- 1Department of Internal Medicine and Cardiology, University Hospital Brno, Brno, Czechia
- 2Department of Internal Medicine and Cardiology, Faculty of Medicine, Masaryk University, Brno, Czechia
- 3Department of Biochemistry, Faculty of Medicine, Masaryk University, Brno, Czechia
- 4Department of Pathophysiology, Faculty of Medicine, Masaryk University, Brno, Czechia
Indirect evidences in reviews and case reports on Takotsubo syndrome (TTS) support the fact that the existence of oxidative stress (OS) might be its common feature in the pre-acute stage. The sources of OS are exogenous (environmental factors including pharmacological and toxic influences) and endogenous, the combination of both may be present, and they are being discussed in detail. OS is associated with several pathological conditions representing TTS comorbidities and triggers. The dominant source of OS electrones are mitochondria. Our analysis of drug therapy related to acute TTS shows many interactions, e.g., cytostatics and glucocorticoids with mitochondrial cytochrome P450 and other enzymes important for OS. One of the most frequently discussed mechanisms in TTS is the effect of catecholamines on myocardium. Yet, their metabolic influence is neglected. OS is associated with the oxidation of catecholamines leading to the synthesis of their oxidized forms – aminochromes. Under pathological conditions, this pathway may dominate. There are evidences of interference between OS, catecholamine/aminochrome effects, their metabolism and antioxidant protection. The OS offensive may cause fast depletion of antioxidant protection including the homocystein-methionine system, whose activity decreases with age. The alteration of effector subcellular structures (mitochondria, sarco/endoplasmic reticulum) and subsequent changes in cellular energetics and calcium turnover may also occur and lead to the disruption of cellular function, including neurons and cardiomyocytes. On the organ level (nervous system and heart), neurocardiogenic stunning may occur. The effects of OS correspond to the effect of high doses of catecholamines in the experiment. Intensive OS might represent “conditio sine qua non” for this acute clinical condition. TTS might be significantly more complex pathology than currently perceived so far.
Introduction
Takotsubo Cardiomyopathy
Takotsubo cardiomyopathy was first described by Sato et al. in 1990 (1). During an acute attack, this heart condition is characterized by transient systolic (and diastolic) dysfunction primarily of the left ventricle, which cannot be explained by coronary angiography. Differential diagnosis must primarily exclude acute myocardial infarction and acute myocarditis (1–3). It is a relatively rare pathology also known by other names, but Takotsubo syndrome (TTS) is recommended as the formal name for this condition (2). Apical ballooning with basal hyperkinesia as a typical regional kinetic abnormality gave the pathology its name (1).
The incidence of TTS is 1–2% of patients with suspected acute coronary syndrome (4, 5). In the USA, this represents 50,000–100,000 cases of TTS per year, and 0.02% of all acute hospitalizations respectively (6, 7). Post-menopausal women are most often affected (89% of all cases), most patients (60%) are older than 65 years (7, 8). Morphologically the most common forms are the apical form with/without involvement of the middle segments of the left ventricle (75–80% of cases) and the midventricular form (10–15%) (2, 7). Several diagnostic criteria have been suggested, including those by the Mayo Clinic (modified in 2008), the Japanese Takotsubo Cardiomyopathy Group, the Gothenburg Group, and the Takotsubo Italian Network (3, 9–12). It is advisable to distinguish two TTS types: primary TTS (heart problems are the primary cause of medical care) and secondary TTS (acute attack occurs in patients originally hospitalized for some other reason) (2).
In a retrospective analysis of 1,109 patients, the most common TTS comorbidities include hypertension (54%), dyslipidemia (32%), mental disorders (24%), smoking (22%), diabetes and obesity (17%), lung disease (15%), malignancies (10%), nervous system and kidney diseases (7%), and thyroid diseases (6%) (13). Smoking, alcohol abuse, hyperlipidemia and anxiety were identified as risk factors for TTS (7).
Acute coronary syndrome has also been described as a possible trigger for TTS. The extent of contractility disorders in these patients with TTS exceeded the supply area from the affected coronary artery (14). However, myocardial infarction due to coronary embolism secondary to TTS has been reported, leading to a cause-and-effect discussion (15, 16).
Oxidative Stress in Takotsubo Syndrome
Data about oxidative stress (OS) in TTS are scanty. There is a significant disproportion in the number of publications on Takotsubo syndrome/cardiomyopathy and oxidative stress: 5,167 articles about “takotsubo” in the Medline database over the last 20 years, but only 20 articles about “takotsubo oxidative stress” over the past 16 years. References were selected thematically using common web browsers, with no special software used.
Sets of genes controlling the antioxidant response (such as Nrf2-induced genes) have been upregulated in biopsies taken from the myocardium of the small group of patients in the acute phase of TTS, but not after functional recovery. According to the authors, this suggests the importance of OS in the pathophysiology of TTS (17). Other data also point to this possible association (18–21). According to case reports, reviews and systematic reviews, the common characteristics of patients with TTS just before an acute attack seems to be OS, the possible sources of OS have been divided according to its origin into exogenous and endogenous ones (22–24) – Table 1.
Due to the biochemical pathways, OS is associated with various pathologies or comorbidities potentially leading to TTS. OS sources that can be combined with each other are discussed in detail.
Oxidative Stress
Oxidative Stress in Medicine
Oxidative stress as a concept in redox biology and medicine represents “an imbalance between oxidants and antioxidants in favor of the oxidants, leading to a disruption of redox signaling and control and/or molecular damage” (22, 23, 25–27). As the ballast of metabolic processes, OS denotes deviation from redox steady state and evokes stress responses (25).
Under aerobic conditions, more than 90% of the oxygen consumed is reduced directly to water by cytochrome oxidase in the electron transport chain (ETC) using a four-electron mechanism (28–30). ETC located in eukaryotic cells on the inner mitochondrial membrane is associated with oxidative phosphorylation and energy production in the form of ATP from various substrates. Less than 10% of the consumed oxygen is reduced by one-electron conversion to the superoxide radical (O2-); by the reduction by another electron and the addition of two protons, hydrogen peroxide (H2O2) is formed (29–31).
Reactive species (RS) associated with OS can adversely affect biologically important molecules – lipids, proteins and nucleic acids. OS is involved in the development of a number of pathologies such as hypertension, dyslipidemia, metabolic diseases, incl. diabetes mellitus, neurodegenerative disorders, respiratory and inflammatory diseases or malignancies. They also represent TTS comorbidities (7, 13, 24, 32, 33).
In healthy adult women aged 19–78 years, significantly higher levels of lipid peroxidation were found by evaluating the levels of malondialdehyde and F2-isoprostanes. Plasma levels of C-reactive protein (CRP) and cholesterol also positively correlated with both biomarkers (34). Autoantibodies to oxidized DNA were 50 percent higher in women than in men (35). In contrast, ascorbic acid in plasma showed a strong inverse relationship with lipid peroxidation (34). Similarly low concentrations of total glutathione (the most important endogenous antioxidant) were found in the blood of healthy individuals of various ages, primarily those aged between 60 and 79 – see also paragraph 7 (36). These facts suggest a relationship between OS, gender and age, which is important for TTS.
Oxidative Stress and Reactive Species
RS arise in the body from exogenous and endogenous sources (22–24). They are divided into radicals (so-called free radicals – FR) containing unpaired electrons and non-radicals (22, 37).
The most important RS include reactive oxygen species (ROS) and reactive nitrogen species (RNS) (23, 37). Reactive species of sulfur, selenium, chlorine, bromine or reactive carbonyl compounds such as aldehydes are also known (22). The chemical reactivity of different RS varies by up to 11 orders of magnitude; it is low, for example, for hydrogen peroxide, extremely high for the hydroxyl radical (22). Nitrosative stress served as a modulator of inflammatory changes in the experimental TTS model (19).
More than 90% of ROS is formed in mitochondria, most of which is generated by the escape of electrons from coenzyme Q to molecular oxygen. These electrons are further spontaneously or enzymatically converted to H2O2 and OH- (29–31). Small amounts of ROS are also produced in the sarco/endoplasmic reticulum, on the plasma and nuclear membranes, and by oxidases (28).
Oxidases oxidizing a number of substrates (carbohydrates, aldehydes, amino acids, heterocyclic compounds, etc.) use electrons to form superoxide radicals (O2-). For example, xanthine oxidase (XO) or NADPH oxidase (Nox) are well-known (28). XO metabolizes purines (catalyzes the oxidation of hypoxanthine to xanthine and uric acid) and may be important in patients treated with allopurinol for hyperuricemia or gout. XO-catalyzed conversion of allopurinol (prodrug) to oxypurinol lead to the production of superoxide radicals (38). Under hypoxic conditions, XO itself may be the major source of ROS (39, 40). Nox is responsible, for example, for the inactivation of bacteria and other pathogens by cells of the immune system (39, 40).
Another source of ROS is the autoxidation of small molecules of endo- and exogenous origin such as adrenaline, noradrenaline and many xenobiotics, including drugs (30, 41–44). Other OS sources are also documented (22).
Under normal conditions, there is a dynamic equilibrium between RS production and the antioxidant capacity (45). The ability of cells to respond properly to mild OS is referred to as eustress (46). Short-term OS is important for inducing a process called mitohormesis, where low concentrations of RS act as signaling molecules that initiate the cascade of cellular events and protect cells from harmful effects (47). In general, it has a beneficial effect with ischemic pre- and post-conditioning and prepares the cell for further stress events (48, 49).
When OS disrupts or depletes the antioxidant protection provided by glutathione, antioxidant vitamins, and enzymes (e.g., catalase, peroxidase, superoxide dismutase), protein/enzyme, lipid and DNA tend to be damaged (27). Based on the intensity of the effect, OS can be classified as basal oxidative stress (BOS), low intensity oxidative stress (LOS), intermediate intensity oxidative stress (IOS) and high intensity oxidative stress (HOS); sometimes strong oxidative stress is also described (SOS) (22, 30). Acute, chronic and recurrent OS are distinguished in terms of duration (22).
Oxidative damage products (oxidized forms of lipids, proteins and nucleic acids) can be identified in a laboratory setting (49). Elevated concentrations of 8-hydroxy-2-deoxyguanosine have been found in the urine of patients in acute phase of TTS as an agent of oxidative DNA damage (18). OS also regulates the function of calcium channels and may disrupt the redox state of regulatory proteins in acute TTS attack (21, 50). Damage of cardiomyocytes by OS is manifested by changes in subcellular organelles with intracellular excess of Ca2+ (18).
In myocardial biopsies taken in the acute phase of TTS, the whole sets of genes have been triggered by oxidative stress, e.g., genes induced by the transcription factor Nrf2 controlling the antioxidant response by induction of superoxide dismutase (SOD), catalase (CAT) and glutathione peroxidase (GPX) (17, 50). At the same time, protein biosynthesis was significantly overrepresented, transcription of GPX1 and CAT increased (17).
Finally, the effect of OS is manifested by impaired myocardial metabolic function with potassium depletion, depletion of macroergic phosphates, increased intracellular calcium concentration, increased myocardial diastolic tension, and reduced heart contractility (51, 52).
Exogenous Sources of Oxidative Stress in Takotsubo Syndrome
Exogenous sources penetrate the body from the external environment, where they are metabolized or lead to another endogenous response – Table 1.
Drugs
A link between drug effects and cardiac pathologies including TTS was mentioned (53). Many molecules interfere with one or more complexes in the respiratory chain, the mitochondrial toxicity of other drugs depends on the production of FR, increased OS, and reduced anti-oxidative capacity (53, 54). The toxic effect of other drugs consists in the impairment of the oxidative phosphorylation, the Krebs cycle, β-oxidation of fatty acids, or in the induction of apoptosis (53).
Drugs are listed as possible triggers of acute attacks of TTS (so-called drug induced TTS) and may represent important indirect evidence of the relationship to OS. Fifty eight cases of TTS have been described in association with twenty different drugs (55). In most cases (77.6%) iatrogenic TTS attack was associated with direct or indirect-acting sympathomimetics (55). Other drugs are presented in this context (55–58). The attention has also been focused on chemotherapy-induced TTS (59, 60). The individual drugs associated with TTS are listed in Table 2.
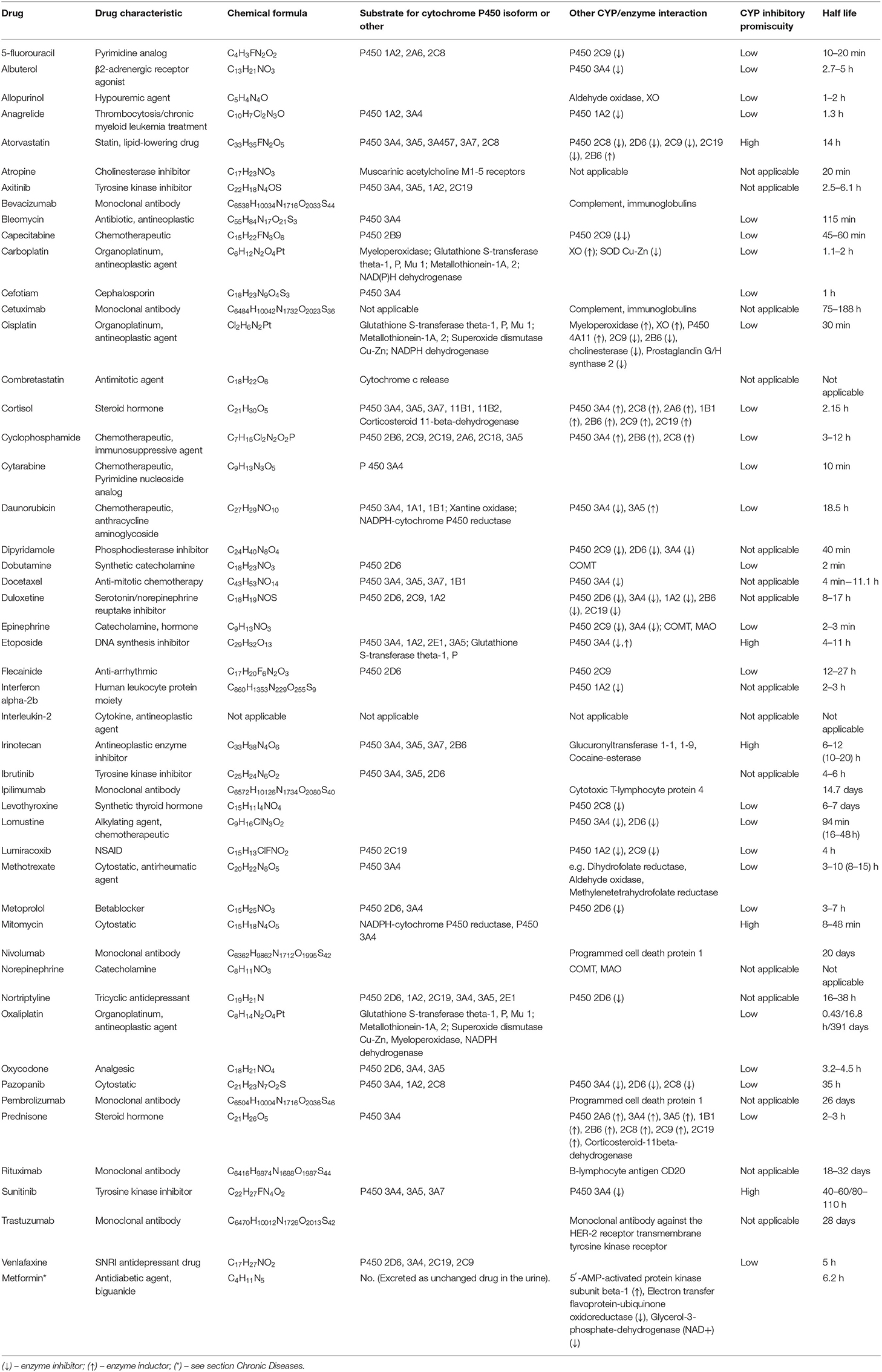
Table 2. Drugs associated with an acute attack of Takotsubo syndrome and their interactions with cytochrome P450 (available from: https://www.drugbank.ca/drugs, https://drug-interactions.medicine.iu.edu/MainTable.aspx).
Comorbidities of TTS are treated with a number of drugs that are not commonly mentioned in the acute phase (55, 59, 60). From the point of view of OS and drug metabolism, there is a significant association with cytochrome P450 function, there are many interactions between cytochrome P450 and drugs as substrates and/or inducers: e.g., 5-fluorouracil, atorvastatin, axitinib, cortisol, cyclophosphamide, daunorubicin, docetaxel, etoposide, irinotecan, ibrutinib, nortriptyline, oxycodone, pazopanib, prednisone, sunitinib, and venlafaxine. Some interactions with cytochromes are dominated by cyclophosphamide and the glucocorticoids cortisol and prednisone (Table 2). Some TTS patients with malignancies have been treated with the combination of several anticancer drugs which potentiates the interference with cytochromes (59, 60). This is not a typical manifestation of cardiotoxicity, because the clinical outcome is the TTS phenotype (61, 62). Glucocorticoids, if administered briefly and in low doses, lead to a slight increase in OS; with long-term administration, the burden of OS increases dramatically (63). Cortisol-related TTS attack has been reported (64).
Monoclonal antibodies and organic platinum derivatives that do not interfere with CYP's are administered as the part of anti-cancer treatment protocols. They can increase the burden of OS by other mechanisms. Their contribution to the development of TTS cannot be ruled out.
Monoclonal antibodies are degraded to protein fragments and gradually eliminated from the body without the involvement of cytochromes (Table 2). Their molecules containing thousands of oxygen and nitrogen atoms are associated with OS by various mechanisms (65).
Organic platinum derivatives represent substrates for enzymes accelerating OS (XO, myeloperoxidase), but also antioxidants (glutathione S-transferase, metallothioneins and superoxide dismutase Cu-Zn). GST has an important role in the protection against OS – it detoxifies a number of electrophilic compounds (e.g., as oxidized lipids, DNA, catechol products) (66, 67). Some alleles for GST (e.g., GSTM1, GSTT1) encoding lower catalytic activity of the enzyme are associated with increased susceptibility to the toxic effects of xenobiotics, but also with malignancies and asthma (66–68). Generally, SOD Cu-Zn (SOD1) is a soluble cytoplasmic and mitochondrial intermembrane protein, acting to convert superoxide radicals to molecular oxygen and hydrogen peroxide. Hydrogen peroxide can then be broken down by another enzyme - catalase (CAT) (69).
Cytochrome P450 and Polymorphisms
The human enzyme metabolizing 50–60% of known drugs is cytochrome P450 (CYP) 3A4 (70–72). It belongs to the group of heme thiolate monooxygenases located in the mitochodria or in the liver microsomes / sarco/endoplasmic reticulum. It is involved in NADPH-dependent electron transport, where it ensures the reduction of molecular oxygen to ROS and water (70, 73). CYP 3A4 catalyzes the oxidation of a number of structurally unrelated compounds, including steroids, fatty acids and xenobiotics (70, 74). As an epoxygenase, it also interferes with the metabolism of arachidonic acid, the derivatives of which are associated with the occurrence of malignancies (74).
In relation to OS, substrates and inducers of cytochromes could be important, which increase the metabolic load and thus the production of RS. Well-known inducers of CYP 3A4 are lipophilic substances (phenobarbital, phenytoin), cigarette smoke and ethanol (risk factors for TTS), glucocorticoids, and a number of drugs (70). In contrast, macrolide antibiotics, anti-HIV drugs, antidepressants, calcium channel blockers, steroid modulators, glitazones, etc. represent clinically important inhibitors of the CYP 3A4 isoenzyme (70–72). Cytochrome P450 shows a polymorphism depending on the race, sex, age and tissue in which it is found (72, 75, 76). It is more often expressed in older age, isoforms CYP 2A6 and 2B6 are more common in women (75).
It is known that CYP 2J2 isoform is most expressed in heart tissue (72, 77, 78). This isoenzyme is also associated with the epoxidation of arachidonic acid to signaling molecules and involved in the biotransformation of xenobiotics (77). Its regulation is ensured by stress stimuli, including pro- and antioxidant effects and pro-inflammatory cytokines (79). Left ventricular tissue samples in women showed higher mRNA expression for CYP 2J2 than in men (77). The isoform is associated with hypertension, type 2 diabetes, respiratory pathologies, and the metabolism of xenobiotics, which are the comorbidities of TTS (77). In contrast, the testing of CYP's with various substrates showed low activity of CYP 3A isoforms in the heart (78).
Catecholamines
The effect of catecholamines on the cardiomyocytes is mediated by the activation of β-adrenergic receptor G protein-coupled receptor kinase 2 (βAR-GRK2). The interaction induces the activation of the coupled G protein into the stimulatory form (Gs protein form) (80). This is followed by the cascade of reactions with the activation of so-called “second messengers,” including enzymes incorporated into the plasma membrane (adenyl cyclase, phospholipase c). The effect of adenyl cyclase is an increase in the level of intracellular cyclic AMP, which activates protein kinase A (PKA) with the subsequent phosphorylation of several intracellular targets, leading to an increased contractile response (80).
Catecholamines in Takotsubo Syndrome
One of the most discussed mechanisms of TTS is the myocardial toxicity of catecholamines. In 2008, it was hypothesized that the high levels of circulating adrenaline led by βadrenergic receptor (βAR) activation to the shift in coupled intracellular signal transmission from the stimulatory form of Gs protein to the inhibitory Gi form (81). The result of this change is a negative inotropy with maximal effect in the apical myocardium causing an apical form of the disease, which was confirmed in an animal model for the high bolus dose of adrenaline (81, 82). Differences in regional left ventricular contractility in different forms of TTS were explained by the existence of an apical-basal gradient in βAR density in the left ventricle (the density of βAR in the myocardium decreases from the apex to the heart base) (81). Reports about recurrences of different forms of TTS in the same patients suggest rather a homogeneous distribution of βAR in the myocardium (83, 84).
In the experiment on age-related rat ventricular myocytes, the attenuation of the contractile response to βARs stimulation was demonstrated. This suggests that the positive inotropic effects of βAR stimulation decrease significantly with the age in rat ventricular myocytes, accompanied by a decrease in receptor density and a decrease in membrane adenyl cyclase activity (85).
Also in pathological conditions, the density of βAR in the myocardium changes. Following acute myocardial infarction without heart failure, βAR density is reduced and this reduction predicts later left ventricular dilatation (86). Decreased expression of the βAR-GRK2 complex in lymphocytes has been experimentally demonstrated in inflammatory processes (e.g., arthritis). OS in lymfocytes induced by the exposure to H2O2 resulted in a 50% reduction in GRK2 protein levels and its activity. The authors consider OS to be a new mechanism for regulating the intracellular activity of GRK2 in inflammation (87).
A review article from 2018 showed that TTS patients have a normal or only a mild to moderate increase in plasma adrenaline at the time of an acute attack (88). Similarly, mild to moderate elevated plasma concentrations of noradrenaline and normetanephrine were found in TTS patients (89). Some TTS patients with heart failure also showed only a mild to moderate elevation of plasma noradrenaline (89). Catecholamines appear to be rather triggers than the cause of an acute attack of TTS (88). There are probably other factors that determine the development of an acute attack of TTS.
In addition to cardiac function and hemodynamics, catecholamines also affect metabolic pathways (90). These effects take place in the context of the “flight and fight” response. In general, catecholamines stimulate aerobic glycolysis, glycogenolysis, gluconeogenesis, and inhibit glycogen synthesis (90). Besides, catecholamines enhance ketogenesis and are involved in proteolysis in order to provide sufficient glucose precursors. Their impact on mitochondria is pronounced during critical illness. Here, catecholamines promote mitochondrial uncoupling, and aggravate OS, either via an accelerated glycolytic pathway, or through the catecholamine oxidation. This may result in exhausted antioxidant defense systems, such as reduced glutathione (GSH) or SOD, and the progression of mitochondrial dysfunction (90). The metabolic effects of catecholamines in TTS may still be neglected.
In terms of cardiac metabolism, catecholamines act by two rnechanisms. One is by the augmenting the supply of oxidizable substrate. Secondly, the utilization of this substrate by cardiac muscle is influenced by the interaction between oxygen and substrate supply and the contractile state of the heart (91). Catecholamine stimulation is accompanied by the increased use of exogenous energy substrates, especially free fatty acids (FFA). These lipolysis products and beta-oxidation substrates are preferentially used in cardiac metabolism (91). In well-oxygenated cardiac tissue with high concentration of ATP, catecholamines do not lead to the utilization of glycogen (91). In relation to OS, in isolated rat cardiac mitochondria operating in the forward electron transport mode, unsaturated fatty acids increase ROS production by partially inhibiting electron transport and possibly altering membrane fluidity (92).
Catecholamines and Oxidative Stress
The biodegradation of catecholamines in the body is ensured by the enzymes COMT and MAO (52, 93). Under normal conditions, 95% of adrenaline and noradrenaline are biotransformed and excreted in the urine in the form of final metabolites (94).
Under pathological conditions (e.g., inflammation), biotransformation of catecholamines via oxidation may predominate (93–97). The resulting products are aminochromes (93, 96). They are formed from their catecholamine precursors by several oxidation pathways, which can be an important source of OS (30, 41, 42, 96). The best known products are adrenochrome and its derivative adrenolutin, that can be detected spectroscopically, chromatographically and by nuclear magnetic resonance spectroscopy (93, 96). Aminochromes in TTS have not yet been investigated.
In the experiments, the hypoxanthine-XO system forming ROS can be used to autooxidize adrenaline (97, 98). Yet, in the presence of uric acid (end product of hypoxanthine oxidation) with/without XO, no reaction occurs; this indicates the importance of ROS (98). In a similar experiment on isolated rat cardiomyocytes, there was a concomitant decrease in GSH (99). A membrane system with NADH/NADPH on separated bovine cardiac sarcolemma also induces adrenochrome formation (100, 101). In this system, Nox contributes to the production of adrenochrome by the formation of ROS and NADPH dramatically stimulates adrenaline oxidation (100). The reaction is strongly suppressed by antioxidants such as SOD, ascorbic acid, α-tocopherol or preincubation of the membrane with the beta-blocker propranolol (101).
Cells of the immune system (e.g., polymorphonuclear cells, monocytes, macrophages, eosinophils) also form RS and oxidize adrenaline to adrenochrome (95). After activation of polymorphonuclears, its metabolism proceeds mainly (more than 80%) via adrenochrome formation (see also section Allergic Reactions). This pathway is inhibited by SOD, CAT or sodium azide (peroxidase and cytochrome oxidase inhibitor) (95). This may be important in infections and inflammation as comorbidities of TTS (13, 102).
Dobutamin as a synthetic catecholamine can also be oxidized to adrenochrome. “In vitro,” this oxidation reaction in the presence of H2O2 is catalyzed by the enzyme horseradish peroxidase, where more than 95% of the substrate is processed in 5 minutes (103). The enzyme is contained, for example, in wasabi, the consumption of which immediately caused an acute TTS (104, 105). In practice, a substrate for the enzymatic oxidation by horseradish peroxidase could be a native catecholamine. Adrenochrome is also likely to occur during organophosphate poisoning, which has been identified as one of the TTS triggers (95, 106).
Aminochromes are cardiotoxic, neurotoxic and psychotomimetic (52, 95, 96, 103, 107). In experiments, adrenochrome acted on various cellular organelles (108). Effects on the sarco/endoplasmic reticulum and mitochondrial Ca2+ transport systems were similar to those of high catecholamine concentrations (108–110). They may explain intracellular and mitochondrial calcium overload with alterations in oxidative phosphorylation, decreased myocardial ATP/AMP ratio, and worsened myocardial contractility (109, 111). Adrenolutin also shows negative inotropy (95). In rat hearts perfused with adrenochrome, signs of necrotic damage such as the dissolution of contractile fibers, disruption and mitochondrial edema, endo/sarcoplasmic reticulum edema were visible by means of electron microscopy (112). These findings are similar to those found in cardiac biopsies of TTS patients (113–115).
In the body, aminochromes are efficiently conjugated to glutathione. The reaction is catalyzed by GST, which prevents redox cycling and shows one of its cytoprotective effects (107). These facts associate the metabolism of catecholamines with OS, and antioxidant protection, respectively.
Food, Drinks, Drugs, Toxins, and Chemicals
A high-fat and energy-dense diet intensifies the OS (49, 116). Glycemic load and glycemic index are associated with lipid peroxidation (49, 117). While saturated fatty acids potentiate OS, monounsaturated fatty acids, antioxidant vitamins and bioactive substances from fruits and vegetables have antioxidant effects (49). Some TTS attacks occurred during parties and are explained by positive emotions as “happy heart syndrome” (118). In these situations, increased energy intake can be expected, which may be related to specific forms of OS - nutritional OS (NOS) or post-prandial OS (POS) (30). Dietary OS (DOS) has also been described (30).
An acute TTS attack after consuming Japanese horseradish - wasabi (Wasabia japonica, Cochlearia wasabi) at a wedding party was described (105). The biologically active substances of wasabi are allyl and other isothiocyanates, which generally have antioxidant, neuroprotective and anticancer effects (119–121). Isothiocyanates are rapidly conjugated to glutathione in the liver, metabolized by the mercapturic acid pathway and excreted in the urine; the enzyme gamma-glutamyltransferase, glutathione and xenobiotic load play an important role (119). This can reduce the pool of free glutathione, which is the major endogenous antioxidant (122). Isothiocyanates are eliminated in parallel. A possible end effect may be an alteration in antioxidant protection. Mercapturic acid is also involved in the metabolism of xenobiotics to form reactive thiols (123). This may be another mechanism of action of wasabi (see also section Catecholamines and Oxidative Stress).
Consumption of energy drinks containing metabolic stimulants (e.g., caffeine) in combination with or without alcohol is also accompanied by the increase in OS parameters, including lipid peroxidation (124). The oxidative load caused by alcohol is associated with its biodegradation, which interferes with the mitochondrial and microsomal systems. Ethanol metabolism is directly involved in the production of ROS and RNS. Alcohol consumption reduces the level of GSH and alters antioxidant protection (125). An acute TTS has been described in a 50-year-old man attacked in front of a bar; the level of ethanol in the blood at the time of examination was 400 mg/L (126).
OS is also associated with tobacco smoking and cocaine abuse (127, 128). A number of metabolites (including formaldehyde) formed during the metabolism of cocaine, which caused an acute TTS, ultimately lead to glutathione depletion (128–130). Smoking and alcohol abuse represent risk factors for TTS (7).
TTS attacks have been described in intoxications with herbicides (glyphosate, glufosinate) and insecticides (organophosphates, fungicide) (106). Those are associated with OS, the decrease in glutathione reductase and SOD activity, and the decrease in the concentration of GSH in the plasma and tissues of experimental animals (131). TTS has also been reported in lithium, venlafaxine, risperidone, barbiturates, benzodiazepines, botulinum, or tetanus toxin poisonings (132–136). Other toxins and allergens are mentioned in section Allergic Reactions.
Photochemical Smog
In a large group of US patients with TTS, the authors identified the late summer months as the period with the highest number of acute attacks (6, 137). This seasonal dependence could be related to the occurrence of so-called photochemical (summer) smog, which was first recognized in Los Angeles during World War II (138). The described form of smog arises in the atmosphere by oxidation of flue gases produced by the combustion of liquid fuels in solar radiation, it promotes the production of nitrogen dioxide and leads to high concentrations of ozone with the participation of other substances in the atmosphere (138, 139). Nowadays, smog episodes are common (not only) in the summer months in various megacities around the world (138). In patients with chronic respiratory disorders, ozone exposure leads to inflammation and lung tissue damage with persistent structural changes (140). About 15% of TTS patients have these comorbidities (13, 102).
Endogenous Sources of Oxidative Stress in Takotsubo Syndrome
Physical and Emotional Stress
The term stress was used by Hans Selye to describe the body's inadequate response to mental, emotional, or physical stimuli (141). The reaction known as the adaptation syndrome has three phases (142). The neuroendocrine response to stressors generally involves activation of the autonomic nervous system, activation of the hypothalamic-pituitary-adrenal axis with the cascade of hormonal messengers (e.g., catecholamines, glucocorticoids), and direct nerve stimulation of the adrenal cortex (143–145). This pathway activates the cardiovascular and immune systems, metabolism and potentiates OS associated with a number pathologies of the CNS (24, 143, 144, 146).
Physical Stress
Physical stress is described as a trigger in 36–53% of TTS patients (8, 147, 148). They also have higher hospitalization morbidity, mortality and worse long-term prognosis compared to patients with emotional stress (148). Nox, XO, phospholipase A2 (PLA2) and lipoxygenases have been identified as sources of ROS in muscle cells (149). During physical activity, ROS are also produced from non-muscular sources. Endurance and strength requiring physical activity induce the production of pro- and anti-inflammatory cytokines, their inhibitors and chemokines (37, 149). Lipid peroxidation leads to the formation of reactive aldehydes (37, 150). Exercise is generally associated with the oxidation of glutathione in skeletal muscle, blood and liver. However, repeated physical activity enhances glutathione-mediated antioxidant protection, whereas chronic inactivity has the opposite effect (151).
Emotional Stress
Emotional stress is mentioned in 21–28% of patients with TTS (8, 147, 148). Various psychological traumas are accompanied by OS in the CNS (144). Compared to patients with acute coronary syndrome, TTS patients have more neurological and psychiatric comorbidities (8). They are also more often neurotic, depressed and anxious (152). Positive emotions such as the so-called “happy heart syndrome” have also been described in TTS (118).
Emotional states are the manifestation of the function of the limbic system. The structures of this part of the CNS include the limbic cortex, hippocampal formation including the hippocampus, amygdala, septal area and hypothalamus (145). While the amygdala is associated with the perception of fear and anxiety, with aggression, emotional memory, and social cognition, the cingulate gyrus is involved in the primary autonomic regulation of heart rate and blood pressure (145). The production of neurotransmitters such as dopamine, noradrenaline or serotonin controls the function of the autonomic nervous system and, indirectly, the endocrine, immune and somatic processes (145).
Limbic System and Oxidative Stress
For many reasons, the nervous system is highly sensitive to OS (143, 144, 153, 154). In the limbic system, glucocorticoids induce OS by directly accelerating cellular respiration and oxidative phosphorylation (155). Neuronal apoptosis, which was not caused by other steroid hormones, was selectively induced in the dexamethasone-exposed hippocampus (156). Dexamethasone can increase the oxidative state of the hippocampus by up to 200%. The highest increase in ROS production was seen after 4 h of dexamethasone incubation, while the antioxidant activity of GPX was reduced; this effect was mitigated by the antioxidant N-acetyl-L-cysteine (156). Corticosterone has a similar effect (143). In the hippocampus, chronic OS promotes gliogenesis over neurogenesis in neural stem cell progenitors (157).
These facts may be related to the findings of homogeneous structural and functional alterations detected by magnetic resonance imaging in the limbic and central autonomic nervous systems of patients with TTS, who also show increased sympathetic nerve activity associated with decreased spontaneous baroreflex control (158, 159). This may indicate the importance of the brain-heart interaction in TTS (158). The relationship between catecholamines and OS has already been discussed in the section Catecholamines.
Epileptic Seizures
Secondary TTS was diagnosed in 0.1% of hospitalizations with epilepsy. Caucasians at higher cardiovascular risk were more commonly affected (160). Impaired antioxidant protection, mitochondrial dysfunction, redox-active metals, activation of the arachidonic acid pathway and aging represent critical factors in the genesis of epilepsy. In the CNS, OS is associated with a decrease in GPX activity, a decrease in GPX and GSH/GSSG ratios, and an increase in GSSG, lipid peroxidation, and protein oxidation (161). The high content of polyunsaturated fatty acids in neuronal membranes may be another reason for their high sensitivity to oxidative damage (161, 162). Antiepileptics can also increase OS parameters in erythrocytes, leukocytes, blood, and plasma (161).
Fenton Reaction
The Fenton reaction can be the source of OS for all TTS conditions associated with bleeding, including injuries, operations, or instrumental procedures. The reaction described by H. J. Fenton takes place in tissues in the presence of hydrogen peroxide, hydroxyl radicals and a suitable ion catalyst (163–165). These are most often iron, copper or cobalt for their ability to cyclically oxidize and reduce in the body (153, 163, 164). Iron is commonly transported and stored in specific proteins that minimize its reactivity. This situation changes under pathological conditions, as blood leaks into tissue outside the vasculature (165). Iron released from erythrocytes catalyzes the autoxidation of physiological compounds or xenobiotics to form highly reactive and toxic substances, which can lead to a number of biological damages (164, 165). TTS has been described after cardiac surgery, shortly after pacemaker implantation, but also after a blood transfusion (165–168). Other case reports related to surgery are also known, some arising between the first and seventh post-operative days (169). Patients with perioperative TTS were younger and less likely to have ECG changes than TTS patients without surgery (169).
Chronic Diseases
Chronic diseases and comorbidities of TTS such as hypertension, diabetes mellitus, dyslipidemia, metabolic, neurodegenerative and inflammatory diseases, respiratory pathologies and malignancies are associated with OS (13, 24, 32, 33). Some of the drugs used in their treatment are listed in Table 2.
A retrospective analysis of 33,894 patients with TTS and diabetes mellitus (DM) showed the twice lower prevalence of DM compared to the general population (169). This effect is explained by the presence of autonomic neuropathy and the hyposecretion of catecholamines, which accompany more severe forms of DM (169). No potential relationship with the treatment has been assessed yet.
The preferred initial pharmacologic agent for the treatment of type 2 diabetes (first-line therapy) is metformin, which accumulates in the mitochondria at concentrations up to 1,000 times higher than extracellularly (170). Metformin is not metabolized in the body and is excreted unchanged in the urine (Table 2). The drug has been shown to act via both AMP-activated protein kinase (AMPK)-dependent and AMPK-independent mechanisms, by the inhibition of mitochondrial respiration, but also perhaps by the inhibition of mitochondrial glycerophosphate dehydrogenase, and by a mechanism involving the lysosome (170, 171). Metformin has a beneficial effect on OS and inflammatory parameters in diabetics (172). Cytochrome inhibitors could have a similar effect (70–72).
Immune- and Immunopathological Reactions
These reactions accelerate OS through various mechanisms (23, 24, 32, 94, 96). The goal of the inflammatory immune response is to inactivate and eliminate the antigen (173). Acute TTS attacks have been reported in patients with the infections of organ systems, including sepsis, chronic inflammation, autoimmune and demyelinating diseases (174–182). Immunopathological mechanisms have also been detected behind the attacks induced by allopurinol, influenza B, transfusion reaction, or influenza vaccination (56, 57, 183–185).
Allergic Reactions
Allergic reactions including anaphylaxis with/without resuscitation, have been described in case reports about TTS (186–190). Various toxins caused acute attacks; some patients have received corticosteroids, tetanus toxoid, or antibiotics during treatment (187, 191–193). A young patient with a shellfish allergy underwent an unspecified dental procedure with the administration of adrenaline before the development of a TTS attack (194). TTS was also described 3 days after contact with the putative antigen which may indicate type IV of immunopathological reaction (delayed type hypersensitivity – DTH) (195).
Delayed Type Hypersensitivity
As many as 97% of patients with TTS showed pathological immune reactivity of the DTH type to metals of dental restoration materials (especially amalgam inorganic mercury) evaluated by the lymphocyte transformation test (102). These reactions can be an important hidden (asymptomatic) source of OS. Seventy-nine percentage of patients of this small cohort also had at least one known immunopathological disorder (102). Metals may catalyze the oxidation of catecholamines and accelerate OS (96).
Kounis Syndrome
The syndrome described by Kounis and Zavras in 1991 is an “allergic” acute coronary syndrome caused by allergic, hypersensitivity, anaphylactic, or anaphylactoid conditions associated with the activation of platelets, mast cells, macrophages, and T-lymphocytes (196, 197). There are three types of disease: type I is caused by a coronary artery spasm, type II affects patients with the pre-existing atherosclerotic changes in the coronary arteries, where an allergic reaction induces/accelerates the erosion or rupture of the plaque and intracoronary thrombosis. Type III is associated with a hypersensitivity reaction to the coronary stent material leading to “in stent” thrombosis (196, 198).
Inflammatory cells activate each other via multidirectional signals (196). Inflammatory mediators in these reactions include histamine, platelet-activating factor, arachidonic acid products, neutral proteases, cytokines, and chemokines released during the allergic activation process (196). The triggering factors, similar to TTS, represent food, xenobiotics incl. drugs, poisons, environmental influences, allergic and other pathological conditions (196, 198, 199). In 2009, TTS was described in a patient with asthma and Kounis syndrome (200).
Malignancies
According to data from the International Registry, the prevalence of malignancies in patients with TTS is 16.6% (201). The most common are malignancies of the breast, gastrointestinal and respiratory systems, internal genitals and skin. As regards the comorbidities, TTS patients (compared to patients with acute coronary syndrome) were significantly more likely to suffer from COPD, asthma, and neurological pathologies (201). As a trigger of an acute attack, physical stimuli were more frequent than emotional (201). Some children with tumors had TTS too (202, 203). Anticancer drugs as a potential source of OS are listed in Table 2.
Sarco/Endoplasmic Reticulum—Mitochondria Complex and Calcium Overload—Energy Failure
Sarco/Endoplasmic Reticulum—Mitochondria Complex
Mitochondria play a central role in the life and death of cells. They provide not only energy metabolism, but also anabolic and catabolic processes, calcium fluxes and various signaling pathways. Mitochondria maintain cellular homeostasis by interacting with ROS and RNS and responding adequately to various stimuli (54). The emission of ROS from mitochondria can induce further release of ROS from neighboring mitochondria, referred to as ROS-induced release of ROS. Mutual communication between different ROS sources represents a (cellular) network of redox signaling (204).
Sarco/endoplasmic reticulum (SER) is a complex organelle formed by a continuous membrane system as a network of interconnected cisterns and tubules (205). Its morphology is determined by integral membrane proteins (e.g., CYP's) and relationships with other organelles such as mytochondria, Golgi apparatus, cell nucleus or cytoskeleton (205, 206). SER consists of 2 parts: the longitudinal SER, composed of tubular structures surrounding the myofilaments, and the junctional SER, which is associated with the transverse tubules to form specialized compartments, where Ca2+-induced Ca2+ release occurs (204, 207). Close contact points between the SER and mitochondria are controlled by SER-located mitofusin 2 (Mfn2) tethering with Mfn2 and Mfn1 on the outer mitochondrial membrane, respectively, creating microdomains of high Ca2+ concentration in the vicinity of mitochondria (204, 207).
Metabolic processes such as glycolysis, the citric acid cycle, β-oxidation of fatty acids, or the metabolism of xenobiotics depend on the continuous recycling of coenzymes (NADH and FADH) into oxidized forms (208). In aerobic organisms, this recovery is ensured by the transport of electrons through the respiratory chain formed by a sequence of redox centers containing CYP's (208). SER complex with mitochondria (the sarco/endoplasmic reticulum - mitochondria complex) regulates protein, lipid, carbohydrate metabolism, steroid synthesis, calcium homeostasis and also myocardial contractility (205, 209).
Sarco/Endoplasmic Reticulum Stress
Sarco/endoplasmatic reticulum stress occurs when the workload increases (210). This condition is accompanied by the accumulation of degraded proteins in the lumen. It negatively affects cellular homeostasis (206, 211). The cascade of reactions known as the unfolded protein response (UPR) serves to restore it (211). With long-term and/or severe SER stress, UPR becomes cytotoxic and can lead to apoptosis (206). Among other things, foci of bound necrosis have been found in myocardial biopsies of TTS patients (113–115).
The main source of OS in the SER are microsomal monooxygenases (so called MMO system), the function of CYP 2E1 is emphasized (206). The efficiency/degree of electron transfer binding from NADPH to cytochrome P450 is usually <50–60% and often is as low as 0.5–3.0%. This electron leakage is important for the cellular production of ROS (206). Interactions with various redox mediators such as protein disulfide isomerase, sarco/endoplasmic reticulum oxidoreductin, glutathione, Nox4, NADPH-P450 reductase, and calcium occur during SER oxidative stress (206). These pathways are also associated with pathogenesis of various diseases (206).
Calcium Overload and Myocardium Energy Failure
OS interferes with the energy and metabolic processes in the cardiomyocyte. Among the numerous mechanisms proposed for myocardial stunning, three appear to be most likely: the generation of ROS, calcium overload, and the excitation-contraction uncoupling caused by an inadequate calcium output from SER were described (51).
Calcium turnover and OS are closely related to the function of mitochondria as the main source of ATP; calcium represents the critical regulator of their function. During β-adrenergic stimulation, cell respiration and Krebs cycle activity are controlled by ADP and mitochondrial Ca2+ uptake which regulates the adaptation of cardiomyocytes to continuous acute, chronic, physiological or pathological changes, including increased contractility or apoptosis. Physiological variations of workload lead to mitochondrial Ca2+ uptake, which is required to match energy supply to demand, but also to keep the antioxidative capacity in a reduced state to prevent the excessive emission of ROS (204). For the influx of Ca2+ into mitochondria is essential the mitochondrial calcium uniporter (MCU), which is the part of a multiprotein complex in the inner mitochondrial membrane (204). Some mitochondria reside in a close apposition to the junctional SER, which is important for excitation–metabolism coupling and apoptosis (207, 212).
Under normal condition, the action potential opens voltage-gated L-type Ca2+ channels. The ensuing Ca2+ entry triggers even greater release of Ca2+ from the SER via ryanodine receptors type 2 (RyR2). This Ca2+-induced Ca2+ release generates a transient increase of the cytoplasmic Ca2+ concentration that activates myofilament cross-bridge formation (213, 214). To terminate contraction, Ca2+ is removed from the cytoplasm into the SER by the sarco/endoplasmic reticulum calcium ATPase (SERCA) and to the extracellular space by the Na+/Ca2+ exchanger (NCX) (213–215). While intracellular Ca2+ is a mediator of contraction, its influx into the mitochondrial matrix regulates the energy production by modulating pyruvate dehydrogenase and other tricarboxylic acid (TCA) cycle enzymes (216). In the matrix, Ca2+ activates Krebs cycle dehydrogenases to regenerate the reduced form of NADH, which donates electrons to the respiratory chain (204). Superoxide generated at complexes I and III of the respiratory chain, is transformed to H2O2 by SOD and eliminated by GPX and the peroxiredoxin/thioredoxin system (204). Reduced NADPH regenerates glutathione (GSH) from its oxidized form (GSSG) via glutathione reductase and thioredoxin reductase, respectively (204).
Under pathological conditions with excitation–contraction uncoupling (e.g., heart failure, TTS), the sarcoplasmic reticulum Ca2+ load is reduced (SERCA inhibition) together with the mitochondrial Ca2+ concentration (due to the alteration of the MCU and NCX activities); the cytoplasmic Ca2+ concentration increases (204, 215, 217). This Ca2+ overload activates the mitochondrial permeability transition pore (mPTP), which leads to mitochondrial swelling, outer mitochondrial membrane rupture, and cell death (apoptotic or necrotic) (218). Respiratory chain blockade may occur (219). In the myocardium, the effect is manifested by the depletion of macroergic phosphates with the disruption of myocardial metabolic function and the reduction of contractile force (51, 220).
Changes in intracellular calcium turnover with impaired contractility have been demonstrated in TTS (221). The two homologous proteins sarcolipin (SLN) and phospholamban (PLN) bound to SER are potentially critical regulators of cardiac contractility. While SLN regulates SERCA activity by decreasing calcium affinity, PLN represents a major substrate for PKA in cardiomyocyte. In the unphosphorylated state, PLN is an inhibitor of SERCA, leading to the accumulation of cytoplasmic Ca2+ (222, 223). In cardiac biopsies of patients with TTS, SLN expression was significantly increased compared to healthy controls (221). Expression of SERCA2a was significantly down-regulated, PLN dephosphorylation was documented. No changes were demonstrated for NCX and RyR2. This suggests an alteration of Ca2+ handling proteins, which might be crucial for the contractile dysfunction (221).
These findings are consistent with the idea of myocardial energy failure in TTS associated with OS (20, 224). Examination of TTS patients with radioisotopes showed an alteration in glucose metabolism during normal myocardial perfusion in an affected left ventricle known as the “mismatch with inverse flow metabolism” (225).
Neurogenic Stunned Myocardium
Neurogenic stunned myocardium (NSM) can be defined as an acute and reversible myocardial dysfunction, occurring after different types of neurologic events, with the participation of the autonomic nervous system (226, 227). That develops during/after subarachnoid hemorrhage (33% of cases), cerebral trauma (22%), ischemic stroke, encephalitis, epilepsy, or tumors (226, 228, 229). Some authors consider NSM as a Takotsubo-like pathology or a secondary TTS (230).
NSM resembles TTS and is explained by limbic-autonomic dysregulation, where the main role is attributed to the insular cortex (231). This visceral-somatic area is one of the least understood parts of the brain. There is a direct structural connection between the insular cortex, the motoric cortex, the limbic system and the autonomic nervous system (232). The severity of NSM depends on the type and severity of the neurologic disease and on involvement of some neurologic structures (insular cortex, hypothalamus, etc.) (230). Diagnostic criteria for NSM are not defined as regards TTS, but include common clinical and echocardiographic characteristics (230). The OS could link the two pathologies.
In patients with TTS, cardiac sympathetic innervation disorder was demonstrated by scintigraphy with I123 MIBG in affected areas of the left ventricle (233). This noradrenaline analog was rather poorly absorbed in segments with contractile disorder, unmasking “sympathetic denervation” (233). Similarly, positron emission tomography (PET) with C11 hydroxy-ephedrine detected cardiac sympathetic nerve endings. Indicator uptake was reduced in the affected segments, indicating an abnormality of sympathetic innervation as a possible manifestation of stunning in TTS (233).
Cardiac wall contractility disorders in TTS have characteristic patterns corresponding to individual forms of the disease (10). They appear to correlate with the anatomy of sympathetic innervation from the left or right ganglion stellatum or from the caudal ganglion (233–235). In humans, the blockade of the right ganglion stellatum has led to a significant prolongation of the QT interval, which is also found in patients with TTS (3, 236). Recent case reports in a woman with four attacks and three variants of TTS and gastroparesis suggest that stunning could affect a larger neurovisceral area (237).
Takotsubo Syndrome, Inflammation, Histological Findings
Takotsubo Syndrome and Inflammation
Cardiac magnetic resonance (CMR) imaging in the acute phase of TTS showed the typical pattern of left ventricle dysfunction, corresponding to wall motion abnormalities, markers for myocardial inflammation with hyperemia and tissue edema, and the absence of significant necrosis/fibrosis (238–240). During follow-up, there is a complete normalization of the left ventricular ejection fraction and inflammatory parameters (238). According to the findings from positron emission tomography/computer tomography (PET CT), the coronary flow reserve and myocardial blood flow in TTS are disrupted globally and not only in the areas of the contractile disorder (241). Magnetic resonance with multiparametric myocardial imaging detected inflammatory macrophage infiltration in the affected areas (242). In the blood of these patients, classical CD14++ CD16- monocytes and serum concentrations of interleukin-6 and chemokine (C-X-C motif) ligand 1 were increased simultaneously whereas intermediate CD14++CD16+ and non-classic CD14+CD16++ monocytes were reduced (242).
Some of these changes persisted for at least 5 months, suggesting a low intensity of chronic inflammation in patients with TTS (242). Monocyte activation is also associated with OS – see sections Oxidative Stress in Medicine and Catecholamines and Oxidative Stress, respectively. In another study, patients in the acute phase of TTS had higher concentrations of interleukins 2, 4, 10, TNF-α, IFN-γ, and EGF, but lower levels of interleukin 6 compared to individuals with acute coronary syndrome (243).
Histological Findings in Takotsubo Syndrome
Histological findings in patients with TTS were described from endomyocardial biopsies, but also from autopsies. In biopsies taken from the myocardium in the acute phase of TTS, sets of genes controlling the antioxidant response were triggered by OS by the induction of SOD, CAT and GPX1 (17). Areas of interstitial edema with irregular infiltrates of monocytes, macrophages, rarely with polymorphonuclear lymphocytes, mast cells and eosinophils are present in the tissue sections (114, 115). The activation of immune cells is associated with OS (94–96). Tissue edema could be the final manifestation of energy production blockade (see section Calcium Overload and Myocardium Energy Failure). Myocytes or groups of myocytes show increased eosinophilic staining, ruptures, and necrosis (114). Epicardial impairment, inflammatory changes, and contraction bands distinguish TTS from coagulation necrosis in acute myocardial infarction (114, 115). Both ventricles may be affected, there is no myocardial fibrosis or correlation with coronary anatomy (114).
In six of the nine fatal cases of TTS, a disease with an immunopathological background was present as (chronic) comorbidity - rheumatoid arthritis, Hodgkin's disease, multiple myeloma, bronchial asthma, and pneumonia (114). Acute inflammation, sepsis or adrenaline intake were the most common triggers of TTS (114). These pathologies are also associated with OS (23, 24, 33).
Takotsubo Syndrome and Antioxidants
Plasma concentrations of certain biomarkers well reflect the redox state in tissues including the heart (244). In women of a small TTS group, high prevalence of vitamin D insufficiency was found associated with worse hemodynamic parameters (245). In addition to interfering with calcium metabolism, vitamin D is also an important antioxidant.
The administration of α-lipoic acid vs. placebo in TTS patients resulted in the significant reduction in CRP, TNF-α and nitrotyrosine (OS marker) (246). This effect is explained by the adjustment of sympathetic-vagal vegetative alteration or the regulation of sympathetic tone (246, 247). Alpha-lipoic acid as an antioxidant plays a crucial role in mitochondrial dehydrogenase reactions, reacts with various RS, protects membranes by interacting with vitamin C and glutathione, which in turn recycles vitamin E (248). Its administration has had beneficial effects in many OS models (248). Another antioxidant, N-acetyl-L-cysteine, reduced OS levels in the hypocampus upon exposure to dexamethasone (156). Further experimental works has shown a beneficial effect of other antioxidants (SOD, ascorbic acid, α-tocopherol) on the synthesis of adrenochrome (100, 101).
Interactions with glutathione and substances/enzymes related to its metabolism have been repeatedly reported in the above-mentioned sources of OS, including physical and mental stress. Glutathione as a product of the methionine-homocysteine cycle is a major endogenous antioxidant (122). The cytoplasm, mitochondria and cell nuclei contain mainly a reduced form of glutathione (GSH) representing up to 99% of total glutathione pool. SER with MMO system contains up to 30% of glutathione in oxidized form as GSSG. A reduced GSH/GSSG ratio is considered an indicator of OS (122). Reduced glutathione performs antioxidant, detoxifying and chelating functions (122). The methionine-homocysteine cycle also provides methyl group transfer for COMT-catalyzed reactions involved in the biodegradation of catecholamines (122, 249). The reduced efficiency of this system could lead to alterations in the biotransformation of catecholamines, including oxidized forms.
Significantly low concentrations of total glutathione were found in the blood of healthy individuals of various ages, especially in the age group of 60–79, which is most often affected by TTS (7, 8, 36). These individuals may be at the risk due to reduced ability to maintain glutathione-mediated metabolic and detoxification pathways (36, 122). Patients with chronic diseases also have significantly lower GSH levels (250). Under OS stress, lower glutathione concentrations are easier to deplete. In contrast, in women of childbearing age, a positive correlation was found between blood estrogen and GSH levels, and CAT activity, respectively (251).
Discussion
Takotsubo syndrome is one of the greatest mysteries of cardiology in the last 30 years. Based on the evidence presented, it can be hypothesized that TTS may occur in neuro-myocardial areas with escalated OS, typically in post-menopausal women. For the development of an acute attack of TTS, the OS could represent an essential metabolic regulator as a “conditio sine qua non” ultimately leading to the sarco/endoplasmic reticulum – mitochondria complex stress with blockade of energy production, calcium overload and Takotsubo phenotype – Figure 1. Subsequent adjustment of metabolic functions may lead to restoration of dynamic equilibrium. An example could be the recent case report describing the development of three forms of TTS in a single patient with intracerebral hemorrhage, where several sources of OS can be identified (252). Possible relationships between TTS, OS and cardiovascular pathologies are shown in Figure 2.
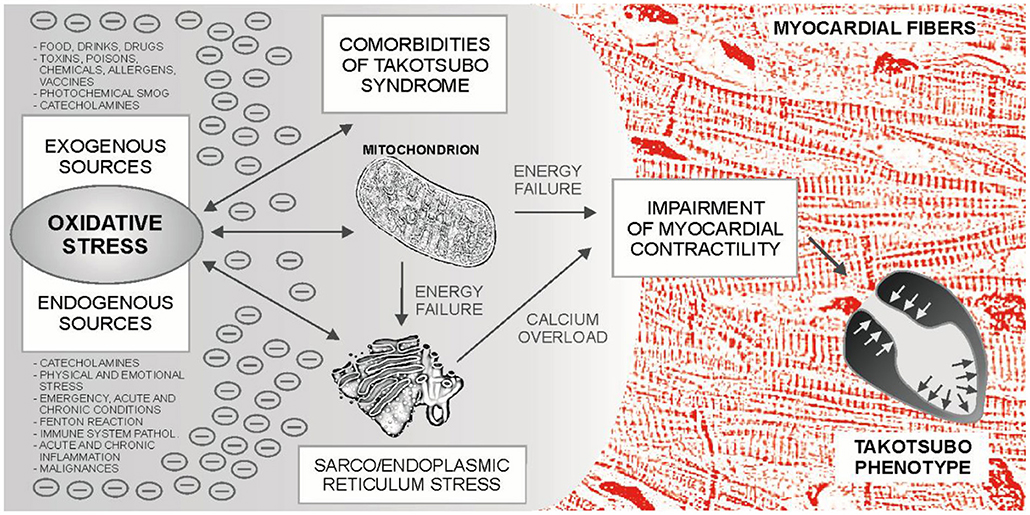
Figure 1. Sources and effects of oxidative stress (OS) in Takotsubo syndrome (TTS). Prior to the development of an acute attack of TTS, various sources of OS can be identified, which can be combined with each other. The main sources of oxidative stress in the body are mitochondria and the mitochondria - sarco/endoplasmic reticulum complex, which are involved in xenobiotic metabolism, calcium turnover and myocardial contractility, among others. Catecholamines can act as metabolic accelerators in this system. Finally, accumulated oxidative stress could lead to the blockade of energy production, disruption of calcium turnover in the cardiomyocyte, impaired contractility, and to the development of the Takotsubo phenotype.
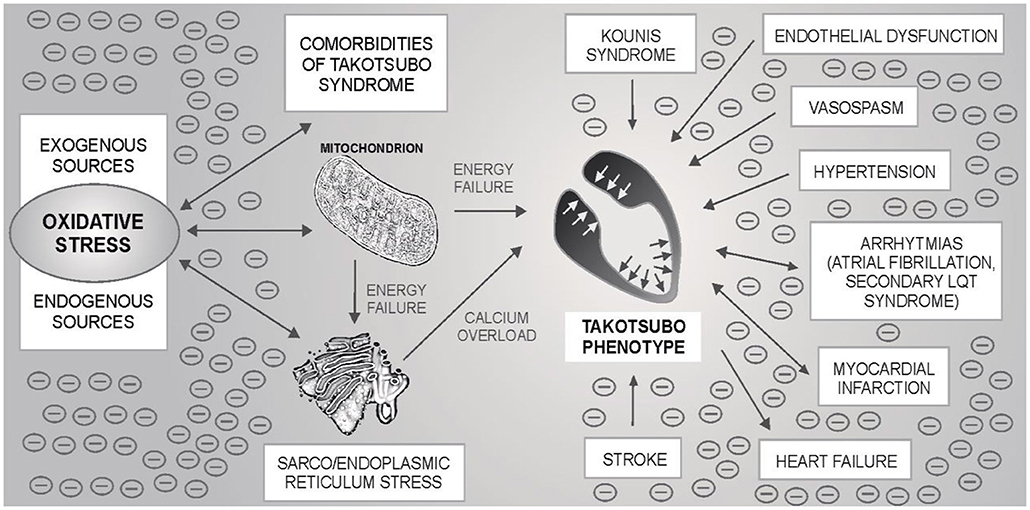
Figure 2. Possible relationship between oxidative stress (OS), mitochondria-sarco/endoplasmic reticulum complex, Takotsubo syndrome (TTS) and other cardiovascular pathologies. By the mechanisms described in the text, OS can lead to the blockade of energy production and calcium overload of myocytes with the subsequent development of an acute attack of TTS. OS is also associated with some TTS comorbidities - one of the most common is hypertension, but also e.g., atrial fibrillation. Secondary long QT syndrome and/or heart failure may be present in TTS. Stroke (ischemic or hemorrhagic) and, exceptionally, myocardial infarction have been described as the triggers of an acute TTS attack. If TTS is associated with OS, an acute attack itself can be an important source of OS. The oxidation load is likely to be potentiated. OS may affect other cardiac structures, incl. coronary (micro) circulation or cardiac conduction system. Endothelial dysfunction and vasospasm have previously been mentioned as possible pathophysiological mechanisms in TTS. Under these circumstances, an acute TTS attack could trigger an acute myocardial infarction. Kounis syndrome is an “allergic” acute coronary syndrome caused by allergic, hypersensitivity, anaphylactic or anaphylactoid conditions. There are three types of the disease: caused by a coronary artery spasm, by the acceleration of the pre-existing atherosclerotic changes in the coronary arteries, and by hypersensitivity reactions to the coronary stent material leading to “in stent” thrombosis, respectively.
Our approach can help in the complex description of the circumstances leading to an acute attack. OS could link three similar heart pathologies with similar or identical triggers – Takotsubo syndrome, Kounis syndrome and neurogenic stunned myocardium.
The correctness of this hypothesis should be confirmed by a detailed analysis of clinical cases, direct biochemical evidence in patients with acute TTS attack and subsequent modeling of TTS in the experiment. TTS can be a much more complex pathology than it currently seems to be.
Data Availability Statement
The original contributions presented in the study are included in the article/supplementary material, further inquiries can be directed to the corresponding author.
Author Contributions
All authors listed have made a substantial, direct and intellectual contribution to the work, and approved it for publication.
Funding
This work was supported by the Ministry of Health of the Czech Republic – conceptual development of research organization (FNBr, 65269705; funding was given to University Hospital Brno).
Conflict of Interest
The authors declare that the research was conducted in the absence of any commercial or financial relationships that could be construed as a potential conflict of interest.
Publisher's Note
All claims expressed in this article are solely those of the authors and do not necessarily represent those of their affiliated organizations, or those of the publisher, the editors and the reviewers. Any product that may be evaluated in this article, or claim that may be made by its manufacturer, is not guaranteed or endorsed by the publisher.
Acknowledgments
We dedicate this article to all health professionals, paramedics, and volunteers working against the COVID-19 pandemic.
Abbreviations
AMP, adenosine monophosphate; AMPK, AMP-activated protein kinase; ADP, adenosine diphosphate; ATP, adenosine triphosphate; βAR-GRK2, βadrenergic receptor Gprotein-coupled receptor kinase 2 complex; βAR, βadrenergic receptors; BOS, basal oxidative stress; cADPR, cyclic adenosine monophosphate ribose; CAT, catalase; CMR, cardiac magnetic resonance; CNS, central nervous system; COMT, catechol-o-methyltransferase; COPD, chronic obstructive pulmonary disease; CRP, C reactive protein; CYP, cytochrome P450; DM, diabetes mellitus; DNA, deoxyribonucleic acid; DOS, dietary oxidative stress; DTH, delayed-type hypersensitivity; EGF, epidermal growth factor; ETC, electron transport chain; FADH, flavin adenine dinucleotide hydrogen; FFA, free farry acids; FR, free radicals; GPX, glutathione peroxidase; GSH, reduced glutathione; GSSG, glutathione disulfide; GST, glutathione S-transferase; HER2, human epidermal growth factor receptor 2; HIV, human immunodeficiency virus; HOS, high intensity oxidative stress; IFN-γ, interferon gamma; IOS, intermediate intensity oxidative stress; LOS, low intensity oxidative stress; MAO, monoamino oxidase; MCU, mitochondrial calcium uniporter; MIBG, meta-iodobenzylguanidine; Mfn1, 2, SER-located mitofusin 1, 2; MMO system, microsomal monooxigenase system; mPTP, mitochondrial permeability transition pore; NADH, nicotinamide adenine dinucleotide hydrogen; NADPH, nicotinamide adenine dinucleotide phosphate hydrogen; NCX, Na+/Ca2+ exchanger; NOS, nutritional oxidative stress; Nox, NADPH oxidase; NSAID, non-steroid antiinflammatory drug; NSM, neurogenic stunned myocardium; Nrf2, Nuclear factor erythroid-2-related factor 2; OS, oxidative stress; PET CT, positron emission tomography/computer tomography; PKA, protein kinase A; PLA2, phospholipase A2; PLN, phospholamban; POS, post-prandial oxidative stress; ROS, reactive oxygen species; RNS, reactive nitrogen species; RS, reactive species; RyR2, ryanodine receptors type 2; SER, sarco/endoplasmic reticulum; SERCA, sarco/endoplasmic reticulum calcium ATPase; SLN, sarcolipin; SOD, superoxide dismutase; SOS, severe (strong) oxidative stress; SNRI, serotonin-norepinephrin reuptake inhibitor; TNF-α, tumor necrosis factor alpha; TTS, Takotsubo syndrome; UPR, unfolded protein response; XO, xanthine oxidase.
References
1. Sato H, Uchida T, Dote K, Ishihara M. Tako-Tsubo-like left ventricular dysfunction due to multivessel coronary spasm. In: Kodama K, Haze K, Hori M, editors. Clinical Aspect of Myocardial Injury: From Ischemia to Heart Failure (in Japanese). Tokyo: Kagakuhyoronsha Publishing Co (1990). p 56–64.
2. Lyon AR, Bossone E, Schneider B, Sechtem U, Citro R, Underwood SR, et al. Current state of knowledge on Takotsubo syndrome: a position statement from the taskforce on takotsubo syndrome of the Heart Failure Association of the European Society of Cardiology: current state of knowledge on Takotsubo syndrome. Eur J Heart Fail. (2016) 18:8–27. doi: 10.1002/ejhf.424
3. Bybee KA, Kara T, Prasad A, Lerman A, Barsness GW, Wright RS, et al. Systematic review: transient left ventricular apical ballooning: a syndrome that mimics ST-segment elevation myocardial infarction. Ann Intern Med. (2004) 141:858–65. doi: 10.7326/0003-4819-141-11-200412070-00010
4. Gianni M, Dentali F, Grandi AM, Sumner G, Hiralal R, Lonn E. Apical ballooning syndrome or takotsubo cardiomyopathy: a systematic review. Eur Heart J. (2006) 27:1523–9. doi: 10.1093/eurheartj/ehl032
5. Kurowski V, Kaiser A, von Hof K, Killermann DP, Mayer B, Hartmann F, et al. Apical and midventricular transient left ventricular dysfunction syndrome (Tako-Tsubo Cardiomyopathy) frequency, mechanisms, and prognosis. Chest. (2007) 132:809–16. doi: 10.1378/chest.07-0608
6. Deshmukh A, Kumar G, Pant S, Rihal C, Murugiah K, Mehta JL. Prevalence of Takotsubo cardiomyopathy in the United States. Am Heart J. (2012) 164:66–71.e1. doi: 10.1016/j.ahj.2012.03.020
7. Brinjikji W, El-Sayed AM, Salka S. In-hospital mortality among patients with takotsubo cardiomyopathy: a study of the National Inpatient Sample 2008 to (2009). Am Heart J. (2012) 164:215–21. doi: 10.1016/j.ahj.2012.04.010
8. Templin C, Ghadri JR, Diekmann J, Napp LC, Bataiosu DR, Jaguszewski M, et al. Clinical features and outcomes of takotsubo (stress) cardiomyopathy. N Engl J Med. (2015) 373:929–38. doi: 10.1056/NEJMoa1406761
9. Omerovic E. How to think about stress-induced cardiomyopathy? Think “out of the box”! Scand Cardiovasc J. (2011) 45:67–71. doi: 10.3109/14017431.2011.565794
10. Prasad A, Lerman A, Rihal CS. Apical ballooning syndrome (Tako-Tsubo or stress cardiomyopathy): a mimic of acute myocardial infarction. Am Heart J. (2008) 155:408–17. doi: 10.1016/j.ahj.2007.11.008
11. Kawai S, Kitabatake A, Tomoike H. Takotsubo cardiomyopathy study grou. Guidelines for diagnosis of takotsubo (ampulla) cardiomyopathy. Circ J. (2007) 71:990–2. doi: 10.1253/circj.71.990
12. Parodi G, Citro R, Bellandi B, Provenza G, Marrani M, Bossone E, et al. Revised clinical diagnostic criteria for Tako-tsubo syndrome: the Tako-tsubo Italian Network proposal. Int J Cardiol. (2014) 172:282–3. doi: 10.1016/j.ijcard.2013.12.239
13. Pelliccia F, Parodi G, Greco C, Antoniucci D, Brenner R, Bossone E, et al. Comorbidities frequency in Takotsubo syndrome: an international collaborative systematic review including (1109). Patients. Am J Med. (2015) 128:654.e11–654.e19. doi: 10.1016/j.amjmed.2015.01.016
14. Department of Cardiology Karolinska Institute at Karolinska University Hospital Sweden S Y-H. Takotsubo syndrome triggered by acute coronary syndrome in a cohort of 20 patients: an often missed diagnosis. Int J Cardiol Res. (2015) 2:28–33. doi: 10.19070/2470-4563-150007
15. Angulo-Llanos R, Sanz-Ruiz R, Solis J, Fernández-Avilés F. Acute myocardial infarction: an uncommon complication of takotsubo cardiomyopathy. Catheter Cardiovasc Interv. (2013) 82:909–13. doi: 10.1002/ccd.24846
16. Y-Hassan S. Myocardial infarction in patients with takotsubo syndrome: trigger and consequence. Am J Med. (2018) 131:e217. doi: 10.1016/j.amjmed.2017.09.030
17. Nef HM, Möllmann H, Troidl C, Kostin S, Böttger T, Voss S, et al. Expression profiling of cardiac genes in Tako-Tsubo cardiomyopathy: insight into a new cardiac entity. J Mol Cell Cardiol. (2008) 44:395–404. doi: 10.1016/j.yjmcc.2007.10.015
18. Nanno T, Kobayashi S, Oda S, Ishiguchi H, Myoren T, Oda T, et al. Relationship between cardiac sympathetic hyperactivity and myocardial oxidative stress in patients with takotsubo cardiomyopathy. J Card Fail. (2015) 21:S146. doi: 10.1016/j.cardfail.2015.08.012
19. Surikow SY, Nguyen TH, Stafford I, Chapman M, Chacko S, Singh K, et al. Nitrosative stress as a modulator of inflammatory change in a model of takotsubo syndrome. JACC Basic Transl Sci. (2018) 3:213–26. doi: 10.1016/j.jacbts.2017.10.002
20. Mao S, Luo X, Li Y, He C, Huang F, Su C. Role of PI3K/AKT/mTOR pathway associated oxidative stress and cardiac dysfunction in takotsubo syndrome. Curr Neurovasc Res. (2020) 17:35–43. doi: 10.2174/1567202617666191223144715
21. Roshanzamir S, Showkathali R. Takotsubo cardiomyopathy a short review. Curr Cardiol Rev. (2013) 9:191–6. doi: 10.2174/1573403X11309030003
22. Sies H, Berndt C, Jones DP. Oxidative stress. Annu Rev Biochem. (2017) 86:715–48. doi: 10.1146/annurev-biochem-061516-045037
23. Betteridge DJ. What is oxidative stress? Metabolism. (2000) 49:3–8. doi: 10.1016/S0026-0495(00)80077-3
24. Phaniendra A, Jestadi DB, Periyasamy L. Free radicals: properties, sources, targets, and their implication in various diseases. Indian J Clin Biochem. (2015) 30:11–26. doi: 10.1007/s12291-014-0446-0
25. Sies H. Oxidative stress: a concept in redox biology and medicine. Redox Biol. (2015) 4:180–3. doi: 10.1016/j.redox.2015.01.002
26. Finkel T, Holbrook NJ. Oxidants, oxidative stress and the biology of ageing. Nature. (2000) 408:239–47. doi: 10.1038/35041687
27. Gagné F. Oxidative stress. In: Biochemical Ecotoxicology. Elsevier (2014). p. 103–15. doi: 10.1016/B978-0-12-411604-7.00006-4
28. Ott M, Gogvadze V, Orrenius S, Zhivotovsky B. Mitochondria, oxidative stress and cell death. Apoptosis. (2007) 12:913–22. doi: 10.1007/s10495-007-0756-2
29. Skulachev VP. Mitochondria-targeted antioxidants as promising drugs for treatment of age-related brain diseases. J Alzheimers Dis. (2012) 28:283–9. doi: 10.3233/JAD-2011-111391
30. Lushchak VI. Free radicals, reactive oxygen species, oxidative stress and its classification. Chem Biol Interact. (2014) 224:164–75. doi: 10.1016/j.cbi.2014.10.016
31. Gille L, Nohl H. The ubiquinol/bc1 redox couple regulates mitochondrial oxygen radical formation. Arch Biochem Biophys. (2001) 388:34–8. doi: 10.1006/abbi.2000.2257
32. Schulz E, Wenzel P, Münzel T, Daiber A. Mitochondrial redox signaling: interaction of mitochondrial reactive oxygen species with other sources of oxidative stress. Antioxid Redox Signal. (2014) 20:308–24. doi: 10.1089/ars.2012.4609
33. Hopps E, Noto D, Caimi G, Averna MR. A novel component of the metabolic syndrome: the oxidative stress. Nutr Metab Cardiovasc Dis. (2010) 20:72–7. doi: 10.1016/j.numecd.2009.06.002
34. Block G. Factors associated with oxidative stress in human populations. Am J Epidemiol. (2002) 156:274–85. doi: 10.1093/aje/kwf029
35. Mooney LA, Perera FP, Van Bennekum AM, Blaner WS, Karkoszka J, Covey L, et al. Gender differences in autoantibodies to oxidative DNA base damage in cigarette smokers. Cancer Epidemiol Biomark Prev. (2001) 10:641–8.
36. Lang CA, Naryshkin S, Schneider DL, Mills BJ, Lindeman RD. Low blood glutathione levels in healthy aging adults. J Lab Clin Med. (1992) 120:720–5.
37. Simioni C, Zauli G, Martelli AM, Vitale M, Sacchetti G, Gonelli A, et al. Oxidative stress: role of physical exercise and antioxidant nutraceuticals in adulthood and aging. Oncotarget. (2018) 9:17181–98. doi: 10.18632/oncotarget.24729
38. Galbusera C, Orth P, Fedida D, Spector T. Superoxide radical production by allopurinol and xanthine oxidase. Biochem Pharmacol. (2006) 71:1747–52. doi: 10.1016/j.bcp.2006.02.008
39. Griguer CE, Oliva CR, Kelley EE, Giles GI, Lancaster JR, Gillespie GY. Xanthine oxidase-dependent regulation of hypoxia-inducible factor in cancer cells. Cancer Res. (2006) 66:2257–63. doi: 10.1158/0008-5472.CAN-05-3364
40. Nanduri J, Vaddi DR, Khan SA, Wang N, Makerenko V, Prabhakar NR. Xanthine oxidase mediates hypoxia-inducible factor-2α degradation by intermittent hypoxia. Eltzschig HK, editor. PLoS ONE. (2013) 8:e75838. doi: 10.1371/journal.pone.0075838
41. Miller JW, Selhub J, Joseph JA. Oxidative damage caused by free radicals produced during catecholamine autoxidation: protective effects of O-methylation and melatonin. Free Radic Biol Med. (1996) 21:241–9. doi: 10.1016/0891-5849(96)00033-0
42. Saller S, Merz-Lange J, Raffael S, Hecht S, Pavlik R, Thaler C, et al. Norepinephrine, active norepinephrine transporter, and norepinephrine-metabolism are involved in the generation of reactive oxygen species in human ovarian granulosa cells. Endocrinology. (2012) 153:1472–83. doi: 10.1210/en.2011-1769
43. Renaud HJ, Rutter A, Winn LM. Assessment of xenobiotic biotransformation including reactive oxygen species generation in the embryo using benzene as an example. In: Harris C, Hansen JM, editors. Developmental Toxicology. Totowa, NJ: Humana Press (2012). p. 253–63. (Methods in Molecular Biology; vol. 889). Available online at: http://link.springer.com/10.1007/978-1-61779-867-2_15 (accessed November 2, 2020).
44. Michail K, Baghdasarian A, Narwaley M, Aljuhani N, Siraki AG. Scavenging of free-radical metabolites of aniline xenobiotics and drugs by amino acid derivatives: toxicological implications of radical-transfer reactions. Chem Res Toxicol. (2013) 26:1872–83. doi: 10.1021/tx4002463
45. Granot E, Kohen R. Oxidative stress in childhood-in health and disease states. Clin Nutr. (2004) 23:3–11. doi: 10.1016/S0261-5614(03)00097-9
46. Moldogazieva NT, Mokhosoev IM, Feldman NB, Lutsenko SV. ROS and RNS signalling: adaptive redox switches through oxidative/nitrosative protein modifications. Free Radic Res. (2018) 52:507–43. doi: 10.1080/10715762.2018.1457217
48. Di Lisa F, Canton M, Carpi A, Kaludercic N, Menabò R, Menazza S, et al. Mitochondrial injury and protection in ischemic pre- and postconditioning. Antioxid Redox Signal. (2011) 14:881–91. doi: 10.1089/ars.2010.3375
49. Vetrani C, Costabile G, Di Marino L, Rivellese AA. Nutrition and oxidative stress: a systematic review of human studies. Int J Food Sci Nutr. (2013) 64:312–26. doi: 10.3109/09637486.2012.738651
50. Giordano FJ. Oxygen, oxidative stress, hypoxia, and heart failure. J Clin Invest. (2005) 115:500–8. doi: 10.1172/JCI200524408
51. Bolli R. Mechanism of myocardial ‘stunning'. Circulation. (1990) 82:723–38. doi: 10.1161/01.CIR.82.3.723
52. Dhalla NS. Formation of aminochrome leads to cardiac dysfunction and sudden cardiac death. Circ Res. (2018) 123:409–11. doi: 10.1161/CIRCRESAHA.118.313416
53. Finsterer J, Ohnsorge P. Influence of mitochondrion-toxic agents on the cardiovascular system. Regul Toxicol Pharmacol RTP. (2013) 67:434–45. doi: 10.1016/j.yrtph.2013.09.002
54. Scatena R. Mitochondria and drugs. Adv Exp Med Biol. (2012) 942:329–46. doi: 10.1007/978-94-007-2869-1_15
55. Amariles P. A comprehensive literature search: drugs as possible triggers of takotsubo cardiomyopathy. Curr Clin Pharmacol. (2011) 6:1–11. doi: 10.2174/157488411794941340
56. Bernards J, Van Kolen K, Govaerts E, Somville FJ, von Stritzky M. Transient left ventricular apical ballooning syndrome in a patient with allopurinol induced skin rash: a case report. Int J Cardiol. (2014) 177:e53–5. doi: 10.1016/j.ijcard.2014.09.130
57. Abbasi D, Mannan H, Patel V, Farhan Hasni S. Rare cardiac manifestation of a commonly prescribed drug: takotsubo cardiomyopathy caused by allopurinol induced dress syndrome. J Heart Cardiovasc Res. (2018) 2:1–6. doi: 10.21767/2576-1455.1000116
58. Coen M, Rigamonti F, Roth A, Koessler T. Chemotherapy-induced Takotsubo cardiomyopathy, a case report and review of the literature. BMC Cancer. (2017) 17:394. doi: 10.1186/s12885-017-3384-4
59. Desai A, Noor A, Joshi S, Kim AS. Takotsubo cardiomyopathy in cancer patients. Cardio-Oncol. (2019) 5:7. doi: 10.1186/s40959-019-0042-9
60. Budnik M, Kucharz J, Wiechno P, Demkow T, Kochanowski J, Górska E, et al. Chemotherapy-induced takotsubo syndrome. In: Pokorski M, editor. Clinical Pulmonary Research. Cham: Springer International Publishing (2018). p. 19–29. (Advances in Experimental Medicine and Biology; vol. 1114). Available online at: http://link.springer.com/10.1007/5584_2018_222 (accessed November 3, 2020).
61. Pai VB, Nahata MC. Cardiotoxicity of chemotherapeutic agents: incidence, treatment and prevention. Drug Saf. (2000) 22:263–302. doi: 10.2165/00002018-200022040-00002
62. Angsutararux P, Luanpitpong S, Issaragrisil S. Chemotherapy-induced cardiotoxicity: overview of the roles of oxidative stress. Oxid Med Cell Longev. (2015) 2015:1–13. doi: 10.1155/2015/795602
63. Costantini D, Marasco V, Møller AP. A meta-analysis of glucocorticoids as modulators of oxidative stress in vertebrates. J Comp Physiol B. (2011) 181:447–56. doi: 10.1007/s00360-011-0566-2
64. Wickboldt N, Pache J-C, Dietrich P-Y, Toso C, Gallay C, Brochard L, et al. Takotsubo syndrome secondary to adrenal adenocarcinoma: cortisol as a possible culprit. Am J Respir Crit Care Med. (2012) 186:1061–2. doi: 10.1164/ajrccm.186.10.1061
65. Teppo H-R, Soini Y, Karihtala P. Reactive oxygen species-mediated mechanisms of action of targeted cancer therapy. Oxid Med Cell Longev. (2017) 2017:1–11. doi: 10.1155/2017/1485283
66. Hayes JD, Strange RC. Glutathione S-transferase polymorphisms and their biological consequences. Pharmacology. (2000) 61:154–66. doi: 10.1159/000028396
67. Strange RC, Fryer AA. The glutathione S-transferases: influence of polymorphism on cancer susceptibility. IARC Sci Publ. (1999) 148:231–49.
68. Tamer L, Calikoglu M, Ates NA, Yildirim H, Ercan B, Saritas E, et al. Glutathione-S-transferase gene polymorphisms (GSTT1, GSTM1, GSTP1) as increased risk factors for asthma. Respirology. (2004) 9:493–8. doi: 10.1111/j.1440-1843.2004.00657.x
69. Mondola P, Damiano S, Sasso A, Santillo M. The Cu, Zn superoxide dismutase: not only a dismutase enzyme. Front Physiol. (2016) 7:594. doi: 10.3389/fphys.2016.00594
70. Zhou S-F. Drugs behave as substrates, inhibitors and inducers of human cytochrome P450 3A4. Curr Drug Metab. (2008) 9:310–22. doi: 10.2174/138920008784220664
71. Zhou S-F, Xue CC, Yu X-Q, Li C, Wang G. Clinically important drug interactions potentially involving mechanism-based inhibition of cytochrome P450 3A4 and the role of therapeutic drug monitoring. Ther Drug Monit. (2007) 29:687–710. doi: 10.1097/FTD.0b013e31815c16f5
72. Preissner SC, Hoffmann MF, Preissner R, Dunkel M, Gewiess A, Preissner S. Polymorphic cytochrome P450 enzymes (CYPs) and their role in personalized therapy. Nie D, editor. PLoS ONE. (2013) 8:e82562. doi: 10.1371/journal.pone.0082562
73. Shet MS, Fisher CW, Holmans PL, Estabrook RW. Human cytochrome P450 3A4: enzymatic properties of a purified recombinant fusion protein containing NADPH-P450 reductase. Proc Natl Acad Sci. (1993) 90:11748–52. doi: 10.1073/pnas.90.24.11748
74. Fleming I. The pharmacology of the cytochrome P450 epoxygenase/soluble epoxide hydrolase axis in the vasculature and cardiovascular disease. Simonsen U, editor. Pharmacol Rev. (2014) 66:1106–40. doi: 10.1124/pr.113.007781
75. Zanger UM, Schwab M. Cytochrome P450 enzymes in drug metabolism: regulation of gene expression, enzyme activities, and impact of genetic variation. Pharmacol Ther. (2013) 138:103–41. doi: 10.1016/j.pharmthera.2012.12.007
76. Sotaniemi EA, Arranto AJ, Pelkonen O, Pasanen M. Age and cytochrome P450-linked drug metabolism in humans: an analysis of 226 subjects with equal histopathologic conditions*. Clin Pharmacol Ther. (1997) 61:331–9. doi: 10.1016/S0009-9236(97)90166-1
77. Xu M, Ju W, Hao H, Wang G, Li P. Cytochrome P450 2J2: distribution, function, regulation, genetic polymorphisms and clinical significance. Drug Metab Rev. (2013) 45:311–52. doi: 10.3109/03602532.2013.806537
78. Evangelista EA, Kaspera R, Mokadam NA, Jones JP, Totah RA. Activity, Inhibition, and induction of cytochrome P450 2J2 in adult human primary cardiomyocytes. Drug Metab Dispos. (2013) 41:2087–94. doi: 10.1124/dmd.113.053389
79. Murray M. CYP2J2 - regulation, function and polymorphism. Drug Metab Rev. (2016) 48:351–68. doi: 10.1080/03602532.2016.1188938
80. Alberts B. Chapter 16 - cell signaling. In: Morales M, editor. Essential Cell Biology. 4th ed. New York, NY: Garland Science (2014). p. 539–51.
81. Lyon AR, Rees PS, Prasad S, Poole-Wilson PA, Harding SE. Stress (Takotsubo) cardiomyopathy-a novel pathophysiological hypothesis to explain catecholamine-induced acute myocardial stunning. Nat Clin Pract Cardiovasc Med. (2008) 5:22–9. doi: 10.1038/ncpcardio1066
82. Paur H, Wright PT, Sikkel MB, Tranter MH, Mansfield C, O'Gara P, et al. High levels of circulating epinephrine trigger apical cardiodepression in a β2-adrenergic receptor/Gi-dependent manner: a new model of Takotsubo cardiomyopathy. Circulation. (2012) 126:697–706. doi: 10.1161/CIRCULATIONAHA.112.111591
83. Koeth O, Mark B, Zahn R, Zeymer U. Midventricular form of takotsubo cardiomyopathy as a recurrence 1 year after typical apical ballooning: a case report. Cases J. (2008) 1:331. doi: 10.1186/1757-1626-1-331
84. Blessing E, Steen H, Rosenberg M, Katus H, Frey N. Recurrence of takotsubo cardiomyopathy with variant forms of left ventricular dysfunction. J Am Soc Echocardiogr. (2007) 20:439.e11–2. doi: 10.1016/j.echo.2006.10.021
85. Xiao RP, Tomhave ED, Wang DJ, Ji X, Boluyt MO, Cheng H, et al. Age-associated reductions in cardiac beta1- and beta2-adrenergic responses without changes in inhibitory G proteins or receptor kinases. J Clin Invest. (1998) 101:1273–82. doi: 10.1172/JCI1335
86. Spyrou N, Rosen SD, Fath-Ordoubadi F, Jagathesan R, Foale R, Kooner JS, et al. Myocardial beta-adrenoceptor density one month after acute myocardial infarction predicts left ventricular volumes at six months. J Am Coll Cardiol. (2002) 40:1216–24. doi: 10.1016/S0735-1097(02)02162-9
87. Lombardi MS, Kavelaars A, Penela P, Scholtens EJ, Roccio M, Schmidt RE, et al. Oxidative stress decreases G protein-coupled receptor kinase 2 in lymphocytes via a calpain-dependent mechanism. Mol Pharmacol. (2002) 62:379–88. doi: 10.1124/mol.62.2.379
88. Y-Hassan S. Plasma Epinephrine Level and its Causal Link to Takotsubo Syndrome revisited: critical review with a diverse conclusion. Cardiovasc Revasc Med. (2019) 20:907–14. doi: 10.1016/j.carrev.2018.10.026
89. Y-Hassan S, Henareh L. Plasma catecholamine levels in patients with takotsubo syndrome: implications for the pathogenesis of the disease. Int J Cardiol. (2015) 181:35–8. doi: 10.1016/j.ijcard.2014.11.149
90. Hartmann C, Radermacher P, Wepler M, Nußbaum B. Non-hemodynamic effects of catecholamines. Shock. (2017) 48:390–400. doi: 10.1097/SHK.0000000000000879
91. Mayer SE. Effect of catecholamines on cardiac metabolism. Circ Res. (1974) 35(Suppl. 3):129–37. doi: 10.1161/res.35.3_supplement.iii-129
92. Schönfeld P, Wojtczak L. Fatty acids decrease mitochondrial generation of reactive oxygen species at the reverse electron transport but increase it at the forward transport. Biochim Biophys Acta BBA Bioenerg. (2007) 1767:1032–40. doi: 10.1016/j.bbabio.2007.04.005
93. Sirota TV. A novel approach to study the reaction of adrenaline autooxidation: a possibility for polarographic determination of superoxide dismutase activity and antioxidant properties of various preparations. Biochem Mosc Suppl Ser B Biomed Chem. (2011) 5:253–9. doi: 10.1134/S1990750811030139
94. Odajima T, Onishi M. Formation of adrenochrome from epinephrine by myeloperoxidase via a free radical: its biological significance. Jpn J Oral Biol. (1997) 39:297–303. doi: 10.2330/joralbiosci1965.39.297
95. Behonick GS, Novak MJ, Nealley EW, Baskin SI. Toxicology update: the cardiotoxicity of the oxidative stress metabolites of catecholamines (aminochromes). J Appl Toxicol. (2001) 21:S15–22. doi: 10.1002/jat.793
96. Heacock RA, Powell WS. 6 adrenochrome and related compounds. In: Progress in Medicinal Chemistry. Amsterdam: Elsevier (1973). p. 275–340. Available online at: https://linkinghub.elsevier.com/retrieve/pii/S0079646808704016 (accessed November 14, 2020).
97. Matthews S, Henderson A, Campbell A. The adrenochrome pathway: the major route for adrenalin catabolism by polymorphonuclear leucocytes. J Mol Cell Cardiol. (1985) 17:339–48. doi: 10.1016/S0022-2828(85)80133-4
98. Valerino DM, McCormack JJ. Xanthine oxidase-mediated oxidation of epinephrine. Biochem Pharmacol. (1971) 20:47–55. doi: 10.1016/0006-2952(71)90470-9
99. Costa VM, Silva R, Ferreira LM, Branco PS, Carvalho F, Bastos ML, et al. Oxidation process of adrenaline in freshly isolated rat cardiomyocytes: formation of adrenochrome, quinoproteins, and GSH adduct. Chem Res Toxicol. (2007) 20:1183–91. doi: 10.1021/tx7000916
100. Guarnieri C, Ventura C. Formation of adrenochrome by bovine cardiac sarcolemma. Biochem Biophys Res Commun. (1984) 120:22–7. doi: 10.1016/0006-291X(84)91408-6
101. Guarnieri C, Ventura C, Georgountzos A, Muscari C, Budini R. Involvement of superoxide radicals on adrenochrome formation stimulated by arachidonic acid in bovine heart sarcolemmal vesicles. Biochim Biophys Acta BBA Gen Subj. (1985) 838:355–60. doi: 10.1016/0304-4165(85)90234-X
102. Manousek J, Stejskal V, Kubena P, Jarkovsky J, Nemec P, Lokaj P, et al. Delayed-type hypersensitivity to metals of environmental burden in patients with takotsubo syndrome - is there a clinical relevance? Andò G, editor. PLoS ONE. (2016) 11:e0164786. doi: 10.1371/journal.pone.0164786
103. Karon BS, Daly TM, Scott MG. Mechanisms of dopamine and dobutamine interference in biochemical tests that use peroxide and peroxidase to generate chromophore. Clin Chem. (1998) 44:155–60. doi: 10.1093/clinchem/44.1.155
104. Kinae N, Masuda H, Shin IS, Furugori M, Shimoi K. Functional properties of wasabi and horseradish. BioFactors. (2000) 13:265–9. doi: 10.1002/biof.5520130140
105. Finkel-Oron A, Olchowski J, Jotkowitz A, Barski L. Takotsubo cardiomyopathy triggered by wasabi consumption: can sushi break your heart? BMJ Case Rep. (2019) 12:e230065. doi: 10.1136/bcr-2019-230065
106. Jeon U, Park S, Park S, Lee E, Gil H-W. Clinical characteristics of stress cardiomyopathy in patients with acute poisoning. Sci Rep. (2018) 8:223. doi: 10.1038/s41598-017-18478-5
107. Baez S, Segura-Aguilar J, Widersten M, Johansson A-S, Mannervik B. Glutathione transferases catalyse the detoxication of oxidized metabolites (o-quinones) of catecholamines and may serve as an antioxidant system preventing degenerative cellular processes. Biochem J. (1997) 324:25–8. doi: 10.1042/bj3240025
108. Fliegel L, Takeo S, Beamish RE, Dhalla NS. Adrenochrome uptake and subcellular distribution in the isolated perfused rat heart. Can J Cardiol. (1985) 1:122–7.
109. Takeo S, Taam GM, Beamish RE, Dhalla NS. Effects of adrenochrome on calcium accumulating and adenosine triphosphatase activities of the rat heart microsomes. J Pharmacol Exp Ther. (1980) 214:688–93.
110. Tappia PS, Hata T, Hozaima L, Sandhu MS, Panagia V, Dhalla NS. Role of oxidative stress in catecholamine-induced changes in cardiac sarcolemmal Ca2+ transport. Arch Biochem Biophys. (2001) 387:85–92. doi: 10.1006/abbi.2000.2234
111. Taam GM, Takeo S, Ziegelhoffer A, Singal PK, Beamish RE, Dhalla NS. Effect of adrenochrome on adenine nucleotides and mitochondrial oxidative phosphorylation in rat heart. Can J Cardiol. (1986) 2:88–93.
112. Yates JC, Beamish RE, Dhalla NS. Ventricular dysfunction and necrosis produced by adrenochrome metabolite of epinephrine: relation to pathogenesis of catecholamine cardiomyopathy. Am Heart J. (1981) 102:210–21. doi: 10.1016/S0002-8703(81)80012-9
113. Mitchell A, Marquis F. Can takotsubo cardiomyopathy be diagnosed by autopsy? Report of a presumed case presenting as cardiac rupture. BMC Clin Pathol. (2017) 17:4. doi: 10.1186/s12907-017-0045-0
114. Kawai S. Pathology of takotsubo (ampulla) cardiomyopathy. In: Veselka J, editor. Cardiomyopathies - From Basic Research to Clinical Management. InTech (2012). Available online at: http://www.intechopen.com/books/cardiomyopathies-from-basic-research-to-clinical-management/pathology-of-takotsubo-ampulla-cardiomyopahty (accessed January 2, 2021).
115. Pascual I, Abó AI, Piqué M. Histological findings in tako-tsubo syndrome. Rev Esp Cardiol Engl Ed. (2015) 68:625. doi: 10.1016/j.rec.2014.08.013
116. Devaraj S, Wang-Polagruto J, Polagruto J, Keen CL, Jialal I. High-fat, energy-dense, fast-food-style breakfast results in an increase in oxidative stress in metabolic syndrome. Metabolism. (2008) 57:867–70. doi: 10.1016/j.metabol.2008.02.016
117. Hu Y, Block G, Norkus EP, Morrow JD, Dietrich M, Hudes M. Relations of glycemic index and glycemic load with plasma oxidative stress markers. Am J Clin Nutr. (2006) 84:70–6. doi: 10.1093/ajcn/84.1.70
118. Ghadri JR, Sarcon A, Diekmann J, Bataiosu DR, Cammann VL, Jurisic S, et al. Happy heart syndrome: role of positive emotional stress in takotsubo syndrome. Eur Heart J. (2016) 37:2823–9. doi: 10.1093/eurheartj/ehv757
119. Hinchman CA, Ballatori N. Glutathione conjugation and conversion to mercapturic acids can occur as an intrahepatic process. J Toxicol Environ Health. (1994) 41:387–409. doi: 10.1080/15287399409531852
120. Lenzi M, Cocchi V, Malaguti M, Barbalace MC, Marchionni S, Hrelia S, et al. 6-(Methylsulfonyl) hexyl isothiocyanate as potential chemopreventive agent: molecular and cellular profile in leukaemia cell lines. Oncotarget. (2017) 8:111697–714. doi: 10.18632/oncotarget.22902
121. Nomura T, Shinoda S, Yamori T, Sawaki S, Nagata I, Ryoyama K, et al. Selective sensitivity to wasabi-derived 6-(methylsulfinyl)hexyl isothiocyanate of human breast cancer and melanoma cell lines studied in vitro. Cancer Detect Prev. (2005) 29:155–60. doi: 10.1016/j.cdp.2004.07.010
122. Lushchak VI. Glutathione homeostasis and functions: potential targets for medical interventions. J Amino Acids. (2012) 2012:1–26. doi: 10.1155/2012/736837
123. Bakke J, Gustafsson J-Å. Mercapturic acid pathway metabolites of xenobiotics: generation of potentially toxic metabolites during enterohepatic circulation. Trends Pharmacol Sci. (1984) 5:517–21. doi: 10.1016/0165-6147(84)90532-7
124. Reis R, Charehsaz M, Sipahi H, Ekici AID, Macit Ç, Akkaya H, et al. Energy drink induced lipid peroxidation and oxidative damage in rat liver and brain when used alone or combined with alcohol: energy drink induced oxidative damage in rat combined with alcohol. J Food Sci. (2017) 82:1037–43. doi: 10.1111/1750-3841.13662
125. Das SK, Vasudevan DM. Alcohol-induced oxidative stress. Life Sci. (2007) 81:177–87. doi: 10.1016/j.lfs.2007.05.005
126. Meyer CG, Gabasha S, Gurujal R, Vacek TP. Down on your luck: cardiomyopathy precipitated by a bar fight. Oxf Med Case Rep. (2018) 2018:omy012. doi: 10.1093/omcr/omy012
127. Ozguner F, Koyu A, Cesur G. Active smoking causes oxidative stress and decreases blood melatonin levels. Toxicol Ind Health. (2005) 21:21–6. doi: 10.1191/0748233705th211oa
128. Kovacic P. Role of oxidative metabolites of cocaine in toxicity and addiction: oxidative stress and electron transfer. Med Hypotheses. (2005) 64:350–6. doi: 10.1016/j.mehy.2004.06.028
129. Gill D, Sheikh N, Ruiz VG, Liu K. Case report: cocaine-induced takotsubo cardiomyopathy. Hell J Cardiol HJC Hell Kardiologike Epitheorese. (2018) 59:129–32. doi: 10.1016/j.hjc.2017.05.008
130. Sundboll J, Pareek M, Hogsbro M, Madsen EH. Iatrogenic takotsubo cardiomyopathy induced by locally applied epinephrine and cocaine. Case Rep. (2014) 2014:bcr2013202401-bcr2013202401. doi: 10.1136/bcr-2013-202401
131. Vanova N, Pejchal J, Herman D, Dlabkova A, Jun D. Oxidative stress in organophosphate poisoning: role of standard antidotal therapy: oxidative stress in organophosphate poisoning: role of antidotes. J Appl Toxicol. (2018) 38:1058–70. doi: 10.1002/jat.3605
132. Kitami M, Oizumi H, Kish SJ, Furukawa Y. Takotsubo cardiomyopathy associated with lithium intoxication in bipolar disorder: a case report. J Clin Psychopharmacol. (2014) 34:410–1. doi: 10.1097/JCP.0b013e3182a95a27
133. Schroeder I, Zoller M, Angstwurm M, Kur F, Frey L. Venlafaxine intoxication with development of takotsubo cardiomyopathy: successful use of extracorporeal life support, intravenous lipid emulsion and cytosorb®. Int J Artif Organs. (2017) 40:358–60. doi: 10.5301/ijao.5000595
134. Romanò M, Zorzoli F, Bertona R, Villani R. Takotsubo cardiomyopathy as an early complication of drug-induced suicide attempt. Case Rep Med. (2013) 2013:1–4. doi: 10.1155/2013/946378
135. Tonomura S, Kakehi Y, Sato M, Naito Y, Shimizu H, Goto Y, et al. Takotsubo-like myocardial dysfunction in a patient with botulism. Intern Med. (2017) 56:2925–7. doi: 10.2169/internalmedicine.8968-17
136. Jung JM, Kim YH, Park MH, Kwon DY. Takotsubo cardiomyopathy following severe tetanus. Neurology Asia. (2012) 17:75–8. doi: 10.2169/internalmedicine.2494-18
137. Lemor A, Lee S, Gongora C, Gholitabar F, Mehta D. Stress induce cardiomyopathy (takotsubo cardiomyopathy): mortality, gender, and admission month based on a nationwide sample during (2013). Abstract 1297-320. In: Presented at: the 66th Scientific Session & Expo of the American College of Cardiology. Washington, DC (2017). Available oniline at: https://www.thecardiologyadvisor.com/acc-2017-meeting-highlights/seasonal-takotsubo-cardiomyopathy/article/646521/ (accessed June 29, 2021).
138. Brimblecombe P. Air pollution episodes. In: Encyclopedia of Environmental Health. Elsevier (2011). p. 39–45. Available online at: https://linkinghub.elsevier.com/retrieve/pii/B9780444522726000581 (accessed November 14, 2020).
139. Jaffe DA. The nitrogen cycle. In: International Geophysics. Elsevier (2000). p. 322–42. Available online at: https://linkinghub.elsevier.com/retrieve/pii/S0074614200801187 (accessed November 14, 2020).
140. Amann M. Health Risks of Ozone From Long-Range Transboundary Air Pollution. Edited by: Frank Theakston. WHO Regional Office Europe (2008). P. xi. Available online at: https://www.euro.who.int/__data/assets/pdf_file/0005/78647/E91843.pdf (accessed June 29, 2021).
141. Selye H. A syndrome produced by diverse nocuous agents. Nature. (1936) 138:32. doi: 10.1038/138032a0
142. Schwarzer R, Schulz U. Stressful life events. In: Weiner IB, editor. Handbook of Psychology. Hoboken, NJ: John Wiley & Sons, Inc. (2003). p. wei0902. Available online at: http://doi.wiley.com/10.1002/0471264385.wei0902 (accessed November 15, 2020).
143. Spiers JG, Chen H-JC, Sernia C, Lavidis NA. Activation of the hypothalamic-pituitary-adrenal stress axis induces cellular oxidative stress. Front Neurosci. (2015) 8:456. doi: 10.3389/fnins.2014.00456
144. Schiavone S, Jaquet V, Trabace L, Krause K-H. Severe life stress and oxidative stress in the brain: from animal models to human pathology. Antioxid Redox Signal. (2013) 18:1475–90. doi: 10.1089/ars.2012.4720
145. RajMohan V, Mohandas E. The limbic system. Indian J Psychiatry. (2007) 49:132. doi: 10.4103/0019-5545.33264
146. Teague CR, Dhabhar FS, Barton RH, Beckwith-Hall B, Powell J, Cobain M, et al. Metabonomic studies on the physiological effects of acute and chronic psychological stress in sprague–dawley rats. J Proteome Res. (2007) 6:2080–93. doi: 10.1021/pr060412s
147. Akashi YJ, Goldstein DS, Barbaro G, Ueyama T. Takotsubo cardiomyopathy: a new form of acute, reversible heart failure. Circulation. (2008) 118:2754–62. doi: 10.1161/CIRCULATIONAHA.108.767012
148. Giannakopoulos K, El-Battrawy I, Schramm K, Ansari U, Hoffmann U, Borggrefe M, et al. Comparison and outcome analysis of patients with takotsubo cardiomyopathy triggered by emotional stress or physical stress. Front Psychol. (2017) 8:527. doi: 10.3389/fpsyg.2017.00527
149. Hellsten Y, Frandsen U, Orthenblad N, Sjødin B, Richter EA. Xanthine oxidase in human skeletal muscle following eccentric exercise: a role in inflammation. J Physiol. (1997) 498:239–48. doi: 10.1113/jphysiol.1997.sp021855
150. Anthonymuthu TS, Kim-Campbell N, Bayir H. Oxidative lipidomics: applications in critical care. Curr Opin Crit Care. (2017) 23:251–6. doi: 10.1097/MCC.0000000000000419
151. Sen CK. Glutathione homeostasis in response to exercise training and nutritional supplements. In: Das DK, editor. Stress Adaptation, Prophylaxis and Treatment. Boston, MA: Springer (1999). p. 31–42. Available online at: http://link.springer.com/10.1007/978-1-4615-5097-6_4 (accessed November 15, 2020).
152. Christensen TE, Bang LE, Holmvang L, Hasbak P, Kjær A, Bech P, et al. Neuroticism, depression and anxiety in takotsubo cardiomyopathy. BMC Cardiovasc Disord. (2016) 16:118. doi: 10.1186/s12872-016-0277-4
153. Friedman J. Why is the nervous system vulnerable to oxidative stress? In: Gadoth N, Göbel HH, editors. Oxidative Stress and Free Radical Damage in Neurology. Totowa, NJ: Humana Press (2011). p. 19–27. Available online at: http://link.springer.com/10.1007/978-1-60327-514-9_2 (accessed November 15, 2020).
154. Cobley JN, Fiorello ML, Bailey DM. 13 reasons why the brain is susceptible to oxidative stress. Redox Biol. (2018) 15:490–503. doi: 10.1016/j.redox.2018.01.008
155. Du J, Wang Y, Hunter R, Wei Y, Blumenthal R, Falke C, et al. Dynamic regulation of mitochondrial function by glucocorticoids. Proc Natl Acad Sci. (2009) 106:3543–8. doi: 10.1073/pnas.0812671106
156. You J-M, Yun S-J, Nam KN, Kang C, Won R, Lee EH. Mechanism of glucocorticoid-induced oxidative stress in rat hippocampal slice cultures. Can J Physiol Pharmacol. (2009) 87:440–7. doi: 10.1139/Y09-027
157. Chetty S, Friedman AR, Taravosh-Lahn K, Kirby ED, Mirescu C, Guo F, et al. Stress and glucocorticoids promote oligodendrogenesis in the adult hippocampus. Mol Psychiatry. (2014) 19:1275–83. doi: 10.1038/mp.2013.190
158. Klein C, Hiestand T, Ghadri J-R, Templin C, Jäncke L, Hänggi J. Takotsubo Syndrome - Predictable from brain imaging data. Sci Rep. (2017) 7:5434. doi: 10.1038/s41598-017-05592-7
159. Vaccaro A, Despas F, Delmas C, Lairez O, Lambert E, Lambert G, et al. Direct evidences for sympathetic hyperactivity and baroreflex impairment in tako tsubo cardiopathy. Baumert M, editor. PLoS ONE. (2014) 9:e93278. doi: 10.1371/journal.pone.0093278
160. Desai R, Singh S, Patel U, Fong HK, Kaur VP, Varma Y, et al. Frequency of takotsubo cardiomyopathy in epilepsy-related hospitalizations among adults and its impact on in-hospital outcomes: a national standpoint. Int J Cardiol. (2020) 299:67–70. doi: 10.1016/j.ijcard.2019.07.034
161. Shin E-J, Jeong JH, Chung YH, Kim W-K, Ko K-H, Bach J-H, et al. Role of oxidative stress in epileptic seizures. Neurochem Int. (2011) 59:122–37. doi: 10.1016/j.neuint.2011.03.025
162. Halliwell B. Reactive oxygen species and the central nervous system. J Neurochem. (1992) 59:1609–23. doi: 10.1111/j.1471-4159.1992.tb10990.x
163. Ameta R, Chohadia AK, Jain A, Punjabi PB. Fenton and photo-fenton processes. In: Advanced Oxidation Processes for Waste Water Treatment. Elsevier (2018). p. 49–87. Available online at: https://linkinghub.elsevier.com/retrieve/pii/B9780128104996000036 (accessed November 15, 2020).
164. Winterbourn CC. Toxicity of iron and hydrogen peroxide: the Fenton reaction. Toxicol Lett. (1995) 82–83:969–74. doi: 10.1016/0378-4274(95)03532-X
165. Gutteridge JMC. Iron promoters of the Fenton reaction and lipid peroxidation can be released from haemoglobin by peroxides. FEBS Lett. (1986) 201:291–5. doi: 10.1016/0014-5793(86)80626-3
166. Li S, Koerner MM, El-Banayosy A, Soleimani B, Pae WE, Leuenberger UA. Takotsubo's syndrome after mitral valve repair and rescue with extracorporeal membrane oxygenation. Ann Thorac Surg. (2014) 97:1777–8. doi: 10.1016/j.athoracsur.2013.08.032
167. Wever-Pinzon O, Tami L. Takotsubo cardiomyopathy following a blood transfusion: takotsubo cardiomyopathy following a blood transfusion. Congest Heart Fail. (2009) 16:129–31. doi: 10.1111/j.1751-7133.2009.00134.x
168. Postema PG, Wiersma JJ, van der Bilt IaC, Dekkers P, van Bergen PFMM. Takotsubo cardiomyopathy shortly following pacemaker implantation-case report and review of the literature Neth Heart. J Mon J Neth Soc Cardiol Neth Heart Found. (2014) 22:456–9. doi: 10.1007/s12471-012-0320-8
169. Madias JE. Low prevalence of diabetes mellitus in patients with Takotsubo syndrome: a plausible ‘protective' effect with pathophysiologic connotations. Eur Heart J Acute Cardiovasc Care. (2016) 5:164–70. doi: 10.1177/2048872615570761
170. Rena G, Hardie DG, Pearson ER. The mechanisms of action of metformin. Diabetologia. (2017) 60:1577–85. doi: 10.1007/s00125-017-4342-z
171. Li M, Li X, Zhang H, Lu Y. Molecular mechanisms of metformin for diabetes and cancer treatment. Front Physiol. (2018) 9:1039. doi: 10.3389/fphys.2018.01039
172. Chakraborty A, Chowdhury S, Bhattacharyya M. Effect of metformin on oxidative stress, nitrosative stress and inflammatory biomarkers in type 2 diabetes patients. Diabetes Res Clin Pract. (2011) 93:56–62. doi: 10.1016/j.diabres.2010.11.030
173. Marshall JS, Warrington R, Watson W, Kim HL. An introduction to immunology and immunopathology. Allergy Asthma Clin Immunol. (2018) 14:49. doi: 10.1186/s13223-018-0278-1
174. De Giorgi A, Fabbian F, Pala M, Parisi C, Misurati E, Molino C, et al. Takotsubo cardiomyopathy and acute infectious diseases: a mini-review of case reports. Angiology. (2015) 66:257–61. doi: 10.1177/0003319714523673
175. Galea F, Abela G, Felice H. Takotsubo cardiomyopathy in chronic infection. Scott Med J. (2013) 58:e11–4. doi: 10.1177/0036933013508044
176. Mori K, Yagi M, Oe K, Shimojima M, Yamagishi M. Pericarditis-complicated takotsubo cardiomyopathy in a patient with rheumatoid arthritis. Cardiovasc Diagn Ther. (2018) 8:520–4. doi: 10.21037/cdt.2018.05.02
177. Ugurlucan M, Zorman Y, Ates G, Arslan AH, Yildiz Y, Karahan Zor A, et al. Takotsubo cardiomyopathy in a patient with multiple autoimmune disorders and hyperthyroidism. Res Cardiovasc Med. (2013) 2:145–8. doi: 10.5812/cardiovascmed.10023
178. Rathish D, Karalliyadda M. Takotsubo syndrome in patients with myasthenia gravis: a systematic review of previously reported cases. BMC Neurol. (2019) 19:281. doi: 10.1186/s12883-019-1523-z
179. Bayer AD, Cahill JF, Rizvi SA. Multiple sclerosis relapse presenting as an acute cardiomyopathy. Mult Scler Relat Disord. (2019) 27:7–8. doi: 10.1016/j.msard.2018.09.021
180. Boon M, Dennesen PJW, Veldkamp RF. A rare stress cardiomyopathy in a patient with Guillain-Barré syndrome. Neth J Med. (2016) 74:86–8.
181. Manfredini R, Fabbian F, Giorgi AD, Pala M, Menegatti AM, Parisi C, et al. Heart and lung, a dangerous liaison-Tako-tsubo cardiomyopathy and respiratory diseases: a systematic review. World J Cardiol. (2014) 6:338–44. doi: 10.4330/wjc.v6.i5.338
182. Mirijello A, D'Errico MM, Curci S, Bossa F, d'Angelo C, Vendemiale G, et al. Takotsubo syndrome and inflammatory bowel diseases: does a link exist? Dig Dis. (2020) 38:204–10. doi: 10.1159/000502088
183. Elikowski W, Malek-Elikowska M, Lisiecka M, Trypuč Z, Mozer-Lisewska I. Takotsubo cardiomyopathy triggered by influenza B. Pol Med J. (2018) 266:67–70.
184. Zhou JQ, Choe E, Ang L, Schnittger I, Rockson SG, Tremmel JA, et al. Stress-induced cardiomyopathy associated with a transfusion reaction: a case of potential crosstalk between the histaminic and adrenergic systems. Exp Clin Cardiol. (2011) 16:30–2.
185. Singh K, Marinelli T, Horowitz JD. Takotsubo cardiomyopathy after anti-influenza vaccination: catecholaminergic effects of immune system. Am J Emerg Med. (2013) 31:1627.e1–.e4. doi: 10.1016/j.ajem.2013.06.039
186. Vultaggio A, Matucci A, Del Pace S, Simonetti I, Parronchi P, Rossi O, et al. Tako-Tsubo-like syndrome during anaphylactic reaction. Eur J Heart Fail. (2007) 9:209–11. doi: 10.1016/j.ejheart.2006.05.011
187. Ghanim D, Adler Z, Qarawani D, Kusniec F, Amir O, Carasso S. Takotsubo cardiomyopathy caused by epinephrine-treated bee sting anaphylaxis: a case report. J Med Case Reports. (2015) 9:247. doi: 10.1186/s13256-015-0722-5
188. Kajander OA, Virtanen MPO, Sclarovsky S, Nikus KC. Iatrogenic inverted takotsubo syndrome following intravenous adrenaline injections for an allergic reaction. Int J Cardiol. (2013) 165:e3–5. doi: 10.1016/j.ijcard.2012.09.157
189. Khoueiry G, Abi Rafeh N, Azab B, Markman E, Waked A, AbouRjaili G, et al. Reverse takotsubo cardiomyopathy in the setting of anaphylaxis treated with high-dose intravenous epinephrine. J Emerg Med. (2013) 44:96–9. doi: 10.1016/j.jemermed.2011.09.032
190. Imran MA, Khalid H, Karim S, Gierer SA. Broken heart syndrome (takotsubo cardiomyopathy) induced by epinephrine. J Allergy Clin Immunol. (2016) 137:AB47. doi: 10.1016/j.jaci.2015.12.156
191. Aono J, Saito M, Inaba S, Kurata A, Uetani T, Annen S, et al. Multiple bee sting-induced life-threatening takotsubo cardiomyopathy. Circ J. (2019) 83:489. doi: 10.1253/circj.CJ-18-0047
192. Murase K, Takagi K. Takotsubo cardiomyopathy in a snake bite victim: a case report. Pan Afr Med J. (2012) 13:51.
193. Alexakis L-C, Arapi S, Stefanou I, Gargalianos P, Astriti M. Transient reverse takotsubo cardiomyopathy following a spider bite in greece: a case report. Medicine (Baltimore). (2015) 94:e457. doi: 10.1097/MD.0000000000000457
194. Gupta P, Zhu N. Epinephrine or no. epinephrine: shellfish allergy in patient with takotsobu cardiomyopathy. Ann Allergy Asthma Immunol. (2018) 121:S117. doi: 10.1016/j.anai.2018.09.389
195. Yew KL, Kok VSL. Exotic food anaphylaxis and the broken heart: sago worm and takotsubo cardiomyopathy. Med J Malaysia. (2012) 67:540–1.
196. Kounis NG. Kounis syndrome: an update on epidemiology, pathogenesis, diagnosis and therapeutic management. Clin Chem Lab Med CCLM. (2016) 54:10. doi: 10.1515/cclm-2016-0010
197. Kounis NG, Zavras GM. Histamine-induced coronary artery spasm: the concept of allergic angina. Br J Clin Pract. (1991) 45:121–8.
198. Lopez PR, Peiris AN. Kounis syndrome. South Med J. (2010) 103:1148–55. doi: 10.1097/SMJ.0b013e3181f8c56f
199. Kounis NG. Mast Cells and the Heart: Kounis Syndrome and Takotsubo Cardiomyopathy (2016). Available online at: http://dev.ministersofdesign.com/tms2/expert-information/mast-cells-heart-kounis-syndrome-takotsubo-cardiomyopathy/ (accessed June 29, 2021).
200. Yanagawa Y, Nishi K, Tomiharu N, Kawaguchi T. A case of takotsubo cardiomyopathy associated with Kounis syndrome. Int J Cardiol. (2009) 132:e65–7. doi: 10.1016/j.ijcard.2007.08.022
201. Cammann VL, Sarcon A, Ding KJ, Seifert B, Kato K, Di Vece D, et al. Clinical features and outcomes of patients with malignancy and takotsubo syndrome: observations from the international takotsubo registry. J Am Heart Assoc. (2019) 8:e00881. doi: 10.1161/JAHA.118.010881
202. Wittekind SG, Yanay O, Johnson EM, Gibbons EF. Two pediatric cases of variant neurogenic stress cardiomyopathy after intracranial hemorrhage. Pediatrics. (2014) 134:e1211–7. doi: 10.1542/peds.2013-1881
203. Schoof S, Bertram H, Hohmann D, Jack T, Wessel A, Yelbuz TM. Takotsubo cardiomyopathy in a 2-year-old girl: 3-dimensional visualization of reversible left ventricular dysfunction. J Am Coll Cardiol. (2010) 55:e5. doi: 10.1016/j.jacc.2009.08.050
204. Bertero E, Maack C. Calcium signaling and reactive oxygen species in mitochondria. Circ Res. (2018) 122:1460–78. doi: 10.1161/CIRCRESAHA.118.310082
205. Schwarz DS, Blower MD. The endoplasmic reticulum: structure, function and response to cellular signaling. Cell Mol Life Sci CMLS. (2016) 73:79–94. doi: 10.1007/s00018-015-2052-6
206. Zeeshan H, Lee G, Kim H-R, Chae H-J. Endoplasmic reticulum stress and associated ROS. Int J Mol Sci. (2016) 17:327. doi: 10.3390/ijms17030327
207. Pinali C, Bennett H, Davenport JB, Trafford AW, Kitmitto A. Three-dimensional reconstruction of cardiac sarcoplasmic reticulum reveals a continuous network linking transverse-tubules: this organization is perturbed in heart failure. Circ Res. (2013) 113:1219–30. doi: 10.1161/CIRCRESAHA.113.301348
208. Nantes IL, Rodrigues T, Yokomizo CH, Araújo-Chaves J, Pessoto FS, Kisaki MK, et al. Antioxidant action of mobile electron carriers of the respiratory chain. In: Clark K, editor. Bioenergetics. InTech (2012). Available online at: http://www.intechopen.com/books/bioenergetics/antioxidant-action-of-mobile-electron-carriers-of-the-respiratory-chain (accessed November 21, 2020).
209. Lopez-Crisosto C, Pennanen C, Vasquez-Trincado C, Morales PE, Bravo-Sagua R, Quest AFG, et al. Sarcoplasmic reticulum-mitochondria communication in cardiovascular pathophysiology. Nat Rev Cardiol. (2017) 14:342–60. doi: 10.1038/nrcardio.2017.23
210. Scriven P, Brown NJ, Pockley AG, Wyld L. The unfolded protein response and cancer: a brighter future unfolding? J Mol Med Berl Ger. (2007) 85:331–41. doi: 10.1007/s00109-006-0150-5
211. Adams CJ, Kopp MC, Larburu N, Nowak PR, Ali MMU. Structure and molecular mechanism of ER stress signaling by the unfolded protein response signal activator IRE1. Front Mol Biosci. (2019) 6:11. doi: 10.3389/fmolb.2019.00011
212. Lu X, Ginsburg KS, Kettlewell S, Bossuyt J, Smith GL, Bers DM. Measuring local gradients of intramitochondrial [Ca 2+] in cardiac myocytes during sarcoplasmic reticulum Ca 2+ release. Circ Res. (2013) 112:424–31. doi: 10.1161/CIRCRESAHA.111.300501
213. Eisner DA, Caldwell JL, Kistamás K, Trafford AW. Calcium and excitation-contraction coupling in the heart. Circ Res. (2017) 121:181–95. doi: 10.1161/CIRCRESAHA.117.310230
214. Bers DM. Cardiac excitation-contraction coupling. Nature. (2002) 415:198–205. doi: 10.1038/415198a
215. Bers DM. Altered cardiac myocyte ca regulation in heart failure. Physiology. (2006) 21:380–7. doi: 10.1152/physiol.00019.2006
216. Williams GSB, Boyman L, Lederer WJ. Mitochondrial calcium and the regulation of metabolism in the heart. J Mol Cell Cardiol. (2015) 78:35–45. doi: 10.1016/j.yjmcc.2014.10.019
217. Hobai IA, O'Rourke B. Decreased sarcoplasmic reticulum calcium content is responsible for defective excitation-contraction coupling in canine heart failure. Circulation. (2001) 103:1577–84. doi: 10.1161/01.CIR.103.11.1577
218. Lemasters JJ, Theruvath TP, Zhong Z, Nieminen A-L. Mitochondrial calcium and the permeability transition in cell death. Biochim Biophys Acta BBA Bioenerg. (2009) 1787:1395–401. doi: 10.1016/j.bbabio.2009.06.009
219. Jekabsone A. Nitric oxide and calcium together inactivate mitochondrial complex I and induce cytochrome c release. J Mol Cell Cardiol. (2003) 35:803–9. doi: 10.1016/S0022-2828(03)00137-8
220. Dhalla NS, Temsah RM. Netticadan T. Role of oxidative stress in cardiovascular diseases. J Hypertens. (2000) 18:655–73. doi: 10.1097/00004872-200018060-00002
221. Nef HM, Mollmann H, Troidl C, Kostin S, Voss S, Hilpert P, et al. Abnormalities in intracellular Ca2+ regulation contribute to the pathomechanism of Tako-Tsubo cardiomyopathy. Eur Heart J. (2009) 30:2155–64. doi: 10.1093/eurheartj/ehp240
222. Asahi M, Sugita Y, Kurzydlowski K, De Leon S, Tada M, Toyoshima C, et al. Sarcolipin regulates sarco(endo)plasmic reticulum Ca2+-ATPase (SERCA) by binding to transmembrane helices alone or in association with phospholamban. Proc Natl Acad Sci USA. (2003) 100:5040–5. doi: 10.1073/pnas.0330962100
223. MacLennan DH, Asahi M, Tupling AR. The regulation of SERCA-type pumps by phospholamban and sarcolipin. Ann N Y Acad Sci. (2003) 986:472–80. doi: 10.1111/j.1749-6632.2003.tb07231.x
224. Nef HM, Möllmann H, Akashi YJ, Hamm CW. Mechanisms of stress (Takotsubo) cardiomyopathy. Nat Rev Cardiol. (2010) 7:187–93. doi: 10.1038/nrcardio.2010.16
225. Cimarelli S, Imperiale A, Ben-Sellem D, Rischner J, Detour J, Morel O, et al. Nuclear medicine imaging of takotsubo cardiomyopathy: typical form and midventricular ballooning syndrome. J Nucl Cardiol. (2008) 15:137–41. doi: 10.1007/BF02976903
226. Nguyen H, Zaroff JG. Neurogenic stunned myocardium. Curr Neurol Neurosci Rep. (2009) 9:486–91. doi: 10.1007/s11910-009-0071-0
227. Biso S, Wongrakpanich S, Agrawal A, Yadlapati S, Kishlyansky M, Figueredo V, et al. Review of neurogenic stunned myocardium. Cardiovasc Psychiatry Neurol. (2017) 2017:5842182. doi: 10.1155/2017/5842182
228. Guglin M, Novotorova I. Neurogenic stunned myocardium and takotsubo cardiomyopathy are the same syndrome: a pooled analysis. Congest Heart Fail Greenwich Conn. (2011) 17:127–32. doi: 10.1111/j.1751-7133.2011.00210.x
229. Kenigsberg BB, Barnett CF, Mai JC, Chang JJ. Neurogenic stunned myocardium in severe neurological injury. Curr Neurol Neurosci Rep. (2019) 19:90. doi: 10.1007/s11910-019-0999-7
230. Gherasim L. Takotsubo syndrome versus neurogenic stunned myocardium. Maedica. (2020) 15:288–96. doi: 10.26574/maedica.2020.15.3.288
231. Nagai M, Hoshide S, Kario K. The insular cortex and cardiovascular system: a new insight into the brain-heart axis. J Am Soc Hypertens. (2010) 4:174–82. doi: 10.1016/j.jash.2010.05.001
232. Uddin LQ, Nomi JS, Hébert-Seropian B, Ghaziri J, Boucher O. Structure and function of the human insula. J Clin Neurophysiol. (2017) 34:300–6. doi: 10.1097/WNP.0000000000000377
233. Y-Hassan S, De Palma R. Contemporary review on the pathogenesis of takotsubo syndrome: the heart shedding tears. Int J Cardiol. (2017) 228:528–36. doi: 10.1016/j.ijcard.2016.11.086
234. Zaroff JG, Rordorf GA, Ogilvy CS, Picard MH. Regional patterns of left ventricular systolic dysfunction after subarachnoid hemorrhage: evidence for neurally mediated cardiac injury. J Am Soc Echocardiogr. (2000) 13:774–9. doi: 10.1067/mje.2000.105763
235. Coote JH, Chauhan RA. The sympathetic innervation of the heart: important new insights. Auton Neurosci Basic Clin. (2016) 199:17–23. doi: 10.1016/j.autneu.2016.08.014
236. Cinca J, Evangelista A, Montoyo J, Barutell C, Figueras J, Valle V, et al. Electrophysiologic effects of unilateral right and left stellate ganglion block on the human heart. Am Heart J. (1985) 109:46–54. doi: 10.1016/0002-8703(85)90414-4
237. Frankel E, Prior P, Mehta H, Rajagopalan P, Wiener DH, Rose A, et al. One patient, three variants, four episodes of takotsubo cardiomyopathy. CASE Phila PA. (2020) 4:204–7. doi: 10.1016/j.case.2020.01.009
238. Eitel I, von Knobelsdorff-Brenkenhoff F, Bernhardt P, Carbone I, Muellerleile K, Aldrovandi A, et al. Clinical characteristics and cardiovascular magnetic resonance findings in stress (takotsubo) cardiomyopathy. JAMA. (2011) 306:92. doi: 10.1001/jama.2011.992
239. Eitel I, Behrendt F, Schindler K, Kivelitz D, Gutberlet M, Schuler G, et al. Differential diagnosis of suspected apical ballooning syndrome using contrast-enhanced magnetic resonance imaging. Eur Heart J. (2008) 29:2651–9. doi: 10.1093/eurheartj/ehn433
240. Eitel I, Lücke C, Grothoff M, Sareban M, Schuler G, Thiele H, et al. Inflammation in takotsubo cardiomyopathy: insights from cardiovascular magnetic resonance imaging. Eur Radiol. (2010) 20:422–31. doi: 10.1007/s00330-009-1549-5
241. Ghadri JR, Dougoud S, Maier W, Kaufmann PA, Gaemperli O, Prasad A, et al. A PET/CT-follow-up imaging study to differentiate takotsubo cardiomyopathy from acute myocardial infarction. Int J Cardiovasc Imaging. (2014) 30:207–9. doi: 10.1007/s10554-013-0311-x
242. Scally C, Abbas H, Ahearn T, Srinivasan J, Mezincescu A, Rudd A, et al. Myocardial and systemic inflammation in acute stress-induced (takotsubo) cardiomyopathy. Circulation. (2019) 139:1581–92. doi: 10.1161/CIRCULATIONAHA.118.037975
243. Santoro F, Costantino MD, Guastafierro F, Triggiani G, Ferraretti A, Tarantino N, et al. Inflammatory patterns in Takotsubo cardiomyopathy and acute coronary syndrome: a propensity score matched analysis. Atherosclerosis. (2018) 274:157–61. doi: 10.1016/j.atherosclerosis.2018.05.017
244. Margaritelis NV, Veskoukis AS, Paschalis V, Vrabas IS, Dipla K, Zafeiridis A. Blood reflects tissue oxidative stress: a systematic review. Biomarkers. (2015) 20:97–108. doi: 10.3109/1354750X.2014.1002807
245. Dande AS, Sena SF, Wasserman HS, Warshofsky MK, Belsky JL. Prevalence and consequences of vitamin d insufficiency in women with takotsubo cardiomyopathy. J Clin Endocrinol Metab. (2013) 98:E872–6. doi: 10.1210/jc.2013-1082
246. Marfella R, Barbieri M, Sardu C, Rizzo MR, Siniscalchi M, Paolisso P, et al. Effects of α-lipoic acid therapy on sympathetic heart innervation in patients with previous experience of transient takotsubo cardiomyopathy. J Cardiol. (2016) 67:153–61. doi: 10.1016/j.jjcc.2015.07.012
247. Vinik AI, Maser RE, Ziegler D. Autonomic imbalance: prophet of doom or scope for hope? Diabet Med J Br Diabet Assoc. (2011) 28:643–51. doi: 10.1111/j.1464-5491.2010.03184.x
248. Packer L, Witt EH, Tritschler HJ. Alpha-lipoic acid as a biological antioxidant. Free Radic Biol Med. (1995) 19:227–50. doi: 10.1016/0891-5849(95)00017-R
249. James SJ, Cutler P, Melnyk S, Jernigan S, Janak L, Gaylor DW, et al. Metabolic biomarkers of increased oxidative stress and impaired methylation capacity in children with autism. Am J Clin Nutr. (2004) 80:1611–7. doi: 10.1093/ajcn/80.6.1611
250. Lang CA, Mills BJ, Mastropaolo W, Liu MC. Blood glutathione decreases in chronic diseases. J Lab Clin Med. (2000) 135:402–5. doi: 10.1067/mlc.2000.105977
251. Sheng-Huang C, Chieh-Hsin C, Mu-Chun Y, Wen-Tung H, Chia-Ying H, Ya-Ting H, et al. Effects of estrogen on glutathione and catalase levels in human erythrocyte during menstrual cycle. Biomed Rep. (2015) 3:266–8. doi: 10.3892/br.2014.412
Keywords: Takotsubo syndrome, oxidative stress, catecholamines, cytochrome P450, sarco(endo)plasmic reticulum - mitochondria complex, calcium overload, energy failure, antioxidants
Citation: Manousek J, Kala P, Lokaj P, Ondrus T, Helanova K, Miklikova M, Brazdil V, Tomandlova M, Parenica J, Pavkova Goldbergova M and Hlasensky J (2021) Oxidative Stress in Takotsubo Syndrome—Is It Essential for an Acute Attack? Indirect Evidences Support Multisite Impact Including the Calcium Overload—Energy Failure Hypothesis. Front. Cardiovasc. Med. 8:732708. doi: 10.3389/fcvm.2021.732708
Received: 29 June 2021; Accepted: 16 September 2021;
Published: 19 October 2021.
Edited by:
Nicola Montano, University of Milan, ItalyReviewed by:
Owais Bhat, Virginia Commonwealth University, United StatesGayani Kanchana Nanayakkara, The University of Utah, United States
Copyright © 2021 Manousek, Kala, Lokaj, Ondrus, Helanova, Miklikova, Brazdil, Tomandlova, Parenica, Pavkova Goldbergova and Hlasensky. This is an open-access article distributed under the terms of the Creative Commons Attribution License (CC BY). The use, distribution or reproduction in other forums is permitted, provided the original author(s) and the copyright owner(s) are credited and that the original publication in this journal is cited, in accordance with accepted academic practice. No use, distribution or reproduction is permitted which does not comply with these terms.
*Correspondence: Jiri Hlasensky, aGxhc2Vuc2t5LmppcmlAZm5icm5vLmN6