- Heart and Muscle Metabolism Laboratory, Department of Health and Exercise Physiology, Ursinus College, Collegeville, PA, United States
Ketone bodies have been identified as an important, alternative fuel source in heart failure. In addition, the use of ketone bodies as a fuel source has been suggested to be a potential ergogenic aid for endurance exercise performance. These findings have certainly renewed interest in the use of ketogenic diets and exogenous supplementation in an effort to improve overall health and disease. However, given the prevalence of ischemic heart disease and myocardial infarctions, these strategies may not be ideal for individuals with coronary artery disease. Although research studies have clearly defined changes in fatty acid and glucose metabolism during ischemia and reperfusion, the role of ketone body metabolism in the ischemic and reperfused myocardium is less clear. This review will provide an overview of ketone body metabolism, including the induction of ketosis via physiological or nutritional strategies. In addition, the contribution of ketone body metabolism in healthy and diseased states, with a particular emphasis on ischemia-reperfusion (I-R) injury will be discussed.
Introduction
Ketone body metabolism has become an important topic in the scientific and medical communities over the last several years. The identification of elevated ketone body oxidation in hypertrophied and failing hearts (1, 2) and the potential of exogenous ketone body supplements to promote exercise performance (3) have been a critical driver in research. Since ketone bodies are suggested to be more energy efficient than glucose or fatty acids (4, 5), the utilization of ketone bodies as an energy source may be advantageous for failing myocardium or exercising skeletal muscle. Certainly, the therapeutic and ergogenic potential of nutritional or pharmacological strategies that promote cardiac and muscular ketone body metabolism are of increased interest.
Ketogenic diets (KD) are high fat, low carbohydrates that were originally developed for the treatment of epilepsy (6). KDs can also be an effective treatment for inherited metabolic disorders of glucose metabolism (7). Although KDs appear to be effective strategies for weight loss, at least in the short-term, their ability to promote exercise performance appears to be limited (8). The use of exogenous ketone body supplementation has been of increased interest in exercise and sport performance, but the effectiveness of supplements remains controversial (9, 10). Although the KD or supplementation strategies appear to be recognized as plausible treatments in models of cardiac dysfunction and failure (11), there is less discussion regarding the role of ketone body metabolism in ischemic heart disease.
Research studies have uncovered critical changes in cardiac substrate metabolism under conditions of stress, especially in pathological hypertrophy (12, 13), heart failure (14, 15), ischemia-reperfusion (16–18), and obesity/diabetes (19, 20). Although the importance of ketone body metabolism to the hypertrophied and failing heart has been well-discussed, the contribution of ketone body oxidation to the myocardium following ischemia has received less attention. In this review, ketone body metabolism in the healthy and diseased myocardium will be discussed, with a particular focus on the impact of ketone body metabolism in the functional recovery from ischemic injury.
Metabolic Pathways of Ketone Bodies
Ketone bodies are 4-carbon molecules that are synthesized through the process known as ketogenesis and broken down and utilized within tissues via the ketolysis pathway (also referred to as ketone body oxidation). Three ketone bodies exist: acetone, acetoacetate, and beta-hydroxybutyrate (β-OHB). β-OHB is the ketone body which is in highest concentration in the blood and is typically measured in many commercially available assay kits or handheld meters used in the research setting. The liver is the primary site of ketogenesis (Figure 1), which generates acetyl-CoA via beta-oxidation of fatty acids. The acetyl-CoA is ultimately converted into acetoacetone or β-OHB via reactions that require several enzymes, namely mitochondrial acetyl-CoA acetyltransferase 1 (Acat1, commonly referred to as thiolase), 3-hydroxy-3-methylglutaryl-CoA synthase (Hmgcs2), HMGC-CoA lyase (Hmgcl), and mitochondrial beta-hydroxybutyrate dehydrogenase (Bdh1). Of note, acetoacetone is generated as a result of the Hmgcl reaction and can be converted to β-OHB via Bdh1. Once β-OHB enters peripheral tissues via monocarboxylate transporters, MCT1 (encoded by the Slc16a1 gene) or MCT2 (encoded by the Slc16a7 gene) (21), the ketolytic process commences (Figure 1). Acetyl CoA is produced through rapid oxidation of β-OHB by Bdh1, succinyl-CoA:3-oxoacid-CoA transferase (Scot, encoded by the Oxct1 gene), and Acat1, which then is available for entry into the tricarboxylic acid (TCA) cycle. These ketolytic enzymes are present in the heart and the reactions are primarily based on the substrate availability. However, the Scot reaction is also dependent upon the availability of succinyl CoA.
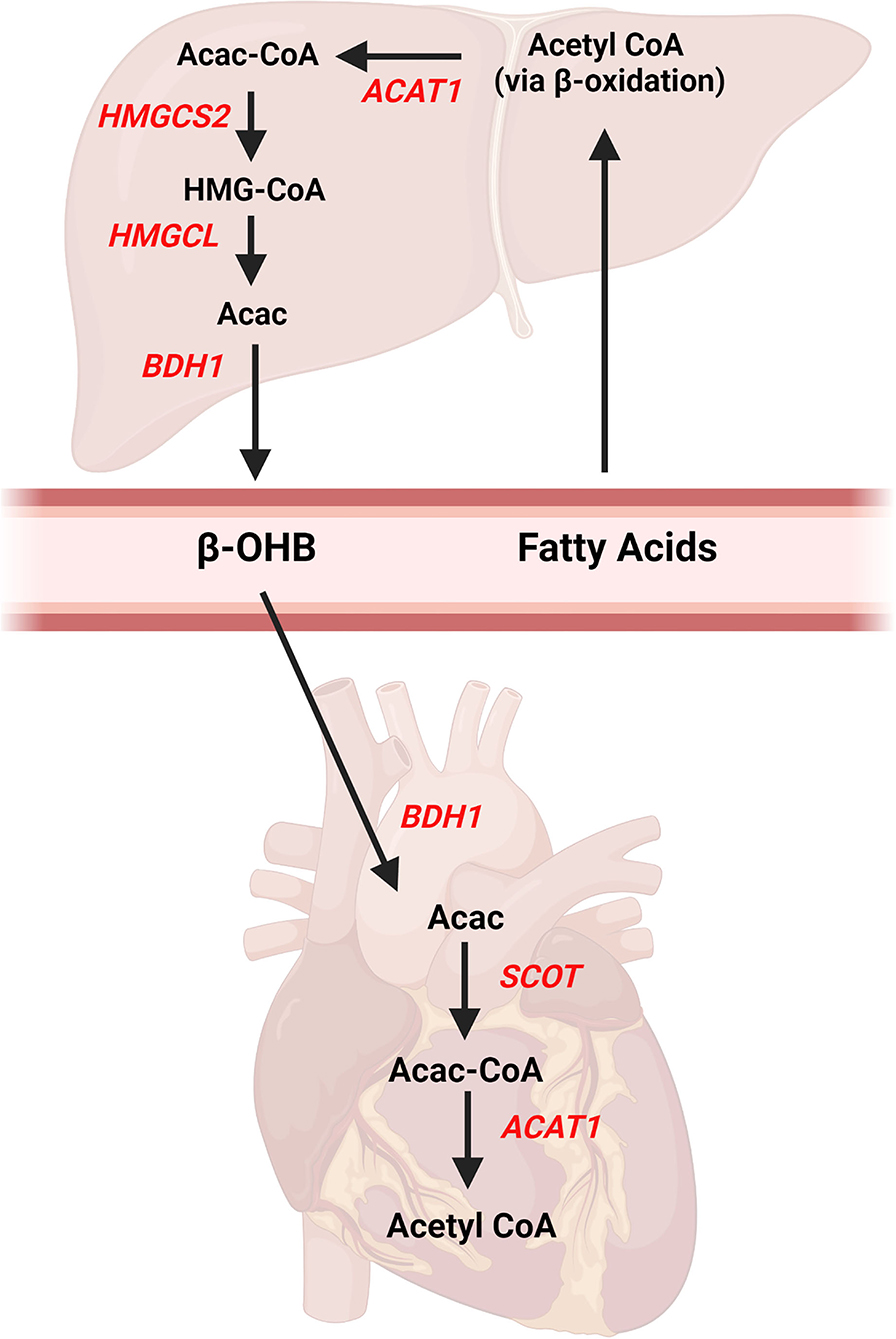
Figure 1. Ketone body pathways in the liver and heart. Ketogenesis occurs primarily in the liver from fatty acids obtained from the blood. Acetyl CoA is formed from hepatic beta-oxidation and ultimately converted to beta-hydroxybutyrate (β-OHB) via multiple enzymatic reactions. Once β-OHB enters the heart, a series of reactions forms Acetyl CoA for entry into Kreb's Cycle. AcAc, acetoacetate; Acac CoA, acetoacetyl CoA; ACAT1, acetyl-coenzyme A acetyltransferase 1, βOHB, beta-hydroxybutyrate; BDH1, mitochondrial beta-hydroxybutyrate dehydrogenase; HMGCS2, 3-hydroxy-3-methylglutaryl-CoA synthase 2; HMGCL, HMGC-CoA lyase; SCOT, succinyl-CoA:3-oxoacid-CoA transferase. Created with BioRender.com.
Older texts often discussed the presence of ketone bodies as negative consequences of abnormal metabolic processes (22, 23). However, since serum ketone bodies are measurable in the fed and fasted condition of healthy humans and animals, there must be some metabolic value. In fact, the use of ketone bodies as a metabolic fuel is suggested to be more energy efficient (4, 5). However, the total ATP yield per carbon atom for ketone bodies is slightly above glucose (~5.4 vs. ~5.2), while both substrates lag behind fatty acids (~6.7) (24, 25). In terms of ATP per oxygen (i.e., P/O ratio), ketone bodies are slightly lower than glucose (~2.50 vs. ~2.58), with both yielding higher ratios than fatty acids (~2.33) (24, 25). Based on these values, ketone bodies would rank second on both scales in relation to glucose and fatty acids. However, in isolated perfused heart experiments in rodents, the presence of ketone bodies in the perfusate increased cardiac efficiency (26, 27) and ATP production (28). Therefore, the relationship of ketone bodies to overall cardiac metabolism may require more intricate examination.
Physiological and Nutritional Ketosis
Ketosis is defined as a physiological condition where serum ketone body concentrations are elevated acutely. Studies in humans and rodents commonly report serum ketone body concentrations in the range of ~0.1 to 0.5 mM, so ketosis is generally identified as serum concentrations above 0.5 mM. Conditions that result in nutrient deprivation or low glucose availability, such as fasting/starvation and exercise (29, 30), are commonly associated with elevated serum ketone body concentrations, which is termed “physiological ketosis.” Diabetics, particularly Type I diabetics, may also exhibit elevated serum ketone body levels (31, 32). However, when in the 3.8–25.0 mM range and accompanied by low arterial pH values, the term “ketoacidosis” is used to reflect a potentially dangerous pathological state (33–35). More recently, the term “nutritional ketosis” has been used to better identify a state where ketosis was induced by nutritional or supplementation strategies (36–38). The use of these specific terms could help differentiate between the physiological/pathological and/or intentional/unintentional causes of ketosis.
In our recent study, we explored physiological ketosis in response to both fasting and endurance exercise (29). Female mice fasted up to 8 h, demonstrated gradual increases in serum ketone body concentrations, which peaked at ~ 0.8 mM (29). Our unpublished data showed that male mice respond similarly, with peak serum ketone body concentrations of ~0.7 mM. In response to endurance exercise of up to 2.5 h, serum ketone body levels rose to ~1.2 mM in female mice (29). Interestingly, this “exercise-induced ketosis” was blunted in fasted female mice (29). These values of physiological ketosis related to fasting or exercise are fairly consistent across the literature in both humans (39–41) and rodents (30, 42, 43). However, whether physiological ketosis is consistent between the sexes is relatively unexplored.
To induce nutritional ketosis, one strategy involves the consumption of the ketogenic diet (KD), a diet that is typically low in carbohydrates with high fat and adequate protein. The KD was originally used as a treatment for individuals with diabetes, epilepsy, and other neurological conditions approximately 100 years ago (6, 44, 45). This classical KD called for a fat to protein plus carbohydrate ratio of 4:1 (46), which typically results in a dietary intake of 80-90% of calories from fat, 10-15% calories from protein, and <5% of calories from carbohydrate sources (5). Although the use of the KD in research has increased significantly over the last several years, the dietary composition can deviate quite substantially from the classical KD. In some human studies, dietary regimens referred to as low-carbohydrate KD (LCKD) or very low-carbohydrate KDs (VLCKD) are used with carbohydrates ranges from ~10% to 45% of total calories (47–50). Despite the varied ranges of carbohydrate intake, all of these diets tend to be included in the KD category. Therefore, careful attention to the details of the dietary composition is needed to adequately interpret experimental findings.
Although the KD has been successful for weight reduction in mice (30, 51) and humans (52, 53), the high fat and low carbohydrate intake may be problematic for long-term adherence. Moreover, KDs are associated with negative consequences such as abnormal glucose homeostasis (54–56), hyperlipidemia (29, 54, 57, 58), and liver steatosis (29, 55). Therefore, another strategy to induce nutritional ketosis has been developed, which involves exogenous ingestion of ketone bodies as a nutritional supplement. These ketone body supplements are now commercially available and have been tested in several studies evaluating the potential ergogenic aid on exercise performance (3, 59–63). However, special attention must be paid to the exact formulation of these supplements as they are available in ketone salts (KS) or ketone esters (KE). Although more readily available and inexpensive, the use of KS supplements can be problematic, depending on the exact formulation, which may include β-OHB or 1,3-butandeniol (BD). In the salt form, β-OHB is present in both the active (D) and non-metabolizable (L) form, which may result in a less effective increase in serum ketone bodies (64, 65). KS supplements that contain BD may also be a less desirable method to induce ketosis since dehydrogenase enzymes in the liver are required to create β-OHB (64, 66). Although several formulations of KE supplements are available, the (R)-3-hydroxybutyl (R)-3-hydroxybutyrate ketone monoester, which converts to D-β-OHB and BD (67) has become the most commonly used. This KE, when combined with carbohydrates, elicited an increase in exercise performance in trained cyclists (3). Of note, other studies using KE supplementation have not consistently shown an increase in exercise performance (61–63).
Ketone Body Metabolism in the Healthy Heart
The term, metabolic flexibility, has become synonymous with the cardiometabolic profile of the healthy heart. Due to the enormous requirement for continual replenishment of energy stores, the heart must possess an ability to utilize any carbon-based substrate available. To this end, the heart can metabolize the exogenous substrates fatty acids, glucose, lactate, amino acids, and ketone bodies to produce energy. Research suggests that the normal healthy heart generates approximately 60-80% of its energy requirements from fatty acids, with approximately 10-20% from glucose (68–70). Lactate can also be an important fuel, particularly during instances of increased workload, such as exercise (71, 72). The contribution of amino acids to cardiac energy metabolism is reported to be low, ~1-3% (73, 74). However, this low contribution to oxidative metabolism should not be interpreted as insignificant as disruption of amino acid catabolism in genetic models can be detrimental, particularly in stressed conditions (75).
Although previously considered to be a relatively minor substrate, ketone body oxidation is reported to contribute ~10-20% to cardiac energy metabolism (28, 43). Interestingly, ketone body oxidation may not be essential to the healthy myocardium as cardiac-specific deletion of Bdh1 (76) or Scot (Oxct1) (77) virtually eliminates ketone body oxidation with no myocardial phenotype in unstressed conditions. In contrast, cardiomyocyte overexpression of Bdh1 increases ketone body oxidation by approximately 70% without negatively affecting cardiac function (78). These studies in transgenic mice would suggest that low or high ketone body oxidation is relatively inconsequential to the heart in unchallenged situations. However, a potential concern is the effect of changes in ketone body availability and delivery on the usage of other fuels, particularly glucose and fatty acids, on cardiac metabolism. Indeed, increased delivery of β-OHB suppresses fatty acid oxidation but does not significantly affect glucose oxidation in animal models (30, 76, 79). Increased ketone bodies also decreased myocardial glucose uptake in humans (80). However, increasing β-OHB concentrations in working heart preparation in mice did not affect fatty acid oxidation and tended to enhance glucose oxidation (28). In relation to this, increasing glucose uptake via overexpression of glucose transporter 4 (Glut4) suppressed ketone body oxidation in isolated perfused mouse hearts (81). These seemingly discrepant findings are likely due to differences in the experimental logistics, particularly in the concentrations of ketone bodies employed. However, the influence of changes in ketone body oxidation on oxidation of other substrates appears to be essentially meaningless, as cardiac function remains relatively normal in these situations, thus highlighting the metabolic flexibility of the heart under normal, baseline conditions.
Ketone Body Metabolism in the Obese and Diabetic Heart
The discussion of ketone bodies in settings of obesity and diabetes are traditionally thought to represent a pathophysiological state due to the condition of diabetic ketoacidosis (DKA) that can result in serious clinical complications (33–35). Indeed, Type 1 diabetic (T1D) patients without insulin therapy quickly develop ketonemia within 5 h (32). Therefore, the American Diabetes Association recommends regular monitoring of both glucose and ketone bodies for T1D (82). However, blood levels of ketone bodies are also increased in Type 2 diabetics (T2D) patients (31), indicating that systemic disruptions in glucose homeostasis, either via insulin deficiency or insulin resistance, result in changes in systemic ketone body concentrations. Whether the elevation in ketone body availability contributes directly to the cardiac dysfunction often associated with the obese and diabetic heart is not known.
The cardiometabolic profile of the diabetic and obese heart has been generally described as increased reliance on fatty acid oxidation with a concomitant reduction in glucose oxidation (83), which in is contrast to the hypertrophied and failing heart discussed in the next section. However, studies conducted over the last several years also demonstrate alterations in cardiac ketone body metabolism in diabetes. In T2D patients, myocardial uptake of the ketone bodies, β-OHB and acetoacetate were higher than non-diabetic controls, inferring a possible increase of ketone body oxidation in human hearts (84). However, ketone body oxidation was shown to be reduced in isolated hearts from diabetic (db/db) mice (20). Consistent with this, T1D, induced by streptozotocin (STZ), or T2D, induced by 12-weeks of high fat diet, was associated with reduced mRNA expression and protein level of myocardial Bdh1 (81). Moreover, STZ hearts demonstrated accumulation of cardiac β-OHB with a tendency for reduced myocardial β-OHB oxidation (81). One potential interpretation of these animal studies is that the reduction in ketone body oxidation is a hallmark of the diabetic heart, which might serve as a therapeutic target. In support of this, a recent study demonstrated improved systolic and diastolic function as well as increased expression of ketone body oxidation enzymes in db/db mice fed a diet supplemented with KE (85). Future research can help to clarify the impact of enhanced ketone body metabolism in the obese and diabetic myocardium.
Ketone Body Metabolism in Hypertrophy and FailurE
Over the last 5 years, the importance of cardiac ketone body metabolism in the pathological setting has gained great attention (1). Although the metabolic derangements that occur in the hypertrophied and failing heart, including significant decreases in fatty acid oxidation concomitant with increased glycolysis, are well established (14, 69, 86), the role of ketone body oxidation previously received little focus. However, seminal studies in humans (87) and mice (88) have underscored the contribution of ketone body metabolism to cardiac pathologies, particularly in heart failure. These publications have led to a host of recent studies that have examined the role of ketone body metabolism in cardiac disease (28, 76, 78, 85, 89, 90).
Findings from recent studies have indicated that the hypertrophied and failing heart demonstrates elevated ketone body oxidation (76, 87, 88), which provides an alternative fuel source to maintain cardiac function. These studies have provided the framework for targeting ketone body metabolism as a potential therapeutic treatment for cardiac dysfunction. Indeed, cardiac-specific deletion of Bdh1 in mice exacerbates cardiac dysfunction and remodeling in a heart failure model (76), while overexpression of Bdh1 protects against cardiac dysfunction and adverse myocardial remodeling (78). These provocative findings could support the use of the KD as a nutritional strategy to treat heart failure. In support of this idea, mice fed a KD of ~80% fat, ~20% protein, ~0% carbohydrates for 4-weeks had reduced pathological remodeling in a combined pressure-overload/myocardial infarction heart failure model (76). However, long term KD treatment (i.e., 62 weeks) may worsen diabetic cardiomyopathy in rats (89). Considering the high fat content of KDs, this may not be a suitable long-term strategy so ketone body supplementation might offer a better alternative. In agreement with this notion, a diet supplemented with KEs improved cardiac function, in part by increased mitochondrial oxygen consumption, in type 2 diabetic mice (85). However, although β-OHB infusion has been reported to increase cardiac function in failing canine hearts (76), provision of β-OHB in the perfusate to hypertrophied and/or failing mouse hearts in isolated heart experiments may not improve cardiac efficiency (28) or myocardial energetics (90). Certainly, experimental conditions, including in-vivo vs. ex-vivo situation and the concentration of ketone bodies used, are a concern.
Ketone Body Metabolism During Ischemia and Reperfusion
Consistent with the cardiac metabolic profile in hypertrophied and failing hearts, the research literature has clearly defined changes that occur during ischemia-reperfusion (I-R). During reperfusion following ischemia, excessive rates of fatty acid oxidation (16, 18) and uncoupling of glycolysis from glucose oxidation (17, 91) have been reported, which may contribute to the reduced functional recovery from ischemic injury. In support, stimulation of glucose oxidation (18, 92, 93) or inhibition of fatty acid oxidation (94–96) improves functional recovery after cardiac ischemia. Oxidation of ketone bodies is suggested to supplant fatty acid oxidation (30, 76, 79), and as a result, upregulation of ketone body metabolism may decrease reliance on fatty acid oxidation and/or improve the coupling of glycolysis to glucose oxidation. Therefore, investigations examining the relationship of the ketogenic diet, ketone body metabolism, and functional recovery from ischemia are needed.
Although the role of ketone body metabolism in heart failure has gained significant attention in the research and medical communities as a therapeutic intervention (2, 97), the role of ketone body metabolism in the ischemia and post-ischemic heart has been less discussed. However, a recent publication identified that circulating ketone bodies were significantly elevated in patients presenting with ST-Segment Elevation Myocardial Infarction (STEMI) (98). Interestingly, elevations in ketone body concentrations 24 h after percutaneous coronary intervention (PCI) were independently associated with greater infarct size and lower LV ejection fraction after 4-months (98). β-OHB concentrations were higher in blood from acute MI patients and mice following left anterior descending (LAD) ligation surgery, which was negatively associated with left ventricular ejection fraction in both models (99). Ketone bodies, particularly β-OHB, was associated with higher odds ratios for myocardial infarction and ischemic stroke (100). Increased ketone body concentrations have also been reported in heart failure patients (87, 101, 102), after fasting and exercise (29, 30), and diabetes (31, 32). Therefore, speculation could arise as to whether elevations in serum ketone body concentrations are a hallmark of metabolic stress.
Since ischemia represents a condition with low oxygen and nutrient availability, the likelihood that ketone bodies contribute to metabolic processes would be quite low. Consistent with this, a recent report demonstrated that ketone body utilization was suppressed by myocardial ischemia in patients presenting with chest pain (103). However, β-OHB has been shown to accumulate in rat hearts exposed to low-flow ischemia (104), and since the perfusate was devoid of ketone bodies, this suggests that ketogenesis was active in the ischemic heart. Supporting this, inhibition of Hmgcs2 decreased β-OHB accumulation and improved functional recovery during reperfusion (104). This reported finding is somewhat curious, as extrahepatic tissues have been considered incapable of ketogenesis. Interestingly, recent studies have reported changes in gene and/or protein levels of Hmgcs2 in the hearts of mice and human patients (81, 87, 88). However, the role of Hmgcs2 in the normal heart or in the pathogenesis of cardiac disease is relatively unknown. In terms of ischemia-reperfusion injury, whether improvements in recovery are due to changes in ketone body oxidation or changes in oxidation of glucose or fatty acids is not clear and requires more extensive research.
Several studies from the same laboratory evaluated the effects of a low-carbohydrate diet (LCD) on tolerance to myocardial ischemia (105–108). The LCD diet consisted of 60% of the total calories from fat, 30% of total calories from protein, and 10% of the total calories from carbohydrates. Consumption of LCD was shown to induce a mild nutritional ketosis (108). Isolated hearts from lean and obese rats fed the diet for 2 weeks showed decreased recovery from low flow ischemia (105, 108). The 2-week LCD strategy also resulted in poor function, decreased survival, and increased arrhythmias in rats exposed to left anterior descending (LAD) ligation (107). One potential critique of these studies is the relatively short duration of the dietary intervention as the adaptation to KDs is purported to require 4–6 weeks (8). Since ketosis was relatively mild (~0.6 mM), perhaps, greater concentrations of serum ketone bodies would be required to cause positive adaptations. However, increasing the ketone body concentration to 1.2 mM did not improve the poor recovery in these animals (106, 108). Surprisingly, increasing ketone body concentrations in the perfusate did not appear to alter myocardial ketone body oxidation either in baseline or I-R conditions; however, there was a mild positive correlation between ketone body oxidation and recovery in all groups (106). Overall, these studies would suggest that a short-term (i.e., 2 weeks) diet of high fat and low carbohydrates would render the heart vulnerable to ischemic stress.
A recent study also evaluated the effectiveness of a KD on cardiac function following MI in mice (99). In this study, mice were fed a KD consisting of ~94% calories from fat and ~2% calories from carbohydrates for 4 weeks and then were subjected to LAD ligation. Four weeks following LAD ligation, infarct size was significantly greater and fractional shortening (FS) was significantly lower in mice fed the KD (99). MI hearts fed the KD also had lower protein content of glucose transporter 1 (Glut1) and hypoxia-inducible factor 1 alpha (HIF-1α) (99). The findings of this study suggest that elevations in serum ketone bodies have the potential to suppress glucose utilization in the ischemic heart, which may enhance myocardial injury.
In contrast to the above studies, fasting-induced ketosis (109) or long term LCKD (110) were associated with improved responses to ischemia in rats. Extreme fasting of 72 h increased β-OHB nearly 15-fold, which reduced infarct size and ventricular arrhythmias in Wistar rats subjected to occlusion of the LAD (109). Wistar rats fed a LCKD for 19-weeks demonstrated improved recovery following global ischemia compared to a control or high carbohydrate diet, which could be due to maintenance of mitochondrial number (110). Infusion of β-OHB to fed rats for 60 min prior to left coronary artery occlusion did not improve infarct size or functional recovery; however, β-OHB in rats fasted for 84 h led to reduced infarct size and higher functional recovery (111). Although the findings are promising, the severity of the fasting period or duration of the dietary intervention could be a bit impractical. Therefore, alternative methods of elevating ketone bodies may offer a more realistic treatment option.
Exogenous delivery of ketone bodies, especially β-OHB, may represent a suitable cardioprotective strategy for ischemia. Rat hearts reperfused with a glucose buffer containing a 5 mM ketone body concentration had improved LV contractility following 10 min of ischemia (27). Isolated mouse hearts provided with 3 mM β-OHB in the perfusate during reperfusion led to a significant improvement in functional recovery following 30 min of ischemia (112). These studies certainly highlight the potential of acute administration of β-OHB in supraphysiologic levels to improve recovery from ischemia. Mice implanted with an osmotic mini-pump with continuous delivery of β-OHB prior to reperfusion had reduced infarct size and preserved cardiac function (113). KE supplemented to rats, either immediately after or 2 weeks post, left coronary artery ligation resulted in an attenuation of pathological cardiac remodeling and improved ejection fraction compared to chow fed rats (114). Overall, the action of β-OHB or KE in the reperfused myocardium or ischemic myocardium may function via improved mitochondrial energetics (27, 114), reduced inflammation (112), and protection against oxidative stress (113). Certainly, additional investigations are required to further evaluate the therapeutic potential of ketone bodies from ischemic injury. In addition, studies examining long-term treatments would be more practical.
Recently, the use of sodium-glucose co-transporter 2 (SGLT2) inhibitors have been of increased interest in medicine and research due to decreased cardiovascular mortality and heart failure in diabetic patients (115). Although a variety of mechanisms have been proposed regarding the benefits of SGLT2 inhibitor on the heart (116), some studies suggest that SGLT2 inhibitors promote ketone body utilization (117, 118). In animal models, SGLT2 inhibitors are associated with beneficial outcomes in the non-diabetic ischemic heart (117–119). Administration of the SGLT2 inhibitor, dapagliflozin, prior to the onset of ischemia was associated with reduced infarct size and improved recovery in a rat model of in-vivo ischemia-reperfusion injury (119). Rats treated with empagliflozin for 10 weeks after MI induced by coronary artery ligation had improved cardiac function, an effect that was observed if treatment occurred 2 days prior to or 2 weeks after the surgical procedure (118). Of note, the positive functional outcomes with empagliflozin treatment were associated with elevated serum ketone bodies, upregulation of Bdh1 and Scot (Oxct1), and higher cardiac ATP content, suggesting that enhanced cardiac ketone body utilization was involved (118). In non-diabetic pigs, 2 months of empagliflozin treatment improved cardiac remodeling and LV function after proximal LAD occlusion, which was also associated with increased protein content of Bdh1 and Scot in the myocardium (117). Although these studies in animals are promising and appear to indicate that enhanced cardiac ketone body metabolism is mediating the response, additional work is clearly needed to evaluate the efficacy of SGLT2 inhibitors in ischemic heart disease.
Conclusions And Perspectives
Despite an increase in the number of studies focused on cardiac ketone body metabolism in pathological hypertrophy and heart failure, there seems to be a paucity of research elucidating the role of ketone body metabolism in the ischemic myocardium. Given the reported potential of ketone bodies to affect the use of fatty acids and glucose in the healthy myocardium, their delivery to the heart following bouts of ischemia could influence functional recovery. A summary of the findings from various strategies to enhance ketone body metabolism in models of ischemia are presented in Figure 2. Unfortunately, the current literature does not allow for definitive conclusions to be drawn, due to the relatively low number of studies in animal models. Studies investigating the effects of nutritional ketosis on recovery from ischemia appear to suggest that this strategy is detrimental. However, most of these studies are limited to short-term interventions. Physiological ketosis induced by fasting appears to be promising, but these animal studies seem impractical with fasting of 3 days or more. Delivery of exogenous ketone bodies (i.e., β-OHB) in ex-vivo perfused heart preparations have positive reports, but the concentrations used in these studies may be unrealistic compared to the in-vivo situation. Although the use of continuous β-OHB delivery via osmotic mini-pumps in mice demonstrates positive effects on functional recovery, the recent report that demonstrated an association between poor outcomes and high ketone body concentrations in humans following MI are equivocal. The use of SGLT2 inhibitors in non-diabetic hearts also appear to be promising. However, additional investigations to further evaluate all of these treatment strategies are warranted. For studies in animal models, careful attention should be paid to the concentrations used either in in-vivo or ex-vivo experimental conditions to ensure that the ranges of ketone bodies are within achievable concentrations consistent with nutritional or physiological ketosis. In human studies, a focus on the specific etiology of the disease (i.e., ischemic vs. non-ischemic) could help to delineate important contributions of ketone body metabolism to cardiac pathologies.
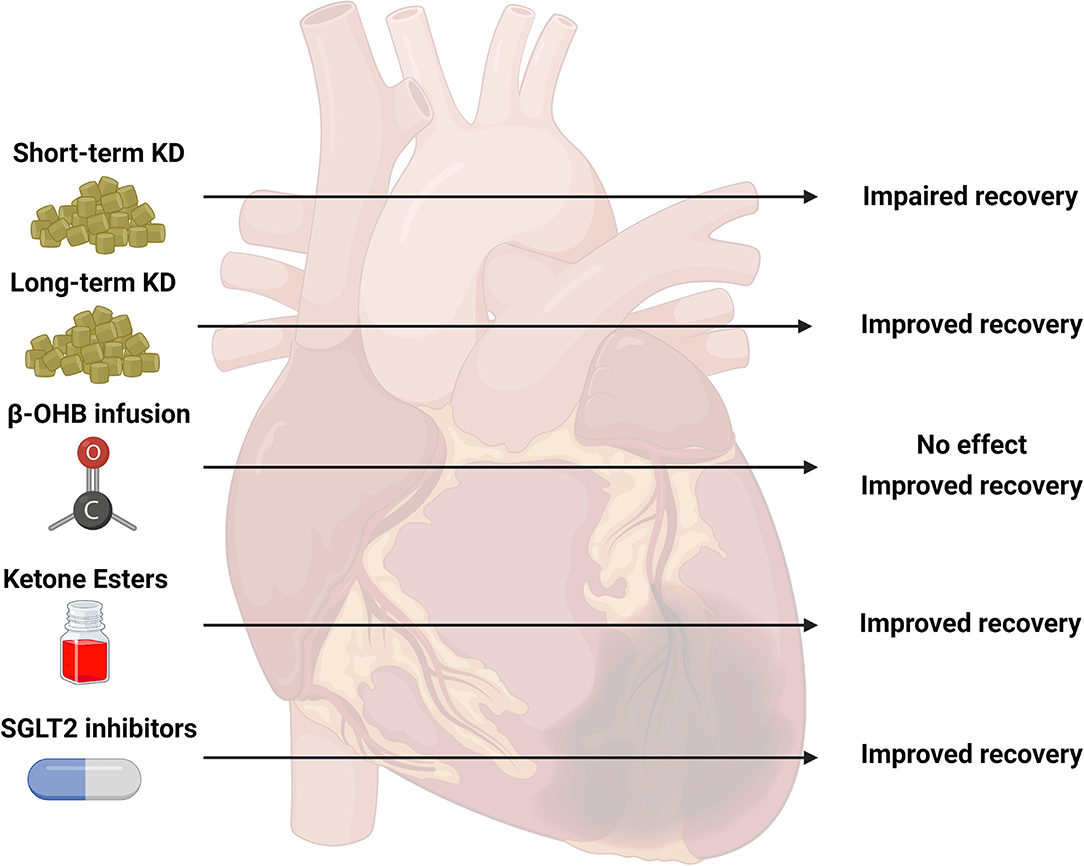
Figure 2. Summary of studies investigating ketone body metabolism in the ischemic heart. Various strategies to target ketone body metabolism in models of cardiac ischemia have been attempted including short-term ketogenic diets (KD, 2–4 weeks), long-term KD (19 weeks), infusion of beta-hydroxybutyrate (β-OHB), ketone ester (KE) supplementation, and sodium-glucose co-transporter 2 (SGLT2) inhibitors. The observed outcomes of these various interventions are presented as impaired, improved, or no effect. The current literature is limited on the effectiveness of these specific strategies: short-term KD (five studies), long-term KD (1 study), β-OHB infusion (four studies), KE supplementation (1 study), SGLT2 inhibitors (three studies). Created with BioRender.com.
Author Contributions
The author confirms being the sole contributor of this work and has approved it for publication.
Funding
This work was supported by an AHA Institutional Research Enhancement Award from the American Heart Association (Grant Number: 20AIREA35080151).
Conflict of Interest
The author declares that the research was conducted in the absence of any commercial or financial relationships that could be construed as a potential conflict of interest.
Publisher's Note
All claims expressed in this article are solely those of the authors and do not necessarily represent those of their affiliated organizations, or those of the publisher, the editors and the reviewers. Any product that may be evaluated in this article, or claim that may be made by its manufacturer, is not guaranteed or endorsed by the publisher.
References
1. Kolwicz SC, Jr., Airhart S, Tian R. Ketones step to the plate: a game changer for metabolic remodeling in heart failure? Circulation. (2016) 133:689–91. doi: 10.1161/CIRCULATIONAHA.116.021230
2. Lopaschuk GD, Karwi QG, Ho KL, Pherwani S, Ketema EB. Ketone metabolism in the failing heart. Biochim Biophys Acta Mol Cell Biol Lipids. (2020) 1865:158813. doi: 10.1016/j.bbalip.2020.158813
3. Cox PJ, Kirk T, Ashmore T, Willerton K, Evans R, Smith A, et al. Nutritional ketosis alters fuel preference and thereby endurance performance in athletes. Cell Metab. (2016) 24:256–68. doi: 10.1016/j.cmet.2016.07.010
4. Cotter DG, Schugar RC, Crawford PA. Ketone body metabolism and cardiovascular disease. Am J Physiol Heart Circ Physiol. (2013) 304:H1060–76. doi: 10.1152/ajpheart.00646.2012
5. Veech RL. The therapeutic implications of ketone bodies: the effects of ketone bodies in pathological conditions: ketosis, ketogenic diet, redox states, insulin resistance, and mitochondrial metabolism. Prostaglandins Leukot Essent Fatty Acids. (2004) 70:309–19. doi: 10.1016/j.plefa.2003.09.007
6. Wheless JW. History of the ketogenic diet. Epilepsia. (2008) 49 Suppl 8:3–5. doi: 10.1111/j.1528-1167.2008.01821.x
7. Scholl-Burgi S, Holler A, Pichler K, Michel M, Haberlandt E, Karall D. Ketogenic diets in patients with inherited metabolic disorders. J Inherit Metab Dis. (2015) 38:765–73. doi: 10.1007/s10545-015-9872-2
8. Harvey KL, Holcomb LE, Kolwicz SC Jr. Ketogenic diets and exercise performance. Nutrients. (2019) 11:2296. doi: 10.3390/nu11102296
9. Valenzuela PL, Morales JS, Castillo-Garcia A, Lucia A. Acute ketone supplementation and exercise performance: a systematic review and meta-analysis of randomized controlled trials. Int J Sports Physiol Perform. (2020) 1–11. doi: 10.1123/ijspp.2019-0918
10. Yao A, Li Z, Lyu J, Yu L, Wei S, Xue L, et al. On the nutritional and therapeutic effects of ketone body D-beta-hydroxybutyrate. Appl Microbiol Biotechnol. (2021) 105:6229–43. doi: 10.1007/s00253-021-11482-w
11. Abdul Kadir A, Clarke K, Evans RD. Cardiac ketone body metabolism. Biochim Biophys Acta Mol Basis Dis. (2020) 1866:165739. doi: 10.1016/j.bbadis.2020.165739
12. Choi YS, de Mattos AB, Shao D, Li T, Nabben M, Kim M, et al. Preservation of myocardial fatty acid oxidation prevents diastolic dysfunction in mice subjected to angiotensin II infusion. J Mol Cell Cardiol. (2016) 100:64–71. doi: 10.1016/j.yjmcc.2016.09.001
13. Kolwicz SC Jr, Olson DP, Marney LC, Garcia-Menendez L, Synovec RE, et al. Cardiac-specific deletion of acetyl CoA carboxylase 2 prevents metabolic remodeling during pressure-overload hypertrophy. Circ Res. (2012) 111:728–38. doi: 10.1161/CIRCRESAHA.112.268128
14. Fillmore N, Levasseur JL, Fukushima A, Wagg CS, Wang W, Dyck JRB, et al. Uncoupling of glycolysis from glucose oxidation accompanies the development of heart failure with preserved ejection fraction. Mol Med. (2018) 24:3. doi: 10.1186/s10020-018-0005-x
15. Lahey R, Wang X, Carley AN, Lewandowski ED. Dietary fat supply to failing hearts determines dynamic lipid signaling for nuclear receptor activation and oxidation of stored triglyceride. Circulation. (2014) 130:1790–9. doi: 10.1161/CIRCULATIONAHA.114.011687
16. Kudo N, Barr AJ, Barr RL, Desai S, Lopaschuk GD. High rates of fatty acid oxidation during reperfusion of ischemic hearts are associated with a decrease in malonyl-CoA levels due to an increase in 5'-AMP-activated protein kinase inhibition of acetyl-CoA carboxylase. J Biol Chem. (1995) 270:17513–20. doi: 10.1074/jbc.270.29.17513
17. Liu B, el Alaoui-Talibi Z, Clanachan AS, Schulz R, Lopaschuk GD. Uncoupling of contractile function from mitochondrial TCA cycle activity and MVO2 during reperfusion of ischemic hearts. Am J Physiol. (1996) 270(1 Pt 2):H72–80. doi: 10.1152/ajpheart.1996.270.1.H72
18. Liu Q, Docherty JC, Rendell JC, Clanachan AS, Lopaschuk GD. High levels of fatty acids delay the recovery of intracellular pH and cardiac efficiency in post-ischemic hearts by inhibiting glucose oxidation. J Am Coll Cardiol. (2002) 39:718–25. doi: 10.1016/S0735-1097(01)01803-4
19. Ussher JR, Koves TR, Jaswal JS, Zhang L, Ilkayeva O, Dyck JR, et al. Insulin-stimulated cardiac glucose oxidation is increased in high-fat diet-induced obese mice lacking malonyl CoA decarboxylase. Diabetes. (2009) 58:1766–75. doi: 10.2337/db09-0011
20. Verma S, Rawat S, Ho KL, Wagg CS, Zhang L, Teoh H, et al. Empagliflozin increases cardiac energy production in diabetes: novel translational insights into the heart failure benefits of SGLT2 inhibitors. JACC Basic Transl Sci. (2018) 3:575–87. doi: 10.1016/j.jacbts.2018.07.006
21. Halestrap AP, Wilson MC. The monocarboxylate transporter family–role and regulation. IUBMB Life. (2012) 64:109–19. doi: 10.1002/iub.572
22. Peters JP, Van Slyke DD. Quantitative Clinical Chemistry. 2d ed. Baltimore: Williams & Wilkins (1946).
24. Karwi QG, Uddin GM, Ho KL, Lopaschuk GD. Loss of Metabolic Flexibility in the Failing Heart. Front Cardiovasc Med. (2018) 5:68. doi: 10.3389/fcvm.2018.00068
25. Opie LH. Heart Physiology: From Cell to Circulation. 4th ed. Philadelphia: Lippincott Williams & Wilkins; 2004. p. xix, 648.
26. Kashiwaya Y, King MT, Veech RL. Substrate signaling by insulin: a ketone bodies ratio mimics insulin action in heart. Am J Cardiol. (1997) 80:50A−64A. doi: 10.1016/S0002-9149(97)00458-X
27. Sato K, Kashiwaya Y, Keon CA, Tsuchiya N, King MT, Radda GK, et al. Insulin, ketone bodies, and mitochondrial energy transduction. FASEB J. (1995) 9:651–8. doi: 10.1096/fasebj.9.8.7768357
28. Ho KL, Zhang L, Wagg C, Al Batran R, Gopal K, Levasseur J, et al. Increased ketone body oxidation provides additional energy for the failing heart without improving cardiac efficiency. Cardiovasc Res. (2019) 115:1606–16. doi: 10.1093/cvr/cvz045
29. Holcomb LE, O'Neill CC, DeWitt EA, Kolwicz SC Jr. The effects of fasting or ketogenic diet on endurance exercise performance and metabolism in female mice. Metabolites. (2021) 11:397. doi: 10.3390/metabo11060397
30. Wentz AE, d'Avignon DA, Weber ML, Cotter DG, Doherty JM, Kerns R, et al. Adaptation of myocardial substrate metabolism to a ketogenic nutrient environment. J Biol Chem. (2010) 285:24447–56. doi: 10.1074/jbc.M110.100651
31. Avogaro A, Crepaldi C, Miola M, Maran A, Pengo V, Tiengo A, et al. High blood ketone body concentration in type 2 non-insulin dependent diabetic patients. J Endocrinol Invest. (1996) 19:99–105. doi: 10.1007/BF03349844
32. Guerci B, Benichou M, Floriot M, Bohme P, Fougnot S, Franck P, et al. Accuracy of an electrochemical sensor for measuring capillary blood ketones by fingerstick samples during metabolic deterioration after continuous subcutaneous insulin infusion interruption in type 1 diabetic patients. Diabetes Care. (2003) 26:1137–41. doi: 10.2337/diacare.26.4.1137
33. Gosmanov AR, Gosmanova EO, Dillard-Cannon E. Management of adult diabetic ketoacidosis. Diabetes Metab Syndr Obes. (2014) 7:255–64. doi: 10.2147/DMSO.S50516
34. Kanikarla-Marie P, Jain SK. Hyperketonemia and ketosis increase the risk of complications in type 1 diabetes. Free Radic Biol Med. (2016) 95:268–77. doi: 10.1016/j.freeradbiomed.2016.03.020
35. Lebovitz HE. Diabetic ketoacidosis. Lancet. (1995) 345:767–72. doi: 10.1016/S0140-6736(95)90645-2
36. Gershuni VM, Yan SL, Medici V. Nutritional ketosis for weight management and reversal of metabolic syndrome. Curr Nutr Rep. (2018) 7:97–106. doi: 10.1007/s13668-018-0235-0
37. Poff AM, Koutnik AP, Egan B. Nutritional ketosis with ketogenic diets or exogenous ketones: features, convergence, and divergence. Curr Sports Med Rep. (2020) 19:251–9. doi: 10.1249/JSR.0000000000000732
38. Roberts MD, Holland AM, Kephart WC, Mobley CB, Mumford PW, Lowery RP, et al. A putative low-carbohydrate ketogenic diet elicits mild nutritional ketosis but does not impair the acute or chronic hypertrophic responses to resistance exercise in rodents. J Appl Physiol. (1985) (2016) 120:1173–85. doi: 10.1152/japplphysiol.00837.2015
39. Evans M, Cogan KE, Egan B. Metabolism of ketone bodies during exercise and training: physiological basis for exogenous supplementation. J Physiol. (2017) 595:2857–71. doi: 10.1113/JP273185
40. Reichard GA Jr, Owen OE, Haff AC, Paul P, Bortz WM. Ketone-body production and oxidation in fasting obese humans. J Clin Invest. (1974) 53:508–15. doi: 10.1172/JCI107584
41. Volek JS, Freidenreich DJ, Saenz C, Kunces LJ, Creighton BC, Bartley JM, et al. Metabolic characteristics of keto-adapted ultra-endurance runners. Metabolism. (2016) 65:100–10. doi: 10.1016/j.metabol.2015.10.028
42. Kennedy AR, Pissios P, Otu H, Roberson R, Xue B, Asakura K, et al. A high-fat, ketogenic diet induces a unique metabolic state in mice. Am J Physiol Endocrinol Metab. (2007) 292:E1724–39. doi: 10.1152/ajpendo.00717.2006
43. Yan J, Young ME, Cui L, Lopaschuk GD, Liao R, Tian R. Increased glucose uptake and oxidation in mouse hearts prevent high fatty acid oxidation but cause cardiac dysfunction in diet-induced obesity. Circulation. (2009) 119:2818–28. doi: 10.1161/CIRCULATIONAHA.108.832915
45. Woodyatt R. Objects and methods of diet adjustment in diabetics. Arch Intern Med. (1921) 28:125–41. doi: 10.1001/archinte.1921.00100140002001
46. Peterman M. The ketogenic diet in epilepsy. JAMA. (1925) 84:1979–83. doi: 10.1001/jama.1925.02660520007003
47. de Souza RJ, Bray GA, Carey VJ, Hall KD, LeBoff MS, Loria CM, et al. Effects of 4 weight-loss diets differing in fat, protein, and carbohydrate on fat mass, lean mass, visceral adipose tissue, and hepatic fat: results from the POUNDS LOST trial. Am J Clin Nutr. (2012) 95:614–25. doi: 10.3945/ajcn.111.026328
48. Shai I, Schwarzfuchs D, Henkin Y, Shahar DR, Witkow S, Greenberg I, et al. Weight loss with a low-carbohydrate, Mediterranean, or low-fat diet. N Engl J Med. (2008) 359:229–41. doi: 10.1056/NEJMoa0708681
49. Sondike SB, Copperman N, Jacobson MS. Effects of a low-carbohydrate diet on weight loss and cardiovascular risk factor in overweight adolescents. J Pediatr. (2003) 142:253–8. doi: 10.1067/mpd.2003.4
50. Sacks FM, Bray GA, Carey VJ, Smith SR, Ryan DH, Anton SD, et al. Comparison of weight-loss diets with different compositions of fat, protein, and carbohydrates. N Engl J Med. (2009) 360:859–73. doi: 10.1056/NEJMoa0804748
51. Badman MK, Kennedy AR, Adams AC, Pissios P, Maratos-Flier E. A very low carbohydrate ketogenic diet improves glucose tolerance in ob/ob mice independently of weight loss. Am J Physiol Endocrinol Metab. (2009) 297:E1197–204. doi: 10.1152/ajpendo.00357.2009
52. Partsalaki I, Karvela A, Spiliotis BE. Metabolic impact of a ketogenic diet compared to a hypocaloric diet in obese children and adolescents. J Pediatr Endocrinol Metab. (2012) 25:697–704. doi: 10.1515/jpem-2012-0131
53. Samaha FF, Iqbal N, Seshadri P, Chicano KL, Daily DA, McGrory J, et al. A low-carbohydrate as compared with a low-fat diet in severe obesity. N Engl J Med. (2003) 348:2074–81. doi: 10.1056/NEJMoa022637
54. Ellenbroek JH, van Dijck L, Tons HA, Rabelink TJ, Carlotti F, Ballieux BE, et al. Long-term ketogenic diet causes glucose intolerance and reduced beta- and alpha-cell mass but no weight loss in mice. Am J Physiol Endocrinol Metab. (2014) 306:E552–8. doi: 10.1152/ajpendo.00453.2013
55. Garbow JR, Doherty JM, Schugar RC, Travers S, Weber ML, Wentz AE, et al. Hepatic steatosis, inflammation, and ER stress in mice maintained long term on a very low-carbohydrate ketogenic diet. Am J Physiol Gastrointest Liver Physiol. (2011) 300:G956–67. doi: 10.1152/ajpgi.00539.2010
56. Kinzig KP, Honors MA, Hargrave SL. Insulin sensitivity and glucose tolerance are altered by maintenance on a ketogenic diet. Endocrinology. (2010) 151:3105–14. doi: 10.1210/en.2010-0175
57. Dashti HM, Al-Zaid NS, Mathew TC, Al-Mousawi M, Talib H, Asfar SK, et al. Long term effects of ketogenic diet in obese subjects with high cholesterol level. Mol Cell Biochem. (2006) 286:1–9. doi: 10.1007/s11010-005-9001-x
58. Nilsson J, Ericsson M, Joibari MM, Anderson F, Carlsson L, Nilsson SK, et al. A low-carbohydrate high-fat diet decreases lean mass and impairs cardiac function in pair-fed female C57BL/6J mice. Nutr Metab. (2016) 13:79. doi: 10.1186/s12986-016-0132-8
59. Scott BE, Laursen PB, James LJ, Boxer B, Chandler Z, Lam E, et al. The effect of 1,3-butanediol and carbohydrate supplementation on running performance. J Sci Med Sport. (2019) 22:702–6. doi: 10.1016/j.jsams.2018.11.027
60. DC Harvey CJ, Schofield GM, Williden M, McQuillan JA. The Effect of Medium Chain Triglycerides on Time to Nutritional Ketosis and Symptoms of Keto-Induction in Healthy Adults: A Randomised Controlled Clinical Trial. J Nutr Metab. (2018) 2018:2630565. doi: 10.1155/2018/2630565
61. Dearlove DJ, Faull OK, Rolls E, Clarke K, Cox PJ. Nutritional Ketoacidosis During Incremental Exercise in Healthy Athletes. Front Physiol. (2019) 10:290. doi: 10.3389/fphys.2019.00290
62. Evans M, Egan B. Intermittent Running and Cognitive Performance after Ketone Ester Ingestion. Med Sci Sports Exerc. (2018) 50:2330–8. doi: 10.1249/MSS.0000000000001700
63. Leckey JJ, Ross ML, Quod M, Hawley JA, Burke LM. Ketone Diester Ingestion Impairs Time-Trial Performance in Professional Cyclists. Front Physiol. (2017) 8:806. doi: 10.3389/fphys.2017.00806
64. Kesl SL, Poff AM, Ward NP, Fiorelli TN, Ari C, Van Putten AJ, et al. Effects of exogenous ketone supplementation on blood ketone, glucose, triglyceride, and lipoprotein levels in Sprague-Dawley rats. Nutr Metab. (2016) 13:9. doi: 10.1186/s12986-016-0069-y
65. Stubbs BJ, Cox PJ, Evans RD, Santer P, Miller JJ, Faull OK, et al. On the Metabolism of Exogenous Ketones in Humans. Front Physiol. (2017) 8:848. doi: 10.3389/fphys.2017.00848
66. Tate RL, Mehlman MA, Tobin RB. Metabolic fate of 1,3-butanediol in the rat: conversion to -hydroxybutyrate. J Nutr. (1971) 101:1719–26. doi: 10.1093/jn/101.12.1719
67. Clarke K, Tchabanenko K, Pawlosky R, Carter E, Todd King M, Musa-Veloso K, et al. Kinetics, safety and tolerability of (R)-3-hydroxybutyl (R)-3-hydroxybutyrate in healthy adult subjects. Regul Toxicol Pharmacol. (2012) 63:401–8. doi: 10.1016/j.yrtph.2012.04.008
68. Kolwicz SC Jr, Purohit S, Tian R. Cardiac metabolism and its interactions with contraction, growth, and survival of cardiomyocytes. Circ Res. (2013) 113:603–16. doi: 10.1161/CIRCRESAHA.113.302095
69. Kolwicz SC Jr, Tian R. Glucose metabolism and cardiac hypertrophy. Cardiovasc Res. (2011) 90:194–201. doi: 10.1093/cvr/cvr071
70. Lopaschuk GD, Ussher JR. Evolving Concepts of Myocardial Energy Metabolism: More Than Just Fats and Carbohydrates. Circ Res. (2016) 119:1173–6. doi: 10.1161/CIRCRESAHA.116.310078
71. Goodwin GW, Taegtmeyer H. Improved energy homeostasis of the heart in the metabolic state of exercise. Am J Physiol Heart Circ Physiol. (2000) 279:H1490–501. doi: 10.1152/ajpheart.2000.279.4.H1490
72. Kaijser L, Berglund B. Myocardial lactate extraction and release at rest and during heavy exercise in healthy men. Acta Physiol Scand. (1992) 144:39–45. doi: 10.1111/j.1748-1716.1992.tb09265.x
73. Fillmore N, Wagg CS, Zhang L, Fukushima A, Lopaschuk GD. Cardiac branched-chain amino acid oxidation is reduced during insulin resistance in the heart. Am J Physiol Endocrinol Metab. (2018) 315:E1046–E52. doi: 10.1152/ajpendo.00097.2018
74. Ichihara K, Neely JR, Siehl DL, Morgan HE. Utilization of leucine by working rat heart. Am J Physiol. (1980) 239:E430–6. doi: 10.1152/ajpendo.1980.239.6.E430
75. Li T, Zhang Z, Kolwicz SC Jr, Abell L, Roe ND, et al. Defective Branched-Chain Amino Acid Catabolism Disrupts Glucose Metabolism and Sensitizes the Heart to Ischemia-Reperfusion Injury. Cell Metab. (2017) 25:374–85. doi: 10.1016/j.cmet.2016.11.005
76. Horton JL, Davidson MT, Kurishima C, Vega RB, Powers JC, Matsuura TR, et al. The failing heart utilizes 3-hydroxybutyrate as a metabolic stress defense. JCI Insight. (2019) 4:e124079. doi: 10.1172/jci.insight.124079
77. Schugar RC, Moll AR, Andre d'Avignon D, Weinheimer CJ, Kovacs A, Crawford PA. Cardiomyocyte-specific deficiency of ketone body metabolism promotes accelerated pathological remodeling. Mol Metab. (2014) 3:754–69. doi: 10.1016/j.molmet.2014.07.010
78. Uchihashi M, Hoshino A, Okawa Y, Ariyoshi M, Kaimoto S, Tateishi S, et al. Cardiac-specific Bdh1 overexpression ameliorates oxidative stress and cardiac remodeling in pressure overload-induced heart failure. Circ Heart Fail. (2017) 10:e004417. doi: 10.1161/CIRCHEARTFAILURE.117.004417
79. Stanley WC, Meadows SR, Kivilo KM, Roth BA, Lopaschuk GD. Beta-Hydroxybutyrate inhibits myocardial fatty acid oxidation in vivo independent of changes in malonyl-CoA content. Am J Physiol Heart Circ Physiol. (2003) 285:H1626–31. doi: 10.1152/ajpheart.00332.2003
80. Gormsen LC, Svart M, Thomsen HH, Sondergaard E, Vendelbo MH, Christensen N, et al. Ketone body infusion with 3-hydroxybutyrate reduces myocardial glucose uptake and increases blood flow in humans: a positron emission tomography study. J Am Heart Assoc. (2017) 6:e005066. doi: 10.1161/JAHA.116.005066
81. Brahma MK, Ha CM, Pepin ME, Mia S, Sun Z, Chatham JC, et al. Increased glucose availability attenuates myocardial ketone body utilization. J Am Heart Assoc. (2020) 9:e013039. doi: 10.1161/JAHA.119.013039
82. Chiang JL, Kirkman MS, Laffel LM, Peters AL, Type 1 Diabetes Sourcejournal. A Type 1 diabetes through the life span: a position statement of the American Diabetes Association. Diabetes Care. (2014) 37:2034–54. doi: 10.2337/dc14-1140
83. Sowton AP, Griffin JL, Murray AJ. Metabolic profiling of the diabetic heart: toward a richer picture. Front Physiol. (2019) 10:639. doi: 10.3389/fphys.2019.00639
84. Mizuno Y, Harada E, Nakagawa H, Morikawa Y, Shono M, Kugimiya F, et al. The diabetic heart utilizes ketone bodies as an energy source. Metabolism. (2017) 77:65–72. doi: 10.1016/j.metabol.2017.08.005
85. Thai PN, Miller CV, King MT, Schaefer S, Veech RL, Chiamvimonvat N, et al. Ketone ester D-beta-hydroxybutyrate-(r)-1,3 butanediol prevents decline in cardiac function in type 2 diabetic mice. J Am Heart Assoc. (2021) 10:e020729. doi: 10.1161/JAHA.120.020729
86. Arnett DK, Blumenthal RS, Albert MA, Buroker AB, Goldberger ZD, Hahn EJ, et al. 2019 ACC/AHA Guideline on the Primary Prevention of Cardiovascular Disease. Circulation. (2019) 140:e596–e646. doi: 10.1161/CIR.0000000000000678
87. Bedi KC Jr, Snyder NW, Brandimarto J, Aziz M, Mesaros C, et al. Evidence for intramyocardial disruption of lipid metabolism and increased myocardial ketone utilization in advanced human heart failure. Circulation. (2016) 133:706–16. doi: 10.1161/CIRCULATIONAHA.115.017545
88. Aubert G, Martin OJ, Horton JL, Lai L, Vega RB, Leone TC, et al. The failing heart relies on ketone bodies as a fuel. Circulation. (2016) 133:698–705. doi: 10.1161/CIRCULATIONAHA.115.017355
89. Abdurrachim D, Teo XQ, Woo CC, Chan WX, Lalic J, Lam CSP, et al. Empagliflozin reduces myocardial ketone utilization while preserving glucose utilization in diabetic hypertensive heart disease: A hyperpolarized (13) C magnetic resonance spectroscopy study. Diabetes Obes Metab. (2019) 21:357–65. doi: 10.1111/dom.13536
90. Carley AN, Maurya SK, Fasano M, Wang Y, Selzman CH, Drakos SG, et al. Short-chain fatty acids outpace ketone oxidation in the failing heart. Circulation. (2021) 143:1797–808. doi: 10.1161/CIRCULATIONAHA.120.052671
91. Lopaschuk GD, Wambolt RB, Barr RL. An imbalance between glycolysis and glucose oxidation is a possible explanation for the detrimental effects of high levels of fatty acids during aerobic reperfusion of ischemic hearts. J Pharmacol Exp Ther. (1993) 264:135–44.
92. Hafstad AD, Khalid AM, How OJ, Larsen TS, Aasum E. Glucose and insulin improve cardiac efficiency and postischemic functional recovery in perfused hearts from type 2 diabetic (db/db) mice. Am J Physiol Endocrinol Metab. (2007) 292:E1288–94. doi: 10.1152/ajpendo.00504.2006
93. Ussher JR, Wang W, Gandhi M, Keung W, Samokhvalov V, Oka T, et al. Stimulation of glucose oxidation protects against acute myocardial infarction and reperfusion injury. Cardiovasc Res. (2012) 94:359–69. doi: 10.1093/cvr/cvs129
94. Dyck JR, Cheng JF, Stanley WC, Barr R, Chandler MP, Brown S, et al. Malonyl coenzyme a decarboxylase inhibition protects the ischemic heart by inhibiting fatty acid oxidation and stimulating glucose oxidation. Circ Res. (2004) 94:e78–84. doi: 10.1161/01.RES.0000129255.19569.8f
95. Dyck JR, Hopkins TA, Bonnet S, Michelakis ED, Young ME, Watanabe M, et al. Absence of malonyl coenzyme A decarboxylase in mice increases cardiac glucose oxidation and protects the heart from ischemic injury. Circulation. (2006) 114:1721–8. doi: 10.1161/CIRCULATIONAHA.106.642009
96. Lopaschuk GD, Barr R, Thomas PD, Dyck JR. Beneficial effects of trimetazidine in ex vivo working ischemic hearts are due to a stimulation of glucose oxidation secondary to inhibition of long-chain 3-ketoacyl coenzyme a thiolase. Circ Res. (2003) 93:e33–7. doi: 10.1161/01.RES.0000086964.07404.A5
97. Yurista SR, Chong CR, Badimon JJ, Kelly DP, de Boer RA, Westenbrink BD. Therapeutic potential of ketone bodies for patients with cardiovascular disease: JACC state-of-the-art review. J Am Coll Cardiol. (2021) 77:1660–9. doi: 10.1016/j.jacc.2020.12.065
98. de Koning MLY, Westenbrink BD, Assa S, Garcia E, Connelly MA, van Veldhuisen DJ, et al. Association of circulating ketone bodies with functional outcomes after ST-Segment elevation myocardial infarction. J Am Coll Cardiol. (2021) 78:1421–32. doi: 10.1016/j.jacc.2021.07.054
99. Ma X, Dong Z, Liu J, Ma L, Sun X, Gao R, et al. Beta-Hydroxybutyrate exacerbates hypoxic injury by inhibiting HIF-1alpha-dependent glycolysis in cardiomyocytes-adding fuel to the fire? Cardiovasc Drugs Ther. (2021). doi: 10.1007/s10557-021-07267-y
100. Holmes MV, Millwood IY, Kartsonaki C, Hill MR, Bennett DA, Boxall R, et al. Lipids, lipoproteins, and metabolites and risk of myocardial infarction and stroke. J Am Coll Cardiol. (2018) 71:620–32. doi: 10.1016/j.jacc.2017.12.006
101. Du Z, Shen A, Huang Y, Su L, Lai W, Wang P, et al. 1H-NMR-based metabolic analysis of human serum reveals novel markers of myocardial energy expenditure in heart failure patients. PLoS ONE. (2014) 9:e88102. doi: 10.1371/journal.pone.0088102
102. Lommi J, Kupari M, Koskinen P, Naveri H, Leinonen H, Pulkki K, et al. Blood ketone bodies in congestive heart failure. J Am Coll Cardiol. (1996) 28:665–72. doi: 10.1016/0735-1097(96)00214-8
103. Arima Y, Izumiya Y, Ishida T, Takashio S, Ishii M, Sueta D, et al. Myocardial ischemia suppresses ketone body utilization. J Am Coll Cardiol. (2019) 73:246–7. doi: 10.1016/j.jacc.2018.10.040
104. Lindsay RT, Dieckmann S, Krzyzanska D, Manetta-Jones D, West JA, Castro C, et al. Beta-hydroxybutyrate accumulates in the rat heart during low-flow ischaemia with implications for functional recovery. Elife. (2021) 10:e71270. doi: 10.7554/eLife.71270
105. Liu J, Lloyd SG. High-fat, low-carbohydrate diet alters myocardial oxidative stress and impairs recovery of cardiac function after ischemia and reperfusion in obese rats. Nutr Res. (2013) 33:311–21. doi: 10.1016/j.nutres.2013.02.005
106. Liu J, Wang P, Douglas SL, Tate JM, Sham S, Lloyd SG. Impact of high-fat, low-carbohydrate diet on myocardial substrate oxidation, insulin sensitivity, and cardiac function after ischemia-reperfusion. Am J Physiol Heart Circ Physiol. (2016) 311:H1–H10. doi: 10.1152/ajpheart.00809.2015
107. Liu J, Wang P, Zou L, Qu J, Litovsky S, Umeda P, et al. High-fat, low-carbohydrate diet promotes arrhythmic death and increases myocardial ischemia-reperfusion injury in rats. Am J Physiol Heart Circ Physiol. (2014) 307:H598–608. doi: 10.1152/ajpheart.00058.2014
108. Wang P, Tate JM, Lloyd SG. Low carbohydrate diet decreases myocardial insulin signaling and increases susceptibility to myocardial ischemia. Life Sci. (2008) 83:836–44. doi: 10.1016/j.lfs.2008.09.024
109. Snorek M, Hodyc D, Sedivy V, Durisova J, Skoumalova A, Wilhelm J, et al. Short-term fasting reduces the extent of myocardial infarction and incidence of reperfusion arrhythmias in rats. Physiol Res. (2012) 61:567–74. doi: 10.33549/physiolres.932338
110. Al-Zaid NS, Dashti HM, Mathew TC, Juggi JS. Low carbohydrate ketogenic diet enhances cardiac tolerance to global ischaemia. Acta Cardiol. (2007) 62:381–9. doi: 10.2143/AC.62.4.2022282
111. Zou Z, Sasaguri S, Rajesh KG, Suzuki R. dl-3-Hydroxybutyrate administration prevents myocardial damage after coronary occlusion in rat hearts. Am J Physiol Heart Circ Physiol. (2002) 283:H1968–74. doi: 10.1152/ajpheart.00250.2002
112. Byrne NJ, Soni S, Takahara S, Ferdaoussi M, Al Batran R, Darwesh AM, et al. Chronically elevating circulating ketones can reduce cardiac inflammation and blunt the development of heart failure. Circ Heart Fail. (2020) 13:e006573. doi: 10.1161/CIRCHEARTFAILURE.119.006573
113. Yu Y, Yu Y, Zhang Y, Zhang Z, An W, Zhao X. Treatment with D-beta-hydroxybutyrate protects heart from ischemia/reperfusion injury in mice. Eur J Pharmacol. (2018) 829:121–8. doi: 10.1016/j.ejphar.2018.04.019
114. Yurista SR, Matsuura TR, Sillje HHW, Nijholt KT, McDaid KS, Shewale SV, et al. Ketone ester treatment improves cardiac function and reduces pathologic remodeling in preclinical models of heart failure. Circ Heart Fail. (2021) 14:e007684. doi: 10.1161/CIRCHEARTFAILURE.120.007684
115. Zinman B, Wanner C, Lachin JM, Fitchett D, Bluhmki E, Hantel S, et al. Empagliflozin, cardiovascular outcomes, and mortality in type 2 diabetes. N Engl J Med. (2015) 373:2117–28. doi: 10.1056/NEJMoa1504720
116. Lopaschuk GD, Verma S. Mechanisms of cardiovascular benefits of sodium glucose co-transporter 2 (SGLT2) inhibitors: a state-of-the-art review. JACC Basic Transl Sci. (2020) 5:632–44. doi: 10.1016/j.jacbts.2020.02.004
117. Santos-Gallego CG, Requena-Ibanez JA, San Antonio R, Ishikawa K, Watanabe S, Picatoste B, et al. Empagliflozin ameliorates adverse left ventricular remodeling in nondiabetic heart failure by enhancing myocardial energetics. J Am Coll Cardiol. (2019) 73:1931–44. doi: 10.1016/j.jacc.2019.01.056
118. Yurista SR, Sillje HHW, Oberdorf-Maass SU, Schouten EM, Pavez Giani MG, Hillebrands JL, et al. Sodium-glucose co-transporter 2 inhibition with empagliflozin improves cardiac function in non-diabetic rats with left ventricular dysfunction after myocardial infarction. Eur J Heart Fail. (2019) 21:862–73. doi: 10.1002/ejhf.1473
Keywords: ischemia, reperfusion, hypoxia, beta-hydroxybutyrate, ketosis
Citation: Kolwicz SC Jr (2021) Ketone Body Metabolism in the Ischemic Heart. Front. Cardiovasc. Med. 8:789458. doi: 10.3389/fcvm.2021.789458
Received: 04 October 2021; Accepted: 16 November 2021;
Published: 07 December 2021.
Edited by:
Haiyang Tang, University of Arizona, United StatesReviewed by:
Christopher Q. Rogers, University of South Florida, United StatesRami Al Batran, Université de Montréal, Canada
Copyright © 2021 Kolwicz. This is an open-access article distributed under the terms of the Creative Commons Attribution License (CC BY). The use, distribution or reproduction in other forums is permitted, provided the original author(s) and the copyright owner(s) are credited and that the original publication in this journal is cited, in accordance with accepted academic practice. No use, distribution or reproduction is permitted which does not comply with these terms.
*Correspondence: Stephen C. Kolwicz Jr, c2tvbHdpY3pAdXJzaW51cy5lZHU=