- 1Institute of Physiology and Pharmacology, Lithuanian University of Health Sciences, Kaunas, Lithuania
- 2Department of Pediatrics, Medical Faculty, Lithuanian University of Health Sciences, Kaunas, Lithuania
Since early 2020, SARS-CoV-2-induced infection resulted in global pandemics with high morbidity, especially in the adult population. COVID-19 is a highly prothrombotic condition associated with subsequent multiorgan failure and lethal outcomes. The exact mechanism of the prothrombotic state is not well understood and might be multifactorial. Nevertheless, platelets are attributed to play a crucial role in COVID-19-associated thrombosis. To date, platelets' role was defined primarily in thrombosis and homeostasis. Currently, more focus has been set on their part in inflammation and immunity. Moreover, their ability to release various soluble factors under activation as well as internalize and degrade specific pathogens has been highly addressed in viral research. This review article will discuss platelet role in COVID-19-associated thrombosis and their role in the cholinergic anti-inflammatory pathway. Multiple studies confirmed that platelets display a hyperactivated phenotype in COVID-19 patients. Critically ill patients demonstrate increased platelet activation markers such as P-selectin, PF4, or serotonin. In addition, platelets contain acetylcholine and express α7 nicotinic acetylcholine receptors (α7nAchR). Thus, acetylcholine can be released under activation, and α7nAchR can be stimulated in an autocrine manner and support platelet function. α7 receptor is one of the most important mediators of the anti-inflammatory properties as it is associated with humoral and intrinsic immunity and was demonstrated to contribute to better outcomes in COVID-19 patients when under stimulation. Hematopoietic α7nAchR deficiency increases platelet activation and, in experimental studies, α7nAchR stimulation can diminish the pro-inflammatory state and modulate platelet reactiveness via increased levels of NO. NO has been described to inhibit platelet adhesion, activation, and aggregation. In addition, acetylcholine has been demonstrated to decrease platelet aggregation possibly by blocking the e p-38 pathway. SARS-CoV-2 proteins have been found to be similar to neurotoxins which can bind to nAChR and prevent the action of acetylcholine. Concluding, the platelet role in COVID-19 thrombotic events could be explained by their active function in the cholinergic anti-inflammatory pathway.
Introduction
Since early 2020, severe acute respiratory syndrome coronavirus 2 (SARS-CoV-2)-induced infection resulted in global pandemics with high morbidity, especially in the adult population (1, 2). Coronavirus disease 19 (COVID-19) presented with a specific organ and system involvement, such as severe acute respiratory syndrome (SARS) which was already observed in other viral infections (3). In addition, this infection demonstrated very specific SARS-CoV-2-unique pathological phenotypes which raised a lot of concern and unanswered questions with regard to evidence-based management options (4, 5). Those clinical phenotypes do differ in patient clinical data on admission, complications, comorbidities, and clinical outcomes; thus, treatment might be tailored based on the clinical course and previous risk (5). Moreover, it emerged that COVID-19 is a highly prothrombotic condition associated with subsequent multiorgan failure and lethal outcomes (6–8). Multiorgan failure is still under investigation, yet different mechanisms such as endothelial cell damage, immune response, dysregulation of the renin-angiotensin-aldosterone system, and thromboinflammation have been involved (9, 10). A new type of COVID-19-associated multiorgan failure—a multisystem inflammatory syndrome (MISC) was described in children (11, 12). It closely resembles Kawasaki disease, known for several decades for its coronary complications (13). Up to 68% of affected children are treated in the pediatric intensive care unit (PICU) (14). Also, the increasing incidence of MISC is reported in young adults (15, 16). In most cases, MISC is characterized and investigated with the main focus on hyperinflammation, meanwhile, coagulation and thrombosis are less understood. Still, a study by Buonsenso et al. found D-dimers (fibrin degradation products) as an independent predictor of the outcomes of MISC (17). From the beginning of the COVID-19 pandemics, various data revealed that 20–50% of all COVID-19 hospitalized cases show abnormal coagulation results (18). An increase in D-dimer concentrations has been shown in a high percentage of severe COVID-19 cases. Elevated D-dimer values are associated with more severe diseases course and unfavorable outcomes of COVID-19 (8, 19–22). Platelets are another important marker in COVID-19. The most common finding in severe SARS-CoV-2-induced infection is thrombocytopenia. A meta-analysis by Jiang et al. demonstrated that lower platelet counts were detected in severe COVID-19 cases compared to milder ones (23). Thrombocytopenia has been reported to be associated with an increased risk of severe disease (24–26). Also, more studies analyse platelet activation role in the prothrombotic phenotype of COVID-19 patients. The exact mechanism of the prothrombotic state is not well understood and might be multifactorial. Nevertheless, platelets are attributed to play a crucial role in COVID-19-associated thrombosis. In this review, we will summarize the platelet role in COVID-19-associated thrombosis. Moreover, we will provide more insight into the role of the platelet alpha7 nicotinic acetylcholine receptor (α7nAChR) in the COVID-19-associated inflammation leading to thrombotic events.
COVID-19 inflammation and thrombotic events: Clinical picture
The global pandemic of COVID-19 caused by SARS-CoV-2 started in 2020 and continues nowadays with the new disease entities. Initially, COVID-19 was thought to cause mainly respiratory symptoms which for the most affected were mild, subsequently, it had shown to be associated with a higher number of different complications.
Thrombosis plays a crucial part in the pathogenesis of COVID-19. In the beginning, SARS-CoV-2 infection induces a tremendous inflammatory reaction leading to uncontrolled or disrupted anti-inflammatory response (27). Interaction between SARS-CoV-2 and host cells, and prolonged inflammation cause endothelial damage and dysfunction with the result of excessive prothrombotic factor production contributing to an increased coagulation state. Moreover, COVID-19-induced hypoxia can further stimulate thrombosis through blood viscosity and hypoxia-inducible transcription factors (28). Nevertheless, DNA and histones from neutrophil extracellular traps (NETs) can additionally contribute to pro-thrombotic pathway activation (9, 29).
Up to 4.7% of severe COVID-19 cases progress to critically ill patients (30), and a significant number—approximately 79% result in severe thrombotic complications associated with a high mortality rate (8, 31, 32). Despite prophylactic anticoagulation treatment, almost one-third of the patients experience thrombotic events as demonstrated by the study of Lodigiani et al. (33). Moreover, a systematic review by Alahyari et al. revealed that thromboembolic events, such as deep vein thrombosis (DVT) or pulmonary embolism are most frequent of all the COVID-19-associated hematologic complications (34). Globally, a wide spectrum of incidence (10.9–58%) of DVT in COVID-19 patients was reported by several studies (33, 35, 36) with a higher percentage in critically ill patients (37). When compared to non-COVID acute respiratory distress syndrome (ARDS), COVID-19 ARDS demonstrated higher rates of pulmonary embolism (2.1 vs. 11.7%, respectively) (38, 39). A post-mortem study by Wichmann et al. revealed an important interplay between COVID-19 and venous thrombosis events (37). Most importantly, the unique feature of COVID-19-induced thrombosis is that it can be, both arterial and venous (32). Arterial thrombotic complications are less common (40), nevertheless, they can cause severe and devastating outcomes even with prescribed prophylactic anticoagulation therapy (41). A plethora of thrombotic complications are affecting cardiovascular and cerebrovascular systems (9, 10), myocardial infarction being the most prominent event (40, 42), and reaching 21% in the most recent meta-analysis study (43). Few studies suggested that ischemic stroke affects COVID-19 diseased younger people (6, 44, 45). Furthermore, the latest study by Xie et al. showed significantly higher cardiovascular outcomes after COVID-19 exposure (46), and cardiac complications have been linked to poor outcomes (43). Additionally, mesenteric ischemia is being reported in 1–5% of the cases with COVID-19 (38, 47). Besides macrovascular complications, more evidence demonstrates COVID-19-associated microvascular thrombotic events. Alveolar-capillary microthrombi have been found in severe COVID-19-induced ARDS cases (48–50). Nevertheless, more evidence shows that microangiopathy can cause complications in COVID-19 asymptomatic patients or patients with mild respiratory symptoms. An international study in perinatology recently demonstrated that pregnant women with mild COVID-19 symptoms resulted in placentitis leading to widespread placental insufficiency with subsequent fetal hypoxia and even lethal outcomes (51). In 37% of the examined placenta samples, multiple intervillous thrombi formations were identified and suggested as one of the contributing mechanisms to severe placental malperfusion. Another hypothesis of the possible presentation of SARS-CoV-2-induced microangiopathy was reported in several studies of case series showing increased incidence of “chilblains like” skin lesions during COVID-19 (52, 53). Moreover, this was supported by histological reports of skin biopsies where microthrombi were detected (54–56). The underlying hypothesis was SARS-CoV-2-associated epithelial damage, and secondary ischemia leading to the microangiopathic lesions (54). However, the clear confirmed pathogenesis and association of these skin lesions to COVID-19, especially in asymptomatic forms of the disease, is still under debate (57, 58). In general, the cause of various thrombosis in SARS-CoV-2 infection is closely related to coagulopathy, inflammation, platelet hyperactivity, thrombocytopathy, and endotheliopathy (9).
Role of the platelets in the immune system, inflammation, and COVID-19-associated thrombosis
For a long time, platelets have been known as cells playing role in thrombosis and hemostasis. It is noteworthy that recently they have been attributed a significant role as immune mediators (59, 60). Platelets are a nucleate blood cells derived from megakaryocytes that reside primarily within the bone marrow (61). Additionally, studies have shown that the lung can be a potential site for platelet biogenesis. In the lung, platelets function as antiseptic cells when released in the vicinity of potential pathogen entry (62).
Patients with acute COVID-19 tend to be in a prothrombotic state and have severe inflammation (63). COVID-19 thrombosis encompasses both arterial and venous thromboembolic events, and they frequently co-occur with thrombocytopenia (32, 39). Systemic inflammation often leads to sepsis and septic shock and may present with increased platelet-leukocyte aggregates and thrombocytopenia (64–66). Genes encoding transcription factors involved in hematopoiesis and megakaryocyte biogenesis, such as Runt-related transcription factor 1 (RUNX1), GATA-binding factor 1 (GATA1), and others, have an impact on variations in platelet count (67–69). The liver produces thrombopoietin (TPO), which activates the TPO receptor in megakaryocytes to cause the creation of platelets through a process that is triggered by thrombocytopenia (70). In the final stage of platelet production, some of the transcription factors play a negative feedback role on TPO (71). Numerous cytokines can initiate megakaryopoiesis (e.g., interleukins 3, 6, and 11 (IL-3, IL-6, IL-11), fibroblast growth factor 4 (FGF4), and others) (72). Viruses can activate the host's cytokine profile to alter platelet formation through hepatic TPO synthesis. The simian immunodeficiency virus (SIV), which increases tumor growth factor (TGF), causes the synthesis of TPO (73); human papilloma virus-6 (HPV6) may prevent the development of TPO-induced megakaryocytic colonies (74). Meanwhile, SARS-CoV-2 via its spike protein may trigger the production of antibodies that cross-react with human TPO, to induce thrombocytopenia (75). Conversely, SAR2-CoV-2 stimulates angiotensin-converting enzyme (ACE) expression, which leads to induced inflammation via angiotensin II (Ang II) resulting in IL-6 stimulated TPO augmentation (76). In inflammation, IL-6 raises the levels of TPO to promote the creation of platelets (77), therefore, it can be considered that an inflammatory environment is required for COVID-19-induced thrombosis.
Currently, more studies demonstrate direct viral-platelet interaction in platelet thrombotic and inflammatory function modulation (78, 79). Platelets do express pattern recognition receptors (PRRs), such as Toll-like receptors (TLR), Nod-like receptors, or C-type lectin receptors (80). Those receptors are crucial in damage-associated molecular patterns (DAMPs) and exogenous pathogen-associated molecular patterns (PAMPs) recognition. DAMPs and PAMPs are referred to as virus-associated molecular patterns (80–83). The attachment to DAMPs and PAMPs can initiate different intracellular pathways resulting in various pro-inflammatory cytokine production (84–87). In addition, platelet expression of functional TLR2 can further contribute to thrombotic pathway activation (84) (Figure 1). In the case of flu, the influenza virus has been proven to activate platelets via TLR-7 and Fcγ receptor IIa (FcγRIIa). The platelet expression of FcγRIIa leads to the activation of immune complexes (88). Antibodies against self-antigens, such as antiphospholipidic antibodies, have been reported in COVID-19 patients (89). However, thrombus formation was seen in COVID-19 patients' serum that had low levels of antiphospholipidic antibodies (90). Nevertheless, aberrant glycosylation of anti-SARS-CoV-2 spike immunoglobulin G(IgG) complexes was found to be a significant factor in the ability of these complexes to increase thrombus formation (91). Activation of TLR-7 evokes platelet degranulation, platelet-leucocyte aggregation, and NETosis stimulation leading to thrombus formation (79, 92) (Figure 1). Plasma from hospitalized COVID-19 patients demonstrated increased circulating platelet-neutrophil aggregates (93). Additionally, the autopsy of COVID-19 patients showed that microvascular thrombi composed of platelets and neutrophil extracellular traps were present (94). Also, recent studies show that platelets can internalize virus particles, and after viral ssRNA, dsRNA, or CpG DNA attachment to TLRs downstream signaling is initiated leading to platelet activation, platelet granule release, and P-selectin exposure (95, 96). P-selectin is a platelet receptor that has been linked to platelet activation. Platelets can bind to leukocytes via the P-selectin glycoprotein ligand-1 to mediate neutrophil rolling and intracellular leukocyte signaling (97, 98). Their depletion or blocking of the P-selectin-mediated interaction with neutrophils may reduce lung injury in COVID-19 (99). P-selectin, soluble CD40 ligand and others are released under platelet stimulation (100, 101). Their increased levels are observed in COVID-19 patients and P-selectin stimulates monocyte tissue factor (TF) expression contributing to a prothrombotic phenotype (102, 103).
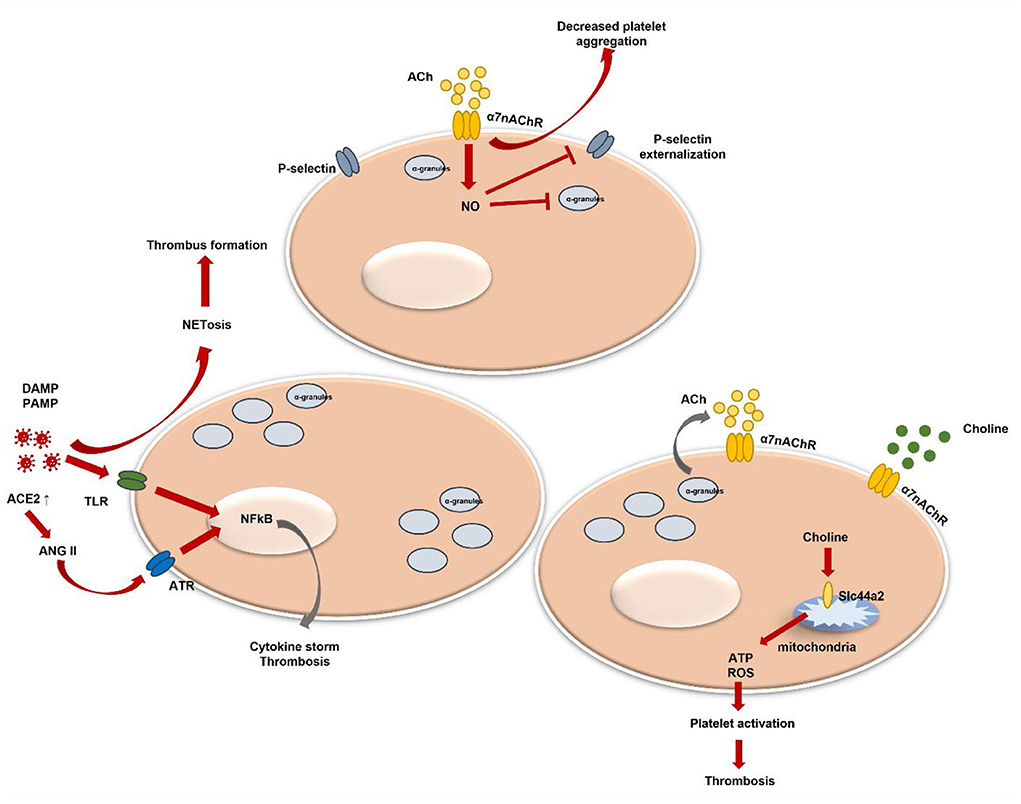
Figure 1. Platelet activation and α7nAChR involvement in thrombosis regulation. DAMPs, danger associated molecular patterns; PAMPs, pathogen associated molecular patterns; TLR, toll-like receptor; NF-kB, nuclear factor kappa-light-chain-enhancer of activated B cells; ACh, acetylcholine; NETosis, neutrophil extracellular trap formation; NO, nitric oxide; ACE2, angiotensin-converting enzyme-2; ANG II, angiotensin II; ATR, ANG II receptor; ATP, adenosine triphosphate; ROS, reactive oxygen species; α7nAChR, alpha7 nicotinic acetylcholine receptor.
Systemic levels of pro-inflammatory cytokines, such as TNFα, IL-1, and IL-6, are markedly elevated in severe COVID-19 (104). Moreover, the expression of pro-inflammatory cytokines, including TNFα, and IL-6 is dependent on Ang II (105, 106) (Figure 1), which amount is increased in SARS-CoV-2 infection (107). Angiotensin II (Ang II) contributes to endothelial dysfunction and the development of microvascular thrombosis (108), it stimulates TF expression, which is the physiological initiator of blood coagulation (109). Also, Ang II triggers platelet-derived growth factors (PDGF) production (110) and increases platelet aggregation (111). In addition, P-selectin expression levels are enhanced by activated platelets and by endothelial cells during Ang II stimulation (112). The relationship between Ang II and α7nAchR has been determined when activation of α7nAChR alleviated Ang II-mediated vascular smooth muscle senescence (113). Furthermore, it was suggested that decreased expression of α7nAchR might contribute to TNFα-induced vascular tissue inflammation, which was previously described as related to Ang II-mediated microvascular thrombosis (114). α7nAchR and the cholinergic system have been already studied regarding their beneficial role in COVID-19-induced hyperinflammation and disease outcomes (115, 116). Moreover, it has been shown that vagal stimulation via α7nAchR improves COVID-19-induced lung infection and inflammation, as well as systemic hyperinflammation (117–119). Additionally, patients lacking α7nAchR levels presented with higher C-reactive protein (CRP) values, more pronounced lymphopenia, extended pulmonary lesions, and increased expression of the TNFα pathway (115). Despite that α7nAchR role in platelets is still not widely studied and not well summarized, thus, we will analyse currently present data and platelet α7nAchR role in COVID-19-induced thrombosis.
The cholinergic system, α7nAChR, and platelet role in COVID-19-induced hyperthrombosis
More and more data suggest that the autonomous nervous system plays a crucial role in inflammation via a cholinergic anti-inflammatory pathway (CAP). CAP is mainly composed of the parasympathetic nerves with the vagal nerve being most important together with acetylcholine (ACh) and its receptors (120). This pathway bridges the autonomic nervous system and immune system. Recently, the alleviating effect of COVID-19-induced hyperinflammation has been widely described in several studies (115, 117, 121–123). Under direct activation of the afferent vagal nerve, the main neurotransmitter ACh is released which further stimulates α7nAChR (124, 125) resulting in an anti-inflammatory response. Non-neuronal ACh was demonstrated to have an anti-inflammatory potential as well. α7nAChR is widely present on different immune cells and a variety of other cells, such as neuronal, endothelial cells, and platelets (126–128). Increased levels of TNFα, IL-6, and CRP have been detected in α7nAChR knockout mice (124, 129). Moreover, endothelial cell activation as well as leucocyte recruitment can be inhibited via α7nAChR stimulation (126). In addition, platelet function can be modulated during the inflammation process (128, 130).
Several studies have shown that platelets do contain some components of a non-neuronal cholinergic system, e.g., ACh, choline acetyltransferase, and acetylcholinestares (131–133). It is known that acetylcholinestares (AChE), for instance, hydrolyses the neurotransmitter ACh in the nervous system. Under AChE excess, an inflammatory process can be promoted (134). Three C-terminal variants of AChE have been identified (135). One of them—is a read-through transcript which is formed through the continuous transcription through intron I-4. This variant has been demonstrated to play an active role in the hematopoietic system and could be linked with its regulation under specific conditions, such as development or stress (132). Moreover, few studies found RNA signals of nAChR subunits, as well as α7 subunit in platelets (136, 137). Schedel et al. described functional α7nAChR Ca2+ channels in human platelets and in the megakaryocytic lineage and proposed an autocrine regulation mechanism via released stored ACh (128). Platelets are known to store various molecules in their granules. Those different cargo molecules are released under stimulation and contribute to coagulation, inflammation, or facilitating adhesion to other cells (138, 139). Nevertheless, ACh could be presented via other cells, such as endothelial cells, which are in close contact with platelets (131). A study by Bennett et al. indicated that endogenous ACh produced by platelets does inhibit platelet activation (140). It was demonstrated that via elevated nitric oxide (NO), ACh inhibits platelet degranulation, inhibits P-selectin externalization, and glycoprotein IIb IIIa (GPIIbIIIa) activation (141, 142) (Figure 1). Moreover, platelets express nitric oxide synthase 3 (NOS3) which may regulate platelets by an endogenous NO pathway (143). Few studies have shown that inhaled NO downregulates P-selectin, platelet aggregation, and fibrinogen binding in severe ARDS (144, 145). Kooijman et al. confirmed that mice lacking α7nAChR showed increased platelet aggregation ex vivo (130). Still, the hypothesis by authors has been raised that only a lack of both, platelet and endothelial α7nAChR could be associated with a significant impact on inflammation. Afterall, the role of endothelial cells in a cholinergic anti-inflammatory pathway is not well studied. Platelet and endothelial cell interaction is clearly described and shown to be important in various inflammatory conditions. Endothelial cell disruption during COVID-19 and released cytokines can be a possible mechanism of thrombosis (146). In addition, it has been demonstrated that platelets do play a crucial role in hypercoagulation during COVID-19 (147). Additionally, few studies revealed that monomeric C-reactive protein (mCRP) is linked with platelet activation which is mediated via p38 mitogen-activated protein kinase (MAPK) and Jun N-terminal kinase (JNK) (148). Interestingly, ACh has been shown to block mCRP binding and related pro-inflammatory action (149). MAPK is highly important in platelet activation, aggregation, and thrombus formation (150, 151). Moreover, the involvement and activation of p38 MAPK has already been widely described in SARS-CoV-2 (152). In addition, p38 MAPK can facilitate viral entry via ACE2 (153).
Interestingly, α7nAChR can be activated via choline as well (154). Choline is a precursor of ACh and phosphocholine (PC). It can efficiently act and is a relatively selective α7nAChR agonist (155). In COVID-19 patients, choline has been found to be downregulated, particularly in severe cases (156). Meanwhile, the intermediate product phosphatidylcholine has been detected to be upregulated. The possible underlying mechanism could be macrophage polarization associated with pathogen presentation (157). This further results in various cytokine secretion as a response to a COVID-19 infection. Another study showed that higher choline levels in pregnant women were associated with protective action against COVID-19 in fetal brain development (158). A recent study identified choline's role in platelet activation and thrombosis. The genetic loci including Slc44a2 have been already studied in thrombosis (159). Slc44a2 was demonstrated to mediate choline transport into mitochondria which results in mitochondrial oxygen consumption and ATP production (159). Mitochondrial dysfunction induces ATP decrease which results in decreased ATP release from platelets. Moreover, decreased ADP causes lower activation of platelets. Slc44a2 was already associated with venous thromboembolism (160, 161). In addition, Slc44a2 is well defined as a human neutrophil antigen (162). Moreover, it was proven to directly interact with platelet integrin αIIbβ3 and trigger NETosis leading to thrombosis (163, 164) (Figure 1). PC is nAChR agonist as well (165). Studies showed its inhibitory potential for IL-1beta release from monocytes in α7nAChR dependent manner (166). Furthermore, PC epitopes are exposed on various pathogens and their interaction with host proteins, such as platelet-activating factor receptors (167, 168) leads to pathogen adhesion to the surface of the host cell and cell invasion (169, 170). Nevertheless, less is known regarding PC function and excretion from platelets and involvement in SARS-CoV-2 or other viral pathogen-induced inflammation and/or thrombosis. To date, only one study defined that platelets could release choline metabolites under stimulation (171).
Conclusion
Concluding, the prothrombotic state of COVID-19 is multifactorial, nevertheless, platelets do play an important role in inducing COVID-19 hypercoagulation and thrombosis. Due to their different secretory factors which induce coagulation and inflammation, they participate in thrombosis induction via different pathways. One of the possible and less studied is the cholinergic system and platelet α7nAChR which has been less studied but could be a very significant part in SARS-CoV-2-induced infection. As previously shown, nervus vagus stimulation can benefit COVID-19-associated hyperinflammation, thus, via platelet α7nAChR it might decrease coagulation and thrombotic process together with decreased inflammatory factors (which additionally activate platelets) and benefit COVID-19 patients. Different studies show that platelets can produce choline products under stimulation, thus, α7nAChR could be stimulated leading to its beneficial anti-inflammatory and possible anti-thrombotic effect. However, more studies are necessary to confirm this hypothesis.
Author contributions
LJ: hypothesis, editing, visualization, and supervision. LJ, MM, and AS: analysis, writing original draft, and review. All authors contributed to the article and approved the submitted version.
Funding
Publishing costs were partially covered by a local fund of Scientific center of Lithuanian University of Health Sciences.
Acknowledgments
We kindly thank Prof. E. Kaniušas for the initial knowledge and for giving us motivation to further analyse data with regard to vagal involvement in COVID-19. Moreover, we are grateful for Saravanan Subramaniam for inviting us to contribute.
Conflict of interest
The authors declare that the research was conducted in the absence of any commercial or financial relationships that could be construed as a potential conflict of interest.
Publisher's note
All claims expressed in this article are solely those of the authors and do not necessarily represent those of their affiliated organizations, or those of the publisher, the editors and the reviewers. Any product that may be evaluated in this article, or claim that may be made by its manufacturer, is not guaranteed or endorsed by the publisher.
References
1. Pijls BG, Jolani S, Atherley A, Derckx RT, Dijkstra JIR, Franssen GHL, et al. Demographic risk factors for COVID-19 infection, severity, ICU admission and death: a meta-analysis of 59 studies. BMJ Open. (2021) 11:e044640. doi: 10.1136/bmjopen-2020-044640
2. Viner RM, Mytton OT, Bonell C, Melendez-Torres GJ, Ward J, Hudson L, et al. Susceptibility to SARS-CoV-2 infection among children and adolescents compared with adults: a systematic review and meta-analysis. JAMA Pediatr. (2021) 175:143–56. doi: 10.1001/jamapediatrics.2020.4573
4. Rello J, Storti E, Belliato M, Serrano R. Clinical phenotypes of SARS-CoV-2: implications for clinicians and researchers. Eur Respir J. (2020) 55:1182–96. doi: 10.1183/13993003.01028-2020
5. Lusczek ER, Ingraham NE, Karam BS, Proper J, Siegel L, Helgeson ES, et al. Characterizing COVID-19 clinical phenotypes and associated comorbidities and complication profiles. PLoS ONE. (2021) 16:e0248956. doi: 10.1371/journal.pone.0248956
6. Klok FA, Kruip MJHA, van der Meer NJM, Arbous MS, Gommers DAMPJ, Kant KM, et al. Incidence of thrombotic complications in critically ill ICU patients with COVID-19. Thromb Res. (2020) 191:145–7. doi: 10.1016/j.thromres.2020.04.013
7. Middeldorp S, Coppens M, van Haaps TF, Foppen M, Vlaar AP, Müller MCA, et al. Incidence of venous thromboembolism in hospitalized patients with COVID-19. J Thromb Haemost. (2020) 18:1995–2002. doi: 10.1111/jth.14888
8. Tang N, Li D, Wang X, Sun Z. Abnormal coagulation parameters are associated with poor prognosis in patients with novel coronavirus pneumonia. J Thromb Haemost. (2020) 18:844–7. doi: 10.1111/jth.14768
9. Gu SX, Tyagi T, Jain K, Gu VW, Lee SH, Hwa JM, et al. Thrombocytopathy and endotheliopathy: crucial contributors to COVID-19 thromboinflammation. Nat Rev Cardiol. (2021) 18:194–209. doi: 10.1038/s41569-020-00469-1
10. Miesbach W, Makris M. COVID-19: coagulopathy, risk of thrombosis, and the rationale for anticoagulation. Clin Appl Thromb Hemost. (2020) 26:1076029620938149. doi: 10.1177/1076029620938149
11. Levy M, Recher M, Hubert H, Javouhey E, Fléchelles O, Leteurtre S, et al. Multisystem inflammatory syndrome in children by COVID-19 vaccination status of adolescents in France. JAMA. (2022) 327:281–3. doi: 10.1001/jama.2021.23262
12. Riphagen S, Gomez X, Gonzalez-Martinez C, Wilkinson N, Theocharis P. Hyperinflammatory shock in children during COVID-19 pandemic. Lancet. (2020) 395:1607–8. doi: 10.1016/S0140-6736(20)31094-1
13. Verdoni L, Mazza A, Gervasoni A, Martelli L, Ruggeri M, Ciuffreda M, et al. An outbreak of severe Kawasaki-like disease at the Italian epicentre of the SARS-CoV-2 epidemic: an observational cohort study. Lancet. (2020) 395:1771–8. doi: 10.1016/S0140-6736(20)31103-X
14. Radia T, Williams N, Agrawal P, Harman K, Weale J, Cook J, et al. Multi-system inflammatory syndrome in children and adolescents (MIS-C): a systematic review of clinical features and presentation. Paediatr Respir Rev. (2021) 38:51–7. doi: 10.1016/j.prrv.2020.08.001
15. Morris SB, Schwartz NG, Patel P, Abbo L, Beauchamps L, Balan S, et al. Case series of multisystem inflammatory syndrome in adults associated with SARS-CoV-2 infection—United Kingdom and United States, March–August 2020. MMWR Morb Mortal Wkly Rep. (2020) 69:1450–6. doi: 10.15585/mmwr.mm6940e1
16. Patel P, Decuir J, Abrams J, Campbell AP, Godfred-Cato S, Belay ED. Clinical characteristics of multisystem inflammatory syndrome in adults: a systematic review. JAMA Netw Open. (2021) 4: e146614. doi: 10.1001/jamanetworkopen.2021.26456
17. Buonsenso D, Mariani F, Pierri L, Morello R, Yock-Corrales A, del Aguila O, et al. Association between coagulation profile and clinical outcome in children with SARS-CoV-2 infection or MIS-C: a multicenter cross-sectional study. Children. (2022) 9:279. doi: 10.3390/children9020279
18. Gómez-Mesa JE, Galindo-Coral S, Montes MC, Muñoz Martin AJ. Thrombosis and coagulopathy in COVID-19. Curr Probl Cardiol. (2021) 46:100742. doi: 10.1016/j.cpcardiol.2020.100742
19. Czupryna P, Moniuszko-Malinowska A, Rogalska M, Zarebska-Michaluk D, Lorenc B, Rorat M, et al. Inflammatory and thrombotic parameters associated with the COVID-19 course in Poland (SARSTer study). Adv Med Sci. (2022) 67:291. doi: 10.1016/j.advms.2022.07.003
20. Ozen M, Yilmaz A, Cakmak V, Beyoglu R, Oskay A, Seyit M, et al. D-Dimer as a potential biomarker for disease severity in COVID-19. Am J Emerg Med. (2021) 40:55–9. doi: 10.1016/j.ajem.2020.12.023
21. Yao Y, Cao J, Wang Q, Shi Q, Liu K, Luo Z, et al. D-dimer as a biomarker for disease severity and mortality in COVID-19 patients: a case control study. J Intensive Care. (2020) 8:1097–8. doi: 10.1186/s40560-020-00466-z
22. Zhang L, Yan X, Fan Q, Liu H, Liu X, Liu Z, et al. D-dimer levels on admission to predict in-hospital mortality in patients with COVID-19. J Thromb Haemost. (2020) 18:1324–9. doi: 10.1111/jth.14859
23. Jiang SQ, Huang QF, Xie WM, Lv C, Quan XQ. The association between severe COVID-19 and low platelet count: evidence from 31 observational studies involving 7613 participants. Br J Haematol. (2020) 190:e29–33. doi: 10.1111/bjh.16817
24. Guan WJ, Ni ZY, Hu Y, Liang WH, Ou CQ, He JX, et al. Clinical Characteristics of Coronavirus Disease 2019 in China. N Engl J Med. (2020) 382:1708–20. doi: 10.1056/NEJMoa2002032
25. Lippi G, Plebani M, Henry BM. Thrombocytopenia is associated with severe coronavirus disease 2019 (COVID-19) infections: a meta-analysis. Clin Chim Acta. (2020) 506:145–8. doi: 10.1016/j.cca.2020.03.022
26. Yang X, Yang Q, Wang Y, Wu Y, Xu J, Yu Y, et al. Thrombocytopenia and its association with mortality in patients with COVID-19. J Thromb Haemost. (2020) 18:1469–72. doi: 10.1111/jth.14848
27. Tan LY, Komarasamy TV, RMT Balasubramaniam V. Hyperinflammatory immune response and COVID-19: a double edged sword. Front Immunol. (2021) 12:3981. doi: 10.3389/fimmu.2021.742941
28. Tang N, Bai H, Chen X, Gong J, Li D, Sun Z. Anticoagulant treatment is associated with decreased mortality in severe coronavirus disease 2019 patients with coagulopathy. J Thromb Haemost. (2020) 18:1094–9. doi: 10.1111/jth.14817
29. Barnes BJ, Adrover JM, Baxter-Stoltzfus A, Borczuk A, Cools-Lartigue J, Crawford JM, et al. Targeting potential drivers of COVID-19: neutrophil extracellular traps. J Exp Med. (2020) 217:e20200652. doi: 10.1084/jem.20200652
30. Becker RC. COVID-19 update: Covid-19-associated coagulopathy. J Thromb Thrombolysis. (2020) 50:54. doi: 10.1007/s11239-020-02134-3
31. Nahum J, Morichau-Beauchant T, Daviaud F, Echegut P, Fichet J, Maillet JM, et al. Venous thrombosis among critically ill patients with coronavirus disease 2019 (COVID-19). JAMA Netw Open. (2020) 3:e2010478–e2010478. doi: 10.1001/jamanetworkopen.2020.10478
32. Levi M, Thachil J, Iba T, Levy JH. Coagulation abnormalities and thrombosis in patients with COVID-19. Lancet Haematol. (2020) 7:e438–40. doi: 10.1016/S2352-3026(20)30145-9
33. Lodigiani C, Iapichino G, Carenzo L, Cecconi M, Ferrazzi P, Sebastian T, et al. Venous and arterial thromboembolic complications in COVID-19 patients admitted to an academic hospital in Milan, Italy. Thromb Res. (2020) 191:9–14. doi: 10.1016/j.thromres.2020.04.024
34. Alahyari S, Moradi M, Rajaeinejad M, Jalaeikhoo H. Post-COVID-19 hematologic complications: a systematic review. Expert Rev Hematol. (2022) 15:539–46. doi: 10.1080/17474086.2022.2080051
35. Mumoli N, Dentali F, Conte G, Colombo A, Capra R, Porta C, et al. Upper extremity deep vein thrombosis in COVID-19: incidence and correlated risk factors in a cohort of non-ICU patients. PLoS One. (2022) 17:e0262522. doi: 10.1371/journal.pone.0262522
36. Baccellieri D, Bertoglio L, Apruzzi L, Ardita V, D'Angelo A, Bossi M, et al. Incidence of deep venous thrombosis in COVID-19 hospitalized patients during the first peak of the Italian outbreak. Phlebology. (2021) 36:375–83. doi: 10.1177/0268355520975592
37. Wichmann D, Sperhake JP, Lütgehetmann M, Steurer S, Edler C, Heinemann A, et al. Autopsy findings and venous thromboembolism in patients with COVID-19. Ann Intern Med. (2020) 173:268–77. doi: 10.7326/M20-2003
38. Helms J, Tacquard C, Severac F, Leonard-Lorant I, Ohana M, Delabranche X, et al. High risk of thrombosis in patients with severe SARS-CoV-2 infection: a multicenter prospective cohort study. Intensive Care Med. (2020) 46:1089–98. doi: 10.1007/s00134-020-06062-x
39. Klok FA, Kruip MJHA, van der Meer NJM, Arbous MS, Gommers D, Kant KM, et al. Confirmation of the high cumulative incidence of thrombotic complications in critically ill ICU patients with COVID-19: an updated analysis. Thromb Res. (2020) 191:148–50. doi: 10.1016/j.thromres.2020.04.041
40. Bilaloglu S, Aphinyanaphongs Y, Jones S, Iturrate E, Hochman J, Berger JS. Thrombosis in hospitalized patients with COVID-19 in a New York City health system. JAMA. (2020) 324:799–801. doi: 10.1001/jama.2020.13372
41. Kashi M, Jacquin A, Dakhil B, Zaimi R, Mahé E, Tella E, et al. Severe arterial thrombosis associated with COVID-19 infection. Thromb Res. (2020) 192:75–7. doi: 10.1016/j.thromres.2020.05.025
42. Katsoularis I, Fonseca-Rodríguez O, Farrington P, Lindmark K, Fors Connolly AM. Risk of acute myocardial infarction and ischaemic stroke following COVID-19 in Sweden: a self-controlled case series and matched cohort study. Lancet. (2021) 398:599–607. doi: 10.1016/S0140-6736(21)00896-5
43. Jafari-Oori M, Moradian ST, Ebadi A, Jafari M, Dehi M. Incidence of cardiac complications following COVID-19 infection: an umbrella meta-analysis study. Heart Lung. (2022) 52:136–45. doi: 10.1016/j.hrtlng.2022.01.001
44. Mao L, Jin H, Wang M, Hu Y, Chen S, He Q, et al. Neurologic Manifestations of hospitalized patients with coronavirus disease 2019 in Wuhan, China. JAMA Neurol. (2020) 77:683–90. doi: 10.1001/jamaneurol.2020.1127
45. Oxley TJ, Mocco J, Majidi S, Kellner CP, Shoirah H, Singh IP, et al. Large-vessel stroke as a presenting feature of COVID-19 in the Young. N Engl J Med. (2020) 382:e60. doi: 10.1056/NEJMc2009787
46. Xie Y, Xu E, Bowe B, Al-Aly Z. Long-term cardiovascular outcomes of COVID-19. Nat Med. (2022) 28:583–90. doi: 10.1038/s41591-022-01689-3
47. Gorog DA, Storey RF, Gurbel PA, Tantry US, Berger JS, Chan MY, et al. Current and novel biomarkers of thrombotic risk in COVID-19: a consensus statement from the international COVID-19 thrombosis biomarkers colloquium. Nat Rev Cardiol. (2022) 19:475–95. doi: 10.1038/s41569-021-00665-7
48. Ackermann M, Verleden SE, Kuehnel M, Haverich A, Welte T, Laenger F, et al. Pulmonary vascular endothelialitis, thrombosis, and angiogenesis in COVID-19. N Engl J Med. (2020) 383:120–8. doi: 10.1056/NEJMoa2015432
49. Fox SE, Akmatbekov A, Harbert JL Li G, Quincy Brown J, vander Heide RS. Pulmonary and cardiac pathology in African American patients with COVID-19: an autopsy series from New Orleans. Lancet Respir Med. (2020) 8:681–6. doi: 10.1016/S2213-2600(20)30243-5
50. Dolhnikoff M, Duarte-Neto AN, de Almeida Monteiro RA, da Silva LFF, de Oliveira EP, Saldiva PHN, et al. Pathological evidence of pulmonary thrombotic phenomena in severe COVID-19. J Thromb Haemost. (2020) 18:1517–9. doi: 10.1111/jth.14844
51. Schwartz DA, Hyg M, Avvad-Portari E, Baba P, Baldewijns M, Blomberg M, et al. Placental tissue destruction and insufficiency from COVID-19 causes stillbirth and neonatal death from hypoxic-ischemic injury: study of 68 cases with SARS-CoV-2 placentitis from 12 countries. Arch Pathol Lab Med. (2022) 146:660–76. doi: 10.5858/arpa.2022-0029-SA
52. Piccolo V, Neri I, Filippeschi C, Oranges T, Argenziano G, Battarra VC, et al. Chilblain-like lesions during COVID-19 epidemic: a preliminary study on 63 patients. J Eur Acad Dermatol Venereol. (2020) 34:e291–3. doi: 10.1111/jdv.16526
53. Fernandez-Nieto D, Jimenez-Cauhe J, Suarez-Valle A, Moreno-Arrones OM, Saceda-Corralo D, Arana-Raja A, et al. Characterization of acute acral skin lesions in nonhospitalized patients: A case series of 132 patients during the COVID-19 outbreak. J Am Acad Dermatol. (2020) 83:e61–3. doi: 10.1016/j.jaad.2020.04.093
54. Colmenero I, Santonja C, Alonso-Riaño M, Noguera-Morel L, Hernández-Martín A, Andina D, et al. SARS-CoV-2 endothelial infection causes COVID-19 chilblains: histopathological, immunohistochemical and ultrastructural study of seven paediatric cases. Br J Dermatol. (2020) 183:729–37. doi: 10.1111/bjd.19327
55. Rocha KO, Zanuncio VV, Freitas BAC de, Lima LM. “COVID toes”: a meta-analysis of case and observational studies on clinical, histopathological, and laboratory findings. Pediatr Dermatol. (2021) 38:1143–9. doi: 10.1111/pde.14805
56. Kolivras A, Dehavay F, Delplace D, Feoli F, Meiers I, Milone L, et al. Coronavirus (COVID-19) infection-induced chilblains: a case report with histopathologic findings. JAAD Case Rep. (2020) 6:489–92. doi: 10.1016/j.jdcr.2020.04.011
57. le Cleach L, Dousset L, Assier H, Fourati S, Barbarot S, Boulard C, et al. Most chilblains observed during the COVID-19 outbreak occur in patients who are negative for COVID-19 on polymerase chain reaction and serology testing*. Br J Dermatol. (2020) 183:866–74. doi: 10.1111/bjd.19377
58. de Masson A, Bouaziz JD, Sulimovic L, Cassius C, Jachiet M, Ionescu MA, et al. Chilblains is a common cutaneous finding during the COVID-19 pandemic: A retrospective nationwide study from France. J Am Acad Dermatol. (2020) 83:667–70. doi: 10.1016/j.jaad.2020.04.161
59. Mezger M, Nording H, Sauter R, Graf T, Heim C, von Bubnoff N, et al. Platelets and immune responses during thromboinflammation. Front Immunol. (2019) 10:1731. doi: 10.3389/fimmu.2019.01731
60. Hottz ED, Bozza FA, Bozza PT. Platelets in immune response to virus and immunopathology of viral infections. Front Med (Lausanne) (2018) 5:121. doi: 10.3389/fmed.2018.00121
61. Sim X, Poncz M, Gadue P, French DL. Understanding platelet generation from megakaryocytes: implications for in vitro-derived platelets. Blood. (2016) 127:1227. doi: 10.1182/blood-2015-08-607929
62. Lefrançais E, Looney MR. Platelet biogenesis in the lung circulation. Physiology. (2019) 34:392–401. doi: 10.1152/physiol.00017.2019
63. Abou-Ismail MY, Diamond A, Kapoor S, Arafah Y, Nayak L. The hypercoagulable state in COVID-19: Incidence, pathophysiology, and management. Thromb Res. (2020) 194:101. doi: 10.1016/j.thromres.2020.06.029
64. Kälsch T, Elmas E, Nguyen XD, Suvajac N, Klüter H, Borggrefe M, et al. Endotoxin-induced effects on platelets and monocytes in an in vivo model of inflammation. Basic Res Cardiol. (2007) 102:460–6. doi: 10.1007/s00395-007-0667-y
65. Gawaz M, Dickfeld T, Bogner C, Fateh-Moghadam S, Neumann FJ. Platelet function in septic multiple organ dysfunction syndrome. Intens Care Med. (1997) 23:379–85. doi: 10.1007/s001340050344
66. Gawaz M, Fateh-Moghadam S, Pilz G, Gurland H.-J., Werdan K. Platelet activation and interaction with leucocytes in patients with sepsis or multiple organ failure. Eur J Clin Invest. (1995) 25:843–51. doi: 10.1111/j.1365-2362.1995.tb01694.x
67. Palma-barqueros V, Revilla N, Sánchez A, Cánovas AZ, Rodriguez-alén A, Marín-quílez A, et al. Inherited platelet disorders: an updated overview. Int J Mol Sci. (2021) 22:4521. doi: 10.3390/ijms22094521
68. Balduini CL, Melazzini F, Pecci A. Inherited thrombocytopenias-recent advances in clinical and molecular aspects. Platelets. (2017) 28:3–13. doi: 10.3109/09537104.2016.1171835
69. Almazni I, Stapley R, Morgan N. Inherited thrombocytopenia: update on genes and genetic variants which may be associated with bleeding. Front Cardiovasc Med. (2019) 6:80. doi: 10.3389/fcvm.2019.00080
70. Grozovsky R, Giannini S, Falet H, Hoffmeister KM. Regulating billions of blood platelets: glycans and beyond. Blood. (2015) 126:1877–84. doi: 10.1182/blood-2015-01-569129
71. Guo T, Wang X, Qu Y, Yin Y, Jing T, Zhang Q. Megakaryopoiesis and platelet production: insight into hematopoietic stem cell proliferation and differentiation. Stem Cell Investig. (2015) 2. doi: 10.3978/j.issn.2306-9759.2015.02.0
72. de Graaf CA, Metcalf D. Thrombopoietin and hematopoietic stem cells. 10:1582–9. doi: 10.4161/cc.10.10.15619
73. Pate KAM, Lyons CE, Dorsey JL, Queen SE, Adams RJ, Morrell CN, et al. TGFβ-mediated downregulation of thrombopoietin is associated with platelet decline in asymptomatic SIV infection. J Acquir Immune Defic Syndr. (2014) 65:510–6. doi: 10.1097/QAI.0000000000000048
74. Yamanishi K, Mori Y. Human herpesvirus 6 and human herpesvirus 7. Clinical Virology. (2016) 7:511–22. doi: 10.1128/9781555819439.ch24
75. Nunez-Castilla J, Stebliankin V, Baral P, Balbin CA, Sobhan M, Cickovski T, et al. Potential autoimmunity resulting from molecular mimicry between SARS-CoV-2 Spike and human proteins. bioRxiv. (2022) 33199:2021.08.10.455737. doi: 10.1101/2021.08.10.455737
76. Sriram K, Insel PA. Inflammation and thrombosis in covid-19 pathophysiology: proteinase-activated and purinergic receptors as drivers and candidate therapeutic targets. Physiol Rev. (2021) 101:545–67. doi: 10.1152/physrev.00035.2020
77. Kaser A, Brandacher G, Steurer W, Kaser S, Offner FA, Zoller H, et al. Interleukin-6 stimulates thrombopoiesis through thrombopoietin: role in inflammatory thrombocytosis. Blood. (2001) 98:2720–5. doi: 10.1182/blood.V98.9.2720
78. Assinger A. Platelets and infection—an emerging role of platelets in viral infection. Front Immunol. (2014). doi: 10.3389/fimmu.2014.00649
79. Koupenova M, Clancy L, Corkrey HA, Freedman JE. Circulating platelets as mediators of immunity, inflammation, and thrombosis. Circ Res. (2018) 122:337–51. doi: 10.1161/CIRCRESAHA.117.310795
80. Dib PRB, Quirino-Teixeira AC, Merij LB, Pinheiro MBM, Rozini SV, Andrade FB, et al. Innate immune receptors in platelets and platelet-leukocyte interactions. J Leukoc Biol. (2020) 108:1157–82. doi: 10.1002/JLB.4MR0620-701R
81. Zhang S, Zhang S, Hu L, Zhai L, Xue R, Ye J, et al. Nucleotide-binding oligomerization domain 2 receptor is expressed in platelets and enhances platelet activation and thrombosis. Circulation. (2015) 131:1160–70. doi: 10.1161/CIRCULATIONAHA.114.013743
82. Schattner M. Platelet TLR4 at the crossroads of thrombosis and the innate immune response. J Leukoc Biol. (2019) 105:873–80. doi: 10.1002/JLB.MR0618-213R
83. Clemetson KJ, Clemetson JM. Platelet receptors. In: Platelets. New York: Academic Press (2019). p. 169–92. doi: 10.1016/B978-0-12-813456-6.00009-6
84. Blair P, Rex S, Vitseva O, Beaulieu L, Tanriverdi K, Chakrabarti S, et al. Stimulation of toll-like receptor 2 in human platelets induces a thromboinflammatory response through activation of phosphoinositide 3-kinase. Circ Res. (2009) 104:346–54. doi: 10.1161/CIRCRESAHA.108.185785
85. Rivadeneyra L, Carestia A, Etulain J, Pozner RG, Fondevila C, Negrotto S, et al. Regulation of platelet responses triggered by Toll-like receptor 2 and 4 ligands is another non-genomic role of nuclear factor-kappaB. Thromb Res. (2014) 133:235–43. doi: 10.1016/j.thromres.2013.11.028
86. Wu J. Chen ZJ. Innate Immune Sensing and Signaling of Cytosolic Nucleic Acids. (2014) 32:461–88. doi: 10.1146/annurev-immunol-032713-120156
87. Zhang G, Han J, Welch EJ, Ye RD, Voyno-Yasenetskaya TA, Malik AB, et al. Lipopolysaccharide stimulates platelet secretion and potentiates platelet aggregation via TLR4/MyD88 and the cGMP-dependent protein kinase pathway. J Immunol. (2009) 182:7997–8004. doi: 10.4049/jimmunol.0802884
88. Jevtic SD, Nazy I. The COVID complex: a review of platelet activation and immune complexes in COVID-19. Front Immunol. (2022) 13:807934. doi: 10.3389/fimmu.2022.807934
89. Serrano M, Espinosa G, Serrano A, Cervera R. Antigens and antibodies of the antiphospholipid syndrome as new allies in the pathogenesis of COVID-19 coagulopathy. Int J Mol Sci. (2022) 23:4946. doi: 10.3390/ijms23094946
90. Espinosa G, Zamora-Martínez C, Pérez-Isidro A, Neto D, Bravo-Gallego LY, Prieto-González S, et al. Persistent antiphospholipid antibodies are not associated with worse clinical outcomes in a prospective cohort of hospitalised patients with SARS-CoV-2 infection. Front Immunol. (2022) 13:911979. doi: 10.3389/fimmu.2022.911979
91. Bye AP, Hoepel W, Mitchell JL, Jégouic S, Loureiro S, Sage T, et al. Aberrant glycosylation of anti-SARS-CoV-2 spike IgG is a prothrombotic stimulus for platelets. Blood. (2021) 138:1481–9. doi: 10.1182/blood.2021011871
92. Koupenova M, Vitseva O, MacKay CR, Beaulieu LM, Benjamin EJ, Mick E, et al. Platelet-TLR7 mediates host survival and platelet count during viral infection in the absence of platelet-dependent thrombosis. Blood. (2014) 124:791–802. doi: 10.1182/blood-2013-11-536003
93. Taus F, Salvagno G, Canè S, Fava C, Mazzaferri F, Carrara E, et al. Platelets promote thromboinflammation in SARS-CoV-2 pneumonia. Arterioscler Thromb Vasc Biol. (2020) 40:2975–89. doi: 10.1161/ATVBAHA.120.315175
94. Middleton EA, He XY, Denorme F, Campbell RA, Ng D, Salvatore SP, et al. Neutrophil extracellular traps contribute to immunothrombosis in COVID-19 acute respiratory distress syndrome. Blood. (2020) 136:1169–79. doi: 10.1182/blood.2020007008
95. Banerjee M, Huang Y, Joshi S, Popa GJ, Mendenhall MD, Wang QJ, et al. Platelets endocytose viral particles and are activated via TLR (toll-like receptor) signaling. Arterioscler Thromb Vasc Biol. (2020) 40:1635–50. doi: 10.1161/ATVBAHA.120.314180
96. Koupenova M, Corkrey HA, Vitseva O, Manni G, Pang CJ, Clancy L, et al. The role of platelets in mediating a response to human influenza infection. Nat Commun. (2019) 10:1–18. doi: 10.1038/s41467-019-09607-x
97. Frenette PS, Denis C, Weiss L, Jurk K, Subbarao S, Kehrel B, et al. P-Selectin glycoprotein ligand 1 (PSGL-1) is expressed on platelets and can mediate platelet-endothelial interactions in vivo. J Exp Med. (2000) 191:1413–22. doi: 10.1084/jem.191.8.1413
98. Evangelista V, Manarini S, Coller BS, Smyth SS. Role of P-selectin, beta2-integrins, and Src tyrosine kinases in mouse neutrophil-platelet adhesion. J Thromb Haemost. (2003) 1:1048–54. doi: 10.1046/j.1538-7836.2003.00214.x
99. Barale C, Melchionda E, Morotti A, Russo I. Prothrombotic phenotype in COVID-19: focus on platelets. Int J Mol Sci. (2021) 22:13638. doi: 10.3390/ijms222413638
100. Cunin P, Nigrovic PA. Megakaryocytes as immune cells. J Leukoc Biol. (2019) 105:1111–21. doi: 10.1002/JLB.MR0718-261RR
101. Jenne CN, Urrutia R, Kubes P. Platelets: bridging hemostasis, inflammation, and immunity. Int J Lab Hematol. (2013) 35:254–61. doi: 10.1111/ijlh.12084
102. Goshua G, Pine AB, Meizlish ML, Chang CH, Zhang H, Bahel P, et al. Endotheliopathy in COVID-19-associated coagulopathy: evidence from a single-centre, cross-sectional study. Lancet Haematol. (2020) 7:e575–82. doi: 10.1016/S2352-3026(20)30216-7
103. Frantzeskaki F, Armaganidis A, Orfanos SE. Immunothrombosis in acute respiratory distress syndrome: cross talks between inflammation and coagulation. Respiration. (2017) 93:212–25. doi: 10.1159/000453002
104. Huang C, Wang Y, Li X, Ren L, Zhao J, Hu Y, et al. Clinical features of patients infected with 2019 novel coronavirus in Wuhan, China. Lancet. (2020) 395:497–506. doi: 10.1016/S0140-6736(20)30183-5
105. Luther JM, Gainer J, Murphey LJ, Yu C, Vaughan DE, Morrow JD, et al. Angiotensin II induces interleukin-6 in humans through a mineralocorticoid receptor-dependent mechanism. Hypertension. (2006) 48:1050–7. doi: 10.1161/01.HYP.0000248135.97380.76
106. Sturgis LC, Brands MW. Role of TNF-α in the dependency of angiotensin II hypertension on IL-6. FASEB J. (2007) 21:A590–A590. doi: 10.1096/fasebj.21.5.A590-a
107. Camargo RL, Bombassaro B, Monfort-Pires M, Mansour E, Palma AC, Ribeiro LC, et al. Plasma angiotensin II is increased in critical coronavirus disease 2019. Front Cardiovasc Med. (2022) 9:847809. doi: 10.3389/fcvm.2022.847809
108. Senchenkova EY, Russell J, Almeida-Paula LD, Harding JW, Granger DN. Angiotensin II-mediated microvascular thrombosis. Hypertension. (2010) 56:1089–95. doi: 10.1161/HYPERTENSIONAHA.110.158220
109. Celi A, Cianchetti S, Dellomo G, Pedrinelli R. Angiotensin II, tissue factor and the thrombotic paradox of hypertension. Exp Rev Cardiovasc Therapy. (2014) 8:1723–9. doi: 10.1586/erc.10.161
110. Deguchi JO, Makuuchi M, Nakaoka T, Collins T, Takuwa Y. Angiotensin II stimulates platelet-derived growth factor-B chain expression in newborn rat vascular smooth muscle cells and neointimal cells through Ras, extracellular signal-regulated protein kinase, and c-Jun N-terminal protein kinase mechanisms. Circ Res. (1999) 85:565–74. doi: 10.1161/01.RES.85.7.565
111. López-Farré A, Sánchez de Miguel L, Montón M, Jiménez A, Lopez-Bloya A, Gómez J, et al. Angiotensin II AT1 receptor antagonists and platelet activation. Nephrol Dial Transpl. (2001) 16(suppl_1):45–9. doi: 10.1093/ndt/16.suppl_1.45
112. Liu G, Liang B, Song X, Bai R, Qin W, Sun X, et al. P-selectin increases angiotensin II-induced cardiac inflammation and fibrosis via platelet activation. Mol Med Rep. (2016) 13:5021–8. doi: 10.3892/mmr.2016.5186
113. Li DJ, Huang F, Ni M, Fu H, Zhang LS, Shen FM. α7 nicotinic acetylcholine receptor relieves angiotensin II-induced senescence in vascular smooth muscle cells by raising nicotinamide adenine dinucleotide-dependent SIRT1 activity. Arterioscler Thromb Vasc Biol. (2016) 36:1566–76. doi: 10.1161/ATVBAHA.116.307157
114. Chen JK, Zhao T, Ni M, Li DJ, Tao X, Shen FM. Downregulation of alpha7 nicotinic acetylcholine receptor in two-kidney one-clip hypertensive rats. BMC Cardiovasc Disord. (2012) 12:1–7. doi: 10.1186/1471-2261-12-38
115. Courties A, Boussier J, Hadjadj J, Yatim N, Barnabei L, Péré H, et al. Regulation of the acetylcholine/α7nAChR anti-inflammatory pathway in COVID-19 patients. Sci Rep. (2021) 11:1–8. doi: 10.1038/s41598-021-91417-7
116. Alexandris N, Lagoumintzis G, Chasapis CT, Leonidas DD, Papadopoulos GE, Tzartos SJ, et al. Nicotinic cholinergic system and COVID-19: in silico evaluation of nicotinic acetylcholine receptor agonists as potential therapeutic interventions. Toxicol Rep. (2021) 8:73–83. doi: 10.1016/j.toxrep.2020.12.013
117. Seitz T, Szeles JC, Kitzberger R, Holbik J, Grieb A, Wolf H, et al. Percutaneous auricular vagus nerve stimulation reduces inflammation in critical covid-19 patients. Front Physiol. (2022) 13:897257. doi: 10.3389/fphys.2022.897257
118. Wu H, Li L, Su X. Vagus nerve through α7 nAChR modulates lung infection and inflammation: models, cells, and signals. Biomed Res Int. (2014) 2014:283525. doi: 10.1155/2014/283525
119. Caravaca AS, Gallina AL, Tarnawski L, Shavva VS, Colas RA, Dalli J, et al. Vagus nerve stimulation promotes resolution of inflammation by a mechanism that involves Alox15 and requires the α7nAChR subunit. Proc Natl Acad Sci USA. (2022) 119:e2023285119. doi: 10.1073/pnas.2023285119
120. van Maanen MA, Vervoordeldonk MJ, Tak PP. The cholinergic anti-inflammatory pathway: towards innovative treatment of rheumatoid arthritis. Nat Rev Rheumatol. (2009) 5:229–32. doi: 10.1038/nrrheum.2009.31
121. Qin Z, Xiang K, Su DF, Sun Y, Liu X. Activation of the cholinergic anti-inflammatory pathway as a novel therapeutic strategy for COVID-19. Front Immunol. (2021) 11:3870. doi: 10.3389/fimmu.2020.595342
122. Kaniusas E, Szeles JC, Kampusch S, Alfageme-Lopez N, Yucuma-Conde D, Li X, et al. Non-invasive auricular vagus nerve stimulation as a potential treatment for covid19-originated acute respiratory distress syndrome. Front Physiol. (2020) 11:890. doi: 10.3389/fphys.2020.00890
123. Jankauskaite L, Malinauskas M, Mickeviciute GC. HMGB1: a potential target of nervus vagus stimulation in pediatric SARS-CoV-2-induced ALI/ARDS. Front Pediatr. (2022) 10:884539. doi: 10.3389/fped.2022.884539
124. Wang H, Yu M, Ochani M, Amelia CA, Tanovic M, Susarla S, et al. Nicotinic acetylcholine receptor α7 subunit is an essential regulator of inflammation. Nature. (2003) 421:384–8. doi: 10.1038/nature01339
125. Vieira-Alves I, Coimbra-Campos LMC, Sancho M, da Silva RF, Cortes SF, Lemos VS. Role of the α7 nicotinic acetylcholine receptor in the pathophysiology of atherosclerosis. Front Physiol. (2020) 11:1719. doi: 10.3389/fphys.2020.621769
126. Saeed RW, Varma S, Peng-Nemeroff T, Sherry B, Balakhaneh D, Huston J, et al. Cholinergic stimulation blocks endothelial cell activation and leukocyte recruitment during inflammation. J Exp Med. (2005) 201:1113–23. doi: 10.1084/jem.20040463
127. Steinlein O. New functions for nicotinic acetylcholine receptors? Behav Brain Res. (1998) 95:31–5. doi: 10.1016/S0166-4328(97)00207-6
128. Schedel A, Thornton S, Schloss P, Klüter H, Bugert P. Human platelets express functional alpha7-nicotinic acetylcholine receptors. Arterioscler Thromb Vasc Biol. (2011) 31:928–34. doi: 10.1161/ATVBAHA.110.218297
129. Wilund KR, Rosenblat M, Chung HR, Volkova N, Kaplan M, Woods JA, et al. Macrophages from alpha 7 nicotinic acetylcholine receptor knockout mice demonstrate increased cholesterol accumulation and decreased cellular paraoxonase expression: a possible link between the nervous system and atherosclerosis development. Biochem Biophys Res Commun. (2009) 390:148–54. doi: 10.1016/j.bbrc.2009.09.088
130. Kooijman S, Meurs I, van der Stoep M, Habets KL, Lammers B, Berbée JFP, et al. Hematopoietic α7 nicotinic acetylcholine receptor deficiency increases inflammation and platelet activation status, but does not aggravate atherosclerosis. J Thromb Haemost. (2015) 13:126–35. doi: 10.1111/jth.12765
131. Wessler I, Kirkpatrick CJ. Acetylcholine beyond neurons: the non-neuronal cholinergic system in humans. Br J Pharmacol. (2008) 154:1558–71. doi: 10.1038/bjp.2008.185
132. Deutsch VR, Pick M, Perry C, Grisaru D, Hemo Y, Golan-Hadari D, et al. The stress-associated acetylcholinesterase variant AChE-R is expressed in human CD34 hematopoietic progenitors and its C-terminal peptide ARP promotes their proliferation. Exp Hematol. (2002) 30:1153–61. doi: 10.1016/S0301-472X(02)00900-1
133. Pick M, Perry C, Lapidot T, Guimaraes-Sternberg C, Naparstek E, Deutsch V, et al. Stress-induced cholinergic signaling promotes inflammation-associated thrombopoiesis. Blood. (2006) 107:3397–406. doi: 10.1182/blood-2005-08-3240
134. Pick M, Flores-Flores C, Grisaru D, Shochat S, Deutsch V, Soreq H. Blood-cell-specific acetylcholinesterase splice variations under changing stimuli. Int J Dev Neurosci. (2004) 22:523–31. doi: 10.1016/j.ijdevneu.2004.07.016
135. Aziz-Aloya R ben, Sternfeld M, Soreq H. Chapter 16: promoter elements and alternative splicing in the human ACHE gene. Prog Brain Res. (1993) 98:147–53. doi: 10.1016/S0079-6123(08)62392-4
136. Bugert P, Klüter H. Profiling of gene transcripts in human platelets: an update of the platelet transcriptome. Platelets. (2009) 17:503–4. doi: 10.1080/09537100600901491
137. Bugert P, Dugrillon A, Günaydin A, Eichler H, Klüter H. Messenger RNA profiling of human platelets by microarray hybridization. Thromb Haemost. (2003) 90:738–48. doi: 10.1055/s-0037-1613622
138. Heijnen H, van der Sluijs P. Platelet secretory behaviour: as diverse as the granules … or not? J Thromb Haemostasis. (2015) 13:2141–51. doi: 10.1111/jth.13147
139. Blair P, Flaumenhaft R. Platelet α-granules: basic biology and clinical correlates. Blood Rev. (2009) 23:177. doi: 10.1016/j.blre.2009.04.001
140. Bennett JA, Ture SK, Schmidt RA, Mastrangelo MA, Cameron SJ, Terry LE, et al. Acetylcholine inhibits platelet activation. J Pharmacol Exp Therap. (2019) 369:182–7. doi: 10.1124/jpet.118.253583
141. Bennett J, Cameron SJ. Acetylcholine inhibits platelet activation and regulates hemostasis. BioRxiv, 324319.
142. Jeske WP, Walenga JM, Szatkowski E, Ero M, Herbert JM, Haas S, et al. Effect of glycoprotein IIb/IIIa antagonists on the HIT serum induced activation of platelets. Thromb Res. (1997) 88:271–81. doi: 10.1016/S0049-3848(97)00254-5
143. Sase K, Michel T. Expression of constitutive endothelial nitric oxide synthase in human blood platelets. Life Sci. (1995) 57:2049–55. doi: 10.1016/0024-3205(95)02191-K
144. Gries A, Bode C, Peter K, Herr A, Böhrer H, Motsch J, et al. Inhaled nitric oxide inhibits human platelet aggregation, P-selectin expression, and fibrinogen binding in vitro and in vivo. Circulation. (1998) 97:1481–7. doi: 10.1161/01.CIR.97.15.1481
145. Chung A, Wildhirt SM, Wang S, Koshal A, Radomski MW. Combined administration of nitric oxide gas and iloprost during cardiopulmonary bypass reduces platelet dysfunction: a pilot clinical study. J Thorac Cardiovasc Surg. (2005) 129:782–90. doi: 10.1016/j.jtcvs.2004.06.049
146. Escher R, Breakey N, Lämmle B. Severe COVID-19 infection associated with endothelial activation. Thromb Res. (2020) 190:62. doi: 10.1016/j.thromres.2020.04.014
147. Litvinov RI, Evtugina NG, Peshkova AD, Safiullina SI, Andrianova IA, Khabirova AI, et al. Altered platelet and coagulation function in moderate-to-severe COVID-19. Sci Rep. (2021) 11:1–14. doi: 10.1038/s41598-021-95397-6
148. Molins B, Pea E, de La Torre R, Badimon L. Monomeric C-reactive protein is prothrombotic and dissociates from circulating pentameric C-reactive protein on adhered activated platelets under flow. Cardiovasc Res. (2011) 92:328–37. doi: 10.1093/cvr/cvr226
149. Slevin M, Iemma RS, Zeinolabediny Y, Liu D, Ferris GR, Caprio V, et al. Acetylcholine inhibits monomeric C-reactive protein induced inflammation, endothelial cell adhesion, and platelet aggregation; a potential therapeutic? Front Immunol. (2018) 9:2124. doi: 10.3389/fimmu.2018.02124
150. Flaumenhaft R. Platelets and thrombopoiesis: stressed platelets ASK1 for a MAPK. Blood. (2017) 129:1066. doi: 10.1182/blood-2017-01-760546
151. Li Z, Xi X, Du X. A mitogen-activated protein kinase-dependent signaling pathway in the activation of platelet integrin alpha IIbbeta3. J Biol Chem. (2001) 276:42226–32. doi: 10.1074/jbc.M106129200
152. Battagello DS, Dragunas G, Klein MO, Ayub ALP, Velloso FJ, Correa RG. Unpuzzling COVID-19: tissue-related signaling pathways associated with SARS-CoV-2 infection and transmission. Clin Sci (Lond). (2020) 134:2137–60. doi: 10.1042/CS20200904
153. Grimes JM, Grimes K. p38 MAPK inhibition: a promising therapeutic approach for COVID-19. J Mol Cell Cardiol. (2020) 144:63. doi: 10.1016/j.yjmcc.2020.05.007
154. Mike A, Castro NG, Albuquerque EX. Choline and acetylcholine have similar kinetic properties of activation and desensitization on the alpha7 nicotinic receptors in rat hippocampal neurons. Brain Res. (2000) 882:155–68. doi: 10.1016/S0006-8993(00)02863-8
155. Alkondon M, Pereira EFR, Cortes WS, Maelicke A, Albuquerque EX. Choline is a selective agonist of alpha7 nicotinic acetylcholine receptors in the rat brain neurons. Eur J Neurosci. (1997) 9:2734–42. doi: 10.1111/j.1460-9568.1997.tb01702.x
156. Shen B, Yi X, Sun Y, Bi X, Du J, Zhang C, et al. Proteomic and metabolomic characterization of COVID-19 patient sera. Cell. (2020) 182:59–72.e15. doi: 10.1016/j.cell.2020.05.032
157. Sanchez-Lopez E, Zhong Z, Stubelius A, Sweeney SR, Booshehri LM, Antonucci L, et al. Choline uptake and metabolism modulate macrophage IL-1β and IL-18 production. Cell Metab. (2019) 29:1350–62.e7. doi: 10.1016/j.cmet.2019.03.011
158. Chowdhury P, Pathak P. Neuroprotective immunity by essential nutrient “Choline” for the prevention of SARS CoV2 infections: an in silico study by molecular dynamics approach. Chem Phys Lett. (2020) 761:138057. doi: 10.1016/j.cplett.2020.138057
159. Bennett JA, Mastrangelo MA, Ture SK, Smith CO, Loelius SG, Berg RA, et al. The choline transporter Slc44a2 controls platelet activation and thrombosis by regulating mitochondrial function. Nat Commun. (2020) 11:1–9. doi: 10.1038/s41467-020-17254-w
160. Germain M, Chasman DI, de Haan H, Tang W, Lindström S, Weng LC, et al. Meta-analysis of 65,734 individuals identifies TSPAN15 and SLC44A2 as two susceptibility loci for venous thromboembolism. Am J Hum Genet. (2015) 96:532–42. doi: 10.1016/j.ajhg.2015.01.019
161. Hinds DA, Buil A, Ziemek D, Martinez-Perez A, Malik R, Folkersen L, et al. Genome-wide association analysis of self-reported events in 6135 individuals and 252 827 controls identifies 8 loci associated with thrombosis. Hum Mol Genet. (2016) 25:1867–74. doi: 10.1093/hmg/ddw037
162. Flesch BK, Reil A. Molecular genetics of the human neutrophil antigens. Transf Med Hemother. (2018) 45:300–9. doi: 10.1159/000491031
163. Constantinescu-Bercu A, Grassi L, Frontini M, Salles-Crawley II, Woollard KJ, Crawley JTB. Activated αiibβ3 on platelets mediates flow-dependent netosis via slc44a2. Elife. (2020) 9:53353. doi: 10.7554/eLife.53353
164. Kenny M, Schoen I. A handshake between platelets and neutrophils might fuel deep vein thrombosis. Platelets. (2020) 31:624–6. doi: 10.1080/09537104.2020.1769053
165. Richter K, Mathes V, Fronius M, Althaus M, Hecker A, Krasteva-Christ G, et al. Phosphocholine—an agonist of metabotropic but not of ionotropic functions of α9-containing nicotinic acetylcholine receptors. Sci Rep. (2016) 6:1–13. doi: 10.1038/srep28660
166. Hecker A, Küllmar M, Wilker S, Richter K, Zakrzewicz A, Atanasova S, et al. Phosphocholine-modified macromolecules and canonical nicotinic agonists inhibit ATP-induced IL-1β release. J Immunol. (2015) 195:2325–34. doi: 10.4049/jimmunol.1400974
167. Cundell DR, Gerard NP, Gerard C, Idanpaan-Heikkila I, Tuomanen EI. Streptococcus pneumoniae anchor to activated human cells by the receptor for platelet-activating factor. Nature. (1995) 377:435–8. doi: 10.1038/377435a0
168. Iuchi H, Ohori J, Kyutoku T, Ito K, Kurono Y. Role of phosphorylcholine in Streptococcus pneumoniae and nontypeable Haemophilus influenzae adherence to epithelial cells. Auris Nasus Larynx. (2019) 46:513–9. doi: 10.1016/j.anl.2018.11.003
169. Pang B, Winn D, Johnson R, Hong W, West-Barnette S, Kock N, et al. Lipooligosaccharides containing phosphorylcholine delay pulmonary clearance of nontypeable Haemophilus influenzae. Infect Immun. (2008) 76:2037–43. doi: 10.1128/IAI.01716-07
170. Ring A, Weiser JN, Tuomanen EI. Pneumococcal trafficking across the blood-brain barrier. Molecular analysis of a novel bidirectional pathway. J Clin Invest. (1998) 102:347–60. doi: 10.1172/JCI2406
Keywords: COVID-19, SARS-CoV-2, platelets, inflammation, alpha7 nicotinic acetylcholine receptor (α7nAchR), thrombosis
Citation: Jankauskaite L, Malinauskas M and Snipaitiene A (2022) Effect of stimulated platelets in COVID-19 thrombosis: Role of alpha7 nicotinic acetylcholine receptor. Front. Cardiovasc. Med. 9:1037369. doi: 10.3389/fcvm.2022.1037369
Received: 05 September 2022; Accepted: 26 September 2022;
Published: 14 October 2022.
Edited by:
Luca Spiezia, University of Padua, ItalyReviewed by:
Hanan Salah El-Abhar, Cairo University, EgyptDenis Kudryavtsev, Institute of Bioorganic Chemistry (RAS), Russia
Copyright © 2022 Jankauskaite, Malinauskas and Snipaitiene. This is an open-access article distributed under the terms of the Creative Commons Attribution License (CC BY). The use, distribution or reproduction in other forums is permitted, provided the original author(s) and the copyright owner(s) are credited and that the original publication in this journal is cited, in accordance with accepted academic practice. No use, distribution or reproduction is permitted which does not comply with these terms.
*Correspondence: Lina Jankauskaite, bGluYS5qYW5rYXVza2FpdGVAbHNtdW5pLmx0