Corrigendum: The Regulation Mechanisms and Clinical Application of MicroRNAs in Myocardial Infarction: A Review of the Recent 5 Years
- Xiamen Cardiovascular Hospital of Xiamen University, School of Medicine, Xiamen University, Xiamen, China
Myocardial infarction (MI) is the most frequent end-point of cardiovascular pathology, leading to higher mortality worldwide. Due to the particularity of the heart tissue, patients who experience ischemic infarction of the heart, still suffered irreversible damage to the heart even if the vascular reflow by treatment, and severe ones can lead to heart failure or even death. In recent years, several studies have shown that microRNAs (miRNAs), playing a regulatory role in damaged hearts, bring light for patients to alleviate MI. In this review, we summarized the effect of miRNAs on MI with some mechanisms, such as apoptosis, autophagy, proliferation, inflammatory; the regulation of miRNAs on cardiac structural changes after MI, including angiogenesis, myocardial remodeling, fibrosis; the application of miRNAs in stem cell therapy and clinical diagnosis; other non-coding RNAs related to miRNAs in MI during the past 5 years.
Introduction
Myocardial infarction (MI) is one of the most common cardiovascular diseases globally, which can easily cause arrhythmia, ventricular remodeling, and heart failure. In 2019, millions of death patients were attributed to MI globally, which amounted to more than 20% from 2010 (1). Emerging studies demonstrated that miRNAs were critical players in MI since they participate in the genetic regulation of hundreds of essential proteins involved in signaling pathways (2–4).
MicroRNAs (miRNAs), the endogenous non-coding RNAs (ncRNAs), contain 20–24 nucleotides and bind to mRNA to block subsequent corresponding protein synthesis pathways, thereby regulating nearly all of the biological pathways (5). Studies have shown that there are at least 1,500 miRNAs in the human genome, and one miRNA can regulate multiple target genes, and the expression of one gene may also be regulated by multiple miRNAs (6, 7). This also reflects the complexity and rigor of life activities in the human body. The occurrence and development of diseases are often closely related to miRNAs. In the past decades, many scholars have proved that miRNAs as the key targets, regulated multiple cardiovascular diseases, including MI, heart failure, atherosclerosis, diabetes (8).
In this review, we summarized the effect of miRNAs on MI with different mechanisms, such as apoptosis, autophagy, proliferation, inflammatory. We further summarized the regulation of miRNAs on cardiac structural changes after MI, including angiogenesis, myocardial remodeling, fibrosis. And the application of miRNAs in stem cell therapy and clinical diagnosis is introduced (Figure 1). Finally, some other ncRNAs involved in miRNA regulation of MI, such as long ncRNAs (lncRNAs), circular RNAs (circRNAs) were summarized. This study only reviewed the research on the regulation of MI by miRNAs in the past 5 years, tracing the frontier reports and time-sensitive information.
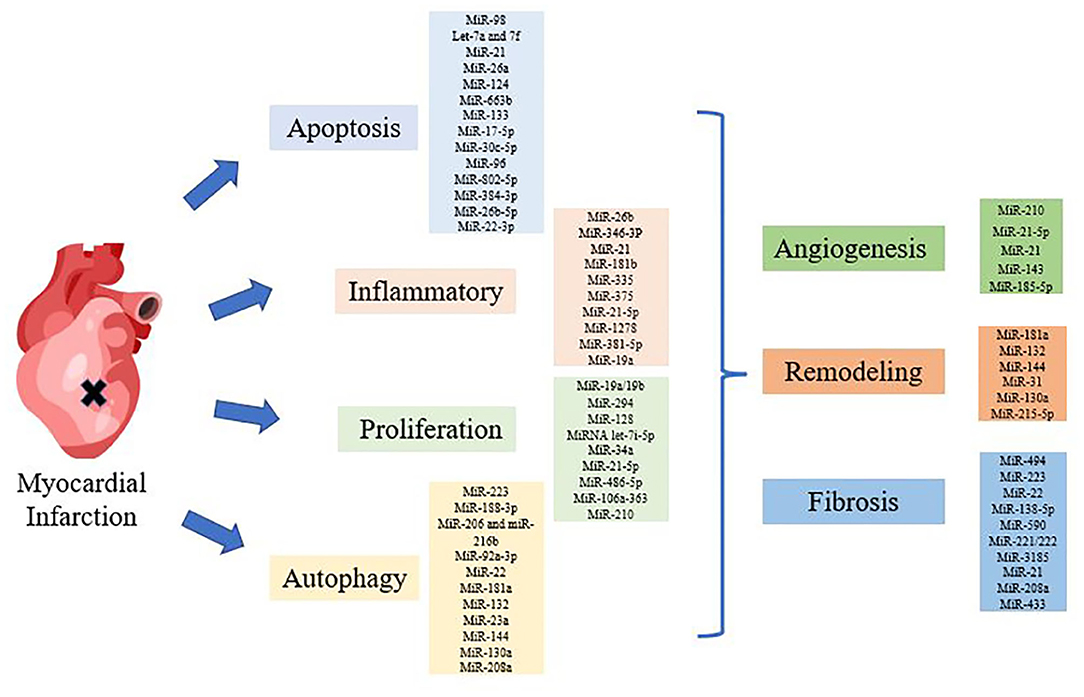
Figure 1. Diagram of miRNAs regulating myocardial infarction (MI). Various miRNAs regulated MI through apoptosis, inflammation, autophagy, proliferation and structural changes, such as angiogenesis, remodeling, and fibrosis.
miRNAs and Mechanism of MI
miRNAs Regulated Cardiomyocyte Apoptosis During MI
Apoptosis is the autonomous and orderly death of cells controlled by genes to maintain the stability of the internal environment. Apoptosis can remove unnecessary or abnormal cells in the body, which has important biological significance and molecular biological mechanisms (9). Apoptosis in the cardiomyocytes occurs throughout all stages of MI. MiRNAs have emerged as potent regulators of cardiomyocyte apoptosis (7). Here we summarized the effect of miRNAs on cardiomyocyte apoptosis in MI.
The let-7 family, one of the earliest miRNAs discovered, were found to be an essential regulator of cardiomyocyte apoptosis in MI (10). The expression of miR-98, one member of the let-7 family, was reduced during MI. Further studies have found that overexpression of MiR-98 inhibited apoptosis by down-regulating Fas/Caspase3, thereby alleviating MI (11). In addition, another group showed that the expressions of let-7a and let-7f were reduced in infarcted myocardium and blood of pigs, which accelerated apoptosis, led to cardiac hypertrophy and decreased ejection fraction, and the TGFBR3 was one target of let-7 (12).
MiR-21, one of the first miRNAs discovered, regulated many signaling pathways and was regarded as an apoptotic regulator in the circulatory system (13). In H2O2-induced cardiomyocytes, miR-21 exerted its anti-apoptosis role by targeting programmed cell death 4 (PDCD4) and activator protein 1 (AP-1) pathway (14). In addition, another group discovered that miR-21 reduced inflammation, dysfunction, and myocardial remodeling after MI by targeting KBTBD7 and inhibiting the activation of p38 and NF-κB (15). However, miR-21 may be a two-sided sword. One study examined that the expression of miR-21 was up-regulated in MI mice and miR-21 regulates human peripheral blood-derived sEVs (PB-sEVs), which consisted with H9C2 cells exposed to hypoxia. And down-regulated miR-21 level by PB-sEVs improved MI mice's survival rates and cardiac function (16).
MiR-26a is downregulated in ST-elevation MI (STEMI) patients and had a strong correlation with myocardial enzyme levels. Up-regulation of miR-26a attenuated apoptosis and fibrosis and improved cardiac function by targeting ataxia–telangiectasia mutated (ATM) (17). Experiment in vivo and in vitro showed that the expression level of MiR-124 increased during MI, and down-regulating miR-124 expression reduced apoptotic cell death and improved mitochondrial dysfunction, and decreased the infarct area, as well as cardiac function in MI mice by targeting STAT3 (18). In cell-culture studies, the authors found that miR-663b inhibition reduced cardiomyocyte damage induced by hypoxia by targeting anti-apoptosis related genes (19).
The apoptosis of cardiomyocytes promotes the process of MI. We have summarized the miRNAs and their mechanisms that regulate MI through the apoptosis pathway (Table 1). MiRNA can interfere with the expression of its target genes. According to the role of the target in the process of apoptosis, the miRNA mentioned above can alleviate or aggravate myocardial infarction. MiR-663b, miR-17-5p, miR-30c-5p, miR-96, miR-802-5p, miR-384-3p, and miR-22-3p promoted MI process by targeting anti-apoptotic genes, thereby causing cardiomyocyte apoptosis (19, 24, 26, 28). Other miRNAs, such as miR-98 and miR-21, can also reduce the damage of MI by regulating different apoptotic signaling pathways (11).
miRNAs Regulated Myocardial Autophagy During MI
Moderate conditions of nutrient deprivation, ischemia, or hypoxia lead to autophagy slightly in the basal rate, which enables cardiomyocytes to conserve the adequate energy (29). But when myocardial ischemia is severe and lasts too long, intracellular autophagy increases sharply, which further induces cell death (30). This is also the key reason for the deterioration of the structure and function of the heart caused by autophagy (31, 32). Thus, this section will focus on the regulation of miRNAs on autophagy during MI.
Apoptosis and autophagy often occur together, and are closely related to each other in signaling pathways. MiR-223 can protect myocardial tissue from ischemic damage and myocardial cell hypoxia damage by inhibiting apoptosis and autophagy related to Akt/mTOR pathway (33). In addition, autophagy-related genes (ATGs) play an important role in regulating the occurrence and subsequent processes of autophagy (34, 35). A study revealed that miR-188-3p targeted ATG7, which led to the autophagic program and MI, while another one long non-coding RNA, AK079427, down-regulated miR-188-3p by combining with each other (36). Other research have suggested that the activities of miR-206 and miR-216b were significantly enhanced by histamine, which further inhibited autophagy. The specific regulatory mechanism was related to the inhibition of ATG13, which in turn increased the activity of the inflammatory factor caspase-8 (37). Another recent study showed that miR-92a down-regulation ameliorated endothelial cell function and alleviated MI damage by augmenting autophagy of endothelial cell via ATG4a and regulating energy metabolism of cardiac cells via Cd36 and Abca8b (38).
Generally, autophagy plays a role in protecting cells, while apoptosis is responsible for clearing aging damaged or mutated cells, thereby protecting the health of the body.
In addition to regulating apoptosis, some miRNAs can influence the process of MI by regulating autophagy (Table 2). Interestingly, miR-188-3p relieved MI by inhibiting autophagy, while miR-92a-3p aggravated MI by inhibiting autophagy (36, 38). When less affected by environmental conditions, autophagy inhibits cell apoptosis. However, in a highly stressed state, when autophagy consumes excessive amounts of intracellular proteins and organelles, making the cell unable to survive, the cell gradually develops into apoptosis. Therefore, autophagy is a double-edged sword that regulates the operation of the body.
miRNAs Regulated Cardiomyocyte Proliferation During MI
Generally, MI patients are relieved of their dilemma through medication, intervention or surgery. However, since the cardiomyocytes in the adult mammalian heart are highly differentiated and basically do not have the ability to proliferate, pathological damage to the heart will gradually develop into irreversible damage (46). Therefore, we need effective treatments for this condition. MiRNAs have already appeared as important regulators of cardiomyocytes differentiation, proliferation, and cardiac function.
In vivo studies have shown that the overexpression of heart-specific miR-199a restored the proliferation and differentiation of cardiomyocytes and enhanced heart function, thereby significantly reducing the heart damage in pigs with myocardial infarction (47). The miR-17~92 cluster was a crucial driving factors of cardiomyocyte proliferation and was considered as promising targets for cardiac regeneration and repair (48). In particular, miR-19, members of the miR-17~92 cluster, reduced MI-induced cell death and preserved cardiac function by enhanced cardiomyocyte proliferation and repressed the immune in the early stage of heart attack. Therefore, miR-19 could be a candidate for valuable method for cardiovascular diseases and significantly relieve the patient's pain (49). MiR-92a, one part of the miR-17–92 cluster, is proved to be able to ameliorate the function of endothelial cell and alleviate acute MI damage (38). Studies have shown that the regenerative dysfunction of the heart of neonatal mice was often accompanied by up-regulated miR-34a, while knockout of miR-34a in the heart of adult mice restored cardiac regeneration and reduced ventricular remodeling. Further in vitro studies have found that miR-34a activated cell cycle activities through Cyclin D1 and Sirt1 and restored cardiomyocyte regeneration (50).
MiR-302–367 cluster is another group of miRNAs that have a regulatory effect on heart regeneration. In vivo studies have shown that miR-302–367 promoted the proliferation of cardiomyocytes by regulating the Hippo signaling pathway (51). Besides, miR-290–295 also played a crucial role in regulating cell cycle, DNA methylation, apoptosis, and pluripotency transcription factors. Recently, miR-294 was reported to significantly improve MI and reduce apoptosis associated with MI. The cardioprotective mechanism of miR-294 was related to multiple genes involved in the cell cycle, such as Wee1 and CyclinB1, to further restore cell cycle operation, promote angiogenesis, and maintain cardiac function (52). A study found that the heart regeneration function of neonatal mice was negatively affected by miR-128. Further research found that miR-128 inhibition in the heart of adult mice enhanced heart regeneration and improved cardiac dysfunction, which was related to the regulatory effect of miR-128 on the chromatin regulator SUZ12, further improving cell cycle activity (53).
Not only in regulating cardiomyocytes apoptosis, let-7i-5p also plays a vital role in the cardiomyocyte cell cycle and proliferation processes. Increased expression of let-7i-5p during heart growth is negatively correlated to cardiomyocyte proliferation, and knockdown of let-7i-5p enhances cardiac proliferation by regulating CCND2 and E2F2 both in vitro and in vivo (54). In addition, miR-210 restored cardiac function after MI by improving angiogenesis, cardiomyocyte proliferation, and cell survival by upregulated VEGF, Bcl-2, and β-catenin and downregulated APC, p16, and caspase-3 (55).
During MI, myocardial cells lack oxygen and energy, leading to cell death, which in turn causes cardiac dysfunction. Methods to alleviate MI include reducing the apoptosis of myocardial cells and promoting cell proliferation to restore the heart's original structure and function as much as possible. However, most mammalian cardiomyocytes have lost the ability to regenerate. Some miRNAs can regulate the cell cycle of cardiomyocytes, thereby promoting or inhibiting the proliferation of cardiomyocytes after damage (Table 3). We summarized various miRNA-related signal pathways that affect cell proliferation, including miR-294-Wee1/CyclinB-CDK1 pathway, miR-128-SUZ12-p27-Cyclin E/CDK2 pathway, miR-486-5p-MMP19-VEGFA cleavage signaling pathway, and miR302-367/Hippo signal pathway (52, 53, 57). Therefore, the research of miRNA on improving cell proliferation is very promising.
miRNAs Regulated Inflammation During MI
MI (MI) initiates an inflammatory response that promotes both beneficial and harmful effects. In the first minutes after injury, pro-inflammatory cytokines in the ischemic zone synthesis and release efficiently. And the massive production of inflammatory factors affects the survival of cardiomyocytes in the infarcted area. Some miRNA control inflammation after MI to reduce apoptosis of myocytes and relieve cardiac remodeling (Table 4).
Ge et al. demonstrated that overexpression of miR-26b alleviated myocardial remodeling caused by MI, and prostaglandin endoperoxide synthase 2 (PTGS2) acted as a target of miR-26b to mediate the MAPK signaling pathway (59). On the contrary, some studies have shown that down-regulation of miR-26a enhanced the heart function of MI mice, which was related to the inhibitory effect of miR-26a on angiogenesis (68). Garikipati et al. found that antagonistic miR-375 exerted a protective effect on the heart by promoting angiogenesis, reducing inflammation and inhibiting cell apoptosis, which involved the PDK-1/AKT signaling pathway (63). Compared with healthy people, the expression of MiR-181b in heart failure patients decreased, while the expression of hypersensitive C-reactive protein (hsCRP) levels increased. The expression of MiR-181b in heart failure rats was negatively correlated with IL-1, TNF-α, and IL-6 (61). Wang et al. proved up-regulation of miR-335 reduced MI damage in rats by reducing oxidative stress, inflammation, and apoptosis, and MAP3K2 was an essential target for miR-335 to exert cardioprotection (62).
MI triggers an inflammatory response, which can remove debris from damaged tissues, and different inflammatory response eventually induces cardiomyocyte apoptosis. Therefore, inhibiting inflammation and reducing apoptosis are the fundamental mechanisms to alleviate the damage of cardiac structure and function after MI. Several key targets are involved in the inflammatory response to initiate or promote the inflammatory process. Some miRNAs negatively regulated the expression of inflammation-related factors, such as NF-κB, MAPK, IL-22, MAP3K2, and CHI3L1, and affected the operation of MI (Table 4). It is of great significance to study the mechanism of these miRNAs in regulating inflammation response.
miRNAs and Heart Structural Changes After MI
The Effect of miRNAs on Angiogenesis After MI
In addition to large blood vessels, obstruction of microvessels can also cause or accelerate cardiovascular diseases, so angiogenesis has great potential in the treatment of ischemic diseases (69). The miRNAs related to angiogenesis potential on ischemic cardiovascular diseases are summarized following (Table 5).
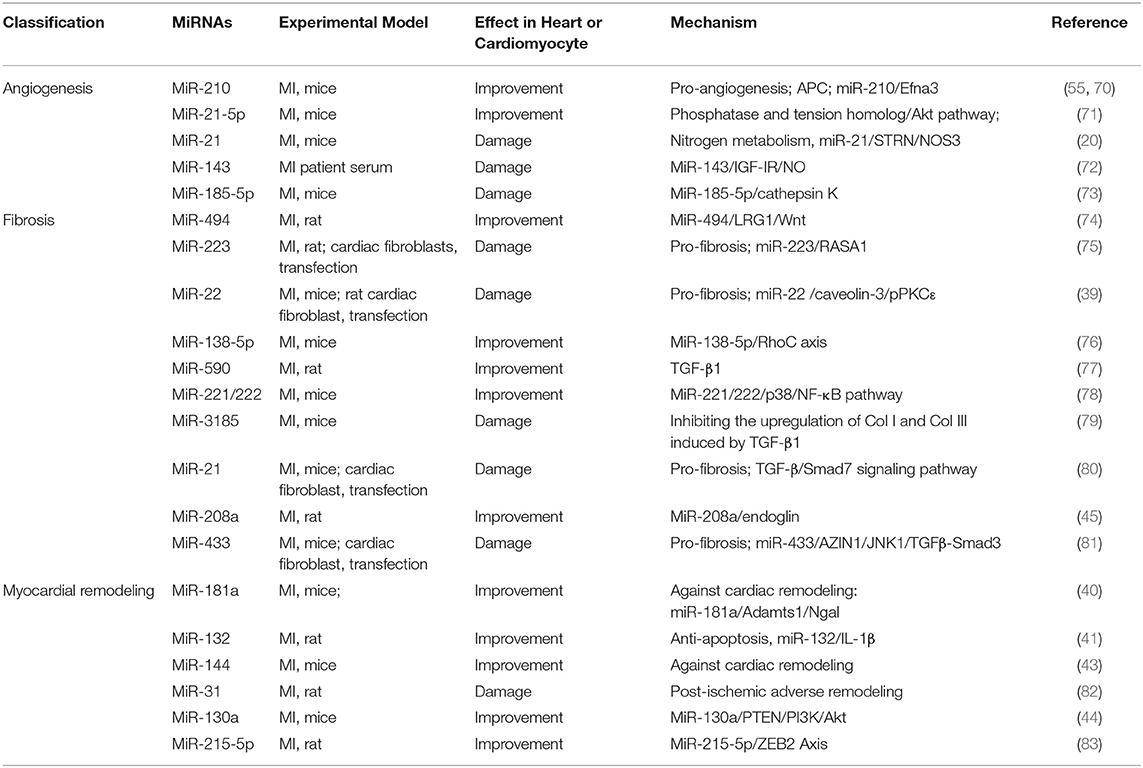
Table 5. Summary of studies on miRNA regulating angiogenesis, fibrosis, and myocardial remodeling after MI in the past 5 years.
MiR-21, one of the first miRNAs discovered, regulates many signaling pathways and plays a vital role in the cardiovascular system (13). A study shows that miR-21-5p is a component of exosomes, which can enhance angiogenesis and cardiomyocyte survival through augmented phosphorylation of Akt via the inhibition of PTEN in the recipient cells (71). Extracellular vesicles (EVs)/exosomes, which are responsible for cell-cell information transmission (84), can mediate the cardioprotective effects of stem cells through paracrine action (85, 86). The study showed exosomes derived from serum of MI patients accelerated cardiac angiogenesis. Furthermore, microarray assays demonstrated that the regulation of exosomes on cardiac angiogenesis was related to the inhibitory effect of miR-143, which bind to IGF-IR and enhanced the production of NO (72). The function of endothelial cells plays an indispensable role in the process of MI. In human umbilical vein endothelial cells (HUVECs), miR-185-5p down-regulates the expression of cathepsin K, thereby hindering angiogenesis, leading to further impairment of cardiac function in MI mice (73).
During MI, blood vessel damage of the heart accelerates energy metabolism disorder, so vascular regeneration is significant. MiR-210 and miR-21-5p promoted vascular regeneration through different pathways, while miR-143 and miR-185-5p blocked cardiac angiogenesis and aggravated heart damage (Table 5). Therefore, it is necessary to conduct in-depth research on the mechanism of miRNA regulating vascular regeneration after MI.
The Effect of miRNAs on Fibrosis After MI
Even after interventional therapy opens MI patients to block the blood vessels, which greatly reduces the mortality of MI patients, the development of cardiac injury to myocardial fibrosis in MI patients cannot be curbed. Myocardial fibrosis can also be regarded as an important signal for heart remodeling, which further leads to arrhythmia, cardiac dysfunction and even heart failure (87, 88). So, the study of myocardial fibrosis has predominant clinical significance, and miRNAs may become promising candidates for regulating myocardial fibrosis.
The study reported that increased miR-22 levels promoted fibrosis process by enlarged proliferation of cardiac fibroblasts. In addition, others reported that in MI mice models and cardiac fibroblasts, miR-21 promoted cardiac fibrosis via the TGF-β/Smad7 signaling pathway (80). The research showed that miR-494 targeted and downregulated leucine-richalpha-2-glycoprotein 1(LRG1), resulting in the inactivation of the Wnt signaling pathway and promoting proliferation and migration and invasion ability of fibroblasts and VECs (74). Inhibition of miR-223, as a pro-fibrosis factor, can accelerate the transformation of cardiac fibroblasts into myofibroblasts through the RASA1/TGF-β1 signaling pathway (75). In contrast, the downregulation of miR-22 level reduced cardiac fibroblasts via miR-22/Cav3/p-PKCε signaling (39). Decreased levels of miR-22 in MI elderly mice significantly enhanced heart function and prevented ventricular remodeling by promoting autophagy activity. However, the regulatory effect of miR-22 on young mice was not significant. Clinical related studies have also shown that the level of miR-22 in patients with heart failure was positively correlated with patient mortality (89). Tao et al. reported that elevated levels of miR-433 also appeared in three cardiovascular disease models with fibrotic transition trends, and especially expressed in fibroblasts in heart tissue. In comparison, inhibition of miR-433 attenuates cardiac fibroblast proliferation and myofibroblast differentiation in vitro. Mechanistically, mitogen-activated protein kinase 8 (JNK1) and antizyme inhibitor 1 (AZIN1) were determined as important targets of miR-433 (81). A previous study reported miR-208 is a marker of myocardial necrosis (90). In another research, Shyu et al. demonstrated that antagonistic miR-208a prevented myocardial fibrosis after MI via endoglin (45).
There are also reports that miR-138-5p, miR-590, and miR-221/222 inhibited fibrosis after MI through RhoC, TGF-β1, and p38/NF-κB, respectively. And miR-3185 promoted myocardial fibrosis by increasing collagen deposition. The above results indicated that different miRNAs targeted multiple mRNAs to encourage or inhibit myocardial fibrosis after MI (Table 5).
The Effect of miRNAs on Myocardial Remodeling After MI
Despite stent technology, procedural techniques, and adjunctive pharmacotherapy reduced death rate of MI, survivors of MI also experienced ventricular remodeling and even heart failure (HF) (91). Cardiac remodeling is a process that involves cellular, molecular, and physiological changes, including inflammation, fibrosis, and ventricular dysfunction and is an important factor affecting the occurrence of cardiovascular events, long-term survival rate, and quality of life (92).
The occurrence and development of cardiac remodeling involved a variety of miRNAs. This section focuses on summarizing the role of miRNAs in cardiac remodeling caused by MI (Table 5). MiR-181a, upregulated in MI, is an important regulatory factor of the aldosterone–mineralocorticoid receptor (Aldo–MR) pathway, which drive cardiac arterial stiffness, fibrosis, hypertrophy, and cardiac remodeling by promoting inflammation and oxidative stress, which were involved in its downstream factors such as NGAL (lipocalin-2) (40, 93, 94). Interestingly, other miRNAs derived from the miR-181 family have differential effects on heart function regulation. Study showed that miR-181b-5p has a deleterious cardiac impact and can decrease MI damage via PI3K/Akt signaling pathway (95). Another study shows that miR-132 inhibition was meaningful for suppressing cardiac remodeling by regulating IL-1β-related apoptosis (41). Pan et al. reported the restoration of miR-101a improves cardiac function in rats after MI by inhibiting interstitial fibrosis (96). In another study, the researchers demonstrated that in mice's MI (MI) model, the level of miR-144 decreased, which contributes to the LV remodeling via modification of local inflammatory and auto-phagocytic pathways (43). Martinez et al. revealed that the time-dependent upregulation of miR-31 plays a significant role in adverse remodeling following rat MI, inhibition of miR-31 modulates several genes, such as Tnnt2, Timp4, and E2f6, which is vital to the recovery of cardiac function and structure after MI (82). Besides, Lu et al. showed that miR-130a remarkably enhanced cardiac function and prevented remodeling after MI by activating PTEN/PI3K/Akt signaling pathway (44).
Myocardial remodeling manifests ventricular cavity enlargement, progressive hypofunction, collagen deposition outside myocardial cells, inflammatory cell infiltration, and apoptosis. Some miRNAs, for example, miR-181, miR-144, miR-130a, regulate phenotypes related to ventricular remodeling by down-regulating the expression of related genes (40, 43, 44), which can be regarded as potential targets for anti-ventricular remodeling after MI (Table 5).
The Involvement of miRNAs in the Diagnosis and Treatment of MI
Stem Cell Therapy on MI
External stimulation triggers the myocardial self-defense system, which reduces the loss of myocardial cells to a certain extent and increases the proliferation of myocardial cells, but it is not enough to achieve cardiac repair (85, 97, 98). Cell therapy is to achieve tissue repair by transplanting stem cells or their derived cells into damaged tissues, which has gradually become an alternative therapy that has attracted much attention (Table 6) (109, 110).
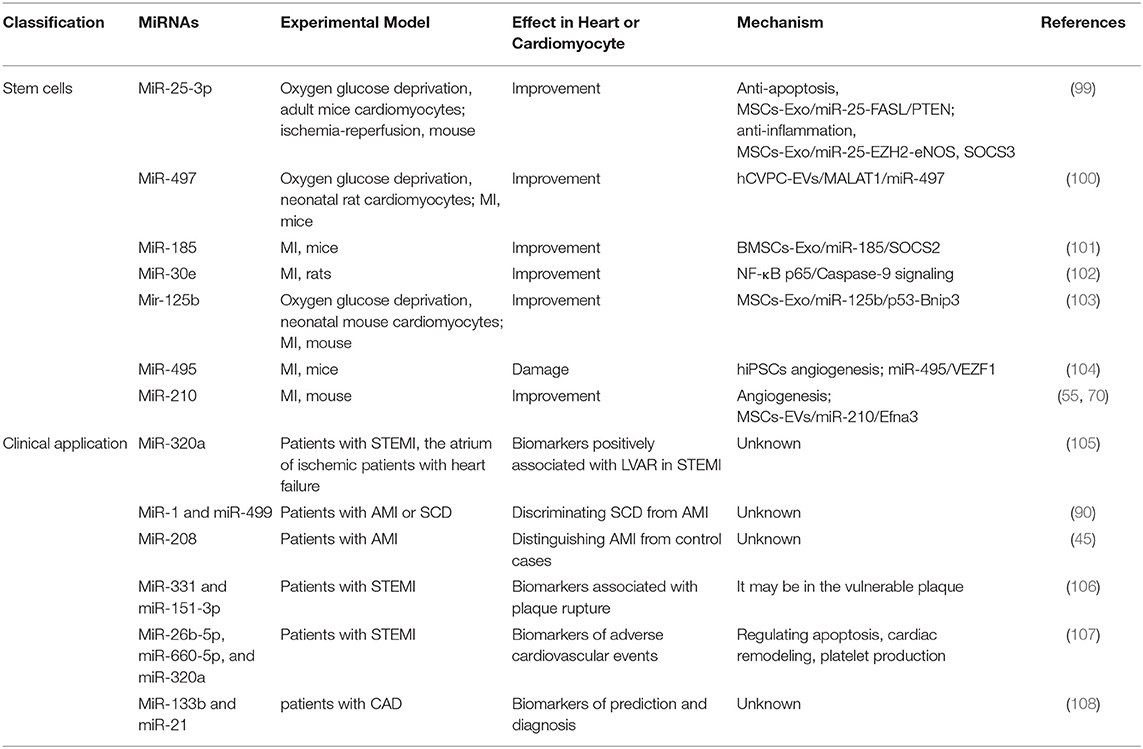
Table 6. Summary of studies on miRNA's involvement in the diagnosis and treatment of MI in the past 5 years.
Mesenchymal stem cells (MSCs) in multiple MI models have been shown to inhibit apoptosis, regenerative, and inflammatory (111, 112), which was involved in over 50 miRNAs in MSC-derived exosomes (113). MiR-25-3p, one of the exosomal miRNA from MSCs, decreased the level of apoptosis directly by targeting FASL and PTEN (114). The other mechanism is that MiR-25-3p reduced the expression of EZH2 and H3K27, which led to the anti-inflammatory gene SOCS3 promoter was decreased (99), leading to SOCS3 restoration and decreased proinflammatory cytokines (115). Thus, exosomes carrying miR-25-3p may become a promising way to treat MI.
MSCs are derived from mesenchymal tissues like bone marrow (BMSC), which are stromal cells with proliferation function (116). The expression level of MiR-185, bone marrow mesenchymal stem cells-derived exosomal (BMSCs-Exo), reduced in the mouse heart tissues after MI. BMSCs-Exo containing miR-185 in MI mice exerted cardiac remolding inhibitory effect by targeting suppressors of cytokine signaling 2 (SOCS2) (101). In addition, another study reported that MSCs treatment recovered damage to the structure and function of the heart in MI by reducing apoptosis and autophagy via miR-125-5p/p53/Bnip3 signaling pathway (103). As a paracrine product of MSC, MSC-EVs play a role in promoting vascular regeneration and functional recovery in the damaged heart. Further studies have shown that the angiogenesis effect of MSC-EVs was highly related to miR-210/Efna3 (70). Human pluripotent stem cells derived from cardiovascular progenitor cells are regarded as a valued method for myocardial repair (110, 117). A study in vivo has reported injection of human cardiovascular progenitor cells extracellular vesicles into infracted myocardial tissue enhanced cardiac function, accelerated vascularization and prevented fibrosis via targeting miR-497 (100). In another study, Liang et al. revealed that miR-495 represented a new target for improving EC generation from human induced pluripotent stem cells (hiPSCs) and promoting angiogenesis and transplant of hiPSC-derived ECs in infarcted heart. The cardiac regulatory mechanism was that miR-495 affected EC-related gene expression by targeting VEZF1 (104).
Clinical Application of MI
A clinical study on the analysis of miRNA arrays in humans was used to screen out human heart-specific miRNAs for the diagnosis of heart-related diseases. The results showed that miR-499, which was mainly enriched in the heart, was highly expressed in the plasma of patients with acute MI (118). In the other study, the investigator found miR-499 and miR-1 were the most sensitive markers in discriminating sudden cardiac death (SCD) from acute MI, whereas miR-208 showed the highest specificity in distinguishing acute MI from control cases (90). A multicenter prospective study showed that miR-320a, miR-660-5p, and miR-26b-5p to be highly correlated with patients with ST-segment-elevation MI (STEMI), and these three miRNAs might be further used for the improvement of risk assessment and follow-up intervention in STEMI patients (Table 6). Significantly, apoptosis, ventricular remodeling, and platelet production were primary regulatory mechanism of these miRNAs (107).
The formation of atherosclerotic plaque has the risk of causing occlusion of arteries and blood vessels. Once the vulnerable plaque ruptures, thrombus will form, which can further develop into ischemic infarction, stroke and other complications (119, 120). A study design contributes to screening out differential miRNAs in acute coronary artery occlusion due to atherosclerotic plaque rupture. The results suggest that Elevated miR-151-3p and miR-331 appeared even earlier than myocardial infarction markers, indicating that these two miRNAs have higher sensitivity to atherosclerotic plaque rupture (106). Atherosclerosis is regarded as a pathological change in the early stage of coronary syndrome, and it is difficult to be detected in the early stage. Therefore, there is still a lack of fast and effective methods for early detection of atherosclerosis. In a clinical study, investigators recruited 147 subjects, including 78 topics with angiographically proven coronary artery disease (CAD), 15 normal coronary arteries issues with atherosclerosis, and 54 healthy individuals. They found miR-133b was decreased in plasma of CAD patients, whereas miR-21 was elevated. Notably, both of these miRNAs have a significant correlation with the deterioration of heart disease (108).
Another study about circulating miRNA-1 (miR-1) in acute MI (AMI) diagnosis and prognosis. Another clinical trial recruited 337 patients with acute chest pain, including 174 patients with acute MI and 163 patients with non-acute MI. The results of the study showed that miR-1 was significantly different between the two groups. The miR-1 in the plasma samples of patients with acute MI was significantly increased, indicating that the diagnosis results of miR-1 and the myocardial infarction marker cTnI were similar. In addition, miR-1 combined with CK-MB, cTnI, and other clinical and laboratory indicators (including BMI, HR, TG, and LDL-C, smoking history, and renal dysfunction) had the highest diagnostic efficiency (121). Other investigators revealed that circulating miR-1 and miR-133b, cardiac-specific miRNA, may as early alternative markers for STEMI patients (122). Another clinical study of 20 STEMI patients found that after the coronary blood flow was restored, the levels of miR-1 and miR-133b increased rapidly either alone or at the same time, and the increase of miR-1 was associated with left ventricular dysfunction (123). MiR-320a was determined inpatient with STEMI and positively associated with left ventricular adverse remodeling (LVAR) (105).
The clinical diagnosis of MI is mainly based on the patient's clinical symptoms, abnormalities in the electrocardiogram, and specific biomarkers. Among them, cTnT and cTnI are currently the preferred MI markers. However, due to the relatively delayed release of troponin, finding new biomarkers is incredibly beneficial to the early diagnosis of MI. According to the above introduction, miRNA can be detected more sensitively and quickly and used as a potential early diagnosis method for MI.
The Regulation of Other ncRNAs on miRNAs During MI
In addition to miRNAs, there are some other ncRNAs, such as lncRNAs and circRNAs, which also play an important role in the occurrence and development of MI (124). A further summary found that lncRNAs and circRNA acted as sponges of miRNAs, and regulated MI by affecting the function of miRNAs (125).
LncRNAs as Sponges of miRNAs
LncRNAs are composed of more than 200 nucleotides and involved in cell growth, differentiation, proliferation and other processes (126). During MI, some lncRNAs can interact with miRNAs to regulate the target molecules corresponding to miRNAs (Table 7). We have previously summarized that miR-26a reduced MI through regulation of apoptosis and inflammation (17). Su et al. found that lncRNA MIRF acted on the sponge of miR-26a and reduces apoptosis during MI by regulating the pro-apoptotic protein Bak1 (139). In addition, there is also a report proving that autophagy was a key mechanism after the interaction between lncRNA MIRF and miR-26a (127). Notably, lncRNA MALAT1 acted on miR-320 to aggravate cell apoptosis (130), while the interaction between lncRNA MALAT1 and miR-26b-5p promoted microcirculation repair after MI (27). These studies showed that lncRNA MALAT1 acted on different miRNAs to have different effects on MI. Otherwise, lncRNA GAS5 acted as the sponges of miR-21, miR-525-5p, and miR-142-5p, which can aggravate MI through different mechanisms (131–133). In summary, one type of lncRNA, as the sponges for multiple miRNAs, inhibited the function of miRNAs, and then exerted different physiological and pathological regulatory effects.
CircRNAs as Sponges of miRNAs
Similar to lncRNAs, circRNAs also acted as the sponges for miRNAs during MI, thereby reducing/aggravating MI (Table 8). Studies have shown that circRNA MFACR inhibited the protective effect of miR-652-3p on myocardial mitochondrial function in MI mice (140). At the same time, circRNA MFACR also promoted cell apoptosis in MI region by binding miR-125b (141). In addition, circRNA POSTN, as a sponge of miR-96-5p, led to increased myocardial infarction area and decreased cardiac function by targeting BNIP3 (142). Some circRNAs can aggravate MI, such as ACAP2, MAT2B, Cdr1as, but there are also some circRNAs that improve MI injury (143–145). Zhu et al. found that circRNA SNRK targeting miR-103-3p reduced apoptosis and promoted proliferation, thereby improving cardiac function, through the GSK3β/β-catenin pathway (146). Studies have also shown that overexpression of circRNA Hipk3 improved endothelial cell function, promoted angiogenesis, and reduced MI damage through the Notch1/miR-133a axis (147). In short, the regulatory effect of circRNAs on MI mainly depends on the regulatory mechanism of miRNAs' targets.
Conclusions
MiRNAs are highly conservative in evolution, with tissue specificity and timing in expression, so they play an important role in various life activities of organisms. During the pathogenesis of MI, the corresponding miRNA changes and regulates the processes of cardiomyocyte apoptosis, myocardial fibrosis, angiogenesis, and inflammation after MI. Therefore, the identification of miRNA related to MI is crucial for developing miRNA diagnosis and treatment methods, and the corresponding clinical research is also ongoing.
This study concluded that miRNAs could reduce or aggravate MI by regulating apoptosis, autophagy, cell proliferation, and inflammation. MiRNAs can also regulate myocardial fibrosis, cardiac vascular regeneration, and ventricular remodeling after MI. The specific mechanism of miRNAs regulating MI was introduced. At present, the mechanisms for miRNAs to regulate MI were also very different. Some miRNAs may affect the process of MI through a variety of signaling pathways. MiR-21 can inhibit apoptosis and inflammation by targeting KBTBD7, regulate angiogenesis through STRN/NOS3, and promote myocardial fibrosis through the TGF-β/Smad7 signaling pathway. This also shows that miRNAs can target a variety of mRNAs, affecting the characteristics of downstream pathways. In addition, some MiRNAs, such as miR-320a and miR-208, can also be used as potential biomarkers for the diagnosis of MI. In addition to the above mechanisms, abnormal lipid metabolism is involved in the occurrence and development of atherosclerosis, and is also the inducement and main driver of MI (148). Current studies have shown that miRNAs regulated lipid metabolism through lipoprotein synthesis and reverse cholesterol transport (149). However, there are few reports related to MI, and the knowledge system needs to be further expanded (150). In fact, the regulation of MI by miRNAs is also affected by other ncRNAs, such as lncRNAs, circRNAs, which change the regulation of miRNAs on downstream pathways by binding to the sponge region of miRNAs. Therefore, miRNAs are potential for basic research and clinical development of MI.
Although miRNAs have made some progress in studying the mechanism of the occurrence and development of MI, there are still some limitations that need to be further investigated. For example, the specific regulation pathways of most miRNAs in vivo are not yet clear; most of the current researches are limited to animal experiments, and a large number of clinical trials are needed for verification; although different methods can knock down miRNA, most miRNA overexpression still requires viral transfection; the off-target effect of miRNA hinders potential transformation studies in humans. But it is worthy of recognition that the discovery of miRNAs opened up a new path for MI research. With the advancement of science and technology and the research of countless researchers, I believe that these problems will be overcome one by one. Thus, more efforts are required to deepen our understanding of the role of miRNA networks in MI. This will be particularly important when seeking safe and effective diagnosis and treatment.
Author Contributions
GL and RW designed experiments. CW and BL carried out investigations. CW and RW wrote the manuscript. GL supervised the entire study, revised the manuscript, and provided the funding. All authors contributed to the writing and editing of the manuscript.
Funding
This study was supported by a Joint Fund for Science and Technology Cooperation across the Taiwan Straits from the National Natural Science Foundation and Fujian Province, China (Grant No. U1605226), a Science and Technology Project from Xiamen Science and Technology Bureau, Fujian Province, China (Grant Nos. 3502Z20184025 and 3502Z20184024).
Conflict of Interest
The authors declare that the research was conducted in the absence of any commercial or financial relationships that could be construed as a potential conflict of interest.
Publisher's Note
All claims expressed in this article are solely those of the authors and do not necessarily represent those of their affiliated organizations, or those of the publisher, the editors and the reviewers. Any product that may be evaluated in this article, or claim that may be made by its manufacturer, is not guaranteed or endorsed by the publisher.
References
1. Virani SS, Alonso A, Benjamin EJ, Bittencourt MS, Callaway CW, Carson AP, et al. Heart disease and stroke statistics-2020 update. A Report From the American Heart Association. Circulation. (2020) 141:e139–596. doi: 10.1161/CIR.0000000000000746
2. Bang C, Batkai S, Dangwal S, Gupta SK, Foinquinos A, Holzmann A, et al. Cardiac fibroblast-derived microRNA passenger strand-enriched exosomes mediate cardiomyocyte hypertrophy. J Clin Investig. (2014) 124:2136–46. doi: 10.1172/JCI70577
3. Thum T, Condorelli G. Long noncoding RNAs and microRNAs in cardiovascular pathophysiology. Circ Res. (2015) 116:751–62. doi: 10.1161/CIRCRESAHA.116.303549
4. Ye Y, Perez-Polo JR, Qian J, Birnbaum Y. The role of microRNA in modulating myocardial ischemia-reperfusion injury. Physiol Genomics. (2011) 43:534–42. doi: 10.1152/physiolgenomics.00130.2010
5. Bartel DP. MicroRNAs: genomics, biogenesis, mechanism, and function. Cell. (2004) 116:281–97. doi: 10.1016/S0092-8674(04)00045-5
6. Saito Y, Saito H. Role of CTCF in the regulation of microRNA expression. Front Genet. (2012) 3:186. doi: 10.3389/fgene.2012.00186
7. Bartel DP. MicroRNAs: target recognition and regulatory functions. Cell. (2009) 136:215–33. doi: 10.1016/j.cell.2009.01.002
8. Condorelli G, Latronico MV, Cavarretta E. microRNAs in cardiovascular diseases: current knowledge and the road ahead. J Am Coll Cardiol. (2014) 63:2177–87. doi: 10.1016/j.jacc.2014.01.050
9. Whelan RS, Kaplinskiy V, Kitsis RN. Cell death in the pathogenesis of heart disease: mechanisms and significance. Ann Rev Physiol. (2010) 72:19–44. doi: 10.1146/annurev.physiol.010908.163111
10. Reinhart BJ, Slack FJ, Basson M, Pasquinelli AE, Bettinger JC, Rougvie AE, et al. The 21-nucleotide let-7 RNA regulates developmental timing in Caenorhabditis elegans. Nature. (2000) 403:901–6. doi: 10.1038/35002607
11. Sun C, Liu H, Guo J, Yu Y, Yang D, He F Du Z. MicroRNA-98 negatively regulates myocardial infarction-induced apoptosis by down-regulating Fas and caspase-3. Sci Rep. (2017) 7:7460. doi: 10.1038/s41598-017-07578-x
12. Chen CY, Choong OK, Liu LW, Cheng YC, Li SC, Yen CYT, et al. MicroRNA let-7-TGFBR3 signalling regulates cardiomyocyte apoptosis after infarction. EBioMedicine. (2019) 46:236–247. doi: 10.1016/j.ebiom.2019.08.001
13. Krzywińska O, Bracha M, Jeanniere C, Recchia E, Kedziora Kornatowska K, Kozakiewicz M. Meta-analysis of the potential role of miRNA-21 in cardiovascular system function monitoring. BioMed Res Int. (2020) 2020:4525410. doi: 10.1155/2020/4525410
14. Cheng Y, Liu X, Zhang S, Lin Y, Yang J, Zhang C. MicroRNA-21 protects against the H(2)O(2)-induced injury on cardiac myocytes via its target gene PDCD4. J Mol Cell Cardiol. (2009) 47:5–14. doi: 10.1016/j.yjmcc.2009.01.008
15. Yang L, Wang B, Zhou Q, Wang Y, Liu X, Liu Z, et al. MicroRNA-21 prevents excessive inflammation and cardiac dysfunction after myocardial infarction through targeting KBTBD7. Cell Death Dis. (2018) 9:769. doi: 10.1038/s41419-018-0805-5
16. Maries L, Marian C, Sosdean R, Goanta F, Sirbu IO, Anghel A. MicroRNAs-the heart of post-myocardial infarction remodeling. Diagnostics. (2021) 11:1675. doi: 10.3390/diagnostics11091675
17. Chiang MH, Liang CJ, Lin LC, Yang YF, Huang CC, Chen YH, et al. miR-26a attenuates cardiac apoptosis and fibrosis by targeting ataxia-telangiectasia mutated in myocardial infarction. J Cell Physiol. (2020) 235:6085–102. doi: 10.1002/jcp.29537
18. He F, Liu H, Guo J, Yang D, Yu Y, Yu J, et al. Inhibition of MicroRNA-124 reduces cardiomyocyte apoptosis following myocardial infarction via targeting STAT3. Cell Physiol Biochem. (2018) 51:186–200. doi: 10.1159/000495173
19. Yu F, Zhang X, Sun C, Xu W, Xia J. Downregulation of miRNA-663b protects against hypoxia-induced injury in cardiomyocytes by targeting BCL2L1. Exp Ther Med. (2020) 19:3581–8. doi: 10.3892/etm.2020.8644
20. Kang JY, Kim H, Mun D, Yun N, Joung B. Therapeutic potential of miR-21 regulation by human peripheral blood derived-small extracellular vesicles in myocardial infarction. Clin Sci. (2020) 134:985–99. doi: 10.1042/CS20191077
21. Zhang B, Zhou H, Qian C, Huang N, Gu Y, Huang Y, et al. Effect of MiR-133 on myocardial cell apoptosis in rats with myocardial infarction through the Notch1 signaling pathway. Minerva Med. (2021) 112:303–5. doi: 10.23736/S0026-4806.19.06226-8
22. Zhao L, Jiang S, Wu N, Shi E, Yang L, Li Q. MiR-17-5p-mediated endoplasmic reticulum stress promotes acute myocardial ischemia injury through targeting Tsg101. Cell Stress Chaperones. (2021) 26:77–90. doi: 10.1007/s12192-020-01157-2
23. Meng S, Hu Y, Zhu J, Feng T, Quan X. miR-30c-5p acts as a therapeutic target for ameliorating myocardial ischemia-reperfusion injury. Am J Transl Res. (2021) 13:2198–212.
24. Wang J, Dong G, Chi W, Nie Y. MiR-96 promotes myocardial infarction-induced apoptosis by targeting XIAP. Biomed Pharmacother. (2021) 138:111208. doi: 10.1016/j.biopha.2020.111208
25. Li SH, Zhang YY, Sun YL, Zhao HJ, Wang Y. Inhibition of microRNA-802-5p inhibits myocardial apoptosis after myocardial infarction via Sonic Hedgehog signaling pathway by targeting PTCH1. Eur Rev Med Pharmacol Sci. (2021) 25:326–34. doi: 10.26355/eurrev_202101_24398
26. Huang C, Deng H, Zhao W, Xian L. Knockdown of miR-384-3p protects against myocardial ischemia-reperfusion injury in rats through targeting HSP70. Heart Surg Forum. (2021) 24:E143–50. doi: 10.1532/hsf.3449
27. Chen Y, Li S, Zhang Y, Wang M, Li X, Liu S, et al. The lncRNA Malat1 regulates microvascular function after myocardial infarction in mice via miR-26b-5p/Mfn1 axis-mediated mitochondrial dynamics. Redox Biol. (2021) 41:101910. doi: 10.1016/j.redox.2021.101910
28. Wang L, Wang L, Wang Q. Constitutive activation of the NEAT1/miR-22-3p/Ltb4r1 signaling pathway in mice with myocardial injury following acute myocardial infarction. Aging. (2021) 13:15307–19. doi: 10.18632/aging.203089
29. Matsui Y, Takagi H, Qu X, Abdellatif M, Sakoda H, Asano T, et al. Distinct roles of autophagy in the heart during ischemia and reperfusion: roles of AMP-activated protein kinase and Beclin 1 in mediating autophagy. Circ Res. (2007) 100:914–22. doi: 10.1161/01.RES.0000261924.76669.36
30. Takagi H, Matsui Y, Hirotani S, Sakoda H, Asano T, Sadoshima J. AMPK mediates autophagy during myocardial ischemia in vivo. Autophagy. (2007) 3:405–7. doi: 10.4161/auto.4281
31. Lavandero S, Troncoso R, Rothermel BA, Martinet W, Sadoshima J, Hill JA. Cardiovascular autophagy: concepts, controversies, and perspectives. Autophagy. (2013) 9:1455–66. doi: 10.4161/auto.25969
32. Zhu H, Tannous P, Johnstone JL, Kong Y, Shelton JM, Richardson JA, et al. Cardiac autophagy is a maladaptive response to hemodynamic stress. J Clin Investig. (2007) 117:1782–93. doi: 10.1172/JCI27523
33. Liu X, Deng Y, Xu Y, Jin W, Li H. MicroRNA-223 protects neonatal rat cardiomyocytes and H9c2 cells from hypoxia-induced apoptosis and excessive autophagy via the Akt/mTOR pathway by targeting PARP-1. J Mol Cell Cardiol. (2018) 118:133–46. doi: 10.1016/j.yjmcc.2018.03.018
34. Moreau K, Ravikumar B, Renna M, Puri C, Rubinsztein DC. Autophagosome precursor maturation requires homotypic fusion. Cell. (2011) 146:303–17. doi: 10.1016/j.cell.2011.06.023
35. Mizushima N, Sugita H, Yoshimori T, Ohsumi Y. A new protein conjugation system in human. The counterpart of the yeast Apg12p conjugation system essential for autophagy. J Biol Chem. (1998) 273:33889–92. doi: 10.1074/jbc.273.51.33889
36. Wang K, Liu CY, Zhou LY, Wang JX, Wang M, Zhao B, et al. APF lncRNA regulates autophagy and myocardial infarction by targeting miR-188-3p. Nat Commun. (2015) 6:6779. doi: 10.1038/ncomms7779
37. Ding S, Abudupataer M, Zhou Z, Chen J, Li H, Xu L, et al. Histamine deficiency aggravates cardiac injury through miR-206/216b-Atg13 axis-mediated autophagic-dependant apoptosis. Cell Death Dis. (2018) 9:694. doi: 10.1038/s41419-018-0723-6
38. Rogg EM, Abplanalp WT, Bischof C, John D, Schulz MH, Krishnan J, et al. Analysis of cell type-specific effects of MicroRNA-92a provides novel insights into target regulation and mechanism of action. Circulation. (2018) 138:2545–58. doi: 10.1161/CIRCULATIONAHA.118.034598
39. Zhang L, Yin H, Jiao L, Liu T, Gao Y, Shao Y, et al. Abnormal downregulation of caveolin-3 mediates the pro-fibrotic action of MicroRNA-22 in a model of myocardial infarction. Cell Physiol Biochem. (2018) 45:1641–53. doi: 10.1159/000487732
40. Garg A, Foinquinos A, Jung M, Janssen-Peters H, Biss S, Bauersachs J, et al. MiRNA-181a is a novel regulator of aldosterone-mineralocorticoid receptor-mediated cardiac remodelling. Eur J Heart Fail. (2020) 22:1366–77. doi: 10.1002/ejhf.1813
41. Zhao Z, Du S, Shen S, Wang L. microRNA-132 inhibits cardiomyocyte apoptosis and myocardial remodeling in myocardial infarction by targeting IL-1β. J Cell Physiol. (2020) 235:2710–21. doi: 10.1002/jcp.29175
42. Wang L, Li Q, Diao J, Lin L, Wei J. MiR-23a is involved in myocardial ischemia/reperfusion injury by directly targeting CX43 and regulating mitophagy. Inflammation. (2021) 44:1581–91. doi: 10.1007/s10753-021-01443-w
43. Li J, Cai SX, He Q, Zhang H, Friedberg D, Wang F, et al. Intravenous miR-144 reduces left ventricular remodeling after myocardial infarction. Basic Res Cardiol. (2018) 113:36. doi: 10.1007/s00395-018-0694-x
44. Lu C, Wang X, Ha T, Hu Y, Liu L, Zhang X, et al. Attenuation of cardiac dysfunction and remodeling of myocardial infarction by microRNA-130a are mediated by suppression of PTEN and activation of PI3K dependent signaling. J Mol Cell Cardiol. (2015) 89:87–97. doi: 10.1016/j.yjmcc.2015.10.011
45. Shyu KG, Wang BW, Cheng WP, Lo HM. MicroRNA-208a increases myocardial endoglin expression and myocardial fibrosis in acute myocardial infarction. Can J Cardiol. (2015) 31:679–90. doi: 10.1016/j.cjca.2014.12.026
46. Roth GA, Johnson C, Abajobir A, Abd-Allah F, Abera SF, Abyu G, et al. Global, regional, and national burden of cardiovascular diseases for 10 causes, 1990 to 2015. J Am Coll Cardiol. (2017) 70:1–25. doi: 10.1016/j.jacc.2017.04.052
47. Gabisonia K, Prosdocimo G, Aquaro GD, Carlucci L, Zentilin L, Secco I, et al. MicroRNA therapy stimulates uncontrolled cardiac repair after myocardial infarction in pigs. Nature. (2019) 569:418–22. doi: 10.1038/s41586-019-1191-6
48. Chen J, Huang ZP, Seok HY, Ding J, Kataoka M, Zhang Z, et al. mir-17-92 cluster is required for and sufficient to induce cardiomyocyte proliferation in postnatal and adult hearts. Circ Res. (2013) 112:1557–66. doi: 10.1161/CIRCRESAHA.112.300658
49. Gao F, Kataoka M, Liu N, Liang T, Huang ZP, Gu F, et al. Therapeutic role of miR-19a/19b in cardiac regeneration and protection from myocardial infarction. Nat Commun. (2019) 10:1802. doi: 10.1038/s41467-019-09530-1
50. Yang Y, Cheng HW, Qiu Y, Dupee D, Noonan M, Lin YD, et al. MicroRNA-34a plays a key role in cardiac repair and regeneration following myocardial infarction. Circ Res. (2015) 117:450–9. doi: 10.1161/CIRCRESAHA.117.305962
51. Tian Y, Liu Y, Wang T, Zhou N, Kong J, Chen L, et al. A microRNA-Hippo pathway that promotes cardiomyocyte proliferation and cardiac regeneration in mice. Sci Transl Med. (2015) 7:279ra38. doi: 10.1126/scitranslmed.3010841
52. Borden A, Kurian J, Nickoloff E, Yang Y, Troupes CD, Ibetti J, et al. Transient introduction of miR-294 in the heart promotes cardiomyocyte cell cycle reentry after injury. Circ Res. (2019) 125:14–25. doi: 10.1161/CIRCRESAHA.118.314223
53. Huang W, Feng Y, Liang J, Yu H, Wang C, Wang B, et al. Loss of microRNA-128 promotes cardiomyocyte proliferation and heart regeneration. Nat Commun. (2018) 9:700. doi: 10.1038/s41467-018-03019-z
54. Hu Y, Jin G, Li B, Chen Y, Zhong L, Chen G, et al. Suppression of miRNA let-7i-5p promotes cardiomyocyte proliferation and repairs heart function post injury by targetting CCND2 and E2F2. Clin Sci. (2019) 133:425–41. doi: 10.1042/CS20181002
55. Arif M, Pandey R, Alam P, Jiang S, Sadayappan S, Paul A, et al. MicroRNA-210-mediated proliferation, survival, and angiogenesis promote cardiac repair post myocardial infarction in rodents. J Mol Med. (2017) 95:1369–85. doi: 10.1007/s00109-017-1591-8
56. Liao Z, Chen Y, Duan C, Zhu K, Huang R, Zhao H, et al. Cardiac telocytes inhibit cardiac microvascular endothelial cell apoptosis through exosomal miRNA-21-5p-targeted silencing to improve angiogenesis following myocardial infarction. Theranostics. (2021) 11:268–91. doi: 10.7150/thno.47021
57. Li Q, Xu Y, Lv K, Wang Y, Zhong Z, Xiao C, et al. Small extracellular vesicles containing miR-486-5p promote angiogenesis after myocardial infarction in mice and nonhuman primates. Sci Transl Med. (2021) 13:eabb0202. doi: 10.1126/scitranslmed.abb0202
58. Jung JH, Ikeda G, Tada Y, von Bornstädt D, Santoso MR, Wahlquist C, et al. miR-106a-363 cluster in extracellular vesicles promotes endogenous myocardial repair via Notch3 pathway in ischemic heart injury. Basic Res Cardiol. (2021) 116:19. doi: 10.1007/s00395-021-00858-8
59. Ge ZW, Zhu XL, Wang BC, Hu JL, Sun JJ, Wang S, et al. MicroRNA-26b relieves inflammatory response and myocardial remodeling of mice with myocardial infarction by suppression of MAPK pathway through binding to PTGS2. Int J Cardiol. (2019) 280:152–9. doi: 10.1016/j.ijcard.2018.12.077
60. Zheng X, Li J, Fan Q, Zhao X, Chen K. Dexmedetomidine alleviates myocardial ischemia/reperfusion-induced injury and Ca overload via the microRNA-346-3p/CaMKIId axis. Int J Cardiol. (2021) 338:185–95. doi: 10.1016/j.ijcard.2021.03.016
61. Yang H, Shan L, Gao Y, Li L, Xu G, Wang B, et al. MicroRNA-181b serves as a circulating biomarker and regulates inflammation in heart failure. Dis Mark. (2021) 2021:4572282. doi: 10.1155/2021/4572282
62. Wang D, Dai LF, Yang L, Wang YS, Hao XH, Liu ZC, et al. Upregulation of miR-335 reduces myocardial injury following myocardial infarction via targeting MAP3K2. Eur Rev Med Pharmacol Sci. (2021) 25:344–52. doi: 10.26355/eurrev_202101_24401
63. Garikipati VNS, Verma SK, Jolardarashi D, Cheng Z, Ibetti J, Cimini M, et al. Therapeutic inhibition of miR-375 attenuates post-myocardial infarction inflammatory response and left ventricular dysfunction via PDK-1-AKT signalling axis. Cardiovasc Res. (2017) 113:938–49. doi: 10.1093/cvr/cvx052
64. Shen D, He Z. Mesenchymal stem cell-derived exosomes regulate the polarization and inflammatory response of macrophages via miR-21-5p to promote repair after myocardial reperfusion injury. Ann Transl Med. (2021) 9:1323. doi: 10.21037/atm-21-3557
65. Liu D, Qiao C, Luo H. MicroRNA-1278 ameliorates the inflammation of cardiomyocytes during myocardial ischemia by targeting both IL-22 and CXCL14. Life Sci. (2021) 269:118817. doi: 10.1016/j.lfs.2020.118817
66. Deng Y, Cai L, Wang F, Huang J, Wang H, Li L, et al. Upregulated microRNA-381-5p strengthens the effect of dexmedetomidine preconditioning to protect against myocardial ischemia-reperfusion injury in mouse models by inhibiting CHI3L1. Int Immunopharmacol. (2021) 92:107326. doi: 10.1016/j.intimp.2020.107326
67. Chen C, Chen T, Li Y, Xu Y. miR-19a/19b improves the therapeutic potential of mesenchymal stem cells in a mouse model of myocardial infarction. Gene Ther. (2021) 28:29–37. doi: 10.1038/s41434-020-0122-3
68. Icli B, Dorbala P, Feinberg MW. An emerging role for the miR-26 family in cardiovascular disease. Trends Cardiovasc Med. (2014) 24:241–8. doi: 10.1016/j.tcm.2014.06.003
69. Masumura M, Watanabe R, Nagashima A, Ogawa M, Suzuki J, Shichiri M, et al. Anti-salusin-β antibody enhances angiogenesis after myocardial ischemia reperfusion injury. Expert Opin Ther Targets. (2013) 17:1003–9. doi: 10.1517/14728222.2013.819852
70. Wang N, Chen C, Yang D, Liao Q, Luo H, Wang X, et al. Mesenchymal stem cells-derived extracellular vesicles, via miR-210, improve infarcted cardiac function by promotion of angiogenesis. Biochim Biophys Acta Mol Basis Dis. (2017) 1863:2085–92. doi: 10.1016/j.bbadis.2017.02.023
71. Qiao L, Hu S, Liu S, Zhang H, Ma H, Huang K, et al. microRNA-21-5p dysregulation in exosomes derived from heart failure patients impairs regenerative potential. J Clin Investig. (2019) 129:2237–50. doi: 10.1172/JCI123135
72. Geng T, Song ZY, Xing JX, Wang BX, Dai SP, Xu ZS. Exosome derived from coronary serum of patients with myocardial infarction promotes angiogenesis through the miRNA-143/IGF-IR pathway. Int J Nanomed. (2020) 15:2647–58. doi: 10.2147/IJN.S242908
73. Li CC, Qiu XT, Sun Q, Zhou JP, Yang HJ, Wu WZ, et al. Endogenous reduction of miR-185 accelerates cardiac function recovery in mice following myocardial infarction via targeting of cathepsin K. J Cell Mol Med. (2019) 23:1164–73. doi: 10.1111/jcmm.14016
74. Su Q, Lv XW, Sun YH, Ye ZL, Kong BH, Qin ZB. MicroRNA-494 inhibits the LRG1 expression to induce proliferation and migration of VECs in rats following myocardial infarction. Mol Ther Nucleic Acids. (2019) 18:110–22. doi: 10.1016/j.omtn.2019.08.007
75. Liu X, Xu Y, Deng Y, Li H. MicroRNA-223 regulates cardiac fibrosis after myocardial infarction by targeting RASA1. Cell Physiol Biochem. (2018) 46:1439–54. doi: 10.1159/000489185
76. Wang Y, Li C, Zhao R, Qiu Z, Shen C, Wang Z, et al. CircUbe3a from M2 macrophage-derived small extracellular vesicles mediates myocardial fibrosis after acute myocardial infarction. Theranostics. (2021) 11:6315–33. doi: 10.7150/thno.52843
77. Sun Q, Luo M, Gao Z, Han X, Yan Z, Xie S, et al. TUG1 knockdown suppresses cardiac fibrosis after myocardial infarction. Mamm Genome. (2021) 32:435–42. doi: 10.1007/s00335-021-09895-z
78. Lee TL, Lai TC, Lin SR, Lin SW, Chen YC, Pu CM, et al. Conditioned medium from adipose-derived stem cells attenuates ischemia/reperfusion-induced cardiac injury through the microRNA-221/222/PUMA/ETS-1 pathway. Theranostics. (2021) 11:3131–49. doi: 10.7150/thno.52677
79. Lang M, Ou D, Liu Z, Li Y, Zhang X, Zhang F. LncRNA MHRT promotes cardiac fibrosis via miR-3185 pathway following myocardial infarction. Int Heart J. (2021) 62:891–9. doi: 10.1536/ihj.20-298
80. Yuan J, Chen H, Ge D, Xu Y, Xu H, Yang Y, et al. Mir-21 promotes cardiac fibrosis after myocardial infarction via targeting Smad7. Cell Physiol Biochem. (2017) 42:2207–19. doi: 10.1159/000479995
81. Tao L, Bei Y, Chen P, Lei Z, Fu S, Zhang H, et al. Crucial role of miR-433 in regulating cardiac fibrosis. Theranostics. (2016) 6:2068–83. doi: 10.7150/thno.15007
82. Martinez EC, Lilyanna S, Wang P, Vardy LA, Jiang X, Armugam A, et al. MicroRNA-31 promotes adverse cardiac remodeling and dysfunction in ischemic heart disease. J Mol Cell Cardiol. (2017) 112:27–39. doi: 10.1016/j.yjmcc.2017.08.013
83. Tu J, Ma L, Zhang M, Zhang J. Long non-coding RNA SOX2 overlapping transcript aggravates H9c2 cell injury via the miR-215-5p/ZEB2 axis and promotes ischemic heart failure in a rat model. Tohoku J Exp Med. (2021) 254:221–31. doi: 10.1620/tjem.254.221
84. Meldolesi J. Exosomes and ectosomes in intercellular communication. Curr Biol. (2018) 28:R435–44. doi: 10.1016/j.cub.2018.01.059
85. Sahoo S, Losordo DW. Exosomes and cardiac repair after myocardial infarction. Circ Res. (2014) 114:333–44. doi: 10.1161/CIRCRESAHA.114.300639
86. Kishore R, Khan M. More than tiny sacks. Stem cell exosomes as cell-free modality for cardiac repair. Circ Res. (2016) 118:330–43. doi: 10.1161/CIRCRESAHA.115.307654
87. Tomasek JJ, Gabbiani G, Hinz B, Chaponnier C, Brown AR. Myofibroblasts and mechano-regulation of connective tissue remodelling. Nat Rev Mol Cell Biol. (2002) 3:349–63. doi: 10.1038/nrm809
88. van den Borne SW, Diez J, Blankesteijn WM, Verjans J, Hofstra L, Narula J. Myocardial remodeling after infarction: the role of myofibroblasts. Nat Rev Cardiol. (2010) 7:30–7. doi: 10.1038/nrcardio.2009.199
89. Gupta SK, Foinquinos A, Thum S, Remke J, Zimmer K, Bauters C, et al. Preclinical development of a MicroRNA-based therapy for elderly patients with myocardial infarction. J Am Coll Cardiol. (2016) 68:1557–71. doi: 10.1016/j.jacc.2016.07.739
90. Pinchi E, Frati P, Aromatario M, Cipolloni L, Fabbri M, La Russa R, et al. miR-1, miR-499 and miR-208 are sensitive markers to diagnose sudden death due to early acute myocardial infarction. J Cell Mol Med. (2019) 23:6005–16. doi: 10.1111/jcmm.14463
91. Wang X, Meng H, Chen P, Yang N, Lu X, Wang ZM, et al. Beneficial effects of muscone on cardiac remodeling in a mouse model of myocardial infarction. Int J Mol Med. (2014) 34:103–11. doi: 10.3892/ijmm.2014.1766
92. Cohn JN, Ferrari R, Sharpe N. Cardiac remodeling–concepts and clinical implications: a consensus paper from an international forum on cardiac remodeling. Behalf of an International Forum on Cardiac Remodeling. J Am Coll Cardiol. (2000) 35:569–82. doi: 10.1016/S0735-1097(99)00630-0
93. Buonafine M, Bonnard B, Jaisser F. Mineralocorticoid receptor and cardiovascular disease. Am J Hypertens. (2018) 31:1165–74. doi: 10.1093/ajh/hpy120
94. Tarjus A, Martínez-Martínez E, Amador C, Latouche C, El Moghrabi S, Berger T, et al. Neutrophil gelatinase-associated lipocalin, a novel mineralocorticoid biotarget, mediates vascular profibrotic effects of mineralocorticoids. Hypertension. (2015) 66:158–66. doi: 10.1161/HYPERTENSIONAHA.115.05431
95. Yuan L, Fan L, Li Q, Cui W, Wang X, Zhang Z. Inhibition of miR-181b-5p protects cardiomyocytes against ischemia/reperfusion injury by targeting AKT3 and PI3KR3. J Cell Biochem. (2019) 120:19647–59. doi: 10.1002/jcb.29271
96. Pan Z, Sun X, Shan H, Wang N, Wang J, Ren J, et al. MicroRNA-101 inhibited postinfarct cardiac fibrosis and improved left ventricular compliance via the FBJ osteosarcoma oncogene/transforming growth factor-β1 pathway. Circulation. (2012) 126:840–50. doi: 10.1161/CIRCULATIONAHA.112.094524
97. Senyo SE, Steinhauser ML, Pizzimenti CL, Yang VK, Cai L, Wang M, et al. Mammalian heart renewal by pre-existing cardiomyocytes. Nature. (2013) 493:433–6. doi: 10.1038/nature11682
98. Nakada Y, Canseco DC, Thet S, Abdisalaam S, Asaithamby A, Santos CX, et al. Hypoxia induces heart regeneration in adult mice. Nature. (2017) 541:222–7. doi: 10.1038/nature20173
99. Jo D, Liu D, Yao S, Collins RD, Hawiger J. Intracellular protein therapy with SOCS3 inhibits inflammation and apoptosis. Nat Med. (2005) 11:892–8. doi: 10.1038/nm1269
100. Wu Q, Wang J, Tan WLW, Jiang Y, Wang S, Li Q, et al. Extracellular vesicles from human embryonic stem cell-derived cardiovascular progenitor cells promote cardiac infarct healing through reducing cardiomyocyte death and promoting angiogenesis. Cell Death Dis. (2020) 11:354. doi: 10.1038/s41419-020-2508-y
101. Li Y, Zhou J, Zhang O, Wu X, Guan X, Xue Y, et al. Bone marrow mesenchymal stem cells-derived exosomal microRNA-185 represses ventricular remolding of mice with myocardial infarction by inhibiting SOCS2. Int Immunopharmacol. (2020) 80:106156. doi: 10.1016/j.intimp.2019.106156
102. Pu L, Kong X, Li H, He X. Exosomes released from mesenchymal stem cells overexpressing microRNA-30e ameliorate heart failure in rats with myocardial infarction. Am J Transl Res. (2021) 13:4007–25.
103. Xiao C, Wang K, Xu Y, Hu H, Zhang N, Wang Y, et al. Transplanted mesenchymal stem cells reduce autophagic flux in infarcted hearts via the exosomal transfer of miR-125b. Circ Res. (2018) 123:564–78. doi: 10.1161/CIRCRESAHA.118.312758
104. Liang J, Huang W, Cai W, Wang L, Guo L, Paul C, et al. Inhibition of microRNA-495 enhances therapeutic angiogenesis of human induced pluripotent stem cells. Stem Cells. (2017) 35:337–50. doi: 10.1002/stem.2477
105. Galeano-Otero I, Del Toro R, Guisado A, Díaz I, Mayoral-González I, Guerrero-Márquez F, et al. Circulating miR-320a as a predictive biomarker for left ventricular remodelling in STEMI patients undergoing primary percutaneous coronary intervention. J Clin Med. (2020) 9:1051. doi: 10.3390/jcm9041051
106. Horváth M, Horváthová V, Hájek P, Štěchovský C, Honěk J, Šenolt L, et al. MicroRNA-331 and microRNA-151-3p as biomarkers in patients with ST-segment elevation myocardial infarction. Sci Rep. (2020) 10:5845. doi: 10.1038/s41598-020-62835-w
107. Jakob P, Kacprowski T, Briand-Schumacher S, Heg D, Klingenberg R, Stähli BE, et al. Profiling and validation of circulating microRNAs for cardiovascular events in patients presenting with ST-segment elevation myocardial infarction. Eur Heart J. (2017) 38:511–5. doi: 10.1093/eurheartj/ehw563
108. Kumar D, Narang R, Sreenivas V, Rastogi V, Bhatia J, Saluja D Srivastava K. Circulatory miR-133b and miR-21 as novel biomarkers in early prediction and diagnosis of coronary artery disease. Genes. (2020) 11:164. doi: 10.3390/genes11020164
109. Mauritz C, Martens A, Rojas SV, Schnick T, Rathert C, Schecker N, et al. Induced pluripotent stem cell (iPSC)-derived Flk-1 progenitor cells engraft, differentiate, and improve heart function in a mouse model of acute myocardial infarction. Eur Heart J. (2011) 32:2634–41. doi: 10.1093/eurheartj/ehr166
110. Ye L, Chang YH, Xiong Q, Zhang P, Zhang L, Somasundaram P, et al. Cardiac repair in a porcine model of acute myocardial infarction with human induced pluripotent stem cell-derived cardiovascular cells. Cell Stem Cell. (2014) 15:750–61. doi: 10.1016/j.stem.2014.11.009
111. Shafei E, Ali MA, Ghanem HG, Shehata AI, Abdelgawad AA, Handal HR, et al. Mesenchymal stem cell therapy: a promising cell-based therapy for treatment of myocardial infarction. J Gene Med. (2017) 19:e2995. doi: 10.1002/jgm.2995
112. Miao C, Lei M, Hu W, Han S Wang Q. A brief review: the therapeutic potential of bone marrow mesenchymal stem cells in myocardial infarction. Stem Cell Res Ther. (2017) 8:242. doi: 10.1186/s13287-017-0697-9
113. Ferguson SW, Wang J, Lee CJ, Liu M, Neelamegham S, Canty JM Nguyen J. The microRNA regulatory landscape of MSC-derived exosomes: a systems view. Sci Rep. (2018) 8:1419. doi: 10.1038/s41598-018-19581-x
114. Viré E, Brenner C, Deplus R, Blanchon L, Fraga M, Didelot C, et al. The polycomb group protein EZH2 directly controls DNA methylation. Nature. (2006) 439:871–4. doi: 10.1038/nature04431
115. Peng Y, Zhao JL, Peng ZY, Xu WF, Yu GL. Exosomal miR-25-3p from mesenchymal stem cells alleviates myocardial infarction by targeting pro-apoptotic proteins and EZH2. Cell Death Dis. (2020) 11:317. doi: 10.1038/s41419-020-2545-6
116. Mead B, Tomarev S. Bone marrow-derived mesenchymal stem cells-derived exosomes promote survival of retinal ganglion cells through miRNA-dependent mechanisms. Stem Cells Transl Med. (2017) 6:1273–85. doi: 10.1002/sctm.16-0428
117. Mummery CL, Zhang J, Ng ES, Elliott DA, Elefanty AG, Kamp TJ. Differentiation of human embryonic stem cells and induced pluripotent stem cells to cardiomyocytes: a methods overview. Circ Res. (2012) 111:344–58. doi: 10.1161/CIRCRESAHA.110.227512
118. Adachi T, Nakanishi M, Otsuka Y, Nishimura K, Hirokawa G, Goto Y, et al. Plasma microRNA 499 as a biomarker of acute myocardial infarction. Clin Chem. (2010) 56:1183–5. doi: 10.1373/clinchem.2010.144121
119. Townsend N, Wilson L, Bhatnagar P, Wickramasinghe K, Rayner M Nichols M. Cardiovascular disease in Europe: epidemiological update 2016. Eur Heart J. (2016) 37:3232–45. doi: 10.1093/eurheartj/ehw334
120. Muller JE, Tofler GH, Stone PH. Circadian variation and triggers of onset of acute cardiovascular disease. Circulation. (1989) 79:733–43. doi: 10.1161/01.CIR.79.4.733
121. Su T, Shao X, Zhang X, Yang C, Shao X. Value of circulating miRNA-1 detected within 3 h after the onset of acute chest pain in the diagnosis and prognosis of acute myocardial infarction. Int J Cardiol. (2020) 307:146–51. doi: 10.1016/j.ijcard.2019.09.050
122. Ludman PF. British cardiovascular intervention society registry for audit and quality assessment of percutaneous coronary interventions in the United Kingdom. Heart. (2011) 97:1293–7. doi: 10.1136/heartjnl-2011-300299
123. Coelho-Lima J, Mohammed A, Cormack S, Jones S, Ali A, Panahi P, et al. Kinetics analysis of circulating MicroRNAs unveils markers of failed myocardial reperfusion. Clin Chem. (2019) 66:247–56. doi: 10.1373/clinchem.2019.308353
124. Duenas A, Aranega A, Franco D. The role of non-coding RNA in congenital heart diseases. J Cardiovasc Dev Dis. (2019) 6:15. doi: 10.3390/jcdd6020015
125. Xiong W, Qu Y, Chen H, Qian J. Insight into long noncoding RNA-miRNA-mRNA axes in myocardial ischemia-reperfusion injury-the implications for mechanism and therapy. Epigenomics. (2019) 11:1733–48. doi: 10.2217/epi-2019-0119
126. Xie L, Zhang Q, Mao J, Zhang J, Li L. The roles of lncRNA in myocardial infarction: molecular mechanisms, diagnosis biomarkers, therapeutic perspectives. Front Cell Dev Biol. (2021) 9:680713. doi: 10.3389/fcell.2021.680713
127. Liang H, Su X, Wu Q, Shan H, Lv L, Yu T, et al. 2810403D21Rik/MirfLncRNA promotes ischemic myocardial injury by regulating autophagy through targeting. Autophagy. (2020) 16:1077–91. doi: 10.1080/15548627.2019.1659610
128. Shu L, Zhang W, Huang C, Huang G, Su G, Xu J. lncRNA ANRIL protects H9c2 cells against hypoxia-induced injury through targeting the miR-7-5p/SIRT1 axis. J Cell Physiol. (2020) 235:1175–83. doi: 10.1002/jcp.29031
129. Wang QS, Zhou J, Li X. LncRNA UCA1 protects cardiomyocytes against hypoxia/reoxygenation induced apoptosis through inhibiting miR-143/MDM2/p53 axis. Genomics. (2020) 112:574–80. doi: 10.1016/j.ygeno.2019.04.009
130. Hu H, Wu J, Li D, Zhou J, Yu H, Ma L. Knockdown of lncRNA MALAT1 attenuates acute myocardial infarction through miR-320-Pten axis. Biomed Pharmacother. (2018) 106:738–46. doi: 10.1016/j.biopha.2018.06.122
131. Zhou XH, Chai HX, Bai M, Zhang Z. LncRNA-GAS5 regulates PDCD4 expression and mediates myocardial infarction-induced cardiomyocytes apoptosis via targeting MiR-21. Cell Cycle. (2020) 19:1363–77. doi: 10.1080/15384101.2020.1750257
132. Zhang Y, Hou YM, Gao F, Xiao JW, Li CC, Tang Y. lncRNA GAS5 regulates myocardial infarction by targeting the miR-525-5p/CALM2 axis. J Cell Biochem. (2019) 120:18678–88. doi: 10.1002/jcb.29156
133. Du J, Yang ST, Liu J, Zhang KX, Leng YJ. Silence of LncRNA GAS5 protects cardiomyocytes H9c2 against hypoxic injury via sponging miR-142-5p. Mol Cells. (2019) 42:397–405. doi: 10.14348/molcells.2018.0180
134. Sun F, Zhuang Y, Zhu H, Wu H, Li D, Zhan L, et al. LncRNA PCFL promotes cardiac fibrosis via miR-378/GRB2 pathway following myocardial infarction. J Mol Cell Cardiol. (2019) 133:188–98. doi: 10.1016/j.yjmcc.2019.06.011
135. Lin B, Xu J, Wang F, Wang J, Zhao H, Feng D. LncRNA XIST promotes myocardial infarction by regulating FOS through targeting miR-101a-3p. Aging. (2020) 12:7232–47. doi: 10.18632/aging.103072
136. Wang J, Zhang S, Li X, Gong M. LncRNA SNHG7 promotes cardiac remodeling by upregulating ROCK1 via sponging miR-34-5p. Aging. (2020) 12:10441–56. doi: 10.18632/aging.103269
137. Zhang D, Wang B, Ma M, Yu K, Zhang Q, Zhang X. lncRNA HOTAIR protects myocardial infarction rat by sponging miR-519d-3p. J Cardiovasc Transl Res. (2019) 12:171–83. doi: 10.1007/s12265-018-9839-4
138. Su Q, Liu Y, Lv XW, Dai RX, Yang XH, Kong BH. LncRNA TUG1 mediates ischemic myocardial injury by targeting miR-132-3p HDAC3 axis. Am J Physiol Heart Circ Physiol. (2020) 318:H332–44. doi: 10.1152/ajpheart.00444.2019
139. Su X, Lv L, Li Y, Fang R, Yang R, Li C, et al. lncRNA MIRF promotes cardiac apoptosis through the miR-26a-Bak1 Axis. Mol Ther Nucleic Acids. (2020) 20:841–50. doi: 10.1016/j.omtn.2020.05.002
140. Wang K, Gan TY, Li N, Liu CY, Zhou LY, Gao JN, et al. Circular RNA mediates cardiomyocyte death via miRNA-dependent upregulation of MTP18 expression. Cell Death Differ. (2017) 24:1111–20. doi: 10.1038/cdd.2017.61
141. Wang S, Li L, Deng W, Jiang M. CircRNA MFACR is upregulated in myocardial infarction and downregulates miR-125b to promote cardiomyocyte apoptosis induced by hypoxia. J Cardiovasc Pharmacol. (2021) 78:802–8. doi: 10.1097/FJC.0000000000001123
142. Cheng N, Wang MY, Wu YB, Cui HM, Wei SX, Liu B, et al. Circular RNA POSTN promotes myocardial infarction-induced myocardial injury and cardiac remodeling by regulating miR-96-5p/BNIP3 axis. Front Cell Dev Biol. (2020) 8:618574. doi: 10.3389/fcell.2020.618574
143. Zhang J, Tang Y, Zhang J, Wang J, He J, Zhang Z, et al. CircRNA ACAP2 is overexpressed in myocardial infarction and promotes the maturation of miR-532 to induce the apoptosis of cardiomyocyte. J Cardiovasc Pharmacol. (2021) 78:247–52. doi: 10.1097/FJC.0000000000001065
144. Zhu Y, Zou C, Jia Y, Zhang H, Ma X, Zhang J. Knockdown of circular RNA circMAT2B reduces oxygen-glucose deprivation-induced inflammatory injury in H9c2 cells through up-regulating miR-133. Cell Cycle. (2020) 19:2622–30. doi: 10.1080/15384101.2020.1814025
145. Geng HH, Li R, Su YM, Xiao J, Pan M, Cai XX, et al. The circular RNA Cdr1as promotes myocardial infarction by mediating the regulation of miR-7a on its target genes expression. PLoS ONE. (2016) 11:e0151753. doi: 10.1371/journal.pone.0151753
146. Zhu Y, Zhao P, Sun L, Lu Y, Zhu W, Zhang J, et al. Overexpression of circRNA SNRK targets miR-103-3p to reduce apoptosis and promote cardiac repair through GSK3beta/beta-catenin pathway in rats with myocardial infarction. Cell Death Discov. (2021) 7:84. doi: 10.1038/s41420-021-00467-3
147. Si X, Zheng H, Wei G, Li M, Li W, Wang H, et al. circRNA Hipk3 induces cardiac regeneration after myocardial infarction in mice by binding to Notch1 and miR-133a. Mol Ther Nucleic Acids. (2020) 21:636–55. doi: 10.1016/j.omtn.2020.06.024
148. Feinberg MW, Moore KJ. MicroRNA regulation of atherosclerosis. Circ Res. (2016) 118:703–20. doi: 10.1161/CIRCRESAHA.115.306300
149. Flowers E, Froelicher ES, Aouizerat BE. MicroRNA regulation of lipid metabolism. Metabolism. (2013) 62:12–20. doi: 10.1016/j.metabol.2012.04.009
150. Agbu P, Carthew RW. MicroRNA-mediated regulation of glucose and lipid metabolism. Nat Rev Mol Cell Biol. (2021) 22:425–38. doi: 10.1038/s41580-021-00354-w
151. Gan J, Yuan J, Liu Y, Lu Z, Xue Y, Shi L, et al. Circular RNA_101237 mediates anoxia/reoxygenation injury by targeting let7a5p/IGF2BP3 in cardiomyocytes. Int J Mol Med. (2020) 45:451–60. doi: 10.3892/ijmm.2019.4441
Keywords: microRNAs, myocardial infarction, mechanisms, clinical application, review
Citation: Wu C, Liu B, Wang R and Li G (2022) The Regulation Mechanisms and Clinical Application of MicroRNAs in Myocardial Infarction: A Review of the Recent 5 Years. Front. Cardiovasc. Med. 8:809580. doi: 10.3389/fcvm.2021.809580
Received: 05 November 2021; Accepted: 24 December 2021;
Published: 17 January 2022.
Edited by:
Ding-Sheng Jiang, Huazhong University of Science and Technology, ChinaCopyright © 2022 Wu, Liu, Wang and Li. This is an open-access article distributed under the terms of the Creative Commons Attribution License (CC BY). The use, distribution or reproduction in other forums is permitted, provided the original author(s) and the copyright owner(s) are credited and that the original publication in this journal is cited, in accordance with accepted academic practice. No use, distribution or reproduction is permitted which does not comply with these terms.
*Correspondence: Ruiying Wang, d2FuZ3J1aXlpbmc4ODY2JiN4MDAwNDA7MTYzLmNvbQ==; Gang Li, bGlnYW5nJiN4MDAwNDA7eG11LmVkdS5jbg==