- 1Department of Cardiology, The Second Affiliated Hospital, Zhejiang University School of Medicine, Hangzhou, China
- 2Cardiovascular Key Lab of Zhejiang Province, Hangzhou, China
Although great advances have been made, the problem of irreversible myocardium loss due to the limited regeneration capacity of cardiomyocytes has not been fully solved. The morbidity and mortality of heart disease still remain high. There are many therapeutic strategies for treating heart disease, while low efficacy and high cost remain challenging. Abundant evidence has shown that both acute and chronic inflammations play a crucial role in heart regeneration and repair following injury. Macrophages, a primary component of inflammation, have attracted much attention in cardiac research in recent decades. The detailed mechanisms of the roles of macrophages in heart regeneration and repair are not completely understood, in part because of their complex subsets, various functions, and intercellular communications. The purpose of this review is to summarize the progress made in the understanding of macrophages, including recent reports on macrophage differentiation, polarization and function, and involvement in heart regeneration and repair. Also, we discuss progress in treatments, which may suggest directions for future research.
Introduction
Heart disease is one of the leading causes of death worldwide. Previous studies have predicted that there will be over 8 million adults suffering from heart failure within about 10 years (1). Heart disease is also a great financial burden to society. According to the recent data on heart disease and stroke, the costs of cardiovascular disease and stroke were $351.3 billion from 2014 to 2015 in the United States (2). While in China, the total cost of hospitalization for ischemic heart disease was ¥111.982 billion in 2018, with an average annual growth rate of over 20% (3). Diseases like arrhythmia, valvulopathies, and cardiomyopathies can lead to heart failure and ultimately the loss of mobility. Due to minimal regenerative capacity, mature cardiomyocytes have little ability to compensate for the loss of cells following ischemia, mechanical stress, hypothermia, and other injuries. Therefore, to maintain normal pumping function and integrity under such circumstances, cardiomyocytes tend to become thicker and longer with increased contractility. Furthermore, cardiac remodeling is also characterized by an abnormal increase in the numbers of cardiac fibroblasts and extracellular matrix (ECM) (4, 5). However, when myocytes are overloaded, the heart will ultimately exhibit contractile and diastolic dysfunction. At present, there are many ways to treat heart disease, including drugs, surgery, and heart transplantation, whereas each of these has limitations in terms of therapeutic effectiveness and economic cost. Therefore, the identification of new therapeutic targets seems to be crucial for slowing disease progression and improving the quality of life. The roles of inflammation and macrophages have received much attention. This review explores the current progress in understanding the relationship between macrophages and myocardial regeneration, and summarizes the potential therapeutic targets for stimulating the heart muscle repair following cardiac injury.
Heart Regeneration and Repair
Cardiomyocytes were used to be identified as non-renewable cells with low proliferative ability. It seems to be difficult for cardiomyocytes to repair the injured heart by regeneration. However, in 2002, Poss et al. first reported that 1- to 2-year-old adult zebrafish could completely regenerate their hearts over a 2-month period instead of producing scar tissue in response to apical resection (6, 7). In addition to fish, another experimental model was reported by Porrello et al. (8). The same surgery was performed on 1-day-old mice and complete heart regeneration was observed at 21 days after the operation (8). In other words, mammals could also have the capacity to replenish cardiomyocytes and regenerate myocardium. However, this regenerative ability was extremely attenuated 7 days after birth (7, 8). In humans, mitosis has been observed in cardiomyocytes of adult hearts under both normal and pathological conditions, thus indicating myocyte proliferation and the potential of myocardial renewal (9, 10). The proportion of cardiomyocytes undergoing mitosis was reported to be 0.3–1%, and it could be slightly higher in the hearts after myocardial infarction (11). Considering the lower rate of regeneration in the adult mammalian heart, fibrosis and hypertrophy still seem to be the dominant processes during the cardiac repair.
The Relationships Between Inflammation, Heart Regeneration, and Repair
Inflammation, defined as the defensive reactions to external or internal injury, is a process in which inflammatory cells infiltrate the injured tissue, remove damaged cellular debris, release various chemokines and cytokines, and stimulate cells like fibroblasts to participate in tissue repair. In the heart, inflammation plays a central role in post-injury repair (12). When an ischemic injury occurs, acute inflammation is initiated within a few hours (13–15). Leukocytes, mainly neutrophils, then accumulate in the damaged area, remove cell fragments, secrete cytokines to modify the microenvironment, and recruit other immune cells (16, 17). In previous studies, early and acute inflammation was thought to play a negative role in myocardial repair, which resulted in collagen deposition and scar formation, and even a decrease in contractility. However, in recent decades, it has gradually become apparent that treatments that suppress the immune system, such as dexamethasone (18) or microinjection of immunogenic particles (19), may damage the mouse heart following cardiac ischemic injury. If neonatal mice received such therapy, the regenerative capacity of the heart would undoubtedly be greatly reduced. Consistent with this concern, suppression of interleukin-6 (IL-6) and its downstream target, signal transducer and activator of transcription 3 (STA3), resulted in greatly reduced cardiomyocyte proliferation following apical resection of hearts in 1-day old mice (18). Therefore, acute inflammation can be considered a double-edged sword; it is necessary to initiate proliferation and regeneration, but an excessive immune response can amplify scar tissue formation and lead to irreversible damage and dysfunction. Chronic inflammation has been shown to play a vital role in cardiac remodeling and dysfunction following heart failure, but therapies that suppress the inflammatory response have had a little curative effect (20, 21).
Macrophages and Heart Regeneration and Repair
Origins, Development, and Differentiation of Macrophages
Macrophages are a part of the innate immune system and mononuclear phagocytic population, participating in phagocytosis, chemotaxis, secretion, and antigen presentation for immune defense and tissue healing (17, 22–24). With the development of tools like lineage tracing and fate mapping, researchers began to realize that contrary to the idea that macrophages are derived from blood monocytes, different macrophage lineages were resident in tissues, including precursors of monocytes in the yolk sac and fetal liver (25–28). It is known that the generation of macrophages in rodents can generally be described as occurring in three stages, including primitive hematopoiesis in the yolk sac, followed by differentiation into erythro-myeloid progenitors (EMPs) and hematopoietic stem cells (HSCs) (29). Macrophages from both the yolk sac and HSC can be found in the heart, and their proportions are stable in the healthy tissue (29, 30).
To highlight the different roles in the inflammatory response of these subsets of macrophages, Mills has in recent decades developed the concept of M1 and M2 macrophages in mice, where M1 acts to inhibit and M2 acts to heal (31, 32). Later, based on this framework, researchers began to combine the M1 type with macrophages that are classically activated by interferon γ (IFN-γ) and lipopolysaccharide (LPS) in vitro and combine the M2 type with those that are alternatively activated by cytokines like interleukin-4 (IL-4) and interleukin-13 (IL-13) in vitro (33–38). On the one hand, this classification is not entirely consistent with the ideas of Mills who defined macrophages based on their function, and the macrophage types defined in these two ways actually have different gene signatures as shown by comparing transcriptomes between the M1/M2 and classically/alternatively activated types (39, 40). On the other hand, it is an oversimplification to describe the spectrum of macrophage subsets using only two categories (34, 41–43), which neglect the diversity of macrophages in distinct tissues and conditions (33, 44, 45). Some suggest that M1 and M2 are more like two extremes of macrophage polarization and that the observation of the complete process is limited by experimental methods (46). Nevertheless, this classification provides a basic framework for further studies of the dynamic differentiation of the macrophages.
In contrast to blood cells, macrophages can also be categorized as resident and monocyte-derived types. This kind of classification emphasizes their different origins and functions. In general, almost all resident macrophages originate from yolk sac progenitor cells that have the ability to self-renew until adulthood, although their numbers and proliferative ability decrease with age (30). Tissue-resident macrophages play a crucial role in embryogenesis, participating in the formation of various structures (29). Monocyte-derived macrophages, which usually originate from HSCs, can be replaced by adult cells that are also monocyte-derived, and contribute greatly to inflammatory responses. In most situations, these two types of macrophages have different transcriptomes. However, the boundary between these two populations is not very firm. Some studies have shown that macrophages derived from monocytes can compensate for the loss of the other type of macrophages, presenting a very similar transcriptome to resident cells with little change in the original markers (47, 48). According to Zaman et al., macrophages in mouse heart can be divided into three types based on their markers and functions (49): (1) T-cell immunoglobulin and mucin domain-containing protein 4 (TIMD4) + CC-chemokine receptor 2 (CCR2) -macrophages that have the ability to self-renew, (2) major histocompatibility complex II (MHC II)hi CCR2- macrophages, 30% of which are replaced by monocytes, and (3) MHC II hi CCR2+ macrophages, which are the monocyte-derived cells mentioned above. Dick et al. used fate mapping and transcriptomes to distinguish four populations classified on the basis of markers like TIMD4, lymphatic vessel hyaluronan receptor-1 (LYVE1) (48, 50–53), MHC-II, and CCR2. These macrophage subtypes are replaced in different proportions by monocytes in the adult myocardium (48). Although the M1/M2 and other classifications discussed above seem different, where the former emphasizes function and the latter the origin, some similarities and overlap can be found between them. M1 and monocyte-derived macrophages tend to function as tissue destroyers, while M2 and resident macrophages promote the reparative process and maintain stability. Similarly, macrophages in humans can also be divided into two subsets according to CCR2, CCR2–, and CCR2+ macrophages, which have analogical functions of those in mice (54).
Distribution of Macrophages in the Heart Tissue
Immune cells are distributed throughout the human body. It has been reported that nearly all types of white blood cells are present among cardiomyocytes and in other soft tissues in adult mouse hearts (55). Among these, macrophages have been shown to be plentiful, widespread, and carry out a variety of functions (35, 54). To distinguish the different roles of macrophages, we consider them in terms of the following tissue function: electrical conduction, blood flow, and pumping.
In the cardiac electrical conduction system, the atrioventricular node connects atrial with ventricular electrical signals to maintain atrioventricular synchronization. Hulmans et al. found that at the distal atrioventricular node, macrophages are electrically connected to cardiomyocytes via the gap junction protein connexin 43. Ablation of connexin 43 in macrophages or elimination of macrophages disrupts the cardiac rhythm and leads to atrioventricular block (56).
Macrophages also play a crucial role in the formation and maturation of coronary arteries. Embryo-derived macrophages, located close to coronary arteries, facilitate maturation of the coronary artery system and increase blood perfusion and coronary plexus remodeling (57). Gula et al. found that during embryonic development in mice, the first cardiac tissue macrophages are located in the subepicardial area at the E10 stage, where the formation of blood and lymphatic vessels occurs (58). In 1-day-old neonatal mouse hearts, embryo-derived macrophages also play a vital role in the promotion of cardiac recovery and angiogenesis after myocardial infarction (59, 60).
With regard to structures associated with the pumping of blood, there are steady numbers of macrophages among cardiomyocytes and valves under normal conditions (55, 61). During myocardial infarction, the quantity of macrophages rapidly increases in remote zones (62, 63), which may be due to an altered microenvironment in the presence of high ventricular stress (63).
In addition to the above locations, macrophages exist in the serous fluid in the middle of the pericardium where they have essential functions that include defense against the accumulation of pathogens and leukocytes (64–66). After myocardial infarction, GATA binding protein 6 (GATA6) + macrophages in the pericardial cavity are recruited to the remote infarct area to function in a cardioprotective role (67).
Macrophages and Heart Homeostasis
Cardiac macrophages contribute to heart homeostasis by supporting microenvironmental balance and mechanical contraction. In addition, they function in defense against pathogens, clearance of aging and dead cell debris, regulation of the extracellular matrix (ECM), and response to tissue stress (55). In Swirski and Nahrendorf's studies, they proposed that macrophages might interact with the nervous system since they could influence signals passing through synaptic junctions (55). In the cardiac electrical conduction system, macrophages can also influence the membrane potential of cardiomyocytes and conduction through connexin 43 (also known as gap junction protein alpha 1, GJA1) (68).
Role of Macrophages in Heart Regeneration and Repair
Macrophages Are Crucial in Tissue Regeneration and Repair
Numerous factors can influence tissue regeneration, including oxygen stress, immune response, sympathetic and parasympathetic signaling, ECM, metabolism, and various micro RNAs (miRNAs) (69–72). In previous studies, it has been shown that regeneration of salamander limbs is blocked when macrophages are deleted, and amputated limbs do not regenerate if the severed end is covered by fibrotic tissue. When the loss of macrophages was reversed by restoring macrophages, salamanders regained the ability to regenerate body parts (73). In addition to limb regeneration, Godwin et al. also reported an indispensable role for macrophages in salamander heart regeneration (74), consistent with the results of Aurora's studies (59). These studies have shown that macrophages activate fibroblasts and regulate lysyl oxidase activity soon after heart ischemic or cryogenic injury (59, 74). In later studies using gene editing, such as Tribbles homolog 1, or drug delivery, such as clodronate liposomes, it was shown that macrophage deficiency is lethal to mammals and increases the risk of cardiac rupture (75–78).
Monocytes Coordinate With Macrophages During Repair
Monocytes derived from myeloid progenitors are also an important component of the immune system owing to their potential to differentiate into dendritic cells and macrophages. They can be recruited from bone marrow and extramedullary hematopoietic sites, migrating to specific destinations in response to chemokine binding to CCR2 (79). In general, monocytes in the mouse can be divided into two subsets, classical [expressing high lymphocyte antigen 6 complex (Ly6C), positive for CCR2 and low in chemokine C-X3-C motif receptor 1 (CX3CR1)] and non-classical (expressing low Ly6C, negative for CCR2 and high in CX3CR1) (36). The corresponding subsets in humans are a cluster of differentiation (CD) 14++CD16− and CD14+CD16+ monocytes (79, 80). After an ischemic injury, abundant circulating Ly6Chigh (CD14++CD16−) monocytes are rapidly recruited to the infarcted area, where they contribute to the initiation of neutrophil infiltration and differentiate into macrophages that remove cell debris and degrade the extracellular matrix (79, 81). In the later stage of the response, Ly6Clow (CD14+CD16+) monocytes, which can be derived from Ly6Chigh monocytes (81), tend to accumulate in the area and express high levels of growth factors like vascular endothelial growth factor (VEGF) (36), contributing to tissue healing (81). Nuclear receptor subfamily four group A member 1 (Nr4a1) is crucially important for the development of Ly6Clow monocytes and regulation of monocyte behavior and cytokine expression by Ly6Chigh monocytes (81, 82). In a clinical study on the association between different monocyte subsets and prognosis of patients with acute myocardial infarction (AMI), it was found that CD14++CD16− monocytes were negatively related to the myocardial repair and recovery of left ventricular function (83).
Macrophages Function in Heart Regeneration and Repair
In general, macrophages are crucial for repair after injury. During tissue repair, macrophages can aggravate an ischemic injury by disturbing the microenvironment and metabolism and causing cell death. On the other hand, cytokines like vascular endothelial growth factor-α (VEGF-α), insulin-like growth factor-1 (IGF-1), produced by macrophages promote the proliferation of various cells (84).
In terms of heart regeneration and repair, macrophage functions can be divided into three primary categories (Figure 1). First, macrophages can stimulate the proliferation of endothelial cells, smooth muscle cells, and fibroblasts by releasing growth factors (85, 86) and promoting angiogenesis for tissue restoration. In the study of Fantin et al., cardiac tissue macrophages were shown to participate in the formation of capillary networks in the myocardium, which might be mediated by the neuropilin1 signaling pathway. Following this, macrophages can work as chaperones in tip cell fusion targeted by vascular endothelial growth factor (VEGF) (85). Moreover, it has been reported that macrophages can differentiate into endothelial cells or endothelial precursors (87, 88).
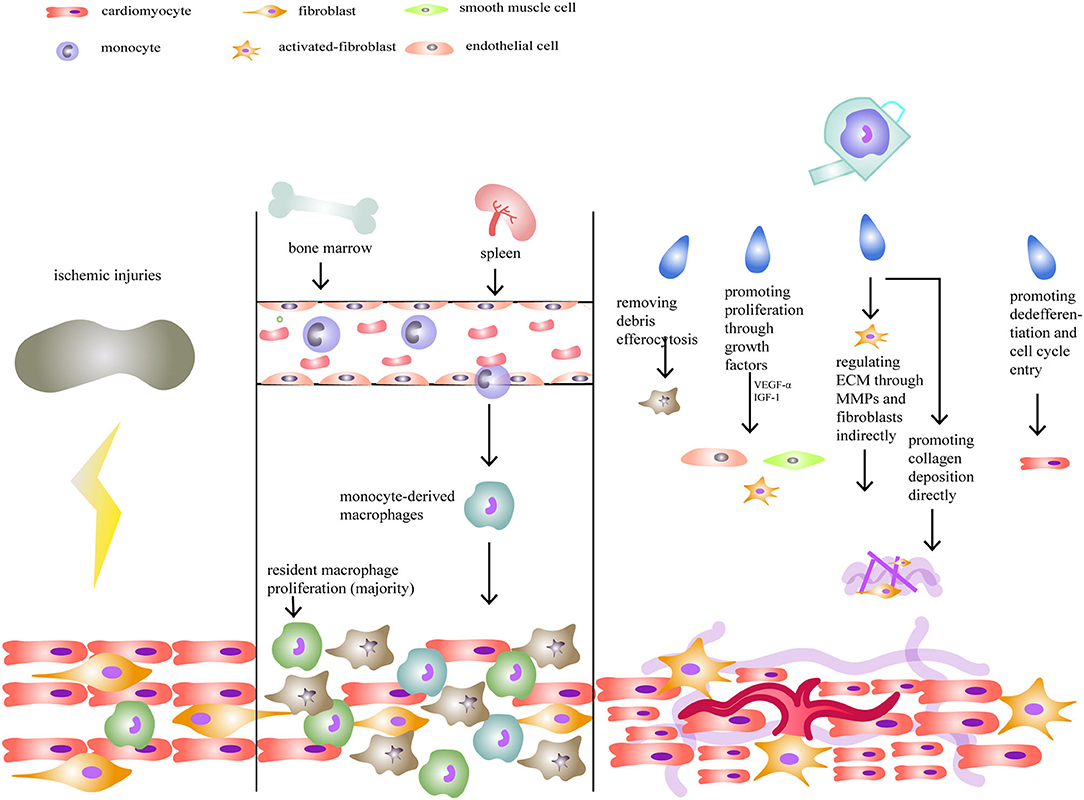
Figure 1. Macrophages and neonatal heart regeneration. After injuries, resident macrophages play a more important role in heart regeneration compared to monocyte-derived macrophages. Their functions include removing debris, promoting cellular proliferation, regulating extracellular matrix (ECM), and stimulating cardiomyocytes to enter the cell cycle.
Second, ECM composition is regulated by macrophages. In an axolotl cryogenic cardiac model, researchers found that fibrosis was disrupted when macrophages were depleted, showing that macrophages are crucial for fibrosis and maturation of the ECM (74). On the one hand, by secreting TGF-β and fibroblast growth factors, macrophages indirectly activate myofibroblasts to produce collagen and work together with them to release matrix metalloproteases (MMPs) that modify ECM composition (84, 89). On the other hand, many studies have discovered that macrophages can directly promote fibrosis. In 1999, Weitkamp et al. first found macrophages, isolated from human samples, could secrete Type VIII collagen in vitro (90). Later, other studies also identified that macrophages could synthesize proteoglycans and related proteins (91), Type VI collagen (92, 93), which were important components of ECM. In a research published in 2020, by analyzing the transcriptome from zebrafish hearts following injury (1 day, 5 days, and 14 days) and comparing P1 and P7 neonatal mouse hearts 7 days after myocardial infarction, collagen and ECM-associated genes were found in macrophages, indicating their autonomous and direct role in fibrosis (94). In mice, macrophages produced about 6.8% of total collagen (94). Since macrophages could exhibit a fibrosis-associated phenotype, some researchers proposed that macrophages could go through a fibroblast-like transition after ischemic injury (93, 95, 96).
Third, macrophages can stimulate cardiomyocytes to dedifferentiate and enter the cell cycle (25). In neonatal humans and mice, it was revealed that resident macrophages play an important role in augmenting cardiac proliferation under low oxygen conditions (97). In salamanders, however, when macrophages were depleted in a heart cryo-injury model, whole heart regeneration was blocked when the activation of fibroblasts and remodeling of the ECM was disturbed. In contrast, cardiomyocyte proliferation was unaffected (74). Given these disparate results, the ability of macrophages to directly or indirectly promote cardiomyocyte proliferation in mice and humans requires further study.
Different Macrophage Subsets Have Distinct Roles in Heart Repair
When the heart is injured, different types of macrophages usually respond in a variety of ways over different time scales (98). In the early response to ischemic damage signals in mouse, numerous Ly6Chigh monocytes are recruited from blood and lead to further M1-type macrophage activation, which provides the injured area with a “spring-cleaning” (36, 99, 100). In addition, M1-type macrophages can significantly upregulate MMP1, 3, 7, 10, 14, and 25 to modulate the ECM (41, 81, 101). Meanwhile, Ly6Chigh monocytes and other immune cells, mainly neutrophils, secrete a series of chemokines and cytokines like IL-1β, IL-6, tumor necrosis factor (TNF)-α, and chemokine (C-C motif) ligand (CCL)-3, and CCL-4 to amplify inflammation and activate cardiomyocytes and endothelial cells (102). At later times during tissue repair, changes in the microenvironment stimulate macrophages to polarize toward the M2 type and to increase the concentration of VEGF, and myeloid-derived growth factor (MYDGF) (75, 103), which increases cell proliferation and production of new vessels (23, 59, 78). In some organs, M2 macrophages seem to be more essential for repair than the M1 type (75), and in the heart, a reduced M1/M2 ratio is associated with increased numbers of vessels and more stable ECM. However, contrary to earlier results, a recent study reported simultaneous pro-inflammatory and anti-inflammatory effects in response to cardiac cryoinjury, but not a transition from M1 to M2 macrophages (104). Also, crosstalk between M1 and M2 macrophages should not be neglected as M1 polarization may be essential for the activation of M2 (46, 105). Since lipopolysaccharide (LPS) and IL-4 can elicit different types of macrophages in vitro, Munoz-Rojas et al. co-stimulated macrophages with LPS+IFN-γ and IL-4, and by single-cell sequencing, they found that this kind of macrophage contained a new transcriptome. Interestingly, some cytokines like IL-6, IL12b, arginase-1 (Arg1), and chitinase-3-like protein 1 (Chi3l1) began to be exclusively expressed in macrophages (106).
As we discussed earlier, macrophages can be classified as resident or monocyte-derived. These two kinds of macrophages have distinct functions as well (47, 48, 107). Normally, both resident and monocyte-derived macrophages exist in neonatal and adult hearts, but their subtypes and quantities differ. Neonatal hearts, which can renew themselves, will selectively amplify the resident population with little increase in the numbers of monocyte-derived cells (Figure 1). In contrast, adult hearts contain increased numbers of monocyte-derived macrophages, although the percentage of resident cells is initially similar to neonates (30, 60, 63). In a cardiomyocyte ablation experiment, it was found that resident macrophages could produce less inflammation and promote cell proliferation and the formation of new coronary vessels, while monocyte-derived macrophages only had inflammatory effects (60). Also, it was shown that macrophage amplification following injury in neonatal mice occurred rapidly, over no more than 5 days, while inflammation and repair tended to be slower in adult hearts (60, 97). However, since monocyte-derived macrophages can replace the loss of resident cells, the differences between responses to injuries in neonatal and adult hearts require further study to elucidate mechanisms.
Different Roles of Macrophages in Ischemic and Non-ischemic Heart Disease
In ischemic heart disease, different responses are observed in infarct, peri-infarct, and remote regions (49). In the infarct zone, the number of resident macrophages is greatly reduced and numerous monocytes are recruited for the clearance of cell debris. In the peri-infarct zone, the rapid proliferation of resident macrophages contributes to myocardial repair, the loss of which can lead to negative remodeling. Finally, in the remote zone, little proliferation or change in the numbers of resident macrophages is observed. After an injury, CCR2– and CCR2+ resident macrophages contribute to the recruitment of monocytes (47). CCR2+ macrophages promote monocyte and neutrophil recruitment through damage-associated molecular patterns (DAMPS)-toll-like receptor (TLR)-myeloid differentiation factor 88 (MyD88) signaling, but in contrast, CCR2– macrophages may reduce the aggregation of monocytes (47, 108).
Macrophages play various roles in non-ischemic heart diseases, such as pressure overload hypertrophy and chronic heart failure. In a hypertension model where macrophages were depleted by clodronate liposomes, loss of heart function and fibrosis were clearly attenuated (109). When mechanical strain is exerted on the myocardium, the mitogen-activated protein kinase (MAPK) pathway can be activated, promoting re-entry to the cell cycle and increasing the proliferation of macrophages (63). Pressure-overload hypertrophy is often accompanied by the activation of sympathetic nerves and angiotensin-family proteins. It has been shown that the CXCL1-CXCR2 axis participates in the regulation of monocyte recruitment during hypertrophy (110). In the early stages of hypertrophy, resident macrophages proliferate under the influence of the transcription factor krüppel-like factor 4 (KLF4), leading to an increase in the formation of new vessels (49, 111). Monocytes then take over the role of the resident macrophages to function in cardiac protection (49).
New Targets for Enlisting Macrophages for Cardiac Regeneration and Repair
Since macrophages play a crucial role in heart regeneration, various factors affect the process. Cells like neutrophils (112), Foxp3+CD4+ regulatory T cells (113), cytokines like IL-4 (75), IL-35 (114), IL-10 (33, 105, 115, 116), other proteins like Dectin-2 (98), matrix metalloproteinase-28 (117), and serum-glucocorticoid regulated kinase 1 (118) can all affect the activation of macrophages or the M1/M2 ratio, and thus likely influence heart regeneration. Nuclear receptor subfamily 4 group A member 1 (NR4A1) inhibits the expression of IL-6 and TNF to promote phenotype transitions of macrophages. In recent years, great progress has been made in revealing metabolic transformations in macrophages. It has been reported that changes in energy sources, such as glucose or lipids, can affect the utilization of substrates by macrophages, as can macrophage polarization (46, 119, 120).
Given the crucial role of macrophages in cell proliferation and heart regeneration, many studies seek new targets for stimulating macrophages to more efficiently exert positive effects. Some studies have reported that anti-TNF therapy had a certain effect on cardiac function in tumor patients with heart failure (121), thus demonstrating an effect of specific anti-immune therapy. Clinically, many drugs, such as trimetazidine or sodium-glucose cotransporter-2 (SGLT2) inhibitors and anti-inflammatory therapies, such as IL-1β inhibition are proven to have an effect on improving cardiac function (46, 122–124). However, whether they may target macrophages is uncertain. In recent years, many studies have tried to investigate the potential therapy methods, such as simple compounds, nanoparticles, exosomes from macrophages (engineered and unprocessed), and mesenchymal stem cells (MSCs), targeting macrophages during cardiac injury (both ischemic and non-ischemic injuries) in animal models (77, 125–130). Compared to simple compounds, nanoparticles and engineered exosomes provide for more precise targeting, thus decreasing the side effects and enhancing the effectiveness (25). In a recent study, Rangasami et al. used nanoparticles derived from hyaluronic acid (HA) to deliver anti-cancer drugs, strengthening the effectiveness while avoiding side effects (130). This offers a new therapeutic tool as the contents of the particles can be changed to make full use of the HA shell. However, considering the fact that many markers and characteristics of macrophages are known from experiments in vitro, the current classification of macrophages may not allow for precisely targeting specific subsets that exist in vivo. In addition, the tissue environment is not the same for mice and humans. Therefore, more basic research and clinical studies are needed to explore therapeutic strategies.
Discussion
Macrophages play an indispensable role in maintaining homeostasis and repair of injuries. It has been reported that macrophages constitute the majority of leukocytes that accumulate in the heart after myocardial infarction (63). Therefore, probing the functions of macrophages and their molecular mechanisms is necessary for the study of heart injuries. Historically, there has been disagreement among researchers about the origins, subtypes, and functions of macrophages. Now, through more advanced techniques, a much clearer understanding is emerging. Compared with M1 and monocyte-derived macrophages, M2 and resident macrophages seem to have more positive effects on heart regeneration and repair. Among their many functions, macrophages also support arterial elasticity and tension (52) and promote angiogenesis and myofibroblast activation. However, from our perspective, crosstalk between different types of macrophages should not be neglected in the context of heart repair. In the future, we look forward to more therapeutic strategies being developed to target macrophages directly or indirectly, strengthen heart regeneration in neonatal mammals, and improve cardiac repair in adult hearts following injury.
Author Contributions
YG: conceptualization and writing the original draft. NQ: data collection and paper correction. JX: data collection and paper correction. YW: writing and review and editing. All authors contributed to the article and approved the submitted version.
Funding
This study was supported by the National Natural Science Foundation of China (No. 81670235 to YW).
Conflict of Interest
The authors declare that the research was conducted in the absence of any commercial or financial relationships that could be construed as a potential conflict of interest.
Publisher's Note
All claims expressed in this article are solely those of the authors and do not necessarily represent those of their affiliated organizations, or those of the publisher, the editors and the reviewers. Any product that may be evaluated in this article, or claim that may be made by its manufacturer, is not guaranteed or endorsed by the publisher.
References
1. Truby LK, Rogers JG. Advanced heart failure: epidemiology, diagnosis, and therapeutic approaches. JACC Heart Fail. (2020) 8:523–36. doi: 10.1016/j.jchf.2020.01.014
2. Virani SS, Alonso A, Benjamin EJ, Bittencourt MS, Callaway CW, Carson AP, et al. Heart disease and stroke statistics-2020 update: a report from the american heart association. Circulation. (2020) 141:e139–596. doi: 10.1161/CIR.0000000000000757
3. China TWCotRoCHaDi. China cardiovascular health and disease report 2020. Chin J Circ. (2021) 36:521–45.
4. Genet M, Lee LC, Baillargeon B, Guccione JM, Kuhl E. Modeling pathologies of diastolic and systolic heart failure. Ann Biomed Eng. (2016) 44:112–27. doi: 10.1007/s10439-015-1351-2
5. Prabhu SD, Frangogiannis NG. The biological basis for cardiac repair after myocardial infarction: from inflammation to fibrosis. Circ Res. (2016) 119:91–112. doi: 10.1161/CIRCRESAHA.116.303577
6. Poss KD, Wilson LG, Keating MT. Heart regeneration in zebrafish. Science. (2002) 298:2188–90. doi: 10.1126/science.1077857
8. Porrello ER, Mahmoud AI, Simpson E, Hill JA, Richardson JA, Olson EN, et al. Transient regenerative potential of the neonatal mouse heart. Science. (2011) 331:1078–80. doi: 10.1126/science.1200708
9. Kajstura J, Leri A, Finato N, Di Loreto C, Beltrami CA, Anversa P. Myocyte proliferation in end-stage cardiac failure in humans. Proc Natl Acad Sci USA. (1998) 95:8801–5. doi: 10.1073/pnas.95.15.8801
10. Beltrami AP, Urbanek K, Kajstura J, Yan SM, Finato N, Bussani R, et al. Evidence that human cardiac myocytes divide after myocardial infarction. N Engl J Med. (2001) 344:1750–7. doi: 10.1056/NEJM200106073442303
11. Bergmann O, Bhardwaj RD, Bernard S, Zdunek S, Barnabe-Heider F, Walsh S, et al. Evidence for cardiomyocyte renewal in humans. Science. (2009) 324:98–102. doi: 10.1126/science.1164680
12. Lai SL, Marin-Juez R, Stainier DYR. Immune responses in cardiac repair and regeneration: a comparative point of view. Cell Mol Life Sci. (2019) 76:1365–80. doi: 10.1007/s00018-018-2995-5
13. Frangogiannis NG. The immune system and cardiac repair. Pharmacol Res. (2008) 58:88–111. doi: 10.1016/j.phrs.2008.06.007
14. Frangogiannis NG. Regulation of the inflammatory response in cardiac repair. Circ Res. (2012) 110:159–73. doi: 10.1161/CIRCRESAHA.111.243162
15. Marchant DJ, Boyd JH, Lin DC, Granville DJ, Garmaroudi FS, McManus BM. Inflammation in myocardial diseases. Circ Res. (2012) 110:126–44. doi: 10.1161/CIRCRESAHA.111.243170
16. Mescher AL, Neff AW. Regenerative capacity and the developing immune system. Adv Biochem Eng Biotechnol. (2005) 93:39–66. doi: 10.1007/b99966
17. Koh TJ, DiPietro LA. Inflammation and wound healing: the role of the macrophage. Expert Rev Mol Med. (2011) 13:e23. doi: 10.1017/S1462399411001943
18. Han C, Nie Y, Lian H, Liu R, He F, Huang H, et al. Acute inflammation stimulates a regenerative response in the neonatal mouse heart. Cell Res. (2015) 25:1137–51. doi: 10.1038/cr.2015.110
19. Hammerman H, Kloner RA, Hale S, Schoen FJ, Braunwald E. Dose-dependent effects of short-term methylprednisolone on myocardial infarct extent, scar formation, and ventricular function. Circulation. (1983) 68:446–52. doi: 10.1161/01.CIR.68.2.446
20. Ong SB, Hernandez-Resendiz S, Crespo-Avilan GE, Mukhametshina RT, Kwek XY, Cabrera-Fuentes HA, et al. Inflammation following acute myocardial infarction: multiple players, dynamic roles, and novel therapeutic opportunities. Pharmacol Ther. (2018) 186:73–87. doi: 10.1016/j.pharmthera.2018.01.001
21. Riehle C, Bauersachs J. Key inflammatory mechanisms underlying heart failure. Herz. (2019) 44:96–106. doi: 10.1007/s00059-019-4785-8
22. Motwani MP, Gilroy DW. Macrophage development and polarization in chronic inflammation. Semin Immunol. (2015) 27:257–66. doi: 10.1016/j.smim.2015.07.002
23. Leor J, Palevski D, Amit U, Konfino T. Macrophages and regeneration: lessons from the heart. Semin Cell Dev Biol. (2016) 58:26–33. doi: 10.1016/j.semcdb.2016.04.012
24. Park JE, Barbul A. Understanding the role of immune regulation in wound healing. Am J Surg. (2004) 187:11S−6. doi: 10.1016/S0002-9610(03)00296-4
25. de Couto G. Macrophages in cardiac repair: environmental cues and therapeutic strategies. Exp Mol Med. (2019) 51:1–10. doi: 10.1038/s12276-019-0269-4
26. Yona S, Kim KW, Wolf Y, Mildner A, Varol D, Breker M, et al. Fate mapping reveals origins and dynamics of monocytes and tissue macrophages under homeostasis. Immunity. (2013) 38:79–91. doi: 10.1016/j.immuni.2012.12.001
27. Ginhoux F, Greter M, Leboeuf M, Nandi S, See P, Gokhan S, et al. Fate mapping analysis reveals that adult microglia derive from primitive macrophages. Science. (2010) 330:841–5. doi: 10.1126/science.1194637
28. Chakarov S, Lim HY, Tan L, Lim SY, See P, Lum J, et al. Two distinct interstitial macrophage populations coexist across tissues in specific subtissular niches. Science. (2019) 363:eaau0964. doi: 10.1126/science.aau0964
29. Wu Y, Hirschi KK. Tissue-resident macrophage development and function. Front Cell Dev Biol. (2020) 8:617879. doi: 10.3389/fcell.2020.617879
30. Epelman S, Lavine KJ, Beaudin AE, Sojka DK, Carrero JA, Calderon B, et al. Embryonic and adult-derived resident cardiac macrophages are maintained through distinct mechanisms at steady state and during inflammation. Immunity. (2014) 40:91–104. doi: 10.1016/j.immuni.2013.11.019
31. Mills CD, Kincaid K, Alt JM, Heilman MJ, Hill AM. M-1/M-2 macrophages and the Th1/Th2 paradigm. J Immunol. (2000) 164:6166–73. doi: 10.4049/jimmunol.164.12.6166
32. Mills CD, Ley K. M1 and M2 macrophages: the chicken and the egg of immunity. J Innate Immun. (2014) 6:716–26. doi: 10.1159/000364945
33. Murray PJ, Allen JE, Biswas SK, Fisher EA, Gilroy DW, Goerdt S, et al. Macrophage activation and polarization: nomenclature and experimental guidelines. Immunity. (2014) 41:14–20. doi: 10.1016/j.immuni.2014.06.008
34. Gordon S, Martinez FO. Alternative activation of macrophages: mechanism and functions. Immunity. (2010) 32:593–604. doi: 10.1016/j.immuni.2010.05.007
35. Pinto AR, Paolicelli R, Salimova E, Gospocic J, Slonimsky E, Bilbao-Cortes D, et al. An abundant tissue macrophage population in the adult murine heart with a distinct alternatively-activated macrophage profile. PLoS ONE. (2012) 7:e36814. doi: 10.1371/journal.pone.0036814
36. Nahrendorf M, Swirski FK, Aikawa E, Stangenberg L, Wurdinger T, Figueiredo JL, et al. The healing myocardium sequentially mobilizes two monocyte subsets with divergent and complementary functions. J Exp Med. (2007) 204:3037–47. doi: 10.1084/jem.20070885
37. Nathan CF, Murray HW, Wiebe ME, Rubin BY. Identification of interferon-gamma as the lymphokine that activates human macrophage oxidative metabolism and antimicrobial activity. J Exp Med. (1983) 158:670–89. doi: 10.1084/jem.158.3.670
38. Stein M, Keshav S, Harris N, Gordon S. Interleukin 4 potently enhances murine macrophage mannose receptor activity: a marker of alternative immunologic macrophage activation. J Exp Med. (1992) 176:287–92. doi: 10.1084/jem.176.1.287
39. Ley K. M1 Means kill; M2 means heal. J Immunol. (2017) 199:2191–3. doi: 10.4049/jimmunol.1701135
40. Orecchioni M, Ghosheh Y, Pramod AB, Ley K. Macrophage polarization: different gene signatures in M1(LPS+) vs. classically and M2(LPS-) vs. alternatively activated macrophages. Front Immunol. (2019) 10:1084. doi: 10.3389/fimmu.2019.01084
41. Wang G, Shi Y, Jiang X, Leak RK, Hu X, Wu Y, et al. HDAC inhibition prevents white matter injury by modulating microglia/macrophage polarization through the GSK3beta/PTEN/Akt axis. Proc Natl Acad Sci USA. (2015) 112:2853–8. doi: 10.1073/pnas.1501441112
42. Lee S, Huen S, Nishio H, Nishio S, Lee HK, Choi BS, et al. Distinct macrophage phenotypes contribute to kidney injury and repair. J Am Soc Nephrol. (2011) 22:317–26. doi: 10.1681/ASN.2009060615
43. Yue Y, Huang S, Wang L, Wu Z, Liang M, Li H, et al. M2b macrophages regulate cardiac fibroblast activation and alleviate cardiac fibrosis after reperfusion injury. Circ J. (2020) 84:626–35. doi: 10.1253/circj.CJ-19-0959
44. Zhang C, Cheng N, Qiao B, Zhang F, Wu J, Liu C, et al. Age-related decline of interferon-gamma responses in macrophage impairs satellite cell proliferation and regeneration. J Cachexia Sarcopenia Muscle. (2020) 11:1291–305. doi: 10.1002/jcsm.12584
45. Gordon S, Pluddemann A, Martinez Estrada F. Macrophage heterogeneity in tissues: phenotypic diversity and functions. Immunol Rev. (2014) 262:36–55. doi: 10.1111/imr.12223
46. Mouton AJ, Li X, Hall ME, Hall JE. Obesity, hypertension, and cardiac dysfunction: novel roles of immunometabolism in macrophage activation and inflammation. Circ Res. (2020) 126:789–806. doi: 10.1161/CIRCRESAHA.119.312321
47. Bajpai G, Bredemeyer A, Li W, Zaitsev K, Koenig AL, Lokshina I, et al. Tissue resident CCR2- and CCR2+ cardiac macrophages differentially orchestrate monocyte recruitment and fate specification following myocardial injury. Circ Res. (2019) 124:263–78. doi: 10.1161/CIRCRESAHA.118.314028
48. Dick SA, Macklin JA, Nejat S, Momen A, Clemente-Casares X, Althagafi MG, et al. Self-renewing resident cardiac macrophages limit adverse remodeling following myocardial infarction. Nat Immunol. (2019) 20:29–39. doi: 10.1038/s41590-018-0272-2
49. Zaman R, Hamidzada H, Epelman S. Exploring cardiac macrophage heterogeneity in the healthy and diseased myocardium. Curr Opin Immunol. (2021) 68:54–63. doi: 10.1016/j.coi.2020.09.005
50. Mrdjen D, Pavlovic A, Hartmann FJ, Schreiner B, Utz SG, Leung BP, et al. High-dimensional single-cell mapping of central nervous system immune cells reveals distinct myeloid subsets in health, aging, and disease. Immunity. (2018) 48:599. doi: 10.1016/j.immuni.2018.02.014
51. Mass E, Ballesteros I, Farlik M, Halbritter F, Gunther P, Crozet L, et al. Specification of tissue-resident macrophages during organogenesis. Science. (2016) 353:aaf4238. doi: 10.1126/science.aaf4238
52. Lim HY, Lim SY, Tan CK, Thiam CH, Goh CC, Carbajo D, et al. Hyaluronan receptor LYVE-1-expressing macrophages maintain arterial tone through hyaluronan-mediated regulation of smooth muscle cell collagen. Immunity. (2018) 49:1191. doi: 10.1016/j.immuni.2018.12.009
53. Han X, Wang R, Zhou Y, Fei L, Sun H, Lai S, et al. Mapping the mouse cell atlas by microwell-Seq. Cell. (2018) 173:1307. doi: 10.1016/j.cell.2018.05.012
54. Bajpai G, Schneider C, Wong N, Bredemeyer A, Hulsmans M, Nahrendorf M, et al. The human heart contains distinct macrophage subsets with divergent origins and functions. Nat Med. (2018) 24:1234–45. doi: 10.1038/s41591-018-0059-x
55. Swirski FK, Nahrendorf M. Cardioimmunology: the immune system in cardiac homeostasis and disease. Nat Rev Immunol. (2018) 18:733–44. doi: 10.1038/s41577-018-0065-8
56. Hulsmans M, Clauss S, Xiao L, Aguirre AD, King KR, Hanley A, et al. Macrophages facilitate electrical conduction in the heart. Cell. (2017) 169:510–22 e20. doi: 10.1016/j.cell.2017.03.050
57. Leid J, Carrelha J, Boukarabila H, Epelman S, Jacobsen SE, Lavine KJ. Primitive embryonic macrophages are required for coronary development and maturation. Circ Res. (2016) 118:1498–511. doi: 10.1161/CIRCRESAHA.115.308270
58. Gula G, Ruminski S, Niderla-Bielinska J, Jasinska A, Kiernozek E, Jankowska-Steifer E, et al. Potential functions of embryonic cardiac macrophages in angiogenesis, lymphangiogenesis and extracellular matrix remodeling. Histochem Cell Biol. (2020) 155:117–32. doi: 10.1007/s00418-020-01934-1
59. Aurora AB, Porrello ER, Tan W, Mahmoud AI, Hill JA, Bassel-Duby R, et al. Macrophages are required for neonatal heart regeneration. J Clin Invest. (2014) 124:1382–92. doi: 10.1172/JCI72181
60. Lavine KJ, Epelman S, Uchida K, Weber KJ, Nichols CG, Schilling JD, et al. Distinct macrophage lineages contribute to disparate patterns of cardiac recovery and remodeling in the neonatal and adult heart. Proc Natl Acad Sci USA. (2014) 111:16029–34. doi: 10.1073/pnas.1406508111
61. Leuschner F, Rauch PJ, Ueno T, Gorbatov R, Marinelli B, Lee WW, et al. Rapid monocyte kinetics in acute myocardial infarction are sustained by extramedullary monocytopoiesis. J Exp Med. (2012) 209:123–37. doi: 10.1084/jem.20111009
62. Ismahil MA, Hamid T, Bansal SS, Patel B, Kingery JR, Prabhu SD. Remodeling of the mononuclear phagocyte network underlies chronic inflammation and disease progression in heart failure: critical importance of the cardiosplenic axis. Circ Res. (2014) 114:266–82. doi: 10.1161/CIRCRESAHA.113.301720
63. Sager HB, Hulsmans M, Lavine KJ, Moreira MB, Heidt T, Courties G, et al. Proliferation and recruitment contribute to myocardial macrophage expansion in chronic heart failure. Circ Res. (2016) 119:853–64. doi: 10.1161/CIRCRESAHA.116.309001
64. Horckmans M, Bianchini M, Santovito D, Megens RTA, Springael JY, Negri I, et al. Pericardial adipose tissue regulates granulopoiesis, fibrosis, and cardiac function after myocardial infarction. Circulation. (2018) 137:948–60. doi: 10.1161/CIRCULATIONAHA.117.028833
65. Butts B, Goeddel LA, George DJ, Steele C, Davies JE, Wei CC, et al. Increased inflammation in pericardial fluid persists 48 hours after cardiac surgery. Circulation. (2017) 136:2284–6. doi: 10.1161/CIRCULATIONAHA.117.029589
66. Kohlgruber AC, LaMarche NM, Lynch L. Adipose tissue at the nexus of systemic and cellular immunometabolism. Semin Immunol. (2016) 28:431–40. doi: 10.1016/j.smim.2016.09.005
67. Deniset JF, Belke D, Lee WY, Jorch SK, Deppermann C, Hassanabad AF, et al. Gata6(+) pericardial cavity macrophages relocate to the injured heart and prevent cardiac fibrosis. Immunity. (2019) 51:131–40.e5. doi: 10.1016/j.immuni.2019.06.010
68. Zhang YL, Cao HJ, Han X, Teng F, Chen C, Yang J, et al. Chemokine receptor CXCR-2 initiates atrial fibrillation by triggering monocyte mobilization in mice. Hypertension. (2020) 76:381–92. doi: 10.1161/HYPERTENSIONAHA.120.14698
69. Deshmukh V, Wang J, Martin JF. Leading progress in heart regeneration and repair. Curr Opin Cell Biol. (2019) 61:79–85. doi: 10.1016/j.ceb.2019.07.005
70. Uygur A, Lee RT. Mechanisms of cardiac regeneration. Dev Cell. (2016) 36:362–74. doi: 10.1016/j.devcel.2016.01.018
71. Cardoso AC, Pereira AHM, Sadek HA. Mechanisms of neonatal heart regeneration. Curr Cardiol Rep. (2020) 22:33. doi: 10.1007/s11886-020-01282-5
72. Mahmoud AI, O'Meara CC, Gemberling M, Zhao L, Bryant DM, Zheng R, et al. Nerves regulate cardiomyocyte proliferation and heart regeneration. Dev Cell. (2015) 34:387–99. doi: 10.1016/j.devcel.2015.06.017
73. Godwin JW, Pinto AR, Rosenthal NA. Macrophages are required for adult salamander limb regeneration. Proc Natl Acad Sci USA. (2013) 110:9415–20. doi: 10.1073/pnas.1300290110
74. Godwin JW, Debuque R, Salimova E, Rosenthal NA. Heart regeneration in the salamander relies on macrophage-mediated control of fibroblast activation and the extracellular landscape. NPJ Regen Med. (2017) 2:22. doi: 10.1038/s41536-017-0027-y
75. Shiraishi M, Shintani Y, Shintani Y, Ishida H, Saba R, Yamaguchi A, et al. Alternatively activated macrophages determine repair of the infarcted adult murine heart. J Clin Invest. (2016) 126:2151–66. doi: 10.1172/JCI85782
76. Heidt T, Courties G, Dutta P, Sager HB, Sebas M, Iwamoto Y, et al. Differential contribution of monocytes to heart macrophages in steady-state and after myocardial infarction. Circ Res. (2014) 115:284–95. doi: 10.1161/CIRCRESAHA.115.303567
77. Ben-Mordechai T, Holbova R, Landa-Rouben N, Harel-Adar T, Feinberg MS, Abd Elrahman I, et al. Macrophage subpopulations are essential for infarct repair with and without stem cell therapy. J Am Coll Cardiol. (2013) 62:1890–901. doi: 10.1016/j.jacc.2013.07.057
78. van Amerongen MJ, Harmsen MC, van Rooijen N, Petersen AH, van Luyn MJ. Macrophage depletion impairs wound healing and increases left ventricular remodeling after myocardial injury in mice. Am J Pathol. (2007) 170:818–29. doi: 10.2353/ajpath.2007.060547
79. Peet C, Ivetic A, Bromage DI, Shah AM. Cardiac monocytes and macrophages after myocardial infarction. Cardiovasc Res. (2020) 116:1101–12. doi: 10.1093/cvr/cvz336
80. Ingersoll MA, Spanbroek R, Lottaz C, Gautier EL, Frankenberger M, Hoffmann R, et al. Comparison of gene expression profiles between human and mouse monocyte subsets. Blood. (2010) 115:e10–9. doi: 10.1182/blood-2009-07-235028
81. Hilgendorf I, Gerhardt LM, Tan TC, Winter C, Holderried TA, Chousterman BG, et al. Ly-6Chigh monocytes depend on Nr4a1 to balance both inflammatory and reparative phases in the infarcted myocardium. Circ Res. (2014) 114:1611–22. doi: 10.1161/CIRCRESAHA.114.303204
82. Hanna RN, Carlin LM, Hubbeling HG, Nackiewicz D, Green AM, Punt JA, et al. The transcription factor NR4A1 (Nur77) controls bone marrow differentiation and the survival of Ly6C- monocytes. Nat Immunol. (2011) 12:778–85. doi: 10.1038/ni.2063
83. Tsujioka H, Imanishi T, Ikejima H, Kuroi A, Takarada S, Tanimoto T, et al. Impact of heterogeneity of human peripheral blood monocyte subsets on myocardial salvage in patients with primary acute myocardial infarction. J Am Coll Cardiol. (2009) 54:130–8. doi: 10.1016/j.jacc.2009.04.021
84. Vannella KM, Wynn TA. Mechanisms of organ injury and repair by macrophages. Annu Rev Physiol. (2017) 79:593–617. doi: 10.1146/annurev-physiol-022516-034356
85. Fantin A, Vieira JM, Gestri G, Denti L, Schwarz Q, Prykhozhij S, et al. Tissue macrophages act as cellular chaperones for vascular anastomosis downstream of VEGF-mediated endothelial tip cell induction. Blood. (2010) 116:829–40. doi: 10.1182/blood-2009-12-257832
86. Frantz S, Nahrendorf M. Cardiac macrophages and their role in ischaemic heart disease. Cardiovasc Res. (2014) 102:240–8. doi: 10.1093/cvr/cvu025
87. Fernandez Pujol B, Lucibello FC, Gehling UM, Lindemann K, Weidner N, Zuzarte ML, et al. Endothelial-like cells derived from human CD14 positive monocytes. Differentiation. (2000) 65:287–300. doi: 10.1046/j.1432-0436.2000.6550287.x
88. Rehman J, Li J, Orschell CM, March KL. Peripheral blood “endothelial progenitor cells” are derived from monocyte/macrophages and secrete angiogenic growth factors. Circulation. (2003) 107:1164–9. doi: 10.1161/01.CIR.0000058702.69484.A0
89. Weinberger T, Schulz C. Myocardial infarction: a critical role of macrophages in cardiac remodeling. Front Physiol. (2015) 6:107. doi: 10.3389/fphys.2015.00107
90. Weitkamp B, Cullen P, Plenz G, Robenek H, Rauterberg J. Human macrophages synthesize type VIII collagen in vitro and in the atherosclerotic plaque. FASEB J. (1999) 13:1445–57. doi: 10.1096/fasebj.13.11.1445
91. Chang MY, Chan CK, Braun KR, Green PS, O'Brien KD, Chait A, et al. Monocyte-to-macrophage differentiation: synthesis and secretion of a complex extracellular matrix. J Biol Chem. (2012) 287:14122–35. doi: 10.1074/jbc.M111.324988
92. Schnoor M, Cullen P, Lorkowski J, Stolle K, Robenek H, Troyer D, et al. Production of type VI collagen by human macrophages: a new dimension in macrophage functional heterogeneity. J Immunol. (2008) 180:5707–19. doi: 10.4049/jimmunol.180.8.5707
93. Mouton AJ, DeLeon-Pennell KY, Rivera Gonzalez OJ, Flynn ER, Freeman TC, Saucerman JJ, et al. Mapping macrophage polarization over the myocardial infarction time continuum. Basic Res Cardiol. (2018) 113:26. doi: 10.1007/s00395-018-0686-x
94. Simoes FC, Cahill TJ, Kenyon A, Gavriouchkina D, Vieira JM, Sun X, et al. Macrophages directly contribute collagen to scar formation during zebrafish heart regeneration and mouse heart repair. Nat Commun. (2020) 11:600. doi: 10.1038/s41467-019-14263-2
95. Haider N, Bosca L, Zandbergen HR, Kovacic JC, Narula N, Gonzalez-Ramos S, et al. Transition of macrophages to fibroblast-like cells in healing myocardial infarction. J Am Coll Cardiol. (2019) 74:3124–35. doi: 10.1016/j.jacc.2019.10.036
96. Wen Y, Yan HR, Wang B, Liu BC. Macrophage heterogeneity in kidney injury and fibrosis. Front Immunol. (2021) 12:681748. doi: 10.3389/fimmu.2021.681748
97. Liu B, Zhang HG, Zhu Y, Jiang YH, Luo GP, Tang FQ, et al. Cardiac resident macrophages are involved in hypoxiainduced postnatal cardiomyocyte proliferation. Mol Med Rep. (2017) 15:3541–8. doi: 10.3892/mmr.2017.6432
98. Yan X, Zhang H, Fan Q, Hu J, Tao R, Chen Q, et al. Dectin-2 deficiency modulates Th1 differentiation and improves wound healing after myocardial infarction. Circ Res. (2017) 120:1116–29. doi: 10.1161/CIRCRESAHA.116.310260
99. Nahrendorf M, Pittet MJ, Swirski FK. Monocytes: protagonists of infarct inflammation and repair after myocardial infarction. Circulation. (2010) 121:2437–45. doi: 10.1161/CIRCULATIONAHA.109.916346
100. Swirski FK, Nahrendorf M, Etzrodt M, Wildgruber M, Cortez-Retamozo V, Panizzi P, et al. Identification of splenic reservoir monocytes and their deployment to inflammatory sites. Science. (2009) 325:612–6. doi: 10.1126/science.1175202
101. Mack M. Inflammation and fibrosis. Matrix Biol. (2018) 68–69:106–21. doi: 10.1016/j.matbio.2017.11.010
102. Kim Y, Nurakhayev S, Nurkesh A, Zharkinbekov Z, Saparov A. Macrophage polarization in cardiac tissue repair following myocardial infarction. Int J Mol Sci. (2021) 22:2715. doi: 10.3390/ijms22052715
103. Harel-Adar T, Ben Mordechai T, Amsalem Y, Feinberg MS, Leor J, Cohen S. Modulation of cardiac macrophages by phosphatidylserine-presenting liposomes improves infarct repair. Proc Natl Acad Sci USA. (2011) 108:1827–32. doi: 10.1073/pnas.1015623108
104. Walter W, Alonso-Herranz L, Trappetti V, Crespo I, Ibberson M, Cedenilla M, et al. Deciphering the dynamic transcriptional and post-transcriptional networks of macrophages in the healthy heart and after myocardial injury. Cell Rep. (2018) 23:622–36. doi: 10.1016/j.celrep.2018.03.029
105. Hulsmans M, Sager HB, Roh JD, Valero-Munoz M, Houstis NE, Iwamoto Y, et al. Cardiac macrophages promote diastolic dysfunction. J Exp Med. (2018) 215:423–40. doi: 10.1084/jem.20171274
106. Munoz-Rojas AR, Kelsey I, Pappalardo JL, Chen M, Miller-Jensen K. Co-stimulation with opposing macrophage polarization cues leads to orthogonal secretion programs in individual cells. Nat Commun. (2021) 12:301. doi: 10.1038/s41467-020-20540-2
107. Honold L, Nahrendorf M. Resident and monocyte-derived macrophages in cardiovascular disease. Circ Res. (2018) 122:113–27. doi: 10.1161/CIRCRESAHA.117.311071
108. Li W, Hsiao HM, Higashikubo R, Saunders BT, Bharat A, Goldstein DR, et al. Heart-resident CCR2(+) macrophages promote neutrophil extravasation through TLR9/MyD88/CXCL5 signaling. JCI Insight. (2016) 1:e87315. doi: 10.1172/jci.insight.87315
109. Kain D, Amit U, Yagil C, Landa N, Naftali-Shani N, Molotski N, et al. Macrophages dictate the progression and manifestation of hypertensive heart disease. Int J Cardiol. (2016) 203:381–95. doi: 10.1016/j.ijcard.2015.10.126
110. Wang L, Zhang YL, Lin QY, Liu Y, Guan XM, Ma XL, et al. CXCL1-CXCR2 axis mediates angiotensin II-induced cardiac hypertrophy and remodelling through regulation of monocyte infiltration. Eur Heart J. (2018) 39:1818–31. doi: 10.1093/eurheartj/ehy085
111. Liao X, Shen Y, Zhang R, Sugi K, Vasudevan NT, Alaiti MA, et al. Distinct roles of resident and nonresident macrophages in nonischemic cardiomyopathy. Proc Natl Acad Sci USA. (2018) 115:E4661–9. doi: 10.1073/pnas.1720065115
112. Horckmans M, Ring L, Duchene J, Santovito D, Schloss MJ, Drechsler M, et al. Neutrophils orchestrate post-myocardial infarction healing by polarizing macrophages towards a reparative phenotype. Eur Heart J. (2017) 38:187–97. doi: 10.1093/eurheartj/ehw002
113. Weirather J, Hofmann UD, Beyersdorf N, Ramos GC, Vogel B, Frey A, et al. Foxp3+ CD4+ T cells improve healing after myocardial infarction by modulating monocyte/macrophage differentiation. Circ Res. (2014) 115:55–67. doi: 10.1161/CIRCRESAHA.115.303895
114. Jia D, Jiang H, Weng X, Wu J, Bai P, Yang W, et al. Interleukin-35 promotes macrophage survival and improves wound healing after myocardial infarction in mice. Circ Res. (2019) 124:1323–36. doi: 10.1161/CIRCRESAHA.118.314569
115. Krishnamurthy P, Rajasingh J, Lambers E, Qin G, Losordo DW, Kishore R. IL-10 inhibits inflammation and attenuates left ventricular remodeling after myocardial infarction via activation of STAT3 and suppression of HuR. Circ Res. (2009) 104:e9–18. doi: 10.1161/CIRCRESAHA.108.188243
116. Jung M, Ma Y, Iyer RP, DeLeon-Pennell KY, Yabluchanskiy A, Garrett MR, et al. IL-10 improves cardiac remodeling after myocardial infarction by stimulating M2 macrophage polarization and fibroblast activation. Basic Res Cardiol. (2017) 112:33. doi: 10.1007/s00395-017-0622-5
117. Ma Y, Halade GV, Zhang J, Ramirez TA, Levin D, Voorhees A, et al. Matrix metalloproteinase-28 deletion exacerbates cardiac dysfunction and rupture after myocardial infarction in mice by inhibiting M2 macrophage activation. Circ Res. (2013) 112:675–88. doi: 10.1161/CIRCRESAHA.111.300502
118. Yang M, Zheng J, Miao Y, Wang Y, Cui W, Guo J, et al. Serum-glucocorticoid regulated kinase 1 regulates alternatively activated macrophage polarization contributing to angiotensin II-induced inflammation and cardiac fibrosis. Arterioscler Thromb Vasc Biol. (2012) 32:1675–86. doi: 10.1161/ATVBAHA.112.248732
119. Saha S, Shalova IN, Biswas SK. Metabolic regulation of macrophage phenotype and function. Immunol Rev. (2017) 280:102–11. doi: 10.1111/imr.12603
120. Ma J, Wei K, Liu J, Tang K, Zhang H, Zhu L, et al. Glycogen metabolism regulates macrophage-mediated acute inflammatory responses. Nat Commun. (2020) 11:1769. doi: 10.1038/s41467-020-15636-8
121. Martini E, Kunderfranco P, Peano C, Carullo P, Cremonesi M, Schorn T, et al. Single-cell sequencing of mouse heart immune infiltrate in pressure overload-driven heart failure reveals extent of immune activation. Circulation. (2019) 140:2089–107. doi: 10.1161/CIRCULATIONAHA.119.041694
122. Rosano GM, Vitale C. Metabolic modulation of cardiac metabolism in heart failure. Card Fail Rev. (2018) 4:99–103. doi: 10.15420/cfr.2018.18.2
123. Everett BM, Cornel JH, Lainscak M, Anker SD, Abbate A, Thuren T, et al. Anti-Inflammatory therapy with canakinumab for the prevention of hospitalization for heart failure. Circulation. (2019) 139:1289–99. doi: 10.1161/CIRCULATIONAHA.118.038010
124. Zelniker TA, Wiviott SD, Raz I, Im K, Goodrich EL, Bonaca MP, et al. SGLT2 inhibitors for primary and secondary prevention of cardiovascular and renal outcomes in type 2 diabetes: a systematic review and meta-analysis of cardiovascular outcome trials. Lancet. (2019) 393:31–9. doi: 10.1016/S0140-6736(18)32590-X
125. Leuschner F, Panizzi P, Chico-Calero I, Lee WW, Ueno T, Cortez-Retamozo V, et al. Angiotensin-converting enzyme inhibition prevents the release of monocytes from their splenic reservoir in mice with myocardial infarction. Circ Res. (2010) 107:1364–73. doi: 10.1161/CIRCRESAHA.110.227454
126. Majmudar MD, Keliher EJ, Heidt T, Leuschner F, Truelove J, Sena BF, et al. Monocyte-directed RNAi targeting CCR2 improves infarct healing in atherosclerosis-prone mice. Circulation. (2013) 127:2038–46. doi: 10.1161/CIRCULATIONAHA.112.000116
127. Leuschner F, Dutta P, Gorbatov R, Novobrantseva TI, Donahoe JS, Courties G, et al. Therapeutic siRNA silencing in inflammatory monocytes in mice. Nat Biotechnol. (2011) 29:1005–10. doi: 10.1038/nbt.1989
128. Cho DI, Kim MR, Jeong HY, Jeong HC, Jeong MH, Yoon SH, et al. Mesenchymal stem cells reciprocally regulate the M1/M2 balance in mouse bone marrow-derived macrophages. Exp Mol Med. (2014) 46:e70. doi: 10.1038/emm.2013.135
129. Zlatanova I, Pinto C, Silvestre JS. Immune modulation of cardiac repair and regeneration: the art of mending broken hearts. Front Cardiovasc Med. (2016) 3:40. doi: 10.3389/fcvm.2016.00040
130. Rangasami VK, Samanta S, Parihar VS, Asawa K, Zhu K, Varghese OP, et al. Harnessing hyaluronic acid-based nanoparticles for combination therapy: a novel approach for suppressing systemic inflammation and to promote antitumor macrophage polarization. Carbohydr Polym. (2021) 254:117291. doi: 10.1016/j.carbpol.2020.117291
Keywords: inflammation, macrophages, subsets, heart injury, heart repair
Citation: Gao Y, Qian N, Xu J and Wang Y (2021) The Roles of Macrophages in Heart Regeneration and Repair After Injury. Front. Cardiovasc. Med. 8:744615. doi: 10.3389/fcvm.2021.744615
Received: 20 July 2021; Accepted: 20 September 2021;
Published: 25 October 2021.
Edited by:
Rajesh Katare, University of Otago, New ZealandReviewed by:
Jason Johnson, University of Bristol, United KingdomAnita Coral Thomas, University of Bristol, United Kingdom
Copyright © 2021 Gao, Qian, Xu and Wang. This is an open-access article distributed under the terms of the Creative Commons Attribution License (CC BY). The use, distribution or reproduction in other forums is permitted, provided the original author(s) and the copyright owner(s) are credited and that the original publication in this journal is cited, in accordance with accepted academic practice. No use, distribution or reproduction is permitted which does not comply with these terms.
*Correspondence: Yaping Wang, eWFwaW5nd2FuZyYjeDAwMDQwO3pqdS5lZHUuY24=