- 1National Heart and Lung Institute, Imperial College London, London, United Kingdom
- 2Department of Biomedical Sciences, Florida State University College of Medicine, Tallahassee, FL, United States
In cardiac muscle the action of adrenaline on β1 receptors of heart muscle cells is essential to adjust cardiac output to the body’s needs. Adrenergic activation leads to enhanced contractility (inotropy), faster heart rate (chronotropy) and faster relaxation (lusitropy), mainly through activation of protein kinase A (PKA). Efficient enhancement of heart output under stress requires all of these responses to work together. Lusitropy is essential for shortening the heartbeat when heart rate increases. It therefore follows that, if the lusitropic response is not present, heart function under stress will be compromised. Current literature suggests that lusitropy is primarily achieved due to PKA phosphorylation of troponin I (TnI) and phospholamban (PLB). It has been well documented that PKA-induced phosphorylation of TnI releases Ca2+ from troponin C faster and increases the rate of cardiac muscle relaxation, while phosphorylation of PLB increases SERCA activity, speeding up Ca2+ removal from the cytoplasm. In this review we consider the current scientific evidences for the connection between suppression of lusitropy and cardiac dysfunction in the context of mutations in phospholamban and thin filament proteins that are associated with cardiomyopathies. We will discuss what advances have been made into understanding the physiological mechanism of lusitropy due to TnI and PLB phosphorylation and its suppression by mutations and we will evaluate the evidence whether lack of lusitropy is sufficient to cause cardiomyopathy, and under what circumstances, and consider the range of pathologies associated with loss of lusitropy. Finally, we will discuss whether suppressed lusitropy due to mutations in thin filament proteins can be therapeutically restored.
Introduction
It is 10 years since we wrote a review, based mainly on in vitro work, proposing that a major defect commonly connected with inherited cardiomyopathies (and perhaps some others) was their lack of response to TnI phosphorylation (1). The modulation of cardiac muscle relaxation rate due to TnI and phospholamban phosphorylation is a key determinant of the lusitropic response. This lead us to propose that suppression of lusitropy could be a disease mechanism in cardiomyopathies. This review revisits the question in the light of recent research.
Calcium ions (Ca2+), released from the sarcoplasmic reticulum, bind to troponin C to switch the thin filament on, however, in cardiac muscle a more graded form of regulation is essential to tailor cardiac output to the body’s needs. The level of contractility of heart muscle is determined by three factors: the initial sarcomere length (preload), the force against which the muscle must shorten (afterload), and the speed and force of contraction. Speed and force of contraction can be modulated independently of preload and afterload by changing the inotropic state. This is controlled largely, but not exclusively, by stimuli from the sympathoadrenal system and the parasympathetic nervous system.
Adrenergic activation acts mainly on β1 receptors to trigger a coordinated response of the heart during exercise or “flight-or-flight” that increases cardiac output up to fivefold. This is achieved by an increase in heart rate of up to threefold (chronotropy) and an increase in the force of contraction (inotropy). Ventricular pressure rises quicker and higher arterial pressure is produced. At the same time the duration of systole grows briefer and relaxation is faster (lusitropy).
The increased speed of relaxation is essential for the adrenergic response since the heart beat must become shorter if the heart rate is increased to avoid successive beats overlapping which would reduce stroke volume. Nevertheless, lusitropy is not often given the attention it deserves since heart rate and magnitude of contraction are obvious and easily measured parameters whilst relaxation rates are not commonly recorded. It is the objective of this review to consider the evidence that defects in lusitropy can be a significant contributor to heart disease.
The biochemical and physiological process of lusitropy is well understood. β-1 receptor activation leads to adenylate cyclase activation and cAMP production. cAMP acts directly on membrane channels and also activates the cyclic AMP-dependent protein kinase (PKA). PKA itself phosphorylates a variety of ion channels, ion pumps in the sarcolemma and sarcoplasmic reticulum (SR) and contractile proteins.
The SR in cardiac myocytes stores large quantities of Ca2+. The SR is a complex cellular compartment that allows intracellular Ca2+ cycling that is coordinated with other cellular systems such as myofilament and sarcolemma proteins. Ca2+ release from the SR is driven by the opening of Ryanodine Receptor whilst Ca2+ reuptake by the SR is mostly dictated by the Ca2+ pump called sarco/endoplasmic reticulum Ca2 + -ATPase (SERCA2a). In the sarcoplasmic reticulum PKA phosphorylates Phospholamban (PLB), an accessory protein crucial in the regulation of SERCA2a activity.
There are two phosphorylation sites in PLB, one at serine 16 and another at threonine 17 and the kinases involved are PKA for serine 16 and Ca2+/CaM kinase for threonine 17. Unphosphorylated PLB is an inhibitor of SERCA2a; PKA-mediated phosphorylation of phospholamban relieves the inhibition, thus activating SERCA2a, resulting in faster sequestration of Ca2+ (lusitropy) and increased filling of the sarcoplasmic reticulum that contributes to positive inotropy. In addition, positive lusitropism is achieved by a PKA-mediated phosphorylation of troponin I, exclusively at serines 22 and 23 (2, 3); phosphorylation of TnI at these two serine sites increases the rate of Ca2+-release from troponin C (4). To terminate these events when plasma concentrations of β-agonists fall, cyclic adenosine monophosphate (cAMP) is hydrolyzed by the cyclic nucleotide phosphodiesterases, and protein phosphatases hydrolyze the protein-bound phosphate. As will be discussed later, it is probable that both PLB and TnI phosphorylation are necessary for effective positive lusitropy. It is possible other targets of PKA, such as the L-type Ca2+ channel (5), may influence lusitropy, but comprehensive data is lacking. Recent publications have described the molecular mechanisms of troponin and phospholamban phosphorylation modulation of function (6, 7).
Evidence that lusitropy is necessary from PLB loss of function models
The key studies of the role of phospholamban in cardiac muscle regulation are based on a PLB knockout (KO) mouse model (8). In unloaded assays (e.g., myocytes) the relaxation rate is completely unresponsive to isoprenaline (Iso) compared with a 30% increase in WT myocytes. However, in isometric contractions lusitropy is merely blunted: decrease in tau relax was 17% vs. 30–50% in non-transgenic littermates (NTG). PLB KO has additional actions, since it activated SERCA2a: tau is lower than NTG and there is a substantial inotropic effect. However, it can be concluded that PLB phosphorylation is a mechanism for lusitropy. Pathogenic, exonic variants have been identified in the PLB gene associated with DCM; to date, there are six known PLN mutations linked to dilated cardiomyopathy (p.R9C, R9L, R9H, R14del, R25C, L39X) (9–11) with several more candidate mutations suggested from large scale surveys [e.g., (12)].
The PLB R9C variant associated with DCM has been extensively studied for its inotropic and lusitropic effects in transgenic mice, cardiomyocytes and human iPSC-derived cardiomyocytes. Cardiomyocytes isolated from transgenic mice bearing the PLB R9C in the heart display prolonged Ca2+ transients. Upon further investigation it was found that the PLB R9C mutation “traps” PKA and inactivates it, preventing phosphorylation. This has an constitutive inotropic and lusitropic effect but yields negative consequences of impaired frequency potentiation and blunted β-adrenergic responsiveness (9). Another report suggested that acute expression of PLB R9C in cardiomyocytes enhances inotropic and lusitropic responses of the transfected cells but also blunts the response to the frequency of stimulation and isoproterenol stimulation (13). The PLB R9C mutation was also shown to cause a blunted β-agonist response in human iPSC-CMs in experiments performed using 3D human EHTs (14).
Evidence that lusitropy is necessary from TnI phosphorylation sites serine 22/23 loss of function models
The role of TnI phosphorylation in modulating contractility has been studied in several transgenic mouse models either substituting the unphosphorylatable slow skeletal TnI for native cardiac TnI or by modifying the phosphorylatable serines, to non-phosphorylated (Ala substitution) or pseudophosphorylated (Asp or Glu substitution) forms.
One of the most extensively studied models is the slow skeletal troponin overexpression model where cTnI in the heart is completely replaced with ssTnI that does not have the N-terminal phosphorylatable peptide (15–17). The substitution increases Ca2+ sensitivity of isometric tension in myofibrils (pCa50 is 5.81 WT, 6.13 TG) but also blunts the effect of PKA phosphorylation (Δ pCa50 = 0.146 in WT but 0.08 in TG). In myocytes, isoprenaline stimulation accelerated relaxation 1.7-fold in WT but only 1.3-fold in TG indicating a blunting of the lusitropic response that is also indicated by measurements of contraction kinetics on intact heart muscle strips (fmin). In Langendorf working heart studies the effect of Iso on LVdp/dtmin is also blunted and the blunting of lusitropy is also evident in PV loops. The inotropic effect of Iso is not greatly altered in unloaded studies but, like the PLB KO, is partially blunted in loaded (auxotonic) muscles (Figure 1).
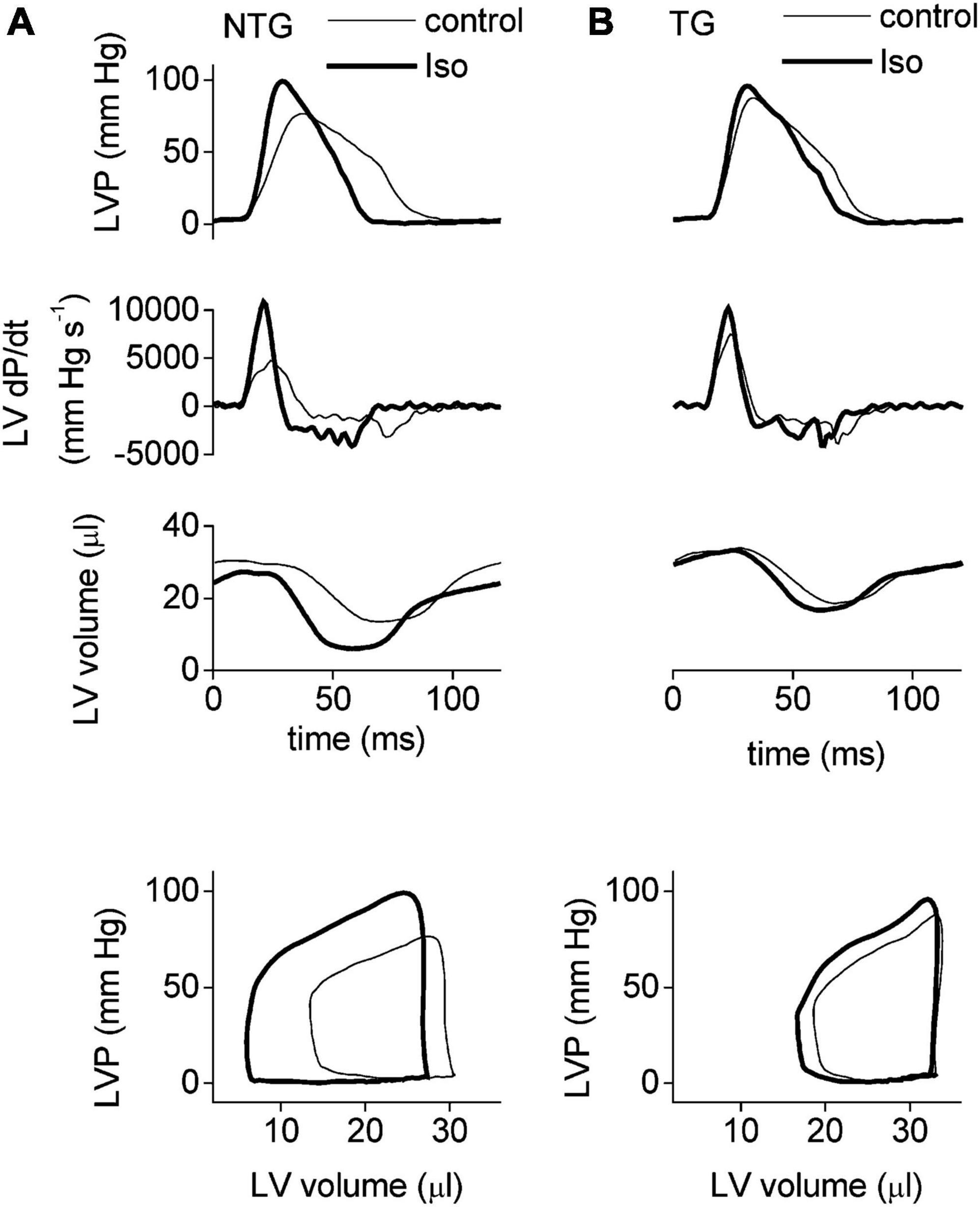
Figure 1. The adrenergic response in NTG (A) and ssTnI (B) TG mice. Examples of LV pressure traces (top set of panels), their derivatives (second set of panels), and the corresponding LV volume (third set of panels) recorded by a Millar microconductance catheter-manometer during ejecting heart experiments at 20 cm H2O preload. The bottom set of panels illustrate the steady-state pressure–volume loops derived from the instantaneous LV pressure and volume traces above (first and third panels). Thin lines indicate baseline conditions and thick lines indicate the signal during stimulation with isoprenaline (10 nm). Faster relaxation in presence of Iso is apparent in NTG but the effect is suppressed In the unphosphorylatable mutant TG mouse heart. Reproduced from Layland et al. (17) with permission.© The Physiological Society 2004.
Substituting a non-native TnI and overexpression may have unforeseen physiological off target effects. Better models have been produced by mutating the phosphorylatable serines in native cTnI. The model described by Pi et al. overexpressed cTnI with ser22 and 23 mutated to Alanine (unphosphorylatable); moreover the transgenic mice were bred with cTnI null mice to avoid any interactions between native and transgenic cTnI (18, 19). The blunting of lusitropy was clear: as measured by ATPase in myofibrils or tension in skinned muscle fibers, the Ca2+-sensitivity was shifted 1.3-fold in NTG by Iso but no shift was detected in TG (18). In intact myocytes 2.5 nM Iso decreased relaxation time by 37% in WT but only 18% in TG. Yasuda et al. also studied Ser > Ala replacement in transfected myocytes, finding Iso decreased relaxation time by 36% in NTG but only 9% in TG (20).
The alternative model- substituting aspartic acid for the two serines to create a pseudophosphorylated troponin I has also been studied in transgenic mice and transfected myocytes. The first study used a mouse model overexpressing Asp 22/23 (21) and 95% replacement. It was noted that the mouse model was healthy but had enhanced cardiac function (as measured by Millar catheter) with faster contraction and slower relaxation, however, the response to β- adrenergic stimulation was not significantly different from NTG. Another group produced a similar model with either Asp 22/23 or Asp 22/23/43/45/144 overexpressed and studied lines with almost complete protein replacement (22). In vitro the Asp22/23 mouse myofibrillar Ca2+ sensitivity was lower than NTG, as expected for pseudophosphorylation (ΔpCa50 = 0.20), but upon PKA phosphorylation the Ca2+ sensitivity was not changed compared with ΔpCa50 of 0.23 in NTG. In the intact beating heart (Millar catheter), the rate of relaxation (dP/dTmin) was increased from –6,869 to –8,651 mmHg/s by Iso in NTG and –10,598 to –12,099 in Asp22/23 mouse. Although the studies of Takimoto et al. and Sakthivel et al. show little evidence that loss of the TnI Ca2+-sensitivity shift causes lusitropy, the study by Yasuda et al. (20) using the same Asp22/23 model did show a blunting of lusitropy in myocytes. Myocyte twitch tension was measured by attaching microfibre probes. In Wild-type, ttb75 was decreased from 69 to 39 ms on treatment with Iso, a 44% change, whilst in Asp22/23 myocytes, ttb75 was decreased from 44 to 38 ms, a 14% change. A similar blunting was measured in myocytes transfected with Asp22/23: Iso produced a 33% reduction in relaxation time in WT but only 16% in Asp22/23. In addition, this study recapitulated the previous results with Ala22/23 and with ssTnI substitutions in both TG mouse and transfected myocytes (Table 1).
“Is PLB or TnI the prima donna in β adrenergic induced lusitropy?”
This question was raised in a key Circulation Research editorial that discussed the findings of Yasuda in relation to the other published work at the time (23). It is still a very pertinent question. Essentially we need to know whether the release of Ca2+ from troponin or the removal of Ca2+ from the sarcoplasm by SERCA is rate limiting for relaxation. The answer to the question depends on the balance of rates of these two processes, which themselves depend on conditions, especially temperature since many experiments are conducted at below normal body temperature but also force, since these rates are different in unloaded and isometric muscle. Measurement technique, experimental medium and most likely also the animal model that is used are also critical.
Two studies have indicated that (in unloaded rat myofibrils at least) Ca2+ dissociating from TnC becomes rate limiting at 37°C whereas SERCA activity is limiting at lower, non-physiological temperatures (24, 25). Experiments do not support a dominating role for either PLB or TnI phosphorylation. Li et al. (8) proposed TnI phosphorylation contributes just 14–18% of lusitropy in unloaded muscle but nothing in isometric muscle based on comparison of WT with PLB-KO mouse. Yasuda et al. (20) predicted an opposite pattern with 75% contributed by TnI phosphorylation in unloaded myocytes. Layland et al. (17) argue that cTnI has the pivotal role in the positive inotropic response of the murine heart to β-adrenergic stimulation, under all loading conditions but agree that it is most evident in the auxotonically loaded ejecting heart.
It seems unlikely that either TnI or phospholamban phosphorylation is actually the “prima donna” but rather that the control of lusitropy is a duet. Wolska et al. (26) studied a transgenic mouse with both a PLB KO and ssTnI substitution. In isolated papillary muscle, the study confirmed that single mutant PLB KO enhances relaxation rate but uncouples it from Iso stimulation whilst single mutant ssTnI replacement slows relaxation and also uncouples. In the double mutant (PLBKO/ssTnI) relaxation is enhanced relative to wild-type (like the PLBKO) but the relaxation rate is still uncoupled from Iso stimulation. Thus, in the double KO TnI and PLB appear to act independently and additively. It is likely that phosphorylation of both PLB and cTnI contribute to the increased rate of relaxation during β-adrenergic stimulation and that deficiency of either will lead to suppression of lusitropy (23).
Somewhat disappointingly, none of these studies looked at the effects of chronic adrenergic stimulation. The transgenic mouse models often showed no cardiac phenotype at rest and this is entirely to be expected since sedentary mouse in the typical animal cage environment are not subjected to any stress. However, studies on mutations in troponin and other thin filament proteins that are demonstrated to be causative of dilated cardiomyopathy have provided a link between suppression of lusitropy and heart failure.
Mutations can also suppress lusitropy
Many in vitro studies have demonstrated that mutations in thin filament proteins associated with inherited cardiomyopathies abolish the relationship between Ca2+ sensitivity and TnI phosphorylation by PKA and would therefore likely impair lusitropy. This trait, which we have termed “uncoupling,” was first noted in 2001 (27, 28). In 2007-8 three publications studying the recently discovered TNNC1 G159D mutation showed uncoupling with recombinant mutant troponin, with troponin extracted from a patient with the mutation and with rat trabecula with the mutation exchanged in myocytes (29–31). Subsequently, almost every thin filament mutation that was tested proved to be uncoupled. This was summarized by Messer and Marston (1) and Table 2 lists all the reports of uncoupling to date. In wild-type the mean ΔpCa50 is –0.31 (mean of 8 studies) (32) whereas it is close to zero in the mutations studied. It is relevant to note that in in vitro experiments, uncoupling is always complete, irrespective of the mutation and that uncoupling is associated with both HCM and DCM-causing mutations. In the case of DCM mutations a case can be made that this is causative of the disease since it is the only mutation-related property that is common to every thin-filament related DCM mutation (1, 33, 38), whereas for HCM, the increase in Ca2+-sensitivity is probably the key driver of the cardiomyopathy. Mechanistically, uncoupling usually involves an impaired response of the thin filaments to TnI phosphorylation (34), however, the cTnI R21C mutation has a mechanism whereby the mutation interferes with the phosphorylation process itself (35). Another mechanism that could generate impaired lusitropy is if the balance of phosphorylation of TnI and PLB in response to Iso is disturbed as suggested by Najafi et al. (36).
There are a few reported cases of uncoupling were the mutation is in thick filament or cytoskeletal proteins or occurs with no known mutation (50, 54, 55), however, there is also clear evidence that DCM-associated titin truncation (TTNtv) mutations are fully coupled and that abnormal stiffness and disabled length-dependent activation may be more important for the pathology (56, 57).
Uncoupling implies impaired lusitropy but this has only been tested in a few instances, listed in Table 1. Methodology varies widely: myofibril, iPSC contractility and myocyte contractility, trabecula and papillary muscles and Langendorf-mounted heart. In intact animal, Millar catheter, Echocardiography and Cine-MRI have been used. It is remarkable that mostly these techniques give a uniform result: a 30–50% reduction in ttb90 on adding PKA or β1 adrenergic stimulation in the WT compared to a 10–20% reduction in the HCM or DCM mutant animal. Figure 2 shows an example from the work of Wilkinson et al. A possible exception is the DCM-causing D230N mutations in tropomyosin (58) which is uncoupled in single filament assays (Table 2) but shows very little blunting of lusitropy in unloaded myocytes. In general, the range of the lusitropy parameter in cardiomyopathic mutant studies is very similar to the phosphomimetic transgenic animals, confirming that uncoupling due to mutations suppresses lusitropy (59) and revealing this important role for cTnI phosphorylation in cardiac muscle regulation.
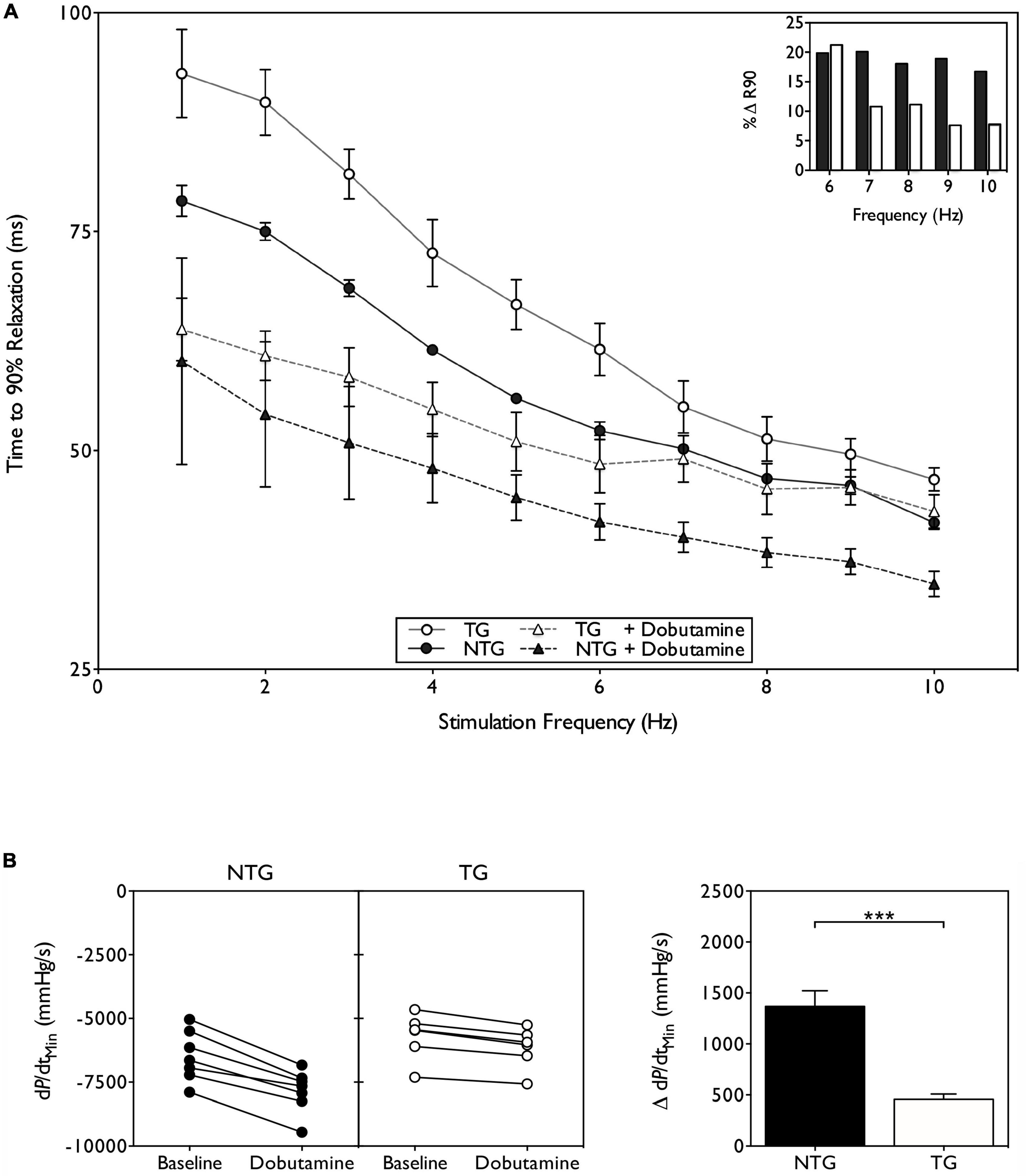
Figure 2. Suppression of lusitropy in ACTC E361G transgenic mouse. (A) Effect of dobutamine on intact papillary muscle contractility. Papillary muscles, isolated from both ACTC E361G (n = 5) and NTG mice (n = 5), were stimulated with the addition of 10 μM dobutamine to the perfusion solution at 37°C. Time to 90% relaxation is plotted against stimulation frequency; (inset) percent change of ttb90 with dobutamine treatment at physiologically relevant frequencies. (B) The effect of acute dobutamine treatment on cardiac performance determined by Millar catheter. Data are presented as mean ± SE of 6 ACTC E361G (open bars) mice and 7 NTG (solid bars) mice. The change of rate of pressure decline (dP/dtmin) on treatment with dobutamine is plotted (left) and mean values plotted in a bar chart (right). ***P = 0.001, unpaired student’s t-test. Data from Wilkinson et al. (59).
The next stage is to determine whether impaired lusitropy can lead to cardiac dysfunction. The transgenic mice studies have found that the majority of DCM mutations in thin filament proteins produce little or no cardiac phenotype, with the exception of a few TnT and Tm mouse models (41, 67, 68). This is to be expected if the defect is in the response to stress.
Very few studies have considered whether chronic stress could induce cardiac dysfunction. The most well documented study is by Wilkinson et al. (59). This study used the ACTC E361G DCM-associated mouse model with the mutant expressed at 50% of total actin, as was commonly found in patients. Initial studies indicated that the loss of lusitropy was the only cardiac dysfunction linked to the mutation and that the mice had a normal phenotype at rest up to 22 months (37). It was hypothesized that the loss of cardiac reserve due to suppressed lusitropy would predispose the ACT E361G mouse hearts to failure under chronic adrenergic stress. Mice were treated with high doses of Angiotensin II applied by osmotic minipump for 4 weeks. In E361G mice the angiotensin II treatment induced mild systolic dysfunction, as measured by Millar catheter, whilst having no effect on the NTG controls. Compared with the NTG mice ACTC E361G mice had significantly lower rates of pressure increase and decrease as well as reduced end-systolic pressure. As a result cardiac output and ejection fraction were approximately half the value in NTG, indicating that uncoupling had induced contractile dysfunction under chronic stress, characteristic of the early stages of DCM.
Restoration of lusitropy as a potential treatment for cardiomyopathies
Studies on the effects of mutations that uncouple TnI phosphorylation from the Ca2+-sensitivity shift indicate that they probably act by inducing a subtle, phosphorylation-dependent change in the dynamics of troponin (69–74), most clearly indicated by a recent study on the TnC G159D mutation (34). Remarkably, it has been found that several small molecules are capable of fully restoring the Ca2+-sensitivity shift in vitro and restoring lusitropy to mutated cardiomyocytes (7, 45, 75, 76). These small molecules (EGCG, SilybinB, Resveratrol) therefore have potential for treatment of cardiac dysfunction due to suppression of lusitropy, particularly by thin filament mutations but also as a tool for probing for cardiac dysfunction due to suppression of lusitropy. For instance, Tadano et al. in HCM mouse (77) and Mou et al. (78), using a rat abdominal aortic constriction model of heart failure, have demonstrated that EGCG corrects cardiac systolic and diastolic dysfunction and prevents cardiac remodeling.
Is there a connection between suppression of lusitropy and human cardiomyopathy?
Based on the animal studies of the consequences of uncoupling, we have proposed that patients with uncoupling mutations that are associated with DCM would have impaired lusitropy and that this could contribute to the heart failure phenotype. A thorough investigation of the state of knowledge of idiopathic DCM around this possibility has revealed no direct evidence for the possibility since the question has not been investigated.
There is indirect evidence compatible with our hypothesis, although, of course, other mechanisms may play a part. Mutations that cause suppressed lusitropy also have a reduced contractile reserve when challenged with β1 agonists in transgenic mouse models (59, 79). A significant association between the absence of left ventricular contractile reserve and increased rate of cardiovascular events, cardiac death and all- cause mortality has been demonstrated (80). Several studies have reported that IDCM patient cohorts tend to segregate in to two groups: those that respond normally to dobutamine and those with a reduced response, usually measured as contractile reserve (Table 3). Moreover, the patients with a reduced response to dobutamine have a more severe prognosis that the other group and also do not respond to standard heart failure medication [see meta-analysis by Waddingham et al. (81)].
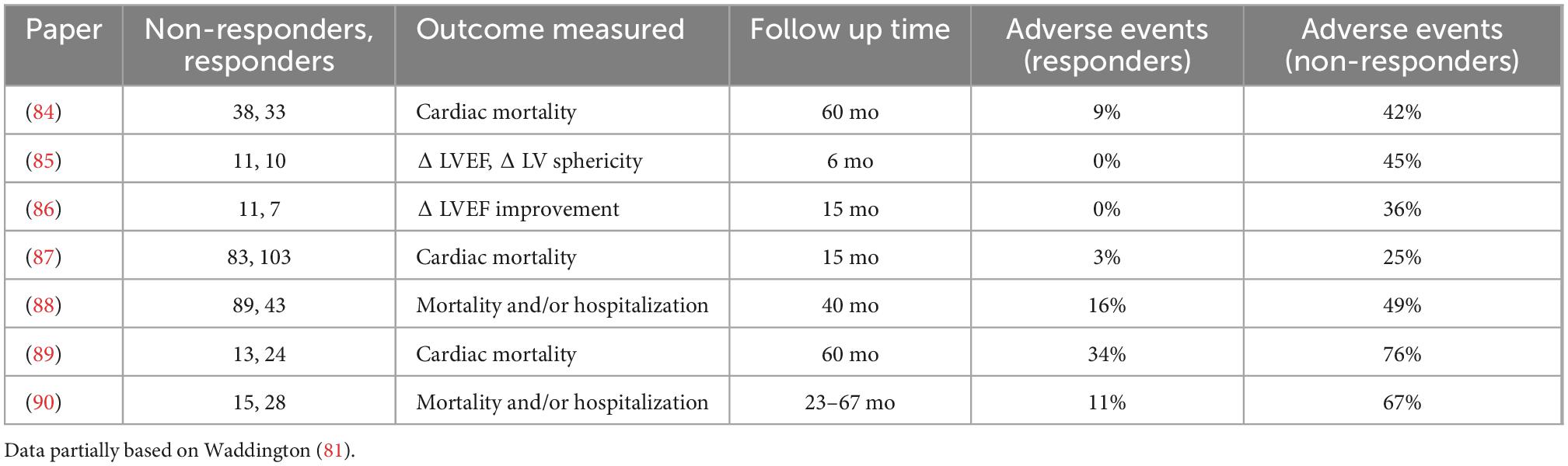
Table 3. Studies showing that reduced response to adrenergic stimulation is correlated with cardiac adverse outcome in patients with idiopathic DCM.
The behavior of the non-responders matches that which would be expected if they had familial DCM mutations that suppressed lusitropy whereas the responders probably correspond to patients with idiopathic DCM caused by non-genetic factors where, both at the single filament and patient levels, lusitropy has been demonstrated to be normal (82, 83).
Unfortunately this hypothesis has not been tested in IDCM patients. There is no clinical study that correlates thin filament mutations with reduced response to β1 stimulation, nor is lusitropy (relaxation rate increase or twitch duration decrease) commonly measured in Echo or MRI analysis of patients although protocols to do so are available. To test this hypothesis we need to know whether cases of familial DCM caused by mutations correlate with the non-responders group and also whether lusitropy is suppressed in the non-responders.
It is possible that suppression of lusitropy could also play a role in other cardiomyopathies. It is consistently observed that thin filament mutations that cause HCM are associated with uncoupling (Table 2) and loss of lusitropy in cells and intact animals (see ACTC E99K, TNNT2 R92Q in Table 1). Enhanced Ca2+-sensitivity is the predominant effect of HCM mutations (91–95). Impaired relaxation is characteristic of HCM. Suppression of lusitropy may play a role here, however, it would be very difficult to unravel what contribution loss of lusitropy makes to the HCM phenotype since the increased Ca2+ sensitivity and the suppressed lusitropy would both compromise relaxation.
HFpEF is a heterogeneous disease associated with diastolic dysfunction. HFpEF patients have a high prevalence of a blunted response to exercise which may be linked to suppressed lusitropy. However, this has not been measured in any of the studies to date (96, 97).
Conclusion
Basic and animal studies indicate that specific suppression of lusitropy can induce symptoms of heart failure under stress. In the human heart, where stress is far more common than in laboratory animals, there is considerable circumstantial evidence that impaired lusitropy whether due to cardiomyopathic mutation or other causes, can contribute to heart failure. It is predicted that such symptoms of heart failure would not be amenable to conventional heart failure therapy but may benefit from small molecules that have been shown to restore lusitropy in laboratory studies. We believe these issues should be taken into account in clinical investigations of cardiomyopathy.
Author contributions
Both authors listed have made a substantial, direct, and intellectual contribution to the work, and approved it for publication.
Funding
This work was funded by the British Heart Foundation Programme (grant RG/11/20/29266) to SM and the National Institutes of Health, National Heart, Lung and Blood Institute (grant RO1 HL128683, RO1 HL160966 and RO1 AR077802) awarded to JP.
Acknowledgments
We are grateful to Profs. M. Kaski (University College London), J. Cleland (University of Glasgow), and G. Limongelli (University Campania) for informed discussions.
Conflict of interest
The authors declare that the research was conducted in the absence of any commercial or financial relationships that could be construed as a potential conflict of interest.
Publisher’s note
All claims expressed in this article are solely those of the authors and do not necessarily represent those of their affiliated organizations, or those of the publisher, the editors and the reviewers. Any product that may be evaluated in this article, or claim that may be made by its manufacturer, is not guaranteed or endorsed by the publisher.
References
1. Messer A, Marston S. Investigating the role of uncoupling of troponin I phosphorylation from changes in myofibrillar Ca2+-sensitivity in the pathogenesis of cardiomyopathy. Front Physiol. (2014) 5:315. doi: 10.3389/fphys.2014.00315
2. Zabrouskov V, Ge Y, Schwartz J, Walker JW. Unraveling molecular complexity of phosphorylated human cardiac troponin I by top down electron capture dissociation/electron transfer dissociation mass spectrometry. Mol Cell Proteomics. (2008) 7:1838–49. doi: 10.1074/mcp.M700524-MCP200
3. Chen Y-C, Sumandea MP, Larsson L, Moss RL, Ge Y. Dissecting human skeletal muscle troponin proteoforms by top-down mass spectrometry. J Musc Res Cell Motil. (2015) 36:169–81. doi: 10.1007/s10974-015-9404-6
4. Robertson SP, Johnson JD, Holroyde MJ, Kranias EG, Potter JD, Solaro RJ. The effect of troponin I phosphorylation on the Ca2+-binding properties of the Ca2+-regulatory site of bovine cardiac troponin. J Biol Chem. (1982) 257:260–3.
5. Weiss S, Oz S, Benmocha A, Dascal N. Regulation of cardiac L-Type Ca2+ channel CaV1.2 via the beta-adrenergic-cAMP-protein kinase A pathway. Circ Res. (2013) 113:617–31. doi: 10.1161/circresaha.113.301781
6. Ablorh N-AD, Thomas DD. Phospholamban phosphorylation, mutation, and structural dynamics: a biophysical approach to understanding and treating cardiomyopathy. Biophys Rev. (2015) 7:63–76. doi: 10.1007/s12551-014-0157-z
7. Marston S. Recent studies of the molecular mechanism of lusitropy due to phosphorylation of cardiac troponin I by protein kinase A. J Muscle Res Cell Motil. (2022) 43: doi: 10.1007/s10974-022-09630-4
8. Li L, Chu G, Kranias EG, Bers DM. Cardiac myocyte calcium transport in phospholamban knockout mouse: relaxation and endogenous CaMKII effects. Am J Physiol. (1998) 274(4 Pt 2):H1335–47. doi: 10.1152/ajpheart.1998.274.4.H1335
9. Schmitt JP, Kamisago M, Asahi M, Li GH, Ahmad F, Mende U, et al. Dilated cardiomyopathy and heart failure caused by a mutation in phospholamban. Science. (2003) 299:1410–3.
10. Medeiros A, Biagi DG, Biagi DG, Sobreira TJP, de Oliveira PSL, Negrão CE, et al. Mutations in the human phospholamban gene in patients with heart failure. Am Heart J. (2011) 162:1088.e–95.e. doi: 10.1016/j.ahj.2011.07.028
11. Fish M, Shaboodien G, Kraus S, Sliwa K, Seidman CE, Burke MA, et al. Mutation analysis of the phospholamban gene in 315 South Africans with dilated, hypertrophic, peripartum and arrhythmogenic right ventricular cardiomyopathies. Sci Rep. (2016) 6:22235. doi: 10.1038/srep22235
12. Walsh R, Thomson KL, Ware JS, Funke BH, Woodley J, McGuire KJ, et al. Reassessment of mendelian gene pathogenicity using 7,855 cardiomyopathy cases and 60,706 reference samples. Genet Med. (2017) 19:192–203. doi: 10.1038/gim.2016.90
13. Abrol N, de Tombe PP, Robia SL. Acute inotropic and lusitropic effects of cardiomyopathic R9C mutation of phospholamban. J Biol Chem. (2015) 290:7130–40. doi: 10.1074/jbc.M114.630319
14. Ceholski DK, Turnbull IC, Kong C-W, Koplev S, Mayourian J, Gorski PA, et al. Functional and transcriptomic insights into pathogenesis of R9C phospholamban mutation using human induced pluripotent stem cell-derived cardiomyocytes. J Mol Cell Cardiol. (2018) 119:147–54. doi: 10.1016/j.yjmcc.2018.05.007
15. Fentzke RC, Buck SH, Patel JR, Lin H, Wolska BM, Stojanovic MO, et al. Impaired cardiomyocyte relaxation and diastolic function in transgenic mice expressing slow skeletal troponin I in the heart. J Physiol (Lond). (1999) 517(Pt 1):143–57. doi: 10.1111/j.1469-7793.1999.0143z.x
16. Kentish JC, McCloskey DT, Layland J, Palmer S, Leiden JM, Martin AF, et al. Phosphorylation of troponin I by protein kinase A accelerates relaxation and crossbridge cycle kinetics in mouse ventricular muscle. Circ Res. (2001) 88:1059–65. doi: 10.1161/hh1001.091640
17. Layland J, Grieve DJ, Cave AC, Sparks E, Solaro RJ, Shah AM. Essential role of troponin I in the positive inotropic response to isoprenaline in mouse hearts contracting auxotonically. J Physiol. (2004) 556:835–47. doi: 10.1113/jphysiol.2004.061176
18. Pi Y-Q, Kemnitz KR, Zhang D, Kranias EG, Walker JW. Phosphorylation of troponin I controls cardiac twitch dynamics. Evidence from phosphorylation site mutants expressed on a troponin I-null background in mice. Circ Res. (2002) 90:649–56. doi: 10.1161/01.res.0000014080.82861.5f
19. Pi Y, Zhang D, Kemnitz KR, Wang H, Walker JW. Protein kinase C and A sites on troponin I regulate myofilament Ca2+ sensitivity and ATPase activity in the mouse myocardium. J Physiol. (2003) 552:845–57. doi: 10.1113/jphysiol.2003.045260
20. Yasuda S, Coutu P, Sadayappan S, Robbins J, Metzger JM. Cardiac transgenic and gene transfer strategies converge to support an important role for troponin I in regulating relaxation in cardiac myocytes. Circ Res. (2007) 101:377–86. doi: 10.1161/CIRCRESAHA.106.145557
21. Takimoto E, Soergel DG, Janssen PM, Stull LB, Kass DA, Murphy AM. Frequency- and afterload-dependent cardiac modulation in vivo by troponin I with constitutively active protein kinase A phosphorylation sites. Circ Res. (2004) 94:496–504. doi: 10.1161/01.res.0000117307.57798.f5
22. Sakthivel S, Finley NL, Rosevear PR, Lorenz JN, Gulick J, Kim S, et al. In vivo and in vitro analysis of cardiac troponin I phosphorylation. J Biol Chem. (2005) 280:703–14. doi: 10.1074/jbc.M409513200
23. Ramirez-Correa GA, Murphy AM. Is phospholamban or troponin I the “prima donna” in beta-adrenergic induced lusitropy? Circ Res. (2007) 101:326–7. doi: 10.1161/CIRCRESAHA.107.158873
24. Janssen PML, Stull LB, Marbaìn E. Myofilament properties comprise the rate-limiting step for cardiac relaxation at body temperature in the rat. Am J Physiol Heart Circ Physiol. (2002) 282:H499–507. doi: 10.1152/ajpheart.00595.2001
25. Little SC, Biesiadecki BJ, Kilic A, Higgins RSD, Janssen PML, Davis JP. The rates of Ca2+ dissociation and cross-bridge detachment from ventricular myofibrils as reported by a fluorescent cardiac troponin C. J Biol Chem. (2012) 287:27930–40. doi: 10.1074/jbc.M111.337295
26. Wolska BM, Arteaga GM, Peña JR, Nowak G, Phillips RM, Sahai S, et al. Expression of slow skeletal troponin I in hearts of phospholamban knockout mice alters the relaxant effect of beta-adrenergic stimulation. Circ Res. (2002) 90:882–8. doi: 10.1161/01.res.0000016962.36404.04
27. Deng Y, Schmidtmann A, Redlich A, Westerdorf B, Jaquet K, Thieleczek R. Effects of phosphorylation and mutation R145G on human cardiac troponin I function. Biochemistry. (2001) 40:14593–602. doi: 10.1021/bi0115232
28. Deng Y, Schmidtmann A, Kruse S, Filatov V, Heilmeyer LM Jr., Jaquet K, et al. Phosphorylation of human cardiac troponin I G203S and K206Q linked to familial hypertrophic cardiomyopathy affects actomyosin interaction in different ways. J Mol Cell Cardiol. (2003) 35:1365–74. doi: 10.1016/j.yjmcc.2003.08.003
29. Biesiadecki BJ, Kobayashi T, Walker JS, John Solaro R, de Tombe PP. The troponin C G159D mutation blunts myofilament desensitization induced by troponin I Ser23/24 phosphorylation. Circ Res. (2007) 100:1486–93. doi: 10.1161/01.RES.0000267744.92677.7f
30. Dong W, Xing J, Ouyang Y, An J, Cheung HC. Structural kinetics of cardiac troponin C mutants linked to familial hypertrophic and dilated cardiomyopathy in troponin complexes. J Biol Chem. (2008) 283:3424–32. doi: 10.1074/jbc.M703822200
31. Dyer E, Jacques A, Burch M, Kaski J, Marston S. *Functional effects of DCM mutation G159D in troponin C from an explanted heart. J Mol Cell Cardiol. (2008) 44:729–30.
32. Marston S. Why is there a limit to the changes in myofilament Ca2+-sensitivity associated with myopathy causing mutations? Front Physiol. (2016) 7:415. doi: 10.3389/fphys.2016.00415
33. Memo M, Messer AE, Leung M-C, Marston SB. Mutations in thin filament proteins that cause familial dilated cardiomyopathyuncouple troponin I phosphorylation from changes in myofibrillar Ca2+-Sensitivity. Biophys J. (2012) 102:614a. doi: 10.1016/j.bpj.2011.11.3348
34. Yang Z, Marston S, Gould I. Modulation of structure and dynamics of cardiac troponin by phosphorylation and mutations revealed by molecular dynamics simulations. ChemRxiv [Preprint]. (2022). doi: 10.26434/chemrxiv-2022-kngl7
35. Wang Y, Pinto J, Sancho Solis R, Dweck D, Liang J, Diaz-Perez Z, et al. The generation and functional characterization of knock in mice harboring the cardiac troponin I-R21C mutation associated with hypertrophic cardiomyopathy. J Biol Chem. (2011) 287:2156–67. doi: 10.1074/jbc.M111.294306
36. Najafi A, Sequeira V, Helmes M, Bollen IAE, Goebel M, Regan JA, et al. Selective phosphorylation of PKA targets after β-adrenergic receptor stimulation impairs myofilament function in Mybpc3-targeted HCM mouse model. Cardiovasc Res. (2016) 110:200–14. doi: 10.1093/cvr/cvw026
37. Song W, Dyer E, Stuckey D, Leung M-C, Memo M, Mansfield C, et al. Investigation of a transgenic mouse model of familial dilated cardiomyopathy. J Mol Cell Cardiol. (2010) 49:380–9. doi: 10.1016/j.yjmcc.2010.05.009
38. Memo M, Leung M-C, Ward DG, dos Remedios C, Morimoto S, Zhang L, et al. Mutations in thin filament proteins that cause familial dilated cardiomyopathy uncouple troponin I phosphorylation from changes in myofibrillar Ca2+-sensitivity. Cardiovasc Res. (2013) 99:65–73. doi: 10.1093/cvr/cvt071
39. Dyer E, Jacques A, Hoskins A, Ward D, Gallon C, Messer A, et al. Functional analysis of a unique troponin C mutation, Gly159Asp that causes familial dilated cardiomyopathy, studied in explanted heart muscle. Circ Heart Fail. (2009) 2:456–64. doi: 10.1161/CIRCHEARTFAILURE.108.818237
40. Pinto JR, Siegfried JD, Parvatiyar MS, Li D, Norton N, Jones MA, et al. Functional characterization of TNNC1 rare variants identified in dilated cardiomyopathy. J Biol Chem. (2011) 286:34404–12. doi: 10.1074/jbc.M111.267211
41. Du CK, Morimoto S, Nishii K, Minakami R, Ohta M, Tadano N, et al. Knock-in mouse model of dilated cardiomyopathy caused by troponin mutation. Circ Res. (2007) 101:185–94.
42. Inoue T, Kobirumaki-Shimozawa F, Kagemoto T, Fujii T, Terui T, Kusakari Y, et al. Depressed frank-starling mechanism in the left ventricular muscle of the knock-in mouse model of dilated cardiomyopathy with troponin T deletion mutation ΔK210. J Mol Cell Cardiol. (2013) 63:69–78. doi: 10.1016/j.yjmcc.2013.07.001
43. Carballo S, Robinson P, Otway R, Fatkin D, Jongbloed JD, de Jonge N, et al. Identification and functional characterization of cardiac troponin I as a novel disease gene in autosomal dominant dilated cardiomyopathy. Circ Res. (2009) 105:375–82. doi: 10.1161/CIRCRESAHA.109.196055
44. Song W, Dyer E, Stuckey D, Copeland O, Leung M, Bayliss C, et al. Molecular mechanism of the Glu99lys mutation in cardiac actin (ACTC gene) that causes apical hypertrophy in man and mouse. J Biol Chem. (2011) 286:27582–93. doi: 10.1074/jbc.M111.252320
45. Papadaki M, Vikhorev PG, Marston SB, Messer AE. Uncoupling of myofilament Ca2+ sensitivity from troponin I phosphorylation by mutations can be reversed by epigallocatechin-3-gallate. Cardiovasc Res. (2015) 108:99–110. doi: 10.1093/cvr/cvv181
46. Alves ML, Dias FAL, Gaffin RD, Simon JN, Montminy EM, Biesiadecki BJ, et al. Desensitization of myofilaments to Ca2+ as a therapeutic target for hypertrophic cardiomyopathy with mutations in thin filament proteins. Circ Cardiovasc Genet. (2014) 7:132–43. doi: 10.1161/CIRCGENETICS.113.000324
47. Schmidtmann A, Lindow C, Villard S, Heuser A, Mügge A, Gessner R, et al. Cardiac troponin C-L29Q, related to hypertrophic cardiomyopathy, hinders the transduction of the protein kinase A dependent phosphorylation signal from cardiac troponin I to C. FEBS J. (2005) 272:6087–97. doi: 10.1111/j.1742-4658.2005.05001.x
48. Li AY, Stevens CM, Liang B, Rayani K, Little S, Davis J, et al. Familial hypertrophic cardiomyopathy related cardiac troponin C L29Q mutation alters length-dependent activation and functional effects of phosphomimetic troponin I*. PLoS One. (2013) 8:e79363. doi: 10.1371/journal.pone.0079363
49. Messer AE, Bayliss CR, El-Mezgueldi M, Redwood CS, Ward DG, Papadaki M, et al. Mutations in troponin T associated with hypertrophic cardiomyopathy increase Ca2+-sensitivity and suppress the modulation of Ca2+-sensitivity by troponin I phosphorylation. Arch Biochem Biophys. (2016) 601:113–20. doi: 10.1016/j.abb.2016.03.027
50. Bayliss CR, Jacques AM, Leung M-C, Ward DG, Redwood CS, Gallon CE, et al. Myofibrillar Ca2+-sensitivity is uncoupled from troponin I phosphorylation in hypertrophic obstructive cardiomyopathy due to abnormal troponin T. Cardiovasc Res. (2012) 97:500–8. doi: 10.1093/cvr/cvs322
51. Cheng Y, Rao V, Tu A-Y, Lindert S, Wang D, Oxenford L, et al. Troponin I mutations R146G and R21C alter cardiac troponin function, contractile properties and modulation by PKA-mediated phosphorylation. J Biol Chem. (2015) 290:27749–66. doi: 10.1074/jbc.M115.683045
52. Dvornikov AV, Smolin N, Zhang M, Martin JL, Robia SL, de Tombe PP. Restrictive cardiomyopathy troponin-I R145W mutation does not perturb myofilament length dependent activation in human cardiac sarcomeres. J Biol Chem. (2016) 291:21817–28. doi: 10.1074/jbc.M116.746172
53. Cheng Y, Lindert S, Oxenford L, Tu A-Y, McCulloch AD, Regnier M. Effects of cardiac troponin I mutation P83S on contractile properties and the modulation by PKA-mediated phosphorylation. J Physi Chem B. (2016) 120:8238–53. doi: 10.1021/acs.jpcb.6b01859
54. Knoell R, Kostin S, Klede S, Savvatis K, Klinge L, Stehle I, et al. A common MLP (muscle LIM protein) variant is associated with cardiomyopathy. Circ Res. (2010) 106:695–704. doi: 10.1161/CIRCRESAHA.109.206243
55. Stöhr A, Friedrich FW, Flenner F, Geertz B, Eder A, Schaaf S, et al. Contractile abnormalities and altered drug response in engineered heart tissue from Mybpc3-targeted knock-in mice. J Mol Cell Cardiol. (2013) 63:189–98. doi: 10.1016/j.yjmcc.2013.07.011
56. Vikhorev PG, Smoktunowicz N, Munster AB, Copeland O, Kostin S, Montgiraud C, et al. Abnormal contractility in human heart myofibrils from patients with dilated cardiomyopathy due to mutations in TTN and contractile protein genes. Sci Rep. (2017) 7:14829. doi: 10.1038/s41598-017-13675-8
57. Vikhorev PG, Vikhoreva NN, Yeung W, Li A, Lal S, Remedios CGD, et al. Titin-truncating mutations associated with dilated cardiomyopathy alter length-dependent activation and its modulation via phosphorylation. Cardiovasc Res. (2020) 118:241–53. doi: 10.1093/cvr/cvaa316
58. Lynn ML, Grinspan LT, Holeman TA, Jimenez J, Strom J, Tardiff JC. The structural basis of alpha-tropomyosin linked (Asp230Asn) familial dilated cardiomyopathy. J Mol Cell Cardiol. (2017) 108:127–37. doi: 10.1016/j.yjmcc.2017.06.001
59. Wilkinson R, Song W, Smoktunowicz N, Marston S. A dilated cardiomyopathy mutation blunts adrenergic response and induces contractile dysfunction under chronic angiotensin II stress. Am J Physiol Heart Circ Physiol. (2015) 309:H1936–46. doi: 10.1152/ajpheart.00327.2015
60. Li L, Desantiago J, Chu G, Kranias EG, Bers DM. Phosphorylation of phospholamban and troponin I in β-adrenergic-induced acceleration of cardiac relaxation. Am J Physiol Heart Circ Physiol. (2000) 278:H769–79. doi: 10.1152/ajpheart.2000.278.3.h769
61. Schober T, Huke S, Venkataraman R, Gryshchenko O, Kryshtal D, Hwang HS, et al. Myofilament Ca sensitization increases cytosolic Ca binding affinity, alters intracellular Ca homeostasis, and causes pause-dependent Ca triggered arrhythmia. Circ Res. (2012) 111:170–9. doi: 10.1161/CIRCRESAHA.112.270041
62. Sirenko SG, Potter JD, Knollmann BC. Differential effect of troponin T mutations on the inotropic responsiveness of mouse hearts–role of myofilament Ca2+ sensitivity increase. J Physiol. (2006) 575(Pt 1):201–13. doi: 10.1113/jphysiol.2006.107557
63. Javadpour MM, Tardiff JC, Pinz I, Ingwall JS. Decreased energetics in murine hearts bearing the R92Q mutation in cardiac troponin T. J Clin Invest. (2003) 112:768–75.
64. Moore RK, Grinspan LT, Jimenez J, Guinto PJ, Ertz-Berger B, Tardiff JC. HCM-linked Δ160E cardiac troponin T mutation causes unique progressive structural and molecular ventricular remodeling in transgenic mice. J Mol Cell Cardiol. (2013) 58:188–98. doi: 10.1016/j.yjmcc.2013.02.004
65. Dweck D, Sanchez-Gonzalez MA, Chang AN, Dulce RA, Badger CD, Koutnik AP, et al. Long term ablation of protein kinase A (PKA)-mediated cardiac troponin I phosphorylation leads to excitation-contraction uncoupling and diastolic dysfunction in a knock-in mouse model of hypertrophic cardiomyopathy. J Biol Chem. (2014) 289:23097–111. doi: 10.1074/jbc.M114.561472
66. Vikhorev PG, Song W, Wilkinson R, Copeland O, Messer AE, Ferenczi MA, et al. The dilated cardiomyopathy-causing mutation ACTC E361G in cardiac muscle myofibrils specifically abolishes modulation of Ca(2+) regulation by phosphorylation of troponin I. Biophys J. (2014) 107:2369–80. doi: 10.1016/j.bpj.2014.10.024
67. Juan F, Wei D, Xiongzhi Q, Ran D, Chunmei M, Lan H, et al. The changes of the cardiac structure and function in cTnTR141W transgenic mice. Int J Cardiol. (2008) 128:83–90. doi: 10.1016/j.ijcard.2008.03.006
68. Tal L, Jimenez J, Tardiff JC. DCM-linked D230N tropomyosin mutation results in early dilatation and systolic dysfunction in mice. Biophys J. (2012) 102:356A.
69. Baryshnikova OK, Li MX, Sykes BD. Modulation of cardiac troponin C function by the cardiac-specific N-terminus of troponin I: influence of PKA phosphorylation and involvement in cardiomyopathies. J Mol Biol. (2008) 375:735–51. doi: 10.1016/j.jmb.2007.10.062
70. Dong X, Sumandea CA, Chen Y-C, Garcia-Cazarin ML, Zhang J, Balke CW, et al. Augmented phosphorylation of cardiac troponin I in hypertensive heart failure. J Biol Chem. (2012) 287:848–57. doi: 10.1074/jbc.M111.293258
71. Matsuo T, Takeda S, Oda T, Fujiwara S. Structures of the troponin core domain containing the cardiomyopathy-causing mutants studied by small-angle X-ray scattering. Biophys Physicobiol. (2015) 12:145–58. doi: 10.2142/biophysico.12.0_145
72. Mahmud Z, Dhami PS, Rans C, Liu PB, Hwang PM. Dilated cardiomyopathy mutations and phosphorylation disrupt the active orientation of cardiac troponin C. J Mol Biol. (2021) 433:167010. doi: 10.1016/j.jmb.2021.167010
73. Kekenes-Huskey PM, Lindert S, McCammon JA. Molecular basis of calcium-sensitizing and desensitizing mutations of the human cardiac troponin C regulatory domain: a multi-scale simulation study. PLoS Comput Biol. (2012) 8:e1002777. doi: 10.1371/journal.pcbi.1002777
74. Stevens CM, Rayani K, Singh G, Lotfalisalmasi B, Tieleman DP, Tibbits GF. Changes in the dynamics of the cardiac troponin C molecule explain the effects of Ca 2+-sensitizing mutations. J Biol Chem. (2017) 292:11915–26. doi: 10.1074/jbc.M116.770776
75. Sheehan A, Messer AE, Papadaki M, Choudhry A, Kren V, Biedermann D, et al. Molecular defects in cardiac myofilament Ca2+-regulation due to cardiomyopathy-linked mutations can be reversed by small molecules binding to troponin. Front Physiol. (2018) 9:243. doi: 10.3389/fphys.2018.00243
76. Alsulami K, Marston S. Small molecules acting on myofilaments as treatments for heart and skeletal muscle diseases. IJMS. (2020) 21:9599. doi: 10.3390/ijms21249599
77. Tadano N, Du C, Yumoto F, Morimoto S, Ohta M, Xie M, et al. Biological actions of green tea catechins on cardiac troponin C. Br J Pharmacol. (2010) 161:1034–43. doi: 10.1111/j.1476-5381.2010.00942.x
78. Mou Q, Jia Z, Luo M, Liu L, Huang X, Quan J, et al. Epigallocatechin-3-gallate exerts cardioprotective effects related to energy metabolism in pressure overload-induced cardiac dysfunction. Arch Biochem Biophys. (2022) 723:109217. doi: 10.1016/j.abb.2022.109217
79. Haddad F, Vrtovec B, Ashley EA, Deschamps A, Haddad H, Denault AY. The concept of ventricular reserve in heart failure and pulmonary hypertension: an old metric that brings us one step closer in our quest for prediction. Curr Opin Cardiol. (2011) 26:123–31. doi: 10.1097/hco.0b013e3283437485
80. Thein PM, Mirzaee S, Cameron JD, Nasis A. Left ventricular contractile reserve as a determinant of adverse clinical outcomes: a systematic review. Intern Med J. (2022) 52:186–97. doi: 10.1111/imj.14995
81. Waddingham PH, Bhattacharyya S, Zalen JV, Lloyd G. Contractile reserve as a predictor of prognosis in patients with non-ischaemic systolic heart failure and dilated cardiomyopathy: a systematic review and meta-analysis. Echo Res Pract. (2018) 5:1–9. doi: 10.1530/erp-17-0054
82. Messer AE, Jacques AM, Marston SB. Troponin phosphorylation and regulatory function in human heart muscle: dephosphorylation of Ser23/24 on troponin I could account for the contractile defect in end-stage heart failure. J Mol Cell Cardiol. (2007) 42:247–59. doi: 10.1016/j.yjmcc.2006.08.017
83. Parker JD, Landzberg JS, Bittl JA, Mirsky I, Colucci WS. Effects of beta-adrenergic stimulation with dobutamine on isovolumic relaxation in the normal and failing human left ventricle. Circulation. (2018) 84:1040–8. doi: 10.1161/01.cir.84.3.1040
84. Nagaoka H, Isobe N, Kubota S, Iizuka T, Imai S, Suzuki T, et al. Myocardial contractile reserve as prognostic determinant in patients with idiopathic dilated cardiomyopathy without overt heart failure. Chest. (1997) 111:344–50. doi: 10.1378/chest.111.2.344
85. Naqvi TZ, Goel RK, Forrester JS, Siegel RJ. Myocardial contractile reserve on dobutamine echocardiography predicts late spontaneous improvement in cardiac function in patients with recent onset idiopathic dilated cardiomyopathy. J Am Coll Cardiol. (1999) 34:1537–44.
86. Kitaoka H, Takata J, Yabe T, Hitomi N, Furuno T, Doi YL. Low dose dobutamine stress echocardiography predicts the improvement of left ventricular systolic function in dilated cardiomyopathy. Heart. (1999) 81:523. doi: 10.1136/hrt.81.5.523
87. Pratali L, Picano E, Otasevic P, Vigna C, Palinkas A, Cortigiani L, et al. Prognostic significance of the dobutamine echocardiography test in idiopathic dilated cardiomyopathy. Am J Cardiol. (2001) 88:1374–8. doi: 10.1016/s0002-9149(01)02116-6
88. Rigo F, Gherardi S, Galderisi M, Sicari R, Picano E. The independent prognostic value of contractile and coronary flow reserve determined by dipyridamole stress echocardiography in patients with idiopathic dilated cardiomyopathy. Am J Cardiol. (2007) 99:1154–8.
89. Stipac AV, Otašević P, Popović ZB, Čvorović V, Putniković B, Stanković I, et al. Prognostic significance of contractile reserve assessed by dobutamine-induced changes of Tei index in patients with idiopathic dilated cardiomyopathy. Eur J Echo. (2010) 11:264–70. doi: 10.1093/ejechocard/jep208
90. Parthenakis F, Patrianakos A, Nyktari E, Arfanakis D, Zacharis E, Vardas P. Prognostic value of NT-pro BNP, left ventricular inotropic reserve and cardiopulmonary exercise test in patients with non-ischemic dilated cardiomyopathy. Int J Cardiol. (2011) 147:326–8. doi: 10.1016/j.ijcard.2010.12.079
91. Huke S, Knollmann BC. Increased myofilament Ca2+-sensitivity and arrhythmia susceptibility. J Mol Cell Cardiol. (2010) 48:824–33. doi: 10.1016/j.yjmcc.2010.01.011
92. Willott RH, Gomes AV, Chang AN, Parvatiyar MS, Pinto JR, Potter JD. Mutations in troponin that cause HCM, DCM AND RCM: what can we learn about thin filament function? J Mol Cell Cardiol. (2010) 48:882–92. doi: 10.1016/j.yjmcc.2009.10.031
93. Marston SB. How do mutations in contractile proteins cause the primary familial cardiomyopathies? J Cardiovasc Transl Res. (2011) 4:245–55.
94. Spudich JA. Hypertrophic and dilated cardiomyopathy: four decades of basic research on muscle lead to potential therapeutic approaches to these devastating genetic diseases. Biophys J. (2014) 106:1236–49. doi: 10.1016/j.bpj.2014.02.011
95. Tadros HJ, Life CS, Garcia G, Pirozzi E, Jones EG, Datta S, et al. Meta-analysis of cardiomyopathy-associated variants in troponin genes identifies loci and intragenic hot spots that are associated with worse clinical outcomes. J Mol Cell Cardiol. (2020) 142:118–25. doi: 10.1016/j.yjmcc.2020.04.005
96. Kitzman DW, Higginbotham MB, Cobb FR, Sheikh KH, Sullivan MJ. Exercise intolerance in patients with heart failure and preserved left ventricular systolic function: failure of the Frank-Starling mechanism. J Am Coll Cardiol. (1991) 17:1065–72. doi: 10.1016/0735-1097(91)90832-t
Keywords: adrenergic stimulation, lusitropy, contractility, protein kinase A, hypertrophic cardiomyopathy, dilated cardiomyopathy
Citation: Marston S and Pinto JR (2023) Suppression of lusitropy as a disease mechanism in cardiomyopathies. Front. Cardiovasc. Med. 9:1080965. doi: 10.3389/fcvm.2022.1080965
Received: 26 October 2022; Accepted: 19 December 2022;
Published: 09 January 2023.
Edited by:
Kenneth Scott Campbell, University of Kentucky, United StatesReviewed by:
Farid Moussavi-Harami, University of Washington, United StatesChiara Tesi, University of Florence, Italy
Copyright © 2023 Marston and Pinto. This is an open-access article distributed under the terms of the Creative Commons Attribution License (CC BY). The use, distribution or reproduction in other forums is permitted, provided the original author(s) and the copyright owner(s) are credited and that the original publication in this journal is cited, in accordance with accepted academic practice. No use, distribution or reproduction is permitted which does not comply with these terms.
*Correspondence: Steven Marston, S.marston@imperial.ac.uk
†ORCID: Steven Marston, orcid.org/0000-0001-6054-6070; Jose Renato Pinto, orcid.org/0000-0001-9092-4976