1002/cne.1423
64. van Dijk CG, Nieuweboer FE, Pei JY, Xu YJ, Burgisser P, van Mulligen E, et al. The complex mural cell: pericyte function in health and disease. Int J Cardiol. (2015) 190:75–89. doi: 10.1016/j.ijcard.2015.03.258
65. Brighton CT, Lorich DG, Kupcha R, Reilly TM, Jones AR, Woodbury RA 2nd. The pericyte as a possible osteoblast progenitor cell. Clin Orthop Relat Res. 1992:287–99. doi: 10.1097/00003086-199202000-00043
66. Boström K, Watson KE, Horn S, Wortham C, Herman IM, Demer LL. Bone morphogenetic protein expression in human atherosclerotic lesions. J Clin Invest. (1993) 91:1800–9. doi: 10.1172/JCI116391
67. Lin CS, Lue TF. Defining vascular stem cells. Stem Cells Dev. (2013) 22:1018–26. doi: 10.1089/scd.2012.0504
68. Guimarães-Camboa N, Cattaneo P, Sun Y, Moore-Morris T, Gu Y, Dalton ND, et al. Pericytes of multiple organs do not behave as mesenchymal stem cells in vivo. Cell Stem Cell. (2017) 20:345–59.e5. doi: 10.1016/j.stem.2016.12.006
69. Deng J, Ni Z, Gu W, Chen Q, Nowak WN, Chen T, et al. Single-cell gene profiling and lineage tracing analyses revealed novel mechanisms of endothelial repair by progenitors. Cell Mol Life Sci. (2020) 77:5299–320. doi: 10.1007/s00018-020-03480-4
70. Chen Q, Yang M, Wu H, Zhou J, Wang W, Zhang H, et al. Genetic lineage tracing analysis of c-kit(+) stem/progenitor cells revealed a contribution to vascular injury-induced neointimal lesions. J Mol Cell Cardiol. (2018) 121:277–86. doi: 10.1016/j.yjmcc.2018.07.252
71. Ouyang S, Li Y, Wu X, Wang Y, Liu F, Zhang J, et al. GPR4 signaling is essential for the promotion of acid-mediated angiogenic capacity of endothelial progenitor cells by activating STAT3/VEGFA pathway in patients with coronary artery disease. Stem Cell Res Ther. (2021) 12:149. doi: 10.1186/s13287-021-02221-z
72. Xie Y, Potter CMF, Le Bras A, Nowak WN, Gu W, Bhaloo SI, et al. Leptin induces Sca-1(+) progenitor cell migration enhancing neointimal lesions in vessel-injury mouse models. Arterioscler Thromb Vasc Biol. (2017) 37:2114–27. doi: 10.1161/ATVBAHA.117.309852
73. Inoue T, Sata M, Hikichi Y, Sohma R, Fukuda D, Uchida T, et al. Mobilization of CD34-positive bone marrow-derived cells after coronary stent implantation: impact on restenosis. Circulation. (2007) 115:553–61. doi: 10.1161/CIRCULATIONAHA.106.621714
74. Dai Z, Zhu MM, Peng Y, Jin H, Machireddy N, Qian Z, et al. Endothelial and smooth muscle cell interaction via FoxM1 signaling mediates vascular remodeling and pulmonary hypertension. Am J Respir Crit Care Med. (2018) 198:788–802. doi: 10.1164/rccm.201709-1835OC
75. Shankman LS, Gomez D, Cherepanova OA, Salmon M, Alencar GF, Haskins RM, et al. KLF4-dependent phenotypic modulation of smooth muscle cells has a key role in atherosclerotic plaque pathogenesis. Nat Med. (2015) 21:628–37. doi: 10.1038/nm.3866
76. Wu J, Montaniel KR, Saleh MA, Xiao L, Chen W, Owens GK, et al. Origin of matrix-producing cells that contribute to aortic fibrosis in hypertension. Hypertension. (2016) 67:461–8. doi: 10.1161/HYPERTENSIONAHA.115.06123
77. Zou S, Ren P, Zhang L, Azares AR, Zhang S, Coselli JS, et al. Activation of bone marrow-derived cells and resident aortic cells during aortic injury. J Surg Res. (2020) 245:1–12. doi: 10.1016/j.jss.2019.07.013
78. Hansson GK. Inflammation, atherosclerosis, and coronary artery disease. N Engl J Med. (2005) 352:1685–95. doi: 10.1056/NEJMra043430
79. Baeyens N, Bandyopadhyay C, Coon BG, Yun S, Schwartz MA. Endothelial fluid shear stress sensing in vascular health and disease. J Clin Invest. (2016) 126:821–8. doi: 10.1172/JCI83083
80. Gimbrone MA, García-Cardeña G. Endothelial cell dysfunction and the pathobiology of atherosclerosis. Circ Res. (2016) 118:620–36. doi: 10.1161/CIRCRESAHA.115.306301
81. Moore KJ, Sheedy FJ, Fisher EA. Macrophages in atherosclerosis: a dynamic balance. Nat Rev Immunol. (2013) 13:709–21. doi: 10.1038/nri3520
82. Wolf D, Ley K. Immunity and inflammation in atherosclerosis. Circ Res. (2019) 124:315–27. doi: 10.1161/CIRCRESAHA.118.313591
83. Ross R, Glomset JA. Atherosclerosis and the arterial smooth muscle cell: proliferation of smooth muscle is a key event in the genesis of the lesions of atherosclerosis. Science. (1973) 180:1332–9. doi: 10.1126/science.180.4093.1332
84. Chistiakov DA, Orekhov AN, Bobryshev YV. Effects of shear stress on endothelial cells: go with the flow. Acta Physiol (Oxf). (2017) 219:382–408. doi: 10.1111/apha.12725
85. Li JH, Li Y, Huang D, Yao M. Role of stromal cell-derived factor-1 in endothelial progenitor cell-mediated vascular repair and regeneration. Tissue Eng Regen Med. (2021) 18:747–58. doi: 10.1007/s13770-021-00366-9
86. Jolly AJ, Lu S, Strand KA, Dubner AM, Mutryn MF, Nemenoff RA, et al. Heterogeneous subpopulations of adventitial progenitor cells regulate vascular homeostasis and pathological vascular remodeling. Cardiovasc Res. (2021). doi: 10.1093/cvr/cvab174. [Epub ahead of print].
87. Karamariti E, Zhai C, Yu B, Qiao L, Wang Z, Potter CMF, et al. DKK3 (Dickkopf 3) alters atherosclerotic plaque phenotype involving vascular progenitor and fibroblast differentiation into smooth muscle cells. Arterioscler Thromb Vasc Biol. (2018) 38:425–37. doi: 10.1161/ATVBAHA.117.310079
88. Chen WT, Hsu WT, Yen MH, Changou CA, Han CL, Chen YJ, et al. Alteration of mesenchymal stem cells polarity by laminar shear stimulation promoting β-catenin nuclear localization. Biomaterials. (2019) 190–1:1–10. doi: 10.1016/j.biomaterials.2018.10.026
89. Colmegna I, Stochaj U. MSC - targets for atherosclerosis therapy. Aging (Albany NY). (2018) 11:285–6. doi: 10.18632/aging.101735
90. Wang SS, Hu SW, Zhang QH, Xia AX, Jiang ZX, Chen XM. Mesenchymal stem cells stabilize atherosclerotic vulnerable plaque by anti-inflammatory properties. PLoS One. (2015) 10:e0136026. doi: 10.1371/journal.pone.0136026
91. Tannock LR. Advances in the management of hyperlipidemia-induced atherosclerosis. Expert Rev Cardiovasc Ther. (2008) 6:369–83. doi: 10.1586/14779072.6.3.369
92. Chen T, Wu Y, Gu W, Xu Q. Response of vascular mesenchymal stem/progenitor cells to hyperlipidemia. Cell Mol Life Sci. (2018) 75:4079–91. doi: 10.1007/s00018-018-2859-z
93. Pickering JG, Ford CM, Chow LH. Evidence for rapid accumulation and persistently disordered architecture of fibrillar collagen in human coronary restenosis lesions. Am J Cardiol. (1996) 78:633–7. doi: 10.1016/S0002-9149(96)00384-0
94. Jukema JW, Ahmed TA, Verschuren JJ, Quax PH. Restenosis after PCI. Part 2: prevention and therapy. Nat Rev Cardiol. (2011) 9:79–90. doi: 10.1038/nrcardio.2011.148
95. Jukema JW, Verschuren JJ, Ahmed TA, Quax PH. Restenosis after PCI. Part 1: pathophysiology and risk factors. Nat Rev Cardiol. (2011) 9:53–62. doi: 10.1038/nrcardio.2011.132
96. Toutouzas K, Colombo A, Stefanadis C. Inflammation and restenosis after percutaneous coronary interventions. Eur Heart J. (2004) 25:1679–87. doi: 10.1016/j.ehj.2004.06.011
97. Kipshidze N, Dangas G, Tsapenko M, Moses J, Leon MB, Kutryk M, et al. Role of the endothelium in modulating neointimal formation: vasculoprotective approaches to attenuate restenosis after percutaneous coronary interventions. J Am Coll Cardiol. (2004) 44:733–9. doi: 10.1016/S0735-1097(04)01083-6
98. Smith SA, Newby AC, Bond M. Ending restenosis: inhibition of vascular smooth muscle cell proliferation by cAMP. Cells. (2019) 8:1447. doi: 10.3390/cells8111447
99. Yang F, Chen Q, He S, Yang M, Maguire EM, An W, et al. miR-22 Is a novel mediator of vascular smooth muscle cell phenotypic modulation and neointima formation. Circulation. (2018) 137:1824–41. doi: 10.1161/CIRCULATIONAHA.117.027799
100. Yu B, Wong MM, Potter CM, Simpson RM, Karamariti E, Zhang Z, et al. Vascular stem/progenitor cell migration induced by smooth muscle cell-derived chemokine (C-C Motif) ligand 2 and chemokine (C-X-C motif) ligand 1 contributes to neointima formation. Stem Cells. (2016) 34:2368–80. doi: 10.1002/stem.2410
101. Renna NF, de Las Heras N, Miatello RM. Pathophysiology of vascular remodeling in hypertension. Int J Hypertens. (2013) 2013:808353. doi: 10.1155/2013/808353
102. Mei X, Cui XB, Li Y, Chen SY. CircSOD2: a novel regulator for smooth muscle proliferation and neointima formation. Arterioscler Thromb Vasc Biol. (2021) 41:2961–73. doi: 10.1161/ATVBAHA.121.316911
103. Issa Bhaloo S, Wu Y, Le Bras A, Yu B, Gu W, Xie Y, et al. Binding of Dickkopf-3 to CXCR7 enhances vascular progenitor cell migration and degradable graft regeneration. Circ Res. (2018) 123:451–66. doi: 10.1161/CIRCRESAHA.118.312945
104. Le Bras A, Yu B, Issa Bhaloo S, Hong X, Zhang Z, Hu Y, et al. Adventitial Sca-1+ cells transduced with ETV2 are committed to the endothelial fate and improve vascular remodeling after injury. Arterioscler Thromb Vasc Biol. (2018) 38:232–44. doi: 10.1161/ATVBAHA.117.309853
105. Messerli FH, Williams B, Ritz E. Essential hypertension. Lancet. (2007) 370:591–603. doi: 10.1016/S0140-6736(07)61299-9
106. Drummond GR, Vinh A, Guzik TJ, Sobey CG. Immune mechanisms of hypertension. Nat Rev Immunol. (2019) 19:517–32. doi: 10.1038/s41577-019-0160-5
107. Intengan HD, Schiffrin EL. Vascular remodeling in hypertension: roles of apoptosis, inflammation, and fibrosis. Hypertension. (2001) 38(3 Pt 2):581–7. doi: 10.1161/hy09t1.096249
108. Takayanagi T, Forrester SJ, Kawai T, Obama T, Tsuji T, Elliott KJ, et al. Vascular ADAM17 as a novel therapeutic target in mediating cardiovascular hypertrophy and perivascular fibrosis induced by angiotensin II. Hypertension. (2016) 68:949–55. doi: 10.1161/HYPERTENSIONAHA.116.07620
109. Cardoso CRL, Salles GF. Prognostic value of changes in aortic stiffness for cardiovascular outcomes and mortality in resistant hypertension: a cohort study. Hypertension. (2022) 79:447–56. doi: 10.1161/HYPERTENSIONAHA.121.18498
110. Jana S, Hu M, Shen M, Kassiri Z. Extracellular matrix, regional heterogeneity of the aorta, and aortic aneurysm. Exp Mol Med. (2019) 51:1–15. doi: 10.1038/s12276-019-0286-3
111. Nienaber CA, Clough RE, Sakalihasan N, Suzuki T, Gibbs R, Mussa F, et al. Aortic dissection. Nat Rev Dis Primers. (2016) 2:16053. doi: 10.1038/nrdp.2016.71
112. Guo DC, Papke CL, He R, Milewicz DM. Pathogenesis of thoracic and abdominal aortic aneurysms. Ann N Y Acad Sci. (2006) 1085:339–52. doi: 10.1196/annals.1383.013
113. Wang X, Khalil RA. Matrix metalloproteinases, vascular remodeling, and vascular disease. Adv Pharmacol. (2018) 81:241–330. doi: 10.1016/bs.apha.2017.08.002
114. López-Candales A, Holmes DR, Liao S, Scott MJ, Wickline SA, Thompson RW. Decreased vascular smooth muscle cell density in medial degeneration of human abdominal aortic aneurysms. Am J Pathol. (1997) 150:993–1007.
115. Sinha S, Santoro MM. New models to study vascular mural cell embryonic origin: implications in vascular diseases. Cardiovasc Res. (2018) 114:481–91. doi: 10.1093/cvr/cvy005
116. Cai D, Sun C, Zhang G, Que X, Fujise K, Weintraub NL, et al. A novel mechanism underlying inflammatory smooth muscle phenotype in abdominal aortic aneurysm. Circ Res. (2021) 129:e202–e14. doi: 10.1161/CIRCRESAHA.121.319374
117. Quintana RA, Taylor WR. Cellular mechanisms of aortic aneurysm formation. Circ Res. (2019) 124:607–18. doi: 10.1161/CIRCRESAHA.118.313187
118. Pinard A, Jones GT, Milewicz DM. Genetics of thoracic and abdominal aortic diseases. Circ Res. (2019) 124:588–606. doi: 10.1161/CIRCRESAHA.118.312436
119. Fletcher AJ, Syed MBJ, Aitman TJ, Newby DE, Walker NL. Inherited thoracic aortic disease: new insights and translational targets. Circulation. (2020) 141:1570–87. doi: 10.1161/CIRCULATIONAHA.119.043756
120. Judge DP, Dietz HC. Marfan's syndrome. Lancet. (2005) 366:1965–76. doi: 10.1016/S0140-6736(05)67789-6
121. Yeh JSM, Rubens M, Nienaber CA. Novel reconstruction of a vascular aneurysm in Marfan syndrome. Eur Heart J. (2017) 38:1521. doi: 10.1093/eurheartj/ehw520
122. Yin X, Wanga S, Fellows AL, Barallobre-Barreiro J, Lu R, Davaapil H, et al. Glycoproteomic analysis of the aortic extracellular matrix in Marfan patients. Arterioscler Thromb Vasc Biol. (2019) 39:1859–73. doi: 10.1161/ATVBAHA.118.312175
123. Chen PY, Qin L, Li G, Malagon-Lopez J, Wang Z, Bergaya S, et al. Smooth muscle cell reprogramming in aortic aneurysms. Cell Stem Cell. (2020) 26:542–57.e11. doi: 10.1016/j.stem.2020.02.013
124. Gong J, Zhou D, Jiang L, Qiu P, Milewicz DM, Chen YE, et al. In vitro lineage-specific differentiation of vascular smooth muscle cells in response to SMAD3 deficiency: implications for SMAD3-related thoracic aortic aneurysm. Arterioscler Thromb Vasc Biol. (2020) 40:1651–63. doi: 10.1161/ATVBAHA.120.313033
125. Teti G, Chiarini F, Mazzotti E, Ruggeri A, Carano F, Falconi M. Cellular senescence in vascular wall mesenchymal stromal cells, a possible contribution to the development of aortic aneurysm. Mech Ageing Dev. (2021) 197:111515. doi: 10.1016/j.mad.2021.111515
126. Ciavarella C, Gallitto E, Ricci F, Buzzi M, Stella A, Pasquinelli G. The crosstalk between vascular MSCs and inflammatory mediators determines the pro-calcific remodelling of human atherosclerotic aneurysm. Stem Cell Res Ther. (2017) 8:99. doi: 10.1186/s13287-017-0554-x
Keywords: vascular stem cells, atherosclerosis, restenosis, hypertension, aortic aneurysm, vascular injury, vascular remodeling
Citation: Tao J, Cao X, Yu B and Qu A (2022) Vascular Stem/Progenitor Cells in Vessel Injury and Repair. Front. Cardiovasc. Med. 9:845070. doi: 10.3389/fcvm.2022.845070
Received: 29 December 2021; Accepted: 17 January 2022;
Published: 10 February 2022.
Edited by:
Zhanpeng Huang, The First Affiliated Hospital of Sun Yat-sen University, ChinaReviewed by:
Qingzhong Xiao, Queen Mary University of London, United KingdomCopyright © 2022 Tao, Cao, Yu and Qu. This is an open-access article distributed under the terms of the Creative Commons Attribution License (CC BY). The use, distribution or reproduction in other forums is permitted, provided the original author(s) and the copyright owner(s) are credited and that the original publication in this journal is cited, in accordance with accepted academic practice. No use, distribution or reproduction is permitted which does not comply with these terms.
*Correspondence: Baoqi Yu, YmFvcWl5dUBjY211LmVkdS5jbg==; Aijuan Qu, YWlqdWFucXVAY2NtdS5lZHUuY24=
†These authors have contributed equally to this work
Disclaimer: All claims expressed in this article are solely those of the authors and do not necessarily represent those of their affiliated organizations, or those of the publisher, the editors and the reviewers. Any product that may be evaluated in this article or claim that may be made by its manufacturer is not guaranteed or endorsed by the publisher.
Research integrity at Frontiers
Learn more about the work of our research integrity team to safeguard the quality of each article we publish.