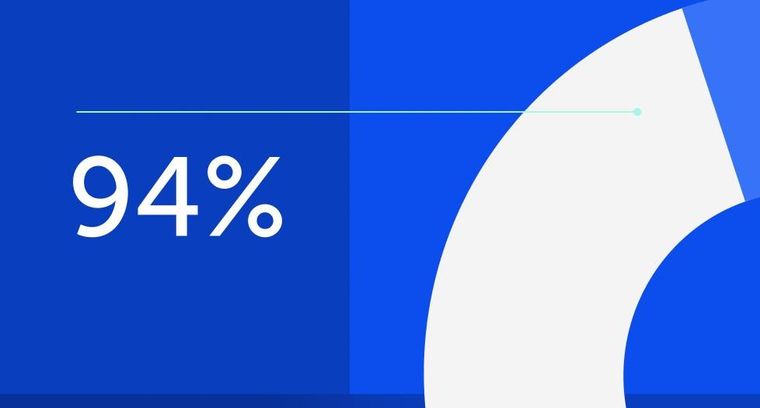
94% of researchers rate our articles as excellent or good
Learn more about the work of our research integrity team to safeguard the quality of each article we publish.
Find out more
MINI REVIEW article
Front. Cardiovasc. Med., 26 July 2021
Sec. Cardiovascular Biologics and Regenerative Medicine
Volume 8 - 2021 | https://doi.org/10.3389/fcvm.2021.715903
This article is part of the Research TopicRole of Molecular Modulators in Combatting Cardiac Injury and Disease: Prevention, repair and regenerationView all 6 articles
Inflammation plays a central role in cardiovascular diseases (CVD). One pathway under investigation is the innate immune DNA sensor cyclic GMP-AMP synthase (cGAS) and its downstream receptor stimulator of interferon genes (STING). cGAS-STING upregulates type I interferons in response to pathogens. Recent studies show that also self-DNA may activate cGAS-STING, for instance, DNA released from nuclei or mitochondria during obesity or myocardial infarction. Here, we focus on emerging evidence describing the interaction of cGAS-STING with cardiovascular risk factors and disease. We also touch on translational therapeutic opportunities and potential further investigations.
Cardiovascular diseases (CVD) are one of the large health problems in our societies. The 2015 incidence for CVD in the European Society of Cardiology member countries was 11 million people with a prevalence of around 83.5 million (1). CVDs are the major global cause of death. Worldwide, more than 17 million people die from CVD each year (2, 3), 4 million of which in Europe (2). The relation between inflammatory and immune phenomena and the pathophysiology of CVD is receiving increasing attention recently. Its role in disease progression is evident in several conditions (3–5). For example, immune phenomena comprising both innate and adaptive responses are central in the development of atherosclerosis (6) and a landmark clinical trial demonstrated for the first time in 2017 that specifically targeting inflammation reduces cardiovascular events in high-risk subjects (7).
Inflammation and immune phenomena are also intricately involved in myocardial infarction healing and the progression of heart failure (8, 9). Early myocardial infarction remodeling is a wound healing response with the massive influx of myeloid cells from extra-cardiac reservoirs (10). These innate immune cells clear necrosis and pave the way for the establishment of a functional scar. Exuberant responses, e.g., after inhibiting regulatory cytokines such as TGF-β, are detrimental, and preclinical trials demonstrate the benefit of suppressing these (11, 12). However, clinical trials that sought to translate this to clinical use failed (13). Inflammatory processes are not only detrimental in injured tissues. In fact, they are often a requisite for repair and regeneration. The key is the right balance of proinflammatory and antiinflammatory responses in terms of magnitude and timing (12, 14). For instance, macrophages can be proinflammatory (M1 like) and required to clear damaged tissue, while pro-reparative M2 like macrophages mitigate inflammatory responses and are essential for ensuing healing processes (15, 16). In chronic heart failure, proinflammatory cytokines such as IL-1, IL-6, and TNFα are elevated and may contribute to disease progression (17), however, clinical studies targeting cytokines such as TGFα in heart failure patients failed in the past (18).
The innate immune system is our first-line defense and consists of humoral and cellular parts. Cellular components are phagocytes like neutrophils, eosinophils, and macrophages, as well as natural killer cells and dendritic cells (19, 20). The humoral system includes the complement system and natural antibodies as well as cytokines like interferons (IFNs), interleukins (ILs), tumor necrosis factors (TNFs), and transforming growth factors (TGFs) (20–22). Triggers initiating inflammation are pathogen-associated molecular patterns (PAMPs) derived from infectious agents, or non-infectious damage-associated molecular patterns (DAMPs). These molecular patterns can consist of different components like cell wall components, proteoglycans, or nucleic acids. DNA e.g., can originate from extracellular sources such as viruses, bacteria, or dying cells. However, DNA can also be derived from intracellular sources such as damaged nuclei or mitochondria. What these sources have in common is that DNA is present in compartments where it is out-of-place. DNA sensors are, for instance, the Toll-like receptor 9 (TLR9), absent in melanoma 2 (AIM2), or interferon gamma-induced 16 (IFI16) (23). cGAS is another example for a DNA sensor.
cGAS is a 63kDa protein predominantly localized in the cytoplasm during the cell cycle's interphase (24). It was initially described as a defense mechanism against viral and bacterial infections by binding foreign DNA and transforming ATP and GTP to the second messenger cyclic-GMP-AMP (cGAMP) (Figure 1) (25–36). cGAMP activates the STING receptor (36–38). STING is a receptor protein with three isoforms ranging from 9–34 kDa and is localized at the endoplasmic reticulum (39). STING activates the TANK-binding kinase 1 (TBK1), which phosphorylates the transcription factor interferon releasing factor 3 (IRF3) (35–37). Ultimately, IRF3 induces the transcription of type I interferons (25, 37), which in turn activate several signaling cascades including activation of the IFN-α receptor 1 (IFNAR1) and the transcription of IFN-stimulated genes (ISGs) (40, 41).
Regulatory mechanisms are necessary to attenuate excessive proinflammatory stimulation. This is also true for the cGAS-STING pathway. For instance, cytosolic deoxyribonuclease degrades cytosolic DNA and ensures that minute amounts of free DNA do not trigger the full inflammatory cascade (42). Additionally, an intact cell's compartmentalization restricts nuclear or mitochondrial DNA sensing by cytosolic sensors (42). However, the regulatory capacity is limited. Genomic instability and nucleic damage can release DNA in considerable amounts into the cytosolic compartment activating cGAS (36, 38, 43). ER stress also actives STING and IRF3 (31, 44) and promotes autophagy in stressed cells, e.g., through direct interaction of cGAS with Beclin-1(31, 45). STING activated T-cells can further induce a type I IFN response eliciting apoptosis (46). Interestingly, even in T cell-derived cancer cells, this process is still functional and represents a therapeutic approach (47). Similarly, STING may induce apoptosis in malignant B cells (48). Activating the cGAS-STING pathway also improves the outcome of solid tumors, for instance in metastatic breast cancer, by enhancing the immune response against tumor cells (49, 50).
Another area where cGAS-STING is under investigation is infectious disease. For instance, virus infections like hepatitis B (51), Dengue (52), and HIV (31, 38) or bacterial infections like tuberculosis (53) and Streptococcus pyogenes infections (38).
An increased amount of self-DNA released into the cytosol by autoimmune diseases activates the cGAS-STING pathway as well, e.g., in Systemic Lupus Erythematosus or Aicardi-Goutières syndrome (31, 34, 43, 54, 55). Another autoinflammatory disease without an increased amount of self-DNA is the STING-associated vasculopathy with onset in infancy (SAVI) with gain-of-function mutations in the STING gene (56).
Self-DNA can activate cGAS-STING in non-communicable, non-immune disorders such as CVD. Here, we give a concise overview of evidence linking CVD risk factors and disease to cGAS-STING (Figure 2).
Liu et al. (57) showed that side-steam smoke exposure (SSE), a model for second-hand smoking (SHS), reduced fractional shortening (FS) in mice and increased left ventricular (LV) mass. Additionally, they investigated these effects on mice haploinsufficient for the autophagy protein Beclin 1 (Becn+/−). They found no difference between wild-type (WT) mice and Becn+/− without SSE, but a significant reduction in FS and an increase in LV mass in Becn+/− with SSE. On the cellular level, myocyte hypertrophy was present, myocardial TNFα and IL-1β increased, and cardiomyocyte peak shortening was reduced. This was associated with an increase of cGAS and STING protein expression, suggesting that this pathway is involved in the inflammatory process of SHS in WT and Becn+/− mice and that this is exacerbated with impaired autophagy. Furthermore, the authors tested the cGAS inhibitor (PF-06928215) and STING inhibitor (Astin C) in their study. In WT mice with SSE, the inhibitors improved peak shortening significantly, while this effect was lost in Becn+/− mice (57). Chronic ozone exposure, which mimics smoke-induced chronic obstructive pulmonary disease (COPD) and induces reactive oxygen species (ROS) and mitochondrial damage, may also be associated with the cGAS-STING signaling in humans (58).
Another major risk factor for CVDs is obesity. Obesity is associated with endothelial inflammation (59) and induces proinflammatory responses in M1 macrophages, e.g., through elevated levels of palmitic acid (PA) in the blood (60). Mao et al. (61) investigated the influence of PA on cardiovascular endothelia and STING's role in this interaction. In-vitro experiments in human aortic endothelial cells demonstrate PA-induced mitochondrial damage and release of mitochondrial DNA (mtDNA) into the cytosol leading to cGAS STING pathway activation and IFN production. Silencing STING or IRF responses via small interfering RNA (siRNA) attenuates this response. These results were reiterated in-vivo as well. Wild-type mice on a high-fat diet (HFD) had a significant increase of IRF3 in adipose tissue and the aortic wall, which was reduced in STING-deficient (STINGgt/gt) mice (61).
A recent study from Gong et al. supports cGAS-STING's effect on HFD associated cardiovascular dysfunction. They showed that WT mice with HFD had significantly reduced FS in-vivo, peak shorting in isolated cardiomyocytes, and cardiomyocyte hypertrophy. This was accompanied by elevation of TNFα, IL-1β, STING, and cGAS. Deletion of Akt2 and Ampkα2 (double knock-out, DKO), decreased phosphorylation of Unc-51 like autophagy activation kinase (ULK1) (62), which phosphorylates Beclin1 and thereby induces autophagy (63). Furthermore, cGAS/STING activation on HFD was amplified in DKO (62).
Inflammageing describes low-grade, chronic, and sterile inflammation that occurs with aging and is associated with CVDs (64). One trigger for this inflammatory process is the degeneration of DNA during aging.
Quan et al. (65) demonstrated that cGAS-STING regulates the senescence-associated secretory phenotype (SASP). SASP in aged hearts is primed by an increase in proinflammatory cytokines like IL-1β, IL-6, and IL-8, and release of mtDNA into the cytosol may induce SASP via cGAS-STING. Circulating mtDNA associated with age increases inflammatory SASP in aged hearts (66, 67).
Interestingly, patients suffering from the accelerated aging disease Hutchinson Gilford Progeria Syndrome (HGPS) often die from CVDs like myocardial infarction (MI) or stroke (68) and HGPS is associated with amplified interferon responses potentially via the cGAS-STING pathway (69–71). However, mutations can also be protective. The single nucleotide polymorphism (SNP) R293Q of the STING gene is protective in obesity-associated CVDs and other age-related diseases (72, 73).
Heart Failure (HF) is a clinical syndrome with symptoms and structural and/or functional cardiac abnormalities (68). It represents end stage disease in many CVDs like ischemia or hypertension.
In a model of non-ischemic pressure-overload induced heart failure (transverse aortic constriction, TAC) exhibiting hypertrophy, cardiac dysfunction, and fibrosis expression of STING, IFNα and IFNβ were increased (74). In STING knock-out (STING-KO) mice, levels returned to baseline levels (74). Neonatal rat cardiomyocytes treated with angiotensin II had increased levels of STING, IFNα, and IFNβ. STING inhibition via siRNA resulted in a significant reduction of IL-6, IL-1β, TNFα, IFNα, and IFNβ in these cells. Increased levels of STING, IFNα, and IFNβ were also seen in human samples of dilative and hypertrophic cardiomyopathies (74).
Another study confirmed these findings: the expression levels of cGAS, STING, IFN, and the IFN induced chemokines CXCL10, IFIT3, and ISG15 were significantly increased 3 days after TAC (75). Silencing cGAS via adeno-associated virus 9 (AAV9) resulted in a significant decrease of LV remodeling and fibrosis (75).
Two independent groups investigated the relevance of cGAS-STING in myocardial infarction healing. They demonstrate increased IFNβ1 expression and IRF3 phosphorylation and an increase in the expression levels of CXCL10, IRF7, STING, and cGAS after myocardial infarction (76, 77). This was attenuated by using knockout models for pathway members such as cGAS, STING, or IRF3. Interestingly, cGAS knock-out (cGAS−/−) did not reduce the universal proinflammatory cytokines IL-1β, TNFα, and IL-6 (76).
By using fluorescence reporter tagged cells, parabiosis experiments, and scRNAseq King et al. (77) demonstrated that cardiomyocyte cell death after MI leads to recruitment of interferon-inducible cells (IFNICs) with increased expression of IRF3-dependent genes from the blood to the heart and they identify these IFNICs as monocyte-derived cardiac macrophages that phagocytose cell debris. Disruption of pathway activation via genetic or pharmacologic means improves outcomes.
Cao et al. (76) treated WT and cGAS−/− human macrophages with IFN stimulatory DNA. As expected, cGAS−/− macrophages produced no cGAS and also no CXCL10 (76). CXCL10 was expressed in WT macrophages and associated with M1-like polarization. In contrast, M2 marker expression like CD163, IL-10, and CCL17 was increased in cGAS−/− animals (76).
Both groups observed improved outcomes in cGAS−/− mice compared to WT in terms of LV function and survival (76, 77), although, the survival benefit was more pronounced in INFAR and IRF3 knockout animals. IFNAR neutralization via antibodies mirrored survival and functional benefit.
Interestingly, Cao et al. showed increased myofibroblast activation and collagen deposition in cGAS−/− mice after MI and propose this enhances functional scar generation.
Cao et al. also provide data demonstrating high myocardial levels of cGAS and CXCL10 in human end stage ischemic heart failure patients that are decreased back to near normal levels by unloading the left ventricle by means of mechanical circulatory support via left ventricular assist devices (LVADs).
Li et al. (78) showed that the cGAS-STING pathway is also involved in stroke in an in-vivo model with middle cerebral artery occlusion (MCAO). They observed increased levels of cGAS and STING in the infarcted brain area. Using a small synthetic oligodeoxynucleotide, A151 (TTAGGG), which inhibits cGAS, this was reduced to the levels of sham-operated mice. Additionally, A151 reduced IL-1β levels, reduced infarct size and improved cognitive function (78).
Li et al. (79) described upregulation of STING and phosphorylated IRF3 in an in vitro model of sepsis induced cardiomyopathy (SIC) using neonatal rat cardiomyocytes. Treating cells with siRNA against STING resulted in a decrease in IRF3 phosphorylation. In an in vivo model of SIC using LPS injection STING-KO reduced CK-MB, IL-1β, and TNFα levels and improved EF, FS, and survival. Likewise, other investigators found that the small cGAS inhibitor molecule RU.521 improved LPS induced SIC (IRF3 phosphorylation, IL-1β, IL-6, TNFα expression, apoptosis, left ventricular function, and survival) (80). Lastly, selenium supplementation appeared to ameliorate LPS-induced SIC via STING (81).
In Chagas cardiomyopathy, Choudhuri et al. showed that extracellular vesicles from Trypanosoma cruzi infected cells lead to increased levels of IL-1β, IL-6, and TNFα in macrophages. Using different inhibitors, including the cGAS inhibitor PF-06928215, they detected a significant decrease in the levels of IL-1β, IL-6c, and TNFα (82).
Further, there is speculation that COVID-19 infection may lead to prolonged cGAS-STING pathway activation in leucocytes (83) and increased leucocyte infiltration was present in the majority COVID-19 patient's hearts in an autopsy studie (84).
Radiation produces DNA damage, which can be sensed in the cytosol by cGAS (85). Phillipp et al. (86) studied the effect of radiation on cultured human coronary artery endothelial cells. With increasing radiation up to 10 Gy, the expression levels of STING and ISG15 increased continuously after 1 week as well as ISG15 and cGAS up to a dose of 2 Gy. This may have clinical implications as radiation therapy for breast cancer may result in up to 20 Gy delivered to the left anterior descending coronary artery (LAD) (87).
cGAS-STING is involved in the pathophysiology of cardiovascular disease and risk factors. This ranges from conditions with cell death and massive release of DAMPs such as myocardial infarction or stroke to chronic conditions where inflammatory responses are mildly increased over longer periods such as heart failure. This may have translational implications, as pharmacologic agents are available and have been tested for non-cardiovascular diseases. Inhibitors of cGAS include PF-06928215, A151, RU.521, J014, G140, or X6 (80, 88–90). Direct STING inhibition also seems promising (91) and antagonists include Astin C, C-176, C178, and H-151 (88, 92, 93). However, potential adverse effects need to be studied. As cGAS and STING agonists are used for cancer and viral infection treatments (48–51), inhibition may promote these conditions. Furthermore, pathways are more complex and promiscuous than mentioned here, and inhibitors targeting other molecules may impact cGAS-STING too, for instance the ALK inhibitor LDK378 (94).
In conclusion, CVD and risk factors modulate cGAS-STING and this may contribute to disease progression. Targeting pathway members may be useful to attenuate excessive inflammation, e.g., ischemic injury to the heart or brain.
LR and PR wrote the manuscript. All authors contributed to the article and approved the submitted version.
PR is supported by the Austrian Science Fund, ERA-CVD (AIR-MI, I 4168-B), and the Austrian Society of Cardiology.
The authors declare that the research was conducted in the absence of any commercial or financial relationships that could be construed as a potential conflict of interest.
All claims expressed in this article are solely those of the authors and do not necessarily represent those of their affiliated organizations, or those of the publisher, the editors and the reviewers. Any product that may be evaluated in this article, or claim that may be made by its manufacturer, is not guaranteed or endorsed by the publisher.
Figures have been created with BioRender.com.
1. Timmis A, Townsend N, Gale CP, Torbica A, Lettino M, Petersen SE, et al. European Society of cardiology: cardiovascular disease statistics 2019. Eur Heart J. (2020) 41:12–85. doi: 10.1093/eurheartj/ehz859
2. Wilkins E, Wilson L, Wickramasinghe K, Bhatnagar P, Leal J, Luengo-Fernandez R, et al. European Cardiovascular Disease Statistics 2017. Brussels: European Heart Network AISBL (2017).
3. Golia E, Limongelli G, Natale F, Fimiani F, Maddaloni V, Pariggiano I, et al. Inflammation and cardiovascular disease: from pathogenesis to therapeutic target. Curr Atheroscler Rep. (2014) 16:435. doi: 10.1007/s11883-014-0435-z
4. Libby P, Ridker PM, Hansson GK. Inflammation in atherosclerosis: from pathophysiology to practice. J Am Coll Cardiol. (2009) 54:2129–38. doi: 10.1016/j.jacc.2009.09.009
5. Taleb S. Inflammation in atherosclerosis. Arch Cardiovasc Dis. (2016) 109:708–15. doi: 10.1016/j.acvd.2016.04.002
6. Wolf D, Ley K. Immunity and inflammation in atherosclerosis. Circ Res. (2019) 124:315–27. doi: 10.1161/CIRCRESAHA.118.313591
7. Ridker PM, Everett BM, Thuren T, MacFadyen JG, Chang WH, Ballantyne C, et al. Antiinflammatory therapy with canakinumab for atherosclerotic disease. N Engl J Med. (2017) 377:1119–31. doi: 10.1056/NEJMoa1707914
8. Shirazi LF, Bissett J, Romeo F, Mehta JL. Role of inflammation in heart failure. Curr Atheroscler Rep. (2017) 19:27. doi: 10.1007/s11883-017-0660-3
9. Frangogiannis NG. The inflammatory response in myocardial injury, repair, and remodelling. Nat Rev Cardiol. (2014) 11:255–65. doi: 10.1038/nrcardio.2014.28
10. Nahrendorf M, Swirski FK, Aikawa E, Stangenberg L, Wurdinger T, Figueiredo J-L, et al. The healing myocardium sequentially mobilizes two monocyte subsets with divergent and complementary functions. J Exp Med. (2007) 204:3037–47. doi: 10.1084/jem.20070885
11. Frantz S, Hu K, Adamek A, Wolf J, Sallam A, Maier SKG, et al. Transforming growth factor beta inhibition increases mortality and left ventricular dilatation after myocardial infarction. Basic Res Cardiol. (2008) 103:485–92. doi: 10.1007/s00395-008-0739-7
12. Epelman S, Liu PP, Mann DL. Role of innate and adaptive immune mechanisms in cardiac injury and repair. Nat Rev Immunol. (2015) 15:117–29. doi: 10.1038/nri3800
13. Silverman HS, Pfeifer MP. Relation between use of antiinflammatory agents and left ventricular free wall rupture during acute myocardial infarction. Am J Cardiol. (1987) 59:363–4. doi: 10.1016/0002-9149(87)90817-4
14. Ong S-B, Hernández-Reséndiz S, Crespo-Avilan GE, Mukhametshina RT, Kwek X-Y, Cabrera-Fuentes HA, et al. Inflammation following acute myocardial infarction: multiple players, dynamic roles, and novel therapeutic opportunities. Pharmacol Ther. (2018) 186:73–87. doi: 10.1016/j.pharmthera.2018.01.001
15. de Couto G. Macrophages in cardiac repair: environmental cues and therapeutic strategies. Exp Mol Med. (2019) 51:1–10. doi: 10.1038/s12276-019-0269-4
16. Das A, Sinha M, Datta S, Abas M, Chaffee S, Sen CK, et al. Monocyte and macrophage plasticity in tissue repair and regeneration. Am J Pathol. (2015) 185:2596–606. doi: 10.1016/j.ajpath.2015.06.001
17. Adamo L, Rocha-Resende C, Prabhu SD, Mann DL. Reappraising the role of inflammation in heart failure. Nat Rev Cardiol. (2020) 17:269–85. doi: 10.1038/s41569-019-0315-x
18. Chung ES, Packer M, Lo KH, Fasanmade AA, Willerson JT. Randomized, double-blind, placebo-controlled, pilot trial of infliximab, a chimeric monoclonal antibody to tumor necrosis factor-alpha, in patients with moderate-to-severe heart failure: results of the anti-TNF Therapy Against Congestive Heart Failure (ATTACH) trial. Circulation. (2003) 107:3133–40. doi: 10.1161/01.CIR.0000077913.60364.D2
19. Beutler B. Innate immunity: an overview. Mol Immunol. (2004) 40:845–59. doi: 10.1016/j.molimm.2003.10.005
20. Riera Romo M, Pérez-Martínez D, Castillo Ferrer C. Innate immunity in vertebrates: an overview. Immunology. (2016) 148:125–39. doi: 10.1111/imm.12597
21. Tomar N, De RK. A brief outline of the immune system. Meth Mol Biol. (2014) 1184:3–12. doi: 10.1007/978-1-4939-1115-8_1
22. Medzhitov R. Origin and physiological roles of inflammation. Nature. (2008) 454:428–35. doi: 10.1038/nature07201
23. Briard B, Place DE, Kanneganti T-D. DNA sensing in the innate immune response. Physiology. (2020) 35:112–24. doi: 10.1152/physiol.00022.2019
24. Zhong L, Hu M-M, Bian L-J, Liu Y, Chen Q, Shu H-B. Phosphorylation of cGAS by CDK1 impairs self-DNA sensing in mitosis. Cell Discov. (2020) 6:26. doi: 10.1038/s41421-020-0162-2
25. Sun L, Wu J, Du F, Chen X, Chen ZJ. Cyclic GMP-AMP synthase is a cytosolic DNA sensor that activates the type I interferon pathway. Science. (2013) 339:786–91. doi: 10.1126/science.1232458
26. Schoggins JW, MacDuff DA, Imanaka N, Gainey MD, Shrestha B, Eitson JL, et al. Pan-viral specificity of IFN-induced genes reveals new roles for cGAS in innate immunity. Nature. (2014) 505:691–5. doi: 10.1038/nature12862
27. Li X-D, Wu J, Gao D, Wang H, Sun L, Chen ZJ. Pivotal roles of cGAS-cGAMP signaling in antiviral defense and immune adjuvant effects. Science. (2013) 341:1390–4. doi: 10.1126/science.1244040
28. Collins AC, Cai H, Li T, Franco LH, Li X-D, Nair VR, et al. Cyclic GMP-AMP synthase is an innate immune DNA sensor for mycobacterium tuberculosis. Cell Host Microbe. (2015) 17:820–8. doi: 10.1016/j.chom.2015.05.005
29. Civril F, Deimling T, Oliveira Mann CC de, Ablasser A, Moldt M, Witte G, et al. Structural mechanism of cytosolic DNA sensing by cGAS. Nature. (2013) 498:332–7. doi: 10.1038/nature12305
30. Wu J, Sun L, Chen X, Du F, Shi H, Chen C, et al. Cyclic GMP-AMP is an endogenous second messenger in innate immune signaling by cytosolic DNA. Science. (2013) 339:826–30. doi: 10.1126/science.1229963
31. Wan D, Jiang W, Hao J. Research advances in how the cGAS-STING pathway controls the cellular inflammatory response. Front Immunol. (2020) 11:615. doi: 10.3389/fimmu.2020.00615
32. Liu H, Moura-Alves P, Pei G, Mollenkopf H-J, Hurwitz R, Wu X, et al. cGAS facilitates sensing of extracellular cyclic dinucleotides to activate innate immunity. EMBO Rep. (2019) 20:e46293. doi: 10.15252/embr.201846293
33. Yang H, Wang H, Ren J, Chen Q, Chen ZJ. cGAS is essential for cellular senescence. PNAS. (2017) 114:E4612–20. doi: 10.1073/pnas.1705499114
34. Cai X, Chiu Y-H, Chen ZJ. The cGAS-cGAMP-STING pathway of cytosolic DNA sensing and signaling. Mol Cell. (2014) 54:289–96. doi: 10.1016/j.molcel.2014.03.040
35. Paludan SR, Bowie AG. Immune sensing of DNA. Immunity. (2013) 38:870–80. doi: 10.1016/j.immuni.2013.05.004
36. Chen Q, Sun L, Chen ZJ. Regulation and function of the cGAS-STING pathway of cytosolic DNA sensing. Nat Immunol. (2016) 17:1142–9. doi: 10.1038/ni.3558
37. Banete A, Seaver K, Bakshi D, Gee K, Basta S. On taking the STING out of immune activation. J Leukoc Biol. 103:1189–95. (2018). doi: 10.1002/JLB.2MIR0917-383R
38. Barber GN. STING: infection, inflammation, and cancer. Nat Rev Immunol. (2015) 15:760–70. doi: 10.1038/nri3921
39. Ishikawa H, Barber GN. STING is an endoplasmic reticulum adaptor that facilitates innate immune signalling. Nature. (2008) 455:674–8. doi: 10.1038/nature07317
40. Meager A, editor. The Interferons: Characterization and Application. Weinheim: Wiley-VCH (2006). doi: 10.1002/3527608206
41. Schneider WM, Chevillotte MD, Rice CM. Interferon-stimulated genes: a complex web of host defenses. Annu Rev Immunol. (2014) 32:513–45. doi: 10.1146/annurev-immunol-032713-120231
42. Ablasser A, Chen ZJ. cGAS in action: expanding roles in immunity and inflammation. Science. (2019) 363:eaat8657. doi: 10.1126/science.aat8657
43. Li T, Chen ZJ. The cGAS-cGAMP-STING pathway connects DNA damage to inflammation, senescence, and cancer. J Exp Med. (2018) 215:1287–99. doi: 10.1084/jem.20180139
44. Liu Y-P, Zeng L, Tian A, Bomkamp A, Rivera D, Gutman D, et al. Endoplasmic reticulum stress regulates the innate immunity critical transcription factor IRF3. J Immunol. (2012) 189:4630–9. doi: 10.4049/jimmunol.1102737
45. Liang Q, Seo GJ, Choi YJ, Kwak M-J, Ge J, Rodgers MA, et al. Crosstalk between the cGAS DNA sensor and Beclin-1 autophagy protein shapes innate antimicrobial immune responses. Cell Host Microb. (2014) 15:228–38. doi: 10.1016/j.chom.2014.01.009
46. Larkin B, Ilyukha V, Sorokin M, Buzdin A, Vannier E, Poltorak A. Cutting edge: activation of STING in T cells induces type I IFN responses and cell death. J Immunol. (2017) 199:397–402. doi: 10.4049/jimmunol.1601999
47. Gulen MF, Koch U, Haag SM, Schuler F, Apetoh L, Villunger A, et al. Signalling strength determines proapoptotic functions of STING. Nat Commun. (2017) 8:1–10. doi: 10.1038/s41467-017-00573-w
48. Tang C-HA, Zundell JA, Ranatunga S, Lin C, Nefedova Y, Del Valle JR, et al. Agonist-mediated activation of STING induces apoptosis in malignant B cells: single nucleotide polymorphisms of human STING can affect innate immune response to cyclic dinucleotides. Cancer Res. (2016) 76:2137–52. doi: 10.1158/0008-5472.CAN-15-1885
49. Corrales L, Gajewski TF. Molecular pathways: targeting the stimulator of interferon genes (STING) in the immunotherapy of cancer. Clin Cancer Res. (2015) 21:4774–9. doi: 10.1158/1078-0432.CCR-15-1362
50. Weiss JM, Guérin MV, Regnier F, Renault G, Galy-Fauroux I, Vimeux L, et al. The STING agonist DMXAA triggers a cooperation between T lymphocytes and myeloid cells that leads to tumor regression. Oncoimmunology. (2017) 6:e1346765. doi: 10.1080/2162402X.2017.1346765
51. Guo F, Han Y, Zhao X, Wang J, Liu F, Xu C, et al. STING agonists induce an innate antiviral immune response against Hepatitis B virus. Antimicrob Agents Chemother. (2015) 59:1273–81. doi: 10.1128/AAC.04321-14
52. Aguirre S, Luthra P, Sanchez-Aparicio MT, Maestre AM, Patel J, Lamothe F, et al. Dengue virus NS2B protein targets cGAS for degradation and prevents mitochondrial DNA sensing during infection. Nat Microbiol. (2017) 2:17037. doi: 10.1038/nmicrobiol.2017.37
53. Wiens KE, Ernst JD. The mechanism for type I interferon induction by mycobacterium tuberculosis is bacterial strain-Dependent. PLoS Pathog. (2016) 12:e1005809. doi: 10.1371/journal.ppat.1005809
54. Gao D, Li T, Li X-D, Chen X, Li Q-Z, Wight-Carter M, et al. Activation of cyclic GMP-AMP synthase by self-DNA causes autoimmune diseases. PNAS. (2015) 112:E5699–705. doi: 10.1073/pnas.1516465112
55. Härtlova A, Erttmann SF, Am Raffi F, Schmalz AM, Resch U, Anugula S, et al. DNA damage primes the type I interferon system via the cytosolic DNA sensor STING to promote anti-microbial innate immunity. Immunity. (2015) 42:332–43. doi: 10.1016/j.immuni.2015.01.012
56. Liu Y, Jesus AA, Marrero B, Yang D, Ramsey SE, Sanchez GAM, et al. Activated STING in a vascular and pulmonary syndrome. N Engl J Med. (2014) 371:507–18. doi: 10.1056/NEJMoa1312625
57. Liu F, Liu Y, Zhuang Z, Ma J, Xu X, Zhang W, et al. Beclin1 haploinsufficiency accentuates second-hand smoke exposure -induced myocardial remodeling and contractile dysfunction through a STING-mediated mechanism. J Mol Cell Cardiol. (2020) 148:78–88. doi: 10.1016/j.yjmcc.2020.08.016
58. Wiegman CH, Li F, Ryffel B, Togbe D, Chung KF. Oxidative stress in ozone-induced chronic lung inflammation and emphysema: a facet of chronic obstructive pulmonary disease. Front. Immunol. (2020) 11:1957. doi: 10.3389/fimmu.2020.01957
59. Villaret A, Galitzky J, Decaunes P, Estève D, Marques M-A, Sengenès C, et al. Adipose tissue endothelial cells from obese human subjects: differences among depots in angiogenic, metabolic, and inflammatory gene expression and cellular senescence. Diabetes. (2010) 59:2755–63. doi: 10.2337/db10-0398
60. Korbecki J, Bajdak-Rusinek K. The effect of palmitic acid on inflammatory response in macrophages: an overview of molecular mechanisms. Inflamm Res. (2019) 68:915–32. doi: 10.1007/s00011-019-01273-5
61. Mao Y, Luo W, Zhang L, Wu W, Yuan L, Xu H, et al. STING-IRF3 triggers endothelial inflammation in response to free fatty acid-induced mitochondrial damage in diet-induced obesity. Arterioscler Thromb Vasc Biol. (2017) 37:920–9. doi: 10.1161/ATVBAHA.117.309017
62. Gong Y, Li G, Tao J, Wu NN, Kandadi MR, Bi Y, et al. Double knockout of Akt2 and AMPK accentuates high fat diet-induced cardiac anomalies through a cGAS-STING-mediated mechanism. Biochim Biophys Acta Mol Basis Dis. (2020) 1866:165855. doi: 10.1016/j.bbadis.2020.165855
63. Russell RC, Tian Y, Yuan H, Park HW, Chang Y-Y, Kim J, et al. ULK1 induces autophagy by phosphorylating Beclin-1 and activating VPS34 lipid kinase. Nat Cell Biol. (2013) 15:741–50. doi: 10.1038/ncb2757
64. Franceschi C, Garagnani P, Parini P, Giuliani C, Santoro A. Inflammaging: a new immune-metabolic viewpoint for age-related diseases. Nat Rev Endocrinol. (2018) 14:576–90. doi: 10.1038/s41574-018-0059-4
65. Quan Y, Xin Y, Tian G, Zhou J, Liu X. Mitochondrial ROS-Modulated mtDNA: a potential target for cardiac aging. Oxid Med Cell Longev. (2020) 2020:9423593. doi: 10.1155/2020/9423593
66. Padilla-Sánchez SD, Navarrete D, Caicedo A, Teran E. Circulating cell-free mitochondrial DNA levels correlate with body mass index and age. Biochim Biophys Acta Mol Basis Dis. (2020) 1866:165963. doi: 10.1016/j.bbadis.2020.165963
67. Pinti M, Cevenini E, Nasi M, de Biasi S, Salvioli S, Monti D, et al. Circulating mitochondrial DNA increases with age and is a familiar trait: Implications for “inflamm-aging”. Eur J Immunol. (2014) 44:1552–62. doi: 10.1002/eji.201343921
68. Ponikowski P, Voors AA, Anker SD, Bueno H, Cleland JGF, Coats AJS, et al. 2016 ESC Guidelines for the diagnosis treatment of acute chronic heart failure: The Task Force for the diagnosis treatment of acute chronic heart failure of the European Society of Cardiology (ESC) developed with the special contribution of the Heart Failure Association (HFA) of the ESC. Eur Heart J. (2016) 37:2129–200. doi: 10.1093/eurheartj/ehw128
69. Gonzalo S, Coll-Bonfill N. Genomic instability and innate immune responses to self-DNA in progeria. Geroscience. (2019) 41:255–66. doi: 10.1007/s11357-019-00082-2
70. Graziano S, Kreienkamp R, Coll-Bonfill N, Gonzalo S. Causes and consequences of genomic instability in laminopathies: replication stress and interferon response. Nucleus. (2018) 9:258–75. doi: 10.1080/19491034.2018.1454168
71. Kreienkamp R, Graziano S, Coll-Bonfill N, Bedia-Diaz G, Cybulla E, Vindigni A, et al. A cell-intrinsic interferon-like response links replication stress to cellular aging caused by progerin. Cell Rep. (2018) 22:2006–15. doi: 10.1016/j.celrep.2018.01.090
72. Hamann L, Ruiz-Moreno JS, Szwed M, Mossakowska M, Lundvall L, Schumann RR, et al. STING SNP R293Q is associated with a decreased risk of aging-related diseases. Gerontology. (2019) 65:145–54. doi: 10.1159/000492972
73. Hamann L, Szwed M, Mossakowska M, Chudek J, Puzianowska-Kuznicka M. First evidence for STING SNP R293Q being protective regarding obesity-associated cardiovascular disease in age-advanced subjects - a cohort study. Immun Ageing. (2020) 17:7. doi: 10.1186/s12979-020-00176-y
74. Zhang Y, Chen W, Wang Y. STING is an essential regulator of heart inflammation and fibrosis in mice with pathological cardiac hypertrophy via endoplasmic reticulum (ER) stress. Biomed Pharmacother. (2020) 125:110022. doi: 10.1016/j.biopha.2020.110022
75. Hu D, Cui Y-X, Wu M-Y, Li L, Su L-N, Lian Z, et al. Cytosolic DNA sensor cGAS plays an essential pathogenetic role in pressure overload-induced heart failure. Am J Physiol Heart Circ Physiol. (2020) 318:H1525–37. doi: 10.1152/ajpheart.00097.2020
76. Cao DJ, Schiattarella GG, Villalobos E, Jiang N, May HI, Li T, et al. Cytosolic DNA sensing promotes macrophage transformation and governs myocardial ischemic injury. Circulation. (2018) 137:2613–34. doi: 10.1161/CIRCULATIONAHA.117.031046
77. King KR, Aguirre AD, Ye Y-X, Sun Y, Roh JD Ng, et al. IRF3 and type I interferons fuel a fatal response to myocardial infarction. Nat Med. (2017) 23:1481–7. doi: 10.1038/nm.4428
78. Li Q, Cao Y, Dang C, Han B, Han R, Ma H, et al. Inhibition of double-strand DNA-sensing cGAS ameliorates brain injury after ischemic stroke. EMBO Mol Med. (2020) 12:e11002. doi: 10.15252/emmm.201911002
79. Li N, Zhou H, Wu H, Wu Q, Duan M, Deng W, et al. STING-IRF3 contributes to lipopolysaccharide-induced cardiac dysfunction, inflammation, apoptosis and pyroptosis by activating NLRP3. Redox Biol. (2019) 24:101215. doi: 10.1016/j.redox.2019.101215
80. Xu Q, Xiong H, Zhu W, Liu Y, Du Y. Small molecule inhibition of cyclic GMP-AMP synthase ameliorates sepsis-induced cardiac dysfunction in mice. Life Sci. (2020) 260:118315. doi: 10.1016/j.lfs.2020.118315
81. Wang X, Yang B, Cao H-L, Wang R-Y, Lu Z-Y, Chi R-F, et al. Selenium supplementation protects against lipopolysaccharide-induced heart injury via STING pathway in mice. Biol Trace Elem Res. (2020) 199:1885–92. doi: 10.1007/s12011-020-02295-5
82. Choudhuri S, Garg NJ. PARP1-cGAS-NF-kappa B pathway of proinflammatory macrophage activation by extracellular vesicles released during Trypanosoma cruzi infection and chagas disease. PLoS Pathogens. (2020) 16:e1008474. doi: 10.1371/journal.ppat.1008474
83. Berthelot J-M, Lioté F, Maugars Y, Sibilia J. Lymphocyte changes in severe COVID-19: delayed over-activation of STING? Front Immunol. (2020) 11:607069. doi: 10.3389/fimmu.2020.607069
84. Basso C, Leone O, Rizzo S, Gaspari M de, van der Wal AC, Aubry M-C, et al. Pathological features of COVID-19-associated myocardial injury: a multicentre cardiovascular pathology study. Eur Heart J. (2020) 41:3827–35. doi: 10.1093/eurheartj/ehaa664
85. Durante M, Formenti SC. Radiation-induced chromosomal aberrations and immunotherapy: micronuclei, cytosolic DNA, and interferon-production pathway. Front Oncol. (2018) 8:192. doi: 10.3389/fonc.2018.00192
86. Philipp J, Le Gleut R, Toerne CV, Subedi P, Azimzadeh O, Atkinson MJ, et al. Radiation response of human cardiac endothelial cells reveals a central role of the cGAS-STING Pathway in the development of inflammation. Proteomes. (2020) 8:30. doi: 10.3390/proteomes8040030
87. Aznar MC, Korreman S-S, Pedersen AN, Persson GF, Josipovic M, Specht L. Evaluation of dose to cardiac structures during breast irradiation. Br J Radiol. (2011) 84:743–6. doi: 10.1259/bjr/12497075
88. Vanpouille-Box C, Hoffmann JA, Galluzzi L. Pharmacological modulation of nucleic acid sensors - therapeutic potential and persisting obstacles. Nat Rev Drug Discov. (2019) 18:845–67. doi: 10.1038/s41573-019-0043-2
89. An J, Woodward JJ, Lai W, Minie M, Sun X, Tanaka L, et al. Inhibition of cyclic GMP-AMP synthase using a novel antimalarial drug derivative in Trex1-deficient mice. Arthritis Rheumatol. (2018) 70:1807–19. doi: 10.1002/art.40559
90. Lama L, Adura C, Xie W, Tomita D, Kamei T, Kuryavyi V, et al. Development of human cGAS-specific small-molecule inhibitors for repression of dsDNA-triggered interferon expression. Nat Commun. (2019) 10:1–14. doi: 10.1038/s41467-019-08620-4
91. Sheridan C. Drug developers switch gears to inhibit STING. Nat Biotechnol. (2019) 37:199–201. doi: 10.1038/s41587-019-0060-z
92. Li S, Hong Z, Wang Z, Li F, Mei J, Huang L, et al. The cyclopeptide astin c specifically inhibits the innate immune CDN sensor STING. Cell Rep. (2018) 25:3405–3421.e7. doi: 10.1016/j.celrep.2018.11.097
93. Haag SM, Gulen MF, Reymond L, Gibelin A, Abrami L, Decout A, et al. Targeting STING with covalent small-molecule inhibitors. Nature. (2018) 559:269–73. doi: 10.1038/s41586-018-0287-8
Keywords: STING, interferon, DAMPs, cardiovascular, inflammation, cGAS
Citation: Rech L and Rainer PP (2021) The Innate Immune cGAS-STING-Pathway in Cardiovascular Diseases – A Mini Review. Front. Cardiovasc. Med. 8:715903. doi: 10.3389/fcvm.2021.715903
Received: 27 May 2021; Accepted: 01 July 2021;
Published: 26 July 2021.
Edited by:
Lisandra de Castro Brás, East Carolina University, United StatesReviewed by:
Nazareno Paolocci, Johns Hopkins University, United StatesCopyright © 2021 Rech and Rainer. This is an open-access article distributed under the terms of the Creative Commons Attribution License (CC BY). The use, distribution or reproduction in other forums is permitted, provided the original author(s) and the copyright owner(s) are credited and that the original publication in this journal is cited, in accordance with accepted academic practice. No use, distribution or reproduction is permitted which does not comply with these terms.
*Correspondence: Peter P. Rainer, cGV0ZXIucmFpbmVyQG1lZHVuaWdyYXouYXQ=
Disclaimer: All claims expressed in this article are solely those of the authors and do not necessarily represent those of their affiliated organizations, or those of the publisher, the editors and the reviewers. Any product that may be evaluated in this article or claim that may be made by its manufacturer is not guaranteed or endorsed by the publisher.
Research integrity at Frontiers
Learn more about the work of our research integrity team to safeguard the quality of each article we publish.