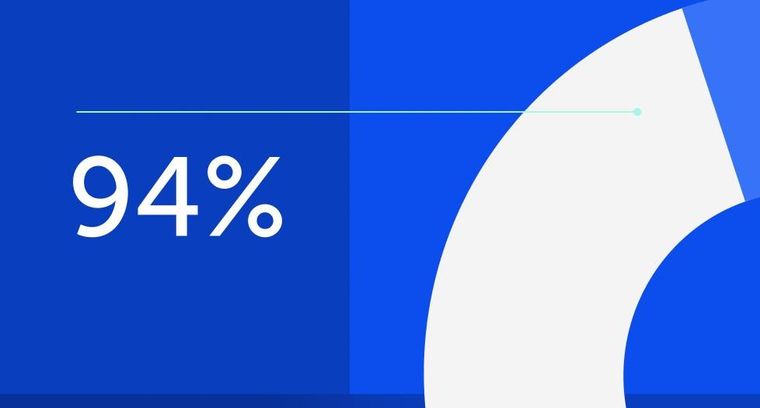
94% of researchers rate our articles as excellent or good
Learn more about the work of our research integrity team to safeguard the quality of each article we publish.
Find out more
REVIEW article
Front. Cardiovasc. Med., 16 March 2022
Sec. Atherosclerosis and Vascular Medicine
Volume 9 - 2022 | https://doi.org/10.3389/fcvm.2022.802783
This article is part of the Research TopicCerebrovasculature in Health and DiseasesView all 13 articles
Cardiocerebrovascular diseases (CCVDs) are the leading cause of death worldwide; therefore, to deeply explore the pathogenesis of CCVDs and to find the cheap and efficient strategies to prevent and treat CCVDs, these are of great clinical and social significance. The discovery of nitric oxide (NO), as one of the endothelium-derived relaxing factors and its successful utilization in clinical practice for CCVDs, provides new ideas for us to develop drugs for CCVDs: “gas medicine” or “medical gases.” The endogenous gas molecules such as carbon monoxide (CO), hydrogen sulfide (H2S), sulfur dioxide (SO2), methane (CH4), and hydrogen (H2) have essential biological effects on modulating cardiocerebrovascular homeostasis and CCVDs. Moreover, it has been shown that noble gas atoms such as helium (He), neon (Ne), argon (Ar), krypton (Kr), and xenon (Xe) display strong cytoprotective effects and therefore, act as the exogenous pharmacologic preventive and therapeutic agents for CCVDs. Mechanistically, besides the competitive inhibition of N-methyl-D-aspartate (NMDA) receptor in nervous system by xenon, the key and common mechanisms of noble gases are involved in modulation of cell death and inflammatory or immune signals. Moreover, gases interaction and reduction in oxidative stress are emerging as the novel biological mechanisms of noble gases. Therefore, to investigate the precise actions of noble gases on redox signals, gases interaction, different cell death forms, and the emerging field of gasoimmunology, which focus on the effects of gas atoms/molecules on innate immune signaling or immune cells under both the homeostatic and perturbed conditions, these will help us to uncover the mystery of noble gases in modulating CCVDs.
Cardiovascular diseases (CVDs) are a group of disorders of heart and blood vessels, CVDs include primary hypertension, pulmonary arterial hypertension, abdominal aortic aneurysm, coronary heart disease (CHD) (especially myocardial ischemia, which is primarily mediated by the buildup of atherosclerotic plaque in the blood vessels that supply oxygen and nutrients to the heart, coronary artery vasospasm, and coronary microvascular dysfunction) (1–4), congenital heart disease, valvular heart disease (e.g., rheumatic heart disease), myocarditis and inflammatory cardiomyopathy, diabetic cardiomyopathy, and other conditions, ultimately cardiac arrhythmias and/or heart failure; additionally, cerebrovascular diseases (CBVDs), a range of conditions influencing brain and cerebral arteries, e.g., ischemic stroke, also belong to CVDs; therefore, CVDs also refer to as cardiocerebrovascular diseases (CCVDs) (5–13). Heart attack and stroke are the representative diseases of CCVDs (14). CCVDs are the leading cause of death globally and the statistical data from the WHO indicate that CCVDs take an estimated 17.9 million lives each year (15). Therefore, to deeply explore the pathogenesis of CCVDs and to find the cheap and efficient strategies to prevent/treat CCVDs, these are of great clinical and social significance.
The discovery of nitric oxide (NO), as one of the endothelium-derived relaxing factors (for which the Nobel Prize in Physiology or Medicine was awarded in 1998) and its successful clinical application in CCVDs, opens a new direction for the scientists to discover drugs for treating CCVDs: “medical gases” or “gas medicine” (16–18). For example, the endogenous gases, including carbon monoxide (CO), hydrogen sulfide (H2S), sulfur dioxide (SO2), methane (CH4), and hydrogen (H2, which is primarily produced by intestinal flora), have been shown to prevent or treat CCVDs in animals or in human body (19–32). Recently, noble gas family has emerged as the novel exogenous pharmacologic preventive and therapeutic agents for CCVDs (33–38). The aim of this comprehensive review is to summarize and discuss the current understanding of the biological effects and mechanisms of noble gases on CCVDs.
The noble gases refer to the gas atoms corresponding to all the group 18 elements on the periodic table of the elements. This family constitutes six naturally occurring gases: helium (He), neon (Ne), argon (Ar), krypton (Kr), xenon (Xe), and the radioactive radon (Rn) (38) (Figure 1). Xe was first shown to possess anesthetic properties in 1951, whereas none of the other five noble gases show anesthetic properties under normobaric conditions (38, 39). At normal temperature and normal pressure, noble gases are odorless, colorless, and monatomic gases that are characterized by a filled outer shell of valence electrons, making them “inert” or at least less capable of interaction with other compounds; therefore, they are also known as “inert gases” (34–36, 38). However, some of these noble gases have strong biological activities such as the properties of neuroprotection and cardioprotection (34–36, 38).
Figure 1. Noble gases have emerged as the novel preventive and therapeutic agents for cardiocerebrovascular diseases (CCVDs). The noble gas family includes helium (He), neon (Ne), argon (Ar), krypton (Kr), xenon (Xe), and the radioactive radon (Rn). They are monatomic gases at the far right of the periodic table and are chemically inert. Last but not least, it has been shown that most of noble gases have essential biological effects, including modulation of cell death, immunity/inflammation, gases interaction, and oxidative stress. They have been acted as protectants for alleviating the injuries of heart, brain, blood vessels (e.g., endothelial cells), liver, kidney, and intestine in animal models or in human body. Therefore, noble gases therapy provides a novel idea for the prevention and treatment of CCVDs.
Helium is the second most abundant element in the universe after H2; however, He is only sixth element in the composition of dry air (0.00052%) (40). It is the lightest noble gas with an atomic weight of 4 g/mol and has the lowest melting (−458°F, −272.2°C) and boiling (−452.1°F, −268.9°C) points of all the elements (36, 40). Due to the lower density and viscosity, heliox-21 (21% oxygen and 79% helium), which weight is one-third compared with air, can reduce work of breathing; therefore, heliox has been reported to be effective in a variety of respiratory conditions, including asthma exacerbation, post-extubation stridor, croup, upper airway obstruction, bronchiolitis, acute respiratory distress syndrome (ARDS), chronic obstructive pulmonary disease (COPD), and pulmonary function testing (36, 40, 41). He has the lower solubility than nitrogen; the mixture of helium and oxygen rather than nitrogen and oxygen decreases the formation of nitrogen bubbles and, therefore, alleviating decompression illness in deep-sea divers (41). Moreover, He is safe for abdominal insufflation and may be the insufflating agent of choice in patients with significant cardiopulmonary disease and laparoscopic renal surgery (42–44). He inhalation enhanced vasodilator effect of inhaled NO on pulmonary vessels in hypoxic dogs; this enhanced vasodilatory effect of NO on He might be associated with facilitated diffusion of NO diluted in the gas mixture with He (45). In the past decade, a series of studies showed that He has essential cytoprotective effects on endothelial cells (ECs) (46–48), heart (49–60), brain (59, 61–67), liver (68), and intestine (69).
Caveolin-1 (Cav-1) was secreted after He exposure in vitro, altered the cytoskeleton, and increased the adherent junction protein vascular endothelial-cadherin (VE-cadherin) and gap junction protein connexin 43 (Cx43) expression thus, resulting in decreased permeability in ECs (47). These indicated that He protected endothelium by maintaining barrier function and preventing leakage and tissue edema and ultimately preserving endothelial function (47). Furthermore, the plasma of healthy volunteers breathing He protected ECs against hypoxic cell damage by increasing Cav-1 expression and Cav-1 knockdown in ECs abolished this effect (48); the interesting question is what contents from the plasma contribute to these effects. However, another study showed that pretreating with He increased ECs damage in vitro under the stimulation of tumor necrosis factor-α (TNF-α) or hydrogen peroxide (H2O2) (70).
Helium can induce preconditioning in human endothelium in vivo: inhalation of 3 cycles of heliox21 for 5 min, followed by 5 min of normal air breathing either directly before forearm ischemia (20 min) or 24 h before forearm ischemia (20 min), attenuated ischemia-reperfusion (I/R)-induced endothelial dysfunction independent of endothelial NO synthase (eNOS), as that the protection of He was not abolished after blockade of eNOS (46). However, Eliana Lucchinetti et al. (71) showed that heliox-50 (50% helium and 50% oxygen), breathing from 15 min before ischemia until 5 min after the onset of reperfusion, provided modest anti-inflammatory effects, but did not restore endothelial dysfunction of the forearm in humans in vivo. A case report indicated that accidental inhalation of He under high pressure can cause symptomatic cerebral and coronary artery gas embolism (72). Therefore, the concentration, time, and mode (continuously or intermittently) of supplying He, the different pathological stimuli, and in vivo and in vitro might be responsible for the above controversies.
Helium preconditioning (HePC) can considerably reduce infarct size in myocardial I/R injury model of rabbits, young rats but not aged rats, Zucker lean rat but not Zucker obese rats (49–51, 73) (Table 1). These He-induced cardioprotection are related to activating phosphoinositide 3-kinase (PI3K), p44/42 mitogen-activated protein kinase (MAPK) (ERK1/2), p70S6 kinase (p70s6K), cyclic AMP (cAMP)-dependent protein kinase (PKA), cyclooxygenase-2 (COX-2), opioid receptors, mitochondrial Ca2+-sensitive potassium channel, and mitochondrial ATP-regulated potassium (KATP) channels (possibly producing small quantities of ROS); inhibiting mitochondrial permeability transition pore (mPTP) opening and NO production by eNOS (49, 50, 53, 73–76, 78). Moreover, suppression of glycogen synthase kinase-3 (GSK-3) or p53 lowered the threshold of He-induced preconditioning via the mPTP-dependent mechanism in vivo (77). He also induced post-conditioning in the myocardial I/R injury model of Zucker lean rats or male Wistar rats, these protective effects on Wistar rats are related to increasing genes involved in autophagy, inhibiting genes involved in apoptosis, increasing protein levels of Cav-1/3, and activating ERK1/2 and Akt (51, 56, 57) (Table 1). However, inhaled 30 or 60 min of 70% He during reperfusion dose does not induce cardioprotection in male adult Wistar rats (55). This process was not accompanied by reducing the hyperacute burst of inflammatory cytokines, but the prolonged He inhalation might contribute to the proinflammatory response, such as increasing cytokine-induced neutrophil chemoattractant 3 (CINC-3) and interleukin-1β (IL-1β) in myocardium from area at risk, but not from area not at risk (55). Moreover, one clinical investigation indicated that HePC (3 ×5 min of 70% He and 30% oxygen was applied before aortic cross-clamping), helium post-conditioning (15 min of He was applied before release of the aortic cross-clamp and was continued for 5 min after begin of reperfusion) or the combination had no effects on the activation of p38 MAPK, ERK1/2, or on the levels of protein kinase C-epsilon (PKC-ε) and heat-shock protein 27 (HSP27) in patient hearts undergoing coronary artery bypass graft surgery; HePC and helium post-conditioning did not affect postoperative troponin release in these patients (58). In contrast to the healthy Wistar Kyoto rats (WKRs), only a triple intervention of He conditioning can reduce cell damage after myocardial I/R in spontaneous hypertensive rats (SHR), suggesting the presence of a threshold in the hypertensive heart (54) (Table 1). An in-vitro study indicated that He conditioning contributed to cardioprotection by increasing fibroblast migration, but not by releasing protective medium extracellular vesicles or soluble factors from the cardiac fibroblasts (88). Our recent study indicated that intraperitoneal injection of 99.999% He gas improved lipopolysaccharide (LPS)-induced left ventricular dysfunction and cavity enlargement in a dose-dependent manner, it is better at the dose of 1.0 ml/100 g (89). Mechanistically, He inhibited Toll-like receptor 4 (TLR4) expression, reduced the phosphorylation of nuclear factor-kappa B (NF-κB), and subsequently alleviated interleukin-18 (IL-18) and TNF-α expression in heart (89). The dose effect of He gas has also been confirmed in intestine; the HePC profile consisting of three cycles of 10 or 15 min He breathing interspersed with three 5-min washout periods by breathing room air reduced I/R-induced intestinal injury, inflammatory response, and cell apoptosis; however, the 2- or 5-min He breathing dose does not confer any protective effects (69).
Helium displayed neuroprotective effects on a traumatic brain injury model in vitro (61) and in a decompression-induced neurological deficits model in vivo (90). Breathing 70% He during a middle cerebral artery occlusion (MCAO) for 2 h and early reperfusion (1 h) reduced infarct volume and improved neurological deficits 24 h after MCAO in rats (91). Seventy-five percentage He treatment from 1 h after reperfusion to 4 h after reperfusion also provided neuroprotection by producing hypothermia in rats (62). In a rat resuscitation model, HePC and He post-conditioning (received 70% He and 30% oxygen for 5 min before cardiac arrest and for 30 min after restoration of spontaneous circulation) reduced apoptosis in brain, but had no influence on viable neuron count and no beneficial effects were seen on neurofunctional outcome (59). He-PC-induced NO production and subsequent NO-mediated up-regulation of antioxidases (e.g, nuclear factor E2-related factor 2), angiogenesis, and inhibition of inflammation and apoptosis, all contributed to the neuroprotective effect of helium in a neonatal cerebral hypoxia/ischemia model (63, 65, 66). However, in a clinical perspective for the treatment of acute ischemic stroke, He should not be administered before or together with tissue plasminogen activator therapy due to the risk of inhibiting the benefit of tissue plasminogen activator-induced thrombolysis; He therapy could be an efficient neuroprotective agent, if given after tissue plasminogen activator-induced reperfusion (64).
Fukuda et al. have confirmed that inhalation of H2 gas (1–4% at 10 min before reperfusion until the end of reperfusion) suppressed hepatic I/R (90/180 min) injury through reducing oxidative stress in male C57 BL/6N mice (4–5 weeks old, 15–18 g); however, 4% He gas showed no protective effect (92). Similarly, HePC (three cycles of ventilation with inhalation of mixture of 70% He and 30% oxygen for 5 min, each followed by 5-min washout with inhalation of mixture of 30% oxygen and 70% nitrogen) did not attenuate hepatic I/R (45/240 min) injury in male Wistar rats (300 ± 30 g), although there was evidence for a modulation of the inflammatory response (93). In contrast, Zhang et al. have revealed that HePC (70% He-30% oxygen mixture inhalation for three 5-min periods interspersed with three 5-min washout periods using room air) alleviated 90 min ischemia-induced liver injury at 1, 3, and 6 h after reperfusion in male BALB/c mice (25–30 g); mechanistically, activation of hepatic adenosine A2A receptor-PI3K-Akt axis, alleviation of necrosis and apoptosis, reduction of IκBα phosphorylation, and TNF-α, interleukin-6 (IL-6), monocyte chemotactic protein-1 (MCP-1) and chemokine (C-X-C motif) ligand 10 (CXCL10, IP-10) expression, and inhibition of inflammatory cell infiltration in liver all contributed to this protective effects of HePC (68). The difference of animal strains, the time of I/R, and even the gas mixture used in washout periods might be responsible for these controversy. Furthermore, Zhang et al. have confirmed that HePC-induced protection in hepatic I/R injury and Akt activation were dependent on the interaction between He inhalation and air gaps, but not any of the two factors alone (68). As the protection of the intermittent pattern of He inhalation, drinking hydrogen-rich water, or intermittent hydrogen gas exposure, but not lactulose or continuous hydrogen gas exposure, prevented 6-hydroxydopamine-induced Parkinson's disease in rats (94). Therefore, the continuous heliox inhalation, rather than intermittent pattern, might be responsible for the none alteration of myocardial infarct size or the extent of no reflow in rabbits with continuous heliox breathing during 30 min of ischemia and 180 min of reperfusion (95).
When 7-day-old postnatal Sprague-Dawley rats subjected to hypoxic-ischemia (moderate) injury, 2 h after hypoxic insult, exposure of He, Ar, and Xe (70% noble gas balanced with oxygen) for 90 min improved cell survival, brain structural integrity, and neurologic function on postnatal day 40 compared with nitrogen, whereas only Ar and Xe reduced infarct volume after more severe hypoxic-ischemic injury (96). The in-vivo and in-vitro studies indicated that Ar acted as a protector for cerebral ischemia injury, brain trauma, and cardiac arrest-induced neurological damage (97–110). The neuroprotective effects of Ar were involved in inhibiting microglia/macrophage activation and enhancing M2 microglia/macrophage polarization (107, 109, 110), reducing stress-activated protein kinase/c-Jun N-terminal kinase (SAPK/JNK) activation and high mobility group protein B1 (HMGB1) expression (106), inhibiting TLR2/4-mediated activation of signal transducer and activator of transcription 3 (STAT3) and NF-κB, and subsequently decreasing IL-8 expression (111).
Argon displayed cardioprotective effects both in vitro and in vivo (73, 79, 106, 112, 113). Pre-treatment with 30 or 50% Ar for 90 min before oxygen-glucose deprivation protected human cardiac myocyte-like progenitor cells against apoptosis via activation of ERK, Akt, and biphasic regulation of JNK (113). Preconditioning with three cycles of 50% Ar (50% Ar, 21% oxygen, and 29% nitrogen) for 5 min, interspersed with 5 min of 79% nitrogen-21% oxygen in vivo, enhanced post-ischemic cardiac functional recovery following cardioplegic arrest and global cold ischemia in vitro; this protective effect of Ar was related to improving cardiac energy metabolism, inhibiting JNK phosphorylation, and HMGB1 expression (106). The cardioprotection of Ar on ischemia was also confirmed in rabbit in vivo (73) (Table 1). Lemoine et al. have further revealed the therapeutic effect of Ar on left ventricular dysfunction in myocardial I/R injury in vivo, in which Ar activated PI3K/Akt mitogen-activated protein kinase kinase (MEK)-ERK1/2 signaling, inhibited the opening of mitochondrial permeability transition pore (79) (Table 1).
Argon is the key modulator of IL-6 expression in different liver injury models. Under the physiological conditions, IL-6 is essential for proper hepatic tissue homeostasis, liver regeneration, infection defense, and fine-tuning of metabolic functions, while persistent activation of IL-6 seems to be detrimental, impairs liver regeneration and can even lead to the development of liver cancer (114, 115). Inhalation of 50% Ar inhibited liver regeneration after hepatic I/R or after partial hepatectomy in rats, the former may be related to upregulation of IL-1β and IL-6 in liver, and the latter may be related to the downregulation of hepatocyte growth factor (HGF) and IL-6 (116, 117). Breathing 70% Ar in a rabbit model of abdominal aorta occlusion for 30 min and reperfusion for 300 min also reduced the plasma concentrations of IL-6 and HMGB1, improved hepatic and renal injuries (118). The detail mechanisms of Ar-mediated IL-6 expression are not clear.
As that of Ar, Xe also has essential neuroprotective effects and it has been extensively investigated in the animal models of ischemia- and/or hypoxia-induced nervous system damage, such as, stroke, brain trauma, and hypoxic-ischemic injury in rat hippocampus (61, 96, 119–135). Glutamate mediates most excitatory neurotransmission in the mammalian central nervous system; normal activation of glutamate receptors mediates, in large measure, physiological excitatory synaptic transmission in the brain and is, therefore, crucial for the normal functioning of nervous system (136, 137). However, among three classical glutamate-gated ion channels, excessive activation of N-methyl-D-aspartate receptor (NMDA-R) leads to increasing intracellular calcium concentrations and the consequent production of damaging free radicals and activation of proteolytic processes that contribute to cell injury or death (136, 138). Xe has been identified to competitively inhibit the glycine site of NMDA-R, thus contributing to neuroprotective effects (128, 133, 139, 140) and it has carried out several clinical trials on brain–heart injury after cardiac arrest and achieved the positive results (141–143).
Xenon is a new type of gaseous anesthetic with minimal hemodynamic side effects, thus, it is an ideal anesthetic for patients with heart damage (80, 144), while it has been suggested that Xe should be used with caution in patients with known intracranial hypertension (145–148). Global administration of 50 or 70% Xe only significantly reduced left ventricular systolic pressure and the maximum rate of pressure increase (dP/dtmax), the regional myocardial function and coronary blood flow in left anterior descending coronary artery- and left circumflex coronary artery-dependent myocardium were not changed; regional administration of 50 or 70% Xe only to the left anterior descending-perfused myocardium had no influence in global hemodynamics, regional myocardial function, and coronary blood flow in the circumflex coronary artery-dependent myocardium, while 70% xenon, rather than 50% xenon, reduced systolic wall thickening by 7.2 ± 4.0% and mean velocity of systolic wall thickening by 8.2 ± 4.0% in the left anterior descending coronary artery-perfused area, resulting in a small but consistent negative inotropic effect on beagle dog heart in vivo (149). Forty or 80% Xe did not significantly alter NO-dependent flow response, the electrical, mechanical, or metabolic effects in isolated guinea pig hearts, possibly due to no alteration of major cation currents in cardiomyocytes by Xe (150). Moreover, breathing 70% Xe had only minimal negative inotropic effects on rabbits with left ventricular dysfunction after coronary artery ligation (151). Schroth et al. also showed that 65% Xe did not alter myocardial contractility and the response to inotropic stimuli such as calcium, isoproterenol, or increase in pacing frequency in isolated guinea pig ventricular muscle bundles (144). The biological mechanisms of cardiovascular stability and unchanged muscle sympathetic activity during Xe anesthesia have been revealed by the Peter Kienbaum group; they found that the increased concentrations of norepinephrine at the synaptic cleft and in plasma by Xe in an NMDA-R-dependent mechanism contributed to the hemodynamic stability of patients during Xe anesthesia (152).
However, Xe (0, 20, 50, and 65%), in addition to basic intravenous anesthesia, has been shown to elicit downregulation of heart rate and cardiac output with no change in mean arterial pressure, decrease portal venous blood flow with no change in hepatic arterial blood flow, and reduce total hepatic oxygen delivery and venous hepatic oxygen saturation, but did not impair intestinal oxygenation in pigs (153, 154). 73–78% Xe with additional supplementation of pentobarbital and buprenorphine increased oxygen contents of hepatic venous blood in pigs (155). These indicate that the basic intravenous anesthesia might influence the effects of Xe on cardiovascular activities and hepatic oxygen contents.
Under pathological conditions, 70% Xe inhalation in the early stage of reperfusion can reduce infarct size after myocardial ischemia in rabbits (81); combined application of 20% Xe and 34°C hypothermia in early reperfusion can also reduce myocardial infarction size in rats (82) (Table 1). The mechanisms of Xe in cardioprotection have been relatively clear. Xe first activates mitochondrial KATP channel and phosphatidylinositol-dependent kinase-1 (PDK-1); these two activates PKC-ε, PKC-ε activates p38 MAPK, subsequently, two downstream targets of p38 MAPK, MAPK-activated protein kinase-2 (MAPKAPK-2/MK-2) and HSP27, are phosphorylated, and then, induces the translocation of HSP27 to particulate fraction and increases F-actin polymerization (80, 83, 84). Besides p38 MAPK, ERK1/2, and COX-2 are essential mediators of Xe preconditioning (85, 86); Xe can also induce the phosphorylation of Akt and GSK-3β, inhibit Ca2+-induced opening of mPTP, and preserve mitochondrial function (87). Similar to Ar, Xe also acts as an inhibitor of NF-κB activation and prevents adhesion molecule expression in TNF-α-treated ECs in vitro (156). The saturation point of Xe in water without a cage vehicle for encapsulation of xenon was 0.22 mM; when the cage molecule 2-hydroxypropyl-β-cyclodextrin (HPCD) was added, Xe solubility increased from 0.22 to 0.67 mM; supplement of this Xe-enriched solutions by gavage improved hypertension and left ventricular hypertrophy and dysfunction in aged apolipoprotein E (ApoE)-knockout mice fed high-fat diet (HFD) for 6 weeks (157).
Both the Ar and Xe have been shown as renoprotectants in kidney transplantation (158, 159). In addition, 70% Xe has been reported to improve kidney function and renal histology and decrease neutrophil chemoattractants expression in kidney, thereby attenuating the glomerular neutrophil infiltration in an accelerated and severe lupus nephritis model in female NZB/W F1 mice (160). This protective effects of Xe on kidney was mediated by enhancing renal hypoxia inducible factor 1-α expression; decreasing serum levels of antidouble-stranded DNA autoantibody; and inhibiting ROS production, glomerular deposition of IgG and C3 and apoptosis, nucleotide-binding oligomerization domain (NOD)-like receptor family protein 3 (NLRP3) inflammasome and NF-κB activation, and intercellular cell adhesion molecule-1 (CD54 or ICAM-1) expression in kidney (160). The role of Xe and other noble gases on the activation or inhibition of other forms of inflammasomes still need further investigation.
The biological effects of Ne and Kr have been relatively few investigated in the past. Similar to He and Ar, Ne has also been shown to reduce the infarct area in rabbit model of myocardial I/R injury (73) (Table 1). Kr gas can promote the survival rate of Japanese quails embryos under acute hypoxia, Kr partial pressure of 5–5.5 kg/cm2 produces the narcotic effect on adult Japanese quails (161). However, in hypoxia/glucose deficiency injury model and in focal mechanical injury model of mouse hippocampal slices, only Ar and Xe showed the neuroprotective effects, while He, Ne, and Kr did not show neuroprotective effects (128, 133). Thus, the biological effects and mechanisms of Ne and Kr are worthy of further exploration.
Radon is an imperceptible natural occurring radioactive noble gas that exists in soil, water, and outdoor and indoor air; exposure to Rn accounts for more than 50% of the annual effective dose of natural radioactivity, it contributes as the largest single fraction to radiation exposure from natural sources (162, 163). Rn is a recognized pathogenic factor of human lung cancer, it is the second leading cause of lung cancer death after tobacco smoke (162). However, a certain dose of Rn has been reported for treating chronic musculoskeletal diseases, e.g., ankylosing spondylitis, osteoarthritis, or rheumatoid arthritis, these effects may be related to the regulation of oxidative stress and inflammation (163).
The noble gases are chemically inert because their outer electron orbitals are completely filled; however, they have been found to be very biologically active (159, 164). The noble gas family has emerged as the essential cellular or organic protectants such as in ECs, heart, brain, liver, kidney, and intestine; therefore, it protects against CCVDs (Figure 1).
Helium, Ar, and Xe displayed the neuroprotective effects on acute brain I/R injury models in vivo or in vitro. He, Ne, Ar, and Xe can reduce infarct size; Ar can improve the impaired left ventricular function in myocardial I/R injury animal models; however, the roles of other noble gases on left ventricular function under I/R or other pathological conditions still need further investigation (Table 1). It has been reported that oral administration of 6 weeks of Xe-enriched solution can be a promising nutraceutical strategy for cardiovascular protection (157). However, the effects of noble gases on chronic CCVDs and the side effects of long-time supplement of noble gases still need further investigation.
Besides competitively inhibiting NMDA-R by Xe in nervous system, modulation of cell death (mainly apoptosis), inflammatory or immune signals, oxidative damage, and gases interaction are the essential mechanisms of noble gases (Figure 1). The detail roles of noble gases on redox signaling, necrosis, autophagy, pyroptosis, and ferroptosis, which all play essential roles in CCVDs (165–167), and on other novel cell death types, such as alkaliptosis (168) and oxeiptosis (169), still need further investigation. The modulation of TLR4 signaling by He (89), NLRP3 inflammasome by Xe (160), and TLR2/4-mediated signaling by Ar (111) indicated that noble gases might act as essential modulators of innate immune signaling. Innate immune signaling is a complex cascade that quickly recognizes pathogen-associated molecular patterns (PAMPs) or damage-associated molecular patterns (DAMPs) through multiple germline-encoded cell surface or cytoplasmic pattern recognition receptors (PRRs), then, transmits signals through adaptors, kinases, and transcription factors, resulting in the production of cytokines (170–174). The mammalian host innate defense system utilizes more than 50 PRRs, which can be divided into two classes: the membrane-bound PRRs [including TLRs, C-type lectin receptors (CLRs), and receptors for advanced glycation end-products (RAGE)] and the cytosolic PRRs [including RIG-I-like receptors (RLRs), NOD-like receptors (NLRs), absent in melanoma 2 (AIM2)-like receptors (ALRs), and other nucleic acid-sensing receptors] (173, 174). Gasoimmunology, which investigates the effects of medical gases (such as NO, CO, H2S, SO2, H2, CH4, and noble gases) on innate immune signaling or on immune cells under both the homeostatic and perturbed conditions, will help us to open a novel door for medical gases investigation. Moreover, NO, CO, H2S, SO2, H2, and CH4 are essential endogenous gas molecules in modulating cardiocerebrovascular homeostasis (19–32). The cardioprotection of He is partially mediated by inducing NO production through eNOS in rabbits (78). It is not clear whether other noble gases can influence the levels and/or activities of these endogenous gases, if they can, what will happen to cardiocerebrovascular homeostasis and CCVDs?
It is not known the action forms of noble gases in vivo, by gases directly (in the alveolus where a gas phase exists) or dissolved non-electrolytes at very low concentration and with extremely weak interactions with other atoms/molecules. Therefore, as that of the small molecule signaling agents NO, CO, H2S, and their derived species, the physical or chemical interactions between noble elements and biological targets will be an important factor in their roles as signaling agents; thus, a fundamental understanding of the physics, chemistry, and biochemistry of noble gas atoms will be essential to understand their biological, physiological, or pathophysiological utility (175).
YZ: conceptualization. JZ and YZ: writing-original draft preparation. YZ, HY, WL, and JX: writing-review and editing. MB and YZ: visualization. YZ and HY: supervision. All authors have read and agreed to the published version of the manuscript.
This study was funded by the National Natural Science Foundation of China (Grant nos. 81900376 and 81673772), the Natural Science Foundation of Guangdong Province (Grant nos. 2017A030313738 and 2018A030313657), Project of administration of Traditional Chinese Medicine of Guangdong province (20221116), the key discipline construction project for traditional Chinese Medicine in Guangdong province, and the construction project of inheritance studio of national famous and old traditional Chinese Medicine experts in 2021.
The authors declare that the research was conducted in the absence of any commercial or financial relationships that could be construed as a potential conflict of interest.
All claims expressed in this article are solely those of the authors and do not necessarily represent those of their affiliated organizations, or those of the publisher, the editors and the reviewers. Any product that may be evaluated in this article, or claim that may be made by its manufacturer, is not guaranteed or endorsed by the publisher.
We apologize to all of the authors whose invaluable work we could not discuss or cite in this review due to space constraints.
1. Crea F, Camici PG, Bairey Merz CN. Coronary microvascular dysfunction: an update. Eur Heart J. (2014) 35:1101–11. doi: 10.1093/eurheartj/eht513
2. Khera AV, Kathiresan S. Genetics of coronary artery disease: discovery, biology and clinical translation. Nat Rev Genet. (2017) 18:331–44. doi: 10.1038/nrg.2016.160
3. Levy BI, Heusch G, Camici PG. The many faces of myocardial ischaemia and angina. Cardiovasc Res. (2019) 115:1460–70. doi: 10.1093/cvr/cvz160
5. Hill JA, Olson EN. Cardiac plasticity. N Engl J Med. (2008) 358:1370–80. doi: 10.1056/NEJMra072139
6. Nordon IM, Hinchliffe RJ, Loftus IM, Thompson MM. Pathophysiology and epidemiology of abdominal aortic aneurysms. Nat Rev Cardiol. (2011) 8:92–102. doi: 10.1038/nrcardio.2010.180
7. Simonneau G, Gatzoulis MA, Adatia I, Celermajer D, Denton C, Ghofrani A, et al. Updated clinical classification of pulmonary hypertension. J Am Coll Cardiol. (2013) 62:D34–41. doi: 10.1016/j.jacc.2013.10.029
8. Bastami M, Choupani J, Saadatian Z, Zununi Vahed S, Mansoori Y, Daraei A, et al. miRNA polymorphisms and risk of cardio-cerebrovascular diseases: a systematic review and meta-analysis. Int J Mol Sci. (2019) 20:293. doi: 10.3390/ijms20020293
9. Ritchie RH, Abel ED. Basic mechanisms of diabetic heart disease. Circ Res. (2020) 126:1501–25. doi: 10.1161/CIRCRESAHA.120.315913
10. Schiffrin EL. How structure, mechanics, and function of the vasculature contribute to blood pressure elevation in hypertension. Can J Cardiol. (2020) 36:648–58. doi: 10.1016/j.cjca.2020.02.003
11. Coffey S, Roberts-Thomson R, Brown A, Carapetis J, Chen M, Enriquez-Sarano M, et al. Global epidemiology of valvular heart disease. Nat Rev Cardiol. (2021) 18:853–64. doi: 10.1038/s41569-021-00570-z
12. Tschope C, Ammirati E, Bozkurt B, Caforio ALP, Cooper LT, Felix SB, et al. Myocarditis and inflammatory cardiomyopathy: current evidence and future directions. Nat Rev Cardiol. (2021) 18:169–93. doi: 10.1038/s41569-020-00435-x
13. Virani SS, Alonso A, Aparicio HJ, Benjamin EJ, Bittencourt MS, Callaway CW, et al. Heart disease and stroke statistics-2021 update: a report from the american heart association. Circulation. (2021) 143:e254–743. doi: 10.1161/CIR.0000000000000950
14. Jeong YW. Blood pressure awareness and knowledge of cardio-cerebrovascular diseases in south korean women with hypertension. Healthcare. (2021) 9:360. doi: 10.3390/healthcare9030360
15. World Health Organization. Cardiovascular Disease (CVDs). WHO (2020). Available online at: https://www.who.int/health-topics/cardiovascular-diseases#tab=tab_1 (accessed December 21, 2020).
16. Furchgott RF, Zawadzki JV. The obligatory role of endothelial cells in the relaxation of arterial smooth muscle by acetylcholine. Nature. (1980) 288:373–6. doi: 10.1038/288373a0
17. Furchgott RF. Endothelium-derived relaxing factor: discovery, early studies, and identification as nitric oxide. Biosci Rep. (1999) 19:235–51. doi: 10.1023/A:1020537506008
18. Farah C, Michel LYM, Balligand JL. Nitric oxide signalling in cardiovascular health and disease. Nat Rev Cardiol. (2018) 15:292–316. doi: 10.1038/nrcardio.2017.224
19. Otterbein LE, Zuckerbraun BS, Haga M, Liu F, Song R, Usheva A, et al. Carbon monoxide suppresses arteriosclerotic lesions associated with chronic graft rejection and with balloon injury. Nat Med. (2003) 9:183–90. doi: 10.1038/nm817
20. Ohsawa I, Ishikawa M, Takahashi K, Watanabe M, Nishimaki K, Yamagata K, et al. Hydrogen acts as a therapeutic antioxidant by selectively reducing cytotoxic oxygen radicals. Nat Med. (2007) 13:688–94. doi: 10.1038/nm1577
21. True AL, Olive M, Boehm M, San H, Westrick RJ, Raghavachari N, et al. Heme oxygenase-1 deficiency accelerates formation of arterial thrombosis through oxidative damage to the endothelium, which is rescued by inhaled carbon monoxide. Circ Res. (2007) 101:893–901. doi: 10.1161/CIRCRESAHA.107.158998
22. Du SX, Jin HF, Bu DF, Zhao X, Geng B, Tang CS, et al. Endogenously generated sulfur dioxide and its vasorelaxant effect in rats. Acta Pharmacol Sin. (2008) 29:923–30. doi: 10.1111/j.1745-7254.2008.00845.x
23. Ghyczy M, Torday C, Kaszaki J, Szabo A, Czobel M, Boros M. Hypoxia-induced generation of methane in mitochondria and eukaryotic cells: an alternative approach to methanogenesis. Cell Physiol Biochem. (2008) 21:251–8. doi: 10.1159/000113766
24. Yang G, Wu L, Jiang B, Yang W, Qi J, Cao K, et al. H2S as a physiologic vasorelaxant: hypertension in mice with deletion of cystathionine gamma-lyase. Science. (2008) 322:587–90. doi: 10.1126/science.1162667
25. Liu W, Wang D, Tao H, Sun X. Is methane a new therapeutic gas? Med Gas Res. (2012) 2:25. doi: 10.1186/2045-9912-2-25
26. Hine C, Harputlugil E, Zhang Y, Ruckenstuhl C, Lee BC, Brace L, et al. Endogenous hydrogen sulfide production is essential for dietary restriction benefits. Cell. (2015) 160:132–44. doi: 10.1016/j.cell.2014.11.048
27. Yetik-Anacak G, Sorrentino R, Linder AE, Murat N. Gas what: NO is not the only answer to sexual function. Br J Pharmacol. (2015) 172:1434–54. doi: 10.1111/bph.12700
28. Huang Y, Tang C, Du J, Jin H. Endogenous sulfur dioxide: a new member of gasotransmitter family in the cardiovascular system. Oxid Med Cell Longev. (2016) 2016:8961951. doi: 10.1155/2016/8961951
29. Otterbein LE, Foresti R, Motterlini R. Heme oxygenase-1 and carbon monoxide in the heart: the balancing act between danger signaling and pro-survival. Circ Res. (2016) 118:1940–59. doi: 10.1161/CIRCRESAHA.116.306588
30. Jia Y, Li Z, Liu C, Zhang J. Methane medicine: a rising star gas with powerful anti-inflammation, antioxidant, antiapoptosis properties. Oxid Med Cell Longev. (2018) 2018:1912746. doi: 10.1155/2018/1912746
31. Zhang Y, Tan S, Xu J, Wang T. Hydrogen therapy in cardiovascular and metabolic diseases: from bench to bedside. Cell Physiol Biochem. (2018) 47:1–10. doi: 10.1159/000489737
32. Zhang Y, Liu H, Xu J, Zheng S, Zhou L. Hydrogen gas: a novel type of antioxidant in modulating sexual organs homeostasis. Oxid Med Cell Longev. (2021) 2021:8844346. doi: 10.1155/2021/8844346
33. Hollig A, Schug A, Fahlenkamp AV, Rossaint R, Coburn M, Argon Organo-Protective N. Argon: systematic review on neuro- and organoprotective properties of an “inert” gas. Int J Mol Sci. (2014) 15:18175–96. doi: 10.3390/ijms151018175
34. Smit KF, Weber NC, Hollmann MW, Preckel B. Noble gases as cardioprotectants - translatability and mechanism. Br J Pharmacol. (2015) 172:2062–73. doi: 10.1111/bph.12994
35. Weber NC, Smit KF, Hollmann MW, Preckel B. Targets involved in cardioprotection by the non-anesthetic noble gas helium. Curr Drug Targets. (2015) 16:786–92. doi: 10.2174/1389450116666150120104459
36. Weber NC, Preckel B. Gaseous mediators: an updated review on the effects of helium beyond blowing up balloons. Intensive Care Med Exp. (2019) 7:73. doi: 10.1186/s40635-019-0288-4
37. Maze M, Laitio T. Neuroprotective properties of xenon. Mol Neurobiol. (2020) 57:118–24. doi: 10.1007/s12035-019-01761-z
38. Hollig A, Coburn M. Noble gases and neuroprotection: summary of current evidence. Curr Opin Anaesthesiol. (2021) 34:603–6. doi: 10.1097/ACO.0000000000001033
39. Cullen SC, Gross EG. The anesthetic properties of xenon in animals and human beings, with additional observations on krypton. Science. (1951) 113:580–2. doi: 10.1126/science.113.2942.580
40. Harris PD, Barnes R. The uses of helium and xenon in current clinical practice. Anaesthesia. (2008) 63:284–93. doi: 10.1111/j.1365-2044.2007.05253.x
41. Berganza CJ, Zhang JH. The role of helium gas in medicine. Med Gas Res. (2013) 3:18. doi: 10.1186/2045-9912-3-18
42. Fleming RY, Dougherty TB, Feig BW. The safety of helium for abdominal insufflation. Surg Endosc. (1997) 11:230–4. doi: 10.1007/s004649900332
43. Makarov DV, Kainth D, Link RE, Kavoussi LR. Physiologic changes during helium insufflation in high-risk patients during laparoscopic renal procedures. Urology. (2007) 70:35–7. doi: 10.1016/j.urology.2007.03.010
44. Carmona M, Lopes RI, Borba M, Omokawa M, Naufal R, Miyaji K, et al. Comparison of the effects of carbon dioxide and helium pneumoperitoneum on renal function. J Endourol. (2008) 22:1077–82. doi: 10.1089/end.2007.0369
45. Nie M, Kobayashi H, Sugawara M, Tomita T, Ohara K, Yoshimura H. Helium inhalation enhances vasodilator effect of inhaled nitric oxide on pulmonary vessels in hypoxic dogs. Am J Physiol Heart Circ Physiol. (2001) 280:H1875–81. doi: 10.1152/ajpheart.2001.280.4.H1875
46. Smit KF, Oei GT, Brevoord D, Stroes ES, Nieuwland R, Schlack WS, et al. Helium induces preconditioning in human endothelium in vivo. Anesthesiology. (2013) 118:95–104. doi: 10.1097/ALN.0b013e3182751300
47. Smit KF, Konkel M, Kerindongo R, Landau MA, Zuurbier CJ, Hollmann MW, et al. Helium alters the cytoskeleton and decreases permeability in endothelial cells cultured in vitro through a pathway involving caveolin-1. Sci Rep. (2018) 8:4768. doi: 10.1038/s41598-018-23030-0
48. Smit KF, Oei G, Konkel M, Augustijn QJJ, Hollmann MW, Preckel B, et al. Plasma from volunteers breathing helium reduces hypoxia-induced cell damage in human endothelial cells-mechanisms of remote protection against hypoxia by helium. Cardiovasc Drugs Ther. (2019) 33:297–306. doi: 10.1007/s10557-019-06880-2
49. Heinen A, Huhn R, Smeele KM, Zuurbier CJ, Schlack W, Preckel B, et al. Helium-induced preconditioning in young and old rat heart: impact of mitochondrial Ca(2+) -sensitive potassium channel activation. Anesthesiology. (2008) 109:830–6. doi: 10.1097/ALN.0b013e3181895aa0
50. Huhn R, Heinen A, Weber NC, Hieber S, Hollmann MW, Schlack W, et al. Helium-induced late preconditioning in the rat heart in vivo. Br J Anaesth. (2009) 102:614–9. doi: 10.1093/bja/aep042
51. Huhn R, Heinen A, Weber NC, Kerindongo RP, Oei GT, Hollmann MW, et al. Helium-induced early preconditioning and postconditioning are abolished in obese zucker rats in vivo. J Pharmacol Exp Ther. (2009) 329:600–7. doi: 10.1124/jpet.108.149971
52. Oei GT, Weber NC, Hollmann MW, Preckel B. Cellular effects of helium in different organs. Anesthesiology. (2010) 112:1503–10. doi: 10.1097/ALN.0b013e3181d9cb5e
53. Huhn R, Weber NC, Preckel B, Schlack W, Bauer I, Hollmann MW, et al. Age-related loss of cardiac preconditioning: impact of protein kinase A. Exp Gerontol. (2012) 47:116–21. doi: 10.1016/j.exger.2011.11.003
54. Oei GT, Huhn R, Heinen A, Hollmann MW, Schlack WS, Preckel B, et al. Helium-induced cardioprotection of healthy and hypertensive rat myocardium in vivo. Eur J Pharmacol. (2012) 684:125–31. doi: 10.1016/j.ejphar.2012.03.045
55. Oei GT, Aslami H, Kerindongo RP, Steenstra RJ, Beurskens CJ, Tuip-de Boer AM, et al. Prolonged helium postconditioning protocols during early reperfusion do not induce cardioprotection in the rat heart in vivo: role of inflammatory cytokines. J Immunol Res. (2015) 2015:216798. doi: 10.1155/2015/216798
56. Oei GT, Heger M, van Golen RF, Alles LK, Flick M, van der Wal AC, et al. Reduction of cardiac cell death after helium postconditioning in rats: transcriptional analysis of cell death and survival pathways. Mol Med. (2015) 20:516–26. doi: 10.2119/molmed.2014.00057
57. Flick M, Albrecht M, Oei G, Steenstra R, Kerindongo RP, Zuurbier CJ, et al. Helium postconditioning regulates expression of caveolin-1 and−3 and induces RISK pathway activation after ischaemia/reperfusion in cardiac tissue of rats. Eur J Pharmacol. (2016) 791:718–25. doi: 10.1016/j.ejphar.2016.10.012
58. Smit KF, Brevoord D, De Hert S, de Mol BA, Kerindongo RP, van Dieren S, et al. Effect of helium pre- or postconditioning on signal transduction kinases in patients undergoing coronary artery bypass graft surgery. J Transl Med. (2016) 14:294. doi: 10.1186/s12967-016-1045-z
59. Aehling C, Weber NC, Zuurbier CJ, Preckel B, Galmbacher R, Stefan K, et al. Effects of combined helium pre/post-conditioning on the brain and heart in a rat resuscitation model. Acta Anaesthesiol Scand. (2018) 62:63–74. doi: 10.1111/aas.13041
60. Weber NC, Schilling JM, Warmbrunn MV, Dhanani M, Kerindongo R, Siamwala J, et al. Helium-Induced changes in circulating caveolin in mice suggest a novel mechanism of cardiac protection. Int J Mol Sci. (2019) 20:2640. doi: 10.3390/ijms20112640
61. Coburn M, Maze M, Franks NP. The neuroprotective effects of xenon and helium in an in vitro model of traumatic brain injury. Crit Care Med. (2008) 36:588–95. doi: 10.1097/01.CCM.0B013E3181611F8A6
62. David HN, Haelewyn B, Chazalviel L, Lecocq M, Degoulet M, Risso JJ, et al. Post-ischemic helium provides neuroprotection in rats subjected to middle cerebral artery occlusion-induced ischemia by producing hypothermia. J Cereb Blood Flow Metab. (2009) 29:1159–65. doi: 10.1038/jcbfm.2009.40
63. Liu Y, Xue F, Liu G, Shi X, Liu Y, Liu W, et al. Helium preconditioning attenuates hypoxia/ischemia-induced injury in the developing brain. Brain Res. (2011) 1376:122–9. doi: 10.1016/j.brainres.2010.12.068
64. Haelewyn B, David HN, Blatteau JE, Vallee N, Meckler C, Risso JJ, et al. Modulation by the noble gas helium of tissue plasminogen activator: effects in a rat model of thromboembolic stroke. Crit Care Med. (2016) 44:e383–9. doi: 10.1097/CCM.0000000000001424
65. Li Y, Liu K, Kang ZM, Sun XJ, Liu WW, Mao YF. Helium preconditioning protects against neonatal hypoxia-ischemia via nitric oxide mediated up-regulation of antioxidases in a rat model. Behav Brain Res. (2016) 300:31–7. doi: 10.1016/j.bbr.2015.12.001
66. Li Y, Zhang P, Liu Y, Liu W, Yin N. Helium preconditioning protects the brain against hypoxia/ischemia injury via improving the neurovascular niche in a neonatal rat model. Behav Brain Res. (2016) 314:165–72. doi: 10.1016/j.bbr.2016.08.015
67. Deng RM, Li HY, Li X, Shen HT, Wu DG, Wang Z, et al. Neuroprotective effect of helium after neonatal hypoxic ischemia: a narrative review. Med Gas Res. (2021) 11:121–3. doi: 10.4103/2045-9912.314332
68. Zhang R, Zhang L, Manaenko A, Ye Z, Liu W, Sun X. Helium preconditioning protects mouse liver against ischemia and reperfusion injury through the PI3K/Akt pathway. J Hepatol. (2014) 61:1048–55. doi: 10.1016/j.jhep.2014.06.020
69. Du L, Zhang R, Luo T, Nie M, Bi J. Effects of helium preconditioning on intestinal ischemia and reperfusion injury in rats. Shock. (2015) 44:365–70. doi: 10.1097/SHK.0000000000000418
70. Smit KF, Kerindongo RP, Böing A, Nieuwland R, Hollmann MW, Preckel B, et al. Effects of helium on inflammatory and oxidative stress-induced endothelial cell damage. Exp Cell Res. (2015) 337:37–43. doi: 10.1016/j.yexcr.2015.06.004
71. Lucchinetti E, Wacker J, Maurer C, Keel M, Harter L, Zaugg K, et al. Helium breathing provides modest antiinflammatory, but no endothelial protection against ischemia-reperfusion injury in humans in vivo. Anesth Analg. (2009) 109:101–8. doi: 10.1213/ane.0b013e3181a27e4b
72. Tretjak M, Gorjup V, Mozina H, Horvat M, Noc M. Cerebral and coronary gas embolism from the inhalation of pressurized helium. Crit Care Med. (2002) 30:1156–7. doi: 10.1097/00003246-200205000-00034
73. Pagel PS, Krolikowski JG, Shim YH, Venkatapuram S, Kersten JR, Weihrauch D, et al. Noble gases without anesthetic properties protect myocardium against infarction by activating prosurvival signaling kinases and inhibiting mitochondrial permeability transition in vivo. Anesth Analg. (2007) 105:562–9. doi: 10.1213/01.ane.0000278083.31991.36
74. Pagel PS, Krolikowski JG, Pratt PF Jr, Shim YH, Amour J, Warltier DC, et al. Reactive oxygen species and mitochondrial adenosine triphosphate-regulated potassium channels mediate helium-induced preconditioning against myocardial infarction in vivo. J Cardiothorac Vasc Anesth. (2008) 22:554–9. doi: 10.1053/j.jvca.2008.04.005
75. Pagel PS, Krolikowski JG. Transient metabolic alkalosis during early reperfusion abolishes helium preconditioning against myocardial infarction: restoration of cardioprotection by cyclosporin A in rabbits. Anesth Analg. (2009) 108:1076–82. doi: 10.1213/ane.0b013e318193e934
76. Pagel PS, Krolikowski JG, Amour J, Warltier DC, Weihrauch D. Morphine reduces the threshold of helium preconditioning against myocardial infarction: the role of opioid receptors in rabbits. J Cardiothorac Vasc Anesth. (2009) 23:619–24. doi: 10.1053/j.jvca.2008.12.020
77. Pagel PS, Krolikowski JG, Pratt P. F. Jr., Shim YH, Amour J, et al. Inhibition of glycogen synthase kinase or the apoptotic protein p53 lowers the threshold of helium cardioprotection in vivo: the role of mitochondrial permeability transition. Anesth Analg. (2008) 107:769–75. doi: 10.1213/ane.0b013e3181815b84
78. Pagel PS, Krolikowski JG, Pratt PF Jr, Shim YH, Amour J, Warltier DC, et al. The mechanism of helium-induced preconditioning: a direct role for nitric oxide in rabbits. Anesth Analg. (2008) 107:762–8. doi: 10.1213/ane.0b013e3181815995
79. Lemoine S, Blanchart K, Souplis M, Lemaitre A, Legallois D, Coulbault L, et al. Argon exposure induces postconditioning in myocardial ischemia-reperfusion. J Cardiovasc Pharmacol Ther. (2017) 22:564–73. doi: 10.1177/1074248417702891
80. Weber NC, Toma O, Wolter JI, Obal D, Mullenheim J, Preckel B, et al. The noble gas xenon induces pharmacological preconditioning in the rat heart in vivo via induction of PKC-epsilon and p38 MAPK. Br J Pharmacol. (2005) 144:123–32. doi: 10.1038/sj.bjp.0706063
81. Preckel B, Müllenheim J, Moloschavij A, Thämer V, Schlack W. Xenon administration during early reperfusion reduces infarct size after regional ischemia in the rabbit heart in vivo. Anesth Analg. (2000) 91:1327–32. doi: 10.1097/00000539-200012000-00003
82. Schwiebert C, Huhn R, Heinen A, Weber NC, Hollmann MW, Schlack W, et al. Postconditioning by xenon and hypothermia in the rat heart in vivo. Eur J Anaesthesiol. (2010) 27:734–9. doi: 10.1097/EJA.0b013e328335fc4c
83. Weber NC, Toma O, Wolter JI, Wirthle NM, Schlack W, Preckel B. Mechanisms of xenon- and isoflurane-induced preconditioning - a potential link to the cytoskeleton via the MAPKAPK-2/HSP27 pathway. Br J Pharmacol. (2005) 146:445–55. doi: 10.1038/sj.bjp.0706324
84. Weber NC, Toma O, Damla H, Wolter JI, Schlack W, Preckel B. Upstream signaling of protein kinase C-epsilon in xenon-induced pharmacological preconditioning. Implication of mitochondrial adenosine triphosphate dependent potassium channels and phosphatidylinositol-dependent kinase-1. Eur J Pharmacol. (2006) 539:1–9. doi: 10.1016/j.ejphar.2006.03.054
85. Weber NC, Stursberg J, Wirthle NM, Toma O, Schlack W, Preckel B. Xenon preconditioning differently regulates p44/42 MAPK (ERK 1/2) and p46/54 MAPK (JNK 1/2 and 3) in vivo. Br J Anaesth. (2006) 97:298–306. doi: 10.1093/bja/ael153
86. Weber NC, Frassdorf J, Ratajczak C, Grueber Y, Schlack W, Hollmann MW, et al. Xenon induces late cardiac preconditioning in vivo: a role for cyclooxygenase 2? Anesth Analg. (2008) 107:1807–13. doi: 10.1213/ane.Ob013e31818874bf
87. Mio Y, Shim YH, Richards E, Bosnjak ZJ, Pagel PS, Bienengraeber M. Xenon preconditioning: the role of prosurvival signaling, mitochondrial permeability transition and bioenergetics in rats. Anesth Analg. (2009) 108:858–66. doi: 10.1213/ane.0b013e318192a520
88. Jelemensky M, Kovacshazi C, Ferenczyova K, Hofbauerova M, Kiss B, Pallinger E, et al. Helium conditioning increases cardiac fibroblast migration which effect is not propagated via soluble factors or extracellular vesicles. Int J Mol Sci. (2021) 22:10504. doi: 10.3390/ijms221910504
89. Zhang Y, Zhang J, Xu K, Chen Z, Xu X, Xu J, et al. Helium protects against lipopolysaccharide-induced cardiac dysfunction in mice via suppressing toll-like receptor 4-nuclear factor kappaB-Tumor necrosis factor-alpha/ interleukin-18 signaling. Chin J Physiol. (2020) 63:276–85. doi: 10.4103/CJP.CJP_66_20
90. Zhang R, Yu Y, Manaenko A, Bi H, Zhang N, Zhang L, et al. Effect of helium preconditioning on neurological decompression sickness in rats. J Appl Physiol. (2019) 126:934–40. doi: 10.1152/japplphysiol.00275.2018
91. Pan Y, Zhang H, VanDeripe DR, Cruz-Flores S, Panneton WM. Heliox and oxygen reduce infarct volume in a rat model of focal ischemia. Exp Neurol. (2007) 205:587–90. doi: 10.1016/j.expneurol.2007.03.023
92. Fukuda K, Asoh S, Ishikawa M, Yamamoto Y, Ohsawa I, Ohta S. Inhalation of hydrogen gas suppresses hepatic injury caused by ischemia/reperfusion through reducing oxidative stress. Biochem Biophys Res Commun. (2007) 361:670–4. doi: 10.1016/j.bbrc.2007.07.088
93. Braun S, Plitzko G, Bicknell L, van Caster P, Schulz J, Barthuber C, et al. Pretreatment with helium does not attenuate liver injury after warm ischemia-reperfusion. Shock. (2014) 41:413–9. doi: 10.1097/SHK.0000000000000125
94. Ito M, Hirayama M, Yamai K, Goto S, Ito M, Ichihara M, et al. Drinking hydrogen water and intermittent hydrogen gas exposure, but not lactulose or continuous hydrogen gas exposure, prevent 6-hydorxydopamine-induced Parkinson's disease in rats. Med Gas Res. (2012) 2:15. doi: 10.1186/2045-9912-2-15
95. Hale SL, Vanderipe DR, Kloner RA. Continuous heliox breathing and the extent of anatomic zone of noreflow and necrosis following ischemia/reperfusion in the rabbit heart. Open Cardiovasc Med J. (2013) 8:1–5. doi: 10.2174/1874192401408010001
96. Zhuang L, Yang T, Zhao H, Fidalgo AR, Vizcaychipi MP, Sanders RD, et al. The protective profile of argon, helium, and xenon in a model of neonatal asphyxia in rats. Crit Care Med. (2012) 40:1724–30. doi: 10.1097/CCM.0b013e3182452164
97. Loetscher PD, Rossaint J, Rossaint R, Weis J, Fries M, Fahlenkamp A, et al. Argon: neuroprotection in in vitro models of cerebral ischemia and traumatic brain injury. Crit Care. (2009) 13:R206. doi: 10.1186/cc8214
98. Ryang YM, Fahlenkamp AV, Rossaint R, Wesp D, Loetscher PD, Beyer C, et al. Neuroprotective effects of argon in an in vivo model of transient middle cerebral artery occlusion in rats. Crit Care Med. (2011) 39:1448–53. doi: 10.1097/CCM.0b013e31821209be
99. David HN, Haelewyn B, Degoulet M, Colomb D. G. Jr., Risso JJ, et al. Ex vivo and in vivo neuroprotection induced by argon when given after an excitotoxic or ischemic insult. PLoS ONE. (2012) 7:e30934. doi: 10.1371/journal.pone.0030934
100. Brucken A, Cizen A, Fera C, Meinhardt A, Weis J, Nolte K, et al. Argon reduces neurohistopathological damage and preserves functional recovery after cardiac arrest in rats. Br J Anaesth. (2013) 110 (Suppl. 1):i106–112. doi: 10.1093/bja/aes509
101. Brucken A, Kurnaz P, Bleilevens C, Derwall M, Weis J, Nolte K, et al. Dose dependent neuroprotection of the noble gas argon after cardiac arrest in rats is not mediated by K(ATP)-channel opening. Resuscitation. (2014) 85:826–32. doi: 10.1016/j.resuscitation.2014.02.014
102. Ristagno G, Fumagalli F, Russo I, Tantillo S, Zani DD, Locatelli V, et al. Postresuscitation treatment with argon improves early neurological recovery in a porcine model of cardiac arrest. Shock. (2014) 41:72–8. doi: 10.1097/SHK.0000000000000049
103. Brucken A, Kurnaz P, Bleilevens C, Derwall M, Weis J, Nolte K, et al. Delayed argon administration provides robust protection against cardiac arrest-induced neurological damage. Neurocrit Care. (2015) 22:112–20. doi: 10.1007/s12028-014-0029-1
104. Brucken A, Bleilevens C, Fohr P, Nolte K, Rossaint R, Marx G, et al. Influence of argon on temperature modulation and neurological outcome in hypothermia treated rats following cardiac arrest. Resuscitation. (2017) 117:32–9. doi: 10.1016/j.resuscitation.2017.05.029
105. Grusser L, Blaumeiser-Debarry R, Krings M, Kremer B, Hollig A, Rossaint R, et al. Argon attenuates the emergence of secondary injury after traumatic brain injury within a 2-hour incubation period compared to desflurane: an in vitro study. Med Gas Res. (2017) 7:93–100. doi: 10.4103/2045-9912.208512
106. Kiss A, Shu H, Hamza O, Santer D, Tretter EV, Yao S, et al. Argon preconditioning enhances postischaemic cardiac functional recovery following cardioplegic arrest and global cold ischaemia. Eur J Cardiothorac Surg. (2018) 54:539–46. doi: 10.1093/ejcts/ezy104
107. Liu J, Nolte K, Brook G, Liebenstund L, Weinandy A, Hollig A, et al. Post-stroke treatment with argon attenuated brain injury, reduced brain inflammation and enhanced M2 microglia/macrophage polarization: a randomized controlled animal study. Crit Care. (2019) 23:198. doi: 10.1186/s13054-019-2493-7
108. Ma S, Chu D, Li L, Creed JA, Ryang YM, Sheng H, et al. Argon inhalation for 24 hours after onset of permanent focal cerebral ischemia in rats provides neuroprotection and improves neurologic outcome. Crit Care Med. (2019) 47:e693–9. doi: 10.1097/CCM.0000000000003809
109. Fumagalli F, Olivari D, Boccardo A, De Giorgio D, Affatato R, Ceriani S, et al. Ventilation with argon improves survival with good neurological recovery after prolonged untreated cardiac arrest in pigs. J Am Heart Assoc. (2020) 9:e016494. doi: 10.1161/JAHA.120.016494
110. Moro F, Fossi F, Magliocca A, Pascente R, Sammali E, Baldini F, et al. Efficacy of acute administration of inhaled argon on traumatic brain injury in mice. Br J Anaesth. (2021) 126:256–64. doi: 10.1016/j.bja.2020.08.027
111. Ulbrich F, Lerach T, Biermann J, Kaufmann KB, Lagreze WA, Buerkle H, et al. Argon mediates protection by interleukin-8 suppression via a TLR2/TLR4/STAT3/NF-kappaB pathway in a model of apoptosis in neuroblastoma cells in vitro and following ischemia-reperfusion injury in rat retina in vivo. J Neurochem. (2016) 138:859–73. doi: 10.1111/jnc.13662
112. Mayer B, Soppert J, Kraemer S, Schemmel S, Beckers C, Bleilevens C, et al. Argon induces protective effects in cardiomyocytes during the second window of preconditioning. Int J Mol Sci. (2016) 17:1159. doi: 10.3390/ijms17071159
113. Qi H, Soto-Gonzalez L, Krychtiuk KA, Ruhittel S, Kaun C, Speidl WS, et al. Pretreatment with argon protects human cardiac myocyte-like progenitor cells from oxygen glucose deprivation-induced cell death by activation of akt and differential regulation of mapkinases. Shock. (2018) 49:556–63. doi: 10.1097/SHK.0000000000000998
114. Wustefeld T, Rakemann T, Kubicka S, Manns MP, Trautwein C. Hyperstimulation with interleukin 6 inhibits cell cycle progression after hepatectomy in mice. Hepatology. (2000) 32:514–22. doi: 10.1053/jhep.2000.16604
115. Schmidt-Arras D, Rose-John S. IL-6 pathway in the liver: from physiopathology to therapy. J Hepatol. (2016) 64:1403–15. doi: 10.1016/j.jhep.2016.02.004
116. Ulmer TF, Fragoulis A, Dohmeier H, Kroh A, Andert A, Stoppe C, et al. Argon delays initiation of liver regeneration after partial hepatectomy in rats. Eur Surg Res. (2017) 58:204–15. doi: 10.1159/000466690
117. Schmitz SM, Dohmeier H, Stoppe C, Alizai PH, Schipper S, Neumann UP, et al. Inhaled argon impedes hepatic regeneration after ischemia/reperfusion injury in rats. Int J Mol Sci. (2020) 21:5457. doi: 10.3390/ijms21155457
118. de Roux Q, Lidouren F, Kudela A, Slassi L, Kohlhauer M, Boissady E, et al. Argon attenuates multiorgan failure in relation with HMGB1 inhibition. Int J Mol Sci. (2021) 22:3257. doi: 10.3390/ijms22063257
119. Homi HM, Yokoo N, Ma D, Warner DS, Franks NP, Maze M, et al. The neuroprotective effect of xenon administration during transient middle cerebral artery occlusion in mice. Anesthesiology. (2003) 99:876–81. doi: 10.1097/00000542-200310000-00020
120. Schmidt M, Marx T, Gloggl E, Reinelt H, Schirmer U. Xenon attenuates cerebral damage after ischemia in pigs. Anesthesiology. (2005) 102:929–36. doi: 10.1097/00000542-200505000-00011
121. Martin JL, Ma D, Hossain M, Xu J, Sanders RD, Franks NP, et al. Asynchronous administration of xenon and hypothermia significantly reduces brain infarction in the neonatal rat. Br J Anaesth. (2007) 98:236–40. doi: 10.1093/bja/ael340
122. Derwall M, Timper A, Kottmann K, Rossaint R, Fries M. Neuroprotective effects of the inhalational anesthetics isoflurane and xenon after cardiac arrest in pigs. Crit Care Med. (2008) 36:S492–5. doi: 10.1097/CCM.0b013e31818a904a
123. Fries M, Nolte KW, Coburn M, Rex S, Timper A, Kottmann K, et al. Xenon reduces neurohistopathological damage and improves the early neurological deficit after cardiac arrest in pigs. Crit Care Med. (2008) 36:2420–6. doi: 10.1097/CCM.0b013e3181802874
124. Hobbs C, Thoresen M, Tucker A, Aquilina K, Chakkarapani E, Dingley J. Xenon and hypothermia combine additively, offering long-term functional and histopathologic neuroprotection after neonatal hypoxia/ischemia. Stroke. (2008) 39:1307–13. doi: 10.1161/STROKEAHA.107.499822
125. Limatola V, Ward P, Cattano D, Gu J, Giunta F, Maze M, et al. Xenon preconditioning confers neuroprotection regardless of gender in a mouse model of transient middle cerebral artery occlusion. Neuroscience. (2010) 165:874–81. doi: 10.1016/j.neuroscience.2009.10.063
126. Fries M, Brucken A, Cizen A, Westerkamp M, Lower C, Deike-Glindemann J, et al. Combining xenon and mild therapeutic hypothermia preserves neurological function after prolonged cardiac arrest in pigs. Crit Care Med. (2012) 40:1297–303. doi: 10.1097/CCM.0b013e31823c8ce7
127. Sheng SP, Lei B, James ML, Lascola CD, Venkatraman TN, Jung JY, et al. Xenon neuroprotection in experimental stroke: interactions with hypothermia and intracerebral hemorrhage. Anesthesiology. (2012) 117:1262–75. doi: 10.1097/ALN.0b013e3182746b81
128. Harris K, Armstrong SP, Campos-Pires R, Kiru L, Franks NP, Dickinson R. Neuroprotection against traumatic brain injury by xenon, but not argon, is mediated by inhibition at the N-methyl-D-aspartate receptor glycine site. Anesthesiology. (2013) 119:1137–48. doi: 10.1097/ALN.0b013e3182a2a265
129. Campos-Pires R, Armstrong SP, Sebastiani A, Luh C, Gruss M, Radyushkin K, et al. Xenon improves neurologic outcome and reduces secondary injury following trauma in an in vivo model of traumatic brain injury. Crit Care Med. (2015) 43:149–58. doi: 10.1097/CCM.0000000000000624
130. Campos-Pires R, Koziakova M, Yonis A, Pau A, Macdonald W, Harris K, et al. Xenon protects against blast-induced traumatic brain injury in an in vitro model. J Neurotrauma. (2018) 35:1037–44. doi: 10.1089/neu.2017.5360
131. Zhao CS, Li H, Wang Z, Chen G. Potential application value of xenon in stroke treatment. Med Gas Res. (2018) 8:116–20. doi: 10.4103/2045-9912.241077
132. Campos-Pires R, Hirnet T, Valeo F, Ong BE, Radyushkin K, Aldhoun J, et al. Xenon improves long-term cognitive function, reduces neuronal loss and chronic neuroinflammation, and improves survival after traumatic brain injury in mice. Br J Anaesth. (2019) 123:60–73. doi: 10.1016/j.bja.2019.02.032
133. Koziakova M, Harris K, Edge CJ, Franks NP, White IL, Dickinson R. Noble gas neuroprotection: xenon and argon protect against hypoxic-ischaemic injury in rat hippocampus in vitro via distinct mechanisms. Br J Anaesth. (2019) 123:601–9. doi: 10.1016/j.bja.2019.07.010
134. Terrando N, Warner DS. Xenon for traumatic brain injury: a noble step forward and a wet blanket. Br J Anaesth. (2019) 123:9–11. doi: 10.1016/j.bja.2019.04.004
135. Campos-Pires R, Onggradito H, Ujvari E, Karimi S, Valeo F, Aldhoun J, et al. Xenon treatment after severe traumatic brain injury improves locomotor outcome, reduces acute neuronal loss and enhances early beneficial neuroinflammation: a randomized, blinded, controlled animal study. Crit Care. (2020) 24:667. doi: 10.1186/s13054-020-03373-9
136. Lipton SA. Paradigm shift in neuroprotection by NMDA receptor blockade: memantine and beyond. Nat Rev Drug Discov. (2006) 5:160–70. doi: 10.1038/nrd1958
137. Hansen KB, Wollmuth LP, Bowie D, Furukawa H, Menniti FS, Sobolevsky AI, et al. Structure, function, and pharmacology of glutamate receptor ion channels. Pharmacol Rev. (2021) 73:298–487. doi: 10.1124/pharmrev.120.000131
138. Lau A, Tymianski M. Glutamate receptors, neurotoxicity and neurodegeneration. Pflugers Arch. (2010) 460:525–42. doi: 10.1007/s00424-010-0809-1
139. Dickinson R, Peterson BK, Banks P, Simillis C, Martin JC, Valenzuela CA, et al. Competitive inhibition at the glycine site of the N-methyl-D-aspartate receptor by the anesthetics xenon and isoflurane: evidence from molecular modeling and electrophysiology. Anesthesiology. (2007) 107:756–67. doi: 10.1097/01.anes.0000287061.77674.71
140. Banks P, Franks NP, Dickinson R. Competitive inhibition at the glycine site of the N-methyl-D-aspartate receptor mediates xenon neuroprotection against hypoxia-ischemia. Anesthesiology. (2010) 112:614–22. doi: 10.1097/ALN.0b013e3181cea398
141. Arola OJ, Laitio RM, Roine RO, Gronlund J, Saraste A, Pietila M, et al. Feasibility and cardiac safety of inhaled xenon in combination with therapeutic hypothermia following out-of-hospital cardiac arrest. Crit Care Med. (2013) 41:2116–24. doi: 10.1097/CCM.0b013e31828a4337
142. Laitio R, Hynninen M, Arola O, Virtanen S, Parkkola R, Saunavaara J, et al. Effect of inhaled xenon on cerebral white matter damage in comatose survivors of out-of-hospital cardiac arrest: a randomized clinical trial. JAMA. (2016) 315:1120–8. doi: 10.1001/jama.2016.1933
143. Arola O, Saraste A, Laitio R, Airaksinen J, Hynninen M, Backlund M, et al. Inhaled xenon attenuates myocardial damage in comatose survivors of out-of-hospital cardiac arrest: the xe-hypotheca trial. J Am Coll Cardiol. (2017) 70:2652–60. doi: 10.1016/j.jacc.2017.09.1088
144. Schroth SC, Schotten U, Alkanoglu O, Reyle-Hahn MS, Hanrath P, Rossaint R. Xenon does not impair the responsiveness of cardiac muscle bundles to positive inotropic and chronotropic stimulation. Anesthesiology. (2002) 96:422–7. doi: 10.1097/00000542-200202000-00030
145. Schmidt M, Marx T, Kotzerke J, Luderwald S, Armbruster S, Topalidis P, et al. Cerebral and regional organ perfusion in pigs during xenon anaesthesia. Anaesthesia. (2001) 56:1154–9. doi: 10.1046/j.1365-2044.2001.02322.x
146. Rylova AV, Lubnin A. [Intracranial pressure changes during xenon anesthesia in neurosurgical patients without intracranial hypertention]. Anesteziol Reanimatol. (2011) 13–17.
147. Rylova AV, Beliaev A, Lubnin A. [Effects of xenon anesthesia on cerebral blood flow in neurosurgical patients without intracranial hypertension]. Anesteziol Reanimatol. (2013) 4–9.
148. Rylova AV, Gavrilov AG, Lubnin A, Potapov AA. [Intracranial and cerebral perfusion pressure in neurosurgical patients during anaesthesia with xenon]. Anesteziol Reanimatol. (2014) 59:19–25.
149. Preckel B, Ebel D, Müllenheim J, Frässdorf J, Thämer V, Schlack W. The direct myocardial effects of xenon in the dog heart in vivo. Anesth Analg. (2002) 94:545–51. doi: 10.1097/00000539-200203000-00012
150. Stowe DF, Rehmert GC, Kwok WM, Weigt HU, Georgieff M, Bosnjak ZJ. Xenon does not alter cardiac function or major cation currents in isolated guinea pig hearts or myocytes. Anesthesiology. (2000) 92:516–22. doi: 10.1097/00000542-200002000-00035
151. Preckel B, Schlack W, Heibel T, Rütten H. Xenon produces minimal haemodynamic effects in rabbits with chronically compromised left ventricular function. Br J Anaesth. (2002) 88:264–9. doi: 10.1093/bja/88.2.264
152. Neukirchen M, Hipp J, Schaefer MS, Brandenburger T, Bauer I, Winterhalter M, et al. Cardiovascular stability and unchanged muscle sympathetic activity during xenon anaesthesia: role of norepinephrine uptake inhibition. Br J Anaesth. (2012) 109:887–96. doi: 10.1093/bja/aes303
153. Vagts DA, Hecker K, Iber T, Roesner JP, Spee A, Otto B, et al. Effects of xenon anaesthesia on intestinal oxygenation in acutely instrumented pigs. Br J Anaesth. (2004) 93:833–41. doi: 10.1093/bja/aeh271
154. Iber T, Hecker K, Vagts DA, Roesner JP, Otto B, Steinicke A, et al. Xenon anesthesia impairs hepatic oxygenation and perfusion in healthy pigs. Minerva Anestesiol. (2008) 74:511–9.
155. Reinelt H, Marx T, Kotzerke J, Topalidis P, Luederwald S, Armbruster S, et al. Hepatic function during xenon anesthesia in pigs. Acta Anaesthesiol Scand. (2002) 46:713–6. doi: 10.1034/j.1399-6576.2002.460614.x
156. Weber NC, Kandler J, Schlack W, Grueber Y, Fradorf J, Preckel B. Intermitted pharmacologic pretreatment by xenon, isoflurane, nitrous oxide, and the opioid morphine prevents tumor necrosis factor alpha-induced adhesion molecule expression in human umbilical vein endothelial cells. Anesthesiology. (2008) 108:199–207. doi: 10.1097/01.anes.0000299441.32091.ed
157. Yin X, Moody MR, Hebert V, Klegerman ME, Geng YJ, Dugas TR, et al. Oral delivery of xenon for cardiovascular protection. Sci Rep. (2019) 9:14035. doi: 10.1038/s41598-019-50515-3
158. Faure A, Bruzzese L, Steinberg JG, Jammes Y, Torrents J, Berdah SV, et al. Effectiveness of pure argon for renal transplant preservation in a preclinical pig model of heterotopic autotransplantation. J Transl Med. (2016) 14:40. doi: 10.1186/s12967-016-0795-y
159. Zhao H, Rossaint R, Coburn M, Ma D, Argon Organo-Protective N. The renoprotective properties of xenon and argon in kidney transplantation. Eur J Anaesthesiol. (2017) 34:637–40. doi: 10.1097/EJA.0000000000000632
160. Yang SR, Hua KF, Chu LJ, Hwu YK, Yang SM, Wu CY, et al. Xenon blunts NF-kappaB/NLRP3 inflammasome activation and improves acute onset of accelerated and severe lupus nephritis in mice. Kidney Int. (2020) 98:378–90. doi: 10.1016/j.kint.2020.02.033
161. Kussmaul AR, Gur'eva TS, Dadasheva OA, Pavlov NB, Pavlov BN. [Effect of krypton-containing gas mixture on Japanese quail embryo development]. Aviakosm Ekolog Med. (2008) 42:41–4.
162. Al-Zoughool M, Krewski D. Health effects of radon: a review of the literature. Int J Radiat Biol. (2009) 85:57–69. doi: 10.1080/09553000802635054
163. Maier A, Wiedemann J, Rapp F, Papenfuss F, Rodel F, Hehlgans S, et al. Radon exposure-therapeutic effect and cancer risk. Int J Mol Sci. (2020) 22:316. doi: 10.3390/ijms22010316
164. Sanders RD, Ma D, Maze M. Xenon: elemental anaesthesia in clinical practice. Br Med Bull. (2004) 71:115–35. doi: 10.1093/bmb/ldh034
165. Burchfield JS, Xie M, Hill JA. Pathological ventricular remodeling: mechanisms: part 1 of 2. Circulation. (2013) 128:388–400. doi: 10.1161/CIRCULATIONAHA.113.001878
166. Wang J, Deng B, Liu Q, Huang Y, Chen W, Li J, et al. Pyroptosis and ferroptosis induced by mixed lineage kinase 3 (MLK3) signaling in cardiomyocytes are essential for myocardial fibrosis in response to pressure overload. Cell Death Dis. (2020) 11:574. doi: 10.1038/s41419-020-02777-3
167. Shi H, Gao Y, Dong Z, Yang J, Gao R, Li X, et al. GSDMD-Mediated cardiomyocyte pyroptosis promotes myocardial I/R injury. Circ Res. (2021) 129:383–96. doi: 10.1161/CIRCRESAHA.120.318629
168. Song X, Zhu S, Xie Y, Liu J, Sun L, Zeng D, et al. JTC801 induces ph-dependent death specifically in cancer cells and slows growth of tumors in mice. Gastroenterology. (2018) 154:1480–93. doi: 10.1053/j.gastro.2017.12.004
169. Holze C, Michaudel C, Mackowiak C, Haas DA, Benda C, Hubel P, et al. Oxeiptosis, a ROS-induced caspase-independent apoptosis-like cell-death pathway. Nat Immunol. (2018) 19:130–40. doi: 10.1038/s41590-017-0013-y
170. Zhang Y, Huang Z, Li H. Insights into innate immune signalling in controlling cardiac remodelling. Cardiovasc Res. (2017) 113:1538–50. doi: 10.1093/cvr/cvx130
171. Zhang Y, Li H. Reprogramming interferon regulatory factor signaling in cardiometabolic diseases. Physiology. (2017) 32:210–23. doi: 10.1152/physiol.00038.2016
172. Zhang Y, Zhang XJ, Wang PX, Zhang P, Li H. Reprogramming innate immune signaling in cardiometabolic disease. Hypertension. (2017) 69:747–60. doi: 10.1161/HYPERTENSIONAHA.116.08192
173. Cai J, Xu M, Zhang X, Li H. Innate immune signaling in nonalcoholic fatty liver disease and cardiovascular diseases. Annu Rev Pathol. (2019) 14:153–84. doi: 10.1146/annurev-pathmechdis-012418-013003
174. Xu M, Liu PP, Li H. Innate immune signaling and its role in metabolic and cardiovascular diseases. Physiol Rev. (2019) 99:893–948. doi: 10.1152/physrev.00065.2017
175. Fukuto JM, Carrington SJ, Tantillo DJ, Harrison JG, Ignarro LJ, Freeman BA, et al. Small molecule signaling agents: the integrated chemistry and biochemistry of nitrogen oxides, oxides of carbon, dioxygen, hydrogen sulfide, and their derived species. Chem Res Toxicol. (2012) 25:769–93. doi: 10.1021/tx2005234
Keywords: helium (He), neon (Ne), argon (Ar), krypton (Kr), xenon (Xe), cardiovascular diseases, cerebrovascular disease, gasoimmunology
Citation: Zhang J, Liu W, Bi M, Xu J, Yang H and Zhang Y (2022) Noble Gases Therapy in Cardiocerebrovascular Diseases: The Novel Stars? Front. Cardiovasc. Med. 9:802783. doi: 10.3389/fcvm.2022.802783
Received: 27 October 2021; Accepted: 18 January 2022;
Published: 16 March 2022.
Edited by:
Anne-Clémence Vion, INSERM U1087 L'unité de recherche de l'institut du thorax, FranceReviewed by:
Jerzy Beltowski, Medical University of Lublin, PolandCopyright © 2022 Zhang, Liu, Bi, Xu, Yang and Zhang. This is an open-access article distributed under the terms of the Creative Commons Attribution License (CC BY). The use, distribution or reproduction in other forums is permitted, provided the original author(s) and the copyright owner(s) are credited and that the original publication in this journal is cited, in accordance with accepted academic practice. No use, distribution or reproduction is permitted which does not comply with these terms.
*Correspondence: Yaxing Zhang, emhhbmd5YXhpbmdAZ3p1Y20uZWR1LmNu; Hongzhi Yang, eWFuZ2h6aGlAbWFpbC5zeXN1LmVkdS5jbg==
†Lead Contact
Disclaimer: All claims expressed in this article are solely those of the authors and do not necessarily represent those of their affiliated organizations, or those of the publisher, the editors and the reviewers. Any product that may be evaluated in this article or claim that may be made by its manufacturer is not guaranteed or endorsed by the publisher.
Research integrity at Frontiers
Learn more about the work of our research integrity team to safeguard the quality of each article we publish.