- 1Department of Obstetrics, School of Medicine, Women's Hospital, Zhejiang University, Hangzhou, China
- 2Institute of Reproduction and Development, Obstetrics and Gynecology Hospital, Fudan University, Shanghai, China
- 3Shanghai Ji Ai Genetics and IVF Institute, Institute of Reproduction and Development, Obstetrics and Gynecology Hospital, Fudan University, Shanghai, China
- 4Regional Community Health Service Center of Minzhi Sub-district, Shenzhen, China
- 5Department of General Surgery, School of Medicine, Sir Run Run Shaw Hospital, Zhejiang University, Hangzhou, China
- 6Department of Obstetrics and Gynecology, The First Affiliated Hospital of Nanjing Medical University, Nanjing, China
- 7Department of Cardiology, Beijing Chaoyang Hospital, Capital Medical University, Beijing, China
- 8University of Connecticut School of Medicine, Farmington, CT, United States
Cardiovascular dysfunction in children born after in vitro fertilization (IVF) has been of great concern, the potential molecular mechanisms for such long-term outcomes are still unknown. Here, we found that systolic blood pressure was a little higher in IVF born offspring at 2 years old compared to those born after being naturally conceived. Besides, the expression level of maternally expressed gene 3 (MEG3) was higher in human umbilical vein endothelial cells (HUVECs) from IVF offspring than that in spontaneously born offspring. Pearson correlation test showed that MEG3 relative expression is significantly related to the children's blood pressure (Coefficient = 0.429, P = 0.0262). Furthermore, we found decreased expression of endothelial nitric oxide synthase (eNOS) and vascular endothelial growth factor (VEGF) along with elevated expression of endothelial-1(ET1) in HUVECs from IVF offspring, accompanied by lower secretion of nitrite, VEGF, and higher secretion of ET1 in the umbilical cord serum of IVF offspring. Correlation analysis showed MEG3 expression highly correlated with ET1 and Nitrate concentration. With pyrosequencing technology, we found that elevated expression of MEG3 was the result of hypomethylation of the MEG3 promoter. Therefore, our results provide a potential mechanism addressing the high-risk of hypertension in IVF offspring via MEG3 epigenetic regulation.
Introduction
Since the first in vitro fertilization (IVF) pregnancy was reported in 1978 (1), an estimated seven million pregnancies have been achieved worldwide by IVF. Recently, an increasing number of studies have shown that IVF conceived fetuses are exposed to a high-estradiol environment in utero, which is closely correlated with increased risk of low birth weight (LBW) and small-for-gestational-age (SGA) (2, 3). Disturbed intrauterine environments have been proven to be associated with rapid weight gain in early childhood, increased risk of high blood pressure in late childhood, a higher number of skin folds, and elevated fasting serum glucose level concentrations (4, 5). In our laboratory, we also previously reported that, at the age of 3–13 years old, the blood pressure of IVF-conceived Chinese children was higher than that of spontaneously conceived born children (3). While recent studies have focused on the epidemiological consequences of IVF conceived offspring, few have explored the potential molecular mechanisms for such outcomes.
The “Fetal programming hypothesis” proposed by Barker and Fall suggests that cardiovascular and related disorders derive from fetal adaptions to adverse in utero environments, which could permanently alter offspring's postnatal metabolism and physiology (6). For example, maternal undernutrition induced intrauterine growth retardation, which causes a significant decrease in the number of nephrons within 1 year of birth, could be an underlying mechanism in the early development of hypertension (7, 8). However, the question as to how an intrauterine high-estradiol environment increases the risk of hypertension in IVF offspring later in life remains contentious (4, 9, 10).
Epigenetic modifications, such as DNA methylation, histone modifications, and non-coding RNAs are involved in mediating how early life impacts later health. Long non-coding RNAs (lncRNAs) are non-coding transcripts that are longer than 200 nucleotides in length. So far, abundant studies have demonstrated that dysfunction of lncRNAs is associated with the pathogenesis and progression of a broad range of diseases including cardiovascular disease (11), examples of this include cyclin-dependent kinase inhibitor 2B (CDKN2B) antisense RNA 1 (ANRIL), which have been implicated in atherosclerosis (12), and metastasis associated lung adenocarcinoma transcript 1(MALAT1), which has been shown to stimulate vascular growth in vivo and drive the proliferation of migratory endothelial cells in vitro (13). MEG3 is a long non-coding RNA located at chromosome 14q32.3 in humans. MEG3 is expressed in many normal tissues, and lost in several human tumors and tumor cell lines (14). MEG3 is also expressed in arterial endothelial cells (13), and MEG3 knock-out enhances the expression of VEGF signaling pathway genes in the brain (15). Notably, MEG3 is the only significantly increased lncRNA in senescent HUVEC, which suggests that MEG3 may mediate endothelial dysfunction in aging (16). Hypoxic conditions result in a significantly increased MEG3 expression, accompanied by endothelial cell proliferation, migration and angiogenesis, cell death, and growth arrest (17). Furthermore, the abnormal methylation status of MEG3 contributes to vascular defects, which induce abnormal placentation (18).
In this study, we investigated the role of lncRNA MEG3 in the umbilical cord blood vessels of IVF born offspring, which might offer a potential mechanism for adult chronic cardiovascular diseases of fetal origin.
Methods
Study Population
We reviewed the records of 421 singletons born by natural conception and 482 singletons born by in vitro fertilization-embryo transfer (IVF-ET) in our hospital between 2013 and 2016. We evaluated the fetal growth measurement and ratio of umbilical cord systolic peak velocity over end diastolic velocity (S/D). Furthermore, we recruited 21 singletons born by fresh IVF-ET and 22 singletons who were naturally conceived (NC) from January 1, 2016, to January 1, 2017, for evaluation of the function of human umbilical endothelial cells. The baselines of parental characteristics were collected in the third trimester. These included maternal blood pressure, heart rate, the serum levels of fasting blood glucose, triglycerides, total cholesterol, high-density lipoprotein cholesterol, low-density lipoprotein cholesterol, homocysteine, and serum estradiol concentration.
Children born with cardiovascular diseases, congenital anomalies, premature delivery, who were small for gestational age were excluded. We also excluded children whose mothers had gestational complications such as gestational diabetes, pre-eclampsia, and others.
Tissue Samples
Umbilical vessels and cord blood were collected from twenty-one IVF and twenty-two NC singleton pregnancies immediately after cesarean delivery in the Women's Hospital. The detailed characteristics of these samples are listed in Table 1. Mothers with previous cardiovascular diseases or other gestational complications were also excluded. Mothers of IVF babies had a normal ovarian function and experienced controlled ovarian hyper-stimulation with gonadotropins followed by the standard luteal long gonadotropin-releasing hormone agonist down-regulation protocol for the first IVF cycle. Embryo transfer was performed after 2–3 days of egg retrieval. All babies included met the following criteria: maternal age between 25 and 35 years old; full-term delivery; singleton pregnancy; child's birth weight was between 2,500 and 4,000 g; no indication of pregnancy complication; no birth defects; and no cardiovascular diseases. We examined the E2 levels in cord blood from newborns with the E2 kit (H-Estradiol E2, Abcam, ab108640).
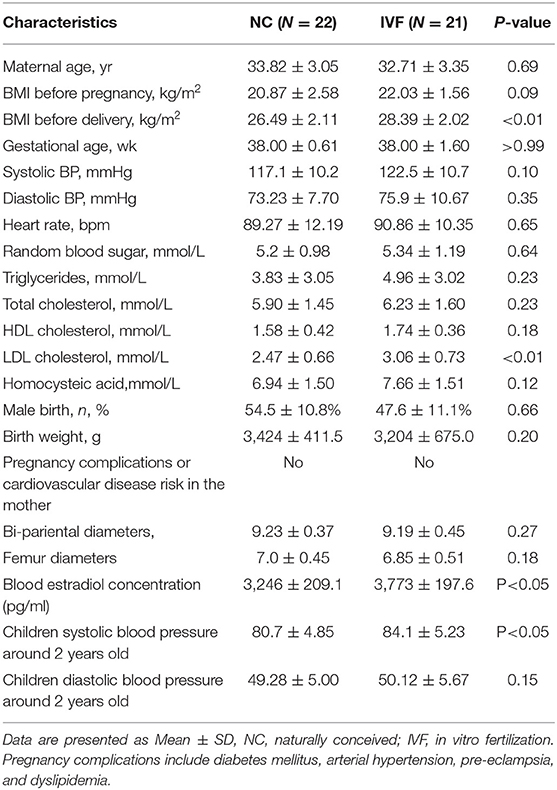
Table 1. The characteristic of the maternal characteristics at the admission to hospital, children at birth, and 2 years-old blood pressure follow up.
Isolation and Culture of Primary Human Umbilical Vein Endothelial Cells
The protocols for HUVEC isolation and culture were performed and modified according to Crampton et al. (19). Briefly, the fresh vein was filled with a solution containing 1 mg/ml collagenase. The cord was incubated in pre-warmed phosphate-buffered saline at 37°C for 30 min, and cells were cultured in 5% fetal bovine serum combined with endothelial cell medium (ECM, ScienCell, cat. #1001). Over 90% of HUVECs in one plate under the microscope were considered to indicate the successful isolation of the HUVECs used between passages 3–5.
Small Interfering RNA-Mediated MEG3 Knock-Down
SiRNA oligonucleotides were purchased from Thermo Fisher Scientific. The sequences of siRNAs targeting MEG3 are as follows (5′-3′): sense, GCUCAUACUUUGACUCUAUTT; anti-sense, AUAGAGUCAAAGUAUGAGCTT. The sequences of negative control (NC) are as follows (5′-3′): sense, UUCUCCGAACGUGUCACGUdTdT; anti-sense, ACGUGACACGUUCGGAGAAdTdT. The siRNAs against MEG3 were reverse-transfected into cells at a dose of 10 nm in 6-wells-plates using the Lipofectamine 3000 reagent (ThermoFisher, Catalog. L3000008) for 48 h.
Quantitative Realtime PCR Analysis
Total RNA was extracted from the tissue sample and primary human umbilical vessel cells using the TRIzol Reagents (Invitrogen Life Technologies, Carlsbad, CA, USA). cDNA was synthesized using PrimerScript RT Reagent Kit (Takara, RR037A, Japan) in a 20 μl reaction containing 0.5–1 ug of total RNA. Real-time quantitative polymerase chain reaction (RT-qPCR) was performed using ABI Prism 7900HT (Applied Biosystem, Foster City, CA). Glyceraldehyde-3-Phosphate Dehydrogenase (GAPDH) was the internal control. The full list of primer sequences is shown in Supplementary Table 1.
Western Blot
The protein was extracted from HUVECs tissues with lysis buffer, which was separated using 10% SDS-PAGE. Western Blots was performed using polyvinylidene fluoride membrane and the antibodies for DNA Methyltransferase 3 Alpha (DNMT3A) (Cell Signaling, 32578, used at a dilution of 1:1000), DNA Methyltransferase 3 Alpha (DNA Methyltransferase 3 Beta) (Cell Signaling, 57868, used at a dilution of 1:1000), beta-ACTIN (Abcam, ab8226, used at a dilution of 1:5000). Protein bands were visualized by the enhanced chemiluminescence system (Pierce, Rockford, IL).
ELISA Assay
Umbilical cord blood and cell supernatants were collected after cesarean delivery. ELISA kits were used for the determination of NO (Nitric Oxide Assay, ab65328, Abcam, UK), VEGF (H-VEGF, DVE00, R&D, USA), and ET-1 (H-Endothelin-1, QET00B, R&D, USA) levels. The procedures were performed according to the manufacturer's protocols. For the cell-line culture, three replicates of data were used for statistical analyses.
DNA Isolation and Bisulfite Conversion
Total genomic DNA was isolated from umbilical vessel tissues using Genomic DNA Purification Kit (Invitrogen, cat. K0512, USA). Bisulfite was converted using the EpiTect bisulfite kit (Qiagen, Valencia, CA) according to the manufacturer's instructions to deaminate cytosine to uracil; 5-methyl-cytosine was protected from deamination. PCRs were performed in an ABI 9700 PCR System (Applied Biosystems, USA) using an annealing temperature of 56°C.
DNA Methylation Analysis by Pyrosequencing
The bisulfite converted DNA was amplified using Hotstart Plus DNA polymerase (Qiagen). PCR products were immobilized on streptavidin-sepharose beads (GE Healthcare), washed, denatured, and released into annealing buffer containing sequencing primer, which is described in Supplementary Table 2. Pyrosequencing was carried out on a PyroMark Q96 instrument (Qiagen) according to the manufacturer's instructions. Percent methylation was calculated using the Pyro Q CpG software (Qiagen).
Statistical Analysis
Data were analyzed using SPSS 18.0 and were presented as mean ± SD. Statistical analysis including unpaired two-tailed Student's t-test was performed as described in the figure legends or Excel legends. Correlations of MEG3 relative expression with the children's blood pressure at 2 years old were performed using the Pearson correlation coefficient. P < 0.05, P < 0.01, or P < 0.001 was considered statistically significant.
Results
Perinatal Characteristics and Children' Blood Pressure at 2 Years-Old
The baseline and perinatal characteristics of the study population are shown in Table 1. Children's systolic blood pressure (SBE) at 2 years old, born by fresh IVF-ET was slightly higher compared with those who were naturally conceived (84.1 ± 5.23 vs. 80.7 ± 4.85, P < 0.05), while there were no differences in the aspect of diastolic blood pressure and birth weight. Besides, by reviewing the baseline and perinatal characteristics of 903 babies (421 NC babies and 482 IVF babies) who were born in the past 4 years (2013–2016) in our hospital (Table 2), we found that there were no significant differences in S/D ratio, which are related to umbilical cord vessel function. Additionally, bi-parietal, femur diameter, and birth weight were smaller in IVF born babies (9.16 ± 0.44 vs. 9.26 ± 0.41, P < 0.01; 6.88 ± 0.44 vs. 7.02 ± 0.41, P < 0.0001; 3,250 ± 478.1 vs. 3,183 ± 643.3, P < 0.05), which might be induced by earlier delivery (37.48 ± 2.41 vs. 38.44 ± 1.84, P < 0.0001; Table 2).
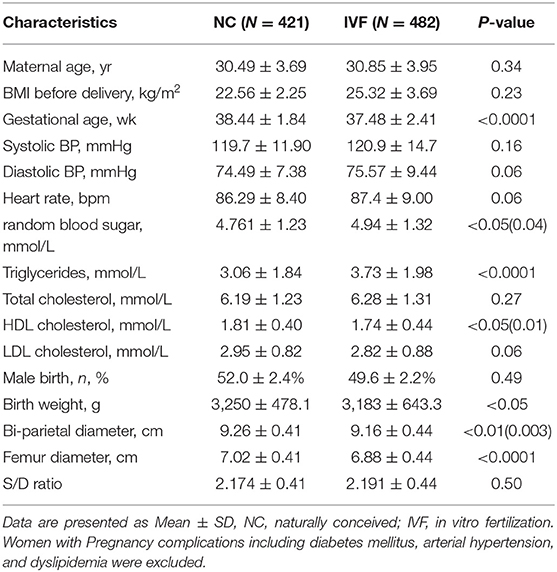
Table 2. The characteristic of children at birth, fetal development index (36–38 weeks ultrasound), and the maternal characteristics at conception.
Up-Regulated Expression of MEG3 in IVF HUVEC
To identify the mRNA expression level of MEG3 in HUVEC, we found that the expression level of MEG3 was significantly higher in IVF born HUVECs (Figure 1A) by qPCR. This suggests that the intrauterine environment of IVF induced abnormal MEG3 expression.
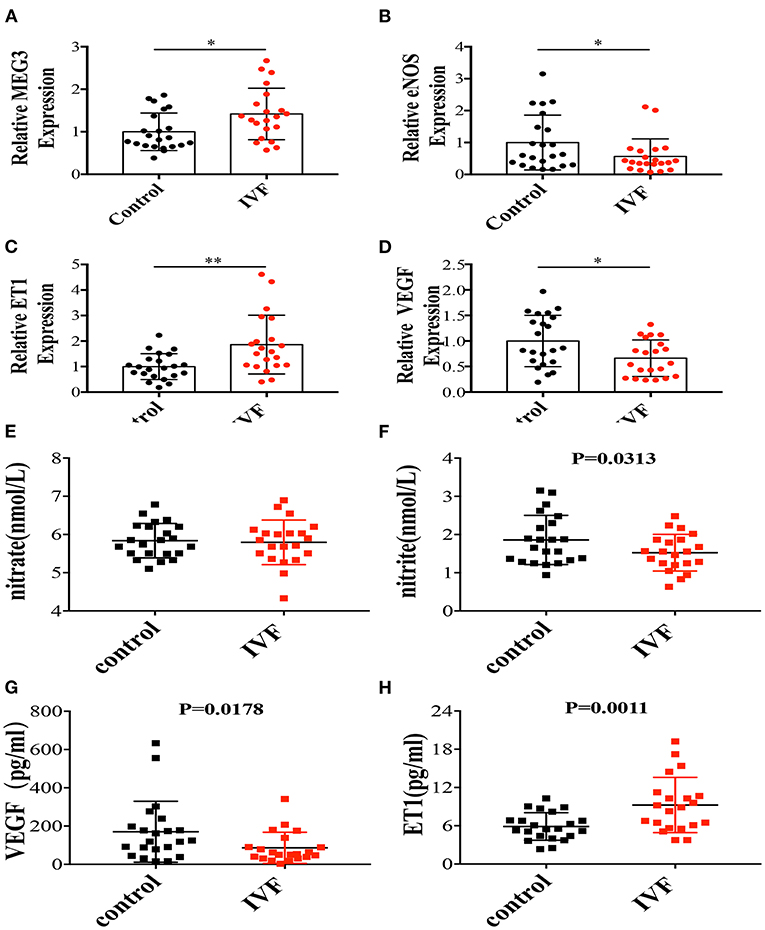
Figure 1. The mRNA expressions of MEG3 and endothelium-derived genes and serum secretions related with endothelial function. (A–D) RNA levels determined by RT-qPCR. Data were analyzed with the Equation 2−ΔΔCT,where ΔΔCT = ΔCT (treatment group) –ΔCT (control group), and ΔCT = CT (sample)–CT (internal control). The values were normalized to GAPDH mRNA levels. (E–H) ELISA was performed to detect the secretion of nitrate (E), nitrite (F), VEGF (G), and ET1 (H). Control has 22 samples and IVF group has 21 samples. In all panels, data are presented as mean ± SD, *P < 0.05, **P < 0.01. Significance was determined by Student t-test.
Endothelium-Derived Factors Altered in HUVEC of IVF Offspring
We previously found that offspring born after IVF have elevated blood pressure between the ages of 3–13 years old (3). This study observed similar results in offspring around 2 years of age (Table 1). Therefore, we focused on the endothelium-derived factors, such as endothelial nitric oxide synthase (eNOS), which is a classical vasodilation factor, endothelin-1(ET1), one of the critical vasoconstriction factors, and vascular endothelial growth factor (VEGF), which is related with endothelial cell proliferation, angiogenesis, and vascular permeability. qPCR showed that eNOS and VEGF expression were significantly decreased in the IVF offspring group (Figures 1B,D), while ET1 expression was significantly increased (Figure 1C). Since NO has a very short half-life of several seconds, using ELISA, we tested the levels of the first and second oxidation products of NO, nitrite and nitrate, respectively. The results showed that nitrite concentration was significantly lower in the umbilical cord serum of IVF offspring (Figure 1F), while there was no difference in nitrate concentration (Figure 1E). Furthermore, ET1 was significantly higher in IVF offspring (Figure 1H) while VEGF secretion was decreased (Figure 1G), which is coincident with the mRNA level. These results suggested that the intra-uterine environment of IVF might lead to endothelial dysfunction associated with vascular activity since HUVECs share a common embryological origin with other fetal vessels.
Knockdown of MEG3 in HUVEC Decreases ET1 Expression and Increases Secretion of Nitrite and VEGF in vitro
Michalik et al. reported that MEG3 was highly rich in the nuclear fraction, and profoundly increased by hypoxia (13). Given this data, we further explored the function of MEG3 in endothelial cells by silencing MEG3 expression with one specific siRNA, which could reduce endogenously expressed MEG3 by 85% (Figure 2A). Through silencing MEG3, we discovered that MEG3 reduction significantly suppressed ET1 mRNA levels and VEGF mRNA levels (Figures 2C,D), but did not arouse any significant change in eNOS expression (Figure 2B). Through ELISA, we demonstrated that nitrite and nitrite secretion was significantly increased with MEG3 suppression (Figures 2E,F), while ET1 secretion was decreased (Figure 2H). We also observed no significant difference in VEGF secretion with MEG3 suppression (Figure 2G), which was consistent with the mRNA synthesis, and might be related to the diverse stimulation mechanisms of VEGF production (20).
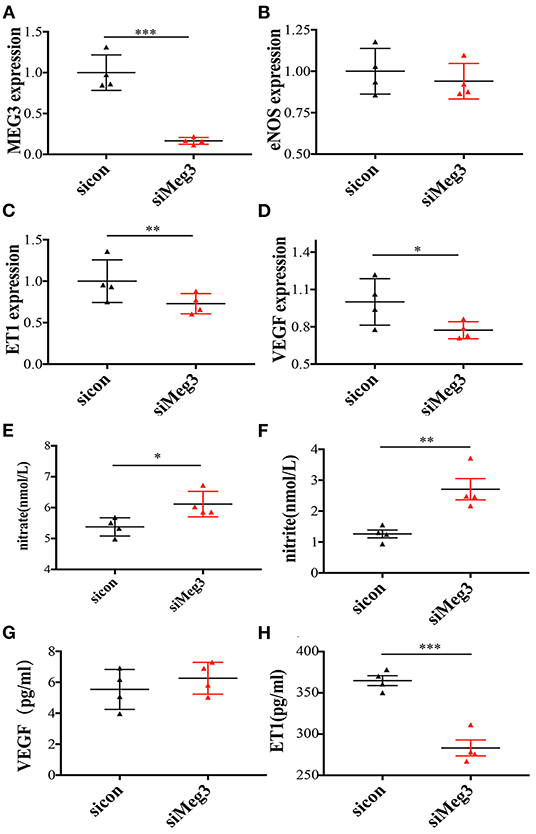
Figure 2. The mRNA expression of endothelium-derived genes in MEG3 knockdown treatment in-vitro primary human umbilical endothelial cells and serum secretions of these target proteins in supernatant liquids. (A–D) RNA levels determined by RT-qPCR. Data were analyzed with the Equation 2−ΔΔCT, where ΔΔCT = ΔCT (treatment group) –ΔCT (control group), and ΔCT = ΔCT (sample)–ΔCT (internal control). The values were normalized to GAPDH mRNA levels. (E–H) ELISA was performed to detect the secretion of nitrate (E), nitrite (F), VEGF (G), and ET1 (H). Both control and IVF group have 4 samples and tried for triple times. In all panels, data are presented as mean ± SD, *P < 0.05, **P < 0.01, ***P < 0.001. Significance was determined by Student t-test.
High Expression Level of MEG3 in Human Umbilical Vein Endothelial Cells Was Controlled by DNA Methylation
As a maternally imprinted gene, MEG3 expression is regulated by two critical different methylation regions (DMR): the intergenic region between DLK1 and MEG3 (IG-DMR), and MEG3 DMR (CG7) (21) (Figure 3A). Pyrosequencing showed no difference in the average methylation status in IG-DMR between IVF offspring and spontaneously born offspring (Figure 3D), while 5 sites were in hypermethylation status from the aspect of single CpG status in the IG-DMR (Figure 3B). However, MEG3-DMR, located in the −287 to −120 region of the human MEG3 promoter (which has eight differentially methylated CpGs), showed significantly reduced methylation status in IVF HUVECs, which consisted of 6 sites of hypomethylation (Figures 3C,E).
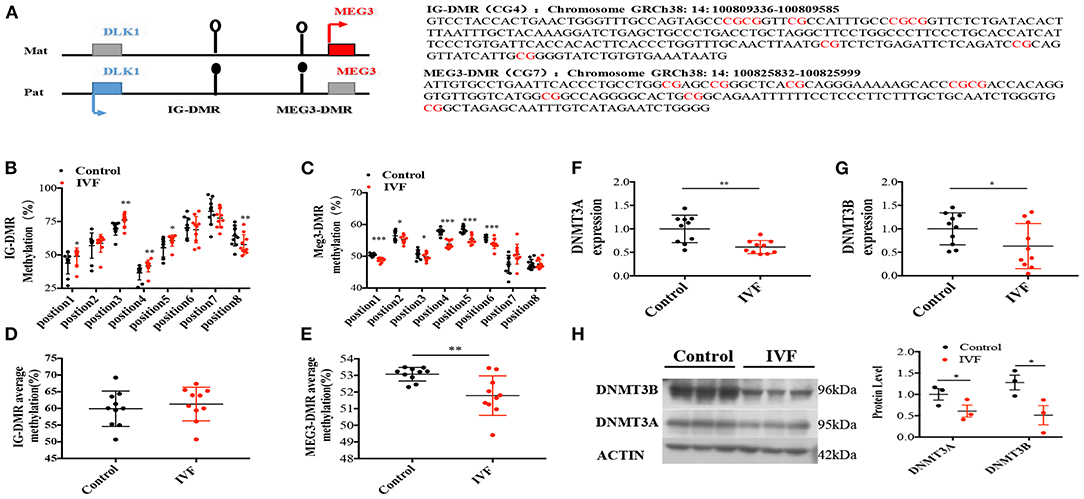
Figure 3. Methylation analysis of IG-DMR/MEG3-DMR by pyrosequencing. (A) Schematic representation of human imprinted locus, showing the relative position of the DLK1 and MEG3 genes and indicating the location of the two DMRs known to contribute to MEG3 imprinting. Exons are known as blue (DLK1 gene) and red (MEG3 gene) rectangles with arrows for transcription start sites. Under the genetic map listed specific gene sequence of the two DMRs. (B) Methylation status of individual DNA strands of IG-DMR containing 8 CpG sites and the average methylation ratio in each CpG site; (C) Methylation status of individual DNA strands of MEG3-DMR containing 8 CpG sites and the average methylation ratio in each CpG site. (D,E) The average methylation status of IG-DMR and MEG3-DMR, ten patients were included in each CpG site. (F,G) Dnmt3A, Dnmt3B RNA levels determined by RT-qPCR. (H) Dnmt3A, Dnmt3B protein levels determined by Western-Blot. Data were analyzed with the Equation 2−ΔΔCT, where ΔΔCT = ΔCT (treatment group) –ΔCT (control group), and ΔCT = ΔCT (sample)–ΔCT (internal control). The values were normalized to GAPDH mRNA levels. For western blot, the values were normalized to β-ACTIN protein levels. For pyrosequencing and RT-qPCR, there were 10 samples both for control and IVF group, for Western-blot, there were 3 samples both for control and IVF group. In all panels, data are presented as mean ± SD, *P < 0.05, **P < 0.01, ***P < 0.001. Significance was determined by Student t-test.
Three DNMTs determine the methylation status of DNA methylation in humans. DNMT3a and DNMT3b are responsible for de novo methylation, while DNMT1 is conservatively expressed and required for maintenance of methylation (22). We performed qPCR and Western-blot to detect the expression of DNMTs, and the results indicated that there was a significant reduction of DNMT3A and DNMT3B in IVF HUVECs compared with spontaneous-born HUVECs (Figures 3F–H) both in mRNA level and protein levels, which was consistent with the DNA methylation status of MEG3 DMR.
Abnormal MEG3 Expression Might Be the Inducer of Endothelial Dysfunction
To support the idea that elevated expression of long non-coding RNA MEG3 leads to abnormal endothelial function, we compared the MEG3 expression on HUVECs derived from IVF born offspring and children's blood pressure at 2 years old. Pearson correlation test showed that MEG3 relative expression was significantly related to the children's blood pressure (Coefficient = 0.429, P = 0.0262; Figure 4A). Furthermore, we also analyzed the correlation of MEG3 expression and endothelial-derived factors in IVF born umbilical cords. In line with the observed higher expression of MEG3 in HUVECs of IVF born offspring, MEG3 expression was also significantly negatively related with nitrate concentration (Coefficient = −0.5279, P = 0.0070; Figure 4C), while positively connected with ET1 concentration (Coefficient = 0.5624, P = 0.0040; Figure 4B).
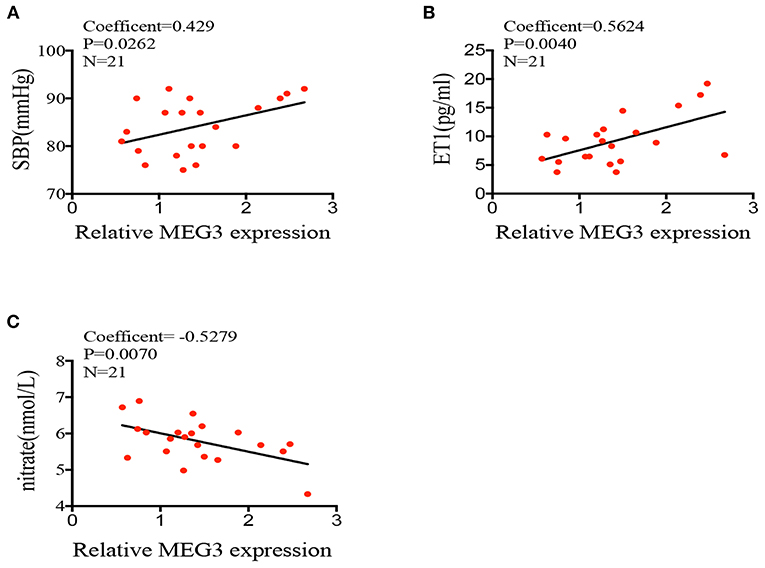
Figure 4. Correlations between MEG3 expression and endothelial-derived factors. (A–C) correlation between MEG3 relative expression and children's blood pressure, serum nitrate, ET1 concentration. Significance was determined by Pearson correlation coefficient.
Effects of High Estradiol Concentration on Primary Human Umbilical Vein Cells
In order to understand the cause of the up-regulation of MEG3, we cultured primary HUVECs in three different estradiol concentrations (10−10, 10−8, 10−6 mmol/L). We found that as estradiol concentration went up, MEG3 and ET1 expression were significantly increased (Figures 5A,D) while eNOS and VEGF expression were decreased (Figures 5B,C), which were in accordance with the in vivo results.
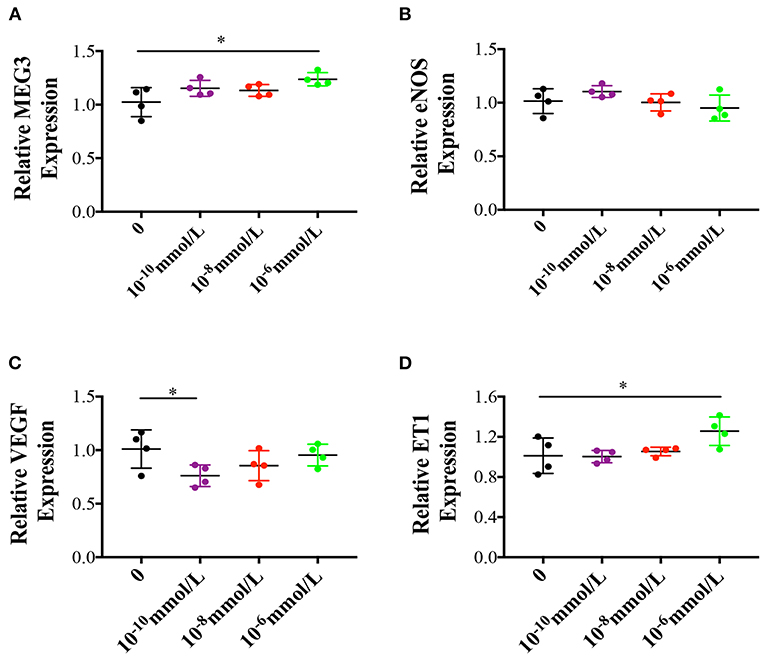
Figure 5. Effects of different estradiol concentration on primary human umbilical vein endothelial cells. (A–D) Represented MEG3, eNOS, VEGF, and ET1 RNA levels which were determined by RT-qPCR. The values were normalized to GAPDH mRNA levels. For different estradiol treatment group, there were 4 samples and tried for triple times. In all panels, data are presented as mean ± SD, *P < 0.05, **P < 0.01. Significance was determined by Student t-test.
Discussion
This study is the first to demonstrate that long coding RNA MEG3 suppresses vasodilation while promoting vasoconstriction in HUVECs derived from IVF born offspring. Our molecular findings were consistent with follow-up data showing that IVF offspring have a higher incidence of hypertension. Meanwhile, these alterations may originate from the regulation of MEG3-DMR at the epigenetic level, leading to higher expression of MEG3. This study may provide a novel mechanism and potential theory for high risk of hypertension in IVF offspring.
The endothelium is one of the largest organ systems by surface area. Normal endothelial function is critical in keeping the symbiotic balance between vasoconstrictive (namely like ET1) and vasodilatory (namely via NO) stimuli, thus a disturbance in normal endothelial function is a predictor of future adverse cardiovascular events (23). Since endothelial cells from the cord may reflect characteristics of the offspring's vascular system (24). In our study, we sought to investigate the function of HUVECs derived from IVF born babies. As we know, the endothelial-dependent response to vasodilate is fundamentally regulated by a release of nitric oxide (NO) synthesized from the amino-acid L-arginine by endothelial nitric oxidase synthase (eNOS) (25). In addition to NO as one critical factor in endothelial function, enhanced activities and levels of ET1 also were related to endothelial dysfunction by stimulating nicotinamide adenine dinucleotide phosphate (NADPH) oxidase-derived ROS production, which inhibits NO-mediated endothelial relaxation and mediating ETA receptors to blunt NO relaxant responses (26). VEGF plays a central role in endothelial function, including stimulating endothelial proliferation, migration, and nitric oxide release. Thus, VEGF might exhibit the same tendency with NO (27). In our study, we found that the IVF group has lower eNOS and VEGF expression, lower secretion of nitrate, nitrite, VEGF concentration, and higher ET1 expression and production, all of which might lead to endothelial dysfunction in IVF born babies. Although certain concentration and time course exposures of estradiol may improve NO product and thus enhance vasodilation (28), as observed in our study, it may be related to extremely elevated concentrations of estradiol in vitro and long-term estradiol exposure in vivo for IVF born babies, which may result in endothelial wall damage.
The recent identification of a novel group of mediators known as long-coding RNAs (lncRNAs) has provided a large quantity of new biology to explore for cardiovascular risk reduction. Several lncRNAs take part in acute myocardial infarction (eg, Novlnc6), heart failure (eg, Myosin Heavy Chain Associated RNA Transcript, Mhrt), control hypertrophy and apoptosis of cardiomyocytes (29), and the regulation of vascular growth and function (e.g., MALAT1) (13). MEG3 is an imprinted gene belonging to the imprinted DLK1-MEG3 locus at chromosome 14q32.3 in humans. The gene expression in this locus is tightly controlled by at least two differentially methylation regions (DMRs): the intergenic DMR (IG-DMR) and the MEG3-DMR. Numerous studies have implicated the involvement of MEG3 in a myriad of biological processes, notably as a tumor suppressor (14, 30). MEG3 is also involved in many cardiovascular functions, including angiogenesis through VEGFA and VEGF1R expression (31, 32) and smooth muscle cell proliferation through the p53 pathway. Knocking-down MEG3 with a nano-polymer wrapped MEG3 short hairpin RNA (shRNA) plasmid conjugated with OX26 antibody (MPO) enhanced endothelial cells migration, tube formation in vitro and reduced the volume of cerebral infarction, capillary density, cerebral cortex micro-vessel in vivo by increasing the angiogenesis associated genes VEGFA and VEGFR (33). In an in vivo mice model of hindlimb ischemia, MEG3 inhibition increased blood flow recovery (16). Besides, MEG3 acts as a miRNA sponge in vascular ECs by negatively regulating miR-9, a key player in angiogenesis and proliferation (34). In this study, we show that HUVECs from IVF born offspring have high expression levels of MEG3. Besides, MEG3 expression was significantly correlated with endothelial-derived factors, including nitrate and ET1, and long-term children's blood pressure at 2 years old. Taken together, these results suggest that MEG3 may be involved in the etiology of cardiovascular diseases of offspring born in an intrauterine high-estradiol environment.
Conclusion
Altogether these data show that IVF neonates have an abnormal endothelium response in human umbilical vein endothelial cells, decreased eNOS expression and synthesis of NO in endothelial cells, and increased ET1 expression and secretion in umbilical cord serum, which may be the result of elevated expression of MEG3. These data suggest that the abnormal expression of MEG3 may contribute to the development of cardiovascular disease in IVF born offspring later in life.
Data Availability Statement
The datasets presented in this study can be found in online repositories. The names of the repository/repositories and accession number(s) can be found at: doi: 10.5061/dryad.hhmgqnkhc.
Ethics Statement
The study protocol (ethical review serial number: 20180046) was approved by the Research and Ethics Committee of the Women's Hospital, School of Medicine, Zhejiang University, China. All participants signed informed consents. We design our study in accordance with the Declaration of Helsinki.
Author Contributions
YJ, HZ, and HC contributed to collection, analysis, and interpretation of data as well as manuscript preparation. YC-Y contributed to data collection and analysis. SN-H, YT-X, and FL contributed to interpretation of data. MS contributed to edit the language. QL and YM-Z contributed to study design and data interpretation and the manuscript preparation. QL is the guarantor of this work and, as such, has full access to all the data in the study and takes responsibility for the integrity of the data and the accuracy of the data analysis.
Funding
This work was supported by the Key Subjects Group of Reproductive Medicine, School of Medicine, Zhejiang University. It was funded by the National Key R&D Program of China (2018YFC1002702), the National Nature Science Foundation of China (Grant Nos. 81571447 and 81501339), Construction of Medical Core Subjects and Innovation Platform in Zhejiang Province (Grant No. 2018RC005), the Medical and Health Technology Program (General Project) in Zhejiang Province (Grant Nos. 2014KYA245 and 2016KYB150), the Fundamental Research Funds for the Central Universities (2018FZA7011), and the Natural Scientific Foundation of Zhejiang Province (LY13H040004).
Conflict of Interest
The authors declare that the research was conducted in the absence of any commercial or financial relationships that could be construed as a potential conflict of interest.
Publisher's Note
All claims expressed in this article are solely those of the authors and do not necessarily represent those of their affiliated organizations, or those of the publisher, the editors and the reviewers. Any product that may be evaluated in this article, or claim that may be made by its manufacturer, is not guaranteed or endorsed by the publisher.
Acknowledgments
The authors thank the staff at the Women's Hospital, Zhejiang University for technical assistance and facility support. This study was presented as an e-poster at RCOG World Congress 2018 meeting, Singapore, March 21–24, 2018.
Supplementary Material
The Supplementary Material for this article can be found online at: https://www.frontiersin.org/articles/10.3389/fcvm.2021.717729/full#supplementary-material
References
1. Steptoe PC, Edwards RG. Birth after the reimplantation of a human embryo. Lancet. (1978) 2:366. doi: 10.1016/S0140-6736(78)92957-4
2. Hu XL, Feng C, Lin XH, Zhong ZX, Zhu YM, Lv PP, et al. High maternal serum estradiol environment in the first trimester is associated with the increased risk of small-for-gestational-age birth. J Clin Endocrinol Metab. (2014) 99:2217–24. doi: 10.1210/jc.2013-3362
3. Gao Q, Pan HT, Lin XH, Zhang JY, Jiang Y, Tian S, et al. Altered protein expression profiles in umbilical veins: insights into vascular dysfunctions of the children born after in vitro fertilization. Biol Reprod. (2014) 91:71. doi: 10.1095/biolreprod.114.120659
4. Ceelen M, van Weissenbruch MM, Vermeiden JP, van Leeuwen FE, Delemarre-van de Waal HA. Cardiometabolic differences in children born after in vitro fertilization: follow-up study. J Clin Endocrinol Metab. (2008) 93:1682–8. doi: 10.1210/jc.2007-2432
5. Ceelen M, Mirjam vWM, Prein J, Smit JJ, Vermeiden JP, Spreeuwenberg M, van Leeuwen FE, et al. Growth during infancy and early childhood in relation to blood pressure and body fat measures at age 8-18 years of IVF children and spontaneously conceived controls born to subfertile parents. Hum Reprod. (2009) 24:2788–95. doi: 10.1093/humrep/dep273
6. Barker DJ, Fall CH. Fetal and infant origins of cardiovascular disease. Arch Dis Child. (1993) 68:797–9. doi: 10.1136/adc.68.6.797
7. Law CM, de Swiet M, Osmond C, Fayers PM, Barker DJ, Cruddas AM, et al. Initiation of hypertension in utero and its amplification throughout life. BMJ. (1993) 306:24–7. doi: 10.1136/bmj.306.6869.24
8. do Carmo Pinho Franco M, Nigro D, Fortes ZB, Tostes RC, Carvalho MH, Lucas SR, et al. Intrauterine undernutrition–renal and vascular origin of hypertension. Cardiovasc Res. (2003) 60:228–34. doi: 10.1016/S0008-6363(03)00541-8
9. Scherrer U, Rimoldi SF, Rexhaj E, Stuber T, Duplain H, Garcin S, et al. Systemic and pulmonary vascular dysfunction in children conceived by assisted reproductive technologies. Circulation. (2012) 125:1890–6. doi: 10.1161/CIRCULATIONAHA.111.071183
10. Xu GF, Zhang JY, Pan HT, Tian S, Liu ME, Yu TT, et al. Cardiovascular dysfunction in offspring of ovarian-hyperstimulated women and effects of estradiol and progesterone: a retrospective cohort study and proteomics analysis. J Clin Endocrinol Metab. (2014) 99:E2494–503. doi: 10.1210/jc.2014-2349
11. Leung A, Trac C, Jin W, Lanting L, Akbany A, Saetrom P, et al. Novel long noncoding RNAs are regulated by angiotensin II in vascular smooth muscle cells. Circ Res. (2013) 113:266–78. doi: 10.1161/CIRCRESAHA.112.300849
12. Holdt LM, Stahringer A, Sass K, Pichler G, Kulak NA, Wilfert W, et al. Circular non-coding RNA ANRIL modulates ribosomal RNA maturation and atherosclerosis in humans. Nat Commun. (2016) 7:12429. doi: 10.1038/ncomms12429
13. Michalik KM, You X, Manavski Y, Doddaballapur A, Zornig M, Braun T, et al. Long noncoding RNA MALAT1 regulates endothelial cell function and vessel growth. Circ Res. (2014) 114:1389–97. doi: 10.1161/CIRCRESAHA.114.303265
14. Zhou Y, Zhang X, Klibanski A. MEG3 noncoding RNA: a tumor suppressor. J Mol Endocrinol. (2012) 48:R45–53. doi: 10.1530/JME-12-0008
15. Gordon FE, Nutt CL, Cheunsuchon P, Nakayama Y, Provencher KA, Rice KA, et al. Increased expression of angiogenic genes in the brains of mouse meg3-null embryos. Endocrinology. (2010) 151:2443–52. doi: 10.1210/en.2009-1151
16. Boon RA, Hofmann P, Michalik KM, Lozano-Vidal N, Berghauser D, Fischer A, et al. Long noncoding RNA meg3 controls endothelial cell aging and function: implications for regenerative angiogenesis. J Am Coll Cardiol. (2016) 68:2589–91. doi: 10.1016/j.jacc.2016.09.949
17. Yu B, Wang S. Angio-LncRs: LncRNAs that regulate angiogenesis and vascular disease. Theranostics. (2018) 8:3654–75. doi: 10.7150/thno.26024
18. Kawahara M, Morita S, Takahashi N, Kono T. Defining contributions of paternally methylated imprinted genes at the Igf2-H19 and Dlk1-Gtl2 domains to mouse placentation by transcriptomic analysis. J Biol Chem. (2009) 284:17751–65. doi: 10.1074/jbc.M109.000299
19. Crampton SP, Davis J, Hughes CC. Isolation of human umbilical vein endothelial cells (HUVEC). J Vis Exp. (2007) 3:183. doi: 10.3791/183
20. Yoshino Y, Aoyagi M, Tamaki M, Duan L, Morimoto T, Ohno K. Activation of p38 MAPK and/or JNK contributes to increased levels of VEGF secretion in human malignant glioma cells. Int J Oncol. (2006) 29:981–7. doi: 10.3892/ijo.29.4.981
21. Kagami M, Sekita Y, Nishimura G, Irie M, Kato F, Okada M, et al. Deletions and epimutations affecting the human 14q32.2 imprinted region in individuals with paternal and maternal upd(14)-like phenotypes. Nat Genet. (2008) 40:237–42. doi: 10.1038/ng.2007.56
22. Jeltsch A, Jurkowska RZ. New concepts in DNA methylation. Trends Biochem Sci. (2014) 39:310–8. doi: 10.1016/j.tibs.2014.05.002
23. Widmer RJ, Lerman A. Endothelial dysfunction and cardiovascular disease. Glob Cardiol Sci Pract. (2014) 2014:291–308. doi: 10.5339/gcsp.2014.43
24. Onat D, Brillon D, Colombo PC, Schmidt AM. Human vascular endothelial cells: a model system for studying vascular inflammation in diabetes and atherosclerosis. Curr Diab Rep. (2011) 11:193–202. doi: 10.1007/s11892-011-0182-2
25. Janssens SP, Shimouchi A, Quertermous T, Bloch DB, Bloch KD. Cloning and expression of a cDNA encoding human endothelium-derived relaxing factor/nitric oxide synthase. J Biol Chem. (1992) 267:14519–22. doi: 10.1016/S0021-9258(18)42066-2
26. Sanchez A, Martinez P, Munoz M, Benedito S, Garcia-Sacristan A, Hernandez M, et al. Endothelin-1 contributes to endothelial dysfunction and enhanced vasoconstriction through augmented superoxide production in penile arteries from insulin-resistant obese rats: role of ETA and ETB receptors. Brit J Pharmacol. (2014) 171:5682–95. doi: 10.1111/bph.12870
27. Kliche S, Waltenberger J. VEGF receptor signaling and endothelial function. IUBMB Life. (2001) 52:61–6. doi: 10.1080/15216540252774784
28. Chambliss KL, Shaul PW. Estrogen modulation of endothelial nitric oxide synthase. Endocr Rev. (2002) 23:665–86. doi: 10.1210/er.2001-0045
29. Uchida S, Dimmeler S. Long noncoding RNAs in cardiovascular diseases. Circ Res. (2015) 116:737–50. doi: 10.1161/CIRCRESAHA.116.302521
30. Wang P, Ren Z, Sun P. Overexpression of the long non-coding RNA MEG3 impairs in vitro glioma cell proliferation. J Cell Biochem. (2012) 113:1868–74. doi: 10.1002/jcb.24055
31. Zhan R, Xu K, Pan J, Xu Q, Xu S, Shen J. Long noncoding RNA MEG3 mediated angiogenesis after cerebral infarction through regulating p53/NOX4 axis. Biochem Biophys Res Commun. (2017) 490:700–6. doi: 10.1016/j.bbrc.2017.06.104
32. Ruan W, Zhao F, Zhao S, Zhang L, Shi L, Pang T. Knockdown of long noncoding RNA MEG3 impairs VEGF-stimulated endothelial sprouting angiogenesis via modulating VEGFR2 expression in human umbilical vein endothelial cells. Gene. (2018) 649:32–9. doi: 10.1016/j.gene.2018.01.072
33. Shen J, Zhao Z, Shang W, Liu C, Zhang B, Xu Z, et al. Fabrication of a nano polymer wrapping Meg3 ShRNA plasmid for the treatment of cerebral infarction. Artif Cells Nanomed Biotechnol. (2018) 46:894–903. doi: 10.1080/21691401.2018.1471483
Keywords: long non-coding RNA, endothelial dysfunction, IVF, epigenetic, fetal-origin adult diseases
Citation: Jiang Y, Zhu H, Chen H, Yu Y-C, Xu Y-T, Liu F, He S-N, Sagnelli M, Zhu Y-M and Luo Q (2022) Elevated Expression of lncRNA MEG3 Induces Endothelial Dysfunction on HUVECs of IVF Born Offspring via Epigenetic Regulation. Front. Cardiovasc. Med. 8:717729. doi: 10.3389/fcvm.2021.717729
Received: 31 May 2021; Accepted: 22 November 2021;
Published: 03 January 2022.
Edited by:
Michele Ciccarelli, University of Salerno, ItalyReviewed by:
Elise Peery Gomez-Sanchez, University of Mississippi Medical Center, United StatesKirtikar Shukla, Wake Forest School of Medicine, United States
Copyright © 2022 Jiang, Zhu, Chen, Yu, Xu, Liu, He, Sagnelli, Zhu and Luo. This is an open-access article distributed under the terms of the Creative Commons Attribution License (CC BY). The use, distribution or reproduction in other forums is permitted, provided the original author(s) and the copyright owner(s) are credited and that the original publication in this journal is cited, in accordance with accepted academic practice. No use, distribution or reproduction is permitted which does not comply with these terms.
*Correspondence: Yi-Min Zhu, emh1eWltQHpqdS5lZHUuY24=; Qiong Luo, bHVvcUB6anUuZWR1LmNu
†These authors have contributed equally to this work