- 1ENDomics Lab, Department of Medicine, Center of Oncology, University Medical Center Hamburg-Eppendorf, Hamburg, Germany
- 2Division of Pneumology and Center for Pulmonary Arterial Hypertension Hamburg, University Medical Center Hamburg-Eppendorf, Hamburg, Germany
Pulmonary arterial hypertension (PAH) is a disease characterized by elevated pulmonary vascular resistance and pulmonary artery pressure. Mortality remains high in severe cases despite significant advances in management and pharmacotherapy. Since currently approved PAH therapies are unable to significantly reverse pathological vessel remodeling, novel disease-modifying, targeted therapeutics are needed. Pathogenetically, PAH is characterized by vessel wall cell dysfunction with consecutive remodeling of the pulmonary vasculature and the right heart. Transcription factors (TFs) regulate the process of transcribing DNA into RNA and, in the pulmonary circulation, control the response of pulmonary vascular cells to macro- and microenvironmental stimuli. Often, TFs form complex protein interaction networks with other TFs or co-factors to allow for fine-tuning of gene expression. Therefore, identification of the underlying molecular mechanisms of TF (dys-)function is essential to develop tailored modulation strategies in PAH. This current review provides a compendium-style overview of TFs and TF complexes associated with PAH pathogenesis and highlights their potential as targets for vasculoregenerative or reverse remodeling therapies.
Introduction
Pulmonary arterial (PA) hypertension (PAH), whether idiopathic (IPAH), hereditary (HPAH), or associated with other conditions (APAH), is a rare, serious and progressive pulmonary vascular disease. Despite improvements in the management of PAH, overall 5-year mortality remains around 30% (1).
PAH is characterized by elevated resistance and pressure in precapillary pulmonary vessels leading to right heart failure, if untreated (2, 3). Pathophysiologically, PAH is characterized by an initial loss of small pulmonary microvessels via endothelial cell (EC) apoptosis in combination with neointima formation through the uncontrolled growth of smooth muscle cell (SMC)-like cells, adventitial fibroblasts (AF), pericytes and mesenchymally transdifferentiated endothelial cells (endothelial-mesenchymal transition, EndMT) (4–6). Although the origin of hyperproliferative neointimal cells in PAH is still not fully understood, recent lineage-tracing studies suggest that the neointima mainly consists of propagating SMC, while EndMT can be detected in a smaller fraction of pathologically remodeled lung vessels (7). Upon persistent vascular inflammation, PAECs also undergo a phenotypic switch from initially increased propensity to apoptosis toward a more apoptosis-resistant and hyperproliferative state thereby further contributing to intraluminal PA obstruction (4, 8).
Currently available pharmacological options in PAH comprise vasodilatory drugs with selectivity for the pulmonary vasculature that attenuate disease progression; namely endothelin receptor antagonists (ERA: bosentan, ambrisentan, and macitentan), phosphodiesterase 5 inhibitors (PDE5i: sildenafil, tadalafil), or soluble guanylate cyclase (sGC: riociguat) stimulator in addition to prostanoids/prostacyclin receptor agonist (epoprostenol, iloprost, treprostinil, selexipag) (9, 10). However, all of the currently available drugs fail to meaningfully reverse PAH-associated structural remodeling of pulmonary blood vessels and lung transplantation remains the only cure. Therefore, novel therapeutic approaches are needed to attenuate PAH progression but also reverse prevalent structural remodeling of the pulmonary vasculature.
In this light, disease-modifying drugs have been an important research focus in PAH over the last few years. Bone morphogenic protein receptor type II (BMPR2) has evolved as a promising molecular target (11, 12). BMPR2 is a transmembrane serine/threonine receptor kinase and a member of the transforming growth factor (TGF)-β superfamily and is a pivotal player in differentiation, inflammation, apoptosis, and proliferation pathways of the pulmonary vasculature (4, 13–15). Pathogenic variants in the BMPR2 gene account for approx. 75% of HPAH cases and for ∼20% of IPAH cases (16, 17). In addition to germline mutations, BMPR2 expression and BMPR2 signal transduction is universally impaired in all PAH forms, including APAH (16, 18–20) and other precapillary PH forms such as chronic thromboembolic PH and interstitial lung disease associated PH (21, 22) by a plethora of pathological mechanisms [reviewed in (23)]. Pharmacological strategies to re-activate or re-balance BMPR2 signaling in the pulmonary vasculature have been able to restore PA endothelial function, suppress PASMC proliferation and successfully treat PH in experimental models (24–28) and early clinical trials (29–31).
Downstream of BMPR2, non-canonical transcription factors (TFs) can be pharmacologically harnessed to reverse experimental PH (28, 32–35) and repair prevalent DNA damage in PAEC from PAH patients harboring BMPR2 mutations (28) uncovering an additional BMPR2-dependent disease-modifying approach.
This review, therefore, summarizes the current knowledge regarding the role of TFs in PAH pathogenesis and explores their therapeutic potential as disease modifiers in PAH.
Transcription factors: Molecular basics
TFs are key cellular components that—as molecular switches—control gene expression: TFs are DNA-binding proteins that relay external and internal cellular stimuli to a molecular function enabling gene transcription (36). These processes require modification in chromatin structure by chemical modification of DNA and histones as well as other ribonucleoproteins. Therefore, TFs are part of a finely tuned interaction network with chromatin remodeling or histone-modifying proteins to regulate gene transcription (37). TFs bind to highly specific regulatory DNA elements, so called “motifs,” within promoter or enhancer regions of their target genes to either activate or repress transcription (38, 39). TFs can regulate transcription either by recruiting chromatin remodeling proteins to induce conformational changes of chromatin to provide DNA accessibility or by directly binding to promotors and enhancers to facilitate the recruitment of additional components of the transcriptional machinery for transcription initiation (37). In this regard, TFs have much higher (> 1,000-fold) affinity to their specific DNA-binding sites within a target gene (= TF-binding site, TFBS) than to surrounding, non-specific DNA sequences (40). These TFBS (or “motifs”) are usually found as DNA repeats in cis-regulatory and non-coding DNA elements (see above) (41). As TFs are pivotal to integrating a plethora of cellular processes, TF dysfunction, e.g., through mutations, or (epigenetic) inactivation, contributes to the pathogenesis of numerous diseases (41–45).
Transcription factors: Key regulators in PAH pathogenesis
In the pulmonary vasculature, TFs regulate crucial cellular functions such as proliferation, differentiation, inflammation, cell death, repair, and regenerative programs (39, 45). In PAH, TFs are responsible for altered expression of multiple disease-related genes thereby contributing to defective cellular homeostasis and vascular remodeling (46, 47). Members from eight out of ten TF superclasses (48) are crucially involved in PAH pathophysiology. In this section, we provide a short compendium of the most relevant TFs of each superclass with relevance to PAH (please also see Table 1 and Figures 1A,B).
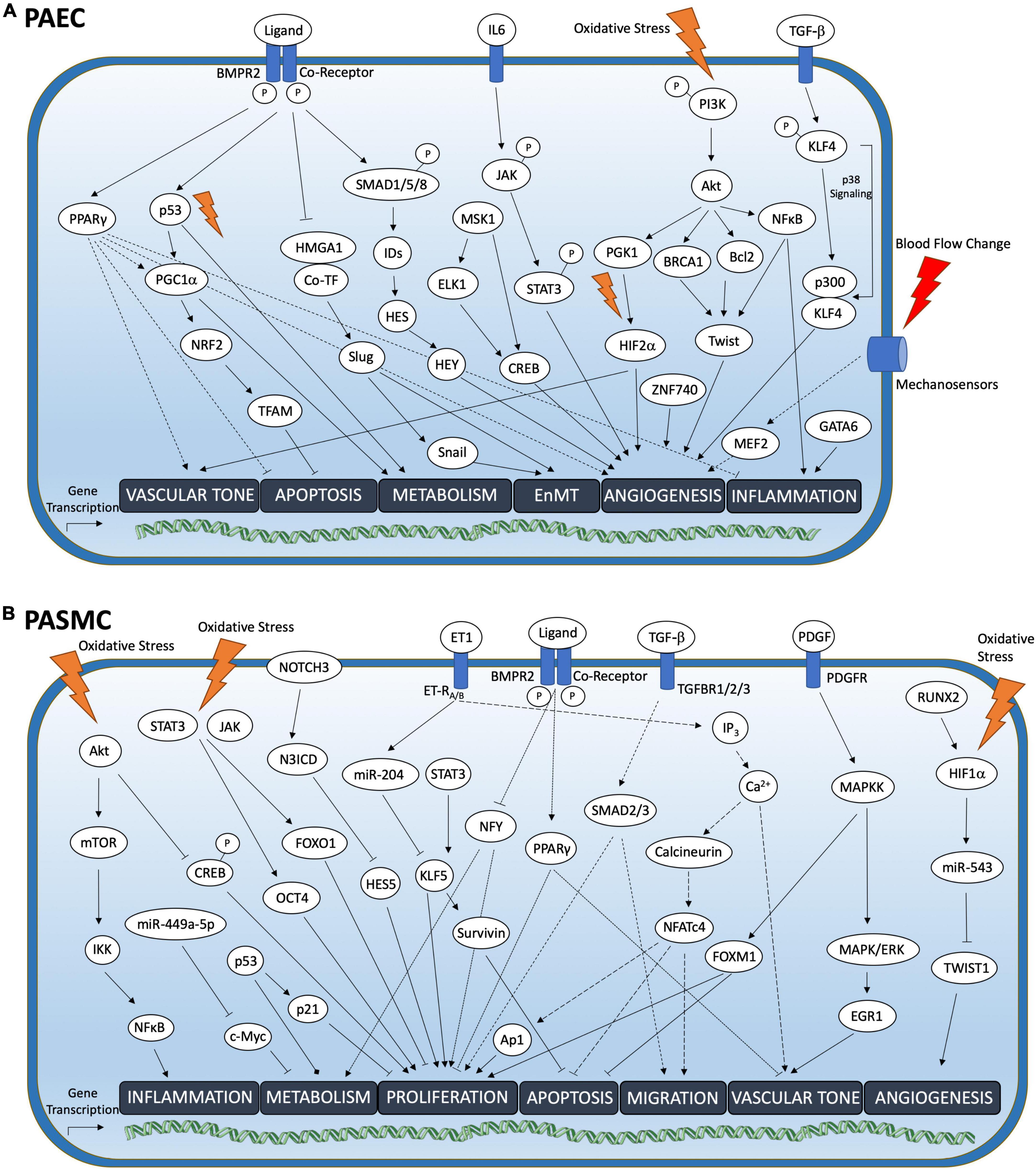
Figure 1. Transcription factor pathways in PAEC and PASMC associated with PAH. Overview of pathogenetically relevant transcription factor (TF) pathways in (A) pulmonary arterial endothelial cells (PAEC) and (B) pulmonary arterial smooth muscle cells (PASMC) upon activation by cell membrane anchored receptor signaling or cellular stress events. Depending on the pulmonary vascular cell type TF activation mediated gene transcription elicits cell-type specific downstream responses. Blue box represents cell membrane. Ca2+, Calcium; IP3, Inositol 1,4,5-trisphosphate; ET-RA/B, Endothelin Receptor Type A and B; TGFBR1/2/3, TGF-ß receptor 1/2/3; −p, Phosphorylation.
TF superclass 1
TFs belonging to the basic domains group superclass (S1) bind DNA through a basic region which becomes folded in an alpha-helically manner if added to DNA (48). At least six members of this superclass contribute to PAH pathogenesis.
CREB
Cyclic adenosine monophosphate (cAMP) response element binding protein (CREB) functions as an anti-proliferative TF in healthy PASMC. In PAH and associated oxidative stress with excessive production of reactive oxygen species (ROS) like H2O2, CREB is downregulated leading to enhanced proliferation of PASMC (49).
HES5
The hes family bHLH transcription factor 5 (HES5) binds to the Notch-receptor and promotes proliferative signals (50). In PASMCs, HES5 inactivation reverses the proliferative effect of NOTCH3 and induces a shift in gene expression toward a more differentiated phenotype (51).
MYC
MicroRNAs (miR/miRNA) regulate numerous disease pathways in the pulmonary vasculature and have been linked with PAH development (52–54). In this context, Zhang et al. showed that miR-449a-5p, which is downregulated in PAH, represses the activity of the TF MYC proto-oncogene (MYC). Lack of MYC repression in PAH PASMC is associated with mitochondrial and metabolic dysfunction as well as phenotype transformation (55).
TWIST1
Expression of Twist-related protein 1 (TWIST1) is increased in the lungs of PAH patients and TWIST1 has been shown to mediate EndMT thereby contributing to pathological vascular remodeling in PAECs (56, 57).
TF superclass 2
The TF superclass 2 contains TFs with Zinc-coordination DNA-binding domains. Such zinc fingers, consisting of a repetitive pattern of cysteine and histidine residue, represent the most frequent DNA-binding motifs found in eukaryotic TFs (48). The frequency of zinc fingers among DNA-binding motifs is also represented by the many members of the TF superclass 2 that play a role in PAH.
PPARG
Peroxisome proliferator-activated receptor gamma (PPARγ) is a member of the nuclear hormone receptor superfamily of ligand-activated TFs. It is pivotal for the regulation of mulitple central processes in pulmonary vascular cells (47, 58–61). PPARγ, which is ubiquitously expressed, represents probably the best-studied TF in pulmonary hypertension. Norbert Voelkel and his group were first to demonstrate that PPARγ is downregulated in lungs from PAH patients and in PAH-associated vascular lesions (62). PPARγ dysfunction in PAEC or PASMC facilitates the hyperproliferative vascular phenotype typical for PAH (47, 63).
In PASMC, a downregulation of PPARγ by short interfering RNA leads to increased proliferation, decreased mitochondrial mass and increased mitochondrial ROS generation (47, 63), which is in part mediated by decreased levels of TFAM, GRP75, and MFN2 (47) and by NF-kB dependent NOX4 upregulation (64). In contrast, pharmacological PPARγ activation is sufficient to reverse experimental PH (58, 65).
In this regard, Hansmann et al. showed that PPARγ-mediated anti-proliferative BMP-2 signaling in PASMC and that loss of PPARγ function in PASMC was associated with the spontaneous onset of experimental PH. PPARγ agonists were able to restore anti-proliferative signaling in wildtype and BMPR2-mutant PASMC, suggesting early on that activation of PPARγ signaling may reverse PAH (66). Mechanistically, in PASMC this is mediated by BMP2-dependent upregulation of a protective autocrine PPARγ—Apolipoprotein E (ApoE)—Low density lipoprotein receptor-related protein 1 (LRP1) axis (66) and inhibition of TGF1-mediated SMAD3/4 and STAT3-FOXO1 signaling (see below) (67). In these studies, Chakraborty et al. used Cre-constructs driven by the Tagln/Sm22-promoter to delete PPARγ in SMC instead of more SMC-specific promoters such as Myh11 (68). The Tagln/Sm22 promoter has been shown to also be active in cardiomyocytes and non-muscle tissues such as myeloid cells and platelets [reviewed in: (68)]. Therefore, future studies need to evaluate to what extent PPARγ’s protective function to reverse experimental PH relates to rehabilitation of SMC-specific signaling or also includes effects on additional cell types such as cardiomyocytes as suggested by a recent study of the same group (32).
In PAECs, Vattulainen-Collanus et al. suspected that a lack of PPARγ could result in increased expression of E2F1, which is associated with a dysregulated Wnt pathway and disturbed angiogenesis and migration (69). PPARγ may also play a role in PAEC’s response to DNA damage (70). In cellular studies, depletion of PPARγ was sufficient to promote the development of a PH phenotype by upregulation of cell cycle- and angiogenesis-related genes (71). In an EC-specific PPARγ knockout mouse model (using the Tie2 promoter), experimental PAH developed spontaneously (63). Additional information on the beneficial effects of PPARγ on the pulmonary vasculature can be found further down in the section on PPARγ TF complexes.
SNAI2
Snail family transcriptional repressor 2 (SNAI2), also known as Slug, a highly conserved zinc finger TF, has been implicated in epithelial-mesenchymal transition (EMT) and EndMT (72). In PAEC, loss of BMPR2 leads to increased expression of High-mobility group protein 1 (HMGA1) and Slug, which is associated with upregulation of SMC markers and EndMT (57).
EGR1
Expression of early growth response protein 1 (EGR1) is increased in plexiform lesions of PAH (73, 74), is triggered by tissue damage and is associated with pathological remodeling of the lung vessel wall (75). Interestingly, EGR-1 is negatively regulated by PPARγ agonists (75).
ZNF740
Zinc finger proteins (ZNFs) bind classically to DNA, RNA, proteins, and other small molecules and are highly conserved in their binding specificity of a particular protein. Yu et al. identified a novel signaling pathway involved in proliferation and angiogenesis of PAECs and in vascular remodeling in vitro. This new signaling axis consists of ZNF740, GDF11, TGF-β-receptor I, and SMAD, which is also involved in the imbalance of pulmonary vascular homeostasis in PAH (76).
KLF2
Krüppel-like Factor 2 (KLF2) is a vasculoprotective factor expressed in endothelial cells that is activated by laminar shear stress and is pivotal for normal lung vessel formation (77). Heterozygous germline missense mutations in KLF2 have recently been associated with HPAH (Table 2) (78–80) and KLF2 mRNA expression is strongly downregulated in lungs from rodents and humans with PAH (80, 81). Loss of KLF2 impairs NO synthesis and thereby contributes to the severity of hypoxia-induced PH in Apelin-deficient mice (82). In contrast, adenoviral transduction mediates anti-inflammatory, anti-apoptotic, and anti-proliferative effects in PAEC under nutrient stress (80). Additionally, miRNA isolated from exosomes derived from KLF2-overexpressing PAEC can be therapeutically harnessed to attenuate experimental PH in the Sugen/hypoxia mouse model (80).
KLF4
Krüppel-like Factor 4 (KLF4), a protective PAEC maintenance factor, is inactivated by posttranslational modification upon nitrosative stress, thereby disabling its protective function in the pulmonary vasculature (83). Recently, KLF4 was identified as an interaction partner of the SWI/SNF complex to increase accessibility of enhancer sites which regulate genes essential for endothelial homeostasis under laminar shear stress (84).
KLF5
Krüppel-like Factor 5 (KLF5) has been linked with an apoptosis-resistant and proliferative phenotype in PASMCs (85), as an upstream regulator of HIF1 in PASMC (86). In addition, KLF5 and HIF1 might form a TF complex with yet unknown function (86).
PGC1A
PPARγ coactivator-1α (PGC1A/PPARGC1A), which normally regulates oxidative metabolism and mitochondrial biogenesis, was found to regulate inflammation in blood cells of IPAH patients by activating cytochrome complex (CYTC) under hypoxia (87, 88). In PASMC, PGC1A regulates the expression of the Mitofusin-2 gene MFN2 to maintain mitochondrial integrity. PAH PASMC lacking PGC1A and MFN2 show heightened mitochondrial fragmentation associated with increased PASMC proliferation (88). In PAEC, PGC1A promotes EC survival and sustains mitochondrial membrane potential upon oxidative stress downstream of a non-canonical BMPR2-p53 axis (20).
GATA6
GATA sequence binding protein 6 (GATA6), a member of the ZNF TF family, is upregulated in inactive vasculature and downregulated during vascular injury (89). In PAECs, GATA6 directly regulates ET1 receptor type A (ETA), a gene for controlling vascular tone, as well as pro-inflammatory genes like 5-lipoxygenase-activating protein PAI-1, which is involved in vascular remodeling and increased vascular muscularization (89).
TF superclass 3
The helix-turn-helix superclass (S3) of TFs comprises a DNA-recognition helix that fits into the major DNA groove. Some important TFs regarding their relevance to PAH belong to this superclass.
FOXO1 and FOXM1
Forkhead box proteins O1 (FOXO1) and M1 (FOXM1) have opposing roles in PAH pathogenesis. While FOXM1 is overexpressed in PASMC of PAH patients and promotes hypoxia-induced proliferation as well as resistance against apoptosis and DNA repair (90–92), FOXO1, which integrates multiple vasculoprotective pathways, shows reduced expression and/or is inactivated in PAH PASMC (93).
ELK1
ETS Like-1 protein (ELK1) is a member of the E-twenty-six (Ets) ternary complex family of TFs known to stimulate the expression of immediate early response genes involved in cellular proliferation and apoptosis (94). Phosphorylation of Elk-1 in concert with p38-mitogen-activated protein kinase (MAPK) induces PAEC proliferation (95).
MSX1
Msh homeobox 1 (MSX1) is upregulated in lymphocytes of IPAH patients and EC of BMPR2-deficicent mice. Lack of BMPR2-mediated suppression derepressed MSX1 expression which correlates with upregulation of MSX1 target genes in IPAH (96).
OCT4
The octamer-binding TF 4 (OCT4) is a marker for undifferentiated cells, highly expressed in human embryonic stem cells. Even though OCT4 is frequently silenced in differentiated somatic cells (97), Firth et al. detected weak expression of OCT4 isoforms A and/or B mRNA and strong expression of OCT4 pseudogene (PSG) 1 and 5 mRNA in PASMC from healthy controls. In PASMCs under hypoxia or isolated from IPAH patients, mRNA expression of OCT4A/B is upregulated, whereas OCT4 PSG 1 and 5 are downregulated (98). OCT4A/B upregulation in IPAH PASMC might be mediated by HIF2α, which has been shown to directly bind to the OCT4 promoter (99), and is a key regulator of the pro-proliferative response in PAAF (100). This is in line with a study by Bertero et al. showing that HIF2α-dependent OCT4 activation promotes early vascular stiffening as a central pathological event in PAH via induction of microRNA 130/301 (53). Therefore, hypoxia-associated OCT4 upregulation might also contribute to a hyperproliferative, dedifferentiated PASMC phenotype in IPAH.
TF superclass 4
TF superclass 4 comprises transcription factors with alpha-helical DNA-binding domains. At least three members of this superclass have important functions in PAH pathogenesis.
SOX17
SRY-related HMG-box (SOX) 17 is an endothelial-specific TF pivotal for cardiac and pulmonary development by integrating and regulating VEGF, WNT and NOTCH signaling [reviewed in (101)]. Activation of SOX17 represses PA remodeling in the monocrotaline PH model (102). Using genome-wide association studies in PAH, rare pathogenic variants within the coding region of SOX17 and SNPs in an enhancer region have been associated with PAH (103–105).
TFAM
Transcription Factor A, Mitochondrial (TFAM) is a crucial modulator of the inflammatory response to oxidative stress and maintains mitochondrial DNA integrity and cell survival in PAEC under oxidant stress downstream of the non-canonical BMPR2-p53 signaling axis (20).
NFY
Nuclear factor Y (NFY) is epigenetically activated in PASMC isolated from PAH patients to induce pro-proliferative and glycolysis genes to facilitate the cancer-like hyperproliferative and glycolytic-switch phenotype of PAH PASMC (106).
TF superclass 5
Members of the alpha-helices exposed by beta-structures (S5), as the name suggests, possess alpha helices exposed by a scaffold of beta-strands (48). To our knowledge, there is a single TF of this group with a well-established role in PAH.
MEF2
Transcriptional activity of myocyte enhancer factor 2 (MEF2) is inhibited in PAEC isolated from PAH patients by nuclear accumulation of histone deacetylases 4 and 5. Thereby, expression of vasculoprotective factors miR-424, miR-503, connexins 37 and 40 as well as KLF2 and 4 is impaired contributing to PAH pathogenesis (107).
TF superclass 6
The Immunoglobulin fold TF superclass (S6) comprises TFs that are characterized by a beta-core structure that induces a DNA contact. Many TFs of this group play a role in the context of PAH.
NFAT
Nuclear factor of activated T cells (NFAT), discovered approx. three decades ago (108), is increased in PAH and regulates PASMC calcium homeostasis in conjunction with calcineurin (CaN) as interaction partner (109). Increased CaN/NFAT promotes PASMC proliferation, survival and migration in chronic hypoxia and MCT-induced PAH (109). In addition, NFAT is upregulated by DNA-damage mediated PARP-1 activation facilitating pulmonary vascular remodeling which was reversible by PARP-1 inhibitors (110).
RUNX1
Liang et al. reported that bone-marrow derived endothelial progenitor cells (EPC) undergo endothelial-to-hematopoietic transition (EHT) to promote pulmonary arterial hypertension. Inhibition of the critical hematopoietic transcription factor Runt-related transcription factor 1 (RUNX1), also known as acute myeloid leukemia 1 protein (AML1), blocked EHT in vivo, and attenuated progression of experimental PH by preventing bone-marrow egression of EPC (111). In addition, RUNX1 mediates expression of neutrophil elastase in PASMC contributing to ECM remodeling in the pulmonary vasculature (112).
RUNX2
RUNX family transcription factor 2 (RUNX2) promotes vascular remodeling and stiffening in vascular disease (113–115). RUNX2 activation promotes vascular calcification. Excessive proliferation of PASMCs in PAH is sustained over time by the loss of miR-204-mediated upregulation of RUNX2 contributing to the development of proliferative and calcified PA lesions (116).
TP53
Tumor protein p53 (p53), the Guardian of the Genome (117), is a crucial TF highly conserved in multicellular vertebrates, where it functions as a tumor suppressor by maintaining genome integrity and stability (118). In general, p53 controls many central cellular functions such as cell cycle, DNA repair, apoptosis as well as inflammatory and metabolic homeostasis via its numerous (direct) target genes (119, 120). In the vasculature, depending on the context, p53 exerts both, detrimental (121–123) and regenerative effects (124, 125). In the pulmonary vasculature, p53 fulfills protective functions: Mizuno et al. demonstrated that mice with global p53 knockout developed more severe PH upon chronic hypoxia (126). This is in concert with data showing that pharmacological inhibition of p53 transcriptional activity by Pifithrin-α was sufficient to spontaneously induce PH in rats and to aggravate MCT-induced PH (127). In addition, Wakasugi et al. found that reduced p53 expression in PASMC led to increased aerobic glycolysis and downregulation of mitochondrial respiration thereby contributing to the cancer-like hyper-proliferative “Warburg phenotype” found in PASMC isolated from PAH patients. PASMC-specific p53-knockout, however, did not aggravate hypoxia-induced PH (128). Activation of p53 in PASMC by Nutlin-3, on the other hand, prevented and reversed experimental PH mice (129). In PAEC, p53 is a non-canonical effector downstream of BMPR2 (20, 28). Under oxidative stress, BMPR2-defective PAEC are unable to stabilize and transcriptionally activate p53 and p53-dependent TFs PGC1A, nuclear factor erythroid 2-related factor 2 (NRF2), and mitochondrial transcription factor A (TFAM). Loss of BMPR2-p53 signaling destabilizes mitochondrial DNA integrity and biogenesis causing adenosine triphosphate (ATP)-crises-mediated PAEC apoptosis which is associated with an inability to recover from hypoxia-induced PH (20). While, strictly speaking, p53 itself is a TF complex by auto-multimerization, fine-tuning of cellular effects depends on additional context-specific interaction partners (120). In the pulmonary vasculature, in response to oxidative stress and other DNA-damaging agents, p53 forms a transcriptionally active, vasculoprotective complex with PPARγ in PAEC, PASMC, and PAAF which is BMPR2-dependent (28). This is discussed further in the section on TF complexes of this review.
NF-kB
Strictly speaking, nuclear factor kappa-light-chain-enhancer of activated B cells (NF-κB) resembles a TF complex, best studied in cancer, that mediates transcription of proinflammatory cytokines and thus promotes unfavorable cell phenotypes (130). In advanced PAH, NF-κB is active in PAEC, PASMC and perivascular macrophages and lymphocytes of large and small pre-capillary vessels and is correlated with expression of pro-inflammatory cytokines (131). In PAEC, NF-κB contributes to leucocyte adhesion and inflammation facilitating EndMT (132). In contrast, genetic and pharmacological inhibition of NF-κB reversed and prevented experimental PAH in rodent models, respectively (133, 134). This indicates that targeting might be a reasonable therapeutic strategy.
TBX4
T-box TF 4 (TBX4) is necessary in embryonal development and a gene mutation leads into an autosomal-dominant disorder called small patella syndrome (135). Kerstjens-Frederikse et al. showed that genetically depleted TBX4 is associated with childhood-onset PAH, which, with 0.7 cases per million, is an even rarer disease than PAH (136).
STAT3
Several physiological processes, like cell growth and apoptosis, are affected by the pro-survival TF signal transducers and activators of transcription-3 (STAT3) and an inhibition always leads to dramatic changes in biological processes. In PASMCs, Paulin et al. demonstrated that STAT3 activation induces proliferation and resistance to apoptosis by activating NFAT (137).
STAT1
Gairhe et al. showed that Signal Transducer and Activator of Transcription 1 (STAT1) is elevated in PAECs with caveolin1 loss-of-function. This results in a proliferative, hypermigratory phenotype (138). Also Otsuki et al. showed, that PAECs stimulated with human endogenous retrovirus K (HERV-K) dUTPase have a TLR4-STAT1-dependent inflammatory response (132).
TF superclass 7
This superclass features an alpha-/beta-scaffold in the DNA-binding domain (48).
SMAD3
SMADs, in particular phospho-SMAD1/5/8 are important downstream TF of BMPR2 signaling in the pulmonary vasculature (23). SMAD family member 3 (SMAD3) is downregulated in lungs from PAH patients or animal models. Loss of SMAD3 is associated with a hyperproliferative and pro-migratory PASMC and PAEC phenotype in a myocardin-related transcription factor (MRTF)-dependent manner (139). In PAEC stimulated with HERV-K dUTPase, activation of SMAD3 can induce EndMT via SNAIL (132). More details about SMAD signaling can be found in the section on TF complexes of this review.
TF superclass 8
In this superclass, DNA binding occurs through β-sheets (48). A single TF from this superclass has been associated with PAH pathogenesis.
HMGA1
High Mobility Group AT-hook 1 (HMGA1) is upregulated in PAECs of PAH patients, which is associated with a loss of BMPR2. By inducing SLUG expression, HMGA1 promotes EndMT of PAECs into an SMC-like mesenchymal phenotype in the vasculature of BMPR2-mutant PAH patients (54).
Newly identified transcription factors with unknown impact
In addition to the above-listed TFs with well-established implications for PAH, there are other TFs that might contribute to the disease. A recent comprehensive analysis of chromatin remodeling in PAEC identified 18 novel TFs with differential activity in PAH compared with healthy control donors (more active in PAH: ATF1, ATF7, E4F1, CREB5, RFX3, RFX4, FOSL1, FOSL2, JUN, JUND, BATF; more active in controls: ARI3A, FOXG1, FOXJ3, FOXL1, TBX3, PITX2). These TFs are not discussed further in this review as the exact molecular mechanisms and associated pathophysiological ramifications remain to be elucidated (140).
Pathogenic genetic transcription factor variants in pulmonary arterial hypertension
Specific variants in at least 22 genes have been associated with PAH pathogenesis (78, 105, 141, 142). Of these, six genes code for TFs, namely KLF2, SMAD1, SMAD4, SMAD9, SOX17, and TBX4. Variants in two of these genes show definitive associations with PAH pathogenesis as classified by independent expert panel working groups (Table 2).
Transcription factor complexes: Basics
Finely tuned regulation of transcription requires sequence-specific DNA binding of TFs and co-factors. The combination of multiple TFs is termed combinational control. Cooperation between multiple copies of the same TF, or between different TFs, can stimulate transcriptional synergy in which the regulatory effect of TFs working together is greater than the sum of the individual TFs (143). Cooperative TFs typically generate transcriptional output through such multi-protein complexes (144). A distinction must be made between (1) complexes that consist of several TFs, i.e., individual TFs that have a potentially transcriptional modulatory effect on their own, and (2) complexes in which TFs are influenced in their function by cofactors. Depending on the binding partner and cell type, a single TF can thus influence various signaling pathways through complex formation (145). Whether a TF complex consisting of at least two TFs is referred to as a TF complex, TF dimer, or TF multimer, or to what extent a distinction is made between TF-TF complex and TF-co-factor complexes has not yet been defined uniformly.
Transcription factor complexes in the pathogenesis of pulmonary arterial hypertension
Although a multitude of TF complexes are known to affect pulmonary cells, only a few of them have been identified to play a pivotal role in the pathogenesis of PAH or harbor therapeutic potential. A descriptive overview of the best-described TF complexes in PAEC and PASMC is given below and summarized in Figure 2.
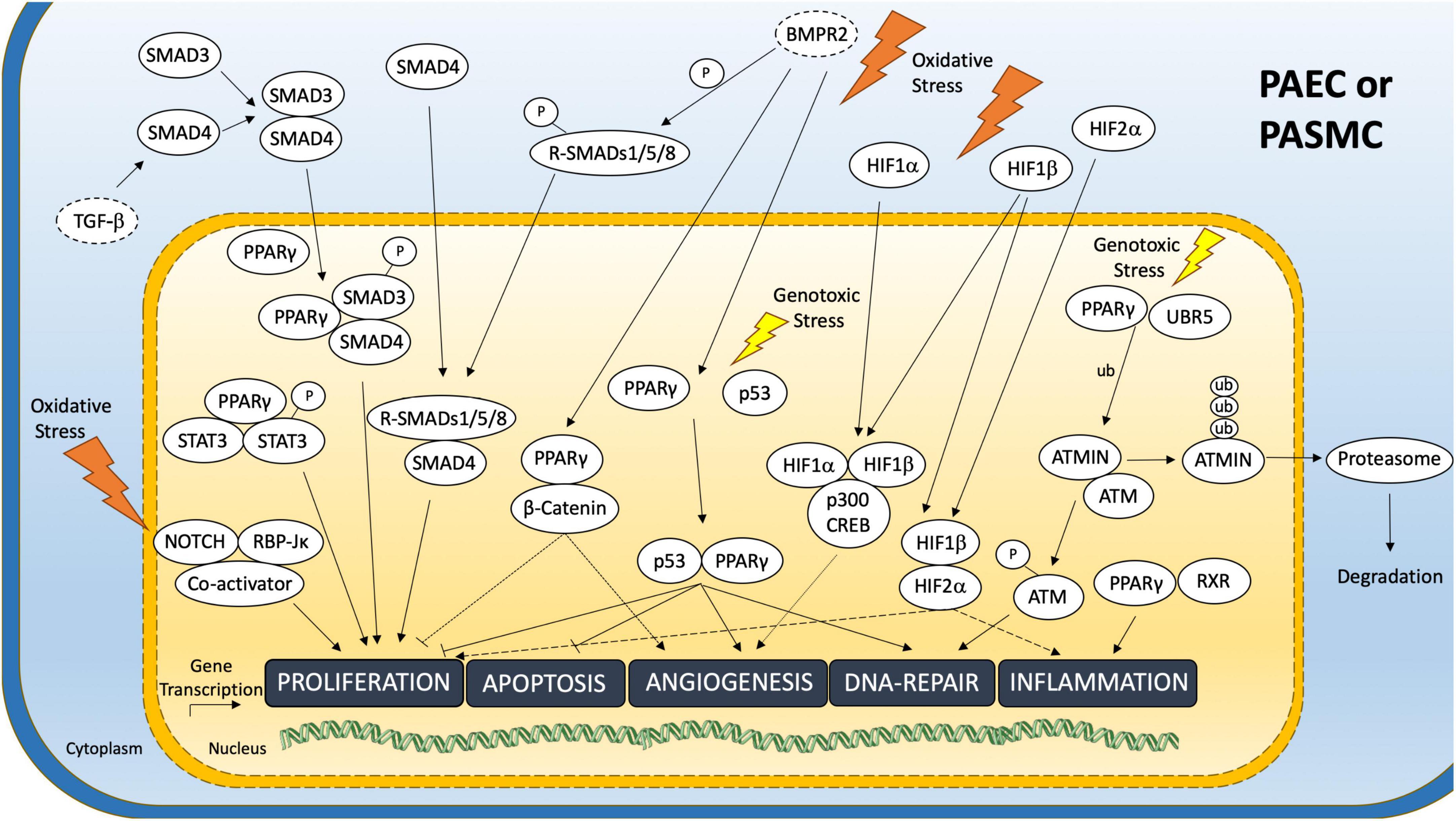
Figure 2. Transcription Factor Complexes in the Pulmonary Vasculature related to PAH. Overview of most relevant TF complex pathways in the pulmonary vasculature. TF complex formation upon complex inducing microenvironmental stimuli and downstream TF complex mediated gene transcription programs are shown. Yellow box represents nucleus. Blue box represents cell membrane. Dotted ovals indicate cell membrane anchored receptors. ub, Ubiquitin residue/Ubiquitination; –p, Phosphorylation.
AP1 complex
The activator protein 1 (AP1) TF complex, which is composed of c-JUN-c-FOS and c-JUN–c-JUN dimers, is regulated by many extracellular stimuli like peptide growth factors, pro-inflammatory cytokines, and other forms of cellular stress (146). In the vessel wall of lungs from IPAH patients, higher levels of total and phosphorylated c-FOS and c-JUN were detected, which results in an altered proliferative response in PASMCs mediated by the potent vasoconstrictor endothelin-1 (ET1) (147).
NOTCH1/RBP-Jκ TF-complex
The transmembrane protein Neurogenic locus notch homolog protein 1 (NOTCH1), which can be activated by extracellular ligands or hypoxia, releases the Notch intracellular domain (NICD) through proteolytic cleavage, which then translocates to the nucleus. There, NICD binds to the Recombinant Signal Sequence Binding Protein J kappa (RBP-Jκ) to form a heterodimeric TF complex. This complex positively influences the proliferation of PAEC and exerts anti-apoptotic effects (148). In addition, PAEC-PASMC contact, mediated by BMPR2-activated NOTCH1, induces transcription of endothelial regeneration genes, and coordinates the link between PAEC metabolism and chromatin remodeling to activate vascular homeostasis and repair in response to endothelial injury (149).
Hypoxia-inducible factor complexes
Hypoxia-inducible factor (HIF) represents a TF complex that is highly responsive to subtle changes in the environmental oxygen content of the lung. The HIF complex is permanently formed and degraded under normoxic conditions (150) and HIF complex dynamics are finely tuned: With decreasing ambient oxygen content the complex is rapidly stabilized and is also degraded within minutes when reoxygenation occurs (150, 151). In the lung, HIF isoforms HIF1α and HIF2α, individually form a TF complex with HIF1β (also known as aryl hydrocarbon receptor nuclear translocator, ARNT) (152–154).
Under hypoxia, HIF1 (= HIF1α/HIF1β complex) recruits another co-factor, CREB/p300 to bind to the hypoxia-responsive element (HRE) in the promoter region of its target genes (151) to transcriptionally regulate angiogenesis, vascular tone and remodeling (150, 151, 155, 156).
HIF1 is the predominant hypoxia sensor in PASMC and promotes a hyperproliferative PASMC phenotype (157). HIF1α expression is increased in pulmonary arteries of PAH patients (158) and contributes to the hyperproliferation of PASMC by modulating the vascular tone through altered expression of membrane ion channels in PASMC (159, 160). HIF1 directly induces expression of angiogenetic genes like VEGF (156) via nitric oxide (NO) synthases 2 (NOS2) which synthesizes the most potent vasodilator NO (158, 161, 162). Under conditions of reduced NOS2 expression or impaired activity, the relaxing effect of NO on the PA vascular bed is attenuated thereby facilitating vascular remodeling and neointima formation via increased PASMC proliferation and resistance to apoptosis (163, 164). In addition to NO synthesis, Wang and Ying describe another mechanism influencing vascular tone: Loss of HIF1α induces expression of miRNA-543, which then downregulates Twist1 resulting in increased expression of the potent vasoconstrictor ET-1 in PASMC (44). Mitogenic effects of ET-1 have been shown to be associated with PA remodeling (165). Vascular remodeling in the lung is further facilitated by HIF1 via transcriptional repression of miRNA-223 in PASMCs leading to increased PARP-1 expression (166), via a feedback loop with KLF5 (see above, 86) and by HIF1-dependent upregulation of plasminogen activator inhibitor-1 (PAI-1) (167) and Ras association domain-containing protein 1A (RASSF1A) to promote hypoxia-signaling to PASMC in PAH thereby likely conferring a cancer-like PASMC phenotype (168). Interestingly, PASMC proliferation caused by the transient HIF1 activation is attenuated by treatment with PPARγ activator rosiglitazone (169). HIF1α also mediates a metabolic shift to a cancer-like Warburg phenomenon in PAEC (158). Nevertheless, in PAEC HIF2α appears to be the predominant HIF isoform (147, 148).
HIF2α is increased in lung vascular ECs (LVECs) of IPAH patients which was associated with downregulation of HIF2α degrading enzyme prolyl hydroxylase domain protein 2 (PHD2). This resulted in induction of SNAI1/2 expression facilitating EndMT and formation of pulmonary vascular lesions (170). Endothelial-specific KO of PDH2 leads to experimental PH under normoxia which was dependent on HIF2α but not HIF1α (171). HIF2α also influences vascular resistance in the pulmonary vasculature. Mice with heterozygous global KO of HIF2α were protected from hypoxia-induced PH in an ET-1- and plasma catecholamine-dependent manner (172). Endothelial HIF2α disturbs EC NO homeostasis by upregulation of Arginase and mice with endothelial deletion of HIF2α were protected from hypoxia-induced PH (173). On the other hand, an activating mutation in the HIF2α gene is associated with erythrocytosis and pulmonary hypertension (174, 175), which, interestingly, seems to be mostly related to a phenotypic switch of PASMC but not PAEC (176).
It is likely that the HIF complex also interacts with additional TFs in the pulmonary vasculature to fine-tune hypoxia-associated gene expression. In this light, Palmer et al. suggest that HIF1α cooperates with activating Transcription Factor 1 (ATF-1) and/or CREB-1 either in the form of a complex or to functionally replace the two TFs in hypoxia (151). Additional interaction partners and their role in PA maintenance and remodeling remain to be elucidated.
SMAD complexes
Dysfunction of the BMPR2 signal transduction is found in all forms of PAH (24, 177, 178). Normally, BMPR2 activation triggers a canonical signaling pathway resulting in phosphorylation of Receptor-regulated Small Mothers Against Decapentaplegic Homolog Family members (R-SMADs, SMAD 1/5/8) (23). Activated R-SMADs form a heteromeric complex with common mediators (Co-SMADs, SMAD4). The R-SMAD-Co-SMAD complex translocates into the nucleus (179). SMAD proteins are crucial for cell development, the transcription of specific vasculoprotective target genes (180) and growth regulation by activating the TGF-β superfamily. While R/Co-SMADs activate the TGF-β pathway, I-SMADs disrupt the TGF-β pathway. Disturbed SMAD signaling leads to increased MSX1, which seems to be associated with IPAH and HPAH pathogenesis (96). The BMPR2-Smad axis is a promising therapeutic target as SMAD signaling can be pharmacologically reactivated on the BMPR2 level by tacrolimus (24, 181).
STAT3 homodimer
Signal transducer and activators of transcription-3 (STAT3) is a cytoplasmatic transcription factor, which is activated in response to cytokines (IL-6), growth factors (PDGF), and agonists (ET1) and mediates its function as a homodimer (182). It plays an important role in regulating the expression of multiple proteins and TFs associated with the pathogenesis of PAH such as HIF1α, Pim1, and NFAT. STAT3 signaling confers a cancer-like, hyperproliferative, anti-apoptotic phenotype to PAH PASMC (183). Functionally, STAT3 promotes pro-inflammatory processes by increasing the recruitment of inflammatory cells through induction of interleukin-6 (IL-6). In addition, STAT3 activation increases proliferation and migration of vascular SMCs in response to vascular injuries (184). STAT3 also regulates the miR-cluster17/92 and miR-204, which regulates BMPR2 translation. Via induction of KLF5, STAT3 augments transcription of the anti-apoptotic gene BIRC5 (survivin) to increase PASMC proliferation (184, 185). Another way, STAT3 promotes a pro-proliferative PASMC phenotype found in PAH patients is by increasing PIM1 gene expression and Nuclear Factor of Activated T Cells 2 (NFATC2) activity (183, 185).
YAP/TAZ/TEAD complex
Transcriptional co-regulators Yes-associated protein 1 (YAP) and Transcriptional Co-Activator with PDZ-Binding Motif (TAZ, official gene symbol: WWTR1) form complexes with TEA domain (TEAD) transcription factors and function as mechanotransducers and -effectors of the Hippo signaling cascade (186). Altered mechanobiological properties are a well-established pathological feature of PAH and stiffening of the ECM initiates a vicious circle of vessel wall remodeling that is further promoting ECM rigidity [reviewed in: (187)]. In this context, Bertero et al. have shown that ECM remodeling activates YAP/TAZ, which then induces expression of miRNA-130/301 independent of TEAD (188). miRNA-130/301 then promoted PA collagen deposition, lysyl oxidase (LOX) activation with subsequent release of pro-fibrotic factors causing proliferation of PAEC, PASMC, and PAAF and subsequent vessel wall remodeling, ECM stiffening and thus further YAP/TAZ activation (188). In addition, pulmonary vascular stiffening-associated YAP/TAZ activation also promoted metabolic reprogramming of PAEC through direct transcriptional regulation of the key enzyme of glutaminolysis, GLS1 (189). YAP/TAZ activation also contributes to PAH severity by suppressing anti-inflammatory and vasodilatory cyclooxygenase-2 and prostaglandin F1α in a TEAD-dependent fashion in PASMC (190).
PPARγ/RXRα complex
Peroxisome proliferator-activated receptors (PPARs) belong to a family of nuclear hormone receptors called nuclear factors. Different PPAR isoforms (α, β/δ, γ) are ubiquitously expressed, while PPARγ represents the main isoform in pulmonary vascular cells (66, 191). PPARs usually bind to a nuclear receptor response element (NRRE) in the promoter region of their target genes in complex with a co-repressor or co-activator and a histone deacetylase. Interaction with PPAR ligands forces co-repressor dissociation to activate the transcription machinery (192). Likewise, co-activators heavily influence the cellular response of this TF-complex by chromatin acetylation thereby making it accessible to RNA polymerase II (61). Typically, PPARs form heterodimers with their canonical interaction partner, retinoid X receptor (RXR), to control transcription of target genes that play a critical role in energy balance, including triglyceride and fatty acid metabolism and glucose homeostasis: processes that are dysregulated in obesity, diabetes, and atherosclerosis (58, 60, 61, 193, 194). It is highly likely, that most anti-inflammatory and vasculoprotective PPARγ effects in the pulmonary vasculature for which no exclusive PPARγ interaction partners have been identified are mediated by the PPARγ/RXRα complex (34, 59, 194).
PPARγ/MRN and PPARγ/UBR5 complexes
Although not a classical TF-TF complex, another DNA-associated PPARγ protein complex is of special interest for PAH pathogenesis. Upon genotoxic stress, PPARγ interacts with the DNA damage-sensing heterotrimer MRE11-RAD50-NBS1 (MRN) to facilitate DNA repair via the ATM pathway. This also requires the interaction of PPARγ with UBR5, an E3 ubiquitin-protein ligase, responsible for damage-associated degradation of ATM inhibitor (ATMIN) (70). Interestingly, the PPARγ-UBR5 interaction is disturbed in PAEC of PAH patients. This corresponds to an inability to activate the DNA damage response pathway upon genotoxic stress and to repair DNA damage (70).
PPARγ/β-catenin complex
The protein β-catenin is normally involved in cell adhesion and gene transcription. In PAECs, PPARγ forms a BMPR2-mediated TF complex with β-catenin (PPARγ/β-catenin complex). In PAH patients with a dysfunctional BMPR2-signaling, the expression of PPARγ//β-catenin inducible vasculoprotective genes such as Apelin is reduced. Apelin-deficient PAECs are prone to apoptosis and promote PASMC proliferation (46).
PPARγ/SMAD3 and PPARγ/STAT3 complexes
Two other interesting complexes are related to PASMC proliferation and metabolism: PPARγ/SMAD3 and PPARγ/STAT3. On the one hand, PPARγ inhibits the well-known canonical TGF-β1-pSMAD3/4 signaling pathway through interactions with SMAD3 and, on the other hand, the non-canonical TGF-β/STAT3-FoxO1 signaling, which is mostly unknown (33). Interestingly, the direct interaction between PPARγ-SMAD3 (cytoplasm) and PPARγ-STAT3 (nucleus) inhibits TGF-induced phosphorylation and shuttling of SMAD3/4 and STAT3/FoxO1 through pioglitazone which resulted in altered proliferation and metabolism (67).
PPARγ/p53 complex
Under conditions of genotoxic stress PPARγ and p53 form a TF complex in various cell types (28, 195). In the pulmonary vasculature, PPARγ and p53 interact physically in all cell types across the vessel wall, namely PAEC, PASMC, and PA adventitial fibroblasts, to activate a vasculo-regenerative gene transcription program, which in PAEC is BMPR2-dependent (20, 28). Of note, the PPARγ-p53 TF complex can be harnessed pharmacologically as Nutlin-3, a p53-stabilizing compound, induces complex formation even under conditions of dysfunctional or lacking BMPR2, thereby salvaging impaired transcription of vasculoprotective genes including but not limited to genes promoting EC metabolism, survival, angiogenesis, and DNA repair (28). In a genetic PAH model with endothelial cell-specific BMPR2 knockout Nutlin-3 induces formation of the PPARγ-p53 complex and upregulation of complex target genes in lung microvascular EC was associated with reversal of persistent pulmonary hypertension, PA remodeling, and regeneration of pulmonary microvessels (28).
Therapeutic potential of transcription factors in pulmonary arterial hypertension
Despite the many advances in recent decades, PAH remains a disease with a poor long-term prognosis. If left untreated, around 2–10% of patients die in the first year after diagnosis (196). Current therapies can only delay, but not prevent or reverse progression to right heart failure (11). Currently approved PAH-specific therapies target four different pathways: (1) the endothelin pathway promotes vasoconstriction and proliferation, therefore endothelin receptor blockers (ERA) are used, (2) prostacyclins or prostanoid receptor agonists directly promote vasodilatation and partially exert anti-proliferative effects, (3) activation of the NO-sGC-cGMP pathway has vasodilatory and anti-proliferative effects, and (4) in the subset of vasoresponsive PAH patients voltage-dependent calcium-channel blocker are used (196, 197). An early and upfront combination of these drugs is recommended to improve long-term outcomes (198). Thus, although long-term mortality has significantly improved, it remains high (199, 200).
Currently approved pharmacological options for PAH mainly influence the vascular tone. Therefore, current medication cannot significantly reverse the pathologically dysregulated signaling pathways that lead to vascular remodeling through inflammation, growth factor signaling, and metabolic dysfunction (10). New treatment options for PAH patients are therefore needed to further improve outcome.
In this light, TF-based therapies might pave way for reverse remodeling strategies. TFs are involved in numerous pathological conditions like cancer, diabetes, or cardiovascular diseases. However, TFs were long deemed “undruggable,” yet targeting transcription factors for therapy has become reality (201). Strategies include the use of small molecule compounds to modulate TF activity, e.g., by inhibition of TF (-co-factor) complex formation or DNA binding or promotion of TF degradation [reviewed in (202)]. For some diseases, TF targeting therapies are clinically well established like TZD therapy in type 2 diabetes mellitus (203). For PAH, multiple novel compounds are currently in clinical trials with promising candidate TF pathways still in preclinical phases (204).
One such candidate is FOXO1 (205). Loss of FOXO1 function in PASMCs promoted a disease phenotype in vitro and in vivo and caused experimental PAH. On the other hand, pharmacological activation of FOXO1 was associated with reconstitution of a healthy PASMC phenotype and reversal of experimental PH (93). The multitude of routes and options for pharmacological FOXO1 activation (206) augurs well for FOXO1-based PAH treatment strategies (93, 206).
HIF has been a TF of interest as a therapeutic target for PAH for many years (150, 157). Early evidence indicated that pharmacological inhibition of HIF1 and HIF2α attenuated hypoxia-induced pulmonary hypertension, RV hypertrophy and PA remodeling by inhibiting intracellular Ca2+ release and pH changes upon hypoxia in PASMC (207). In general, at least 12 different pharmacological inhibitors of HIF1 and HIF2α were able to attenuate, prevent or reverse experimental pulmonary hypertension in rats or mice [reviewed in (157)] and multiple strategies appear feasible: Besides pharmacological inhibition of HIF signaling, destabilization of HIF via activation of HIF-degrading enzyme cascades or disruption of HIF complexes have all shown promising results as potential therapeutic strategies in experimental PH (and, partly, in ECs isolated from PAH patients) (208–210). In addition, HIF augurs well for novel combination therapies since pharmacological inhibition of HIF2α with simultaneous activation of p53 was more effective in reversing experimental PH and vascular remodeling than either treatment alone (211). This is particularly interesting, as pharmacological activation of p53 has been shown to reverse experimental PH by PASMC- (129) and PAEC (28)-specific mechanisms (see below).
The YAP/TAZ/TEAD pathway can also be harnessed as a therapeutic target in PAH. Pharmacological blunting of YAP/TAZ activation by glutaminase inhibitors, LOX inhibitors, ApoE activators or gene therapy using adeno-associated viruses expressing shRNA against the newly identified YAP/TAZ upstream regulator HSP110 attenuated or reversed experimental PH in rodent models (188, 189, 212).
Despite recent evidence that emphasizes the beneficial role of HIF2α inhibition (in endothelial cells) as a therapeutic target for pulmonary hypertension (208–210), thorough selection of patients to test proof-of-concept of these results in humans will be necessary as endothelial HIF2α appears to be crucial for vascular survival and maintenance of a functional alveolar structure (213, 214). As an alternative, targeting HIF1 in PASMC might be a reasonable approach (157).
As mentioned before, PPARγ is pivotal for maintaining pulmonary vascular homeostasis via complex formation with various interaction partners. PPARγ activation through endogenous ligands or pharmacological compounds has been shown to convey a broad spectrum of beneficial functions in the pulmonary vasculature from facilitation of normal cell signaling to maintaining pulmonary vascular cell homeostasis and promoting reverse remodeling of pathological vascular changes associated with PAH (28, 32–34, 46, 53, 63, 66, 67, 69, 215–219). In PAH studies, pharmacological activation of PPARγ is achieved by using thiazolidinediones (TZD, including Rosiglitazone and Pioglitazone), a class of drugs that has been under scrutiny for some time due to unwanted and potentially harmful side effects (34).
Earlier studies mostly used Rosiglitazone showing beneficial effects in various animal models of PH (53, 66, 216, 219). The first evidence in PAH came from Hansmann et al. who showed a complete reversal of right ventricular and pulmonary arterial remodeling by inhibition of proliferation and promotion of insulin sensitization in PASMC (66, 216). This was further substantiated in additional PH animal models by Liu et al. (219) as well as Bertero et al. (53). In a PAEC-dependent mechanism, Rosiglitazone restored miR-98 expression to attenuate ET-1-mediated hypoxia-induced PH (220). Due to the more favorable side effect profile, recent PH studies have used Pioglitazone (34). Pioglitazone also reversed PA and RV remodeling through beneficial effects on PASMC and cardiomyocytes (32, 33, 67, 217) by inhibiting canonical and non-canonical TGFβ1 signaling (33, 67), restoring mitochondrial homeostasis and improving cellular energy production by optimization of β-oxidation and glucose utilization (32).
Recently, we have also shown that PPARγ signaling is essential for Nutlin-3-mediated vasculoregeneration by modulating PAEC-protective p53 signaling (28). The small molecule compound Nutlin, currently in clinical trials for various cancers (221–223), induces activation of the PPARγ/p53 complex and a vasculo-regenerative gene transcription program in PAEC, PASMC, and PAAF (28). This resulted in reversal of persistent pulmonary hypertension in mice with endothelial-specific loss of BMPR2 via restoration of endothelial function and regeneration of pulmonary microvessels (28). In PAECs harboring BMPR2 mutations that were isolated from patients with PAH, Nutlin-induced PPARγ/p53 target genes facilitated the repair of prevalent DNA damage (28). In PASMC-based PH models, Nutlin-3 was also successful in preventing and reversing experimental PH by inhibiting PASMC proliferation through induction of a quasi-senescent phenotype (129). Since the PPARγ/p53 TF complex is also formed in PASMC (28) it would be interesting to see to which extent the beneficial effects of Nutlin-3 on PASMC are mediated by PPARγ/p53 complex target genes.
In addition to the direct targeting of TFs, the therapeutic potential of modulating TF co-factors or epigenetic factors which alter TF DNA is currently being investigated [reviewed in (45)].
In light of the recent advances in molecular strategies to modulate TF function, it appears to be only a matter of time before TF-based therapies will become a clinical reality in PAH treatment regimens (35).
Conclusion
A growing body of evidence highlights the central role of TFs in the pathogenesis of PAH. Currently approved therapies mainly modulate vascular tone, but fail to significantly reverse pathological vascular changes in pulmonary arteries and microvessels or the right ventricle/heart. Dysregulation of TF function is closely associated with pathological remodeling of the pulmonary vasculature and the right heart. Multiple TFs have been identified that are related to either maintaining pulmonary vessel homeostasis or, if dysfunctional, contributing to vascular pathology. Even well-studied, pathways commonly dysbalanced in PAH such as BMPR2 and TGFβ signaling, often disembogue in a highly intertwined network of downstream TFs. These TFs often form complex protein-protein interaction networks (e.g., PPARγ) to elicit cell-specific, in part opposing functions, adding complexity.
However, critical knowledge gaps remain. Most importantly, available data suggest that TFs operate through elaborate networks comprising multiple TFs and Co-factors (28, 53, 70). Investigation of individual TFs may not fully reflect their biological function in the pulmonary vasculature and how they integrate a multitude of intracellular and extracellular signals. Therefore, additional systems biology approaches are needed to dissect the pathobiology of complex TF networks. An additional layer of complexity is added by chromatin remodeling phenomena in PAH, which directly affect TF activity (84, 140). In the pulmonary vasculature, it is thus necessary (a) to fully understand the underlying epigenetic mechanisms that facilitate three-dimensional chromatin conformation and accessibility and (b) how exactly epigenetic modifications affect TF networks. This is especially important in light of epigenetic modifiers emerging as druggable targets in PAH (224, 225).
While current data suggest that transcriptional dysfunction is an early event in PAH pathogenesis (4, 226, 227), the spatial resolution of TF dysfunction is less understood. Even though there is growing evidence that (microvascular) endothelial dysfunction precedes the pathological changes in PASMC and PAAF (228), it remains unclear what microniche-specific factors contribute to cell-type specific TF functions. In this regard, the application of single-cell epigenomics and multi-omics technologies will help uncover cell-type specific TF networks.
Therefore, the characterization of molecular TF functions, binding partners, and modes of action are essential for understanding PAH pathogenesis and identification of new therapeutic targets. Current experimental TF-based therapeutic strategies focus on modulating individual TF function, stability, or TF interaction partner network formation with very promising results.
Although specific targeting of dysregulated TF pathways in PAH is advantageous over the currently available broad and rather symptomatic therapeutic approaches, off-target effects need to be mitigated when using systemic drug strategies (229). Hence, utilizing gene therapy approaches with high selectivity (tropism) for specific pulmonary vascular cell types might be useful to overcome this (230, 231).
In summary, recent advances in our understanding of the underlying molecular mechanisms as well as tailored modulation of TF function pave the way for TF-based vasculo-regenerative or reverse remodeling therapies. The clinical usability of TF-based therapies needs to be validated in upcoming clinical trials.
Author contributions
CM, JR, and JKl reviewed the literature and collected the data. CM made the illustrations and drafted the manuscript with support of JKl, JKö, and JKH. LH and HK helped to revise the manuscript and contributed to important intellectual content. JKö and JKH were responsible for oversight, design, manuscript preparation, and revision. All authors have read and approved the final manuscript.
Funding
JKH was part of the Clinician Scientist Career Development Program of the University of Hamburg/University Medical Center Hamburg-Eppendorf.
Acknowledgments
We greatly acknowledge expert technical assistance by Julia Mienert, MSc.
Conflict of interest
The authors declare that the research was conducted in the absence of any commercial or financial relationships that could be construed as a potential conflict of interest.
Publisher’s note
All claims expressed in this article are solely those of the authors and do not necessarily represent those of their affiliated organizations, or those of the publisher, the editors and the reviewers. Any product that may be evaluated in this article, or claim that may be made by its manufacturer, is not guaranteed or endorsed by the publisher.
References
1. Hoeper MM, Kramer T, Pan Z, Eichstaedt CA, Spiesshoefer J, Benjamin N, et al. Mortality in pulmonary arterial hypertension: prediction by the 2015 European pulmonary hypertension guidelines risk stratification model. Eur Respir J. (2017) 50:1700740. doi: 10.1183/13993003.00740-2017
2. Badesch DB, Champion HC, Gomez Sanchez MA, Hoeper MM, Loyd JE, Manes A, et al. Diagnosis and assessment of pulmonary arterial hypertension. J Am Coll Cardiol. (2009) 54:S55–66. doi: 10.1016/j.jacc.2009.04.011
3. Simonneau G, Montani D, Celermajer DS, Denton CP, Gatzoulis MA, Krowka M, et al. Haemodynamic definitions and updated clinical classification of pulmonary hypertension. Eur Respir J. (2019) 53:1801913. doi: 10.1183/13993003.01913-2018
4. Humbert M, Guignabert C, Bonnet S, Dorfmuller P, Klinger JR, Nicolls MR, et al. Pathology and pathobiology of pulmonary hypertension: state of the art and research perspectives. Eur Respir J. (2019) 53:1801887. doi: 10.1183/13993003.01887-2018
5. Ranchoux B, Antigny F, Rucker-Martin C, Hautefort A, Pechoux C, Bogaard HJ, et al. Endothelial-to-mesenchymal transition in pulmonary hypertension. Circulation. (2015) 131:1006–18. doi: 10.1161/CIRCULATIONAHA.114.008750
6. Ricard N, Tu L, Le Hiress M, Huertas A, Phan C, Thuillet R, et al. Increased pericyte coverage mediated by endothelial-derived fibroblast growth factor-2 and interleukin-6 is a source of smooth muscle-like cells in pulmonary hypertension. Circulation. (2014) 129:1586–97. doi: 10.1161/CIRCULATIONAHA.113.007469
7. Crnkovic S, Marsh LM, El Agha E, Voswinckel R, Ghanim B, Klepetko W, et al. Resident cell lineages are preserved in pulmonary vascular remodeling. J Pathol. (2018) 244:485–98. doi: 10.1002/path.5044
8. Tian W, Jiang X, Sung YK, Shuffle E, Wu TH, Kao PN, et al. Phenotypically silent bone morphogenetic protein receptor 2 mutations predispose rats to inflammation-induced pulmonary arterial hypertension by enhancing the risk for neointimal transformation. Circulation. (2019) 140:1409–25. doi: 10.1161/CIRCULATIONAHA.119.040629
9. LeVarge BL. Prostanoid therapies in the management of pulmonary arterial hypertension. Ther Clin Risk Manag. (2015) 11:535–47. doi: 10.2147/TCRM.S75122
10. Galie N, Ghofrani AH. New horizons in pulmonary arterial hypertension therapies. Eur Respir Rev. (2013) 22:503–14. doi: 10.1183/09059180.00006613
11. Spiekerkoetter E, Kawut SM, de Jesus Perez VA. New and emerging therapies for pulmonary arterial hypertension. Annu Rev Med. (2019) 70:45–59. doi: 10.1146/annurev-med-041717-085955
12. Dannewitz Prosseda S, Ali MK, Spiekerkoetter E. Novel advances in modifying BMPR2 signaling in PAH. Genes. (2020) 12:8. doi: 10.3390/genes12010008
13. Hennigs JK, Luneburg N, Stage A, Schmitz M, Korbelin J, Harbaum L, et al. The P2-receptor-mediated Ca(2+) signalosome of the human pulmonary endothelium - implications for pulmonary arterial hypertension. Purinergic Signal. (2019) 15:299–311. doi: 10.1007/s11302-019-09674-1
14. Pousada G, Lupo V, Castro-Sanchez S, Alvarez-Satta M, Sanchez-Monteagudo A, Baloira A, et al. Molecular and functional characterization of the BMPR2 gene in pulmonary arterial hypertension. Sci Rep. (2017) 7:1923. doi: 10.1038/s41598-017-02074-8
15. Rabinovitch M. Molecular pathogenesis of pulmonary arterial hypertension. J Clin Invest. (2012) 122:4306–13. doi: 10.1172/JCI60658
16. Atkinson C, Stewart S, Upton PD, Machado R, Thomson JR, Trembath RC, et al. Primary pulmonary hypertension is associated with reduced pulmonary vascular expression of type II bone morphogenetic protein receptor. Circulation. (2002) 105:1672–8. doi: 10.1161/01.cir.0000012754.72951.3d
17. Evans JD, Girerd B, Montani D, Wang XJ, Galie N, Austin ED, et al. BMPR2 mutations and survival in pulmonary arterial hypertension: an individual participant data meta-analysis. Lancet Respir Med. (2016) 4:129–37. doi: 10.1016/S2213-2600(15)00544-5
18. Lavoie JR, Ormiston ML, Perez-Iratxeta C, Courtman DW, Jiang B, Ferrer E, et al. Proteomic analysis implicates translationally controlled tumor protein as a novel mediator of occlusive vascular remodeling in pulmonary arterial hypertension. Circulation. (2014) 129:2125–35. doi: 10.1161/CIRCULATIONAHA.114.008777
19. Richter A, Yeager ME, Zaiman A, Cool CD, Voelkel NF, Tuder RM. Impaired transforming growth factor-beta signaling in idiopathic pulmonary arterial hypertension. Am J Respir Crit Care Med. (2004) 170:1340–8. doi: 10.1164/rccm.200311-1602OC
20. Diebold I, Hennigs JK, Miyagawa K, Li CG, Nickel NP, Kaschwich M, et al. BMPR2 preserves mitochondrial function and DNA during reoxygenation to promote endothelial cell survival and reverse pulmonary hypertension. Cell Metab. (2015) 21:596–608. doi: 10.1016/j.cmet.2015.03.010
21. Feng YX, Liu D, Sun ML, Jiang X, Sun N, Mao YM, et al. BMPR2 germline mutation in chronic thromboembolic pulmonary hypertension. Lung. (2014) 192:625–7. doi: 10.1007/s00408-014-9580-y
22. Chen NY, D Collum S, Luo F, Weng T, Le TT, M Hernandez A, et al. Macrophage bone morphogenic protein receptor 2 depletion in idiopathic pulmonary fibrosis and Group III pulmonary hypertension. Am J Physiol Lung Cell Mol Physiol. (2016) 311:L238–54. doi: 10.1152/ajplung.00142.2016
23. Andruska A, Spiekerkoetter E. Consequences of BMPR2 deficiency in the pulmonary vasculature and beyond: contributions to pulmonary arterial hypertension. Int J Mol Sci. (2018) 19:2499. doi: 10.3390/ijms19092499
24. Spiekerkoetter E, Tian X, Cai J, Hopper RK, Sudheendra D, Li CG, et al. FK506 activates BMPR2, rescues endothelial dysfunction, and reverses pulmonary hypertension. J Clin Invest. (2013) 123:3600–13. doi: 10.1172/JCI65592
25. Yung LM, Yang P, Joshi S, Augur ZM, Kim SSJ, Bocobo GA, et al. ACTRIIA-Fc rebalances activin/GDF versus BMP signaling in pulmonary hypertension. Sci Transl Med. (2020) 12:eaaz5660. doi: 10.1126/scitranslmed.aaz5660
26. Long L, Ormiston ML, Yang X, Southwood M, Graf S, Machado RD, et al. Selective enhancement of endothelial BMPR-II with BMP9 reverses pulmonary arterial hypertension. Nat Med. (2015) 21:777–85. doi: 10.1038/nm.3877
27. Nickel NP, Spiekerkoetter E, Gu M, Li CG, Li H, Kaschwich M, et al. Elafin reverses pulmonary hypertension via caveolin-1-dependent bone morphogenetic protein signaling. Am J Respir Crit Care Med. (2015) 191:1273–86. doi: 10.1164/rccm.201412-2291OC
28. Hennigs JK, Cao A, Li CG, Shi M, Mienert J, Miyagawa K, et al. PPARgamma-p53-mediated vasculoregenerative program to reverse pulmonary hypertension. Circ Res. (2021) 128:401–18. doi: 10.1161/CIRCRESAHA.119.316339
29. Humbert M, McLaughlin V, Gibbs JSR, Gomberg-Maitland M, Hoeper MM, Preston IR, et al. Sotatercept for the treatment of pulmonary arterial hypertension. N Engl J Med. (2021) 384:1204–15. doi: 10.1056/NEJMoa2024277
30. Spiekerkoetter E, Sung YK, Sudheendra D, Bill M, Aldred MA, van de Veerdonk MC, et al. Low-Dose FK506 (tacrolimus) in end-stage pulmonary arterial hypertension. Am J Respir Crit Care Med. (2015) 192:254–7. doi: 10.1164/rccm.201411-2061LE
31. Spiekerkoetter E, Sung YK, Sudheendra D, Scott V, Del Rosario P, Bill M, et al. Randomised placebo-controlled safety and tolerability trial of FK506 (tacrolimus) for pulmonary arterial hypertension. Eur Respir J. (2017) 50:1602449. doi: 10.1183/13993003.02449-2016
32. Legchenko E, Chouvarine P, Borchert P, Fernandez-Gonzalez A, Snay E, Meier M, et al. PPARgamma agonist pioglitazone reverses pulmonary hypertension and prevents right heart failure via fatty acid oxidation. Sci Transl Med. (2018) 10:eaao0303. doi: 10.1126/scitranslmed.aao0303
33. Calvier L, Chouvarine P, Legchenko E, Kokeny G, Mozes MM, Hansmann G. Chronic TGF-beta1 signaling in pulmonary arterial hypertension induces sustained canonical smad3 pathways in vascular smooth muscle cells. Am J Respir Cell Mol Biol. (2019) 61:121–3. doi: 10.1165/rcmb.2018-0275LE
34. Hansmann G, Calvier L, Risbano MG, Chan SY. Activation of the metabolic master regulator PPARgamma: a potential pioneering therapy for pulmonary arterial hypertension. Am J Respir Cell Mol Biol. (2020) 62:143–56. doi: 10.1165/rcmb.2019-0226PS
35. Olschewski A, Berghausen EM, Eichstaedt CA, Fleischmann BK, Grunig E, Grunig G, et al. Pathobiology, pathology and genetics of pulmonary hypertension: update from the Cologne consensus conference 2018. Int J Cardiol. (2018) 272S:4–10. doi: 10.1016/j.ijcard.2018.09.070
36. Vaquerizas JM, Kummerfeld SK, Teichmann SA, Luscombe NM. A census of human transcription factors: function, expression and evolution. Nat Rev Genet. (2009) 10:252–63. doi: 10.1038/nrg2538
37. Xie Z, Hu S, Qian J, Blackshaw S, Zhu H. Systematic characterization of protein-DNA interactions. Cell Mol Life Sci. (2011) 68:1657–68. doi: 10.1007/s00018-010-0617-y
38. Fulton DL, Sundararajan S, Badis G, Hughes TR, Wasserman WW, Roach JC, et al. TFCat: the curated catalog of mouse and human transcription factors. Genome Biol. (2009) 10:R29. doi: 10.1186/gb-2009-10-3-r29
39. Spitz F, Furlong EE. Transcription factors: from enhancer binding to developmental control. Nat Rev Genet. (2012) 13:613–26. doi: 10.1038/nrg3207
40. Geertz M, Shore D, Maerkl SJ. Massively parallel measurements of molecular interaction kinetics on a microfluidic platform. Proc Natl Acad Sci U.S.A. (2012) 109:16540–5. doi: 10.1073/pnas.1206011109
41. Lambert SA, Jolma A, Campitelli LF, Das PK, Yin Y, Albu M, et al. The human transcription factors. Cell. (2018) 172:650–65. doi: 10.1016/j.cell.2018.01.029
42. Han J, Kaufman RJ. Physiological/pathological ramifications of transcription factors in the unfolded protein response. Genes Dev. (2017) 31:1417–38. doi: 10.1101/gad.297374.117
43. Martin-Martin N, Carracedo A, Torrano V. Metabolism and transcription in cancer: merging two classic tales. Front Cell Dev Biol. (2017) 5:119. doi: 10.3389/fcell.2017.00119
44. Wang CC, Ying L, Barnes EA, Adams ES, Kim FY, Engel KW, et al. Pulmonary artery smooth muscle cell HIF-1alpha regulates endothelin expression via microRNA-543. Am J Physiol Lung Cell Mol Physiol. (2018) 315:L422–31. doi: 10.1152/ajplung.00475.2017
45. Pullamsetti SS, Perros F, Chelladurai P, Yuan J, Stenmark K. Transcription factors, transcriptional coregulators, and epigenetic modulation in the control of pulmonary vascular cell phenotype: therapeutic implications for pulmonary hypertension (2015 Grover conference series). Pulm Circ. (2016) 6:448–64. doi: 10.1086/688908
46. Alastalo TP, Li M, Perez Vde J, Pham D, Sawada H, Wang JK, et al. Disruption of PPARgamma/beta-catenin-mediated regulation of apelin impairs BMP-induced mouse and human pulmonary arterial EC survival. J Clin Invest. (2011) 121:3735–46. doi: 10.1172/JCI43382
47. Yeligar SM, Kang BY, Bijli KM, Kleinhenz JM, Murphy TC, Torres G, et al. PPARgamma regulates mitochondrial structure and function and human pulmonary artery smooth muscle cell proliferation. Am J Respir Cell Mol Biol. (2018) 58:648–57. doi: 10.1165/rcmb.2016-0293OC
48. Wingender E, Schoeps T, Haubrock M, Krull M, Donitz J. TFClass: expanding the classification of human transcription factors to their mammalian orthologs. Nucleic Acids Res. (2018) 46:D343–7. doi: 10.1093/nar/gkx987
49. Klemm DJ, Majka SM, Crossno JT Jr, Psilas JC, Reusch JE, Garat CV. Reduction of reactive oxygen species prevents hypoxia-induced CREB depletion in pulmonary artery smooth muscle cells. J Cardiovasc Pharmacol. (2011) 58:181–91. doi: 10.1097/FJC.0b013e31821f2773
50. Karlsson C, Jonsson M, Asp J, Brantsing C, Kageyama R, Lindahl A. Notch and HES5 are regulated during human cartilage differentiation. Cell Tissue Res. (2007) 327:539–51. doi: 10.1007/s00441-006-0307-0
51. Li X, Zhang X, Leathers R, Makino A, Huang C, Parsa P, et al. Notch3 signaling promotes the development of pulmonary arterial hypertension. Nat Med. (2009) 15:1289–97. doi: 10.1038/nm.2021
52. Kim J, Kang Y, Kojima Y, Lighthouse JK, Hu X, Aldred MA, et al. An endothelial apelin-FGF link mediated by miR-424 and miR-503 is disrupted in pulmonary arterial hypertension. Nat Med. (2013) 19:74–82. doi: 10.1038/nm.3040
53. Bertero T, Lu Y, Annis S, Hale A, Bhat B, Saggar R, et al. Systems-level regulation of microRNA networks by miR-130/301 promotes pulmonary hypertension. J Clin Invest. (2014) 124:3514–28. doi: 10.1172/JCI74773
54. Hong Z, Chen KH, DasGupta A, Potus F, Dunham-Snary K, Bonnet S, et al. MicroRNA-138 and MicroRNA-25 down-regulate mitochondrial calcium uniporter, causing the pulmonary arterial hypertension cancer phenotype. Am J Respir Crit Care Med. (2017) 195:515–29. doi: 10.1164/rccm.201604-0814OC
55. Zhang C, Ma C, Zhang L, Zhang L, Zhang F, Ma M, et al. MiR-449a-5p mediates mitochondrial dysfunction and phenotypic transition by targeting Myc in pulmonary arterial smooth muscle cells. J Mol Med. (2019) 97:409–22. doi: 10.1007/s00109-019-01751-7
56. Mammoto T, Muyleart M, Konduri GG, Mammoto A. Twist1 in hypoxia-induced pulmonary hypertension through transforming growth factor-beta-Smad signaling. Am J Respir Cell Mol Biol. (2018) 58:194–207. doi: 10.1165/rcmb.2016-0323OC
57. Hopper RK, Moonen JR, Diebold I, Cao A, Rhodes CJ, Tojais NF, et al. In pulmonary arterial hypertension, reduced BMPR2 promotes endothelial-to-mesenchymal transition via HMGA1 and its target slug. Circulation. (2016) 133:1783–94. doi: 10.1161/CIRCULATIONAHA.115.020617
58. Green DE, Sutliff RL, Hart CM. Is peroxisome proliferator-activated receptor gamma (PPARgamma) a therapeutic target for the treatment of pulmonary hypertension? Pulm Circ. (2011) 1:33–47. doi: 10.4103/2045-8932.78101
59. Hansmann G, Zamanian RT. PPARgamma activation: a potential treatment for pulmonary hypertension. Sci Transl Med. (2009) 1:12s14. doi: 10.1126/scitranslmed.3000267
60. Bradley EA, Bradley D. Pulmonary arterial hypertension and insulin resistance. J Mol Genet Med. (2014) 2:15. doi: 10.4172/1747-0862.S1-015
61. Yu S, Reddy JK. Transcription coactivators for peroxisome proliferator-activated receptors. Biochim Biophys Acta. (2007) 1771:936–51. doi: 10.1016/j.bbalip.2007.01.008
62. Ameshima S, Golpon H, Cool CD, Chan D, Vandivier RW, Gardai SJ, et al. Peroxisome proliferator-activated receptor gamma (PPARgamma) expression is decreased in pulmonary hypertension and affects endothelial cell growth. Circ Res. (2003) 92:1162–9. doi: 10.1161/01.RES.0000073585.50092.14
63. Guignabert C, Alvira CM, Alastalo TP, Sawada H, Hansmann G, Zhao M, et al. Tie2-mediated loss of peroxisome proliferator-activated receptor-gamma in mice causes PDGF receptor-beta-dependent pulmonary arterial muscularization. Am J Physiol Lung Cell Mol Physiol. (2009) 297:L1082–90. doi: 10.1152/ajplung.00199.2009
64. Bijli KM, Kleinhenz JM, Murphy TC, Kang BY, Adesina SE, Sutliff RL, et al. Peroxisome proliferator-activated receptor gamma depletion stimulates Nox4 expression and human pulmonary artery smooth muscle cell proliferation. Free Radic Biol Med. (2015) 80:111–20. doi: 10.1016/j.freeradbiomed.2014.12.019
65. Green DE, Murphy TC, Kang BY, Searles CD, Hart CM. PPARgamma ligands attenuate hypoxia-induced proliferation in human pulmonary artery smooth muscle cells through modulation of MicroRNA-21. PLoS One. (2015) 10:e0133391. doi: 10.1371/journal.pone.0133391
66. Hansmann G, de Jesus Perez VA, Alastalo TP, Alvira CM, Guignabert C, Bekker JM, et al. An antiproliferative BMP-2/PPARgamma/apoE axis in human and murine SMCs and its role in pulmonary hypertension. J Clin Invest. (2008) 118:1846–57. doi: 10.1172/JCI32503
67. Calvier L, Chouvarine P, Legchenko E, Hoffmann N, Geldner J, Borchert P, et al. PPARgamma links BMP2 and TGFbeta1 pathways in vascular smooth muscle cells, regulating cell proliferation and glucose metabolism. Cell Metab. (2017) 25:1118–34.e7. doi: 10.1016/j.cmet.2017.03.011
68. Chakraborty R, Saddouk FZ, Carrao AC, Krause DS, Greif DM, Martin KA. Promoters to study vascular smooth muscle. Arterioscler Thromb Vasc Biol. (2019) 39:603–12. doi: 10.1161/ATVBAHA.119.312449
69. Vattulainen-Collanus S, Akinrinade O, Li M, Koskenvuo M, Li CG, Rao SP, et al. Loss of PPARgamma in endothelial cells leads to impaired angiogenesis. J Cell Sci. (2016) 129:693–705. doi: 10.1242/jcs.169011
70. Li CG, Mahon C, Sweeney NM, Verschueren E, Kantamani V, Li D, et al. PPARgamma interaction with UBR5/ATMIN promotes DNA repair to maintain endothelial homeostasis. Cell Rep. (2019) 26:1333–43.e7. doi: 10.1016/j.celrep.2019.01.013
71. Tian J, Smith A, Nechtman J, Podolsky R, Aggarwal S, Snead C, et al. Effect of PPARgamma inhibition on pulmonary endothelial cell gene expression: gene profiling in pulmonary hypertension. Physiol Genomics. (2009) 40:48–60. doi: 10.1152/physiolgenomics.00094.2009
72. Kokudo T, Suzuki Y, Yoshimatsu Y, Yamazaki T, Watabe T, Miyazono K. Snail is required for TGFbeta-induced endothelial-mesenchymal transition of embryonic stem cell-derived endothelial cells. J Cell Sci. (2008) 121:3317–24. doi: 10.1242/jcs.028282
73. Bhattacharyya S, Fang F, Tourtellotte W, Varga J. Egr-1: new conductor for the tissue repair orchestra directs harmony (regeneration) or cacophony (fibrosis). J Pathol. (2013) 229:286–97. doi: 10.1002/path.4131
74. Kwapiszewska G, Chwalek K, Marsh LM, Wygrecka M, Wilhelm J, Best J, et al. BDNF/TrkB signaling augments smooth muscle cell proliferation in pulmonary hypertension. Am J Pathol. (2012) 181:2018–29. doi: 10.1016/j.ajpath.2012.08.028
75. Dickinson MG, Kowalski PS, Bartelds B, Borgdorff MA, van der Feen D, Sietsma H, et al. A critical role for Egr-1 during vascular remodelling in pulmonary arterial hypertension. Cardiovasc Res. (2014) 103:573–84. doi: 10.1093/cvr/cvu169
76. Yu X, Chen X, Zheng XD, Zhang J, Zhao X, Liu Y, et al. Growth differentiation factor 11 promotes abnormal proliferation and angiogenesis of pulmonary artery endothelial cells. Hypertension. (2018) 71:729–41. doi: 10.1161/HYPERTENSIONAHA.117.10350
77. Doddaballapur A, Michalik KM, Manavski Y, Lucas T, Houtkooper RH, You X, et al. Laminar shear stress inhibits endothelial cell metabolism via KLF2-mediated repression of PFKFB3. Arterioscler Thromb Vasc Biol. (2015) 35:137–45. doi: 10.1161/ATVBAHA.114.304277
78. Eichstaedt CA, Song J, Viales RR, Pan Z, Benjamin N, Fischer C, et al. First identification of Kruppel-like factor 2 mutation in heritable pulmonary arterial hypertension. Clin Sci. (2017) 131:689–98. doi: 10.1042/CS20160930
79. Eichstaedt CA, Sassmannshausen Z, Shaukat M, Cao D, Xanthouli P, Gall H, et al. Gene panel diagnostics reveals new pathogenic variants in pulmonary arterial hypertension. Respir Res. (2022) 23:74. doi: 10.1186/s12931-022-01987-x
80. Sindi HA, Russomanno G, Satta S, Abdul-Salam VB, Jo KB, Qazi-Chaudhry B, et al. Therapeutic potential of KLF2-induced exosomal microRNAs in pulmonary hypertension. Nat Commun. (2020) 11:1185. doi: 10.1038/s41467-020-14966-x
81. Rhodes CJ, Wharton J, Boon RA, Roexe T, Tsang H, Wojciak-Stothard B, et al. Reduced microRNA-150 is associated with poor survival in pulmonary arterial hypertension. Am J Respir Crit Care Med. (2013) 187:294–302. doi: 10.1164/rccm.201205-0839OC
82. Chandra SM, Razavi H, Kim J, Agrawal R, Kundu RK, de Jesus Perez V, et al. Disruption of the apelin-APJ system worsens hypoxia-induced pulmonary hypertension. Arterioscler Thromb Vasc Biol. (2011) 31:814–20. doi: 10.1161/ATVBAHA.110.219980
83. Ban Y, Liu Y, Li Y, Zhang Y, Xiao L, Gu Y, et al. S-nitrosation impairs KLF4 activity and instigates endothelial dysfunction in pulmonary arterial hypertension. Redox Biol. (2019) 21:101099. doi: 10.1016/j.redox.2019.101099
84. Moonen JR, Chappell J, Shi M, Shinohara T, Li D, Mumbach MR, et al. KLF4 recruits SWI/SNF to increase chromatin accessibility and reprogram the endothelial enhancer landscape under laminar shear stress. Nat Commun. (2022) 13:4941. doi: 10.1038/s41467-022-32566-9
85. Courboulin A, Tremblay VL, Barrier M, Meloche J, Jacob MH, Chapolard M, et al. Kruppel-like factor 5 contributes to pulmonary artery smooth muscle proliferation and resistance to apoptosis in human pulmonary arterial hypertension. Respir Res. (2011) 12:128. doi: 10.1186/1465-9921-12-128
86. Li X, He Y, Xu Y, Huang X, Liu J, Xie M, et al. KLF5 mediates vascular remodeling via HIF-1alpha in hypoxic pulmonary hypertension. Am J Physiol Lung Cell Mol Physiol. (2016) 310:L299–310. doi: 10.1152/ajplung.00189.2015
87. Mata M, Sarrion I, Milian L, Juan G, Ramon M, Naufal D, et al. PGC-1alpha induction in pulmonary arterial hypertension. Oxid Med Cell Longev. (2012) 2012:236572. doi: 10.1155/2012/236572
88. Ryan JJ, Marsboom G, Fang YH, Toth PT, Morrow E, Luo N, et al. PGC1alpha-mediated mitofusin-2 deficiency in female rats and humans with pulmonary arterial hypertension. Am J Respir Crit Care Med. (2013) 187:865–78. doi: 10.1164/rccm.201209-1687OC
89. Ghatnekar A, Trojanowska M. GATA-6 is a novel transcriptional repressor of the human Tenascin-C gene expression in fibroblasts. Biochim Biophys Acta. (2008) 1779:145–51. doi: 10.1016/j.bbagrm.2007.11.012
90. Bourgeois A, Lambert C, Habbout K, Ranchoux B, Paquet-Marceau S, Trinh I, et al. FOXM1 promotes pulmonary artery smooth muscle cell expansion in pulmonary arterial hypertension. J Mol Med. (2018) 96:223–35. doi: 10.1007/s00109-017-1619-0
91. Li Z, You Q, Zhang X. Small-molecule modulators of the hypoxia-inducible factor pathway: development and therapeutic applications. J Med Chem. (2019) 62:5725–49. doi: 10.1021/acs.jmedchem.8b01596
92. Raghavan A, Zhou G, Zhou Q, Ibe JC, Ramchandran R, Yang Q, et al. Hypoxia-induced pulmonary arterial smooth muscle cell proliferation is controlled by forkhead box M1. Am J Respir Cell Mol Biol. (2012) 46:431–6. doi: 10.1165/rcmb.2011-0128OC
93. Savai R, Al-Tamari HM, Sedding D, Kojonazarov B, Muecke C, Teske R, et al. Pro-proliferative and inflammatory signaling converge on FoxO1 transcription factor in pulmonary hypertension. Nat Med. (2014) 20:1289–300. doi: 10.1038/nm.3695
94. Oikawa T. ETS transcription factors: possible targets for cancer therapy. Cancer Sci. (2004) 95:626–33. doi: 10.1111/j.1349-7006.2004.tb03320.x
95. Patel M, Predescu D, Tandon R, Bardita C, Pogoriler J, Bhorade S, et al. A novel p38 mitogen-activated protein kinase/Elk-1 transcription factor-dependent molecular mechanism underlying abnormal endothelial cell proliferation in plexogenic pulmonary arterial hypertension. J Biol Chem. (2013) 288:25701–16. doi: 10.1074/jbc.M113.502674
96. Austin ED, Menon S, Hemnes AR, Robinson LR, Talati M, Fox KL, et al. Idiopathic and heritable PAH perturb common molecular pathways, correlated with increased MSX1 expression. Pulm Circ. (2011) 1:389–98. doi: 10.4103/2045-8932.87308
97. Lengner CJ, Welstead GG, Jaenisch R. The pluripotency regulator Oct4: a role in somatic stem cells? Cell Cycle. (2008) 7:725–8. doi: 10.4161/cc.7.6.5573
98. Firth AL, Yao W, Remillard CV, Ogawa A, Yuan JX. Upregulation of Oct-4 isoforms in pulmonary artery smooth muscle cells from patients with pulmonary arterial hypertension. Am J Physiol Lung Cell Mol Physiol. (2010) 298:L548–57. doi: 10.1152/ajplung.00314.2009
99. Covello KL, Kehler J, Yu H, Gordan JD, Arsham AM, Hu CJ, et al. HIF-2alpha regulates Oct-4: effects of hypoxia on stem cell function, embryonic development, and tumor growth. Genes Dev. (2006) 20:557–70. doi: 10.1101/gad.1399906
100. Eul B, Rose F, Krick S, Savai R, Goyal P, Klepetko W, et al. Impact of HIF-1alpha and HIF-2alpha on proliferation and migration of human pulmonary artery fibroblasts in hypoxia. FASEB J. (2006) 20:163–5. doi: 10.1096/fj.05-4104fje
101. Wu Y, Wharton J, Walters R, Vasilaki E, Aman J, Zhao L, et al. The pathophysiological role of novel pulmonary arterial hypertension gene SOX17. Eur Respir J. (2021) 58:2004172. doi: 10.1183/13993003.04172-2020
102. Sun X, Sun BL, Babicheva A, Vanderpool R, Oita RC, Casanova N, et al. Direct extracellular NAMPT involvement in pulmonary hypertension and vascular remodeling. transcriptional regulation by SOX and HIF-2alpha. Am J Respir Cell Mol Biol. (2020) 63:92–103. doi: 10.1165/rcmb.2019-0164OC
103. Graf S, Haimel M, Bleda M, Hadinnapola C, Southgate L, Li W, et al. Identification of rare sequence variation underlying heritable pulmonary arterial hypertension. Nat Commun. (2018) 9:1416. doi: 10.1038/s41467-018-03672-4
104. Zhu N, Welch CL, Wang J, Allen PM, Gonzaga-Jauregui C, Ma L, et al. Rare variants in SOX17 are associated with pulmonary arterial hypertension with congenital heart disease. Genome Med. (2018) 10:56. doi: 10.1186/s13073-018-0566-x
105. Rhodes CJ, Batai K, Bleda M, Haimel M, Southgate L, Germain M, et al. Genetic determinants of risk in pulmonary arterial hypertension: international genome-wide association studies and meta-analysis. Lancet Respir Med. (2019) 7:227–38. doi: 10.1016/S2213-2600(18)30409-0
106. Li D, Shao NY, Moonen JR, Zhao Z, Shi M, Otsuki S, et al. ALDH1A3 coordinates metabolism with gene regulation in pulmonary arterial hypertension. Circulation. (2021) 143:2074–90. doi: 10.1161/CIRCULATIONAHA.120.048845
107. Kim J, Hwangbo C, Hu X, Kang Y, Papangeli I, Mehrotra D, et al. Restoration of impaired endothelial myocyte enhancer factor 2 function rescues pulmonary arterial hypertension. Circulation. (2015) 131:190–9. doi: 10.1161/CIRCULATIONAHA.114.013339
108. Muller MR, Rao A. NFAT, immunity and cancer: a transcription factor comes of age. Nat Rev Immunol. (2010) 10:645–56. doi: 10.1038/nri2818
109. He RL, Wu ZJ, Liu XR, Gui LX, Wang RX, Lin MJ. Calcineurin/NFAT signaling modulates pulmonary artery smooth muscle cell proliferation, migration and apoptosis in monocrotaline-induced pulmonary arterial hypertension rats. Cell Physiol Biochem. (2018) 49:172–89. doi: 10.1159/000492852
110. Meloche J, Pflieger A, Vaillancourt M, Paulin R, Potus F, Zervopoulos S, et al. Role for DNA damage signaling in pulmonary arterial hypertension. Circulation. (2014) 129:786–97. doi: 10.1161/CIRCULATIONAHA.113.006167
111. Liang OD, So EY, Egan PC, Goldberg LR, Aliotta JM, Wu KQ, et al. Endothelial to haematopoietic transition contributes to pulmonary arterial hypertension. Cardiovasc Res. (2017) 113:1560–73. doi: 10.1093/cvr/cvx161
112. Wigle DA, Thompson KE, Yablonsky S, Zaidi SH, Coulber C, Jones PL, et al. AML1-like transcription factor induces serine elastase activity in ovine pulmonary artery smooth muscle cells. Circ Res. (1998) 83:252–63. doi: 10.1161/01.res.83.3.252
113. Lin ME, Chen T, Leaf EM, Speer MY, Giachelli CM. Runx2 expression in smooth muscle cells is required for arterial medial calcification in mice. Am J Pathol. (2015) 185:1958–69. doi: 10.1016/j.ajpath.2015.03.020
114. Lin ME, Chen TM, Wallingford MC, Nguyen NB, Yamada S, Sawangmake C, et al. Runx2 deletion in smooth muscle cells inhibits vascular osteochondrogenesis and calcification but not atherosclerotic lesion formation. Cardiovasc Res. (2016) 112:606–16. doi: 10.1093/cvr/cvw205
115. Sun Y, Byon CH, Yuan K, Chen J, Mao X, Heath JM, et al. Smooth muscle cell-specific Runx2 deficiency inhibits vascular calcification. Circ Res. (2012) 111:543–52. doi: 10.1161/CIRCRESAHA.112.267237
116. Ruffenach G, Chabot S, Tanguay VF, Courboulin A, Boucherat O, Potus F, et al. Role for runt-related transcription factor 2 in proliferative and calcified vascular lesions in pulmonary arterial hypertension. Am J Respir Crit Care Med. (2016) 194:1273–85. doi: 10.1164/rccm.201512-2380OC
117. Toufektchan E, Toledo F. The guardian of the genome revisited: p53 downregulates genes required for telomere maintenance, DNA repair, and centromere structure. Cancers. (2018) 10:135. doi: 10.3390/cancers10050135
118. Hafner A, Bulyk ML, Jambhekar A, Lahav G. The multiple mechanisms that regulate p53 activity and cell fate. Nat Rev Mol Cell Biol. (2019) 20:199–210. doi: 10.1038/s41580-019-0110-x
119. Fischer M. Census and evaluation of p53 target genes. Oncogene. (2017) 36:3943–56. doi: 10.1038/onc.2016.502
120. Kastenhuber ER, Lowe SW. Putting p53 in context. Cell. (2017) 170:1062–78. doi: 10.1016/j.cell.2017.08.028
121. Chavala SH, Kim Y, Tudisco L, Cicatiello V, Milde T, Kerur N, et al. Retinal angiogenesis suppression through small molecule activation of p53. J Clin Invest. (2013) 123:4170–81. doi: 10.1172/JCI67315
122. Gogiraju R, Xu X, Bochenek ML, Steinbrecher JH, Lehnart SE, Wenzel P, et al. Endothelial p53 deletion improves angiogenesis and prevents cardiac fibrosis and heart failure induced by pressure overload in mice. J Am Heart Assoc. (2015) 4:e001770. doi: 10.1161/JAHA.115.001770
123. Secchiero P, Corallini F, Gonelli A, Dell’Eva R, Vitale M, Capitani S, et al. Antiangiogenic activity of the MDM2 antagonist nutlin-3. Circ Res. (2007) 100:61–9. doi: 10.1161/01.RES.0000253975.76198.ff
124. Heo KS, Lee H, Nigro P, Thomas T, Le NT, Chang E, et al. PKCzeta mediates disturbed flow-induced endothelial apoptosis via p53 SUMOylation. J Cell Biol. (2011) 193:867–84. doi: 10.1083/jcb.201010051
125. Lee CL, Moding EJ, Cuneo KC, Li Y, Sullivan JM, Mao L, et al. P53 functions in endothelial cells to prevent radiation-induced myocardial injury in mice. Sci Signal. (2012) 5:ra52. doi: 10.1126/scisignal.2002918
126. Mizuno S, Bogaard HJ, Kraskauskas D, Alhussaini A, Gomez-Arroyo J, Voelkel NF, et al. P53 gene deficiency promotes hypoxia-induced pulmonary hypertension and vascular remodeling in mice. Am J Physiol Lung Cell Mol Physiol. (2011) 300:L753–61. doi: 10.1152/ajplung.00286.2010
127. Jacquin S, Rincheval V, Mignotte B, Richard S, Humbert M, Mercier O, et al. Inactivation of p53 is sufficient to induce development of pulmonary hypertension in rats. PLoS One. (2015) 10:e0131940. doi: 10.1371/journal.pone.0131940
128. Wakasugi T, Shimizu I, Yoshida Y, Hayashi Y, Ikegami R, Suda M, et al. Role of smooth muscle cell p53 in pulmonary arterial hypertension. PLoS One. (2019) 14:e0212889. doi: 10.1371/journal.pone.0212889
129. Mouraret N, Marcos E, Abid S, Gary-Bobo G, Saker M, Houssaini A, et al. Activation of lung p53 by Nutlin-3a prevents and reverses experimental pulmonary hypertension. Circulation. (2013) 127:1664–76. doi: 10.1161/CIRCULATIONAHA.113.002434
130. Aggarwal BB. Nuclear factor-kappaB: the enemy within. Cancer Cell. (2004) 6:203–8. doi: 10.1016/j.ccr.2004.09.003
131. Price LC, Caramori G, Perros F, Meng C, Gambaryan N, Dorfmuller P, et al. Nuclear factor kappa-B is activated in the pulmonary vessels of patients with end-stage idiopathic pulmonary arterial hypertension. PLoS One. (2013) 8:e75415. doi: 10.1371/journal.pone.0075415
132. Otsuki S, Saito T, Taylor S, Li D, Moonen J-R, Marciano DP, et al. Monocyte released HERV-K dUTPase engages TLR4 and MCAM causing endothelial mesenchymal transition. JCI Insight. (2021) 6:e146416. doi: 10.1172/jci.insight.146416
133. Farkas D, Alhussaini AA, Kraskauskas D, Kraskauskiene V, Cool CD, Nicolls MR, et al. Nuclear factor kappaB inhibition reduces lung vascular lumen obliteration in severe pulmonary hypertension in rats. Am J Respir Cell Mol Biol. (2014) 51:413–25. doi: 10.1165/rcmb.2013-0355OC
134. Li L, Wei C, Kim IK, Janssen-Heininger Y, Gupta S. Inhibition of nuclear factor-kappaB in the lungs prevents monocrotaline-induced pulmonary hypertension in mice. Hypertension. (2014) 63:1260–9. doi: 10.1161/HYPERTENSIONAHA.114.03220
135. Bongers EM, Duijf PH, van Beersum SE, Schoots J, Van Kampen A, Burckhardt A, et al. Mutations in the human TBX4 gene cause small patella syndrome. Am J Hum Genet. (2004) 74:1239–48. doi: 10.1086/421331
136. Kerstjens-Frederikse WS, Bongers EM, Roofthooft MT, Leter EM, Douwes JM, Van Dijk A, et al. TBX4 mutations (small patella syndrome) are associated with childhood-onset pulmonary arterial hypertension. J Med Genet. (2013) 50:500–6. doi: 10.1136/jmedgenet-2012-101152
137. Paulin R, Courboulin A, Meloche J, Mainguy V, Dumas de la Roque E, Saksouk N, et al. Signal transducers and activators of transcription-3/pim1 axis plays a critical role in the pathogenesis of human pulmonary arterial hypertension. Circulation. (2011) 123:1205–15. doi: 10.1161/CIRCULATIONAHA.110.963314
138. Gairhe S, Awad KS, Dougherty EJ, Ferreyra GA, Wang S, Yu Z-X, et al. Type I interferon activation and endothelial dysfunction in caveolin-1 insufficiency-associated pulmonary arterial hypertension. Proc Natl Acad Sci U.S.A. (2021) 118:e2010206118. doi: 10.1073/pnas.2010206118
139. Zabini D, Granton E, Hu Y, Miranda MZ, Weichelt U, Breuils Bonnet S, et al. Loss of SMAD3 promotes vascular remodeling in pulmonary arterial hypertension via MRTF disinhibition. Am J Respir Crit Care Med. (2018) 197:244–60. doi: 10.1164/rccm.201702-0386OC
140. Reyes-Palomares A, Gu M, Grubert F, Berest I, Sa S, Kasowski M, et al. Remodeling of active endothelial enhancers is associated with aberrant gene-regulatory networks in pulmonary arterial hypertension. Nat Commun. (2020) 11:1673. doi: 10.1038/s41467-020-15463-x
141. Garcia-Rivas G, Jerjes-Sanchez C, Rodriguez D, Garcia-Pelaez J, Trevino V. A systematic review of genetic mutations in pulmonary arterial hypertension. BMC Med Genet. (2017) 18:82. doi: 10.1186/s12881-017-0440-5
142. Aldred MA, Morrell NW, Guignabert C. New mutations and pathogenesis of pulmonary hypertension: progress and puzzles in disease pathogenesis. Circ Res. (2022) 130:1365–81. doi: 10.1161/CIRCRESAHA.122.320084
143. Maston GA, Evans SK, Green MR. Transcriptional regulatory elements in the human genome. Annu Rev Genomics Hum Genet. (2006) 7:29–59. doi: 10.1146/annurev.genom.7.080505.115623
144. Aguilar D, Oliva B. Topological comparison of methods for predicting transcriptional cooperativity in yeast. BMC Genomics. (2008) 9:137. doi: 10.1186/1471-2164-9-137
145. Amoutzias GD, Robertson DL, Van de Peer Y, Oliver SG. Choose your partners: dimerization in eukaryotic transcription factors. Trends Biochem Sci. (2008) 33:220–9. doi: 10.1016/j.tibs.2008.02.002
146. Wisdom R, Johnson RS, Moore C. C-Jun regulates cell cycle progression and apoptosis by distinct mechanisms. EMBO J. (1999) 18:188–97. doi: 10.1093/emboj/18.1.188
147. Biasin V, Chwalek K, Wilhelm J, Best J, Marsh LM, Ghanim B, et al. Endothelin-1 driven proliferation of pulmonary arterial smooth muscle cells is c-fos dependent. Int J Biochem Cell Biol. (2014) 54:137–48. doi: 10.1016/j.biocel.2014.06.020
148. Dabral S, Tian X, Kojonazarov B, Savai R, Ghofrani HA, Weissmann N, et al. Notch1 signalling regulates endothelial proliferation and apoptosis in pulmonary arterial hypertension. Eur Respir J. (2016) 48:1137–49. doi: 10.1183/13993003.00773-2015
149. Miyagawa K, Shi M, Chen PI, Hennigs JK, Zhao Z, Wang M, et al. Smooth muscle contact drives endothelial regeneration by BMPR2-Notch1-mediated metabolic and epigenetic changes. Circ Res. (2019) 124:211–24. doi: 10.1161/CIRCRESAHA.118.313374
150. Yu AY, Frid MG, Shimoda LA, Wiener CM, Stenmark K, Semenza GL. Temporal, spatial, and oxygen-regulated expression of hypoxia-inducible factor-1 in the lung. Am J Physiol. (1998) 275:L818–26. doi: 10.1152/ajplung.1998.275.4.L818
151. Palmer LA, Semenza GL, Stoler MH, Johns RA. Hypoxia induces type II NOS gene expression in pulmonary artery endothelial cells via HIF-1. Am J Physiol. (1998) 274:L212–9. doi: 10.1152/ajplung.1998.274.2.L212
152. Jiang BH, Rue E, Wang GL, Roe R, Semenza GL. Dimerization, DNA binding, and transactivation properties of hypoxia-inducible factor 1. J Biol Chem. (1996) 271:17771–8. doi: 10.1074/jbc.271.30.17771
153. Semenza GL. Hypoxia-inducible factors: roles in cardiovascular disease progression, prevention, and treatment. Cardiovasc Res. (2022) doi: 10.1093/cvr/cvac089 [Epub ahead of print].
154. Urrutia AA, Aragones J. HIF oxygen sensing pathways in lung biology. Biomedicines. (2018) 6:68. doi: 10.3390/biomedicines6020068
155. Abe H, Semba H, Takeda N. The roles of hypoxia signaling in the pathogenesis of cardiovascular diseases. J Atheroscler Thromb. (2017) 24:884–94. doi: 10.5551/jat.RV17009
156. Ahmad A, Ahmad S, Malcolm KC, Miller SM, Hendry-Hofer T, Schaack JB, et al. Differential regulation of pulmonary vascular cell growth by hypoxia-inducible transcription factor-1alpha and hypoxia-inducible transcription factor-2alpha. Am J Respir Cell Mol Biol. (2013) 49:78–85. doi: 10.1165/rcmb.2012-0107OC
157. Pullamsetti SS, Mamazhakypov A, Weissmann N, Seeger W, Savai R. Hypoxia-inducible factor signaling in pulmonary hypertension. J Clin Invest. (2020) 130:5638–51. doi: 10.1172/JCI137558
158. Fijalkowska I, Xu W, Comhair SA, Janocha AJ, Mavrakis LA, Krishnamachary B, et al. Hypoxia inducible-factor1alpha regulates the metabolic shift of pulmonary hypertensive endothelial cells. Am J Pathol. (2010) 176:1130–8. doi: 10.2353/ajpath.2010.090832
159. Wang J, Weigand L, Lu W, Sylvester JT, Semenza GL, Shimoda LA. Hypoxia inducible factor 1 mediates hypoxia-induced TRPC expression and elevated intracellular Ca2+ in pulmonary arterial smooth muscle cells. Circ Res. (2006) 98:1528–37. doi: 10.1161/01.RES.0000227551.68124.98
160. Shimoda LA, Fallon M, Pisarcik S, Wang J, Semenza GL. HIF-1 regulates hypoxic induction of NHE1 expression and alkalinization of intracellular pH in pulmonary arterial myocytes. Am J Physiol Lung Cell Mol Physiol. (2006) 291:L941–9. doi: 10.1152/ajplung.00528.2005
161. Ignarro LJ, Buga GM, Wood KS, Byrns RE, Chaudhuri G. Endothelium-derived relaxing factor produced and released from artery and vein is nitric oxide. Proc Natl Acad Sci U.S.A. (1987) 84:9265–9. doi: 10.1073/pnas.84.24.9265
162. Semenza GL. Perspectives on oxygen sensing. Cell. (1999) 98:281–4. doi: 10.1016/s0092-8674(00)81957-1
163. Tonelli AR, Haserodt S, Aytekin M, Dweik RA. Nitric oxide deficiency in pulmonary hypertension: pathobiology and implications for therapy. Pulm Circ. (2013) 3:20–30. doi: 10.4103/2045-8932.109911
164. Xue C, Rengasamy A, Le Cras TD, Koberna PA, Dailey GC, Johns RA. Distribution of NOS in normoxic vs. hypoxic rat lung: upregulation of NOS by chronic hypoxia. Am J Physiol. (1994) 267:L667–78. doi: 10.1152/ajplung.1994.267.6.L667
165. Pullamsetti SS, Schermuly RT. Endothelin receptor antagonists in preclinical models of pulmonary hypertension. Eur J Clin Invest. (2009) 39(Suppl. 2):3–13. doi: 10.1111/j.1365-2362.2009.02115.x
166. Meloche J, Le Guen M, Potus F, Vinck J, Ranchoux B, Johnson I, et al. MiR-223 reverses experimental pulmonary arterial hypertension. Am J Physiol Cell Physiol. (2015) 309:C363–72. doi: 10.1152/ajpcell.00149.2015
167. Diebold I, Djordjevic T, Hess J, Gorlach A. Rac-1 promotes pulmonary artery smooth muscle cell proliferation by upregulation of plasminogen activator inhibitor-1: role of NFkappaB-dependent hypoxia-inducible factor-1alpha transcription. Thromb Haemost. (2008) 100:1021–8.
168. Dabral S, Muecke C, Valasarajan C, Schmoranzer M, Wietelmann A, Semenza GL, et al. A RASSF1A-HIF1alpha loop drives Warburg effect in cancer and pulmonary hypertension. Nat Commun. (2019) 10:2130. doi: 10.1038/s41467-019-10044-z
169. Blum JI, Bijli KM, Murphy TC, Kleinhenz JM, Hart CM. Time-dependent PPARgamma modulation of HIF-1alpha signaling in hypoxic pulmonary artery smooth muscle cells. Am J Med Sci. (2016) 352:71–9. doi: 10.1016/j.amjms.2016.03.019
170. Tang H, Babicheva A, McDermott KM, Gu Y, Ayon RJ, Song S, et al. Endothelial HIF-2alpha contributes to severe pulmonary hypertension due to endothelial-to-mesenchymal transition. Am J Physiol Lung Cell Mol Physiol. (2018) 314:L256–75. doi: 10.1152/ajplung.00096.2017
171. Kapitsinou PP, Rajendran G, Astleford L, Michael M, Schonfeld MP, Fields T, et al. The endothelial prolyl-4-hydroxylase domain 2/hypoxia-inducible factor 2 axis regulates pulmonary artery pressure in mice. Mol Cell Biol. (2016) 36:1584–94. doi: 10.1128/MCB.01055-15
172. Brusselmans K, Compernolle V, Tjwa M, Wiesener MS, Maxwell PH, Collen D, et al. Heterozygous deficiency of hypoxia-inducible factor-2alpha protects mice against pulmonary hypertension and right ventricular dysfunction during prolonged hypoxia. J Clin Invest. (2003) 111:1519–27. doi: 10.1172/JCI15496
173. Cowburn AS, Crosby A, Macias D, Branco C, Colaco RD, Southwood M, et al. HIF2alpha-arginase axis is essential for the development of pulmonary hypertension. Proc Natl Acad Sci U.S.A. (2016) 113:8801–6. doi: 10.1073/pnas.1602978113
174. Gale DP, Harten SK, Reid CD, Tuddenham EG, Maxwell PH. Autosomal dominant erythrocytosis and pulmonary arterial hypertension associated with an activating HIF2 alpha mutation. Blood. (2008) 112:919–21. doi: 10.1182/blood-2008-04-153718
175. Tan Q, Kerestes H, Percy MJ, Pietrofesa R, Chen L, Khurana TS, et al. Erythrocytosis and pulmonary hypertension in a mouse model of human HIF2A gain of function mutation. J Biol Chem. (2013) 288:17134–44. doi: 10.1074/jbc.M112.444059
176. Chan XY, Volkova E, Eoh J, Black R, Fang L, Gorashi R, et al. HIF2A gain-of-function mutation modulates the stiffness of smooth muscle cells and compromises vascular mechanics. iScience. (2021) 24:102246. doi: 10.1016/j.isci.2021.102246
177. Humbert M, Morrell NW, Archer SL, Stenmark KR, MacLean MR, Lang IM, et al. Cellular and molecular pathobiology of pulmonary arterial hypertension. J Am Coll Cardiol. (2004) 43:13–24S. doi: 10.1016/j.jacc.2004.02.029
178. Nasim MT, Ghouri A, Patel B, James V, Rudarakanchana N, Morrell NW, et al. Stoichiometric imbalance in the receptor complex contributes to dysfunctional BMPR-II mediated signalling in pulmonary arterial hypertension. Hum Mol Genet. (2008) 17:1683–94. doi: 10.1093/hmg/ddn059
179. Nohe A, Keating E, Underhill TM, Knaus P, Petersen NO. Dynamics and interaction of caveolin-1 isoforms with BMP-receptors. J Cell Sci. (2005) 118:643–50. doi: 10.1242/jcs.01402
180. Massague J, Wotton D. Transcriptional control by the TGF-beta/Smad signaling system. EMBO J. (2000) 19:1745–54. doi: 10.1093/emboj/19.8.1745
181. Gomez-Puerto MC, Iyengar PV, Garcia de Vinuesa A, Ten Dijke P, Sanchez-Duffhues G. Bone morphogenetic protein receptor signal transduction in human disease. J Pathol. (2019) 247:9–20. doi: 10.1002/path.5170
182. Harada M, Qin Y, Takano H, Minamino T, Zou Y, Toko H, et al. G-CSF prevents cardiac remodeling after myocardial infarction by activating the Jak-Stat pathway in cardiomyocytes. Nat Med. (2005) 11:305–11. doi: 10.1038/nm1199
183. Paulin R, Meloche J, Bonnet S. STAT3 signaling in pulmonary arterial hypertension. JAKSTAT. (2012) 1:223–33. doi: 10.4161/jkst.22366
184. Dutzmann J, Daniel JM, Bauersachs J, Hilfiker-Kleiner D, Sedding DG. Emerging translational approaches to target STAT3 signalling and its impact on vascular disease. Cardiovasc Res. (2015) 106:365–74. doi: 10.1093/cvr/cvv103
185. Bonnet S, Rochefort G, Sutendra G, Archer SL, Haromy A, Webster L, et al. The nuclear factor of activated T cells in pulmonary arterial hypertension can be therapeutically targeted. Proc Natl Acad Sci U.S.A. (2007) 104:11418–23. doi: 10.1073/pnas.0610467104
186. Panciera T, Azzolin L, Cordenonsi M, Piccolo S. Mechanobiology of YAP and TAZ in physiology and disease. Nat Rev Mol Cell Biol. (2017) 18:758–70. doi: 10.1038/nrm.2017.87
187. Dieffenbach PB, Maracle M, Tschumperlin DJ, Fredenburgh LE. Mechanobiological feedback in pulmonary vascular disease. Front Physiol. (2018) 9:951. doi: 10.3389/fphys.2018.00951
188. Bertero T, Cottrill KA, Lu Y, Haeger CM, Dieffenbach P, Annis S, et al. Matrix remodeling promotes pulmonary hypertension through feedback mechanoactivation of the YAP/TAZ-miR-130/301 circuit. Cell Rep. (2015) 13:1016–32. doi: 10.1016/j.celrep.2015.09.049
189. Bertero T, Oldham WM, Cottrill KA, Pisano S, Vanderpool RR, Yu Q, et al. Vascular stiffness mechanoactivates YAP/TAZ-dependent glutaminolysis to drive pulmonary hypertension. J Clin Invest. (2016) 126:3313–35. doi: 10.1172/JCI86387
190. Dieffenbach PB, Haeger CM, Coronata AMF, Choi KM, Varelas X, Tschumperlin DJ, et al. Arterial stiffness induces remodeling phenotypes in pulmonary artery smooth muscle cells via YAP/TAZ-mediated repression of cyclooxygenase-2. Am J Physiol Lung Cell Mol Physiol. (2017) 313:L628–47. doi: 10.1152/ajplung.00173.2017
191. Sutliff RL, Kang BY, Hart CM. PPARgamma as a potential therapeutic target in pulmonary hypertension. Ther Adv Respir Dis. (2010) 4:143–60. doi: 10.1177/1753465809369619
192. Lehrke M, Lazar MA. The many faces of PPARgamma. Cell. (2005) 123:993–9. doi: 10.1016/j.cell.2005.11.026
193. Chandra V, Huang P, Hamuro Y, Raghuram S, Wang Y, Burris TP, et al. Structure of the intact PPAR-gamma-RXR- nuclear receptor complex on DNA. Nature. (2008) 456:350–6. doi: 10.1038/nature07413
194. Plutzky J. The PPAR-RXR transcriptional complex in the vasculature: energy in the balance. Circ Res. (2011) 108:1002–16. doi: 10.1161/CIRCRESAHA.110.226860
195. Khandekar MJ, Banks AS, Laznik-Bogoslavski D, White JP, Choi JH, Kazak L, et al. Noncanonical agonist PPARgamma ligands modulate the response to DNA damage and sensitize cancer cells to cytotoxic chemotherapy. Proc Natl Acad Sci U.S.A. (2018) 115:561–6. doi: 10.1073/pnas.1717776115
196. Thenappan T, Ormiston ML, Ryan JJ, Archer SL. Pulmonary arterial hypertension: pathogenesis and clinical management. BMJ. (2018) 360:j5492. doi: 10.1136/bmj.j5492
197. Boucly A, Savale L, Jais X, Bauer F, Bergot E, Bertoletti L, et al. Association between Initial treatment strategy and long-term survival in pulmonary arterial hypertension. Am J Respir Crit Care Med. (2021) 204:842–54. doi: 10.1164/rccm.202009-3698OC
198. Humbert M, Lau EM, Montani D, Jais X, Sitbon O, Simonneau G. Advances in therapeutic interventions for patients with pulmonary arterial hypertension. Circulation. (2014) 130:2189–208. doi: 10.1161/CIRCULATIONAHA.114.006974
199. Benza RL, Miller DP, Barst RJ, Badesch DB, Frost AE, McGoon MD. An evaluation of long-term survival from time of diagnosis in pulmonary arterial hypertension from the REVEAL Registry. Chest. (2012) 142:448–56. doi: 10.1378/chest.11-1460
200. Hoeper MM, Pausch C, Grunig E, Staehler G, Huscher D, Pittrow D, et al. Temporal trends in pulmonary arterial hypertension: results from the COMPERA registry. Eur Respir J. (2022) 59:2102024. doi: 10.1183/13993003.02024-2021
201. Bushweller JH. Targeting transcription factors in cancer - from undruggable to reality. Nat Rev Cancer. (2019) 19:611–24. doi: 10.1038/s41568-019-0196-7
202. Henley MJ, Koehler AN. Advances in targeting ‘undruggable’ transcription factors with small molecules. Nat Rev Drug Discov. (2021) 20:669–88. doi: 10.1038/s41573-021-00199-0
203. Defronzo RA, Inzucchi S, Abdul-Ghani M, Nissen SE. Pioglitazone: the forgotten, cost-effective cardioprotective drug for type 2 diabetes. Diab Vasc Dis Res. (2019) 16:133–43. doi: 10.1177/1479164118825376
204. Sitbon O, Gomberg-Maitland M, Granton J, Lewis MI, Mathai SC, Rainisio M, et al. Clinical trial design and new therapies for pulmonary arterial hypertension. Eur Respir J. (2019) 53:1801908. doi: 10.1183/13993003.01908-2018
205. Paulin R, Michelakis ED. Addressing complexity in pulmonary hypertension: the FoxO1 case. Circ Res. (2015) 116:1732–5. doi: 10.1161/CIRCRESAHA.115.305773
206. Calissi G, Lam EW, Link W. Therapeutic strategies targeting FOXO transcription factors. Nat Rev Drug Discov. (2021) 20:21–38. doi: 10.1038/s41573-020-0088-2
207. Abud EM, Maylor J, Undem C, Punjabi A, Zaiman AL, Myers AC, et al. Digoxin inhibits development of hypoxic pulmonary hypertension in mice. Proc Natl Acad Sci U.S.A (2012) 109:1239–44. doi: 10.1073/pnas.1120385109
208. Macias D, Moore S, Crosby A, Southwood M, Du X, Tan H, et al. Targeting HIF2α-ARNT hetero-dimerisation as a novel therapeutic strategy for pulmonary arterial hypertension. Eur Respir J. (2021) 57:1902061. doi: 10.1183/13993003.02061-2019
209. Hu CJ, Poth JM, Zhang H, Flockton A, Laux A, Kumar S, et al. Suppression of HIF2 signalling attenuates the initiation of hypoxia-induced pulmonary hypertension. Eur Respir J. (2019) 54:1900378. doi: 10.1183/13993003.00378-2019
210. Ghosh MC, Zhang DL, Ollivierre WH, Noguchi A, Springer DA, Linehan WM, et al. Therapeutic inhibition of HIF-2alpha reverses polycythemia and pulmonary hypertension in murine models of human diseases. Blood. (2021) 137:2509–19. doi: 10.1182/blood.2020009138
211. Zheng Q, Lu W, Yan H, Duan X, Chen Y, Zhang C, et al. Established pulmonary hypertension in rats was reversed by a combination of a HIF-2alpha antagonist and a p53 agonist. Br J Pharmacol. (2022) 179:1065–81. doi: 10.1111/bph.15696
212. Liu H, Zhang S, Liu Y, Ma J, Chen W, Yin T, et al. Knockdown of HSP110 attenuates hypoxia-induced pulmonary hypertension in mice through suppression of YAP/TAZ-TEAD4 pathway. Respir Res. (2022) 23:209. doi: 10.1186/s12931-022-02124-4
213. Torres-Capelli M, Marsboom G, Li QO, Tello D, Rodriguez FM, Alonso T, et al. Role Of Hif2alpha oxygen sensing pathway in bronchial epithelial club cell proliferation. Sci Rep. (2016) 6:25357. doi: 10.1038/srep25357
214. Pasupneti S, Tian W, Tu AB, Dahms P, Granucci E, Gandjeva A, et al. Endothelial HIF-2alpha as a key endogenous mediator preventing emphysema. Am J Respir Crit Care Med. (2020) 202:983–95. doi: 10.1164/rccm.202001-0078OC
215. Klinke A, Moller A, Pekarova M, Ravekes T, Friedrichs K, Berlin M, et al. Protective effects of 10-nitro-oleic acid in a hypoxia-induced murine model of pulmonary hypertension. Am J Respir Cell Mol Biol. (2014) 51:155–62. doi: 10.1165/rcmb.2013-0063OC
216. Hansmann G, Wagner RA, Schellong S, Perez VA, Urashima T, Wang L, et al. Pulmonary arterial hypertension is linked to insulin resistance and reversed by peroxisome proliferator-activated receptor-gamma activation. Circulation. (2007) 115:1275–84. doi: 10.1161/CIRCULATIONAHA.106.663120
217. Behringer A, Trappiel M, Berghausen EM, Ten Freyhaus H, Wellnhofer E, Odenthal M, et al. Pioglitazone alleviates cardiac and vascular remodelling and improves survival in monocrotaline induced pulmonary arterial hypertension. Naunyn Schmiedebergs Arch Pharmacol. (2016) 389:369–79. doi: 10.1007/s00210-015-1205-3
218. Kozlowska H, Baranowska-Kuczko M, Schlicker E, Kozlowski M, Kloza M, Malinowska B. Relaxation of human pulmonary arteries by PPARgamma agonists. Naunyn Schmiedebergs Arch Pharmacol. (2013) 386:445–53. doi: 10.1007/s00210-013-0846-3
219. Liu Y, Tian XY, Mao G, Fang X, Fung ML, Shyy JY, et al. Peroxisome proliferator-activated receptor-gamma ameliorates pulmonary arterial hypertension by inhibiting 5-hydroxytryptamine 2B receptor. Hypertension. (2012) 60:1471–8. doi: 10.1161/HYPERTENSIONAHA.112.198887
220. Kang BY, Park KK, Kleinhenz JM, Murphy TC, Green DE, Bijli KM, et al. Peroxisome proliferator-activated receptor gamma and microRNA 98 in hypoxia-induced endothelin-1 signaling. Am J Respir Cell Mol Biol. (2016) 54:136–46. doi: 10.1165/rcmb.2014-0337OC
221. Zauli G, Celeghini C, Melloni E, Voltan R, Ongari M, Tiribelli M, et al. The sorafenib plus nutlin-3 combination promotes synergistic cytotoxicity in acute myeloid leukemic cells irrespectively of FLT3 and p53 status. Haematologica. (2012) 97:1722–30. doi: 10.3324/haematol.2012.062083
222. Mascarenhas J, Lu M, Kosiorek H, Virtgaym E, Xia L, Sandy L, et al. Oral idasanutlin in patients with polycythemia vera. Blood. (2019) 134:525–33. doi: 10.1182/blood.2018893545
223. Italiano A, Miller WH Jr, Blay JY, Gietema JA, Bang YJ, Mileshkin LR, et al. Phase I study of daily and weekly regimens of the orally administered MDM2 antagonist idasanutlin in patients with advanced tumors. Invest New Drugs. (2021) 39:1587–97. doi: 10.1007/s10637-021-01141-2
224. Gamen E, Seeger W, Pullamsetti SS. The emerging role of epigenetics in pulmonary hypertension. Eur Respir J. (2016) 48:903–17. doi: 10.1183/13993003.01714-2015
225. Ho L, Hossen N, Nguyen T, Vo A, Ahsan F. Epigenetic mechanisms as emerging therapeutic targets and microfluidic chips application in pulmonary arterial hypertension. Biomedicines. (2022) 10:170. doi: 10.3390/biomedicines10010170
226. Rhodes CJ, Im H, Cao A, Hennigs JK, Wang L, Sa S, et al. RNA sequencing analysis detection of a novel pathway of endothelial dysfunction in pulmonary arterial hypertension. Am J Respir Crit Care Med. (2015) 192:356–66. doi: 10.1164/rccm.201408-1528OC
227. Ainscough AJ, Smith TJ, Haensel M, Rhodes CJ, Fellows A, Whitwell HJ, et al. An organ-on-chip model of pulmonary arterial hypertension identifies a BMPR2-SOX17-prostacyclin signalling axis. Commun Biol. (2022) 5:1192. doi: 10.1038/s42003-022-04169-z
228. Spiekerkoetter E, Goncharova EA, Guignabert C, Stenmark K, Kwapiszewska G, Rabinovitch M, et al. Hot topics in the mechanisms of pulmonary arterial hypertension disease: cancer-like pathobiology, the role of the adventitia, systemic involvement, and right ventricular failure. Pulm Circ. (2019) 9:2045894019889775. doi: 10.1177/2045894019889775
229. Hennigs JK, Matuszcak C, Trepel M, Korbelin J. Vascular endothelial cells: heterogeneity and targeting approaches. Cells. (2021) 10:2712. doi: 10.3390/cells10102712
230. Korbelin J, Sieber T, Michelfelder S, Lunding L, Spies E, Hunger A, et al. Pulmonary targeting of adeno-associated viral vectors by next-generation sequencing-guided screening of random capsid displayed peptide libraries. Mol Ther. (2016) 24:1050–61. doi: 10.1038/mt.2016.62
231. Remes A, Korbelin J, Arnold C, Rowedder C, Heckmann M, Mairbaurl H, et al. Adeno-associated virus-mediated gene transfer of inducible nitric oxide synthase to an animal model of pulmonary hypertension. Hum Gene Ther. (2022) 33:959–67. doi: 10.1089/hum.2021.230
232. Hu H, Miao YR, Jia LH, Yu QY, Zhang Q, Guo AY. AnimalTFDB 3.0: a comprehensive resource for annotation and prediction of animal transcription factors. Nucleic Acids Res. (2019) 47:D33–8. doi: 10.1093/nar/gky822
233. Sysol JR, Natarajan V, Machado RF. PDGF induces SphK1 expression via Egr-1 to promote pulmonary artery smooth muscle cell proliferation. Am J Physiol Cell Physiol. (2016) 310:C983–92. doi: 10.1152/ajpcell.00059.2016
234. Shatat MA, Tian H, Zhang R, Tandon G, Hale A, Fritz JS, et al. Endothelial Kruppel-like factor 4 modulates pulmonary arterial hypertension. Am J Respir Cell Mol Biol. (2014) 50:647–53. doi: 10.1165/rcmb.2013-0135OC
235. Li Y, Wu F, Tan Q, Guo M, Ma P, Wang X, et al. The multifaceted roles of FOXM1 in pulmonary disease. Cell Commun Signal. (2019) 17:35. doi: 10.1186/s12964-019-0347-1
Keywords: epigenetics, epigenetics (chromatin remodeling), transcriptomics, targeted therapy, reverse remodeling, pathogenesis, pulmonary hypertension (PAH)
Citation: Körbelin J, Klein J, Matuszcak C, Runge J, Harbaum L, Klose H and Hennigs JK (2023) Transcription factors in the pathogenesis of pulmonary arterial hypertension—Current knowledge and therapeutic potential. Front. Cardiovasc. Med. 9:1036096. doi: 10.3389/fcvm.2022.1036096
Received: 03 September 2022; Accepted: 21 November 2022;
Published: 06 January 2023.
Edited by:
Carmen Brás-Silva, University of Porto, PortugalReviewed by:
Liming Yu, The University of Texas Health Science Center at San Antonio, United StatesDustin Fraidenburg, University of Illinois at Chicago, United States
Copyright © 2023 Körbelin, Klein, Matuszcak, Runge, Harbaum, Klose and Hennigs. This is an open-access article distributed under the terms of the Creative Commons Attribution License (CC BY). The use, distribution or reproduction in other forums is permitted, provided the original author(s) and the copyright owner(s) are credited and that the original publication in this journal is cited, in accordance with accepted academic practice. No use, distribution or reproduction is permitted which does not comply with these terms.
*Correspondence: Jakob Körbelin, ai5rb2VyYmVsaW5AdWtlLmRl; Jan K. Hennigs, ai5oZW5uaWdzQHVrZS5kZQ==