- 1Bristol Heart Institute, University of Bristol, Bristol, United Kingdom
- 2Department of Cardiothoracic Surgery, Faculty of Medicine, Assiut University, Asyut, Egypt
Pericytes surround capillaries in every organ of the human body. They are also present around the vasa vasorum, the small blood vessels that supply the walls of larger arteries and veins. The clinical interest in pericytes is rapidly growing, with the recognition of their crucial roles in controlling vascular function and possible therapeutic applications in regenerative medicine. Nonetheless, discrepancies in methods used to define, isolate, and expand pericytes are common and may affect reproducibility. Separating pure pericyte preparations from the continuum of perivascular mesenchymal cells is challenging. Moreover, variations in functional behavior and antigenic phenotype in response to environmental stimuli make it difficult to formulate an unequivocal definition of bona fide pericytes. Very few attempts were made to develop pericytes as a clinical-grade product. Therefore, this review is devoted to appraising current methodologies’ pros and cons and proposing standardization and harmonization improvements. We highlight the importance of developing upgraded protocols to create therapeutic pericyte products according to the regulatory guidelines for clinical manufacturing. Finally, we describe how integrating RNA-seq techniques with single-cell spatial analysis, and functional assays may help realize the full potential of pericytes in health, disease, and tissue repair.
1. Background
Benjamin Rouget was the first to describe and give his name to a particular type of cell within the capillary basement membrane with projections protruding to enwrap the vessel wall (1). In 1923, Zimmerman renamed these Rouget cells “Pericytes.” Under this broad name, they included many forms of cells from different tissue origins located around capillaries (2). Then, research on pericytes entered a hibernation period until 20 years ago, when the pericytes’ regenerative potential started attracting translational interest (3, 4). Nonetheless, a discrepancy persists in the literature regarding pericytes vs. other cardiovascular cells. For instance, pericytes are plentiful in the heart. However, there were only 73 publications on cardiac pericytes in PubMed during 2021 (out of 687 publications retrievable using the general term “pericytes”) compared with 5,400 for cardiomyocytes and 1,150 for coronary endothelial cells. This data highlights the need for more research on this cell population.
Several teams have reported technologies for the isolation and derivation of pericytes. Culture expansion allows the generation of stocks for use as a product for cell therapy and tissue engineering. Nonetheless, the consensus on pericyte-related techniques remains far behind that of human endothelial cells (ECs) (5–7). In particular, the diversity of pericytes’ properties and antigenic expression in different organs, together with the overlapping with other perivascular mesenchymal cells, represents a significant obstacle to using one method that fits all experimental conditions. Ad hoc standard operating procedures (SOP) may better accomplish distinct scientific/medical goals. For instance, pure pericyte products are necessary for obtaining regulatory approval for patients’ treatment. Semi-purified preparations are more suitable for deciphering the transition of pericytes across different antigenic and functional phenotypes in response to environmental stimuli. The main characteristics of pericytes are illustrated in Figure 1 and relevant text sections.
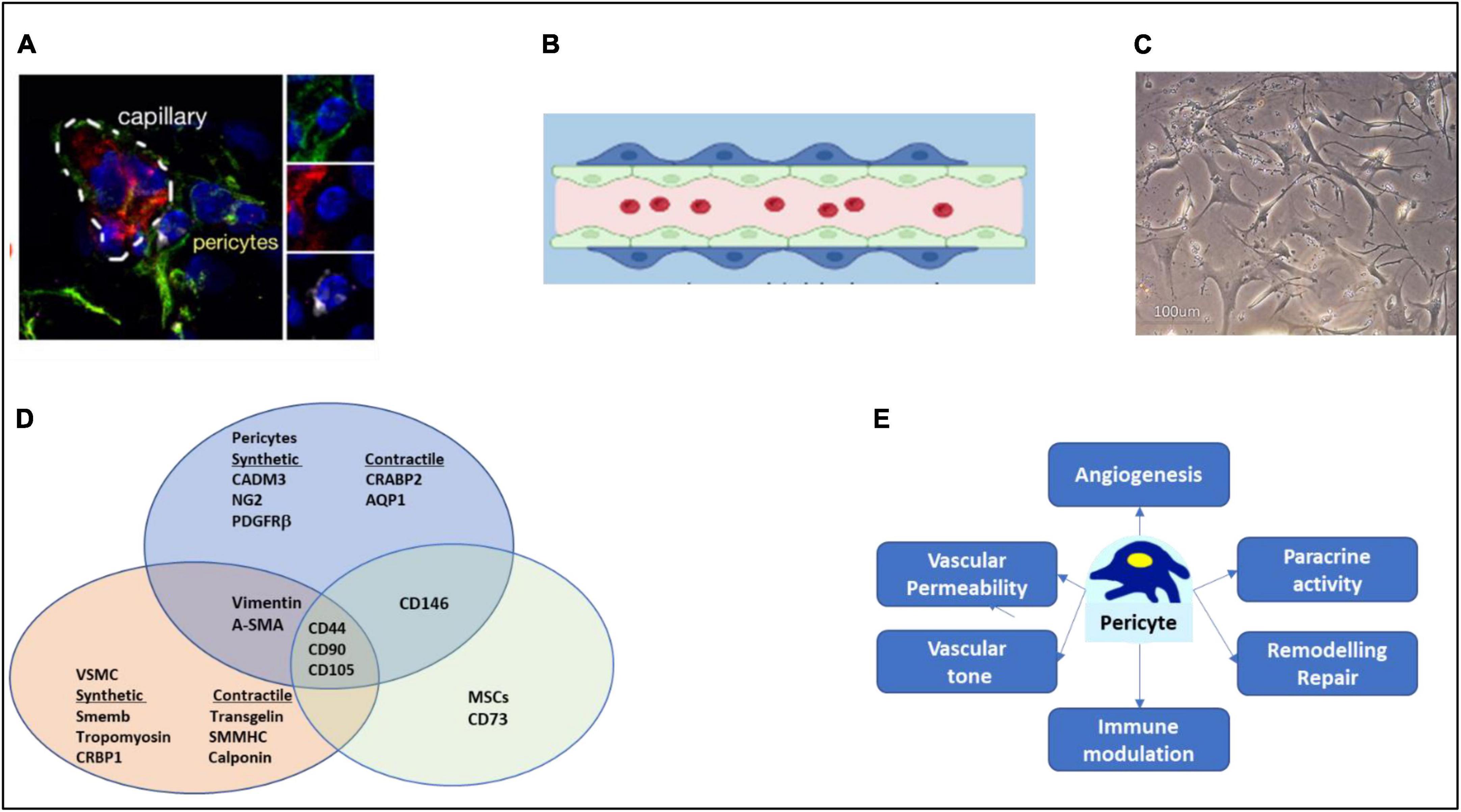
Figure 1. Characteristics of pericytes. (A) Immunofluorescence microscopy image of NG2 positive (green) pericytes, with endothelial cells labeled in red and nuclei in blue. (B) Cartoon of pericyte around a capillary lined internally by endothelial cells. (C) Aspect of pericytes in culture. (D) Overlapping of antigens with other mesenchymal cells. For clarity, refer to text for the overlapping with perivascular fibroblasts. (E) Key pericyte functions. Image in (A) has been reproduced with permission from the original article in Avolio et al. (3).
2. Aims
This review article aims to present, compare, and discuss the experience of deriving pericytes from different organs and species. We initially report current nomenclature, functional roles, and antigenic profiles that distinguish pericytes from other perivascular cell populations. The main body of the review is then devoted to (i) documenting the pros and cons of current techniques for isolation, expansion, and characterization of bona fide pericytes, (ii) suggesting avenues for standardization and harmonization, (iii) upgrading protocols for clinical manufacturing, and (iv) prospecting the potential of novel single-cell technologies in advancing pericyte research.
3. Origin and nomenclature of pericytes
Developmentally, pericytes in the cephalic region and thymus originate from the neuroectoderm. The ones found in the liver, lungs, and heart derive from mesothelium. The mesoderm gives rise to pericytes in other organs (such as kidneys, liver, and pancreas) (8–10). Intriguingly, Yamazaki et al. proposed that a subgroup of pericytes may also derive from the hematopoietic cell lineage (11). Tracing studies may help reveal additional embryological contributors to vascular pericytes.
In the adult organism, microvascular pericytes are classically located within the basement membrane in direct contact with capillary ECs in different organs. In addition, pericytes are present in the vascular adventitia, the blood vessel’s outer layer near the vasa vasorum (3, 12). The nomenclature of these intravascular pericytes is inconsistent: the term “adventitial stromal cells/CD34 + cells” refers to the in situ expression of CD34, a transmembrane glycoprotein, while “adventitial pericyte-like progenitors” denotes their plasticity and positivity for typical stem cell markers (12–15). For clarity, we will refer to these cells as adventitial pericytes.
4. Functional roles of pericytes
Pericytes are essential for vasculature’s physiological stability, as exemplified by the prominent pericyte-endothelial interactions forming the blood-brain barrier (16). Reduction in pericytes abundance, pericyte detachment from capillaries, and accrual of senescence markers are associated with microvascular fragility and dysfunction, as observed in aging, ischemic disease, fibrotic and neurodegenerative disorders, diabetes, tumors, and COVID-19 (17–21). Moreover, capillary pericytes modulate blood flow in different organs. Mice with brain pericyte ablation reportedly developed an acute blood-brain barrier breakdown, severe blood flow decrease, and rapid neuron loss (22). Pericyte contraction participates in the no-reflow phenomenon, the failure of blood to reperfuse an ischemic tissue after the vascular obstruction has been removed or bypassed (23–26).
In addition to their vascular functions, pericytes exert immunomodulatory tasks, sensing inflammatory stimuli through pattern-recognition receptors, and promoting monocyte and neutrophil migration and homing via a PDGFRβ signaling mechanism (27, 28). They also express ligands interacting with activated T cell receptors during adaptive immunity responses (29).
Lastly, pericytes play critical roles in tissue restoration, promoting, and stabilizing reparative neovascularization, as reviewed in Navarro et al. (30). Accordingly, regenerative therapies using exogenous pericytes have been preclinically tested (31–37). Pericytes were delivered either as dispersed preparations or as a part of a “tissue-engineered” product to models of myocardial infarction (MI) (33, 36, 37), congenital heart disease (34, 35), limb ischemia (38), muscular dystrophy and skeletal muscle injuries (39–42), blood-brain barrier disorders (43–48), diabetic retinopathy (49–51), and kidney fibrosis (52). These preclinical studies have paved the way for future therapeutic applications in human diseases.
5. Antigenic profile of pericytes in relation to function and localization
Like other mesenchymal cells, pericytes are positive for CD105, CD29, CD44, and CD90 and negative for CD45 and CD31. They typically express Platelet-Derived Growth Factor Receptor beta (PDGFRβ), the cell surface glycoprotein MUC18 (CD146), GTPase signaling 5 (RGS5), chondroitin sulfate proteoglycan 4 (NG2), myosin heavy chain 11 (Myh11), and the transmembrane metalloprotease CD13 (12, 53–55).
The expression of antigenic markers can vary according to organ location. Capillary and adventitial pericytes express both NG2 and PDGFRβ. However, capillary pericytes are positive for CD146, whereas adventitial pericytes are positive for CD34 and negative for CD146 (3, 12, 56, 57). Kramann et al. and Crisan et al. illustrated a difference in the antigenic profile of pericytes according to their vascular site. They classified the pericytes into two types. Capillary (type I) pericytes (NG2+/Desmin–/α-SMA–) and pre-capillary (type II) pericytes (NG2+/Desmin+/α-SMA+) (58, 59). Bergers et al. identified post-capillary (type III) pericytes (NG2–/αSMA+) (60). Moreover, coronary artery pericytes were classified as endocardial-derived (NG2–/ PDGFRβ+/αSMA–) and epicardial-derived (NG2+/PDGFRβ+/αSMA+) (61, 62). Three different subgroups of aortic pericytes were identified based on similarities to fibroblasts (PDGFRβ+/PDGFRα+/CD34+), VSMCs (PDGFRβ+/αSMA+), and neural cells (PDGFRβ+/PLP1+/CNP+) (63).
Pericytes can change phenotype when transiting from a quiescent to an activated state. NG2 distinguishes topical pericyte properties such as the capacity to control vascular permeability in the brain (64–66), participation in myofibroblast differentiation during the process of cardiac and renal fibrosis (67, 68), and progenitor cell activity in the coronary microvasculature (69). The concurrent expression of CD133, CD34, and Kinase Insert Domain Receptor (KDR) identifies fetal aorta-derived pericytes’ ability to differentiate into endothelial or muscular lineages (15). Adult aortic pericytes express the stemness marker SRY (sex determining region Y)-box 2 (Sox2) (12). and microvascular cardiac pericytes express OCT3/4, GATA−4, and Homeobox protein NANOG (32). The upregulation of another pluripotency gene, Krüppel-like factor 4 (KLF4), marked the switch of perivascular cells to a less differentiated state in tumors (70).
In angiogenesis, the transition from quiescent to actively proliferating pericytes is accompanied by profound changes in the antigenic and secretory profile (71). The expression of alpha-smooth muscle actin (α-SMA) and the downregulation of protein tyrosine kinase Tie2 reportedly mark the pericyte participation in pathological angiogenesis (72–74). Using RNA-Seq transcriptomics, we unveiled new markers distinguishing the differentiation stages of human cardiac pericytes into pro-angiogenic, contractile cells. Naive pericytes express the Cell Adhesion Molecule 3 (CADM3). The expression of the angiogenesis-related Cellular Retinoic Acid–Binding Protein 2 (CRABP2) and Aquaporin 1 (AQP1) distinguished contractile pericytes from naive pericytes and coronary VSMCs (55). In addition, we reported that epigenetic modifications can influence the pericyte pro-angiogenic activity (75). Transplantation of human adventitial pericytes to a murine limb ischemia model improved perfusion recovery and muscular angiogenesis. The therapeutic effect varied among different donor lines. Whole genome screening showed that this variation was significantly associated with the DNA methylation state at the promoter and gene body levels (75).
Gubernator et al. identified two types of skeletal muscle pericytes relevant to adipogenesis and myogenesis (76). Type I pericytes’ antigenic profile (NG2+/Nestin–/PDGFRα+) is linked to a differentiation fate to adipocytes and is associated with fat accumulation (77). Type II expressional profile (NG2+/Nestin+/PDGFRα–) is linked to myocyte commitment and associated with skeletal muscle regeneration (78).
6. Distinction from other cells within the perivascular niche
The microenvironment within or in proximity to the blood vessel wall, the perivascular niche, contains mesenchymal stromal cells (MSCs), fibroblasts, and pericytes. Perivascular MSCs express CD146, CD105, alkaline phosphatase (ALP)+, Stro-1, and VCAM1 in humans, and CD105, PDGFRα, Sca1, CD44, CD29, and VCAM1 in the mouse (79). It remains unclear whether MSCs and pericytes have a lineage relationship or represent functionally distinct mesenchymal populations that share a perivascular location. Some studies support the possibility that pericytes constitute a more primitive version of MSCs (15, 80), while others suggest that all MSCs are pericytes with some narrow exceptions (81). In line with the latter possibility, a study from Crisan demonstrated that pericytes isolated from different organs express almost all the known markers of MSCs, especially CD10, CD13, CD44, CD73, CD90, and CD105 (82).
Moreover, a strict relationship exists between fibroblasts and pericytes. Fibroblasts are mesenchymal cells in the interstitial space of all organs (83). They express mesenchymal markers such as vimentin, PDGFRα, and type I collagen, along with some tissue-specific markers, such as Transcription Factor 21 (TCF21) and periostin (84). In the brain, perivascular fibroblasts surround cortical penetrating arterioles and their branches in the arteriole-capillary transition zone. Still, they are absent in the capillary zone where pericytes prevail (85). Recent single-cell transcriptomics studies in mice showed that perivascular fibroblast-like cells in the adult mouse brain express unique markers compared with other mural cells (86–88). In the heart, the periostin-expressing myofibroblast originates from tissue-resident fibroblasts and plays critical roles in adaptive healing and fibrosis, according to genetic lineage tracing of cardiac cells (84). A study in the Zebrafish, using a combination of in vivo live imaging, genetic ablation, and CRISPR mutant analysis, demonstrated that perivascular fibroblasts play dual roles in stabilizing nascent intersegmental vessel sprouts from the dorsal aorta and later functioning as pericyte progenitors (89). Furthermore, Birbrair et al. distinguished two bona fide pericyte subtypes in the skeletal muscle interstitium of mice, naming them type-1 (Nestin-GFP–/NG2-DsRed+) and type-2 (Nestin-GFP+/NG2-DsRed+) pericytes. In vitro, type-1 pericytes were profibrotic, and type-2 pericytes were myogenic. The latter fate was confirmed after transplantation of type-2 pericytes in young mice and, to a lesser extent, in older mice. Moreover, type-2 pericytes participate in normal and tumoral angiogenesis (77, 78). Therefore, transition states and shared functions between pericytes and perivascular fibroblasts may coexist in disease states.
Given that there is no single-specific pericyte marker, adopting the concept of “Identifying Package’ seems more plausible (82).
7. Current protocols for the derivation of pericyte populations
The different steps of pericyte derivation and purification are illustrated in Figure 2.
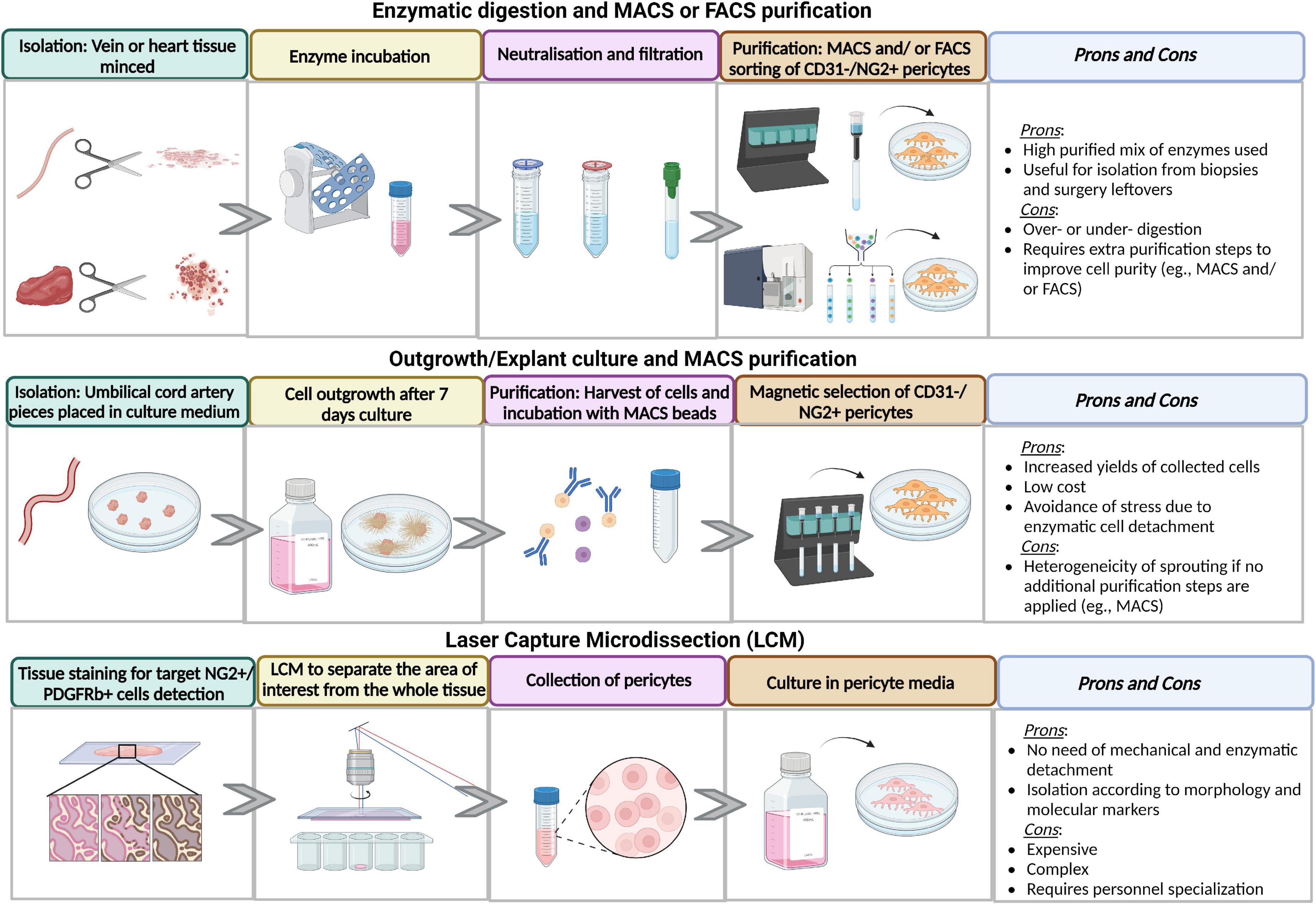
Figure 2. Advantages and disadvantages of different methods of isolation and purification methods. Figure created in Biorender.com source.
7.1. Tissue harvesting and pericyte extraction
The harvesting procedure can affect the subsequent isolation steps. For instance, cardiac surgeons use two techniques to prepare saphenous veins for coronary artery bypass grafting. Removal of perivascular tissue from the vein may damage the adventitia, thereby precluding the isolation of resident pericytes. We prefer to isolate pericytes from veins obtained through the “no-touch” technique (90, 91). The optimal time from harvesting to cell isolation varies according to the tissue source. Blood vessels can be stored for up to 7 days, while cardiac samples must be processed for digestion within 48 h.
The isolation is generally performed using enzymatic digestion or microdissection techniques. The enzymatic digestion approach is particularly useful for pericyte isolation from biopsies and surgery leftovers. The tissue is finely chopped and subjected to one or multiple rounds of enzymatic digestion. Samples with a high collagen content should undergo mechanical dissociation to increase the cell yield (39, 46, 47, 92, 93). Quality control of reagents’ purity and stability includes checking the batch enzymatic activity, determining the activity decay with storage, and ensuring that the final concentration is appropriate to the tissue source and sample quantity. A highly purified mix of Collagenase I and II, commercially available as Liberase TM, is used to digest and isolate pericytes from human and swine saphenous veins and heart (12, 32–35). A mix of Collagenase Dispase, Dnase I, and Tosyl-L-lysyl-chloromethane hydrochloride (TLCK) is used to isolate swine and bovine pericytes from the brain (45). A Papain and Dnase I are employed to isolate mouse brain pericytes (43). A precise tuning of the enzyme volume in relation to the tissue weight is essential to ensure efficient cell isolation (3, 12). An excessively aggressive procedure can impact cell viability, while incomplete tissue processing can compromise cell yield. The incubation time for tissue digestion is also critical. For pericytes extraction from the heart, we treat the chopped tissue with Liberase for up to 1 h at 37°C under gentle rotation.
Microdissection is usually applied to outgrowth/explant cultures. The tissue is dissected into small pieces using a scalpel or scissors, washed to avoid red blood cell contamination, and distributed over the surface of a culture dish with or without extracellular matrix (ECM) coating. Pre-warmed media is then added to cover the fragments completely. Explanted cultures are kept undisturbed for a few days until pericytes become visible as migrated cells from the tissue pieces (31, 41, 94). The advantages of this isolation technique are the increased yield of collected cells, low cost, and avoidance of the destructive stress caused by enzymes (95). However, enzymatic pre-treatment may sometimes become necessary, as in the case of micro-vessels from the placenta (91).
Laser capture microdissection (LCM) enables the operator to separate the cells of interest from the whole tissue specimen under the microscope using a cutting beam of UV laser (96, 97). This separation strategy does not require prior mechanical or enzymatic digestion. Thus, it allows for preserving the native cell phenotype, gene expression, and function (98). The disadvantage is represented by costs, complexity, and personnel specialization (99, 100). LCM was used to isolate pericytes from snap-frozen human and mouse brains (101).
Enzymatic dissociation and microdissection are generally followed by separation of antigenically defined cell populations by FACS or immunomagnetic columns. This could be associated with using selective culture media during cell expansion (31, 102).
7.2. Purification
Table 1 shows the negative and positive markers used for sorting, while Table 2 illustrates the methods for enhancing purity.
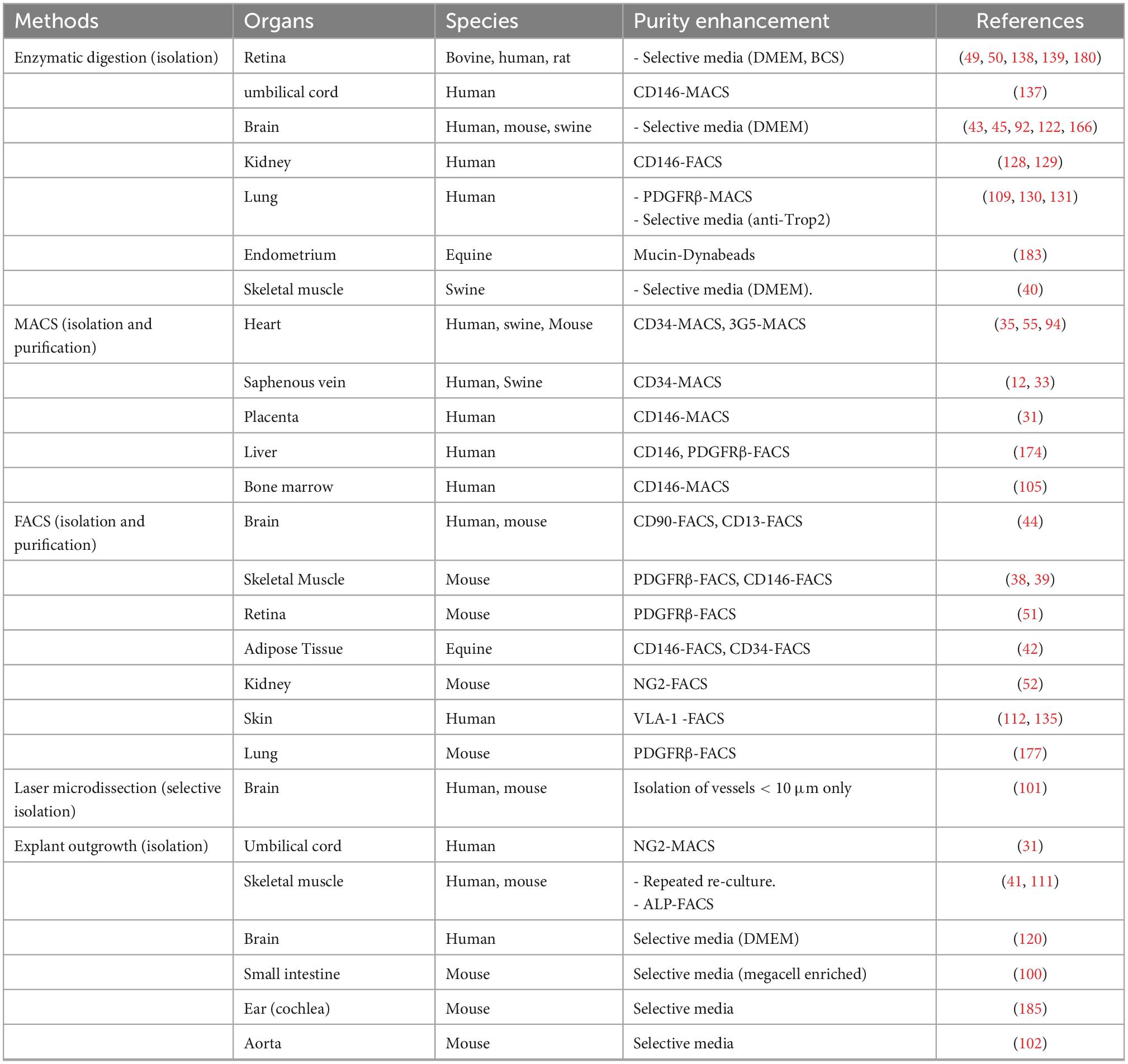
Table 2. Different strategies and methods for enhancing purity in isolation and purification of pericytes from different species and tissues.
In preparation for immunomagnetic sorting, the crude preparation from digestion or microdissection is passed through 70-, 40-, and 30-μm strainers to eliminate debris. Using immunomagnetic columns, cells are labeled with magnetic microbeads conjugated with antibodies and exposed to a magnetic field (90). Three platforms are available for this purpose: the magnetic separator, a column-free magnetic separation fitting tube containing cells labeled with antibodies, and magnetic particles. The labeled cells are retained in the tube walls, and the unlabeled cells are discarded. MACS columns and Dynabeads are widely used to isolate pericytes due to the simplicity and rapidity of the procedure (32–35, 94, 103). The harsh magnetic field may cause damage or rupture of the cell membranes of fragile cells. Furthermore, column-based separations generally employ a limited number of positive and negative markers, resulting in a semi-purified cell population.
Fluorescent-Activated Cells Sorting (FACS) is more reliable than immunomagnetic columns. The final population is highly pure for the selected antigens, but cell yields may be insufficient for immediate applications requiring large cell quantities. The strategy for gating cells can make a profound difference in the outcomes (12, 39, 41, 42, 44, 52, 90, 104, 105).
7.3. Expansion
This step requires fine-tuning environmental conditions for pericytes’ rapid and consistent growth and suppression of contaminant cells. Essential elements and components include appropriate cell seeding density, flasks/plates coating, media, growth factors (GFs), and antibiotics.
Pericytes have elongated cytoplasmic processes which enable the in vitro cell-cell communication necessary for the production and secretion of their “sensome,” a novel term used to indicate different GFs sensed by these cells (106). Hence, calculating the correct cell seeding density is essential to avoid growth arrest and senescence caused by the lack of physical contact. To support pericytes adherence to the culture surface, 0.1–0.2% (w/v) gelatin solution is widely used for precoating.
Dulbecco’s Modified Eagle Medium (DMEM) has been used for the expansion of pericytes sourced from the brain (107), kidney (108), lung (109), skeletal muscle (110, 111), and skin (112). A DMEM version including many additional factors (e.g., zinc, thymidine) could help improve the growth rate (113). The addition of Fetal Bovine Serum (FBS) is debated due to the reagent’s complexity (114–116). Additionally, pericytes from the heart (32), saphenous vein (117), and umbilical cord (31) were expanded in Endothelial Cell Growth Medium 2 (EGM-2), while bone marrow pericytes were cultured with α-MEM containing FBS (105). Antibiotic treatment with penicillin and streptomycin is usually added to the culture medium to reduce contamination.
The expansion by single-cell cloning allows for enriching progenitor cells within the bulk preparation of sorted pericytes (118, 119). Single cells are sorted into a Terasaki multi-well plate to give rise to clones and subclones (12, 111). Mechanical and chemical stress during the sorting procedure can result in poor recovery and reduced clonogenic expansion. Moreover, the FACS system requires a high level of daily maintenance and sterility to preserve the cell culture environment from possible contamination.
Even adopting a very stringent expansion protocol, expansion efficiency may vary depending on the donor’s tissue source, age, and clinical conditions. Using a good manufacturing practices-compliant SOP, we expanded dozens of pericyte lines from the saphenous vein and heart. Neonatal cardiac pericytes grew faster and better than adult pericytes. From a sample weighing less than 100 mg, we could obtain ≈20 million cells at passage 5, in 4–6 weeks of culture expansion. Ten times larger amounts of the vein were necessary to reach these quantities (32). Moreover, older patients’ pericytes undergo proliferative senescence at earlier passages (75). Experts generally consider a cell preparation’s optimum purity and viability above 90%.
8. Isolation of pericytes from human tissues
Pericytes have been isolated from different tissues.
8.1. Brain
Brain pericytes could be isolated from the white and the gray matter using enzymatic digestion and explant culture (92, 107, 120–122). They express PDGFRβ, αSMA, CD13, NG2, CD146, and desmin (121). Brain pericytes are expanded in culture with DMEM/F12 supplemented with 10% (v/v) FBS (120).
8.2. Heart
Cardiac pericytes were isolated from atria and ventricles using enzymatic digestion, filtration, and immunomagnetic separation to obtain CD31–/CD34+ cells (21, 32, 55). Cardiac pericytes are expanded in a dedicated medium supplemented with human recombinant GFs (ECGM2) and 2% FBS. This medium was superior to mesenchymal stromal cell media such as MACS + 10%FBS and DMEM + 20%FBS (32).
8.3. Skeletal muscle
Skeletal muscle-derived pericytes were mainly isolated by explant culture. Distinct populations, such as alkaline phosphatase/CD56– cells and CD146+/NG2+/αSMA+/ CD45–/CD31–/CD34– cells, could be purified by FACS or immunomagnetic microbeads (77, 78, 93, 123, 124). Type I collagen-coated plates are expanded in DMEM containing 5% (v/v) FBS (41, 111).
8.4. Bone marrow
Bone marrow pericytes can be isolated immunomagnetically using a microbead cell sorting strategy. Bone marrow samples are treated with Ficoll Histopaque 1,077 to obtain mononuclear cells. Firstly, cell populations are negatively purified from CD45+/CD34+ fractions. Then, CD146+ cells are selected and further expanded, with final confirmation of purity for the CD45–/CD34–/CD146+ combination by flow cytometry and immunocytochemistry (105). The bone marrow pericytes can be grown in α-MEM basal medium supplemented with 20% FBS (105, 125).
8.5. Kidney
Kidney pericytes are essential in regulating permeability, formation of myofibroblasts, angiogenesis, and renal medullary blood flow (126–128). After tissue digestion using a collagenase cocktail (IA, II, and IV), cells are FACSorted to obtain a population of CD146+/CD34–/CD45–/CD56– pericytes, which also express the classical makers PDGFRβ and NG2. Culture expansion is performed on gelatin-coated dishes (108, 128, 129).
8.6. Lung
The methods for human lung pericyte isolation include enzymatic digestion, magnetic microbead sorting, and FACS selection (109, 130–132). Then, cells are sorted to collect the CD45–/CD31–/CD326–/PDGFRβ+ fraction (109, 130). In addition, Kramann et al. isolated lung pericytes using a selection medium that contained AY16 (an anti-Trop2 monoclonal antibody)-DT3C conjugate to eliminate epithelial stem cells and endothelial cells (131). The modified medium selected cells express PDGFRβ and chondroitin sulfate proteoglycan 4 (CSPG4).
8.7. Skin
Pericytes have been isolated from the neonatal foreskin or adult female breast skin (133). After digestion with type II collagenase or dispase (134), a VLA-1bri/CD45– population was sorted by FACS or immunomagnetic microbeads (112, 133, 135). In addition, skin pericytes express Human high molecular weight-melanoma-associated antigen (HMW-MAA), PDGFRβ, desmin, αSMA, α1β1-integrin, α- and γ-actin isoforms, and myosin (136).
8.8. Umbilical cord
Human umbilical cord pericytes can be isolated by enzymatic digestion and explant culture (31). Helmbold et al. performed immunomagnetic sorting of cells using CD146-conjugated microbeads to separate the CD146+ population (137). Cathery et al. isolated pericytes by explant culture. The migrated cells underwent immunomagnetic sorting, and the CD31–/NG2+ population was acquired (31). All the isolated populations showed the characteristic negative expression of CD34, and positive expression of CD146 and NG2.
8.9. Retina
After separation from the pigmented layer, the human retinal pericytes are isolated by enzymatic dissociation. The cells are seeded on gelatin-precoated dishes and cultured with DMEM/F-12 containing FBS. The characteristic morphology of retinal pericytes is identified by immunofluorescent staining of NG2, αSMA, glial fibrillary acidic protein, and cytoskeletal structural proteins (138, 139).
9. Isolation and expansion of pericytes from animal tissues
Although human cells constitute the final therapeutic product for patients, animal-derived cells represent a suitable surrogate in preclinical safety and efficacy studies (140). Using donors and recipients from the same species, ideally from the same litter, eliminates the need for immunosuppression, which is instead required when transplanting human cells into an animal model (33). Recent technological progress makes swine-derived cells, tissues, and organs attractive for immune-compatible xenotransplantation. The cells are genetically modified to remove the immunogenic factors. Human transgenes can be expressed to suppress rejection (141).
Lack of uniformity in cell manufacturing between human and animal species might influence the interpretation and translatability of preclinical results. Therefore, robust approaches for isolation, culture, expansion, characterization, and appropriate in vivo testing of pericytes in larger animal species are of utmost importance.
9.1. Protocols for isolation and expansion
Animal primary cells may incur bacterial or fungal contamination because of the poor sterile conditions used to collect the starting material. Therefore, using antibiotics and Amphotericin B and manipulating cells in the laminar flow cabinets is fundamental to maintaining sterile conditions and avoiding the spread of cross-infection to the cells. An accurate selection of the proper culture medium, GFs, percentage of serum, and plate coating is key to simulating the in vivo environment and allowing adequate expansion (142). Significant changes may need to be introduced to adapt protocols initially devised for human pericytes. For instance, swine pericytes grown in autologous or allogeneic serum displayed a 100% success of expansion and high doubling time compared with the standard xenogeneic FBS employed for the growth of human pericytes (12, 32, 33).
10. Pericyte generation from iPSCs
Induced Pluripotent Stem Cells (iPSC) derived through reprogramming somatic cells with pluripotency factors (OCT3/4, SOX2, c-MYC, KLF4) can be differentiated to a specific cell line, including pericytes. 3D-culture systems of iPSC-derived cardiovascular cells have provided a novel in vitro model to study the interplay among different cell types and potentially develop new personalized treatments for congenital and acquired cardiac diseases (116, 143–145).
The developmental origin of cardiac pericytes is thought to be the epicardium. Differentiation of iPSCs to epicardial cells can be achieved through the modulation of Wnt/β-catenin signaling and treatment with pericyte growth factors, such as PDGF-BB (144, 146, 147). Szepes et al. applied a mesodermal induction using BMP4, VEGFA, and activation of Wnt/β-catenin signaling with further VEGFA and TGFβ inhibition. FACS staining and sorting for PDGFRβ and CD31 markers and further expansion were carried out for antigenic characterization, gene expression, and function in an endothelial tube formation assay. A tri-cultured bioartificial cardiac tissue model was generated using iPSC-derived pericytes, cardiomyocytes, and ECs to study vascularization and fibrosis in vitro (145). Faal et al. reported two protocols using mesoderm and neural crest to obtain brain pericytes from iPSCs generated from healthy subjects and Alzheimer’s disease patients. Briefly, human iPSCs were cultured on a Matrigel-coated plate in a mesodermal induction medium with growth factors. The activation of Wnt/β-catenin signaling initiated neural crest differentiation through specific medium and supplements. Mesodermal and neural crest-derived cells were differentiated in pericytes seeded on gelatin or Matrigel-coated plate. Both pericyte types were passaged, split, and kept in the pericyte medium for their characterization and functional trans-endothelial electrical resistance assay with ECs (146). Jamieson et al. generated pericytes from human iPSCs obtained from mesodermal lineage. In brief, iPSCs were cultured on a feeder layer of mouse embryonic fibroblasts and gelatin-coated plates. For the differentiation, iPSCs were detached with EDTA, strained, and seeded on a collagen-coated plate using α-MEM, FBS, and β-mercaptoethanol. Following further detachment, they were grown in EC growth medium, TGFβ inhibitor, and VEGF to promote vascular cell specification and proliferation. After an enzymatic dissociation, DMEM + FBS was added to an uncoated plate to support the pericyte selection. Generated iPSC-derived pericytes were used for developing a microvessel model of the blood-brain barrier (148).
11. Standardization and harmonization
It has been shown that fewer than one-third of biomedical papers can be reproduced, resulting in associated costs of about $28 billion each in the US only, mainly attributable to the wasted follow-on work that the initial study has inspired (149, 150). Reproducibility of results from peer-reviewed papers by researchers at pharmaceutical companies failed at rates upwards of 75% (151). This reflects the inconsistent use and communication of standardized processes to achieve efficiency, quality, and uniformity of performance. The main body of information on pericytes methodologies can be retrieved from original papers, which show large variability regarding the depth of details. The following paragraphs are devoted to suggesting improvements to current methodologies.
11.1. Increasing reproducibility
It is highly recommended that the entire SOP is published as an accompanying material or companion paper and deposited in an accessible database (152). Open access public repositories, such as Zenodo,1 SEEK (153), OpenAIRE,2 FAIRsharing3 are available for SOP submission.
We have previously reported the SOP for GMP production of human adventitial pericytes, which consists of: (i) Cell Isolation, (ii) Cell expansion (iii) Cell splitting, (iv) Cell freezing/thawing, and (v) Extraction of serum from blood. Each section contains a checklist of 6 to 44 operational steps that the named operator has to do (154). An attached document reports details of the collection, the code associates the sample to the NHS patient’s records and informed consent form and ethical approval, code and storage conditions for reagents, equipment, and deviations from the protocol. An electronic copy was stored in a dedicated folder of the institutional server at the University of Bristol.
11.2. Upgrading
GMP manufacture is a quality assurance system pharmaceutical companies use to ensure that the final medicinal product meets proper specifications. GMP covers both the manufacturing and testing of the final product. Translation of the GMP concept to cell therapy and tissue engineering remains vague, mainly because most cell products are early stage prototypes studied in academic laboratories (155). To the best of our knowledge, our previous study remains the only one to have upgraded human adventitial pericytes to GMP (154). We have verified that the clinical-grade, xeno-free reagents worked like the research-grade ones. Then, the whole production process was transferred to the GMP facilities at the NHS Blood and Transplant (NHSBT) Unit in Bristol. NHSBT is authorized by the Human Tissue Authority (HTA) and Medicine and Healthcare products Regulatory Agency (MHRA) to manufacture advanced therapy products for cell therapy trials.
11.3. Quality control
Introducing quality and quantity control (QQc) systems to produce pericytes provides two significant improvements. Namely, it reduces production costs and certifies the cell product. We process only samples that exceed a minimum weight. Using this quantity control criterion, we calculated a 33% cost saving thanks to avoiding expansion failure.
Quality and quantity control analyses include a cell viability/death test by Calcein/EDthIII assay and Trypan Blue, repeated antigenic characterization using both positive and negative markers to exclude that contaminant cells had emerged during expansion, karyotyping to ensure that cells do not accumulate chromosomic damage and functional characterization (33, 75). Among the most widely used tests, the Matrigel assay demonstrates the pericytes’ capacity to improve the network-forming activity of ECs, while measuring angiogenic factors in the media allows recognizing the pericytes’ ability to release typical angiogenic factors such as Hepatocyte Growth Factor (HGF) and Angiopoietin 2 (Ang-2) (32). More sophisticated tests aiding the characterization of pericytes include the assessment of contraction and relaxation using the Electrical Cell Intuitive Sensing (ECIS) platform, barrier integrity by analyzing membrane current, permeability using co-culture of ECs and pericytes on Transwell filters and microfluidic models, mechano-transduction properties, and circadian rhythms regulating the crosstalk between pericytes and endothelial cells (32, 55, 156–159).
11.4. Integrating methodologies
All the derivation techniques described above have pros and cons. Appropriate combinations could increase the purity and yield of production. For instance, repeating immunomagnetic separation can help maintain a high purity level during expansion. When massive production of pericytes is needed, using Multi-Flasks provides an optimal cell culture environment, simplifies the workflow by eliminating multiple steps, and reduces the risk of contamination. A step forward, automated pericyte culture systems could deliver high throughput, high purity, and high yield cell growth, perform large-scale screening, and run several experiments in parallel, thereby allowing for reducing inter-assay variability.
Another attractive approach is based on the use of multi-omics. Potent technologies such as single-cell RNAseq have been applied to pericyte research, providing a spatial atlas of the vascular niche in different organs, and revealing organ-specific pericyte markers and identities (88, 160, 161). In the brain, a continuum phenotypic change (zonation) along the arteriovenous axis for ECs was contraposed by a punctuated continuum for mural cells. Among the latter population, pericytes coexisted with a population of perivascular fibroblast-like cells localized at all vessel types except capillaries (88). In the heart, large-scale single-cell and single-nucleus transcriptome analyses identified 6 anatomical regions (162). The vascular compartment included 17 distinct populations of ECs, VSMC, pericytes, and mesothelial cells. Pericytes formed 4 clusters, of which one was transcriptionally distant from other vascular cells, while another constituted a transitional state between pericytes and ECs. The relevance of this new sub-classification should be validated at the level of the functionome. Multifunctional microwell arrays that enable single-cell functional analysis have already been used to study lymphocytes (163). Live-cell imaging and analysis unveiled cell transition dynamics that could not be captured by classical snapshot imaging (164). Other emerging single-cell methodologies promise to define cell heterogeneity on the protein and DNA epigenetics levels (165).
12. Conclusion
This review highlighted the significant efforts made by different teams to develop effective protocols of isolation and in vitro expansion of human pericytes. A consensus on these protocols and the integration of methodologies will help speed up research at the highest level of reproducibility and translatability. Moreover, additional research is needed to dissect the functional diversification of pericytes according to their zonal distribution and the surrounding microenvironment. More work is also necessary to trace pericytes in tissues during vascular remodeling. Expanding and refining the atlas of different perivascular niches at the single-cell level will clarify the enigmatic phenotype of pericytes and the relevance of expressional deviations in response to organ injury. This novel technological approach should be integrated into the dimensions of space and time, for instance, by clustering dynamic gene expression profiles from single-cell RNA-Sequencing databases and associating transcripts to functionomics analysis. Most studies on pericytes have been performed in rodent models. More research in large animal models is needed to demonstrate therapeutic utility.
Data availability statement
The original contributions presented in this study are included in the article/supplementary material, further inquiries can be directed to the corresponding author.
Author contributions
PM was responsible for the final writing and the data presented. All authors conceived and designed the article, read, and approved the final manuscript.
Funding
This work was supported by grants from (i) the British Heart Foundation (PG/22//10843, Transferring healthy longevity gene to improve age-related heart dysfunction), (ii) Ph.D. scholarship from the Ministry of Higher Education of the Arabic Republic of Egypt (MM24/21, Pericyte tissue engineering for prevention of aortic aneurysm development and rupture), (iii) British Heart Foundation studentship (FS/16/64/32480, Derivation of swine pericytes to enable fit-for-purpose clinical translation), (iv) British Heart Foundation (grant PG/19/49/34440, A novel pericyte mechanism involving the transcriptional activator Nrf2), and (v) Heart Research UK translational project grant “Targeting pericytes for halting pulmonary hypertension in infants with congenital heart disease” (RG2697/21/23).
Conflict of interest
The authors declare that the research was conducted without any commercial or financial relationships that could be construed as a potential conflict of interest.
Publisher’s note
All claims expressed in this article are solely those of the authors and do not necessarily represent those of their affiliated organizations, or those of the publisher, the editors and the reviewers. Any product that may be evaluated in this article, or claim that may be made by its manufacturer, is not guaranteed or endorsed by the publisher.
Footnotes
- ^ https://zenodo.org
- ^ https://explore.openaire.eu/participate/deposit-publications
- ^ https://fair-dom.org/platform/
References
1. Attwell D, Mishra A, Hall CN, O’Farrell FM, Dalkara T. What is a pericyte? J Cereb Blood Flow Metab. (2016) 36:451–5. doi: 10.1177/0271678X15610340
2. Zimmermann KW. Der feinere bau der blutcapillaren. Z Anat Entwicklungsgeschichte. (1923) 68:29–109. doi: 10.1007/BF02593544
3. Avolio E, Madeddu P. Discovering cardiac pericyte biology: from physiopathological mechanisms to potential therapeutic applications in ischemic heart disease. Vascul Pharmacol. (2016) 86:53–63. doi: 10.1016/j.vph.2016.05.009
4. Meijer EM, van Dijk CGM, Kramann R, Verhaar MC, Cheng C. Implementation of pericytes in vascular regeneration strategies. Tissue Eng Part B Rev. (2022) 28:1–21. doi: 10.1089/ten.TEB.2020.0229
5. Jaffe EA, Nachman RL, Becker CG, Minick CR. Culture of human endothelial cells derived from umbilical veins. Identification by morphologic and immunologic criteria. J Clin Invest. (1973) 52:2745–56. doi: 10.1172/JCI107470
6. Gimbrone MA Jr., Cotran RS, Folkman J. Human vascular endothelial cells in culture. Growth and DNA synthesis. J Cell Biol. (1974) 60:673–84. doi: 10.1083/jcb.60.3.673
7. Nachman RL, Jaffe EA. Endothelial cell culture: beginnings of modern vascular biology. J Clin Invest. (2004) 114:1037–40. doi: 10.1172/JCI23284
8. Korn J, Christ B, Kurz H. Neuroectodermal origin of brain pericytes and vascular smooth muscle cells. J Comp Neurol. (2002) 442:78–88. doi: 10.1002/cne.1423
9. Muller SM, Stolt CC, Terszowski G, Blum C, Amagai T, Kessaris N, et al. Neural crest origin of perivascular mesenchyme in the adult thymus. J Immunol. (2008) 180:5344–51. doi: 10.4049/jimmunol.180.8.5344
10. Dias Moura Prazeres PH, Sena IFG, Borges IDT, de Azevedo PO, Andreotti JP, de Paiva AE, et al. Pericytes are heterogeneous in their origin within the same tissue. Dev Biol. (2017) 427:6–11. doi: 10.1016/j.ydbio.2017.05.001
11. Yamazaki T, Nalbandian A, Uchida Y, Li W, Arnold TD, Kubota Y, et al. Tissue myeloid progenitors differentiate into pericytes through TGF-beta signaling in developing skin vasculature. Cell Rep. (2017) 18:2991–3004. doi: 10.1016/j.celrep.2017.02.069
12. Campagnolo P, Cesselli D, Al Haj Zen A, Beltrami AP, Krankel N, Katare R, et al. Human adult vena saphena contains perivascular progenitor cells endowed with clonogenic and proangiogenic potential. Circulation. (2010) 121:1735–45. doi: 10.1161/CIRCULATIONAHA.109.899252
13. Wang Y, Xu J, Chang L, Meyers CA, Zhang L, Broderick K, et al. Relative contributions of adipose-resident CD146(+) pericytes and CD34(+) adventitial progenitor cells in bone tissue engineering. NPJ Regen Med. (2019) 4:1. doi: 10.1038/s41536-018-0063-2
14. Howson KM, Aplin AC, Gelati M, Alessandri G, Parati EA, Nicosia RF. The postnatal rat aorta contains pericyte progenitor cells that form spheroidal colonies in suspension culture. Am J Physiol Cell Physiol. (2005) 289:C1396–407. doi: 10.1152/ajpcell.00168.2005
15. Invernici G, Emanueli C, Madeddu P, Cristini S, Gadau S, Benetti A, et al. Human fetal aorta contains vascular progenitor cells capable of inducing vasculogenesis, angiogenesis, and myogenesis in vitro and in a murine model of peripheral ischemia. Am J Pathol. (2007) 170:1879–92. doi: 10.2353/ajpath.2007.060646
16. Armulik A, Genové G, Mäe M, Nisancioglu MH, Wallgard E, Niaudet C, et al. Pericytes regulate the blood-brain barrier. Nature. (2010) 468:557–61. doi: 10.1038/nature09522
17. Hellström M, Gerhardt H, Kalén M, Li X, Eriksson U, Wolburg H, et al. Lack of pericytes leads to endothelial hyperplasia and abnormal vascular morphogenesis. J Cell Biol. (2001) 153:543–53. doi: 10.1083/jcb.153.3.543
18. Winkler EA, Bell RD, Zlokovic BV. Central nervous system pericytes in health and disease. Nat Neurosci. (2011) 14:1398–405. doi: 10.1038/nn.2946
19. Schrimpf C, Teebken OE, Wilhelmi M, Duffield JS. The role of pericyte detachment in vascular rarefaction. J Vasc Res. (2014) 51:247–58. doi: 10.1159/000365149
20. Berthiaume AA, Schmid F, Stamenkovic S, Coelho-Santos V, Nielson CD, Weber B, et al. Pericyte remodeling is deficient in the aged brain and contributes to impaired capillary flow and structure. Nat Commun. (2022) 13:5912. doi: 10.1038/s41467-022-33464-w
21. Avolio E, Carrabba M, Milligan R, Kavanagh Williamson M, Beltrami AP, Gupta K, et al. The SARS-CoV-2 Spike protein disrupts human cardiac pericytes function through CD147 receptor-mediated signalling: a potential non-infective mechanism of COVID-19 microvascular disease. Clin Sci. (2021) 135:2667–89. doi: 10.1042/CS20210735
22. Nikolakopoulou AM, Montagne A, Kisler K, Dai Z, Wang Y, Huuskonen MT, et al. Pericyte loss leads to circulatory failure and pleiotrophin depletion causing neuron loss. Nat Neurosci. (2019) 22:1089–98. doi: 10.1038/s41593-019-0434-z
23. Hall CN, Reynell C, Gesslein B, Hamilton NB, Mishra A, Sutherland BA, et al. Capillary pericytes regulate cerebral blood flow in health and disease. Nature. (2014) 508:55–60.
24. Hartmann DA, Berthiaume AA, Grant RI, Harrill SA, Koski T, Tieu T, et al. Brain capillary pericytes exert a substantial but slow influence on blood flow. Nat Neurosci. (2021) 24:633–45. doi: 10.1038/s41593-020-00793-2
25. O’Farrell FM, Mastitskaya S, Hammond-Haley M, Freitas F, Wah WR, Attwell D. Capillary pericytes mediate coronary no-reflow after myocardial ischaemia. Elife. (2017) 6:e29280.
26. Costa MA, Paiva AE, Andreotti JP, Cardoso MV, Cardoso CD, Mintz A, et al. Pericytes constrict blood vessels after myocardial ischemia. J Mol Cell Cardiol. (2018) 116:1–4.
27. Stark K, Eckart A, Haidari S, Tirniceriu A, Lorenz M, von Bruhl ML, et al. Capillary and arteriolar pericytes attract innate leukocytes exiting through venules and ‘instruct’ them with pattern-recognition and motility programs. Nat Immunol. (2013) 14:41–51. doi: 10.1038/ni.2477
28. Olson LE, Soriano P. PDGFRbeta signaling regulates mural cell plasticity and inhibits fat development. Dev Cell. (2011) 20:815–26. doi: 10.1016/j.devcel.2011.04.019
29. Navarro R, Compte M, Alvarez-Vallina L, Sanz L. Immune regulation by pericytes: modulating innate and adaptive immunity. Front Immunol. (2016) 7:480. doi: 10.3389/fimmu.2016.00480
30. Cathery W, Faulkner A, Maselli D, Madeddu P. Concise review: the regenerative journey of pericytes toward clinical translation. Stem Cells. (2018) 36:1295–310. doi: 10.1002/stem.2846
31. Cathery W, Faulkner A, Jover E, Rodriguez-Arabaolaza I, Thomas AC, Avolio E, et al. Umbilical cord pericytes provide a viable alternative to mesenchymal stem cells for neonatal vascular engineering. Front Cardiovasc Med. (2020) 7:609980. doi: 10.3389/fcvm.2020.609980
32. Avolio E, Rodriguez-Arabaolaza I, Spencer HL, Riu F, Mangialardi G, Slater SC, et al. Expansion and characterization of neonatal cardiac pericytes provides a novel cellular option for tissue engineering in congenital heart disease. J Am Heart Assoc. (2015) 4:e002043. doi: 10.1161/JAHA.115.002043
33. Alvino VV, Fernandez-Jimenez R, Rodriguez-Arabaolaza I, Slater S, Mangialardi G, Avolio E, et al. Transplantation of allogeneic pericytes improves myocardial vascularization and reduces interstitial fibrosis in a swine model of reperfused acute myocardial infarction. J Am Heart Assoc. (2018) 7:e006727. doi: 10.1161/JAHA.117.006727
34. Alvino VV, Kilcooley M, Thomas AC, Carrabba M, Fagnano M, Cathery W, et al. In vitro and in vivo preclinical testing of pericyte-engineered grafts for the correction of congenital heart defects. J Am Heart Assoc. (2020) 9:e014214. doi: 10.1161/JAHA.119.014214
35. Alvino VV, Thomas AC, Ghorbel MT, Rapetto F, Narayan SA, Kilcooley M, et al. Reconstruction of the swine pulmonary artery using a graft engineered with syngeneic cardiac pericytes. Front Bioeng Biotechnol. (2021) 9:715717. doi: 10.3389/fbioe.2021.715717
36. Katare R, Riu F, Mitchell K, Gubernator M, Campagnolo P, Cui Y, et al. Transplantation of human pericyte progenitor cells improves the repair of infarcted heart through activation of an angiogenic program involving micro-RNA-132. Circ Res. (2011) 109:894–906. doi: 10.1161/CIRCRESAHA.111.251546
37. Avolio E, Meloni M, Spencer HL, Riu F, Katare R, Mangialardi G, et al. Combined intramyocardial delivery of human pericytes and cardiac stem cells additively improves the healing of mouse infarcted hearts through stimulation of vascular and muscular repair. Circ Res. (2015) 116:e81–94. doi: 10.1161/CIRCRESAHA.115.306146
38. Hayes KL, Messina LM, Schwartz LM, Yan J, Burnside AS, Witkowski S. Type 2 diabetes impairs the ability of skeletal muscle pericytes to augment postischemic neovascularization in db/db mice. Am J Physiol Cell Physiol. (2018) 314:C534–44. doi: 10.1152/ajpcell.00158.2017
39. Nirwane A, Gautam J, Yao Y. Isolation of type I and type II pericytes from mouse skeletal muscles. J Vis Exp. (2017) 123:55904.
40. Fuoco C, Sangalli E, Vono R, Testa S, Sacchetti B, Latronico MV, et al. 3D hydrogel environment rejuvenates aged pericytes for skeletal muscle tissue engineering. Front Physiol. (2014) 5:203. doi: 10.3389/fphys.2014.00203
41. Ausems CRM, Raaijmakers RHL, van den Broek W, Willemse M, van Engelen BGM, Wansink DG, et al. Intrinsic myogenic potential of skeletal muscle-derived pericytes from patients with myotonic dystrophy type 1. Mol Ther Methods Clin Dev. (2019) 15:120–32. doi: 10.1016/j.omtm.2019.09.002
42. Esteves CL, Sheldrake TA, Mesquita SP, Pesantez JJ, Menghini T, Dawson L, et al. Isolation and characterization of equine native MSC populations. Stem Cell Res Ther. (2017) 8:80. doi: 10.1186/s13287-017-0525-2
43. Tigges U, Welser-Alves JV, Boroujerdi A, Milner R. A novel and simple method for culturing pericytes from mouse brain. Microvasc Res. (2012) 84:74–80.
44. Crouch EE, Doetsch F. FACS isolation of endothelial cells and pericytes from mouse brain microregions. Nat Protoc. (2018) 13:738–51. doi: 10.1038/nprot.2017.158
45. Vandenhaute E, Culot M, Gosselet F, Dehouck L, Godfraind C, Franck M, et al. Brain pericytes from stress-susceptible pigs increase blood-brain barrier permeability in vitro. Fluids Barriers CNS. (2012) 9:11. doi: 10.1186/2045-8118-9-11
46. Bernard-Patrzynski F, Lecuyer MA, Puscas I, Boukhatem I, Charabati M, Bourbonniere L, et al. Isolation of endothelial cells, pericytes and astrocytes from mouse brain. PLoS One. (2019) 14:e0226302.
47. Mehra A, Dehouck L, Vandenhaute E, Fatar M, Fenart L, Gosselet F. A high output method to isolate cerebral pericytes from mouse. J Vis Exp. (2020). doi: 10.3791/60588
48. Yamamoto S, Muramatsu M, Azuma E, Ikutani M, Nagai Y, Sagara H, et al. A subset of cerebrovascular pericytes originates from mature macrophages in the very early phase of vascular development in CNS. Sci Rep. (2017) 7:3855. doi: 10.1038/s41598-017-03994-1
49. Liu G, Meng C, Pan M, Chen M, Deng R, Lin L, et al. Isolation, purification, and cultivation of primary retinal microvascular pericytes: a novel model using rats. Microcirculation. (2014) 21:478–89. doi: 10.1111/micc.12121
50. Primo VA, Arboleda-Velasquez JF. Isolation and transfection of primary culture bovine retinal pericytes. Methods Mol Biol. (2016) 1430:107–17. doi: 10.1007/978-1-4939-3628-1_7
51. Rangasamy S, Monickaraj F, Legendre C, Cabrera AP, Llaci L, Bilagody C, et al. Transcriptomics analysis of pericytes from retinas of diabetic animals reveals novel genes and molecular pathways relevant to blood-retinal barrier alterations in diabetic retinopathy. Exp Eye Res. (2020) 195:108043. doi: 10.1016/j.exer.2020.108043
52. Ajay AK, Zhao L, Vig S, Fujiwara M, Thakurela S, Jadhav S, et al. Deletion of STAT3 from Foxd1 cell population protects mice from kidney fibrosis by inhibiting pericytes trans-differentiation and migration. Cell Rep. (2022) 38:110473. doi: 10.1016/j.celrep.2022.110473
53. Corliss BA, Ray HC, Doty RW, Mathews C, Sheybani N, Fitzgerald K, et al. Pericyte bridges in homeostasis and hyperglycemia. Diabetes. (2020) 69:1503–17. doi: 10.2337/db19-0471
54. Ozen I, Roth M, Barbariga M, Gaceb A, Deierborg T, Genove G, et al. Loss of regulator of G-protein signaling 5 leads to neurovascular protection in stroke. Stroke. (2018) 49:2182–90. doi: 10.1161/STROKEAHA.118.020124
55. Avolio E, Katare R, Thomas AC, Caporali A, Schwenke D, Carrabba M, et al. Cardiac pericyte reprogramming by MEK inhibition promotes arteriologenesis and angiogenesis of the ischemic heart. J Clin Invest. (2022) 132:e152308. doi: 10.1172/JCI152308
56. Corselli M, Chen C-W, Sun B, Yap S, Rubin JP, Péault B. The tunica adventitia of human arteries and veins as a source of mesenchymal stem cells. Stem Cells Dev. (2012) 21:1299–308.
57. Kramann R, Goettsch C, Wongboonsin J, Iwata H, Schneider RK, Kuppe C, et al. Adventitial MSC-like cells are progenitors of vascular smooth muscle cells and drive vascular calcification in chronic kidney disease. Cell Stem Cell. (2016) 19:628–42. doi: 10.1016/j.stem.2016.08.001
58. Crisan M, Corselli M, Chen WC, Péault B. Perivascular cells for regenerative medicine. J Cell Mol Med. (2012) 16:2851–60.
59. Kumar A, D’Souza SS, Moskvin OV, Toh H, Wang B, Zhang J, et al. Specification and diversification of pericytes and smooth muscle cells from mesenchymoangioblasts. Cell Rep. (2017) 19:1902–16. doi: 10.1016/j.celrep.2017.05.019
60. Bergers G, Song S. The role of pericytes in blood-vessel formation and maintenance. Neuro Oncol. (2005) 7:452–64.
61. Chen Q, Zhang H, Liu Y, Adams S, Eilken H, Stehling M, et al. Endothelial cells are progenitors of cardiac pericytes and vascular smooth muscle cells. Nat Commun. (2016) 7:12422.
62. Volz KS, Jacobs AH, Chen HI, Poduri A, McKay AS, Riordan DP, et al. Pericytes are progenitors for coronary artery smooth muscle. Elife. (2015) 4:e10036.
63. He D, Mao A, Zheng CB, Kan H, Zhang K, Zhang Z, et al. Aortic heterogeneity across segments and under high fat/salt/glucose conditions at the single-cell level. Natl Sci Rev. (2020) 7:881–96. doi: 10.1093/nsr/nwaa038
64. Chiaverina G, di Blasio L, Monica V, Accardo M, Palmiero M, Peracino B, et al. Dynamic interplay between pericytes and endothelial cells during sprouting angiogenesis. Cells. (2019) 8:1109. doi: 10.3390/cells8091109
65. Zhu X, Bergles DE, Nishiyama A. NG2 cells generate both oligodendrocytes and gray matter astrocytes. Development. (2008) 135:145–57.
66. Milesi S, Boussadia B, Plaud C, Catteau M, Rousset M-C, De Bock F, et al. Redistribution of PDGFRβ cells and NG2DsRed pericytes at the cerebrovasculature after status epilepticus. Neurobiol Dis. (2014) 71:151–8. doi: 10.1016/j.nbd.2014.07.010
67. Su H, Zeng H, Liu B, Chen JX. Sirtuin 3 is essential for hypertension-induced cardiac fibrosis via mediating pericyte transition. J Cell Mol Med. (2020) 24:8057–68. doi: 10.1111/jcmm.15437
68. Feng X, Su H, He X, Chen J-X, Zeng H. SIRT3 deficiency sensitizes angiotensin-II-Induced renal fibrosis. Cells. (2020) 9:2510. doi: 10.3390/cells9112510
69. Ozerdem U, Grako KA, Dahlin-Huppe K, Monosov E, Stallcup WB. NG2 proteoglycan is expressed exclusively by mural cells during vascular morphogenesis. Dev Dyn. (2001) 222:218–27. doi: 10.1002/dvdy.1200
70. Murgai M, Ju W, Eason M, Kline J, Beury DW, Kaczanowska S, et al. KLF4-dependent perivascular cell plasticity mediates pre-metastatic niche formation and metastasis. Nat Med. (2017) 23:1176–90. doi: 10.1038/nm.4400
71. van Dijk CG, Nieuweboer FE, Pei JY, Xu YJ, Burgisser P, van Mulligen E, et al. The complex mural cell: pericyte function in health and disease. Int J Cardiol. (2015) 190:75–89.
72. Ozerdem U, Monosov E, Stallcup WB. NG2 proteoglycan expression by pericytes in pathological microvasculature. Microvasc Res. (2002) 63:129–34.
73. Ozerdem U, Stallcup WB. Early contribution of pericytes to angiogenic sprouting and tube formation. Angiogenesis. (2003) 6:241–9. doi: 10.1023/B:AGEN.0000021401.58039.a9
74. Teichert M, Milde L, Holm A, Stanicek L, Gengenbacher N, Savant S, et al. Pericyte-expressed Tie2 controls angiogenesis and vessel maturation. Nat Commun. (2017) 8:16106. doi: 10.1038/ncomms16106
75. Gubernator M, Slater SC, Spencer HL, Spiteri I, Sottoriva A, Riu F, et al. Epigenetic profile of human adventitial progenitor cells correlates with therapeutic outcomes in a mouse model of limb ischemia. Arterioscler Thromb Vasc Biol. (2015) 35:675–88. doi: 10.1161/ATVBAHA.114.304989
76. Birbrair A, Zhang T, Wang ZM, Messi ML, Enikolopov GN, Mintz A, et al. Role of pericytes in skeletal muscle regeneration and fat accumulation. Stem Cells Dev. (2013) 22:2298–314. doi: 10.1089/scd.2012.0647
77. Birbrair A, Zhang T, Wang Z-M, Messi ML, Mintz A, Delbono O. Type-1 pericytes participate in fibrous tissue deposition in aged skeletal muscle. Am J Physiol Cell Physiol. (2013) 305:C1098–113. doi: 10.1152/ajpcell.00171.2013
78. Birbrair A, Zhang T, Wang Z-M, Messi ML, Olson JD, Mintz A, et al. Type-2 pericytes participate in normal and tumoral angiogenesis. Am J Physiol Cell Physiol. (2014) 307:C25–38. doi: 10.1152/ajpcell.00084.2014
79. Mendez-Ferrer S, Michurina TV, Ferraro F, Mazloom AR, Macarthur BD, Lira SA, et al. Mesenchymal and haematopoietic stem cells form a unique bone marrow niche. Nature. (2010) 466:829–34. doi: 10.1038/nature09262
80. Zengin E, Chalajour F, Gehling UM, Ito WD, Treede H, Lauke H, et al. Vascular wall resident progenitor cells: a source for postnatal vasculogenesis. Development. (2006) 133:1543–51.
82. Crisan M, Yap S, Casteilla L, Chen C-W, Corselli M, Park TS, et al. A perivascular origin for mesenchymal stem cells in multiple human organs. Cell Stem Cell. (2008) 3:301–13.
83. Di Carlo SE, Peduto L. The perivascular origin of pathological fibroblasts. J Clin Invest. (2018) 128:54–63.
84. Kanisicak O, Khalil H, Ivey MJ, Karch J, Maliken BD, Correll RN, et al. Genetic lineage tracing defines myofibroblast origin and function in the injured heart. Nat Commun. (2016) 7:12260. doi: 10.1038/ncomms12260
85. Bonney SK, Sullivan LT, Cherry TJ, Daneman R, Shih AY. Distinct features of brain perivascular fibroblasts and mural cells revealed by in vivo two-photon imaging. J Cereb Blood Flow Metab. (2022) 42:966–78. doi: 10.1177/0271678X211068528
86. Marques S, Zeisel A, Codeluppi S, van Bruggen D, Mendanha Falcao A, Xiao L, et al. Oligodendrocyte heterogeneity in the mouse juvenile and adult central nervous system. Science. (2016) 352:1326–9.
87. Saunders A, Macosko EZ, Wysoker A, Goldman M, Krienen FM, de Rivera H, et al. Molecular diversity and specializations among the cells of the adult mouse brain. Cell. (2018) 174:1015–30 e16.
88. Vanlandewijck M, He L, Mae MA, Andrae J, Ando K, Del Gaudio F, et al. A molecular atlas of cell types and zonation in the brain vasculature. Nature. (2018) 554:475–80.
89. Rajan AM, Ma RC, Kocha KM, Zhang DJ, Huang P. Dual function of perivascular fibroblasts in vascular stabilization in zebrafish. PLoS Genet. (2020) 16:e1008800. doi: 10.1371/journal.pgen.1008800
90. Dore-Duffy P, Esen N. The microvascular pericyte: approaches to isolation, characterization, and cultivation. Adv Exp Med Biol. (2018) 1109:53–65.
91. Maier CL, Shepherd BR, Yi T, Pober JS. Explant outgrowth, propagation and characterization of human pericytes. Microcirculation. (2010) 17:367–80. doi: 10.1111/j.1549-8719.2010.00038.x
92. Smyth LCD, Rustenhoven J, Park TI, Schweder P, Jansson D, Heppner PA, et al. Unique and shared inflammatory profiles of human brain endothelia and pericytes. J Neuroinflammat. (2018) 15:138. doi: 10.1186/s12974-018-1167-8
93. Birbrair A, Zhang T, Wang ZM, Messi ML, Mintz A, Delbono O. Pericytes: multitasking cells in the regeneration of injured, diseased, and aged skeletal muscle. Front Aging Neurosci. (2014) 6:245. doi: 10.3389/fnagi.2014.00245
94. Cao Z, Minnier J, Liu L, Scott KLL, Reddy AP, Wilmarth PA, et al. Proteomic profiling of concurrently isolated primary microvascular endothelial cells, pericytes, and vascular smooth muscle cells from adult mouse heart. Sci Rep. (2022) 12:8835. doi: 10.1038/s41598-022-12749-6
95. Hendijani F. Explant culture: an advantageous method for isolation of mesenchymal stem cells from human tissues. Cell Prolif. (2017) 50:e12334.
96. Sonne SB, Dalgaard MD, Nielsen JE, Hoei-Hansen CE, Rajpert-De Meyts E, Gjerdrum LM, et al. Optimizing staining protocols for laser microdissection of specific cell types from the testis including carcinoma in situ. PLoS One. (2009) 4:e5536. doi: 10.1371/journal.pone.0005536
97. Wang H, Owens JD, Shih JH, Li MC, Bonner RF, Mushinski JF. Histological staining methods preparatory to laser capture microdissection significantly affect the integrity of the cellular RNA. BMC Genomics. (2006) 7:97. doi: 10.1186/1471-2164-7-97
98. Podgorny OV, Lazarev VN. Laser microdissection: a promising tool for exploring microorganisms and their interactions with hosts. J Microbiol Methods. (2017) 138:82–92. doi: 10.1016/j.mimet.2016.01.001
99. Liu H, McDowell TL, Hanson NE, Tang X, Fujimoto J, Rodriguez-Canales J. Laser capture microdissection for the investigative pathologist. Vet Pathol. (2014) 51:257–69.
100. Perin S, McCann CJ, De Coppi P, Thapar N. Isolation and characterisation of mouse intestinal mesoangioblasts. Pediatr Surg Int. (2019) 35:29–34. doi: 10.1007/s00383-018-4373-7
101. Song HW, Foreman KL, Gastfriend BD, Kuo JS, Palecek SP, Shusta EV. Transcriptomic comparison of human and mouse brain microvessels. Sci Rep. (2020) 10:12358.
102. Montiel-Eulefi E, Nery AA, Rodrigues LC, Sánchez R, Romero F, Ulrich H. Neural differentiation of rat aorta pericyte cells. Cytometry Part A. (2012) 81A:65–71. doi: 10.1002/cyto.a.21152
103. Su X, Huang L, Qu Y, Xiao D, Mu D. Pericytes in cerebrovascular diseases: an emerging therapeutic target. Front Cell Neurosci. (2019) 13:519. doi: 10.3389/fncel.2019.00519
104. Hindle P, Baily J, Khan N, Biant LC, Simpson AH, Peault B. Perivascular mesenchymal stem cells in sheep: characterization and autologous transplantation in a model of articular cartilage repair. Stem Cells Dev. (2016) 25:1659–69. doi: 10.1089/scd.2016.0165
105. Mangialardi G, Ferland-McCollough D, Maselli D, Santopaolo M, Cordaro A, Spinetti G, et al. Bone marrow pericyte dysfunction in individuals with type 2 diabetes. Diabetologia. (2019) 62:1275–90.
106. Eltanahy AM, Koluib YA, Gonzales A. Pericytes: intrinsic transportation engineers of the CNS microcirculation. Front Physiol. (2021) 12:719701. doi: 10.3389/fphys.2021.719701
107. Park TI, Feisst V, Brooks AE, Rustenhoven J, Monzo HJ, Feng SX, et al. Cultured pericytes from human brain show phenotypic and functional differences associated with differential CD90 expression. Sci Rep. (2016) 6:26587. doi: 10.1038/srep26587
108. Stefanska A, Kenyon C, Christian HC, Buckley C, Shaw I, Mullins JJ, et al. Human kidney pericytes produce renin. Kidney Int. (2016) 90:1251–61.
109. Wilson CL, Stephenson SE, Higuero JP, Feghali-Bostwick C, Hung CF, Schnapp LM. Characterization of human PDGFR-beta-positive pericytes from IPF and non-IPF lungs. Am J Physiol Lung Cell Mol Physiol. (2018) 315:L991–1002. doi: 10.1152/ajplung.00289.2018
110. Spinazzola JM, Gussoni E. Isolation of primary human skeletal muscle cells. Bio Protoc. (2017) 7:e2591.
111. Dellavalle A, Sampaolesi M, Tonlorenzi R, Tagliafico E, Sacchetti B, Perani L, et al. Pericytes of human skeletal muscle are myogenic precursors distinct from satellite cells. Nat Cell Biol. (2007) 9:255–67. doi: 10.1038/ncb1542
112. Paquet-Fifield S, Schluter H, Li A, Aitken T, Gangatirkar P, Blashki D, et al. A role for pericytes as microenvironmental regulators of human skin tissue regeneration. J Clin Invest. (2009) 119:2795–806. doi: 10.1172/JCI38535
113. Chaudhry MA, Vitalis TZ, Bowen BD, Piret JM. Basal medium composition and serum or serum replacement concentration influences on the maintenance of murine embryonic stem cells. Cytotechnology. (2008) 58:173–9.
114. Sotiropoulou PA, Perez SA, Salagianni M, Baxevanis CN, Papamichail M. Cell culture medium composition and translational adult bone marrow-derived stem cell research. Stem Cells. (2006) 24:1409–10.
115. van der Valk J, Bieback K, Buta C, Cochrane B, Dirks WG, Fu J, et al. Fetal Bovine Serum (FBS): past - present - future. ALTEX. (2018) 35: 99–118.
116. Orlova VV, van den Hil FE, Petrus-Reurer S, Drabsch Y, Ten Dijke P, Mummery CL. Generation, expansion and functional analysis of endothelial cells and pericytes derived from human pluripotent stem cells. Nat Protoc. (2014) 9:1514–31. doi: 10.1038/nprot.2014.102
117. Slater SC, Jover E, Martello A, Mitic T, Rodriguez-Arabaolaza I, Vono R, et al. MicroRNA-532-5p regulates pericyte function by targeting the transcription regulator BACH1 and angiopoietin-1. Mol Ther. (2018) 26:2823–37. doi: 10.1016/j.ymthe.2018.08.020
118. Ito M, Kawa Y, Watabe H, Ono H, Ooka S, Nakamura M, et al. Establishment by an original single-cell cloning method and characterization of an immortal mouse melanoblast cell line (NCCmelb4). Pigment Cell Res. (2004) 17:643–50. doi: 10.1111/j.1600-0749.2004.00184.x
119. Yim M, Shaw D. Achieving greater efficiency and higher confidence in single-cell cloning by combining cell printing and plate imaging technologies. Biotechnol Prog. (2018) 34:1454–9. doi: 10.1002/btpr.2698
120. Jansson D, Rustenhoven J, Feng S, Hurley D, Oldfield RL, Bergin PS, et al. A role for human brain pericytes in neuroinflammation. J Neuroinflammat. (2014) 11:104.
121. Smyth LCD, Rustenhoven J, Scotter EL, Schweder P, Faull RLM, Park TIH, et al. Markers for human brain pericytes and smooth muscle cells. J Chem Neuroanat. (2018) 92:48–60. doi: 10.1016/j.jchemneu.2018.06.001
122. Gibbons HM, Hughes SM, Van Roon-Mom W, Greenwood JM, Narayan PJ, Teoh HH, et al. Cellular composition of human glial cultures from adult biopsy brain tissue. J Neurosci Methods. (2007) 166:89–98.
123. Birbrair A, Delbono O. Pericytes are essential for skeletal muscle formation. Stem Cell Rev Rep. (2015) 11:547–8. doi: 10.1007/s12015-015-9588-6
124. Kostallari E, Baba-Amer Y, Alonso-Martin S, Ngoh P, Relaix F, Lafuste P, et al. Pericytes in the myovascular niche promote post-natal myofiber growth and satellite cell quiescence. Development. (2015) 142:1242–53. doi: 10.1242/dev.115386
125. Mangialardi G, Cordaro A, Madeddu P. The bone marrow pericyte: an orchestrator of vascular niche. Regen Med. (2016) 11:883–95. doi: 10.2217/rme-2016-0121
126. Kramann R, Humphreys BD. Kidney pericytes: roles in regeneration and fibrosis. Semin Nephrol. (2014) 34:374–83.
127. Schrimpf C, Duffield JS. Mechanisms of fibrosis: the role of the pericyte. Curr Opin Nephrol Hypertens. (2011) 20:297–305. doi: 10.1097/MNH.0b013e328344c3d4
128. Lemos DR, Marsh G, Huang A, Campanholle G, Aburatani T, Dang L, et al. Maintenance of vascular integrity by pericytes is essential for normal kidney function. Am J Physiol Renal Physiol. (2016) 311:F1230–42. doi: 10.1152/ajprenal.00030.2016
129. Stefanska A, Briski A, Khan NS, Kenyon C, Peault BM, Mullins JJ. Role of pericytes in the development of the renin/angiotensin system: induction of functional renin in cultures of pericytes. Methods Mol Biol. (2021) 2235:169–80. doi: 10.1007/978-1-0716-1056-5_12
130. Stephenson SE, Wilson CL, Bond NG, Kaur A, Alvarez X, Midkiff CC, et al. Pericytes as novel targets for HIV/SIV infection in the lung. Am J Physiol Lung Cell Mol Physiol. (2020) 319:L848–53. doi: 10.1152/ajplung.00296.2020
131. Yamaguchi M, Hirai S, Tanaka Y, Sumi T, Tada M, Takahashi H, et al. Pericyte-myofibroblast transition in the human lung. Biochem Biophys Res Commun. (2020) 528:269–75.
132. Bichsel CA, Hall SR, Schmid RA, Guenat OT, Geiser T. Primary human lung pericytes support and stabilize in vitro perfusable microvessels. Tissue Eng Part A. (2015) 21:2166–76. doi: 10.1089/ten.TEA.2014.0545
133. Zhuang L, Visalakshan RM, Kaur P. Dermal pericytes exhibit declined ability to promote human skin regeneration with ageing in 3D organotypic culture models. Cells (2021) 10:3051. doi: 10.3390/cells10113051
134. Helmbold P, Nayak RC, Marsch WC, Herman IM. Isolation and in vitro characterization of human dermal microvascular pericytes. Microvasc Res. (2001) 61:160–5. doi: 10.1006/mvre.2000.2292
135. Zhuang L, Lawlor KT, Schlueter H, Pieterse Z, Yu Y, Kaur P. Pericytes promote skin regeneration by inducing epidermal cell polarity and planar cell divisions. Life Sci Alliance. (2018) 1:e201700009. doi: 10.26508/lsa.201700009
136. Sundberg C, Kowanetz M, Brown LF, Detmar M, Dvorak HF. Stable expression of angiopoietin-1 and other markers by cultured pericytes: phenotypic similarities to a subpopulation of cells in maturing vessels during later stages of angiogenesis in vivo. Lab Invest. (2002) 82:387–401. doi: 10.1038/labinvest.3780433
137. Gokcinar-Yagci B, Karaosmanoglu B, Taskiran EZ, Celebi-Saltik B. Transcriptome and proteome profiles of human umbilical cord vein CD146+ stem cells. Mol Biol Rep. (2020) 47:3833–56. doi: 10.1007/s11033-020-05474-8
138. Miller AG, Smith DG, Bhat M, Nagaraj RH. Glyoxalase I is critical for human retinal capillary pericyte survival under hyperglycemic conditions. J Biol Chem. (2006) 281:11864–71. doi: 10.1074/jbc.M513813200
139. Lin H, Zhang Z, Zhang H, Yan P, Wang Q, Bai L. Primary culture of human blood-retinal barrier cells and preliminary study of APOBEC3 expression: an in vitro study. Invest Ophthalmol Vis Sci. (2009) 50:4436–43. doi: 10.1167/iovs.08-3169
140. Esteves CL, Donadeu FX. Pericytes in veterinary species: prospective isolation, characterization and tissue regeneration potential. Adv Exp Med Biol. (2018) 1109:67–77.
142. Mushahary D, Spittler A, Kasper C, Weber V, Charwat V. Isolation, cultivation, and characterization of human mesenchymal stem cells. Cytometry A. (2018) 93:19–31.
143. Thomas D, Choi S, Alamana C, Parker KK, Wu JC. Cellular and engineered organoids for cardiovascular models. Circ Res. (2022) 130: 1780–802.
144. Yu B, Zhao SR, Yan CD, Zhang M, Wu JC. Deconvoluting the cells of the human heart with iPSC technology: cell types, protocols, and uses. Curr Cardiol Rep. (2022) 24:487–96. doi: 10.1007/s11886-022-01670-z
145. Szepes M, Melchert A, Dahlmann J, Hegermann J, Werlein C, Jonigk D, et al. Dual function of iPSC-derived pericyte-like cells in vascularization and fibrosis-related cardiac tissue remodeling in vitro. Int J Mol Sci. (2020) 21:8947. doi: 10.3390/ijms21238947
146. Faal T, Phan DTT, Davtyan H, Scarfone VM, Varady E, Blurton-JONES M, et al. Induction of mesoderm and neural crest-derived pericytes from human pluripotent stem cells to study blood-brain barrier interactions. Stem Cell Rep. (2019) 12:451–60. doi: 10.1016/j.stemcr.2019.01.005
147. Stebbins MJ, Gastfriend BD, Canfield SG, Lee MS, Richards D, Faubion MG, et al. Human pluripotent stem cell-derived brain pericyte-like cells induce blood-brain barrier properties. Sci Adv. (2019) 5:eaau7375.
148. Jamieson JJ, Linville RM, Ding YY, Gerecht S, Searson PC. Role of iPSC-derived pericytes on barrier function of iPSC-derived brain microvascular endothelial cells in 2D and 3D. Fluids Barriers CNS. (2019) 16:15.
149. Harris JK, Wondmeneh SB, Zhao Y, Leider JP. Examining the reproducibility of 6 published studies in public health services and systems research. J Public Health Manag Pract. (2019) 25:128–36. doi: 10.1097/PHH.0000000000000694
150. Freedman LP, Cockburn IM, Simcoe TS. The economics of reproducibility in preclinical research. PLoS Biol. (2015) 13:e1002165. doi: 10.1371/journal.pbio.1002165
151. Begley CG, Ellis LM. Drug development: raise standards for preclinical cancer research. Nature. (2012) 483:531–3. doi: 10.1038/483531a
152. Hollmann S, Frohme M, Endrullat C, Kremer A, D’Elia D, Regierer B, et al. Ten simple rules on how to write a standard operating procedure. PLoS Comput Biol. (2020) 16:e1008095. doi: 10.1371/journal.pcbi.1008095
153. Wolstencroft K, Owen S, Krebs O, Nguyen Q, Stanford NJ, Golebiewski M, et al. SEEK: a systems biology data and model management platform. BMC Syst Biol. (2015) 9:33. doi: 10.1186/s12918-015-0174-y
154. Spencer HL, Slater SC, Rowlinson J, Morgan T, Culliford LA, Guttridge M, et al. A journey from basic stem cell discovery to clinical application: the case of adventitial progenitor cells. Regen Med. (2015) 10:39–47. doi: 10.2217/rme.14.64
155. Bedford P, Jy J, Collins L, Keizer S. Considering cell therapy product “good manufacturing practice” Status. Front Med. (2018) 5:118. doi: 10.3389/fmed.2018.00118
156. Lee LL, Khakoo AY, Chintalgattu V. Cardiac pericytes function as key vasoactive cells to regulate homeostasis and disease. FEBS Open Bio. (2021) 11:207–25. doi: 10.1002/2211-5463.13021
157. Rolle IG, Crivellari I, Zanello A, Mazzega E, Dalla E, Bulfoni M, et al. Heart failure impairs the mechanotransduction properties of human cardiac pericytes. J Mol Cell Cardiol. (2021) 151:15–30. doi: 10.1016/j.yjmcc.2020.10.016
158. Rogers MT, Gard AL, Gaibler R, Mulhern TJ, Strelnikov R, Azizgolshani H, et al. A high-throughput microfluidic bilayer co-culture platform to study endothelial-pericyte interactions. Sci Rep. (2021) 11:12225. doi: 10.1038/s41598-021-90833-z
159. Mastrullo V, van der Veen DR, Gupta P, Matos RS, Johnston JD, McVey JH, et al. Pericytes’ circadian clock affects endothelial cells’ synchronization and angiogenesis in a 3d tissue engineered scaffold. Front Pharmacol. (2022) 13:867070. doi: 10.3389/fphar.2022.867070
160. He L, Vanlandewijck M, Mae MA, Andrae J, Ando K, Del Gaudio F, et al. Single-cell RNA sequencing of mouse brain and lung vascular and vessel-associated cell types. Sci Data. (2018) 5:180160. doi: 10.1038/sdata.2018.160
161. Baek SH, Maiorino E, Kim H, Glass K, Raby BA, Yuan K. Single cell transcriptomic analysis reveals organ specific pericyte markers and identities. Front Cardiovasc Med. (2022) 9:876591. doi: 10.3389/fcvm.2022.876591
162. Litvinukova M, Talavera-Lopez C, Maatz H, Reichart D, Worth CL, Lindberg EL, et al. Cells of the adult human heart. Nature. (2020) 588:466–72. doi: 10.1038/s41586-020-2797-4
163. Park H, Kim H, Doh J. Multifunctional microwell arrays for single cell level functional analysis of lymphocytes. Bioconjug Chem. (2018) 29:672–9. doi: 10.1021/acs.bioconjchem.7b00620
164. Wang W, Douglas D, Zhang J, Kumari S, Enuameh MS, Dai Y, et al. Live-cell imaging and analysis reveal cell phenotypic transition dynamics inherently missing in snapshot data. Sci Adv. (2020) 6:eaba9319. doi: 10.1126/sciadv.aba9319
165. Chavkin NW, Hirschi KK. Single cell analysis in vascular biology. Front Cardiovasc Med. (2020) 7:42. doi: 10.3389/fcvm.2020.00042
166. Bernard-Patrzynski F, Lécuyer MA, Puscas I, Boukhatem I, Charabati M, Bourbonnière L, et al. Isolation of endothelial cells, pericytes and astrocytes from mouse brain. PLoS One. (2019) 14:e0226302. doi: 10.1371/journal.pone.0226302
167. Hill RA, Tong L, Yuan P, Murikinati S, Gupta S, Grutzendler J. Regional blood flow in the normal and ischemic brain is controlled by arteriolar smooth muscle cell contractility and not by capillary pericytes. Neuron. (2015) 87:95–110. doi: 10.1016/j.neuron.2015.06.001
168. Ma Q, Zhao Z, Sagare AP, Wu Y, Wang M, Owens NC, et al. Blood-brain barrier-associated pericytes internalize and clear aggregated amyloid-β42 by LRP1-dependent apolipoprotein E isoform-specific mechanism. Mol Neurodegener. (2018) 13:57. doi: 10.1186/s13024-018-0286-0
169. Warmke N, Platt F, Bruns AF, Ozber CH, Haywood NJ, Abudushalamu Y, et al. Pericyte insulin receptors modulate retinal vascular remodeling and endothelial angiopoietin signaling. Endocrinology. (2021) 162:bqab182. doi: 10.1210/endocr/bqab182
170. Cheng J, Korte N, Nortley R, Sethi H, Tang Y, Attwell D. Targeting pericytes for therapeutic approaches to neurological disorders. Acta Neuropathol. (2018) 136:507–23. doi: 10.1007/s00401-018-1893-0
171. Teng YC, Porfírio-Sousa AL, Ribeiro GM, Arend MC, da Silva Meirelles L, Chen ES, et al. Analyses of the pericyte transcriptome in ischemic skeletal muscles. Stem Cell Res Ther. (2021) 12:183. doi: 10.1186/s13287-021-02247-3
172. Sacchetti B, Funari A, Remoli C, Giannicola G, Kogler G, Liedtke S, et al. No identical “mesenchymal stem cells” at different times and sites: human committed progenitors of distinct origin and differentiation potential are incorporated as adventitial cells in microvessels. Stem Cell Rep. (2016) 6:897–913. doi: 10.1016/j.stemcr.2016.05.011
173. Lee LL, Khakoo AY, Chintalgattu V. Isolation and purification of murine cardiac pericytes. J Vis Exp. (2019). doi: 10.3791/59571
174. Meng YM, Jiang X, Zhao X, Meng Q, Wu S, Chen Y, et al. Hexokinase 2-driven glycolysis in pericytes activates their contractility leading to tumor blood vessel abnormalities. Nat Commun. (2021) 12:6011. doi: 10.1038/s41467-021-26259-y
175. Yuan K, Shamskhou EA, Orcholski ME, Nathan A, Reddy S, Honda H, et al. Loss of endothelium-derived Wnt5a is associated with reduced pericyte recruitment and small vessel loss in pulmonary arterial hypertension. Circulation. (2019) 139:1710–24. doi: 10.1161/CIRCULATIONAHA.118.037642
176. Hannan RT, Miller AE, Hung RC, Sano C, Peirce SM, Barker TH. Extracellular matrix remodeling associated with bleomycin-induced lung injury supports pericyte-to-myofibroblast transition. Matrix Biol Plus. (2021) 10:100056. doi: 10.1016/j.mbplus.2020.100056
177. Hung CF, Mittelsteadt KL, Brauer R, McKinney BL, Hallstrand TS, Parks WC, et al. Lung pericyte-like cells are functional interstitial immune sentinel cells. Am J Physiol Lung Cell Mol Physiol. (2017) 312:L556–67. doi: 10.1152/ajplung.00349.2016
178. Li P, Zhou Y, Goodwin AJ, Cook JA, Halushka PV, Zhang XK, et al. Fli-1 governs pericyte dysfunction in a murine model of sepsis. J Infect Dis. (2018) 218:1995–2005. doi: 10.1093/infdis/jiy451
179. Shi H, Koronyo Y, Rentsendorj A, Regis GC, Sheyn J, Fuchs DT, et al. Identification of early pericyte loss and vascular amyloidosis in Alzheimer’s disease retina. Acta Neuropathol. (2020) 139:813–36. doi: 10.1007/s00401-020-02134-w
180. Bryan BA, D’Amore PA. Pericyte isolation and use in endothelial/pericyte coculture models. Methods Enzymol. (2008) 443: 315–31.
181. Khan NS, West CC, Rossi F, Crisan M. Assessment of pericyte phenotype by flow cytometry. Methods Mol Biol. (2021) 2235:27–35. doi: 10.1007/978-1-0716-1056-5_3
182. Patel JJ, Srivastava S, Siow RC. Isolation, culture, and characterization of vascular smooth muscle cells. Methods Mol Biol. (2016) 1430:91–105. doi: 10.1007/978-1-4939-3628-1_6
183. Rink BE, Amilon KR, Esteves CL, French HM, Watson E, Aurich C, et al. Isolation and characterization of equine endometrial mesenchymal stromal cells. Stem Cell Res Ther. (2017) 8:166. doi: 10.1186/s13287-017-0616-0
184. Schrimpf C, Xin C, Campanholle G, Gill SE, Stallcup W, Lin SL, et al. Pericyte TIMP3 and ADAMTS1 modulate vascular stability after kidney injury. J Am Soc Nephrol. (2012) 23:868–83. doi: 10.1681/ASN.2011080851
Keywords: pericytes, regenerative medicine, methods, isolation and expansion, cardiovascular
Citation: Alvino VV, Mohammed KAK, Gu Y and Madeddu P (2023) Approaches for the isolation and long-term expansion of pericytes from human and animal tissues. Front. Cardiovasc. Med. 9:1095141. doi: 10.3389/fcvm.2022.1095141
Received: 10 November 2022; Accepted: 22 December 2022;
Published: 10 January 2023.
Edited by:
Narasimman Gurusamy, Nova Southeastern University, United StatesReviewed by:
Christian Göritz, Karolinska Institutet (KI), SwedenBruce A. Corliss, University of Virginia, United States
Copyright © 2023 Alvino, Mohammed, Gu and Madeddu. This is an open-access article distributed under the terms of the Creative Commons Attribution License (CC BY). The use, distribution or reproduction in other forums is permitted, provided the original author(s) and the copyright owner(s) are credited and that the original publication in this journal is cited, in accordance with accepted academic practice. No use, distribution or reproduction is permitted which does not comply with these terms.
*Correspondence: Paolo Madeddu, bWRwcm1AYnJpc3RvbC5hYy51aw==
†These authors have contributed equally to this work