- 1School of Pharmacy, Anhui University of Chinese Medicine, Hefei, China
- 2Anhui Province Key Laboratory of Chinese Medicinal Formula, Hefei, China
- 3Institute of Traditional Chinese Medicine Resources Protection and Development, Anhui Academy of Chinese Medicine, Hefei, China
- 4Anhui Province Key Laboratory of Traditional Chinese Medicine Decoction Pieces of New Manufacturing Technology, Hefei, China
Non-alcoholic fatty liver disease (NAFLD) is rapidly prevalent due to its strong association with increased metabolic syndrome such as cardio- and cerebrovascular disorders and diabetes. Few drugs can meet the growing disease burden of NAFLD. Salvia miltiorrhiza Bge. (Danshen) have been used for over 2,000 years in clinical trials to treat NAFLD and metabolic syndrome disease without clarified defined mechanisms. Metabolic targets restored metabolic homeostasis in patients with NAFLD and improved steatosis by reducing the delivery of metabolic substrates to liver as a promising way. Here we systematic review evidence showing that Danshen against NAFLD through diverse and crossing mechanisms based on metabolic targets. A synopsis of the phytochemistry and pharmacokinetic of Danshen and the mechanisms of metabolic targets regulating the progression of NAFLD is initially provided, followed by the pharmacological activity of Danshen in the management NAFLD. And then, the possible mechanisms of Danshen in the management of NAFLD based on metabolic targets are elucidated. Specifically, the metabolic targets c-Jun N-terminal kinases (JNK), sterol regulatory element-binding protein-1c (SREBP-1c), nuclear translocation carbohydrate response element–binding protein (ChREBP) related with lipid metabolism pathway, and peroxisome proliferator-activated receptors (PPARs), cytochrome P450 (CYP) and the others associated with pleiotropic metabolism will be discussed. Finally, providing a critical assessment of the preclinic and clinic model and the molecular mechanism in NAFLD.
Introduction
NAFLD refers to a spectrum of liver diseases, which defined by steatosis in more than 5% of hepatocytes with little or no alcohol consumption (1). NAFLD is composed of non-alcoholic fatty liver (NAFL) and non-alcoholic steatohepatitis (NASH), which is characterized by steatosis, hepatocellular ballooning and lobular inflammation and accompanied by varying degrees of liver fibrosis (2). With the worsening of the fibrosis, some patients with NASH deteriorate to hepatic cirrhosis or even hepatic carcinoma. Currently, almost 25% of the general population worldwide suffer from NAFLD, and 20% of them will progress to hepatocirrhosis as the disease deteriorates (3). NAFLD is a metabolic stress liver injury, and its main pathogenesis is insulin resistance and lipid metabolism disorders. Metabolic syndromes such as obesity, insulin resistance, hyperglycemia, dyslipidemia, and hypertension are the main risk factors for NAFLD. However, drugs that interfere with NASH have side effects such as pioglitazone for insulin resistance, vitamin E for antioxidants, (4), and the only approved treatment is hygiene and dietary measures to date. At present, the prescription of traditional Chinese medical (TCM) contribute to the improvement of lipid infiltration of the liver and of the related anthropometric, and biochemical parameters (5). Besides, TCM may be promising treatment strategies for NAFLD due to their relatively cost-effective, multiple targets and few side effects.
Danshen has been used widely to treat metabolic syndromes such as hypertension, dyslipidemia, and hyperglycemia, which is consisted of active constituents such as hydrophilic phenols, lipophilic diterpenoids and polysaccharides (6). Accumulating evidence has suggested that the preventive and therapeutic potential of Danshen on NAFLD is related to reducing the risk of metabolic disorder in preclinic and clinic trials (7–10). What counts is, the frequency of clinical use of Danshen reached 7.28% in treatment with NAFLD, second only to the peach kernel (11). A case in point is that 8 randomized controlled trials with 800 patients of NAFLD showed that Danshen relieved the degree of hepatosteatosis by significantly reducing the plasma levels of transaminases (9).
The pathogenic driver of NAFLD is that the imbalance between glucose and lipid metabolism leads to excessively accumulated lipids within the liver (12, 13). Metabolic targets can rebalance the metabolic disorders and improve steatosis by reducing the delivery of metabolic substrates to the liver in patients with NAFLD (14–16). Additionally, a large number of pharmacological studies have shown that drugs targeting metabolic targets can effectively treat NASH, which proves that metabolic targets would be a promising prospect therapy for the treatment of NAFLD (4, 17, 18). Previous reviews discussed the prominent metabolic targets of NAFLD pharmacotherapy, including lipid metabolism pathway modulator acetyl-CoA carboxylase (acetyl-CoA), nuclear receptors, thyroid hormone receptors, glycemic modulator, sodium-glucose co-transporter 2 and fibroblast growth factors, etc. (12, 19). Herein we focus primarily on the significant metabolic targets associated with Danshen, including c-Jun N-terminal kinases (JNKs), SREBP-1c, ChREBP, PPARs, CYPs and the others, to provide theoretical basis for the development of a new way of Danshen treatment of NAFLD.
Phytochemistry of Danshen
We reviewed phytochemistry of Danshen to fully understand its roles in NAFLD. Over 200 chemical components including phenolic acids, diterpenoids and polysaccharides have been identified from this plant (20).
The phenolic acids of Danshen contain a core skeleton of phenylpropanoid (C6-C3). It is generally believed that the phenolic acids are mostly conjugated with (R)-3- (3,4-Dihydroxyphenyl)-2-hydroxy- propanoic acid and derivatives or dimer of caffeic acid (21). For instance, Salvianolic acid A (SAA) is composed of tanshinol (TSL) and two molecules of caffeic acid. Previous studies have clarified the phenolic acids bioactivities such as anti-inflammatory, anti-oxidative, cardio-protection activity (22).
As a representative of diterpenoids, tanshinones are comprised of naphthalene or tetrahydronaphthalene rings A and B, ortho- or para-naphthoquinone or lactone ring C, and a furan or dihydrofuran ring D (21). Among these, tanshinone IIA (TSIIA) and cryptotanshinone (CT), which are characterized by an ortho-quinone C-ring. TSIIA, CT, tanshinone I (TSI), and dihydrotanshinone I; (TI) as primary and marker constituents in the official Chinese Pharmacopoeia, which exhibit the biological activity of anti-oxidative, anti-inflammatory, and anti-diabetic (23).
Besides, polysaccharides, flavonoids, steroids, and phenanthrenequinone have been identified in Danshen, which proved that a variety of pharmacological activity. For instance, the polysaccharides of Danshen exhibits immunomodulatory and antitumor effects (21).
In this article, we briefly presented the identified chemical structure in Danshen, which related to the pharmacological effects of NAFLD as Figure 1. Interested readers are inspired to know more compounds of Danshen refer to related reviews.
Pharmacokinetics Characteristics of Danshen
Pharmacokinetics is a bridge linking herbal medicine and pharmacological responses. The active ingredients of Danshen exert therapeutic effects of NAFLD through entering the systemic circulation to reach the target organ or tissue. Painstaking efforts directed at mechanisms influencing the pharmacokinetics of Danshen can aid in improving their intended pharmacological activity. The major topics cover (i) Danshen ability to enter a system and how to metabolite and eliminate through various tissues, and (ii) what factors determine the course of Danshen bioavailability and how to improve it.
Phenolic Acids
Extensive research performed the complicated absorption mechanism of phenolic acids of Danshen. The gastrointestinal absorption of rosmarinic acid (RA), salvianolic acid B (SAB), protocatechuic aldehyde (PCA) and TSL were characterized by passive diffusion in the intestine (24, 25). Besides, the paracellular absorption transport pathway also existed in SAB and TSL (26). The phenolic acids were of low bioavailability in animal or human plasma after oral administration, except for TSL. For instance, the oral bioavailability of SAB, salvianolic acid C (SAC), lithospermic acid (LA), salvianolic acid D (SAD), magnesium lithospermate B and miltirone were 1.07, 0.29, 1.15, 4.16, 0.02, and 3.4% in rats, respectively (27–31). Moreover, the concentration of Danshen preparations in plasma after oral administration slightly detectable in human subjects. Li et al. found that the Cmax of TSL, PCA and protocatechuic acid (PAA) in humans were 134.1 ± 48.4, 10.5 ± 5.6, and 59.0 ± 18.6 ng/mL after oral administration of Compound Danshen Dripping Pills (32). Researches showed that the poor bioavailability of phenolic acids was due to their adverse properties, including molecular mass, hydrogen bonding capacity and molecular flexibility (33). TSL has good properties and its bioavailability reaches to 30–40% in animals (34). Injection is more effective than oral administration. The TSL, SAD, LA, RA, PCA, and TSL exhibited considerable exposure in human subjects and rats after intravenous administration Danshen (35, 36).
Polyphenol compounds were transformed by colonic microflora and decomposed into simple phenolic acid derivatives, easily absorbed from the intestine. The major metabolites of phenolic acids were the methylated and their sulfated and glucuronide products in humans, rats and dogs, which may be responsible for the biological activity (37, 38). SAA, SAB, PCA, and LA were subject to extensive elimination mainly via hepatobiliary excretion of glucuronide and renal excretion (37). For example, SAB seemed to enter liver cells rapidly after oral or intravenous dosing, and methylated metabolites may exert antioxidant action. PCA was transformed into PAA in the liver and then eliminated by conversion into sulfates and methylated glucuronides followed by renal excretion (36). However, RA, TSL, and SAD were eliminated mainly via renal excretion, rather than hepatobiliary excretion (34, 39). Among these, RA could be broken down into smaller phenolic acids such as TSL and caffeic acid and eliminated by renal excretion in healthy human subjects (40). Further studies with the metabolic pathways of other component in Danshen preparations are required.
Several presystemic processes, including gastrointestinal solubility, membrane permeability, gastrointestinal degradation, transporter-mediated intestinal efflux, systemic intestinal wall metabolism, and hepatic metabolism, could lead to the low bioavailability of phenolic acids. Researchers have been trying to improve the bioavailability of Danshen such as ameliorating dosage form (e.g., sodium caprate and lipid nanoparticle formulations). For instance, the sodium caprate could significantly enhance the bioavailability of both TSL and SAB by improving intestinal permeability (26). When encapsulated into liposomes and chitosan nanoparticles, the t1/2 and AUC0–∞ of SAB in beagle dogs were higher than that of free SAB (41, 42). Certain components in Danshen preparations can affect the pharmacokinetic behavior of other coexisting ingredients, which contributed to synergistic or metabolic transformation. Chang et al. observed that the AUC0–8, CL and t1/2 of TSL and SAB were significantly influenced by the content variation of the other major components in the Danshen injection (43). Moreover, the tanshinones affected the bioavailability and distribution of RA, SAB, and TSL, and accelerated the biotransformation of Sal B to TSL. Similarly, the phenolic acids could influence the biotransformation of CT to TSIIA and improve the bioavailability of TSIIA (44). Additionally, the common Danshen compound compatibility herbs such as borneol can also improve the bioavailability of Danshen (45). Therefore, it will be interesting to focus on the transformation or interaction of components between Danshen preparation in the future.
Tanshinones
Few studies have reported the absorption mechanism of tanshinones. Yan et al. elucidated that the transport mechanisms of TSIIA and CT were active transport or facilitated diffusion (46). Zhang et al. observed that CT was transported primarily via an active mechanism across the intestinal epithelium, a saturable process that could pump CT into the luminal side (47). Meanwhile, other studies reported that TSIIA might not exclude a passive transport process (48, 49) due to its low molecular weight (294 D) and high lipophilicity (logP = 6.1) (50). The plasma level of tanshinones is low, generally in the nM to the sub-μM range in animals after oral or injection. Here offers instances that, Yu et al. found that the oral bioavailability of tanshinone IIB (TSIIB) was about 3% in rats (51), and the Cmax of 0.274 μg/mL of TSI after intravenous injection at 3 mg/kg dose in rats (52). Additionally, the blood concentration of Danshen mixture was poor in rats, either. The Cmax of TSI, TI, TSIIA, and CT were 1.63 ± 0.78, 3.23 ± 1.40, 2.78 ± 0.96, and 0.66 ± 0.27 ng/mL after oral administration of PF2401-SF (the standardized fraction of Danshen, equivalent to TSI: TI: TSIIA: CT = 1.15:1.10:4.1:1.91 mg/kg) to rats (53). When a mixture of phenolic acids and tanshinones including TSL (10.25 mg/kg), RA (6.39 mg/kg), CT (9.82 mg/kg), TI (13.58 mg/kg), TSI (3.90 mg/kg), and TSIIA (5.79 mg/kg) were orally administered to rats, the Cmax of each constituent was 72, 37, 43, 11, 55, and 22 ng/ml, respectively (54). Exposure to tanshinones of granular powder formulation after a single oral administration in healthy volunteers, the Cmax of TSI, TSIIA, and CT was 6.57, 25.8, and 146.7 ng/mL, respectively (55). The low bioavailability of tanshinone is mainly due to the lack of molecules with high logP or optimal HLB values and permeability. Therefore, bioavailability enhancement improved the application of tanshinones in the clinic.
Previous investigation indicated that the concentration of tanshinones in bile was much higher than in plasma and urine, suggesting that the tanshinones are mainly metabolized in the liver (56). TSIIA is preferentially distributed into the liver after either intravenous or oral doses, which is due to the self-association or self-assembly of highly hydrophobic compound to form macromolecules or polymers and can be recognized and taken up by the reticuloendothelial system (48). TSIIA underwent extensive CYP-mediated oxidation, and then subject to glucuronidation, after the glucuronides hydrolysis, aglycones are reabsorbed from the intestine and excreted into bile as a conjugate (57). TI was initially biotransformed into TSI and then shared the metabolic pathways, including glucuronidation, hydroxylation, reduction, methylation, and the O-sulfate conjugated reaction (58, 59). Hence, methylation, demethylation, dehydrogenation, hydrogenation, and hydroxylation were the major metabolic transformation of tanshinones.
The factors including poor solubility, poor permeability, and formulation, etc. resulting in low bioavailability of tanshinones. Similar to phenolic acids, the ultrafine powder and solid lipid nanoparticles formulations could improve the bioavailability of CT, TSIIA, TI, and TSI by enhancing their stability and permeability (60–62). On the other hands, components in tanshinone extracts also affected the pharmacokinetics of monomer constituents. Song et al. reported that tanshinone extracts synergically promoted the absorption of TSIIA and CT and accelerated the transformation of CT to TSIIA (63). The mechanism of promoting absorption and biotransformation between components in TCM formula should be further studied.
The Polysaccharides of Danshen
In addition to phenolic acids and tanshinones, polysaccharides of Danshen exhibited antioxidant, immunoregulatory, ameliorating metabolic disease, hepato-protective, and against NAFLD (64). Few studies involved pharmacokinetics of Danshen polysaccharides compared with the pharmacodynamics. This is related to the complex molecular structure, which lacks both chromogenic and light absorption groups, and susceptible to the interference of biological substances in concentration detection. What’s worse, it is well known that polysaccharides are poorly absorbed. Generally, there are three theories for the mechanism of oral absorption of polysaccharides: direct absorption, intestinal microflora absorption and intestinal Peyer collection lymph node absorption (65). Wang et al. concluded that ganoderma lucidum polysaccharide was ingested by pinocytosis in Caco-2 cells (66). And the elimination of polysaccharides is mostly by the kidney (67). Some researchers believe that polysaccharides work through direct absorption in the gut. A novel polysaccharide of Danshen, SMWP-U&E, improved the absorption of weaned piglets by increasing the diversity and evenness of the intestinal microflora after doses at 1.5 g/kg to the pigs (68). Lack of evidence regarding the mechanism of the pharmacokinetics of polysaccharides is a major barrier to realizing the full potential pharmacological activity.
Discuss the Activity Against Non-Alcoholic Fatty Liver Disease of Danshen Compounds With Low Bioavailability
The phenolic acids, tanshinones, and polysaccharides isolated from Danshen exhibited various biological activity such as against NAFLD. However, how some Danshen compounds with low bioavailability exhibit their pharmacological activity against NAFLD. Here we attempt to interpret this from four aspects: (i) the chemical structure-activity of Danshen, (ii) the bioavailability of Danshen compounds in comparison between physiological and physiological condition, (iii) pharmacological activity of the improved bioavailability compound of Danshen, (iiii) Danshen induced gut microbiota alteration.
The Chemical Structure-Activity of Danshen
The preventive and therapeutic potential of Danshen on NAFLD is related to reducing the risk of metabolic disorders, including antioxidant, anti-inflammatory, immunoregulatory, etc. Normally, the chemical structures of phenolic acids influence their redox potential. Specially, polyphenols with two o-hydroxyl groups on an aromatic residue are more capable of scavenging free radicals than polyphenols with only one hydroxyl group (69). The other active constituents of Danshen, diterpenoids, were demonstrated to exert anti-inflammatory, immunosuppressive and antitumor activity. Quinone group and aromatic ring are the basic structure of tanshinone activity, and carbonyl may play an important role in its antitumor activity (37). Zheng et al. confirmed that the antiproliferative effects of abietane quinone diterpenoids against five cancer cell lines (70). On the other hand, in addition to the parent compounds, the metabolites usually exhibited pharmacological activity. For instance, the sulfate esters and glucuronides metabolites of phenolic acids were shown to retain part of their antioxidant properties (71). Therefore, many of the biological effects observed in animal or clinical studies may be explained by the microbial metabolites of those compounds. Much research effort is needed to evaluate the biological effects of the conjugated derivatives and microbial metabolites of polyphenolic acids and diterpenoids. Generally, the structure-activity relationship between polysaccharides and intestinal flora from molecular weight, glycosidic bond, and monosaccharide composition (72). However, Danshen polysaccharide has not been studied in this respect.
The Bioavailability of Danshen Compounds in Comparison Between Physiological and Physiological Condition
The pharmacokinetics of Danshen formulation varies with pathological and physiological conditions due to the biological environment in the body. Accumulative studies have shown that the bioavailability of Danshen increased under pathological conditions compared to normal conditions. Shi et al. found that the plasma concentrations of TSL, PCA, PAL, SAC, SAB, and TSIIA and the AUC0–t, MRT0–t, and t1/2 of SAB and TSL in the middle cerebral artery occlusion rats were significantly increased compared with the sham-operated group (73). The CL of STS was lower in coronary heart disease patients than in healthy volunteers in a population pharmacokinetic model study (74). Moreover, several metabolites of TSIIA presented differences in the distribution between the sham control and the Alzherimer’s Disease Rat model (75). The pharmacokinetics of Danshen in the NAFLD disease state needs to be studied since several studies have demonstrated the altered pharmacokinetics of drugs in NASH patients (76).
Pharmacological Activity of the Improved Bioavailability Danshen Compound
The modified Danshen compounds improved bioavailability and pharmacological activity. Li et al. reported that STS was a promising drug for treating NAFLD by decreasing lipid accumulation and suppressing inflammation (77). Moreover, TSIIA encapsulated into globin to form nanoparticles that could markedly attenuate the progression of hepatic fibrosis (78). The nanoparticle-containing TSIIA was significantly more effective in inhibiting tumor growth in mice with hepatoma than free TSIIA (79). The pharmacological activity of modified other compounds from Danshen need further study.
Danshen Induced Gut Microbiota Alteration
Hypothesized mechanisms of how the gut microbiome contributes to the development of NAFLD by increasing intestinal permeability, leading to the release of lipopolysaccharide into the host, which can trigger tissue and systemic inflammation, and metabolite produced by microorganisms that can influence immunity (80, 81). Currently, increasing evidences showed that Danshen preparations and the monomer active components regulated intestinal flora. For example, the aerial parts of Danshen could imbalance the intestinal microflora disorder caused by diabetes (82). Wang et al. observed that SAA modulated gut microbiota imbalance during colitis by increasing the gut microbial diversity as well as selectively promoting some probiotic populations (83). Many preparations containing Danshen balanced the intestinal flora such as SMWP -U&E (68), BuZangTongLuo decoction (84), the mixture of yeyachun and Danshen (85). Among these, Danshensu Bingpian Zhi not only reversed HFD-induced intestinal microbiota dysbiosis but also stimulated brown adipose tissue browning and maintains intestinal barrier integrity (86). Previous studies indicated that microbiota composition is related to insulin resistance (87, 88). Probiotics and Danshen polysaccharide combination has the potential to be used as a therapeutic for ameliorating NAFLD via regrouping the composition of the intestinal microbiota and improvement of insulin resistance. In addition, Bacteroidetes/Firmicutes ratio can be increased by Danshen polysaccharide, which is a compositional biomarker for obesity and type 1 diabetes mellitus (89). Danshen preparation including Jian-Gan-Xiao-Zhi (90), DLT-SM (91), and GuanXinNing Tablet Decoction (92) decreased the Firmicutes/Bacteroidetes ratio through regulation of host metabolism. Recent study has found the genera Muribaculaceae might be concerned with the resistance effect of lean mice to HFD (93). Meanwhile, the reduction of Erysipelotrichaceae may be beneficial to lipid metabolism (94). Dingxin Recipe IV increased the relative abundance of Muribaculaceae and decreased Erysipelotrichaceae, which is beneficial to lipid metabolism (95). However, the mechanism of those bacteria in the development of lipid metabolism remains unclear. Besides, there is an intense correlation between fecal microbiota and inflammatory factors. For example, TI enriched bacterial species which promote butyric acid metabolism or negatively correlated with inflammatory factors (89). Interestingly, the effect of water extract on root and rhizome of Danshen was stronger than that of alcohol extract, which further confirmed that Danshen could improve intestinal microflora disorder (96). Most evidence in this field comes from animal experiments and further human study is needed.
Danshen in Management of Disease Based on Metabolic Targets Related With Non-Alcoholic Fatty Liver Disease
Mechanisms of Metabolic Targets Regulating the Progression of Non-alcoholic Fatty Liver Disease
From the theory of “second two-hit” to “multiple hits,” the pathogenesis of NAFLD only partially understood so far. When determining the pathogenicity drivers of NAFL and NASH, elucidating the source and fate of fatty acids in hepatocytes is vital to interpret the metabolic basis of NAFLD. The liver acquires free fatty acids through de novo lipogenesis (DNL) and triglyceride lipolysis in adipocytes, as shown in Figure 2. Primarily, fatty acids are delivered from the blood to the liver through the lipolysis of triglycerides in adipose tissue, regulated by the insulin on adipocytes. Phosphorylation of JNKs in adipocytes dramatically impacts insulin signaling, which contributes to excessive delivery of lipids to the liver and leads to NASH. Secondly, the increased peripheral fatty acids and DNL also elevated liver lipid content in NAFLD. DNL can be pharmacologically inhibited by targeting synthetic enzymes, such as acetyl-CoA, SREBP-1c, ChREBP (12, 97).
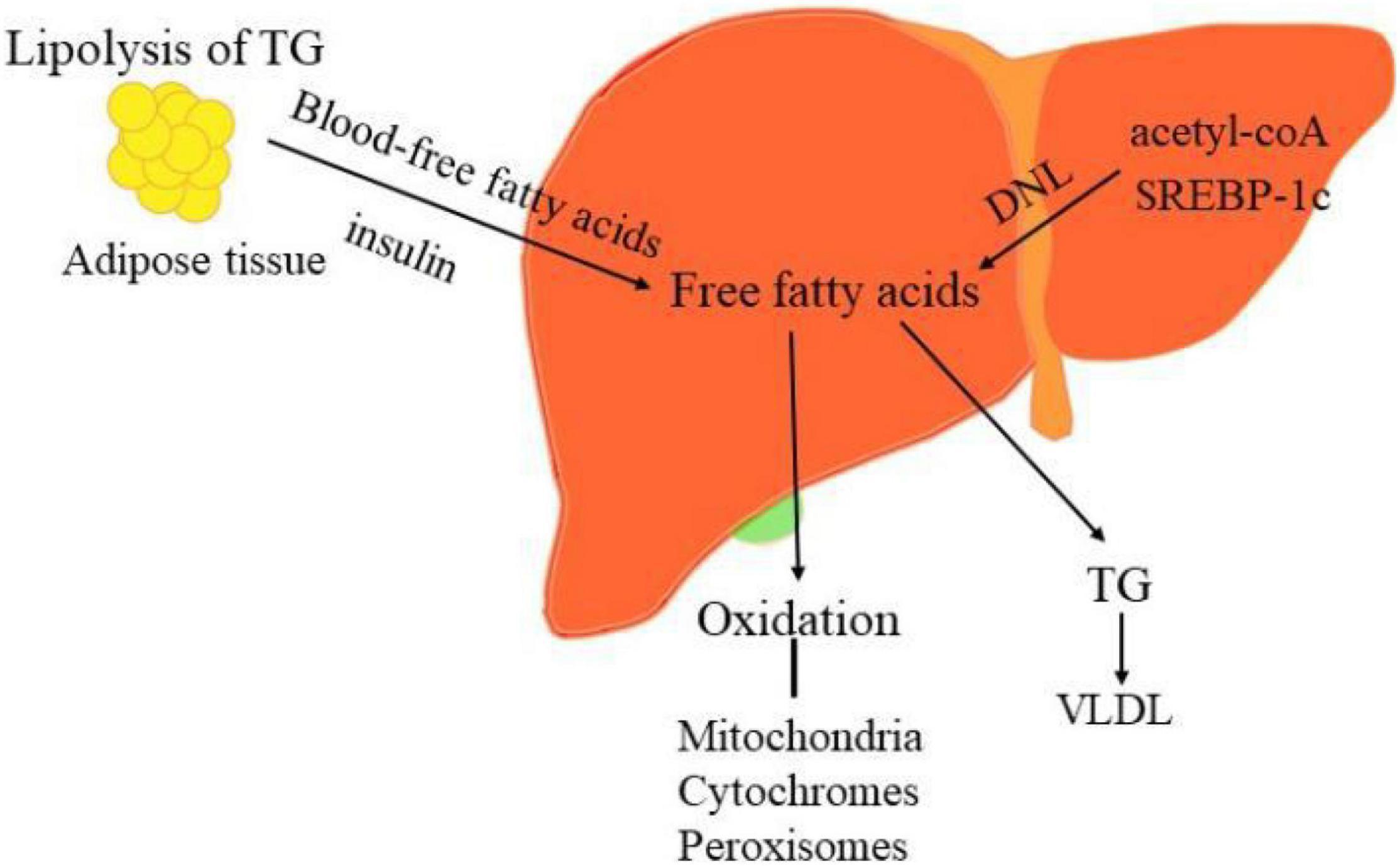
Figure 2. The sources and disposal of hepatic free fatty acids. The key pathogenesis of NASH are hepatic free fatty acids. The liver acquires free fatty acids through DNL and lipolysis of triglyceride in adipocytes. Phosphorylation of JNKs in adipose tissue dramatically impacts insulin signaling, which contributes to excessive delivery of lipids to the liver and leads to NASH. Moreover, increased DNL also result in the elevated liver lipid content in NAFLD. DNL can be pharmacologically inhibited by targeting its synthetic enzymes acetyl-CoA, SREBP-1c, and ChREBP. Conversely, the disposal of fatty acids including oxidation in the mitochondria, cytochromes, and peroxisomes and formation of triglyceride (TG).
Besides, nuclear receptors including PPARs, retinoid X receptorα (RXRα), liver-X-receptorα (LXRα), farnesoid X receptor (FXR), pregnane X receptor (PXR), and constitutive androstane receptor (CAR) regulated fatty acids (Figure 3) (98). Moreover, NAFLD is poised to have an appreciable impact on the expression and function of ATP-binding cassette efflux transport proteins (e.g., MRPs), uptake transporters (e.g., OATPs), and metabolic enzymes such as CYP, UDP-glucuronosyltransferases (UGT) and sulfotransferases (SULT) (99–101).
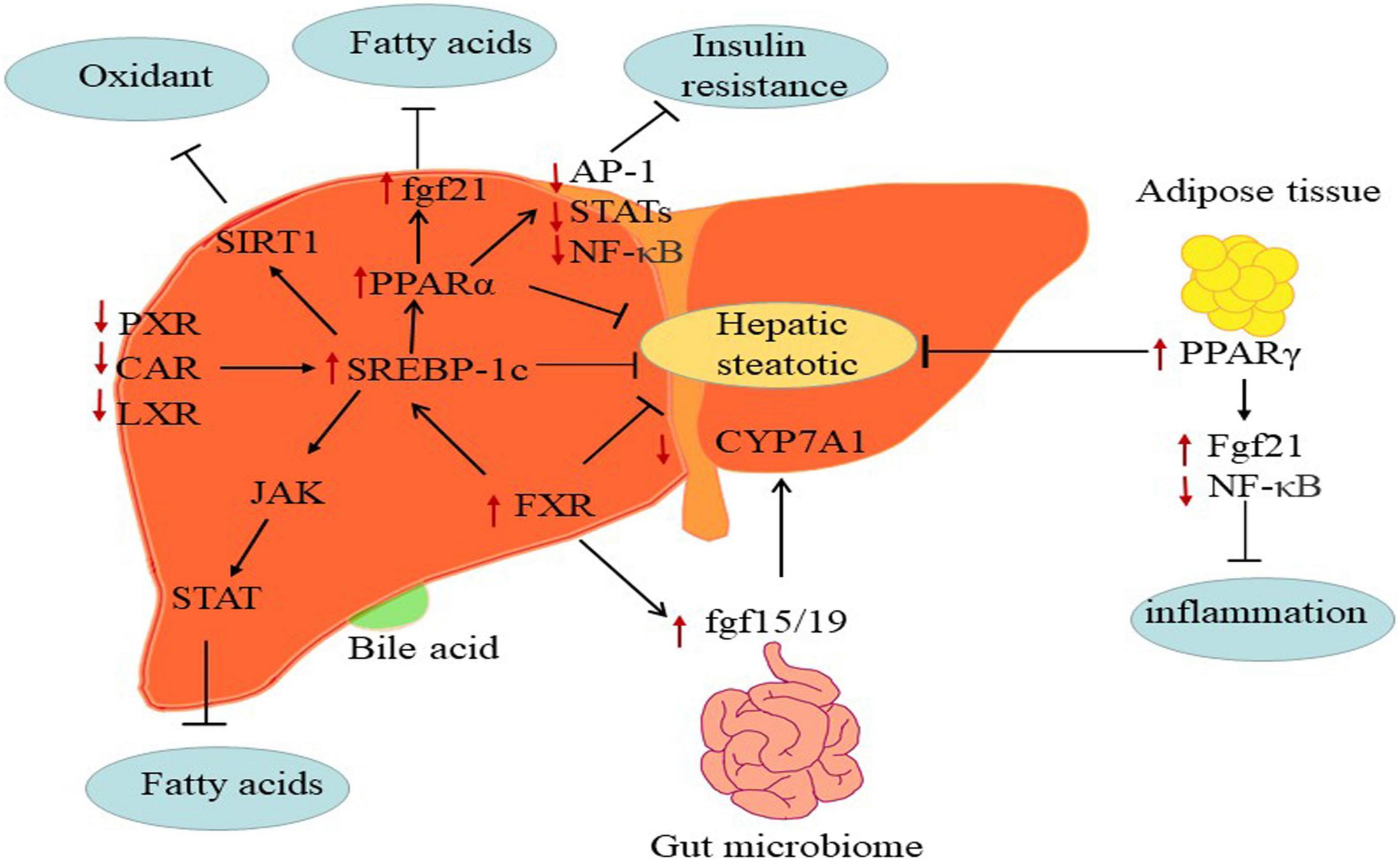
Figure 3. The mechanisms of major metabolic targets regulating the progression of NAFLD. PPARs: The FGF21 directly targeting PPARs key pathogenesis of NASH are hepatic free fatty acids. And activated PPARα may negatively interfere with the activity of pro-inflammatory transcription factors AP-1, STATs, and NF-κB. PPARγ attenuates inflammation via inhibiting NF-κB activity and elevating FGF21 in adipose tissue. FXR: FXR regulates hepatic bile salt synthesis through stimulating FGF15 (FGF19 in humans) expression in the intestinal and repressing CYP7A1 in the liver. LXR: Inhibition of LXRα via SREBP-1c contributes to alleviate progress of hepatic steatosis. PXR and CAR: PXR and CAR regulates SREBP1 involved in metabolic homeostasis.
The PPARα, PPARβ/δ, and PPARγ are members of the nuclear receptor PPAR super-family. PPARα, which is expressed at the high levels in the liver. The deletion of this gene gradually as NASH progresses accelerated the development of NAFLD in preclinic and clinic (102). The activation of PPARβ/δ regulated hepatic glycolipid metabolism in NAFLD (103). PPARγ is mainly in adipose tissues, where its ligands enhanced adipocyte storage of fat and contributed to improve NASH (104). In short, PPARs exerted functions in glycose and lipid metabolism, inflammation, and fibrosis by modulating the expression of specific target genes (105). Indeed, the fibroblast growth factor 21 (FGF21) is an effective metabolic modulator of hepatic glycolipid homeostasis and insulin sensitivity, and PPARs directly induces FGF21 expression in the rodent liver (106, 107). Besides, activated PPARs may negatively interfere with the activity of pro-inflammatory transcription factors such as activator protein-1 (AP-1), signal transducers and activators of transcription (STATs), and nuclear factor-κB (NF-κB) and therefore repressing fibrosis progression (108–110). For instance, PPARα-agonists, fibrates, have shown vital benefit in treating cardiovascular disease in clinical (111) and NAFLD in rodents (112). However, the efficacy of fibrates on NAFLD treatment in humans has not been clarified. Moreover, PPARs modulated lipid dysregulation and inflammatory by adiponectin. Overexpression of PPARγ detected in NAFLD, which is potentially due to adiponectin (113, 114). Researches showed that the agonists of PPARγ against NAFLD might attributed to induce adiponectin (115, 116).
FXR, the bile acid receptor, negatively modulated bile acid synthesis and reduced hepatic gluconeogenesis, lipogenesis and steatosis at both hepatic and extrahepatic tissues (117). The hepatic expression of FXR downregulated in NAFLD patients. Activated FXR appears to influence insulin sensitivity and lipoprotein transport at multiple levels, which indicated that FXR holds promise as targeted therapeutic (118). FXR regulated hepatic bile salt synthesis by stimulating FGF15 (a hormone with direct glycogen synthesis in the liver and distant organs, FGF19 in humans) expression in the intestinal and repressing CYP7A1 in the liver (119, 120). Activated FXR improved hepatic glycogen synthesis via inducing FGF15 in mice (121). Of note, FXR agonist CDCA affected glucose homeostasis by controlling the expression of glucose transporter 4 (GLUT-4) (122). Meanwhile, activation of FXR also suppressed the formation of hepatic lipid and stimulated FAβ-oxidation to limit lipid accumulation. At present, BA-derivative OCA (FXR agonists) has been studied in patients with NAFLD, and further studies are needed to better define the clinical usefulness of OCA in NASH (123).
LXR regulated liver cholesterol homeostasis, inflammation, and fibrosis in vitro and in patients with NAFLD (124, 125). LXRα is responsible for lipid metabolism mainly in the liver, whereas LXRβ expressed ubiquitously (126). Higuchi et al. observed that the expression of LXRαincreased in patients with NAFLD (127). Morin, a dual antagonist of LXRα and LXRβ, alleviated hepatic steatosis and metabolic disorders via the suppression of LXR signaling (128). Namely, LXR antagonism may be productive for attenuating hepatic steatosis and ensuing fibrosis.
PXR and CAR are xenobiotic-sensing nuclear receptors that modulated the expression of genes such as CYPs, UGTs, and OATPs (129). More recently, PXR and CAR participated in regulating glucose, lipid, and bile acid metabolism, and highly expressed in the liver and gut (130). Previous studies have shown that activated PXR and CAR worsen the hepatic steatosis and insulin resistance in NAFLD by suppressing gluconeogenesis and β-oxidation and increasing hepatic fatty acids uptake (131, 132). Indeed, PXR also regulated SREBP1 and PPARα involved in metabolic homeostasis (133, 134), and CAR targeted CYP2B6 (99). For example, a study demonstrated that SREBP-1 inhibited drug-mediated induction of CYP2B and CYP3A via activating PXR and CAR in rodents, which indicated that PXR and CAR respond to lipid accumulation through direct interaction with SREBP-1 (135).
Due to its progressive nature and its significant impacts on hepatic histopathology, NAFLD is expected to significantly affect transporters and metabolic enzymes such as CYPs, UGTs, and SULTs. The expression and function of transporters such as OATPs and MRP changed with NAFLD progressing in humans and animals. This change affected the plasma and tissue disposition of endogenous and exogenous compounds (136). It seems to be that the CYP2E1 and CYP3A have been identified as the most relevant enzyme due to the vital role of oxidative stress in NAFLD (137). The induction of CYP2E1 is an adaptive response, which prevented lipid overload in NAFLD. While the increased CYP2E1 and improved insulin resistance appear to stimulate each other, that may ultimately worsen the process of steatosis with the increase in oxidative stress (138). However, the mechanism is not clarified yet. CYP3A genes appear to be regulated by various signaling pathways such as CCAAT-enhancer-binding proteins (C/EBP), HNF, PXR, and CAR, Janus kinase/signal transducers and activators of transcription (JAK/STAT), and mitogen-activated protein kinases (MAPK) pathway (19). However, few study have been reported that elucidating the shifts in the drug metabolism and bile acid associated with UGTs and SULTs enzymes in NAFLD to date. Hardwick et al. showed minimal alterations in the activity of UGTs while several changes in the expression and function of specific SULT during human NAFLD progression (101). The bile acid-related enzymes UGT and SULT2A1 were strongly suppressed in high-fat-cholesterol-fed males (139, 140). Expression and functional of transporters changed in NASH patients. OATP1B1 and OATP1B3 downregulated, MRP3 and MRP4 upregulated and MRP2 mislocalized in NAFLD (141). The mechanism is that the loss of glycosylation of the transporters (142).
The Pharmacological Activity of Danshen in the Management of Non-alcoholic Fatty Liver Disease
Concerning the therapy for NAFLD, we focus mostly on risk factors such as metabolic syndrome, including overweight, hyperglycemia, insulin resistance, dyslipidemia, and hypertension (143). Conversely, the NAFLD may strengthen some features and comorbidities of the metabolic syndrome. Especially, cardiovascular diseases are the main causes of mortality of the patients with NAFLD (5). Thus, effective treatment of NAFLD and metabolic syndrome could have mutual benefits for each other. Danshen is a highly versatile and multi-activity herb that can significantly limit the processes of liver diseases. Clinical trials of Danshen effectively treat NAFLD and metabolic syndrome (9, 10, 144). More evidence indicated that Danshen and their derived compounds limited the progression of hepatic steatosis of NASH in animals and in vitro (145–147). For example, Danshen tablets combined with Alovastatin significantly alleviated fatty liver cirrhosis and cardiovascular atherosclerosis caused by abnormal lipid metabolism in NAFLD treatment (148). Danshen polysaccharides combined with Probiotics regulated lipid metabolism disorders and protected the liver in NAFLD mice (8). Conclusively, the preventive and therapeutic potential of Danshen for NAFLD is related to reducing the risk of metabolic disorders.
A variety of mechanisms in inhibiting hepatic steatosis and modulating lipid metabolism have been shown by the compounds of Danshen and its preparations (Table 1), including the regulation of hepatocyte apoptosis via inducing autophagy in an AMPK-dependent way (149), the modulation of lipid metabolism via activating ChREBP (146), PPARα (7), PXR (150) or inhibiting PPARγ (151) and LXR (95), anti-inflammation through inhibiting Nrf2 (152) and JNK (153), anti-oxidative stress by activating Nrf2 and suppressing CYP2E1 (154), anti-fibrosis through inhibiting MAPK (155), regulating NF-κB/IκBα (156), activating Wnt/β-catenin (157) and SIRT1/HSF1 (158) and improving the insulin resistance (159). For instance, SAB against hepatocyte apoptosis by upregulating the mortalin, a protein of maintaining mitochondrial homeostasis (160). CT exhibits a hepatoprotective effect by activating Nrf2 and AMPK/SIRT1 and inhibiting CYP2E1 (161). SAB could effectively inhibit liver fibrosis with 60 patients (162). Potentially, the combination of Danshen Polysaccharide and Probiotics ameliorated NAFLD via controlling the gut microbiota and insulin resistance (8). In particular, the genes including JNKs, SREBP-1c, ChREBP, PPARs, CYPs, and the others have been highlighted as crucial molecular targets for Danshen treating patients with NAFLD (Figure 4).
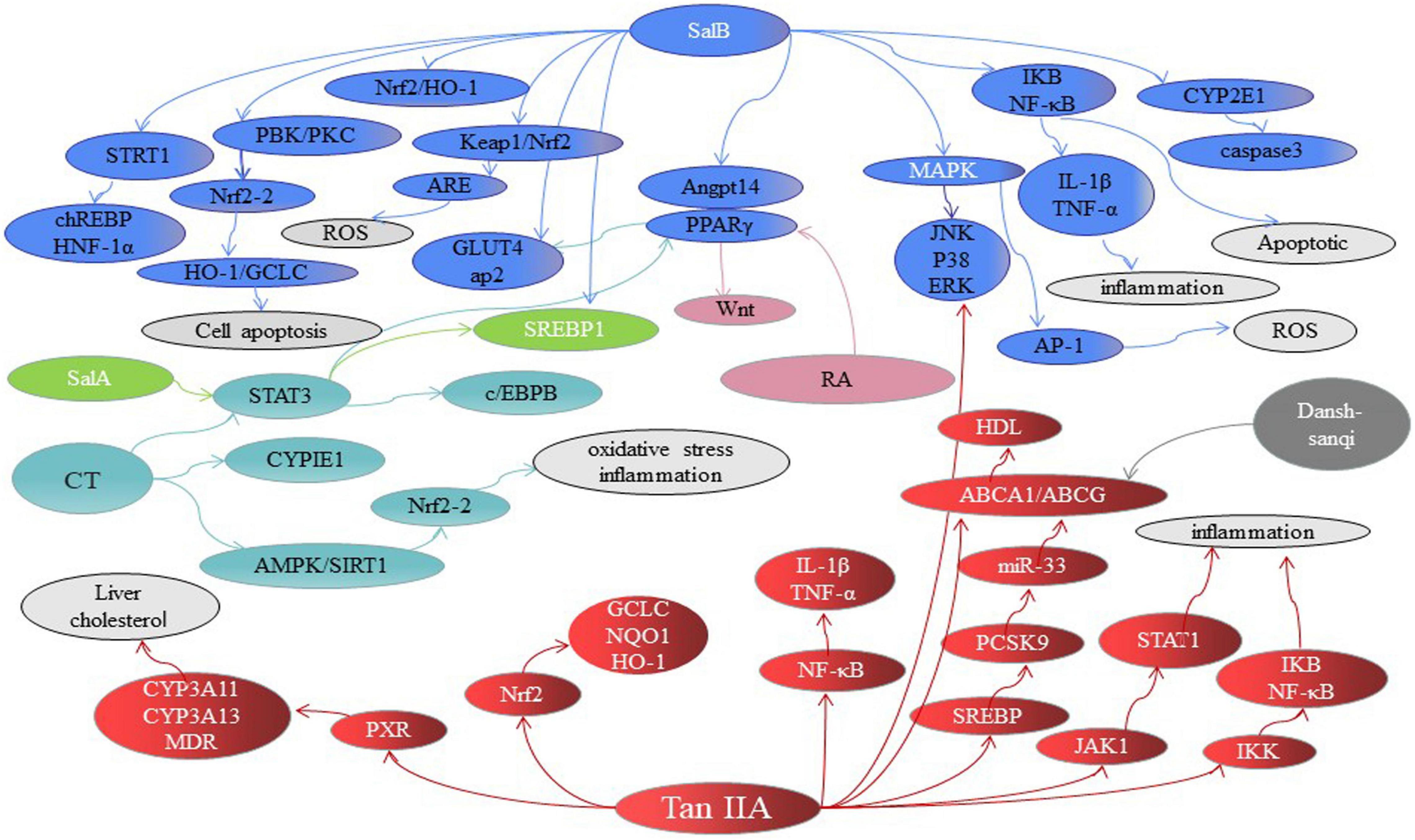
Figure 4. The major signaling pathways involved in treating NAFLD of Danshen compounds. SAB, Salvianolic acid B; SAA, Salvianolic acid A; SAC, Salvianolic acid C; RA, Rosmarinic acid; TSIIA, tanshinone IIA; CT, Cryptotanshinone. FA, fatty acids.
Possible Mechanisms of Danshen in Management of Non-Alcoholic Fatty Liver Disease Based on Metabolic Targets
Fatty Acids in the Non-alcoholic Fatty Liver Disease Control the Activity or Expression of Key Metabolic Targets
c-Jun N-terminal kinases
JNK is a critical mediator of insulin resistance, which leads to NASH through dysregulated lipolysis causing excessive transmit of fatty acids to the liver and intracellular accumulation of toxic lipid products that impair insulin signaling and activate inflammatory pathways (163). Several compounds of Danshen exhibits hepatoprotection and anti-inflammatory and subsequently against NAFLD through JNK-related signaling pathways. TSIIA, TSL, and SAB alleviated NAFLD progression through targeting PPARα and PPARα/JNK signaling pathways. TSIIA inhibited phosphorylation of JNK on lipopolysaccharide-induced rat hepatic stellate cells, and TSIIA exhibited stronger effects of hepatoprotection than SAB (228). Similarly, CT effectively against apoptosis by blocking the activation of extracellular signal-regulated protein kinase (ERK)1/2, NF-κB and JNK signaling pathways (165). Additionally, Tanshinlactone A, a new diterpenoid tanshinone compound from Danshen, which against inflammatory through inhibiting the ERK, p38 and JNK in cells (166). NF-κB regulated inflammation by phosphorylation of IκB via activation of the p38, ERK1/2 and JNK (167). TSIIA (168) and SAC (169) presented the anti-inflammatory activity through the signaling pathways mentioned above.
Sterol Regulatory Element-Binding Protein-1c
Free fatty acids are central to the pathogenesis of NASH. The main source of fatty acids from food or synthesized by acetyl-CoA through complex reactions including glycolysis and citric acid (170). AMPK is an energy mediator, which controlled cellular lipid metabolism. Phosphorylation of acetyl-CoA promoted adipogenesis by deactivating AMPK and inhibiting lipid oxidation (171). TI potently enhanced acetyl-CoA phosphorylation which caused decreased lipogenesis (172). Moreover, PPARα is one of the major downstream targets of AMPK (173). SAB relieved dyslipidemia and hyperglycemia partly by modulating the acetyl-CoA and AMPK/PPARα in db/db mice (174). Moreover, AMPK interacted with SREBP-1c and SREBP-2 and directly phosphorylated in diet-induced insulin-resistant mice (175). SREBP-1C, a major transcriptional regulator of the acetyl-CoA in the process of synthesis, could benefit the treatment of NASH (12). And signal transducer and activator of transcription (STAT-3) related to lipid synthesis by modulating the expression of SREBP-1C (176). Salvianolic acids significantly balanced the lipid metabolism disorders by inhibiting STAT-3 via suppressing the expression of SREBP1 (177). Meanwhile, SREBP-2 regulated the expression of Pcsk9 in hepatic cholesterol (178). A miRNA located in the SREBP-2 gene, microRNA (miR)-33, modulated lipid metabolism through regulation of ATP-binding cassette transporter A1 (ABCA1) and G1 (ABCG1) (179). TSIIA ameliorated lipid accumulation in the hyperlipidemic rats via modulating the miR-33a and SREBP2/Pcsk9 pathway without impacting the lipid profile serum (180). Besides, the combination of herbs usually exerted synergistic effects. Danshen-Sanqi prescription increased the expression of hepatic glycogen synthesis gene, GLUT-1, GK, and GLUT-4, and reduced SREBP-1c gene related to fat and cholesterol anabolism (181). Generally, downregulation of SREBP-1C expression is likely due to the modulate of FXR (12). Besides, LXRα, SREBP-1c and acetyl-CoA regulated fatty acid and cholesterol synthesis through the tricarboxylate transport system (182). For instance, Dingxin Recipe containing Danshen regulated lipid metabolism by LXRα/SREBP1 but not LXRβ and SREBP2 in ApoE-/- mice fed with a high-fat diet (95).
Carbohydrate Response Element–Binding Protein
Theoretically, ChREBP induced the expression of the acetyl-CoA involved in DNL in NAFLD conditions (183). SAA alleviated hepatic lipid accumulation of high-fat diet-induced NAFLD rats partially due to the cross-talk mechanism between ChREBP and Thioredoxin-interacting protein (TXNIP) (146). SAB played a critical role in antisteatotic and anti-inflammatory by activating SIRT1-mediated suppression of CRP (C-reactive protein, HNF-1α promoter) and ChREBP expression in rats (184). However, Benhamed et al. observed that ChREBP expression is increased in patients with NASH, 50% decreased in livers of severe insulin resistance patients, which suggested that ChREBP-mediated steatosis is not strongly associated with high insulin resistance (185), so it is uncertain whether ChREBP would be a viable target for therapeutics of NASH patients with severe insulin resistance.
Peroxisome Proliferator-Activated Receptors
Diversion of fatty acids away from liver into other tissues such as peripheral adipose tissue that reduced their transport to liver. PPARs have emerged as lipid sensors that transcriptionally modulated the metabolic process (104). PPARα and PPARγ are PPAR isotypes, which exhibited distinct functions.
PPARα is activated by fibrates and modulated the adaptive response to nutritional inputs by regulating fatty acid delivery in the liver. Furthermore, the mRNA level for PPARα progressively reduced as NASH progresses in humans (102). Hence, PPARα activation is central to the remission of hepatic steatosis and NAFLD progression. Recent studies demonstrated that the active constituents of Danshen and related preparations enhanced PPARα in the livers of rodents and thus reduced lipid accumulation. For instance, a pharmacological network analysis suggested that the mechanism of hepatoprotective effects of TSIIA, TSL, and SAB may involve modulating lipid metabolism and anti-fibrogenesis via PPARα, CYP1A2, and MMP2 (7). Besides, Danhong injection significantly enhanced lipolysis and diminished fatty acids’ synthesis in the liver by increasing the mRNA transcription of PPARα in hyperlipidemia rats (186). Of note, cellular protection is associated with activated PPARα involvement in 4-Hydroxynonenal metabolism (187). Ding et al. found that Danshen injection played a mechanistic role in hepatoprotection in mice via inducing the activation of PPARα and subsequent degradation of 4-Hydroxynonenal (188). Worth the whistle, 4-Hydroxynonenal degradation promoted by PPARα activation is likely to relate with multiple enzyme systems, which deserves further study. In addition, the action of RXRα as a heterodimer partner with PPARα promoted the expression of RXRα that mediated fatty acid transport to mitochondria and oxidation (189). Yin et al. found that TSIIA reversed lipopolysaccharide-induced the decreased gene expression of RXRα (190). Nevertheless, the proof of other ingredients of Danshen has not been brought yet.
In contrast to PPARα, the expression of PPARγ in the liver increased as steatosis develop in rodents. SAA combined with prednisone exhibited therapeutic and antiproteinuric effects on adriamycin-induced minimal change disease rat model through PPARγ/Angptl4 and Nrf2/Heme oxygenase-1 (HO-1) pathways (191). Further, PPARγ is considered as a downstream transcriptional target of Nrf2 in adipocytes differentiation (192). Hence, the influence of SAA modulated PPARγ/Angptl4 pathway is directly associated with Nrf2 could be studied in future. Wnt10b is a direct target of necdin, and necdin-Wnt pathway induced trans-differentiation of hepatic stellate cells through epigenetic inhibition of PPARγ (193). RA against liver fibrosis via inhibiting the expression and signaling of canonical Wnts/PPARγ in hepatic stellate cells (151). Similarly, it has been shown that SAB moderated lipid disorders by suppressing PPARγ-mediated adipogenesis in mice with high-fat diet-induced obesity (194). TSIIA inhibited adipogenesis as a natural antagonist of PPARγ in high-fat diet-induced obese mice (195). CT was demonstrated as an effectively anti-adipogenesis candidate through a multimodal signaling pathway- related to PPARγ (196). Moreover, PCA,2-(3-methoxy-4-hydroxy-phenyl)-6-(3-hydroxypropyl)-5-methoxybenzo[b]furan (an active compound identified from Danshen) and Danshensu Bingpian Zhi are efficient natural PPARγ agonists that exhibited excellent effects on insulin resistance, antiadipogenic, hepatic steatosis and inflammation (197, 198).
The Role of CYP450 Enzymes in the Pathogenesis of Non-alcoholic Fatty Liver Disease
Patients with NAFLD are more susceptible to drug- induced toxicity due to altered drug metabolism (19). Previous workers reviewed the change of human hepatic CYP450 enzymes in NAFLD, such as CYP2E1,3A4, 1A2, 2A6, 2B6, 2C8, 2C9, and 2C19 (199). Generally, it seems to be that the alter in CYP2E1 and 3A4 activity is predominant in clinic studies (19, 200). CYP2E1 is related to the regulation of oxidant stress, insulin resistance and fatty acids, and the expression of hepatic CYP2E1 enhanced in patients with NAFLD (201). Danshen aqueous extracts containing TSL and SAB protected hepatocytes from Paracetamol-induced injury via remaining mitochondrial metabolic activity and suppressing the activity of CYP2E1 and total glutathione depletion (202). As mentioned above, CT and SAC reduced the content of CYP2E1 (161, 169). Previous studies about the expression and function of CYP3A4 associated with NAFLD are a contradiction. Recently, the recent consensus is more in favor of the protein expressions and mRNA of CYP3A decreased in NAFLD (203, 204). CYP3A4 is in charge of the oxidative metabolism of over 50% of all drugs prescribed in NAFLD (203). CT and TSIIA activated PXR and subsequently induced CYP3A4 (205). CYP1A2 promoted the generation of ROS to facilitate oxidative damage further. And the active ingredients of Danshen, isoimperatorin and oleanolic acid attenuating oxidative stress by modulating the expression of CYP1A2, 2B6, and 1B1 in the liver (7). TSIIA protected against lithocholic acid-induced liver cholestasis due to the upregulation of PXR, as well as CYP3A11, CYP3A13, and MDR1 (150). Additionally, Danshen polysaccharides exhibited preventive success on the injury of chicken hepatocytes via reducing the contents of ALT, AST, and malondialdehyde and upregulating GSH and CYP450 (206).
The Role of the Other Proteins in the Pathogenesis of Non-alcoholic Fatty Liver Disease
Similar to CYPs, Phase II drug metabolic enzymes including UGTs, SULTs, and transporters (e.g., OATPs, MRPs) play various regulatory roles in NAFLD. UGT1A6, UGT1A9, and SULT1A1, SULT2A1 protein levels were downregulated in the liver of NASH patients (101, 139). Literature reports that the tanshinones are the inhibitors/substrates of UGT1A6 and UGT1A9 (207). However, whether Danshen treating NAFLD through UGTs and SULTs remains unclear. Besides, the induction of phase II detoxification enzymes HO-1 and glutamate-Lcysteine ligase catalytic subunit (GCLC) plays an antioxidant role in the Nrf2 pathway in NAFLD (208). TSIIA alleviated acetaminophen-induced liver injury by activating Nrf2 and its target genes such as GCLC and HO-1 (209). Additionally, the expression and function of OATPs changed with the disease progression of NAFLD, and LA, RA, SAA, SAB, SAD, and TSL are potent inhibitors of OATP1 and OATP3 (210, 211). It is worth mentioning that the other metabolizing enzymes and transporters of drugs altered in NASH patients when compared with healthy human livers, and the change deserves to explore to prevent adverse drug reactions.
Clinic Studies
Clinical trials have shown that Danshen improved NAFLD therapy. Danshen prescriptions such as Danshen powder injection, Danning tablet and Sangming Mixture are effective in patients with NAFLD by improving the symptoms, liver function and blood lipids with no serious side effects (10, 212, 213, 226). Hu et al. observed that Yindan Xinnaotong Soft Capsule is effective in the treatment of NAFLD by regulating lipid and insulin resistance and liver function and anti-inflammatory (214). Hence, the herbal formula that have proved to take positive effects on the physiological features of NAFLD. Clinically, the combination therapy of Danshen and other agents including probiotics (e.g., Bacillus subtilis enterococcus dual living bacteria capsule) (164), hepatic protectants (e.g., Magnesium isoglycyrrhizinate, Reduced glutathione, Tiopronin) (215–217), statins (e.g., Alvastatin) (148), and even the apparatus with liver disease treatment (227) has been already practiced, which presented better beneficial effects than interference alone. For instance, compound Danshen dripping pill combined with polyene phosphatidyl choline exhibited more effective in patients with NAFLD (218). Although claimed to be hepatoprotective in NAFLD, the molecular mechanisms of combination therapy with Danshen have not been clarified, further multicenter large-sample randomized clinical trials are required to confirm the therapeutic and safety.
SAB regulated insulin resistance mainly through the AMPK/GLUT4 and/or SREBP-1/PPARγ signaling pathway, and modulated fatty acids through PPAR-mediated pathways, and exerted the effect of anti-inflammatory by STRT1/chREBP. TSIIA exhibited the role of anti-inflammatory anti-oxidant by regulating signals such as MAPK, JAK/STAT, and PXR/CYP and modulated fatty acids through PPARγ and SREBP pathways. CT exerted the effect of anti-oxidant by CYP2E1 and regulated fatty acids through AMPK and PPAR-mediated pathways. SalA and SalC rebalance the lipid metabolism by PPARγ and CYP2E1, respectively. RA prevented liver fibrosis by PPARγ pathways. Each color represents a compound, and dotted line indicates inhibition effects.
Future Prospects
Although steady progress has been made in elucidating the pathogenesis of NAFLD, determining therapeutic targets and advancing drug development, there are still unresolved challenges. To determine the molecular mechanisms, discern potential therapy targets, and guide the preclinic use of single or drug combinations, more studies are urgently needed using in vitro and animal NAFLD models. Many advances in the development of preclinic models such as in vitro models and genetic and dietary animal models for NAFLD have been made that have provided valuable insights on disease pathogenesis. However, only a few models resemble the key elements needed to be representative of NAFLD, while there exists a substantial difference between rodents and humans, such as the metabolic and transcriptomic characteristics, including the altered immune system and the altered glycolipid metabolism (219). Furthermore, no published data indicate that if drugs are effective in a given model, they consistently translate into efficacy in humans (220, 221). In addition, the heterogeneity of NAFLD according to sex, ethnicity and geographic region should be taken into consideration by researchers for clinic trials (222). Besides, the poor bioavailability, the most appropriate doses, the optimal route of administration, frequency of drug administration and the duration of treatment by Danshen are needed for various animal models to study comprehensively. Specifically, it is vital to focus on the experiment design, such as group size, controls and animal species.
Moreover, the tendency of this field is moving rapidly toward combination therapies, likely due to concern about insufficient ability to attack a single target, as shown that combining herbal medicine with other interventions presented better beneficial effects than interference alone (223). Besides, it is expected that researchers should discover more potent interplayed targets to increase synergy effects in future, such as PPARα agonist + PPARγ agonist, FXR agonist + PPARγ agonist, and PPAR pan-agonist + LXR antagonist (224).
Finally, the verification of predictive biomarkers of disease risk is lacking and urgently needed. Importantly, genetic risk factors such as the PNPLA3 gene have been identified that increase the NASH risk (225).
Author Contributions
JL, YS, DP, NY, GW, LW, and WC wrote and contributed to the writing of the manuscript. All authors contributed to the article and approved the submitted version.
Funding
This work was supported in part by the Program of National Key Research and Development (2017YFC17016000), the Special Project of Anhui Province’s Central Government to Guide Local Scientific and Technological Development (20107d0700002), the Natural Science Foundation of Anhui Province (1908085QH351), and the University Natural Science Research Project of Anhui Province (KJ2021A0586).
Conflict of Interest
The authors declare that the research was conducted in the absence of any commercial or financial relationships that could be construed as a potential conflict of interest.
Publisher’s Note
All claims expressed in this article are solely those of the authors and do not necessarily represent those of their affiliated organizations, or those of the publisher, the editors and the reviewers. Any product that may be evaluated in this article, or claim that may be made by its manufacturer, is not guaranteed or endorsed by the publisher.
Acknowledgments
We thank the members of the Laboratory YS, and the teachers DP, NY, GW, LW, and WC for guideline and discussion during this work.
Abbreviations
ABC, ATP-binding cassette transporter A1; AP-1, activator protein-1; CAR, constitutive androstane receptor; C/EBP, CCAAT-enhancer-binding proteins; ChREBP, carbohydrate response element–binding protein; CT, cryptotanshinone; CYP, cytochrome P450; DNL, de novo lipogenesis; ERK, extracellular signal-regulated protein kinase; FGF21, fibroblast growth factor 21; FXR, farnesoid X receptor; GLUT-4, glucose transporter 4; JNK, c-JunN-terminal kinases; LA, lithospermic acid; LXR α, liver-X-receptor α; MAPK, mitogen-activated protein kinases; NAFL, non-alcoholic fatty liver; NAFLD, Non-alcoholic fatty liver disease; NASH, non-alcoholic steatohepatitis; NF- κ B, nuclear factor- κ B; OATPs, organic anion transporting polypeptides; PAA, protocatechuic acid; PCA, protocatechuic aldehyde; PPARs, peroxisome proliferator-activated receptors; PXR, pregnane X receptor; RA, rosmarinic acid; RXR α, retinoid X receptor α; SHP, small heterodimer partner; SAA, salvianolic acid A; SAB, salvianolic acid B; SAC, salvianolic acid C; SAD, salvianolic acid D; SREBP-1c, sterol regulatory element-binding protein-1c; SULT, sulfotransferases; STATs, signal transducers and activators of transcription; TCM, traditional Chinese medical; TI, dihydrotanshinone I; TSI, tanshinone I; TSIIA, tanshinone IIA; TSL, tanshinol; UGT, UDP-glucuronosyltransferases.
References
1. Sanyal AJ, Brunt EM, Kleiner DE, Kowdley KV, Chalasani N, Lavine JE, et al. Endpoints and clinical trial design for nonalcoholic steatohepatitis. Hepatology. (2011) 54:344–53. doi: 10.1002/hep.24376
2. Kleiner DE, Makhlouf HR. Histology of nonalcoholic fatty liver disease and nonalcoholic steatohepatitis in adults and children. Clin Liver Dis. (2016) 20:293–312. doi: 10.1016/j.cld.2015.10.011
3. Araújo AR, Rosso N, Bedogni G, Tiribelli C, Bellentani S. Global epidemiology of non-alcoholic fatty liver disease/non-alcoholic steatohepatitis: what we need in the future. Liver Int. (2018) 38(Suppl. 1):47–51. doi: 10.1111/liv.13643
4. Sanyal AJ, Chalasani N, Kowdley KV, McCullough A, Diehl AM, Bass NM, et al. Pioglitazone, vitamin E, or placebo for nonalcoholic steatohepatitis. N Engl J Med. (2010) 362:1675–85.
5. Cicero A, Colletti A, Bellentani S. Nutraceutical approach to non-alcoholic fatty liver disease (NAFLD): the available clinical evidence. Nutrients. (2018) 10:1153. doi: 10.3390/nu10091153
6. Tan Y, Kamal MA, Wang ZZ, Xiao W, Seale JP, Qu X. Chinese herbal extracts (SK0506) as a potential candidate for the therapy of the metabolic syndrome. Clin Sci (Lond). (2011) 120:297–305. doi: 10.1042/CS20100441
7. Hong M, Li S, Wang N, Tan HY, Cheung F, Feng Y. A biomedical investigation of the hepatoprotective effect of radix salviae miltiorrhizae and network pharmacology-based prediction of the active compounds and molecular targets. Int J Mol Sci. (2017) 18:620. doi: 10.3390/ijms18030620
8. Wang W, Xu AL, Li ZC, Li Y, Xu SF, Sang HC, et al. Combination of probiotics and Salvia miltiorrhiza Polysaccharide alleviates hepatic steatosis via gut microbiota modulation and insulin resistance improvement in high fat-induced NAFLD mice. Diabetes Metab J. (2020) 44:336–48. doi: 10.4093/dmj.2019.0042
9. Peng H, He Y, Zheng G, Zhang W, Yao Z, Xie W. Meta-analysis of traditional herbal medicine in the treatment of nonalcoholic fatty liver disease. Cell Mol Biol. (2016) 62:88–95.
10. Sang XF. Effect evaluation of hepatopathy therapeutic apparatus combined with compound danshen injection in treatment of non-alcoholic steatohepatitis. China Mod. Med. (2013) 20:23–5.
11. Guo MY. Based on the Literature Review of Non-alcoholic Fatty Liver, the Relevant Research Between Prescription and Syndrome. Harbin: Heilongjiang University of Chinese Medicine (2019).
12. Friedman SL, Neuschwander-Tetri BA, Rinella M, Sanyal AJ. Mechanisms of NAFLD development and therapeutic strategies. Nat Med. (2018) 24:908–22. doi: 10.1038/s41591-018-0104-9
13. Musso G, Cassader M, Gambino R. Non-alcoholic steatohepatitis: emerging molecular targets and therapeutic strategies. Nat Rev Drug Discov. (2016) 15:249–74. doi: 10.1038/nrd.2015.3
14. Mummadi RR, Kasturi KS, Chennareddygari S, Sood GK. Effect of bariatric surgery on nonalcoholic fatty liver disease: systematic review and meta-analysis. Clin Gastroenterol Hepatol. (2008) 6:1396–402. doi: 10.1016/j.cgh.2008.08.012
15. Huang MA, Greenson JK, Chao C, Anderson L, Peterman D, Jacobson J, et al. One-year intense nutritional counseling results in histological improvement in patients with non-alcoholic steatohepatitis: a pilot study. Am J Gastroenterol. (2005) 100:1072–81. doi: 10.1111/j.1572-0241.2005.41334.x
16. Vilar-Gomez E, Martinez-Perez Y, Calzadilla-Bertot L, Torres-Gonzalez A, Gra-Oramas B, Gonzalez-Fabian L, et al. Weight loss through lifestyle modifcation signifcantly reduces features of nonalcoholic steatohepatitis. Gastroenterology. (2015) 149:367–78. doi: 10.1053/j.gastro.2015.04.005
17. Neuschwander-Tetri BA, Loomba R, Sanyal AJ, Lavine JE, Van Natta ML, Abdelmalek MF, et al. Farnesoid X nuclear receptor ligand obeticholic acid for non-cirrhotic, non-alcoholic steatohepatitis (FLINT): a multicentre, randomised, placebo-controlled trial. Lancet. (2015) 385:956–65. doi: 10.1016/S0140-6736(14)61933-4
18. FDA. Safety Announcement: “FDA Adds Boxed Warning to Highlight Correct Dosing of Ocaliva (Obeticholic Acid) for Patients With a Rare Chronic Liver Disease” Released on 1 Feb. Washington, DC: US Food and Drug Administration. (2018).
19. Cobbina E, Akhlaghi F. Non-alcoholic fatty liver disease (NAFLD)-pathogenesis, classification, and effect on drug metabolizing enzymes and transporters. Drug Metab Rev. (2017) 49:197–211. doi: 10.1080/03602532.2017.1293683
20. Liu M, Ma Y, Du Q, Hou X, Wang M, Lu S. Functional analysis of polyprenyl diphosphate synthase genes involved in plastoquinone and ubiquinone biosynthesis in Salvia miltiorrhiza. Front Plant Sci. (2019) 10:893. doi: 10.3389/fpls.2019.00893
21. Pang H, Wu L, Tang Y, Zhou G, Qu C, Duan JA. Chemical analysis of the herbal medicine Salviae miltiorrhizae radix et rhizoma (Danshen). Molecules. (2016) 21:51. doi: 10.3390/molecules21010051
22. Jiang RW, Lau KM, Hon PM, Mak TC, Woo KS, Fung KP. Chemistry and biological activities of caffeic acid derivatives from Salvia miltiorrhiza. Curr Med Chem. (2005) 12:237–46. doi: 10.2174/0929867053363397
23. Fraga BM, Díaz CE, Guadaño A, González-Coloma A. Diterpenes from Salvia broussonetii transformed roots and their insecticidal activity. J Agric Food Chem. (2005) 53:5200–6. doi: 10.1021/jf058045c
24. Shu Y, Li Y, Li L. Comparison of effective ingredients and absorption in rats’ intestine of compound Salvia preparations. Pharmacol Clin Chin Mater Med. (2011) 27:76–9.
25. Zhao J, Xu XL, Yi HZ, Zhang HM, Liu XQ, Zhu JJ, et al. Absorption of aqueous extracts from Salviae miltiorrhizae radix et rhizoma by everted intestinal sac method. China J Chin Mater Med. (2015) 40:3088–93.
26. Zhou L, Chow MS, Zuo Z. Effect of sodium caprate on the oral absorptions of danshensu and salvianolic acid B. Int J Pharm. (2009) 379:109–18. doi: 10.1016/j.ijpharm.2009.06.016
27. Guo L, Duan L, Dong X, Dou LL, Zhou P, Liu EH, et al. A simple and sensitive LC-MS/MS method for determination of miltirone in rat plasma and its application to pharmacokinetic studies. Anal Technol Biomed Life Sci. (2014) 973C:33–8. doi: 10.1016/j.jchromb.2014.10.008
28. Song J, Zhang W, Sun J, Xu X, Zhang X, Zhang L, et al. Pharmacokinetic study of salvianolic acid D after oral and intravenous administration in rats. Acta Pharm Sin B. (2015) 5:246–53. doi: 10.1016/j.apsb.2015.03.015
29. Song J, Zhang W, Sun J, Zhang X, Xu X, Zhang L, et al. Determination of salvianolic acid C in rat plasma using liquid chromatography-mass spectrometry and its application to pharmacokinetic study. Biomed Chromatogr. (2016) 30:376–83. doi: 10.1002/bmc.3558
30. Wang L, Zhang Q, Li X, Lu Y, Xue Z, Xuan L, et al. Pharmacokinetics and metabolism of lithospermic acid by LC/MS/MS in rats. Int J Pharm. (2008) 350:240–6. doi: 10.1016/j.ijpharm.2007.09.001
31. Zhang Y, Akao T, Nakamura N, Duan CL, Hattori M, Yang XW, et al. Extremely low bioavailability of magnesium lithospermate B, an active component from Salvia miltiorrhiza, in rat. Planta Med. (2004) 70:138–42. doi: 10.1055/s-2004-815490
32. Li W, Zhou H, Chu Y, Wang X, Luo R, Yang L, et al. Simultaneous determination and pharmacokinetics of danshensu, protocatechuic aldehyde, 4-hydroxy-3-methyloxyphenyl lactic acid and protocatechuic acid in human plasma by LC-MS/MS after oral administration of compound danshen dripping pills. J Pharm Biomed Anal. (2017) 145:860–4. doi: 10.1016/j.jpba.2017.06.014
33. Veber DF, Johnson SR, Cheng HY, Smith BR, Ward KW, Kopple KD. Molecular properties that influence the oral bioavailability of drug candidates. J Med Chem. (2002) 45:2615–23. doi: 10.1021/jm020017n
34. Lu T, Yang J, Gao X, Chen P, Du F, Sun Y, et al. Plasma and urinary tanshinol from Salvia miltiorrhiza (Danshen) can be used as pharmacokinetic markers for cardiotonic pills, a cardiovascular herbal medicine. Drug Metab Disposit. (2008) 36:1578–86. doi: 10.1124/dmd.108.021592
35. Li X, Yu C, Cai Y, Liu G, Jia J, Wang Y. Simultaneous determination of six phenolic constituents of danshen in human serum using liquid chromatography/tandem mass spectrometry. J Chromatogr B Analyt Technol Biomed Life Sci. (2005) 820:41–7. doi: 10.1016/j.jchromb.2005.03.005
36. Li M, Wang F, Huang Y, Du F, Zhong C, Olaleye OE, et al. Systemic exposure to and disposition of catechols derived from Salvia miltiorrhiza roots (Danshen) after intravenous dosing DanHong injection in human subjects, rats, and dogs. Drug Metab Disposit. (2015) 43:679–90. doi: 10.1124/dmd.114.061473
37. Hügel HM, Jackson N. Danshen diversity defeating dementia. Bioorgan Med Chem Lett. (2014) 24:708–16. doi: 10.1016/j.bmcl.2013.12.042
38. Cai H, Su S, Li Y, Zhu Z, Guo J, Zhu Y, et al. Danshen can interact with intestinal bacteria from normal and chronic renal failure rats. Biomed Pharmacother. (2019) 109:1758–71. doi: 10.1016/j.biopha.2018.11.047
39. Baba S, Osakabe N, Natsume M, Terao J. Orally administered rosmarinic acid is present as the conjugated and/or methylated forms in plasma, and is degraded and metabolized to conjugated forms of caffeic acid, ferulic acid and m-coumaric acid. Life Sci. (2004) 75:165–78. doi: 10.1016/j.lfs.2003.11.028
40. Jia JY, Lu YL, Li XC, Liu GY, Li SJ, Liu Y, et al. Pharmacokinetics of depside salts from Salvia miltiorrhiza in healthy Chinese volunteers: a randomized, open-label, single-dose study. Curr Ther Res Clin Exp. (2010) 71:260–71. doi: 10.1016/j.curtheres.2010.08.004
41. Peng Q, Gong T, Zuo J, Liu J, Zhao D, Zhang Z. Enhanced oral bioavailability of salvianolic acid B by phospholipid complex loaded nanoparticles. Pharmazie. (2008) 63:661–6.
42. Jin X, Zhang SB, Li SM, Liang K, Jia ZY. Influence of chitosannanoparticlesas the absorption enhancers on salvianolic acid B in vitro and in vivoevaluation. Pharmacogn Mag. (2016) 12:57–63. doi: 10.4103/0973-1296.176047
43. Chang BB, Zhang L, Cao WW, Cao Y, Yang WL, Wang Y, et al. Pharmacokinetic interactions induced by content variation of major water-soluble components of Danshen preparation in rats. Acta Pharmacol Sin. (2010) 31:638–46. doi: 10.1038/aps.2010.27
44. Ren H, Qian D, Su S, Guan H, Zhang W, Shang E, et al. Simultaneous determination of tanshinones and polyphenolics in rat plasma by UPLC-MS/MS and its application to the pharmacokinetic interaction between them. Drug Test Anal. (2016) 8:744–54. doi: 10.1002/dta.1840
45. Lai XJ, Zhang L, Li JS, Liu HQ, Liu XH, Di LQ, et al. Comparative pharmacokinetic and bioavailability studies of three salvianolic acids after the administration of Salvia miltiorrhizae alone or with synthetical borneol in rats. Fitoterapia. (2011) 82:883–8. doi: 10.1016/j.fitote.2011.04.015
46. Yan H, Wu Q, Du S, Yang Y, Zhou L, Li X. Absorption mechanism of tanshinone II A, cryptotanshinone, tanshinone I and tanshinones extract in rat small intestine in vivo. China J Chin Mater Med. (2010) 35:2917–22.
47. Zhang J, Huang M, Guan S, Bi HC, Pan Y, Duan W, et al. A mechanistic study of the intestinal absorption of cryptotanshinone, the major active constituent of Salvia miltiorrhiza. J Pharmacol Exp Ther. (2006) 317:1285–94. doi: 10.1124/jpet.105.100701
48. Hao H, Wang G, Cui N, Li J, Xie L, Ding Z. Pharmacokinetics, absorption and tissue distribution of tanshinone IIA solid dispersion. Planta Med. (2006) 72:1311–7. doi: 10.1055/s-2006-951698
49. Ling W, Rui LC, Hua JX. In situ intestinal absorption behaviors of tanshinone IIA from its inclusion complex with hydroxypropyl-beta-cyclodextrin. Biol Pharm Bull. (2007) 30:1918–22. doi: 10.1248/bpb.30.1918
50. Chen X, Zhou ZW, Xue CC, Li XX, Zhou SF. Role of P-glycoprotein in restricting the brain penetration of tanshinone IIA, a major active constituent from the root of Salvia miltiorrhiza bunge, across the blood-brain barrier. Xenobiotica. (2007) 37:635–78. doi: 10.1080/00498250701411258
51. Yu XY, Lin SG, Zhou ZW, Chen X, Liang J, Duan W, et al. Tanshinone IIB, a primary active constituent from Salvia miltiorrhza, exhibits neuro-protective activity in experimentally stroked rats. Neurosci Lett. (2007) 417:261–5. doi: 10.1016/j.neulet.2007.02.079
52. Du W, Wei YH, Zhao G, Qin HY, Wu XA. Determination of tanshinone I in rat plasma by high-performance liquid chromatography and its application to pharmacokinetic studies. J Chromatogr Sci. (2008) 46:730–4. doi: 10.1093/chromsci/46.8.730
53. Park EJ, Ji HY, Kim NJ, Song WY, Kim YH, Kim YC, et al. Simultaneous determination of tanshinone I, dihydrotanshinone I, tanshinone IIA and cryptotanshinone in rat plasma by liquid chromatography-tandem mass spectrometry: application to a pharmacokinetic study of a standardized fraction of Salvia miltiorrhiza, PF2401-SF. Biomed Chromatogr. (2008) 22:548–55. doi: 10.1002/bmc.968
54. Liu Y, Li X, Li Y, Wang L, Xue M. Simultaneous determination of danshensu, rosmarinic acid, cryptotanshinone, tanshinone IIA, tanshinone I and dihydrotanshinone I by liquid chromatographic-mass spectrometry and the application to pharmacokinetics in rats. J Pharm Biomed Anal. (2010) 53:698–704. doi: 10.1016/j.jpba.2010.03.041
55. Xing L, Tan ZR, Cheng JL, Huang WH, Zhang W, Deng W, et al. Bioavailability and pharmacokinetic comparison of tanshinones between two formulations of Salvia miltiorrhiza in healthy volunteers. Sci Rep. (2017) 7:4709. doi: 10.1038/s41598-017-02747-4
56. Sun JH, Yang M, Wang XM, Xu M, Liu AH, Guo DA. Identification of tanshinones and their metabolites in rat bile after oral administration of TTE-50, a standardized extract of Salvia miltiorrhiza by HPLC-ESI-DAD-MSn. J Pharm Biomed Anal. (2007) 44:564–74. doi: 10.1016/j.jpba.2006.11.003
57. Bi HC, Zuo Z, Chen X, Xu CS, Wen YY, Sun HY, et al. Preclinical factors affecting the pharmacokinetic behaviour of tanshinone IIA, an investigational new drug isolated from Salvia miltiorrhiza for the treatment of ischaemic heart diseases. Xenobiotica. (2008) 38:185–222. doi: 10.1080/00498250701767675
58. Wang M, Dai H, Li X, Li Y, Wang L, Xue M. Structural elucidation of metabolites of tanshinone I and its analogue dihydrotanshinone I in rats by HPLC-ESI-MSn. J Chromatogr B Anal Technol Biomed Life Sci. (2010) 878:915–24. doi: 10.1016/j.jchromb.2010.02.014
59. Zhao WJ, Huang MR, Shang ZP, Wang ZJ, Wang ZB, Zhang JY. Metabolites of tanshinone I and tanshinone II A in vivo in rats. China J Chin Mater Med. (2018) 43:174–82. doi: 10.19540/j.cnki.cjcmm.2018.0005
60. Ashour AA, Ramadan AA, Abdelmonsif DA, El-Kamel AH. Enhanced oral bioavailability of tanshinone IIA using lipid nanocapsules: formulation, in-vitro appraisal and pharmacokinetics. Int J Pharmaceut. (2020) 586:119598. doi: 10.1016/j.ijpharm.2020.119598
61. Hu L, Xing Q, Meng J, Shang C. Preparation and enhanced oral bioavailability of cryptotanshinone-loaded solid lipid nanoparticles. AAPS PharmSciTech. (2010) 11:582–7. doi: 10.1208/s12249-010-9410-3
62. Zhi H, Li Z, Deng Y, Qi P, Hou J, Deng W, et al. Comparative in vivo constituents and pharmacokinetic study in rats after oral administration of ultrafine granular powder and traditional decoction slices of Chinese Salvia. Biomed Chromatogr. (2019) 33:e4385. doi: 10.1002/bmc.4385
63. Song M, Hang TJ, Zhang Z, Chen HY. Effects of the coexisting diterpenoid tanshinones on the pharmacokinetics of cryptotanshinone and tanshinone IIA in rat. Eur J Pharm Sci. (2007) 32:247–53. doi: 10.1016/j.ejps.2007.07.007
64. Xiang X, Sha X, Su S, Zhu Z, Guo S, Yan H, et al. Simultaneous determination of polysaccharides and 21 nucleosides and amino acids in different tissues of Salvia miltiorrhiza from different areas by UV-visible spectrophotometry and UHPLC with triple quadrupole MS/MS. J Sep Sci. (2018) 41:996–1008. doi: 10.1002/jssc.201700802
65. Han QB. Critical problems stalling progress in natural bioactive polysaccharide research and development. J Agric Food Chem. (2018) 66:4581–3. doi: 10.1021/acs.jafc.8b00493
66. Wang Z, Zhang H, Shen Y, Zhao X, Wang X, Wang J, et al. Characterization of a novel polysaccharide from Ganoderma lucidum and its absorption mechanism in Caco-2 cells and mice model. Int J Biol Macromol. (2018) 118:320–6. doi: 10.1016/j.ijbiomac.2018.06.078
67. Xu X, Wang R, Gao J, Peng JY, Li H, Wang L. Progress in pharmacokinetics of polysaccharides. Chin J Clin Pharmacol Ther. (2021) 26:230–5.
68. Jiang YY, Li YB, Yu J, Chen H, Zhou J, Wang L, et al. Preliminary structure and bioactivities of polysaccharide SMWP-U&E isolated from Salvia miltiorrhiza bunge residue. Int J Biol Macromol. (2020) 157:434–43. doi: 10.1016/j.ijbiomac.2020.04.092
69. Rice-Evans CA, Miller NJ, Paganga G. Structure-antioxidant activity relationships of flavonoids and phenolic acids. Free Radic Biol Med. (1996) 20:933–56. doi: 10.1016/0891-5849(95)02227-9
70. Zheng X, Kadir A, Zheng G, Jin P, Qin D, Maiwulanjiang M, et al. Antiproliferative abietane quinone diterpenoids from the roots of Salvia deserta. Bioorgan Chem. (2020) 104:104261. doi: 10.1016/j.bioorg.2020.104261
71. Manach C, Mazur A, Scalbert A. Polyphenols and prevention of cardiovascular diseases. Curr Opin Lipidol. (2005) 16:77–84. doi: 10.1097/00041433-200502000-00013
72. Huang YY, Chen HG, Xie W, Tang T, Zhao C, Gong XJ, et al. Interaction between polysaccharide and intestinal flora and its structure effect relationship: a review. Chin Soc Microbiol. (2022) 1–29.
73. Shi B, Li Q, Feng Y, Dai X, Zhao R, Zhao Y, et al. Pharmacokinetics of 13 active components in a rat model of middle cerebral artery occlusion after intravenous injection of radix salviae miltiorrhizae-lignum dalbergiae odoriferae prescription. J Sep Sci. (2020) 43:531–46. doi: 10.1002/jssc.201900748
74. Qin WW, Wang L, Jiao Z, Wang B, Wang CY, Qian LX, et al. Lower clearance of sodium tanshinone IIA sulfonate in coronary heart disease patients and the effect of total bilirubin: a population pharmacokinetics analysis. Chin J Nat Med. (2019) 17:218–26. doi: 10.1016/S1875-5364(19)30024-X
75. Liang S, Wang Z, Yuan J, Zhang J, Dai X, Qin F, et al. Rapid Identification of Tanshinone IIA Metabolites in an Amyloid-β1-42 Induced Alzherimer’s disease rat model using UHPLC-Q-exactive qrbitrap mass spectrometry. Molecules. (2019) 24:2584. doi: 10.3390/molecules24142584
76. Li H, Toth E, Cherrington NJ. Asking the right questions with animal models: methionine- and choline-deficient model in predicting adverse drug reactions in human NASH. Toxicol Sci. (2018) 161:23–33. doi: 10.1093/toxsci/kfx253
77. Li XX, Lu XY, Zhang SJ, Chiu AP, Lo LH, Largaespada DA, et al. Sodium tanshinone IIA sulfonate ameliorates hepatic steatosis by inhibiting lipogenesis and inflammation. Biomed Pharmacother. (2019) 111:68–75. doi: 10.1016/j.biopha.2018.12.019
78. Meng Z, Meng L, Wang K, Li J, Cao X, Wu J, et al. Enhanced hepatic targeting, biodistribution and antifibrotic efficacy of tanshinone IIA loaded globin nanoparticles. Eur J Pharm Sci. (2015) 73:35–43. doi: 10.1016/j.ejps.2015.03.002
79. Li Q, Wang Y, Feng N, Fan Z, Sun J, Nan Y. Novel polymeric nanoparticles containing tanshinone IIA for the treatment of hepatoma. J Drug Target. (2008) 16:725–32. doi: 10.1080/10611860802374303
80. Aron-Wisnewsky J, Vigliotti C, Witjes J, Le P, Holleboom AG, Verheij J, et al. Gut microbiota and human NAFLD: disentangling microbial signatures from metabolic disorders. Nat Rev Gastroenterol Hepatol. (2020) 17:279–97. doi: 10.1038/s41575-020-0269-9
81. Leung C, Rivera L, Furness JB, Angus PW. The role of the gut microbiota in NAFLD. Nat Rev Gastroenterol Hepatol. (2016) 13:412–25. doi: 10.1038/nrgastro.2016.85
82. Gu JF, Su SL, Guo JM, Zhu Y, Zhao M, Duan JA. The aerial parts of Salvia miltiorrhiza Bge. strengthen intestinal barrier and modulate gut microbiota imbalance in streptozocin-induced diabetic mice. J Funct Foods. (2017) 36:362–74. doi: 10.1016/j.jff.2017.06.010
83. Wang K, Yang Q, Ma Q, Wang B, Wan Z, Chen M, et al. Protective effects of salvianolic acid A against dextran sodium sulfate-induced acute colitis in rats. Nutrients. (2018) 10:791. doi: 10.3390/nu10060791
84. Zheng J, Chen M, Ye C, Sun X, Jiang N, Zou X, et al. BuZangTongLuo decoction improved hindlimb ischemia by activating angiogenesis and regulating gut microbiota in diabetic mice. J Ethnopharmacol. (2020) 248:112330. doi: 10.1016/j.jep.2019.112330
85. Tan Y, Li Y, Zhou F, Guo J, Wang T, Shi Y, et al. Administration of a mixture of triterpenoids from yeyachun and phenolic acids from danshen ameliorates carbon tetrachloride-induced liver fibrosis in mice by the regulation of intestinal flora. J Pharmacol Sci. (2020) 143:165–75. doi: 10.1016/j.jphs.2020.04.007
86. Xu P, Hong F, Wang J, Wang J, Zhao X, Wang S, et al. DBZ is a putative PPARγ agonist that prevents high fat diet-induced obesity, insulin resistance and gut dysbiosis. Biochim Biophys Acta Gen Subj. (2017) 1861:2690–701. doi: 10.1016/j.bbagen.2017.07.013
87. Saad MJ, Santos A, Prada PO. Linking gut microbiota and inflammation to obesity and insulin resistance. Physiology (Bethesda). (2016) 31:283–93. doi: 10.1152/physiol.00041.2015
88. Kahleova H, Rembert E, Alwarith J, Yonas WN, Tura A, Holubkov R, et al. Effects of a low-fat vegan diet on gut microbiota in overweight individuals and relationships with body weight, body composition, and insulin sensitivity. A randomized clinical trial. Nutrients. (2020) 12:2917. doi: 10.3390/nu12102917
89. Wang L, Wang R, Wei GY, Wang SM, Du GH. Dihydrotanshinone attenuates chemotherapy-induced intestinal mucositis and alters fecal microbiota in mice. Biomed Pharmacother. (2020) 128:110262. doi: 10.1016/j.biopha.2020.110262
90. Liao J, Xie X, Gao J, Zhang Z, Qu F, Cui H, et al. Jian-Gan-Xiao-Zhi decoction alleviates inflammatory response in nonalcoholic fatty liver disease model rats through modulating gut microbiota. Evid Based Complement Altern Med. (2021) 2021:5522755. doi: 10.1155/2021/5522755
91. Zhao X, Chen Y, Li L, Zhai J, Yu B, Wang H, et al. Effect of DLT-SML on chronic stable angina through ameliorating inflammation, correcting dyslipidemia, and regulating gut microbiota. J Cardiovasc Pharmacol. (2021) 77:458–69. doi: 10.1097/FJC.0000000000000970
92. Zhang F, Xu Y, Shen L, Huang J, Xu S, Li J, et al. GuanXinNing tablet attenuates Alzheimer’s disease via improving gut microbiota, host metabolites, and neuronal apoptosis in rabbits. Evid Based Complement Altern Med. (2021) 2021:9253281. doi: 10.1155/2021/9253281
93. Cao W, Chin Y, Chen X, Mi Y, Xue C, Wang Y, et al. The role of gutmicrobiota in the resistace to obesity in mice fed a high fat diet. Int J Food Sci Nutr. (2019) 11:1–11.
94. Zhang H, Li C, Xin Y, Cui X, Cui J, Zhou G. Suppression of NSDHL attenuates adipogenesis with a downregulation of LXR-SREBP1 pathway in 3T3 L1cells. Biosci Biotechnol Biochem. (2020) 84:980–8. doi: 10.1080/09168451.2020.1719823
95. Zhang Y, Gu Y, Chen Y, Huang Z, Li M, Jiang W, et al. Dingxin Recipe IV attenuates atherosclerosis by regulating lipid metabolism through LXR-α/SREBP1 pathway and modulating the gut microbiota in ApoE-/- mice fed with HFD. J Ethnopharmacol. (2021) 266:113436. doi: 10.1016/j.jep.2020.113436
96. Cai HD, Su SL, Guo JM, Duan JA. Effects of salvia miltiorrhiza on intestinal microflora diversity in diabetic kidney injury rats. China J Chin Mater Med. (2021) 46:426–35. doi: 10.19540/j.cnki.cjcmm.20200723.402
97. Donnelly KL, Smith CI, Schwarzenberg SJ, Jessurun J, Boldt MD, Parks EJ. Sources of fatty acids stored in liver and secreted via lipoproteins in patients with nonalcoholic fatty liver disease. J Clin Investig. (2005) 115:1343–51. doi: 10.1172/JCI23621
98. Jump DB. N-3 polyunsaturated fatty acid regulation of hepatic gene transcription. Curr Opin Lipidol. (2008) 19:242–7. doi: 10.1097/MOL.0b013e3282ffaf6a
99. Hardwick RN, Fisher CD, Canet MJ, Scheffer GL, Cherrington NJ. Variations in ATP-binding cassette transporter regulation during the progression of human nonalcoholic fatty liver disease. Drug Metab Disposit. (2011) 39:2395–402. doi: 10.1124/dmd.111.041012
100. Fisher CD, Lickteig AJ, Augustine LM, Oude Elferink RP, Besselsen DG, Erickson RP, et al. Experimental non-alcoholic fatty liver disease results in decreased hepatic uptake transporter expression and function in rats. Eur J Pharmacol. (2009) 613:119–27. doi: 10.1016/j.ejphar.2009.04.002
101. Hardwick RN, Ferreira DW, More VR, Lake AD, Lu Z, Manautou JE, et al. Altered UDP-glucuronosyl-transferase and sulfotransferase expression and function during progressive stages of human nonalcoholic fatty liver disease. Drug Metab Disposit. (2013) 41:554–61. doi: 10.1124/dmd.112.048439
102. Francque S, Verrijken A, Caron S, Prawitt J, Paumelle R, Derudas B, et al. PPARα gene expression correlates with severity and histological treatment response in patients with non-alcoholic steatohepatitis. J Hepatol. (2015) 63:164–73. doi: 10.1016/j.jhep.2015.02.019
103. Chen J, Montagner A, Tan NS, Wahli W. Insights into the Role of PPARβ/δ in NAFLD. Int J Mol Sci. (2018) 19:1893. doi: 10.3390/ijms19071893
104. Gross B, Pawlak M, Lefebvre P. PPARs in obesity-induced T2DM, dyslipidaemia and NAFLD. Nat Rev Endocrinol. (2017) 13:36–49. doi: 10.1038/nrendo.2016.135
105. Chinetti G, Fruchart JC, Staels B. Peroxisome proliferator-activated receptors and inflammation: from basic science to clinical applications. Int J Obes Relat Metab Disord. (2003) 27(Suppl. 3):S41–5. doi: 10.1038/sj.ijo.0802499
106. Montagner A, Polizzi A, Fouché E, Ducheix S, Lippi Y, Lasserre F, et al. Liver PPARα is crucial for whole-body fatty acid homeostasis and is protective against NAFLD. Gut. (2016) 65:1202–14. doi: 10.1136/gutjnl-2015-310798
107. Oishi K, Tomita T. Thiazolidinediones are potent inducers of fibroblast growth factor 21 expression in the liver. Biol Pharm Bull. (2011) 34:1120–1. doi: 10.1248/bpb.34.1120
108. Jiang C, Ting AT, Seed B. PPAR-gamma agonists inhibit production of monocyte inflammatory cytokines. Nature. (1998) 391:82–6. doi: 10.1038/34184
109. Mukohda M, Lu KT, Guo DF, Wu J, Keen HL, Liu X, et al. Hypertension-causing mutation in peroxisome proliferator-activated receptor γ impairs nuclear export of nuclear factor-κB p65 in vascular smooth muscle. Hypertension. (2017) 70:174–82. doi: 10.1161/HYPERTENSIONAHA.117.09276
110. Yoo SH, Park O, Henderson LE, Abdelmegeed MA, Moon KH, Song BJ. Lack of PPARα exacerbates lipopolysaccharide-induced liver toxicity through STAT1 inflammatory signaling and increased oxidative/nitrosative stress. Toxicol Lett. (2011) 202:23–9. doi: 10.1016/j.toxlet.2011.01.013
111. Saha SA, Kizhakepunnur LG, Bahekar A, Arora RR. The role of fibrates in the prevention of cardiovascular disease–a pooled meta-analysis of long-term randomized placebo-controlled clinical trials. Am Heart J. (2007) 154:943–53. doi: 10.1016/j.ahj.2007.07.011
112. Honda Y, Kessoku T, Ogawa Y, Tomeno W, Imajo K, Fujita K, et al. Pemafibrate, a novel selective peroxisome proliferator-activated receptor alpha modulator, improves the pathogenesis in a rodent model of nonalcoholic steatohepatitis. Sci Rep. (2017) 7:42477. doi: 10.1038/srep42477
113. Hui Y, Yu YL, Yu QN, Wei HS, Yan LD, Xiao BL, et al. Effect of peroxisome proliferator-activated receptors-gamma and co-activator-1alpha genetic polymorphisms on plasma adiponectin levels and susceptibility of non-alcoholic fatty liver disease in Chinese people. Liver Int. (2008) 28:385–92. doi: 10.1111/j.1478-3231.2007.01623.x
114. Pan X, Wang P, Luo J, Wang Z, Song Y, Ye J, et al. Adipogenic changes of hepatocytes in a high-fat diet-induced fatty liver mice model and non-alcoholic fatty liver disease patients. Endocrine. (2015) 48:834–47. doi: 10.1007/s12020-014-0384-x
115. de Mendonça M, Dos Santos BAC, de Sousa É, Rodrigues AC. Adiponectin is required for pioglitazone-induced improvements in hepatic steatosis in mice fed a high-fat diet. Mol Cell Endocrinol. (2019) 493:110480. doi: 10.1016/j.mce.2019.110480
116. Zarzour RHA, Alshawsh MA, Asif M, Al-Mansoub MA, Mohamed Z, Ahmad M, et al. Adipocytokine regulation and antiangiogenic activity underlie the molecular mechanisms of therapeutic effects of Phyllanthus niruri against non-alcoholic fatty liver disease. Nutrients. (2018) 10:1057. doi: 10.3390/nu10081057
117. Wang H, Chen J, Hollister K, Sowers LC, Forman BM. Endogenous bile acids are ligands for the nuclear receptor FXR/BAR. Mol Cell. (1999) 3:543–53. doi: 10.1016/s1097-2765(00)80348-2
118. Ijssennagger N, Janssen AWF, Milona A, Ramos Pittol JM, Hollman DAA, Mokry M, et al. Gene expression profiling in human precision cut liver slices in response to the FXR agonist obeticholic acid. J Hepatol. (2016) 64:1158–66. doi: 10.1016/j.jhep.2016.01.016
119. de Boer JF, Schonewille M, Boesjes M, Wolters H, Bloks VW, Bos T, et al. Intestinal farnesoid X receptor controls transintestinal cholesterol excretion in mice. Gastroenterology. (2017) 152:1126–38.e6. doi: 10.1053/j.gastro.2016.12.037
120. Inagaki T, Choi M, Moschetta A, Peng L, Cummins CL, McDonald JG, et al. Fibroblast growth factor 15 functions as an enterohepatic signal to regulate bile acid homeostasis. Cell Metab. (2005) 2:217–25. doi: 10.1016/j.cmet.2005.09.001
121. Zhang Y, Lee FY, Barrera G, Lee H, Vales C, Gonzalez FJ, et al. Activation of the nuclear receptor FXR improves hyperglycemia and hyperlipidemia in diabetic mice. Proc Natl Acad Sci USA. (2006) 103:1006–11. doi: 10.1073/pnas.0506982103
122. Carr RM, Reid AE. FXR agonists as therapeutic agents for non-alcoholic fatty liver disease. Curr Atheroscler Rep. (2015) 17:500. doi: 10.1007/s11883-015-0500-2
123. Mudaliar S, Henry RR, Sanyal AJ, Morrow L, Marschall HU, Kipnes M, et al. Efficacy and safety of the farnesoid X receptor agonist obeticholic acid in patients with type 2 diabetes and nonalcoholic fatty liver disease. Gastroenterology. (2013) 145:574–82. doi: 10.1053/j.gastro.2013.05.042
124. Ahn SB, Jang K, Jun DW, Lee BH, Shin KJ. Expression of liver X receptor correlates with intrahepatic inflammation and fibrosis in patients with nonalcoholic fatty liver disease. Digest Dis Sci. (2014) 59:2975–82. doi: 10.1007/s10620-014-3289-x
125. Lin YN, Chang HY, Wang CCN, Chu FY, Shen HY, Chen CJ, et al. Oleanolic acid inhibits liver X receptor alpha and pregnane X receptor to attenuate ligand-induced lipogenesis. J Agric Food Chem. (2018) 66:10964–76. doi: 10.1021/acs.jafc.8b03372
126. Repa JJ, Mangelsdorf DJ. The role of orphan nuclear receptors in the regulation of cholesterol homeostasis. Annu Rev Cell Dev Biol. (2000) 16:459–81. doi: 10.1146/annurev.cellbio.16.1.459
127. Higuchi N, Kato M, Shundo Y, Tajiri H, Tanaka M, Yamashita N, et al. Liver X receptor in cooperation with SREBP-1c is a major lipid synthesis regulator in nonalcoholic fatty liver disease. Hepatol Res. (2008) 38:1122–9. doi: 10.1111/j.1872-034X.2008.00382.x
128. Gu M, Zhang Y, Liu C, Wang D, Feng L, Fan S, et al. Morin, a novel liver X receptor α/β dual antagonist, has potent therapeutic efficacy for nonalcoholic fatty liver diseases. Br J Pharmacol. (2017) 174:3032–44. doi: 10.1111/bph.13933
129. Wallace BD, Redinbo MR. Xenobiotic-sensing nuclear receptors involved in drug metabolism: a structural perspective. Drug Metab Rev. (2013) 45:79–100. doi: 10.3109/03602532.2012.740049
130. Banerjee M, Robbins D, Chen T. Targeting xenobiotic receptors PXR and CAR in human diseases. Drug Discov Today. (2015) 20:618–28. doi: 10.1016/j.drudis.2014.11.011
131. Dong B, Saha PK, Huang W, Chen W, Abu-Elheiga LA, Wakil SJ, et al. Activation of nuclear receptor CAR ameliorates diabetes and fatty liver disease. Proc Natl Acad Sci USA. (2009) 106:18831–6. doi: 10.1073/pnas.0909731106
132. Zhang J, Wei Y, Hu B, Huang M, Xie W, Zhai Y. Activation of human stearoyl-coenzyme A desaturase 1 contributes to the lipogenic effect of PXR in HepG2 cells. PLoS One. (2013) 8:e67959. doi: 10.1371/journal.pone.0067959
133. Bitter A, Rümmele P, Klein K, Kandel BA, Rieger JK, Nüssler AK, et al. Pregnane X receptor activation and silencing promote steatosis of human hepatic cells by distinct lipogenic mechanisms. Arch Toxicol. (2015) 89:2089–103. doi: 10.1007/s00204-014-1348-x
134. Li Y, Cheng SL, Bammler TK, Lee JS, Kim WY, Choi SH, et al. Editor’s highlight: neonatal activation of the xenobiotic-sensors PXR and CAR results in acute and persistent down-regulation of PPARα-signaling in mouse liver. Toxicol Sci. (2016) 153:282–302. doi: 10.1093/toxsci/kfw127
135. Roth A, Looser R, Kaufmann M, Meyer UA. Sterol regulatory element binding protein 1 interacts with pregnane X receptor and constitutive androstane receptor and represses their target genes. Pharmacogenet Genomics. (2008) 18:325–37. doi: 10.1097/FPC.0b013e3282f706e0
136. Chatterjee S, Mukherjee S, Sankara Sivaprasad LVJ, Naik T, Gautam SS, Murali BV, et al. Transporter activity changes in nonalcoholic steatohepatitis: assessment with plasma coproporphyrin I and III. J Pharmacol Exp Ther. (2021) 376:29–39. doi: 10.1124/jpet.120.000291
137. Abdelmegeed MA, Banerjee A, Yoo SH, Jang S, Gonzalez FJ, Song BJ. Critical role of cytochrome P450 2E1 (CYP2E1) in the development of high fat-induced non-alcoholic steatohepatitis. J Hepatol. (2012) 57:860–6. doi: 10.1016/j.jhep.2012.05.019
138. Leung TM, Nieto N. CYP2E1 and oxidant stress in alcoholic and non-alcoholic fatty liver disease. J Hepatol. (2013) 58:395–8. doi: 10.1016/j.jhep.2012.08.018
139. Yetti H, Naito H, Yuan Y, Jia X, Hayashi Y, Tamada H, et al. Bile acid detoxifying enzymes limit susceptibility to liver fibrosis in female SHRSP5/Dmcr rats fed with a high-fat-cholesterol diet. PLoS One. (2018) 13:e0192863. doi: 10.1371/journal.pone.0192863
140. Schiöth HB, Boström A, Murphy SK, Erhart W, Hampe J, Moylan C, et al. A targeted analysis reveals relevant shifts in the methylation and transcription of genes responsible for bile acid homeostasis and drug metabolism in non-alcoholic fatty liver disease. BMC Genomics. (2016) 17:462. doi: 10.1186/s12864-016-2814-z
141. Thakkar N, Slizgi JR, Brouwer KLR. Effect of liver disease on hepatic transporter expression and function. J Pharmacol Sci. (2017) 106:2282–94. doi: 10.1016/j.xphs.2017.04.053
142. Clarke JD, Novak P, Lake AD, Hardwick RN, Cherrington NJ. Impaired N-linked glycosylation of uptake and efflux transporters in human non-alcoholic fatty liver disease. Liver Int. (2017) 37:1074–81. doi: 10.1111/liv.13362
143. Sanyal AJ, Friedman SL, McCullough AJ, Dimick-Santos L. Challenges and opportunities in drug and biomarker development for nonalcoholic steatohepatitis: findings and recommendations from an American association for the study of liver diseases-U.S food and drug administration joint workshop. Hepatology. (2015) 61:1392–405. doi: 10.1002/hep.27678
144. Lyu J, Xue M, Li J, Lyu W, Wen Z, Yao P, et al. Clinical effectiveness and safety of salvia miltiorrhiza depside salt combined with aspirin in patients with stable angina pectoris: a multicenter, pragmatic, randomized controlled trial. Phytomedicine. (2021) 81:153419. doi: 10.1016/j.phymed.2020.153419
145. Lee HS, Son WC, Ryu JE, Koo BA, Kim YS. Standardized Salvia miltiorrhiza extract suppresses hepatic stellate cell activation and attenuates steatohepatitis induced by a methionine-choline deficient diet in mice. Molecules. (2014) 19:8189–211. doi: 10.3390/molecules19068189
146. Ding C, Zhao Y, Shi X, Zhang N, Zu G, Li Z, et al. New insights into salvianolic acid A action: regulation of the TXNIP/NLRP3 and TXNIP/ChREBP pathways ameliorates HFD-induced NAFLD in rats. Sci Rep. (2016) 6:28734. doi: 10.1038/srep28734
147. Lee MM, Kim HG, Lee SB, Lee JS, Kim WY, Choi SH, et al. CGplus, a standardized herbal composition ameliorates non-alcoholic steatohepatitis in a tunicamycin-induced mouse model. Phytomedicine. (2018) 41:24–32. doi: 10.1016/j.phymed.2018.01.020
148. Zhang XJ, Tian Y. Efficacy and safety of Alovastatin combined with compound Danshen tablets in the treatment of non-alcoholic fatty liver disease. Liver. (2019) 24:839–41.
149. Park IJ, Yang WK, Nam SH, Hong J, Yang KR, Kim J, et al. Cryptotanshinone induces G1 cell cycle arrest and autophagic cell death by activating the AMP-activated protein kinase signal pathway in HepG2 hepatoma. Apoptosis. (2014) 19:615–28. doi: 10.1007/s10495-013-0929-0
150. Zhang X, Ma Z, Liang Q, Tang X, Hu D, Liu C, et al. Tanshinone IIA exerts protective effects in a LCA-induced cholestatic liver model associated with participation of pregnane X receptor. J Ethnopharmacol. (2015) 164:357–67. doi: 10.1016/j.jep.2015.01.047
151. Yang MD, Chiang YM, Higashiyama R, Asahina K, Mann DA, Mann J, et al. Rosmarinic acid and baicalin epigenetically derepress peroxisomal proliferator-activated receptor γ in hepatic stellate cells for their antifibrotic effect. Hepatology. (2012) 55:1271–81. doi: 10.1002/hep.24792
152. Li GH, Li YR, Jiao P, Zhao Y, Hu HX, Lou HX, et al. Therapeutic Potential of Salviae miltiorrhizae radix et rhizoma against human diseases based on activation of Nrf2-mediated antioxidant defense system: bioactive constituents and mechanism of action. Oxid Med Cell Longev. (2018) 2018:7309073. doi: 10.1155/2018/7309073
153. Mahesh R, Jung HW, Kim GW, Kim YS, Park YK. Cryptotanshinone from Salviae miltiorrhizae radix inhibits sodium-nitroprusside-induced apoptosis in neuro-2a cells. Phytother Res PTR. (2012) 26:1211–9. doi: 10.1002/ptr.3705
154. Tsai MK, Lin YL, Huang YT. Effects of salvianolic acids on oxidative stress and hepatic fibrosis in rats. Toxicol Appl Pharmacol. (2010) 242:155–64. doi: 10.1016/j.taap.2009.10.002
155. Lv Z, Song Y, Xue D, Zhang W, Cheng Y, Xu L. Effect of salvianolic-acid B on inhibiting MAPK signaling induced by transforming growth factor-β1 in activated rat hepatic stellate cells. J Ethnopharmacol. (2010) 132:384–92. doi: 10.1016/j.jep.2010.05.026
156. Wang R, Yu XY, Guo ZY, Wang YJ, Wu Y, Yuan YF. Inhibitory effects of salvianolic acid B on CCl(4)-induced hepatic fibrosis through regulating NF-κB/IκBα signaling. J Ethnopharmacol. (2012) 144:592–8. doi: 10.1016/j.jep.2012.09.048
157. Yu F, Guo Y, Chen B, Shi L, Dong P, Zhou M, et al. LincRNA-p21 Inhibits the Wnt/β-catenin pathway in activated hepatic stellate cells via sponging microRNA-17-5p. Cell Physiol Biochem. (2017) 41:1970–80. doi: 10.1159/000472410
158. Zhu J, Wang R, Xu T, Zhang S, Zhao Y, Li Z, et al. Salvianolic acid A attenuates endoplasmic reticulum stress and protects against cholestasis-induced liver fibrosis via the SIRT1/HSF1 pathway. Front Pharmacol. (2018) 9:1277. doi: 10.3389/fphar.2018.01277
159. Wang W. Study on the Prevention and Treatment Effects and Mechanism of Lactobacillus plantarum, Bifidobacterium bifidum and Salvia miltiorrhiza Polysaccharides on Non-alcoholic Fatty Liver Disease. Guangzhou: Southern Medical University (2020).
160. Liu Y, Hu Y, E Q, Zuo J, Yang L, Liu W. Salvianolic acid B inhibits mitochondrial dysfunction by up-regulating mortalin. Sci Rep. (2017) 7:43097. doi: 10.1038/srep43097
161. Nagappan A, Kim JH, Jung DY, Jung MH. Cryptotanshinone from the Salvia miltiorrhiza bunge attenuates ethanol-induced liver injury by activation of AMPK/SIRT1 and Nrf2 signaling pathways. Int J Mol Sci. (2019) 21:265. doi: 10.3390/ijms21010265
162. Liu P, Hu YY, Liu C, Zhu DY, Xue HM, Xu ZQ, et al. Clinical observation of salvianolic acid B in treatment of liver fibrosis in chronic hepatitis B. World J Gastroenterol. (2002) 8:679–85. doi: 10.3748/wjg.v8.i4.679
163. Lomonaco R, Ortiz-Lopez C, Orsak B, Webb A, Hardies J, Darland C, et al. Effect of adipose tissue insulin resistance on metabolic parameters and liver histology in obese patients with nonalcoholic fatty liver disease. Hepatology. (2012) 55:1389–97. doi: 10.1002/hep.25539
164. Liu SJ, Zhang HM, Jia AP, Zhou JK, Lu LF. Effect of bacillus subtilis enterococcus capsule combined with compound Salvia miltiorrhiza injection on non-alcoholic fatty liver. Chin J Clin Ration Drug Use. (2014) 7:32–3.
165. Chen W, Liu L, Luo Y, Odaka Y, Awate S, Zhou H, et al. Cryptotanshinone activates p38/JNK and inhibits Erk1/2 leading to caspase-independent cell death in tumor cells. Cancer Prev Res (Phila). (2012) 5:778–87. doi: 10.1158/1940-6207.CAPR-11-0551
166. Wu MH, Tsai WJ, Don MJ, Chen YC, Chen IS, Kuo YC. Tanshinlactone A from Salvia miltiorrhiza modulates interleukin-2 and interferon-gamma gene expression. J Ethnopharmacol. (2007) 113:210–7. doi: 10.1016/j.jep.2007.05.026
167. Kwon KH, Kim KI, Jun WJ, Shin DH, Cho HY, Hong BS. In vitro and in vivo effects of macrophage-stimulatory polysaccharide from leaves of Perilla frutescens var. crispa. Biol Pharm Bull. (2002) 25:367–71. doi: 10.1248/bpb.25.367
168. Jang SI, Kim HJ, Kim YJ, Jeong SI, You YO. Tanshinone IIA inhibits LPS-induced NF-kappaB activation in RAW 264.7 cells: possible involvement of the NIK-IKK, ERK1/2, p38 and JNK pathways. Eur J Pharmacol. (2006) 542:1–7. doi: 10.1016/j.ejphar.2006.04.044
169. Wu CT, Deng JS, Huang WC, Shieh PC, Chung MI, Huang GJ. Salvianolic acid C against acetaminophen-induced acute liver injury by attenuating inflammation, oxidative stress, and apoptosis through inhibition of the Keap1/Nrf2/HO-1 signaling. Oxid Med Cell Longev. (2019) 2019:9056845. doi: 10.1155/2019/9056845
170. Wakil SJ, Abu-Elheiga LA. Fatty acid metabolism: target for metabolic syndrome. J Lipid Res. (2009) 50(Suppl.):S138–43.
171. Hardie DG. The AMP-activated protein kinase pathway–new players upstream and downstream. J Cell Sci. (2004) 117:5479–87. doi: 10.1242/jcs.01540
172. Liu Q, Zhang Y, Lin Z, Shen H, Chen L, Hu L, et al. Danshen extract 15,16-dihydrotanshinone I functions as a potential modulator against metabolic syndrome through multi-target pathways. J Steroid Biochem Mol Biol. (2010) 120:155–63. doi: 10.1016/j.jsbmb.2010.03.090
173. He XW, Yu D, Li WL, Zheng Z, Lv CL, Li C, et al. Anti-atherosclerotic potential of baicalin mediated by promoting cholesterol efflux from macrophages via the PPARγ-LXRα-ABCA1/ABCG1 pathway. Biomed Pharmacother. (2016) 83:257–64. doi: 10.1016/j.biopha.2016.06.046
174. Huang MQ, Zhou CJ, Zhang YP, Zhang XQ, Xu W, Lin J, et al. Salvianolic acid B ameliorates hyperglycemia and dyslipidemia in db/db mice through the AMPK pathway. Cell Physiol Biochem. (2016) 40:933–43. doi: 10.1159/000453151
175. Li Y, Xu S, Mihaylova MM, Zheng B, Hou X, Jiang B, et al. AMPK phosphorylates and inhibits SREBP activity to attenuate hepatic steatosis and atherosclerosis in diet-induced insulin-resistant mice. Cell Metab. (2011) 13:376–88. doi: 10.1016/j.cmet.2011.03.009
176. Zeng Z, He W, Jia Z, Hao S. Lycopene improves insulin sensitivity through inhibition of STAT3/Srebp-1c-mediated lipid accumulation and inflammation in mice fed a high-fat diet. Exp Clin Endocrinol Diabetes. (2017) 125:610–7. doi: 10.1055/s-0043-101919
177. Chen J, Yue J, Liu J, Liu Y, Jiao KL, Teng MY, et al. Salvianolic acids improve liver lipid metabolism in ovariectomized rats via blocking STAT-3/SREBP1 signaling. Chin J Nat Med. (2018) 16:838–45. doi: 10.1016/S1875-5364(18)30125-0
178. Browning JD, Horton JD. Fasting reduces plasma proprotein convertase, subtilisin/kexin type 9 and cholesterol biosynthesis in humans. J Lipid Res. (2010) 51:3359–63. doi: 10.1194/jlr.P009860
179. Gerin I, Clerbaux LA, Haumont O, Lanthier N, Das AK, Burant CF, et al. Expression of miR-33 from an SREBP2 intron inhibits cholesterol export and fatty acid oxidation. J Biol Chem. (2010) 285:33652–61. doi: 10.1074/jbc.M110.152090
180. Jia L, Song N, Yang G, Ma Y, Li X, Lu R, et al. Effects of tanshinone IIA on the modulation of miR?33a and the SREBP?2/Pcsk9 signaling pathway in hyperlipidemic rats. Mol Med Rep. (2016) 13:4627–35. doi: 10.3892/mmr.2016.5133
181. Xie Z, Loi Truong T, Zhang P, Xu F, Xu X, Li P. Dan-Qi prescription ameliorates insulin resistance through overall corrective regulation of glucose and fat metabolism. J Ethnopharmacol. (2015) 172:70–9. doi: 10.1016/j.jep.2015.05.041
182. Chen Q, Wang T, Li J, Wang S, Qiu F, Yu H, et al. Effects of natural products on fructose-induced nonalcoholic fatty liver disease (NAFLD). Nutrients. (2017) 9:96. doi: 10.3390/nu9020096
183. Strable MS, Ntambi JM. Genetic control of de novo lipogenesis: role in diet-induced obesity. Crit Rev Biochem Mol Biol. (2010) 45:199–214. doi: 10.3109/10409231003667500
184. Zhang N, Hu Y, Ding C, Zeng W, Shan W, Fan H, et al. Salvianolic acid B protects against chronic alcoholic liver injury via SIRT1-mediated inhibition of CRP and ChREBP in rats. Toxicol Lett. (2017) 267:1–10. doi: 10.1016/j.toxlet.2016.12.010
185. Benhamed F, Denechaud PD, Lemoine M, Robichon C, Moldes M, Bertrand-Michel J, et al. The lipogenic transcription factor ChREBP dissociates hepatic steatosis from insulin resistance in mice and humans. J Clin Investig. (2012) 122:2176–94. doi: 10.1172/JCI41636
186. Chen J, Deng J, Zhang Y, Yang J, He Y, Fu W, et al. Lipid-lowering effects of Danhong injection on hyperlipidemia rats. J Ethnopharmacol. (2014) 154:437–42. doi: 10.1016/j.jep.2014.04.023
187. Dou X, Li S, Wang Z, Gu D, Shen C, Yao T, et al. Inhibition of NF-κB activation by 4-hydroxynonenal contributes to liver injury in a mouse model of alcoholic liver disease. Am J Pathol. (2012) 181:1702–10. doi: 10.1016/j.ajpath.2012.08.004
188. Ding L, Wo L, Du Z, Tang L, Song Z, Dou X. Danshen protects against early-stage alcoholic liver disease in mice via inducing PPARα activation and subsequent 4-HNE degradation. PLoS One. (2017) 12:e0186357. doi: 10.1371/journal.pone.0186357
189. IJpenberg A, Tan NS, Gelman L, Kersten S, Seydoux J, Xu J, et al. In vivo activation of PPAR target genes by RXR homodimers. EMBO J. (2004) 23:2083–91. doi: 10.1038/sj.emboj.7600209
190. Yin HQ, Kim YS, Choi YJ, Kim YC, Sohn DH, Ryu SY, et al. Effects of tanshinone IIA on the hepatotoxicity and gene expression involved in alcoholic liver disease. Arch Pharm Res. (2008) 31:659–65. doi: 10.1007/s12272-001-1209-2
191. Wang X, Qi D, Fu F, Li X, Liu Y, Ji K, et al. Therapeutic and antiproteinuric effects of salvianolic acid A in combined with low-dose prednisone in minimal change disease rats: involvement of PPARγ/Angptl4 and Nrf2/HO-1 pathways. Eur J Pharmacol. (2019) 858:172342. doi: 10.1016/j.ejphar.2019.04.023
192. Pi J, Leung L, Xue P, Wang W, Hou Y, Liu D, et al. Deficiency in the nuclear factor E2-related factor-2 transcription factor results in impaired adipogenesis and protects against diet-induced obesity. J Biol Chem. (2010) 285:9292–300. doi: 10.1074/jbc.M109.093955
193. Zhu NL, Wang J, Tsukamoto H. The Necdin-Wnt pathway causes epigenetic peroxisome proliferator-activated receptor gamma repression in hepatic stellate cells. J Biol Chem. (2010) 285:30463–71. doi: 10.1074/jbc.M110.156703
194. Wang P, Xu S, Li W, Wang F, Yang Z, Jiang L, et al. Salvianolic acid B inhibited PPARγ expression and attenuated weight gain in mice with high-fat diet-induced obesity. Cell Physiol Biochem. (2014) 34:288–98. doi: 10.1159/000362999
195. Gong Z, Huang C, Sheng X, Zhang Y, Li Q, Wang MW, et al. The role of tanshinone IIA in the treatment of obesity through peroxisome proliferator-activated receptor gamma antagonism. Endocrinology. (2009) 150:104–13. doi: 10.1210/en.2008-0322
196. Rahman N, Jeon M, Song HY, Kim YS. Cryptotanshinone, a compound of Salvia miltiorrhiza inhibits pre-adipocytes differentiation by regulation of adipogenesis-related genes expression via STAT3 signaling. Phytomedicine. (2016) 23:58–67. doi: 10.1016/j.phymed.2015.12.004
197. Sung HY, Jun JG, Kang SW, Kim HS, Shin D, Kang IJ, et al. Novel danshen methoxybenzo[b]furan derivative antagonizing adipogenic differentiation and production of inflammatory adipokines. Chem Biol Interact. (2010) 188:457–66. doi: 10.1016/j.cbi.2010.09.017
198. Byun JW, Hwang S, Kang CW, Kim JH, Chae MK, Yoon JS, et al. Therapeutic effect of protocatechuic aldehyde in an in vitro model of graves’ orbitopathy. Investig Ophthalmol Vis Sci. (2016) 57:4055–62. doi: 10.1167/iovs.15-19037
199. Jamwal R, Barlock BJ. Nonalcoholic fatty liver disease (NAFLD) and hepatic cytochrome P450 (CYP) enzymes. Pharmaceuticals. (2020) 13:222. doi: 10.3390/ph13090222
200. Jamwal R, de la Monte SM, Ogasawara K, Adusumalli S, Barlock BB, Akhlaghi F. Nonalcoholic fatty liver disease and diabetes are associated with decreased CYP3A4 protein expression and activity in human liver. Mol Pharm. (2018) 15:2621–32. doi: 10.1021/acs.molpharmaceut.8b00159
201. Abdelmegeed MA, Ha SK, Choi Y, Akbar M, Song BJ. Role of CYP2E1 in mitochondrial dysfunction and hepatic injury by alcohol and non-alcoholic substances. Curr Mol Pharmacol. (2017) 10:207–25. doi: 10.2174/1874467208666150817111114
202. Zhou X, Cheung CM, Yang JM, Or PM, Lee WY, Yeung JH. Danshen (Salvia miltiorrhiza) water extract inhibits paracetamol-induced toxicity in primary rat hepatocytes via reducing CYP2E1 activity and oxidative stress. J Pharm Pharmacol. (2015) 67:980–9. doi: 10.1111/jphp.12381
203. Woolsey SJ, Mansell SE, Kim RB, Tirona RG, Beaton MD. CYP3A activity and expression in nonalcoholic fatty liver disease. Drug Metab Disposit. (2015) 43:1484–90. doi: 10.1124/dmd.115.065979
204. Woolsey SJ, Beaton MD, Mansell SE, Leon-Ponte M, Yu J, Pin CL, et al. A fibroblast growth factor 21-pregnane X receptor pathway downregulates hepatic CYP3A4 in nonalcoholic fatty liver disease. Mol Pharmacol. (2016) 90:437–46. doi: 10.1124/mol.116.104687
205. Yu C, Ye S, Sun H, Liu Y, Gao L, Shen C, et al. PXR-mediated transcriptional activation of CYP3A4 by cryptotanshinone and tanshinone IIA. Chem Biol Interact. (2009) 177:58–64. doi: 10.1016/j.cbi.2008.08.013
206. Han C, Wei Y, Wang X, Ba C, Shi W. Protective effect of Salvia miltiorrhiza polysaccharides on liver injury in chickens. Poult Sci. (2019) 98:3496–503. doi: 10.3382/ps/pez153
207. Zhang XX, Cao YF, Wang LX, Yuan XL, Fang ZZ. Inhibitory effects of tanshinones towards the catalytic activity of UDP-glucuronosyltransferases (UGTs). Pharma Biol. (2017) 55:1703–9. doi: 10.3109/13880209.2015.1045621
208. Barve A, Khor TO, Nair S, Lin W, Yu S, Jain MR, et al. Pharmacogenomic profile of soy isoflavone concentrate in the prostate of Nrf2 deficient and wild-type mice. J Pharm Sci. (2008) 97:4528–45. doi: 10.1002/jps.21311
209. Wang W, Guan C, Sun X, Zhao Z, Li J, Fu X, et al. Tanshinone IIA protects against acetaminophen-induced hepatotoxicity via activating the Nrf2 pathway. Phytomedicine. (2016) 23:589–96. doi: 10.1016/j.phymed.2016.02.022
210. Wang L, Sweet DH. Competitive inhibition of human organic anion transporters 1 (SLC22A6), 3 (SLC22A8) and 4 (SLC22A11) by major components of the medicinal herb Salvia miltiorrhiza (Danshen). Drug Metab Pharmacokinet. (2013) 28:220–8. doi: 10.2133/dmpk.dmpk-12-rg-116
211. Jia W, Du F, Liu X, Jiang R, Xu F, Yang J, et al. Renal tubular secretion of tanshinol: molecular mechanisms, impact on its systemic exposure, and propensity for dose-related nephrotoxicity and for renal herb-drug interactions. Drug Metab Dispos. (2015) 43:669–78. doi: 10.1124/dmd.114.062000
212. An YG, Li XC. Clinical observation of salvia miltiorrhiza injection in treatment of nonalcoholic fatty liver disease. J Anhui Med. (2011) 15:885–6.
213. Ji G, Fan JG, Chen JJ. Clinical study on treatment of non-alcoholic fatty liver of damp-heat syndrome type by Danning tablet. Chin J Integr Med. (2005) 25:485–8.
214. Hu S, Gu N, Jing SB, Ma S, Chen W, Shi B. Effect of Yindan Xinnaotong soft capsule on nonalcoholic fatty liver and its mechanism. Chin J Trad Chin Med. (2019) 37:2229–32.
215. Wu QB. Magnesium isoglycyrrhizinate combined with Salvia miltiorrhiza in treating nonalcoholic fatty hepatitis control observation. J Pract Trad Chin Med. (2012) 26:41–2.
216. Wu H, Chen JJ. Treatment of 32 cases of non-alcoholic fatty liver disease with reduced glutathione and Salvia miltiorrhiza injection. Chin J Integr Trad Western Med Digest. (2010) 18:400–1.
217. Shao SQ. Tiopronin combined with Salvia miltiorrhiza injection in the treatment of non-alcoholic steatohepatitis. Clin Med. (2005) 9:59–60.
218. Fan HX, Lv LS. Effect of compound danshen dripping pill combined with polyene phosphatidyl choline in treatment of nonalcoholic fatty liver disease. Drug Eval Res. (2017) 40:1327–30.
219. Tsuchida T, Lee YA, Fujiwara N, Ybanez M, Allen B, Martins S, et al. A simple diet- and chemical-induced murine NASH model with rapid progression of steatohepatitis, fibrosis and liver cancer. Hepatology. (2018) 69:385–95. doi: 10.1016/j.jhep.2018.03.011
220. Santhekadur PK, Kumar DP, Sanyal AJ. Preclinical models of non-alcoholic fatty liver disease. Hepatology. (2018) 68:230–7.
221. Van Herck MA, Vonghia L, Francque SM. Animal models of nonalcoholic fatty liver disease-A starter’s guide. Nutrients. (2017) 9:1072. doi: 10.3390/nu9101072
222. Eslam M, Sanyal AJ, George J. MAFLD: a consensus-driven proposed nomenclature for metabolic associated fatty liver disease. Gastroenterology. (2020) 158:1999–2014.e1. doi: 10.1053/j.gastro.2019.11.312
223. Tanaka N, Aoyama T, Kimura S, Gonzalez FJ. Targeting nuclear receptors for the treatment of fatty liver disease. Pharmacol Ther. (2017) 179:142–57. doi: 10.1016/j.pharmthera.2017.05.011
224. Heinrich M, Appendino G, Efferth T, Fürst R, Izzo AA, Kayser O, et al. Best practice in research-Overcoming common challenges in phytopharmacological research. J Ethnopharmacol. (2020) 246:112230. doi: 10.1016/j.jep.2019.112230
225. Eslam M, Valenti L, Romeo S. Genetics and epigenetics of NAFLD and NASH: clinical impact. J Hepatol. (2018) 68:268–79. doi: 10.1016/j.jhep.2017.09.003
226. Ling MZ. Sangming mixture in treatment of 60 cases of nonalcoholic fatty liver disease. Sichuan J Trad Chin Med. (2007) 2007:42–4.
227. Liu YX. Clinical observation of compound Salvia miltiorrhiza injection combined with liver disease therapeutic apparatus in the treatment of non-alcoholic fatty liver disease. J Pract Trad Chin Med. (2017) 33:612–3.
Keywords: Danshen, non-alcoholic fatty liver disease, metabolic targets, pharmacokinetic, molecular mechanism
Citation: Liu J, Shi Y, Peng D, Wang L, Yu N, Wang G and Chen W (2022) Salvia miltiorrhiza Bge. (Danshen) in the Treating Non-alcoholic Fatty Liver Disease Based on the Regulator of Metabolic Targets. Front. Cardiovasc. Med. 9:842980. doi: 10.3389/fcvm.2022.842980
Received: 24 December 2021; Accepted: 28 March 2022;
Published: 22 April 2022.
Edited by:
Hongliang Li, Wuhan University, ChinaReviewed by:
Di Ren, University of South Florida, United StatesXiaoqing Yan, Wenzhou Medical University, China
Copyright © 2022 Liu, Shi, Peng, Wang, Yu, Wang and Chen. This is an open-access article distributed under the terms of the Creative Commons Attribution License (CC BY). The use, distribution or reproduction in other forums is permitted, provided the original author(s) and the copyright owner(s) are credited and that the original publication in this journal is cited, in accordance with accepted academic practice. No use, distribution or reproduction is permitted which does not comply with these terms.
*Correspondence: Lei Wang, d2FuZ2xlaUBhaHRjbS5lZHUuY24=; Weidong Chen, d2RjaGVuQGFodGNtLmVkdS5jbg==